- Agroécologie, INRAE, Institut Agro Dijon, CNRS, Univ. Bourgogne, Univ. Bourgogne Franche-Comté, Dijon, France
Under agroforestry practices, inter-specific facilitation between tree rows and cultivated alleys occurs when plants increase the growth of their neighbors especially under nutrient limitation. Owing to a coarse root architecture limiting soil inorganic phosphate (Pi) uptake, walnut trees (Juglans spp.) exhibit dependency on soil-borne symbiotic arbuscular mycorrhizal fungi that extend extra-radical hyphae beyond the root Pi depletion zone. To investigate the benefits of mycorrhizal walnuts in alley cropping, we experimentally simulated an agroforestry system in which walnut rootstocks RX1 (J. regia x J. microcarpa) were connected or not by a common mycelial network (CMN) to maize plants grown under two contrasting Pi levels. Mycorrhizal colonization parameters showed that the inoculum reservoir formed by inoculated walnut donor saplings allowed the mycorrhization of maize recipient roots. Relative to non-mycorrhizal plants and whatever the Pi supply, CMN enabled walnut saplings to access maize Pi fertilization residues according to significant increases in biomass, stem diameter, and expression of JrPHT1;1 and JrPHT1;2, two mycorrhiza-inducible phosphate transporter candidates here identified by phylogenic inference of orthologs. In the lowest Pi supply, stem height, leaf Pi concentration, and biomass of RX1 were significantly higher than in non-mycorrhizal controls, showing that mycorrhizal connections between walnut and maize roots alleviated Pi deficiency in the mycorrhizal RX1 donor plant. Under Pi limitation, maize recipient plants also benefited from mycorrhization relative to controls, as inferred from larger stem diameter and height, biomass, leaf number, N content, and Pi concentration. Mycorrhization-induced Pi uptake generated a higher carbon cost for donor walnut plants than for maize plants by increasing walnut plant photosynthesis to provide the AM fungus with carbon assimilate. Here, we show that CMN alleviates Pi deficiency in co-cultivated walnut and maize plants, and may therefore contribute to limit the use of chemical P fertilizers in agroforestry systems.
1 Introduction
Inorganic phosphate (Pi) availability, as the orthophosphate anion PO4−, is a major factor constraining plant growth and metabolism in many soils worldwide (Bates and Lynch, 2001). Consequently, large amounts of Pi fertilizers are used to ensure plant productivity in most conventional agricultural systems (Smith et al., 2011). However, only approximately 10%–30% of the P fertilizer is taken up by the roots, with a substantial part accumulated in the soil as residual P not readily available for plants (Syers et al., 2008). In addition, injudicious and untimely application of chemical fertilizers in agricultural field has generated environmental concerns, including soil degradation and water eutrophication (Tilman, 2001; Foley, 2005; Conley et al., 2009). To mitigate environmental damages, it is crucial to enhance P-use efficiency in crop production through the diminution of the application of P fertilizers and utilization of residual P and other P pools from soils (Xue et al., 2016). As a substitute to conventional cropping systems, which rely on large inputs of chemical fertilizers to sustain production, agroforestry is a low-input design that combines trees with annual crops in various combinations or sequences (Nair, 1993). Agroforestry systems aim at reducing the need for inputs through minimizing losses and maximizing internal cycling of nutrients (Smith, 2010). One of the major arguments in favor of agroforestry is the fact that a mixed system of trees and annual crops uses natural resources such as light, water, and nutrients more efficiently than a monoculture. As resources are used more efficiently, less chemical fertilizers are required to the benefit of the overall environment (Postma, 2005).
A fundamental aspect of agroforestry is to favor inter-specific facilitation between tree rows and cultivated alleys, where plants increase the growth and survival of their neighbors (Callaway, 1998), particularly regarding limited resources such as nutrients (Schoeneberger et al., 2012; Battie-Laclau et al., 2020). In the maintenance of soil P fertility under alley cropping systems, the role of roots is at least as important as that of aboveground biomass (van Noordwijk et al., 2015). Belowground facilitation occurs when one species makes previously unavailable P available to the other, due to the exudation of organic acids, phosphatases, or rhizosphere acidification resulting in increased P availability (Hinsinger et al., 2011). Since a decade ago, numerous studies have also pointed to the importance of soil-borne arbuscular mycorrhizal (AM) fungi of the phylum Glomeromycota (Tedersoo et al., 2020) in mediating inter-plant facilitation processes (van der Heijden et al., 2008; Montesinos-Navarro et al., 2016). In soils with strong Pi-fixing capacity, or where Pi is limiting, plant demand for this nutrient exceeds the rate at which it diffuses into the root zone, resulting in zones of Pi depletion surrounding the roots (Smith and Read, 1997). AM fungi help to overcome this limitation by extending their extra-radical hyphae from root surfaces to areas beyond the P depletion zone (Hayman, 1983; Javot et al., 2007). When roots are colonized by AM fungi, soil Pi is acquired at the soil–fungus interface through high-affinity fungal phosphate transporters (PHTs) located in the extra-radical mycelium (Rui et al., 2022). In the plant interfacial apoplast of fungal arbuscules, Pi is acquired by plant PHT located on the peri-arbuscular membrane (Harrison et al., 2002; Javot et al., 2007). The Pi uptake pathway of mycorrhizae may dominate Pi uptake in AM symbiosis, which is heavily dependent on AM-induced PHT1 members (Casieri et al., 2013; Rui et al., 2022). Consequently, AM fungi have the potential to maintain crop yields at low soil P levels through a more effective exploitation of available P sources. It has been estimated that inoculation with AM fungi might reach a reduction of approximately 80% of the recommended fertilizer P rates under certain conditions (Jakobsen et al., 1992). In return, AM fungi are supplied with sugars and lipids from the host plant (Gutjahr and Parniske, 2013; Roth and Paszkowski, 2017). Conversely, the importance of the role of mycorrhizal symbiosis in providing Pi to the plant usually declines when soil phosphate concentration is elevated (Kiers et al., 2011).
Many trees species used in tropical and temperate alley cropping systems such as ash, cocoa, coffee, eucalyptus, olive tree, paulownia, poplar, rubber (Cardoso, 2002; Janos, 2007; de Kroon et al., 2012), and walnut (the common name given to the species of deciduous trees belonging to the genus Juglans) exhibit a high dependency on symbiotic AM fungi for their development. Coarse roots and relatively few roots per plant limit soil Pi uptake (Brundrett, 1991; Comas and Eissenstat, 2009; Comas et al., 2014). By providing wood, ornament, and nutrition value to human beings, and food and a habitat to wildlife, walnuts belong to the most important trees in the northern hemisphere, ecologically and economically (Bernard et al., 2018). Because of its short growing season, sparse canopy, and deep rooting system, walnut is an ideal species for agroforestry and belongs to the dominant trees used in temperate alley cropping (Wolz and DeLucia, 2018). Intercropping of Juglans spp. is aimed at increasing growth and quality of walnut trees together with providing an early financial return to help offset the costs associated with establishing walnut orchards (van Sambeek and Garrett, 2004; Mohni et al., 2009; Wolz and DeLucia, 2019). Before walnut plantations reach economic maturity, intercrops—winter cereals (Triticum spp.), alfafa (Medicago sativa), soybean (Glycine max), or summer crops (e.g., maize)—are the only source of income during the first 5 to 10 years; thereafter, both trees and intercrops produce simultaneously (Mary et al., 1998). Among these AM-dependent intercrops, maize (Zea mays L.) is one of the most cultivated crops for both staple food and industrial usage, in tropical and temperate soils worldwide, with phosphorus being a major growth-limiting factor for commercial maize production (Brady and Weil, 2008).
The role of AM fungi in mixed plant communities is applicable due to their low host specificity: extra-radical hyphae can connect the roots of different plant species to form a common mycelial network (CMN) (Ingleby et al., 2007). This inter-plant mycorrhizal connection promotes facilitation through differential access to the nutrient pool from the common hyphal network and plant-to-plant nutrient transfers (Fitter et al., 1998; Martins and Cruz, 1998; Leake et al., 2004; Simard and Durall, 2004; Hauggaard-Nielsen and Jensen, 2005; Ingleby et al., 2007; van der Heijden and Horton, 2009; de Carvalho et al., 2010; Walder et al., 2012; Fellbaum et al., 2014; Gorzelak et al., 2015; Montesinos-Navarro et al., 2016). Focusing on the effect of intercrops on tree growth, trees display a larger growth than that observed in simple forestry systems, due to a better mineral nutrition even in low input systems (Jose et al., 2000; Rivest et al., 2010). To date, improved tree growth has been ascribed to the likely ability of tree roots to intercept and uptake a significant proportion of nutrient fertilizer residues from the crop rooting zone (Smith, 2010), but the contribution of inter-plant mycorrhizal connections to this process remains poorly investigated. Because improving tree mineral nutrition through agroforestry systems is a low-cost means to increase tree growth and to shorten the development duration before tree harvesting (Chifflot et al., 2009), this information is of importance to assess the overall costs or benefits of growing trees and annuals.
In this study, we investigated the extent to which intercropping with maize contributes to the mineral nutrition and biomass production of young hybrid walnut plants through the formation of a common AM fungal mycelial network. To this aim, we experimentally simulated an agroforestry system using a homemade experimental device in which young walnut hybrid rootstocks were connected or not by a common mycelial network to maize plants grown under contrasting Pi supply levels. This experimental device was made of two separate compartments in which walnut and maize were planted and connected by a third compartment in which the extra-radical mycelium responsible for mineral nutrient supply for the plants was separated by fine nylon nets from the associated host roots. Two months after plant sowing, we analyzed plant growth parameters, nutrient contents, and expression of plant genes coding mycorrhiza-inducible PHT1 transporters, here identified by phylogenic inference of orthologs. We hypothesized that (1) the inoculum reservoir formed by walnut roots would allow the mycorrhization of maize roots through the formation of a CMN, thereby giving access to maize fertilization residues; (2) hyphal connections under P-deficient conditions would result in a benefit comparable to P-sufficient conditions without a CMN; and (3) due to a slower growth rate than maize, walnut would invest more carbon in the development of the mycorrhizal fungus than maize.
2 Materials and methods
2.1 Biological material
Micropropagated plantlets of the walnut hydrid rootstock RX1 (J. regia x J. microcarpa) (Browne et al., 2015) were provided by Dr. Laurent Jouve (Tarnagas, Brive-la-Gaillarde, France). Rootstocks were received 7 weeks after root induction and placed for ex vitro acclimatization in closed mini-greenhouses (Rapid Grow, Nortene) filled with a sterilized (120°C, 12 h) quartz sand (Biot, France): zeolite (Symbion, Czech Republic) substrate (1:1, v:v). They were sprayed twice a week with tap water to maintain humidity and fertilized once a week with 15 ml per plant of an NPK 6-4-6 solution containing 2% of magnesium and oligoelements (Algoflash, France). Walnut cultures were maintained in a growth chamber under controlled conditions (16 h photoperiod, 23°C/18°C day/night, 100% relative humidity, 220 µE m−2 s−1). After 2 months of growth, plantlets were gradually exposed to reduced relative humidity by progressively removing the box covers during a further month, then RX1 rootstocks were planted in microcosms.
Maize (Z. mays L. var. Pompeo) seeds were surface-disinfected for 15 min in a commercial solution of sodium hypochlorite (1% available chlorine) and rinsed thoroughly. After imbibition in sterile water for 12 h in the dark at 4°C, seeds were germinated at 20°C with 16 h of fluorescent light (Osram Fluora L36W/77) on filter papers moist with sterile water. Maize plantlets were sown in microcosms at the third leaf stage.
The isolate DAOM197198 (also known as DAOM181602) of the model AM fungus Rhizophagus irregularis originally isolated from Pont Rouge, Canada (Stockinger et al., 2009), and commercialized by Agronutrition (Labège, France), was purchased as an axenic spore suspension (200 spores/ml).
2.2 Microcosm setup, plant inoculation, and phosphate fertilization
Microcosms were set up in a homemade device comprising tripartite compartments consisting of two root hyphal compartments, each connected to one central hyphal pipe (Figure 1). Root hyphal compartments were made out of PVC tubes, each having a hole cut to allow the insertion of the horizontal central PVC tube (7.5 cm length, 5 cm inner diameter) containing the hyphal compartment. The walnut mycorrhizal donor plant compartment was designed wider (30 cm height, 12 cm inner diameter) than the maize plant receiver compartment (30 cm height, 7.5 cm inner diameter) to allow maize plants to explore beyond their own compartment (Teste et al., 2015). To create an air gap, a PVC ring (3.5 cm length, 5 cm external diameter) was vertically inserted in the central PVC tube and distributed at equal distance from each root hyphal compartment. In order to prevent a substrate-mediated diffusion of solutes between both sides of the PVC ring, the air gap was sandwiched between two 1-mm wire meshes using two PVC seals. Both sides of the air gap had a 40-µm nylon membrane (Anachem, Bedfordshire, UK) through which hyphae, but not plant roots, can grow (Wang et al., 2016). Two additional plastic boxes were placed to the base of each of the root hyphal compartments to collect drainage solutions. Root hyphal and central compartments were filled with the corresponding volume of a sterilized (120°C, 12 h) quartz sand (Biot, France): zeolite (Symbion, Czech Republic) substrate (1:1, v:v). Walnut rootstocks were planted into the wider root hyphal compartment and maize seedlings were planted into the other one. To assess whether mycorrhizal walnuts can benefit maize plant growth through a CMN, four treatments were compared. For the mycorrhizal modality, to check whether inoculated saplings in the nursery should serve as infection sources for the interrow crop via a CMN, only donor walnut plants were inoculated (AM_Jug) or not (NM_Jug) with 1 ml of the spore suspension of R. irregularis. For the Pi modality, only recipient maize plants were fertilized in order to simulate nutrient fertilizer residues in the crop rooting zone. To this aim, maize plant received once a week 10 ml of either a low-Pi (Zea_P/10: 0.13 mM NaH2PO4, 2H2O) or a full-strength Pi solution (Zea_P: 1.3 mM NaH2PO4, 2H2O) Hoagland solution (Bonneau et al., 2013), while walnut donor saplings received 10 ml of tap water. Plants were maintained in a growth chamber under controlled conditions with 16 h of light (220 µE m−2 s−1) at 23°C and 8 h of dark at 19°C for 2 months before harvest. For each treatment, the experimental setup was replicated at least six times.
