- 1Department of Biological Sciences, Graduate School of Science, The University of Tokyo, Tokyo, Japan
- 2Department of Biology, Faculty of Science, Chiba University, Chiba, Japan
- 3Department of Biology, Graduate School of Science, Chiba University, Chiba, Japan
- 4Center for Molecular Biodiversity Research, National Museum of Nature and Science, Tsukuba, Ibaraki, Japan
Allopolyploidization often leads to disruptive conflicts among more than two sets of subgenomes, leading to genomic modifications and changes in gene expression. Although the evolutionary trajectories of subgenomes in allopolyploids have been studied intensely in angiosperms, the dynamics of subgenome evolution remain poorly understood in ferns, despite the prevalence of allopolyploidization. In this study, we have focused on an allotetraploid fern—Phegopteris decursivepinnata—and its diploid parental species, P. koreana (K) and P. taiwaniana (T). Using RNA-seq analyses, we have compared the gene expression profiles for 9,540 genes among parental species, synthetic F1 hybrids, and natural allotetraploids. The changes in gene expression patterns were traced from the F1 hybrids to the natural allopolyploids. This study has revealed that the expression patterns observed in most genes in the F1 hybrids are largely conserved in the allopolyploids; however, there were substantial differences in certain genes between these groups. In the allopolyploids compared with the F1 hybrids, the number of genes showing a transgressive pattern in total expression levels was increased. There was a slight reduction in T-dominance and a slight increase in K-dominance, in terms of expression level dominance. Interestingly, there is no obvious bias toward the T- or K-subgenomes in the number and expression levels overall, showing the absence of subgenome dominance. These findings demonstrated the impacts of the substantial transcriptome change after hybridization and the moderate modification during allopolyploid establishment on gene expression in ferns and provided important insights into subgenome evolution in polyploid ferns.
1 Introduction
Polyploidy, or whole genome duplication, is pervasive in eukaryotes (Van De Peer et al., 2017). It is especially common in plants (Wood et al., 2009), and ancient polyploidization has occurred in most lineages of land plants including mosses (Gao et al., 2022), lycophytes (Xia et al., 2022; Li et al., 2023), ferns (Huang et al., 2020; Pelosi et al., 2022; Chen et al., 2023), gymnosperms (Li et al., 2015), and angiosperms (e.g. Landis et al., 2018). Polyploids are generally categorized into two types: autopolyploids, which arise from simple genome duplication within a single species, and allopolyploids, which result from the merging of entire genomes from more than two species. Allopolyploids possess evolutionary advantages over autopolyploids due to the benefits of both genome duplication and hybridization (Barker et al., 2016). However, the merging of formerly independent genomes may also result in genomic obstacles for allopolyploid species.
Newly formed allopolyploids often experience various deleterious effects due to the rapidity of the genomic change, commonly referred to as “genomic shock” (McClintock, 1984) and “transcriptomic shock” (Hegarty et al., 2006; Buggs et al., 2011). These shocks lead to further genomic modifications and reorganization. The abrupt change in ploidy immediately increases DNA content, resulting in physical changes, such as expanded cell size (Beaulieu et al., 2008) and delayed cell cycle progression (Šímová and Herben, 2012). Genome duplication may also cause dosage imbalances that adversely affect the regulation of dosage-sensitive genes. Beyond the effects of simple genome duplication, allopolyploids may also suffer from the effect of hybridization—a disruptive conflict between the two genomes that have evolved independently but are now merged into one genome. This conflict ranges from negative epistatic interactions (Orr, 1996) and regulatory interference between genes (Kaltenegger and Ober, 2015) to alterations in the DNA methylation pattern (Song and Chen, 2015; Rigal et al., 2016) and changes in gene expression (Yoo et al., 2014). Polyploids must mitigate the adverse effects of polyploidization and hybridization to enable their survival and long-term success (Hegarty and Hiscock, 2008). The necessity for this can trigger subsequent transcriptomic and genomic reorganizations. These reorganizations encompass modifications of gene expression, neo- and sub-functionalization (Lynch and Conery, 2000; Birchler and Yang, 2022), or loss of the duplicated genes (De Smet et al., 2013), and genome shrinkage (Wang et al., 2021), ultimately resulting in complete diploidization of the duplicated genomes (Mandáková and Lysak, 2018; Li et al., 2021).
The aforementioned genomic changes usually initially start with changes in gene expression in response to genomic duplication and hybridization (Hegarty et al., 2006; Buggs et al., 2011; Yoo et al., 2014), and the patterns may vary among polyploids derived from phylogenetically distinct groups. There are two major phenomena that have been broadly reported across taxa (Grover et al., 2012). The first is “expression level dominance (ELD),” which is the phenomenon where the total expression level of a pair of homoeologous genes is similar to that exhibited by only one of the two diploid parents (Rapp et al., 2009; Flagel and Wendel, 2010; Bardil et al., 2011; Sigel et al., 2019; Chen et al., 2021). The second is “homoeolog expression bias (HEB),” which is the phenomenon where two homoeologous genes are expressed unequally compared to prior expectations based on the progenitor diploid expression levels (Chaudhary et al., 2009; Koh et al., 2010; Edger et al., 2017; Sigel et al., 2019; Chen et al., 2021; Vasudevan et al., 2023). Among the studies focusing on angiosperms, while some studies reported that ELD and/or HEB occur randomly on the homoeologous genes from both parental subgenomes (e.g. Boatwright et al., 2018; Boatwright et al., 2021; Burns et al., 2021), the other studies revealed that ELD and/or HEB tend to preferentially biased towards the subgenome from one parental species (e.g. Chaudhary et al., 2009; Koh et al., 2010; Edger et al., 2017; Chen et al., 2021; Vasudevan et al., 2023). The latter phenomenon, known as “subgenome dominance” (Bird et al., 2018; Cheng et al., 2018), is expected to accelerate genome shrinkage by causing a more pronounced fractionation of the recessive subgenome due to relaxed selective constraints. In contrast, gymnosperms, characterized by their highly stable and large genomes, show no subgenome dominance in the cases examined to date (Wu et al., 2021). Consequently, patterns of gene expression that change in subgenomes following polyploidization may differ across several aspects, including the scale and the direction, among different land plant lineages that exhibit the distinct consequences of genomic evolution.
Ferns exhibit the highest frequencies of polyploidization and hybridization among vascular plants (Whitney et al., 2010), with more than 30% of speciation events in ferns estimated to involve polyploidy (Wood et al., 2009). Not only does the frequent formation of neo-polyploids contribute to the statistics, but there is also increasing evidence for paleo-polyploid origins in the majority of lineages (Huang et al., 2020; Pelosi et al., 2022; Chen et al., 2023). However, the genomic consequences of polyploid evolution in ferns appear to differ from those in angiosperms (Barker, 2013). In angiosperms, most lineages conserve small genome sizes and chromosome numbers despite frequent WGDs in the evolutionary history, suggesting fast diploidization including dysploidal reduction and genome downsizing in this group (Wang et al., 2021). In contrast to angiosperms, in the ferns, the genome is generally highly stable in terms of chromosome number and genome size (Clark et al., 2016). The evolutionary trajectory of the polyploid fern genome is thought to be characterized by slow reductions in chromosome numbers and gradual genome downsizing (Barker, 2013; Haufler, 2014). This is exemplified by the positive correlation between chromosome number and genome size observed in whole ferns (Clark et al., 2016; Fujiwara et al., 2023). These differences in the macroevolutionary patterns of genome evolution between angiosperms and ferns indicate the potential variations in the short-term consequences of gene expression changes in their subgenomes following allopolyploid formation. However, our current understanding of ferns from this perspective remains limited, as gene expression patterns have been examined for only one allotetraploid fern to date (Sigel et al., 2019). Sigel et al. demonstrated that the allotetraploid fern, Polypodium hesperium Maxon, exhibits a strong subgenome dominance, with the number of genes showing ELD and HEB being extremely biased toward one parental subgenome. However, it remains unclear whether the reported pattern is universal across all allopolyploid ferns. Additionally, as the study explored the transcriptome-wide patterns only through comparisons of the parental species and allopolyploids, it is unknown whether the observed pattern was primarily driven by the effects of hybridization or polyploidization (Hegarty et al., 2006; Edger et al., 2017; Duan et al., 2023). To achieve a comprehensive understanding of gene expression changes during allopolyploidization in ferns, more case studies involving diverse allopolyploid fern species will be required. Furthermore, comparing expression patterns between homoploid hybrids and allopolyploids of the same parental species pair could help to distinguish the effects of interspecific hybridization and those of the events that follow polyploidization.
To explore gene expression pattern changes in response to hybridization and polyploidization in ferns, we have focused on Phegopteris decursivepinnata (H. C. Hall) Fée. This species, which is tetraploid (2n = 4x = 120), is mainly distributed across East Asia. While this fern was previously presumed to be an autopolyploid of a diploid P. decursivepinnata strain (Nakato et al., 2012; Kawakami et al., 2019), a recent phylogenetic study revealed that the species is actually an allotetraploid resulting from hybridization between P. koreana B. Y. Sun & C. H. Kim (previously treated as diploid P. decursivepinnata) (2n = 2x = 60) and P. taiwaniana T. Fujiw., Ogiso & Seriz. (2n = 2x = 60) (Fujiwara et al., 2021). Although the two parental species occupy different ecological niches, cool temperate forests and warm temperate to subtropical forests, they are in close geographical proximity to each other in the Japanese archipelago. Considering that both parental species still exist and are geographically close to the allopolyploid species, it is speculated that the two parental species hybridized, producing the allotetraploid relatively recently. Therefore, it provides an opportunity to examine the initial gene expression changes that occur in allopolyploid speciation. This species is also relatively easy to cultivate in a laboratory setting, as evidenced by previous studies on the mating system of gametophytes and artificial manipulation of ploidy (Masuyama, 1979; Masuyama, 1986; Kawakami et al., 2019; Nakato and Masuyama, 2021). Artificial F1 hybrids can also be created between the parental species, facilitating comparisons with natural allopolyploids. This study system thus offers the potential to discern the effects of hybridization and polyploidization on gene expression patterns in allopolyploid species.
In this study, we have conducted a comparison of the transcriptome profiles to investigate gene expression changes between the F1 hybrids and natural allopolyploids. Because there is no available published genome for Phegopteris, we construct de-novo reference transcriptomes using only single-copy genes with a one-to-one correspondence between the parental species and focused on co-expressed genes among the parental species, F1 hybrids, and allopolyploids. Using the dataset, we aimed to clarify the evolutionary implications of hybridization and polyploidization on gene expression changes. In particular, the phenomena of ELD and HEB were assessed to explore the evolutionary trends that occur during fern subgenome evolution.
2 Materials and methods
2.1 Plant materials
To create F1 hybrids, gametophytes were cultivated from the parental diploid species, Phegopteris koreana and P. taiwaniana, on agar media in petri dishes. The spores sown were collected from two samples of P. koreana and two samples of P. taiwaniana (Supplementary Table S1). The culture conditions were primarily based on those outlined by Watano and Masuyama (1991). From each of the two species, one gametophyte (total of two) was transplanted into the same well of a 12-well petri dish. Sporophytic outcrossing or gametophytic selfing (Haufler et al., 2016) was then promoted with the addition of two to three drops of sterilized water to each well once a week. The resulting sporophytes were transferred into pots individually and cultivated in the experimental field at the Nishi-chiba campus of Chiba University. To evaluate the hybridity of the produced sporophytes, Sanger sequencing was conducted for the plastid DNA marker, rbcL, and the single-copy nuclear gene, PgiC. Subsequently, phylogenetic analyses were performed for each gene by incorporating the sequences into the previous sequence matrix used by Fujiwara et al. (2021). The experimental procedures, from PCR to phylogenetic analysis, followed the methods described by Fujiwara et al. (2021). As a result, three F1 hybrid individuals with P. koreana as a maternal parent (referred as to F1K) and two F1 hybrid individuals with P. taiwaniana as a maternal parent (referred as to F1T) were obtained. The maternal diploid ancestor of the allotetraploid P. decursivepinnata has been proven to be P. koreana (Fujiwara et al., 2021), and thus the F1K hybrids were verified to be the products of crossing in the same direction as P. decursivepinnata, while the F1T hybrids were in the opposite direction. The sporophytes genetically identified as F1 hybrids were used for subsequent analyses (Supplementary Table S2).
Sporophytes of the parental diploid species, Phegopteris koreana and P. taiwaniana, were also produced by artificial fertilization of the gametophytes. This was necessary as the parental sporophytes of the F1 hybrids (Supplementary Table S1) were not cultured. Two gametophytes of the same species were transplanted into the same well of a 12-well petri dish, and sporophytic selfing or gametophytic selfing was promoted as previously described. Genotypes of the resulting sporophytes were determined using the same methods as described for F1 hybrid identification. Ultimately, three P. koreana and three P. taiwaniana sporophytes were selected for subsequent analyses (Supplementary Table S2).
