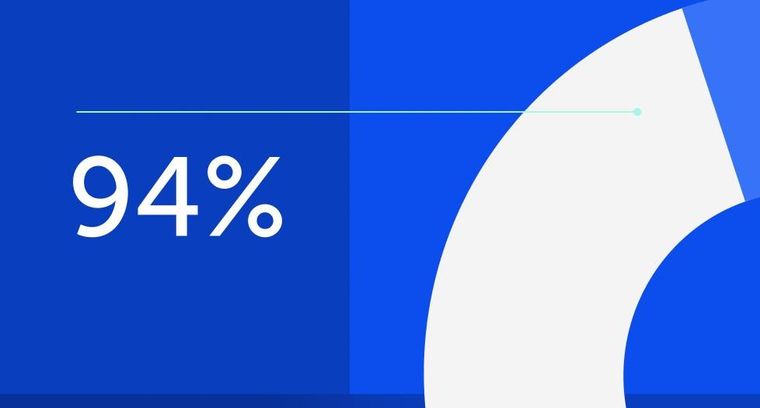
94% of researchers rate our articles as excellent or good
Learn more about the work of our research integrity team to safeguard the quality of each article we publish.
Find out more
ORIGINAL RESEARCH article
Front. Plant Sci., 08 August 2023
Sec. Plant Cell Biology
Volume 14 - 2023 | https://doi.org/10.3389/fpls.2023.1208285
This article is part of the Research TopicMeiosis in Plants: sexual reproduction, genetic variation and crop improvementView all 8 articles
Effective chromosome synapsis and crossover formation during meiosis are essential for fertility, especially in grain crops such as wheat. These processes function most efficiently in wheat at temperatures between 17-23 °C, although the genetic mechanisms for such temperature dependence are unknown. In a previously identified mutant of the hexaploid wheat reference variety ‘Chinese Spring’ lacking the long arm of chromosome 5D, exposure to low temperatures during meiosis resulted in asynapsis and crossover failure. In a second mutant (ttmei1), containing a 4 Mb deletion in chromosome 5DL, exposure to 13 °C led to similarly high levels of asynapsis and univalence. Moreover, exposure to 30 °C led to a significant, but less extreme effect on crossovers. Previously, we proposed that, of 41 genes deleted in this 4 Mb region, the major meiotic gene TaDMC1-D1 was the most likely candidate for preservation of synapsis and crossovers at low (and possibly high) temperatures. In the current study, using RNA-guided Cas9, we developed a new Chinese Spring CRISPR mutant, containing a 39 bp deletion in the 5D copy of DMC1, representing the first reported CRISPR-Cas9 targeted mutagenesis in Chinese Spring, and the first CRISPR mutant for DMC1 in wheat. In controlled environment experiments, wild-type Chinese Spring, CRISPR dmc1-D1 and backcrossed ttmei1 mutants were exposed to either high or low temperatures during the temperature-sensitive period from premeiotic interphase to early meiosis I. After 6-7 days at 13 °C, crossovers decreased by over 95% in the dmc1-D1 mutants, when compared with wild-type plants grown under the same conditions. After 24 hours at 30 °C, dmc1-D1 mutants exhibited a reduced number of crossovers and increased univalence, although these differences were less marked than at 13 °C. Similar results were obtained for ttmei1 mutants, although their scores were more variable, possibly reflecting higher levels of background mutation. These experiments confirm our previous hypothesis that DMC1-D1 is responsible for preservation of normal crossover formation at low and, to a certain extent, high temperatures. Given that reductions in crossovers have significant effects on grain yield, these results have important implications for wheat breeding, particularly in the face of climate change.
Like many plants, wheat (Triticum aestivum L) is highly sensitive to temperatures that fall outside the range typically experienced during a growing season. The optimum temperature range for wheat growth over an entire season is generally considered to be around 17-23 °C, with temperatures above or below this range significantly reducing grain yield (Porter and Gawith, 1999). The extent to which temperature stresses affect yield is dependent on developmental stage, with reproductive stages more sensitive to high temperatures than vegetative growth stages (Fischer and Maurer, 1976). Even quite short periods of high temperature (20-24 hours at 30 °C) during meiosis can reduce grain number (Saini and Aspinall, 1982; Draeger and Moore, 2017). Meiosis has also been identified as the stage most sensitive to low temperature stress (Thakur et al., 2010), with reductions in grain yield occurring when low temperatures coincide with the ‘booting’ stage, which broadly corresponds to meiosis (Ji et al., 2017). Bread wheat is an allohexaploid (2n = 6x = 42), made up of three related (homeologous) sub-genomes (A, B and D). During wheat meiosis, chromosome behaviour is tightly controlled by the TaZIP4-B2 (Ph1) gene, which promotes synapsis and crossover between homologous chromosomes (homologs), rather than between homeologous chromosomes (homeologs) from related genomes (Riley and Chapman, 1958; Sears and Okamoto, 1958; Martín et al., 2014 and Martín et al., 2017; Rey et al., 2017; Draeger et al., 2023). This allows the three genomes to behave as separate diploids during meiosis, thus maintaining genome stability and fertility.
Meiosis is a highly dynamic process during which parental chromosomes pair and recombine. It is essential for gamete formation in sexually reproducing organisms. During early meiosis, the parental chromosomes align alongside each other as pairs, enabling a proteinaceous structure, the synaptonemal complex (SC), to assemble between them, linking the chromosomes along their entire lengths in a process called synapsis (Page and Hawley, 2004). The SC is thought to provide the structural framework for meiotic recombination to take place. Recombination initiates by programmed double-strand breaks (DSBs) in the DNA. A small minority of these are repaired as crossovers, forming physical connections between each chromosome pair, enabling genetic information to be reciprocally exchanged, and potentially creating new advantageous allelic combinations. These physical connections can be seen cytologically as chiasmata.
Assembly of the SC completes at pachytene, and by diplotene crossovers are fully formed. The SC then disassembles, leaving homologs connected only by their chiasmata. At metaphase I, the homolog pairs align on the equatorial plate, and the chiasmata connecting the chromosomes can be seen using light microscopy. At this stage, in hexaploid wheat, the chromosomes of each parent are normally bound together as 21 bivalent pairs, most forming as ring bivalents with two chiasmata linking them, one in each arm, usually towards the distal ends. Occasionally, the homologs are bound together by chiasmata in one arm only, forming a rod bivalent. In wheat, on average, there are around 2.3 crossovers per homolog pair (Miller and Reader, 1985). At least one crossover (the ‘obligate’ crossover) must form between each bivalent pair to ensure accurate chromosome segregation and balanced gametes in daughter cells (Zickler and Kleckner, 1999; Jones and Franklin, 2006), which is vital for maintaining genome stability and fertility.
Assembly of the SC is a highly temperature-sensitive process (Bilgir et al., 2013). Both high and low temperatures can lead to disruption of synapsis, resulting in unpaired univalent chromosomes that segregate randomly or are lost completely (reviewed in Bomblies et al., 2015 and Morgan et al., 2017). Relatively small changes in temperature can alter the frequency and distribution of crossovers (Elliott, 1955; Dowrick, 1957; Bayliss and Riley, 1972a; Higgins et al., 2012). In wheat, high and low temperatures have been found to generally decrease chiasma frequency. In the wheat cultivar ‘Chinese Spring’, this temperature sensitivity is under the genetic control of a major gene located on chromosome 5D (Riley, 1966). In Chinese Spring plants lacking chromosome 5D, numbers of chiasmata progressively decrease as the temperature falls below the optimum range (Riley, 1966; Bayliss and Riley, 1972a). Chiasma frequency is greatly reduced at 15 °C, and pronounced chromosome pairing failure occurs at 12 °C, resulting in complete male sterility (Riley, 1966; Hayter and Riley, 1967). This reduction in chiasma frequency is due to failure of chromosome synapsis at zygotene, although the temperature-sensitive phase is earlier, during premeiotic interphase, prior to DNA synthesis (Bayliss and Riley, 1972b).
These studies led to the proposal that there must be a gene on chromosome 5D that stabilizes chromosome ‘pairing’ (synapsis) at low temperatures. This putative gene, named low-temperature pairing (Ltp) (Hayter and Riley, 1967), was further defined to the long arm of chromosome 5D (Hayter, 1969) and later renamed Ltp1 (Queiroz et al., 1991). In plants lacking chromosome 5D, chiasma frequency also appears to decrease progressively at temperatures of ~30°C and above (Bayliss and Riley, 1972a), suggesting that chromosome 5D may also be associated with tolerance to high temperatures. However, this suggestion was based on the scoring of a few cells only, because high temperature treatments for 3 days made the chromosomes too sticky for accurate scoring.
