- 1Provincial Key Laboratory of Forestry Ecological Engineering of Sichuan Province, College of Forestry, Sichuan Agricultural UR.A.niversity, Chengdu, China
- 2School of Environment, Sichuan Agricultural University, Chengdu, China
Salicylic acid (SA) has been recognized as a promising molecule for improving abiotic stress tolerance in plants due to its ability to enhance antioxidant defense system, and promote root architecture system. Recent research has focused on uncovering the mechanisms by which SA confers abiotic stress tolerance in horticultural crops. SA has been shown to act as a signaling molecule that triggers various physiological and morphological responses in plants. SA regulates the production of reactive oxygen species (ROS). Moreover, it can also act as signaling molecule that regulate the expression of stress-responsive genes. SA can directly interact with various hormones, proteins and enzymes involved in abiotic stress tolerance. SA regulates the antioxidant enzymes activities that scavenge toxic ROS, thereby reducing oxidative damage in plants. SA can also activate protein kinases that phosphorylate and activate transcription factors involved in stress responses. Understanding these mechanisms is essential for developing effective strategies to improve crop resilience in the face of changing environmental conditions. Current information provides valuable insights for farmers and plant researchers, offering new strategies to enhance crop resilience and productivity in the face of environmental challenges. By harnessing the power of SA and its signaling pathways, farmers can develop more effective stress management techniques and optimize crop performance. Plant researchers can also explore innovative approaches to breed or engineer crops with enhanced stress tolerance, thereby contributing to sustainable agriculture and food security.
Introduction
Abiotic stresses are major threats to the production of horticulture crops globally (Kreps et al., 2002). Nevertheless, significant abiotic stresses like heavy metals (HMs), drought, ozone, salinity, ultra-violet radiation, nutrients, temperature fluctuations (low and high), and anthropogenic activities in the developmental period have exacerbated the agriculture system (Figure 1) (Khan et al., 2015). From the earliest stage of seed germination through maturity, the imposed abiotic stresses can affect practically all the molecular, physiological, biochemical, and metabolic functions in plants (Jia et al., 2013). This could ultimately result in serious losses in the economic output of horticulture crops (Chaudhry and Sidhu, 2022; Liu et al., 2022). The approach that plants interact with numerous environmental prompts is a furthermost vital question for plant researchers. Drought, heat, and salt stresses are the most prevalent and vital environmental stresses which have adverse effects on plant growth, yield, and quality (Altaf et al., 2022a). Phytohormones are crucial in coordinating several signal transduction pathways under stress environments (Khan et al., 2020). These hormones perform a vigorous function in the plants’ development and play an important part in the plants’ external and internal factors (Ryu and Cho, 2015). Phytohormones are recognized as environmentally acceptable for improving horticulture crops’ tolerance against abiotic stress (Mantri et al., 2012). Plants’ growth is regulated by the phytohormones as they are released in small quantities as a chemical regulator (Altaf et al., 2022b).
SA is the hormone that has been shown to activate several stress-reactive genes. Every aspect of the plant’s life is controlled by the biological substances known as plant hormones containing seed germination and distribution as well as the development of fruit (Klessig and Malamy, 1994). Under abiotic stress tolerance and regulation of the hormones involves in the cascade of the composite signaling from the stimulus sensitivity to the expression of the gene (Souza et al., 2017). According to previous research, the ROS-dependent signaling system and hormone responses interact to allow plants to acclimatize to biotic and abiotic challenges by improving performance (Tognetti et al., 2012).
It is challenging to exaggerate the importance of SA spray in preventing abiotic stresses on horticultural crops. SA is essential for increasing crop resistance to numerous abiotic stresses. SA causes a series of physiological processes i.e. better water retention, higher antioxidant activity, and reinforced cell walls. These reactions aid the plants in preventing damage brought on by stress, preserving metabolic balance, and boosting the growth (Figure 2) (Xu et al., 2015a). SA has a crucial role in protecting horticulture crops, increasing productivity, and maintaining food security in the face of unfavorable environmental conditions by lessening the harmful effects of abiotic stress. The current review aimed to provide a comprehensive understanding of the mechanisms by which SA confers abiotic stress tolerance in horticultural crops and its potential as a tool for improving crop productivity and resilience in the face of changing environmental conditions.
Overview of SA and its potential as a tool for improving plant growth and development
SA sometimes referred to technically as 2-hydroxybenzoic acid, is an aromatic phenolic molecule produced by plants that contains a hydroxyl group or its functional derivative. Phenolic compounds, particularly systemic acquired resistance (SAR), have a role in trendy activating plant defenses (Vlot et al., 2009). SA improves flowering and fruit set even under normal and stress conditions (Pacheco et al., 2013), promoting root growth, stomatal closure, and reducing transpiration (Gorni and Pacheco, 2016), reversing ABA effects (Davies, 2004), regulating gravitropism, and inhibiting fruit ripening (Srivastava and Dwivedi, 2000). Additionally, it has also been observed that plants of pepper and tomato treated with 05 - 10 µM of SA had increased weight of the dry plant shoots along with the area of the leaf (Sardar et al., 2021).
Nevertheless, on plants growth and gas exchange parameters, the SA stimulatory effects depend on the application technique, exposure time, and ontogenetic plants stages and effective concentrations of the SA vary reliant on species (Horváth et al., 2007; Sardar et al., 2021). SA can indirectly affect flowering because it changes production and plant hormone signaling directions such as auxin, ethylene, and jasmonic acid (Vlot et al., 2009). The exogenous use of SA might cause an uprise in the quantity of endogenous bioactive GA in response, altering the plant’s hormonal condition (Mukherjee and Kumar, 2007). Exogenous application improved the level of phytohormones involved in enhanced plant growth, flowering, fruit set, and ultimately yield (Kim et al., 2009).
SA is the hormone that controls defense of the plants against pathogens and is connected to phenolic chemicals (Houston et al., 2016). To modify the tolerance to different abiotic stimuli, SA is also crucial. SA encourages defensive photosynthetic processes, which leads to regulation in stomatal conductance, carbon dioxide (CO2) assimilation, ribulose-1,5-bisphosphate carboxylase/oxygenase activity, and chlorophyll and carotenoid content (Feng et al., 2020). By lowering cellular lipid peroxidation and hydrogen peroxide (H2O2) buildup, SA application to plants with insufficient water lower cell membrane damage in leaves. Additionally, SA-treated plants have less H2O2 buildup, which boosts the activity of antioxidant enzymes like catalase (CAT), superoxide dismutase (SOD), and guaiacol peroxidase (GPOD) (Ahmad et al., 2023).
SA performs an important part in the plants’ developmental processes (Table 1). Research has shown its functions in stomatal conductance, seeds development, glycolysis, the flowering of the plants, production of the fruit, ion transpiration and accumulation (Harper and Balke, 1981; Sardar et al., 2022), and the rate of photosynthesis (Khan, et al., 2003). SA can regulate the antioxidant defenses and reduce oxidative damage in plants (Figure 3) (Banu et al., 2009). SA controlled the plant nitrogen, glycine betaine (GB) formation, photosynthesis, proline metabolism, and plant water interactions under abiotic stress (Nazar et al., 2011; Miura and Tada, 2014). Additionally, abiotic stressors have been shown to induce defense-related genes and stress tolerance (Rivas-San and Plasencia, 2011; Kumar, 2014).
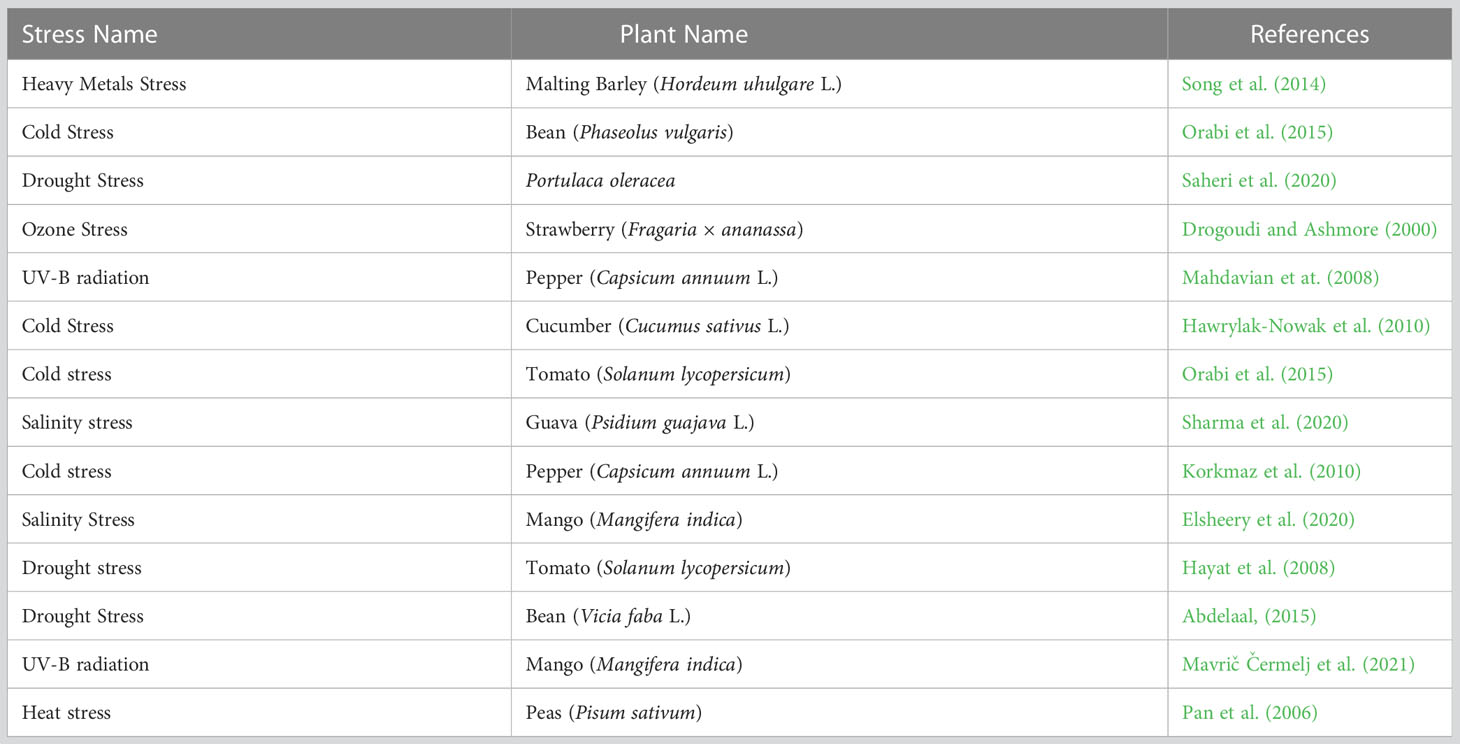
Table 1 Role of salicylic acid in abiotic stresses in horticultural crops focusing on sustainable productivity.
