- 1Anhui Provincial Engineering Laboratory for Efficient Utilization of Featured Resource Plants, College of Life Sciences, Huaibei Normal University, Huaibei, Anhui, China
- 2College of Agronomy & Resources and Environment, Tianjin Agricultural University, Tianjin, China
Drought is one of the major abiotic stresses limiting agricultural production, particularly for shallow-rooted plants like Pinellia ternata. It damages plants via oxidative burst, but this effect could be mitigated by catalase (CAT). However, no studies have been reported on CAT homologs in P. ternata, a drought-sensitive plant species. In the present study, a novel CAT gene, PtCAT2, was functionally characterized via overexpression in Arabidopsis and analysis of cis-elements in its promoter. The isolated CAT gene was 1479 bp and encoded a protein containing 242 amino acids. The protein contains the CAT activity motif and the heme-binding site of a typical CAT, and the subcellular analysis indicated that the protein localizes at the cytoplasm and membrane. Moreover, the quantitative real-time reverse transcription PCR indicated that PtCAT2 is expressed ubiquitously in P. ternata and is strongly induced by drought stress and abscisic acid (ABA) signals. PtCAT2 overexpression enhanced the drought tolerance of Arabidopsis, as shown by the 30% increase in plant survival and a five-fold- increase in CAT activity. Moreover, PtCAT2-transgenic plants increased superoxide dismutase and peroxidase activities and reduced malondialdehyde, membrane leakage, and hydrogen peroxide (H2O2) (P<0.05). Furthermore, PtCAT2-transgenic plants showed higher tolerance to oxidative stress caused by exogenous H2O2 and retained higher chlorophyll and water contents than the WT. The mitochondria function was better maintained as presented by the higher oxygen consumption rate in transgenics under drought stress (P<0.05). The endogenous CATs and drought response-related genes were also upregulated in transgenic lines under drought stress, indicating that PtCAT2 confers drought stress tolerance by enhancing the H2O2 scavenging ability of plants to maintain their membrane integrity. These results improve our understanding of the drought response mechanisms and provide a potential breeding strategy for P. ternata genetic improvement.
1 Introduction
Being sessile organisms, plants face various environmental stresses, including abiotic (such as drought, heat, salinity, and freezing) and biotic (such as fungi, bacteria, and viruses) factors, throughout their life cycles. Among these stresses, drought causes more annual loss in crop yield, making it the most limiting factor in agricultural production (Gupta et al., 2020; Yang and Qin, 2023). The shallow-rooted plants are sensitive to water deficit since their roots are distributed in the superficial soil layer.
Pinellia ternata is an Araceae plant species that has been broadly used in China, Japan, and Korea for thousands of years as a medicinal herb. Its tubers contain various bioactive compounds, including alkaloids, organic acids and lectins (Jin et al., 2012; Lee et al., 2016; Duan et al., 2019), conferring them with antitussive, antiemetic and anticancer activities (Lu et al., 2013). These multiple uses have ranked P. ternata among the top ten bulk Chinese medicinal materials (Zhang et al., 2021). Naturally, P. ternata grows in shaded environments. However, artificial cultivation has reduced the available shaded conditions for P. ternata growth. Thus, being a shallow-rooted plant, P. ternata suffers severe water deficit stress under artificial cultivation (Li et al., 2020; Zhou et al, 2020). Water deficit reduces tuber biomass, plant height, leaf area and propagation index (Chen et al., 2021), further decreasing productivity. Various plant growth regulators, including brassinolide (Guo et al., 2022), betaine (Zhang et al., 2014) and uniconazole (Cheng, 2010), have been applied to prevent the effects of water deficit in P. ternata production. However, the molecular mechanism underlying drought response in drought-sensitive plant species remains elusive.
Plants generate excessive reactive oxygen species (ROS), particularly the superoxide radical (O2-) and hydrogen peroxide (H2O2) (Kohli et al., 2019) under drought conditions, which, when not timely scavenged, accelerate the peroxidation of membrane lipids and even lead to cell death (Vijayaraghavareddy et al., 2022). Therefore, plants have evolved various ROS scavenging systems via enzymatic or non-enzymatic approaches (Mittler, 2017). Among the ROS, H2O2 is comparatively stable and diffuses freely in plants, severely threatening plant productivity under drought stress (Tian et al., 2016). Catalase (CAT, EC1.11.1.6), a tetrameric iron porphyrin protein present in all aerobically living organisms (Zaid and Wani, 2019), is an efficient ROS scavenger that directly catalyzes H2O2 degradation into O2 and H2O. This protects the plant cells against H2O2-caused membrane peroxidation. Therefore, modulating the CAT gene expression could be a promising approach for overcoming stress-caused H2O2 damage. Various studies have shown that plant CAT genes respond to various abiotic and biotic stresses (Yong et al., 2017; Sun et al., 2018); however, the responses are species-dependent. Moreover, the CATs responding to drought stress have not been fully investigated, particularly in drought-sensitive plants.
In this study, we functionally characterized a novel CAT gene, PtCAT2, through expression pattern analysis, subcellular location and overexpression analysis in Arabidopsis. The results will help understand the response mechanisms of P. ternata under water deficit conditions and developing drought-resistant germplasms.
2 Materials and methods
2.1 Plant materials and growth conditions
P. ternata (Thunb) Breit tubers were collected from the Experimental Farm of Huaibei University (E116.8, N34.0) and germinated in potted soil. The potted plants were kept at 25°C under a 16-h photoperiod and light intensity of 35 μmol m-2s-1. The leaves, roots, petioles, tubers and flowers were collected separately from the uniform-sized three-leaf stage seedlings treated with various abiotic stresses using previously published methods (Zhang et al., 2021). For temperature treatment, the potted plants were moved to RGC-1500C artificial climate incubators (Hefei Youke Instrument Equipment Co., Ltd, Hefei, China) at lower (4°C) and higher (40°C) temperatures, with those incubated at 25°C as control. The incubators had an illumination scheme of 16 h/8 h (light/dark) with a light intensity of 35 μmol m-2s-1. For other treatments, the three-leaf stage plants were rinsed in ddH2O and cultured in half-strength MS liquid medium containing 150 mM NaCl, 25% polyethylene glycol (PEG6000), or 100 μM ABA for salt, drought and abscisic acid (ABA) treatments, respectively, with those maintained in solely half-strength MS liquid medium as control. Whole plants were collected at 0, 6, 12, 24 and 72 h of treatment and rinsed in ddH2O. Three plants were bulked as one sample and three biological replicates were contained for each treatment. The sampled tissues or whole plants were frozen immediately in liquid nitrogen and preserved at –80°C for further analyses.
Arabidopsis Col-0 plants were grown in 2-L pots and cultured in RGC-1500C artificial climate incubators (Hefei Youke Instrument Equipment Co., Ltd, Hefei, China) at 22°C under a 16-h photoperiod and light intensity of 50 μmol m-2s-1.
2.2 Cloning of the PtCAT2 gene
A putative cDNA sequence of the PtCAT2 gene was obtained from our previously published full-length transcriptome data (Xue et al., 2019) and amplified using the primer pair PtCAT2-Full-F/PtCAT2-Full-R (Table S1). The PCR products were purified and cloned into pEASY-T1 Simple Cloning Vector (Transgen, Beijing, China). The PtCAT2 gene was also subjected to RT-PCR analysis at 94°C for 2 min, followed by 35 cycles of 94°C for 30 s, 58°C for 30 s, 72°C for 2 min, and a final extension at 72°C for 5 min.
2.3 Bioinformatics analysis of the PtCAT2 gene and its promoter
The open reading frame (ORF) of the PtCAT2 gene was obtained using the ORFfinder program (https://www.ncbi.nlm.nih.gov/orffinder/) and used to analyze the putative PtCAT2 protein sequence. The isoelectric point (pI), molecular weight (MW) and the grand average of hydropathicity (GRAVY) of the protein were then determined using the ExPASy ProtParam program (http://web.expasy.org/protparam/). Thereafter, the NCBI Conserved Domain (https://www.ncbi.nlm.nih.gov/Structure/cdd/wrpsb.cgi) and InterPro (http://www.ebi.ac.uk/interpro/) databases and the Motif Scan tool (http://myhits.isb-sib.ch/cgi-bin/motif_scan) were used to detect the catalytic active and heme-binding sites of PtCAT2. The subcellular localization of PtCAT2 was determined using the ProtComp (http://www.softberry.com/), CELLO (http://cello.life.nctu.edu.tw/) and PlantmPLoc (http://www.csbio.sjtu.edu.cn/bioinf/plant-multi/). Furthermore, SWISS-MODEL (http://swissmodel.expasy.org) was used to simulate the three-dimensional (3D) structure of PtCAT2. Functionally characterized CAT proteins (14) were used to construct the phylogenetic tree with the MEGA 7.0 program via the neighbor-joining method with 1000 bootstrap replicates.
The promoter sequence of PtCAT2 was obtained from our genomic data (unpublished data), and a 2 kb sequence upstream of its transcription initiation site (ATG) was analyzed using the PlantCARE program (http://bioinformatics.psb.ugent.be/webtools/plantcare/html/) to predict the promoter cis-elements.
2.4 Subcellular localization assay
The ORF fragment of PtCAT2 was amplified from the pEASY-T1-PtCAT2 vector using primers PtCAT2-EcoRI-F/PtCAT2-SmaI-R (Table S1). The amplified product was then transformed into the multiple cloning sites upstream of the green fluorescent protein (GFP) of the pCAMBIA1302 vector via double enzyme digestion and ligation. Thereafter, the obtained pCAMBIA1302-PtCAT2-GFP construct was verified by enzyme digestion, and the expression vector was transformed into onion cells according to a previously published method (Xu et al., 2014). The GFP fluorescence of the onion cells was then observed under a confocal microscope (FV1000SP, Olympus, Japan) 24 h later.