More recently, we used wheat (Chinese Spring) lines with terminal deletions of 5DL to delimit the Ltp1 locus to the proximal half of chromosome 5DL (Draeger et al., 2020). KASP markers specific to 5DL, that mapped within this delimited region, were used to screen ~2500 gamma-irradiated deletion lines, from which 16 plants were identified with 5DL deletions. Mapping and candidate gene identification were facilitated by resources including the Chinese Spring IWGSC RefSeq v1.0 genome assembly (International Wheat Genome Sequencing Consortium (IWGSC), 2018), the Wheat 820 K Axiom® Breeders’ Array probe set (Winfield et al., 2015) and the Ensembl Plants database (Bolser et al., 2016). The 16 mutant plants were then exposed to low temperature (13°C) for 7 days, during a period lasting from premeiotic interphase to early meiosis I. From this, we identified a deletion mutant with meiotic chromosomes exhibiting extremely high levels of asynapsis and chromosome univalence after the low temperature treatment. This was very similar to the phenotype previously described for Ltp1 when the whole of chromosome 5D was absent. Exposure of this same ltp1-like deletion mutant to 30°C for 24 hours during the same developmental period, also led to a reduced number of crossovers and increased univalence, although the effect was less pronounced than that observed following exposure to 13 °C. However, as the deletion had a clear effect on chromosome pairing at 30 °C as well as at 13 °C, we renamed the mutant line temperature tolerant meiosis 1 (ttmei1), to reflect its reduced tolerance to high temperature in addition to its loss of the low temperature pairing gene Ltp1.
Using KASP genotyping, we then mapped the ttmei1 deletion to a 4 Mb region of chromosome 5DL. Of the 41 genes deleted in this region, 18 were expressed during meiosis, of which 12 were high confidence genes. Of these, the strongest candidate for the observed effects on meiosis was the D-genome homeolog of the meiotic recombination gene DMC1 (TaDMC1-D1), which had a ten-fold higher expression level in meiotic tissues compared with non-meiotic. For the remaining 17 genes expressed during meiosis, expression was proportionally higher in non-meiotic tissues, and none had any previously known meiotic function. Moreover, TaDMC1-D1 was expressed most highly during early prophase I, which in wheat coincides with the ‘telomere bouquet’ stage, when synapsis is initiated (Martín et al., 2017). Consistent with this, in the ttmei1 mutant, synapsis was abnormal after exposure to 13 °C and did not complete. Therefore, we proposed that DMC1-D1 was probably responsible for the Ltp1/ttmei1 phenotype. DMC1 (Disrupted Meiotic cDNA 1) is a recombinase that plays a central role in meiotic recombination, performing homology search, strand invasion and strand exchange during repair of meiotic DSBs (Bishop et al., 1992). The process of strand invasion is fundamentally dynamic, which makes it vulnerable to disruption by temperature stress.
In the current study, we have developed a CRISPR dmc1-D1 mutant in the hexaploid wheat variety Chinese Spring. Until recently, very few wheat genotypes have been transformable, but the development of a GRF-GIF chimeric protein (Debernardi et al., 2020) has addressed this issue, and this technology, combined with our efficient transformation system (Hayta et al., 2019; Hayta et al., 2021), has allowed us to generate the first CRISPR mutants in Chinese Spring. Chinese Spring is used as the hexaploid wheat reference genome by most wheat researchers, as it has a fully annotated sequenced genome (International Wheat Genome Sequencing Consortium (IWGSC), 2018), with all data integrated into the Ensembl Plants database (Bolser et al., 2016). Thus, the successful transformation of Chinese Spring is a major step forward. We have also backcrossed ttmei1 mutants with wild-type Chinese Spring plants, to reduce background mutations. We have exposed the ttmei1 and CRISPR dmc1-D1 mutants to high (30 °C) and low (13 °C) temperatures during the sensitive period of premeiosis to meiosis I, to determine whether the D-genome copy of DMC1 has a stabilizing effect on chromosome synapsis and crossover in wheat.
Mutants for DMC1-D1 (TraesCS5D02G141200) and TTMEI1 were derived from the hexaploid wheat cultivar ‘Chinese Spring’ (Triticum aestivum L., 2n = 6x = 42; AABBDD), which was also used as a wild-type control. The CRISPR Tadmc1-D1 mutant was developed within the Crop Transformation Facility (BRACT) at the John Innes Centre, using RNA-guided Cas9. The Tattmei1 deletion mutant was generated previously by gamma irradiation (Draeger et al., 2020). Tattmei1 has a 4 Mb interstitial deletion on chromosome 5DL, with 41 genes deleted, including TaDMC1-D1. For the current study, ttmei1 M2 plants were backcrossed twice with Chinese Spring to produce Bc2F2 plants with fewer background mutations.
The genomic DNA sequences of the Chinese Spring TaDMC1 homeologs were aligned using the software Geneious Prime, version 2020.2.4 (Biomatters). Two guide RNAs, TaDMC1-D Guide1: 5’-GCTCATGGAGGCCGACCGGG-3’ and TaDMC1-D Guide2: 5’-CAAGCAGCTCATCAAGCGTT-3’, were designed to target the D genome copy of TaDMC1, and were cloned into a binary vector. Only the D-genome copy of DMC1 contained the PAM sequences utilised by the guide RNAs. Additionally, the guide RNA2 target sequence was D-genome specific, differing from the A- and B-genome sequences by 12 nucleotides. Supplementary Figure 1 shows an alignment of all three wheat DMC1 sequences, including positions of the guide RNAs and the D-genome specific PAM sequences.
The binary vector was prepared for wheat transformation using standard Golden Gate MoClo assembly (Werner et al., 2012; Smedley et al., 2021). Construct assembly incorporated GRF4-GIF1 technology (Debernardi et al., 2020). The guide RNAs were cloned between the TaU6 promoter and the guide scaffold for Streptococcus pyogenes Cas9 in the Level 1 acceptor plasmids pL1P3-TaU6 (Addgene #165599) and pL1P4-TaU6 (Addgene #165600), as described in Smedley et al. (2021). These level 1 plasmids were sequenced, before proceeding to the Level 2 assembly. Level 2 assembly was performed using the Level 2 acceptor pGoldenGreenGate-M (pGGG-M) (Addgene #165422) binary vector (Smedley et al., 2021). The Level 1 plasmids pL1P1OsActinP:hpt-int:35sT selection cassette (Addgene #165423), pL1P2OsUbiP : Cas9:NosT (Addgene #165424), pL1P5ZmUbiP : GRF-GIF : NosT (Addgene #198046) and guide cassettes were assembled into pGGG-M, along with end linker pELE-5 (Addgene #48020). The resulting plasmid was named pGGG-TaDMC1-D, and was sequenced to ensure authenticity before transferring to Agrobacterium.
The hypervirulent Agrobacterium tumefaciens strain AGL1 (Lazo et al., 1991) was used for the wheat transformation experiments. The pGGG-TaDMC1-D vector was electroporated into A. tumefaciens AGL1 competent cells and standard Agrobacterium inoculums prepared, as described previously (Hayta et al., 2021). Wheat transformation was performed with 10 mg L–1 hygromycin used for selection. Transgenesis was confirmed and transgene copy number analysis performed using Taqman qPCR and probe (Hayta et al., 2019). Values obtained were used to calculate transgene copy number, according to published methods (Livak and Schmittgen, 2001).
Six T0 lines were chosen for analysis, and 12 plants per T1 line grown and analysed. A further 32 plants (T2) from one T1 edited plant were analysed for the presence of gene edits. Two sets of primers were designed to amplify the two target regions within the TaDMC1-D1 CDS. For amplicon 1 (TaDMC1-D Guide1 target area), primers were TaDMC1-D F1 5′-GAGCGTGGGCTTGGTGTTAC-3′ and TaDMC1-D R1 5′-GAGGCGGAAGCACCCGGG-3′. For Amplicon 2 (TaDMC1-D Guide2 target area), TaDMC1-D F2 5′-TGCGATAGAATCTTCTGAAGTTTGTGTA-3′ and TaDMC1-D R2 5′-TCAATCCCT CCTTCAAATTACGC-3′ were used. PCR amplification was performed using GoTaq® Master Mix (Promega, M7122), with the following conditions: 3 min 94 °C, 40 cycles of 30 s at 94 °C, 15 s at 58 °C, 1 min at 72 °C and 5 min at 72 °C. Amplicons were Sanger sequenced directly (using their respective forward primers) by the Molecular Genetics Platform at the John Innes Centre. A and B homeologs of DMC1 were sequenced to ensure that no off-target editing had occurred in these copies.
