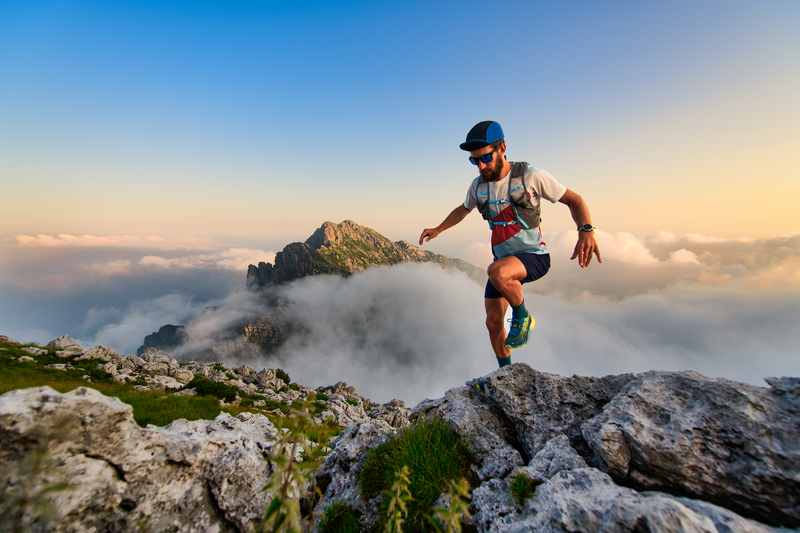
95% of researchers rate our articles as excellent or good
Learn more about the work of our research integrity team to safeguard the quality of each article we publish.
Find out more
ORIGINAL RESEARCH article
Front. Plant Sci. , 12 July 2023
Sec. Functional and Applied Plant Genomics
Volume 14 - 2023 | https://doi.org/10.3389/fpls.2023.1212528
Glossiness is an important quality-related trait of Chinese cabbage, which is a leafy vegetable crop in the family Brassicaceae. The glossy trait is caused by abnormal cuticular wax accumulation. In this study, on the basis of a bulked segregant analysis coupled with next-generation sequencing (BSA-seq) and fine-mapping, the most likely candidate gene responsible for the glossy phenotype of Chinese cabbage was identified. It was subsequently named Brcer2 because it is homologous to AtCER2 (At4g24510). A bioinformatics analysis indicated a long interspersed nuclear element 1 (LINE-1) transposable element (named BrLINE1-RUP) was inserted into the first exon of Brcer2 in HN19-G via an insertion-mediated deletion mechanism, which introduced a premature termination codon. Gene expression analysis showed that the InDel mutation of BrCER2 reduced the transcriptional expression levels of Brcer2 in HN19-G. An analysis of cuticular waxes suggested that a loss-of-function mutation to BrCER2 in Chinese cabbage leads to a severe decrease in the abundance of very-long-chain-fatty-acids (> C28), resulting in the production of a cauline leaf, inflorescence stem, flower, and pistil with a glossy phenotype. These findings imply the insertion of the LINE-1 transposable element BrLINE1-RUP into BrCER2 can modulate the waxy traits of Chinese cabbage plants.
Chinese cabbage (Brassica rapa L. ssp. pekinensis) is an important vegetable crop in the family Brassicaceae that is widely cultivated in northeastern Asia. Leaf and stalk glossiness is a commercially important quality-related trait among Brassica species, including Brassica rapa and Brassica oleracea. Remarkably, compared with waxy leaf and stalk, glossy leaf and stalk are more attractive to consumers. Previous studies showed that the glossy phenotype is due to defective cuticular wax biosynthesis (Liu et al., 2018; Ji et al., 2021; Yang et al., 2022a; Yang et al., 2022b). Cuticular waxes are classified as intracuticular waxes and epicuticular waxes based on their location. Intracuticular waxes are deposited within the cutin matrix, while epicuticular waxes cover on top of intracuticular wax (Haslam et al., 2012). The structural and chemical characteristics of cuticular wax vary greatly among plant species, tissues, genotypes, and developmental stages (Arya et al., 2021). Cuticular waxes are formed by a complex mixture of C20–C40 very-long-chain-fatty-acids (VLCFAs) and their derivatives, including alkanes, ketones, aldehydes, primary and secondary alcohols, and esters (Samuels et al., 2008; Isaacson et al., 2009). Besides, triterpenoids are also present in cuticular waxes and are main components of cuticular waxes in some species such as olives and grapes (Diarte et al., 2019; Arand et al., 2021).
In Arabidopsis thaliana, the related genes and enzymes involved in VLCFA biosynthesis have been thoroughly characterized. The C16 and C18 fatty acids are synthesized in the plastids of epidermal cells and then elongated to VLCFAs in the endoplasmic reticulum by fatty acid elongase complexes consisting of the following four enzymes: β-ketoacyl-CoA synthase, β-ketoacyl-CoA reductase, β-hydroxyacyl-CoA dehydratase, and β-enoyl-CoA reductase (Millar and Kunst, 1997; Samuels et al., 2008). Two functionally redundant genes (KCS2 and KCS20) encode the proteins responsible for the elongation of C20 fatty acids to C22 fatty acids (Lee et al., 2009). Additionally, KCS9 mediates the elongation of C22 fatty acids to C24 fatty acids, whereas KCS1 is required for the elongation of C24 VLCFAs (Todd et al., 1999; Kim et al., 2013). The silencing of KCS1 expression decreases the C26–C30 wax alcohol and aldehyde levels by up to 80% (Todd et al., 1999). Moreover, KCS5/CER60 and KCS6/CER6 play redundant roles during the production of the C26–C28 fatty acids involved in wax biosynthesis (Millar et al., 1999; Fiebig et al., 2000; Trenkamp et al., 2004). Two BAHD acyltransferases (CER2 and CER26) contribute to C28 and C30 fatty acid elongation (Haslam et al., 2012; Pascal et al., 2013).
Bulked segregant analysis (BSA) is an efficient approach to rapidly mine genes responsible for mutant phenotypes (Liu et al., 2012). Main procedure of BSA includes selecting two types of segregating individuals with extremely opposing phenotypes, pooling respectively the DNA of all individuals to form two bulks of DNA pools, and identifying genetic markers strongly associated with targeted genes (Giovannoni et al., 1991; Zou et al., 2016). The recent and rapid advance of next-generation sequencing (NGS) technology promotes the development and application of BSA-seq technology (BSA coupled with whole-genome sequencing), which has been extensively applied to identify trait-related genes in plants (Zou et al., 2016). In Chinese cabbage, three waxy genes (BrWAX1, BrWAX2, and BrWAX3) have been mapped and cloned by BSA-seq and fine mapping. They were involved in epidermal wax biosynthesis and responsive for waxy phenotype (Zhang et al., 2013; Liu et al., 2021; Yang et al., 2022a; Yang et al., 2022b).