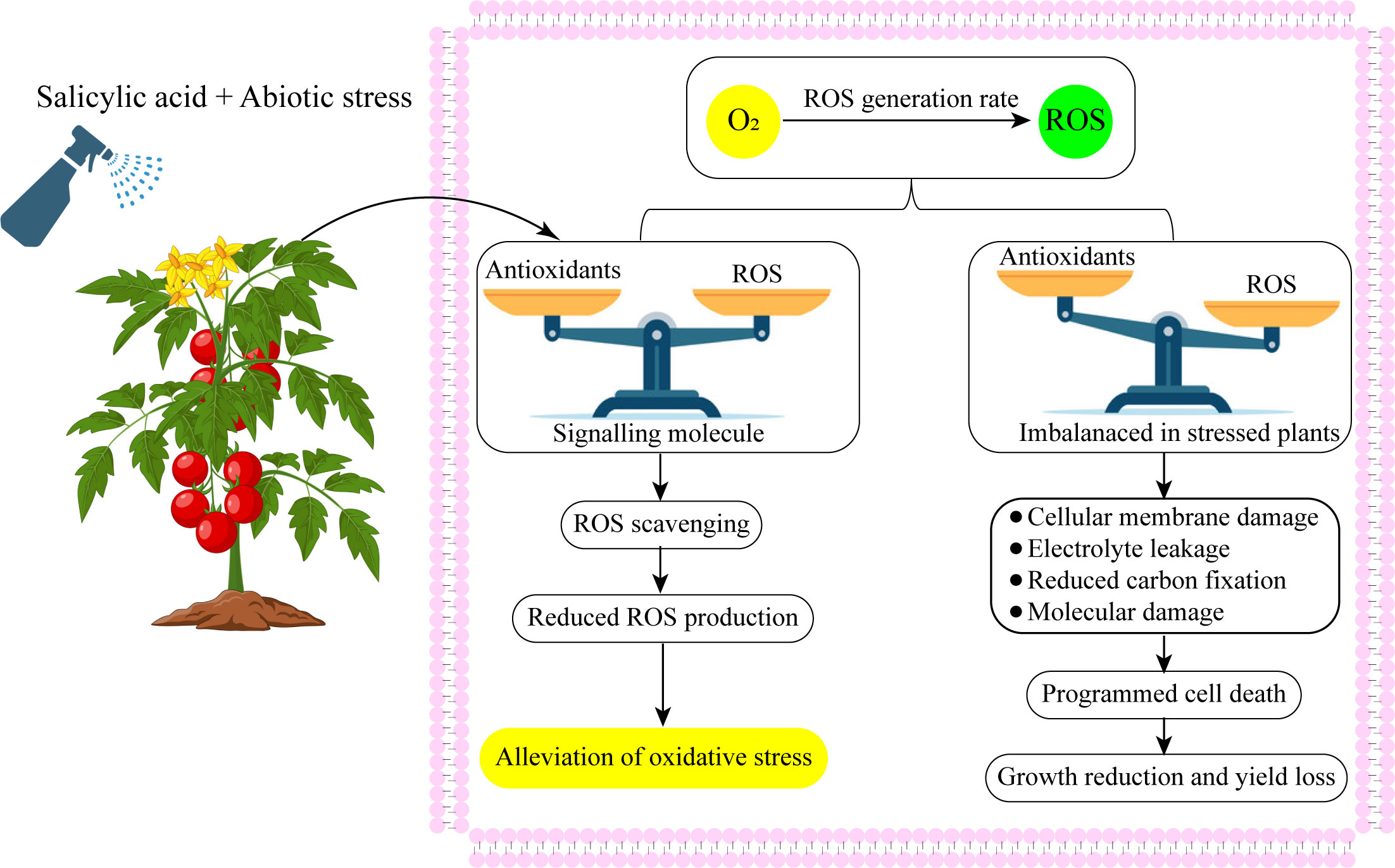
Figure 3 Exogenous salicylic acid application up-regulated antioxidant defense system and balanced redox homeostasis.
SA and drought stress
The most important abiotic stressor limiting crop output is thought to be drought (Table 2). Only 10 - 30% of the available irrigation water is used each year (Szilagyi, 2003). The rise of the drought was further aided by the amalgamation of high temperatures and low precipitation (Yu et al., 2013). Global food security is facing a challenge from climate change which pollutes water resources and declining crop production (Hedhly et al., 2009; Gomes et al., 2019). Water shortages would impact agricultural land by up to 50% by 2050 (Hasanuzzaman et al., 2019). Moreover, lack of water is the main environmental barrier to the production of horticulture crops (Ullah et al., 2018; Sirisuntornlak et al., 2019). In arid and semiarid environments, the two most significant environmental stresses drought and salinity occur simultaneously (Slama et al., 2008). Extreme drought and salt stress levels affect a large portion of the earth and harm crop plant output (FAO, 2017). Drought negatively affects plant development growth, and other metabolic functions, and is a limiting factor in the effective production of crop plants worldwide (Osuagwu et al., 2010). The weight of the dry roots can be increased due to the drought stress and also had an impact on nutrient uptake, resulting in a decline in the potassium content of the plant’s roots and shoots.
Phytohormone supplementation is regarded as an efficient adaptation technique (El-Mageed et al., 2017). SA affects the absorption of ions, the development of the plant, and the transport of substances. It is the crucial plant signaling molecule regarding the plants’ biotic and abiotic stress tolerance (Kazemi, 2014; Gorni et al., 2017). SA treatment had a progressive effect on Portulaca oleracea growth and biomass accumulation during water deficit by improving photosynthetic pigments and CO2 absorption (Saheri et al., 2020). According to Ahmad et al. (2023), the application of SA on Carthamus tinctorius L. during a water shortage increased the plants’ phenylalanine lyase (PAL) enzyme activity and anthocyanin contents but had no impact on the dry matter (Ahmad et al., 2023). Recent findings indicate that foliar SA spray on tomato mitigates water deficits (Chakma et al., 2021). The necessity of verifying SA dosages in scenarios that farmers can use is raised by the fact that grape tomatoes are not the most widely farmed tomato in the world and that the investigational circumstances of this research cannot be reconstructed in the field.
Previous research suggested using SA, which is intricate in the stress signaling compared to abiotic and biotic stresses to combat drought problems (Rabnawaz et al., 2020). SA applied topically to plants stated to cut their susceptibility to stress such as drought and the maximum temperature (Wang and Li, 2006). Opening of the stomata, ion transport regulation, and cleaning of the HMs adverse effects are all functions of SA (Rai, 2002).
SA and cold stress
Environmental stresses including heat, salinity, cold, and drought harm plant growth and production and cause several physiological, molecular, morphological, and biochemical changes in plants that ultimately cause the plants to become unsustainable (Ahmad and Prasad, 2012). Cold stress inhibits the growth of a plant its development and plant metabolism (Lichtenthaler, 1998). The sensitivity and intolerance of many crops to low temperatures (cold stress) have an impact on crop yield. A sudden temperature change harms the plants (Schwartz et al., 2006). According to Ruelland and Zachowski, (2010), plants typically succumb to chilling and freezing stress as a result of membrane damage, change in enzyme activity, necrosis, changes in cytoplasm viscosity, and chlorosis.
Low-temperature stress is most dangerous for vegetable crops regarding their productivity and quality (Yadav, 2010). The low temperature of about 0 - 15 °C is vulnerable for summer vegetables, for the whole plant life cycle including germination of seeds, vegetative growth, and reproduction. Cold stress plants reproductive stages, cause them to blossom later and produce sterile pollen, which has a significant negative impact on agricultural productivity (Suzuki et al., 2008; Yadav, 2010). Among abiotic stresses, the low temperature has a significant impact on the existence and geographic spread of the plants, as well as on the agricultural production of plants in mountainous terrain. Disruptions to plant growth and productivity lead to significant crop failure (Hatfield and Prueger, 2015). Low-temperature initial effect on the plant is the membrane fluidity decrease may be the harm perception of the potential site (Orvar et al., 2000). ROS damaged the membrane due to freezing temperature with cell wall and membrane adhesions and causes the rupture of the cell (Olien and Smith, 1997).
SA application enhanced the number of fruits in cucumber and pepper (Elvwan and Hamahyomy, 2009; Hayat et al., 2010). SA applied on the cucumber leaves enhanced their resistance to cold (Sayyari et al., 2013). Lower acetyl SA and SA concentrations (0.1 mM and 0.5 mM) have been revealed to be highly effective against low-temperature stress in bean and tomato (Orabi et al., 2015).
SA and heat stress
When plants are subjected to abiotic stress, they cannot relocate to more hospitable conditions, which has a substantial adverse effect on the growth of the plant, development, and yield (Lippmann et al., 2019). High temperatures (HT) are a significant stress, and in recent decades, global warming has increased the rise in air temperatures (Lippmann et al., 2019). The processes by which plants adapt to extreme temperatures are therefore quite interesting. One of the most harmful pressures among the continually shifting environmental factors is the steadily rising ambient temperature. By 2100, temperatures are expected to be 1.8 – 4.0°C greater than they are now due to the global air temperature increase of 0.2°C every decade (IPCC, 2007). Plants that are subjected to heat stress (HS) experience significant, and occasionally fatal, negative impacts. Plants have developed sophisticated HS response mechanisms to deal with such circumstances. HS influences many essential physiological functions of plants, including photosynthesis, water metabolism, and respiration. Characterizing HS reactive genes, non-coding RNAs, DNA methylation, and histone amendments have made significant strides (Ruelland and Zachowski, 2010).
For plant scientists, developing new crop cultivars resistant to HT is a big task (Zhang et al., 2006; Moreno and Orellana, 2011). HT affects plants in numerous means, depending on their intensity, duration, the kinds of plants present, and other environmental conditions (Rodríguez et al., 2005; Wahid et al., 2007). However, the features that confer resistance to HT have yet to be identified and confirmed. Researchers studying plants under HT stress are attempting to understand the plant retorts that result in heat tolerance as well as how plants might be controlled in HT situations. Omics techniques and the development of transgenic plants by modifying target genes are two recently highly explored molecular approaches (Schoffl et al., 1999; Kosová et al., 2011; Duque et al., 2013). Investigating these underlying molecular mechanisms may help to develop stress-resistant crop variants and enable the HT cultivation of vital crop plants.
SA has a role in altering resistance in plants against biotic stress and abiotic stress due to the part that plays in immune system activation (Kawano and Bouteau, 2013). Researchers are very interested in the use of SA to enhance plant thermo tolerance as well as the physiological and biochemical mechanisms involved. SA foliar spraying promoted heat tolerance in essential horticultural plants like cucumbers and grapes (Wang et al., 2010; Yang et al., 2022). By controlling enzymatic activity, SA application boosts the rate of photosynthesis and preserves cell membrane stability (Waqas et al., 2021). The formation of cardenolides and antioxidants is also stimulated by SA treatments, which may be crucial in boosting resistance to HT stress (Zhang et al., 2020).
SA and salinity stress
Salinity had numerous detrimental effects on the germination of seeds, plant development, and crop yield (Munns and Tester, 2008). Various irrigated regions get salinized, primarily as a result of the usage of saline water. Approximately, 45 million hectares of land are damaged due to salinity (Munns and Tester, 2008). Plants are impacted by high salinity in a variety of aspects, including oxidative stress, water stress, nutritional issues, metabolic process changes, ion toxicity, decreased expansion and cell division, membrane disruption, and genotoxicity (Hasegawa et al., 2000; Munns, 2002; Zhu, 2007). These effects collectively by inhibiting the survival of the plant, its growth, and its development (Sardar et al., 2023).
