- 1International Institute of Tropical Agriculture (IITA), Nairobi, Kenya
- 2Agro-Systems Research Group, Wageningen University and Research, Wageningen, Netherlands
- 3Department of Plant Science and Crop Protection, The University of Nairobi, Nairobi, Kenya
- 4International Centre of Insect Physiology and Ecology (icipe), Nairobi, Kenya
- 5Real IPM Co. (Kenya) LTD., Thika, Kenya
- 6The Norwegian Institute of Bioeconomy Research (NIBIO), Ås, Norway
Coffee is an important commodity for Kenya, where production is steadily declining, despite a global rise in demand. Of the various constraints affecting production, plant-parasitic nematodes are a significant, but often overlooked, threat. As a perennial crop, treating plantations once infected with nematodes becomes difficult. The current study evaluated the drenching application of two biocontrol agents, Trichoderma asperellum and Purpureocillium lilacinum, for their nematode control efficacy, as well as their impact on the soil nematode community structure on mature, established coffee trees in Kenya. Seven Arabica coffee field trials were conducted over two years on trees of various ages. All the fields were heavily infested with Meloidogyne hapla, the first report of the species on coffee in Kenya. Both fungal biocontrol agents were detected endophytically infecting roots and recovered from soil but not until six months after initial applications. The population densities of M. hapla had significantly declined in roots of treated trees 12 months after the initial application, although soil nematode density data were similar across treatments. Based upon the maturity index and the Shannon index, treatment with T. asperellum led to improved soil health conditions and enrichment of diversity in the microbial community. Application of P. lilacinum, in particular, led to an increased abundance of fungivorous nematodes, especially Aphelenchus spp., for which P. lilacinum would appear to be a preferred food source. The soils in the trials were all stressed and denuded, however, which likely delayed the impact of such treatments or detection of any differences between treatments using indices, such as the functional metabolic footprint, over the period of study. A longer period of study would therefore likely provide a better indication of treatment benefits. The current study positively demonstrates, however, the potential for using biologically based options for the environmentally and climate-smart management of nematode threats in a sustainable manner on established, mature coffee plantations.
1 Introduction
Worldwide, the trade in coffee (Coffea arabica, C. canephora) is rising, driven by its increasing consumption as a beverage, including in Africa (International Coffee Organization, 2022). This global increase in demand is largely supported by rapidly expanding plantations in Asia (Krishnan, 2017). So, with increasing demand, why is Africa’s coffee production declining? In Africa, coffee supports the livelihoods of some 10.9 million African farmers, who produce 15.7 million bags per crop year, and which accounts for approximately 13% of global production (International Coffee Organization, 2015). Across East Africa, a general decline in coffee production is attributed to various factors, including loss of land to real estate. But for remaining plantations, pests and diseases rank high among the reasons for production losses (Gitonga and Townsend, 2019). Plant-parasitic nematodes, however, are rarely indicated as a threat, or as a cause for concern even. There is in general, an almost complete lack of knowledge of nematodes as pests of coffee within the coffee sector in East Africa (Souza, 2008; Coyne et al., 2018; Villain et al., 2018). This is despite being considered amongst the most serious production constraints of coffee in other parts of the world, such as in Latin America and Asia (Souza, 2008; Villain et al., 2018).
In Africa, this declining trend in coffee production is particularly evident in Kenya, where coffee has traditionally been one of the country’s most important export crops (Coffee Research Institute - Kenya Agricultural and Livestock Research Organization, 2022). Although demand for Kenyan coffee remains high on the global market, due to the high quality of the Arabica type primarily grown, investments in new plantations are limited, with yields sliding in the ageing plantations. With perennial crops, pest and disease issues often build up gradually over time, especially nematode pests (Campos and Silva, 2008). The hallmark of nematode infection in mature plantations is the gradual suppression of growth and productivity over time, without necessarily causing plant death. Nematodes are especially aggressive on transplanted young seedlings, while on mature plants they can induce nutrient deficiencies, defoliation, stunting and ultimately impact yield (Villain et al., 2018). Above-ground symptoms of nematode damage, however, are notoriously difficult to diagnose, as they are otherwise indistinguishable from general plant malaise and stunting due to low soil fertility, nutrient deficiencies or low water availability and drought susceptibility (Coyne et al., 2018). Considering the damage that nematodes are known to cause on coffee in Latin America and Asia, the likelihood that they are also imposing a sizable damage on Kenyan coffee production is high.
Globally, root-knot nematodes (RKN; Meloidogyne spp.) are viewed as the number one nematode pest across crops (Jones et al., 2013) and considered as the greatest biotic threat to agriculture in sub-Saharan Africa (Coyne et al., 2018). On coffee, they are also the most commonly occurring and are generally regarded as the most important nematode pest (Villain et al., 2018).
There are several RKN species that infect coffee, as well as other genera of plant-parasitic nematodes (Humphreys-Pereira et al., 2014; Nzesya et al., 2014; Janssen et al., 2016; Gitonga, 2020). Among the coffee-parasitic RKN species, 17 have been recognized worldwide: M. exigua, M. Africana, M. arabicida, M. arenaria, M. coffeicola, M. decalineata, M. hapla, M. incognita, M. inornate, M. izalcoensis, M. javanica, M. kikuyensis, M. konaensis, M. mayaguensis, M. megadora, M. oteigae and M. paranaensis (Carneiro and Cofcewicz, 2008). Nematologists and extensionists must identify the species of parasitic nematodes infesting a coffee field at the species level in order to determine the appropriate management strategies, especially with regards to which resistance sources should be employed (Campos and Silva, 2008).
In East Africa, the information on species distribution and incidence in the region is scant and erratic, with most records documenting their detection only. Data on the levels of damage nematodes cause to coffee in the region, or their economic importance, is effectively non-existent and yet to be determined. Coffee yield losses due to nematode infection is based mostly on data from Latin America and documented as between 10 to 35%, depending on species (Barriga, 1976; Bertrand et al., 1997; López-Lima et al., 2015), but with some indications of wholescale plantation destruction in Brazil (Lordello, 1984). Considering the perennial nature of coffee, however, accurately estimating the economic losses due to nematodes is difficult. In addition, loss estimates should also consider the impact of infection on plantation longevity and the replant cost of non-performing trees.
The management of soil-borne pests and diseases on perennial crops presents numerous challenges. Once established, they become extremely difficult to eliminate, including nematodes. Reducing infection through the use of clean, healthy, resistant and protected seedlings is therefore an advised nematode management strategy, and a policy which is being actively promoted in Latin America and Asia (Villain et al., 2018). In Africa, with an almost total ignorance of coffee nematode pests, few if any management practices are observed or followed. With limited recognition and costly or unsuitable management options available, such as total renovation of aged plantations, the outlook does not look positive. Smallholder farmers also represent a high proportion of coffee farmers in the region, who are less able or flexible to incorporate nematode management practices.
In addition to the practical difficulties of nematode management in coffee fields, sustainability aspects also need to be taken into consideration. Nematicides are often a first line of defense, especially in more commercial farming systems. Biological control options, however, offer environmentally sensitive alternatives, especially under organically oriented systems. And in regard to this, there is increasing interest in the use of biologically based products for pest and disease management and improving coffee production (Alwora and Gichuru, 2014). Various microbial antagonists have been identified for use on coffee, such as the bacterium Pasteuria penetrans (Sharma and Lordello, 1992; Maximiniano et al., 2001) and egg parasitic fungi (Naves and Campos, 1991; Ribeiro and Campos, 1993). Trichoderma spp. is the most studied biocontrol fungi and has been extensively investigated and utilized for their capacity to compete and parasitize phytopathogens as well as to mitigate unfavorable growth conditions (Poveda, 2021). Purpureocillium lilacinum (=Paecillomyces lilacinus) is also a well-recognized fungal biocontrol agent for use against root-knot nematodes (Holland et al., 1999; Kiewnick and Sikora, 2006; Heidari and Olia, 2016). On coffee, Campos and Campos (1997) demonstrated the potential of P. lilacinum against Meloidogyne exigua on seedlings in Brazil. Additionally, Trichoderma harzianum (Arita et al., 2020; Zinger et al., 2021) and P. lilacinum (Arita et al., 2020) have been evaluated for efficacy against RKN on coffee in Brazil. Although they were ineffective against the nematodes, P. lilacinum promoted coffee plant growth (Arita et al., 2020). In Kenya, both P. lilacinum and Trichoderma asperellum are registered for use against nematodes and are commercially available, where they have been proven effective against RKN on pineapple (Kiriga et al., 2018) and T. asperellum against Radopholus similis on banana (Kisaakye et al., 2023).
Given the current scarcity of suitable or sustainable nematode management options amongst a rising global demand for nematode control, there is much current interest to identify options including biologically based alternatives to synthetic chemicals (Campos and Silva, 2008; Coyne et al., 2018). However, how the long-term and intensive application of biological products to perennial crops impacts on the soil food web and soil ecosystem functions has been little studied. Some studies have demonstrated the positive effect of Trichoderma spp. on enhancing the species richness and evenness of microbial distribution (Alexander and Phin, 2014) as well as the improved availability of nutrients for microbial consumption and ultimately promotion of plant health (Cai et al., 2015; Carvalhais et al., 2015). Conversely, some studies have indicated that application of microbial products disrupts the microbial community structure in the rhizosphere leading to impaired nutrient absorption (Ros et al., 2017; Halifu et al., 2019). Free-living nematodes have long been recognized as useful bioindicators of soil health due to their high abundance, rapid response to new resources and food specificity (Yeates et al., 1993). Since the development of nematode community indices (Bongers, 1990; Ferris et al., 2001; Ferris and Bongers, 2009), attention towards the use of nematodes as a quantitative measure to evaluate the impact of management practices on soil health (Okada and Harada, 2007) and measure the ecological impact (Krashevska et al., 2019) has increased.
