- 1Postharvest Research Division, National Institute of Horticultural and Herbal Science, Wanju, Republic of Korea
- 2Department of Plant Biotechnology, College of Life Sciences and Biotechnology, Korea University, Seoul, Republic of Korea
- 3Planning and Coordination Division, National Institute of Horticultural & Herbal Science, Wanju, Republic of Korea
Introduction: Paprika (Capsicum annuum L.) is prone to chilling injury (CI) during low-temperature storage. Although recent findings suggest that CO2 treatment may protect against CI, the effects of short-term CO2 treatment on CI and the underlying molecular mechanisms in paprika remain unknown. Therefore, this study aimed to examine the effect of short-term CO2 treatment on CI and postharvest quality in paprika during storage at cold storage and retail condition at physio-biochemical-molecular level.
Methods: Paprika was treated with 20 and 30% CO2 for 3 h and stored at 4°C for 14 days, followed by additional storage for 2 days at 20°C (retail condition). Fruit quality parameters, including weight loss, firmness, color, and pitting were assessed, and the molecular mechanism of the treatment was elucidated using transcriptomic and metabolomic analyses.
Results: Short-term treatment with 20 and 30% CO2 effectively maintained paprika quality during cold storage and retailer conditions, with reduced surface pitting, a common symptom of CI. Additionally, transcriptomic and metabolomic analyses revealed that 20% CO2 treatment induced genes associated with biosynthesis of phosphatidic acid (PA), diacylglycerol, triacylglycerol, and stress response, metabolites associated with phasphatidyl inositol signaling, inositol phosphate metabolism, and starch and sucrose metabolism.
Conclusion: CO2 treatment activates PA biosynthesis through PLD and PLC-DGK pathways, and induces inositol phosphate, starch, and sucrose metabolism, thereby regulating chilling stress response via the ICE-CBF pathway. These findings suggest that short-term CO2 treatment enhances resistance to cold-induced injury and preserves postharvest quality in non-climacteric fruits, such as paprika, through activation of PA signaling, which improves membrane stability during cold storage and distribution.
1 Introduction
Postharvest storage and transportation of fresh produce are crucial stages in the supply chain that substantially affect product quality and shelf life. Among the various factors affecting the postharvest quality of fruits and vegetables, chilling injury (CI) remains a persistent challenge, particularly for chilling-sensitive crops, such as paprika (Capsicum annuum L.). CI is a physiological disorder characterized by the development of various symptoms, including tissue softening, water soaking, discoloration, and increased susceptibility to decay, leading to considerable economic losses for producers and retailers (Biswas et al., 2016; Park et al., 2021; Rai et al., 2022). Conventional approaches to mitigate CI often involve controlling storage temperatures above the chilling threshold, which is typically around 10°C for paprika (Lim et al., 2007). However, maintaining high temperatures during storage can result in accelerated deterioration and reduced shelf life (Rao et al., 2011). Therefore, it is critical to explore alternative strategies that can effectively alleviate CI, while preserving the quality attributes and extending the postharvest life of paprika.
Recently, carbon dioxide (CO2) treatment has emerged as a promising strategy for preserving the post-harvest quality of various horticultural products. For instance, continuous exposure to CO2 (5%) effectively maintained the postharvest quality of tomatoes during storage at 10°C (Taye et al., 2017). Similarly, strawberries exposed to 18% CO2 for 48 h prior to storage at 1°C exhibited enhanced resistance to softening and oxidative stress (del Olmo et al., 2022). Additionally, treatment with 95% CO2 for 36 h prior to storage at 1°C reduced the susceptibility of persimmons to CI (Besada et al., 2015). Moreover, treatment with 10% CO2 for 24 h in combination with modified atmosphere packaging effectively maintained the quality of sweet peppers stored at 10°C (Afolabi et al., 2023). Furthermore, treatment with 30% CO2 for 6 h prior to storage at 0°C reduced CI, extended storability, and preserved the sensory quality and antioxidant capacity of Madoka peach fruit (Tilahun et al., 2022). However, it is imperative to minimize the treatment duration to enhance the feasibility and cost-effectiveness of postharvest treatments for producers and distributors. Notably, studies have shown that exposing strawberries and tomatoes to 30% CO2 for only 3 h can effectively maintain quality and mitigate CI (Eum et al., 2021; Park et al., 2021).
The mechanism underlying CO2-induced postharvest quality preservation is attributed to its ability to reduce respiration rate and ethylene production. For instance, apples, melons, tomatoes, and bananas showed respiratory reduction following high CO2 treatment (Kubo et al., 1989; Park et al., 2021). CO2 pretreatment coupled with cold storage synergistically reduced ethylene production, leading to delayed ripening in tomatoes (Park et al., 2021). At the molecular level, ethylene biosynthesis and signaling genes are suppressed by CO2 pretreatment in tomatoes (Rothan et al., 1997; Park et al., 2021), and CO2 treatment can modulate genes encoding cell wall-degrading enzymes in strawberries (Eum et al., 2021). Additionally, CO2 pretreatment triggers the expression of genes involved in stress and the activity of antioxidant enzymes in several fruits and vegetables, including tomatoes, grapes, peaches, and strawberries (Rothan et al., 1997; Romero et al., 2016; Park et al., 2021; del Olmo et al., 2022; Tilahun et al., 2022). CI often disrupts membrane integrity due to altered fluidity and rigidity caused by temperature fluctuations during storage, resulting in cellular leakage, compromised physiological functions, and decrease in overall quality (Biswas et al., 2016; Valenzuela et al., 2017). Moreover, chilling stress can induce oxidative stress by triggering the production of reactive oxygen species (ROS) owing to disrupted electron transport chains and impaired antioxidant systems (Biswas et al., 2016; Valenzuela et al., 2017). Therefore, developing postharvest technologies that target membrane lipid metabolism and stress responses would be highly beneficial for inhibiting CI and maintaining the postharvest quality of fresh produce.
Despite the positive effects of short-term CO2 treatment in tomato and strawberry, its impact on the quality of stored paprika remains unexplored. Moreover, the molecular mechanisms of CO2-induced quality preservation and CI resistance remains unclear, particularly in non-climacteric fruits, such as paprika. Therefore, this study aimed to comprehensively evaluate the effect and molecular mechanism of short-term CO2 pretreatment on postharvest quality in paprika under cold storage and retail conditions, using transcriptomic and metabolomic analyses.
2 Materials and methods
2.1 Plant materials and treatments
Paprika fruits (cv. Sirocco, red color) were harvested at approximately 80–85% maturity stage. After arrival to the laboratory, the fruits were immediately treated with 20 and 30% CO2 (mixed with ambient air) or left untreated in a commercial cardboard box for 3 h in a closed chamber at room temperature (~20°C). After the treatment period, the chamber was flushed with air to remove CO2. In total, 30 boxes per treatment were used for the study, with each box containing 30 fruits. The CO2 concentration in the closed chamber was measured using a portable headspace analyzer (Dansensor, Ringsted, Denmark). Samples in the control group were flushed with ambient air, and the damaged fruits were discarded. The fruits were stored in a covered cardboard box at 4°C (cold storage) for 14 d or at 4°C for 14 d, followed by additional 2 d at 20°C (14 + 2 d; retail condition). Relative humidity was maintained at 90 ± 5% during the storage period.
2.2 Fruit quality evaluation
Briefly, 20 fruits were sampled per treatment for fruit quality assessment. The fruits were weighed to determine weight loss using an electronic weighing balance. Skin color was monitored using a color meter (Minolta CR-400; Konica Minolta, Osaka, Japan), and values were reported based on Hunter’s redness scale (a*). Firmness was analyzed using a texture analyzer (TA Plus Lloyd Instruments Ltd., Fareham, Hamshire, UK) equipped with a 5-mm plunger head (diameter) at a speed of 2 mm/s. Total soluble solid content (SSC) was analyzed using a digital refractometer (PAL-1, Atago Co. Ltd., Tokyo, Japan). Fruit pitting was expressed as the percentage of fruits that exhibited pitting. The final reported quality attributes were obtained from three independent replicates per treatment per day.
2.3 Light microscopy for tissue structure analysis
Tissue analysis was performed as previously described (Clément et al., 1996), with some modifications. Briefly, paprika tissues were fixed in 2.5% glutaraldehyde (v/v in a 0.1 M phosphate buffer) at pH of 7.2 with 4% sucrose (w/v) for 24 h. After three rinses with the above fixing buffer (30 min each), the samples were post-fixed with 1% OsO4 w/v in the same buffer with 4% sucrose (w/v) for 4 h. After rinsing three times (30 min each), the tissues were dehydrated in alcohol gradient series, transferred to propylene oxide, and embedded in Epon epoxy resin. Semi-thin sections (2.5 µm) were prepared using an ultra-microtome and placed on glass slides. The polysaccharide-specific reaction was performed using periodic acid-Schiff (PAS) and the tissue structures are shown in red. Sections for staining were first immersed in 1% periodic acid (w/v) for 30 min, followed by immersion in Schiff’s reagent for 40 min and in 5% sodium bisulfite (w/v) for 35 min. Thereafter, the sections were rinsed with distilled water, dried on a warm plate, and mounted on Histomount. The negative control was prepared by omitting the oxidation step using periodic acid. The samples were observed under a light microscope (Axioscop 2; Carl Zeiss, Germany).
