- Key Laboratory of Saline-Alkali Vegetation Ecology Restoration, Ministry of Education, College of Life Sciences, Northeast Forestry University, Harbin, China
Betula platyphylla, belonging to the cold-specialized lineage Betulaceae, exhibits a unique reproductive strategy where staminate catkins emerge in the first summer and undergo an overwintering process, culminating in flowering in the following year. However, the underlying regulatory mechanism remains unclear. In this study, we investigated the male germline development of B. platyphylla in four distinct stages: microsporocytes in Oct. (S1), uninuclear microspores from Dec. (S2) to Mar. of the following year (S3), and bicellular microspores in Apr. (S4). We performed RNA sequencing on mature pollen and the four stages of staminate catkins. Using weighted gene co-expression network analysis (WGCNA), we identified five highly correlated gene modules with distinct expression profiles. These modules exhibited strong correlations with sugar metabolism, cell cycle, flowering, and cell wall dynamics, highlighting their dynamic roles during male germline developmental stages. During the overwintering process, we observed that the expression of transcription factors such as BpDUO1 and BpAMS at the appropriate developmental stages, suggests their significant roles in male germline development. The expression patterns of BpFLC and BpFT suggest their potential involvement in temperature perception during male reproductive development. These findings offer valuable insights into the reproductive success of plants adapting to cold environments.
1 Introduction
Angiosperm undergo a conserved male germline developmental process in the anther, where primary sporogenous cells differentiate into microsporocytes (Gómez et al., 2015). These microsporocytes then undergo meiosis to form tetrads, which eventually release four uninuclear microspores. Subsequently, the uninuclear microspores divide and develop into mature pollen grains consisting of a vegetative cell and two generative cells (Hafidh et al., 2016). The genetic regulation of these developmental processes is critical for successful male reproduction (Mandaokar et al., 2006; Wilson and Zhang, 2009). Many key genes, including DUO1 (DUO POLLEN 1), EMS1 (EXCESS MICROSPOROCYTES1), and AMS (ABORTED MICROSPORES), have been identified as essential regulators of male germline development (Zhao et al., 2002; Borg et al., 2011; Ferguson et al., 2017).
Cold stress often disrupts the male germline developmental process in cold-susceptible plants by decreasing reducing sugar availability and altering phytohormone levels, ultimately resulting in abnormal tapetal programmed cell death (PCD) and male sterility (Nayyar et al., 2005; Oliver et al., 2005; Oliver et al., 2007). In contrast, moderately low temperature induce the transition from vegetative to reproductive growth through a process called vernalization (Luo and He, 2020). Vernalization promotes the expression of flowing related genes, such as FT (FLOWERING LOCUS T), by downregulating the expression of FLC (FLOWERING LOCUS C), a key repressor of FT (Helliwell et al., 2006). The decline of FLC expression, mediated by epigenetic modification at the FLC locus, enables the transition from vegetative to reproductive growth in response to prolonged cold exposure (Bouché et al., 2017; Zhu et al., 2021). The dormancy phenomenon in trees shares similarities with vernalization (Chouard, 1960; Cooke et al., 2012).
Cold-tolerant plants have evolved adaptive strategies to cope with cold temperature during reproductive development (Campoy et al., 2011; Viti et al., 2013; Sharma and Nayyar, 2016). The genus Betula species, which is predominantly found in the northern hemisphere, exhibit staminate catkins that undergo a prolonged overwintering process and eventually achieve pollen dispersal (Chen et al., 1999; Wang et al., 2021). The reproductive processes in Betulaceae species have adapted to long-term low-temperature environments, including the slow development and dehydration of overwintering staminate catkins (Miller-Rushing and Primack, 2008). Climate factors, particularly air temperature, greatly influence the pollen concentration of birch (Jochner et al., 2013; Ranpal et al., 2022; Ranpal et al., 2023). Thus, it is essential to understand the molecular regulatory network underlying temperature response in this species for safeguarding long-term survival under climate change.
In this study, we investigated the developmental process of staminate catkins in Betula platyphylla trees during overwintering process. Throughout this process, male germline cells experienced a range of cold temperatures, from microsporocyte stages at chilling temperature to prolonged uninuclear microspore stages at freezing temperature, culminating in mature bicellular pollen. Weighted gene co-expression network analysis (WGCNA) of mature pollen and four stages of staminate catkins based on RNA sequencing (RNA-seq) revealed related biological processes during male germline development. Our results reveal that temperature fluctuation governs gene expression patterns of male germline development and pollen viability of B. platyphylla during the overwintering process.
2 Materials and methods
2.1 Plant materials
The staminate catkins and pollen were collected from outdoor B. platyphylla trees located at the Northeast Forestry University (45.7662°N, 126.6247°E). Staminate catkin samples were collected on Oct. 20 and Dec. 20, 2021, as well as on Mar. 20 and Apr. 20, 2022, respectively. Pollen samples were collected on May 1, 2022. Each sample was collected from three trees, and three biological replicates were used for each time point.
2.2 Sectioning and DAPI staining
The collected staminate catkins were fixed in FAA (Formalin-Aceto-Alcohol) with 70% ethanol: acetic acid: 37% formaldehyde = 18: 1: 1, for 48 hours, followed by five rounds of vacuuming for 1.5 hours each. The catkins were then dehydrated using a series of ethanol solutions with increasing concentrations: 50%, 70%, 85%, and 100%, with each concentration step lasting for 3.5 hours. Subsequently, the catkins were dehydrated using a series of xylene solutions with increasing concentrations: 50%, 70%, 85%, and 100%, with each concentration step lasting for 4 hours. The dehydrated material was gradually saturated with paraffin wax. The paraffin mass was sectioned into sections of 7 µm by ultramicrotome. The sections were then stained with 1% toluidine blue for visualization.
The staminate catkins were ground into powder to obtain germline cells. Germline cells were stained with 0.1 μg/mL DAPI (4’,6-diamidino-2-phenylindole) for 30 min and washed three times with PBS (phosphate buffered saline, pH = 7.4). The stained germline cells were observed using a DX51 fluorescence microscope (Olympus, Japan) under bright fields and ultraviolet illumination.
2.3 RNA extraction and cDNA library preparation
Samples were instantly frozen in liquid nitrogen and stored at -80°C. Total RNA was extracted using RNAprep Pure Plant Kit (DP441, Tiangen, China). The quality of the extracted RNA was assessed using a NanoPhotometer (Implen). The KAPA Stranded RNA-seq Kits (Roche) were employed for sequencing library preparation, and the library quality was evaluated using Agilent Bioanalyzer 2100 system (Agilent Technologies, CA, USA). Illumina RNA-Seq was performed by Metware Biotechnology Co. (Wuhan, China) using the Illumina Novaseq6000 system.
2.4 RNA-seq data profiling
The Betula platyphylla v1.1 reference genome was obtained from Phytozome (https://phytozome-next.jgi.doe.gov/info/Bplatyphylla_v1_1). Low-quality reads (Q20 ≤ 50%) were filtered out and Illumina adapters were removed using Cutadapt (Martin, 2011). The quality of the resulting clean reads was assessed using FastQC (Andrews, 2010).
Alignment of the clean reads to the reference genome was performed using Bowtie2 (Langmead and Salzberg, 2012). Gene counts were obtained using featureCounts, and fragments per kilobase of exon per million mapped reads (FPKM) values were calculated using the countToFPKM R package (Liao et al., 2014; Alhendi, 2019). Differentially expressed genes (DEGs) were identified using the DESeq2 R package with the following thresholds: adjusted p-value (p.adjust, FDR method) < 0.05 and absolute log2 fold change (|log2FC|) > 1 (Love et al., 2014).
Gene Ontology (GO) enrichment analysis was performed using the clusterProfiler R package (Yu et al., 2012). Gene functional annotations were based on the best match of BLAST searches against TAIR (https://www.arabidopsis.org/) and Swissprot protein databases (https://www.sib.swiss/swiss-prot) of genes. Weighted gene co-expression network analysis (WGCNA) was performed with soft thresholding power β = 8 using the WGCNA R package (Langfelder and Horvath, 2008). The similarity between genes (1-TOM) was calculated, and the genes with similar expression profiles were grouped into the same gene modules using hierarchical clustering and dynamic tree-cut, with a minimum size requirement of 30.
