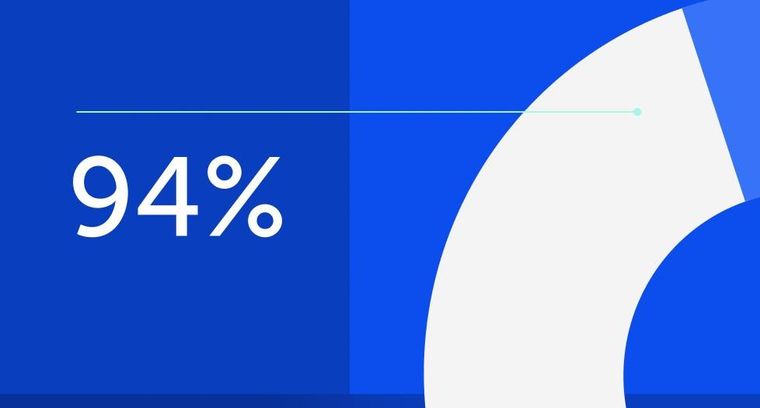
94% of researchers rate our articles as excellent or good
Learn more about the work of our research integrity team to safeguard the quality of each article we publish.
Find out more
ORIGINAL RESEARCH article
Front. Plant Sci., 07 December 2023
Sec. Plant Abiotic Stress
Volume 14 - 2023 | https://doi.org/10.3389/fpls.2023.1301560
This article is part of the Research TopicDeciphering Mechanisms of Plant Adaptation and Resistance Under Cold Temperature StressView all 8 articles
Introduction: With the climate warming, the occurrence of freezing events is projected to increase in late spring and early autumn in the Northern Hemisphere. Observation of morphological traits showed that Cycas panzhihuaensis was more tolerant to unexpected freezing stress than C. bifida. Energy balance is crucial for plant tolerance to stress. Here, we aimed to determine whether the different responses of the two species to the unpredicted freezing stress were associated with the metabolism of energy and related substances.
Methods: The effects of unexpected freezing temperatures on C. panzhihuaensis and C. bifida were studied by measuring chlorophyll fluorescence parameters, energy charge and the profile of nonstructural carbohydrates (NSC) and lipids.
Results: C. panzhihuaensis exhibited higher stability of photosynthetic machinery than C. bifida under unpredicted freezing events. Significant interaction between species and treatments were observed in the energy charge, the level of NSC and its most components and the amount of most lipid categories and lipid classes. The decrease of soluble sugar and the increase of neutral glycerolipids at the early freezing stage, the accumulation of membrane glycerolipids at the late freezing stage and the continuous decrease of energy charge during the freezing period were the characteristics of C. panzhihuaensis responding to unexpected freezing stress. The degradation of membrane glycerolipids and the continuous decrease of soluble sugar during the freezing period and the accumulation of neutral glycerolipids and energy charge at the late freezing stage represented the characteristics of C. bifida responses.
Discussion: The different freezing sensitivity between C. panzhihuaensis and C. bifida might be associated with the differential patterns of the metabolism of energy, NSC and lipids. C. panzhihuaensis possesses the potential to be introduced to the areas of higher latitudes and altitudes.
Freezing stress can decrease membrane fluidity and the freezing-induced extracellular ice crystals can cause dehydration and physical stress, which result in the collapse of cell structure (Webb et al., 1994; Uemura et al., 1995; Barnes et al., 2016). Photosynthesis is one of the physiological processes that is highly sensitive to cold temperatures (Ensminger et al., 2006). It has been reported that freezing stress can cause the rupture of thylakoid membranes where the photosynthetic apparatus are located (Hincha et al., 1987; Goral et al., 2012). Therefore, photoinhibition and photodamage might occur to various extents depending on plant sensitivity to freezing temperatures (Cavender-Bares, 2007). The precise control of energy homeostasis that depends on the coordination of photosynthesis, photorespiration, and respiration is crucial for plant development and tolerance to stress conditions (Dröge-Laser and Weiste, 2018; Shameer et al., 2019). However, low temperatures can induce an imbalance between the light energy absorbed by photosystems and the energy consumed by metabolic sinks (Huner et al., 1996; Ensminger et al., 2006). Correspondingly, the metabolism of carbohydrates and lipids that represent two major forms of energy storage in plants is likely to be regulated by freezing stress. However, how plants regulate the metabolism of energy, carbohydrates, and lipids under freezing stress is poorly understood.
The dynamics of nonstructural carbohydrates (NSCs) including starch and soluble sugars are key determinent of plant growth, productivity, and resistance ability under environmental stress (Dietze et al., 2014; Martínez-Vilalta et al., 2016; Thalmann and Santelia, 2017). Starch is a major energy storage polysaccharide in most plants, and soluble sugars can serve as osmolytes, antioxidants, and signaling molecules (Couée et al., 2006; Stitt and Zeeman, 2012; Vitova et al., 2015; Saddhe et al., 2021). Soluble sugars can decrease the cytoplasmic freezing point and prevent the cellular dehydration induced by the intercellular ice formation (Burg and Ferraris, 2008; Ouellet and Charron, 2013). The starch degradation and accumulation of soluble sugars is involved in the acquisition of freezing tolerance for some plants (Yano et al., 2005; Thalmann and Santelia, 2017). Zareei et al. (2021) showed that the dynamic change of soluble sugars with the decrease of freezing temperatures (−5°C to −25°C) was dependent on cultivars and plant organs. The carbohydrate concentration of Pinus brutia also dynamically changed with the decrease of freezing temperatures (−5°C to −20°C), which was related to the provenances and growth stages (Yildiz et al., 2014).
Lipids are used not only as energy sources but also as principal constituents of biological membranes and signaling molecules in plants (Hou et al., 2016; Yang and Benning, 2018; Chng et al., 2022). Membrane is the major site of damage induced by low temperatures in plants (Hincha et al., 1987). For some plants, freezing stress can lead to the degradation of the membrane lipids including phospholipids and saccharolipids (Zheng et al., 2016). However, some other plants can remodel the membrane lipids to survive the low temperatures (Thomashow, 1999). Studies have shown that the levels of plastidic and extraplastidic membrane lipids and the unsaturation of these lipids can increase under chilling and freezing conditions to maintain the integrity and fluidity of membranes (Welti et al., 2002; Zheng et al., 2021). In general, the accumulation of the bilayer-stabilizing lipids such as phosphatidylcholine (PC), digalactosyldiacylglycerol (DGDG), and oligogalactolipids is conducive to the acquirement of freezing tolerance (Moellering et al., 2010; Zhang et al., 2013; Arisz et al., 2018).
For temperate plants, many species can acquire freezing tolerance through a period of cold acclimation at low but non-freezing temperatures (Chang et al., 2021). In addition, subsequent exposure to moderate freezing temperatures can further enhance freezing tolerance (Junttila and Kaurin, 1990; Herman et al., 2006). However, due to the lengthening of the growing season of plants induced by climate warming, the freezing events occurring in early autumn and late spring are likely to increase in some areas of the Northern Hemisphere (Liu et al., 2018; Zohner et al., 2020). The unpredicted freezing events might have devastating effects on the growth and survival for the plants unprepared for the changing environments (Seydel et al., 2022). For the plants distributed in the tropical and subtropical areas, some of them are vulnerable to low temperatures above 0°C, but some species can be introduced to the higher latitude areas and survive the freezing temperatures there (Huang, 2011). However, very little is known about the response of tropical and subtropical plants to unexpected freezing stress.
Cycas species possess high ornamental and scientific research values, which are mainly distributed in tropical and subtropical areas (Hoffmann et al., 2010). Among these species, C. panzhihuaensis is limited to the dry-hot valleys of the Jinsha River in southwest China where the northern latitudinal limits of Cycas species are observed. The population of this species in Panzhihua National Nature Reserve is well conserved, but other wild populations are very small (Yu et al., 2007). C. bifida is restricted to some areas of Yunnan and Guangxi provinces, with extremely small populations (Yin, 2013). Despite the different distribution, both the species can experience more than 40°C in their habitats. Studies have shown that the continuing climate warming will pose higher threat to tropical species than the species distributed in the areas of higher latitude (Doughty and Goulden, 2008; Sentinella et al., 2020). Considering the narrow geographic distributions and occurrence of extreme high temperatures, Cycas species might be introduced into areas with lower temperatures to avoid the potential risk of endangerment. However, how these plants including C. panzhihuaensis and C. bifida respond to the unpredicted freezing stress is poorly understood. On the basis of the observation of morphological traits, C. panzhihuaensis was more tolerant to unexpected freezing shock than C. bifida. We speculated that this difference between C. panzhihuaensis and C. bifida might be related to the metabolism of energy, NSC, and lipids. To explore the effects of unexpected freezing stress on the metabolism of energy and related substances and provide theoretical basis for the introduction, the energy status, the photosynthetic activity, NSC concentration, and lipid profiles were determined in the two species.