A major factor restricting agricultural production is salinity (Slama et al., 2008). It will be difficult to feed the 9.3 billion people on the planet in 2050. According to estimates, agricultural production must increase by more than 60% from 2005 to 2007 levels by 2050. (Fita et al., 2015). However, stress factors like salinity are a constant danger to effective agricultural productivity. Climate change, groundwater penetration, seawater backflow, and human activities like fertilizer application and irrigation have all contributed to an increase in soil salinity in recent years (Arani et al., 2023). The salinity of the soil reduces plant product quality, productivity, and yield (Yang and Guo, 2018a). Salinity stress affects the land by about 950 million hectares, although the percentage of soil salinity is rising in the future (Yang and Guo, 2018b).
When salt stress initially appears, excessive salt and chloride ions restrict root water because it decreases the water potential surrounding the roots of the plants (Negrão et al., 2016). Second, too much salt and chloride in plants cause toxicity of the ion. It not only interferes with the homeostasis of ions like Na+ and K+ but also averts the efficient uptake of nutrients like Ca2+, leaving plants deficient in vital nutrients (Zhu, 2002; Wu, 2018). Plants experience oxidative stress due to the overabundance of ROS brought on by osmotic and ionic stressors (Zhu, 2016). In chloroplasts and mitochondria, excessive H2O2 accumulation impairs respiration and photosynthesis under salt stress (Balal et al., 2011).
To address the salt issue, a variety of phenolic compounds and plant growth regulators (PGRs) are used to decrease their effects. SA is an endogenous signal molecule that functions in plants’ ability to withstand environmental challenges. Plants must be able to respond to abiotic and biotic stresses (Moustafa-Farag et al., 2020). SA also promotes several physiological processes important for plant growth (Basit et al., 2018; Guo et al., 2022). Antioxidant SA is regarded as having hormone-like properties. Abiotic stressors can raise the SA concentration in the plant, strengthening its ability to withstand these challenging circumstances (Yuan and Lin, 2008). SA is essential for stomatal conductance, photosynthesis, and transpiration. Additionally, it decreases Na+ and Cl- buildup in plant tissues, enhancing antioxidant defense (Sardar et al., 2022). SA can thereby increase salt tolerance, reducing the adverse effects that occur on plants (Manaa et al., 2014; Semida et al., 2014). The upregulation of anti-stress mechanisms and the restoration of the development process are two examples of SA’s beneficial effects (Mahmood, 2006).
SA and heavy metals
HMs have surpassed pesticides and eminent pollutants like sulfur dioxide (SO2) and CO2 (Chen et al., 2007). It is expected that HMs could overtake solid waste and nuclear waste as the most harmful contaminant (Lajayer et al., 2017). Eating is the primary way that 70% of all HMs and their derivatives enter human bodies (Jaishankar et al., 2014). HMs are emitted into the air, land, and water from numerous sources and as a result of human activity (Tchounwou et al., 2012). HMs toxicity has grown to be a significant issue in many terrestrial ecosystems all over the globe. Recently, excessive industrialization damages soil quality and agricultural yield by collecting HMs (Shahid et al., 2015).
Changes in the soil pH which damage the texture of the soil, the presence of various elements, and the HMs accumulation, may delay a variety of molecular as well as plant physiological processes, which may either directly or indirectly limit plant development (Panuccio et al., 2009). Several developmental and biological processes need HMs such as Cu, Mo, Ni, Mn, Co, and Zn at very low amounts (Salla et al., 2011; Shahid et al., 2015). However, when their concentration exceeds suboptimal levels, these metals along with four other extremely poisonous heavy metals, Be (beryllium), As (arsenic), Al (aluminum), Cd (cadmium), Pb (lead), and Hg (mercury) can significantly decrease the crop yield (Pierart et al., 2015). These harmful substances result in aberrant plant morphology and metabolic issues that lower plant production (Amari et al., 2017). ROS such as OH-, O2-, and H2O2 are produced as a result of these irregularities, which disturb the redox balance of the cells (Ibrahim et al., 2015; Shahid et al., 2015). This redox state imbalance is known to have a significant role in the HMs toxicity in plants. Earlier studies showed that HMs accumulation in horticulture products harms human health (Uzu et al., 2011; Shahid et al., 2015).
Metal absorption and sequestration capabilities vary across plant types and plant regions. There are considerable changes in the absorption and transport of metals across species of plants and numerous varieties of the same species plant species (Shahid et al., 2017). The majority of cereal crop plants’ roots are located 25 cm below the surface of the soil, where the plant absorbs HMs. Metals like Cd and Pb are primarily collected in roots, which are the major target for metal accumulation (Kumar et al., 2012). The cellular transport system is one method that plants have progressed to lessen the toxicity of the metal ion.
Phytohormones are small signaling molecules that have a significant impact on virtually every aspect of plant development and growth. Distinct hormones can have quite different mechanisms of action for various functions. So, although several hormones may be involved in the regulation of a single function, this is shown that a single hormone may govern the range of developmental and cellular processes (Gray, 2004). Phytohormones such as IAA (indole-3-acetic acid), also known as auxin, abscisic acid (ABA), cytokinin (CK), gibberellin (GA), ethylene (ET), jasmonic acid (JA), brassinosteroids (BRs), cytokinin (CK), salicylic acid (SA) and the most recent discovered strigolactones (SLs) are essential for plant growth and development, signaling, and crosstalk. Phytohormones are much important for plants to protect them from biotic stress as well as abiotic stress (Nishiyama et al., 2011; Colebrook et al., 2014; Xu et al., 2016; Bücker-Neto et al., 2017). In agronomical crop management strategies, phytohormones have been used as regulators of HM absorption to reduce metal toxicity (Piotrowska-Niczyporuk et al., 2012). Exogenous phytohormone application demonstrated to be safe to employ and to produce favorable outcomes for plants exposed to HM toxicity (Zhu et al., 2012; Zhu et al., 2016; Masood et al., 2016). Phytohormones like IAAs, BRs, SAs, CKs, GAs, and much more play important signaling roles, metabolic and defense pathways, but the way they alleviate HM stress is at this time a subject of great attention worldwide (Bücker-Neto et al., 2017). Phytohormone priming is carried out to promote imminent research for crop stress treatment.
Under HM stress, naturally accruing SA phenolic molecule is associated with the defense response in plants (Table 3). SA contributes to coordinating plant development, ripening, and abiotic stress responses (Rivas-San and Plasencia, 2011). Both the ABA and SA are engaged in managing the HMs response (Miura and Tada, 2014). The presence of SA can also control a plant’s tolerance to salt, heat, and cell death under heavy metals toxicity (Naz et al., 2022). SA shown to activate the antioxidant defense system and regulate Cd detoxification pathways, hence reducing Cd toxicity. Cd exposure increases barley SA content (Hordeum vulgare) roots. SA and other phytohormones, such as JA and ET, commonly interact with one another (Jia et al., 2013). Plant stress response as a part of hormone production, transport, and storage result in a cascade of signaling pathways (Matilla-Vazquez and Matilla, 2014). ET biosynthesis enzyme activity increases in the presence of HM, and MAPKs phosphorylate ACS2 and ACS6 (Skottke et al., 2011; Bücker-Neto et al., 2017; Ahmad and Anjum, 2023).
SA and ozone stress
Tropospheric ozone (O3) is a naturally occurring element of the atmosphere; however, since industrialization, anthropogenic emissions of NO (nitrogen oxides), NMVOCs (Non-methane volatile organic compounds), CO (Carbon monoxide), and CH4 have caused the O3 to be produced as a result of photochemical reactions. O3 levels have increased as a result, rising from pre-industrial levels of less than 20 ppb to contemporary levels, which have boosted the average of Northern Mid-Latitude O3 concentrations (30-50 ppb) (Cooper et al., 2014). Since the 1950s, mean O3 levels have been increasing, with the Northern Hemisphere (NH) seeing an increase of 1 to 5 ppb/decade and the Southern Hemisphere (SH) seeing an increase of 2 ppb/decade (Cooper et al., 2014). Over the past 50 years, evidence of O3 harm and damage to vegetation as a result of O3 phytotoxicity has increased significantly along with these concentrations (Grulke and Heath, 2020). O3 is a secondary pollutant, so its concentrations vary a lot in space and time. This is because of things like the season, interactions between land and coast, monsoon systems, and the exchange of air between the stratosphere and troposphere. Accordingly, in semi-rural and rural areas O3 concentrations are typically maximum that are downwind of the industrial and the urbanization sources of precursor emissions, i.e., in areas vital to agriculture, forestry, and grasslands and the ecosystem services they provide (Mills et al., 2013). When O3 degradation (via chemical transformation and deposition) is slower than O3 creation, background O3 concentrations will be increased. O3 and its precursors are also carried around the planet in air masses. The duration, frequency, and magnitude of O3 levels are all crucial factors in determining how plants will react, and these global processes have an impact on local to regional O3 concentrations.
Limited research has been done on the ozone effects on plant reproductive characteristics. Leisner and Ainsworth (2012) examined 128 peer-reviewed studies to assess the detrimental effects of present ozone levels on the reproductive development and production of several crops. However, current levels of ambient ozone considerably reduced the number of seeds (16%), fruits (9%), and fruit weight (22%). The development and makeup of fruits vary with the growing environment and may be impacted by other environmental factors. Ozone can affect reproductive development at many distinct stages (Black et al., 2000). It was demonstrated that strawberry fruiting plants bare to ozone (184 g/m3 O3 for 69 days) experienced an initial acceleration of inflorescence formation, monitored by a decrease in inflorescence production rate and fruit setting rate (Drogoudi and Ashmore, 2000). Moreover, ozone treatment (156 g m3 ozone for 2 months of exposure) reduced ascorbic acid content, increased peroxidation of lipids, and decreased strawberry fruit taste because it altered the fruit’s carbohydrate pool (Keutgen and Pawelzik, 2008; Yi et al., 2021). This research showed that ozone modifies the development of reproductive organs to influence fruit yield and quality. Consumers’ demands for higher-quality fruit have grown.
In response to relating the ozone effects to concentrations averaged at one-hour intervals, which might serve as a substitute for dosage, it is crucial to summarize concentrations in a physiologically significant way (Lefohn, 1992). The exposure index should, in theory, be based on the idea of effective dose (Runeckles, 1974), that is, it should capture the exposure features that are most closely related to the quantity of ozone that is absorbed by the plants. In this case, the absorbed dosage would be the integral of the rate of absorption (flux) over time, which could be determined for ozone by dividing the concentration at the leaf surface by the leaf conductance (Fowler and Cape, 1982).
The conduction of the atmosphere could be incorporated into this conception (Grunhage and Jager, 1994), when there is adequate air mixing (high air conductivity), ozone concentration, and conductance of the leaf control the diurnal pattern of ozone flux. In open-top contact chambers, this is the case. In crops, the use of radiation as a substitute for leaf conductance has been proposed due to the lack of data on leaf conductance (Rawlings et al., 1988). It is necessary to characterize exposure by using ozone concentrations during the daylight hours measured (for example, >50 W/m2 global radiation). However, no such distinction should be established for species that exhibit significant leaf conductivity at night. Although it is also known that other parameters, such as air humidity, soil water availability, and temperature affect leaf conductance, these factors have not yet been employed in long-term research to quantify ozone uptake or dose.
