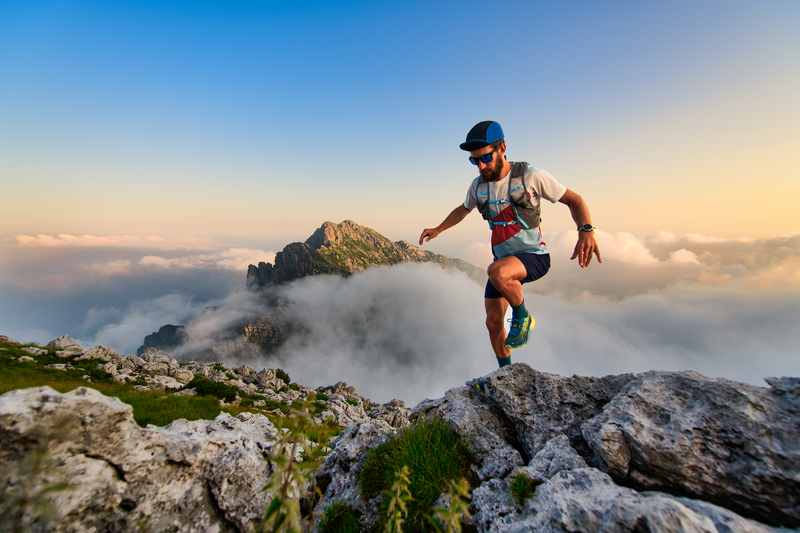
95% of researchers rate our articles as excellent or good
Learn more about the work of our research integrity team to safeguard the quality of each article we publish.
Find out more
EDITORIAL article
Front. Plant Sci. , 26 May 2023
Sec. Plant Cell Biology
Volume 14 - 2023 | https://doi.org/10.3389/fpls.2023.1211807
This article is part of the Research Topic Regulation of Plant Organelle Biogenesis and Trafficking View all 8 articles
Editorial on the Research Topic
Regulation of plant organelle biogenesis and trafficking
The emergence of membrane-bounded organelles is a hallmark of eukaryotic cells, allowing multiple and incompatible biochemical processes to occur simultaneously (Gomes and Shorter, 2019; Mathur, 2020). Each organelle contains a specific set of proteins, lipids, and cofactors that define its characteristic morphology and function. Meanwhile, the activities and functions of cellular organelles must be well orchestrated for the cell to function properly as a biological unit (Cohen et al., 2018; Perico and Sparkes, 2018). The process of organelle biogenesis involves the coordinated expression of genes, signaling pathways, and molecular interactions that result in the formation and maintenance of organelle structure and function. Furthermore, proper regulations of organelle subcellular localization, trafficking, and interactions are crucial for cell growth, development, and homeostasis (Lippincott-Schwartz et al., 2000; Noack and Jaillais, 2017). Thus, organelle biogenesis, function, and diversity are fundamental and critical to every cell.
The organelles play essential roles in various physiological and metabolic processes, including photosynthesis, cell wall construction, hormonal distribution, and cell signaling (Saftig and Klumperman, 2009; Kang et al., 2011; Perico and Sparkes, 2018; Li et al., 2020; Robinson, 2020; Shimizu et al., 2021). Moreover, the trafficking and interactions of organelles within cells are dynamic processes that involve coordinated action of various components, including motor proteins, cytoskeletal elements, and membrane trafficking regulators such as small GTPases and soluble N-ethylmaleimide-sensitive-factor attachment protein receptors (SNAREs). They are also influenced by environmental cues such as light, temperature, and various other biotic and abiotic stresses (Uemura and Ueda, 2014; Luo et al., 2017; Yun and Kwon, 2017; Rosquete and Drakakaki, 2018; Won and Kim, 2020). Understanding the mechanisms underlying plant organelle biogenesis and trafficking is essential for improving plant growth and development, as well as for devising new biotechnological applications that rely on the targeted delivery of molecules to specific plant cells or tissues (Miao et al., 2008; Michoux et al., 2011; Ou et al., 2014; Lomonossoff and D’Aoust, 2016).