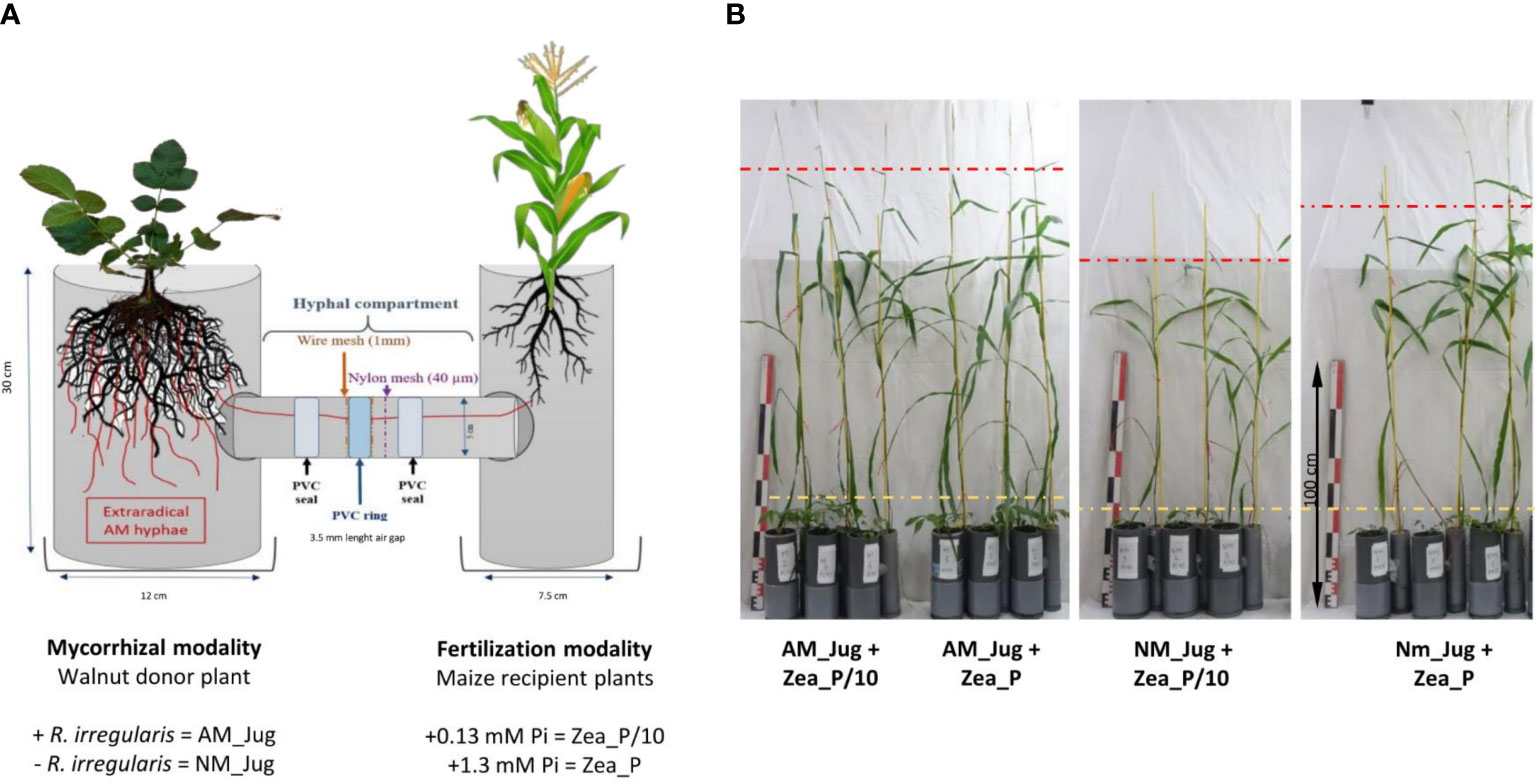
Figure 1 Schematic representation (A) and pictures (B) of the experimental device in which young walnut hybrid rootstocks (Jug) were connected (AM) or not (NM) by a common mycelial network to maize plants (Zea) grown under two contrasting Pi supply levels (Zea_P/10 and Zea_P). Yellow and red dashed lines show the stem height reached by walnut and maize plants, respectively. Pictures in panel B were taken 2 months after plant sowing in microcosms.
2.3 Measurement of plant growth and nutritional parameters
Before harvest, the leaf chlorophyll a fluorescence was quantified on attached donor and recipient leaves using a Pulse–Amplitude–Modulation (PAM) Chlorophyll Fluorimeter (Junior PAM, Walz, Effeltrich, Germany). Plants were dark-adapted for at least 1 h and measurements were further performed on leaf adaxial side. From these measurements, the maximum photochemical quantum yield of PSII (Fv/Fm) and the effective photochemical quantum yield of PSII (YII) were calculated as described in the operator’s guide. For plant growth parameters, plant height (cm), branch or leaf number per plant, stem diameter at the soil line (mm), fresh shoot and root weights (g), and primary root length (cm) were measured 2 months after sawing. To determine dry matter weights (DW), shoot and root subsamples were separately weighed fresh, and subsequently dried for 2 days at 80°C and weighed. For soluble Pi, C, and N measurements, dried root and leaf samples were finely ground using a Retsch Mixer ball mill (Haan, Germany). Soluble Pi was extracted from 5 mg of dried samples as described by Grunwald et al. (2009). Free Pi was determined by measuring absorbance at 650 nm using the BIOMOL Green™ Reagent (Enzo Life Sciences, Villeurbanne, France) according to the instructions of the manufacturer. Root and leaf dried aliquots (5 mg) were analyzed for their C and N contents at the Biogeosciences Laboratory of the University of Bourgogne Franche-Comté (Dijon, France) on a FlashSmart Elemental Analyzer (Thermo Scientific).
2.4 Staining of AM fungal propagules and quantification of root colonization
At harvest, root samples were randomly collected from each plant and dried for 2 days at 80°C (Hetrick et al., 1989). After overnight rehydratation in water (Tarkalson et al., 1998), they were cleared at 90°C for 30 min in 10% (w/v) KOH (Phillips and Hayman, 1970). Walnut roots were additionally bleached with three drops of 30% H2O2 to remove any phenolic compounds left in cleared roots (Kormanik and McGraw, 1982). Roots were rinsed with tap water before staining in a 0.05% (w/v) trypan blue (Sigma-Aldrich) solution in lactoglycerol (lactic acid-glycerol demineralized water, 1:1:1) for 30 min at 90°C. Thirty fragments, ca. 10 mm long, were randomly collected from each sample, mounted 15 per slide in glycerol and observed at a magnification of 200 as described in Trouvelot et al. (1986). Calculations of frequency of mycorrhization (F%), percentage of root cortex colonization (M%), and percentage of arbuscules (A%) were carried out with the MycoCalc program (http://www.dijon.inra.fr/mychintec/Mycocalc-prg/download.html). Similar to roots but without drying, clearing, and bleaching steps, the 40-µm nylon mesh and the substrate collected in the half-hyphal pipes connecting the maize compartment were also stained in trypan blue for 30 min at room temperature and rinsed with tap water before examination.
2.5 Identification and in silico characterization of proteins orthologous to the AM-inducible phosphate transporter MtPT4 of Medicago truncatula in J. regia, J. microcarpa, and Z. mays
To identify walnut RX1 and maize plant PHT1 transporters orthologous to the AM-inducible phosphate transporter MtPT4 of the model legume Medicago truncatula (Harrison et al., 2002), we used Orthofinder (version 2.2.6; Emms and Kelly, 2015) through standard mode parameters in the Diamond tool (v0.9.22.123) all-versus-all. To this aim, the complete predicted proteomes of J. regia, J. microcarpa, M. truncatula, and Z. mays were download at: https://treegenesdb.org/FTP/Genomes/Jure/v2.0/annotation/Jure.2_0.pep.fa.gz, https://treegenesdb.org/FTP/Genomes/Jumi/v1.0/annotation/jumi.1_0.peptides.fa, https://genome.jgi.doe.gov/portal/pages/dynamicOrganismDownload.jsf?organism=Mtruncatula/Mtruncatula_198_protein.fa.gz, and https://ftp.ensemblgenomes.org/pub/release-43/plants/fasta/zea_mays/pep/Zea_mays.B73_RefGen_v4.pep.all.fa.gz, respectively. Bioinformatics tools available at NGPhylogeny.fr (https://ngphylogeny.fr) were used according to the one click mode with MAFFT for multiple alignment, BGME for alignment refinement, FastME for tree inference, Newick Display for tree rendering, and iTOL for phylogenic tree display and annotation (Lemoine et al., 2019).
Protein sequences were aligned using MultAlin (http://multalin.toulouse.inra.fr/multalin/) alpha-helical trans-membrane (TM) regions together with cyto- and exoplasmic sequence orientations and were predicted according to DeepTMHMM (https://dtu.biolib.com/DeepTMHMM).
2.6 Primer design
On the basis of the plant orthologous proteins identified using Orthofinder, corresponding nucleotide sequences were retrieved to design oligonucleotide primers with Primer-BLAST (https://www.ncbi.nlm.nih.gov/tools/primer-blast). To monitor Pi acquisition through the fungal side, the expression pattern of the gene coding the high-affinity transporter RiPT1 previously found to be regulated by external Pi concentrations in R. irregularis (Calabrese et al., 2019) was monitored in mRNA extracts of walnut and maize roots. All primers used are listed in Supplementary Table 1.
2.7 RNA extraction, reverse transcription, and qRT-PCR
At harvest, maize and walnut root subsamples (1.5 g) were immediately frozen in liquid nitrogen and kept at −80°C before use. They were ground to a fine powder with a pre-cooled mortar and pestle before mRNA extraction. The SV Total RNA Isolation System (Promega, Madison, USA), which incorporates a DNase treatment step, was used to recover total root RNA from 50 mg of ground tissues, according to the supplier instructions. For walnut, 50 mg of insoluble polyvinylpyrrolidone was added to root samples before RNA extraction in order to remove polyphenols (Hu et al., 2002). Purified RNAs were quantified by measuring the absorbance at 260 nm with a Nanodrop (ND-1000 spectrophotometer, Isogen Life Sciences, Maarssen, Netherlands). Complementary DNAs (cDNAs) were obtained using the High-Capacity cDNA Reverse Transcription Kit (Thermo Fisher Scientific) using 300 ng of total RNA per reaction. Quantitative RT-PCRs were run in a 7500 real-time PCR system (Roche) using the following settings: 95°C for 3 min and then 40 cycles of 95°C for 30 s, 60°C for 1 min, and 72°C for 30 s. These analyses comprised at least five biologicals and three technical replicates per treatment. Transcript levels were expressed as normalized expression to previously described reference genes (Table S1).
2.8 Statistical analyses
For each plant, mycorrhizal colonization parameters were compared between the two fertilization regimes with the Welch’s t-test (unequal variances t-test), after arcsin square root transformation of percentages. For comparison of growth, nutritional, and gene expression parameters between treatments, the Kruskal–Wallis H-test with post-hoc Tukey HSD (Honestly Significant Difference) was used under the Rstudio environment (version 4.03) due to non-Gaussian distribution of data and/or inequality of variances, as checked by the Kolmogrov–Smirnov’s and Levene’s tests, respectively. p-values less than 0.05 were used for statistical significance.
3 Results
3.1 Mycorrhizal colonization and extra-radical hypha development
In walnut roots inoculated with R. irregularis, the frequency of colonization (F%), the intensity of root cortex colonization (M%), and arbuscule abundance (A%) in the Zea_P/10 treatment reached 100%, 20%, and 10%, respectively (Figure 2A). The intensity of root cortex colonization and arbuscule abundance in AM-Jug roots were significantly (p < 0.05) higher in the Zea_P/10 treatment when compared to Zea_P (Figure 2A). In non-inoculated maize roots connected to AM walnut plants, F%, M%, and A% values reached 100%, 30%, and 25%, respectively, in both Pi fertilization regimes (Figure 2B). Whatever the fertilization regime applied to maize plants, extra-radical hyphae were visualized after trypan blue staining of the nylon mesh (Figure 2C) and the substrate (Figure 2D) contained in the hyphal pipes close to the maize root compartment. In both Pi fertilization regimes, walnut and maize plants were not colonized when the walnut donor plant was not inoculated (negative controls).
