- 1College of Agriculture and Veterinary Medicine, Jimma University, Jimma, Ethiopia
- 2Hawkesbury Institute for the Environment, Western Sydney University, Penrith, NSW, Australia
- 3R H Smith Institute of Plant Sciences and Genetics in Agriculture, The Hebrew University of Jerusalem, Rehovot, Israel
The uptake and accumulation of silicon (Si) in grass plants play a crucial role in alleviating both biotic and abiotic stresses. Si supplementation has been reported to increase activity of defence-related antioxidant enzyme, which helps to reduce oxidative stress caused by reactive oxygen species (ROS) following herbivore attack. Atmospheric CO2 levels are known to affect Si accumulation in grasses; reduced CO2 concentrations increase Si accumulation whereas elevated CO2 concentrations often decrease Si accumulation. This can potentially affect antioxidant enzyme activity and subsequently insect herbivory, but this remains untested. We examined the effects of Si supplementation and herbivory by Helicoverpa armigera on antioxidant enzyme (catalase, CAT; superoxide dismutase, SOD; and ascorbate peroxidase, APX) activity in tall fescue grass (Festuca arundinacea) grown under CO2 concentrations of 200, 410, and 640 ppm representing reduced, ambient, and elevated CO2 levels, respectively. We also quantified foliar Si, carbon (C), and nitrogen (N) concentrations and determined how changes in enzymes and elemental chemistry affected H. armigera relative growth rates and plant consumption. Rising CO2 concentrations increased plant mass and foliar C but decreased foliar N and Si. Si supplementation enhanced APX and SOD activity under the ranging CO2 regimes. Si accumulation and antioxidant enzyme activity were at their highest level under reduced CO2 conditions and their lowest level under future levels of CO2. The latter corresponded with increased herbivore growth rates and plant consumption, suggesting that some grasses could become more susceptible to herbivory under projected CO2 conditions.
Introduction
Most grasses are silicon (Si) accumulators, which can account for up to 10% of their dry mass (Epstein, 1994). Si uptake and accumulation are a functional trait with multiple implications for plant biology and ecology (Epstein, 2009). Si is taken up as silicic acid [Si(OH)4] via the roots and, after being transported into plant tissues, is deposited within and between plant cells, the cell wall, and silicified structures such as trichomes or other phytoliths (Perry et al., 1984; Kumar et al., 2017). Although the role of Si in protecting plants against multiple biotic (e.g., herbivores and pathogens) and abiotic (e.g., drought and salinity) stresses has been widely reported (Cooke and Leishman, 2011; Debona et al., 2017), an understanding of the exact mechanisms underpinning such protection remains incomplete (Coskun et al., 2019). However, the consensus is that Si supplementation enhances plant physical defences and integrates with the regulation of secondary metabolite defences (Reynolds et al., 2016; Alhousari and Greger, 2018; Hall et al., 2019; Waterman et al., 2020).
In terms of physical defences, it is well established that Si confers plant resistance and reduces plant damage caused by both vertebrate and invertebrate herbivores (Massey and Hartley, 2009; Hartley et al., 2015; Alhousari and Greger, 2018). Si deposition within and around plant cells makes plant tissues tougher and abrasive, causing wear on herbivore mouthparts, damages digestive organs, inhibits movement, and reduces the feeding efficiency of insect herbivores (Massey et al., 2006; Reynolds et al., 2009). Moreover, Si is known to alter grass physical defences such as macrohairs, silica cells, and prickle cells, which are linked to reduced feeding by insect herbivores (Hartley et al., 2015; Hall et al., 2020; Biru et al., 2021). Si uptake and accumulation have also been shown to be induced following herbivory (Massey et al., 2007; Islam et al., 2020; Biru et al., 2022).
In addition to direct physical defences, Si potentially protects plants against herbivores by influencing production of plant biochemical defences (Reynolds et al., 2016; Yang et al., 2017), although there is much uncertainty about this since Si has limited chemical reactivity within the plant. Herbivore attack is associated with the induction of oxidative stress in plants, resulting from overproduction of reactive oxygen species (ROS) (Bi and Felton, 1995; Kerchev et al., 2012). For instance, insect herbivore attacks can induce various ROS such as hydrogen peroxide (H2O2), superoxide (O2•−), singlet oxygen (1O2), or hydroxyl radicals (•OH) in cells (Sharma et al., 2012; Das and Roychoudhury, 2014). While ROS have signalling roles under physiological setup (Hasanuzzaman et al., 2020), (biotic) stress-induced ROS overproduction damages cell structures and functionality (Tripathy and Oelmüller, 2012; Das and Roychoudhury, 2014; Fichman and Mittler, 2020). In order to reduce excessive ROS content caused by the imbalance between free radical formation and the capability of cells to detoxify them (Pizzino et al., 2017), plants have developed efficient antioxidant enzymatic machinery to scavenge ROS (Tripathy and Oelmüller, 2012). The antioxidant defence system in the plant cell includes both enzyme constituents such as superoxide dismutase (SOD), catalase (CAT), ascorbate peroxidase (APX), glutathione reductase (GR), and non-enzyme constituents like cysteine (Cys), reduced glutathione (GSH), and ascorbic acid (AsA) (Farooq et al., 2013; Kim et al., 2017). Plants possess either constitutive or induced antioxidants (Sudhakar et al., 2001) and the increased activities of these enzymes in plant cells appear to better control oxidative stress (Das and Roychoudhury, 2014; Huang et al., 2019).
Exogenous Si application has been linked to enhanced activity of antioxidant enzyme defences such as CAT, APX, and SOD (Kim et al., 2016; Hasanuzzaman et al., 2018; Ahanger et al., 2020). However, the mode of action by which Si regulates antioxidant capacities is poorly understood. Previous work has shown that Si enhances SOD, CAT, APX, and peroxidase activities in rice (Oryza sativa) (Han et al., 2016), wheat (Triticum aestivum L.) (Gong et al., 2005), and maize (Zea mays L.) (Moussa, 2006). Si-mediated regulation of antioxidant defences, therefore, reduces the harmful effects of herbivore-induced oxidative stress (Ahanger et al., 2020; Acevedo et al., 2021).
Atmospheric concentrations of carbon dioxide (CO2) have emerged as an important environmental driver of Si accumulation (Johnson and Hartley, 2018). In general, several studies report that elevated CO2 concentrations (eCO2) decrease Si accumulation (Ryalls et al., 2017; Johnson and Hartley, 2018; Johnson et al., 2023), but see Frew et al. (2017) and Fulweiler et al. (2014). In contrast, reduced levels of atmospheric CO2 can lead to increased Si accumulation (Biru et al., 2020; Biru et al., 2021). These effects are likely due to carbon (C) being either more available under eCO2 (Johnson and Hartley, 2018; Johnson et al., 2022) or less available under reduced CO2 conditions (Biru et al., 2020). Si accumulation is often negatively correlated with C potentially due to stoichiometric dilution or Si substitution for C-based structural or defensive compounds (Raven, 1983; Hodson et al., 2005).
Given CO2 is such an important driver of Si accumulation, which has been shown to influence enzymatic responses (e.g., Kim et al., 2016; Ahanger et al., 2020; Acevedo et al., 2021), CO2 may also affect production of plant biochemical defences (i.e., antioxidant enzyme), potentially via enhanced plant susceptibility to herbivore-induced oxidative stress. To our knowledge, no studies have yet investigated the effects of variable rates of Si accumulation on antioxidant enzyme activity and regulation of herbivore-induced oxidative stress under different CO2 concentrations. Using tall fescue (Festuca arundinacea) and the generalist insect herbivore, cotton bollworm [Helicoverpa armigera (Hübner)], we investigated the effect of Si treatments on antioxidant enzyme activities and foliar chemistry of plants grown under three CO2 concentrations (200, 410, and 640 ppm) and the consequences for insect herbivory. The objective of this study was to determine how Si treatments (+Si or −Si) under different CO2 concentrations affect antioxidant enzyme activity and foliar chemistry (e.g., C, N) in tall fescue and the consequences for insect herbivore growth rate and feeding efficiency. We hypothesised that (1) +Si and reduced CO2 decrease shoot C concentrations but increase shoot N concentration, i.e., decreasing shoot C-to-N ratio, and (2) +Si together with reduced CO2 treatments increases antioxidant enzyme activity, whereas −Si together with elevated CO2 would decrease antioxidant enzyme activity.
Materials and methods
Plant material and growth conditions
Tall fescue (Festuca arundinacea) is a common pasture grass and a high Si accumulator (Hodson et al., 2005). Seeds of tall fescue (accession T 9627), obtained from Margot Forde Germplasm Centre (Palmerston North, New Zealand), were sterilised in a solution of 0.9% sodium hypochlorite and 0.1% Triton X-100 for 30 min, followed by washing several times with water before being inserted into perlite irrigated with water. Seeds were stratified at 4°C for 3 days, and plants were grown in the glasshouse for 2 weeks using a rectangular plastic tray to achieve uniform seedling growth. Two weeks after germination, individual plants were transferred to hydroponics cups. The hydroponics setup consisted of two nested disposable plastic cups as per Hall et al. (2019). Each cup was filled with approximately 350 mL of full-strength standard hydroponic solutions following the protocol of Jung et al. (2015). Seedlings were grown in three plant growth chambers (TPG-1260TH, Thermoline Scientific, NSW, Australia), maintained at a reduced CO2 of 200 ppm, an ambient CO2 of 410 ppm, and an elevated CO2 of 640 ppm CO2 concentrations; the latter CO2 concentration predicted for 2100 under the RCP6.0 scenario outlined by the IPCC (2014). Chambers were illuminated with five 400-W Sunmaster Dual Spectrum High-Pressure Sodium globes at 350 µmol m−2 s−1 at the plant canopy level. Daytime air temperature was regulated at 26°C and fell to 18°C at night on a 15L:9D photoperiod cycle. Humidity was controlled at 50% ( ± 6%). Carbon dioxide within the chambers was monitored by a Li-Cor LI-820 CO2 gas analyser, with CO2 (food grade, Air Liquide, NSW, Australia) injected from pressurised cylinders through solenoid valves. For reduced CO2 treatment in the chamber, the computer controller constantly monitors CO2 and powers fans to direct chamber air through the scrubbers filled with Sodasorb® (W.R. Grace & Co, Chicago, USA).