For the allotetraploid species, Phegopteris decursivepinnata, three sporophyte samples were collected (Supplementary Table S1), and then cultivated in an experimental field alongside those of the F1 hybrids and parental species, for the period from April 2021 to April 2022 prior to RNA extraction.
2.2 RNA extraction
Approximately 2 × 2 cm squares of the unfolding young leaves were collected in April 2021 from three Phegopteris koreana, three P. taiwaniana, and three F1K individuals, and in April to May 2022 from two F1T and three P. decursivepinnata individuals. Additionally, individuals representing each parental species (K1 and T1) were resampled in April 2022 to assess the reproducibility of results across the two years (Supplementary Table S2). The collected leaf samples were rapidly frozen in liquid nitrogen and subsequently crushed using zirconia beads. Total RNA was extracted using the PureLink™ Plant RNA Reagent (ThermoFisher Scientific). The RNA-seq libraries were constructed using NEBNext® Poly(A) mRNA Magnetic Isolation Module (for PolyA selection) and NEBNext® Ultra™ II Directional RNA Library Prep Kit according to the manufacturer’s instructions. Illumina 150 bp paired-end sequencing was performed in NovaSeq6000. The raw read data was deposited in the DDBJ Sequence Read Archive under the accession numbers (DRR498230–DRR498244).
2.3 De novo assembly and ortholog inference among P. koreana, P. taiwaniana, their F1 hybrids, and the allotetraploids
For all RNA-seq reads, adapter sequences and low-quality bases (Q < 20) were trimmed using Trimmomatic v.0.39 (Bolger et al., 2014). After trimming, the filtered reads from biological replicates of each species (Supplementary Table S2) were assembled using Trinity v.2.9.1 (Grabherr et al., 2011). To remove isoform redundancy in the Trinity assembly outputs, the highest expressed isoforms within genes estimated by RSEM (Li and Dewey, 2011) were selected using the “filter_low_expr_transcripts.pl” provided in Trinity v.2.9.1. TransDecoder v.5.5.0 was used to recognize the ORFs, and only the longest ORF per transcript was retained. The obtained sequences were annotated with TAIR IDs based on the best BLASTP hit with a cutoff E-value of 10–5 against the Arabidopsis protein sequence database (Araport11_pep_20220914_representative_gene_model). Organelle-encoded genes were removed from subsequent analyses. After the above filtering steps, orthogroups were constructed among parental species, F1K, F1T, and Phegopteris decursivepinnata using OrthoFinder v.2.5.2 (Emms and Kelly, 2019). The venn diagrams showing the numbers of coexpressed orthologs within orthogroups were drawn using the venn package v1.11 in R software (R Core Team, 2022). The orthogroups containing a single gene each in parental species were used as reference gene sets (Supplementary Table S3).
2.4 Differential expression analysis among species
For the differentially expressed gene (DEG) analyses of the homoeologs in the F1 hybrids and the allotetraploids, the reads of the parental species were mapped to their own reference gene sets and the reads from the F1 hybrids and the allotetraploids were then mapped to the reference genes in both P. koreana and P. taiwaniana using bowtie2 v.2.4.5 (Langmead and Salzberg, 2012). Considering that the F1 hybrids and the allotetraploids retain homoeologs from both parental species, the output bam files from bowtie2 were used as input for the file for EAGLE-RC (Kuo T. et al., 2018), a tool that identifies the parental origin of the reads from the F1 hybrids and the allotetraploids. The number of mapped reads was counted using eXpress v.1.5.1 (Roberts and Pachter, 2013). The edgeR package v.3.40.2 (Robinson et al., 2010) in R, was used to determine the read count data for the replicates and this was normalized using the trimmed mean of M values (TMM) methods, and differential expression analyses were performed between parental species, between the F1 hybrids and each parent, and between the allotetraploids and each parent. In the present study, the DEGs were identified using Fisher’s exact test, with the following criteria: |log2(fold change (FC))| >1 and false discovery rate (FDR) < 0.05. To evaluate the consistency of the trends of the expression patterns of the entire genomes/subgenomes, we conducted the three patterns of transcripts per million (TPM) filtering, TPM > 0.0, TPM > 0.5, and TPM > 1.0, in all five groups.
2.5 Analysis of expression level dominance
Genes identified as DEGs in the F1 hybrids and the allotetraploids relative to their diploid parents were classified into 12 expression-level categories (Figure 1), using the differential expression classification outlined by Rapp et al. (2009). These categories include the following: additivity (I and XII), Phegopteris taiwaniana ELD (T-dominance; II and XI), P. koreana ELD (K-dominance; IV and IX), transgressive expression lower than either parent (Transgressive Down-regulation; III, VII, and X), and transgressive expression higher than either parent (Transgressive Up-regulation; V, VI, and VIII). Genes that did not show significant differential expression levels were categorized as “No Change.” When comparing the F1 hybrids to the allotetraploids, the 12 categories were grouped into five broader categories (K-dominance, T-dominance, Additivity, Transgressive Up-regulation, and Transgressive Down-regulation). The changes among the five differential expression categories from the F1 hybrids to the allotetraploids were visualized using the qgraph package (v.1.9.5) in R.
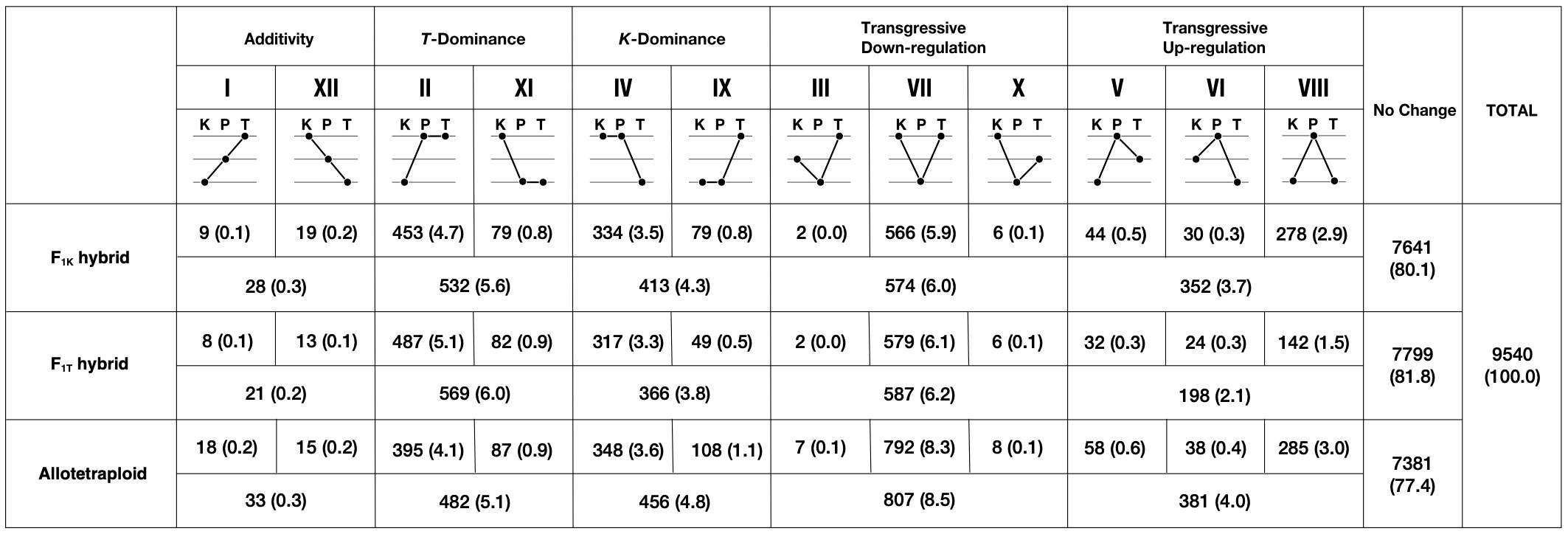
Figure 1 Twelve categories for differential expression states in the F1 hybrids (F1K and F1T) and the allotetraploids, Phegopteris decursivepinnata relative to the diploid parents. Roman numerals indicate categories as described by Rapp et al. (2009). Numbers show the number of genes assigned to each category and the rates are shown in parentheses. Schematic figures show the gene expression levels relative to the parents (K: P. koreana, P: F1K, F1T, or P. decursivepinnata, and T: P. taiwaniana).
2.6 Detection of homoeolog expression bias
Using the edgeR package in R, the expression level ratio, log2(FC), of the orthologous genes from Phegopteris taiwaniana and P. koreana was calculated. This calculation involved comparing the mean count-per-million (CPM) of the alleles from P. taiwaniana (Pt) replicates with the mean CPM of those from the P. koreana replicates (Pk), as follows: log2(Pt_ortholog/Pk_ortholog). The same method was used to determine the expression level ratios of the parental alleles in the F1 hybrids, as follows: log2(F1_T-homoeolog/F1_K-homoeolog), and those of the homoeologs in the allotetraploid P. decursivepinnata (Pd): log2(Pd_T-homoeolog/Pd_K-homoeolog). Subsequently, the distribution of the log2(FC) was compared among the parental species, the F1 hybrids, and the allotetraploids.
The expression bias of the homoeologs in the F1 hybrids and the allotetraploids was analyzed using the existing difference in gene expression levels between the parental species. To achieve this, the ratio of the mean CPM of the orthologous genes in the parental species and the ratio of the mean CPM of homoeologous genes in each of the F1 hybrids or the allotetraploids was compared using Fisher’s exact test. The ratio of the log2 (FC) for the orthologous genes in the parental species and that of the homoeologs in the F1 hybrids was then determined as follows: log2((F1_T-homoeolog/F1_K-homoeolog)/(Pt_ortholog/Pk_ortholog)). Similar comparisons were conducted between the parental species and the allotetraploids, as follows: log2((Pd_T-homoeolog/Pd_K-homoeolog)/(Pt_ortholog/Pk_ortholog)). The ratio of log2(FC) was referred to as the “magnitude” of the bias. The HEB was detected for each of the homoeologous gene pairs using the following criteria: P-value <0.05 and FDR <0.05 with the Fisher’s exact test, and the absolute value of the “magnitude” >1. The homoeologous gene pairs with a “magnitude” >1 and <−1 are considered as HEB toward P. taiwaniana (T-bias) and P. koreana (K-bias), respectively. The relationship of the gene sets exhibiting the HEB between the F1 hybrids and the allotetraploids are shown using Circular layout graphs using the qgraph packages in R. The plots that show the relationship between the log2(FC) values of parental orthologs and those of homoeologs and the histograms showing the difference in the expression bias between the F1 hybrids or the allotetraploids and the parents were generated using the ggplot package in R.
3 Results
3.1 Artificial F1 hybrid production
The artificial crossing experiment successfully produced several sporophyte individuals. The nuclear PgiC tree confirmed that five of these were interspecific F1 hybrids between Phegopteris koreana and P. taiwaniana (Supplementary Figure S1). The plastid rbcL tree showed that there were three and two hybrids with P. koreana and P. taiwaniana as their mother, respectively, given that maternal inheritance of organelle genomes in ferns (reviewed in Kuo L.-Y. et al., 2018) (Supplementary Figure S1; Supplementary Table S2).
3.2 Transcriptome profiles and inference of parental orthologous pairs
Approximately, 45–72 millions of 150 bp pair-end raw reads were sequenced in each studied sample. After filtering the RNA-seq reads for quality, the initial transcripts were assembled and filtered to 26,663–31,796 nuclear genes containing complete or partial ORFs across five groups containing three species and reciprocal F1 hybrids of the parental species (Supplementary Table S3). The ortholog identification using these filtered genes yielded 31,936 orthogroups among the five groups. Of these, 12,380 genes that were detected in both parental species and in which no gene duplication was identified for each parental species, were retained as mapping references for subsequent gene expression analysis (Supplementary Figure S2). To reveal the effects of the low-expressed genes, we performed three levels of TPM filtering for all five groups, and 12,380 orthologous genes were retained with TPM > 0, 9,540 with TPM > 0.5, and 9,003 with TPM > 1.0 (Supplementary Table S3). MDS plot of the expression matrix (Supplementary Figure S3) showed that the replicates of each parental species, F1K hybrids, F1T hybrids, and allotetraploids, Phegoteris decursivepinnnata are closely clustered together, suggesting that the expression pattern is highly consistent among the replicate samples of each group. The samples from each parental species include those collected in different years (2021 and 2022), but all are closely clustered, and there is no apparent influence due to the difference in years. Furthermore, the clustering result is congruent among the datasets with different TPM filtering. In the comparison of expression levels across species, genes showing stable expression should be used because genes that respond to transient environmental changes should be excluded from the analyses. Therefore, we mainly showed the results obtained from the dataset with TPM > 0.5 for the subsequent analysis.
