- 1Department of Agronomy, Sher-e-Bangla Agricultural University, Dhaka, Bangladesh
- 2Department of Horticulture, Sher-e-Bangla Agricultural University, Dhaka, Bangladesh
- 3Agronomy Department of Superior School Engineering, University of Almería, Almería, Spain
- 4Department of Agricultural Botany, Sher-e-Bangla Agricultural University, Dhaka, Bangladesh
- 5Department of Agronomy, Kansas State University, Manhattan, KS, United States
Ozone (O3) levels on Earth are increasing because of anthropogenic activities and natural processes. Ozone enters plants through the leaves, leading to the overgeneration of reactive oxygen species (ROS) in the mesophyll and guard cell walls. ROS can damage chloroplast ultrastructure and block photosynthetic electron transport. Ozone can lead to stomatal closure and alter stomatal conductance, thereby hindering carbon dioxide (CO2) fixation. Ozone-induced leaf chlorosis is common. All of these factors lead to a reduction in photosynthesis under O3 stress. Long-term exposure to high concentrations of O3 disrupts plant physiological processes, including water and nutrient uptake, respiration, and translocation of assimilates and metabolites. As a result, plant growth and reproductive performance are negatively affected. Thus, reduction in crop yield and deterioration of crop quality are the greatest effects of O3 stress on plants. Increased rates of hydrogen peroxide accumulation, lipid peroxidation, and ion leakage are the common indicators of oxidative damage in plants exposed to O3 stress. Ozone disrupts the antioxidant defense system of plants by disturbing enzymatic activity and non-enzymatic antioxidant content. Improving photosynthetic pathways, various physiological processes, antioxidant defense, and phytohormone regulation, which can be achieved through various approaches, have been reported as vital strategies for improving O3 stress tolerance in plants. In plants, O3 stress can be mitigated in several ways. However, improvements in crop management practices, CO2 fertilization, using chemical elicitors, nutrient management, and the selection of tolerant crop varieties have been documented to mitigate O3 stress in different plant species. In this review, the responses of O3-exposed plants are summarized, and different mitigation strategies to decrease O3 stress-induced damage and crop losses are discussed. Further research should be conducted to determine methods to mitigate crop loss, enhance plant antioxidant defenses, modify physiological characteristics, and apply protectants.
1 Introduction
Ozone (O3) is a major environmental stressor that affects crop production negatively. The concentration of O3 is predicted to increase by 25% by 2050 and 60% by 2100 (Meehl et al., 2007; Jimenez-Montenegro et al., 2021). Ozone is present both in the stratosphere and the troposphere, where the stratospheric O3 is known as the “good O3” or “O3 layer” because it absorbs the harmful ultraviolet (UV-B) rays of sunlight and acts as a UV-ray filter, preventing its harmful effects on living organisms on Earth including plants. Tropospheric or ground-level O3 is responsible for promoting damaging effects on living cells, organs, and species; therefore, it is known as “bad O3” (Xu, 2021). Tropospheric O3, namely ground-level O3, is an air pollutant and secondary air pollutant recommended by air quality guidelines in all countries. Although they are generated in the troposphere by sunlight-driven chemical processes that combine nitrogen oxides (NOx) and volatile organic carbons (VOCs), the use of fossil fuels by the industrial and transport sectors has significantly increased the level of O3 in the troposphere, mainly due to the release upon combustion of several O3 precursors (Zhang et al., 2019; Hasan et al., 2021). Consequently, the phytotoxic effects of increased tropospheric O3 on many plant species, losses in global crop production, especially in industrial and urban areas, and damage to ecological health and environmental sustainability in the long term are well known (Monks et al., 2015; Ramya et al., 2023). However, the generation of O3 occurs in the troposphere through photochemical reactions between precursors emitted by anthropogenic, natural, and agronomic sources, such as NOx, carbon monoxide, methane, VOCs, and peroxyacetyl nitrate (Vainonen and Kangasjärvi, 2015).
Plants take up O3 through the stomata, and it is later converted into reactive oxygen species (ROS) in the apoplast. The accumulation of ROS damages the photosynthetic machinery, causes stomatal closure, and degrades ribulose-1,5-bis-phosphate carboxylase/oxygenase (RuBisCO) (Ren, 2021). However, the damage caused by O3 to plants depends on the dose and exposure time. Ozone exposure can be categorized as acute or chronic. High doses of O3 over short periods (acute damage) may lead to programmed cell death and leaf damage, particularly in sensitive plant species. However, a lower dose of O3 for a longer duration (chronic damage) affects the photosynthetic rate, causing growth reduction and rapid leaf senescence, with or without visible damage to the leaves (Chen et al., 2018; Emberson et al., 2018). Increased O3 exposure results in higher yield loss through foliar damage, suppression of photosynthesis with altered carbon translocation, and consequently earlier plant senescence occurs. Numerous distinct alterations in gene expression, metabolic profiles, and enzyme activity occur in plants exposed to O3. Acute O3 exposure in sensitive accessions led to increased cell death, lesion formation, and reduced photosynthesis (Morales et al., 2021). Owing to its high reactivity and instability, O3 can cause oxidative stress in apoplast plant cells by chemically altering different components such as proteins to form short-lived ROS. Plants activate antioxidant defense mechanisms to scavenge ROS and prevent their negative effects. Ozone-tolerant plants have different characteristics that suppress cellular toxic elements, such as peroxidation, and maintain cell membrane stability, which is made possible by the activation of both enzymatic and non-enzymatic antioxidant components (Dhevagi et al., 2021; Ramya et al., 2021a). Thus, plants can become tolerant to O3, which ultimately safeguards their yield under adverse circumstances.
The generation of O3 has a strong relationship with different meteorological factors, being positively correlated with sunshine hours and negatively correlated with wind speed and relative humidity (Markovic and Markovic, 2005). Consequently, the level of O3 changes over the season and even during the day, with high values in the day and dry months and low values in the night and wet months (Jain et al., 2005). It has been reported that the diurnal variation in O3 coincides with the intensity of solar radiation and higher air temperatures. Nevertheless, ground-level O3 concentrations remain low under nighttime conditions because there is no photolysis of nitrogen dioxide (NO2) or photooxidation of O3 precursors (Ma et al., 2021). Upon exposure to ultraviolet (UV) radiation, NO2 dissociated into nitric oxide (NO) and oxygen (O). The short lifetime of O3 is related to its ability to react with NO to produce NO2 and O2 again (Simon et al., 2014).
In this review, we explored the processes involved in O3 uptake by plants and their perception of this pollutant. In this study, we investigated the multivariate effects of O3 on plant growth, nutrition, physiology, yield, and oxidative stress. In addition, we discuss strategies for mitigating the phytotoxic effects of O3 and enhancing the performance of O3-affected plants. These strategies include crop management practices, carbon dioxide (CO2) fertilization, using chemical elicitors, proper nutrient management, and the selection of tolerant crop varieties. Therefore, this review aims to provide a comprehensive understanding of O3-induced damage in plants and techniques for improving O3 tolerance, thereby shedding light on O3-related research.
2 Ozone uptake by plants
Ozone pollution has been perceived mostly as a daytime problem because gas generation occurs through complex photolytic reactions, and the leaf stomata are open during this period, allowing O3 uptake into plants. Nevertheless, O3 uptake may also occur at night because the stomata are not completely closed (Dawson et al., 2007; Rannik et al., 2009). Furthermore, O3 can enter the leaves by direct absorption through the leaf exterior surfaces, albeit less than the amount of O3 entering the stomata (Pleijel et al., 2004). Besides the uptake of O3 via the stomata, O3 can also be deposited onto agricultural systems via non-stomatal pathways (e.g., soil and cuticular deposition) (Morales et al., 2021). Stomata are the first barrier to overcome the damage in plants caused by O3 because stomatal aperture control is responsible for O3 flux into the leaves (Fiscus et al., 2005). The size of the stomatal aperture is controlled by the activity of the guard cell ion channels and transporters responsible for the movement of osmolytes across the tonoplast and plasma membrane (Kollist et al., 2011; Hedrich, 2012). Ion channel regulation is controlled by reversible protein phosphorylation by protein kinases and phosphatases (Evans et al., 2005).
Three levels of O3 concentration have been established: low (20–70 nL O3 L-1), moderate (70–150 nL O3 L-1), and high (>150 nL O3 L-1) (Grulke and Heath, 2020). According to European Environmental Legislation, the threshold O3 concentration should not exceed 40 ppb to protect crops during the growing season (Proietti et al., 2021). The chronological and spatial sequences of these actions define how O3 exposure affects plant physiology. First, O3 enters the leaf apoplast via the stomata, where it is degraded into secondary ROS at two different locations (mesophyll and guard cell walls) by triggering specific calcium (Ca) signatures in the cytosol. High concentrations of Ca and ROS in the cytosol of guard cells result in stomatal closure and, consequently restriction of O3. There is a high influx of O3 in the apoplast of mesophyll cells, inducing excessive ROS accumulation that is not scavenged by apoplastic antioxidants, such as ascorbate (AsA) (Grulke and Heath, 2020). Higher accumulation of ROS in the apoplast triggers several downstream signaling pathways that work in parallel, in series, or both (Roychowdhury et al., 2019; Li et al., 2023). Reactive oxygen species signaling and apoplast propagation in the apoplast are related to the induction of respiratory burst oxidase homolog (RBOH) activity and type III peroxidases. The signaling process in the apoplast is then quickly relayed to the chloroplast, where the ROS signal is amplified by chloroplastic ROS formation. This process is regulated by heterotrimeric G proteins (Joo et al., 2005; Booker et al., 2012). The intracellular pathways promoted by ROS involve the activation of mitogen-activated protein kinase (MAPK) cascades, modification of intracellular redox homeostasis, and generation of NO (Morales et al., 2021).
The absence of α- or β-subunits of the G-protein in gpa1 or agb1 mutants resulted in the first early peak of ROS not being generated, while only the G α-subunit was required for the second peak of ROS accumulation (Joo et al., 2005; Vainonen and Kangasjärvi, 2015). These results indicate that ROS synthesis in the chloroplasts may be involved in apoplast signaling process (Shapiguzov et al., 2012; Sierla et al., 2013). Retrograde signaling from the chloroplasts to the nucleus may cause modifications in nuclear gene expression (Leister, 2012; Estavillo et al., 2013). This signaling process may be achieved via ABI4 (Koussevitzky et al., 2007), WHIRLY 1 (Isemer et al., 2012), and PTM (PHD-type transcription factor with transmembrane domains) transcription factors (Sun et al., 2011) as well as metabolites such as Mg-protoporphyrin IX (Strand et al., 2003), heme (Woodson et al., 2011), 3′-phosphoadenosine-5′-phosphate (PAP) (Xiao et al., 2012) and β-cyclocitral (Ramel et al., 2012).
3 Plant sensing and indication of O3 stress
Plants respond differentially to ozone exposure. The response pattern of ozone-tolerant and sensitive plants has been reported in several studies. Ozone-responsive proteins and signaling molecules are primarily involved in ozone sensing. The mechanisms involved in O3 sensing are as follows: a) recognition by an apoplastic receptor protein, which can be changed by the ROS synthesis related to O3 breakdown, b) oxidation of plasma membrane lipids resulting in the generation of lipid-based signaling molecules that are further sensed, and c) modification in the redox homeostasis due to the participation of ascorbic acid (AsA), glutathione (GSH) or the ratio NAD(P)H/NAD(P) (nicotinamide adenine dinucleotide phosphate-with and without hydrolase) (Kangasjärvi et al., 2005).
Excess ROS production due to O3 exposure causes cellular damage; however, ROS initially act as signaling molecules (Jaspers and Kangasjärvi, 2010). ROS can alter signal transduction proteins within membranes in response to O3 stress (Rossard et al., 2006). Consequently, several cellular changes occur, such as a) depolarization and dysfunction of the membrane, b) modification of cell wall compounds, c) promotion of MAP kinase protein cascades to generate new proteins through transcription factor activation, d) ozonolysis of double bonds in the unsaturated fatty acids of cell membranes, and e) lipid peroxidation in the membrane (Evans et al., 2005; Iriti and Faoro, 2008; Sharma et al., 2012; Emberson et al., 2018).
Baier et al. (2005) noted that the signaling process of O3 from the chemical reaction sites in the apoplast or plasma membrane to the cytosol can be associated with O3 induced ROS production, particularly H2O2, which functions as a diffusible messenger (Laloi et al., 2004) and modulates cytosolic AsA and GSH (Gomez et al., 2004). Extracellular peroxidases (PRX) and plasma membrane-bound NADPH oxidases (RBOH) enhance ROS generation under O3 stress (Kangasjärvi et al., 2005). In addition to H2O2, ethylene and salicylic acid (SA) have been reported as secondary or tertiary messenger molecules involved in O3 sensing, because O3 or O3-derived ROS can activate them. Both ethylene and SA can increase oxidative signaling (Sandermann, 2000; Kangasjärvi et al., 2005).
Exposure of plants to O3 also triggers SA-induced cell death because SA can inhibit the main H2O2-scavenging enzymes such as catalase (CAT) and ascorbate peroxidase (APX) (Faoro and Iriti, 2009). Under severe exposure to O3, NO synthesis increased. This molecule plays a crucial role in the signaling of plants subjected to stress conditions. Moreover, NO can react with H2O2 and NO donors such as sodium nitroprusside (SNP), leading to the accumulation of H2O2 in plants (Astier et al., 2018). Another consequence of long-term exposure to O3 is increased cell wall lignification in many plants, which helps decrease O3 penetration (Cabané et al., 2012).
The physiological damage caused by O3 exposure is related to photosynthetic reduction, ROS generation, increased dark respiration, and reduced crop yield (Cailleret et al., 2018). More accurately, the main effects of chronic exposure to O3 are a reduction in the photosynthetic rate, growth reduction, and premature senescence without visible symptoms (Krupa, 2003). In contrast, acute exposure to O3 results in cell death and other adverse effects (Kangasjärvi et al., 2005). Symptoms of O3 exposure can be observed between the veins on the adaxial leaf surfaces of older and middle-aged leaves; however, symptoms can appear on both leaf surfaces (adaxial and abaxial) in some species if the damage is severe (Cho et al., 2011; Vaultier and Jolivet, 2015). Induced chlorosis and bronzing (several spots on the leaves) are the most frequent symptoms of chronic O3 exposure, and acute exposure may result in a higher number of visible lesions (Kangasjärvi et al., 2005).