2.5 RNA isolation, cDNA synthesis and quantitative reverse transcription PCR analysis
RNA isolation and cDNA synthesis were performed according to our previously published method (Zhang et al., 2021). Briefly, RNA from P. ternata and Arabidopsis plants was extracted using the standard TRIzol method. The obtained RNA was used as the template for cDNA synthesis, conducted using the 5×All-In-One MasterMix transcription and AccuRT Genomic DNA Removal Kits (Cat#G492) (ABM, Richmond, BC, Canada), following the manufacturer’s instructions.
Quantitative real-time RT-PCR (qRT-PCR) was performed on an ABI7500 fast Real-Time PCR system (Applied Biosystems, Foster City, CA, USA) using a 2×AceQ qPCR SYBR Green Master Mix (Vazyme, Piscataway, NJ, USA). The ribosomal 18S gene (Pt18S) and β-tubulin (AtTUB) were used as internal controls for P. ternata and Arabidopsis, respectively, and the relative expression of the target genes was calculated using the 2−ΔΔCT method (Livak and Schmittgen, 2001). Three technical replicates and three biological replicates were used for each treatment. The primer sequences used for the qRT-PCR are listed in Supplementary (Table S1).
2.6 Arabidopsis transformation and selection of the homozygous lines
The PtCAT2 cDNA, amplified from the pEASY-T1-PtCAT2 vector using the primer pair PtCAT2-Smal I-F/PtCAT2-Xba I-R, was purified and inserted into the multiple cloning sites of the pCAMBIA2300-35S-GUS-CaMVterm vector (Duan et al., 2015). The obtained recombinant vector and the empty vector pCAMBIA2300-35S-GUS-CaMVterm were verified by double enzyme digestion and then transformed into Agrobacterium-tumefaciens strain EHA105. Thereafter, Arabidopsis (Col-0) was transformed with the Agrobacterium cells containing the vectors via the floral-dip method (Clough and Bent, 1998). The transgenic plants were screened by plating the surface-disinfected seeds onto half-strength MS media containing 10 mg·L-1 kanamycin, and the positive plants were transplanted into pot soil for seed harvesting. The seeds obtained from individual plants were then screened on 10 mg·L-1 kanamycin until the T3 homozygous lines were obtained.
2.7 Drought tolerance analysis of the transgenic plants
The T3 transgenic seeds were surface-disinfected, vernalized and germinated on half-strength MS media containing 25% (m/v) polyethylene glycol (PEG)-6000. Their germination rates were recorded, and the images were captured two weeks after the treatments.
For the water deficit treatment, 2-week-old seedlings from T3 homozygous transgenic Arabidopsis lines and WT were transplanted into six-hole plastic plates filled with a mixture of soil and vermiculite (3:1). Five plates containing 30 plants were included for each treatment in triplicate. The seedlings were maintained at 22°C under 70% humidity and a 16 h/8 h light/dark photoperiod and subjected to water deficit treatment after one week. Leaves were harvested after seven days of drought stress, frozen in liquid nitrogen, and preserved at −80°C for further analysis. After ten days of drought stress, the survival rates of the seedlings were recorded, and the images were captured.
2.8 Determination of ROS, antioxidant enzyme activity and membrane integrity
The activities of antioxidant enzymes superoxide dismutase (SOD), peroxidase (POD), CAT and the content of malondialdehyde (MDA) were measured as described previously (Tian et al., 2022). Histochemical staining with diaminobezidin (DAB) and nitrotetrazolium blue chloride (NBT) was performed using the method by Zhang et al. (2021). H2O2 and O2·- levels were analyzed by using the detection kits (Nanjing Jiancheng Bioengineering Institute, Jiangsu, China).
The electrolyte leakage (El) was measured following a previously published method (Zhang et al., 2021). Briefly, the leaves were sliced, immersed in deionized water, and incubated at room temperature on a shaker (20×g) for 1 h. The initial conductivities of the treatment (C1) and blank (CK1) samples were measured by a conductivity meter (DDSJ-318, INESA Scientific Instrument Co., Ltd., China) before boiling. Thereafter, the samples were boiled for 10 min and cooled down to room temperature, and their second conductivities (C2 and CK2) were recorded. The El was presented as the relative conductance (C) calculated using the following equation: C (%) = (C1 − CK1)/(C2 − CK2) × 100.
The water loss rate was measured by weighing the leaf samples at 0, 1, 2, 3, 4 and 5 h after their detachment from the stems, and the relative water loss rate was presented as the ratio of leaf weight at each time point relative to that at 0 h.
2.9 Mitochondria isolation and respiration assays
The mitochondria isolation and respiratory measurements were performed following a previously published method (Lyu et al., 2018). The leaves of two-week-old wild-type and transgenic Arabidopsis seedlings grown at 22°C were used to isolate the mitochondria. The respiration of the isolated mitochondria was determined using a Clark-type oxygen electrode (Hansatech, King’s Lynn, England). The reaction buffer contained 0.3 M mannitol, 10 mM TES-KOH (pH 7.5), 3 mM MgSO4, 10 mM NaCl, 5 mM KH2PO4 and 0.1% BSA. Succinate (SA) at 5 mM and NADH at 1 mM were the respiratory substrates. The buffer was added with 5 mM SA and 1 mM NADH, and then incubated at 25°C for reaction.
2.10 Measurement of chlorophyll content under oxidative stress
The exogenous H2O2-caused oxidative damage on chlorophyll was measured following the method by Tian et al. (2022). Briefly, whole leaves obtained from the same positions on 2-week-old transgenic and WT Arabidopsis plants were incubated in 10 mL solution containing 0, 0.5% and 1% H2O2 for 24 h at 25°C under a 16 h/8 h light/dark photoperiod. Thereafter, the images were captured, and the chlorophyll content of the leaves was determined, as described previously (Lichtenthaler, 1987).
2.11 Statistical analysis
Data were analyzed using the Statistical Analysis System software (IBM SPSS Statistics 21.0, IBM Corp., NY, USA), and the significant differences among treatments were determined using the Students’ t-test or Duncan’s multiple range test (P < 0.05). The data are presented as means ± SD values of three biological replicates (n=3).
3 Results
3.1 Bioinformatics analysis of the PtCAT2
From a P. ternata transcriptome data (Xue et al., 2019), 13 CAT homologs were identified. Of them, i1_HQ_Pts1_c90512/f3p3/1940 (annotated as PtCAT2), showed a remarkable drought-inducible expression profile (Figure S1). PtCAT2 cDNA consisted of 1479 bp long ORF (Figure S2) encoding 492 amino acids. Its molecular weight and isoelectric points were 57 KDa and 6.91, respectively. Moreover, the protein had an aliphatic index of 37.65 and a GRAVY of –0.570, suggesting that PtCAT2 is a hydrophilic protein. The sequence alignment analysis identified the CAT activity motif (FARERIPERVVHARGAS) and heme-binding site (RIFAYADTQ) of PtCAT2 at positions 54–70 and 344–352, respectively, consistent with the other analyzed CATs (Figure S3). This implies that PtCAT2 is a typical CAT.
Furthermore, the SWISS-MODEL analysis showed that the three-dimensional (3D) structure of PtCAT2 has four typical subunits (Figure S4). The PtCAT2 aligned with the template protein 4qol.1.A from amino acids 11 to 490, covering 97.36% of the template. Moreover, the PtCAT2 protein shared a high homology (48.54%) with the template protein, and it had a GMQE value and QMEAN Z-Score of 0.81 and –1.08, respectively, confirming the high reliability of the predicted structure.
Phylogenetic analysis suggested that CATs from Triticum monococcum, Triticum aestivum, Brassica oleracea, Saccharum hybrid, Zea mays, Oryza sativa, Setaria italica, Nelumbo nucifera, Selenicereus undatus, Arabidopsis thaliana, Hordeum vulgare, Sorghum bicolor and Aeluropus littoralis could be divided into two classes, consistent with previous publications (Sun et al., 2018). PtCAT2 clustered with class II CATs, though it did not have a high similarity with other CATs (the highest homology was 82% homology with the T. aestivum TaCAT) (Figure S5), demonstrating that PtCAT2 is a hypothetical class II CAT.
3.2 PtCAT2 expresses ubiquitously in P. ternata and is highly induced by drought and ABA
Quantitative real-time RT-PCR indicated that PtCAT2 was ubiquitously expressed in P. ternata (Figure 1A) and had a 5.5-fold higher expression in the leaves, followed by flowers (4.9-fold), tubers (4.1-fold) and petioles (2.8-fold), compared with that in roots.