SA and UV-B radiation
Sunlight, the supreme energy source on Earth, is required for all vital biological activities. On the earth’s surface, UV light is one of the principal solar spectrum components (Dotto et al., 2018). The geomagnetic field and stratospheric ozone layer are only two examples of the many natural barriers that exist to lessen the effects of hazardous natural radiations on biotic components (Herndon et al., 2018). Approximately, 90% of (290-320 nm) of UV-B and 100% of (100-290 nm) light cannot reach the Earth’s surface due to the stratospheric ozone layer. Although over the past two decades, the thinning of the stratospheric ozone layer has gained attention due to the massive buildup of environmental pollutants that eventually cause UV-B rays to reach the earth’s surface (Barnes et al., 2019; Neale et al., 2021). As a result, concern over UV-B radiation’s effects on plants has increased due to its increased penetration.
UV-B-induced morphological alterations in plants may have an impact on how well they compete for light. Deformed morphological characteristics are the result of UV-B radiation’s harmful impacts. UV-B exposure decreased plant leaf area, height, and dry weight while increasing auxiliary branching and leaf curling (Greenberg et al., 1997; Furness et al., 1999). After a few weeks of UV-B exposure, the leaf area, and plant dry weight of rice plants significantly reduced. Weih et al. (1998) found that improved UV-A decreased the amount of leaf area per unit plant biomass (leaf area ratio), but increased the amount of biomass produced per unit leaf area (leaf area productivity) and per unit leaf nitrogen (leaf nitrogen productivity). It was clear that these variables affected the relative growth rate and nitrogen productivity since high UV-B levels decreased the leaf area ratio, leaf area productivity, and leaf nitrogen productivity (Manzoor et al., 2023). The principal causes of UV-B-induced the loss of photosynthetic pigments, thylakoid membrane integrity, reduced rubisco enzymatic activity, and the down-regulation of photosynthesis-related gene transcript levels (Jansen et al., 1996; Hollósy, 2002). Adverisites occur from abiotic stress tolerance can be mitigated by SA application (Figure 4; Table 4).
Conclusion and future horizon
SA works as a signaling molecule and regulates physiological activities in plants such as photosynthesis, metabolic, and growth processes (Figure 5). Numerous studies indicate that SA plays a significant part in regulating how plants react to different abiotic stressors. Depending on the plant type, the SA was proven to be efficacious in various foliar sprays/incorporation with growing media treatment forms. SA helps crops withstand the effects of salinity, cold, heat, heavy metals, and drought stressors. Foliar SA treatment proved effective in reducing the negative effects of water deficiency on plants. By boosting the activities of antioxidant enzymes, SA administered to drought-stressed seedlings improved physiological traits and decreased ROS generation, which lessened drought stress and increased plant growth. One method to reduce the low-temperature stress on crops is the application of PGRs and the unitization of genetic tools and plant breeding. PGRs are more important in increasing resistance to cold stress. There has been a lot of research on the use of exogenous SA to reduce heat exhaustion.
Future research should focus on how SA controls cross-talk with PGR operating at high, medium, and short ranges (auxins, gibberellins, cytokinins, ethylene, etc.). It will be possible to identify plant systems devoted to maintaining an optimum balance between growth and defense with further study into the double roles in the development and stress response of salicylic acid. Moreover, this innovative growth regulator has prodigious potential as a running tool for allowing crops to attain greater yields.
Author contributions
HY and RF: Conceptualization, Literature survey, Writing major original draft, Review structure. LL and WY: Literature survey, Writing- review and editing, Figure designing. QH: Literature survey, Writing- review and editing. CY: Reviewing and editing, References collection. HY: Supervision, WH and WG, JW: Preparing figures and tables: All authors contributed to the article and approved the submitted version.
Funding
This work was supported financially by the National Key Research and Development Program of China (Program No. 2020YFD1000700) and the Sichuan Characteristic Economic Crops Innovation Team Project of National Modern Agricultural Technology Systems (sccxtd-2020-12).
Conflict of interest
The authors declare that the research was conducted in the absence of any commercial or financial relationships that could be construed as a potential conflict of interest.
Publisher’s note
All claims expressed in this article are solely those of the authors and do not necessarily represent those of their affiliated organizations, or those of the publisher, the editors and the reviewers. Any product that may be evaluated in this article, or claim that may be made by its manufacturer, is not guaranteed or endorsed by the publisher.
References
Abdelaal, K. A. (2015). Effect of salicylic acid and abscisic acid on morpho-physiological and anatomical characters of faba bean plants (Vicia faba L.) under drought stress. J. Plant Prod. 6 (11), 1771–1788. doi: 10.21608/jpp.2015.52096
Ahmad, R., Anjum, M. A. (2023). “Mineral nutrition management in fruit trees under salt stress: a review,” Erwerbs-Obstbau, 1–9. doi: 10.1007/s10341-023-00830-x
Ahmad, R., Manzoor, M., Muhammad, H. M. D., Altaf, M. A., Shakoor, A. (2023). Exogenous melatonin spray enhances salinity tolerance in Zizyphus germplasm: a brief theory. Life 13, 493. doi: 10.3390/life13020493
Ahmad, P. M., Prasad, N. V. (2012). Abiotic stress responses in plants: metabolism, productivity and sustainability (New York, NY, USA: Springer). doi: 10.1007/978-1-4614-0634-1
Altaf, M. A., Shahid, R., Altaf, M. M., Kumar, R., Naz, S., Kumar, A., et al. (2022a). Melatonin: first-line soldier in tomato under abiotic stress current and future perspective. Plant Physiol. Biochem. 185, 188–197. doi: 10.1016/j.plaphy.2022.06.004
Altaf, M. A., Shahid, R., Ren, M. X., Naz, S., Altaf, M. M., Khan, L. U., et al. (2022b). Melatonin mitigates cadmium toxicity by promoting root architecture and mineral homeostasis of tomato genotypes. J. Soil Sci. Plant Nutr. 22 (1), 1112–1128. doi: 10.1007/s42729-021-00720-9
Amari, T., Ghnaya, T., Abdelly, C. (2017). Nickel, cadmium and lead phytotoxicity and potential of halophytic plants in heavy metal extraction. S. Afr. J. Bot. 111, 99–110. doi: 10.1016/j.sajb.2017.03.011
Arani, E. A., Hosseini, S. A., Javadi, S., Ghazavi, R. (2023). Field studies, ion analysis, and modeling of Kashan plain aquifer to predict the source of salinization. Environ. Monit. Assess. 195 (1), 23. doi: 10.1007/s10661-022-10644-1
Ashraf, Q. H., Weil, D. N., Wilde, J. (2013). The effect of fertility reduction on economic growth. Popul Dev. Rev. 39 (1), 97–130. doi: 10.1111/j.1728-4457.2013.00575.x
Balal, R. M., Ashraf, M. Y., Khan, M. M., Jaskani, M. J., Ashfaq, M. (2011). Influence of salt stress on growth and biochemical parameters of citrus rootstocks. Pak. J. Bot. 43, 2135–2141. Available at: https://www.pakbs.org/pjbot/PDFs/43(4)/PJB43(4)2135.pdf
Bano, C., Singh, N. B. (2017). Interactive effects of UV-B radiation and salicylic acid on Vigna radiata L. Russian Agri Sci. 43, 128–133. doi: 10.3103/S1068367416060057
Banu, M. N. A., Hoque, M. A., Watanabe-Sugimoto, M., Matsuoka, K., Nakamura, Y., Shimoishi, Y., et al. (2009). Proline and glycinebetaine induce antioxidant defense gene expression and suppress cell death in cultured tobacco cells under salt stress. J. Plant Physiol. 166 (2), 146–156. doi: 10.1016/j.jplph.2008.03.002
Barnes, P. W., Williamson, C. E., Lucas, R. M. (2019). Ozone depletion, ultraviolet radiation, climate change and prospects for a sustainable future. Nat. Sustain. 2 (7), 569–579. doi: 10.1038/s41893-019-0314-2
Basit, A., Shah, K., Rahman, M. U., Xing, L., Zuo, X., Han, M., et al. (2018). Salicylic acid an emerging growth and flower inducing hormone in marigold (Tagetes sp. L.). Pure Appl. Biol. 7 (4), 1301–1308. doi: 10.19045/bspab.2018.700151
Black, V. I., Black, C. R., Roberts, I. A., Stewart, C. A. (2000). Impact of ozone on the reproductive development of plants. New Phytol. 147, 421–447. doi: 10.1046/j.1469-8137.2000.00721.x
Bücker-Neto, L., Paiva, A. L. S., MaChado, R. D., Arenhart, R. A., Margis-Pinheiro, M. (2017). Interactions between plant hormones and HMs responses. Genet. Mol. Biol. 40, 373–386. doi: 10.1590/1678-4685-gmb-2016-0087
Çanakci-Gülengül, S., Yildiz, T. U. Ğ. B. A., Deveci, D. (2017). Some physiological and biochemical responses to copper of detached cucumber (Cucumis sativus L.) cotyledons pre-floated in salicylic acid. Pak. J. Bot. 49 (2), 487–492. Available at: https://www.pakbs.org/pjbot/PDFs/49(2)/13.pdf
Chakma, R., Biswas, A., Saekong, P., Ullah, H., Datta, A. (2021). Foliar application and seed priming of salicylic acid affect growth, fruit yield, and quality of grape tomato under drought stress. Sci. Hortic. 280, 109904. doi: 10.1016/j.scienta.2021.109904
Chaudhry, S., Sidhu, G. P. S. (2022). Climate change regulated abiotic stress mechanisms in plants: a comprehensive review. Plant Cell Rep. 41 (1), 1–31. doi: 10.1007/s00299-021-02759-5
Chavoushi, M., Najafi, F., Salimi, A., Angaji, S. A. (2020). Effect of salicylic acid and sodium nitroprusside on growth parameters, photosynthetic pigments and secondary metabolites of safflower under drought stress. Sci. Hortic. 259, 108823. doi: 10.1016/j.scienta.2019.108823
Chen, T. M., Gokhale, J., Shofer, S., Kuschner, W. G. (2007). Outdoor air pollution: nitrogen dioxide, sulfur dioxide, and carbon monoxide health effects. Am. J. Med. Sci. 333, 249–256. doi: 10.1097/MAJ.0b013e31803b900f
Colebrook, E. H., Thomas, S. G., Phillips, A. L., Hedden, P. (2014). The role of gibberellin signalling in plant responses to abiotic stress. J. Exp. Biol. 217, 67–75. doi: 10.1242/jeb.089938
Cooper, O. R., Parrish, D. D., Ziemke, J., Balashov, N. V., Cupeiro, M., Galbally, I. E., et al. (2014). Global distribution and trends of tropospheric ozone: an observationbased review. Elementa 2, 1–28. doi: 10.12952/journal.elementa.000029
Davies, P. J. (2004). “Plant hormones: their nature, occurrence and functions,” in Plant hormones: biosynthesis, signal transduction, action. Ed. Davies, P. J. (London: Kluwer Academic Publishers), 1–15.