4 Ozone as a Prooxidant and O3-induced oxidative stress
Ozone can directly generate ROS in the leaf mesophyll and guard cell walls after entering the stomata (Grulke and Heath, 2020). Higher exposure activates the pro-oxidant activity of O3 by increasing ROS accumulation and decreasing the antioxidant machinery in plant cells (Dhevagi et al., 2021; Ramya et al., 2021a). Moreover, the overgeneration of ROS and the reaction between O3 and plasma membrane lipids results in peak levels of superoxide and thiobarbituric acid (Marchica et al., 2019). This phenomenon confirmed the oxidative stress in plant cells upon O3 exposure. Under O3 exposure, plants suffer several physiological damages, resulting in a lower photosynthetic rate, which in turn results in growth reduction, premature senescence, and cell death, mainly due to the generation of ROS. To counteract these harmful effects, plants activate different antioxidant systems to scavenge the reactive molecules (Figure 1).
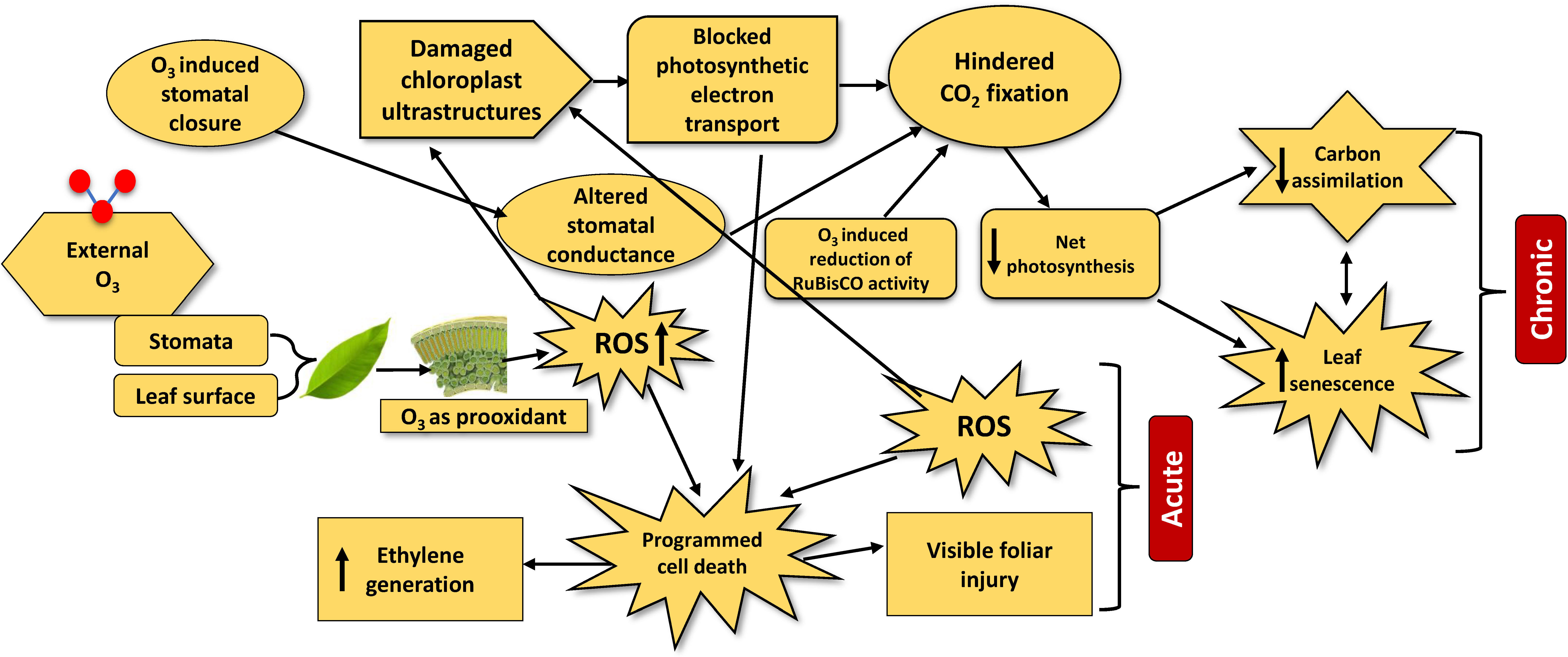
Figure 1 The major physiological processes and mechanisms involved in leaf injury under chronic and acute ozone exposure. The upward arrow inside boxes indicates increase and downward arrow indicates decrease.
Several studies have investigated the pro-oxidant effects of O3 and the respective changes in redox homeostasis. Rice (Oryza sativa L.) seedlings of three different cultivars, Nipponbare and BRRI dhan28 (sensitive to O3), and L81 (O3-tolerant introgression line), were grown from April to October 2016 in a controlled climate greenhouse. Plants were sprayed with 80 ppb O3 for 7 hours daily, five weeks after transplantation to induce acute stress. Owing to tolerance differences between cultivars, malondialdehyde (MDA) content increased in sensitive cultivars but not in the tolerant line. However, the total leaf AsA did not show any significant differences among the cultivars (Ashrafuzzaman et al., 2017). Tobacco (Nicotiana tabacum L.) plants exposed to 300 nmol mol-1 for 4 h at midday showed clear symptoms of leaf necrosis and a reduced net photosynthetic rate. Oxidative stress caused by exposure to O3 results in an increase in H2O2 and MDA contents, and ion leakage (Guo et al., 2009). In another experiment, two cultivars of bean (Phaseolus vulgaris L.) with different O3 tolerance levels (O3-sensitive “Cannellino” and O3-tolerant “Top Crop”) were subjected to a severe O3 stress (165 nL L-1). Biochemical characterization of different cultivars showed that exposure to this phytotoxic air pollutant increased superoxide dismutase (SOD) and CAT activities in both cultivars, which was more pronounced in the tolerant genotype (Guidi et al., 2010). Similarly, two cultivars of soybean (Glycine max L.) with opposed degrees of O3 tolerance (O3-sensitive “Mandarin (Ottawa)” and O3-tolerant “Fiskeby III”) were treated with 70 ppb for 4 days (7 h day-1). Histochemical assays performed on these cultivars showed an accumulation of H2O2 via 3,3′-diaminobenzidine (DAB) in the sensitive cultivar, whereas no spots were detected in the tolerant cultivar. Nevertheless, superoxide anion (O2•-) generation showed different trends in the two cultivars when tested by nitro blue tetrazolium chloride (NBT) reduction. There were no significant differences in SOD and glutathione reductase (GR) activities between the genotypes or O3 treatments (Chutteang et al., 2016). Similarly, Szpunar-Krok et al. (2020) tested the effects of two doses of O3 (5 and 10 ppm), different exposure times (2, 4, 8, 12, and 16 min), and two application periods (21 and 28 d after sowing) on potatoes (Solanum tuberosum L.). This experiment revealed a significant decrease in the total antioxidant activity based on ABTS•+ and DPPH• radical assays. Table 1 includes additional information on studies that investigated the pro-oxidant effects of O3 on different crops.
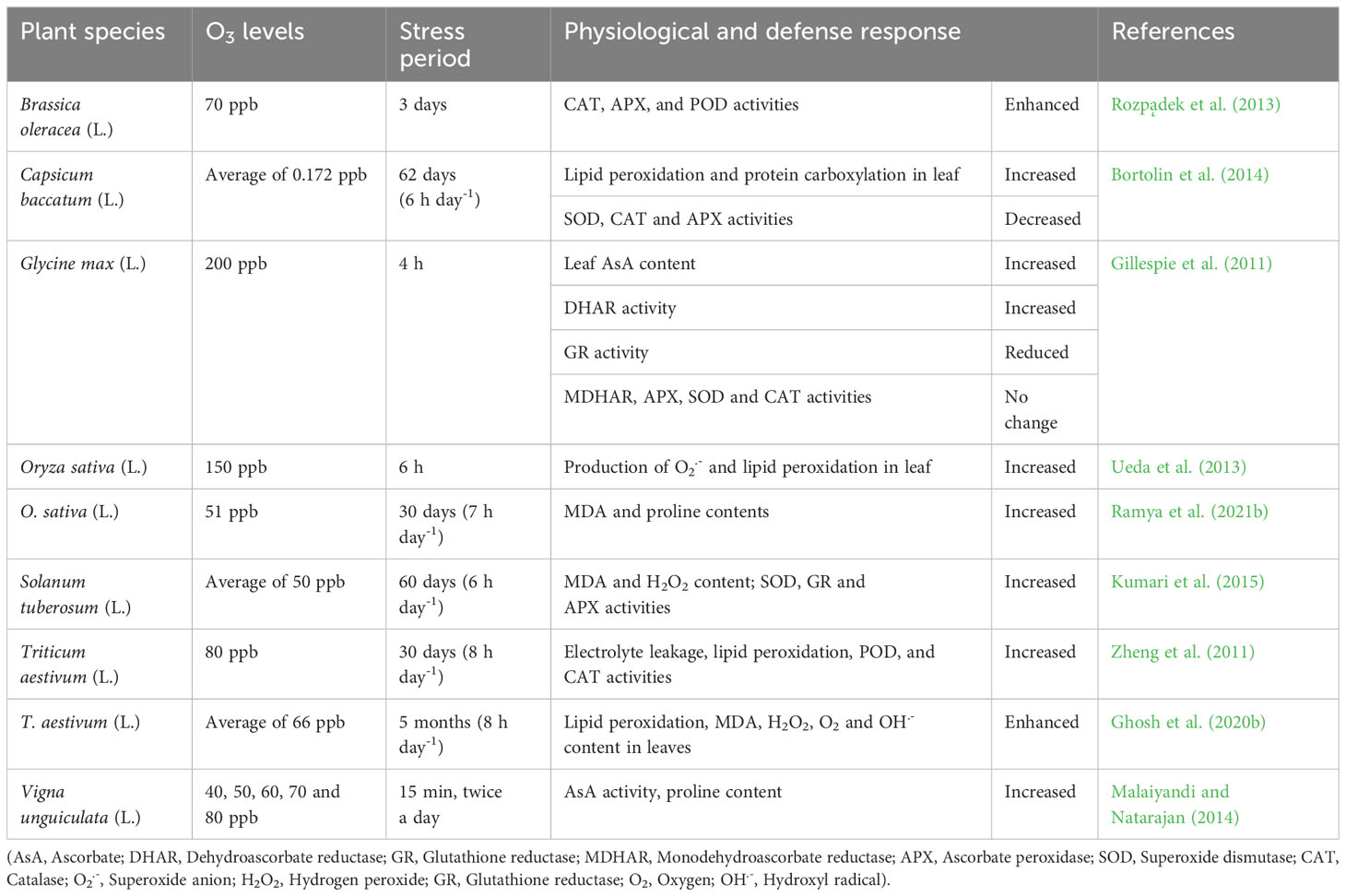
Table 1 Effects of ozone stress in crops and the different defense responses triggered in each species.
5 Plant responses to O3
The response of plants to O3 stress depends on the concentration and duration of exposure. Ozone may also be deposited in plant cells by non-stomatal channels, such as soil and cuticular deposits, in addition to being taken up by the stomata (Morales et al., 2021). Because stomatal opening and closing regulate the flow of O3 into the leaves, stomata act as an initial defense against O3 damage in plants. However, the activities of transporters and ion channels in guard cells that move osmolytes through the tonoplast and plasma membrane regulate the size of the stomatal aperture. After accumulation in plant cells, O3 induces short-term responses in plants depending on the frequency of stress exposure, such as the production of noticeable fine bronze or pale-yellow spots on the upper surface of the leaves. However, O3-induced phytotoxicity begins when O3 diffuses into the vacuolar space of the leaves through stomatal openings, promoting oxidative stress by encouraging the rapid production of ROS in the apoplast (Figure 2). Additionally, O3 can directly diffuse into the cytosol through the cell membrane, generating ROS that alters stomatal conductance (gs) (Ainsworth, 2017. ) Numerous studies have used external O3 to investigate the responses of plants to stress in terms of growth, biomass, reproduction, and yield (Figure 2).
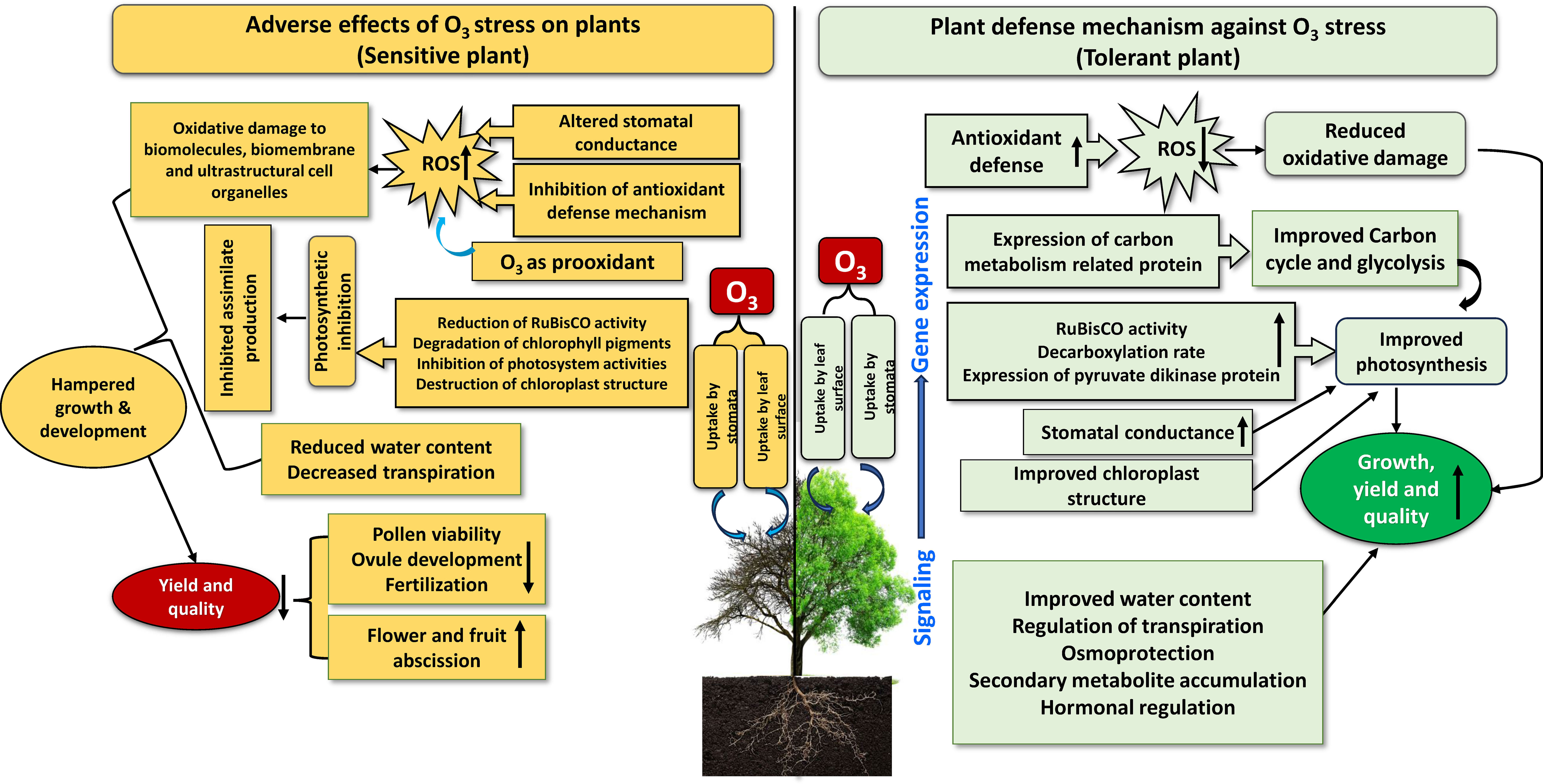
Figure 2 A schematic illustration of the response of sensitive and tolerant plants to ozone. The upward arrow inside boxes indicates increase and downward arrow indicates decrease.