Transposable elements (TEs) are major drivers of plant genome evolution. In plants, TEs facilitate the duplication or deletion of genes, modulate gene expression or function, and combine genes from different genomic locations (i.e., gene fusions) (Bennetzen, 2000; Krasileva, 2019). More specifically, TEs are mobile DNA segments that are capable of replicating and changing positions in the genome. They are generally divided into two categories (DNA transposons and retrotransposons) according to how they are mobilized (Wicker et al., 2007). Briefly, DNA transposons move via a cut-and-paste mechanism, whereas retrotransposons move via a copy-and-paste process that involves the duplication and incorporation of a sequence into a new genomic location through an RNA intermediate (Kim et al., 2012). Non-long terminal repeat (LTR) retrotransposons include long interspersed nuclear elements (LINEs) and short interspersed nuclear elements (SINEs). Of these two elements, LINEs usually comprise two open reading frames (ORFs). The first ORF (i.e., ORF1) encodes an RNA-binding protein, whereas ORF2 encodes a protein that has endonuclease and reverse transcriptase activities. Additionally, LINEs contain a poly(A) stretch, poly(T) stretch, or simple sequence motifs at the 3′ end and are flanked by a sequence modified by a target site duplication (TSD) (Wicker et al., 2007).
In this study, the VLCFA biosynthesis-related gene (BrCER2) on chromosome A01 of Chinese cabbage was identified by BSA-seq and fine mapping. A loss-of-function mutation to BrCER2 caused the waxy phenotype of the cauline leaf, inflorescence stem, flower, and pistil to change to a glossy phenotype. A partial LINE-1 retrotransposon (BrLINE1-RUP) sequence inserted itself into the first exon of BrCER2 in an insertion-mediated deletion manner, resulting in a mutated BrCER2 gene. Our findings have clarified the molecular mechanism underlying the BrCER2-mediated regulation of the biosynthesis of the VLCFAs in the cuticular waxes of Chinese cabbage. Specifically, we confirmed that BrLINE1-RUP is an active LINE-1 retrotransposon and revealed that its insertion into the BrCER2 exon is the cause of the glossy phenotype of Chinese cabbage.
Lines QM19 and HN19-G of Chinese cabbage (B. rapa L. ssp. pekinensis) respectively have a traditional waxy phenotype and a glossy phenotype (cauline leaf, inflorescence stem, flower, and pistil) (Figure 1A). An F2 population (896 plants) derived from the QM19 × HN19-G hybridization was used for the BSA-seq and fine-mapping of the Brwax gene. The chi-square test (IBM SPSS Statistics 26.0) was used to determine the fit of the segregation ratio of the F2 generation to the expected ratio.
Figure 1 Phenotype of HN19-G (glossy) and QM19 (waxy) plants (A). Scanning electron microscopy images of the cauline leaves from HN19-G (glossy) and QM19 (waxy) plants (B). The arrowhead indicates cuticular wax crystals.
Two fresh cauline leaves (having similar size) obtained from HN19-G and QM19 plants at the flowering stage were fixed for 2-4 hours by 2.5% glutaraldehyde fixing solution and rinsed 3 times with 0.1 M phosphate buffer (pH 7.0), subsequently fixed for 1-3 hours with 1% osmic acid · 0.1 M phosphate buffer (pH 7.0) and washed 3 times by 0.1 M phosphate buffer (pH 7.0). The samples were dehydrated by 50%, 70%, 80%, 90%, 95%, and 100% alcohol (two times) for 15 minutes each time and permeated with 100% alcohol: isoamyl acetate (1:1) for 30 minutes and permeated overnight by pure isoamyl acetate. The samples were dried and transferred to a preparation chamber under vacuum for coating. The photographs of the adaxial and abaxial surface of the sample were taken using scanning electron microscopy (SEM) system (Hitachi 8100, Tokyo, Japan).
The BSA-seq analysis was conducted using two pooled samples of 50 glossy phenotype (G-bulk) and 50 waxy phenotype (W-bulk) F2 segregants as well as the two parental lines (HN19-G and QM19). The Illumina Nova 6000 platform was used to generate 150-base paired-end reads for the four pools by Biomarker technologies Co., Ltd. (Beijing, China). The raw data was deposited in the Sequence Read Archive (SRA) in NCBI as PRJNA967584. The B. rapa reference genome v3.0 and GATK were used to identify high-quality single nucleotide polymorphisms (SNPs) and insertions/deletions (InDels). The SNP-index and InDel-index were calculated at each position for the G-bulk and W-bulk. The ΔSNP-index of each SNP position was calculated by subtracting the SNP-index of the G-bulk from the SNP-index of the W-bulk (Fekih et al., 2013; Hill et al., 2013). The ΔInDel-index of each InDel position was similarly calculated. Significant linkage disequilibrium was used to identify the candidate region for the glossy trait (correlation threshold = 0.54) (BIOMARKER; Beijing, China). The intersection of the linked regions (ΔSNP-index and ΔInDel-index) was selected as the final candidate linked region (Table S1).
Polymorphic primer sets (Table S2) were used to analyze the genotype of the plants in the F2 population with glossy and waxy phenotypes. The recombination events were assayed to delimit the region containing Brwax.
The PCR products produced using the primers for the M81 marker were examined by 1% agarose gel electrophoresis. The amplified fragments differed between the glossy and waxy F2 plants derived from the HN19-G × QM19 hybridization. One fragment (198 bp) was amplified for the glossy plants (BrwaxBrwax). In contrast, one fragment (108 bp) and two fragments (198/108 bp) were amplified for the homozygous waxy plants (BrWAXBrWAX) and the heterozygous waxy plants (BrWAXBrwax), respectively.
The sequences and chromosome position information of these genes within the target region were obtained from B. rapa genome v3.0 deposited in the Brassica database (http://brassicadb.cn/). Function of each gene was anotated based on the corresponding Arabidopsis homolog, deduced by the BLAST analysis from the National Center for Biotechnology Information (http://blast.ncbi.nlm.nih.gov/Blast.cgi). The sequences of the candidate genes of HN19-G and QM19 were acquired from resequencing data and were aligned by ClustalX software. The InDel fragment in HN19-G was verified by PCR with the M81 marker.
The BrCER2 sequence in QM19 and the Brcer2 sequence in HN19-G were analyzed using the raw resequencing data for QM19 and HN19-G. The 130-bp inserted fragment in Brcer2 of HN19-G was retrieved from the B. rapa genome v3.0 in BRAD (http://brassicadb.cn/#/) to determine its origin (Zhang et al., 2018; Priyam et al., 2019). The potential TE was further analyzed and grouped according to the B. rapa genome v3.0 TE database in BRAD (http://brassicadb.cn/#/) (Zhang et al., 2018). Target site duplications were analyzed using REPuter in BiBiserv2 (https://bibiserv.cebitec.uni-bielefeld.de/sessionTimeout.jsf) (Kurtz et al., 2001). The TE ORFs were analyzed by aligning BrLINE1-RUP with other annotated LINE-1 sequences (http://repeatmasker.org). The position of BrLINE1-RUP in the B. rapa genome v3.0 was determined using JBrowse (http://brassicadb.cn/#/) (Zhang et al., 2018). A PCR amplification was performed using specific primer pairs (Table S2) to clarify the mechanism mediating the transposition of BrLINE1-RUP. BioEdit was used to analyze BrLINE1-RUP in the B. oleracea, B. rapa, A. thaliana, Raphanus sativus, and Brassica nigra genomes as well as in 18 other representative B. rapa genomes (http://brassicadb.cn/#/) (Cai et al., 2021).