Six-year-old plants of C. panzhihuaensis and C. bifida were cultivated in a 1:1:1 (v/v/v) mixture of sand, humus, and laterite soil in a greenhouse where the temperature and light intensity cannot be automatically controlled. The temperature was about 15°C to 25°C, and the daytime maximum photosynthetic photon flux density was approximately 600 μmol m−2 s−1 during the period that we conducted the experiment. The different intensity of freezing stress is achieved by different subzero temperatures with the same treatment duration or achieved by the same subzero temperature with different treatment durations (Lu et al., 2019; Zareei et al., 2021). The temperature and duration of freezing stress were chosen on the basis of our preliminary study. The application of freezing temperature was conducted referring to the procedure described by Arisz et al. (2018) with several modifications. Freezing treatment was conducted in the light (300 μmol m−2 s−1) by lowering the temperature from 4°C to −1°C at a rate of 2°C h−1. After 1 h at −1°C, ice crystals were sprinkled to induce freezing and prevent supercooling. Temperature was then decreased at the same rate to −5°C and maintained for 1.5 h (F1) and 6 h (F2), respectively. Plants were sampled immediately after freezing treatment for measurement of some metabolites. Plants that were not treated at freezing temperatures were used as the controls. To evaluate the freezing tolerance, plants previously subjected to F1 and F2 treatments were transferred to 4°C and thawed for 12 h in the dark and then placed back to the control conditions for 3 days. The chlorophyll fluorescence parameters were measured for both the thawed and recovered plants, and morphological traits of leaves were observed for the recovered plants.
The plants that were subjected to freezing-thawing stress and subsequent 3 days of recovery were used to measure chlorophyll fluorescence parameters. Measurements were taken after a 30-min dark adaptation of plants at room temperature. Chlorophyll fluorescence was measured in leaves of five plants per treatment with a PAM-2500 portable chlorophyll fluorometer (Walz, Germany). The saturating light pulse was set at about 8,000 µmol m–2 s–1 for 0.8 s. The actinic light was set at 617 µmol m–2 s–1, and the plants were adapted to such actinic light for more than 5 min. All the chlorophyll fluorescence parameters were given automatically by the instrument.
After being frozen in liquid nitrogen, leaf sample was ground and mixed with cold 1:2:2 (v/v/v) H2O/methanol/acetonitrile. The mixture was centrifuged at 4°C for 20 min (14,000g) and dried in a vacuum centrifuge. The samples were dissolved in 1:1 (v/v) acetonitrile/water and centrifuged again at 4°C for 15 min. The supernatants were used for determination of adenosine monophosphate (AMP), adenosine diphosphate (ADP), and adenosine triphosphate (ATP) by liquid chromatography tandem mass spectrometry (LC-MS/MS) analysis using an ultra high performance liquid chromatography (UHPLC) (1290 Infinity LC, Agilent Technologies) coupled to a QTRAP (AB Sciex 5500). Multiquant software was used for data acquisition and processing. Energy charge (EC) was calculated as follows: EC = (ATP + 0.5 × ADP)/(ATP + ADP + AMP).
The concentration of soluble sugars was measured using the protocols described by Salvador et al. (2000) and Wang et al. (2018). Briefly, following the hydrolysis in trifluoroacetic acid (2 M), the leaf sample was dried with nitrogen and then washed two to three times with methanol. Thereafter, the residue was dissolved in deionized water and filtered for measurement. The soluble sugar composition and content of the sample extracts were determined by high-performance anion-exchange chromatography. The starch content was measured using a starch assay kit (Comin, China). The concentration of soluble sugars was calculated by summing the concentrations of each composition of soluble sugars. The concentration of NSC was calculated by summing the concentrations of soluble sugars and starch.
The protocols of lipid extraction and detection were described by Zhu et al. (2019). The freeze-dried samples were ground, mixed with 200 µL of cold water and 800 µL of cold methyl tert-butyl ether, and vortexed for 30 s. Thereafter, the mixture was added to 240 µL of methanol, sonicated at 4°C for 20 min and stand for 30 min, and then centrifuged at 14,000g for 15 min at 10°C to extract lipids. The upper organic layer was dried in a vacuum centrifuge. Lipid extracts were resuspended in 200 µL of isopropanol acetonitrile 9:1 (v/v) before LC-MS analysis. For untargeted lipidomics analysis, lipids were separated on a Waters ACQUITY PREMIER CSH C18 Column (1.7 µm, 2.1 mm × 100 mm). MS detection was performed using a Thermo Scientific™ Q Exactive mass spectrometer, equipped with an electrospray ionization (ESI) ion source. Lipidsearch 4.0 software was used for peak detection and annotation of lipids or internal standards. The main parameters are as follows: precursor tolerance, 5 ppm; product tolerance, 5 ppm; and product ion threshold, 5%.
Five replicates for each measurement were arranged for each treatment. For measurement of chlorophyll fluorescence, three leaves from each plant were measured, and the average of these values represented the respective plant. For analysis of lipidomics, the amount of NSCs and energy substances, sample collection was performed from five plants for each replicate. All statistical analyses were performed with SPSS 15.0 (IBM Corp., Armonk, NY). To analyze the significance (P ≤ 0.05) of species, treatment and their interactive effects, the data were assessed using two-way analysis of variance (ANOVA). To evaluate the significance of difference among treatments within one species, the data were assessed using one-way ANOVA. To evaluate the significance of difference between species within one treatment, the data were evaluated by the independent sample T-test.
For C. panzhihuaensis, all the chlorophyll fluorescence parameters except the non-regulated energy dissipation of photosystem II (PSII)–[Y(NO)] maintained unchanged immediately following freezing stress (Table 1). Y(NO) of F2-treated plants increased significantly compared to that of the control but recovered to the control level after 3 days of recovery. For C. bifida, compared to those of the non-treated plants, the potential quantum yield of PSII (Fv/Fm), effective quantum yield of PSII [Y(II)], photochemical quenching coefficient (qP), and the relative electron transport rate (rETR) declined by 4.7%, 41.3%, 46.8%, and 42.0%, respectively, after F1 treatment and decreased by 22.8%, 83.7%, 84.9%, and 84.7%, respectively, after F2 treatment. Following 3 days of recovery, Fv/Fm, Y(II), qP, and rETR increased significantly in F1-treated plants but decreased significantly in F2-treated plants in comparison with those of plants sampled immediately from F1 and F2 treatments, respectively. Compared to the control, the photoprotective regulated energy dissipation of PSII [Y(NPQ)] increased significantly after F1 treatment but decreased significantly after F2 treatment. After 3 days of recovery, Y(NPQ) maintained in F1-treated plants and further decreased significantly in F2-treated plants. Compared to that of the control, Y(NO) increased significantly following F1 and F2 treatments, by 22.1% and 216.8%, respectively. This parameter recovered to the control level in F1-treated plants but continued to increase significantly in F2-treated plants following recovery. Compared to that of the control, the non-photochemical quenching coefficient (NPQ) remained unchanged in F1-treated plants but decreased significantly by 96.4% in F2-treated plants. Compared to that of the corresponding freezing-treated plants, NPQ increased significantly in F1-treated plants and did not change significantly in F2-treated plants after recovery. Significant interaction was observed between species and treatment on all the chlorophyll fluorescence parameters. These results showed that photosynthetic machinery of C. panzhihuaensis was more stable than that of C. bifida under unexpected freezing stress. After 3 days of recovery, the leaf morphological traits did not change visibly for all the freezing-treated C. panzhihuaensis and F1-treated C. bifida (Supplementary Figure S1). However, the leaves of F2-treated C. bifida plants turned yellow and curled up after recovery.