2.5 Quantitative real-time polymerase chain reaction
cDNA was synthesized with TransScript® One-Step gDNA Removal and cDNA Synthesis SuperMix (AT311, Transgen). The primers were listed in Supplementary Table 1. qRT-PCR was performed according to the protocol using TransStart® Top Green qPCR SuperMix (AQ131, Transgen), and three biological replicates were used for each experiment. The relative expressions of all tested candidate genes were normalized to the inner reference genes BpTUB (BPChr11G09309). The relative gene expression level was calculated by the 2-ΔΔCT method.
3 Results
3.1 Phenotypes of staminate catkins and male germline development
The staminate catkins of B. platyphylla, which emerged in June 2021 and dispersed pollen in early May 2022, were subjected to a long period of low temperature during development. To investigate the staminate catkins development across winter, we selected four distinct stages. At S1, staminate catkins were subjected to chilling temperature (0-15°C) in the monthly average temperature from 1°C to 13°C. Staminate catkins at S2-S3 were subjected to freezing temperature (< 0°C) (Figure 1A). While at S4, the temperature increased to above zero temperature, and the mean length of staminate catkins increased from 34.2 mm at S1 to 59.6 mm at S4. During freezing temperature, staminate catkins at S2 and S3 exhibited a dehydrated state, which enhanced their freezing tolerance. As the temperature rises, staminate catkins at S4 underwent significant swelling in size and the anthers in catkins approached the pollen-releasing stage (Figure 1B; Supplementary Table 2).
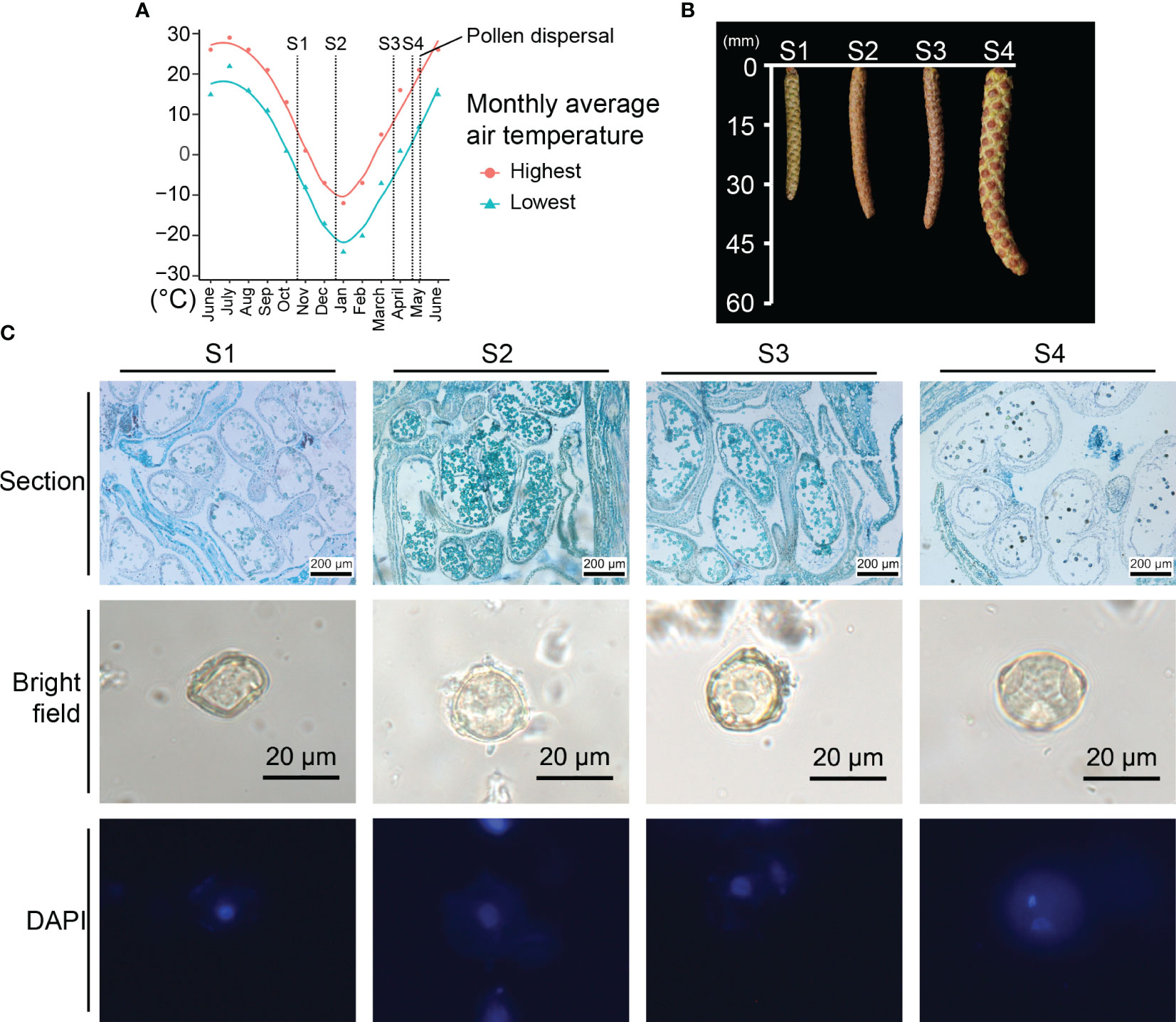
Figure 1 Phenotype and temperature condition of prolonged Betula platyphylla male development. (A) Monthly average temperature and development stages. y-axis: monthly average temperature of Harbin in °C from National Meteorological Information Center (http://data.cma.cn/). (B) Appearance and mean length of staminate catkins. (C) Phenotypic comparison of male germline stages.
We investigated the male germline development from B. platyphylla by performing sectioning and DAPI staining. The paraffin section and DAPI staining demonstrated a slow development across the four stages. Each anther of B. platyphylla often contains two locules. At S1, the locules contained interconnected microsporocytes. As the meiosis progresses from S1 to S2, uninuclear microspores differentiated and divided from microsporocytes, which significantly increased the cell numbers within the locules. From S2 to S3, uninuclear microspores with three pores were observed, and the phenotype had no significant changes during three months. At S4, the anther dehiscence occurred, and bicellular microspores were observed (Figure 1C).
Our findings suggest a microsporogenesis from S1 to S2, resulting in the formation of uninuclear microspores. From S2 to S3, uninuclear microspore development experiences a freeze-induced slowdown. Finally, at S4, the uninuclear microspores have developed into bicellular pollen, which is ready for release.
3.2 RNA-seq and differentially expressed genes analysis
RNA-seq was performed on pollen and staminate catkins from S1 to S4. The sequencing yielded a total of 1.2 billion high-quality clean reads. All the clean reads were aligned against the B. platyphylla reference genome (Chen et al., 2021), of which more than 91% were mapped and more than 82% were uniquely mapped, respectively (Supplementary Table 3). To obtain gene expression data, we calculated the fragments per kilobase of exon per kilobase million (FPKM) values of genes from the RNA-seq data for further analysis.
Principal component analysis (PCA) using the gene expression data revealed a strong correlation within three replicates of each experimental group along PC1 (41.99%) and PC2 (19.4%). In contrast to the other experimental groups that could be clearly distinguished from each other, the S2 and S3 experimental groups showed significant overlap, suggesting limited differentiation between these two groups (Figure 2A).