Dong, C. J., Li, L., Shang, Q. M., Liu, X. Y., Zhang, Z. G. (2014). Endogenous salicylic acid accumulation is required for chilling tolerance in cucumber (Cucumis sativus L.) seedlings. Planta 240, 687–700. doi: 10.1007/s00425-014-2115-1
Dotto, M., Go´mez, M. S., Soto, M. S., Casati, P. (2018). UV-B radiation delays flowering time through changes in the PRC2 complex activity and miR156 levels in Arabidopsis thaliana. Plant Cell Environ. 41 (6), 1394–1406. doi: 10.1111/pce.13166
Drogoudi, P. D., Ashmore, M. R. (2000). Does elevated ozone have differing effects in flowering and deblossomed strawberry? New Phytol. 147, 561–569. doi: 10.1046/j.1469-8137.2000.00718.x
Duque, A. S., de Almeida, A. M., da Silva, A. B., da Silva, J. M., Farinha, A. P., Santos, D., et al. (2013). “Abiotic stress responses in plants: Unraveling the complexity of genes and networks to survive,” in Abiotic Stress—Plant Responses and Applications in Agriculture. Eds. Vahdati, K., Leslie, C. (Rijeka, Croatia: InTech), 3–23.
Eisalou, A. V., Namdjoyan, S., Soorki, A. A. (2021). The combined effect of melatonin and salicylic acid improved the tolerance of safflower seedlings to zinc toxicity. Acta Physiologiae Plantarum 43, .1–14. doi: 10.1007/s11738-021-03311-4
El-Mageed, T. A., Semida, W. M., Rady, M. M. (2017). Moringa leaf extract as bio stimulant improves water use efficiency, physio-biochemical attributes of squash plants under deficit irrigation. Agric. Water Manage. 193, 46–54. doi: 10.1016/j.agwat.2017.08.004
Elsheery, N. I., Helaly, M. N., El-Hoseiny, H. M., Alam-Eldein, S. M. (2020). Zinc oxide and silicone nanoparticles to improve the resistance mechanism and annual productivity of salt-stressed mango trees. Agronomy 10 (4), 558. doi: 10.3390/agronomy10040558
Elvwan, M. W. M., Hamahyomy, M. A. M. (2009). Improved productivity and quality associated with salicylic acid application in greenhouse pepper. Sci. Hortic. 122, 521–526. doi: 10.1016/j.scienta.2009.07.001
Eraslan, F., Inal, A., Gunes, A., Alpaslan, M. (2007). Impact of exogenous salicylic acid on the growth, antioxidant activity and physiology of carrot plants subjected to combined salinity and boron toxicity. Sci. Hortic. 113, 120–128. doi: 10.1016/j.scienta.2007.03.012
Eraslan, F., Inal, A., Pilbeam, D. J., Gunes, A. (2008). Interactive effects of salicylic acid and silicon on oxidative damage and antioxidant activity in spinach (Spinacia oleracea L. cv. Matador) grown under boron toxicity and salinity. Plant Growth Regul. 55, 207–219. doi: 10.1007/s10725-008-9277-4
Ervin, E. H., Zhang, X. Z., Fike, J. H. (2004). Ultraviolet-B radiation damage on Kentucky Bluegrass II: hormone supplement effects. Hortic. Sci. 39, 1471–1474. doi: 10.21273/HORTSCI.39.6.1471
Feng, B. H., Li, G. Y., Islam, M., Fu, W. M., Zhou, Y. Q., Chen, T. T., et al. (2020). Strengthened antioxidant capacity improves photosynthesis by regulating stomatal aperture and ribulose-1, 5-bisphosphate carboxylase/oxygenase activity. Plant Sci. 290, 110245. doi: 10.1016/j.plantsci.2019.110245
Fita, A., Rodriguez-Burruezo, A., Boscaiu, M., Prohens, J., Vicente, O. (2015). Breeding and domesticating crops adapted to drought and salinity: a new paradigm for increasing food production. Front. Plant Sci. 6, 978. doi: 10.3389/fpls.2015.00978
Fowler, D., Cape, J. N. (1982). “Air pollutants in agriculture and horticulture,” in Effects of gaseous air pollution in agriculture and horticulture. Eds. Unsworth, M. H., Ormrod, D. P. (London: Butterworth Scientific), 3–26.
Furness, N., Upadhyaya, M. K., Ormrod, D. P. (1999). Seedling growth and leaf surface morphological responses of three rangeland weeds to ultraviolet-B radiation. Weed Sci. 47, 427–434. doi: 10.1017/S0043174500092031
Gilani, M., Danish, S., Ahmed, N., Rahi, A. A., Akrem, A., Younis, U., et al. (2020). Mitigation of drought stress in spinach using individual and combined applications of salicylic acid and potassium. Pak. J. Bot. 52, 1505–1513. doi: 10.30848/PJB2020-5(18)
Gomes, A. M. F., Nhantumbo, N., Ferreira-Pinto, M., Massinga, R., Ramalho, J. C., Ribeiro-Barros, A. (2019). “Breeding elite cowpea [Vigna unguiculata (L.) walp] varieties for improved food security and income in Africa: opportunities and challenges,” in Legume Crops—Characterization and Breeding for Improved Food Security (London, UK: IntechOpen), 626–640.
Gorni, P. H., Brozulato, M. O., Lourenção, R. S., Konrad, E. C. G. (2017). Increased biomass and salicylic acid elicitor activity in fennel (Foeniculum vulgare Miller). Braz. J. Food Technol. 20, e2016172. doi: 10.1590/1981-6723.17216
Gorni, P. H., Pacheco, A. C. (2016). Growth promotion and elicitor activity of salicylic acid in Achillea millefolium L. Afr. J. Biotechnol. 15 (16), 657–665. Available at: https://www.ajol.info/index.php/ajb/article/view/134362
Gray, W. M. (2004). Hormonal regulation of plant growth and development. PloS Biol. 2, e311. doi: 10.1371/journal.pbio.0020311
Greenberg, B. M., Wilson, M. I., Huang, X. D., Duxbury, C. L., Gerhaddt, K. E., Gensemer, R. W. (1997). “The effects of ultraviolet-B radiation on higher plants,” in Plants for environmental studies, vol. 1–35 . Eds. Wang, W., Goursuch, J., Hughes, J. S. (Boca Raton, FL: CRC Press).
Grulke, N. E., Heath, R. L. (2020). Ozone effects on plants in natural ecosystems. Plant Biol. 22 (S1), 12–37. doi: 10.1111/plb.12971
Grunhage, L., Jager, H. J. (1994). Influence of the atmospheric conductivity on the ozone exposure of plants under ambient conditions: considerations for establishing ozone standards to protect vegetation. Environ. pollut. 85, 125–129. doi: 10.1016/0269-7491(94)90078-7
Guo, Y., Khan, R. A. A., Xiong, Y., Fan, Z. (2022). Enhanced suppression of soil-borne phytopathogenic bacteria Ralstonia solanacearum in soil and promotion of tomato plant growth by synergetic effect of green synthesized nanoparticles and plant extract. J. App. Microbiol. 132 (5), 3694–3704. doi: 10.1111/jam.15459
Harper, J. R., Balke, N. E. (1981). Characterization of the inhibition of K+ absorption in oats roots by salicylic acid. Plant Physiol. 68, 1349–1353. doi: 10.1104/pp.68.6.1349
Hasanuzzaman, M., Fugita, M., Oku, H., Islam, M. T. (2019). Plant Tolerance to Environmental Stress: Role of Phytoprotectants. 1st ed (Boca Raton, FL, USA: CRC Press), 488.
Hasegawa, P. M., Bressan, R. A., Zhu, J. K., Bohnert, H. J. (2000). Plant cellular and molecular responses to high salinity. Annu. Rev. Plant Physiol. Plant Mol. 51, 463–499. doi: 10.1146/annurev.arplant.51.1.463
Hatfield, J. L., Prueger, J. H. (2015). Temperature extremes: effect on plant growth and development. Weather Clim. Extrem. 10, 4–10. doi: 10.1016/j.wace.2015.08.001
Hawrylak-Nowak, B., Matraszek, R., Szymańska, M. (2010). Selenium modifies the effect of short-term chilling stress on cucumber plants. Biol. Trace Elem. Res. 138, 307–315. doi: 10.1007/s12011-010-8613-5
Hayat, S., Hasan, S. A., Fariduddin, Q., Ahmad, A. (2008). Growth of tomato (Lycopersicon esculentum) in response to salicylic acid under water stress. J. Plant Interact. 3, 297–304. doi: 10.1080/17429140802320797
Hayat, Q., Hayat, S., Irfan, M., Ahmad, A. (2010). Effect of exogenous salicylic acid under changing environment-a review. Environ. Experi. Bot. 68, 14–25. doi: 10.1016/j.envexpbot.2009.08.005
Hedhly, A., Hormaza, J., Herrero, M. (2009). Global warming and sexual plant reproduction. Trends Plant Sci. 14, 30–36. doi: 10.1016/j.tplants.2008.11.001
Herndon, J. M., Hoisington, R. D., Whiteside, M. (2018). Deadly ultraviolet UV-C and UV-B penetration to Earth’s surface: human and environmental health implications. J. Geog. Environ. Earth Sci. Intn. 14 (2), 1–11. doi: 10.9734/jgeesi/2018/40245
Hollósy, F. (2002). Effects of ultraviolet radiation on plant cells. Micron. 33 (2), 179–197. doi: 10.1016/S0968-4328(01)00011-7
Horváth, E., Szalai, G., Janda, T. (2007). Induction of abiotic stress tolerance by salicylic acid signaling. J. Plant Growth Regul. 26, 290–300. doi: 10.1007/s00344-007-9017-4
Houston, K., Tucker, M. R., Chowdhury, J., Shirley, N., Little, A. (2016). The plant cell wall: a complex and dynamic structure as revealed by the responses of genes under stress conditions. Front. Plant Sci. 7, 984. doi: 10.3389/fpls.2016.00984
Ibrahim, M. A., Khan, P. R., Hegazy, S. S., Hashim, E. A., Azamal, H., Ansari, M. K. A., et al. (2015). Improving the phytoextraction capacity of plants to scavenge heavy-metal infested sites. Environ. Rev. 23, 1–22.
Idrees, M., Naeem, M., Aftab, T., Khan, M. M. A. (2013). Salicylic acid restrains nickel toxicity, improves antioxidant defence system and enhances the production of anticancer alkaloids in Catharanthus roseus (L.). J. Hazard. Mat. 252, 367–374. doi: 10.1016/j.jhazmat.2013.03.005
Intergovernmental Panel on Climate Change (IPCC) (2007). “Climate change 2007–The physical science basis,” in Contribution of Working Group I to the Fourth Assessment Report of the Intergovernmental Panel on Climate Change (Cambridge, UK: Cambridge University Press).