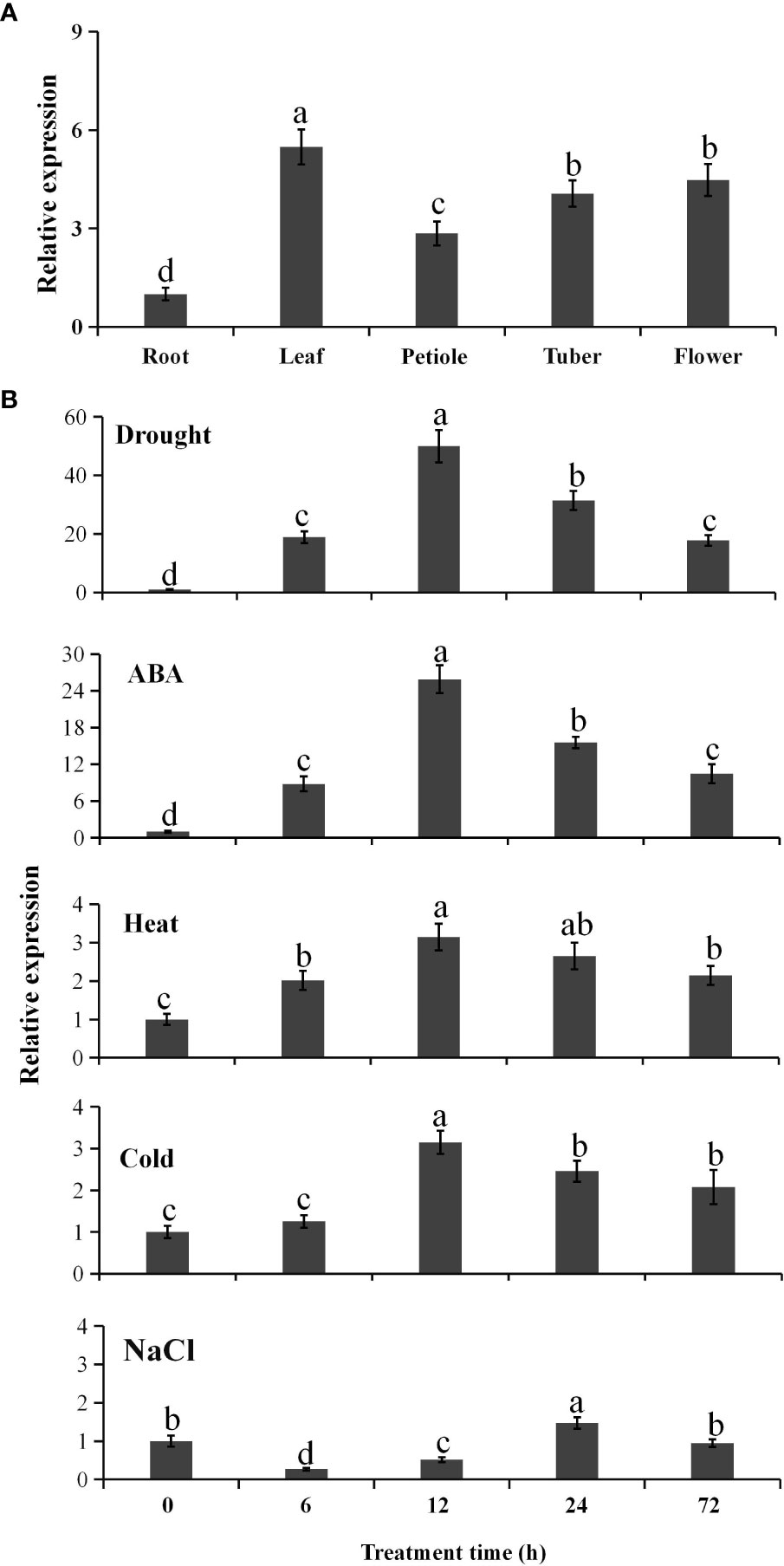
Figure 1 Spatial expression profile of PtCAT2 and its inducible expression pattern under abiotic stresses. (A) The root, leaf, petiole, tuber, and flower from two-month-old Pinellia ternata plants (three-leaf stage) were collected for analysis. The relative expressions in each tissue were calculated using the 2−ΔΔCT method. (B) The 3-week-old seedlings were cultured in 1/2 MS liquid medium containing 150 mM NaCl or 25% polyethylene glycol (PEG6000) or 100 μM abscisic acid (ABA) for salt, drought, and ABA treatment respectively. The potted 3-week-old seedlings exposed to 4°C or 40°C for cold or heat stress respectively. Whole seedlings were collected at the indicated time points for RNA isolation. Pt18SrRNA was used as an internal control to normalize the relative expressions. Values are presented as means ± SD (n = 3). Different lower cases indicate significant differences at P<0.05.
To identify the abiotic stress response of PtCAT2, we exposed the P. ternata plants to various abiotic stresses and used qRT-PCR to analyze the relative expression level of PtCAT2. When stressed with 25% PEG, we observed that the PtCAT2 mRNA level significantly increased within 72 h of the treatment, with the expression peak at 12 h (a 51-fold induction) (Figure 1B). PtCAT2 was also remarkably induced by 100 μM ABA, with a 25-fold expression at 12 h. The PtCAT2 mRNA abundance also increased to some extent when treated with salt (150 mM NaCl), low temperature (4°C) and heat (40°C); however, the inductions were less than 3.5-fold (Figure 1B). Thus, PtCAT2 could be remarkably inducible by drought and ABA but not by the other abiotic stresses tested in this study.
To further analyze the drought-induced expression of PtCAT2, we used the PlantCARE software to predict the possible acting cis-elements in its promoter. We identified various cis-elements in the PtCAT2 promoter (Figure S6), which regulate its response to abiotic stresses. Among these were three MYCCONSENSUSAT (CANNTG), two MYCATRD22 (CACATG) and one ABRE, which may be involved in drought and ABA response. There were also GT, ACGTATERD1 and GAATTC cis-elements, which may be associated with the weak response of PtCAT2 to salt, cold and heat stresses. These results imply that the significant induction of PtCAT2 under drought could be attributed to MYCCONSENSUSAT or MYCATRD22 and probably mediated by ABA.
3.3 Subcellular location of PtCAT2
The plant expression vector harboring the fused PtCAT2-GFP cassete (Figure S7) was transformed into onion epidermal cells. The fluorescence results showed the presence of GFP signals in the cell membrane and cytoplasm, not in the nucleus (Figure 2). This is consistent with the results of the protein subcellular localization prediction tool (PSORT), which showed a 78.7% probability of localization in the cytoplasm and 4.3% of localization in the plasma membrane.
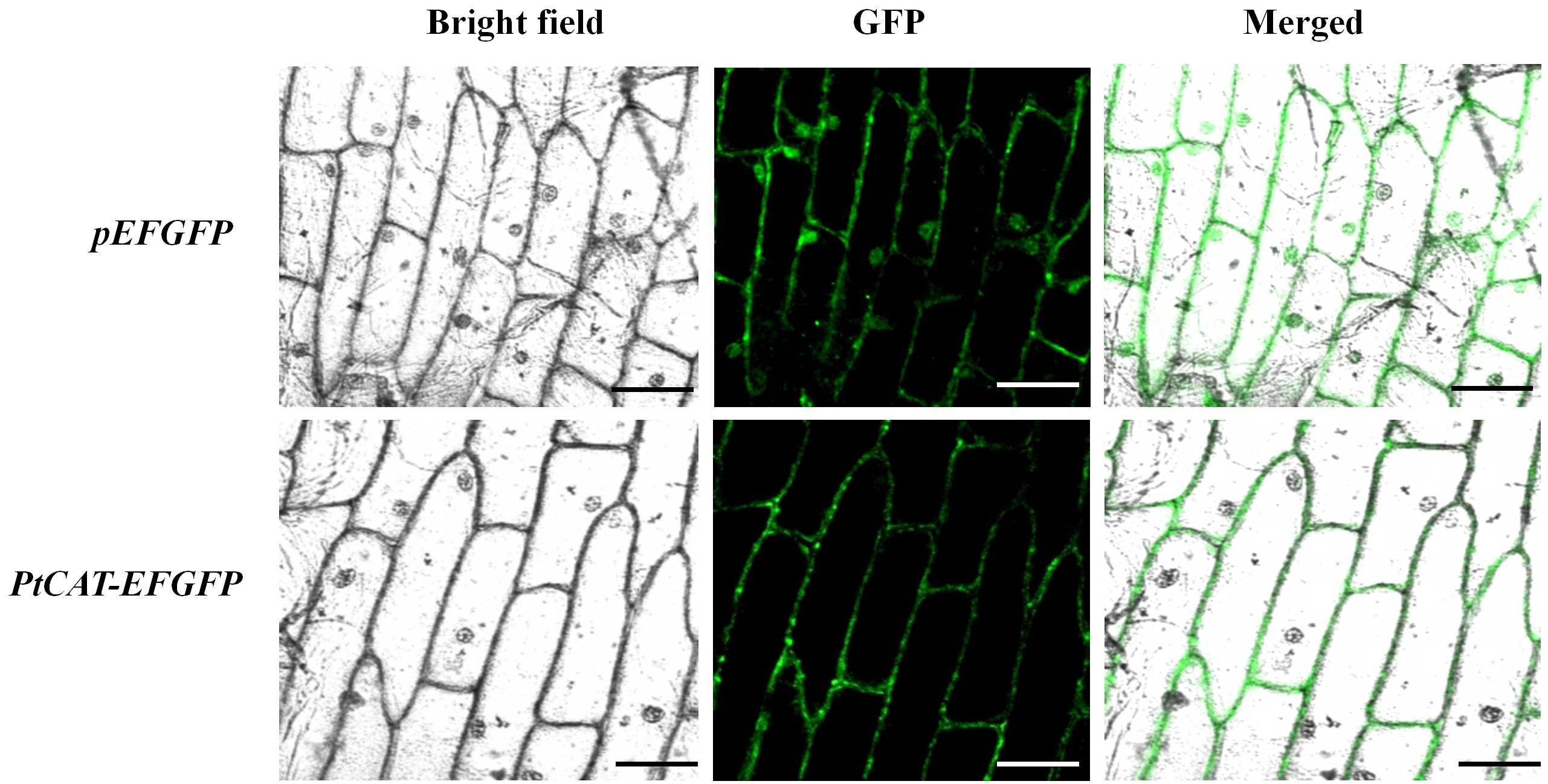
Figure 2 Subcellular localization of PtCAT2 and empty vector pEFGFP in onion after 12 h of infiltration. Images of epidermal cells were captured under visible, green fluorescence and merged light. Bar: 50 μm.