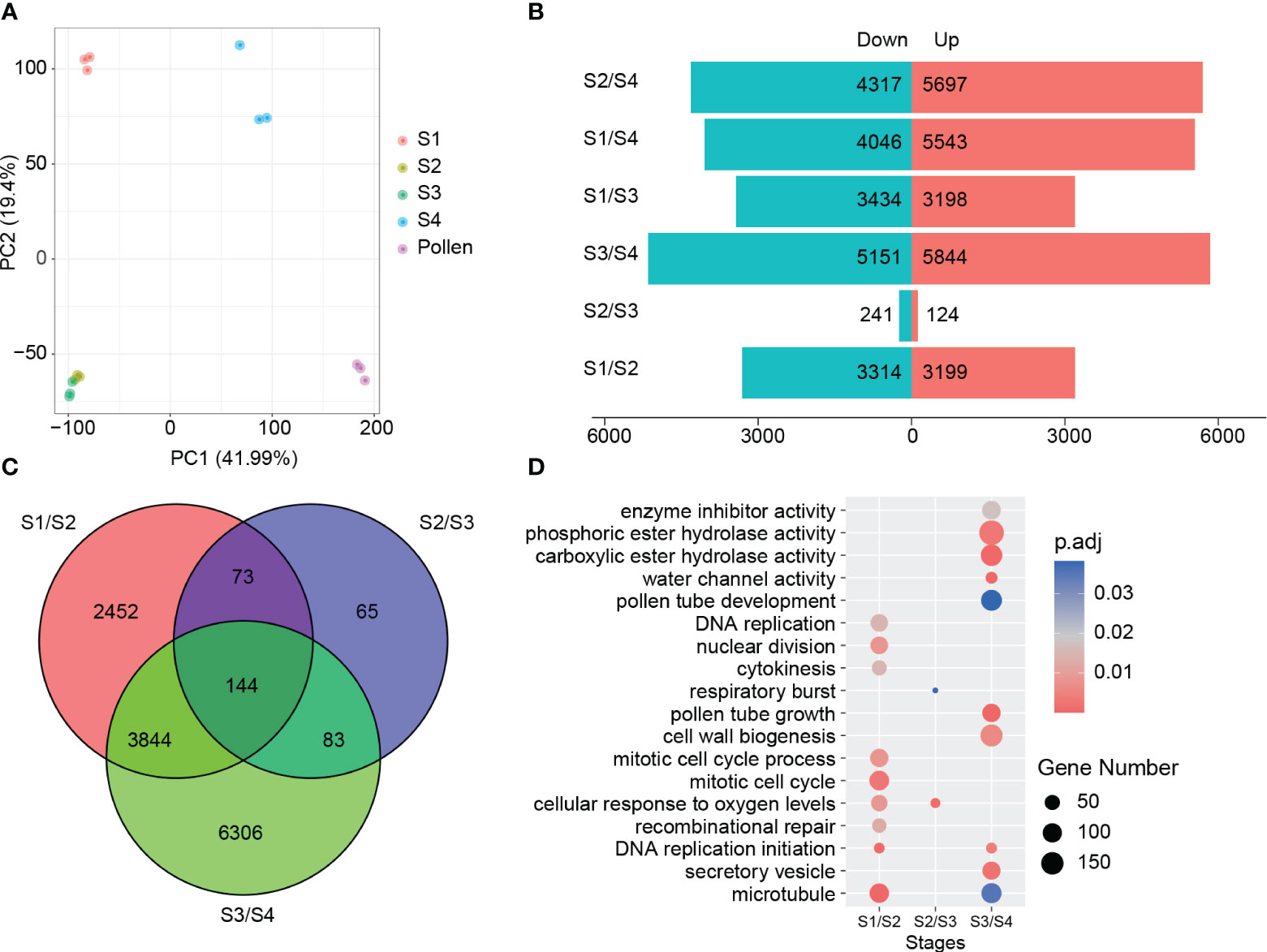
Figure 2 Overview of RNA-seq data. (A) PCA of 15 samples. (B, C) DEGs of four stages. (D) GO enrichment analysis of DEGs, gene counts representation in circular form.
We identified 6,513, 365, and 10,377 differentially expressed genes (DEGs) between adjacent stages in the comparisons of S2 vs S1, S3 vs S2, and S4 vs S3, respectively. A total of 12,967 DEGs were found to be differentially expressed in at least one of the examined periods. From S1 to S2, more DEGs (3,314) were down-regulated than up-regulated (3,199). In contrast, more DEGs were up-regulated (5,844) than down-regulated (5,151) from S3 to S4. Only 365 DEGs were found in the comparison of S3 vs S2, indicating a relatively smaller degree of regulatory changes in gene expression at freezing temperature (Figures 2B, C). In comparison of S4 and pollen, 4,392 DEGs were upregulated and 6,686 were downregulated (Supplementary Table 4). These findings exhibit the profound impact of substantial temperature fluctuations on male reproduction development, resulting in significant gene expression alteration.
GO enrichment analysis of the DEGs revealed the biological processes associated with different stages of male germline development in B. platyphylla. In the DEGs between S1 and S2, biological processes were enriched in “DNA replication” and “mitotic cell cycle”, suggesting activated cell division and growth during this process. On the other hand, the DEGs between S2 and S3 were found to be enriched in biological processes related to “cellular response to oxygen levels” and “respiratory burst”, suggesting a potential involvement of reactive oxygen species (ROS) in the response to prolonged cold temperature. Between S3 and S4, biological processes related to “cell wall biogenesis” and “water channel activity” were significantly enriched, indicating a focus on cellular structure and water transport in preparation for pollen release (Figure 2D). These findings suggested dynamic gene expression patterns and associated biological processes during male germline development in B. platyphylla during overwintering.
3.3 WGCNA analysis and key modules in temperature-related male germline development
To identify gene modules associated with male germline development in B. platyphylla from the four stages to mature pollen, we performed WGCNA to explore relationships between trait factors and gene co-expression modules. The trait matrix represented the developmental characterization in the five experiment groups (S1-S4 and pollen) using binary values. We defined four traits: recovery phase (“00011”) indicates the development process of S4 and pollen; microspore development (“01100”) indicates the development process of S2 and S3; microsporocyte development (“10000”) indicates the development process of S1; cold-inhibited development (“00010”) indicates the development process of S4, respectively. A total of 19,165 genes, filtered based on a mean FPKM value > 0.5, were grouped into 28 co-expression modules (Figure 3A; Supplementary Figure 1). We calculated pearson correlation coefficients and p-values for each module to assess their association with each trait. The modules significantly correlated with the four traits were identified.
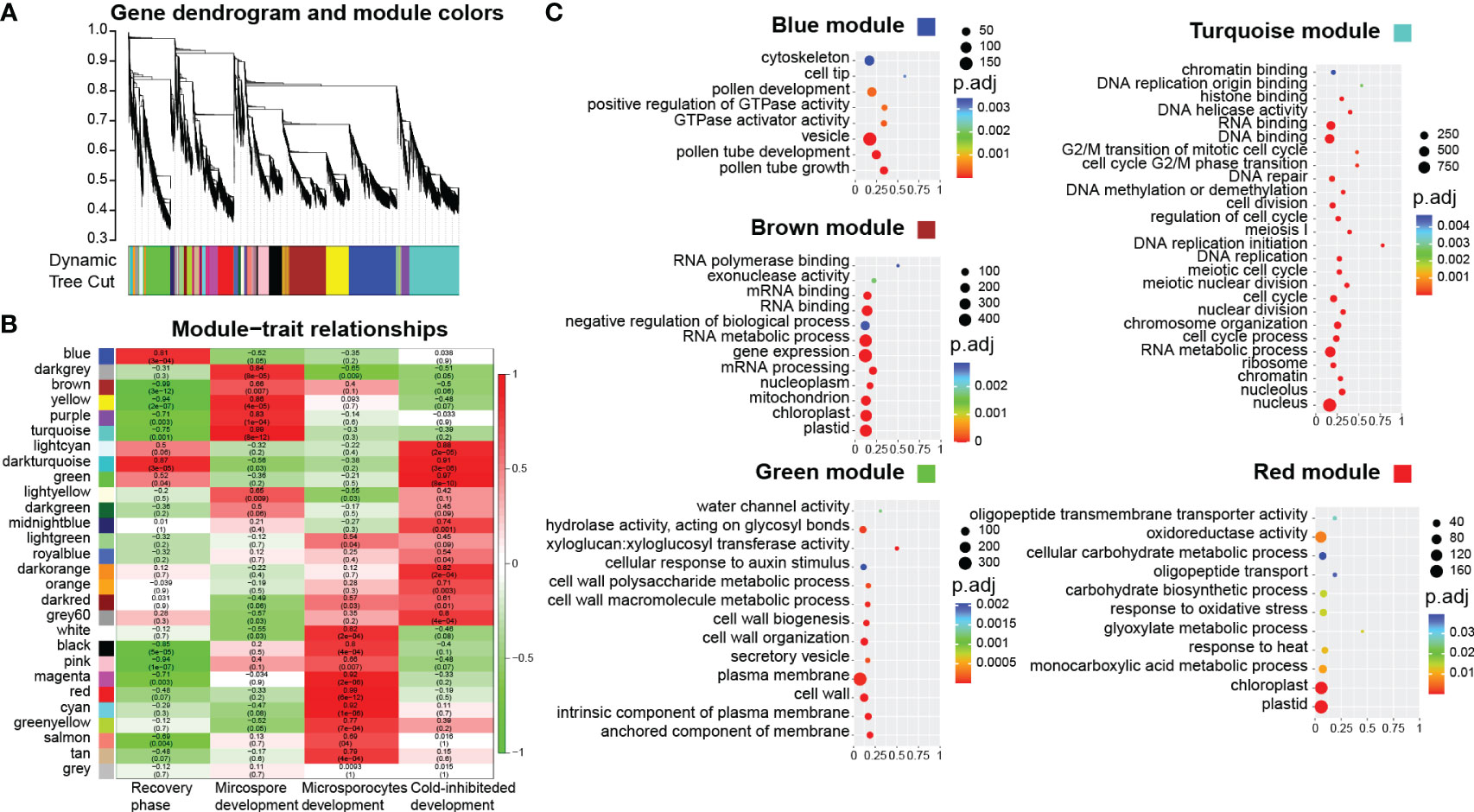
Figure 3 Co-expression network analysis. (A) Cluster dendrogram of different genes in co-expression modules. (B) Relationships between modules (left) and trait (bottom). (C) GO enrichment analysis of five modules. x-axis: rich factor.
