- 1Indian Institute of Pulses Research (IIPR), Indian Council of Agricultural Research (ICAR), Kanpur, Uttar Pradesh, India
- 2Department of Botany, Panjab University, Chandigarh, India
- 3Department of Plant Pathology, Pulse Research Station, S.D. Agricultural University SK Nagar, SK Nagar, Gujarat, India
- 4Department of Plant Physiology, College of Agriculture, Vellayani, Kerala Agricultural University (KAU), Thiruvananthapuram, Kerala, India
- 5Dryland Agriculture Research Station, Sher-e-Kashmir University of Agricultural Sciences and Technology (SKUAST)-Kashmir, Srinagar, India
- 6Department of Agricultural Biotechnology and Molecular Biology, Dr. Rajendra Prasad Central Agricultural University, Samatipur, Bihar, India
- 7Shandong Academy of Agricultural Sciences, Jinan, Shandong, China
- 8Center for Crop Health, University of Southern Queensland, Toowoomba, QLD, Australia
- 9Department of Agronomy, Kansas State University, Manhattan, KS, United States
- 10Indian Council of Agricultural Research, New Delhi, India
- 11The University of Western Australia (UWA) Institute of Agriculture, The University of Western Australia, Perth, WA, Australia
Grain legumes play a crucial role in human nutrition and as a staple crop for low-income farmers in developing and underdeveloped nations, contributing to overall food security and agroecosystem services. Viral diseases are major biotic stresses that severely challenge global grain legume production. In this review, we discuss how exploring naturally resistant grain legume genotypes within germplasm, landraces, and crop wild relatives could be used as promising, economically viable, and eco-environmentally friendly solution to reduce yield losses. Studies based on Mendelian and classical genetics have enhanced our understanding of key genetic determinants that govern resistance to various viral diseases in grain legumes. Recent advances in molecular marker technology and genomic resources have enabled us to identify genomic regions controlling viral disease resistance in various grain legumes using techniques such as QTL mapping, genome-wide association studies, whole-genome resequencing, pangenome and ‘omics’ approaches. These comprehensive genomic resources have expedited the adoption of genomics-assisted breeding for developing virus-resistant grain legumes. Concurrently, progress in functional genomics, especially transcriptomics, has helped unravel underlying candidate gene(s) and their roles in viral disease resistance in legumes. This review also examines the progress in genetic engineering-based strategies, including RNA interference, and the potential of synthetic biology techniques, such as synthetic promoters and synthetic transcription factors, for creating viral-resistant grain legumes. It also elaborates on the prospects and limitations of cutting-edge breeding technologies and emerging biotechnological tools (e.g., genomic selection, rapid generation advances, and CRISPR/Cas9-based genome editing tool) in developing virus-disease-resistant grain legumes to ensure global food security.
Introduction
Grain legumes, rich in essential amino acids, vitamins, and minerals, are a crucial component of agroecosystems and vital for combating protein- and micronutrient-related malnutrition problems in the growing human population (Graham and Vance, 2003; Jha et al., 2022a). However, changing global climate trends have increased the incidence of various biotic and abiotic stresses, including viral diseases that cause significant yield losses in grain legumes worldwide. It has been estimated that plant viral diseases alone cause 50% of plant disease globally with economic losses measuring $30 billion annually (Hilaire et al., 2022). To address this challenge, researchers are developing sustainable and ecofriendly approaches to design the next generation of virus-disease-resistant grain legumes. Plants have sophisticated innate immune systems that respond to attacking viral pathogens using pattern-triggered immunity (PTI) and effector-triggered immunity (ETI) (Jones and Dangl, 2006). Mendelian-based genetic approaches initially helped uncover the major genetic determinants controlling these diseases in various legumes. Subsequently, molecular marker technologies facilitated the identification of causative virus-disease-resistant genomic regions/QTL in various grain legumes using biparental QTL analysis and genome-wide association studies (GWAS). Decoding various grain legume genome sequences enabled the discovery of novel single nucleotide sequence (SNP) markers for GWAS to explore marker-trait associations/haplotypes for disease resistance at the whole-genome level (for details see Ha and Lee, 2020). Whole-genome resequencing and the availability of pangenomes in various legumes and viruses offer novel opportunities to explore presence/absence variations, structural variations conferring viral disease resistance, and novel resistance (R) gene(s) and virulence gene(s) across the whole genome (Zhao et al., 2020; Jha et al., 2022b). These approaches also offer novel insights into the various effector molecules of virulent viruses to design virus-disease-resistant grain legumes. In addition, advances in functional genomics using RNA-seq-based transcriptome analysis technologies have enriched our understanding of possible candidate genes and regulatory non-coding RNAs attributing to resistance against various virus diseases with putative functions. In recent years, researchers have made progress in designing virus-resistant grain legumes using genetic-engineering-driven approaches, such as RNA interference (RNAi) and virus-induced gene silencing (VIGS) (Cruz and Aragão, 2014; Gao et al., 2015; Xun et al., 2019; Gao et al., 2020). Moreover, the benefits of emerging novel breeding approaches, such as rapid generation advancement protocol, genomic selection, and genome editing tools, could be harnessed for developing virus-resistant grain legumes. Thus, amalgamation of various ‘omics’ technologies with various novel breeding approaches could greatly benefit us designing future grain legumes cultivars with improved virus resistance (Weckwerth et al., 2020; Vahabi and Michailidis, 2022).
