- 1College of Agronomy, Anhui Agricultural University, Hefei, Anhui, China
- 2School of Environmental and Life Sciences, The University of Newcastle, Newcastle, NSW, Australia
- 3Centre for Crop Science, Queensland Alliance for Agriculture and Food Innovation, The University of Queensland, Brisbane, QLD, Australia
Securing maize grain yield is crucial to meet food and energy needs for the future growing population, especially under frequent drought events and elevated CO2 (eCO2) due to climate change. To maximize the kernel setting rate under drought stress is a key strategy in battling against the negative impacts. Firstly, we summarize the major limitations to leaf source and kernel sink in maize under drought stress, and identified that loss in grain yield is mainly attributed to reduced kernel set. Reproductive drought tolerance can be realized by collective contribution with a greater assimilate import into ear, more available sugars for ovary and silk use, and higher capacity to remobilize assimilate reserve. As such, utilization of CO2 fertilization by improved photosynthesis and greater reserve remobilization is a key strategy for coping with drought stress under climate change condition. We propose that optimizing planting methods and mining natural genetic variation still need to be done continuously, meanwhile, by virtue of advanced genetic engineering and plant phenomics tools, the breeding program of higher photosynthetic efficiency maize varieties adapted to eCO2 can be accelerated. Consequently, stabilizing maize production under drought stress can be achieved by securing reproductive success by harnessing CO2 fertilization.
Introduction
Maize (Zea mays L.) is one of the most important cereal crops worldwide, serving as a major source of food, feed, and biofuel, with a total production of 1.16 billion tons from 201.98 million hectares cultivated (FAOSTAT, 2021). Maize production is predominantly influenced by climatic conditions during the growing season, with drought stress having a significant impact on grain yield, comparable to the cumulative effects of all other environmental factors (Boyer and Westgate, 2004). In past climate, maize plants have suffered from drought stress during the individual or multiple growth stages, grain yield losses are most pronounced when drought stress occurs during early reproductive stage (Saini and Westgate, 1999; Kadam et al., 2014; Messina et al., 2019). That can lead to several reproductive development failure irreversibly even though the parent remains alive, especially ovary abortion in maize (Boyer and Westgate, 2004; Shen et al., 2020; Sinha et al., 2021). Yield losses from drought stress at early reproductive stage are foreseen to be as much as 30% based on modelling studies (Lobell et al., 2014). Consequently, securing reproductive success in maize under drought stress is essential for increasing stability of food system.
During the early reproductive stage, drought stress reduces grain numbers in maize are often ascribed to a lack of egg fertilization, resulting in undeveloped ovules (Boyer and Westgate, 2004). Due to pollen water potential always remains lower than parent or silk, female florets show more sensitive to drought stress than male florets, suggesting that the abortion is controlled by female inflorescence under drought stress (Westgate and Boyer, 1986a; Westgate and Boyer, 1986b). A study on the drought tolerance of 18 maize hybrids released during the 1953-2001 period (Campos et al., 2006) showed that genetic yield gains are associated with increased kernels per ear and reduced anthesis–silking interval (ASI) under drought stress at flowering stage. Later in early filling stage, drought stress also reduces kernel number due to less available carbon supply (Boyer and Westgate, 2004; Cakir, 2004). There is abundant evidence that drought stress inhibits photosynthesis (Tang et al., 2023), impairs carbon metabolism (Zinselmeier et al., 1995b; Muller et al., 2011; Shen et al., 2020), and ultimately triggers ovary abortion due to sugar starvation (Boyle et al., 1991; Zinselmeier et al., 1995a; Tang et al., 2023).
With climate change, drought stress is projected to become more frequent, longer, and more severe, posing a huge challenge to sustainable maize production (Harrison et al., 2014; Lobell et al., 2014; Yuan et al., 2023). According to the latest AR6 Synthesis Report, ambient CO2 concentration has increased from the preindustrial level of 280 to 410 ppm today (IPCC, 2023), and is considered a major driving force of drought stress (Kadam et al., 2014; Yuan et al., 2023). However, a recent meta-analysis by Ainsworth and Long (2021) has found that elevated CO2 (eCO2) can enhance the productive capacity of C4 crops under drought stress, as eCO2 significantly improves water use efficiency (WUE), based on over 250 observations from free-air CO2 enrichment (FACE) experiments worldwide. Consequently, rising CO2 concentration provides a unique opportunity to maintain maize productivity under drought stress (Leakey et al., 2004; Lobell et al., 2014; Gonsamo et al., 2021). Given the limitations of FACE experiments, far less is known about how maize plants achieve their reproductive success under the interactive effects of eCO2 and drought stress, and what the contribution of CO2 fertilization? In this review, we summarize studies that have explored the photosynthetic production capacity and reproductive development in maize under drought stress, and propose strategies to secure reproductive success under drought stress in maize by harnessing CO2 fertilization for greater productivity.
Effects of drought stress during reproductive stage on leaf photosynthesis and grain yield in maize
The reproductive stage is critical for maize as it determines kernel setting and final yield potential. Drought stress during this stage can cause a significant reduction in both photosynthesis and grain yield in maize, the extent to which depends on the severity and duration of the stress period (Table 1). It is evident that reproductive stage of maize is more sensitive to drought than vegetative stage and the concurrent decrease in photosynthesis and yield under drought stress occurs consistently in maize.
Photosynthesis under drought stress
The response of maize photosynthesis to drought stress during the reproductive period is more intense than during the vegetative period, as the drought recovery capacity of leaf photosynthesis after the tasseling stage is relatively poor (Cai et al., 2020). Moreover, the influence on photosynthesis is more profound at the tasseling stage than at the jointing and milk stages, even at the same drought level (Myers et al., 2017). These results suggest that drought stress during reproductive stage, particularly at the flowering stage, threatens kernel setting by limiting leaf photosynthesis and thus carbohydrate supply (Table 1). This limitation results from a decrease in leaf expansion, impaired photosynthetic machinery, premature leaf senescence, and a related decrease in assimilate production (Cai et al., 2020).
Both stomatal and non-stomatal limitations on leaf photosynthesis occur under drought stress (Figure 1), and photosynthesis is prone to non-stomatal limitations to photosynthesis in maize (Farooq et al., 2009; Lopes et al., 2011; Song et al., 2020b). This suggests that processes other than CO2 uptake are being affected. Drought stress typically limits CO2 uptake by inducing stomatal closure in leaves (Muller et al., 2011), but the ability of maize to concentrate CO2 around Rubisco in the bundle sheath cells mitigates this effect (Leakey et al., 2019). Regarding non-stomatal limitation, drought stress causes changes in photosynthetic pigments and components (Manderscheid et al., 2014; Ye et al., 2020; Bheemanahalli et al., 2022), damages photosynthetic apparatus (Ye et al., 2020), diminishes activities of Calvin cycle enzymes (Kakani et al., 2011; Cai et al., 2020; Correia et al., 2021), and induces photorespiration (Sicher and Barnaby, 2012), all of which contribute to reduced photosynthesis. In addition, to survive under drought stress, maize expends a considerable amount of energy to cope with it through respiration (Farooq et al., 2009). Another critical effect of drought stress is the imbalance between reactive oxygen species (ROS) production and antioxidant defense (Sicher and Barnaby, 2012; Ye et al., 2020; Sinha et al., 2021), which results in ROS accumulation and induces oxidative stress in proteins, membrane lipids and other cellular components. Variation in these non-stomatal limitations affects photosynthesis and assimilate accumulation, providing potential targets for increasing maize yield under drought stress (Figure 1).
Grain yield under drought stress
Many yield-determining physiological processes in plants respond to drought stress (Figure 1). In maize, early reproductive stage is highly susceptible to drought stress, resulting in a pronounced loss of kernel number and, consequently, grain yield (Saini and Westgate, 1999; Boyer and Westgate, 2004; Setter et al., 2011). As presented in Table 1, drought stress occurring during the rapid vegetative growth period causes a little loss of final grain yield by 9-17%, while more significant losses during the filling stage by 22-39% are mostly through reducing kernel size, and severe losses during early reproductive stage by 36-99% result from reductions in kernel numbers. Large agricultural losses can occur during the whole reproductive stage, but the irreversibility of the early events is particularly damaging. When drought stress occurs during the early reproductive stage, losses in kernel number are attributed to long anthesis-silking interval (ASI) (Boyer and Westgate, 2004; Duvick, 2005; Fuad-Hassan et al., 2008) and disruption of ovarian carbon metabolism (Muller et al., 2011; Liu et al., 2022); while during filling stage, grain yield losses are attributed to the reduced kernel weight that limited by insufficient assimilate supply (Boyer and Westgate, 2004). Consequently, reproductive success in maize can be mitigated by increasing photo-assimilation supply (Figure 1).
Reproductive drought tolerance mechanisms in maize
In past decades, breeding has led to the development of maize genotypes with increased drought tolerance, primarily attributable to enhanced reproductive resilience (Zinselmeier et al., 1995b; Lobell et al., 2014; Messina et al., 2019; Sinha et al., 2021). For example, conventional breeding has produced hybrid lines less susceptible to drought stress, with the improvements primarily resulting from increased assimilate accumulation in reproductive organs and rapidly silking (Bänziger et al., 2002; Cattivelli et al., 2008; Araus et al., 2012; Nuccio et al., 2015). Consequently, constitutive drought tolerance mechanisms may exist in maize and are closely related to the establishment of reproductive structures (Bänziger et al., 2002). Under drought stress, reproductive organ expansion being affected earlier and more intensively than photosynthesis and metabolism (Fuad-Hassan et al., 2008; Muller et al., 2011), resulting in reproductive failure, i.e., failure to pollinate or post-pollination ovary abortion (Zinselmeier et al., 1995b; Boyer and Westgate, 2004; Fuad-Hassan et al., 2008; Oury et al., 2016; Turc et al., 2016; Yang et al., 2018; Bheemanahalli et al., 2022). Therefore, securing ovary and silking success poses a critical importance for enhancing reproductive drought tolerance in maize under drought stress.
