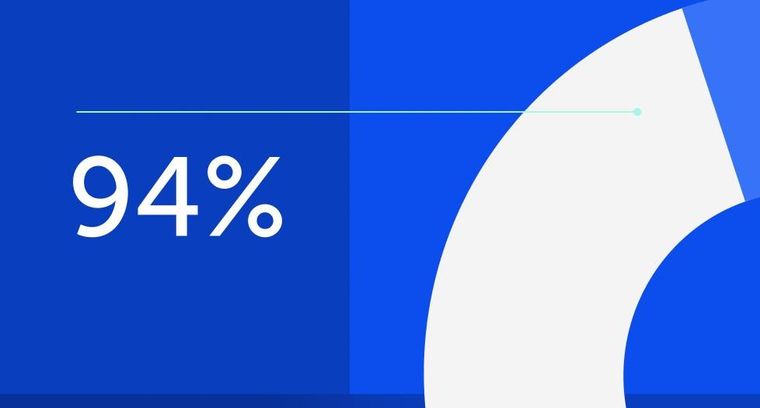
94% of researchers rate our articles as excellent or good
Learn more about the work of our research integrity team to safeguard the quality of each article we publish.
Find out more
ORIGINAL RESEARCH article
Front. Plant Sci., 23 November 2023
Sec. Plant Abiotic Stress
Volume 14 - 2023 | https://doi.org/10.3389/fpls.2023.1239008
The duration and intensity of freezing stress are the most critical factors determining injury in autumn chickpeas, limiting their production and development. To evaluate the effects of freezing temperature and duration on the survival rate (SU%), as well as the physiological and biochemical characteristics of autumn chickpea seedlings, a study was conducted using five different temperatures (0, -6, -8, -10, and -12°C) and five different durations (1 h, 2 h, 3 h, 4 h, and 5 h) of exposure to freezing stress. The SU% of chickpea seedlings decreased to zero after exposure to temperatures of -10°C and -12°C for 5 hours. As the temperature decreased from -8°C to -12°C and the duration of exposure to freezing stress increased from 1 to 5 hours, the leaf membrane stability index decreased by 33%, 48%, 46%, 57%, and 58%, respectively. The highest and lowest total pigment contents were observed after 1 hour at 0°C and 5 hours at -12°C, respectively. The maximum photochemical efficiency of photosystem II (Fv’/Fm’) was not affected by temperatures as low as -8°C in any of the time treatments during the recovery period. However, this parameter’s value decreased as the freezing stress duration increased. At -12°C, the activity of ascorbate peroxidase, catalase, and peroxidase increased by 44.6%, 38.3%, and 33.0%, respectively, as the duration of stress was increased from 1 hour to 5 hours. A positive and significant correlation was observed between plant dry weight, membrane stability index, photosynthetic pigment content, and Fv’/Fm’ with SU% after exposure to freezing stress. The minimum temperature and the maximum duration of freezing stress tolerance in chickpea seedlings were observed at -12°C for two hours. Our findings confirm that prolonging the freezing duration disrupts the defense mechanisms of chickpea seedlings. Therefore, future studies on breeding chickpeas tolerant to freezing stress should concentrate on attributes strongly correlated with SU%.
The nutritional value of chickpeas (Cicer arietinum L.) in terms of vitamins, nutrition, and body health has been recently emphasized often by nutritionists in health and food areas in many countries worldwide (Merga and Haji, 2019). The production of 80% of chickpeas by low-income food-deficit countries reveals the unique role of this product as an alternative source of animal protein (FAOSTAT, 2020). Also, this crop is a vital source of protein, calcium, iron, and phosphorus, and it forms an essential part of the diet of vegetarians. Hence, identifying and eliminating the limiting factors of its cultivation worldwide is one of the most critical research objectives of ICRISAT and ICARDA.
Climate change has increased extreme temperature events, restricting the production and development of many plant species. Freezing stress is one of the crucial constraints limiting plant growth and development worldwide (Hasanfard et al., 2022). The growth response of plants in exposure to freezing stress is affected by physiological, biochemical, and morphological processes. Thus, the change in these attributes leads to different yield and yield-related variables (Liliane and Charles, 2020). Nezami et al. (2022) reported that decreasing the temperature to -12°C led to significant modifications in the survival (SU%) and physiological and biochemical attributes of chickpeas, including the content of photosynthetic pigments, anthocyanin, and water-soluble carbohydrates. Karimzadeh Soureshjani et al. (2022) reported that chickpea genotypes exhibit varying responses at different levels, including molecular (gene expression), biochemical, and physiological modifications, to mitigate freezing damages. The damage resulting from freezing stress can be so severe that it leads to the death of the plant (Gull et al., 2019). Therefore, it is necessary to accurately evaluate the damage caused by freezing stress and the mechanisms involved in the tolerance to freezing stress to predict the SU% of the plant species in winter and its recovery capacity. Breeders will also be able to improve plant tolerance by gene manipulation after determining traits effective in tolerance to freezing stress.