Experimental design
The experimental design consisted of 252 hydroponically grown tall fescue plants. The experiment comprised a factorial combination of CO2 concentrations (200, 410, or 640 ppm), Si (+Si or −Si), and herbivore (herbivory, +H; no herbivory, −H) treatments (see Figure 1). Si treatments (+Si) used liquid potassium silicate (K2SiO3) (Agsil32, PQ Australia, SA, Australia) at a concentration equivalent to 2 mM SiO2. Chemically, silicic acid polymerises to form silica gel when the concentration of silicic acid exceeds 2 mM (Ma and Yamaji, 2006). Potassium chloride was added to the control (−Si) cups to balance additional K+ ions in +Si treatments. The pH of both +Si and −Si solutions was adjusted to 5.6 using hydrochloric acid to reduce silicate polymerisation (Ma and Yamaji, 2006). Solutions were replaced weekly for the first 2 weeks and then three times a week afterwards. Cups were rotated and chambers were swapped weekly to minimise chamber effects and pseudo-replication as previously described by Johnson et al. (2018). Plants were grown hydroponically for a further 6 weeks (42 days, Figure 1) before insect inoculation.
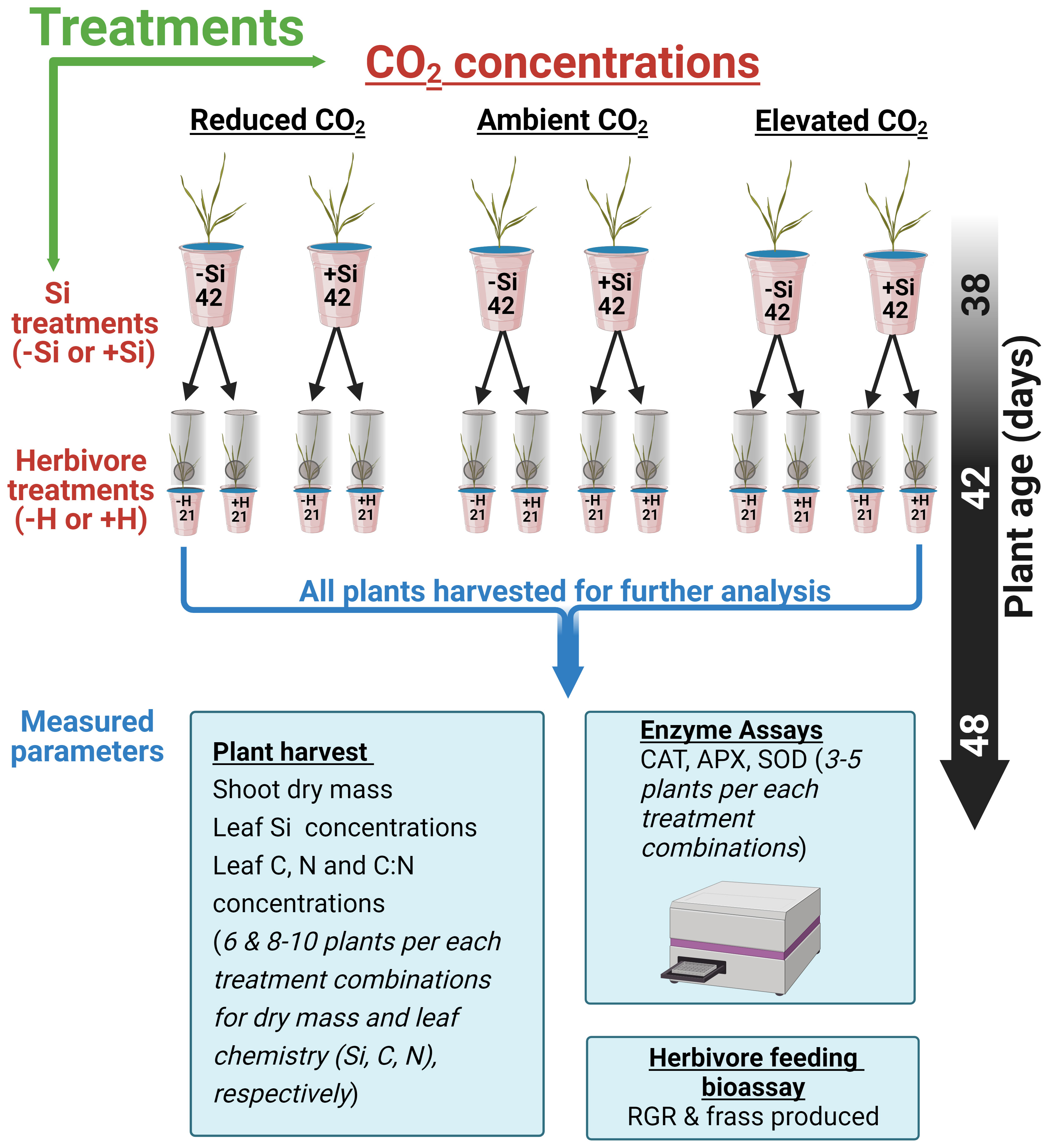
Figure 1 Schematic showing 252 (42*6) fescue grass grown under three CO2 concentrations and Si treatments (−Si or +Si). Six weeks after transplant, plants were either inoculated with herbivore, (+H) or kept in control (−H). The specific arrangement of the cup within each chamber was randomised and swiped within the chambers every 2 weeks.
Herbivore performance
To assess the impacts of different CO2 concentrations and Si supplementation on the growth of H. armigera larvae, a feeding performance assay was conducted. Helicoverpa armigera third instar larvae supplied by CSIRO Agriculture & Food, Narrabri, Australia, reared on an artificial diet (Teakle and Jensen, 1985), were used for feeding assays. Initially, larvae were starved for 24 h and weighed, and a single larva was either applied for each plant shoot [herbivory (+H)]) or kept control [no herbivory (−H)] (see Figure 1 for details). Each plant was then caged with transparent Perspex sheaths and herbivores were allowed to feed on shoot parts of the plants for 6 days, after which they were removed and starved for a further 24 h to allow the frass to pass, before being reweighed. All frass were collected, dried, and weighed. Frass production was used as a surrogate for plant consumption. RGR was calculated according to Massey and Hartley (2009). RGR estimates the change in larval fresh mass relative to initial mass and was calculated as mass gained (mg)/initial mass (mg)/time (days).
Antioxidant enzyme activity assays
Immediately after herbivore removal, plants from all treatment groups were harvested into liquid nitrogen and stored at −80°C until analysis. For the measurement of enzymatic activities, ca. 0.05 g of leaf tissue was ground in liquid N and homogenised in 3 mL of ice-cold 100 mM K-phosphate buffer (pH 6.8) containing 0.1 mM EDTA. The homogenate was centrifuged at 16,000 g for 15 min and the supernatant was used as the source of crude extracts. The supernatant was utilised to measure the activity of antioxidant enzyme such as CAT, APX, and SOD.
CAT activity was measured spectrophotometrically following the method of Fimognari et al. (2020) and Maksimović and Živanović (2012). Reaction mixture consists of 50 mM potassium phosphate buffer, pH 7.0, 20 mM H2O2, and crude extract. Absorbance at 240 nm was recorded for 130s using CLARIOstar® plate reader (BMG Labtech, Ortenberg, Germany) in 96-well plates (96-well Flat Bottom Plate, Greiner Bio-one, Australia). For control reactions, H2O2 was omitted. Enzyme activity was calculated using the molar extinction coefficient 36 × 103 mM−1 m−1 and expressed as µmol H2O2 oxidised g−1 FW min−1.
APX activity was assayed according to Hartmann and Asch (2019). The reaction mixture consists of 50 mM potassium phosphate buffer, pH 7.0, 0.2 mM ascorbate, 0.2 mM H2O2 and crude extract. Absorbance at 290 nm was recorded for 130 s using CLARIOstar® in 96-well plates. APX activity was calculated according to Hartmann and Asch (2019); one unit of APX is defined as the amount of enzyme required to oxidise 1 μmol of ascorbic acid per minute.
SOD activity was estimated following the inhibition of photochemical reduction of nitroblue tetrazolium (NBT) by the enzyme according to Hartmann and Asch (2019). The reaction mixture contained 0.05 M sodium carbonate, 13.3 mM methionine, 1.3 μM riboflavin, 21 μM NBT, and plant extract (Hartmann and Asch, 2019). The reaction took place in a chamber under illumination of a 30-W fluorescent lamp at 25°C. The reaction was started by turning the fluorescent lamp on and stopped 5 min later by turning it off. The blue formazan produced by NBT photoreduction was measured as increase in absorbance at 560 nm. The blank solution had the same complete reaction mixture but was kept in the dark. One SOD unit was defined as the amount of enzyme required to inhibit 50% of the NBT photoreduction in comparison with wells lacking the plant extract and expressed as units of enzyme activity g−1 FW min−1 (Cavalcanti et al., 2004).
Analyses of foliar chemistry
Harvested sample leaves were oven-dried for 3 days at 60°C and ball milled for further analysis (see sample size from Figure 1). For foliar Si analysis, roughly 80 mg of ground leaf material was analysed using x-ray fluorescence spectrometry (Epsilon 3x, PANalytical, EA Almelo, The Netherlands) as per Reidinger et al. (2012). Si measurements were calibrated against a certified plant reference material of known Si concentrations (Hiltpold et al., 2017). For foliar C and N concentration, approximately 7 mg of ground leaf material was analysed using Elementar Vario EL Cube, CHNOS elemental analyser (Elementar Analysensysteme GmbH, Hanau, Germany), at a combustion temperature of 950°C.