In this Research Topic issue, several essential aspects of organelle biogenesis, membrane trafficking, and protein sorting in both model and non-model plants have been advanced. In eukaryotic cells, new proteins and lipids are delivered from their site of synthesis in the endoplasmic reticulum (ER) to various subcellular destinations. The Golgi apparatus is a central organelle in secretory membrane traffic and sorting. In plant cells, the Golgi additionally serves as a major biosynthetic organelle for synthesizing polysaccharides, which are key elements for the plant cell wall construction (Reyes et al., 2011; Chung and Zeng, 2017). The morphogenesis and maintenance of the stacked cisternal structure of the Golgi body are critical for its biological functions. Rui et al. provide an update on key regulators that mediate ER-Golgi, intra-Golgi, and post-Golgi trafficking pathways. Furthermore, they focus on functional molecules that participate in retrograde vesicular transport from trans-Golgi to cis-Golgi cisternae, including Arf1, coatomer, the COG complex, SYP31 and 32, Rab GTPases and Golgi matrix proteins.
In addition, soluble N-ethylmaleimide-sensitive factor attachment protein receptor (SNARE) proteins are a family of proteins that are essential to mediate membrane fusion in eukaryotic cells. They also play a crucial role in various cellular processes, including organelle interactions, vesicular trafficking, cytokinesis, and are involved in growth, development and stress responses in plants (Grefen and Blatt, 2008; Kwon et al., 2020). VPS45 is a protein that belongs to the Sec1/Munc18 family and interacts with and regulates Qa-SNARE function during membrane fusion. Mugume et al.identified a mutant of VPS45, which is caused by a point mutation in the VPS45 gene that differs from the lethal vps45 knockout mutation in Arabidopsis. They further revealed that impaired VPS45 function causes vacuolar defects and leads to a loss of turgor pressure that is needed for proper tip growth. Besides, Luo et al. summarized recent progress in understanding the biological functions and signaling network of SNAREs in vesicle trafficking and the regulation of root growth and development in Arabidopsis.
Proteins of the secretory pathway are transported from the Golgi stack to the trans-Golgi network (TGN) for sorting and trafficking to different subcellular localizations. In plant cells, the TGN has been identified as an independent organelle that also functions like the early endosome (EE) of animal cells and is therefore also referred to as TGN/EE (Dettmer et al., 2006; Lam et al., 2007; Richter et al., 2009; McKay et al., 2022). It is also the source for the biogenesis of the multivesicular bodies (MVBs), the late endosomes (LEs) that facilitate the trafficking to the lytic vacuole (Scheuring et al., 2011). Shimizu and Uemura review the recent results from fast live imaging by spinning disk confocal microscopy and from 3D reconstructions by electron tomography that allowed to distinguish the Golgi-associated TGN (GA-TGN) from the Golgi-independent TGN (GI-TGN) and also specialized domains within the GA-TGN. The markers AP-1, Epsin1, clathrin and VAMP721 are associated with a domain involved in trafficking to the plasma membrane, while AP-4, MTV1 and VAMP727 are associated with another domain involved in trafficking to the vacuole. Whether clathrin is involved in the latter pathway is unclear. The GI-TGN derives from the former domain of the GA-TGN and produces AP-1/clathrin-coated vesicles, which may play a role in retrograde trafficking. The secretory trafficking is further complicated by the separate sorting and transport of proteins to different domains of the plasma membrane, as is seen in root endodermal cells. Inhibitors differently affect transport to the two target membranes, but this sorting has not yet been localized within the TGN. Finally, not all protein trafficking to the vacuoles may implicate the TGN, as AP-3 mediated sorting of several proteins may well already occur at the trans-Golgi.
Plant adaptation relies on neofunctionalization of the endomembrane system (ES) to acquire new organelles, which may serve for secondary metabolism. This approach is often ignored due to the intricacy of angiosperms. Bryophytes, with their simple cellular structures and unique organelles such as oil bodies (OBs), are great models for studying the role of the endomembrane system (ES) in the production of plant secondary metabolites (PSMs), as well as how new organelles are acquired during evolution (He et al., 2013; Kanazawa et al., 2020; Romani et al., 2020; Romani et al., 2022). Liverwort’s OBs are single-membrane organelles containing lipophilic globules and PSMs in a protein matrix, and are typically found in gametophyte cells (He et al., 2013; Romani et al., 2022). Recent studies on OBs in Marchantia polymorpha have identified several key transcriptional factors, including ERF, MYB and HDZ, which coordinate the redirection of the secretory pathway toward OB formation (Romani et al., 2020). Research on the M. polymorpha SNARE protein (MpSYP12B) found in OBs suggests that these organelles may have originated from the expansion of secretory trafficking systems in plants (Kanazawa et al., 2020). Furthermore, the same research group recently identified that maintaining the shape of the OB is a complex process that involves the COPI components (Kanazawa et al.). Systemic research on OB provides compelling evidence to support the notion that the redirection of the secretory pathway contributes to OB formation and shape, although the physiological significance of maintaining OB shape requires further study (Kanazawa et al., 2020). This research underscores the importance of studying OBs in bryophytes and highlights the need for similar future studies on non-model organisms to maximize our understanding of organelles and trafficking. Ultimately, such studies can enrich our knowledge in this field.