2.4 Transcriptome analysis
Paprika fruits were sampled at days 0, 7, and 14 + 2 from the untreated control and 20% CO2-treated groups. Thereafter, five fruits were pooled from each sample, and the peel tissue was used for RNA isolation using the Qiagen RNA mini prep (Qiagen, USA). RNA purity and integrity were verified using an Agilent 2100 Bioanalyzer (Agilent Technologies, Santa Clara, CA, USA USA), and only RNA with an RNA integrity value (RIN) > 8 were used for library preparation. Library preparation and RNA sequencing (RNA-seq) were performed at C&K Genomics in Seoul, South Korea. The processed reads were aligned to the sequence of Capsicum annuum (AVRZ02) using HISAT v2.1.0 (Kim et al., 2015). Aligned reads were counted using featureCounts in the Subread package version 1.6.0100 (Liao et al., 2014). Count data were analyzed for differential gene expression using the EdgeR package (Robinson et al., 2010). The expression level of each transcript was normalized to the TMM (trimmed mean) using the M-value normalization method (Robinson and Oshlack, 2010). The filtered data were log2-transformed and subjected to quantile normalization. Differentially expressed genes (DEGs) were selected using p ≤ 0.05 and log2-fold change (FC) ≥ 1 as thresholds. Gene ontology (GO) enrichment sets of the DEGs were obtained using the DAVID (Databank for Annotation, Visualization, and Integrated Discovery) database (Dennis et al., 2003).
2.5 Quantitative real-time PCR
Quantitative real-time PCR (qRT-PCR) was performed as described by Park et al. (2021). Target genes were amplified on a CFX96 TouchTM Real-Time PCR Detection System (Bio-Rad, USA) using the iQTM SYBR Green Supermix (Bio-Rad) with specific primers (Supplementary Table S1). The qRT-PCR conditions were as follows: 95°C for 30 s, followed by 40 cycles of 95°C for 10 s and 55°C or 58°C for 40 s. The relative gene expression was calculated using the ΔΔCt method and normalized to that of the housekeeping genes actin and elongation factor 1. The qRT-PCR was performed using at least three biological replicates and two technical replicates.
2.6 Metabolome analysis using gas chromatography–mass spectrometry
Samples were prepared for primary metabolites profiling following previously described methods (Lisec et al., 2015; Song and Ku, 2021), with some modifications. Briefly, freeze-dried paprika powder was extracted in methanol. Ribitol and tetracosane were used as internal standards for water- and lipid-soluble compounds, respectively. Water- and lipid-soluble compounds were separated into two phases via liquid-to-liquid extraction using deionized water and chloroform, respectively. Each organic phase was fully dried using a SpeedVac. Thereafter, methoxyamide (in anhydrous pyridine) was added to a tube containing dried water-soluble phase and incubated at 37°C for 90 min under constant shaking at 800 rpm. For derivatization of the water-soluble metabolites, N-methyl-N-(trimethylsilyl)trifluoroacetamide and 1% trimethylchlorosilane (TMCS) were added, and the sample was incubated at 50°C for 20 min under constant shaking at 800 rpm. For derivatization of the lipid-soluble metabolites, N, O-bis (trimethylsilyl)trifluoroacetamide + TMCS was added to the sample. The mixture was incubated at 60°C for 60 min under constant shaking at 800 rpm. The sample was transferred to vials with an insert and 1 µL was injected into a gas chromatograph (Nexis GC-2030, Shimadzu, Kyoto, Japan) coupled to a gas chromatograph–mass spectrometer (GC/MS-QP 2020 NX, Shimadzu) and an autosampler with injector (AOC-20i PLUS, Shimadzu). Chromatographic separation was performed in a capillary column (DB-5MS, Agilent, CA, USA; 30 m × 0.25 mm coated with 0.25 µm film). The flow rate of the carrier gas (helium) was set to 1.2 mL·min-1. The mass spectrophotometry parameters were as follows: ion source temperature, interface temperature, and mass scan range were set to 300°C, 250°C, and 40-600 m/z, respectively. For analysis of water-soluble metabolites, the initial oven temperature was set at 80°C for 2 min, then increased to 330°C at a rate of 12°C·min-1, and maintained at 330°C for 5 min. For analysis of lipid-soluble metabolites, the initial oven temperature was set at 150°C for 1 min, then increased to 320°C at a rate of 12°C·min-1, and maintained at 320°C for 7 min. Metabolites were identified based on the library from National Institute of Standards and Technology (NIST) or standard compounds (Supplementary Table S4).
2.7 Statistical analyses
Data are presented as the mean ± standard error. Significant differences were determined using analysis of variance (ANOVA), followed by t-test for comparisons between groups. Partial least squares discriminant analysis (PLS–DA) and pathway analysis were performed using MetaboAnalyst (https://www.metaboanalyst.ca/). All analyses were performed using SAS v.9.2 (SAS Institute, Cary, NC, USA).
3 Results
3.1 CO2 treatment reduces chilling injury and maintains quality in paprika
Compared with that in the control group, treatment with 20 and 30% CO2 increased the respiration rate at day 0, indicating the successful absorption of CO2 in treated paprika (Supplementary Figure S1). However, there was a decrease in respiration rate during cold storage (4°C) for 14 days with respiration rate peaking at day 5 of storage at 20°C (Supplementary Figure S1). Notably, there was no significant difference in respiration rate between the treatment and control groups during storage at 4 and 20°C regardless of the CO2 treatment concentration. Additionally, treatment with 20% CO2 caused a decrease in hue value and fresh weight during cold storage (Supplementary Table S2). Figure 1A represents the images of CO2 -treated and untreated fruits stored at 14 days of cold storage at 4°C and additional 2 days of storage under retails condition at 20°C. Moreover, 20% CO2 - and 30% CO2 -treated paprika were significantly firmer than untreated fruits at 14 days cold storage at 4°C and 5 days at 20°C (Figure 1B; Supplementary Table S2). Specifically, fruits treated with 20% CO2 were 18.2% firmer than those in the control group after 14 days of cold storage and additional 2 days of storage under retails condition (14 + 2 days) (Figure 1B). There was no significant difference in SSC between CO2-treated and untreated fruits (Supplementary Table S2). The Pitting rate is a primary symptom of CI in paprika, fruits treated with 20 and 30% CO2 (Supplementary Figure S3) showed significantly lower surface pitting after transfer from cold storage to retail conditions. Specifically, only 39% of fruits treated with 20% CO2 showed surface pitting at day 2 after transfer from cold storage (14 days) to retail conditions compared with a rate of 51% in the control group (Figure 1C). CO2 treatment reduced the loss rate by about 12% during distribution, which is an economic benefit depending on the market price (Supplementary Table S3) Overall, these results suggest that CO2 treatment effectively delayed ripening and senescence and maintained fruit firmness during storage, which improved quality and reduced CI in paprika. As there were no notable differences between 20 and 30% CO2 treatments, 20% CO2 treatment was selected for further experiments.