Statistical analysis
All data were analysed using SPSS (version 27) statistical software. Before analysis, all data were checked for assumptions of normality for residuals according to inspection of quantile–quantile plots. Plant dry mass was analysed on square-root transformed data whereas CAT, C:N ratio, and RGR were analysed on log10 transformed data, as they did not meet the assumptions of normality. Foliar Si was analysed using two-way analysis of variance (ANOVA) type = II, comparing CO2, and herbivory (larval fed vs. undamaged controls) as treatments and their interaction. For foliar Si analyses, control (−Si) plants were omitted since −Si plants had Si concentrations lower than the machine detection limits (Reidinger et al., 2012). Plant dry mass, antioxidant enzyme activities (CAT, APX, and SOD), foliar C, N, and C-to-N ratio concentrations were all analysed using three-way ANOVA type = II, comparing CO2, Si (Si-supplemented vs. non-Si-supplemented plants) and herbivory as treatments and their interaction. Additionally, we tested the independent effects of CO2 on antioxidant enzyme using a one-way ANCOVA, with CO2 levels as a fixed factor and foliar Si concentration fitted as a covariate. For herbivore RGR and frass produced, three insects escaped, so data were analysed using type = III ANOVAs due to the unbalanced design. Bonferroni post hoc test (Aslam and Albassam, 2020) was applied for pairwise multiple comparisons when interaction terms were statistically significant. Potential relationships between foliar Si and herbivore RGR, frass produced, CAT, APX, and SOD enzymes activity were investigated using Spearman’s rank correlation test.
Results
Plant dry mass and foliar chemistry
Averaged across CO2 treatments, Si supply increased plant dry mass by 160% relative to those grown without Si supply, whereas elevated CO2 increased plant dry mass by twofold and threefold compared to reduced CO2 and ambient CO2, respectively (Figure 2A; Table 1). Furthermore, herbivory decreased plant dry mass in Si-free (control) plants by 1.5-, 2-, and 2-fold under reduced, ambient, and elevated CO2, respectively, compared to Si-supplemented plants (Figure 2A). Si supplementation decreased foliar C concentrations under all CO2 regimes. This effect was reversed when herbivores were present and foliar C concentrations increased to levels observed in Si-free plants (Figure 2B; Table 1). Si supply decreased foliar C by 149%, 177%, and 107% under reduced, ambient, and elevated CO2, respectively, regardless of herbivore treatments (Figure 2B). Reduced CO2 significantly decreased foliar C (Figure 2B; Table 1). Si supply decreased foliar N under all CO2 regimes. However, there was also an effect of CO2 whereby reduced CO2 increased foliar N by twofold and threefold compared to ambient and elevated CO2, respectively, irrespective of herbivore treatments (Figure 2C; Table 1). In addition to variations in C and N concentrations, there was also an effect on their ratio. Si supply increased foliar C:N ratio especially when plants were damaged by herbivores or in herbivore-free plants only under elevated CO2 (Figure 2D; Table 1). While herbivory increased foliar C:N ratio regardless of CO2 levels, reduced CO2 decreased foliar C:N ratio relative to elevated CO2 (Figure 2D; Table 1). Foliar Si accumulation [% dry weight (DW)] was significantly higher under reduced CO2 relative to ambient and elevated CO2 (Figure 3; Table 1).
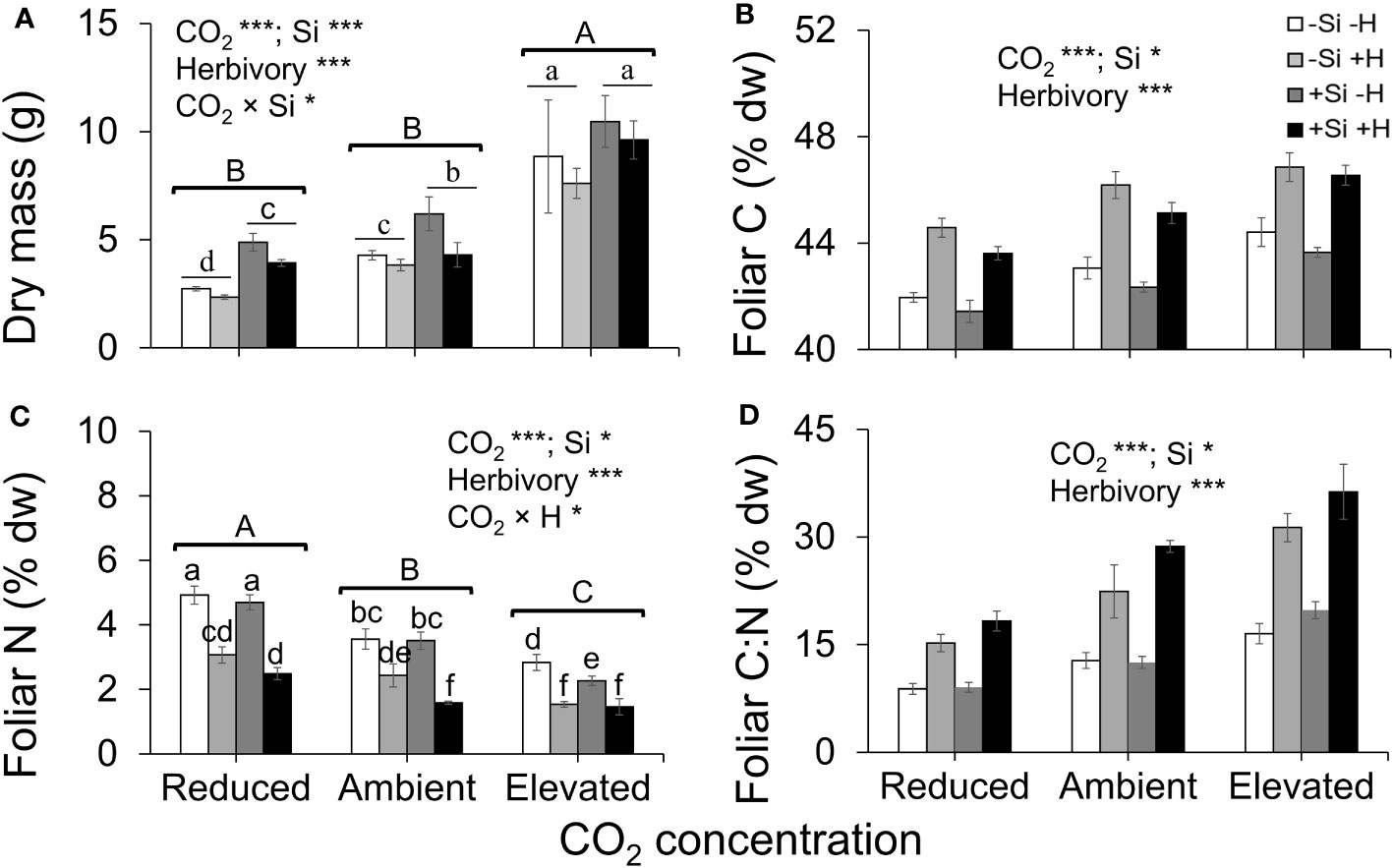
Figure 2 Effects of Si supply (+Si or –Si) and herbivory treatments (+H or –H) on (A) plant dry mass, (B) foliar C, (C) foliar N, and (D) foliar C:N of tall fescue grass grown under reduced, ambient, and elevated CO2 concentration. Means ± SE shown. N = 6–8. Uppercase letters represent differences between CO2 concentrations whereas lowercase letters indicate differences between Si treatments (Panel 1A) and herbivore treatments (Panel 1C). Statistically significant effects are indicated *p < 0.05, and ***p < 0.001.
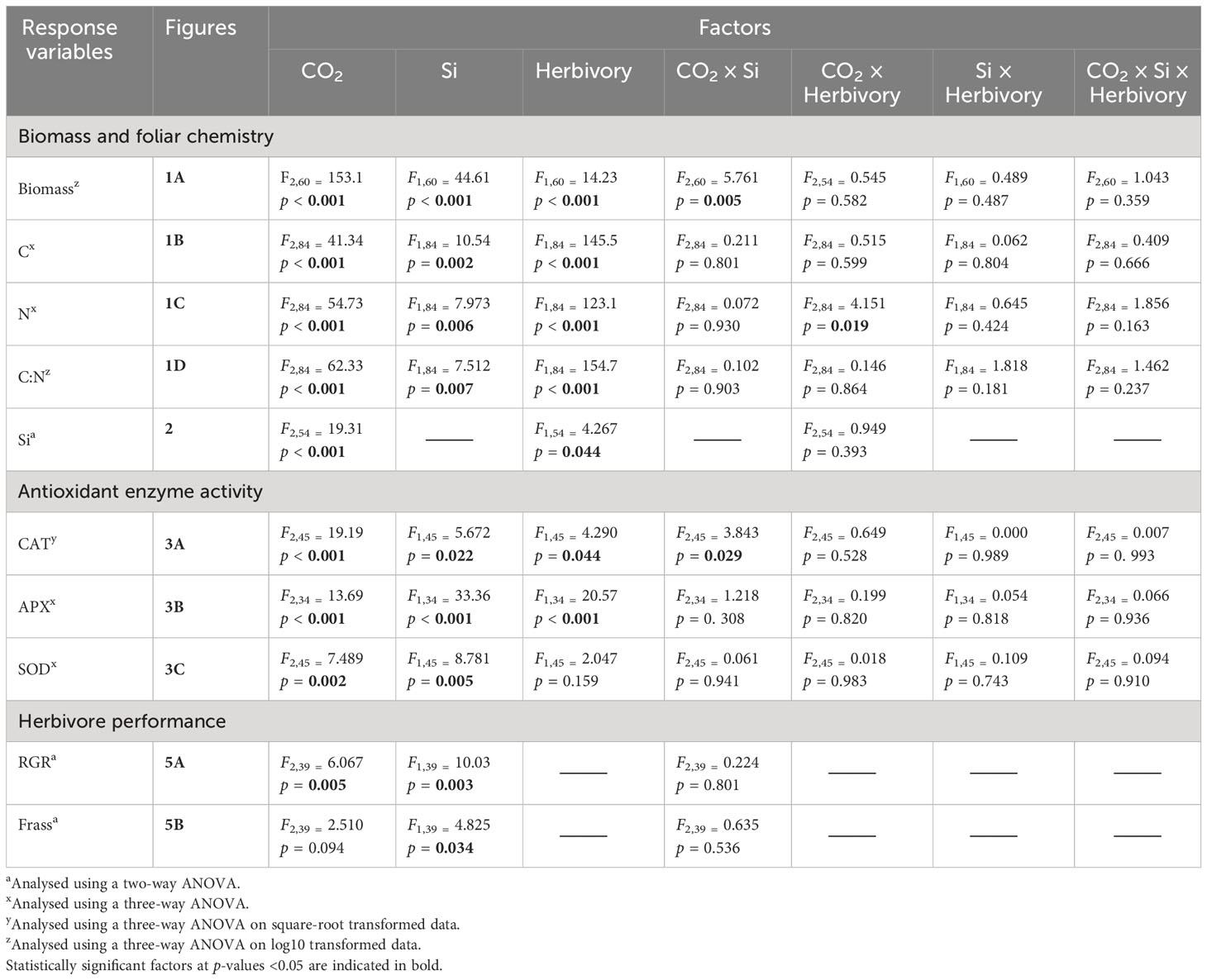
Table 1 Results of ANOVA for plant biomass, foliar chemistry, and antioxidant enzyme as affected by CO2 levels, Si treatment, and herbivore presence and their interactive effects.