The duration of exposure to freezing stress is also an important factor in the successful wintering of plant species. For example, Parvanova et al. (2004) reported that freezing stress for 24 h resulted in more severe damage in wild-type tobacco compared to 12 h. The B189 and DE15 triticale (× Triticosecale Wittmack) seedlings took 11 and 13 days, respectively, to reach 50% mortality when kept at -12°C (Gusta et al., 1997). Nevertheless, if the seedlings were held at -15°C, the time was reduced to 2 and 10 days, respectively. Therefore, a plant species can respond differently to freezing stress depending on the temperature patterns of each year and region because the duration and intensity of freezing are often different for each year and region.
Objective evidence has indicated that the freezing injury of winter chickpeas is affected by extreme freezing temperatures and freezing durations during winter. However, what is the tolerance threshold for chickpea freezing stress? How long can chickpeas tolerate freezing stress? Which morphophysiological and biochemical attributes of chickpea change under freezing stress? Can a single freezing stress tolerance threshold be determined for chickpea seedlings? Does the tolerance threshold vary depending on the duration of stress exposure? The responses to these queries can determine the ideal areas for growing this plant worldwide. Most importantly, there are seldom reports on the interaction effect of the duration and intensity of freezing stress on chickpea seedlings. Hence, this study was conducted to determine the impact of the duration and intensity of freezing stress on chickpea seedlings’ response and to clarify the ambiguities mentioned above.
The experiments were conducted in November 2021 at Ferdowsi University of Mashhad (FUM), Iran. The study area is located in Northeast Iran, where the climate is arid, with cold and relatively wet winters and hot and dry summers. The mean annual temperature and precipitation are 14.6°C and 250 mm, respectively.
Seeds were provided from the Mashhad chickpea collection (Research Center for Plant Science) at the FUM. Ten seeds were planted in 14-cm diameter plastic pots filled with sand, soil, and peat moss (1:1:1, v/v). Immediately after emergence, seedlings were thinned to keep eight plants pot-1. For cold acclimation, the pots were kept outdoors (Figure 1). Pots were regularly watered with potable water. No fertilizer was applied to the crop at any growth stage.
Figure 1 Minimum and maximum daily air temperatures at the experimental site. Relative Humidity ~50% under the natural photoperiod. SD, Sowing date; FST, Freezing stress treatment; SUE, Survival evaluation. The average minimum and maximum long-term temperatures in Mashhad during the period of low temperature application are -5°C and 6°C, respectively.
Chickpea seedlings were exposed to freezing stress at the six-leaf stage. The treatments of this study include freezing temperatures (0, -6, -8, -10, and -12°C) and the duration of exposure of chickpea seedlings to freezing (1, 2, 3, 4, and 5 h). This experiment used a completely randomized design in factorial arrangement (5×5) with four replications.
Freezing treatment was applied using a thermogradient freezer with the ability to adjust the duration and intensity of freezing. The temperature drop slope was adjusted based on the long-term meteorological statistics of Mashhad. The initial temperature of the thermogradient freezer was +5°C. However, the freezer was gradually cooled down at a rate of 2°C per hour. Seedlings were kept at each freezing temperature at the five mentioned times. After the freezing treatment, the pots were transferred to the growth chamber at 5 ± 1°C for approximately 24 hours to slow down the thawing process. Afterward, the pots were transferred to the glasshouse, where they were exposed to natural light conditions, a photoperiod of 16 hours, and a temperature of 16/22°C (day/night) for 30 days. The relative humidity in the glasshouse was maintained at 50% to facilitate recovery.
Freezing stress injury to the cell membrane was measured by electrolyte leakage (EL) using an electrical conductivity meter (Jenway Model 4510, UK). The youngest fully expanded leaves and crown were harvested for the electrolyte leakage assays. The membrane stability index (MSI) was calculated using Eq. 1 and 2.
Where EC1 is the initial conductivity, EC2 is the conductivity of the killed samples (using autoclave 121°C at a pressure of 1.2 atm for 30 min).
Thirty days after freezing treatment, the plants’ SU% in each pot was calculated as SU% = (s1/s2) × 100. Where s1 and s2 are the number of alive plants four weeks after freezing treatment and the number of alive plants before freezing treatment, respectively. Plant dry weight was measured after oven-drying at 75°C for 72 h. Three-parameter logistic model (Eq. 3) was used to calculate the lethal temperature for 50% of plants according to the survival (LT50 value).
Where Y is the response rate (survival %) at temperature x, d is the upper limit for Y, b is the slope of the curve around e, and e is the temperature (°C). Here, e is replaceable with LT50.