This current study evaluated the application of two commercial products based on P. lilacinum (IMPEDE®, RealIPM, Kenya) and T. asperellum (SUSTAIN®, RealIPM, Kenya) over an 18-month period on Kenyan Arabica coffee suffering from Meloidogyne infection. The study focused on in-field application to established coffee fields of various ages. The objectives were to evaluate the efficacy of T. asperellum and P. lilacinum to suppress field densities of plant-parasitic nematodes, and to evaluate these products for their impact on the soil food web and soil health using nematode community assemblages as a basis.
2 Materials and methods
2.1 Study sites and experimental design
The field trials were conducted on Chania Estate, Kiambu County, Kenya (1°01’36”S, 37°01’20”E). The study comprised trials in seven Arabica coffee fields, three with cv. Ruiru 11 and four with cv. French Mission. Fields were planted between 1926 to 2012 and the age varied by field. Two fields were planted in 1926, three were planted in 2012, and the rest were planted in 1960 and 1992. The coffee plants are pruned after every harvest. The average temperature and rainfall ranged from 20 °C (May in 2021) to 24 °C (October in 2020) and from 0 mm (June, September in 2021, January in 2022) to 230.8 mm (April in 2021). The fields received no irrigation. In each field, three treatment plots were demarcated, each consisting of four replications of five trees each, arranged in a line, giving 12 experimental units per field and 84 experimental units in total. The three treatments included T. asperellum, P. lilacinum and untreated control. Treatments were applied around the base of each tree after creating a ~1 m diam. basin with a hand hoe, which helped contain the applied microbial suspension to the tree base. Treatments were consistently applied using the same volumes on each occasion: 20 L of T. asperellum suspension (2x107 cfu/L), 20 L of P. lilacinum suspension (2x107 cfu/L) according to the manufacturer’s recommendation and 20 L water (control). The regularity of these applications reduced over time. For the first month, treatments were applied weekly to initially immerse the root system with the products and to encourage the fungal colonization in soil and roots. From the second month, treatments were applied bi-weekly for 11 months until fungal colonization was confirmed in roots. From 12 months onwards, treatments were applied monthly for six months. The field trial was conducted over a total of 25 months from February 2020 to March 2022. For the latter seven months of the study, half of each plot in each field received no treatment application to evaluate the treatment effect persistence in soil and roots.
2.2 Data collection
Soil and root samples were collected from each trial site prior to the beginning of the study. Data were not collected for the following six months due to Covid-19 restrictions. Thereafter, soil samples were collected from each experimental unit monthly and root samples every six months, except for the final root sampling, which was collected eight months after the previous sampling. Samples were collected from the root zone of each of the five trees per replication for soil and three trees per replication for the newly developed lateral roots from 5 to 20 cm depth and combined for each replication. Soil and root samples were processed separately using the modified Baermann plate method for nematode extraction from 100 cm3 soil and 5 g fresh root sub-samples (Coyne et al., 2014; Saikai et al., 2021). All plant-parasitic nematodes were identified to genus level and counted at 40X magnification using a ZEISS Primo Star binocular microscope (Carl Zeiss AG, Oberkochen, Germany) for all monthly samples. Meloidogyne spp. infecting roots were further identified to species level morphologically, based on the perineal patterns of mature females, and molecularly, based on COI mtDNA (Janssen et al., 2016). Every second month, free-living nematodes in the samples were identified to genus level and counted at 40X or 100X magnifications from the first 100 encountered nematode individuals in each sample. When nematodes were unable to be assigned to genus level morphologically, they were assigned to family level (i.e. Cephalobidae, Rhabditidae and Tylenchidae). The nematodes were assigned to their trophic groups according to Yeates et al. (1993). The total abundance of each genus and each trophic group were calculated based on the total abundance of all the nematodes and the abundance of each genera/trophic group in 100 identified nematodes. These data were uploaded to NINJA (http://spark.rstudio.com/bsierieb/ninja/; Sieriebriennikov et al., 2014) to calculate the footprints, the relative abundance of total or free-living nematodes for each trophic group and each colonizer-persister group (c-p group), as well as nematode indices (i.e. Basal Index, Channel Index, Enrichment Index, Maturity Index, Maturity Index 2-5, Sigma Maturity Index and Structure Index) for each of the data entries. On a six-monthly basis, plant-parasitic nematodes were assessed from the roots and fungal colonization assessed from soil and root samples. Colony forming units of T. asperellum and P. lilacinum were quantified from ~5 g fresh root and 1 g soil sub-samples using the spread plate culture technique (Hohmann et al., 2011) on Potato Dextrose Agar with Acid.
2.3 Data analysis
Nematode data from root samples were analyzed using the generalized linear mixed model (PROC GLIMMIX, SAS Institute, Cary, NC) to evaluate the effect of treatments on nematodes infecting roots at each of the five different time points. Studentized residual plots were generated by PLOTS = STUDENTPANEL. The Satterthwaite approximation was used to adjust the degrees of freedom. Appropriate distributions were selected for each of the response variables after comparing the diagnostic plots and model fitness for preliminary model runs using multiple distributions. Effects of cultivar, field nested within cultivar, and replication nested within cultivar and field were included as random (G-side) effects. Tests of covariance parameters using the Restricted Likelihood were conducted for each combination of the random effects using COVTEST option. The effect of treatment was compared by performing the mean separation test using the least square means. For the root data for March 2022, in addition to the model to assess differences between treatments in the fixed (R-side) effect, an additional generalized linear mixed model was performed, which included the binary variable for trees that were treated or not treated, in addition to the treatment effect, and the interaction of the two. The remainder of the model options remained the same.
The soil samples were analyzed separately for samples before the fungal isolation from roots (Oct. 2020~Feb. 2021: 4 time points) and after isolation (Mar. 2021~Apr. 2022: 11 time points). Each time set of the data points was analyzed using the generalized linear mixed model (PROC GLIMMIX, SAS Institute, Cary, NC). The same options as the models for the root samples were applied for the residual plots and the degrees of freedom adjustment. The effect of treatment, time and the interaction of treatment and time on the parasitic nematode density in soil were included as fixed effects. The effects of cultivar, field nested within cultivar, were included as random and the interaction of replication, cultivar, field and treatment were added in the random side of the model as a whole plot error. Time was treated as a repeated measure. The first order autoregressive covariance structure was selected after evaluating the model fitness using other covariance structures in the preliminary model runs and based on the fact that these different time point data were collected at equally spaced time points. The effect of treatment was compared at each time point by performing a partitioned analysis of the least square means with p-value adjusted by the Tukey procedure.
Pearson correlation coefficient was also computed between the nematode density in soil and in roots using cor() function of the basic package in R (R Core Team, 2020). The regression analysis was performed between the nematode density in soil and roots after transforming by log10(N+1) for both variables to satisfy the model assumptions using lm() function of the basic R package.
Similar to the assessment of soil samples for parasitic nematodes, the nematode community data were analyzed separately for samples before fungal isolation from roots (Oct. 2020~Feb. 2021: 3 time points) and after isolation (Apr. 2021~Apr. 2022: 7 time points) to evaluate the effects of the treatments on the abundance parameters and nematode indices that were computed by NINJA using the same model and model options used to analyze the soil samples.
Principle component analysis (PCA) was performed separately on the early (Oct. 2020) and on the final nematode community data (Apr. 2022) using facto extra package. The 10 most common genera detected were included in the analysis, with the less common genera omitted from analysis. Before the analysis, the nematode counts were standardized to have mean=0 and standard deviation=1 for each genus at the individual time points. PCA values and associated Eigenvalues were computed by prcomp() and get_eigenvalue() functions in the package. The bi-graph was generated for the individuals (i.e. the nematode genera) and the vectors (i.e. the treatments) by fviz_pca_biplot() function.
Functional metabolic footprints of nematodes in the soil food webs were visualized by plotting Structure index on X-axis against Enrichment index on Y-axis. The visualized plots were interpreted according to Ferris et al. (2001) and Ferris (2010).
3 Results
3.1 Effects of biocontrol agents on Meloidogyne spp. densities
All seven fields used in the study were infested with RKN, which was identified as Meloidogyne hapla. This was the most dominant nematode genus throughout the study period, especially from roots with densities of second stage infective juveniles (J2) ranging between 0-1,828 per 100 cm3 soil and 0-3,492 per 5 g roots. Other parasitic nematodes (Helicotylenchus spp. (0-20 per 100 cm3 soil), Hemicycliophora spp. (0-5 per 100 cm3 soil), Trichodorus spp. (0-213 per 100 cm3 soil), Tylenchorhynchus spp. (0-4 per 100 cm3 soil), Pratylenchus spp. (0-19 per 100 cm3 soil), and Rotylenchulus spp. (0-766 per 100 cm3 soil)) were also recovered but their distribution was sporadic and in general were not prevalent.
No effect of either biocontrol agent was observed on the RKN root density until 2021 August, six months after first detecting fungal colonization in roots (Figure 1). RKN root densities were lower in plots treated with either biocontrol agent on 2021 August and on 2022 April sampling (P ≤ 0.05) than in the control plots. Densities were lower in P. lilacinum treated plots than T. asperellum plots on 2021 August (P ≤ 0.05) but RKN root densities between the two biocontrol agents were similar for the 2022 April samples (Figure 1).