Greater assimilate import into ear
Assimilate partitioning and transportation under drought stress contribute significantly to reproductive growth and development of maize, particularly when an inadequate assimilate supply to ear causes severe grain yield losses (Mclaughlin and Boyer, 2004b; Boyer and Mclaughlin, 2007; Sinha et al., 2021). Multiple lines of evidence suggest that maintaining ear development under drought stress and achieving high seed set are related to maintenance of sugar supply (Zinselmeier et al., 1995a; Boyer and Mclaughlin, 2007). For example, a recent study by Tang et al. (2023) demonstrated that varying degrees of drought stress decreased carbohydrate supply in developing ear by 12%-63%, resulting in ovary abortion, particularly apparent on the tip of maize ear. In addition, two prominent studies have shown that overexpression of trehalose-6-phosphate phosphatase in developing maize ear using a floral promoter decreased trehalostrehalose-6-phosphatephosphate, leading to increased sucrose concentration by regulating assimilate partitioning, and the engineered trait improved yields from 31% to 123% under drought stress (Nuccio et al., 2015; Oszvald et al., 2018). Taken together, reproductive success is closely related to the assimilate flux to the young ear around flowering under drought stress, and concurrent photosynthesis is required to maintain this flux (Bänziger et al., 2002). In maize, overexpression of ZmNF-YB16 (Wang et al., 2018) and Nicotiana protein kinase (NPK1) (Shou et al., 2004) can improve drought tolerance and yield by enhancing photosynthesis (Figure 2).
However, the relationship between sugar content and reproductive growth is intricate, and ovary abortion is not solely result from limitations in sugar supply (Lopes et al., 2011; Muller et al., 2011). Ovary abortion can also occur due to disturbed sugar-to-starch synthesis, even when there is an adequate sucrose supply (Zinselmeier et al., 1999). The reason is that factors i.e. phytohormones and plant metabolites other than an inadequate supply of assimilates also initiate the ovary abortion process (Feng et al., 2011a; Shabbaj et al., 2022). For example, the addition of 1-aminocyclopropane-1-carboxylic acid to the culture medium results in the evolution of ethylene in vitro, which causes abortion and reduces mature kernel mass in maize (Cheng and Lur, 1996). Amino acids and their derived metabolism e.g. polyamine is also found that play a crucial role in the regulation of early endosperm development under eCO2 (Liang and Lur, 2002; Geng et al., 2020). The ovary abortion caused by phytohormones and plant metabolites largely due to a complex relationship with sugar stream and availability (Feng et al., 2011a). It is clear that increasing assimilate supply by photosynthesis to ear will increase maize reproductive drought tolerance, while maintaining a steady sugar stream and its use in maize reproductive tissues are equally important for reproductive drought tolerance (Figure 2).
More available sugars for ovary and silk use
Sugar availability in the developing ovary and silk have been correlated with the incidence of ovary abortion under drought stress (Andersen et al., 2002; Feng et al., 2011b; Kakumanu et al., 2012; Oury et al., 2016). In this process, invertases (INVs) serve as an important part of hexose supply that maintains growth and developmental processes for ovary and silk (Andersen et al., 2002; Oury et al., 2016). Generally, drought stress reduces INVs activity in the ovaries, leading to a decrease in hexose, depletion of starch reserves in ovary, and abortion of ovaries (Boyer and Mclaughlin, 2007; Sinha et al., 2021). Not just that, the decreased invertase activity accounts for the slower sucrose uptake in ovary under drought stress (Boyer and Westgate, 2004). A related work showed that increased INVs in drought-stressed maize can maintain reproductive organ hexose concentration at an “unstressed” level, contributing to enhance reproductive drought tolerance (Kakumanu et al., 2012). Specifically, the ivr2 gene, which encodes an acid-soluble invertase, is verified to be functional in enhancing maize ovary drought tolerance (Qin et al., 2004; Li et al., 2011). Additionally, the decreased sugar availability can delay silk growth under drought stress, leading to an increased ASI and ultimately resulting in ovary abortion (Muller et al., 2011; Oury et al., 2016; Messina et al., 2019). It follows that the differences of sugar utilization in ovary and silk determine the “live or die” fate of maize ovary siblings during sugar competition under drought stress (Shen et al., 2020; Shen et al., 2023).
In addition to sucrose availability itself, the signaling of sucrose availability is critically important for ovary development under drought stress (Andersen et al., 2002; Bledsoe et al., 2017). Trehalose-6-phosphate (Tre6P) plays a central role in sugar sensing, leading to the proposal that Tre6P acts a signal of sucrose availability, and in turn regulates sucrose production and utilization (Bledsoe et al., 2017). In maize, several works showed that expression of Tre6P phosphatase in ovary improves its drought tolerance (Nuccio et al., 2015; Bledsoe et al., 2017; Oszvald et al., 2018). Overall, sugar utilization in ovary is as important as the continuous sugar supply for reproductive drought tolerance (Figure 2).
Higher capacity to remobilize assimilate reserve
Drought stress causes maize plants scarcely any assimilate accumulated, but kernels can continue to fill for some time results from the assimilate reserves (Mcpherson and Boyer, 1977). A further study by Sinclair et al. (1990) found kernel yield is closely related to assimilate accumulation in stem under drought stress. Therefore, assimilate reserves in maize are especially critical for drought tolerance. Although stem serves as a major sink for assimilate during the vegetative phase of maize growth, assimilate stored in the stem can be remobilized to reproductive organs (Ribaut et al., 2009). This remobilization and its contribution to final kernel yield are significantly affected by drought stress (Mclaughlin and Boyer, 2004a; Boyer and Mclaughlin, 2007; Ribaut et al., 2009; Golzardi et al., 2017). Inhibition of pre-fertilization ear growth due to drought can be in partially overcome by sugar supply via the stem, which is transported to the ear (Mclaughlin and Boyer, 2004a; Boyer and Mclaughlin, 2007). Early seminal studies by Boyle et al. (1991) and Zinselmeier et al. (1995a) suggested that re-establishing the sugar stream via the stem can prevent ovary abortion, and seed set is largely preserved in maize. Despite remobilization of stem assimilates to ear is possible in maize, remobilization efficiency still requires further investigation. As demonstrated in sorghum (Borrell et al., 2006), an increase in stem diameter may favor assimilate reserve and remobilization to developing grains under drought stress. Consequently, maize genotypes with a better ability to store and remobilize assimilates in stem will exhibit greater drought stress tolerance (Figure 2).
Securing reproductive success by harnessing CO2 fertilization
As summarized above, maize reproductive success depends on three aspects: (i) greater assimilate import into ear; (ii) more available sugars for ovary and silk use; (iii) higher capacity to remobilize assimilate reserve. Several CO2 enrichment studies with maize conducted under controlled environment conditions have demonstrated an increase in photosynthesis and grain yield under drought stress (Leakey et al., 2004; Markelz et al., 2011; Manderscheid et al., 2014), but no significant effect was observed when the plant was not experiencing drought stress (Leakey et al., 2006; Markelz et al., 2011). As a result, a strong correlation exists between reproductive success under drought stress and CO2 fertilization.
Boosting leaf photosynthesis by harnessing CO2 fertilization under drought stress
Drought stress can inhibit maize photosynthesis by limiting the water availability and inducing a set of related limitations. FACE experiments with drought stress have indicated that eCO2 can indirectly enhance maize photosynthesis through improving plant water relations, thus mitigating the negative effects of water scarcity on growth and photosynthetic system, (Kimball et al., 2002; Leakey et al., 2006; Manderscheid et al., 2014; Ainsworth and Long, 2021). WUE is a constraint and target for improving crop resilience and productivity (Leakey et al., 2019). In maize, photosynthesis is saturated at the current ambient CO2 level due to its CO2 concentrating mechanism, so there is no direct stimulation of photosynthetic carbon gain and yield (Manderscheid et al., 2014). However, eCO2 decreases maize stomatal conductance (gs) by 22%, mostly through reduced aperture (Ainsworth and Rogers, 2007), resulting in a 16-68% decrease in transpiration (Kimball and Idso, 1983), greatly improving WUE and conserving soil moisture (Leakey et al., 2006; Leakey, 2009). This indicates that the potential benefits of greater WUE associated with lower gs and equivalent photosynthesis can be realized in maize; such mechanisms may counteract the development of drought stress under eCO2 and prevented the inhibition of photosynthesis observed under ambient CO2.
There is also evidence that eCO2 directly stimulate maize leaf photosynthesis by reducing the time needed for stomatal opening under dynamic irradiance (Leakey et al., 2005), decreasing stomatal aperture (Ainsworth and Rogers, 2007), increasing intercellular CO2 concentration (Ci) and leaf temperature, and altering diurnal CO2 fixation patterns (Ghannoum et al., 2000), resulting in increased photosynthesis. Decreasing respiration has been a target for improving photosynthesis (Joshi et al., 2023), early studies attributed eCO2 enhanced photosynthesis of immature fully exposed leaves to suppressed photorespiration and enhanced energy use efficiency from decreased leakage of CO2 from bundle sheath cells and reduced over-cycling of the C4 pump (Cousins et al., 2001; Ainsworth and Rogers, 2007). A more recent study showed that eCO2 led to an 8.4% reduction in day respiration rate and a 16.2% reduction in dark respiration, as decreased leaf N and chlorophyll contents (Sun et al., 2022). In addition to these physiological traits, eCO2 promotes leaf area (Kadam et al., 2014) and decreases leaf thickness (Leakey et al., 2006), combining with low leaf chlorophyll concentration, thereby providing more surface area for light interception and allowing more light penetration to lower layers of a dense canopy, ultimately resulting in higher maize canopy photosynthesis and biomass accumulation (Kim et al., 2006; Allen et al., 2011). eCO2 maintains water balance by increasing the accumulation of compatible solutes including glucose, fructose, β-alanine, and γ-aminobutyric acid (GABA) in addition to enhancing photosynthetic properties, thereby improving drought tolerance (Abdelhakim et al., 2022). Consequently, the increase in photosynthesis under eCO2 and drought stress involves a complex process with multiple mechanisms (Figure 3). Although not yet fully understood, these mechanisms offer promising avenues for providing adequate supply of assimilate by enhancing leaf photosynthesis under drought stress and mitigating the negative effects of water scarcity on reproductive growth and productivity.