3.4 Overexpression of the PtCAT2 gene enhanced the drought tolerance of the transgenic Arabidopsis
The T3 homozygous lines of transgenic Arabidopsis were identified with RT-PCR, and three lines (OE1, OE2 and OE3) were selected for further analysis (Figure 3A). The seeds of the three lines were subjected to drought stress via germination on a half-strength MS medium containing 25% PEG. We found that the PtCAT2-transgenic and WT seeds had no significant difference during germination under normal conditions. However, the germination rate of the PtCAT2-transgenic Arabidopsis seeds was remarkably higher than that of the WT under PEG-simulated drought conditions (Figure 3B).
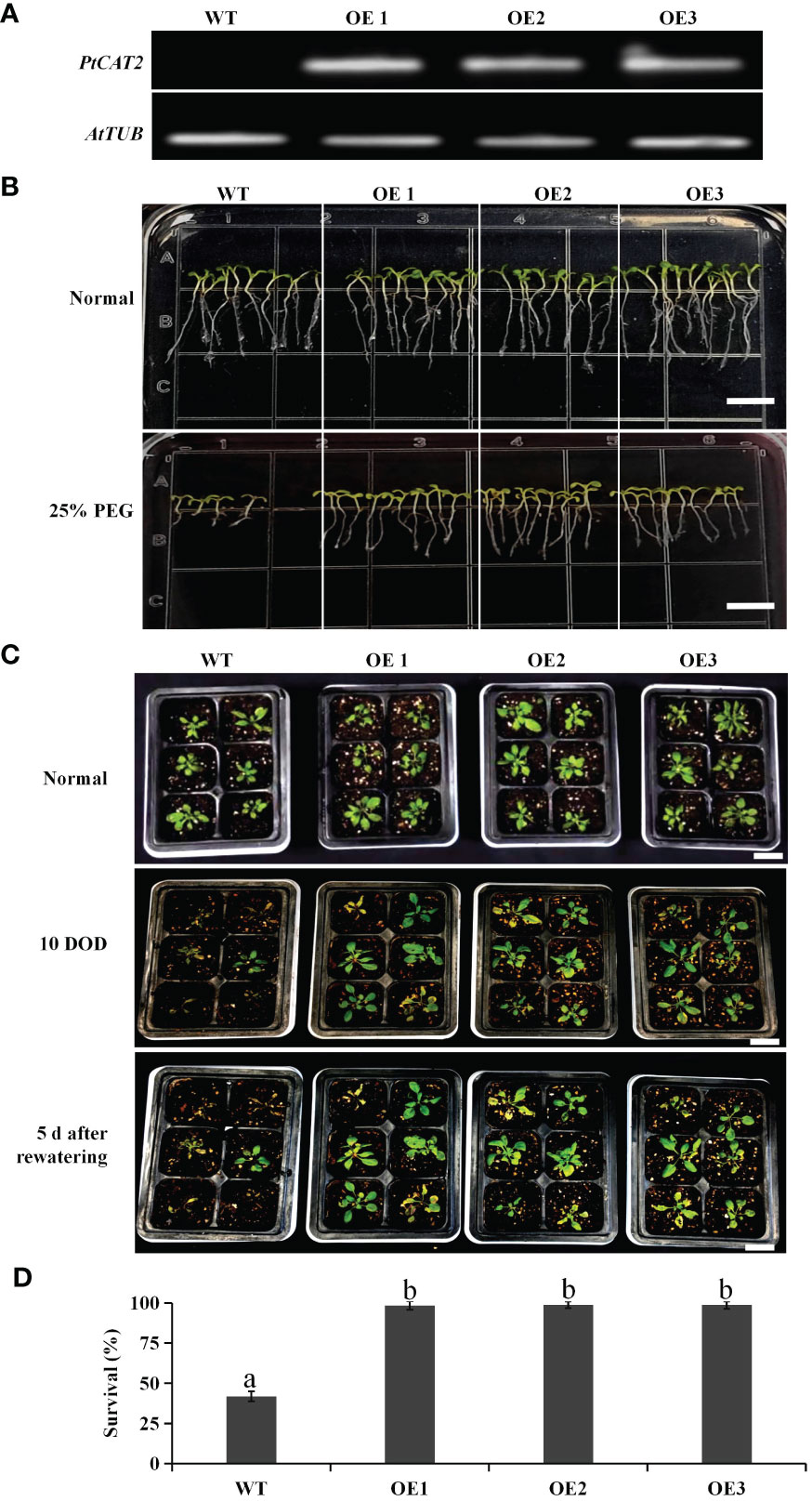
Figure 3 Identification of PtCAT2-transgenic Arabidopsis lines and drought tolerance analysis of wild-type (WT) and transgenic seedlings. (A) The relative expression of PtCAT2 was assessed by RT-PCR using Arabidopsis β-tubulin (AtTUB) as an internal control. (B) Germination performance of WT and transgenics on 1/2MS medium with or without 25% PEG. The pictures were taken at 14 d after culture. Bar: 0.5 cm. (C) Three-week-old Arabidopsis seedlings were subjected to water deficit treatment for 10 d and a followed 5 d of rewatering culture. Bar: 3 cm. (D) Survival of Arabidopsis plants recorded after 5 d of rewatering following 10 d of water deficit. Data are the mean of three biological replicates. OE1, OE2 and OE3 represent three independent transgenic lines. Values are presented as means ± SD values (n = 3). Different letters indicate significant differences at P < 0.05.
The drought tolerance of the transgenic Arabidopsis plants was further evaluated through water deficit treatment (Figure 3C). We found that most WT plants withered or even died after 10 days of water deficit treatment, while the transgenic plants maintained normal growth. Following a 5 d recovery after rewatering, over 95% of the transgenic plants recovered, compared to the 41% survival of the WT (Figure 3D). Thus, these results indicate that overexpressing PtCAT2 significantly enhanced the drought tolerance of Arabidopsis plants.
3.5 PtCAT2 overexpression alleviated free radical damage on the membrane under drought stress
Since the Arabidopsis plants overexpressing PtCAT2 had enhanced drought tolerance, we subjected their leaves and those of the WT to DAB and NBT staining. In DAB staining, the transgenic leaves had lighter stains than the WT under drought stress but did not differ from the WT under normal conditions (Figure 4A). However, the NBT-stained leaves showed no much difference between transgenics and WT (Figure 4B). The H2O2 content was lower in transgenic leaves than in WT under normal and drought conditions (P<0.05) (Figure 4C), though O2·- content did not show significant difference between transgenics and WT (Figure 4D) except OE1. The results further confirm that overexpressing PtCAT2 efficiently degrades H2O2 in Arabidopsis under drought stress but not significantly affects O2·- production.
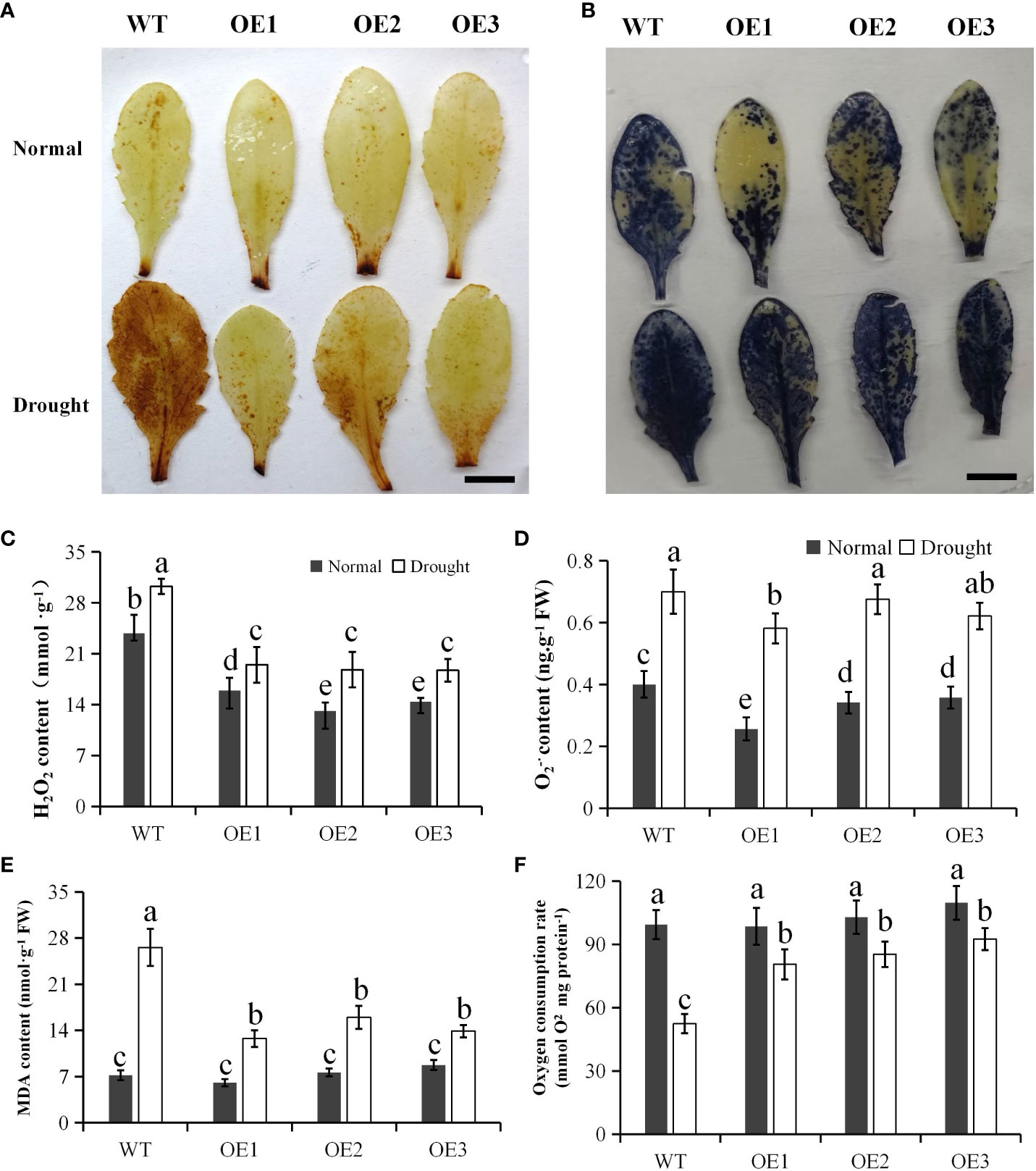
Figure 4 Measurements of ROS and oxygen consumption rate in WT and transgenic plants under heat stress. The leaves were sampled from 3-week-old plants grown under normal growth conditions and subjected to 7 d of water deficit stress. (A) DAB staining of intact leaves. Bar: 1 cm. (B) NBT staining of intact leaves. Bar: 1 cm. (C) H2O2 content. (D) O2-· content. (E) MDA content. (F) Oxygen consumption rate of mitochondria.