Wild-type Chinese Spring and ttmei1 mutant plants were grown to the 2-3 leaf stage, and DNA extracted from leaf material, as in Draeger et al., 2020 (adapted from Pallotta et al., 2003). Final DNA template concentrations were between 15-30 ng. KASP genotyping was performed using 5D chromosome-specific KASP primers with homeologous SNPs at the 3′ end, previously selected from the Wheat Breeders’ 820 K Axiom® array (Winfield et al., 2015), available at www.cerealsdb.uk.net, and aligned with the Chinese Spring reference sequence assembly, IWGSC RefSeq v1.0, (International Wheat Genome Sequencing Consortium (IWGSC), 2018). Two KASP primers were used to identify the ttmei1 deletion region: BA00822801, based on a marker mapping proximal to DMC1-D1, and BA00750321, mapping distal to DMC1-D1. Primer sequences are shown in Table 1. The allele-specific forward primers and common reverse primers were synthesized by Merck https://www.merckgroup.com/. Allele-specific primers were synthesized with standard FAM or VIC compatible tails at their 5’ ends (see Table 1).
The KASP reaction and its components were as recommended by LGC Genomics Ltd and described at https://www.biosearchtech.com/support/education/kasp-genotyping-reagents/how-does-kasp-work.
Assays were set up as 5 μl reactions in a 384-well format, and included 2.5 μl genomic DNA template (15-30 ng of DNA), 2.5 μl of KASP 2x Master Mix (LGC Genomics) and 0.07 μl primer mix. Primer mix consisted of 12 μl of each tailed primer (100 μM), 30 μl common primer (100 μM) and 46 μl dH2O. PCR amplification was performed using the following programme: Hotstart at 94°C for 15 min, followed by ten touchdown cycles (94°C for 20 s; touchdown from 65-57°C for 1 min, decreasing by 0.8°C per cycle), followed by 30 cycles of amplification (94°C for 20 s; 57°C for 1 min). Fluorescent signals from PCR products were read in a PHERAstar microplate reader (BMG LABTECH Ltd.). If tight genotyping clusters were not obtained, additional rounds of amplification were performed. Genotyping data was analysed using KlusterCaller software (LGC Genomics).
CRISPR dmc1-D1 and ttmei1 (Bc2F2) mutants and Chinese Spring control plants were initially grown in pots in a controlled environment room at 20°C day and 15°C night, with a 16-hour photoperiod and 70% humidity, until development of the main shoot or tiller to be sampled had progressed to Zadoks growth stage 39 (Zadoks et al., 1974; Tottman, 1987), when the flag leaf ligule was just visible and meiocytes were deemed to be at premeiotic interphase. At this stage, the immature spikes enclosed within the leaf sheaths were between 3.5-6.5 cm in length (average 4.8 cm). Plants were then transferred to growth cabinets under continuous light and exposed to a low temperature (13°C) for 6-7 days (with 70% humidity) or a high temperature (30°C) for 24 h (75% humidity). For the high temperature experiments, treatments were initiated at a similar time of day, between 11.00 and 11.30 am, with the plant pots placed in trays of water to prevent dehydration.
Immediately following treatment, to identify anthers with metaphase I meiocytes, one anther from each floret was stained with acetocarmine and squashed to extrude the meiocytes, which were then examined using a DM2000 light microscope (Leica Microsystems). As the three anthers within a floret are synchronized in meiotic development, when metaphase I chromosomes were identified in one anther, the two remaining anthers from the same floret were prepared for cytological analysis by Feulgen staining with Schiff’s reagent, as described by Draeger et al. (2020). Anthers were sampled from three plants of each genotype, and images of metaphase I chromosomes captured using a DM2000 microscope equipped with a DFC450 camera and controlled by LAS v4.4 system software (Leica Microsystems). Images were captured in up to 8 different focal planes to aid scoring.
For each plant, a minimum of 30 meiocytes were blind scored from digital images. This involved counting the following different meiotic chromosome configurations in each meiocyte: unpaired univalents (0 chiasmata), rod bivalents (1-2 chiasmata), ring bivalents (2-3 chiasmata), trivalents (2–3 chiasmata), tetravalents (3 chiasmata) and pentavalents (4 chiasmata). Previous studies have suggested that ‘double’ chiasmata may sometimes occur in these chromosome configurations (Gennaro et al., 2012; Dreissig et al., 2017). For example, in some bivalents it is difficult or impossible to distinguish whether one (single) chiasma or two (double) chiasmata are present in the same arm, and in such cases, the number of chiasmata can be interpreted according to the shape of the bivalent at metaphase I, as described in Sybenga, 1975. However, such interpretations can be subjective, so chiasma frequency per meiocyte was calculated separately using two different methods (as in Rey et al., 2018), with single chiasmata scores representing the minimum number of chiasmata per cell and double chiasmata scores representing the maximum. Figure 1 shows examples of the scored structures.
Figure 1 Representative images of Feulgen-stained metaphase I chromosomes from meiocytes of wild-type Chinese Spring, CRISPR dmc1-D1 and ttmei1 mutant plants treated at different temperatures. (A) wild type, (B) CRISPR dmc1-D1 and (C) ttmei1 at normal temperatures; (D) wild type, (E) CRISPR dmc1-D1 and (F) ttmei1 after 24 h at 30°C; (G) wild type, (H) CRISPR dmc1-D1 and (I) ttmei1 after 6-7 days at 13 °C. Examples of univalent chromosomes (univ), rod bivalents (rod), ring bivalents (ring), single chiasma (X) and possible double chiasmata (XX) are indicated with arrows; note complete univalence in CRISPR dmc1-D1 and ttmei1 mutants after treatment at 13 °C. Scale bar, 10 μm.
Statistical analyses were performed using STATISTIX 10.0 software (Analytical Software, Tallahassee, FL, USA). All treatments were analysed by the Kruskal–Wallis test (nonparametric one-way analysis of variance). Means were separated using Dunn’s test with a probability level of 0.05. Statistical analysis was carried out between genotypes (Table 2), and between temperatures (Table 3). A column chart (Figure 2), showing the effects of the temperature treatments, was plotted using Microsoft Excel (2016).
Table 2 Effects of genotype on meiotic metaphase I chromosomes of wild-type Chinese Spring, CRISPR Tadmc1-D1 and ttmei1 mutant plants after treatment at 20°C, 13°C and 30°C.
Table 3 Effects of three different temperature treatments on meiotic metaphase I chromosomes of wild-type Chinese Spring, CRISPR Tadmc1-D1 and ttmei1 mutant plants.
Figure 2 Column chart showing the effects of three different temperature treatments (20°C, 13°C and 30°C) on meiotic metaphase I chromosomes of three hexaploid wheat genotypes: Chinese Spring wild type (CS WT) plants, CRISPR dmc1-D1 mutants and ttmei1 mutants. The mean numbers of univalent chromosomes, rod bivalents and ring bivalents per meiocyte are shown, along with mean numbers of single chiasmata per meiocyte. Numbers of multivalent chromosomes and double chiasmata are not shown. Note reduced chiasma frequencies in dmc1-D1 and ttmei1 mutants at 30°C, and almost total univalence at 13°C.
From each of 6 selected T0 lines, 12 T1 lines were analysed for edits in the TaDMC1-D1 target region. Sanger sequencing revealed that none of these lines had a homozygous edit, but one T1 plant had a heterozygous edit in the target region. The edited T1 plant was self-fertilized, and 32 T2 progeny plants were sequenced. In 9 of the T2 generation plants, sequencing revealed a 39 bp homozygous deletion within the DMC1-D1 target region. Examples of the T2 sequence chromatograms are shown in Supplementary Figure 2. The sequences of the DMC1-A1 and DMC1-B1 copies were also analysed, and this confirmed that there were no off-target edits in these copies. The 9 T2 plants were used in the temperature treatment experiments: 3 plants were treated at 13°C for 6-7 days; 3 were treated at 30°C for 24 h; and 3 control plants remained under normal conditions of 20°C day, 15°C night.
