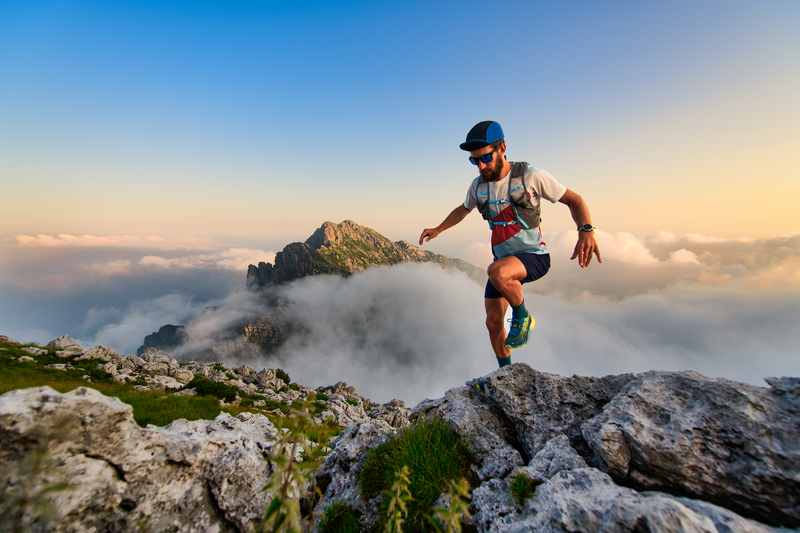
94% of researchers rate our articles as excellent or good
Learn more about the work of our research integrity team to safeguard the quality of each article we publish.
Find out more
MINI REVIEW article
Front. Plant Sci. , 29 September 2023
Sec. Plant Breeding
Volume 14 - 2023 | https://doi.org/10.3389/fpls.2023.1260102
A correction has been applied to this article in:
Corrigendum: Genetic amelioration of fruit and vegetable crops to increase biotic and abiotic stress resistance through CRISPR Genome Editing
Environmental changes and increasing population are major concerns for crop production and food security as a whole. To address this, researchers had focussed on the improvement of cereals and pulses and have made considerable progress till the beginning of this decade. However, cereals and pulses together, without vegetables and fruits, are inadequate to meet the dietary and nutritional demands of human life. Production of good quality vegetables and fruits is highly challenging owing to their perishable nature and short shelf life as well as abiotic and biotic stresses encountered during pre- and post-harvest. Genetic engineering approaches to produce good quality, to increase shelf life and stress-resistance, and to change the time of flowering and fruit ripening by introducing foreign genes to produce genetically modified crops were quite successful. However, several biosafety concerns, such as the risk of transgene-outcrossing, limited their production, marketing, and consumption. Modern genome editing techniques, like the CRISPR/Cas9 system, provide a perfect solution in this scenario, as it can produce transgene-free genetically edited plants. Hence, these genetically edited plants can easily satisfy the biosafety norms for crop production and consumption. This review highlights the potential of the CRISPR/Cas9 system for the successful generation of abiotic and biotic stress resistance and thereby improving the quality, yield, and overall productivity of vegetables and fruits.
Vegetables, fruits, nuts, ornamental, aromatic, and medicinal plants are grouped under horticultural crops. Vegetables and fruits are essential dietary components of our meals as they are excellent sources of carbohydrates, fibres, proteins, vitamins, organic acids, antioxidants, minerals, and trace elements. Climate change and global warming render various dreadful effects on agriculture resulting in loss of crop yield and its nutritional value owing to abiotic factors (heat waves, irregular and uneven precipitation patterns) and biotic factors (increase in plant pathogens and pests). Vegetable and fruit crops are generally more vulnerable to abiotic and biotic stresses leading to huge loss of productivity and nutritional value. Traditional crop breeding such as crossbreeding and mutation-breeding has been successful for a long time to introduce required genetic variations in horticultural crops (Schaart et al., 2016). However, with increasing population, conventional breeding methods, being time-consuming and laborious, are not been able to live up to the growing market demands (Gao, 2021). Transgenic breeding surely provides an alternative by creating genetically modified crops with desired traits in less time but their use is strictly restricted or legally prohibited by government agencies of several countries under safety regulations (Voytas and Gao, 2014). The discovery of genome-editing technology, particularly CRISPR/Cas, has enabled researchers to make precise modifications at specific sites in the DNA to generate horticultural crops possessing novel and desired traits within a short time (Tian et al., 2021) (Figure 1).
Figure 1 Schematic representation of comparison between traditional, modern and advanced methods of plant breeding for the production of biotic and abiotic stress-resistant vegetable and fruit crops.
With the advent of genome editing technology in the 1990s and significant advancements made in the years thereafter, different methods of gene editing have revolutionized the area of research involving functional genomics and crop improvement. Genome editing technology involves the recognition of particular DNA sequences, followed by the induction of double-stranded breaks (DSBs) at specific sites in the targeted DNA using synthetic sequence-specific nucleases (SSNs) (Yin and Qiu, 2019). To maintain genome integrity, cells of almost all living organisms can detect and repair DSBs via either non-homologous end-joining (NHEJ) or homology-directed repair (HDR) (Sonoda et al., 2006). NHEJ is the most common but error-prone DNA-repair process, where DNA ligase IV joins DSBs with minimal DNA end processing in the absence of a repair template, often causing random insertion or deletion of base pairs, leading to a frameshift mutation and thereby resulting in gene knock-out or knockdown. Contrarily, HDR is a high-fidelity repair pathway that uses a homologous repair template DNA to ligate DSBs leading to gene modification or insertion of a new gene (Kumari et al., 2022).
Zinc Finger Nucleases (ZFNs) were the first generation SSNs that were synthesized artificially by the fusion of dimers of Cys2-His2 zinc finger (ZF) domains and FokI restriction endonuclease domains and have been successfully used in Arabidopsis, Nicotiana, and Zea mays. However, ZFNs face problems over high-expense, moderate complexity in design, low specificity, difficulty in multiplexing, and consuming time (Kamburova et al., 2017). Later, the identification of transcription activator-like effectors (TALEs) from Xanthomonas spp. led to the synthesis of TALENs by joining dimers of TALE domains with FokI domains. TALENs have been used with great success in rice, wheat, Arabidopsis, potato, and tomato, but high cost, difficulty in multiplexing, complex designing modules, low specificity during screening, and labour-intensiveness pose challenges to their usage (Tavakoli et al., 2021). In 2012, the invention of the CRISPR/Cas9 system from Streptococcus pyogenes by Jennifer Doudna and Emmanuelle Charpentier (Jinek et al., 2012), followed by several other discoveries on different CRISPR/Cas systems and rapid advancements and modifications in the technology over the last decade have completely transformed the universe of genome-editing, with multiple gene targeting (multiplexing) becoming a reality. CRISPR/Cas technology possesses several advantages over ZFNs and TALENs for being cheaper, simpler, time-saving, reproducible, and highly efficient in high-yield multiplexing. This article reviews the application of different types of CRISPR/Cas systems in horticultural crops to alleviate biotic and abiotic stresses and discusses challenges and probable solutions.
In nature, CRISPR/Cas system serves as an adaptive immune system of bacteria and archaea against the invading foreign DNA originating from plasmids and bacteriophages. The CRISPR/Cas9 system was the first to be discovered and modified into an efficient genome editing tool (Ishino et al., 1987; Jinek et al., 2012). The CRISPR/Cas9 technology comprises two components: the Cas9 endonuclease and the single guide RNA (sgRNA), produced by linking CRISPR RNA (crRNA) with trans-activating crRNA (tracrRNA) and is generally designed with a specific 20-nucleotide spacer sequence complementary to the ‘target’ DNA. For CRISPR/Cas9-mediated genome editing, sgRNA first binds to the DNA target and then recruits Cas9 endonuclease. Next, Cas9 endonuclease thoroughly searches a protospacer-adjacent motif (PAM) sequence, i.e., 5′-NGG-3′ (for S. pyogenes), in the target DNA and snips the complementary and the non-complementary strands using its two nuclease domains, HNH and RuvC, respectively, producing a blunt DSB (Ran et al., 2013). To expand the range of this technology, several subsequent modifications were introduced into the SpCas9 for recognition of different PAM sequences present in the target DNA, like SpCas9-VQR (5’-NGAN-3′ or 5’-NGNG-3′), SpCas9-EQR (5’-NGAG-3′) SpCas9-VRER (5’-NGCG-3′) and xCas9 (5’-NG-3′, 5’-GAA-3′, and 5’-GAT-3′) (Hu et al., 2018; Yamamoto et al., 2019). Moreover, the discoveries of different PAM site-recognizing Cas9 endonucleases from several other prokaryotes, viz., Cas9 from Streptococcus thermophilus, Neisseria meningitidis, Brevibacillus laterosporus, Staphylococcus aureus, and Geobacillus stearothermophilus identifies 5’-NNAGAAW-3′ and 5′-NGGNG-3′, 5’-NNNNGATT-3′, 5’-NNNCND-3′, 5’-NNGRRT-3′, and 5′-NNNNCRAA-3′ PAM sites, respectively, have also increased the puissance of the technology (Kim et al., 2021). Another CRISPR/Cas technology, CRISPR/Cas12a (previously known as CRISPR from Prevotella and Francisella1) system uses a 24-nucleotide spacer complementary sequence for crRNA-DNA/gRNA-DNA binding, doesn’t require a tracrRNA and possesses a smaller endonuclease than most Cas9 orthologs. CRISPR/Cas12a generates staggered-end DSBs after recognizing an AT-rich PAM sequence with a high frequency in the genome and is proving to be a better substitute for CRISPR/Cas9 (Moon et al., 2018). Besides, CRISPR/Cas13a is a single RNA-guided RNA-editing system that needs a crRNA containing a 28-nucleotide spacer complementation sequence to bind adjacent to a protospacer flanking sequence (3′-A/U/C-5′) for Cas13a-mediated target recognition. Cas13a was first identified from Leptotrichia shahii and was demonstrated to cleave single-stranded RNAs without the requirement of a tracrRNA (Robertson et al., 2022).