Table 1 The chlorophyll fluorescence parameters of Cycas panzhihuaensis and C. bifida exposed to unexpected freezing stress and subsequent 3 days of recovery.
Compared to those of the control, the relative amount of AMP increased significantly but that of ADP and ATP decreased significantly in F2-treated C. panzhihuaensis (Figure 1). The energy charge of C. panzhihuaensis significantly declined with the duration of freezing treatment. For C. bifida, AMP, ADP, and ATP presented different change trend during freezing treatment. When compared to those in the control, the relative amount of AMP increased significantly in F1-treated plants and decreased significantly in F2-treated plants; ADP significantly increased in F2-treated plants, and ATP significantly declined in F1-treated plants. The energy charge remained unchanged in F1-treated plants but increased significantly in F2-treated plants. Significant interaction was observed between species and treatment on the relative amounts of AMP, ADP, ATP, and energy charge.
Figure 1 The relative level of adenosine monophosphate (AMP), adenosine diphosphate (ADP), adenosine triphosphate (ATP) and energy charge of Cycas panzhihuaensis and C. bifida exposed to unexpected freezing stress. Two-way ANOVA analysis was performed in the general linear model. * indicates P ≤ 0.05. ns indicates not significant. For the same species, different letters in the same column are significantly different among treatments according to One-way ANOVA at P ≤ 0.05. For the same treatment, * is significantly different between species according to independent samples T-test at P ≤ 0.05. Values shown are the mean ± SD, n = 5. F1, freezing treatment at -5 °C for 1.5 h; F2, freezing treatment at -5 °C for 6 h.
For C. panzhihuaensis, compared to that of the corresponding control, the amount of glucose, fructose, sucrose, raffinose, and the total soluble sugars decreased significantly following F1 treatment (Figure 2). F2 treatment induced the significant decrease of galactose, glucose, and fructose and significant increase of sucrose and raffinose but did not change the total level of soluble sugars. Starch amount remained unchanged following both F1 and F2 treatments. NSC level significantly declined, by 19.6% in F1-treated plants and then increased to the original level in F2-treated plants. For C. bifida, the amount of glucose, fructose, sucrose, and the total soluble sugars decreased significantly, and raffinose level significantly increased in F1-treated plants, compared to the control. However, the level of glucose increased significantly and that of fructose, sucrose, raffinose, and the total soluble sugars decreased significantly following F2 treatment, compared to the control. During the freezing period, the starch amount significantly declined, by 23.0% in the early stage and then recovered to the control plant level in the late stage. Compared to that of the non-treated plants, the NSC amount decreased significantly in F1-treated and F2-treated plants, by 16.4% and 27.1%, respectively. Significant interaction was observed between species and treatment on the levels of all the components of NSC except fructose.
Figure 2 The content of the nonstructural carbohydrates of Cycas panzhihuaensis and C. bifida exposed to unexpected freezing stress. Two-way ANOVA analysis was performed in the general linear model. * indicates P ≤ 0.05. ns indicates not significant. For the same species, different letters in the same column are significantly different among treatments according to One-way ANOVA at P ≤ 0.05. For the same treatment, * is significantly different between species according to independent samples T-test at P ≤ 0.05. Values shown are the mean ± SD, n = 5. F1, freezing treatment at -5 °C for 1.5 h; F2, freezing treatment at -5 °C for 6 h.
For C. panzhihuaensis, when compared to that of the non-treated plants, the amount of the total lipid increased with the duration of freezing, by 20.0% and 59.2%, respectively, after F1 and F2 treatments (Figure 3). For C. bifida, the total lipid level decreased significantly, by 33.80% in F1-treated plants, but then increased to the control level in F2-treated plants. Significant interaction was observed between species and treatment on the amount of the total lipids.
Figure 3 The content of the total lipids of Cycas panzhihuaensis and C. bifida exposed to unexpected freezing stress. Two-way ANOVA analysis was performed in the general linear model. * indicates P ≤ 0.05. ns indicates not significant. For the same species, different letters in the same column are significantly different among treatments according to One-way ANOVA at P ≤ 0.05. For the same treatment, * is significantly different between species according to independent samples T-test at P ≤ 0.05. Values shown are the mean ± SD, n = 5. F1, freezing treatment at -5 °C for 1.5 h; F2, freezing treatment at -5 °C for 6 h.
For C. panzhihuaensis, the amount of DAG and the total neutral glycerolipids increased by 45.3% and 41.70%, respectively, after F1 treatment, which then recovered to the control level after F2 treatment (Figure 4). Freezing stress did not induce significant change of the TAG amount. For C. bifida, compared to those of the non-treated plants, the amounts of DAG, TAG, and the total neutral glycerolipids remained unchanged in F1-treated plants but increased significantly in F2-treated plants, by 161.0%, 114.9%, and 158.4%, respectively. Significant interaction was observed between species and treatment on the amounts of DAG, TAG, and the total neutral glycerolipids.
Figure 4 The content of the neutral glycerolipids of Cycas panzhihuaensis and C. bifida exposed to unexpected freezing stress. Two-way ANOVA analysis was performed in the general linear model. * indicates P ≤ 0.05. ns indicates not significant. For the same species, different letters in the same column are significantly different among treatments according to One-way ANOVA at P ≤ 0.05. For the same treatment, * is significantly different between species according to independent samples T-test at P ≤ 0.05. Values shown are the mean ± SD, n = 5. DAG, diacylglycerol; TAG, triacylglycerol; F1, freezing treatment at -5 °C for 1.5 h; F2, freezing treatment at -5 °C for 6 h.
For C. panzhihuaensis, compared to those of the control, phosphatidylinositol (PI) phosphate (PIP) and cardiolipin (CL) increased significantly in F1-treated plants, and the total phospholipids and all the phospholipid classes except phosphatidylethanolamine (PE) and phosphatidylglycerol (PG) increased significantly in amount after F2 treatment (Figure 5). For C. bifida, the levels of phosphatidic acid (PA), PC, PE, phosphatidylserine (PS), CL, and the total phospholipids decreased significantly following both F1 and F2 treatments. Compared to that of the control, PG amount remained unchanged after F1 treatment and decreased significantly after F2 treatment and the amount of PI and PIP decreased significantly after F1 treatment and increased up to the control level after F2 treatment. Significant interaction was observed between species and treatment on the amounts of each component of phospholipids and the total phospholipids.
Figure 5 The content of the phospholipids of Cycas panzhihuaensis and C. bifida exposed to unexpected freezing stress. Two-way ANOVA analysis was performed in the general linear model. * indicates P ≤ 0.05. ns indicates not significant. For the same species, different letters in the same column are significantly different among treatments according to One-way ANOVA at P ≤ 0.05. For the same treatment, * is significantly different between species according to independent samples T-test at P ≤ 0.05. Values shown are the mean ± SD, n = 5. F1, freezing treatment at -5 °C for 1.5 h; F2, freezing treatment at -5 °C for 6 h; PA, phosphatidic acid; PC, phosphatidylcholine; PE, phosphatidylethanolamine; PG, phosphatidylglycerol; PI, phosphatidylinositol; PIP, phosphatidylinositol phosphate; PS, phosphatidylserine; CL, cardiolipin.
For C. panzhihuaensis, F2 treatment induced the significant decline of lysophosphatidylcholine (LPC) amount and significant increase of lysophosphatidylethanolamine (LPE) amount (Figure 6). For C. bifida, LPC amount significantly increased in F1-treated plants and LPE significantly increased in F2-treated plants. The amounts of lysophosphatidylglycerol (LPG) and the total lysophospholipids remained unchanged following both F1 and F2 treatments in the two species. Significant interaction was observed between species and treatment on the levels of LPC and LPE.
Figure 6 The content of the lysophospholipids of Cycas panzhihuaensis and C. bifida exposed to unexpected freezing stress. Two-way ANOVA analysis was performed in the general linear model. * indicates P ≤ 0.05. ns indicates not significant. For the same species, different letters in the same column are significantly different among treatments according to One-way ANOVA at P ≤ 0.05. For the same treatment, * is significantly different between species according to independent samples T-test at P ≤ 0.05. Values shown are the mean ± SD, n = 5. F1, freezing treatment at -5 °C for 1.5 h; F2, freezing treatment at -5 °C for 6 h; LPC, lysophosphatidylcholine; LPE, lysophosphatidylethanolamine; LPG, lysophosphatidylglycerol.
