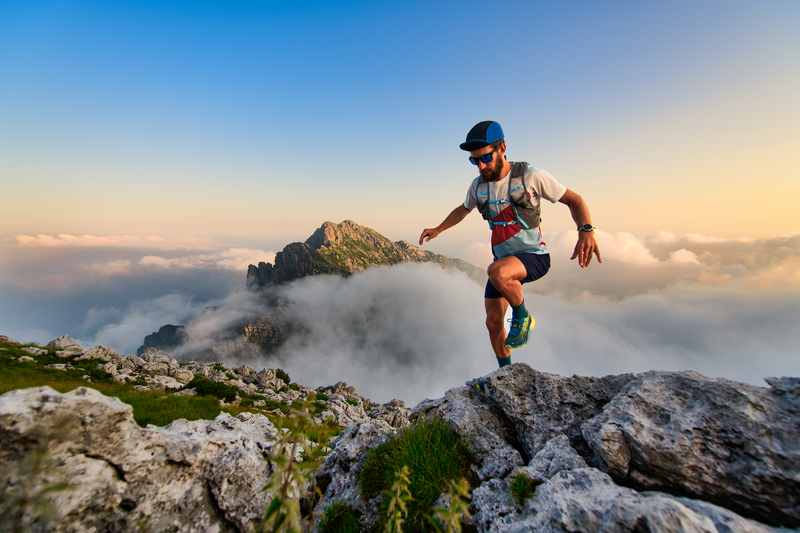
94% of researchers rate our articles as excellent or good
Learn more about the work of our research integrity team to safeguard the quality of each article we publish.
Find out more
ORIGINAL RESEARCH article
Front. Plant Sci. , 18 January 2024
Sec. Plant Abiotic Stress
Volume 14 - 2023 | https://doi.org/10.3389/fpls.2023.1244555
Resources in water-limited ecosystems are highly variable and unpredictable, and the maintenance of functional diversity among coexisting species is a crucial ecological strategy through which plants mitigate environmental stress. The comparison of differential eco-physiological responses among co-occurring plants in harsh environments could help provide deep insights into the coexistence mechanisms of competing species. Two coexisting desert shrubs with different photosynthetic pathways (Haloxylon ammodendron and Tamarix ramosissima) were selected in the Gurbantunggut Desert located in northwest China. This study detected variations in the water sources, photosynthetic parameters, stem water status, and non-structural carbohydrates of the two shrubs at three sites with different groundwater table depths during the growing seasons of 2015 and 2016 to identify distinct eco-physiological performances in coexisting plants with different functional types under fluctuating water conditions. The water sources of H. ammodendron shifted from soil water to groundwater, while T. ramosissima extracted water mainly from deep soil layers at both sites. Significant reductions in carbon assimilation and stomatal conductance in H. ammodendron with deeper groundwater table depth were detected during most drought periods, but no significant decreases in transpiration rate were detected with declining groundwater table depth. For T. ramosissima, all of these gas exchange parameters decreased with the progression of summer drought, and their relative reduction rates were larger compared with those of H. ammodendron. The stem water status of H. ammodendron deteriorated, and the relative reduction rates of water potential increased with deeper groundwater, whereas those of T. ramosissima did not differ with greater groundwater depth. These findings indicated that prolonged drought would intensify the impact of declining groundwater depth on the eco-physiology of both shrubs, but the extent to which the shrubs would respond differed. The two shrubs were segregated along the water–carbon balance continuum: the C3 shrub T. ramosissima maximized its carbon fixation at an enormous cost of water, while greater carbon fixation was achieved with far greater water economy for H. ammodendron. These results demonstrated that the two shrubs prioritized carbon gain and water loss differently when faced with limited water sources. These mechanisms might mitigate competitive stress and enable their coexistence.
Water source availability is the most determining factor in plant survival and ecosystem functioning in arid ecosystems. As a main water input, precipitation is typically insufficient, unpredictable, and inconsistent in arid environments (Schwinning and Ehleringer, 2001), causing the soil moisture to fluctuate with precipitation. As a result, desert plants heavily rely on groundwater as one of the few reliable sources of water (Wu et al., 2019). However, the groundwater resources of arid regions face critical threats such as overexploitation and contamination due to climate change and anthropogenic activities (Panneerselvam et al., 2022). General circulation models have predicted the unprecedented duration, intensity, and seasonality of future droughts, which may alter the precipitation patterns of arid areas (Huang et al., 2016; Bhusal et al., 2020). This trend can significantly affect water resource accessibility, which may alter the composition and function of arid ecosystems (Hoover et al., 2014; Grossiord et al., 2017). Obtaining a deeper understanding of desert ecosystem responses to varying water conditions will be essential to ecosystem protection and the assessment of ecosystem adaptation strategies under future climate change scenarios.
In drylands, diversity in functional types is essential for the coexistence of plants, through which plants can use limited resources efficiently, as well as differently in space and time to cope with periodic and chronic drought events (Chesson et al., 2004; Araya et al., 2011; Pardos et al., 2021). Differential mechanisms adopted by coexisting plants have been examined extensively to explain how competing species in water-limited environments achieve stable coexistence (Angert et al., 2009; Adler et al., 2013; Bermúdez and Retuerto, 2014). Resource acquisition traits (root functioning) are among the most important mechanisms that can help desert plants partition their water sources spatially and temporally to alleviate competition stress for limited water resources (Filella and Penuelas, 2003; Eggemeyer et al., 2008; Ellsworth and Sternberg, 2015). Plant coexistence mechanisms are also closely related to the resource use strategies (leaf and shoot function and traits) of different plants (Moreno-Gutierrez et al., 2012). The species-specific adaptations of different plants to a certain environment are modulated by their own evolutionary trajectories; water-use strategies and trade-offs are, consequently, inherent to different species (Filella and Penuelas, 2003). Therefore, the knowledge of variations in eco-physiological responses and the water–carbon trade-offs of coexisting plants help to reveal the differential eco-physiological acclimation and coexistence mechanisms of competing species.
