- Alson H. Smith Jr. Agricultural Research and Extension Center, School of Plant and Environmental Sciences, Virginia Tech, Winchester, VA, United States
Low temperature stress significantly threatens crop productivity and economic sustainability. Plants counter this by deploying advanced molecular mechanisms to perceive and respond to cold stress. Transmembrane proteins initiate these responses, triggering a series of events involving secondary messengers such as calcium ions (Ca2+), reactive oxygen species (ROS), and inositol phosphates. Of these, calcium signaling is paramount, activating downstream phosphorylation cascades and the transcription of cold-responsive genes, including cold-regulated (COR) genes. This review focuses on how plants manage freeze-induced damage through dual strategies: cold tolerance and cold avoidance. Tolerance mechanisms involve acclimatization to decreasing temperatures, fostering gradual accumulation of cold resistance. In contrast, avoidance mechanisms rely on cryoprotectant molecules like potassium ions (K+), proline, glycerol, and antifreeze proteins (AFPs). Cryoprotectants modulate intracellular solute concentration, lower the freezing point, inhibit ice formation, and preserve plasma membrane fluidity. Additionally, these molecules demonstrate antioxidant activity, scavenging ROS, preventing protein denaturation, and subsequently mitigating cellular damage. By forming extensive hydrogen bonds with water molecules, cryoprotectants also limit intercellular water movement, minimizing extracellular ice crystal formation, and cell dehydration. The deployment of cryoprotectants is a key adaptive strategy that bolsters plant resilience to cold stress and promotes survival in freezing environments. However, the specific physiological and molecular mechanisms underlying these protective effects remain insufficiently understood. Therefore, this review underscores the need for further research to elucidate these mechanisms and assess their potential impact on crop productivity and sustainability, contributing to the progressive discourse in plant biology and environmental science.
1 Introduction
Spring frost is a major environmental stress caused by low temperature combined with dewpoints below freezing points (≤ 0°C), posing substantial economic threat on plants. While the terms “frost damage” and “freeze damage” are commonly used interchangeably, there are slight distinctions between them. Freeze damage is attributed to temperature below freezing points, whereas frost damage is caused by dewpoints below freezing points irrespective of temperature being at or below freezing points (Perry, 1998). However, the damage mechanisms at organ level are comparable in both cases. Consequently, for the purpose of this review, the term “freeze damage” will be employed. At the organ level, the damage occurs when water within plant tissues freezes, forming extracellular ice crystals that result in cell dehydration. Freezing-induced cellular dehydration is the predominant cause of damage in which the cell membranes are disrupted when the dehydration exceeds cell dehydration-tolerance (Pearce, 2001). The severity of damage is determined by multiple factors including plants species, their genetic makeup, dewpoint, surface moisture, probability of an extracellular ice nucleation event, pre-frost environmental conditions, and developmental stage – with flowering being the most susceptible developmental stage (Centinari et al., 2016). Crops can be exposed to two types of frost: radiation and advective – with the former being more common (Liu and Sherif, 2019). Radiation frost is a meteorological phenomenon resulting from the release of thermal energy stored in the soil. This thermal energy is radiated back into the atmosphere during the nocturnal period, characterized by a stable atmosphere and a low wind regime, allowing the formation of a temperature inversion. This atmospheric condition, in conjunction with a reduced dewpoint temperature, leads to a sharp reduction in the air temperature, which falls below the freezing point of water. Advective frost, on the other hand, occurs when a massive system of cold air moves into an area from polar and arctic regions, typically accompanied by wind ≥ 8 km/h. The resultant wind-driven turbulent mixing and cold advection lead to a significant reduction in the air temperature, resulting in the formation of frost.
Crop losses resulting from frost damage represent a significant economic threat to the crop production industry, with frost-related yield reduction being ranked highest among all weather-related disasters in the United States (Centinari et al., 2016; Snyder and de Melo-Abreu, 2005). For example, the one-week Easter freeze in 2007 led to over 2 billion dollars in economic losses due to reduced production of wheat (Triticum aestivum) by 19%, peach (Prunus persica) by 75%, apple (Malus domestica) by 67%, and pecan (Carya illinoinensis) by 66% (Liu et al., 2018). Additionally, based on our observations, even a brief frost event persisting only for a few hours can cause substantial damage to various crops. A recent hard freeze event in 2022, lasting 2.5 hours in Virginia, U.S., resulted in an 80% rate of damage to the sweet cherry crop, 15 – 35% damage to apple floral buds, and 15% damage to peach crops (Sherif, 2023). Climate change, leading to increasing global temperatures, exacerbates this threat (Liu et al., 2018), causing alterations in plant phenology such as shortened dormancy and early budburst and flowering (Augspurger, 2013; Ma et al., 2019). Economic losses vary in severity, ranging from partial to complete loss of valuable crops, and can have detrimental impacts on crop quality. Frost-related losses are not limited to specific regions or species; rather, they are a global phenomenon. Notable incidents such as the Easter freeze of 2007 (Liu et al., 2018), the Mother’s Day Freeze (2010), the Killer Frost (2012), and the Polar Vortex (2014) have inflicted billions of dollars in damages on the agricultural industry across North America.
The critical temperature at which crop damage occurs depends on multiple factors, including species specificity, duration of the frost event, and developmental stage (Centinari et al., 2016). For instance, the lower temperature limit at which 90% of floral buds are damaged ranges from -17.6˚C to -3.0˚C for tree fruits like apples, -19.4˚C to -2.8˚C for grapes, -6.1˚C to -0.6˚C for small fruits like strawberries, and -2.8˚C to -0.8˚C for citrus (Snyder and de Melo-Abreu, 2005). Plants at the dormant stage are less susceptible to low temperatures compared to advanced developmental stages. For example, apple floral buds at the silver-tip stage, characterized by slight separation and a shimmery gray color, can tolerate temperatures as low as -17.6˚C. However, at the post-bloom stage, 90% flower mortality can occur at -3˚C. Furthermore, the duration and severity of a frost event have a significant impact on the extent of damage – the more severe and longer it persists, the more damage occurs. Our research, involving a series of experiments investigating frost mitigation strategies, showed that a deleterious frost event in 2023, persisting for 1.5 hours, resulted in approximately 87% damage to apple floral buds, while a 2-hour long frost event in 2021 caused around 65% bud mortality. However, a relatively longer frost event in 2022, lasting around 2.5 hours, resulted in approximately 15 – 35% damage to floral buds. Interestingly, although the duration of frost in 2022 was relatively longer, it coincided with the early developmental stage of the crops, resulting in comparatively lower damage to the crops (Sherif, 2023). These observations suggest that crops at an early developmental stage exhibit increased resilience to extended frost exposure.
Frost protection methods for plants can be categorized into passive and active methods. Passive methods are considered preventive measures applied before the occurrence of frost events (Liu and Sherif, 2019). These methods are more economically efficient compared to active ones and are intended to provide protection over an extended period. Examples of passive methods include site selection, enhancing plant tolerance to frost through the application of cryoprotectant compounds and growth regulators, and the selection of frost-tolerant varieties (cold-hardy and/or late-blooming varieties). On the other hand, active frost protection methods are those applied during or immediately before a frost event with the aim of preventing the temperature from dropping below the freezing point. These methods involve various techniques including heaters (i.e., solid fuel, propane), wind machines, helicopters, surface irrigation, and sprinklers (i.e., over- and under-tree sprinklers). These approaches are designed to actively counteract the effects of frost in real-time.
Specific weather conditions, including wind speed, humidity, and the severity and duration of a frost event, significantly influence the efficacy of both active and passive methods of frost mitigation (Unterberger et al., 2018). For example, wind machines are beneficial for countering radiation frost, but they fail to provide protection against advective frost. Moreover, active methods frequently carry considerable costs, such as those involved in the acquisition and upkeep of necessary equipment. Implementing heaters is one such method, which, while effective, necessitates energy consumption and regular maintenance expenses. Another example is the use of helicopters, the operation and fueling of which can be significantly costly. Certain practical constraints may also emerge, causing some methods to be unsuitable in particular agricultural contexts. An example is the implementation of surface irrigation or sprinkler systems, which might not be feasible in regions with scarce water resources. In addition to these considerations, the environmental impact of these methods, particularly in terms of their energy and water usage, should be evaluated to determine their sustainability. A comprehensive understanding of these limitations is indispensable for making informed decisions about frost protection strategies, as this allows for the consideration of the specific needs and restrictions unique to each agricultural system.
A relatively recent strategy to mitigate frost damage in plants involves the application of chemical compounds, specifically plant growth regulators (PGRs). Over the past half-century, researchers have extensively probed PGRs for their potential in bolstering plant frost tolerance and delaying blooming periods, thereby averting late-spring freezes. Ethephon (2-chloroethylphosphonic acid), for example, is an ethylene-based PGR compound that has shown promising results in delaying bloom and increasing cold hardiness in various fruit and nut trees (Liu and Sherif, 2019; Liu et al., 2021a). Our research involved conducting a set of experiments to investigate the impact of ethephon applications on bloom delay. Our findings revealed that applying ethephon in the fall resulted in a bloom delay of 5-6 days in peaches, varying based on application timing and concentration (Liu et al., 2021a). Such a delay could notably lower the risk of frost damage. Furthermore, our results indicated that ethephon could enhance the cold hardiness of dormant buds, thereby promoting their survival through winter. However, ethephon application does have its drawbacks. For instance, it has been associated with adverse effects like gummosis, terminal dieback, and yield reduction (Liu et al., 2021a). Another frost damage mitigation approach involves applying exogenous cryoprotectant compounds. Yet, the specific physiological and molecular mechanisms that enable these compounds to offer frost protection remain relatively unclear. In this article, we present an in-depth overview of the physiological and molecular mechanisms associated with cryoprotectant compounds. However, gaining a robust understanding of these mechanisms requires a comprehensive grasp of plant cold-sensing mechanisms, natural plant defenses against freezing, and the damaging effects of freezing on plant tissues. Therefore, we also provide a detailed examination of these defense mechanisms and the impact of freezing on plants.
2 Cold sensing mechanisms
Plants have evolved intricate mechanisms to identify and respond to abiotic stresses such as cold stress. They perceive shifts in their physical and chemical surroundings like water availability, ion concentration, and temperature, and translate these changes into a biological signal via primary sensory mechanisms (Lamers et al., 2020). Each sensor is typically designed to detect a specific feature of the stress, potentially playing a role in a unique branch of the stress signaling pathway. In this context, the cold-signaling pathway is our focal point for this article. Plants deploy sophisticated multilevel processes in response to a decrease in temperature (Örvar et al., 2000). At the cellular level, cold stress signals are perceived via a variety of receptors that include plasma membrane (PM) rigidification, PM-bound G-protein-associated receptors, and other cold sensors such as Ca2+ influx channels, two-component histidine kinases, and changes in protein and nucleic acid conformations or metabolite concentrations (Figure 1) (Kazemi-Shahandashti and Maali-Amiri, 2018). These signals give rise to secondary messengers like Ca2+, ROS, and inositol phosphates. These messengers subsequently adjust the intracellular Ca2+ level. Changes in the cytosolic Ca2+ level are detected by calcium-binding proteins, also known as Ca2+ sensors. These sensors engage with their target proteins to relay the calcium signal within the cell. These proteins coordinate the transfer of the cold stress signal, trigger protein phosphorylation cascades, and manage the expression of transcription factors and cold-regulated (COR) genes in plants (Figure 1, Table 1) (Drerup et al., 2013; Zhang et al., 2014; Ma et al., 2015; Zhu, 2016; Guo et al., 2018).
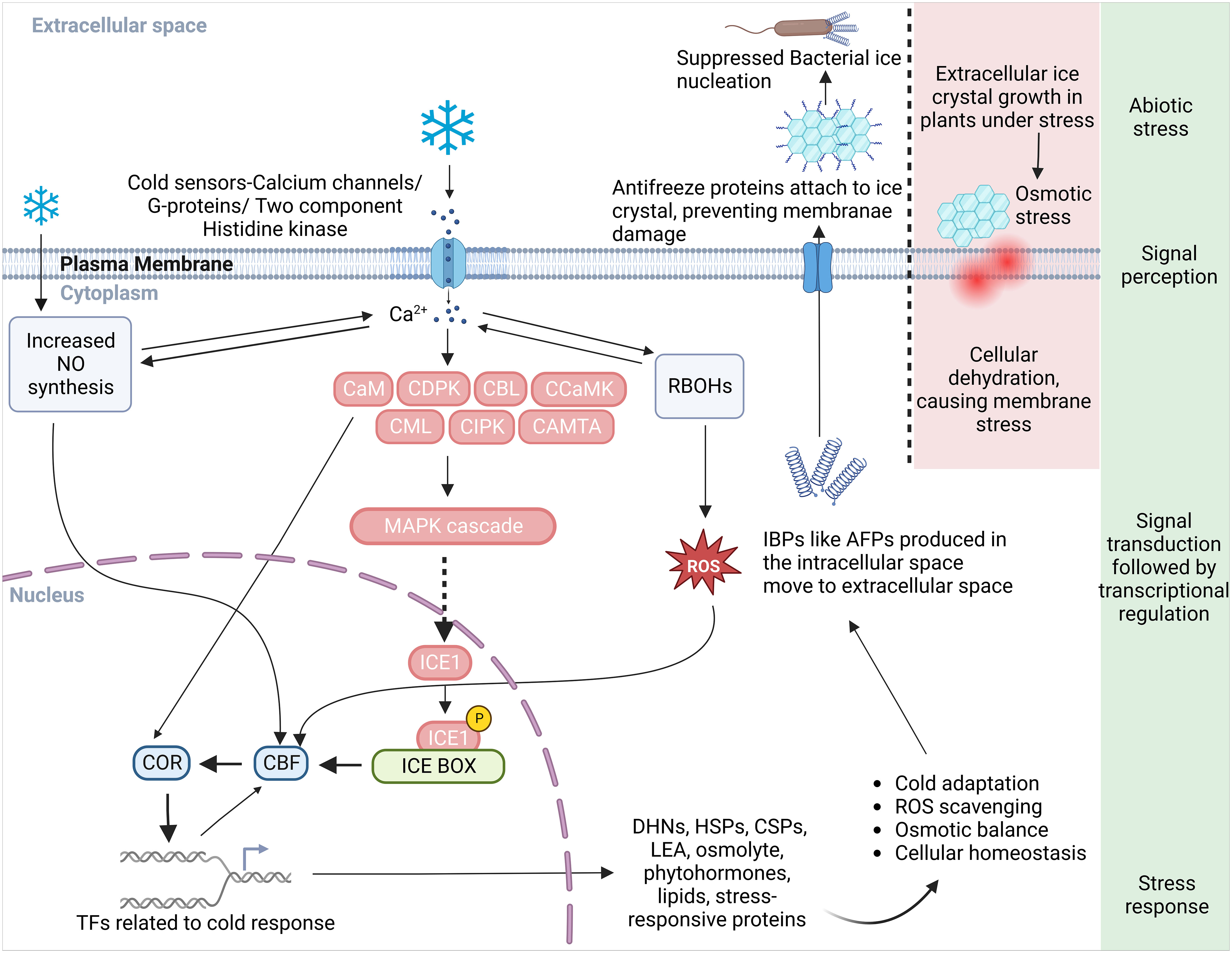
Figure 1 A schematic diagram illustrating an overview of the cold perception and the Ca2+ mediated cold responsive signal transduction and response pathways in plant. Plasma membrane receptors and membrane rigidification sense the cold stress and initiate a series of downstream reactions. This cascade activates calcium channels/G-proteins, a two-component histidine kinase, and increases Ca2+ influx in the cytoplasm, subsequently stimulating Ca2+-related proteins such as CaM, CDPK, CBL, CCaMK, CML, CIPK, CaMTA, and the MAPK signaling pathway. ICE1 interaction with the signaling cascade instigates the ICE-CBF-COR transcriptome machinery’s response. Proteins responsible for the synthesis of cryoprotectants, PKs, phytohormones, and protective proteins like DHNs, AFPs, HSPs, and CSPs, all crucial in cold adaptation, are encoded by the COR genes. For freeze protection in cold-acclimated plants, the process involves IBP induction, secretion of AFPs into the extracellular space, and attachment to ice crystals to inhibit their growth and to prevent freezing linked with bacterial ice nucleation. In non-acclimated plants lacking IBP, large damaging ice crystals form that can rupture the plasma membrane due to cellular dehydration caused by an osmotic gradient that sequesters intracellular water. An increase in Ca2+ level due to cold stress also activates RBOHs to produce more ROS and prompts NO synthesis necessary for cold adaptation response. The crosstalk between ROS and Ca2+ controls the expression of defense related genes in nucleus. ROS, reactive oxygen species; NO, nitric oxide; RBOH, respiratory burst oxidase homologues; CaM, calmodulin; CDPKs, Ca2+ dependent protein kinases; CBLs, calcineurin B-like proteins; CCaMK, Ca2+/calmodulin-dependent protein kinase; CML, CaM like; CIPK, CBL-interacting protein kinases; CaMTA, CaM-binding transcription activator; MAPK, mitogen-activated protein kinase; CBFs, C-repeat binding factors; ICE, inducer of CBF expression; COR, cold-responsive; AFP, anti-freeze proteins; IBP, ice-binding proteins; DHN, dehydrin, HSP, heat shock proteins; CSP, cold shock protein; LEA, late embryogenesis abundant protein; TFs, transcription factors.
