- College of Horticulture, South China Agricultural University, Guangzhou, China
The escalating impact of global warming on crop yield and quality poses a significant threat to future food supplies. Breeding heat-resistant crop varieties holds promise, but necessitates a deeper understanding of the molecular mechanisms underlying plant heat tolerance. Recent studies have shed light on the initial events of heat perception in plants. In this review, we provide a comprehensive summary of the recent progress made in unraveling the mechanisms of heat perception and response in plants. Calcium ion (Ca2+), hydrogen peroxide (H2O2), and nitric oxide (NO) have emerged as key participants in heat perception. Furthermore, we discuss the potential roles of the NAC transcription factor NTL3, thermo-tolerance 3.1 (TT3.1), and Target of temperature 3 (TOT3) as thermosensors associated with the plasma membrane. Additionally, we explore the involvement of cytoplasmic HISTONE DEACETYLASE 9 (HDA9), mRNA encoding the phytochrome-interacting factor 7 (PIF7), and chloroplasts in mediating heat perception. This review also highlights the role of intranuclear transcriptional condensates formed by phytochrome B (phyB), EARLY FLOWERING 3 (ELF3), and guanylate-binding protein (GBP)-like GTPase 3 (GBPL3) in heat perception. Finally, we raise the unresolved questions in the field of heat perception that require further investigation in the future.
1 Introduction
According to the recently released evaluation report from the Intergovernmental Panel on Climatic Change (IPCC) in 2023, global warming is accelerating and it is projected to rise by 1.5°C in the near term (2021–2040) due to the elevated levels of greenhouse gas emissions resulting from human activities (IPCC, 2023) (Figure 1A). The frequency of extreme heat-induced damage to crops has significantly risen, posing a critical threat to global food security. Furthermore, the global population is expected to reach 9.7-10 billion by 2050, up from the current population of 7.5 billion (Gupta et al., 2020). Enhancing plants’ ability to acclimate and endure high temperatures holds great promise as a solution to these challenges. Thermomorphogenesis and thermotolerance are the two primary responses employed by plants to cope with elevated temperatures (Figure 1B). As sessile organisms, plants rapidly response to heat by undergoing heat perception, signal transduction, and physiological changes to establish thermotolerance.
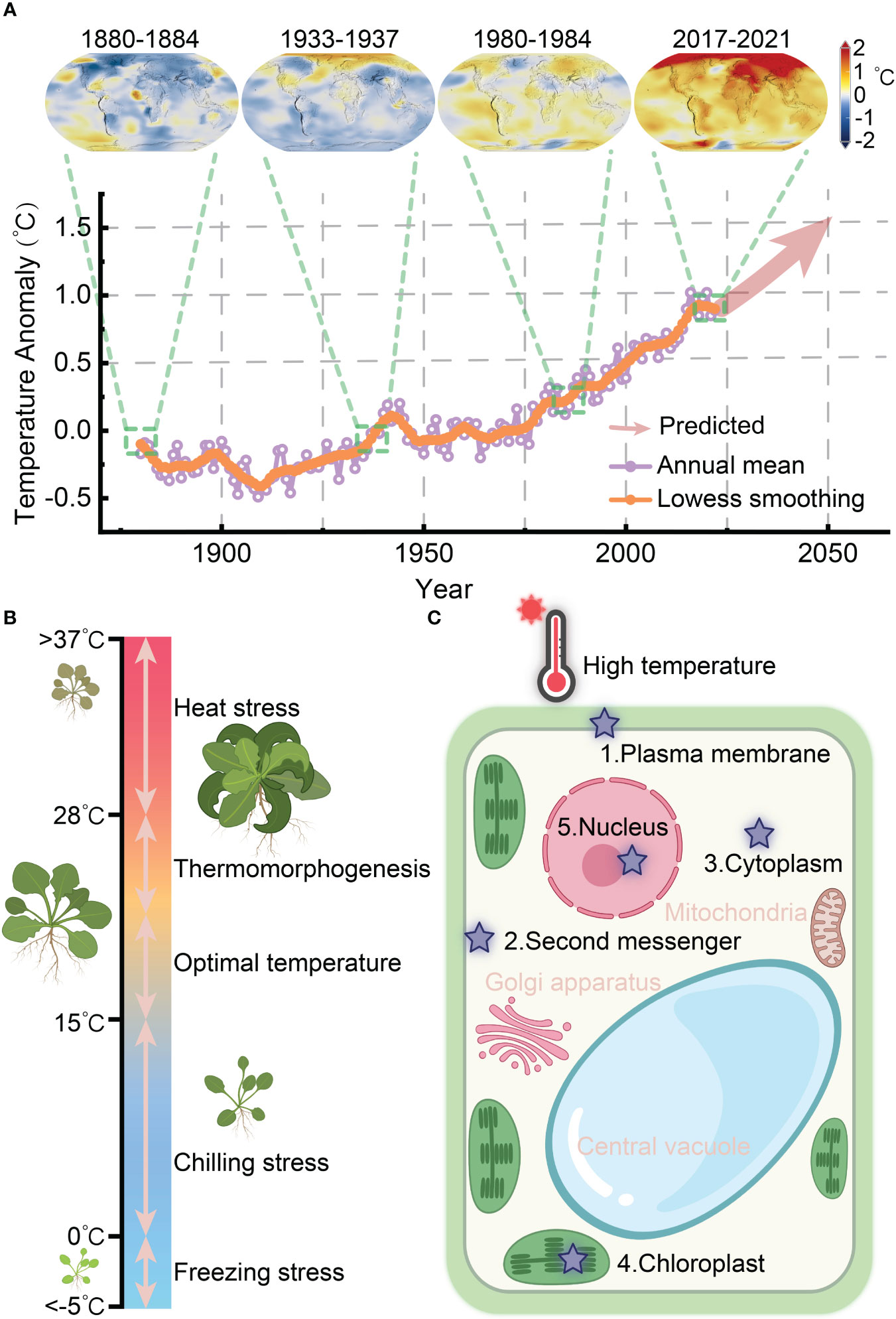
Figure 1 Heat perception and response of plants in coping with global warming. (A) The global surface temperature anomaly from 1880 to 2021 and expected warming trend up to 2050 (the data source: NASA/GISS https://climate.nasa.gov/vital-signs/global-temperature/). (B) Schematic representation of plant growth in response to temperature ranges, spanning from freezing stress to heat stress. (C) Potential heat perception sites within plant cells.
Heat perception serves as the initial event in the heat response process, decoding the initial stimulus to initiate specific cellular responses, known as the themosensory mechanism. However, the precise primary thermosensor(s) in plants have not been definitively identified. Essentially, a thermosensor would perceive elevated temperature by directly modifying its biochemical properties, transmit this information to downstream thermal responses, and ultimately generate physiological and morphological changes as outputs (Dai Vu et al., 2019; Kerbler and Wigge, 2023). Generally, heat can be sensed throughout a plant cell, and multiple thermosensing and overlapping downstream signaling pathways operate cocurrently. Proposed mechanisms for plant thermosensation include plasma membrane fluidity, photoreceptors, DNA/chromatin structures, alternative splicing, protein translation, protein stability, and phase separation (Kerbler and Wigge, 2023).
The quest for a bona fide thermosensor presents challenges due to technical limitations, genetic functional redundancy, and the complexity of phenotypic responses (Lamers et al., 2020). Nevertheless, significant progress has been made in recent years in characterizing components involved in heat perception, including phytochrome B (phyB), EARLY FLOWERING 3 (ELF3), thermo-tolerance 3.1 (TT3.1), and guanylate-binding protein (GBP)-like GTPase 3 (GBPL3) (Jung et al., 2016; Jung et al., 2020; Kim et al., 2022; Zhang et al., 2022). In this review, we specifically focus on the recent advances in understanding the heat perception mechanisms in plants, categorizing the initial process into extracellular and intracellular levels. This division is warranted because extracellular perception not only triggers a fast transcription-independent physiological response but also initiates a slower transcription-dependent response through the activation of signaling pathways. We particularly emphasize the involvement of second messengers, such as calcium ions (Ca2+), hydrogen peroxide (H2O2), and nitric oxide (NO), as well as plasma membrane-associated proteins, cytoplasmic and chloroplast components, and intranuclear transcriptional condensates that mediate heat perception and trigger response mechanisms in plants (Figure 1C).
2 Heat perception is mediated by second messenger
2.1 Ca2+ signal
As the most prominent second messenger, Ca2+ serves as a crucial link between extracellular abiotic and biotic stimuli and specific intracellular biological responses. Stress sensors recognize these stimuli and activate Ca2+–permeable cation channels, resulting in transient Ca2+ currents from the apoplastic space or intracellular vacuoles to the cytosol (Tian et al., 2020; Dindas et al., 2021). Various Ca2+-binding sensor proteins, including calcium dependent kinases (CDPKs), Calmodulin (CaM), CaM-like proteins (CMLs), and calcineurin B-like proteins (CBLs), decode the cytosolic Ca2+ signal and modulate downstream physiological responses (Luan, and Wang, 2021; Dong et al., 2022). Recent advances have shed light on early Ca2+-mediated signaling events that regulate pollen tube reception, low potassium responses, and immune response initiation (Jacob et al., 2021; Gao et al., 2022; Li et al., 2023a; Zhao et al., 2023). Notably, the combination of genetically encoded Ca2+ indicators (GECI) and forward genetics has facilitated the successful discovery of key stress sensors by screening mutants with altered cytosolic [Ca2+] (Yuan et al., 2014; Jiang et al., 2019; Wu et al., 2020; Grenzi et al., 2021; Jacob et al., 2021; Sun et al., 2021).
In plants, the cytosolic calcium concentration ([Ca2+]cyt) rapidly increases to initiate the earliest signaling events when exposed to low temperature conditions (Knight et al., 1991; Liu et al., 2021; Ding et al., 2022). This temperature-dependent transient burst of Ca2+ response may be attributed to either decreased plasma membrane fluidity or mediated through membrane-localized Ca2+ channels (Zhu, 2016; Gong et al., 2020). The plasma membrane protein CHILLING-TOLERANCE DIVERGENCE 1 (COLD1) has been identified as a potential cold sensor in rice. It is thought to interact with cold-responsive Ca2+ channels to stimulate apoplastic Ca2+ flux into the cytosol (Ma et al., 2015). Additionally, the mechanosensitive (MS) ion channels MID1-COMPLEMENTING ACTIVITY (MCA) proteins, namely MCA1 and MCA2, have been identified as responsive to cold stimuli and capable of inducing cytosolic Ca2+ influx (Mori et al., 2018; Yoshimura et al., 2021). The glutamate receptor channel-like Ca2+ channels protein GLR1.2, GLR1.3 and GLR3.4 are also sensitive to cold (Meyerhoff et al., 2005; Zheng et al., 2018). Cyclic nucleotide-gated calcium channels 9 (CNGC9) respond to cold treatment by triggering Ca2+ influx into the cytosol (Wang et al., 2021a). Ca2+-dependent phospholipid-binding proteins known as annexins also possess Ca2+ channel characteristic. ANN1, ANN3 and ANN5 have been described as positive regulators that mediate cold-induced calcium signaling in plants (Shen et al., 2017; Liu et al., 2021). These cold sensitive Ca2+ gating proteins may serve as potential sensory mechanisms in response to external cold stimulation, activating downstream signaling cascades.