5.1 Crop growth
The response of plants to O3 varies depending on species, cultivar, and developmental stage (Table 2). A few varieties of wheat (Triticum aestivum L.) and rice show visible signs of foliage injury during their early growth stages (Ramya et al., 2021b). Ozone causes early leaf senescence and abscission, which can affect biomass and growth by allocating carbon to edible plant portions. Additionally, the O3-induced reduction in root growth is greater than the reduction in shoot growth because of impaired carbohydrate partitioning in the roots (Witting et al., 2009). However, it adversely affects shoot growth. Yadav et al. (2021) observed significant effects on plant growth when experimenting with four Indian wheat cultivars (early-sown cultivars HUW468 and HD3086; late-sown cultivars HUW234 and HD3118). They reported a greater reduction (26%) in the aboveground biomass of early-sown cultivars than that of late-sown cultivars (21%) under ambient and elevated O3 (ambient+20 ppb).
5.2 Plant physiology and metabolism
Ozone-induced phytotoxicity negatively affects plant physiology and metabolism, including photosynthesis, respiration, transpiration, relative water content, and secondary metabolite accumulation in various crop plants (Cho et al., 2011; Ainsworth et al., 2012; Hassan et al., 2017). In particular, evaluating physiological processes is a more accurate method for assessing intrinsic O3-induced injuries in plants because physiological damage can start at lower O3 concentrations and before the onset of visible impairment (Pandey et al., 2019). Several studies have evaluated the detrimental effects of ambient and elevated O3 on the physiological processes in plants (Table 3). O3-induced injuries are also related to reduced dry mass accumulation in leaves, lower leaf area-based antioxidant levels, and altered gs. However, the O3 uptake is linked to gs,which varies according to the absorption capacity of the cuticle and stem in different plant species, such as the cuticle and stem. For example, increasing the amount of O3 leads to a reduction in gsand stomatal pore area in tomatoes (Solanum lycopersicum L.) (Thwe et al., 2014). Moreover, Yendrek et al. (2015) reported that legume crops, such as peas (Pisum sativum L.), soybeans, and beans, display reduced net photosynthetic rates and leaf longevity. Cabbage (Brassica oleracea var. capitata) (cv. Tekila and Primero) plants showed decreased photosynthetic rates (71.2%), stomatal conductance (81.03%), and chlorophyll content (32.98%) (Ramakrishnan et al., 2023) when exposed to O3 (200 ppb). Ozone-induced stomatal closure occurs because of the inhibition of carbon assimilation in chloroplasts, which leads to an accelerated internal carbon dioxide (CO2) concentration (Ainsworth et al., 2012). Moreover, O3 exposure directly impacted the net CO2 assimilation rate and CO2 fixation ability negatively. Total carbon sequestration and transpiration rates were also related to reduced gsand photosynthetic rates. It has also been observed that O3-induced alterations in the CO2 assimilation rate further influence plant respiration, leading to reduced crop growth and productivity (Ainsworth, 2017). In addition to ROS production, the concentration of secondary metabolites in plant cells is altered by O3-induced stress. Higher concentrations of O3 activate the first enzyme of the phenylpropanoid pathway, leading to a higher accumulation of flavonoids, phenolic acids, monolignols, GSH, gamma-aminobutyric acid (GABA), terpenoids, and volatile organic compounds such as isopropanoids (Mikkelsen et al., 2015).
Ozone exposure also causes a nutrient imbalance in plant cells by altering the allocation of nutritional elements and their ratios in the belowground (root) and aboveground parts (stem and leaves) and disrupting other physiological activities. For example, the potassium (K), calcium (Ca), sodium (Na), iron (Fe), and zinc (Zn) contents in potato tubers are lower under elevated O3 than under ambient conditions, which lowers tuber quality (Kumari and Agrawal, 2014). Similarly, Ghosh et al. (2020a) revealed that the concentrations of nitrogen (N), phosphorus (P), K, magnesium (Mg), and Ca were reduced in the leaves and shoots of wheat under ambient and elevated (ambient+20 ppb) O3 stress from 2 weeks after germination to maturity for 4 h. However, carbon was enhanced under the same stressful conditions, which led to an increase in the C:N and C:K ratios in the leaves. Similarly, as O3 alters the absorption and distribution of macronutrients owing to changes in organic matter mineralization, the uptake of other nutrients is also influenced. Under O3 stress, copper (Cu) concentration was significantly reduced in the leaves, shoots, and roots, although the reduction was higher in the shoots than in the roots. Ozone-induced reduction in leaf N has also been observed in previous studies (Chen et al., 2015; Pandey et al., 2018).
5.3 Reproductive development
Reproductive development is the key determinant of plant productivity and species distribution. Increased O3 has a detrimental effect on the reproductive system, primarily because it alters the allocation of carbon among tissues and directly affects plant reproductive processes (Gillespie et al., 2015). Several studies have demonstrated the effects of O3 on flower initiation, floral development, pod formation, seed quality, seedling germination, and seedling vigor have been demonstrated in several studies. For example, Gillespie et al. (2015) demonstrated that O3 adversely affected pollen viability, pollen germination, germ tube growth, pollen-stigma interactions, and fertilization in tomatoes, leading to decreased seed size, weight, and quality. Consequently, O3-induced lower pollen viability or ovule development leads to reduced fertilization. Moreover, elevated O3 levels promoted flower and pod abscission. For example, decreased pod production without an effect on flower production was observed in soybeans when plants were exposed to 150 ppb of O3 inside O3 chambers. Fruit number, fruit size, seed number, and seed size also decrease at this level of O3 stress in soybeans (Leisner et al., 2014). Additionally, research suggests that the effects of O3 exposure vary depending on the stage of plant growth, affecting flowering patterns in various ways and affecting the pollination and reproduction of annual crops and wild species. According to a recent investigation by Duque et al. (2021a), wild mustard (Sinapis arvensis L.) plants exposed to 120 ppb O3 for 6 h day-1 at earlier stages had more open flowers than the corresponding controls, whereas plants exposed to the same concentrations of O3 at later stages tended to have fewer open flowers. Similarly, when S. arvensis plants were exposed to 120 ppb O3 for 6 h day−1 during the flower initiation stage, flowering accelerated, increasing the proportion of open flowers in O3-exposed plants at the start of the flowering phase (Duque et al., 2021b). Furthermore, the O3-induced reduction in the photosynthesis rate inhibits the accumulation of carbohydrates in pollen in some plant species, conferring adverse effects on pollen germination (Zhang et al., 2017). O3-induced reductions in the number, size, weight, and quality of grains in cereal crops such as rice and wheat have also been observed in some studies (Banerjee and Roychoudhury, 2019; Schauberger et al., 2019). Although O3 has been found to have detectable effects on reproductive growth, the precise sites of action and mechanisms underlying these effects remain unknown.
5.4 Crop yield and quality
A significant proportion of crop yield losses is caused by tropospheric O3, which is a transient, volatile, secondary air pollutant and a powerful phytotoxic compound (Ainsworth, 2017). According to the results of several controlled environmental and field studies, current O3 concentrations in the environment have been found to negatively influence the yield and quality of several crop species worldwide, according to the results of several controlled environment and field studies (Table 4). For example, according to McGrath et al. (2015), yield losses of 10% and 20% were observed in soybean and maize (Zea mays L.), respectively, in combination with dry conditions and high seasonal temperatures, and 5% and 10%, respectively, under rainfed conditions at field levels from 1980 to 2011 due to O3 exposure. Additionally, when two cultivars of tropical maize were exposed to two different doses of O3 (ambient+15 ppb and ambient+30 ppb), a reduction in the test weight of kernel plant-1 of 6% and 10%, respectively, in DHM117, and 4% and 6%, respectively, in HQPM1 was observed (Singh et al., 2014a). Another long-term investigation conducted by Sinha et al. (2015) revealed that yield losses in rice, wheat, and maize were 21–26%, 27–41%, and 3–5%, respectively, under elevated O3. Additionally, according to the Intergovernmental Panel on Climate Change’s Special Report on Emission Scenarios (IPCC SRES) A2 Scenario, O3-induced worldwide yield losses in 2030 will range from 5 to 26% for wheat and 4 to 9% for maize (Avnery et al., 2011). Moreover, a recent study by Ghosh et al. (2020a) revealed that the grain yield was reduced by 45% in wheat cv. HD 2967 under ambient and elevated (ambient+20 ppb) O3 stress for 4 hours day−1 from 2 weeks after germination to maturity. Baqasi et al. (2018) reported a 49% decline in grain dry mass in wheat after 50 ppb O3 exposure. Ozone also influences the quality of crops in terms of starch, protein, nutrients, and oil content. For example, it affects grain quality by decreasing starch content and increasing the protein and nutritional contents of crops such as wheat and rice (Broberg et al., 2015; Frei, 2015).
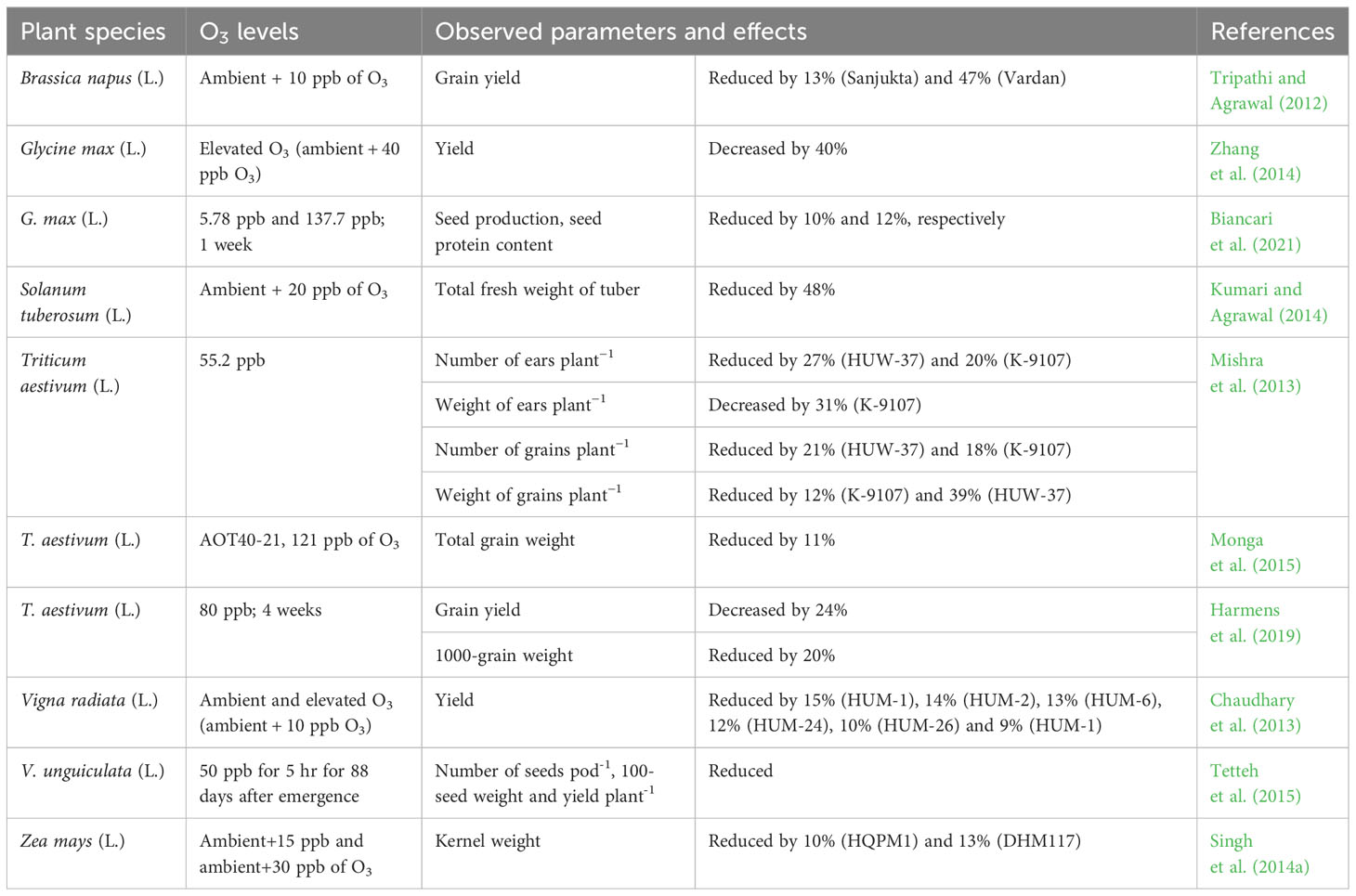
Table 4 Overview of recent studies on O3 responses in crop yield and quality of different plant species.
6 Developing plant tolerance to O3
Higher O3 exposure causes greater yield losses through foliar damage, inhibition of photosynthesis with altered carbon translocation, and faster plant senescence (Osborne et al., 2019). There are opportunities to develop plant tolerance to O3 which ultimately protects the yield under stressful conditions. Breeding for stress tolerance and variety development can be time consuming and costly. Using physiological gateways such as the photosynthetic pathway, antioxidant defense mechanisms, and hormonal regulation to enhance plant tolerance to O3 could be a short-term option (Parankusam et al., 2019; Emberson, 2020). It is mandatory to identify the available options, followed by the most suitable option, to increase plant productivity where mitigation actions can be implemented. In this section, we present an overall discussion focusing on various approaches for developing plant tolerance to O3 and how O3 sensitivity can be lowered.