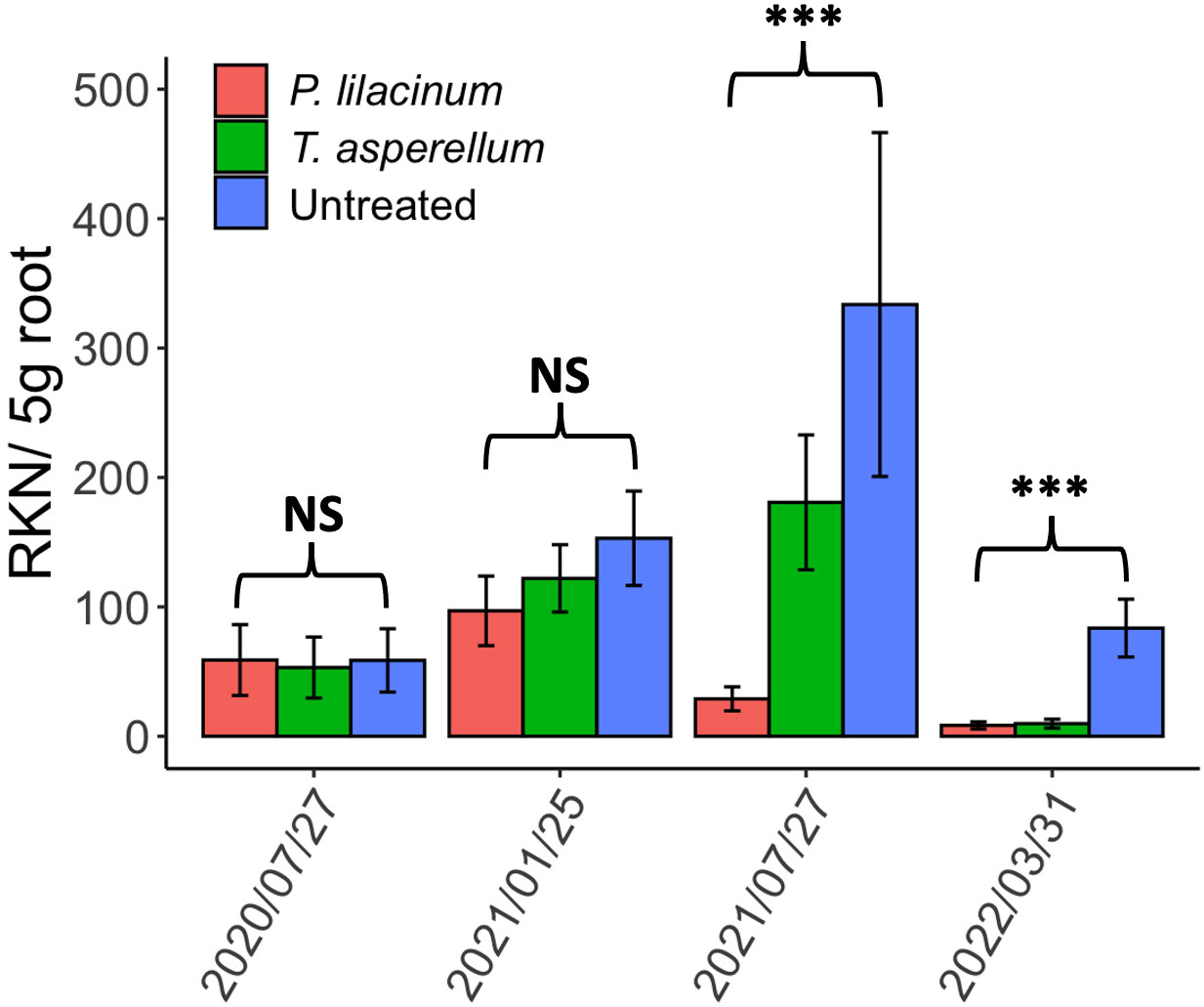
Figure 1 The bar plot of Meloidogyne hapla in 5g roots from August 2020 to April 2022 for T. asperellum, P. lilacinum and the untreated. Asterisks represent the statistically significant differences among the treatments. NS, not significant.
The tests of covariance parameters based on the restricted likelihood demonstrated the significant contribution of the cultivar effect in G-side effects (P ≤ 0.05) for the 2021 August sampling but not for the 2022 April sampling. Other random effects, such as tree age, field, and replication, were not significant. When the cultivar effect and the interaction between the effects of cultivar and treatment were included in the R-side of the generalized linear mixed model, both the cultivar effect and its interaction with the treatment effect were insignificant without alternating the data interpretation of the treatment effect above. This confirms that both ‘French mission’ and ‘Ruiru 11’ are equally susceptible to M. hapla and react similarly to the biocontrol agents. Rotylenchulus spp. was sporadically found cohabiting with RKN in roots in low to medium relative densities (mean=6.8, range 0 to 256.9/5 g root) but no significant effects of the biocontrol agents were observed on these nematodes.
The RKN soil density was not affected by treatment with either biocontrol agent, except for the 2021 March samples, when densities were lower in P. lilacinum treated plot soil than in the untreated and T. asperellum plots (P ≤ 0.05). The correlation coefficient between the RKN soil density and the root density was 0.30 and the relationship was positive between the log (base 10)-transformed densities in soil and roots (P ≤ 0.01). Based on the regression model, a unit increase in RKN soil density will result in an increase in RKN root density by 0.13, although just 8% of the variation in RKN root density can be explained by the soil densities based on adjusted R2.
3.2 Fungal colonization of soil and roots
The fungal colonization in soil and roots were confirmed 6 months and 12 months after the initial treatments, respectively. The fungal soil colonization for both treatments fluctuated by season and ranged from 1.26 x 102 (Aug. 2021) to 3.22 x 103 cfu/g soil (Feb. 2022) for P. lilacinum and 1.38 x 102 (Aug. 2021) to 2.45 x 106 cfu/g soil (Feb. 2022) for T. asperellum, which were unaffected by the tree age (P > 0.05). There was no difference observed in fungal colonization of T. asperellum treated roots and the soil during the final 8 months between the plots that received the application and the plots that did not (P > 0.05). Conversely, the P. lilacinum colonization in soil became undetectable (0 cfu/g soil) in five of the seven fields. The other two fields remained colonized at the same level between plots with and without the application, although this did not seem to correlate to age or cultivar. For both biocontrol agents, the fungal colonization in roots at the final sampling (Feb. 2022) was significantly lower (P ≤ 0.05) than the previous sampling (Aug. 2021), where P. lilacinum had 2.23 x 104 cfu/g root and T. asperellum had 1.98 x 104 cfu/g root. In the final sampling, the fungal colonization in roots decreased to 7.0 x 10-1 cfu/g root for P. lilacinum on plots received no application and 5.29 x 102 cfu/g root for T. asperellum without no significant difference between plots with and without applications. The effect of T. asperellum persisted in coffee roots for at least eight months after the final application given that there was no significant difference in the RKN root density between with and without applications.
3.3 Effect of biocontrol agents on the soil nematode community
In total, 59 genera and family of free-living nematodes were recovered from soil samples over the two years of study (Table 1). The ten most abundant nematode genera, in descending order, were: Cephalobus, Pseudacrobeles, Aphelenchus, Aphelenchoides, Meloidogyne, Prismatolaimus, Rotylenchulus, Mesorhabditis, Tylenchidae, Panagrellus. Total mean abundance was 51.2% (38.3 - 63.9%) for bacterivores, 30.9% (16.7 - 49.3%) for fungivores, 16.0% (8.9 - 27.0%) for herbivores and 6.9% (2.5 - 12.0%) for omnivores and predators.
The generalized linear mixed model with time as a repeated measure revealed that application of the biocontrol agents before the fungal isolation from roots had no significant effect on the nematode indices (Table 2).
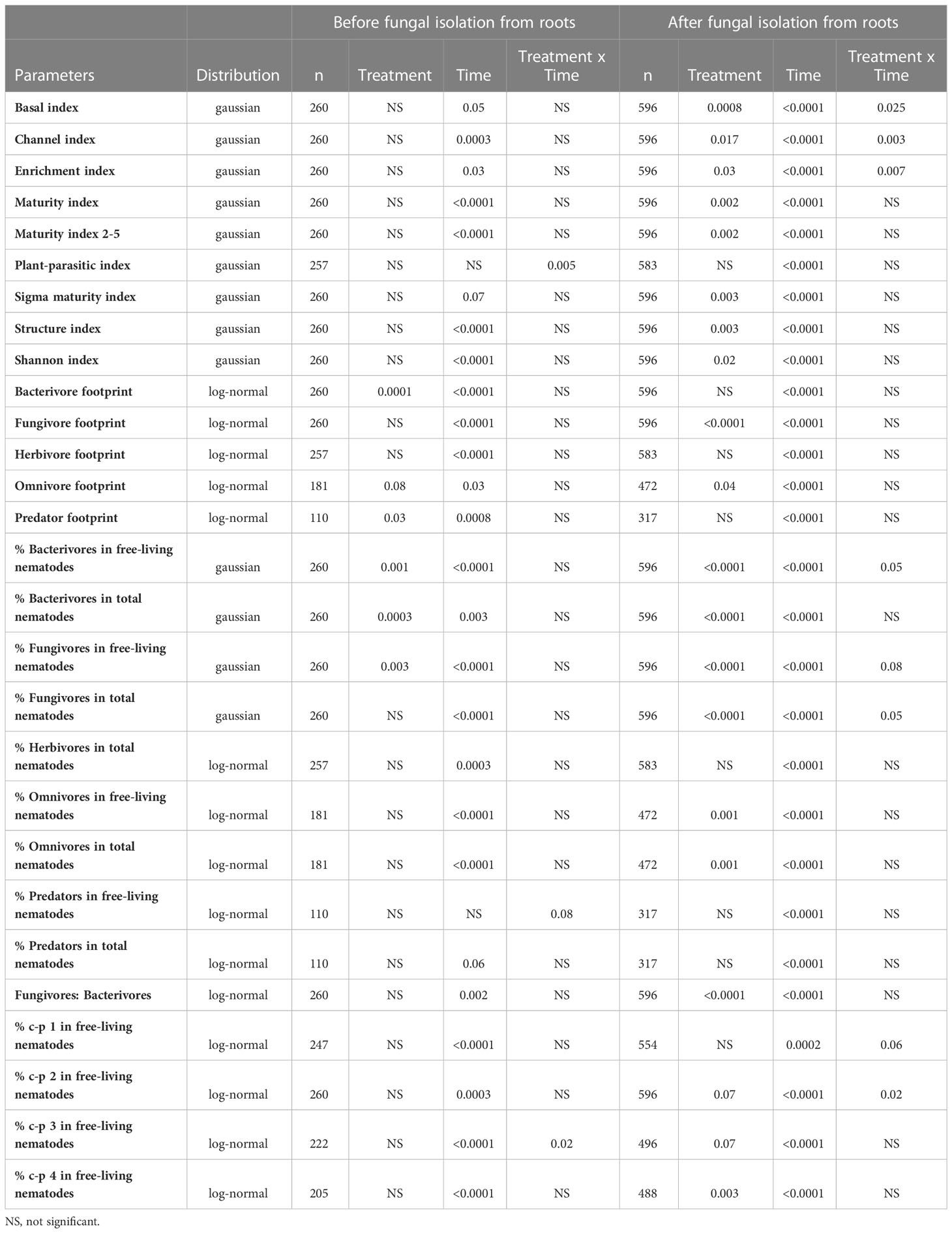
Table 2 The p-values of the effects of treatment, time and their interaction from models based on the data points before fungal isolation from roots and after fungal isolation from roots for nematode community parameters.