Viral diseases in soybean (Glycine max)
Soybean mosaic virus (SMV; genus Potyvirus, family Potyviridae) is one of the most devastating seed-borne viral diseases, causing severe yield and quality losses annually (Cho et al., 1977; Ma et al., 2002). As a vector, SMV is transmitted by soybean aphids (Aphis glycines) (Wang et al., 2006a). SMV also acts synergistically with bean pod mottle virus (BPMV) (genus Comovirus, family Comoviridae), decreasing yields by up to 85% (Ross, 1968). SMV virus particles contain a linear, positive sense, single-stranded RNA ~9.6 kb in length (Tolin, 1999). Cho and Goodman (1979); Cho and Goodman (1982) first established a classification system for grouping SMV isolates, reporting seven strain groups (G1–G7) based on the differential reactions of soybean cultivars conferring resistance to a common strain. In Japan, five main strains (A–E) of SMV isolates have been reported (Takahashi et al., 1963; Takahashi et al., 1980). In other studies, SMV was classified into 11 groups in South Korea (Cho et al., 1983; Seo et al., 2009) and 22 groups (SC1–SC22) in China (Guo et al., 2005; Moon et al., 2009; Li et al., 2010; Wang et al., 2013; for details see Usovsky et al., 2022). Stunted growth, mosaic leaf pattern, leaf curling, and seed coat mottling are the major symptoms of SMV (Bowers and Goodman, 1979; Tu, 1992) (see Table 1). Early mosaic symptoms appear 5–7 days after infection, and late mosaic symptoms appear 2–3 weeks after infection (Hill, 1999). Tobacco ringspot virus (TRSV), a single-stranded bipartite RNA virus, causes significant yield losses (25–100%) in soybean (Crittenden et al., 1966; Hartman and Domier, 2015), with stunted plant growth, dwarf and rolled leaflets, brownish, necrotic, and brittle buds, and bud death the major symptoms (Crittenden et al., 1966).
Viral diseases in common bean (Phaseolus vulgaris)
Major viral diseases of common bean resulting in significant yield losses include bean common mosaic virus (BCMV), bean common mosaic necrosis virus (BCMNV), bean yellow mosaic virus (BYMV), clover yellow vein virus (ClYVV), cowpea aphid-borne mosaic virus (CABMV), and watermelon mosaic virus-2 (WMV-2), with ssRNA belonging to family Potyviridae and genus Potyvirus (for details see Meziadi et al., 2017), and bean golden yellow mosaic virus (BGYMV) and bean dwarf mosaic virus (BDMV), with ssDNA belonging to family Geminiviridae and genus Begomovirus (Seo et al., 2004). Eight pathogenicity groups in the BCMV complex have been reported (Drijfhout et al., 1978; Drijfhout and Morales, 2005; Feng et al., 2015). BCMV is predominant worldwide in all legume-cultivation areas (Drijfhout, 1978; McKern et al., 1992; Makkouk et al., 2012); however, BCMNV is restricted to Africa, Europe, and North and South America (Worrall et al., 2015). Yield losses due to BCMV and BCMNV infection range from 6–98% (Hagedorn and Inglis, 1986; Worrall et al., 2015). BCMV and BCMNV show similar symptoms, including mosaic, dwarfing, chlorosis, and leaf curling (Flores-Estévez et al., 2003). BYMV, another viral disease of common bean, produces leaf mosaic symptoms and 30–40% yield losses (Swenson, 1968; Hagedorn and Inglis, 1986). For CIYVV, the notable symptoms are yellow mosaic, malformation, and reduced plant size (Tracy et al., 1992) (see Table 1).