C3 and C4 plants are two differential functional types that respond differently to environmental stress due to contrasting water–carbon balance. C4 photosynthesis refers to various modifications in anatomy, biochemistry, and physiology that strategically concentrate CO2 in the bundle sheath, which leads to the saturation of Rubisco at ambient CO2 concentrations (Sage and Pearcy, 1987; Taylor et al., 2011; Osborne and Sack, 2012; Taylor et al., 2014). C4 plants are able to increase their maximum net photosynthesis rates with less stomatal conductance compared to C3 species by almost eliminating photorespiration. As a result, they can reduce transpiration and conserve water, which is particularly beneficial in hot conditions with high evaporative demand (Pearcy and Ehleringer, 1984; Osborne and Sack, 2012; Taylor et al., 2014). Therefore, C4 photosynthetic traits and resultant increased water-use efficiency are likely to provide C4 plants with selective and competitive advantages over C3 plants in hot and arid climates (Ripley et al., 2007; Ripley et al., 2010). These aforementioned observations bring up necessary inquiries regarding the benefits and costs of C4 photosynthesis in water-limited ecosystems.
Tamarix ramosissima and Haloxylon ammodendron are the dominant desert shrubs with C3 and C4 photosynthetic pathways, respectively, that are distributed in the Gurbantunggut Desert of Central Asia. These two desert shrubs play critical roles in maintaining desert ecosystem structure and functioning, biodiversity conservation, and soil protection. Despite the importance of the shrubs, there remains a paucity of extensive evidence on the responses of C3 and C4 desert shrubs to the changing groundwater depth. Previous works have mainly focused on observations of the precipitation responses and water resource use of these C3 and C4 desert shrubs, thus limiting the understanding of these shrubs and hindering the ability of researchers to predict future impacts on the Central Asian Desert ecosystem. With differential photosynthetic pathways, the two shrubs might develop contrasting forms of eco-physiological acclimation, as well as different response patterns under changing water conditions. This study investigated the eco-physiological performances of the two shrubs under declining groundwater depths to detect their divergent survival mechanisms in an unfavorable environment. This study aimed to address the following questions: 1) to what degree do the two shrubs rely on groundwater? 2) To what extent does the varying groundwater depth affect the eco-physiological performance of the two desert shrubs? 3) Does the C4 pathway have any advantages under drought stress?
The study area was on the southern border of the Gurbantunggut Desert located in the Central Asian continental desert, which was near the Fukang Station of Desert Ecology, Chinese Academy of Sciences (44°22′N, 87°55′E). The research area has a typical temperate continental arid climate, with a hot, dry summer lasting from June to the end of August, and a cold, snow-covered winter lasting from December to March. The mean annual temperature is 6.6°C (1997–2016), the annual potential evaporation is approximately 1,000 mm, and the annual precipitation ranges from 70 to 180 mm (Fan et al., 2014; Xu et al., 2016). The C3 and C4 shrubs are the dominant vegetation types distributed across the area from the fringe of the alluvial plain to the interior of the desert.
Three shrub communities with distinct vegetation structures were selected along the groundwater gradient from the southern border to the interior of the desert to identify the divergent eco-physiological performances of two different C3 and C4 shrubs to deteriorating soil water conditions. The C4 shrub community (C4 site hereafter) was chosen from the interior of the sandy desert, where the groundwater depth was approximately 10 m. The C3 and C4 mixed shrub community (C3/C4 site hereafter) and the C3 shrub community (C3 site hereafter) were chosen from the fringe of the alluvial plain, where the groundwater depths were 3.5 m and 6.5 m, respectively (Figure 1). The selected three sites had comparable climatic characteristics and weather patterns and were 5–8 km apart. The C4 shrub H. ammodendron (C. A. Mey.) Bunge was chosen in the C4 site, and the C3 shrub T. ramosissima Ledeb. Fl. Alt. was selected in the C3 site, and both shrubs were chosen from the C3/C4 site for comparison. The characteristics of these shrubs are presented in Supplementary Table 1. The study was conducted for two consecutive years, 2015 and 2016, with similar annual precipitation amounts (approximately 170 mm) and mean annual temperatures (Figure 1). The soil moisture differed with the groundwater depth in the selected three shrub communities, with the C3/C4 site having the highest soil moisture and the C4 site having the lowest soil moisture (Figure 1).
Figure 1 Variations in monthly total precipitation (mm) and monthly average temperature (°C) of 2015 (A) and 2016 (B) in the southern edge of Gurbantunggut Desert and gravimetric soil water content of the three different study sites (C represents the C4 site; D represents C3/C4 site; E represents C3 site) during the growing seasons of 2015 and 2016.
Precipitation, soil, plant stems, and groundwater samples were collected to determine the plant water sources. Soil, stem, and groundwater samples were collected in April, June, August, and October 2015 and in April, July, and September 2016. Precipitation samples were collected after each rainfall (or snowfall) event using a collector installed in the ground of each site. The meteorological data were collected from the weather station located in the study area. Detailed information on the soil and stem sample collection method and soil water content measurement were described by Tiemuerbieke et al. (2018). Soil and xylem water were extracted using a cryogenic vacuum distillation system, and the oxygen and hydrogen isotope compositions of all the water samples were analyzed using a liquid water isotope analyzer (LWIA, DLT-100, Los Gatos Research Inc., Mountain View, CA, USA), with precision levels of 0.25‰ and 0.1‰ for δ18O and δ2H, respectively. The specific procedures were described by Tiemuerbieke et al. (2018) and Wu et al. (2014).
A portable gas exchange system (Li-6400; Li-Cor, Lincoln, NE, USA) was used to measure the leaf gas exchange parameters of C3 and C4 shrubs monthly from June to September. This study detected the responses of leaf gas exchange to photosynthetically active radiation and intercellular CO2 concentration from 08:00 to 14:00 on clear sunny days. To measure the light response of plants, the light source leaf chamber (20 × 30 mm2) was used to measure the light response of three to four mature, fully extended sunlit leaves from each tree, and the light intensities were set at 0 μmol m−2 s−1, 20 μmol m−2 s−1, 50 μmol m−2 s−1, 100 μmol m−2 s−1, 150 μmol m−2 s−1, 200 μmol m−2 s−1, 400 μmol m−2 s−1, 600 μmol m−2 s−1, 800 μmol m−2 s−1, 1,200 μmol m−2 s−1, 1,600 μmol m−2 s−1, 1,800 μmol m−2 s−1, 2,000 μmol m−2 s−1, and 2,200 μmol m−2 s−1. The chamber temperature was adjusted to 30°C to keep the ambient air temperature and relative humidity, and the gas flow rate was set at 400 μmol/s with a 400 μmol/mol reference CO2 concentration. A modified rectangular hyperbola model (Ye and Yu, 2008) was used to derive the light saturation point (LSP) maximum net photosynthetic rate (Pnmax):
where Pnmax represents the maximum net photosynthetic rate; I represents the PPFDi; α represents the initial slope of the photosynthetic light-response curve when PPFDi approaches zero, namely, the apparent quantum efficiency; β and γ are coefficients that are independent of I and obtained by curve fitting (Ye & Yu, 2008); and Rd represents the dark respiration. The stomatal conductance (gs) and transpiration rate (Tr) were measured at PPFDi = 1,800 μmol m−2 s−1. Nine measurements were performed for each species, with three duplicates for each species at each site.