For the recovery phase, the blue module exhibited a significant positive correlation (R = 0.81, p < 0.001, Figure 3B; Supplementary Figure 2). GO enrichment on the genes in the blue module revealed that GO terms of “pollen tube” and “GTPase activity” were enriched (Figure 3C). As bicellular microspores emerge, pollen-specific genes such as BpPRK3 (BPChr06G30681), BpPRK4 (BPChr07G02858), BpCPK17 (BPChr04G27193), and BpPPME1 (BPChr03G09691) were upregulated at S4 and pollen (Figure 4). These genes play crucial roles in pollen tube growth (Tian et al., 2006; Myers et al., 2009; Takeuchi and Higashiyama, 2016). CALS5 encodes a callose synthase involved in the synthesis and deposition of callose in the pollen exine wall (Dong et al., 2005). The low expression of BpCALS5 (BPChr13G16035) from S1 to S3, followed by its upregulation at S4, suggested that pollen wall development was achieved by S4 (Figure 4).
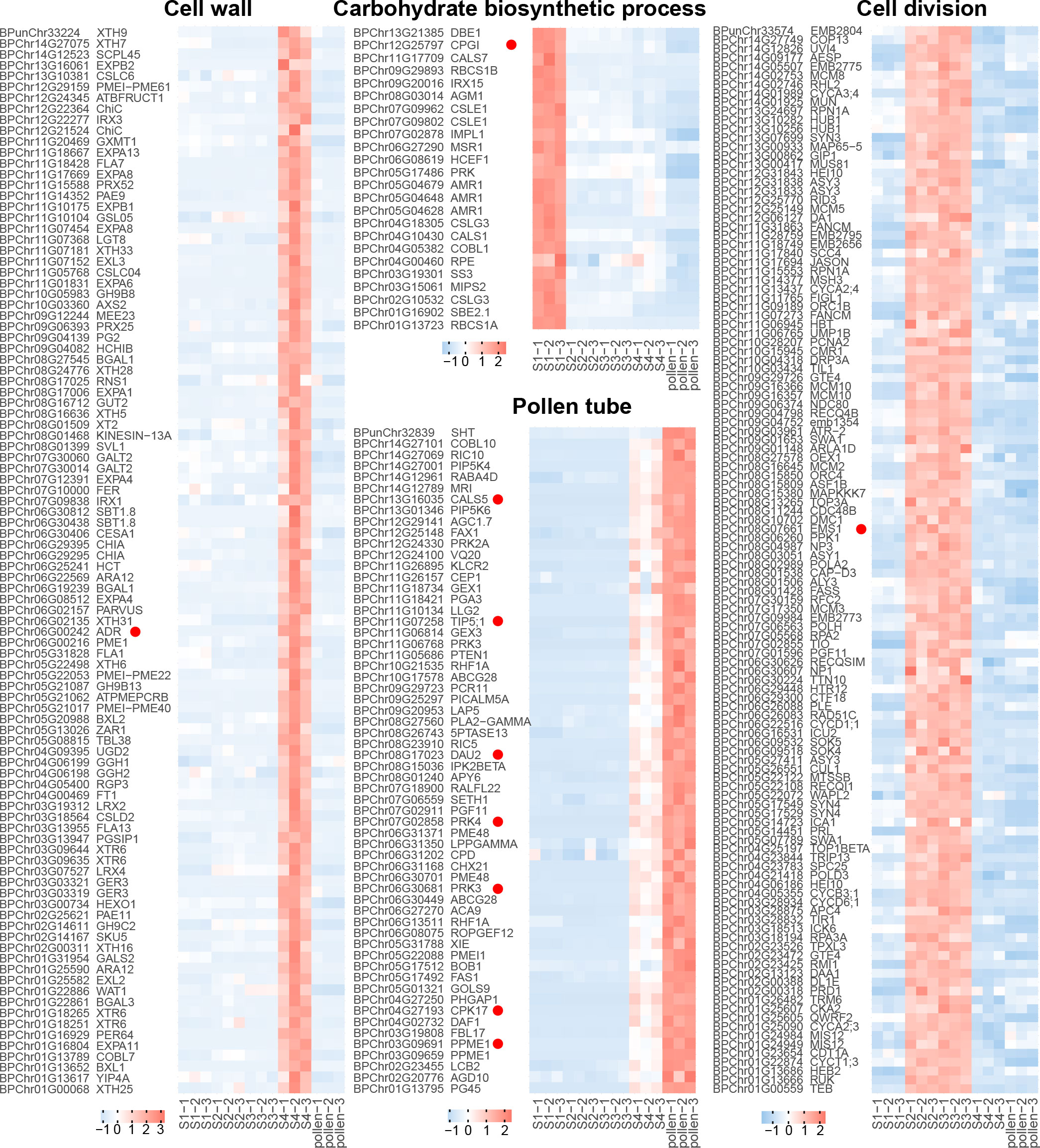
Figure 4 Gene expression of different biological processes. Each row represents a gene, and each column corresponds to different developmental stages (S1-S4 and pollen). The color scale indicates the standardized expression levels, with warmer colors representing higher expression and cooler colors indicating lower expression.
On the other hand, the brown module exhibited a significant negative correlation with the recovery phase (R = -0.99, p < 0.001, Figure 3B; Supplementary Figure 2). This module showed significantly enriched in GO terms related to “gene expression”, “mRNA processing”, and “RNA metabolic process” (Figure 3C). This implies that cold temperature stimulates the upregulation of gene expression during male reproduction development.
For microspore development, the turquoise module exhibited a significant positive correlation under freezing temperature (R = 0.99, p < 0.001, Figure 3B; Supplementary Figure 2). GO enrichment analysis revealed that the terms associated with “cell division”, “DNA replication”, and “cell cycle G2/M phase transition” were significantly enriched within the turquoise module (Figure 3C). During overwintering, cell division emerges as a crucial metabolic process (Perry, 1971). The upregulation of BpEMS1 (BPChr08G07661) at S2 and S3 highlights its essential role in microspore development (Figure 4). The ems1 mutant exhibits male sterility and a lack of tapetal cells, indicating its essential function in microspore development (Zhao et al., 2002). The absence of modules significantly negatively correlated with microspore development suggests that cold temperature primarily regulated male germline development in an activating manner.