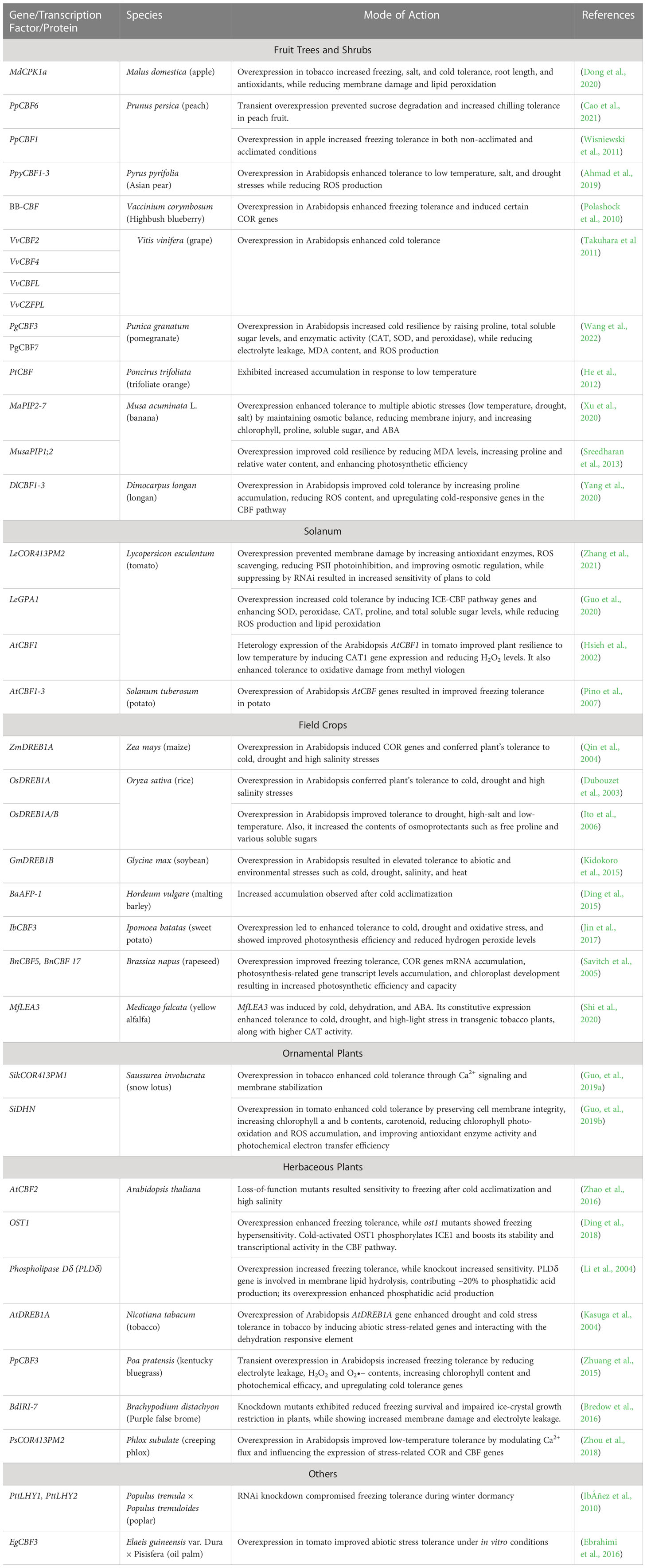
Table 1 A comprehensive list of cold acclimatization genes, transcription factors, and proteins, along with the species of identification, their mode of action, and references, is provided.
Plant cold signaling perception and transduction have been extensively studied in recent years, leading to significant advances in our understanding of this complex process (Zhang et al., 2017; Zhao et al., 2017; Ding et al., 2018; Guo et al., 2018). Plants perceive the environmental stimuli and transduce the signal into downstream biological responses by decreasing membrane fluidity, which in turn affects membrane-associated cellular functions (Hou et al., 2014). Different microdomains with specific lipid compositions, including sphingolipids in the plasma membrane, have been identified for their crucial roles in sensing particular temperature ranges (Fabri et al., 2020). Additionally, multiple cold sensors associated with sending temperature changes and cold signaling, such as putative calcium channels, PM-bound G-protein-associated receptors, and plasma membrane-localized receptor-like kinases (RLKs), have been identified in plants (Figure 1). Calcium ions enter the cell primarily through transmembrane proteins complex, known as calcium channels, which are crucial sensors for abiotic stress (Görlach et al., 2015). The rigidification of the membrane due to cold stress activates mechanosensitive or ligand-activated calcium channels, leading to a transient Ca2+ influx into the cytosol (Wei et al., 2021).
After perceiving cold stress, plants initiate a series of intricate signal transduction events within the cytosol and nucleus. These events rely on secondary messengers like Ca2+, ROS, and nitric oxide (NO) to facilitate intracellular signaling and cell-to-cell communication (Marcec et al., 2019). Cold stress-induced Ca2+ signatures are deciphered through various pathways involving specific groups of Ca2+ sensors such as CaM (calmodulin) and CMLs (CaM-like), CDPKs (Ca2+-dependent protein kinases), CCaMK (Ca2+-and Ca2+/CaM-dependent protein kinase), CAMTA (CaM-binding transcription activator), CBLs (calcineurin B-like proteins) and CIPKs (CBL-interacting protein kinases) (Kudla et al., 2018). The Calcium/CaM-Regulated Receptor-Like Kinase 1, CRLK1, actively modulates MAPK kinase activity and exerts regulatory control over C-repeat Binding Factors (CBFs) regulons, as well as freezing tolerance (Yang et al., 2010). Subsequent downstream reactions in plants are triggered by cold stress encompass the ICE-CBF/DREB1 pathway, the intricate regulation of cold-responsive genes (Miura and Furumoto, 2013), and post-transcriptional and post-translational modifications (Vyse et al., 2020).
In addition to the plasma membrane, chloroplasts may also serve as important sensors for ambient temperature. When temperatures drop, the equilibrium between the ability to capture light energy and the ability to disperse this energy through metabolic processes is disturbed. This disruption results in enhanced excitation pressure on photosystem II (PSII) and subsequently hinders the photosynthetic capacity by inhibiting it (Ivanov et al., 2012). This phenomenon, known as photoinhibition, results in redox imbalances in photosynthetic electron transport and photosynthetic carbon reduction cycles. Eventually, the photosynthetic apparatus is destroyed, and ROS are generated, serving as a secondary messenger (Bhattacharjee, 2019). Disruption of the photosynthesis process under cold-stress conditions significantly reduces crop yield through various mechanisms. The maximum attainable yield, which represents the optimal conversion of captured light energy into biomass, known as photosynthetic efficiency, is compromised under cold stress (Simkin et al., 2019). This reduction in photosynthetic efficiency is primarily attributed to the impairment of key enzymes involved in the carbon fixation cycle, namely fructose-1,6-bisphosphatase (FBPase) and sedoheptulose-1,7-bisphosphatase (SBPase) (Sassenrath et al.,1990; Kingston-Smith et al., 1997; Hutchison et al., 2000). The decreased activity of ribulose-1,5-bisphosphate carboxylase/oxygenase (Rubisco), the primary enzyme involved in carbon fixation, and the reduction in bisphosphate (RuBP) levels are processes observed at low temperatures. Multiple causes contribute to the diminished RuBP regeneration, such as limitations in linear electron transport, chronic photoinactivation, deactivation of stromal bisphosphatases, and end-product limitation. In the case of end-product limitation, the limited synthesis of sucrose, starch, and amino acids leads to the accumulation of phosphorylated intermediates, depletion of the inorganic phosphate pool, and inhibition of ATP synthesis (Leegood and Furbank, 1986; Sharkey et al., 1986; Lemoine et al., 2013). Furthermore, imbalances in the source-sink relationship, where the plant’s source activity surpasses the sink demand, result in decreased mRNA levels, degradation of photosynthetic proteins, and ultimately reduced productivity (Adams et al., 2013; Kurepin et al., 2015). These combined effects contribute to lower crop yields under cold stress conditions.
3 Freeze-induced damage in plants: mechanisms and consequences
At freezing temperatures, the aggregation of water molecules within plant cells leads to the formation of stable ice nuclei, which is a critical step in initiating the freezing process. Ice nucleation occurs when these small ice nuclei form a group of membrane proteins known as ice-nucleating proteins (INPs), which act as nucleation sites. These sites promote the formation of extracellular ice crystals by facilitating the proper arrangement of water molecules. In the absence of ice-binding proteins (IBPs), large ice crystals form in the apoplast, which can physically damage plasma membranes. The formation of ice crystals and the subsequent sequestration of intracellular water create an osmotic gradient, leading to cellular dehydration. This loss of cell volume can result in cell collapse or rupture (Pearce, 2001; Larcher, 2003; Snyder and de Melo-Abreu 2005; Zhang et al., 2019). Freeze damage also occurs due to alterations in membrane fluidity caused by the transition of lipid components from liquid into a gel state. This transition reduces membrane selectivity, increases permeability, destabilizes metabolic activities, and inhibits photosynthesis (Larcher, 2003; Zhang et al., 2019).
The magnitude of damage depends on the intensity and duration of freezing conditions and the developmental stage of the plant (Larcher, 2003; Centinari et al., 2016). In some cases, plants can delay the formation of extracellular ice crystals through a process called supercooling, where water in plant tissues remain in a liquid state below the freezing point of water. Supercooling in plants is a sophisticated process, involving various mechanisms working synergistically. It prevents the transition of intercellular water from a liquid to a solid state, effectively suppressing water nucleation and thereby avoiding ice crystallization (Wisniewski et al., 2004; Londo and Kovaleski, 2019). Supercooling is a common phenomenon in woody plants, manifesting in both leaves and the living cells of the xylem, including the xylem ray parenchyma cells. This phenomenon readily takes place in small volumes of water, where the free energy of water is influenced by surface properties, especially in the absence of nucleation particles or agents responsible for initiating ice-crystal formation. This process allows cells to maintain their function, albeit at a relatively reduced rate, and become more tolerant to freeze damage (Cavender-Bares, 2005). However, even in supercooled parts of the plant, progressive dehydration can still occur, indicating that the avoidance of freezing-induced dehydration through deep supercooling is only partial (Pearce, 2001; Larcher, 2003).
Another equally important factor that influences the extent of damage caused by dehydration is the ratio of bound water to free water within plant cells. As freezing-induced dehydration progresses, it can disrupt cell structures associated with the bound water compartment, which is involved in the structural organization of membranes, organelles, and proteins. This disruption can result in irreversible injury to the cells (Elliott et al., 2017). Negative osmotic pressure induces a net movement of water towards the extracellular space, reducing cell volume. In non-acclimated cells, this reduction in cell volume leads to invagination of the plasma membrane and formation of endocytic vesicles, resulting in loss of surface area of the plasma membrane. Upon rewarming, melted water from the extracellular space re-enters the cell, causing the cell to burst before it can regain its original volume (Ambroise et al., 2020).
The antioxidant system in plants also scavenges the excessive amount of ROS, including superoxide (O2•−), hydroxyl radicals (OH•), hydrogen peroxide (H2O2) and singlet oxygen (1O2) in response to cold stress. The antioxidant compounds play a critical role in maintaining redox homeostasis during cold stress, as optimal levels of ROS are necessary for normal progression of fundamental biological processes including cellular proliferation and differentiation (Tsukagoshi et al., 2010; Mittler, 2017). Under normal conditions, excessive ROS is neutralized through various enzymatic and non-enzymatic antioxidative defense mechanisms. However, when plants are exposed to cold stress, the equilibrium between ROS production and scavenging is disrupted, leading to mitochondrial membrane rigidification (Saha et al., 2015), loss of complex IV (Prasad et al., 1994), DNA damage, and impaired regulation of physiological cell death (Xie et al., 2014; Berni et al., 2019). These disruptive events can cause severe damage to plant cells and their functions, which may affect their survival and reproduction.
4 Plant defense mechanisms against freezing: insights and perspectives
Plants possess avoidance and tolerance strategies to mitigate the detrimental effects of freezing temperatures and prevent damage to their cellular structures (Larcher, 2003; Hoermiller et al., 2018). Avoidance mechanisms rely on a variety of cryoprotectant molecules to reduce the intracellular freezing temperature through a plant-specific process called supercooling (Francko et al., 2011). These molecules help maintain the intracellular liquids at sub-zero temperatures supercooled and delay or inhibit the formation of extracellular ice crystals (Larcher, 2003; Wisniewski et al., 2018). Certain plant species have developed sophisticated mechanisms to mitigate freezing damage by utilizing IBPs as a part of their survival strategy. IBPs, also known as antifreeze proteins (AFPs) or ice recrystallization inhibition (IRI) proteins, are a group of low temperature-associated proteins found in various cold-adapted organisms, including plants such as Lolium perenne, Loliurn perenne and Ammopiptanthus nanus (Yu et al., 2021), animals, and microorganisms. Plant IBPs possess unique structural features and functional properties that enable them to interact with ice crystals, thereby impeding their growth and inhibiting the movement of water molecules from the intracellular to extracellular space. This binding ability helps prevent cell dehydration and maintains cellular integrity during freezing conditions. Additionally, IBPs exhibit the ability to suppress ice recrystallization, a process that can lead to tissue damage and disrupt plasma membranes. By effectively limiting ice crystal growth and inhibiting ice recrystallization, IBPs play a crucial role in safeguarding plant tissues against freezing temperatures (Bredow and Walker, 2017; Wisniewski et al., 2020). Numerous AFPs have been identified in various plant species, highlighting the diversity of these ice-binding proteins. For example, fpAFP has been discovered in Festuca pratensis (Muthukumaran et al., 2011), LpAFP and LpIRI2/3 in Lolium perenne (Bredow et al., 2017), BdIRI1-7 in Brachypodium distachyon (Bredow et al., 2016), daAFP in Daucus carota (Cid et al., 2018) and rsAFP in Raphanus sativus (Wisniewski et al., 2020). These specific IBPs exemplify the adaptations of plants to withstand freezing stress and highlight the intricate molecular strategies employed by these organisms to protect themselves from the detrimental effects of low temperatures.
