- 1Microbial Technology Department, CSIR-Central Institute of Medicinal and Aromatic Plants, Lucknow, India
- 2Division of Plant Breeding and Genetic Resource Conservation, CSIR-Central Institute of Medicinal and Aromatic Plants, Lucknow, India
- 3Faculty of Education, Teerthanker Mahaveer University, Moradabad, India
- 4Academy of Scientific and Innovative Research (AcSIR), Ghaziabad, India
The global healthcare market in the post-pandemic era emphasizes a constant pursuit of therapeutic, adaptogenic, and immune booster drugs. Medicinal plants are the only natural resource to meet this by supplying an array of bioactive secondary metabolites in an economic, greener and sustainable manner. Driven by the thrust in demand for natural immunity imparting nutraceutical and life-saving plant-derived drugs, the acreage for commercial cultivation of medicinal plants has dramatically increased in recent years. Limited resources of land and water, low productivity, poor soil fertility coupled with climate change, and biotic (bacteria, fungi, insects, viruses, nematodes) and abiotic (temperature, drought, salinity, waterlogging, and metal toxicity) stress necessitate medicinal plant productivity enhancement through sustainable strategies. Plants evolved intricate physiological (membrane integrity, organelle structural changes, osmotic adjustments, cell and tissue survival, reclamation, increased root-shoot ratio, antibiosis, hypersensitivity, etc.), biochemical (phytohormones synthesis, proline, protein levels, antioxidant enzymes accumulation, ion exclusion, generation of heat-shock proteins, synthesis of allelochemicals. etc.), and cellular (sensing of stress signals, signaling pathways, modulating expression of stress-responsive genes and proteins, etc.) mechanisms to combat stresses. Endophytes, colonizing in different plant tissues, synthesize novel bioactive compounds that medicinal plants can harness to mitigate environmental cues, thus making the agroecosystems self-sufficient toward green and sustainable approaches. Medicinal plants with a host set of metabolites and endophytes with another set of secondary metabolites interact in a highly complex manner involving adaptive mechanisms, including appropriate cellular responses triggered by stimuli received from the sensors situated on the cytoplasm and transmitting signals to the transcriptional machinery in the nucleus to withstand a stressful environment effectively. Signaling pathways serve as a crucial nexus for sensing stress and establishing plants’ proper molecular and cellular responses. However, the underlying mechanisms and critical signaling pathways triggered by endophytic microbes are meager. This review comprehends the diversity of endophytes in medicinal plants and endophyte-mediated plant-microbe interactions for biotic and abiotic stress tolerance in medicinal plants by understanding complex adaptive physiological mechanisms and signaling cascades involving defined molecular and cellular responses. Leveraging this knowledge, researchers can design specific microbial formulations that optimize plant health, increase nutrient uptake, boost crop yields, and support a resilient, sustainable agricultural system.
1 Introduction
Medicinal plants are crucial in the pharmaceutical and drug industries for providing many pharmaceutically vital bioactive molecules for herbal medicine. Rising consumer demand for herbal drugs and natural products has significantly increased the cultivation acreage of medicinal plants, competing with fixed land resources for cereals and other horticultural crops. The intent of increasing productivity per unit area from the limited land resources has led to excessive usage of agrochemicals (fertilizers, insecticides, pesticides, weedicides, etc.) consumption over the past few decades. Their redundant usage has critically affected soil microbiome and environmental health. Therefore, developing green, efficient, affordable, and eco-friendly agrotechnologies is essential for improving medicinal plants’ health and productivity. Sustainable agricultural production is a significant challenge in the global climate change paradigm. In this context, harnessing endophytic microbes as biostimulants can be an effective, sustainable approach. Endophytes are microorganisms (bacteria or fungi) that spend at least a portion of their life cycle forming an association with an asymptomatic plant (Vanessa and Christopher, 2004). Medicinal plants are strongly influenced by microbial endophyte association. In general, endophytic microbes can modify their structure and diversity depending on genotypes, organs, health conditions, and growth stages of host medicinal plants in order to obtain a constant supply of nutrients. Medicinal plants have a range of physiological characteristics, metabolites, and growth patterns that influence their ability to attract different endophytic microbes. Environmental factors considerably impact the quality and yield of medicinal plants. They not only affect the distribution of a medicinal plant but also determine the species of microbial endophytes that can colonize the host during its life cycle.
Plants grown in biologically diverse soil abundant with beneficial microbes have better survival under harsh conditions. The plant’s roots anchor it to the soil, enabling it to absorb minerals and essential nutrients and synthesize chemical substances mediating various plant-microbe interactions. These interactions comprise mutualistic relationships with beneficial microbes; however, parasitism occurs with harmful microbes (Badri et al., 2009). The plant deploys surface-localized receptor proteins to recognize self-modified or microbe-derived molecules to recognize microbial invaders are potentially harmful or beneficial microbes. The recognition of β-glucan chains and plant immunity depends on the degree of polymerization and β-1,3-glucan receptor systems perception by a specific plant species (Wanke et al., 2020). The positive interactions have practical implications useful in pharmaceutical, biotechnological, and agricultural applications, but the negative interactions lead to severe plant diseases that endanger global agricultural productivity. Utilizing plant-microbe interactions eliminates the need for synthetic inorganic pesticides and fertilizers, which lowers input costs and, thus, minimizes the impact of synthetic agrochemicals on vital existing ecological communities (Whipps and Gerhardson, 2007). Furthermore, plant-microbe symbiosis produces crucial compounds of industrial and pharmaceutical interest, which eliminates the need for costly catalysts and synthetic derivatives (Wu et al., 2007).
Integrating plant-associated microbes into farming to support agricultural production mitigates a series of biotic and abiotic perturbations (Tanaka et al., 2005; Vega et al., 2008; Wani et al., 2016; Lata et al., 2018; Mukherjee et al., 2021; Siddique et al., 2022). Biotic and abiotic factors influence many morpho-physiological disturbances in plants, including stunted growth and development, senescence, altered gene expression, cellular metabolism, etc., reducing overall crop yield and quality (Purohit et al., 2019). Abiotic stresses are caused by non-living factors such as drought, salinity, waterlogging, temperature extremes (heat, cold, and freezing), metal toxicity, etc., while biotic stresses (caused by living organisms, especially bacteria, fungi, viruses, insects, nematodes, and weeds, etc.), directly starve the hosts of their nutrients limiting the growth or plant death resulting in the pre- and post-harvest crop losses. Plants can mitigate biotic stressors even if they lack an adaptive immune system by adjusting to specific, sophisticated strategies such as antibiosis, hypersensitivity, allelochemical synthesis, membrane integrity, organelle modifications, etc. Plants’ genetic makeup controls the defensive schemes that respond to these stresses. Numerous genes in the plant genome are either tolerant or resistant to various biotic stressors. Being sessile, plants have no choice to escape these environmental cues; however, they alter their genetic architecture for stress adaptation. Specifically, by inducing immunological responses, generating antioxidants, and inhibiting pathogen growth, endophytic microorganisms help plants cope with biotic and abiotic stress. Notably, the interaction between plants and microbes results in the production of a wide range of bioactive substances, including artemisinin, taxol, phenolic acid, huperzine, azadirachtin, vindoline, guanosine, inosine, serpentine ajmalicine, curcumin, and camptothecin, which are profoundly utilized in agriculture and medicine.
Endophytes modulate levels and activity of phytohormones, viz., gibberellins, cytokinins, ethylene (ET), abscisic acid (ABA), jasmonic acid (JA), and salicylic acid (SA), which play a crucial role in plant growth, fitness, and stress amelioration (Barnawal et al., 2016; Egamberdieva et al., 2017; Xu et al., 2018; Sabagh et al., 2021; Chaudhary et al., 2022; Tripathi A. et al., 2022). In stressful conditions, plant defense systems trigger appropriate cellular responses by responding to stimuli from sensors situated on the cytoplasm or cell surface and transmitting signals to the transcriptional machinery in the nucleus with the help of various signaling pathways. Signaling pathways are crucial for sensing stress and establishing the proper molecular and cellular responses (Mir et al., 2022). Phytohormones are an integral part of the plant defense system, commonly known as the plant’s systemic acquired resistance (SAR) and induced systemic resistance (ISR). These plant hormones operate as plant protective agents against different phytopathogens. In addition to regulating plant physiological and morphological responses, phytohormones also shape the plant microbiome. Different phytohormones induce distinct effects on plant microbiomes. Plants constantly face a wide range of biotic and abiotic stresses that lead to specific transcriptional variations at the individual gene level, with high variability and stress specificity. Therefore, more practical and fundamental studies are required to address the processes and functioning of hormonal signaling and crosstalk. Hence, this review focuses on a detailed overview of the diversity of endophytes in medicinal plants and defense mechanisms at the cellular level associated with endophyte-mediated plant-microbe interactions for biotic-abiotic stress alleviation, including different signaling pathways.
2 Diversity of endophytic microbes in medicinal plants
Endophytic microbes live in various plant habitats that communally shape the plant endomicrobiome and are most frequently found in plant roots, stems, leaves, fruits, and seeds. Generally, they establish communities in intercellular spaces; nevertheless, certain species can penetrate cells (Toubal et al., 2018). The primary habitat and colonization of endophytic microbes are roots, and their preferred entry points are root hairs, cracks, or wounds caused by phytopathogen infection; this permits the leakage of metabolites that attract more endophytes. Nevertheless, the other vital regions for root colonization are the cortex and epidermis intercellular gaps (Compant et al., 2005). For instance, the root colonization of Piriformospora indica, commences in the cortical area with a biotrophic development stage and proceeds to a cell death-dependent step. Rhizospheric microbes associated with Fenugreek (Trigonella foenumgraecum) stimulate host plant growth via soil nutrient uptake and recycling (Kumari et al., 2020). Different endophytes may serve as the primary root mutualistic symbionts in stressful situations where mycorrhizae are often scarce (Mandyam et al., 2010; Rat et al., 2021). Sometimes, endophytes enter within the xylem vessels that migrate from the root zones; several harbor-diversified communities penetrate the aerial regions utilizing the soil surface. The majority of endophytic microorganisms embrace an array of entryways, especially the leaves (phyllosphere), above ground stem (caulosphere), below ground stem (laimosphere), flowers (anthosphere), fruits (carposphere), and seeds (spermasphere) (Lindow and Brandl, 2003; Ritpitakphong et al., 2016; Abdullaeva et al., 2020; Sun et al., 2023). Upon arriving leaves and stems from openings like stomata, they grow and create a thin biofilm (Frank et al., 2017). In addition, several microbes might penetrate the inner regions and establish where other microorganisms may invade the xylem. They continue to colonize and grow in various organs, such as the caulosphere, phylloplane, anthrosphere, and carposphere (Meyer and Leveau, 2012). These microbes are inherently advantageous in that they serve as a marker for the beginning of the community structure in the seedling and the end of the community assemblage in the seed (Shahzad et al., 2018). They are pretty intriguing since they transmit their personalities to subsequent generations vertically and can generate endospores, uphold plant growth, control cell motility, and regulate endogenous phytohormones, which improve the structure of the soil, disrupt seed dormancy, and degrade xenobiotics. However, seed endophytes developed multiple paths; few penetrate through the xylem, stigma, and the extrinsic route, wherein an external factor contaminates seeds. The floral components of plants have not been comprehensively investigated to study endophytic diversity; nevertheless, Qian et al. (2014) isolated an endophytic fungus, Lasiodiplodia sp., from floral parts of Viscum coloratum, which is involved in the synthesis of vital metabolites. Therefore, the diversity of endophytic communities is primarily determined by a series of transforming factors, including the host genetic makeup and immune system, the environment, microbe-microbe interactions, types of soil, and nutrition. Figure 1 depicts the schematic representation of the diversity of endophytic microbes in various plant parts.
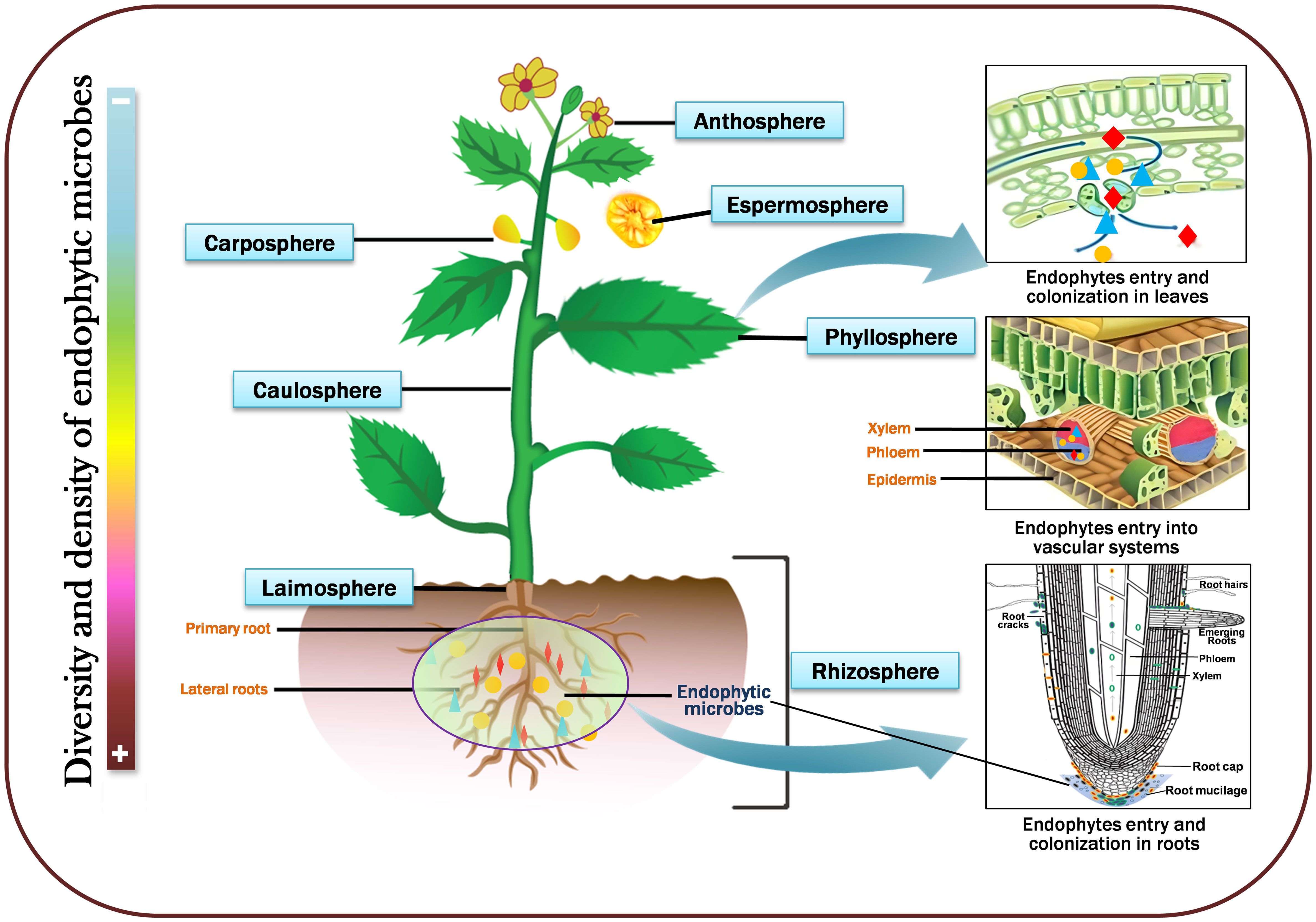
Figure 1 A simplified diagramm showing microbial diversity in various plant parts viz., leaves (phyllosphere), above ground stem (caulosphere), below ground stem (laimosphere), flowers (anthosphere), fruits (carposphere), and seeds (spermasphere). Sidebar color intensities represent microbial density and diversity; dark red represents high, and light blue indicates low diversity and density.
3 The complexity of the plants-microbes relationship
Plant-microbe interactions bear a complex relationship depending on the biological and physicochemical ecology of soil, seed surface, phyllosphere, and rhizosphere. While “obligate” microbes interact with living cells in order to develop and complete their life cycle, “epiphytes” grow upon another plant merely for physical support, and “opportunistic” microbes occasionally penetrate the endosphere of plants (Hardoim et al., 2008). The plant and the endophyte coexist in this interaction and greatly benefit one another (Ting et al., 2009). These endophytes are frequently rhizospheric; basal root zones with tiny crevices and the apical root zone may be the ideal sites for their linkage and subsequent entrance into the host (Gagne et al., 1987). They multiply throughout the host plant (Hallmann et al., 1997) and dwell in the cells, vascular system, or intercellular regions (Bell et al., 1995). While roots have the most excellent chance of colonization through the epidermis created by the lateral root system, endophytic microbes could penetrate through the stomata and transmit vertically to offspring via maternal seeds (Agarwal and Shende, 1987). It is indisputable from the “balanced antagonism” during asymptomatic colonization among the host and endophytic microorganisms that endophytes can survive inside the host without invoking any innate immunity and enhance their ability to sustain themselves by producing substances that are similar to those of plants (Schulz and Boyle, 2005). According to extensive research on the symbiotic association between endophytic microbes and their host plant, the plant safeguards and sustains the endophytes, which ‘in return’ deliver natural compounds with therapeutic potential (antiviral, antifungal, antibacterial, insecticidal, etc.) to uplift the former’s productivity and sustainability in their natural habitat. Additionally, they defend host plants from phytopathogens by triggering the synthesis of plant secondary metabolites under adverse conditions (Azevedo et al., 2000; Strobel, 2003). Hence, they are now considered an essential component of biodiversity; the distribution of endophytic microflora varies depending on the host. They have been found inside nearly all vascular plants, notably those with medicinal properties that have been assumed to be linked to drug synthesis; several studies have shown that these endophytes represent a significant source of medicinal compounds (Zhang et al., 2006).
Endophytic microbes have a wide and diverse niche in plants, which leads to a complex relationship that implies mutualism, antagonism, and rarely parasitism (Nair and Padmavathy, 2014). They reside within the plant tissue, wherein numerous bacteria and fungi species constitute the “plant endomicrobiome,” capable of triggering a number of cellular and physiological changes in the plant. Some relationships between plants and microbes are commensalism, whereby the plant incurs no harm, but the microbe benefits. The microbes and the plant interact through chemical signaling molecules released by the plants and discharge of corresponding microbial substances (phenols, steroids, taxol, xanthones, terpenoids, benzopyranones, isocoumarins, chinones, tetralones, cytochalasins, and enniatines, etc.), resulting in a two-way “crosstalk” that employs signal transduction. Once a link between plant and microbe is established, both organisms continue to monitor each others’ physiology and adjust their behavior accordingly. Endophytic bacteria have a considerable advantage over plants’ rhizospheric bacteria and provide more benefits than microorganisms outside of the plants and in the rhizosphere because they are in direct contact with the plant tissues (Araujo et al., 2002; Hardoim et al., 2015). Fungal endophytes spread into progeny via hyphal fragments or spores in above-ground tissues by pathogens (biotic dispersal agents) or air or water (abiotic dispersal agents) through parent plants, whereby the progeny become infected (Hodgson et al., 2014; Gagic et al., 2018), growing in the rhizosphere’s nutrient-rich environment, harboring airborne pathogenic organisms (Sasse et al., 2018), enabling transmission of fungal endophytes across different host species (Wiewiora et al., 2015).
4 Interaction of secondary metabolites of the host and metabolites from endophytic microbes
The interaction between secondary metabolites of the host and metabolites from endophytic microbes is a complex and dynamic process that can result in diversified effects from beneficial to detrimental. One of the most fascinating aspects of endophytic microbes is their potential to synthesize bioactive compounds that might interact with secondary metabolites of their host. Plant secondary metabolites perform diverse functions in plants, including growth and development, inherent immunity (Piasecka et al., 2015), defense responses (Isah, 2019), stress adaptation (Yang et al., 2018), phytopathogen control, operating as signals for plant-microbe symbiosis, and transforming microbial communities linked to hosts (Guerrieri et al., 2019). Similarly, plant microbiomes are involved in many of the abovementioned processes, directly or indirectly modulating plant metabolism (Trivedi et al., 2020; Adeleke and Babalola, 2021; Ayilara et al., 2022). Plants can shape their microbiome by secreting an array of metabolites; consequently, the microbiome could affect the host plants’ metabolome. Perhaps in medicinal plants, the stimulation of secondary metabolites through endophytes is a common phenomenon that can transform the rhizobiome (Sasse et al., 2018; Cotton et al., 2019). Recent research suggested that interactions between plants and their microbiomes could increase the biomass of Salvia miltiorrhiza, having a unique microbiome (Sphingomonas, Pantoea, Dothideomycetes, and Pseudomonas), as well as affect the synthesis of a novel bioactive compound “tanshinone” (Chen et al., 2018; Huang A. C. et al., 2019). Similarly, Marmoricola sp. and Acinetobacter sp. enhanced morphine content in Papaver somniferum via modulating expression of morphine biosynthesis genes (Ray et al., 2019), and Phialemoniopsis cornearis, Fusarium redolens, and Macrophomina pseudophaseolina influenced forskolin biosynthesis in a medicinal plant Coleus forskohlii (Mastan et al., 2019). Using a chemical recognition framework, plants can also recognize specific molecules released by microbiomes that trigger plants to build signaling networks, modify associated gene functions, and accumulate specific secondary metabolites (Tidke et al., 2019). Nevertheless, it is likely that a portion of these so-called “secondary metabolites” are actually the metabolic by-products of their endophytic microbes. Endophytic microbes can synthesize numerous secondary metabolites, such as paclitaxel (taxol), podophyllotoxin, camptothecin, and deoxypodophyllotoxin, which are also generated by plants (Etalo et al., 2018; Furtado et al., 2019; Pang et al., 2021). Consequently, it is crucial to distinguish which metabolites originated from the plant microbiome and which ones from the host.
The effects of microbial secondary metabolites on plants have been well-documented. Even though some pathogenic microbes secrete toxins that harm plants, such as fumonisins and AAL-toxins made by the Fusarium sp. and Alternaria alternata f. sp. (Chen et al., 2020), many microbes synthesize valuable secondary metabolites that promote plant growth; for example, Bacillus tequilensis SSB07 produces several phytohormones viz., gibberellins, IAA, and ABA which boosted growth and thermotolerance in soybean (Kang et al., 2019). Plant microbiomes can also produce numerous volatile organic compounds (aldehydes, alcohols, ammonia, ketones, terpenes, esters, etc.) that can influence plant development, communication, pathogen defense, and prevent herbivorous insects and parasitic nematodes (Kai et al., 2009; Ortíz-Castro et al., 2009; Zhang et al., 2020). Maggini et al. (2017) reported that the influence of the interaction between the medicinal plant Echinacea purpurea (L.) Moench and its endophytic microbes revealed that microbes could affect the synthesis of volatile organic compounds, phenylpropanoid, and alkamides in the host. Besides, plant-derived non-volatile secondary metabolites like flavonoids and coumarins shape the root microbiota. Furthermore, secondary metabolite “benzoxazinoids” could act as allelochemicals and natural pesticides on the root microbiome (Hu et al., 2018; Schütz et al., 2019; Jacoby et al., 2020). The symbiotic relationships of plants and endophytic microbes enable them to sustain safely, regardless of extremely harsh environments. The long-term coevolution within ecosystems due to this mutual association, each endophyte evolved a distinct range of hosts, allowing them to colonize a specific host group. The production of secondary metabolites, crucial for endophyte-host communication for mutual survival and their sensitivity to various habitats, is hypothesized to be influenced by the coevolution of endophytes and their host (Lind et al., 2017). Endophytes and their host plants share precursors in their corresponding secondary metabolite in biosynthesis pathways. However, endophytes may mimic the host pathways to establish their own metabolic route for secondary metabolites (Alam et al., 2021). Overall, it has been confirmed that despite their diversity, secondary metabolites are synthesized via a few shared biosynthetic, and the metabolomic pathways of endophytic microbes and their host are similar. Determining whether these secondary metabolites are produced by plants or due to symbiosis with endophytic microorganisms remains disputed. Therefore, understanding the processes influencing plant-microbiome assembly, signaling crosstalk in plant-microbiome communications, genetic controls on secondary metabolites, and how microbiomes and environment alter them are exciting research areas for the future.
5 Endophytes-mediated plant-microbe interactions to mitigate environmental cues
Plant phenotypic performance is determined by its genotype, environment, and interactions between genotype x environment. The phenotypic potential of a crop is fully expressed in a stress-free environment with no interference from any environmental factors. However, plants endure a range of perturbations categorized into two major groups: (i) weather extremes or abiotic stresses (drought, soil salinity, waterlogging, low and high temperatures, etc.) and (ii) pathogenesis or biotic stresses (bacteria, viruses, fungi, insects, nematodes, etc.). Endophytes improve plants’ stress tolerance by stimulating the synthesis of secondary metabolites (comprising or clinically useful molecules) through various sophisticated strategies (Tripathi A. et al., 2022; Liu et al., 2023). Moreover, they decrease the pressure caused by toxic heavy metals, reduce hazardous greenhouse gases, and limit pests’ growth on plants through a plethora of other specific methods (through extracellular sequestration, modulating antioxidative enzyme activities, mineral nutrient uptake, degradation of pathways for reducing phytotoxicity, etc.) (Azevedo et al., 2000; Stępniewska and Kuźniar, 2013). Remediation by conventional strategies is quite expensive, laborious, and unsustainable, whereas plant-microbe-based approaches for remediation are remarkably potent, less intrusive, and sustainable (Anderson et al., 1993; Radwan, 2009). Additionally, endophytic plants with pertinent metabolic frameworks and degradation pathways toward diminishing phytotoxicity and optimizing decay can rejuvenate groundwater and wastelands (Weyens et al., 2009). Polyaromatic hydrocarbon (PAH) removal by endophytes is also successful; decreasing atmospheric carbon by storing carbon in plants’ rhizospheres is likely a viable strategy (Wu et al., 2009). The schematic representation of the impact of biotic and abiotic perturbations on plants and how the integration of endophytic microbes helps to alleviate these perturbations is illustrated in Figure 2.
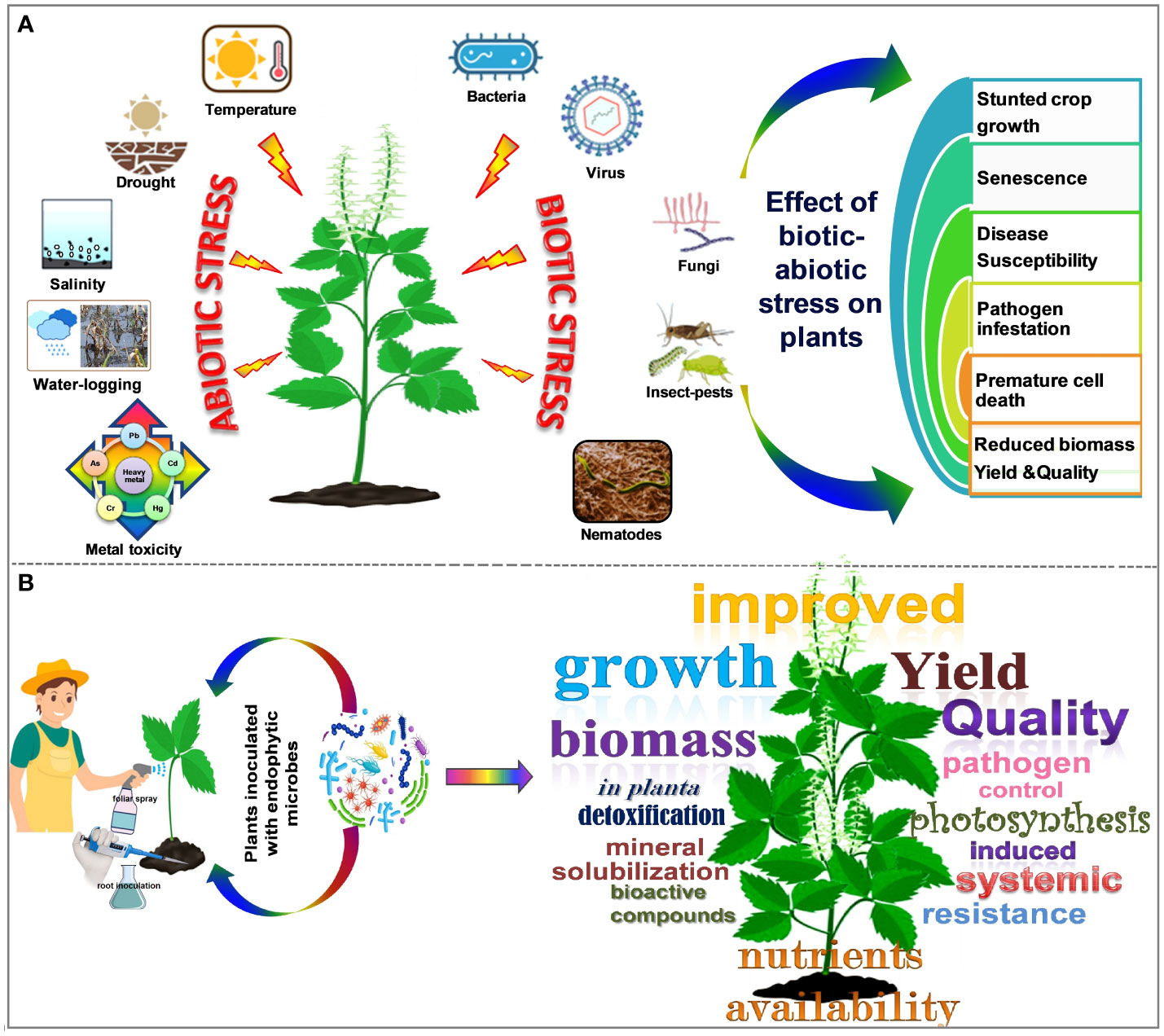
Figure 2 Impact of biotic and abiotic stresses on plants (A), integration of endophytic microbes in plants for improving yield quality and tolerance against different stresses (B).