The bacterivore footprint was larger for the control treatment, before fungal isolation from roots (P = 0.0001), whereas the difference in the footprint became insignificant following fungal isolation. However, the relative abundance of bacterivores in the nematode community (% bacterivores of total nematodes) and of free-living nematodes (% bacterivores of free-living nematodes) remained significant after the fungal isolation (Table 2). The relative abundance of bacterivores in the free-living nematode community had a significant interaction between the treatment and time, in which the two biocontrol agents occasionally had similar relative abundance of bacterivores. The control treatment had the highest and P. lilacinum had the lowest values for the relative abundance of bacterivores in free-living nematode community. Conversely, the relative abundance of fungivores in the free-living nematode community (% fungivores of free-living nematodes) was greater for P. lilacinum than the other treatments (P = 0.003), which was not affected by the sampling time points.
The fungivore footprint was not affected by treatment before fungal isolation from roots but it significantly increased for P. lilacinum after (P < 0.0001) (Table 2). The relative abundance of fungivores in both free-living and total nematode communities was consistently high for P. lilacinum among the three treatments, although there were occasionally significant differences for the other two treatments with the untreated generally having the lowest values. Purpureocillium lilacinum had a lower omnivore (P = 0.08) and predator footprint (P = 0.03), relative to the other two treatments, before the fungal isolation (Table 2). The omnivore and predator footprints were lower for P. lilacinum before fungal isolation (P <0.1). However, a higher omnivore footprint was associated with both biocontrol agents after fungal isolation (P = 0.04) but not for the predator footprint.
The models based on the soil samples after the fungal isolation from roots demonstrated that the Maturity index, Maturity index 2-5 and Sigma maturity index, structure index, and Shannon index all significantly increased for T. asperellum compared to the control, while for P. lilacinum they did not differ from T. asperellum or the control. Before fungal isolation, none of the treatments had any effect on these nematode based indices (Table 2). Although the treatment effect was significant for basal, channel and enrichment indices after the fungal isolation, there was no consistency in how treatments influenced the indices (Table 2). The ratio of fungivores to bacterivores significantly increased for P. lilacinum after the fungal isolation from roots. There was no significant effect of either biocontrol agent on any of the c-p groups before the fungal isolation in roots, however, T. asperellum increased the number of nematodes belonging to c-p 3 and the c-p 4 groups after the fungal isolation (Table 2). The number of nematodes in c-p 2 was also significantly affected by the treatment but inconsistently after the fungal isolation, indicated by the significant interaction between the treatment and the time effects. Both the abundance of Cephalobus and Pseudocrobeles were greater for the control than for P. lilacinum after the fungal isolation in roots (P ≤ 0.05) but not affected before (P > 0.1). Interestingly, Aphelenchus density was significantly higher for P. lilacinum after the fungal isolation (P ≤ 0.0001), whereas Aphelenchoides, another dominant fungivore in samples, was unaffected. Other abundant genera were not affected by the treatment before nor after the fungal isolation. The time effect was significant for all the parameters except for the plant-parasitic index and the abundance of predators in the free-living nematode community before fungal isolation from roots, indicating that these values fluctuate significantly with time.
The footprint of all the trophic groups, the relative abundances in total and in free-living nematodes for herbivore, omnivore and predator, and the relative abundances in free-living nematodes of all the c-p group fitted lognormal distributions to satisfy the model assumptions, especially homoscedasticity. Other parameters were described by Gaussian distribution. The covariance test on the random effects revealed that the cultivar effect was significantly contributing in the G-side. The exceptions were the channel index, the enrichment index, the relative abundances of fungivores and predators in total nematodes and free-living nematodes before fungal isolation from roots, and the abundance of c-p 1 group in free-living nematodes before and after fungal isolation, which had no significant effect in G-side. The predator footprint and the ratio of fungivores to bacterivores before fungal isolation and the channel index after the fungal isolation had a significant contribution of the replication but not for the field or variety in G-side. The relative abundances of fungivores and herbivores in the total nematode community after the fungal isolation from roots demonstrated that all the random effects in G-side were significant.
PCA on the early (Oct. 2020) and final nematode community data (Apr. 2022) revealed distinguished nematode community profiles of the biocontrol agents, with significant changes in nematode genera associated with each of the treatments between the time points (Figures 2A, B). The Oct. 2020 nematode community data showed strong associations with Cephalobus, Aphelenchoides, Pseudacrobeles for the untreated, Tylenchidae, Meloidogyne and Prismatolaimus for P. lilacinum, and Mesorhabditis for T. asperellum (Figure 2A). The Apr. 2022 nematode community data, however, revealed a strong association of Pseudacrobeles, Prismatolaimus and Meloidogyne with the control and Mesorhabditis, Aphelenchus, Rotylenchulus and Panagrellus with P. lilacinum (Figure 2B). The first and second principal components explained 64.5% (Eigenvalue = 5.18) and 35.5% (Eigenvalue = 2.84) of the total variance of the Oct. 2020 nematode community data, respectively. For the Apr. 2022 nematode community data, the first principal component explained 57.8% (Eigenvalue = 4.22) and the second principal component explained 42.2% (Eigenvalue = 4.22) of the total variance.
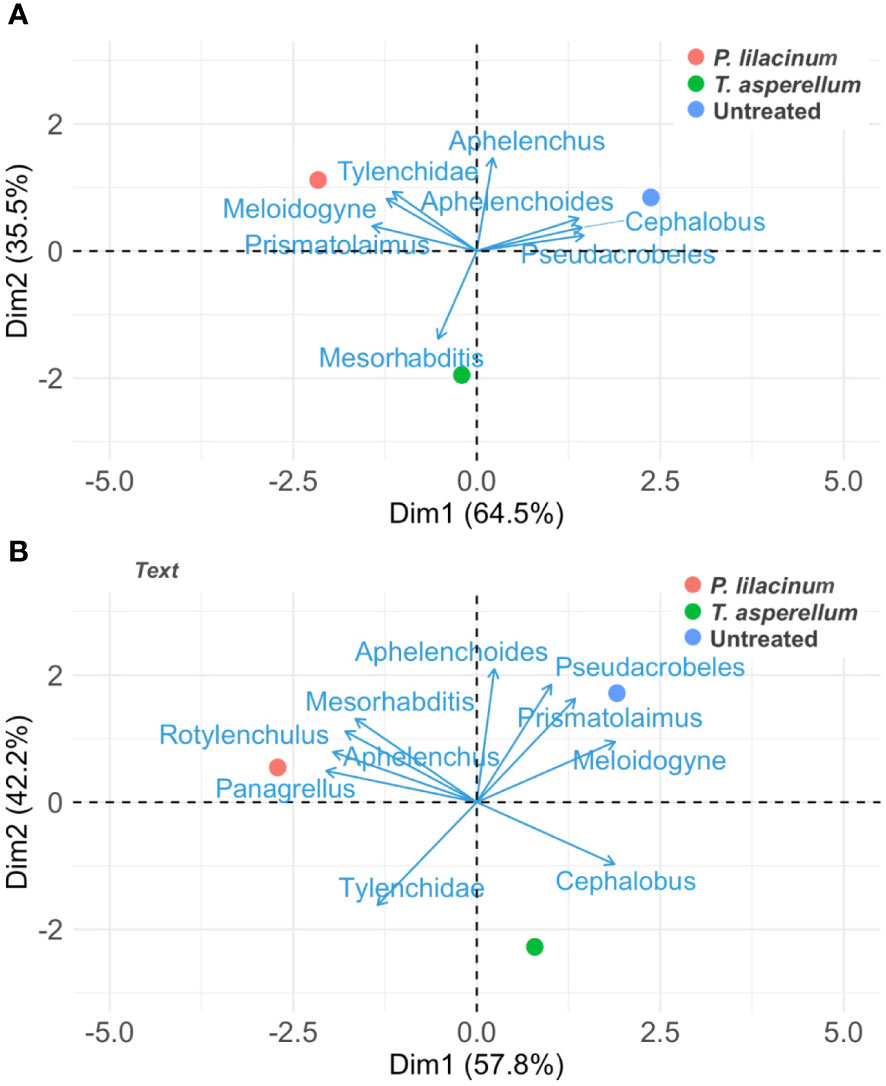
Figure 2 Principal component analysis of the treatments as related to nematode genera in the early nematode community data sampled on Oct. 2020 (A) and the final nematode community data sampled on Apr. 2022 (B).