A pressure chamber (Model 3005, PMS Instrument Company, Albany, NY, USA) was used to test the stem water potential for three duplicates of shrubs at each site (a total of nine measurements were taken for each species) from June to September of 2015 and in June, August, and September of 2016. The specific procedures were described by Tiemuerbieke et al. (2018).
The leaf sample collection for leaf non-structural carbohydrate (NSC) analysis was conducted simultaneously with gas exchange measurements. After being collected, the plant leaves were frozen immediately in liquid nitrogen to halt any enzymatic activity. Then, samples were dried in the laboratory at 65°C for 48 h before being ground to a fine powder to measure NSC concentrations, including leaf solute sugars and starch. This study used a modified microplate method (Zhao et al., 2010) to analyze foliar NSC, including foliar solute sucrose and starch content. To extract ethanol-soluble sucrose, the samples were heated in an 80°C water bath for 15 minutes. A mixture of 70 mg of ground tissue and 2 mL of 80% (v/v) ethanol was utilized for the extraction process. The starch concentration was determined by measuring the glucose in an aliquot of the supernatant following the hydrolysis of starch in the sample residue remaining after the EtOH extraction of soluble NSC fractions. The specific procedures were described by Zhao et al. (2010).
The inter- and intraspecific differences, seasonal (or monthly) differences in water potential, gas exchange parameters, and foliar non-structural carbohydrate content between the two plants were tested using univariate analysis based on a general linear model (GLM) and one-way analysis of variance (ANOVA), in which the species, season (or month), and sites were set as fixed effects. Prior to analysis, normality and homoscedasticity tests were conducted on all data, and transformations (logarithmic or square root) were applied as needed. For post hoc tests, Tukey’s honestly significant difference (HSD) was utilized. Pearson’s correlation coefficient was employed for correlation analysis. All data analyses were carried out using SPSS 19.0 (SPSS Inc., Chicago, IL, USA), while linear and non-linear regression analyses and graphing were performed using Origin 8.5 (Origin Lab Corp., Northampton, MA, USA).
The relative reduction ratios (RRRs) for some of the parameters were calculated to evaluate the impact of groundwater decline on the plant’s physiological performance:
where B is the mean value of the parameters at the shallower groundwater depth site and A is the mean value of the parameters at the deeper groundwater depth site. Therefore, this method evaluates the relatively reduction ratio of given parameters at the site with deeper groundwater table depth compared to those of the site with shallower groundwater table depth.
The water sources of the H. ammodendron differed at the two sites with different groundwater table depths (Figure 2). The percentage of groundwater use was higher for the H. ammodendron at the C4 site, whereas the percentage of soil water was higher for the H. ammodendron at the C3/C4 site (Figure 2). However, the water sources of T. ramosissima did not differ between the two sites with different groundwater table depths, and the shrub mainly used soil water over groundwater at both sites (Figure 2).
Figure 2 The percentage of groundwater (A, B) and soil water use (C, D) of the Haloxylon ammodendron and Tamarix ramosissima at the sites with different groundwater table depths during the growing seasons of 2015 and 2016.
Significant differences were detected in the gas exchange parameters between the shrubs at different sites for both H. ammodendron and T. ramosissima (Figure 3). The significant differences in the Pnmax (Equation 1) and gs of H. ammodendron in the two sites varied with months, with the Pnmax and gs of H. ammodendron in the C4 site reduced significantly during August and September compared with the H. ammodendron in the C3/C4 site (Figure 3). The LSP and Tr of H. ammodendron at the C4 site exhibited a decreasing trend from June to September, but the differences were not statistically significant (Figure 3). For the T. ramosissima, decreases in the Pnmax, gs, Tr, and LSP of T. ramosissima in the drier site (C3 site) were detected during the entire study period of both years, and the reductions in Pnmax, gs, and LSP went greater during the drier months of both years (Figure 2).
Figure 3 Variations in maximum net photosynthetic rate (Pnmax) and stomata conductance (gs), transpiration rate (Tr), and LSP of Haloxylon ammodendron (A, C, E, G) and Tamarix ramosissima (B, D, F, H) in different sites during the growing seasons of 2015 and 2016. The different uppercase and lowercase letters represent monthly significant differences for given shrubs at different sites. * represents significant intraspecific differences in different sites (p< 0.05). Data are presented as mean ± 1 standard error (n = 6).
The Ψpd and Ψmd of H. ammodendron differed significantly at the two sites. The H. ammodendron at the drier site (C4 site) had more negative water potential than the H. ammodendron at the wetter site from June to September. Particularly, the Ψpd of H. ammodendron at the drier site dropped significantly during the drier months (Figure 4). However, the water potential of T. ramosissima did not differ significantly between the two sites during the study period, except for in June and August of 2016 (Figure 4).
Figure 4 Variations in stem predawn (Ψmd) and midday water potentials (Ψpd) of Haloxylon ammodendron (A, C) and Tamarix ramosissima (B, D) in different sites during the growing seasons of 2015 and 2016. The different uppercase and lowercase letters represent monthly significant differences for given shrubs at different sites. * represents significant intraspecific differences in different sites (p< 0.05). Data are presented as mean ± 1 standard error (n = 6).
The RRR (Equation 2) values of the Pnmax, gs, and LSP of both shrubs increased gradually with increasing monthly cumulative non-precipitation days (MCND) and monthly cumulative high temperature days before sampling (MCHT), as well as with the decreasing monthly total precipitation amount (MTPA) (Figure 5). The RRR values of the parameters were higher for T. ramosissima than for H. ammodendron. The RRR of Ψpd was higher in August and September than in June for H. ammodendron, whereas there was no such trend in the Ψpd for T. ramosissima (Figure 5).