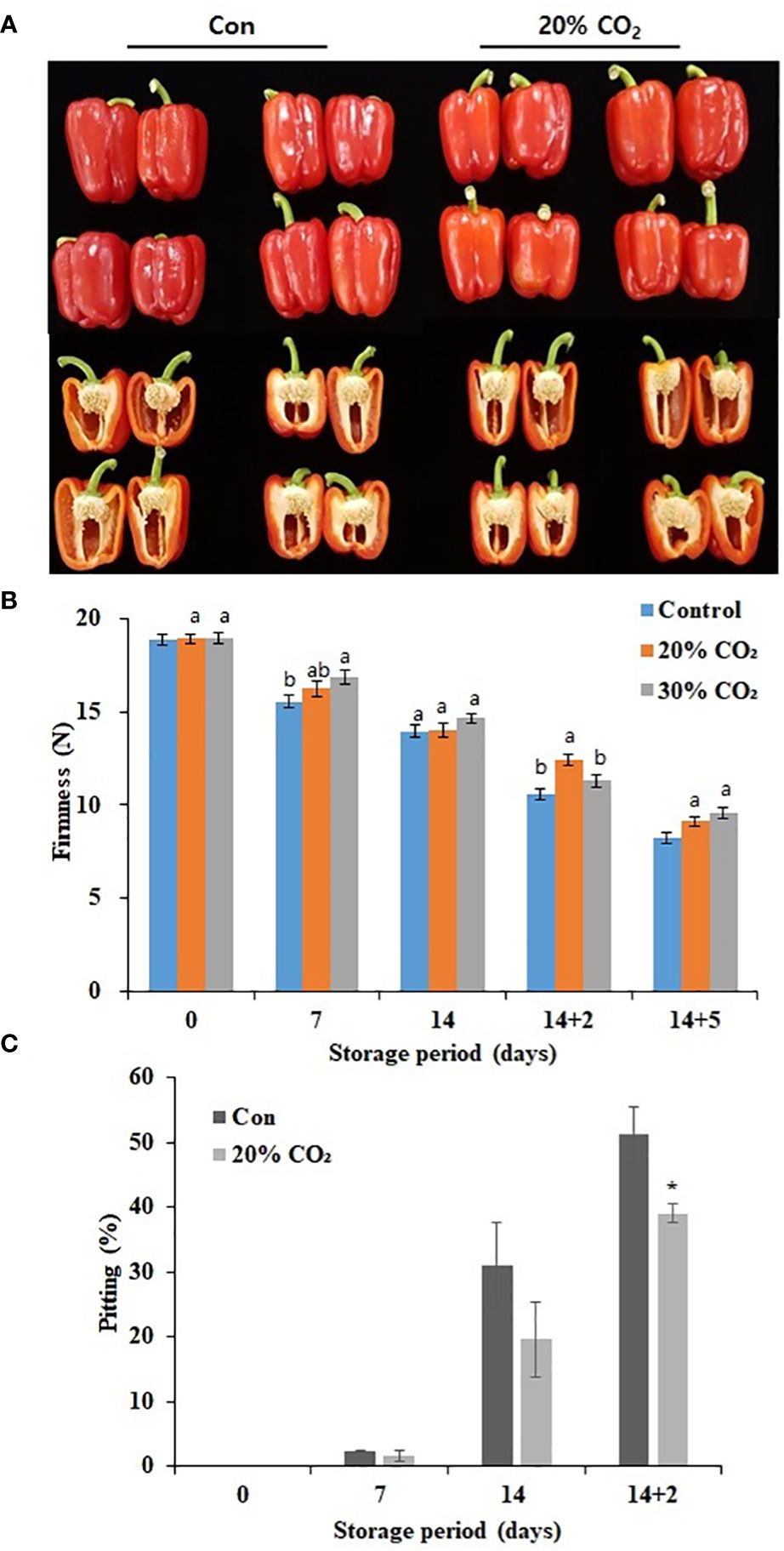
Figure 1 Effect of short-term CO2 treatment on postharvest quality and chilling injury in paprika. Representative images of CO2-treated and untreated fruits at 4°C for 14 days, followed by storage for 2 days at 20°C (A), firmness (B), and pitting (C) in paprika treated with CO2 and stored at 4°C for 14 days, and retail condition. Data represents the mean ± standard error of three replicates. At (B), different letters on the graphs represent significant differences between the control and CO2 treatments (DMRT, P < 0.05). and at (C), * represent t-test for comparisons between groups(p<0.1,**p < 0.05 and ***p < 0.0005).
3.2 CO2 treatment affects the transcriptome profile of paprika
RNA sequencing was performed at days 0, 14, and 14 + 2 after CO2 treatment using pericarp tissues. Heatmap revealed remarkable changes in the transcriptome of the fruits following 20% CO2 treatment (Figure 2A). Differential expression analysis identified 3,511 DEGs in the treated vs. untreated groups, among which 2,996 DEGs were expressed at day 0, 56 DEGs at day 14, and 459 DEGs at day 14 + 2 after CO2 treatment (Figure 2B). GO functional annotation showed that the DEGs were enriched in different functional terms in the cellular components, biological processes, and molecular functions categories (Figure 2C). Kyoto Encyclopedia of Genes and Genomes (KEGG) enrichment analysis showed that the DEGs were enriched in amino sugar and nucleotide sugar metabolism, fatty acid degradation, fatty acid metabolism, alpha-linoleic acid metabolism, plant hormone signal transduction, and metabolic pathways (Figure 2D). All upregulated and downregulated genes in response to CO2 treatment are shown in Tables S5, S6, S7.
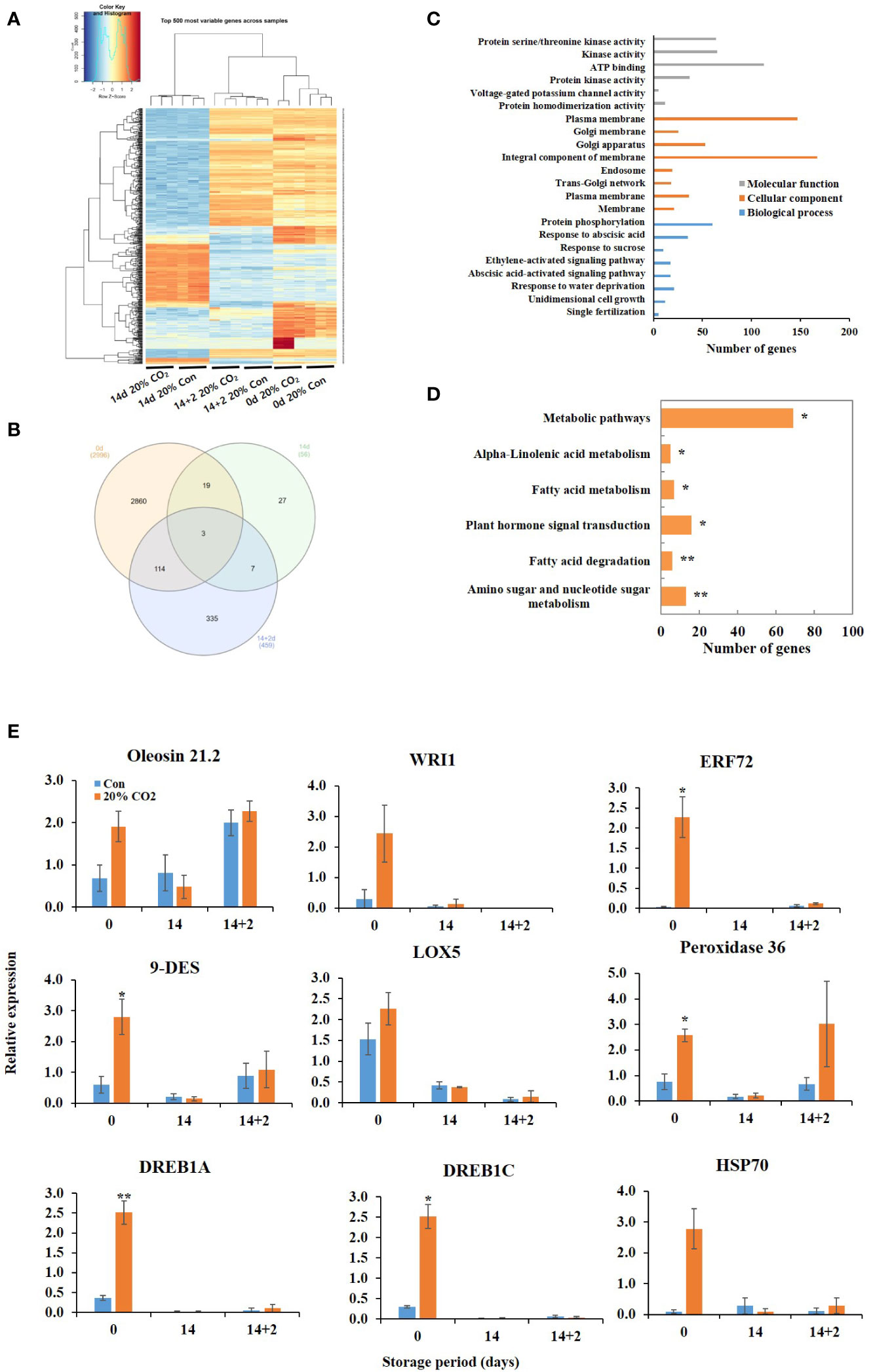
Figure 2 Transcriptome analysis of CO2-treated tomatoes. Heatmap (A) and Venn diagram (B) of differentially expressed genes (DEGs); Gene ontology functional categorization of DEGs (C); KEGG pathway enrichment analysis of DEGs (D); quantitative real-time PCR validation of lipid metabolism- and stress-related genes (E) in paprika treated with CO2 and stored at 4°C for 14 days, followed by storage for 2 days at 20°C (14 + 2). *p < 0.1, and **p < 0.01.
Membrane lipid metabolism plays an important role in the cold stress response. DEGs involved in lipid processes, such as phospholipases (phospholipase D delta and phospholipase A1-II 1), diacylglycerol kinase 5 (DGK5), diacylglycerol O-acyltransferase 1 (DGAT1), omega-6 fatty acid desaturase (FAD), GDSL esterase/lipases (GDSL esterase/lipase At5g18430, GDSL esterase/lipase At1g71250), non-specific lipid-transfer proteins, oleosins (oleosin 21.2 kDa, oleosin 18 kDa, oleosin 18.5 kDa), and WRI1 were upregulated at day 0 (Table 1). Additionally, DEGs encoding the lipid-body membrane proteins, including oleosins (oleosin 21.2 kDa, oleosin 18 kDa, oleosin 18.5 kDa), were strongly induced at day 0. Moreover, DEGs encoding glycerol-3-phosphate acyltransferase 3, FAD, enoyl-CoA delta isomerase 1, and lipid phosphate phosphatase 3 (LPP3) were upregulated in the CO2-treated fruits at day 14 + 2 (Table 1). At day 14, only 18 DEGs showed expression of more than 1.5 fold, and where mainly involved in encoding unknown proteins, except for cardiolipin synthase, which was downregulated. Additionally, DEG related to lipid-derived molecules, including oxylipins and jasmonates (alpha-dioxygenase 1, 9-divinyl ether synthase, linolenate hydroperoxide lyase, and linoleate 9S-lipoxygenase), were induced by CO2 treatment at days 0 and 14 + 2 after treatment (Table 1).
