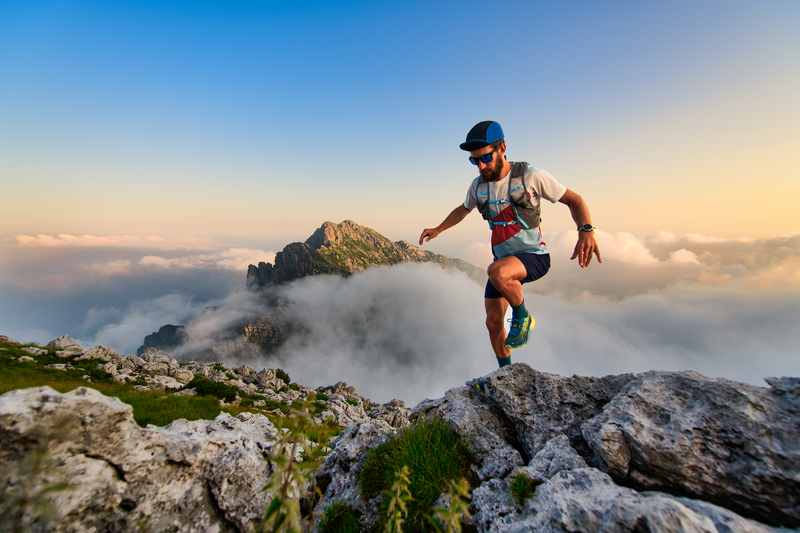
95% of researchers rate our articles as excellent or good
Learn more about the work of our research integrity team to safeguard the quality of each article we publish.
Find out more
REVIEW article
Front. Plant Sci. , 08 January 2024
Sec. Plant Biotechnology
Volume 14 - 2023 | https://doi.org/10.3389/fpls.2023.1331258
Gene editing technologies have opened up the possibility of manipulating the genome of any organism in a predicted way. CRISPR technology is the most used genome editing tool and, in agriculture, it has allowed the expansion of possibilities in plant biotechnology, such as gene knockout or knock-in, transcriptional regulation, epigenetic modification, base editing, RNA editing, prime editing, and nucleic acid probing or detection. This technology mostly depends on in vitro tissue culture and genetic transformation/transfection protocols, which sometimes become the major challenges for its application in different crops. Agrobacterium-mediated transformation, biolistics, plasmid or RNP (ribonucleoprotein) transfection of protoplasts are some of the commonly used CRISPR delivery methods, but they depend on the genotype and target gene for efficient editing. The choice of the CRISPR system (Cas9, Cas12), CRISPR mechanism (plasmid or RNP) and transfection technique (Agrobacterium spp., PEG solution, lipofection) directly impacts the transformation efficiency and/or editing rate. Besides, CRISPR/Cas technology has made countries rethink regulatory frameworks concerning genetically modified organisms and flexibilize regulatory obstacles for edited plants. Here we present an overview of the state-of-the-art of CRISPR technology applied to three important crops worldwide (citrus, coffee and sugarcane), considering the biological, methodological, and regulatory aspects of its application. In addition, we provide perspectives on recently developed CRISPR tools and promising applications for each of these crops, thus highlighting the usefulness of gene editing to develop novel cultivars.
Since the advent of genetic engineering with the creation of the first recombinant DNA molecules in the 1970s (Jackson et al., 1972; Cohen et al., 1973), recombinant DNA technology has evolved to reach a new phase with the field of synthetic biology (Benner and Sismour, 2005). Although synthetic biology has its roots traced to a landmark publication in 1961 (Jacob and Monod, 1961), it significantly matured and scaled-up in the period from 2008 to 2013 (Cameron et al., 2014), in which novel and remarkable molecular cloning techniques could be used to generate complex gene constructs (Engler et al., 2008; Gibson et al., 2009; Engler et al., 2014), thus paving the way for wide-ranging applications through genome engineering tools.
These tools have been incorporated into plant biotechnology over the last decades, finally enabling the advent of New Breeding Techniques (NBTs) as the future of plant genetic manipulation (Ricroch et al., 2022). Hence, meganucleases (Chilton and Que, 2003), ZFNs (Zinc-finger nucleases) (Wright et al., 2005) and TALENs (Transcription activator-like effector nucleases) (Cermak et al., 2011; Mahfouz et al., 2011) had their use consecutively inaugurated in plants until the most recent generation of gene editor tools, based on CRISPR/Cas (Clustered regularly interspaced short palindromic repeats/CRISPR-associated protein) systems. CRISPR technology (Jinek et al., 2012) is the most sophisticated and practical genome editing approach and was firstly applied to the development of edited plants ten years ago (Feng et al., 2013; Shan et al., 2013).
CRISPR/Cas systems remain the most modern editing tool to date, and it has been increasingly improved or adapted (Cardi et al., 2023), being boosted even by nanotechnology-based delivery systems (Demirer et al., 2021). CRISPR systems in biotechnology are derived from the natural adaptive ‘immune system’ of bacteria and archaea, based on RNA-guided endonucleases that bind and cleave foreign nucleic acids. In nature, these nucleases called Cas effectors are guided to target genome sites as a complex by coupling to a pair of CRISPR RNAs (crRNAs) and trans-activating crRNAs (tracrRNAs), thus cleaving the target site located next to a PAM (protospacer adjacent motif) sequence after forming an RNA/DNA heteroduplex between crRNA and host DNA strand (Jinek et al., 2012; Anzalone et al., 2020). This molecular system was engineered for biotech purposes by fusing both crRNA and tracrRNA into a single guide RNA (sgRNA), and the editing mechanism is based on the indels (insertions/deletions) or mutagenesis triggered by repair mechanisms occurring in the host cell after target cleavage by double-strand breaks (DSBs), which are mostly mediated by non-homologous end-joining (NHEJ), but also homology-directed repair (HDR) (Jinek et al., 2012).
Currently, CRISPR/Cas systems are known as ‘genetic scissors’ and make use of a high diversity of nucleases (Figure 1), either naturally occurring from different bacterial species or artificial/engineered variants, each one with their respective PAM sequence (Pickar-Oliver and Gersbach, 2019; Anzalone et al., 2020). CRISPR/SpCas9 (from Streptococcus pyogenes) is by far the most used one in any living host (Anzalone et al., 2020; Cardi et al., 2023), including plants (Abdul Aziz et al., 2022), but CRISPR/Cas12a (formerly Cpf1) editing systems have been discovered, optimized and applied to plants as well (Bernabé-Orts et al., 2019) with many advantages and very promising applications. Likewise, other potential successors or novel CRISPR-like systems (Figure 1) with very similar activity and mechanisms have been discovered in the last years and have also great potential for plant biotechnology applications, such as the new class of prokaryote transposon-encoded TnpB RNA-guided system named OMEGA (Obligate mobile element-guided activity) (Altae-Tran et al., 2021) and the newly discovered Fanzor (Fz) OMEGA-like programmable system existing in eukaryotes (Saito et al., 2023), both of them phylogenetically related to Cas12 proteins.
Figure 1 Panorama of CRISPR/Cas and CRISPR-like systems, strategies, applications and optimizations. Methodologies of CRISPR technology vary according to many parameters, as indicated in each box: PAM site complexity, which can be very stringent or even near-PAMless, and then is related to the frequency of on or off-targets in the host genome; the CRISPR system chosen, based on different Cas variants (which can be native or artificial) used to perform gene editing; sgRNA setups, which make use of traditional or optimized scaffolds (in this last case, sgRNA can be structurally and/or chemically modified in order to increase stability and/or gene editing efficiency); strategies/mechanisms adopted for the delivery of CRISPR components, which can be plasmid-based or DNA-free (RNPs, IVTs or viral replicons); and applications (e.g., genome editing, epigenome editing for transcriptional modulation, RNA editing, nucleic acid probing). Moreover, recently discovered and characterized CRISPR-like systems (OMEGA) may be promising for use in agriculture, such as TnpB from prokaryotes and Fanzor (Fz) from eukaryotes, both based on ωRNA scaffolds, showed in the box “Novel discovered systems”. Image created with BioRender.com.
Regardless of the system of choice, CRISPR technology can be used for many strategies, such as gene knockout or knock-in, transcriptional regulation, epigenetic modification, base editing, RNA editing, prime editing and nucleic acid probing or detection (Figure 1) (Pickar-Oliver and Gersbach, 2019; Anzalone et al., 2020). However, experimental parameters for the CRISPR strategy depend on the intended application and desired organism trait: (a) cell type or explant used, (b) effector nuclease, (c) CRISPR mechanism (plasmid or DNA-free) (d) delivery method and (e) transfection/transformation technique (Anzalone et al., 2020). In general, strategies based on DNA-free mechanisms are the most suitable when the edited plant is wished to reach the market. This is because a transgene-free product may turn the licensing and market approval processes more feasible if considered as non-GMO in a case-by-case analysis, thus making DNA-free editing the most desirable scenario (Kocsisova and Coneva, 2023). In this context, we can classify CRISPR/Cas systems according to the SDN (site-directed nuclease) approach: SDN-1 refers to the introduction of simple random mutations (i.e., substitutions, insertions or deletions) into the host genome through NHEJ mechanism; SDN-2 denotes the replacement of small segments or even single nitrogen bases at the cleaved target site through template recombination carrying desired mutations by using HDR mechanism; and SDN-3 stands for the insertion of at least one large fragment/genetic element (e.g., promoter, CDS and/or terminator) at the target site also using HDR and, differently to what occurs for SDN-1 and SDN-2, SDN-3 is based on the introduction of exogenous sequences in the host genome (Molinari et al., 2021; Rostoks, 2021; Abdul Aziz et al., 2022; Cardi et al., 2023).
In this review, we first present an overview on the state-of-the-art in CRISPR technology applied to agriculture, mainly involving regulatory aspects of plant gene editing around the world, and proceed focusing on three of the most economically and industrially important crops worldwide (citrus, coffee and sugarcane). At this point, we emphasize the aimed traits with CRISPR-mediated crop breeding by giving many research examples reported in the literature. Moreover, we point out the methods for genetic transformation of each crop and correlate them to the main techniques and strategies already employed for their genome editing, without neglecting the main drawbacks and bottlenecks usually faced by researchers for this purpose. We also provide perspectives on recently developed CRISPR tools and promising applications for each crop and what the novel variants and optimizations of CRISPR technology could supply. Finally, we discuss the development of next-generation edited plants based on what is most urgent and feasible for improving these crops.
Undoubtedly, gene editing approaches bring numerous potential applications to agriculture. To our knowledge, the first use of gene editing via CRISPR in crop species were published in 2013 (Feng et al., 2013; Shan et al., 2013), just a few months after the inception of the technology in bacteria and animal cells. Thus, fortuitously, the power of this genetic tool was rapidly harnessed and transferred to plant species as a successful example of technical democratization, which is a key feature of CRISPR.
Since then, several genetic modifications via CRISPR have been performed in various economically important plant species (Table 1). Among them, we may cite soybean (Glycine max) (Jacobs et al., 2015), cassava (Manihot esculenta) (Odipio et al., 2017), banana (Musa spp.) (Kaur et al., 2018), coffee (Coffea canephora) (Casarin et al., 2022), grapevine (Vitis vinifera) (Malnoy et al., 2016), rice (Oryza sativa) (Lowder et al., 2015), wheat (Triticum aestivum) (Upadhyay et al., 2013) and sweet orange (Citrus sinensis) (Wang et al., 2014). And the list grows quickly, reaching species once intractable by conventional genetic methodologies. Thus, the possibility of precisely manipulating the genome of any plant in a predicted way, based on limited DNA information (a guide sequence of 20 nucleotides, usually), launches new hope for scientists working with recalcitrant species.
Similarly to most biotechnological developments, the first reports on using CRISPR in plants were “proof-of-principle” studies, i.e., knocking out genes whose null phenotypes were easily observed. Most of them rely on phytoene desaturase (PDS) gene knockout, which disrupts the synthesis of carotenoids, resulting in an albino phenotype (Shan et al., 2013). Following these first publications on gene editing protocols for crop species, reports analyzing the phenotypical effects of specific genes began to be released. During the first decade (2013-2022), the technology was applied for various agronomical purposes.
For example, the knockout of Oryza sativa cytokinin oxidase/dehydrogenase (OsCKX11), an enzyme involved in cytokinin inactivation, was shown to result in a significant increase in branch, tiller and grain number compared with the wild type (Zhang et al., 2021). Transgenic barley, cassava, banana, soybean, rice, potato, and grapevine have been engineered to directly target DNA and RNA viruses. In contrast, cucumber and wheat plants have undergone editing of endogenous genes (host factors) for virus resistance (Robertson et al., 2022). In soybean, knockout of the E1 gene resulted in the production of plants with early flowering under long-day conditions (Han et al., 2019). In banana, modifications in the MaACO1 gene delayed natural fruit ripening from 21 days to 80 days, thus extending its shelf life (Hu et al., 2021). In sorghum, changes in an alpha-kafirin gene family increased digestibility and protein quality (Li et al., 2018). Low-gluten, non-transgenic wheat was developed via CRISPR engineering of the α-gliadin gene family (Sánchez-León et al., 2018), while a reduction in the toxic steroidal glycoalkaloids content in potato was achieved by knocking out the sterol side chain reductase 2 gene (Zheng et al., 2021).
Tomato has also been one of the most frequently edited species via CRISPR technology. For example, mutations in the MAX-1 gene yielded plants resistant to the root parasitic weed Phelipanche aegyptiaca (Bari et al., 2021), while simultaneous knockout of the SlINVINH1 and SlVPE5 genes increased fructose and glucose levels for sweetness enhancement (Wang et al., 2021). Furthermore, mutating the ENO gene resulted in plants that yielded larger multilocular fruits (Yuste-Lisbona et al., 2020); knock-in of the salt-tolerant SlHKT1;2 allele conferred tolerance to germination in 100 mM NaCl (Yuste-Lisbona et al., 2020); and single or multiple mutants for SGR1, LCY-E, Blc, and LCY-B2 genes had their lycopene content increased (Li et al., 2018). Finally, mutations in the SlAMS gene promoted male sterility, which reduces the cost of F1 seed production (Bao et al., 2022).
Crop species are just beginning to be engineered via CRISPR. The next decade promises the generation of numerous agronomically interesting phenotypes via precise gene editing in many other species. Nevertheless, it is worth remarking that, despite the groundbreaking application of CRISPR in molecular plant breeding, only a few CRISPR-based crops have been approved for commercialization, such as soybean (Waltz, 2018), canola (Waltz, 2018), maize (Waltz, 2016), tomato (Waltz, 2022), and camelina (Waltz, 2018). Therefore, while scientists wield the needed molecular tool to sow the crop field, other social and legal hurdles remain to be overcome.
Crop plants can be categorized according to their life cycle lengths, such as annuals (e.g., soybean, maize), perennials (e.g., coffee, citrus, vines and the semi-perennial sugarcane), and biennials (e.g., beets, carrots, onions). Mostly, we see examples of CRISPR application in annual plants, such as cereals (Matres et al., 2021) and horticultural crops, such as tomato (Kumari et al., 2022). Among the characteristics that facilitate genome editing in annual plants is the rapid development of a new generation with the possibility of segregating the transgenes encoding the CRISPR/Cas system and other genetic elements from the gene construct in a short time (Lobato-Gómez et al., 2021) (Figure 2). Perennial plants, however, have a significant advantage, which consists of the possibility of fixing a mutation/editing that would hardly be carried forward in one generation (Lobato-Gómez et al., 2021). Also, due to the search for more sustainable agriculture, with greater potential for carbon fixation in the soil, there is an interest in converting traditionally annual crops into perennial ones, such as wheat (DeHaan et al., 2020). In these cases, genome editing has accelerated redomestication research, allowing the desired traits to be fixed in cultivars with better performance (Hanak et al., 2022).
Figure 2 Plasmid-based CRISPR strategy for annual and perennial crops. Comparison of time needed for developing transgene-free events from annual and perennial crops when using a plasmid-based CRISPR strategy. Genetic transformation achieved using a transfection technique (e.g., Agrobacterium tumefaciens) yields CRISPR-edited transgenic plants in the T0 generation. Mendelian inheritance allows segregation for transgenes (yellow triangles) elimination while selecting for CRISPR-triggered mutation (DNA with scissor/cut DNA) over the next generations (T1, T2, T3). Plants can harbor transgenes without (triangle only) or with (triangle and cut DNA) gene editing, harbor only the gene editing without transgenes (cut DNA) or neither. Whereas annual crops can be selected for a DNA-free event within a few years, perennials usually take decades. In this case, DNA-free strategies (i.e., RNPs, IVTs, viral replicons) should be employed to accelerate this development process, possibly reducing the time to approximately four years, depending on the species. Image created with BioRender.com.
Nonetheless, the long juvenile stage of perennial plants poses an additional obstacle when transgene segregation is needed due to delayed generational advancement (Figure 2), which usually takes up to decades for transgenes elimination aiming to keep CRISPR-induced mutations without the presence of plasmid backbone. Therefore, when considering this need, it is interesting to use a DNA-free method for delivering the editing machinery (Molinari et al., 2021). In this case, in order to ensure that the ribonucleoprotein complexes (RNPs) edit all genetic material (and not produce chimeras or mosaics), it is recommended that the particles are delivered into protoplasts (Woo et al., 2015). The difficulty of this procedure lies in the recalcitrance of protoplast regeneration in many plants (Reed and Bargmann, 2021). In these cases, other transgene-free methods can be used (Molinari et al., 2021), such as particle bombardment of either the mRNA-based CRISPR machinery (in vitro transcripts, IVTs) (Zhang et al., 2016) or ribonucleoproteins (Woo et al., 2015), as well as the use of virus-induced genome editing (VIGE) through viral replicon systems (Oh et al., 2021; Gentzel et al., 2022; Zhang et al., 2022) or transient expression via Agrobacterium spp. (i.e. agroinfiltration) (Kaur et al., 2021).
However, there are two major challenges when editing either annual or perennial plants. The first is its high heterozygosity rate, which makes it difficult to edit all gene alleles (Savadi et al., 2021). Prior sequencing of the target regions is necessary to circumvent this difficulty with the objective of a rational design of sgRNAs. Furthermore, since the regeneration process of post-transformation plants via in vitro tissue culture is highly dependent on the genotype, regeneration protocols still need to be established or even find recalcitrance in some plants (Chapman et al., 2022). In some cases, regeneration has been achieved by methods involving meristematic induction via genomic editing (Maher et al., 2020; Lian et al., 2022).
Regardless, the next topics provide an overview of the main parameters, achievements and bottlenecks for gene editing of three of the most economically important crops worldwide among perennial and semi-perennial plants. In this way, we could correlate and clearly show how plasmid-based strategies are being applied to them and how DNA-free approaches are promising and might overcome issues concerning transgene segregation and market feasibility.
The Citrus genus and related genera belong to the Rutaceae family and Aurantioideae subfamily (Talon et al., 2020), whose center of diversity extends from Tropical Africa through Southeast Asia and Eastern Australasia to Polynesia (Swingle and Reece, 1967). Sweet orange (C. sinensis) constitutes the most economically important citrus species in the world. In 2021, Brazil dominated global orange production, yielding approximately 16.2 million tons, followed by India and China (FAO, 2023). In addition to orange juice production, Citrus spp. are noteworthy for their potential in essential oil production (González-Mas et al., 2019) and pharmacological biomolecules (Ademosun et al., 2018).
Citrus species can be transformed through different techniques (Conti et al., 2021) (Figure 3), although Agrobacterium-mediated gene transfer is the most commonly used transformation method (Boscariol et al., 2006; Dutt and Grosser, 2009; Caserta et al., 2014; Souza-Neto et al., 2022). Different explants can be used to transform citrus species, mostly obtained from juvenile tissues, such as epicotyls and embryogenic cells, besides protoplasts (Omar et al., 2007; Dutt et al., 2020; Li et al., 2022; Mahmoud et al., 2022; Su et al., 2023). However, mature tissues are also possible sources of explants for citrus genetic engineering (Almeida et al., 2003; Peng et al., 2019). Li et al. (2022) have described the transformation of epicotyls in Carrizo citrange, whose explants were pre-treated with cytokinin (6-benzylaminopurine) and auxins (2,4-dichlorophenoxyacetic acid and 1-naphthaleneacetic acid), thus increasing the transformation efficiency from 11.5% to 31.8%.
Figure 3 Citrus genome engineering scenario. Illustration of the genetic transformation methods already reported either for stable or transient expression, main explants (juvenile or mature) used for in vitro tissue culture, methods and strategies used for genome editing of citrus species, and the desired traits achieved through gene editing. Image created with BioRender.com.