Similarly, a transient increase in [Ca2+]cyt following a heat stimulus serves as an early signaling event necessary for the positive regulation of thermotolerance. In mammals, the perception of high temperatures is mediated by the TRANSIENT RECEPTOR POTENTIAL (TRP) cationic channel family and the TWIK-RELATED POTASSIUM (TREK) channel family, which promote the influx of extracellular Ca2+ (Basbaum et al., 2009). In plants, exposure to heat activates the cyclic nucleotide-gated calcium channels CNGCb and CNGC2/4/6, resulting in their transient opening and subsequent entry of extracellular calcium, resulting in the induction of heat shock protein (HSP) gene expression and the acquisition of thermotolerance (Saidi et al., 2009; Finka et al., 2012; Gao et al., 2012). Notably, CNGC6 has been demonstrated to positively regulate the generation of H2O2 and NO by increasing [Ca2+]cyt upon heat exposure (Peng et al., 2019; Wang et al., 2021b). Additionally, CNGC14 and CNGC16 can enhance both chilling and heat tolerance in rice (Cui et al., 2020). While lacking genetic evidence, a study proposed that glutamate receptor-like (GLR) calcium channels, which mediate calcium signaling, may enhance heat tolerance and have the potential to function as thermosensors (Li et al., 2019). GLRs are known to facilitate systemic leaf–to–leaf wound signaling through the vasculature, and it is plausible that plants can also transmit systemic Ca2+ signals from a heated leaf to neighboring leaves (Mousavi et al., 2013; Nguyen et al., 2018; Simon et al., 2022; Yu et al., 2022). Furthermore, the Ca2+-dependent phospholipid-binding annexins ANN1 and ANN5 have been identified as Ca2+-permeable channels that respond to heat, with their transcriptional capacity being repressed by the R2R3-MYB transcription factor MYB30 (Qiao et al., 2015; Liao et al., 2017). These findings propose the hypothesis that calcium channels and/or their associated regulators present at the plasma membrane may function as thermosensors (Figure 2).
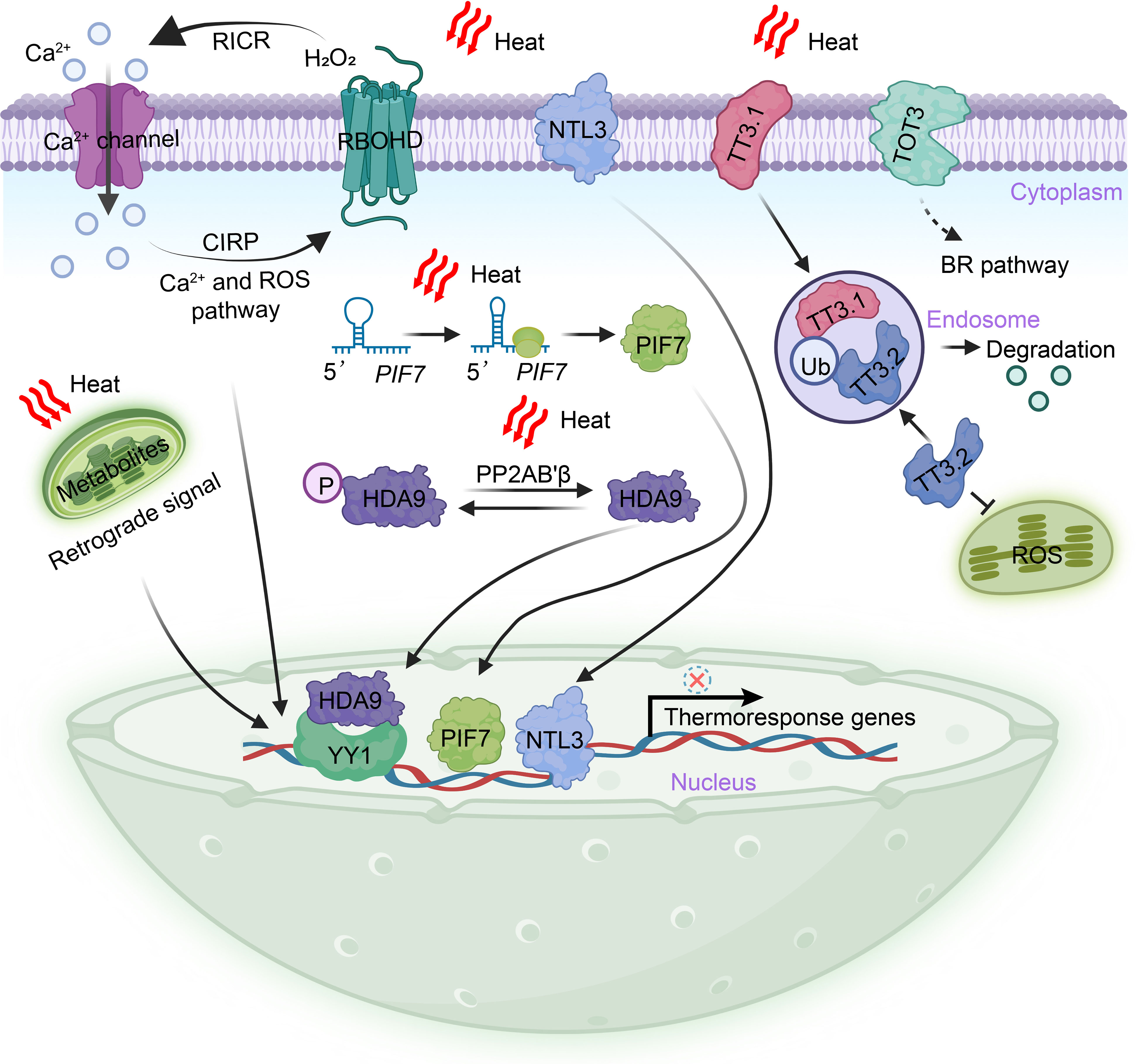
Figure 2 Extranuclear heat perception and triggering mechanisms in plants. The perception of heat in plants occurs outside the nucleus and involves various mechanisms. Heat stimuli are transmitted to the nucleus through a burst of heat-induced second messengers, including Ca2+ and H2O2. These second messengers play a crucial role in inducing the expression of thermoresponse genes. Additionally, regulatory circuits or homeostats can be formed by H2O2 and Ca2+, which provide feedback functions. During heat stress, specific cell surface-localized proteins, namely NTL3, TOT3, and TT3.1, mediate heat perception. These proteins translocate into the nucleus, where they induce the expression of heat response genes, activate the BR signal pathway, and maintain the integrity of chloroplasts. In the cytoplasm, the dephosphorylation of HDA9 by the PP2AB’β subunit of PROTEIN PHOSPHATASE 2A results in the stabilization and translocation of HDA9 into the nucleus. Once in the nucleus, HDA9 represses the expression of target genes through pathways that are both dependent and independent of the transcription factor YY1. The hairpin structure of PIF7 mRNA is capable of perceiving heat and leads to an increased abundance of the PIF7 protein in the nucleus. This, in turn, activates the expression of thermoresponse genes. Heat stress also induces extensive metabolic remodeling, some of which can regulate gene expression in the nucleus through retrograde signaling. BR, brassinosteroids; CIRP, Ca2+-induced ROS-production; HDA9, HISTONE DEACETYLASE 9; NTL3, NAC transcription factor NTM1-like 3; P, phosphate; PP2AB′β, ′β regulatory subunit of PROTEIN PHOSPHATASE 2A; PIF7, Phytochrome interacting factor 7; RICR, ROS–induced Ca2+-release; RBOHD, respiratory burst oxidase homologue D; TT3.1, thermo-tolerance 3.1; TT3.2, thermo-tolerance 3.2; Ub, Ubiquitination; TOT3, TARGET OF TEMPERATURE 3; YY1, YIN YANG 1.
2.2 H2O2 and NO signal
Reactive oxygen species (ROS) and reactive nitrogen species (RNS) play pivotal roles as second messengers in the initial stages of the heat response. It remains critical to further explore how ROS signals are perceived and transduced into the nucleus during heat responses. In plants, H2O2 is generated by the plasma membrane-bound respiratory burst oxidase homologue D (RBOHD), which is sensitive to various abiotic stresses, including heat (Wang et al., 2014). Both H2O2 and Ca2+ can form regulatory circuits or homeostatic feedback mechanisms (Gilroy et al., 2014). The N terminus of RBOHD contains EF-hand motifs capable of binding to Ca2+, indicating that the production of H2O2 by RBOHD can be directly activated by changes of [Ca2+]cyt (Gilroy et al., 2014). Apoplastic H2O2 can induce the release of Ca2+ by directly modulating the activity of calcium channels or pumps. For instance, ANN Ca2+ channels and the vacuolar cation channel TWO PORE CHANNEL 1 (TPC1) are activated by ROS, leading to an increase in [Ca2+]cyt (Laohavisit et al., 2010; Evans et al., 2016). In Arabidopsis, H2O2-induced elevation of [Ca2+]cyt is mediated by HYDROGEN-PEROXIDE-INDUCED CALCIUM INCREASES 1 (HPCA1) (Wu et al., 2020). HPCA1 undergoes oxidation by H2O2, enhancing its kinase activity. This modified HPCA1 subsequently phosphorylates membrane-localized Ca2+ channels, facilitating calcium influx (Wu et al., 2020). ROS and Ca2+ have been demonstrated to propagate leaf-to-leaf long-distance signals rapidly in response to local stimuli, including heat, and are thus critical for systemic acquired acclimation (SAA) (Miller et al., 2009; Fichman et al., 2022) (Figure 2).
NO, a free radical of RNS, primarily functions as a signaling molecule that regulates plant adaptation to biotic and abiotic stresses (Fancy et al., 2017). Previous studies have demonstrated that heat exposure leads to an increase in NO levels, and both external application and genetic modification experiments support a positive role of NO in thermotolerance (Song et al., 2006; Peng et al., 2019; Rai et al., 2020; He et al., 2022). It is believed that NO acted downstream of Ca2+ and H2O2 signaling pathways to promote the accumulation of heat response proteins (Wang et al., 2014; Peng et al., 2019). A recent investigation uncovered that heat induces a burst of NO in the inflorescence apex, which then reacts with glutathione to form S-nitrosoglutathione (GSNO). GSNO rapidly travels through the vascular system to the root, where it acts as a signal to initiate whole-plant cellular heat responses through the S-nitrosylation of the GT-1 transcription factor. GT-1 subsequently binds to the promoter of the heat-inducible transcription factor HsfA2, thereby activating its expression and rapidly triggering downstream thermotolerance responses (He et al., 2022). These findings provide valuable insights into the precise mechanism by which NO functions in thermosensing. However, the regulatory relationships and mechanisms of interaction between NO, Ca2+, and H2O2 still require further elucidation.
3 Heat perception via cell surface–localized proteins
Changes in plasma membrane fluidity directly affected by heat can be sensed by membrane-localized proteins, although these potential heat transducers remain poorly understood. In addition to proteins involved in Ca2+ release and H2O2 production, recent studies have revealed that plasma membrane-embedded protein kinases, transcription factors, and E3 ligases can also sense heat and initiate downstream heat signaling responses (Liu et al., 2020; Vu et al., 2021; Zhang et al., 2022). NAM, ATAF, and CUC (NAC) transcription factors, known to be induced by abiotic stress and capable of enhancing stress tolerance, have emerged as key players in heat perception (Nakashima et al., 2012). For instance, the plasma membrane-localized NAC transcription factor NTL3 has been identified as a positive regulator of rice thermotolerance. A loss-of-function mutant of rice NTL3 displayed increased sensitivity to heat (Liu et al., 2020). In response to heat-induced changes in membrane fluidity, the plasma membrane-localized OsNTL3 is partially processed and translocate into the nucleus, where it activates the transcription of OsbZIP74, leading to the extensive expression of heat response genes (Liu et al., 2020). Such mechanisms promote the unfolded protein response of the endoplasmic reticulum (ER) and the detoxification of ROS (Liu et al., 2020) (Figure 2). These findings highlight the significance of NTL3 in facilitating communication among the plasma membrane, ER, and nucleus during heat perception. Additionally, the Arabidopsis NAC transcription factor NTL4, as well as rice NAC127 and NAC129, have also been shown to contribute positively to the heat response (Lee et al., 2014; Ren et al., 2021).
The accumulation of ROS due to photoinhibition can cause severe damage to chloroplast components under stress conditions, resulting in reduced crop yield and quality (Yang et al., 2019). Through quantitative trait locus (QTL) mapping, the first potential thermosensor in rice, TT3.1, was recently identified (Zhang et al., 2022). The TT3.1-TT3.2 genetic module is located at a single QTL locus and is crucial for linking plasma membrane heat perception to the maintenance of chloroplast quality, significantly reducing rice yield losses at high temperatures. TT3.1 is a RING-type E3 ligase localized to the plasma membrane, while TT3.2 binds to the chloroplast membrane and disrupts the core photoprotective apparatus under high temperatures. Overexpression of TT3.1 or mutation of TT3.2 significantly enhances thermotolerance capacity. Zhang et al. (2022) found that TT3.1 is released from the cell surface into the cytosol, where it recruits newly synthesized TT3.2 precursors into endosomes and polyubiquitinates TT3.2 for degradation in the vacuole. This study elucidates an important mechanism in which a plasma membrane-localized E3 ligase mediates heat perception and signal transduction, thereby preserving chloroplast integrity under high temperatures (Figure 2). Intriguingly, both NTL3 and TT3.1 are membrane-associated proteins that relocate from the plasma membrane to regulate downstream organelles in response to heat, indicating their pivotal roles as thermosensors in rice (Li & Liu, 2022).