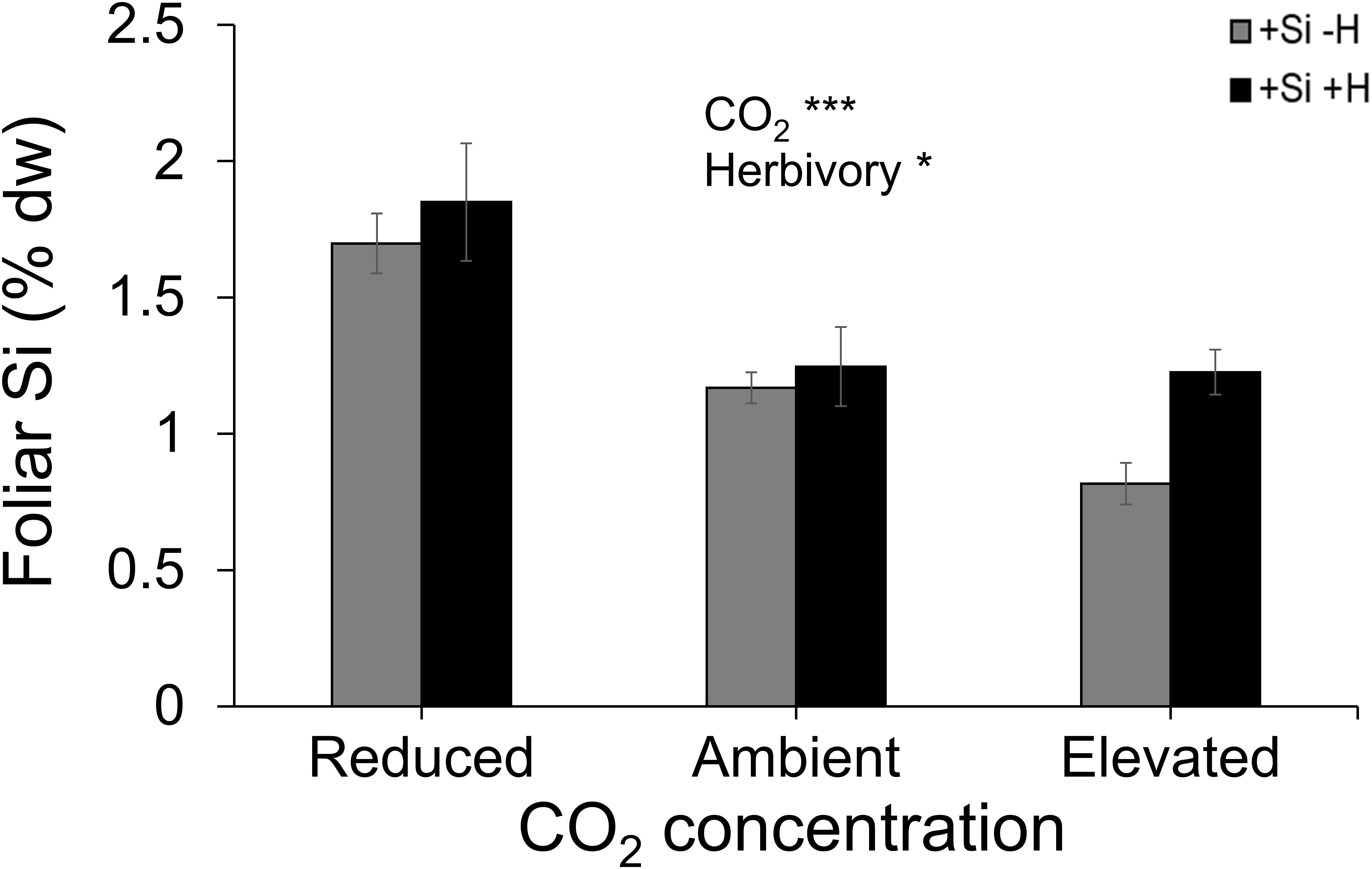
Figure 3 Effects of Si supply and herbivory (+H or –H) on foliar Si concentration of tall fescue grass grown under reduced, ambient, and elevated CO2 concentration. Means ± SE shown. N = 10. Statistically significant effects are indicated *p < 0.05, and ***p < 0.001.
Antioxidant enzyme activity was enhanced by Si uptake and reduced CO2
CAT activity increased in response to Si supply and herbivore damage overall, although this was only apparent under reduced CO2 concentrations (Table 1; Figure 4A). The significant interaction between Si and CO2 reflects that Si impacts were only apparent under reduced CO2 concentrations (Table 1). In contrast, Si supply increased APX enzymes activity under all CO2 regimes and SOD enzyme activity under reduced and ambient CO2 (Table 1; Figures 4B, C). Herbivory caused higher APX activity specifically in Si-supplemented plants (Table 1; Figure 4B); however, it had no significant effect on SOD enzyme activity (Table 1; Figure 4C). Overall, antioxidant enzyme activity (CAT, APX, and SOD) declined with increasing CO2 concentrations (Table 1; Figures 3A–C). Including foliar Si as a covariate in ANCOVA indicated that the changes in antioxidant enzyme activity were linked to CO2 levels, which fully explained the observed changes in CAT (F1,53 = 11.67, p = 0.001) and APX (F1,42 = 5.79, p = 0.021) but not in SOD (F1,53 = 1.130, p = 0.258). There was a positive correlation between foliar Si concentrations and concentration of CAT under reduced CO2, and concentration of APX under elevated CO2 (Figures 5A, B). Interestingly, frass produced was negatively correlated with SOD (r = −0.310, p = 0.038), but had marginally non-significant negative correlation with APX (r = −0.286, p = 0.057). However, there was no such relationship observed between CAT and frass produced (r = −0.191, p = 0.208) (data not shown).