24 h after each freezing temperature, the youngest fully expanded leaves were harvested for the biochemical assays. All samples were stored at -20°C before being analyzed biochemically. Content of photosynthetic pigments (CPPs) (Dere et al., 1998), anthocyanins (Wagner, 1979), free radical scavenging activity by DPPH (Brand-Williams et al., 1995), flavonoids (Chun et al., 2003), total phenol content (Singleton and Rossi, 1965) and enzymatic antioxidants activity, including ascorbate peroxidase (APX), catalase (CAT), and peroxidase (POD) (Lee and Lee, 2000) were measured by spectrophotometer (Jenway UV-Visible Spectrophotometer Model 6305, UK). Chlorophyll fluorescence parameters (before stress and at 24, 72, and 120 hours after stress) were measured under stable environmental conditions from the youngest fully developed leaf using a pulse-modulated fluorometer (model OS1-FL, Opti-Sciences, Hudson, NH, USA) (Hasanfard et al., 2023a). All measurements were taken on one leaf per plant using sun-exposed leaves under similar environmental conditions between 11:00 and 14:00 on sunny days (Leakey et al., 2006; Li et al., 2019). In this study, Fv’ and Fm’ were used to represent the variable and maximum fluorescence of light-adapted leaves, respectively (Baker, 2008). The leaf osmotic potential was measured using an osmometer (OM802-D; Vogel, Germany).
Data were analyzed using the SAS 9.4 software (v. 9.4, SAS Institute Inc, Cary, NC, USA) and means were compared using Fisher’s LSD (least significant difference) test at a 5% probability level. GraphPad Prism software (v. 8.00; GraphPad, CA, USA) was used to draw the graphs.
The lowest SU% of chickpea seedlings was observed at -12°C (Figure 2A). Thus, chickpea seedlings were exposed to -12°C for one to 5 h, and their SU decreased to 75%, 78%, 25%, 8%, and 0%, respectively. The estimated LT50 was -12.1, -12.1, -10.5, -11, and -9°C during the freezing stress periods of 1, 2, 3, 4, and 5 hours, respectively (Figure 2A).
Figure 2 Effect of freezing temperatures on the survival percentage (A) and dry weight of the aerial parts (B) in chickpeas. Vertical bars indicate the standard error of means.
A significant decrease in the dry weight of the plant began during the 5-h treatment at -6°C (Figure 2B). In this way, the dry weight of the plant decreased by 51% at -8°C and by 100% at -10 and -12°C. The lowest dry weight of the plant in other periods of freezing stress (1 h to 5 h) was also observed at -12°C.
The maximum decrease in the MSI of the leaf during the stress period began at -8°C (Figure 3A). Thus, by decreasing the temperature from -8 to -12°C and increasing the duration of exposure to freezing stress from 1 h to 5 h, the MSI of the leaf decreased by 33%, 48%, 46%, 57%, and 58%, respectively. In all stress periods, the crown membrane’s lowest stability index was observed at -12°C (Figure 3B). The decrease in this index was more intense when exposed to stress for 5 h. Thus, exposing the seedlings for 5 h at -12°C decreased the MSI of the crown by 61% compared to -6°C.
Figure 3 Effect of freezing temperatures on the membrane stability index (MSI) of the leaf (A) and crown (B) in chickpeas. Vertical bars indicate the standard error of means.
Duration and intensity of freezing stress significantly affected CPPs (Figure 4). The highest CPPs were observed in the control conditions (0°C for 1 h). Although freezing stress decreased CPPs, the percentage and decrease trend differed depending on the duration of exposure to stress. The lowest chlorophyll a content was observed when exposed to -12°C for 4 and 5 h. Thus, the chlorophyll a content in the mentioned conditions was 61% lower than in the control. In general, a similar trend was observed in other photosynthetic pigments. In this way, with decreasing temperature and increasing the duration of exposure to freezing stress, the CPPs in leaves decreased further. Therefore, there was a 50% difference between the highest total pigment content (0°C for 1 h) and the lowest (-12°C for 5 h) (Figure 4).
Figure 4 Content of photosynthetic pigments response to duration and intensity of freezing stress. Vertical bars indicate the standard error of means, and bars with the same letter indicate no significant differences according to LSD at P ≤ 0.05.
A significant decrease in the maximum photochemical efficiency of photosystem II (Fv’/Fm’) was clear from -10°C. The lowest Fv’/Fm’ was observed in all temperature treatments under stress for 5 h (Figure 5). Thus, Fv’/Fm’ in seedlings reached zero 120 h after freezing stress (-12°C for 5 h). By decreasing the temperature to -8°C, Fv’/Fm’ was not affected in any of the time treatments in the recovery period. With increasing exposure time to freezing stress, other chlorophyll fluorescence parameters (Fq’/Fm’, Fq’/Fv,’ and qL’) also decreased (Supplementary Table S1). Generally, the parameters’ lowest values were observed when chickpea seedlings were exposed to -12°C for 5 h.
Figure 5 Changes in the maximum photochemical efficiency of photosystem II (Fv’/Fm’) of chickpea seedlings under freezing temperature and duration in the recovery period. The error bars indicate standard error. BS (before stress) and AS (after stress).