The G-bulk and W-bulk were respectively prepared by mixing equal amounts of entire cauline leaves (having similar size, 5-7 cm2) at the flowering stage from 20 glossy (BrwaxBrwax) and 20 waxy F2 individuals (BrWAXBrWAX : BrWAXBrwax=7:13). Three biological replicates of the W-bulk and G-bulk were assessed. The pictures of cauline leaves were taken to determine surface area of cauline leaf using ImageJ. The total cuticular waxes were collected by soaking the leaves in chloroform for 30 s and 2 µL tetracosane (10 mg/mL) (C24, SUPERLCO, Sigma) was added as an internal standard. The chloroform was evaporated under a stream of gaseous nitrogen. The sample was dissolved with 100μL hexane, subsequently incubated for 60 min at 70°C after adding 100 μL-bis(trimethylsilyl)fuoroacetamide (BSTFA, SUPERLCO, sigma). These derivatized samples were analyzed using a GC-MS system (Agilent 7890B-5977B GC–MS) at Shanghai Jiao Tong University. The initial temperature of 50°C was held for 2 min, increased at 20°C/min to 200°C, increased again at 3°C/min to 310°C, and held for 10 min at 310°C. Compounds were quantified according to the flame ionization detector peak areas and the internal standard (C24 alkane) (Yang et al., 2022a; Yang et al., 2022b). Cuticular wax content was calculated across three biological replications per composition and indicated as mean + standard deviation (SD) (n=3). Statistical analysis was performed using Student’s t-test.
To analyze BrCER2 and Brcer2 expression in a common genetic background, we constructed the HN19-G near isogenic line, which was subsequently named HN19-W. The detailed scheme for HN19-W development was described in Figure S1. Details regarding the primer sets are provided in Table S2. Relative gene expression levels in the root, rosette leaf, cauline leaf, inflorescence stem, flower, and pistil were determined using the ABI StepOne™ Real-Time PCR System (Applied Biosystems) and the 2−ΔΔCt method. Relative expression levels were normalized first against the BrACT7 transcript level (i.e., internal control) and then against the expression level in the flower of HN19-W. The relative fold-change in the expression of each gene was calculated across all biological and technical replicates. Relative gene expression levels are presented herein as the mean + standard deviation.
The cauline leaves of HN19-G and HN19-W were sampled at the same developmental stage. Total RNA was extracted and sequenced by the Illumina Nova 6000 platform (BIOMARKER; Beijing, China). The raw data, which was composed of 150-base paired-end reads, was deposited in the Sequence Read Archive (SRA) in NCBI as PRJNA968036. The clean reads for each sample were aligned to the B. rapa genome v3.0 (http://brassicadb.cn/#/Download/). Gene expression levels were determined in terms of FPKM values. Differentially expressed genes (DEGs) (i.e., fold-change ≥ 2 and false discovery rate < 0.01) were identified.
The examination of the cauline leaf, inflorescence stem, flower, and pistil indicated QM19 has the traditional waxy phenotype, which is in contrast to the glossy phenotype of HN19-G (Figure 1A). The SEM analysis indicated that unlike QM19, HN19-G has less cuticular wax crystals, which are composed of VLCFAs and their derivatives (Figure 1B). These findings suggested that VLCFA biosynthesis was affected in HN19-G. All F1 plants, which were derived from a cross between a glossy parent (HN19-G) and a waxy parent (QM19), had a waxy phenotype. Of the 896 F2 plants, 675 had a waxy phenotype and 221 had a glossy phenotype. The F2 segregation ratio corresponded to the expected Mendelian ratio of 3:1 (χ2 < χ2[df = 1, P = 0.05]) according to the χ2 test (Table S3). Accordingly, the glossy phenotype of HN19-G is likely controlled by a recessive nuclear gene (i.e., Brwax).
To preliminarily map the Brwax gene, a BSA-seq analysis was performed using the waxy (W-bulk) and glossy (G-bulk) F2 segregants and the two parental lines (HN19-W and QM19). In total, 65229700 and 82759172 clean reads were generated from the G-bulk and W-bulk, respectively. The Q30 (those reads with an average quality score >30) was >91%, indicating that the sequencing results was highly accurate (Table S4). Using the B. genome v3.0 as a reference, average sequencing depth of G-bulk and W-bulk was 53× and 67×, respectively (Table S4). Moreover, B. rapa genome v3.0 was used to identify SNPs and InDels in the W-bulk and the G-bulk. The ΔSNP-index of each SNP position and the ΔInDel-index of each InDel position were calculated via a sliding window analysis. The correlation threshold was set as 0.54. The final target regions were located on chromosome A01: 6,210,000–8,680,000 bp and 19,120,000–19,170,000 bp (Figure 2A; Table S1).
Figure 2 Gene mapping and candidate gene analysis for the glossy phenotype gene Brwax. (A) Preliminary mapping of Brwax on the basis of ΔSNP-index and ΔInDel-index (threshold value = 0.54), which were calculated at 4-Mb intervals with a 10-kb sliding window. (B) Fine-mapping of Brwax according to recombination events using molecular markers. (C) Comparative analysis of the genotypes and phenotypes of F2 plants using the M81 marker.
To narrow the target region, 869 F2 plants were selected as the fine-mapping population. The primer pairs used for detecting recombination events revealed that Brwax was flanked by M70, M73, M79, and M80 on one side and M81.3, M81.7, M87, M94, M104, and M124 on the other side. The Brwax gene was delimited to a 130.1-kb region (A01: 8,006,264–8,136,374) flanked by the M80 and M81.3 markers (Figure 2B). The M81 marker was used to compare the F2 plant genotypes and phenotypes. This marker co-segregated with Brwax (Figure 2C).
By screening the B. rapa genome v3.0, we identified and annotated 20 genes in the target region (Table 1). BraA01g015290.3C was identified as the most likely gene responsible for the glossy phenotype. Because BraA01g015290.3C was revealed as a homolog of AtCER2 (At4g24510) in A. thaliana, it was named BrCER2. In A. thaliana, AtCER2 is involved in the biosynthesis of cuticular wax and contributes to VLCFA biosynthesis. Specifically, its expression is required for C28 fatty acid elongation in the stem (Haslam et al., 2012; Pascal et al., 2013).
The sequencing of Brcer2 in HN19-G and BrCER2 in QM19 indicated that BrCER2 in the waxy parent QM19 comprises 3,349 bp and contains two exons and one intron (Figure 3A). The BrCER2 coding sequence in QM19 is 1,254 bp long and is similar to AtCER2 (At4g24510) in A. thaliana (80.7% sequence identity). However, Brcer2 in the glossy parent HN19-G consists of 3,438 bp, which includes a 1,344-bp coding sequence. A 40-bp deletion and a 130-bp insertion were identified in the first exon of Brcer2 in HN19-G (Figure 3A). A premature termination codon was detected in the 130-bp insertion, resulting in the expression of a non-functional truncated protein (Figure 3A). A functional marker (M81) for BrCER2 and Brcer2 co-segregated with Brwax (Figure 2C).