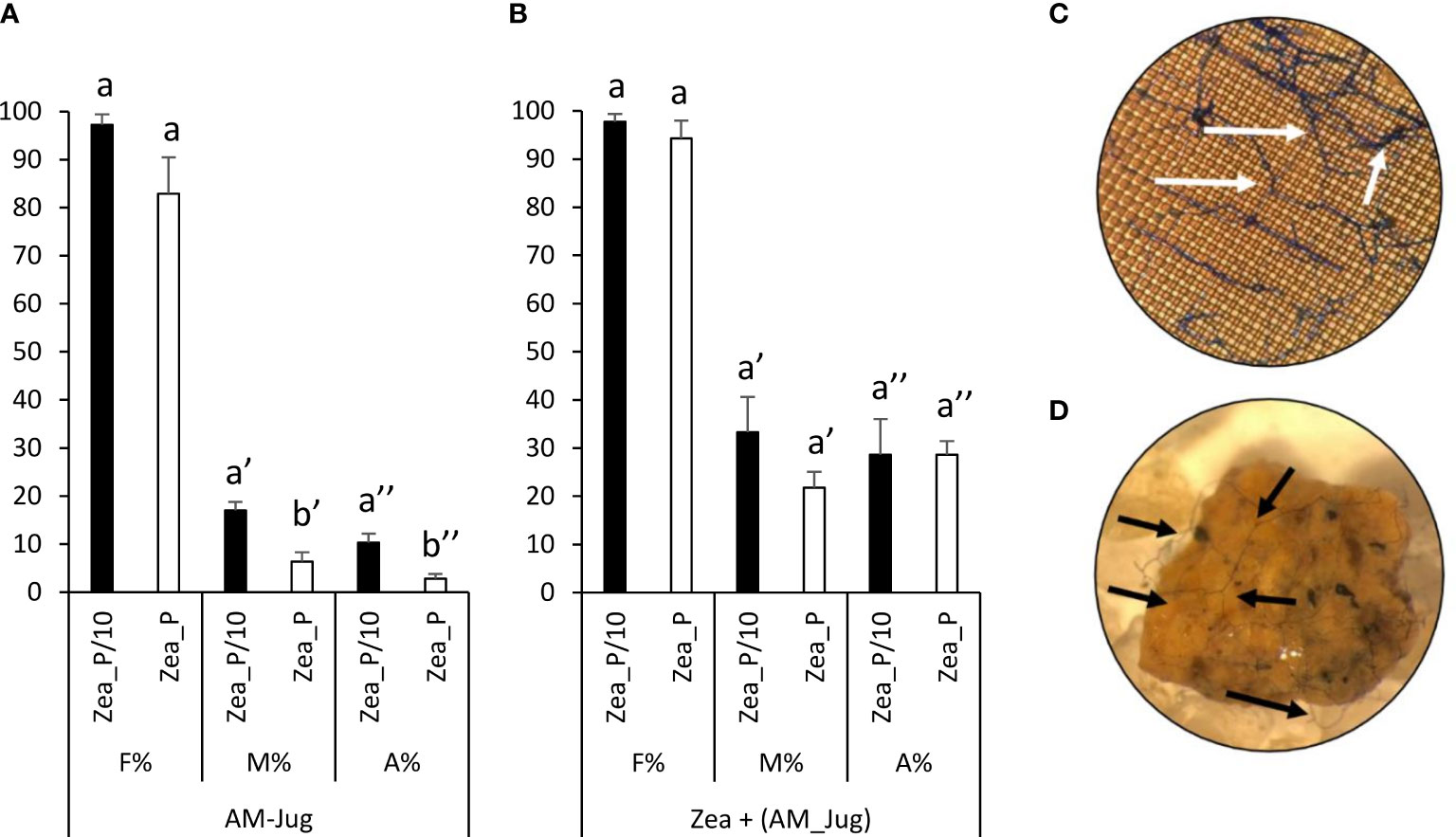
Figure 2 Mycorrhizal colonization parameters (frequency, F%; mycorrhizal intensity, M%; arbuscule abundance, A%, in the whole root) measured 2 months after inoculation of walnut RX1 plants with R. irregularis under contrasting Pi fertilization regimes of maize (Zea_P/10, Zea_P) in (A) walnut donor and (B) non-inoculated maize recipient roots. Values correspond to the mean ( ± SE) of six replicates per treatment. Different lowercase letters indicate significant difference (Welch’s t-test after arcsin square root transformation; p < 0.05). Whatever the Pi fertilization regime of maize, white and black arrows show the development of the extra-radical mycelium on the nylon mesh (C) and the substrate (D) in the hyphal compartment close to maize roots after trypan blue staining and observation at a magnification of 100 and 16, respectively.
3.2 Effect of walnut mycorrhization and maize Pi fertilization on rootstock growth and nutritional parameters
In walnut roots, in both Pi-limited (P/10) and Pi-replete (P) fertilization regimes of maize, mycorrhization of walnut (AM_Jug) led to a significant increase (p < 0.05) in root collar, root, and shoot dry weights (DW), relative to non-mycorrhizal (NM_Jug) plants (Figure 3A). Under the maize Pi-limited condition, but not in the Pi-replete condition, AM walnut plants displayed a higher (p < 0.05) stem height, leaf Pi concentration, and effective photochemical quantum yield of PSII (YII), together with a decreased root carbon content and shoot-to-root biomass ratio when compared to NM saplings (Figure 3B). All the other parameters measured in walnut plants [the maximum photochemical quantum yield of PS II (Fv/Fm), primary root length, the number of branches, root soluble Pi, leaf C content, plant N content, and leaf C/N ratio] were not significantly modified by either walnut mycorrhization or Pi fertilization regime applied to maize plants (Supplementary Table 2).
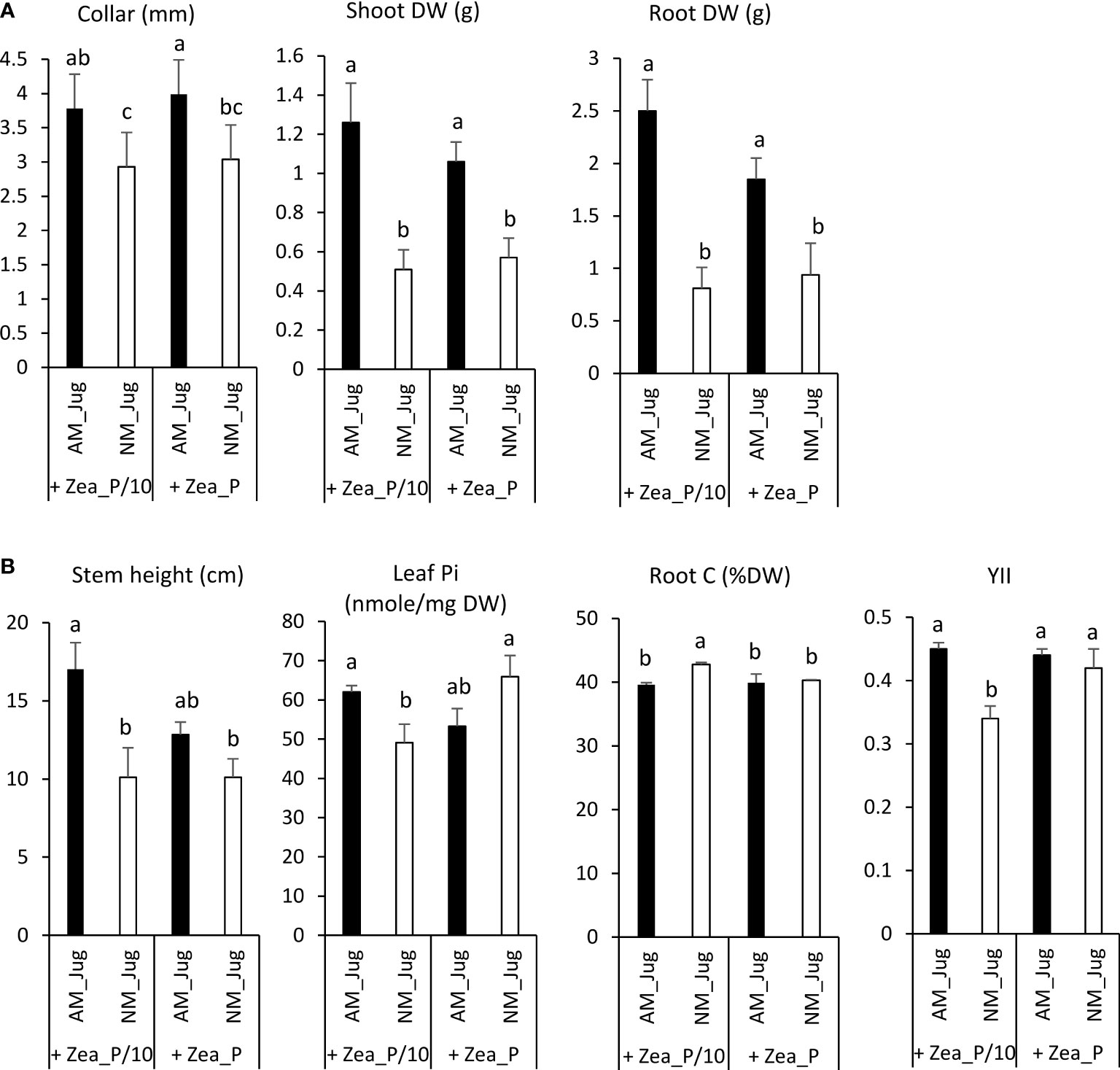
Figure 3 Effect walnut mycorrhization (AM) as compared to non-mycorrhizal controls (NM), on the growth and nutritional parameters of RX1, irrespective of Pi fertilization of Z. mays (A), or only recorded in the P/10 condition (B). Values correspond to the mean ( ± SE) of six replicates per treatment. Different lowercase letters indicate significant difference (Kruskal–Wallis H-test with post-hoc Tukey HSD; p < 0.05).
3.3 Effect of walnut mycorrhization and maize Pi fertilization on Z. mays growth and nutritional parameters
In maize plants, walnut mycorrhization with R. irregularis (AM_Jug) as compared to NM plants led to a significant increase in root collar diameter, height, leaf number, root and shoot DW, leaf N content, and Pi concentration, and to a decrease in leaf C/N ratio under the maize Pi-limited condition, but not in the Pi-replete condition (Figures 1 and 4). The other parameters measured in maize plants, namely, YII, Fv/Fm, primary root length, shoot-to-root biomass ratio, root Pi, and C and N root and leaf content, were not significantly modified by the mycorrhization of walnut with R. irregularis (Table S2). As regards Pi availability, the maximum photochemical quantum yield of PSII (Fv/Fm) was significantly but slightly decreased only in NM plants under the low-Pi condition, when compared to the Pi-replete fertilization regime (Table S2).
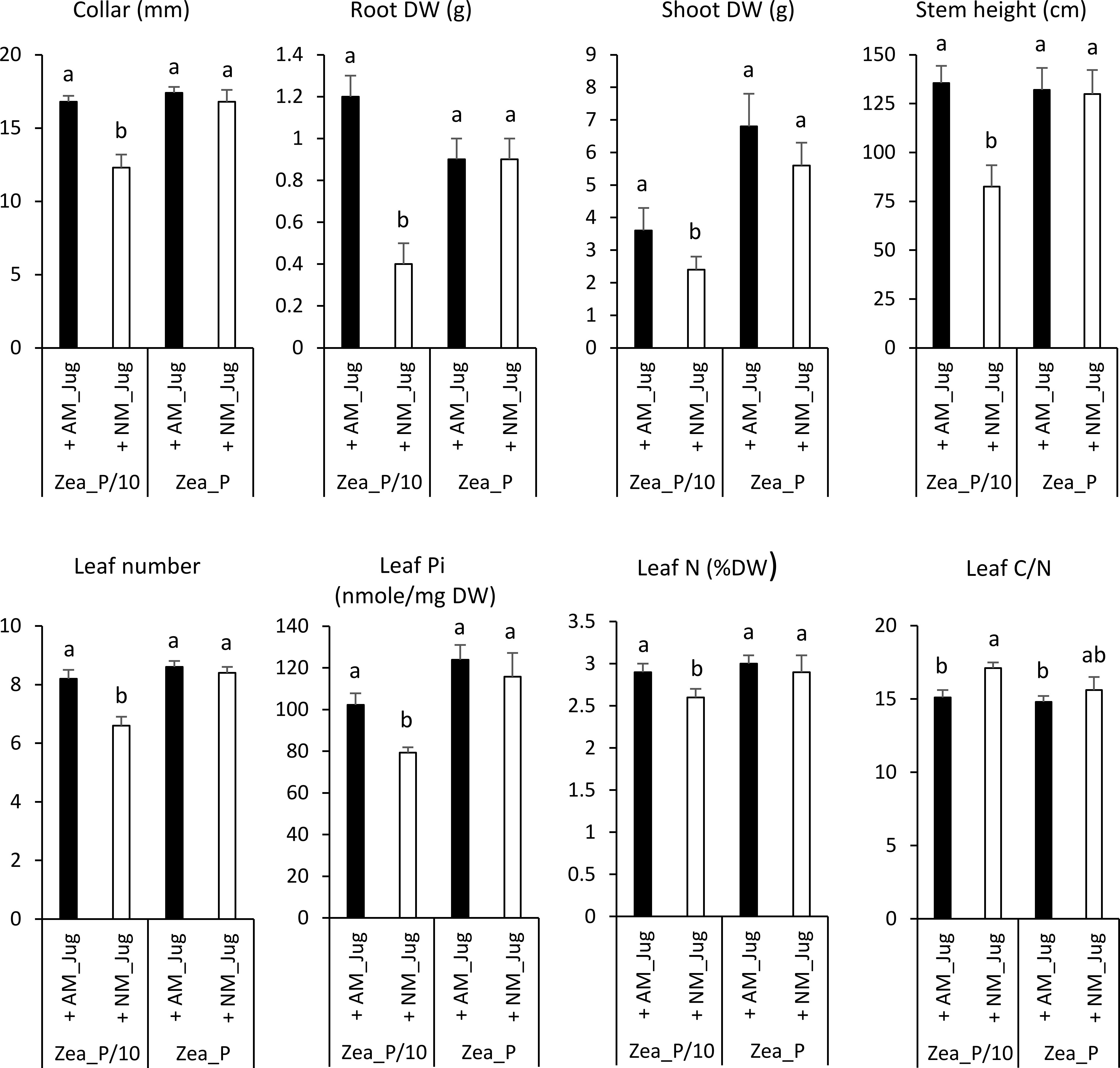
Figure 4 Effect of walnut mycorrhization (AM) as compared to non-mycorrhizal controls (NM), on the growth and nutritional parameters of maize plants only recorded under the P/10 condition. Values correspond to the mean ( ± SE) of six replicates per treatment. Different lowercase letters indicate significant difference (Kruskal–Wallis H-test with post-hoc Tukey HSD; p < 0.05).