Protein kinases play crucial roles in plant resistance and adaptation to unfavorable growing environments by sensing stress and transmitting signals. Various types of protein kinases, including CDPKs, mitogen-activated protein kinase (MAPK) cascades, receptor-like cytoplasmic kinases (RLCKs), and sucrose nonfermenting1 (SNF1)-related protein kinases (SnRKs), have been widely reported to regulate plant growth, development, and stress tolerance (Liang and Zhou, 2018; Chen et al., 2021; Li et al., 2022). These kinases influence the activity, localization, interaction, and stability of proteins through dynamic and reversible processes. For instance, the plasma membrane localized cold-responsive protein kinase (CRPK) can phosphorylate and transport 14-3-3 proteins to the nucleus, fine-tuning CBF signaling to regulate cold responses (Liu et al., 2017; Praat et al., 2021). However, our understanding of kinase-mediated phosphorylation events in the heat response remains limited. Therefore, the identification of plasma membrane-localized protein kinases that perceive heat and transmit perception signals to promote downstream physiological responses is an area of great interest. In rice, a plasma membrane-localized leucine-rich repeat receptor-like kinase (LRR-RLK) named Thermo-Sensitive Genic Male Sterile 10 (TMS10) was identified through a 60Co γ-ray radiation mutagenesis screen (Yu et al., 2017). Typically, an LRR-RLK protein consists of a transmembrane domain, a cytoplasmic kinase domain for signal transduction, and an extracellular domain for cell surface signal perception. Yu et al. (2017) demonstrated that both TMS10 and its redundant paralog, TMS10-Like (TMS10L), are important for controlling rice male fertility under lower temperatures, while only TMS10 is required for normal male fertility under heat conditions. A recent phosphoproteomics study identified the plasma membrane-localized mitogen-activated protein kinase kinase kinase kinase (MAP4K), known as TARGET OF TEMPERATURE 3 (TOT3), as an important and conserved thermoregulator in flowering plants (Vu et al., 2021) (Figure 2). TOT3 may perceive and transmit heat signals to control the activity of BRASSINAZOLE-RESISTANT 1 (BZR1), acting in parallel with the phyB-PIF4 pathway to regulate plant thermomorphogenesis (Vu et al., 2021). Nevertheless, further studies are required to fully understand how plasma membrane-localized protein kinases respond to heat.
4 Heat perception and signal transduction in cytoplasm
In addition to protein kinase-mediated phosphorylation, histone modifications play a crucial role in rapidly bridging heat signals to transcriptional programs (Han et al., 2022; Hou et al., 2022; Perrella et al., 2022; Bai et al., 2023). A recent study by Niu et al. (2022) demonstrated that HISTONE DEACETYLASE 9 (HDA9) acts as a novel and evolutionarily conserved cytoplasmic thermosensor responsible for transmitting heat signals to the nucleus. Previous research has shown that HDA9 participates in thermomorphogenesis by regulating H2A.Z-nucleosome dynamics, although the precise mechanism of the heat response remains unclear (Shen et al., 2019; Van Der Woude et al., 2019). Niu et al. (2022) identified that heat induces the interaction and dephosphorylation of HDA9 by the ′β regulatory subunit of PROTEIN PHOSPHATASE 2A (PP2AB’β) in the cytoplasm. This interaction leads to the stabilization of HDA9 and its rapid translocation into the nucleus. The accumulation of HDA9 in the nucleus is facilitated by the nuclear pore complex component HIGH EXPRESSION OF OSMOTICALLY RESPONSIVE GENE1 (HOS1) (Tasset et al., 2018; Niu et al., 2022). Within just 5 minutes of a temperature increase from 22°C to 37°C, the abundance of HDA9 in the nucleus significantly rises, underscoring its role as a typical signal transducer (Tasset et al., 2018; Niu et al., 2022). In the nucleus, HDA9 binds to and deacetylates the promoters of target genes, repressing their expression through pathways that are both dependent and independent of the transcription factor YIN YANG 1 (YY1). This intricate mechanism helps balance developmental and heat responses (Niu et al., 2022) (Figure 2). Given its fundamental properties, HDA9 has been emerged as a complex mediator in the heat response, serving as a potential cytoplasmic thermosensor in plants.
In the cytoplasm, mRNA translation efficiency is dynamically regulated by temperature fluctuations, primarily through the formation of thermolabile secondary structures in the 5′ untranslated region (UTR) (de Smit & Van Duin, 1990; Kortmann & Narberhaus, 2012). These secondary structures, resulting from intricate base pairing, expand the functional repertoire of mRNA beyond its DNA coding sequence (Vandivier et al., 2016). The formation of RNA secondary structures is highly responsive to abiotic stresses, and posttranscriptional covalent modifications may act as cytoplasmic thermosensors by modulating protein abundance (Assmann et al., 2023). Indeed, the concept of “RNA thermometers” has been previously proposed in bacteria and viruses and more recently observed in plants (Kortmann and Narberhaus, 2012; Chung et al., 2020; Meyer et al., 2020). For instance, the mRNA of phytochrome interacting factor 7 (PIF7) undergoes a temperature-dependent reversible change in secondary structure. Specifically, a hairpin structure consisting of 31 nucleotides in the 5′ UTR partially unfolds as the temperature increases, leading to enhanced translation efficiency of PIF7 mRNA and subsequent elevation of protein levels in the nucleus. Consequently, this activation triggers the transcription of key genes associated with thermomorphogenesis (Chung et al., 2020; Fiorucci et al., 2020) (Figure 2). PIF7 has been identified as the predominant transcriptional regulator of thermomorphogenesis and the shade avoidance syndrome in plants (Burko et al., 2022; Yang et al., 2023). These findings illustrate a thermosensory mechanism in which the hairpin structure of PIF7 mRNA functions as a heat sensor in the cytoplasm, initiating a conformational change that transduces the thermal signal to the nucleus, ultimately leading to the induction of downstream transcriptional responses. Similar phenomena observed in WRKY22 and HSFA2 mRNA suggest that a conserved cytoplasmic thermosensory mechanism may operate widely in plants (Chung et al., 2020).
5 Heat perception in chloroplast
In addition to its primary role in energy and matter production, the chloroplast may also function as a critical center for heat perception and signal transduction. Leaves, with their high surface area/volume ratio, act as cooling mechanisms for plants. However, the intricate processes of chloroplast photosynthetic electron transport and metabolic reactions are highly sensitive to environmental fluctuations. Heat stress typically leads to the excessive accumulation of ROS in chloroplasts, which not only directly damages nearby microregions, particularly the photosynthetic apparatus and thylakoid membrane, but also initiates retrograde signaling to regulate cellular responses (Li, and Kim, 2021; Zahra et al., 2023) (Figure 2). Under heat stress conditions, chloroplasts undergo swelling, disorganization of thylakoid ultrastructure, an increase in the size and number of plastoglobules, and extensive metabolic remodeling (Djanaguiraman et al., 2018; Wang et al., 2018). Lipidome research has shown that heat stress significantly reduces the content of galactolipids and unsaturated phospholipids in tomato leaves, while increasing the content of triacylglycerols and decreasing lipid fatty acyl saturation in wheat leaf thylakoid membranes (Spicher et al., 2016; Djanaguiraman et al., 2018). This lipid composition remodeling plays a crucial role in protecting the photosynthetic apparatus by maintaining membrane stability. The proteins required for an intact chloroplast (approximately 2000–3000 proteins) are encoded by two spatially separate genomes: the chloroplast genomes, which encodes only around 100 proteins, and the nuclear genome, which encodes the majority of these proteins. Stressed chloroplasts undergo rapid protein turnover to restore homeostasis, necessitating the effective degradation of damaged proteins and the import of newly synthesized proteins. These processes heavily rely on chloroplast retrograde signaling (Woodson, 2022). Herein, we emphasize the crucial role of chloroplasts as both energy and matter-producing organelles, capable of acting as a potential center for heat signal sensing and transduction.
Retrograde signals serve as a communication language between the chloroplast and the nucleus, enabling the adjustment of cellular activities according to the extent of chloroplast damage (Chan et al., 2016; Sun and Guo, 2016). In plants, the absorption of excess light energy in photosystem (PSII) and photosystem I (PSI) leads to the production of ROS such as the superoxide anion (O2−), H2O2, hydroxyl radical (OH−), and highly reactive singlet oxygen (1O2) (Li and Kim, 2021). Among these, 1O2 is the most prominent ROS–associated retrograde signal and can modulate nuclear gene expression and stress response. Two spatially distinct sensors, EXECUTER proteins and β-carotene, are involved in mediating 1O2-triggered signaling (Dogra and Kim, 2020). For example, the shuttle protein EX1 detects 1O2 in the chloroplast and translocates to the nucleus, where it facilitates the expression of 1O2–responsive genes by interacting with WRKY transcription factors (Li et al., 2023b). Additionally, various chloroplast metabolites function as retrograde signals to induce the expression of stress-responsive nuclear genes. These metabolites include methylerythritol cyclodiphosphate (MEcPP), tetrapyrroles, oxidative products of β-carotene, and phosphonucleotide 3′-phosphoadenosine 5′-phosphate (PAP) (Chan et al., 2016). While it is hypothesized that chloroplasts act as heat-sensing organelles, the specific identification of thermosensor proteins, lipids, or other metabolites in the chloroplast requires further confirmation.
6 Intranuclear transcriptional condensates mediate heat perception
6.1 PhyB
The photoreceptor phyB is the first identified and the best-known thermosensor in plants, initiating both photomorphogenesis and thermomorphogenesis through specific signaling pathways (Jung et al., 2016; Legris et al., 2016). It was originally identified as a red/far-red light receptor, and has been the subject of extensive research in plants for many years (Butler et al., 1959). PhyB, consisting of a C-terminal output module (OPM) and a N-terminal photosensory module (PSM), functions in two forms: an inactive Pr form that absorbs red light and an active Pfr form that absorbs far-red light. Pr form and Pfr form could convert mutually according to the light quality. Upon exposure to red light, Pr converts to the active Pfr form, while far-red light irradiation reverts Pfr back to Pr (Cheng et al., 2021) (Figure 3A). In the absence of light, active Pfr can revert to the inactive Pr form, and this reversible conformational switch, known as thermal reversion, can also be induced by changes in temperature (Jung et al., 2016; Legris et al., 2016). When seedlings are subjected to high temperatures in the dark, the fraction of active phyB decreases, leading to an increase in the Pr/Pfr ratio. This higher Pr/Pfr ratio subsequently promotes hypocotyl elongation in seedlings (Jung et al., 2016; Legris et al., 2016) (Figure 3B).