Figure 5 Variations of the relative reduction ratio of average Pnmax (A), gs (B), LSP (C), and Ψpd (D) of Haloxylon ammodendron and Tamarix ramosissima with the monthly cumulative non-precipitation days (MCND, panel E), monthly cumulative high-temperature days before sampling (MCHTD, panel E), and monthly total precipitation amount (MTPA, panel E) during growing seasons of 2015 and 2016.
The leaf starch content and leaf soluble sugar content of H. ammodendron did not vary significantly with sites (Figure 6). However, there were significant monthly differences observed in these two parameters at both sites. Specifically, the leaf starch content decreased significantly during drier months, whereas the leaf soluble sugar content tended to increase significantly during the drier period (p< 0.05, Figure 6). For T. ramosissima, the leaf starch content tended to increase during the drier months of both years and increased significantly during August 2016 (Figure 6). However, the leaf-soluble sugar content of T. ramosissima showed a decreasing trend at the drier site, but a statistically significant decrease was only detected in August 2015 (Figure 6). The NSCs of T. ramosissima did not differ by month in both sites during both years (Figure 6).
Figure 6 Variations in leaf starch content and leaf solute sugar content of Haloxylon ammodendron (A, C) and Tamarix ramosissima (C, D) in different communities in 2015 and 2016. The different uppercase and lowercase letters represent monthly significant differences for given shrubs at different sites. * represents significant intraspecific differences in different sites (p< 0.05). Data are presented as mean ± 1 standard error (n = 6).
This study demonstrated that the two studied Central Asian desert shrubs with differential photosynthetic pathways performed differently with declining groundwater depth.
The diminishing groundwater had a great impact on the water use, gas exchange, and stem water status of the Central Asian desert shrubs. The water sources of H. ammodendron reverted from the soil water (at the C3/C4 site) to the groundwater (at the C4 site), and the percentage of groundwater use increased with the month in response to the declining groundwater depth (Figure 2). These results were in line with the findings of earlier reports conducted on the same species in the same study area (Dai et al., 2015; Wu et al., 2019), which suggest that this shrub could adjust its rooting depth flexibly in space and time to cope with the water stress caused by fluctuating water conditions (Tiemuerbieke et al., 2018). In addition, the capacity to extract water from relatively stable groundwater via a strong root system would account for the ability of H. ammodendron to maintain relatively constant eco-physiological performance under changing water conditions (Drake and Franks, 2003; Xu and Li, 2006).
The responses of the eco-physiological parameters of H. ammodendron to the declining groundwater depth were manifested during the prolonged summer drought, indicating that the prolonged summer drought further intensified the effect of declining groundwater depth. At the start of the summer drought (during June), the response of H. ammodendron to the declining groundwater depth in terms of gas exchange was not pronounced. The RRR values of the Pnmax and gs of the shrubs at the drier site were the lowest during this period (Figure 5). However, with the progress of the summer drought, with elevated high temperatures and an increasing number of non-precipitation days, the RRRs of Pnmax, gs, and LSP increased substantially during the following months, and the highest values were observed in September (Figure 5). The same trends were detected in the predawn water potential of H. ammodendron, which tended to be more negative at the C4 site during August and September (Figure 4). The RRR of the predawn water potential was greater during the chronic summer drought (Figure 5), suggesting that the ability of H. ammodendron to recover daytime water status was impaired by the prolonged summer drought at the site with deeper groundwater depth (Figures 4, 5). Similar results were reported in previous studies conducted on the same species (Wu et al., 2019), indicating the pronounced impacts of the summer drought on the plant responses under changing water conditions. Moreover, the ΔΨ reflects the hydraulic balance between water loss from the leaves and supply from the roots and soil, with smaller ΔΨ at the C4 site (Supplementary Figure 2), indicating that nighttime rehydration under severe drought was insufficient to bring the Ψpd into equilibrium with the soil water potential (Taylor et al., 2014). The present study did not detect a significant reduction in the transpiration rate of H. ammodendron at the site with lower groundwater depth. It can be inferred that H. ammodendron sustains the transpiration rate and stomatal conductance essential for growth by relying on stable groundwater. This might be a water-saving strategy for this shrub, as it allows H. ammodendron to respond quickly to changes in the ambient vapor pressure deficit (VPD) to prevent excessive dehydration (Chen et al., 2015).
The carbon assimilation, stomatal conductance, and water transpiration were all suppressed at the site with deeper groundwater depth, and the relative reductions in the average photosynthetic rate and stomatal conductance were greater at the site with deeper groundwater depth and accelerated with drought, indicating that the eco-physiological performance of T. ramosissima was greatly affected by the combined effects of declining groundwater depth and chronic summer drought. However, unlike H. ammodendron, the water sources of T. ramosissima did not shift, and this shrub mainly relied on deep soil water at both sites (Figure 2). The stem water status did not differ significantly with declining groundwater depth but deteriorated with the progression of the summer drought at both sites (Figure 4). These results suggested that the deeper soil water might be the reliable water source that enabled this shrub to maintain comparable water status with the wetter site, but the monthly declining midday water potential indicated that the water status of this shrub would be significantly affected by progressive atmospheric desiccation at both sites.
Both species exhibited a decline in eco-physiological performance due to the reduction in groundwater depth, especially when coupled with prolonged summer drought, and the response patterns differed between the two shrubs. With declining groundwater table depth, the water sources shifted, carbon fixation and stomatal conductance were suppressed, the water status of stems further deteriorated, and the transpiration became less sensitive in H. ammodendron. The water sources and water status responded less sensitively in T. ramosissima, indicating that the two shrubs prioritized water consumption and carbon gain differently when faced with changing water conditions.
The reduced transpiration rate and stomatal conductance and increased instantaneous water use efficiency of H. ammodendron (Table 1, Supplementary Figure 1) together with its deep root system reflected the adoption of a typical conservative water use mechanism by the C4 shrub. The deep roots of H. ammodendron at the deeper groundwater site would guarantee a reliable water source to maintain its normal eco-physiological performance. Such a strategy seemed to be beneficial for maintaining its hydraulic functions (Zhu and Cao, 2009; Chen et al., 2015) during the dry season with high temperatures and evaporative demand. The smaller stomatal aperture and higher efficiency of water use in H. ammodendron were consistent with the typical pattern and classical understanding of the traits of C4 grass species discussed in previous reports and developed models (Kocacinar and Sage, 2003; Taylor et al., 2011; Osborne and Sack, 2012; Taylor et al., 2014). This is also the case for the Central Asian C4 shrub based on the present study.