6.1 Improving photosynthetic pathways
Leaf health, gs, photosynthesis, and photosynthetic machinery are hampered by elevated O3 levels. Improving photosynthesis may be an important approach for attaining higher plant tolerance to O3 exposure. Ozone-mediated chlorophyll decline causes early senescence, but ethylene diurea (EDU) supplementation delays senescence in maize by increasing the chlorophyll content (Gupta et al., 2020; Poornima et al., 2022; Dhevagi et al., 2023). Increased expression of carbon metabolism-related proteins, which are part of the Calvin cycle and glycolysis, contributed to higher O3 tolerance by accumulating more starch, which was reflected in better biomass production in EDU-treated maize. In addition, EDU-induced elevation of RuBisCO activity also supports higher photosynthesis in combating O3 stress. Exogenous EDU increases the C3 and C4 photosynthesis rates by increasing the decarboxylation rate and expression of pyruvate phosphate dikinase protein, respectively, leading to higher O3 tolerance (Alfonso and Brüggemann, 2012). Sensitive wheat cultivars showed higher photosynthetic rates with exogenous EDU application under elevated O3 stress, which was correlated with EDU-induced higher chlorophyll content (Fatima et al., 2019). Therefore, improved photosynthesis and chlorophyll content may explain the higher biomass and yield, which later resulted in increased O3 tolerance due to EDU supplementation.
Exogenous catechin (5 mM) supplementation in rice under elevated O3 conditions reversed O3-induced damage by enhancing chlorophyll content and its precursor (Mg2+ content), gs, which resulted in higher grain production (Kittipornkul et al., 2020). Catechins can improve photosynthetic processes, thereby improving O3 tolerance. Recently, calcium acetate application was shown to increase photosystem (PS)-II efficiency and improve the yield performance of rice under O3 stress (Lakaew et al., 2022). There is a lack of knowledge regarding the mechanisms involved in improving the photosynthetic pathways to regulate plant growth and yield under O3 stress conditions. Further in-depth research is required to elucidate these mechanisms and the associated pathways.
6.2 Enhancing antioxidant defense
Plant antioxidant defenses must be upregulated to scavenge O3-induced excess ROS, thereby protecting cellular functions (Gupta et al., 2020). The inhibition of cellular component peroxidation and the maintenance of the subsequent stability of the cell membrane under O3-induced ROS overgeneration of ROS are distinct features of O3-tolerant plants, which are made possible by the activation of defense mechanisms, including both enzymatic and non-enzymatic antioxidant components (Gill and Tuteja, 2010; Noctor et al., 2014; Pellegrini et al., 2019; Hasanuzzaman et al., 2020). Similarly, Czarnocka and Karpińsky (2018) stated that insufficient responses to plant antioxidants cause oxidative damage and strengthen the defense responses of plants, which are required to develop tolerance to O3.
Both AsA and GSH are major antioxidants in the AsA-GSH cycle and are involved in regulating oxidative damage by scavenging ROS and maintaining cellular redox balance (Hasanuzzaman et al., 2020). Biogenic AgNPs and EDU-mediated higher antioxidant content (AsA and GSH) and enzymatic activities (SOD, CAT, APX, and GR) in wheat resulted in improved O3 tolerance, which was correlated with lower H2O2 and MDA accumulation (Pellegrini et al., 2019).
Fatima et al. (2018) evaluated the ROS-scavenging capability of O3-sensitive and tolerant wheat genotypes through their antioxidant responses. Kharchiya 65 (tolerant) displayed a maximum level of AsA, GSH, and flavonoids along with high free radical scavenging activities as well as lower ROS content than genotypes such as HD 2987 (sensitive) and PBW 502 (intermediately sensitive). At high O3 (ambient+30 ppb), both enzymatic and non-enzymatic antioxidant responses varied among the three cultivars. The highest SOD, peroxidase (POD), GR, and GPX activities were observed in HD 2987, whereas Kharchiya 65 and PBW 502 showed the lowest increases. The maximum APX and the lowest CAT activity was observed in HD 2987 and Kharchiya 65. Sensitive cultivars showed higher enzymatic antioxidant responses when they suffered from O3-induced elevated ROS levels. However, lowered SOD and POD activities were required in the tolerant cultivars, where ROS levels were lower than those in the sensitive ones. Higher free radical scavenging activities were observed in the tolerant (Kharchiya 65) cultivar than in the sensitive cultivar. Non-enzymatic antioxidant levels, such as those of AsA, GSH, and flavonoids, were also higher in the tolerant cultivar than in the sensitive cultivar, which is probably the most efficient mechanism for combating the elevated O3. This may be because higher O3 tolerance is highly associated with the genetic competence to preserve high AsA/DHA (Burkey et al., 2003). In cabbage (cv. Tekila and Primero), proline content was increased by 32.24%, ascorbic acid by 64.75%, CAT activity by 3.58%, and POD activity by 56%, which helped to reduce oxidative stress under O3 stress (200 ppb) (Ramakrishnan et al., 2023). Therefore, variations in antioxidant responses to counteract O3-induced oxidative stress are highly dependent on the crop species and the cultivars of the same crop species (Singh et al., 2014b).
Plant researchers are becoming increasingly interested in selecting appropriate techniques to increase plant tolerance by stimulating antioxidant activity. The supplementation of exogenous chemical substances to O3-exposed plants is one of the most efficient approaches for reducing oxidative stress and cellular damage through the enrichment of antioxidant defense systems (Qiu et al., 2019).
Ethylene diurea is widely used as an anti-ozonant to increase plant tolerance to O3 phytotoxicity and protect plants from damage (Manning et al., 2011). EDU-induced plant protection under O3 stress depends on the activation of antioxidant activity (Gupta et al., 2021). Therefore, EDU supplementation in wheat revealed an EDU-mediated active role of apoplastic SOD, CAT, and amino methyltransferase, which facilitated the maintenance of ROS at optimum levels and decreased O3-induced damage (Gupta et al., 2021).
Foliar spraying of catechin and SA can significantly affect the overexpression of APX and CAT genes, followed by their higher enzymatic activities, leading to lower lipid peroxidation (MDA), and thus increased tolerance of rice to O3 (Kittipornkul et al., 2020).
6.3 Phytohormone regulation
Similar to ROS and Ca, phytohormones, such as abscisic acid (ABA), SA, jasmonic acid (JA), and ethylene (ET), are involved in the regulation of stomatal aperture movement upon O3 exposure, leading to increased plant tolerance, which is mainly related to the cell signaling cascade (Pellegrini et al., 2016). Moreover, O3-induced stomatal movement is controlled by anion channels, such as slow anion channel 1 and open stomata 1 (Vahisalu et al., 2010). These channels are regulated by ABA (Negi et al., 2008). However, variations in O3 tolerance have been attributed to gs and other protective mechanisms involved (Castagna and Ranieri, 2009). How phytohormones are associated with plant tolerance to O3 exposure needs to be explored to better understand plant responses to stressful conditions. It was reported that the O3-mediated increases in JA, asmonoyl-l-isoleucine, and ABA reduce leaf damage in Habataki rice (Tsukahara et al., 2015). Stress-induced apoplastic ROS exacerbates SA synthesis, which contributes to OsORAP1 expression and causes O3 sensitivity and tolerance (Ueda et al., 2015).
Salicylic acid is essential for maintaining antioxidant defense mechanisms and cellular redox responses in plants upon O3 exposure (Hasan et al., 2021). Ozone-induced leaf damage accompanying ET biosynthesis has been confirmed by the inhibition of ET biosynthesis in tobacco following O3 treatment (Bandurska et al., 2009). This suggests that the suppression of ET biosynthesis can increase O3 tolerance in plants. Abscisic acid controls ET and ABA biosynthesis by limiting ABI1 phosphatase activity and ROS homeostasis to induce O3 tolerance (Pellegrini et al., 2016). Jasmonic acid is responsible for suppressing ROS-dependent leaf damage under O3 stress (Hasan et al., 2021). Ozone exposure induces ET-dependent damage, which can be inhibited by JA when AT2G24850 and AT5G24770 are induced by JA (Wang et al., 2017). The significant role of SA in increasing the tolerance of rice to O3 was studied by Kittipornkul et al. (2020), where exogenous 100 µM SA supplementation decreased MDA due to higher activity of CAT, APX upon 100–150 ppb (8 h day−1) O3. Additionally, plants inhibit O3 uptake by increasing SA under O3 exposure as a mechanism of O3 tolerance (Pheomphun et al., 2019). Therefore, a comprehensive research on exogenous phytohormones is required to understand the mechanisms underlying their protective roles in the development of O3 tolerance in cultivated crops.
7 Mitigation of O3 stress in crops
7.1 Improving crop management practices
Because abiotic stress is inevitable, it is crucial to develop strategies to combat stress-induced losses in crop production. Agronomic practices, such as changing the cropping season, air quality management, proper irrigation, and adequate plant protection measures, can be used to manage O3-induced damage in crops. As O3 is strongly linked to seasonal and regional changes, shifting the crop growing season by manipulating the sowing time has been suggested by Teixeira et al. (2011). Seasonal variation in crops can influence the physiological responses of plants by altering their gas exchange capacity, PS I function, and stomatal density upon exposure to O3 stress. The generation of adaptive measures has been recorded through decreased gs, increased stomatal density, and increased PS I activity when plants are exposed to stress at a later stage in their life cycle (Fusaro et al., 2016). Moreover, early sown crops exhibit higher sensitivity to O3 owing to their longer life cycles, lengthy post-anthesis stages, higher gs, and lower threshold levels. In contrast, the comparatively higher enzymatic antioxidant activity of late-grown crops, with increased energy allocation toward growth, facilitates reduced O3-induced damage in crops (Yadav et al., 2019; Yadav et al., 2021). Air quality management to check for O3 precursors is beneficial during O3 exposure. For instance, decreasing methane, an important precursor of O3 and greenhouse gases, has proven to be beneficial in combating O3 (Shindell et al., 2012). Furthermore, controlling nitrogen oxide emissions in air is beneficial for reducing O3 stress in northern China (Lu et al., 2021). By calculating the O3 depletion potential of the substances (responsible for O3 depletion), Ravishankara et al. (2009) reported that nitrous oxide (N2O) is one of the most important greenhouse gases responsible for O3 layer depletion. Nitrous oxide production can be mitigated using mulches (e.g., rice straw) and by minimizing fertilizer requirements in the soil. It is evident that soil water-filled pore spaces play an important role in N2O emissions in the field; therefore, altering nitrogenous fertilizer with mulch can contribute to N2O mitigation (Wu et al., 2018). Intercultural operations, such as mulching, can also reduce O3-induced losses.
Although irrigation is desirable for improving crop production, it can enhance the susceptibility of crops to O3 toxicity. In irrigated crops, the widely open leaf pores are subjected to stimulated gs with elevated uptake of O3 (Mills et al., 2018). This phenomenon is in agreement with another study (Harmens et al., 2019) that concluded that reduced irrigation could be an effective strategy to mitigate O3-induced negative impacts partially or completely by delaying adversities on flag leaves at the time of flower initiation or during the gain-filling period of wheat. Therefore, it is imperative to manage irrigation properly to protect crops from the adverse effects of O3 without causing water stress. For example, alternate wetting and drying irrigation was found to be beneficial for increasing rice productivity with decreasing gs (Carrijo et al., 2017) and was effective in mitigating the adverse impacts of O3. In addition, protective measures should be implemented to control plant competition and prevent plant injury in response to O3 stress. It has been reported that when weed infestation is coupled with O3, it enhances the susceptibility of crops to increased losses compared with O3 exposure alone (Li et al., 2016). Ghosh et al. (2020b) reported a higher yield loss in wheat under O3 stress owing to weed competition and concluded that strong weed management should be introduced to combat O3 stress-affected production loss.
7.2 Nutrient management
Although nutrient supplementation is important for enriching soil fertility, improper nutrient maintenance can increase crop vulnerability to various stressors, including O3 (Tiwari and Agrawal, 2018). Zhang et al. (2018) conducted an experiment to assess the O3 risk management capacity of plant nutrients, where N fertilizer was recorded to elevate the sensitivity of plants to stress; conversely, P improved tolerance by increasing the critical level of O3 exposure in crops. Biomass loss caused by O3 also differed between the two nutrients. Nitrogen fertilization caused the maximum gs, which increased the sensitivity to O3 and, in turn, resulted in a loss of biomass production, whereas P fertilization decreased gs. In another study (Tatsumi et al., 2019), it was claimed that a lack of N supplementation in rice caused N deficiency while giving rise to photosynthetic assimilate translocation to the roots in an attempt to increase nutrient uptake, thereby protecting against O3-induced damage to plant growth. Nutrient supplementation contributes to detoxification of ROS-induced O3. Both N and P were recorded to encourage the mobilization of integrated participation of antioxidant compounds (carotenoid and AsA) and osmoprotectant (proline) and consequently reduce oxidative stress by keeping minimum O2•− and H2O2 while maintaining membrane integrity (Podda et al., 2019). Moreover, N addition can participate in diversifying stored carbohydrates and photosynthates to synthesize amino acids that help repair damage caused by O3 (Podda et al., 2019). Although some studies have shown the negative impacts of O3 and nutrient interactions, improved plant nutrient management can help mitigate O3 stress to some extent (Gautam and Tiwari, 2020).
7.3 Carbon dioxide (CO2) fertilization
Plant growth can be stimulated by the subsidiary carbon supply provided by CO2, which is known as CO2 fertilization. This phenomenon can help relieve stress by reducing ROS production during the oxidative damage caused by various stressors, including O3 (AbdElgawad et al., 2016). When CO2 is used under O3 concentrations in chickpeas (Cicer arietinum L.), a source-sink imbalance changes with the accumulation of photosynthates in leaves and, subsequently, an alteration of phenological characteristics that ultimately accelerate the crop life cycle to early maturity to escape the damage caused by O3 exposure (Singh et al., 2021). Elevated CO2 can counteract the damaging effects of O3 by increasing shoot biomass and pod weight compared to O3-exposed plants alone. Moreover, CO2 fertilization can increase protein, starch, and certain mineral nutrients, even under O3 exposure, thus combatting the negative impacts of imposed stress (Bhatia et al., 2021). A similar compensation tendency was observed in maize (Yadav et al., 2020). Interactive treatment with CO2 and O3 resulted in a positive result, as this combination resulted in a higher photosynthesis rate with improved growth attributes and, consequently, an increased yield component compared to the O3 treatment alone. Carbon dioxide fertilization also results in improved gs and carbon assimilation (Yadav et al., 2020). When elevated CO2 levels were coupled with elevated O3, reduced lipid peroxidation and solute leakage decreased, indicating improved cell membrane integrity. Enhanced antioxidant enzyme activity, which indicates reduced oxidative stress, has also been observed (Kumari et al., 2015). Therefore, CO2 fertilization can help mitigate O3-induced damage to some extent by reducing O3 uptake, increasing carbon assimilation, and reducing oxidative damage, which helps to overcome the detrimental effects on plant growth, physiology (especially photosynthesis), and yield.
