- 1College of Forestry and Prataculture, Ningxia University, Yinchuan, China
- 2Inner Mongolia Pratacultural Technology Innovation Center Co., Ltd, Hohhot, China
- 3Ningxia Grassland and Animal Husbandry Engineering Technology Research Center, Yinchuan, China
- 4Key Laboratory for Model Innovation in Forage Production Efficiency, Ministry of Agriculture and Rural Affairs, Yinchuan, China
Alfalfa is an excellent leguminous forage crop that is widely cultivated worldwide, but its yield and quality are often affected by drought and soil salinization. Hyperosmolality-gated calcium-permeable channel (OSCA) proteins are hyperosmotic calcium ion (Ca2+) receptors that play an essential role in regulating plant growth, development, and abiotic stress responses. However, no systematic analysis of the OSCA gene family has been conducted in alfalfa. In this study, a total of 14 OSCA genes were identified from the alfalfa genome and classified into three groups based on their sequence composition and phylogenetic relationships. Gene structure, conserved motifs and functional domain prediction showed that all MsOSCA genes had the same functional domain DUF221. Cis-acting element analysis showed that MsOSCA genes had many cis-regulatory elements in response to abiotic or biotic stresses and hormones. Tissue expression pattern analysis demonstrated that the MsOSCA genes had tissue-specific expression; for example, MsOSCA12 was only expressed in roots and leaves but not in stem and petiole tissues. Furthermore, RT–qPCR results indicated that the expression of MsOSCA genes was induced by abiotic stress (drought and salt) and hormones (JA, SA, and ABA). In particular, the expression levels of MsOSCA3, MsOSCA5, MsOSCA12 and MsOSCA13 were significantly increased under drought and salt stress, and MsOSCA7, MsOSCA10, MsOSCA12 and MsOSCA13 genes exhibited significant upregulation under plant hormone treatments, indicating that these genes play a positive role in drought, salt and hormone responses. Subcellular localization results showed that the MsOSCA3 protein was localized on the plasma membrane. This study provides a basis for understanding the biological information and further functional analysis of the MsOSCA gene family and provides candidate genes for stress resistance breeding in alfalfa.
Introduction
Alfalfa (Medicago sativa L.) is a perennial forage grass that is widely grown worldwide and is highly adaptable to the environment. It is known as the ‘king of forages’ due to its high nutritional content and palatability. However, a number of adverse biotic (such as thrips, aphids, brown spot and downy mildew) and abiotic (such as drought, saline-alkali conditions and extreme temperature) factors severely constrain alfalfa production and have a serious negative impact on alfalfa yield and quality (He et al., 2022). For example, drought resulted in poor crop growth due to impeded water and nutrient uptake and excessive salt can lead to crop root death. Therefore, it is necessary to identify superior genes to breed alfalfa varieties with strong environmental adaptability through gene editing.
As an important secondary messenger in plants, calcium ions (Ca2+) play a key role in regulating plant growth and development and abiotic stress responses (Kolukisaoglu et al., 2004; Hepler, 2005). Studies have demonstrated that when plants are subjected to osmotic stress, this leads to a temporary increase in Ca2+ in the cytoplasm, which triggers the expression of osmotic stress-related genes that regulate plant tolerance to osmotic stress (Zelman et al., 2012; Kaur et al., 2018; Chen et al., 2021). Ca2+ mainly relies on calcium channels to enter cells. The major calcium channels in plants include cyclic nucleotide-gated channels (CNGCs) (Galione et al., 2009; Zelman et al., 2012), twin pore channels (TPCS) (Plattner and Verkhratsky, 2015), force-sensitive channels (MCAS), glutamate receptor channels (GLRS) (Hou et al., 2012), and hyperosmolality-gated calcium-permeable channels (OSCA) (Alaimo and Villarroel, 2018). Plants sense calcium signals delivered to effectors via three receptors, calmodulin (CAM) (Batistic and Kudla, 2009; Cheval et al., 2013), calcium-regulated neurophosphatase B-like protein (CBL) (Komatsu et al., 2001; Shabala et al., 2021) and Ca2+-dependent protein kinase (CDPK) (Ito et al., 2011; Vivek et al., 2017), and trigger the activation of protective mechanisms. OSCA is a family of calcium-permeable cation channel proteins that respond to hyperosmotic stress (Yuan et al., 2014; Zhang et al., 2023). OSCA family proteins have three conserved functional domains, namely, RSN1_TM (PF13967), DUF4463 (PHM7_cyt, PF14703), and DUF221 (RSN1_7TM, PF02714) (Li et al., 2022). The DUF4463 domain is usually located before the DUF221 domain. The DUF221 structural domain is essential for all OSCA family genes, and it is a representative and decisive structural domain that plays a role in the calcium channel during osmosis (Hou et al., 2014).
OSCA is located on the plasma membrane and plays an important role in the regulation of signal transduction during vegetative and reproductive growth in plants (Li et al., 2015). The OSCA family genes play specific roles in leaf, flower and root development in Arabidopsis, as evidenced by the high expression levels of Solanum habrochaite OSCA genes at specific developmental stages (Yuan et al., 2014; Miao et al., 2022). AtOSCA1.2 is highly permeable to calcium, potassium and sodium ions, which play an important role in ion absorption and exchange (Liu et al., 2018a). In Arabidopsis, OSCA1.3 has the specific function of participating in stomatal closure during immune signal transduction, while OSCA1.3 does not participate in erythranilic acid-induced stomatal closure (Thor et al., 2020). In wheat, TaOSCA1.4 is related to the number of grain ears. TaOSCA1.4 haplotype Hap-1B-C had more spikelets than Hap-1B-A (Lv, 2015). In addition, OsOSCA1.1/-1.2/-2.4/-3.1/-4.1 play more essential roles in the development of rice roots, buds, mature stems, mature leaves, stamens, pistils and mature seeds, as their expression levels are significantly higher in these tissues than in other tissues (Li et al., 2015).
As the only osmoreceptors in plants, OSCAs are capable of responding to abiotic stress, such as salt and drought, and have been confirmed in a variety of crops, such as rice (Li et al., 2015), sunflower (Shan et al., 2023), cucumber (Yang et al., 2022), soybean (Liu et al., 2022) and maize (Cao et al., 2020). In Arabidopsis, OSCA1 functions as an osmotic sensing receptor that mediates the elevation of Ca2+ concentration in response to osmotic stress (Liu et al., 2018b). It was found that silencing the ShOSCA3 gene decreased the activities of SOD, POD and APX while enhancing the ability of Solanum habrochaites to cope with high levels of ABA-induced stress (Miao et al., 2022). Transcriptomic analysis revealed that OsOSCA1.1 was involved in regulating stomatal closure and promoting seedling survival in rice roots under hyperosmotic and salt stresses (Li et al., 2015). Overexpression of ZmOSCA2.4 in Arabidopsis resulted in upregulation of the MYB44, DREB2A and NCED3 genes, while decreasing the expression of senescence-related genes and improving drought tolerance in Arabidopsis (Cao et al., 2020). Overall, OSCA genes have important roles in plant resistance to high osmotic stress environments. However, little is known about the response of OSCA family genes to abiotic stress in alfalfa.
In this study, we identified OSCA gene family members in alfalfa by analyzing the evolutionary relationships, gene structure, protein motifs and cis-acting elements of MsOSCA genes within the whole alfalfa genome. The expression patterns of the MsOSCA family members were analyzed in root, stem, leaf and petiole tissues and under salt, drought, abscisic acid (ABA), jasmonic acid (JA) and salicylic acid (SA) stresses. The results of this study lay a foundation for further understanding the functional verification of the MsOSCA gene family and provide a reference for exploring the mechanism of OSCA genes under abiotic stress in the future.
Materials and methods
OSCA gene identification in alfalfa
The protein sequences of 15 Arabidopsis OSCA genes were downloaded from TAIR. The reference genome of alfalfa (Zhongmu No. 1) and annotation files (https://figshare.com/articles/dataset/Medicago_sativa_genome_and_ annotation_files/12623960) were used in this study. First, 15 AtOSCA protein sequences were used to perform a BLAST search against the alfalfa protein database with the threshold of E-value < 1 × 10 −5 (Chen et al., 2021). Furthermore, the hidden Markov model (HMM) profile of DUF221 was used as a query to search against the alfalfa protein database using the simple HMM search in TBtools (Chen et al., 2020). Subsequently, the results of the BLAST and HMMER searches were merged, and redundancies were removed manually. Then, the Inter Pro (https://www.ebi.ac.uk/interpro/search/sequence/) function was used to confirm whether the candidate MsOSCAs had the conserved DUF221 domain and other typical domains. The gene without the typical functional domain was deleted.
Basic analysis of MsOSCA proteins
We analyzed the protein length (aa), instability index, molecular weight (MW), isoelectric point (pI) and grand average of hydropathicity (GRAVY) of MsOSCA proteins using the ProtParam ExPASy online website (https://web.expasy.org/protparam/) (Wilkins et al., 1999). SOPMA (https://npsa-prabi.ibcp.fr/cgi-bin/secpred_sopma.pl) was used to analyze the secondary structure of MsOSCA proteins (Ahmad et al., 2021). The subcellular location of MsOSCA proteins was predicted using the online tool WoLF PSORT (https://wolfpsort.hgc.jp) and Cell-PLOC (http://www.csbio.sjtu.edu.cn/bioinf/Cell-PLOC-2/) (Horton et al., 2007). We used the AlphaFold database to analyze the three-dimensional (3D) structure of MsOSCA proteins and PyMOL software to label the typical functional domain (El Khoury et al., 2023).
Phylogenetic analysis
The phylogenetic tree of 67 OSCA protein sequences, including 14 MsOSCAs from alfalfa, 15 AtOSCAs from Arabidopsis, 12 CaOSCAs from Cicer arietinum, 13 VrOSCAs from Vigna radiata and 13 CcOSCAs from Cajanus cajan, was constructed using MEGA 11 software with the maximum likelihood (ML) estimate, 1000 bootstrap replicates and other parameters set to default (Gregory et al., 2017; Yan et al., 2021). 67 OSCA protein sequences on Supplementary Table S1.
Functional domain, conserved motif and gene structure analysis
InterPro (https://www.ebi.ac.uk/interpro/) online website was used to analyze the functional domains of MsOSCA proteins. We used MEME (https://meme-suite.org/meme/tools/meme) to analyze conserved motifs and set the maximum number of predicted patterns to 10, and the screening threshold was E < e-10 (Bailey et al., 2009). The gene structure was analyzed using the alfalfa genome annotation files and visualized with TBtools.
Analysis of promoter Cis-Acting element and MsOSCA protein interactions
The 2 kb genomic DNA sequence upstream of the start codon (ATG) of each MsOSCA gene was extracted from the alfalfa genome annotation files using TBtools. The cis-regulatory elements in the promoter sequences of MsOSCA genes were analyzed using PlantCare (http://bioinformatics.psb.ugent.be/webtools/plantcare/html/) (Rombauts et al., 1999; Lescot et al., 2002). Arabidopsis was used as a model plant for query, and STRING online website (http://cn.string-db.org/) was used to analyze the protein–protein interactions of MsOSCAs.
Chromosome location, gene duplication and collinearity analysis
The genomic data for Arabidopsis and maize were downloaded from the EnsemblPlants database (https://plants.ensembl.org/index.html). The chromosome distribution of MsOSCA genes was analyzed and localized using TBtools software (https://github.com/CJ-Chen/TBtools) (Yin et al., 2021). A multiple collinear scanning toolkit (MCScanX) was utilized to analyze the collinear blocks of OSCA genes across alfalfa, Arabidopsis and Medicago truncatula and visualized by TBtools. Synonymous (Ka), nonsynonymous (Ks) substitutions, and Ka/Ks ratios were calculated by the Simple Ka/Ks Calculator (NG) (Li et al., 2023).
Analysis of MsOSCA gene expression using transcriptome data
The transcriptome data of alfalfa (Xinjiangdaye) were downloaded from the NCBI website (https://www.ncbi.nlm.nih.gov/sra/?term=SRP055547) (O'Rourke et al., 2015). The most closely matching transcript sequences of MsOSCAs were identified in the Alfalfa Breeder’s Toolbox database using the BLAST alignment tool (https://www.alfalfatoolbox.org/), and the expression profiles of MsOSCA genes in different tissues of alfalfa were obtained. The data for each MsOSCA were normalized by logarithm (FPKM) and displayed as a heatmap using TBtools.
Plant materials and stress treatments
Alfalfa (Zhongmu No. 1) seeds were sterilized with 75% ethanol for 30 s and 50% sodium hypochlorite for 5-6 min, rinsed 10 times with distilled water and incubated on wet filter paper in petri dishes at 25°C. After 3 days of growth in petri dishes, alfalfa seedlings were transferred to a plastic container (25 cm × 18 cm × 14 cm) with Hoagland nutrient solution for hydroponics under controlled conditions: temperature 24 ± 1°C, 16 hours of light, 8 hours of darkness, and 60% relative humidity. After 30 days of growth, the seedlings were exposed to nutrient solutions supplemented with 20% PEG-6000, 200 mM NaCl, 100 μM JA, 100 μM SA, or 100 μM ABA for different treatments (Luo et al., 2019). The seedlings cultured with normal nutrient solution without adding any substance served as the control. Alfalfa leaf samples from the control and treatment groups were collected at five time points of 0, 2, 4, 8, and 12 h for gene expression analysis. The roots, stems, leaves and petioles of the untreated seedlings were collected for gene expression analysis of different tissues. All samples were rapidly frozen in liquid nitrogen and stored at -80°C for further RNA extraction.
