- 1Department of Systems Biotechnology, Konkuk University, Seoul, Republic of Korea
- 2Division of Biological Science and Technology, Yonsei University, Wonju, Republic of Korea
In multicellular organisms, including higher plants, asymmetric cell divisions (ACDs) play a crucial role in generating distinct cell types. The Arabidopsis root ground tissue initially has two layers: endodermis (inside) and cortex (outside). In the mature root, the endodermis undergoes additional ACDs to produce the endodermis itself and the middle cortex (MC), located between the endodermis and the pre-existing cortex. In the Arabidopsis root, gibberellic acid (GA) deficiency and hydrogen peroxide (H2O2) precociously induced more frequent ACDs in the endodermis for MC formation. Thus, these findings suggest that GA and H2O2 play roles in regulating the timing and extent of MC formation. However, details of the molecular interaction between GA signaling and H2O2 homeostasis remain elusive. In this study, we identified the PEROXIDASE 34 (PRX34) gene, which encodes a class III peroxidase, as a molecular link to elucidate the interconnected regulatory network involved in H2O2- and GA-mediated MC formation. Under normal conditions, prx34 showed a reduced frequency of MC formation, whereas the occurrence of MC in prx34 was restored to nearly WT levels in the presence of H2O2. Our results suggest that PRX34 plays a role in H2O2-mediated MC production. Furthermore, we provide evidence that SCARECROW-LIKE 3 (SCL3) regulates H2O2 homeostasis by controlling transcription of PRX34 during root ground tissue maturation. Taken together, our findings provide new insights into how H2O2 homeostasis is achieved by SCL3 to ensure correct radial tissue patterning in the Arabidopsis root.
Introduction
Plants dynamically integrate environmental signals into their genetic programs to provide flexibility for growth and development. Plant hormones have been shown to play key roles in such signaling pathways (Achard et al., 2006; Wolters and Jürgens, 2009; Verma et al., 2016). Despite the complexity of the pathways, signals are ultimately relayed to transcription factors (TFs) to spatiotemporally regulate cellular behaviors and responses (Srivastava et al., 2010; Moreno-Risueno et al., 2015; Barah et al., 2016). Identifying and characterizing transcriptional regulatory networks are important for understanding the cellular processes involved in plant growth and development (Moreno-Risueno et al., 2015; Chaiwanon et al., 2016; Dhar et al., 2022).
Multicellular organisms such as animals and plants require asymmetric cell divisions (ACDs) to generate distinct cell types during development (Horvitz and Herskowitz, 1992; Knoblich, 2008; Ten Hove and Heidstra, 2008; Abrash and Bergmann, 2009; De Smet and Beeckman, 2011; Smolarkiewicz and Dhonukshe, 2013). Therefore, the timing and extent of ACDs should be precisely controlled to ensure correct cell/tissue patterning. In the Arabidopsis (Arabidopsis thaliana) root, stem cells of the ground tissue (known as cortex/endodermis initial; CEI) undergo anticlinal ACDs to generate both self-renewed CEI and its daughter cell (known as cortex/endodermis initial daughter; CEID). Subsequent periclinal ACDs of the CEID cells produce two ground tissue layers (endodermis and cortex) from embryogenesis onwards (Benfey et al., 1993; Dolan et al., 1993; Scheres et al., 1994; Scheres et al., 1995; Di Laurenzio et al., 1996; Helariutta et al., 2000; Cui et al., 2007; Cruz-Ramirez et al., 2012). Therefore, in the early developmental phases, the Arabidopsis root has an endodermis (inside) and a cortex (outside) in the ground tissue. Around 7 days post-germination (dpg), another round of endodermal ACDs occurs to generate both endodermis and additional cortex (termed middle cortex; MC), resulting in three ground tissue layers: endodermis, MC, and cortex (inside to outside) (Baum et al., 2002; Paquette and Benfey, 2005). Therefore, the onset of endodermal ACDs has been used to assess the maturation of the Arabidopsis root ground tissue by measuring the proportion of plants with MC (Baum et al., 2002; Paquette and Benfey, 2005; Cui and Benfey, 2009a; Cui and Benfey, 2009b; Heo et al., 2011; Koizumi et al., 2012a; Koizumi et al., 2012b; Gong et al., 2016; Lee et al., 2016; Bertolotti et al., 2021; Xie et al., 2023).
Accumulating evidence has revealed that developmental pathways and plant hormones interact to modulate the timing and extent of endodermal ACDs for MC formation (Paquette and Benfey, 2005; Cui and Benfey, 2009a; Cui and Benfey, 2009b; Heo et al., 2011; Pauluzzi et al., 2012; Petricka et al., 2012; Koizumi et al., 2012a; Koizumi et al., 2012b; Cui et al., 2014; Choi and Lim, 2016; Cui, 2016; Gong et al., 2016; Lee et al., 2016; Di Ruocco et al., 2018; Bertolotti et al., 2021; Hernández-Coronado and Ortiz-Ramírez, 2021; Shtin et al., 2022; Xie et al., 2023). Previous studies have highlighted the role of GA in controlling MC generation (Paquette and Benfey, 2005; Cui and Benfey, 2009a; Cui and Benfey, 2009b; Heo et al., 2011; Koizumi et al., 2012b; Gong et al., 2016; Lee et al., 2016; Bertolotti et al., 2021). Under GA-deficient conditions caused by the GA biosynthesis inhibitor paclobutrazol (PAC) or the loss-of-function mutation in the key GA biosynthesis gene (e.g., ga1-3), the endodermis undergoes more excessive periclinal ACDs for MC production than in the wild-type (WT) (Paquette and Benfey, 2005; Cui and Benfey, 2009a; Cui and Benfey, 2009b; Heo et al., 2011; Koizumi et al., 2012b; Gong et al., 2016; Lee et al., 2016). Interestingly, SCARECROW-LIKE 3 (SCL3), a member of the GRAS transcription factor family, acts as a tissue-specific GA signaling integrator in GA-mediated MC formation (Heo et al., 2011; Gong et al., 2016; Lee et al., 2016). The loss of SCL3 function mutant (scl3-1) displayed precocious endodermal ACDs, whereas the overexpression of SCL3 (SCL3-OX) resulted in a reduced frequency of MC formation (Heo et al., 2011; Lee et al., 2016). Therefore, these findings suggest that transcriptional inputs integrated by SCL3 play key roles in GA regulation of endodermal ACDs for MC formation (Heo et al., 2011; Choi and Lim, 2016; Gong et al., 2016; Lee et al., 2016).
Reactive oxygen species (ROS) are inevitably generated as undesirable byproducts in aerobic organisms (Mittler et al., 2004; Livanos et al., 2012). ROS also act as important signaling molecules that control diverse processes in plant growth and development (Apel and Hirt, 2004; Gapper and Dolan, 2006; Tsukagoshi et al., 2010; Xia et al., 2015; Tsukagoshi, 2016; Zhou et al., 2020; Mase and Tsukagoshi, 2021). In particular, H2O2, a relatively stable type of ROS, regulates MC formation (Cui et al., 2014; Li et al., 2020). For instance, when supplemented with H2O2, ACDs were more frequently observed in the endodermis (Cui et al., 2014). Conversely, the occurrence of MC was reduced in roots treated with the H2O2 scavenger, potassium iodide (KI), compared to untreated roots (Li et al., 2020). In addition, previous work implied that a subset of class III peroxidases (PRXs) might play a role in the H2O2-mediated modulation of MC generation (Cui et al., 2014). Class III PRXs are plant-specific secretory peroxidases, which belong to multigene families with a diverse range of functions (Tognolli et al., 2002; Valério et al., 2004; Dunand et al., 2007; Cosio and Dunand, 2009; Francoz et al., 2015; Shigeto and Tsutsumi, 2016). Apoplastic PRXs have different functions and reactivities that facilitate either ROS generation or scavenging in the context of reactions (Tognolli et al., 2002; Valério et al., 2004; Dunand et al., 2007; Cosio and Dunand, 2009; Francoz et al., 2015; Shigeto and Tsutsumi, 2016). However, their roles in H2O2-mediated MC formation are currently unknown. Furthermore, the molecular link between H2O2 and GA-mediated periclinal ACDs in the endodermis remains unclear.
In this study, to better understand H2O2-mediated endodermal ACDs for MC formation, we identified and characterized a transcriptional regulatory network in ground tissue maturation. Our results have confirmed that H2O2 generation facilitates MC production in the Arabidopsis root. Importantly, we provide convincing evidence that SCL3 plays a role in H2O2 homeostasis through transcriptional regulation of the PRX34 gene in H2O2-mediated MC formation.
Materials and methods
Plant material and growth conditions
Arabidopsis thaliana ecotype Columbia (Col-0) was used as the WT control. The mutant and transgenic lines used in this study were pCO2::H2B-YFP (Heidstra et al., 2004; Heo et al., 2011), ga1-3 (Sun and Kamiya, 1994; Heo et al., 2011; Zhang et al., 2011), prx34 (SALK_051769) (Passardi et al., 2006; Han et al., 2015), scl3-1 and SCL3-OX (Heo et al., 2011; Zhang et al., 2011). As described previously (Heo et al., 2011; Lee et al., 2016), seeds were surface-sterilized, imbibed at 4°C in the dark, and grown on half-strength of Murashige-Skoog (MS) agar plates (1/2 MS salt mixture, 0.5 mM MES, pH5.7-5.8, 1% sucrose, and 1% agar). To verify homozygous plants from genetic crosses, PCR-based genotyping was performed as previously described (Heo et al., 2011; Lee et al., 2016). For genetic crosses and seed multiplication, seedlings on 1/2 MS agar plates were transferred to soil and grown under long-day conditions (16 h light/8 h dark cycles) as described previously (Heo et al., 2011; Lee et al., 2016). Sequences of the PCR primers used for genotyping are listed in Supplementary Table S1.
MC formation analysis
For phenotypic analysis of MC formation, approximately 36-40 hours post-germination (hpg) seeds were transferred to new 1/2 MS agar plates supplemented with different chemicals, including H2O2 (100 μM; Sigma-Aldrich, USA), KI (1 mM; Duchefa Biochemie, Netherlands), or PAC (1 µM; Duchefa Biochemie, Netherlands). The same batches of the seeds were transferred to new 1/2 MS agar plates with no supplemented chemicals as controls. More than 300 roots from three replicate experiments (each experiment, n > 100 roots per treatment or genotype) were observed using an Axio Imager.A1 microscope (Carl Zeiss, Germany), and the frequency of endodermal ACDs for MC formation was measured, as previously described (Heo et al., 2011; Lee et al., 2016). Simultaneously, seedlings (n > 30 roots per treatment or genotype) grown on 1/2 MS agar plates with different supplements were observed using a Zeiss LSM 800 confocal laser scanning microscope (Carl Zeiss, Germany), as previously described (Lee et al., 2016; Yoon et al., 2016; Dhar et al., 2022). Student’s t-test was performed using Microsoft Excel (Microsoft, USA) and the data presented herein are means values ± standard error (SEM) as described previously (Heo et al., 2011; Lee et al., 2016; Yoon et al., 2016; Dhar et al., 2022).