Table 1 Inter-specific differences in the eco-physiological parameters between Haloxylon ammodendron and Tamarix ramosissima at same sites during the growing seasons of 2015 and 2016.
As discussed above, strict stomatal regulation of the C4 shrub could be the key water conservation strategy to limit excessive water loss. H. ammodendron may obtain a great advantage by adopting this water-saving mechanism. On the one hand, the effective regulation of stomata in H. ammodendron is essential for sustaining hydraulic function in hot and dry conditions. Additionally, the transpiration rate in H. ammodendron remained constant at its lowest point across the growing season, which might aid this shrub conserve water in the prolonged dry season and decrease the likelihood of stem embolism (Meinzer and McCulloh, 2013; Chen et al., 2015). On the other hand, the consequence of this avoidance of hydraulic failure is the subsequent limitation of carbon gain that occurs with tight stomatal control (McDowell and Sevanto, 2010; Silvertown et al., 2015). Nevertheless, the CO2-concentrating mechanism within the C4 shrub seemed to compensate for the CO2 limitation caused by tight stomatal control (Ripley et al., 2007; Osborne and Sack, 2012). According to previous studies, rapid reduction in atmospheric CO2 concentrations and elevated ambient temperatures have selected the evolution of the C4 pathway (Taylor et al., 2010; Osborne and Sack, 2012), which eliminates the limitation of photorespiration by elevating CO2 concentrations at the site of the bundle sheath where Rubisco fixes CO2 (Ripley et al., 2010; Sage, 2014). This allows C4 plants to maintain the same rate of photosynthetic efficiency at a lower stomatal conductance compared with C3 ancestors (Taylor et al., 2012; Liu et al., 2019). As revealed in this study, the Pnmax of H. ammodendron did not differ significantly compared with T. ramosissima at the same site during the rapid growth month (June) in both years (Figure 3, Table 1), demonstrating that H. ammodendron exhibited higher carbon fixation, with stomatal conductance being approximately half that of T. ramosissima. Additionally, it can be inferred from the A–Ci curves that the reduced Pnmax of H. ammodendron might result from metabolic limitations. H. ammodendron seemed to be more susceptible to metabolic limitations under declining groundwater depth than T. ramosissima (Supplementary Figure 3), which aligns with the results of previous reports on C3 and C4 grasses (Ripley et al., 2007).
Contrary to H. ammodendron, the C3 shrub T. ramosissima adopted a contrasting water–carbon balance. T. ramosissima maintained higher stomatal conductance during the growing season. Consequently, the transpiration rate and photosynthetic rate were higher (Table 1), indicating that T. ramosissima might guarantee a higher carbon fixation rate at the great cost of higher water loss and lower water use efficiency (Figure 7). This was also consistent with the earlier research on the same shrub in the same study region, which suggested that T. ramosissima has the tendency to assimilate carbon at its maximum level, at the risk of greater water loss (Xu et al., 2007). However, the higher RRR in gas exchange parameters (Figure 5) indicated the downregulation of its eco-physiological performance with declining groundwater depth. Retaining greater stomatal conductance under a declining groundwater table gradient might make this shrub more susceptible to hydraulic failure, which might be the main factor determining its absence in the central areas of the desert, where the groundwater table is even lower. Therefore, H. ammodendron achieved a higher rate of carbon fixation with greater water use efficiency (lower stomatal conductance) than the C3 shrub T. ramosissima to which a higher rate of carbon assimilation comes with far greater stomatal conductance.
Figure 7 Relationships of δ13C (A) and intrinsic water use efficiency (B) with stomatal conductance, transpiration rate, and predawn water potential of the Haloxylon ammodendron and Tamarix ramosissima during the growing seasons of 2015 and 2016. Data were pooled for all individuals of both shrubs at two sites during the whole study period. R2 and p-values are given (p< 0.05).
Additionally, the stem water status of the two shrubs was affected differently by declining groundwater depth. The stem water status of T. ramosissima was more sensitive to the progressing summer drought than the declining groundwater table, whereas both the groundwater table and the prolonged summer drought strongly affected the water status of H. ammodendron. It could be inferred that the two shrubs might adopt different stem hydraulic and morphological properties to guarantee the water transport in stems under changing water conditions. H. ammodendron could tolerate greater negative water potential with the decline in groundwater table depth, which might be associated with specific stem morphology and hydraulic properties (such as different xylem morphological traits, stem hydraulic conductance, and stem vulnerability). Previous reports have pointed out that plants with lower water potentials have smaller and thicker xylem conduits with fewer pits specialized in membranes (Lens et al., 2013). These plants are able to handle low water potentials without experiencing embolism, which allows them to safely transport water to the leaves even in extremely dry environments at near-maximum rates (Manzoni et al., 2013). These embolism-resistant stems would guarantee hydraulic safety for these shrubs under unfavorable conditions.
When data were pooled for all individuals of both shrubs at two sites during the entire study period, the greater negative stem water potential of H. ammodendron coupling with lower transpiration rate and stomatal conductance were associated with improved instantaneous water use efficiency and more positive δ13C values. The higher water potential of T. ramosissima coupled with its higher stomatal conductance and transpiration rate was associated with lower instantaneous water use efficiency and more negative δ13C values (Figure 7). Therefore, it could be inferred that the evaluated carbon fixation of T. ramosissima was accompanied by the coordination with more favorable stem water status and higher water conductance from the leaf to the atmosphere. However, the progressive atmospheric desiccation during summer drought might greatly inhibit this process. For H. ammodendron, the water conservation mechanism seemed to rely on the coordination of the stomatal behavior, photosynthetic pathway, and stem hydraulic properties. Tight stomatal regulation was coordinated with an embolism-resistant stem that could tolerate greater negative water potentials. This was in line with previous reports that have proposed that plants’ regulation of stomatal behavior could be in balance with the leaf water potential above a threshold value that limits excessive water loss to avoid hydraulic failure in the xylem to maintain the water-carbon balance (Sperry, 2000; Meinzer et al., 2009; Klein, 2014; Roman et al., 2015). Furthermore, the reduction in stomatal conductance would not necessarily give rise to a marked reduction in photosynthetic assimilation due to the C4 pathway (Figure 5). Therefore, it is suggested that the evolution of stem hydraulic traits occurred in coordination with the evolution of photosynthetic pathways so that water conductance would keep pace with water consumption. As suggested by Taylor et al. (2014), the diversification in plants’ hydraulic traits may have been crucial in the colonization and adaptation of C4 grasses to arid and open environments. This was also the case for C4 and C3 desert shrubs in the Gurbantunggut Desert, where Haloxylon species were mainly distributed at the fringe of the desert, whereas the C3 species were distributed at the edge of the desert.