3.4 Identification of walnut and maize proteins orthologous to the AM-inducible phosphate transporter MtPT4 of M. truncatula
To identify walnut RX1 (J. regia x J. microcarpa) and maize plant PHT1 transporters orthologous to the AM-inducible phosphate transporter MtPT4 of M. truncatula, we performed an OrthoFinder analysis that included the predicted proteomes of J. microcarpa, J. regia, and Z. mays, and the reference proteome of M. truncatula. OrthoFinder outputs (Supplementary Table 3; Supplementary Figure 1) indicated that the MtPT4 orthogroup contained three proteins of J. microcarpa (Jumi_00855.t1, Jumi_15132.t1, and Jumi_21692.t1, further named JmPHT1;1, JmPHT1;2, and JmPHT1;3, respectively), three proteins of J. regia (Jr13_30200_p1, Jr13_30210_p1, and Jr16_00830_p1, further named JrPHT1;1; JrPHT1;2, and JrPHT1;3, respectively), and one protein of Z. mays (Zm00001d011498_P001). The latter protein turned out to be the AM-inducible Pi transporter ZmPHT1;6 previously described in maize (Glassop et al., 2005; Tian et al., 2013). Figure S1 shows that all the seven proteins retrieved in the MtPT4 orthogroup display the PHT1 signature consisting of the conserved sequence GGDYPLSATIxSE of 12 amino acid residues (Karandashov and Bucher, 2005). Hydrophobicity analysis (Figure S1) further indicated that they also share a common topology with PHT1 transporters, as inferred from 12 membrane-spanning domains, which are separated into two groups of six domains by one intracellular central loop to transfer the phosphate molecule (Poirier and Bucher, 2002). As expected, both C- and N-termini were predicted to be oriented inside the cell (Nussaume et al., 2011). According to the nomenclature of Nagy et al. (2015), the phylogenetic tree of the PHT1 protein family built with 81 additional amino acid sequences with substantial diversity and redundancy within dicots and monocots (Table S3) showed that all the protein candidates retrieved as MtPT4 orthologs in J. regia and J. microcarpa are associated with the AM-inducible subfamily I (Figure 5). The latter cluster includes the mycorrhiza-specific Pi transporters OsPT11, which, similar to MtPT4 (Pumplin and Harrison, 2009), has been localized at the arbuscule-branch domain of the peri-arbuscular membrane (Kobae and Hata, 2010). Reduced fungal growth and altered arbuscule morphology in Medicago pt4 and rice pt11 loss-of-function mutants also indicated that PT4 and PT11 are essential for AM-mediated phosphate uptake and arbuscule maintenance (Javot et al., 2007; Yang et al., 2012). Likewise, ZmPt1;6 is a mycorrhiza-specific Pi transporter of maize, and a knock-down pht1;6 mutant showed reduced mycorrhiza formation (Willmann et al., 2013). In dicot plant species, the other AM-inducible Pi transporters that cluster in subfamily III (Figure 5), such as StPT3 in potato (Rausch et al., 2001), are over-expressed in mycorrhizal roots but are also expressed in non-symbiotic conditions (Casieri et al., 2013).
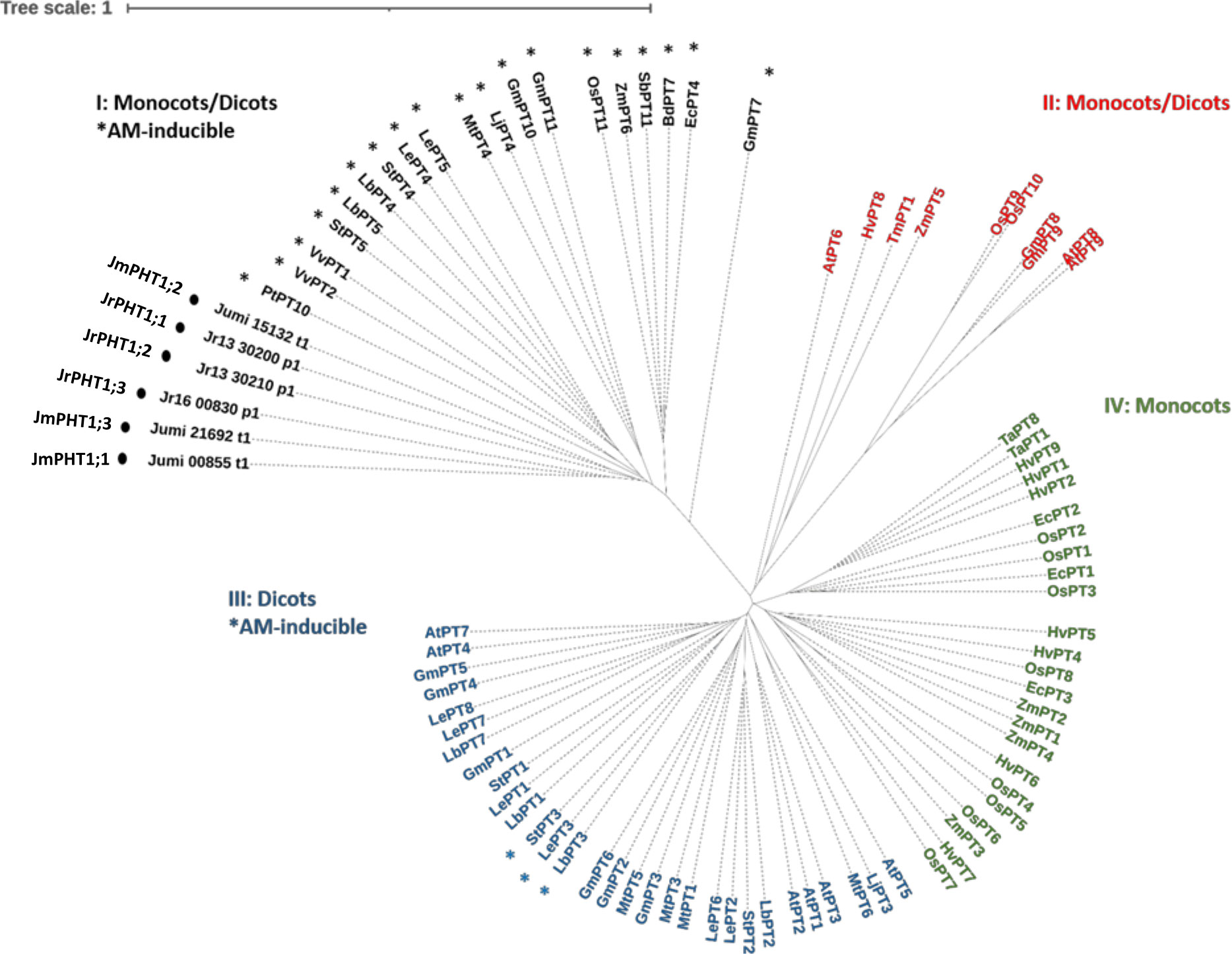
Figure 5 Unrooted phylogenic tree for the amino acid sequences of plant PHT1 transporters. Accessions are provided in Table S3. Roman numerals indicate the four PHT1 subfamilies thought to differ in evolutionary age (Nagy et al., 2005). Asterisks refer to AM-inducible PHT1 genes (Table S3). Circles indicate the putative PHT1 transporters of J. microcarpa and J. regia orthologous to MtPT4. Abbreviations for plant species: At, Arabidopsis thaliana; Bd, Brachypodium distachyon; Ec, Eleusine coracana; Gm, Glycine max; Hv, Hordeum vulgare; Jm, J. microcarpa; Jr, J. regia; Lb, Lycium barbarum; Le, Lycopersicon esculentum; Lj, Lotus japonicus; Mt, Medicago truncatula; Os, Oryza sativa; Pt, Populus trichocarpa; Sb, Sorghum bicolor; St, Solanum tuberosum; Ta, Triticum aestivum; Tm, Triticum monococcum; Vv, Vitis vinifera; and Zm, Zea mays.
3.5 Phosphate transporter gene expression
With respect to plant Pi acquisition through the mycorrhizal pathway, we analyzed the expression of the maize AM-essential phosphate transporter ZmPHT1;6 owing to its unique identification through Orthofinder. As expected, analysis of transcript levels in non-mycorrhizal (NM_Jug) maize roots showed no expression of ZmPHT1;6 (Figure 6). By contrast, inoculation of the donor walnut RX1 (AM_Jug) with R. irregularis resulted in a significant (p < 0.05) induction of ZmPHT1;6 in recipient maize roots whatever the Pi fertilization provided to maize. These results showed that in our experimental conditions, the expression of the gene ZmPHT1;6 is induced by the colonization of maize roots through the formation of a common mycelial network irrespective of the Pi supply.
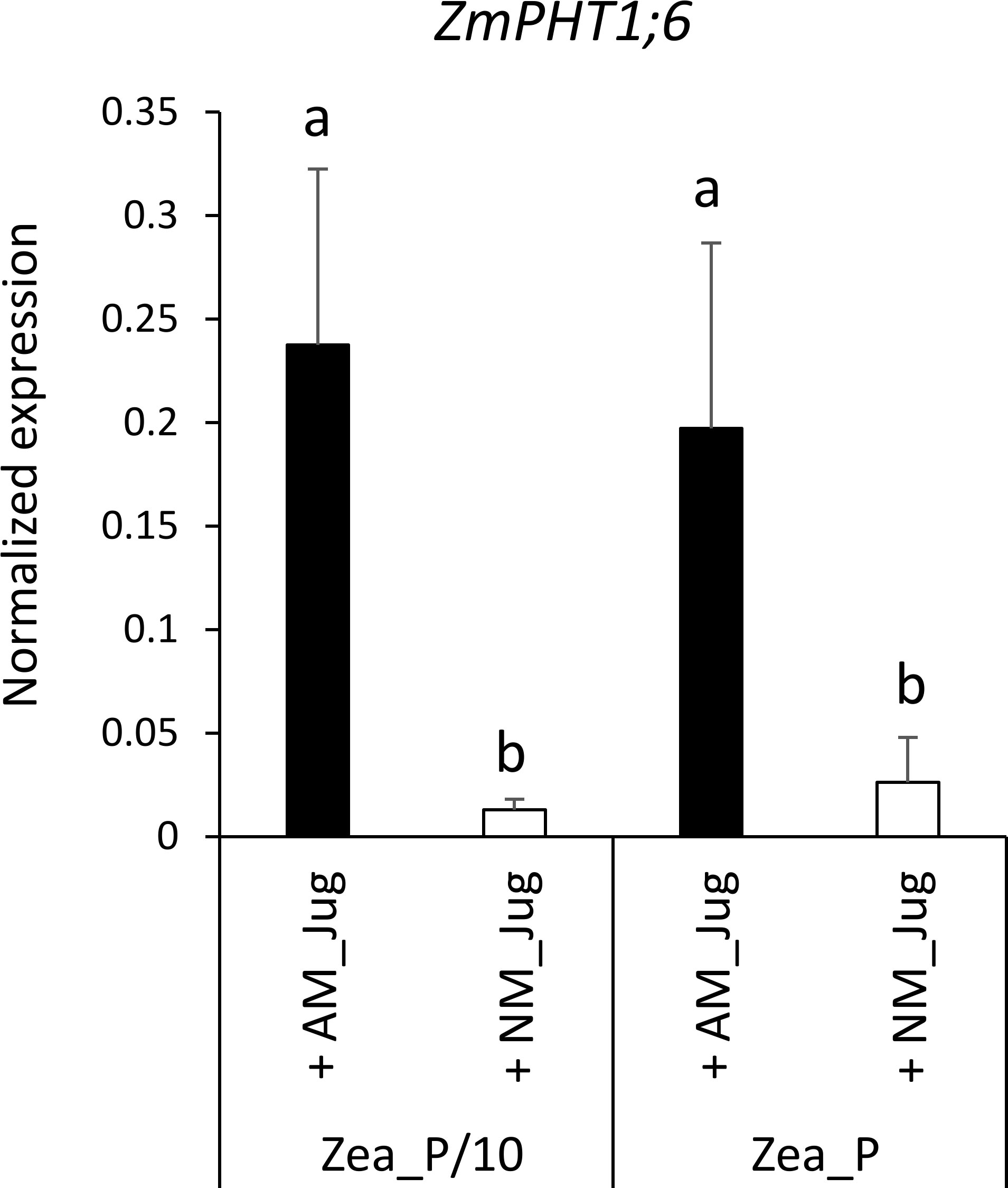
Figure 6 Expression pattern of the gene ZmPHT1;6 coding the phosphate transporter orthologous to MtPT4 in recipient maize roots 2 months after the inoculation (AM_Jug) of donor walnut RX1 with R. irregularis rootstocks or not (NM_Jug) under contrasting Pi fertilization regimes of maize (Zea_P/10, Zea_P). Gene expression was quantified by RT-qPCR and expressed as normalized expression to the maize reference genes described in Table S1. Values correspond to the mean ( ± SE) of five replicates per treatment. Different lowercase letters indicate significant difference (p < 0.05) according to the Kruskal–Wallis H-test with post-hoc Tukey HSD.
Whatever the treatment of walnut donor plants, the results displayed in Figure S2 show a very low expression in the roots of the hybrid rootstock RX1 of each of the three putative J. microcarpa Pi transporters retrieved in the MtPT4 orthogroup (JmPHT1;1, JmPHT1;2, and JmPHT1;3). The analysis of the transcript abundance of JrPHT1;1; JrPHT1;2, and JrPHT1;3 of J. regia in the roots of the hybrid walnut RX1 showed only weak expression levels of JrPHT1;3 in the four conditions tested (Figure 7). On the opposite, inoculation of RX1 (AM_Jug) with R. irregularis resulted in a significant (p < 0.05) induction of JrPHT1;1 and JrPHT1;2 in AM-walnut roots, relative to NM_Jug controls whatever the Pi fertilization provided to maize plants (Figure 7). Expression levels of both JrPHT1;1 and JrPHT1;2 tend to be higher, although not significantly, under the P/10 than under the P supply to maize roots. Together, these results demonstrated that the expression of JrPHT1;1 and JrPHT1;2 is induced in the roots of the hybrid walnut rootstock RX1 by R. irregularis irrespective of the Pi level supplied to maize recipient plants.