The highest anthocyanin content in each temperature treatment was observed when the seedlings were exposed to freezing stress for 5 h (Table 1). In other words, the content of anthocyanin increased at each temperature with increasing duration of exposure to stress. The anthocyanin content increased by 23%, 20%, 29%, and 38%, respectively, when exposed to -6, -8, -10, and -12°C for 5 h compared to the control (stress for 1 h).
Table 1 Changes in the anthocyanins (ACNs), free radical scavenging activity (DPPH), flavonoid content, phenol content, and leaf osmotic potential (OP) of chickpea seedlings under freezing temperature and duration.
Increasing the duration of freezing stress increased DPPH (Table 1). In contrast, DPPH decreased with an increase in the stress period at -12°C. In the most extreme conditions of freezing stress (-12°C for 5 h), DPPH was 45% lower than the control.
The highest flavonoid content was observed in the control (Table 1). Generally, the flavonoid content decreased with decreasing temperature and increased exposure duration to freezing compared to the control. In the most extreme conditions of freezing stress (-12°C for 5 h), the flavonoid content was 51% lower than the control.
The trend of increasing the phenol content at -12°C was clear with increasing the duration of stress (Table 1). The phenol content increased by 11%, 15%, 17%, and 20% when exposed to -12°C for 2 to 5 h compared to the control (stress for 1 h).
The osmotic adjustment was almost done by exposing the seedlings to -6 to -12°C for 1 to 4 h (Table 1). During the mentioned conditions, the osmotic potential of the leaves of the seedlings was almost in the same range. The highest osmotic potential among the treatments was observed when the seedlings were exposed to -12°C for 5 h. The osmotic potential in these conditions was 37% higher than the control (stress for 1 h).
Significantly, with the decrease in temperature and the increase in the duration of freezing stress, the activity of antioxidants increased (Figure 6). The activity of APX increased by 43, 35, 46, 44, and 45%, respectively, when exposed to temperatures from zero to -12°C for 5 h compared to the control (stress for 1 h). CAT activity increased by 63%, 38%, 54%, 36%, and 38%, and POD activity by 33, 44, 35, 5, and 33%, respectively, compared to the control.
Figure 6 Changes in the antioxidant activity of chickpea seedlings under freezing temperature and duration. APX, ascorbate peroxidase; CAT, catalase; POD, peroxidase. Vertical bars indicate the standard error of means, and bars with the same letter indicate no significant differences according to LSD at P ≤ 0.05.
Pearson’s correlation analysis was performed among chickpea seedlings’ morphological, physiological, and biochemical parameters under freezing stress (Figure 7). Under freezing stress, SU% had a positive and significant correlation with MSI% leaf (0.84***), MSI% crown (0.84***), and plant dry weight (0.85***). Between SU% and the content of photosynthetic pigments including Chl a (0.85***), Chl b (0.54***), Chl a/Chl b (0.77***), and total pigment content (0.78***), a positive and significant correlation was observed. Further, a significantly stronger correlation was exhibited between SU% and Fv'/Fm' (0.88***), while APX (-0.64***) and CAT (-0.70***) were negatively associated with SU%. Interestingly, MSI% leaf was positively and highly correlated with Fv'/Fm' (0.70***); however, APX (-0.52***) and CAT (-0.63***) had a strong negative correlation with MSI% leaf.
Figure 7 Pearson correlation coefficient matrix between chickpea seedlings’ biochemical, physiological, and morphological attributes under freezing temperature and duration. Asterisks denote significant differences: *p ≤ 0.05; **p ≤ 0.01 and ***p ≤ 0.001.
Determining SU% is one of the most common and accurate methods of evaluating plant species’ tolerance to freezing stress. The ability of plant species to successfully overwinter leads to the development of their cultivation (Nezami et al., 2023). Therefore, the expansion of geographical distribution and autumn cultivation of valuable plants such as chickpeas requires screening traits most physiologically and biochemically related to the SU%. In fact, a higher winter SU% ensures the successful cultivation of chickpeas in autumn. The spring season, due to its shorter duration, reduces the productivity of chickpeas compared to longer winter seasons. Autumn cultivation provides more time for crops to grow, resulting in higher yields (Rani et al., 2020). In our study, the SU% of chickpea seedlings decreased with increasing duration of exposure to freezing stress (Figure 2A). Thus, with the increase in the duration of exposure to freezing stress, the LT50 of chickpea seedlings increased (Figure 2A). A similar study on winter cereals revealed that LT50 was negatively affected by the length of exposure to -4°C (Willick et al., 2021). Analyzing chickpea seedlings’ response under freezing stress showed that their maximum capacity (SU=100%) was at -8°C for 5 h. The chickpea plants maintained a high survival rate even under unfavorable conditions, as they were able to withstand exposure to -12°C for two hours (Figure 2A). The exposure of chickpeas to -10 and -12°C for 5 h led to their mortality (SU=0%).