Freezing stress did not change the amounts of monogalactosyldiacylglycerol (MGDG) and DGDG of C. panzhihuaensis (Figure 7). Compared to those in the control group, the levels of monogalactosylmonoacylglycerol (MGMG) and sulphoquinovosyldiacylglycerol (SQDG) and the total saccharolipids remained unchanged in F1-treated plants but significantly increased in F2-treated plants. For C. bifida, the level of the total saccharolipids and its each lipid class decreased significantly following both F1 and F2 treatments. Significant interaction was observed between species and treatment on the level of MGMG, DGDG, and SQDG and the total saccharolipids.
Figure 7 The content of the saccharolipids of Cycas panzhihuaensis and C. bifida exposed to unexpected freezing stress. Two-way ANOVA analysis was performed in the general linear model. * indicates P ≤ 0.05. ns indicates not significant. For the same species, different letters in the same column are significantly different among treatments according to One-way ANOVA at P ≤ 0.05. For the same treatment, * is significantly different between species according to independent samples T-test at P ≤ 0.05. Values shown are the mean ± SD, n = 5. DGDG, digalactosyldiacylglycerol; F1, freezing treatment at -5 °C for 1.5 h; F2, freezing treatment at -5 °C for 6 h; MGDG, monogalactosyldiacylglycerol; MGMG, monogalactosylmonoacylglycerol; SQDG, sulphoquinovosyldiacylglycerol.
The amount of sphingolipids, sterol lipids, and prenol lipids remained unchanged in the freezing-treated C. panzhihuaensis (Figure 8). For C. bifida, the level of prenol lipids did not change but that of both sphingolipids and sterol lipids significantly declined in F1-treated plants but increased to the control level in F2-treated plants. Significant interaction was observed between species and treatment on the amounts of sphingolipids and sterol lipids.
Figure 8 The content of sphingolipids, sterol lipids and prenol lipids of Cycas panzhihuaensis and C. bifida exposed to unexpected freezing stress. Two-way ANOVA analysis was performed in the general linear model. * indicates P ≤ 0.05. ns indicates not significant. For the same species, different letters in the same column are significantly different among treatments according to One-way ANOVA at P ≤ 0.05. For the same treatment, * is significantly different between species according to independent samples T-test at P ≤ 0.05. Values shown are the mean ± SD, n = 5. F1, freezing treatment at -5 °C for 1.5 h; F, freezing treatment at -5 °C for 6 h.
The maximum freezing tolerance of plants can be acquired by cold acclimation followed by freezing temperatures (Junttila and Kaurin, 1990; Herman et al., 2006). Some studies have investigated the effects of freezing temperatures following cold acclimation on the metabolic process of plants including carbohydrates, enzyme activity, amino acid, and lipids (Tan et al., 2018; Zareei et al., 2021) and found the metabolic adjustment of plants. However, how plants respond to unexpected freezing temperatures is not well understood.
Plants acquire carbon and energy through photosynthesis, which, however, is extremely sensitive to various stresses (Gao et al., 2020). Chlorophyll fluorescence is generally used to evaluate the photosynthetic activity under environmental stress (Hanachi et al., 2014; Kalaji et al., 2016). The maintenance and recovery of all the chlorophyll fluorescence parameters of C. panzhihuaensis (Table 1) suggest that the photosynthetic machinery of this species was stable when exposed to unpredicted freezing stress. This was consistent with the maintenance of the leaf morphological traits following recovery (Supplementary Figure S1). The dramatic decline of Fv/Fm, Y(II), qP, and rETR shows that the process of light reaction of photosynthesis in C. bifida was inhibited by freezing stress, being more severely with the extension of freezing time. Considering the partial or complete recovery of Fv/Fm, Y(II), qP, and rETR in F1-treated plants following 3 days of recovery, the photoinhibition induced by F1 treatment can be reversible. However, the further decrease of Fv/Fm, Y(II), qP, rETR and Y(NPQ) and the increase of Y(NO) in F2-treated plants after recovery suggest that C. bifida could not avoid photodamage of PSII induced by excessive light energy. On the basis of the yellowing of leaves in the recovered plants (Supplementary Figure S1), the photosynthetic disorders of F2-treated C. bifida were likely due to the injury of the photosynthetic apparatus that are related to overproduction of reactive oxygen species (ROS) in chloroplasts (Nishiyama et al., 2001). Low temperature has an immediate effect on enzymatic activities, and the chemical reaction rates are reduced by a factor of 2–3 per 10°C according to the van’t Hoff rule (Reyes et al., 2008; Seydel et al., 2022). Therefore, the reduced photosynthetic capacity might be also related to the freezing-induced lowered activities of enzymes involved in CO2 assimilation. Taken together, the photosynthetic machinery of C. panzhihuaensis exhibited higher tolerance to unpredicted freezing stress compared to C. bifida. Therefore, the unexpected freezing event will impose more negative effects on plant growth and survival of C. bifida. This demonstrates that C. panzhihuaensis possesses the potential to be introduced to the areas of higher latitudes and altitudes. However, the degree of its freezing tolerance needs to be ascertained.
Low temperatures can potentially disturb the energy balance through inhibiting photochemical processes and decreasing the rates of the enzymatic reactions (Huner et al., 1998; Ensminger et al., 2006). Energy charge is generally used to reflect the status of energy metabolism (Wang T. et al., 2020). The different change trend of energy charge between the two species (Figure 1) suggest that the two species adopted different strategies of energy metabolism under unexpected freezing stress that reflected the different cellular process and stress response. The respiration capacity of the alternative pathway will increase under adverse conditions to avoid the photodamage of photosystems (Zhang et al., 2012). Considering the stability of photosynthetic machinery in C. panzhihuaensis, the decrease of energy charge with the prolongation of freezing treatment might be due to the increased utilization of energy in the stress defense or/and the enhanced photoprotection by mitochondrial alternative pathway. Considering the recovery of starch level and the accumulation of neutral glycerolipids following F2 treatment in C. bifida (Figures 2, 4), the significant increase of energy charge might imply that the energy accumulated substantially in leaves. Niu et al. (2021) reported that the degree of postharvest yellowing of the tobacco leaves had a significant positive correlation with energy charge and the tobacco variety that is more prone to postharvest yellowing and browning maintained higher level of energy charge. However, the causal relationship between energy status and leaf damage and senescence under various environmental conditions needs to be explored. As only the leaves of F2-treated C. bifida cannot recover and ultimately died, whether the accumulation of energy charge can be used as a biomarker of freezing-induced severe damage should be further confirmed.
Carbohydrates are direct products of photosynthetic CO2 assimilation, and the tight regulation of carbohydrate and photosynthesis metabolism is needed to stabilize photosynthetic efficiency and energy metabolism (Seydel et al., 2022; Kitashova et al., 2023). The accumulation of soluble sugars is one of adaptive mechanisms adopted by some plants to cope with low temperatures (Yano et al., 2005; Thalmann and Santelia, 2017). However, the level of soluble sugars do not change or even decrease for some other plants subjected to low temperatures (Yildiz et al., 2014; Jiang et al., 2016; Ding et al., 2023). The research by Yildiz et al. (2014) and Zareei et al. (2021) suggest that the carbohydrate amount of some plant species/cultivars presents dynamic change with the increase of severity of freezing stress. Studies have shown that sugar phosphates accumulate at low temperatures, which can regulate carbon distribution between structural compounds, starch, and biosynthesis of soluble intermediates (Gray and Heath, 2005; Guy et al., 2008; Domon et al., 2013). For C. panzhihuaensis, considering the stability of photosynthetic machinery, significant decrease of the total soluble sugar level following F1 treatment (Figure 2) might be an defense response to cope with the sudden decrease of temperatures, and more soluble sugars might be converted to some other protective substances or structures. The stems of Cycas species are rich in starch, and their fleshy roots are rich in proteins, lipids, and carbohydrates (Lu, 2006). Whether the accumulated soluble sugars in F2-treated plants in comparison with that of the F1-treated plants were transferred from the fleshy roots and/or stems of C. panzhihuaensis needs to be verified. Although the soluble sugars recovered to the control level, sugar remodeling occurred in F2-treated plants of C. panzhihuaensis in comparison with the control group. Studies show that the contributing sugar in the freezing tolerance depends on plant species (Knaupp et al., 2011; Taïbi et al., 2018). Both sucrose and raffinose are non-reducing sugars, which can stabilize membranes (Strauss and Hauser, 1986; Nägele and Heyer, 2013). The significant accumulation of sucrose and raffinose might be conducive to the acquirement of tolerance to freezing stress in F2-treated plants of C. panzhihuaensis.