Biolistics (particle bombardment) is also an alternative method, although less commonly used due to its low transformation efficiency (Yao et al., 1996; Wu et al., 2016; Wu et al., 2019). In order to increase its transformation efficiency and reduce shoot escapes, Boscariol et al. (2003) and Wu et al. (2019) developed and applied, respectively, a methodology based on E. coli manA as a selection marker gene. The manA gene confers to the transformed shoots the ability to metabolize mannose and its intermediates through the phosphomannose isomerase (PMI) enzyme (Stoykova and Stoeva-Popova, 2011).
The polyethylene glycol (PEG) method has been used for many years for citrus genetic transformation (Huang et al., 2020; Huang et al., 2022a; Su et al., 2023). Although PEG-based transformation has limitations related to cytotoxicity and low transformation efficiency (Vardi et al., 1990; Mahmoud et al., 2022), this method allows the transfection of exogenous macromolecules into the cell via endocytosis (Vardi et al., 1990; Couchoud et al., 2019). Based on this, sweet orange protoplasts were also edited through plasmid transfection mediated by cationic lipids (Mahmoud et al., 2022). The vector harboring sgRNA targeting the Nonexpressor of Pathogenesis-Related 3 gene (CsNPR3) was designed to promote a greater induction of systemic acquired resistance (SAR). For this, Mahmoud et al. (2022) used the technique called ‘lipofection’, which uses liposomes for the delivery of exogenous material. Liposomes fuse to the plasma membrane and their cargo is released into the cytoplasm. Lipofectamine LTX coupled to PLUS reagent composed the nanostructured vehicle that resulted in the highest transformation efficiency, keeping 90% of protoplasts viability. Furthermore, they verified that under the presence of the Arg9 CPPs (Cell-Penetrating Peptides), the transformation efficiency could be even more increased. Therefore, boosted cationic lipids may be an alternative to the use of PEG solution as a transfection agent for protoplasts and should be tested in plants for the transfection of RNP complexes. Electroporation is also a suitable alternative, since it does not have host range limitations. Nevertheless, in recent years, this technique has not been used for citrus transformation (Hidaka and Omura, 1993; Niedz et al., 2003).
In some techniques, transformation is not necessary for citrus genome editing (Alquézar et al., 2022; Huang et al., 2022b). Accordingly, Su et al. (2023) performed gene editing of the CsLOB1 gene by using a DNA-free strategy based on RNPs, thus achieving a high rate of biallelic mutations and no off-target effect. In the same way, Huang et al. (2022) employed PEG-mediated transfection to trigger mutagenesis in protoplasts of C. sinensis, targeting the same gene. Additionally, they employed a highly efficient editing mechanism that modifies one or more base pairs through Cas nickase (nCas) with high specificity and low error rate (Azameti and Dauda, 2021; Molla et al., 2021).
It is known that citrus cultivars have a low regeneration rate and transformation efficiency (Peng et al., 2019; Wu et al., 2019). Furthermore, mosaicism during regeneration of citrus plants is a frequently reported issue (Alquézar et al., 2022), especially when epicotyls are used as explants. Due to these problems, protocols are often optimized for each variety (Dutt and Grosser, 2009; Oliveira et al., 2009) and, in order to minimize mosaicism rate, the use of embryogenic cells has been a feasible alternative to obtain engineered plants with a relatively high transformation efficiency (Dutt and Grosser, 2010; Dutt et al., 2020). Juvenility is another commonly faced problem for the evaluation of citrus phenotypes. Hence, in order to reduce flowering and fruiting time, Cervera et al. (2009) developed APETALA1 (AP1) transgenic citrange plants with short tree cycle which showed early flowering and fruiting. Low levels of polyembryony can also be a barrier to genetic transformation, since some species may produce a small number of nucellar embryos (Omar et al., 2007; Alquézar et al., 2017).
Regardless of the bottlenecks for genetic engineering and genome editing of citrus species, there are key traits that are interesting for genetic breeding that could already be improved or modulated in order to yield more desirable varieties. Disease resistance is one of the most important characteristics sought for citrus species. Huanglongbing/HLB (caused by Candidatus Liberibacter spp. – CLs) and citrus canker (caused by Xanthomonas citri subsp. citri – Xac) are the main diseases in citrus orchards worldwide (Coletta-Filho et al., 2004; Ference et al., 2018; Bassanezi et al., 2020; Naqvi et al., 2022). Boscariol-Camargo et al. (2016) and Robertson et al. (2018) observed that transgenic plants overexpressing the Arabidopsis NPR1 protein displayed tolerance to HLB and resistance to citrus canker. Souza-Neto et al. (2022) observed that sweet orange and Carrizo citrange overexpressing the mqsR gene from Xyllela fastidiosa showed resistance to citrus canker and citrus variegated chlorosis. Potential genes of interest for genetic transformation and/or gene editing have been studied in order to obtain plants resistant to HLB (Curtolo et al., 2020), such as AtPs21 and CsACD2, which act in repellency against the psyllid Diaphorina citri and as a susceptibility gene, respectively (Alquézar et al., 2017; Pang et al., 2020). Viral and fungal pathogens are important targets in citrus genetic engineering as well. Muccilli et al. (2020), for instance, developed a transgenic lemon expressing chit12 gene, which confers tolerance to fungal pathogens in post-harvest conditions.
Abiotic stresses, such as drought and salinity, are also important problems faced in citrus orchards. In this case, genes coding for osmoprotectants (Longhi et al., 2022; Barichello et al., 2017) or transcriptional factors (Romero-Romero et al., 2020) can be interesting for genetic transformation or gene editing. In the field, modulation of genes involved in the architecture of the canopy and fruit quality (Dutt et al., 2022) are also interesting strategies to facilitate management and commercialization of citrus fruits.
Regarding achievements already made in citrus genome editing, reporter genes allowing easy identification of the resulting knockout phenotype, such as CsPDS (C. sinensis phytoene desaturase gene) (Jia and Wang, 2014; Jia et al., 2017a; Zhang et al., 2017; Dutt et al., 2020; Tang et al., 2021) and CsALS (C. sinensis acetolactate synthase gene) (Alquézar et al., 2022; Huang et al., 2022b), have been used to optimize gene editing protocols. Until now, the citrus species targeted for genome editing are Carrizo citrange (Poncirus trifoliata x C. sinensis), grapefruit (Citrus paradisi), pummelo (Citrus maxima) and sweet orange (C. sinensis). After optimizing these protocols, researchers have been editing target genes to mainly provide resistance against citrus canker. In this context, the CsLOB1 (Citrus Lateral Organ Boundaries 1) gene has been the most studied because it encodes a transcription factor related to citrus canker susceptibility (Jia et al., 2016; Jia et al., 2017b; Peng et al., 2017; Huang et al., 2020; Jia and Wang, 2020; Huang et al., 2022a; Huang et al., 2022b; Su et al., 2023).
Jia et al. (2017b) obtained six independent Duncan grapefruit events edited for the CsLOB1 gene, from which two (DLOB9 and DLOB10) did not develop pustules typical of citrus canker symptoms, and their mutation rates were the highest among the events (89.36% and 88.79%, respectively). In pummelo, eight transgenic events were obtained, but only those with homozygous and biallelic mutations showed resistance to citrus canker. The same was observed for Hamlin sweet orange events that have biallelic mutations in the EBE (Effector Binding Element) (Huang et al., 2022a) and TATA box regions (Huang et al., 2022b) of the CsLOB1 gene promoter.
It is worth to mention that, although HLB is the most devastating citrus disease worldwide, there are no reports yet regarding resistant GE or even GM varieties. However, Curtolo et al. (2020) have identified key genes that are promising to achieve this trait, among them endochitinases B (ChiB) genes, which showed to be upregulated in a resistant pool made up from hybrids of Citrus sunki and P. trifoliata (an HLB-tolerant citrus species), and encoding vacuolar enzymes displaying putative antimicrobial activity (Medeiros et al., 2018) that should be evaluated against CLs. Furthermore, HLB tolerance and resistance is also able to be achieved by disrupting susceptibility or sensitivity genes. In the latter case, Granato et al. (2019) observed that two out of nine callose synthase (CsCalS) genes, named CsCalS7 and CsCalS12, were highly upregulated in C. sinensis during HLB infection and could significantly contribute to callose deposition in the phloem during bacterial colonization. This mechanism is known to trigger symptoms of this disease by blocking translocation of sap nutrientes, and could be enhanced when CsCalS7 and CsCalS12 are overexpressed. Hence, genome silencing of these genes could lead to symptoms attenuation or even avoid their arising, and edited plants could be HLB-tolerant.
Moreover, other sensitivity genes involved in the same mechanism of phloem obstruction during host response to bacterial infection are described in the literature and could be silenced as well. Batailler et al. (2012) and Ernst et al. (2012) have described the function of SEO (Sieve Element Occlusion) genes in encoding phloem proteins (P-proteins) that could aggregate and promote wound sealing in the sieve elements of Arabidopsis thaliana and Nicotiana tabacum, respectively. Based on this, Curtolo et al. (2020) evaluated SEO orthologs in C. sinensis and discovered that SEOc and SEOd genes were largely upregulated in all HLB-susceptible plants studied, as well as in some of the tolerant hybrids, thus indicating their analog function of phloem wound sealing by crystalloid proteins and suitability for gene silencing. Similarly, Boava et al. (2017) have discussed the function of the PP2 (Phloem Protein 2) gene family during HLB infection and found that these conserved phloem lectins encoded by pp2 genes consequently block the transport of photoassimilates from sap to plant organs, leading to symptoms and tissues death, which thus suggests pp2 genes as strong candidates for gene editing.
Regarding explants used for this intent, they are the same as those used for transgenic plant production (i.e. epicotyls, embryogenic cell suspensions and protoplasts) (Table 2). However, using epicotyls has the disadvantage of regenerating mosaic shoots for editing as well, as demonstrated by Zhang et al. (2017), which edited PDS gene and obtained mosaic, albino and green shoots from explants. Furthermore, the use of epicotyls depends on the availability of viable seeds. In contrast, shoots regenerated from embryogenic cell suspensions or protoplasts are derived from single cells, which eliminates mosaicism or chimerism (Dutt et al., 2020; Mahmoud et al., 2022).
Table 2 Summary of reports on citrus genome editing and details concerning genetic engineering and CRISPR/Cas parameters.
In general, plasmid vectors used in citrus editing harbor kanamycin or hygromycin selectable marker genes, as well as employ GUS or GFP as reporter genes. Multiplex editing systems are mostly based on Csy4 endoribonuclease or polycistronic tRNA-gRNA for sgRNA units processing (Jia and Wang, 2014; Jia et al., 2017a; Zhang et al., 2017; Dutt et al., 2020; Huang et al., 2020; Tang et al., 2021; Alquézar et al., 2022; Huang et al., 2022a; Huang et al., 2022b). Yang et al. (2023) optimized the transfection of callus-derived protoplasts through the PEG method, evaluating multifactorial conditions, and proved the functionality of the polycistronic tRNA-gRNA system in protoplasts for transient expression. Huang et al., 2020 demonstrated that in Carrizo citrange the tRNA-gRNA multiplex system had a higher editing efficiency than the Csy4 system, possibly due to the fact that the tRNA-gRNA system depends on an endogenous processing machinery (i.e. native tRNA expressed by the host), in contrast to the heterologous processing machinery provided when using the Csy4 system. Nevertheless, Dutt et al. (2020) edited the CsPDS gene in embryogenic cell suspensions using two sgRNAs through the Csy4 processing system. The transformation rate obtained was 36.5% and from the 12 events evaluated, all of them were edited by sgRNA1 and 11 had mutations triggered by sgRNA2, thus demonstrating high editing efficiencies. As expected, they also demonstrated the absence of mosaic embryos due to the use of cell suspensions.
Concerning the CRISPR system chosen, plant codon-optimized SpCas9 (Streptococcus pyogenes Cas9) is a recommended nuclease to be used, but Jia et al., 2017a demonstrated that it is possible to perform gene editing in Carrizo citrange and Duncan grapefruit using the native Cas9 from Staphylococcus aureus (SaCas9). This nuclease has the advantage of reducing the number of off-targets due to its stringent PAM sequence (NNGRRT). In the genome of C. sinensis, for example, the SaCas9 PAM occurs every 79 bp, whereas the SpCas9 PAM occurs every 32 bp, which makes SaCas9 a more specific nuclease for its gene editing.
Additionally, efforts have been made to develop protocols that allow DNA-free genome editing. This approach is very useful because it facilitates the release of genetically modified events by circumventing regulatory issues related to GM development (Ishii and Araki, 2016), since mutations occur without inserting exogenous DNA into the host genome (Molinari et al., 2021). For this, C. sinensis protoplasts have been transfected with three subtypes of Cas nucleases composing RNPs: ErCas12a, LbCas12a and LbCas12aU (Zhang et al., 2022; Su et al., 2023). Zhang et al. (2022) tested different RNP concentrations using LbCas12a nuclease to edit the CsPH5 gene and concluded that 0.1 µM allows the best editing efficiency (90.8%). Su et al. (2023) performed a protocol for developing DNA-free edited plants within 10 months and showed that both ErCas12a and LbCas12aU were efficient to generate biallelic/homozygous CsPDS mutations.
Coffee is one of the most consumed beverages worldwide and its production reached a volume equivalent to 167 million bags of 60 kg in the year 2020-2021 (https://www.embrapa.br/). Coffee plants belong to the genus Coffea, being Coffea arabica and Coffea canephora the main species responsible for the production of grains consumed all over the world. C. canephora species is diploid, allogamous, highly productive and resistant to some pests and diseases. On the other hand, C. arabica is an allopolyploid (4n) resulting from a natural hybridization between C. canephora and C. eugenioides, preferentially autogamous, susceptible to several pathogens, and highly productive. Arabica grains result in a high cup quality, including specialty coffees (Carvalho et al., 1991).
Breeding of Coffea species guaranteed the availability of commercial cultivars adapted to different environments, easily managed, highly productive, resistant to biotic and abiotic stress, and most importantly with high organoleptic quality and specific chemical attributes. However, traditional coffee breeding is time-consuming and limited by the low genetic diversity of C. arabica germplasm. Therefore, the use of novel genome-based methodologies, such as MAS and genome editing, represents an opportunity to introduce novel traits into this culture in a faster and more controlled manner (Guerreiro-Filho and Maluf, 2019).
The in vitro cultivation of Coffea species started in the early 1970s, with the development of protocols of somatic embryogenesis, aiming to use in vitro strategies to multiply coffee seedlings from elite cultivars, special hybrids and potential F1 (Campos et al., 2017). Later, with the advancements in plant transformation, embryogenic callus could be selected for plant regeneration (Etienne et al., 2018). Those studies also indicated that embryogenesis and regeneration efficiency depend on the genetic background, media composition, type of explants and cultivation conditions.
Indirect somatic embryogenesis is the most promising tissue culture technique in coffee, since regeneration into viable adult plants is well-established, although it still needs to be optimized for large-scale production of seedlings (Mishra and Slater, 2012; Etienne et al., 2018). However, embryogenic calli are suitable for coffee transformation. Ribas et al. (2011) compared different cultivation and selection methods and determined that a four-month established embryogenic callus culture has a high transformation efficiency. Also, they found that color and type of callus, as well as culture media composition, are key parameters to improve transformation efficiency.
Methods for efficient transformation of both C. arabica and C. canephora species mostly include particle bombardment (Ribas et al., 2005; Albuquerque et al., 2009; De Guglielmo-Cróquer et al., 2010), Agrobacterium-mediated transformation of embryogenic callus (Ribas et al., 2011), hairy roots (Alpizar et al., 2006), agroinfiltration of mature leaves (Vargas-Guevara et al., 2018) and protoplast electroporation (Fernandez and Menéndez-Yuffá, 2003) (Figure 4). Overall transformation efficiency depends on several parameters, reviewed by Mishra and Slater (2012) and Etienne et al. (2018), and is comparable to other plant species. Despite these initiatives, coffee transformation is not a regular strategy, neither for the development of novel cultivars nor to evaluate the effect of any given gene over selected traits. Major limitations for the use of transformation in coffee culture include the allopolyploid nature of C. arabica, the long-life cycle and high cost to maintain evaluation areas. Also, another major drawback is the long and laborious process of coffee in vitro regeneration, which does not always result in mature transformed plants. On the other hand, molecular analysis indicated that regenerated transformed plants display very low or no somaclonal variation (Landey et al., 2013; Oliveira et al., 2019).
Figure 4 Coffee genome engineering scenario. Illustration of the genetic transformation methods reported, the genomic complexity and low number of identified gene targets as major challenges, the low regeneration rate as a limitation on in vitro tissue culture, the genome-wide association studies as a strategy to identify genes associated with agronomic traits, and the possibilities on coffee genome editing. Image created with BioRender.com.
Most of the genetic transformation events of Coffea obtained so far aimed to develop plants resistant to diseases and pests. To introduce the Cry1Ac gene of Bacillus thuringiensis (Bt) into C. arabica, biolistics was used in somatic embryos to confer resistance to Leucoptera coffeella. However, out of a total of 12 embryos, only one expressed the gene of interest (De Guglielmo-Cróquer et al., 2010). The same gene was also successfully introduced into C. canephora through A. tumefaciens (Perthuis et al., 2005). Looking for resistance to the coffee berry borer (CBB), Hypotheneumus hampei, Valencia-Lozano et al. (2021) developed transgenic C. arabica var. Typica expressing Bt Cry10Aa by biolistics. The authors achieved 16.7% transformation efficiency, and seeds harvested from the T1 generation, derived from three transformed plants, expressed Cry10Aa and successfully controlled CBB. Fruits of genetically transformed plants inhibited the development and infestation capacity of CBB females (Valencia-Lozano et al., 2021). Similarly, particle bombardment was used to transform embryogenic calli of C. arabica with the α-amylase-1 inhibitor gene (α-AI1) that triggers resistance to CBB (Albuquerque et al., 2015). The authors evaluated 54 plants and observed that the α-AI1 transgene was stably inherited in the T2 progeny and expressed in a tissue-specific manner in seeds by using the PHA-L promoter. Despite these efforts, there is no GM coffee cultivar available for cultivation yet.
Regardless of the limitations of in vitro tissue culture and the genetic transformation described here, genome editing of coffee represents a promising strategy to develop novel cultivars. Breeding perennial plants is time-consuming, and the possibility to modify specific traits by editing target genes with no other potential change in the genetic background is very appealing. In coffee, gene editing studies available so far (Table 3) are merely proof of concepts in C. canephora plants (Breitler et al., 2018; Casarin et al., 2022). In both studies, PDS was used as a target gene and the CRISPR machinery was delivered to embryogenic callus by A. tumefaciens. The overall editing efficiencies were 30.4% (Breitler et al., 2018) and 76.9% (Casarin et al., 2022), with 7.6% and 54% of homozygous mutations, respectively. Besides albine and variegated seedlings, Casarin et al. (2022) also observed seedlings with abnormal cotyledon and root development, and limited growth. Based on the mutation rates obtained in those studies, the use of editing strategies by breeding programs is encouraging. However, editing of C. arabica remains a challenge due to its allopolyploid nature, which means that four alleles must be edited simultaneously to obtain a homozygous mutated trait.
Table 3 Summary of reports on coffee genome editing and details concerning genetic engineering and CRISPR/Cas parameters.
Nonetheless, CRISPR technology remains an important and strongly promising tool to improve the quality of coffee beverage by reducing caffeine content, among other desired traits. In this way, genomic selection and association studies have been useful to accelerate molecular breeding and to reduce the delivery time of more adapted coffee cultivars in C. arabica (Carvalho et al., 2020; Sousa et al., 2021; Carvalho et al., 2023) and C. canephora (Ferrão et al., 2018; Adunola, et al., 2023). Advances in scale, resolution and analysis of “omics” techniques help to reveal possible targets for genome editing. However, high-impact coffee challenges such as drought and heat tolerance, as well as resistance against CBB and to aggressive nematodes such as Meloidogyne spp. and Pratylenchus spp. require further study of the molecular mechanisms behind their regulation and plant interactions. Anyway, we can point out interesting traits for coffee breeding that may be a closer reality due to the availability of potential targets for genome editing.