7.4 Selecting tolerant crop varieties
The use of tolerant cultivars can be an effective strategy that needs to be expanded by including agricultural practices, particularly in O3 risk areas (Tiwari and Agrawal, 2018). A noticeable variation in rice genotypes in response to stress was observed by Arshad (2021), in which plant height, dry mass, leaf area, plant damage, and leaf damage were visible between susceptible and tolerant genotypes. Biochemical attributes such as total amino acids, sugar, protein profile, and phenolic content were not affected in varieties tolerant to O3 stress, indicating tolerance capacity, whereas a trend of reduction was observed in susceptible genotypes. The susceptible varieties showed early visual symptoms through yellow to brown spots, which later turned into necrosis and early leaf senescence; however, this phenomenon was slower in the tolerant varieties (Arshad, 2021). In wheat, the tolerant variety (HD2967) sensed O3 stressors at an early vegetative stage through increased MDA and initiated ROS scavenging activity, and consequently uplifted better antioxidant defense prior to the reproductive stage to protect against yield losses compared to the sensitive genotype (Sonalika), which was later in sensing (Pandey et al., 2018). Moreover, they added that the tolerant variety has tended to translocate more photosynthetic assimilates to increase biomass and husk weight with better resource partitioning, thus ensuring better protection of the reproductive parts under higher levels of O3 exposure. These findings agree with those of Dhevagi et al. (2021), who concluded that the tolerant variety of mung beans (Vigna mungo L.) had higher AsA under exaggerated O3 concentrations, which resulted in better morphological, physiological, biochemical performance, and antioxidant defense than the susceptible variety. Bailey et al. (2019) demonstrated the distinctiveness of a soybean-tolerant variety in the exclusion of O3 uptake through stomata by lowering gs and the transpiration rate, followed by higher water-use efficiency than the sensitive variety. Furthermore, under O3 exposure, variations in phosphoenolpyruvate, carboxylase activity, and RuBisCO content and activity controlled the tolerance mechanism of maize hybrids more than the antioxidant defense mechanism of gs. Stable leaf N content and RuBisCO activity under reduced O3 exposure indicated better tolerance to late senescence and better yield than sensitive hybrids (Choquette et al., 2020). Therefore, the selection of tolerant varieties under O3 exposure could be an effective approach for adaptation to O3.
7.5 Using chemical elicitors
Elicitors with different chemical structures can be used against different stressors through exogenous application to crops or incorporation of transcription factors through breeding (Chakraborty et al., 2019). The use of various chemical elicitors to protect against O3-induced phytotoxicity has also been demonstrated. For example, chitosan positively influenced wheat growth under O3 exposure (Picchi et al., 2021). In addition to improving crop yield and quality, chitosan serves as a protectant that enhances the defense metabolism of plants by increasing the concentration of AsA within a short period by activating the APX enzyme to control H2O2 and oxidative stress. It also showed limited symptoms on the leaf surface area of chitosan-treated plants compared to O3-stressed plants. The same trend of increasing antioxidant enzyme levels under stress conditions was reported by Kittipornkul et al. (2020), using catechins in rice. Application of catechin to rice under O3 exposure activated antioxidant enzymes and helped to maintain chlorophyll, gs, and Mg contents at the vegetative stage, which consecutively resulted in increased panicle number, filled grain weight, and starch, conferring protection against stress. These chemical elicitors protect plants by forming chemical barriers that detoxify O3 (Li et al., 2018). It has been shown that trichomes have the capacity to deplete O3 near the leaf surface, resulting in reduced O3 uptake through stomata (Li et al., 2018). In addition, stress-induced lipoxygenase (LOX) activity (a consequence of O3 uptake) were maintained through trichome density, providing plant tolerance to O3 exposure. Ethylene diurea is widely used to enhance tolerance to O3 exposure, and its effectiveness has been demonstrated in rice (Ashrafuzzaman et al., 2018), maize (Gupta et al., 2020), groundnuts (Arachis hypogaea L.; Chaudhary and Rathore, 2020) and castors (Ricinus communis L.; Rathore and Chaudhary, 2018). As EDU actively participates in the upregulation of crop growth, photosynthesis, and maintenance of improved membrane properties by activating defense metabolism by increasing AsA and flavonoid content, it can protect against O3 stress (Rathore and Chaudhary, 2018; Chaudhary and Rathore, 2020). Furthermore, in addition to improving plant mechanisms (increased SOD, CAT, and APX activities) to regulate the defense system, EDU enhances protein accumulation in plants, which in turn enhances metabolic functions to mitigate damage under O3 stress (Gupta et al., 2020). Foliar spraying with calcium acetate and calcium chloride improved tolerance through protectant-induced mechanisms of the antioxidant defense system in O3-stressed rice plants (Lakaew et al., 2022). Calcium acetate-treated plants tolerated longer periods of O3 exposure by augmenting NAD kinase and NADPH activities. This calcium acetate-mediated increase in NADPH content was associated with higher AsA and GSH levels, and higher APX and GR activities, resulting in an approximately 29% reduction in MDA generation. This calcium acetate-mediated oxidative stress mitigation contributes to improved plant growth and yield (Lakaew et al., 2022).
8 Conclusion
Over the past few decades, several studies on the effects of O3 on plants have demonstrated that elevated levels of O3 hamper overall plant growth and productivity. Ozone can be degraded into ROS in the mesophyll and guard cell walls, which damage the chloroplast ultrastructure and block photosynthetic electron transport after entering directly through the leaves. Leading to stomatal closure and modification of stomatal conductance O3 hinder CO2 fixation. Ozone induces leaf chlorosis, necrosis, and abscission. Reduced photosynthesis, altered respiration and transpiration, decreased water uptake, disrupted nutrient homeostasis, and the assimilate translocation caused by O3 lead to reduced growth. Both chronic and severe O3 stress can lead to growth reduction, anomalous reproductive development, yield loss, and crop quality deterioration. Approaches for protecting plant physiological pathways such as photosynthesis, antioxidant defense mechanisms, and hormonal regulation have been reported to enhance plant tolerance to elevated O3. Agronomic approaches, such as adjusting planting dates and cropping systems, nutrient management, CO2 fertilization, and the use of several chemical stress elicitors have been shown to improve plant performance under elevated O3. However, it is difficult to conduct research on ambient O3 because it is difficult to measure the amount of ambient O3 and the amount of O3 entering plants. An appropriate method should be developed to understand these issues, and research on meteorological, biochemical, and physiological aspects should be considered. Literature on the effects of O3 on various aspects of plants is readily available, but there is limited availability of literature on strategies for mitigating O3-induced stress. Therefore, various agronomic approaches that may mitigate O3 stress in plants should be determined. Understanding the biochemistry and physiology of O3-stressed plants is vital for developing O3-tolerant plants. Therefore, integrated research themes and their implementation are vital for reducing O3-induced damage and developing O3-tolerant cultivars.
Author contributions
MH and PV conceived and designed the project; FN, AS, KP, PG, and KN wrote the MS. MH and PV edited the manuscript. All authors contributed to the article and approved the submitted version.
Funding
The author(s) declare financial support was received for the research, authorship, and/or publication of this article. This work was supported by the Future Innovation Lab for Collaborative Research on Sustainable Intensification (Grant No. AID-OAA-L-14-00006), funded by the United States Agency for International Development. The authors would like to thank the Feed the Future Innovation Lab for Collaborative Research on Sustainable Intensification (Grant no. AID-OAA-L-14-00006), funded by the United States Agency for International Development for supporting this research.
Acknowledgments
Contribution no. 24-100-J from the Kansas Agricultural Experiment Station. We thank Farha Naz for drafting this manuscript.
Conflict of interest
The authors declare that the research was conducted in the absence of any commercial or financial relationships that could be construed as a potential conflict of interest.
Publisher’s note
All claims expressed in this article are solely those of the authors and do not necessarily represent those of their affiliated organizations, or those of the publisher, the editors and the reviewers. Any product that may be evaluated in this article, or claim that may be made by its manufacturer, is not guaranteed or endorsed by the publisher.
Author disclaimer
The contents of this publication are the sole responsibility of the authors and do not reflect the views of funding agencies or representative organizations.
References
AbdElgawad, H., Zinta, G., Beemster, G. T. S., Janssens, I. A., Asard, H. (2016). Future climate CO2 levels mitigate stress impact on plants: Increased defense or decreased challenge? Front. Plant Sci. 7. doi: 10.3389/fpls.2016.00556
Ainsworth, E. A. (2017). Understanding and improving global crop response to ozone pollution. Plant J. 90, 886–897. doi: 10.1111/tpj.13298
Ainsworth, E. A., Yendrek, C. R., Sitch, S., Collins, W. J., Emberson, L. D. (2012). The effects of tropospheric ozone on net primary productivity and implications for climate change. Annu. Rev. Plant Biol. 63, 637–661. doi: 10.1146/annurev-arplant-042110-103829
Alfonso, S. U., Brüggemann, W. (2012). Photosynthetic responses of a C3 and three C4 species of the genus Panicum (s.l.) with different metabolic subtypes to drought stress. Photosynth. Res. 112, 175–191. doi: 10.1007/s11120-012-9763-4
Alves, E. S., Moura, B. B., Pedroso, A. N. V., Tresmondi, F., MaChado, S. R. (2016). Cellular markers indicative of ozone stress on bioindicator plants growing in a tropical environment. Ecologic. Indicat. 67, 417–424. doi: 10.1016/j.ecolind.2016.03.011
Arshad, A. (2021). A growth and biochemistry of ten high yielding genotypes of Pakistani rice (Oryza sativa L.) at maturity under elevated tropospheric ozone. Heliyon. 7, e08198. doi: 10.1016/j.heliyon.2021.e08198
Ashrafuzzaman, M., Haque, Z., Ali, B., Mathew, B., Yu, P., Hochholdinger, F., et al. (2018). Ethylenediurea (EDU) mitigates the negative effects of ozone in rice: Insights into its mode of action. Plant Cell Environ. 41, 2882–2898. doi: 10.1111/pce.13423
Ashrafuzzaman, M., Lubna, F. A., Holtkamp, F., Manning, W. J., Kraska, T., Frei, M. (2017). Diagnosing ozone stress and differential tolerance in rice (Oryza sativa L.) with ethylenediurea (EDU). Environ. Pollut. 230, 339–350. doi: 10.1016/j.envpol.2017.06.055
Astier, J., Gross, I., Durner, J. (2018). Nitric oxide production in plants: An update. J. Exp. Bot. 69, 3401–3411. doi: 10.1093/jxb/erx420
Avnery, S., Mauzerall, D. L., Liu, J., Horowitz, L. W. (2011). Global crop yield reductions due to surface ozone exposure: 1. Year 2000 crop production losses and economic damage. Atmos. Environ. 45, 2284–2296. doi: 10.1016/j.atmosenv.2010.11.045
Baier, M., Kandlbinder, A., Golldack, D., Dietz, K. J. (2005). Oxidative stress and ozone: perception, signaling and response. Plant Cell Environ. 28, 1012–1020. doi: 10.1111/j.1365-3040.2005.01326.x
Bailey, A., Burkey, K., Taggart, M., Rufty, T. (2019). Leaf traits that contribute to differential ozone response in ozone-tolerant and sensitive soybean genotypes. Plants 8, 235. doi: 10.3390/plants8070235
Bandurska, H., Borowiak, K., Miara, M. (2009). Effect of two different ambient ozone concentrations on antioxidative enzymes in leaves of two tobacco cultivars with contrasting ozone sensitivity. Acta Biol. Crac. Ser. Bot. 51, 37–44.
Banerjee, A., Roychoudhury, A. (2019). “Rice responses and tolerance to elevated ozone,” in Advances in rice research for abiotic stress tolerance. Eds. Hasanuzzaman, M., Fujita, M., Nahar, K., Biswas, J. K. (United Kingdom: Woodhead Publishing), 399–411.
Baqasi, L. A., Qari, H. A., Al-Nahhas, N., Badr, R. H., Taia, W. K., El-Dakkak, R., et al. (2018). Effects of low concentration of ozone (O3) on metabolic and physiological attributes in wheat (Triticum aestivum L.) plants. Biomed. Pharmacol. J. 11, 929–934. doi: 10.13005/bpj/1450
Bhatia, A., Mina, U., Kumar, V., Tomer, R., Kumar, A., Chakrabarti, B., et al. (2021). Effect of elevated ozone and carbon dioxide interaction on growth, yield, nutrient content and wilt disease severity in chickpea grown in Northern India. Heliyon 7, e06049. doi: 10.1016/j.heliyon.2021.e06049
Biancari, L., Cerrotta, C., Menéndez, A. I., Gundel, P. E., Martínez-Ghersa, M. A. (2021). Episodes of high tropospheric ozone reduce nodulation, seed production and quality in soybean (Glycine max (L.) merr.) on low fertility soils. Environ. pollut. 269, 116117. doi: 10.1016/j.envpol.2020.116117
Booker, F., Burkey, K., Morgan, P., Fiscus, E., Jones, A. (2012). Minimal influence of G-protein null mutations on ozone-induced changes in gene expression, foliar injury, gas exchange and peroxidase activity in Arabidopsis thaliana L. Plant Cell Environ. 35, 668–681. doi: 10.1111/j.1365-3040.2011.02443.x
Bortolin, R. C., Caregnato, F. F., Divan, J. A. M., Reginatto, F. H., Gelain, D. P., Moreira, J. C. F. (2014). Effects of chronic elevated ozone concentration on the redox state and fruit yield of red pepper plant Capsicum baccatum. Ecotoxicol. Environ. Saf. 100, 114–121. doi: 10.1016/j.ecoenv.2013.09.035
Bortolin, R. C., Caregnato, F. F., Junior, A. M. D., Zanotto-Filho, A., Moresco, K. S., de Oliveira Rios, A., et al. (2016). Chronic ozone exposure alters the secondary metabolite profile, antioxidant potential, anti-inflammatory property, and quality of red pepper fruit from Capsicum baccatum. Ecotoxicol. Environ. Saf. 129, 16–24. doi: 10.1016/j.ecoenv.2016.03.004
Broberg, M. C., Feng, Z., Xin, Y., Pleijel, H. (2015). Ozone effects on wheat grain quality–A summary. Environ. pollut. 197, 203–213. doi: 10.1016/j.envpol.2014.12.009
Burkey, K. O., Eason, G., Fiscus, E. L. (2003). Factors that affect leaf extracellular ascorbic acid content and redox status. Physiol. Plant 117, 51–57. doi: 10.1034/j.1399-3054.2003.1170106.x
Cabané, M., Afif, D., Hawkins, S. (2012). Lignins and abiotic stresses. Adv. Bot. Res. 61, 219–262. doi: 10.1016/B978-0-12-416023-1.00007-0
Cailleret, M., Ferretti, M., Gessler, A., Rigling, A., Schaub, M. (2018). Ozone effects on European forest growth-Towards an integrative approach. J. Ecol. 106, 1377–1389. doi: 10.1111/1365-2745.12941
Carrijo, D. R., Lundy, M. E., Linquist, B. A. (2017). Rice yields and water use under alternate wetting and drying irrigation: A meta-analysis. Field Crops Res. 203, 173–180. doi: 10.1016/j.fcr.2016.12.002
Castagna, A., Ranieri, A. (2009). Detoxification and repair process of ozone injury: From O3 uptake to gene expression adjustment. Environ. pollut. 157, 1461–1469. doi: 10.1016/j.envpol.2008.09.029
Chakraborty, N., Sarkar, A., Acharya, K. (2019). ““Elicitor-mediated amelioration of abiotic stress in plants,”,” in Molecular plant abiotic stress: biology and biotechnology. Eds. Roychoudhury, D. A., Tripathi, D. D. (United States: Wiley Online Library), 105–122.