For C. bifida, the continuous decrease of soluble sugar level with the extension of freezing time was likely to be related to the increasing photoinhibition. The regulation of starch turnover is regulated by the rate of starch synthesis and degradation (Thalmann et al., 2016; Fernandez et al., 2017). Under adverse environmental conditions, starch is generally degraded to supply soluble sugars, energy, and other derived metabolites, which can mitigate the effects of stress (Thalmann and Santelia, 2017). The decreased starch level of C. bifida after F1 treatment might be due to the declined synthesis rate and/or enhanced degradation. However, the mechanism of the recovery of starch to the control level again after F2 treatment is not understood. It might be associated with a decreased sink capacity for photosynthate and relatively more soluble sugars converted to starch. In addition, cell wall polysaccharides can decrease in amount under low temperatures, and they can maintain energy homeostasis during senescence of green leaves serving as carbon source (Bilska-Kos et al., 2017; Biswal and Pandey, 2018). However, whether the recovery of starch in F2-treated C. bifida is related to the adjustment of cell wall polysaccharides is not sure. The continuous decline of NSC during freezing period might be an indicator of freezing sensitivity in Cycas species, which should be further confirmed.
Carbohydrates and lipids can interact in the modulation of carbon and energy homeostasis (Yu et al., 2018; Huang et al., 2019). Some studies suggest that more carbon flow is driven to lipid synthesis under some environmental conditions (Sun et al., 2016; Rai et al., 2017). As the amount of other lipid categories maintained unchanged, whether the significant accumulation of DAG in the F1-treated plants of C. panzhihuaensis (Figure 4) was associated with the depletion of the soluble sugars is not clear. DAG is an important intermediate in metabolism of both TAG and membrane lipids (Sergeeva et al., 2021). For C. panzhihuaensis, the transient accumulation of DAG at the early freezing stage (F1) might tend to provide signaling molecules, energy, and carbon, which enable plants to respond to subsequent stress rapidly. TAG is often accumulated in plants that are subjected to challenging environmental conditions (Gasulla et al., 2013; Higashi et al., 2015). For C. bifida, the levels of both DAG and TAG increased significantly after F2 treatment. In addition to the enhanced de novo synthesis, the metabolism of preexisting membrane lipids contributes to DAG formation and then TAG accumulation under various stress (Sun et al., 2016; Mueller et al., 2017; Rai et al., 2017; Young et al., 2022). The accumulated DAG and TAG after F2 treatment might be derived from the soluble sugars and/or membrane lipids that reduced concomitantly.
The membrane damage induced by stresses is generally accompanied with the degradation of membrane lipids (Zheng and Li, 2017; Wang Y. et al., 2020). Saccharolipids and PG are crucial for the maintenance of the photosynthetic machinery (Sakurai et al., 2003; Kobayashi et al., 2013). For C. bifida, the degradation of saccharolipids and phospholipids (Figures 5, 7) following freezing treatments might be a key factor leading to photoinhibition. The origin of the accumulated membrane lipids in F2-treated C. panzhihuaensis was not determined. However, the accumulation of membrane glycerolipids might be conducive to stabilizing both the plastidic and extraplastidic membranes of C. panzhihuaensis under prolonged freezing temperatures.
Sphingolipids and sterol lipids can preferentially interact with each other and segregate to form microdomains that dubbed the membrane raft (Simons and Toomre, 2000). The levels and composition of sterols and sphingolipids can affect membrane stability, the activity of membrane proteins, and signal transduction processes (Lynch and Dunn, 2004; Sharfman et al., 2014; Yu et al., 2021). Considering the strong freezing tolerance of C. panzhihuaensis, the maintenance of the levels of sphingolipids and sterol lipids (Figure 8) might be associated with the membrane stability. The consistent change of sterol lipids and sphingolipids might reflect the dynamic change of membrane rafts during the freezing treatment in C. bifida. However, the specific roles of these changes are not clear and need to be further explored.
With the prolongation of freeing time, the amounts of most categories of the membrane lipids presented different change trend between C. panzhihuaensis and C. bifida, which might be related to their differential tolerance to unpredicted freezing stress.
The photosynthetic machinery of C. panzhihuaensis was more stable under the freezing stress in comparison with that of C. bifida. The energy charge showed a different change trend with the extension of freezing treatment time between C. panzhihuaensis and C. bifida. Moreover, with the prolongation of freezing time, the most components of NSC and most lipid categories and lipid classes changed differently in amount between the two species. These results suggest that the different freezing tolerance between the two species might be related to the differential patterns of the metabolism of energy and related substances. The continuous decrease of soluble sugars, the degradation of membrane glycerolipids, and the increment of neutral glycerolipids and energy charge might be used as predictors of freezing sensitivity in Cycas species, which should be further confirmed. The results show that C. panzhihuaensis can well cope with the unexpected freezing temperatures and possesses the potential to be introduced to the areas of higher latitudes and altitudes. However, several questions still remain to be answered, so further work will be undertaken to explore the dynamic change of physiological processes including photosynthesis, respiration, and photorespiration; the cooperation of different organs in carbon metabolism; and the molecular mechanisms underlying the changes of energy and related substances under unexpected freezing stress.
The original contributions presented in the study are included in the article/Supplementary Material. Further inquiries can be directed to the corresponding author.
YW: Data curation, Formal analysis, Writing – review & editing. HZ: Data curation, Formal analysis, Writing – review & editing. ZL: Formal analysis, Writing – review & editing. EL: Formal analysis, Writing – review & editing. XP: Writing – review & editing. YZ: Formal analysis, Funding acquisition, Writing – original draft, Conceptualization.
The author(s) declare financial support was received for the research, authorship, and/or publication of this article. This work was supported by the National Natural Science Foundation of China (32060094, 31560093).
We acknowledge Shuangzhi Li for identification of C. panzhihuaensis and C. bifida.
The authors declare that the research was conducted in the absence of any commercial or financial relationships that could be construed as a potential conflict of interest.
All claims expressed in this article are solely those of the authors and do not necessarily represent those of their affiliated organizations, or those of the publisher, the editors and the reviewers. Any product that may be evaluated in this article, or claim that may be made by its manufacturer, is not guaranteed or endorsed by the publisher.
The Supplementary Material for this article can be found online at: https://www.frontiersin.org/articles/10.3389/fpls.2023.1301560/full#supplementary-material
DAG, diacylglycerol; DGDG, digalactosyldiacylglycerol; Fv/Fm, the maximum quantum yield of photosystem II (PSII); MGDG, monogalactosyldiacylglycerol; MGMG, monogalactosylmonoacylglycerol; NPQ, non-photochemical quenching coefficient; PA, phosphatidic acid; PC, phosphatidylcholine; PE, phosphatidylethanolamine; PG, phosphatidylglycerol; PI, phosphatidylinositol; PIP, phosphatidylinositol phosphate; PS, phosphatidylserine; qP, photochemical quenching coefficient; rETR, relative electron transport rate; SQDG, sulphoquinovosyldiacylglycerol; TAG, triacylglycerol; Y(II), effective quantum yield of PS II; Y(NO), non-regulated non-photochemical energy loss in PS II; Y(NPQ), regulated non-photochemical energy loss in PS II.