The main viruses in genus Begomovirus that cause serious yield losses in common bean are BGYMV, tomato yellow leaf curl virus (TYLCV), and BDMV (Blair and Morales, 2008). The main symptoms of BGYMV are intense yellowing, pod deformation, stunting, and flower or pod abortion, causing 40–100% yield losses (Morales and Anderson, 2001; Morales, 2006; Blair et al., 2007; Subramanya, 2013; Aragão and Faria, 2009). The characteristic symptoms of TYLCV are leaf thickening and crumpling (Hedesh et al., 2011), while those of BDMV are stunted plant growth and leaf mosaic and mottle symptoms (Seo et al., 2004).
Viral disease in pigeonpea
Pigeonpea sterility mosaic virus (PPSMV) belongs to genus Emaravirus, causing sterility mosaic disease in pigeonpea (Cajanus cajan), resulting in yield losses >US$300 million in India (Patil and Kumar, 2015). An eriophyid mite Aceria cajani transmits the disease (Patil and Kumar, 2015). The visual symptoms include bushy and pale green appearance, mosaic leaf mottling, reduced leaf size, and partial/complete failure or no formation of reproductive structures (Daspute et al., 2014; Patil and Kumar, 2015).
Viral diseases in mungbean (Vigna radiata)
Two species cause yellow mosaic disease—mungbean yellow mosaic virus (MYMV) and mungbean yellow mosaic India virus (MYMIV)—which significantly affect mungbean productivity (Tsai et al., 2013). MYMIV is a major yield constraint in mungbean in South and Southeast Asia (Selvi et al., 2006; Iqbal et al., 2011), causing up to 100% yield losses under congenial conditions (Bashir et al., 2006). MYMV and MYMIV are begomoviruses with genomes comprising circular single-stranded DNA-A and DNA-B components known as ‘legumoviruses’ (Ilyas et al., 2009). This disease is transmitted by white fly (Bemisia tabaci Genn.) (Nariani, 1960). The disease features bright yellow mosaic symptoms on infected leaves, with few flowers, yellow-spotted pods, and immature and deformed seeds causing up to 100% yield losses (Hussain et al., 2004; Malathi and John, 2009; Biswas et al., 2012; Akbar et al., 2019) (see Table 1).
Viral diseases in urdbean (Vigna mungo)
Yellow mosaic disease (YMD) caused by MYMIV (Mayo, 2005) is the most destructive viral disease of urdbean, causing significant yield losses (Singh, 1980). MYMIV belongs to the group Geminiviridae and is transmitted by whitefly (B. tabaci Genn.) (Nariani, 1960). The characteristic symptoms of YMD include scattered yellow chlorotic spots on leaves, which enlarge and coalesce, resulting in conspicuous systemic bright patches, with the leaves eventually turning yellow (Kundu and Pal, 2012). Infected plants exhibit stunted growth, delayed maturity, and reduced flower and pod numbers (Grewal, 1978). Urdbean leaf crinkle virus (ULCV) disease decreases yields by 35–81% depending on host genotype and infection condition (Bashir et al., 1991). ULCV is transmitted by seed and sap inoculation, with white fly and aphid insect pests acting as vectors (Beniwal and Bharathan, 1980). The characteristic symptoms of this disease are leaf crinkling, curling, puckering, rugosity, stunted plant growth, and deformed floral parts (Nene, 1972) (see Table 1).