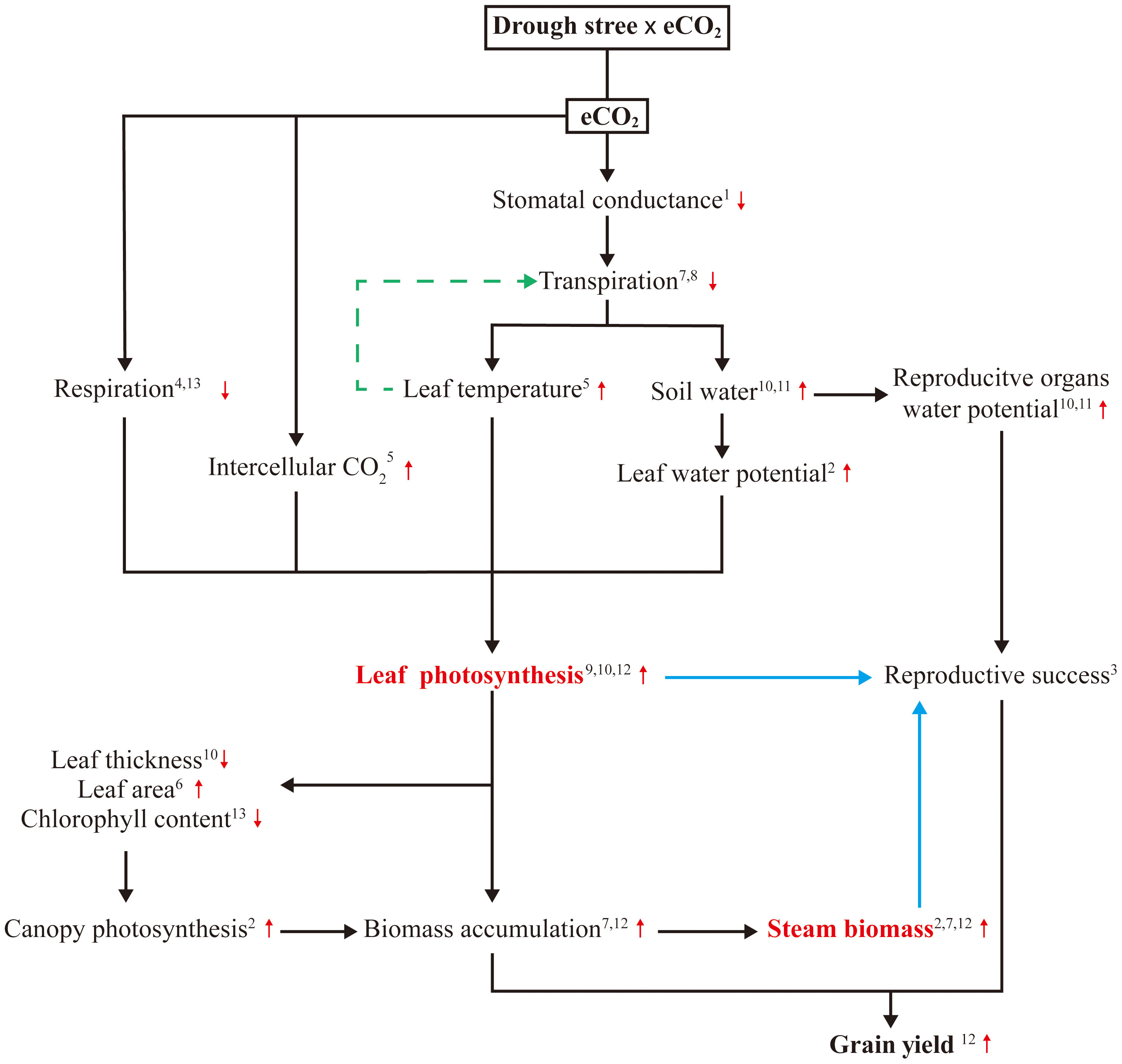
Figure 3 Summary of the main mechanisms contributing to reproductive success in maize exposed to eCO2 under drought stress. 1(Ainsworth and Rogers, 2007); 2(Allen et al., 2011); 3(Boyer and Westgate, 2004); 4(Cousins et al., 2001); 5(Ghannoum et al., 2000); 6(Kadam et al., 2014); 7(Kim et al., 2006); 8(Kimball and Idso, 1983); 9(Kimball et al., 2002); 10(Leakey et al., 2006); 11(Leakey, 2009); 12(Manderscheid et al., 2014); 13(Sun et al., 2022).
The impact of eCO2 on maize productivity was commonly positive when soil water content be limiting for growth and evaporative demand under ambient CO2. The response of maize to eCO2 is therefore likely to vary among different periods. For example, under limited water conditions, in the experiments by enriching CO2 since emergence, eCO2 increased maize canopy daily photosynthesis by 9% and reduced canopy transpiration by 22% on 26 days after emergence (DAE) (Allen et al., 2011), and caused an enhancement of leaf photosynthesis by 21% on 37 DAE and 11% on 48 DAE (Leakey et al., 2004). When CO2 enrichment occurred from 23 DAE to harvest, eCO2 significantly increased final biomass by 24% and grain yield by 41% (Manderscheid et al., 2014). However, eCO2 may negatively affect maize yields when eCO2 partially offsets the yield gaps caused climate extremes (drought, heat) due to excessively high CO2 concentrations. A simulation study demonstrated that severe drought pattern caused by eCO2 (550 ppm) accelerated early maturity and had the greatest impacts on maize yield (Harrison et al., 2014). Under eCO2 (845 ppm) scenario, high temperature and heat stress replace the dominant stress of drought on maize, resulting in prominent yield losses (Jin et al., 2017). These results highlight the importance of considering interaction of eCO2 with other environmental variables, especially extreme events, in future agronomic adaptation and mitigation strategies.
Increasing and remodeling assimilate reserves in stem
When drought stress occurs during reproductive stage in maize, having the adequate assimilate reserves in stem appears to be particularly important, as reproductive cellular activities and respiration continue to demand substrates (Boyer and Mclaughlin, 2007; Farooq et al., 2009). Assimilate accumulation of maize stem under different drought stress conditions has been shown to decrease by 16-24% (Kamara et al., 2003), 16-44% (Ge et al., 2011) and 12-63% (Tang et al., 2023) due to decreased photosynthesis capacity, and the corresponding yield decreased by 49-99%, 20.4-84.5%, and 46-99%, respectively. Can this reduction be reversed? As previously mentioned, eCO2 can increase assimilate supply in maize by enhancing photosynthesis under drought stress. Based on CO2 enrichment experiments, significant increases in biomass accumulation in maize stem under drought stress have been reported, range from 9% (Manderscheid et al., 2014) to 20% (Allen et al., 2011). These results imply that eCO2 allows maize to produce and store more assimilate in stem before flowering stage under drought stress. When drought stress occurred during the reproductive period, stress can stimulate the remobilization of pre-stored assimilate reserves (Yang et al., 2001), subsequently, large amounts of carbohydrate were moved from the stems to the grain, compensating for the lack of current photosynthesis (Boyer and Westgate, 2004). These evidences suggest that eCO2 improves the potential for remobilization of assimilate reserves in maize stem to support kernel growth under drought stress, providing a promising strategy for enhancing maize productivity and food security under drought stress (Figure 3).
The effect of eCO2 on grain quality
Although eCO2 increases photosynthesis and carbohydrate content, but not mineral elements, thus alters the stoichiometric balance of nutrients in crop grains and has a significant impact on human nutrition (Loladze, 2002). Gradually, the effect of eCO2 on crop quality is becoming a hot topic of research (Myers et al., 2017; Zhu et al., 2018). Most studies are in favour of the idea that eCO2 leads to an increase in carbohydrate content, which dilutes mineral and protein concentrations in plant tissues (Taub et al., 2008). For example, eCO2 decreased the mineral concentrations such as nitrogen and phosphorus in maize grains (Abebe et al., 2016), iron and zinc in rice grains (Zhu et al., 2018), and magnesium, copper, calcium, and manganese in wheat grains (Guo et al., 2021). Protein content decreased in wheat and rice, but not significantly in soybean and pea, indicating the ability of legumes to convert excess carbon for nitrogen fixation (Myers et al., 2014). In maize, eCO2 did not significantly affect the protein content of maize grains, but increased the oil content (Qiao et al., 2019). Interestingly, a recent study found that the negative effects of eCO2 on grain quality in rice and wheat grains were compensated by the positive effects of elevated temperature (Guo et al., 2021). Limited by the FACE facilities, there remains substantial uncertainty about the interacting consequences of eCO2, multiple environmental factors, and cropping practices on crop quality. More comprehensive replicated experiments are needed to clarify the mechanisms and environmental conditions that lead to lower nutrient levels in eCO2.
Adapting to rising CO2 concentration
Rising CO2 concentration has the potential to boost photosynthesis and assimilate accumulation, and thus secure reproductive success and productivity in maize under drought stress as elaborated above. However, environmental factors and leaf photosynthetic capacity determine the extent to which photosynthesis responds to eCO2. To capitalize on this potential, optimizing planting methods and selecting maize varieties that can adapt to rising CO2 concentration hold the same importance. In the following sections, we will explore strategies for achieving such adaptation, enabling to harness the enhanced productivity from rising CO2 concentration while mitigating the various detrimental impacts of climate change on global food supply.
Optimizing planting methods
Optimizing cropping systems to adapt to an elevated CO2 environment involves integrating the implications of the various technological possibilities associated with the system components and identifying and exploiting their interactions at the population scale (Hammer et al., 2021). Water availability directly restricts maize growth and development. Fortunately, conservation agriculture (e.g., minimum tillage (Lobell et al., 2014), mulching (Niu et al., 2020), and cover cropping (Manderscheid et al., 2014)) and precision irrigation techniques (e.g., surface drip irrigation (Liu et al., 2023), shallow‐buried drip irrigation (Ayars et al., 2015), alternate furrow irrigation (Golzardi et al., 2017), and micro-sprinkling irrigation (Li et al., 2021a)) have been shown to help retain soil moisture, reduce evaporation, and improve water use efficiency. In a future eCO2 scenario, these water-saving measures can allocate limited water supplies to irrigate larger area of maize, potentially increasing overall maize yields in water-limited areas. Given the large amount of nitrogen invested by plants in Rubisco and Rubisco’s role as a C fixing enzyme (Evans and Clarke, 2019), it is not surprising that the balance between photosynthetic utilization and nitrogen status plays an important role in shaping the plant’s response to eCO2. Ainsworth and Long (2005) reported that the stimulation of light-saturated CO2 uptake (Asat) and maximum carboxylation rate (Vcmax) at eCO2 was 23% and 85% lower in plants grown with a low nitrogen supply, respectively. Furthermore, Markelz et al. (2011) pointed out that drought damage to maize photosynthesis is exacerbated by nitrogen limitation and improved by eCO2. Nitrogen limitation can cause carbon sink limitation at the individual plant level, thereby reducing the actual growth achieved by eCO2 (Ainsworth and Rogers, 2007). Matching the increased C supply with additional nitrogen at eCO2 is key to avoiding sink limitation (Leakey et al., 2009). It follows that adequate nitrogen supply is an effective measure for maize to adapt to eCO2. While these planting methods and agricultural practices can help adapt maize production to eCO2 and drought stress, they still need to be further tested in relation to local conditions and specific crop requirements.