Jaishankar, M., Tseten, T., Anbalagan, N., Mathew, B. B., Beeregowda, K. N. (2014). Toxicity, mechanism and health effects of some HMs. Interdiscip. Toxicol. 7 (2), 60–72. doi: 10.2478/intox-2014-0009
Jansen, M. A., Greenberg, B. M., Edelman, M., Mattoo, A. K., Gaba, V. (1996). Accelerated degradation of the D2 protein of photosystem II under ultraviolet radiation. Photochem. Photobiol. 63 (6), 814 817. doi: 10.1111/j.1751-1097.1996.tb09636.x
Jia, C., Zhang, L., Liu, L., Wang, J., Li, C., Wang, Q. (2013). Multiple phytohormone signalling pathways modulate susceptibility of tomato plants to Alternaria alternate f. sp. lycopersici. J. Exp. Bot. 64 (2), 637–650. doi: 10.1093/jxb/ers360
Kaur, G., Sharma, P., Rathee, S., Singh, H. P., Batish, D. R., Kohli, R. K. (2021). Salicylic acid pre-treatment modulates Pb2+-induced DNA damage vis-à-vis oxidative stress in Allium cepa roots. Environ. Sci. Poll Res. 28, 51989–52000. doi: 10.1007/s11356-021-14151-7
Kawano, T., Bouteau, F. (2013). Crosstalk between intracellular and extracellular salicylic acid signaling events leading to long-distance spread of signals. Plant Cell Rep. 32 (7), 1125–1138. doi: 10.1007/s00299-013-1451-0
Kaya, C., Sarıoglu, A., Ashraf, M., AlYemeni, M. N., Ahmad, P. (2022). The combined supplementation of melatonin and salicylic acid effectively detoxifies arsenic toxicity by modulating phytochelatins and nitrogen metabolism in pepper plants. Environ. Poll 297, 118727. doi: 10.1016/j.envpol.2021.118727
Kazemi, N., Khavari-Nejad, R. A., Fahimi, H., Saadatmand, S., Nejad-Sattari, T. (2010). Effects of exogenous salicylic acid and nitric oxide on lipid peroxidation and antioxidant enzyme activities in leaves of Brassica napus L. under nickel stress. Sci. Hortic. 126 (3), 402–407. doi: 10.1016/j.scienta.2010.07.037
Kazemi, M. (2014). Effect of foliar application with salicylic acid and methyl jasmonate on growth, flowering, yield and fruit quality of tomato. Bull. Environ. Pharmacol. Life Sci. 3, 154–158. doi: 10.12816/0031770
Keutgen, A. J., Pawelzik, E. (2008). Influence of pre-harvest ozone exposure on quality of strawberry fruit under simulated retail conditions. Postharvest Biol. Tech. 49, 10–18. doi: 10.1016/j.postharvbio.2007.12.003
Khalil, R., Haroun, S., Bassyoini, F., Nagah, A., Yusuf, M. (2021). Salicylic acid in combination with kinetin or calcium ameliorates heavy metal stress in Phaseolus vulgaris plant. J. Agri Food Res. 5, 100182. doi: 10.1016/j.jafr.2021.100182
Khan, W., Prithiviraj, B., Smith, D. L. (2003). Photosynthetic responses of corn and soybean to foliar application of salicylates. J. Plant Physiol. 160 (5), 485–492. doi: 10.1078/0176-1617-00865
Khan, N., Bano, A., Ali, S., Babar, M. A. (2020). Crosstalk amongst phytohormones from planta and PGPR under biotic and abiotic stresses. Plant Growth Regul. 90, 189–203. doi: 10.1007/s10725-020-00571-x
Khan, M. I. R., Fatma, M., Per, T. S., Anjum, N. A., Khan, N. A. (2015). Salicylic acid-induced abiotic stress tolerance and underlying mechanisms in plants. Front. Plant Sci. 6, 462. doi: 10.3389/fpls.2015.00462
Kohli, S. K., Handa, N., Bali, S., Arora, S., Sharma, A., Kaur, R., et al. (2018). Modulation of antioxidative defense expression and osmolyte content by co-application of 24-epibrassinolide and salicylic acid in Pb exposed Indian mustard plants. Ecotoxicol. Environ. Saf. 147, 382–393. doi: 10.1016/j.ecoenv.2017.08.051
Kim, Y. H., Hamayun, M., Khan, A. L., Kang, S. M., Han, H. H., Lee, I. J. (2009). Exogenous application of plant growth regulators increased the total flavonoid content in Taraxacum officinale (Wigg). Afr. J. Biotechnol. 8, 5727–5732. doi: 10.5897/AJB09.927
Klessig, D. F., Malamy, J. (1994). The salicylic acid signal in plants. Plant Mol. Biol. 26, 1439–1458. doi: 10.1007/BF00016484
Korkmaz, A., Korkmaz, Y., Demirkıran, A. R. (2010). Enhancing chilling stress tolerance of pepper seedlings by exogenous application of 5-aminolevulinic acid. Environ. Exp. Bot. 67 (3), 495–501. doi: 10.1016/j.envexpbot.2009.07.009
Kosová, K., Vítámvás, P., Prášil, I. T., Renaut, J. (2011). Plant proteome changes under abiotic stress-contribution of proteomics studies to understanding plant stress response. J. Proteom. 274, 1301–1322. doi: 10.1016/j.jprot.2011.02.006
Kreps, J. A., Wu, Y., Chang, H. S., Zhu, T., Wang, X., Harper, J. F. (2002). Transcriptome changes for Arabidopsis in response to salt, osmotic, and cold stress. Plant Physiol. 130, 2129–2141. doi: 10.1104/pp.008532
Kumar, D. (2014). Salicylic acid signaling in disease resistance. Plant Sci. 228, 127–124. doi: 10.1016/j.plantsci.2014.04.014
Kumar, A., Prasad, M. N. V., Sytar, O. (2012). Lead toxicity, defense strategies and associated indicative biomarkers in Talinum triangulare grown hydroponically. Chemosphere 89, 1056–1065. doi: 10.1016/j.chemosphere.2012.05.070
Lajayer, A. B., Ghorbanpour, M., Nikabadi, S. (2017). HMs in contaminated environment: Destiny of secondary metabolite biosynthesis, oxidative status and phytoextraction in medicinal plants. Ecotoxicol. Environ. Saf. 145, 377–390. doi: 10.1016/j.ecoenv.2017.07.035
Lefohn, A. S. (1992). “The characterization of ambient ozone exposures,” in Surface level ozone exposures and their effects on vegetation. Ed. Lefohn, A. S. (Chelsea, MI: Lewis Publishers), 31–92.
Leisner, C. P., Ainsworth, E. A. (2012). Quantifying the effects of ozone on plant reproductive growth and development. Glob. Change Biol. 18, 606–616. doi: 10.1111/j.1365-2486.2011.02535.x
Li, Q., Wang, G., Wang, Y., Yang, D., Guan, C., Ji, J. (2019). Foliar application of salicylic acid alleviate the cadmium toxicity by modulation the reactive oxygen species in potato. Ecotoxicol. Environ. Saf. 172, 317–325. doi: 10.1016/j.ecoenv.2019.01.078
Lichtenthaler, H. K. (1998). The stress concept in plants: an introduction. Ann. NY Acad. Sci. 851, 187–198. doi: 10.1111/j.1749-6632.1998.tb08993.
Lippmann, R., Babben, S., Menger, A., Delker, C., Quint, M. (2019). Development of wild and cultivated plants under global warming conditions. Curr. Biol. 29, R1326–R1338. doi: 10.1016/j.cub.2019.10.016
Liu, J., Qiu, G., Liu, C., Li, H., Chen, X., Fu, Q., et al. (2022). Salicylic acid, a multifaceted hormone, combats abiotic stresses in plants. Life 12 (6), 886. doi: 10.3390/life12060886
Mahdavian, K., Ghorbanli, M., Kalantari, K. (2008). The effects of ultraviolet radiation on the contents of chlorophyll, flavonoid, anthocyanin and proline in Capsicum annuum L. Turk. J. Bot. 32 (1), 25–33. doi: 10.1007/s10535-008-0037-0
Mahmood, R. (2006). In vitro effect of salt on the vigor of potato (Solanum tuberosum L.) plantlets. Int. J. Biotechnol. 1, 73–77.
Manaa, A., Gharbi, E., Mimouni, H., Wasti, S., AschiSmiti, S., Lutts, S., et al. (2014). Simultaneous application of salicylic acid and calcium improves salt tolerance in two contrasting tomato (Solanum lycopersicum) cultivars. S. Afr. J. Bot. 95, 32–39. doi: 10.1016/j.sajb.2014.07.015
Mantri, N., Patade, V., Penna, S., Ford, R. (2012). “Abiotic stress responses in plants: present and future,” in Abiotic Stress Responses in Plants. Eds. Ahmad, P., Prasad, M. N. V. (New York, NY: Springer), 1–19. doi: 10.1007/978-1-4614-0634-1_1
Manzoor, M., Naz, S., Muhammad, H. M. D., Ahmad, R. (2023). Smart reprogramming of jujube germplasm against salinity tolerance through molecular tools. Funct. Integ. Genom. 23, 222. doi: 10.1007/s10142-023-01140-x
Masood, A., Khan, M. I., Fatma, M., Asgher, M., Per, T. S., Khan, N. A. (2016). Involvement of ethylene in gibberellic acid-induced sulfur assimilation, photosynthetic responses, and alleviation of cadmium stress in mustard. Plant Physiol. Biochem. 104, 1–10. doi: 10.1016/j.plaphy.2016.03.017
Matilla-Vazquez, M. A., Matilla, A. J. (2014). “Ethylene:Role in plants under environmental stress,” in Physiological mechanisms and adaptation strategies in plants under changing environment, vol. 2 . Eds. Ahmad, P., Wani, M. R. (New York: Springer), 189–222.
Mavrič Čermelj, A., Golob, A., Vogel-Mikuš, K., Germ, M. (2021). Silicon mitigates negative impacts of drought and UV-b radiation in plants. Plants 11 (1), 91. doi: 10.3390/plants11010091
Mills, G., Wagg, S., Harmens, H. (2013) Ozone pollution: Impacts on ecosystem services and biodiversity. Available at: http://nora.nerc.ac.uk/id/eprint/502675/1/N502\penalty-\@M675CR.