RNA extraction and quantitative real-time PCR analysis
Total RNA was extracted from the samples using the RNA Extraction Kit (Promega, Shanghai, China) according to the instructions. A total of 1 μg RNA was used for reverse transcription, and RT–qPCR was carried out according to the method provided by ChamQ Universal SYBR qPCR Master Mix (Vazyme, Nanjing, China). The MsActin gene (Li et al., 2019) was used as an internal reference gene, and all primers used in this study are listed in Supplementary Table S6. All primer concentrations were 10 μg/ml. The RT–qPCR system was 20 μl, containing 2 μl of cDNA, 0.6 μl of forward and reverse primers, 10 μl of ChamQ Universal SYBR enzyme and 6.8 μl of ddH2O (No RNA enzyme water). The reaction procedure was as follows: 3 min at 95°C; 10 s at 95°C, 30 s at 60°C for 40 cycles; 5 s at 65°C and 5 s at 95°C. Each treatment for RT–qPCR consisted of three independent biological replicates and three technical replicates. The melting curves of all primers used in this study are shown in Figure S1. The expression values of root tissue or 0 h were normalized, and the relative expression values of other tissues or time points were evaluated. The relative expression levels of genes were calculated by 2-ΔΔCT (Livak and Schmittgen, 2001).
Subcellular localization of MsOSCA proteins
Based on the results of MsOSCA gene response to abiotic stress, MsOSCA3 was selected for amplification, and the primers are shown in Supplementary Table S6. After PCR product recovery and purification, the CDS clones were inserted into the EcoRI cloning site in the pCAMBIA-1300-GFP vector. The recombinant vector was separately transformed into Agrobacterium tumefaciens GV3101. To validate the transient expression of MsOSCAs in tobacco, Agrobacterium carrying the recombinant vector was injected into tobacco leaves grown for one month. After incubation in the dark for 48 h, the leaf epidermal cells were used for microscopic (Leica SP8, Germany) observation and image acquisition by the laser confocal method.
Statistical analysis
Origin 2023 software was used to test the differences between groups by one-way analysis of variance (ANOVA), and Tukey’s multiple range test was used to assess the significant differences, where P < 0.05 indicated significance. Data are expressed as the mean or mean ± standard deviation (SD).
Results
Genome-wide identification of OSCA members in alfalfa
To identify all OSCA family members in alfalfa, the protein sequences of 15 OSCA genes in Arabidopsis were downloaded from TAIR as queries to search the alfalfa genome (https://figshare.com/articles/dataset/Medicago_sativa_genome_and_annotation_files/12623960). After removing identical and incomplete gene sequences, a total of 14 OSCA genes containing RSN1_7TM (PF02714) conserved functional domains were identified. These members were named MsOSCA1 to MsOSCA14 according to their position on the chromosome (Table 1). The nucleic acid and protein sequences of MsOSCA gene family members are shown in Supplementary Table S1. The lengths of MsOSCA protein sequences ranged from 205 (MsOSCA8) to 1265 aa (MsOSCA7), with corresponding protein molecular weights (MWs) of 23.55 to 143.01 kDa. The theoretical isoelectric point (pI) and instability index of MsOSCAs ranged from 5.43~9.32 and 37.03~53.18, respectively, with a GRAVY of (-0.229) ~0.297. Secondary structure analysis of the MsOSCA proteins showed that the α-helix, extended strand, β-turn and random coils ranged from 28.57% (MsOSCA12) to 60.57% (MsOSCA2), 11.79% (MsOSCA2) to 20.40% (MsOSCA6), 1.68% (MsOSCA3) to 9.83% (MsOSCA6), and 26.16% (MsOSCA11) to 46.18% (MsOSCA12), respectively. Subcellular localization prediction of WoLF PSORT showed that most MsOSCA proteins were located on the plasma membrane, whereas MsOSCA5, MsOSCA8, and MsOSCA14 were located in the chloroplast. Subcellular localization predicted using Cell-PLOC showed that only six MsOSCA proteins were located in the cell membrane.
Phylogenetic analysis of MsOSCA proteins in alfalfa
To investigate the evolutionary relationships of OSCA proteins, a phylogenetic tree was constructed using OSCA family members from M. sativa, Arabidopsis, Cicer arietinum, Vigna radiata and Cajanus cajan. As shown in Figure 1, a total of 67 OSCA proteins were classified into four clades (I, II, III and IV) based on the similarity of the full-length sequences. Clade I consisted of 8 AtOSCAs, 5 MsOSCAs, 5 CcOSCAs, 5 VrOSCAs and 4 CaOSCAs. Clade II consisted of 5 AtOSCAs, 7 MsOSCAs, 6 CcOSCAs, 6 VrOSCAs and 6 CaOSCAs. Clade III had 5 OSCA proteins, with one (MsOSCA3) alfalfa members. Clade IV contained one MsOSCA protein (MsOSCA13) of alfalfa. Furthermore, the OSCAs of alfalfa and Arabidopsis, C. arietinum, V. radiata and C. cajan were closer together in clades, indicating that they had higher homology. Overall, these results indicated that OSCA family proteins were highly conserved among plant species.
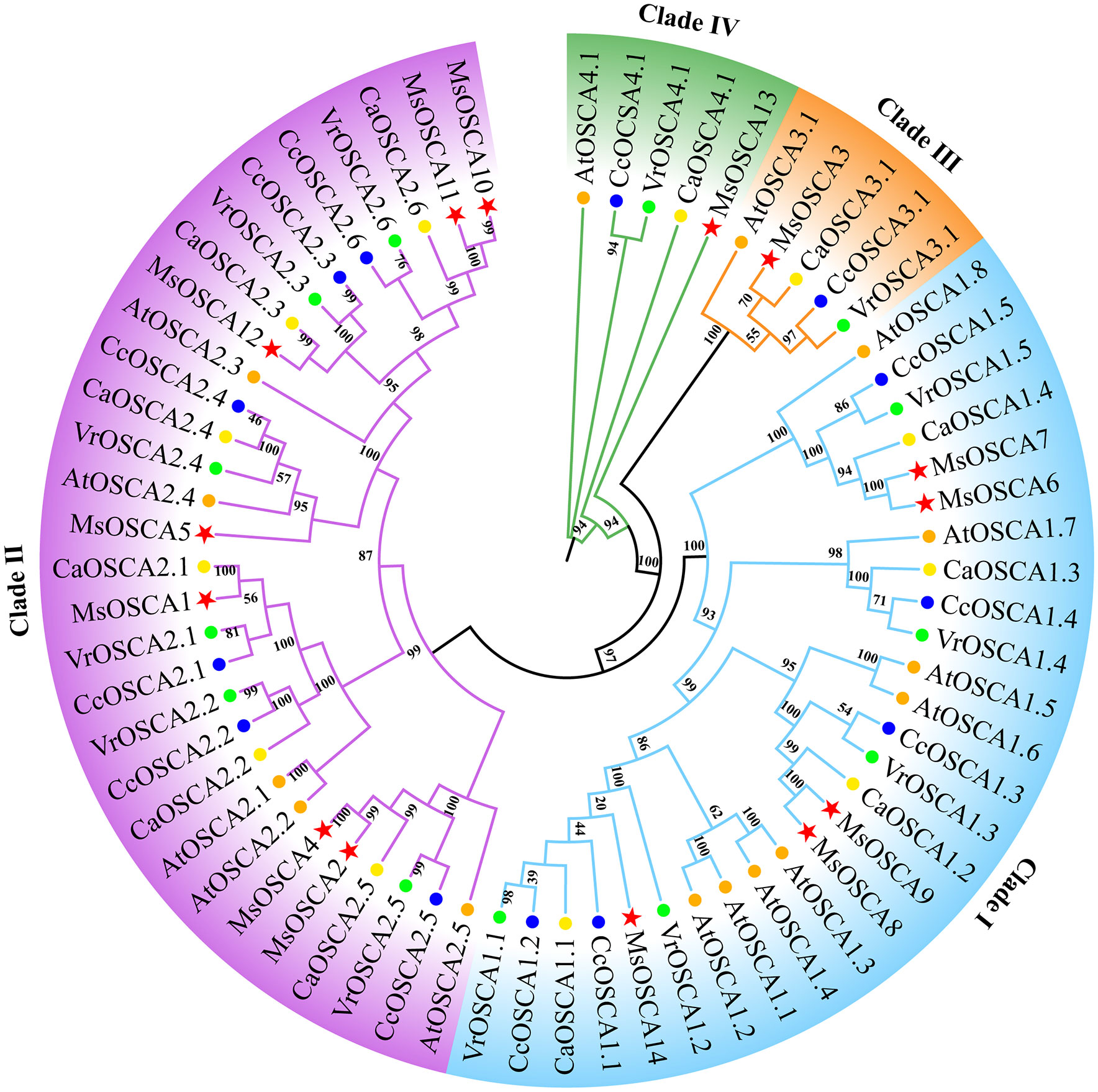
Figure 1 Phylogenetic tree analysis of OSCA proteins in M. sativa, Arabidopsis, Cicer arietinum, Vigna radiata and Cajanus cajan. The phylogenetic tree was constructed using OSCA protein sequences by the maximum likelihood estimate (ML) in MEGA 11 with 1000 bootstrap replicates. The red stars, blue circles, green circles, yellow circles and orange circles represent the M. sativa, Cajanus cajan, Vigna radiata, Cicer arietinum, and A. thaliana OSCA proteins, respectively.
Functional domains, conserved motifs and gene structure analysis of MsOSCA genes
The RSN1_7TM (PF02714) functional domain is the signature domain of the OSCA family and represents the seven transmembrane functional domains of the calcium-dependent channel (Liu et al., 2018b). All 14 MsOSCA members of alfalfa contained the conserved RSN1_7TM (PF02714) functional domain (Figure 2B). In addition, MsOSCA1/-2/-3/-4/-6/-7/-9/-10/-13 had RSN1_TM and PHM7_cyt functional domains, and the PHM7_cyt functional domain was located in the middle of the RSN1_TM and RSN1_7TM functional domains. Furthermore, similar conserved structural domains were found in MsOSCA proteins that were closely related on the evolutionary branches (Figures 2A, B). The 3D structures of the MsOSCA proteins are shown in Figure S2. All MsOSCA proteins contained the PHM7_cyt functional domain, which existed in linear and helical forms. MsOSCA6 and MsOSCA7 were closely related in the evolutionary tree, and both contained the AMP-binding domain. Interestingly, only MsOSCA14 contained the AUX_IAA structural domain.