Mining natural genetic variation
Genetic variation in crops responses to eCO2 is crucial for future breeding efforts aimed at improving productivity (Ainsworth and Long, 2021). Under eCO2 environment, notable variation in grain yield has been observed, ranging from 46% to 127% for three maize cultivars (Vanaja et al., 2015), 3% to 36% for eight rice cultivars (Hasegawa et al., 2013) and from 0% to 24% for nine soybean genotypes (Bishop et al., 2015). Both studies demonstrated a similar dependence of yield response to eCO2 on sink capacity, indicating that sink capacity in these seed crops is a key limitation to yield responsiveness to eCO2 in the field. However, the mechanisms driving greater yields at eCO2 in wheat differ from those in soybean and rice. A study by Tausz-Posch et al. (2015) found that eCO2 stimulated grain yield increase in a freely tillering cultivar exclusively due to an increase in fertile tiller number, while yield stimulation in a restricted tillering cultivar is additionally associated with increased kernel weight and kernel numbers per spike. In addition, a greater performance response to eCO2 is observed in wheat cultivars selected for higher transpiration efficiency (Tausz-Posch et al., 2012). As a result, more comprehensive screening of the vast genetic variation in essential crops will likely reveal significant differences in CO2 response that can be utilized in breeding programs. Attaining the theoretical yield response offered by rising CO2 levels may be vital for addressing the anticipated supply-demand gap as the century unfolds (Ray et al., 2013).
Utilizing genetic engineering approach
Though the sufficient natural genetic variability in crops can be used in breeding to increase sink strength to counteract the feedbacks from increased photosynthetic potential under eCO2, there is limited time for conventional breeding to adjust to rapidly rising CO2 concentration (Ainsworth and Rogers, 2007; Ainsworth and Long, 2021). Advanced genetic engineering tools may be necessary to design and implement new photosynthetic system for better efficiency under eCO2 (Long et al., 2015; Zhu et al., 2022). Rubisco catalyzes ribulose bisphosphate (RuBP) carboxylation and oxygenation, representing an evolutionary preferred choice in optimizing photosynthesis (Long et al., 2015; Zhu et al., 2022). Under eCO2, leaf Rubisco content decreases by 20% due to reduced leaf nitrogen content (Ainsworth et al., 2002). A recent transgenic study by Yoon et al. (2020) found that upregulation of Rubisco content in rice causes increased photosynthesis and yield, suggesting that advanced genetic engineering technology has potential to overcome the reduced Rubisco content under eCO2. In scenario with simultaneous increases in CO2 and temperature, the limitation of CO2 assimilation has a tendency to RuBP regeneration instead of Rubisco (Perdomo et al., 2017; Ainsworth and Long, 2021). Sedoheptulose-1,7-bisphosphatase (SBPase) has been reported to be related to RuBP regeneration (Long et al., 2015) can thus be a target for manipulation to increase assimilation without additional resources. Transgenic upregulation of SBPase in soybean, allowing to enhance photosynthesis, thereby protecting against temperature-induced yield loss under eCO2 (Köhler et al., 2017). In addition, upregulation of the Rieske Fe-S protein of electron transport (Simkin et al., 2017) and the H-protein of the glycine cleavage system (López-Calcagno et al., 2019) can also increase RuBP regeneration rates. Taken together, these results highlight genetic engineering can efficiently modify key targets to further maximizing photosynthesis under eCO2.
Combining with plant phenomics
To fully exploit the potential of genetic engineering tools, greater emphasis should be placed on applying appropriate secondary traits and high-throughput phenotyping tools to identify germplasm with high photosynthetic capacity under eCO2 (Araus et al., 2012; Meacham-Hensold et al., 2020; Zhu et al., 2022; Munne-Bosch and Villadangos, 2023). Complex traits, such as grain yield, drought tolerance, and high photosynthetic efficiency, appear to have low heritability due to significant genotype × environment interactions (Lopes et al., 2011; Leakey et al., 2019). Using secondary traits as the primary phenotypic traits may be a viable alternative for selecting high photosynthetic efficiency. This approach can improve the selection efficiency and precision because the heritability of some secondary traits remains higher than that of complex traits, has exhibits sufficient genetic variability, and is genetically correlated with complex traits (Araus et al., 2012; Munne-Bosch and Villadangos, 2023). Advanced plant phenotyping technologies allow to predict physiological and anatomical traits related to photosynthetic efficiency. For example, typical gas exchange system (Kumagai et al., 2022), emerging multispectral (Fu et al., 2022), hyperspectral (Yendrek et al., 2017; Meacham-Hensold et al., 2020), fluorescence (Li et al., 2020; Xia et al., 2023), and thermal (Munne-Bosch and Villadangos, 2023) sensors.
Based on LI-6800 Portable Photosynthesis System, combining with PACiR (Stinziano et al., 2017) and DAT (Saathoff and Welles, 2021) techniques, measurements of Vcmax and maximal linear electron transport rate (Jmax) can be obtained in 5 min, and possibly even faster compared to typical Steady State technique. To make the measurement more convenient, Xia et al. (2023) developed an least-squares SVM (LSSVM) model that can obtain Fv/Fm from chlorophyll a fluorescence signals measured without dark adaptation. Although these methods have greatly improved their efficiency compared to traditional methods, they still do not allow for the rapid measurement of more species or genotypes within a species to enable the study of genetic diversity. Interestingly, at leaf level, Kumagai et al. (2022) constructed a predictive model for Vcmax and Jmax by coupling spectral vegetation indices and machine learning methods. The results showed that hyperspectral reflectance captured the biochemical acclimation of leaf photosynthesis to high temperature in the field. Using a similar method, Yendrek et al. (2017) accurately predicted chlorophyll content, N content, specific leaf area and Vcmax of maize leaf, enabling to phenotyping over 1000 rows during midday hours in only 2 to 4 days. The widespread application of PAM fluorescence in quantitative photosynthesis has further stimulated interest in passive detection of chlorophyll fluorescence under solar irradiation (Fu et al., 2022), namely solar-induced fluorescence (SIF). Camino et al. (2019) estimated Vcmax for both rainfed and irrigated wheat trials by combining SIF and hyperspectral images through the inversion of the SCOPE model. At plot level, Based on time-synchronized hyperspectral images and irradiance measurements, Fu et al. (2021) purposed an alternative yet promising approach to monitor tobacco photosynthetic capacity (Vcmax and Jmax). At canopy level, Li et al. (2020) used solar induced fluorescence (SIF) and hyperspectral imagery to characterize the maize canopy photosynthetic light use efficiency. To detect the effect of drought stress on maize and soybean leaf physiology, Sobejano-Paz et al. (2020) used thermal imaging and machine learning techniques (PLS-R) to assess canopy evapotranspiration, leaf transpiration, stomatal conductance, photosynthesis, chlorophyll content and morphological properties. The results showed that this method can help to parameterize canopy photosynthesis or evapotranspiration models, and identify different photosynthetic processes in response to drought. Despite these techniques can high-throughput phenotyping secondary traits that link leaf photosynthetic capacity to underlying genetics, and thus improve the efficiency of crop photosynthesis improvement in target CO2 concentration environment; it is not yet clear whether they have the precision needed to infer small changes in photosynthesis.
Modelling assists breeding
Exploiting genetic variation in crop yield responses to eCO2 necessitates screening diverse germplasm and structured populations to identify the genomic regions associated with greater yield quantity and quality under such conditions (Leakey et al., 2009; Kimball, 2016). Although conducting experiments can be challenging due to the size of individual FACE plots and potential variation between and within them, these obstacles can be overcome by applying a multi-scale modelling approach (Toreti et al., 2020). Nearly 40 years of FACE experiments have generated a vast database and insight into potential mechanisms of plant responses to eCO2, which can be invaluable for constructing such models (Leakey et al., 2009; Toreti et al., 2020). Relevant studies have been reported, combining gene network, metabolic and leaf-level models, Kannan et al. (2019) predicted the impacts of Gm-GATA2 gene regulatory change on soybean photosynthesis under eCO2. Furthermore, Song et al. (2020a) used a 3D canopy model to reveal synergistic effects of CO2 and light on soybean photosynthesis, found that eCO2 improved canopy photosynthesis through increased leaf area index at early developmental stages, while canopy photosynthesis was associated with a higher proportion of leaves in a canopy limited by Rubisco carboxylation at later developmental stages. This suggests modifying Rubisco can further routes for maximizing photosynthesis under eCO2. Constructing multi-scale models facilitates the connection between genomics and phenomics (Hammer et al., 2021), and increases the predictability of plant systems (Messina et al., 2018). In addition to eCO2 effects, the complex interactions of eCO2, temperature, water and nitrogen on crop processes should also be considered in crop models (Toreti et al., 2020). For example, Castaño-Sánchez et al. (2020) used three crop models (CropSyst, DSSAT-M and IFSM) to assess the response of maize yield and evapotranspiration to eCO2, and found that models using radiation use efficiency (DSSAT-M, IFSM) and models using transpiration use efficiency (CropSyst) to limit crop growth both overestimated maize growth. However, by coupling photosynthesis, stomatal conductance and transpiration models, Li et al. (2021b) suggested the use of a coupled model predicted rice canopy gas exchange processes under eCO2 and warming temperature conditions more accurately than an uncoupled photosynthesis/transpiration model. These results indicate that photosynthesis and transpiration processes should be coupled in models, rather than be simulated separately, in order to precise simulation used in crop breeding. Modelling therefore enables the translation of plant biology understanding and measurement systems into decisions that improve human well-being. Modelling can also generate testable hypotheses to advance plant science, providing a blueprint for future eCO2 studies aimed at future-proofing crops.
Conclusion
Confronted with the challenge of sustaining global food security, a thorough understanding of the complex interaction between maize reproductive process, eCO2 and drought stress is clarified. Here, we demonstrated that the potential of harnessing CO2 fertilization to secure reproductive success and enhance maize productivity under drought stress. eCO2 can enhance maize reproductive resilience to drought stress, including increasing photosynthetic efficiency and optimizing assimilate reserves in stems. These mechanisms contribute to maintaining or even increasing maize yields under drought-stressed environment, ensuring food security for a growing global population. To capitalize on the potential benefits of eCO2, we have discussed the importance of optimizing planting methods, mining natural genetic variation and utilizing genetic engineering techniques to develop crop varieties with improved sink strength and optimized photosynthetic systems. Additionally, we have highlighted the value of integrating advanced plant phenomics and modelling techniques in crop breeding programs, which can streamline the identification of target traits and facilitate the translation of plant biology understanding into practical applications. Ultimately, the successful adaptation of maize to elevated CO2 and drought stress will play a vital role in ensuring global food security in facing a rapidly changing climate.