Viral diseases in cowpea (Vigna unguiculata)
Twenty viruses have been reported in cowpea (Hampton et al., 1997; Lima et al., 2005); however, the major viruses causing yield limitations are (1) cowpea severe mosaic virus (CPSMV; family Comoviridae, genus Comovirus); (2) cowpea aphid-borne mosaic virus (CABMV; family Potyviridae, genus Potyvirus); (3) cucumber mosaic virus (CMV; family Bromoviridae, genus Cucumovirus); (4) cowpea golden mosaic virus (CGMV; family Geminiviridae, genus Begomovirus) (Lima et al., 2005). Leaf crinkling and severe mottling of newly emerging leaves are characteristic symptoms of CPSMV, with stunting in severe cases (Umaharan et al., 1997b). CABMV is a seed-borne disease causing 13–87% yield losses under field conditions (Bashir et al., 2002). Virus-infected seed provides the initial inoculum, with aphids contributing secondary spread of the disease (Bashir et al., 2002). The characteristic features of CMV-caused disease in cowpea are chlorosis, vein clearing, necrosis, leaf deformation, and mild to severe mosaic and mottle (Hughes et al., 2003) (see Table 1). For CGMV infection, plants show mosaic, mottling, necrosis, and stunting, with low seed production (Boukar et al., 2013).
Viral diseases in groundnut
The most notable viral diseases in groundnut are peanut bud necrosis virus (PBNV), tobacco streak virus (TSV), peanut mottle virus (PeMoV), and Indian peanut clump virus, causing significant yield losses (Radhakrishnan et al., 2016). PBNV, belonging to genus Tospovirus, is transmitted by Thrips palmi, causing 30–90% yield losses (Vemana et al., 2015). Necrosis of terminal buds is a characteristic feature of this disease (Radhakrishnan et al., 2016). Peanut stem necrosis disease, caused by TSV, belongs to genus Ilarvirus of family Bromoviridae (Prasada Rao et al., 2003). Characteristic symptoms of this disease are complete stem necrosis and necrotic lesions on terminal leaflets (Radhakrishnan et al., 2016).
Viral diseases in chickpea
Chickpea is susceptible to several viral diseases including chickpea stunt disease (CSD). It is an emerging concern, posing a serious challenge to chickpea production and causes up to 95% yield loss (Abraham and Vetten, 2022). Interestingly, two different pathogens, viz., Chickpea chlorotic stunt virus (CpCSV), a member of Polerovirus (Abraham and Vetten, 2022) and Chickpea chlorotic dwarf virus (CpCDV), a member of Mastrevirus, family Geminiviridae (Kanakala and Kuria, 2018) are found to be associated with this disease. Small leaves, discolotration and bushy stunted appearance are the major symptoms of this disease. Stunt [bean (pea) leaf roll virus] is an important viral disease in chickpea mostly prevalent in chickpea-growing regions across the world. Stunting and phloem browning are the most visible symptom of this disease, the leaflets of infected plants become yellow, brown or orange in color (Nene et al., 2012). Of the other viruses, cucumber mosaic virus (CMV) causes significant yield losses in chickpea, reportedly 45%, when CMV incidence reached 75% (Jones et al., 2008). CMV symptoms occur on shoots, pods, and seeds. CMV also decreases seed quality (Jones et al., 2008). Mosaic [alfalfa mosaic virus] is a minor viral disease primarily found in Algeria, India, Iran, and Morocco, which causes terminal bud chlorosis followed by necrosis (Nene et al., 2012). Necrosis, caused by lettuce necrotic yellow virus, is characterized by twisted main and axillary shoots and necrotic tip burn on leaves (Nene et al., 2012).
Plant genetic resources for developing virus-resistant legumes
Various approaches, from breeding and plant protection to integrated approaches, have been embraced to develop virus-resistant legume crops (Bag et al., 2014; Meziadi et al., 2017). For example, plant breeding is a viable and sustainable approach for identifying grain legume landraces, accessions, and breeding lines, requiring no chemical pesticides that adversely affect the environment (Jha et al., 2020). Further, equipping grain legume cultivars with virus-resistant gene(s) using pre-breeding approaches, backcross breeding, and other modern breeding tools could help design virus-resistant grain legumes.