The increase in the intensity and duration of freezing stress is effective on morphological attributes, including the accumulation of dry matter. In our study, dry matter accumulation decreased under increasing freezing stress duration. The ability to accumulate dry matter during the recovery period indicates the activity of the plant’s photosynthetic apparatus. Nabati et al. (2021) showed that a decrease in temperature from 0°C to -4°C caused a 20% decrease in the dry weight of the aerial parts of faba bean (Vicia faba L.) seedlings as a result of a disturbance in gas exchange variables.
Understanding the physiological and biochemical attributes effective in increasing tolerance to freezing stress and breeding chickpeas based on these factors will increase the cultivation of this plant worldwide. The plasma membrane is the primary target of freezing stress, affecting its conformation and properties and disturbing cellular homeostasis. Degradation of cellular membranes is commonly observed following freeze-thaw stress to plant tissue. Therefore, any disturbance in the membrane structure causes severe damage to the plant. Our objective evidence from various studies has shown that the destruction of the crown of chickpea seedlings is highly likely to result in their mortality. However, there is a high possibility of regrowth of leaves after freezing stress, and the plant can recover. This mindset was aligned with the findings of the study. In this study, the reduction of MSI (leaf and crown) increased with a decrease in temperature and an increasing duration of exposure to freezing stress (Figure 3A, B). Increasing the duration of exposure to freezing stress (-12°C) probably changed the membrane structure in terms of the quantity and quality of fatty acids and proteins. Subsequently, the plant growth regulation and mediating responses to stress were affected. Willick et al. (2021) reported that due to global climate change in Western Canada, winter cereals would be exposed to a higher frequency of winter thaws, increasing crown water and reducing freezing tolerance.
Photosynthetic pigments participating in the absorption, transformation, and transfer of light energy play a crucial role in plant growth (Hasanfard et al., 2023b). Under freezing stress, lipid peroxidation and reactive oxygen species (ROS) are important in damaging photosynthetic membranes. The results of our study confirmed that the CPPs could be an advantageous screening technique to investigate the tolerance to freezing in chickpea seedlings (Figure 4). Improvement in pigment content and photosystem functions in plant species increases photosynthetic efficiency. In this way, breeders can concentrate on CPPs to decrease the effects of freezing stress. For example, An et al. (2020) reported that using some modifiers can reduce damage to CPPs and therefore decrease the effects of stress in plants.
The sensitivity of photosystem II activity to freezing stress has made chlorophyll fluorescence analysis a practical technique for understanding photosynthetic mechanisms. Our study revealed that chlorophyll’s fluorescence parameters could be suitable criteria for determining the activity of the plant’s photosynthetic apparatus (Figure 5). Freezing stress decreased the quantum yield of the electron transport flux from QA to QB of photosystem II. The longer chickpea seedlings were exposed to low temperatures, the more their recovery ability decreased. Increasing the duration of exposure to freezing stress led to a decrease in the amount of electron transfer to electron acceptors of photosystem II and disruption of the activity of the photosystem II electron transfer chain. Therefore, the ability to recover and the high performance of the photosynthetic apparatus of a species after exposure to freezing stress indicate the high adaptability of that species to harsh winter conditions.
Contrary to our assumption, flavonoid content did not increase with increasing freezing stress. This result showed that all biochemical attributes in plant species do not primarily function in stress tolerance. Hence, according to the results of this study, we could not prove the role of flavonoids in tolerance to freezing stress, and we propose it as an ambiguity.
Anthocyanins are a class of water-soluble flavonoids that have many biological functions in higher plants. To adapt to freezing stress, plants have adaptive responses, including the accumulation of anthocyanins. Here we demonstrated that freezing stress-induced anthocyanins contribute substantially to the low-temperature tolerance of chickpea seedlings (Table 1). We also concluded that anthocyanin accumulation is ineffective in plant tolerance if freezing stress is prolonged.
The increase in free radicals and, as a result, cell destruction has caused “oxidative stress.” This phenomenon destroys proteins and lipids. The increase in DPPH in conditions of severe freezing stress indicates the increase in free radicals. In the most severe freezing stress treatment, the increase of DPPH has stopped, so inhibition of free radical activity by DPPH as a single defense mechanism against freezing stress in chickpea seedlings is impossible. With the increase in reactive oxygen species, various mechanisms are activated in plants. Our study determined that the activity of antioxidant enzymes (APX, CAT, and POD) increased with exposure to freezing stress (Figure 6). In other words, ROS-scavenging enzymes have increased to decrease the toxic effects caused by oxidative stress resulting from freezing stress. Freezing stress can cause damage to plant cells through the formation of ice crystals, which can disrupt cellular structures and membranes. ROS production increases during freezing stress due to the disruption of cellular homeostasis. However, plants have evolved mechanisms to counteract the harmful effects of ROS and mitigate the damage caused by freezing stress. One of the main ways in which ROS helps reduce the effects of freezing stress is through its involvement in signaling pathways that activate various defense mechanisms. ROS can act as signaling molecules to trigger the expression of genes involved in stress responses, including the production of protective proteins and antioxidants. In this study, it is likely that these defense mechanisms help chickpea plants maintain cellular integrity and minimize damage caused by freezing stress.