Reverse transcription-associated quantitative PCR (RT-qPCR)
Total RNA samples were extracted from WT, mutant, and transgenic seedling roots grown on 1/2 MS agar plates, and used for cDNA synthesis and RT-qPCR as described previously (Heo et al., 2011; Lee et al., 2016; Yoon et al., 2016; Dhar et al., 2022). ACTIN2 (ACT2; AT3G18780) was used as an internal reference (Yoon et al., 2016; Dhar et al., 2022). Each experiment was independently performed with at least three biological replicates, as previously described (Heo et al., 2011; Lee et al., 2016; Yoon et al., 2016; Dhar et al., 2022). Student’s t-test was performed using Microsoft Excel (Microsoft, USA).
H2O2 assays
Qualitative and quantitative assays were conducted to analyze H2O2 concentrations in seedling roots. DAB staining (3,3′-diaminobenzidine; Sigma-Aldrich, USA) was used to qualitatively assess H2O2 concentration, as previously described (Thordal-Christensen et al., 1997; Vanacker et al., 2000; Bindschedler et al., 2006; Daudi et al., 2012) with minor modifications. DAB solution (final concentration: 1 mg/mL) was prepared in 0.05% Triton X-100 (v/v) and 10 mM sodium phosphate buffer. The seedlings were vacuum-infiltrated in the DAB solution for 10 min and subsequently incubated at 30°C for 30 min in the dark. After termination of the staining reaction, samples were fixed in the bleaching solution [ethanol:acetic acid:glycerol, 3:1:1 (v/v/v)] at 95°C for 15 min. Seedling roots were observed using an Axio Imager.A1 microscope equipped with an AxioCam MRc5 digital camera (Carl Zeiss, Germany). The intensity of DAB staining in the seedling roots was quantified by NIH Image J software (http://rsb.info.nih.gov/ij; Schneider et al., 2012), as described previously (Li et al., 2020). To quantitatively measure H2O2 concentration, we used the Amplex® Red Hydrogen Peroxide Assay Kit (cat. #A22188, Invitrogen), according to the manufacturer’s instructions. The 7 dpg seedling roots grown on 1/2 MS agar plates with or without supplemented chemicals were pulverized in liquid nitrogen. Subsequently, five volumes of 50 mM sodium phosphate buffer (pH 7.4) were added, mixed thoroughly, and incubated on ice for 10 min. The samples were centrifuged at 12,000 rpm for 20 min at 4°C, and supernatants were used for the measurement of H2O2 concentration as previously described (Cui et al., 2014). Each experiment was independently repeated at least three times and data were analyzed using Microsoft Excel (Microsoft, USA).
Results
Induction of H2O2 by GA deficiency facilitates MC formation
Previously, it has been shown that H2O2 promotes periclinal ACDs in the endodermis for MC production (Cui et al., 2014; Li et al., 2020). These findings suggest that H2O2 homeostasis plays a role in modulating the timing and extent of MC formation in the root ground tissue. We thus verified that the frequency of MC occurrence in Columbia wild-type (hereafter referred to as WT) roots was elevated by H2O2. Under normal growth conditions, cells in the endodermis of 4 dpg WT seedlings barely divided to generate the MC layer, whereas endodermal ACDs were frequently observed under H2O2 (100 μM) treatment (~3.7% vs. ~10%) (Supplementary Figures 1A–C). In the presence of KI (1 mM), an efficient scavenger of H2O2 (Dunand et al., 2007), MC formation in 7 dpg WT seedlings was attenuated compared to that in untreated roots (~10.7% vs. ~22%) (Supplementary Figures 1D–F). Interestingly, the application of H2O2 in the presence of KI almost restored the frequency of MC formation to that in untreated WT roots (Supplementary Figure 1F). Therefore, consistent with previous studies (Cui et al., 2014; Li et al., 2020), our findings strongly support the notion that H2O2 induces ACDs in the endodermis for MC production in the root ground tissue.
GA-deficient conditions caused by the loss-of-function mutant ga1-3 or by the GA biosynthesis inhibitor PAC facilitate endodermal ACDs for the MC layers (Paquette and Benfey, 2005; Cui and Benfey, 2009a; Heo et al., 2011; Koizumi et al., 2012a; Koizumi et al., 2012b; Gong et al., 2016; Lee et al., 2016). Thus, we hypothesized that frequent MC formation might be due to elevated H2O2 levels induced by GA deficiency. To test this, we first analyzed H2O2 concentration in roots by DAB staining in the absence or presence of PAC. DAB staining was more intense in PAC-treated WT roots than untreated controls (Figures 1A–C). In addition, we quantitatively assessed H2O2 levels in PAC-treated roots relative to those in untreated controls. Consistent with the DAB staining results, H2O2 accumulation was higher in PAC-treated roots than untreated controls (Figure 1D). Likewise, H2O2 was more accumulated in ga1-3 than WT (Figures 1A, E). In the presence of KI, ga1-3 roots showed reduced H2O2 accumulation (Figures 1F–H). These findings indicate that GA-deficient conditions promoted H2O2 generation. Next, we assessed the frequency of MC formation in ga1-3 with or without KI. As expected, the occurrence of the MC layers was substantially reduced in KI-treated ga1-3 compared to that in untreated ga1-3 (Figures 1J–L). Intriguingly, the KI-treated ga1-3 phenotype was almost indistinguishable from that of the WT control (Figures 1I, K, L; Supplementary Figures 1D, F). This observation indicates that KI treatment reduced H2O2 accumulation in ga1-3, which resulted in decreased MC formation.
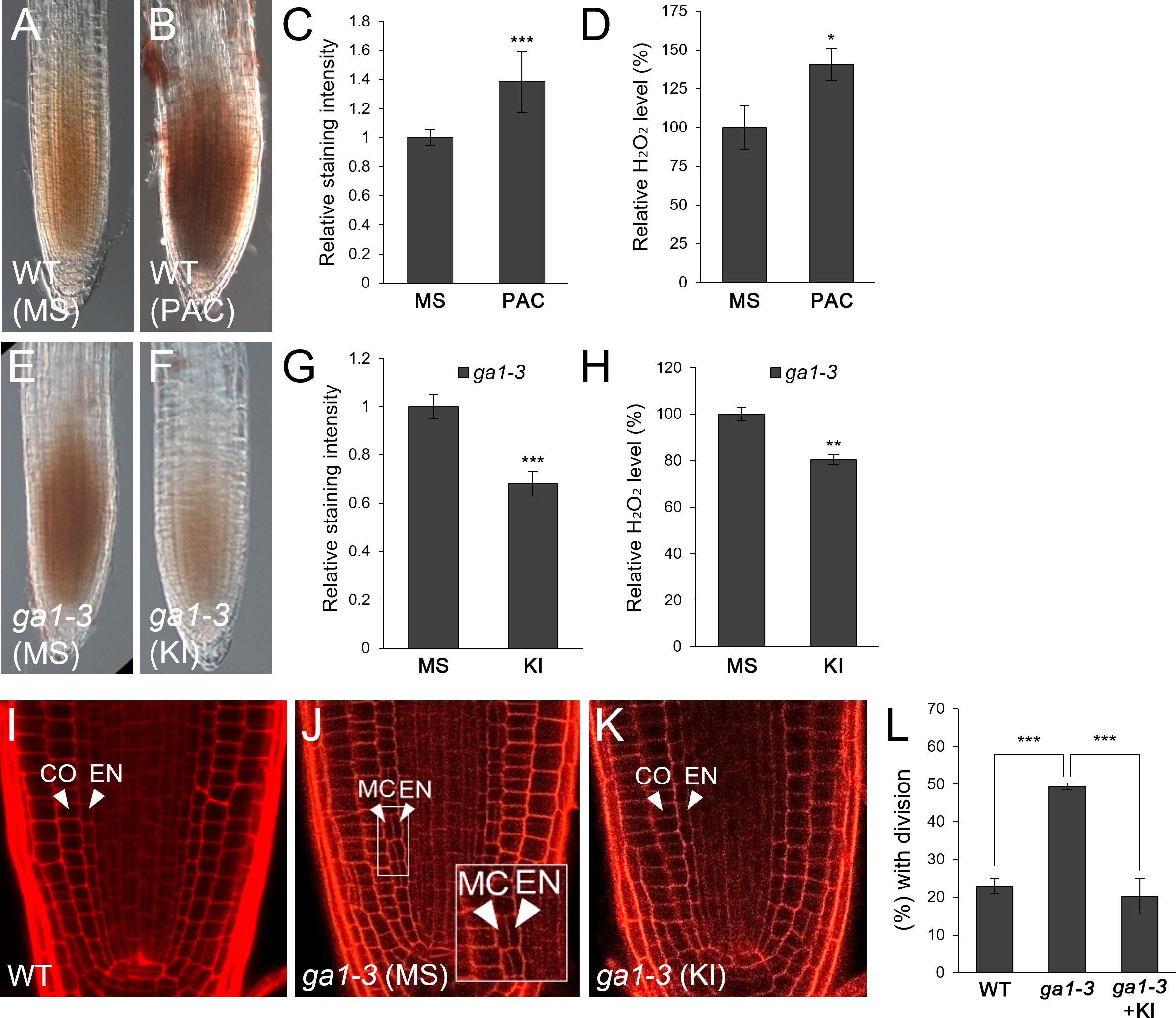
Figure 1 H2O2 generation by GA deficiency facilitates MC formation in the Arabidopsis root. (A, B) DAB staining of WT roots in the absence (A) or presence (B) of PAC. (C) Quantification of DAB staining of the WT roots with or without PAC. (D) Measurement of H2O2 in PAC-treated or -untreated WT roots. (E, F) DAB staining of ga1-3 roots in the absence (E) or presence (F) of KI. (G) Quantification of DAB staining of ga1-3 roots with or without KI. (H) Measurement of H2O2 in KI-treated or -untreated ga1-3 roots. (I-K) Confocal images of WT (I) and ga1-3 roots in the absence (J) or presence (K) of KI. The inset in (J) shows endodermal ACDs for MC formation. The endodermis (EN), middle cortex (MC), and cortex (CO) layers are indicated with white arrowheads. (L) Proportion of WT and ga1-3 plants with MC in the absence or presence of KI. Significance of difference was statistically determined by Student’s t-test (*P < 0.05; **P < 0.01; ***P < 0.001).
Taken together, our results strongly suggest that the induction of H2O2 under GA-deficient conditions causes more frequent MC production in the root ground tissue.