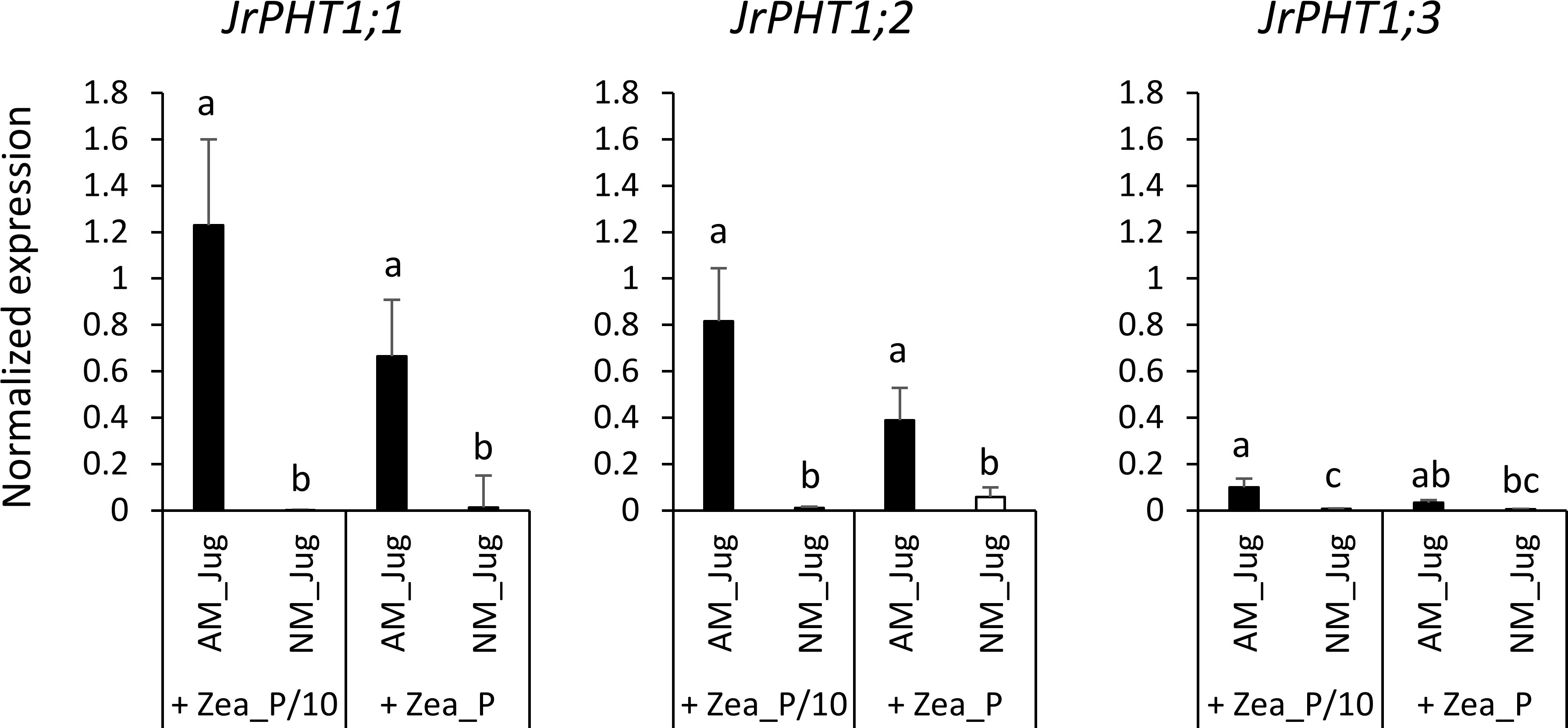
Figure 7 Expression patterns of the genes JrPHT1;1; JrPHT1;2, JrPHT1;3 coding proteins orthologous to the phosphate transporter MtPT4 in donor walnut RX1 roots 2 months after their inoculation with R. irregularis (AM_Jug) or not (NM_Jug) under contrasting Pi fertilization regimes of maize (Zea_P/10, Zea_P). Gene expression was quantified by RT-qPCR and expressed as normalized expression to the walnut reference genes described in Table S1. Values correspond to the mean ( ± SE) of five replicates per treatment. Different lowercase letters indicate significant difference (p < 0.05) according to the Kruskal–Wallis H-test with post-hoc Tukey HSD.
To monitor soil Pi acquisition through the fungal side, we monitored in walnut RX1 and maize roots the expression of the gene coding the high-affinity phosphate transporter RiPT1 of R. irregularis. As expected, analysis of transcript levels in non-inoculated (NM_Jug) walnut and maize roots showed very low expression of RiPT1 (Figures 8A, B). By contrast, inoculation of RX1 (AM_Jug) with R. irregularis resulted in a significant (p < 0.05) induction of the expression of RiPT1 not only in walnut (Figure 8A), but also in maize roots (Figure 8B), whatever the Pi fertilization provided to maize. These results indicated that the expression of RiPT1 is induced not only in the mycorrhizated donor RX1 but also in the recipient maize roots. Further Welch’s t-test comparison between AM walnut and AM maize roots indicated a higher expression of the RiPT1 gene in maize than in walnut roots both under the lowest (Zea_P/10, p < 0.08) and the highest Pi supply (Zea_P, p < 0.03). This showed that the contribution of the extra-radical mycelium of AM maize roots to Pi acquisition is higher than that of AM walnut roots.
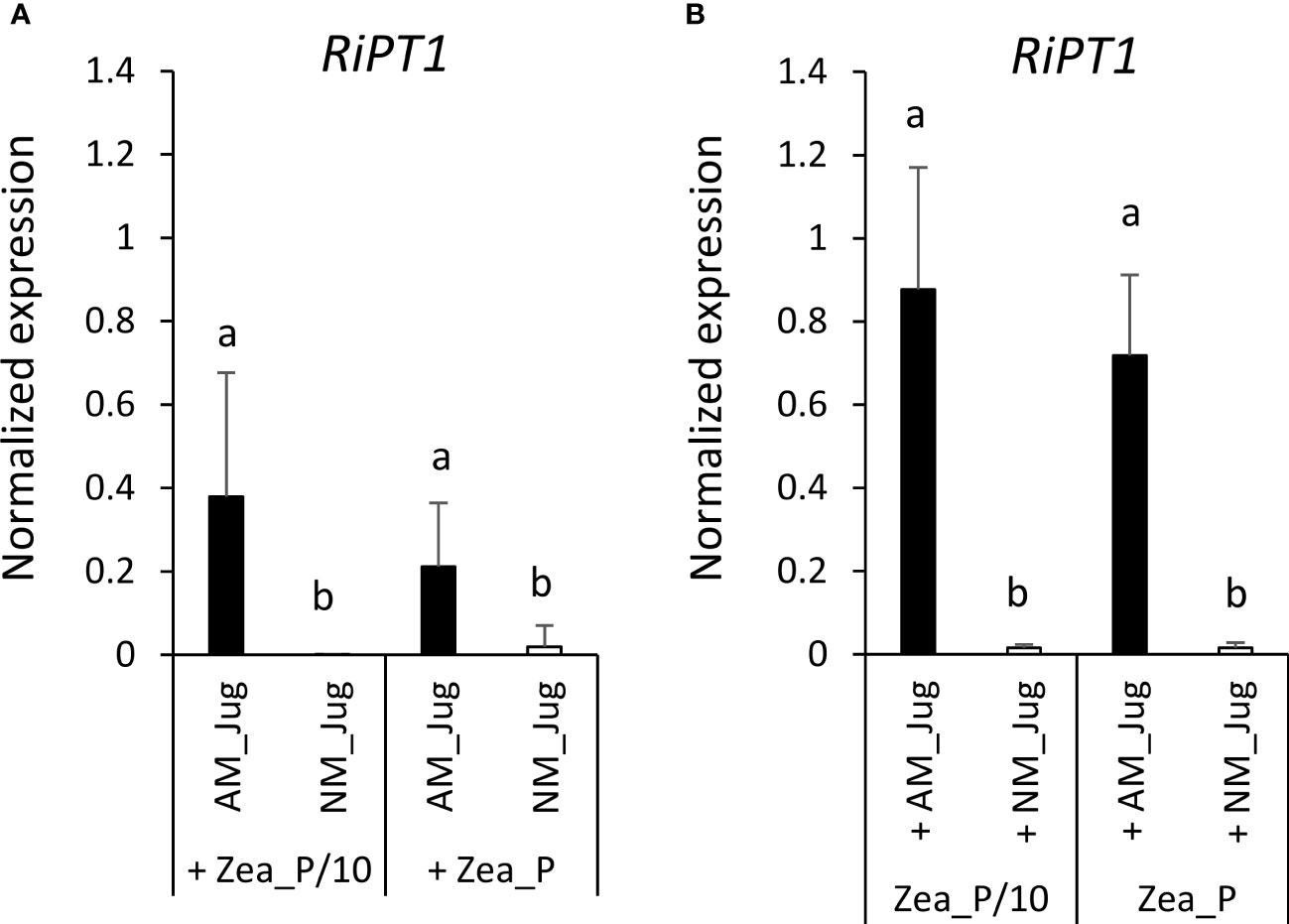
Figure 8 Expression pattern of the gene RiPT1 of R. irregularis 2 months after its inoculation (AM_Jug) as compared to not inoculated (NM_Jug) of walnut RX1 rootstocks under contrasting Pi fertilization regimes of maize (Zea_P/10, Zea_P) in walnut donor (A), and non-inoculated maize recipient roots (B). Gene expression was quantified by RT-qPCR and expressed as normalized expression to the two fungal reference genes described in Table S1. Values correspond to the mean ( ± SE) of five replicates per treatment. Different lowercase letters indicate significant difference (p < 0.05) according to the Kruskal–Wallis H-test with post-hoc Tukey HSD.
4 Discussion
4.1 The inoculum reservoir formed by walnut roots allows the mycorrhization of maize through the formation of a CMN that gives access to maize fertilization residues
Irrespective of the Pi supplied to Z. mays recipient plants, non-inoculated maize roots connected to mycorrhizal walnut rootstocks displayed F%, M%, and A% values reaching 100%, 30%, and 25%, respectively. These results demonstrated that whatever the Pi fertilization regime applied to maize, the inoculum reservoir formed by AM walnut roots enables the mycorrhization of maize recipient roots through the development of a common mycelial network (CMN). Consistently, extra-radical hyphae emanating from walnut root were visualized in the two Pi treatments after trypan blue staining of the nylon mesh and substrate contained in the fungal pipes close to Z. mays roots. This observation showed that the extra-radical mycelium that developed outside AM walnut roots have crossed the 40-µm nylon mesh to reach the recipient compartment and further colonize maize roots. Consistently, the high-affinity phosphate transporter RiPT1 of R. irregularis and the maize phosphate transporter ZmPHT1;6 were only expressed in maize connected to mycorrhizal RX1 donor plants.
There was also evidence that AM walnut plants connected to recipient roots have access to maize Pi fertilization residues via the CMN as shown by higher stem diameter, shoot, and root dry biomasses recorded in mycorrhizal RX1 saplings as compared to non-inoculated walnuts in both Pi treatments. This finding demonstrated that walnut developing AM symbiosis gains growth benefit from the Pi supplied to maize through hyphal connections with walnut sapling. In addition, AM walnuts connected to recipient plants happened to be responsive to the extent of maize fertilization, as inferred from significantly lower M% and A% in the P condition relative to the P/10 treatment. This showed that Pi shortage in the maize compartment sustains walnut mycorrhization, while a 10-fold higher Pi fertilization restricts the intra-radical development of R. irregularis in RX1. Earlier studies have reported a significant suppression of AM symbiosis upon high Pi supplies (Rausch et al., 2001; Breuillin et al., 2010; Bonneau et al., 2013; Kobae et al., 2016). When plants consume more Pi to provide photosynthetically fixed carbon than that furnished by mycorrhizal hyphae, the host limits soil Pi uptake through the mycorrhizal pathway by reducing carbon allocation to the symbiont in order to save energy (Treseder and Allen, 2002; Blanke et al., 2005; Gavito et al., 2019). In agreement with this line, a decreased root carbon content, along with an increased leaf soluble Pi concentration and effective photochemical quantum yield of PSII in AM walnuts compared to NM plants, was only significant in the Pi-limiting condition. In the process of phosphate suppression of mycorrhization, Pi acts systemically to repress the expression of symbiotic genes, including phosphate transporters (Breuillin et al., 2010), resulting in lower AMF colonization (Salmeron-Santiago et al., 2021). However, in the present study, the transcript abundance of JrPHT1;1 and JrPHT1;2 in the roots of RX1 was similar irrespective of the Pi supply to maize plants. Because the cumulative Pi amount acquired through the mycorrhizal pathway and its translation into biomass yield did not differ in AM walnut saplings between the two Pi fertilization regimes, it is likely possible that the expression of mycorrhiza-inducible PHT1 transporters measured after symbiosis establishment may not actually reflect the Pi flux at the symbiotic interface (Grace et al., 2009; Walder et al., 2015; Sawers et al., 2017; Watts-Williams et al., 2019). In this respect, it has been proposed that the regulation of the abundance of Pi transporters in the periarbuscular membrane is probably more dependent on post-transcriptional and post-translational regulation events (Grace et al., 2009; Smith et al., 2011; Walder et al., 2015). As previously observed in maize plants (Tian et al., 2013; Polcyn et al., 2019; Liang et al., 2022), increasing Pi fertilization did not significantly decrease mycorrhizal colonization, suggesting that phosphate inhibition of internal mycorrhization may not be a general paradigm, depending on host and fungal genotypes (Peña Venegas et al., 2021).