5.1 Endophytic microbes for abiotic stress tolerance in host medicinal plants
Abiotic factors like drought, salt, heat, freezing, heavy metal toxicity, hypoxia and anoxia, waterlogging, and nutritional imbalance are the most severe constraints leading to a drastic decline in crop production (about 51–82%), which hampers global food and nutritional security (Khare and Arora, 2015; Cooke and Leishman, 2016; Yadav et al., 2020; Del Buono, 2021; Raza et al., 2022; Kaur et al., 2023). These stressors have become more common over the past several decades, mainly as a result of the aberrant weather fluctuations triggered by climate change. Plants tolerate these stresses by modifying their physiological, molecular, and biochemical architecture to maintain homeostasis, including osmotic adjustment, nutrient absorption and assimilation, enzyme activity, membrane integrity, metabolic alterations, and most notably, photosynthesis (Moradtalab et al., 2018; Ahanger et al., 2019; Raza, 2021). Most of these imbalances in response to stress conditions are linked to phytohormone synthesis and distribution in plants’ underground and aerial regions (Verma et al., 2016; Arif et al., 2021). Plants generate reactive oxygen species (ROS) as a consequence of these abiotic stresses, which cause severe cell injuries (Oktem et al., 2008; Hasanuzzaman et al., 2020). To counteract the damaging effects of these cues, plants respond physiologically and molecularly, which includes the synthesis of essential proteins associated with metabolism, stimulation of cell signaling, and transcription factors governed through the expression of the majority of stress-tolerant genes that, in turn, are driven by multifaceted biomolecules (Hasanuzzaman et al., 2020; Raza, 2022).
Drought stress has a detrimental effect on plant growth and development, physiological, biochemical, and cellular metabolism, viz., cell membrane elasticity, fluidity, integrity, stomatal conductance, water potential, the structure of enzymes, proteins, amino acids, nucleic acids, etc. and, as well as the homeostasis of the agroecosystems (Kutasy et al., 2022; Noor et al., 2022). Plants modulate diverse cellular signaling pathways, including phytohormones, stress response proteins, osmolytes, and antioxidant enzymes for drought adaptation (Kosar et al., 2021). Numerous endophytes generate ACC deaminase (1-Amino Cyclopropane-1-Carboxylate), which assists its host plant in combating drought by interrupting the ET biosynthesis pathway and diminishing the ET levels, which in turn restricts stress signals. Bacillus licheniformis K11, having auxin and ACC deaminase-producing activities, mitigated drought’s detrimental effects without using synthetic agrochemicals (Lim and Kim, 2013). Nevertheless, drought drastically reduces photosynthesis compared to plants’ respiration (Vanlerberghe et al., 2016). Crop plants activate regulons like dehydration-responsive element-binding protein (DREB2) in response to temperature and drought stress (Nakashima et al., 2012). Furthermore, plants produce defensive chemicals in response to drought by mobilizing the metabolites critical for their osmotic adjustment. ABA-mediated stomatal closure may be crucial in controlling plant development by lowering other abiotic stressors, including osmotic stress (Waqas et al., 2012). An endophytic microbe, Sinorhizobium meliloti increased FeSOD and CU/ZnSOD, improving drought tolerance in alfalfa (Naya et al., 2007). Likewise, Meng and He (2011) reported an arbuscular mycorrhizal fungus (AMF) maximizes nutrient uptake and modulates metabolic activities (soluble sugar, chlorophyll, leaf subsurface, total phosphorous, total underground nitrogen and tanshinone content, and decreases the content of total aerial nitrogen) to boost drought tolerance in Salvia. Moreover, Trichoderma hamatum promoted drought tolerance in the Theobroma cacao plant by delaying drought-related stomatal conductance and net photosynthesis adjustments (Bae et al., 2009). Sziderics et al. (2007) claim that a fungus called Piriformospora indica increases resistance to osmotic stress by expressing the enzymes ACC-oxidase and lipid transfer protein. The synthesis of ROS under drought conditions often leads to premature cell death (Cruz de Carvalho, 2008), and antioxidant enzymes like catalase (CAT), polyphenol oxidase (PPO), and peroxidase (POD) scavenge ROS to prevent stress-induced damage (Zandalinas et al., 2018). These antioxidants also facilitate rejuvenation from water deficit and dehydration (Laxa et al., 2019). Similarly, Bacillus amyloliquefaciens and Pseudomonas fluorescens improved drought tolerance in Mentha piperita (L.) by enhancing antioxidant enzymes, total phenolic content, and decreasing malondialdehyde (MDA) and proline content (Chiappero et al., 2019). Therefore, antioxidant-producing endophyte microbes are being explored further for favorable eco-friendly gains. Recent research demonstrates the beneficial effects of antioxidant enzymes in peppermint under severe drought (Chiappero et al., 2019; Asghari et al., 2020). Proline accumulation is a key strategy for promoting drought tolerance as it helps in the maintenance of protein structure and function to preserve membrane integrity (Kishor et al., 2005). Besides enhancing antioxidant activity, Pseudomonas strains and Bacillus subtilis also considerably increased proline levels and total soluble sugars in sweet corn (Zarei et al., 2020). Endophytic microbes have an inherent property to produce phytohormones such as gibberellins (GA), auxin, JA, SA, and ABA. These hormones could also be directly responsible for stimulating various defensive systems in host plants. It has been demonstrated that SA performs an important role in drought stress by altering nitrogen metabolism, inducing the generation of antioxidants, and glycine betaine accumulation, thereby conferring protection from stress (Khan et al., 2022). Shah et al. (2019) reported that Piriformospora indica promotes drought tolerance by synthesizing auxins and bioactive compounds in Cymbidium aloifolium (L.) Sw. Similarly, Azospirillum brasilense and A. Chroococcum enhanced drought stress tolerance via improving ABA, proteins, phenolic, soluble sugars, flavonoid, and oxygenated monoterpenes while reducing the activity of CAT and GPX in Peppermint (Asghari et al., 2020). An endophyte, Paenibacillus polymyxa strain CR1, increased Arabidopsis’s dehydration-responsive genes (RD29), enabling the plants to face drought environments effectively (Liu et al., 2020). Likewise, the GOT9 strain of Bacillus subtilis in Arabidopsis stimulated the upregulation of several genes related to drought stress, specifically response-to-desiccation (RD20 and 29B), encodes dehydrin protein (RAB18), as well as 9-cis epoxy carotenoid dioxygenase (NCED3), consequently mitigating the physiological damage caused by drought (Woo et al., 2020). An erratic rainfall pattern due to climate change often functions as an acute stressor, leading to a rapid increase in available soil water, ultimately resulting in premature plant death. Wang et al. (2009) showed that Penicillium griseofulvin reduces water stress injury by improving the function of protective enzymes and osmotic levels, thereby increasing the ability to withstand salt, drought, and water stress in Glycyrrhiza uralensis. Furthermore, Orchard et al. (2016) claimed that the AMF Glomus tenue enhanced the tolerance of ryegrass (Lolium rigidum) plants during waterlogging stress. Pseudomonas putida inoculation in Arabidopsis regulated linked to key polyamine synthetic genes [ADC (arginine decarboxylase), CPA (N-carbamoyl putrescine amidohydrolase, AIH (agmatine iminohydrolase), SPMS (spermine synthase), SPDS (spermidine synthase) and SAMDC (S-adenosyl methionine decarboxylase)] affecting the amounts of polyamine in cells. The higher level of putrescine and free cellular spermidine is positively linked with water stress (Sen et al., 2018). Recently, Endostemon obtusifolius plant inoculated with Paenibacillus polymyxa and Fusarium oxysporum showed enhanced drought tolerance (Ogbe et al., 2023). In other studies Streptomyces dioscori SF1 strain enhanced drought, salinity and phytopathogen resistance in Glycyrrhiza uralensis via the production of ammonia, IAA, enzyme activities, potassium solubilization, nitrogen fixation and Sphingomonas paucimobilis ZJSH1 strain ameliorate drought, salt, and heavy metal toxicity in Dendrobium officinale plants (Li X. et al., 2023; Li J. et al., 2023). Fungal endophytes, Acrocalymma aquatic and Alternaria alstroemeriae provide tolerance against drought-induced damage in Isatis indigotica simply because of synergistic effects on soil enzymatic activity, soil organic material, the biomass of roots, as well as epigoitrin levels (Li W. et al., 2023).
Salinity stress is the most critical abiotic stress that limits crop growth, development, and metabolism, resulting in reduced yield and productivity (Khan et al., 2020; Han et al., 2021). Worldwide, over 6% of the land is classified as saline; this percentage by 2050 is predicted to rise drastically owing to climate change, further aggravating the situation for farming systems. Salinity triggers osmotic pressure, inadequate nutrient supply, and increased ion accumulation beyond critical levels (Hasegawa et al., 2000; Hafeez et al., 2021; Saddiq et al., 2021). Human-generated causes such as irrigation with saline water, industrial pollution, and excessive use of harmful agrochemicals often increase salt stress (Zhu et al., 2019). Different strategies for enhancing plant development under salt stress are triggered by microbial inoculation, including the synthesis of ACC-deaminase, antioxidant enzymes, phytohormones, volatile organic compounds, osmoprotectant metabolites (glycine, proline, alanine, glutamic acid, threonine, serine, choline, betaine, aspartate, and organic acids), modifying ion transporters, which in turn preserves ionic, osmotic, and water homeostasis (Choudhary et al., 2022; Gamalero and Glick, 2022; Kumawat et al., 2022). When sodium ions accumulation reaches toxic levels, ROS is produced that severely damages cellular organelles, viz., mitochondria, chloroplasts, cell membranes, and peroxisomes, impairing plants’ metabolic systems (Munns and Gilliham, 2015). Furthermore, high salinity declined the plant’s water absorption capacity, resulting in poor stomatal activity and reduced cell growth as a consequence of lower cellular water levels. According to Liu et al. (2011), during salt stress, soluble protein content and peroxidase activity (POD) are modulated by endophytic fungi Botrytis sp. and Chaetomium globosum in Chrysanthemum morifolium. Recently, Jan et al. (2019) claimed salt stress tolerance in Euphorbia milii is promoted by the fungus Yarrowia lipolytica. An endophyte, Brachybacterium paraconglomeratu strain SMR20, ameliorates salt stress in Chlorophytum borivilianum via delaying chlorosis and senescence, enhanced foliar nutrient uptake, deamination of ACC, modifying ET, IAA, ABA, proline, and MDA (Barnawal et al., 2016). Similarly, Glutamicibacter halophytocola enhanced tolerance to high NaCl levels in Limonium sinense (Qin et al., 2018). de Zélicourt et al. (2018) have demonstrated that an endophyte Enterobacter sp. conquers the root and shoot tissues of Arabidopsis and promotes salt stress tolerance via producing 2-keto-4-methylthiobutyric. For instance, a bacterial endophyte, Burkholderia phytofirmans modified the gene expression for encoding signaling of cell surface component that signals bacteria of environmental stimuli and subsequently enhances their metabolism (Pinedo et al., 2015; Sheibani-Tezerji et al., 2015). Additionally, numerous bacteria in the plant endosphere modify ABA-mediated cell signaling systems as well as their production during salt stress, which may promote plant development. Similarly, Pseudomonas PS01 induced salinity tolerance by modulating the expression of stress-responsive genes LOX2 (lipoxygenase) while reducing GLY17 (glycogen synthase 17) and APX2 (ascorbate peroxidase 2) in Arabidopsis (Chu et al., 2019). A critical factor in managing the nutrient profile and promoting plant growth during salt stress is enhanced microbe-mediated soil enzymatic activity (Shabaan et al., 2022). Recent research revealed that applying Kosakonia sacchari to soil can lower antioxidants like CAT, APX, GR (glutathione reductase), and SOD (superoxide dismutase) levels and oxidative stress markers like proline, MDA, and H2O2 (Shahid et al., 2021). Similarly, Pseudomonas putida, Klebsiella sp., Alcaligenes sp., and P. cedrina enhanced salt stress tolerance by decreasing the accumulation of MDA, proline, and H2O2 in Medicago sativa (Tirry et al., 2021). Karthikeyan et al. (2012) demonstrated that the inoculation of Achromobacter xylosoxidans in Catharanthus roseus reduced ET levels and increased the content of antioxidants such as APX, CAT, and SOD under salinity stress. Moreover, halophilic microorganisms control critical stress signaling pathways, such as proline, ABA, and MDA synthesis, ultimately minimizing stress impacts (Ayaz et al., 2022). Likewise, Semwal et al. (2023) reported that Bacillus strains NBRI HYL5, NBRIHYL8, and NBRIHYL9 with ACC deaminase activity, biofilm, phosphate solubilization, exo-polysaccharide and alginate generation properties enhanced abiotic stress tolerance in Gloriosa superb. Endophytic microbes, Streptomyces umbrinus EG1 and S. carpaticus EG2 promote root-shoot growth and chlorophyll content, thereby enhancing salt tolerance in Iris persica and Echium amoenum plants (Oloumi et al., 2023).
Like drought, salinity, and water stress, global agricultural production is greatly constrained by temperature extremes (heat, cold, and freezing). Heat stress alters the rate of osmotic adjustment, resulting in a disparity in water potential and a negative impact on metabolism and tissue damage. Plants have developed several tolerance mechanisms to cope with such temperature extremes, including the synthesis of heat-shock proteins (HSPs), pathways for eliminating ROS, and the stimulation of certain phytohormones (Khan et al., 2020; Haider et al., 2021; Raza et al., 2021a). The consequences of cold stress, including chilling temperatures of 15°C and freezing temperatures below 0°C, also severely impact the growth and development of plants (Habibi, 2015). Cold-induced abiotic stress profoundly affects all cellular processes in plants, including several signal transduction pathways by which these stressors are transduced, such as ABA, protein kinase, Ca2+, protein phosphate, ROS components, etc. The plants’ gene expression is altered in response to surviving cold stress, which modifies osmolytes levels, membrane lipids, phytohormones, proteins, ROS scavenging enzymes, and phenolic content (Ritonga et al., 2021; Saleem et al., 2021; Hwarari et al., 2022; Wei et al., 2022). For example, Fernandez et al. (2012) demonstrated that by balancing carbohydrate metabolism, stress-induced gene expression, and increased metabolite levels, Burkholderia phytofirmans PsJN bacterized grapevine showed enhanced tolerance against low temperature. Similarly, Su et al. (2015)) discovered that treating Arabidopsis thaliana with Burkholderia phytofirmans PsJN during cold stress curtailed the plasmalemmas’ disruption and strengthened the mesophyll cell wall. In other studies, PsJN ameliorated cold tolerance in Vitis vinifera with an improved accumulation of proline, aldehydes (ALD), and MDA along with PAL (phenylalanine ammonia-lyase) and STS (stilbene synthase) genes (Theocharis et al., 2012) as well as improved CO2 fixation, starch and phenolics (Barka et al., 2006). However, the Dichanthelium lanuginosum plant relies on endophytic fungi Curvularia protuberate in three-way mutualistic interactions with a virus (virus-fungal endophyte-plant) for survival at high soil temperatures (Márquez et al., 2007).
Metal toxicity is increasing globally due to anthropogenic activities that have not only polluted the soil but also pose a severe threat to human health when they reach the food chain and are biomagnified. Heavy metals like arsenic (As), cadmium (Cd), lead (Pb), mercury (Hg), aluminum (Al), copper (Cu), and zinc (Zn) supplied through irrigation significantly influenced soil dynamics (Nazli et al., 2020; Mehmood et al., 2019; Bashir et al., 2021; Haseeb et al., 2022). The deleterious effects of heavy metal ions on tissues, such as the stimulation of necrosis and chlorosis, inhibition of chlorophyll biosynthesis, and membrane lipid degradation, may significantly impact crop productivity (Takasaki et al., 2010; Raza et al., 2021b; Raza et al., 2022). Plants have evolved sophisticated mechanisms, including hyperaccumulation, tolerance, exclusion, and chelation with organic compounds as the fundamental strategies. Research findings have suggested that endophytic microorganisms play a significant role in boosting resilience to metal toxicity via complex mechanisms, including intracellular accumulation, sequestration, extracellular precipitation, and conversion of toxic metals to a negligible or non-toxic form (Rajkumar et al., 2009; Ma et al., 2016; Mishra et al., 2017). Interestingly, Domka et al. (2019) discovered a fungal endophyte called Mucor sp. significantly strengthens the ability of Arabidopsis arinosa to tolerate metal toxicity. Furthermore, an endophyte, Bacillus sp. SLS18 diminishes the toxicity of heavy metals by accumulating biomass in the root tillers and leaves of Solanum nigrum and Phytolacca acinosa (Luo et al., 2012). Similarly, microbial endophytes Paenibacillus hunanensis strain CIMAP-A4 and BAC-7 improved arsenic tolerance in Bacopa monnieri (L.) via IAA production and biofilm formation (Tripathi P. et al., 2022). Xu et al. (2016) claimed that Agrobacterium spp. and Bacillus spp. reduced arsenate to arsenite in Pteris vittata (L.). An endophyte, Paenibacillus relieved heavy metal toxicity in Tridax procumbens (Govarthanan et al., 2016) as well as helped in the removal of PAHs phytotoxicity via biodegradation of phenanthrene through co-metabolism in Plantago asiatica (Zhu et al., 2016). Endophytic microorganisms can also diminish heavy metal-induced oxidative-stress damage (Wan et al., 2012). The toxic effects of Cd accumulation were synergistically controlled by various plant metabolic defensive systems, including hyperaccumulators, detoxification routes, and antioxidative processes by bacterial endophytes, Klenkia, Modestobacter, Sphingomonas in Lonicera japonica (Xie et al., 2023) Pseudomonas strain E3 in Solanum nigrum (Chi et al., 2023).
5.2 Endophytic microbes for biotic stress tolerance in host medicinal plants
Biotic stresses are known to be affected by abiotic stress conditions in terms of their incidence and dissemination (Scherm and Coakley, 2003; McDonald et al., 2009; Ziska et al., 2010; Peters et al., 2014). Through modifications to plant physiology and defense mechanisms, these stress conditions also directly impact plant-pest interactions (Scherm and Coakley, 2003; Duveiller et al., 2007; Gimenez et al., 2018). Several biological agents, including bacteria, fungi, viruses, weeds, insects, and nematodes, are the major stress factors that tend to increase ROS, affecting how well plants operate physiologically and molecularly and decreasing agricultural productivity. Plant-parasitic nematodes can attack all parts of the plant, although they predominantly harm the root system and spread disease through the soil. They cause stunting and wilting, which are symptoms of inadequate nutrition. Although they seldom kill, their hosts’ viruses can harm plants systemically, producing stunting, chlorosis, and malformations in different regions of the plant. Piercing-sucking insects can spread viruses to plants via their styles. In combination with bacteria, fungi cause a more severe impact, resulting in vascular wilts, leaf spots, and cankers (Schlenker and Roberts, 2009). Insects may physically harm plants severely, including the leaves, stems, bark, and flowers, while infected plants can transmit viruses and bacteria to healthy plants via insects.
In many cases, weeds can take over habitats faster than certain attractive plants because they proliferate and generate many viable seeds. Inhibiting the growth of desirable plants, such as crops or flowers, is not done directly by weeds, which are viewed as undesired and unproductive plants, but rather through competing with the desirable plants for nutrients and space. Through antagonistic action, endophytic microbes can strengthen plants’ defense systems against pathogen invasion (Miller et al., 2002; Gunatilaka, 2006). Additionally, they are said to improve the health of the soil and crops by assisting plants in coping with biotic stress. Therefore, using endophytic microbes as biofertilizers and biocontrol agents has established a natural alternative to harmful chemicals for crop production and alleviating biotic stress. In general, two mechanisms, systemic-acquired resistance (SAR) and induced systemic resistance (ISR), confer plant resistance to pathogens. ISR is defined as the plants’ innate resistance primarily mediated by beneficial microbes via modulating root immunity, root colonization, and the production of specific elicitors like volatile organic compounds, siderophores, polysaccharides, enzymes, and phytohormones, whereas SAR is considered as the plants’ acquired resistance (Olowe et al., 2020; Hamid et al., 2021).
A wide range of pests and pathogens can be successfully combated using the SAR and ISR mechanisms (Vlot et al., 2021; Meena et al., 2022; Yu et al., 2022). Even though multiple studies have shown that endophytic microbes regulate diversified physiological, cellular, and molecular functions in plants and aid in their survival when attacked by pathogens (Teixeira et al., 2019; Olowe et al., 2020; Castiglione et al., 2021; Yu et al., 2022), unfortunately, the fundamental mode of action of pathogenesis has yet to be discovered. The results of comprehensive investigations show that developing resistance to several pathogens, such as bacteria, viruses, and fungi, relies on complex mechanisms that may operate simultaneously (Yu et al., 2022), including stimulation of several defense response genes and enzymes (CAT, GPX (guaiacol peroxidase), APX, GR, SOD, and POD), accumulation of hormones (auxin, GA, ET, JA, and SA), glucanases, sugars, chitinases, PR proteins, secondary metabolites and osmolytes which in turn play a direct role in limiting the growth and spread of pathogens (Baxter et al., 2014; Pieterse et al., 2014; Conrath et al., 2015; Camejo et al., 2016; Guo et al., 2019; Olowe et al., 2020; Luo et al., 2022). Previous research confirmed that endophytes significantly control the host’s gene expression, physiological responses, and defense-related processes in plants (Van Bael et al., 2012; Estrada et al., 2013; Salam et al., 2017). For instance, JA and SA prove to be very helpful in plant stress responses against phytopathogens (Mejía et al., 2008; Ren and Dai, 2012; Khare et al., 2016). Furthermore, the gibberellins synthesized by endophytes boost insect and pathogens’ resistance via SA and JA pathways (Waqas et al., 2015a). Fusarium solani, an endophyte, induces systemic resistance to the pathogenic fungi Septoria lycopersici by promoting the expression of genes associated with the pathogenesis (Kavroulakis et al., 2007). Additionally, some endophytic microbes produce an array of bioactive compounds that might improve the plants’ resistance to different phytopathogens such as Macrophomina phaseolina, which causes charcoal rot disease via siderophores-synthesizing (Arora et al., 2001), Vertcillium wilt (Mercado-Blanco et al., 2004), Cadosporium sphaerospermum and C. cladosporioides through the synthesis of pathogen-toxic cadinane sesquiterpenoids (Silva et al., 2006), antagonistic to pathogenic fungi by toxic chemical “trichothecin” (Zhang et al., 2010), Fusarium oxysporum and F. Solani (Yang et al., 2012), Rhizoctonia solani, Pythium myriotylum, Phytophthora capsici, Colletotrichum gloeosporioides, and Radopholus similis by producing volatile substances(Sheoran et al., 2015) as well as inhibiting pathogenic fungi by releasing some toxins (Wang et al. (2012). According to Strobel et al. (1999), an endophytic microbe Cryptosporiopsis cf. quercina in Triptergyium wilfordii (thunder god vine) produces “cryptocin” and “cryptocandin,” which are poisonous to the host plant’s pathogenic fungus Pyricularia oryzae. Moreover, Cao et al. (2009) reported endophytes, Stachybotrys elegans, Choiromyces aboriginum, and Cylindrocarpon linked with cell wall-disruptive enzymes combat pathogenic fungi in Phragmites australis plant. Microbial endophytes viz., Cohnella sp., Paenibacillus sp., and Pantoea sp. induced plant defense mechanism against anthracnose disease in Centella asiatica (Rakotoniriana et al., 2013). In other studies, Bacillus amyloliquefaciens improved tolerance to root-rot in Panax notoginseng (Ma et al., 2013), phytophthora blight resistance in Ginkgo biloba (Yang et al., 2014), and inhibited multiple phytopathogens in Curcuma longa via synthesizing ‘iturin’ and ‘surfactin’ (Jayakumar et al., 2019). Hong et al. (2018) reported that microbial endophytes, Stenotrophomonas maltophilia and Bacillus sp. suppressed phytopathogens growth in Panax ginseng. Fungal endophytes, Penicillium chrysogenum and Alternaria alternate enhanced tolerance against pathogenic microorganisms in Asclepias sinaica by producing extracellular enzymes viz., amylase, pectinase, xylanase, cellulase, gelatinase, and tyrosinase (Fouda et al., 2015). In another study, Withania somnifera plants inoculated with Talaromyces trachyspermus effectively combat phytopathogens which resulted from the antagonistic activity of endophytes and enhanced IAA, phosphate solubilization, and siderophore synthesis (Sahu et al., 2019). Similarly, Jiang et al. (2018) showed that Bacillus velezensis increased plants’ resistance to gray mold disease caused by Botrytis cinerea by activating antioxidant-mediated defense signaling genes SOD, POD, CAT, and SA-signaling genes viz., NPR1 (non-expressor of pathogenesis-related genes) and PR1 (pathogenesis-related protein1). These findings suggest that endophyte priming triggers molecular and biochemical changes that prevent pathogen invasions of plants. Interestingly, Kumar et al. (2016) identified that the inoculation of the endophyte Peanibacillus lentimorbus in Nicotiana tabacum reduced the prevalence of CMV (cucumber mosaic virus) by augmenting the expression of genes related to stress PR1, AsSyn (asparagine synthetase), Gluc (b-1,3-glucanase), BR-SK1(brassinosteroid signaling kinase 1), TCAS (tetra-hydrocannabinolic acid synthase), ZF-HD (zinc finger-homeodomain), RdRP2 (RNA dependent RNA polymerase), and antioxidants (CAT, SOD, APX, and GPX). Recently, Azabou et al. (2020) reported that an endophyte Bacillus velezensis OEE1 prevents Verticillium wilt disease in olive plants by producing antifungal volatile organic molecules (benzene acetic acid, 1-decene, phenyl ethyl alcohol, tetradecane, and benzaldehyde). Likewise, Microbacterium sp. SMR1 enhanced downy mildew tolerance in Papaver somniferum (L.) via protein modification, differential expression of transcripts related to signal transduction, transcription factors, and SA-dependent defense pathway (Ray et al., 2021). Many researchers showed the ability of both bacterial and fungal endophytes to control diseases and phytopathogens by synthesized volatile and non-volatile compounds, soluble antifungal metabolites and by specific mechanisms including activation of defense enzymes and PR proteins associated with ISR, JA/ET mediated disease resistance, antagonism, antimicrobial, antioxidant, and anti-proliferative properties, production of IAA, siderophores.and β-1,3-glucanase, proteolytic activity, chitinase and cellulose synthesis in diverse medicinal plants including Chloranthus elatior, Taxillus chinensis, Salvia miltiorrhiza, Curcuma longa, Dioscorea bulbifera, Viola odorata, Cremastra appendiculata, Angelica sinensis, Cornus florida, Nicotiana tabacum, Zingiber zerumbet and Piper betle (Harsha et al., 2023; Jiao et al., 2023; Manasa et al., 2023; Mei et al., 2023; Rotich and Mmbaga, 2023; Salwan et al., 2023; Santra and Banerjee, 2023; Sharma et al., 2023; Song et al., 2023; Thankam and Manuel, 2023; Wang et al., 2023; Yehia, 2023; Zou et al., 2023).