Functional metabolic footprints demonstrated no separation among the treatments during the two years of the field trial (Figure 3). The three treatments all clustered to the left bottom quadrant, inferring a degraded food web condition and soils that are stressed, depleted, fungal decomposition channels, and a high C:N ratio.
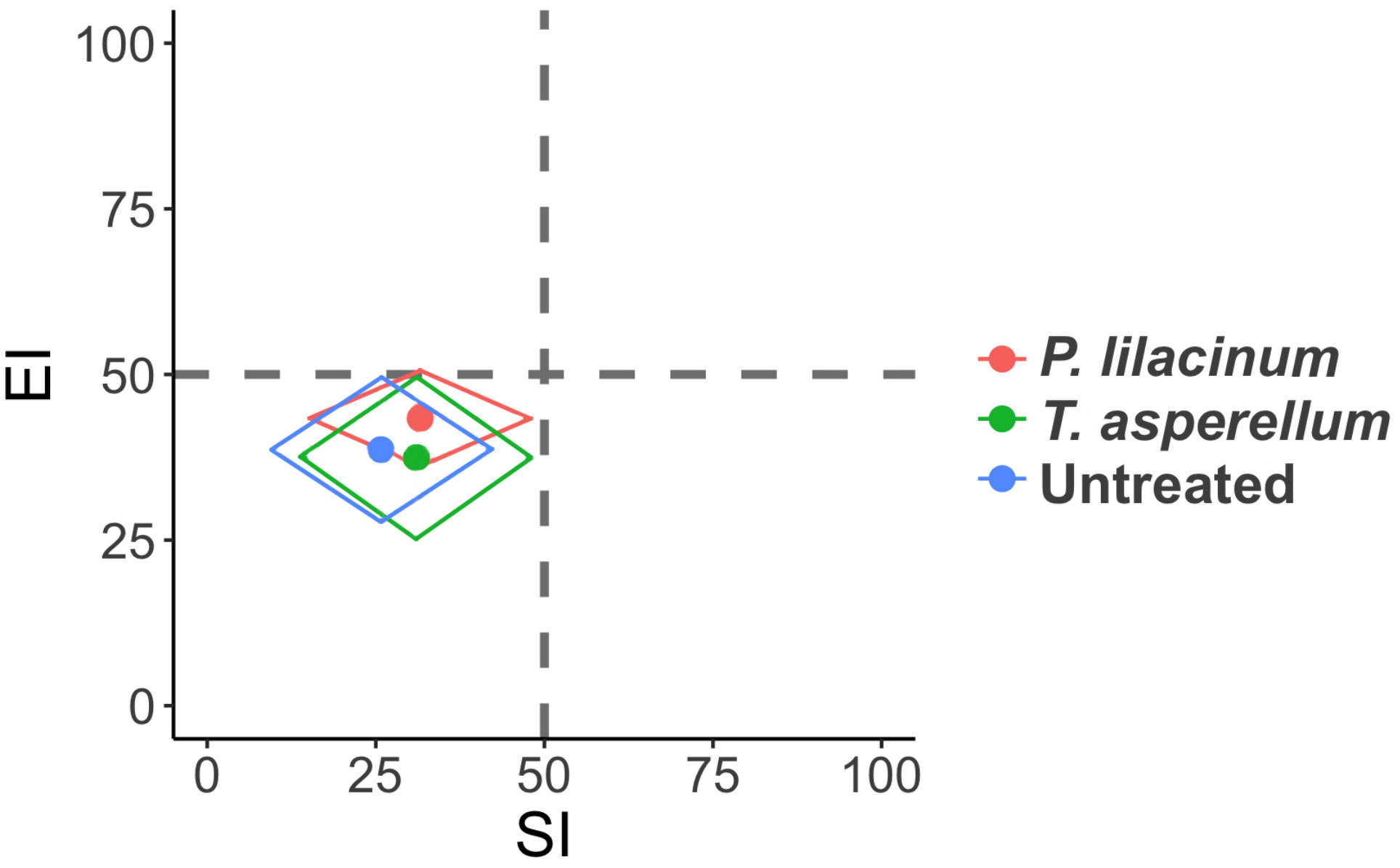
Figure 3 Functional metabolic footprints of nematodes with different treatments. Vertical axis represents the enrichment footprint and horizontal axis the structure footprint.
4 Discussion
Over a two-year period, the application of T. asperellum and P. lilacinum products demonstrated that even over this relatively short period, RKN densities, and consequently damage, could be suppressed on established Arabica coffee plantations. Some positive changes in the soil food web structure were also observed following the application of these biocontrol agents. How sustainable this is, in terms of reversing such damage, yet needs to be determined, as does the economic viability of such a practice. But this study demonstrates the potential for the use of biocontrol agents on perennial crops. It took up to one year before we detected endophytic colonization of the fungi in coffee roots, which was longer than we anticipated. However, this could have been affected by tree age, some of which are approaching 100 years, while unfavorable weather could have affected fungal establishment. For example, excess rainfall at the beginning of the study (March 2020 = 241 mm and April 2020 = 370 mm) could have washed out the applications, while a following long dry period between June to September in 2020 with less than 10 days of rain during the four months, without irrigation, may have restricted penetration of the drench to lower depths. Abiotic factors are well known to influence the colonization of biocontrol agents in soil and roots (Timper, 2011). In a similar study on mature coffee trees in Brazil, Zinger et al. (2021), evaluated the efficacy of T. harzianum against RKN. Unlike our finding, a significant reduction in M. incognita was observed in roots after just two months of the application, although no record of fungal colonization in roots was provided. On the other hand, Arita et al. (2020) reported a poor efficacy of P. lilacinum and T. harzianum to suppress M. paranaensis on Arabica coffee in Brazil. The differences in these findings might derive from the different application dosage, methods, RKN species and tested cultivars. Moreover, the use of organic amendments and thus the soil condition can also affect biocontrol colonization, which are often recommended to enhance biocontrol efficacy (Garbeva et al., 2004; Chilosi et al., 2020). In the current study, assessment of the various nematode based indices showed that the soils in which the trials were situated were all generally depleted and stressed, and consequently not ideal for biocontrol establishment. For example, low soil microbial diversity in coffee fields were associated negatively with soil functions and reduced coffee production (Zhao et al., 2018). Application of organic amendments may therefore help in enhancing the efficacy and speed of activity of the biocontrol agents.
The biocontrol agents, T. asperellum and P. lilacinum, are both common soil inhabitants and suppress RKN in a similar manner, which is mainly through the parasitism of nematode eggs and J2, although Trichoderma spp. also produces metabolites, which are nematicidal (Sharon et al., 2001). And yet, in our study, P. lilacinum reduced the RKN root population densities more rapidly than T. asperellum but then disappeared from soil and roots faster, once the application was stopped. The persistence of biocontrol agents in soil or roots mainly depends on how well they can multiply, where various external factors affect their ability to proliferate, such as edaphic factors, the microbial community structure, and the abundance of the host organisms (Abd-Elgawad and Askary, 2020). It is possible that P. lilacinum failed to adapt in the soil and root habitats and thus could not multiply efficiently in the fields of our study. It was not clear from our study as to which factors specifically prevented the longer-term establishment of P. lilacinum but for it to proliferate it would likely demand a higher frequency application in order to maintain a similar control efficacy of RKN that T. asperellum showed.
The nematode community assay in our study revealed different responses of the nematode fauna to the application of T. asperellum and P. lilacinum. As both products are fungal based, an increase of fungivores for both treatments was anticipated; however, an increase in fungivores was only obvious for P. lilacinum. Furthermore, of the two dominant fungivores, Aphelenchoides and Aphelenchus, only Aphelenchus was responsive to the P. lilacinum application, which may imply that P. lilacinum is a preferable food source to some genera than others. The increase in fungivores by the P. lilacinum application is not necessarily an ideal change to the soil health conditions, however, as the decomposition channel shifts from bacterial to fungal, which results in a slower decomposition process. Nonetheless, it would be important to understand the mechanism behind this phenomenon towards optimizing P. lilacinum application. An increased abundance of omnivores, dominated by Dorylaimid, and an improvement in maturity indices (i.e. maturity index, maturity index 2-5 and sigma maturity index), Structure index and Shannon index following T. asperellum application indicated less stressed, less disturbed and more stable environments with higher diversity (Ferris and Bongers, 2009). The enhanced diversity richness with T. asperellum applications also reflects previous studies (e.g. Alexander and Phin, 2014). Despite the more rapid suppression of RKN in roots by P. lilacinum, the nematode community assay revealed that T. asperellum had more positive impacts on soil health conditions, as demonstrated through the various indices.
The PCA was useful in highlighting the difference in nematode assemblages between the treatments as well as a temporal effect on the genera associated with the treatments. A few observations in the PCA supported the observation above, such as the shift of Aphelenchus spp. early in the study from a weak association across treatments to a strong association with the P. lilacinum treatment and the strong association of Meloidogyne in the control later in the study. On the other hand, the PCA revealed perspectives that were not clear from the generalized linear mixed models. For example, the genus Mesorhabditis, which is a c-p 1 group nematode, was initially associated with T. asperellum but later showed a strong association with P. lilacinum. Nematodes in c-p 1 group are indicative of food-enriched conditions (Bongers and Bongers, 1998), and that the application of P. lilacinum may enrich the environmental quality. Furthermore, Rotylenchulus showed a strong association with P. lilacinum later in the study, which was not confirmed using generalized linear mixed models. The analysis of the nematode community at the genus level, also highlighted the distinguished assemblage associated with the T. asperellum treatment, which showed less association with the most abundant nematode genus of this study. The evaluation of the impact of treatments at the genus, or even at species level, can be more insightful to better understand the soil ecosystem as opposed to analysis based on feeding type (Brussaard et al., 2004). The analysis of the nematode community data at multiple levels therefore facilitates an integrated understanding of the soil health conditions and should be considered in similar studies.