Meiotic metaphase I chromosomes were blind scored in 3 plants of each genotype at each of 13°C, 30°C and normal temperatures. A minimum of 30 meiocytes were scored for each plant (at least 100 meiocytes per genotype). At normal temperatures, wild-type plants contained ~20 ring bivalents and a single rod bivalent per meiocyte as usual, with univalents occurring only occasionally (Figure 1A; Table 2; Figure 2). Mean numbers of single and double chiasmata were ~41 and ~44 respectively. However, under the same temperature conditions, in the dmc1-D1 and ttmei1 mutants, univalents (~4) and rod bivalents (6-7) occurred significantly more frequently than in the wild-type plants, whereas ring bivalents (12-13), single chiasmata (31-32) and double chiasmata (33-34) were significantly fewer than in the wild type (Figures 1B, C; Table 2; Figure 2). Chiasma/crossover frequency was thus reduced by 22-24% compared to the wild type. No multivalent chromosomes were observed at normal temperatures in either wild-type or dmc1-D1 meiocytes. In one ttmei1 mutant plant, two trivalents were observed, but this was not significantly different to the wild-type or dmc1-D1 scores.
After 24 hours at 30°C, in wild type plants, mean numbers of univalents per meiocyte remained the same as at normal temperatures (< 1), whereas numbers of rod bivalents increased from around one to around two, ring bivalents decreased from ~20 to ~19, and single and double chiasmata were correspondingly reduced, which were all significant differences, albeit small (Figures 1A, D; Table 3; Figure 2). In the dmc1-D1 mutants, numbers of univalents and rod bivalents increased from ~4 and ~6 respectively at normal temperatures to ~11 and ~8 respectively after treatment at 30°C; numbers of ring bivalents decreased from ~13 to ~7, single chiasmata numbers decreased from ~32 to ~23 (a reduction of ~27%) and double chiasmata from ~34 to ~26 (a reduction of ~25%), which were all significant differences (Figures 1B, E; Table 3; Figure 2). In ttmei1 mutants, numbers of univalents and rod bivalents also increased at 30°C, from ~4 to ~21 and from ~7 to ~8 respectively; ring bivalents decreased from 13 to ~3, single chiasmata dropped from ~31 to ~14 (a reduction of ~57%) and double chiasmata from ~33 to ~15 (a reduction of ~56%) (Figures 1C, F; Table 3; Figure 2). Again, all differences were significant. Although numbers of rod bivalents were similar in both mutants at 30°C, differences between numbers of univalents, ring bivalents and chiasmata at normal temperatures and at 30°C were larger in ttmei1 mutants than in dmc1-D1 mutants. At 30°C, a small but significant number of trivalents were observed in dmc1-D1 and ttmei1 mutants, but not in wild-type plants. Most of the trivalents were observed in a single ttmei1 plant. A single tetravalent and two pentavalents were observed in the same ttmei1 mutant, but this was not a significant difference. No tetravalents or pentavalents were observed in any other plants.
After 6-7 days treatment at 13°C, in wild-type plants, numbers of univalents, rod and ring bivalents and chiasmata per meiocyte were similar those seen at normal temperatures (Figures 1A, C; Table 3; Figure 2). However, in the dmc1-D1 mutants, after treatment at 13°C, there was a dramatic increase in the number of univalents from ~4 to ~39; ring bivalent numbers decreased from ~13 to almost none (< 1), rod bivalents decreased from ~6 to ~2 and chiasma frequency decreased dramatically from ~32 (single chiasmata) and ~34 (double chiasmata) to only ~2. Almost all observed chromosomes (~39 out of 42) were univalents (Figure 1H; Table 3; Figure 2). Scores for ttmei1 mutants were similar to those for dmc1-D1 mutants: 37 out of 42 chromosomes were univalents, there were almost no ring bivalents (< 1), around two rod bivalents and an average of only 2-3 chiasmata per meiocyte (Figure 1I; Table 3; Figure 2). This means that, at 13 °C, crossover was reduced by ~96% in dmc1-D1 mutants and by ~94% in ttmei1 mutants, compared to levels seen in wild-type plants at the same temperature. Furthermore, in dmc1-D1 mutants, exposing plants to 13 °C for 6-7 days reduced crossover by ~95% compared to levels observed at normal temperatures. In ttmei1 mutants, the reduction in crossover was 92%. No multivalent chromosomes were observed in any plants at 13°C.
Using RNA-guided Cas9, we have developed new CRISPR mutants containing a 39 bp deletion in the 5D copy of the DMC1 gene in the hexaploid wheat reference variety Chinese Spring. Until recently, wheat transformation has remained genotype dependent, therefore limiting the potential use of genomic tools such as CRISPR-Cas technologies. However, recent development and deployment of the morphological gene fusion GRF-GIF (Debernardi et al., 2020), coupled with our efficient and robust transformation system (Hayta et al., 2019; Hayta et al., 2021), has reduced genotype dependence in wheat and enabled us to report this first CRISPR-Cas9 targeted mutagenesis in Chinese Spring. These CRISPR dmc1-D1 mutants, along with backcrossed ttmei1 mutants (containing a 4 Mb deletion of DMC1-D1), were used to determine whether meiosis is stabilized by DMC1-D1 at high and/or low temperatures.
In wild type plants grown at normal temperatures, chromosomes aligned on the equatorial plate as normal, pairing as bivalents, mostly rings, but with the occasional rod bivalent (Figure 1A). However, in the ttmei1 and CRISPR dmc1-D1 mutants there were significantly more univalents and rod bivalents, and significantly fewer ring bivalents and chiasmata (Figures 1B, C; Table 2; Figure 2), with a reduction in chiasma (and therefore crossover) frequency of 22-24%. Two multivalents were observed in one ttmei1 mutant, but none in the other ttmei1 mutants or in any of the dmc1-D1 mutants, so the deletion of DMC1-D1 does not seem linked to the occurrence of multivalents.
Clearly, disruption of DMC1-D1 has a significant effect on meiosis, but this effect is not severe at normal temperatures, probably due to gene redundancy. There are two other homeologs of the DMC1 gene in hexaploid wheat: TaDMC1-A1 (TraesCS5A02G133000) on chromosome 5A and TaDMC1-B1 (TraesCS5B02G131900) on 5B. All three copies are expressed in wheat (Devisetty et al., 2010). As described in Draeger et al. (2020), the A- and D-genome copies of TaDMC1 are highly conserved when each gene copy is compared to that of its ancestral homeolog, suggesting that these copies are functional. The B-genome copy shows relatively lower conservation, primarily due to a substantial insertion of 294 bp within intron 7. However, this study also showed that, in Chinese Spring, homeologs of the DMC1 gene on chromosomes 5A and 5B are present and expressed, and although the expression level of DMC1-B1 is lower than in DMC1-D1, it is higher than in DMC1-A1, which suggests that the B-genome copy may also be functional. Furthermore, when grown under normal temperature conditions, dmc1 mutants in Arabidopsis, rice and barley show mostly univalents at meiotic metaphase I (Couteau et al., 1999; Wang et al., 2016; Colas et al., 2019). This indicates that the presence of a functional DMC1 gene is crucial for crossover formation. Since our dmc1-D1 mutants show only a slight reduction in crossovers at normal temperatures, this implies that at least one of the other DMC1 copies retains its functionality. However, the fact that our dmc1-D1 mutants have some meiotic defects at normal temperatures suggests that redundancy with the other DMC1 homeologs is only partial.
In the current study, Chinese Spring wild-type plants and dmc1-D1 and ttmei1 mutants were exposed to a low temperature treatment of 13 °C for 6-7 days during premeiotic interphase to early meiosis I. Exposure to the low temperature had no significant effect on metaphase I chromosomes in the wild type plants (Table 3, Figure 2), but in the CRISPR dmc1-D1 mutants, almost all chromosomes observed were univalents (Figure 1H), and crossover decreased by over 95% compared to that seen in the wild-type plants (Table 2, Figure 2). Similar results were obtained for the ttmei1 mutants (Figure 1I). In the dmc1-D1 mutants, exposure to the low temperature reduced crossover by ~95% compared to that observed at normal temperatures, and in the ttmei1 mutants crossover was reduced by 92%. The low chiasma frequencies and high numbers of univalent chromosomes observed in the dmc1-D1 and ttmei1 mutants at low temperatures were similar to those observed when the whole of chromosome 5D is deleted in Chinese Spring (Draeger et al., 2020).
This high number of univalent chromosomes suggests a major problem with crossover formation. Consistent with this, previously we showed that ttmei1 mutants exhibit significant abnormalities of synapsis at low temperatures (Draeger et al., 2020): after exposure to a low temperature of 13 °C, in both wild type and ttmei1 mutant meiocytes, synapsis initiates normally at one pole of the nucleus at early zygotene, but in the mutant, synapsis does not complete at pachytene. These experiments confirm our previous hypothesis that, in wheat, DMC1-D1 is responsible for the preservation of normal synapsis and crossover at lower temperatures, and is therefore equivalent to the Ltp1 locus first described by Hayter and Riley (1967), providing an answer to a question that has existed for over 55 years.