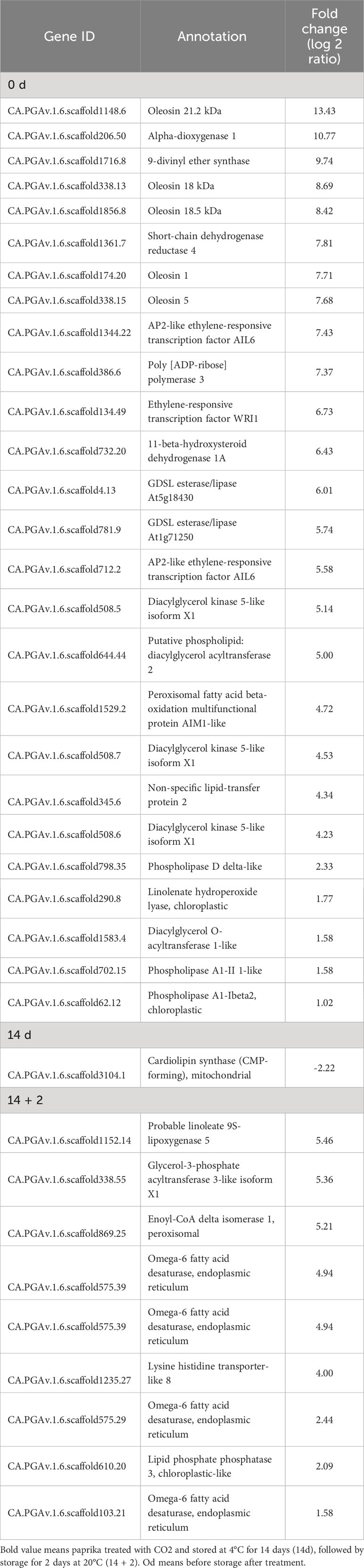
Table 1 Differentially expressed genes (DEGs) involved in lipid processes in paprika treated with CO2..
Furthermore, CO2 treatment modulated the expression of various stress-related genes, including dehydration-responsive element-binding proteins (DREB1A and DREB1C), MYBs, heat shock 70 kDa protein, peroxidase 36, proline-rich protein 4, desiccation-related protein, and pathogenesis-related proteins, at days 0 and 14 + 2 (Table 2). However, one DEG encoding NAC domain-containing protein 7 was suppressed at day 14 after CO2 treatment (Table 2). qRT-PCR was performed to confirm the effects of CO2 treatment on oleosin, 9-DES, LOX5, ERF72, WRI1, peroxidase 36, HSP70, DREB1A, and DREB1C. There were no significant differences in the expression levels of lipoxygenase, oleosin, WRI, and HSP70 between CO2-treated and untreated fruits (Figure 2E). Notably, these genes, except for oleosin and peroxidase36, were specifically induced in CO2-treated fruits at day 0 (Figure 2E).
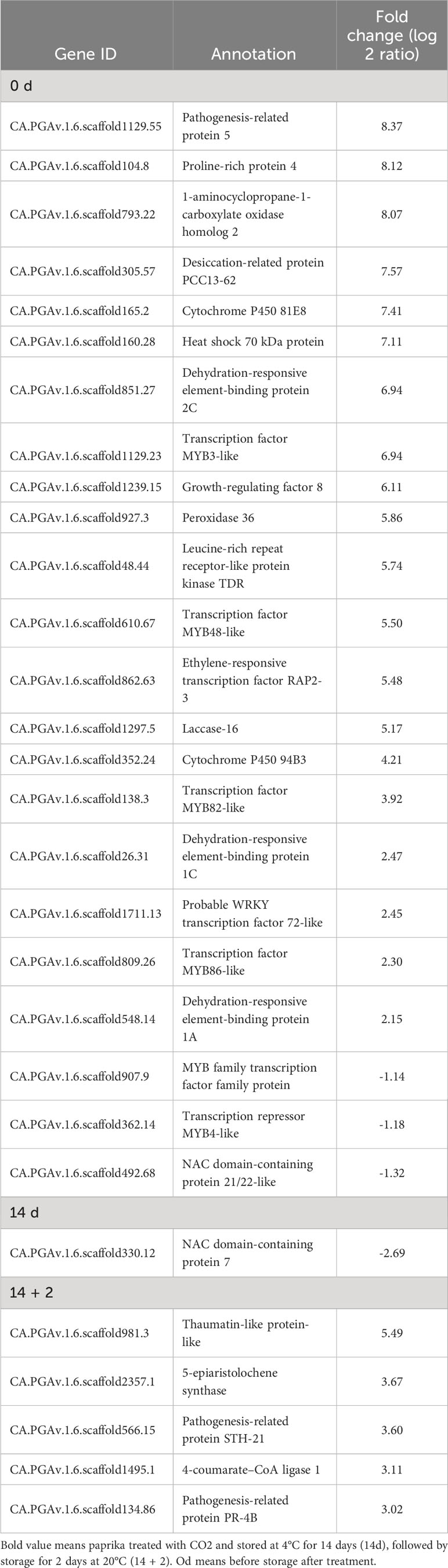
Table 2 Differentially expressed genes (DEGs) involved in stress response in paprika treated with CO2..
3.3 CO2 treatment affects the metabolome of paprika
The metabolite profiles of CO2-treated and untreated fruits were analyzed at days 0, 14, and 14 + 2 after CO2 treatment. In total, 36 metabolites, including 28 water-soluble and 8 lipid-soluble metabolites including 2 internal standards, were identified (Supplementary Table S4). PLS-DA was conducted to explore the effect of CO2 and the relationship between the metabolites. The two PLS-DA components collectively accounted for 85.4 and 86.4% of the total variance in the dataset at days 14 and 14 + 2, respectively (Figures 3A, B). Additionally, there was a clear separation of the two clusters, indicating the significant impact of CO2 on metabolites in paprika during storage (Figures 3A, B).
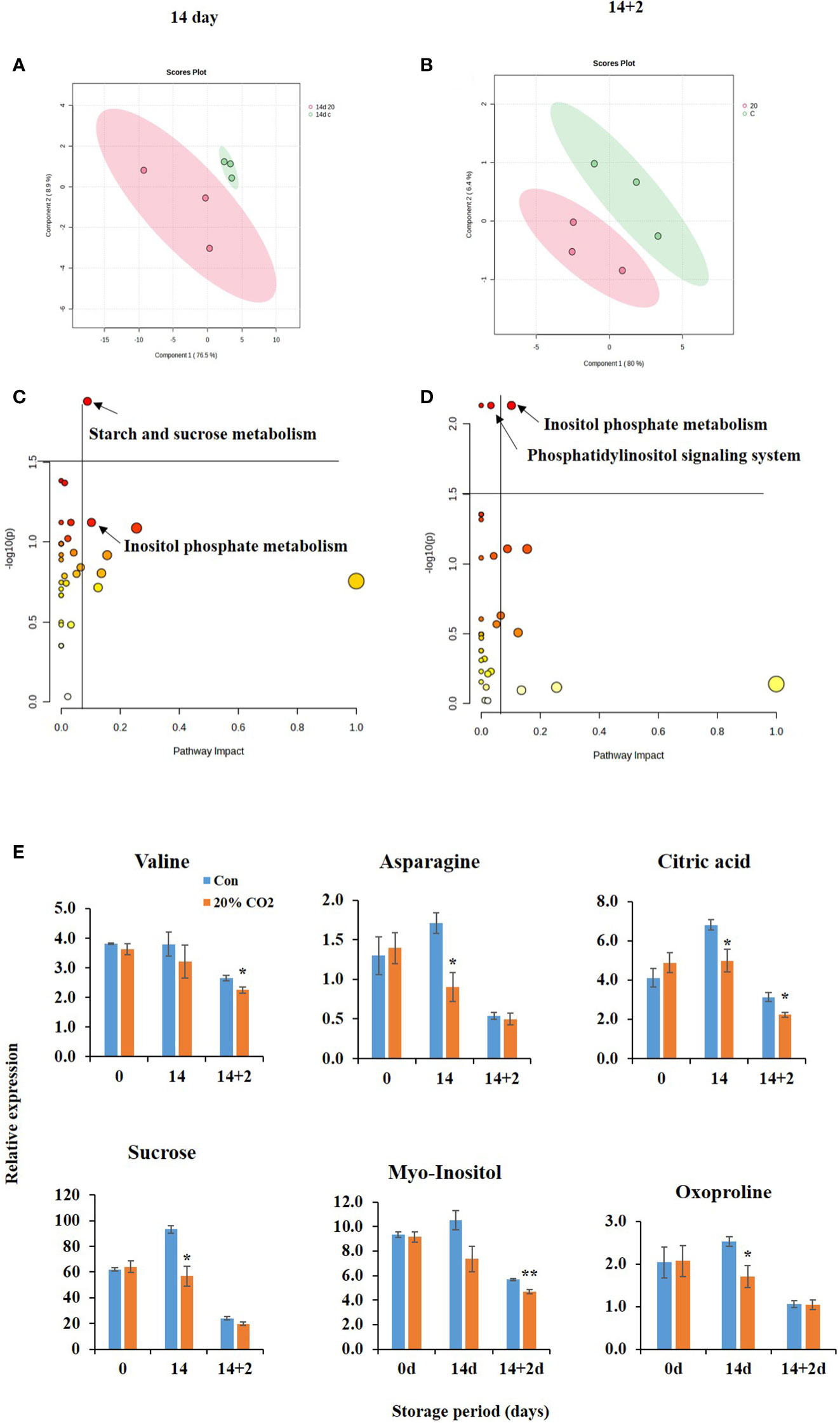
Figure 3 Metabolic changes in of CO2-treated paprika. Scores plot of partial least squares discriminant analysis of water soluble and lipid soluble metabolites at days 14 (A) and 14 + 2 (B); Pathway analysis of water soluble and lipid soluble metabolites on days 14 (C) and 14 + 2 (D); differential accumulation of metabolites (E) in paprika treated with CO2 and stored at 4°C for 14 days, followed by storage for 2 days at 20°C (14 + 2). *p < 0.1, and **p < 0.01.