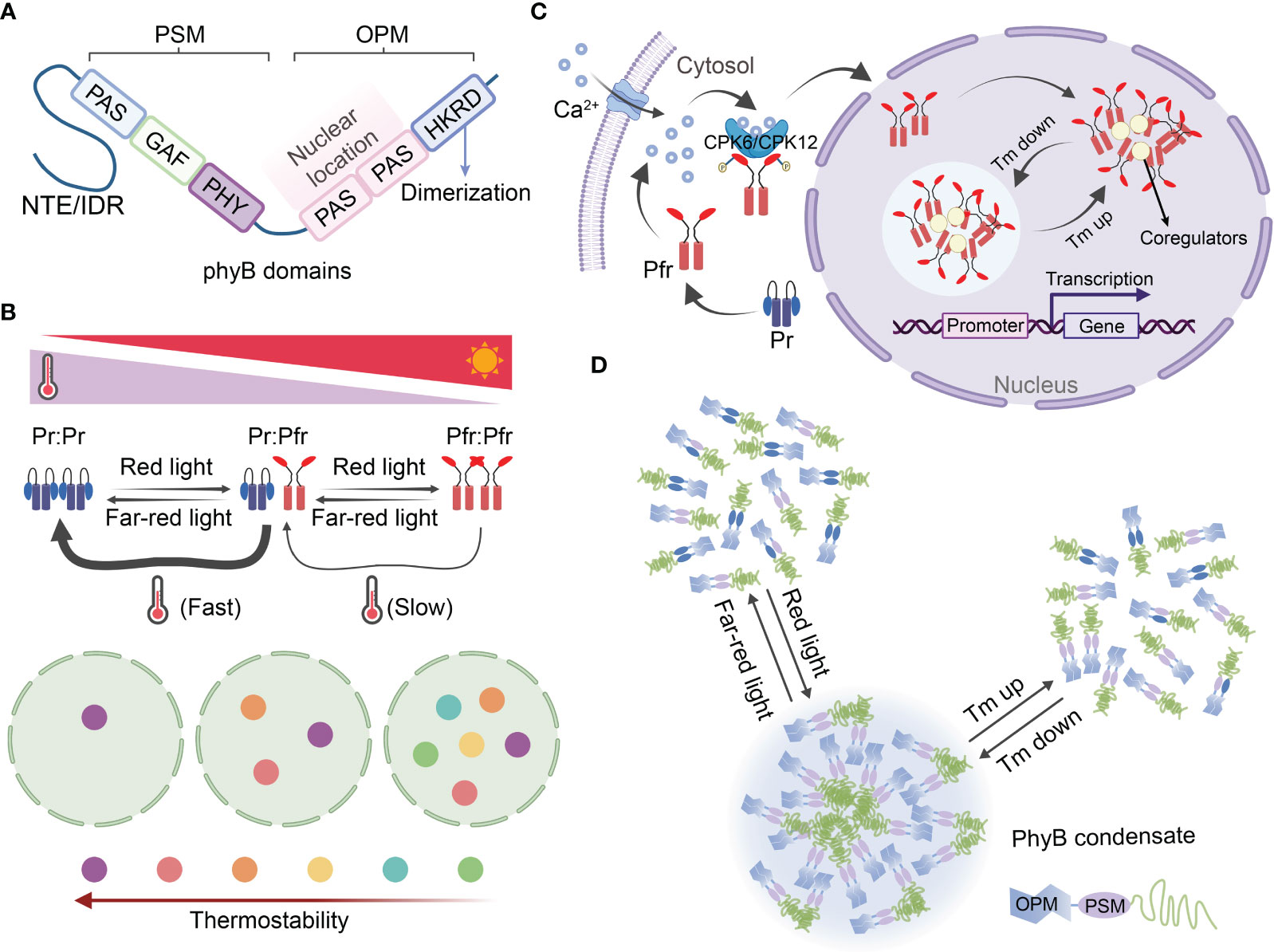
Figure 3 Photosensor phyB functions as themosensor in plants through reversible condensate formation. (A) Structural domains of phyB. The phyB protein can be divided into two structural domains: the C-terminal output module (OPM) and the N-terminal photosensory module (PSM). (B) The reversible photoconversion and thermal reversion of Pr-to-Pfr are key processes that contribute to the dynamics of phyB photobodies. Phytochromes are dimers that exhibit a highly dynamic nature due to photoconversion and thermal reversion of Pr-to-Pfr. Red light promotes the formation of the active dimer (Pfr-Pfr), while far-red light favors the inactive dimer (Pr-Pr). The active dimer pool is also influenced by thermal reversion, a two-step process that converts Pfr-Pfr back to the inactive Pr-Pr state. The kinetics of thermal reversion from Pfr : Pr to Pr : Pr are approximately two orders of magnitude faster than those from Pfr : Pfr to Pfr : Pr. The model depicting temperature-dependent phyB photobody (PB) dynamics is presented in the schematic below. The colored circles represent PBs with different thermostabilities, while the circle with a dotted line represents the nucleolus. Less thermostable PBs are only formed at lower temperatures, whereas increasing temperatures progressively induce the disassembly of individual PBs, which is dependent on the thermostability of phyB photobodies. Overall, this model provides insights into the reversible photoconversion and thermal reversion mechanisms of phyB, as well as the temperature-dependent dynamics of phyB photobodies. (C) A potential mechanism role of phyB import into the nucleus and photobody biogenesis through LLPS. The inactive Pr converting to active Pfr, and the activated Pfr was phosphorylated by CPK6/12 that induced by Ca2+, followed by rapidly import from the cytoplasm into the nucleus to form thermal reversion phyB photobodies. Red light can activate the Ca2+–CPK6/12 pathway via phyB, leading to the phosphorylation of the Pfr conformer and subsequent nuclear import. This phyB–Ca2+–CPK6/12–phyB regulatory loop, which operates in light signaling. Ultimately formed thermal reversion condensate through LLPS. Potential mechanism for the role of phyB in nuclear import and photobody biogenesis through liquid-liquid phase separation (LLPS). The inactive Pr form of phyB converts to the active Pfr form, which is then phosphorylated by CPK6/12 in response to Ca2+ signaling. Subsequently, the phosphorylated Pfr is rapidly imported from the cytoplasm into the nucleus, where it forms thermal reversion phyB photobodies. This phyB–Ca2+–CPK6/12–phyB regulatory loop operates in light signaling and ultimately leads to the formation of thermal reversion condensates through LLPS. (D) The light and temperature sensing mechanism of phyB involves the assembly and deformation of photobodies through LLPS. In its Pr form, phyB exists as a dimer and is unable to form a condensate. However, upon activation by red light, the conformational changes in the Pfr form allow for high-order assembly and drive condensate formation. Conversely, far-red light facilitates the disassembly of these condensates. Additionally, the multivalent interactions of phyB can be reversibly altered by changes in temperature, resulting in the assembly or disassembly of condensates. For a more comprehensive understanding, please refer to the study conducted by Chen et al. (2022). CPK6/CPK12, calcium-dependent protein kinase 6/calcium-dependent protein kinase 12; GAF, cGMP phosphodiesterase/adenylyl cyclase/FhlA domain; HKRD, histidine kinase-related domain; IDR, intrinsically disordered region; PAS, period/arnt/single-minded domain; OPM, output module; PHY, phytochrome-specific domain; PSM, photosensory module; Pr, biologically inactive red-light absorbing form of phyB; Pfr, biologically active far-red light-absorbing form of phyB; NTE, N-terminal extension; Tm, temperature.
PhyB, a dimeric chromoprotein, exhibits a highly dynamic nature due to the photoconversion and thermal reversion of Pr-to-Pfr. The kinetics of thermal reversion from Pfr : Pr to Pr : Pr are nearly two orders of magnitude faster than those from Pfr : Pfr to Pfr : Pr. Moreover, it is only the Pfr : Pfr pool in the nucleus that can initiate light and thermal responses (Klose et al., 2015; Legris et al., 2016; Klose et al., 2020). Biophysical analysis has demonstrated that phyB undergoes thermal reversion at a rate approximately ten times faster than other phy isoforms found in Arabidopsis, potato (Solanum tuberosum), and maize (Zea mays), suggesting that phyB is the primary thermosensor among the five isoforms in plants (Burgie et al., 2021) (Figure 3B). The rapid import of activated Pfr from the cytoplasm to the nucleus serves as an early gating step in information delivery (Chen & Chory, 2011; Van Buskirk et al., 2012). While the exact mechanism underlying phyB imported into the nucleus has remained unclear, a recent discovery by Zhao et al. (2023) revealed that red light can activate the Ca2+–CPK6/12 pathway via phyB, leading to the phosphorylation of the Pfr conformer and subsequent nuclear import. This phyB–Ca2+–CPK6/12–phyB regulatory loop, which operates in light signaling, likely participates in heat perception, as Ca2+ plays a ubiquitous role in plant responses to various stimuli (Figure 3C).
Phytochromes have the ability to homodimerize or heterodimerize with each other. As a result, the imported active Pfr forms small aggregates initially, which then assemble into subnuclear speckles known as photobodies (PBs). Conversely, the inactivated Pr is disassembled from photobodies. These subnuclear compartments have been referred to as speckles, foci, and nuclear bodies, but their biogenesis, components, and function have yet to be clearly confirmed. The size, number, and potentially the function of phyB photobodies are dynamically regulated by light and temperature. Under typical ambient temperatures, photobodies disappear, and phyB localizes to the numerous smaller subnuclear foci in the dark (or under far-red light) conditions, but not under red light. However, even under red light, the number of photobodies decreases at higher temperatures (Legris et al., 2016; Hahm et al., 2020). The intrinsically disordered region (IDR) of phyB contributes to the liquid-liquid phase separation (LLPS) process, resulting in the assembly of photobodies (Burgie et al., 2021; Pardi & Nusinow, 2021). Recently, a possible light and heat sensing mechanism of phyB through LLPS was proposed, suggesting that it plays a role in the assembly and deformation of photobodies (Chen et al., 2022). The intrinsically disordered N-terminal extension (NTE) and C terminus of phyB facilitate the spontaneous LLPS and oligomerization of phyB, allowing for its compartmentalization with transcription factors for signal transduction. The NTE acts as a biophysical modulator, directly influencing the temperature-dependent formation and dissolution of phyB droplets. Its conformational properties enable phyB to function both as a photoreceptor and a thermosensor (Viczián et al., 2020; Chen et al., 2022). Recent findings also support the notion that phyB photobodies can be regarded as “thermobodies” (TBs), given their characteristics related to heat perception (Figure 3D).
6.2 ELF3
The plasticity of plant morphology must dynamically respond to circadian rhythm (Silva et al., 2020; Xu et al., 2022). Recently, an important protein component of the circadian evening complex (EC), ELF3, was identified as a thermosensor in plant. Upon exposure to heat, ELF3 undergoes reversible liquid droplet formation driven by LLPS. These ELF3 droplets temporarily inhibit EC function, leading to the induction of PIF4 transcription (Jung et al., 2020). The C-terminal prion-like domain (PrD) of ELF3 plays a specific role in enabling the protein to aggregate into multiple speckles through LLPS in response to heat. Importantly, this process is reversible when temperatures return to normal (Jung et al., 2020; Lindsay et al., 2023). PrD regions are intrinsically disordered regions (IDRs) with low sequence complexity that are known to promote LLPS (Hennig et al., 2015; Dorone et al., 2021). Analysis of hypocotyl elongation and condensate formation has revealed a correlation between thermal responsiveness and the length of the polyglutamine repeat (PolyQ) within the PrD (Jung et al., 2020; Lindsay et al., 2023). A recent study further elucidated the potential biophysical basis for the thermal sensing mechanism of ELF3 involving the PrD region. It identified three processes contributing to ELF3 aggregation: the formation of temperature-sensitive helices adjacent to PolyQ tracts, increased solvent accessibility of expanded PolyQ tracts, and conformational changes that enhance the exposure of several aromatic residues at higher temperatures (Lindsay et al., 2023) (Figure 4). This study also proposed a potential mechanism for destabilizing the EC and facilitating the dissociation of ELF3.