Most reported SC failures involve high temperatures, but, as in wheat, low-temperature failures have also been reported in Hyacinthus orientalis and two species of Solanum (Elliott, 1955; Karihaloo, 1991). Moreover, in the ectothermic Japanese red-bellied newt, Cynops pyrrhogaster, low temperatures during meiosis also give rise to univalent chromosomes, indicating failure of chromosome pairing due to asynapsis, and leading to abnormal spermatozoa production (Yazawa et al., 2003). DMC1 expression in C. pyrrhogaster also decreases under the same low-temperature conditions (8°C or 12°C), suggesting its low level of expression may contribute to the temperature-dependent abnormalities seen in spermatogenesis. This study supports our suggestion that DMC1 is involved in the maintenance of normal chromosome synapsis at low temperatures.
In the current study, exposure of dmc1-D1 mutants to a high temperature of 30 °C for just 24 hours during premeiotic interphase to meiosis I, resulted in a reduced number of crossovers and increased univalence, though to a lesser extent than that seen after a low temperature treatment of 13 °C. Similar results were obtained for ttmei1 mutants, although there was more variation between scores for individual plants. Previously, high variation between chiasma frequency scores was also observed in the original ttmei1 mutant plants (prior to backcrossing), following treatment at 30 °C for 24 hours (Draeger et al., 2020). It was suggested that this variation could be linked to a high level of background mutations due to gamma irradiation. In the current study, backcrossing these mutants with Chinese Spring for two generations (Bc2F2), should have substantially reduced the large numbers of undesirable background mutations, but variation between scores was still high, suggesting that this variation is less likely to be due to background mutations.
High variation between scores for different plants may have occurred if the heat applied reached the meiocytes at slightly different developmental stages or for longer or shorter durations. When Chinese Spring plants are grown at 20 °C under continuous light, meiosis is estimated to take around 24 hours to complete (Bennett et al., 1971; Bennett et al., 1973), although at higher temperatures (25 °C), meiosis is accelerated and completes in around 18 hours (Bennett et al., 1972). Although assigning meiosis to specific growth stages by assessing the external morphology of a plant is unreliable (Barber et al., 2015), in the current study, the 24-hour high temperature treatments should have been of sufficient duration to coincide with the temperature-sensitive period from premeiotic interphase to early meiosis I (as described in Bayliss and Riley, 1972b, and Draeger and Moore, 2017). Other studies have suggested that it might be difficult to deliver a heat stress treatment to cells such as meiocytes at a specific time, because within an anther they are surrounded by many different cell layers, such as the tapetum layer and the epidermis, which are able to buffer the high temperatures. However, in Arabidopsis thaliana, tracking the deposition of stress granules which form at elevated temperatures has demonstrated that the ambient temperature reaches the meiocytes in less than 15 minutes (De Jaeger-Braet et al., 2022). Even so, since the structures in wheat are much larger, it is still possible that a change in temperature may take longer to reach different meiocytes, due to their varying level of insulation according to the location of their anther within a spike.
TaDMC1-D1 expression is at its highest during early meiotic prophase I (Draeger et al., 2020), which in wheat, is when synapsis initiates at the ‘telomere bouquet’ stage (Martín et al., 2017). DMC1 has a central role in synapsis and homologous recombination. It is a meiosis-specific protein, structurally similar to the bacterial strand-exchange recombinase, RecA (Bishop et al., 1992). Homologous recombination is initiated by programmed DNA DSBs at leptotene, which results in single-stranded DNA ‘overhangs’ at the break sites. DMC1 and RAD51 (another RecA homolog) form helical nucleoprotein filaments by polymerizing on the single-stranded overhangs. These filaments perform homology searches and carry out strand invasion and strand exchange between homologous chromosomes (Neale and Keeney, 2006; reviewed in Brown and Bishop, 2015, and in Emmenecker et al., 2022). Repair of these interhomolog invasion events results in crossovers or non-crossovers, although only a small minority of DSBs are repaired as crossovers (reviewed in Lambing et al., 2017). RAD51 has a role in both somatic and meiotic repair, and is essential for maintaining chromosomal integrity in mitotic cells. DMC1 is the main catalytically active strand-exchange protein during meiosis, but it is also thought to suppress RAD51-mediated recombination in plant meiosis (Da Ines et al., 2022).
DMC1 homologs are found in a wide variety of organisms. In yeast (Saccharomyces cerevisiae) and mice, DMC1 deficiency results in defective meiotic recombination and chromosome synapsis, with cells arresting in prophase, leading to sterility (Bishop et al., 1992; Pittman et al., 1998; Yoshida et al., 1998; Bannister et al., 2007). Similarly, in wheat Tadmc1-D1 (ttmei1) mutants, synapsis does not complete, and meiosis appears to arrest before pachytene in late prophase, although this was after a treatment at 13°C (Draeger et al., 2020), whereas the phenotypes observed in yeast and mice were at ambient temperatures. Disruption of DMC1 also leads to sterility in most diploid plant species. Arabidopsis thaliana has a single copy of the DMC1 gene, and synapsis is disrupted in Atdmc1 mutants, which also show high levels of univalence, and drastically reduced fertility (Couteau et al., 1999). Rice (Oryza sativa) has two DMC1 homologs, OsDMC1A and OsDMC1B (Ding et al., 2001; Wang et al., 2016). A mutation in either one of these homologs does not lead to serious chromosome pairing defects, but in Osdmc1a Osdmc1b double mutants, synapsis is abnormal, crossover is greatly reduced, and there are high numbers of univalent chromosomes at metaphase I, leading to complete sterility (Wang et al., 2016). Barley (Hordeum vulgare) has a single DMC1 homolog, HvDMC1, and mutations in this gene lead to abnormal synapsis, multiple univalents and chromosome mis-segregation (Colas et al., 2019; Szurman-Zubrzycka et al., 2019). As in yeast and mice, disruption of the barley orthologue of DMC1 at ambient temperatures leads to a phenotype similar to that of Tadmc1 at low temperatures.
Based on our findings, it appears that TaDMC1-D1 in Chinese Spring, provides low temperature tolerance, and possibly high temperature tolerance. However, the underlying mechanism behind this phenomenon remains unclear. One possible explanation could be a transcription-related effect, where a low-level of DMC1 is sufficient under normal temperature conditions. However, when plants experience thermal stress, an increased level of DMC1 becomes necessary, and this requirement is fulfilled by the D-genome copy. Maintaining the proper dosage of meiotic proteins is crucial, as emerging evidence suggests that even “heterozygous mutants” can exhibit minor meiotic defects (Su et al., 2017). Previously, we found differences in gene expression levels between the three DMC1 homeologs in meiotic anthers, with DMC1-D1 having the highest meiotic gene expression levels and DMC1-A1 the lowest (Draeger et al., 2020). It is not yet known how the 5A and 5B copies contribute to meiosis, but these differences in gene expression could be related to differences in the abilities of these three genes to stabilize the genome at low temperatures.
Tetraploid wheat (AABB) has only two copies of DMC1 (Tang et al., 2017), but synapsis at 12°C is normal, despite the absence of chromosome 5D (Riley et al., 1966). This is probably due to the presence of a dominant Ltp allele on chromosome 5A, with a similar chromosome stabilizing activity to that of chromosome 5D in hexaploid wheat (Hayter and Riley, 1967). Interestingly, some other varieties and subspecies of wheat differ from Chinese Spring, in that the gene responsible for stabilizing chromosome pairing at low temperatures is located on chromosome 5A rather than 5D (Chapman and Miller, 1981). Future research will require development of dmc1-A1 and dmc1-B1 mutants, along with double and triple mutants in different hexaploid and tetraploid wheat varieties, to determine how each of the DMC1 homeologs contributes to stabilizing synapsis and crossover at high and low temperatures. This could be achieved using CRISPR/Cas9, which, in addition to its high specificity, can be used to simultaneously target multiple copies of a gene, a technology that has already enabled the production of loss-of-function triple wheat mutants (Taagen et al., 2020; Li et al., 2021). Recently, another genome editing system, transcription activator-like effector nucleases (TALENs), has been used to disrupt all six CmDMC1 loci in the hexaploid flower, Chrysanthemum morifolium (Shinoyama et al., 2020).