Author contributions
YS conceived this idea, and YL and PZ drafted the manuscript. ZZ and WS helped in drafting and collecting references. RR helped in polishing the draft. YL, PZ and YS finalized the manuscript. All authors contributed to the article and approved the submitted version.
Funding
This work was supported by the Natural Science Foundation of Anhui Province of China (No. 2208085MC59), Provincial Grant No. 2021H254, and Uni Grant No. rc312212. We are also grateful for the support of the Anhui Agricultural University Elite Postdoctoral Project for YL.
Conflict of interest
The authors declare that the research was conducted in the absence of any commercial or financial relationships that could be construed as a potential conflict of interest.
Publisher’s note
All claims expressed in this article are solely those of the authors and do not necessarily represent those of their affiliated organizations, or those of the publisher, the editors and the reviewers. Any product that may be evaluated in this article, or claim that may be made by its manufacturer, is not guaranteed or endorsed by the publisher.
References
Abdelhakim, L. O. A., Mendanha, T., Palma, C. F. F., Vrobel, O., Štefelová, N., Ćavar Zeljković, S., et al. (2022). Elevated CO2 improves the physiology but not the final yield in spring wheat genotypes subjected to heat and drought stress during anthesis. Front. Plant Sci. 13. doi: 10.3389/fpls.2022.824476
Abebe, A., Pathak, H., Singh, S. D., Bhatia, A., Harit, R. C., Kumar, V. (2016). Growth, yield and quality of maize with elevated atmospheric carbon dioxide and temperature in north–west India. Agric. Ecosyst. Environ. 218, 66–72. doi: 10.1016/j.agee.2015.11.014
Ainsworth, E. A., Davey, P. A., Bernacchi, C. J., Dermody, O. C., Heaton, E. A., Moore, D. J., et al. (2002). A meta-analysis of elevated [CO2] effects on soybean (Glycine max) physiology, growth and yield. Global Change Biol. 8, 695–709. doi: 10.1046/j.1365-2486.2002.00498.x
Ainsworth, E. A., Long, S. P. (2005). What have we learned from 15 years of free-air CO2 enrichment (FACE)? A meta-analytic review of the responses of photosynthesis, canopy properties and plant production to rising CO2. New Phytol. 165, 351–372. doi: 10.1111/j.1469-8137.2004.01224.x
Ainsworth, E. A., Long, S. P. (2021). 30 years of free-air carbon dioxide enrichment (FACE): What have we learned about future crop productivity and its potential for adaptation? Global Change Biol. 27, 27–49. doi: 10.1111/gcb.15375
Ainsworth, E. A., Rogers, A. (2007). The response of photosynthesis and stomatal conductance to rising [CO2]: mechanisms and environmental interactions. Plant Cell Environ. 30, 258–270. doi: 10.1111/j.1365-3040.2007.01641.x
Allen, L. H., Kakani, V. G., Vu, J. C. V., Boote, K. J. (2011). Elevated CO2 increases water use efficiency by sustaining photosynthesis of water-limited maize and sorghum. J. Plant Physiol. 168, 1909–1918. doi: 10.1016/j.jplph.2011.05.005
Andersen, M. N., Asch, F., Wu, Y., Jensen, C. R., Naested, H., Mogensen, V. O., et al. (2002). Soluble invertase expression is an early target of drought stress during the critical, abortion-sensitive phase of young ovary development in maize. Plant Physiol. 130, 591–604. doi: 10.1104/pp.005637
Araus, J. L., Serret, M. D., Edmeades, G. O. (2012). Phenotyping maize for adaptation to drought. Front. Physiol. 3. doi: 10.3389/fphys.2012.00305
Ayars, J., Fulton, A., Taylor, B. (2015). Subsurface drip irrigation in California—Here to stay? Agric. Water Manage. 157, 39–47. doi: 10.1016/j.agwat.2015.01.001
Bänziger, M., Edmeades, G., Lafitte, H. (2002). Physiological mechanisms contributing to the increased N stress tolerance of tropical maize selected for drought tolerance. Field Crops Res. 75, 223–233. doi: 10.1016/s0378-4290(02)00028-x
Bheemanahalli, R., Ramamoorthy, P., Poudel, S., Samiappan, S., Wijewardane, N., Reddy, K. R. (2022). Effects of drought and heat stresses during reproductive stage on pollen germination, yield, and leaf reflectance properties in maize (Zea mays L.). Plant Direct. 6, e434. doi: 10.1002/pld3.434
Bishop, K. A., Betzelberger, A. M., Long, S. P., Ainsworth, E. A. (2015). Is there potential to adapt soybean (Glycine max Merr.) to future [CO2]? An analysis of the yield response of 18 genotypes in free-air CO2 enrichment. Plant Cell Environ. 38, 1765–1774. doi: 10.1111/pce.12443
Bledsoe, S. W., Henry, C., Griffiths, C. A., Paul, M. J., Feil, R., Lunn, J. E., et al. (2017). The role of Tre6P and SnRK1 in maize early kernel development and events leading to stress-induced kernel abortion. BMC Plant Biol. 17, 74. doi: 10.1186/s12870-017-1018-2
Borrell, A., Jordan, D., Mullet, J., Henzell, B., Hammer, G. L. (2006). “Drought Adaptation in Sorghum,” in Drought adaptation in cereals. Ed. Ribaut, J.-M. (Binghamton, NY, USA: The Haworth Press, Inc), 335–400.
Boyer, J. S., Mclaughlin, J. E. (2007). Functional reversion to identify controlling genes in multigenic responses: analysis of floral abortion. J. Exp. Bot. 58, 267–277. doi: 10.1093/jxb/erl177
Boyer, J., Westgate, M. (2004). Grain yields with limited water. J. Exp. Bot. 55, 2385–2394. doi: 10.1093/jxb/erh219
Boyle, M. G., Boyer, J. S., Morgan, P. W. (1991). Stem infusion of liquid culture medium prevents reproductive failure of maize at low water potential. Crop Sci. 31, 1246–1252. doi: 10.2135/cropsci1991.0011183X003100050033x
Cai, F., Zhang, Y., Mi, N., Ming, H., Zhang, S., Zhang, H., et al. (2020). Maize (Zea mays L.) physiological responses to drought and rewatering, and the associations with water stress degree. Agric. Water Manage. 241, 106379. doi: 10.1016/j.agwat.2020.106379
Cairns, J. E., Crossa, J., Zaidi, P., Grudloyma, P., Sanchez, C., Araus, J. L., et al. (2013). Identification of drought, heat, and combined drought and heat tolerant donors in maize. Crop Sci. 53, 1335–1346. doi: 10.2135/cropsci2012.09.0545
Cakir, R. (2004). Effect of water stress at different development stages on vegetative and reproductive growth of corn. Field Crops Res. 89, 1–16. doi: 10.1016/j.fcr.2004.01.005
Camino, C., Gonzalez-Dugo, V., Hernandez, P., Zarco-Tejada, P. J. (2019). Radiative transfer Vcmax estimation from hyperspectral imagery and SIF retrievals to assess photosynthetic performance in rainfed and irrigated plant phenotyping trials. Remote Sens. Environ. 231, 111186. doi: 10.1016/j.rse.2019.05.005
Campos, H., Cooper, M., Edmeades, G., Loffler, C., Schussler, J., Ibanez, M. (2006). Changes in drought tolerance in maize associated with fifty years of breeding for yield in the US corn belt. Maydica 51, 369–381. doi: 10.1300/J064v27n04_08
Castaño-Sánchez, J. P., Rotz, C. A., Karsten, H. D., Kemanian, A. R. (2020). Elevated atmospheric carbon dioxide effects on maize and alfalfa in the Northeast US: A comparison of model predictions and observed data. Agric. For. Meteorol. 291, 108093. doi: 10.1016/j.agrformet.2020.108093
Castiglioni, P., Warner, D., Bensen, R. J., Anstrom, D. C., Harrison, J., Stoecker, M., et al. (2008). Bacterial RNA chaperones confer abiotic stress tolerance in plants and improved grain yield in maize under water-limited conditions. Plant Physiol. 147, 446–455. doi: 10.1104/pp.108.118828
Cattivelli, L., Rizza, F., Badeck, F.-W., Mazzucotelli, E., Mastrangelo, A. M., Francia, E., et al. (2008). Drought tolerance improvement in crop plants: an integrated view from breeding to genomics. Field Crops Res. 105, 1–14. doi: 10.1016/j.fcr.2007.07.004
Cheng, C. Y., Lur, H. S. (1996). Ethylene may be involved in abortion of the maize caryopsis. Physiologia Plantarum. 98, 245–252. doi: 10.1034/j.1399-3054.1996.980205.x
Claasen, M., Shaw, R. H. (1970). Water deficit effects on corn. II. Grain components. Agron. J. 62, 652–655. doi: 10.2134/agronj1970.00021962006200050032x
Correia, P. M., Da Silva, A. B., Vaz, M., Carmo-Silva, E., Marques Da Silva, J. (2021). Efficient regulation of CO2 assimilation enables greater resilience to high temperature and drought in maize. Front. Plant Sci. 12. doi: 10.3389/fpls.2021.675546
Cousins, A. B., Adam, N. R., Wall, G. W., Kimball, B. A., Pinter, P. J., Jr., Leavitt, S. W., et al. (2001). Reduced photorespiration and increased energy-use efficiency in young CO2-enriched sorghum leaves. New Phytol. 150, 275–284. doi: 10.2307/1353735
Duvick, D. N. (2005). The contribution of breeding to yield advances in maize (Zea mays L.). Adv. Agron. 86, 83–145. doi: 10.1016/s0065-2113(05)86002-x
Evans, J. R., Clarke, V. C. (2019). The nitrogen cost of photosynthesis. J. Exp. Bot. 70, 7–15. doi: 10.1093/jxb/ery366
Faostat (2021). Food and Agriculture Organization of the United Nations Statistical Database (Rome, Italy: Food and Agriculture Organization of the United Nations Statistical Database; Statistical Division; FAO). Available at: https://www.fao.org/faostat/en/#data/QCL.