3.3 Expression level dominance in the F1 hybrids and the allotetraploid P. decursivepinnata
To identify additivity, transgressive expression, and ELD in the F1 hybrids and the allotetraploids, in comparison to the expression levels in the parental species, the 9,540 orthologous genes were classified into 12 categories, following the categorization system described by Rapp et al. (2009) (Figure 1). The analysis revealed that most genes from the F1 hybrids and the allotetraploids did not display significant differential expression levels when compared to the orthologs of their parents (denoted as “No Change” in Figure 1; 80.1% in the F1K hybrids, 81.8% in the F1T hybrids, and 77.4% in the allotetraploids), with a lesser proportion being observed in the allotetraploids (2.7% and 4.4% decreases compared to the F1 hybrids). Few differences were found in the count for “No Change” genes between the hybrids with Phegopteris koreana (F1K) and P. taiwaniana (F1T) as their mothers, respectively. For “Additivity,” the category I and XII showed one of the lowest proportions among the all of categories in the F1T hybrids, the F1K hybrids, and the allotetraploids, and the proportion of the categorized genes was almost consistent between the F1 hybrids and the allotetraploids.
For transgressive expression, a substantial number of genes were found to display lower and higher expression levels when compared to both parents (Transgressive Down- and Up-regulation) in the F1 hybrids and the allotetraploids. The number of genes in “Transgressive Down-regulation” always outnumbers the number in “Transgressive Up-regulation” across both the F1 hybrids and the allotetraploids. Interestingly, the number of genes exhibiting transgressive expression was increased in the allotetraploids, and also the difference in the number of genes between “Transgressive Down-regulation” and “Transgressive Up-regulation” became more pronounced in the allotetraploids: from 574 in the F1K hybrids and 587 in the F1T hybrids to 807 genes in the allotetraploids across “Transgressive Down-regulation” categories (III, VII and X), and from 352 in the F1K hybrids and 198 in the F1T hybrids to 381 genes in the allotetraploids across “Transgressive Up-regulation” categories (V, VIII, and VI).
The analyses have also revealed significant trends in ELD. There were significantly more instances where the F1 hybrids and the allotetraploids exhibited the same expression level as the parent with higher expression, regardless of either “T-dominance” or “K-dominance” categories. For instance, for the category “T-dominance” in the F1K hybrids, there were 453 instances where Phegoteirs taiwaniana showed higher expression than P. koreana (category II), compared to only 79 instances where the opposite was observed (category XI). A similar trend was observed in the F1T hybrids and the allotetraploids (Figure 1). In addition, while a higher number of the genes exhibited “T-dominance” when compared to “K-dominance” in both the F1K and F1T hybrids, the allotetraploids showed a similar proportion of the genes exhibiting “T-dominance” and “K-dominance”. In the allotetraploids, 395 and 87 genes in categories II and XI, respectively, exhibited “T-dominance”, and 348 and 108 genes in categories IV and IX, respectively, exhibited “K-dominance”.
The changes in the expression level categories for each gene from the F1K hybrids to the allotetraploids were then examined (Figure 2). The F1K hybrids are the product of crossing in the same direction as the natural allotetraploids. Most genes that were categorized as “No Change” (89.4%), “T-dominance” (63.3%), and “K-dominance” (67.3%) in the F1 hybrids also belonged to the same categories in the allotetraploids. The transition between categories occurred the most frequently between “No Change” and “Transgressive-Up-regulation” or “Transgressive Down-regulation” (208–403 genes). Especially, the transition from “No Change” to “Transgressive Down-regulation” detected almost twice as many cases (403 genes) compared to other transitions between “No Change” and transgressive expressions (208–215 genes). In the genes showing ELD, the number of genes that transitioned between “No Change” and “T-dominance” are almost the same in both directions (71 and 79 genes), while the number of genes that transitioned from “No-Change” to “K-dominance” (92 genes) was almost double that of the other direction (47 genes).
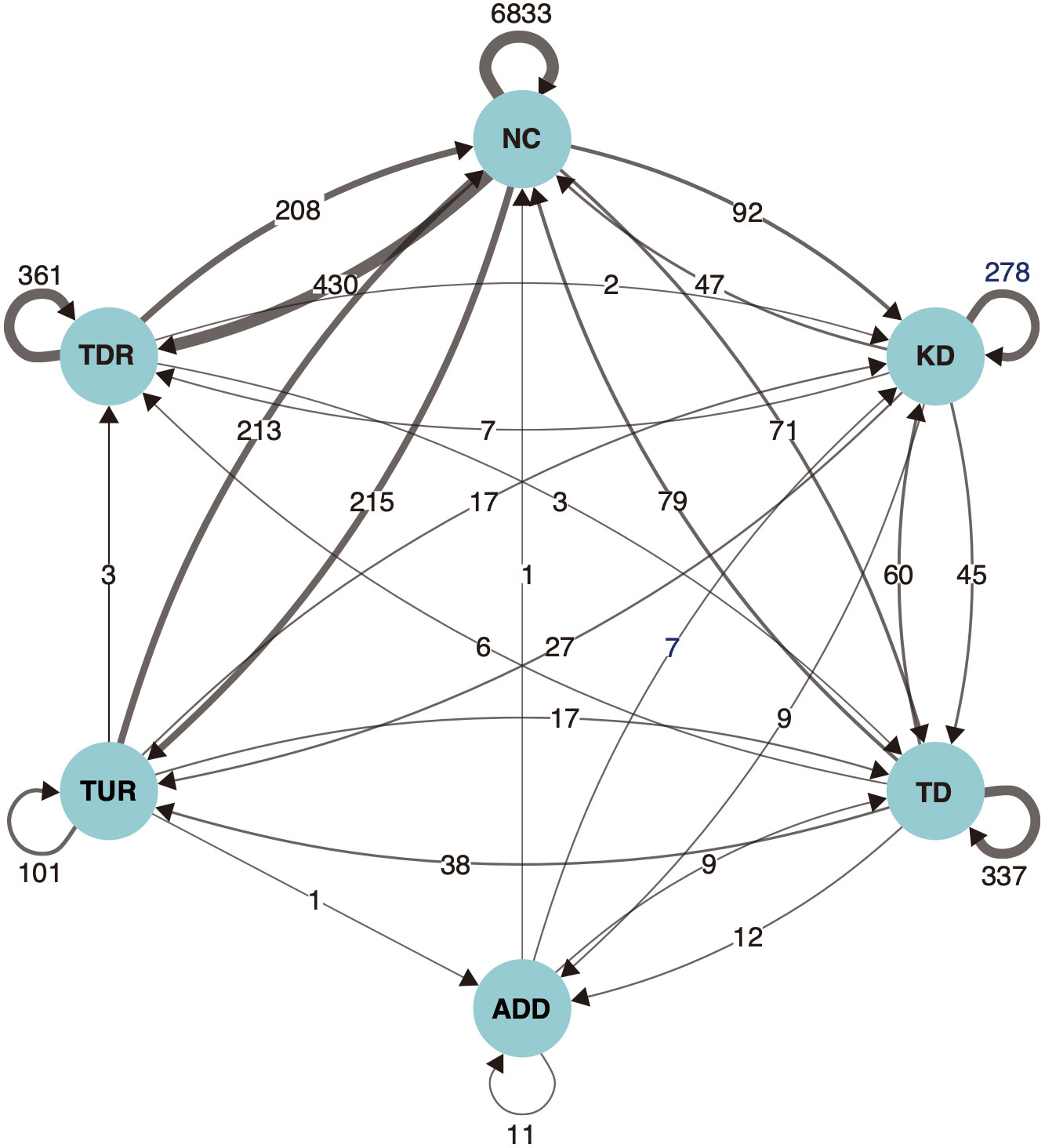
Figure 2 Diagram showing categorical changes in the differential expression of genes from the F1K hybrids to the allotetraploids, Phegopteris decursivepinnata.NC, KD, TD, ADD, TUR, and TDR are the abbreviations of “No Change”, “K-dominance”, “T-dominance”, “Additivity”, “Transgressive Up-Regulation”, and “Transgressive Down-Regulation” respectively. Numbers with arrows indicate the number of genes whose categories changed from the F1K hybrids to the allotetraploids.
3.4 Relative homoeolog contributions and expression biases in the F1 hybrids and the allotetraploid P. decursivepinnata
To test the subgenome dominance of Phegopteris koreana or P. taiwaniana in the F1 hybrids and the allotetraploids, the relative homoeolog contributions and distributions of the expression level ratios for all 9,540 orthologous gene pairs were examined (Figure 3). Although significant deviations from the mean of 0 were detected in the parental pairs, the F1K hybrids, and the allotetraploids, an apparent expression bias toward either subgenome was not observed in all cases, as the mean and median values were close to 0 (Table 1; Figure 3). However, the kurtosis of the distribution was highest in the parental species, and lowest in the allotetraploids. This suggests that the genes with biased expression increased in number after the acquisition of hybridization and polyploidization.
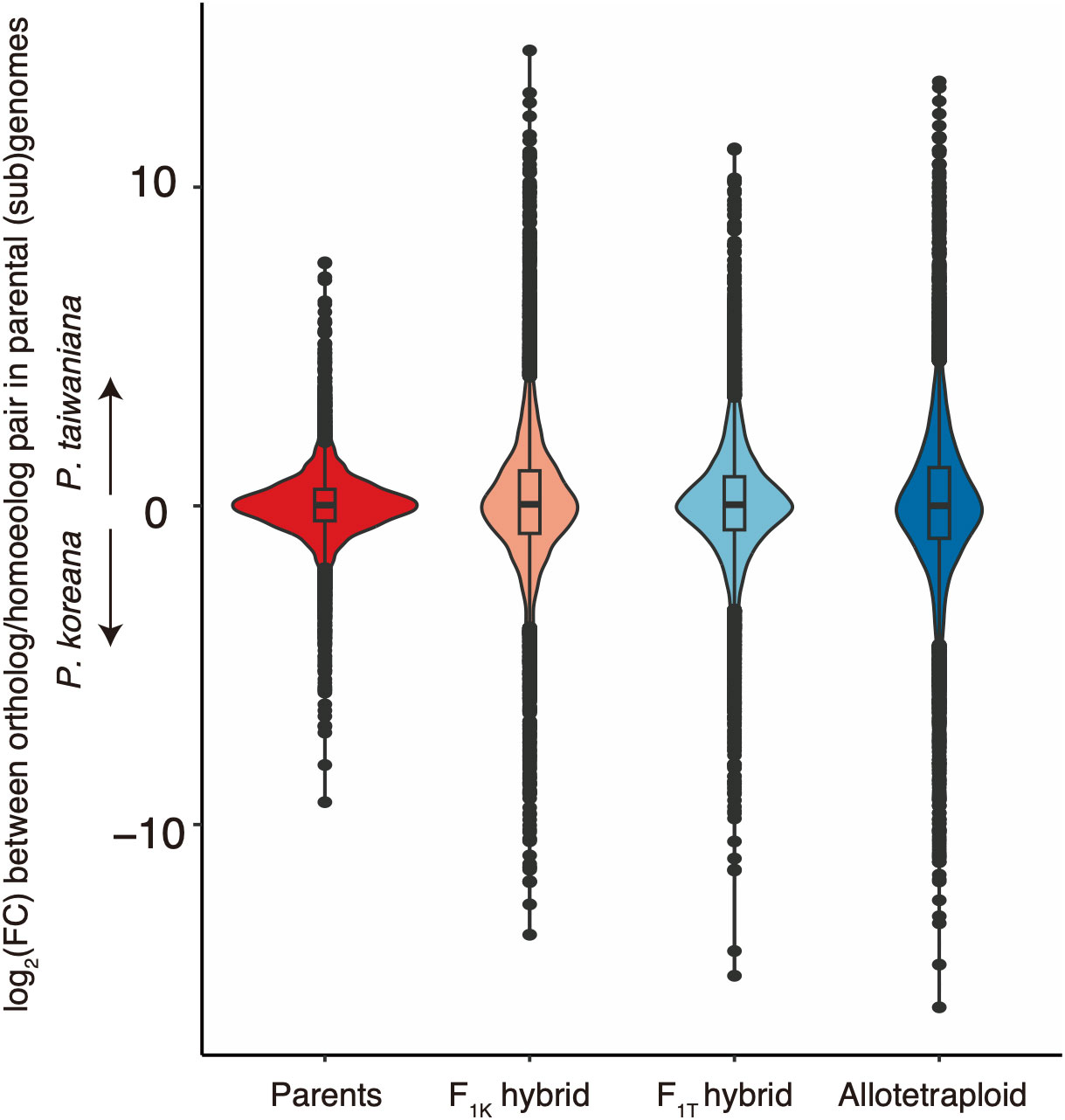
Figure 3 Patterns in the expression level ratio between homoeologs derived from Phegopteris koreana and P. taiwaniana (sub)genomes. Violin plots showing the log2(fold change (FC)) between the orthologous genes of the parental species (P. koreana and P. taiwaniana), the parental alleles of the F1K and F1T hybrids, and the homoeologous genes of the allotetraploids, P. decursivepinnata. The center line indicates the median and the box limits represent the interquartile range. The whiskers represent the largest and smallest values within 1.5 times the interquartile range above and below the 75th and 25th percentiles, respectively.