Chaudhary, I. J., Rathore, D. (2020). Relative effectiveness of ethylenediurea, phenyl urea, ascorbic acid and urea in preventing groundnut (Arachis hypogaea L.) crop from ground level ozone. Environ. Technol. Innov. 19, 100963. doi: 10.1016/j.eti.2020.100963
Chaudhary, N., Singh, S., Agrawal, S. B., Agrawal, M. (2013). Assessment of six Indian cultivars of mung bean against ozone by using foliar injury index and changes in carbon assimilation, gas exchange, chlorophyll fluorescence and photosynthetic pigments. Environ. Monit. Assess. 185, 7793–7807. doi: 10.1007/s10661-013-3136-0
Chen, Z., Cao, J., Yu, H., Shang, H. (2018). Effects of elevated ozone levels on photosynthesis, biomass and non-structural carbohydrates of Phoebe bournei and Phoebe zhennan in subtropical China. Front. Plant Sci. 9. doi: 10.3389/fpls.2018.01764
Chen, Z., Wang, X., Shang, H. (2015). Structure and function of rhizosphere and non-rhizosphere soil microbial community respond differently to elevated ozone in field-planted wheat. J. Environ. Sci. 32, 126–134. doi: 10.1016/j.jes.2014.12.018
Cho, K., Tiwari, S., Agrawal, S. B., Torres, N. L., Agrawal, M., Sarkar, A., et al. (2011). Tropospheric ozone and plants: absorption, responses, and consequences. Rev. Environ. Contam. Toxicol. 212, 61–111. doi: 10.1007/978-1-4419-8453-1_3
Choquette, N. E., Ainsworth, E. A., Bezodis., W., Cavanagh, A. P. (2020). Ozone tolerant maize hybrids maintain Rubisco content and activity during long-term exposure in the field. Plant Cell Environ. 43, 3033–3047. doi: 10.1111/pce.13876
Chutteang, C., Booker, F. L., Na-Ngern, P., Burton, A., Aoki, M., Burkey, K. O. (2016). Biochemical and physiological processes associated with the differential ozone response in ozone-tolerant and sensitive soybean genotypes. Plant Biol. 18, 28–36. doi: 10.1111/plb.12347
Czarnocka, W., Karpińsky, S. (2018). Friend or foe? Reactive oxygen species production, scavenging and signaling in plant response to environmental stresses. Free Radic. Biol. Med. 122, 4–20. doi: 10.1016/j.freeradbiomed.2018.01.011
Dawson, T. E., Burgess, S. S. O., Tu, K. P., Oliveira, R. S., Santiago, L. S., Fisher, J. B., et al. (2007). Night-time transpiration in woody plants from contrasting ecosystems. Tree Physiol. 27, 561–575. doi: 10.1093/treephys/27.4.561
Dhevagi, P., Ramya, A., Poornima, R., Chandrakumar, K. (2023). Effectiveness of ethylene diurea in ameliorating ozone stress in blackgram varieties (Vigna mungo L.). Arch. Agron. Soil Sci. 69, 1503–1518. doi: 10.1080/03650340.2022.2099542
Dhevagi, P., Ramya, A., Priyatharshini, S., Poornima, R. (2021). Effect of elevated tropospheric ozone on Vigna mungo L. Varieties. Ozone: Sci. Eng. 44, 566–586. doi: 10.1080/01919512.2021.2009332
Duque, L., Poelman, E. H., Steffan-Dewenter, I. (2021a). Plant age at the time of ozone exposure affects flowering patterns, biotic interactions and reproduction of wild mustard. Sci. Rep. 11, 1–10. doi: 10.1038/s41598-021-02878-9
Duque, L., Poelman, E. H., Steffan-Dewenter, I. (2021b). Effects of ozone stress on flowering phenology, plant-pollinator interactions and plant reproductive success. Environ. pollut. 272, 15953. doi: 10.1016/j.envpol.2020.115953
Emberson, L. (2020). Effects of ozone on agriculture, forests and grasslands. Philos. Trans. R. Soc 378, 20190327. doi: 10.1098/rsta.2019.0327
Emberson, L. D., Pleijel, H., Ainsworth, E. A., Van den Berg, M., Ren, W., Osborne, S., et al. (2018). Ozone effects on crops and consideration in crop models. Eur. J. Agron. 100, 19–34. doi: 10.1016/j.eja.2018.06.002
Estavillo, G. M., Chan, K. X., Phua, S. Y., Pogson, B. J. (2013). Reconsidering the nature and mode of action of metabolite retrograde signaling from the chloroplast. Front. Plant Sci. 3, 300. doi: 10.3389/fpls.2012.00300
Evans, N. H., McAinsh, M. R., Hetherington, A. M., Knight, M. R. (2005). ROS perception in Arabidopsis thaliana: the ozone-induced calcium response. Plant J. 41, 615–626. doi: 10.1111/j.1365-313X.2004.02325.x
Faoro, F., Iriti, M. (2009). Plant cell death and cellular alterations induced by ozone: key studies in Mediterranean conditions. Environ. pollut. 157, 1470–1477. doi: 10.1016/j.envpol.2008.09.026
Fatima, A., Singh, A. A., Mukherjee, A., Agrawal, M., Agrawal, S. B. (2018). Variability in defence mechanism operating in three wheat cultivars having different levels of sensitivity against elevated ozone. Environ. Exp. Bot. 155, 66–78. doi: 10.1016/j.envexpbot.2018.06.015
Fatima, A., Singh, A. A., Mukherjee, A., Dolker, T., Agrawal, M., Agrawal, S. B. (2019). Assessment of ozone sensitivity in three wheat cultivars using ethylenediurea. Plants 8, 80. doi: 10.3390/plants8040080
Fiscus, E. L., Booker, F. L., Burkey, K. O. (2005). Crop responses to ozone: uptake, modes of action, carbon assimilation and partitioning. Plant Cell Environ. 28, 997–1011. doi: 10.1111/j.1365-3040.2005.01349.x
Frei, M. (2015). Breeding of ozone resistant rice: Relevance, approaches and challenges. Environ. pollut. 197, 144–155. doi: 10.1016/j.envpol.2014.12.011
Fusaro, L., Gerosa, G., Salvatori, E., Marzuoli, R., Monga, R., Kuzminsky, E., et al. (2016). Early and late adjustments of the photosynthetic traits and stomatal density in Quercus ilex L. grown in an ozone-enriched environment. Plant Biol. 18, 13–21. doi: 10.1111/plb.12383
Gautam, A. K., Tiwari, S. (2020). ““Use of different agronomic practices to minimize ozone injury in plants: A step toward sustainable agriculture”,” in New frontiers in stress management for durable agriculture. Eds. Rakshit, A., Singh, H., Singh, A., Singh, U., Fraceto, L. (Singapore: Sringer), 213–229.
Ghosh, A., Agrawal, M., Agrawal, S. B. (2020a). Effect of water deficit stress on an Indian wheat cultivar (Triticum aestivum L. HD 2967) under ambient and elevated level of ozone. Sci. Total Environ. 714, 136837. doi: 10.1016/j.scitotenv.2020.136837
Ghosh, A., Pandey, B., Agrawal, M., Agrawal, S. B. (2020b). Interactive effects and competitive shift between Triticum aestivum L. (wheat) and Chenopodium album L. (fat-hen) under ambient and elevated ozone. Environ. pollut. 265, 114764. doi: 10.1016/j.envpol.2020.114764
Gill, S. S., Tuteja, N. (2010). Reactive oxygen species and antioxidant machinery in abiotic stress tolerance in crop plants. Plant Physiol. Biochem. 48, 909–930. doi: 10.1016/j.plaphy.2010.08.016
Gillespie, C., Stabler, D., Tallentire, E., Goumenaki, E., Barnes, J. (2015). Exposure to environmentally-relevant levels of ozone negatively influence pollen and fruit development. Environ. pollut. 206, 494–501. doi: 10.1016/j.envpol.2015.08.003
Gillespie, K. M., Rogers, A., Ainsworth, E. A. (2011). Growth at elevated ozone or elevated carbon dioxide concentration alters antioxidant capacity and response to acute oxidative stress in soybean (Glycine max). J. Exp. Bot. 62, 2667–2678. doi: 10.1093/jxb/erq435
Gomez, L. D., Noctor, G., Knight, M. R., Foyer, C. H. (2004). Regulation of calcium signaling and gene expression by glutathione. J. Exp. Bot. 55, 1851–1859. doi: 10.1093/jxb/erh202
Grulke, N. E., Heath, R. L. (2020). Ozone effects on plants in natural ecosystems. Plant Biol. 22, 12–37. doi: 10.1111/plb.12971
Guidi, L., Degl’Innocenti, E., Giordano, C., Biricolti, S., Tattini, M. (2010). Ozone tolerance in Phaseolus vulgaris depends on more than one mechanism. Environ. pollut. 158, 3164–3171. doi: 10.1016/j.envpol.2010.06.037
Guo, J., Li, X. F., Qi, D. M., Chen, S. Y., Li, Z. Q., Nijs, I., et al. (2009). Effects of ozone on wild type and transgenic tobacco. Biol. Plant 53, 670–676. doi: 10.1007/s10535-009-0121-0
Gupta, S. K., Sharma, M., Majumder, B., Maurya, V. K., Deeba, F., Zhang, J. L. (2020). Effects of ethylenediurea (EDU) on regulatory proteins in two maize (Zea mays L.) varieties under high tropospheric ozone phytotoxicity. Plant Physiol. Biochem. 154, 675–688. doi: 10.1016/j.plaphy.2020.05.037
Gupta, S. K., Sharma, M., Maurya, V. K., Deeba, F., Pandey, V. (2021). Effects of ethylenediurea (EDU) on apoplast and chloroplast proteome in two wheat varieties under high ambient ozone: An approach to investigate EDU’s mode of action. Protoplasma 258, 1009–1028. doi: 10.1007/s00709-021-01617-1
Han, Y. J., Gharibeshghi, A., Mewis, I., Förster, N., Beck, W., Ulrichs, C. (2020). Plant responses to ozone: Effects of different ozone exposure durations on plant growth and biochemical quality of Brassica campestris L. ssp. Chinensis. Sci. Hortic. 262, 108921. doi: 10.1016/j.scienta.2019.108921
Harmens, H., Hayes, F., Sharps, K., Radbourne, A., Mills, G. (2019). Can reduced irrigation mitigate ozone impacts on an ozone-sensitive African wheat variety? Plants 8, 220. doi: 10.3390/plants8070220
Hasan, M. M., Rahman, M. A., Skalicky, M., Alabdallah, N. M., Waseem, M., Jahan, M. S., et al. (2021). Ozone induced stomatal regulations, MAPK and phytohormone signaling in plants. Int. J. Mol. Sci. 22, 6304. doi: 10.3390/ijms22126304
Hasanuzzaman, M., Bhuyan, M. H. M., Zulfiqar, F., Raza, A., Mohsin, S. M., Mahmud, J. A., et al. (2020). Reactive oxygen species and antioxidant defense in plants under abiotic stress: Revisiting the crucial role of a universal defense regulator. Antioxidants 9, 681. doi: 10.3390/antiox9080681
Hassan, I. A., Haiba, N. S., Badr, R. H., Basahi, J. M., Almeelbi, T., Ismail, I. M., et al. (2017). Effects of ambient ozone on reactive oxygen species and antioxidant metabolites in leaves of pea (Pisum sativum L.) plants. Pak. J. Bot. 49, 47–55.