Further analysis indicated changes in pathways analysis at days 14 and 14 + 2 after CO2 treatment (Figures 3C, D). At day 14, the most significantly affected pathway was starch and sucrose metabolism’ pathway. At day 14 + 2, the most significantly affected pathway was the phosphatidylinositol signaling system and inositol phosphate metabolism. Furthermore, CO2 treatment significantly reduced the levels of the metabolites valine, asparagine, citric acid, sucrose, myoinositol, and oxyproline at days 14 and 14 + 2 after CO2 treatment (Figure 3E), indicating potential alteration of the TCA cycle, electron transport chain, and stress tolerance mechanisms in CO2-treated fruits. Decreased citric acid and sucrose levels in CO2-treated fruits (Figure 3E) suggest their channelizing into GABA shunt pathway.
3.4 Anatomical analysis of pericarp of paprika treated with CO2
In this study, surface pitting was observed on the fruits at day 14 + 2 of storage (Figure 1C). Microscopic examination of cross-sections of pericarp tissues of the fruits at days 0 and 14 + 2 showed that the epidermis and hypodermis of the pericarp tissues appeared to have a compact cell size and shrinking cell morphology, suggesting severe water loss in the hypodermal layer (Figure 4). Notably, hypodermal cells were substantially shirked and appeared to collapse compared with epidermal cells (Figure 4), suggesting cell membrane impairment in hypodermal cells during long-term low-temperature storage.
4 Discussion
4.1 Short-term CO2 treatment enhances postharvest quality and reduces CI in paprika
The primary CI symptoms in paprika such as surface pitting, calyx discoloration remains a major concern, leading to substantial economic losses. CI is initiated in fruits exposed to cold temperatures; however, the symptoms are more evident when the fruits are shifted from cold storage temperatures to non-chilling temperatures (Biswas et al., 2016). In the present study, short-term treatment with 20 and 30% CO2 for 3 h prior to cold storage delayed ripening progression, enhanced firmness, reduced weight loss, and minimized surface pitting (Figure 1), which was consistent with previous findings in CO2-treated crops, including tomatoes, strawberries, persimmons, peaches, and sweet peppers (Besada et al., 2015; Eum et al., 2021; Park et al., 2021; Tilahun et al., 2022; Afolabi et al., 2023). Notably, our study differs from previous approaches that utilized longer treatment durations, ranging from 6 to 48 h or continuous. Although the effect of CO2 treatment for 3 h on tomato and strawberries has been previously examined, this is the first study to best of our knowledge to examine the effects of short-term CO2 treatment on postharvest quality and CI in paprika. The results of the present study are attributed to the ability of CO2 to modulate respiration rates and ethylene production, as observed in studies on tomatoes and other fruits (Kubo et al., 1989; Taye et al., 2017; Park et al., 2021; Tilahun et al., 2022).
4.2 Short-term CO2 treatment activates genes associated with phosphatidic acid biosynthesis and stress response
Transcriptomic and metabolomic analyses revealed the intricate molecular responses triggered by CO2 treatment in paprika. Specifically, CO2 treatment activated specific DEGs and metabolites associated with lipid processes and stress responses, shedding light on the underlying mechanisms. Particularly, CO2 treatment activated genes involved in phosphatidic acid (PA) biosynthesis, a central precursor for glycerophospholipids, galactolipids, and triacylglycerol (TAG) biosynthesis; moreover, PA plays a pivotal role in cellular responses to stress conditions (Hong et al., 2016; Perlikowski et al., 2016; Yu et al., 2019; Wu et al., 2022). PA is produced through the acylation of lysophosphatidic acid (LPA), which is derived from glycerol 3-phosphate by the enzyme glycerol 3-phosphate acyltransferase (GPAT) (Nakamura, 2017). PA biosynthesis via acylation steps is the start of the glycerolipid de novo biosynthesis. In this study, CO2 treatment induced the expression of GPAT (Table 1), indicating the induction of PA production in these fruits. Alternatively, PA levels are controlled by phospholipase (PL) D and PLC-DGK (diacylglycerol kinase) pathways, involving PLs, phosphates, and lipid kinases (Wu et al., 2022). In the PLD pathway, the structural phospholipids are hydrolyzed by PLD to produce PA and soluble head groups (Wu et al., 2022). In the PLC-DGK pathway, PLC acts on phosphatidylinositol 4,5-bisphosphate (PtdInsP2) to produce DAG and inositol phosphate (IP) 3. Notably, DAG can be phosphorylated by DGK to form PA (Wu et al., 2022). Additionally, PA can be dephosphorylated back into DAG by lipid phosphate phosphatases (LPP) (Craddock et al., 2017; Su et al., 2021). In this study, CO2 treatment enhanced the expression of PLDδ, DGK5s, and LPP3, indicating the activation of PLD and PLC-DGK pathways. These pathways are the two principal routes that produce signaling PA and have been extensively studied for their early response to cold stress (Vergnolle et al., 2005; Wu et al., 2022). For instance, PLDs and DGKs were responsive to low temperature in peppers (Kong et al., 2019). Deactivating PLDδ makes Arabidopsis plants more sensitive to freezing, while its overexpression enhances freezing tolerance (Li et al., 2004). Furthermore, DAG can serve as a substrate for the synthesis of various lipids, including membrane phospholipids and TAGs. Diacylglycerol O-acyltransferase 1 (DGAT) transfers a fatty acyl group from a fatty acyl-CoA molecule to a DAG molecule, resulting in the formation of TAG (Wu et al., 2022). In this study, the expression of DEGs encoding DGAT and oleosins, the structural proteins of TAGs, were induced in the CO2-treated fruits (Table 1; Shimada et al., 2008). The dgat1 mutant lines exhibited reduced cold tolerance, and DAG and PA levels were significantly increased in Arabidopsis (Tan et al., 2018). Moreover, the dynamic balance of PA, DAG, and TAG is an important protective strategy to combat freezing temperatures (Tan et al., 2018).
The ICE–CBF/DREB1 transcriptional cascade has been extensively studied for its role in cold signaling (Chinnusamy et al., 2007). ICE1 transcription factor directly activates cold-responsive genes by binding to cis-elements in the CBF3/DREB1a and CBF2 (DREB1C) promoters (Chinnusamy et al., 2007). In the present study, DREB1A and DREB1C were expressed at day 0 after CO2 treatment (Table 2; Figure 2E), indicating the potent impact of CO2 treatment on the early activation of crucial components of the ICE–CBF/DREB1 pathway. Moreover, PLC and PLD pathways function upstream of the ICE–CBF/DREB1 pathway (Vergnolle et al., 2005). These results indicate that CO2 treatment may trigger ICE–CBF/DREB1 pathway to regulate chilling stress. Furthermore, the jasmonate signaling pathway acts as a pivotal upstream regulator of the ICE–CBF/DREB1 pathway, and plays a pivotal role in enhancing freezing tolerance in Arabidopsis (Hu et al., 2013). Remarkably, CO2 treatment induced the expression of jasmonate synthesis-related genes, specifically alpha-dioxygenase 1, 9-divinyl ether synthase (DES), 9S-lipoxygenase (LOX5), in the treated fruits (Table 1; Figure 2E). The concurrent induction of DREBs and jasmonic acid biosynthesis-related genes strongly suggests the activation of the ICE–CBF pathway following CO2 treatment. Moreover, CO2 treatment triggered the expression of known stress-responsive genes, such as peroxidase 32, heatshok protein 70, pathogenesis related proteins (Table 2; Figure 2E), and promoted stress defense mechanisms. The upregulation of stress-related genes may have amplified antioxidant enzyme activity, as confirmed by ABTS and DPPH assays, and enhanced polyphenol content (Supplementary Figure S2). Overall, these results indicate that CO2 enhances resistance to freezing stress in fruits through a multifaceted approach, thereby improving postharvest quality.