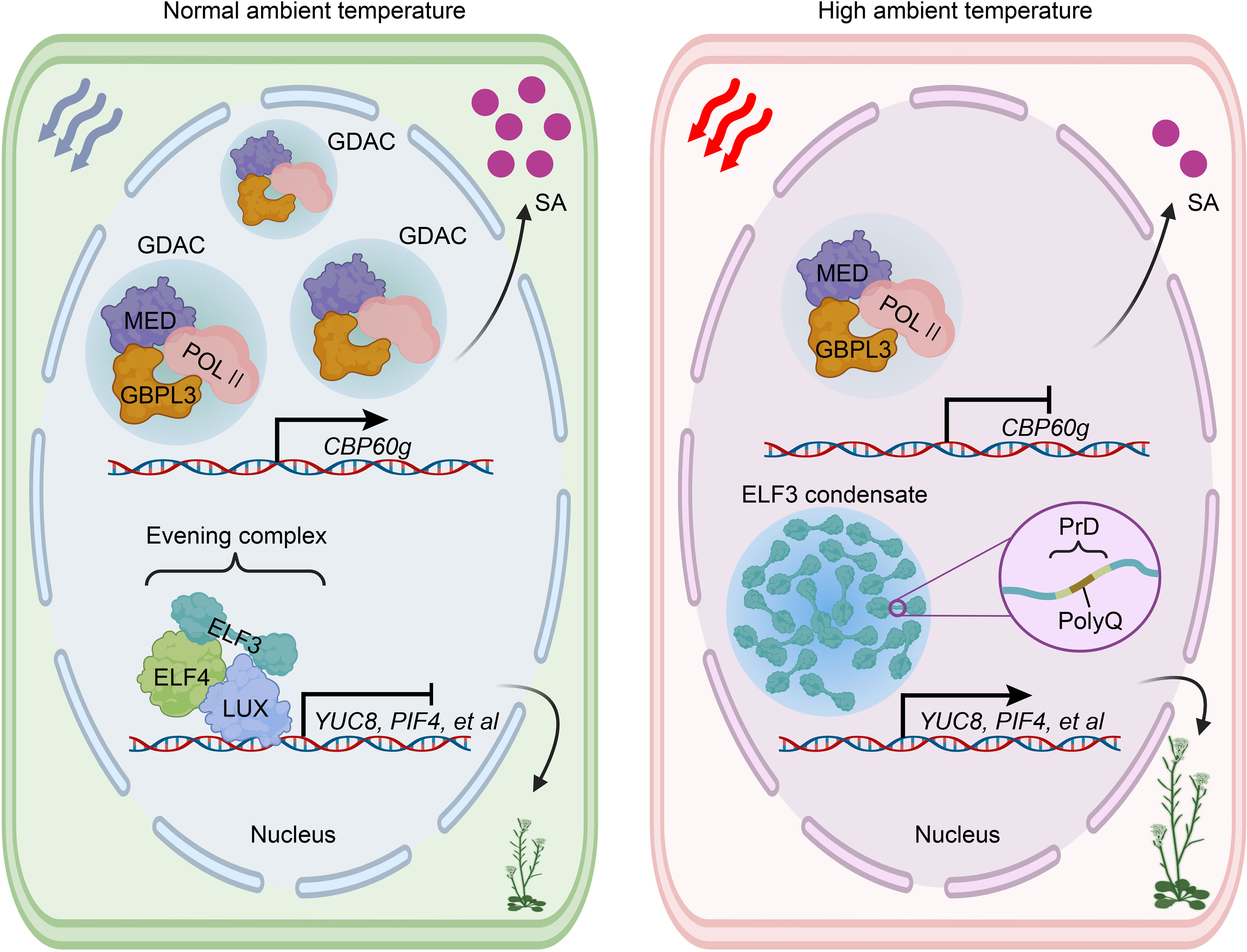
Figure 4 ELF3 and GBPL3 condensates play a crucial role in plants’ heat perception. Elevated ambient temperature inhibits the accumulation of GDAC, consequently repressing the transcription of CBP60g. This repression ultimately leads to the downregulation of SA biosynthesis and compromised immunity. Notably, ELF3 undergoes reversible condensate formation mediated by LLPS upon exposure to heat. Under normal ambient temperature conditions, ELF3 can form an evening complex by interacting with ELF4 and LUX, which subsequently represses the expression of YUC8, PIF4, and other relevant factors. However, the formation of ELF3 condensate induced by heat temporarily disrupts the functioning of the evening complex, consequently triggering thermalmorphogenesis. ELF3, EARLY FLOWERING 3; ELF4, EARLY FLOWERING 4; GDAC, GBPL defense-activated condensate; GBPL3, GUANYLATE BINDING PROTEIN-LIKE 3; LUX, LUX ARRYTHMO; MED, Mediator complex; POL II, RNA polymerase II; PIF4, phytochrome interacting factor 4; PolyQ, polyglutamine repeat; PrD, prion-like domain; YUC8, auxin biosynthetic protein YUCCA8; SA, salicylic acid.
It is noteworthy that there are multiple molecular mechanisms involved in the function of ELF3 in heat perception. For instance, N6-methyladenosine (m6A) RNA modification has been proposed as a facilitator of heat-induced formation of ELF3 condensates, independent of ELF3 phase separation (Lee et al., 2022). Recent studies have reported inconsistent results regarding the increased ELF3 nuclear condensates that disassemble the EC at warm temperatures. A genetic study demonstrated that ELF3 can act as a Zeitnehmer to transmit temperature cues to the central oscillator controlling thermoresponsive behaviors, independent of the EC (Anwer et al., 2020; Zhu et al., 2022). Another study found that ELF3 multimerization in the nucleus was reduced at warm temperatures, consistent with reports of ELF3 degradation through the ubiquitination degradation system (UPS) at warm temperatures (Ronald et al., 2021; Zhang et al., 2021a; Zhang et al., 2021b). These inconsistent findings may be attributed to variations in experimental conditions, molecular methods, or the length of the polyQ in the PrD of ELF3 among different Arabidopsis populations (Ronald et al., 2021). Moreover, the recent elucidation of the regulation of ELF3 protein stability through UPS revealed that the nuclear-localized proteins B-box 18 (BBX18) and BBX23 interact with ELF3 and the E3 ubiquitin ligase CONSTITUTIVE PHOTOMORPHOGENIC 1 (COP1) to promote ELF3 degradation at high temperatures in Arabidopsis. Additionally, the functionally redundant XB3 ORTHOLOG 1 IN ARABIDOPSIS THALIANA (XBAT31) and XBAT35 proteins perform a similar role (Ding et al., 2018; Zhang et al., 2021a; Zhang et al., 2021b).
6.3 GBPL3
The temperature sensor GUANYLATE BINDING PROTEIN-LIKE 3 (GBPL3), known for its ability to form GBPL defense-activated condensates (GDACs) through LLPS, has been identified recently. This unique property allows GBPL3 to connect external elevated temperatures to internal immunity by inducing a massive reprogramming of host disease-resistance gene expression (Huang et al., 2021; Kim et al., 2022; Tang et al., 2022). Under non-induced conditions, the higher-affinity chromatin-binding protein GBPL1 may compete directly with GBPL3 for chromatin binding. However, this competition can be reversed upon immune activation after phytopathogen infection. For instance, phytopathogen-induced salicylic acid (SA) can trigger the formation of GBPL3 condensates in the nucleus (Huang et al., 2021). In Arabidopsis, the nucleocytoplasmic relocation of GBPL3, along with C-terminal IDR- and N-terminal GTPase-dependent LLPS, plays a critical role in the formation of transcriptional condensates that activate defense gene expression and immunity. GBPL3 recruits the RNA polymerase II (POL II) apparatus and Mediator complex (MED) to form GDACs directly at the promoters of several major defense genes (Huang et al., 2021) (Figure 4).
Heat stress can directly impact various aspects of cellular physiology, making the plant immune system more vulnerable to pathogen attacks compared to normal growth temperatures (Janda et al., 2019; Saijo & Loo, 2020; Hua & Dong, 2022). SA, a versatile signaling molecule and essential defense phytohormone, plays a crucial role in activating plant immune gene expression and inducing resistance against diverse phytopathogens (van Butselaar and Van den, 2020; Peng et al., 2021; Jia et al., 2023). Higher temperatures or heatwaves have been shown to inhibit both basal and pathogen-induced levels of SA (Huot et al., 2017; Castroverde & Dina, 2021). Recently, Kim et al. (2022) demonstrated that elevated temperatures specifically suppress the recruitment of GBPL3, transcriptional coactivators, and RNA polymerase II (POL II) to form GDACs at the promoters of CBP60g and SARD1, which are crucial targets of SA suppression under high temperatures. Notably, this suppression occurs independently of the phyB and ELF3 thermosensors mentioned earlier. The GBPL3-dependent thermosensory mechanism offers a new framework that can be optimized to maintain immune capacity and ensure crop yields in the face of global warming (Dhar & Lee, 2022; Hua & Dong, 2022; Prasad et al., 2022).
7 Conclusions and perspectives
The escalating threat of higher temperatures owing to global warming poses a serious challenge to the survival of plants. Despite significant research efforts over the past few decades focusing on heat signal transduction and thermophysiological responses, there remains a challenging task of accurately defining thermosensors and conducting rigorous screening. Nonetheless, substantial progress has been made in unraveling heat sensing mechanisms at various levels within plant cells. Notably, the discovery in 2016 that the photoreceptor phyB also functions as a thermosensor has opened new avenues for research. Moreover, several potential thermosensors have been successfully identified in model plants such as Arabidopsis thaliana and rice. In this review, we have summarized the current understanding of heat perception and triggering mechanisms in plants, specifically categorizing them as extra- and intranuclear mechanisms. The insights discussed herein contribute to a deeper comprehension of plant thermotolerance mechanisms at the molecular level and hold promise for accelerating the production of new, more thermotolerant crop varieties by breeders.
Despite these breakthroughs in the field of plant heat perception, numerous unresolved questions still need further investigation. Several areas require attention and investigation in the near future are listed below:
a) Heat-induced alterations in physical forces may trigger cell wall integrity signaling, suggesting that the cell wall could also be serve as a site for heat perception.
b) We propose that the chloroplast may function as a heat perception organelle. However, identifying the specific sensory components within the chloroplast remains challenging.
c) The formation of LLPS–driven transcriptional condensates may represent a common mechanism underlying heat responses in plants. It is conceivable that new phase separation-mediated thermosensors may be discovered in the future. Further characterization is also necessary for different sensing mechanisms.
d) Multiple thermosensors are involved in mediating different physiological responses to meet the requirements of specific plant developmental stages. The coordination of these thermosensors in regulating downstream responses and determining the primary actor is yet to be determined.
e) Plant heat sensing and response should be examined from both temporal and spatial perspectives. How systemic heat signals are sensed and transmitted, as well as how thermal memory is stored, need to be unveiled.
f) Multiple stresses occur simultaneously or sequentially during plant growth, and it remains unclear how plants perceive and integrate heat signals with responses to other stresses.
Author contributions
XY: Conceptualization, Visualization, Writing – original draft, Data curation, Funding acquisition, Investigation, Methodology, Software, Validation, Writing – review & editing. HG: Writing – review & editing, Formal Analysis, Methodology. YY: Validation, Writing – review & editing. YZ: Validation, Resources, Supervision, Writing – review & editing. WS: Resources, Project administration, Writing – review & editing. SS: Resources, Supervision, Writing – review & editing. HL: Resources, Supervision, Writing – review & editing. RC: Funding acquisition, Project administration, Supervision, Resources, Writing – review & editing. YH: Conceptualization, Funding acquisition, Project administration, Supervision, Validation, Writing – review & editing, Writing – original draft.
Funding
The authors declare financial support was received for the research, authorship, and/or publication of this article. This study was supported by the National Natural Science Foundation of China (Grant No. 32202576; 31902013 and 31870286), the Natural Science Foundation of Guangdong Province (Grant No. 2023A1515012674; 2023A1515010497; 2022A1515012278 and 2021A1515010528).
Acknowledgments
We appreciated all the research works published in this filed. Due to the space limitation, we are sorry for the involving research not citing here. Some of components in the figures created with BioRender.com.
Conflict of interest
The authors declare that the research was conducted in the absence of any commercial or financial relationships that could be construed as a potential conflict of interest.
Publisher’s note
All claims expressed in this article are solely those of the authors and do not necessarily represent those of their affiliated organizations, or those of the publisher, the editors and the reviewers. Any product that may be evaluated in this article, or claim that may be made by its manufacturer, is not guaranteed or endorsed by the publisher.