Malonaldehyde (MDA) is an important indicator of the degree of lipid peroxidation, commonly used to estimate membrane damage under oxidative stress (Ashraf et al., 2017). Although there was no difference between the MDA content of the WT and transgenic plants under normal conditions, the transgenic plants had significantly lower MDA content than WT plants under drought stress (P< 0.05) (Figure 4E). Further, the oxygen consumption rate was investigated to test the effect of PtCAT2 expression on the mitochondrial function. Three transgenic lines maintained higher oxygen consumption rate under drought stress in comparison with WT (P< 0.05) (Figure 4F). These data indicate that overexpression of PtCAT2 alleviates the membrane damage and maintain the mitochondrial function under drought stress.
We also determined the CAT activity of the transgenic lines and WT, and found that similar to the mRNA level, the CAT activities of the three transgenic lines were significantly higher than WT under normal and drought stress conditions (Figure 5A). This showed the enzymatic correlation between PtCAT2 and drought tolerance. We also quantified the activities of SOD and POD. The result showed that the POD and SOD activities were similar between WT and transgenic plants (P>0.05) under normal conditions. However, when stressed with the water deficit treatment, the POD and SOD activities increased in WT or transgenic plants, but the increment was significantly higher in transgenic plants compared to WT plants (P<0.05) (Figures 5B, C). The El was measured to determine the membrane integrity of the plants under water deficit stress. We found no significant differences between the El values of the WT and transgenic leaves under normal conditions; however, the transgenic leaves presented significantly lower El values under drought treatment (P< 0.05) (Figure 5D). These data suggest that PtCAT2 overexpression could alleviate the membrane damage by enhancing the free radical scavenging ability of Arabidopsis, thus improving its drought stress tolerance.
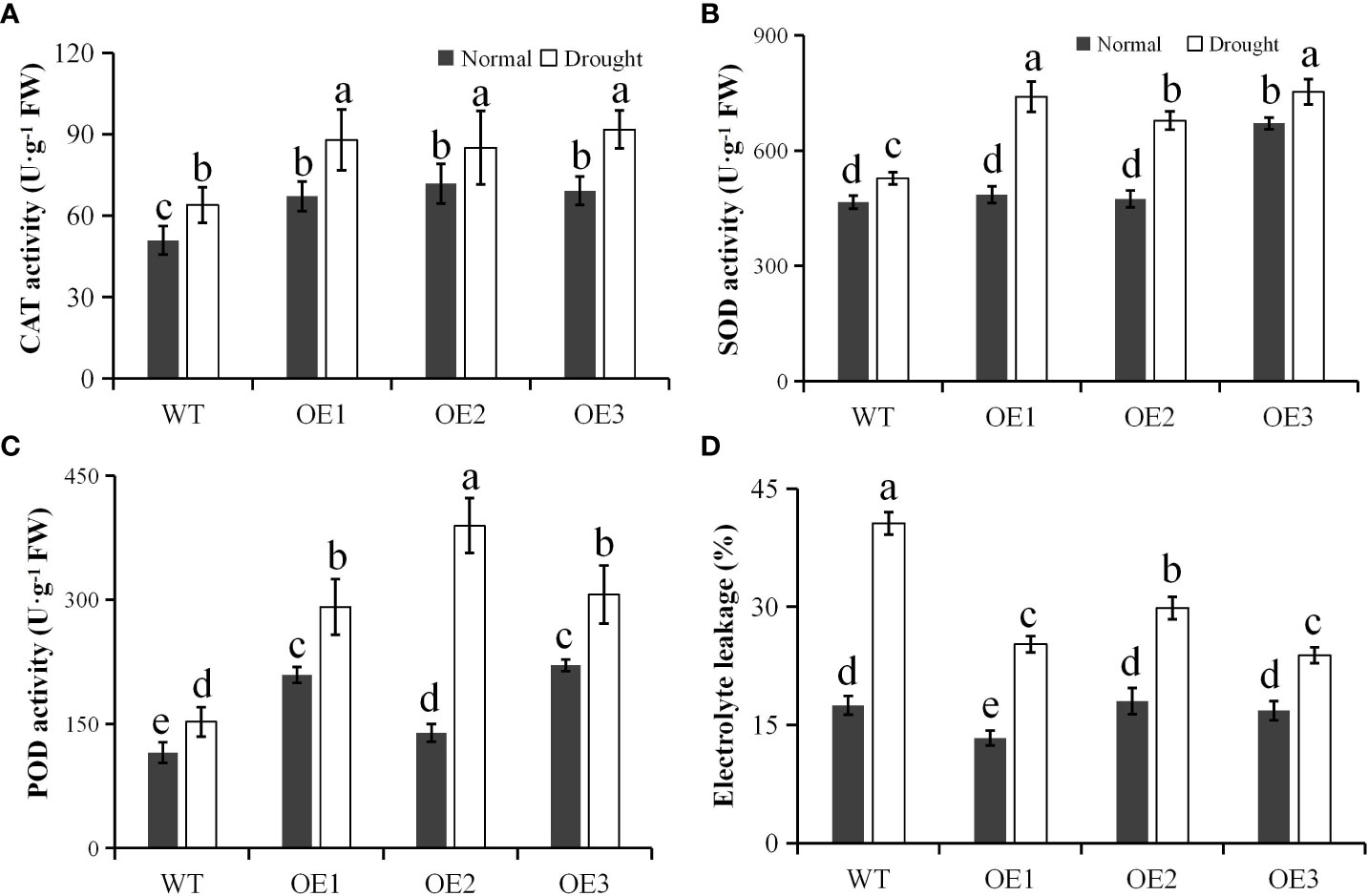
Figure 5 Antioxidant enzyme activities and membrane integrity. (A) CAT activity. (B) SOD activity. (C) POD activity. (D) Electrolyte leakage. OE1, OE2 and OE3 represent three independent transgenic lines. Values are presented as means ± SD values (n = 3). Different letters indicate significant differences at P < 0.05. Bar: 1 cm.
3.6 PtCAT2 overexpression enhanced the antioxidative and water retention capacities of Arabidopsis
To reveal the effect of PtCAT2 overexpression on H2O2 accumulation under oxidative stress, we incubated the leaves of WT and transgenic Arabidopsis plants in different concentrations of H2O2 solution. When exposed to either 0.5% or 1.0% H2O2, the leaves of three transgenic lines were lusher than WT (Figure 6A) and had higher chlorophyll content (Figure 6B), indicating that PtCAT2 overexpression enables plants to degrade H2O2 and maintain their chlorophyll content.