Intricate stress signaling might induce lipid processes, as evidenced by enhanced expression of the membrane integrity associated gene fatty acid desaturases (FADs) in CO2-treated fruits (Table 1; Figure 2E), leading to improved membrane stability (Figure 4), which may contribute to extending the shelf life of the fruits. This is of particular significance, considering that the balance of unsaturated fatty acids within the lipid bilayer plays a pivotal role in plant responses to CI (Zhang and Tian, 2010). Moreover, heterologous overexpression of Eriobotrya japonica’s FAD8 in Arabidopsis increased the expression of ICE-CBF-cold regulated genes in response to low temperatures (Xu et al., 2023), indicating intricate interaction between lipid process and stress signaling during chilling stress.
4.3 Short-term CO2 treatment activates metabolites associated with inositol phosphate metabolism and starch and sucrose metabolism
At day 14 of cold storage, starch and sucrose metabolism were remarkably altered, suggesting that high CO2 exposure may play a role in modulating carbohydrate metabolism in CO2-treated paprika during cold storage. Starch and sucrose, the principal carbohydrates in plants, play critical roles in energy storage and transfer (Zhu et al., 2023), and alterations in this pathway indicates that short-term CO2 treatment increases the tricarboxylic acid (TCA) cycle, potentially affecting fruit respiration. Exposing agricultural produce to elevated levels of CO2, especially for short durations may promote anaerobic metabolism or stress responses. Under these conditions, a reduction in mitochondrial respiration, which in turn limits the availability of ATP for energy-demanding processes. In response to this energy crisis, fruit metabolism adapts by enhancing substrate-level ATP production. This adaptation involves various processes, including the breakdown of soluble sugars and the degradation of starch (Gorin et al., 1978; Planchet et al., 2017; Brizzolara et al., 2020).
Terzoudis et al. (2022) reported that a decrease in oxygen levels was associated with reduced levels of sucrose, citrate, and valine in postharvest peach fruits. Furthermore, under anaerobic conditions, certain amino acids, including valine, can be metabolized via the branched-chain amino acid degradation pathway to produce compounds that feed into the TCA cycle or the electron transport chain (Araújo et al., 2011). This might lead to a decrease in valine levels in CO2-treated fruits (Figure 3E). Another possible explanation is that a sudden elevation in CO2 may affect protein synthesis and degradation in cells (Brizzolara et al., 2020). Notably, Tanou et al. (2017) reported differential accumulation of valine was associated with CI tolerance in peach fruits. Similarly, the reduction in citric acid and sucrose levels can be interpreted as continuous metabolism under elevated CO2 levels. Although it is known that high levels of CO2 and low O2 inhibit cell respiration (Kubo et al., 1989), metabolism might increase through the GABA shunt pathway under low O2 conditions (Li et al., 2021).
IPs and phosphatidylinositol (PI) signaling pathways are involved in several biological processes, including chilling stress. IP3 is generated by PLC-mediated hydrolysis of PtdInsP2, and triggers a set of cellular processes by releasing calcium, which acts as a secondary messenger to transduce cold signals (Sun et al., 2021). In this study, inositol phosphate metabolism was identified at both days 14 and 14 + 2, emphasizing its importance and potential sensitivity to CO2 exposure. Phosphatidylinositol signaling is pivotal for various cellular processes, including cell growth, differentiation, and motility (Sun et al., 2021). The phosphatidylinositol signaling system and inositol phosphate metabolic pathways are linked to freezing tolerance and CI regulation in plants (Zakharian et al., 2010; Sun et al., 2021). Notably, metabolite changes in CO2-treated fruits were associated with phosphatidylinositol signaling and inositol phosphate metabolism (Figure 3D), suggesting their potential role in CO2-induced chilling tolerance in paprika.
Notably, the cellular response to CO2 in non-climacteric fruits appears to differ from that in climacteric fruits. For instance, treatment with 30% CO2 for 3 h maintained the quality of tomatoes and protected against CI through associated with transcriptional changes in ethylene-related genes and respiratory-related metabolism (Park et al., 2021), whereas CO2 treatment maintained the quality of paprika, a non-climacteric fruit, by modulating mainly lipid-related processes, stress responses, and metabolism of starch and inositol phosphates.
5 Conclusion
Short-term CO2 treatment reduced CI and improved postharvest quality in paprika by activating PA synthesis and its signaling via the PLD and PLC–DK pathways, inducing stress signaling via the ICE–CBF pathway, and enhancing lipid processes and antioxidant defense mechanisms, thereby promoting membrane stability (Figure 5). Overall, these findings contribute to the advancement of innovative strategies for preserving postharvest quality in paprika and minimizing losses. However, further studies are necessary to examine the effects of short-term CO2 treatment on the postharvest quality of other crop species under cold storage.
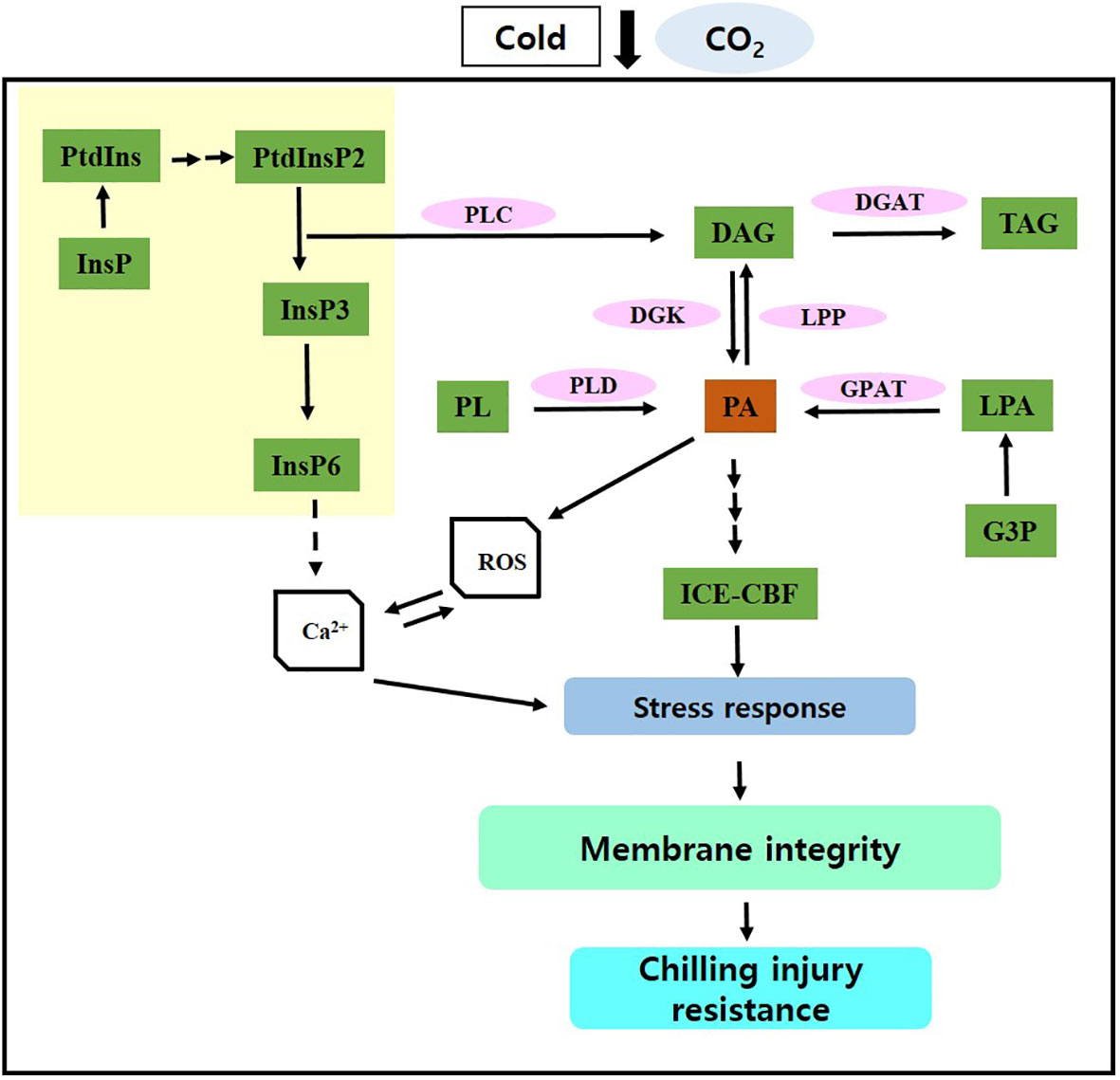
Figure 5 Potential molecular mechanism through which CO2 maintains postharvest quality and protects against chilling injury. InsP, inositol phosphate; PtdIns, phosphatidylinositol; PtdInsP, phosphatidylinositol phosphate; PL, phospholipid; PLD, phospholipase D; PLC, phospholipase C; PA, phosphatidic acid; DAG, diacylglycerol; TAG, triacylglycerol; G3P, glycerol-3-phosphate, LPA, lysophosphatidic acid; DGK, DAG kinase; GPAT, G3P acyltransferase; LPP, LPA phosphatase. Solid arrows represent established pathways; dashed arrows indicate potential pathways; array of arrows indicate multiple steps; yellow region indicate inositol phosphate metabolism.