References
Anwer, M. U., Davis, A., Davis, S. J., Quint, M. (2020). Photoperiod sensing of the circadian clock is controlled by EARLY FLOWERING 3 and GIGANTEA. Plant J. 101, 1397–1410. doi: 10.1111/tpj.14604
Assmann, S. M., Chou, H. L., Bevilacqua, P. C. (2023). Rock, scissors, paper: How RNA structure informs function. Plant Cell. 35, 1671–1707. doi: 10.1093/plcell/koad026
Bai, J., Shi, Z., Zheng, S. (2023). The role of histone modifications in heat signal transduction in plants. Adv. Biol., 2200323. doi: 10.1002/adbi.202200323
Basbaum, A. I., Bautista, D. M., Scherrer, G., Julius, D. (2009). Cellular and molecular mechanisms of pain. Cell 139, 267–284. doi: 10.1016/j.cell.2009.09.028
Burgie, E. S., Gannam, Z. T., McLoughlin, K. E., Sherman, C. D., Holehouse, A. S., Stankey, R. J., et al. (2021). Differing biophysical properties underpin the unique signaling potentials within the plant phytochrome photoreceptor families. P. Natl. Acad. Sci. U.S.A. 118, e2105649118. doi: 10.1073/pnas.2105649118
Burko, Y., Willige, B. C., Seluzicki, A., Novák, O., Ljung, K., Chory, J. (2022). PIF7 is a master regulator of thermomorphogenesis in shade. Nat. Commun. 13, 4942. doi: 10.1038/s41467-022-32585-6
Butler, W. L., Norris, K. H., Siegelman, H. W., Hendricks, S. B. (1959). Detection, assay, and preliminary purification of the pigment controlling photoresponsive development of plants. P. Natl. Acad. Sci. U.S.A. 45, 1703–1708. doi: 10.1073/pnas.45.12.1703
Castroverde, C. D. M., Dina, D. (2021). Temperature regulation of plant hormone signaling during stress and development. J. Exp. Bot. 72, 7436–7458. doi: 10.1093/jxb/erab257
Chan, K. X., Phua, S. Y., Crisp, P., McQuinn, R., Pogson, B. J. (2016). Learning the languages of the chloroplast: retrograde signaling and beyond. Annu. Rev. Plant Biol. 67, 25–53. doi: 10.1146/annurev-arplant-043015-111854
Chen, M., Chory, J. (2011). Phytochrome signaling mechanisms and the control of plant development. Trends. Cell. Biol. 21, 664–671. doi: 10.1016/j.tcb.2011.07.002
Chen, X., Ding, Y., Yang, Y., Song, C., Wang, B., Yang, S., et al. (2021). Protein kinases in plant responses to drought, salt, and cold stress. J. Integr. Plant Biol. 63, 53–78. doi: 10.1111/jipb.13061
Chen, D., Lyu, M., Kou, X., Li, J., Yang, Z., Gao, L., et al. (2022). Integration of light and temperature sensing by liquid-liquid phase separation of phytochrome B. Mol. Cell. 82, 3015–3029. doi: 10.1016/j.molcel.2022.05.026
Cheng, M. C., Kathare, P. K., Paik, I., Huq, E. (2021). Phytochrome signaling networks. Annu. Rev. Plant Biol. 72, 217–244. doi: 10.1146/annurev-arplant-080620-024221
Chung, B. Y., Balcerowicz, M., Antonio., M., Jaeger, K. E., Geng, F., Franaszek, K., et al. (2020). An RNA thermoswitch regulates daytime growth in Arabidopsis. Nat. Plants. 6, 522–532. doi: 10.1038/s41477-020-0633-3
Cui, Y., Lu, S., Li, Z., Cheng, J., Hu, P., Zhu, T., et al. (2020). Cyclic Nucleotide-Gated Ion Channels 14 and 16 promote tolerance to heat and chilling in rice. Plant Physiol. 183, 1794–1808. doi: 10.1104/pp.20.00591
Dai Vu, L., Gevaert, K., De Smet., I. (2019). Feeling the heat: searching for plant thermosensors. Trends. Plant Sci. 24, 210–219. doi: 10.1016/j.tplants.2018.11.004
de Smit, M. H., Van Duin., J. (1990). Secondary structure of the ribosome binding site determines translational efficiency: a quantitative analysis. P. Natl. Acad. Sci. U.S.A. 87, 7668–7672. doi: 10.1073/pnas.87.19.766
Dhar, S., Lee, J. Y. (2022). How does global warming sabotage plant immunity? Mol. Cells 45, 883–885. doi: 10.14348/molcells.2022.0150
Dindas, J., Dreyer, I., Huang, S., Hedrich, R., Roelfsema, M. R. G. (2021). A voltage-dependent Ca2+ homeostat operates in the plant vacuolar membrane. New. Phytol. 230, 1449–1460. doi: 10.1111/nph.17272
Ding, L., Wang, S., Song, Z. T., Jiang, Y., Han, J. J., Lu, S. J., et al. (2018). Two B-box domain proteins, BBX18 and BBX23, interact with ELF3 and regulate thermomorphogenesis in Arabidopsis. Cell. Rep. 25, 1718–1728. doi: 10.1016/j.celrep.2018.10.060
Ding, Y., Yang, H., Wu, S., Fu, D., Li, M., Gong, Z., et al. (2022). CPK28-NLP7 module integrates cold-induced Ca2+ signal and transcriptional reprogramming in Arabidopsis. Sci. Adv. 8, eabn7901. doi: 10.1126/sciadv.abn7901
Djanaguiraman, M., Boyle, D. L., Welti, R., Jagadish, S. V. K., Prasad, P. V. V. (2018). Decreased photosynthetic rate under high temperature in wheat is due to lipid desaturation, oxidation, acylation, and damage of organelles. BMC. Plant Biol. 18, 1–17. doi: 10.1186/s12870-018-1263-z
Dogra, V., Kim, C. (2020). Singlet oxygen metabolism: from genesis to signaling. Front. Plant Sci. 10. doi: 10.3389/fpls.2019.01640
Dong, Q., Wallrad, L., Almutairi, B. O., Kudla, J. (2022). Ca2+ signaling in plant responses to abiotic stresses. J. Integr. Plant Biol. 64, 287–300. doi: 10.1111/jipb.13228
Dorone, Y., Boeynaems, S., Flores, E., Jin, B., Hateley, S., Bossi, F., et al. (2021). A prion-like protein regulator of seed germination undergoes hydration-dependent phase separation. Cell 184, 4284–4298. doi: 10.1016/j.cell.2021.06.009
Evans, M. J., Choi, W. G., Gilroy, S., Morris, R. J. (2016). A ROS-assisted calcium wave dependent on the AtRBOHD NADPH oxidase and TPC1 cation channel propagates the systemic response to salt stress. Plant Physiol. 171, 1771–1784. doi: 10.1104/pp.16.00215
Fancy, N. N., Bahlmann, A. K., Loake, G. J. (2017). Nitric oxide function in plant abiotic stress. Plant Cell. Environ. 40, 462–472. doi: 10.1111/pce.12707
Fichman, Y., Zandalinas, S. I., Peck, S., Luan, S., Mittler, R. (2022). HPCA1 is required for systemic reactive oxygen species and calcium cell-to-cell signaling and plant acclimation to stress. Plant Cell. 34, 4453–4471. doi: 10.1093/plcell/koac241
Finka, A., Cuendet, A. F. H., Maathuis, F. J., Saidi, Y., Goloubinoff, P. (2012). Plasma membrane cyclic nucleotide gated calcium channels control land plant thermal sensing and acquired thermotolerance. Plant Cell. 24, 3333–3348. doi: 10.1105/tpc.112.095844
Fiorucci, A. S., Galvão, V. C., Ince, Y. C., Boccaccini, A., Goyal, A., Allenbach Petrolati, L., et al. (2020). PHYTOCHROME INTERACTING FACTOR 7 is important for early responses to elevated temperature in Arabidopsis seedlings. New. Phytol. 226, 50–58. doi: 10.1111/nph.16316
Gao, F., Han, X., Wu, J., Zheng, S., Shang, Z., Sun, D., et al. (2012). A heat-activated calcium-permeable channel-Arabidopsis cyclic nucleotide-gated ion channel 6-is involved in heat shock responses. Plant J. 70, 1056–1069. doi: 10.1111/j.1365-313X.2012.04969.x
Gao, Q., Wang, C., Xi, Y., Shao, Q., Li, L., Luan, S. (2022). A receptor–channel trio conducts Ca2+ signalling for pollen tube reception. Nature 607, 534–539. doi: 10.1038/s41586-022-04923-7
Gilroy, S., Suzuki, N., Miller, G., Choi, W. G., Toyota, M., Devireddy, A. R., et al. (2014). A tidal wave of signals: calcium and ROS at the forefront of rapid systemic signaling. Trends. Plant Sci. 19, 623–630. doi: 10.1016/j.tplants.2014.06.013
Gong, Z., Xiong, L., Shi, H., Yang, S., Herrera-Estrella, L. R., Xu, G., et al. (2020). Plant abiotic stress response and nutrient use efficiency. Sci. China. Life. Sci. 63, 635–674. doi: 10.1007/s11427-020-1683-x
Grenzi, M., Resentini, F., Vanneste, S., Zottini, M., Bassi, A., Costa, A. (2021). Illuminating the hidden world of calcium ions in plants with a universe of indicators. Plant Physiol. 187, 550–571. doi: 10.1093/plphys/kiab339
Gupta, A., Rico-Medina, A., Caño-Delgado, A. I. (2020). The physiology of plant responses to drought. Science 368, 266–269. doi: 10.1126/science.aaz7614
Hahm, J., Kim, K., Qiu, Y., Chen, M. (2020). Increasing ambient temperature progressively disassembles Arabidopsis phytochrome B from individual photobodies with distinct thermostabilities. Nat. Commun. 11, 1660. doi: 10.1038/s41467-020-15526-z
Han, D., Yu, Z., Lai, J., Yang, C. (2022). Post-translational modification: a strategic response to high temperature in plants. Abiotech 3, 49–64. doi: 10.1007/s42994-021-00067-w
He, N. Y., Chen, L. S., Sun, A. Z., Zhao, Y., Yin, S. N., Guo, F. Q. (2022). A nitric oxide burst at the shoot apex triggers a heat-responsive pathway in Arabidopsis. Nat. Plants. 8, 434–450. doi: 10.1038/s41477-022-01135-9
Hennig, S., Kong, G., Mannen, T., Sadowska, A., Kobelke, S., Amanda, B., et al. (2015). Prion-like domains in RNA binding proteins are essential for building subnuclear paraspeckles. J. Cell. Biol. 210 (4), 529–539. doi: 10.1083/jcb.201504117
Hou, Y., Yan, Y., Cao, X. (2022). Epigenetic regulation of thermomorphogenesis in Arabidopsis thaliana. Abiotech 3, 12–24. doi: 10.1007/s42994-022-00070-9
Hua, J., Dong, X. (2022). Sustaining plant immunity in rising temperature. Cell. Res. 32, 1038–1039. doi: 10.1038/s41422-022-00710-1
Huang, S., Zhu, S., Kumar, P., MacMicking, J. D. (2021). A phase-separated nuclear GBPL circuit controls immunity in plants. Nature 594, 424–429. doi: 10.1038/s41586-021-03572-6
Huot, B., Castroverde, C. D. M., Velásquez, A. C., Hubbard, E., Pulman, J. A., Yao, J., et al. (2017). Dual impact of elevated temperature on plant defence and bacterial virulence in Arabidopsis. Nat. Commun. 8, 1808. doi: 10.1038/s41467-017-01674-2
IPCC (2023) This synthesis report (SYR) of the IPCC sixth assessment report (AR6). Available at: https://www.ipcc.ch/report/sixth-assessment-report-cycle/.