Different dosages of the TaDMC1 alleles can affect the stability of synapsis and crossover at low temperatures. In Chinese Spring plants lacking DMC1-D1, the chromosome 5A and 5B homeologs DMC1-A1 and DMC1-B1, are unable to compensate for the lack of DMC1-D1, and cannot stabilize synapsis and crossover at low temperatures. In a previous study, even when chromosome 5B was present as a double dose in Chinese Spring plants, it was still unable to compensate for the lack of 5D, but when chromosome 5A was present as a double dose, chromosome synapsis at 12°C was normal (Riley et al., 1966). Since TaDMC1-A1 has the lowest meiotic gene expression of the three DMC1 homeologs in Chinese Spring, this suggests that when DMC1-A1 is present as a double dose, an increase in its expression compensates for the loss of DMC1-D1 to preserve low temperature tolerance. This supports our suggestion that DMC1-A1 is functional and shows the importance of gene expression in stabilizing synapsis and crossover at low temperatures. Since the Tadmc1-D1 mutation makes wheat less tolerant to low and high temperatures, it is possible that overexpression of TaDMC1-D1 might make wheat more tolerant to variations in temperature above and below the normal range. This would be a useful addition to the array of tools being developed to combat the effects of climate change on wheat crop production.
Climate change is likely to have a negative effect on meiosis, and therefore on wheat fertility and ultimately crop yields, so screening of germplasm collections to identify heat-tolerant genotypes is a high priority for future crop improvement. Moving forward, it will be important to determine the relative meiotic temperature tolerance of plants carrying these specific TaDMC1 alleles growing under natural conditions. To further our understanding of the mechanisms by which DMC1 could be involved in stabilizing crossovers under temperature stress, it would be very informative to carry out future experiments involving transcription analysis and immunolocalization of DMC1 under normal conditions and after cold and heat stress.
For example, if the transcription levels of the DMC1-A1 and/or DMC1-B1 copies decreases under temperature stress in the dmc1-D1 mutant, it is likely that DMC1 foci would also be reduced, suggesting a direct transcription-related effect. However, if transcription of the A and B copies remains unchanged between normal and stress conditions, but the number of DMC1 foci decreases, this could indicate a defect in nucleoprotein filament formation, associated with the stressful environment. These results should be compared or evaluated in relation to the expression levels of DMC1-D1 and the number of DMC1 foci in Chinese Spring. Such analyses can provide us with a better understanding of the dynamics and dosage effect of the different TaDMC1 copies, and serve as a starting point for investigating the molecular mechanisms underlying DMC1’s role in stabilizing crossovers during episodes of temperature stress.
The datasets presented in this study can be found in online repositories. The names of the repository/repositories and accession number(s) can be found below: NCBI Biosample database (http://www.ncbi.nlm.nih.gov/biosample) under accession number PRJNA975063.
TD grew and maintained the plants, made the crosses, carried out the KASP genotyping, sampled anthers and collected metaphase I images for scoring the phenotype, produced the corresponding figure and wrote the manuscript. M-DR scored chromosome crossover, performed the statistical analysis and produced the column chart. SH and MS developed the CRISPR Tadmc1-D1 mutant in ‘Chinese Spring’ using RNA-guided Cas9 and produced the supplementary figures; GM provided the concept, and with AM, provided thoughts and guidance, and revised and edited the manuscript. All authors contributed to the article and approved the submitted version.
This work was supported by the UK Biological and Biotechnology Research Council (BBSRC) through a grant as part of the ‘Designing Future Wheat’ (DFW) Institute Strategic Programme (BB/P016855/1) and response mode grant (BB/R0077233/1). MD-R thanks the contract “Ayudas Juan de la Cierva-Formación (FJCI-2016-28296)” of the Spanish Ministry of Science, Innovation and Universities.
We thank the following John Innes Centre staff members: Martha Clarke of the Crop Transformation facility for her excellent technical support in the development of the CRISPR dmc1-D1 mutant; Saleha Bakht, Molecular Genetics Platform Manager for sequencing services; the Germplasm Resource Unit for providing seed and the Horticultural Services Department for plant maintenance. We also acknowledge The International Atomic Energy Agency, Vienna for gamma irradiation of Chinese Spring seed to produce the original ttmei1 mutant.
The authors declare that the research was conducted in the absence of any commercial or financial relationships that could be construed as a potential conflict of interest.
All claims expressed in this article are solely those of the authors and do not necessarily represent those of their affiliated organizations, or those of the publisher, the editors and the reviewers. Any product that may be evaluated in this article, or claim that may be made by its manufacturer, is not guaranteed or endorsed by the publisher.
The Supplementary Material for this article can be found online at: https://www.frontiersin.org/articles/10.3389/fpls.2023.1208285/full#supplementary-material
Bannister, L. A., Pezza, R. J., Donaldson, J. R., de Rooij, D. G., Schimenti, K. J., Camerini-Otero, R. D., et al. (2007). A dominant, recombination-defective allele of Dmc1 causing male-specific sterility. PloS Biol. 5, e105. doi: 10.1371/journal.pbio.0050105
Barber, H. M., Carney, J., Alghabari, F., Gooding, M. J. (2015). Decimal growth stages for precision wheat production in changing environments? Ann. Appl. Biol. 166, 355–371. doi: 10.1111/aab.12207
Bayliss, M., Riley, R. (1972a). An analysis of temperature-dependent asynapsis in Triticum aestivum. Genet. Res. 20 (2), 193–200. doi: 10.1017/S0016672300013707
Bayliss, M., Riley, R. (1972b). Evidence of premeiotic control of chromosome pairing in Triticum aestivum. Genet. Res. 20 (2), 201–212. doi: 10.1017/S0016672300013719
Bennett, M. D., Chapman, V., Riley, R. (1971). The duration of meiosis in pollen mother cells of wheat, rye and Triticale. Proc. R. Soc Lond. B. 178, 259–275. doi: 10.1098/rspb.1971.0065
Bennett, M. D., Rao, M. K., Smith, J. B., Bayliss, M. W. (1973). The duration of meiosis in pollen mother cells of wheat, rye and Triticale. Phil. Trans. R. Soc B. 266, 39–81. doi: 10.1098/rspb.1971.0065
Bennett, M. D., Smith, J. B., Kemble, R. (1972). The effect of temperature on meiosis and pollen development in wheat and rye. Can. J. Genet. Cytol. 14 (3), 615–624. doi: 10.1139/g72-076
Bilgir, C., Dombecki, C. R., Chen, P. F., Villeneuve, A. M., Nabeshima, K. (2013). Assembly of the synaptonemal complex is a highly temperature-sensitive process that is supported by PGL-1 during Caenorhabditis elegans meiosis. G3-Genes Genom. Genet. 3, 585–595. doi: 10.1534/g3.112.005165
Bishop, D. K., Park, D., Xu, L., Kleckner, N. (1992). DMC1: a meiosis-specific yeast homolog of E. coli recA required for recombination, synaptonemal complex formation, and cell cycle progression. Cell 69, 439–456. doi: 10.1016/0092-8674(92)90446-J
Bolser, D., Staines, D. M., Pritchard, E., Kersey, P. (2016). “Ensembl plants: integrating tools for visualizing, mining, and analyzing plant genomics data,” in Ed. Edwards, D. Plant Bioinformatics. Methods Mol. Biol., vol. vol 1374. (New York, NY: Humana Press). doi: 10.1007/978-1-4939-3167-5_6
Bomblies, K., Higgins, J. D., Yant, L. (2015). Meiosis evolves: adaptation to external and internal environments. New Phytol. 208, 306–323. doi: 10.1111/nph.13499
Brown, M. S., Bishop, D. K. (2015). DNA strand exchange and RecA homologs in meiosis. Cold Spring Harb. Perspect. Biol. 7, a016659. doi: 10.1101/cshperspect.a016659
Chapman, V., Miller, T. E. (1981). The location of a gene affecting meiotic chromosome pairing at low temperature in Triticum aestivum. Z. Pflanzenzüchtg 86, 50–55.