The partitioning pattern of leaf NSCs differed between the two shrubs. The significant monthly variations in the non-structural carbohydrates of the two shrubs demonstrated different carbon allocation processes in the two shrubs. The significant negative association of leaf soluble sugar content with midday water potential in H. ammodendron (Figure 8) revealed that the reduction of leaf starch content and the significant increase in leaf solute sugar content in drier months may be the result of osmotic or turgor regulation of the leaf. This suggested that when leaf water potentials gradually became negative during dry periods, more osmotically active carbohydrates such as sugars were partitioned to the leaf to maintain leaf turgor and leaf hydraulic water status in H. ammodendron (Woodruff et al., 2015).
Figure 8 Relationship between leaf soluble sugar content and midday water potential of Haloxylon ammodendron (A) and Tamarix ramosissima (B) in 2015 and 2016. Data were pooled for the two plants. R2 and p-values are given (p< 0.05).
The two studied shrubs demonstrated contrasting trade-offs between water loss and carbon gain under water stress and changing water conditions. Tight stomatal regulation was beneficial for H. ammodendron due to its C4 photosynthetic pathway, which seemed to compensate for the constraints on carbon fixation caused by lower stomatal conductance during the growing season. A relatively strong rooting system made this shrub more resilient with the declining groundwater table. On the contrary, T. ramosissima maximized carbon gain at the cost of lower water use efficiency by maintaining constantly greater stomatal conductance across the growing season. However, this might make T. ramosissima more vulnerable to hydraulic failure under the declining groundwater gradient. Marked differences in water status between the two shrubs suggested that different stomatal behaviors might coordinate with contrasting hydraulic properties in the two shrubs to maintain the balance between the water conductance and water loss, which would mitigate competitive stress by separating their hydrological niches and facilitate their coexistence.
The original contributions presented in the study are included in the article/Supplementary Material. Further inquiries can be directed to the corresponding author.
BT: Conceptualization, Funding acquisition, Investigation, Methodology, Formal analyses, Writing – original draft, Visualization. J-YM: Conceptualization, Funding acquisition, Provision of measurements and data, Writing – review & editing. WS: Writing – review and editing. All authors contributed to the article and approved the submitted version.
The author(s) declare financial support was received for the research, authorship, and/or publication of this article. This work was financially supported by the Natural Sciences Foundation of Xinjiang Uygur Autonomous Region (No. 2021D01C056), the Natural Science Foundation of Jilin Province (No. 20230101171), and by the National Natural Science Foundation of China (No. 41671207).
We thank all the staff at the Fukang Station of Desert Ecology for their assistance; special thanks to Yong-Xin Zang and Xiao-Jun Min for their help in the laboratory analysis and field experiments. We thank LetPub (www.letpub.com) for its linguistic assistance during the preparation of this manuscript.
The authors declare that the research was conducted in the absence of any commercial or financial relationships that could be construed as a potential conflict of interest.
All claims expressed in this article are solely those of the authors and do not necessarily represent those of their affiliated organizations, or those of the publisher, the editors and the reviewers. Any product that may be evaluated in this article, or claim that may be made by its manufacturer, is not guaranteed or endorsed by the publisher.
The Supplementary Material for this article can be found online at: https://www.frontiersin.org/articles/10.3389/fpls.2023.1244555/full#supplementary-material
Adler, P. B., Fajardo, A., Kleinhesselink, A. R., Kraft, N. J. B. (2013). Trait-based tests of coexistence mechanisms. Ecol. Lett. 16, 1294–1306. doi: 10.1111/ele.12157
Angert, A. L., Huxman, T. E., Chesson, P., Venable, D. L. (2009). Functional tradeoffs determine species coexistence via the storage effect. Proc. Natl. Acad. Sci. U. S. A. 106, 11641–11645. doi: 10.1073/pnas.0904512106
Araya, Y. N., Silvertown, J., Gowing, D. J., McConway, K. J., Peter Linder, H., Midgley, G. (2011). A fundamental, eco-hydrological basis for niche segregation in plant communities. New Phytol. 189, 253–258. doi: 10.1111/j.1469-8137.2010.03475.x
Bermúdez, R., Retuerto, R. (2014). Together but different: co-occurring dune plant species differ in their water- and nitrogen-use strategies. Oecologia 174, 651–663. doi: 10.1007/s00442-013-2820-7
Bhusal, N., Lee, M., Reum Han, A., Han, A., Kim, H. S. (2020). Responses to drought stress in Prunus sargentii and Larix kaempferi seedlings using morphological and physiological parameters. For. Ecol. Manage. 465, 118099. doi: 10.1016/j.foreco.2020.118099
Chen, Y., Cao, K., Schnitzer, S. A., Fan, Z., Zhang, J., Bongers, F. (2015). Water-use advantage for lianas over trees in tropical seasonal forests. New Phytol. 205, 128–136. doi: 10.1111/nph.13036
Chesson, P., Gebauer, R. L. E., Schwinning, S., Huntly, N., Wiegand, K., Ernest, M. S. K., et al. (2004). Resource pulses, species interactions, and diversity maintenance in arid and semi-arid environments. Oecologia 141, 236–253. doi: 10.1007/s00442-004-1551-1