It is well documented that endophytic microbes improve host plant resistance to insect herbivores primarily by synthesizing a variety of alkaloid-based protective chemicals in the plant tissue or by changing the nutritional quality of the plant. Eventually, endophytes such as Chaetomium cochliodes, Trichoderma viride, and Cladosporium cladosporioide are known to facilitate insect resistance in creeping thistle (Gange et al., 2012) and red spruce (Sumarah et al., 2010). An endophyte, Epichloë coenophiala AR584, showed enhanced herbivore resistance in Lolium arundinaceum (Schreb.) via the production of alkaloids which provide anti-herbivore defenses, stoichiometry, photosynthesis, and transpiration rates, and stomatal conductance (Johnson et al., 2023). Endophytes function as an acquired plant immune system, taking up space, fighting diseases that may otherwise attack the host, and delaying or deterring herbivores’ infection. For instance, Bittleston et al. (2011) showed that an endophyte Leucocoprinus gongylophorus produces compounds that are antagonistic fungal-ants’ symbionts to boost insect resistance. Furthermore, an endophyte Chaetomium Ch1001 increases resistance to the root-knot nematode by synthesizing ABA that affects the insect juveniles’ second-stage motility (Yan et al., 2011). Additionally, endophytes Beauveria bassiana and Lecanicillium dimorphum improve insect resistance by altering cell division-related protein expressions in the host plant (Gómez-Vidal et al., 2009). Daungfu et al. (2019) found that bacterial endophytes Bacillus subtilis LE24, B. amyloliquefaciens LE109, and B. tequilensis PO80 from the citrus plant with antagonistic properties against phytopathogens might be helpful in the biocontrol of diseases. Diab et al. (2023) recently claimed that endophytic microbes, Streptomyces sp. ES2, Streptomyces, Nocardioides, and Pseudonocardia produce metabolites that act as natural biocontrol agents against insects in Artemisia herba-alba and A. judaica plants. A list of endophytic microbes enhancing abiotic and biotic stress tolerance and associated mechanisms in the host plants are shown in Table 1 (bacterial endophytes) and Table 2 (fungal endophytes).
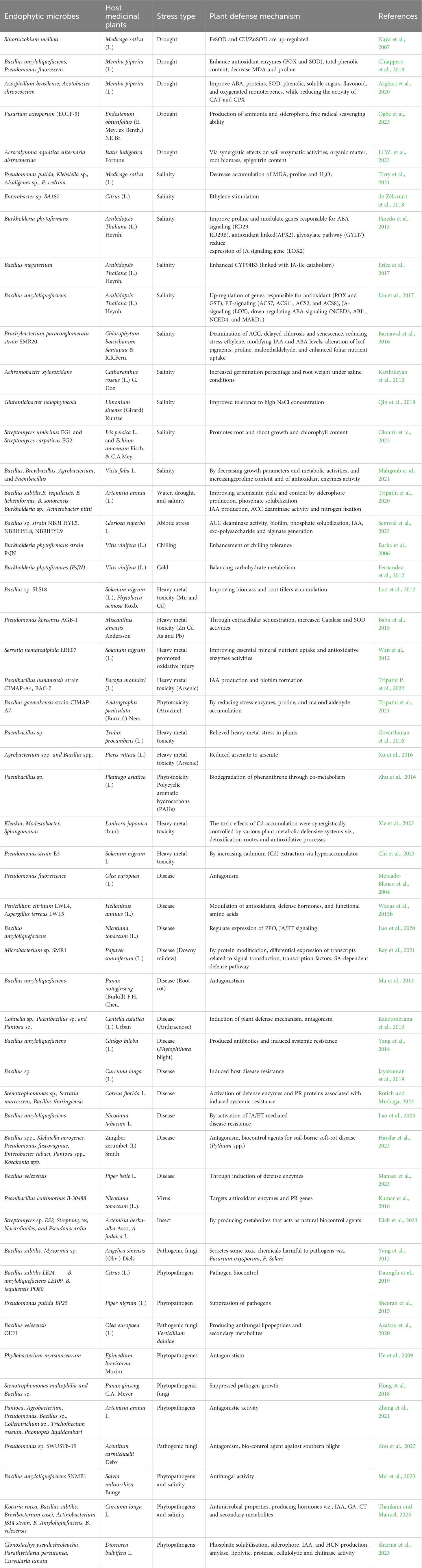
Table 1 Biotic-abiotic stress tolerance and plant defense mechanism conferred by endophytic bacteria in host medicinal plants.
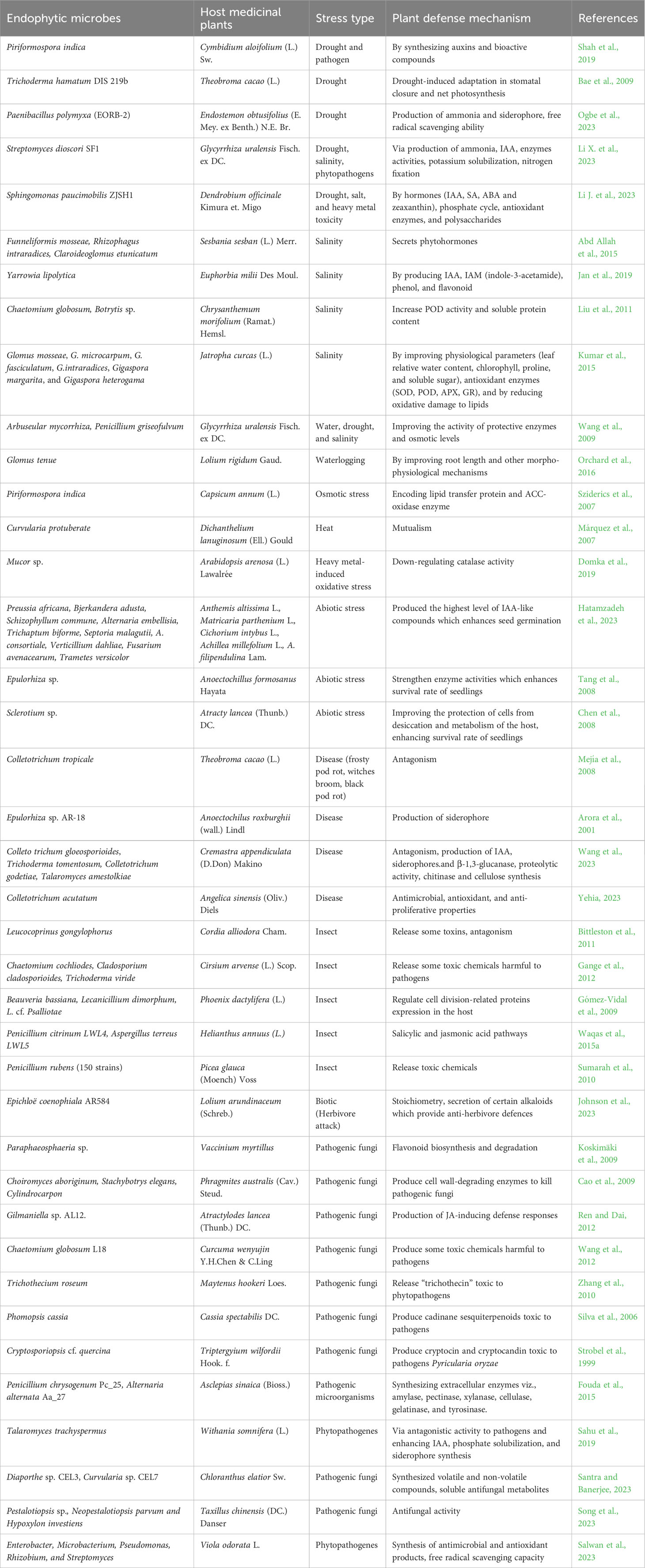
Table 2 Biotic-abiotic stress tolerance and plant defense mechanism conferred by endophytic fungi in host medicinal plants.
These studies confirm that endophytes may increase the hosts’ tolerance to pathogens through diverse methods. In summary, while endophytes invade plant tissues, they impact the interactions between both the endophytes and the pathogens, perhaps causing facilitation (positive stimulation of pathogens), negatively reinforcing host resistance, or exhibiting merely no effect (Suryanarayanan et al., 2009; Adame-Alvarez et al., 2014; Schmidt et al., 2014). Nevertheless, it is unclear how endophytic entomopathogenic fungi invade and are colonized; this requires additional research for confirmation. Plants sense the information signal of stresses and respond accordingly to activate specific molecules to combat such stressors. Furthermore, the behavior of a given plant species or cultivar may vary, plant responses are frequently organ-dependent, and findings acquired with whole plants are sometimes misleading.
6 Mechanisms mediating plant-microbe interactions to alleviate biotic-abiotic stresses
Plants have developed a multitude of physiological (membrane integrity, organelle structural changes, osmotic adjustments, photosynthesis, and respiration, cell and tissue survival, reclamation, increased root-shoot ratio, increased root hair length and density, photosynthates translocations, antibiosis, hypersensitivity, etc.), biochemical (phytohormones synthesis, proline, protein levels, increased chlorophyll accumulation, ACC-deaminase production, antioxidant enzymes accumulation, ion exclusion, generation of heat-shock proteins, protein denaturation, membrane lipid saturation/unsaturation, synthesis of allelochemicals. etc.), and cellular (sensing of stress signals, signaling pathways, ROS generation, SAR, ISR, modulating expression of stress-responsive genes and proteins, regulation of transcriptional factors, etc.) adaptive mechanisms to withstand stressful environments (Figure 3). Endophytes live close interactions with plants and penetrate host plants through their roots, seeds, leaves, and stems to colonize their internal tissues. During the initial phases of colonization, endophytes produce exopolysaccharides (EPS), which aid in adhesion to the root surface and shield them from oxidative damage (Wan et al., 2012). During the fungal transmission of phosphate and nitrogen, the AMF mycelial system mainly spreads around plant roots and facilitates nutrient intake that promotes plant growth in adverse circumstances. Moreover, by maintaining plants’ homeostasis, endophytes diminish water stress damage and trigger regulons like DREB2, stress-induced gene expression, better CO2 fixation, starch and phenolics, HSPs generation, balancing carbohydrate metabolism, disrupting plasmalemmas, and reinforced cell walls to face of drought and temperature (heat and cold) and strengthen the functioning of protective enzymes and osmosis delivering plants more resilience plants to various abiotic stressors including drought, waterlogging and salinity (Barka et al., 2006; Nakashima et al., 2012; Raza et al., 2021a). Different strategies for enhancing salt stress tolerance triggered by microbial inoculation are synthesis of antioxidant enzymes, phytohormones, ACC-deaminase, volatile organic compounds, osmoprotectant compounds (glycine, proline, alanine, glutamic acid, threonine, serine, choline, betaine, aspartate, and organic acids), altering ion transporters, resulting in water, ionic, and osmotic homeostasis. They further strengthen plant resistance to heavy metal toxicity through transport, cell wall development, redox communication, and intra/extra-cellular trapping. Most of these abnormalities in reaction to stressful situations are attributed to the creation and dissemination phytohormones in plants’ subterranean and aerial parts (Verma et al., 2016; Arif et al., 2021). Phytohormones also operate as signal molecules between endophytic microbes and plants, regulating structural and morphological changes necessary for plant growth and to accelerating total root biomass through expanding root length and surface (Spaepen et al., 2007). For instance, Sphingomonas sp. isolated from Tephrosia apollinea augment host plant growth through IAA production (Khan et al., 2014), Pseudomonas spadiceum lowers osmotic stress by producing GA (Waqas et al., 2012) and Pseudomonas, Sphingomonas, Stenotrophomonas, and Arthrobacter sp. generate cytokinins that perform an indispensable function in plants including apical dominance, chloroplast development, cell growth and transformation, senescence prevention, and plant-pathogen interactions (De Hita et al., 2020). Endophytes, including Rhizobium sp., Azospirillum brasilense, Burkholderia cepacia, Acetobacter diazotrophicus, and Klebsiella oxytoca have the potential of biological nitrogen fixation that supply alternate nitrogen for farming (Kong and Hong, 2020). Additionally, some endophytes, such as Pseudomonas fluorescens have the potential to dissolve insoluble phosphates or to liberate organic phosphates through the manufacturing of citric, malic, and gluconic acids (Otieno et al., 2015). Endophytes are also successful in bioremediation (Ayilara et al., 2023) through various methods, such as reducing heavy metal stress (Zhang et al., 2012) and removing dangerous greenhouse gases (Stępniewska and Kuźniar, 2013). In heavy metal-contaminated soil, bacterial root endophytes associated with the medicinal plant Festuca rubra produce siderophores (hydroxamate and catechol) that accelerate host plant development (Grobelak and Hiller, 2017).
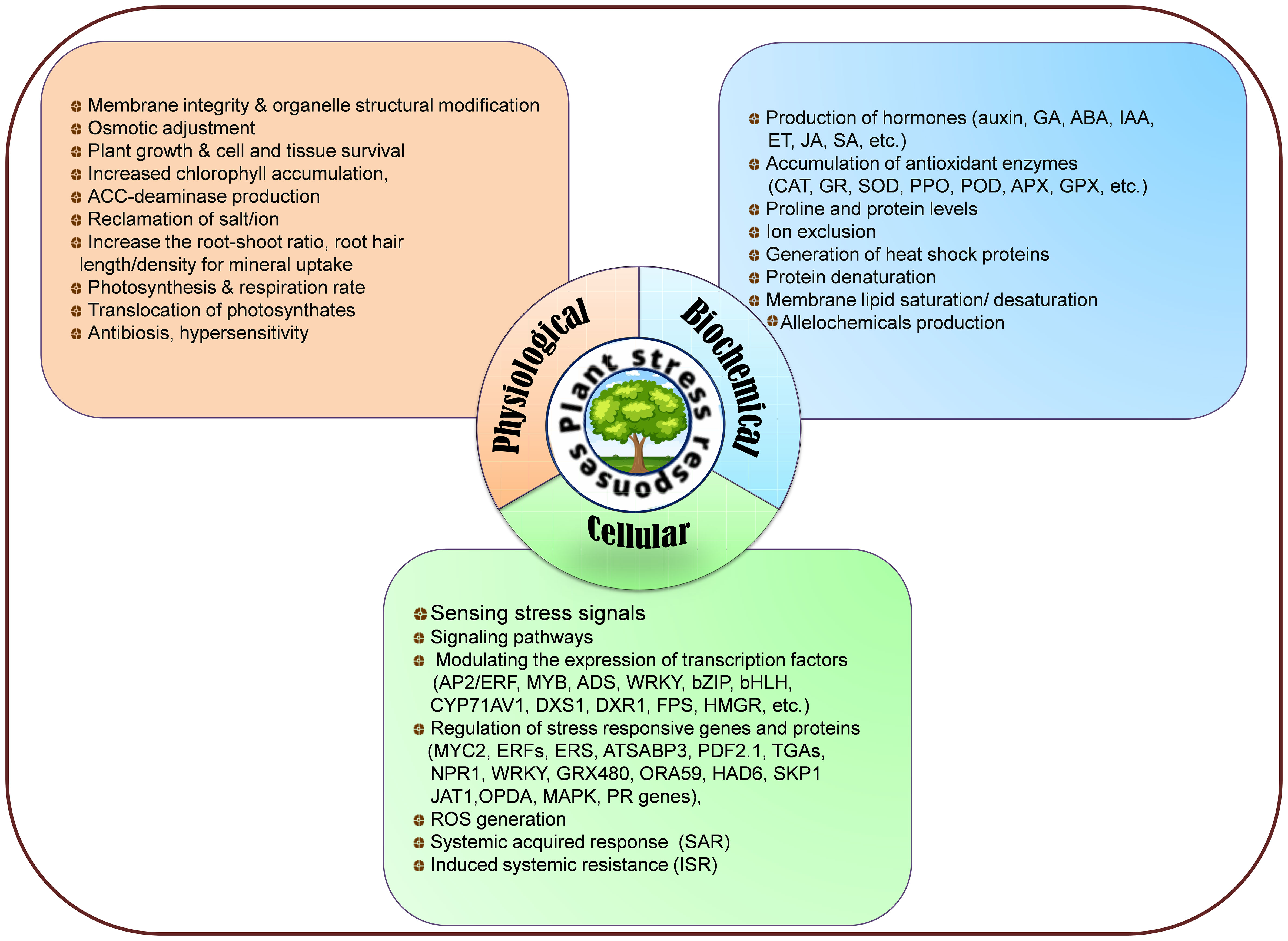
Figure 3 Physiological, biochemical, and cellular responses to mitigate biotic and abiotic stresses.
Biocontrol strategies by endophytic microbes exist directly through pathogen control or indirectly utilizing systemic plant resistance (Santoyo et al., 2016). They produce different kinds of siderophores (phenolate, hydroxamate, carboxylate, etc.) to converse security against pathogens (Rajkumar et al., 2010). Competition for habitats and food resources, the formation of cell wall-degrading enzymes, lytic enzymes, antibiotic compounds, the commencement of ISR, and the quenching of pathogens’ quorum sensing, among some of the other mechanisms (Rajesh and Rai, 2014). The majority of endophytes are recognized for synthesizing secondary metabolites, notably phenols, terpenoids, alkaloids, flavonoids, steroids, and peptides, which have potent antifungal and antibacterial effects and restrict the spread of harmful pathogens. There have been numerous reports of endophytes producing a variety of lytic enzymes, including chitinase, amylase, proteases, cellulose, and hemicelluloses (Bodhankar et al., 2017). Lytic enzymes are critical for establishing endophytes in host cells by the formation of protein biofilms as well as polysaccharides, which lend phytopathogens’ cell walls structural rigidity (Limoli et al., 2015). Nevertheless, it is also beneficial in managing plant diseases through cell wall breakdown while causing cell death (Cao et al., 2009). The virulence-associated factors, viz., biofilm creation, toxin synthesis, antibiotic resistance, and secretions of degradative exoenzymes, are closely governed by quorum sensing. Several pathogenic microbes, Pseudomonas and Ralstonia, effectively employ acylated homoserine lactones for communication, causing significant crop damage (Mansfield et al., 2012). In order to prevent infection, the antiquorum sensing mechanism could be employed (Chen et al., 2013). Moreover, once a pathogen attacks, the inherent immune system is triggered, which blocks the pathogen’s invasion and stops its spread. It is an early defense system against phytopathogens, which involves physical barriers like trichomes, stiff cell walls, and waxy cuticles. Plants release exudates from their roots, comprising proteins, amino acids, and organic acids, which interact among the host plant and endophytes (Kawasaki et al., 2016; Shen et al., 2019; Inbaraj, 2021). Hyperparasitism is a novel biocontrol mechanism where the parasitic host is a plant pathogen; probably the most common hyperparasite is a well-known necrotrophic mycoparasite called Trichoderma species that feeds on host mycelium (Qualhato et al., 2013).
In summary, plant-microbe interactions are an efficient, eco-friendly way for plants to cope with severe environmental conditions. Plants evolved multifaceted relationships with diverse groups of microbes to combat biotic-abiotic stresses. Generally, microbes stimulate plant growth by optimizing the physiology and metabolism of the host through different mechanisms. The symbiosis relationships of microbes on host plants might encourage their recruitment through responsive feedback regarding plant health. Endophytes strengthen crop yield by promoting plant growth via regulating nutrient supply and metabolism, enhancing abiotic stresses (heat, drought, waterlogging, salinity, metal-toxicity etc.) tolerance by generating phytohormones, osmotic adjustment, photosynthesis, and respiration rate while controlling biotic stresses (phytopathogens) through antibiosis, SAR, ISR, competition with pathogens, hyperparasitism, and synthesizing toxins and currently extensively utilized in sustainable agriculture. The mechanism strategies whereby endophytic microbes promote plant growth and control phytopathogens, resulting in increased yields, have been schematically illustrated in Figure 4.
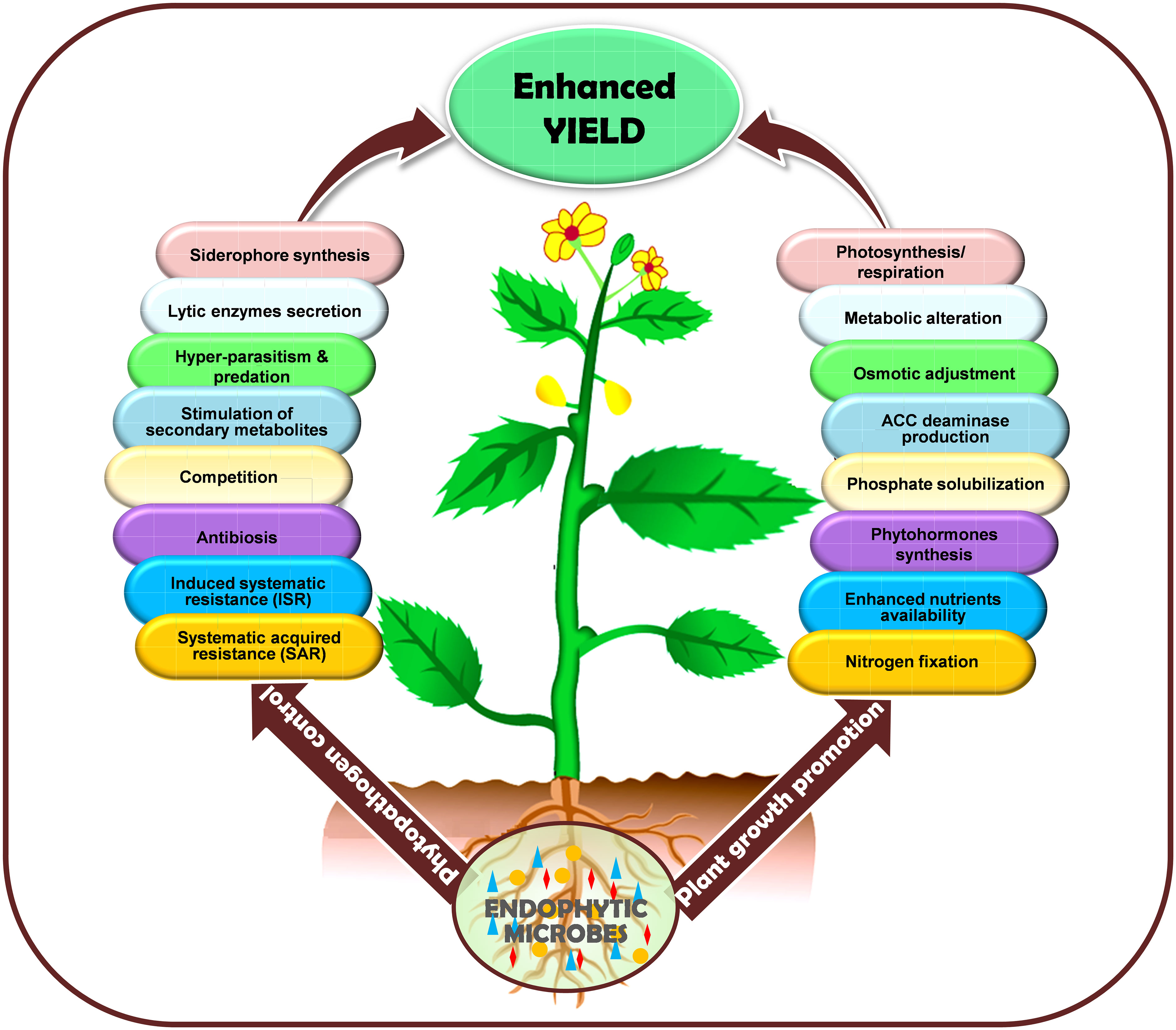
Figure 4 Schematic representation of the endophytes mediated mechanisms in biotic-abiotic stress amelioration in plants. The figure depicts endophytes boosting crop yield through enhancing abiotic stress tolerance by promoting plant growth via regulating nutrient supply and metabolism, phytohormones, osmotic adjustment, photosynthesis, and respiration rate while controlling biotic stress (phytopathogens) through antibiosis, SAR, ISR, competition with pathogens, hyperparasitism, and synthesizing toxins.
7 Hormonal signaling and crosstalk to mitigate biotic-abiotic stresses
Plants’ defense mechanism is influenced by many factors, primarily genetic makeup and the physiological condition of the plant. Each cell in a plant’s defense system has figured out how and where to respond to stressors, thereby creating an inherent immunity. Among these strategies, phytohormones substantially impact plants’ ability to endure stresses. Generally, cytokinins, gibberellins (GAs), and auxins (IAAs) are linked to plant growth and development, whereas ET, JA, and SA are related to plant defense (Koo et al., 2020; Hossain et al., 2021). GAs and IAAs play a significant role in abiotic and biotic stress tolerance, whereas ET, JA, and SA promote abiotic stress tolerance (Kazan, 2013; Santino et al., 2013; Colebrook et al., 2014). When carried directly to the appropriate cells or transmitted to distant tissues, these hormones influence various physiological networks at low concentrations, increasing resistance to environmental stresses (Colebrook et al., 2014). A comprehensive phytohormone network’s tweaking enables plants to respond in a balanced way to developmental and environmental stimuli.
7.1 Ethylene signaling
ET, the gaseous phytohormone, has diversified functions in plants, including cell division and elongation (Love et al., 2009), apical dominance (Yeang and Hillman, 1984; De Martinis, 2000), senescence and abscission (Pierik et al., 2006), flowering (Ogawara et al., 2003; Wang et al., 2013), fruit ripening (Barry and Giovannoni, 2007), breaking seed dormancy and promoting seed germination (Corbineau et al., 2014; Wang et al., 2018; Ahammed et al., 2020), as well as a critical role in programmed cell death (Bouchez et al., 2007). It is a crucial player in both harmful and advantageous plant-microbe interactions (Pierik et al., 2006; Schaller, 2012; Ravanbakhsh et al., 2018; Liu et al., 2019), either through interactions with other phytohormones (Leon-Reyes et al., 2009; Leon-Reyes et al., 2010; Zander et al., 2010) or by controlling the expression of ethylene-responsive genes (Broekaert et al., 2006; Teixeira et al., 2019). Since many biotic and abiotic perturbations influence plants’ physiological and developmental processes, ET synthesis plays a pivotal role in the plant’s adaptation to these environmental threats (Arraes et al., 2015; Sun et al., 2016; Fröhlich et al., 2023). The sensing of ET signaling occurs at the endoplasmic reticulum membrane, triggering a signaling cascade that controls the transcription of ethylene-responsive genes in the nucleus via ERFs (ethylene-responsive factors) (Ju and Chang, 2015). However, the ET-signaling pathway in Arabidopsis is negatively regulated by the ET-receptors viz., ethylene response sensors (ERS1, ERS2), ethylene response (ETR1, ETR2), and ethylene insensitive4 (EIN4) (Liu and Wen, 2012). These ET-receptors stimulate constitutive triple response1 (CTR1) in the absence of ET-signaling, which restricts EIN2, a positive regulator of ET-signaling, through phosphorylating EIN2’s C-terminus. Conversely, the presence of ET renders the ET receptors inactive, thereby preventing CTR1 activation. Subsequently, dephosphorylated and cleaved EIN2 C-terminus (CEND) reaches the nucleus, where it stimulates the function of ethylene-insensitive3/ethylene-insensitive3-like1 (EIN3/EIL1), which modulates the expression of ethylene-responsive genes like ERFs. ERFs constitute transcription factors (TFs) with AP2domains that control various genes associated with stress tolerance, growth, development, and hormone-related pathways (Chen et al., 2010; Shakeel et al., 2015; Zhao et al., 2021).
The up-regulation of ET-biosynthesis genes following interactions with advantageous microbes reveals that ET-signaling is activated not only in response to pathogenic microbes but also to helpful endophytic microbes before they are recognized as friends, possibly to optimize the colonization of adequate levels of beneficial microbes (Ravanbakhsh et al., 2018; Eichmann et al., 2021). Owing to inherent physiological reactions to abiotic stressors, plants can instantly produce an enormous amount of ET, which helps the plants to withstand external challenges, but it can also jeopardize growth and development, thereby reducing crop yield and productivity since increased ET levels can cause senescence, abscission, and chlorosis. Research on plant growth-promoting rhizobacteria (PGPR) has shown that they can prevent soil-borne pathogen infections in plants in an ET-dependent way. Furthermore, beneficial microbes can stimulate ISR and SAR in plants to control diseases (Ton et al., 2001).