Jacob, P., Kim, N. H., Wu, F., El-Kasmi, F., Chi, Y., Walton, W. G., et al. (2021). Plant “helper” immune receptors are Ca2+-permeable nonselective cation channels. Science 373, 420–425. doi: 10.1126/science.abg7917
Janda, M., Lamparová, L., Zubíková, A., Burketová, L., Martinec, J., Krčková, Z. (2019). Temporary heat stress suppresses PAMP-triggered immunity and resistance to bacteria in Arabidopsis thaliana. Mol. Plant Pathol. 20, 1005–1012. doi: 10.1111/mpp.12799
Jia, X., Wang, L., Zhao, H., Zhang, Y., Chen, Z., Xu, L., et al. (2023). The origin and evolution of salicylic acid signaling and biosynthesis in plants. Mol. Plant 16, 245–259. doi: 10.1016/j.molp.2022.12.002
Jiang, Z., Zhou, X., Tao, M., Yuan, F., Liu, L., Wu, F., et al. (2019). Plant cell-surface GIPC sphingolipids sense salt to trigger Ca2+ influx. Nature 572, 341–346. doi: 10.1038/s41586-019-1449-z
Jung, J. H., Barbosa, A. D., Hutin, S., Kumita, J. R., Gao, M., Derwort, D., et al. (2020). A prion-like domain in ELF3 functions as a thermosensor in Arabidopsis. Nature 585, 256–260. doi: 10.1038/s41586-020-2644-7
Jung, J. H., Domijan, M., Klose, C., Biswas, S., Ezer, D., Gao, M., et al. (2016). Phytochromes function as thermosensors in Arabidopsis. Science 354, 886–889. doi: 10.1126/science.aaf6005
Kerbler, S. M., Wigge, P. A. (2023). Temperature sensing in plants. Annu. Rev. Plant Biol. 74, 20.1–20.26. doi: 10.1146/annurev-arplant-102820-102235
Kim, J. H., Castroverde, C. D. M., Huang, S., Li, C., Hilleary, R., Seroka, A., et al. (2022). Increasing the resilience of plant immunity to a warming climate. Nature 607, 339–344. doi: 10.1038/s41586-022-04902-y
Klose, C., Nagy, F., Schäfer, E. (2020). Thermal reversion of plant phytochromes. Mol. Plant 13, 386–397. doi: 10.1016/j.molp.2019.12.004
Klose, C., Venezia, F., Hussong, A., Kircher, S., Schäfer, E., Fleck, C. (2015). Systematic analysis of how phytochrome B dimerization determines its specificity. Nat. Plants. 1, 1–9. doi: 10.1038/nplants.2015.90
Knight, M. R., Campbell, A. K., Smith, S. M., Trewavas, A. J. (1991). Transgenic plant aequorin reports the effects of touch and cold-shock and elicitors on cytoplasmic calcium. Nature 352, 524–526. doi: 10.1038/352524a0
Kortmann, J., Narberhaus, F. (2012). Bacterial RNA thermometers: molecular zippers and switches. Nat. Rev. Microbiol. 10, 255–265. doi: 10.1038/nrmicro2730
Lamers, J., van der Meer, T., Testerink, C. (2020). How plants sense and respond to stressful environments. Plant Physiol. 182, 1624–1635. doi: 10.1104/pp.19.01464
Laohavisit, A., Brown, A. T., Cicuta, P., Davies, J. M. (2010). Annexins: components of the calcium and reactive oxygen signaling network. Plant Physiol. 152, 1824–1829. doi: 10.1104/pp.109.145458
Lee, H. G., Kim, J., Seo, P. J. (2022). N6-methyladenosine-modified RNA acts as a molecular glue that drives liquid–liquid phase separation in plants. Plant Signal. Behavior. 17, 2079308. doi: 10.1080/15592324.2022.2079308
Lee, S., Lee, H. J., Huh, S. U., Paek, K. H., Ha, J. H., Park, C. M. (2014). The Arabidopsis NAC transcription factor NTL4 participates in a positive feedback loop that induces programmed cell death under heat stress conditions. Plant Sci. 227, 76–83. doi: 10.1016/j.plantsci.2014.07.003
Legris, M., Klose, C., Burgie, E. S., Rojas, C. C. R., Neme, M., Hiltbrunner, A., et al. (2016). Phytochrome B integrates light and temperature signals in Arabidopsis. Science 354, 897–900. doi: 10.1126/science.aaf56
Li, M., Kim, C. (2021). Chloroplast ROS and stress signaling. Plant Commun. 3, 100264. doi: 10.1016/j.xplc.2021.100264
Li, J. Y., Liu, J. X. (2022). TT3. 1: a journey to protect chloroplasts upon heat stress. Stress. Biol. 2, 27. doi: 10.1007/s44154-022-00051-4
Li, Y., Liu, H., Ma, T., Li, J., Yuan, J., Xu, Y. C., et al. (2023b). Arabidopsis EXECUTER1 interacts with WRKY transcription factors to mediate plastid-to-nucleus singlet oxygen signaling. Plant Cell. 35, 827–851. doi: 10.1093/plcell/koac330
Li, K. L., Tang, R. J., Wang, C., Luan, S. (2023a). Potassium nutrient status drives posttranslational regulation of a low-K response network in Arabidopsis. Nat. Commun. 14, 360. doi: 10.1038/s41467-023-35906-5
Li, Y., Xue, J., Wang, F. Z., Huang, X., Gong, B. Q., Tao, Y., et al. (2022). Plasma membrane-nucleo-cytoplasmic coordination of a receptor-like cytoplasmic kinase promotes EDS1-dependent plant immunity. Nat. Plants. 8, 802–816. doi: 10.1038/s41477-022-01195-x
Li, Z. G., Ye, X. Y., Qiu, X. M. (2019). Glutamate signaling enhances the heat tolerance of maize seedlings by plant glutamate receptor-like channels-mediated calcium signaling. Protoplasma 256, 1165–1169. doi: 10.1007/s00709-019-01351-9
Liang, X., Zhou, J. M. (2018). Receptor-like cytoplasmic kinases: central players in plant receptor kinase–mediated signaling. Annu. Rev. Plant Biol. 69, 267–299. doi: 10.1146/annurev-arplant-042817-040540
Liao, C., Zheng, Y., Guo, Y. (2017). MYB30 transcription factor regulates oxidative and heat stress responses through ANNEXIN-mediated cytosolic calcium signaling in Arabidopsis. New. Phytol. 216, 163–177. doi: 10.1111/nph.14679
Lindsay, R. J., Wigge, P. A., Hanson, S. M. (2023). Molecular basis of polyglutamine-modulated ELF3 phase change in Arabidopsis temperature response. BioRxiv, 2023–2003. doi: 10.1101/2023.03.15.532793
Liu, Q., Ding, Y., Shi, Y., Ma, L., Wang, Y., Song, C., et al. (2021). The calcium transporter ANNEXIN1 mediates cold-induced calcium signaling and freezing tolerance in plants. EMBO. J. 40, e104559. doi: 10.15252/embj.2020104559
Liu, Z., Jia, Y., Ding, Y., Shi, Y., Li, Z., Guo, Y., et al. (2017). Plasma membrane CRPK1-mediated phosphorylation of 14-3-3 proteins induces their nuclear import to fine-tune CBF signaling during cold response. Mol. Cell. 66, 117–128.e5. doi: 10.1016/j.molcel.2017.02.016
Liu, X. H., Lyu, Y. S., Yang, W., Yang, Z. T., Lu, S. J., Liu, J. X. (2020). A membrane-associated NAC transcription factor OsNTL3 is involved in thermotolerance in rice. Plant Biotech. J. 18, 1317–1329. doi: 10.1111/pbi.13297
Luan, S., Wang, C. (2021). Calcium signaling mechanisms across kingdoms. Annu. Rev. Cell. Dev. Biol. 37, 311–340. doi: 10.1146/annurev-cellbio-120219-035210
Ma, Y., Dai, X., Xu, Y., Luo, W., Zheng, X., Zeng, D., et al. (2015). COLD1 confers chilling tolerance in rice. Cell 160, 1209–1221. doi: 10.1016/j.cell.2015.01.046
Meyer, A., Freier, M., Schmidt, T., Rostowski, K., Zwoch, J., Lilie, H., et al. (2020). An RNA thermometer activity of the west Nile virus genomic 3′-terminal stem-loop element modulates viral replication efficiency during host switching. Viruses 12, 104. doi: 10.3390/v12010104
Meyerhoff, O., Müller, K., Roelfsema, M. R. G., Latz, A., Lacombe, B., Hedrich, R., et al. (2005). AtGLR3. 4, a glutamate receptor channel-like gene is sensitive to touch and cold. Planta 222, 418–427. doi: 10.1007/s00425-005-1551-3
Miller, G., Schlauch, K., Tam, R., Cortes, D., Torres, M. A., Shulaev, V., et al. (2009). The plant NADPH oxidase RBOHD mediates rapid systemic signaling in response to diverse stimuli. Sci. Signal. 2, ra45–ra45. doi: 10.1126/scisignal.2000448
Mori, K., Renhu, N., Naito, M., Nakamura, A., Shiba, H., Yamamoto, T., et al. (2018). Ca2+-permeable mechanosensitive channels MCA1 and MCA2 mediate cold-induced cytosolic Ca2+ increase and cold tolerance in Arabidopsis. Sci. Rep. 8, 550. doi: 10.1038/s41598-017-17483-y
Mousavi, S. A., Chauvin, A., Pascaud, F., Kellenberger, S., Farmer, E. E. (2013). GLUTAMATE RECEPTOR-LIKE genes mediate leaf-to-leaf wound signalling. Nature 500, 422–426. doi: 10.1038/nature12478
Nakashima, K., Takasaki, H., Mizoi, J., Shinozaki, K., Yamaguchi-Shinozaki, K. (2012). NAC transcription factors in plant abiotic stress responses. BBA-Gene. Regul. Mech. 1819, 97–103. doi: 10.1016/j.bbagrm.2011.10.005
Nguyen, C. T., Kurenda, A., Stolz, S., Chételat, A., Farmer, E. E. (2018). Identification of cell populations necessary for leaf-to-leaf electrical signaling in a wounded plant. P. Natl. Acad. Sci. U.S.A. 115, 10178–10183. doi: 10.1073/pnas.1807049115
Niu, Y., Bai, J., Liu, X., Zhang, H., Bao, J., Zhao, W., et al. (2022). HISTONE DEACETYLASE 9 transduces heat signal in plant cells. P. Natl. Acad. Sci. U.S.A. 119, e2206846119. doi: 10.1073/pnas.2206846119
Pardi, S. A., Nusinow, D. A. (2021). Out of the dark and into the light: a new view of phytochrome photobodies. Front. Plant Sci. 12. doi: 10.3389/fpls.2021.732947
Peng, Y., Yang, J., Li, X., Zhang, Y. (2021). Salicylic acid: biosynthesis and signaling. Annu. Rev. Plant Biol. 72, 761–791. doi: 10.1146/annurev-arplant-081320-092855
Peng, X., Zhang, X., Li, B., Zhao, L. (2019). Cyclic nucleotide-gated ion channel 6 mediates thermotolerance in Arabidopsis seedlings by regulating nitric oxide production via cytosolic calcium ions. BMC. Plant Biol. 19, 1–13. doi: 10.1186/s12870-019-1974-9
Perrella, G., Bäurle, I., van Zanten, M. (2022). Epigenetic regulation of thermomorphogenesis and heat stress tolerance. New. Phytol. 234, 1144–1160. doi: 10.1111/nph.17970
Praat, M., De Smet, I., van Zanten, M. (2021). Protein kinase and phosphatase control of plant temperature responses. J. Exp. Bot. 72, 7459–7473. doi: 10.1093/jxb/erab345
Prasad, A., Chirom, O., Prasad, M. (2022). Shedding light on immune suppression at high temperature. Trends. Microbiol. 30, 918–919. doi: 10.1016/j.tim.2022.07.010
Qiao, B., Zhang, Q., Liu, D., Wang, H., Yin, J., Wang, R., et al. (2015). A calcium-binding protein, rice annexin OsANN1, enhances heat stress tolerance by modulating the production of H2O2. J. Exp. Bot. 66, 5853–5866. doi: 10.1093/jxb/erv294
Rai, K. K., Pandey, N., Rai, S. P. (2020). Salicylic acid and nitric oxide signaling in plant heat stress. Physiol. Plantarum. 168, 241–255. doi: 10.1111/ppl.12958
Ren, Y., Huang, Z., Jiang, H., Wang, Z., Wu, F., Xiong, Y., et al. (2021). A heat stress responsive NAC transcription factor heterodimer plays key roles in rice grain filling. J. Exp. Bot. 72, 2947–2964. doi: 10.1093/jxb/erab027