Biotic stress involves the interaction of plants with plant pathogens such as bacteria, viruses, fungi, oomycetes, nematodes, etc., that hampers the normal growth and development of plants leading to a huge loss in quality and yield (around 30% of crop production worldwide) (van Esse et al., 2020). The generation of disease-resistant crop varieties using CRISPR/Cas technologies has proven to be effective in combating plant diseases in fruits and vegetables (Table 1).
Table 1 CRISPR/Cas-mediated targeting of genes in fruits and vegetables for imparting resistance against biotic stress.
Plant viruses are perilous pathogens causing massive destruction of productivity in horticultural crops. Based on genome composition, plant viruses are grouped into five major categories: double-stranded RNA (dsRNA) viruses, positive sense single-stranded RNA (+ssRNA) viruses, negative sense single-stranded RNA (-ssRNA) viruses, single-stranded DNA (ssDNA) viruses and double-stranded DNA (dsDNA) viruses (Roossinck et al., 2015). Researchers have employed two main strategies to design sgRNAs for engineering virus-resistant plants. The first strategy involves direct targeting of the virus genome. For example, Tomato yellow leaf curl virus (TYLCV) is a whitefly-transmitted monopartite Begomovirus that causes significant loss of worldwide tomato production. Engineering tomato plants with CRISPR/Cas9 targeting the sequences encoding intergenic region (IR) and coat protein (CP) produced TYLCV-resistant plants where Cas9 was cloned under a virus-inducible rgsCaM promoter to overcome any potential off-target effects of Cas9 (Ghorbani Faal et al., 2020). In the plantain banana ‘Gonja Manjaya’, the presence of endogenous Banana streak virus (eBSV) as integrative viral elements in the genome limits banana production. CRISPR/Cas9 was utilized to introduce mutations that prevent proper transcription and/or translation into functional viral proteins to produce eBSV-resistant banana plants (Tripathi et al., 2019). Another devastating pathogen, Potato virus Y (PVY), belonging to the +ssRNA genome containing genus Potyvirus, brings about an 80% decrease in yield and poor tuber quality in potatoes. Targeting the PVY genome with CRISPR/LshCas13 system at the sequences encoding potyviral membrane protein (P3), cytoplasmic inclusion bodies laminating protein (CI), RNA-dependent RNA polymerase (NIb) or coat protein (CP) resulted in resistance against multiple strains of PVY in transgenic potato plants (Zhan et al., 2019). To enhance immunity in edited sweet potato plants against sweet potato virus disease, CRISPR/RfxCas13d system was used to target the Sweet potato chlorotic stunt virus genome encoding pathogenesis-related factor, RNase III endoribonuclease (Yu et al., 2022).
The second strategy requires the manipulation of plant genes responsible for rendering plants susceptible to viruses. For instance, TYLCV-resistant tomatoes were obtained by CRISPR/Cas9-mediated mutagenesis of SlPelo that encodes a mRNA surveillance factor (Pramanik et al., 2021). CRISPR/Cas9-mediated multiplexed targeting of susceptibility genes in tomato plants also induces strong resistance to multiple viral diseases. Tomato Tobamovirus Multiplication 1a-d are functionally redundant genes essential for Tobamovirus multiplication in tomato. The generation of quadruple-mutant of SlTOM1a-d through CRISPR/Cas9 multiplexed genome editing exhibited enhanced resistance to Tobamoviruses (Ishikawa et al., 2022). RNA viruses hijack host cellular machinery, including eIF4E, eIF4G, and their isoforms, to complete their life cycle (Sanfaçon, 2015). Different isoforms of eIF4E were targeted by CRISPR/Cas9 to generate resistant varieties in tomato, potato, and cucumber against viruses belonging to Potyviridae (Chandrasekaran et al., 2016; Atarashi et al., 2020; Yoon et al., 2020; Lucioli et al., 2022; Noureen et al., 2022). Cassava is a tuberous root crop and a staple source of food for sub-Saharan African people. South African cassava mosaic virus (SACMV) is a whitefly-transmitted bipartite Begomovirus that causes regional pandemics in cassava production in East and Central Africa. Transformation of SACMV-susceptible and -resistant varieties of cassava protoplasts with a ribonucleoprotein complex containing the Cas9 nuclease and sgRNA (CRISPR/Cas9 RNPs) targeting the Ubiquitin E3 Ligase gene resulted in less virus titre in protoplasts of susceptible variety, similar to that of the resistant variety protoplasts (Chatukuta and Rey, 2020). Another group used RNP-mediated delivery of CRISPR/Cas9 machinery to enable editing of at least one allele of Coilin gene in potato. The edited Coilin gene-containing potato plants of the Chicago cultivar showed increased resistance to PVY (Makhotenko et al., 2019).
Citrus canker, caused by Xanthomonas citri subsp. citri (Xcc), is one of the most economically destructive bacterial disease owing to its appearance at pre- and post-harvest stages. To suppress the immunity of citrus plants, Xcc secretes a TALE, PthA4 that binds to effector-binding elements present at the promoter region of Lateral Organ Boundaries 1 (LOB1) (Jia et al., 2016). Citrus canker resistance was first reported in Duncan grapefruit, where CRISPR/Cas9-mediated alteration of PthA4 effector binding elements of one allele of biallelic gene LOB1 gave partial resistance (Jia et al., 2016), and of two alleles gave complete resistance to Xcc (Jia et al., 2022a). In Wanjincheng orange, a multiplexed CRISPR/Cas9 genome editing strategy was applied to delete the entire PthA4 effector binding elements from the promoter region of all the alleles of CsLOB1 to produce citrus canker-resistant varieties (Peng et al., 2017) In Arabidopsis, Downy Mildew Resistance 6 (DMR6) encodes for 2-oxoglutarate Fe(II)-dependent oxygenase that facilitates pathogen infection. In tomato, targeting DMR6 ortholog, SlDMR6-1, via CRISPR/Cas9 resulted in broad-spectrum resistance against multiple pathogens, Pseudomonas syringae pv. tomato DC3000, Xanthomonas gardneri, X. perforans, Pseudoidium neolycopersici, and Phytophthora capsici (Thomazella et al., 2021). Likewise, CRISPR/Cas9-mediated mutagenesis of MusaDMR6 conferred resistance to Xanthomonas campestris pv. musacearum, which causes banana Xanthomonas wilt that accounts for huge losses in banana production in East and Central Africa (Tripathi et al., 2021). In apple, two independent research groups using two different transgene-free CRISPR/Cas9 genome editing strategies have demonstrated that editing of one or more genes belonging to the susceptibility gene family, DspA/E-Interacting Proteins From Malus, could alleviate fire blight susceptibility to Erwinia amilovora (Malnoy et al., 2016; Pompili et al., 2020).