7.2 Salicylic acid signaling
SA, a key phytohormone, has crucial physiological and cellular impacts on plants, including membrane permeability and photosynthetic metabolism, and absorption and transport of ions during stress (Noreen et al., 2009). Furthermore, SA is recognized to outwit various abiotic stresses like ROS, pathogens attacks, drought, and salinity (Hara et al., 2012). Additionally, it regulates plant responses to infection by diversified pathogens, viz., bacteria, fungi, viruses, etc. (Fujita et al., 2006; Loake and Grant, 2007), and is necessary for developing resistance strategies like host cell death, ISR, and SAR. The expression of various genes, including those encoding PR-proteins (pathogenesis-related proteins), might be a mechanism whereby SA induces stress tolerance (Nakashima et al., 2009). The cytoplasm contains an oligomer of NPR1, a crucial regulator of SA-induced plant resistance. Once a disease has occurred, it monomerizes and transports to the nucleus, activating a series of genes involved in pathogenesis (Kinkema et al., 2000). But in normal plants, Cys156’s S-nitrosylation, which prevents its monomerization, controls the oligomer to monomer switch. Following infection, nitrous oxide (NO) accretion causes the Arabidopsis thaliana SA-binding protein 3 (ATSABP3) to become S-nitrosylated at Cys280, which reduces the protein’s capacity to bind to SA and inhibits its carbonic anhydrase function (Wang F. et al., 2019). In contrast, S-nitrosylation regulates SAR by focusing on the NPR1/TGA1 system. As mentioned earlier, SA activates thioredoxin (TRX), which helps denitrosylate NPR1 so that it may be monomerized throughout the plant immune response (Kneeshaw et al., 2014). This facilitates NPR1 to enter the nucleus and interact with the primary leucine zipper transcription factor TGA, which in turn makes it easier for TGA to bind to the gene-expression promoters. Upon sensing and detecting stimuli of stresses, mitogen-activated protein kinase (MAPK) cascades are triggered that regulate the stress-modulatory systems and are responsible for the signaling of diverse cellular activities under different stressors (Brader et al., 2007). SA facilitates the activation of MAPK pathways driven by pathogen infection and the subsequent production of PR genes for host defense (Xiong and Yang, 2003). Following MPK3 phosphorylation, the Arabidopsis protein VIP1 is translocated into the nucleus and functions as a covert inducer of PR1 genes (Pitzschke et al., 2009). Similarly, MAPKs such as MPK3, MPK4, and MPK6 are confronted with different stresses (Ichimura et al., 2000; Gudesblat et al., 2007). Moreover, pathogen-associated molecular patterns (PAMPs), such as flagellin, activate MAPK cascades to develop pathogen response signaling (Chinchilla et al., 2007). In addition to interacting with ABA-signaling pathways and ROS to improve plant defense, MAPK cascades also play a crucial role in modulating cross-tolerance (Miura and Tada, 2014; Zhou et al., 2014).
7.3 Jasmonic acid signaling
JA is another hormone crucial for eliciting responses against various biotic and abiotic perturbations by triggering plant defense signaling systems (Berendsen et al., 2012; Broekgaarden et al., 2015; Wang J. et al., 2020; Yadav et al., 2021). It is ubiquitously present in plants, having multiple regulatory functions, notably root growth inhibition (Han et al., 2023), axis elongation and root formation (Huang P. et al., 2019), leaf senescence (Wang T. et al., 2020), stomatal opening (Suhita et al., 2003), and flower formation (Niwa et al., 2018). Research findings have shown that JAs boost plant growth and development and various adverse environmental circumstances using JA-signaling pathways. Microbe-associated molecular patterns (MAMPs), damage-associated molecular patterns (DAMPs), and herbivore-associated molecular patterns (HAMPs), which are predominantly derived from attacking organisms, cell damage, and abiotic stresses, are some plant-environment interaction models linked to JA-signaling pathways (Newman et al., 2013; Basu et al., 2018; Hou et al., 2019). The most functional JAs in plants’ cells is jasmonyl isoleucine (JA-Ile); however, under normal conditions, its concentration is relatively low (Fonseca et al., 2009). It is recognized that the formation of JA-Ile in plant leaves during stressful situations serves as a physiological defensive system. Jasmonates are transported to the apoplast and nucleus from the cytoplasm by JA-transfer protein1 (JAT1), located in both cell and nuclear membranes (Wang Y. et al., 2019). Even in distant regions, the presence of JAs in the apoplast triggers the JA-signaling system, and the signals are sent to neighboring cells via the vascular bundles and air transmission (Thorpe et al., 2007). Different JAs synthases are localized in the sieve component of vascular bundles, which enables the re-syncretization of JAs throughout their movement (Heil and Ton, 2008). The biosynthesis of the JA precursor 12-oxo-PDA (OPDA) in the phloem sieve component has confirmed the theory of re-synthesis. Owing to the reduced level of JA-Ile under normal situations, specific transcription factors (TFs) are unable to activate the promoters of jasmonates-responsive genes. Owing to the reduced level of JA-Ile under typical conditions, specific transcription factors (TFs) cannot trigger the promoters of jasmonates-responsive genes.
The expression of the jasmonates sensitive genes is inhibited by the efficient transcriptional repression complex, composed of the proteins rendering and the putative JAZ (jasmonate-zim domain) interactor. This complex is further activated by histone deacetylase 6 (HAD 6), which closes the open complex (Hause et al., 2003). Thirteen JAZ proteins from Arabidopsis have been identified to contain the main ZIM domain and the C-terminal JA-associated domain. Different parts of JAZ proteins promote protein complexes (Gimenez-Ibanez et al., 2015). JAZ links with TFs and NINJA (novel interactor of JAZ) [comprising ethylene-responsive element binding factor associated with amphiphilic repression (EAR) motif and recruits TPL (topless)] to form the JAZ-NINJA-TPL repressor complex (Pauwels and Goossens, 2011). The amino acid sequence, JAZ degron, known as JAZ degron seems to have a bipartite structure with a loop and amphipathic alpha hexyl that bind coronatine or JA-Ile and coronatine insensitive 1 (COI1), respectively (Sheard et al., 2010). SKP1 (Suppressor of kinetochore protein1) and SCF (cullin-F-box) create the ubiquitin-proteasome complex. Establishing an SCF-type E3 ubiquitin ligase is the outcome of the interaction between SKP1 and cullin with the F-box protein. In stressful conditions, this F-box protein COI can identify the JA-Ile and deliver it to the nucleus. JA-Ile facilitates JAZ and COI1 communication inside the SCF complex, with inositol pentakisphosphate functioning as a cofactor in the formation of the CO1-JAZ co-receptor complex (Mosblech et al., 2011). JAs-mediated defenses are modulated by the proteasome-mediated degradation of the JAZ protein and the release of transcription factors (TFs) under environmental perturbations. According to Qi et al. (2011), there is solid proof that the expression of the genes that respond to jasmonates is primarily dependent on the linkage of transcription factors (TFs) with JAZ repressors.
7.4 Crosstalk between ethylene, jasmonic and salicylic acid
Hormonal signaling crosstalk triggers plants to develop certain specific traits that make them tolerant against the plethora of biotic and abiotic stresses via distinct molecular pathways with a complex network of regulatory interactions (complementary, antagonistic, and or synergistic). Specifically, ET modulates plant defense by controlling the levels of JA and SA (Leon-Reyes et al., 2009; Zander et al., 2010). In such defense responses, ET and JA act synergistically (Penninckx et al., 1998; Zhu, 2014), nevertheless, it has also been reported that they mutually antagonize functions of each other in some specific circumstances (Turner et al., 2002; Bodenhausen and Reymond, 2007). Lorenzo et al. (2003) documented that the ERFs integrate signals from ET and JA. Eventually, other prominent genes that are expressed following the detection of ET and JA include PDF1.2, POTLX3, ACS (ethylene synthesis gene), THI2.1 (thionin), PR-3 (chitinase), PR-4 (hevein-like protein), PR-6 (proteinase inhibitor), and PR-9 (peroxidase) (Kolomiets et al., 2000; Norman-Setterbald et al., 2000; Kondo et al., 2007; Chen et al., 2009). However, ET shows antagonistic effects with SA, and they can both suppress each other’s biosynthetic pathways. The direct interaction between NPR1 and EIN3 prevents the transcription of genes activated by EIN3, a crucial element of SA signaling (Huang P. et al., 2019). As a result, EIN3 and EIL1 bind directly to the SID2 promotor, decreasing pathogen-induced SA production and increasing disease susceptibility in host plants (Chen et al., 2009).
Likewise, it is quite interesting that the crosstalk between the antagonistic pathways of hormones JA and SA also results in plant tolerance to various stresses. Several genes, including MYC2, plant defensin 2.1 (PDF2.1), TGAs, MAPK, NPR1, ERF1, WRKY62, WRKY70, glutaredoxin 480 (GRX480), and octadecanoid-responsive Arabidopsis (ORA59), play a critical role in JA-SA inter-modulation (Wang et al., 2021). Three NAC (TF family) genes-ANAC019, ANAC055, and ANAC072 interact with MYC2 in different ways to prevent SA accumulation. These TFs also regulate the expression of genes that produce SA. GRX480 preferentially binds to TGAs, modulating PR1 gene expression, and MPK4 controls GRX480 positively (SA-signaling pathway), while MYC2 is negatively regulated (JA-signaling pathway). However, GRX genes can prevent the activation of the JA response gene ORA59 (Wang et al., 2020). The hormonal changes between interactions of JA and SA enhance plants’ tolerance against chilling, drought, and oxidative stress. Methyl jasmonate (MeJA) possesses excellent permeability to cell membranes than JA and is very volatile by nature, and it might quickly diffuse nearby plants (Munemasa et al., 2011). External MeJA supplementation controls the formation of ROS and the immune systems by promoting antioxidant enzyme activity in Panax ginseng (Wahab et al., 2022). Following stress sensing, plants rapidly generate ROS (Wojtaszek, 1997; Foyer and Noctor, 2005). Furthermore, the plant meticulously regulates ROS synthesis to prevent tissue damage (Vinocur and Altman, 2005; Mittler et al., 2011; Bhattacharjee, 2008). It has been recognized that although higher levels of ROS are toxic and harmful to organisms and can cause permanent cell death, its lower levels are primarily responsible for controlling stresses. Perhaps ROS could be the critical factor facilitating cross-tolerance between biotic and abiotic stress-responsive stimuli (Choudhury et al., 2013; Kissoudis et al., 2014). A diagrammatic representation of ET, JA, and SA signaling cascade and pathway genes for biotic and abiotic stress tolerance is illustrated in Figure 5.
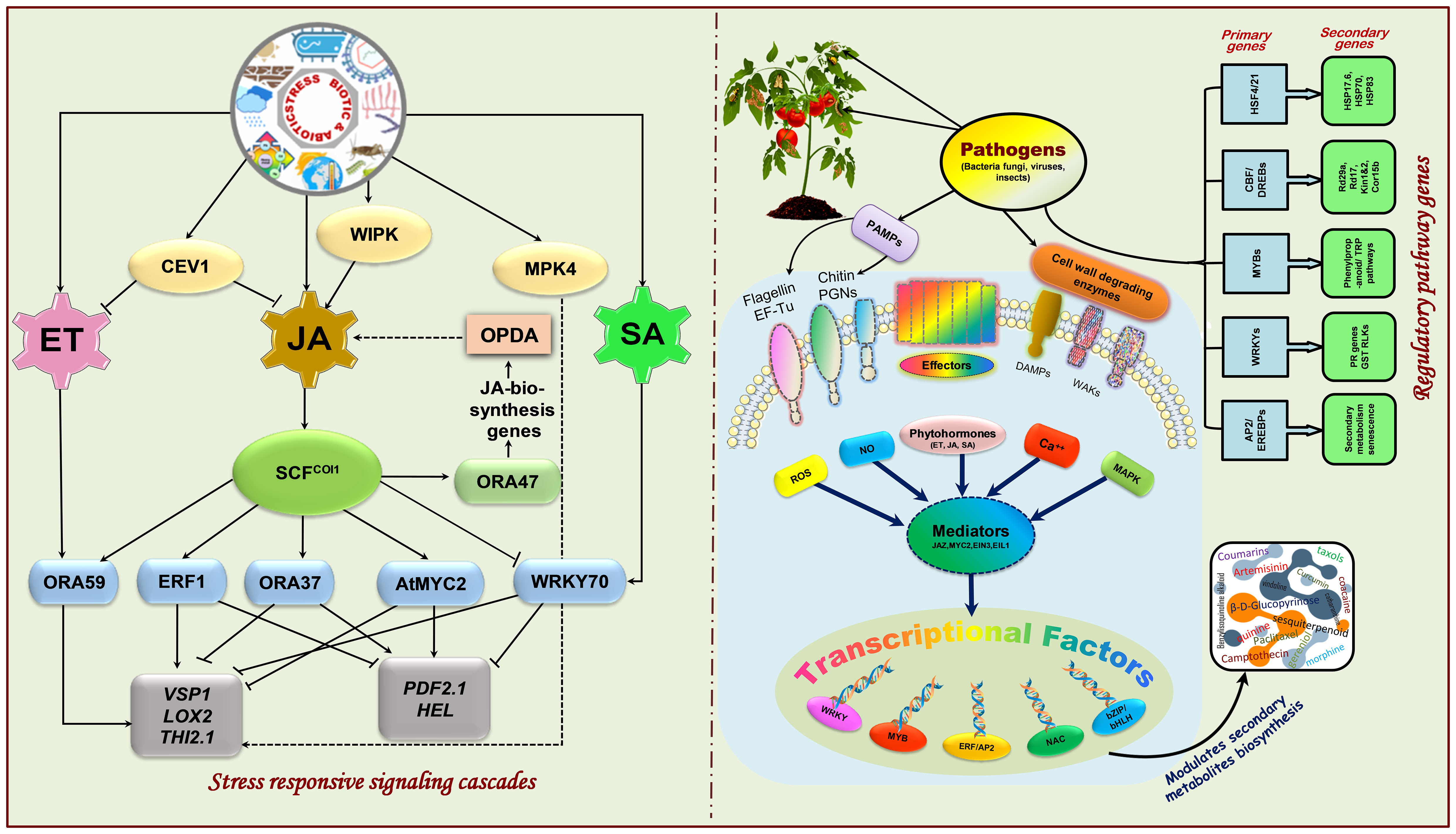
Figure 5 Signaling pathways and regulatory genes to mitigate biotic and abiotic stresses. This figure show a simplified depiction of biotic/abiotic stress-induced signaling pathways like jasmonic acid (JA), salicylic acid (SA), and ethylene (ET) signal transduction and their cross-talk with each other. JA has a central hub position acting with ET and SA. ET, in turn, primarily regulates SCF biosynthesis, transport, and signaling, which is crucial for establishing other genes, like ORA59, ORA37, ERF1, AtMMYC2, and WRKY70, and activation of downstream signaling genes for resistance to different type stresses. Furthermore, a cascade of early (primary) and late (secondary) genes is activated in response to pathogen and insect-induced damage. Genes of herbivory resistance, plant disease resistance, JAs, and endogenous signaling molecules are not only involved in the pathogen resistance mechanism of plants but also have an apparent defensive effect on necrotrophic pathogens. Significant changes in defensive enzymes and secondary metabolites occur, which play essential roles in plant resistance against pathogens. CEV1, Cellulose synthase family protein; WIPK, wound-induced protein kinase; OPDA, 12-oxo-PDA; SCFCOI1, (Skp, Cullin, F-box containing complex); ORAs, octadecanoid-responsive Arabidopsis; ERFs, ethylene-responsive genes, AtMYC2, Arabidopsis thaliana MYC2; VSP1, vegetative storage protein1; LOX2, lysyl oxidase-like 2; THI2.1, thionin 2.1; PDF2.1, plant defensin2.1; HEL=, AP2: adipocyte protein 2, EREBPs, ethylene-responsive element binding proteins; MYBs, Myeloblastosis; CBF, C-repeat binding factors; DREBs, dehydration responsive element binding protein; HSF4/21, heat shock factor protein, GST, plant glutathione S-transferases; RLKs, receptor-like kinases; TRP, transient receptor potential; Rd, responsive to desiccation, Kln, kallikreins; Cor15b, cold-responsive15b.
8 Endophytic microbes as biostimulants in sustainable agriculture
8.1 Benefits
Endophytes are an array of ubiquitous microorganisms that inhabit different niches in plant tissues. In addition to the fact that endophytic microbes can help plants to lessen the negative effects of abiotic stresses, research has shown that endophytes have functional traits with linked detrimental impacts of environmental factors on the continued existence and development of susceptible plant species by synthesizing bioactive compounds, triggering resistance that results from gene expression, and altering the metabolism of certain enzymes. They can inhibit the growth of phytopathogens via the production of antifungal compounds, thereby augmenting crop yields by facilitating plants to acquire nutrients while synthesizing phytohormones. Moreover, they reduce heavy metal stress, eliminate hazardous greenhouse gases, and degrade PAHs in the bioremediation process (Stępniewska and Kuźniar, 2013). Additionally, in recent years, endophytes have gained more recognition for their use in the phytoremediation of a range of environmental pollutants and could be helpful in developing effective cleanup systems (McGuinness and Dowling, 2009; Weyens et al., 2009; Segura and Ramos, 2013; Anyasi and Atagana, 2018; Adeleke et al., 2022). The diversity of endophytes, their ability for stress adaptation, and their synthesis of metabolites make them an endless supply of novel metabolites that can reduce harmful chemicals in agriculture. To illustrate, several studies have reported the beneficial effects of microbial endophytes on a wide range of medicinal plants, including Withania somnifera, Artemisia annua, Papaver somniferum, Cymbidium aloifolium, Salvia miltiorrhiza, Catharanthus roseus, Bacopa monnieri, Nicotiana tobaccum, Andrographis paniculata, Chlorophytum borivilianum, Panax ginseng, Panax notoginseng, Curcuma longa, Curcuma wenyujin, etc. (Meng and He, 2011; Karthikeyan et al., 2012; Wang et al., 2012; Ma et al., 2013; Barnawal et al., 2016; Kumar et al., 2016; Hong et al., 2018; Jayakumar et al., 2019; Sahu et al., 2019; Shah et al., 2019; Jiao et al., 2020; Ray et al., 2021; Zheng et al., 2021; Mei et al., 2023; Salwan et al., 2023; Sharma et al., 2023; Song et al., 2023; Wang et al., 2023; Zou et al., 2023). Thus, unquestionably, these endophytes have demonstrated tremendous potential as a green and eco-friendly alternative for boosting food production in sustainable agricultural systems.
8.2 Potential applications
Biostimulants are a class of substances or microbes derived from natural resources that are applied to soil or plants to boost crop yield and quality by stimulating plants’ biological processes or enriching the soil microbiome for better nutrition and stress tolerance. Biostimulants have emerged as a boon for sustainable agriculture because they significantly accelerate the process of agronomic trait advancement in plants without jeopardizing yield, quality, or biodiversity. In recent years, endophytic microorganisms have been thoroughly explored for the possibility of being utilized as biostimulants for minimizing the usage of harmful chemicals in agriculture, thereby fulfilling the WHO’s envisioned sustainable development goals while ensuring food and nutritional security (Omotayo and Babalola, 2020). To exemplify, investigations using endophytic microorganisms have demonstrated their potential roles as biostimulants (Kumar et al., 2015; Wani et al., 2016; Hashem et al., 2017; Vyas et al., 2018; Saia et al., 2021; Tharek et al., 2022), biofertilizers (Arora and Mishra, 2016; Santoyo et al., 2016), biopesticides (Gange et al., 2012; Waqas et al., 2015a; Lugtenberg et al., 2016), and biocontrol agents (Hashem et al., 2017; Halecker et al., 2020; Jiao et al., 2020). Likewise, da Silva et al. (2017) developed an inexpensive and efficient biostimulant formulation made with endophytic diazotrophic bacteria and humic acids that boosts crop production while ensuring the finest use of fertilizers. Considering the practical implications, microbial formulations promote plant growth and development by restoring soil minerals, improving plant nutrient uptake, or making nutrients easily accessible (Bashan et al., 2014; Mishra et al., 2015). In addition, they also affect the host’s other beneficial effects, such as osmotic adjustment, stomatal regulation, shaping root architecture, and adjustment of nitrogen accumulation and metabolism (Compant et al., 2005). Bioinoculants facilitate seed treatment by distributing inoculants evenly over seeds, causing systemic acquired resistance (Ma, 2019), and assisting in bioremediation using a metabolic engineering approach (Dangi et al., 2019). In terms of agrochemical and metal pollutants solubilization, bioabsorption, and mineralization, endophytes have also proven effective in environmental remediation (Gavrilaş et al., 2022). Studies have advanced further the potential implementation of microorganisms as traditional biological control agents (BCAs) by inundating inoculation in plants. Tahir et al. (2017) found that Bacillus subtilis volatiles negatively impact Ralstonia solanacearum’s physiology and ultrastructure and elicit systemic resistance in tobacco against bacterial wilt. The best characterized and most frequently microbial endophytes in biological control programs are Beauveria bassiana and Metarhizium anisopliae have antagonistic activities on plant pathogens via an array of mechanisms, including the synthesis of metabolites (volatile compounds, antibiotics, and enzymes), competition, parasitic relationships, triggering systemic resistance by the plant, and improvements in plant growth (Vidal and Jaber, 2015; Vega, 2018; Moraga, 2020; Baron and Rigobelo, 2021). In another study, endophytes frequently assist plants in reinforcing their defense mechanisms by facilitating the stimulation of induced systemic resistance, which occasionally overlaps with those of acquired systemic resistance, considering both of them may foster the growth and development of plants (Busby et al., 2016) and protect against phytopathogens (Chadha et al., 2015). Therefore, implementing microbial formulations as biocontrol or biofertilizers might be an effective alternative to the overuse of agrochemicals. Perhaps the most environmentally and farmer-friendly step toward sustainability might be developing consortia from aspiring endophytic strains from native agricultural fields, resulting in multifaceted bio-solutions.
8.3 Challenges
Despite the widespread interest in endophyte research, there are still certain challenges in designing efficient microbial formulations, such as:
i. Endophytes are tissue-specific; identifying suitable host plants, their healthy tissues or organs is critical.
ii. Isolating novel endophytes and investigating the relevant complementary or antagonistic signaling pathways during symbiosis.
iii. Pecularity of microbial consortia in terms of their modes of action. Some endophytes have aseptic or uncultivable properties, making synthetic cultivation challenging. Therefore, developing new bioengineering systems or modifying traditional isolation methods is crucial.
iv. The biological constraint still exists even though some endophytes’ facultative nature offers the possibility of continued colonization, provided they can survive in the rhizosphere.
v. The interactions of microbial biostimulants with the micro-climate (temperature, pH, water, humidity, nutrients, etc.), host plants (defense system and exudates), and native microbes should also be considered.
vi. The inoculants’ concentration, functionality, and survivability during storage as well as maintaining sterility, are critical for designing efficient formulations.
vii. Limitation of biological adjuvants as bio-careers.
viii. Artificially inoculated endophytes may begin acting as latent pathogens by disseminating toxins through the food chain.
ix. The potential of exogenously applied endophytic microbes to establish a habitat beneficial to both entities is contingent upon their ability to compete successfully with native microbes. Thus, inoculating crops with consortia rather than a single strain will increase their persistence.
x. Licensing/registration of formulations before arriving on the market is complicated.
Screening of endophytic microbes in a greenhouse, either solely or in combined applications, has proven to be efficient in maximizing crop yields. Designing formulations with high microbial concentrations and survivability is crucial for developing potent biostimulants. However, finding the most critical factors and ensuring sterility during the formulation process is challenging because testing every possible combination is not feasible. Therefore, the commercial success of endophyte-based biostimulants requires a comprehensive knowledge of molecular plant-microbe interaction, methods of transmission, and strategies for establishing a symbiotic relationship between the endophyte and host plant. The research efforts aimed at discovering microbial biostimulants are beginning, which might result in significant advancement in this emerging field. In modern agriculture, methods to increase the use of endophytic microorganisms are desired to use these microbes alone or in combination with bioprospecting as bioinoculants in crop systems. The most effective methods for using endophytic microorganisms in agriculture have not yet been identified. However, applying endophytes as seed dressings or directly into the soil is the most frequent and common method utilized by farmers. Meanwhile, the implementation of these endophytes-based inoculations is unsuccessful on field sites owing to issues with the endophytes’ establishment.
Therefore, the manifold characteristics of endophytes make them possible alternatives to harmful agrochemicals, and thus, they are now being utilized more frequently throughout the world. Endophyte-based biostimulants are cost-effective, preserve natural soil microbiota, have few or no hazardous byproducts, enrich soil organic matter, and ensure ecosystem sustainability. Utilizing improved microbial inoculants can be one of the best input components for green farming. Although endophytic microorganisms can be engineered, little is known about their use as bioinoculants in contemporary farming situations. Therefore, more research is required to determine the effectiveness of microbial bio-input for commercialization before these endophytes can be used as bioinoculants to improve soil health and crop yield.
9 Conclusion
The yield and quality of medicinal plants are considerably influenced by various edaphic and climatic factors such as soil characteristics, soil microbiota, light, humidity, temperature, drought, salinity, etc. To adapt to a stressful environment, plants acclimatize themselves by modulating the genes responsive to stress, transcriptional factors, and biosynthesis signaling pathways. Furthermore, in stressful conditions, plant defense systems trigger appropriate cellular responses by stimuli from the sensors situated on the cytoplasm or cell surface and transmitting signals to the transcriptional machinery in the nucleus with the help of various signaling pathways. Sustainable production is still a significant challenge; perhaps specific strategies might be helpful in such scenarios as rescue measures like integrating plant-associated microbes into farming systems, supporting agricultural production through various interventions, and mitigating biotic and abiotic perturbations. Utilizing endophytic microbes as biostimulants not only eliminates the need for synthetic inorganic pesticides and fertilizers but also lowers input costs and, more importantly, minimizes the impact of these agrochemicals on vital existing ecological communities. Nevertheless, its practical application suffers some limitations, viz., endophytes are tissue-specific, and tissue type, the host, and the environment mainly influence their functionality. However, the information gap of their multifaceted nature in plant tissues has hampered the advancement of endophyte research in various fields. Furthermore, the underlying mechanisms governing these interactions are still not fully explored; several studies have raised the hope of their potential exploitation of plant-microbe interactions in managing various stresses. Therefore, to promote the practicality of endophyte-assisted biological applications as biostimulants, particularly in the field, comprehensive research is necessitated to demonstrate an insight into the microorganisms in its host medicinal plants. Modern high-throughput genomic studies have revolutionized the field of microbiome research by unveiling the enigmatic realms of endophytism, facilitating the pursuit of endophytes, enabling the sequencing of a broader range of microbes, and enticing a comprehensive examination of microbial ecosystems by taxonomic classification, phylogeny, and evolutionary studies, In the future, advanced omics approaches such as genomics, transcriptomics, proteomics, and metabolomics can support an in-depth knowledge of plant-microbe interactions and stress signaling pathways, leading to its potential exploitation in agriculture for improving yield, quality, and resistance of medicinal plants, drug development, and management of the environment.
Author contributions
PP: Conceptualization, Writing—original draft preparation, Writing - review and editing, Visualization. AT: Conceptualization, Writing—original draft preparation. SD: Writing - review and editing. KL: Writing - review and editing. TJ: Writing - review and editing. All authors contributed to the article and approved the submitted version.
Funding
The author(s) declare that no financial support was received for the research, authorship, and/or publication of this article.
Acknowledgments
We are thankful to the Director, Central Institute of Medicinal and Aromatic Plants (CSIR-CIMAP), Lucknow, India. We also thank the peer reviewers for their critical review and valuable suggestions for improving this manuscript. The CIMAP publication communication number is CIMAP/PUB/2023//007.
Conflict of interest
The authors declare that the research was conducted in the absence of any commercial or financial relationships that could be construed as a potential conflict of interest.
Publisher’s note
All claims expressed in this article are solely those of the authors and do not necessarily represent those of their affiliated organizations, or those of the publisher, the editors and the reviewers. Any product that may be evaluated in this article, or claim that may be made by its manufacturer, is not guaranteed or endorsed by the publisher.
Glossary
References
Abd Allah, E. F., Hashem, A., Alqarawi, A. A., Bahkali, A. H., Alwhibi, M. S. (2015). Enhancing growth performance and systemic acquired resistance of medicinal plant Sesbania sesban (L.) merr using arbuscular mycorrhizal fungi under salt stress. Saudi J. Biol. Sci. 22 (3), 274–283. doi: 10.1016/j.sjbs.2015.03.004
Abdullaeva, Y., Ambika Manirajan, B., Honermeier, B., Schnell, S., Cardinale, M. (2020). Domestication affects the composition, diversity, and co-occurrence of the cereal seed microbiota. J. Adv. Res. 31, 75–86. doi: 10.1016/j.jare.2020.12.008
Adame-Alvarez, R. M., Mendiola-Soto, J., Heil, M. (2014). Order of arrival shifts endophyte-pathogen interactions in bean from resistance induction to disease facilitation. FEMS Microbiol. Lett. 355, 100–107. doi: 10.1111/1574-6968.12454
Adeleke, B. S., Babalola, O. O. (2021). Roles of endosphere microbes in agriculture - a review. J. Plant Growth Regul. 41, 1411–1428. doi: 10.1007/s00344-021-10406-2
Adeleke, B. S., Fadiji, A. E., Ayilara, M. S., Igiehon, O. N., Nwachukwu, B. C., Babalola, O. O. (2022). Strategies to enhance the use of endophytes as bioinoculants in agriculture. Horticulturae 8, 498. doi: 10.3390/horticulturae8060498
Agarwal, S., Shende, S. T. (1987). Tetrazolium reducing microorganisms inside the root of brassica species. Curr. Sci. 56, 187–188.