Several resistance sources are available for soybean SMV, including PI 96983 (Kiihl and Hartwig, 1979; Chen et al., 1991), ‘Columbia’ (Ma et al., 2002), PI 88788 (Gunduz et al., 2004), L29 (Buss et al., 1999), and PI 486355 (Ma et al., 1995), along with several near-isogenic lines, including L96-1676, L96-1680, L96-1683, and L96-1687 carrying the Rsv1 resistance gene developed from Williams × Buffalo, V97-9001 and V97-9003 carrying the Rsv4 resistance gene developed from Essex × PI 486355, and L88-8431 and L88-8440 carrying the Rsv1-r resistance gene developed from Williams × Raiden (Wang et al., 2006b; for details, see Usovsky et al., 2022). In addition, soybean genotypes harboring the Rsv1Rsv3 gene conferring resistance against SMV include ‘Hourei’ (Gunduz et al., 2002), ‘OX670’ (Gunduz et al., 2001), ‘Tousan’ (Gunduz et al., 2002), ‘J05’ (Zheng et al., 2006), ‘Zao18’ (Liao et al., 2002), and ‘Jiodou1’ (Shi et al., 2008) (see Table 2). Likewise, the ‘8101’ genotype harboring the Rsv1Rsv3Rsv4 gene (Liao et al., 2011) could be used to pyramid various genes conferring resistance against SMV. Intending to transfer SMV resistance into high-yielding soybean genotypes, Kato et al. (2016) introgressed genes conferring resistance against Japanese strains SMV-C and D into ‘Fukuibuki’ from ‘Harosoy’ donor parents via backcross breeding.
The common bean genotype ‘Redlands Greenleaf C’ harbors the bc-1 resistance gene against BCMV (Drijfhout, 1978; Strausbaugh et al., 1999; Miklas et al., 2000). Other soybean genotypes confer resistance against combined BCMV/BCMNV, including ‘Olathe’ harboring the bc-u gene (Drijfhout, 1978; Strausbaugh et al., 1999; Miklas et al., 2000), ‘Nodak’ harboring the bc-12 gene (Drijfhout, 1978; Miklas et al., 2000), Michelite’ harboring the bc-2 gene (Drijfhout, 1978; Miklas et al., 2000), ‘N85120’ harboring the bc-22 gene (Drijfhout, 1978; Kelly et al., 1995), and ‘B85009’ harboring the bc-3 gene (Drijfhout, 1978; Mukeshimana et al., 2005). In addition, Hart and Griffiths (2013) reported that ‘Clipper’ and ‘Jolanda’ soybean genotypes harbor the cyv and desc genes, respectively, conferring resistance against ClYVV (see Table 2), while ‘A429’ or ‘9236-6’ harboring the bgm-1 gene (Urrea et al., 1996; Blair et al., 2007) and ‘DOR303’ harboring the bgm-2 gene (Velez et al., 1998) confer resistance against BGYMV.
A multilocation evaluation of mungbean genotypes across India explored the resistance source against MYMV. The mungbean line ‘NM 94’ was identified as resistant against MYMV in the eastern state of Odisha but only moderately resistant in the southern state of Tamil Nadu. The evaluation identified ‘ML 1628’ as a source of high resistance against MYMV (Nair et al., 2017). Basavaraj et al. (2019) screened 14 mungbean genotypes over three seasons for MYMV resistance, reporting five genotypes (AVMU 1698, AVMU 1699, AVMU 16100, AVMU 16101, and KPS) with resistance. A rigorous field screening of a diverse set of 344 urdbean genotypes was tested for YMV resistance under field conditions for two years (Bag et al., 2014). Eight resistant genotypes were tested further in a glasshouse, identifying IC144901 and IC001572 as highly resistant against YMV (Bag et al., 2014).