The successful application of CRISPR/Cas technology to mutate the genes responsible for susceptibility has led to the generation of horticultural crops resistant to fungal diseases. Oidium neolycopersici and Erysiphe necator cause powdery mildew in tomato and grapevine plants, respectively. Mildew Resistant Locus O (Mlo) encodes a seven-transmembrane domain-containing protein that is conserved throughout angiosperms and negatively regulates immunity to powdery mildew disease (Acevedo-Garcia et al., 2014). In tomato, two independent reports revealed CRISPR/Cas9-mediated targeting of SlMlo1 confer enhanced resistance to powdery mildew (Nekrasov et al., 2017; Pramanik et al., 2021). Further, (Nekrasov et al., 2017) tested and selfed a specific line of T0 transformants to obtain T-DNA-free slmlo1 plants. Transgene-free powdery mildew-resistant grapevine protoplasts were obtained by knocking out VvMlo7 via RNP-mediated delivery of CRISPR/Cas9 genome editing machinery (Malnoy et al., 2016). Another report showed CRISPR/Cas9-based alteration of VvMlo3 was able to produce powdery mildew-resistant grapevine plants (Wan et al., 2020). The multi-host fungal pathogen, Botrytis cinerea that causes grey mold disease in tomatoes and noble rot disease in grapevines, poses a serious threat to tomato and grapevine production at both pre- and post-harvest levels. The function of several tomato genes, including the well-characterized genes like Mitogen Activated Protein Kinase 3 (MAPK3), SlMYC2, Pectate Lyase, Acetylase 1a and 1b, and genes of unknown functions like Solyc12g100250, and Solyc12g100270, were identified during Botrytis cinerea infection through the generation of deletion mutants by CRISPR/Cas9. While knock-out mutants of SlMAPK3 and SlMYC2 were susceptible to Botrytis cinerea, loss-of-function of the other above-mentioned genes rendered tomato plants resistant to grey mold disease (Zhang et al., 2018; Jeon et al., 2020; Shu et al., 2020; Silva et al., 2021). In addition, both the single and double knock-out mutants of tomato Histone H3 Lysine Methyltransferases Set Domain Group33 and 34 (SDG33 and SDG34) produced via CRISPR/Cas9 exhibited alterations in H3K36 and H3K4 methylations. However, only the sdg33sdg34 plants and not sdg33 and sdg34 plants exhibited resistance to Botrytis cinerea (Bvindi et al., 2022). The fungal pathogen Colletotrichum spp. causes anthracnose of chilli resulting in major pre- and post-harvest losses. In chilli, CRISPR/Cas9-mediated modification Ethylene Response Factor 28 produced mutant lines with elevated resistance against anthracnose (Mishra et al., 2021). The causal organism of ring rot disease in apple is Botryosphaeria dothidea. CRISPR/Cas9-produced apple Cyclic Nucleotide-Gated Ion Channels 2 knock-out mutant calli exhibited significantly lower growth of B. dothidea, increased levels of salicylic acid accumulation and elevated expression of several defense-related genes including Pathogenesis-related genes, MdPR1, MdPR2, MdPR4, MdPR5, MdPR8, and MdPR10a compared to wild-type calli (Zhou et al., 2020).
The most-well studied oomycete, Phytophthora infestans is famous for causing the devastating Irish potato famine of the 1840s, killing over a million people. Phytophthora infestans causes late blight in potato and tomato plants. Independent studies showing CRISPR/Cas9-targeted mutagenesis of five potato susceptibility genes, Defense, No Death1, CIB1/HBI1-like 1, DMR6-1, Caffeoyl-CoA O-methyltransferase and Signal Responsive 4, conferred resistance to late blight disease (Hegde et al., 2021; Kieu et al., 2021; Moon et al., 2022). However, knocking-out of MYB transcription factor S2 by CRISPR/Cas9 produced tomato mutants susceptible to P. infestans with increased necrotic cells, lesion sizes, disease index, and reduced expression of defense-related genes, indicating that SlMYBS2 acts as a positive regulator of resistance to P. infestans (Liu et al., 2021). CRISPR/Cas9-engineered cacao plants for the susceptible gene, Non-Expressor of Pathogenesis-Related 3, exhibited enhanced resistance to Phytophthora tropicalis (Fister et al., 2018).
Editing of the virulent genes in oomycete pathogens provide an alternative strategy to achieve disease resistance. Phytophthora palmivora causes worldwide destruction of papaya plantations and reduction in yield. Extracellular cystatin-like cysteine protease inhibitor 8 is unique to P. palmivora and contributes to the virulence of the pathogen by inhibiting papain. CRISPR/Cas9-mediated PpalEPIC8 editing produced a less virulent version of the pathogen with reduced pathogenicity (Gumtow et al., 2018). Another oomycete, Peronophythora litchii causes downy blossom blight of litchi that results in tremendous economic loss in litchi production every year (Situ et al., 2020). Two independent research groups designed CRISPR/Cas9-based modification of two different virulent genes, Pectin Acetylesterase 5 and Avh142, from P. litchii. PlPAE5 encodes pectin acetylesterases that cause deacetylation of pectin and Avh142 is an RXLR effector that induces plant cell-death. Both strategies resulted in the production of less invasive and less virulent variants of P. litchii that are less capable of infecting litchi plants (Kong et al., 2019; Situ et al., 2020).
Plants experience single or multiple abiotic stresses simultaneously, which can lead to 50%-70% loss of crop productivity and poses a direct threat to achieving global food security. (Francini and Sebastiani, 2019). Apart from drought, salinity and temperature, the presence of heavy metals in the soil and excessive use of herbicides and weedicides also contribute to abiotic stress factors (Hamdan et al., 2022). Targeting one or more genes concomitantly utilising CRISPR/Cas technologies could be propitious for engineering abiotic stress-resilient varieties of fruits and vegetables (Table 2).
Table 2 CRISPR/Cas-mediated targeting of genes in fruits and vegetables for imparting resistance against abiotic stress.
Plants under drought stress manifest complex symptoms at multiple morphological, physiological, and biochemical levels that consequently lower the quality and yield of the produce. Knocking-out of tomato Auxin Response Factor 4 (ARF4) via CRISPR/Cas9 technology resulted in increased drought resistance and revival ability with induced morphological changes in stomata and vascular bundles, higher content of antioxidant substances, and up-regulated expression of ABA signal transduction pathway genes, like Scarecrow-Like 3 and Abscisic acid Insensitive 5 (Chen et al., 2021). Pipecolic acid is known to play an important role in salicylic acid-mediated plant immune response. CRISPR/Cas9-mediated targeting of tomato pipecolic acid biosynthetic pathway gene, Aberrant Growth and Death2-like Defense Response Protein 1, has led to increased drought resistance with enhanced CO2 assimilation, photosystems activities, antioxidant enzymes activities, ascorbate and glutathione content, and reduced reactive oxygen species accumulation, lipid peroxidation, and protein oxidation than the wild-type tomato plants (Wang et al., 2021a). Interestingly, the sdg33 and sdg34 tomato plants were drought-resistant and exhibited high water retention capacity during drought and improved recovery and survival, while sdg33sdg34 plants were superior drought stress-resistant (Bvindi et al., 2022).
Contrarily, the positive regulators of drought stress were also identified by using CRISPR/Cas technology. For example, CRISPR/Cas9-generated SlMAPK3 mutant plants were drought-sensitive showing severe wilting symptoms, higher hydrogen peroxide content, and lower antioxidant enzymes activities as compared to the wild-type tomato plants (Wang et al., 2017). Knock-out mutant of another tomato pipecolic acid-related gene, Flavin-dependent Monooxygenase 1, exhibited damaged photosystems and impaired antioxidant systems and thus were more sensitive to drought (Wang et al., 2021a). In potato, Cycling Dof Factor 1 together with a long non-coding RNA (lncRNA) counterpart, StFLORE, regulate vegetative reproduction and water homeostasis. CRISPR/Cas9-mediated editing of StFLORE promoter region rendered potato mutant plants sensitive to drought due to disruption of stomatal growth and diurnal opening of stomata in an ABA-dependent manner (Ramírez Gonzales et al., 2021).
Salinity stress impedes water absorption by altering the osmotic balance between plant roots and surrounding soil and severely affects the yield and quality of crops. CRISPR/Cas9-based precise removal of PRD domain, 8CM domain, or both of Hybrid Proline-rich Protein 1, resulted in high salinity tolerance at the germination and vegetative stages of tomato plants (Tran et al., 2021). CRISPR/Cas9 slarf4 mutants displayed decreased leaf area, CO2 assimilation, stomatal conductance, enhanced water use efficiency, and salt and osmotic stress resistance in comparison to wild-type tomato plants (Bouzroud et al., 2020). Transgene-free CRISPR/Cas12a-mediated HDR-based editing of one allele of tomato High-affinity K+ Transporter 1;2 has led to increased salinity stress tolerance, which was completely inherited by theprogeny plants (Vu et al., 2020). Another transgene-free genome editing approach was successful when potato plants manifested tolerance to salt and osmotic stress following CRISPR/Cas9 RNP-based editing of Coilin gene (Makhotenko et al., 2019).
Alternatively, CRISPR/Cas9-mediated alteration of Salt Overlay Sensitive 1 resulted in increased sensitivity to salinity stress in tomato (Wang et al., 2021b). CRISPR/Cas9 slmapk3 plants, were showcasing salt stress-sensitivity with increased salinity-induced cell death, chlorophyll degradation, and reduced activities of antioxidant enzymes than wild-type tomato plants (Shu et al., 2022). In Cucurbita moschata, CRISPR/Cas9-based editing of Respiratory Burst Oxidase Homolog D showed salt stress hypersensitive phenotype with a decrease in root apex H2O2 and K+ content (Huang et al., 2019).