For microsporocyte development, the red module showed a positive correlation under chilling temperature (R = 0.97, p < 0.001, Figure 3B; Supplementary Figure 2). These genes belong to GO terms, including “cellular carbohydrate metabolic process” and “glyoxylate metabolic process” (Figures 3C, 4). Among them, BpCPGI (BPChr12G25797), a cytosolic phosphoglucose isomerase was highly expressed at S1, whose homolog in Arabidopsis thaliana is essential for microsporogenesis (Liu et al., 2023) (Figure 4). These findings imply that sugar metabolism plays a crucial role in microsporogenesis under chilling temperature, and ensures sugar supply for subsequent microspore development.
For cold-inhibited development, the green module showed a strong positive correlation (R = 0.99, p < 0.001, Figure 3B; Supplementary Figure 2). The enrichment of GO terms within the green module, including “cell wall” and “water channel activity”, suggested their potential involvement in the late development of staminate catkins (Figure 3C). ADR (ANTHER DEHISCENCE REPRESSOR) acts as a key regulator of anther dehiscence by controlling ROS accumulation during secondary thickening in the anther cell wall (Dai et al., 2019). During the key stage of cell wall development, BpADR (BPChr06G00242) was upregulated specifically at S4 (Figure 4). When resource availability was limited, B. platyphylla might prioritize resource allocation towards crucial cellular processes necessary for microspore development and viability by suppressing specific genes and modifying expression patterns in response to low temperature.
Our findings suggest an active sugar metabolism under chilling temperature at S1, which meets the developmental requirements of the microsporocyte and subsequent microspore development. Under freezing temperature from S2 to S3, genes involved in cell division are upregulated, facilitating the development and preparation of uninuclear microspores for division. From S3 to S4, the expression of genes associated with cell wall metabolism and water transport is activated, enabling the successful development of uninuclear microspores into bicellular pollen.
3.4 Identification of hub transcription factors in WGCNA
Hub genes, which serve as important regulators within the gene co-expression network, were identified based on thresholds with module membership > 0.8 and gene significance > 0.2. We extracted hub TFs, which may act as central regulators within the network. The blue module encompasses 17 hub TFs potentially involved in male germline development. We found that BpDUO1 (BPChr13G10264) and its potential downstream targets, BpDAZ2 (BPChr09G17992), BpTIP5;1 (BPChr11G07258), and BpDAU2 (BPChr08G17023) exhibited high expression at S4 and pollen, while their expression was low from S1 to S3 (Figures 4, 5). DUO1, a member of the R2R3 MYB family, is reported to promote the cell division of uninuclear microspores (Borg et al., 2011). The expression pattern of BpDUO1 explains the insufficient cell division of uninuclear microspores under the freezing temperature from S2 to S3.
In the brown module, hub TFs including BpSHI (BPChr05G17937) and BpPDF2 (BPChr14G27036) suggest their essential involvement in the long-term development of staminate catkins from S1 to S3 (Figure 5). These findings align with the well-established roles of SHI (SHORT INTERNODES) and PDF2 (PROTODERMAL FACTOR 2) in regulating stamen development (Ståldal et al., 2012; Kamata et al., 2013). We observed increased expression of BpICE1 (BPChr11G12210) under cold temperature (Figure 5). ICE1 (INDUCER OF CBF EXPRESSION 1) serves as an inducer of CBFs (C-repeat binding factors) and activates various processes related to cold tolerance and adaptation (Chinnusamy et al., 2007). ICE1 is required for maintaining the dehydrated state of anthers, thereby enhancing cold tolerance during pollen development (Wei et al., 2018).
The hub TFs are specifically upregulated at S2 and S3 in the turquoise module (Figure 5). BpAMS (BPChr09G16382), whose homolog in Arabidopsis is known to be involved in anther and microspore development (Ferguson et al., 2017), exhibited specific upregulation under freezing temperature (Figure 5). Moreover, we observed an upregulation of BpMYB65 (BPChr13G00912), BpARF8 (BPChr11G26339), and BpARF17 (BPChr08G06267) during uninuclear microspore development from S2 to S3 (Figure 5). These genes, MYB65 (MYB DOMAIN PROTEIN 65) and ARFs (AUXIN RESPONSE FACTORs) are involved in gibberellic acid (GA)- and auxin-mediated anther development, respectively (Millar and Gubler, 2005; Wang et al., 2017; Ghelli et al., 2023). Furthermore, the increased expression of BpCBF1 (BPChr02G23333) may enhance cold tolerance and the survival of B. platyphylla by regulating the response to cold temperature (Figure 5).
In the red module, BpGAI (BPChr03G02378), is upregulated under chilling temperature at S1 (Ito et al., 2018) (Figure 5). GAI (GIBBERELLIC ACID INSENSITIVE) encoding a DELLA protein, acts as a negative regulator of GA signaling and regulates microsporogenesis and anther dehiscence (Liu et al., 2017; Huang et al., 2022). The upregulation of BpGAI suggests a diminished GA signaling response under chilling temperature at S1. The subsequent downregulation of BpGAI from S2 to S4 suggests that the activation of the GA signaling pathway contributes to the adaption of microspore development to freezing temperature.
In the green module, flowering-associated hub TFs, including BpAP1 (BPChr08G11263), BpMYB24 (BPChr11G26891), and BpWUS (BPChr05G04645), showed a low expression pattern from S1 to S3, and a high expression level at S4 (Figure 5). These TFs have been previously characterized as crucial regulators of stamen development and male identity determination (Deyhle et al., 2007; Song et al., 2011; Huang et al., 2014; Wang et al., 2019). NST1 (NAC SECONDARY WALL THICKENING PROMOTING FACTOR1) and MYB108 are involved in anther dehiscence and pollen release (Mitsuda et al., 2005; Xu et al., 2019). BpNST1 (BPChr09G20586) and BpMYB108 (BPChr08G15721) showed significant upregulation at S4 (Figure 5), implying an activated cell wall metabolism occurred at this stage during anther development.
Our findings reveal the dynamic temperature-dependent regulation of microspore and anther development by GA and auxin responses. At chilling temperature, there is a low GA response at S1, which transitions to a high auxin and GA response from S2 to S3 under freezing temperature. Among the hub TFs, BpAMS, BpMYB65, BpARF8, and BpARF17 may regulate microspore and anther development under freezing temperature from S2 to S3. The hub TFs involved in anther dehiscence and uninuclear microspore division show a low expression from S1 to S3 and a high expression at S4.
3.5 The expression profiles of genes related to vernalization pathway
Vernalization pathway play a crucial role in the inflorescence bud dormancy in addition to triggering the transition from vegetative to reproductive growth (Lu et al., 2022). We examined the expression of genes related to vernalization pathway. The expression level of BpFLC (BPChr08G03183), was induced by freezing temperature from S1 to S2, then gradually decreased from S2 to S4, along with BpFRI (BPChr13G03877). FRI (FRIGIDA) activates the transcription of FLC, and this activation was reported to be progressively repressed by epigenetic modification at the FLC locus (Li et al., 2018; Zhu et al., 2021). VIP4 (VERNALIZATION INDEPENDENCE 4) was reported to positively regulate FLC expression, and its mutation renders the absence of FLC expression (Zhang and Van Nocker, 2002). BpVIP4 (BPChr04G09387) exhibited high expression from S2 to S3. Specifically, BpFT (BPChr05G17496) showed high expression at S4, low expression at S1, and was undetectable at S2 and S3, indicating its repression under freezing temperature, possibly due to the high expression of its repressor, BpFLC (Figure 6). These observations suggest the involvement of vernalization pathway in the overwintering process of staminate catkins in B. platyphylla.
3.6 qRT-PCR validation of candicate genes
We used qRT-PCR to verify the reliability of the RNA-seq data. A total of eight genes that may be involved in the development of staminate catkins in B. platyphylla during overwintering were selected. These genes included BpADR, BpAMS, BpAP1, BpDUO1, BpFT, BpINV4, BpMYB65 and BpNST1. We found that the expression patterns of these genes obtained from qRT-PCR were consistent with the RNA-seq data (Figure 7; Supplementary Figure 3). The results revealed the reliability of the RNA-seq data.