Phenolic compounds play their antioxidant function with several mechanisms, such as free radical scavenging activity and interrupting oxidation chain reactions, donating hydrogen, and acting as a substrate for peroxidase enzymes. In this study, the role of these compounds was observed at -12°C with increasing stress intensity. The phenol content had almost the same trend at different temperatures during the freezing stress period.
Plants could often adjust the reduced potential without water loss, except when the freezing was very severe. The exposure of plants to freezing stress for 5 h disturbed the osmotic adjustment. In other words, the mechanism to prevent water loss (the process of maintaining cellular turgescence) decreased with the increased exposure to stress (stress for 5 h).
The damage of cells under freezing stress depends on the production of reactive oxygen species and the efficiency of detoxification mechanisms in plants. To deal with the created oxidative stress, plants have a highly efficient antioxidant defense system that can neutralize or scavenge free radicals. In this study, the antioxidant enzymes likely significantly reduced the levels of superoxide and hydrogen peroxide in chickpea seedlings. The increase in the levels of antioxidants was more evident at -10 and -12°C and during the stress periods of 4 and 5 h. It can be concluded that the protective function of antioxidant enzymes increases with increasing intensity and duration of freezing stress.
The positive and significant correlation between SU% and MSI% showed that chickpea seedlings could withstand freezing stress with more cell membrane integrity. Plant cell membranes are critical sites for the perception of abiotic stress factors; therefore, plant breeders should endeavor to target these sites in the breeding of plants to tolerate freezing stress. Membrane lipids changed drastically in plants when they suffered from freezing stress. Campos et al. (2003) reported that electrolyte leakage and lipid degradation account for cold sensitivity in the leaves of plants. Freezing stress decreases the contents of total lipids and stimulates lipid degradation, accumulating free fatty acids. In previous studies, researchers reported that the more successful the plant species were in maintaining the integrity of their cell membranes, the fewer electrolytes leaked from their cell membranes, and their SU was higher (Nabati et al., 2020; Angmo et al., 2022).
Photosynthetic pigments are essential in all parts of the photosynthesis system because their quantity and quality play vital roles in plant assimilation (Sayyad-Amin et al., 2016). The positive and significant correlation of photosynthetic pigments with SU% reveals their crucial function in tolerance to freezing stress. When the intensity and duration of freezing stress increased, CPPs in chickpea seedlings decreased significantly (Figure 4). In other words, the plant’s photosynthetic apparatus has been disturbed, and the ability of CO2 assimilation has decreased. These interactions resulted in the instability of the photosystem and fluorescence parameters of Chl a and the decrease of Rubisco carboxylation efficiency, which led to the decrease of SU% of chickpea seedlings.
Fv’/Fm’ indicates the maximum quantum efficiency of photosystem II photochemistry and is used for early stress detection in plants. Its high and positive correlation with the SU% in this study showed that this parameter could be considered as one of the most reliable techniques for detecting damage to photosynthetic apparatus and tolerance to freezing stress in plants. The high Fv’/Fm’ values in exposure to freezing stress reveal that the electron transfer chain is established in the thylakoid membrane, and the plant can recover adequately after the stress.
Contrary to our expectation, the correlation between SU% and antioxidant enzymes was negative (Figure 7). Antioxidant enzymes are plants’ most important defense mechanisms in exposure to stress. This negative association is only due to a severe decrease in SU% and a severe increase in the activity of antioxidants at low temperatures. In this study, the increase in the activity of antioxidants was significant in the most severe freezing treatment. However, this defense mechanism did not lead to the SU of chickpea seedlings.
The duration of freezing stress was influential in determining the SU of chickpea seedlings, so increasing the stress time (-12°C) from 1 to 5 h led to a decrease in SU% from 75 to zero. The results of our study prove that the interactions of coping with freezing stress in chickpea seedlings are complex. Therefore, a combination of defense mechanisms (morphological, physiological, and biochemical) in plants leads to high tolerance to exposure to freezing stress. Although chickpea seedlings with high antioxidant potential could survive and sustain their growth under freezing stress, this ability was insufficient to cope with freezing stress. Cell membrane stability, CPPs, and the maximum quantum efficiency of photosystem II were effective characteristics in tolerance to freezing stress in chickpea seedlings. Accordingly, chickpea breeders should concentrate on the mentioned attributes in breeding for freezing stress tolerance.
The original contributions presented in the study are included in the article/Supplementary Files, further inquiries can be directed to the corresponding author.
JN: Methodology, Reviewing, Editing. AN: Conceptualization, Supervision. AH: Conceptualization, Methodology, Laboratory work, Data collection, Data analysis, Writing. ZN: Laboratory work, Data collection. NK: Laboratory work, Data collection. All authors contributed to the article and approved the submitted version.
The author(s) declare that no financial support was received for the research, authorship, and/or publication of this article.
We are grateful to Ferdowsi University of Mashhad, for providing financial support for the present research.
The authors declare that the research was conducted in the absence of any commercial or financial relationships that could be construed as a potential conflict of interest.