Cold tolerance mechanisms involve acquiring tolerance to low, non-freezing temperatures through a process known as cold acclimation. It is a complex physiological process where plants gradually adapt to decreasing temperatures, resulting in enhanced hardiness and adaptive responses that enable them to withstand freezing conditions. Cold acclimation encompasses coordinated molecular, physiological, and biochemical changes that enhance plant tolerance to cold stress, crucial for survival under low temperature conditions. These adaptations include diverse morphological modifications such as reduced plant height, decreased leaf number, and increased epidermal thickness. Biochemical alterations involve the accumulation of sugars, amino acids, and secondary metabolites. Physiological adjustments encompass decreased photosynthesis, reduced water use efficiency, and altered pigment synthesis. Collectively, these mechanisms enhance a plant’s ability to thrive in cold environments (Fürtauer et al., 2019; Wang et al., 2020; Fang et al., 2021; Guo et al., 2021; Liu et al., 2021b; Satyakam et al., 2022). The regulation of gene expression during cold stress response is a complex network triggered by multiple factors including Ca2+ and plant hormones, particularly brassinosteroids (BRs), gibberellins, abscisic acid, jasmonic acid, and ethylene (Arias et al., 2015; Eremina et al., 2016; Hu et al., 2017; Li et al., 2017:1; Juurakko et al., 2021; Mishra et al., 2022; Sarkar and Sadhukhan, 2022). Additionally, plants synthesize secondary metabolites in response to cold stress, such as proline, betaine, and putrescine. These osmolytes act as protective compounds, helping to mitigate the effects of cold stress on plant cells (Lee et al., 2019). Plants also accumulate low-molecular-weight compatible solutes or osmolyte cryoprotectants, such as sucrose, glucose, raffinose, fructose and trehalose. These molecules assist in maintaining cellular osmotic balance and protect cellular structures from cold-induced damage (Karabudak et al., 2014; Lunn et al., 2014; Judy and Kishore, 2016; Roychoudhury and Banerjee, 2016; Suo et al., 2017; Siddique et al., 2018; Liu et al., 2019; Chen et al., 2022). In addition, plants respond to cold stress by increasing the accumulation of suberin and lignin, which contribute to the reinforcement of cell walls and the protection of plant tissues against freezing injury. These modifications are essential for reducing the adverse effects of cold stress on plant growth and development (Trache et al., 2017; Jian et al., 2020; Sun et al., 2021).
The process of cold acclimation in plants also involves plasma membrane rigidification and rearrangement of the cytoskeleton (Knight and Knight, 2012; Ambroise et al., 2020; Satyakam et al., 2022). These alterations trigger increased metabolic activities, initiating signaling pathways that regulate the expression of COR genes (Iqbal et al., 2022; Satyakam et al., 2022). The induction of COR genes is mediated by transcriptional activators known as C-repeat Binding Factors (CBFs), which are controlled by the regulatory module involving the Inducer of CBF Expression (ICE). This regulatory module, referred to as ICE-CBF-COR, represents a central pathway responsible for initiating the cold response in plants (Chinnusamy et al., 2003; Jin et al., 2018). Upon activation, COR genes regulate the synthesis of various protective molecules, including compatible solutes such as soluble sugars and proline, pigments like xanthophylls and carotenoids, amino acids, and cold-responsive proteins such as AFPs, late embryogenesis abundant (LEA) proteins, heat shock proteins (HSPs), cold shock proteins (CSPs), as well as antioxidants and stabilizing proteins like chaperones and dehydrins (DHNs) (Rinehart et al., 2007; Latowski et al., 2011; Yu et al., 2017; Meena et al., 2019; Ambroise et al., 2020). The synthesis and accumulation of these molecules collectively contribute to the development of cold tolerance in plants.
5 Cryoprotectants: mechanisms of action and insights in frost mitigation
Over the past two decades, agrochemical companies have developed multiple cryoprotectants (such as KDL, Glacier, diKap, Anti-Stress 550, ThermoMax, FrostGard, etc.) for frost protection – a comprehensive list of the artificial cryoprotectants has recently been summarized in a review by (Román‐Figueroa et al., 2021). These compounds, when externally applied to plants, enhance plant’s freezing avoidance ability through various methods. They create a physical barrier around plant tissues, increase the concentration of endogenous cryoprotectants like metabolites and proteins, and boost the intercellular solute concentration. This elevated solute concentration leads to a depression in freezing point, preventing the formation of extracellular ice crystals and reducing cell dehydration-induced damage caused by apoplastic freezing. It has also been suggested that solute accumulation stabilizes cell membranes and macromolecules, either through direct interaction with the membrane surface or through strong interplay with the surrounding water. The precise mechanism by which these molecules prevent ice crystal formation and cell dehydration is intricate and varies depending on the specific type of cryoprotectant. In this summary, we outline some general mechanisms through which cryoprotectants alleviate freezing damage to plant tissues.
5.1 Physical barrier: plant tissue protection using cellulose nanocrystals
Cellulose nanocrystals (CNCs) are nanoscale biomaterials with unique structural characteristics and impressive physicochemical properties. These properties include biocompatibility, biodegradability, renewability, low density, versatile surface chemistry, optical transparency, and enhanced mechanical properties (Trache et al., 2017). CNCs serve as the foundational polymeric motifs of macroscopic cellulosic fibers produced through the acid hydrolysis of cellulose materials. CNCs exhibit a needle-like or rod-like shape and have dimensions on the nanometer scale, typically ranging from a few to several hundred nanometers in length and a few nanometers in width. These materials can be obtained from various sources such as higher plants, marine animals (such as tunicates), and, to a lesser extent, algae, fungi, bacteria, invertebrates, and even amoeba like Dictyostelium discoideum (Habibi et a., 2010; Grishkewich et al., 2017). Due to their exceptional attributes, CNCs find applications in diverse fields, including automotive, medicine, construction, marine, aerospace, barrier materials, flexible displays, antimicrobial coatings, biomedical implants, transparent films, pharmaceuticals, drug delivery, electronic component templates, fibers and textiles, separation membranes, supercapacitors, batteries, and electroactive polymers (Trache et al., 2017).
Recently, researchers have explored the use of CNCs as a cryoprotectant for grape and sweet cherry reproductive buds (Alhamid et al., 2018). The application of sprayable CNCs forms a thermal insulation coating around the buds and flowers, resulting in enhanced cold hardness and effective mitigation of frost damage. The protective properties of CNCs can be attributed to their low thermal conductivity (0.061 W m-1 K-1), which surpasses that of other commonly used frost protection materials. In this study, CNC treatment significantly reduced the damage temperature of grape and sweet cherry buds and improved their resistance to frost damage by 2 – 4°C. Moreover, the application of CNCs delayed the formation of ice nucleation in the buds when exposed to freezing temperatures. The freezing temperature at which ice nucleation occurred was lowered by approximately 3°C in CNC-treated buds compared to untreated buds (Alhamid and Mo 2021). These findings highlight the extensive potential of CNCs as cryoprotectants in enhancing plants’ protective mechanisms and their capacity for freeze protection.
5.2 Reducing freezing point
Certain cryoprotectants exhibit cryohydricity, which refers to their ability to depress the freezing point of water. This phenomenon enables plants to endure lower temperatures by modifying the colligative properties of water, including osmotic potential and increased intracellular solute concentration. Solute compounds, such as sugars, salts, and other dissolved substances, disrupt ice crystal formation and impede the progress of freezing. As a result, the freezing temperature of water within plant tissues is reduced below the standard freezing point of pure water (0°C), known as freezing point depression (Ginot et al., 2020) (Figure 2). The extent of this depression depends on the concentration and nature of the solutes involved, with higher solute concentrations yielding greater reductions in freezing point. This reduced freezing point enables plant cells to remain in a liquid state even at sub-zero temperatures, thus preventing or delaying ice crystal formation. At the cellular level, cryoprotectants modify water properties through hydrogen bonding interactions between water and cryoprotectant molecules. This hydrogen bonding plays a crucial role in hindering the movement of intracellular water into the extracellular space, effectively reducing ice crystal formation and minimizing cellular damage (Weng et al., 2011; Elliott et al., 2017) (Figure 2).
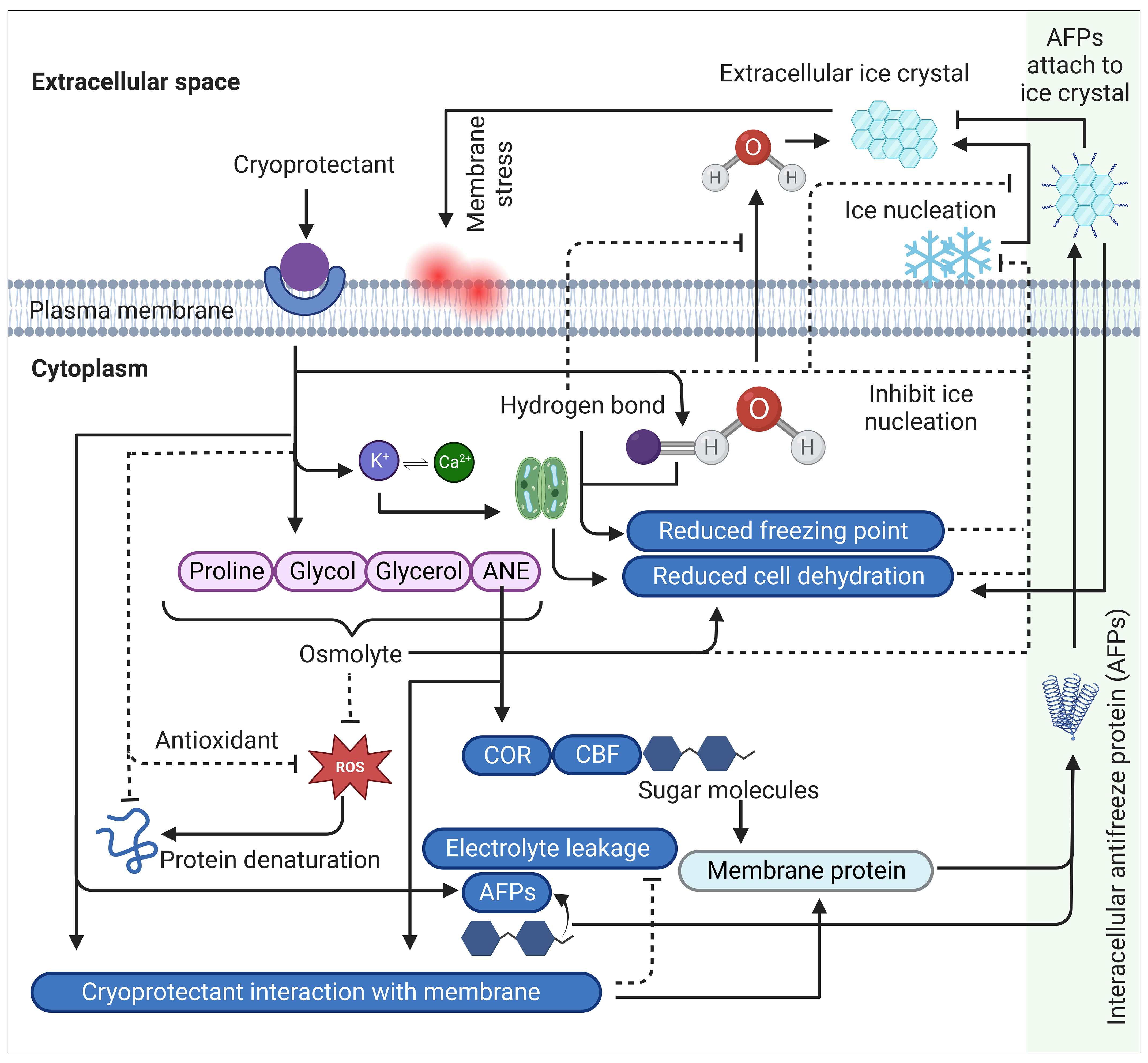
Figure 2 A comprehensive schematic summary of cryoprotectants mechanism of action and cold stress modulation in plants. Cryoprotectants regulate osmolytes such as proline, glycol, glycerol and ANE. The increased accumulation of these osmolytes triggers the induction of cold regulating (COR) genes through the activation of transcriptional activators called C-repeat Binding Factors (CBFs), which are involved in reducing cell dehydration, regulating membrane proteins and synthesizing antifreeze proteins (AFPs). Sugar molecules also participate in this regulation by reducing cell dehydration and modulating membrane proteins, including antifreeze proteins (AFPs). AFPs are secreted into extracellular spaces, attaching to ice crystals to prevent ice aggregation, resulting in suppressing recrystallization and reducing cell dehydration. Additionally, cryoprotectants trigger K+ interacting with Ca2+ that plays an important role in the hydrodynamic stomatal closure mechanism, leading to reduced cell dehydration. Furthermore, cryoprotectants establish hydrogen bonds with intracellular water molecules, restricting their movement into extracellular spaces and inhibiting its conversion into ice crystals. This hydrogen bonding lowers the freezing point of water. In addition, certain cryoprotectants scavenge ROS either through their antioxidant properties or through its osmoregulatory properties that contributes to maintaining cellular osmotic balance and integrity by regulating water movement across the membrane, resulting in enhanced plasma membrane stability. Collectively, these mechanisms prevent the formation of ice-nucleation sites on the membrane surface, which is a critical step in the initiation of extracellular ice crystal formation and subsequent freezing stress. Arrows indicate positive regulation while dashed line with a perpendicular line at the end indicates negative regulation. ROS, reactive oxygen species; CBF, C-repeat Binding Factors, COR, cold-responsive, AFP, antifreeze proteins, ANE, Ascophyllum nodosum (Seaweed) extract.
An example of an exogenous cryoprotectant capable of lowering the freezing point of plant tissues is FreezePruf, a commercially available product. FreezePruf is formulated using polyethylene glycol, potassium silicate, glycerol, silicone polyether surfactant, and a bicyclic oxazolidine anti-desiccant. These constituent molecules act as solutes or osmolytes, and their application increases solute concentration in the intracellular cytoplasmic compartments, leading to a reduction in the freezing point of these cellular regions. Francko et al. (2011) conducted a study to evaluate the effectiveness of FreezePruf on various monocot herbaceous and dicot fruit crops. Their experimental results demonstrated that the treatment with FreezePruf significantly decreased freezing-induced injury and lowered the mortality temperature by approximately 7 degrees when applied to foliage and about 1 degree when applied to open flowers. This reduction in freezing temperature is primarily attributed to the active ingredients present in FreezePruf, which act colligatively via freezing point depression as solutes. Moreover, these ingredients exert non-colligative effects by stabilizing cell membranes, thereby providing protection against freeze damage caused by ice crystal formation (Francko et al., 2011).