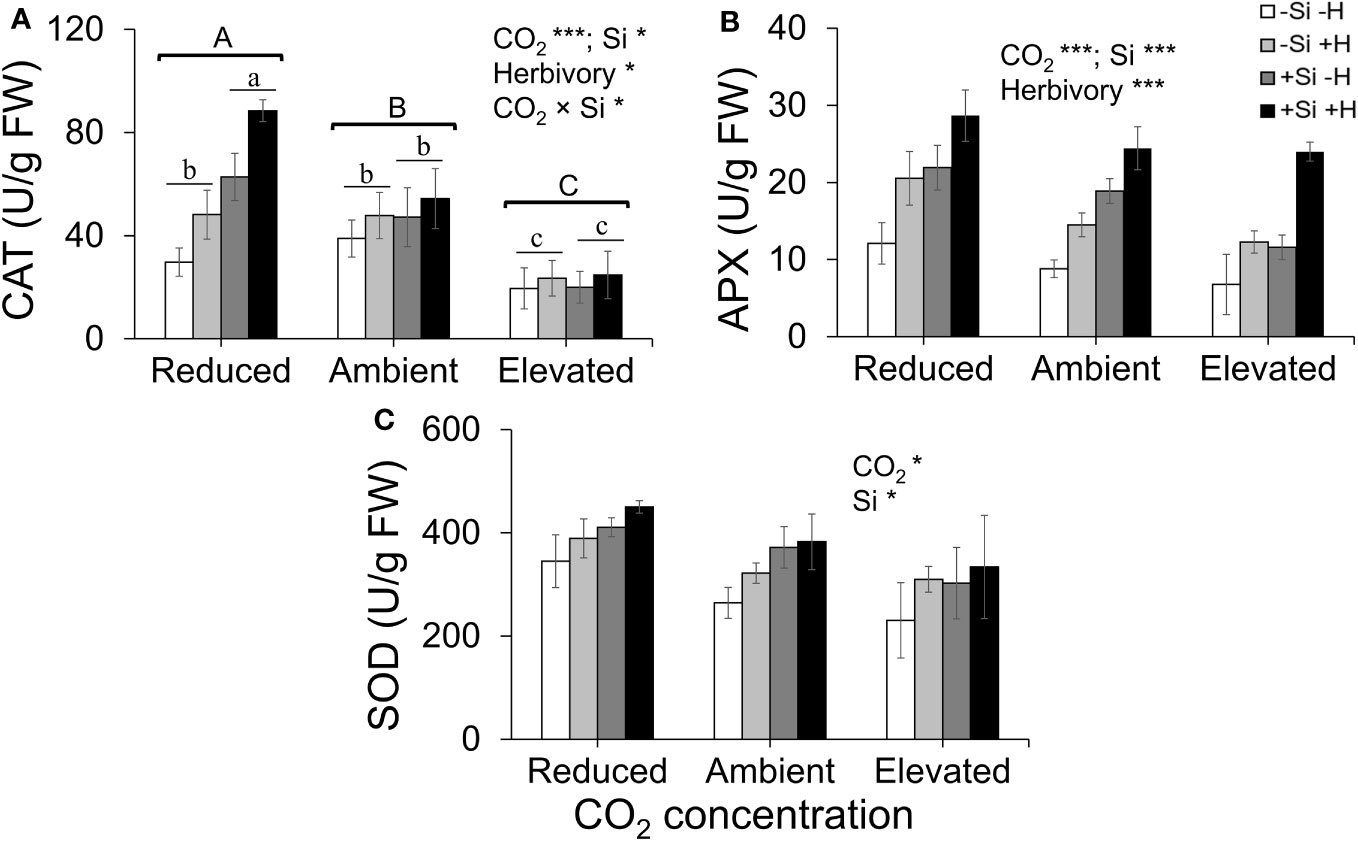
Figure 4 Effects of Si supply (+Si or –Si) and herbivory (+H or –H) on (A) CAT, (B) APX, and (C) SOD enzyme activity of tall fescue grass grown under reduced, ambient, and elevated CO2 concentration. Means ± SE shown. N = 3–5. Uppercase letters represent differences between CO2 concentrations whereas lowercase letters indicate differences between Si treatments (Panel 1A). Statistically significant effects are indicated *p < 0.05, and ***p < 0.001
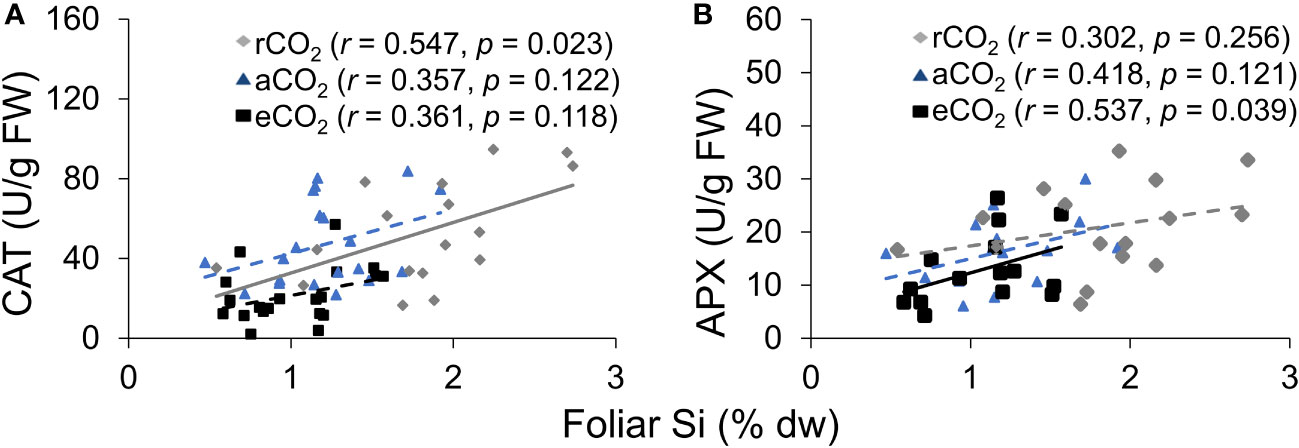
Figure 5 The relationship between (A) CAT and foliar Si, and (B) APX and foliar Si. The solid line represents linear regression through all data points and dashed lines indicate that no significant relationship was observed.
Si supply and low CO2 environment suppressed herbivore RGR and feeding efficiency
Si supplementation decreased RGR under all CO2 regimes; RGR was significantly lower under reduced CO2 compared to elevated CO2 (Table 1; Figure 6A). Si supply decreased the amount of frass produced by caterpillars (indicative of feeding efficiency) under all CO2 levels; CO2 had no significant effect on frass production, although there was a large increase in production in Si-free plants grown under elevated CO2 (Table 1; Figure 6B). While herbivore RGR and frass produced were negatively correlated with foliar Si under ambient CO2, there was no such relationship observed under the other two CO2 regimes (Figures 6C, D). Here, the correlation between rate of herbivore feeding on foliar tissues of tall fescue grown under different CO2 concentrations potentially indicates a new perspective towards mitigating challenges of CO2 enriched environment on plant defences.
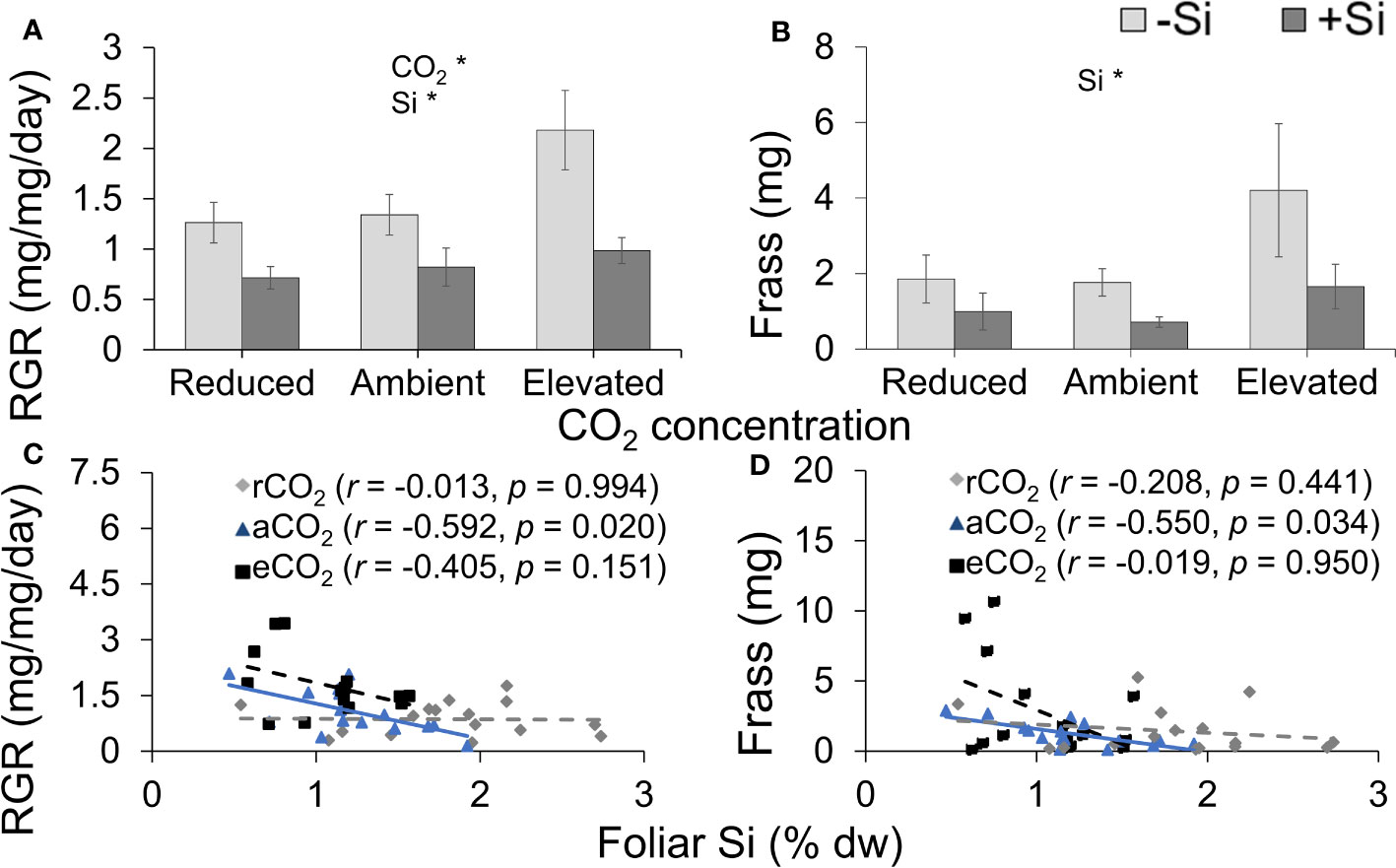
Figure 6 Effects of Si supply (+Si or –Si) and CO2 level on (A) relative growth rate (RGR) of Helicoverpa armigera fed on tall fescue grass, (B) frass produced, and the relationship between foliar Si concentrations and (C) RGR and (D) frass produced. Means ± SE shown. N = 7–8. For (C) and (D), the solid line represents linear regression through all data points and dashed lines indicate that no significant relationship was observed. Statistically significant effects are indicated *p < 0.05.
Discussion
We demonstrated that reduced levels of atmospheric CO2 caused plants to accumulate more Si and produce higher levels of antioxidant enzyme relative to future levels of atmospheric CO2. These increased levels of Si and antioxidant enzyme concentrations under reduced levels of CO2 were associated with reduced insect herbivore performance. In contrast, herbivore performance and plant consumption (frass production as proxy) were highest under elevated atmospheric CO2 conditions, which typically had the lowest levels of Si and antioxidant enzyme. To our knowledge, this is the first study to address the relationship between Si defences and antioxidant enzyme production in the context of variable atmospheric CO2 conditions, which we summarise in Figure 7.