Involvement of PRX34 in H2O2-mediated MC formation
In an attempt to identify the molecular component(s) underlying the H2O2-mediated regulation of MC production, we encountered an interesting report that the expression levels of class III peroxidase (PRX) genes were significantly changed in the-loss-of-function spindly (spy) mutants (2-fold enrichment with P < 0.05; Cui et al., 2014), which have been shown to play a role in GA signaling (Jacobsen and Olszewski, 1993; Jacobsen et al., 1996; Sun and Gubler, 2004). Of the differentially expressed class III peroxidase (PRX) genes (Cui et al., 2014), we focused on PRX34 because this gene fulfilled our criteria: i) its expression levels were induced by GA-deficient conditions (PAC and ga1-3) and H2O2 (Supplementary Figures 2A–C), ii) its expression was enriched in roots (Supplementary Figure 2D), and iii) its T-DNA insertion mutant was publicly available (Passardi et al., 2006; Daudi et al., 2012; O’Brien et al., 2012). Therefore, in this study, we aimed to elucidate the role of PRX34 in H2O2-mediated MC formation.
Because PRX34 plays a role in H2O2 homeostasis (Daudi et al., 2012; O’Brien et al., 2012), we first investigated whether H2O2 levels were altered in the-loss-of-function prx34 mutant. Indeed, accumulation of H2O2 decreased in prx34 compared to that in WT roots (Figures 2A–D). Next, we analyzed the frequency of endodermal ACDs in WT and prx34 roots. Under normal conditions, prx34 showed a reduced frequency of MC formation compared to WT (Figures 2E, F, H). In the presence of H2O2, the occurrence of endodermal ACDs for MC generation in prx34 was almost restored to that in WT (Figures 2E, G, H).
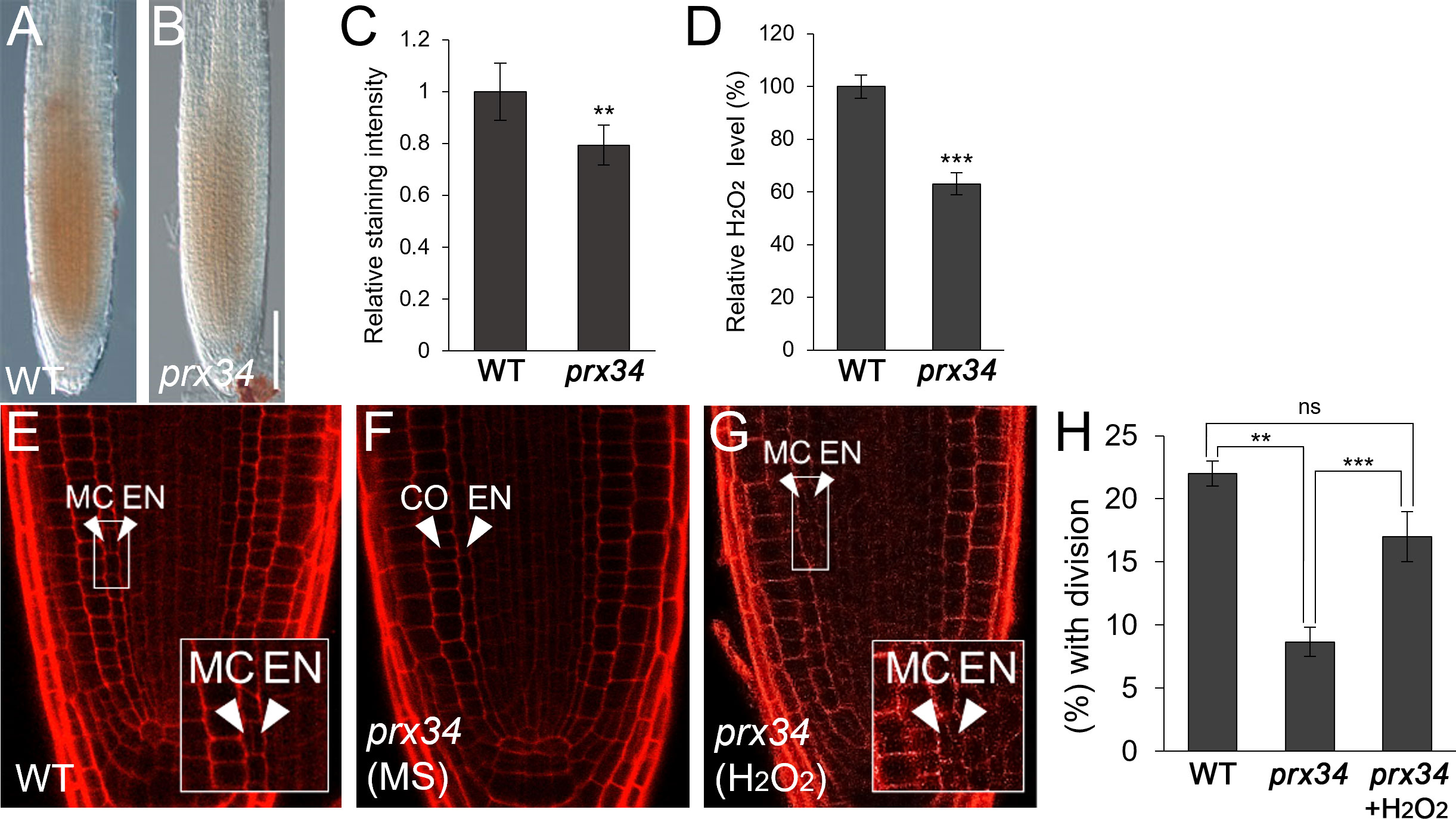
Figure 2 prx34 roots exhibit reductions of H2O2 accumulation and MC formation. (A, B) DAB staining of WT (A) and prx34 (B) roots. (C) DAB staining quantification of WT and prx34 roots. (D) Measurement of H2O2 in WT and prx34. (E-G) Confocal images of WT (E) and prx34 in the absence (F) or presence (G) of H2O2. The insets in (E) and (G) illustrate periclinal ACDs in the endodermis for MC formation. The endodermis (EN), middle cortex (MC), and cortex (CO) layers are indicated with white arrowheads. (H) Proportion of WT and prx34 plants with MC in the absence or presence of H2O2. Significance of difference was determined by Student’s t-test (**P < 0.01; ***P < 0.001; ns: statistically not significant).
Taken together, our findings indicate that PRX34 likely plays a role in H2O2-mediated MC production in the root ground tissue.
Involvement of PRX34 in the GA-mediated regulation of MC formation
Considering the results that i) GA deficiency induced H2O2 accumulation; ii) PRX34 expression was promoted by GA deficiency and H2O2; and iii) both H2O2 level and MC occurrence were substantially attenuated in prx34, we hypothesized that PRX34 might be involved in GA-mediated MC formation in the root ground tissue. To test this, we assessed MC production in prx34 under GA-deficient conditions. In the presence of PAC, prx34 exhibited a reduced occurrence of the MC layers compared to the WT (Figures 3A–C). Next, we performed a genetic analysis using prx34 ga1-3 double mutants. Compared with ga1-3 single mutants, prx34 ga1-3 displayed attenuated MC formation, which was similar to WT (Figures 3D–G). To investigate whether the less frequent MC layers in prx34 under GA-deficient conditions (PAC or ga1-3) was due to lower H2O2 production, we measured the level of H2O2 in prx34 ga1-3 roots. The accumulation of H2O2 in prx34 ga1-3 was indeed lower than that in ga1-3 (Figures 3H–L). These findings are consistent with our phenotypic analyses in prx34 roots under GA-deficient conditions.
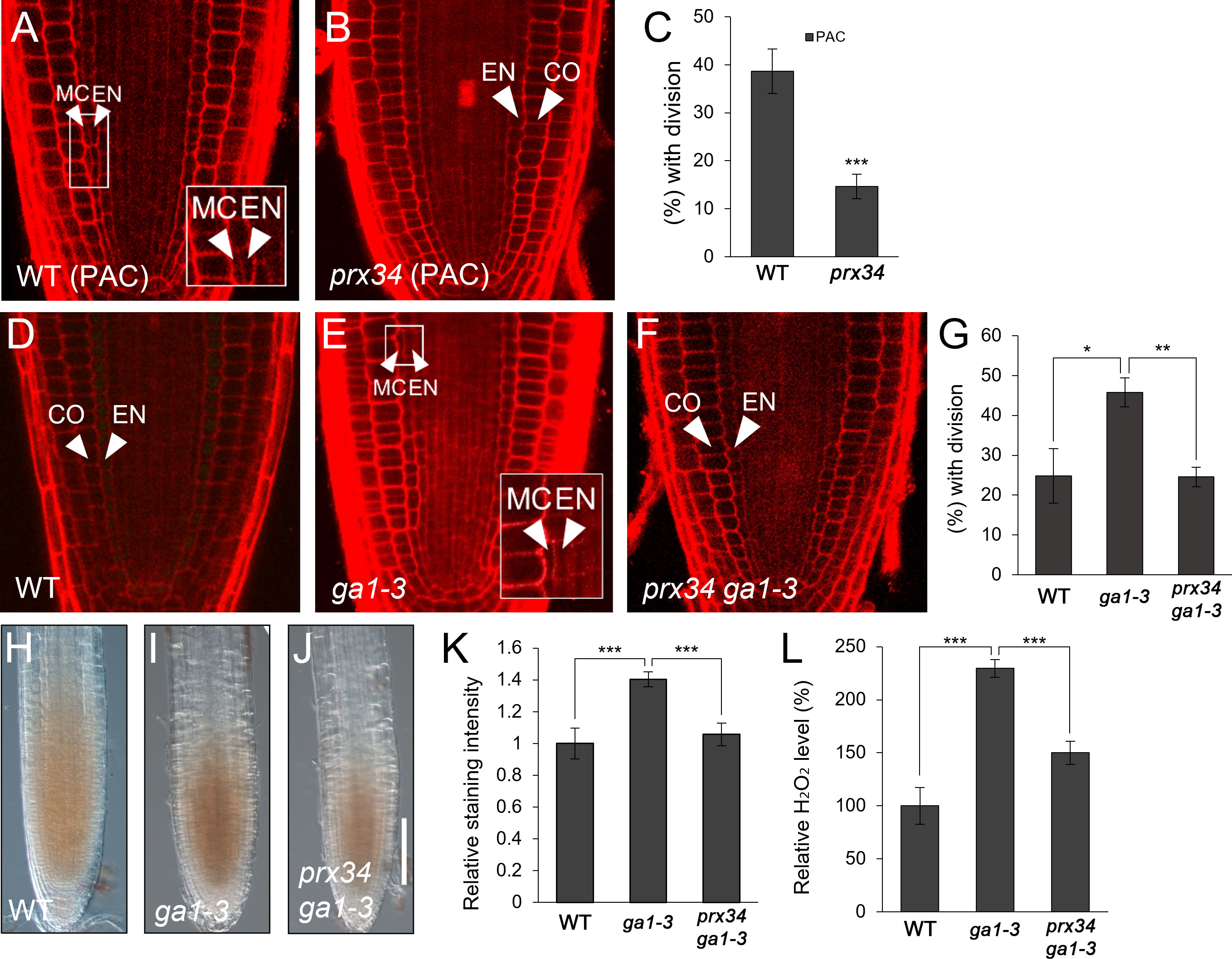
Figure 3 prx34 roots exhibit reductions of H2O2 accumulation and MC formation under GA-deficient conditions. (A, B) Confocal images of WT (A) and prx34 (B) in the presence of PAC. (C) Proportion of WT and prx34 plants with MC in the presence of PAC. (D-F) Confocal images of WT (D), ga1-3 (E) and prx34 ga1-3 (F). The insets in (A) and (E) illustrate endodermal ACDs for MC formation. The endodermis (EN), middle cortex (MC), and cortex (CO) layers are indicated with white arrowheads. (G) Proportion of WT, ga1-3 and prx34 ga1-3 plants with the MC layers. (H-J) DAB staining of WT (H), ga1-3 (I) and prx34 ga1-3 (J). (K) DAB staining quantification of WT, ga1-3 and prx34 ga1-3 roots. (L) Measurement of H2O2 in WT, ga1-3 and prx34 ga1-3. Significance of difference was determined using Student’s t-test (*P < 0.05; **P < 0.01; ***P < 0.001).