5.3 Stabilizing cellular structures
Plasma membranes are highly vulnerable to damage caused by freezing. This damage arises from several factors, including increased levels of ROS, the transition of the lipid bilayer from a fluid state to a solid-gel state, cell dehydration, and the formation of extracellular ice crystals. Collectively, these factors compromise the integrity, fluidity, and functionality of the membrane, leading to cellular damage and impaired plant survival under freeze stress. The temperature-dependent phase transition of the lipid bilayer plays a critical role in freeze-induced membrane damage. This transition reduces water diffusion across the membrane (Eze, 1991) and disrupts lipid-protein interactions, impacting membrane integrity and function (Iivonen et al., 2004). The composition of lipids, specifically the number of carbon atoms and double bonds in the lipid tail, influences the phase transition temperature. Lipids with higher unsaturation, characterized by an increased number of double bonds, exhibit a lower phase transition temperature, thereby reducing the occurrence of phase transition, leading to reduced freeze-induced membrane damage (Ambroise et al., 2020).
Cryoprotectants have been demonstrated to stabilize cellular structures and plasma membranes through various mechanisms. These mechanisms include modulating the membrane lipid phase transition, preventing protein denaturation, interacting with membrane proteins to stabilize their conformation and function, inducing membrane repair mechanisms, enhancing membrane flexibility, reducing mechanical stress, and acting as osmoregulators (Fürtauer et al., 2019). Furthermore, the osmoregulatory properties of these compounds contribute to maintaining cellular osmotic balance and integrity by regulating water movement across the membrane, resulting in enhanced plasma membrane stability (Sami et al., 2016; Arakawa et al., 2018; Fürtauer et al., 2019).
Certain commercially available cryoprotectants, such as KDL, FrostGard, and Superkelp/Vitazyme, utilize Ascophyllum nodosum (Seaweed) extract (ANE) as an active ingredient. ANE has been reported to alleviate low-temperature stress in plants by mitigating freezing-induced electrolyte leakage through the preservation of membrane integrity (Figure 2). Notably, ANE application on winter barley has been found to enhance winter hardiness and augment cold resistance (Shukla et al., 2019). Additionally, treatment with the lipophilic fraction (LPC) of ANE in Arabidopsis plants improved cold tolerance, as evidenced by the suppression of chlorosis and recovery from freezing-induced tissue damage (Rayirath et al., 2009).
Furthermore, a comprehensive analysis of the transcriptome and metabolome of LPC-treated Arabidopsis plants revealed that ANE treatment induces the expression of cold-responsive genes, including COR15A, RD29A, and CBF (Rayirath et al., 2009), as well as genes associated with sugar accumulation and lipid metabolism (Nair et al., 2012). Metabolite profiling of LPC-treated plants showed that the observed protection against freezing stress is mediated by the regulation of soluble sugars, sugar alcohols, organic acids, and lipophilic components such as fatty acids (Nair et al., 2012). The accumulation of sugars plays a crucial role in mitigating freezing stress by stabilizing various biological components, such as cellular membranes and membrane-bound organelles (Tarkowski and Van den Ende 2015). Interestingly, ANE treatment did not improve freezing tolerance in the sfr4 (sensitive to freezing) mutant of Arabidopsis, which is defective in the accumulation of free sugars, suggesting that ANE-induced accumulation of soluble sugars prior to freezing stress exposure is critical for its protective effects (Nair et al., 2012).
At the molecular level, ANE-mediated cold tolerance in plants is attributed to the regulation of key transcription factors and genes encoding cryoprotective proteins. For instance, ANE-treated Arabidopsis plants showed increased cold tolerance due to the downregulation of chlorophyll degradation genes, including AtCLH1 and AtCLH2, resulting in an increased chlorophyll content. ANE-mediated cryoprotectants also upregulated genes responsible for proline biosynthesis, such as 5CS1 and P5CS2, while downregulating ProDH, a gene involved in proline degradation. Moreover, ANE treatment upregulated genes responsible for polysaccharide degradation (9SEX1 and SEX4) and carbohydrate biosynthesis (GOLS2 and GOLS3), while downregulating genes involved in sucrose degradation. ANE treatment also induced key genes involved in freezing tolerance, including galactinol synthase 2, pyrroline 5-carboxylate synthase, and acetyl-CoA carboxylase (Zamani-Babgohari et al., 2019; Ali et al., 2021). These findings provide compelling evidence supporting the role of ANE-based cryoprotectants in enhancing cellular stabilization in plants through molecular, biochemical, and physiological mechanisms.
Another class of cryoprotectants, such as Superkelp/Vitazyme, confer cold protection in plants by increasing the levels of endogenous proline. Proline is naturally accumulated compound in plants in response to cold stress as it is water-soluble and electrically neutral at neutral pH, which helps maintaining cell osmotic potential and protecting against cold damage (Verbruggen and Hermans, 2008). The application of exogenous cryoprotectants containing proline significantly elevates the endogenous proline levels and enhances cold tolerance. Studies have shown that proline-mediated cryoprotectant treatments in rapeseed shoots increase cold tolerance and reduce electrolyte leakage, which is an indicator of membrane injury (Jonytiene et al., 2012). Additionally, proline-treated Brassica juncea has been shown to protect isolated thylakoid membranes from photoinhibition (Bhandari and Nayyar, 2014) and improve the maintenance of chloroplast ultrastructure and membrane integrity in tobacco plants (Van Rensburg et al., 1993). Moreover, proline-stimulating cryoprotectants exert their protective effects by interacting with plasma membrane-bound proteins and enzymes. These compounds act as hydrotropes to stabilize enzymes and biomembranes, owing to the ability of proline to form hydrophilic colloids with water and a hydrophobic backbone for protein interactions. This helps maintain the structure and conformation of proteins necessary for their functional integrity. Additionally, proline functions as a chaperone to prevent membrane proteins from denaturation (Rajendrakumar et al., 1994; Zhang et al., 2011).
5.4 Cryoprotectant interactions with ice crystals: inhibiting nucleation and their growth
Certain cryoprotectants possess the ability to inhibit the formation of ice nuclei, which serve as the initial points for ice crystal growth. These cryoprotectants also prevent the fusion of smaller ice crystals into larger ones, which could otherwise lead to cellular damage. For example, cryoprotectants containing antifreeze proteins (AFPs) have been shown to bind to the surface of ice crystals and inhibit their growth, thus preventing the formation of large ice crystals in the extracellular space (Figure 2). These molecules can also inhibit ice recrystallization, a phenomenon that can occur during repeated freeze-thaw cycles or prolonged exposure to low temperatures (Meyer et al., 1999; Wisniewski and Fuller, 1999; Griffith and Yaish, 2004). The effect of three synthetic analogues based on naturally occurring antifreeze peptides, AFPW, DCR26, DCR39, was investigated on carrot and cherry fruits (Kong et al., 2016; Kong et al., 2017). The in vitro experimental results showed that these compounds modified ice crystal morphology, resulting in reduced cell damage by ice crystals (Kong et al., 2017). Interestingly, certain plant AFPs share sequence homology with plant pathogenesis-related (PR) proteins including endochitinases (PR3), endo-β-1,3-glucanases (PR2), and thaumatines (PR5) (Antikainen et al., 1996; Pearce, 2001). This suggests that AFPs may serve a dual role in cold survival by mitigating freeze-related damage and providing defense against psychrophilic pathogens (e.g., ice-nucleating bacteria) (Griffith and Yaish, 2004).
The precise molecular mechanism underlying the interaction between cryoprotectants, particularly those containing AFPs, and ice crystals is not yet fully understood. Several factors that facilitate the binding of AFPs to ice nuclei have been identified. These include the amino acid composition of AFPs, with specific residues playing a role in the binding process (Davies and Sykes, 1997). Additionally, the secondary structure of these compounds, such as beta-strand-rich proteins, has been implicated in their ice-binding ability (Lu et al., 2002). Motifs such as the presence of N-acetyl groups at C-2 peptide chains with O-glycosidic linkages and gamma-methyl groups at threonine have also been identified as potential contributors to the ice-binding mechanism (Urbańczyk et al., 2017; Chakraborty and Jana, 2018). These ice-binding sites are primarily hydrophobic, although recent molecular studies have shown that both hydrophobic and hydrophilic properties contribute to the ice-binding mechanism (Hudait et al., 2018). Furthermore, AFPs and AFP-mediated cryoprotectants are known to form cages around the methyl groups on the ice-binding surface, organizing surrounding water molecules into an ice-like lattice and creating a quasi-liquid-like layer between water and the already formed ice crystals (Satyakam et al., 2022). However, despite these insights, the precise molecular mechanism by which certain cryoprotectants interact with ice crystals remains to be fully elucidated, and further research is needed to better understand this intriguing phenomenon.
In some cases, exogenously applied cryoprotectants can inhibit ice-nucleating bacteria by using inorganic salts or organic polymers to create a vapor barrier on the leaf surface, offering modest frost and freeze protection (Francko et al., 2011). An example of such cryoprotectants is a successful patent by Glenn et al. (2001), which describes a slurry comprising particulate materials that prevent ice crystal formation on the leaf surface at freezing temperatures. Ice-nucleating active (INA) bacteria, such as Pseudomonas syringae van Hall and Erwinia herbicola (Lihnis) Dye, possess a unique capability to catalyze ice formation at temperatures slightly below the freezing point (approximately -2°C) (Lindow et al., 1982). The ice-nucleation capability of INA bacteria is attributed to their specialized hydrophilic-hydrophobic ice-active sites (Pandey et al., 2016). In natural conditions, frost damage to plants typically occurs between -2°C and -5°C. Within this temperature range, ice crystals form from supercooled water within the plants, propagating throughout the intercellular and intracellular spaces and causing damage. In the absence of ice nucleation sites, water in plant tissues can remain in a supercooled state without freezing until the temperature becomes low enough for the most active ice nucleation site associated with the plant to catalyze the crystallization of supercooled water (Lindow et al., 1982). INA bacteria, found ubiquitously on plant surfaces, prevent supercooling by initiating the ice nucleation process using specialized ice-nucleating proteins (INPs) attached to their outer cellular membrane (Pandey et al., 2016), ultimately leading to freeze injury alleviation (Roeters et al., 2021; Lukas et al., 2022).
5.5 Reducing cell dehydration
At sub-zero temperatures, freeze-induced dehydration stress triggers changes in the lipid bilayer configuration of cell membranes, leading to a transition from a lamellar phase to a hexagonal II phase. The formation of hexagonal II phase membranes is facilitated by decreased water content, an increased number of intracellular membranes, and the presence of specific lipids such as phosphatidylethanolamine and sterols in the bilipid membrane (Uemura et al., 1995). Lipid unsaturation in plant cells plays a pivotal role in mitigating freeze damage and enhancing cold resistance. This is largely due to the properties of unsaturated fatty acids within the phospholipids of the cell membrane. These fatty acids have double bonds that introduce kinks in the fatty acid chains, preventing them from packing tightly and solidifying at low temperatures. Consequently, a higher degree of lipid unsaturation typically decreases freeze damage in plants. This is an adaptive strategy that many cold-tolerant plant species employ, synthesizing and accumulating unsaturated fatty acids under low-temperature exposure.
Alongside the structural changes in the lipid bilayer, dehydration stress can also induce expansion-induced lysis, where negative osmotic pressure drives water movement from the cell interior towards the extracellular space, resulting in a reduction in cell volume. This phenomenon causes the plasma membrane to invaginate and form endocytic vesicles, leading to a loss of plasma membrane surface area. Upon rewarming, the melted extracellular water reenters the cell, causing the cell to burst before regaining its original volume. In contrast, in cold-acclimated cell protoplasts, the volume reduction triggers the formation of exocytotic extrusions that do not decrease the membrane surface area and do not cause cell bursting upon rewarming (Uemura et al., 1995; Ambroise et al., 2020).
The application of specific exogenous cryoprotectants, such as Anti-Stress 2000 (Terra Tech, Eugene, OR) and Wilt-Pruf (Wilt-Pruf Products, Essex, CT), has been demonstrated to mitigate dehydration risk in plants during freezing and thawing cycles. This protective effect is attributed to the anti-desiccant properties of these compounds, which reduce water loss from plant tissues resulting from osmotic stress induced by ice crystal formation (Figure 2). By preserving cellular integrity and preventing dehydration-induced damage, these cryoprotectants play a vital role in plant protection. As discussed earlier, certain cryoprotectants contain molecules capable of lowering the freezing points of plant tissues. Additionally, these compounds contribute significantly to the prevention of cellular dehydration. For instance, potassium, a key component of several cryoprotectants, plays a crucial role in the hydrodynamic stomatal closure mechanism and helps maintain cellular osmotic balance (Figure 2). This regulatory mechanism of potassium is essential for preserving cellular integrity during freezing and thawing cycles (Cakmak, 2005; Wang et al., 2013).
5.6 Scavenging reactive oxygen species
Reactive Oxygen Species (ROS) are partially reduced or excited derivatives of molecular oxygen (O2), which naturally arise as a normal byproduct of aerobic life (Mittler, 2017; Sies and Jones, 2020). In contrast to O2, ROS are highly reactive and autonomously produced in various cellular compartments, leading to the oxidation of lipids, proteins, DNA, RNA, and many small cellular molecules. The high reactivity of ROS is attributed to their altered chemical composition, enabling them to engage in electron donation or transfer an excited energy states to accepter molecules (Mittler et al., 2022). The major forms of ROS in cells include free radicals like superoxide anion radical (O2•−) and hydroxyl radical (OH•), and non-radicals like hydrogen peroxide (H2O2) and singlet oxygen (1O2), and various forms of organic and inorganic peroxides (Mittler, 2017; Sies and Jones, 2020). ROS are primarily formed in chloroplast, mitochondria and peroxisomes. There are secondary sites including endoplasmic reticulum, cell membrane, cell wall and the apoplast, typically equipped with molecules having a high redox potential to reduced or excite molecular oxygen (Das and Roychoudhury, 2014; Mittler, 2017). ROS can be produced both passively by housekeeping enzyme or as by-products of metabolic pathways such as photosynthesis and respiration, and actively by specialized oxidase enzymes such as the respiratory burst oxidase homologues (RBOHs) proteins, which are the functional equivalent of mammalian NADPH oxidase (NOX proteins) (Mittler et al., 2022). Superoxide radical is the first by-product produced at the apoplast through the function of RBOHs proteins, which is subsequently dismutated by the action of superoxide dismutase (SOD) to H2O2. The membrane permeable H2O2 is the premier signaling molecule, playing an important role in regulating various cellular metabolic activities associated with growth, development, cell expansion and response to environmental stimuli (Baxter et al., 2014; Smirnoff and Arnaud, 2019). Hydrogen peroxide interacts with ferrous ions (Fe2+), forming OH•, the highly reactive form of ROS, through Fenton reaction (Mittler, 2017).