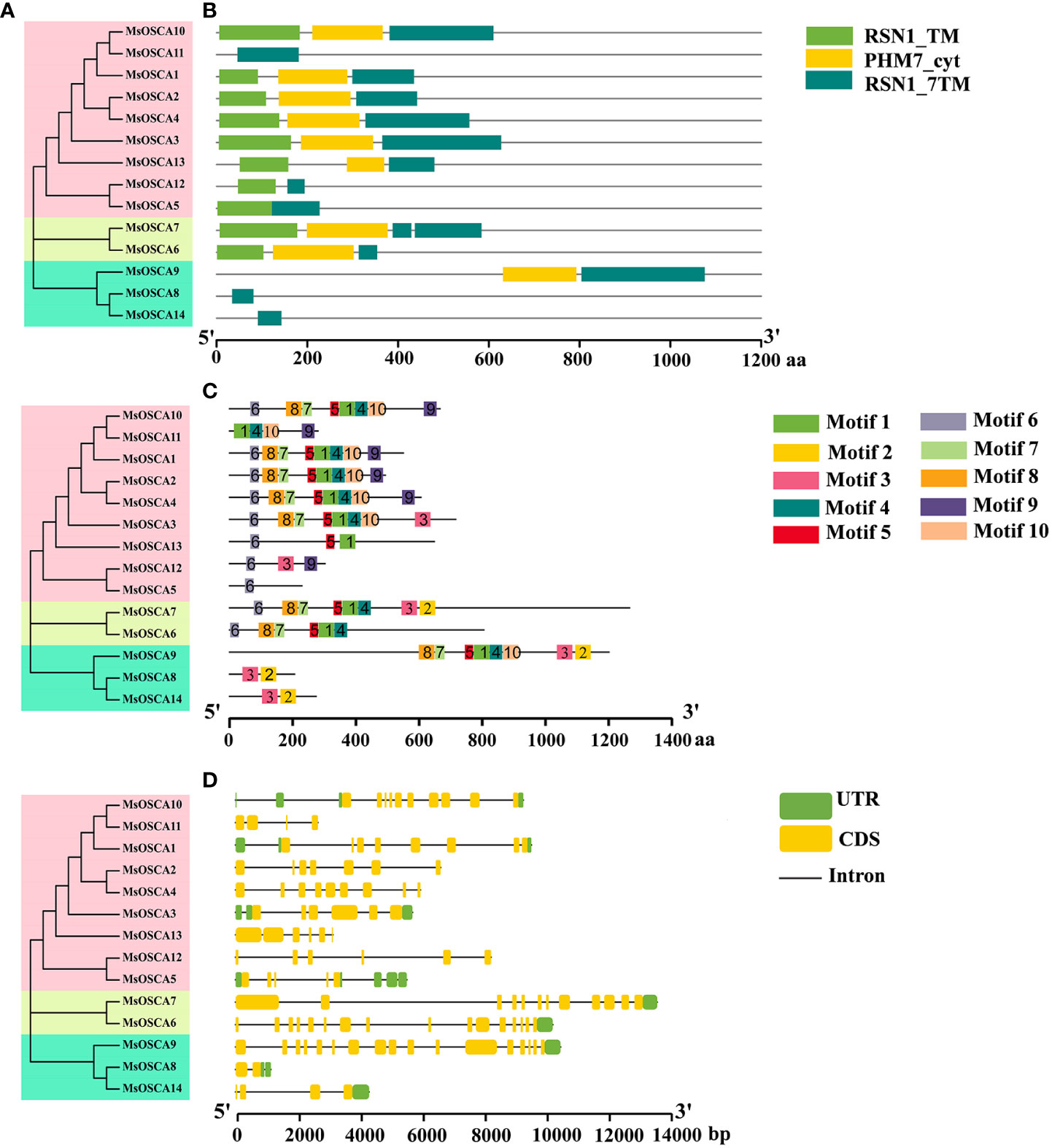
Figure 2 Analysis of the conserved domains, motifs and gene structure of alfalfa MsOSCA genes. (A) Phylogenetic tree of MsOSCA gene family. (B) Functional domains distribution of MsOSCAs. The colored rectangles represent the protein conserved domains. (C) Motifs of MsOSCA proteins. Different motifs are annotated by boxes of different colors and numbered 1–10. (D) Exon-intron structure of MsOSCA genes. The untranslated regions (UTRs) are indicated by green boxes. Yellow boxes represent exons and grey lines represent introns.
To better understand the conserved structure of MsOSCA proteins, the distribution of conserved motifs was identified and visualized using the MEME program and TBtools software, respectively. As shown in Figure 2C, a total of 10 conserved motifs were identified in alfalfa MsOSCA proteins. The sequence information for each motif is provided in Supplementary Table S2 and Figure S3. The results showed that the number of motifs contained in the 14 MsOSCAs varied widely. For example, MsOSCA1/-2/-3/-4/-6/-7/-9/-10 contained more than 5 motifs, of which MsOSCA7 contained 9 motifs. In contrast, MsOSCA8 and MsOSCA14 had only two motifs, and MsOSCA5 only contained motif 6. Moreover, MsOSCA1/-2/-4/proteins had the same quantity and type motifs, and correspondingly, they were close to each other on the phylogenetic tree, indicating high homology (Figure 2A).
Gene structure plays an important role in the regulation of gene expression (Oudelaar and Higgs, 2020). By analyzing the MsOSCA gene structure, we found that all members of the MsOSCA gene family had introns and exons, but the number varied (Figure 2D). For instance, MsOSCA9 contained 16 introns and 17 exons, while MsOSCA8 only contained 1 intron and 2 exons. MsOSCA12 and MsOSCA13 contained the same number of introns and exons. In addition, some of the MsOSCAs contained UTRs, the number of which varied between 1 and 5. Among them, MsOSCA6, MsOSCA7, MsOSCA9 and MsOSCA14 contained only one UTR, whereas MsOSCA5 contained five UTRs.
Analysis of cis-acting elements in the MsOSCA gene promoters
The cis-acting elements in the promoters of MsOSCA members were analyzed by PlantCare. As shown in Figure 3, the results showed that a total of 13 different types of cis-acting elements were predicted in the MsOSCA genes, which were widely involved in growth processes, hormone responsiveness, light responsiveness, metabolic regulation, and stress responses (Supplementary Table S3). The number of CGTCA motif, ARE, GT1 motif, TCA element and ABREs was relatively high in the MsOSCA genes, followed by GARE motif, TGA element, ABRE, circadian and MBS elements. Of these, stress response-related elements were found in almost all MsOSCAs. All 14 MsOSCAs contained AREs of stress-related cis-elements. Furthermore, the drought response element MBS was found in MsOSCA1/-3/-4/-5/-11 (Figure 3). These results indicated that MsOSCA genes may play an active role in the regulation of various stresses and plant hormone responses.
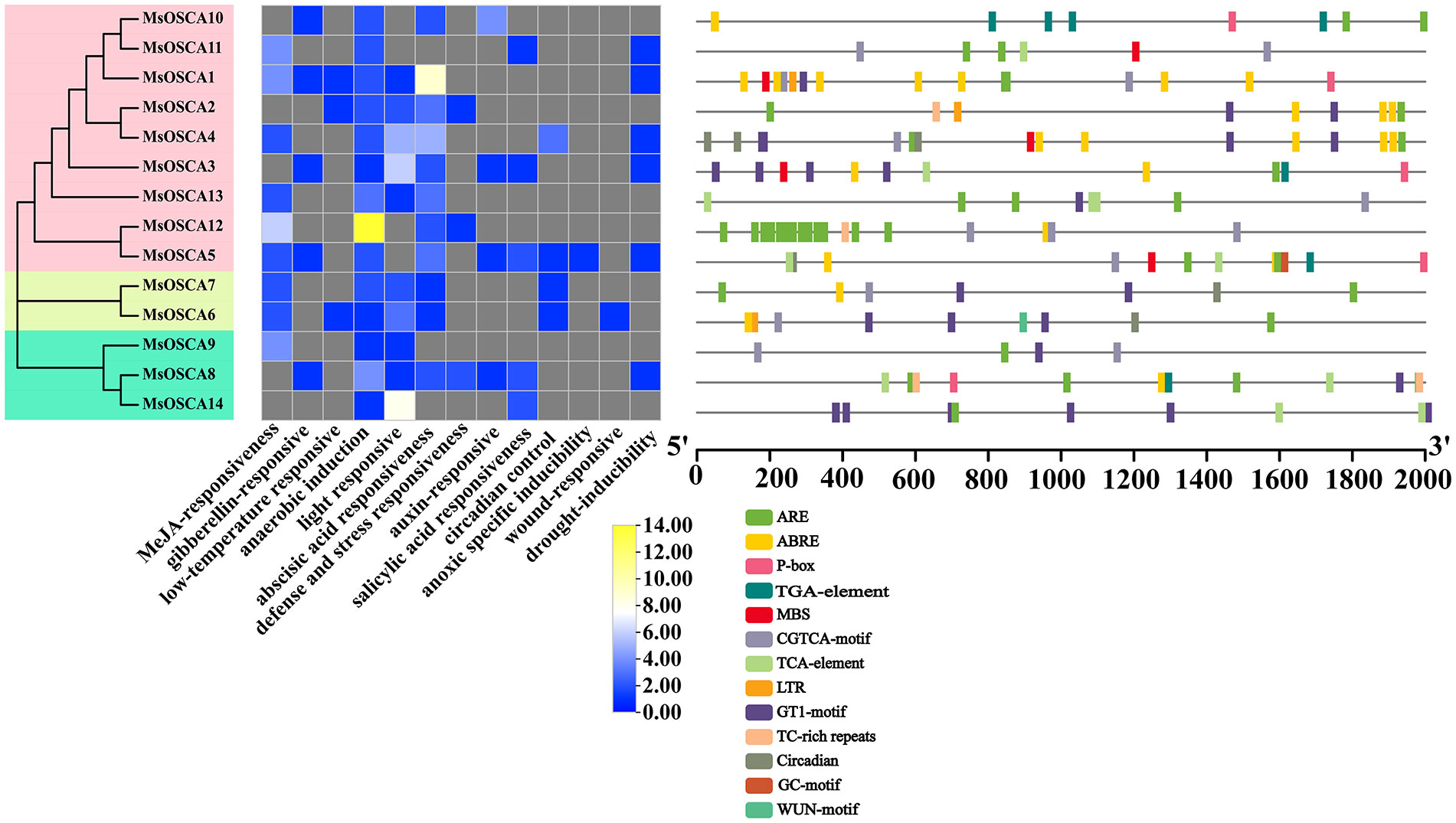
Figure 3 Distribution of cis-acting elements in the promoter region of MsOSCA genes in alfalfa. The cis-elements are represented by rectangles of different colors. ARE: responsive element for the anaerobic induction; ABRE: abscisic acid responsive element; P-box: gibberellin-responsive element; TGA-element: auxin-responsive element; MBS: MYB binding site involved in drought-inducibility; CGTCA-motif: cis-acting regulatory element involved in the MeJA-responsiveness; TCA-element: cis-acting element involved in salicylic acid responsiveness; LTR: cis-acting element involved in low-temperature responsiveness; GT1-motif:light responsive element; TC-rich repeats: cis-acting element involved in defense and stress responsiveness; Circadian: cis-acting regulatory element involved in circadian control; GC-motif: enhancer-like element involved in anoxic specific inducibility; WUN-motif: wound-responsive element.
Analysis of the chromosomal localization and collinearity of MsOSCA genes
The position of MsOSCA genes on each chromosome was determined by alfalfa genome annotation. As shown in Figure 4A, 14 MsOSCA genes were unevenly distributed on 8 chromosomes of alfalfa. Chromosomes 2, 3, 4 and 7 each contained one MsOSCA gene, chromosomes 1, 6 and 8 each contained two MsOSCA genes, and chromosome 5 contained four MsOSCA genes. To further investigate the evolutionary mechanisms and orthologous relationship of the MsOSCA gene family, we analyzed tandem and segmental duplication events. We found that MsOSCA2 and MsOSCA4 were segmental duplications, and MsOSCA8 and MsOSCA9 were tandem duplications (Figure 4A; Table 2). In addition, we analyzed the collinearity of OSCA genes from alfalfa, Medicago truncatula and Arabidopsis (Figure 4B). Notably, nine direct homologous pairs were found between alfalfa and Arabidopsis, and fifteen direct homologous pairs were observed between alfalfa and Medicago truncatula. The results showed that eleven MsOSCA genes (MsOSCA1/-2/-3/-4/-5/-6/-8/-9/-10/-12/-14) had a collinear relationship with Medicago truncatula, while five genes (MsOSCA3/-6/-10/-13/-14) were found to have a collinear relationship with Arabidopsis (Figure 4B).
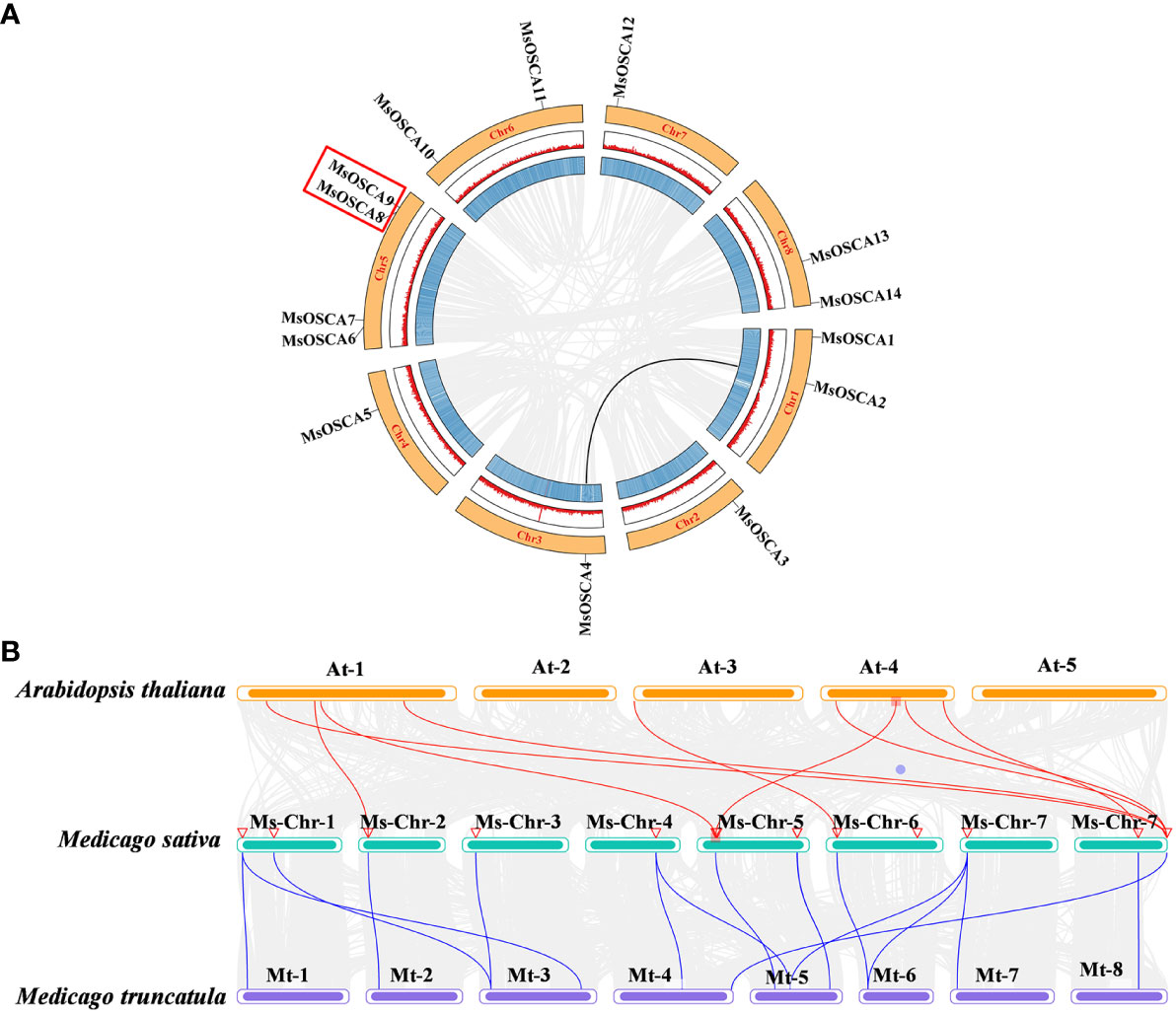
Figure 4 Chromosomal distribution and colinearity analysis of MsOSCA genes. (A) Chromosomal localization and interchromosomal relationships in alfalfa. The black lines represented the MsOSCA collinearity genes. (B) Colinearity analysis of OSCA genes in alfalfa, Medicago truncatula and Arabidopsis. The grey lines in the background represent collinear blocks between alfalfa and Arabidopsis/Medicago truncatula, and the red/blue lines represent collinear OSCA gene pairs.