Taken together, our results suggest that PRX34, via H2O2 production, is involved in the GA-mediated regulation of MC formation.
Involvement of SCL3 in H2O2-mediated MC formation
Previously, it has been demonstrated that SCL3 plays a role in GA-mediated MC production and thus, the loss-of-function scl3-1 results in more frequent MC formation than in WT roots (Heo et al., 2011; Zhang et al., 2011; Lee et al., 2016). Therefore, we hypothesized that the frequent MC generation phenotype in scl3-1 roots might be due to the elevated H2O2 level. To test this, we assessed H2O2 concentration in scl3-1 in comparison with WT roots and found that scl3-1 accumulated more H2O2 than the WT (Figures 4A–D). We then analyzed the occurrence of MC in the presence of KI. The frequency of MC formation was substantially reduced in KI-treated scl3-1 roots compared to untreated controls (Figures 4E–H). These findings indicate that increased H2O2 levels are likely a causative factor in the frequent generation of MC in scl3-1 roots.
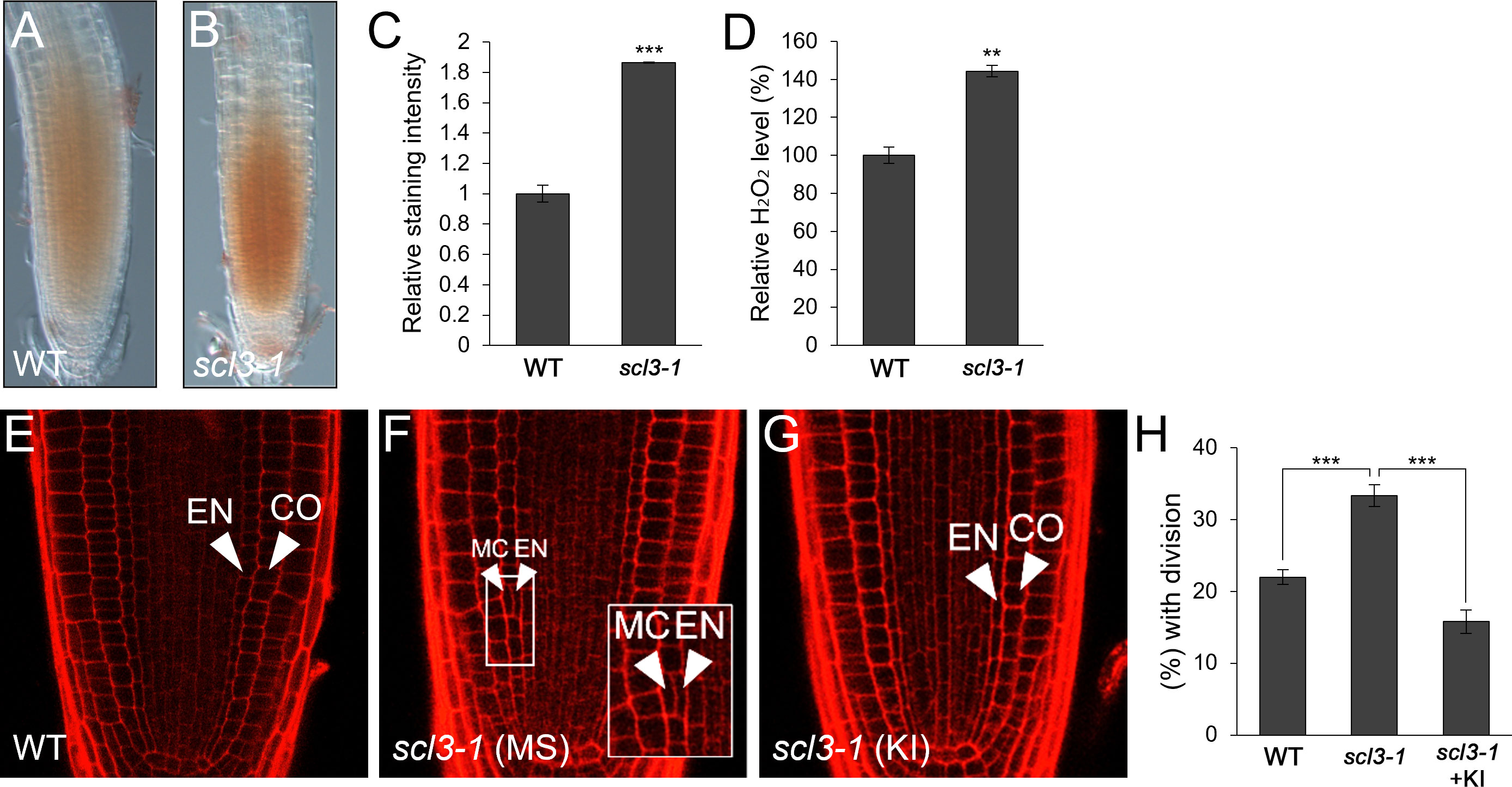
Figure 4 scl3 plays a role in the regulation of H2O2-mediated MC formation. (A, B) DAB staining of WT (A) and scl3-1 (B) roots. (C) Quantification of DAB staining in WT and scl3-1. (D) Measurement of H2O2 in WT and scl3-1. (E-G) Confocal images of WT (E) and scl3-1 roots in the absence (F) or presence (G) of KI. The inset in (F) illustrates periclinal ACDs in the endodermis for MC formation. The endodermis (EN), middle cortex (MC), and cortex (CO) layers are indicated with white arrowheads. (H) Proportion of WT and scl3-1 plants with MC in the absence or presence of KI. Significance of difference was determined by Student’s t-test (**P < 0.01; ***P < 0.001).
SCL3 acts upstream of PRX34 in H2O2-mediated MC formation
Because SCL3 is likely involved in H2O2-mediated MC production, we investigated the relationship between SCL3 and PRX34. First, we performed a genetic analysis by generating prx34 scl3-1 double mutants. Under normal conditions, prx34 and scl3-1 single mutants showed opposite phenotypes in MC generation: a decreased occurrence in prx34 and an increase in scl3-1 (Figures 5A–C, E). Unexpectedly, the MC phenotype of prx34 scl3-1 double mutants resembled that of prx34 single mutants; in that the frequency of MC formation was significantly attenuated (Figures 5B, D, E). Therefore, our genetic analysis indicates that prx34 is epistatic to scl3. Next, we assessed H2O2 levels in prx34 scl3-1 and demonstrated that H2O2 accumulation in prx34 scl3-1 was reduced to a level similar to prx34 (Figures 5F–K).
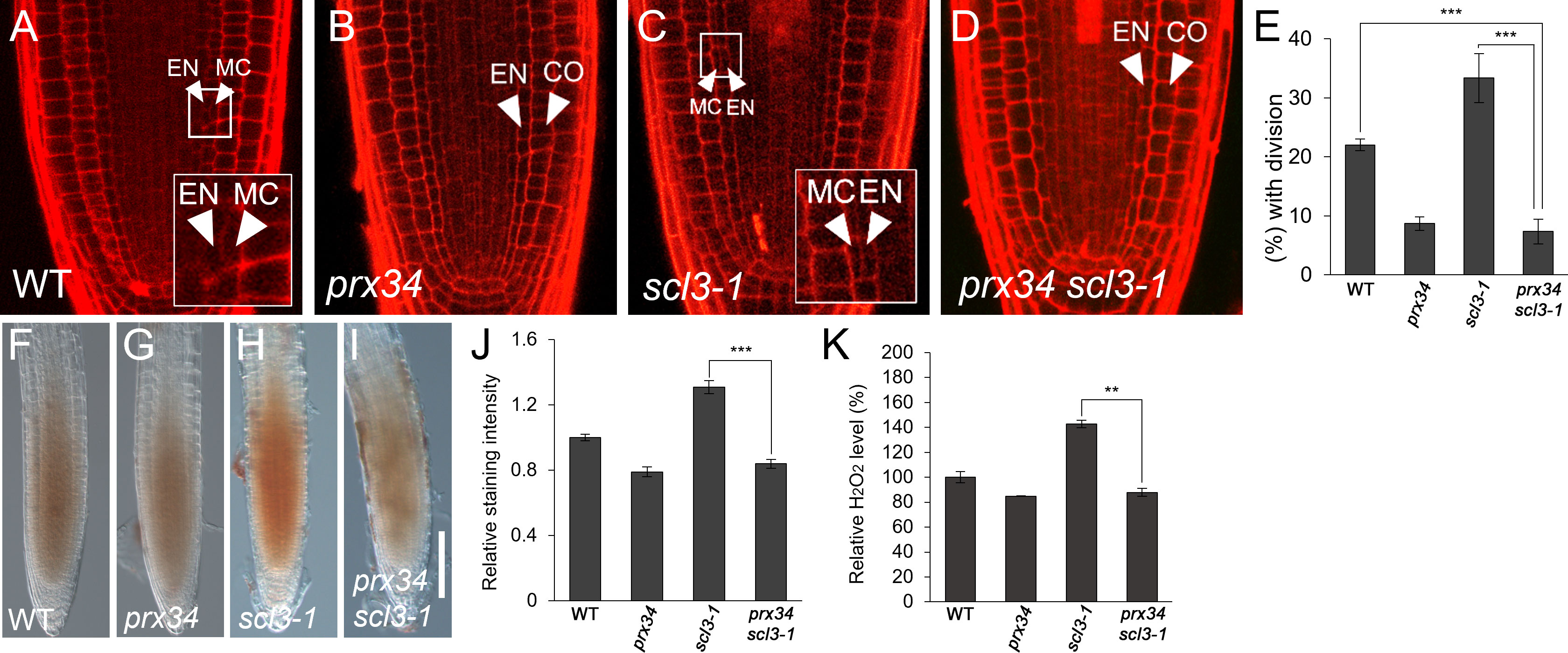
Figure 5 scl3 roots exhibit reductions of H2O2 accumulation and MC formation in the loss of PRX34 function. (A-D) Confocal images of WT (A), prx34 (B), scl3-1 (C) and prx34 scl3-1 (D) roots. The insets in (A) and (C) illustrate endodermal ACDs for MC formation. The endodermis (EN), middle cortex (MC), and cortex (CO) layers are indicated with white arrowheads. (E) Proportion of WT, prx34, scl3-1 and prx34 scl3-1 plants with the MC layers. (F-I). DAB staining of WT (F), prx34 (G), scl3-1 (H) and prx34 scl3-1 (I) roots. (J) Quantification of DAB staining in WT, prx34, scl3-1 and prx34 scl3-1. (K) Measurement of H2O2 in WT, prx34, scl3-1 and prx34 scl3-1. Significance of difference was determined by Student’s t-test (**P < 0.01; ***P < 0.001).