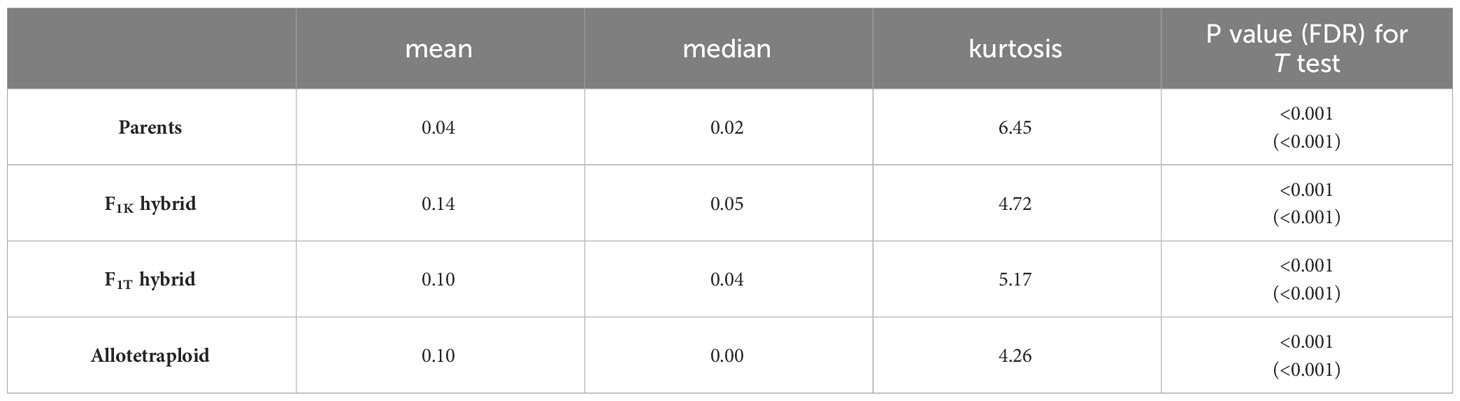
Table 1 Statistics of the distribution of log2(fold change (FC)) between orthologous or homologous gene pairs.
To understand the influence of the expression bias of the homoeologous gene pairs on gene expression, while considering the pre-existing differences in the parental species, the expression level ratios between the homoeologs in the F1 hybrids and the allotetraploids were compared with those between the parental species. Among the 9,540 orthologous gene pairs, in both the F1 hybrids and the allotetraploids, approximately 1,000–1,600 pairs exhibited different expression level ratios when compared with the parental ortholog pairs, a phenomenon referred to as HEB (Table 2). Notably, there is no substantial difference between the proportions of T- or K-biased gene pairs observed in both the F1 hybrids and the allotetraploids (1,400 “T-bias” vs 1,339 “K-bias” in the F1K hybrids, and 1,020 vs 991 in the F1T hybrids, 1,619 vs 1,558 in the allotetraploids). Of the gene pairs showing HEB, 60.5% (1658 gene pairs) were shared between the F1K hybrids and the allotetraploids (Figure 4). The majority of the genes in the other 40% varied between the F1K hybrids and the allotetraploids by transitioning to or from the “Non-bias” category, while a limited number of gene pairs, only 40 or 41 gene pairs, showed a direct switch between the “T-bias” and “K-bias” categories (Figure 4). More gene pairs altered their expression ratios from “Non-bias” in the F1K hybrids to “T-bias” or “K-bias” in the allotetraploids than from “T-bias” or “K-bias” to “Non-bias.”
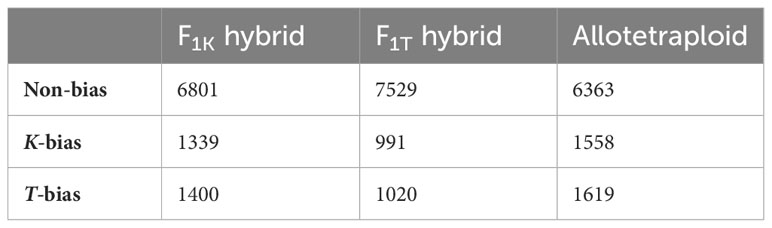
Table 2 The number of genes showing homoeolog expression bias in the F1 hybrids and the allotetraploids, Phegopteris decursivepinnata.
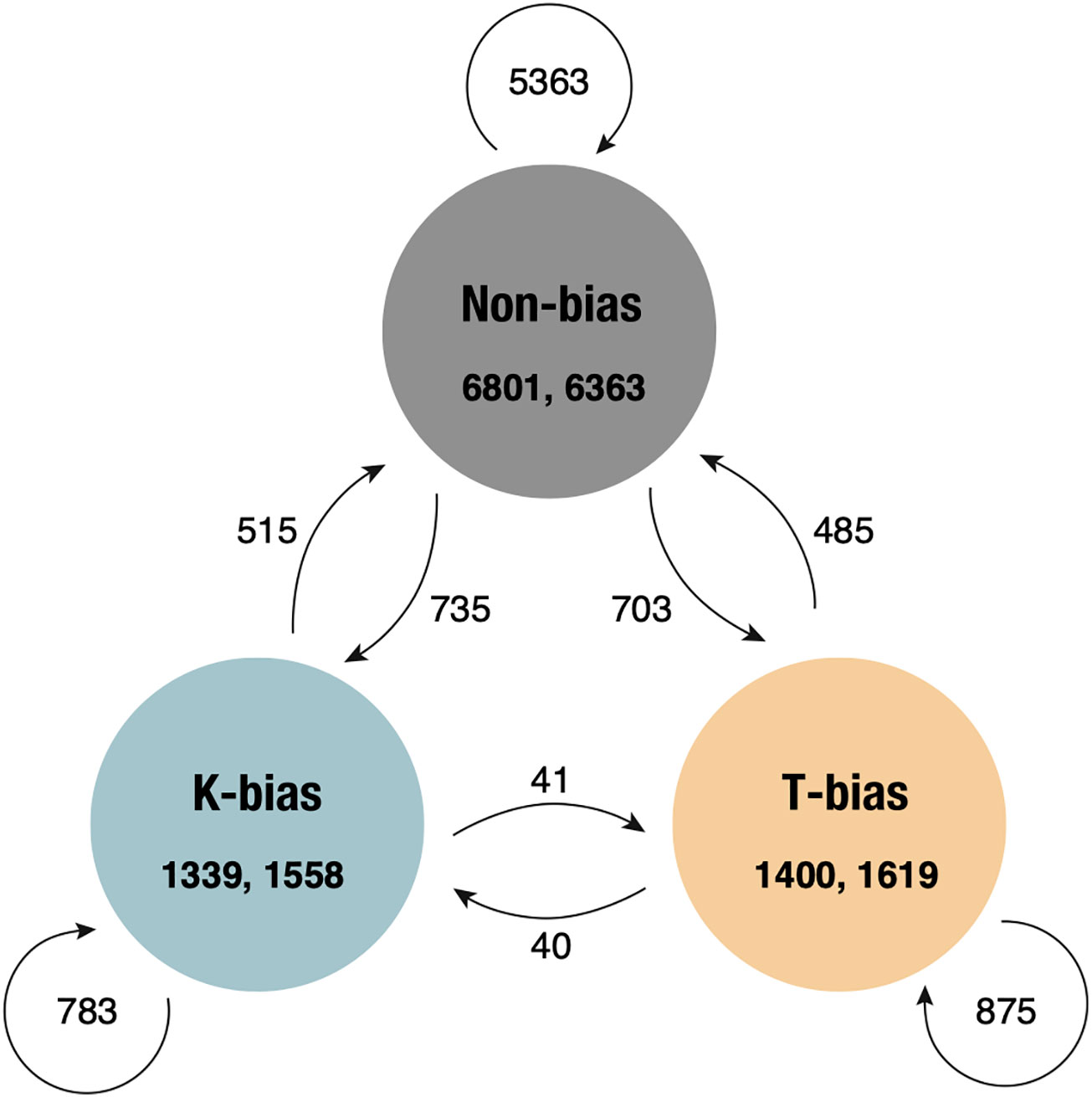
Figure 4 Categorical changes in homoeolog expression bias genes between the F1K hybrids and the allotetraploids, Phegopteris decursivepinnata. Numbers with arrows indicate the number of genes that show changes in homoeolog expression bias between the F1K and the allotetraploids. The numbers in each category show the number of genes in the F1K hybrids and the allotetraploids on the left and right, respectively.
To examine the differences in strength of the HEB between the F1K hybrids and the allotetraploids, the relative expression levels of the homoeolog pairs in the F1 hybrids and the allotetraploids were compared with the orthologous gene pairs between the parental species (Figure 5). The F1 hybrids and the allotetraploids showed a similar trend whereas both the T-biased and K-biased gene pairs showed a similar distribution in the scatter plot and histogram (Figure 5). In the allotetraploids, along with increases in both subgenome-biased gene pairs, the strength of both biases also increased (Table 3; Figure 5). The number of genes with a “magnitude” > |10| for T- and K-biased gene pairs increased from nine in the F1K hybrids and none in the F1T hybrids to 11 in the allotetraploids for T-biased genes, and five in the F1K hybrids and two in the F1T hybrids to 13 in the allotetraploids for K-biased genes, respectively (Table 3). Additionally, the mean values of “magnitude” for T- and K-biased genes were also amplified from 2.72 in the F1K hybrids and 2.39 in the F1T hybrids to 2.83 in the allotetraploids for T-biased genes, and −2.36 in the F1K hybrids and −2.19 in the F1T hybrids to −2.64 in the allotetraploids for K-biased genes (Table 3). Finally, we explored the functional differences associated with HEB, showing both T-biased and K-biased expression in the F1K hybrids and the allotetraploids. Among the five K-biased genes and nine T-biased genes in the the F1K hybrids, and 13 K-biased genes and 11 T-biased genes in the allotetraploids (a “magnitude” >|10|), four, nine, 11 and nine genes were successfully identified using blast analysis against the Arabidopsis protein sequences for K- and T-bias of the F1K hybrids and the allotetraploids, respectively (Supplementary Tables S4–S7).