Ashfaq et al. (2007) screened 87 urdbean genotypes for ULCV resistance over two seasons under field conditions. Based on the disease severity index, nine genotypes (2cm-703, 90cm-015, 93cm-006, 94cm-019, 99cm-001, IAM 382-1, IAM382-9, IAM382-15, and IAM133) were highly resistant to this disease. Likewise, a field screening of 40 urdbean genotypes identified M-6206, IAM-382-15, IAM-133, and Mash-1 as ULCV resistant (Binyamin et al., 2011). Sravika et al. (2019) conducted a field screening of 107 mungbean genotypes, reporting RME-16-3, RME-16-12, MLT-GG R-16-007, and MLT-GG R-16-009 as highly resistant to ULCV in rabi-sown mungbean but no resistant genotypes in kharif-sown mungbean. Cowpea genotypes with resistance against blackeye cowpea mosaic virus and CABMV include TVu401, Tvu1453, and Tvu1948, and advanced breeding lines IT82D-885, IT28D-889, and IT82E-60 (Gumedzoe et al., 1998) (see Table 2).
Besides cultivated plant species, crop wild relatives (CWRs) are a valuable source of novel genes associated with biotic and abiotic stresses. Kumar et al. (2005) reported sterility mosaic disease (SMD) resistance in several pigeonpea accessions belonging to six CWRs, including C. albicans, C. platycarpus, C. cajanifolius, C. lineatus, C. scarabaeoides, and C. sericeus. Among them, 15 accessions, including ICP 15614, 15615, 15626, 15684, 15688, 15700, and 15701, had SMD resistance. Mallikarjuna et al. (2011) reported that lines derived from C. acutifolius and C. platycarpus exhibited resistance against pigeonpea SMD under field conditions. Similarly, resistance to MYMV was found in V. radiata var. sublobata Roxb. Verde., a progenitor of mungbean, with resistance genes transferred to commercial mungbean cultivars (Singh and Ahuja, 1977). In chickpea, C. echinospermum and other chickpea CWRs (Kahraman et al., 2017; Rajpal et al., 2023) have the potential to transfer viral disease resistance to cultivated species.
Genetics of viral disease resistance in legumes
Mendelian genetics provides preliminary information on the genetic resistance of viral diseases in grain legumes. Kiihl and Hartwig (1979) developed the gene symbol for controlling resistance against SMV-1 in soybean as Rsv Rsv (resistance), rsvt rsvt (partial resistance), and rsv rsv (susceptible). Eight allelic-dominant genes (Rsv1, Rsv1-y, Rsv1-m, Rsv1-t, Rsv1-k, Rsv1-s, Rsv1-r, and Rsv1-h) for SMV resistance have been reported at the most common locus, Rsv1 (Chen et al., 1991; Chen et al., 1994; Ma et al., 1995; Chen et al., 2001; Chen et al., 2002). The Rsv1 gene identified in PI 96983 was mapped to the soybean molecular linkage group ‘F’ (Yu et al., 1994; Yu et al., 1996). The Rsv2 gene derived from the Raiden cultivar and allelic to the Rsv1 locus was assigned a new gene symbol Rsv1-r (Chen et al., 2002). Subsequently, a new resistant gene Rsv3, independent of Rsv1 and Rsv2, was reported (Buzzell and Tu, 1989) and mapped to the molecular linkage group ‘B2’ (Jeong et al., 2002). Ma et al. (2002) shed further light on the genetic resistance of SMV, confirming the presence of two independent resistant R3 and R4 genes working in a complementary fashion in the soybean genotype ‘Columbia’. A new resistance gene independent of Rsv1 and Rsv3 was reported in PI 486355 and mapped on ‘D1b’ (Hayes et al., 2000). The presence of Rsv1 and Rsv3 in ‘OX670’ soybean cultivar conferring resistance to SMV-G1 through G7 was reported (Gunduz et al., 2001). Gunduz et al. (2004) identified resistance against SMV strains G1 and G7. A genetic analysis of resistance in PI 88788 revealed that a single, partially dominant gene controlled SMV-G1; however, the same gene was dominant for SMV-G7 (Gunduz et al., 2004). The authors also confirmed that the resistance gene in PI 88788 was independent of Rsv1 and Rsv3, and the dominant resistance gene in PI 88788 was allelic to the SMV-resistant gene at the Rsv4 locus in V94-5152. The Rsv4 gene contributed resistance to all SMV strains (SMV-G1 to SMV-G7) (Chen et al., 1993; Ma et al., 1995). Recently, Klepadlo et al. (2017) assigned a new gene symbol Rsv5 to the resistance gene in ‘York’ to substitute the old allele named Rsv1-y on chromosome 13. Subsequently, several other researchers advocated that a single dominant gene governed the genetic control of SMV resistance (Zheng et al., 2014; Ma et al., 2016; Karthikeyan et al., 2017; Rui et al., 2017; Karthikeyan et al., 2018; Wu et al., 2019; Jin et al., 2022; Liu et al., 2022a).