All claims expressed in this article are solely those of the authors and do not necessarily represent those of their affiliated organizations, or those of the publisher, the editors and the reviewers. Any product that may be evaluated in this article, or claim that may be made by its manufacturer, is not guaranteed or endorsed by the publisher.
The Supplementary Material for this article can be found online at: https://www.frontiersin.org/articles/10.3389/fpls.2023.1239008/full#supplementary-material
An, M., Wei, C., Wang, K., Fan, H., Wang, X. (2020). Study on the effects of polymer modifiers and phloem girdling on cotton in cadmium-contaminated soil in Xinjiang Province, China. Sci. Rep. 10, 6356. doi: 10.1038/s41598-020-63421-w
Angmo, D., Sharma, S. P., Kalia, A., Brar, N. S., Bhardwaj, V. (2022). Effect of cold stress on field performance, chlorophyll fluorescence, electrolyte leakage and leaf gas exchange parameters of potato (Solanum tuberosum L.) genotypes. Potato Res. 66 (3), 641–661. doi: 10.1007/s11540-022-09593-6
Baker, N. R. (2008). Chlorophyll fluorescence: a probe of photosynthesis in vivo. Annu. Rev. Plant Biol. 59, 89–113. doi: 10.1146/annurev.arplant.59.032607.092759
Brand-Williams, W., Cuvelier, M. E., Berset, C. (1995). Use of a free radical method to evaluate antioxidant activity. LWT-Food Sci. Technol. 28, 25–30. doi: 10.1016/S0023-6438(95)80008-5
Campos, P. S., nia Quartin, V., chicho Ramalho, J., Nunes, M. A. (2003). Electrolyte leakage and lipid degradation account for cold sensitivity in leaves of Coffea sp. plants. J. Plant Physiol. 160, 283–292. doi: 10.1078/0176-1617-00833
Chun, O. K., Kim, D. O., Lee, C. Y. (2003). Superoxide radical scavenging activity of the major polyphenols in fresh plums. J. Agric. Food Chem. 51, 8067–8072. doi: 10.1021/jf034740d
Dere, S., Güneş, T., Sivaci, R. (1998). Spectrophotometric determination of chlorophyll-A, B and total carotenoid contents of some algae species using different solvents. Turk J. Bot. 22, 13–18.
FAOSTAT (2020). Food and agriculture Organization (Statistical database of the United Nations Food and Agriculture Organization (FAO) statistical division).
Gull, A., Lone, A. A., Wani, N. U. I. (2019). “Biotic and abiotic stresses in plants,” in Abiotic and Biotic Stress in Plants. Ed. De Oliveira, A. B. (London, UK: IntechOpen), 1–19.
Gusta, L. V., O’Connor, B. J., MacHutcheon, M. G. (1997). The selection of superior winter-hardy genotypes using a prolonged freeze test. Can. J. Plant Sci. 77, 15–21. doi: 10.4141/P95-197
Hasanfard, A., Nabati, J., Nezami, A., Farooq, M. (2023b). Antioxidant potential and osmotic adjustment modulates growth and yield formation in kabuli-type chickpea genotypes under freezing stress. J. Plant Growth Regul. 1–11. doi: 10.1007/s00344-023-11040-w
Hasanfard, A., Rastgoo, M., Darbandi, E. I., Nezami, A., Chauhan, B. S. (2022). Freezing stress affects the efficacy of clodinafop-propargyl and 2,4-D plus MCPA on wild oat (Avena ludoviciana Durieu) and turnipweed [Rapistrum rugosum (L.) All.] in wheat (Triticum aestivum L.). PloS One 17 (10), e0274945. doi: 10.1371/journal.pone.0274945
Hasanfard, A., Rastgoo, M., Izadi-Darbandi, E., Nezami, A., Chauhan, B. S. (2023a). Freezing stress affects herbicide efficacy on sterile oat and turnipweed in wheat. Gesunde Pflanz, 1–8. doi: 10.1007/s10343-023-00842-3
Karimzadeh Soureshjani, H., Nezami, A., Nabati, J., Oskoueian, E., Ahmadi-Lahijani, M. J. (2022). The physiological, biochemical, and molecular modifications of chickpea (Cicer arietinum L.) seedlings under freezing stress. J. Plant Growth Regul., 1–16. doi: 10.1007/s00344-021-10369-4
Leakey, A. D., Uribelarrea, M., Ainsworth, E. A., Naidu, S. L., Rogers, A., Ort, D. R., et al. (2006). Photosynthesis, productivity, and yield of maize are not affected by open-air elevation of CO2 concentration in the absence of drought. Plant Physiol. 140 (2), 779–790. doi: 10.1104/pp.105.073957
Lee, D. H., Lee, C. B. (2000). Chilling stress-induced changes of antioxidant enzymes in the leaves of cucumber: in gel enzyme activity assays. Plant Sci. 159, 75–85. doi: 10.1016/S0168-9452(00)00326-5
Li, S., Courbet, G., Ourry, A., Ainsworth, E. A. (2019). Elevated ozone concentration reduces photosynthetic carbon gain but does not alter leaf structural traits, nutrient composition or biomass in switchgrass. Plants 8 (4), 85. doi: 10.3390/plants8040085
Liliane, T. N., Charles, M. S. (2020). Factors affecting yield of crops. Agronomy-climate change and food security 9. doi: 10.5772/intechopen.90672
Merga, B., Haji, J. (2019). Economic importance of chickpea: Production, value, and world trade. Cogent. Food Agricult. 5, 1615718. doi: 10.1080/23311932.2019.1615718
Nabati, J., Hasanfard, A., Nezami, A., Ahmadi-Lahijani, M. J., Boroumand Rezazadeh, E. (2021). Gas exchange variables as promising criteria for screening freezing-tolerant faba bean (Vicia faba L.) landraces at early growth stages. Legume Sci. 3 (1), e72. doi: 10.1002/leg3.72
Nabati, J., Nezami, A., Mirmiran, S. M., Hasanfard, A. R., Hojjat, S. S., Bagheri, A. (2020). Freezing tolerance in some lentil genotypes under controlled conditions. Seed Plant J. 36, 183–205.