Ronald, J., Wilkinson, A. J., Davis, S. J. (2021). EARLY FLOWERING3 sub-nuclear localization responds to changes in ambient temperature. Plant Physiol. 187, 2352–2355. doi: 10.1093/plphys/kiab423
Saidi, Y., Finka, A., Muriset, M., Bromberg, Z., Weiss, Y. G., Maathuis, F. J., et al. (2009). The heat shock response in moss plants is regulated by specific calcium-permeable channels in the plasma membrane. Plant Cell. 21, 2829–2843. doi: 10.1105/tpc.108.065318
Saijo, Y., Loo, E. P. I. (2020). Plant immunity in signal integration between biotic and abiotic stress responses. New. Phytol. 225, 87–104. doi: 10.1111/nph.15989
Shen, Y., Lei, T., Cui, X., Liu, X., Zhou, S., Zheng, Y., et al. (2019). Arabidopsis histone deacetylase HDA15 directly represses plant response to elevated ambient temperature. Plant J. 100, 991–1006. doi: 10.1111/tpj.14492
Shen, C., Que, Z., Xia, Y., Tang, N., Li, D., He, R., et al. (2017). Knock out of the annexin gene OsAnn3 via CRISPR/Cas9-mediated genome editing decreased cold tolerance in rice. J. Plant Biol. 60, 539–547. doi: 10.1007/s12374-016-0400-1
Silva, C. S., Nayak, A., Lai, X., Hutin, S., Hugouvieux, V., Jung, J. H., et al. (2020). Molecular mechanisms of Evening Complex activity in Arabidopsis. P. Natl. Acad. Sci. U.S.A. 117, 6901–6909. doi: 10.1073/pnas.1920972117
Simon, A. A., Navarro-Retamal, C., Feijó, J. A. (2022). Merging signaling with structure: functions and mechanisms of plant glutamate receptor ion channels. Annu. Rev. Plant Biol. 74, 415–452. doi: 10.1146/annurev-arplant-070522-033255
Song, L., Ding, W., Zhao, M., Sun, B., Zhang, L. (2006). Nitric oxide protects against oxidative stress under heat stress in the calluses from two ecotypes of reed. Plant Sci. 171, 449–458. doi: 10.1016/j.plantsci.2006.05.002
Spicher, L., Glauser, G., Kessler, F. (2016). Lipid antioxidant and galactolipid remodeling under temperature stress in tomato plants. Front. Plant Sci. 7. doi: 10.3389/fpls.2016.00167
Sun, A. Z., Guo, F. Q. (2016). Chloroplast retrograde regulation of heat stress responses in plants. Front. Plant Sci. 7. doi: 10.3389/fpls.2016.00398
Sun, S., Zhang, X., Chen, K., Zhu, X., Zhao, Y. (2021). Screening for Arabidopsis mutants with altered Ca2+ signal response using aequorin-based Ca2+ reporter system. STAR. Protoc. 2, 100558. doi: 10.1016/j.xpro.2021.100558
Tang, Y., Ho, M. I., Kang, B. H., Gu, Y. (2022). GBPL3 localizes to the nuclear pore complex and functionally connects the nuclear basket with the nucleoskeleton in plants. PloS Biol. 20, e3001831. doi: 10.1371/journal.pbio.3001831
Tasset, C., Yadav., A.S., Sureshkumar, S., Singh, R., van der Woude, L., Nekrasov, M., et al. (2018). POWERDRESS-mediated histone deacetylation is essential for thermomorphogenesis in Arabidopsis thaliana. PLoS. Genet. 14, e1007280. doi: 10.1371/journal.pgen.1007280
Tian, W., Wang, C., Gao, Q., Li, L., Luan, S. (2020). Calcium spikes, waves and oscillations in plant development and biotic interactions. Nat. Plants. 6, 750–759. doi: 10.1038/s41477-020-0667-6
Van Buskirk, E. K., Decker, P. V., Chen, M. (2012). Photobodies in light signaling. Plant Physiol. 158, 52–60. doi: 10.1104/pp.111.186411
van Butselaar, T., Van den Ackerveken, G. (2020). Salicylic acid steers the growth–immunity tradeoff. Trends. Plant Sci. 25, 566–576. doi: 10.1016/j.tplants.2020.02.002
Van Der Woude, L. C., Perrella, G., Snoek, B. L., van Hoogdalem, M., Novak, O., van Verk, M. C., et al. (2019). HISTONE DEACETYLASE 9 stimulates auxin-dependent thermomorphogenesis in Arabidopsis thaliana by mediating H2A.Z depletion. P. Natl. Acad. Sci. U.S.A. 116, 25343–25354. doi: 10.1073/pnas.1911694116
Vandivier, L. E., Anderson, S. J., Foley, S. W., Gregory, B. D. (2016). The conservation and function of RNA secondary structure in plants. Annu. Rev. Plant Biol. 67, 463–488. doi: 10.1146/annurev-arplant-043015-111754
Viczián, A., Ádám, É., Staudt, A. M., Dorothee, L., Klement, E., Montepaone, S. R., et al. (2020). Differential phosphorylation of the N-terminal extension regulates phytochrome B signaling. New. Phytol. 225, 1635–1650. doi: 10.1111/nph.16243
Vu, L. D., Xu, X., Zhu, T., Pan, L., van Zanten, M., de Jong, D., et al. (2021). The membrane-localized protein kinase MAP4K4/TOT3 regulates thermomorphogenesis. Nat. Commun. 12, 2842. doi: 10.1038/s41467-021-23112-0
Wang, Q. L., Chen, J. H., He, N. Y., Guo, F. Q. (2018). Metabolic reprogramming in chloroplasts under heat stress in plants. Int. J. Mol. Sci. 19, 849. doi: 10.3390/ijms19030849
Wang, L., Guo, Y., Jia, L., Chu, H., Zhou, S., Chen, K., et al. (2014). Hydrogen peroxide acts upstream of nitric oxide in the heat shock pathway in Arabidopsis seedlings. Plant Physiol. 164, 2184–2196. doi: 10.1104/pp.113.229369
Wang, J., Ren, Y., Liu, X., Luo, S., Zhang, X., Liu, X., et al. (2021a). Transcriptional activation and phosphorylation of OsCNGC9 confer enhanced chilling tolerance in rice. Mol. Plant 14, 315–329. doi: 10.1016/j.molp.2020.11.022
Wang, W., Zhang, J., Ai, L., Wu, D., Li, B., Zhang, L., et al. (2021b). Cyclic nucleotide-gated ion channel 6 mediates thermotolerance in Arabidopsis seedlings by regulating hydrogen peroxide production via cytosolic calcium ions. Front. Plant Sci. 12. doi: 10.3389/fpls.2021.708672
Woodson, J. D. (2022). Control of chloroplast degradation and cell death in response to stress. Trends. Biochem. Sci. 47, 851–864. doi: 10.1016/j.tibs.2022.03.010
Wu, F., Chi, Y., Jiang, Z., Xu, Y., Xie, L., Huang, F., et al. (2020). Hydrogen peroxide sensor HPCA1 is an LRR receptor kinase in Arabidopsis. Nature 578, 577–581. doi: 10.1038/s41586-020-2032-3
Xu, X., Yuan, L., Xie, Q. (2022). The circadian clock ticks in plant stress responses. Stress. Biol. 2, 15. doi: 10.1007/s44154-022-00040-7
Yang, X., Li, Y., Qi, M., Liu, Y., Li, T. (2019). Targeted control of chloroplast quality to improve plant acclimation: from protein import to degradation. Front. Plant Sci. 10. doi: 10.3389/fpls.2019.00958
Yang, C., Zhu, T., Zhou, N., Huang, S., Zeng, Y., Jiang, W., et al. (2023). PIF7-mediated epigenetic reprogramming promotes the transcriptional response to shade in Arabidopsis. EMBO J. 42, e111472. doi: 10.15252/embj.2022111472
Yoshimura, K., Iida, K., Iida, H. (2021). MCAs in Arabidopsis are Ca2+-permeable mechanosensitive channels inherently sensitive to membrane tension. Nat. Commun. 12, 6074. doi: 10.1038/s41467-021-26363-z
Yu, J., Han, J., Kim, Y. J., Song, M., Yang, Z., He, Y., et al. (2017). Two rice receptor-like kinases maintain male fertility under changing temperatures. P. Natl. Acad. Sci. U.S.A. 114, 12327–12332. doi: 10.1073/pnas.1705189114
Yu, B., Wu, Q., Li, X., Zeng, R., Min, Q., Huang, J. (2022). GLUTAMATE RECEPTOR-like gene OsGLR3. 4 is required for plant growth and systemic wound signaling in rice (Oryza sativa). New. Phytol. 233, 1238–1256. doi: 10.1111/nph.17859
Yuan, F., Yang, H., Xue., Y., Kong, D., Ye, R., Li, C., et al. (2014). OSCA1 mediates osmotic-stress-evoked Ca2+ increases vital for osmosensing in Arabidopsis. Nature 514, 367–371. doi: 10.1038/nature13593
Zahra, N., Hafeez, M. B., Ghaffar, A., Kausar, A., Al Zeidi, M., Siddique, K. H., et al. (2023). Plant photosynthesis under heat stress: Effects and management. Environ. Exp. Bot. 206, 105178. doi: 10.1016/j.envexpbot.2022.105178
Zhang, L. L., Li, W., Tian, Y. Y., Davis, S. J., Liu, J. X. (2021a). The E3 ligase XBAT35 mediates thermoresponsive hypocotyl growth by targeting ELF3 for degradation in Arabidopsis. J. Integr. Plant Biol. 63, 1097–1103. doi: 10.1111/jipb.13107
Zhang, L. L., Shao, Y. J., Ding, L., Wang, M. J., Davis, S. J., Liu, J. X. (2021b). XBAT31 regulates thermoresponsive hypocotyl growth through mediating degradation of the thermosensor ELF3 in Arabidopsis. Sci. Adv. 7, eabf4427. doi: 10.1126/sciadv.abf4427
Zhang, H., Zhou, J. F., Kan, Y., Shan, J. X., Ye, W. W., Dong, N. Q., et al. (2022). A genetic module at one locus in rice protects chloroplasts to enhance thermotolerance. Science 376, 1293–1300. doi: 10.1126/science.abo5721
Zhao, Y., Shi, H., Pan, Y., Lyu, M., Yang, Z., Kou., X., et al. (2023). Sensory circuitry controls cytosolic calcium-mediated phytochrome B phototransduction. Cell 186, 1230–1243. doi: 10.1016/j.cell.2023.02.011
Zheng, Y., Luo, L., Wei, J., Chen, Q., Yang, Y., Hu, X., et al. (2018). The glutamate receptors AtGLR1. 2 and AtGLR1.3 increase cold tolerance by regulating jasmonate signaling in Arabidopsis thaliana. Biochem. Bioph. Res. Co. 506, 895–900. doi: 10.1016/j.bbrc.2018.10.153
Zhu, J. K. (2016). Abiotic stress signaling and responses in plants. Cell 167, 313–324. doi: 10.1016/j.cell.2016.08.029
Keywords: global warming, heat stress, thermosensor, GBPL3, phyB, liquid-liquid phase separation, transcriptional condensates
Citation: Yang X, Guan H, Yang Y, Zhang Y, Su W, Song S, Liu H, Chen R and Hao Y (2023) Extra- and intranuclear heat perception and triggering mechanisms in plants. Front. Plant Sci. 14:1276649. doi: 10.3389/fpls.2023.1276649
Received: 12 August 2023; Accepted: 20 September 2023;
Published: 04 October 2023.
Edited by:
Silvana Scalon, Federal University of Grande Dourados, BrazilReviewed by:
Jean-David Rochaix, University of Geneva, SwitzerlandDaisuke Urano, Temasek Life Sciences Laboratory, Singapore
Copyright © 2023 Yang, Guan, Yang, Zhang, Su, Song, Liu, Chen and Hao. This is an open-access article distributed under the terms of the Creative Commons Attribution License (CC BY). The use, distribution or reproduction in other forums is permitted, provided the original author(s) and the copyright owner(s) are credited and that the original publication in this journal is cited, in accordance with accepted academic practice. No use, distribution or reproduction is permitted which does not comply with these terms.
*Correspondence: Riyuan Chen, cnljaGVuQHNjYXUuZWR1LmNu; Yanwei Hao, eWFud2VpaGFvQHNjYXUuZWR1LmNu
†These two authors contributed equally to this work.