Dai, Y., Zheng, X.-J., Tang, L.-S., Li, Y. (2015). Stable oxygen isotopes reveal distinct water use patterns of two Haloxylon species in the Gurbantonggut Desert. Plant Soil 389, 73–87. doi: 10.1007/s11104-014-2342-z
Drake, P. L., Franks, P. J. (2003). Water resource partitioning, stem xylem hydraulic properties, and plant water use strategies in a seasonally dry riparian tropical rainforest. Oecologia 137, 321–329. doi: 10.1007/s00442-003-1352-y
Eggemeyer, K. D., Awada, T., Harvey, F. E., Wedin, D. A., Zhou, X., Zanner, C. W. (2008). Seasonal changes in depth of water uptake for encroaching trees Juniperus virginiana and Pinus ponderosa and two dominant C4 grasses in a semiarid grassland. Tree Physiol. 29, 157–169. doi: 10.1093/treephys/tpn019
Ellsworth, P. Z., Sternberg, L. S. L. (2015). Seasonal water use by deciduous and evergreen woody species in a scrub community is based on water availability and root distribution: Deciduous and Evergreen Water Uptake. Ecohydrology 8, 538–551. doi: 10.1002/eco.1523
Fan, L. L., Tang, L. S., Wu, L. F., Ma, J., Li, Y., Güsewell, S. (2014). The limited role of snow water in the growth and development of ephemeral plants in a cold desert. J. Veg. Sci. 25, 681–690. doi: 10.1111/jvs.12121
Filella, I., Penuelas, J. (2003). Partitioning of water and nitrogen in co-occurring Mediterranean woody shrub species of different evolutionary history. Oecologia 137, 51–61. doi: 10.1007/s00442-003-1333-1
Grossiord, C., Sevanto, S., Dawson, T. E., Adams, H. D., Collins, A. D., Dickman, L. T., et al. (2017). Warming combined with more extreme precipitation regimes modifies the water sources used by trees. New Phytol. 213, 584–596. doi: 10.1111/nph.14192
Hoover, D. L., Knapp, A. K., Smith, M. D. (2014). Resistance and resilience of a grassland ecosystem to climate extremes. Ecology 95, 2646–2656. doi: 10.1890/13-2186.1
Huang, J., Yu, H., Guan, X., Wang, G., Guo, R. (2016). Accelerated dryland expansion under climate change. Nat. Clim. Change 6, 166–171. doi: 10.1038/nclimate2837
Klein, T. (2014). The variability of stomatal sensitivity to leaf water potential across tree species indicates a continuum between isohydric and anisohydric behaviours. Funct. Ecol. 28, 1313–1320. doi: 10.1111/1365-2435.12289
Kocacinar, F., Sage, R. F. (2003). Photosynthetic pathway alters xylem structure and hydraulic function in herbaceous plants. Plant Cell Environ. 26, 2015–2026. doi: 10.1111/j.1365-2478.2003.01119.x
Lens, F., Tixier, A., Cochard, H., Sperry, J. S., Jansen, S., Herbette, S. (2013). Embolism resistance as a key mechanism to understand adaptive plant strategies. Curr. Opin. Plant Biol. 16, 287–292. doi: 10.1016/j.pbi.2013.02.005
Liu, H., Taylor, S. H., Xu, Q., Lin, Y., Hou, H., Wu, G., et al. (2019). Life history is a key factor explaining functional trait diversity among subtropical grasses, and its influence differs between C3 and C4 species. J. Exp. Bot. 70, 1567–1580. doi: 10.1093/jxb/ery462
Manzoni, S., Vico, G., Katul, G., Palmroth, S., Jackson, R. B., Porporato, A. (2013). Hydraulic limits on maximum plant transpiration and the emergence of the safety–efficiency trade-off. New Phytol. 198, 169–178. doi: 10.1111/nph.12126
McDowell, N. G., Sevanto, S. (2010). The mechanisms of carbon starvation: how, when, or does it even occur at all? New Phytol. 186, 264–266. doi: 10.1111/j.1469-8137.2010.03232.x
Meinzer, F. C., Johnson, D. M., Lachenbruch, B., McCulloh, K. A., Woodruff, D. R. (2009). Xylem hydraulic safety margins in woody plants: coordination of stomatal control of xylem tension with hydraulic capacitance. Funct. Ecol. 23, 922–930. doi: 10.1111/j.1365-2435.2009.01577.x
Meinzer, F. C., McCulloh, K. A. (2013). Xylem recovery from drought-induced embolism: where is the hydraulic point of no return? Tree Physiol. 33, 331–334. doi: 10.1093/treephys/tpt022
Moreno-Gutierrez, C., Dawson, T. E., Nicolas, E., Querejeta, J. I. (2012). Isotopes reveal contrasting water use strategies among coexisting plant species in a Mediterranean ecosystem. New Phytol. 196 (2), 489–496. doi: 10.1111/j.1469-8137.2012.04276.x
Osborne, C. P., Sack, L. (2012). Evolution of C 4 plants: a new hypothesis for an interaction of CO 2 and water relations mediated by plant hydraulics. Philos. Trans. R. Soc B Biol. Sci. 367, 583–600. doi: 10.1098/rstb.2011.0261
Panneerselvam, B., Pande, D., Muniraj, K., Balasubramanian, A., Ravichandran, N. (2022). Climate Change Impact on Groundwater Resources. Cham: Springer. doi: 10.1007/978-3-031-04707-7
Pardos, M., del RÃo, M., Pretzsch, H., Jactel, H., Bielak, K., Bravo, F., et al. (2021). The greater resilience of mixed forests to drought mainly depends on their composition: Analysis along a climate gradient across Europe. For. Ecol. Manage. 481, 118687. doi: 10.1016/j.foreco.2020.118687
Pearcy, R. W., Ehleringer, J. (1984). Comparative ecophysiology of C3 and C4 plants. Plant Cell Environ. 7, 1–13. doi: 10.1111/j.1365-3040.1984.tb01194.x
Ripley, B., Frole, K., Gilbert, M. (2010). Differences in drought sensitivities and photosynthetic limitations between co-occurring C3 and C4 (NADP-ME) Panicoid grasses. Ann. Bot. 105, 493–503. doi: 10.1093/aob/mcp307
Ripley, B. S., Gilbert, M. E., Ibrahim, D. G., Osborne, C. P. (2007). Drought constraints on C4 photosynthesis: stomatal and metabolic limitations in C3 and C4 subspecies of Alloteropsis semialata. J. Exp. Bot. 58, 1351–1363. doi: 10.1093/jxb/erl302
Roman, D. T., Novick, K. A., Brzostek, E. R., Dragoni, D., Rahman, F., Phillips, R. P. (2015). The role of isohydric and anisohydric species in determining ecosystem-scale response to severe drought. Oecologia 179, 641–654. doi: 10.1007/s00442-015-3380-9