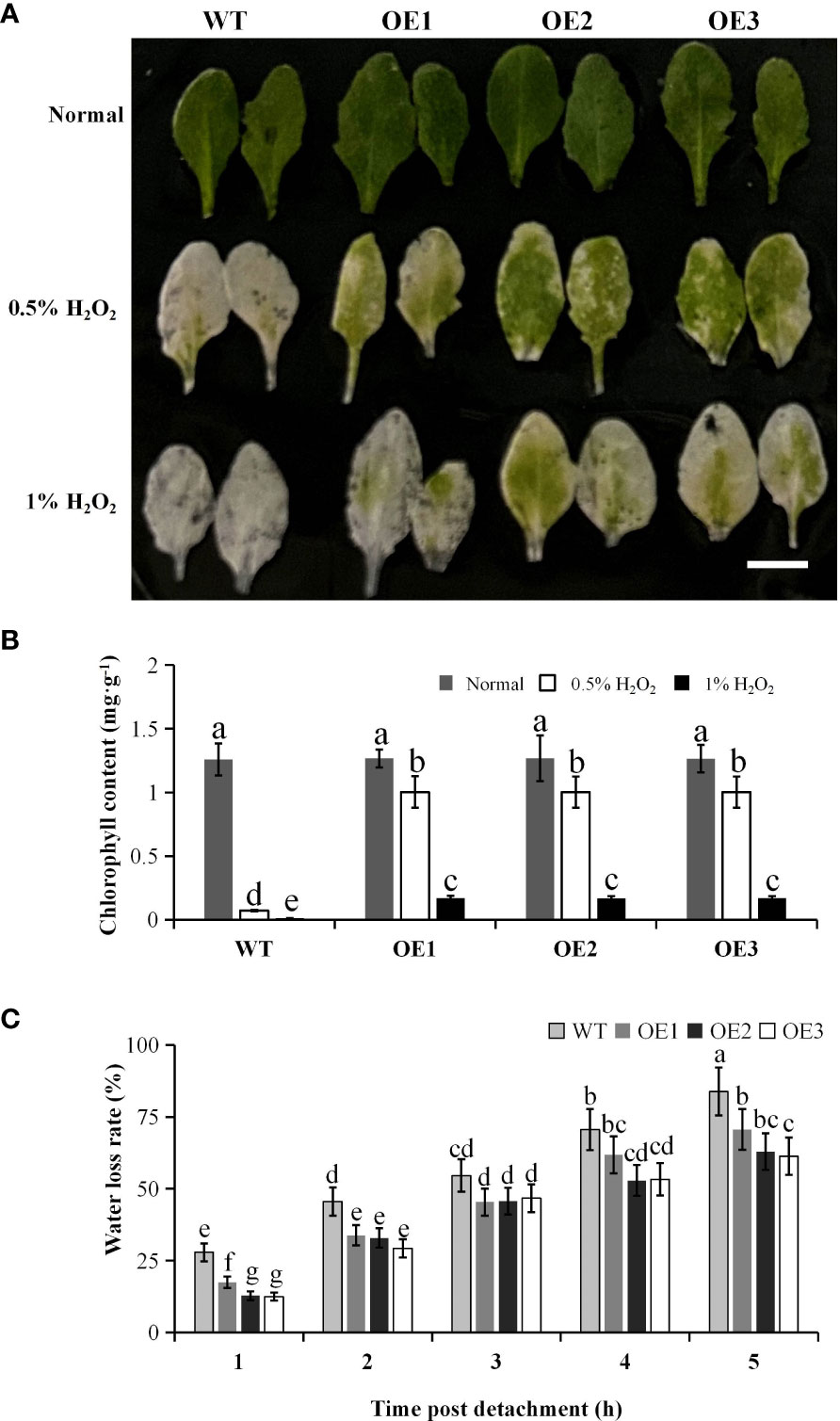
Figure 6 Oxidative stress tolerance experiment and water loss rate of 3-week-old transgenic lines and wild-type (WT) plants. (A) Intact leaves with petioles from 3-week-old plants were exposed to 0, 0.5%, and 1% H2O2 solutions for 24 (h) Bar: 1 cm. (B) Chlorophyll content in leaves was determined at 24 h after treatment. (C) The water loss rate of the leaves was measured by recording the weights of leaves at the indicated time points after they detached. OE1, OE2 and OE3 represent three independent transgenic lines. Values are presented as means ± SD values (n = 3). Different letters indicate a significant difference at P < 0.05.
We also monitored the water loss rate of the WT and transgenic leaves within 5 h after their detachment from the stems. The results showed that though the water content decreased in the WT and transgenic leaves, the transgenic lines maintained higher water content than the WT (P<0.05). This suggested that the PtCAT2- transgenic plants exhibited an improved water retention capacity (Figure 6C).
3.7 PtCAT2 altered the expression levels of the endogenous genes
Since PtCAT2 overexpression enhanced drought tolerance in transgenic Arabidopsis plants, expression patterns of the drought stress-related genes and endogenous CATs were quantified in the WT and transgenic Arabidopsis lines under normal and drought conditions. The expression levels of drought stress-related genes, AtRAB18, AtRD22, AtRD29B and AtRD29A, were similar between WT and transgenic lines under normal conditions but were significantly upregulated in the transgenic lines compared to the WT under drought treatment (P<0.05) (Figure 7A). Moreover, the expression levels of the Arabidopsis endogenous CATs (AtCAT1, AtCAT2 and AtCAT3) were also significantly upregulated in the transgenic lines than the WT under drought stress. In particular, PtCAT3 had >10 folds upregulation in the three transgenic lines compared to the WT (Figure 7B). These results suggest that PtCAT2 overexpression activates the expressions of drought stress-related genes and endogenous CATs to confer drought tolerance in plants.
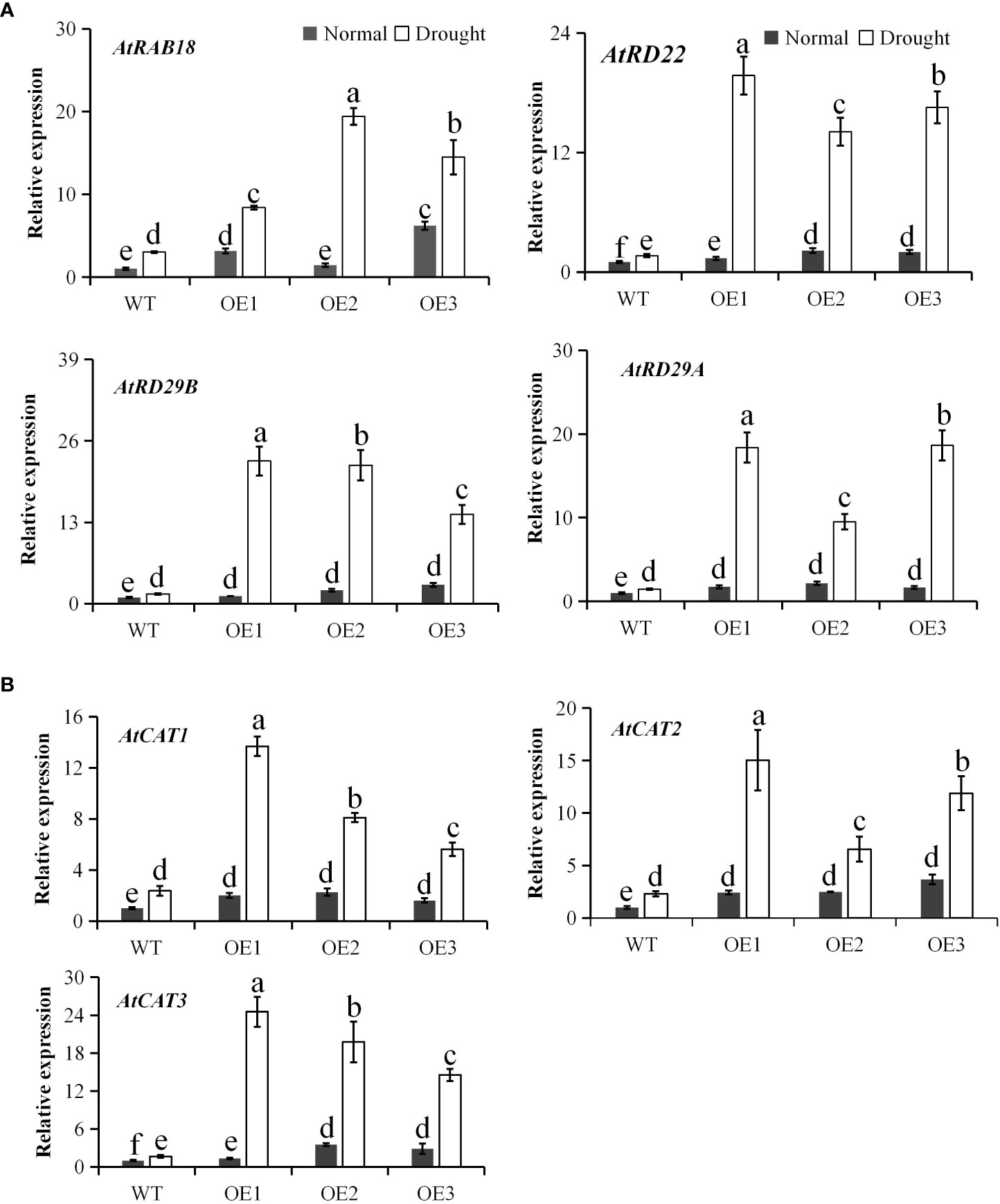
Figure 7 Expression of endogenous genes involved in drought stress response and CAT homologs in Arabidopsis. Real time RT-PCR analysis of wild-type (WT) and transgenic lines before and after 7 d of water deficit. (A) Drought stress related genes AtRAB18, RESPONSIVE TO DEHYDRATION 22 (AtRD22), RESPONSIVE TO DEHYDRATION 29B (AtRD29B), RESPONSIVE TO DEHYDRATION 29A (AtRD29A). (B) Three endogenous CAT homologs (AtCAT1, AtCAT2 and AtCAT3) in Arabidopsis. OE1, OE2 and OE3 represent three independent transgenic lines. Values are presented as means ± SD (n = 3). Different letters indicate a significant difference at P < 0.05.
4 Discussion
In this study, a novel class II CAT gene PtCAT2 from P. ternata was functionally demonstrated to regulate plant drought tolerance. This effect was attributed to the enhanced H2O2-scavenging capacity and intact membrane integrity maintenance induced by PtCAT2 overexpression and upregulation of the endogenous genes involved in drought stress.