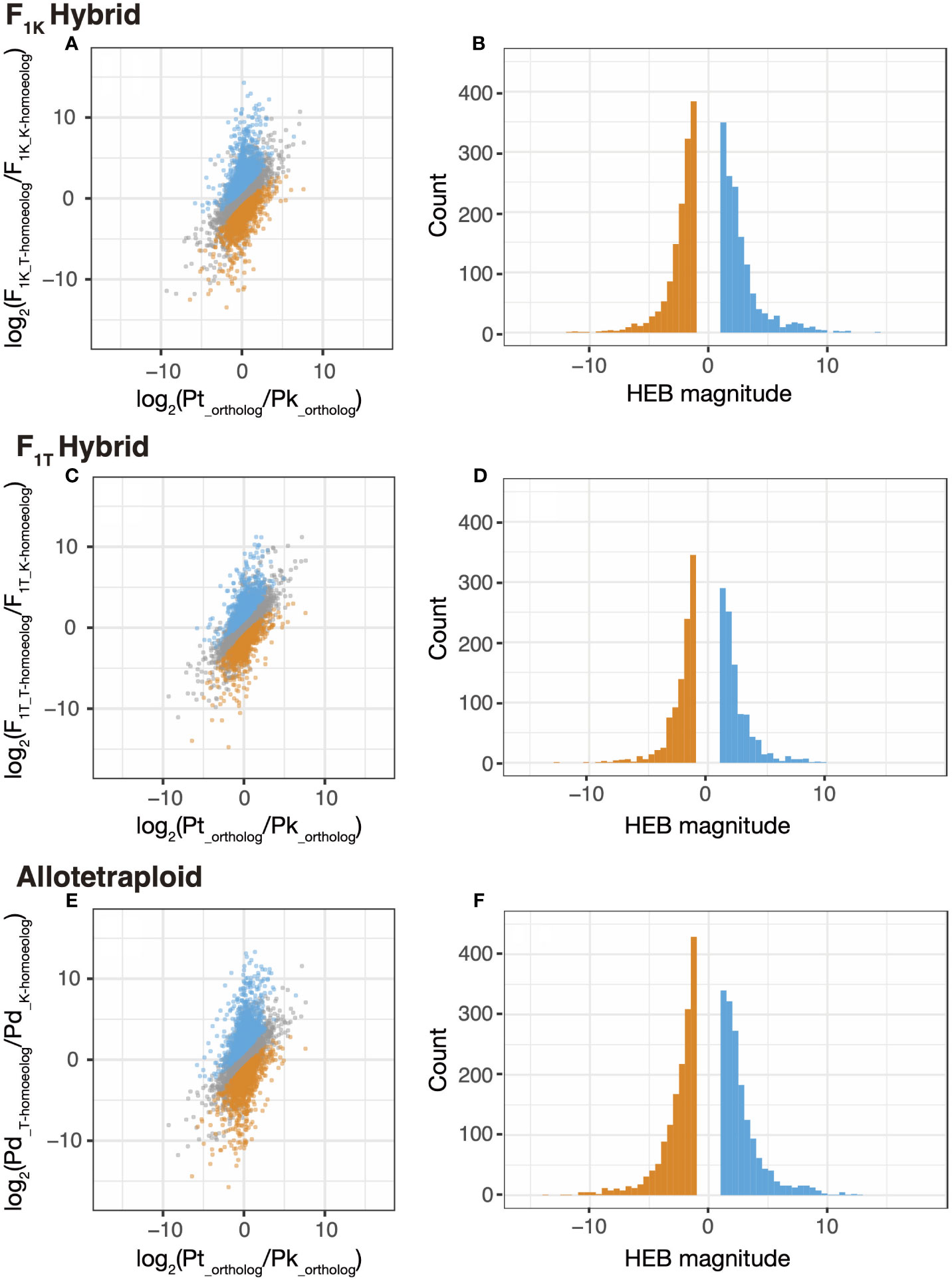
Figure 5 Homoeolog expression bias (HEB) in the F1K hybrids, the F1T hybrids, and the allotetraploids, Phegopteris decursivepinnata. (A, B) the F1K hybrids, (C, D) F1T hybrids. (E, F) Allotetraploids. (A, C, E) Scatter plots of gene pairs showing HEB in F1K hybrids (A), F1T hybrids (C), and allotetraploids (E). The horizontal axis is log2(fold change (FC)) between the orthologous genes of P. koreana and P. taiwaniana and the vertical axis is log2(FC) of the homoeologous genes in F1 hybrids or allotetraploids; gray, blue, and orange points indicate gene pairs exhibiting no HEB, T-bias, and K-bias, respectively. (B, D, F) Histograms showing the “magnitude” of HEB calculated from the formula; log2((F1K_T-homoeolog/F1K_K-homoeolog)/(Pt_ortholog/Pk_ortholog)) (B), log2((F1T_T-homoeolog/F1T_K-homoeolog)/(Pt_ortholog/Pk_ortholog)) (D), and log2((Pd_T-homoeolog/Pd_K-homoeolog)/(Pt_ortholog/Pk_ortholog)) (F). Blue and orange bars indicate T- and K-biased gene pairs, respectively. Pd, P. decursivepinnata; Pk, P. koreana; Pt, P. taiwaniana.
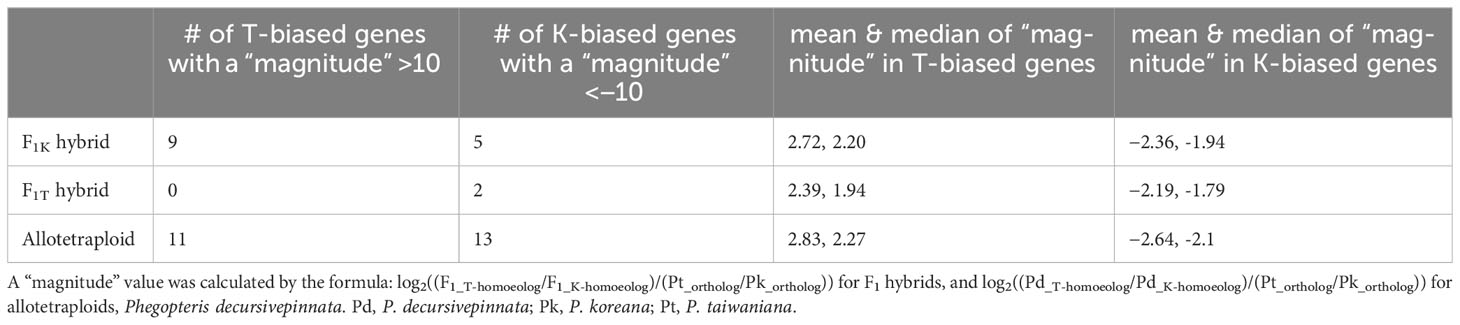
Table 3 Magnitude of homoeolog expression bias in the F1 hybrids and the allotetraploids, Phegopteris decursivepinnata.
4 Discussion
4.1 Changes in gene expression levels between the F1 hybrids and the allotetraploid Phegopteris decursivepinnata
Remarkable changes were observed in the gene expression levels of the F1 hybrids and the allotetraploids when compared to the parental species (Figure 1). Notably, more than half of genes (71.14%) showing non-additive expression in the F1K hybrids were also detected as non-additive in allotetraploids (Figure 2). These findings suggest that the merger of divergent genomes through hybridization itself had a substantial impact on gene expression changes, which is consistent with previous studies (Hegarty et al., 2006; Chaudhary et al., 2009; Flagel and Wendel, 2010; Buggs et al., 2011; Duan et al., 2023). However, we also observed substantial differences in expression levels between the F1 hybrids and the allotetraploids. In the allotetraploids, the proportion of genes categorized as “No Change” was decreased compared to those in the F1 hybrids (77.4% in the allotetraploid vs. 80.1% and 81.8% in the F1 hybrids).
The gene showing transgressive expression was more frequent in the allotetraploids than in the F1 hybrids (1,188 genes in the allopolyploids vs. 926 and 785 genes in the F1 hybrids) (Figure 1). Interestingly, although very low rates of transgressive expression patterns in allopolyploid fern species (approximately 1% or less in both “Transgressive Up-regulation” and “Transgressive Down-regulation”) have been reported in Polypodium hesperium (Sigel et al., 2019), the proportion in this study is rather consistent with the reports from allopolyploids of flowering plants: 12.5% in this study, 8.9–10.9% in Achillea (Chen et al. 2021), 16–18% in Coffea (Bardil et al., 2011), 13.2–30.4% in Gossypium (Flagel and Wendel, 2010) and 5.5–8.9% in Tragopogon (Shan et al., 2020). Tracing the category transition of genes between the F1K hybrids and the allotetraploids, the transition from the category “No-Change” to “Transgressive Down-regulation” mostly accounted for the increase in the number of genes showing transgressive expression (Figure 2). This result indicates that additional expression changes that had occurred after polyploidization contributed to the higher rate of transgressive expression genes in the allotetraploids. A similar phenomenon including the category transition from “No change” to transgressive expression has been reported in angiosperms, and was likely to be caused by epigenetic modification or cis- and trans-regulatory evolution (Flagel and Wendel, 2010; Hu and Wendel, 2019).
ELD is a well-known phenomenon observed in numerous allopolyploids as demonstrated in several previous studies (Rapp et al., 2009; Flagel and Wendel, 2010; Bardil et al., 2011; Sigel et al., 2019; Chen et al., 2021). In this study, a substantial number of genes showing “K-dominance” or “T-dominance” in the F1K hybrids was also highly conserved in the allotetraploids: 67.3% in “K-dominance” and 63.3% in “T-dominance” (Figure 2). Therefore, hybridization plays a major role in shaping the pattern of ELD in this study. In many allopolyploid species, unbalanced ELD has been reported, with a tendency for expression levels of more genes to resemble those of one parental species (Rapp et al., 2009; Wu et al., 2018; Chen et al., 2021). Similarly, in the allopolyploid fern Polypodium, almost all genes showing ELD are reportedly biased toward one parental species (Sigel et al., 2019). In this study, however, although the number of genes showing “T-dominance” was slightly higher than those showing “K-dominance” in both the F1K hybrids (5.6% vs. 4.3%) and the F1T hybrids (6.0% vs. 3.8%), the allotetraploids showed the same extent in the proportion of genes exhibiting either “T-dominance” and “K-dominance” (5.1% vs. 4.8%). This pattern of ELD in the allotetraploids was shaped by larger outflows from “T-dominance” to other categories than inflows and larger inflows from other categories into “K-dominance” than outflows (Figure 2). This suggests that the hybridization between Phegopteris koreana and P. taiwaniana contributed to “T-dominance”, and the alleviation of “T-dominance” and the activation of “K-dominance” occurred over evolutionary time after polyploidization.
4.2 Homoeolog expression bias in the F1 hybrids and the allotetraploid Phegopteris decursivepinnata
HEB is the phenomenon where F1 or allopolyploid homoeologous gene pairs exhibit expression ratios that differ from those of orthologous gene pairs of their parent species (Yoo et al., 2013). HEB has been frequently documented in allopolyploid species (Chaudhary et al., 2009; Koh et al., 2010; Edger et al., 2017; Boatwright et al., 2018; Sigel et al., 2019; Boatwright et al., 2021; Chen et al., 2021; Vasudevan et al., 2023). In this study, 21.1–33.3% of the 9,540 gene pairs showed HEB in both the F1 hybrids and the allotetraploids (Table 2). Importantly, a significant number of genes displaying HEB were shared between the F1 hybrids and the allotetraploids. More than half of the genes showing HEB in the F1 hybrids (60.5%) were also identified with HEB in the allotetraploids. The transition from “K-bias” to “T-bias” or vice versa between the F1 hybrids and the allotetraploids was infrequently observed (Figure 4). These findings demonstrate that HEB primarily originated in the response to hybridization before the occurrence of polyploidization. However, there was also a substantial change in the pattern between the F1 hybrids and the allotetraploids—the intensification of HEB in the allotetraploids compared to the F1 hybrids. This intensification was evident not only in the number of genes showing HEB but also in the “magnitude” of bias for both “K-bias” and “T-bias” in the allotetraploids (see Tables 2, 3; Figure 5). These changes resulted in the lowest kurtosis in the distribution of the expression level ratio between homoeologs in the allotetraploids (Table 1; Figure 3). Therefore, this observation suggests that HEB gradually intensified in the number of genes and the “magnitude” of bias after polyploidization. Unbalanced HEB is commonly reported across allopolyploids (Chaudhary et al., 2009; Koh et al., 2010; Edger et al., 2017; Sigel et al., 2019; Chen et al., 2021; Vasudevan et al., 2023). In this study, there was no apparent bias toward either parent in the F1 hybrids, and the allotetraploids (1,339 “K-bias” vs. 1,400 “T-bias” in the F1K hybrids, 1,020 vs. 991 in the F1T hybrids, and 1,619 vs. 1,558 in the allotetraploids) (Table 2). This pattern of HEB aligns with the previous reports of allopolyploids exhibiting a balanced HEB (Boatwright et al., 2018; Boatwright et al., 2021; Burns et al., 2021; Wu et al., 2021). The balanced HEB obtained in this study contrasts with the previous study focusing on allotetraploid fern, which reported an exceptionally strong HEB towards one parent (744–923 genes vs. 39–100 genes) (Sigel et al., 2019). Therefore, the strong unbalanced HEB observed in the previous study is not a common pattern in transcriptomic change after allopolyploidization among ferns.
The HEB is expected to be partly attributed to cytonuclear gene coordination (Sharbrough et al., 2017), although its significance for gene expression patterns in allopolyploids remains inconsistent among studies (Gong et al., 2012; Gong et al., 2014; Sehrish et al., 2015; Ferreira De Carvalho et al., 2019). In allopolyploid plants, the nuclear genome is contributed from both parental species, but organellar genomes are inherited only from maternal parents, including ferns (Gastony and Yatskievych, 1992; reviewed in Kuo L.-Y. et al., 2018). Therefore, cytonuclear coordination should occur following hybridization, where the expression of nuclear-encoded organelle-targeted genes is biased toward the maternal parent, or the function of the paternal homoeolog is lost (Sharbrough et al., 2017). Given that the maternal parent of Phegopteris decursivepinnta is P. koreana (Fujiwara et al., 2021), it is suggested that cytonuclear interactions might favor higher expressions of organelle-targeted homoeologs derived from P. koreana. In this study, among genes exhibiting extremely strong HEB (“magnitude” > |10|), whereas one out of five K-biased genes and two out of nine T-biased genes in the F1K hybrids were associated with chloroplast and mitochondria-related functions (Supplementary Tables S6, S7), four out of 13 K-biases genes and four out of 11 T-biased genes in the allotetraploids were detected to be functionally related to organelle (Supplementary Tables S4, S5). Two of four organelle-related K-biased genes detected in the allotetraploids are orthologue to AtRBCX1 (AT4G04330), which functions in RuBisCO assembly (Kolesiński et al., 2011), and to NDU9/B14.5b, a subunit of mitochondrial Complex I (Subrahmanian et al., 2016) (Supplementary Table S4). Consistent with our initial prediction, our results support the idea that cytonuclear coordination favors the higher expression of the maternal homologue in organelle-targeted genes, particularly those directly related to organelle function and structure. Therefore, this finding indicates that the increased HEB in the allotetraploids observed in this study can be partly explained by the concerted action between the duplicated nuclear genome and the increased copy of organelle genomes in cells.