Our results strongly support the notion that the reduced production of MC in prx34 scl3-1 is due to the attenuated levels of H2O2 in the double mutant roots. Furthermore, because prx34 is epistatic to scl3, PRX34 is likely to act downstream of SCL3 in the H2O2-mediated pathway for MC formation in the root ground tissue. To test this, we analyzed the expression levels of PRX34 in the loss (scl3-1) and gain (SCL3-OX) of SCL3 function plants. Interestingly, the abundance of PRX34 mRNA was elevated in scl3-1, whereas its level was reduced in SCL3-OX (Figure 6). This finding indicates that SCL3 likely acts as a negative regulator to modulate the PRX34 expression, thereby maintaining H2O2 homeostasis.
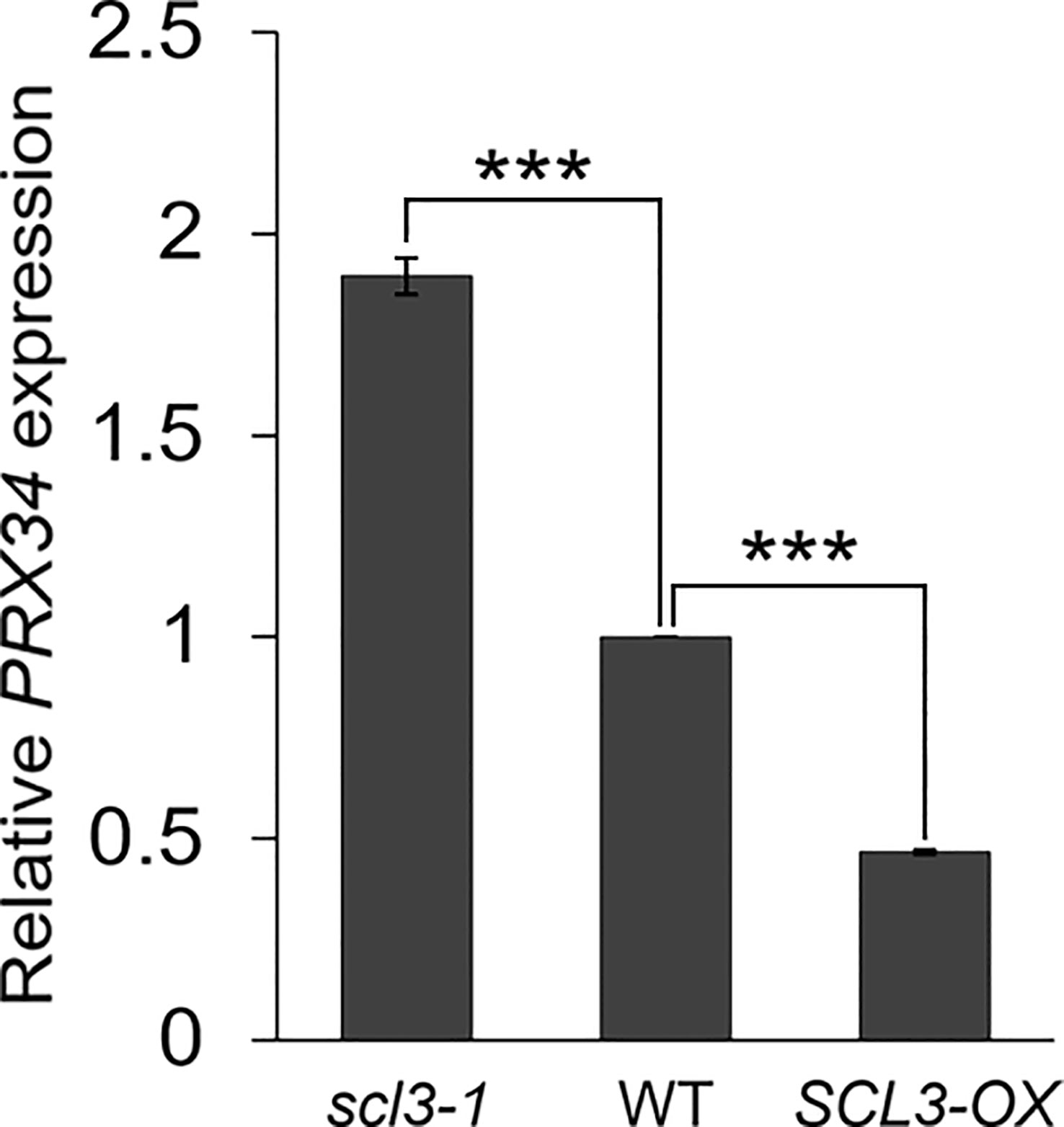
Figure 6 SCL3 negatively regulates the expression levels of PRX34 in roots. The PRX34 expression is upregulated in scl3-1, whereas its expression is attenuated in SCL3-OX. Error bars indicate ± SEM from three independent replicates. Significance of difference was determined by Student’s t-test (***P < 0.001).
Discussion
Previously, it has been reported that ROS, particularly H2O2, induce periclinal ACDs in the endodermis for MC formation in the Arabidopsis root (Cui et al., 2014; Li et al., 2020). In agreement with previous reports, we also found that H2O2 facilitated the occurrence of MC. In addition, the plant hormone GA has been shown to regulate the timing and extent of MC formation (Paquette and Benfey, 2005; Cui and Benfey, 2009a; Cui and Benfey, 2009b; Heo et al., 2011; Pauluzzi et al., 2012; Koizumi et al., 2012a; Koizumi et al., 2012b; Cui et al., 2014; Choi and Lim, 2016; Cui, 2016; Gong et al., 2016; Lee et al., 2016; Bertolotti et al., 2021). Here, we demonstrated that H2O2 accumulated more in ga1-3 roots than in WT, resulting in excessive endodermal ACDs for MC production. However, the molecular link between the GA pathway and H2O2 homeostasis during Arabidopsis ground tissue maturation remains elusive.
To better understand the molecular events in H2O2- and GA-mediated MC formation, we attempted to identify a candidate molecular link using three criteria: i) expression in both GA deficiency and H2O2, ii) enrichment of expression in the root, and iii) availability of a T-DNA insertion mutant. Previously, it was demonstrated that SPY regulated expression of some class III PRX genes (Cui et al., 2014). In particular, the expression levels of PRX33 and PRX34, which are closely related and tandemly located in the Arabidopsis genome, were substantially reduced in the spy root. Both PRX33 and PRX34 were shown to generate H2O2, which conferred resistance to pathogens during the Arabidopsis defense response (Daudi et al., 2012; O’Brien et al., 2012). In addition, both PRXs were reported to be involved in root elongation, possibly modifying cell walls (Passardi et al., 2006). However, the roles of these PRXs in H2O2-mediated MC formation remain unknown. In this study, we focused our efforts on PRX34, which fulfilled our criteria; further investigation of other PRX genes is the subject of another study, which is not discussed here. Interestingly, prx34 roots exhibited a reduction of H2O2 accumulation, resulting in less frequent endodermal ACDs for MC formation than in WT roots. When applied with H2O2, the frequency of MC production in prx34 was restored to WT levels. Furthermore, prx34 showed reduced MC production in GA-deficient conditions caused by ga1-3 or PAC, compared to the mutant under normal conditions. These findings strongly support the idea that GA deficiency induces H2O2 generation via PRX34, and, in turn, H2O2 accumulation promotes endodermal ACDs for MC formation during Arabidopsis ground tissue maturation.
SHORT-ROOT (SHR) and SCARECROW (SCR) play key roles in MC formation (Paquette and Benfey, 2005; Cui and Benfey, 2009a; Cui and Benfey, 2009b; Heo et al., 2011; Koizumi et al., 2012a; Koizumi et al., 2012b; Gong et al., 2016). The shr mutant has no endodermis or MC, whereas scr exhibits excessive MC production (Paquette and Benfey, 2005; Cui and Benfey, 2009a; Cui and Benfey, 2009b; Heo et al., 2011; Koizumi et al., 2012a; Koizumi et al., 2012b; Gong et al., 2016). Both SHR and SCR directly regulate the expression of the cell cycle regulator CYCLIND6;1 (CYCD6;1) in CEID cells, giving rise to the endodermis and cortex in the root (Sozzani et al., 2010; Cruz-Ramirez et al., 2012). During ground tissue maturation, CYCD6;1 is upregulated in the endodermis, triggering periclinal ACDs for MC formation (Koizumi et al., 2012a; Koizumi et al., 2012b; Gong et al., 2016; Lee et al., 2016). Recently, it has been reported that SHR promotes H2O2 accumulation in the Arabidopsis root by transcriptionally activating the RESPIRATORY BURST OXIDASE HOMOLOG (RBOH) genes, which encode NADPH oxidases (Li et al., 2020). In particular, seedling roots ectopically expressing an inducible version of SHR (pG1090-XVE::SHR; Yu et al., 2017) exhibited increased H2O2 accumulation and CYCD6;1 activity, resulting in the frequent occurrence of MC (Li et al., 2020). Therefore, this study suggests that SHR, acting as a positive regulator, plays a role in H2O2 generation via the transcriptional regulation of the RBOH genes.