ROS display a dual role in plant cells, depending on their accumulation levels. A moderate, “steady-state” level of ROS is required for the progression of various fundamental biological processes, including cellular proliferation, differentiation and important signaling reactions in cells, but are also the unavoidable toxic byproducts of aerobic metabolism. As signaling molecules, ROS enables cells to rapidly respond to environmental stimuli and triggers plant’s cellular defense signaling cascade (Chi et al., 2013). Under optimal growth conditions, plants maintain a basal level of ROS serving as signaling molecules to sense elevated atmospheric oxygen levels and to monitor different metabolic reactions. However, different biotic and abiotic stress (i.e., freezing stress) disrupt the cellular homeostasis, uncouple metabolic pathways and leads to an increased production of ROS (Suzuki and Mittler, 2006; Miller et al., 2010). The elevated accumulation of ROS in cells leads to toxic oxidative stress, which adversely damages cellular components including protein denaturation, membrane lipids degradation through peroxidation (Heidarvand and Maali-Amiri, 2013) and oxidation and damaging of DNA, RNA, protein and membrane (Mittler, 2002; Mittler, 2017; Sharma et al., 2021), leading to cell death. The ROS-induced cell death is mediated through a programmed physiological and genetic pathway such as ferroptosis or regulated necrosis (Mittler, 2017). Plants pose ROS-scavenging mechanisms to detoxify the excessive ROS and maintain the balance between scavenging and production. This include the various antioxidative enzymes such as superoxide dismutase (SOD), ascorbate peroxidase (APX), catalase (CAT), glutathione peroxidase (GPX), peroxiredoxin (PRX), monodehydroascorbate reductase (MDHAR), and dehydroascorbate reductase (DHAR), and non-enzymatic antioxidants including ascorbic acid (AsA), reduced glutathione (GSH), α-tocopherol, carotenoids, flavonoids, and the osmolyte proline. The presence of these antioxidative systems in plants helps maintain a basal non-toxic level of ROS in cells, which serve as signaling molecules and promotes normal cellular processes (Miller et al., 2010; Das and Roychoudhury, 2014).
The application of certain cryoprotectants, such as Basfoliar® Frost Protect or COMPO® Frost Protect, has been shown to effectively mitigate ROS toxicity in plant cells. These cryoprotectants contain α-tocopherol, a lipophilic antioxidant that exhibit ROS and lipid radicals scavenging properties (Gill and Tuteja, 2010; Das and Roychoudhury, 2014) (Figure 2). Among the four isoforms of tocopherol (α-, β-, γ-, δ-), α-tocopherol has the highest antioxidant capability (Das and Roychoudhury, 2014). The antioxidant activity of tocopherol is primarily attributed to its ability of donating phenolic hydrogens to lipid free-radicals (Kamal-Eldin and Appelqvist, 1996). These compounds are known for their ability to inhibit lipid peroxidation, preserve the integrity and fluidity of photosynthesizing membranes and prevent membrane damage by interacting with O2 molecule and quenching reactive oxidative anions in which the reactivity of the highly reactive ROS, such as 1O2, is substantially reduced. It has been estimated that a single molecule of α-tocopherol can neutralize up to 120 molecules of 1O2 through resonance energy transfer (Gill and Tuteja, 2010; Das and Roychoudhury, 2014; Sadiq et al., 2019). Multiple studies demonstrated that increased accumulation of α-tocopherol in winter wheat leaves was associated with enhanced frost tolerance (Janeczko et al., 2018). Additionally, the application of exogenous α-tocopherol cryoprotectants has been found to enhance abiotic stress tolerance in onions by reducing the level of endogenous H2O2 and lipid peroxidation, and increasing the activity of antioxidative enzymes (i.e., SAD, CAT, APX, and GPX) and non-enzymatic antioxidants (i.e., AsA and GSH) (Semida et al., 2016).
Additionally, cryoprotectants, such as Frost Shield® (Maz-Zee S.A. International) and CROPAID® NPA® (Natural Plant Antifreeze, Bimas, Turkey), can enhance plant tolerance to excessive ROS levels through sequestering or replacing cellular free iron with other minerals. These cryoprotectants contain minerals and amino acids in their composition. Minerals, such as Mn2+ that is found in complex with amino acid, peptides, nucleotides and carbohydrates, can replace iron to prevent its toxicity during oxidative stress (Mittler, 2017). The presence of free iron in the form of Fe2+ is considered crucial for ROS toxicity due to its role in the Fenton reaction, where it catalyzes the generation of highly reactive hydroxyl radicals. By sequestering or substituting free iron with alternative minerals (i.e., Mn2+), cryoprotectants can disrupt the Fenton reaction and attenuate the production of damaging hydroxyl radicals. In addition, these manganese complexes are shown to effectively scavenge O2•−, H2O2 and OH• (Slade and Radman, 2011; Mittler, 2017). Overall, cryoprotectants modulate the equilibrium between ROS production through NADPH and ROS scavenging through various enzymatic and non-enzymatic antioxidants. However, it should be acknowledged that the specific molecular mechanisms can vary based on the type of cryoprotectant, plant species, and cellular conditions. Further research is required to gain a comprehensive understanding of these mechanisms.
6 Conclusion
Plants utilize advanced molecular mechanisms to perceive and respond to cold stress. Transmembrane proteins function as initial sensors for cold stress signals, triggering a cascade of molecular events that involve the generation of secondary messengers such as calcium ions (Ca2+), reactive oxygen species (ROS), and inositol phosphates. Among these, calcium signaling plays a critical role, activating downstream phosphorylation cascades and inducing the transcription of cold-responsive genes, including the cold-regulated (COR) genes. Cold stress imposes significant challenges on plant tissues, leading to membrane rigidification and dehydration caused by freeze-induced damage. In an effort to mitigate these adverse effects, plants have evolved strategies encompassing both avoidance and tolerance mechanisms. Tolerance mechanisms involve the gradual acclimatization of plants to decreasing temperatures, allowing them to incrementally accumulate cold tolerance. In contrast, avoidance mechanisms are predicted on the presence of cryoprotectant molecules such as potassium ions (K+), proline, glycerol, and antifreeze proteins (AFPs).
Cryoprotectants operate by increasing intracellular solute concentration, thereby lowering the freezing point and obstructing ice formation. These molecules also exhibit potent antioxidant properties, efficiently scavenging ROS and preventing protein denaturation. By forming extensive hydrogen bonds with water molecules, cryoprotectants can also alter water properties, limiting intercellular water movement, and minimizing extracellular ice crystal formation (Figure 2). This action consequently reduces cell dehydration and mitigates freeze-induced cellular damage. In addition, cryoprotectants aid in the preservation of plasma membrane fluidity and the reduction of electrolyte leakage, effectively shielding plant cells against freeze-induced injury. The intricate relationship between these cryoprotectant molecules and cellular processes enhances the plant’s resilience to cold stress and bolsters survival in freezing environments. Taken together, the deployment of cryoprotectants represents a sophisticated adaptive strategy utilized by plants to counter the damaging effects of cold stress, thereby facilitating their acclimation and survival in freezing conditions. Nevertheless, a comprehensive understanding of these mechanisms warrants further research. It is essential to elucidate the physiological and molecular mechanisms of these compounds and evaluate their potential impact on crop productivity and sustainability. Such efforts will undoubtedly contribute to the ongoing advancement of plant biology and the broader field of environmental science.
Author contributions
KJ conducted literature searches, prepared schematic diagrams, and wrote the first draft. AS contributed to figure preparation and literature review. SS oversaw the study, provided edits to improve clarity and readability, and ensured the overall quality of the manuscript. All authors contributed to the article and approved the submitted version.
Funding
This research was supported partially by funding to SMS from VA-Specialty Crop Block Grant Program (SCBGP) (Grant # 419842) and NIFA-AFRI (Grant# 13367799).
Conflict of interest
The authors declare that the research was conducted in the absence of any commercial or financial relationships that could be construed as a potential conflict of interest.
Publisher’s note
All claims expressed in this article are solely those of the authors and do not necessarily represent those of their affiliated organizations, or those of the publisher, the editors and the reviewers. Any product that may be evaluated in this article, or claim that may be made by its manufacturer, is not guaranteed or endorsed by the publisher.
References
Adams, W. W., Muller, O., Cohu, C. M., Demmig-Adams, B. (2013). May photoinhibition be a consequence, rather than a cause, of limited plant productivity? Photosynthesis Res. 117 (1), 31–44. doi: 10.1007/s11120-013-9849-7
Ahmad, M., Li, J., Yang, Q., Jamil, W., Teng, Y., Bai, S. (2019). Phylogenetic, molecular, and functional characterization of ppyCBF proteins in asian pears (Pyrus pyrifolia). Int. J. Mol. Sci. 20 (9), 2074. doi: 10.3390/ijms20092074
Alhamid, J. O., Mo, C. (2021). Numerical analysis of cellulose nanocrystals CNC for reducing cold damage to reproductive buds in fruit crops.” Thermal Sci. Eng. Prog. 26, 101123. doi: 10.1016/j.tsep.2021.101123
Alhamid, J. O., Mo, C., Zhang, X., Wang, P., Whiting, M. D., Zhang, Q. (2018). Cellulose nanocrystals reduce cold damage to reproductive buds in fruit crops.” Biosyst. Eng. 172, 124–133 doi: 10.1016/j.biosystemseng.2018.06.006
Ali, O., Ramsubhag, A., ayaraman, J. J. (2021). Biostimulant properties of seaweed extracts in plants: implications towards sustainable crop production. Plants 10 (3), 531. doi: 10.3390/plants10030531
Ambroise, V., Legay, S., Guerriero, G., Hausman, J.-F., Cuypers, A., Sergeant, K. (2020). The roots of plant frost hardiness and tolerance. Plant Cell Physiol. 61 (1), 3–20. doi: 10.1093/pcp/pcz196
Antikainen, M., Griffith, M., Zhang, J., Hon, W.-C., Yang, D. S. C., Pihakaski-Maunsbach, K. (1996). Immunolocalization of antifreeze proteins in winter rye leaves, crowns, and roots by tissue printing. Plant Physiol. 110 (3), 845–857. doi: 10.1104/pp.110.3.845
Arakawa, K., Kasuga, J., Takata, N. (2018). “Mechanism of overwintering in trees,” in Survival strategies in extreme cold and desiccation: adaptation mechanisms and their applications, advances in experimental medicine and biology. Eds. Iwaya-Inoue, M., Sakurai, M., Uemura, M. (Singapore: Springer), 129–147.
Arias, N. S., Bucci, S. J., Scholz, F. G., Goldstein, G. (2015). Freezing avoidance by supercooling in olea europaea cultivars: the role of apoplastic water, solute content and cell wall rigidity. Plant Cell Environ. 38 (10), 2061–2070. doi: 10.1111/pce.12529
Augspurger, C. K. (2013). Reconstructing patterns of temperature, phenology, and frost damage over 124 years: spring damage risk is increasing. Ecology 94 (1), 41–50. doi: 10.1890/12-0200.1
Baxter, A., Mittler, R., Suzuki, N. (2014). ROS as key players in plant stress signalling. J. Exp. Bot. 65 (5), 1229–1240. doi: 10.1093/jxb/ert375
Berni, R., Luyckx, M., Xu, X., Legay, S., Sergeant, K., Hausman, J.-F., et al. (2019). Reactive oxygen species and heavy metal stress in plants: impact on the cell wall and secondary metabolism.” Environ. Exp. Bot. 161, 98–106. doi: 10.1016/j.envexpbot.2018.10.017
Bhandari, K., Nayyar, H. (2014). “Low temperature stress in plants: an overview of roles of cryoprotectants in defense,” in Physiological mechanisms and adaptation strategies in plants under changing environment, vol. 1 . Eds. Ahmad, P., Wani, M. R. (New York, NY: Springer), 193–265.
Bhattacharjee, S. (2019). “ROS and regulation of photosynthesis,” in Reactive oxygen species in plant biology, (New Delhi: Springer India) 107–125.