Temperature stress occurs due to continuous rise or drop in temperature above or below the optimum temperature necessary for plant growth over a period of time. Both heat and cold stresses seriously affect the growth and productivity of crops. Despite being sensitive to drought and salinity stresses, tomato CRISPR/Cas9 slmapk3 displayed heat stress resistance accompanied by reduced wilting, membrane damage, and enhanced expression of transcripts of heat stress transcription factors and heat shock proteins (Yu et al., 2019). Conversely, CRISPR/Cas9-based modification of Calcium-Dependent Protein Kinase 28 rendered tomato plants sensitive to heat stress with increased levels of protein oxidation and lower antioxidant enzymes activities than wild-type plants (Hu et al., 2021). In lettuce, CRISPR/Cas9-generated knock-out mutants of 9-cis-Epoxycarotenoid Dioxygenase 4 were capable of seed germination above optimum temperature (Bertier et al., 2018).
Compared to wild-type tomato plants, CRISPR/Cas9-produced C-repeat Binding Factor 1 knock-out plants demonstrated sensitivity to cold stress accompanied by more severe chilling-injury symptoms, higher electrolyte leakage, and less proline and protein contents, and antioxidant enzymes activities (Li et al., 2018).
Excessive use of herbicides and weedicides causes herbicide stress that leaves a major effect on the physiology of the non-targeted crop plants. CRISPR/Cas technology was applied to prepare herbicide-resistant plants. For example, the tomato Acetolactate Synthase (ALS) gene was targeted using CRISPR/Cas9 technology to create herbicide-resistant plants (Yang et al., 2022). Moreover, transgene-free HDR-mediated genome editing of SlALS1 using Agrobacterium-assisted CRISPR/Cas9 delivery produced herbicide resistance in tomato plants (Danilo et al., 2019). Transgene-free herbicide-resistant watermelon plants were obtained via CRISPR/Cas9-mediated genome editing of ClALS (Tian et al., 2018). Likewise, PcALS gene of pear plant was manipulated by CRISPR/Cas9-based base-editing to obtain resistance to the herbicide, chlorosulfuron (Malabarba et al., 2021). Also, CRISPR/Cas9 base-editing of ALS gene was used to produce T-DNA-free imazapyr-resistant citrus Carrizo citrange plants (Alquézar et al., 2022).
The application of CRISPR/Cas technology has proven to be quite successful in modern agriculture and in improving the agronomic traits of crops. However, barring tomato plants, the usage of CRISPR/Cas technology is very limited among horticultural crops. Though this technology is now being extended to different horticultural crops, researchers are experiencing several challenges during implementation. The success of CRISPR/Cas application depends on the availability of information about the whole-genome sequence, annotation of genes, and their functions in a particular crop. Therefore, more genome sequencing and functional genomics studies on vegetable and fruit plants are required due to the availability of limited data regarding the identification and characterization of genes controlling important traits, such as quality, yield, biotic, and abiotic stress tolerance (Li et al., 2022). Furthermore, polyploidy is common in vegetable and fruit crops, which make it difficult to study the genome due to their highly diverse and complex nature (Chen et al., 2019). Also, CRISPR/Cas technology has mostly been used to create loss-of-function mutations, which limits its application as gain-of-function mutations in positive regulators controlling important agronomic traits might prove to be advantageous in obtaining desired phenotype(s) in horticultural crops (Kim et al., 2021). Interestingly, a recent report demonstrating successful single or multiplexed activation of gene(s) present in anthocyanin and lignin biosynthesis pathways of pear calli via a third-generation CRISPR activation-mediated gain-of-function mutation system, CRISPR-Act3.0, raised the prospect of further broadening its application to other vegetable and fruit crops (Ming et al., 2022). Another major challenge resides in the perception of common people that do not make any distinction between genetically-modified and genome-edited crops and considers the production and consumption of both crop types to be hazardous to the environment and human health. Additionally, the absence of a common and unified legislative framework that differentiates genome-edited crops from genetically-modified crops and facilitates the production and marketing of genome-edited plants also aggravates the challenge (Bhatta and Malla, 2020). Every nation has drafted its legal framework for the release of genome-edited crops. In general, most countries of North and South America (USA, Canada, Argentina, Colombia), some countries of Asia (India, China, Japan), and Australia consider case-by-case procedures for the release of genome-edited crops and products, while most countries of Europe and New Zealand maintain a conservative approach by adhering to the same restrictive laws framed for production and release of both genetically-modified and genome-edited crops (Wang et al., 2022; Rukavtsova et al., 2023). The presence of the foreign DNA in GMOs is the main reason behind their non-acceptance in society, while the CRISPR/Cas-edited crops could be made transgene-free depending on the mode of delivery of CRISPR/Cas machinery during transformation and the choice of propagation method thereafter. Transgene can easily be removed from CRISPR/Cas-edited crops by self-pollinated sexual reproduction and off-target threat can be reduced by expressing CRISPR/Cas machinery under inducible promoters (Nekrasov et al., 2017; Ghorbani Faal et al., 2020). However, most horticultural crops are propagated vegetatively, especially fruit trees due to their long juvenile period. Moreover, propagation by sexual reproduction would produce transgene-free horticultural crops with undesirable traits as most of the important traits are best expressed when the corresponding genes are present in heterozygous conditions (Tsanova et al., 2021). For the generation of CRISPR/Cas-edited transgene-free horticultural crops, RNP-mediated delivery of CRISPR/Cas machinery through particle bombardment or protoplast transformation using PEG remains the most reliable method, closely followed by Agrobacterium-mediated transient expression of CRISPR/Cas cassette. Interestingly, transgene-free genome-edited plants can be produced from Agrobacterium-mediated stably transformed horticultural plants by using FLP/FRT and CRE/LOX site-specific recombination systems or by adding two additional Cas9 cleavage target sites at the T-DNA borders (Li et al., 2020b; Wan et al., 2021). The efficiency of transformation and regeneration of horticultural plants in case of both stable and transient transformation are quite low due to their recalcitrance nature towards regeneration protocols. Regeneration process involves tissue culture methods, which are quite difficult, expensive, time-consuming, and labour-intensive (Kumari et al., 2022). Therefore, invention of new technology is required that can bypass the regeneration phase of transformed plants. Plant virus-mediated delivery of CRISPR/Cas machinery has the potency to bypass the regeneration phase as this method takes advantage of virus replication and translocation within plants. The only disadvantage of this process is the limited cargo capacity of most DNA and +ssRNA viruses, which makes delivery of large DNA sequences deletion-prone and thereby preferred replication and translocation of deletion mutant viral vectors over the original vectors (Tsanova et al., 2021). Nevertheless, reports orchestrating the delivery of complete CRISPR/Cas9 machinery by Rhabdovirus and potato virus X and consequent successful editing of Nicotiana benthamiana genome enhances the possibility of extending the technology to horticultural plants (Ariga et al., 2020; Ma et al., 2020). Thus, transgene-free CRISPR/Cas-mediated gene/genome-edited mutants of horticultural crops are technically the same as those obtained in nature or from mutation breeding (Li et al., 2022). Conventional breeding and mutation breeding, besides being time-consuming, laborious, and expensive, lack specificity and cause introgression of undesired traits due to linkage drag. Conversely, CRISPR/Cas technology is simple, fast, versatile, and introduces changes in the target gene/genome in a well-defined and efficient manner (Wolter et al., 2019). Moreover, the ability of CRISPR/Cas technology to target multiple genes at one go with maximum precision compared to other existing technologies, and that too in a cost-effective manner, actually makes this technology truly exceptional for genome-edited plant breeding. The upcoming advancements in the CRISPR/Cas system itself and the associated technologies, such as delivery methods with or without regeneration phase, high-throughput sequence-based target analyses, whole genome sequencing, and other omics-based approaches, will simplify the process of identification of key genes controlling biotic and abiotic stress resistance pathways and thereby generation of biotic and abiotic stress-resistant plants.
AS: Conceptualization, Data curation, Writing – original draft, Writing – review & editing.
The author declares that no financial support was received for the research, authorship, and/or publication of this article.
The author declares that the research was conducted in the absence of any commercial or financial relationships that could be construed as a potential conflict of interest.
All claims expressed in this article are solely those of the authors and do not necessarily represent those of their affiliated organizations, or those of the publisher, the editors and the reviewers. Any product that may be evaluated in this article, or claim that may be made by its manufacturer, is not guaranteed or endorsed by the publisher.