4 Discussion
Trees, as perennial plants, have evolved various reproductive strategies such as flower dormancy to withstand prolonged cold exposure (Nilsson, 2022). Such phenomena have evolved distinct stages of male germline development in the face of different environments. Some plants undergo microsporogenesis after overwintering, e.g. Prunus armeniaca, while some occur before overwintering, e.g. Rhododendron luteum (Julian et al., 2011; Mirgorodskaya et al., 2015). Our observation of male germline development being arrested at the uninuclear microspore stage during overwintering in B. platyphylla indicates a unique adaptation strategy for reproductive processes in accordance with environmental conditions.
The overwintering process of trees involves intricate transcriptional regulation, including growth cessation, bud dormancy, cryoprotective process, and energy metabolism alteration (Chang et al., 2021). Similar to previous reports, the development of staminate catkins of B. platyphylla during winter may be involved in the regulation of dehydrins (DHNs), FLC, and FT, and energy metabolism related genes. Previous investigations have indicated that dehydrins expression in birch overwintering buds is temperature-responsive and may contributes to cryoprotection (Welling et al., 2004). In this study, the expression of BpDHN1 (BPChr05G17956) in staminate catkins was upregulated at freezing temperature (Supplementary Figure 4).
Cold-tolerant plants employ diverse strategies to strengthen their resistance to low temperature, ensuring the proper development of male germline under cold stress (Sharma and Nayyar, 2016). In cold-susceptible plants, cold exposure typically leads to an increase in ABA levels, which inhibits PCD in the tapetum and leads to pollen abortion. INV4 encodes a cell wall-localized invertase and is expressed in the tapetum. The expression of INV4 is suppressed by a high level of ABA under cold exposure, which leads to pollen sterility by affecting the synthesis and accumulation of reducing sugars in the anthers. On the contrary, in cold-tolerant plants, cold stress upregulats the expression of ABA-8-hydroxylase genes (ABA8ox1 and ABA8ox2), which are involved in decreasing ABA accumulation and improving reducing sugars (Oliver et al., 2005; Oliver et al., 2007; Ji et al., 2011). BpINV4 (BPChr05G08787) and BpABA8OX1 (BPChr02G19591) were upregulated during uninuclear microspore stages under freezing temperature, indicating the importance of low ABA content and increasing free reducing sugar content for microspore development under freezing temperature (Supplementary Figures 3, 5). Additionally, our analysis indicates the potential involvement of related biological processes in cold adaptation, such as GA and auxin signaling pathways, and anther dehydration. The enrichment of GO terms associated with microsporocyte development before overwintering, including sugar biosynthesis and glyoxylate metabolism, supporting the resource storage hypothesis (Bogdziewicz et al., 2020). These metabolic adaptations in cold-tolerant species provide the necessary regulatory mechanisms for successful male germline development under cold temperature.
Temperature plays a significant role in the reproduction and development of trees, influencing the dormancy cycles and fulfilling the developmental requirements of overwintering flowers (Perry, 1971; Campoy et al., 2011; Viti et al., 2013). Previous studies have reported a negative correlation between temperature and birch pollen production (Jochner et al., 2013). Specifically, elevated temperature correlated with the upregulation of key genes such as BpDUO1, BpADR, BpNST1, and BpMYB108. These genes may be associated with uninuclear microspore division and anther dehiscence, thereby facilitating the effective release of pollen. Furthermore, the exclusive upregulation of potential key genes, such as BpEMS1, BpAMS, and BpMYB65, are involved in uninuclear microspore development under freezing temperature, suggests that insufficient cold accumulation may result in partial pollen sterility. These findings suggest a hypothetical gene regulatory pattern of the temperature fluctuation on pollen viability in B. platyphylla.
5 Conclusion
Our study provides a comprehensive transcriptomic analysis of staminate catkin and pollen development in Betula platyphylla under specific temperature fluctuation conditions. Our findings offer valuable insights into the molecular networks governing cold tolerance, male germline development, hormone signaling, flowering control, and the vernalization pathway. This research contributes to a better understanding of the adaptive traits in B. platyphylla and serves as a valuable resource for future studies in tree breeding and ecological management.
Data availability statement
The data presented in the study are deposited in the NCBI Sequence Read Archive (SRA) repository, accession number PRJNA994611.
Author contributions
JZ, JS, and XL conceived the study and research plans. JZ and MC collected plant materials and performed the experiments. JS and KZ analyzed the data. JS organized figures and tables. JS drafted the manuscript. XL revised the manuscript. All authors contributed to the article and approved the submitted version.
Funding
This research was supported by the Fundamental Research Funds for the Central Universities (No. 2572022DX11), National Key R&D Program of China during the 14th Five-year Plan Period (2021YFD2200105), and Heilongjiang Touyan Innovation Team Program (Tree Genetics and Breeding Innovation Team).
Conflict of interest
The authors declare that the research was conducted in the absence of any commercial or financial relationships that could be construed as a potential conflict of interest.
Publisher’s note
All claims expressed in this article are solely those of the authors and do not necessarily represent those of their affiliated organizations, or those of the publisher, the editors and the reviewers. Any product that may be evaluated in this article, or claim that may be made by its manufacturer, is not guaranteed or endorsed by the publisher.
Supplementary material
The Supplementary Material for this article can be found online at: https://www.frontiersin.org/articles/10.3389/fpls.2023.1249122/full#supplementary-material
Supplementary Figure 1 | Gene number of each module.
Supplementary Figure 2 | The correlation between gene significance and module membership in the five modules.
Supplementary Figure 3 | Integrative Genomics Viewer tracks displaying of gene expression coverage.
Supplementary Figure 4 | Gene expression of BpDHN1.
Supplementary Figure 5 | Gene expression of BpINV4 and BpABA8OX1.
Supplementary Table 1 | Primer of qRT-PCR.
Supplementary Table 2 | Sampling time and mean length of staminate catkins.
Supplementary Table 3 | RNA-seq and alignment summary statistics.
Supplementary Table 4 | DEG data of RNA-seq.
References
Alhendi, A. S. N. (2019) countToFPKM: Convert Counts to Fragments per Kilobase of Transcript per Million (FPKM). Available at: https://CRAN.R-project.org/package=countToFPKM (Accessed May 10, 2023).
Andrews, S. (2010) FastQC: a quality control tool for high throughput sequence data. Available at: http://www.bioinformatics.babraham.ac.uk/projects/fastqc (Accessed May 10, 2023).