Expression patterns of MsOSCA genes in different tissues
To further study the function of MsOSCAs in growth and development, we analyzed the expression of MsOSCA genes in six different tissues (roots, leaves, flowers, elongating stems, postelongating stems, and nodules) of alfalfa “Xinjiangdaye” based on transcriptomic data (Supplementary Table S4). In this study, the expression values of 11 MsOSCAs were determined and comprehensively investigated (Figure 5). The results showed that the expression of 11 MsOSCA genes changed remarkably, and they were obviously divided into two groups. As a whole, MsOSCA1, MsOSCA3, MsOSCA8, MsOSCA10, MsOSCA13 and MsOSCA14 were highly expressed in all tissues, while other genes had lower expression levels, except for the high expression of MsOSCA5 in roots and MsOSCA6 in flowers. The expression levels of MsOSCA2 and MsOSCA4 in leaves and roots were significantly higher than those in other tissues. The expression of MsOSCA12 was high in flowers, indicating that it is a specific expression gene for flower development. MsOSCA5 was not expressed in nodules or postelongating stems (Figure 5). These results suggested that MsOSCA family genes had tissue-specific expression.
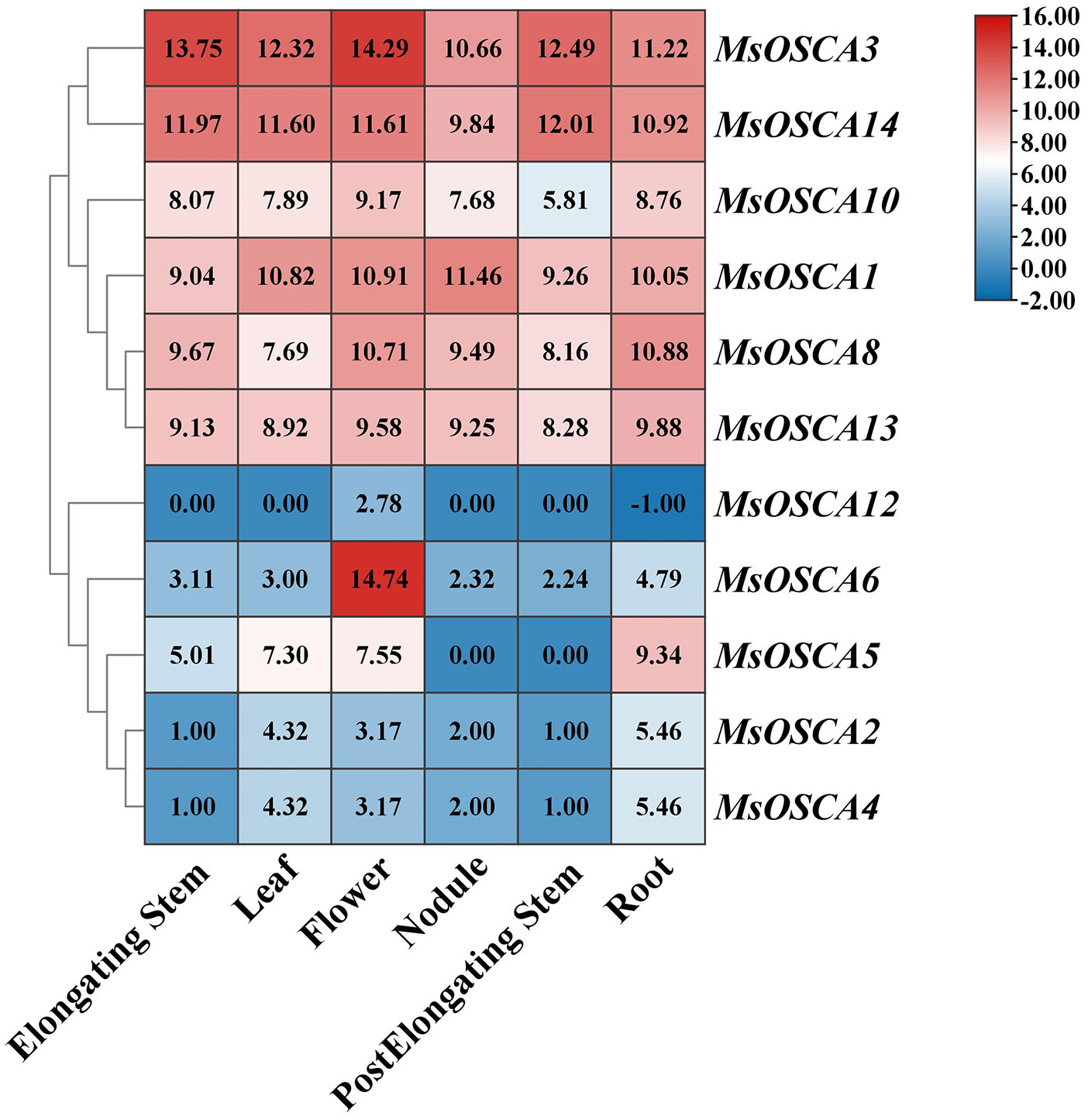
Figure 5 Expression patterns of MsOSCA genes in different tissues at various growth stages of alfalfa. The values in the heat map represent the amount of gene expression (FPKM).
We determined the relative expression levels of MsOSCA genes in roots, stems, leaves and petioles by RT–qPCR. As shown in Figure 6, the expression levels of MsOSCA genes varied considerably in four different tissues but were not completely consistent with the results of the transcriptome data. Except for MsOSCA12, other MsOSCA genes had different expression values in all tissues. The results showed that MsOSCA12 had no detectable expression in stems and petioles. MsOSCA2/-3/-6/-7/-11/-13/-14 had significantly higher expression levels in stems than in other tissues. The expression levels of the MsOSCA5 and MsOSCA12 genes were significantly higher in leaves than in other tissues. In addition, it is worth noting that MsOSCA8 and MsOSCA9 were highly expressed in roots (Figure 6). These results suggest that MsOSCA genes play different roles in the regulation of growth and development in alfalfa.
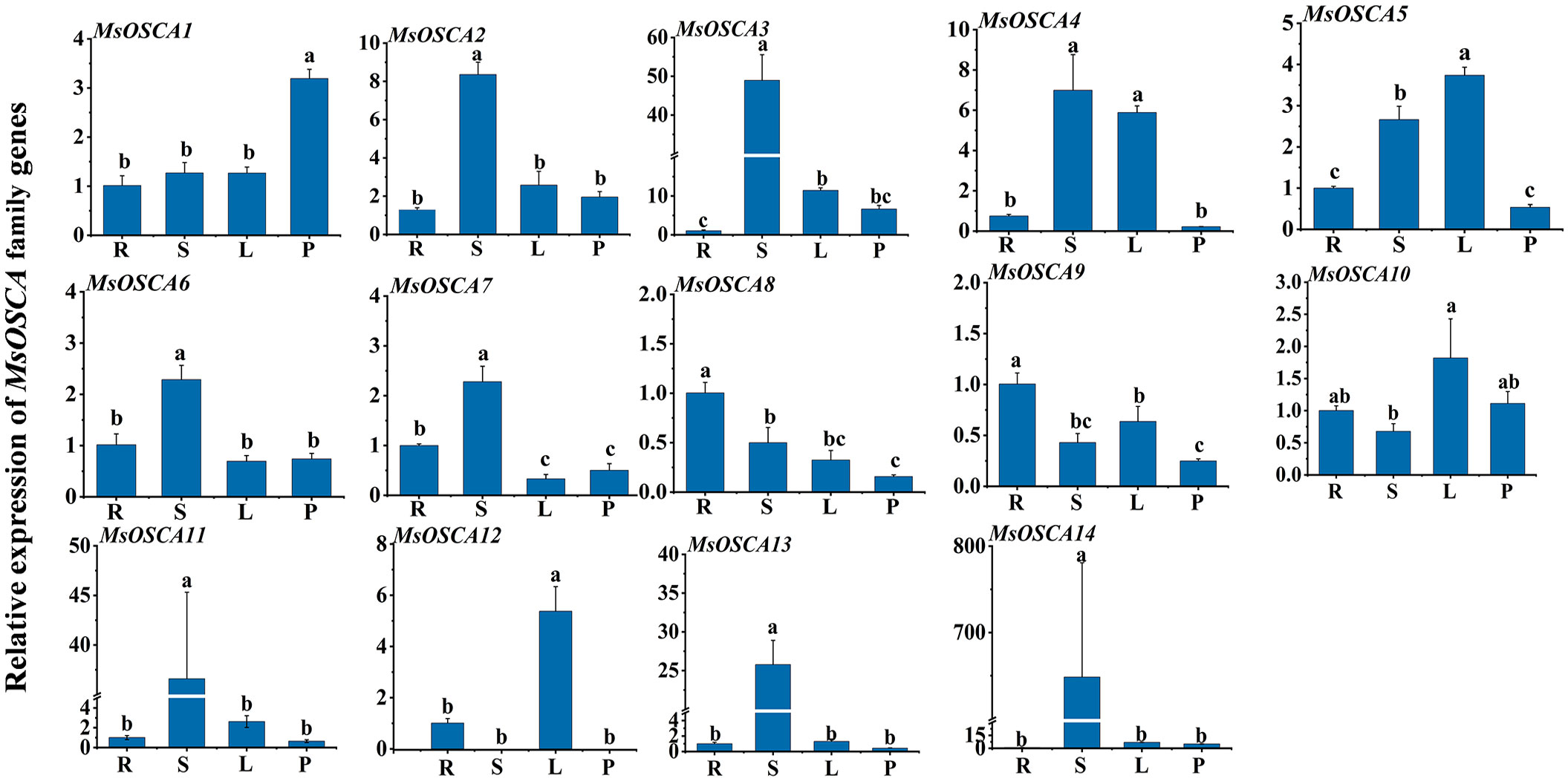
Figure 6 Relative expression levels analysis of MsOSCA genes in different tissues by RT-qPCR. Mean expression values relative to roots were calculated from three independent biological replicates and triple technique repetition. R, Root; S, Stem; L, Leaf; P, Petiole. Standard deviation is shown as bars above columns. Lower case letters indicate significant differences at P < 0.05.
Expression patterns of MsOSCA genes in response to abiotic stress
To investigate the potential function of MsOSCA genes under different abiotic stresses, the expression profiles of MsOSCA genes in alfalfa leaves under drought and salt stress were analyzed by RT–qPCR. With the exception of MsOSCA2 and MsOSCA6, 12 MsOSCA members showed positive responses to drought stress under PEG-simulated drought stress conditions (Figure 7A). As shown in Figure 7A, the expression levels of the MsOSCA3 and MsOSCA5 genes initially increased and subsequently declined during drought stress. Further analysis showed that the MsOSCA4 and MsOSCA12 genes were dramatically upregulated at 4 h, with >3-fold of the control. The expression levels of MsOSCA3/-5/-10/-13/-14 were more than 4-30-fold higher at 8 h than at 0 h. In the whole drought stress period, the expression levels of MsOSCA7/-8/-11 genes showed the highest value at 12 h, and MsOSCA7 was upregulated with an increase of >30-fold compared with the control. In addition, MsOSCA2 and MsOSCA6 decreased first and then increased under drought stress and finally maintained the same expression levels at 0 h (Figure 7A).