Mitochondria, the cell’s “powerhouse”, produce respiratory ATP and are essential for eukaryotic life. Most mitochondrial proteins are encoded by the nuclear genome, synthesized in the cytosol, and translocated to the mitochondria (Møller et al., 2021). Nevertheless, mitochondria are known as semi-independent organelles, which also contain their own mitochondrial genome (mitogenome) (Barrera-Paez and Moraes, 2022). Understanding the plant mitogenome can help us better understand the function of mitochondria in plant cells, and develop strategies to improve plant health and crop yields by editing the mitochondrial genome (Yang et al., 2022). Mitogenomes from different plant species can be used to study the evolution of plant lineages, as well as to investigate the relationships between plant species and other organisms such as fungi and bacteria. In addition, plant mitogenomes can also serve as a tool for studying the phylogenetics of different plant species (Zardoya, 2020). In recent years, high-throughput sequencing techniques have accelerated the sequencing of mitogenomes and uncovered the great diversity of organizations, gene contents, and modes of replication and transcription found in living eukaryotes. Feng et al. have assembled complete mitogenomes of 23 species that cover seven families of Fagales. By their analysis of their mitogenomic structures and capacity in phylogeny, they offer a fresh perspective on the evolution of mitochondrial genomes and the variations in their size. Furthermore, Bi et al. completed the assembly of the complete mitochondrial genome of Populus simonii and provide insights into the stability of genome sizes and gene contents in the genus Populus.
Together, the biogenesis and trafficking of plant organelles involve complex cellular processes that are coordinated by various signaling pathways and molecular interactions. Recent advances in our understanding of the regulation of plant organelle biogenesis and trafficking have provided new insights into the complex cellular processes underlying plant growth and development. Further research in this fascinating and challenging area will undoubtedly uncover new regulatory pathways and molecular mechanisms, providing new targets for plant/crop improvement, environmental sustainability, and biotechnological applications.
All authors listed have made a substantial, direct, and intellectual contribution to the work and approved it for publication.
Research in the authors’ laboratories is funded by the East China Normal University and Shanghai Pujiang Program (20PJ1403200) to QZ, the National Natural Science Foundation of China (31970185.P.P and 32270742.P.P), the Shenzhen Science and Technology Program (No. KQTD20190929173906742), and the Key Laboratory of Molecular Design for Plant Cell Factory of Guangdong Higher Education Institutes (2019KSYS006) to PP, the National Natural Science Foundation of China (31770196 and 91954110) and the Natural Science Foundation of Guangdong Province (2021A1515012066) to HW.
The authors declare that the research was conducted in the absence of any commercial or financial relationships that could be construed as a potential conflict of interest.
All claims expressed in this article are solely those of the authors and do not necessarily represent those of their affiliated organizations, or those of the publisher, the editors and the reviewers. Any product that may be evaluated in this article, or claim that may be made by its manufacturer, is not guaranteed or endorsed by the publisher.
Barrera-Paez, J. D., Moraes, C. T. (2022). Mitochondrial genome engineering coming-of-age. Trends Genet. 38, 869–880. doi: 10.1016/j.tig.2022.04.011
Chung, K. P., Zeng, Y. (2017). An overview of protein secretion in plant cells. Methods Mol. Biol. 1662, 19–32. doi: 10.1007/978-1-4939-7262-3_2
Cohen, S., Valm, A. M., Lippincott-Schwartz, J. (2018). Interacting organelles. Curr. Opin. Cell Biol. 53, 84–91. doi: 10.1016/j.ceb.2018.06.003