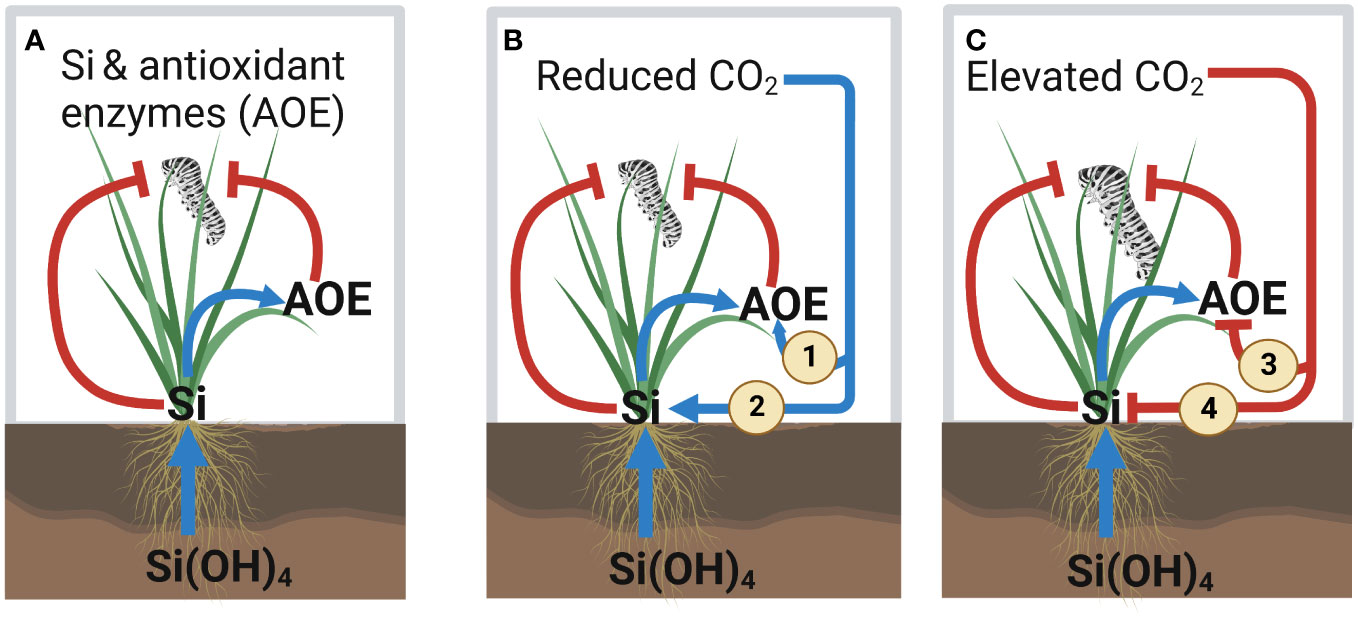
Figure 7 Summary of the effect of silicon (Si) and variable atmospheric CO2 concentrations on the Si and antioxidant enzyme defences against herbivory. (A) Current knowledge of the interaction effect between Si and antioxidant enzyme (AOE) activity on insect herbivory. Our key results from this study are indicated in panels (B, C). (B) Reduced CO2 enhances AOE defences by (1) directly enhancing AOE activity and (2) indirectly increasing Si uptake, which leads to reduced herbivore performance. (C) Elevated CO2 reduces AOE defences by (3) directly reducing AOE activity and (4) indirectly decreasing Si uptake, which leads to increased herbivore performance. Positive and negative effects for both plants and insects are indicated by blue arrows and red lines, respectively.
Direct and Si-mediated impacts of CO2 on the activity of antioxidant enzyme
The effects of CO2 in this study on Si concentrations are broadly similar to the few studies exploring this, whereby elevated CO2 leads to decreased Si accumulation (Ryalls et al., 2017; Johnson and Hartley, 2018; Biru et al., 2022; Johnson et al., 2022; Johnson et al., 2023), whereas reduced CO2 leads to increased Si accumulation. To the best of our knowledge, the current study is only the third study to investigate the latter (Biru et al., 2020; Biru et al., 2021). Si has been hypothesised to act as a structural substitute for C at a lower metabolic cost, particularly when CO2 concentrations were lower in the Miocene (Craine, 2009).
Exogenous application of Si has been shown to increase antioxidant enzyme including CAT, APX, and SOD (Kim et al., 2016; Hasanuzzaman et al., 2018; Ahanger et al., 2020). It seems likely that changes in Si in response to CO2 growing conditions influenced activity of antioxidant enzyme in the current study. Additionally, the ANCOVA results indicated that CO2 affects antioxidant enzyme activity (e.g., CAT and APX), which were mostly explained by the direct impacts of CO2 levels on antioxidant enzyme. However, the observed positive correlation of leaf Si with CAT and APX under reduced CO2 and elevated CO2, respectively, suggests that CAT defence response may be linked to higher levels of Si under reduced CO2, whereas APX defence response may be associated with increased induction of defence responses following herbivore attack under elevated CO2. Overall, our results demonstrated significant augmentation of antioxidant enzyme responses via increased Si uptake under reduced CO2 as well as by the direct effect of CO2 levels (see Figure 7). In contrast, previous studies have reported that elevated CO2 increases activity of antioxidant enzyme in different plants (Wang et al., 2003; Moghimifam et al., 2020). For example, Moghimifam et al. (2020) found that elevated CO2 enhances CAT, polyphenol oxidase (PPO), SOD, and proline activity in algae (Dunaliella sp.). Our result may reflect that reduced CO2 often increases photorespiration (Moroney et al., 2013; Voss et al., 2013) and since photorespiration is the key source for ROS production (Voss et al., 2013), reduced CO2 may cause increased antioxidant enzyme activity in order to scavenge excessive ROS produced. However, these studies did not address whether Si played a role in these changes, while our findings suggest Si as an important influencer of the activity of these enzymes.
Herbivory and Si enhanced antioxidant enzyme activity
In addition to CO2 having direct and Si-mediated impacts on antioxidant enzyme activity, it is also possible that the amount of herbivore damage played a role in antioxidant enzyme activity. Herbivores induced higher activity of CAT and APX, so these enzymes activity is at least associated with the levels of damage done to the plant, which has been similarly reported in previous studies (Leitner et al., 2005; Yang et al., 2017). Increased levels of antioxidant enzyme under reduced CO2 may reflect the fact that insects were doing less damage to the plant under these conditions and, therefore, there was less ROS oxidative stress that may have persisted in plant tissues to react with higher levels of antioxidant enzyme. Results from the ANCOVA and correlation tests also revealed that CO2 directly changed antioxidant enzyme activity and altered feeding efficiency (leaf consumption), which eventually reduced the growth rate of herbivores.
Diminishing Si defence with rising CO2
The recent finding by Biru et al. (2021) showed that H. armigera RGR was lowest when fed on the model grass Brachypodium distachyon grown under reduced CO2 due to these plants having higher levels of Si defences compared to plants grown under ambient and elevated CO2 concentrations. In the current study, we observed that tall fescue had the highest concentrations of foliar N when grown under reduced CO2, which, in theory, could have promoted herbivore RGR because N is frequently the limiting factor in insect herbivore diets (Mattson, 1980; Huberty and Denno, 2006). The lower production of frass under reduced CO2, which we used as a proxy for plant consumption, suggests that herbivores were deterred from feeding; thus, they would have not been able to access these N resources.
Understanding the diminishing levels of Si-based plant defences against herbivores under future elevated atmospheric CO2 concentrations has received limited attention. Previous studies have shown that elevated CO2 concentrations decrease Si accumulation in different Poaceae genera (e.g., grass species and wheat) (Johnson and Hartley, 2018; Biru et al., 2022; Johnson et al., 2022), and this was associated with reduced Si defences while increasing herbivore performance; however, this effect is not always reproducible (Frew et al., 2017). The impact of elevated CO2 on Si defences reflects that plants switch from Si defences to C-based defences due to higher C availability under this scenario (Johnson and Hartley, 2018; Johnson et al., 2022). Although not examined in the context of Si defences, previous studies have demonstrated that elevated atmospheric CO2 increased consumption and growth rate of the generalist (Pseudaletia unipuncta) and specialist (Spodoptera frugiperda) insect herbivores when fed on C3 grass relative to lower atmospheric CO2 conditions (Barbehenn et al., 2004). Johnson et al. (2020) also reported that H. armigera RGR increased under elevated CO2 as a result of lower plant defence signalling and minimal reductions in the nutritional quality of lucerne (Medicago sativa).
Conclusions
The present study provides further evidence that CO2 concentrations are strong drivers of Si accumulation in an important plant species, not previously reported on. We found strong evidence that reduced CO2 increased foliar Si concentration and antioxidant enzyme levels, which potentially linked to suppressed insect herbivore performance. This suggests that the negative effects of silicification, whether via physical or biochemical mechanisms, are stronger under reduced CO2. We showed a strong linkage between Si supplementation and activity of antioxidant enzyme, which may help in alleviating the harmful effects of herbivore-induced oxidative stress on plant defence responses. Although Si defences are minimal under elevated atmospheric CO2 conditions, many agricultural soils can become deficient in bioavailable Si (Haynes, 2017), which points to the importance of maintaining Si levels in soils under future projected atmospheric CO2 conditions.
Data availability statement
The raw data supporting the conclusions of this article will be made available by the authors, without undue reservation.
Author contributions
FB: Conceptualization, Data curation, Formal Analysis, Methodology, Validation, Writing – original draft. CC: Supervision, Writing – review & editing. RE: Supervision, Writing – review & editing. SJ: Conceptualization, Supervision, Writing – review & editing.
Funding
The author(s) declare financial support was received for the research, authorship, and/or publication of this article. FB is the holder of a scholarship as part of an Australian Research Council Future Fellowship (grant no. FT170100342) awarded to SJ.
Acknowledgments
Special thank you to Tarikul Islam for assisting with larvae inoculation. We thank Ximena Cibils-Stewart for providing the F. arundinacea seeds. We also express sincere thanks to Andrew Gherlenda for the technical assistance with this work.
Conflict of interest
The authors declare that the research was conducted in the absence of any commercial or financial relationships that could be construed as a potential conflict of interest.
Publisher’s note
All claims expressed in this article are solely those of the authors and do not necessarily represent those of their affiliated organizations, or those of the publisher, the editors and the reviewers. Any product that may be evaluated in this article, or claim that may be made by its manufacturer, is not guaranteed or endorsed by the publisher.