Hedrich, R. (2012). Ion channels in plants. Physiol. Rev. 92, 1777–1811. doi: 10.1152/physrev.00038.2011
Iriti, M., Faoro, F. (2008). Oxidative stress, the paradigm of ozone toxicity in plants and animals. Water Air Soil pollut. 187, 285–301. doi: 10.1007/s11270-007-9517-7
Isemer, R., Mulisch, M., Schäfer, A., Kirchner, S., Koop, H. U., Krupinska, K. (2012). Recombinant Whirly1 translocates from transplastomic chloroplasts to the nucleus. FEBS Lett. 586, 85–88. doi: 10.1016/j.febslet.2011.11.029
Jain, S. L., Arya, B. C., Kumar, A., Ghude, S. D., Kulkarni, P. S. (2005). Observational study of surface ozone at New Delhi, India. Int. J. Remote Sens. 26, 3515–3524. doi: 10.1080/01431160500076616
Jaspers, P., Kangasjärvi, J. (2010). Reactive oxygen species in abiotic stress signaling. Physiol. Plant 138, 405–413. doi: 10.1111/j.1399-3054.2009.01321.x
Jimenez-Montenegro, L., Lopez-Fernandez, M., Gimenez, E. (2021). Worldwide research on the ozone influence in plants. Agron. 11 1504. doi: 10.3390/agronomy11081504
Joo, J. H., Wang, S., Chen, J. G., Jones, A. M., Fedoroff, N. V. (2005). Different signaling and cell death roles of heterotrimeric G protein alpha and beta subunits in the Arabidopsis oxidative stress response to ozone. Plant Cell. 17, 957–970. doi: 10.1105/tpc.104.029603
Kangasjärvi, J., Jaspers, P., Kollist, H. (2005). Signalling and cell death in ozone-exposed plants. Plant Cell Environ. 28, 1021–1036. doi: 10.1111/j.1365-3040.2005.01325.x
Kittipornkul, P., Treesubsuntorn, C., Thiravetyan, P. (2020). Effect of exogenous catechin and salicylic acid on rice productivity under ozone stress: the role of chlorophyll contents, lipid peroxidation, and antioxidant enzymes. Environ. Sci. pollut. Res. 27, 25774–25784. doi: 10.1007/s11356-020-08962-3
Kollist, H., Jossier, M., Laanemets, K., Thomine, S. (2011). Anion channels in plant cells. FEBS J. 278, 4277–4292. doi: 10.1111/j.1742-4658.2011.08370.x
Koussevitzky, S., Nott, A., Mockler, T. C., Hong, F., Sachetto-Martins, G., Surpin, M., et al. (2007). Signals from chloroplasts converge to regulate nuclear gene expression. Science. 316, 715–719. doi: 10.1126/science.1140516
Krupa, S. V. (2003). Joint effects of elevated levels of ultraviolet-B radiation, carbon dioxide and ozone on plants. Photochem. Photobiol. 78, 535–542. doi: 10.1562/0031-8655(2003)078<0535:JEOELO>2.0.CO;2
Kumari, S., Agrawal, M. (2014). Growth, yield and quality attributes of a tropical potato variety (Solanum tuberosum L. cv Kufri chandramukhi) under ambient and elevated carbon dioxide and ozone and their interactions. Ecotoxicol. Environ. Saf. 101, 146–156. doi: 10.1016/j.ecoenv.2013.12.021
Kumari, S., Agrawal, M., Singh, A. (2015). Effects of ambient and elevated CO2 and ozone on physiological characteristics, antioxidative defense system and metabolites of potato in relation to ozone flux. Environ. Exp. Bot. 109, 276–287. doi: 10.1016/j.envexpbot.2014.06.015
Lakaew, K., Akeprathumchai, S., Thiravetyan, P. (2022). Effect of calcium acetate and calcium chloride on grain morphology and antioxidant regulation in rice under ozone stress. J. Plant Growth Regul. 41, 3138–3152. doi: 10.1007/s00344-021-10501-4
Laloi, C., Mestres-Ortega, D., Marco, Y., Meyer, Y., Reichheld, J. P. (2004). The Arabidopsis cytosolic thioredoxin h5 gene induction by oxidative stress and its W-Box mediated response to pathogen elicitor. Plant Physiol. 134, 1006–1016. doi: 10.1104/pp.103.035782
Leisner, C. P., Ming, R., Ainsworth, E. A. (2014). Distinct transcriptional profiles of ozone stress in soybean (Glycine max L.) flowers and pods. BMC Plant Biol. 14. doi: 10.1186/s12870-014-0335-y
Leister, D. (2012). Retrograde signaling in plants: from simple to complex scenarios. Front. Plant Sci. 3. doi: 10.3389/fpls.2012.00135
Li, C., Meng, J., Guo, L., Jiang, G. (2016). Effects of ozone pollution on yield and quality of winter wheat under flixweed competition. Environ. Exp. Bot. 129, 77–84. doi: 10.1016/j.envexpbot.2015.11.011
Li, Y., Roychowdhury, R., Govta, L., Jaiwar, S., Wei, Z. Z., Shams, I., et al. (2023). Intracellular reactive oxygen species-aided localized cell death contributing to immune responses against wheat powdery mildew pathogen. Phytopathology 113, PHYTO–PHY07. doi: 10.1094/PHYTO-07-22-0271-FI
Li, S., Tosens, T., Harley, P. C., Jiang, Y., Kanagendran, A., Grosberg, M., et al. (2018). Glandular trichomes as a barrier against atmospheric oxidative stress: Relationships with ozone uptake, leaf damage, and emission of LOX products across a diverse set of species. Plant Cell Environ. 41, 1263–1277. doi: 10.1111/pce.13128
Lu, X., Ye, X., Zhou, M., Zhao, Y., Weng, H., Kong, H., et al. (2021). The underappreciated role of agricultural soil nitrogen oxide emissions in ozone pollution regulation in North China. Nat. Commun. 12, 5021. doi: 10.1038/s41467-021-25147-9
Ma, M., Yao, G., Guo, J., Bai, K. (2021). Distinct spatiotemporal variation patterns of surface ozone in China due to diverse influential factors. J. Environ. Manage. 288, 112368. doi: 10.1016/j.jenvman.2021.112368
Malaiyandi, M., Natarajan, M. (2014). Impact of ozone on morphological, physiological, and biochemical changes in cow pea (Vigna unguiculata L. Walp.). Ozone Sci. Eng. 36, 36–42. doi: 10.1080/01919512.2013.824817
Manning, W. J., Paoletti, E. C., OMMAJ.R.X.X.X, H. S., Ernst, D. (2011). Ethylenediurea (EDU): A research tool for assessment and verification of the effects of ground level ozone on plants under natural conditions. Environ. pollut. 159, 3283–3293. doi: 10.1016/j.envpol.2011.07.005
Marchica, A., Lorenzini, G., Papini, R., Bernardi, R., Nali, C., Pellegrini, E. (2019). Signalling molecules responsive to ozone-induced oxidative stress in Salvia officinalis. Sci. Total Environ. 657, 568–576. doi: 10.1016/j.scitotenv.2018.11.472
Markovic, D. M., Markovic, D. A. (2005). The relationship between some meteorological parameters and the tropospheric concentrations of ozone in the urban area of Belgrade. J. Serb. Chem. Soc 70, 1487–1495. doi: 10.2298/JSC0512487M
McGrath, J. M., Betzelberger, A. M., Wang, S., Shook, E., Zhu, X. G., Long, S. P., et al. (2015). An analysis of ozone damage to historical maize and soybean yields in the United States. Proc. Natl. Acad. Sci. 112, 14390–14395. doi: 10.1073/pnas.1509777112
Meehl, G. A., Stocker, T. F., Collins, W. D., Friedlingstein, P., Gaye, A. T., Gregory, J. M., et al. (2007). “Global climate projections,” in Climate change 2007: the physical science basis. Contribution of working group I to the fourth assessment report of the intergovernmental panel on climate change, vol. 747-846 . Eds. Solomon, S., Qin, D., Manning, M., Chen, Z., Marquis, M., Avery, K. B., Tignor, M., Miller, H. L. (Cambridge: Cambridge University Press).
Mikkelsen, B. L., Olsen, C. E., Lyngkjær, M. F. (2015). Accumulation of secondary metabolites in healthy and diseased barley, grown under future climate levels of CO2, ozone and temperature. Phytochemistry 118, 162–173. doi: 10.1016/j.phytochem.2015.07.007
Mills, G., Sharps, K., Simpson, D., Pleijel, H., Broberg, M., Uddling, J., et al. (2018). Ozone pollution will compromise efforts to increase global wheat production. Glob. Change Biol. 24, 3560–3574. doi: 10.1111/gcb.14157
Mishra, A. K., Rai, R., Agrawal, S. B. (2013). Differential response of dwarf and tall tropical wheat cultivars to elevated ozone with and without carbon dioxide enrichment: growth, yield and grain quality. Field Crops Res. 145, 21–32. doi: 10.1016/j.fcr.2013.02.007
Monga, R., Marzuoli, R., Alonso, R., Bermejo, V., González-Fernández, I., Faoro, F., et al. (2015). Varietal screening of ozone sensitivity in Mediterranean durum wheat (Triticum durum, Desf.). Atmos. Environ. 110, 18–26. doi: 10.1016/j.atmosenv.2015.03.040
Monks, P. S., Archibald, A. T., Colette, A., Cooper, O., Coyle, M., Derwent, R., et al. (2015). Tropospheric ozone and its precursors from the urban to the global scale from air quality to short-lived climate forcer. Atmos. Chem. Phys. 15, 8889–8973. doi: 10.5194/acp-15-8889-2015
Morales, L. O., Shapiguzov, A., Safronov, O., Leppälä, J., Vaahtera, L., Yarmolinsky, D., et al. (2021). Ozone responses in Arabidopsis: beyond stomatal conductance. Plant Physiol. 186, 180–192. doi: 10.1093/plphys/kiab097
Negi, J., Matsuda, O., Nagasawa, T., Oba, Y., Takahashi, H., Kawai-Yamada, M., et al. (2008). CO2 regulator SLAC1 and its homologues are essential for anion homeostasis in plant cells. Nature 452, 483–486. doi: 10.1038/nature06720
Noctor, G., Mhamdi, A., Foyer, C. H. (2014). The roles of reactive oxygen metabolism in drought: Not so cut and dried. Plant Physiol. 164, 1636–1648. doi: 10.1104/pp.113.233478
Osborne, S., Pandey, D., Mills, G., Hayes, F., Harmens, H., Gillies, D., et al. (2019). New insights into leaf physiological responses to ozone for use in crop modelling. Plants 8, 84. doi: 10.3390/plants8040084
Pandey, A. K., Ghosh, A., Agrawal, M., Agrawal, S. B. (2018). Effect of elevated ozone and varying levels of soil nitrogen in two wheat (Triticum aestivum L.) cultivars: Growth, gas-exchange, antioxidant status, grain yield and quality. Ecotoxicol. Environ. Saf. 158, 59–68. doi: 10.1016/j.ecoenv.2018.04.014
Pandey, A. K., Ghosh, A., Rai, K., Fatima, A., Agrawal, M., Agrawal, S. B. (2019). “Abiotic stress in plants: A general outline” in Approaches for enhancing abiotic stress tolerance in plants. Eds. Hasanuzzaman, M., Nahar, K., Fujita, M., Oku, H., Islam, T. (Florida, USA: CRC Press), 1–46.
Parankusam, S., Adimulam, S. S., Bhatnagar-Mathur, P., Sharma, K. K. (2019). “Plant responses to ozone stress: Actions and adaptations” in Approaches for enhancing abiotic stress tolerance in plants. Eds. Hasanuzzaman, M., Nahar, K., Fujita, M., Oku, H., Islam, T. (Florida: CRC Press), 193–218.
Pellegrini, E., Hoshika, Y., Dusart, N., Cotrozzi, L., Gérard, J., Nali, C., et al. (2019). Antioxidative responses of three oak species under ozone and water stress conditions. Sci. Total Environ. 647, 390–399. doi: 10.1016/j.scitotenv.2018.07.413
Pellegrini, E., Trivellini, A., Cotrozzi, L., Vernieri, P., Nali, C. (2016). “Involvement of phytohormones in plant responses to ozone,” in Plant hormones under challenging environmental factors. Eds. Ahammed, G. J., Yu, J. Q. (New York: Springer), 215–245.
Pheomphun, P., Treesubsuntorn, C., Thiravetyan, P. (2019). Effect of exogenous catechin on alleviating O3 stress: the role of catechin-quinone in lipid peroxidation, salicylic acid, chlorophyll contents, and antioxidant enzymes of Zamioculcas zamiifolia. Ecotoxicol. Environ. Saf. 180, 374–383. doi: 10.1016/j.ecoenv.2019.05.002
Picchi, V., Gobbi, S., Fattizzo, M., Zefelippo, M., Faoro, F. (2021). Chitosan nanoparticles loaded with N-acetyl cysteine to mitigate ozone and other possible oxidative stresses in durum wheat. Plants 10, 691. doi: 10.3390/plants10040691
Pleijel, H., Broberg, M. C., Uddling, J., Mills, G. (2018). Current surface ozone concentrations significantly decrease wheat growth, yield and quality. Sci. Total Environ. 613, 687–692. doi: 10.1016/j.scitotenv.2017.09.111
Pleijel, H., Danielsson, H., Ojanperä, K., Temmerman, L. D., Högy, P., Badiani, M. (2004). Relationships between ozone exposure and yield loss in European wheat and potato – a comparison of concentration – and flux-based exposure indices. Atmospheric Environ. 38, 2259–2269. doi: 10.1016/j.atmosenv.2003.09.076
Podda, A., Pisuttu, C., Hoshika, Y., Pellegrini, E., Carrari, E., Lorenzini, G., et al. (2019). Can nutrient fertilization mitigate the effects of ozone exposure on an ozone-sensitive poplar clone? Sci. Total Environ. 657, 340–350. doi: 10.1016/j.scitotenv.2018.11.459
Poornima, R., Dhevagi, P., Ramya, A., Maheswari, M., Karthikeyan, S., Jayabalakrishnan, R. M. M. (2022). Efficiency of protectants in alleviating ozone stress on rice cultivars (Oryza sativa L.). Atmosph. pollut. Res. 13, 101593. doi: 10.1016/j.apr.2022.101593
Proietti, C., Fornasier, M. F., Sicard, P., Anav, A., Paoletti, E., De Marco, A. (2021). Trends in tropospheric ozone concentrations and forest impact metrics in Europe over the time period 2000–2014. J. For. Res. 32, 543–551. doi: 10.1007/s11676-020-01226-3
Qiu, Y., An, K., Sun, J., Chen, X., Gong, X., Ma, L., et al. (2019). Investigating the effect of methyl jasmonate and melatonin on resistance of Malus crabapple ‘Hong Jiu’to ozone stress. Environ. Sci. pollut. Res. 26, 27761–27768. doi: 10.1007/s11356-019-05946-w
Ramakrishnan, S., Dhevagi, P., Poornima, R., Ramya, A., Kannan, B., Chandrakumar, K., et al. (2023). Impact of elevated ozone on cabbage. Int. J. Environ. Clim. Change 13 (11), 176–186. doi: 10.9734/ijecc/2023/v13i113157
Ramel, F., Birtic, S., Ginies, C., Soubigou-Taconnat, L., Triantaphylidès, C., Havaux, M. (2012). Carotenoid oxidation products are stress signals that mediate gene responses to singlet oxygen in plants. Proc. Natl. Acad. Sci. U.S.A. 109, 5535–5540. doi: 10.1073/pnas.1115982109
Ramya, A., Dhevagi, P., Poornima, R., Avudainayagam, S., Watanabe, M., Agathokleous, E. (2023). Effect of ozone stress on crop productivity: A threat to food security. Environ. Res. 116816. doi: 10.1016/j.envres.2023.116816
Ramya, A., Dhevagi, P., Priyatharshini, S., Chandrasekhar, C. N., Valliappan, K., Venkataramani, S. (2021a). Physiological and biochemical response of rice cultivars (Oryza sativa L.) to elevated ozone. Ozone Sci. Eng. 43, 363–377. doi: 10.1080/01919512.2020.1796585
Ramya, A., Dhevagi, P., Priyatharshini, S., Saraswathi, R., Avudainayagam, S., Venkataramani, S. (2021b). Response of rice (Oryza sativa L.) cultivars to elevated ozone stress. Environ. Monit. Assess. 193, 808. doi: 10.1007/s10661-021-09595-w
Rannik, U., Mammarella, I., Keronen, P., Vesala, T. (2009). Vertical advection and nocturnal deposition of ozone over a boreal pine forest. Atmos. Chem. Phys. 9, 2089–2095. doi: 10.5194/acp-9-2089-2009
Rathore, D., Chaudhary, I. J. (2018). Ozone risk assessment of castor (Ricinus communis L.) cultivars using open top chamber and ethylenediurea (EDU). Environ. pollut. 244, 257–269. doi: 10.1016/j.envpol.2018.10.036
Ravishankara, A. R., John, S. D., Robert, W. P. (2009). Nitrous oxide (N2O): the dominant ozone-depleting substance emitted in 21st century. Science 326, 123–125. doi: 10.1126/science.1176985
Ren, J. (2021). Effects of O3 pollution near formation on crop yield and economic loss. Environ. Technol. Innov. 22, 101446. doi: 10.1016/j.eti.2021.101446
Rossard, S., Luini, E., Pérault, J. M., Bonmort, J., Roblin, G. (2006). Early changes in membrane permeability, production of oxidative burst and modification of PAL activity induced by ergosterol in cotyledons of Mimosa pudica. J. Exp. Bot. 57, 1245–1252. doi: 10.1093/jxb/erj090
Roychowdhury, R., Khan, M. H., Choudhury, S. (2019). “Physiological and molecular responses for metalloid stress in rice—A Comprehensive Overview,” in Advances in rice research for abiotic stress tolerance. Eds. Hasanuzzaman, M., Fujita, M., Nahar, K., Biswas, J. K. (Amsterdam: Elsevier), 341–369.