Arisz, S. A., Heo, J.-Y., Koevoets, I. T., Zhao, T., Van Egmond, P., Meyer, A. J., et al. (2018). DIACYLGLYCEROL ACYLTRANSFERASE1 contributes to freezing tolerance. Plant Physiol. 177, 1410–1424. doi: 10.1104/pp.18.00503
Barnes, A. C., Benning, C., Roston, R. L. (2016). Chloroplast membrane remodeling during freezing stress is accompanied by cytoplasmic acidification activating SENSITIVE TO FREEZING2. Plant Physiol. 171, 2140–2149. doi: 10.1104/pp.16.00286
Bilska-Kos, A., Solecka, D., Dziewulska, A., Ochodzki, P., Jończyk, M., Bilski, H., et al. (2017). Low temperature caused modifications in the arrangement of cell wall pectins due to changes of osmotic potential of cells of maize leaves (Zea mays L.). Protoplasma 254, 713–724. doi: 10.1007/s00709-016-0982-y
Biswal, B., Pandey, J. K. (2018). Loss of photosynthesis signals a metabolic reprogramming to sustain sugar homeostasis during senescence of green leaves: Role of cell wall hydrolases. Photosynthetica 56, 404–410. doi: 10.1007/s11099-018-0784-x
Burg, M. B., Ferraris, J. D. (2008). Intracellular organic osmolytes: function and regulation. J. Biol. Chem. 283, 7309–7313. doi: 10.1074/jbc.R700042200
Cavender-Bares, J. (2007). Chilling and freezing stress in live oaks (Quercus section Virentes): intra- and inter-specific variation in PS II sensitivity corresponds to latitude of origin. Photosynth. Res. 94, 437. doi: 10.1007/s11120-007-9215-8
Chang, C. Y., Bräutigam, K., Hüner, N. P. A., Ensminger, I. (2021). Champions of winter survival: cold acclimation and molecular regulation of cold hardiness in evergreen conifers. New Phytol. 229, 675–691. doi: 10.1111/nph.16904
Chng, C., Wang, K., Ma, W., Hsia, K. J., Huang, C. (2022). Chloroplast membrane lipid remodeling protects against dehydration by limiting membrane fusion and distortion. Plant Physiol. 188, 526–539. doi: 10.1093/plphys/kiab512
Couée, I., Sulmon, C., Gouesbet, G., El Amrani, A. (2006). Involvement of soluble sugars in reactive oxygen species balance and responses to oxidative stress in plants. J. Exp. Bot. 57, 449–459. doi: 10.1093/jxb/erj027
Dietze, M. C., Sala, A., Carbone, M. S., Czimczik, C. I., Mantooth, J. A., Richardson, A. D., et al. (2014). Nonstructural carbon in woody plants. Annu. Rev. Plant Biol. 65, 667–687. doi: 10.1146/annurev-arplant-050213-040054
Ding, Y., Wang, X., Wang, F., Shao, Y., Zhang, A., Chang, W. (2023). The effects of chilling stress on antioxidant enzymes activities and proline, malondialdehyde, soluble sugar contents in three Paphiopedilum species. Russian J. Plant Physiol. 70, 61. doi: 10.1134/S1021443722603184
Domon, J. M., Baldwin, L., Acket, S., Caudeville, E., Arnoult, S., Zub, H., et al. (2013). Cell wall compositional modifications of Miscanthus ecotypes in response to cold acclimation. Phytochemistry 85, 51–61. doi: 10.1016/j.phytochem.2012.09.001
Doughty, C. E., Goulden, M. L. (2008). Are tropical forests near a high temperature threshold? J. Geophysical Res. 113, G00B07. doi: 10.1029/2007JG000632
Dröge-Laser, W., Weiste, C. (2018). The C/S1 bZIP network: A regulatory hub orchestrating plant energy homeostasis. Trends Plant Sci. 23, 422–433. doi: 10.1016/j.tplants.2018.02.003
Ensminger, I., Busch, F., Huner, N. P. A. (2006). Photostasis and cold acclimation: sensing low temperature through photosynthesis. Physiol. Plant 126, 28–44. doi: 10.1111/j.1399-3054.2006.00627.x
Fernandez, O., Ishihara, H., George, G. M., Mengin, V., Flis, A., Sumner, D., et al. (2017). Leaf starch turnover occurs in long days and in falling light at the end of the day. Plant Physiol. 174, 2199–2212. doi: 10.1104/pp.17.00601
Gao, T., Zhang, Z., Liu, X., Wu, Q., Chen, Q., Liu, Q., et al. (2020). Physiological and transcriptome analyses of the effects of exogenous dopamine on drought tolerance in apple. Plant Physiol. Bioch. 148, 260–272. doi: 10.1016/j.plaphy.2020.01.022
Gasulla, F., Vom Dorp, K., Dombrink, I., Zähringer, U., Gisch, N., Dörmann, P., et al. (2013). The role of lipid metabolism in the acquisition of desiccation tolerance in Craterostigma plantagineum: a comparative approach. Plant J. 75, 726–741. doi: 10.1111/tpj.12241
Goral, T. K., Johnson, M. P., Duffy, C. D. P., Brain, A. P. R., Ruban, A. V., Mullineaux, C. W. (2012). Light-harvesting antenna composition controls the macrostructure and dynamics of thylakoid membranes in Arabidopsis. Plant J. 69, 289–301. doi: 10.1111/j.1365-313X.2011.04790.x
Gray, G. R., Heath, D. (2005). A global reorganization of the metabolome in Arabidopsis during cold acclimation is revealed by metabolic fingerprinting. Physiol. Plantarum 124, 236–248. doi: 10.1111/j.1399-3054.2005.00507.x
Guy, C., Kaplan, F., Kopka, J., Selbig, J., Hincha, D. K. (2008). Metabolomics of temperature stress. Physiol. Plantarum 132, 220–235. doi: 10.1111/j.1399-3054.2007.00999.x
Hanachi, S., Van Labeke, M. C., Mehouachi, T. (2014). Application of chlorophyll fluorescence to screen eggplant (Solanum melongena L.) cultivars for salt tolerance. Photosynthetica 52, 57–62. doi: 10.1007/s11099-014-0007-z
Herman, E. M., Rotter, K., Premakumar, R., Elwinger, G., Bae, H., Ehler-King, L., et al. (2006). Additional freeze hardiness in wheat acquired by exposure to –3 °C is associated with extensive physiological, morphological, and molecular changes. J. Exp. Bot. 57, 3601–3618. doi: 10.1093/jxb/erl111
Higashi, Y., Okazaki, Y., Myouga, F., Shinozaki, K., Saito, K. (2015). Landscape of the lipidome and transcriptome under heat stress in Arabidopsis thaliana. Sci. Rep. 5, 10533. doi: 10.1038/srep10533
Hincha, D. K., Höfner, R., Schwab, K. B., Heber, U., Schmitt, J. M. (1987). Membrane rupture is the common cause of damage to chloroplast membranes in leaves injured by freezing or excessive wilting. Plant Physiol. 83, 251–253. doi: 10.1104/pp.83.2.251
Hoffmann, M., Hilton-Taylor, C., Angulo, A., Böhm, M., Brooks, T. M., Butchart, S. H. M., et al. (2010). The impact of conservation on the status of the world’s vertebrates. Science 330, 1503–1509. doi: 10.1126/science.1194442
Hou, Q., Ufer, G., Bartels, D. (2016). Lipid signalling in plant responses to abiotic stress. Plant Cell Environ. 39, 1029–1048. doi: 10.1111/pce.12666
Huang, A., Wu, S., Gu, W., Li, Y., Xie, X., Wang, G. (2019). Provision of carbon skeleton for lipid synthesis from the breakdown of intracellular protein and soluble sugar in Phaeodactylum tricornutum under high CO2. BMC Biotechnol. 19, 53. doi: 10.1186/s12896-019-0544-4
Huang, X. X. (2011). Primary study on cold tolerance feature of Trachycarpus fortunei (China: Beijing Forestry University).