Bredow, M., Vanderbeld, B., Walker, V. K. (2016). Knockdown of ice-binding proteins in brachypodium distachyon demonstrates their role in freeze protection. PloS One 11 (12), e0167941. doi: 10.1371/journal.pone.0167941
Bredow, M., Vanderbeld, B., Walker, V. K. (2017). Ice-binding proteins confer freezing tolerance in transgenic arabidopsis thaliana. Plant Biotechnol. J. 15 (1), 68–81. doi: 10.1111/pbi.12592
Bredow, M., Walker, V. K. (2017). Ice-binding proteins in plants. Front. Plant Sci. 8. doi: 10.3389/fpls.2017.02153
Cakmak, I. (2005). The role of potassium in alleviating detrimental effects of abiotic stresses in plants. J. Plant Nutr. Soil Sci. 168 (4), 521–530. doi: 10.1002/jpln.200420485
Cao, K., Wei, Y., Chen, Yi, Jiang, S., Chen, X., Wang, X., et al. (2021). PpCBF6 is a low-temperature-sensitive transcription factor that binds the ppVIN2 promoter in peach fruit and regulates sucrose metabolism and chilling injury. Postharvest Biol. Technol. 181, 111681. doi: 10.1016/j.postharvbio.2021.111681
Cavender-Bares, J. (2005). Impacts of freezing on longdistance transport in woody plants. In: NM, Holbrook, M, Zwieniecki, eds. Vascular transport in plants. (Oxford, UK: Elsevier) 401–424. doi: 10.1016/B978-012088457-5/50021-6
Centinari, M., Smith, M. S., Jason P., L. (2016). Assessment of freeze injury of grapevine green tissues in response to cultivars and a cryoprotectant product. HortScience 51 (7), 856–860. doi: 10.21273/HORTSCI.51.7.856
Chakraborty, S., Jana, B. (2018). Antifreeze proteins: an unusual tale of structural evolution, hydration and function. Proc. Indian Natl. Sci. Acad. 100, 169–187. doi: 10.16943/ptinsa/2018/49553
Chen, D., Mubeen, B., Hasnain, A., Rizwan, M., Adrees, M., Naqvi, S. A. H., et al. (2022). Role of promising secondary metabolites to confer resistance against environmental stresses in crop plants: current scenario and future perspectives. Front. Plant Sci. 13. doi: 10.3389/fpls.2022.881032
Chi, Y. H., Paeng, S. Ki, Kim, M. Ji, Hwang, G. Y., Melencion, S. M., Oh, H. T., et al. (2013). Redox-dependent functional switching of plant proteins accompanying with their structural changes. Front. Plant Sci. 4. doi: 10.3389/fpls.2013.00277
Chinnusamy, V., Ohta, M., Kanrar, S., Lee, B.-h., Hong, X., Agarwal, M., et al. (2003). ICE1: A regulator of cold-induced transcriptome and freezing tolerance in arabidopsis. Genes Dev. 17 (8), 1043–1054. doi: 10.1101/gad.1077503
Cid, F. P., Maruyama, F., Murase, K., Graether, S. P., Larama, G., Bravo, L. A., et al. (2018). Draft genome sequences of bacteria isolated from the deschampsia Antarctica phyllosphere. Extremophiles 22 (3), 537–552. doi: 10.1007/s00792-018-1015-x
Das, K., Roychoudhury, A. (2014). Reactive oxygen species (ROS) and response of antioxidants as ROS-scavengers during environmental stress in plants.” Front. Environ. Sci. 2. doi: 10.3389/fenvs.2014.00053
Davies, P. L., Sykes, B. D. (1997). Antifreeze proteins. Curr. Opin. Struct. Biol. 7 (6), 828–834. doi: 10.1016/S0959-440X(97)80154-6
Ding, Y., Jia, Y., Shi, Y., Zhang, X., Song, C., Gong, Z., et al. (2018). OST1-mediated BTF3L phosphorylation positively regulates CBFs during plant cold responses. EMBO J. 37 (8), e98228. doi: 10.15252/embj.201798228
Ding, X., Zhang, H., Chen, H., Wang, Li, Qian, H., Qi, X. (2015). Extraction, purification and identification of antifreeze proteins from cold acclimated malting barley (Hordeum vulgare L.).” Food Chem. 175, 74–81. doi: 10.1016/j.foodchem.2014.11.027
Dong, H., Wu, C., Luo, C., Wei, M., Qu, S., Wang, S. (2020). Overexpression of mdCPK1a gene, a calcium dependent protein kinase in apple, increase tobacco cold tolerance via scavenging ROS accumulation. PloS One 15 (11), e0242139. doi: 10.1371/journal.pone.0242139
Drerup, M. M., Schlücking, K., Hashimoto, K., Manishankar, P., Steinhorst, L., Kuchitsu, K., et al. (2013). The calcineurin B-like calcium sensors CBL1 and CBL9 together with their interacting protein kinase CIPK26 regulate the arabidopsis NADPH oxidase RBOHF. Mol. Plant 6 (2), 559–569. doi: 10.1093/mp/sst009
Dubouzet, J. G., Sakuma, Y., Ito, Y., Kasuga, M., Dubouzet, E. G., Miura, S., et al. (2003). OsDREB genes in rice, oryza sativa L., encode transcription activators that function in drought-, high-salt- and cold-responsive gene expression. Plant J. 33 (4), 751–763. doi: 10.1046/j.1365-313X.2003.01661.x
Ebrahimi, M., Abdullah, S. N. A., Aziz, M. A., Namasivayam, P. (2016). Oil palm egCBF3 conferred stress tolerance in transgenic tomato plants through modulation of the ethylene signaling pathway. J. Plant Physiol. 202, 107–120. doi: 10.1016/j.jplph.2016.07.001
Elliott, G. D., Wang, S., Fuller, B. J. (2017). Cryoprotectants: A review of the actions and applications of cryoprotective solutes that modulate cell recovery from ultra-low temperatures. Cryobiology 76, 74–91. doi: 10.1016/j.cryobiol.2017.04.004
Eremina, M., Unterholzner, S. J., Rathnayake, A. I., Castellanos, M., Khan, M., Kugler, K. G., et al. (2016). Brassinosteroids participate in the control of basal and acquired freezing tolerance of plants. Proc. Natl. Acad. Sci. 113 (40), E5982–E5991. doi: 10.1073/pnas.1611477113
Eze, M. O. (1991). Phase transitions in phospholipid bilayers: lateral phase separations play vital roles in biomembranes. Biochem. Educ. 19 (4), 204–208. doi: 10.1016/0307-4412(91)90103-F
Fabri, JoãoH. T. M., de Sá, N. P., Malavazi, I., Poeta, M. D. (2020). The dynamics and role of sphingolipids in eukaryotic organisms upon thermal adaptation. Prog. Lipid Res. 80, 101063. doi: 10.1016/j.plipres.2020.101063
Fang, J.-C., Tsai, Y.-C., Chou, W.-L., Liu, H.-Y., Chang, C.-C., Wu, S.-J., et al. (2021). A CCR4-associated factor 1, osCAF1B, confers tolerance of low-temperature stress to rice seedlings. Plant Mol. Biol. 105 (1), 177–192. doi: 10.1007/s11103-020-01079-8
Francko, D. A., Wilson, K. G., Li, Q. Q., Equiza, M. A. (2011). A topical spray to enhance plant resistance to cold injury and mortality. HortTechnology 21 (1), 109–118. doi: 10.21273/HORTTECH.21.1.109
Fürtauer, L., Weiszmann, J., Weckwerth, W., Nägele, T. (2019). Dynamics of plant metabolism during cold acclimation. Int. J. Mol. Sci. 20 (21), 5411. doi: 10.3390/ijms20215411
Gill, S. S., Tuteja, N. (2010). Reactive oxygen species and antioxidant machinery in abiotic stress tolerance in crop plants. Plant Physiol. Biochem. 48 (12), 909–930. doi: 10.1016/j.plaphy.2010.08.016
Ginot, Félix, Lenavetier, Théo, Dedovets, D., Deville, S. (2020). Solute strongly impacts freezing under confinement. Appl. Phys. Lett. 116 (25), 253701. doi: 10.1063/5.0008925
Glenn, D. M., Wisniewski, M., Puterka, G. J., Sekutowski, D. G. (2001). Method for enhanced supercooling of plants to provide frost protection.
Görlach, A., Bertram, K., Hudecova, S., Krizanova, O. (2015). Calcium and ROS: A mutual interplay. Redox Biol. 6, 260–271. doi: 10.1016/j.redox.2015.08.010
Griffith, M., Yaish, M. W.F. (2004). Antifreeze proteins in overwintering plants: A tale of two activities. Trends Plant Sci. 9 (8), 399–405. doi: 10.1016/j.tplants.2004.06.007
Grishkewich, N., Mohammed, N., Tang, J., Tam, K. C. (2017). Recent advances in the application of cellulose nanocrystals. Curr. Opin. Colloid Interface Sci. 29, 32–45. doi: 10.1016/j.cocis.2017.01.005
Guo, Q., Li, X., Niu, Li, Jameson, P. E., Zhou, W. (2021). Transcription-associated metabolomic adjustments in maize occur during combined drought and cold stress. Plant Physiol. 186 (1), 677–695. doi: 10.1093/plphys/kiab050
Guo, X., Li, J., Zhang, Li, Zhang, Z., He, P., Wang, W., et al. (2020). Heterotrimeric G-protein α Subunit (LeGPA1) confers cold stress tolerance to processing tomato plants (Lycopersicon esculentum mill). BMC Plant Biol. 20 (1), 394. doi: 10.1186/s12870-020-02615-w
Guo, X., Liu, D., Chong, K. (2018). Cold signaling in plants: insights into mechanisms and regulation. J. Integr. Plant Biol. 60 (9), 745–756. doi: 10.1111/jipb.12706
Guo, X., Zhang, Li, Dong, G., Xu, Z., Li, G., Liu, N., et al. (2019a). A novel cold-regulated protein isolated from saussurea involucrata confers cold and drought tolerance in transgenic tobacco (Nicotiana tabacum). Plant Sci. 289, 110246. doi: 10.1016/j.plantsci.2019.110246
Guo, X., Zhang, Li, Wang, X., Zhang, M., Xi, Y., Wang, A., et al. (2019b). Overexpression of saussurea involucrata dehydrin gene siDHN promotes cold and drought tolerance in transgenic tomato plants. PloS One 14 (11), e0225090. doi: 10.1371/journal.pone.0225090
Habibi, Y., Lucia, L. A., Rojas, O. J. (2010). Cellulose nanocrystals: chemistry, self-assembly, and applications. Chem. Rev. 110 (6), 3479–3500. doi: 10.1021/cr900339w
He, L. G., Wang, H. L., Liu, D. C., Zhao, Y. J., Xu, M., Zhu, M., et al. (2012). Isolation and expression of a cold-responsive gene ptCBF in poncirus trifoliata and isolation of citrus CBF promoters. Biol. Plantarum 56 (3), 484–492. doi: 10.1007/s10535-012-0059-5
Heidarvand, L., Maali-Amiri, R. (2013). Physio-biochemical and proteome analysis of chickpea in early phases of cold stress. J. Plant Physiol. 170 (5), 459–469. doi: 10.1016/j.jplph.2012.11.021
Hoermiller, I. I., Ruschhaupt, M., Heyer, A. G. (2018). Mechanisms of frost resistance in arabidopsis thaliana. Planta 248 (4), 827–835. doi: 10.1007/s00425-018-2939-1
Hou, C., Tian, W., Kleist, T., He, K., Garcia, V., Bai, F., et al. (2014). DUF221 proteins are a family of osmosensitive calcium-permeable cation channels conserved across eukaryotes. Cell Res. 24 (5), 632–635. doi: 10.1038/cr.2014.14
Hsieh, T.-H., Lee, J.-T., Yang, P.-T., Chiu, L.-H., Charng, Y.-y., Wang, Y.-C., et al. (2002). Heterology expression of the arabidopsis C-repeat/dehydration response element binding factor 1 gene confers elevated tolerance to chilling and oxidative stresses in transgenic tomato. Plant Physiol. 129 (3), 1086–1094. doi: 10.1104/pp.003442
Hu, Y., Jiang, Y., Han, X., Wang, H., Pan, J., Yu, D. (2017). Jasmonate regulates leaf senescence and tolerance to cold stress: crosstalk with other phytohormones. J. Exp. Bot. 68 (6), 1361–1369. doi: 10.1093/jxb/erx004
Hudait, A., Odendahl, N., Qiu, Y., Paesani, F., Molinero, V. (2018). Ice-nucleating and antifreeze proteins recognize ice through a diversity of anchored clathrate and ice-like motifs. J. Am. Chem. Soc. 140 (14), 4905–4912. doi: 10.1021/jacs.8b01246
Hutchison, R. S., Groom, Q., Ort, D. R. (2000). Differential effects of chilling-induced photooxidation on the redox regulation of photosynthetic enzymes. Biochemistry 39 (22), 6679–6688. doi: 10.1021/bi0001978
IbÁñez, C., Kozarewa, I., Johansson, M., Ögren, E., Rohde, A., Eriksson, M. E. (2010). Circadian clock components regulate entry and affect exit of seasonal dormancy as well as winter hardiness in populus trees. Plant Physiol. 153 (4), 1823–1833. doi: 10.1104/pp.110.158220
Iivonen, S., Saranpää, P., Sutinen, M.-L., Vapaavuori, E. (2004). Effects of temperature and nutrient availability on plasma membrane lipid composition in scots pine roots during growth initiation. Tree Physiol. 24 (4), 437–446. doi: 10.1093/treephys/24.4.437
Iqbal, Z., Memon, A. G., Ahmad, A., Iqbal, M. S. (2022). Calcium mediated cold acclimation in plants: underlying signaling and molecular mechanisms. Front. Plant Sci. 13. doi: 10.3389/fpls.2022.855559
Ito, Y., Katsura, K., Maruyama, K., Taji, T., Kobayashi, M., Seki, M., et al. (2006). Functional analysis of rice DREB1/CBF-type transcription factors involved in cold-responsive gene expression in transgenic rice. Plant Cell Physiol. 47 (1), 141–153. doi: 10.1093/pcp/pci230
Ivanov, A. G., Allakhverdiev, S. I., Huner, N. P. A., Murata, N. (2012). Genetic decrease in fatty acid unsaturation of phosphatidylglycerol increased photoinhibition of photosystem I at low temperature in tobacco leaves. Biochim. Biophys. Acta (BBA) - Bioenergetics 1817 (8), 1374–1379. doi: 10.1016/j.bbabio.2012.03.010
Janeczko, A., Dziurka, Michał, Pociecha, E. (2018). Increased leaf tocopherol and β-carotene content is associated with the tolerance of winter wheat cultivars to frost. J. Agron. Crop Sci. 204 (6), 594–602. doi: 10.1111/jac.12287
Jian, H., Xie, L., Wang, Y., Cao, Y., Wan, M., Lv, D., et al. (2020). Characterization of cold stress responses in different rapeseed ecotypes based on metabolomics and transcriptomics analyses. PeerJ 8, e8704. doi: 10.7717/peerj.8704
Jin, R., Kim, B. H., Ji, C. Y., Kim, Ho S., Li, H. M., Ma, D. Fu, et al. (2017). Overexpressing ibCBF3 increases low temperature and drought stress tolerance in transgenic sweetpotato. Plant Physiol. Biochem. 118, 45–54. doi: 10.1016/j.plaphy.2017.06.002
Jin, Ya’nan, Zhai, S., Wang, W., Ding, X., Guo, Z., Bai, L., et al. (2018). Identification of genes from the ICE–CBF–COR pathway under cold stress in aegilops–triticum composite group and the evolution analysis with those from triticeae. Physiol. Mol. Biol. Plants 24 (2), 211–229. doi: 10.1007/s12298-017-0495-y
Jonytiene, V., Burbulis, N., Kuprienė, Ramunė, Blinstrubienė, Aušra (2012). Effect of exogenous proline and de-acclimation treatment on cold tolerance in brassica napus shoots cultured in vitro. J. Food Agric. Environ. 1010, 327–330.
Judy, E., Kishore, N. (2016). Biological wonders of osmolytes: the need to know more. Biochem. Anal. Biochem. 5 (4), 1–5. doi: 10.4172/2161-1009.1000304
Juurakko, C. L., diCenzo, G. C., Walker, V. K. (2021). Cold acclimation and prospects for cold-resilient crops. Plant Stress 2, 100028. doi: 10.1016/j.stress.2021.100028
Kamal-Eldin, A., Appelqvist, Lars-Åke (1996). The chemistry and antioxidant properties of tocopherols and tocotrienols. Lipids 31 (7), 671–701. doi: 10.1007/BF02522884
Karabudak, T., Bor, M., Özdemir, F., Türkan, İ. (2014). Glycine betaine protects tomato (Solanum lycopersicum) plants at low temperature by inducing fatty acid desaturase7 and lipoxygenase gene expression. Mol. Biol. Rep. 41 (3), 1401–1410. doi: 10.1007/s11033-013-2984-6
Kasuga, M., Miura, S., Shinozaki, K., Yamaguchi-Shinozaki, K. (2004). A combination of the arabidopsis DREB1A gene and stress-inducible rd29A promoter improved drought- and low-temperature stress tolerance in tobacco by gene transfer. Plant Cell Physiol. 45 (3), 346–350. doi: 10.1093/pcp/pch037
Kazemi-Shahandashti, S.-S., Maali-Amiri, R. (2018). Global insights of protein responses to cold stress in plants: signaling, defence, and degradation. J. Plant Physiol. 226, 123–135. doi: 10.1016/j.jplph.2018.03.022
Kidokoro, S., Watanabe, K., Ohori, T., Moriwaki, T., Maruyama, K., Mizoi, J., et al. (2015). Soybean DREB1/CBF-type transcription factors function in heat and drought as well as cold stress-responsive gene expression. Plant J. 81 (3), 505–518. doi: 10.1111/tpj.12746
Kingston-Smith, A. H., Harbinson, J., Williams, J., Foyer, C. H. (1997). Effect of chilling on carbon assimilation, enzyme activation, and photosynthetic electron transport in the absence of photoinhibition in maize leaves. Plant Physiol. 114 (3), 1039–1046. doi: 10.1104/pp.114.3.1039
Knight, M. R., Knight, H. (2012). Low-temperature perception leading to gene expression and cold tolerance in higher plants. New Phytol. 195 (4), 737–751. doi: 10.1111/j.1469-8137.2012.04239.x
Kong, C. H. Z., Hamid, N., Liu, T., Sarojini, V. (2016). Effect of antifreeze peptide pretreatment on ice crystal size, drip loss, texture, and volatile compounds of frozen carrots. J. Agric. Food Chem. 64 (21), 4327–4335. doi: 10.1021/acs.jafc.6b00046
Kong, C. H.Z., Hamid, N., Ma, Q., Lu, J., Wang, B.-G., Sarojini, V. (2017). Antifreeze peptide pretreatment minimizes freeze-thaw damage to cherries: an in-depth investigation. LWT 84, 441–448. doi: 10.1016/j.lwt.2017.06.002
Kudla, Jörg, Becker, D., Grill, E., Hedrich, R., Hippler, M., Kummer, U., et al. (2018). Advances and current challenges in calcium signaling. New Phytol. 218 (2), 414–431. doi: 10.1111/nph.14966
Kurepin, L. V., Ivanov, A. G., Zaman, M., Pharis, R. P., Allakhverdiev, S. I., Hurry, V., et al. (2015). Stress-related hormones and glycinebetaine interplay in protection of photosynthesis under abiotic stress conditions. Photosynthesis Res. 126 (2), 221–235. doi: 10.1007/s11120-015-0125-x
Lamers, J., Meer, T. v. d., Testerink, C. (2020). How plants sense and respond to stressful environments1. Plant Physiol. 182 (4), 1624–1635. doi: 10.1104/pp.19.01464
Larcher, W. (2003). Physiological plant ecology: ecophysiology and stress physiology of functional groups (Berlin, Germany: Springer Science & Business Media).