Since being proposed by Ferris (2010), the functional metabolic footprint is gaining increasing recognition for the description of food web form and function, and to evaluate the impact of land use (Krashevska et al., 2019), farming systems (Briar et al., 2011; Ilieva-Makulec et al., 2016) and management options (Gupta et al., 2019) on food web conditions. In our study, the analysis was not sensitive enough to capture any differences between treatments. All three treatments fell into the quadrant that indicates stressed conditions, while the temporal factor failed to influence the food web conditions over the two-year period. It is possible that this is due to the high abundance of fungivores, as well as c-p 2 nematodes across our coffee fields. For example, the two most abundant nematode families, Cephalobidae and Aphelenchoididae, are c-p 2 group bacterial scavengers and fungal feeders (Bongers and Bongers, 1998). These general opportunists can quickly adapt to and are associated with stressed conditions (Ferris et al., 2001). The high ratio of fungivores to bacterivores also indicated a fungal based slower decomposition channel rather than bacterial. This thereby reflects depleted, poor quality nutrient availability, resulting in a low Enrichment index value (Ferris et al., 2001). An increased abundance of c-p 3 and c-p 4 group nematodes was observed, however, after fungal colonization was recorded in roots for T. asperellum. This indicates that the shift in the food web condition following application of such biocontrol agents is gradual and requires longer-term monitoring to better appreciate the influence of biocontrol applications, especially when dealing with stressed, degraded soils.
In respect to plant-parasitic nematodes, the most commonly occurring and dominant genus was Meloidogyne, which was identified solely as M. hapla. No other Meloidogyne species was detected from any of the coffee fields in the study. Meloidogyne hapla has been detected in Kenya on other vegetable crops (Karuri et al., 2017; Chitambo et al., 2018) and has also been identified from neighboring coffee estates (Saikai, personal observation). Among the Meloidogyne species reported on coffee globally, M. exigua, M. incognita, and M. paranaensis are considered the most important (Villain et al., 2018); M. africana and M. decalineata were reported as important from a study in Tanzania (Bridge, 1984). All the fields in our current study were heavily infested with M. hapla with significant root damage observed, unlike the reports of Villain et al. (2013). It has been recorded from coffee elsewhere in the region, such as Democratic Republic of the Congo (Villain et al., 2018) and Tanzania (Bridge, 1984; dos Santos et al., 2019), with some occasional reports from higher altitudes in Latin America (Villain et al., 2013) but has generally been considered to be of limited economic importance on coffee (Lordello, 1982; Bridge, 1984). It is, however, reported to be of significant importance on coffee in Hawaii (Handoo et al., 2005). The current study indicates that M. hapla is likely of significant importance on coffee, at least in Kenya, although no studies have so far been conducted to determine this. During our study, RKN soil densities were not necessarily reflective of the actual disease pressure observed on the roots, with which there was a poor correlation between the RKN soil densities and RKN root densities. We often recovered low or even zero RKN densities from soil, even when high RKN densities were observed in roots, and vice versa. Consequently, this might be associated with weather conditions to some extent, but there is also potential for some improvements in sampling and nematode extraction techniques. This is particularly relevant as the modified Baermann plate method relies heavily on nematode mobility. Nonetheless, based on our study, assessment of the RKN infection in roots was preferable to soil, to better appreciate pest pressure.
Globally, RKN is considered as the most significant nematode threat to the coffee production (Villain et al., 2018) but there is relatively little knowledge on the distribution of species or damage levels in Africa (Coyne et al., 2018). Bridge (1984) estimated a ~20% loss in yield on coffee due to nematode pests, mainly RKN, in Tanzania, while a survey in Ethiopia (Mekete et al., 2008) found only a limited occurrence of RKN on Ethiopian coffee. Some concern regarding RKN damage to coffee in Kenya has been raised (Nzesya et al., 2014) but in general, there is little understanding of the issue across the coffee sector. In our study, the mean total abundance of herbivores, including the root hair feeders, was just 7%, whereas Nzesya et al. (2014) reported a 64% total abundance of herbivores in their samples. Despite some extensive root galling damage observed in our fields, recovered nematodes from samples were at times relatively low. In general, poorly managed coffee farms tend to be more affected by RKN, compared to well-managed commercial farms (Nzesya et al., 2014).
For practical usage of these biocontrol agents by farmers in the region, the financial and economic viability needs to be established. The frequency of application in our study was conducted to initially establish the fungal antagonists in the soil through a high frequency of application and then to maintain them. More in-depth assessment is required to determine effective and economical establishment rates, and for different soil types. We estimate an approximate product cost of $23.43 USD per tree over the two years of study, plus labor fee. At current 2022 rates, farmers receive approximately $5.00 USD for grade AA dried beans per kilogram. To break even therefore, a yield improvement of approximately 4.7 kg (28 kg fresh cherries) of the best quality dry beans per tree over two years is needed. However, the additional merits of using these biocontrol products would be useful to assess, such as an impact on other pests and diseases and long-term soil health improvement that would improve nutrient use efficiency, for example, due to improved root development and feeder root mass. During sampling of roots towards the latter part of the study, more prolific feeder root growth was visually observed in the biocontrol treatment plots than the control, indicating their influence on root recovery and development. This proved difficult to validate empirically but should be looked at in future studies. Application regimes could also be developed to optimize the application timing, such as targeted application to coincide with the period of root flush, to reduce the frequency. We were unable to measure yield in the current study, as the pruning program/cycle rendered yield data of little value. Instead, yield needs to be monitored over a longer time period, such as five years and a mean per year calculated, to negate the influence of the pruning program. Considering the observed damage caused by RKN alone, it was apparent that root efficiency was significantly compromised and not functioning effectively to access nutrients or water. Neither replacing existing coffee trees with new seedlings nor applying synthetic chemical pesticides are attractive strategies. However, our study has demonstrated that biocontrol agents provide an alternative option for managing nematode diseases on mature and established coffee farms.
5 Conclusions
Reversing or arresting even, long-term nematode damage inflicted on the root systems of perennial crops is not readily addressed, nor easily implemented. The decades-long manifestation of nematode pests, such as RKN on mature coffee plantations, has major implications to productivity and the economic viability of aging plantations. Replacing these plantations with new plants has huge financial implications, while the blanket treatment of the soil with synthetic pesticides has major environmental and cost considerations. This current study therefore assessed the potential of drenching biocontrol agents on to established, mature coffee fields in Kenya, all of which were affected by nematode infection and damage. Our study has shown that two locally available biocontrol agents, Trichoderma asperellum and Purpureocillium lilacinum, can effectively suppress RKN infection on mature coffee plants and improve soil health. These biocontrol agents offer a potential sustainable alternative to chemical inputs for coffee farmers in the region. During a two-year period, the drenching application of T. asperellum and P. lilacinum products showed a significant reduction in the population density of Meloidogyne hapla in roots of established Arabica coffee plantations. Additionally, some improvements were observed in the soil food web structure after the application of these biocontrol agents, even over this relatively short period. Our study showed that P. lilacinum was more effective than T. asperellum in reducing root population densities of M. hapla, although it disappeared from the soil and roots faster once the application was stopped. However, despite the more rapid suppression of M. hapla by P. lilacinum, the nematode community assay revealed that T. asperellum had a more positive impact on soil health conditions, as demonstrated through various nematode based indices. Contrary to previous reports in other regions, our current study suggests that M. hapla may be significant in coffee cultivation, particularly in Kenya, although no previous studies have investigated its importance in this context.
Data availability statement
The raw data supporting the conclusions of this article will be made available by the authors, without undue reservation.
Author contributions
KS designed the experiment as the project lead. KS implemented and managed the field trials and the sample processing in collaboration with CO and ES. The data collection, especially for the nematode community data, was conducted by KS. KS analyzed the data. The manuscript was drafted by KS. CO contributed to the experimental design and assisted the implementation and management of the field trial with KS. CO counted and identified the plant-parasitic nematodes. ES assisted the implementation and management of the field trials and the sample processing. SN supported the implementation and management of the field trials as well as the fungal isolation from soil and roots. RM provided the biocontrol products evaluated in the study. SH supervised the lead author at icipe and provided the research facilities. SH contributed to draft the article and approved the version submitted to the journal. DC supervised the lead author at IITA and provided the research facilities. DC contributed to design of the experiment, draft the article and approved the version submitted to the journal. All authors contributed to the article and approved the submitted version.
Funding
The field trial was implemented with the support by the Real IPM. Japan Society for the Promotion of Science funded the lead author to conduct the study in Kenya. This work was supported by International Institute of Tropical Agriculture (IITA) in Nairobi, Kenya and International Centre of Insect Physiology and Ecology (ICIPE) in Nairobi, Kenya.
Conflict of interest
Authors SN and RM are employed by the company RealIPM.
The remaining authors declare that the research was conducted in the absence of any commercial or financial relationships that could be construed as a potential conflict of interest.
JWK and DWM supervised CO at University of Nairobi for her MSc and contributed to the experimental design and helped revise the original thesis.
Publisher’s note
All claims expressed in this article are solely those of the authors and do not necessarily represent those of their affiliated organizations, or those of the publisher, the editors and the reviewers. Any product that may be evaluated in this article, or claim that may be made by its manufacturer, is not guaranteed or endorsed by the publisher.