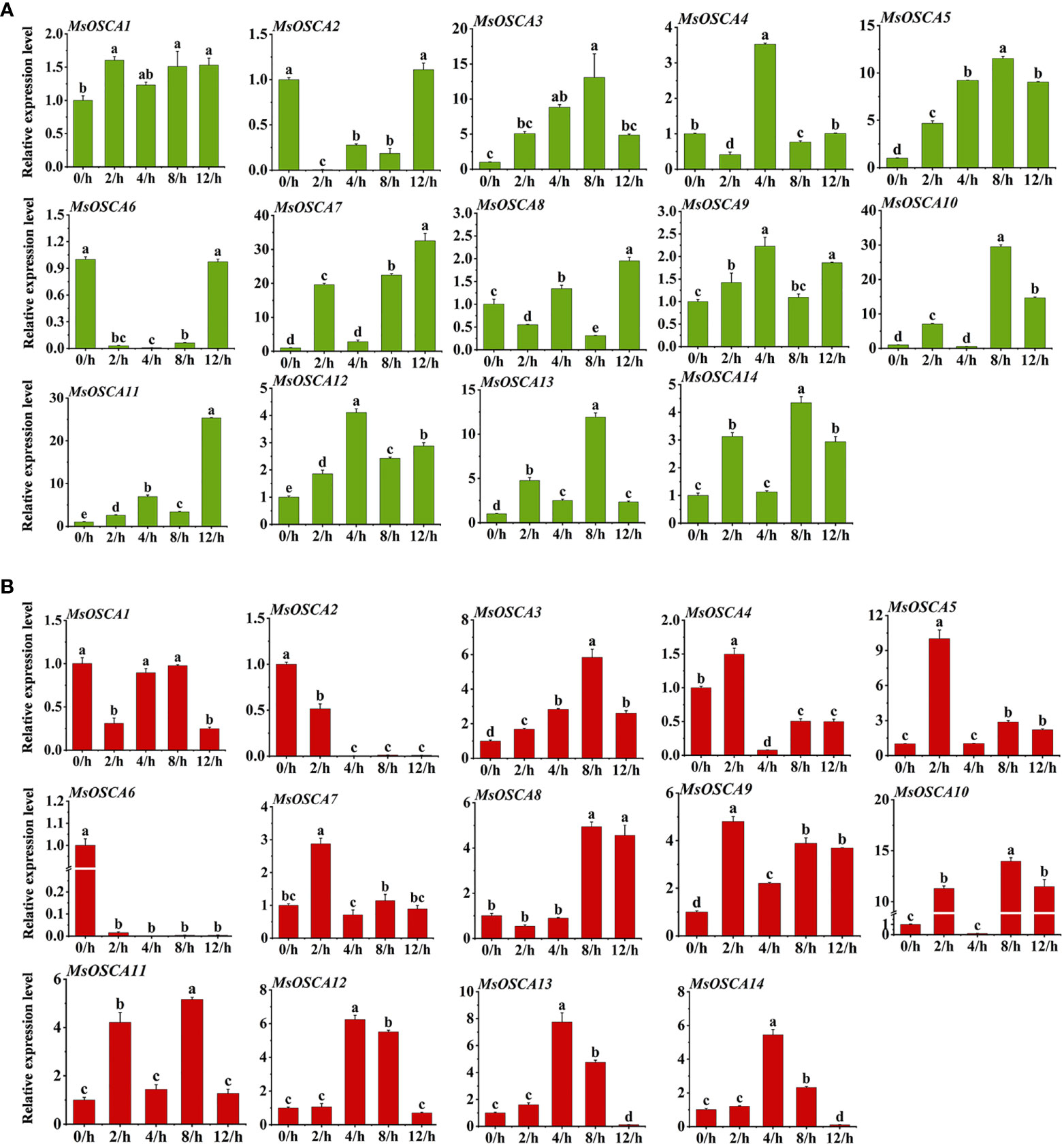
Figure 7 Relative expression levels analysis of MsOSCA genes under drought and salt treatments by RT-qPCR. (A) Relative expression levels of MsOSCA genes at 0, 2, 4, 8 and 12 h after drought treatment. (B) Relative expression levels of MsOSCA genes at 0, 2, 4, 8 and 12 h after salt treatment. Data represent the mean ± SD of three independent biological replicates and triple technique repetition. Standard deviation is shown as bars above columns. Lower case letters indicate significant differences at P < 0.05.
Compared with the control plants, all members of the MsOSCA gene family were differentially expressed under salt treatment, among which MsOSCA1, MsOSCA2, and MsOSCA6 genes were significantly downregulated, and other genes were highly upregulated (Figure 7B). Among these differentially upregulated MsOSCAs, except for MsOSCA8, the expression of other genes showed an initial elevation and subsequent decrease under salt stress. The expression levels of MsOSCA 12, MsOSCA13, and MsOSCA 14 reached their maximum at 4 h after salt stress, whereas the expression levels of MsOSCA3/-8/-10/-11 reached their maximum at 8 h after salt stress (Figure 7B). In addition, the expression levels of the MsOSCA5, MsOSCA7 and MsOSCA9 genes were increased by 2-10-fold at 2 h under salt stress compared to 0 h. Compared with 0 h, the expression levels of MsOSCA3/-8/-10/-11 were increased by 5-15-fold at 8 h. MsOSCA10 and MsOSCA11 showed similar expression trends under salt stress. However, MsOSCA2 and MsOSCA6 gene expression gradually decreased with the duration of salt stress (Figure 7B). Further analysis showed that MsOSCA3, MsOSCA5, MsOSCA12 and MsOSCA13 responded strongly to drought and salt stress. These results suggest that MsOSCA genes may play different roles under drought and salt stress.
Expression patterns of MsOSCA genes in response to exogenous phytohormones
Plant hormones are important stress signals because they stimulate the synthesis of phytohormones to regulate the expression of many stress-responsive genes in plants in response to abiotic stress (Putranto et al., 2015; Liu et al., 2022). To determine whether MsOSCA family genes were affected by different phytohormone treatments, the expression levels of MsOSCA genes were determined by RT–qPCR in the presence of JA, SA and ABA phytohormones (Figure 8; Supplementary Table S5). We found that the MsOSCA genes showed diverse expression patterns during JA treatment. Under JA stress, seven out of fourteen MsOSCA genes were significantly upregulated and peaked at 4, 8 or 12 h (Figure 8A). For example, the expression level of MsOSCA12 at 4 h was 5-fold higher than that at 0 h. MsOSCA3/-7/-8/-10 expression levels reached the highest at 8 h, while MsOSCA13 and MsOSCA14 expression levels were highest at 12 h, more than 5-fold higher than at 0 h. Interestingly, MsOSCA8, MsOSCA10 and MsOSCA14 showed initial fluctuation, with downregulated expression levels at 4 h. Furthermore, MsOSCA1/-2/-4/-6 showed a decreasing trend expression pattern under JA treatment in alfalfa (Figure 8A).
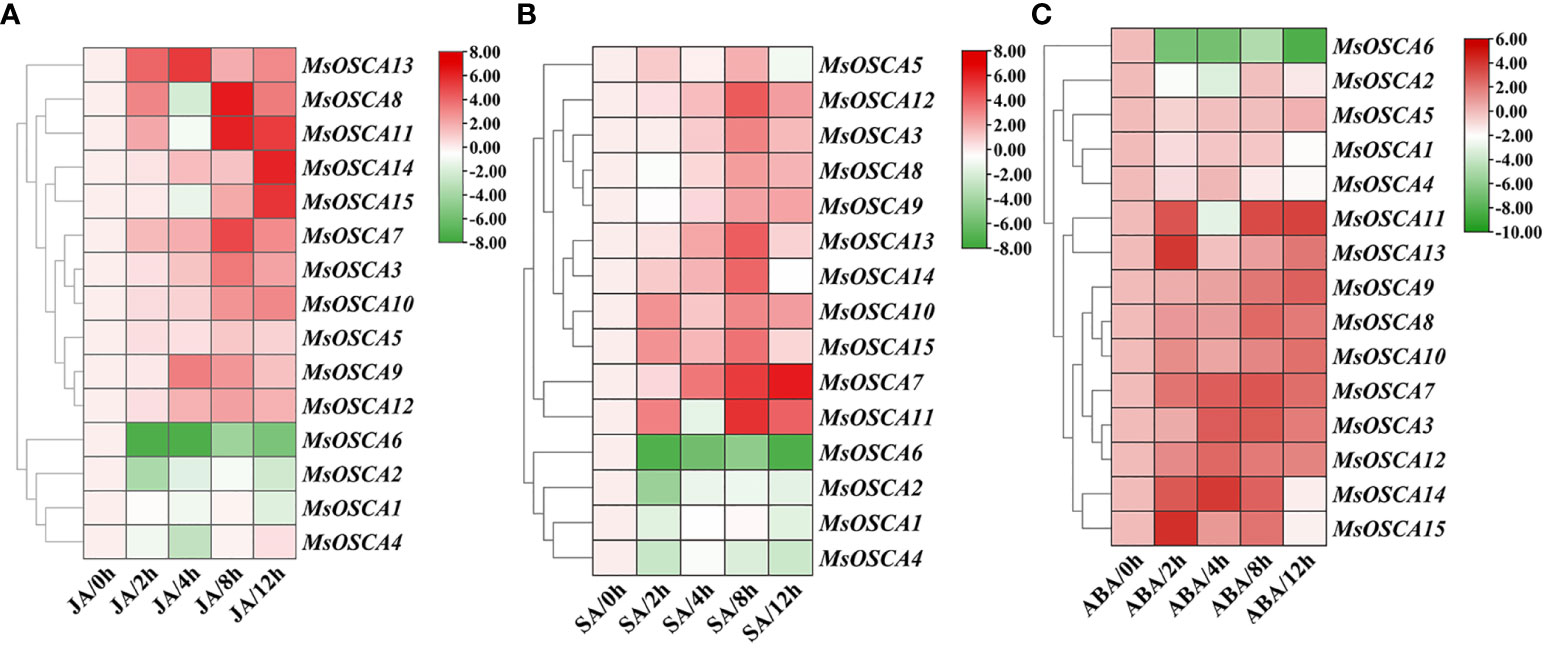
Figure 8 Expression patterns of MsOSCA genes under different phytohormone treatments. (A) Expression patterns of MsOSCAs under JA treatment. (B) Expression patterns of MsOSCAs under SA treatment. (C) Expression patterns of MsOSCAs under ABA treatment. The color scale at the right of the heatmap represents the log2 expression value, and the gradient from green to red represents low to high expression level.
The MsOSCCA expression levels were markedly triggered by SA treatment (Figure 8B). Most MsOSCA genes showed a positive response, and the expression levels of MsOSCA3/-7/-8/-9/-11/-12/-14 increased significantly after treatment of alfalfa with SA (Figure 8B). In contrast, MsOSCA1/-2/-4/-6 showed negative regulation in response to SA treatment. The expression levels of MsOSCA8 first decreased slightly and then increased and peaked at 8 h. Further analysis showed that MsOSCA3/-9/-11/-12/-14 expression reached a maximum at 8 h and was more than 3-fold higher than that of the control, while MsOSCA7 expression was highest at 12 h and was 6-fold higher than that of the control (Figure 8B).
We found that the expression levels of MsOSCA genes were strongly associated with ABA hormone treatment, but the expression profiles varied in MsOSCAs (Figure 8C). The heatmap showed that the expression levels of nine genes (MsOSCA-3/-7/-8/-9/-10/-11/-12/-13/-14) were significantly upregulated in the presence of ABA, reaching the maximum expression values at 2 h, 4 h, 8 h and 12 h, respectively. In addition, MsOSCA1, MsOSCA2 and MsOSCA6 genes were downregulated, and MsOSCA5 gene expression showed no significant change under ABA treatment (Figure 8C). Overall, the MsOSCA7, MsOSCA10, MsOSCA12 and MsOSCA13 genes were more likely to be strongly expressed under phytohormone treatments (Figure 8).
MsOSCA protein interaction network prediction
To provide more evidence for the function of the MsOSCA proteins, we used the online STRING to predict the MsOSCA protein interaction network. The results showed that MsOSCA3 interacted with MsOSCA13 and MsOSCA14 (Figure S4). MsOSCA1 interacted with myelin transcription factor-like protein (A0JPZ2_ARATH) and transmembrane protein (Q5XV37_ARATH). MsOSCA3/-4/-5/-6/-13 interacted with ureide permease 4 (UPS4). In addition, MsOSCA13 interacted with PX domain-containing protein (EREL1), DnaJ homolog subfamily C (GRV2), and ADP-ribosylation factor (ARFC1).