In the GA signaling pathway, SCL3 antagonizes the function of DELLA proteins, which are the major negative regulators (Silverstone et al., 1997; Silverstone et al., 1998; Sun and Gubler, 2004; Zentella et al., 2007; Heo et al., 2011; Zhang et al., 2011; Yoshida and Ueguchi-Tanaka, 2014; Weng et al., 2020; Ito and Fukazawa, 2021). The scl3-1 mutant in the ga1-3 background showed enhanced GA-deficient phenotypes in growth and development, whereas loss-of-function mutations in the DELLA genes restored the ga1-3 phenotypes (Silverstone et al., 1997; Silverstone et al., 1998; Dill and Sun, 2001; Heo et al., 2011; Zhang et al., 2011; Yoshida and Ueguchi-Tanaka, 2014; Weng et al., 2020; Ito and Fukazawa, 2021). In particular, GA-deficient conditions (ga1-3 or PAC) exacerbate the MC phenotype of scl3-1, resulting in excessive endodermal ACDs for MC formation (Heo et al., 2011). When overexpressed, SCL3-OX reduced the occurrence of MC, even under GA-deficient conditions (Heo et al., 2011). Thus, SCL3 plays a crucial role in GA-mediated MC formation (Heo et al., 2011). In this study, we demonstrated that H2O2 accumulation was higher in scl3-1 than WT. In the presence of KI, the frequency of MC formation in scl3-1 was attenuated. Furthermore, the prx34 scl3-1 double mutant showed lower H2O2 accumulation than scl3-1. Consistent with the low level of H2O2 in prx34 scl3-1, the double mutant displayed less frequent MC production than scl3-1. Taken together, the prx34 scl3-1 double mutant was indistinguishable from the prx34 single mutant in both H2O2 accumulation and MC phenotype. Thus, our genetic analysis led us to the conclusion that prx34 is epistatic to scl3. In addition, the abundance of PRX34 transcripts was higher in scl3-1 and lower in SCL3-OX than that in WT. These results strongly suggest that SCL3 serves as a negative regulator of PRX34 expression to maintain H2O2 homeostasis during root ground tissue maturation.
SCL3, acting downstream of the SHR/SCR regulatory module, is uniquely positioned in controlling the timing and extent of MC formation (Heo et al., 2011; Choi and Lim, 2016; Gong et al., 2016; Lee et al., 2016). Recently, it has been reported that the SHR/SCR module physically interacted with NAC1 to restrict excessive periclinal ACDs in the endodermis during root ground tissue maturation (Xie et al., 2023). NAC1 directly inhibited the transcription of CYCD6;1 with the transcriptional co-repressor TOPLESS (TPL), resulting in reduced MC generation (Xie et al., 2023). Thus, it is tempting to speculate that the SHR/SCR regulatory module, together with NAC1, is also involved in H2O2- and GA-mediated MC formation, impinging on the transcriptional regulation of SCL3.
The physiological function of MC formation remains elusive. Except for the Arabidopsis root, most plant roots have multiple cortex layers (Esau, 1953; Esau, 1977; Benfey et al., 1993; Dolan et al., 1993; Scheres et al., 1994; Scheres et al., 1995; Di Laurenzio et al., 1996; Helariutta et al., 2000; Cui et al., 2007; Cruz-Ramirez et al., 2012; Wu et al., 2014; Choi and Lim, 2016). However, as the individual Arabidopsis plant grows, periclinal ACDs in the endodermis produce an additional cortex layer, namely MC, in the root ground tissue. (Baum et al., 2002; Paquette and Benfey, 2005; Cui and Benfey, 2009a; Cui and Benfey, 2009b; Heo et al., 2011; Pauluzzi et al., 2012; Koizumi et al., 2012a; Koizumi et al., 2012b; Cui et al., 2014; Choi and Lim, 2016; Cui, 2016; Gong et al., 2016; Lee et al., 2016; Di Ruocco et al., 2018; Bertolotti et al., 2021; Hernández-Coronado and Ortiz-Ramírez, 2021; Shtin et al., 2022; Xie et al., 2023). Our results, together with those of previous studies, imply that MC formation caused by GA deficiency and H2O2 might be a consequence of plant adaptation to environmental stimuli, such as stresses (Cui et al., 2014; Cui, 2015; Choi and Lim, 2016).
Taken together, in a simplified model (Figure 7), the plant hormone GA negatively regulates the expression of SCL3. Hence, SCL3 acts as a convergent point for the interaction between GA and ROS. SCL3 plays a role in H2O2 homeostasis via the transcriptional regulation of PRX34 in H2O2-mediated MC formation. Therefore, we have not only provided new insights into the crosstalk between GA and ROS but also unveiled a novel role for PRX34 in the maintenance of H2O2 homeostasis during root ground tissue maturation. Thus, it is tempting to speculate that diverse transcriptional inputs from hormonal (e.g., GA) and developmental (e.g., SHR/SCR) pathways impinge on the tissue-specific integrator SCL3 to modulate the timing and extent of MC formation during root ground tissue maturation.
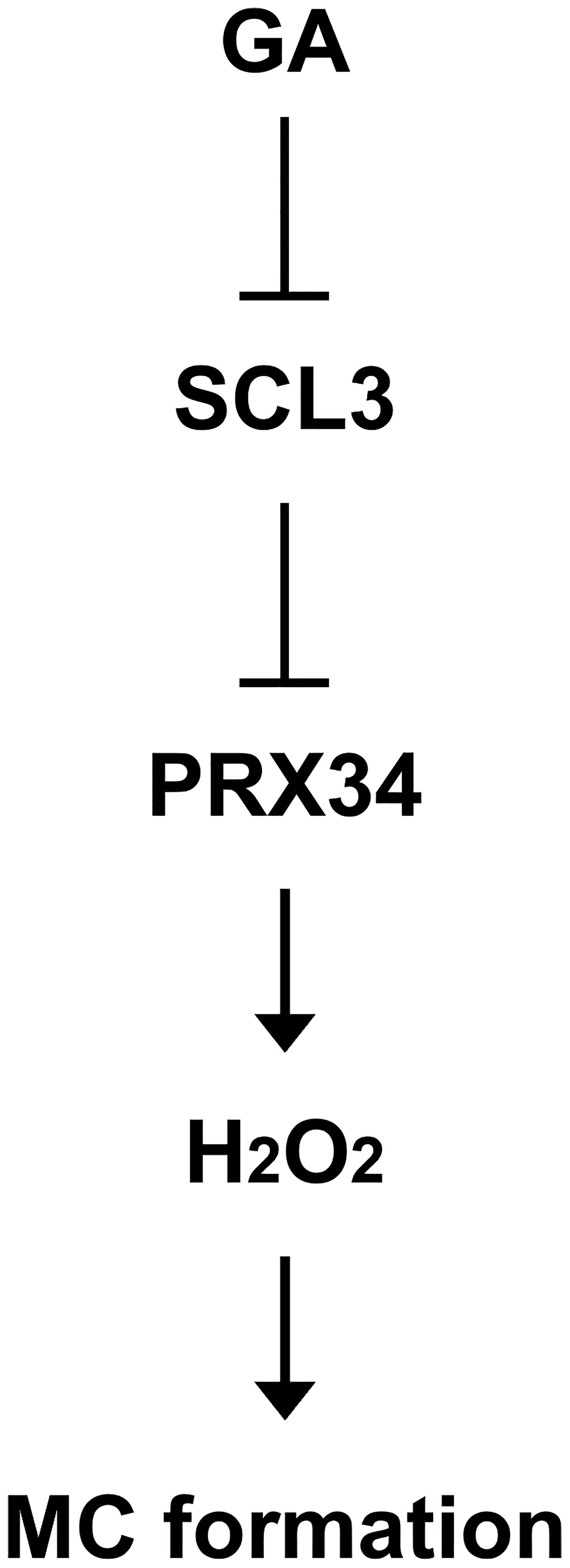
Figure 7 Schematic model for the transcriptional regulation of H2O2-mediated MC formation in the Arabidopsis root. The SCL3 transcription factor, whose expression is modulated by GA, negatively regulates transcription of PRX34 to maintain H2O2 homeostasis. The class III peroxidase PRX34, which is positioned downstream of SCL3, produces H2O2. In turn, H2O2 accumulation induced by PRX34 promotes periclinal ACDs in the endodermis for MC formation during root ground tissue maturation.
Data availability statement
The raw data supporting the conclusions of this article will be made available by the authors, without undue reservation.
Author contributions
JO, JWC, SJ and JL conceived, designed, and performed the experiments. SWK, J-OH, and EKY analyzed the data and performed plant work including genotyping. S-HK contributed new analytical tools and reagents and provided critical comments and suggestions on the experiments. JO, JWC, SJ, and JL wrote the manuscript with contributions from all the authors. All authors contributed to the article and approved the submitted version.
Funding
This work was supported by the Konkuk University Research Fund 2017.
Acknowledgments
We thank the members of the Lim laboratory for their comments on the manuscript. We are grateful to Arabidopsis Biological Resource Center (ABRC) for providing the plant lines. We would also like to thank Editage (www.editage.co.kr) for English language editing.
Conflict of interest
The authors declare that the research was conducted in the absence of any commercial or financial relationships that could be construed as a potential conflict of interest.
Publisher’s note
All claims expressed in this article are solely those of the authors and do not necessarily represent those of their affiliated organizations, or those of the publisher, the editors and the reviewers. Any product that may be evaluated in this article, or claim that may be made by its manufacturer, is not guaranteed or endorsed by the publisher.