References
Abd-Elgawad, M. M. M., Askary, T. H. (2020). Factors affecting success of biological agents used in controlling the plant-parasitic nematodes. Egyptian J. Biol. Pest Control. 30, 17. doi: 10.1186/s41938-020-00215-2
Alexander, A., Phin, C. K. (2014). Combination of biological agents in suppressing colonization of Ganoderma boninenseof basal stem rot. Am. Eurasian J. Sustain Agric. 8, 1–7.
Alwora, G., Gichuru, E. K. (2014). Advances in the management of coffee berry disease and coffee leaf rust in Kenya. J. Renew. Agric. 2, 5–10. doi: 10.12966/jra.03.02.2014
Arita, L. Y., da Silva, S. A., Machado, A. C. Z. (2020). Efficacy of chemical and biological nematicides in the management of Meloidogyne paranaensis in coffee arabica. Crop Prot. 131, 105099. doi: 10.1016/j.cropro.2020.105099
Barriga, R. (1976). “Reports by regional investigators: b. South America,” in International meloidogyne project. proceedings of the research planning conference on root-knot nematodes meloidogyne (North Carolina, USA: North Carolina State University), 7–9.
Bertrand, B., Anzueto, F., Peña, M., Anthony, F., Eskes, A. B., Aguilar, G., et al. (1997). Comportement agronomique et résistance aux principaux déprédateurs des lignées de sarchimors et catimors au Costa Rica. Plante Recherche Développement 4, 312–321.
Bongers, T. (1990). The maturity index: an ecological measure of environmental disturbance based on nematode species composition. Oecologia 83, 14–19. doi: 10.1007/BF00324627
Bongers, T., Bongers, M. (1998). Functional diversity of nematodes. Appl. Soil Ecol. 10, 239–251. doi: 10.1016/S0929-1393(98)00123-1
Briar, S. S., Fonte, S. J., Park, I., Six, J., Scow, K., Ferris, H. (2011). The distribution of nematodes and soil microbial communities across soil aggregate fractions and farm management systems. Soil Biol. Biochem. 43, 905–914. doi: 10.1016/j.soilbio.2010.12.017
Bridge, J. (1984). Coffee nematode survey of tanzania. report on a visit to examine plant-parasitic nematodes of coffee in Tanzania (St Albans, UK: Commonwealth Institute of Parasitology).
Brussaard, L., Kuyper, T. W., Didden, W. A. M., de Goede, R. G. M., Bloem, J. (2004). “Biological soil quality from biomass to biodiversity – importance and resilience to management stress and disturbance,” in Managing soil quality: challenges in modern agriculture. Eds. schionning, P., Elmholt, S., Christensen, B. T. (Wallingford, UK: CAB International), 139–158.
Cai, F., Chen, W., Wei, Z., Pang, G., Li, R., Ran, W., et al. (2015). Colonization of Trichoderma harzianum strain SQR-T037 on tomato roots and its relationship to plant growth, nutrient availability and soil microflora. Plant Soil 388, 337–350. doi: 10.1007/s11104-014-2326-z
Campos, H. D., Campos, V. P. (1997). Effect of timing and forms of application of Arthrobotrys conoides, arthrobotrys musiformis, paecilomyces lilacinus and Verticillium chlamydosporium in the soil for the control of Meloidogyne exigua of coffee. Fitopatologia Bras. 22, 361–365.
Campos, V., Silva, J. (2008). “Management of meloidogyne spp. in coffee plantations,” in Plant-parasitic nematodes of coffee. Ed. Souza, R. M. (Berlin, Germany: Springer Science), 149–164.
Carneiro, R. M. D. G., Cofcewicz, E. T. (2008). “Taxonomy of coffee-parasitic root-knot nematodes, meloidogyne spp,” in Plant-parasitic nematodes of coffee. Ed. Souza, R. M. (Berlin, Germany: Springer Science), 87–122.
Carvalhais, L. C., Dennis, P. G., Badri, D. V., Kidd, B. N., Vivanco, J. M., Schenk, P. M. (2015). Linking jasmonic acid signaling, root exudates, and rhizosphere microbiomes. Mol. Plant-Microbe Interact. 28, 1049–1058. doi: 10.1094/MPMI-01-15-0016-R
Chilosi, G., Aleandri, M. P., Luccioli, E., Stazi, S. R., Marabottini, R., Morales-Rodríguez, C., et al. (2020). Suppression of soil-borne plant pathogens in growing media amended with espresso spent coffee grounds as a carrier of Trichoderma spp. Scientia Hortic. 259, 108666. doi: 10.1016/j.scienta.2019.108666
Chitambo, O., Haukeland, S., Fiaboe, K. K. M., Grundler, F. M. W. (2018). First report of Meloidogyne hapla and M. javanica coinfection on Parthenium hysterophorus in Kenya. Plant Dis. 103, 162. doi: 10.1094/PDIS-06-18-0964-PDN
Coffee Research Institute - Kenya Agricultural and Livestock Research Organization (2022) Coffee research institution: history. Available at: https://www.kalro.org/coffee (Accessed March 1, 2023).
Coyne, D. L., Cortada, L., Dalzell, J. J., Claudius-Cole, A. O., Haukeland, S., Luambano, N., et al. (2018). Plant-parasitic nematodes and food security in Sub-Saharan Africa. Annu. Rev. Phytopathol. 56, 381–403. doi: 10.1146/annurev-phyto-080417-045833
Coyne, D. L., Nicol, J. M., Claudius-Cole, B. (2014). Practical plant nematology: a field and laboratory guide. 2nd edition (Contou, Benin: SP-IPM Secretariat).
dos Santos, M. F. A., da Silva Mattos, V., Monteiro, J. M. S., Almeidaa, M. R. A., Jorge, A. S., Cares, J. E., et al. (2019). Diversity of meloidogyne spp. from peri-urban areas of sub-Saharan Africa and their genetic similarity with populations from the Latin America. Physiol. Mol. Plant Pathol. 105, 110–118. doi: 10.1016/j.pmpp.2018.08.004
Ferris, H. (2010). Form and function: metabolic footprints of nematodes in the soil food web. Eur. J. Soil Biol. 46, 97–104. doi: 10.1016/j.ejsobi.2010.01.003
Ferris, H., Bongers, T. (2009). “Indices developed specifically for analysis of nematode assemblages,” in Nematodes as environmental indicators. Eds. Wilson, M. J., Kakouli-Duarte, T. (Wallingford, UK: CAB International), 124–145.
Ferris, H., Bongers, T., Goede, R. G. M. (2001). A framework for soil food web diagnostics: extension of the nematode faunal analysis concept. Appl. Soil Ecol. 18, 13–29. doi: 10.1016/S0929-1393(01)00152-4
Garbeva, P. V., Van Veen, J. A., Van Elsas, J. D. (2004). Microbial diversity in soil: selection of microbial populations by plant and soil type and implications for disease suppressiveness. Annu. Rev. Phytopathol. 42, 243–270. doi: 10.1146/annurev.phyto.42.012604.135455
Gitonga, D. (2020). Diversity of plant-parasitic nematodes associated with coffee and soybean in Kenya with description of known and putative new species (Ghent, Belgium: Ghent University).
Gitonga, K., Townsend, S. (2019) Kenya Coffee sector to decline as reforms fall short. GAIN report. Available at: https://apps.fas.usda.gov/newgainapi/api/report/downloadreportbyfilename?filename=Coffee%20Annual_Nairobi_Kenya_5-21-2019.pdf (Accessed March 1, 2023).
Gupta, D., Bhandari, S., Bhusal, D. R. (2019). Variation of nematode indices under contrasting pest management practices in a tomato growing agro-ecosystem. Heliyon. 5, e02621. doi: 10.1016/j.heliyon.2019.e02621
Halifu, S., Deng, X., Song, X., Song, R. (2019). Effects of two trichoderma strains on plant growth, rhizosphere soil nutrients, and fungal community of pinus sylvestris var. mongolica annual seedlings. Forests 10, 758. doi: 10.3390/f10090758
Handoo, Z. A., Skantar, A. M., Carta, L. K., Schmitt, D. P. (2005). Morphological and molecular evaluation of a Meloidogyne hapla population damaging coffee (Coffea arabica) in maul, Hawaii. J. Nematol. 37, 136–145.
Heidari, F., Olia, M. (2016). Biological control of root-knot nematode, Meloidogyne javanica, using vermicompost and fungus Trichoderma harzianum on tomato. Iran. J. Plant Pathol. 52, 109–124.
Hohmann, P., Jones, E. E., Hill, R. A., Stewart, A. (2011). Understanding Trichoderma in the root system of Pinus radiata: associations between rhizosphere colonization and growth promotion for commercially grown seedlings. Fungal Biol. 115, 759–767. doi: 10.1016/j.funbio.2011.05.010
Holland, R. J., Williams, K. L., Khan, A. (1999). Infection of Meloidogyne javanica by Paecilomyces lilacinus. Nematology 1, 131–139. doi: 10.1163/156854199508090
Humphreys-Pereira, D. A., Flores-Chaves, L., Gómez, M., Salazar, L., Gómez-Alpízar, L., Elling, A. A. (2014). Meloidogyne lopezi n. sp. (Nematoda: meloidogynidae), a new root-knot nematode associated with coffee (Coffea arabica l.) in Costa Rica, its diagnosis and phylogenetic relationship with other coffee-parasitising Meloidogyne species. Nematology 16, 643–661. doi: 10.1163/15685411-00002794
Ilieva-Makulec, K., Tyburski, J., Makulec, G. (2016). Soil nematodes in organic and conventional farming system: a comparison of the taxonomic and functional diversity. Pol. J. Ecol. 64, 547–563. doi: 10.3161/15052249pje2016.64.4.010
International Coffee Organization (2015) Sustainability of the coffee sector in Africa. Available at: https://www.ico.org/documents/cy2014-15/icc-114-5e-overview-coffee-sector-africa.pdf (Accessed March 1, 2023).