Latowski, D., Kuczyńska, P., Strzałka, K. (2011). Xanthophyll cycle – a mechanism protecting plants against oxidative stress. Redox Rep. 16 (2), 78–90. doi: 10.1179/174329211X13020951739938
Lee, J.-H., Kwon, M. C., Jung, E. S., Lee, C. H., Oh, M.-M. (2019). Physiological and metabolomic responses of kale to combined chilling and UV-A treatment. Int. J. Mol. Sci. 20 (19), 4950. doi: 10.3390/ijms20194950
Leegood, R. C., Furbank, R. T. (1986). Stimulation of photosynthesis by 2% Oxygen at low temperatures is restored by phosphate. Planta 168 (1), 84–93. doi: 10.1007/BF00407013
Lemoine, R., Camera, S. La, Atanassova, R., Dédaldéchamp, F., Allario, T., Pourtau, N., et al. (2013). Source-to-sink transport of sugar and regulation by environmental factors. Front. Plant Sci. 4. doi: 10.3389/fpls.2013.00272
Li, W., Li, M., Zhang, W., Welti, R., Wang, X. (2004). The plasma membrane–bound phospholipase Dδ Enhances freezing tolerance in arabidopsis thaliana. Nat. Biotechnol. 22 (4), 427–433. doi: 10.1038/nbt949
Li, H., Ye, K., Shi, Y., Cheng, J., Zhang, X., Yang, S. (2017). BZR1 positively regulates freezing tolerance via CBF-dependent and CBF-independent pathways in arabidopsis. Mol. Plant 10 (4), 545–559. doi: 10.1016/j.molp.2017.01.004
Lindow, S. E., Arny, D. C., Upper, C. D. (1982). Bacterial ice nucleation: A factor in frost injury to plants. Plant Physiol. 70 (4), 1084–1089. doi: 10.1104/pp.70.4.1084
Liu, X., Fu, L., Qin, P., Sun, Y., Liu, J., Wang, X. (2019). Overexpression of the wheat trehalose 6-phosphate synthase 11 gene enhances cold tolerance in arabidopsis thaliana. Gene 710, 210–217. doi: 10.1016/j.gene.2019.06.006
Liu, J., Islam, Md T., Sapkota, S., Ravindran, P., Kumar, P. P., Artlip, T. S., et al. (2021a). Ethylene-mediated modulation of bud phenology, cold hardiness, and hormone biosynthesis in peach (Prunus persica). Plants 10 (7), 1266. doi: 10.3390/plants10071266
Liu, J., Islam, Md T., Sapkota, S., Ravindran, P., Kumar, P. P., Artlip, T. S., et al. (2021b). Ethylene-mediated modulation of bud phenology, cold hardiness, and hormone biosynthesis in peach (Prunus persica). Plants 10 (7), 1266. doi: 10.3390/plants10071266
Liu, Q., Piao, S., Janssens, I. A., Fu, Y., Peng, S., Lian, Xu, et al. (2018). Extension of the growing season increases vegetation exposure to frost. Nat. Commun. 9 (1), 426. doi: 10.1038/s41467-017-02690-y
Liu, J., Sherif, S. M. (2019). Combating spring frost with ethylene. Front. Plant Sci. 10. doi: 10.3389/fpls.2019.01408
Londo, J. P., Kovaleski, A. P. (2019). Deconstructing cold hardiness: variation in supercooling ability and chilling requirements in the wild grapevine vitis riparia. Aust. J. Grape Wine Res. 25 (3), 276–285. doi: 10.1111/ajgw.12389
Lu, M., Wang, B., Li, Zh., Fei, Y., Wei, L., Gao, Sh. (2002). Differential scanning calorimetric and circular dichroistic studies on plant antifreeze proteins. J. Thermal Anal. Calorimetry 67 (3), 689–698. doi: 10.1023/A:1014369208229
Lukas, M., Schwidetzky, R., Eufemio, R. J., Bonn, M., Meister, K. (2022). Toward understanding bacterial ice nucleation. J. Phys. Chem. B 126 (9), 1861–1867. doi: 10.1021/acs.jpcb.1c09342
Lunn, J. E., Delorge, I., Figueroa, C. María, Dijck, P. V., Stitt, M. (2014). Trehalose metabolism in plants. Plant J. 79 (4), 544–567. doi: 10.1111/tpj.12509
Ma, Y., Dai, X., Xu, Y., Luo, W., Zheng, X., Zeng, D., et al. (2015). COLD1 confers chilling tolerance in rice. Cell 160 (6), 1209–1221. doi: 10.1016/j.cell.2015.01.046
Ma, Q., Huang, J.-G., Hänninen, H., Berninger, F. (2019). Divergent trends in the risk of spring frost damage to trees in europe with recent warming. Global Change Biol. 25 (1), 351–360. doi: 10.1111/gcb.14479
Marcec, M. J., Gilroy, S., Poovaiah, B. W., Tanaka, K. (2019). Mutual interplay of ca2+ and ROS signaling in plant immune response. Plant Sci. 283, 343–354. doi: 10.1016/j.plantsci.2019.03.004
Meena, M., Divyanshu, K., Kumar, S., Swapnil, P., Zehra, A., Shukla, V., et al. (2019). Regulation of L-proline biosynthesis, signal transduction, transport, accumulation and its vital role in plants during variable environmental conditions. Heliyon 5 (12), e02952. doi: 10.1016/j.heliyon.2019.e02952
Meyer, K., Keil, M., Naldrett, M. J. (1999). A leucine-rich repeat protein of carrot that exhibits antifreeze activity. FEBS Lett. 447 (2), 171–178. doi: 10.1016/S0014-5793(99)00280-X
Miller, G., Suzuki, N., Ciftci-Yilmaz, S., Mittler, R. (2010). Reactive oxygen species homeostasis and signalling during drought and salinity stresses. Plant Cell Environ. 33 (4), 453–467. doi: 10.1111/j.1365-3040.2009.02041.x
Mishra, B. S., Sharma, M., Laxmi, A. (2022). Role of sugar and auxin crosstalk in plant growth and development. Physiologia Plantarum 174 (1), e13546. doi: 10.1111/ppl.13546
Mittler, R. (2002). Oxidative stress, antioxidants and stress tolerance. Trends Plant Sci. 7 (9), 405–410. doi: 10.1016/S1360-1385(02)02312-9
Mittler, R. (2017). ROS are good. Trends Plant Sci. 22 (1), 11–19. doi: 10.1016/j.tplants.2016.08.002
Mittler, R., Zandalinas, S. I., Fichman, Y., Breusegem, F. V. (2022). Reactive oxygen species signalling in plant stress responses. Nat. Rev. Mol. Cell Biol. 23 (10), 663–679. doi: 10.1038/s41580-022-00499-2
Miura, K., Furumoto, T. (2013). Cold signaling and cold response in plants. Int. J. Mol. Sci. 14 (3), 5312–5337. doi: 10.3390/ijms14035312
Muthukumaran, J., Manivel, P., Kannan, M., Jeyakanthan, J., Krishna, R. (2011). A framework for classification of antifreeze proteins in over wintering plants based on their sequence and structural features. J. Bioinform. Seq. Anal. 3, 70–88. doi: 10.5897/JBSA11.003
Nair, P., Kandasamy, S., Zhang, J., Ji, X., Kirby, C., Benkel, B., et al. (2012). Transcriptional and metabolomic analysis of ascophyllum nodosum mediated freezing tolerance in arabidopsis thaliana. BMC Genomics 13 (1), 643. doi: 10.1186/1471-2164-13-643
Örvar, BjörnLárus, Sangwan, V., Omann, F., Dhindsa, R. S. (2000). Early steps in cold sensing by plant cells: the role of actin cytoskeleton and membrane fluidity. Plant J. 23 (6), 785–794. doi: 10.1046/j.1365-313x.2000.00845.x
Pandey, R., Usui, K., Livingstone, R. A., Fischer, S. A., Pfaendtner, J., Backus, E. H.G., et al. (2016). Ice-nucleating bacteria control the order and dynamics of interfacial water. Sci. Adv. 2 (4), e1501630. doi: 10.1126/sciadv.1501630
Pearce, R. (2001). Plant freezing and damage. Ann. Bot. 87 (4), 417–424. doi: 10.1006/anbo.2000.1352
Perry, K. B. (1998). Basics of frost and freeze protection for horticultural crops. HortTechnology 8 (1), 10–15. doi: 10.21273/HORTTECH.8.1.10
Pino, María-Teresa, Skinner, J. S., Park, E.-J., Jeknić, Z., Hayes, P. M., Thomashow, M. F., et al. (2007). Use of a stress inducible promoter to drive ectopic atCBF expression improves potato freezing tolerance while minimizing negative effects on tuber yield. Plant Biotechnol. J. 5 (5), 591–604. doi: 10.1111/j.1467-7652.2007.00269.x
Polashock, J. J., Arora, R., Peng, Y., Naik, D., Rowland, L. J. (2010). Functional identification of a C-repeat binding factor transcriptional activator from blueberry associated with cold acclimation and freezing tolerance. J. Am. Soc. Hortic. Sci. 135 (1), 40–48. doi: 10.21273/JASHS.135.1.40
Prasad, T. K., Anderson, M. D., Stewart, C. R. (1994). Acclimation, hydrogen peroxide, and abscisic acid protect mitochondria against irreversible chilling injury in maize seedlings. Plant Physiol. 105 (2), 619–627. doi: 10.1104/pp.105.2.619
Qin, F., Sakuma, Y., Li, J., Liu, Q., Li, Y.-Q., Shinozaki, K., et al. (2004). Cloning and functional analysis of a novel DREB1/CBF transcription factor involved in cold-responsive gene expression in zea mays L. Plant Cell Physiol. 45 (8), 1042–1052. doi: 10.1093/pcp/pch118
Rajendrakumar, C. S. V., Reddy, B. V. B., Reddy, A. R. (1994). Proline-protein interactions: protection of structural and functional integrity of M4 lactate dehydrogenase. Biochem. Biophys. Res. Commun. 201 (2), 957–963. doi: 10.1006/bbrc.1994.1795
Rayirath, P., Benkel, B., Hodges, D.M., Allan-Wojtas, P., MacKinnon, S., Critchley, A. T., et al. (2009). Lipophilic components of the brown seaweed, ascophyllum nodosum, enhance freezing tolerance in arabidopsis thaliana. Planta 230 (1), 135–147. doi: 10.1007/s00425-009-0920-8
Rinehart, J. P., Li, A., Yocum, G. D., Robich, R. M., Hayward, S. A.L., Denlinger, D. L. (2007). Up-regulation of heat shock proteins is essential for cold survival during insect diapause. Proc. Natl. Acad. Sci. 104 (27), 11130–11137. doi: 10.1073/pnas.0703538104
Roeters, S. J., Golbek, T. W., Bregnhøj, M., Drace, T., Alamdari, S., Roseboom, W., et al. (2021). Ice-nucleating proteins are activated by low temperatures to control the structure of interfacial water. Nat. Commun. 12 (1), 1183. doi: 10.1038/s41467-021-21349-3
Román-Figueroa, Celián, Bravo, León, Paneque, M., Navia, R., Cea, M. (2021). Chemical products for crop protection against freezing stress: A review. J. Agron. Crop Sci. 207 (3), 391–403. doi: 10.1111/jac.12489
Roychoudhury, A., Banerjee, A. (2016). Endogenous glycine betaine accumulation mediates abiotic stress tolerance in plants. Trop. Plant Res. 3 (1), 105–111.
Sadiq, M., Akram, N. A., Ashraf, M., Al-Qurainy, F., Ahmad, P. (2019). Alpha-tocopherol-induced regulation of growth and metabolism in plants under non-stress and stress conditions. J. Plant Growth Regul. 38 (4), 1325–1340. doi: 10.1007/s00344-019-09936-7
Saha, J., Brauer, E. K., Sengupta, A., Popescu, S. C., Gupta, K., Gupta, B. (2015). Polyamines as redox homeostasis regulators during salt stress in plants. Front. Environ. Sci. 3. doi: 10.3389/fenvs.2015.00021
Sami, F., Yusuf, M., Faizan, M., Faraz, A., Hayat, S. (2016). Role of sugars under abiotic stress. Plant Physiol. Biochem. 109, 54–61. doi: 10.1016/j.plaphy.2016.09.005
Sarkar, A. K., Sadhukhan, S. (2022). Imperative role of trehalose metabolism and trehalose-6-phosphate signaling on salt stress responses in plants. Physiologia Plantarum 174 (1), e13647. doi: 10.1111/ppl.13647
Sassenrath, G. F., Ort, D. R., Portis, A. R. (1990). Impaired reductive activation of stromal bisphosphatases in tomato leaves following low-temperature exposure at high light. Arch. Biochem. Biophysics 282 (2), 302–308. doi: 10.1016/0003-9861(90)90121-e
Satyakam, G. Z., Singh, R. K., Kumar, R. (2022). Cold adaptation strategies in plants—An emerging role of epigenetics and antifreeze proteins to engineer cold resilient plants. Front. Genet. 13. doi: 10.3389/fgene.2022.909007
Savitch, L. V., Allard, G., Seki, M., Robert, L. S., Tinker, N. A., Huner, N. P.A., et al. (2005). The effect of overexpression of two brassica CBF/DREB1-like transcription factors on photosynthetic capacity and freezing tolerance in brassica napus. Plant Cell Physiol. 46 (9), 1525–1539. doi: 10.1093/pcp/pci165
Semida, W. M., Abd, E.-M. T. A., Howladar, S. M., Rady, M. M. (2016). Foliar-applied alpha-tocopherol enhances salt-tolerance in onion plants by improving antioxidant defence system. Aust. J. Crop Sci. 10 (7), 1030–1039. doi: 10.3316/informit.327621977858527
Sharkey, T. D., Stitt, M., Heineke, D., Gerhardt, R., Raschke, K., Heldt, H. W. (1986). Limitation of photosynthesis by carbon metabolism 1: II. O2-insensitive CO2 uptake results from limitation of triose phosphate utilization. Plant Physiol. 81 (4), 1123–1129. doi: 10.1104/pp.81.4.1123
Sharma, P., Jha, A. B., Dubey, R. S., Pessarakli, M. (2021). “Reactive oxygen species generation, hazards, and defense mechanisms in plants under environmental (Abiotic and biotic) stress conditions,” in Handbook of plant and crop physiology (Boca Raton, FL, USA: CRC Press) (2021). pp. 509–548.