4.3 The absence of subgenome dominance in Phegopteris decursivepinnata and its implication for genome evolution in ferns
Our results showed that neither ELD nor HEB showed a pronounced bias toward one of the subgenomes in the F1 hybrids and the natural allotetraploids, Phegopteris decursivepinnata. The pattern observed in P. decursivepinnata can be interpreted as the absence of subgenome dominance when compared with the previous studies (Flagel and Wendel, 2010; Edger et al., 2017; Bird et al., 2018; Sigel et al., 2019; Chen et al., 2021; Glombik et al., 2021; Vasudevan et al., 2023). The absence of subgenome dominance in P. decursivepinnata contrasts with the extreme case of subgenome dominance observed in Polypodium hesperium, the only fern species for which DEG analysis has been conducted to date (Sigel et al., 2019). The difference in transcriptomic consequence of allopolyploidy between the two ferns may be explained by two possible reasons.
Firstly, the age of allopolyploid ferns may reflect the degree of subgenome dominance. In other words, it is likely that subgenome dominance has not extensively proceeded in Phgopteris decursivepinnata due to its relatively recent origin. The previous phylogenetic analysis showed no genetic differentiation from parental species for cpDNA gene (rbcL) and nuclear DNA (pgiC), indicating the recent origin of the allotetraploid species (Fujiwara et al., 2021). However, because the parental species currently do not co-occur due to their ecological differentiation, the allopolyploid speciation is not an ongoing process, but rather more likely to have occurred during the climatic oscillations in the Quaternary, as suggested for the other allopolyploid ferns in Japan (Fujiwara and Watano, 2020; Fujiwara et al., 2022). However, the allopolyploid fern showing strong subgenome dominance, Polypodium hesperium is also considered to have originated very recently during glacial cycles in the Pleistocene (Sigel et al., 2014). Therefore, the difference in the degree of subgenome dominance between the two allopolyploid ferns with recent origins cannot be attributed to the difference in their age since polyploidization. Secondly, the degree of subgenome dominance may be linked to genomic differentiation between parental species, known as parental legacy (Buggs et al., 2014). In other words, the genomic properties inherited from the parental species may predetermine the tendency of subgenome dominance. Indeed, in allopolyploid species that exhibit strong subgenome dominance, the bias toward one subgenome is already evident in the F1 hybrids and the first generation of synthetic allopolyploids and subsequently tends to be strengthened in the later generations of allopolyploids (Flagel and Wendel, 2010; Edger et al., 2017; Bird et al., 2021; Vasudevan et al., 2023). Increasing evidence suggests that one significant aspect of the genomic difference between parental species that contributes to subgenome dominance is the variation in the abundance of transposable elements (TEs) between them (Bird et al., 2018; Alger and Edger, 2020). TEs can be activated during allopolyploidization, but subsequently, most TEs are rapidly silenced. This process affects the expression of the neighboring coding genes, resulting in a lower expression or silencing in the homoeologous genes of a subgenome possessing more TEs when compared with another subgenome. According to the hypothesis, the observed difference in subgenome dominance between the two allopolyploid ferns may be explained by the difference between the two species in genomic differentiation in TE abundance between the parental species. Future studies will require an investigation into the differences in the genomic composition of these species by focusing on the abundance of TEs.
Although we found the contrasting result of subgenome dominance between two allopolyploid ferns, several lines of genomic evidence can predict that the absence of subgenome dominance may be more common, particularly in homosporous ferns. Unlike angiosperms, ferns are hypothesized to exhibit low TE activity, and this is supported by the old insertion time of LTR retrotransposons (Baniaga and Barker, 2019) and genome size stability over long periods (Schneider et al., 2015; Clark et al., 2016; Fujiwara et al., 2023). Therefore, ferns may maintain a highly similar genomic landscape (Szövényi et al., 2021; Huang et al., 2022), even between species from different genera (Rothfels et al., 2015), and the relatively less divergent genome in ferns could potentially generate subtle or absence of subgenome dominance in most homosporous fern allopolyploids, as observed in this study. Furthermore, subgenome dominance is hypothesized to trigger a biased fractionation of the recessive subgenome, leading to rapid diploidization and genome-downsizing in polyploid genomes (Cheng et al., 2018; Wendel et al., 2018; Alger and Edger, 2020). In ferns, however, it is traditionally believed that the diploidization process is slower when compared with angiosperms (Barker, 2013). Instead of the physical loss of genes, the diploidization in ferns is hypothesized to involve gene silencing, leading to slow rates of chromosome reduction and genome downsizing (Haufler, 2014). The limited empirical evidence based on genome sequencing also supports this hypothesis on slow genome evolution in homosporous ferns (Huang et al., 2022; Zhong et al., 2022), with one exception (Marchant et al., 2022). Therefore, the observed absence of subgenome dominance in Phegopteris decursivepinnata is consistent with the slow genome downsizing in ferns and could partially explain the process of post-polyploid evolution seen in the broad range of homosporous ferns. Also in homosporous lycophyte, a lineage of land plants considered to exhibit a similar genome evolution as homosporous ferns (Sessa and Der, 2016), a recent finding from ancient allotetraploid Huperzia showed a limited subgenome dominance and slow genome evolution following polyploidization (Li et al., 2023), which support our prediction. To test the validity of our prediction, we still need to examine transcriptomic change and the pattern of genomic differentiation including TE abundance in more diverse allopolyploid fern species.
4.4 Limitations in this study and future directions
In this study, we successfully demonstrated the dynamics of gene expression change between the F1 hybrids and the allotetraploids of Phegopteris fern, highlighting the absence of subgenome dominance in the allotetraploid. Nevertheless, it is essential to note several limitations in our study design and technical approaches that need to be addressed in future investigations.
Firstly, one of the limitations is the relatively limited number of genes examined in this study. We targeted 9,540 genes co-expressed among the parental species, the F1 hybrids, and the allotetraploids. This represents less than half of the total number of protein-coding genes reported in homosporous ferns (36,857 genes in Ceratopteris, Marchant et al., 2022; 31,244 genes in Adiantum, Fang et al., 2022). However, this is because the necessity of focusing on co-expressed genes arose from the absence of available genome sequence data for Phegopteris fern and the specific goal of tracing the transition of genes exhibiting ELD and HEB from the F1 hybrids to the allotetraploids. Although our study successfully provided a robust overview of the transcriptomic changes in the allotetraploids as evidenced by the consistency across three datasets with TPM filtering thresholds of > 0, > 0.5, and > 1.0 (Supplementary Figures S4–S7), it should be noted that our results are solely based on co-expressed genes and thus might have led to the oversight of critical changes, such as gene silencing, and reactivation of genes suppressed in the parental species. To comprehensively examine genome-wide expression changes following allopolyploidization, future studies should consider analyzing transcriptome data using parental genome sequences as references.
Secondly, this study is solely based on gene expression in leaf tissues, possibly overlooking tissue-specific expression differences. Previous studies comparing homoeolog gene expression among different tissues reported substantial differentiation of homoeolog expression bias in direction and expression level for each gene among the examined tissues (Adams et al., 2003; Buggs et al., 2010; Huang et al., 2022). On the other hand, recent studies focusing on the whole transcriptome-wide pattern of homoeolog gene expression suggest that the overall trend of homoeolog expression bias is highly conserved among different tissues (Edger et al., 2017; Griffiths et al., 2019; Chen et al., 2021; Vasudevan et al., 2023). Therefore, while the trend obtained in this study could be consistent among other tissues, we might have missed key changes in homoeolog gene expression occurring in different tissues, such as tissue-specific silencing and tissue-specific homoeolog bias, as reported in other studies. Thus, in future studies, it is required to examine gene expression patterns using different tissue types of Phegopteris species.
Lastly, the pattern of gene expression in the allotetraploids might change in response to environmental conditions (Shimizu, 2022). In Cardamine, studies have shown that allopolyploids in each dry and humid environment tend to exhibit gene expression patterns resembling those of each parental species specialized for each environment, which enables the allopolyploids to obtain adaptive plasticity to diverse niches (Shimizu-Inatsugi et al., 2016; Akiyama et al., 2021). Phegopteris decursivepinnata has a wider distribution than those of its parental species (Fujiwara et al., 2021) and thus is expected to have a higher tolerance to a wide range of environmental conditions than its parental species. Given that, it is likely that P. decursivepinnata could also show different patterns of homoeolog gene expression from that observed in this study according to different environmental conditions. Thus, it is essential to trace the change in the pattern of gene expression in response to different environmental conditions in this species.
Data availability statement
The raw sequence data used in this study were deposited in the DDBJ sequence Read Archive (DRA) under the accession numbers (DRR498230–DRR498244).
Author contributions
NK: Conceptualization, Data curation, Formal analysis, Methodology, Writing – original draft, Writing – review & editing. TY: Data curation, Formal analysis, Writing – review & editing. SA: Data curation, Formal analysis, Writing – review & editing. YW: Conceptualization, Project administration, Supervision, Writing – review & editing. TF: Conceptualization, Formal Analysis, Funding acquisition, Project administration, Supervision, Writing – original draft, Writing – review & editing.
Funding
The author(s) declare financial support was received for the research, authorship, and/or publication of this article. This study was supported by a grant-in-aid from the Japan Society for the Promotion of Science (JSPS) KAKENHI (22K15178), awarded to TF.
Acknowledgments
We thank Mr. Junki Ogiso for providing spores of the parental diploid species, Phegopteris taiwaniana and P. koreana, and Mr. Sosuke Ishii for collecting P. decursivepinnnata sporophytes.
Conflict of interest
The authors declare that the research was conducted in the absence of any commercial or financial relationships that could be construed as a potential conflict of interest.
Publisher’s note
All claims expressed in this article are solely those of the authors and do not necessarily represent those of their affiliated organizations, or those of the publisher, the editors and the reviewers. Any product that may be evaluated in this article, or claim that may be made by its manufacturer, is not guaranteed or endorsed by the publisher.