Figure 3 The mutation in Brcer2 of HN19-G was due to the retrotransposition of BrLINE1-RUP. (A) A 40-bp deletion (black background) and a 130-bp insertion (yellow background) were detected in the first exon of Brcer2 in HN19-G. The sequence of the 130-bp insertion was identical to a sequence on chromosome A08 (19,840,059–19,840,188). (B) Sequence and structure of a LINE-1 retrotransposon on chromosome A08 (19,838,382–19,840,203). The ORF2 sequence of LINE-1 (endonuclease and reverse transcriptase) is indicated with an orange background. The 130-bp sequence of LINE-1 that was identical to that in Brcer2 of HN19-G is marked by a yellow underline. (C) Schematic diagram of BrLINE1-RUP. (D) Products of the PCR amplification of BrLINE1-RUP and Brcer2-LINE1 in HN19-G and QM19. TSD, target site duplication.
The 130-bp insertion was used as the query for a BLAST search of the B. rapa genome v3.0, which detected 184 homologous copies dispersed on all 10 chromosomes in the B. rapa genome (Table S5). This finding suggested that the 130-bp insertion in Brcer2 of HN19-G was probably from a TE that replicated itself within genomes. The 130-bp insertion was potentially derived from the transposition of another TE. Among the 184 homologous fragments, the unique sequence on chromosome A08 (19,840,059–19,840,188) was identical to the 130-bp insertion in Brcer2 (Figure 3A). Hence, we speculated that the fragment on chromosome A08 may be a TE that replicated itself and produced the mutated Brcer2 in HN19-G. To verify this possibility, we analyzed a 10-kb sequence containing the fragment on chromosome A08 (19,840,059–19,840,188). The fragment was flanked by a TSD site, which is a characteristic of transposons. The potential transposon contained homologous sequences encoding an endonuclease and reverse transcriptase and a poly(A) sequence (Figure 3B), which is required for the target-primed reverse transcription of the LINE-1 element. It was localized to the promoter of a gene encoding a RING/U-box superfamily protein (RUP; BraA08g028400.3C) (Figure 3C). According to its structure and location, the TE was considered to be a LINE-1 retrotransposon and named BrLINE1-RUP. Thus, the 130-bp insertion on chromosome A01 (Brcer2-LINE1) was derived from the transposition of BrLINE1-RUP on chromosome A08.
To identify the mechanism mediating the transposition of BrLINE1-RUP, PCR amplifications were performed using primer pairs designed for detecting BrLINE1-RUP on chromosome A08 and Brcer2-LINE1 on chromosome A01. The PCR results indicated BrLINE1-RUP is present in QM19, whereas Brcer2-LINE1 is not (control). However, two PCR products (Brcer2-LINE1 on chromosome A01 and BrLINE1-RUP on chromosome A08) were detected for HN19-G, indicating BrLINE1-RUP remained in its original position after the transposition of BrLINE1-RUP into Brcer2 (Figure 3D). Because of this copy-and-paste mechanism, BrLINE1-RUP is probably a retrotransposon. The transposition of BrLINE1-RUP into Brcer2 on chromosome A01 did not result in TSDs flanking the inserted fragment, but Brcer2 was missing a 40-bp fragment. In previous studies, researchers detected LINE-1 insertion-mediated deletions (L1IMDs) and suggested the LINE-1 element size may be correlated with the size of the corresponding deleted fragment (Han et al., 2005). The results of the current study also indicated that the retrotransposition of BrLINE1-RUP involved an insertion-mediated deletion, resulting in a lack of TSDs in Brcer2-LINE1 (Figure 3A).
To investigate the origins of LINE1-RUP and cer2-LINE1, Brassica species were screened for RUP, LINE1-RUP, cer2, and cer2-LINE1. Although RUP genes were detected in B. oleracea and B. rapa, they were undetectable in A. thaliana, R. sativus, and B. nigra. In contrast, LINE1-RUP was exclusive to B. rapa (Figure 4A). Both RUP and LINE1-RUP were also detected in 18 representative B. rapa genomes, implying that RUP is present in all 18 B. rapa genomes. However, 10 B. rapa genomes contained BrLINE1-RUP, whereas eight B. rapa genomes only contained BrRUP (Figure 4B; Table S6). The comparison of the BrRUP and BrLINE1-RUP sequences indicated the LINE-1 transposition into the RUP promoter led to the production of a new LINE1-RUP TE in the A genome (Figures 4C, S2). A PCR analysis of 56 B. rapa L. ssp. pekinensis lines identified seven lines carrying BrLINE1-RUP in their genome (Figure S3). Furthermore, CER2 genes were detected in A. thaliana, R. sativus, B. nigra, B. oleracea, and B. rapa, but cer2-LINE1 was not detected in the genomes of these species. Similarly, cer2-LINE1 was also absent in 18 representative B. rapa genomes (Figure 4B).
Figure 4 LINE1-RUP and CER2-LINE1 in the genomes of five Brassica species (A) and 18 representative B. rapa genomes (B). The phylogenetic relationships among Brassicaceae crops were obtained from a previous study (Cheng et al., 2017). Orange and green square blocks indicate presence and absence, respectively. (C) Structural comparison of BrLINE1-RUP (LINE-1 insertion in BrRUP) and BrRUP (no transposition) in B. rapa.
To elucidate the mechanism underlying the glossy trait of HN19-G, cuticular wax from the W-bulk and G-bulk was collected for the GC-MS analysis. The average total wax content was considerably higher for the waxy leaves (792.23 µg/dm2 surface area) than for the glossy leaves (231.85 µg/dm2 surface area). Hence, the wax content was 71% lower for the G-bulk than for the W-bulk (Figure 5; Table S7). The wax composition analysis revealed that the major waxes in the W-bulk were C29 alkane, C29 ketone, and C30 aldehyde, whereas they were C26 and C28 primary alcohols, C28 aldehyde, and C26 fatty acid in the G-bulk. The C29 alkane, C30 aldehyde, and C29 ketone contents in the leaves of the G-bulk were respectively only 4.7%, 3.5%, and 4.8% of the corresponding levels in the leaves of the W-bulk (Figure 5; Table S7). However, the C26 fatty acid, C27 alkane, C28 primary alcohol, and C28 aldehyde contents were higher in the G-bulk than in the W-bulk (Figure 5; Table S7). Overall, the abundance of the long-chain waxes (> C28) decreased substantially in the glossy plants. Conversely, the VLCFA (< C28) contents were greater in the glossy plants than in the waxy plants. These findings suggested that BrCER2 encodes the protein responsible for C28 fatty acid elongation, similar to AtCER2 in A. thaliana.
Figure 5 Cuticular wax composition in the cauline leaves of the W-bulk and G-bulk. (A) Total wax contents in the W-bulk and G-bulk, calculated as average values for three biological replicates. (B) Wax compositions in the W-bulk and G-bulk, measured as average values for three biological replicates. Error bars indicate SD (n = 3); **P<0.01 (Student’s t test). *0.01<P<0.05 (Student’s t test).