Ahammed, G. J., Gantait, S., Mitra, M., Yang, Y., Li, X. (2020). Role of ethylene crosstalk in seed germination and early seedling development: a review. Plant Physiol. Biochem. 151, 124–131. doi: 10.1016/j.plaphy.2020.03.016
Ahanger, M. A., Qin, C., Maodong, Q., Dong, X. X., Ahmad, P., Abd_Allah, E. F., et al. (2019). Spermine application alleviates salinity induced growth and photosynthetic inhibition in Solanum lycopersicum by modulating osmolyte and secondary metabolite accumulation and differentially regulating antioxidant metabolism. Plant Physiol. Biochem. 144, 1–13. doi: 10.1016/j.plaphy.2019.09.021
Alam, B., Lï, J., Gě, Q., Khan, M. A., Gōng, J., Mehmood, S., et al. (2021). Endophytic fungi: from symbiosis to secondary metabolite communications or vice versa? Front. Plant Sci. 12. doi: 10.3389/fpls.2021.791033
Anderson, T. A., Guthrie, E. A., Walton, B. T. (1993). Bioremediation in the rhizosphere. Environ. Sci. Technol. 27 (13), 2630–2636. doi: 10.1021/es00049a001
Anyasi, R. O., Atagana, H. I. (2018). Profiling of plants at petroleum contaminated site for phytoremediation. Int. J. Phytorem. 20, 352–361. doi: 10.1080/15226514.2017.1393386
Araujo, W. L., Marcon, J., Maccheroni, W., van Elsas, J. D., van Vuurde, J. W., Azevedo, J. L. (2002). Diversity of endophytic bacterial populations and their interaction with Xylella fastidiosa in citrus plants. Appl. Environ. Microbiol. 68 (10), 4906–4914. doi: 10.1128/AEM.68.10.4906-4914.2002
Arif, Y., Singh, P., Bajguz, A., Alam, P., Hayat, S. (2021). Silicon mediated abiotic stress tolerance in plants using physio-biochemical, omic approach and cross-talk with phytohormones. Plant Physiol. Biochem. 166, 278–289. doi: 10.1016/j.plaphy.2021.06.002
Arora, N. K., Kang, S. C., Maheshwari, D. K. (2001). Isolation of siderophore-producing strains of rhizobium meliloti and their biocontrol potential against Macrophomina phaseolina that causes charcoal rot of groundnut. Curr. Sci. 81, 673–677.
Arora, N. K., Mishra, J. (2016). Prospecting the roles of metabolites and additives in future bioformulations for sustainable agriculture. Appl. Soil Ecol. 107, 405–407. doi: 10.1016/j.apsoil.2016.05.020
Arraes, F. B. M., Beneventi, M. A., Lisei de Sa, M. E., Paixao, J. F. R., Albuquerque, E. V. S., Marin, S. R. R., et al. (2015). Implications of ethylene biosynthesis and signaling in soybean drought stress tolerance. BMC Plant Biol. 15, 1–20. doi: 10.1186/s12870-015-0597-z
Asghari, B., Khademian, R., Sedaghati, B. (2020). Plant growth promoting rhizobacteria (PGPR) confer drought resistance and stimulate biosynthesis of secondary metabolites in pennyroyal (Mentha pulegium L.) under water shortage condition. Sci. Hortic. 263, 109132. doi: 10.1016/j.scienta.2019.109132
Ayaz, M., Ali, Q., Jiang, Q., Wang, R., Wang, Z., Mu, G., et al. (2022). Salt tolerant Bacillus strains improve plant growth traits and regulation of phytohormones in wheat under salinity stress. Plants 11, 2769. doi: 10.3390/plants11202769
Ayilara, M. S., Adeleke, B. S., Adebajo, M. T., Akinola, S. A., Fayose, C. A., Adeyemi, U. T., et al (2023). Enhanced natural attenuation, an environment-friendly remediation approach. Front. Environ. Sci. 11, 1182586. doi: 10.3389/fenvs.2023.1182586
Ayilara, M. S., Adeleke, B. S., Babalola, O. O. (2022). Bioprospecting and challenges of plant microbiome research for sustainable agriculture, a review on soybean endophytic bacteria. Microb. Ecol. 86, 1454. doi: 10.1007/s00248-022-02141-2
Azabou, M. C., Gharbi, Y., Medhioub, I., Ennouri, K., Barham, H., Tounsi, S., et al. (2020). The endophytic strain Bacillus velezensis OEE1: An efficient biocontrol agent against verticillium wilt of olive and a potential plant growth promoting bacteria. Biol. Control 142, 104168. doi: 10.1016/j.biocontrol.2019.104168
Azevedo, J. L., Maccheroni, W., Jr., Pereira, J. O., de Araújo, W. L. (2000). Endophytic microorganisms: a review on insect control and recent advances on tropical plants. Electron. J. Biotechnol. 3 (1), 15–16. doi: 10.2225/vol3-issue1-fulltext-4
Babu, A. G., Shea, P. J., Sudhakar, D., Jung, I. B., Oh, B. T. (2015). Potential use of pseudomonas koreensis AGB-1 in association with miscanthus sinensis to remediate heavy metal (loid)-contaminated mining site soil. J. Environ. Manage. 151, 160–166. doi: 10.1016/j.jenvman.2014.12.045
Badri, D. V., Weir, T. L., van der Lelie, D., Vivanco, J. M. (2009). Rhizosphere chemical dialogues: plant–microbe interactions. Curr. Opin. Biotechnol. 20 (6), 642–650. doi: 10.1016/j.copbio.2009.09.014
Bae, H., Sicher, R. C., Kim, M. S., Kim, S. H., Strem, M. D., Melnick, R. L., et al. (2009). The beneficial endophyte Trichoderma hamatum isolate DIS 219b promotes growth and delays the onset of the drought response in Theobroma cacao. J. Exp. Bot. 60 (11), 3279–3295. doi: 10.1093/jxb/erp165
Barka, E. A., Nowak, J., Clément, C. (2006). Enhancement of chilling resistance of inoculated grapevine plantlets with a plant growth-promoting rhizobacterium, Burkholderia phytofirmans strain PsJN. Appl. Environ. Microbiol. 72 (11), 7246–7252. doi: 10.1128/AEM.01047-06
Barnawal, D., Bharti, N., Tripathi, A., Pandey, S. S., Chanotiya, C. S., Kalra, A. (2016). ACC-deaminase-producing endophyte Brachybacterium paraconglomeratum strain SMR20 ameliorates Chlorophytum salinity stress via altering phytohormone generation. J. Plant Growth Regul. 35, 553–564. doi: 10.1007/s00344-015-9560-3
Baron, N. C., Rigobelo, E. C. (2021). Endophytic fungi: a tool for plant growth promotion and sustainable agriculture. Mycology 13, 39–55. doi: 10.1080/21501203.2021.1945699
Barry, C. S., Giovannoni, J. J. (2007). Ethylene and fruit ripening. J. Plant Growth Regul. 26, 143. doi: 10.1007/s00344-007-9002-y
Bashan, Y., de-Bashan, L. E., Prabhu, S. R., Hernandez, J. P. (2014). Advances in plant growth-promoting bacterial inoculant technology: formulations and practical perspectives, (1998–2013). Plant Soil 378, 1–33. doi: 10.1007/s11104-013-1956-x
Bashir, M. A., Wang, X., Naveed, M., Mustafa, A., Ashraf, S., Samreen, T. (2021). Biochar mediated-alleviation of chromium stress and growth improvement of different maize cultivars in tannery polluted soils. Int. J. Environ. Res. Public Health 18, 4461. doi: 10.3390/ijerph18094461
Basu, S., Varsani, S., Louis, J. (2018). Altering plant defenses: herbivore-associated molecular patterns and effector arsenal of chewing herbivores. Mol. Plant Microbe Interact. 31, 13–21. doi: 10.1094/MPMI-07-17-0183-FI
Baxter, A., Mittler, R., Suzuki, N. (2014). ROS as key players in plant stress signaling. J. Exp. Bot. 65, 1229–1240. doi: 10.1093/jxb/ert375
Bell, C. R., Dickie, G. A., Harvey, W. L. G., Chan, J. W. Y. F. (1995). Endophytic bacteria in grapevine. Can. J. Microbiol. 41 (1), 46–53. doi: 10.1139/m95-006
Berendsen, R. L., Pieterse, C. M., Bakker, P. A. (2012). The rhizosphere microbiome and plant health. Trends Plant Sci. 17 (8), 478–486. doi: 10.1016/j.tplants.2012.04.001
Bhattacharjee, R. B., Singh, A., Mukhopadhyay, S. N. (2008). Use of nitrogen-fixing bacteria as biofertiliser for non-legumes: prospects and challenges. Appl. Microbiol. Biotechnol. 80 (2), 199–209. doi: 10.1007/s00253-008-1567-2
Bittleston, L. S., Brockmann, F., Wcislo, W., Van Bael, S. A. (2011). Endophytic fungi reduce leaf-cutting ant damage to seedlings. Biol. Lett. 7 (1), 30–32. doi: 10.1098/rsbl.2010.0456
Bodenhausen, N., Reymond, P. (2007). Signaling pathways controlling induced resistance to insect herbivores in Arabidopsis. Mol. Plant Microbe Interact. 20, 1406–1420. doi: 10.1094/MPMI-20-11-1406
Bodhankar, S., Grover, M., Hemanth, S., Reddy, G., Rasul, S., Yadav, S. K., et al. (2017). Maize seed endophytic bacteria: Dominance of antagonistic, lytic enzyme-producing Bacillus spp. 3 Biotech. 7, 1–13. doi: 10.1007/s13205-017-0860-0
Bouchez, O., Huard, C., Lorrain, S., Roby, D., Balagué, C. (2007). Ethylene is one of the key elements for cell death and defense response control in the Arabidopsis lesion mimic mutant vad1. Plant Physiol. 145 (2), 465–477. doi: 10.1104/pp.107.106302
Brader, G., Djamei, A., Teige, M., Palva, E. T., Hirt, H. (2007). The MAP kinase kinase MKK2 affects disease resistance in Arabidopsis. Mol. Plant Microbe Interact. 20 (5), 589–596. doi: 10.1094/MPMI-20-5-0589
Broekaert, W. F., Delauré, S. L., De Bolle, M. F., Cammue, B. P. (2006). The role of ethylene in host-pathogen interactions. Annu. Rev. Phytopathol. 44, 393–416. doi: 10.1146/annurev.phyto.44.070505.143440
Broekgaarden, C., Caarls, L., Vos, I. A., Pieterse, C. M., Van Wees, S. C. (2015). Ethylene: traffic controller on hormonal crossroads to defense. Plant Physiol. 169 (4), 2371–2379. doi: 10.1104/pp.15.01020
Busby, P. E., Ridout, M., Newcombe, G. (2016). Fungal endophytes: modifiers of plant disease. Plant Mol. Biol. 90, 645–655. doi: 10.1007/s11103-015-0412-0
Camejo, D., Guzmán-Cedeño, Á., Moreno, A. (2016). Reactive oxygen species, essential molecules, during plant–pathogen interactions. Plant Physiol. Biochem. 103, 10–23. doi: 10.1016/j.plaphy.2016.02.035
Cao, R., Liu, X., Gao, K., Mendgen, K., Kang, Z., Gao, J., et al. (2009). Mycoparasitism of endophytic fungi isolated from reed on soilborne phytopathogenic fungi and production of cell wall-degrading enzymes in vitro. Curr. Microbiol. 59 (6), 584–592. doi: 10.1007/s00284-009-9477-9
Castiglione, A. M., Mannino, G., Contartese, V., Bertea, C. M., Ertani, A. (2021). Microbial biostimulants as response to modern agriculture needs: Composition, role and application of these innovative products. Plants 10, 1533. doi: 10.3390/plants10081533
Chadha, N., Mishra, M., Rajpal, K., Bajaj, R., Choudhary, D. K., Varma, A. (2015). An ecological role of fungal endophytes to ameliorate plants under biotic stress. Arch. Microbiol. Sep; 197 (7), 869–881. doi: 10.1007/s00203-015-1130-3
Chaudhary, P., Agri, U., Chaudhary, A., Kumar, A., Kumar, G. (2022). Endophytes and their potential in biotic stress management and crop production. Front. Microbiol. 13. doi: 10.3389/fmicb.2022.933017
Chen, J. X., Dai, C. C., Li, X., Tian, L. S., Xie, H. (2008). Endophytic fungi screening from Atracty lancea and inoculating into the host plantlet. Guihaia 28 (2), 256–260. doi: 10.3969/j.issn.1000-3142.2008.02.022
Chen, F., Gao, Y., Chen, X., Yu, Z., Li, X. (2013). Quorum quenching enzymes and their application in degrading signal molecules to block quorum sensing-dependent infection. Int. J. Mol. Sci. 14, 17477–17500. doi: 10.3390/ijms140917477
Chen, Y. F., Gao, Z., Kerris, R. J., III, Wang, W., Binder, B. M., Schaller, G. E. (2010). Ethylene receptors function as components of high-molecular-mass protein complexes in Arabidopsis. PloS One 5 (1), e8640. doi: 10.1371/journal.pone.0008640
Chen, J., Li, Z. M., Cheng, Y., Gao, C. S., Guo, L. T., Wang, T. H., et al. (2020). Sphinganine-analog mycotoxins (SAMs): chemical structures, bioactivities, and genetic controls. J. Fungi 6, 312. doi: 10.3390/jof6040312
Chen, H., Wu, H., Yan, B., Zhao, H., Liu, F., Zhang, H., et al. (2018). Core microbiome of medicinal plant salvia miltiorrhiza seed: a rich reservoir of beneficial microbes for secondary metabolism? Int. J. Mol. Sci. 19, 672. doi: 10.3390/ijms19030672
Chen, H., Xue, L., Chintamanani, S., Germain, H., Lin, H., Cui, H., et al. (2009). Ethylene insensitive3 and ethylene insensitive3-like1 repress salicylic acid induction defi-cient2 expression to negatively regulate plant innate immunity in Arabidopsis. Plant Cell. 21, 2527–2540. doi: 10.1105/tpc.108.065193
Chi, Y., Ma, X., Wu, J., Wang, R., Zhang, X., Chu, S., et al. (2023). Plant growth promoting endophyte promotes cadmium accumulation in Solanum nigrum L. by regulating plant homeostasis. J. Hazard. Mater. 457, 131866. doi: 10.1016/j.jhazmat.2023.131866
Chiappero, J., del Rosario Cappellari, L., Alderete, L. G. S., Palermo, T. B., Banchio, E. (2019). Plant growth promoting rhizobacteria improve the antioxidant status in Mentha piperita grown under drought stress leading to an enhancement of plant growth and total phenolic content. Ind. Crop Prod. 139, 111553. doi: 10.1016/j.indcrop.2019.111553
Chinchilla, D., Zipfel, C., Robatzek, S., Kemmerling, B., Nürnberger, T., Jones, J. D. G., et al. (2007). A flagellin-induced complex of the receptor FLS2 and BAK1 initiates plant defence. Nature 448, 497–500. doi: 10.1038/nature05999
Choudhary, M., Chandra, P., Dixit, B., Nehra, V., Choudhary, U., Choudhary, S. (2022). Plant growth-promoting microbes: Role and prospective in amelioration of salt stress. Commun. Soil Sci. Plant Anal. 53, 1692–1711. doi: 10.1080/00103624.2022.2063316
Choudhury, S., Panda, P., Sahoo, L., Panda, S. K. (2013). Reactive oxygen species signaling in plants under abiotic stress. Plant Signal. Behav. 4), e23681. doi: 10.4161/psb.23681
Chu, T. N., Tran, B. T. H., Van Bui, L., Hoang, M. T. T. (2019). Plant growth-promoting rhizobacterium Pseudomonas PS01 induces salt tolerance in Arabidopsis thaliana. BMC Res. Notes 12, 1–7. doi: 10.1186/s13104-019-4046-1
Colebrook, E. H., Thomas, S. G., Phillips, A. L., Hedden, P. (2014). The role of gibberellin signaling in plant responses to abiotic stress. J. Exp. Biol. 217 (1), 67–75. doi: 10.1242/jeb.089938
Compant, S., Reiter, B., Sessitsch, A., Nowak, J., Clément, C., Ait Barka, E. (2005). Endophytic colonization of Vitis vinifera L. by plant growth-promoting bacterium Burkholderia sp. strain PsJN. Appl. Environ. Microbiol. 71, 1685–1693. doi: 10.1128/AEM.71.4.1685-1693.2005
Conrath, U., Beckers, G. J., Langenbach, C. J., Jaskiewicz, M. R. (2015). Priming for enhanced defense. Annu. Rev. Phytopathol. 53, 97–119. doi: 10.1146/annurev-phyto-080614-120132
Cooke, J., Leishman, M. R. (2016). Consistent alleviation of abiotic stress with silicon addition: a metaanalysis. Funct. Ecol. 30 (8), 1340–1357. doi: 10.1111/1365-2435.12713
Corbineau, F., Xia, Q., Bailly, C., El-Maarouf-Bouteau, H. (2014). Ethylene, a key factor in the regulation of seed dormancy. Front. Plant Sci. 5. doi: 10.3389/fpls.2014.00539
Cotton, T. E. A., Pétriacq, P., Cameron, D. D., Meselmani, M. A., Schwarzenbacher, R., Rolfe, S. A., et al. (2019). Metabolic regulation of the maize rhizobiome by benzoxazinoids. ISME J. 13, 1647–1658. doi: 10.1038/s41396-019-0375-2
Cruz de Carvalho, M. H. (2008). Drought stress and reactive oxygen species: production, scavenging and signaling. Plant Signal. Behav. 3, 156–165. doi: 10.4161/psb.3.3.5536
Dangi, A. K., Sharma, B., Hill, R. T., Shukla, P. (2019). Bioremediation through microbes: systems biology and metabolic engineering approach. Crit. Rev. Biotechnol. 39 (1), 79–98. doi: 10.1080/07388551.2018.1500997
da Silva, S. F., Olivares, F. L., Canellas, L. P. (2017). The biostimulant manufactured using diazotrophic endophytic bacteria and humates is effective to increase sugarcane yield. Chem. Biol. Technol. Agric. 4, 24. doi: 10.1186/s40538-017-0106-8
Daungfu, O., Youpensuk, S., Lumyong, S. (2019). Endophytic bacteria isolated from citrus plants for biological control of citrus canker in lime plants. Trop. Life Sci. Res. 30 (1), 73. doi: 10.21315/tlsr2019.30.1.5
De Hita, D., Fuentes, M., Zamarreño, A. M., Ruiz, Y., Garcia-Mina, J. M. (2020). Culturable bacterial endophytes from sedimentary humic acid-treated plants. Front. Plant Sci. 11. doi: 10.3389/fpls.2020.00837
Del Buono, D. (2021). Can biostimulants be used to mitigate the effect of anthropogenic climate change on agriculture? It is time to respond. Sci. Total Environ. 751, 141763. doi: 10.1111/nph.13519
De Martinis, D. (2000). “Modification of plant development by genetic manipulation of the ethylene biosynthesis and action pathway,” in Developments in plant genetics and breeding, vol. 6 . Eds. de Vries, G. E., Metzlaff, K. (Rome, Italy: Elsevier B.V.), 123–132.
de Zélicourt, A., Synek, L., Saad, M. M., Alzubaidy, H., Jalal, R., Xie, Y., et al. (2018). Ethylene induced plant stress tolerance by enterobacter sp. SA187 is mediated by 2-keto-4-methylthiobutyric acid production. PLoS. Genet. 14 (3), e1007273. doi: 10.1371/journal.pgen.1007273
Diab, M. K., Mead, H. M., Khedr, M. A., Nafie, M. S., Abu-Elsaoud, A. M., Hanora, A., et al. (2023). Endophytic actinobacteria from wild medicinal plants are a natural source of insecticide to control the African cotton leafworm (Spodoptera littoralis). A.M.B. Express 13, 47. doi: 10.1186/s13568-023-01550-x
Domka, A., Rozpądek, P., Ważny, R., Turnau, K. (2019). Mucor sp.–an endophyte of brassicaceae capable of surviving in toxic metal-rich sites. J. Basic Microbiol. 59 (1), 24–37. doi: 10.1002/jobm.201800406
Duveiller, E., Singh, R. P., Nicol, J. M. (2007). The challenges of maintaining wheat productivity: pests, diseases, and potential epidemics. Euphytica 157 (3), 417–430. doi: 10.1007/s10681-007-9380-z
Egamberdieva, D., Wirth, S. J., Shurigin, V. V., Hashem, A., Abd_Allah, E. F. (2017). Endophytic bacteria improve plant growth, symbiotic performance of chickpea (Cicer arietinum L.) and induce suppression of root rot caused by Fusarium solani under salt stress. Front. Microbiol. 8. doi: 10.3389/fmicb.2017.01887
Eichmann, R., Richards, L., Schäfer, P. (2021). Hormones as go-betweens in plant microbiome assembly. Plant J. 105, 518–541. doi: 10.1111/tpj.15135
Erice, G., Ruíz-Lozano, J. M., Zamarreño, Á. M., García-Mina, J. M., Aroca, R. (2017). Transcriptomic analysis reveals the importance of JA-ile turnover in the response of Arabidopsis plants to plant growth promoting rhizobacteria and salinity. Environ. Exp. Bot. 143, 10–19. doi: 10.1016/j.envexpbot.2017.08.006
Estrada, G. A., Baldani, V. L. D., de Oliveira, D. M., Urquiaga, S., Baldani, J. I. (2013). Selection of phosphate-solubilizing diazotrophic herbaspirillum and burkholderia strains and their effect on rice crop yield and nutrient uptake. Plant Soil 369 (1-2), 115–129. doi: 10.1007/s11104012-1550-7
Etalo, D. W., Jeon, J. S., Raaijmakers, J. M. (2018). Modulation of plant chemistry by beneficial root microbiota. Nat. Prod. Rep. 35, 398–409. doi: 10.1039/c7np00057j
Fernandez, O., Theocharis, A., Bordiec, S., Feil, R., Jacquens, L., Clément, C., et al. (2012). Burkholderia phytofirmans PsJN acclimates grapevine to cold by modulating carbohydrate metabolism. Mol. Plant Microbe Interact. 25 (4), 496–504. doi: 10.1094/MPMI-09-11-0245
Fonseca, S., Chini, A., Hamberg, M., Adie, B., Porzel, A., Kramell, R., et al. (2009). (+)-7-iso-Jasmonoyl-L-isoleucine is the endogenous bioactive jasmonate. Nat. Chem. Biol. 5 (5), 344–350. doi: 10.1038/nchembio.161
Fouda, A. H., Hassan, S. E. D., Eid, A. M., Ewais, E. E. D. (2015). Biotechnological applications of fungal endophytes associated with medicinal plant Asclepias sinaica (Bioss.). Ann. Agric. Sci. 60 (1), 95–104. doi: 10.1016/j.aoas.2015.04.001
Foyer, C. H., Noctor, G. (2005). Redox homeostasis and antioxidant signaling: a metabolic interface between stress perception and physiological responses. Plant Cell 17 (7), 1866–1875. doi: 10.1105/tpc.105.033589
Frank, A. C., Saldierna Guzmán, J. P., Shay, J. E. (2017). Transmission of bacterial endophytes. Microorganisms 5, 70. doi: 10.3390/microorganisms5040070
Fröhlich, K., García-Ramírez, G. X., Trapp, M. A., Hirt, H. (2023). Ethylene: a master regulator of plant–microbe interactions under abiotic stresses. Cells 12, 31. doi: 10.3390/cells12010031
Fujita, M., Fujita, Y., Noutoshi, Y., Takahashi, F., Narusaka, Y., Yamaguchi-Shinozaki, K., et al. (2006). Crosstalk between abiotic and biotic stress responses: a current view from the points of convergence in the stress signaling networks. Curr. Opin. Plant Biol. 9 (4), 436–442. doi: 10.1016/j.pbi.2006.05.014
Furtado, B. U., Golebiewski, M., Skorupa, M., Hulisz, P., Hrynkiewicz, K. (2019). Bacterial and fungal endophytic microbiomes of Salicornia europaea. Appl. Environ. Microbiol. 85, e305–e319. doi: 10.1128/AEM.00305-19
Gagic, M., Faville, M. J., Zhang, W., Forester, N. T., Rolston, M. P., Johnson, R. D., et al. (2018). Seed transmission of epichloë endophytes in Lolium perenne is heavily influenced by host genetics. Front. Plant Sci. 9. doi: 10.3389/fpls.2018.01580
Gagne, S., Richard, C., Rousseau, H., Antoun, H. (1987). Xylem-residing bacteria in alfalfa roots. Can. J. Microbiol. 33 (11), 996–1000. doi: 10.1139/m87-175
Gamalero, E., Glick, B. R. (2022). Recent advances in bacterial amelioration of plant drought and salt stress. Biology 11, 437. doi: 10.3390/biology11030437
Gange, A. C., Eschen, R., Wearn, J. A., Thawer, A., Sutton, B. C. (2012). Differential effects of foliar endophytic fungi on insect herbivores attacking a herbaceous plant. Oecologia 168 (4), 1023–1031. doi: 10.1007/s00442-011-2151-5
Gavrilaş, S., Ursachi, C. Ş., Perţa-Crişan, S., Munteanu, F. D. (2022). Recent trends in biosensors for environmental quality monitoring. Sensors 22, 1513. doi: 10.3390/s22041513
Gimenez, E., Salinas, M., Manzano-Agugliaro, F. (2018). Worldwide research on plant defense against biotic stresses as improvement for sustainable agriculture. Sustainability 10 (2), 391. doi: 10.3390/su10020391
Gimenez-Ibanez, S., Boter, M., Solano, R. (2015). Novel players fine-tune plant trade-offs. Essays Biochem. 58 (83), 10–1042. doi: 10.1042/bse0580083
Gómez-Vidal, S., Salinas, J., Tena, M., Lopez-Llorca, L. V. (2009). Proteomic analysis of date palm (Phoenix dactylifera L.) responses to endophytic colonization by entomopathogenic fungi. Electrophoresis 30 (17), 2996–3005. doi: 10.1002/elps.200900192
Govarthanan, M., Mythili, R., Selvankumar, T., Kamala-Kannan, S., Rajasekar, A., Chang, Y. C. (2016). Bioremediation of heavy metals using an endophytic bacterium Paenibacillus sp. RM isolated from the roots of Tridax procumbens. 3 Biotech. 6, 242. doi: 10.1007/s13205-016-0560-1
Grobelak, A., Hiller, J. (2017). Bacterial siderophores promote plant growth: Screening of catechol and hydroxamate siderophores. Int. J. Phytoremed. 19, 825–833. doi: 10.1080/15226514
Gudesblat, G. E., Iusem, N. D., Morris, P. C. (2007). Guard cell-specific inhibition of Arabidopsis MPK3 expression causes abnormal stomatal responses to abscisic acid and hydrogen peroxide. New Phytol. 173 (4), 713–721. doi: 10.1111/j.1469-8137.2006.01953.x
Guerrieri, A., Dong, L., Bouwmeester, H. J. (2019). Role and exploitation of underground chemical signaling in plants. Pest Manage. Sci. 75, 2455–2463. doi: 10.1002/ps.5507
Gunatilaka, A. L. (2006). Natural products from plant-associated microorganisms: distribution, structural diversity, bioactivity, and implications of their occurrence. J. Natural Prod. 69 (3), 509–526. doi: 10.1021/np058128n
Guo, Q., Li, Y., Lou, Y., Shi, M., Jiang, Y., Zhou, J., et al. (2019). Bacillus amyloliquefaciens Ba13 induces plant systemic resistance and improves rhizosphere microecology against tomato yellow leaf curl virus disease. Appl. Soil Ecol. 137, 154–166. doi: 10.1016/j.apsoil.2019.01.015
Habibi, G. (2015). Effects of soil-and foliar-applied silicon on the resistance of grapevine plants to freezing stress. Acta Biol. Szeged. 59 (2), 109–117.
Hafeez, M. B., Raza, A., Zahra, N., Shaukat, K., Akram, M. Z., Iqbal, S., et al. (2021). “Gene regulation in halophytes in conferring salt tolerance,” in Handbook of Bioremediation. Ed. Hasanuzzaman, M. (New York: Academic Press), 341–370.