Acevedo-Garcia, J., Kusch, S., Panstruga, R. (2014). Magical mystery tour: MLO proteins in plant immunity and beyond. J. Physiol. 204 (2), 273–281. doi: 10.1111/nph.12889
Alquézar, B., Bennici, S., Carmona, L., Gentile, A., Peña, L. (2022). Generation of transfer-DNA-free base-edited citrus plants. Front. Plant Science. 13. doi: 10.3389/fpls.2022.835282
Ariga, H., Toki, S., Ishibashi, K. (2020). Potato virus X vector-mediated DNA-free genome editing in plants. Plant Cell Physiol. 61 (11), 1946–1953. doi: 10.1093/pcp/pcaa123
Atarashi, H., Jayasinghe, W. H., Kwon, J., Kim, H., Taninaka, Y., Igarashi, M., et al. (2020). Artificially edited alleles of the eukaryotic translation initiation factor 4E1 gene differentially reduce susceptibility to cucumber mosaic virus and potato virus Y in tomato. Front. Microbiol. 11. doi: 10.3389/fmicb.2020.564310
Bertier, L. D., Ron, M., Huo, H., Bradford, K. J., Britt, A. B., Michelmore, R. W. (2018). High-resolution analysis of the efficiency, heritability, and editing outcomes of CRISPR/Cas9-induced modifications of NCED4 in lettuce (Lactuca sativa). G3: Genes Genomes Genet. 8 (5), 1513–1521. doi: 10.1534/g3.117.300396
Bhatta, B. P., Malla, S. (2020). Improving horticultural crops via crispr/cas9: Current successes and prospects. Plants. 9 (10), 1–19. doi: 10.3390/plants9101360
Bouzroud, S., Gasparini, K., Hu, G., Barbosa, M. A. M., Rosa, B. L., Fahr, M., et al. (2020). Down regulation and loss of auxin response factor 4 function using CRISPR/Cas9 alters plant growth, stomatal function and improves tomato tolerance to salinity and osmotic stress. Genes. 11, (3). doi: 10.3390/genes11030272
Butler, N. M., Atkins, P. A., Voytas, D. F., Douches, D. S. (2015). Generation and inheritance of targeted mutations in potato (Solanum tuberosum L.) Using the CRISPR/Cas System. PloS One 10, (12). doi: 10.1371/journal.pone.0144591
Bvindi, C., Lee, S., Tang, L., Mickelbart, M. V., Li, Y., Mengiste, T. (2022). Improved pathogen and stress tolerance in tomato mutants of SET domain histone 3 lysine methyltransferases. New Phytologist. 235 (5), 1957–1976. doi: 10.1111/NPH.18277
Chandrasekaran, J., Brumin, M., Wolf, D., Leibman, D., Klap, C., Pearlsman, M., et al. (2016). Development of broad virus resistance in non-transgenic cucumber using CRISPR/Cas9 technology. Mol. Plant pathology. 17 (7), 1140–1153. doi: 10.1111/mpp.12375
Chatukuta, P., Rey, M. E. C. (2020). A cassava protoplast system for screening genes associated with the response to South African cassava mosaic virus. Virol. J. 17, (1). doi: 10.1186/s12985-020-01453-4
Chen, F., Song, Y., Li, X., Chen, J., Mo, L., Zhang, X., et al. (2019). Genome sequences of horticultural plants: past, present, and future. Horticulture Res. 6 (1), 112. doi: 10.1038/s41438-019-0195-6
Chen, M., Zhu, X., Liu, X., Wu, C., Yu, C., Hu, G., et al. (2021). Knockout of auxin response factor SlARF4 improves tomato resistance to water deficit. Int. J. Mol. Sci. 22, (7). doi: 10.3390/ijms22073347
Danilo, B., Perrot, L., Mara, K., Botton, E., Nogué, F., Mazier, M. (2019). Efficient and transgene-free gene targeting using Agrobacterium-mediated delivery of the CRISPR/Cas9 system in tomato. Plant Cell Rep. 38 (4), 459–462. doi: 10.1007/s00299-019-02373-6
Fister, A. S., Landherr, L., Maximova, S. N., Guiltinan, M. J. (2018). Transient expression of CRISPR/Cas9 machinery targeting TcNPR3 enhances defense response in theobroma cacao. Front. Plant Science. 9. doi: 10.3389/fpls.2018.00268
Francini, A., Sebastiani, L. (2019). Abiotic stress effects on performance of horticultural crops. Horticulturae 5 (4), 67. doi: 10.3390/horticulturae5040067
Gao, C. (2021). Genome engineering for crop improvement and future agriculture. Cell. 184 (6), 1621–1635. doi: 10.1016/j.cell.2021.01.005
Ghorbani Faal, P., Farsi, M., Seifi, A., Mirshamsi Kakhki, A. (2020). Virus-induced CRISPR-Cas9 system improved resistance against tomato yellow leaf curl virus. Mol. Biol. Rep. 47 (5), 3369–3376. doi: 10.1007/s11033-020-05409-3
Gomez, M. A., Lin, Z. D., Moll, T., Chauhan, R. D., Hayden, L., Renninger, K., et al. (2019). Simultaneous CRISPR/Cas9-mediated editing of cassava eIF4E isoforms nCBP-1 and nCBP-2 reduces cassava brown streak disease symptom severity and incidence. Plant Biotechnol. J. 17 (2), 421–434. doi: 10.1111/pbi.12987
Gumtow, R., Wu, D., Uchida, J., Tian, M. (2018). A Phytophthora palmivora extracellular cystatin-like protease inhibitor targets papain to contribute to virulence on papaya. Mol. Plant-Microbe Interactions. 31 (3), 363–373. doi: 10.1094/MPMI-06-17-0131-FI
Hamdan, M. F., Karlson, C. K. S., Teoh, E. Y., Lau, S. E., Tan, B. C. (2022). Genome editing for sustainable crop improvement and mitigation of biotic and abiotic stresses. Plants. 11 (19), 2625. doi: 10.3390/plants11192625
Hegde, N., Joshi, S., Soni, N., Kushalappa, A. C. (2021). The caffeoyl-CoA O-methyltransferase gene SNP replacement in Russet Burbank potato variety enhances late blight resistance through cell wall reinforcement. Plant Cell Rep. 40 (1), 237–254. doi: 10.1007/s00299-020-02629-6
Hong, Y., Meng, J., He, X., Zhang, Y., Liu, Y., Zhang, C., et al. (2021). Editing mir482b and mir482c simultaneously by crispr/cas9 enhanced tomato resistance to phytophthora infestans. Phytopathology. 111 (6), 1008–1016. doi: 10.1094/PHYTO-08-20-0360-R
Hu, Z., Li, J., Ding, S., Cheng, F., Li, X., Jiang, Y., et al. (2021). The protein kinase CPK28 phosphorylates ascorbate peroxidase and enhances thermotolerance in tomato. Plant Physiol. 186 (2), 1302–1317. doi: 10.1093/PLPHYS/KIAB120
Hu, J. H., Miller, S. M., Geurts, M. H., Tang, W., Chen, L., Sun, N., et al. (2018). Evolved Cas9 variants with broad PAM compatibility and high DNA specificity. Nature. 556 (7699), 57–63. doi: 10.1038/nature26155
Hua, D., Ma, M., Ge, G., Suleman, M., Li, H. (2020). The role of cyanide-resistant respiration in Solanum tuberosum L. against high light stress. Plant Biol. 22 (3), 425–432. doi: 10.1111/PLB.13098
Huang, Y., Cao, H., Yang, L., Chen, C., Shabala, L., Xiong, M., et al. (2019). Tissue-specific respiratory burst oxidase homolog-dependent H2O2 signaling to the plasma membrane H+-ATPase confers potassium uptake and salinity tolerance in Cucurbitaceae. J. Exp. Botany. 70 (20), 5879–5893. doi: 10.1093/jxb/erz328
Hummel, A. W., Chauhan, R. D., Cermak, T., Mutka, A. M., Vijayaraghavan, A., Boyher, A., et al. (2018). Allele exchange at the EPSPS locus confers glyphosate tolerance in cassava. Plant Biotechnol. J. 16 (7), 1275–1282. doi: 10.1111/pbi.12868
Illouz-Eliaz, N., Nissan, I., Nir, I., Ramon, U., Shohat, H., Weiss, D. (2020). Mutations in the tomato gibberellin receptors suppress xylem proliferation and reduce water loss under water-deficit conditions. J. Exp. Botany. 71 (12), 3603–3612. doi: 10.1093/jxb/eraa137
Ishikawa, M., Yoshida, T., Matsuyama, M., Kouzai, Y., Kano, A., Ishibashi, K. (2022). Tomato brown rugose fruit virus resistance generated by quadruple knockout of homologs of TOBAMOVIRUS MULTIPLICATION1 in tomato. Plant Physiol. 189 (2), 679–686. doi: 10.1093/plphys/kiac103
Ishino, Y., Shinagawa, H., Makino, K., Amemura, M., Nakata, A. (1987). Nucleotide Sequence of the iap Gene, Responsible for Alkaline Phosphatase Isozyme Conversion in Escherichia coli, and Identification of the Gene Product. J. Bacteriol. 169 (12), 5429–5433. doi: 10.1128/jb.169.12.5429-5433.1987
Jeon, J. E., Kim, J. G., Fischer, C. R., Mehta, N., Dufour-Schroif, C., Wemmer, K., et al. (2020). A pathogen-responsive gene cluster for highly modified fatty acids in tomato. Cell. 180 (1), 176–187.e19. doi: 10.1016/j.cell.2019.11.037
Jia, H., Omar, A. A., Orbovic, V., Wang, N. (2022a). Biallelic editing of the LOB1 promoter via CRISPR/Cas9 creates canker-resistant ‘Duncan’ Grapefruit. Phytopathology. 112 (2), 308–314. doi: 10.1094/PHYTO-04-21-0144-R
Jia, H., Orbovic, V., Jones, J. B., Wang, N. (2016). Modification of the PthA4 effector binding elements in Type I CsLOB1 promoter using Cas9/sgRNA to produce transgenic Duncan grapefruit alleviating XccΔpthA4: DCsLOB1.3 infection. Plant Biotechnol. J. 14 (5), 1291–1301. doi: 10.1111/pbi.12495
Jia, H., Orbović, V., Wang, N. (2019). CRISPR-LbCas12a-mediated modification of citrus. Plant Biotechnol. J. 17 (10), 1928–1937. doi: 10.1111/pbi.13109
Jia, H., Wang, Y., Su, H., Huan, X., Wang, N. (2022b). LbCas12a-D156R efficiently edits LOB1 effector binding elements to generate canker-resistant citrus plants. Cells. 11, (3). doi: 10.3390/cells11030315
Jinek, M., Chylinski, K., Fonfara, I., Hauer, M., Doudna, J. A., Charpentier, E. (2012) A programmable dual-RNA-guided DNA endonuclease in adaptive bacterial immunity. Available at: https://www.science.org.