Huner, N. P. A., Maxwell, D. P., Gray, G. R., Savitch, L. V., Krol, M., Ivanov, A. G., et al. (1996). Sensing environmental temperature change through imbalances between energy supply and energy consumption: redox state of photosystem II. Physiol. Plant 98, 358–364. doi: 10.1034/j.1399-3054.1996.980218.x
Huner, N. P. A., Öquist, G., Sarhan, F. (1998). Energy balance and acclimation to light and cold. Trends Plant Sci. 3, 224–230. doi: 10.1016/S1360-1385(98)01248-5
Jiang, H., Gao, Y., Wang, W., He, B. (2016). Evaluation of cold resistance in grapevines via photosynthetic characteristics, carbohydrate metabolism and gene expression levels. Acta Physiol. Plant 38, 251. doi: 10.1007/s11738-016-2267-7
Junttila, O., Kaurin, Å. (1990). Environmental control of cold acclimation in Salix pentandra. Scand. J. For. Res. 5, 195–204. doi: 10.1080/02827589009382605
Kalaji, H. M., Jajoo, A., Oukarroum, A., Brestic, M., Zivcak, M., Samborska, I. A., et al. (2016). Chlorophyll a fluorescence as a tool to monitor physiological status of plants under abiotic stress conditions. Acta Physiol. Plant 38, 102. doi: 10.1007/s11738-016-2113-y
Kitashova, A., Adler, S. O., Richter, A. S., Eberlein, S., Dziubek, D., Klipp, E., et al. (2023). Limitation of sucrose biosynthesis shapes carbon partitioning during plant cold acclimation. Plant Cell Environ. 46, 464–478. doi: 10.1111/pce.14483
Knaupp, M., Mishra, K. B., Nedbal, L., Heyer, A. G. (2011). Evidence for a role of raffinose in stabilizing photosystem II during freeze-thaw cycles. Planta 234, 477–486. doi: 10.1007/s00425-011-1413-0
Kobayashi, K., Narise, T., Sonoike, K., Hashimoto, H., Sato, N., Kondo, M., et al. (2013). Role of galactolipid biosynthesis in coordinated development of photosynthetic complexes and thylakoid membranes during chloroplast biogenesis in Arabidopsis. Plant J. 73, 250–261. doi: 10.1111/tpj.12028
Liu, Q., Piao, S., Janssens, I. A., Fu, Y., Peng, S., Lian, X., et al. (2018). Extension of the growing season increases vegetation exposure to frost. Nat. Commun. 9, 426. doi: 10.1038/s41467-017-02690-y
Lu, Y. F. (2006). Studies on root system and fleshy root structure of Cycas [master’s thesis]. (Nanning: Guangxi University).
Lu, H., Xue, L., Cheng, J., Yang, X., Xie, H., Song, X., et al. (2020). Polyploidization-driven differentiation of freezing tolerance in Solidago canadensis. Plant Cell Environ. 43, 1394–1403. doi: 10.1111/pce.13745
Lynch, D. V., Dunn, T. M. (2004). An introduction to plant sphingolipids and a review of recent advances in understanding their metabolism and function. New Phytol. 161, 677–702. doi: 10.1111/j.1469-8137.2004.00992.x
Martínez-Vilalta, J., Sala, A., Asensio, D., Galiano, L., Hoch, G., Palacio, S., et al. (2016). Dynamics of nonstructural carbohydrates in terrestrial plants: A global synthesis. Ecol. Monogr. 86, 495–516. doi: 10.1002/ecm.1231
Moellering, E. R., Muthan, B., Benning, C. (2010). Freezing tolerance in plants requires lipid remodeling at the outer chloroplast membrane. Science 330, 226–228. doi: 10.1126/science.1191803
Mueller, S. P., Unger, M., Guender, L., Fekete, A., Mueller, M. J. (2017). Phospholipid:diacylglycerol acyltransferase-mediated triacylglyerol synthesis augments basal thermotolerance. Plant Physiol. 175, 486–497. doi: 10.1104/pp.17.00861
Nägele, T., Heyer, A. G. (2013). Approximating subcellular organization of carbohydrate metabolism during cold acclimation in different natural accessions of Arabidopsis thaliana. New Phytol. 198, 777–787. doi: 10.1111/nph.12201
Nishiyama, Y., Yamamoto, H., Allakhverdiev, S. I., Inaba, M., Yokota, A., Murata, N. (2001). Oxidative stress inhibits the repair of photodamage to the photosynthetic machinery. EMBO J. 20, 5587–5594. doi: 10.1093/emboj/20.20.5587
Niu, H., Duan, W., Wang, D., Bai, J., Peng, J., Song, Z., et al. (2021). The relationship between the color change of postharvest tobacco leaves and energy metabolism. Acta Tabacaria Sin. 27, 51–58. doi: 10.16472/j.chinatobacco.2021.003
Ouellet, F., Charron, J.-B. (2013). Cold acclimation and freezing tolerance in plants (Chichester: John Wiley & Sons, Ltd). doi: 10.1002/9780470015902.a0020093.pub2
Rai, V., Muthuraj, M., Gandhi, M. N., Das, D., Srivastava, S. (2017). Real-time iTRAQ-based proteome profiling revealed the central metabolism involved in nitrogen starvation induced lipid accumulation in microalgae. Sci. Rep. 7, 45732. doi: 10.1038/srep45732
Reyes, B. A., Pendergast, J. S., Yamazaki, S. (2008). Mammalian peripheral circadian oscillators are temperature compensated. J. Biol. Rhythms. 23, 95–98. doi: 10.1177/0748730407311855
Saddhe, A. A., Manuka, R., Penna, S. (2021). Plant sugars: Homeostasis and transport under abiotic stress in plants. Physiol. Plantarum 171, 739–755. doi: 10.1111/ppl.13283
Sakurai, I., Hagio, M., Gombos, Z., Tyystjärvi, T., Paakkarinen, V., Aro, E.-M., et al. (2003). Requirement of phosphatidylglycerol for maintenance of photosynthetic machinery. Plant Physiol. 133, 1376–1384. doi: 10.1104/pp.103.026955
Salvador, L. D., Suganuma, T., Kitahara, K., Tanoue, H., Ichiki, M. (2000). Monosaccharide composition of sweetpotato fiber and cell wall polysaccharides from sweetpotato, cassava, and potato analyzed by the high-performance anion exchange chromatography with pulsed amperometric detection method. J. Agr. Food Chem. 48, 3448–3454. doi: 10.1021/jf991089z
Sentinella, A. T., Warton, D. I., Sherwin, W. B., Offord, C. A., Moles, A. T. (2020). Tropical plants do not have narrower temperature tolerances, but are more at risk from warming because they are close to their upper thermal limits. Global Ecol. Biogeogr. 29, 1387–1398. doi: 10.1111/geb.13117
Sergeeva, A., Mettler-Altmann, T., Liu, H., Mai, H., Bauer, P. (2021). Glycerolipid profile differences between perennial and annual stem zones in the perennial model plant Arabis alpina. Plant Direct 5, e00302. doi: 10.1002/pld3.302
Seydel, C., Kitashova, A., Fürtauer, L., Nägele, T. (2022). Temperature-induced dynamics of plant carbohydrate Metabolism. Physiol. Plantarum 174, e13602. doi: 10.1111/ppl.13602
Shameer, S., Ratcliffe, R. G., Sweetlove, L. J., Notes, A. (2019). Leaf energy balance requires mitochondrial respiration and export of chloroplast NADPH in the Light. Plant Physiol. 180, 1947–1961. doi: 10.1104/pp.19.00624
Sharfman, M., Bar, M., Schuster, S., Leibman, M., Avni, A. (2014). Sterol-dependent induction of plant defense responses by a microbe-associated molecular pattern from Trichoderma viride. Plant Physiol. 164, 819–827. doi: 10.1104/pp.113.230136
Simons, K., Toomre, D. (2000). Lipid rafts and signal transduction. Nat. Rev. Mol. Cell Biol. 1, 31–39. doi: 10.1038/35036052
Stitt, M., Zeeman, S. C. (2012). Starch turnover: pathways, regulation and role in growth. Curr. Opin. Plant Biol. 15, 282–292. doi: 10.1016/j.pbi.2012.03.016
Strauss, G., Hauser, H. (1986). Stabilization of lipid bilayer vesicles by sucrose during freezing. PNAS 83, 2422–2426. doi: 10.1073/pnas.83.8.2422
Sun, Z., Chen, Y., Du, J. (2016). Elevated CO2 improves lipid accumulation by increasing carbon metabolism in Chlorella sorokiniana. Plant Biotechnol. J. 14, 557–566. doi: 10.1111/pbi.12398
Taïbi, K., Del Campo, A. D., Vilagrosa, A., Bellés, J. M., López-Gresa, M. P., López-Nicolás, J. M., et al. (2018). Distinctive physiological and molecular responses to cold stress among cold-tolerant and cold-sensitive Pinus halepensis seed sources. BMC Plant Biol. 18, 236. doi: 10.1186/s12870-018-1464-5