Plants respond to water deficit stress by producing drought stress signals such as ROS and ABA (Yang et al., 2021). These signals lead to morphological and physiological changes, presenting as retarded growth, withering, or even plant death. Under drought stress, the balance between ROS production and clearance is disrupted (Yang et al., 2021), resulting in multiple cytological effects. One of these effects is plasma membrane lipid peroxidation (Noctor et al., 2014), which directly correlates with the integrity degree of the plasma membrane. In this study overexpression of PtCAT2, located at the membrane and cytoplasm, successfully enhanced the drought tolerance of Arabidopsis. CAT genes cloned from various plant species like Arabidopsis (Yang et al., 2019), Ipomoea batatas (Yong et al., 2017) and Brassica oleracea (Chiang et al., 2014) reportedly responded to multiple abiotic conditions. However, unlike the previously reported CATs involved in multiple abiotic responses (Yong et al., 2017; Zhou et al., 2017), PtCAT2 overexpression specifically enhanced drought tolerance but not other abiotic stresses. Since mitochondria and chloroplast are the active sources of ROS (Mittler, 2017), the capacity of transgenic Arabidopsis to maintain the functions of these two organelles was evaluated under oxidative stress. Transgenic plants performed better oxygen consumption rate of mitochondria and chlorophyll under drought or exogenous H2O2 treatments, as well as the lower water loss rate, and membrane integrity. Our results provided insights into the biochemical mechanisms of CAT in response to drought stress, through alleviating the damage of ROS-producing organelles and maintaining membrane integrity.
The inducible expression profile of genes is usually determined by the cis-regulatory elements (Zemlyanskaya et al., 2021). An in silico analysis was performed to identify cis-elements in the promoter region. Known drought-responsive elements MYCCONSENSUSAT and MYCATRD22, which can bind the upstream bHLH transcription factors, were identified (Zhu et al., 2017). ABRE, the ABA-responding cis-element (Fujita et al., 2005), was also identified in the promoter region. This suggests that PtCAT2 might respond to drought stress via an ABA-mediated signaling pathway. The RESPONSIVE TO DEHYDRATION 22 (RD22) gene acts as a molecular link between ABA signaling and drought stress response (Harshavardhan et al., 2014). RABs are also known for their involvement in ABA accumulation under drought stress (Yang et al., 2012; Li et al., 2017). qRT-PCR analysis suggested that the AtRD22 and AtRAB18 levels were much higher in the PtCAT2-transgenic lines than in the WT under drought stress, suggesting that PtCAT2 overexpression probably activates the linker between drought and ABA signaling. Moreover, the drought memory genes Response to Desiccation (RD) 29A and RD29B (Liu et al., 2020) were also upregulated in transgenics compared with the WT. This implies that the enhanced drought tolerance in PtCAT2-transgenic plants was probably mediated by ABA signaling. Unlike the studies on drought tolerance regulation via stomata closure (Huber et al., 2019; Li et al., 2019), which may compromise the yield, our study provides a potentially useful approach for genetic improvement. Interestingly, the endogenous CAT genes (AtCAT1, AtCAT2 and AtCAT3) were also upregulated in transgenic lines, and their induction folds were much higher than WT under drought treatment. Similar phenomenon has been observed previously (Yu et al., 1999), by ectopically expressing tobacco class II CAT gene CatNt to activate the endogenous homologous genes which regulate disease resistance in potatoes. This is explained by a positive and negative feedback loops within the salicylic acid signalling pathways (Yu et al., 1999). Thus, we speculate that PtCAT2 overexpression may activate a similar signal transduction on its endogenous homologs under drought stress. The detailed mechanisms involved in this interaction necessitate further study.
5 Conclusion
This study functionally characterized a class II CAT gene, PtCAT2, from P. ternata, which specifically enhanced the tolerance of Arabidopsis plants to drought. This specific response could be attributed to its role in scavenging H2O2 to maintain the functions of ROS-producing organelles and membrane integrity, and upregulating its endogenous genes involved in drought stress responses. These results may facilitate the genetic breeding of P. ternata for enhanced drought tolerance.
Data availability statement
The original contributions presented in the study are included in the article/Supplementary Material. Further inquiries can be directed to the corresponding authors.
Author contributions
FLZ, YBD and JPX conceived this study. JJX, ND, TCD and HZ performed most of the experiments. JJX and TX analyzed the data. FZ performed the bioinformatics analysis. FLZ, YBD and JPX wrote the manuscript, with contribution and approval from all authors. All authors contributed to the article and approved the submitted version.
Funding
This work was supported by the National Natural Science Foundation of China (82373993; 82274048), the Key Project of Natural Science Research for Colleges and Universities in Anhui Province (KJ2021A0533; 2023AH050345), and Excellent Scientific Research and Innovation Team of University in Anhui Province (2022AH010029).
Conflict of interest
The authors declare that the research was conducted in the absence of any commercial or financial relationships that could be construed as a potential conflict of interest.
Publisher’s note
All claims expressed in this article are solely those of the authors and do not necessarily represent those of their affiliated organizations, or those of the publisher, the editors and the reviewers. Any product that may be evaluated in this article, or claim that may be made by its manufacturer, is not guaranteed or endorsed by the publisher.
Supplementary material
The Supplementary Material for this article can be found online at: https://www.frontiersin.org/articles/10.3389/fpls.2023.1206798/full#supplementary-material
References
Ashraf, U., Kanu, A. S., Deng, Q., Mo, Z., Pan, S., Tian, H., et al. (2017). Lead (Pb) toxicity; physio-biochemical mechanisms, grain yield, quality, and Pb distribution proportions in scented rice. Front. Plant Sci. 8. doi: 10.3389/fpls.2017.00259
Chen, Y., Chen, Y., Guo, Q. S., Zhu, G. S., Wang, C., Liu, Z. (2021). Effects of drought stress on the growth, physiology and secondary metabolite production in Pinellia ternata Thunb. Pak. J. Bot. 53, 833–840. doi: 10.30848/PJB2021-3(25
Cheng, S. M. (2010). Effects of uniconazole on the stage bulbil of drought-tolerant characteristics of Pinellia ternata (Chengdu (Sichuan: Sichuan Agricultural University).
Chiang, C. M., Chen, S. P., Chen, L. F., Chiang, M. C., Chen, H. L., Lin, K. H. (2014). Expression of the broccoli catalase gene (BoCAT) enhances heat tolerance in transgenic Arabidopsis. J. Plant Biochem. Biotechnol. 23, 266–277. doi: 10.1007/s13562-013-0210-1
Clough, S. J., Bent, A. F. (1998). Floral dip: A simplified method for Agrobacterium-mediated transformation of Arabidopsis thaliana. Plant J. 16, 735–743. doi: 10.1046/j.1365-313x.1998.00343.x
Duan, Y. B., Zhang, H., Meng, X., Huang, M. M., Zhang, Z. Y., Huang, C. H., et al. (2019). Accumulation of salicylic acid-elicited alkaloid compounds in in vitro cultured Pinellia ternata microtubers and expression profiling of genes associated with benzoic acid-derived alkaloid biosynthesis. Plant Cell Tiss. Org. 139, 317–325. doi: 10.1007/s11240-019-01685-5
Duan, Y., Zhao, F., Li, Q., Shen, S., Li, Z., Teng, J., et al. (2015). High efficiency Agrobacterium-mediated transformation of Pinellia ternata using petiole explants from submerged cultures. Biologia 70, 1351–1358. doi: 10.1515/biolog-2015-0159
Fujita, Y., Fujita, A. M., Satoh, C. R., Maruyama, C. K., Parvez, A., Seki, A. M. (2005). AREB1 is a transcription activator of novel ABRE-dependent ABA signaling that enhances drought stress tolerance in Arabidopsis. Plant Cell 17, 3470–3488. doi: 10.1105/tpc.105.035659
Guo, C., Zhang, Y., Wu, D., Wang, M., Du, Y., Chu, J. (2022). Principal component analysis to assess the changes of yield and quality in Pinellia ternata at different stages after brassinolide treatments. Int. J. Mol. Sci. 23, 15375. doi: 10.3390/ijms232315375
Gupta, A., Rico-Medina, A., Cao-Delgado, A. I. (2020). The physiology of plant responses to drought. Science 368, 266–269. doi: 10.1126/science.aaz7614
Harshavardhan, V. T., Van Son, L. E., Seiler, C., Junker, A., Weigelt-Fischer, K., Klukas, C., et al. (2014). AtRD22 and AtUSPL1, members of the plant-specific BURP domain family involved in Arabidopsis thaliana drought tolerance. PloS One 9, e110065. doi: 10.1371/journal.pone.0110065
Huber, A. E., Melcher, P. J., Piñeros, M. A., Setter, T. L., Bauerle, T. L. (2019). Signal coordination before, during and after stomatal closure in response to drought stress. New Phytol. 224, 675–688. doi: 10.1111/nph.16082
Jin, S. X., Zhang, X. L., Daniell, H. (2012). Pinellia ternata agglutinin expression in chloroplasts confers broad spectrum resistance against aphid, whitefly, lepidopteran insects, bacterial and viral pathogens. Plant Biotechonol. J. 10, 313–327. doi: 10.1111/j.1467-7652.2011.00663.x
Kohli, S. K., Khanna, K., Bhardwaj, R., Abd Allah, E. F., Ahmad, P., Corpas, F. J. (2019). Assessment of subcellular ROS and NO metabolism in higher plants: multifunctional signaling molecules. Antioxidants 8, 641. doi: 10.3390/antiox8120641
Lee, J. Y., Park, N. H., Lee, W., Kim, E. H., Jin, Y. H. (2016). Comprehensive chemical profiling of Pinellia species tuber and processed Pinellia tuber by gas chromatography–mass spectrometry and liquid chromatography–atmospheric pressure chemical ionization–tandem mass spectrometry. J. Chromatogr. A 1471, 164–177. doi: 10.1016/j.chroma.2016.10.033
Li, B., Tang, Z. S., Wang, N., Sun, X. C., He, Y. H., Song, Z. X., et al (2020). Transcriptome analysis of stems of Pinellia ternata under drought stress simulated by PEG. Chin. Trad. Herb. Drugs 51, 5579–5589.