Kamburova, V. S., Nikitina, E. V., Shermatov, S. E., Buriev, Z. T., Kumpatla, S. P., Emani, C., et al. (2017). Genome editing in plants: an overview of tools and applications. Int. J. Agronomy. 2017, 15. doi: 10.1155/2017/7315351
Kieu, N. P., Lenman, M., Wang, E. S., Petersen, B. L., Andreasson, E. (2021). Mutations introduced in susceptibility genes through CRISPR/Cas9 genome editing confer increased late blight resistance in potatoes. Sci. Rep. 11, (1). doi: 10.1038/s41598-021-83972-w
Kim, Y.-C., Kang, Y., Yang, E.-Y., Cho, M.-C., Schafleitner, R., Lee, J. H., et al. (2021). Applications and major achievements of genome editing in vegetable crops: A review. Front. Plant Science. 12. doi: 10.3389/fpls.2021.688980
Klap, C., Yeshayahou, E., Bolger, A. M., Arazi, T., Gupta, S. K., Shabtai, S., et al. (2017). Tomato facultative parthenocarpy results from SlAGAMOUS-LIKE 6 loss of function. Plant Biotechnol. J. 15 (5), 634–647. doi: 10.1111/pbi.12662
Kong, G., Wan, L., Deng, Y. Z., Yang, W., Li, W., Jiang, L., et al. (2019). Pectin acetylesterase PAE5 is associated with the virulence of plant pathogenic oomycete Peronophythora litchii. Physiol. Mol. Plant Pathology. 106, 16–22. doi: 10.1016/j.pmpp.2018.11.006
Kumari, C., Sharma, M., Kumar, V., Sharma, R., Kumar, V., Sharma, P., et al. (2022). Genome editing technology for genetic amelioration of fruits and vegetables for alleviating post-harvest loss. Bioengineering 9 (4), 176. doi: 10.3390/bioengineering9040176
Li, M. Y., Jiao, Y. T., Wang, Y. T., Zhang, N., Wang, B. B., Liu, R. Q., et al. (2020a). CRISPR/Cas9-mediated VvPR4b editing decreases downy mildew resistance in grapevine (Vitis vinifera L.). Horticulture Res. 7, (1). doi: 10.1038/s41438-020-00371-4
Li, J., Li, Y., Pawlik, K. M., Napierala, J. S., Napierala, M. (2020b). A CRISPR-Cas9, crelox, and Flp-FRT cascade strategy for the precise and efficient integration of exogenous DNA into cellular genomes. CRISPR J. 3 (6), 470–486. doi: 10.1089/crispr.2020.0042
Li, R., Liu, C., Zhao, R., Wang, L., Chen, L., Yu, W., et al. (2019). CRISPR/Cas9-Mediated SlNPR1 mutagenesis reduces tomato plant drought tolerance. BMC Plant Biol. 19, (1). doi: 10.1186/s12870-018-1627-4
Li, Y., Wu, X., Zhang, Y., Zhang, Q. (2022). CRISPR/Cas genome editing improves abiotic and biotic stress tolerance of crops. Front. Genome Editing. 4. doi: 10.3389/fgeed.2022.987817
Li, R., Zhang, L., Wang, L., Chen, L., Zhao, R., Sheng, J., et al. (2018). Reduction of tomato-plant chilling tolerance by CRISPR-Cas9-mediated SlCBF1 mutagenesis. J. Agric. Food Chem. 66 (34), 9042–9051. doi: 10.1021/acs.jafc.8b02177
Liu, C., Zhang, Y., Tan, Y., Zhao, T., Xu, X., Yang, H., et al. (2021). Crispr/cas9-mediated slmybs2 mutagenesis reduces tomato resistance to phytophthora infestans. Int. J. Mol. Sci. 22 (21), 110683. doi: 10.3390/ijms222111423
Liu, L., Zhang, J., Xu, J., Li, Y., Guo, L., Wang, Z., et al. (2020). CRISPR/Cas9 targeted mutagenesis of SlLBD40, a lateral organ boundaries domain transcription factor, enhances drought tolerance in tomato. Plant Sci. 301, 110683. doi: 10.1016/j.plantsci.2020.110683
Lucioli, A., Tavazza, R., Baima, S., Fatyol, K., Burgyan, J., Tavazza, M. (2022). CRISPR-Cas9 Targeting of the eIF4E1 Gene Extends the Potato Virus Y Resistance Spectrum of the Solanum tuberosum L. cv. Desirée. Front. Microbiol. 13. doi: 10.3389/fmicb.2022.873930
Ma, X., Zhang, X., Liu, H., Li, Z. (2020). Highly efficient DNA-free plant genome editing using virally delivered CRISPR–Cas9. Nat. Plants. 6 (7), 773–779. doi: 10.1038/s41477-020-0704-5
Makhotenko, A. V., Khromov, A. V., Snigir, E. A., Makarova, S. S., Makarov, V. V., Suprunova, T. P., et al. (2019). Functional Analysis of Coilin in Virus Resistance and Stress Tolerance of Potato Solanum tuberosum using CRISPR-Cas9 Editing. Doklady Biochem. Biophysics. 484 (1), 88–91. doi: 10.1134/S1607672919010241
Malabarba, J., Chevreau, E., Dousset, N., Veillet, F., Moizan, J., Vergne, E. (2021). New strategies to overcome present CRISPR/CAS9 limitations in apple and pear: Efficient dechimerization and base editing. Int. J. Mol. Sci. 22 (1), 1–20. doi: 10.3390/ijms22010319
Malnoy, M., Viola, R., Jung, M. H., Koo, O. J., Kim, S., Kim, J. S., et al. (2016). DNA-free genetically edited grapevine and apple protoplast using CRISPR/Cas9 ribonucleoproteins. Front. Plant Science. 7. doi: 10.3389/fpls.2016.01904
Ming, M., Long, H., Ye, Z., Pan, C., Chen, J., Tian, R., et al. (2022). efficient CRISPR systems for loss-of-function and gain-of-function research in pear calli. Horticulture Res. 9, uhac148. doi: 10.1093/hr/uhac148
Mishra, R., Mohanty, J. N., Mahanty, B., Joshi, R. K. (2021). A single transcript CRISPR/Cas9 mediated mutagenesis of CaERF28 confers anthracnose resistance in chilli pepper (Capsicum annuum L.). Planta. 254, (1). doi: 10.1007/s00425-021-03660-x
Moon, S. B., Lee, J. M., Kang, J. G., Lee, N. E., Ha, D. I., Kim, D. Y., et al. (2018). Highly efficient genome editing by CRISPR-Cpf1 using CRISPR RNA with a uridinylate-rich 3′-overhang. Nat. Commun. 9, (1). doi: 10.1038/s41467-018-06129-w
Moon, K. B., Park, S. J., Park, J. S., Lee, H. J., Shin, S. Y., Lee, S. M., et al. (2022). Editing of StSR4 by Cas9-RNPs confers resistance to Phytophthora infestans in potato. Front. Plant Sci. 13. doi: 10.3389/fpls.2022.997888
Nekrasov, V., Wang, C., Win, J., Lanz, C., Weigel, D., Kamoun, S. (2017). Rapid generation of a transgene-free powdery mildew resistant tomato by genome deletion. Sci. Rep. 7, (1). doi: 10.1038/s41598-017-00578-x
Noureen, A., Khan, M. Z., Amin, I., Zainab, T., Mansoor, S. (2022). CRISPR/Cas9-mediated targeting of susceptibility factor eIF4E-enhanced resistance against potato virus Y. Front. Genet. 13. doi: 10.3389/fgene.2022.922019
Ortigosa, A., Gimenez-Ibanez, S., Leonhardt, N., Solano, R. (2019). Design of a bacterial speck resistant tomato by CRISPR/Cas9-mediated editing of SlJAZ2. Plant Biotechnol. J. 17 (3), 665–673. doi: 10.1111/pbi.13006
Peng, A., Chen, S., Lei, T., Xu, L., He, Y., Wu, L., et al. (2017). Engineering canker-resistant plants through CRISPR/Cas9-targeted editing of the susceptibility gene CsLOB1 promoter in citrus. Plant Biotechnol. J. 15 (12), 1509–1519. doi: 10.1111/pbi.12733
Pompili, V., Dalla Costa, L., Piazza, S., Pindo, M., Malnoy, M. (2020). Reduced fire blight susceptibility in apple cultivars using a high-efficiency CRISPR/Cas9-FLP/FRT-based gene editing system. Plant Biotechnol. J. 18 (3), 845–858. doi: 10.1111/pbi.13253