Tan, W. J., Yang, Y. C., Zhou, Y., Huang, L. P., Xu, L., Chen, Q. F., et al. (2018). Diacylglycerol acyltransferase and diacylglycerol kinase modulate tricylglycerol and phosphatidic acid production in the plant response to freezing stress. Plant Physiol. 177, 1303–1318. doi: 10.1104/pp.18.00402
Thalmann, M., Pazmino, D., Seung, D., Horrer, D., Nigro, A., Meier, T., et al. (2016). Regulation of leaf starch degradation by abscisic acid is important for osmotic stress tolerance in plants. Plant Cell 28, 1860–1878. doi: 10.1105/tpc.16.00143
Thalmann, M., Santelia, D. (2017). Starch as a determinant of plant fitness under abiotic stress. New Phytol. 214, 943–951. doi: 10.1111/nph.14491
Thomashow, M. F. (1999). Plant cold acclimation: freezing tolerance genes and regulatory mechanisms. Annu. Rev. Plant Physiol. Plant Mol. Biol. 50, 571–599. doi: 10.1146/annurev.arplant.50.1.571
Uemura, M., Joseph, R. A., Steponkus, P. L. (1995). Cold-acclimation of Arabidopsis thaliana: effect on plasma membrane lipid composition and freeze-induced lesions. Plant Physiol. 109, 15–30. doi: 10.1104/pp.109.1.15
Vitova, M., Bisova, K., Kawano, S., Zachleder, V. (2015). Accumulation of energy reserves in algae: From cell cycles to biotechnological applications. Biotechnol. Adv. 33, 1204–1218. doi: 10.1016/j.biotechadv.2015.04.012
Wang, T., Hu, M., Yuan, D., Yun, Z., Gao, Z., Su, Z., et al. (2020). Melatonin alleviates pericarp browning in litchi fruit by regulating membrane lipid and energy metabolisms. Postharvest Biol. Tech. 160, 111066. doi: 10.1016/j.postharvbio.2019.111066
Wang, Y., Zhang, X., Huang, G., Feng, F., Liu, X., Guo, R., et al. (2020). Dynamic changes in membrane lipid composition of leaves of winter wheat seedlings in response to PEG-induced water stress. BMC Plant Biol. 20, 84. doi: 10.1186/s12870-020-2257-1
Wang, L., Zhang, B., Xiao, J., Huang, Q., Li, C., Fu, X. (2018). Physicochemical, functional, and biological properties of water-soluble polysaccharides from Rosa roxburghii Tratt fruit. Food Chem. 249, 127–135. doi: 10.1016/j.foodchem.2018.01.011
Webb, M. S., Uemura, M., Steponkus, P. L. (1994). A comparison of freezing injury in oat and rye: two cereals at the extremes of freezing tolerance. Plant Physiol. 104, 467–478. doi: 10.1104/pp.104.2.467
Welti, R., Li, W., Li, M., Sang, Y., Biesiada, H., Zhou, H. E., et al. (2002). Profiling membrane lipids in plant stress responses: role of phospholipase D alpha in freezing-induced lipid changes in Arabidopsis. J. Biol. Chem. 277, 31994–32002. doi: 10.1074/jbc.M205375200
Yang, Y., Benning, C. (2018). Functions of triacylglycerols during plant stress and development. Curr. Opin. Biotechnol. 49, 191–198. doi: 10.1016/j.copbio.2017.09.003
Yano, R., Nakamura, M., Yoneyama, T., Nishida, I. (2005). Starch-related α-glucan/water dikinase is involved in the cold-induced development of freezing tolerance in Arabidopsis. Plant Physiol. 138, 837–846. doi: 10.1104/pp.104.056374
Yildiz, D., Nzokou, P., Deligoz, A., Koc, I., Genc, M. (2014). Chemical and physiological responses of four Turkish red pine (Pinus brutia Ten.) provenances to cold temperature treatments. Eur. J. For. Res. 133, 809–818. doi: 10.1007/s10342-014-0798-2
Young, D. Y., Pang, N., Shachar-Hill, Y. (2022). 13C-labeling reveals how membrane lipid components contribute to triacylglycerol accumulation in Chlamydomonas. Plant Physiol. 189, 1326–1344. doi: 10.1093/plphys/kiac154
Yu, L., Fan, J., Yan, C., Xu, C. (2018). Starch deficiency enhances lipid biosynthesis and turnover in leaves. Plant Physiol. 178, 118–129. doi: 10.1104/pp.18.00539
Yu, Z., Yang, Y., Liu, J., Gong, L., Zhan, C. (2007). Preliminary experiment on propagation of Cycas panzhihuaensis. J. Southwest Forestry Coll. 27, 36–41.
Yu, L., Zhou, C., Fan, J., Shanklin, J., Xu, C. (2021). Mechanisms and functions of membrane lipid remodeling in plants. Plant J. 107, 37–53. doi: 10.1111/tpj.15273
Zareei, E., Karami, F., Gholami, M., Ershadi, A., Avestan, S., Aryal, R., et al. (2021). Physiological and biochemical responses of strawberry crown and leaf tissues to freezing stress. BMC Plant Biol. 21, 532. doi: 10.1186/s12870-021-03300-2
Zhang, X. D., Wang, R. P., Zhang, F. J., Tao, F. Q., Li, W. Q. (2013). Lipid profiling and tolerance to low-temperature stress in Thellungiella salsuginea in comparison with Arabidopsis thaliana. Biol. Plantarum 57, 149–153. doi: 10.1007/s10535-012-0137-8
Zhang, L., Zhang, Z., Gao, H., Meng, X., Yang, C., Liu, J., et al. (2012). The mitochondrial alternative oxidase pathway protects the photosynthetic apparatus against photodamage in Rumex K-1 leaves. BMC Plant Biol. 12, 40. doi: 10.1186/1471-2229-12-40
Zheng, G. W., Li, W. Q. (2017). Profiling membrane glycerolipids during γ-ray-induced membrane injury. BMC Plant Biol. 17, 203. doi: 10.1186/s12870-017-1153-9
Zheng, G. W., Li, L. X., Li, W. Q. (2016). Glycerolipidome responses to freezing and chilling-induced injuries: examples in Arabidopsis and rice. BMC Plant Biol. 16, 70. doi: 10.1186/s12870-016-0758-8
Zheng, Y. L., Yang, Y. Q., Wang, M., Hu, S. J., Wu, J. R., Yu, Z. X. (2021). Differences in lipid homeostasis and membrane lipid unsaturation confer differential tolerance to low temperatures in two Cycas species. BMC Plant Biol. 21, 377. doi: 10.1186/s12870-021-03158-4
Zhu, Y., Wang, K., Wu, C., Zhao, Y., Yin, X., Zhang, B., et al. (2019). Effect of ethylene on cell wall and lipid metabolism during alleviation postharvest chilling injury in peach. Cells 8, 1612. doi: 10.3390/cells8121612
Keywords: energy metabolism, glycerolipids, starch, soluble sugar, unexpected freezing stress
Citation: Wu Y, Zhu H, Ling Z, Lu E, Peng X and Zheng Y (2023) The metabolism of nonstructural carbohydrates, lipids, and energy in two Cycas species with differential tolerance to unexpected freezing stress. Front. Plant Sci. 14:1301560. doi: 10.3389/fpls.2023.1301560
Received: 25 September 2023; Accepted: 17 November 2023;
Published: 07 December 2023.
Edited by:
Karl J. Niklas, Cornell University, United StatesReviewed by:
Zhaodong Hao, Nanjing Forestry University, ChinaCopyright © 2023 Wu, Zhu, Ling, Lu, Peng and Zheng. This is an open-access article distributed under the terms of the Creative Commons Attribution License (CC BY). The use, distribution or reproduction in other forums is permitted, provided the original author(s) and the copyright owner(s) are credited and that the original publication in this journal is cited, in accordance with accepted academic practice. No use, distribution or reproduction is permitted which does not comply with these terms.
*Correspondence: Yanling Zheng, Zmxhc2hpbmd6eWxAMTYzLmNvbQ==
†These authors have contributed equally to this work and share first authorship
Disclaimer: All claims expressed in this article are solely those of the authors and do not necessarily represent those of their affiliated organizations, or those of the publisher, the editors and the reviewers. Any product that may be evaluated in this article or claim that may be made by its manufacturer is not guaranteed or endorsed by the publisher.
Research integrity at Frontiers
Learn more about the work of our research integrity team to safeguard the quality of each article we publish.