4.2 Common arbuscular mycorrhizal network alleviates Pi shortage in walnut and maize plants
Relative to a 1.3 mM Pi supply, a 0.13 mM Pi fertilization of non-mycorrhizal maize plants led to a significant reduction in stem height and diameter, leaf number, Pi and N content, and shoot and root biomass. As previously observed (Almagrabi and Abdelmoneim, 2012; Ma et al., 2021), this result showed that maize growth is limited by phosphate deficiency. In NM maize exposed to P-sufficient treatments relative to Pi-limiting conditions, higher leaf count, dry mass, plant height, and stem thickness have also been reported: as an indicator of crop growth and development, when N is amply supplied, plant height is known to increase as P availability increases, similar to stem thickness (Coetzee et al., 2016). In contrast to what was observed in NM controls, in AM maize plants cultivated under the P/10 supply, all growth and nutritional parameters rose to a level similar to that recorded for NM plants under the P-sufficient treatment, indicating that mycorrhization of maize plants alleviated Pi deficiency under the P/10 supply. These responses to mycorrhization in low P soil are consistent with previous studies demonstrating that maize crop benefits from mycorrhizal associations (Calderón-Vázquez et al., 2011; Ma et al., 2021). They also fit with the “optimal trade principle” predicting that plant growth will most strongly benefit from symbiosis with AM fungi under conditions of low soil phosphorus supply (Johnson, 2010; Johnson et al., 2015). We concluded that under maize Pi shortage, the mycorrhizal connection between walnut and maize roots alleviates Pi deficiency in the recipient plant through a better mineral nutrition. This resulted in maize growth and nutritional benefits comparable to the P-sufficient condition without a common arbuscular mycorrhizal network.
As expected from the absence of mycorrhiza connections between plants when walnut donor roots were not inoculated with R. irregularis, the Pi fertilization regime of maize had no impact on the parameters measured in NM walnut plants. This result supported the absence of solute diffusion through the air gap. With regard to walnut growth benefits gained from the Pi supplied to maize through hyphal connections relative to non-fertilized NM walnut plants, we observed that a 0.13 mM Pi fertilization led to a significant increase in root collar, stem height, leaf Pi content, and shoot and root DW. This underlines that under maize low Pi fertilization, the mycorrhizal connection between walnut and maize roots alleviates Pi deficiency in the donor plant through a better mineral nutrition. Contrary to maize, mycorrhizal growth benefits of walnut plants in terms of root collar and dry mass were conserved under high Pi fertilization of maize, even though intra-radical colonization was reduced. Altogether, these results support the idea that walnut is more dependent on the Pi uptake mycorrhizal pathway than maize to sustain its development owing to a coarse root architecture that has a limited intrinsic ability to absorb soil nutrients (Bates and Lynch, 2001). We concluded that a common arbuscular mycorrhizal network is able to overcome phosphate shortage in walnut and maize plants.
4.3 R. irregularis acts as a stronger carbon sink in walnut than in maize
When comparing mycorrhizal walnut and maize plants grown under the P/10 supply relative to NM plants, a significant decreased root carbon content along with an increased effective photochemical quantum yield of PSII, was only observed in RX1, while both plants displayed a higher leaf Pi concentration. With respect to the S/R biomass ratio as a measure of the carbon cost for AM establishment (Chen et al., 2010), mycorrhizal colonization decreased the shoot to root biomass partitioning only in RX1 grown under the P/10 supply. These findings indicated that AM fungal colonization enhances walnut investment in root biomass development. Taken together, these results showed that mycorrhization-induced Pi uptake generates a higher carbon cost for walnut donor plants than for maize plants by increasing walnut plant photosynthesis to feed the AM fungus with carbon assimilate. In support of this rationale, when using walnut trees (J. nigra L., C3-plant) and maize (C4-plant), which display distinctly different ratios of 13C/12C (∂ 13C), analysis of ∂ 13C from an AM mycelium taken from the surrounding environment of intercropped walnut and maize roots indicated the transfer of walnut photosynthates to the AM mycelium (van Tuinen et al., 2020). With regard to the potential mechanisms underlying a larger carbon investment in AM symbiosis of walnut than maize, Graham et al. (1997) proposed that a high mycorrhizal dependency, as displayed by walnut, may reflect a greater availability of the carbon supplied by the host. On the opposite, because plants with rapid growth rate need more carbon and absorb more soil nutrients to achieve fast growth (Lovelock, 2008), less photosynthates may thus be available to feed the CMN. According to Elser et al. (2000), plant growth rate is usually negatively related to C/N content ratio. In the current study, when compared to NM plants, mycorrhization of maize with R. irregularis through the CMN led to a significant decrease in leaf C/N ratio under the maize Pi-limited condition, but not in the Pi-replete condition. As this result was not observed in walnut rootstocks, our data support the idea that at low soil fertility, walnut plant growth is limited more by nutrient uptake than by carbon supply (Poorter and de Jong, 1999; Korner, 2003). Under these conditions, it is more advantageous for walnut saplings than for maize plant to allocate carbon assimilates to feed the AM symbiont (van der Heijden and Horton, 2009).
5 Conclusion
In agreement with our working hypotheses, this study provides evidence that (1) the inoculum reservoir formed by walnut roots allows the mycorrhization of maize roots through the formation of a CMN, thereby giving access to maize fertilization residues; (2) hyphal connections under shortage of P result in a benefit comparable to the P-sufficient condition without a CMN; and (3) because of a slower root growth rate than maize, walnut invests more carbon in the development of the AM fungus than maize does. These findings support the idea that in an agroforestry system, the presence of perennial root systems enables the maintenance of an active AM fungal network, which constitutes an AM reservoir allowing root colonization of the annual crop, and authorizing low phosphorus fertilization input. CMN formation is a facilitative mechanisms between trees and companion crops favorable to their growth and development (Ingleby et al., 2007; Bainard et al., 2011; Bainard et al., 2012). These results are also in accordance with the stress-gradient hypothesis that predicts that low-fertilized agricultural lands would benefit more from intercropping than high-fertilized lands (Brooker and Callaghan, 1998; Zhu et al., 2023). Therefore, mycorrhization of tree saplings with a suitable AM fungus able to develop CMN favorable to alley annual crop may result in a benefit comparable to P fertilization and would therefore be a recommendable agronomical practice in the framework of the agroecological transition of agricultural systems.
Data availability statement
The original contributions presented in the study are included in the article/Supplementary Material. Further inquiries can be directed to the corresponding author.
Author contributions
Conceptualization, EM, FM-L, GR, and OL; methodology, EM, AM; JK, GR, and OL; writing—original draft, and writing—review and editing: EM, AM; JK, FM-L, GR, and OL; funding acquisition, FM-L; supervision, FM-L, GR, and OL. All authors contributed to the article and approved the submitted version.
Acknowledgments
EM’s PhD thesis was funded by the French Ministry of Agriculture and benefited from a collaboration with L Jouve’s company. We would like to thank all the people who provided us with invaluable assistance in carrying out this work.
Conflict of interest
The authors declare that the research was conducted in the absence of any commercial or financial relationships that could be construed as a potential conflict of interest.
Publisher’s note
All claims expressed in this article are solely those of the authors and do not necessarily represent those of their affiliated organizations, or those of the publisher, the editors and the reviewers. Any product that may be evaluated in this article, or claim that may be made by its manufacturer, is not guaranteed or endorsed by the publisher.
Supplementary material
The Supplementary Material for this article can be found online at: https://www.frontiersin.org/articles/10.3389/fpls.2023.1206047/full#supplementary-material
Supplementary Figure 1 | Sequence alignment of the mycorrhiza-inducible phosphate transporter M. truncatula PT4 (Medtr1g028600.1) and its corresponding orthologs in J. regia (JrPHT1;1/Jr13_30200_p1, JrPHT1;2/Jr13_30210_p1; JrPHT1;3/Jr16_00830_p1), J. microcarpa (JmPHT1;1/jumi_00855.t1, JmPHT1;2/jumi_15132.t1; JmPHT1;3/jumi_21692.t1), and Z. mays (Zm00001d011498_P001). Protein sequences were aligned using MultAlin (http://multalin.toulouse.inra.fr/multalin/). Green bars indicate predicted transmembrane segments according to DeepTMHMM (https://dtu.biolib.com/DeepTMHMM). Yellow and red bars underline the sequence signature GGDYPLSATIxSE and the intracellular central loop conserved between PHT1 proteins, respectively. Cytoplasmic (In) and exoplasmic (Out) sequence orientations were predicted according to DeepTMHMM (https://dtu.biolib.com/DeepTMHMM).
Supplementary Figure 2 | Expression patterns of the genes JmPHT1;1; JmPHT1;2, JmPHT1;3 coding proteins orthologous to the phosphate transporter MtPT4 in donor walnut RX1 roots 2 months after their inoculation with R. irregularis (AM_Jug) or not (NM_Jug) under contrasting Pi fertilization regimes of maize (Zea_P/10, Zea_P). Gene expression was quantified by RT-qPCR and expressed as normalized expression to the walnut reference genes described in Table S1. Values correspond to the mean ( ± SE) of five replicates per treatment. Different lowercase letters indicate significant difference (p < 0.05) according to the Kruskal–Wallis H-test with post-hoc Tukey HSD.
References
Almagrabi, O. A., Abdelmoneim, T. S. (2012). Using of arbuscular mycorrhizal fungi to reduce the deficiency effect of phosphorous fertilization on maize plants (Zea mays L.). Life Sci. J. 9, 1648–1654.
Bainard, L. D., Klironomos, J. N., Gordon, A. M. (2011). Arbuscular mycorrhizalfungi in tree-based intercropping systems: a review of their abundance and diversity. Pedobiol 54, 57–61. doi: 10.1016/j.pedobi.2010.11.00
Bainard, L. D., Koch, A. M., Gordon, A. M., Klironomos, J. N. (2012). Temporal and compositional differences of arbuscular mycorrhizal fungal communities in conventional monocropping and tree-based intercropping systems. Soil Biol. Biochem. 45, 172–180. doi: 10.1016/j.soilbio.2011.10.008
Bates, T. R., Lynch, J. P. (2001). Root hairs confer a competitive advantage under low phosphorus availability. Plant Soil 236, 243–250. doi: 10.1023/A:1012791706800
Battie-Laclau, P., Taschen, E., Plassard, C., Dezette, D., Abadie, J., Arnal, D., et al. (2020). Role of trees and herbaceous vegetation beneath trees in maintaining arbuscular mycorrhizal communities in temperate alley cropping systems. Plant Soil 453, 153–171. doi: 10.1007/s11104-019-04181-z
Bernard, A., Lheureux, F., Dirlewanger, E. (2018). Walnut: past and future of genetic improvement. Tree Genet. Genomes 14, 1. doi: 10.1007/s11295-017-1214-0
Blanke, V., Renker, C., Wagner, M., Füllner, K., Held, M., Kuhn, A. J., et al. (2005). Nitrogen supply affects arbuscular mycorrhizal colonization of Artemisia vulgaris in a phosphate-polluted field site. New Phytol. 166, 981–992. doi: 10.1111/j.1469-8137.2005.01374.x
Bonneau, L., Huguet, S., Wipf, D., Pauly, P., Truong, H. N. (2013). Combined phosphate and nitrogen limitation generates a nutrient stress transcriptome favorable for arbuscular mycorrhizal symbiosis in Medicago truncatula. New Phytol. 199, 188–202. doi: 10.1111/nph.12234
Brady, N. C., Weil, R. R. (2008). The nature and properties of soil. 14 ed (New Jersey: Prentice-Hall, Upper Saddle River). Available at: https://epsc413.wustl.edu/TOC_Textbook.pdf.
Breuillin, F., Schramm, J., Hajirezaei, M., Ahkami, A., Favre, P., Druege, U., et al. (2010). Phosphate systemically inhibits development of arbuscular mycorrhiza in Petunia hybrida and represses genes involved in mycorrhizal functioning. Plant J. 64, 1002–1017. doi: 10.1111/j.1365-313X.2010.04385.x
Brooker, R. W., Callaghan, T. V. (1998). The balance between positive and negative plant interactions and its relationship to environmental gradients: A model. Oikos 81, 196. doi: 10.2307/354648
Browne, G. T., Leslie, C. A., Grant, J. A., Bhat, R. G., Schmidt, L. S., Hackett, W. P., et al. (2015). Resistance to species of Phytophthora identified among clones of Juglans microcarpa × J. regia. HortScience 50, 1136–1142. doi: 10.21273/HORTSCI.50.8.1136
Brundrett, M. C. (1991). Mycorrhizas in natural ecosystems. Adv. Ecol. Res. 21, 171–313. doi: 10.1016/S0065-2504(08)60099-9
Calabrese, S., Cusant, L., Sarazin, A., Niehl, A., Erban, A., Brulé, D., et al. (2019). Imbalanced regulation of fungal nutrient transports according to phosphate availability in a symbiocosm formed by poplar, sorghum, and Rhizophagus irregularis. Front. Plant Sci. 10. doi: 10.3389/fpls.2019.01617
Calderón-Vázquez, C., Sawers, R. J., Herrera-Estrella, L. (2011). Phosphate deprivation in maize: genetics and genomics. Plant Physiol. 156, 1067–1077. doi: 10.1104/pp.111.174987
allaway, R. M. (1998). Are positive interactions species-specific? Oikos 82, 202–207. doi: 10.2307/3546931
Cardoso, I. M. (2002). Phosphorous in agroforestry systems: a contribution to sustainable agriculture in the Zona da Mata of Minas Gerais, Brazil (Wageningen University). Available at: https://edepot.wur.nl/121343.