Li, J., Han, L., Su, Y., Guo, H., Zhang, H. (2019). Functional identification of Ammopiptanthus mongolicus anion channel AmSLAC1 involved in drought induced stomata closure. Plant Physiol. Biochem. 143, 340–350. doi: 10.1016/j.plaphy.2019.09.012
Li, J., Li, Y., Yin, Z., Jiang, J., Zhang, M., Guo, X., et al. (2017). OsASR5 enhances drought tolerance through a stomatal closure pathway associated with ABA and H2O2 signalling in rice. Plant Biotechn. J. 15, 183–196. doi: 10.1111/pbi.12601
Lichtenthaler, H. K. (1987). Chlorophylls and carotenoids: pigments of photosynthetic biomembranes. Meth. Enzymol. 148, 350–382. doi: 10.1016/0076-6879(87)48036-1
Liu, W., Sikora, E., Park, S. W. (2020). Plant growth-promoting rhizobacterium, Paenibacillus polymyxa CR1, upregulates dehydration-responsive genes, RD29A and RD29B, during priming drought tolerance in Arabidopsis. Plant Physiol. Biochem. 156, 146–154. doi: 10.1016/j.plaphy.2020.08.049
Livak, K. J., Schmittgen, T. D. (2001). Analysis of relative gene expression data using real-time quantitative PCR and the 2(-Delta Delta C(T)) Method. Methods 25, 402–408. doi: 10.1006/meth.2001.1262
Lu, H., Xue, T., Zhang, A., Sheng, W., Zhu, Y., Chang, L., et al. (2013). Construction of an SSH library of Pinellia ternata under heat stress, and expression analysis of four transcripts. Plant Mol. Biol. Rep. 31, 185–194. doi: 10.1007/s11105-012-0488-5
Lyu, W., Selinski, J., Li, L., Day, D., Murcha, M., Whelan, J., et al. (2018). Isolation and respiratory measurements of mitochondria from Arabidopsis thaliana. J. Vis. Exp. 131, e56627. doi: 10.3791/56627
Noctor, G., Mhamdi, A., Foyer, C. H. (2014). The roles of reactive oxygen metabolism in drought: not so cut and dried. Plant Physiol. 164, 1636–1648. doi: 10.1104/pp.113.233478
Sun, T., Liu, F., Wang, W., Wang, L., Wang, Z., Li, J., et al. (2018). The role of sugarcane catalase gene ScCAT2 in the defense response to pathogen challenge and adversity stress. Int. J. Mol. Sci. 19, 2686. doi: 10.3390/ijms19092686
Tian, S., Wang, X., Li, P., Wang, H., Ji, H., Xie, J., et al. (2016). Plant aquaporin AtPIP1;4 links apoplastic H2O2 induction to disease immunity pathways. Plant Physiol. 171, 1635–1650. doi: 10.1104/pp.15.01237
Tian, C., Zhang, Z., Huang, Y., Xu, J., Liu, Z., Xiang, Z., et al. (2022). Functional characterization of the Pinellia ternata cytoplasmic class II small heat shock protein gene PtsHSP17.2 via promoter analysis and overexpression in tobacco. Plant Physiol. Biochem. 177, 1–9. doi: 10.1016/j.plaphy.2022.02.017
Vijayaraghavareddy, P., Lekshmy, S. V., Struik, P. C., Makarla, U., Yin, X. Y., Sreeman, S. (2022). Production and scavenging of reactive oxygen species confer to differential sensitivity of rice and wheat to drought stress. Crop Environ. 1, 15–23. doi: 10.1016/j.crope.2022.03.010
Xu, K., Huang, X., Wu, M., Wang, Y., Chang, Y., Liu, K., et al. (2014). A rapid, highly efficient and economical method of Agrobacterium-mediated in planta transient transformation in living onion epidermis. PloS One 9, e83556. doi: 10.1371/journal.pone.0083556
Xue, T., Zhang, H., Zhang, Y., Wei, S., Chao, Q., Zhu, Y., et al. (2019). Full-length transcriptome analysis of shade-induced promotion of tuber production in Pinellia ternata. BMC Plant Biol. 19, 565. doi: 10.1186/s12870-019-2197-9
Yang, A., Dai, X. Y., Zhang, W. H. (2012). A R2R3-type MYB gene, OsMYB2, is involved in salt, cold, and dehydration tolerance in rice. J. Exp. Bot. 63, 2541–2556. doi: 10.1093/jxb/err431
Yang, X., Lu, M., Wang, Y., Wang, Y., Liu, Z., Chen, S. (2021). Response mechanism of plants to drought stress. Horticulturae 7, 50. doi: 10.3390/horticulturae7030050
Yang, Z., Mhamdi, A., Noctor, G. (2019). Analysis of catalase mutants underscores the essential role of CATALASE2 for plant growth and day length-dependent oxidative signalling. Plant Cell Environ. 42, 688–700. doi: 10.1111/pce.13453
Yang, Z., Qin, F. (2023). The battle of crops against drought: Genetic dissection and improvement. J. Integr. Plant Biol. 65, 496–525. doi: 10.1111/jipb.13451
Yong, B., Wang, X., Xu, P., Zheng, H., Fei, X., Hong, Z., et al. (2017). Isolation and abiotic stress resistance analyses of a catalase gene from Ipomoea batatas (L.) Lam. BioMed. Res. Int. 2017, 6847532. doi: 10.1155/2017/6847532
Yu, D., Xie, Z., Chen, C., Fan, B., Chen, Z. (1999). Expression of tobacco class II catalase gene activates the endogenous homologous gene and is associated with disease resistance in transgenic potato plants. Plant Mol. Biol. 39, 477–488. doi: 10.1023/A:1006180708533
Zaid, A., Wani, S. H. (2019). “Reactive oxygen species generation, scavenging and signaling in plant defense responses,” in Bioactive Molecules in Plant Defense: Signaling in Growth and Stress. Eds. Jogaiah, S., Abdelrahman, M. (Cham: Springer International Publishing), 111–132.
Zemlyanskaya, E. V., Dolgikh, V. A., Levitsky, V. G., Mironova, V. (2021). Transcriptional regulation in plants: using omics data to crack the cis-regulatory code. Curr. Opin. Plant Biol. 63, 102058. doi: 10.1016/j.pbi.2021.102058
Zhang, H. M., Li, J. J., Hei, G. G., Cao, R. X., Zhou, Q. Y., Li, B. B. (2014). Effects of exogenous betaine on the secondary metabolites of Pinellia ternata under drought stress. Acta Patacul Turae Sinica. 23, 229–3236.
Zhang, H., Zhang, Z., Xiong, Y., Shi, J., Chen, C., Pan, Y., et al. (2021). Stearic acid desaturase gene negatively regulates the thermotolerance of Pinellia ternata by modifying the saturated levels of fatty acids. Ind. Crop Prod. 166, 113490. doi: 10.1016/j.indcrop.2021.113490
Zhou, Y., Liu, S. Q., Yang, Z. J., Yang, Y. G., Jiang, L. W., Hu, L. F. (2017). CsCAT3, a catalase gene from Cucumis sativus, confers resistance to a variety of stresses to Escherichia coli. Biotechnol. Biotec. Eq. 31, 886–896. doi: 10.1080/13102818.2017.1360797
Zhou, Yi, X., S., Xu, Q. G., Wang, X. J. (2020). Effects of drought stress on the agronomic characters of different provenance of Pinellia ternata. Crop Res. 34, 331–337.
Keywords: Arabidopsis thaliana, catalase, drought, membrane integrity, Pinellia ternata, reactive oxygen species
Citation: Xu J, Du N, Dong T, Zhang H, Xue T, Zhao F, Zhao F, Duan Y and Xue J (2023) A novel Pinellia ternata catalase gene PtCAT2 regulates drought tolerance in Arabidopsis by modulating ROS balance. Front. Plant Sci. 14:1206798. doi: 10.3389/fpls.2023.1206798
Received: 16 April 2023; Accepted: 19 September 2023;
Published: 02 October 2023.
Edited by:
Yongfeng Guo, Chinese Academy of Agricultural Sciences, ChinaReviewed by:
Viktória Kovács, Centre for Agricultural Research, HungaryJinghua Cui, Children’s Hospital of Capital Institute of Pediatrics, China
Copyright © 2023 Xu, Du, Dong, Zhang, Xue, Zhao, Zhao, Duan and Xue. This is an open-access article distributed under the terms of the Creative Commons Attribution License (CC BY). The use, distribution or reproduction in other forums is permitted, provided the original author(s) and the copyright owner(s) are credited and that the original publication in this journal is cited, in accordance with accepted academic practice. No use, distribution or reproduction is permitted which does not comply with these terms.
*Correspondence: Fenglan Zhao, emhhb2ZlbmdsYW4yMDA0QDE2My5jb20=; Yongbo Duan, eWJvZHVhbkAxNjMuY29t; Jianping Xue, eHVlanBAMTYzLmNvbQ==