Concerning biotic stresses (i.e., pest control and disease resistance), the coffee leaf miner (CLM), L. coffeela, is a major concern for Coffea spp. It is a monophagous insect whose caterpillar feeds on the coffee leaves causing great losses by reducing its productivity. Resistance to CLM derived from Coffea racemosa, a moderately resistant species used in Brazilian breeding programs, is controlled by two complementary dominant genes (Guerreiro-Filho et al., 1999). Microarray analysis was used to unravel the molecular basis of plant mechanisms involved in coffee responses to CLM attacks (Cardoso et al., 2014). The study provides information on molecular aspects of CLM defense mechanisms, describing pathways regularly activated in response to herbivory, primary and secondary metabolism pathways and the expression of genes related to plant antibiosis strategy. The authors concluded that differential expression profiles between resistant and susceptible genotypes are observed in the absence of the leaf miner, indicating that the defense is already built into resistant plants as a priming mechanism. In addition, some potential marker candidate genes were validated by RT-qPCR (Cardoso et al., 2014).
Moreover, GWAS was carried out on an arabica coffee population derived from C. racemosa used for studies on CLM resistance (Nonato et al., 2021). The authors identified four SNPs significantly associated with jasmonic acid metabolism and with LRR-RLK proteins. The first one has an important role in resistance to biotic agents, and the second recognizes pathogen-associated molecular patterns (PAMPs) and herbivore-associated molecular patterns (HAMPs), thus suggesting potential mutagenesis target sites to be mimicked towards achieving CLM resistance in coffee.
Coffee leaf rust (CLR), caused by Hemileia vastatrix, and coffee berry disease (CBD), caused by Colletotrichum kahawae, are diseases limiting coffee productivity. CLR is present in coffee producing regions around the world (Ventura et al., 2019), whereas CBD is restricted to C. arabica in Africa (Van der Vossen and Walyaro, 2009). According to Ventura et al. (2019), genetic resistance to H. vastatrix is observed in diploid species such as C. canephora, Coffea congensis, Coffea dewevrei and Coffea liberica, and is conditioned by at least one of nine dominant genes (SH1 to SH9). Knowledge about the molecular basis of the mechanisms involved in resistance to H. vastatrix advanced through proteomics by identifying markers of resistance to this fungus (Guerra-Guimarães et al., 2015) and transcriptome analyses of the plant-pathogen interaction (Castro et al., 2022; Estanislau, 2022).
Targeting to speed up the breeding programs, DNA markers for CRL and CBD resistance have already been suggested for marker-assisted selection of C. arabica genotypes with introgressed genes from other coffee species (Silva et al., 2018) and for characterization of germplasm diversity (Alkimim et al., 2017). Nonato et al. (2021) found five candidate genes close to SNPs significantly associated with leaf rust according to the type of reaction and type of fungal lesion. Three genes (T, R and k) are involved in resistance to CLB (Van der Vossen and Walyaro, 1980). In addition, Gimase et al. (2020) used GWAS and found associations between two SNP markers (Ck-2 and CK-3) with CBD resistance.
Concerning marketing and quality traits, sensory attributes of coffee beans are influenced by the chemical composition of green beans, which in turn is controlled by genetic factors (Ky et al., 2001; Leroy et al., 2006; Farah, 2009; Tran et al., 2017), environment production, fruit maturation physiology and technological factors such as post-harvest processing (Kitzberger et al., 2020; Wondimkun et al., 2022) or even by different methods of coffee brewing (Novaes et al., 2019; Chavez et al., 2022). It is known that within the species C. arabica (Ky et al., 2001; Scholz et al., 2016) and C. canephora (Mori et al., 2016) there is genetic variability for non-volatile biochemical precursors of coffee aroma such as caffeine and trigonelline, chlorogenic acids, sucrose, and lipids such as cafestol and kahweol diterpenes (Ky et al., 2001; Farah, 2009). GWAS studies performed by Sant’Ana et al. (2018) identified five SNPs associated to lipid content: four with cafestol, three with kahweol and nine with the cafestol/kahweol ratio. As most of these SNPs are located inside or near genes from the metabolic pathways of these chemical compounds in coffee beans, they are potential targets for gene editing approaches.
Among other potential targets for editing in coffee, genes promoting caffeine synthesis are the most promising candidates. A naturally decaffeinated beverage is a recurrent demand from a growing market that seeks coffees with special organoleptic characteristics. Although caffeine is known for its stimulant effects, it can also cause, in sensitive people, unwanted responses such as headaches, tremors and nausea, among others. Caffeine synthesis in coffee is controlled by three genes encoding methyltransferases responsible for converting xanthine into caffeine (Ashihara et al., 2008), which have a simple genetic inheritance (Favoretto et al., 2017).
Caffeine accumulates in all coffee tissues, and since early developmental plant stages, what makes it a valuable biochemical marker. Molecular analysis of a naturally caffeine-free C. arabica plant indicates that the lack of caffeine does not affect other agronomic traits (Guimarães et al., 2021). In this study comparing large-scale gene expression from branches, buds and fruits from regular and caffeine-free coffee plants, the authors identified 171 transcripts out of 65,000 presenting differential expression between both groups. In silico analysis indicated that most of these transcripts are unrelated to caffeine metabolism or plant physiology impairment. Therefore, blocking caffeine synthesis in coffee fruits through gene editing represents a promising strategy to develop caffeine-free events.
Previous studies attempted to block caffeine synthesis through downregulation of the first gene of the caffeine biosynthetic pathway, coding for xanthosine methyltransferase (MXMT), by using RNAi. This strategy led to a 50-70% reduction in caffeine content in transgenic C. canephora leaves, and an almost abolishment of caffeine content in embryogenic tissues of C. arabica (Ogita et al., 2003). As a result of this strategy, a 20% increase in theobromine content and a reduction in transcripts from the XMT (theobromine synthase) and DXMT (caffeine synthase) genes were also observed. Later, the same genetic engineering approach was used to decrease caffeine content by targeting the N-methyl transferase gene family, but this effect was shown to be ineffective over time (Mohanan et al., 2014). Despite the promising results at the seedling stage, none of those events resulted in a mature caffeine-free coffee plant. However, similar approaches based on CRISPR/Cas technology can also be used to achieve this aim in an effective way, thus promoting gene silencing at the genomic level.
Sugarcane (Saccharum spp.) is a C4 grass crop that probably originated in Southeast Asia and New Guinea, where its domestication occurred about 10,000 years ago (Lebot, 1999). Its global economic importance is bolstered by its use as a main feedstock in the production of sugar, bioenergy, and other valuable by-products (e.g., bioplastics, forage). To illustrate, the global sugarcane production in 2020 was around 1.8 billion tons, headed by Brazil, India, and China (FAOSTAT, 2022). Therefore, its enormous potential as a bioenergy feedstock, which is supported by its high photosynthetic efficiency, underscores the importance of sugarcane breeding programs.
Sugarcane breeding programs generally focus on the genetic improvement of major production traits, such as cane yield, biomass and fiber outcomes, sucrose accumulation, uniform tillering, and better stem elongation (Gouy et al., 2013). However, as climate change intensifies, causing severe harm to sugarcane crops (Linnenluecke et al., 2018), other features, such as those providing drought tolerance, have been within the scope of breeders. These features include deep root systems, stay-green phenotype, and erect canopies (Meena et al., 2022), striving for climate-resilient, high-yielding crops. Nevertheless, agronomically essential traits are complex and require genome-wide DNA marker approaches for their genomic predictions (Hayes et al., 2021).
Among other issues threatening sugarcane production and reducing biomass and its by-products is the severity of some pests and diseases. Sugarcane grows well in tropical and subtropical regions in conditions that are also optimal for establishing a range of pathogens (Sanguino, 1998). The major fungal diseases influencing productivity to a greater or lesser extent in different regions are smut, brown and orange rusts, brown spots, pineapple rot, red rot, and fusariosis (Verma et al., 2022). The most common examples of bacteriosis are leaf scald and ratoon stunt disease (Monteiro-Vitorello et al., 2009; Barbasso et al., 2010; Sundar et al., 2015). Also noteworthy, pests such as Plant Parasitic Nematodes (PPN) that attack the roots of living plants have been responsible for widespread yield losses across all sugarcane fields of different soil compositions (Dinardo-Miranda et al., 2019). Besides their regular presence, climate change will impact population dynamics and the overall occurrence of pests and pathogens, contributing to losses in productivity and affecting sugarcane growth and metabolism (Velásquez et al., 2018).
Developing resistant genotypes is the most reliable and durable way to secure plants from pathogens. Beyond the search for ‘major resistance genes’ (R genes), for most diseases, quantitative resistance has many advantages (Pilet-Nayel et al., 2017). Diagnostic markers for quantitative resistance contemplate investigating variation in genes involved directly in pathogen recognition or related processes (‘candidate gene approach’) or an untargeted method such as comparing RNA-Seq data of resistant versus susceptible plants (Mosquera et al., 2016). Furthermore, the exploration of ‘susceptibility genes’ (S genes) with subsequent gene knockout can enhance plant resistance against specific pathogens by abolishing their compatibility with the host (Moniruzzaman et al., 2020). Therefore, the integrated use of modern approaches, such as next-generation sequencing (NGS) and genome editing, might contribute to the prospection of candidate genetic elements for developing high-performance gene-edited sugarcane crops.
The sugarcane genome is huge (>10 gigabases) and has the most polyploidy (2n = 100-120) known among domesticated species (Piperidis and D’Hont, 2020), even with events of aneuploidy, inter-chromosomal translocations, and hybridization between species. The homologous chromosomes of modern commercial hybrids originated mainly from Saccharum officinarum (2n = 80, x = 10) and Saccharum spontaneum (2n = 40–128, x = 8), whose genomes contributions correspond to 80% and 10-15%, respectively (D'Hont et al., 1996; Garsmeur et al., 2018; Piperidis and D’Hont, 2020). Undoubtedly, the complex sugarcane genome represents an obstacle to crop improvement through classical or biotechnological genetic breeding. Additionally, conventional breeding programs face challenges such as dependence on photoperiod and temperature condition in floral induction, lack of pollen fertility and flowering synchrony in specific crosses (Melloni et al., 2015), besides a narrow genetic background (Berding and Roach, 1987). Consequently, it is very costly, extremely laborious, and time-consuming, taking 10–15 years to release a new elite variety (Gazaffi et al., 2010). In contrast, Saccharum vegetative propagation is a feature that favors the improvement of cultivars by conventional transgenesis and genome editing approaches, which overcomes the sexual barriers, difficulties of outcrossing Saccharum species, and transmits intended modifications into the genome without segregation by sexual reproduction (Ingelbrecht et al., 1999; Oz et al., 2021).
While in the mid-1990s the world witnessed a set of approved transgenic crops (James and Krattiger, 1996), two decades passed until the first commercial approval of a transgenic sugarcane crop in 2017. Sugarcane genetic transformation protocols emerged in the 1990s, when the first transgenic sugarcane plant, carrying a selectable marker gene (neomycin phosphotransferase - nptII), was developed using particle bombardment of embryogenic callus (Bower et al., 1996). Sugarcane transformation mediated by intact cell (calli) electroporation was sparse and temporarily reported in the literature (Arencibia et al., 1992; Arencibia et al., 1995; Arencibia et al., 1997). On the other hand, the Agrobacterium tumefaciens-mediated transformation was successfully achieved by Arencibia et al. (1998), using the reporter gene gus (uidA) to optimize the protocol. Shortly thereafter, Agrobacterium-mediated transformation using the bacterial PPT acetyltransferase gene (bar) rendered glufosinate-resistant sugarcane plants (Enríquez-Obregón et al., 1998). Further on, highly efficient protocols have emerged and fostered the industrial-scale production of transgenic plants (Dong et al., 2014; Basso et al., 2017). Furthermore, there are limited reports on sugarcane protoplast transformation that, however, have left the regeneration step as an unsolved problem (Chen et al., 1987; Rathus and Birch, 1992). Thus, transformation via particle bombardment and A. tumefaciens of embryogenic callus or leaf rolls are the most widespread approaches (Figure 5) (Budeguer et al., 2021), which can reach similar transformation efficiencies and level of complexity of foreign DNA insertion (Jackson et al., 2013; Wu et al., 2015) – the nature of complexity refers to positional and copy number of transgene insertion, which promotes a critical condition for silencing and stability of the transgene (Matzke et al., 1994; Iglesias et al., 1997; Waterhouse et al., 2001). Finally, foreign DNA insertion and successful expression of the transgene can be less critical than other factors with higher complexity, such as sugarcane genome size and polyploidy, which comprise a real challenge in this crop.
Figure 5 Sugarcane genome engineering scenario. Illustration of the genetic transformation methods reported, the genomic complexity as a major challenge, the issues on in vitro tissue culture, the high-throughput screening of gene-edited events as a solution, and the prospected strategies for sugarcane genome editing. The genome complexity icon was adapted from Garsmeur et al. (2018). Image created with BioRender.com.
Despite the progress on transformation methodologies in sugarcane (Figure 5), transgene-free genome editing often requires the delivery of RNP complexes or transient expression systems into protoplasts (González et al., 2021; Lin et al., 2022; Sidorov et al., 2022). However, obtaining regenerated sugarcane plants from protoplasts is indeed a herculean task (Taylor et al., 1992), thus demanding efficient protocols for sugarcane protoplast regeneration (Hussin et al., 2022). Alternatively, delivery of CRISPR reagents directly into plant cells can be conducted via particle bombardment of embryogenic cells and zygotes, as reported in maize (Svitashev et al., 2016), wheat (Liang et al., 2017), and rice (Toda et al., 2019). Since their polyploidy and heterozygous nature hinder the use of sugarcane zygotes as targets for bombardment, a possible alternative is the bombardment of CRISPR reagents on somatic embryogenic cells or somatic embryos.
A significant concern in the genetic transformation of Saccharum genotypes lies in genotype-dependent responses to in vitro tissue culture procedures (Figure 5) (Di Pauli et al., 2021; Li et al., 2021). Several elite cultivars are recalcitrant to genetic transformation, thus hindering even conventional transgenesis (Altpeter et al., 2016). Although there are a few dozen genetically engineered Saccharum genotypes in the literature, e.g., ROC22 (China) (Wang et al., 2017), RB855156 and SP80-3280 (Brazil) (Reis et al., 2014; Cristofoletti et al., 2018), Co 86032 (India) (Augustine et al., 2015) and Q117 (Australia) (Jackson et al., 2013), there are many varieties of commercial interest around the world (Cursi et al., 2022; Zhao et al., 2022). To illustrate, our team witnessed energy cane genotypes (S. spontaneum x S. spp. hybrids) that are recalcitrant for callus production, whereas others produce heterogeneous calluses (embryogenic and non-embryogenic) with low regeneration efficiency (unpublished data). Moreover, some calluses regenerate but are incompatible with Agrobacterium-mediated transformation, for which bombardment transformation is encouraged. As a prospect, a versatile platform for simultaneous genome editing and transcription activation of morphogenetic regulators (e.g., BABY BOOM, WUSCHEL2) - CRISPR-Combo (Pan et al., 2022) - would be an innovative strategy to circumvent such plant regeneration barriers as well as to ease the screening of transgene-free genome-edited crops, as reported in rice plants. However, the search for tissue-specific promoters for sugarcane is warranted since these morphogenetic regulators may lead to phenotypic abnormalities and plant sterility under the activity of strong constitutive promoters (Lowe et al., 2018).
Chimerism and mosaicism are other unwanted phenomena derived from tissue culture. In order to avoid this, it is mandatory to regenerate an organism from a single genetically modified cell. The critical concerns for this are: (i) an efficient genetically modified cell selection system in the initial phase of tissue culture; and (ii) regeneration via somatic embryogenesis instead of organogenesis (Dong and McHughen, 1993). In transgenic sugarcane, the high-efficiency selection markers are the geneticin-G418 antibiotic (selectable marker gene: nptII) (Bower et al., 1996) and glufosinate herbicide (selectable marker gene: bar) (Enríquez-Obregón et al., 1998). Additionally, the promoters regulating selectable genes must also be efficient to obtain low transgene-copy plants, as opposed to low-ploidy plants, in which the selectable gene promoter is typical of low expression. Particularly for sugarcane, the use of strong promoters is recommended, such as Zea mays ubiquitin (pZmUbi) (Luo et al., 2022) and the enhanced CaMV 35S promoter (Kim et al., 2017; Guidelli et al., 2018).
Nevertheless, sugarcane tissue culture is time-consuming and plagued with high costs, thus demanding efforts for the application of viral vector-mediated CRISPR delivery. Viral vectors have been frantically tested and prospected towards the delivery of CRISPR machinery into plant cells (Oh et al., 2021); yet, there are no successful reports on sugarcane. Considering the biological nature of these systems, as Agrobacterium-mediated transformation, they rely on compatible biological interactions with the explant. In wheat, the Barley stripe mosaic virus-based sgRNA delivery vector (BSMV-sg) is effective in performing heritable genome editing (Li et al., 2021; Wang et al., 2022), thus raising potential applications in other monocot plants. The recombinant Sugarcane mosaic virus (SCMV) (Beernink et al., 2021) and the Foxtail mosaic virus (FoMV) (Mei et al., 2019) may also be other viable VIGE alternatives for testing in Saccharum spp. plants. Nevertheless, these viral vector-based approaches do not support the efficient delivery of large Cas effectors (more than 1000 amino acids), such as Cas9 (Baltes et al., 2014; Uranga et al., 2021), thus relying on Cas9-overexpressing plants (Li et al., 2021). However, small Cas effectors have been unveiled as efficient tools for VIGE in plants, highlighting the miniature Cas12f (Wu et al., 2021) and the hypercompact Cas12j2 (Liu et al., 2022) as promising candidates. As a prospect, this strategy may promote the accelerated genome editing of recalcitrant sugarcane cultivars in a high-throughput manner.
Finally, the highly-polyploidy nature of the sugarcane genome often impairs the screening of gene-edited events by conventional methods, such as T7 endonuclease 1 (T7E1) (Li et al., 2013), Surveyor nuclease (Cong et al., 2013), high-resolution melting (HRM) analysis (Montgomery et al., 2007) and direct sequencing of PCR-amplified target regions (Brinkman et al., 2014). Alternatively, the preliminary detection of sugarcane mutants by PCR/RE or capillary electrophoresis (Jung and Altpeter, 2016) is feasible but relies on robust validation approaches, such as pyrosequencing (Jung and Altpeter, 2016). Moreover, novel high-throughput and low-cost genotyping methods, such as HI-TOM (Liu et al., 2019) and CRIS.py (Connelly and Pruett-Miller, 2019), are critically relevant for the massive screening of marker-free, genome-edited sugarcane plants.
To date, genome editing technologies are still in their infancy in sugarcane biotechnology (Table 4). However, Altpeter and his colleagues have pioneered elementary molecular strategies employing TALEN and CRISPR/Cas9 systems in these crops (Jung and Altpeter, 2016; Kannan et al., 2018; Eid et al., 2021; Oz et al., 2021). Firstly, they implemented TALEN technology for multiallelic mutagenesis of a lignin biosynthetic gene, caffeic acid O-methyltransferase (COMT), which improved the saccharification efficiency (54%) without impairing biomass yield (Jung and Altpeter, 2016; Kannan et al., 2018). Further on, Altpeter’s team unlocked the application of the CRISPR-Cas9 system in sugarcane. As a proof of concept, a highly evident phenotype was elicited by multiallelic, targeted mutagenesis of magnesium chelatase subunit I (MGCH), a gene encoding a key enzyme for chlorophyll biosynthesis. Furthermore, in this study, they performed a heat treatment of transformed sugarcane calli, which increased the gene editing frequency by 2-fold and enabled the visual identification of the yellow leaf color phenotype (Eid et al., 2021). Strikingly, in another study, a co-editing of multiple alleles was carried out on the acetolactate synthase (ALS) gene involving two amino acid substitutions (W574L and S653I) inserted by template-mediated HDR (Oz et al., 2021). Among the strains that simultaneously bore the W574L and S653I substitutions, the acquired herbicide (nicosulfuron) resistance was displayed in the entire foliage (Oz et al., 2021).
Table 4 Summary of reports on sugarcane genome editing and details concerning genetic engineering and CRISPR/Cas parameters.
In 2022, a massive team of the Brazilian Agricultural Research Corporation (EMBRAPA), was able to deploy a laborious strategy via biolistics by using microparticles carrying RNPs and bombarding onto sugarcane embryogenic calli. They knocked out two genes, BAHD01 and BAHD05, which resulted in increased biomass saccharification and sugarcane concentration, respectively (unpublished data). Although this marker-free selection approach relies on a time-consuming screening of rare gene-edited plants, it can ease the biosafety deregulation process to launch a biotechnological product on the market in some countries, as mentioned before. Collectively, these studies have established early strategies for optimizing sugarcane genome editing, thereby overcoming some hurdles of a highly polyploid genome. Nevertheless, whilst other sophisticated CRISPR systems (e.g., base editing and prime editing) have already been employed in several plant crops (Bharat et al., 2020; Molla et al., 2021), CRISPR-based sugarcane genome editing is still restricted to traditional mutagenesis with Cas9 nucleases (Eid et al., 2021; Oz et al., 2021). Therefore, proof-of-principle studies regarding the usage of other nucleases and robust base- and prime-editing systems in sugarcane are still warranted.