Casieri, L., Ait Lahmidi, N., Doidy, J., Veneault-Fourrey, C., Migeon, A., Bonneau, L., et al. (2013). Biotrophic transportome in mutualistic plant-fungal interactions. Mycorrhiza 23, 597–625. doi: 10.1007/s00572-013-0496-9
Chen, M. M., Yin, H. B., O’Connor, P., Wang, Y. S., Zhu, G. (2010). C: N: P stoichiometry and specific growth rate of clover colonized by arbuscular mycorrhizal fungi. Plant Soil 326, 21–29. doi: 10.1007/s11104-009-9982-4
Chifflot, V., Rivest, D., Olivier, A., Cogliastro, A., Khasa, D. (2009). Molecular analysis of arbuscular mycorrhizal community structure and spores distribution in treebased intercropping and forest systems. Agric. Ecosyst. Environ. 131, 32–39. doi: 10.1016/j.agee.2008.11.010
Coetzee, P. E., Ceronio, G. M., du Preez, C. C. (2016). Evaluation of the effects of phosphorus and nitrogen source on aerial and subsoil parameters of maize (Zea mays L.) during early growth and development. South Afric. J. Plant Soil 33, 237–244. doi: 10.1080/02571862.2015.1112923
Comas, L. H., Callahan, H. S., Midford, P. E. (2014). Patterns in root traits of woody species hosting arbuscular and ectomycorrhizas: implications for the evolution of belowground strategies. Ecol. Evol. 4, 2979–2990. doi: 10.1002/ece3.1147
Comas, L. H., Eissenstat, D. M. (2009). Patterns in root trait variation among 25 co-existing NorthAmerican forest species. New Phytol. 182, 919–928. doi: 10.1111/j.1469-8137.2009.02799.x
Conley, D. J., Paerl, H. W., Howarth, R. W., Boesch, D. F., Seitzinger, S. P., Havens, K. E., et al. (2009). Ecology. Controlling eutrophication: nitrogen and phosphorus. Science 20, 1014–1015. doi: 10.1126/science.1167755
de Carvalho, A. M. X., de Castro, T. R., Cardoso, I. M., Kuyper, T. W. (2010). “Mycorrhizal associations in agroforestry systems,” in Soil biology and agriculture in the tropics, soil biology 21. Ed. Dion, P. (Berlin Heidelberg: Springer-Verlag), 185–208. doi: 10.1007/978-3-642-05076-3_9
de Kroon, H., Hendriks, M., van Ruijven, J., Ravenek, J., Padilla, F. M., Jongejans, E., et al. (2012). Root responses to nutrients and soil biota: drivers of species coexistence and ecosystem productivity. J. Ecol. 100, 6–15. doi: 10.1111/j.1365-2745.2011.01906.x
Elser, J. J., Fagan, W. F., Denno, R. F., Dobberfuhl, D. R., Folarin, A., Huberty, A., et al. (2000). Nutritional constraints in terrestrial and freshwater food webs. Nature 408, 578–580. doi: 10.1038/35046058
Emms, D. M., Kelly, S. (2015). OrthoFinder: solving fundamental biases in whole genome comparisons dramatically improves orthogroup inference accuracy. Genome Biol. 16, 157. doi: 10.1186/s13059-015-0721-2
Fellbaum, C. R., Mensah, J. A., Cloos, A. J., Strahan, G. E., Pfeffer, P. E., Kiers, E. T., et al. (2014). Fungal nutrient allocation in common mycorrhizal networks is regulated by the carbon source strength of individual host plants. New Phytol. 203, 646–656. doi: 10.1111/nph.12827
Fitter, A. H., Graves, J. D., Watkins, N. K., Robinson, D., Scrimgeour, C. (1998). Carbon transfer between plants and its control in networks of arbuscular mycorrhizas. Funct. Ecol. 12, 406–412. doi: 10.1046/j.1365-2435.1998.00206.x
Foley, J. A. (2005). Global consequences of land use. Science 309, 570–574. doi: 10.1126/science.1111772
Gavito, M. E., Jakobsen, I., Mikkelsen, T. N., Mora, F. (2019). Direct evidence for modulation of photosynthesis by an arbuscular mycorrhizainduced carbon sink strength. New Phytol. 223, 896–907. doi: 10.1111/nph.15806
Glassop, D., Smith, S. E., Smith, F. W. (2005). Cereal phosphate transporters associated with the mycorrhizal pathway of phosphate uptake into roots. Planta 222, 688–698. doi: 10.1007/s00425-005-0015-0
Gorzelak, M. A., Asay, A. K., Pickles, B. J., Simmard, S. W. (2015). Inter-plant communication through mycorrhizal networks mediates complex adaptive behavioural plant communities. AoB Plants 7, plv050. doi: 10.1093/aobpla/plv050
Grace, E. J., Cotsaftis, O., Tester, M., Smith, F. A., Smith, S. E. (2009). Arbuscular mycorrhizal inhibition of growth in barley cannot be attributed to extent of colonization, fungal phosphorus uptake or effects on expression of plant phosphate transporter genes. New Phytol. 181, 938–949. doi: 10.1111/j.1469-8137.2008.02720.x
Graham, J. H., Duncan, L. W., Eissenstat, D. M. (1997). Carbohydrate allocation patterns in citrus genotypes as affected by phosphorus nutrition, mycorrhizal colonization and mycorrhizal dependency. New Phytologist 135, 335–343. doi: 10.1046/j.1469-8137.1997.00636.x
Grunwald, U., Guo, W., Fischer, K., Isayenkov, S., Ludwig-Müller, J., Hause, B., et al. (2009). Overlapping expression patterns and differential transcript levels of phosphate transporter genes in arbuscular mycorrhizal, Pi-fertilised and phytohormone-treated Medicago truncatula roots. Planta 229, 1023–1034. doi: 10.1007/s00425-008-0877-z
Gutjahr, C., Parniske, M. (2013). Cell and developmental biology of arbuscular mycorrhiza symbiosis. Annu. Rev. Cell. Dev. Biol. 29, 593–617. doi: 10.1146/annurev-cellbio-101512-122413
Harrison, M. J., Dewbre, G. R., Liu, J. (2002). A phosphate transporter from Medicago truncatula involved in the acquisition of phosphate released by arbuscular mycorrhizal fungi. Plant Cell. 14, 2413–2429. doi: 10.1105/tpc.004861
Hauggaard-Nielsen, H., Jensen, E. S. (2005). Facilitative root interactions in intercrops. Plant Soil 274, 237–250. doi: 10.1007/s11104-004-1305-1
Hayman, D. S. (1983). The physiology of vesicular–arbuscular endomycorrhizal symbiosis. Can. J. Bot. 61, 944–963. doi: 10.1139/b83-105
Hetrick, B. A., Wilson, G. T., Harnett, D. C. (1989). Relationship between mycorrhizal dependence and competitive ability of two tallgrass prairie grasses. Can. J. Bot. 67, 2608–2615. doi: 10.1139/b89-337F
Hinsinger, P., Betencourt, E., Bernard, L., Brauman, A., Plassard, C., Shen, J., et al. (2011). P for two, sharing a scarce resource: soil phosphorus acquisition in the rhizosphere of intercropped species. Plant Physiol. 156, 1078–1086. doi: 10.1104/pp.111.175331
Hu, G. C., Honda, C. H., Kita, M. Y., Zhang, Z., Tsuda, T., Moriguchi, T. (2002). A simple protocol RNA isolation from fruit trees containing high levels of polysaccharides and polyphenol compounds. Plant Molecular Biology Reporter 20, 69a–69g. doi: 10.1007/BF02801935
Ingleby, K., Wilson, J., Munro, R. C., Cavers, S. (2007). Mycorrhizas in agroforestry: spread and sharing of arbuscular mycorrhizal fungi between trees and crops: complementary use of molecular and microscopic approaches. Plant Soil 294, 125–136. doi: 10.1007/s11104-007-9239-z
Jakobsen, I., Abbott, L. K., Robson, A. D. (1992). External hyphae of vesicular-arbuscular mycorrhizal fungi associated with Trifolium subterraneum L. 1. Spread of hyphae and phosphorus inflow into roots. New Phytol. 120, 371–380. doi: 10.1111/j.1469-8137.1992.tb01077.x
Janos, D. P. (2007). Plant responsiveness to mycorrhizas differs from dependence upon mycorrhizas. Mycorrhiza 17, 75–91. doi: 10.1007/s00572-006-0094-1
Javot, H., Pumplin, N., Harrison, M. J. (2007). Phosphate in the arbuscular mycorrhizal symbiosis: transport properties and regulatory roles. Plant Cell Environ. 30, 310–322. doi: 10.1111/j.1365-3040.2006.01617.x
Johnson, N. C. (2010). Resource stoichiometry elucidates the structure and function of arbuscular mycorrhizas across scales. New Phytol. 185, 631–647. doi: 10.1111/j.1469-8137.2009.03110.x
Johnson, N. C., Wilson, G. W. T., Wilson, J. A., Miller, R. M., Bowker, M. A. (2015). Mycorrhizal phenotypes and the law of the minimum. New Phytol. 205, 1473–1484. doi: 10.1111/nph.13172
Jose, S., Gillespie, A. R., Seifert, M. D. B., Pope, P. E. (2000). Defining competition vectors in a temperate alley cropping system in the midwestern USA: 2. Competition for nitrogen and litter decomposition dynamics. Agrofor. Syst. 48, 61–77. doi: 10.1023/A:1006241406462
Karandashov, V, Bucher, M. (2005) Symbiotic phosphate transport in arbuscular mycorrhizas. Trends Plant Sci. 10 (1), 22–29. doi: 10.1016/j.tplants.2004.12.003
Kiers, E. T., Duhamel, M., Beesetty, Y., Mensah, J. A., Franken, O., Verbruggen, E., et al. (2011). Reciprocal rewards stabilize cooperation in the mycorrhizal symbiosis. Science 333, 880–882. doi: 10.1126/science.1208473
Kobae, Y., Hata, S. (2010). Dynamics of periarbuscular membranes visualized with a fluorescent phosphate transporter in arbuscular mycorrhizal roots of rice. Plant Cell Physiol. 51, 341–353. doi: 10.1093/pcp/pcq013
Kobae, Y., Ohmori, Y., Saito, C., Yano, K., Ohtomo, R., Fujiwara, T. (2016). Phosphate treatment strongly inhibits new arbuscule development but not the maintenance of arbuscule in mycorrhizal rice roots. Plant Physiol. 171, 566–579. doi: 10.1104/pp.16.00127
Kormanik, P. P., McGraw, A. C. (1982). Quantification of vesicular arbuscular mycorrhizae in plant roots. Schenck, N. C. Ed. Methods and Principles of Mycorrhizal Research (St. Paul: The American Phytopathological Society), 37–45.
Korner, C. (2003). Carbon limitation in trees. J. Ecol. 91, 4–17. doi: 10.1046/j.1365-2745.2003.00742.x
Leake, J. R., Johnson, D., Donnelly, D., Muckle, G., Boddy, L., Read, D. (2004). Networks of power and influence: the role of mycorrhizal mycelium in controlling plant communities and agroecosystem functioning. Can. J. Bot. 82, 1016–1045. doi: 10.1139/b04-060
Lemoine, F., Correia, D., Lefort, V., Doppelt-Azeroual, O., Mareuil, F., Cohen-Boulakia, S., et al. (2019). NGPhylogeny.fr: new generation phylogenetic services for non-specialists. Nucl. Acids Res. 47(W1), W260–W265. doi: 10.1093/nar/gkz303
Liang, L., Liu, B., Huang, D., Kuang, Q., An, T., Liu, S., et al. (2022). Arbuscular mycorrhizal fungi alleviate low phosphorus stress in maize genotypes with contrasting root systems. Plants 11, 3105. doi: 10.3390/plants11223105
Lovelock, C. E. (2008). Soil respiration and belowground carbon allocation in mangrove forests. Ecosystems 1, 342–354. doi: 10.1007/s10021-008-9125-4
Ma, X., Li, X., Ludewig, U. (2021). Arbuscular mycorrhizal colonization outcompetes root hairs in maize under low phosphorus availability. Ann. Bot. 127, 155–166. doi: 10.1093/aob/mcaa159
Martins, M. A., Cruz, A. F. (1998). The role of the external mycelial networkof arbuslar mycorrhizal fungi. III. A study of nitrogen transfer between plants interconnected by a common mycelium. Rev. Microbiol. 29, 289–294. doi: 10.1590/S0001-37141998000400011
Mary, F., Dupraz, C., Delannoy, E., Liagre, F. (1998). Incorporating agroforestry practices in the management of walnut plantations in Dauphiné, France: an analysis of farmers' motivations. Agrofor. Syst. 43, 243–256. doi: 10.1023/A:1026425307959
Mohni, C. P. F., Pelleri, F., Hemery, G. E. (2009)The modern silviculture of Juglans regia L.: a literature review. In: Die Bodenkultur. Available at: https://pdfs.semanticscholar.org/4f0a/a056625285740786879ac7e02e3bdf9a379c.pdf (Accessed 18 October 2019).