Nezami, A., Mahmoodi, A. A., Nabati, J., Mohammadi, M., Hasanfard, A. (2023). Feasibility study of cultivating desi-type chickpea genotypes in cold and temperate regions. Legume Sci., e179. doi: 10.1002/leg3.179
Nezami, A., Nabati, J., Mirmiran, S. M., Hasanfard, A., Mohammadi, M. (2022). How does the freezing stress in the seedling stage affect the chickpea’s morpho-physiological and biochemical attributes? Gesunde Pflanz, 1–13. doi: 10.1007/s10343-022-00771-7
Parvanova, D., Ivanov, S., Konstantinova, T., Karanov, E., Atanassov, A., Tsvetkov, T., et al. (2004). Transgenic tobacco plants accumulating osmolytes show reduced oxidative damage under freezing stress. Plant Physiol. Biochem. 42, 57–63. doi: 10.1016/j.plaphy.2003.10.007
Rani, A., Devi, P., Jha, U. C., Sharma, K. D., Siddique, K. H., Nayyar, H. (2020). Developing climate-resilient chickpea involving physiological and molecular approaches with a focus on temperature and drought stresses. Front. Plant Sci. 10, 1759. doi: 10.3389/fpls.2019.01759
Sayyad-Amin, P., Jahansooz, M. R., Borzouei, A., Ajili, F. (2016). Changes in photosynthetic pigments and chlorophyll-a fluorescence attributes of sweet-forage and grain sorghum cultivars under salt stress. J. Biol. Phys. 42, 601–620. doi: 10.1007/s10867-016-9428-1
Singleton, V. L., Rossi, J. A. (1965). Colorimetry of total phenolics with phosphomolybdic-phosphotungstic acid reagents. Am. J. Enol. Vitic. 16, 144–158. doi: 10.5344/ajev.1965.16.3.144
Wagner, G. J. (1979). Content and vacuole/extravacuole distribution of neutral sugars, free amino acids, and anthocyanin in protoplasts. Plant Physiol. 64, 88–93. doi: 10.1104/pp.64.1.88
Keywords: autumn chickpea, membrane stability index, peroxidase activity, photosystem II, pigment content
Citation: Nabati J, Nezami A, Hasanfard A, Nemati Z and Kahrom N (2023) Prolonged exposure to freezing stress reduces the ability of chickpea seedlings to effectively tolerate extremely low temperatures. Front. Plant Sci. 14:1239008. doi: 10.3389/fpls.2023.1239008
Received: 12 June 2023; Accepted: 07 November 2023;
Published: 23 November 2023.
Edited by:
Sangeeta Srivastava, Indian Institute of Sugarcane Research (ICAR), IndiaReviewed by:
Khaulood Hemida, Fayoum University, EgyptCopyright © 2023 Nabati, Nezami, Hasanfard, Nemati and Kahrom. This is an open-access article distributed under the terms of the Creative Commons Attribution License (CC BY). The use, distribution or reproduction in other forums is permitted, provided the original author(s) and the copyright owner(s) are credited and that the original publication in this journal is cited, in accordance with accepted academic practice. No use, distribution or reproduction is permitted which does not comply with these terms.
*Correspondence: Jafar Nabati, amFmYXJuYWJhdGlAZmVyZG93c2kudW0uYWMuaXI=
†ORCID: Jafar Nabati, orcid.org/0000-0003-0483-7003
Disclaimer: All claims expressed in this article are solely those of the authors and do not necessarily represent those of their affiliated organizations, or those of the publisher, the editors and the reviewers. Any product that may be evaluated in this article or claim that may be made by its manufacturer is not guaranteed or endorsed by the publisher.
Research integrity at Frontiers
Learn more about the work of our research integrity team to safeguard the quality of each article we publish.