References
Acevedo, F. E., Peiffer, M., Ray, S., Tan, C.-W., Felton, G. W. (2021). Silicon-mediated enhancement of herbivore resistance in agricultural crops. Front. Plant Sci. 12, 631824. doi: 10.3389/fpls.2021.631824
Ahanger, M. A., Bhat, J. A., Siddiqui, M. H., Rinklebe, J., Ahmad, P. (2020). Integration of silicon and secondary metabolites in plants: a significant association in stress tolerance. J. Exp. Bot. 71, 6758–6774. doi: 10.1093/jxb/eraa291
Alhousari, F., Greger, M. (2018). Silicon and mechanisms of plant resistance to insect pests. Plants-Basel 7, 33. doi: 10.3390/plants7020033
Aslam, M., Albassam, M. (2020). Presenting post hoc multiple comparison tests under neutrosophic statistics. J. King Saud Univ. Sci. 32, 2728–2732. doi: 10.1016/j.jksus.2020.06.008
Barbehenn, R. V., Karowe, D. N., Spickard, A. (2004). Effects of elevated atmospheric CO2 on the nutritional ecology of C3 and C4 grass-feeding caterpillars. Oecologia 140, 86–95. doi: 10.1007/s00442-004-1572-9
Bi, J. L., Felton, G. W. (1995). Foliar oxidative stress and insect herbivory: primary compounds, secondary metabolites, and reactive oxygen species as components of induced resistance. J. Chem. Ecol. 21, 1511–1530. doi: 10.1007/bf02035149
Biru, F. N., Cazzonelli, C. I., Elbaum, R., Johnson, S. N. (2020). Contrasting effects of Miocene and Anthropocene levels of atmospheric CO2 on silicon accumulation in a model grass. Biol. Lett. 16, 20200608. doi: 10.1098/rsbl.2020.0608
Biru, F. N., Cazzonelli, C. I., Elbaum, R., Johnson, S. N. (2022). Contrasting impacts of herbivore induction and elevated atmospheric CO2 on silicon defences and consequences for subsequent herbivores. Entomol. Exp. Appl. 170, 681–688. doi: 10.1111/eea.13168
Biru, F. N., Islam, T., Cibils-Stewart, X., Cazzonelli, C. I., Elbaum, R., Johnson, S. N. (2021). Anti-herbivore silicon defences in a model grass are greatest under Miocene levels of atmospheric CO2. Glob. Change Biol. 27, 2959–2969. doi: 10.1111/gcb.15619
Cavalcanti, F. R., Oliveira, J. T. A., Martins-Miranda, A. S., Viégas, R. A., Silveira, J. A. G. (2004). Superoxide dismutase, catalase and peroxidase activities do not confer protection against oxidative damage in salt-stressed cowpea leaves. New Phytol. 163, 563–571. doi: 10.1111/gcb.15619
Cooke, J., Leishman, M. R. (2011). Is plant ecology more siliceous than we realise? Trends Plant Sci. 16, 61–68. doi: 10.1016/j.tplants.2010.10.003
Coskun, D., Deshmukh, R., Sonah, H., Menzies, J., Reynolds, O., Kronzucker, et al. (2019). The controversies of silicon’s role in plant biology. New Phytol. 221, 1340–1357. doi: 10.1111/nph.15343
Craine, J. M. (2009). Resource strategies of wild plants (Princeton, NJ, USA: Princeton University Press). doi: 10.1515/9781400830640
Das, K., Roychoudhury, A. (2014). Reactive oxygen species (ROS) and response of antioxidants as ROS-scavengers during environmental stress in plants. Front. Environ. Sci. 2, 53. doi: 10.3389/fenvs.2014.00053
Debona, D., Rodrigues, F., Datnoff, L. (2017). Silicon’s role in abiotic and biotic plant stresses. Annu. Rev. Phytopathol. 55, 85–107. doi: 10.1146/annurev-phyto-080516-035312
Epstein, E. (1994). The anomaly of silicon in plant biology. PNAS. 91, 11–17. doi: 10.1073/pnas.91.1.11
Epstein, E. (2009). Silicon: its manifold roles in plants. Ann. Appl. Biol. 155, 155–160. doi: 10.1111/j.1744-7348.2009.00343.x
Farooq, M. A., Ali, S., Hameed, A., Ishaque, W., Mahmood, K., Iqbal, Z. (2013). Alleviation of cadmium toxicity by silicon is related to elevated photosynthesis, antioxidant enzymes; suppressed cadmium uptake and oxidative stress in cotton. Ecotoxicol. Environ. Saf. 96, 242–249. doi: 10.1016/j.ecoenv.2013.07.006
Fichman, Y., Mittler, R. (2020). Rapid systemic signaling during abiotic and biotic stresses: is the ROS wave master of all trades? TPJ. 102, 887–896. doi: 10.1111/tpj.14685
Fimognari, L., Dölker, R., Kaselyte, G., Jensen, C. N. G., Akhtar, S. S., Großkinsky, D. K., et al. (2020). Simple semi-high throughput determination of activity signatures of key antioxidant enzymes for physiological phenotyping. Plant Methods 16, 42. doi: 10.1186/s13007-020-00583-8
Frew, A., Allsopp, P. G., Gherlenda, A. N., Johnson, S. N. (2017). Increased root herbivory under elevated atmospheric carbon dioxide concentrations is reversed by silicon-based plant defences. J. Appl. Ecol. 54, 1310–1319. doi: 10.1111/1365-2664.12822
Fulweiler, R. W., Maguire, T. J., Carey, J. C., Finzi, A. C. (2014). Does elevated CO2 alter silica uptake in trees? Front. Plant Sci. 5, 793. doi: 10.3389/fpls.2014.00793
Gong, H. J., Zhu, X. Y., Chen, K. M., Wang, S. M., Zhang, C. L. (2005). Silicon alleviates oxidative damage of wheat plants in pots under drought. Plant Sci. 169, 313–321. doi: 10.1016/j.plantsci.2005.02.023
Hall, C. R., Dagg, V., Waterman, J. M., Johnson, S. N. (2020). Silicon alters leaf surface morphology and suppresses insect herbivory in a model grass species. Plants 9, 643. doi: 10.3390/plants9050643
Hall, C. R., Waterman, J. M., Vandegeer, R. K., Hartley, S. E., Johnson, S. N. (2019). The role of silicon in antiherbivore phytohormonal signalling. Front. Plant Sci. 10, 1132. doi: 10.3389/fpls.2019.01132
Han, Y., Li, P., Gong, S., Yang, L., Wen, L., Hou, M. (2016). Defense responses in rice induced by silicon amendment against infestation by the leaf folder Cnaphalocrocis medinalis. PloS One 11, e0153918. doi: 10.1371/journal.pone.0153918
Hartley, S. E., Fitt, R. N., McLarnon, E. L., Wade, R. N. (2015). Defending the leaf surface: intra- and inter-specific differences in silicon deposition in grasses in response to damage and silicon supply. Front. Plant Sci. 6, 35. doi: 10.3389/fpls.2015.00035
Hartmann, J., Asch, F. (2019). Extraction, storage duration, and storage temperature affect the activity of ascorbate peroxidase, glutathione reductase, and superoxide dismutase in rice tissue. Biology 8, 70. doi: 10.3390/biology8040070
Hasanuzzaman, M., Bhuyan, M. H. M. B., Zulfiqar, F., Raza, A., Mohsin, S. M., Mahmud, J. A., et al. (2020). Reactive oxygen species and antioxidant defense in plants under abiotic stress: revisiting the crucial role of a universal defense regulator. Antioxidants 9, 681. doi: 10.3390/antiox9080681
Hasanuzzaman, M., Nahar, K., Anee, T. I., Khan, M. I. R., Fujita, M. (2018). Silicon-mediated regulation of antioxidant defense and glyoxalase systems confers drought stress tolerance in Brassica napus L. S. Afr. J. Bot. 115, 50–57. doi: 10.1016/j.sajb.2017.12.006
Haynes, R. J. (2017). Chapter 3: Significance and role of Si in crop production. Adv. Agron. 146, 83–166. doi: 10.1016/bs.agron.2017.06.001
Hiltpold, I., Demarta, L., Johnson, S. N., Moore, B. D., Power, S. A., Mitchell, C., et al. (2017). “Silicon and other essential element composition in roots using X-ray fluorescence spectroscopy: A high throughput approach,” in Invertebrate ecology of Australasian grasslands. Ed. Johnson, S. N. (Western Sydney University, Hawkesbury, NSW, Australia:Proceedings of the Ninth ACGIE. Western Sydney University), 191–196).
Hodson, M. J., White, P. J., Mead, A., Broadley, M. R. (2005). Phylogenetic variation in the silicon composition of plants. Ann. Bot. 96, 1027–1046. doi: 10.1093/aob/mci255
Huang, H., Ullah, F., Zhou, D.-X., Yi, M., Zhao, Y. (2019). Mechanisms of ROS regulation of plant development and stress responses. Front. Plant Sci. 10, 800. doi: 10.3389/fpls.2019.00800
Huberty, A. F., Denno, R. F. (2006). Consequences of nitrogen and phosphorus limitation for the performance of two planthoppers with divergent life-history strategies. Oecologia 149, 444–455. doi: 10.1007/s00442-006-0462-8
IPCC (2014). Climate Change 2014: Impacts, Adaptation, and Vulnerability. Part A: Global and sectoral aspects. Contribution of working group II to the fifth assessment report of the intergovernmental panel on climate change. Eds. Field, C. B., Baros, V. R., Dokken, D. J., Mach, K. J., Mastrandrea, M. D., Bilir, T. E., Chatterjee, M., Ebi, K. L., Estrada, Y. O., Genova, R. C., Girma, B., Kissel, E. S., Levy, A. N., MacCracken, S., Mastrandrea, P. R., White, L. L. (Cambridge, UK and New York, NY: Cambridge University Press).