Supplementary material
The Supplementary Material for this article can be found online at: https://www.frontiersin.org/articles/10.3389/fpls.2023.1242211/full#supplementary-material
References
Abrash, E. B., Bergmann, D. C. (2009). Asymmetric cell divisions: a view from plant development. Dev. Cell 16, 783–796. doi: 10.1016/j.devcel.2009.05.014
Achard, P., Cheng, H., De Grauwe, L., Decat, J., Schoutteten, H., Moritz, T., et al. (2006). Integration of plant responses to environmentally activated phytohormonal signals. Science 311, 91–94. doi: 10.1126/science.1118642
Apel, K., Hirt, H. (2004). Reactive oxygen species: metabolism, oxidative stress, and signal transduction. Annu. Rev. Plant Biol. 55, 373–399. doi: 10.1146/annurev.arplant.55.031903.141701
Barah, P., B. N. M. N., Jayavelu, N. D., Sowdhamini, R., Shameer, K., Bones, A. M. (2016). Transcriptional regulatory networks in Arabidopsis thaliana during single and combined stresses. Nucleic Acids Res. 44, 3147–3164. doi: 10.1093/nar/gkv1463
Baum, S. F., Dubrovsky, J. G., Rost, T. L. (2002). Apical organization and maturation of the cortex and vascular cylinder in Arabidopsis thaliana (Brassicaceae) roots. Am. J. Bot. 89, 908–920. doi: 10.3732/ajb.89.6.908
Benfey, P. N., Linstead, P. J., Roberts, K., Schiefelbein, J. W., Hauser, M. T., Aeschbacher, R. A. (1993). Root development in Arabidopsis: four mutants with dramatically altered root morphogenesis. Development 119, 57–70. doi: 10.1242/dev.119.1.57
Bertolotti, G., Unterholzner, S. J., Scintu, D., Salvi, E., Svolacchia, N., Di Mambro, R., et al. (2021). A PHABULOSA-controlled genetic pathway regulates ground tissue patterning in the Arabidopsis root. Curr. Biol. 31, 420–426. doi: 10.1016/j.cub.2020.10.038
Bindschedler, L. V., Dewdney, J., Blee, K. A., Stone, J. M., Asai, T., Plotnikov, J., et al. (2006). Peroxidase-dependent apoplastic oxidative burst in Arabidopsis required for pathogen resistance. Plant J. 47, 851–863. doi: 10.1111/j.1365-313X.2006.02837.x
Chaiwanon, J., Wang, W., Zhu, J. Y., Oh, E., Wang, Z. Y. (2016). Information integration and communication in plant growth regulation. Cell 164, 1257–1268. doi: 10.1016/j.cell.2016.01.044
Choi, J. W., Lim, J. (2016). Control of asymmetric cell divisions during root ground tissue maturation. Mol. Cells 39, 524–529. doi: 10.14348/molcells.2016.0105
Cosio, C., Dunand, C. (2009). Specific functions of individual class III peroxidase genes. J. Exp. Bot. 60, 391–408. doi: 10.1093/jxb/ern318
Cruz-Ramirez, A., Diaz-Trivino, S., Blilou, I., Grieneisen, V. A., Sozzani, R., Zamioudis, C., et al. (2012). A bistable circuit involving SCARECROW-RETINOBLASTOMA integrates cues to inform asymmetric stem cell division. Cell 150, 1002–1015. doi: 10.1016/j.cell.2012.07.017
Cui, H. (2015). Cortex proliferation in the root is a protective mechanism against abiotic stress. Plant Signal. Behav. 10, e1011949. doi: 10.1080/15592324.2015.1011949
Cui, H. (2016). Middle cortex formation in the root: an emerging picture of integrated regulatory mechanisms. Mol. Plant 9, 771–773. doi: 10.1016/j.molp.2016.05.002
Cui, H., Benfey, P. N. (2009a). Interplay between SCARECROW, GA and LIKE HETEROCHROMATIN PROTEIN 1 in ground tissue patterning in the Arabidopsis root. Plant J. 58, 1016–1027. doi: 10.1111/j.1365-313X.2009.03839.x
Cui, H., Benfey, P. N. (2009b). Cortex proliferation: simple phenotype, complex regulatory mechanisms. Plant Signal. Behav. 4, 551–553. doi: 10.4161/psb.4.6.8731
Cui, H., Kong, D., Wei, P., Hao, Y., Torii, K. U., Lee, J. S., et al. (2014). SPINDLY, ERECTA and its ligand STOMAGEN have a role in redox-mediated cortex proliferation in the Arabidopsis root. Mol. Plant 7, 1727–1739. doi: 10.1093/mp/ssu106
Cui, H., Levesque, M. P., Vernoux, T., Jung, J. W., Paquette, A. J., Gallagher, K. L., et al. (2007). An evolutionarily conserved mechanism delimiting SHR movement defines a single layer of endodermis in plants. Science 316, 421–425. doi: 10.1126/science.1139531
Daudi, A., Cheng, Z., O’Brien, J. A., Mammarella, N., Khan, S., Ausubel, F. M., et al. (2012). The apoplastic oxidative burst peroxidase in Arabidopsis is a major component of pattern-triggered immunity. Plant Cell 24, 275–287. doi: 10.1105/tpc.111.093039
De Smet, I., Beeckman, T. (2011). Asymmetric cell division in land plants and algae: the driving force for differentiation. Nat. Rev. Mol. Cell Biol. 12, 177–188. doi: 10.1038/nrm3064
Dhar, S., Kim, J., Yoon, E. K., Jang, S., Ko, K., Lim, J. (2022). SHORT-ROOT controls cell elongation in the etiolated Arabidopsis hypocotyl. Mol. Cells 45, 243–256. doi: 10.14348/molcells.2021.5008
Di Laurenzio, L., Wysocka-Diller, J., Malamy, J. E., Pysh, L., Helariutta, Y., Freshour, G., et al. (1996). The SCARECROW gene regulates an asymmetric cell division that is essential for generating the radial organization of the Arabidopsis root. Cell 86, 423–433. doi: 10.1016/S0092-8674(00)80115-4
Dill, A., Sun, T. (2001). Synergistic derepression of gibberellin signaling by removing RGA and GAI function in Arabidopsis thaliana. Genetics 159, 777–785. doi: 10.1093/genetics/159.2.777
Di Ruocco, G., Di Mambro, R., Dello Ioio, R. (2018). Building the differences: a case for the ground tissue patterning in plants. Proc. Biol. Sci. 285, 20181746. doi: 10.1098/rspb.2018.1746
Dolan, L., Janmaat, K., Willemsen, V., Linstead, P., Poethig, S., Roberts, K., et al. (1993). Cellular organization of the Arabidopsis thaliana root. Development 119, 71–84. doi: 10.1242/dev.119.1.71
Dunand, C., Crevecoeur, M., Penel, C. (2007). Distribution of superoxide and hydrogen peroxide in Arabidopsis root and their influence on root development: possible interaction with peroxidases. New Phytol. 174, 332–341. doi: 10.1111/j.1469-8137.2007.01995.x
Francoz, E., Ranocha, P., Nguyen-Kim, H., Jamet, E., Burlat, V., Dunand, C. (2015). Roles of cell wall peroxidases in plant development. Phytochemistry 112, 15–21. doi: 10.1016/j.phytochem.2014.07.020
Gapper, C., Dolan, L. (2006). Control of plant development by reactive oxygen species. Plant Physiol. 141, 341–345. doi: 10.1104/pp.106.079079
Gong, X., Flores-Vergara, M. A., Hong, J. H., Chu, H., Lim, J., Franks, R. G., et al. (2016). SEUSS integrates gibberellin signaling with transcriptional inputs from the SHR-SCR-SCL3 module to regulate middle cortex formation in the Arabidopsis root. Plant Physiol. 170, 1675–1683. doi: 10.1104/pp.15.01501
Han, S., Fang, L., Ren, X., Wang, W., Jiang, J. (2015). MPK6 controls H2O2-induced root elongation by mediating Ca2+ influx across the plasma membrane of root cells in Arabidopsis seedlings. New Phytol. 205, 695–706. doi: 10.1111/nph.12990
Heidstra, R., Welch, D., Scheres, B. (2004). Mosaic analyses using marked activation and deletion clones dissect Arabidopsis SCARECROW action in asymmetric cell division. Genes Dev. 18, 1964–1969. doi: 10.1101/gad.305504
Helariutta, Y., Fukaki, H., Wysocka-Diller, J., Nakajima, K., Jung, J., Sena, G., et al. (2000). The SHORT-ROOT gene controls radial patterning of the Arabidopsis root through radial signaling. Cell 101, 555–567. doi: 10.1016/S0092-8674(00)80865-X
Heo, J.-O., Chang, K. S., Kim, I. A., Lee, M.-H., Lee, S. A., Song, S. K., et al. (2011). Funneling of gibberellin signaling by the GRAS transcription regulator SCARECROW-LIKE 3 in the Arabidopsis root. Proc. Natl. Acad. Sci. U.S.A. 108, 2166–2171. doi: 10.1073/pnas.1012215108
Hernández-Coronado, M., Ortiz-Ramírez, C. (2021). Root patterning: tuning SHORT ROOT function creates diversity in form. Front. Plant Sci. 12, 745861. doi: 10.3389/fpls.2021.745861
Horvitz, H. R., Herskowitz, I. (1992). Mechanisms of asymmetric cell division: two Bs or not two Bs, that is the question. Cell 68, 237–255. doi: 10.1016/0092-8674(92)90468-R
Ito, T., Fukazawa, J. (2021). SCARECROW-LIKE3 regulates the transcription of gibberellin-related genes by acting as a transcriptional co-repressor of GAI-ASSOCIATED FACTOR1. Plant Mol. Biol. 105, 463–482. doi: 10.1007/s11103-020-01101-z
Jacobsen, S. E., Binkowski, K. A., Olszewski, N. E. (1996). SPINDLY, a tetratricopeptide repeat protein involved in gibberellin signal transduction in Arabidopsis. Proc. Natl. Acad. Sci. U.S.A. 93, 9292–9296. doi: 10.1073/pnas.93.17.9292
Jacobsen, S. E., Olszewski, N. E. (1993). Mutations at the SPINDLY locus of Arabidopsis alter gibberellin signal transduction. Plant Cell 5, 887–896. doi: 10.1105/tpc.5.8.887
Knoblich, J. A. (2008). Mechanisms of asymmetric stem cell division. Cell 132, 583–597. doi: 10.1016/j.cell.2008.02.007
Koizumi, K., Hayashi, T., Gallagher, K. L. (2012a). SCARECROW reinforces SHORT-ROOT signaling and inhibits periclinal cell divisions in the ground tissue by maintaining SHR at high levels in the endodermis. Plant Signal. Behav. 7, 1573–1577. doi: 10.4161/psb.22437
Koizumi, K., Hayashi, T., Wu, S., Gallagher, K. L. (2012b). The SHORT-ROOT protein acts as a mobile, dose-dependent signal in patterning the ground tissue. Proc. Natl. Acad. Sci. U.S.A. 109, 13010–13015. doi: 10.1073/pnas.1205579109
Lee, S. A., Jang, S., Yoon, E. K., Heo, J.-O., Chang, K. S., Choi, J. W., et al. (2016). Interplay between ABA and GA modulates the timing of asymmetric cell divisions in the Arabidopsis root ground tissue. Mol. Plant 9, 870–884. doi: 10.1016/j.molp.2016.02.009
Li, P., Cai, Q., Wang, H., Li, S., Cheng, J., Li, H., et al. (2020). Hydrogen peroxide homeostasis provides beneficial micro-environment for SHR-mediated periclinal division in Arabidopsis root. New Phytol. 228, 1926–1938. doi: 10.1111/nph.16824
Livanos, P., Apostolakos, P., Galatis, B. (2012). Plant cell division: ROS homeostasis is required. Plant Signal. Behav. 7, 771–778. doi: 10.4161/psb.20530