Farooq, M., Wahid, A., Kobayashi, N., Fujita, D., Basra, S. M. A. (2009). Plant drought stress: effects, mechanisms and management. Agron. Sustain. Dev. 29, 185–212. doi: 10.1051/agro:2008021
Feng, H.-Y., Wang, Z.-M., Kong, F.-N., Zhang, M.-J., Zhou, S.-L. (2011). Roles of carbohydrate supply and ethylene, polyamines in maize kernel set. J. Integr. Plant Biol. 53, 388–398. doi: 10.1111/j.1744-7909.2011.01039.x
Fu, P., Meacham-Hensold, K., Siebers, M. H., Bernacchi, C. J. (2021). The inverse relationship between solar-induced fluorescence yield and photosynthetic capacity: benefits for field phenotyping. J. Exp. Bot. 72, 1295–1306. doi: 10.1093/jxb/eraa537
Fu, P., Montes, C. M., Siebers, M. H., Gomez-Casanovas, N., Mcgrath, J. M., Ainsworth, E. A., et al. (2022). Advances in field-based high-throughput photosynthetic phenotyping. J. Exp. Biol. 73, 3157–3172. doi: 10.1093/jxb/erac077
Fuad-Hassan, A., Tardieu, F., Turc, O. (2008). Drought-induced changes in anthesis-silking interval are related to silk expansion: a spatio-temporal growth analysis in maize plants subjected to soil water deficit. Plant Cell Environ. 31, 1349–1360. doi: 10.1111/j.1365-3040.2008.01839.x
Ge, T., Sui, F., Bai, L., Tong, C., Sun, N. (2011). Effects of water stress on growth, biomass partitioning, and water-use efficiency in summer maize (Zea mays L.) throughout the growth cycle. Acta Physiol. Plant 34, 1043–1053. doi: 10.1007/s11738-011-0901-y
Geng, P., Sun, J., Chen, P., Li, Y., Peng, B., Harnly, J. M., et al. (2020). A systematic approach to determine the impact of elevated CO2 levels on the chemical composition of wheat (Triticum aestivum). J. Cereal Sci. 95, 103020. doi: 10.1016/j.jcs.2020.103020
Ghannoum, O., Caemmerer, S. V., Ziska, L. H., Conroy, J. P. (2000). The growth response of C4 plants to rising atmospheric CO2 partial pressure: a reassessment. Plant Cell Environ. 23, 931–942. doi: 10.1046/j.1365-3040.2000.00609.x
Ghooshchi, F., Seilsepour, M., Jafari, P. (2008). Effects of water stress on yield and some agronomic traits of maize (SC 301). Am. Eurasian J. Agric. Environ. Sci. 4, 302–305. doi: api.semanticscholar.org/CorpusID:17
Golzardi, F., Baghdadi, A., Afshar, R. K. (2017). Alternate furrow irrigation affects yield and water-use efficiency of maize under deficit irrigation. Crop Pasture Sci. 68, 726–734. doi: 10.1071/CP17178
Gonsamo, A., Ciais, P., Miralles, D. G., Sitch, S., Dorigo, W., Lombardozzi, D., et al. (2021). Greening drylands despite warming consistent with carbon dioxide fertilization effect. Global Change Biol. 27, 3336–3349. doi: 10.1111/gcb.15658
Guo, X., Huang, B., Zhang, H., Cai, C., Li, G., Li, H., et al. (2021). T-FACE studies reveal that increased temperature exerts an effect opposite to that of elevated CO2 on nutrient concentration and bioavailability in rice and wheat grains. Food Energy Secur. 11, e336. doi: 10.1002/fes3.336
Hammer, G. L., Cooper, M., Reynolds, M. P. (2021). Plant production in water-limited environments. J. Exp. Bot. 72, 5097–5101. doi: 10.1093/jxb/erab273
Harrison, M. T., Tardieu, F., Dong, Z., Messina, C. D., Hammer, G. L. (2014). Characterizing drought stress and trait influence on maize yield under current and future conditions. Global Change Biol. 20, 867–878. doi: 10.1111/gcb.12381
Hasegawa, T., Sakai, H., Tokida, T., Nakamura, H., Zhu, C., Usui, Y., et al. (2013). Rice cultivar responses to elevated CO2 at two free-air CO2 enrichment (FACE) sites in Japan. Funct. Plant Biol. 40, 148–159. doi: 10.1071/fp12357
Hussain, H. A., Men, S., Hussain, S., Chen, Y., Ali, S., Zhang, S., et al. (2019). Interactive effects of drought and heat stresses on morpho-physiological attributes, yield, nutrient uptake and oxidative status in maize hybrids. Sci. Rep. 9, 3890 doi: 10.1038/s41598-019-40362-7
Ipcc (2023). Synthesis Report of the IPCC Sixth Assessment Report (Interlaken, Switzerland: Intergovernmental Panel on Climate Change). Available at: https://www.ipcc.ch/report/sixth-assessment-report-cycle/.
Jin, Z., Zhuang, Q., Wang, J., Archontoulis, S. V., Zobel, Z., Kotamarthi, V. R. (2017). The combined and separate impacts of climate extremes on the current and future US rainfed maize and soybean production under elevated CO2. Global Change Biol. 23, 2687–2704. doi: 10.1111/gcb.13617
Joshi, J., Amthor, J. S., Mccarty, D. R., Messina, C. D., Wilson, M. A., Millar, A. H., et al. (2023). Why cutting respiratory CO2 loss from crops is possible, practicable, and prudential. Modern Agricul. 1, 16–26. doi: 10.1002/moda.1
Kadam, N. N., Xiao, G., Melgar, R. J., Bahuguna, R. N., Quinones, C., Tamilselvan, A., et al. (2014). Agronomic and physiological responses to high temperature, drought, and elevated CO2 interactions in cereals. Adv. Agron. 127, 111–156. doi: 10.1016/B978-0-12-800131-8.00003-0
Kakani, V. G., Vu, J. C., Allen, L. H., Jr., Boote, K. J. (2011). Leaf photosynthesis and carbohydrates of CO2-enriched maize and grain sorghum exposed to a short period of soil water deficit during vegetative development. J. Plant Physiol. 168, 2169–2176. doi: 10.1016/j.jplph.2011.07.003
Kakumanu, A., Ambavaram, M. M., Klumas, C., Krishnan, A., Batlang, U., Myers, E., et al. (2012). Effects of drought on gene expression in maize reproductive and leaf meristem tissue revealed by RNA-Seq. Plant Physiol. 160, 846–867. doi: 10.2307/41694805
Kamara, A. Y., Menkir, A., Badu-Apraku, B., Ibikunle, O. (2003). The influence of drought stress on growth, yield and yield components of selected maize genotypes. J. Agric. Sci. 141, 43–50. doi: 10.1017/S0021859603003423
Kannan, K., Wang, Y., Lang, M., Challa, G. S., Long, S. P., Marshall-Colon, A. (2019). Combining gene network, metabolic and leaf-level models shows means to future-proof soybean photosynthesis under rising CO2. silico Plants 1, diz008. doi: 10.1093/insilicoplants/diz008
Kim, S.-H., Sicher, R. C., Bae, H., Gitz, D. C., Baker, J. T., Timlin, D. J., et al. (2006). Canopy photosynthesis, evapotranspiration, leaf nitrogen, and transcription profiles of maize in response to CO2 enrichment. Global Change Biol. 12, 588–600. doi: 10.1111/j.1365-2486.2006.01110.x
Kimball, B. A. (2016). Crop responses to elevated CO2 and interactions with H2O, N, and temperature. Curr. Opin. Plant Biol. 31, 36–43. doi: 10.1016/j.pbi.2016.03.006
Kimball, B., Idso, S. (1983). Increasing atmospheric CO2: effects on crop yield, water use and climate. Agric. Water Manage. 7, 55–72. doi: 10.1016/0378-3774(83)90075-6
Kimball, B., Kobayashi, K., Bindi, M. (2002). Responses of agricultural crops to free-air CO2 enrichment. Adv. Agron. 77, 293–368. doi: 10.1016/S0065-2113(02)77017-X
Köhler, I. H., Ruiz-Vera, U. M., Vanloocke, A., Thomey, M. L., Clemente, T., Long, S. P., et al. (2017). Expression of cyanobacterial FBP/SBPase in soybean prevents yield depression under future climate conditions. J. Exp. Bot. 68, 715–726. doi: 10.1093/jxb/erw435
Kumagai, E., Burroughs, C. H., Pederson, T. L., Montes, C. M., Peng, B., Kimm, H., et al. (2022). Predicting biochemical acclimation of leaf photosynthesis in soybean under in-field canopy warming using hyperspectral reflectance. Plant Cell Environ. 45, 80–94. doi: 10.1111/pce.14204
Leakey, A. D. (2009). Rising atmospheric carbon dioxide concentration and the future of C4 crops for food and fuel. Proc. R. Soc B. 276, 2333–2343. doi: 10.1098/rspb.2008.1517
Leakey, A. D., Ainsworth, E. A., Bernacchi, C. J., Rogers, A., Long, S. P., Ort, D. R. (2009). Elevated CO2 effects on plant carbon, nitrogen, and water relations: six important lessons from FACE. J. Exp. Bot. 60, 2859–2876. doi: 10.1093/jxb/erp096
Leakey, A. D., Bernacchi, C., Dohleman, F., Ort, D., Long, S. (2004). Will photosynthesis of maize (Zea mays) in the US Corn Belt increase in future [CO2] rich atmospheres? An analysis of diurnal courses of CO2 uptake under free-air concentration enrichment (FACE). Global Change Biol. 10, 951–962. doi: 10.1111/j.1529-8817.2003.00767.x
Leakey, A., Ferguson, J. N., Pignon, C. P., Wu, A., Jin, Z., Hammer, G. L., et al. (2019). Water use efficiency as a constraint and target for improving the resilience and productivity of C3 and C4 crops. Annu. Rev. Plant Biol. 70, 781–808. doi: 10.1146/annurev-arplant-042817040305
Leakey, A., Scholes, J., Press, M. (2005). Physiological and ecological significance of sunflecks for dipterocarp seedlings. J. Exp. Bot. 56, 469–482. doi: 10.1093/jxb/eri055