Islam, T., Ben D, M., S Cott N, J. (2020). Novel evidence for systemic induction of silicon defences in cucumber following attack by a global insect herbivore. Ecol. Entomol. 45, 1373–1381. doi: 10.1111/een.12922
Johnson, S. N., Barton, C. V. M., Biru, F. N., Islam, T., Mace, W. J., Rowe, R. C., et al. (2023). Elevated atmospheric CO2 suppresses silicon accumulation and exacerbates endophyte reductions in plant phosphorus. Funct. Ecol. 37, 1567–1579. doi: 10.1111/1365-2435.14342
Johnson, S. N., Cibils-Stewart, X., Waterman, J. M., Biru, F. N., Rowe, R. C., Hartley, S. E. (2022). Elevated atmospheric CO2 changes defence allocation in wheat but herbivore resistance persists. Proc. R. Soc B: Biol. Sci. 289, 20212536. doi: 10.1098/rspb.2021.2536
Johnson, S. N., Hartley, S. E. (2018). Elevated carbon dioxide and warming impact silicon and phenolic-based defences differently in native and exotic grasses. Glob. Change Biol. 102, 3886–3896. doi: 10.1002/ecy.3250
Johnson, S. N., Ryalls, J. M. W., Gherlenda, A. N., Frew, A., Hartley, S. E. (2018). Benefits from below: silicon supplementation maintains legume productivity under predicted climate change scenarios. Front. Plant Sci. 9, 202. doi: 10.3389/fpls.2018.00202
Johnson, S. N., Waterman, J. M., Hall, C. R. (2020). Increased insect herbivore performance under elevated CO2 is associated with lower plant defence signalling and minimal declines in nutritional quality. Sci. Rep. 10, 14553. doi: 10.1038/s41598-020-70823-3
Jung, H.-I., Yan, J., Zhai, Z., Vatamaniuk, O. (2015). Gene functional analysis using protoplast transient assays. In: Alonso, J., Stepanova, A. (eds) Plant Functional Genomics. Methods in Molecular Biology, vol 1284. Humana Press, New York, NY
Kerchev, P. I., Fenton, B., Foyer, C. H., Hancock, R. D. (2012). Plant responses to insect herbivory: interactions between photosynthesis, reactive oxygen species and hormonal signalling pathways. Plant, Cell Environ. 35, 441–453. doi: 10.1111/j.1365-3040.2011.02399.x
Kim, Y.-H., Khan, A., Waqas, M., Lee, I.-J. (2017). Silicon regulates antioxidant activities of crop plants under abiotic-induced oxidative stress: a review. Front. Plant Sci. 8, 510. doi: 10.3389/fpls.2017.00510
Kim, Y. H., Khan, A. L., Waqas, M., Shahzad, R., Lee, I. J. (2016). Silicon-mediated mitigation of wounding stress acts by up-regulating the rice antioxidant system. Cereal Res. Commun. 44, 111–121. doi: 10.1556/0806.43.2015.031
Kumar, S., Soukup, M., Elbaum, R. (2017). Silicification in grasses: variation between different cell types. Front. Plant Sci. 8, 438. doi: 10.3389/fpls.2017.00438
Leitner, M., Boland, W., Mithöfer, A. (2005). Direct and indirect defences induced by piercing-sucking and chewing herbivores in Medicago truncatula. New Phytol. 167, 597–606. doi: 10.1111/j.1469-8137.2005.01426.x
Ma, J. F., Yamaji, N. (2006). Silicon uptake and accumulation in higher plants. Trends Plant Sci. 11, 392–397. doi: 10.1016/j.tplants.2006.06.007
Maksimović, J. J. D., Živanović, B. D. (2012). Quantification of the antioxidant activity in salt-stressed tissues. Methods Mol. Biol. 913, 237–250. doi: 10.1007/978-1-61779-986-0_16
Massey, F. P., Ennos, A. R., Hartley, S. E. (2006). Silica in grasses as a defence against insect herbivores: contrasting effects on folivores and a phloem feeder. J. Anim. Ecol. 75, 595–603. doi: 10.1111/j.1365-2656.2006.01082.x
Massey, F. P., Hartley, S. E. (2009). Physical defences wear you down: progressive and irreversible impacts of silica on insect herbivores. J. Anim. Ecol. 78, 281–291. doi: 10.1111/j.1365-2656.2008.01472.x
Massey, F. P., Roland Ennos, A., Hartley, S. E. (2007). Herbivore specific induction of silica-based plant defences. Oecologia 152, 677–683. doi: 10.1007/s00442-007-0703-5
Mattson, W. J. (1980). Herbivory in relation to plant nitrogen content. Annu. Rev. Evol. Syst. 11, 119–161. doi: 10.1146/annurev.es.11.110180.001003
Moghimifam, R., Niknam, V., Ebrahimzadeh, H., Hejazi, M. A. (2020). The influence of different CO2 concentrations on the biochemical and molecular response of two isolates of Dunaliella sp. (ABRIINW-CH2 and ABRIINW-SH33). J. Appl. Phycol. 32, 175–187. doi: 10.1007/s10811-019-01914-6
Moroney, J. V., Jungnick, N., DiMario, R. J., Longstreth, D. J. (2013). Photorespiration and carbon concentrating mechanisms: two adaptations to high O2, low CO2 conditions. Photosynth. Res. 117, 121–131. doi: 10.1007/s11120-013-9865-7
Moussa, H. (2006). Influence of exogenous application of silicon on physiological response of salt-stressed maize (Zea mays L.). Int. J. Agric. Biol. 8, 293–297. doi: 10.1007/s12633-015-9372-x
Perry, C. C., Mann, S., Williams, R. J. P. (1984). Structural and analytical studies of the silicified macrohairs from the lemma of the grass Phalaris canariensis L. Proc. R. Soc B. 222, 427–438. doi: 10.1098/rspb.1984.0075
Pizzino, G., Irrera, N., Cucinotta, M., Pallio, G., Mannino, F., Arcoraci, V., et al. (2017). Oxidative stress: harms and benefits for human health. Oxid. Med. Cell. Longev. 2017, 8416763. doi: 10.1155/2017/8416763
Raven, J. A. (1983). The transport and function of silicon in plants. Biol. Rev. 58, 179–207. doi: 10.1111/j.1469-185x.1983.tb00385.x
Reidinger, S., Ramsey, M. H., Hartley, S. E. (2012). Rapid and accurate analyses of silicon and phosphorus in plants using a portable X-ray fluorescence spectrometer. New Phytol. 195, 699–706. doi: 10.1111/j.1469-8137.2012.04179.x
Reynolds, O. L., Keeping, M. G., Meyer, J. H. (2009). Silicon-augmented resistance of plants to herbivorous insects: a review. Ann. Appl. Biol. 155, 171–186. doi: 10.1111/j.1744-7348.2009.00348.x
Reynolds, O. L., Padula, M. P., Zeng, R. S., Gurr, G. M. (2016). Silicon: potential to promote direct and indirect effects on plant defense against arthropod pests in agriculture. Front. Plant Sci. 7, 744. doi: 10.3389/fpls.2016.00744
Ryalls, J. M. W., Hartley, S. E., Johnson, S. N. (2017). Impacts of silicon-based grass defences across trophic levels under both current and future atmospheric CO2 scenarios. Biol. Lett. 13, 20160912. doi: 10.1098/rsbl.2016.0912
Sharma, P., Jha, A. B., Dubey, R. S., Pessarakli, M. (2012). Reactive oxygen species, oxidative damage, and antioxidative defense mechanism in plants under stressful conditions. J. Bot. 2012, 1–26. doi: 10.1155/2012/217037
Sudhakar, C., Lakshmi, A., Giridarakumar, S. (2001). Changes in the antioxidant enzyme efficacy in two high yielding genotypes of mulberry (Morus alba L.) under NaCl salinity. Plant Sci. 161, 613–619. doi: 10.1016/s0168-9452(01)00450-2
Teakle, R., Jensen, J. (1985). “Heliothis punctiger,” in Handbook of insect rearing. Eds. Singh, P., Moore, R. F. (Amsterdam, The Netherlands: Elsevier), 313–322.
Tripathy, B. C., Oelmüller, R. (2012). Reactive oxygen species generation and signaling in plants. Plant Signal. Behav. 7, 1621–1633. doi: 10.4161/psb.22455
Voss, I., Sunil, B., Scheibe, R., Raghavendra, A. S. (2013). Emerging concept for the role of photorespiration as an important part of abiotic stress response. Plant Biol. 15, 713–722. doi: 10.1111/j.1438-8677.2012.00710.x
Wang, S. Y., Bunce, J. A., Maas, J. L. (2003). Elevated carbon dioxide increases contents of antioxidant compounds in field-grown strawberries. J. Agric. Food Chem. 51, 4315–4320. doi: 10.1021/jf021172d
Waterman, J. M., Hall, C. R., Mikhael, M., Cazzonelli, C. I., Hartley, S. E., Johnson, S. N. (2020). Short-term resistance that persists: rapidly induced silicon anti-herbivore defence affects carbon-based plant defences. Funct. Ecol. 35, 82–92. doi: 10.1111/1365-2435.13702
Keywords: antioxidant enzyme, carbon, carbon dioxide, herbivore, physical defences, plant defences, silicon
Citation: Biru FN, Cazzonelli CI, Elbaum R and Johnson SN (2023) Silicon-mediated herbivore defence in a pasture grass under reduced and Anthropocene levels of CO2. Front. Plant Sci. 14:1268043. doi: 10.3389/fpls.2023.1268043
Received: 27 July 2023; Accepted: 17 October 2023;
Published: 01 November 2023.
Edited by:
Eman. A. Mahmoud, Damietta University, EgyptReviewed by:
Mohammad Mukarram, Technical University of Zvolen, SlovakiaKollipara P. M. S. V. Padmasree, University of Hyderabad, India
Copyright © 2023 Biru, Cazzonelli, Elbaum and Johnson. This is an open-access article distributed under the terms of the Creative Commons Attribution License (CC BY). The use, distribution or reproduction in other forums is permitted, provided the original author(s) and the copyright owner(s) are credited and that the original publication in this journal is cited, in accordance with accepted academic practice. No use, distribution or reproduction is permitted which does not comply with these terms.
*Correspondence: Fikadu N. Biru, fikadu.negese@ju.edu.et; f.biru@westernsydney.edu.au