In addition to functional genomics, the commercialization of genome-edited plants is a highly sought-after goal. For almost 30 years, several countries have released transgenic cultivars for commercial planting. So far, 439 events of 32 cultivars of transgenic plants were approved for commercialization in 45 countries, according to the GM approval database from the International Service for the Acquisition of Agri-Biotech Applications (ISAAA, 2022). Most commercialized transgenic plants meet characteristics of interest to the farmer, such as herbicide tolerance and insect resistance (ISAAA, 2018). However, although genetically modified organisms (GMOs) have been on the market for a long time to prove their safety, they still encounter some barriers in society, such as concerns about allergenicity, adverse effects on the environment, and even issues involving intellectual property and hegemony of large companies (Bawa and Anilakumar, 2013; Caserta and de Souza, 2017).
Commercial release of these plants for cultivation obeys careful legislation and different legal frameworks worldwide. Generally, the requirements for releasing a transgenic plant are only sometimes proportional to the risks involved. In other words, regulatory obstacles can be so severe that only large corporations can finance this long process. The legislation of different countries may be oriented toward analyses that consider the process or the product (Turnbull et al., 2021; Ahmad et al., 2023).
The advent of genome editing expanded the possibilities within plant biotechnology, especially due to CRISPR/Cas technology. Since then, countries have been forced to rethink whether their legislation aimed at transgenic plants includes the new possibilities of this toolbox. This demand is because, through genome editing, plants can be generated with precise mutations that could have occurred naturally by spontaneous mutations or even by sexual crossing between compatible species (Custers and Dima, 2022). Moreover, directed mutagenesis and classical breeding, outside the scope of legislation involving transgenic plants, can generate much more significant effects on the genome of plants than genome editing (Ossowski et al., 2010).
The unique characteristics of each gene-edited plant led many countries to elaborate on specific legislation that recommends a case-by-case analysis, as explained below. Additionally, to support their legislation, many countries adopted the definition of Living Modified Organisms (LMOs) included in the Cartagena Protocol on Biosafety of the Convention on Biological Diversity. Thus, some countries interpret that, concerning edited organisms, only organisms that have a modification that results in a new combination of genetic material and would not occur naturally can be treated as LMOs (Whelan and Lema, 2015). This possibility does not classify as LMOs the cases of site-directed nucleases (SDN)-1 and some cases of SDN-2 (Rostoks, 2021). From this, non-LMO organisms can be regulated without following the rules of transgenic plants. Additionally, crops can be distinguished between genetically modified (GM) and genome-edited (GE) plants according to a case-by-case analysis from what each country considers to be or not a GE crop and, in this way, information about some countries and their respective legislation concerning GM and GE crops is summarized in Table 5 and Figure 6 A comprehensive source on the subject, mainly on GE plants, was described by Molinari et al. (2021).
Table 5 Comparison of regulatory distinctions between Genetically Modified (GM) and Genome-Edited (GE) plants.
Figure 6 Current status of global legislation on plant genome editing. Genome-edited (GE) crops are distinctively (particularly) regulated worldwide, as illustrated. Green areas represent countries that address GE and genetically modified (GM) crops with different standards, in which most SDN-1 and SDN-2 are granted a free pass. Although some countries maintain similar regulation between GE and GM crops (in red), these include the USA, which has the largest number of CRISPR-based products released. Other CRISPR-based products have also been released in Japan and Brazil. Meanwhile, the yellow-colored areas represent countries that are still reviewing their deregulation rules for GE crops. Figure elements were obtained from BioRender.com.
Soon after the first cases of genome editing in plants, in 2013, Argentina was the pioneer in establishing specific legislation for edited plants, opting for the case-by-case approach (Whelan and Lema, 2015). Despite not being a signatory country of the Cartagena Protocol, Argentina relied on the definition of LMOs to define its regulatory strategies. Argentinian legislation particularly includes the possibility of previous analyses of the publishing project through the Prior Consultation Instance (PCI) (Goberna et al., 2022). Through these early analyses, the developer can indicate which legislation he must comply with to regulate his product (plant, microorganism or animal). Even so, if the PCI analysis indicates that the product is not characterized as an LMOs, a new consultation is necessary after finalizing the project (Goberna et al., 2022). Due to the possibility of prior consultation, Argentina has generated an increased interest in gene editing by developers.
Biotechnology has evolved rapidly with transgenic plants. However, the most significant breakthrough took place a decade ago, with the discovery of genome editing through the CRISPR/Cas system (Jinek et al., 2012; Gostimskaya, 2022). Since then, worldwide regulatory guidelines are no longer valid to comprehend the plants that originated with this technology. In this way, many countries updated their legislation quickly in search of greater socioeconomic advances. However, as explained above, although often indistinguishable from natural mutations, the mutations caused by genomic editing still face legal barriers and acceptance in some countries. For instance, despite the ultra-restrictive GMO regulation, the European Commission has recently proposed loosening the rules for GE crops to treat them as conventionally bred (Stokstad, 2023). This could not only foster scientific research into GE plants but also accelerate the launch of sustainable products onto the market. However, there are still conservationist counterforces slowing down this progress.
Despite this, founded on analyzes based on science and bioeconomy, several countries already have specific legislation to deal with these cases (Ahmad et al., 2023). In a general context, it is clear that the deregulation of SDN-1 and, in some cases, of SDN-2 in particular countries seeks to meet different purposes and results in different benefits. The Argentinean example of the possibility of prior consultation regarding the deregulation of some edited plants makes it possible to see some of these advantages immediately. While GMOs are mostly restricted to a few large corporations, one of the apparent consequences of the Argentine guidelines on edited plants is the greater interest in investing in this sector by small and medium-sized private companies or public research sectors from the country (Whelan et al., 2020). The increased interest in smaller companies is due to the decrease in the time to produce these plants and the costs of deregulation (Lassoued et al., 2019). Thus, it is common sense that CRISPR/Cas technology democratized plant genetic engineering, making it widely accessible to researchers and companies and making engineered plants accessible to growers and consumers. In addition, the new laws introduced by the regulatory structures of different countries are concerned with facilitating trade, improving the economy and contributing to food security.
Finally, it is important to consider that GE crops acceptance will also depend on clarification of the precision level of genome editing process, by means of assessing unintended or unpredictable off-target edits. In this way, interpreting genome-wide effects should be aligned with comparative evaluation of the degree of mutations with crops developed through traditional breeding. Thus, strategies directed towards avoidance of unintended changes (e.g., ensuring accuracy of gene editing through optimization of on-target activity) can help to clarify regulatory agencies about the possibility to circumvent off-target effects and, consequently, reduce concerns of risks involving GE crops (Zhao and Wolt, 2017). Moreover, differently from human health products derived from genome editing, off-target mutations and deleterious effects in plants tend to be less critical and pose no ethical issues (Schmidt et al., 2020), thus possibly reducing public concern and circumventing regulatory barriers limiting market approval.
CRISPR/Cas technology has revolutionized genetic engineering by enabling precise and efficient modification of DNA sequences in many annual plants. However, its application in perennial/semi-perennial plants presents challenges and promising prospects that deserve thorough exploration.
Unlike annuals, perennial plants have extended life cycles and slower reproduction rates. This characteristic hinders the development of CRISPR-edited plants with desired mutations and their phenotypes, since multiple growing seasons are required to observe the intended phenotypic changes. In addition, perennials interact with their environment differently from annuals, potentially impacting the expression of modified genes. Understanding the intricate relationships between perennial plants and their surroundings is essential to predict the environmental effects of CRISPR modifications. Moreover, CRISPR/Cas systems can introduce unintended genetic alterations, raising concerns about the potential off-target effects that might accumulate over the lifespan of perennial plants. Thus, ensuring accuracy and optimizing on-target activity is crucial to prevent unintended consequences. On the other hand, once the phenotypic changes are characterized, the selected modified plants can be used to insert other genes in the same location. This process avoids several years of selection for events in which the gene may have been inserted in undesirable locations in the genome, as occurs in traditional transgenesis approaches. Therefore, it is expected that, in the future, obtaining edited perennial plants with desirable characteristics will be a less laborious and faster technology to be achieved.
Overall, CRISPR can be employed to improve fruit quality, increase disease resistance, and increase the tolerance of perennial crops to abiotic stresses. These modifications could have significant positive implications for food security and agricultural sustainability. Perennial plants often face several environmental stresses over their long lifespans, and CRISPR technology offers the potential to enhance their adaptability to climate changes and other environmental challenges, making them more resilient. Hence, overcoming the challenges of applying CRISPR to perennial plants will lead to significant scientific insights into plant genetics, gene regulation, and long-term evolutionary processes. In conclusion, the use of CRISPR in perennial plants shows great potential to revolutionize agriculture and contribute to food security. While challenges related to long reproductive cycles, off-target effects, and regulatory considerations exist, the prospects of enhancing fruit yield and stress tolerance make this technology highly promising. As scientific researchers address these challenges, they pave the way for a more sustainable and resilient agricultural future.
GP: Conceptualization, Writing – original draft, Writing – review & editing. DR: Writing – original draft, Writing – review & editing. LS: Writing – original draft, Writing – review & editing. DC: Writing – original draft, Writing – review & editing. PN: Writing – original draft. JS: Writing – original draft. LP: Writing – original draft. MM: Writing – original draft, Writing – review & editing, Funding acquisition, Supervision. GL: Writing – original draft, Writing – review & editing. TP: Writing – review & editing. CV: Writing – original draft. SC: Funding acquisition, Supervision, Writing – review & editing. RB-C: Writing – review & editing. MT: Writing – review & editing. MY: Funding acquisition, Resources, Supervision, Writing – review & editing. AS: Conceptualization, Funding acquisition, Resources, Supervision, Writing – original draft, Writing – review & editing.
The author(s) declare financial support was received for the research, authorship, and/or publication of this article. The authors thank the project fundings (Grant numbers 2020/07045-3, 2021/13478-2, 2022/10505-1 and 2022/11738-0) from FAPESP (The São Paulo Research Foundation, São Paulo-SP, Brazil), the IAC (Agronomic Institute, Campinas-SP, Brazil) and Embrapa (Brazilian Agricultural Research Corporation, Brasília-DF, Brazil).
LP and MM was employed by Brazilian Agricultural Research Corporation.
The remaining authors declare that the research was conducted in the absence of any commercial or financial relationships that could be construed as a potential conflict of interest.
The author(s) declared that they were an editorial board member of Frontiers, at the time of submission. This had no impact on the peer review process and the final decision.
The authors declare that this study received funding from Embrapa (Brazilian Agricultural Research Corporation, Brası́lia-DF, Brazil). The funder had the following involvement with the study: preparation of the manuscript.
All claims expressed in this article are solely those of the authors and do not necessarily represent those of their affiliated organizations, or those of the publisher, the editors and the reviewers. Any product that may be evaluated in this article, or claim that may be made by its manufacturer, is not guaranteed or endorsed by the publisher.
Abdul Aziz, M., Brini, F., Rouached, H., Masmoudi, K. (2022). Genetically engineered crops for sustainably enhanced food production systems. Front. Plant Sci. 13. doi: 10.3389/fpls.2022.1027828
Ademosun, A. O., Oboh, G., Olasehinde, T. A., Adeoyo, O. O. (2018). From folk medicine to functional food: a review on the bioactive components and pharmacological properties of citrus peels. Orient. Pharm. Exp. Med. 18, 9–20. doi: 10.1007/s13596-017-0292-8
Adunola, P., Ferrão, M. A. G., Ferrão, R. G., Fonseca, A. F. A., Volpi, P. S., Comério, M., et al. (2023). Genomic selection for genotype performance and environmental stability in Coffea canephora. Genes Genomes Genet 13 (6), jkad062. doi: 10.1093/g3journal/jkad062
Ahmad, A., Jamil, A., Munawar, N. (2023). GMOs or non-GMOs? CRISPR Conundrum. Front. Plant Sci. 14. doi: 10.3389/fpls.2023.1232938
Albuquerque, E. V. S., Bezerra, C. A., Romero, J. V., Valencia, J. W. A., Valencia-Jiméne, A., Pimenta, L. M., et al. (2015). Seed-specific stable expression of the α-AI1 inhibitor in coffee grains and the in vivo implications for the development of the coffee berry borer. Trop. Plant Biol. 8, 98–107. doi: 10.1007/s12042-015-9153-0
Albuquerque, E. V. S., Cunha, W. G., Barbosa, A. E. A. D., Costa, P. M., Teixeira, J. B., Vianna, G. R., et al. (2009). Transgenic coffee fruits from Coffea arabica genetically modified by bombardment. In Vitro Cell Dev. Biol. Plant 45, 532–539. doi: 10.1007/s11627-009-9254-2
Alkimim, E. R., Caixeta, E. T., Sousa, T. V., Pereira, A. A., Oliveira, A. C. B., Zambolim, L., et al. (2017). Marker-assisted selection provides arabica coffee with genes from other Coffea species targeting on multiple resistance to rust and coffee berry disease. Mol. Breed. 37, 6. doi: 10.1007/s11032-016-0609-1
Almeida, W. A. B., Filho, F. A. A. M., Pino, L. E., Boscariol, R. L., Rodriguez, A. P. M., Mendes, B. M. J. (2003). Genetic transformation and plant recovery from mature tissues of Citrus sinensis L. Osbeck. Plant Sci. 164, 203–2011. doi: 10.1016/S0168-9452(02)00401-6
Alpizar, E., Dechamp, E., Espeout, S., Royer, M., Lecouls, A. C., Nicole, M., et al. (2006). Efficient production of Agrobacterium rhizogenes-transformed roots and composite plants for studying gene expression in coffee roots. Plant Cell Rep. 25 (9), 959–967. doi: 10.1007/s00299-006-0159-9
Alquézar, B., Bennici, S., Carmona, L., Gentile, A., Peña, L. (2022). Generation of transfer-DNA-free base-edited citrus plants. Front. Plant Sci. 13. doi: 10.3389/fpls.2022.835282
Alquézar, B., Volpe, H. X. L., Magnani, R. F., de Miranda, M. P., Santos, M. A., Wulff, N. A., et al. (2017). β-caryophyllene emitted from a transgenic Arabidopsis or chemical dispenser repels Diaphorina citri, vector of Candidatus Liberibacters. Sci. Rep. 7 (1), 5639. doi: 10.1038/s41598-017-06119-w
Altae-Tran, H., Kannan, S., Demircioglu, F. E., Oshiro, R., Nety, S. P., McKay, L. J., et al. (2021). The widespread IS200/IS605 transposon family encodes diverse programmable RNA-guided endonucleases. Science 374 (6563), 57–65. doi: 10.1126/science.abj6856
Altpeter, F., Springer, N. M., Bartley, L. E., Blechl, A. E., Brutnell, T. P., Citovsky, V., et al. (2016). Advancing crop transformation in the era of genome editing. Plant Cell 28, 1510–1520. doi: 10.1105/tpc.16.00196
Andersson, M. H., Turesson, A., Falt, A. S., Samuelsson, M., Hofvander, P. (2017). Efficient targeted multiallelic mutagenesis in tetraploid potato (Solanum tuberosum) by transient CRISPR-Cas9 expression in protoplasts. Plant Cell Rep. 36, 117–128. doi: 10.1007/s00299-016-2062-3
Anzalone, A. V., Koblan, L. W., Liu, D. R. (2020). Genome editing with CRISPR-Cas nucleases, base editors, transposases and prime editors. Nat. Biotechnol. 38, 824–844. doi: 10.1038/s41587-020-0561-9
Arencibia, A. D., Carmona, E. R., Tellez, P., Chan, M.-T., Yu, S.-M., Trujillo, L. E., et al. (1998). An efficient protocol for sugarcane (Saccharum spp. L.) transformation mediated by Agrobacterium tumefaciens. Transgenic Res. 7, 213–222. doi: 10.1023/A:1008845114531
Arencibia, A., Molina, P. R., de la Riva, G., et al. (1995). Production of transgenic sugarcane (Saccharum officinarum L.) plants by intact cell electroporation. Plant Cell Rep. 14, 305–309. doi: 10.1007/BF00232033
Arencibia, A., Molina, P., Gutierrez, C., Fuentes, A., Greenidge, V., Menendez, E., et al. (1992). Regeneration of transgenic sugarcane (Saccharum officinarum L.) plants from intact meristematic tissue transformed by electroporation. Biotecnología Aplicada 9, 156–165.
Arencibia, A., Vasquez, R., Prieto, D., Tellez, P., Carmona, E., Coego, A., et al. (1997). Transgenic sugarcane plants resistant to stem borer attack. Mol. Breed 3, 247–255. doi: 10.1023/A:1009616318854
Ashihara, H., Sano, H., Crozier, A. (2008). Caffeine and related purine alkaloids: biosynthesis, catabolism, function and genetic engineering. Phytochem 69, 841–856. doi: 10.1016/j.phytochem.2007.10.029
Augustine, S. M., Ashwin Narayan, J., Syamaladevi, D. P., Appunu, C., Chakravarthi, M., Ravichandran, V., et al. (2015). Overexpression of EaDREB2 and pyramiding of EaDREB2 with the pea DNA helicase gene (PDH45) enhance drought and salinity tolerance in sugarcane (Saccharum spp. hybrid). Plant Cell Rep. 34 (2), 247–263. doi: 10.1007/s00299-014-1704-6
Awasthi, P., Kocábek, T., Mishra, A. K., Nath, V. S., Shrestha, A., Matoušek, J. (2021). Establishment of CRISPR/Cas9 mediated targeted mutagenesis in hop (Humulus lupulus). Plant Physiol. Biochem. 160, 1–7. doi: 10.1016/j.plaphy.2021.01.006
Azameti, M. K., Dauda, W. P. (2021). Base editing in plants: applications, challenges, and future prospects. Front. Plant Sci. 12. doi: 10.3389/fpls.2021.664997
Baltes, N. J., Gil-Humanes, J., Cermak, T., Atkins, P. A., Voytas, D. F. (2014). DNA replicons for plant genome engineering. Plant Cell 26 (1), 151–163. doi: 10.1105/tpc.113.119792
Bao, H., Ding, Y., Yang, F., Zhang, J., Xie, J., Zhao, C., et al. (2022). Gene silencing, knockout and over-expression of a transcription factor ABORTED MICROSPORES (SlAMS) strongly affects pollen viability in tomato (Solanum lycopersicum). BMC Genomics 23, 346. doi: 10.1186/s12864-022-08549-x
Barbasso, D., Jordão, H., Maccheroni, W., Boldini, J., Bressiani, J., Sanguino, A. (2010). First report of Puccinia kuehnii, causal agent of orange rust of sugarcane, in Brazil. Plant Dis. 94 (9), 1170–1170. doi: 10.1094/PDIS-92-1-0175A
Bari, V. K., Nassar, J. A., Aly, R. (2021). CRISPR/Cas9 mediated mutagenesis of MORE AXILLARY GROWTH 1 in tomato confers resistance to root parasitic weed Phelipanche aEgyptiaca. Sci. Rep. 11, 3905. doi: 10.1038/s41598-021-82897-8
Barichello, D., Farinacio, R., Santos, C. C. N., Andrade, G. A., Nagashima, G. T., Garbúglio, D. D., et al. (2017). Drought-tolerant transgenic Swingle Citrumelo controls accumulation of proline modulating the expression of key genes of the proline metabolism. Citrus Res. Technol. 38 (2), 1–12. doi: 10.4322/crt.icc102
Bassanezi, R. B., Lopes, S. A., De Miranda, M. P., Wulff, N. A., Volpe, H. X. L., Ayres, A. J. (2020). Overview of citrus huanglongbing spread and management strategies in Brazil. Trop. Plant Pathology. 45, 251–264. doi: 10.1007/s40858-020-00343-y
Basso, M. F., da Cunha, B. A. D. B., Ribeiro, A. P., Martins, P. K., de Souza, W. R., de Oliveira, N. G., et al. (2017). Improved genetic transformation of sugarcane (Saccharum spp.) embryogenic callus mediated by Agrobacterium tumefaciens. Curr. Protoc. Plant Biol. 2, 221–239. doi: 10.1002/pb.20055
Batailler, B., Lemaître, T., Vilaine, F., Sanchez, C., Renard, D., Cayla, T., et al. (2012). Soluble and filamentous proteins in Arabidopsis sieve elements. Plant Cell Environ. 35 (7), 1258–1273. doi: 10.1111/j.1365-3040.2012.02487.x
Bawa, A. S., Anilakumar, K. R. (2013). Genetically modified foods: Safety, risks and public concerns - A review. J. Food Sci. Technol. 50, 1035–1046. doi: 10.1007/s13197-012-0899-1
Beernink, B. M., Holan, K. L., Lappe, R. R., Whitham, S. A. (2021). Direct agroinoculation of maize seedlings by injection with recombinant foxtail mosaic virus and sugarcane mosaic virus infectious clones. J. Vis. Exp. 168, e62277. doi: 10.3791/62277
Benner, S. A., Sismour, A. M. (2005). Synthetic biology. Nat. Rev. Genet. 6 (7), 533–543. doi: 10.1038/nrg1637
Berding, N., Roach, B. T. (1987). “Germplasm collection, maintenance, and use,” in Sugarcane improvement through breeding (Amsterdam: Elsevier), 143–210.