Dettmer, J., Hong-Hermesdorf, A., Stierhof, Y. D., Schumacher, K. (2006). Vacuolar h+-ATPase activity is required for endocytic and secretory trafficking in arabidopsis. Plant Cell 18, 715–730. doi: 10.1105/tpc.105.037978
Gomes, E., Shorter, J. (2019). The molecular language of membraneless organelles. J. Biol. Chem. 294, 7115–7127. doi: 10.1074/jbc.TM118.001192
Grefen, C., Blatt, M. R. (2008). SNAREs-molecular governors in signalling and development. Curr. Opin. Plant Biol. 11, 600–609. doi: 10.1016/j.pbi.2008.08.006
He, X. L., Sun, Y., Zhu, R. L. (2013). The oil bodies of liverworts: unique and important organelles in land plants. Crit. Rev. Plant Sci. 32, 293–302. doi: 10.1080/07352689.2013.765765
Kanazawa, T., Morinaka, H., Ebine, K., Shimada, T. L., Ishida, S., Minamino, N., et al. (2020). The liverwort oil body is formed by redirection of the secretory pathway. Nat. Commun. 11, 6152. doi: 10.1038/S41467-020-19978-1
Kang, B. H., Nielsen, E., Preuss, M. L., Mastronarde, D., Staehelin, L. A. (2011). Electron tomography of RabA4b- and PI-4Kbeta1-labeled trans golgi network compartments in arabidopsis. Traffic 12, 313–329. doi: 10.1111/j.1600-0854.2010.01146.x
Kwon, C., Lee, J. H., Yun, H. S. (2020). SNAREs in plant biotic and abiotic stress responses. Mol. Cells 43, 501–508. doi: 10.14348/molcells.2020.0007
Lam, S. K., Siu, C. L., Hillmer, S., Jang, S., An, G. H., Robinson, D. G., et al. (2007). Rice SCAMP1 defines clathrin-coated, trans-golgi-located tubular-vesicular structures as an early endosome in tobacco BY-2 cells. Plant Cell 19, 296–319. doi: 10.1105/tpc.106.045708
Li, S. H., Yan, H., Mei, W. M., Tse, Y. C., Wang, H. (2020). Boosting autophagy in sexual reproduction: a plant perspective. New Phytol. 226, 679–689. doi: 10.1111/nph.16414
Lippincott-Schwartz, J., Roberts, T. H., Hirschberg, K. (2000). Secretory protein trafficking and organelle dynamics in living cells. Annu. Rev. Cell Dev. Biol. 16, 557–589. doi: 10.1146/annurev.cellbio.16.1.557
Lomonossoff, G. P., D’Aoust, M. A. (2016). Plant-produced biopharmaceuticals: a case of technical developments driving clinical deployment. Science 353, 1237–1240. doi: 10.1126/science.aaf6638
Luo, N., Yan, A., Liu, G., Guo, J. Z., Rong, D. Y., Kanaoka, M. M., et al. (2017). Exocytosis-coordinated mechanisms for tip growth underlie pollen tube growth guidance. Nat. Commun. 8, 1687. doi: 10.1038/s41467-017-01452-0
Mathur, J. (2020). Review: morphology, behaviour and interactions of organelles. Plant Sci. 301, 110662. doi: 10.1016/j.plantsci.2020
McKay, D. W., McFarlane, H. E., Qu, Y., Situmorang, A., Gilliham, M., Wege, S. (2022). Plant trans-golgi Network/Early endosome pH regulation requires cation chloride cotransporter (CCC1). Elife 11, e70701. doi: 10.7554/eLife.70701
Miao, Y. S., Ding, Y., Sun, Q. Y., Xu, Z. F., Jiang, L. W. (2008). Plant bioreactors for pharmaceuticals. Biotechnol. Genet. Eng. Rev. 25, 363–380. doi: 10.5661/Bger-25-363
Michoux, F., Ahmad, N., McCarthy, J., Nixon, P. J. (2011). Contained and high-level production of recombinant protein in plant chloroplasts using a temporary immersion bioreactor. Plant Biotechnol. J. 9, 575–584. doi: 10.1111/j.1467-7652.2010.00575.x
Møller, I. M., Rasmusson, A. G., Van Aken, O. (2021). Plant mitochondria - past, present and future. Plant J. 108, 912–959. doi: 10.1111/tpj.15495
Noack, L. C., Jaillais, Y. (2017). Precision targeting by phosphoinositides: how PIs direct endomembrane trafficking in plants. Curr. Opin. Plant Biol. 40, 22–33. doi: 10.1016/j.pbi.2017.06.017
Ou, J. Q., Guo, Z. B., Shi, J. N., Wang, X. H., Liu, J. R., Shi, B., et al. (2014). Transgenic rice endosperm as a bioreactor for molecular pharming. Plant Cell Rep. 33, 585–594. doi: 10.1007/s00299-013-1559-2
Perico, C., Sparkes, I. (2018). Plant organelle dynamics: cytoskeletal control and membrane contact sites. New Phytol. 220, 381–394. doi: 10.1111/nph.15365