Data availability statement
The datasets presented in this study can be found in online repositories. The names of the repository/repositories and accession number(s) can be found here: https://www.ncbi.nlm.nih.gov/bioproject/PRJNA1019830/.
Author contributions
M-HP: Conceptualization, Funding acquisition, Methodology, Project administration, Resources, Supervision, Validation, Visualization, Writing – review & editing. KK: Data curation, Methodology, Supervision, Writing – original draft. K-RD: Data curation, Writing – original draft. HE: Data curation, Software, Writing – original draft. JC: Data curation, Methodology, Writing – original draft. PP: Data curation, Investigation, Resources, Writing – original draft. SM: Conceptualization, Data curation, Formal Analysis, Investigation, Methodology, Software, Supervision, Validation, Visualization, Writing – original draft, Writing – review & editing.
Funding
The author(s) declare financial support was received for the research, authorship, and/or publication of this article. This study was funded by the Cooperative Research Program for Agriculture, Science, and Technology (Project No. PJ01601102) in the Rural Development Administration of the Republic of Korea.
Conflict of interest
The authors declare that the research was conducted in the absence of any commercial or financial relationships that could be construed as a potential conflict of interest.
Publisher’s note
All claims expressed in this article are solely those of the authors and do not necessarily represent those of their affiliated organizations, or those of the publisher, the editors and the reviewers. Any product that may be evaluated in this article, or claim that may be made by its manufacturer, is not guaranteed or endorsed by the publisher.
Supplementary material
The Supplementary Material for this article can be found online at: https://www.frontiersin.org/articles/10.3389/fpls.2023.1287997/full#supplementary-material
References
Afolabi, A. S., Choi, I.-L., Lee, J. H., Kwon, Y. B., Yoon, H. S., Kang, H.-M. (2023). Effect of pre-storage CO2 treatment and modified atmosphere packaging on sweet pepper chilling injury. Plants 12 (3), 671. doi: 10.3390/plants12030671
Araújo, W. L., Tohge, T., Ishizaki, K., Leaver, C. J., Fernie, A. R. (2011). Protein degradation–an alternative respiratory substrate for stressed plants. Trends Plant Sci. 16 (9), 489–498. doi: 10.1016/j.tplants.2011.05.008
Besada, C., Llorca, E., Novillo, P., Hernando, I., Salvador, A. (2015). Short-term high CO2 treatment alleviates chilling injury of persimmon cv. Fuyu by preserving the parenchyma structure. Food Control 51, 163–170. doi: 10.1016/j.foodcont.2014.11.013
Biswas, P., East, A. R., Hewett, E. W., Heyes, J. A. (2016). “Chilling injury in tomato fruit,” in Horticultural reviews, vol. 44, 229–278. doi: 10.1002/9781119281269.ch5
Brizzolara, S., Manganaris, G. A., Fotopoulos, V., Watkins, C. B., Tonutti, P. (2020). Primary metabolism in fresh fruits during storage [Review]. Front. Plant Sci. 11. doi: 10.3389/fpls.2020.00080
Chinnusamy, V., Zhu, J., Zhu, J.-K. (2007). Cold stress regulation of gene expression in plants. Trends Plant Sci. 12 (10), 444–451. doi: 10.1016/j.tplants.2007.07.002
Clément, C., Burrus, M., Audran, J. C. (1996). Floral organ growth and carbohydrate content during pollen development in Lilium. Am. J. Bot. 83 (4), 459–469. doi: 10.1002/j.1537-2197.1996.tb12727.x
Craddock, C. P., Adams, N., Kroon, J. T., Bryant, F. M., Hussey, P. J., Kurup, S., et al. (2017). Cyclin-dependent kinase activity enhances phosphatidylcholine biosynthesis in Arabidopsis by repressing phosphatidic acid phosphohydrolase activity. Plant J. 89 (1), 3–14. doi: 10.1111/tpj.13321
del Olmo, I., Romero, I., Alvarez, M. D., Tarradas, R., Sanchez-Ballesta, M. T., Escribano, M. I., et al. (2022). Transcriptomic analysis of CO2-treated strawberries (Fragaria vesca) with enhanced resistance to softening and oxidative stress at consumption [Original Research]. Front. Plant Sci. 13. doi: 10.3389/fpls.2022.983976
Dennis, G., Sherman, B. T., Hosack, D. A., Yang, J., Gao, W., Lane, H. C., et al. (2003). DAVID: database for annotation, visualization, and integrated discovery. Genome Biol. 4 (9), 1–11. doi: 10.1186/gb-2003-4-9-r60
Eum, H.-L., Han, S.-H., Lee, E.-J. (2021). High-CO2 treatment prolongs the postharvest shelf life of strawberry fruits by reducing decay and cell wall degradation. Foods 10 (7), 1649. doi: 10.3390/foods10071649
Gorin, N., Bonisolli, F., Heidema, F. T., Klop, W., Williams, A. A. (1978). Changes in starch content and amylase zymograms during storage of Golden Delicious and Cox's Orange Pippin apples. Z. fur Lebensmittel-untersuchung und-forschung 166 (3), 157–161. doi: 10.1007/BF01354808
Hong, Y., Zhao, J., Guo, L., Kim, S.-C., Deng, X., Wang, G., et al. (2016). Plant phospholipases D and C and their diverse functions in stress responses. Prog. Lipid Res. 62, 55–74. doi: 10.1016/j.plipres.2016.01.002
Hu, Y., Jiang, L., Wang, F., Yu, D. (2013). Jasmonate regulates the inducer of CBF expression–c-repeat binding factor/DRE binding factor1 cascade and freezing tolerance in Arabidopsis. Plant Cell 25 (8), 2907–2924. doi: 10.1105/tpc.113.112631
Kim, D., Langmead, B., Salzberg, S. L. (2015). HISAT: a fast spliced aligner with low memory requirements. Nat. Methods 12 (4), 357–360. doi: 10.1038/nmeth.3317
Kong, X.-m., Zhou, Q., Luo, F., Wei, B.-d., Wang, Y.-j., Sun, H.-j., et al. (2019). Transcriptome analysis of harvested bell peppers (Capsicum annuum L.) in response to cold stress. Plant Physiol. Biochem. 139, 314–324. doi: 10.1016/j.plaphy.2019.03.033
Kubo, Y., Inaba, A., Nakamura, R. (1989). Effects of high CO2 on respiration in various horticultural crops. J. Japanese Soc. Hortic. Sci. 58 (3), 731–736. doi: 10.2503/jjshs.58.731
Li, W., Li, M., Zhang, W., Welti, R., Wang, X. (2004). The plasma membrane–bound phospholipase Dδ enhances freezing tolerance in Arabidopsis thaliana. Nat. Biotechnol. 22 (4), 427–433. doi: 10.1038/nbt949
Liao, Y., Smyth, G. K., Shi, W. (2014). featureCounts: an efficient general purpose program for assigning sequence reads to genomic features. Bioinformatics 30 (7), 923–930. doi: 10.1093/bioinformatics/btt656
Lim, C. S., Kang, S. M., Cho, J. L., Gross, K. C., Woolf, A. B. (2007). Bell Pepper (Capsicum annuum L.) Fruits are Susceptible to Chilling Injury at the Breaker Stage of Ripeness. HortScience horts 42 (7), 1659–1664. doi: 10.21273/hortsci.42.7.1659
Lisec, J., Schauer, N., Kopka, J., Willmitzer, L., Fernie, A. R. (2015). Corrigendum: Gas chromatography mass spectrometry-based metabolite profiling in plants. Nat. Protoc. 10 (9), 1457. doi: 10.1038/nprot0915-1457a
Nakamura, Y. (2017). Plant phospholipid diversity: emerging functions in metabolism and protein–lipid interactions. Trends Plant Sci. 22 (12), 1027–1040. doi: 10.1016/j.tplants.2017.09.002
Park, M.-H., Kim, S.-J., Lee, J.-S., Hong, Y.-P., Chae, S.-H., Ku, K.-M. (2021). Carbon dioxide pretreatment and cold storage synergistically delay tomato ripening through transcriptional change in ethylene-related genes and respiration-related metabolism. Foods 10 (4), 744. doi: 10.3390/foods10040744
Perlikowski, D., Kierszniowska, S., Sawikowska, A., Krajewski, P., Rapacz, M., Eckhardt, Ä., et al. (2016). Remodeling of leaf cellular glycerolipid composition under drought and re-hydration conditions in grasses from the Lolium-Festuca complex. Front. Plant Sci. 7, 1027. doi: 10.3389/fpls.2016.01027