Miura, K., Tada, Y. (2014). Regulation of water, salinity, and cold stress responses by salicylic acid. Front. Plant Sci. 5. doi: 10.3389/fpls.2014.00004
Monteiro, C. C., Carvalho, R. F., Grata˜o, P. L., Carvalho, G., Tezotto, T., Medici, L. O., et al. (2011). Biochemical responses of the ethylene-insensitive Never ripe tomato mutant subjected to cadmium and sodium stresses. Environ. Exp. Bot. 71, 306–320. doi: 10.1016/j.envexpbot.2010.12.020
Moreno, A. A., Orellana, A. (2011). The physiological role of the unfolded protein response in plants. Biol. Res. 44, 75–80. doi: 10.4067/S0716-97602011000100010
Moustafa-Farag, M., Mohamed, H. I., Mahmoud, A., Elkelish, A., Misra, A. N., Guy, K. M., et al. (2020). Salicylic acid stimulates antioxidant defense and osmolyte metabolism to alleviate oxidative stress in watermelons under excess boron. Plants 9, 724. doi: 10.3390/plants9060724
Mukherjee, D., Kumar, R. (2007). Kinetin regulates plant growth and biochemical changes during maturation and senescence of leaves, flowers, and pods of Cajanus cajan (L.). Biol. Plant 50, 80–85. doi: 10.1007/s10535-007-0016-x
Munns, R. (2002). Comparative physiology of salt and water stress. Plant Cell Environ. 25 (2), 239–250. doi: 10.1046/j.0016-8025.2001.00808.x
Munns, R., Tester, M. (2008). Mechanisms of salinity tolerance. Annu. Rev. Plant Biol. 59, 651–681. doi: 10.1146/annurev.arplant.59.032607.092911
Naz, S., Mushtaq, A., Ali, S., Muhammad, H. M. D., Saddiq, B., Ahmad, R., et al. (2022). Foliar application of ascorbic acid enhances growth and yield of lettuce (Lactuca sativa) under saline conditions by improving antioxidant defence mechanism. Funct. Plant Biol. doi: 10.1071/FP22139
Nazar, R., Iqbal, N., Sayeed, S., Khan, N. A. (2011). Salicylic acid alleviates decreases in photosynthesis under salt stress by enhancing nitrogen and sulfur assimilation and antioxidant metabolism differentially in two mung bean cultivars. J. Plant Physiol. 168, 807–815. doi: 10.1016/j.jplph.2010.11.001
Neale, R. E., Barnes, P. W., Robson, T. M., Neale, P. J., Williamson, C. E., Zepp, R. G., et al. (2021). Environmental effects of stratospheric ozone depletion, UV radiation, and interactions with climate change: UNEP environmental effects assessment panel, update 2020. Photochem. Photobiol. Sci. 20 (1), 1–67. doi: 10.1007/s43630-020-00001-x
Negrão, S., Schmöckel, S. M., Tester, M. (2016). Evaluating physiological responses of plants to salinity stress. Ann. Bot. 119, 1–11. doi: 10.1093/aob/mcw191
Nishiyama, R., Watanabe, Y., Fujita, Y., Le, D. T., Kojima, M., Werner, T., et al. (2011). Analysis of cytokinin mutants and regulation of cytokinin metabolic genes reveals important regulatory roles of cytokinins in drought, salt and abscisic acid responses, and abscisic acid biosynthesis. Plant Cell. 23, 2169–2183. doi: 10.1105/tpc.111.087395
Olien, C. R., Smith, M. N. (1997). Ice adhesions in relation to freeze stress. Plant Physiol. 60, 499–503. doi: 10.1104/pp.60.4.499
Oliveira, K. R., Junior, J. P. S., Bennett, S. J., Checchio, M. V., de Cássia Alves, R., Felisberto, G., et al. (2020). Exogenous silicon and salicylic acid applications improve tolerance to boron toxicity in field pea cultivars by intensifying antioxidant defence systems. Ecotoxicol. Environ. Saf. 201, 110778. doi: 10.1016/j.ecoenv.2020.110778
Orabi, S. A., Dawood, M. G., Saleem, S. R. (2015). Comparative study between the physiological role of hydrogen peroxide and salicylic acid in alleviating low temperature stress on tomato plants grown under sandponic culture. Sci. Agric. 9, 49–59. doi: 10.15192/pscp.sa.2015.1.9.4959
Orvar, B. L., Sangwan, V., Omann, F., Dhindsra, R. S. (2000). Early steps in cold sensing by plant cells: the role of actin cytoskeleton and membrane fluidity. Plant J. 23, 785–794. doi: 10.1046/j.1365-313x.2000.00845.x
Osuagwu, G., Edeoga, H., Osuagwu, A. (2010). The influence of water stress (drought) on the mineral and vitamin potential of the leaves of Ocimum gratissimum (L). Recent Res. Sci. Technol. 2, 27–33. Available at: https://core.ac.uk/download/pdf/236007834.pdf
Pacheco, A. C., Cabral, C. D. S., Fermino, E. S., Aleman, C. C. (2013). Salicylic acid-induced changes to growth, flowering and flavonoids production in marigold plants. J. Med. Plants Res. 7 (42), 3158–3163. Available at: https://citeseerx.ist.psu.edu/document?repid=rep1type=pdf&doi=e7b8c7e567c365897a6f45c4a221b6835dce68c3
Pan, Q., Zhan, J., Liu, H., Zhang, J., Chen, J., Wen, P., et al. (2006). Salicylic acid synthesized by benzoic acid 2-hydroxylase participates in the development of thermotolerance in pea plants. Plant Sci. 171, 226–233. doi: 10.1016/j.plantsci.2006.03.012
Panuccio, M. R., Sorgona, A., Rizzo, M., Cacco, G. (2009). Cadmium adsorption on vermiculite, zeolite and pumice: batch experiment studies. J. Environ. Manage. 90, 364–374. doi: 10.1016/j.jenvman.2007.10.005
Pierart, A., Shahid, M., Séjalon-Delmas, N., Dumat, C. (2015). Antimony bioavailability: knowledge and research perspectives for sustainable agricultures. J. Hazard. Mater. 289, 219–234. doi: 10.1016/j.jhazmat.2015.02.011
Piotrowska-Niczyporuk, A., Bajguz, A., Zambrzycka, E., Godlewska-Żyłkiewicz, B. (2012). Phytohormones as regulators of HM biosorption and toxicity in green alga Chlorella vulgaris (Chlorophyceae). Plant Physiol. Biochem. 52, 52–65. doi: 10.1016/j.plaphy.2011.11.009
Rabnawaz, A., Ahmad, R., Anjum, M. A. (2020). Effect of seed priming on growth, flowering and cut flower quality of carnation. Indian J. Hortic. 77, 527–531. doi: 10.5958/0974-0112.2020.00076.6
Rai, V. K. (2002). Role of amino acids in plant responses to stresses. Biol. Plant 45, 481–487. doi: 10.1023/A:1022308229759
Rawlings, J. O., Lesser, V. M., Heagle, A. S., Heck, W. W. (1988). Alternative ozone dose metrics to characterize ozone impact on crop yield. J. Environ. Qual. 17, 285–291. doi: 10.2134/jeq1988.00472425001700020021x
Rivas-San, V. M., Plasencia, J. (2011). Salicylic acid beyond defence: its role in plant growth and development. J. Exp. Bot. 62 (10), 3321–3338. doi: 10.1093/jxb/err031
Rodríguez, M., Canales, E., Borrás-Hidalgo, O. (2005). Molecular aspects of abiotic stress in plants. Biotechnol. Appl. 22, 1–10. Available at: https://elfosscientiae.cigb.edu.cu/PDFs/Biotecnol20Apl/2005/22/1/BA002201RV001-010.pdf
Ruelland, E., Zachowski, A. (2010). How plants sense temperature. Environ. Exp. Bot. 69, 225–232. doi: 10.1016/j.envexpbot.2010.05.011
Runeckles, V. C. (1974). Dosage of air pollutants and damage to vegetation. Environ. Conserv. 1, 305–308. doi: 10.1017/S0376892900004963
Ryu, H., Cho, Y. G. (2015). Plant hormones in salt stress tolerance. J. Plant Biol. 58, 147–155. doi: 10.1007/s12374-015-0103-z
Saheri, F., Barzin, G., Pishkar, L., Boojar, M. M. A., Babaeekhou, L. (2020). Foliar spray of salicylic acid induces physiological and biochemical changes in purslane (Portulaca oleracea L.) under Drought Stress. Biologia 75, 2189–2200. doi: 10.2478/s11756-020-00571-2
Salla, V., Hardaway, C. J., Sneddon, J. (2011). Preliminary investigation of Spartina alterniflora for phytoextraction of selected heavy metals in soils from Southwest Louisiana. Microchem. J. 97, 207–212. doi: 10.1016/j.microc.2010.09.005
Sardar, H., Nisar, A., Anjum, M. A., Naz, S., Ejaz, S., Ali, S., et al. (2021). Foliar spray of moringa leaf extract improves growth and concentration of pigment, minerals and stevioside in stevia (Stevia rebaudiana Bertoni). Ind. Crops Products 166, 113485. doi: 10.1016/j.indcrop.2021.113485
Sardar, H., Ramzan, M. A., Naz, S., Ali, S., Ejaz, S., Ahmad, R., et al. (2023). Exogenous application of melatonin improves the growth and productivity of two broccoli (Brassica oleracea L.) cultivars under salt stress. J. Plant Growth Regul. 42, 5152–5166. doi: 10.1007/s00344-023-10946-9
Sardar, H., Waqas, M., Naz, S., Ejaz, S., Ali, S., Ahmad, R. (2022). Evaluation of different growing media based on agro-industrial waste materials for the morphological, biochemical and physiological characteristics of stevia. Cleaner Waste Syst. 3, 100038. doi: 10.1016/j.clwas.2022.100038
Sayyari, M., Ghanbari, F., Fatahi, S., Bavandpour, F. (2013). Chilling tolerance improving of watermelon seedling by salicylic acid seed and foliar application. Not. Sci. Biol. 1, 67–73. doi: 10.15835/nsb518293
Schöffl, F., Prandl, R., Reindl, A. (1999). “Molecular responses to heat stress,” in Molecular Responses to Cold, Drought, Heat and Salt Stress in Higher Plants. Eds. Shinozaki, K., Yamaguchi Shinozaki, K. (Austin, TX, USA: R.G. Landes Co), 81–98.
Schwartz, M., Ahas, R., Aasa, A. (2006). Onset of spring starting earlier across the northern hemisphere. Glob. Change Biol. 12, 343–351. doi: 10.1111/j.1365-2486.2005.01097.x
Semida, W. M., Abd El-Mageed, T. A., Mohamed, S. E., El-Sawah, N. A. (2017). Combined effect of deficit irrigation and foliar-applied salicylic acid on physiological responses, yield, and water-use efficiency of onion plants in saline calcareous soil. Arch. Agron. Soil Sci. 63, 1227–1239. doi: 10.1080/03650340.2016.1264579
Semida, W. M., Taha, R. S., Abdelhamid, M. T., Rady, M. M. (2014). Foliar-applied α-tocopherol enhances salt-tolerance in Vicia faba L. plants grown under saline conditions. S. Afr. J. Bot. 95, 24–31. doi: 10.1016/j.sajb.2014.08.005
Shahid, M., Dumat, C., Khalid, S., Schreck, E., Xiong, T., Niazi, N. K. (2017). Foliar heavy metal uptake, toxicity and detoxification in plants: A comparison of foliar and root metal uptake. J. Hazard. Mater. 325, 36–58. doi: 10.1016/j.jhazmat.2016.11.063
Shahid, M., Khalid, S., Abbas, G., Shahid, N., Nadeem, M., Sabir, M., et al. (2015). “Heavy metal stress and crop productivity,” in Crop Production and Global Environmental Issues. Ed. Hakeem, K. R. (Cham: Springer International Publishing), 1–25.