Sherif, S. M. (2023) Tree fruit horticulture updates. Available at: https://blogs.ext.vt.edu/tree-fruit-horticulture/.
Shi, H., He, X., Zhao, Y., Lu, S., Guo, Z. (2020). Constitutive expression of a group 3 LEA protein from medicago falcata (MfLEA3) increases cold and drought tolerance in transgenic tobacco. Plant Cell Rep. 39 (7), 851–860. doi: 10.1007/s00299-020-02534-y
Shukla, P. S., Mantin, E. G., Adil, M., Bajpai, S., Critchley, A. T., Prithiviraj, B. (2019). Ascophyllum nodosum-based biostimulants: sustainable applications in agriculture for the stimulation of plant growth, stress tolerance, and disease management. Front. Plant Sci. 10. doi: 10.3389/fpls.2019.00655
Siddique, A., Kandpal, G., Kumar, P. (2018). Proline accumulation and its defensive role under diverse stress condition in plants: an overview. J. Pure Appl. Microbiol. 12 (3), 1655–1659. doi: 10.22207/JPAM.12.3.73
Sies, H., Jones, D. P. (2020). Reactive oxygen species (ROS) as pleiotropic physiological signalling agents. Nat. Rev. Mol. Cell Biol. 21 (7), 363–383. doi: 10.1038/s41580-020-0230-3
Simkin, A. J., López-Calcagno, P. E., Raines, C. A. (2019). Feeding the world: improving photosynthetic efficiency for sustainable crop production. J. Exp. Bot. 70 (4), 1119–1140. doi: 10.1093/jxb/ery445
Slade, D., Radman, M. (2011). Oxidative stress resistance in deinococcus radiodurans. Microbiol. Mol. Biol. Reviews: MMBR 75 (1), 133–191. doi: 10.1128/MMBR.00015-10
Smirnoff, N., Arnaud, D. (2019). Hydrogen peroxide metabolism and functions in plants. New Phytol. 221 (3), 1197–1214. doi: 10.1111/nph.15488
Snyder, R. L., de Melo-Abreu, J.P. (2005). Frost protection: fundamentals, practice and economics. Food Agric. Organ. United Nations 1, 1–240.
Sreedharan, S., Shekhawat, U. K.S., Ganapathi, T. R. (2013). Transgenic banana plants overexpressing a native plasma membrane aquaporin musaPIP1;2 display high tolerance levels to different abiotic stresses. Plant Biotechnol. J. 11 (8), 942–952. doi: 10.1111/pbi.12086
Sun, S., Fang, J., Lin, M., Hu, C., Qi, X., Chen, J., et al. (2021). Comparative metabolomic and transcriptomic studies reveal key metabolism pathways contributing to freezing tolerance under cold stress in kiwifruit. Front. Plant Sci. 12. doi: 10.3389/fpls.2021.628969
Suo, J., Zhao, Qi, David, L., Chen, S., Dai, S. (2017). Salinity response in chloroplasts: insights from gene characterization. Int. J. Mol. Sci. 18 (5), 1011. doi: 10.3390/ijms18051011
Suzuki, N., Mittler, R. (2006). Reactive oxygen species and temperature stresses: A delicate balance between signaling and destruction. Physiologia Plantarum 126 (1), 45–51. doi: 10.1111/j.0031-9317.2005.00582.x
Takuhara, Y., Kobayashi, M., Suzuki, S. (2011). Low-temperature-induced transcription factors in grapevine enhance cold tolerance in transgenic arabidopsis plants. J. Plant Physiol. 168 (9), 967–975. doi: 10.1016/j.jplph.2010.11.008
Tarkowski, ŁukaszP., Van den Ende, W. (2015). Cold tolerance triggered by soluble sugars: A multifaceted countermeasure. Front. Plant Sci. 6. doi: 10.3389/fpls.2015.00203
Trache, D., Hussin, M.H., Mohamad Haafiz, M. K., Thakur, V. K. (2017). Recent progress in cellulose nanocrystals: sources and production. Nanoscale 9 (5), 1763–1786. doi: 10.1039/C6NR09494E
Tsukagoshi, H., Busch, W., Benfey, P. N. (2010). Transcriptional regulation of ROS controls transition from proliferation to differentiation in the root. Cell 143 (4), 606–616. doi: 10.1016/j.cell.2010.10.020
Uemura, M., Joseph, R. A., Steponkus, P. L. (1995). Cold acclimation of arabidopsis thaliana (Effect on plasma membrane lipid composition and freeze-induced lesions). Plant Physiol. 109 (1), 15–30. doi: 10.1104/pp.109.1.15
Unterberger, C., Brunner, L., Nabernegg, S., Steininger, K. W., Steiner, A. K., Stabentheiner, E., et al. (2018). Spring frost risk for regional apple production under a warmer climate. PloS One 13 (7), e0200201. doi: 10.1371/journal.pone.0200201
Urbańczyk, Małgorzata, Góra, J., Latajka, Rafał, Sewald, N. (2017). Antifreeze glycopeptides: from structure and activity studies to current approaches in chemical synthesis. Amino Acids 49 (2), 209–222. doi: 10.1007/s00726-016-2368-z
Van Rensburg, L., Krüger, G. H. J., Krüger, H. (1993). Proline accumulation as drought-tolerance selection criterion: its relationship to membrane integrity and chloroplast ultrastructure in nicotiana tabacum L. J. Plant Physiol. 141 (2), 188–194. doi: 10.1016/S0176-1617(11)80758-3
Verbruggen, N., Hermans, C. (2008). Proline accumulation in plants: A review. Amino Acids 35 (4), 753–759. doi: 10.1007/s00726-008-0061-6
Vyse, K., Faivre, Léa, Romich, M., Pagter, M., Schubert, D., Hincha, D. K., et al. (2020). Transcriptional and post-transcriptional regulation and transcriptional memory of chromatin regulators in response to low temperature. Front. Plant Sci. 11. doi: 10.3389/fpls.2020.00039
Wang, B., Chai, H., Zhong, Y., Shen, Y., Yang, W., Chen, J., et al. (2020). The DEAD-box RNA helicase SHI2 functions in repression of salt-inducible genes and regulation of cold-inducible gene splicing. J. Exp. Bot. 71 (4), 1598–1613. doi: 10.1093/jxb/erz523
Wang, L., Wang, Sa, Tong, R., Wang, S., Yao, J., Jiao, J., et al. (2022). Overexpression of pgCBF3 and pgCBF7 transcription factors from pomegranate enhances freezing tolerance in arabidopsis under the promoter activity positively regulated by pgICE1. Int. J. Mol. Sci. 23 (16), 9439. doi: 10.3390/ijms23169439
Wang, M., Zheng, Q., Shen, Q., Guo, S. (2013). The critical role of potassium in plant stress response. Int. J. Mol. Sci. 14 (4), 7370–7390. doi: 10.3390/ijms14047370
Wei, X., Liu, S., Sun, C., Xie, G., Wang, L. (2021). Convergence and divergence: signal perception and transduction mechanisms of cold stress in arabidopsis and rice. Plants 10 (9), 1864. doi: 10.3390/plants10091864
Weng, L., Chen, C., Zuo, J., Li, W. (2011). Molecular dynamics study of effects of temperature and concentration on hydrogen-bond abilities of ethylene glycol and glycerol: implications for cryopreservation. J. Phys. Chem. A 115 (18), 4729–4737. doi: 10.1021/jp111162w
Wisniewski, M., Fuller, M. (1999). “Ice nucleation and deep supercooling in plants: new insights using infrared thermography,” in Cold-Adapted Organisms: Ecology, Physiology, Enzymology and Molecular biology. Ed. Margesin and F. Schinner. Berlin, R. (Heidelberg: Springer), 105–118.
Wisniewski, M., Fuller, M. I. C. K., Palta, J., Carter, J., Arora, R. (2004). Ice nucleation, propagation, and deep supercooling in woody plants. J. Crop Improvement 10 (1–2), 5–16. doi: 10.1300/J411v10n01_02
Wisniewski, M., Nassuth, A., Arora, R. (2018). Cold hardiness in trees: A mini-review. Front. Plant Sci. 9. doi: 10.3389/fpls.2018.01394
Wisniewski, M., Norelli, J., Bassett, C., Artlip, T., Macarisin, D. (2011). Ectopic expression of a novel peach (Prunus persica) CBF transcription factor in apple (Malus × Domestica) results in short-day induced dormancy and increased cold hardiness. Planta 233 (5), 971–983. doi: 10.1007/s00425-011-1358-3
Wisniewski, M., Willick, I. R., Duman, J. G., Livingston, D., Newton, S. S. (2020). “Plant Antifreeze Proteins,” in Antifreeze Proteins Volume 1: Environment, Systematics and Evolution. Eds. Ramløv, H., Friis, D. S. (Cham: Springer International Publishing), 189–226.
Xie, H.-T., Wan, Z.-Y., Li, S., Zhang, Y. (2014). Spatiotemporal production of reactive oxygen species by NADPH oxidase is critical for tapetal programmed cell death and pollen development in arabidopsis. Plant Cell 26 (5), 2007–2023. doi: 10.1105/tpc.114.125427
Xu, Yi, Hu, W., Liu, J., Song, S., Hou, X., Jia, C., et al. (2020). An aquaporin gene maPIP2-7 is involved in tolerance to drought, cold and salt stresses in transgenic banana (Musa acuminata L.). Plant Physiol. Biochem. 147, 66–76. doi: 10.1016/j.plaphy.2019.12.011
Yang, T., Ali, G. S., Yang, L., Du, L., Reddy, A. S. N., Poovaiah, B. W. (2010). Calcium/calmodulin-regulated receptor-like kinase CRLK1 interacts with MEKK1 in plants. Plant Signaling Behav. 5 (8), 991–994. doi: 10.4161/psb.5.8.12225
Yang, X., Wang, R., Jing, H., Chen, Q., Bao, X., Zhao, J., et al. (2020). Three novel C-repeat binding factor genes of dimocarpus longan regulate cold stress response in arabidopsis. Front. Plant Sci. 11. doi: 10.3389/fpls.2020.01026
Yu, T.-F., Xu, Z.-S., Guo, J.-K., Wang, Y.-X., Abernathy, B., Fu, J.-D., et al. (2017). Improved drought tolerance in wheat plants overexpressing a synthetic bacterial cold shock protein gene seCspA. Sci. Rep. 7 (1), 44050. doi: 10.1038/srep44050
Yu, H., Zheng, H., Liu, Y., Yang, Q., Li, W., Zhang, Y., et al. (2021). Antifreeze protein from ammopiptanthus nanus functions in temperature-stress through domain A. Sci. Rep. 11 (1), 8458. doi: 10.1038/s41598-021-88021-0
Zamani-Babgohari, M., Critchley, A. T., Norrie, J., Prithiviraj, B. (2019). Increased freezing stress tolerance of nicotiana tabacum L. Cv. Bright yellow-2 cell cultures with the medium addition of ascophyllum nodosum (L.) le jolis extract. In Vitro Cell. Dev. Biol. - Plant 55 (3), 321–333. doi: 10.1007/s11627-019-09972-8
Zhang, Qi, Chen, Q., Wang, S., Hong, Y., Wang, Z. (2014). Rice and cold stress: methods for its evaluation and summary of cold tolerance-related quantitative trait loci. Rice 7 (1), 24. doi: 10.1186/s12284-014-0024-3
Zhang, S., Gottschalk, C., Nocker, S. v. (2019). Genetic mechanisms in the repression of flowering by gibberellins in apple (Malus x domestica borkh.). BMC Genomics 20 (1), 747. doi: 10.1186/s12864-019-6090-6
Zhang, Li, Guo, X., Zhang, Z., Wang, A., Zhu, J. (2021). Cold-regulated gene leCOR413PM2 confers cold stress tolerance in tomato plants.” Gene 764, 145097. doi: 10.1016/j.gene.2020.145097
Zhang, Z., Li, J., Li, F., Liu, H., Yang, W., Chong, K., et al. (2017). OsMAPK3 phosphorylates osbHLH002/osICE1 and inhibits its ubiquitination to activate osTPP1 and enhances rice chilling tolerance. Dev. Cell 43 (6), 731–743.e5. doi: 10.1016/j.devcel.2017.11.016
Zhang, L.-L., Zhao, M.-G., Tian, Q.-Y., Zhang, W.-H. (2011). Comparative studies on tolerance of medicago truncatula and medicago falcata to freezing. Planta 234 (3), 445–457. doi: 10.1007/s00425-011-1416-x
Zhao, C., Wang, P., Si, T., Hsu, C.-C., Wang, Lu, Zayed, O., et al. (2017). MAP kinase cascades regulate the cold response by modulating ICE1 protein stability. Dev. Cell 43 (5), 18–629.e5. doi: 10.1016/j.devcel.2017.09.024
Zhao, C., Zhang, Z., Xie, S., Si, T., Li, Y., Zhu, J.-K. (2016). Mutational evidence for the critical role of CBF transcription factors in cold acclimation in arabidopsis. Plant Physiol. 171 (4), 2744–2759. doi: 10.1104/pp.16.00533
Zhou, A., Liu, E., Li, He, Li, Y., Feng, S., Gong, S., et al. (2018). PsCor413pm2, a plasma membrane-localized, cold-regulated protein from phlox subulata, confers low temperature tolerance in arabidopsis. Int. J. Mol. Sci. 19 (9), 2579. doi: 10.3390/ijms19092579
Zhu, J.-K. (2016). Abiotic stress signaling and responses in plants. Cell 167 (2), 313–324. doi: 10.1016/j.cell.2016.08.029
Keywords: cryoprotectants, low temperature stress, ROS, AFPs, transmembrane proteins, antioxidants, ice crystals, cell dehydration
Citation: Jahed KR, Saini AK and Sherif SM (2023) Coping with the cold: unveiling cryoprotectants, molecular signaling pathways, and strategies for cold stress resilience. Front. Plant Sci. 14:1246093. doi: 10.3389/fpls.2023.1246093
Received: 24 June 2023; Accepted: 31 July 2023;
Published: 15 August 2023.
Edited by:
Shifeng Cao, Zhejiang Wanli University, ChinaReviewed by:
Klára Kosová, Crop Research Institute (CRI), CzechiaJiban Shrestha, Nepal Agricultural Research Council, Nepal
Copyright © 2023 Jahed, Saini and Sherif. This is an open-access article distributed under the terms of the Creative Commons Attribution License (CC BY). The use, distribution or reproduction in other forums is permitted, provided the original author(s) and the copyright owner(s) are credited and that the original publication in this journal is cited, in accordance with accepted academic practice. No use, distribution or reproduction is permitted which does not comply with these terms.
*Correspondence: Sherif M. Sherif, c3NoZXJpZkB2dC5lZHU=