Leakey, A. D., Uribelarrea, M., Ainsworth, E. A., Naidu, S. L., Rogers, A., Ort, D. R., et al. (2006). Photosynthesis, productivity, and yield of maize are not affected by open-air elevation of CO2 concentration in the absence of drought. Plant Physiol. 140, 779–790. doi: 10.2307/4282096
Li, S., Fleisher, D. H., Wang, Z., Barnaby, J., Timlin, D., Reddy, V. R. (2021b). Application of a coupled model of photosynthesis, stomatal conductance and transpiration for rice leaves and canopy. Comput. Electron. Agric. 182, 106047. doi: 10.1016/j.compag.2021.106047
Li, L., Hao, Z., Li, X., Xie, C., Li, M., Zhang, D., et al. (2011). An analysis of the polymorphisms in a gene for being involved in drought tolerance in maize. Genetica 139, 479–487. doi: 10.1007/s10709-011-9568-y
Li, J., Wang, Z., Yao, C., Zhang, Z., Liu, Y., Zhang, Y. (2021a). Micro-sprinkling irrigation simultaneously improves grain yield and protein concentration of winter wheat in the North China Plain. Crop J. 9, 1397–1407. doi: 10.1016/j.cj.2020.12.009
Li, Z., Zhang, Q., Li, J., Yang, X., Wu, Y., Zhang, Z., et al. (2020). Solar-induced chlorophyll fluorescence and its link to canopy photosynthesis in maize from continuous ground measurements. Remote Sens. Environ. 236, 111420. doi: 10.1016/j.rse.2019.111420
Liang, Y. L., Lur, H. S. (2002). Conjugated and free polyamine levels in normal and aborting maize kernels. Crop Sci. 42, 1217–1224. doi: 10.2135/cropsci2002.1217
Liu, G., Yang, Y., Guo, X., Liu, W., Xie, R., Ming, B., et al. (2023). A global analysis of dry matter accumulation and allocation for maize yield breakthrough from 1.0 to 25.0 Mg ha–1. Resour. Conserv. Recycl. 188, 106656. doi: 10.1016/j.resconrec.2022.106656
Liu, X., Yu, Y., Huang, S., Xu, C., Wang, X., Gao, J., et al. (2022). The impact of drought and heat stress at flowering on maize kernel filling: Insights from the field and laboratory. Agric. For. Meteorol. 312, 108733. doi: 10.1016/j.agrformet.2021.108733
Lobell, D. B., Roberts, M. J., Schlenker, W., Braun, N., Little, B. B., Rejesus, R. M., et al. (2014). Greater sensitivity to drought accompanies maize yield increase in the U.S. Midwest. Science 344, 516–519. doi: 10.1126/science.1251423
Loladze, I. (2002). Rising atmospheric CO2 and human nutrition: toward globally imbalanced plant stoichiometry? Trends Ecol. Evol. 17, 457–461. doi: 10.1016/S0169-5347(02)02587-9
Long, S. P., Marshall-Colon, A., Zhu, X. G. (2015). Meeting the global food demand of the future by engineering crop photosynthesis and yield potential. Cell 161, 56–66. doi: 10.1016/j.cell.2015.03.019
Lopes, M. S., Araus, J. L., Van Heerden, P. D., Foyer, C. H. (2011). Enhancing drought tolerance in C4 crops. J. Exp. Bot. 62, 3135–3153. doi: 10.1093/jxb/err105
López-Calcagno, P. E., Fisk, S., Brown, K. L., Bull, S. E., South, P. F., Raines, C. A. (2019). Overexpressing the H-protein of the glycine cleavage system increases biomass yield in glasshouse and field-grown transgenic tobacco plants. Plant Biotechnol. J. 17, 141–151. doi: 10.1111/pbi.12953
Manderscheid, R., Erbs, M., Weigel, H.-J. (2014). Interactive effects of free-air CO2 enrichment and drought stress on maize growth. Eur. J. Agron. 52, 11–21. doi: 10.1016/j.eja.2011.12.007
Markelz, R. C., Strellner, R. S., Leakey, A. D. (2011). Impairment of C4 photosynthesis by drought is exacerbated by limiting nitrogen and ameliorated by elevated [CO2] in maize. J. Exp. Bot. 62, 3235–3246. doi: 10.1093/jxb/err056
Mclaughlin, J. E., Boyer, J. S. (2004a). Glucose localization in maize ovaries when kernel number decreases at low water potential and sucrose is fed to the stems. Ann. Bot. 94, 75–86. doi: 10.1093/aob/mch123
Mclaughlin, J. E., Boyer, J. S. (2004b). Sugar-responsive gene expression, invertase activity, and senescence in aborting maize ovaries at low water potentials. Ann. Bot. 94, 675–689. doi: 10.1093/aob/mch193
Mcpherson, H. G., Boyer, J. S. (1977). Regulation of grain yield by photosynthesis in maize subjected to a water deficiency. Agron. J. 69, 714–718. doi: 10.2134/agronj1977.00021962006900040046x
Meacham-Hensold, K., Fu, P., Wu, J., Serbin, S., Montes, C. M., Ainsworth, E., et al. (2020). Plot-level rapid screening for photosynthetic parameters using proximal hyperspectral imaging. J. Exp. Bot. 71, 2312–2328. doi: 10.1093/jxb/eraa068
Messina, C. D., Hammer, G. L., Mclean, G., Cooper, M., Van Oosterom, E. J., Tardieu, F., et al. (2019). On the dynamic determinants of reproductive failure under drought in maize. silico Plants. 1, diz003. doi: 10.1093/insilicoplants/diz003
Messina, C. D., Technow, F., Tang, T., Totir, R., Gho, C., Cooper, M. (2018). Leveraging biological insight and environmental variation to improve phenotypic prediction: Integrating crop growth models (CGM) with whole genome prediction (WGP). Eur. J. Agron. 100, 151–162. doi: 10.1016/j.eja.2018.01.007
Monneveux, P., Sánchez, C., Beck, D., Edmeades, G. O. (2006). Drought tolerance improvement in tropical maize source populations: Evidence of progress. Crop Sci. 46, 180–191. doi: 10.2135/cropsci2005.04-0034
Muller, B., Pantin, F., Génard, M., Turc, O., Freixes, S., Piques, M., et al. (2011). Water deficits uncouple growth from photosynthesis, increase C content, and modify the relationships between C and growth in sink organs. J. Exp. Bot. 62, 1715–1729. doi: 10.1093/jxb/erq438
Munne-Bosch, S., Villadangos, S. (2023). Cheap, cost-effective, and quick stress biomarkers for drought stress detection and monitoring in plants. Trends Plant Sci. 28, 527–536. doi: 10.1016/j.tplants.2023.01.004
Myers, S. S., Smith, M. R., Guth, S., Golden, C. D., Vaitla, B., Mueller, N. D., et al. (2017). Climate change and global food systems: potential impacts on food security and undernutrition. Annu. Rev. Public Health 38, 259–277. doi: 10.1146/annurev-publhealth-031816-044356
Myers, S. S., Zanobetti, A., Kloog, I., Huybers, P., Leakey, A. D. B., Bloom, A. J., et al. (2014). Increasing CO2 threatens human nutrition. Nature 510, 139–142. doi: 10.1038/nature13179
Niu, L., Yan, Y., Hou, P., Bai, W., Zhao, R., Wang, Y., et al. (2020). Influence of plastic film mulching and planting density on yield, leaf anatomy, and root characteristics of maize on the Loess Plateau. Crop J. 8, 548–564. doi: 10.1016/j.cj.2019.12.002
Nuccio, M. L., Wu, J., Mowers, R., Zhou, H.-P., Meghji, M., Primavesi, L. F., et al. (2015). Expression of trehalose-6-phosphate phosphatase in maize ears improves yield in well-watered and drought conditions. Nat. Biotechnol. 33, 862–869. doi: 10.1038/nbt.3277
Oszvald, M., Primavesi, L. F., Griffiths, C. A., Cohn, J., Basu, S. S., Nuccio, M. L., et al. (2018). Trehalose 6-phosphate regulates photosynthesis and assimilate partitioning in reproductive tissue. Plant Physiol. 176, 2623–2638. doi: 10.1104/pp.17.01673
Oury, V., Tardieu, F., Turc, O. (2016). Ovary apical abortion under water deficit is caused by changes in sequential development of ovaries and in silk growth rate in maize. Plant Physiol. 171, 986–996. doi: 10.1104/pp.15.00268
Perdomo, J. A., Capo-Bauca, S., Carmo-Silva, E., Galmes, J. (2017). Rubisco and rubisco activase play an important role in the biochemical limitations of photosynthesis in rice, wheat, and maize under high temperature and water deficit. Front. Plant Sci. 8. doi: 10.3389/fpls.2017.00490
Qiao, Y., Miao, S., Li, Q., Jin, J., Luo, X., Tang, C. (2019). Elevated CO2 and temperature increase grain oil concentration but their impacts on grain yield differ between soybean and maize grown in a temperate region. Sci. Total Environ. 666, 405–413. doi: 10.1016/j.scitotenv.2019.02.149
Qin, L., Trouverie, J., Chateau-Joubert, S., Simond-Côte, E., Thévenot, C., Prioul, J.-L. (2004). Involvement of the Ivr2-invertase in the perianth during maize kernel development under water stress. Plant Sci. (Amsterdam Neth.). 166, 371–379. doi: 10.1016/j.plantsci.2003.10.003
Ray, D. K., Mueller, N. D., West, P. C., Foley, J. A. (2013). Yield trends are insufficient to double global crop production by 2050. PloS One 8, e66428. doi: 10.1371/journal.pone.0066428
Ribaut, J.-M., Betran, J., Monneveux, P., Setter, T. (2009). “Drought Tolerance in Maize,” in Handbook of Maize: Its Biology. Eds. Bennetzen, J. L., Hake, S. C. (New York, NY: Springer New York), 311–344.