Colas, I., Barakate, A., Macaulay, M., Schreiber, M., Stephens, J., Vivera, S., et al. (2019). desynaptic5 carries a spontaneous semi-dominant mutation affecting Disrupted Meiotic cDNA 1 in barley. J. Exp. Bot. 70, 2683–2698. doi: 10.1093/jxb/erz080
Couteau, F., Belzile, F., Horlow, C., Grandjean, O., Vezon, D., Doutriaux, M.-P. (1999). Random Chromosome Segregation without Meiotic Arrest in Both Male and Female Meiocytes of a dmc1 Mutant of Arabidopsis. Plant Cell. 11 (9), 1623–1634. doi: 10.1105/tpc.11.9.1623
Da Ines, O., Bazile, J., Gallego, M. E., White, C. I. (2022). DMC1 attenuates RAD51-mediated recombination in Arabidopsis. PloS Genet. 18 (8), e1010322. doi: 10.1371/journal.pgen.1010322
Debernardi, J. M., Tricoli, D. M., Ercoli, M. F., Hayta, S., Ronald, P., Palatnik, J. F., et al. (2020). A GRF–GIF chimeric protein improves the regeneration efficiency of transgenic plants. Nat. Biotechnol. 38, 1274–1279. doi: 10.1038/s41587-020-0703-0
De Jaeger-Braet, J., Krause, L., Buchholz, A., Schnittger, A. (2022). Heat stress reveals a specialized variant of the pachytene checkpoint in meiosis of Arabidopsis thaliana. Plant Cell 34, 433–454. doi: 10.1093/plcell/koab257
Devisetty, U. K., Mayes, K., Mayes, S. (2010). The RAD51 and DMC1 homoeologous genes of bread wheat: cloning, molecular characterization and expression analysis. BMC Res. Notes 3, 245. doi: 10.1186/1756-0500-3-245
Ding, Z., Wang, T., Chong, K., Bai, S. (2001). Isolation and characterization of OsDMC1, the rice homologue of the yeast DMC1 gene essential for meiosis. Sex Plant Reprod. 13, 285. doi: 10.1007/s004970100065
Dowrick, G. (1957). The influence of temperature on meiosis. Heredity 11, 37–49. doi: 10.1038/hdy.1957.4
Draeger, T., Martín, A. C., Alabdullah, A. K., Pendle, A., Rey, M. D., Shaw, P., et al. (2020). Dmc1 is a candidate for temperature tolerance during wheat meiosis. Theor. Appl. Genet. 133, 809–828. doi: 10.1007/s00122-019-03508-9
Draeger, T., Moore, G. (2017). Short periods of high temperature during meiosis prevent normal meiotic progression and reduce grain number in hexaploid wheat (Triticum aestivum L.). Theor. Appl. Genet. 130, 1785–1800. doi: 10.1007/s00122-017-2925-1
Draeger, T. N., Rey, M. D., Martín, A. C., Hayta, S., Smedley, M., Alabdullah, A. K., et al. (2023). ZIP4 is required for normal progression of synapsis and for over 95% of crossovers in wheat meiosis. Front. Plant Sci. 14. doi: 10.3389/fpls.2023.1189998
Dreissig, S., Fuchs, J., Himmelbach, A., Mascher, M., Houben, A. (2017). Sequencing of single pollen nuclei reveals meiotic recombination events at megabase resolution and circumvents segregation distortion caused by postmeiotic processes. Front. Plant Sci. 8. doi: 10.3389/fpls.2017.01620
Elliott, C. (1955). The effect of temperature on chiasma frequency. Heredity 9, 385–398. doi: 10.1038/hdy.1955.39
Emmenecker, C., Mézard, C., Kumar, R. (2022). Repair of DNA double-strand breaks in plant meiosis: role of eukaryotic RecA recombinases and their modulators. Plant Reprod. 36, 17–41. doi: 10.1007/s00497-022-00443-6
Fischer, R. A., Maurer, R. (1976). Crop temperature modification and yield potential in a dwarf spring wheat. Crop Sci. 16, 855–859. doi: 10.2135/cropsci1976.0011183X001600060031x
Gennaro, A., Forte, P., Panichi, D., Lafiandra, D., Pagnotta, M. A., D'Egidio, M. G., et al. (2012). Stacking small segments of the 1D chromosome of bread wheat containing major gluten quality genes into durum wheat: transfer strategy and breeding prospects. Mol. Breed. 30, 149–167. doi: 10.1007/s11032-011-9606-6
Hayta, S., Smedley, M. A., Clarke, M., Forner, M., Harwood, W. A. (2021). An efficient Agrobacterium-mediated transformation protocol for hexaploid and tetraploid wheat. Curr. Protoc. 1, e58. doi: 10.1002/cpz1.58
Hayta, S., Smedley, M. A., Demir, S. U., Blundell, R., Hinchliffe, A., Atkinson, N., et al. (2019). An efficient and reproducible Agrobacterium-mediated transformation method for hexaploid wheat (Triticum aestivum L.). Plant Methods 15, 121. doi: 10.1186/s13007-019-0503-z
Hayter, A. M. (1969). “Cytogenetics and cytochemistry of wheat species,” (Cambridge: Cambridge University). PhD thesis.
Hayter, A., Riley, R. (1967). Duplicate Genetic Activities affecting Meiotic Chromosome Pairing at Low Temperatures in Triticum. Nature 216, 1028–1029. doi: 10.1038/2161028a0
Higgins, J. D., Perry, R. M., Barakate, A., Ramsay, L., Waugh, R., Halpin, C., et al. (2012). Spatiotemporal asymmetry of the meiotic program underlies the predominantly distal distribution of meiotic crossovers in barley. Plant Cell 24 (10), 4096–4109. doi: 10.1105/tpc.112.102483
International Wheat Genome Sequencing Consortium (IWGSC) (2018). Shifting the limits in wheat research and breeding using a fully annotated reference genome. Science 362, 7191. doi: 10.1126/science.aar7191
Ji, H., Xiao, L., Xia, Y., Song, H., Liu, B., Tang, L., et al. (2017). Effects of jointing and booting low temperature stresses on grain yield and yield components in wheat. Agric. For. Meteorol. 243, 33–42. doi: 10.1016/j.agrformet.2017.04.016
Jones, G. H., Franklin, F. C. H. (2006). Meiotic crossing-over: Obligation and interference. Cell 126, 246–248. doi: 10.1016/j.cell.2006.07.010
Karihaloo, J. L. (1991). Desynapsis due to temperature stress in three species of Solanum L. Cytologia 56, 603–611. doi: 10.1508/cytologia.56.603
Lambing, C., Franklin, F. C. H., Wang, C.-J. R. (2017). Understanding and manipulating meiotic recombination in plants. Plant Physiol. 173, 1530–1542. doi: 10.1104/pp.16.01530
Lazo, G., Stein, P., Ludwig, R. (1991). A DNA transformation–competent Arabidopsis genomic library in Agrobacterium. Nat. Biotechnol 9, 963–967 doi: 10.1038/nbt1091-963
Li, J., Li, Y., Ma, L. (2021). Recent advances in CRISPR/Cas9 and applications for wheat functional genomics and breeding. aBIOTECH. 2 (4), 375–385. doi: 10.1007/s42994-021-00042-5
Livak, K. J., Schmittgen, T. D. (2001). Analysis of relative gene expression data using real-time quantitative PCR and the 2-ΔΔCT method. Methods 25, 402–408. doi: 10.1006/meth.2001.1262
Martín, A. C., Rey, M.-D., Shaw, P., Moore, G. (2017). Dual effect of the wheat Ph1 locus on chromosome synapsis and crossover. Chromosoma 126, 669–680. doi: 10.1007/s00412-017-0630-0
Martín, A. C., Shaw, P., Phillips, D., Reader, S., Moore, G. (2014). Licensing MLH1 sites for crossover during meiosis. Nat. Commun. 5, 4580. doi: 10.1038/ncomms5580
Miller, T. E., Reader, S. M. (1985). The effect of increased dosage of wheat chromosomes on chromosome pairing and an analysis of the chiasma frequencies of individual wheat bivalents. Can. J. Genet. Cytol. 27, 421–425. doi: 10.1139/g85-062
Morgan, C. H., Zhang, H., Bomblies, K. (2017). Are the effects of elevated temperature on meiotic recombination and thermotolerance linked via the axis and synaptonemal complex? Phil. Trans. R. Soc B. 372, 20160470. doi: 10.1098/rstb.2016.0470
Neale, M. J., Keeney, S. (2006). Clarifying the mechanics of DNA strand exchange in meiotic recombination. Nature 442, 153–158. doi: 10.1038/nature04885
Page, S. L., Hawley, R. S. (2004). The genetics and molecular biology of the synaptonemal complex. Ann. Rev. Cell Dev. Biol. 20, 525–558. doi: 10.1146/annurev.cellbio.19.111301.155141
Pallotta, M. A., Warner, P., Fox, R. L., Kuchel, H., Jefferies, S. J., Langridge, P. (2003). Marker assisted wheat breeding in the southern region of Australia. Proc. 10th Int. Wheat Genet. Symp. 2, 789–791.