The BrCER2 and Brcer2 expression levels were analyzed by completing a quantitative real-time polymerase chain reaction assay (qRT-PCR). In HN19-W, BrCER2 was most highly expressed in the cauline leaf, followed by the flower, rosette leaf, pistil, and inflorescence stem. In HN19-G, Brcer2 expression was highest in the flower, cauline leaf, pistil, and inflorescence stem, followed by the rosette leaf (Figure 6). The 40-bp deletion and 130-bp insertion produced a premature termination codon in Brcer2 in HN19-G (Figure 3A). Premature termination codons in mRNA generally lead to decreased mRNA abundance due to nonsense-mediated decay, which is a post-transcriptional mechanism for regulating gene expression. To analyze the effect of InDel on Brcer2 expression, a comparative expression analysis was performed. The results showed that the Brcer2 expression level in the cauline leaf, flower, rosette leaf, pistil and inflorescence stem of HN19-G was clearly lower than the BrCER2 expression level in the cauline leaf, flower, rosette leaf, pistil and inflorescence stem of HN19-W (Figure 6).
Figure 6 Expression of BrCER2/Brcer2 transcripts in the inflorescence stem, rosette leaf, cauline leaf, flower, and pistil. Error bars indicate SD (n = 3).
A comparative transcriptome analysis of the waxy cauline leaf of HN19-W and the glossy cauline leaf of HN19-G was performed to screen for DEGs and regulatory networks involved in wax biosynthesis. Approximately 121.9 million clean reads were produced for the six samples, ranging from 19.1 to 21.3 million clean reads per library (Table S8). Among the clean reads, 87.69–90.47% were uniquely mapped to B. rapa genome v3.0 (Table S8). There were 301 DEGs (fold change ≥2 and false discovery rate <0.01), among which 129 genes were upregulated and 172 genes were downregulated in the glossy cauline leaf compared with in the waxy cauline leaf (Table S9). RNA-seq results were verified by qRT-PCR analysis (Figure S4).
Gene Ontology (GO) enrichment analysis (biological process) showed that lipid transport processes were enriched. BraA02g011070.3C, BraA02g011080.3C and BraA03g015450.3C, which encode non-specific lipid-transfer proteins, were significantly downregulated in the glossy cauline leaf (Figure 7A). Previous studies indicated that non-specific lipid-transfer proteins may play a role in wax or cutin deposition in epidermal cells (Liu et al., 2014; Deeken et al., 2016).
Figure 7 (A) GO enrichment analysis of DEGs for biological processes. The DEGs were annotated to top 10 GO terms (with smallest Q-value). (B) KEGG pathway enrichment analysis of DEGs for biological processes. Top 5 enriched pathways (with smallest Q-value) were shown with brown dots, whose size represents the number of DEGs enriched in the corresponding pathway.
The main enriched Kyoto Encyclopedia of Genes and Genomes pathways (KEGG) among these DEGs were arachidonic acid metabolism, flavonoid biosynthesis, alpha-linolenic acid metabolism, linoleic acid metabolism, and steroid biosynthesis, and all of them were downregulated in the glossy cauline leaf of HN19-G (Figure 7B). In plant, VLCFA can be converted into other lipids mediated by very long-chain acyl-CoAs, which were produced by fatty acid elongation complexes (Batsale et al., 2021). We deduced that down-regulation of these genes may reduce the conversion from VLCFA into other lipids to compensate for wax loss in glossy plants.
In cutin, suberin and wax biosynthesis, BraA02g026450.3C homologous to CYP86A2 of Arabidopsis thaliana (At4g00360) were significantly upregulated in the glossy cauline leaf. In Arabidopsis thaliana, CYP86A2 is a cytochrome P450 monooxygenase catalyzing fatty acid oxidation. The cutin content is reduced to 30% in cyp86a2 mutants, indicating that CYP86A2 plays a major role in the biosynthesis of extracellular lipids (Xiao et al., 2004). However, BraA01g015290.3C (BrCER2) required for C28 fatty acid elongation was strongly downregulated in HN-19G (Table S9).
In Chinese cabbage, wax-less mutants showed a glossy green phenotype, distinctively different from the waxy glaucous plants. Previous studies showed that three genes have been identified for the glossy phenotype in Chinese cabbage. A single SNP in Brcer1 (Bra032670) results in wax deficiency in Chinese cabbage (B. rapa L. ssp. pekinensis) (Liu et al., 2021). Subsequent research also delimited the locus related to the glossy phenotype to a 100.78-kb interval and showed that the AtCER1 homolog Bra032670 is the most likely candidate gene for BrWAX2 (Yang et al., 2022b). The BrWAX3 locus was fine-mapped to a 161.82-kb region on chromosome A09 of Chinese cabbage, with Bra024749 (BrCER60.A09), encoding a β-ketoacyl-CoA synthase, identified as the candidate gene (Yang et al., 2022a). In a previous study, BrCER2 was identified as the candidate gene for BrWax1 (Zhang et al., 2013). An insertion at the transcription start site essentially silences BrCER2 expression, thereby causing the mutant glossy phenotype of Chinese cabbage plants (Zhang et al., 2013). These candidate genes encode proteins with essential functions related to cuticular wax biosynthesis in Chinese cabbage. In the present study, a physical interval (130.1 kb) containing 20 genes was mapped and BrCER2, which is homologous to AtCER2 (At4g24510), was identified as the candidate gene. Our SEM analysis generated evidence that BrCER2 helps mediate cuticular wax biosynthesis in the cauline leaf. The results of the cuticular wax analysis indicated that a mutation to BrCER2 affects more than C28 VLCFA biosynthesis and is responsible for the glossy phenotype of HN19-G. In A. thaliana, Atcer2 mutant plants lack waxes longer than C28. Moreover, AtCER2 belongs to the BAHD acyltransferase family and is required for C28 elongation by interacting with fatty acid elongation machinery (Haslam et al., 2012). Co-expression of AtCER2 with AtCER6 in yeast results in the production of C30 fatty acids (Haslam et al., 2012). CER2 of Nelumbo nucifera and Oryza sativa also showed similar functions in VLCFA biosynthesis (Wang et al., 2017; Yang et al., 2018), suggesting that the function of CER2 in producing VLCFAs up to C30 is highly conserved across species.