Supplementary material
The Supplementary Material for this article can be found online at: https://www.frontiersin.org/articles/10.3389/fpls.2023.1286320/full#supplementary-material
References
Adams, K. L., Cronn, R., Percifield, R., Wendel, J. F. (2003). Genes duplicated by polyploidy show unequal contributions to the transcriptome and organ-specific reciprocal silencing. Proc. Natl. Acad. Sci. U.S.A. 100, 4649–4654. doi: 10.1073/pnas.0630618100
Akiyama, R., Sun, J., Hatakeyama, M., Lischer, H. E., Briskine, R. V., Hay, A., et al. (2021). Fine-scale empirical data on niche divergence and homeolog expression patterns in an allopolyploid and its diploid progenitor species. New Phytol. 229, 3587–3601. doi: 10.1111/nph.17101
Alger, E. I., Edger, P. P. (2020). One subgenome to rule them all: underlying mechanisms of subgenome dominance. Curr. Opin. Plant Biol. 54, 108–113. doi: 10.1016/j.pbi.2020.03.004
Baniaga, A. E., Barker, M. S. (2019). Nuclear genome size is positively correlated with median LTR-RT insertion time in fern and lycophyte genomes. Am. Fern J. 109, 248. doi: 10.1640/0002-8444-109.3.248
Bardil, A., De Almeida, J. D., Combes, M. C., Lashermes, P., Bertrand, B. (2011). Genomic expression dominance in the natural allopolyploid Coffea arabica is massively affected by growth temperature. New Phytol. 192, 760–774. doi: 10.1111/j.1469-8137.2011.03833.x
Barker, M. S. (2013). “Karyotype and genome evolution in pteridophytes,” in Plant genome diversity, vol. 2 . Eds. Leitch, I. J., Greilhuber, J., Dolezel, J., Wendel, J. F. (Berlin: Springer), 245–253. doi: 10.1007/978-3-7091-1160-4_15
Barker, M. S., Arrigo, N., Baniaga, A. E., Li, Z., Levin, D. A. (2016). On the relative abundance of autopolyploids and allopolyploids. New Phytol 210, 391–398. doi: 10.1111/nph.13698
Beaulieu, J. M., Leitch, I. J., Patel, S., Pendharkar, A., Knight, C. A. (2008). Genome size is a strong predictor of cell size and stomatal density in angiosperms. New Phytol. 179, 975–986. doi: 10.1111/j.1469-8137.2008.02528.x
Birchler, J. A., Yang, H. (2022). The multiple fates of gene duplications: Deletion, hypofunctionalization, subfunctionalization, neofunctionalization, dosage balance constraints, and neutral variation. Plant Cell 34, 2466–2474. doi: 10.1093/plcell/koac076
Bird, K. A., Niederhuth, C. E., Ou, S., Gehan, M., Pires, J. C., Xiong, Z., et al. (2021). Replaying the evolutionary tape to investigate subgenome dominance in allopolyploid Brassica napus. New Phytol. 230, 354–371. doi: 10.1111/nph.17137
Bird, K. A., VanBuren, R., Puzey, J. R., Edger, P. P. (2018). The causes and consequences of subgenome dominance in hybrids and recent polyploids. New Phytol. 220, 87–93. doi: 10.1111/nph.15256
Boatwright, J. L., McIntyre, L. M., Morse, A. M., Chen, S., Yoo, M.-J., Koh, J., et al. (2018). A robust methodology for assessing differential homeolog contributions to the transcriptomes of allopolyploids. Genetics 210, 883–894. doi: 10.1534/genetics.118.301564
Boatwright, J. L., Yeh, C.-T., Hu, H.-C., Susanna, A., Soltis, D. E., Soltis, P. S., et al. (2021). Trajectories of homoeolog-specific expression in allotetraploid Tragopogon castellanus populations of independent origins. Front. Plant Sci. 12. doi: 10.3389/fpls.2021.679047
Bolger, A. M., Lohse, M., Usadel, B. (2014). Trimmomatic: a flexible trimmer for Illumina sequence data. Bioinformatics 30, 2114–2120. doi: 10.1093/bioinformatics/btu170
Buggs, R. J., Elliott, N. M., Zhang, L., Koh, J., Viccini, L. F., Soltis, D. E., et al. (2010). Tissue-specific silencing of homoeologs in natural populations of the recent allopolyploid Tragopogon mirus. New Phytol. 186, 175–183. doi: 10.1111/j.1469-8137.2010.03205.x
Buggs, R. J. A., Wendel, J. F., Doyle, J. J., Soltis, D. E., Soltis, P. S., Coate, J. E. (2014). The legacy of diploid progenitors in allopolyploid gene expression patterns. Phil. Trans. R. Soc B 369, 20130354. doi: 10.1098/rstb.2013.0354
Buggs, R. J. A., Zhang, L., Miles, N., Tate, J. A., Gao, L., Wei, W., et al. (2011). Transcriptomic shock generates evolutionary novelty in a newly formed, natural allopolyploid plant. Curr.Biol 21, 551–556. doi: 10.1016/j.cub.2011.02.016
Burns, R., Mandáková, T., Gunis, J., Soto-Jiménez, L. M., Liu, C., Lysak, M. A., et al. (2021). Gradual evolution of allopolyploidy in Arabidopsis suecica. Nat. Ecol. Evol. 5, 1367–1381. doi: 10.1038/s41559-021-01525-w
Chaudhary, B., Flagel, L., Stupar, R. M., Udall, J. A., Verma, N., Springer, N. M., et al. (2009). Reciprocal silencing, transcriptional bias and functional divergence of homeologs in polyploid cotton (Gossypium). Genetics 182, 503–517. doi: 10.1534/genetics.109.102608
Chen, D., Yan, P.-C., Guo, Y.-P. (2021). Imprints of independent allopolyploid formations on patterns of gene expression in two sibling yarrow species (Achillea, Asteraceae). BMC Genomics 22, 264. doi: 10.1186/s12864-021-07566-6
Chen, H., Fang, Y., Zwaenepoel, A., Huang, S., Van De Peer, Y., Li, Z. (2023). Revisiting ancient polyploidy in leptosporangiate ferns. New Phytol. 237, 1405–1417. doi: 10.1111/nph.18607
Cheng, F., Wu, J., Cai, X., Liang, J., Freeling, M., Wang, X. (2018). Gene retention, fractionation and subgenome differences in polyploid plants. Nat. Plants 4, 258–268. doi: 10.1038/s41477-018-0136-7
Clark, J., Hidalgo, O., Pellicer, J., Liu, H., Marquardt, J., Robert, Y., et al. (2016). Genome evolution of ferns: evidence for relative stasis of genome size across the fern phylogeny. New Phytol. 210, 1072–1082. doi: 10.1111/nph.13833
De Smet, R., Adams, K. L., Vandepoele, K., Van Montagu, M. C. E., Maere, S., Van De Peer, Y. (2013). Convergent gene loss following gene and genome duplications creates single-copy families in flowering plants. Proc. Natl. Acad. Sci. U.S.A. 110, 2898–2903. doi: 10.1073/pnas.1300127110
Duan, T., Sicard, A., Glémin, S., Lascoux, M. (2023). Expression pattern of resynthesized allotetraploid Capsella is determined by hybridization, not whole-genome duplication. New Phytol. 237, 339–353. doi: 10.1111/nph.18542
Edger, P. P., Smith, R., McKain, M. R., Cooley, A. M., Vallejo-Marin, M., Yuan, Y., et al. (2017). Subgenome dominance in an interspecific hybrid, synthetic allopolyploid, and a 140-year-old naturally established neo-allopolyploid monkeyflower. Plant Cell 29, 2150–2167. doi: 10.1105/tpc.17.00010
Emms, D. M., Kelly, S. (2019). OrthoFinder: phylogenetic orthology inference for comparative genomics. Genome Biol. 20, 1–14. doi: 10.1186/s13059-019-1832-y
Fang, Y., Qin, X., Liao, Q., Du, R., Luo, X., Zhou, Q., et al. (2022). The genome of homosporous maidenhair fern sheds light on the euphyllophyte evolution and defenses. Nat. Plants 8, 1024–1037. doi: 10.1038/s41477-022-01222-x
Ferreira De Carvalho, J., Lucas, J., Deniot, G., Falentin, C., Filangi, O., Gilet, M., et al. (2019). Cytonuclear interactions remain stable during allopolyploid evolution despite repeated whole-genome duplications in Brassica. Plant J. 98, 434–447. doi: 10.1111/tpj.14228
Flagel, L. E., Wendel, J. F. (2010). Evolutionary rate variation, genomic dominance and duplicate gene expression evolution during allotetraploid cotton speciation. New Phytol. 186, 184–193. doi: 10.1111/j.1469-8137.2009.03107.x
Fujiwara, T., Egashira, T., Gutiérrez-Ortega, J. S., Hori, K., Ebihara, A., Watano, Y. (2022). Establishment of an allotetraploid fern species, Lepisorus yamaokae Seriz., between two highly niche-differentiated parental species. Am. J. Bot. 109, 1456–1471. doi: 10.1002/ajb2.16043
Fujiwara, T., Liu, H., Meza-Torres, E. I., Morero, R. E., Vega, A. J., Liang, Z., et al. (2023). Evolution of genome space occupation in ferns: linking genome diversity and species richness. Ann. Bot. 131, 59–70. doi: 10.1093/aob/mcab094
Fujiwara, T., Ogiso, J., Ishii, S., Togo, K., Nakato, N., Serizawa, S., et al. (2021). Species delimitation in the Phegopteris decursivepinnata polyploid species complex (Thelypteridaceae). Acta Phytotax. Geobot. 72, 205–226. doi: 10.18942/apg.202102
Fujiwara, T., Watano, Y. (2020). Independent allopatric polyploidizations shaped the geographical structure and initial stage of reproductive isolation in an allotetraploid fern, Lepisorus nigripes (Polypodiaceae). PloS One 15, e0233095. doi: 10.1371/journal.pone.0233095
Gao, B., Chen, M., Li, X., Liang, Y., Zhang, D., Wood, A. J., et al. (2022). Ancestral gene duplications in mosses characterized by integrated phylogenomic analyses. J. Syst. Evol. 60, 144–159. doi: 10.1111/jse.12683
Gastony, G. J., Yatskievych, G. (1992). Maternal inheritance of the chloroplast and mitochondrial genomes in cheilanthoid ferns. Am. J. Bot. 79, 716–722. doi: 10.1002/j.1537-2197.1992.tb14613.x
Glombik, M., Copetti, D., Bartos, J., Stoces, S., Zwierzykowski, Z., Ruttink, T., et al. (2021). Reciprocal allopolyploid grasses (Festuca × Lolium) display stable patterns of genome dominance. Plant J. 107, 1166–1182. doi: 10.1111/tpj.15375
Gong, L., Olson, M., Wendel, J. F. (2014). Cytonuclear evolution of Rubisco in four allopolyploid lineages. Mol. Biol. Evol. 31, 2624–2636. doi: 10.1093/molbev/msu207
Gong, L., Salmon, A., Yoo, M.-J., Grupp, K. K., Wang, Z., Paterson, A. H., et al. (2012). The cytonuclear dimension of allopolyploid evolution: an example from cotton using Rubisco. Mol. Biol. Evol. 29, 3023–3036. doi: 10.1093/molbev/mss110
Grabherr, M. G., Haas, B. J., Yassour, M., Levin, J. Z., Thompson, D. A., Amit, I., et al. (2011). Full-length transcriptome assembly from RNA-Seq data without a reference genome. Nat. Biotechnol. 29, 644–652. doi: 10.1038/nbt.1883
Griffiths, A. G., Moraga, R., Tausen, M., Gupta, V., Bilton, T. P., Campbell, M. A., et al. (2019). Breaking free: the genomics of allopolyploidy-facilitated niche expansion in white clover. Plant Cell 31, 1466–1487. doi: 10.1105/tpc.18.00606
Grover, C. E., Gallagher, J. P., Szadkowski, E. P., Yoo, M. J., Flagel, L. E., Wendel, J. F. (2012). Homoeolog expression bias and expression level dominance in allopolyploids. New Phytol. 196, 966–971. doi: 10.1111/j.1469-8137.2012.04365.x
Haufler, C. H. (2014). Ever since Klekowski: Testing a set of radical hypotheses revives the genetics of ferns and lycophytes. Am. J. Bot. 101, 2036–2042. doi: 10.3732/ajb.1400317
Haufler, C. H., Pryer, K. M., Schuettpelz, E., Sessa, E. B., Farrar, D. R., Moran, R., et al. (2016). Sex and the single gametophyte: Revising the homosporous vascular plant life cycle in light of contemporary research. BioScience 66, 928–937. doi: 10.1093/biosci/biw108
Hegarty, M. J., Barker, G. L., Wilson, I. D., Abbott, R. J., Edwards, K. J., Hiscock, S. J. (2006). Transcriptome shock after interspecific hybridization in Senecio is ameliorated by genome duplication. Curr. Biol. 16, 1652–1659. doi: 10.1016/j.cub.2006.06.071
Hegarty, M. J., Hiscock, S. J. (2008). Genomic clues to the evolutionary success of polyploid plants. Curr. Biol. 18, R435–R444. doi: 10.1016/j.cub.2008.03.043
Hu, G., Wendel, J. F. (2019). Cis–trans controls and regulatory novelty accompanying allopolyploidization. New Phytol. 221, 1691–1700. doi: 10.1111/nph.15515
Huang, C., Qi, X., Chen, D., Qi, J., Ma, H. (2020). Recurrent genome duplication events likely contributed to both the ancient and recent rise of ferns. J. Integr. Plant Biol. 62, 433–455. doi: 10.1111/jipb.12877
Huang, X., Wang, W., Gong, T., Wickell, D., Kuo, L.-Y., Zhang, X., et al. (2022). The flying spider-monkey tree fern genome provides insights into fern evolution and arborescence. Nat. Plants 8, 500–512. doi: 10.1038/s41477-022-01146-6
Kaltenegger, E., Ober, D. (2015). Paralogue interference affects the dynamics after gene duplication. Trends Plant Sci. 20, 814–821. doi: 10.1016/j.tplants.2015.10.003
Kawakami, S. M., Kato, J., Kawakami, S. (2019). Meiosis of dihaploid Thelypteris decursive-pinnata produced artificially by induced apogamy. Cytologia 84, 319–322. doi: 10.1508/cytologia.84.319
Koh, J., Soltis, P. S., Soltis, D. E. (2010). Homeolog loss and expression changes in natural populations of the recently and repeatedly formed allotetraploid Tragopogon mirus (Asteraceae). BMC Genomics 11, 97. doi: 10.1186/1471-2164-11-97
Kolesiński, P., Piechota, J., Szczepaniak, A. (2011). Initial characteristics of RbcX proteins from Arabidopsis thaliana. Plant Mol. Biol. 77, 447–459. doi: 10.1007/s11103-011-9823-8
Kuo, L.-Y., Tang, T.-Y., Li, F.-W., Su, H.-J., Chiou, W.-L., Huang, Y.-M., et al. (2018). Organelle genome inheritance in Deparia ferns (Athyriaceae, Aspleniineae, Polypodiales). Front. Plant Sci. 9. doi: 10.3389/fpls.2018.00486
Kuo, T., Frith, M. C., Sese, J., Horton, P. (2018). EAGLE: explicit alternative genome likelihood evaluator. BMC Med. Genomics 11, 28. doi: 10.1186/s12920-018-0342-1
Landis, J. B., Soltis, D. E., Li, Z., Marx, H. E., Barker, M. S., Tank, D. C., et al. (2018). Impact of whole-genome duplication events on diversification rates in angiosperms. Am. J. Bot. 105, 348–363. doi: 10.1002/ajb2.1060
Langmead, B., Salzberg, S. L. (2012). Fast gapped-read alignment with Bowtie 2. Nat. Method 9, 357–359. doi: 10.1038/nmeth.1923
Li, Z., Baniaga, A. E., Sessa, E. B., Scascitelli, M., Graham, S. W., Rieseberg, L. H., et al. (2015). Early genome duplications in conifers and other seed plants. Sci. Adv. 1, e1501084. doi: 10.1126/sciadv.1501084
Li, B., Dewey, C. N. (2011). RSEM: accurate transcript quantification from RNA-Seq data with or without a reference genome. BMC Bioinf. 12, 323. doi: 10.1186/1471-2105-12-323
Li, C., Wickell, D., Kuo, L. Y., Chen, X., Nie, B., Liao, X., et al. (2023). Extraordinary preservation of gene collinearity over three hundred million years revealed in homosporous lycophytes. bioRxiv. doi: 10.1101/2023.07.24.548637v1
Li, Z., McKibben, M. T. W., Finch, G. S., Blischak, P. D., Sutherland, B. L., Barker, M. S. (2021). Patterns and processes of diploidization in land plants. Annu. Rev. Plant Biol. 72, 387–410. doi: 10.1146/annurev-arplant-050718-100344
Lynch, M., Conery, J. S. (2000). The Evolutionary fate and consequences of duplicate genes. Science 290, 1151–1155. doi: 10.1126/science.290.5494.1151
Mandáková, T., Lysak, M. A. (2018). Post-polyploid diploidization and diversification through dysploid changes. Curr. Opin. Plant Biol. 42, 55–65. doi: 10.1016/j.pbi.2018.03.001
Marchant, D. B., Chen, G., Cai, S., Chen, F., Schafran, P., Jenkins, J., et al. (2022). Dynamic genome evolution in a model fern. Nat. Plants 8, 1038–1051. doi: 10.1038/s41477-022-01226-7
Masuyama, S. (1979). Reproductive biology of the fern Phegopteris decursive-pinnata I. The dissimilar mating systems of diploids and tetraploids. Bot. Mag. Tokyo 92, 275–289. doi: 10.1007/BF02506251
Masuyama, S. (1986). Reproductive biology of the fern Phegopteris decursive-pinnata II. Genetic analyses of self-sterility in diploids. Bot. Mag. Tokyo 99, 107–121. doi: 10.1007/BF02488626
McClintock, B. (1984). The Significance of responses of the genome to challenge. Science 226, 792–801. doi: 10.1126/science.15739260
Nakato, N., Masuyama, S. (2021). Polyploid progeny from triploid hybrids of Phegopteris decursivepinnata (Thelypteridaceae). J. Plant Res. 134, 195–208. doi: 10.1007/s10265-021-01255-x
Nakato, N., Ootsuki, R., Murakami, N., Masuyama, S. (2012). Two types of partial fertility in a diploid population of the fern Thelypteris decursive-pinnata (Thelypteridaceae). J. Plant Res. 125, 465–474. doi: 10.1007/s10265-011-0461-7
Orr, H. A. (1996). Dobzhansky, Bateson, and the genetics of speciation. Genetics 144, 1331–1335. doi: 10.1093/genetics/144.4.1331
Pelosi, J. A., Kim, E. H., Barbazuk, W. B., Sessa, E. B. (2022). Phylotranscriptomics illuminates the placement of whole genome duplications and gene retention in ferns. Front. Plant Sci. 13. doi: 10.3389/fpls.2022.882441
Rapp, R. A., Udall, J. A., Wendel, J. F. (2009). Genomic expression dominance in allopolyploids. BMC Biol. 7, 18. doi: 10.1186/1741-7007-7-18
R Core Team (2022). R: A language and environment for statistical computing (Vienna: R Foundation for Statistical Computing). Available at: https://www.R-project.org.