Sage, R. F. (2014). Stopping the leaks: new insights into C4 photosynthesis at low light. Plant Cell Environ. 37, 1037–1041. doi: 10.1111/pce.12246
Sage, R. F., Pearcy, R. W. (1987). The Nitrogen Use Efficiency of C3 and C4 Plants: II. Leaf Nitrogen Effects on the Gas Exchange Characteristics of Chenopodium album (L.) and Amaranthus retroflexus (L.). Plant Physiol. 84, 959–963. doi: 10.1104/pp.84.3.959
Schwinning, S., Ehleringer, J. R. (2001). Water use trade-offs and optimal adaptations to pulse-driven arid ecosystems. J. Ecol. 89, 464–480. doi: 10.1046/j.1365-2745.2001.00576.x
Silvertown, J., Araya, Y., Gowing, D. (2015). Hydrological niches in terrestrial plant communities: a review. J. Ecol. 103, 93–108. doi: 10.1111/1365-2745.12332
Sperry, J. S. (2000). Hydraulic constraints on plant gas exchange. Agric. For. Meteorol. 104, 13–23. doi: 10.1016/S0168-1923(00)00144-1
Taylor, S. H., Franks, P. J., Hulme, S. P., Spriggs, E., Christin, P. A., Edwards, E. J., et al. (2012). Photosynthetic pathway and ecological adaptation explain stomatal trait diversity amongst grasses. New Phytol. 193, 387–396. doi: 10.1111/j.1469-8137.2011.03935.x
Taylor, S. H., Hulme, S. P., Rees, M., Ripley, B. S., Ian Woodward, F., Osborne, C. P. (2010). Ecophysiological traits in C 3 and C 4 grasses: a phylogenetically controlled screening experiment. New Phytol. 185, 780–791. doi: 10.1111/j.1469-8137.2009.03102.x
Taylor, S. H., Ripley, B. S., Martin, T., De-Wet, L., Woodward, F. I., Osborne, C. P. (2014). Physiological advantages of C 4 grasses in the field: a comparative experiment demonstrating the importance of drought. Glob. Change Biol. 20, 1992–2003. doi: 10.1111/gcb.12498
Taylor, S. H., Ripley, B. S., Woodward, F. I., Osborne, C. P. (2011). Drought limitation of photosynthesis differs between C3 and C4 grass species in a comparative experiment: Drought limitation in C3 and C4 grass species. Plant Cell Environ. 34, 65–75. doi: 10.1111/j.1365-3040.2010.02226.x
Tiemuerbieke, B., Min, X.-J., Zang, Y.-X., Xing, P., Ma, J.-Y., Sun, W. (2018). Water use patterns of co-occurring C3 and C4 shrubs in the Gurbantonggut desert in northwestern China. Sci. Total Environ. 634, 341–354. doi: 10.1016/j.scitotenv.2018.03.307
Woodruff, D. R., Meinzer, F. C., Marias, D. E., Sevanto, S., Jenkins, M. W., McDowell, N. G. (2015). Linking nonstructural carbohydrate dynamics to gas exchange and leaf hydraulic behavior in Pinus edulis and Juniperus monosperma. New Phytol. 206, 411–421. doi: 10.1111/nph.13170
Wu, X., Zheng, X.-J., Li, Y., Xu, G.-Q. (2019). Varying responses of two Haloxylon species to extreme drought and groundwater depth. Environ. Exp. Bot. 158, 63–72. doi: 10.1016/j.envexpbot.2018.11.014
Wu, Y., Zhou, H., Zheng, X.-J., Li, Y., Tang, L.-S. (2014). Seasonal changes in the water use strategies of three co-occurring desert shrubs. Hydrol. Process. 28, 6265–6275.
Xu, G.-Q., McDowell, N. G., Li, Y. (2016). A possible link between life and death of a xeric tree in desert. J. Plant Physiol. 194, 35–44. doi: 10.1016/j.jplph.2016.02.014
Xu, H., Li, Y. (2006). Water-use strategy of three central Asian desert shrubs and their responses to rain pulse events. Plant Soil 285, 5–17. doi: 10.1007/s11104-005-5108-9
Xu, H., Li, Y., Xu, G., Zou, T. (2007). Ecophysiological response and morphological adjustment of two Central Asian desert shrubs towards variation in summer precipitation. Plant Cell Environ. 30, 399–409. doi: 10.1111/j.1365-3040.2006.001626.x
Ye, Z. P., Yu, Q. (2008). A coupled model of stomatal conductance and photosynthesis for winter wheat. Photosynthetica 46, 637–640. doi: 10.1007/s11099-008-0110-0
Zhao, D., MacKown, C. T., Starks, P. J., Kindiger, B. K. (2010). Rapid analysis of nonstructural carbohydrate components in grass forage using microplate enzymatic assays. Crop Sci. 50, 1537–1545. doi: 10.2135/cropsci2009.09.0521
Keywords: C3 and C4 photosynthesis, desert shrubs, eco-physiological acclimation, water-carbon balance, drought stress
Citation: Tiemuerbieke B, Ma J-Y and Sun W (2024) Differential eco-physiological performance to declining groundwater depth in Central Asian C3 and C4 shrubs in the Gurbantunggut Desert. Front. Plant Sci. 14:1244555. doi: 10.3389/fpls.2023.1244555
Received: 22 June 2023; Accepted: 22 December 2023;
Published: 18 January 2024.
Edited by:
Arun K. Shanker, Central Research Institute for Dryland Agriculture (ICAR), IndiaReviewed by:
Xinsheng Liu, Anhui Normal University, ChinaCopyright © 2024 Tiemuerbieke, Ma and Sun. This is an open-access article distributed under the terms of the Creative Commons Attribution License (CC BY). The use, distribution or reproduction in other forums is permitted, provided the original author(s) and the copyright owner(s) are credited and that the original publication in this journal is cited, in accordance with accepted academic practice. No use, distribution or reproduction is permitted which does not comply with these terms.
*Correspondence: Jian-Ying Ma, majy652@nenu.edu.cn
Disclaimer: All claims expressed in this article are solely those of the authors and do not necessarily represent those of their affiliated organizations, or those of the publisher, the editors and the reviewers. Any product that may be evaluated in this article or claim that may be made by its manufacturer is not guaranteed or endorsed by the publisher.
Research integrity at Frontiers
Learn more about the work of our research integrity team to safeguard the quality of each article we publish.