Pramanik, D., Shelake, R. M., Park, J., Kim, M. J., Hwang, I., Park, Y., et al. (2021). CRISPR/Cas9-mediated generation of pathogen-resistant tomato against tomato yellow leaf curl virus and powdery mildew. Int. J. Mol. Sci. 22 (4), 1–18. doi: 10.3390/ijms22041878
Prihatna, C., Barbetti, M. J., Barker, S. J. (2018). A novel tomato Fusarium wilt tolerance gene. Front. Microbiol. 9. doi: 10.3389/fmicb.2018.01226
Ramírez Gonzales, L., Shi, L., Bergonzi, S. B., Oortwijn, M., Franco-Zorrilla, J. M., Solano-Tavira, R., et al. (2021). Potato CYCLING DOF FACTOR 1 and its lncRNA counterpart StFLORE link tuber development and drought response. Plant J. 105 (4), 855–869. doi: 10.1111/TPJ.15093
Ran, F. A., Hsu, P. D., Wright, J., Agarwala, V., Scott, D. A., Zhang, F. (2013). Genome engineering using the CRISPR-Cas9 system. Nat. Protoc. 8 (11), 2281–2308. doi: 10.1038/nprot.2013.143
Robertson, G., Burger, J., Campa, M. (2022). CRISPR/Cas-based tools for the targeted control of plant viruses. Mol. Plant Pathology. 23 (11), 1701–1718. doi: 10.1111/mpp.13252
Roossinck, M. J., Martin, D. P., Roumagnac, P. (2015). Plant virus metagenomics: Advances in virus discovery. Phytopathology 105 (6), 716–727. doi: 10.1094/PHYTO-12-14-0356-RVW
Rukavtsova, E. B., Zakharchenko, N. S., Lebedev, V. G., Shestibratov, K. A. (2023). CRISPR-Cas genome editing for horticultural crops improvement: advantages and prospects. Horticulturae 9 (1), 38. doi: 10.3390/horticulturae9010038
Sanfaçon, H. (2015). Plant translation factors and virus resistance. Viruses 7 (7), 3392–3419 . doi: 10.3390/v7072778
Santillán Martínez, M. I., Bracuto, V., Koseoglou, E., Appiano, M., Jacobsen, E., Visser, R. G. F., et al. (2020). CRISPR/Cas9-targeted mutagenesis of the tomato susceptibility gene PMR4 for resistance against powdery mildew. BMC Plant Biol. 20, (1). doi: 10.1186/s12870-020-02497-y
Schaart, J. G., van de Wiel, C. C. M., Lotz, L. A. P., Smulders, M. J. M. (2016). Opportunities for products of new plant breeding techniques. Trends Plant Science. 21 (5), 438–449. doi: 10.1016/j.tplants.2015.11.006
Shu, P., Li, Y., Li, Z., Sheng, J., Shen, L. (2022). SlMAPK3 enhances tolerance to salt stress in tomato plants by scavenging ROS accumulation and up-regulating the expression of ethylene signaling related genes. Environ. Exp. Botany. 193 (2022), 104698. doi: 10.1016/j.envexpbot.2021.104698
Shu, P., Li, Z., Min, D., Zhang, X., Ai, W., Li, J., et al. (2020). CRISPR/Cas9-mediated SlMYC2 mutagenesis adverse to tomato plant growth and MeJA-induced fruit resistance to botrytis cinerea. J. Agric. Food Chem. 68 (20), 5529–5538. doi: 10.1021/acs.jafc.9b08069
Silva, C. J., Van Den Abeele, C., Ortega-Salazar, I., Papin, V., Adaskaveg, J. A., Wang, D., et al. (2021). Host susceptibility factors render ripe tomato fruit vulnerable to fungal disease despite active immune responses. J. Exp. Botany. 72 (7), 2696–2709. doi: 10.1093/jxb/eraa601
Situ, J., Jiang, L., Fan, X., Yang, W., Li, W., Xi, P., et al. (2020). An RXLR effector PlAvh142 from Peronophythora litchii triggers plant cell death and contributes to virulence. Mol. Plant Pathology. 21 (3), 415–428. doi: 10.1111/mpp.12905
Sonoda, E., Hochegger, H., Saberi, A., Taniguchi, Y., Takeda, S. (2006). Differential usage of non-homologous end-joining and homologous recombination in double strand break repair. DNA Repair. 5 (9–10), 1021–1029. doi: 10.1016/j.dnarep.2006.05.022
Tashkandi, M., Ali, Z., Aljedaani, F., Shami, A., Mahfouz, M. M. (2018). Engineering resistance against Tomato yellow leaf curl virus via the CRISPR/Cas9 system in tomato. Plant Signaling Behavior. 13, (10). doi: 10.1080/15592324.2018.1525996
Tavakoli, K., Pour-Aboughadareh, A., Kianersi, F., Poczai, P., Etminan, A., Shooshtari, L. (2021). Applications of CRISPR-Cas9 as an advanced genome editing system in life sciences. BioTech 10 (3), 14. doi: 10.3390/biotech10030014
Thomazella, D. P. T., Seong, K., Mackelprang, R., Dahlbeck, D., Geng, Y., Gill, U. S., et al. (2021). Loss of function of a DMR6 ortholog in tomato confers broad-spectrum disease resistance. Proc. Natl. Acad. Sci. 118 (27), e2026152118. doi: 10.1073/pnas.2026152118/-/DCSupplemental
Tian, S., Jiang, L., Cui, X., Zhang, J., Guo, S., Li, M., et al. (2018). Engineering herbicide-resistant watermelon variety through CRISPR/Cas9-mediated base-editing. Plant Cell Rep. 37 (9), 1353–1356. doi: 10.1007/s00299-018-2299-0
Tian, S.-W., Xing, S.-N., Xu, Y. (2021). Advances in CRISPR/Cas9-mediated genome editing on vegetable crops. In Vitro Cell. Dev. Biology-Plant. 57, 672–682. doi: 10.1007/s11627-021-10187-z/Published
Tran, M. T., Doan, D. T. H., Kim, J., Song, Y. J., Sung, Y. W., Das, S., et al. (2021). CRISPR/Cas9-based precise excision of SlHyPRP1 domain(s) to obtain salt stress-tolerant tomato. Plant Cell Rep. 40 (6), 999–1011. doi: 10.1007/s00299-020-02622-z
Tripathi, J. N., Ntui, V. O., Ron, M., Muiruri, S. K., Britt, A., Tripathi, L. (2019). CRISPR/Cas9 editing of endogenous banana streak virus in the B genome of Musa spp. overcomes a major challenge in banana breeding. Commun. Biol. 2, (1). doi: 10.1038/s42003-019-0288-7
Tripathi, J. N., Ntui, V. O., Shah, T., Tripathi, L. (2021). CRISPR/Cas9-mediated editing of DMR6 orthologue in banana (Musa spp.) confers enhanced resistance to bacterial disease. Plant Biotechnol. J. 19 (7), 1291–1293. doi: 10.1111/pbi.13614
Tsanova, T., Stefanova, L., Topalova, L., Atanasov, A., Pantchev, I. (2021). DNA-free gene editing in plants: a brief overview. Biotechnol. Biotechnol. Equipment. 35 (1), 131–138. doi: 10.1080/13102818.2020.1858159
van Esse, H. P., Reuber, T. L., van der Does, D. (2020). Genetic modification to improve disease resistance in crops. New Phytologist. 225 (1), 70–86. doi: 10.1111/nph.15967
Veillet, F., Perrot, L., Chauvin, L., Kermarrec, M. P., Guyon-Debast, A., Chauvin, J. E., et al. (2019). Transgene-free genome editing in tomato and potato plants using Agrobacterium-mediated delivery of a CRISPR/Cas9 cytidine base editor. Int. J. Mol. Sci. 20, (2). doi: 10.3390/ijms20020402
Voytas, D. F., Gao, C. (2014). Precision genome engineering and agriculture: opportunities and regulatory challenges. PloS Biol. 12 (6), 1–6. doi: 10.1371/journal.pbio.1001877
Vu, T., Sivankalyani, V., Kim, E. J., Doan, D. T. H., Tran, M. T., Kim, J., et al. (2020). Highly efficient homology-directed repair using CRISPR/Cpf1-geminiviral replicon in tomato. Plant Biotechnol. J. 18 (10), 2133–2143. doi: 10.1111/pbi.13373
Wan, D. Y., Guo, Y., Cheng, Y., Hu, Y., Xiao, S., Wang, Y., et al. (2020). CRISPR/Cas9-mediated mutagenesis of VvMLO3 results in enhanced resistance to powdery mildew in grapevine (Vitis vinifera). Horticulture Res. 7, (1). doi: 10.1038/s41438-020-0339-8