Subcellular localization of MsOSCAs
Research on the subcellular localization of proteins is beneficial for deciphering their functions. As Ca2+ channels, OSCA family members are characterized by multiple transmembrane helices and are localized on membrane structures (Liu et al., 2022). To further verify the accuracy of subcellular localization prediction, the MsOSCA3 gene, which was highly responsive to abiotic stress, was selected for transient expression assays in tobacco. In tobacco leaves injected with the control vector pCAMBIA1300-GFP, green fluorescence could be observed throughout the whole cell (Figure 9). However, in tobacco leaves injected with the pCAMBIA1300-MsOSCA3-GFP fusion vector, fluorescence was observed only in the plasma membrane. In addition, green fluorescence and red fluorescence could be merged by colocalization of the plasma membrane PM marker, indicating that MsOSCA3 encoded a membrane protein, which was in accordance with the predicted results.
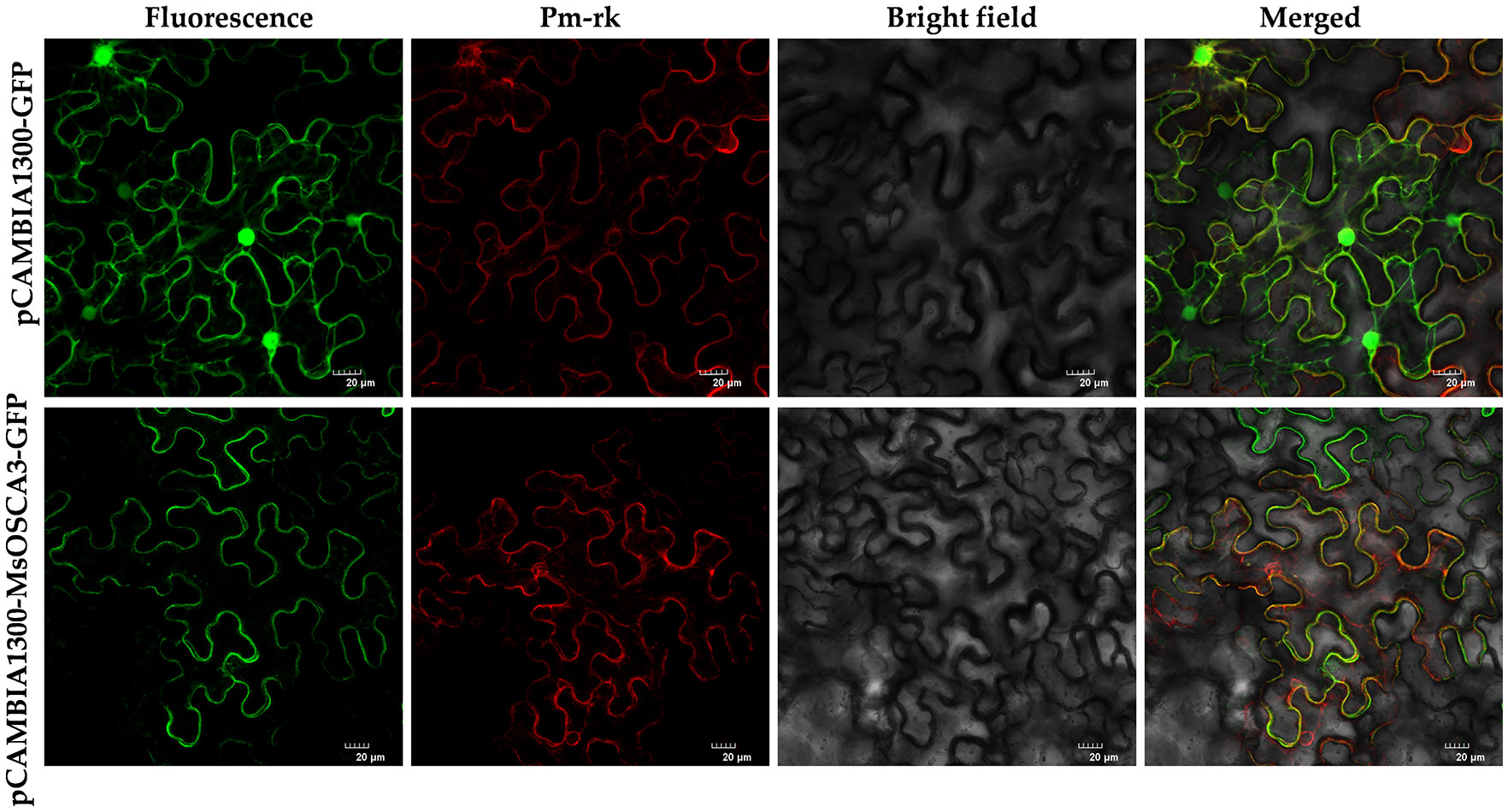
Figure 9 The subcellular localization of MsOSCA3 protein. pCAMBIA1300-MsOSCA3-GFP protein fusions transiently expressed in tobacco leaf cells. Excitation light wavelength: GFP field: 488 nm, PM-rk field: 587 nm. Scale bar, 20 μm.
Discussion
OSCA proteins are regarded as osmotic sensors in plants and play an important role in sensing exogenous and endogenous osmotic changes and regulating plant growth, development and reproduction (Edel and Kudla, 2015; Xiaoyu et al., 2018). Genome-wide investigations and identification of OSCA gene families have been performed in most plant species. In this study, a total of 14 OSCA genes were identified in the alfalfa genome (Table 1), and this number was different from the number of OSCA genes in Arabidopsis (Yuan et al., 2014). The number of OSCA genes varied significantly between homologous species in systematic genome-wide analysis, which typically ranged from 11 to 15 in plants (Cai et al., 2022). However, 42 OSCA genes were identified in the wheat genome, far more than in any other species (Tong et al., 2021), indicating that the wheat OSCA gene family has undergone fragment and tandem duplication events during evolution.
The phylogenetic relationships between species are a fundamental part of biological research (Kapli et al., 2020). We systematically analyzed the phylogenetic relationships of OSCA proteins in alfalfa, Arabidopsis, C. arietinum, V. radiata and C. cajan. The OSCA proteins of alfalfa, Arabidopsis, C. arietinum, V. radiata and C. cajan were clustered into four phylogenetic clades (Figure 1), which was consistent with Solanum habrochaites (Miao et al., 2022), cucumber (Yang et al., 2022), Gossypium hirsutum (Yang et al., 2019), rice (Li et al., 2015) and maize (Cao et al., 2020), possibly due to the conservative evolution of OSCA genes. In addition, on each clade had AtOSCAs, MsOSCAs, CcOSCAs, CaOSCAs and VrOSCAs, suggesting that they might have a common ancestor. The OSCAs of alfalfa and C. arietinum were close to each other in clades I and IV, indicating that these genes were highly homologous. The functional domains of genes are important factors in the regulation of element activity and gene expression. The RSN1_7TM functional domain is the signature domain of the OSCA family. Our results showed that all MsOSCA proteins contained the RSN1_7TM functional domain (Figure 2), which was consistent with maize (Cao et al., 2020), barley (Cai et al., 2022) and legumes (Chakraborty et al., 2023). In addition, some proteins (MsOSCA1/-2/-3/-4/-6/-7/-9/-10/-13) of the MsOSCA family contained RSN1_TM and PHM7_cyt functional domains, similar to wheat (TaOSCA-1/-2/-3/-4/-14/-27) (Tong et al., 2021). This may explain the functional variability of the OSCA genes. The structure of exons and introns affects the function of proteins and the expression and regulation of genes, which means that exons and introns enable genes to play an important role in organisms and thus in the maintenance of life (Xu et al., 2012). MsOSCA genes had different numbers of exons/introns, with some genes having similar exon/intron structures but different sequence lengths, which was similar to those of other plants, such as maize (Cao et al., 2020), Triticum aestivum (Tong et al., 2021) and Populus tomentosa (Jie et al., 2022). Cis-acting elements within the gene promoter region play a crucial role in the regulation of gene expression. The different types of cis-acting elements in the gene promoter predict that the gene may have different functions in response to stress (Chen et al., 2015a). Analysis of cis-acting promoter elements revealed that MsOSCAs contained elements involved in hormone responses (JA, SA, ABA and GA), photoresponses, metabolic regulation and stress responses (low temperature, drought) (Figure 3), suggesting that MsOSCA genes may be involved in the regulation of abiotic and biotic stress responses and plant hormone transduction. For example, the MsOSCA3, MsOSCA5 and MsOSCA11 genes contained MBS elements, suggesting that they may be active in drought stress. The MsOSCA1, MsOSCA10 and MsOSCA12 genes had multiple hormone response elements, indicating that they may play a role in the hormone response and regulation of various physiological metabolic processes in plants. Previous studies have indicated that some OSCA genes are involved in hormonal or abiotic stress responses when specific cis-elements are found in their promoter regions, such as HvOSCA4.1 (She et al., 2022) and ZmOSCA2.5 (Cao et al., 2020). This was in agreement with our predictions. Protein interaction predictions showed that MsOSCA3 interacted with MsOSCA13 and MsOSCA14, suggesting that the three proteins may be functionally similar (Figure S4). MsOSCA1 interacted with myelin transcription factor-like protein (A0JPZ2_ARATH) and transmembrane protein (Q5XV37_ARATH), and we speculated that they function through interactions. These data provide a basis for further studies of the function of MsOSCA proteins.
In this study, the MsOSCA genes were distributed on each chromosome of alfalfa, but their distribution was not uniform, which is similar to that in most species (Jie et al., 2022; Yang et al., 2022). Gene duplication is one of the most important driving forces for the evolution of the genome and the genetics of a species (Magadum et al., 2013). The two main causes of gene family amplification in plants are segmentation and tandem duplication (Lawton-Rauh, 2020). Gene duplication can not only add new members to the gene family but also enrich the function of the gene family, which greatly promotes the genetic evolution of various organisms (Du et al., 2019). Tandem and segmental replication events were observed in the MsOSCA gene family (Figure 4A), indicating that gene replication was also the driving force for the expansion of MsOSCA gene family members. Previous studies have shown that gene pairs with high homology in the gene family may have collinearity, which makes them have similar biological characteristics and expression patterns (Jia et al., 2021). In the present study, we found that MsOSCAs had collinearity with Medicago truncatula and Arabidopsis, suggesting that they might have similar functions, which requires further investigation. However, there were more collinear gene pairs between alfalfa and Medicago truncatula than between Arabidopsis, indicating higher homology between them, which provides a basis for predicting the expression and function of MsOSCA genes.
The tissue specificity of genes is of great importance for the study of life processes and protein functions in tissues (Hwang et al., 2011). Although the tissue specificity of the OSCA gene family has been identified in many plants, the expression level of OSCA genes has significantly changed in different tissues with the evolution of species (She et al., 2022). In this study, we found that all MsOSCA genes of alfalfa had tissue-specific expression (Figure 6), similar to the AtOSCA and CsOSCA gene families (Yuan et al., 2014; Yang et al., 2022). MsOSCAs were expressed in both roots and leaves, indicating that they might play important roles in vegetative growth. However, the expression of MsOSCA12 was not detected in the stems and petioles (Figure 6), and transcriptome data analysis showed that MsOSCA12 was only expressed in flowers (Figure 5), so we hypothesized that the gene may be related to circadian rhythm, osmotic stress sensing site and reproductive growth and development (Cai et al., 2022; Miao et al., 2022). MsOSCA8 was significantly more highly expressed in roots than in other tissues, which may be closely related to the perception and response of osmotic stress. The tissue expression of MsOSCA genes was not completely consistent with the transcriptome results, which may be caused by different alfalfa varieties. These results suggest that the expression of the OSCA gene family in alfalfa is tissue specific.
Drought and salt stress are the most common abiotic stresses encountered by plants. Plants have evolved to adapt to biotic or abiotic stresses by inducing the expression of stress-related genes to modulate physiological response processes and thus improve stress tolerance (Gong et al., 2020; Kaur et al., 2022). In recent years, it has been shown that OSCA genes play important roles in the regulation of plant responses to osmotic stress (Xiaoyu et al., 2018; Tong et al., 2021). In this study, in addition to MsOSCA2 and MsOSCA6, 12 MsOSCA members showed positive responses to drought stress (Figure 7A), indicating that MsOSCA genes may have a regulatory function in osmotic stress. In addition, except for MsOSCA1, MsOSCA2 and MsOSCA6, the expression levels of other genes were significantly increased under salt stress (Figure 7B), suggesting that these genes responded positively to salt stress. Our in-depth analysis revealed that MsOSCA3, MsOSCA5, MsOSCA12 and MsOSCA13 were remarkably upregulated under both drought and stress (Figure 7), which was consistent with the predicted results of the promoter regulatory elements. MsOSCA10 and MsOSCA11 had similar expression trends under salt stress, which correlated with their close relationship in the phylogenetic tree. Researchers found that the ZmOSCA2.4 gene was significantly upregulated in response to drought and salt stress, and overexpression of ZmOSCA2.4 significantly enhanced drought tolerance in Arabidopsis (Cao et al., 2020). The positive regulatory role of the GhOSCA1 gene under salt and drought stress was demonstrated by the increased sensitivity of GhOSCA1.1-silenced plants to salt and drought stress (Yang et al., 2019). Therefore, we speculate that MsOSCA genes may be involved in the regulation of drought and salt-induced osmotic stress.