Haider, S., Iqbal, J., Naseer, S., Yaseen, T., Shaukat, M., Bibi, H., et al. (2021). Molecular mechanisms of plant tolerance to heat stress: current landscape and future perspectives. Plant Cell Rep. 40, 2247–2271. doi: 10.1007/s00299-021-02696-3
Halecker, S., Wennrich, J. P., Rodrigo, S., Andrée, N., Rabsch, L., Baschien, C., et al. (2020). Fungal endophytes for biocontrol of ash dieback: The antagonistic potential of Hypoxylon rubiginosum. Fungal Ecol. 45, 100918. doi: 10.1016/j.funeco.2020.100918
Hallmann, J., Quadt-Hallmann, A., Mahaffee, W. F., Kloepper, J. W. (1997). Bacterial endophytes in agricultural crops. Can. J. Microbiol. 43 (10), 895–914. doi: 10.1139/m97-131
Hamid, B., Zaman, M., Farooq, S., Fatima, S., Sayyed, R. Z., Baba, Z. A., et al. (2021). Bacterial plant biostimulants: A sustainable way towards improving growth, productivity, and health of crops. Sustainability 13, 2856. doi: 10.3390/su13052856
Han, W., Jia, J., Hu, Y., Liu, J., Guo, J., Shi, Y., et al. (2021). Maintenance of root water uptake contributes to salt-tolerance of a wild tomato species under salt stress. Arch. Agron. Soil Sci. 67 (2), 205–217. doi: 10.1080/03650340.2020.1720911
Han, X., Kui, M., He, K., Yang, M., Du, J., Jiang, Y., et al. (2023). Jasmonate-regulated root growth inhibition and root hair elongation. J. Exp. Bot. 74 (4), 1176–1185. doi: 10.1093/jxb/erac441
Hara, M., Furukawa, J., Sato, A., Mizoguchi, T., Miura, K. (2012). “Abiotic stress and role of salicylic acid in plants,” in Abiotic stress responses in plants. Eds. Parvaiz, A., Prasad, M. N. V. (New York: Springer), 235–251.
Hardoim, P. R., Van Overbeek, L. S., Berg, G., Pirttilä, A. M., Compant, S., Campisano, A., et al. (2015). The hidden world within plants: ecological and evolutionary considerations for defining functioning of microbial endophytes. M. M. B. R. 79 (3), 293–320. doi: 10.1128/MMBR.00050-14
Hardoim, P. R., van Overbeek, L. S., van Elsas, J. D. (2008). Properties of bacterial endophytes and their proposed role in plant growth. Trends Microbiol. 16 (10), 463–471. doi: 10.1016/j.tim.2008.07.008
Harsha, K., Shalima, M. V., Nair, A. R., Pillai, P. (2023). Influence of phytochemical and soil characteristics on composition of culturable endophyte from Zingiber zerumbet (L) Smith rhizome. Ecol. Genet. Genomics 26, 100158. doi: 10.1016/j.egg.2022.100158
Hasanuzzaman, M., Bhuyan, M. B., Zulfiqar, F., Raza, A., Mohsin, S. M., Mahmud, J. A., et al. (2020). Reactive oxygen species and antioxidant defense in plants under abiotic stress: Revisiting the crucial role of a universal defense regulator. Antioxidants 9 (8), 681. doi: 10.3390/antiox9080681
Haseeb, M., Iqbal, S., Hafeez, M. B., Saddiq, M. S., Zahra, N., Raza, A., et al. (2022). Phytoremediation of nickel by quinoa: Morphological and physiological response. PloS One 17 (1), e0262309. doi: 10.1371/journal.pone.0262309
Hasegawa, P. M., Bressan, R. A., Zhu, J. K., Bohnert, H. J. (2000). Plant cellular and molecular responses to high salinity. Annu.Rev. Plant Biol. 51 (1), 463–499. doi: 10.1146/annurev.arplant.51.1.463
Hashem, A., Abd_Allah, E. F., Alqarawi, A. A., Radhakrishnan, R., Kumar, A. (2017). Plant defense approach of Bacillus subtilis (BERA 71) against Macrophomina phaseolina (Tassi) Goid in mung bean. J. Plant Interact. 12, 390–401. doi: 10.1080/17429145.2017.1373871
Hatamzadeh, S., Rahnama, K., White, J. F., Oghaz, N. A., Nasrollahnejad, S., Hemmati, K. (2023). Investigation of some endophytic fungi from five medicinal plants with growth promoting ability on maize (Zea mays L.). J. Appl. Microbiol. 134 (1), lxac015. doi: 10.1093/jambio/lxac015
Hause, B., Hause, G., Kutter, C., Miersch, O., Wasternack, C. (2003). Enzymes of jasmonate biosynthesis occur in tomato sieve elements. Plant Cell Physiol. 44 (6), 643–648. doi: 10.1093/pcp/pcg072
He, R., Wang, G., Liu, X., Zhang, C., Lin, F. (2009). Antagonistic bioactivity of an endophytic bacterium isolated from Epimedium brevicornu Maxim. Afr. J. Biotechnol. 8, 191–195. doi: 10.5897/AJB2009.000-9035
Heil, M., Ton, J. (2008). Long-distance signaling in plant defence. Trends Plant Sci. 13 (6), 264–272. doi: 10.1016/j.tplants.2008.03.005
Hodgson, S., de Cates, C., Hodgson, J., Morley, N. J., Sutton, B. C., Gange, A. C. (2014). Vertical transmission of fungal endophytes is widespread in forbs. Ecol. Evol. 4 (8), 1199–1208. doi: 10.1002/ece3.953
Hong, C. E., Jo, S. H., Jo, I., Park, J. M. (2018). Diversity and antifungal activity of endophytic bacteria associated with Panax ginseng seedlings. Plant Biotechnol. Rep. 12, 409–418. doi: 10.1007/s11816-018-0504-9
Hossain, A., Ahmad, Z., Moulik, D., Maitra, S., Bhadra, P., Ahmad, A., et al. (2021). “Jasmonates and salicylates: Mechanisms, transport and signaling during abiotic stress in plants,” in Jasmonates and salicylates signaling in plants. Signaling and Communication in Plants. Eds. Aftab, T., Yusuf, M. (Cham: Springer), 1–29. doi: 10.1007/978-3-030-75805-9_1
Hou, S., Liu, Z., Shen, H., Wu, D. (2019). Damage-associated molecular pattern-triggered immunity in plants. Front. Plant Sci. 10. doi: 10.3389/fpls.2019.00646
Hu, L., Robert, C. A. M., Cadot, S., Zhang, X., Ye, M., Li, B., et al. (2018). Root exudate metabolites drive plant-soil feedbacks on growth and defense by shaping the rhizosphere microbiota. Nat. Commun. 9, 2738. doi: 10.1038/s41467-018-05122-7
Huang, P., Dong, Z., Guo, P., Zhang, X., Qiu, Y., Li, B., et al. (2019). Salicylic acid suppresses apical hook formation via npr1-mediated repression of ein3 and eil1 in Arabidopsis. Plant Cell 32, 612–629. doi: 10.1105/tpc.19.00658
Huang, A. C., Jiang, T., Liu, Y. X., Bai, Y. C., Reed, J., Qu, B., et al. (2019). A specialized metabolic network selectively modulates Arabidopsis root microbiota. Science 364, eaau6389. doi: 10.1126/science.aau6389
Hwarari, D., Guan, Y., Ahmad, B., Movahedi, A., Min, T., Hao, Z., et al. (2022). ICE-CBF-COR signaling cascade and its regulation in plants responding to cold stress. Int. J. Mol. Sci. 23, 1549. doi: 10.3390/ijms23031549
Ichimura, K., Mizoguchi, T., Yoshida, R., Yuasa, T., Shinozaki, K. (2000). Various abiotic stresses rapidly activate Arabidopsis MAP kinases ATMPK4 and ATMPK6. Plant J. 24 (5), 655–665. doi: 10.1046/j.1365-313x.2000.00913.x
Inbaraj, M. P. (2021). Plant-microbe interactions in alleviating abiotic stress—A mini review. Front. Agron. 3. doi: 10.3389/fagro.2021.667903
Isah, T. (2019). Stress and defense responses in plant secondary metabolites production. Biol. Res. 52, 39. doi: 10.1186/s40659-019-0246-3
Jacoby, R., Chen, L., Schwier, M., Koprivova, A., Kopriva, S. (2020). Recent advances in the role of plant metabolites in shaping the root microbiome. F1000Res 9, F1000FacultyRev–151. doi: 10.12688/f1000research.21796.1
Jan, F. G., Hamayun, M., Hussain, A., Jan, G., Iqbal, A., Khan, A., et al. (2019). An endophytic isolate of the fungus yarrowia lipolytica produces metabolites that ameliorate the negative impact of salt stress on the physiology of maize. BMC Microbiol. 19 (1), 1–10. doi: 10.1186/s12866-018-1374-6
Jayakumar, A., Krishna, A., Mohan, M., Nair, I. C., Radhakrishnan, E. K. (2019). Plant growth enhancement, disease resistance, and elemental modulatory effects of plant probiotic endophytic Bacillus sp. Fcl1. Probiotics Antimicrob. Proteins. 11, 526–534. doi: 10.1007/s12602-018-9417-8
Jiang, C. H., Liao, M. J., Wang, H. K., Zheng, M. Z., Xu, J. J., Guo, J. H. (2018). Bacillus velezensis, a potential and efficient biocontrol agent in control of pepper gray mold caused by Botrytis cinerea. Biol. Control 126, 147–157. doi: 10.1016/j.biocontrol.2018.07.017
Jiao, R., Ahmed, A., He, P., Munir, S., Wu, Y., Wang, J., et al. (2023). Bacillus amyloliquefaciens induces resistance in tobacco against powdery mildew pathogen Erysiphe cichoracearum. J. Plant Growth Regul. 42 (10), 6636–6651. doi: 10.1007/s00344-023-10922-3
Jiao, R., Munir, S., He, P., Yang, H., Wu, Y., Wang, J., et al. (2020). Biocontrol potential of the endophytic Bacillus amyloliquefaciens YN201732 against tobacco powdery mildew and its growth promotion. Biol. Control 143, 104160. doi: 10.1016/j.biocontrol.2019.104160
Johnson, S. N., Barton, C. V., Biru, F. N., Islam, T., Mace, W. J., Rowe, R. C., et al. (2023). Elevated atmospheric CO2 suppresses silicon accumulation and exacerbates endophyte reductions in plant phosphorus. Funct. Ecol. 26, 100158. doi: 10.1111/1365-2435.14342
Ju, C., Chang, C. (2015). Mechanistic insights in ethylene perception and signal transduction. Plant Physiol. 169 (1), 85–95. doi: 10.1104/pp.15.00845
Kai, M., Haustein, M., Molina, F., Petri, A., Scholz, B., Piechulla, B. (2009). Bacterial volatiles and their action potential. Appl. Microbiol. Biotechnol. 81, 1001–1012. doi: 10.1007/s00253-008-1760-3
Kang, S. M., Khan, A. L., Waqas, M., Asaf, S., Lee, K. E., Park, Y. G., et al. (2019). Integrated phytohormone production by the plant growth-promoting rhizobacterium Bacillus tequilensis SSB07 induced thermotolerance in soybean. J. Plant Interact. 14, 416–423. doi: 10.1080/17429145.2019.1640294
Karthikeyan, B., Joe, M. M., Islam, M. R., Sa, T. (2012). ACC deaminase containing diazotrophic endophytic bacteria ameliorate salt stress in Catharanthus roseus through reduced ethylene levels and induction of antioxidative defense systems. Symbiosis 56, 77–86. doi: 10.1007/s13199-012-0162-6
Kaur, G., Patel, A., Dwibedi, V., Rath, S. K. (2023). Harnessing the action mechanisms of microbial endophytes for enhancing plant performance and stress tolerance: current understanding and future perspectives. Arch. Microbiol. 205, 303. doi: 10.1007/s00203-023-03643-4
Kavroulakis, N., Ntougias, S., Zervakis, G. I., Ehaliotis, C., Haralampidis, K., Papadopoulou, K. K. (2007). Role of ethylene in the protection of tomato plants against soil-borne fungal pathogens conferred by an endophytic fusarium solani strain. J. Exp. Bot. 58 (14), 3853–3864. doi: 10.1093/jxb/erm230
Kawasaki, A., Donn, S., Ryan, P. R., Mathesius, U., Devilla, R., Jones, A. (2016). Microbiome and exudates of the root and rhizosphere of Brachypodium distachyon, a model for wheat. PloS One 11, e0164533. doi: 10.1371/JOURNAL.PONE.0164533
Kazan, K. (2013). Auxin and the integration of environmental signals into plant root development. Ann. Bot. 112 (9), 1655–1665. doi: 10.1093/aob/mct229
Khan, M., Asaf, S., Khan, A., Adhikari, A., Jan, R., Ali, S., et al. (2020). Plant growth-promoting endophytic bacteria augment growth and salinity tolerance in rice plants. Plant Biol. 22, 850–862. doi: 10.1111/plb.13124
Khan, M. I., Poor, P., Janda, T. (2022). Salicylic acid: A versatile signaling molecule in plants. J. Plant Growth Regul. 41, 1887–1890. doi: 10.1007/s00344-022-10692-4
Khan, A. L., Waqas, M., Kang, S. M., Al-Harrasi, A., Hussain, J., Al-Rawahi, A., et al. (2014). Bacterial endophyte Sphingomonas sp. LK11 produces gibberellins and IAA and promotes tomato plant growth. J. Microbiol. 52, 689–695. doi: 10.1007/s12275-014-4002-7
Khare, E., Arora, N. K. (2015). “Effects of soil environment on field efficacy of microbial inoculants,” in Plant microbes symbiosis: applied facets (New Delhi: Springer), 353–381. doi: 10.1007/978-81-322-2068-8_19
Khare, E., Kim, K., Lee, K. J. (2016). Rice OsPBL1 (Oryza sativa Arabidopsis PBS1-like 1) enhanced defense of arabidopsis against Pseudomonas syringae DC3000. Eur. J. Plant Pathol. 146 (4), 901–910. doi: 10.1007/s10658-016-0968-9
Kinkema, M., Fan, W., Dong, X. (2000). Nuclear localization of NPR1 is required for activation of PR gene expression. Plant Cell 12 (12), 2339–2350. doi: 10.1105/tpc.12.12.2339
Kishor, P. K., Sangam, S., Amrutha, R. N., Laxmi, P. S., Naidu, K. R., Rao, K. S., et al. (2005). Regulation of proline biosynthesis, degradation, uptake and transport in higher plants: Its implications in plant growth and abiotic stress tolerance. Curr. Sci. 88, 424–438. doi: 10.1016/j.heliyon.2019.e02952
Kissoudis, C., van de Wiel, C., Visser, R. G., van der Linden, G. (2014). Enhancing crop resilience to combined abiotic and biotic stress through the dissection of physiological and molecular crosstalk. Front.Plant Sci. 5, 207. doi: 10.3389/fpls.2014.00207
Kneeshaw, S., Gelineau, S., Tada, Y., Loake, G. J., Spoel, S. H. (2014). Selective protein denitrosylation activity of thioredoxin-h5 modulates plant immunity. Mol. Cell 56 (1), 153–162. doi: 10.1016/j.molcel.2014.08.003
Kolomiets, M. V., Chen, H., Gladon, R. J., Braun, E., Hannapel, D. J. (2000). A leaf lipoxygenase of potato induced specifically by pathogen infection. Plant Physiol. 124, 1121–1130. doi: 10.1104/pp.124.3.1121
Kondo, S., Yamada, H., Setha, S. (2007). Effect of jasmonates differed at fruit ripening stages on 1-aminocyclopropane-1-carboxylate (ACC) synthase and ACC oxidase gene expression in pears. J. Am. Soc Hortic. Sci. 132, 120–125. doi: 10.21273/JASHS.132.1.120
Kong, P., Hong, C. (2020). Endophytic Burkholderia sp. SSG as a potential biofertilizer promoting boxwood growth. PeerJ 8, e9547. doi: 10.7717/peerj.9547
Koo, Y. M., Heo, A. Y., Choi, H. W. (2020). Salicylic acid as a safe plant protector and growth regulator. Plant Pathol. J. 36 (1), 1. doi: 10.5423/PPJ.RW.12.2019.0295
Kosar, F., Akram, N. A., Ashraf, M., Ahmad, A., AlYemeni, M. N., Ahmad, P. (2021). Impact of exogenously applied trehalose on leaf biochemistry, achene yield and oil composition of sunflower under drought stress. Physiol. Plant 172, 317–333. doi: 10.1111/ppl.13155
Koskimäki, J. J., Hokkanen, J., Jaakola, L., Suorsa, M., Tolonen, A., Mattila, S., et al. (2009). Flavonoid biosynthesis and degradation play a role in early defense responses of bilberry (Vaccinium myrtillus) against biotic stress. Eur. J. Plant Pathol. 125 (4), 629. doi: 10.1007/s10658-009-9511-6
Kumar, S., Chauhan, P. S., Agrawal, L., Raj, R., Srivastava, A., Gupta, S., et al. (2016). Paenibacillus lentimorbus inoculation enhances tobacco growth and extenuates the virulence of Cucumber mosaic virus. PloS One 11, e0149980. doi: 10.1371/journal.pone.0149980
Kumar, A., Sharma, S., Mishra, S., Dames, J. F. (2015). Arbuscular mycorrhizal inoculation improves growth and antioxidative response of Jatropha curcas (L.) under Na2SO4 salt stress. Plant Biosyst. 149 (2), 260–269. doi: 10.1080/11263504.2013.845268
Kumari, S., Sharma, A., Chaudhary, P., Khati, P. (2020). Management of plant vigor and soil health using two agriusable nanocompounds and plant growth promotory rhizobacteria in Fenugreek. 3Biotech 10, 1–11. doi: 10.1007/s13205-020-02448-2
Kumawat, K. C., Nagpal, S., Sharma, P. (2022). Potential of plant growth-promoting rhizobacteria-plant interactions in mitigating salt stress for sustainable agriculture: A review. Pedosphere 32, 223–245. doi: 10.1016/S1002-0160(21)60070-X
Kutasy, E., Buday-Bódi, E., Virág, I. C., Forgács, F., Melash, A. A., Zsombik, L., et al. (2022). Mitigating the negative effect of drought stress in oat (Avena sativa L.) with silicon and sulphur foliar fertilization. Plants 11 (1), 30. doi: 10.3390/plants11010030
Lata, R., Chowdhury, S., Gond, S. K., White, J. F., Jr (2018). Induction of abiotic stress tolerance in plants by endophytic microbes. Lett. Appl. Microbiol. 66 (4), 268–276. doi: 10.1111/lam.12855
Laxa, M., Liebthal, M., Telman, W., Chibani, K., Dietz, K. J. (2019). The role of the plant antioxidant system in drought tolerance. Antioxidants 8, 94. doi: 10.3390/antiox8040094
Leon-Reyes, A., Du, Y., Koornneef, A., Proietti, S., Körbes, A. P., Memelink, J., et al. (2010). Ethylene signaling renders the jasmonate response of Arabidopsis insensitive to future suppression by salicylic acid. Mol. Plant Microbe Interact. 23 (2), 187–197. doi: 10.1094/MPMI-23-2-0187
Leon-Reyes, A., Spoel, S. H., De Lange, E. S., Abe, H., Kobayashi, M., Tsuda, S., et al. (2009). Ethylene modulates the role of nonexpressor of pathogenesis-related genes1 in crosstalk between salicylate and jasmonate signaling. Plant Physiol. 149, 1797–1809. doi: 10.1104/pp.108.133926
Li, X., Lang, D., Wang, J., Zhang, W., Zhang, X. (2023). Plant-beneficial Streptomyces dioscori SF1 potential biocontrol and plant growth promotion in saline soil within the arid and semi-arid areas. Environ. Sci. pollut. Res. Int. 30, 70194–70212. doi: 10.1007/s11356-023-27362-x
Li, J., Wu, H., Pu, Q., Zhang, C., Chen, Y., Lin, Z., et al. (2023). Complete genome of Sphingomonas paucimobilis ZJSH1, an endophytic bacterium from Dendrobium officinale with stress resistance and growth promotion potential. Arch. Microbiol. 205, 132. doi: 10.1007/s00203-023-03459-2
Li, W., Yao, J., He, C., Ren, Y., Zhao, L., He, X. (2023). The synergy of dark septate endophytes and organic residue on Isatis indigotica growth and active ingredients accumulation under drought stress. Ind. Crops Prod. 203, 117147. doi: 10.3390/plants12173102
Lim, J. H., Kim, S. D. (2013). Induction of drought stress resistance by multi-functional PGPR Bacillus licheniformis K11 in pepper. Plant Pathol. J. 29, 201–208. doi: 10.5423/PPJ.SI.02.2013.0021
Limoli, D. H., Jones, C. J., Wozniak, D. J. (2015). Bacterial extracellular polysaccharides in biofilm formation and function. Microbiol. Spectr. 3, 3–3. doi: 10.1128/microbiolspec.MB-0011-2014
Lind, A. L., Wisecaver, J. H., Lameiras, C., Wiemann, P., Palmer, J. M., Keller, N. P., et al. (2017). Drivers of genetic diversity in secondary metabolic gene clusters within a fungal species. PloS Biol. 15, e2003583. doi: 10.1371/journal.pbio.2003583
Lindow, S. E., Brandl, M. T. (2003). Microbiology of the phyllosphere MINIREVIEW microbiology of the phyllosphere. Appl. Environ. Microbiol. 69, 1875–1883. doi: 10.1128/AEM.69.4.1875
Liu, S., Hao, H., Lu, X., Zhao, X., Wang, Y., Zhang, Y., et al. (2017). Transcriptome profiling of genes involved in induced systemic salt tolerance conferred by Bacillus amyloliquefaciens FZB42 in Arabidopsis thaliana. Sci. Rep. 7, 1–13. doi: 10.1038/s41598-017-11308-8
Liu, H., Khan, M. Y., Carvalhais, L. C., Delgado-Baquerizo, M., Yan, L., Crawford, M., et al. (2019). Soil amendments with ethylene precursor alleviate negative impacts of salinity on soil microbial properties and productivity. Sci. Rep. 9 (1), 6892. doi: 10.1038/s41598-019-43305-4
Liu, W., Sikora, E., Park, S. W. (2020). Plant growth-promoting rhizobacterium, Paenibacillus polymyxa CR1, upregulates dehydration-responsive genes, RD29A and RD29B, during priming drought tolerance in arabidopsis. Plant Physiol. Biochem. 156, 146–154. doi: 10.1016/j.plaphy.2020.08.049
Liu, X., Song, W., Zhang, K., Ye, Y. (2011). Effects of two kinds of endophytic fungi infection on water stress of seedlings of chrysanthemum morifolium. Acta Hortic. Sin. 38 (2), 335–342.
Liu, Q., Wen, C. K. (2012). Arabidopsis ETR1 and ERS1 differentially repress the ethylene response in combination with other ethylene receptor genes. Plant Physiol. 158 (3), 1193–1207. doi: 10.1104/pp.111.187757
Liu, Y., Yue, Z., Sun, Z., Li, C. (2023). Harnessing native Bacillus spp. for sustainable wheat production. Appl. Environ. Microbiol. 89 (2), e0124722. doi: 10.1128/aem.01247-22
Loake, G., Grant, M. (2007). Salicylic acid in plant defence—the players and protagonists. Curr. Opin. Plant Biol. 10 (5), 466–472. doi: 10.1016/j.pbi.2007.08.008
Lorenzo, O., Piqueras, R., Sánchez-Serrano, J. J., Solano, R. (2003). Ethylene response factor1 integrates signals from ethylene and jasmonate pathways in plant defense. Plant Cell. 15, 165–178. doi: 10.1105/tpc.007468
Love, J., Björklund, S., Vahala, J., Hertzberg, M., Kangasjärvim, J., Sundberg, B. (2009). Ethylene is an endogenous stimulator of cell division in the cambial meristem of Populus. Proc. Natl. Acad. Sci. U. S. A. 106 (14), 5984–5989. doi: 10.1073/pnas.0811660106
Lugtenberg, B. J., Caradus, J. R., Johnson, L. J. (2016). Fungal endophytes for sustainable crop production. FEMS Microbiol. Ecol. 92 (12), fiw194. doi: 10.1093/femsec/fiw194
Luo, S., Xu, T., Chen, L., Chen, J., Rao, C., Xiao, X., et al. (2012). Endophyteassisted promotion of biomass production and metal-uptake of energy crop sweet sorghum by plant-growth-promoting endophyte bacillus sp. SLS18. Appl. Microbiol. Biotechnol. 93 (4), 1745–1753. doi: 10.1007/s00253-011-3483-0
Luo, L., Zhao, C., Wang, E., Raza, A., Yin, C. (2022). Bacillus amyloliquefaciens as an excellent agent for biofertilizer and biocontrol in agriculture: An overview for its mechanisms. Microbiol. Res. 259, 127016. doi: 10.1016/j.micres.2022.127016
Ma, L., Cao, Y. H., Cheng, M. H., Huang, Y., Mo, M. H., Wang, Y., et al. (2013). Phylogenetic diversity of bacterial endophytes of Panax notoginseng with antagonistic characteristics towards pathogens of root-rot disease complex. Antonie Van Leeuwenhoek 103, 299–312. doi: 10.1007/s10482-012-9810-3
Ma, Y., Zhang, C., Oliveira, R. S., Freitas, H., Luo, Y. (2016). Bioaugmentation with endophytic bacterium E6S homologous to achromobacter piechaudii enhances metal rhizoaccumulation in host Sedum plumbizincicola. Front. Plant Sci. 7. doi: 10.3389/fpls.2016.00075
Ma, Y. (2019). Seed coating with beneficial microorganisms for precision agriculture. Biotechnol. Adv. 37 (7), 107423. doi: 10.1016/j.biotechadv.2019.107423
Maggini, V., De Leo, M., Mengoni, A., Gallo, E. R., Miceli, E., Reidel, R. V. B., et al. (2017). Plant-endophytes interaction influences the secondary metabolism in Chinacea purpurea (L.) moench: an in vitro model. Sci. Rep. 7, 16924. doi: 10.1038/s41598-017-17110-w
Mahgoub, H. A., Fouda, A., Eid, A. M., Ewais, E. E. D., Hassan, S. E. D. (2021). Biotechnological application of plant growth-promoting endophytic bacteria isolated from halophytic plants to ameliorate salinity tolerance of Vicia faba L. Plant Biotechnol. Rep. 15, 819–843. doi: 10.1007/s11816-021-00716-y
Manasa, T. S., Basavarajappa, M. P., Raghavendra, K. M., Ramangouda, S. H., YC, V., Gurumurthy, S. B. (2023). Endophytic Bacillus velezensis underscore induction of defense enzyme and confers resistance against Xanthomonas axonopodis PV. Betlicola in betel vine. Pharma Innov. 12 (7), 244–249. doi: 10.1007/s11104-023-06152-x
Mandyam, K., Loughin, T., Jumpponen, A. (2010). Isolation and morphological and metabolic characterization of common endophytes in annually burned tallgrass prairie. Mycologia 102, 813–821. doi: 10.3852/09-212
Mansfield, J., Genin, S., Magori, S., Citovsky, V., Sriariyanum, M., Ronald, P., et al. (2012). Top 10 plant pathogenic bacteria in molecular plant pathology. Mol. Plant Pathol. 13, 614–629. doi: 10.1111/j.1364-3703.2012.00804.x
Márquez, L. M., Redman, R. S., Rodriguez, R. J., Roossinck, M. J. (2007). A virus in a fungus in a plant: three-way symbiosis required for thermal tolerance. Science 315 (5811), 513–515. doi: 10.1126/science.1136237
Mastan, A., Bharadwaj, R. K. B., Kushwaha, R. K., Babu, V. C. S. (2019). Functional fungal endophytes in Coleus forskohlii regulate labdane diterpene biosynthesis for elevated forskolin accumulation in roots. Microb. Ecol. 78, 914–926. doi: 10.1007/s00248-019-01376-w
McDonald, A., Riha, S., DiTommaso, A., DeGaetano, A. (2009). Climate change and the geography of weed damage: analysis of US maize systems suggests the potential for significant range transformations. Agric. Ecosyst. Environ. 130 (3-4), 131–140. doi: 10.1016/j.agee.2008.12.007
McGuinness, M., Dowling, D. (2009). Plant-associated bacterial degradation of toxic organic compounds in soil. Int. J. Environ. Res. Public Health 6, 2226–2247. doi: 10.3390/ijerph6082226
Meena, M., Yadav, G., Sonigra, P., Nagda, A., Mehta, T., Swapnil, P., et al. (2022). Role of elicitors to initiate the induction of systemic resistance in plants to biotic stress. Plant Stress 5, 100103. doi: 10.1016/j.stress.2022.100103
Mehmood, A., Hussain, A., Irshad, M., Hamayun, M., Iqbal, A., Khan, N. (2019). In vitro production of IAA by endophytic fungus Aspergillus awamori and its growth promoting activities in zea mays. Symbiosis 77 (3), 225–235. doi: 10.1007/s13199-018-0583-y
Mei, X., Li, Y., Zhang, L., Xu, H., Sha, X. (2023). Multifunctional Radix salvia emiltiorrhizae endophyte and application thereof. CN Patent CN116716210A.