Transposable elements are potent broad-spectrum mutator elements that can increase genomic diversity (Gregory, 2011). Among Brassica species, the insertion of TEs is essential for phenotypic variations, adaptation, and domestication (Cai et al., 2021; Cai et al., 2022). A potential TE insertion was identified in exon 1 of BrCER60.A09 in SD369, which lead to a premature stop codon, thus causing a loss of function of the BrCER60.A09 enzyme and a glossy phenotype in SD369 (Yang et al., 2022a). A copia-like retrotransposon-based marker (BnSHP1.A9R2) has been used for the marker-assisted breeding of oilseed rape with shatter-resistant pods (Liu et al., 2020). In yellowhorn (Xanthoceras sorbifolium), the Xsag1-LINE1-1 fragment inserted in XsAG1 is a LINE-1 transposon; this fragment is responsible for the floral homeotic mutation in yellowhorn (Wang et al., 2022). In the current study, a 130-bp insertion in Brcer2 of HN19-G was the result of the transposition of a sequence from BrLINE1-RUP, which is a LINE1 TE. More precisely, a retrotransposition event introduced a partial BrLINE1-RUP sequence (130 bp) into the first exon of BrCER2 in HN19-G, thereby creating a premature termination codon in the Brcer2 mRNA, ultimately leading to the formation of a truncated protein. A loss-of-function mutation to BrCER2 causes the mutant Chinese cabbage plants to develop glossy cauline leaves rather than the normal waxy cauline leaves. Considered together, the study findings indicate the retrotransposition of BrLINE1-RUP into BrCER2 modifies cuticular wax biosynthesis and affects the waxy phenotype (Figure 8). TE insertions play a crucial role in phenotypic variation and represent a major source of intraspecific variation.
Figure 8 Schematic diagram of how the retrotransposition of BrLINE1-RUP altered the waxy phenotype of Chinese cabbage.
The LINE-1 elements usually contain two ORFs, of which ORF1 encodes a nucleic acid-binding protein necessary for the retrotransposition of LINE1 elements. This protein functions as a nucleic acid chaperone that binds and preferentially mobilizes its own transcript (Callahan et al., 2012). In contrast, ORF2 encodes an endonuclease and a reverse transcriptase, the latter of which is essential for target-primed reverse transcription (Wells and Feschotte, 2020). In the present study, we determined that BrLINE1-RUP is missing ORF1. The insertion of BrLINE1-RUP into BrCER2 of HN19-G suggests that ORF1 is not required for the transposition of BrLINE1-RUP. In accordance with this finding, ORF1 is reportedly dispensable or absent in some groups of non-LTR elements (Burke et al., 1987; Wells and Feschotte, 2020).
A 40-bp fragment was deleted from the first exon of Brcer2. The 130-bp insertion and the 40-bp deletion were localized to the same target site. Moreover, TSDs were not detected. The mechanism facilitating the transposition of BrLINE1-RUP is similar to that of L1IMDs, in which LINE-1 is inserted into a target site, while the target site sequence is removed. Earlier research confirmed L1IMDs occur in Homo sapiens and Pan troglodytes (Han et al., 2005). The insertion-mediated deletion-based transposition of BrLINE1-RUP provides evidence of L1IMDs in eukaryotes, including plants and animals.
The mechanism underlying L1IMDs was proposed to explain how a LINE-1 integration leads to target site deletions (Han et al., 2005). The BrLINE1-RUP sequence includes ORF2 (i.e., endonuclease and reverse transcriptase). The encoded endonuclease usually cleaves DNA at a 5′-TT/AAAA-3′ site, corresponding to genomic regions altered by LINE-1 integration (Richardson et al., 2015). In the present study, the 40-bp deletion in Brcer2 coincided with the location of the integrated BrLINE1-RUP. The plus-strand cleavage site and the minus-strand cleavage site were respectively 5′-CT/AAAG-3′ and 5′-GT/AAGG-3′ (i.e., similar to 5′-TT/AAAA-3′). Moreover, 40-bp overhangs were produced and eliminated by the endonuclease (Figure 9). The poly(A) tail of the BrLINE1-RUP transcript can anneal to the cleavage site, thereby enabling the completion of target-primed reverse transcription. The BrLINE1-RUP sequence is 1,821 bp long, whereas the inserted fragment in Brcer2 comprises 130 bp, suggesting that a partial BrLINE1-RUP RNA sequence was reverse transcribed during the retrotransposition of BrLINE1-RUP (Figure 9). A previous study showed that a hallmark feature of this process is the frequent premature termination of the reverse transcription step. The resulting 5′-truncation generally prevents the propagation of the newly inserted copy (Richardson et al., 2015; Wells and Feschotte, 2020).
Figure 9 Putative model of the BrLINE1-RUP transposition into Brcer2 of HN19-G via an insertion-mediated deletion mechanism.
The data presented in the study are deposited in the NCBI repository, accession number PRJNA967584 and PRJNA968036.
PT performed most of the experiments and wrote the manuscript. BL initiated and directed the study. ZY performed genetic analysis. XD performed partial experiments. YaZ revised the manuscript. JL, YuZ, and QH collected partial data. All authors contributed to the article and approved the submitted version.
This work was supported by the Grand Science and Technology Special Project of Zhejiang Province (2021C02065-5-2), the Zhejiang Provincial Natural Science Foundation of China (LY21C150006) and Zhejiang Province Research and Development Program of “Lingyan” (NO.2022C02030).
We thank Liwen Bianji (Edanz) (www.liwenbianji.cn) for editing the English text of a draft of this manuscript.
The authors declare that the research was conducted in the absence of any commercial or financial relationships that could be construed as a potential conflict of interest.
All claims expressed in this article are solely those of the authors and do not necessarily represent those of their affiliated organizations, or those of the publisher, the editors and the reviewers. Any product that may be evaluated in this article, or claim that may be made by its manufacturer, is not guaranteed or endorsed by the publisher.