Pittman, D. L., Cobb, J., Schimenti, K. J., Wilson, L. A., Cooper, D. M., Brignull, E., et al. (1998). Meiotic prophase arrest with failure of chromosome synapsis in mice deficient for Dmc1, a germline-specific RecA homolog. Mol. Cell 1, 697–705. doi: 10.1016/S1097-2765(00)80069-6
Porter, J. R., Gawith, M. (1999). Temperatures and the growth and development of wheat: a review. Eur. J. Agron. 10, 23–36. doi: 10.1016/S1161-0301(98)00047-1
Queiroz, A., Mello-Sampayo, T., Viegas, W. S. (1991). Identification of low temperature stabilizing genes, controlling chromosome synapsis or recombination, in short arms of chromosomes from the homoeologous group 5 of Triticum aestivum. Hereditas. 115, 37–41. doi: 10.1111/j.1601-5223.1991.tb00344.x
Rey, M.-D., Martín, A. C., Higgins, J., Swarbreck, D., Uauy, C., Shaw, P., et al. (2017). Exploiting the ZIP4 homologue within the wheat Ph1 locus has identified two lines exhibiting homoeologous crossover in wheat-wild relative hybrids. Mol. Breed. 37, 95. doi: 10.1007/s11032-017-0700-2
Rey, M.-D., Martín, A. C., Smedley, M., Hayta, S., Harwood, W., Shaw, P., et al. (2018). Magnesium increases homoeologous crossover frequency during meiosis in ZIP4 (Ph1 Gene) mutant wheat-wild relative hybrids. Front. Plant Sci. 9. doi: 10.3389/fpls.2018.00509
Riley, R. (1966). “Genotype-environmental interaction affecting chiasma frequency in Triticum aestivum,” in Chromosomes today, vol. vol 1 . Eds. Darlington, C. D., Lewis, K. R. (Edinburgh: Oliver & Boyd), 57–64.
Riley, R., Chapman, V. (1958). Genetic control of the cytologically diploid behaviour of hexaploid wheat. Nature 182, 713–715. doi: 10.1038/182713a0
Riley, R., Chapman, V., Young, R. M., Bellfield, A. M. (1966). Control of meiotic chromosome pairing by the chromosomes of homoeologous group 5 of Triticum aestivum. Nature 212, 1475–1477. doi: 10.1038/2121475a0
Saini, H. S., Aspinall, D. (1982). Abnormal sporogenesis in wheat (Triticum aestivum L.) induced by short periods of high temperature. Ann. Bot. 49 (6), 835–846. doi: 10.1093/oxfordjournals.aob.a086310
Sears, E. R., Okamoto, M. (1958). Intergenomic chromosome relationships in hexaploid wheat. Proc. 10th Int. Congr. Genet. 2, 258–259.
Shinoyama, H., Ichikawa, H., Nishizawa-Yokoi, A., Skaptsov, M., Toki, S. (2020). Simultaneous TALEN-mediated knockout of chrysanthemum DMC1 genes confers male and female sterility. Sci. Rep. 10, 16165. doi: 10.1038/s41598-020-72356-1
Smedley, M. A., Hayta, S., Clark, M., Harwood, W. A. (2021). CRISPR-Cas9 based genome editing in wheat. Curr. Protoc. 1, e65. doi: 10.1002/cpz1.65
Su, H., Cheng, Z., Huang, J., Lin, J., Copenhaver, G. P., Ma, H., et al. (2017). Arabidopsis RAD51, RAD51C and XRCC3 proteins form a complex and facilitate RAD51 localization on chromosomes for meiotic recombination. PloS Genet. 13 (5), e1006827. doi: 10.1371/journal.pgen.1006827
Szurman-Zubrzycka, M., Brygida, B., Stolarek-Januszkiewicz, M., Kwaśniewska, J., Szarejko, I., Gruszka, D. (2019). The dmc1 mutant allows an insight into the DNA double-strand break repair during meiosis in barley (Hordeum vulgare L.). Front. Plant Sci. 10. doi: 10.3389/fpls.2019.00761
Taagen, E., Bogdanove, A. J., Sorrells, M. E. (2020). Counting on crossovers: controlled recombination for plant breeding. Trends Plant Sci. 25 (5), 455–465. doi: 10.1016/j.tplants.2019.12.017
Tang, Y., Kang, H. Y., Tang, L., Diao, C. D., Li, D. Y., Zhu, W., et al. (2017). Phylogenetic analysis of tetraploid wheat based on nuclear DMC1 gene. Biochem. Syst. Ecol. 70, 239–246. doi: 10.1016/j.bse.2016.10.022
Thakur, P., Kumar, S., Malik, J. A., Berger, J. D., Nayyar, H. (2010). Cold stress effects on reproductive development in grain crops: an overview. Environ. Exp. Bot. 67, 429–443. doi: 10.1016/j.envexpbot.2009.09.004
Tottman, D. R. (1987). The decimal code for the growth stages of cereals, with illustrations. Ann. Appl. Biol. 110, 441–454. doi: 10.1111/j.1744-7348.1987.tb03275.x
Wang, H., Hu, Q., Tang, D., Liu, X., Du, G., Shen, Y., et al. (2016). OsDMC1 is not required for homologous pairing in rice meiosis. Plant Physiol. 171, 230–241. doi: 10.1104/pp.16.00167
Werner, S., Engler, C., Weber, E., Gruetzner, R., Marillonnet, S. (2012). Fast track assembly of multigene constructs using Golden Gate cloning and the MoClo system. Bioeng. Bugs. 3 (1), 38–43. doi: 10.4161/bbug.3.1.18223
Winfield, M. O., Allen, A. M., Burridge, A. J., Barker, G. L. A., Benbow, H. R., Wilkinson, P. A., et al. (2015). High-density SNP genotyping array for hexaploid wheat and its secondary and tertiary gene pool. Plant Biotechnol. J. 14, 1195–1206. doi: 10.1111/pbi.12485
Yazawa, T., Nakayama, Y., Fujimoto, K., Matsuda, Y., Abe, K., Kitano, T., et al. (2003). Abnormal spermatogenesis at low temperatures in the Japanese red-bellied newt, Cynops pyrrhogaster: Possible biological significance of the cessation of spermatocytogenesis. Mol. Reprod. Dev. 66, 60–66. doi: 10.1002/mrd.10328
Yoshida, K., Kondoh, G., Matsuda, Y., Habu, T., Nishimune, Y., Morita, T. (1998). The mouse RecA-like gene Dmc1 is required for homologous chromosome synapsis during meiosis. Mol. Cell 1, 707–718. doi: 10.1016/S1097-2765(00)80070-2
Zadoks, J. C., Chang, T. T., Konzak, C. F. (1974). A decimal code for the growth stages of cereals. Weed Res. 14, 415–421. doi: 10.1111/j.1365-3180.1974.tb01084.x
Keywords: hexaploid wheat, DMC1, LTP1, meiosis, crossover, high temperature, low temperature, CRISPR
Citation: Draeger TN, Rey M-D, Hayta S, Smedley M, Martin AC and Moore G (2023) DMC1 stabilizes crossovers at high and low temperatures during wheat meiosis. Front. Plant Sci. 14:1208285. doi: 10.3389/fpls.2023.1208285
Received: 18 April 2023; Accepted: 17 July 2023;
Published: 08 August 2023.
Edited by:
Chung-Ju Rachel Wang, Academia Sinica, TaiwanReviewed by:
Fangpu Han, Chinese Academy of Sciences (CAS), ChinaCopyright © 2023 Draeger, Rey, Hayta, Smedley, Martin and Moore. This is an open-access article distributed under the terms of the Creative Commons Attribution License (CC BY). The use, distribution or reproduction in other forums is permitted, provided the original author(s) and the copyright owner(s) are credited and that the original publication in this journal is cited, in accordance with accepted academic practice. No use, distribution or reproduction is permitted which does not comply with these terms.
*Correspondence: Tracie N. Draeger, dHJhY2llLmRyYWVnZXJAamljLmFjLnVr
Disclaimer: All claims expressed in this article are solely those of the authors and do not necessarily represent those of their affiliated organizations, or those of the publisher, the editors and the reviewers. Any product that may be evaluated in this article or claim that may be made by its manufacturer is not guaranteed or endorsed by the publisher.
Research integrity at Frontiers
Learn more about the work of our research integrity team to safeguard the quality of each article we publish.