International Coffee Organization (2022) Coffee market report. Available at: https://www.ico.org/Market-Report-22-23-e.asp (Accessed March 1, 2023).
Janssen, T., Karssen, G., Verhaeven, M., Coyne, D., Bert, W. (2016). Mitochondrial coding genome analysis of tropical root-knot nematodes (Meloidogyne) supports haplotype based diagnostics and reveals evidence of recent reticulate evoluation. Sci. Rep. 6, 22591. doi: 10.1038/srep22591
Jones, J. T., Haegeman, A., Danchin, E. G. J., Gaur, H. S., Helder, J., Jones, M. G. K., et al. (2013). Top 10 plant-parasitic nematodes in molecular plant pathology. Molec. Plant Pathol. 14, 946–961. doi: 10.1111/mpp.12057
Karuri, H. W., Olago, D., Neilson, R., Mararo, E., Villinger, J. (2017). A survey of root knot nematodes and resistance to Meloidogyne incognita in sweet potato varieties from Kenyan fields. Crop Prot. 92, 114–121. doi: 10.1016/j.cropro.2016.10.020
Kiewnick, S., Sikora, R. A. (2006). Biological control of the root-knot nematode Meloidogyne incognita by Paecilomyces lilacinus strain 251. Biol. Control 38, 179–187. doi: 10.1016/j.biocontrol.2005.12.006
Kiriga, A. W., Haukeland, S., Kariuki, G. M., Coyne, D. L., Beek, N. V. (2018). Effect of Trichoderma spp. and Purpureocillium lilacinum on Meloidogyne javanica in commercial pineapple production in Kenya. Biol. Control 119, 27–32. doi: 10.1016/j.biocontrol.2018.01.005
Kisaakye, J., Fourie, H., Coyne, D., Cortada, L., Khamis, F. M., Subramanian, S., et al. (2023). Endophytic fungi improve management of the burrowing nematode in banana (Musa spp.) through enhanced expression of defence-related genes. Nematology 25, 427–442 doi: 10.1163/15685411-bja10229
Krashevska, V., Kudrin, A. A., Widyastuti, R., Scheu, S. (2019). Changes in nematode communities and functional diversity with the conversion of rainforest into rubber and oil palm plantations. Front. Ecol. Evol. 7. doi: 10.3389/fevo.2019.00487
Krishnan, S. (2017). Sustainable coffee production. Oxford research encyclopedia, environmental science. development of the global strategy for conservation of coffee generic resources. doi: 10.1093/acrefore/9780199389414.013.224
López-Lima, D., Sánchez-Nava, P., Carrion, G., Espinosa de los Monteros, A., Villain, L. (2015). Corky-root symptoms for coffee in central veracruz are linked to the root-knot nematode meloidogyne paranaensis, a new report for Mexico. Eur. J. Plant Pathol. 141, 623–629. doi: 10.1007/s10658-014-0564-9
Lordello, L. G. E. (1982). Nova Ocorrência do nematóide Meloidogyne hapla em cafeeiro. Rev. Agricultura 57, 6.
Lordello, L. G. E. (1984). Nematoides das plantas cultivadas. 8th Ed (Sao Paulo: Livraria Nobel S. A. 314).
Maximiniano, C., Campos, V. P., Souza, R. M., Almeida, A. R. (2001). Flutuação populacional de Meloidogyne exigua em cafezal naturalmente infestado por Pasteuria penetrans. Nematologia Bras. 21, 63–69.
Mekete, T., Sikora, R. A., Kiewnick, S., Hallmann, J. (2008). Description of plant-parasitic nematodes associated with coffee in Ethiopia. Nematol. medit. 36, 69–77.
Naves, R. L., Campos, V. P. (1991). Ocorrência de fungos predadores de nematóides no sul de minas gerais e estudo da capacidade predatória e crescimento in vitro de alguns de seus isolados. Nematologia Bras. 15, 152–162.
Nzesya, M. J., Wangai, K. J., Maina, M. W., Peter, W. M., Elijah, G. K. (2014). Plant-parasitic nematodes associated with coffee in Kenya and factors influencing their occurrence, abundance and diversity. J. Biol. Agric. Healthcare 4, 120–129.
Okada, H., Harada, H. (2007). Effects of tillage and fertilizer on nematode communities in a Japanese soybean field. Appl. Soil Ecol. 35, 582–598. doi: 10.1016/j.apsoil.2006.09.008
Poveda, J. (2021). Trichoderma as biocontrol agent against pests: new uses for a mycoparasite. Biol. Control 159, e104634. doi: 10.1016/j.biocontrol.2021.104634
R Core Team (2020) R: a language and environment for statistical computing. Vienna, Austria: R Foundation for Statistical Computing. Available at: https://www.R-project.org/.
RealIPM (2022) RealTrichoderma. Available at: https://realipm.co.za/project/real-trichoderma (Accessed March 1, 2023).
Ribeiro, R. C. F., Campos, V. P. (1993). Isolamento e identificação e efeito da temperatura no crescimento ‘in vitro’ de fungos parasitos de ovos de Meloidogyne spp. no sul de minas gerais. Nematologia Bras. 17, 132–138.
Ros, M., Raut, I., Santisima-Trinidad, A. B., Pascual, J. A. (2017). Relationship of microbial communities and suppressiveness of Trichoderma fortified composts for pepper seed- lings infected by Phytophthora nicotianae. PloS One 12, e0174069. doi: 10.1371/journal.pone.0174069
Saikai, K., Wanjala, S., Oduori, C., Coyne, D. (2021). Revisiting the modified baermann extraction method: extraction efficiency of Radopholus similis using different extraction material. Nematology 23, 1215–1218. doi: 10.1163/15685411-bja10130
Sharma, R. D., Lordello, R. R. A. (1992). “Occurrence of pasteuria penetrans in coffee plantations infested by meloidogyne exigua in the state of são paulo,” in Congresso brasileiro de fitopatologia, XXV, vol. 183. (Gramado, Brazil).
Sharon, E., Bar-Eyal, M., Chet, I., Herrera-Estrella, A., Kleifeld, O., Spiegel, Y. (2001). Biological control of the root-knot nematode Meloidogyne javanica by Trichoderma harzianum. Phytopathol. 91, 687–693. doi: 10.1094/PHYTO.2001.91.7.687
Sieriebriennikov, B., Ferris, H., de Goede, R. G. M. (2014). NINJA: an automated calculation system for nematode-based biological monitoring. Eur. J. Soil Biol. 61, 90–93. doi: 10.1016/j.ejsobi.2014.02.004
Souza, R. M. (2008). “Part V world reports,” in Plant-parasitic nematodes of coffee (Berlin, Germany: Springer Science), 225–313.
Timper, P. (2011). “Utilization of biological control for managing plant-parasitic nematodes,” in Biological control of plant-parasitic nematodes: building coherence between microbial ecology and molecular mechanisms, progress in biological control 11. Eds. Davies, K., Spiegel, Y. (USA: US Government). doi: 10.1007/978-1-4020-9648-8_11
Villain, L., Lima Salgado, S. M., Trinh, P. Q. (2018). “Nematode parasites of coffee and cocoa,” in Plant-parasitic nematodes in subtropical and tropical agriculture, 2nd edition. Eds. Luc, M., Sikora, R., Bridge, J. (Wallingford, UK: CABI International), 529–579.
Villain, L., Sarah, J. L., Hernaíndez, A., Bertrand, B., Anthony, F., Lashermes, P., et al. (2013). Diversity of root-knot nematodes associated with coffee orchards in central America. Nematropica 43, 194–206.
Yeates, G. W., Bongers, T., de Goede, R. G. M., Freckman, D. W., Georgieva, S. S. (1993). Feeding habits in soil nematode families and genera - an outline for soil ecologists. J. Nematol. 25, 315–331.
Zhao, Q., Xiong, W., Xing, Y., Sun, Y., Lin, X., Dong, Y. (2018). Long-term coffee monoculture alters soil chemical properties and microbial communities. Sci. Rep. 8, 6116. doi: 10.1038/s41598-018-24537-2
Keywords: root-knot nematode, Meloidogyne hapla, biological control, nematode management, nematode community
Citation: Saikai KK, Oduori C, Situma E, Njoroge S, Murunde R, Kimenju JW, Miano DW, Haukeland S and Coyne D (2023) Biocontrol-based strategies for improving soil health and managing plant-parasitic nematodes in coffee production. Front. Plant Sci. 14:1196171. doi: 10.3389/fpls.2023.1196171
Received: 29 March 2023; Accepted: 02 May 2023;
Published: 20 June 2023.
Edited by:
Francesca De Luca, National Research Council (CNR), ItalyReviewed by:
Pablo Castillo, Spanish National Research Council (CSIC), SpainDixit Sharma, Central University of Himachal Pradesh, India
Copyright © 2023 Saikai, Oduori, Situma, Njoroge, Murunde, Kimenju, Miano, Haukeland and Coyne. This is an open-access article distributed under the terms of the Creative Commons Attribution License (CC BY). The use, distribution or reproduction in other forums is permitted, provided the original author(s) and the copyright owner(s) are credited and that the original publication in this journal is cited, in accordance with accepted academic practice. No use, distribution or reproduction is permitted which does not comply with these terms.
*Correspondence: Kanan K. Saikai, a2FuYW4uc2Fpa2FpQHd1ci5ubA==