The Supplementary Material for this article can be found online at: https://www.frontiersin.org/articles/10.3389/fpls.2023.1212528/full#supplementary-material
Arand, K., Bieler, E., Dürrenberger, M., Kassemeyer, H. H. (2021). Developmental pattern of grapevine (Vitis vinifera L.) berry cuticular wax: differentiation between epicuticular crystals and underlying wax. PloS One 16, e0246693. doi: 10.1371/journal.pone.0246693
Arya, G. C., Sarkar, S., Manasherova, E., Aharoni, A., Cohen, H. (2021). The plant cuticle: an ancient guardian barrier set against long-standing rivals. Front. Plant Sci. 12, 663165. doi: 10.3389/fpls.2021.663165
Batsale, M., Bahammou, D., Fouillen, L., Mongrand, S., Joubès, J., Domergue, F. (2021). Biosynthesis and functions of very-long-chain fatty acids in the responses of plants to abiotic and biotic stresses. Cells 10, 1284. doi: 10.3390/cells10061284
Bennetzen, J. L. (2000). Transposable element contributions to plant gene and genome evolution. Plant Mol. Biol. 42, 251–269. doi: 10.1023/A:1006344508454
Burke, W., Calalang, C., Eickbush, T. (1987). The site-specific ribosomal insertion element type II of Bombyx mori (R2Bm) contains the coding sequence for a reverse transcriptase-like enzyme. Mol. Cell. Biol. 7, 2221–2230. doi: 10.1128/mcb.7.6.2221-2230.1987
Cai, X., Chang, L., Zhang, T., Chen, H., Zhang, L., Lin, R., et al. (2021). Impacts of allopolyploidization and structural variation on intraspecific diversification in Brassica rapa. Genome Biol. 22, 166. doi: 10.1186/s13059-021-02383-2
Cai, X., Lin, R., Liang, J., King, G. J., Wu, J., Wang, X. (2022). Transposable element insertion: a hidden major source of domesticated phenotypic variation in Brassica rapa. Plant Biotechnol. J. 20, 1298–1310. doi: 10.1111/pbi.13807
Callahan, K. E., Hickman, A. B., Jones, C. E., Ghirlando, R., Furano, A. V. (2012). Polymerization and nucleic acid-binding properties of human L1 ORF1 protein. Nucleic Acids Res. 40, 813–827. doi: 10.1093/nar/gkr728
Cheng, F., Liang, J., Cai, C., Cai, X., Wu, J., Wang, X. (2017). Genome sequencing supports a multi-vertex model for brassiceae species. Curr. Opin. Plant Biol. 36, 79–87. doi: 10.1016/j.pbi.2017.01.006
Deeken, R., Saupe, S., Klinkenberg, J., Riedel, M., Leide, J., Hedrich, R., et al. (2016). The nonspecific lipid transfer protein AtLtpI-4 is involved in suberin formation of Arabidopsis thaliana crown galls. Plant Physiol. 172, 1911–1927. doi: 10.1104/pp.16.01486
Diarte, C., Lai, P. H., Huang, H., Romero, A., Casero, T., Gatius, F., et al. (2019). Insights into olive fruit surface functions: a comparison of cuticular composition, water permeability, and surface topography in nine cultivars during maturation. Front. Plant Sci. 10, 1484. doi: 10.3389/fpls.2019.01484
Fekih, R., Takagi, H., Tamiru, M., Abe, A., Natsume, S., Yaegashi, H., et al. (2013). MutMap+: genetic mapping and mutant identification without crossing in rice. PloS One 8, e68529. doi: 10.1371/journal.pone.0068529
Fiebig, A., Mayfield, J. A., Miley, N. L., Chau, S., Fischer, R. L., Preuss, D. (2000). Alterations in CER6, a gene identical to CUT1, differentially affect long-chain lipid content on the surface of pollen and stems. Plant Cell 12, 2001–2008. doi: 10.1105/tpc.12.10.2001
Giovannoni, J. J., Wing, R. A., Ganal, M. W., Tanksley, S. D. (1991). Isolation of molecular markers from specific chromosomal intervals using DNA pools from existing mapping populations. Nucleic Acids Res. 19, 6553–6558. doi: 10.1093/nar/19.23.6553
Han, K., Sen, S. K., Wang, J., Callinan, P. A., Lee, J., Cordaux, R., et al. (2005). Genomic rearrangements by LINE-1 insertion-mediated deletion in the human and chimpanzee lineages. Nucleic Acids Res. 33, 4040–4052. doi: 10.1093/nar/gki718
Haslam, T. M., Mañas-Fernández, A., Zhao, L., Kunst, L. (2012). Arabidopsis ECERIFERUM2 is a component of the fatty acid elongation machinery required for fatty acid extension to exceptional lengths. Plant Physiol. 160, 1164–1174. doi: 10.1104/pp.112.201640
Hill, J. T., Demarest, B. L., Bisgrove, B. W., Gorsi, B., Su, Y.-C., Yost, H. J. (2013). MMAPPR: mutation mapping analysis pipeline for pooled RNA-seq. Genome Res. 23, 687–697. doi: 10.1101/gr.146936.112
Isaacson, T., Kosma, D. K., Matas, A. J., Buda, G. J., He, Y., Yu, B., et al. (2009). Cutin deficiency in the tomato fruit cuticle consistently affects resistance to microbial infection and biomechanical properties, but not transpirational water loss. Plant J. 60, 363–377. doi: 10.1111/j.1365-313X.2009.03969.x
Ji, J., Cao, W., Tong, L., Fang, Z., Zhang, Y., Zhuang, M., et al. (2021). Identification and validation of an ECERIFERUM2-LIKE gene controlling cuticular wax biosynthesis in cabbage (Brassica oleracea L. var. capitata L.). Theor. Appl. Genet. 134, 4055–4066. doi: 10.1007/s00122-021-03947-3
Kim, J., Jung, J. H., Lee, S. B., Go, Y. S., Kim, H. J., Cahoon, R., et al. (2013). Arabidopsis 3-ketoacyl-coenzyme a synthase9 is involved in the synthesis of tetracosanoic acids as precursors of cuticular waxes, suberins, sphingolipids, and phospholipids. Plant Physiol. 162, 567–580. doi: 10.1104/pp.112.210450
Kim, Y. J., Lee, J., Han, K. (2012). Transposable elements: no more 'Junk DNA'. Genomics Inform 10, 226–233. doi: 10.5808/GI.2012.10.4.226
Krasileva, K. V. (2019). The role of transposable elements and DNA damage repair mechanisms in gene duplications and gene fusions in plant genomes. Curr. Opin. Plant Biol. 48, 18–25. doi: 10.1016/j.pbi.2019.01.004
Kurtz, S., Choudhuri, J. V., Ohlebusch, E., Schleiermacher, C., Stoye, J., Giegerich, R. (2001). REPuter: the manifold applications of repeat analysis on a genomic scale. Nucleic Acids Res. 29, 4633–4642. doi: 10.1093/nar/29.22.4633
Lee, S. B., Jung, S. J., Go, Y. S., Kim, H. U., Kim, J. K., Cho, H. J., et al. (2009). Two arabidopsis 3-ketoacyl CoA synthase genes, KCS20 and KCS2/DAISY, are functionally redundant in cuticular wax and root suberin biosynthesis, but differentially controlled by osmotic stress. Plant J. 60, 462–475. doi: 10.1111/j.1365-313X.2009.03973.x
Liu, D., Dong, X., Liu, Z., Tang, J., Zhuang, M., Zhang, Y., et al. (2018). Fine mapping and candidate gene identification for wax biosynthesis locus, BoWax1 in Brassica oleracea l. var. capitata. Front. Plant Sci. 9, 309. doi: 10.3389/fpls.2018.00309
Liu, C., Song, G., Wang, N., Huang, S., Gao, Y., Fu, W., et al. (2021). A single SNP in Brcer1 results in wax deficiency in Chinese cabbage (Brassica campestris L. ssp. pekinensis). Sci. Hortic. 282, 110019. doi: 10.1016/j.scienta.2021.110019