Wan, L., Wang, Z., Tang, M., Hong, D., Sun, Y., Ren, J., et al. (2021). Crispr-cas9 gene editing for fruit and vegetable crops: Strategies and prospects. Horticulturae 7, (7). doi: 10.3390/horticulturae7070193
Wang, L., Chen, L., Li, R., Zhao, R., Yang, M., Sheng, J., et al. (2017). Reduced drought tolerance by CRISPR/Cas9-mediated SlMAPK3 mutagenesis in tomato plants. J. Agric. Food Chem. 65 (39), 8674–8682. doi: 10.1021/acs.jafc.7b02745
Wang, L., Chen, S., Peng, A., Xie, Z., He, Y., Zou, X. (2019). CRISPR/Cas9-mediated editing of CsWRKY22 reduces susceptibility to Xanthomonas citri subsp. citri in Wanjincheng orange (Citrus sinensis (L.) Osbeck). Plant Biotechnol. Rep. 13 (5), 501–510. doi: 10.1007/s11816-019-00556-x
Wang, T., Deng, Z., Zhang, X., Wang, H., Wang, Y., Liu, X., et al. (2018a). Tomato DCL2b is required for the biosynthesis of 22-nt small RNAs, the resulting secondary siRNAs, and the host defense against ToMV. Horticulture Res. 5, (1). doi: 10.1038/s41438-018-0073-7
Wang, Z., Hardcastle, T. J., Pastor, A. C., Yip, W. H., Tang, S., Baulcombe, D. C. (2018c). A novel DCL2-dependent miRNA pathway in tomato affects susceptibility to RNA viruses. Genes Dev. 32 (17–18), 1155–1160. doi: 10.1101/gad.313601.118
Wang, Z., Hong, Y., Li, Y., Shi, H., Yao, J., Liu, X., et al. (2021b). Natural variations in SlSOS1 contribute to the loss of salt tolerance during tomato domestication. Plant Biotechnol. J. 19 (1), 20–22. doi: 10.1111/pbi.13443
Wang, Z., Hong, Y., Zhu, G., Li, Y., Niu, Q., Yao, J., et al. (2020). Loss of salt tolerance during tomato domestication conferred by variation in a Na+/K+ transporter. EMBO J. 39, (10). doi: 10.15252/embj.2019103256
Wang, P., Luo, Q., Yang, W., Ahammed, G. J., Ding, S., Chen, X., et al. (2021a). A novel role of pipecolic acid biosynthetic pathway in drought tolerance through the antioxidant system in tomato. Antioxidants. 10, (12). doi: 10.3390/antiox10121923
Wang, X., Tu, M., Wang, D., Liu, J., Li, Y., Li, Z., et al. (2018b). CRISPR/Cas9-mediated efficient targeted mutagenesis in grape in the first generation. Plant Biotechnol. J. 16 (4), 844–855. doi: 10.1111/pbi.12832
Wang, Y., Zafar, N., Ali, Q., Manghwar, H., Wang, G., Yu, L., et al. (2022). CRISPR/Cas genome editing technologies for plant improvement against biotic and abiotic stresses: advances, limitations, and future perspectives. Cells 11 (23), 3928. doi: 10.3390/cells11233928
Wolter, F., Schindele, P., Puchta, H. (2019). Plant breeding at the speed of light: The power of CRISPR/Cas to generate directed genetic diversity at multiple sites. BMC Plant Biol. 19 (1), 176. doi: 10.1186/s12870-019-1775-1
Yamamoto, A., Ishida, T., Yoshimura, M., Kimura, Y., Sawa, S. (2019). Developing Heritable Mutations in Arabidopsis thaliana Using a Modified CRISPR/Cas9 Toolkit Comprising PAM-Altered Cas9 Variants and gRNAs. Plant Cell Physiol. 60 (10), 2255–2262. doi: 10.1093/pcp/pcz118
Yang, S. H., Kim, E., Park, H., Koo, Y. (2022). Selection of the high efficient sgRNA for CRISPR-Cas9 to edit herbicide related genes, PDS, ALS, and EPSPS in tomato. Appl. Biol. Chem. 65, (1). doi: 10.1186/s13765-022-00679-w
Yin, Y., Qin, K., Song, X., Zhang, Q., Zhou, Y., Xia, X., et al. (2018). BZR1 transcription factor regulates heat stress tolerance through FERONIA receptor-like kinase-mediated reactive oxygen species signaling in tomato. Plant Cell Physiol. 59 (11), 2239–2254. doi: 10.1093/pcp/pcy146
Yin, K., Qiu, J. L. (2019). Genome editing for plant disease resistance: Applications and perspectives. Philos. Trans. R. Soc. B: Biol. Sci. 374 (1767), 20180322. doi: 10.1098/rstb.2018.0322
Yoon, Y. J., Venkatesh, J., Lee, J. H., Kim, J., Lee, H. E., Kim, D. S., et al. (2020). Genome editing of eIF4E1 in tomato confers resistance to pepper mottle virus. Front. Plant Science. 11. doi: 10.3389/fpls.2020.01098
Yu, Y., Pan, Z., Wang, X., Bian, X., Wang, W., Liang, Q., et al. (2022). Targeting of SPCSV-RNase3 via CRISPR-Cas13 confers resistance against sweet potato virus disease. Mol. Plant Pathology. 23 (1), 104–117. doi: 10.1111/mpp.13146
Yu, W., Wang, L., Zhao, R., Sheng, J., Zhang, S., Li, R., et al. (2019). Knockout of SlMAPK3 enhances tolerance to heat stress involving ROS homeostasis in tomato plants. BMC Plant Biol. 19, (1). doi: 10.1186/s12870-019-1939-z
Zhan, X., Zhang, F., Zhong, Z., Chen, R., Wang, Y., Chang, L., et al. (2019). Generation of virus-resistant potato plants by RNA genome targeting. Plant Biotechnol. J. 17 (9), 1814–1822. doi: 10.1111/pbi.13102
Zhang, M., Liu, Q., Yang, X., Xu, J., Liu, G., Yao, X., et al. (2020). CRISPR/Cas9-mediated mutagenesis of Clpsk1 in watermelon to confer resistance to Fusarium oxysporum f.sp. niveum. Plant Cell Rep. 39 (5), 589–595. doi: 10.1007/s00299-020-02516-0
Zhang, S., Wang, L., Zhao, R., Yu, W., Li, R., Li, Y., et al. (2018). Knockout of SlMAPK3 reduced disease resistance to botrytis cinerea in tomato plants. J. Agric. Food Chem. 66 (34), 8949–8956. doi: 10.1021/acs.jafc.8b02191
Keywords: bacteria, fungi, drought, salinity, fruits, vegetables, CRISPR/Cas9
Citation: Sardar A (2023) Genetic amelioration of fruit and vegetable crops to increase biotic and abiotic stress resistance through CRISPR Genome Editing. Front. Plant Sci. 14:1260102. doi: 10.3389/fpls.2023.1260102
Received: 17 July 2023; Accepted: 28 August 2023;
Published: 29 September 2023.
Edited by:
Chongmei Dong, The University of Sydney, AustraliaReviewed by:
Jianping Zhang, The University of Sydney, AustraliaCopyright © 2023 Sardar. This is an open-access article distributed under the terms of the Creative Commons Attribution License (CC BY). The use, distribution or reproduction in other forums is permitted, provided the original author(s) and the copyright owner(s) are credited and that the original publication in this journal is cited, in accordance with accepted academic practice. No use, distribution or reproduction is permitted which does not comply with these terms.
*Correspondence: Atish Sardar, YXRpc2guamNjY0BnbWFpbC5jb20=
Disclaimer: All claims expressed in this article are solely those of the authors and do not necessarily represent those of their affiliated organizations, or those of the publisher, the editors and the reviewers. Any product that may be evaluated in this article or claim that may be made by its manufacturer is not guaranteed or endorsed by the publisher.
Research integrity at Frontiers
Learn more about the work of our research integrity team to safeguard the quality of each article we publish.