Planchet, E., Lothier, J., Limami, A. M. (2017). Hypoxic respiratory metabolism in plants: reorchestration of nitrogen and carbon metabolisms. Plant respiration: Metab. fluxes carbon balance 43, 209–226. doi: 10.1007/978-3-319-68703-2_10
Rai, A., Kumari, K., Vashistha, P. (2022). Umbrella review on chilling injuries: Post-harvest issue, cause, and treatment in tomato. Scientia Hortic. 293, 110710. doi: 10.1016/j.scienta.2021.110710
Rao, T. V. R., Gol, N. B., Shah, K. K. (2011). Effect of postharvest treatments and storage temperatures on the quality and shelf life of sweet pepper (Capsicum annum L.). Scientia Hortic. 132, 18–26. doi: 10.1016/j.scienta.2011.09.032
Robinson, M. D., McCarthy, D. J., Smyth, G. K. (2010). edgeR: a Bioconductor package for differential expression analysis of digital gene expression data. Bioinformatics 26 (1), 139–140. doi: 10.1093/bioinformatics/btp616
Robinson, M. D., Oshlack, A. (2010). A scaling normalization method for differential expression analysis of RNA-seq data. Genome Biol. 11 (3), 1–9. doi: 10.1186/gb-2010-11-3-r25
Romero, I., Vazquez-Hernandez, M., Escribano, M. I., Merodio, C., Sanchez-Ballesta, M. T. (2016). Expression profiles and DNA-binding affinity of five ERF genes in bunches of Vitis vinifera cv. Cardinal treated with high levels of CO2 at low temperature. Front. Plant Sci. 7, 1748. doi: 10.3389/fpls.2016.01748
Rothan, C., Duret, S., Chevalier, C., Raymond, P. (1997). Suppression of ripening-associated gene expression in tomato fruits subjected to a high CO2 concentration. Plant Physiol. 114 (1), 255–263. doi: 10.1104/pp.114.1.255
Shimada, T. L., Shimada, T., Takahashi, H., Fukao, Y., Hara-Nishimura, I. (2008). A novel role for oleosins in freezing tolerance of oilseeds in Arabidopsis thaliana. Plant J. 55 (5), 798–809. doi: 10.1111/j.1365-313X.2008.03553.x
Song, H.-J., Ku, K.-M. (2021). Optimization of allyl isothiocyanate sanitizing concentration for inactivation of salmonella typhimurium on lettuce based on its phenotypic and metabolome changes. Food Chem. 364, 130438. doi: 10.1016/j.foodchem.2021.130438
Su, W., Raza, A., Zeng, L., Gao, A., Lv, Y., Ding, X., et al. (2021). Genome-wide analysis and expression patterns of lipid phospholipid phospholipase gene family in Brassica napus L. BMC Genomics 22 (1), 1–15. doi: 10.1186/s12864-021-07862-1
Sun, S., Lin, M., Qi, X., Chen, J., Gu, H., Zhong, Y., et al. (2021). Full-length transcriptome profiling reveals insight into the cold response of two kiwifruit genotypes (A. arguta) with contrasting freezing tolerances. BMC Plant Biol. 21 (1), 1–20. doi: 10.1186/s12870-021-03152-w
Tan, W.-J., Yang, Y.-C., Zhou, Y., Huang, L.-P., Xu, L., Chen, Q.-F., et al. (2018). DIACYLGLYCEROL ACYLTRANSFERASE and DIACYLGLYCEROL KINASE modulate triacylglycerol and phosphatidic acid production in the plant response to freezing stress. Plant Physiol. 177 (3), 1303–1318. doi: 10.1104/pp.18.00402
Tanou, G., Minas, I. S., Scossa, F., Belghazi, M., Xanthopoulou, A., Ganopoulos, I., et al. (2017). Exploring priming responses involved in peach fruit acclimation to cold stress. Sci. Rep. 7 (1), 11358. doi: 10.1038/s41598-017-11933-3
Taye, A. M., Tilahun, S., Park, D. S., Seo, M. H., Jeong, C. S. (2017). Effects of continuous application of CO2 on fruit quality attributes and shelf life during cold storage in cherry tomato. Hortic. Sci. Technol. 35 (3), 300–313. doi: 10.12972/kjhst.20170033
Terzoudis, K., Hertog, M. L. A. T. M., Nicolaï, B. M. (2022). Dynamic labelling reveals central carbon metabolism responses to stepwise decreasing hypoxia and reoxygenation during postharvest in pear fruit. Postharvest Biol. Technol. 186, 111816. doi: 10.1016/j.postharvbio.2021.111816
Tilahun, S., Jeong, M. J., Choi, H. R., Baek, M. W., Hong, J. S., Jeong, C. S. (2022). Prestorage high CO2 and 1-MCP treatment reduce chilling injury, prolong storability, and maintain sensory qualities and antioxidant activities of “Madoka” Peach fruit [Original research]. Front. Nutr. 9. doi: 10.3389/fnut.2022.903352
Valenzuela, J. L., Manzano, S., Palma, F., Carvajal, F., Garrido, D., Jamilena, M. (2017). Oxidative stress associated with chilling injury in immature fruit: postharvest technological and biotechnological solutions. Int. J. Mol. Sci. 18 (7), 1467. doi: 10.3390/ijms18071467
Vergnolle, C., Vaultier, M.-N., Taconnat, L., Renou, J.-P., Kader, J.-C., Zachowski, A., et al. (2005). The cold-induced early activation of phospholipase C and D pathways determines the response of two distinct clusters of genes in Arabidopsis cell suspensions. Plant Physiol. 139 (3), 1217–1233. doi: 10.1104/pp.105.068171
Wu, J., Nadeem, M., Galagedara, L., Thomas, R., Cheema, M. (2022). Recent insights into cell responses to cold stress in plants: Signaling, defence, and potential functions of phosphatidic acid. Environ. Exp. Bot. 203, 105068. doi: 10.1016/j.envexpbot.2022.105068
Xu, X., Yang, H., Suo, X., Liu, M., Jing, D., Zhang, Y., et al. (2023). EjFAD8 enhances the low-temperature tolerance of loquat by desaturation of sulfoquinovosyl diacylglycerol (SQDG). Int. J. Mol. Sci. 24 (8), 6946. doi: 10.3390/ijms24086946
Yu, Y., Kou, M., Gao, Z., Liu, Y., Xuan, Y., Liu, Y., et al. (2019). Involvement of phosphatidylserine and triacylglycerol in the response of sweet potato leaves to salt stress. Front. Plant Sci. 10, 1086. doi: 10.3389/fpls.2019.01086
Zakharian, E., Cao, C., Rohacs, T. (2010). Gating of transient receptor potential melastatin 8 (TRPM8) channels activated by cold and chemical agonists in planar lipid bilayers. J. Neurosci. 30 (37), 12526–12534. doi: 10.1523/JNEUROSCI.3189-10.2010
Zhang, C., Tian, S. (2010). Peach fruit acquired tolerance to low temperature stress by accumulation of linolenic acid and N-acylphosphatidylethanolamine in plasma membrane. Food Chem. 120 (3), 864–872. doi: 10.1016/j.foodchem.2009.11.029
Zhu, M., Zang, Y., Zhang, X., Shang, S., Xue, S., Chen, J., et al. (2023). Insights into the regulation of energy metabolism during the seed-to-seedling transition in marine angiosperm Zostera marina L.: Integrated metabolomic and transcriptomic analysis. Front. Plant Sci. 14, 1130292. doi: 10.3389/fpls.2023.1130292
Keywords: Capsicum annum L., chilling injury, membrane integrity, postharvest quality, lipid metabolism, phosphatidic acid, stress response, DREB
Citation: Park M-H, Ku K-M, Do K-R, Eum HL, Cho JH, Park PH and Malka SK (2023) Carbon dioxide treatment modulates phosphatidic acid signaling and stress response to improve chilling tolerance and postharvest quality in paprika. Front. Plant Sci. 14:1287997. doi: 10.3389/fpls.2023.1287997
Received: 03 September 2023; Accepted: 30 October 2023;
Published: 16 November 2023.
Edited by:
Isabel Lara, Universitat de Lleida, SpainReviewed by:
George Manganaris, Cyprus University of Technology, CyprusNatasha Damiana Spadafora, University of Calabria, Italy
Copyright © 2023 Park, Ku, Do, Eum, Cho, Park and Malka. This is an open-access article distributed under the terms of the Creative Commons Attribution License (CC BY). The use, distribution or reproduction in other forums is permitted, provided the original author(s) and the copyright owner(s) are credited and that the original publication in this journal is cited, in accordance with accepted academic practice. No use, distribution or reproduction is permitted which does not comply with these terms.
*Correspondence: Siva Kumar Malka, bWFsa2FAa29yZWEua3I=