Phytohormones play important roles in plant growth, development and stress responses (Chen et al., 2015b; Zhao et al., 2021). Appropriate accumulation of multiple hormones in plants can improve the tolerance level of plants to abiotic and biotic stresses (Singh and Roychoudhury, 2023). JA acts as a signaling molecule that regulates plant responses to biotic and abiotic stresses and induces resistance gene expression (Ruan et al., 2019). SA can counteract the negative effects of abiotic stress on plants (Colebrook et al., 2014). ABA improves plant tolerance to adverse conditions by regulating stomatal opening and closing and the expression of stress response genes (Chen et al., 2014). The OSCA gene family has been reported to be involved in regulating multiple hormone responses (Putranto et al., 2015; Xiaoyu et al., 2018). For instance, the HvOSCA2.2, TaOSCA12 and TaOSCA39 genes exhibited strong responses under ABA stress (Tong et al., 2021; Chakraborty et al., 2023). In this study, all MsOSCAs showed positive responses to JA and SA stress except MsOSCA1/-2/-4/-6 (Figures 8A, B). Most MsOSCA genes were upregulated under ABA treatment, while MsOSCA1, MsOSCA2 and MsOSCA6 were downregulated. Moreover, the MsOSCA7, MsOSCA10, MsOSCA12 and MsOSCA13 genes were more likely to be highly expressed under ABA treatment (Figure 8C). These results indicated that the expression of most MsOSCA genes was consistent with the results of promoter element analysis, implying that these genes may play an important role in the hormone signaling pathway. Similarly, the expression levels of BnOSCA1.1a, BnOSCA1.1b and BnOSCA3.1c were relatively higher under ABA treatment, indicating that these three BnOSCAs respond positively to ABA hormone treatment (Zhang et al., 2023). The subcellular localization of MsOSCA3 on the plasma membrane was consistent with that of some OSCA proteins, such as GmOSCA2.3, GmOSCA3.2 (Liu et al., 2022) and OsOSCA4.1 (Zhai et al., 2020), which further confirmed that the MsOSCA protein may play a vital role in the cell membrane.
Conclusion
In this study, we performed a genome-wide scale analysis of OSCA genes in alfalfa. A total of 14 OSCA genes were identified in the alfalfa reference genome, and the basic molecular characteristics, evolutionary relationships, motifs, gene structure, and duplication events were investigated. The MsOSCA genes were classified into three clades, all of which shared the DUF221 functional domain. Alfalfa was closely related to Arabidopsis and Medicago truncatula, and gene duplication events were found in the MsOSCA gene family. The promoter regions of MsOSCAs contained a large number of abiotic stress and hormone response elements, such as MBS, ABRE, P-box and TCA elements. The expression of MsOSCA genes in alfalfa had tissue specificity. MsOSCA genes could strongly respond to abiotic stress, indicating that MsOSCA genes play important roles in regulating the response of plants to various abiotic stresses. These results will provide the basis for further studies on the function of MsOSCA genes under abiotic stress.
Data availability statement
The datasets presented in this study can be found in online repositories. The names of the repository/repositories and accession number(s) can be found in the article/Supplementary Material.
Author contributions
XL: Conceptualization, Investigation, Methodology, Writing – original draft. XW: Investigation, Methodology, Writing – review and editing. XM: Investigation, Writing – review and editing. WC: Investigation, Writing – review and editing. YL: Investigation, Writing – review and editing. WS: Investigation, Writing – review and editing. BF: Conceptualization, Funding acquisition, Writing – review and editing. SL: Conceptualization, Funding acquisition, Methodology, Supervision, Writing – review and editing.
Funding
The author(s) declare financial support was received for the research, authorship, and/or publication of this article. This research was supported by the Inner Mongolia Pratacultural Technology Innovation Center Co., Ltd (CCPTZX2023B04), the National Natural Science Foundation of China (32101426), and the Natural Science Foundation of Ningxia (2023AAC05019).
Conflict of interest
Author YL was employed by Inner Mongolia Pratacultural Technology Innovation Center Co., Ltd.
The remaining authors declare that the research was conducted in the absence of any commercial or financial relationships that could be construed as a potential conflict of interest.
Publisher’s note
All claims expressed in this article are solely those of the authors and do not necessarily represent those of their affiliated organizations, or those of the publisher, the editors and the reviewers. Any product that may be evaluated in this article, or claim that may be made by its manufacturer, is not guaranteed or endorsed by the publisher.
Supplementary material
The Supplementary Material for this article can be found online at: https://www.frontiersin.org/articles/10.3389/fpls.2023.1285488/full#supplementary-material
References
Ahmad, H. I., Afzal, G., Jamal, A., Kiran, S., Khan, M. A., Mehmood, K., et al. (2021). In silico structural, functional, and phylogenetic analysis of cytochrome (CYPD) protein family. BioMed. Res. Int. 2021, 5574789. doi: 10.1155/2021/5574789
Alaimo, A., Villarroel, A. (2018). Calmodulin: a multitasking protein in Kv7.2 potassium channel functions. Biomolecules. 8, 57. doi: 10.3390/biom8030057
Bailey, T. L., Boden, M., Buske, F. A., Frith, M., Grant, C. E., Clementi, L., et al. (2009). MEME SUITE: tools for motif discovery and searching. Nucleic Acids Res. 37, W202–W208. doi: 10.1093/nar/gkp335
Batistic, O., Kudla, J. (2009). Plant calcineurin B-like proteins and their interacting protein kinases. Biochim. Biophys. Act. 1793, 985–992. doi: 10.1016/j.bbamcr.2008.10.006
Cai, Q., Wang, Y., Ni, S., Mu, J., Liu, M., Wang, Y., et al. (2022). Genome wide identification and analysis of the OSCA gene family in barley (Hordeum vulgare L.). J. Gene. 101, 34. doi: 10.3390/ijms232113027
Cao, L., Zhang, P., Lu, X., Wang, G., Wang, Z., Zhang, Q., et al. (2020). Systematic analysis of the maize OSCA genes revealing ZmOSCA family members involved in osmotic stress and ZmOSCA2.4 confers enhanced drought tolerance in transgenic Arabidopsis. Int. J. Mol. Sci. 21, 351. doi: 10.3390/ijms21010351
Chakraborty, S., Gangwar, R., Zahra, S., Poddar, N., Singh, A., Kumar, S. (2023). Genome-wide characterization and comparative analysis of the OSCA gene family and identification of its potential stress-responsive members in legumes. Sci. Rep. 3, 5914. doi: 10.1038/s41598-023-33226-8
Chen, C., Chen, H., Zhang, Y., Thomas, H. R., Frank, M. H., He, Y., et al. (2020). TBtools: an integrative toolkit developed for interactive analyses of big biological data. Mol. Plant 13, 1194–1202. doi: 10.1016/j.molp.2020.06.009
Chen, J., Wang, B., Chung, J. S., Chai, H., Liu, C., Ruan, Y., et al. (2015a). The role of promoter cis-element, mRNA capping, and ROS in the repression and salt-inducible expression of AtSOT12 in Arabidopsis. Front. Plant Sci. 6. doi: 10.3389/fpls.2015.00974
Chen, K., Li, G. J., Bressan, R. A., Song, C. P., Zhu, J. K., Zhao, Y. (2015b). Abscisic acid dynamics, signaling, and functions in plants. J. Integr. Plant Biol. 62, 25–54. doi: 10.1111/jipb.12899
Chen, X., Wu, X., Qiu, S., Zheng, H., Lu, Y., Peng, J., et al. (2021). Genome-wide identification and expression profiling of the BZR transcription factor gene family in Nicotiana benthamiana. Int. J. Mol. Sci. 22, 10379. doi: 10.3390/ijms221910379
Cheval, C., Aldon, D., Galaud, J. P., Ranty, B. (2013). Calcium/calmodulin-mediated regulation of plant immunity. Biochim. Biophys. Act. 1833, 1766–1771. doi: 10.1016/j.bbamcr.2013.01.031
Colebrook, E. H., Thomas, S. G., Phillips, A. L., Hedden, P. (2014). The role of gibberellin signalling in plant responses to abiotic stress. J. Exp. Biol. 217, 67–75. doi: 10.1242/jeb.089938
Du, H., Zhang, W., Zhang, W., Zhang, W., Pan, H., Pan, Y., et al. (2019). Magnetosome gene duplication as an important driver in the evolution of magnetotaxis in the Alphaproteobacteria. mSystems. 4, e00315–e00319. doi: 10.1128/mSystems.00315-19
Edel, K. H., Kudla, J. (2015). Increasing complexity and versatility: how the calcium signaling toolkit was shaped during plant land colonization. Cell Calcium. 57, 231–246. doi: 10.1016/j.ceca.2014.10.013
El Khoury, G., Azzam, W., Rebehmed, J. (2023). PyProtif: a PyMol plugin to retrieve and visualize protein motifs for structural studies. Amino Acids. 2023, 1–8. doi: 10.1007/s00726-023-03323-z
Galione, A., Evans, A. M., Ma, J., Parrington, J., Arredouani, A., Cheng, X. T., et al. (2009). The acid test: the discovery of two-pore channels (TPCs) as NAADP-gated endolysosomal Ca2+ release channels. Pflugers Arch. – Eur. J. Physio. 458, 869–876. doi: 10.1007/s00424-009-0682-y
Gong, Z., Xiong, L., Shi, H., Yang, S., Herrera-Estrella, L. R., Xu, G., et al. (2020). Plant abiotic stress response and nutrient use efficiency. China Life Sci. 63, 635–674. doi: 10.1007/s11427-020-1683-x
Gregory, L., Carrillo Gaeta, N., Araújo, J., Matsumiya Thomazelli, L., Harakawa, R., Ikuno, A. A., et al. (2017). Bovine leukaemia virus genotypes 5 and 6 are circulating in cattle from the state of São Paulo, Brazil. J. Med. Microbiol. 66, 1790–1797. doi: 10.1099/jmm.0.000639
He, C., Zhao, Y., Wang, Y., Cai, J., Gao, J., Zhang, J. (2022). Forage quality and physiological performance of mowed alfalfa (Medicago sativa L.) subjected to combined light quality and drought. Front. Plant Sci. 13, 1047294. doi: 10.3389/fpls.2022.1047294
Hepler, P. K. (2005). Calcium: a central regulator of plant growth and development. Plant Cell. 17, 2142–2155. doi: 10.1105/tpc.105.032508
Horton, P., Park, K. J., Obayashi, T., Fujita, N., Harada, H., Adams-Collier, C. J., et al. (2007). WoL PSORT: protein localization predictor. Nucleic Acids Res. 35, W585–W587. doi: 10.1093/nar/gkm259
Hou, X., Pedi, L., Diver, M. M., Long, S. B. (2012). Crystal structure of the calcium release-activated calcium channel. Science. 338, 1308–1313. doi: 10.1126/science.1228757
Hou, C., Tian, W., Kleist, T., He, K., Garcia, V., Bai, F., et al. (2014). DUF221 proteins are a family of osmosensitive calcium-permeable cation channels conserved across eukaryotes. Cell Res. 24, 632–637. doi: 10.1038/cr.2014.14
Hwang, P. I., Wu, H. B., Wang, C. D., Lin, B. L., Chen, C. T., Yuan, S., et al. (2011). Tissue-specific gene expression templates for accurate molecular characterization of the normal physiological states of multiple human tissues with implication in development and cancer studies. BMC Genomics 12, 439. doi: 10.1186/1471-2164-12-439
Ito, T., Nakata, M., Ishida, S., Takahashi, Y. (2011). The mechanism of substrate recognition of Ca2+ dependent protein kinases. Plant Signal. Behav. 6, 924–926. doi: 10.4161/psb.6.7.15604
Jia, K., Yan, C., Zhang, J., Cheng, Y., Li, W., Yan, H., et al. (2021). Genome-wide identification and expression analysis of the JAZ gene family in turnip. Sci. Rep. 11, 21330. doi: 10.1038/s41598-021-99593-2
Jie, Z., Chen, X., Pang, D., Anan, D., Ximan, L., Yuhan, S., et al. (2022). Identification of populus tomentosa OSCA gene family and its expression analysis under salt stress. Plant Gene Trait 13, 1–10. doi: 10.5376/pgt.2022.13.0001