Liu, F., Xiong, X., Wu, L., Fu, D., Hayward, A., Zeng, X., et al. (2014). BraLTP1, a lipid transfer protein gene involved in epicuticular wax deposition, cell proliferation and flower development in Brassica napus. PloS One 9, e110272. doi: 10.1371/journal.pone.0110272
Liu, S., Yeh, C. T., Tang, H. M., Nettleton, D., Schnable, P. S. (2012). Gene mapping via bulked segregant RNA-seq (BSR-seq). PloS One 7, e36406. doi: 10.1371/journal.pone.0036406
Liu, J., Zhou, R., Wang, W., Wang, H., Qiu, Y., Raman, R., et al. (2020). A copia-like retrotransposon insertion in the upstream region of the SHATTERPROOF1 gene, BnSHP1.A9, is associated with quantitative variation in pod shattering resistance in oilseed rape. J. Exp. Bot. 71, 5402–5413. doi: 10.1093/jxb/eraa281
Millar, A. A., Clemens, S., Zachgo, S., Giblin, E. M., Taylor, D. C., Kunst, L. (1999). CUT1, an arabidopsis gene required for cuticular wax biosynthesis and pollen fertility, encodes a very-long-chain fatty acid condensing enzyme. Plant Cell 11, 825–838. doi: 10.1105/tpc.11.5.825
Millar, A. A., Kunst, L. (1997). Very-long-chain fatty acid biosynthesis is controlled through the expression and specificity of the condensing enzyme. Plant J. 12, 121–131. doi: 10.1046/j.1365-313X.1997.12010121.x
Pascal, S., Bernard, A., Sorel, M., Pervent, M., Vile, D., Haslam, R. P., et al. (2013). The Arabidopsis cer26 mutant, like the cer2 mutant, is specifically affected in the very long chain fatty acid elongation process. Plant J. 73, 733–746. doi: 10.1111/tpj.12060
Priyam, A., Woodcroft, B. J., Rai, V., Moghul, I., Munagala, A., Ter, F., et al. (2019). Sequenceserver: a modern graphical user interface for custom BLAST databases. Mol. Biol. Evol. 36, 2922–2924. doi: 10.1093/molbev/msz185
Richardson, S. R., Doucet, A. J., Kopera, H. C., Moldovan, J. B., Garcia-Perez, J. L., Moran, J. V. (2015). The influence of LINE-1 and SINE retrotransposons on mammalian genomes. Microbiol. Spectr 3, MDNA3–0061-2014. doi: 10.1128/9781555819217.ch51
Samuels, L., Kunst, L., Jetter, R. (2008). Sealing plant surfaces: cuticular wax formation by epidermal cells. Annu. Rev. Plant Biol. 59, 683–707. doi: 10.1146/annurev.arplant.59.103006.093219
Todd, J., Post-Beittenmiller, D., Jaworski, J. G. (1999). KCS1 encodes a fatty acid elongase 3-ketoacyl-CoA synthase affecting wax biosynthesis in Arabidopsis thaliana. Plant J. 17, 119–130. doi: 10.1046/j.1365-313X.1999.00352.x
Trenkamp, S., Martin, W., Tietjen, K. (2004). Specific and differential inhibition of very-long-chain fatty acid elongases from Arabidopsis thaliana by different herbicides. Proc. Natl. Acad. Sci. 101, 11903–11908. doi: 10.1073/pnas.0404600101
Wang, X., Guan, Y., Zhang, D., Dong, X., Tian, L., Qu, L. Q. (2017). A β-ketoacyl-CoA synthase is involved in rice leaf cuticular wax synthesis and requires a CER2-LIKE protein as a cofactor. Plant Physiol. 173, 944–955. doi: 10.1104/pp.16.01527
Wang, H., Lu, Y., Zhang, T., Liu, Z., Cao, L., Chang, Q., et al. (2022). The double flower variant of yellowhorn is due to a LINE1 transposon-mediated insertion. Plant Physiol. 191, 1122–1137. doi: 10.1093/plphys/kiac571
Wells, J. N., Feschotte, C. (2020). A field guide to eukaryotic transposable elements. Annu. Rev. Genet. 54, 539–561. doi: 10.1146/annurev-genet-040620-022145
Wicker, T., Sabot, F., Hua-Van, A., Bennetzen, J. L., Capy, P., Chalhoub, B., et al. (2007). A unified classification system for eukaryotic transposable elements. Nat. Rev. Genet. 8, 973–982. doi: 10.1038/nrg2165
Xiao, F., Goodwin, S. M., Xiao, Y., Sun, Z., Baker, D., Tang, X., et al. (2004). Arabidopsis CYP86A2 represses Pseudomonas syringae type III genes and is required for cuticle development. EMBO J. 23, 2903–2913. doi: 10.1038/sj.emboj.7600290
Yang, S., Liu, H., Wei, X., Zhao, Y., Wang, Z., Su, H., et al. (2022b). BrWAX2 plays an essential role in cuticular wax biosynthesis in Chinese cabbage (Brassica rapa L. ssp. pekinensis). Theor. Appl. Genet. 135, 693–707. doi: 10.1007/s00122-021-03993-x
Yang, S., Tang, H., Wei, X., Zhao, Y., Wang, Z., Su, H., et al. (2022a). BrWAX3, encoding a β-ketoacyl-CoA synthase, plays an essential role in cuticular wax biosynthesis in Chinese cabbage. Inter J. Mol. Sci. 23, 10938. doi: 10.3390/ijms231810938
Yang, X., Wang, Z., Feng, T., Li, J., Huang, L., Yang, B., et al. (2018). Evolutionarily conserved function of the sacred lotus (Nelumbo nucifera gaertn.) CER2-LIKE family in very-long-chain fatty acid elongation. Planta 248, 715–727. doi: 10.1007/s00425-018-2934-6
Zhang, L., Cai, X., Wu, J., Liu, M., Grob, S., Cheng, F., et al. (2018). Improved Brassica rapa reference genome by single-molecule sequencing and chromosome conformation capture technologies. Hortic. Res. 5, 50. doi: 10.1038/s41438-018-0071-9
Zhang, X., Liu, Z., Wang, P., Wang, Q., Yang, S., Feng, H. (2013). Fine mapping of BrWax1, a gene controlling cuticular wax biosynthesis in Chinese cabbage (Brassica rapa L. ssp. pekinensis). Mol. Breed. 32, 867–874. doi: 10.1007/s11032-013-9914-0
Keywords: LINE-1, Transposable element, retrotransposition, BrCER2, cuticular wax biosynthesis, Brassica rapa L. ssp. pekinensis
Citation: Li B, Yue Z, Ding X, Zhao Y, Lei J, Zang Y, Hu Q and Tao P (2023) A BrLINE1-RUP insertion in BrCER2 alters cuticular wax biosynthesis in Chinese cabbage (Brassica rapa L. ssp. pekinensis). Front. Plant Sci. 14:1212528. doi: 10.3389/fpls.2023.1212528
Received: 26 April 2023; Accepted: 27 June 2023;
Published: 12 July 2023.
Edited by:
Yi-Hong Wang, University of Louisiana at Lafayette, United StatesReviewed by:
Yong Liu, Jiangxi Agricultural University, ChinaCopyright © 2023 Li, Yue, Ding, Zhao, Lei, Zang, Hu and Tao. This is an open-access article distributed under the terms of the Creative Commons Attribution License (CC BY). The use, distribution or reproduction in other forums is permitted, provided the original author(s) and the copyright owner(s) are credited and that the original publication in this journal is cited, in accordance with accepted academic practice. No use, distribution or reproduction is permitted which does not comply with these terms.
*Correspondence: Peng Tao, dGFvcGVuZy04NEAxNjMuY29t
Disclaimer: All claims expressed in this article are solely those of the authors and do not necessarily represent those of their affiliated organizations, or those of the publisher, the editors and the reviewers. Any product that may be evaluated in this article or claim that may be made by its manufacturer is not guaranteed or endorsed by the publisher.
Research integrity at Frontiers
Learn more about the work of our research integrity team to safeguard the quality of each article we publish.