Bernabé-Orts, J. M., Casas-Rodrigo, I., Minguet, E. G., Landolfi, V., Garcia-Carpintero, V., Gianoglio, S., et al. (2019). Assessment of Cas12a-mediated gene editing efficiency in plants. Plant Biotechnol. J. 17 (10), 1971–1984. doi: 10.1111/pbi.13113
Bharat, S. S., Li, S., Li, J., Yan, L., Xia, L. (2020). Base editing in plants: current status and challenges. Crop J. 8 (3), 384–395. doi: 10.1016/j.cj.2019.10.002
Boava, L. P., Cristofani-Yaly, M., MaChado, M. A. (2017). Physiologic, Anatomic, and Gene Expression Changes in Citrus sunki, Poncirus trifoliata, and Their Hybrids After 'Candidatus Liberibacter asiaticus' Infection. Phytopathology 107 (5), 590–599. doi: 10.1094/PHYTO-02-16-0077-R
Bogatyreva, N. V., Gusev, Y. S., Moiseeva, Y. M., Sokolov, A. Y., Chumakov, M. I. (2021). Regulatory status of genome-editing plants: Perspectives for Russian federation. Ecol. Genet. 19, 89–101. doi: 10.17816/ECOGEN42532
Boscariol, R. L., Almeida, W. A., Derbyshire, M. T., Mourão Filho, F. A., Mendes, B. M. (2003). The use of the PMI/mannose selection system to recover transgenic sweet orange plants (Citrus sinensis L. Osbeck). Plant Cell Rep. 22 (2), 122–128. doi: 10.1007/s00299-003-0654-1
Boscariol, R. L., Monteiro, M., Takahashi, E. K., Chabregas, S. M., Vieira, M. L. C., Vieira, L. G. E., et al. (2006). Attacin A Gene from Tricloplusia ni Reduces Susceptibility to Xanthomonas axonopodis pv. citri in Transgenic Citrus sinensis ‘Hamlin’. J. Am. Soc. Hortic. Science. 131 (4), 530–536. doi: 10.21273/JASHS.131.4.530
Boscariol-Camargo, R. L., Takita, M. A., MaChado, M. A. (2016). Bacterial resistance in AtNPR1 transgenic sweet orange is mediated by priming and involves EDS1 and PR2. Trop. Plant Pathol. 41, 341–349. doi: 10.1007/s40858-016-0108-2
Bower, R., Elliott, A. R., Potier, B. A. M., Birch, R. G. (1996). High-efficiency, microprojectile-mediated cotransformation of sugarcane, using visible or selectable markers. Mol. Breed. 2, 239–249. doi: 10.1007/BF00564201
Breitler, J. C., Dechamp, E., Campa, C., Zebral Rodrigues, L. A., Guyot, R., Marraccini, P., et al. (2018). CRISPR/Cas9-mediated efficient targeted mutagenesis has the potential to accelerate the domestication of Coffea canephora. Plant Cell Tiss Organ Cult 134, 383–394. doi: 10.1007/s11240-018-1429-2
Brinkman, E. K., Chen, T., Amendola, M., Van Steensel, B. (2014). Easy quantitative assessment of genome editing by sequence trace decomposition. Nucleic Acids Res. 42 (22), e168–e168. doi: 10.1093/nar/gku936
Budeguer, F., Enrique, R., Perera, M. F., Racedo, J., Castagnaro, A. P., Noguera, A. S., et al. (2021). Genetic transformation of sugarcane, current status and future prospects. Front. Plant Sci. 12. doi: 10.3389/fpls.2021.768609
Cameron, D. E., Bashor, C. J., Collins, J. J. (2014). A brief history of synthetic biology. Nat. Rev. Microbiol. 12 (5), 381–390. doi: 10.1038/nrmicro3239
Campos, N. A., Panis, B., Carpentier, S. C. (2017). Somatic Embryogenesis in Coffee: The evolution of biotechnology and the integration of omics technologies offer great opportunities. Front. Plant Sci. 8. doi: 10.3389/fpls.2017.01460
Cardi, T., Murovec, J., Bakhsh, A., Boniecka, J., Bruegmann, T., Bull, S. E., et al. (2023). CRISPR/Cas-mediated plant genome editing: outstanding challenges a decade after implementation. Trends Plant Sci. 23, 1360–1385. doi: 10.1016/j.tplants.2023.05.012
Cardoso, D. C., Martinati, J. C., Giachetto, P. F., Vidal, R. N. O., Carazzolle, M. F., Padilha, L., et al. (2014). Large-scale analysis of differential gene expression in coffee genotypes resistant and susceptible to leaf miner-toward the identification of candidate genes for marker assisted-selection. BMC Genomics 15, 66. doi: 10.1186/1471-2164-15-66
Carvalho, H. F., Ferrão, L. F. V., Galli, G., Nonato, J. V. A., Padilha, L., Maluf, M. P., et al. (2023). On the accuracy of threshold genomic prediction models for leaf miner and leaf rust resistance in arabica coffee. Tree Genet. Genomics 19, 11. doi: 10.1007/s11295-022-01581-8
Carvalho, H. F., Galli, G., Ferrão, L. F. V., Nonato, J. V. A., Padilha, L., Maluf, M. P., et al. (2020). The effect of bienniality on genomic prediction of yield in arabica coffee. Euphytica 216, 101. doi: 10.1007/s10681-020-02641-7
Carvalho, A., Medina Filho, H. P., Fazuoli, L. C., Guerreiro Filho, O., Lima, M. M. A. (1991). Aspectos genéticos do cafeeiro. Braz. J. Genet. 14, 135–183.
Casarin, T., Freitas, N. C., Pinto, R. T., Breitler, J.-C., Rodrigues, L. A.Z., Marraccini, P., et al. (2022). Multiplex CRISPR/Cas9-mediated knockout of the phytoene desaturase gene in Coffea canephora. Sci. Rep. 12, 17270. doi: 10.1038/s41598-022-21566-w
Caserta, R., de Souza, A. A. (2017). Genetically modified plants: think twice before saying “No”. JSM Genet. Genomics 4 (1), 1021.
Caserta, R., Picchi, S. C., Takita, M. A., Tomaz, J. P., Pereira, W. E. L., MaChado, M. A., et al. (2014). Expression of Xylella fastidiosa RpfF in citrus disrupts signaling in Xanthomonas citri subsp. citri thereby its virulence. Mol. Plant-Microbe Interactions. 27 (11), 1241–1252. doi: 10.1094/MPMI-03-14-0090-R
Castro, I. S. L., Freitas-Lopes, R. L., Ferreira, S. S., Maciel, T. E. F., Florez, J. C., Zambolim, E. M., et al. (2022). Transcriptome analysis uncovers the gene expression profile of Hemileia vastatrix (Race XXXIII) during the interactions with resistant and susceptible coffee. Agronomy 12, 444. doi: 10.3390/agronomy12020444
Cermak, T., Doyle, E. L., Christian, M., Wang, L., Zhang, Y., Schmidt, C., et al. (2011). Efficient design and assembly of custom TALEN and other TAL effector-based constructs for DNA targeting. Nucleic Acids Res. 39 (12), e82. doi: 10.1093/nar/gkr218
Cervera, M., Navarro, L., Peña, L. (2009). Gene stacking in 1-year-cycling APETALA1 citrus plants for a rapid evaluation of transgenic traits in reproductive tissues. J. Biotechnol. 140 (3–4), 278–282. doi: 10.1016/j.jbiotec.2009.01.024
Chapman, E. A., Thomsen, H. C., Tulloch, S., Correia, P. M. P., Luo, G., Najafi, J., et al. (2022). Perennials as future grain crops: opportunities and challenges. Front. Plant Sci. 13. doi: 10.3389/fpls.2022.898769
Chavez, G., Mendoza, M. M., Caetano, A. C. (2022). Antioxidants, phenols, caffeine content and volatile compounds in coffee beverages obtained by different methods. Food Sci. Technol. 42, e47022. doi: 10.1590/fst.47022
Chen, W. H., Gartland, K. M. A., Davey, M. R., Sotak, R., Gartland, J. S., Mulligan, B. J., et al. (1987). Transformation of sugarcane protoplasts by direct uptake of a selectable chimaeric gene. Plant Cell Rep. 6 (4), 297–301. doi: 10.1007/BF00272003
Chilton, M. D., Que, Q. (2003). Targeted integration of T-DNA into the tobacco genome at double-stranded breaks: new insights on the mechanism of T-DNA integration. Plant Physiol. 133 (3), 956–965. doi: 10.1104/pp.103.026104
Cohen, S. N., Chang, A. C., Boyer, H. W., Helling, R. B. (1973). Construction of biologically functional bacterial plasmids in vitro. Proc. Natl. Acad. Sci. U.S.A. 70 (11), 3240–3244. doi: 10.1073/pnas.70.11.3240
Coletta-Filho, H. D., Targon, M. L. P. N., Takita, M. A., De Negri, J. D., Pompeu Junior., J., MaChado, M. A., et al. (2004). First report of the causal agent of huanglongbing ("Candidatus liberibacter asiaticus") in Brazil. Plant Disease. 88 (12), 1382. doi: 10.1094/PDIS.2004.88.12.1382C
Cong, L., Ran, F. A., Cox, D., Lin, S., Barretto, R., Habib, N., et al. (2013). Multiplex genome engineering using CRISPR/Cas systems. Science 339 (6121), 819–823. doi: 10.1126/science.1231143
Connelly, J. P., Pruett-Miller, S. M. (2019). CRIS.py: a versatile and high-throughput analysis program for CRISPR-based genome editing. Sci. Rep. 9 (1), 1–8. doi: 10.1038/s41598-019-40896-w
Conti, G., Xoconostle-Cázares, B., Marcelino-Pérez, G., Hopp, H. E., Reyes, C. A. (2021). Citrus genetic transformation: an overview of the current strategies and insights on the new emerging technologies. Front. Plant Sci. 12. doi: 10.3389/fpls.2021.768197
Couchoud, M., Der, C., Girodet, S., Vernoud, V., Prudent, M., Leborgne-Castel, N. (2019). Drought stress stimulates endocytosis and modifies membrane lipid order of rhizodermal cells of Medicago truncatula in a genotype-dependent manner. BMC Plant Biol. 19 (221), 1–14. doi: 10.1186/s12870-019-1814-y
Cristofoletti, P. T., Kemper, E. L., Capella, A. N., Carmago, S. R., Cazoto, J. L., Ferrari, F., et al. (2018). Development of transgenic sugarcane resistant to sugarcane borer. Trop. Plant Biol. 11 (1), 17–30. doi: 10.1007/s12042-018-9198-y
Cursi, D. E., Hoffmann, H. P., Barbosa, G. V. S., Bressiani, J. A., Gazaffi, R., Chapola, R. G., et al. (2022). History and current status of sugarcane breeding, germplasm development and molecular genetics in Brazil. Sugar Tech 24 (1), 112–133. doi: 10.1007/s12355-021-00951-1
Curtolo, M., Pacheco, I., de, S., Boava, L. P., Takita, M. A., Granato, L. M., et al. (2020). Wide-ranging transcriptomic analysis of Poncirus trifoliata, Citrus sunki, Citrus sinensis and contrasting hybrids reveals HLB tolerance mechanisms. Sci. Rep. 10, 20865. doi: 10.1038/s41598-020-77840-2
Custers, R., Dima, O. (2022). Genome-edited crops and 21st century food system challenges. In-depth analysis. Panel Future Sci. Technol. doi: 10.2861/290440
D'Hont, A., Grivet, L., Feldmann, P., Glaszmann, J. C., Rao, S., Berding, N. (1996). Characterization of the double genome structure of modern sugarcane cultivars (Saccharum spp.) by molecular cytogenetics. Molec. Gen. Genet. 250, 405–413. doi: 10.1007/BF02174028
De Guglielmo-Cróquer, Z., Altosaar, I., Zaidi, M., Menéndez-Yuffá, A. (2010). Transformation of coffee (Coffea arabica L. cv. Catimor) with the cry1ac gene by biolistic, without the use of markers. Braz. J. Biol. 70 (2), 387–393. doi: 10.1590/s1519-69842010000200022
DeHaan, L., Larson, S., López-Marqués, R. L., Wenkel, S., Gao, C., Palmgren, M. (2020). Roadmap for accelerated domestication of an emerging perennial grain crop. Trends Plant Sci. 25, 525–537. doi: 10.1016/j.tplants.2020.02.004
Demirer, G. S., Silva, T. N., Jackson, C. T., Thomas, J. B., W Ehrhardt, D., Rhee, S. Y., et al. (2021). Nanotechnology to advance CRISPR-Cas genetic engineering of plants. Nat. Nanotechnology 16 (3), 243–250. doi: 10.1038/s41565-021-00854-y
Dinardo-Miranda, L. L., Fracasso, J. V., Miranda, I. D. (2019). Damage caused by Meloidogyne javanica and Pratylenchus zeae to sugarcane cultivars. Summa Phytopathologica 45, 146–156. doi: 10.1590/0100-5405/187782
Di Pauli, V., Fontana, P. D., Lewi, D. M., Felipe, A., Erazzú, L. E. (2021). Optimized somatic embryogenesis and plant regeneration in elite Argentinian sugarcane (Saccharum spp.) cultivars. J. Genet. Eng. Biotechnol. 19 (1), 1–8. doi: 10.1186/s43141-021-00270-8
Dong, S., Delucca, P., Geijskes, R. J., Ke, J., Mayo, K., Mai, P., et al. (2014). Advances in agrobacterium-mediated sugarcane transformation and stable transgene expression. Sugar Tech 16, 366–371. doi: 10.1007/s12355-013-0294-x
Dong, J. Z., McHughen, A. (1993). Transgenic flax plants from Agrobacterium mediated transformation: incidence of chimeric regenerants and inheritance of transgenic plants. Plant Sci. 91 (2), 139–148.
Dutt, M., Grosser, J. W. (2009). Evaluation of parameters affecting Agrobacterium-mediated transformation of citrus. Plant Cell Tissue Organ Culture 98 (3), 331–340. doi: 10.1007/s11240-009-9567-1
Dutt, M., Grosser, J. W. (2010). An embryogenic suspension cell culture system for Agrobacterium-mediated transformation of citrus. Plant Cell Rep. 29 (11), 1251–1260. doi: 10.1007/s00299-010-0910-0
Dutt, M., Mahmoud, L. M., Nehela, Y., Grosser, J. W., Killiny, N. (2022). The Citrus sinensis TILLER ANGLE CONTROL 1 (CsTAC1) gene regulates tree architecture in sweet oranges by modulating the endogenous hormone content. Plant Sci. 323, 111401. doi: 10.1016/j.plantsci.2022.111401
Dutt, M., Mou, Z., Zhang, X., Tanwir, S. E., Grosser, J. W. (2020). Efficient CRISPR/Cas9 genome editing with Citrus embryogenic cell cultures. BMC Biotechnol. 20 (58), 1–7. doi: 10.1186/s12896-020-00652-9
Eid, A., Mohan, C., Sanchez, S., Wang, D., Altpeter, F. (2021). Multiallelic, targeted mutagenesis of magnesium chelatase with CRISPR/Cas9 provides a rapidly scorable phenotype in highly polyploid sugarcane. Front. Genome Ed. 3, 654996. doi: 10.3389/fgeed.2021.654996
El-Mounadi, K., Morales-Floriano, M. L., Garcia-Ruiz, H. (2020). Principles, applications, and biosafety of plant genome editing using CRISPR-cas9. Front. Plant Sci. 11. doi: 10.3389/fpls.2020.00056
Engler, C., Kandzia, R., Marillonnet, S. (2008). A one pot, one step, precision cloning method with high throughput capability. PloS One 3 (11), e3647. doi: 10.1371/journal.pone.0003647
Engler, C., Youles, M., Gruetzner, R., Ehnert, T. M., Werner, S., Jones, J. D., et al. (2014). A golden gate modular cloning toolbox for plants. ACS Synthetic Biol. 3 (11), 839–843. doi: 10.1021/sb4001504
Enríquez-Obregón, G. A., Vázquez-Padrón, R. I., Prieto-Samsonov, D. L., Gustavo, A., Selman-Housein, G. (1998). Herbicide-resistant sugarcane (Saccharum officinarum L.) plants by Agrobacterium-mediated transformation. Planta 206, 20–27. doi: 10.1007/s004250050369
Entine, J., Felipe, M. S. S., Groenewald, J. H., Kershen, D. L., Lema, M., McHughen, A., et al. (2021). Regulatory approaches for genome edited agricultural plants in select countries and jurisdictions around the world. Transgenic Res. 30 (4), 551–584. doi: 10.1007/s11248-021-00257-8
Ernst, A. M., Jekat, S. B., Zielonka, S., Müller, B., Neumann, U., Rüping, B., et al. (2012). Sieve element occlusion (SEO) genes encode structural phloem proteins involved in wound sealing of the phloem. Proc. Natl. Acad. Sci. U.S.A. 109 (28), 1980–1989. doi: 10.1073/pnas.1202999109
Estanislau, G. G. (2022). Identificação de análogos a genes de resistência (RGAs) a Hemileia vastatrix no genoma de Coffea arabica e avaliação da expressão gênica na interação. [Dissertation]. ([Viçosa (MG)]: University of Viçosa). doi: 10.1047328/ufvbbt.2022.383
Etienne, H., Breton, D., Breitler, J.-C., Bertrand, B., Déchamp, E., Awada, R., et al. (2018). Coffee Somatic Embryogenesis: How did research, experience gained and innovations promote the commercial propagation of elite clones from the two cultivated species? Front. Plant Sci. 9. doi: 10.3389/fpls.2018.01630
FAO FAOSTAT (2022) Sugarcane production in 2020, Crops/Regions/World list/Production Quantity (pick lists)" (UN Food and Agriculture Organization, Corporate Statistical Database (FAOSTAT) (Accessed December 22, 2022).
FAO FAOSTAT (2023) Orange production in 2021, Crops/Regions/World list/ Production Quantity (pick lists)” (UN Food and Agriculture Organization, Corporate Statistical Database (FAOSTAT) (Accessed August 14, 2023).
Farah, A. (2009). Coffee as a speciality and functional beverage In: Functional and Speciality Beverage Technology. Paqui P, 370–395. doi: 10.1533/9781845695569.3.370
Favoretto, P., Silva, C. C., Tavares, A. G., Giatti, G., Moraes, P. F., Lobato, M. T. V., et al. (2017). Assisted-selection of naturally caffeine-free coffee cultivars: characterization of SNPs from a methyltransferase gene. Mol. Breed. 37, 31. doi: 10.1007/s11032-017-0636-6
Feng, Z., Zhang, B., Ding, W., Liu, X., Yang, D.-L., Wei, P., et al. (2013). Efficient genome editing in plants using a CRISPR/Cas system. Cell Res. 23, 1229–1232. doi: 10.1038/cr.2013.114
Ference, C. M., Gochez, A. M., Behlau, F., Wang, N., Graham, J. H., Jones, J. B. (2018). Recent advances in the understanding of Xanthomonas citri ssp. citri pathogenesis and citrus canker disease management. Mol. Plant Pathol. 19 (6), 1302–1318. doi: 10.1111/mpp.12638
Fernandez-Da Silva, R., Menéndez-Yuffá, A. (2003). Transient gene expression in secondary somatic embryos from coffee tissues electroporated with the genes GUS and BAR. Electronic J. Biotechnol. 6, 29–35. doi: 10.4067/S0717-34582003000100006
Ferrão, L. F. V., Ferrão, R. G., Ferrão, M. A. G., Fonseca, A., Carbonetto, P., Stephens, M., et al. (2018). Accurate genomic prediction of Coffea canephora in multiple environments using whole-genome statistical models. Heredity 122 (3), 261–275. doi: 10.1038/s41437-018-0105-y
Garsmeur, O., Droc, G., Antonise, R., Grimwood, J., Potier, B., Aitken, K., et al. (2018). A mosaic monoploid reference sequence for the highly complex genome of sugarcane. Nat. Commun. 9 (1), 1–10. doi: 10.1038/s41467-018-05051-5
Gatica-Arias, A. (2020). The regulatory current status of plant breeding technologies in some Latin American and the Caribbean countries. Plant Cell. Tissue Organ Cult. 141, 229–242. doi: 10.1007/s11240-020-01799-1
Gazaffi, R., Oliveira, K. M., Souza, A. P., Garcia, A. A. F. (2010). “Sugarcane: Breeding methods and genetic mapping,” in Bioetanol de cana-de-açúcar – P&D para produtividade e sustentabilidade (São Paulo: Blucher), 333–352.