Rigal, M., Becker, C., Pélissier, T., Pogorelcnik, R., Devos, J., Ikeda, Y., et al. (2016). Epigenome confrontation triggers immediate reprogramming of DNA methylation and transposon silencing in Arabidopsis thaliana F1 epihybrids. Proc. Natl. Acad. Sci. U.S.A 113, 2083–2092. doi: 10.1073/pnas.1600672113
Roberts, A., Pachter, L. (2013). Streaming fragment assignment for real-time analysis of sequencing experiments. Nat. Methods 10, 71–73. doi: 10.1038/nmeth.2251
Robinson, M. D., McCarthy, D. J., Smyth, G. K. (2010). edgeR : a Bioconductor package for differential expression analysis of digital gene expression data. Bioinformatics 26, 139–140. doi: 10.1093/bioinformatics/btp616
Rothfels, C. J., Johnson, A. K., Hovenkamp, P. H., Swofford, D. L., Roskam, H. C., Fraser-Jenkins, C. R., et al. (2015). Natural hybridization between genera that diverged from each other approximately 60 million years ago. Am. Nat. 185, 433–442. doi: 10.1086/679662
Schneider, H., Liu, H., Clark, J., Hidalgo, O., Pellicer, J., Zhang, S., et al. (2015). Are the genomes of royal ferns really frozen in time? Evidence for coinciding genome stability and limited evolvability in the royal ferns. New Phytol. 207, 10–13. doi: 10.1111/nph.13330
Sehrish, T., Symonds, V. V., Soltis, D. E., Soltis, P. S., Tate, J. A. (2015). Cytonuclear coordination is not immediate upon allopolyploid formation in Tragopogon miscellus (Asteraceae) allopolyploids. PloS One 10, e0144339. doi: 10.1371/journal.pone.0144339
Sessa, E. B., Der, J. P. (2016). Evolutionary genomics of ferns and lycophytes. Adv. Bot. Res. 78, 215–254. doi: 10.1016/bs.abr.2016.02.001
Shan, S., Boatwright, J. L., Liu, X., Chanderbali, A. S., Fu, C., Soltis, P. S., et al. (2020). Transcriptome dynamics of the inflorescence in reciprocally formed allopolyploid Tragopogon miscellus (Asteraceae). Front. Genet. 11. doi: 10.3389/fgene.2020.00888
Sharbrough, J., Conover, J. L., Tate, J. A., Wendel, J. F., Sloan, D. B. (2017). Cytonuclear responses to genome doubling. Am. J. Bot. 104, 1277–1280. doi: 10.3732/ajb.1700293
Shimizu, K. K. (2022). Robustness and the generalist niche of polyploid species: Genome shock or gradual evolution? Curr. Opin. Plant Biol. 69, 102292. doi: 10.1016/j.pbi.2022.102292
Shimizu-Inatsugi, R., Terada, A., Hirose, K., Kudoh, H., Sese, J., Shimizu, K. K. (2016). Plant adaptive radiation mediated by polyploid plasticity in transcriptomes. Mol. Ecol. 26, 193–207. doi: 10.1111/mec.13738
Sigel, E. M., Der, J. P., Windham, M. D., Pryer, K. M. (2019). Expression level dominance and homeolog expression bias in recurrent origins of the allopolyploid fern Polypodium hesperium. Am. Fern J. 109, 224. doi: 10.1640/0002-8444-109.3.224
Sigel, E. M., Windham, M. D., Pryer, K. M. (2014). Evidence for reciprocal origins in Polypodium hesperium (Polypodiaceae): A fern model system for investigating how multiple origins shape allopolyploid genomes. Am. J. Bot. 101, 1476–1485. doi: 10.3732/ajb.1400190
Šímová, I., Herben, T. (2012). Geometrical constraints in the scaling relationships between genome size, cell size and cell cycle length in herbaceous plants. Proc. R. Soc B. 279, 867–875. doi: 10.1098/rspb.2011.1284
Song, Q., Chen, Z. J. (2015). Epigenetic and developmental regulation in plant polyploids. Curr. Opin. Plant Biol 24, 101–109. doi: 10.1016/j.pbi.2015.02.007
Subrahmanian, N., Remacle, C., Hamel, P. P. (2016). Plant mitochondrial complex I composition and assembly: A review. Biochim. Biophys. Acta - Biomembr. 1857, 1001–1014. doi: 10.1016/j.bbabio.2016.01.009
Szövényi, P., Gunadi, A., Li, F. W. (2021). Charting the genomic landscape of seed-free plants. Nat. Plants 7, 554–565. doi: 10.1038/s41477-021-00888-z
Van De Peer, Y., Mizrachi, E., Marchal, K. (2017). The evolutionary significance of polyploidy. Nat. Rev. Genet. 18, 411–424. doi: 10.1038/nrg.2017.26
Vasudevan, A., Lévesque-Lemay, M., Edwards, T., Cloutier, S. (2023). Global transcriptome analysis of allopolyploidization reveals large-scale repression of the D-subgenome in synthetic hexaploid wheat. Commun. Biol. 6, 426. doi: 10.1038/s42003-023-04781-7
Wang, X., Morton, J. A., Pellicer, J., Leitch, I. J., Leitch, A. R. (2021). Genome downsizing after polyploidy: mechanisms, rates and selection pressures. Plant J. 107, 1003–1015. doi: 10.1111/tpj.15363
Watano, Y., Masuyama, S. (1991). Inbreeding in natural populations of the annual polyploid fern Ceratopteris thalictroides (Parkeriaceae). Syst. Bot. 16, 705–714. doi: 10.2307/2418872
Wendel, J. F., Lisch, D., Hu, G., Mason, A. S. (2018). The long and short of doubling down: polyploidy, epigenetics, and the temporal dynamics of genome fractionation. Curr. Opin. Genet. Dev. 49, 1–7. doi: 10.1016/j.gde.2018.01.004
Whitney, K. D., Ahern, J. R., Campbell, L. G., Albert, L. P., King, M. S. (2010). Patterns of hybridization in plants. Plant Ecol. Evol. Syst. 12, 175–182. doi: 10.1016/j.ppees.2010.02.002
Wood, T. E., Takebayashi, N., Barker, M. S., Mayrose, I., Greenspoon, P. B., Rieseberg, L. H. (2009). The frequency of polyploid speciation in vascular plants. Proc. Natl. Acad. Sci. U.S.A. 106, 13875–13879. doi: 10.1073/pnas.0811575106
Wu, J., Lin, L., Xu, M., Chen, P., Liu, D., Sun, Q., et al. (2018). Homoeolog expression bias and expression level dominance in resynthesized allopolyploid Brassica napus. BMC Genomics 19, 586. doi: 10.1186/s12864-018-4966-5
Wu, H., Yu, Q., Ran, J.-H., Wang, X.-Q. (2021). Unbiased subgenome evolution in allotetraploid species of Ephedra and its implications for the evolution of large genomes in gymnosperms. Genome Biol. Evol. 13, evaa236. doi: 10.1093/gbe/evaa236
Xia, Z. Q., Wei, Z. Y., Shen, H., Shu, J. P., Wang, T., Gu, Y. F., et al. (2022). Lycophyte transcriptomes reveal two whole-genome duplications in Lycopodiaceae: Insights into the polyploidization of Phlegmariurus. Plant Divers. 44, 262–270. doi: 10.1016/j.pld.2021.08.004
Yoo, M.-J., Liu, X., Pires, J. C., Soltis, P. S., Soltis, D. E. (2014). Nonadditive gene expression in polyploids. Annu. Rev. Genet. 48, 485–517. doi: 10.1146/annurev-genet-120213-092159
Yoo, M.-J., Szadkowski, E., Wendel, J. F. (2013). Homoeolog expression bias and expression level dominance in allopolyploid cotton. Heredity 110, 171–180. doi: 10.1038/hdy.2012.94
Keywords: allopolyploid, cytonuclear coordination, expression level dominance, genome evolution, homoeolog expression bias, pteridophyte, subgenome dominance, transcriptome shock
Citation: Katayama N, Yamamoto T, Aiuchi S, Watano Y and Fujiwara T (2024) Subgenome evolutionary dynamics in allotetraploid ferns: insights from the gene expression patterns in the allotetraploid species Phegopteris decursivepinnata (Thelypteridacea, Polypodiales). Front. Plant Sci. 14:1286320. doi: 10.3389/fpls.2023.1286320
Received: 31 August 2023; Accepted: 13 December 2023;
Published: 09 January 2024.
Edited by:
Li-Yaung Kuo, National Tsing Hua University, TaiwanReviewed by:
David Wickell, University of Connecticut, United StatesRie Shimizu-Inatsugi, University of Zurich, Switzerland
Cheng Li, Chinese Academy of Agricultural Sciences (CAAS), China
Copyright © 2024 Katayama, Yamamoto, Aiuchi, Watano and Fujiwara. This is an open-access article distributed under the terms of the Creative Commons Attribution License (CC BY). The use, distribution or reproduction in other forums is permitted, provided the original author(s) and the copyright owner(s) are credited and that the original publication in this journal is cited, in accordance with accepted academic practice. No use, distribution or reproduction is permitted which does not comply with these terms.
*Correspondence: Tao Fujiwara, dGFvLmZ1aml3YXJhQGdtYWlsLmNvbQ==