Shama, M., A., M., Moussa, S., I Abo El Fadel., N. (2016). Salicylic acid efficacy on resistance of garlic plants (Allium sativum, L.) to water salinity stress on growth, yield and its quality. Alexandria Sci. Exchange J. 37 (April-June), 165–174. doi: 10.21608/asejaiqjsae.2016.2238
Sharma, A., Ruiz-Manriquez, L. M., Serrano-Cano, F. I., Reyes-Pérez, P. R., Tovar Alfaro, C. K., Barrón Andrade, Y. E., et al. (2020). Identification of microRNAs and their expression in leaf tissues of guava (Psidium guajava L.) under salinity stress. Agronomy 10 (12), 1920. doi: 10.3390/agronomy10121920
Shi, Q., Bao, Z., Zhu, Z., Ying, Q., Qian, Q. (2006). Effects of different treatments of salicylic acid on heat tolerance, chlorophyll fluorescence, and antioxidant enzyme activity in seedlings of Cucumis sativa L. Plant Growth Regul. 48, 127–135. doi: 10.1007/s10725-005-5482-6
Shi, Q., Zhu, Z. (2008). Effects of exogenous salicylic acid on manganese toxicity, element contents and antioxidative system in cucumber. Environ. Exp. Bot. 63, 317–326. doi: 10.1016/j.envexpbot.2007.11.003
Sirisuntornlak, N., Ghafoori, S., Datta, A., Arirob, W. (2019). Seed priming and soil incorporation with silicon influence growth and yield of maize under water-deficit Stress. Arch. Agron. Soil Sci. 65, 197–207. doi: 10.1080/03650340.2018.1492713
Skottke, K. R., Yoon, G. M., Kieber, J. J., DeLong, A. (2011). Protein phosphatase 2A controls ethylene biosynthesis by differentially regulating the turnover of ACC synthase isoforms. PloS Genet. 7 (4), e1001370. doi: 10.1371/annotation/b4fc15d6-b3ae-4fbb-8d88-b7d674a79697
Slama, I., Ghnaya, T., Savoure, A., Abdelly, C. (2008). Combined effects of long-term salinity and soil drying on growth, water relations, nutrient status and proline accumulation of Sesuvium portulacastrum. C. R. Biol. 331, 442–451. doi: 10.1016/j.crvi.2008.03.006
Soltani Maivan, E., Radjabian, T., Abrishamchi, P., Talei, D. (2017). “Physiological and biochemical responses of Melissa officinalis L. @ to nickel stress and the protective role of salicylic acid,” Arch. Agron. Soil Sci., vol. 63. , 330–343.
Song, W. Y., Yang, H. C., Shao, H. B., Zheng, A. Z., Brestic, M. (2014). The alleviative effects of salicylic acid on the activities of catalase and superoxide dismutase in malting barley (Hordeum uhulgare L.) seedling leaves stressed by heavy metals. Clean - Soil Air Water. 42 (1), 88–97. doi: 10.1002/clen.201200310
Souza, L. A., Monteiro, C. C., Carvalho, R. F., Gratão, P. L., Azevedo, R. A. (2017). Dealing with abiotic stresses: an integrative view of how phytohormones control abiotic stress-induced oxidative stress. Theor. Exp. Plant Physiol. 29, 109–127. doi: 10.1007/s40626-017-0088-8
Srivastava, M. K., Dwivedi, U. N. (2000). Delaying ripening of banana fruits by salicylic acid. Plant Sci. 158, 87–96. doi: 10.1016/S0168-9452(00)00304-6
Suzuki, K., Nagasuga, K., Okada, M. (2008). The chilling injury induced by high root temperature in the leaves of rice seedlings. Plant Cell Physiol. 49, 433–442. doi: 10.1093/pcp/pcn020
Szilagyi, L. (2003). Influence of drought on seed yield components in common bean. Bulg. J. Plant, 320-330. Available at: http://www.bio21.bas.bg/ippg/bg/wp-content/uploads/2011/06/03_essa_320-330.pdf
Talabany, N. K., Albarzinji, I. M. (2023). Effects of Salicylic Acid on some Growth and Physiological Characteristics of Lettuce (Lactuca sativa L.) under Cadmium Stress Conditions. Sci. J. Univ. Zakho 11 (1), 37–44. doi: 10.25271/sjuoz.2023.11.1.987
Tchounwou, P. B., Yedjou, C. G., Patlolla, A. K., Sutton, D. J. (2012). HM toxicity and the environment. EXS 101, 133–164. doi: 10.1007/978-3-7643-8340-4_6
Tognetti, V. B., Muhlenbock, P., Van Breusegem, F. (2012). Stress homeostasis-the redox and auxin perspective. Plant Cell Environ. 35, 321–333. doi: 10.1111/j.1365-3040.2011.02324.x
Ullah, H., Luc, P. D., Gautam, A., Datta, A. (2018). Growth, yield and silicon uptake of rice (Oryza sativa) as influenced by dose and timing of silicon application under water-deficit stress. Arch. Agron. Soil Sci. 64, 318–330. doi: 10.1080/03650340.2017.1350782
Uzu, G., Sauvain, J. J., Baeza-Squiban, A., Riediker, M., Hohl, M. S. S., Val, S., et al. (2011). In vitro assessment of the pulmonary toxicity and gastric availability of lead rich particles from a lead recycling plant. Environ. Sci. Technol. 45, 7888–7895. doi: 10.1021/es200374c
Vlot, A. C., Dempsey, M. A., Klessig, D. F. (2009). Salicylic acid, a multifaceted hormone to combat disease. Annu. Rev. Phytopathol. 47, 177–206. doi: 10.1146/annurev.phyto.050908.135202
Wahid, A., Gelani, S., Ashraf, M., Foolad, M. R. (2007). Heat tolerance in plants: an overview. Environ. Exp. Bot. 61, 199–223. doi: 10.1016/j.envexpbot.2007.05.011
Wang, L., Fan, L., Loescher, W., Duan, W., Liu, G., Cheng, J., et al. (2010). Salicylic acid alleviates decreases in photosynthesis under heat stress and accelerates recovery in grapevine leaves. BMC Plant Biol. 10 (1), 34. doi: 10.1186/1471-2229-10-34
Wang, L. J., Li, S. H. (2006). Salicylic acid-induced heat or cold tolerance in relation to Ca2+ homeostasis and antioxidant systems in young grape plants. Plant Sci. 170, 685–694. doi: 10.1016/j.plantsci.2005.09.005
Waqas, M. A., Wang, X., Zafar, S. A., Noor, M. A., Hussain, H. A., Azher Nawaz, M., et al. (2021). Thermal stresses in maize: effects and management strategies. Plants 10, 293. doi: 10.3390/plants10020293
Wei, T., Lv, X., Jia, H., Hua, L., Xu, H., Zhou, R., et al. (2018). Effects of salicylic acid, Fe (II) and plant growth-promoting bacteria on Cd accumulation and toxicity alleviation of Cd tolerant and sensitive tomato genotypes. J. Environ. Manage. 214, 164–171. doi: 10.1016/j.jenvman.2018.02.100
Weih, M., Johanson, U., Gwynn-Jones, D. (1998). Growth and nitrogen utilization in seedlings of mountain birch (Betula pubescens ssp. tortuosa) as affected by ultraviolet radiation (UV-A and UV-B) under laboratory and outdoor conditions trees. Struct. Funct. 12, 201–204. doi: 10.1007/PL00009711
Wu, H. (2018). Plant salt tolerance and Na+ sensing and transport. Crop J. 6, 215–225. doi: 10.1016/j.cj.2018.01.003
Xu, L. L., Fan, Z. Y., Dong, Y. J., Kong, J., Bai, X. Y. (2015a). Effects of exogenous salicylic acid and nitric oxide on physiological characteristics of two peanut cultivars under cadmium stress. Biol. Plant 59, 171–182. doi: 10.1007/s10535-014-0475-9
Xu, Z., Jiang, Y., Zhou, G. (2015b). Response and adaptation of photosynthesis, respiration, and anti-oxidant systems to elevated CO2 with environmental stress in plants. Front. Plant Sci. 6, 701. doi: 10.3389/fpls.2015.00701
Xu, Y. X., Mao, J., Chen, W., Qian, T. T., Liu, S. C., Hao, W. J., et al. (2016). Identification and expression profiling of the auxin response factors (ARFs) in the tea plant (Camellia sinensis (L.) under various abiotic stresses. Plant Physiol. Biochem. 98, 46–56. doi: 10.1016/j.plaphy.2015.11.014
Yadav, S. (2010). Cold stress tolerance mechanisms in plants. A review. Agron. Sustain. Dev. 30, 515–527. doi: 10.1051/agro/2009050
Yalpani, N., Enyedi, A. J., Leon, J., Raskin, I. (1994). Ultraviolet light and ozone stimulate accumulation of salicylic acid, pathogenesis-related proteins and virus resistance in tobacco. Planta 193, 372–376. doi: 10.1007/BF00201815
Yang, Y., Guo, Y. (2018a). Elucidating the molecular mechanisms mediating plant salt stress responses. New Phytol. 217, 523–539. doi: 10.1111/nph.14920
Yang, Y., Guo, Y. (2018b). Unraveling salt stress signaling in plants. J. Integr. Plant Biol. 60, 796–804. doi: 10.1111/jipb.12689
Yang, L., Zhang, Z., Hu, X., You, L., Khan, R. A. A., Yu, Y. (2022). Phenolic contents, organic acids, and the antioxidant and bio activity of wild medicinal Berberis plants-as sustainable sources of functional food. Molecules 27 (8), 2497. doi: 10.3390/molecules27082497
Yi, X., Guo, Y., Khan, R. A. A., Fan, Z. (2021). Understanding the pathogenicity of Pochonia chlamydosporia to root knot nematode through omics approaches and action mechanism. Biol. Control 162, 104726. doi: 10.1016/j.biocontrol.2021.104726
Yu, O. Y., Raichle, B., Sink, S. (2013). Impact of biochar on the water holding capacity of loamy sand soil. Int. J. Energy Environ. Eng. 4 (1), 44. doi: 10.1186/2251-6832-4-44
Yuan, S., Lin, H. H. (2008). Role of salicylic acid in plant abiotic stress. Z. Naturforsch. C. J. Biosci. 63, 313–320. doi: 10.1515/znc-2008-5-601
Zhang, Z., Lan, M., Han, X., Wu, J., Wang-Pruski, G. (2020). Response of ornamental pepper to high-temperature stress and role of exogenous salicylic acid in mitigating high temperature. J. Plant Growth Reg. 39, 133–146. doi: 10.1007/s00344-019-09969-y
Zhang, Y., Mian, M. A. R., Bouton, J. H. (2006). Recent molecular and genomic studies on stress tolerance of forage and turf grasses. Crop Sci. 46, 497–511. doi: 10.2135/cropsci2004.0572
Zhu, J. K. (2002). Salt and drought stress signal transduction in plants. Annu. Rev. Plant Biol. 53, 247–273. doi: 10.1146/annurev.arplant.53.091401.143329
Zhu, J. K. (2016). Abiotic stress signaling and responses in plants. Cell 167, 313–324. doi: 10.1016/j.cell.2016.08.029
Keywords: poor yield, photosynthetic impairments, stomatal conductance, signaling molecules, stunted growth
Citation: Yang H, Fang R, Luo L, Yang W, Huang Q, Yang C, Hui W, Gong W and Wang J (2023) Uncovering the mechanisms of salicylic acid-mediated abiotic stress tolerance in horticultural crops. Front. Plant Sci. 14:1226041. doi: 10.3389/fpls.2023.1226041
Received: 20 May 2023; Accepted: 28 July 2023;
Published: 28 August 2023.
Edited by:
Mohd Asgher, Baba Ghulam Shah Badshah University, IndiaReviewed by:
Tasir Sharief Per, Aligarh Muslim University, IndiaMuhammad Ahsan Altaf, Hainan University, China
Copyright © 2023 Yang, Fang, Luo, Yang, Huang, Yang, Hui, Gong and Wang. This is an open-access article distributed under the terms of the Creative Commons Attribution License (CC BY). The use, distribution or reproduction in other forums is permitted, provided the original author(s) and the copyright owner(s) are credited and that the original publication in this journal is cited, in accordance with accepted academic practice. No use, distribution or reproduction is permitted which does not comply with these terms.
*Correspondence: Hua Yang, eWFuZ2h1YTE1MTAxN0AxNjMuY29t; eWFuZ2h1YTU0NkBnbWFpbC5jb20=