Mejía, L. C., Rojas, E. I., Maynard, Z., Van Bael, S., Arnold, A. E., Hebbar, P., et al. (2008). Endophytic fungi as biocontrol agents of Theobroma cacao pathogens. Biol. Control 46 (1), 4–14. doi: 10.1016/j.biocontrol.2008.01.012
Meng, J. J., He, X. L. (2011). Effects of AM fungi on growth and nutritional contents of Salvia miltiorrhiza bge. under drought stress. J. Agricult. Univ. Hebei 34 (1), 51–61. doi: 10.3969/j.issn.1000-1573.2011.01.011
Mercado-Blanco, J., Rodrıguez-Jurado, D., Hervás, A., Jiménez-Dıaz, R. M. (2004). Suppression of verticillium wilt in olive planting stocks by root-associated fluorescent pseudomonas spp. Biol. Control 30 (2), 474–486. doi: 10.1016/j.biocontrol.2004.02.002
Meyer, K. M., Leveau, J. H. (2012). Microbiology of the phyllosphere: A playground for testing ecological concepts. Oecologia 168, 621–629. doi: 10.1007/s00442-011-2138-2
Miller, J. D., Mackenzie, S., Foto, M., Adams, G. W., Findlay, J. A. (2002). Needles of white spruce inoculated with rugulosin-producing endophytes contain rugulosin reducing spruce budworm growth rate. Myco. Res. 106 (4), 471–479. doi: 10.1017/S0953756202005671
Mir, R. A., Bhat, B. A., Yousuf, H., Islam, S. T., Raza, A., Rizvi, M. A., et al. (2022). Multidimensional role of silicon to activate resilient plant growth and to mitigate abiotic stress. Front. Plant Sci. 13, 819658. doi: 10.3389/fpls.2022.819658
Mishra, J., Singh, R., Arora, N. K. (2017). Alleviation of heavy metal stress in plants and remediation of soil by rhizosphere microorganisms. Front. Microbiol. 8, 1706. doi: 10.3389/fmicb.2017.01706
Mishra, S., Singh, A., Keswani, C., Saxena, A., Sarma, B. K., Singh, H. B. (2015). “Harnessing plant-microbe interactions for enhanced protection against phytopathogens,” in Plant microbes symbiosis: applied facets (New Delhi: Springer), 111–125.
Mittler, R., Vanderauwera, S., Suzuki, N., Miller, G. A. D., Tognetti, V. B., Vandepoele, K., et al. (2011). ROS signaling: the new wave? Trends Plant Sci. 16 (6), 300–309. doi: 10.1017/S0953756202005671
Miura, K., Tada, Y. (2014). Regulation of water, salinity, and cold stress responses by salicylic acid. Front. Plant Sci. 5, 4. doi: 10.3389/fpls.2014.00004
Moradtalab, N., Weinmann, M., Walker, F., Höglinger, B., Ludewig, U., Neumann, G. (2018). Silicon improves chilling tolerance during early growth of maize by effects on micronutrient homeostasis and hormonal balances. Front. Plant Sci. 9, 420. doi: 10.3389/fpls.2018.00420
Moraga, E. Q. (2020). Entomopathogenic fungi as endophytes: their broader contribution to IPM and crop production. Biocontrol Sci. Technol. 30 (9), 864–877. doi: 10.1080/09583157.2020.1771279
Mosblech, A., Thurow, C., Gatz, C., Feussner, I., Heilmann, I. (2011). Jasmonic acid perception by COI1 involves inositol polyphosphates in Arabidopsis thaliana. Plant J. 65 (6), 949–957. doi: 10.1111/j.1365-313X.2011.04480.x
Mukherjee, A., Gaurav, A. K., Patel, A. K., Singh, S., Chouhan, G. K., Lepcha, A., et al. (2021). Unlocking the potential plant growth-promoting properties of chickpea (Cicer arietinum L.) seed endophytes bio-inoculants for improving soil health and crop production. Land Degrad. Dev. 32, 4362–4374. doi: 10.1002/ldr.4042
Munemasa, S., Mori, I. C., Murata, Y. (2011). Methyl jasmonate signaling and signal crosstalk between methyl jasmonate and abscisic acid in guard cells. Plant Signal Behav. 7), 939–941. doi: 10.4161/psb.6.7.15439
Munns, R., Gilliham, M. (2015). Tolerância à salinidade das culturas. New Phytol. 208, 668–673. doi: 10.1111/nph.13519
Nair, D. N., Padmavathy, S. (2014). Impact of endophytic microorganisms on plants, environment and humans. Sci. World J. 2014, 250693. doi: 10.1155/2014/250693
Nakashima, K., Fujita, Y., Kanamori, N., Katagiri, T., Umezawa, T., Kidokoro, S., et al. (2009). Three Arabidopsis SnRK2 protein kinases, SRK2D/SnRK2. 2, SRK2E/SnRK2. 6/OST1 and SRK2I/SnRK2. 3, involved in ABA signaling are essential for the control of seed development and dormancy. Plant Cell Physiol. 50 (7), 1345–1363. doi: 10.1093/pcp/pcp08
Nakashima, K., Takasaki, H., Mizoi, J., Shinozaki, K., Yamaguchi-Shinozaki, K. (2012). NAC transcription factors in plant abiotic stress responses. Biochim. Biophys. Acta 1819 (2), 97–103. doi: 10.1016/j.bbagrm.2011.10.005
Naya, L., Ladrera, R., Ramos, J., González, E. M., Arrese-Igor, C., Minchin, F. R., et al. (2007). The response of carbon metabolism and antioxidant defenses of alfalfa nodules to drought stress and to the subsequent recovery of plants. Plant Physiol. 144 (2), 1104–1114. doi: 10.1104/pp.107.099648
Nazli, F., Mustafa, A., Ahmad, M., Hussain, A., Jamil, M., Wang, X., et al. (2020). A review on practical application and potentials of phytohormone-producing plant growth-promoting rhizobacteria for inducing heavy metal tolerance in crops. Sustainability 12 (21), 9056. doi: 10.3390/su12219056
Newman, M. A., Sundelin, T., Nielsen, J. T., Erbs, G. (2013). MAMP (microbe-associated molecular pattern) triggered immunity in plants. Front. Plant Sci. 4, 139. doi: 10.3389/fpls.2013.00139
Niwa, T., Suzuki, T., Takebayashi, Y., Ishiguro, R., Higashiyama, T., Sakakibara, H., et al. (2018). Jasmonic acid facilitates flower opening and floral organ development through the upregulated expression of SlMYB21 transcription factor in tomato. Biosci. Biotechnol. Biochem. 82 (2), 292–303. doi: 10.1080/09168451.2017.1422107
Noor, R., Yasmin, H., Ilyas, N., Nosheen, A., Hassan, M. N., Mumtaz, S., et al. (2022). Comparative analysis of iron oxide nanoparticles synthesized from ginger (Zingiber officinale) and cumin seeds (Cuminum cyminum) to induce resistance in wheat against drought stress. Chemosphere 292, 133201. doi: 10.1016/j.chemosphere.2021.133201
Noreen, S., Ashraf, M., Hussain, M., Jamil, A. (2009). Exogenous application of salicylic acid enhances antioxidative capacity in salt stressed sunflower (Helianthus annuus L.) plants. Pak. J. Bot. 41 (1), 473–479. doi: 10.1093/pcp/pcp083
Norman-Setterbald, C., Vidal, S., Palva, E. T. (2000). Interaction signal pathways control defense gene expression in Arabidopsis in response to cell wall-degrading enzymes from Erwinia carotovora. Mol. Plant Microbe Interact. 13, 430–438. doi: 10.1094/MPMI.2000.13.4.430
Ogawara, T., Higashi, K., Kamada, H., Ezura, H. (2003). Ethylene advances the transition from vegetative growth to flowering in Arabidopsis thaliana. J. Plant Physiol. 160, 1335–1340. doi: 10.1078/0176-1617-01129
Ogbe, A. A., Gupta, S., Stirk, W. A., Finnie, J. F., Van Staden, J. (2023). Growth-Promoting characteristics of fungal and bacterial endophytes isolated from a drought-tolerant mint species Endostemon obtusifolius (E. Mey. ex Benth.) NE Br. Plants 12, 638. doi: 10.3390/plants12030638
Oktem, H. A., Eyidogen, F., Selcuk, F., Oz, M. T., da Silva, J. A. T., Yücel, M. (2008). Revealing response of plants to biotic and abiotic stresses with microarray technology. Genes Genomes Genomics 2, 14–48. doi: 10.1016/j.scitotenv.2023.165832
Oloumi, H., Khaleghi, M., Dalvand, A. (2023). Isolation and identification of endophytic actinobacteria from Iris persica and Echium amoenum plants and investigation of their effects on germination and growth of wheat plant. Food Sci. Nutr. 11 (9), 5296–5303. doi: 10.1002/2Ffsn3.3488
Olowe, O. M., Akanmu, A. O., Asemoloye, M. D. (2020). Exploration of microbial stimulants for induction of systemic resistance in plant disease management. Ann. Appl. Biol. 177, 282–293. doi: 10.1111/aab.12631
Omotayo, O. P., Babalola, O. O. (2020). Resident rhizosphere microbiome’s ecological dynamics and conservation: towards achieving the envisioned sustainable development goals, a review. Int. Soil Water Conserv. Res. 9, 127–142. doi: 10.1016/j.iswcr.2020.08.002
Orchard, S., Standish, R. J., Nicol, D., Gupta, V. V. S. R., Ryan, M. H. (2016). The response of fine root endophyte (Glomus tenue) to waterlogging is dependent on host plant species and soil type. Plant Soil 403, 305–315. doi: 10.1007/s11104-016-2804-6
Ortíz-Castro, R., Contreras-Cornejo, H. A., Macías-Rodríguez, L., López- Bucio, J. (2009). The role of microbial signals in plant growth and development. Plant Signal. Behav. 4, 701–712. doi: 10.4161/psb.4.8.9047
Otieno, N., Lally, R. D., Kiwanuka, S., Lloyd, A., Ryan, D., Germaine, K. J., et al. (2015). Plant growth promotion induced by phosphate solubilizing endophytic Pseudomonas isolates. Front. Microbiol. 6. doi: 10.3389/fmicb.2015.00745
Pang, Z., Chen, J., Wang, T., Gao, C., Li, Z., Guo, L., et al. (2021). Linking plant secondary metabolites and plant microbiomes: a review. Front. Plant Sci. 12. doi: 10.3389/fpls.2021.621276
Pauwels, L., Goossens, A. (2011). The JAZ proteins: a crucial interface in the jasmonate signaling cascade. Plant Cell 23 (9), 3089–3100. doi: 10.1105/tpc.111.089300
Penninckx, I. A., Thomma, B. P., Buchala, A., Métraux, J. P., Broekaert, W. F. (1998). Concomitant activation of jasmonate and ethylene response pathways is required for induction of a plant defensin gene in Arabidopsis. Plant Cell 10, 2103–2113. doi: 10.1105/tpc.10.12.2103
Peters, K., Breitsameter, L., Gerowitt, B. (2014). Impact of climate change on weeds in agriculture: a review. Agric. Sustain. Dev. 34, 707–721. doi: 10.1007/s13593-014-0245-2
Piasecka, A., Jedrzejczak-Rey, N., Bednarek, P. (2015). Secondary metabolites in plant innate immunity: conserved function of divergent chemicals. New Phytol. 206, 948–964. doi: 10.1111/nph.13325
Pierik, R., Tholen, D., Poorter, H., Visser, E. J., Voesenek, L. A. C. J. (2006). The Janus face of ethylene: growth inhibition and stimulation. Trends Plant Sci. 11, 176–183. doi: 10.1016/j.tplants.2006.02.006
Pieterse, C. M., Zamioudis, C., Berendsen, R. L., Weller, D. M., Van Wees, S. C., Bakker, P. A. (2014). Induced system resistance by beneficial microbes. Annu. Rev. Phytopath. 52, 347–375. doi: 10.1146/annurev-phyto-082712-102340
Pinedo, I., Ledger, T., Greve, M., Poupin, M. J. (2015). Burkholderia phytofirmans PsJN induces long-term metabolic and transcriptional changes involved in Arabidopsis thaliana salt tolerance. Front. Plant Sci. 6. doi: 10.3389/fpls.2015.00466
Pitzschke, A., Djamei, A., Teige, M., Hirt, H. (2009). VIP1 response elements mediate mitogen-activated protein kinase 3-induced stress gene expression. Proc. Natl. Acad. Sci. U.S.A. 106 (43), 18414–18419. doi: 10.1073/pnas.0905599106
Purohit, A., Ganguly, S., Chaudhuri, R. K., Chakraborti, D. (2019). “Understanding the interaction of molecular factors during the crosstalk between drought and biotic stresses in plants,” in Molecular Plant Abiotic Stress: Biology and Biotechnology (Hoboken: Wiley), 427–446.
Qi, T., Song, S., Ren, Q., Wu, D., Huang, H., Chen, Y., et al. (2011). The Jasmonate-ZIM-domain proteins interact with the WD-Repeat/bHLH/MYB complexes to regulate Jasmonate-mediated anthocyanin accumulation and trichome initiation in Arabidopsis thaliana. Plant Cell 23 (5), 1795–1814. doi: 10.1105/tpc.111.083261
Qian, C. D., Fu, Y. H., Jiang, F. S., Xu, Z. H., Cheng, D. Q., Ding, B., et al. (2014). Lasiodiplodia sp. ME4-2, an endophytic fungus from the floral parts of Viscum coloratum, produces indole-3-carboxylic acid and other aromatic metabolites. BMC Microbiol. 14, 297. doi: 10.1186/s12866-014-0297-0
Qin, S., Feng, W. W., Zhang, Y. J., Wang, T. T., Xiong, Y. W., Xing, K. (2018). Diversity of bacterial microbiota of coastal Halophyte Limonium sinense and amelioration of salinity stress damage by symbiotic plant growth-promoting actinobacterium Glutamicibacter halophytocola KLBMP 5180. Appl. Environ. Microbiol. 84, e01533–e01518. doi: 10.1128/AEM.01533-18
Qualhato, T. F., Lopes, F. A. C., Steindorff, A. S., Brandao, R. S., Jesuino, R. S. A., Ulhoa, C. J. (2013). Mycoparasitism studies of Trichoderma species against three phytopathogenic fungi: Evaluation of antagonism and hydrolytic enzyme production. Biotechnol. Lett. 35, 1461–1468. doi: 10.1007/s10529-013-1225-3
Radwan, S. (2009). “Phytoremediation for oily desert soils,” in Advances in Applied Bioremediation, (Berlin, Heidelberg: Springer), 279–298. doi: 10.1007/978-3-540-89621-0_15
Rajesh, P. S., Rai, R. V. (2014). Quorum quenching activity in cell-free lysate of endophytic bacteria isolated from Pterocarpus santalinus Linn., and its effect on quorum sensing regulated biofilm in Pseudomonas aeruginosa PAO1. Microbiol. Res. 169, 561–569. doi: 10.1016/j.micres.2013.10.005
Rajkumar, M., Ae, N., Freitas, H. (2009). Endophytic bacteria and their potential to enhance heavy metal phytoextraction. Chemosphere 77 (2), 153–160. doi: 10.1016/j.chemosphere.2009.06.047
Rajkumar, M., Ae, N., Prasad, M. N. V., Freitas, H. (2010). Potential of siderophore-producing bacteria for improving heavy metal phytoextraction. Trends Biotechnol. 28, 142–149. doi: 10.1016/j.tibtech.2009.12.002
Rakotoniriana, E. F., Rafamantanana, M., Randriamampionona, D., Rabemanantsoa, C., Urveg-Ratsimamanga, S., El Jaziri, M., et al. (2013). Study in vitro of the impact of endophytic bacteria isolated from Centella asiatica on the disease incidence caused by the hemibiotrophic fungus Colletotrichum higginsianum. Antonie Van Leeuwenhoek 103, 121–133. doi: 10.1007/s10482-012-9791-2
Rat, A., Naranjo, H. D., Krigas, N., Grigoriadou, K., Maloupa, E., Alonso, A. V., et al. (2021). Endophytic bacteria from the roots of the medicinal plant Alkanna tinctoria Tausch (Boraginaceae): Exploration of plant growth promoting properties and potential role in the production of plant secondary metabolites. Front. Microbiol. 12, 113. doi: 10.1016/j.micres.2018.04.006
Ravanbakhsh, M., Sasidharan, R., Voesenek, L. A. C. J., Kowalchuk, G. A., Jousset, A. (2018). Microbial modulation of plant ethylene signaling: ecological and evolutionary consequences. Microbiome 6, 52. doi: 10.1186/s40168-018-0436-1
Ray, T., Pandey, A., Pandey, S. S., Singh, S., Shanker, K., Kalra, A. (2021). Molecular insights into enhanced resistance of Papaver somniferum against downy mildew by application of endophyte bacteria Microbacterium sp. SMR1. Physiol. Plant 173 (4), 1862–1881. doi: 10.1111/ppl.13528
Ray, T., Pandey, S. S., Pandey, A., Srivastava, M., Shanker, K., Kalra, A. (2019). Endophytic consortium with diverse gene-regulating capabilities of benzylisoquinoline alkaloids biosynthetic pathway can enhance endogenous morphine biosynthesis in Papaver somniferum. Front. Microbiol. 10, 925. doi: 10.3389/fmicb.2019.00925
Raza, A. (2021). Eco-physiological and biochemical responses of rapeseed (Brassica napus L.) to abiotic stresses: consequences and mitigation strategies. J. Plant Growth Regul. 40 (4), 1368–1388. doi: 10.1007/s00344-020-10231-z
Raza, A. (2022). Plant biotechnological tools: Solutions for raising climate-resilient crop plants. Modern Phytomorphol. 15, 132–133.
Raza, A., Hussain, S., Javed, R., Hafeez, M. B., Hasanuzzaman, M. (2021a). "Antioxidant defense systems and remediation of metal toxicity in plants" in Approaches to the remediation of inorganic pollutants. Eds. Hasanuzzaman, M. (Singapore: Springer). doi: 10.1007/978-981-15-6221-1_6
Raza, A., Tabassum, J., Kudapa, H., Varshney, R. K. (2021b). Can omics deliver temperature resilient ready-to-grow crops? Crit. Rev. Biotechnol. 41 (8), 1209–1232. doi: 10.1080/07388551.2021.1898332
Raza, A., Tabassum, J., Zahid, Z., Charagh, S., Bashir, S., Barmukh, R., et al. (2022). Advances in “omics” approaches for improving toxic metals/metalloids tolerance in plants. Front. Plant Sci. 12, 794373. doi: 10.3389/fpls.2021.794373
Ren, C. G., Dai, C. C. (2012). Jasmonic acid is involved in the signaling pathway for fungal endophyte-induced volatile oil accumulation of Atractylodes lancea plantlets. BMC Plant Biol. 12 (1), 128. doi: 10.1186/1471-2229-12-128
Ritonga, F. N., Ngatia, J. N., Wang, Y., Khoso, M. A., Farooq, U., Chen, S. (2021). AP2/ERF, an important cold stress-related transcription factor family in plants: A review. Physiol. Mol. Biol. Plants 27, 1953–1968. doi: 10.1007/s12298-021-01061-8
Ritpitakphong, U., Falquet, L., Vimoltust, A., Berger, A., Métraux, J.-P., L’Haridon, F. (2016). The microbiome of the leaf surface of Arabidopsis protects against a fungal pathogen. New Phytol. 210, 1033–1043. doi: 10.1111/nph.13808
Rotich, E., Mmbaga, M. T. (2023). Data on plant defense enzyme activity associated with three endophytes against Cornusflorida Erysiphepulchra powdery mildew. Data Brief 48, 109220. doi: 10.1016/j.dib.2023.109220
Sabagh, A. E., Mbarki, S., Hossain, A., Iqbal, M. A., Islam, M. S., Raza, A., et al. (2021). Potential role of plant growth regulators in administering crucial processes against abiotic stresses. Front. Agron. 3, 648694. doi: 10.3389/fagro.2021.648694
Saddiq, M. S., Afzal, I., Iqbal, S., Hafeez, M. B., Raza, A. (2021). Low leaf sodium content improves the grain yield and physiological performance of wheat genotypes in saline-sodic soil. Pesquisa Agro. Trop. 51, e67663–e67663. doi: 10.1590/1983-40632021v5167663
Sahu, S., Prakash, A., Shende, K. (2019). Talaromyces trachyspermus, an endophyte from Withania somnifera with plant growth promoting attributes. Environ. Sustain. 2, 13–21. doi: 10.1007/s42398-019-00045-5
Saia, S., Corrado, G., Vitaglione, P., Colla, G., Bonini, P., Giordano, M., et al. (2021). An endophytic fungi-based biostimulant modulates volatile and non-volatile secondary metabolites and yield of greenhouse basil (Ocimum basilicum L.) through variable mechanisms dependent on salinity stress level. Pathogens 10 (7), 797. doi: 10.3390/pathogens10070797
Salam, N., Khieu, T. N., Liu, M. J., Vu, T. T., Chu-Ky, S., Quach, N. T., et al. (2017). Endophytic actinobacteria associated with Dracaena cochinchinensis lour.: isolation, diversity, and their cytotoxic activities. Biomed. Res. Int. 2017, 1308563. doi: 10.1155/2017/1308563
Saleem, M., Fariduddin, Q., Janda, T. (2021). Multifaceted role of salicylic acid in combating cold stress in plants: A review. J. Plant Growth Regul. 40, 464–485. doi: 10.1007/s00344-020-10152-x
Salwan, R., Rana, A., Saini, R., Sharma, A., Sharma, M., Sharma, V. (2023). Diversity analysis of endophytes with antimicrobial and antioxidant potential from Viola odorata: An endemic plant species of the Himalayas. Braz. J. Microbiol. 54, 2361–2374. doi: 10.1007/s42770-023-01010-5
Santino, A., Taurino, M., De Domenico, S., Bonsegna, S., Poltronieri, P., Pastor, V., et al. (2013). Jasmonate signaling in plant development and defense response to multiple (a) biotic stresses. Plant Cell Rep. 32, 1085–1098. doi: 10.1007/s00299-013-1441-2
Santoyo, G., Moreno-Hagelsieb, G., Del Carmen Orozco-Mosqueda, M., Glick, B. R. (2016). Plant growth-promoting bacterial endophytes. Microbiol. Res. 183, 92–99. doi: 10.1016/j.micres.2015.11.008
Santra, H. K., Banerjee, D. (2023). Antifungal activity of volatile and non-volatile metabolites of endophytes of Chloranthus elatior Sw. Front. Plant Sci. 14. doi: 10.3389/fpls.2023.1156323
Sasse, J., Martinoia, E., Northen, T. (2018). Feed your friends: do plant exudates shape the root microbiome? Trends Plant Sci. 23 (1), 25–41. doi: 10.1016/j.tplants.2017.09.003
Schaller, G. E. (2012). Ethylene and the regulation of plant development. BMC Biol. 10 (1), 1–3. doi: 10.1186/1741-7007-10-9
Scherm, H., Coakley, S. M. (2003). Plant pathogens in a changing world. Australas. Plant Pathol. 32, 157–165. doi: 10.1071/AP03015
Schlenker, W., Roberts, M. J. (2009). Nonlinear temperature effects indicate severe damages to US crop yields under climate change. Proc. Nat. Acad. Sci. 106 (37), 15594–15598. doi: 10.1073/pnas.0906865106
Schmidt, R., Köberl, M., Mostafa, A., Ramadan, E. M., Monschein, M., Jensen, K. B., et al. (2014). Effects of bacterial inoculants on the indigenous microbiome and secondary metabolites of chamomile plants. Front. Microbiol. 5, 64. doi: 10.3389/fmicb.2014.00064
Schulz, B., Boyle, C. (2005). The endophytic continuum. Mycol. Res. 109 (6), 661–686. doi: 10.1017/S095375620500273X
Schütz, V., Bigler, L., Girel, S., Laschke, L., Sicker, D., Schulz, M. (2019). Conversions of benzoxazinoids and downstream metabolites by soil microorganisms. Front. Ecol. Evol. 7. doi: 10.3389/fevo.2019.00238
Segura, A., Ramos, J. L. (2013). Plant-bacteria interactions in the removal of pollutants. Curr. Opin. Biotechnol. 24, 467–473. doi: 10.1016/j.copbio.2012.09.011
Semwal, P., Misra, S., Misra, A., Kar, S., Majhi, B., Mishra, S. K., et al. (2023). Endophytic Bacillus strains enhance biomass and bioactive metabolites of Gloriosa superba. Ind. Crops Prod. 204, 117296. doi: 10.1016/j.indcrop.2023.117296
Sen, S., Ghosh, D., Mohapatra, S. (2018). Modulation of polyamine biosynthesis in Arabidopsis thaliana by a drought mitigating Pseudomonas putida strain. Plant Physiol. Biochem. 129, 180–188. doi: 10.1016/j.plaphy.2018.05.034
Shabaan, M., Asghar, H. N., Zahir, Z. A., Zhang, X., Sardar, M. F., Li, H. (2022). Salt-tolerant PGPR confer salt tolerance to maize through enhanced soil biological health, enzymatic activities, nutrient uptake and antioxidant defense. Front. Microbiol. 13. doi: 10.3389/fmicb.2022.90186
Shah, S., Thapa, B. B., Chand, K., Pradhan, S., Singh, A., Varma, A., et al. (2019). Piriformospora indica promotes the growth of the in-vitro-raised Cymbidium aloifolium plantlet and their acclimatization. Plant Signal. Behav. 14 (6), 1596716. doi: 10.1080/15592324.2019.1596716
Shahid, M., Ameen, F., Maheshwari, H. S., Ahmed, B., AlNadhari, S., Khan, M. S. (2021). Colonization of Vigna radiata by a halotolerant bacterium Kosakonia sacchari improves the ionic balance, stressor metabolites, antioxidant status and yield under NaCl stress. Appl. Soil Ecol. 158, 103809. doi: 10.1016/j.apsoil.2020.103809
Shahzad, R., Khan, A. L., Bilal, S., Asaf, S., Lee, I. J. (2018). What is there in seeds? Vertically transmitted endophytic resources for sustainable improvement in plant growth. Front. Plant Sci. 9. doi: 10.3389/fpls.2018.00024
Shakeel, S. N., Gao, Z., Amir, M., Chen, Y. F., Rai, M. I., Haq, N. U., et al. (2015). Ethylene regulates levels of ethylene receptor/CTR1 signaling complexes in Arabidopsis thaliana. J. Biol. Chem. 290 (19), 12415–12424. doi: 10.1074/jbc.M115.652503
Sharma, S., Dhar, M. K., Kaul, S. (2023). Antagonistic, plant growth promoting and extracellular hydrolytic enzyme activity of fungal endophytes of Dioscorea bulbifera L. Biocatal. Agric. Biotechnol. 50, 102694. doi: 10.1016/j.bcab.2023.102694
Sheard, L. B., Tan, X., Mao, H., Withers, J., Ben-Nissan, G., Hinds, T. R., et al. (2010). Jasmonate perception by inositol-phosphate-potentiated COI1–JAZ co-receptor. Nature 468 (7322), 400–405. doi: 10.1038/nature09430
Sheibani-Tezerji, R., Rattei, T., Sessitsch, A., Trognitz, F., Mitter, B. (2015). Transcriptome profiling of the endophyte Burkholderia phytofirmans PsJN indicates sensing of the plant environment and drought stress. MBio 6 (5), e00621–e00615. doi: 10.1128/mBio.00621-15
Shen, F. T., Yen, J. H., Liao, C. S., Chen, W. C., Chao, Y. T. (2019). Screening of rice endophytic biofertilizers with fungicide tolerance and plant growth promoting characteristics. Sustainability 11, 1133. doi: 10.3390/su11041133
Sheoran, N., Nadakkakath, A. V., Munjal, V., Kundu, A., Subaharan, K., Venugopal, V., et al. (2015). Genetic analysis of plant endophytic Pseudomonas putida BP25 and chemo-profiling of its antimicrobial volatile organic compounds. Microbiol. Res. 173, 66–78. doi: 10.1016/j.micres.2015.02.001
Siddique, S., Naveed, M., Yaseen, M., Shahbaz, M. (2022). Exploring potential of seed endophytic bacteria for enhancing drought stress resilience in maize (Zea mays L.). Sustainability 14, 673. doi: 10.3390/su14020673
Silva, G. H., Teles, H. L., Zanardi, L. M., Young, M. C. M., Eberlin, M. N., Hadad, R., et al. (2006). Cadinane sesquiterpenoids of Phomopsis cassiae, an endophytic fungus associated with Cassia spectabilis (Leguminosae). Phytochemistry 67 (17), 1964–1969. doi: 10.1016/j.phytochem.2006.06.004
Song, L. S., Huo, J., Wan, L., Pan, L., Jiang, N., Fu, J., et al. (2023). Differences and biocontrol potential of haustorial endophytic fungi from Taxillus chinensis on different host plants. BMC Microbio. 23 (1), 128. doi: 10.1186/s12866-023-02878-x
Spaepen, S., Vanderleyden, J., Remans, R. (2007). Indole-3-acetic acid in microbial and microorganism-plant signaling. FEMS Microbiol. Rev. 31, 425–448. doi: 10.1111/j.1574-6976.2007.00072.x
Stępniewska, Z., Kuźniar, A. (2013). Endophytic microorganisms– promising applications in bioremediation of greenhouse gases. Appl. Microbiol. Biotechnol. 97 (22), 9589–9596. doi: 10.1007/s00253-013-5235-9
Strobel, G. A. (2003). Endophytes as sources of bioactive products. Microb. Inf. 5 (6), 535–544. doi: 10.1016/S1286-4579(03)00073-X
Strobel, G. A., Miller, R. V., Martinez-Miller, C., Condron, M. M., Teplow, D. B., Hess, W. M. (1999). Cryptocandin, a potent antimycotic from the endophytic fungus cryptosporiopsis cf. quercina. Microbiology 145 (8), 1919–1926. doi: 10.1099/13500872-145-8-1919
Su, F., Jacquard, C., Villaume, S., Michel, J., Rabenoelina, F., Clément, C., et al. (2015). Burkholderia phytofirmans PsJN reduces impact of freezing temperatures on photosynthesis in Arabidopsis thaliana. Front. Plant Sci. 6. doi: 10.3389/fpls.2015.00810
Suhita, D., Kolla, V. A., Vavasseur, A., Raghavendra, A. S. (2003). Different signaling pathways involved during the suppression of stomatal opening by methyl jasmonate or abscisic acid. Plant Sci. 164, 481–488. doi: 10.1016/S0168-9452(02)00432-6
Sumarah, M. W., Puniani, E., Sørensen, D., Blackwell, B. A., Miller, J. D. (2010). Secondary metabolites from anti-insect extracts of endophytic fungi isolated from picea rubens. Phytochemistry 71 (7), 760–765. doi: 10.1016/j.phytochem.2010.01.015
Sun, Z., Adeleke, B. S., Shi, Y., Li, C. (2023). The seed microbiomes of staple food crops. Microb. Biotechnol. 00, 1–14. doi: 10.1111/17517915.14352
Sun, X., Zhao, T., Gan, S., Rem, X., Fang, L., Karungo, S. K., et al. (2016). Ethylene positively regulates cold tolerance in grapevine by modulating the expression of ETHYLENE RESPONSE FACTOR 057. Sci. Rep. 6, 24066. doi: 10.1038/srep24066
Suryanarayanan, T. S., Thirunavukkarasu, N., Govindarajulu, M. B., Sasse, F., Jansen, R., Murali, T. S. (2009). Fungal endophytes and bioprospecting. Fungal Biol. Rev. 23, 9–19. doi: 10.1016/j.fbr.2009.07.001
Sziderics, A. H., Rasche, F., Trognitz, F., Sessitsch, A., Wilhelm, E. (2007). Bacterial endophytes contribute to abiotic stress adaptation in pepper plants (Capsicum annuum L.). Can. J. Microbiol. 53 (11), 1195–1202. doi: 10.1139/W07-082
Tahir, H. A. S., Gu, Q., Wu, H., Niu, Y., Huo, R., Gao, X. (2017). Bacillus volatiles adversely affect the physiology and ultra-structure of Ralstonia solanacearum and induce systemic resistance in tobacco against bacterial wilt. Sci. Rep. 7, 40481. doi: 10.1038/srep40481
Takasaki, H., Maruyama, K., Kidokoro, S., Ito, Y., Fujita, Y., Shinozaki, K., et al. (2010). The abiotic stress-responsive NAC-type transcription factor OsNAC5 regulates stress-inducible genes and stress tolerance in rice. Mol. Genet. Genomics 284, 173–183. doi: 10.1007/s00438-010-0557-0
Tanaka, A., Tapper, B. A., Popay, A., Parker, E. J., Scott, B. (2005). A symbiosis expressed non-ribosomal peptide synthetase from a mutualistic fungal endophyte of perennial ryegrass confers protection to the symbiotum from insect herbivory. Mol. Microbiol. 57 (4), 1036–1050. doi: 10.1111/j.1365-2958.2005.04747.x
Tang, M. J., Meng, Z. X., Guo, S. X., Chen, X. M., Xiao, P. G. (2008). Effects of endophytic fungi on the culture and four enzyme activities of anoectochilus roxburghii. Chin. Pharm. J. 43, 890–893. doi: 10.3321/j.issn:1001-2494.2008.12.003
Teixeira, P. J. P., Colaianni, N. R., Fitzpatrick, C. R., Dangl, J. L. (2019). Beyond pathogens: microbiota interactions with the plant immune system. Curr. Opin. Microbiol. 49, 7–17. doi: 10.1016/j.mib.2019.08.003
Thankam, S. R., Manuel, S. G. (2023). Identification and characterization of endophytic bacteria isolated from Curcuma longa. Proc. Nat. Acad. Sci. India Sect. B Biol. Sci. 93, 763–774. doi: 10.100/2Fs13205-016-0393-y
Tharek, M., Abdullahi, S., Mia, M. A. B., Najimudin, N., Ghazali, A. H., Fujita, M. (2022). “Endophytes as potential biostimulants to enhance plant growth for promoting sustainable agriculture,” in Biostimulants for crop production and sustainable agriculture. Eds. Hasanuzzaman, M., Hawrylak-Nowak, B., Islam, T. M. (Cham: Springer), 414–428. doi: 10.1079/9781789248098.0026
Theocharis, A., Bordiec, S., Fernandez, O., Paquis, S., Dhondt-Cordelier, S., Baillieul, F., et al. (2012). Burkholderia phytofirmans PsJN primes Vitis vinifera L. and confers a better tolerance to low nonfreezing temperatures. Mol. Plant Microbe Interact. 25, 241–249. doi: 10.1094/MPMI-05-11-0124
Thorpe, M. R., Ferrieri, A. P., Herth, M. M., Ferrieri, R. A. (2007). 11 C-imaging: methyl jasmonate moves in both phloem and xylem, promotes transport of jasmonate, and of photoassimilate even after proton transport is decoupled. Planta 226, 541–551. doi: 10.1007/s00425-007-0503-5
Tidke, S. A., Kiran, S., Giridhar, P., Gokare, R. A. (2019). “Current understanding and future perspectives of endophytic microbes visa- vis production of secondary metabolites,” in Reference Series in Phytochemistry. Ed. Jha, S. (Cham: Springer). doi: 10.1007/978-3-319-90484-9_12
Ting, A. S. Y., Meon, S., Kadir, J., Radu, S., Singh, G. (2009). Induced host resistance by non-pathogenic fusarium endophyte as a potential defense mechanism in fusarium wilt management of banana. Pest Technol. 3 (1), 67–72.