Bogdziewicz, M., Ascoli, D., Hacket-Pain, A., Koenig, W. D., Pearse, I., Pesendorfer, M., et al. (2020). From theory to experiments for testing the proximate mechanisms of mast seeding: an agenda for an experimental ecology. Ecol. Lett. 23, 210–220. doi: 10.1111/ele.13442
Borg, M., Brownfield, L., Khatab, H., Sidorova, A., Lingaya, M., Twell, D. (2011). The R2R3 MYB transcription factor DUO1 activates a male germline-specific regulon essential for sperm cell differentiation in Arabidopsis. Plant Cell 23, 534–549. doi: 10.1105/tpc.110.081059
Bouché, F., Woods, D. P., Amasino, R. M. (2017). Winter memory throughout the plant kingdom: different paths to flowering. Plant Physiol. 173, 27–35. doi: 10.1104/pp.16.01322
Campoy, J. A., Ruiz, D., Egea, J. (2011). Dormancy in temperate fruit trees in a global warming context: A review. Sci. Hortic. 130, 357–372. doi: 10.1016/j.scienta.2011.07.011
Chang, C. Y., Bräutigam, K., Hüner, N. P. A., Ensminger, I. (2021). Champions of winter survival: cold acclimation and molecular regulation of cold hardiness in evergreen conifers. New Phytol. 229, 675–691. doi: 10.1111/nph.16904
Chen, S., Wang, Y., Yu, L., Zheng, T., Wang, S., Yue, Z., et al. (2021). Genome sequence and evolution of Betula platyphylla. Hortic. Res. 8, 37. doi: 10.1038/s41438-021-00481-7
Chen, Z. D., Manchester, S. R., Sun, H. Y. (1999). Phylogeny and evolution of the Betulaceae as inferred from DNA sequences, morphology, and paleobotany. Am. J. Bot. 86, 1168–1181. doi: 10.2307/2656981
Chinnusamy, V., Zhu, J., Zhu, J. K. (2007). Cold stress regulation of gene expression in plants. Trends Plant Sci. 12, 444–451. doi: 10.1016/j.tplants.2007.07.002
Chouard, P. (1960). Vernalization and its relations to dormancy. Annu. Rev. Plant Physiol. 11, 191–238. doi: 10.1146/annurev.pp.11.060160.001203
Cooke, J. E., Eriksson, M. E., Junttila, O. (2012). The dynamic nature of bud dormancy in trees: environmental control and molecular mechanisms. Plant Cell Environ. 35, 1707–1728. doi: 10.1111/j.1365-3040.2012.02552.x
Dai, S. Y., Hsu, W. H., Yang, C. H. (2019). The gene ANTHER DEHISCENCE REPRESSOR (ADR) controls male fertility by suppressing the ROS accumulation and anther cell wall thickening in arabidopsis. Sci. Rep. 9, 5112. doi: 10.1038/s41598-019-41382-z
Deyhle, F., Sarkar, A. K., Tucker, E. J., Laux, T. (2007). WUSCHEL regulates cell differentiation during anther development. Dev. Biol. 302, 154–159. doi: 10.1016/j.ydbio.2006.09.013
Dong, X., Hong, Z., Sivaramakrishnan, M., Mahfouz, M., Verma, D. P. (2005). Callose synthase (CalS5) is required for exine formation during microgametogenesis and for pollen viability in Arabidopsis. Plant J. 42, 315–328. doi: 10.1111/j.1365-313X.2005.02379.x
Ferguson, A. C., Pearce, S., Band, L. R., Yang, C., Ferjentsikova, I., King, J., et al. (2017). Biphasic regulation of the transcription factor ABORTED MICROSPORES (AMS) is essential for tapetum and pollen development in Arabidopsis. New Phytol. 213, 778–790. doi: 10.1111/nph.14200
Ghelli, R., Brunetti, P., Marzi, D., Cecchetti, V., Costantini, M., Lanzoni-Rossi, M., et al. (2023). The full-length Auxin Response Factor 8 isoform ARF8.1 controls pollen cell wall formation and directly regulates TDF1, AMS and MS188 expression. Plant J. 113, 851–865. doi: 10.1111/tpj.16089
Gómez, J. F., Talle, B., Wilson, Z. A. (2015). Anther and pollen development: A conserved developmental pathway. J. Integr. Plant Biol. 57, 876–891. doi: 10.1111/jipb.12425
Hafidh, S., Fíla, J., Honys, D. (2016). Male gametophyte development and function in angiosperms: a general concept. Plant Reprod. 29, 31–51. doi: 10.1007/s00497-015-0272-4
Helliwell, C. A., Wood, C. C., Robertson, M., James Peacock, W., Dennis, E. S. (2006). The Arabidopsis FLC protein interacts directly in vivo with SOC1 and FT chromatin and is part of a high-molecular-weight protein complex. Plant J. 46, 183–192. doi: 10.1111/j.1365-313X.2006.02686.x
Huang, T. H., Hsu, W. H., Mao, W. T., Yang, C. H. (2022). The oncidium ethylene synthesis gene oncidium 1-aminocyclopropane-1 carboxylic acid synthase 12 and ethylene receptor gene oncidium ETR1 affect GA-DELLA and jasmonic acid signaling in regulating flowering time, anther dehiscence, and flower senescence in arabidopsis. Front. Plant Sci. 13. doi: 10.3389/fpls.2022.785441
Huang, H., Wang, S., Jiang, J., Liu, G., Li, H., Chen, S., et al. (2014). Overexpression of BpAP1 induces early flowering and produces dwarfism in Betula platyphylla × Betula pendula. Physiol. Plant 151, 495–506. doi: 10.1111/ppl.12123
Ito, T., Okada, K., Fukazawa, J., Takahashi, Y. (2018). DELLA-dependent and -independent gibberellin signaling. Plant Signal. Behav. 13, e1445933. doi: 10.1080/15592324.2018.1445933
Ji, X., Dong, B., Shiran, B., Talbot, M. J., Edlington, J. E., Hughes, T., et al. (2011). Control of abscisic acid catabolism and abscisic acid homeostasis is important for reproductive stage stress tolerance in cereals. Plant Physiol. 156, 647–662. doi: 10.1104/pp.111.176164
Jochner, S., Höfler, J., Beck, I., Göttlein, A., Ankerst, D. P., Traidl-Hoffmann, C., et al. (2013). Nutrient status: a missing factor in phenological and pollen research? J. Exp. Bot. 64, 2081–2092. doi: 10.1093/jxb/ert061
Julian, C., Rodrigo, J., Herrero, M. (2011). Stamen development and winter dormancy in apricot (Prunus Armeniaca). Ann. Bot. 108, 617–625. doi: 10.1093/aob/mcr056
Kamata, N., Sugihara, A., Komeda, Y., Takahashi, T. (2013). Allele-specific effects of PDF2 on floral morphology in Arabidopsis thaliana. Plant Signal. Behav. 8, e27417. doi: 10.4161/psb.27417
Langfelder, P., Horvath, S. (2008). WGCNA: an R package for weighted correlation network analysis. BMC Bioinform. 9, 1–13. doi: 10.1186/1471-2105-9-559
Langmead, B., Salzberg, S. L. (2012). Fast gapped-read alignment with Bowtie 2. Nat. Methods 9, 357–359. doi: 10.1038/nmeth.1923
Li, Z., Jiang, D., He, Y. (2018). FRIGIDA establishes a local chromosomal environment for FLOWERING LOCUS C mRNA production. Nat. Plants 4, 836–846. doi: 10.1038/s41477-018-0250-6
Liao, Y., Smyth, G. K., Shi, W. (2014). featureCounts: an efficient general purpose program for assigning sequence reads to genomic features. Bioinformatics 30, 923–930. doi: 10.1093/bioinformatics/btt656
Liu, H. C., Chen, H. C., Huang, T. H., Lue, W. L., Chen, J., Suen, D. F. (2023). Cytosolic phosphoglucose isomerase is essential for microsporogenesis and embryogenesis in Arabidopsis. Plant Physiol. 191, 177–198. doi: 10.1093/plphys/kiac494
Liu, B., De Storme, N., Geelen, D. (2017). Gibberellin induces diploid pollen formation by interfering with meiotic cytokinesis. Plant Physiol. 173, 338–353. doi: 10.1104/pp.16.00480
Love, M. I., Huber, W., Anders, S. (2014). Moderated estimation of fold change and dispersion for RNA-seq data with DESeq2. Genome Biol. 15, 1–21. doi: 10.1186/s13059-014-0550-8
Lu, X., O’neill, C. M., Warner, S., Xiong, Q., Chen, X., Wells, R., et al. (2022). Winter warming post floral initiation delays flowering via bud dormancy activation and affects yield in a winter annual crop. PNAS 119, e2204355119. doi: 10.1073/pnas.2204355119
Luo, X., He, Y. (2020). Experiencing winter for spring flowering: A molecular epigenetic perspective on vernalization. J. Integr. Plant Biol. 62, 104–117. doi: 10.1111/jipb.12896
Mandaokar, A., Thines, B., Shin, B., Lange, B. M., Choi, G., Koo, Y. J., et al. (2006). Transcriptional regulators of stamen development in Arabidopsis identified by transcriptional profiling. Plant J. 46, 984–1008. doi: 10.1111/j.1365-313X.2006.02756.x