Mase, K., Tsukagoshi, H. (2021). Reactive oxygen species link gene regulatory networks during Arabidopsis root development. Front. Plant Sci. 12, 660274. doi: 10.3389/fpls.2021.660274
Mittler, R., Vanderauwera, S., Gollery, M., Van Breusegem, F. (2004). Reactive oxygen gene network of plants. Trends Plant Sci. 9, 490–498. doi: 10.1016/j.tplants.2004.08.009
Moreno-Risueno, M. A., Sozzani, R., Yardımcı, G. G., Petricka, J. J., Vernoux, T., Blilou, I., et al. (2015). Transcriptional control of tissue formation throughout root development. Science 350, 426–430. doi: 10.1126/science.aad1171
O’Brien, J. A., Daudi, A., Finch, P., Butt, V. S., Whitelegge, J. P., Souda, P., et al. (2012). A peroxidase-dependent apoplastic oxidative burst in cultured Arabidopsis cells functions in MAMP-elicited defense. Plant Physiol. 158, 2013–2027. doi: 10.1104/pp.111.190140
Paquette, A. J., Benfey, P. N. (2005). Maturation of the ground tissue of the root is regulated by gibberellin and SCARECROW and requires SHORT-ROOT. Plant Physiol. 138, 636–640. doi: 10.1104/pp.104.058362
Passardi, F., Tognolli, M., De Meyer, M., Penel, C., Dunand, C. (2006). Two cell wall associated peroxidases from Arabidopsis influence root elongation. Planta 223, 965–974. doi: 10.1007/s00425-005-0153-4
Pauluzzi, G., Divol, F., Puig, J., Guiderdoni, E., Dievart, A., Périn, C. (2012). Surfing along the root ground tissue gene network. Dev. Biol. 365, 14–22. doi: 10.1016/j.ydbio.2012.02.007
Petricka, J. J., Winter, C. M., Benfey, P. N. (2012). Control of Arabidopsis root development. Annu. Rev. Plant Biol. 63, 563–590. doi: 10.1146/annurev-arplant-042811-105501
Scheres, B., Di Laurenzio, L., Willemsen, V., Hauser, M.-T., Janmaat, K., Weisbeek, P., et al. (1995). Mutations affecting the radial organization of the Arabidopsis root display specific defects throughout the embryonic axis. Development 121, 53–62. doi: 10.1242/dev.121.1.53
Scheres, B., Wolkenfelt, H., Willemsen, V., Terlouw, M., Lawson, E., Dean, C., et al. (1994). Embryonic origin of the Arabidopsis primary root and root meristem initials. Development 120, 2475–2487. doi: 10.1242/dev.120.9.2475
Schneider, C. A., Rasband, W. S., Eliceiri, K. W. (2012). NIH Image to ImageJ: 25 years of image analysis. Nat. Methods 9, 671–675. doi: 10.1038/nmeth.2089
Shigeto, J., Tsutsumi, Y. (2016). Diverse functions and reactions of class III peroxidases. New Phytol. 209, 1395–1402. doi: 10.1111/nph.13738
Shtin, M., Dello Ioio, R., Del Bianco, M. (2022). It’s time for a change: The role of gibberellin in root meristem development. Front. Plant Sci. 13, 882517. doi: 10.3389/fpls.2022.882517
Silverstone, A., Ciampaglio, C., Sun, T. P. (1998). The Arabidopsis RGA gene encodes a transcriptional regulator repressing the gibberellin signal transduction pathway. Plant Cell 10, 155–169. doi: 10.1105/tpc.10.2.155
Silverstone, A. L., Mak, P. Y., Martínez, E. C., Sun, T. P. (1997). The new RGA locus encodes a negative regulator of gibberellin response in Arabidopsis thaliana. Genetics 146, 1087–1099. doi: 10.1093/genetics/146.3.1087
Smolarkiewicz, M., Dhonukshe, P. (2013). Formative cell divisions: principal determinants of plant morphogenesis. Plant Cell Physiol. 54, 333–342. doi: 10.1093/pcp/pcs175
Sozzani, R., Cui, H., Moreno-Risueno, M. A., Busch, W., Van Norman, J. M., Vernoux, T., et al. (2010). Spatiotemporal regulation of cell-cycle genes by SHORTROOT links patterning and growth. Nature 466, 128–132. doi: 10.1038/nature09143
Srivastava, G. P., Li, P., Liu, J., Xu, D. (2010). Identification of transcription factor’s targets using tissue-specific transcriptomic data in Arabidopsis thaliana. BMC Syst. Biol. 4 (Suppl 2), S2. doi: 10.1186/1752-0509-4-S2-S2
Sun, T.-p., Gubler, F. (2004). Molecular mechanism of gibberellin signaling in plants. Ann. Rev. Plant Biol. 55, 197–223. doi: 10.1146/annurev.arplant.55.031903.141753
Sun, T.-p., Kamiya, Y. (1994). The Arabidopsis GA1 locus encodes the cyclase ent-kaurene synthetase A of gibberellin biosynthesis. Plant Cell 6, 1509–1518. doi: 10.1105/tpc.6.10.1509
Ten Hove, C. A., Heidstra, R. (2008). Who begets whom? Plant cell fate determination by asymmetric cell division. Curr. Opin. Plant Biol. 11, 34–41. doi: 10.1016/j.pbi.2007.11.001
Thordal-Christensen, H., Zhang, Z., Wei, Y., Collinge, D. B. (1997). Subcellular localization of H2O2 in plants, H2O2 accumulation in papillae and hypersensitive response during barley-powdery mildew interaction. Plant J. 11, 1187–1194. doi: 10.1046/j.1365-313X.1997.11061187.x
Tognolli, M., Penel, C., Greppin, H., Simon, P. (2002). Analysis and expression of the class III peroxidase large gene family in Arabidopsis thaliana. Gene 288, 129–138. doi: 10.1016/S0378-1119(02)00465-1
Tsukagoshi, H. (2016). Control of root growth and development by reactive oxygen species. Curr. Opin. Plant Biol. 29, 57–63. doi: 10.1016/j.pbi.2015.10.012
Tsukagoshi, H., Busch, W., Benfey, P. N. (2010). Transcriptional regulation of ROS controls transition from proliferation to differentiation in the root. Cell 143, 606–616. doi: 10.1016/j.cell.2010.10.020
Valério, L., De Meyer, M., Penel, C., Dunand, C. (2004). Expression analysis of the Arabidopsis peroxidase multigenic family. Phytochemistry 65, 1331–1342. doi: 10.1016/j.phytochem.2004.04.017
Vanacker, H., Carver, T. L., Foyer, C. H. (2000). Early H2O2 accumulation in mesophyll cells leads to induction of glutathione during the hyper-sensitive response in the barley-powdery mildew interaction. Plant Physiol. 123, 1289–1300. doi: 10.1104/pp.123.4.1289
Verma, V., Ravindran, P., Kumar, P. P. (2016). Plant hormone-mediated regulation of stress responses. BMC Plant Biol. 16, 86. doi: 10.1186/s12870-016-0771-y
Weng, C. Y., Zhu, M. H., Liu, Z. Q., Zheng, Y. G. (2020). Integrated bioinformatics analyses identified SCL3-induced regulatory network in Arabidopsis thaliana roots. Biotechnol. Lett. 42, 1019–1033. doi: 10.1007/s10529-020-02850-z
Wolters, H., Jürgens, G. (2009). Survival of the flexible: hormonal growth control and adaptation in plant development. Nat. Rev. Genet. 10, 305–317. doi: 10.1038/nrg2558
Wu, S., Lee, C. M., Hayashi, T., Price, S., Divol, F., Henry, S., et al. (2014). A plausible mechanism, based upon SHORT-ROOT movement, for regulating the number of cortex cell layers in roots. Proc. Natl. Acad. Sci. U.S.A. 111, 16184–16189. doi: 10.1073/pnas.1407371111
Xia, X. J., Zhou, Y. H., Shi, K., Zhou, J., Foyer, C. H., Yu, J. Q. (2015). Interplay between reactive oxygen species and hormones in the control of plant development and stress tolerance. J. Exp. Bot. 66, 2839–2856. doi: 10.1093/jxb/erv089
Xie, C., Li, C., Wang, F., Zhang, F., Liu, J., Wang, J., et al. (2023). NAC1 regulates root ground tissue maturation by coordinating with the SCR/SHR-CYCD6;1 module in Arabidopsis. Mol. Plant 16, 709–725. doi: 10.1016/j.molp.2023.02.006
Yoon, E. K., Dhar, S., Lee, M.-H., Song, J. H., Lee, S. A., Kim, G., et al. (2016). Conservation and diversification of the SHR-SCR-SCL23 regulatory network in the development of the functional endodermis in Arabidopsis shoots. Mol. Plant 9, 1197–1209. doi: 10.1016/j.molp.2016.06.007
Yoshida, H., Ueguchi-Tanaka, M. (2014). DELLA and SCL3 balance gibberellin feedback regulation by utilizing INDETERMINATE DOMAIN proteins as transcriptional scaffolds. Plant Signal. Behav. 9, e29726. doi: 10.4161/psb.29726
Yu, Q., Li, P., Liang, N., Wang, H., Xu, M., Wu, S. (2017). Cell-fate specification in Arabidopsis roots requires coordinative action of lineage instruction and positional reprogramming. Plant Physiol. 175, 816–827. doi: 10.1104/pp.17.00814
Zentella, R., Zhang, Z. L., Park, M., Thomas, S. G., Endo, A., Murase, K., et al. (2007). Global analysis of DELLA direct targets in early gibberellin signaling in Arabidopsis. Plant Cell 19, 3037–3057. doi: 10.1105/tpc.107.054999
Zhang, Z. L., Ogawa, M., Fleet, C. M., Zentella, R., Hu, J., Heo, J.-O., et al. (2011). SCARECROW-LIKE 3 promotes gibberellin signaling by antagonizing master growth repressor DELLA in Arabidopsis. Proc. Natl. Acad. Sci. U.S.A. 108, 2160–2165. doi: 10.1073/pnas.1012232108
Keywords: Arabidopsis, class III peroxidase, gibberellic acid, ground tissue, hydrogen peroxide, middle cortex formation, root development
Citation: Oh J, Choi JW, Jang S, Kim SW, Heo J-O, Yoon EK, Kim S-H and Lim J (2023) Transcriptional control of hydrogen peroxide homeostasis regulates ground tissue patterning in the Arabidopsis root. Front. Plant Sci. 14:1242211. doi: 10.3389/fpls.2023.1242211
Received: 18 June 2023; Accepted: 01 August 2023;
Published: 21 August 2023.
Edited by:
Giovanna Serino, Sapienza University of Rome, ItalyReviewed by:
Raffaele Dello Ioio, Sapienza University of Rome, ItalyMaurizio Trovato, Sapienza University of Rome, Italy
Copyright © 2023 Oh, Choi, Jang, Kim, Heo, Yoon, Kim and Lim. This is an open-access article distributed under the terms of the Creative Commons Attribution License (CC BY). The use, distribution or reproduction in other forums is permitted, provided the original author(s) and the copyright owner(s) are credited and that the original publication in this journal is cited, in accordance with accepted academic practice. No use, distribution or reproduction is permitted which does not comply with these terms.
*Correspondence: Jun Lim, amxpbUBrb25rdWsuYWMua3I=
†Present addresses: Ji Won Choi, Plant Biotechnology Research Center, Ghent University Global Campus, Incheon, Republic of Korea
Eun Kyung Yoon, Plant Biotechnology Research Center, Ghent University Global Campus, Incheon, Republic of Korea
Jung-Ok Heo, The Sainsbury Laboratory Cambridge University, Cambridge, United Kingdom
‡These authors have contributed equally to this work and share first authorship