Geng, S., Sohail, H., Cao, H., Sun, J., Chen, Z., Zhou, L., et al. (2022). An efficient root transformation system for CRISPR/Cas9-based analyses of shoot–root communication in cucurbit crops. Hortic. Res. 9, uhab082. doi: 10.1093/hr/uhab082
Gentzel, I. N., Ohlson, E. W., Redinbaugh, M. G., Wang, G.-L.. (2022). VIGE: virus-induced genome editing for improving abiotic and biotic stress traits in plants. Stress Biol. 2, 2. doi: 10.1007/s44154-021-00026-x
Gibson, D. G., Young, L., Chuang, R. Y., Venter, J. C., Hutchison, C. A., Smith, H. O. (2009). Enzymatic assembly of DNA molecules up to several hundred kilobases. Nat. Methods 6 (5), 343–345. doi: 10.1038/nmeth.1318
Gimase, J. M., Thagana, W. M., Chripine, O. O., Cheserek, J. J., Gichimu, B. M., Gichuru, E. K., et al. (2020). Genome-Wide Association Study identify the genetic loci conferring resistance to Coffee Berry Disease (Colletotrichum kahawae) in Coffea arabica var. Rume Sudan. Euphytica 216, 86. doi: 10.1007/s10681-020-02621-x
Goberna, M. F., Whelan, A. I., Godoy, P., Lewi, D. M. (2022). Genomic editing: the evolution in regulatory management accompanying scientific progress. Front. Bioeng. Biotechnol. 10. doi: 10.3389/fbioe.2022.835378
González, M. N., Massa, G. A., Andersson, M., Décima Oneto, C. A., Turesson, H., Storani, L., et al. (2021). Comparative potato genome editing: Agrobacterium tumefaciens-mediated transformation and protoplasts transfection delivery of CRISPR/Cas9 components directed to StPPO2 gene. Plant Cell Tissue Organ Culture (PCTOC) 145 (2), 291–305. doi: 10.1007/s11240-020-02008-9
González-Mas, M. C., Rambla, J. L., López-Gresa, M. P., Blázquez, M. A., Granell, A. (2019). Volatile compounds in citrus essential oils: A comprehensive review. Front. Plant Sci. 10. doi: 10.3389/fpls.2019.00012
Gostimskaya, I. (2022). CRISPR – cas9: A history of its discovery and ethical considerations of its use in genome editing. Biochem 87, 777–788. doi: 10.1134/S0006297922080090
Gouy, M., Rousselle, Y., Bastianelli, D., Lecomte, P., Bonnal, L., Roques, D., et al. (2013). Experimental assessment of the accuracy of genomic selection in sugarcane. Theor. Appl. Genet. 126, 2575–2586. doi: 10.1007/s00122-013-2156-z
Granato, L. M., Galdeano, D. M., D’Alessandre, N. D. R., Breton, M. C., Machado, M. A. (2019). Callose synthase family genes play an important role in the Citrus defense response to Candidatus Liberibacter asiaticus. Eur. J. Plant Pathol. 155, 25–38. doi: 10.1007/s10658-019-01747-6
Guerra-Guimarães, L., Tenente, R., Pinheiro, C., Chaves, I., Silva, M. C., Cardoso, F. M. H., et al. (2015). Proteomic analysis of apoplastic fluid of Coffea arabica leaves highlights novel biomarkers for resistance against Hemileia vastatrix. Front. Plant Sci. 6. doi: 10.3389/fpls.2015.00478
Guerreiro-Filho, O., Maluf, M. P. (2019). “Breeding strategies,” in Coffee: production, quality and chemistry. Ed. Farah, A. (Abingdon: Royal Society of Chemistry), 89–99.
Guerreiro-Filho, O., Silvarolla, M. B., Eskes, A. B. (1999). Expression and mode of inheritance of resistance in coffee to leaf miner Perileucoptera coffeella. Euphytica 105, 7–15. doi: 10.1023/A:1003427613071
Guidelli, G. V., Mattiello, L., Gallinari, R. H., Lucca, P. C. D., Menossi, M. (2018). pGVG: a new Gateway-compatible vector for transformation of sugarcane and other monocot crops. Genet. Mol. Biol. 41, 450–454. doi: 10.1590/1678-4685-GMB-2017-0262
Guimarães, P. G., Martinati-Schenk, J. C., Cintra, L. C., Giachetto, P. F., Silvarolla, M. B., Padilha, L., et al. (2021). Large-scale prospection of genes on caffeine-free Coffea arabica plants – discovery of novel markers associated with development and secondary metabolism. Plant Gene 27, 100314. doi: 10.1016/j.plgene.2021.100314
Han, J., Guo, B., Guo, Y., Zhang, B., Wang, X., Qiu, L.-J. (2019). Creation of early flowering germplasm of soybean by CRISPR/cas9 technology. Front. Plant Sci. 10. doi: 10.3389/fpls.2019.01446
Hanak, T., Madsen, C. K., Brinch-Pedersen, H. (2022). Genome Editing-accelerated Re-Domestication (GEaReD) – A new major direction in plant breeding. Biotechnol. Jour. 17 (7), 2100545. doi: 10.1002/biot.202100545
Hayes, B. J., Wei, X., Joyce, P., Atkin, F., Deomano, E., Yue, J., et al. (2021). Accuracy of genomic prediction of complex traits in sugarcane. Theor. Appl. Genet. 134, 1455–1462. doi: 10.1007/s00122-021-03782-6
Hidaka, T., Omura, M. (1993). Transformation of citrus protoplasts by electroporation. Jour. Japan. Soc Hortic. Sci. 62, 371–376. doi: 10.2503/jjshs.62.371
Hooghvorst, I., López-Cristoffanini, C., Nogués, S. (2019). Efficient knockout of phytoene desaturase gene using CRISPR/Cas9 in melon. Sci. Rep. 9 (1), 1–7. doi: 10.1038/s41598-019-53710-4
Hu, C., Sheng, O., Deng, G., He, W., Dong, T., Yang, Q., et al. (2021). CRISPR/Cas9-mediated genome editing of MaACO1 (aminocyclopropane-1-carboxylate oxidase 1) promotes the shelf life of banana fruit. Plant Biotechnol. Jour 19 (4), 654. doi: 10.1111/pbi.13534
Huang, X., Wang, Y., Xu, J., Wang, N. (2020). Development of multiplex genome editing toolkits for citrus with high efficacy in biallelic and homozygous mutations. Plant Mol. Biol. 104, 3.
Huang, X., Wang, Y., Wang, N. (2022a). Highly efficient generation of canker-resistant sweet orange enabled by an improved CRISPR/cas9 system. Front. Plant Sci. 12, 769907. doi: 10.3389/fpls.2021.769907
Huang, X., Wang, Y., Wang, N. (2022b). Base editors for citrus gene editing. Front. Genome Ed. 4. doi: 10.3389/fgeed.2022.852867
Hussin, S. H., Liu, X., Li, C., Diaby, M., Jatoi, G. H., Ahmed, R., et al. (2022). An Updated Overview on Insights into Sugarcane Genome Editing via CRISPR/Cas9 for Sustainable Production. Sustainability 14 (19), 12285. doi: 10.3390/su141912285
Iglesias, V. A., Moscone, E. A., Papp, I., Neuhuber, F., Michalowski, S., Phelan, T., et al. (1997). Molecular and cytogenetic analyses of stably and unstably expressed transgene loci in tobacco. Plant Cell 9, 1251–1264. doi: 10.1105/tpc.9.8.1251
Ingelbrecht, I. L., Irvine, J. E., Mirkov, T. E. (1999). Posttranscriptional gene silencing in transgenic sugarcane. Dissection of homology-dependent virus resistance in a monocot that has a complex polyploid genome. Plant Physiol. 119, 1187–1197. doi: 10.1104/pp.119.4.1187
ISAAA (2018). Brief, global status of commercialized biotech/GM crops in 2018. ISAAA Br. No. 54. (ISAAA Ithaca, NY).
ISAAA (2022) GM approval database. Available at: https://www.isaaa.org/gmapprovaldatabase/default.asp (Accessed December 24, 2022).
Ishii, T., Araki, M. (2016). Consumer acceptance of food crops developed by genome editing. Plant Cell Rep. 35 (7), 1507–1518. doi: 10.1007/s00299-016-1974-2
Ishii, T., Araki, M. (2017). A future scenario of the global regulatory landscape regarding genome-edited crops. GM Crop Food 8, 44–56. doi: 10.1080/21645698.2016.1261787
Jackson, M. A., Anderson, D. J., Birch, R. G. (2013). Comparison of Agrobacterium and particle bombardment using whole plasmid or minimal cassette for production of high-expressing, low-copy transgenic plants. Transgenic Res. 22, 143–151. doi: 10.1007/s11248-012-9639-6
Jackson, D. A., Symons, R. H., Berg, P. (1972). Biochemical method for inserting new genetic information into DNA of Simian Virus 40: circular SV40 DNA molecules containing lambda phage genes and the galactose operon of Escherichia coli. Proc. Natl. Acad. Sci. U.S.A. 69 (10), 2904–2909. doi: 10.1073/pnas.69.10.2904
Jacob, F., Monod, J. (1961). On the regulation of gene activity. Cold Spring Harb. Symp. Quant. Biol. 26, 193–211. doi: 10.1101/SQB.1961.026.01.024
Jacobs, T. B., LaFayette, P. R., Schmitz, R. J., Parrott, W. A. (2015). Targeted genome modifications in soybean with CRISPR/Cas9. BMC Biotechnol. 15 (1), 1–10. doi: 10.1186/s12896-015-0131-2
James, C., Krattiger, A. F. (1996). Global review of the field testing and commercialization of transgenic plants: 1986 to 1995. ISAAA Briefs. 1,(ISAAA Ithaca, NYs).
Jenkins, D., Dobert, R., Atanassova, A., Pavely, C. (2021). Impacts of the regulatory environment for gene editing on delivering beneficial products. Vitr. Cell. Dev. Biol. - Plant 57, 609–626. doi: 10.1007/s11627-021-10201-4
Jia, H., Orbovic, V, Jones, V. F.B., Wang, N. (2016). Modification of the PthA4 effector binding elements in Type I CsLOB1 promoter using Cas9/sgRNA to produce transgenic Duncan grapefruit alleviating XccΔpthA4:dCsLOB1.3 infection. Plant Biotechnol. J. 14, 5. doi: 10.1111/pbi.12495
Jia, H., Xu, J., Orbović, V., Zhang, Y., Wang, N.. (2017a). Editing citrus genome via saCas9/sgRNA system. Front. Plant Sci. 8, 2135. doi: 10.3389/fpls.2017.02135
Jia, H., Zhang, Y., Orbović, V., Xu, J., White, F. F., Jones, J. B., et al. (2017b). Genome editing of the disease susceptibility gene CsLOB1 in Citrus confers resistance to citrus canker. Plant Biotechnol. J. 15, 817–823. doi: 10.1111/pbi.12677
Jia, H., Omar, A. A., Orbović, V., Wang, N. (2022). Biallelic editing of the LOB1 promoter via CRISPR/cas9 creates canker-resistant 'Duncan' Grapefruit. Phytopathology 112 (2), 308–314. doi: 10.1094/PHYTO-04-21-0144-R
Jia, H., Wang, N. (2014). Targeted genome editing of sweet orange using Cas9/sgRNA. PloS One 9, e93806. doi: 10.1371/journal.pone.0093806
Jia, H., Wang, N. (2020). Generation of homozygous canker-resistant citrus in the T0 generation using CRISPR-SpCas9p. Plant Biotechnol. Journal 18, 1990–1992. doi: 10.1111/pbi.13375
Jinek, M., Chylinski, K., Fonfara, I., Hauer, M., Doudna, J. A., Charpentier, E. (2012). A programmable dual-RNA-guided DNA endonuclease in adaptive bacterial immunity. Science 337, 816–821. doi: 10.1126/science.1225829
Jung, J. H., Altpeter, F. (2016). TALEN mediated targeted mutagenesis of the caffeic acid O-methyltransferase in highly polyploid sugarcane improves cell wall composition for production of bioethanol. Plant Mol. Biol. 92, 131–142. doi: 10.1007/s11103-016-0499-y
Kannan, B., Jung, J. H., Moxley, G. W., Lee, S. M., Altpeter, F. (2018). TALEN-mediated targeted mutagenesis of more than 100 COMT copies/alleles in highly polyploid sugarcane improves saccharification efficiency without compromising biomass yield. Plant Biotechnol. J. 16 (4), 856–866. doi: 10.1111/pbi.12833
Kaur, N., Alok, A., Kaur, N., Pandey, P., Awasthi, P., Tiwari, S. (2018). CRISPR/Cas9-mediated efficient editing in phytoene desaturase (PDS) demonstrates precise manipulation in banana cv. Rasthali genome. Funct. Integr. Genomic 18 (1), 89–99. doi: 10.1007/s10142-017-0577-5
Kaur, M., Manchanda, P., Kalia, A., Ahmed, F. K., Nepovimova, E., Kuca, K., et al. (2021). Agroinfiltration mediated scalable transient gene expression in genome edited crop plants. Int. Journ. Mol. Sci. 22 (19), 10882. doi: 10.3390/ijms221910882
Kim, Y. C., Ahn, W. S., Cha, A., Jie, E. Y., Kim, S. W., Hwang, B.-H., et al. (2022). Development of glucoraphanin-rich broccoli (Brassica oleracea var. italica) by CRISPR/Cas9-mediated DNA-free BolMYB28 editing. Plant Biotechnol. Rep. 16, 123–132. doi: 10.1007/s11816-021-00732-y
Kim, J. Y., Nong, G., Rice, J. D., Gallo, M., Preston, J. F., Altpeter, F. (2017). In planta production and characterization of a hyperthermostable GH10 xylanase in transgenic sugarcane. Plant Mol. Biol. 93 (4), 465–478. doi: 10.1007/s11103-016-0573-5
Kitzberger, C. S. G., Pot, D., Marraccini, P., Pereira, L. F. P., Scholz, M. B. S. (2020). Flavor precursors and sensory attributes of coffee submitted to different post-harvest processing. Agric. Food 5 (4), 700–714. doi: 10.3934/agrfood.2020.4.700
Kocsisova, Z., Coneva, V. (2023). Strategies for delivery of CRISPR/Casmediated genome editing to obtain edited plants directly without transgene integration. Front. Genome Ed. 5. doi: 10.3389/fgeed.2023.1209586
Kuiken, T., Kuzma, J. (2021). Genome editing in latin america: regional regulatory overview. Banco Interam. Desarro 10, 1–30. doi: 10.18235/0003410
Kumari, C., Sharma, M., Kumar, V., Sharma, R., Kumar, V., Sharma, P., et al. (2022). Genome editing technology for genetic amelioration of fruits and vegetables for alleviating post-harvest loss. Bioengineering 9, 1–18. doi: 10.3390/bioengineering9040176
Ky, C. L., Ky, L. J., Dussert, S., Guyot, B., Hamon, S., Noirot, M. (2001). Caffeine, trigonelline, chlorogenic acids and sucrose diversity in wild Coffea arabica L. and C. canephora P. accessions. Food Chem. 75, 223–230. doi: 10.1016/S0308-8146(01)00204-7
Landey, B. R., Cenci, A., Georget, F., Bertrand, B., Camayo, G., Dechamp, E., et al. (2013). High genetic and epigenetic stability in Coffea arabica plants derived from embryogenic suspensions and secondary embryogenesis as revealed by AFLP, MSAP and the phenotypic variation rate. PloS One 8 (2), e56372. doi: 10.1371/journal.pone.0056372
Lassoued, R., Phillips, P. W. B., Smyth, S. J., Hesseln, H. (2019). Estimating the cost of regulating genome edited crops: expert judgment and overconfidence. GM Crop Food 10, 44–62. doi: 10.1080/21645698.2019.1612689
Lebot, V. (1999). Biomolecular evidence for plant domestication in Sahul. Genet. Resour Crop Evol. 46, 619–628. doi: 10.1023/A:1008748504038
Leroy, T., Ribeyre, F., Bertrand, B., Charmetant, P., Dufour, M., Montagnon, C., et al. (2006). Genetics of coffee quality. Braz. J. Plant Physiol. 18 (1), 229–242. doi: 10.1590/S1677-04202006000100016
Li, T., Hu, J., Sun, Y., Li, B., Zhang, D., Li, W., et al. (2021). Highly efficient heritable genome editing in wheat using an RNA virus and bypassing tissue culture. Mol. Plant 14 (11), 1787–1798. doi: 10.1017/S1479262121000198
Li, A., Jia, S., Yobi, A., Ge, Z., Sato, S. J., Zhang, C., et al. (2018). Editing of an alpha-kafirin gene family increases, digestibility and protein quality in sorghum. Plant Physiol. 177, 1425–1438. doi: 10.1104/pp.18.00200
Li, C., Li, X., Lin, X., Qin, W., Lu, X., Mao, J., et al. (2021). Genotypic variation in the response to embryogenic callus induction and regeneration in Saccharum spontaneum. Plant Genet. Resources: Characterization Utilization 19 (2), 153–158. doi: 10.1017/S1479262121000198
Li, C., Liu, C., Qi, X., Wu, Y., Fei, X., Mao, L., et al. (2017). RNA-guided Cas9 as an in vivo desired-target mutator in maize. Plant Biotechnol. J. 15 (12), 1566–1576. doi: 10.1111/pbi.12739
Li, D., Qiu, Z., Shao, Y., Chen, Y., Guan, Y., Liu, M., et al. (2013). Heritable gene targeting in the mouse and rat using a CRISPR-Cas system. Nat. Biotechnol. 31 (8), 681–683. doi: 10.1038/nbt.2661
Li, Y., Tang, D., Liu, Z., Chen, J., Cheng, B., Kumar, R., et al. (2022). An improved procedure for agrobacterium-mediated transformation of ‘Carrizo’ Citrange. Plants 11 (11), 1457. doi: 10.3390/plants11111457
Li, X., Wang, Y., Chen, S., Tian, H., Fu, D., Zhu, B., et al. (2018). Lycopene is enriched in tomato fruit by CRISPR/cas9-mediated multiplex genome editing. Front. Plant Sci. 9. doi: 10.3389/fpls.2018.00559
Lian, Z., Nguyen, C. D., Liu, L., Wang, G., Chen, J., Wang, S., et al. (2022). Application of developmental regulators to improve in planta or in vitro transformation in plants. Plant Biotechnol. J. 20, 1622–1635. doi: 10.1111/pbi.13837
Liang, Z., Chen, K., Li, T., Zhang, Y., Wang, Y., Zhao, Q., et al. (2017). Efficient DNA-free genome editing of bread wheat using CRISPR/Cas9 ribonucleoprotein complexes. Nat. Commun. 8, 14261. doi: 10.1038/ncomms14261
Lin, C. S., Hsu, C. T., Yuan, Y. H., Zheng, P. X., Wu, F. H., Cheng, Q. W., et al. (2022). DNA-free CRISPR-Cas9 gene editing of wild tetraploid tomato Solanum Peruvianum using protoplast regeneration. Plant Physiol. 188 (4), 1917–1930. doi: 10.1093/plphys/kiac022
Linnenluecke, M. K., Nucifora, N., Thompson, N. (2018). Implications of climate change for the sugarcane industry. Wiley Interdiscip. Reviews: Climate Change 9 (1), e498. doi: 10.1002/wcc.498
Liu, S., Sretenovic, S., Fan, T., Cheng, Y., Li, G., Qi, A., et al. (2022). Hypercompact CRISPR–Cas12j2 (CasΦ) enables genome editing, gene activation, and epigenome editing in plants. Plant Commun. 3 (6), 100453. doi: 10.1016/j.xplc.2022.100453
Liu, Q., Wang, C., Jiao, X., Zhang, H., Song, L., Li, Y., et al. (2019). Hi-TOM: a platform for high-throughput tracking of mutations induced by CRISPR/Cas systems. Sci. China Life Sci. 62 (1), 1–7. doi: 10.1007/s11427-018-9402-9
Lobato-Gómez, M., Hewitt, S., Capell, T., Christou, P., Dhingra, A., Girón-Calva, P. S. (2021). Transgenic and genome-edited fruits: background, constraints, benefits, and commercial opportunities. Hortic. Res. 8, 166. doi: 10.1038/s41438-021-00601-3
Longhi, T. V., de Carvalho, D. U., Duin, I. M., da Cruz, M. A., Leite Junior, R. P. (2022). Transgenic sweet orange expressing the sarcotoxin IA gene produces high-quality fruit and shows tolerance to ‘Candidatus liberibacter asiaticus. Int. J. Mol. Sci. 23 (16), 9300. doi: 10.3390/ijms23169300
Lowder, L. G., Zhang, D., Baltes, N. J., Paul, J. W., III, Tang, X., Zheng, X., et al. (2015). A CRISPR/Cas9 toolbox for multiplexed plant genome editing and transcriptional regulation. Plant Physiol. 169 (2), 971–985. doi: 10.1104/pp.15.00636
Lowe, K., La Rota, M., Hoerster, G., Hastings, C., Wang, N., Chamberlin, M., et al. (2018). Rapid genotype “independent” Zea mays L.(maize) transformation via direct somatic embryogenesis. In Vitro Cell. Dev. Biology-Plant 54 (3), 240–252. doi: 10.1007/s11627-018-9905-2
Luo, G., Cao, V. D., Kannan, B., Liu, H., Shanklin, J., Altpeter, F. (2022). Metabolic engineering of energycane to hyperaccumulate lipids in vegetative biomass. BMC Biotechnol. 22 (1), 1–10. doi: 10.1186/s12896-022-00753-7
Maher, M. F., Nasti, R. A., Vollbrecht, M., Starker, C. G., Clark, M. D., Voytas, D. F. (2020). Plant gene editing through de novo induction of meristems. Nat. Biotechnol. 38, 84–89. doi: 10.1038/s41587-019-0337-2.Plant
Mahfouz, M. M., Li, L., Shamimuzzaman, M., Wibowo, A., Fang, X., Zhu, J. K. (2011). De novo-engineered transcription activator-like effector (TALE) hybrid nuclease with novel DNA binding specificity creates double-strand breaks. Proc. Natl. Acad. Sci. U.S.A. 108 (6), 2623–2628. doi: 10.1073/pnas.1019533108
Mahmoud, L. M., Kaur, P., Stanton, D., Grosser, J. W., Dutt, M. (2022). A cationic lipid mediated CRISPR/Cas9 technique for the production of stable genome edited citrus plants. Plant Methods 18 (1), 33. doi: 10.1186/s13007-022-00870-6
Malnoy, M., Viola, R., Jung, M. H., Koo, O. J., Kim, S., Kim, J. S., et al. (2016). DNA-free genetically edited grapevine and apple protoplast using CRISPR/Cas9 ribonucleoproteins. Front. Plant Sci. 7, 1904. doi: 10.3389/fpls.2016.01904
Matres, J. M., Hilscher, J., Datta, A., Armario-Nájera, V., Baysal, C., He, W., et al. (2021). Genome editing in cereal crops: an overview. Transgenic Res. 30, 461–498. doi: 10.1007/s11248-021-00259-6
Matzke, A. J. M., Neuhuber, F., Park, Y. D., Ambros, P. F., Matzke, M. A. (1994). Homology-dependent gene silencing in transgenic plants: Epistatic silencing loci contain multiple copies of methylated transgenes. Mol. Gen. Genet. 244, 219–229. doi: 10.1007/BF00285449
Medeiros, S. C., Monteiro-Júnior, J. E., Sales, G. W.P., Grangeiro, T. B., Nogueira, N. A.P. (2018). Chitinases as antibacterial proteins: a systematic review. J. Young Pharmacists 10 (2), 44–148. doi: 10.5530/jyp.2018.10.33
Meena, M. R., Appunu, C., Arun Kumar, R., Manimekalai, R., Vasantha, S., Krishnappa, G., et al. (2022). Recent advances in sugarcane genomics, physiology, and phenomics for superior agronomic traits. Front. Genet. 13. doi: 10.3389/fgene.2022.854936
Mei, Y., Beernink, B. M., Ellison, E. E., Konečná, E., Neelakandan, A. K., Voytas, D. F., et al. (2019). Protein expression and gene editing in monocots using foxtail mosaic virus vectors. Plant Direct 3 (11), e00181. doi: 10.1002/pld3.181
Melloni, M., Melloni, M., Scarpari, M., Garcia, J., Landell, M., Pinto, L. (2015). Flowering of sugarcane genotypes under different artificial photoperiod conditions. Am. J. Plant Sci. 6, 456–463. doi: 10.4236/ajps.2015.63051
Mishra, M. K., Slater, A. (2012). Recent advances in the genetic transformation of coffee. Biotech. Res. doi: 10.1155/2012/580857
Mohanan, S., Satyanarayana, K. V., Sridevi, V., Gowda, K., Giridhar, P., Chandrashekar, A., et al. (2014). Evaluating the effect and effectiveness of different constructs with a conserved sequence for silencing of Coffea canephora N-methyltransferases. J. Plant Biochem. Biotechnol. 23 (4), 399–409. doi: 10.1007/s13562-013-0224-8
Molinari, H. B. C., Vieira, L. R., Volpi, N., Prado, G. S., Hernandes-Lopes, J. F. (2021). CRISPR technology in plant genome editing - Biotechnology applied to agriculture (Brasília: Embrapa).