Rozpądek, P., Ślesak, I., Cebula, S., Waligórski, P., Dziurka, M., Skoczowski, A., et al. (2013). Ozone fumigation results in accelerated growth and persistent changes in the antioxidant system of Brassica oleracea L. var. capitata f. alba. Plant Physiol. 170, 259–1266. doi: 10.1016/j.jplph.2013.04.018
Saitanis, C. J., Bari, S. M., Burkey, K. O., Stamatelopoulos, D., Agathokleous, E. (2014). Screening of Bangladeshi winter wheat (Triticum aestivum L.) cultivars for sensitivity to ozone. Environ. Sci. pollut. Res. 21, 13560–13571. doi: 10.1007/s11356-014-3286-9
Sandermann, H. J. (2000). Ozone/biotic disease interactions: molecular biomarkers as a new experimental tool. Environ. pollut. 108, 327–332. doi: 10.1016/S0269-7491(99)00211-0
Sarkar, A., Agrawal, S. B. (2010). Elevated ozone and two modern wheat cultivars: an assessment of dose dependent sensitivity with respect to growth, reproductive and yield parameters. Environ. Exp. Bot. 69, 328–337. doi: 10.1016/j.envexpbot.2010.04.016
Schauberger, B., Rolinski, S., Schaphoff, S., Müller, C. (2019). Global historical soybean and wheat yield loss estimates from ozone pollution considering water and temperature as modifying effects. Agric. For. Meteorol. 265, 1–15. doi: 10.1016/j.agrformet.2018.11.004
Shapiguzov, A., Vainonen, J. P., Wrzaczek, M., Kangasjärvi, J. (2012). ROS-talk – how the apoplast, the chloroplast, and the nucleus get the message through. Front. Plant Sci. 3. doi: 10.3389/fpls.2012.00292
Sharma, P., Jha, A. B., Dubey, R. S., Pessarakli, M. (2012). Reactive oxygen species, oxidative damage, and antioxidative defense mechanism in plants under stressful conditions. J. Bot. 217037, 1–26. doi: 10.1155/2012/217037
Shindell, D., Kuylenstierna, J. C., Vignati, E., van Dingenen, R., Amann, M., Klimont, Z., et al. (2012). Simultaneously mitigating near-term climate change and improving human health and food security. Science 335, 183–189. doi: 10.1126/science.1210026
Sierla, M., Rahikainen, M., Salojärvi, J., Kangasjärvi, J., Kangasjärvi, S. (2013). Apoplastic and chloroplastic redox signaling networks in plant stress responses. Antioxid. Redox Signal. 18, 2220–2239. doi: 10.1089/ars.2012.5016
Simon, H., Reff, A., Wells, B., Xing, J., Frank, N. (2014). Ozone trends across the United States over a period of decreasing NOx and VOC emissions. Environ. Sci. Technol. 49, 186–195. doi: 10.1021/es504514z
Singh, A. A., Agrawal, S. B., Shahi, J. P., Agrawal, M. (2014a). Assessment of growth and yield losses in two Zea mays L. cultivars (quality protein maize and non-quality protein maize) under projected levels of ozone. Environ. Sci. pollut. Res. 21, 2628–2641. doi: 10.1007/s11356-013-2188-6
Singh, A. A., Agrawal, S. B., Shahi, J. P., Agrawal, M. (2014b). Investigating the response of tropical maize (Zea mays L.) cultivars against elevated levels of O3 at two developmental stages. Ecotoxicology 23, 1447–1463. doi: 10.1007/s10646-014-1287-6
Singh, R. N., Mukherjee, J., Sehgal, V. K., Krishnan, P., Das, D. K., Dhakar, R. K., et al. (2021). Interactive effect of elevated tropospheric ozone and carbon dioxide on radiation utilisation, growth and yield of chickpea (Cicer arietinum L.). Int. J. Biometeorol. 65, 1939–1952. doi: 10.1007/s00484-021-02150-9
Sinha, B., Sangwan, K. S., Maurya, Y., Kumar, V., Sarkar, C., Chandra, B. P., et al. (2015). Assessment of crop yield losses in Punjab and Haryana using 2 years of continuous in situ ozone measurements. Atmos. Chem. Phys. 15, 9555–9576. doi: 10.5194/acp-15-9555-2015
Strand, Å., Asami, T., Alonso, J., Ecker, J. R., Chory, J. (2003). Chloroplast to nucleus communication triggered by accumulation of Mg-protoporphyrinIX. Nature 421, 79–83. doi: 10.1038/nature01204
Sun, X., Feng, P., Xu, X., Guo, H., Ma, J., Chi, W., et al. (2011). A chloroplast envelope-bound PHD transcription factor mediates chloroplast signals to the nucleus. Nat. Commun. 2 477. doi: 10.1038/ncomms1486
Szpunar-Krok, E., Jańczak-Pieniążek, M., Migut, D., Skrobacz, K., Piechowiak, T., Pawlak, R., et al. (2020). Physiological and biochemical properties of potato (Solanum tuberosum L.) in response to ozone-induced oxidative stress. J. Agron. 10, 1745. doi: 10.3390/agronomy10111745
Tatsumi, K., Abiko, T., Kinose, Y., Inagaki, S., Izuta, T. (2019). Effects of ozone on the growth and yield of rice (Oryza sativa L.) under different nitrogen fertilization regimes. Environ. Sci. pollut. Res. 26, 32103–32113. doi: 10.1007/s11356-019-06358-6
Teixeira, E., Fischer, G., van Velthuizen, H., van Dingenen, R., Dentener, F., Mills, G., et al. (2011). Limited potential of crop management for mitigating surface ozone impacts on global food supply. Atmos. Environ. 45, 2569–2576. doi: 10.1016/j.atmosenv.2011.02.002
Tetteh, R., Yamaguchi, M., Wada, Y., Funada, R., Izuta, T. (2015). Effects of ozone on growth, net photosynthesis and yield of two African varieties of Vigna unguiculata. Environ. pollut. 196, 230–238. doi: 10.1016/j.envpol.2014.10.008
Thwe, A. A., Vercambre, G., Gautier, H., Gay, F., Phattaralerphong, J., Kasemsap, P. (2014). Response of photosynthesis and chlorophyll fluorescence to acute ozone stress in tomato (Solanum lycopersicum Mill.). Photosynthetica 52, 105–116. doi: 10.1007/s11099-014-0012-2
Tiwari, S., Agrawal, M. (2018). “Mitigation of ozone stress” in Tropospheric ozone and its impacts on crop plants. Eds. Tiwari, S., Agrawal, M. (Cham, Switzerland: Springer). 167–189.
Tripathi, R., Agrawal, S. B. (2012). Effects of ambient and elevated level of ozone on Brassica campestris L. with special reference to yield and oil quality parameters. Ecotoxicol. Environ. Saf. 85, 1–12. doi: 10.1016/j.ecoenv.2012.08.012
Tsukahara, K., Sawada, H., Kohno, Y., Matsuura, T., Mori, I. C., Terao, T., et al. (2015). Ozone-induced rice grain yield loss is triggered via a change in panicle morphology that is controlled by ABERRANT PANICLE ORGANIZATION 1 gene. PloS One 10, e0123308. doi: 10.1371/journal.pone.0123308
Ueda, Y., Siddique, S., Frei, M. (2015). A novel gene, Ozone-Responsive Apoplastic Protein1, enhances cell death in ozone stress in rice. Plant Physiol. 169, 873–889. doi: 10.1104/pp.15.00956
Ueda, Y., Uehara, N., Sasaki, H., Kobayashi, K., Yamakawa, T. (2013). Impacts of acute ozone stress on superoxide dismutase (SOD) expression and reactive oxygen species (ROS) formation in rice leaves. Plant Physiol. Biochem. 70, 396–402. doi: 10.1016/j.plaphy.2013.06.009
Vahisalu, T., Puzõrjova, I., Brosché, M., Valg, E., Lepiku, M., Moldau, H., et al. (2010). Ozone-triggered rapid stomatal response involves the production of reactive oxygen species, and is controlled by SLAC1 and OST1. Plant J. 62, 442–453. doi: 10.1111/j.1365-313X.2010.04159.x
Vainonen, J. P., Kangasjärvi, J. (2015). Plant signalling in acute ozone exposure. Plant Cell Environ. 38(2), 240–252. doi: 10.1111/pce.12273
Vaultier, M. N., Jolivet, Y. (2015). Ozone sensing and early signaling in plants: an outline from the cloud. Environ. Exp. Bot. 114, 144–152. doi: 10.1016/j.envexpbot.2014.11.012
Wang, Y., Yuan, J., Yang, W., Zhu, L., Su, C., Wang, X., et al. (2017). Genome wide identification and expression profiling of ethylene receptor genes during soybean nodulation. Front. Plant Sci. 8. doi: 10.3389/fpls.2017.00859
Witting, V. E., Ainsworth, E. A., Naidu, S. L., Karnosky, D. F., Long, S. P. (2009). Quantifying the impact of current and future tropospheric ozone on tree biomass, growth, physiology and biochemistry: a quantitative meta-analysis. Glob. Change Biol. 15, 396–424. doi: 10.1111/j.1365-2486.2008.01774.x
Woodson, J. D., Perez-Ruiz, J. M., Chory, J. (2011). Heme synthesis by plastid ferrochelatase I regulates nuclear gene expression in plants. Curr. Biol. 21, 897–903. doi: 10.1016/j.cub.2011.04.004
Wu, H., Wang, W., Xie, X. L., Yin, C. M., Hou, H. J. (2018). Effects of rice straw mulching on N2O emissions and maize productivity in a rain-fed upland Xiao. Environ. Sci. pollut. Res. 25, 6407–6413. doi: 10.1007/s11356-017-0989-8
Xiao, Y., Savchenko, T., Baidoo, E. E., Chehab, W. E., Hayden, D. M., Tolstikov, V., et al. (2012). Retrograde signaling by the plastidial metabolite MecPP regulates expression of nuclear stress-response genes. Cell J. 149, 1525–1535. doi: 10.1016/j.cell.2012.04.038
Xu, X. (2021). Recent advances in studies of ozone pollution and impacts in China: A short review. Curr. Opin. Environ. Sci. Health 19, 100225. doi: 10.1016/j.coesh.2020.100225
Yadav, D. S., Agrawal, S. B., Agrawal, M. (2021). Ozone flux-effect relationship for early and late sown Indian wheat cultivars: Growth, biomass, and yield. Field Crops Res. 263, 108076. doi: 10.1016/j.fcr.2021.108076
Yadav, A., Bhatia, A., Yadav, S., Singh, A., Tomer, R., Harit, R., et al. (2020). Growth, yield and quality of maize under ozone and carbon dioxide interaction in North West India. Aerosol Air Qual. Res. 21, 200194. doi: 10.4209/aaqr.2020.05.0194
Yadav, D. S., Rai, R., Mishra, A. K., Chaudhary, N., Mukherjee, A., Agrawal, S. B., et al. (2019). ROS production and its detoxification in early and late sown cultivars of wheat under future O3 concentration. Sci. Total Environ. 659, 200–210. doi: 10.1016/j.scitotenv.2018.12.352
Yendrek, C. R., Koester, R. P., Ainsworth, E. A. (2015). A comparative analysis of transcriptomic, biochemical, and physiological responses to elevated ozone identifies species-specific mechanisms of resilience in legume crops. J. Exp. Bot. 66, 7101–7112. doi: 10.1093/jxb/erv404
Zhang, L., Hoshika, Y., Carrari, E., Cotrozzi, L., Pellegrini, E., Paoletti, E. (2018). Effects of nitrogen and phosphorus imbalance on photosynthetic traits of poplar Oxford clone under ozone pollution. J. Plant Res. 131, 915–924. doi: 10.1007/s10265-018-1071-4
Zhang, W., Wang, G., Liu, X., Feng, Z. (2014). Effects of elevated O3 exposure on seed yield, N concentration and photosynthesis of nine soybean cultivars (Glycine max (L.) Merr.) in Northeast China. Plant Sci. 226, 172–181. doi: 10.1016/j.plantsci.2014.04.020
Zhang, J., Wei, Y., Fang, Z. (2019). Ozone pollution: a major health hazard worldwide. Front. Immunol. 10, 2518. doi: 10.3389/fimmu.2019.02518
Zhang, L., Xu, B., Wu, T., Wen, M.-X., Fan, L.-X., Feng, Z.-Z., et al. (2017). Transcriptomic analysis of Pak Choi under acute ozone exposure revealed regulatory mechanism against ozone stress. BMC Plant Biol. 17, 1–15. doi: 10.1186/s12870-017-1202-4
Keywords: abiotic stress, antioxidants, atmospheric pollutants, oxidative stress, photosynthesis, prooxidant, reactive oxygen species
Citation: Nowroz F, Hasanuzzaman M, Siddika A, Parvin K, Caparros PG, Nahar K and Prasad PVV (2024) Elevated tropospheric ozone and crop production: potential negative effects and plant defense mechanisms. Front. Plant Sci. 14:1244515. doi: 10.3389/fpls.2023.1244515
Received: 22 June 2023; Accepted: 15 December 2023;
Published: 09 January 2024.
Edited by:
Juan De Dios Franco-Navarro, Spanish National Research Council (CSIC), SpainReviewed by:
Periasamy Dhevagi, Tamil Nadu Agricultural University, IndiaAnalia Menendez, University of Buenos Aires, Argentina
Copyright © 2024 Nowroz, Hasanuzzaman, Siddika, Parvin, Caparros, Nahar and Prasad. This is an open-access article distributed under the terms of the Creative Commons Attribution License (CC BY). The use, distribution or reproduction in other forums is permitted, provided the original author(s) and the copyright owner(s) are credited and that the original publication in this journal is cited, in accordance with accepted academic practice. No use, distribution or reproduction is permitted which does not comply with these terms.
*Correspondence: Mirza Hasanuzzaman, bWh6c2F1YWdAeWFob28uY29t; P.V. Vara Prasad, dmFyYUBrc3UuZWR1
†These authors have contributed equally to this work