Saathoff, A. J., Welles, J. (2021). Gas exchange measurements in the unsteady state. Plant Cell Environ. 44, 3509–3523. doi: 10.1111/pce.14178
Saini, H. S., Westgate, M. E. (1999). Reproductive development in grain crops during drought. Adv. Agron. 68, 59–96. doi: 10.1016/S0065-2113(08)60843-3
Setter, T. L., Yan, J., Warburton, M., Ribaut, J.-M., Xu, Y., Sawkins, M., et al. (2011). Genetic association mapping identifies single nucleotide polymorphisms in genes that affect abscisic acid levels in maize floral tissues during drought. J. Exp. Bot. 62, 701–716. doi: 10.1093/jxb/erq308
Shabbaj, I. I., Madany, M. M. Y., Balkhyour, M. A., Tammar, A., Abdelgawad, H. (2022). CO2 enrichment differentially upregulated sugar, proline, and polyamine metabolism in young and old leaves of wheat and sorghum to mitigate indium oxide nanoparticles toxicity. Front. Plant Sci. 13. doi: 10.3389/fpls.2022.843771
Shen, S., Liang, X. G., Zhang, L., Zhao, X., Liu, Y. P., Lin, S., et al. (2020). Intervening in sibling competition for assimilates by controlled pollination prevents seed abortion under postpollination drought in maize. Plant Cell Environ. 43, 903–919. doi: 10.1111/pce.13704
Shen, S., Ma, S., Wu, L., Zhou, S. L., Ruan, Y. L. (2023). Winners take all: competition for carbon resource determines grain fate. Trends Plant Sci. 28, 893–901. doi: 10.1016/j.tplants.2023.03.015
Shou, H., Bordallo, P., Wang, K. (2004). Expression of the Nicotiana protein kinase (NPK1) enhanced drought tolerance in transgenic maize. J. Exp. Bot. 55, 1013–1019. doi: 10.1093/jxb/erh129
Sicher, R. C., Barnaby, J. Y. (2012). Impact of carbon dioxide enrichment on the responses of maize leaf transcripts and metabolites to water stress. Physiologia Plantarum. 144, 238–253. doi: 10.1111/j.1399-3054.2011.01555.x
Simkin, A. J., Mcausland, L., Lawson, T., Raines, C. A. (2017). Overexpression of the RieskeFeS protein increases electron transport rates and biomass yield. Plant Physiol. 175, 134–145. doi: 10.1104/pp.17.00622
Sinclair, T. R., Bennett, J. M., Muchow, R. C. (1990). Relative sensitivity of grain yield and biomass accumulation to drought in field-grown maize. Crop Sci. 30, 690–693. doi: 10.2135/cropsci1990.0011183X003000030043x
Sinha, R., Fritschi, F. B., Zandalinas, S. I., Mittler, R. (2021). The impact of stress combination on reproductive processes in crops. Plant Sci. (Amsterdam Neth.). 311, 111007. doi: 10.1016/j.plantsci.2021.111007
Sobejano-Paz, V., Mikkelsen, T. N., Baum, A., Mo, X., Liu, S., Köppl, C. J., et al. (2020). Hyperspectral and thermal sensing of stomatal conductance, transpiration, and photosynthesis for soybean and maize under drought. Remote Sensing. 12, 3182 doi: 10.3390/rs12193182
Song, Q., Srinivasan, V., Long, S. P., Zhu, X. G. (2020a). Decomposition analysis on soybean productivity increase under elevated CO2 using 3-D canopy model reveals synergestic effects of CO2 and light in photosynthesis. Ann. Bot. 126, 601–614. doi: 10.1093/aob/mcz163
Song, X., Zhou, G., He, Q., Zhou, H. (2020b). Stomatal limitations to photosynthesis and their critical water conditions in different growth stages of maize under water stress. Agric. Water Manage. 241, 106330. doi: 10.1016/j.agwat.2020.106330
Stinziano, J. R., Morgan, P. B., Lynch, D. J., Saathoff, A. J., Mcdermitt, D. K., Hanson, D. T. (2017). The rapid A–Ci response: photosynthesis in the phenomic era. Plant Cell Environ. 40, 1256–1262. doi: 10.1111/pce.12911
Sun, Y. R., Ma, W. T., Xu, Y. N., Wang, X., Li, L., Tcherkez, G., et al. (2022). Short- and long-term responses of leaf day respiration to elevated atmospheric CO2. Plant Physiol. 191, 2204–2217. doi: 10.1093/plphys/kiac582
Tang, Y., Guo, J., Jagadish, S. V. K., Yang, S., Qiao, J., Wang, Y., et al. (2023). Ovary abortion in field-grown maize under water-deficit conditions is determined by photo-assimilation supply. Field Crops Res. 293, 108830. doi: 10.1016/j.fcr.2023.108830
Taub, D. R., Miller, B., Allen, H. (2008). Effects of elevated CO2 on the protein concentration of food crops: a meta-analysis. Global Change Biol. 14, 565–575. doi: 10.1111/j.1365-2486.2007.01511.x
Tausz-Posch, S., Dempsey, R. W., Seneweera, S., Norton, R. M., Fitzgerald, G., Tausz, M. (2015). Does a freely tillering wheat cultivar benefit more from elevated CO2 than a restricted tillering cultivar in a water-limited environment? Eur. J. Agron. 64, 21–28. doi: 10.1016/j.eja.2014.12.009
Tausz-Posch, S., Seneweera, S., Norton, R. M., Fitzgerald, G. J., Tausz, M. (2012). Can a wheat cultivar with high transpiration efficiency maintain its yield advantage over a near-isogenic cultivar under elevated CO2? Field Crops Res. 133, 160–166. doi: 10.1016/j.fcr.2012.04.007
Toreti, A., Deryng, D., Tubiello, F. N., Müller, C., Kimball, B. A., Moser, G., et al. (2020). Narrowing uncertainties in the effects of elevated CO2 on crops. Nat. Food. 1, 775–782. doi: 10.1038/s43016-020-00195-4
Turc, O., Bouteille, M., Fuad-Hassan, A., Welcker, C., Tardieu, F. (2016). The growth of vegetative and reproductive structures (leaves and silks) respond similarly to hydraulic cues in maize. New Phytol. 212, 377–388. doi: 10.1111/nph.14053
Vanaja, M., Maheswari, M., Jyothi, L. N., Sathish, P., Yadav, S., Salini, K., et al. (2015). Variability in growth and yield response of maize genotypes at rlevated CO2 concentration. Adv. Plants Agric. Res. 2, 63–66. doi: 10.15406/apar.2015.02.00042
Wang, B., Li, Z., Ran, Q., Li, P., Peng, Z., Zhang, J. (2018). ZmNF-YB16 overexpression improves drought resistance and yield by enhancing photosynthesis and the antioxidant capacity of maize plants. Front. Plant Sci. 9. doi: 10.3389/fpls.2018.00709
Westgate, M. E., Boyer, J. S. (1986a). Reproduction at low and pollen water potentials in maize. Crop Sci. 26, 951–956. doi: 10.2135/cropsci1986.0011183X002600050023x
Westgate, M. E., Boyer, J. S. (1986b). Silk and pollen water potentials in maize. Crop Sci. 26, 947–951. doi: 10.2135/cropsci1986.0011183X002600050022x
Xia, Q., Tang, H., Fu, L., Tan, J., Govindjee, G., Guo, Y. (2023). Determination of fv/fm from chlorophyll a fluorescence without dark adaptation by an LSSVM model. Plant Phenomics. 5, 34. doi: 10.34133/plantphenomics.0034
Yang, L., Fountain, J. C., Ji, P., Ni, X., Chen, S., Lee, R. D., et al. (2018). Deciphering drought-induced metabolic responses and regulation in developing maize kernels. Plant Biotechnol. J. 16, 1616–1628. doi: 10.1111/pbi.12899
Yang, J. C., Zhang, J. H., Wang, Z. Q., Zhu, Q. S., Wang, W. (2001). Remobilization of carbon reserves in response to water deficit during grain filling of rice. Field Crops Res. 71, 47–55. doi: 10.1016/S0378-4290(01)00147-2
Ye, Y. X., Wen, Z. R., Yang, H., Lu, W. P., Lu, D. L. (2020). Effects of post-silking water deficit on the leaf photosynthesis and senescence of waxy maize. J. Integr. Agric. 19, 2216–2228. doi: 10.1016/s2095-3119(20)63158-6
Yendrek, C. R., Tomaz, T., Montes, C. M., Cao, Y., Morse, A. M., Brown, P. J., et al. (2017). High-throughput phenotyping of maize leaf physiological and biochemical traits using hyperspectral reflectance. Plant Physiol. 173, 614–626. doi: 10.1104/pp.16.01447
Yoon, D. K., Ishiyama, K., Suganami, M., Tazoe, Y., Watanabe, M., Imaruoka, S., et al. (2020). Transgenic rice overproducing Rubisco exhibits increased yields with improved nitrogen-use efficiency in an experimental paddy field. Nat. Food. 1, 134–139. doi: 10.1038/s43016-020-0033-x
Yuan, X., Wang, Y., Ji, P., Wu, P., Sheffield, J., Otkin, J. A. (2023). A global transition to flash droughts under climate change. Science 380, 187–191. doi: 10.1126/science.abn6301
Zhu, X. G., Hasanuzzaman, M., Jajoo, A., Lawson, T., Lin, R., Liu, C. M., et al. (2022). Improving photosynthesis through multidisciplinary efforts: The next frontier of photosynthesis research. Front. Plant Sci. 13. doi: 10.3389/fpls.2022.967203
Zhu, C., Kobayashi, K., Loladze, I., Zhu, J., Jiang, Q., Xu, X., et al. (2018). Carbon dioxide (CO2) levels this century will alter the protein, micronutrients, and vitamin content of rice grains with potential health consequences for the poorest rice-dependent countries. Sci. Adv. 4, eaaq1012. doi: 10.1126/sciadv.aaq1012
Zinselmeier, C., Jeong, B. R., Boyer, J. S. (1999). Starch and the control of kernel number in maize at low water potentials. Plant Physiol. 121, 25–36. doi: 10.1104/pp.121.1.25
Zinselmeier, C., Lauer, M. J., Boyer, J. S. (1995a). Reversing drought-induced losses in grain yield: Sucrose mainntains embroyo growth in maize. Crop Sci. 35, 1390–1400. doi: 10.2135/cropsci1995.0011183X003500050022x
Keywords: Zea mays L., reproductive success, drought stress, CO2 fertilization, assimilate allocation, leaf photosynthesis
Citation: Li Y, Zhang P, Sheng W, Zhang Z, Rose RJ and Song Y (2023) Securing maize reproductive success under drought stress by harnessing CO2 fertilization for greater productivity. Front. Plant Sci. 14:1221095. doi: 10.3389/fpls.2023.1221095
Received: 11 May 2023; Accepted: 19 September 2023;
Published: 04 October 2023.
Edited by:
Baris Uzilday, Ege University, TürkiyeReviewed by:
Ashish K. Chaturvedi, Centre for Water Resources Development and Management, IndiaNuria De Diego, Palacký University, Olomouc, Czechia
Copyright © 2023 Li, Zhang, Sheng, Zhang, Rose and Song. This is an open-access article distributed under the terms of the Creative Commons Attribution License (CC BY). The use, distribution or reproduction in other forums is permitted, provided the original author(s) and the copyright owner(s) are credited and that the original publication in this journal is cited, in accordance with accepted academic practice. No use, distribution or reproduction is permitted which does not comply with these terms.
*Correspondence: Youhong Song, eS5zb25nQGFoYXUuZWR1LmNu
†These authors have contributed equally to this work