Martin, M. (2011). Cutadapt removes adapter sequences from high-throughput sequencing reads. EMBnet J 17, 10–12. doi: 10.14806/EJ.17.1.200
Millar, A. A., Gubler, F. (2005). The Arabidopsis GAMYB-like genes, MYB33 and MYB65, are microRNA-regulated genes that redundantly facilitate anther development. Plant Cell 17, 705–721. doi: 10.1105/tpc.104.027920
Miller-Rushing, A. J., Primack, R. B. (2008). Effects of winter temperatures on two birch (Betula) species. Tree Physiol. 28, 659–664. doi: 10.1093/treephys/28.4.659
Mirgorodskaya, O. E., Koteyeva, N. K., Volchanskaya, A. V., Miroslavov, E. A. (2015). Pollen development in Rhododendron in relation to winter dormancy and bloom time. Protoplasma 252, 1313–1323. doi: 10.1007/s00709-015-0764-y
Mitsuda, N., Seki, M., Shinozaki, K., Ohme-Takagi, M. (2005). The NAC transcription factors NST1 and NST2 of Arabidopsis regulate secondary wall thickenings and are required for anther dehiscence. Plant Cell 17, 2993–3006. doi: 10.1105/tpc.105.036004
Myers, C., Romanowsky, S. M., Barron, Y. D., Garg, S., Azuse, C. L., Curran, A., et al. (2009). Calcium-dependent protein kinases regulate polarized tip growth in pollen tubes. Plant J. 59, 528–539. doi: 10.1111/j.1365-313X.2009.03894.x
Nayyar, H., Bains, T., Kumar, S. (2005). Low temperature induced floral abortion in chickpea: relationship to abscisic acid and cryoprotectants in reproductive organs. Environ. Exp. Bot. 53, 39–47. doi: 10.1016/j.envexpbot.2004.02.011
Nilsson, O. (2022). Winter dormancy in trees. Curr. Biol. 32, R630–r634. doi: 10.1016/j.cub.2022.04.011
Oliver, S. N., Dennis, E. S., Dolferus, R. (2007). ABA regulates apoplastic sugar transport and is a potential signal for cold-induced pollen sterility in rice. Plant Cell Physiol. 48, 1319–1330. doi: 10.1093/pcp/pcm100
Oliver, S. N., Van Dongen, J. T., Alfred, S. C., Mamun, E. A., Zhao, X. C., Saini, H. S., et al. (2005). Cold-induced repression of the rice anther-specific cell wall invertase gene OSINV4 is correlated with sucrose accumulation and pollen sterility. Plant Cell Environ. 28, 1534–1551. doi: 10.1111/j.1365-3040.2005.01390.x
Perry, T. O. (1971). Dormancy of trees in winter. Science 171, 29–36. doi: 10.1126/science.171.3966.29
Ranpal, S., Sieverts, M., Wörl, V., Kahlenberg, G., Gilles, S., Landgraf, M., et al. (2022). Is pollen production of birch controlled by genetics and local conditions? Int. J. Environ. Res. Public Health 19. doi: 10.3390/ijerph19138160
Ranpal, S., Von Bargen, S., Gilles, S., Luschkova, D., Landgraf, M., Traidl-Hoffmann, C., et al. (2023). Pollen production of downy birch (Betula pubescens Ehrh.) along an altitudinal gradient in the European Alps. Int. J. Biometeorol. doi: 10.1007/s00484-023-02483-7
Sharma, K. D., Nayyar, H. (2016). Regulatory networks in pollen development under cold stress. Front. Plant Sci. 7. doi: 10.3389/fpls.2016.00402
Song, S., Qi, T., Huang, H., Ren, Q., Wu, D., Chang, C., et al. (2011). The Jasmonate-ZIM domain proteins interact with the R2R3-MYB transcription factors MYB21 and MYB24 to affect Jasmonate-regulated stamen development in Arabidopsis. Plant Cell 23, 1000–1013. doi: 10.1105/tpc.111.083089
Ståldal, V., Cierlik, I., Chen, S., Landberg, K., Baylis, T., Myrenås, M., et al. (2012). The Arabidopsis thaliana transcriptional activator STYLISH1 regulates genes affecting stamen development, cell expansion and timing of flowering. Plant Mol. Biol. 78, 545–559. doi: 10.1007/s11103-012-9888-z
Takeuchi, H., Higashiyama, T. (2016). Tip-localized receptors control pollen tube growth and LURE sensing in Arabidopsis. Nature 531, 245–248. doi: 10.1038/nature17413
Tian, G. W., Chen, M. H., Zaltsman, A., Citovsky, V. (2006). Pollen-specific pectin methylesterase involved in pollen tube growth. Dev. Biol. 294, 83–91. doi: 10.1016/j.ydbio.2006.02.026
Viti, R., Bartolini, S., Andreini, L. (2013). Apricot flower bud dormancy: Main morphological, anatomical and physiological features related to winter climate influence. Adv. Hortic. Sci. 27, 5–17. doi: 10.1400/220117
Wang, S., Huang, H., Han, R., Chen, J., Jiang, J., Li, H., et al. (2019). BpAP1 directly regulates BpDEF to promote male inflorescence formation in Betula platyphylla × B. pendula. Tree Physiol. 39, 1046–1060. doi: 10.1093/treephys/tpz021
Wang, N., Kelly, L. J., Mcallister, H. A., Zohren, J., Buggs, R. J. A. (2021). Resolving phylogeny and polyploid parentage using genus-wide genome-wide sequence data from birch trees. Mol. Phylogenet. Evol. 160, 107126. doi: 10.1016/j.ympev.2021.107126
Wang, B., Xue, J. S., Yu, Y. H., Liu, S. Q., Zhang, J. X., Yao, X. Z., et al. (2017). Fine regulation of ARF17 for anther development and pollen formation. BMC Plant Biol. 17, 243. doi: 10.1186/s12870-017-1185-1
Wei, D., Liu, M., Chen, H., Zheng, Y., Liu, Y., Wang, X., et al. (2018). INDUCER OF CBF EXPRESSION 1 is a male fertility regulator impacting anther dehydration in Arabidopsis. PloS Genet. 14, e1007695. doi: 10.1371/journal.pgen.1007695
Welling, A., Rinne, P., Viherä-Aarnio, A., Kontunen-Soppela, S., Heino, P., Palva, E. T. (2004). Photoperiod and temperature differentially regulate the expression of two dehydrin genes during overwintering of birch (Betula pubescens Ehrh.). J. Exp. Bot. 55, 507–516. doi: 10.1093/jxb/erh045
Wilson, Z. A., Zhang, D. B. (2009). From Arabidopsis to rice: pathways in pollen development. J. Exp. Bot. 60, 1479–1492. doi: 10.1093/jxb/erp095
Xu, X. F., Wang, B., Feng, Y. F., Xue, J. S., Qian, X. X., Liu, S. Q., et al. (2019). AUXIN RESPONSE FACTOR17 directly regulates MYB108 for anther dehiscence. Plant Physiol. 181, 645–655. doi: 10.1104/pp.19.00576
Yu, G., Wang, L. G., Han, Y., He, Q. Y. (2012). clusterProfiler: an R package for comparing biological themes among gene clusters. Omics 16, 284–287. doi: 10.1089/omi.2011.0118
Zhang, H., Van Nocker, S. (2002). The VERNALIZATION INDEPENDENCE 4 gene encodes a novel regulator of FLOWERING LOCUS C. Plant J. 31, 663–673. doi: 10.1046/j.1365-313x.2002.01380.x
Zhao, D.-Z., Wang, G.-F., Speal, B., Ma, H. (2002). The excess microsporocytes1 gene encodes a putative leucine-rich repeat receptor protein kinase that controls somatic and reproductive cell fates in the Arabidopsis anther. Genes Dev. 16, 2021–2031. doi: 10.1101/gad.997902
Keywords: Betula platyphylla, staminate catkins, microspore, transcriptome, weighted gene co-expression network analysis
Citation: Zhang J, Shi J, Zeng K, Cai M and Lan X (2024) Transcriptomic landscape of staminate catkins development during overwintering process in Betula platyphylla. Front. Plant Sci. 14:1249122. doi: 10.3389/fpls.2023.1249122
Received: 28 June 2023; Accepted: 06 September 2023;
Published: 08 January 2024.
Edited by:
Shaojun Dai, Shanghai Normal University, ChinaCopyright © 2024 Zhang, Shi, Zeng, Cai and Lan. This is an open-access article distributed under the terms of the Creative Commons Attribution License (CC BY). The use, distribution or reproduction in other forums is permitted, provided the original author(s) and the copyright owner(s) are credited and that the original publication in this journal is cited, in accordance with accepted academic practice. No use, distribution or reproduction is permitted which does not comply with these terms.
*Correspondence: Xingguo Lan, bGFueGluZ2d1b0BuZWZ1LmVkdS5jbg==
†These authors have contributed equally to this work and share first authorship