Tirry, N., Kouchou, A., Laghmari, G., Lemjereb, M., Hnadi, H., Amrani, K., et al. (2021). Improved salinity tolerance of Medicago sativa and soil enzyme activities by PGPR. Biocatal. Agric. Biotechnol. 31, 101914. doi: 10.1016/j.bcab.2021.101914
Ton, J., Davison, S., Van Wees, S. C., Van Loon, L. C., Pieterse, C. M. (2001). The Arabidopsis ISR1 locus controlling rhizobacteria-mediated induced systemic resistance is involved in ethylene signaling. Plant Physiol. 125 (2), 652–661. doi: 10.1104/pp.125.2.652
Toubal, S., Bouchenak, O., Elhaddad, D., Yahiaoui, K., Boumaza, S., Arab, K. (2018). MALDI-TOF MS detection of endophytic bacteria associated with great nettle (Urtica dioica L.), grown in Algeria. Pol. J. Microbiol. 67, 67–72. doi: 10.5604/01.3001.0011.6145
Tripathi, A., Awasthi, A., Singh, S., Sah, K., Maji, D., Patel, V. K., et al. (2020). Enhancing artemisinin yields through an ecologically functional community of endophytes in Artemisia annua. Ind. Crops Prod. 150, 112375. doi: 10.1016/j.indcrop.2020.112375
Tripathi, A., Pandey, P., Tripathi, S. N., Kalra, A. (2022). Perspectives and potential applications of endophytic microorganisms in cultivation of medicinal and aromatic plants. Front. Plant Sci. 13, 985429. doi: 10.3389/fpls.2022.985429
Tripathi, P., Tripathi, A., Singh, A., Yadav, V., Shanker, K., Khare, P., et al. (2022a). Differential response of two endophytic bacterial strains inoculation on biochemical and physiological parameters of Bacopa monnieri L. under arsenic stress conditions. J. Hazard. Mater. Adv. 6, 100055. doi: 10.1016/j.hazadv.2022.100055
Tripathi, P., Yadav, R., Das, P., Singh, A., Singh, R. P., Kandasamy, P., et al. (2021). Endophytic bacterium CIMAP-A7 mediated amelioration of atrazine induced phyto-toxicity in Andrographis paniculata. Environ. pollut. 287, 117635. doi: 10.1016/j.envpol.2021.117635
Trivedi, P., Leach, J. E., Tringe, S. G., Sa, T., Singh, B. K. (2020). Plant-microbiome interactions: from community assembly to plant health. Nat. Rev. Microbiol. 18 (11), 607–621. doi: 10.1038/s41579-020-0412-1
Turner, J. G., Ellis, C., Devoto, A. (2002). The jasmonate signal pathway. Plant Cell 14 Suppl, S153–S164. doi: 10.1105/tpc.000679
Van Bael, S. A., Seid, M. A., Wcislo, W. T. (2012). Endophytic fungi increase the processing rate of leaves by leaf-cutting ants (Atta). Ecol. Entomol. 37 (4), 318–321. doi: 10.1111/j.1365-2311.2012.01364.x
Vanessa, M. C., Christopher, M. M. F. (2004). Analysis of the endophytic actinobacterial population in the roots of wheat (Triticum aestivum l.) by terminal restriction fragment length polymorphism and sequencing of 16S rRNA clones. Appl. Environ. Microbiol. 70 (3), 1787–1794. doi: 10.1128/AEM.70.3.1787-1794.2004
Vanlerberghe, G. C., Martyn, G. D., Dahal, K. (2016). Alternative oxidase: a respiratory electron transport chain pathway essential for maintaining photosynthetic performance during drought stress. Plant Physiol. 157 (3), 322–337. doi: 10.1111/ppl.12451
Vega, V. (2018). The use of fungal entomopathogens as endophytes in biological control: a review. Mycologia 110, 4–30. doi: 10.1080/00275514.2017.1418578
Vega, F. E., Posada, F., Aime, M. C., Pava-Ripoll, M., Infante, F., Rehner, S. A. (2008). Entomopathogenic fungal endophytes. Biol. Control 46 (1), 72–82. doi: 10.1016/j.biocontrol.2008.01.008
Verma, V., Ravindran, P., Kumar, P. P. (2016). Plant hormone-mediated regulation of stress responses. BMC Plant Biol. 16, 1–10. doi: 10.1186/s12870-016-0771-y
Vidal, S., Jaber, L. R. (2015). Entomopathogenic fungi as endophytes: plant–endophyte–herbivore interactions and prospects for use in biological control. Curr. Sci. 109, 46–54. doi: 10.1016/j.biocontrol.2017.01.018
Vinocur, B., Altman, A. (2005). Recent advances in engineering plant tolerance to abiotic stress: achievements and limitations. Curr. Opin. Biotech. 16 (2), 123–132. doi: 10.1016/j.copbio.2005.02.001
Vlot, A. C., Sales, J. H., Lenk, M., Bauer, K., Brambilla, A., Sommer, A., et al. (2021). Systemic propagation of immunity in plants. New Phytol. 229, 1234–1250. doi: 10.1111/nph.16953
Vyas, P., Kumar, D., Dubey, A., Kumar, A. (2018). Screening and characterization of Achromobacter xylosoxidans isolated from rhizosphere of Jatropha curcas L. (energy crop) for plant-growth-promoting traits. J. Adv. Res. Biotechnol. 3 (1), 1–8. doi: 10.15226/2475-4714/3/1/00134
Wahab, A., Abdi, G., Saleem, M. H., Ali, B., Ullah, S., Shah, W., et al. (2022). Plants’ physio-biochemical and phyto-hormonal responses to alleviate the adverse effects of drought stress: A comprehensive review. Plants 11 (13), 1620. doi: 10.3390/plants11131620
Wan, Y., Luo, S., Chen, J., Xiao, X., Chen, L., Zeng, G., et al. (2012). Effect of endophyte-infection on growth parameters and Cd-induced phytotoxicity of Cd-hyperaccumulator Solanum nigrum L. Chemosphere 89 (6), 743–750. doi: 10.1016/j.chemosphere.2012.07.005
Wang, S., Chen, S., Wang, B., Li, Q., Zu, J., Yu, J., et al. (2023). Screening of endophytic fungi from Cremastra appendiculata and their potential for plant growth promotion and biological control. Folia Microbiol. 68 (1), 121–133. doi: 10.1007/s12223-022-00995-0
Wang, L., Liu, L., Han, S. Z. (2009). Screening and identification of antimicrobe activity of endophytic fungus in glycyrrhiza uralensis. Biotechnol. Bull. 6, 034.
Wang, J., Song, L., Gong, X., Xu, J., Li, M. (2020). Functions of jasmonic acid in plant regulation and response to abiotic stress. Int. J. Mol. Sci. 21 (4), 1446. doi: 10.3390/ijms21041446
Wang, T., Wang, S., Wang, Y., Li, J., Yan, F., Liu, Y., et al. (2020). Jasmonic acid–induced inhibition of root growth and leaf senescence is reduced by GmbHLH3, a soybean bHLH transcription factor. Can. J. Plant Sci. 100 (5), 477–487. doi: 10.1139/cjps-2019-0250
Wang, Y., Xu, H., Liu, W., Wang, N., Qu, C., Jiang, S., et al. (2019). Methyl jasmonate enhances apple’cold tolerance through the JAZ–MYC2 pathway. Plant Cell Tissue Organ Cult. (PCTOC) 136, 75–84. doi: 10.1007/s11240-018-1493-7
Wang, Y., Xu, L., Ren, W., Zhao, D., Zhu, Y., Wu, X. (2012). Bioactive metabolites from chaetomium globosum L18, an endophytic fungus in the medicinal plant curcuma wenyujin. Phytomedicine 19 (3-4), 364–368. doi: 10.1016/j.phymed.2011.10.011
Wang, X., Yesbergenova-Cuny, Z., Biniek, C., Bailly, C., El-Maarouf-Bouteau, H., Corbineau, F. (2018). Revisiting the role of ethylene and N-end rule pathway on chilling-induced dormancy release in Arabidopsis seeds. Int. J. Mol. Sci. 19 (11), 3577. doi: 10.3390/ijms19113577
Wang, F., Yu, G., Liu, P. (2019). Transporter-mediated subcellular distribution in the metabolism and signaling of jasmonates. Front. Plant Sci. 10, 390. doi: 10.3389/fpls.2019.00390
Wang, Q., Zhang, W., Yin, Z., Wen, C. K. (2013). Rice constitutive triple-response2 is involved in the ethylene-receptor signaling and regulation of various aspects of rice growth and development. J. Exp. Bot. 264, 4863–4875. doi: 10.1093/jxb/ert272
Wang, Y., Mostafa, S., Zeng, W., Jin, B. (2021). Function and mechanism of jasmonic acid in plant responses to abiotic and biotic stresses. Int. J. Mol. Sci. 22 (16), 8568. doi: 10.3390/ijms22168568
Wani, S. H., Kumar, V., Shriram, V., Sah, S. K. (2016). Phytohormones and their metabolic engineering for abiotic stress tolerance in crop plants. Crop J. 4 (3), 162–176. doi: 10.1016/j.cj.2016.01.010
Wanke, A., Rovenich, H., Schwanke, F., Velte, S., Becker, S., Hehemann, J. H., et al. (2020). Plant species-specific recognition of long and short β-1, 3-linked glucans is mediated by different receptor systems. Plant J. 102 (6), 1142–1156. doi: 10.1111/tpj.14688
Waqas, M., Khan, A. L., Hamayun, M., Shahzad, R., Kang, S. M., Kim, J. G., et al. (2015a). Endophytic fungi promote plant growth and mitigate the adverse effects of stem rot: an example of Penicillium citrinum and Aspergillus terreus. J. Plant Interact. 10 (1), 280–287. doi: 10.1080/17429145.2015.1079743
Waqas, M., Khan, A. L., Hamayun, M., Shahzad, R., Kim, Y. H., Choi, K. S., et al. (2015b). Endophytic infection alleviates biotic stress in sunflower through regulation of defense hormones, antioxidants and functional amino acids. Eur. J. Plant Pathol. 141 (4), 803–824. doi: 10.1007/s10658-014-0581-8
Waqas, M., Khan, A. L., Kamran, M., Hamayun, M., Kang, S. M., Kim, Y. H., et al. (2012). Endophytic fungi produce gibberellins and indoleacetic acid and promotes host-plant growth during stress. Molecules 17 (9), 10754–10773. doi: 10.3390/molecules170910754
Wei, Y., Chen, H., Wang, L., Zhao, Q., Wang, D., Zhang, T. (2022). Cold acclimation alleviates cold stress-induced PSII inhibition and oxidative damage in tobacco leaves. Plant Signal. Behav. 17, 2013638. doi: 10.1080/15592324.2021.2013638
Weyens, N., van der Lelie, D., Taghavi, S., Vangronsveld, J. (2009). Phytoremediation: plant–endophyte partnerships take the challenge. Curr. Opin. Biotechnol. 20 (2), 248–254. doi: 10.1016/j.copbio.2009.02.012
Whipps, J., Gerhardson, B. (2007). “Biological pesticides for control of seedand soil-borne plant pathogens,” in Modern Soil Microbiol. (Boca Raton: CRC Press), 479–501.
Wiewiora, B., Żurek, G., Pańka, D. (2015). Is the vertical transmission of neotyphodium lolii in perennial ryegrass the only possible way to the spread of endophytes? PloS One 10 (2), e0117231. doi: 10.1371/journal.pone.0117231
Wojtaszek, P. (1997). Oxidative burst: an early plant response to pathogen infection. Biochem. J. 322 (3), 681–692. doi: 10.1042/bj3220681
Woo, O. G., Kim, H., Kim, J. S., Keum, H. L., Lee, K. C., Sul, W. J., et al. (2020). Bacillus subtilis strain GOT9 confers enhanced tolerance to drought and salt stresses in Arabidopsis thaliana and Brassica campestris. Plant Physiol. Biochem. 148, 359–367. doi: 10.1016/j.plaphy.2020.01.032
Wu, C. H., Bernard, S. M., Andersen, G. L., Chen, W. (2009). Developing microbe–plant interactions for applications in plant-growth promotion and disease control, production of useful compounds, remediation and carbon sequestration. Microb. Biotechnol. 2 (4), 428–440. doi: 10.1111/j.1751-7915.2009.00109.x
Wu, J. Y., Ng, J., Shi, M., Wu, S. J. (2007). Enhanced secondary metabolite (tanshinone) production of salvia miltiorrhiza hairy roots in a novel root–bacteria coculture process. Appl. Microbiol. Biotechnol. 77 (3), 543–550. doi: 10.1007/s00253-007-1192-5
Xie, M., Gao, X., Zhang, S., Fu, X., Le, Y., Wang, L. (2023). Cadmium stimulated cooperation between bacterial endophytes and plant intrinsic detoxification mechanism in Lonicera japonica thunb. Chemosphere 325, 138411. doi: 10.1016/j.chemosphere.2023.138411
Xiong, L., Yang, Y. (2003). Disease resistance and abiotic stress tolerance in rice are inversely modulated by an abscisic acid–inducible mitogen-activated protein kinase. Plant Cell 15 (3), 745–759. doi: 10.1105/tpc.008714
Xu, J. Y., Han, Y. H., Chen, Y., Zhu, L. J., Ma, L. Q. (2016). Arsenic transformation and plant growth promotion characteristics of As-resistant endophytic bacteria from As-hyperaccumulator Pteris vittata. Chemosphere 144, 1233–1240. doi: 10.1016/j.chemosphere.2015.09.102
Xu, L., Wu, C., Oelmüller, R., Zhang, W. (2018). Role of phytohormones in Piriformospora indica-induced growth promotion and stress tolerance in plants: More questions than answers. Front. Microbiol. 9. doi: 10.3389/fmicb.2018.01646
Yadav, S., Sella, N., Rather, S. A. (2021). “Role of jasmonates in pathogenesis and crosstalk of jasmonates with other hormones,” in Jasmonates and salicylates signaling in plants. Signaling and Communication in Plants. Eds. Aftab, T., Yusuf, M. (Cham: Springer), 207–237. doi: 10.1007/978-3-030-75805-9_1
Yadav, A., Singh, J., Singh, C., Yadav, N., Ghosh, S., Bhagwat, T., et al. (2020). Endophytic microbiomes and their plant growth promoting attributes for plant health. Curr. Trends Microb. Biotechnol. Sustain. Agric. 11, 245–278. doi: 10.1007/978-981-15-6949-4_11
Yan, X. N., Sikora, R. A., Zheng, J. W. (2011). Potential use of cucumber (Cucumis sativus L.) endophytic fungi as seed treatment agents against root-knot nematode Meloidogyne incognita. J. Zhejiang Univ. Sci. 12 (3), 219–225. doi: 10.1631/jzus.b1000165
Yang, R., Fan, X., Cai, X., Hu, F. (2014). The inhibitory mechanisms by mixtures of two endophytic bacterial strains isolated from Ginkgo biloba against pepper phytophthora blight. Biol. Control. 85, 59–67. doi: 10.1016/j.biocontrol.2014.09.013
Yang, N. Y., Jiang, S., Shang, E. X., Tang, Y. P., Duan, J. A. (2012). A new phenylpentanamine alkaloid produced by an endophyte Bacillus subtilis isolated from angelica sinensis. J. Chem. Res. 36 (11), 647–647. doi: 10.3184/174751912X13469254685262
Yang, L., Wen, K. S., Ruan, X., Zhao, Y. X., Wei, F., Wang, Q. (2018). Response of plant secondary metabolites to environmental factors. Molecules 23, 762. doi: 10.3390/molecules23040762
Yeang, A. Y., Hillman, J. R. (1984). Ethylene and apical dominance. Physiol. Plant 60 (3), 275–282. doi: 10.1111/j.1399-3054.1984.tb06062.x
Yehia, R. S. (2023). Multi-function of a new bioactive secondary metabolite derived from endophytic fungus Colletotrichum acutatum of Angelica sinensis. J. Microbiol. Biotechnol. 33, 806–822. doi: 10.4014/jmb.2206.06010
Yu, Y., Gui, Y., Li, Z., Jiang, C., Guo, J., Niu, D. (2022). Induced systemic resistance for improving plant immunity by beneficial microbes. Plants 11, 386. doi: 10.3390/plants11030386
Zandalinas, S. I., Mittler, R., Balfagón, D., Arbona, V., Gómez-Cadenas, A. (2018). Plant adaptations to the combination of drought and high temperatures. Physiol. Plant 162, 2–12. doi: 10.1111/ppl.12540
Zander, M., La Camera, S., Lamotte, O., Métraux, J. P., Gatz, C. (2010). Arabidopsis thaliana class-II TGA transcription factors are essential activators of jasmonic acid/ethylene-induced defense responses. Plant J. 61, 200–210. doi: 10.1111/j.1365-313X.2009.04044.x
Zarei, T., Moradi, A., Kazemeini, S. A., Akhgar, A., Rahi, A. A. (2020). The role of ACC deaminase producing bacteria in improving sweet corn (Zea mays L. var saccharata) productivity under limited availability of irrigation water. Sci. Rep. 10, 1–12. doi: 10.1038/s41598-020-77305-6
Zhang, Y., Li, S. S., Li, H. X., Wang, R. R., Zhang, K. Q., Xu, J. (2020). Fungal-nematode interactions: diversity, ecology and biocontrol prospects in agriculture. J. Fungi 6, 206. doi: 10.3390/jof6040206
Zhang, X., Li, G., Ma, J., Zeng, Y., Ma, W., Zhao, P. (2010). Endophytic fungus Trichothecium roseum LZ93 antagonizing pathogenic fungi in vitro and its secondary metabolites. J. Microbiol. 48 (6), 784–790. doi: 10.1007/s12275-010-0173-z
Zhang, H. W., Song, Y. C., Tan, R. X. (2006). Biology and chemistry of endophytes. Nat. Prod. Rep. 23 (5), 753–771. doi: 10.1039/b609472b
Zhang, X., Yang, L., Li, Y., Li, H., Wang, W., Ye, B. (2012). Impacts of lead/zinc mining and smelting on the environment and human health in China.Environ. Monit. Assess. 184 (4), 2261–2273. doi: 10.1007/s10661-011-2115-6
Zhao, H., Yin, C. C., Ma, B., Chen, S. Y., Zhang, J. S. (2021). Ethylene signaling in rice and Arabidopsis: New regulators and mechanisms. J. Integr. Plant Biol. 63 (1), 102–125. doi: 10.1111/jipb.13028
Zheng, L. P., Li, X. P., Zhou, L. L., Wang, J. W. (2021). Endophytes in Artemisia annua L.: new potential regulators for plant growth and artemisinin biosynthesis. Plant Growth Regul. 95, 293–313. doi: 10.1007/s10725-021-00751-3
Zhou, J., Xia, X. J., Zhou, Y. H., Shi, K., Chen, Z., Yu, J. Q. (2014). RBOH1-dependent H2O2 production and subsequent activation of MPK1/2 play an important role in acclimation-induced cross-tolerance in tomato. J. Exp. Bot. 65 (2), 595–607. doi: 10.1093/jxb/ert404
Zhu, Z. (2014). Molecular basis for jasmonate and ethylene signal interactions in Arabidopsis. J. Exp. Bot. 65 (20), 5743–5748. doi: 10.1093/jxb/eru349
Zhu, Y. X., Gong, H. J., Yin, J. L. (2019). Role of silicon in mediating salt tolerance in plants: a review. Plants 8 (6), 147. doi: 10.3390/plants8060147
Zhu, X., Jin, L., Sun, K., Li, S., Ling, W., Li, X. (2016). Potential of endophytic bacterium Paenibacillus sp. PHE-3 isolated from Plantago asiatica L. for reduction of PAH contamination in plant tissues. Int. J. Environ. Res. Public Health 13, 633–645. doi: 10.3390/ijerph13070633
Ziska, L. H., Tomecek, M. B., Gealy, D. R. (2010). Competitive interactions between cultivated and red rice as a function of recent and projected increases in atmospheric carbon dioxide. Agron. J. 102 (1), 118–123. doi: 10.2134/agronj2009.0205
Keywords: plant-microbe interaction, medicinal plants, biotic-abiotic stress, signaling pathways, ethylene, salicylic acid, jasmonic acid
Citation: Pandey P, Tripathi A, Dwivedi S, Lal K and Jhang T (2023) Deciphering the mechanisms, hormonal signaling, and potential applications of endophytic microbes to mediate stress tolerance in medicinal plants. Front. Plant Sci. 14:1250020. doi: 10.3389/fpls.2023.1250020
Received: 29 June 2023; Accepted: 30 October 2023;
Published: 15 November 2023.
Edited by:
Mamoona Rauf, Abdul Wali Khan University Mardan, PakistanReviewed by:
Muhammad Yahya Khan, University of Agriculture, Faisalabad, PakistanBartholomew Saanu Adeleke, Olusegun Agagu University of Science and Technology, Nigeria
Copyright © 2023 Pandey, Tripathi, Dwivedi, Lal and Jhang. This is an open-access article distributed under the terms of the Creative Commons Attribution License (CC BY). The use, distribution or reproduction in other forums is permitted, provided the original author(s) and the copyright owner(s) are credited and that the original publication in this journal is cited, in accordance with accepted academic practice. No use, distribution or reproduction is permitted which does not comply with these terms.
*Correspondence: Praveen Pandey, cGFuZGV5cHJhdmVlbjE5ODZAeWFob28uY29t; Tripta Jhang, amhhbmd0QGdtYWlsLmNvbQ==
†These authors have contributed equally to this work