Reyes, F. C., Buono, R., Otegui, M. S. (2011). Plant endosomal trafficking pathways. Curr. Opin. Plant Biol. 14, 666–673. doi: 10.1016/j.pbi.2011.07.009
Richter, S., Voss, U., Jürgens, G. (2009). Post-golgi traffic in plants. Traffic 10, 819–828. doi: 10.1111/j.1600-0854.2009.00916.x
Romani, F., Banic, E., Florent, S. N., Kanazawa, T., Goodger, J. Q. D., Mentink, R. A., et al. (2020). Oil body formation in marchantia polymorpha is controlled by MpC1HDZ and serves as a defense against arthropod herbivores. Curr. Bio 30, 2815–2828. doi: 10.1016/j.cub.2020.05.081
Romani, F., Flores, J. R., Tolopka, J. I., Suarez, G., He, X. L., Moreno, J. E. (2022). Liverwort oil bodies: diversity, biochemistry, and molecular cell biology of the earliest secretory structure of land plants. J. Exp. Bot. 73, 4427–4439. doi: 10.1093/jxb/erac134
Rosquete, M. R., Drakakaki, G. (2018). Plant TGN in the stress response: a compartmentalized overview. Curr. Opin. Plant Biol. 46, 122–129. doi: 10.1016/j.pbi.2018.09.003
Saftig, P., Klumperman, J. (2009). Lysosome biogenesis and lysosomal membrane proteins: trafficking meets function. Nat. Rev. Mol. Cell Biol. 10, 623–635. doi: 10.1038/nrm2745
Scheuring, D., Viotti, C., Krüger, F., Künzl, F., Sturm, S., Bubeck, J., et al. (2011). Multivesicular bodies mature from the trans-golgi Network/Early endosome in arabidopsis. Plant Cell 23, 3463–3481. doi: 10.1105/tpc.111.086918
Shimizu, Y., Takagi, J., Ito, E., Ito, Y., Ebine, K., Komatsu, Y., et al. (2021). Cargo sorting zones in the trans-golgi network visualized by super-resolution confocal live imaging microscopy in plants. Nat. Commun. 12, 1901. doi: 10.1038/S41467-021-22267-0
Uemura, T., Ueda, T. (2014). Plant vacuolar trafficking driven by RAB and SNARE proteins. Curr. Opin. Plant Biol. 22, 116–121. doi: 10.1016/j.pbi.2014.10.002
Won, K. H., Kim, H. (2020). Functions of the plant qbc SNARE SNAP25 in cytokinesis and biotic and abiotic stress responses. Mol. Cells 43, 313–322. doi: 10.14348/molcells.2020.2245
Yang, J., Yang, X., Su, T., Hu, Z., Zhang, M. (2022). The development of mitochondrial gene editing tools and their possible roles in crop improvement for future agriculture. Adv. Genet. 3, 2100019. doi: 10.1002/ggn2.202100019
Yun, H. S., Kwon, C. (2017). Vesicle trafficking in plant immunity. Curr. Opin. Plant Biol. 40, 34–42. doi: 10.1016/j.pbi.2017.07.001
Keywords: plant organelle, organelle biogenesis and trafficking, SNARE, Golgi structure, oil body (OB), mitogenome, vesicle traffic
Citation: Neuhaus J-M, Pimpl P, Zhao Q and Wang H (2023) Editorial: Regulation of plant organelle biogenesis and trafficking. Front. Plant Sci. 14:1211807. doi: 10.3389/fpls.2023.1211807
Received: 25 April 2023; Accepted: 02 May 2023;
Published: 26 May 2023.
Edited and Reviewed by:
Jaideep Mathur, University of Guelph, CanadaCopyright © 2023 Neuhaus, Pimpl, Zhao and Wang. This is an open-access article distributed under the terms of the Creative Commons Attribution License (CC BY). The use, distribution or reproduction in other forums is permitted, provided the original author(s) and the copyright owner(s) are credited and that the original publication in this journal is cited, in accordance with accepted academic practice. No use, distribution or reproduction is permitted which does not comply with these terms.
*Correspondence: Jean-Marc Neuhaus, SmVhbi1NYXJjLk5ldWhhdXNAdW5pbmUuY2g=; Peter Pimpl, cGltcGxAc3VzdGVjaC5lZHUuY24=; Qiong Zhao, cXpoYW9AYmlvLmVjbnUuZWR1LmNu; Hao Wang, d2FuZ2hhb2h3QGdtYWlsLmNvbQ==
Disclaimer: All claims expressed in this article are solely those of the authors and do not necessarily represent those of their affiliated organizations, or those of the publisher, the editors and the reviewers. Any product that may be evaluated in this article or claim that may be made by its manufacturer is not guaranteed or endorsed by the publisher.
Research integrity at Frontiers
Learn more about the work of our research integrity team to safeguard the quality of each article we publish.