Molla, K. A., Sretenovic, S., Bansal, K. C., Qi, Y. (2021). Precise plant genome editing using base editors and prime editors. Nat. Plants. 7 (9), 1166–1187. doi: 10.1038/s41477-021-00991-1
Moniruzzaman, M., Zhong, Y., Yan, H., Yuanda, L., Jiang, B., Zhong, G. (2020). Exploration of susceptible genes with clustered regularly interspaced short palindromic repeats–tissue-specific knockout (CRISPR-TSKO) to enhance host resistance. Crit. Rev. Plant Sci. 39 (5), 387–417. doi: 10.1080/07352689.2020.1810970
Monteiro-Vitorello, C. B., Zerillo, M. M., Van Sluys, M. A., Camargo, L. E. A. (2009). Genome sequence-based insights into the biology of sugarcane pathogen Leifsonia xyli subsp. xyli. In: Plant Pathogenic Bacteria: Genomics and Molecular Biology (Norwich: Horizon Academic Press), 135–146.
Montgomery, J., Wittwer, C. T., Palais, R., Zhou, L. (2007). Simultaneous mutation scanning and genotyping by high-resolution DNA melting analysis. Nat. Protoc. 2 (1), 59–66. doi: 10.1038/nprot.2007.10
Mori, A. L. B., Kalschne, D. L., Ferrão, M. A. G., Fonseca, A. F. A., Ferrão, R. G., Benassi, M. T. (2016). Diterpenes in coffea canephora. J. Food Composition Anal. 52, 52–57. doi: 10.1016/j.jfca.2016.08.004
Mosquera, T., Alvarez, M. F., Jiménez-Gómez, J. M., Muktar, M. S., Paulo, M. J., Steinemann, S., et al. (2016). Targeted and untargeted approaches unravel novel candidate genes and diagnostic SNPs for quantitative resistance of the potato (Solanum tuberosum L.) to Phytophthora infestants causing the late blight disease. PloS One 11 (6), e0156254. doi: 10.1371/journal.pone.0156254
Muccilli, V., Vitale, A., Sheng, L., Gentile, A., Cardullo, N., Tringali, C., et al. (2020). Substantial equivalence of a transgenic lemon fruit showing postharvest fungal pathogens resistance. J. Agric. Food Chem. 68 (12), 3806–3816. doi: 10.1021/acs.jafc.9b07925
Nagamine, A., Ezura, H. (2022). Genome editing for improving crop nutrition. Front. Genome Ed. 4. doi: 10.3389/fgeed.2022.850104
Naqvi, S. A. H., Wang, J., Malik, M. T., Umar, U. U. D., Ateeq-Ur-rehman, Hasnain, A., et al. (2022). Citrus canker - distribution, taxonomy, epidemiology, disease cycle, pathogen biology, detection, and management: A critical review and future research agenda. Agronomy 12 (5), 1075. doi: 10.3390/agronomy12051075
Navet, N., Tian, M. (2020). Efficient targeted mutagenesis in allotetraploid sweet basil by CRISPR/Cas9. Plant Direct 4 (6), e00233. doi: 10.1002/pld3.233
Neelakandan, A. K., Wright, D. A., Traore, S. M., Ma, X., Subedi, B., Veeramasu, S., et al. (2022). Application of CRISPR/cas9 system for efficient gene editing in peanut. Plants 11, 1361. doi: 10.3390/plants11101361
Niedz, R. P., McKendree, W. L., Shatters, R. G. (2003). Electroporation of embryogenic protoplasts of sweet orange (Citrus sinensis (L.) Osbeck) and regeneration of transformed plants. In Vitro Cell. Dev. Biol. - Plant 39 (6), 586–594. doi: 10.1079/IVP2003463
Nonato, J. V. A., Carvalho, H. F., Borges, K. L. R., Padilha, L., Maluf, M. P., Fritsche-Neto, R., et al. (2021). Association mapping reveals genomic regions associated with bienniality and resistance to biotic stresses in arabica coffee. Euphytica 217, 190. doi: 10.1007/s10681-021-02922-9
Novaes, F. M., Bayan, F. C., Aquino Neto, F. R., Rezende, C. M. (2019). The occurrence of cafestol and kahweol diterpenes in different coffee brews. Coffee Science. 14 (2), 265–280. doi: 10.25186/cs.v14i2.1571
Odipio, J., Alicai, T., Ingelbrecht, I., Nusinow, D. A., Bart, R., Taylor, N. J. (2017). Efficient CRISPR/Cas9 genome editing of phytoene desaturase in cassava. Front. Plant Sci. 8, 1780. doi: 10.3389/fpls.2017.01780
Ogita, S., Uefuji, H., Yamaguchi, Y., Koizumi, N., Sano, H. (2003). Producing decaffeinated coffee plants. Nature 423, 823–823. doi: 10.1038/423823a
Oh, Y., Kim, H., Kim, S. G. (2021). Virus-induced plant genome editing. Curr. Opin. Plant Biol. 60, 1–6. doi: 10.1016/j.pbi.2020.101992
Oliveira, M. L. P., Febres, V. J., Costa, M. G. C., Moore, G. A., Otoni, W. C. (2009). High-efficiency Agrobacterium-mediated transformation of citrus via sonication and vacuum infiltration. Plant Cell Rep. 28 (3), 387–395. doi: 10.1007/s00299-008-0646-2
Oliveira, K. C., Guimaraes, P. S., Bazioli, J. M., Martinati, J. C., Santos, M. M., Padilha, L., et al. (2019). Effects of somatic embryogenesis on gene expression of cloned coffee heterozygous hybrids. Acta Physiologiae Plantarum 41, 118. doi: 10.1007/s11738-019-2917-7
Omar, A. A., Song, W. Y., Grosser, J. W. (2007). Introduction of Xa21, a Xanthomonas-resistance, gene from rice, into “Hamlin” sweet orange [Citrus sinensis (L.) Osbeck] using protoplast- GFP co-transformation or single plasmid transformation. J. Hortic. Sci. Biotechnol. 82 (6), 914–923. doi: 10.1080/14620316.2007.11512326
Osakabe, Y., Liang, Z., Ren, C., Nishitani, C., Osakabe, K., Wada, M., et al. (2018). CRISPR–Cas9-mediated genome editing in apple and grapevine. Nat. Protoc. 13 (12), 2844–2863. doi: 10.1038/s41596-018-0067-9
Ossowski, S., Schneeberger, K., Lucas-Lledó, J. I., Warthmann, N., Clark, R. M., Shaw, R. G., et al. (2010). The rate and molecular spectrum of spontaneous mutations in Arabidopsis thaliana. Science 327 (5961), 92–94. doi: 10.1126/science.1180677
Oz, M. T., Altpeter, A., Karan, R., Merotto, A., Altpeter, F. (2021). CRISPR/Cas9-mediated multi-allelic gene targeting in sugarcane confers herbicide tolerance. Front. Genome Ed. 3, 673566. doi: 10.3389/fgeed.2021.673566
Pan, C., Li, G., Malzahn, A. A., Cheng, Y., Leyson, B., Sretenovic, S., et al. (2022). Boosting plant genome editing with a versatile CRISPR-Combo system. Nat. Plants 8 (5), 513–525. doi: 10.1038/s41477-022-01151-9
Pang, Z., Zhang, L., Coaker, G., Ma, W., He, S. Y., Wang, N. (2020). Citrus CsACD2 is a target of Candidatus Liberibacter asiaticus in huanglongbing disease. Plant Physiol. 184 (2), 792–805. doi: 10.1104/pp.20.00348
Peng, A., Chen, S., Lei, T., Xu, L., He, Y., Wu, L., et al. (2017). Engineering canker-resistant plants through CRISPR/Cas9-targeted editing of the susceptibility gene CsLOB1 promoter in Citrus. Plant Biotechnol. J. 15, 1509–1519. doi: 10.1111/pbi.12733
Peng, A., Zou, X., Xu, L., He, Y., Lei, T., Yao, L., et al. (2019). Improved protocol for the transformation of adult Citrus sinensis Osbeck ‘Tarocco’ blood orange tissues. In Vitro Cell. Dev. Biol. - Plant 55 (6), 659–667. doi: 10.1007/s11627-019-10011-9
Perthuis, B., Pradon, J.L., Montagnon, C., Dufour, M, Leroy, T. (2005). Stable resistance against the leaf miner Leucoptera coffeella expressed by genetically transformed Coffea canephora in a pluriannual field experiment in French Guiana. Euphytica 144, 321–339. doi: 10.1007/s10681-005-8003-9
Pickar-Oliver, A., Gersbach, C. A. (2019). The next generation of CRISPR-Cas technologies and applications. Nat. Rev. Mol. Cell Biol. 20 (8), 490–507. doi: 10.1038/s41580-019-0131-5
Pilet-Nayel, M. L., Moury, B., Caffier, V., Montarry, J., Kerlan, M. C., Fournet, S., et al. (2017). Quantitative resistance to plant pathogens in pyramiding strategies for durable crop protection. Front. Plant Sci. 8, 1838. doi: 10.3389/fpls.2017.01838
Piperidis, N., D’Hont, A. (2020). Sugarcane genome architecture decrypted with chromosome-specific oligo probes. Plant J. 103 (6), 2039–2051. doi: 10.1111/tpj.14881
Pramanik, D., Shelake, R. M., Park, J., Kim, M. J., Hwang, I., Park, Y., et al. (2021). CRISPR/cas9-mediated generation of pathogen-resistant tomato against tomato yellow leaf curl virus and powdery mildew. Int. J. Mol. Sci. 22, 1878. doi: 10.3390/ijms22041878
Rathus, C., Birch, R. G. (1992). Stable transformation of callus from electroporated sugarcane protoplasts. Plant Sci. 82, 81–89. doi: 10.1016/0168-9452(92)90010-J
Reed, K. M., Bargmann, B. O. R. (2021). Protoplast regeneration and its use in new plant breeding technologies. Front. Genome Ed 3. doi: 10.3389/fgeed.2021.734951
Reis, R. R., da Cunha, B. A. D. B., Martins, P. K., Martins, M. T. B., Alekcevetch, J. C., Chalfun-Júnior, A., et al. (2014). Induced over-expression of AtDREB2A CA improves drought tolerance in sugarcane. Plant Sci. 221, 59–68. doi: 10.1016/j.plantsci.2014.02.003
Ribas, A., Déchamp, E., Champion, A., Bertrand, B., Combes, M.-C., Verdeil, J.-L., et al. (2011). Agrobacterium-mediated genetic transformation of Coffea arabica (L.) is greatly enhanced by using established embryogenic callus cultures. BMC Plant Biol. 11, 92. doi: 10.1186/1471-2229-11-92
Ribas, A. F., Kobayashi, A. K., Pereira, L. F. P., Vieira, L. G. E. (2005). Genetic transformation of Coffea canephora by particle bombardment. Biol. Plantarum 49 (4), 493–497. doi: 10.1007/s10535-005-0038-1
Ricroch, A. E., Martin-Laffon, J., Rault, B., Pallares, V. C., Kuntz, M. (2022). Next biotechnological plants for addressing global challenges: The contribution of transgenesis and new breeding techniques. New Biotechnol. 66, 25–35. doi: 10.1016/j.nbt.2021.09.001
Robertson, G., Burger, J., Campa, M. (2022). CRISPR/Cas-based tools for the targeted control of plant viruses. Mol. Plant Pathol. 23, 1701–1718. doi: 10.1111/mpp.13252
Robertson, C. J., Zhang, X., Gowda, S., Orbović, V., Dawson, W. O., Mou, Z. (2018). Overexpression of the Arabidopsis NPR1 protein in citrus confers tolerance to Huanglongbing. J. Citrus Pathol. 5, (1). doi: 10.5070/c451038911
Romero-Romero, J. L., Inostroza-Blancheteau, C., Reyes-Díaz, M., Matte, J. P., Aquea, F., Espinoza, C., et al. (2020). Increased drought and salinity tolerance in citrus aurantifolia (Mexican lemon) plants overexpressing arabidopsis CBF3 gene. J. Soil Sci. Plant Nutr. 20 (1), 244–252. doi: 10.1007/s42729-019-00130-y
Rosado, A., Eriksson, D. (2022). Biosafety legislation and the regulatory status of the products of precision breeding in the Latin America and the Caribbean region. Plants People Planet 4, 214–231. doi: 10.1002/ppp3.10243
Rostoks, N. (2021). Implications of the EFSA scientific opinion on site directed nucleases 1 and 2 for risk assessment of genome-edited plants in the EU. Agronomy 11 (3), 572. doi: 10.3390/agronomy11030572
Rowand, G. G. (2009). “The effect of plants with novel traits (PNT) regulation on mutation breeding in Canada,” Induced Plant Mutations in the Genomics Era, Food and Agriculture Organization of the United Stations (Rome), 423–424.
Rozas, P., Kessi-Pérez, E. I., Martínez, C. (2022). Genetically modified organisms: adapting regulatory frameworks for evolving genome editing technologies. Biol. Res. 55, 1–14. doi: 10.1186/s40659-022-00399-x
Saito, M., Xu, P., Faure, G., Maguire, S., Kannan, S., Altae-Tran, H., et al. (2023). Fanzor is a eukaryotic programmable RNA-guided endonuclease. Nature. doi: 10.1038/s41586-023-06356-2
Sánchez-León, S., Gil-Humanes, J., Ozuna, C. V., Giménez, M. J., Sousa, C., Voytas, D. F., et al. (2018). Low-gluten, nontransgenic wheat engineered with CRISPR/Cas9. Plant Biotechnol. J. 16, 902–910. doi: 10.1111/pbi.12837
Sanguino, A. (1998). Situação atual da pesquisa em doenças da cana-de-açúcar. Summa Phytopathol. 24 (1), 90–91.