Kapli, P., Yang, Z., Telford, M. J. (2020). Phylogenetic tree building in the genomic age. Nature reviews. Genetics 21 (7), 428–444. doi: 10.1038/s41576-020-0233-0
Kaur, A., Sharma, A., Dixit, S., Singh, K., Upadhyay, S. K. (2022). OSCA genes in bread wheat: molecular characterization, expression profiling, and interaction analyses indicated their diverse roles during development and stress response. Int. J. Mol. Sci. 23, 14867. doi: 10.3390/ijms232314867
Kaur, H., Sirhindi, G., Bhardwaj, R., AlYemeni, M. N., Siddique, K. H. M., Ahmad, P. (2018). 28-homobrassinolide regulates antioxidant enzyme activities and gene expression in response to salt- and temperature-induced oxidative stress in Brassica juncea. Sci. Rep. 8, 8735. doi: 10.1104/pp.103.033068
Kolukisaoglu, U. N., Weinl, S., Blazevic, D., Batistic, O., Kudla, J. R. (2004). Calcium sensors and their interacting protein kinases: Genomics of the Arabidopsis and rice CBL-CIPK signaling networks. Plant Physiol. 134, 43–58. doi: 10.1104/pp.103.033068
Komatsu, S., Li, W., Konishi, H., Yoshikawa, M., Konishi, T., Yang, G. (2001). Characterization of a Ca2+-dependent protein kinase from rice root: differential response to cold and regulation by abscisic acid. Biol. Pharm. Bull. 24, 1316–1319. doi: 10.1248/bpb.24.1316
Lawton-Rauh, A. (2020). Evolutionary dynamics of duplicated genes in plants. Mol. Phylogenet. Evol. 29, 396–409. doi: 10.1016/j.ympev.2003.07.004
Lescot, M., Déhais, P., Thijs, G., Marchal, K., Moreau, Y., Van de Peer, Y., et al. (2002). Plant CARE, a database of plant cis-acting regulatory elements and a portal to tools for in silico analysis of promoter sequences. Nucleic Acids Res. 30, 325–327. doi: 10.1093/nar/30.1.325
Li, S., Liu, J., An, Y., Cao, Y., Liu, Y., Zhang, J., et al. (2019). MsPIP2; 2, a novel aquaporin gene from Medicago sativa, confers salt tolerance in transgenic Arabidopsis. Environ. Exp. Bot. 165, 39–52. doi: 10.1016/j.envexpbot
Li, L. L., Xiao, Y., Wang, X., He, Z. H., Lv, Y. W., Hu, X. S., et al. (2023). The Ka/Ks and πa/πs ratios under different models of gametophytic and sporophytic selection. Genome Biol. Evol. 15 (8), evad151. doi: 10.1093/gbe/evad151
Li, Y., Yuan, F., Wen, Z., Li, Y., Wang, F., Zhu, T., et al. (2015). Genome-wide survey and expression analysis of the OSCA gene family in rice. BMC Plant Biol. 15, 261. doi: 10.1186/s12870-015-0653-8
Li, Y., Zhang, Y., Li, B., Hou, L., Yu, J., Jia, C., et al. (2022). Preliminary expression analysis of the OSCA gene family in maize and their involvement in temperature stress. Int. J. Mol. Sci. 23, 13658. doi: 10.3390/ijms232113658
Liu, C., Mao, B., Yuan, D., Chu, C., Duan, M. (2022). Salt tolerance in rice: physiological responses and molecular mechanisms. Crop J. 10 (1), 13–25. doi: 10.1016/j.cj.2021.02.010
Liu, X., Wang, J., Sun, L. (2018a). Structure of the hyperosmolality-gated calcium-permeable channel OSCA1.2. Nat. Commun. 9, 5060. doi: 10.1038/s41467-018-07564-5
Liu, C., Wang, H., Zhang, Y., Cheng, H., Hu, Z., Pei, Z. M., et al. (2018b). Systematic characterization of the osca family members in soybean and validation of their functions in osmotic stress. Int. J. Mol. Sci. 23, 10570. doi: 10.3390/ijms231810570
Livak, K. J., Schmittgen, T. D. (2001). Analysis of relative gene expression data using real-time quantitative PCR and the 2(-Delta Delta C(T)) Method. Methods. 25, 402–408. doi: 10.1006/meth.2001.1262
Luo, D., Zhou, Q., Wu, Y., Chai, X., Liu, W., Wang, Y., et al. (2019). Full-length transcript sequencing and comparative transcriptomic analysis to evaluate the contribution of osmotic and ionic stress components towards salinity tolerance in the roots of cultivated alfalfa (Medicago sativa L.). BMC Plant Biol. 19, 32. doi: 10.1186/s12870-019-1630-4
Lv, G. D. (2015). Cloning, marker development and functional analysis of the wheat taosca1.4 gene (Taian, China: Shandong Agricultural University). master’s thesis.
Magadum, S., Banerjee, U., Murugan, P., Gangapur, D., Ravikesavan, R. (2013). Gene duplication as a major force in evolution. J. Gene. 92, 155–161. doi: 10.1007/s12041-013-0212-8
Miao, S., Li, F., Han, Y., Yao, Z., Xu, Z., Chen, X., et al. (2022). Identification of OSCA gene family in Solanum habrochaites and its function analysis under stress. BMC Genomics 23, 547. doi: 10.1186/s12864-022-08675-6
O'Rourke, J. A., Fu, F., Bucciarelli, B., Yang, S. S., Samac, D. A., Lamb, J. F., et al. (2015). The Medicago sativa gene index 1.2: a web-accessible gene expression atlas for investigating expression differences between Medicago sativa subspecies. BMC Genomics 16, 502. doi: 10.1186/s12864-015-1718-7
Oudelaar, A. M., Higgs, D. R. (2020). The relationship between genome structure and function. Nat. Rev. Genet. 22, 154–168. doi: 10.1038/s41576-020-00303-x
Plattner, H., Verkhratsky, A. (2015). The ancient roots of calcium signalling evolutionary tree. Cell Calcium. 57, 123–132. doi: 10.1016/j.ceca.2014.12.004
Putranto, R. A., Herlinawati, E., Rio, M., Leclercq, J., Piyatrakul, P., Gohet, E., et al. (2015). Involvement of ethylene in the latex metabolism and tapping panel dryness of hevea brasiliensis. Int. J. Mol. Sci. 16, 17885–17908. doi: 10.3390/ijms160817885
Rombauts, S., Déhais, P., Van Montagu, M., Rouzé, P. (1999). PlantCARE, a plant cis-acting regulatory element database. Nucleic Acids Res. 27, 295–296. doi: 10.1093/nar/27.1.295
Ruan, J., Zhou, Y., Zhou, M., Yan, J., Khurshid, M., Weng, W., et al. (2019). Jasmonic acid signaling pathway in plants. Int. J. Mol. Sci. 20, 2479. doi: 10.3390/ijms20102479
Shabala, S., Alnayef, M., Bose, J., Chen, Z. H., Venkataraman, G., Zhou, M. X., et al. (2021). Revealing the role of the calcineurin b-like protein-interacting protein kinase 9 (CIPK9) in rice adaptive responses to salinity, osmotic stress, and K+ deficiency. Plants (Basel). 10, 1513. doi: 10.3390/plants10081513
Shan, F., Wu, Y., Du, R., Yang, Q., Liu, C., Wang, Y., et al. (2023). Evolutionary analysis of the OSCA gene family in sunflower (Helianthus annuus L) and expression analysis under NaCl stress. PeerJ. 11, e15089. doi: 10.7717/peerj.15089
She, K., Pan, W., Yan, Y., Shi, T., Chu, Y., Cheng, Y., et al. (2022). Genome-wide identification, evolution and expressional analysis of osca gene family in barley (Hordeum vulgare L.). Int. J. Mol. Sci. 23, 13027. doi: 10.3390/ijms232113027
Singh, A., Roychoudhury, A. (2023). Abscisic acid in plants under abiotic stress: crosstalk with major phytohormones. Plant Cell Rep. 42, 961–974. doi: 10.1007/s00299-023-03013-w
Thor, K., Jiang, S., Michard, E., George, J., Scherzer, S., Huang, S., et al. (2020). The calcium-permeable channel OSCA1.3 regulates plant stomatal immunity. Nature. 585, 569–573. doi: 10.1038/s41586-020-2702-1
Tong, K., Wu, X., He, L., Qiu, S., Liu, S., Cai, L., et al. (2021). Genome-wide identification and expression profile of OSCA gene family members in Triticum aestivum L. Int. J. Mol. Sci. 23, 469. doi: 10.3390/ijms23010469
Vivek, P. J., Resmi, M. S., Sreekumar, S., Sivakumar, K. C., Tuteja, N., Soniya, E. V. (2017). Calcium-dependent protein kinase in ginger binds with importin-α through its junction domain for nuclear localization, and further interacts with NAC transcription factor. Front. Plant Sci. 7. doi: 10.3389/fpls.2016.0
Wilkins, M. R., Gasteiger, E., Bairoch, A., Sanchez, J. C., Williams, K. L., Appel, R. D., et al. (1999). Protein identification and analysis tools in the ExPASy server. Methods Mol. Biol. 112, 531–552. doi: 10.1385/1-59259-584-7:531
Xiaoyu, G., Peng, W., Zhe, L., Li, W., Zhi, H., Shaoling, Z., et al. (2018). Genome-wide identification and expression analysis of the OSCA gene family in Pyrus bretschneideri. Can. J. Plant Sci. 98, 918–929. doi: 10.1139/cjps-2017-0115
Xu, G., Guo, C., Shan, H., Kong, H. (2012). Divergence of duplicate genes in exon-intron structure. Proc. Natl. Acad. Sci.U. S. A. 109, 1187–1192. doi: 10.1073/pnas.1109047109
Yan, L., Pape, T., Meusemann, K., Kutty, S. N., Meier, R., Bayless, K. M., et al. (2021). Monophyletic blowflies revealed by phylogenomics. BMC Biol. 19, 230. doi: 10.1186/s12915-021-01156-4
Yang, X., Xu, Y., Yang, F., Magwanga, R. O., Cai, X., Wang, X., et al. (2019). Genome-wide identification of OSCA gene family and their potential function in the regulation of dehydration and salt stress in Gossypium hirsutum. J. Cotton. Res. 2, 11. doi: 10.1186/s42397-019-0028-z
Yang, S., Zhu, C., Chen, J., Zhao, J., Hu, Z., Liu, S., et al. (2022). Identification and expression profile analysis of the OSCA gene family related to abiotic and biotic stress response in cucumber. Biol. (Basel). 11, 1134. doi: 10.3390/biology11081134
Yin, L., Zhang, M., Wu, R., Chen, X., Liu, F., Xing, B. (2021). Genome-wide analysis of OSCA gene family members in Vigna radiata and their involvement in the osmotic response. BMC Plant Biol. 21, 408. doi: 10.1186/s12870-021-03184-2
Yuan, F., Yang, H., Xue, Y., Kong, D., Ye, R., Li, C., et al. (2014). OSCA1 mediates osmotic-stress-evoked Ca2+ increases vital for osmosensing in Arabidopsis. Nature. 514, 367–371. doi: 10.1038/nature13593
Zelman, A. K., Dawe, A., Gehring, C., Berkowitz, G. A. (2012). Evolutionary and structural perspectives of plant cyclic nucleotide-gated cation channels. Front. Plant Sci. 3. doi: 10.3389/fpls.2012.00095
Zhai, Y., Wen, Z., Han, Y., Zhuo, W., Wang, F., Xi, C., et al. (2020). Heterogeneous expression of plasma-membrane-localised OsOSCA1.4 complements osmotic sensing based on hyperosmolality and salt stress in Arabidopsis osca1 mutant. Cell Calcium. 91, 102261. doi: 10.1016/j.ceca.2020.102261
Zhang, X., Cheng, X., Zhang, C., Ma, X., Zhang, Y., Song, J., et al. (2023). Genome-wide analysis of hyperosmolality-gated calcium-permeable channel (OSCA) family members and their involvement in various osmotic stresses in Brassica napus. Gene. 856, 147137. doi: 10.1016/j.gene.2022.147137
Keywords: alfalfa, OSCA gene family, genome-wide, expression pattern, abiotic stress
Citation: Li X, Wang X, Ma X, Cai W, Liu Y, Song W, Fu B and Li S (2023) Genome-wide investigation and expression analysis of OSCA gene family in response to abiotic stress in alfalfa. Front. Plant Sci. 14:1285488. doi: 10.3389/fpls.2023.1285488
Received: 30 August 2023; Accepted: 23 October 2023;
Published: 03 November 2023.
Edited by:
Debasis Chakrabarty, National Botanical Research Institute (CSIR), IndiaReviewed by:
Madhab Kumar Sen, Czech University of Life Sciences Prague, CzechiaViswanathan Satheesh, Iowa State University, United States
Saurabh Pandey, Guru Nanak Dev University, India
Maharajan Theivanayagam, Rajagiri College of Social Sciences, India
Bharati Pandey, Panjab University, India
Copyright © 2023 Li, Wang, Ma, Cai, Liu, Song, Fu and Li. This is an open-access article distributed under the terms of the Creative Commons Attribution License (CC BY). The use, distribution or reproduction in other forums is permitted, provided the original author(s) and the copyright owner(s) are credited and that the original publication in this journal is cited, in accordance with accepted academic practice. No use, distribution or reproduction is permitted which does not comply with these terms.
*Correspondence: Bingzhe Fu, RmJ6aGUxOUAxNjMuY29t; Shuxia Li, bGlzaHV4aWE2MjBAMTYzLmNvbQ==