Montesinos-Navarro, A., Verdú, M., Querejeta, J. I., Sortibrán, L., Valiente-Banuet, A. (2016). Soil fungi promote nitrogen transfer among plants involved in long-lasting facilitative interactions. Perspect. Plant Ecol. 18, 45–51. doi: 10.1016/j.ppees.2016.01.004
Nagy, R., Karandashov, V., Chague, V., Kalinkevich, K., Tamasloukht, M., Xu, G., et al. (2015). The characterization of novel mycorrhiza-specific phosphate transporters from Lycopersicon esculentum and Solanum tuberosum uncovers functional redundancy in symbiotic phosphate transport in solanaceous species. Plant J. 42(2):236–50. doi: 10.1111/j.1365-313X.2005.02364.x
Nussaume, L., Kanno, S., Javot, H., Marin, E., Pochon, N., Ayadi, A., et al. (2011). Phosphate import in plants: Focus on the PHT1 transporters. Front. Plant Sci. 2. doi: 10.3389/fpls.2011.00083
Peña Venegas, R. A., Lee, S. J., Thuita, M., Mlay, D. P., Masso, C., Vanlauwe, B., et al. (2021). The phosphate inhibition paradigm: Host and fungal genotypes determine arbuscular mycorrhizal fungal colonization and responsiveness to inoculation in cassava with increasing phosphorus supply. Front. Plant Sci. 12. doi: 10.3389/fpls.2021.693037
Phillips, J. M., Hayman, D. S. (1970). Improved procedures for clearing roots and staining parasitic and vesicular-arbuscular mycorrhizal fungi for rapid assessment of infection. Trans. Br. Mycol. Soc 55, 158–161. doi: 10.1016/S0007-1536(70)80110-3
Poirier, Y., Bucher, M. (2002). Phosphate transport and homeostasis in Arabidopsis. Arabidopsis Book 1, e0024. doi: 10.1199/tab.0024
Polcyn, W., Paluch-Lubawa, E., Lehmann, T., Mikuła, R. (2019). Arbuscular mycorrhiza in highly fertilized maize cultures alleviates short-term drought effects but does not improve fodder yield and quality. Front. Plant Sci. 10. doi: 10.3389/fpls.2019.00496
Poorter, H., de Jong, R. (1999). A Comparison of specific leaf area, chemical composition and leaf construction costs of field plants from 15 habitats differing in productivity. New Phytol. 143, 163–176. doi: 10.1046/j.1469-8137.1999.00428.x
Postma, M. (2005). “It’s all in the mix: Agroforestry, a prospective land use system for the Netherlands,” in Thesis Plant Production Systems. Ed. Postma, M. (The Netherlands: Department of Plant Sciences, Wageningen University). Available at: https://www1.montpellier.inra.fr/safe/english/theses/MSc_Thesis_Michel_Postma.pdf.
Pumplin, N., Harrison, M. J. (2009). Live-cell imaging reveals periarbuscular membrane domains and organelle location in Medicago truncatula roots during arbuscular mycorrhizal symbiosis. Plant Physiol. 151, 809–819. doi: 10.1104/pp.109.141879
Rausch, C., Daram, P., Brunner, S., Jansa, J., Laloi, M., Leggewie, G., et al. (2001). A phosphate transporter expressed in arbuscule-containing cells in potato. Nature 22, 462–470. doi: 10.1038/35106601
Rivest, D., Cogliastro, A., Bradley, R. L., Olivier, A. (2010). Intercropping hybrid poplar with soybean increases soil microbial biomass, mineral N supply and tree growth. Agrofor. Syst. 80, 33–40. doi: 10.1007/s10457-010-9342-7
Roth, R., Paszkowski, U. (2017). Plant carbon nourishment of arbuscular mycorrhizal fungi. Curr. Opin. Plant Biol. 39, 50–56. doi: 10.1016/j.pbi.2017.05.008
Rui, W., Mao, Z., Li, Z. (2022). The Roles of phosphorus and nitrogen nutrient transporters in the arbuscular mycorrhizal symbiosis. Int. J. Mol. Sci. 23, 11027. doi: 10.3390/ijms231911027
Salmeron-Santiago, I. A., Martínez-Trujillo, M., Valdez-Alarcón, J. J., Pedraza-Santos, M. E., Santoyo, G., Pozo, M. J., et al. (2021). An updated review on the modulation of carbon partitioning and allocation in arbuscular mycorrhizal plants. Microorganisms 10 (1), 75. doi: 10.3390/microorganisms10010075
Sawers, R. J., Svane, S. F., Quan, C., Grønlund, M., Wozniak, B., Gebreselassie, M. N., et al. (2017). Phosphorus acquisition efficiency in arbuscular mycorrhizal maize is correlated with the abundance of root-external hyphae and the accumulation of transcripts encoding PHT1 phosphate transporters. New Phytol. 214, 632–643. doi: 10.1111/nph.14403
Schoeneberger, M., Bentrup, G., de Gooijer, H., Soolanayakanahally, R., Sauer, T., Brandle, J., et al. (2012). Branching out: Agroforestry as a climate change mitigation and adaptation tool for agriculture. J. Soil Water Conserv. 67, 28A–136A. doi: 10.2489/jswc.67.5.128A
Simard, S. W., Durall, D. M. (2004). Mycorrhizal networks: a review of their extent, function, and importance. Can. J. Bot. 82, 1140–1165. doi: 10.1139/b04-116
Smith, J. (2010). “Agroforestry: Reconciling production with protection of environment,” in A Synopsis of research literature (Berkshire: Elm farm: The organic research centre), 1–24. Available at: https://www.researchgate.net/publication/277754782_Agroforestry_Reconciling_Production_with_Protection_of_the_Environment_A_Synopsis_of_Research_Literature.
Smith, S. E., Jakobsen, I., Grønlund, M., Smith, F. A. (2011). Roles of arbuscular mycorrhizas in plant phosphorus nutrition: Interactions between pathways of phosphorus uptake in arbuscular mycorrhizal roots have important implications for understanding and manipulating plant phosphorus acquisition. Plant Physiol. 156(3), 1050–1057. doi: 10.1104/pp.111.174581
Smith, S. E., Read, D. J. (1997). “Vesicular-arbuscular mycorrhizas,” in Mycorrhizal symbiosis, 2nd edn. Eds. Smith, S. E., Read, D. J. (New York: Academic Press), 9–160.
Stockinger, H., Walker, C., Schußler, A. (2009). Glomus intraradices DAOM197198’, a model fungus in arbuscular mycorrhiza research, is not Glomus intraradices. New Phytol. 183, 1176–1187. doi: 10.1111/j.1469-8137.2009.02874.x
Syers, J. K., Johnston, A. E., Curtin, D. (2008). Efficiency of soil and fertilizer phosphorus Use. FAO Fertilizer and Plant Nutrition Bulletin Number 18 (Rome: Food and Agriculture Organization of the United Nations).
Tarkalson, D. D., von Jolley, D., Charles, W., Robbins, C. W., Terry, R. E. (1998). Mycorrhizal colonization and nutrient uptake of dry bean in manure and compost manure treated subsoil and untreated topsoil and subsoil. J. Plant Nutr. 21, 1867–1878. doi: 10.1080/01904169809365529
Tedersoo, L., Bahram, M., Zobel, M. (2020). How mycorrhizal associations drive plant population and community biology. Science 367 (6480), eaba1223. doi: 10.1126/science.aba1223
Teste, F. P., Veneklaas, E. J., Dixon, K. W., Lambers, H. (2015). Is nitrogen transfer among plants enhanced by contrasting nutrient-acquisition strategies? Plant Cell Environ. 38, 50–60. doi: 10.1111/pce.12367
Tian, H., Drijber, R. A., Li, X., Miller, D. N., Wienhold, B. J. (2013). Arbuscular mycorrhizal fungi differ in their ability to regulate the expression of phosphate transporters in maize (Zea mays L.). Mycorrhiza 23, 507–514. doi: 10.1007/s00572-013-0491-1
Tilman, D. (2001). Forecasting agriculturally driven global environmental change. Science 292, 281–284. doi: 10.1126/science.1057544
Treseder, K. K., Allen, M. F. (2002). Direct nitrogen and phosphorus limitation of arbuscular mycorrhizal fungi: a model and field test. New Phytol. 155, 07–515. doi: 10.1046/j.1469-8137.2002.00470.x
Trouvelot, A., Kough, J. L., Gianinazzi-Pearson, V. (1986). “Mesure du taux de mycorhization VA d’un systeme radiculaire. Recherche de methods d’estimation ayant une signification fonctionnelle,” in Physiological and Genetical Aspects of Mycorrhizae. Eds. Gianinazzi-Pearson, V., Gianinazzi, S. (Paris: INRA), 217–221.
van der Heijden, M. G., Bardgett, R. D., van Straalen, N. M. (2008). The unseen majority: soil microbes as drivers of plant diversity and productivity in terrestrial ecosystems. Ecol. Lett. 11, 296–310. doi: 10.1111/j.1461-0248.2007.01139.x
van der Heijden, M. G., Horton, T. R. (2009). Socialism in soil? The importance of mycorrhizal fungal networks for facilitation in natural ecosystems. J. Ecol. 97, 1139–1150. doi: 10.1111/j.1365-2745.2009.01570.x
van Noordwijk, M., Lawson, G., Hairiah, K., Wilson, J. (2015). “Root distribution of trees and crops: competition and/or complementarity [Chapter 8],” in Tree-crop interactions: agroforestry in a changing climate. 2nd edition. Eds. Ong, C. K., Black, C. R., Wilson, J. (Wallingford, Oxford: CAB International), 221–257. Available at: https://nora.nerc.ac.uk/id/eprint/511997.
van Sambeek, J. W., Garrett, H. E. (2004). Ground cover management in walnut and other hardwood plantings. In: Proceedings of the 6th Walnut Council Research Symposium, Geneneral Techenical. Report NC-243 (Department of Agriculture, Forest Service, North Central Research Station, Saint Paul). Available at: http://www.nrs.fs.fed.us/pubs/gtr/gtr_nc243/gtr_nc243_085.pdf (Accessed 18 October 2019).
van Tuinen, D., Tranchand, E., Hirissou, F., Wipf, D., Courty, P. E. (2020). Carbon partitioning in a walnut-maize agroforestry system through arbuscular mycorrhizal fungi. Rhizosphere 15, 100230. doi: 10.1016/j.rhisph.2020.100230
Walder, F., Brulé, D., Koegel, S., Wiemken, A., Boller, T., Courty, P. E. (2015). Plant phosphorus acquisition in a common mycorrhizal network: regulation of phosphate transporter genes of the Pht1 family in sorghum and flax. New Phytol. 205, 1632–1645. doi: 10.1111/nph.13292
Walder, F., Niemann, H., Natarajan, M., Lehmann, M. F., Boller, T., Wiemken, A. (2012). Mycorrhizal networks: common goods of plants shared under unequal terms of trade. Plant Physiol. 159, 789–797. doi: 10.1104/pp.112.195727
Wang, G., Sheng, L., Zhao, D., Sheng, J., Wang, X., Liaom, H. (2016). Allocation of nitrogen and carbon is regulated by nodulation and mycorrhizal networks in soybean/maize intercropping system. Front. Plant Sci. 16. doi: 10.3389/fpls.2016.01901
Watts-Williams, S., Emmett, B. D., Levesque-Tremblay, V., MacLean, A. M., Sun, X., Satterlee, J. W., et al. (2019). Diverse Sorghum bicolor accessions show marked variation in growth and transcriptional responses to arbuscular mycorrhizal fungi. Plant Cell Environ. 42, 1758–1774. doi: 10.1111/pce.13509
Willmann, M., Gerlach, N., Buer, B., Polatajko, A., Nagy, R., Koebke, E., et al. (2013). Mycorrhizal phosphate uptake pathway in maize: vital for growth and cob development on nutrient poor agricultural and greenhouse soils. Front. Plant Sci. 264. doi: 10.3389/fpls.2013.00533
Wolz, K. J., DeLucia, E. H. (2018). Alley cropping: Global patterns of species composition and function. Agric. Ecosyst. Environ. 252, 61–68. doi: 10.1016/j.agee.2017.10.005
Wolz, K. J., DeLucia, E. H. (2019). Black walnut alley cropping is economically competitive with row crops in the Midwest USA. Ecol. Appl. 29, e01829. doi: 10.1002/eap.1829
Xue, Y., Xia, H., Christie, P., Zhang, Z., Li, L., Tang, C. (2016). Crop acquisition of phosphorus, iron and zinc from soil in cereal/legume intercropping systems: a critical review. Ann. Bot. 117, 363–377. doi: 10.1093/aob/mcv182
Yang, S. Y., Grønlund, M., Jakobsen, I., Grotemeyer, M. S., Rentsch, D., Miyao, A., et al. (2012). Nonredundant regulation of rice arbuscular mycorrhizal symbiosis by two members of the phosphate transporter1 gene family. Plant Cell. 24, 4236–4251. doi: 10.1105/tpc.112.104901
Keywords: agroforestry, phosphate deficiency, microcosm, facilitation, Rhizophagus irregularis, symbiotic phosphate transporters, Juglans spp.
Citation: Mortier E, Mounier A, Kreplak J, Martin-Laurent F, Recorbet G and Lamotte O (2023) Evidence that a common arbuscular mycorrhizal network alleviates phosphate shortage in interconnected walnut sapling and maize plants. Front. Plant Sci. 14:1206047. doi: 10.3389/fpls.2023.1206047
Received: 14 April 2023; Accepted: 24 July 2023;
Published: 10 August 2023.
Edited by:
Katsuharu Saito, Shinshu University, JapanReviewed by:
Roxana Vidican, University of Agricultural Sciences and Veterinary Medicine of Cluj-Napoca, RomaniaYusaku Sugimura, Iwate Biotechnology Research Center, Japan
Copyright © 2023 Mortier, Mounier, Kreplak, Martin-Laurent, Recorbet and Lamotte. This is an open-access article distributed under the terms of the Creative Commons Attribution License (CC BY). The use, distribution or reproduction in other forums is permitted, provided the original author(s) and the copyright owner(s) are credited and that the original publication in this journal is cited, in accordance with accepted academic practice. No use, distribution or reproduction is permitted which does not comply with these terms.
*Correspondence: Ghislaine Recorbet, Z2hpc2xhaW5lLnJlY29yYmV0QGlucmFlLmZy
†ORCID: Arnaud Mounier, orcid.org/0000-0002-4595-0857
Jonathan Kreplak, orcid.org/0000-0001-5563-1932
Fabrice Martin-Laurent, orcid.org/0000-0001-9410-8319
Ghislaine Recorbet, orcid.org/0000-0003-4668-4201
Olivier Lamotte, orcid.org/0000-0002-3721-3769