Sant’Ana, G. C., Pereira, L. F. P., Pot, D., Ivamoto, S. T., Domingues, D. S., Ferreira, R. V., et al. (2018). Genome-wide association study reveals candidate genes influencing lipids and diterpenes contents in Coffea arabica L. Sci. Rep. 8, 46. doi: 10.1038/s41598-017-18800-
Savadi, S., Mangalassery, S., Sandesh, M. S. (2021). Advances in genomics and genome editing for breeding next generation of fruit and nut crops. Genomics 113, 3718–3734. doi: 10.1016/j.ygeno.2021.09.001
Schmidt, S. M., Belisle, M., Frommer, W. B. (2020). The evolving landscape around genome editing in agriculture: Many countries have exempted or move to exempt forms of genome editing from GMO regulation of crop plants. EMBO Rep. 21 (6), e50680. doi: 10.15252/embr.202050680
Scholz, M. B. S., Kitzberger, C. S. G., Pagiatto, N. F., Pereira, L. F. P., Davrieux, F., Pot, D., et al. (2016). Chemical composition in wild Ethiopian Arabica coffee accessions. Euphytica 209, 429–438. doi: 10.1007/s10681-016-1653-y
Selva, C., Shirley, N. J., Houston, K., Whitford, R., Baumann, U., Li, G., et al. (2021). HvLEAFY controls the early stages of floral organ specification and inhibits the formation of multiple ovaries in barley. Plant J. 108, 509–527. doi: 10.1111/tpj.15457
Shan, Q., Wang, Y., Li, J., Zhang, Y., Chen, K., Liang, Z., et al. (2013). Targeted genome modification of crop plants using a CRISPR-Cas system. Nat. Biotechnol. 31, 686–688. doi: 10.1038/nbt.2650
Shi, J., Ni, X., Huang, J., Fu, Y., Wang, T., Yu, H., et al. (2022). CRISPR/cas9-mediated gene editing of bnFAD2 and bnFAE1 modifies fatty acid profiles in brassica napus. Genes 13, 1681. doi: 10.3390/genes13101681
Sidorov, V., Wang, D., Nagy, E. D., Armstrong, C., Beach, S., Zhang, Y., et al. (2022). Heritable DNA-free genome editing of canola (Brassica napus L.) using PEG-mediated transfection of isolated protoplasts. In Vitro Cell. Dev. Biology-Plant 58 (3), 447–456. doi: 10.1007/s11627-021-10236-7
Silva, R. A., Zambolim, L., Castro, I. S. L., Rodrigues, H. S., Cruz, C. D., Caixeta, E. T. (2018). The Híbrido de Timor germplasm: identification of molecular diversity and resistance sources to coffee berry disease and leaf rust. Euphytica 214, 153. doi: 10.1007/s10681-018-2231-2
Smyth, S. J. (2017). Canadian regulatory perspectives on genome engineered crops. GM Crop Food 8, 35–43. doi: 10.1080/21645698.2016.1257468
Sousa, I. C., Nascimento, M., Silva, G. N., Nascimento, A. C. C., Cruz, C. D., Silva, F. F., et al. (2021). Genomic prediction of leaf rust resistance to Arabica coffee using machine learning algorithms. Sci. Agric. 78, e20200021. doi: 10.1590/1678-992X-2020-0021
Souza-Neto, R. R., Carvalho, I. G. B., Martins, P. M. M., Picchi, S. C., Tomaz, J. P., Caserta, R., et al. (2022). MqsR toxin as a biotechnological tool for plant pathogen bacterial control. Sci. Rep. 12, 2794. doi: 10.1038/s41598-022-06690-x
Sprink, T., Wilhelm, R., Hartung, F. (2022). Genome editing around the globe: An update on policies and perceptions. Plant Physiol. 190 (3), 1579–1587. doi: 10.1093/plphys/kiac359
Stokstad, E. (2023). European Commission proposes loosening rules for gene-edited plants. Science 381 (6654), 113. doi: 10.1126/science.adj6428
Stoykova, P., Stoeva-Popova, P. (2011). PMI (manA) as a nonantibiotic selectable marker gene in plant biotechnology. Plant Cell Tissue Organ Culture 105 (2), 141–148. doi: 10.1007/s11240-010-9858-6
Su, H., Wang, Y., Xu, J., Omar, A. A., Grosser, J. W., Calovic, M., et al. (2023). Generation of the transgene-free canker-resistant Citrus sinensis using Cas12a/crRNA ribonucleoprotein in the T0 generation. Nat. Commun. 14 (1), 3957. doi: 10.1038/s41467-023-39714-9
Sundar, A. R., Ashwin, N. M. R., Barnabas, E. L., Malathi, P., Viswanathan, R. (2015). Disease resistance in sugarcane–An overview. Scientia Agraria Paranaensis 14 (4), 200–212.
Svitashev, S., Schwartz, C., Lenderts, B., Young, J. K., Mark Cigan, A. (2016). Genome editing in maize directed by CRISPR–Cas9 ribonucleoprotein complexes. Nat. Commun. 7, 13274. doi: 10.1038/ncomms13274
Swingle, W. T., Reece, P. C. (1967). “The botany of Citrus and its wild relatives,” in The citrus industry, vol. 1, History, world distribution, botany, and varieties (Berkeley: University of California), 190–430.
Syombua, E. D., Zhang, Z., Tripathi, J. N., Ntui, V. O., Kang, M., George, O. O., et al. (2021). A CRISPR/Cas9-based genome-editing system for yam (Dioscorea spp.). Plant Biotechnol. J. 19 (4), 645. doi: 10.1111/pbi.13515
Talon, M., Wu, G. A., Gmitter, F. G., Jr., Rokhsar, D. S. (2020). “The origin of citrus,” in The genus citrus. Eds. Talon, M., Caruso, M., Gmitter, F. (Cambridge: Woodhead Publishing), 9–31. doi: 10.1016/B978-0-12-812163-4.00002-4
Tang, X., Chen, S., Yu, H., Zheng, X., Zhang, F., Deng, X., et al. (2021). Development of a gRNA-tRNA array of CRISPR/Cas9 in combination with grafting technique to improve gene-editing efficiency of sweet orange. Plant Cell Rep. 40, 12. doi: 10.1007/s00299-021-02781-7
Taylor, P. W., Ko, H. L., Adkins, S. W., Rathus, C., Birch, R. G. (1992). Establishment of embryogenic callus and high protoplast yielding suspension cultures of sugarcane (Saccharum spp. hybrids). Plant Cell Tissue Organ Culture 28 (1), 69–78. doi: 10.1007/BF00039917
Thygesen, P. (2019). Clarifying the regulation of genome editing in Australia: situation for genetically modified organisms. Transgenic Res. 28, 151–159. doi: 10.1007/s11248-019-00151-4
Tian, S., Jiang, L., Gao, Q., Zhang, J., Zong, M., Zhang, H., et al. (2017). Efficient CRISPR/Cas9-based gene knockout in watermelon. Plant Cell Rep. 36, 399–406. doi: 10.1007/s00299-016-2089-5
Toda, E., Koiso, N., Takebayashi, A., Ichikawa, M., Kiba, T., Osakabe, K., et al. (2019). An efficient DNA- and selectable-marker-free genome-editing system using zygotes in rice. Nat. Plants 5, 363–368. doi: 10.1038/s41477-019-0386-z
Tran, H. T. M., Vargas, C. A. C., Slade Lee, L., Furtado, A., Smyth, H., Henry, R. (2017). Variation in bean morphology and biochemical composition measured in different genetic groups of arabica coffee (Coffea arabica L.). Tree Genet. Genomes 13, 54. doi: 10.1007/s11295-017-1138-8
Turnbull, C., Lillemo, M., Hvoslef-Eide, T. A. K. (2021). Global regulation of genetically modified crops amid the gene edited crop boom – A review. Front. Plant Sci. 12. doi: 10.3389/fpls.2021.630396
Upadhyay, S. K., Kumar, J., Alok, A., Tuli, R. (2013). RNA-guided genome editing for target gene mutations in wheat. G3: Genes Genomes Genet. 3 (12), 2233–2238. doi: 10.1534/g3.113.008847
Uranga, M., Vazquez-Vilar, M., Orzáez, D., Daròs, J. A. (2021). CRISPR-Cas12a genome editing at the whole-plant level using two compatible RNA virus vectors. CRISPR J. 4 (5), 761–769. doi: 10.1089/crispr.2021.0049
Valencia-Lozano., E., Cabrera-Ponce, J. L., Noa-Carrazana, J. C., Ibarra, J. E. (2021). Coffea arabica L. resistant to coffee berry borer (Hypothenemus hampei) mediated by expression of the Bacillus thuringiensis Cry10Aa protein. Front. Plant Sci. 12. doi: 10.3389/fpls.2021.765292
Van der Vossen, H. A. M., Walyaro, D. J. (1980). Breeding for resistance to coffee berry disease in Coffea arabica L. II. Inheriance of the resistance. Euphytica 29, 777–791. doi: 10.1007/BF00023225
Van der Vossen, H. A. M., Walyaro, D. J. (2009). Additional evidence for oligogenic inheritance of durable host resistance to coffee berry disease (Colletotrichum kahawae) in arabica coffee (Coffea arabica L.). Euphytica 165, 105–111. doi: 10.1007/s10681-008-9769-3
Vardi, A., Bleichman, S., Aviv, D. (1990). Genetic transformation of citrus protoplasts and regeneration of transgenic plants. Plant Sci. 69, 199–206. doi: 10.1016/0168-9452(90)90118-8
Vargas−Guevara, C., Vargas−Segura, C., Villalta−Villalobos, J., Pereira, L. F. P., Gatica−Arias, A. (2018). A simple and efficient agroinfiltration method in coffee leaves (Coffea arabica L.): assessment of factors affecting transgene expression. Biotech 8, 471. doi: 10.1007/s13205-018-1495-5
Velásquez, A. C., Castroverde, C. D. M., He, S. Y. (2018). Plant–pathogen warfare under changing climate conditions. Curr. Biol. 28 (10), 619–634. doi: 10.1016/j.cub.2018.03.054
Ventura, J. A., Costa, H., Lima, I. M. (2019). Conilon cofee disease management (Conilon Coffee. Vitória: Incaper), 535–592, ISBN: ISBN: 978-85-89274-32-6.
Verma, K. K., Song, X. P., Budeguer, F., Nikpay, A., Enrique, R., Singh, M., et al. (2022). Genetic engineering: an efficient approach to mitigating biotic and abiotic stresses in sugarcane cultivation. Plant Signal Behav. 17 (1), 2108253. doi: 10.1080/15592324.2022.2108253
Waltz, E. (2016). CRISPR-edited crops free to enter market, skip regulation. Nat. Biotechnol. 34 (6), 582–583. doi: 10.1038/nbt0616-582
Waltz, E. (2018). With a free pass, CRISPR-edited plants reach market in record time. Nat. Biotechnol. 36 (1), 6–8. doi: 10.1038/nbt0118-6b
Waltz, E. (2022). GABA-enriched tomato is first CRISPR-edited food to enter market. Nat. Biotechnol. 40 (1), 9–11. doi: 10.1038/d41587-021-00026-2
Wang, N., et al. (2014). Targeted genome editing of sweet orange using Cas9/sgRNA. PloS One 9, e93806. doi: 10.1371/journal.pone.0093806
Wang, B., Li, N., Huang, S., Hu, J., Wang, Q., Tang, Y., et al. (2021). Enhanced soluble sugar content in tomato fruit using CRISPR/Cas9-mediated SlINVINH1 and SlVPE5 gene editing. PeerJ 9, e12478. doi: 10.7717/peerj.12478
Wang, W., Liu, J., Wang, H., Li, T., Zhao, H. (2021). A highly efficient regeneration, genetic transformation system and induction of targeted mutations using CRISPR/Cas9 in Lycium ruthenicum. Plant Methods 17, 71. doi: 10.1186/s13007-021-00774-x
Wang, Z., Wang, S., Li, D., Zhang, Q., Li, L., Zhong, C., et al. (2018). Optimized paired-sgRNA/Cas9 cloning and expression cassette triggers high-efficiency multiplex genome editing in kiwifruit. Plant Biotechnol. J. 16, 1424–1433. doi: 10.1111/pbi.12884
Wang, J., Wu, H., Chen, Y., Yin, T. (2020). Efficient CRISPR/Cas9-mediated gene editing in an interspecific hybrid poplar with a highly heterozygous genome. Front. Plant Sci. 11, 996. doi: 10.3389/fpls.2020.00996
Wang, W. Z., Yang, B. P., Feng, X. Y., Cao, Z. Y., Feng, C. L., Wang, J. G., et al. (2017). Development and characterization of transgenic sugarcane with insect resistance and herbicide tolerance. Front. Plant Sci. 8, 1535. doi: 10.3389/fpls.2017.01535
Wang, W., Yu, Z., He, F., Bai, G., Trick, H. N., Akhunova, A., et al. (2022). Multiplexed promoter and gene editing in wheat using a virus-based guide RNA delivery system. Plant Biotechnol. J. 20 (12), 2332–2341. doi: 10.1111/pbi.13910
Waterhouse, P. M., Wang, M.-B., Lough, T. (2001). Gene silencing as an adaptive defense against viruses. Nature 411, 834–842. doi: 10.1038/35081168
Whelan, A. I., Gutti, P., Lema, M. A. (2020). Gene editing regulation and innovation economics. Front. Bioeng. Biotechnol. 8. doi: 10.3389/fbioe.2020.00303
Whelan, A. I., Lema, M. A. (2015). Regulatory framework for gene editing and other new breeding techniques (NBTs) in Argentina. GM Crops Food. 6 (4), 253–265. doi: 10.1080/21645698.2015.1114698
Wondimkun, Y. W., Emire, S. A., Teferra, T. F., Stoecker, B., Esho, T. B. (2022). Influence of genotype and processing on bioactive compounds of Ethiopian specialty Arabica coffee. Int. J. Food Properties 25 (1), 2574–2588. doi: 10.1080/10942912.2022.2147540
Woo, J. W., Kim, J., Kwon, S. I., Corvalán, C., Cho, S. W., Kim, H., et al. (2015). DNA-free genome editing in plants with preassembled CRISPR-Cas9 ribonucleoproteins. Nat. Biotechnol. 33, 1162–1164. doi: 10.1038/nbt.3389
Wright, D. A., Townsend, J. A., Winfrey, R. J., Irwin, P. A., Rajagopal, J., Lonosky, P. M., et al. (2005). High-frequency homologous recombination in plants mediated by zinc-finger nucleases. Plant J. Cell Mol. Biol. 44 (4), 693–705. doi: 10.1111/j.1365-313X.2005.02551.x
Wu, H., Acanda, Y., Canton, M., Zale, J. (2019). Efficient biolistic transformation of immature citrus rootstocks using phosphomannose-isomerase selection. Plants 8 (10), 390. doi: 10.3390/plants8100390
Wu, H., Acanda, Y., Jia, H., Wang, N., Zale, J. (2016). Biolistic transformation of Carrizo citrange (Citrus sinensis Osb. × Poncirus trifoliata L. Raf.). Plant Cell Rep. 35 (9), 1955–1962. doi: 10.1007/s00299-016-2010-2
Wu, H., Awan, F. S., Vilarinho, A., Zeng, Q., Kannan, B., Phipps, T., et al. (2015). Transgene integration complexity and expression stability following biolistic or Agrobacterium-mediated transformation of sugarcane. In Vitro Cell. Dev. Biology-Plant 51 (6), 603–611. doi: 10.1007/s11627-015-9710-0
Wu, Z., Zhang, Y., Yu, H., Pan, D., Wang, Y., Wang, Y., et al. (2021). Programmed genome editing by a miniature CRISPR-Cas12f nuclease. Nat. Chem. Biol. 17 (11), 1132–1138. doi: 10.1038/s41589-021-00868-6
Xin, T., Tian, H., Ma, Y., Wang, S., Yang, L., Li, X., et al. (2022). Targeted creating new mutants with compact plant architecture using CRISPR/Cas9 genome editing by an optimized genetic transformation procedure in cucurbit plants. Hortic. Res. 9, uhab086. doi: 10.1093/hr/uhab086
Yang, W., Ren, J., Liu, W., Liu, D., Xie, K., Zhang, F., et al. (2023). An efficient transient gene expression system for protein subcellular localization assay and genome editing in citrus protoplasts. Hortic Plant J. 9, 425–436. doi: 10.1016/j.hpj.2022.06.006
Yao, J. L., Wub, J. H., Gleave, A. P., Morrisa, B. A. M. (1996). Transformation of citrus embryogenic cells using particle bombardment and production of transgenic embryos. Front. Plant Sci. 113, 175–183. doi: 10.1016/0168-9452(95)04292-X
Yuste-Lisbona, F. J., Fernández-Lozano, A., Pineda, B., Bretones, S., Ortíz-Atienza, A., García-Sogo, B., et al. (2020). ENO regulates tomato fruit size through the floral meristem development network. Proc. Natl. Acad. Sci. U.S.A. 117 (14), 8187–8195. doi: 10.1073/pnas.1913688117
Zhang, F., LeBlanc, C., Irish, V. F., Jacob, Y.. (2017). Rapid and efficient CRISPR/Cas9 gene editing in Citrus using the YAO promoter. Plant Cell Rep. 36, 12. doi: 10.1007/s00299-017-2202-4
Zhang, Y., Cheng, Y., Fang, H., Roberts, N., Zhang, L., Vakulskas, C. A., et al. (2022). Highly efficient genome editing in plant protoplasts by ribonucleoprotein delivery of CRISPR-cas12a nucleases. Front. Genome Ed. 4, 780238. doi: 10.3389/fgeed.2022.780238
Zhang, Y., Liang, Z., Zong, Y., Wang, Y., Liu, J., Chen, K., et al. (2016). Efficient and transgene-free genome editing in wheat through transient expression of CRISPR/Cas9 DNA or RNA. Nat. Commun. 7, 1–8. doi: 10.1038/ncomms12617
Zhang, C., Liu, S., Li, X., Zhang, R., Li, J. (2022). Virus-induced gene editing and its applications in plants. Int. J. Mol. Sci. 23, 10202. doi: 10.3390/ijms231810202
Zhang, W., Peng, K., Cui, F., Wang, D., Zhao, J., Zhang, Y., et al. (2021). Cytokinin oxidase/dehydrogenase OsCKX11 coordinates source and sink relationship in rice by simultaneous regulation of leaf senescence and grain number. Plant Biotechnol. J. 19 (2), 335–350. doi: 10.1111/pbi.13467
Zhang, H., Si, X., Ji, X., Fan, R., Liu, J., Chen, K., et al. (2018). Genome editing of upstream open reading frames enables translational control in plants. Nat. Biotechnol. 36, 894–898. doi: 10.1038/nbt.4202
Zhao, Y., Liu, J., Huang, H., Zan, F., Zhao, P., Zhao, J., et al. (2022). Genetic improvement of sugarcane (Saccharum spp.) contributed to high sucrose content in China based on an analysis of newly developed varieties. Agriculture 12 (11), 1789. doi: 10.3390/agriculture12111789
Zhao, H., Wolt, J. D. (2017). Risk associated with off-target plant genome editing and methods for its limitation. Emerging Topics Life Sci. 1 (2), 231. doi: 10.1042/ETLS20170037
Zheng, Z., Ye, G., Zhou, Y., Pu, X., Su, W., Wang, J. (2021). Editing sterol side chain reductase 2 gene (StSSR2) via CRISPR/Cas9 reduces the total steroidal glycoalkaloids in potato. All Life 14, 401–413. doi: 10.1080/26895293.2021.1925358
Keywords: edited plants, genome editing tools, perennial crops, regulatory scenario, biotechnology
Citation: Prado GS, Rocha DC, Santos LN, Contiliani DF, Nobile PM, Martinati-Schenk JC, Padilha L, Maluf MP, Lubini G, Pereira TC, Monteiro-Vitorello CB, Creste S, Boscariol-Camargo RL, Takita MA, Cristofani-Yaly M and Souza AAd (2024) CRISPR technology towards genome editing of the perennial and semi-perennial crops citrus, coffee and sugarcane. Front. Plant Sci. 14:1331258. doi: 10.3389/fpls.2023.1331258
Received: 31 October 2023; Accepted: 14 December 2023;
Published: 08 January 2024.
Edited by:
Litao Yang, Shanghai Jiao Tong University, ChinaReviewed by:
Phetole Mangena, University of Limpopo, South AfricaCopyright © 2024 Prado, Rocha, Santos, Contiliani, Nobile, Martinati-Schenk, Padilha, Maluf, Lubini, Pereira, Monteiro-Vitorello, Creste, Boscariol-Camargo, Takita, Cristofani-Yaly and Souza. This is an open-access article distributed under the terms of the Creative Commons Attribution License (CC BY). The use, distribution or reproduction in other forums is permitted, provided the original author(s) and the copyright owner(s) are credited and that the original publication in this journal is cited, in accordance with accepted academic practice. No use, distribution or reproduction is permitted which does not comply with these terms.
*Correspondence: Guilherme Souza Prado, Z3NwcmFkbzI1QGdtYWlsLmNvbQ==; Alessandra Alves de Souza, ZGVzb3V6YUBjY3NtLmJy
Disclaimer: All claims expressed in this article are solely those of the authors and do not necessarily represent those of their affiliated organizations, or those of the publisher, the editors and the reviewers. Any product that may be evaluated in this article or claim that may be made by its manufacturer is not guaranteed or endorsed by the publisher.
Research integrity at Frontiers
Learn more about the work of our research integrity team to safeguard the quality of each article we publish.