The inheritance of dominant resistance gene I controlling BCMV has been reported in common bean (Ali, 1950; Kyle et al., 1986), BCMNV resistance controlled by the I gene (Provvidenti, 1974), watermelon mosaic virus-2 resistance controlled by the Hsw and Wmv genes (Provvidenti, 1974), and BYMV controlled by the By-1 gene (Schroeder and Provvidenti, 1968). In contrast, resistance against BCMV and BCMNV is controlled by recessive bc-1, bc-u, bc-12, bc-2, bc-22, and bc-3 (Kelly et al., 1995; Strausbaugh et al., 1999; Miklas et al., 2000; Mukeshimana et al., 2005). Similarly, bgm-1 and bgm-2 recessive genes (Urrea et al., 1996; Velez et al., 1998) governed resistance against BGYMV and cyv and desc recessive genes (Hart and Griffiths, 2013) controlled ClYVV resistance. Monogenic (Bdm) and dominant resistance of BDMV were established by Seo et al. (2004) using disease reaction data from F1, F2, F3, and reciprocal crosses developed from Othello and Topcrop common bean genotypes.
Singh et al. (1983); Sharma et al. (1984), and Srinivas et al. (1997a); Srinivas et al. (1997b) offered initial insights into the genetic inheritance of SMD resistance in pigeonpea. An evaluation of F1 and F2 progenies developed from ICP 7035, ICP 7349, and ICP 8850 (resistant) parents and ICP 8863 (susceptible) parent crosses confirmed monogenic inheritance of disease resistance of two SMD isolates (Srinivas et al., 1997a). Subsequently, Nagaraj and Kulkarni (2004) reported that two genes governed SMD resistance based on F1, F2, BC1, and BC2 populations developed from crossing resistant (ICP 7035 and MAL 14) and susceptible (TTB 7, ICP 8863, and DBN1) parents. Likewise, Daspute et al. (2014) reported that two separate genes (SV1 and SV2) with inhibitory gene action controlled SMD inheritance.
Recessive inheritance of MYMIV resistance in mungbean has been reported (Khattak et al., 2000; Dhole and Reddy, 2012). Six crosses developed from resistant and susceptible parents and F1 and F2 populations revealed that two genes controlled MYMIV resistance, one of which was recessive (Dhole and Reddy, 2012).
Genetics resistance of MYMV in blackgram was analyzed in F1, F2, and backcross populations developed from Pant U-84 × UL-2 and UPU-2 × UL-2 (Singh, 1980), with the results indicating recessive and digenic resistance. The same resistance gene was validated against MYMV by analyzing F1, F2, and F3 developed from Pant U84 and UPU 2 resistant donors (Verma and Singh, 1986). Subsequently, Basak et al. (2005) reported recessive monogenic resistance of YMV based on phenotyping F1, F2, and F3 progenies derived from MYMV-tolerant T-9 × MYMV-susceptible T-9 genotype in response to the YMV reaction.
Molecular mechanisms involved in plant virus resistance
During viral pathogen attack, plants recruit two branches of the immune defense system to evade the pathogen-associated attack: molecular pattern-triggered immunity (PTI) and effector-triggered immunity (ETI) (Jones and Dangl, 2006). For PTI, pattern recognition receptors (PRRs) on the cell surface of host plants perceive the pathogen-associated molecular patterns (PAMPs) to initiate defense responses known as PRR-triggered immunity (Dodds and Rathjen, 2010; Thomma et al., 2011; Win et al., 2012; Jones et al., 2016; Langner et al., 2018). For ETI, intracellular nucleotide-binding domain and leucine-rich repeat-containing (NB-LRR) encoded by host disease resistance (R) genes inside cells recognize the viral effector, mediating viral growth and disease in host plants (Jones and Dangl, 2006) and initiating the NLR-mediated response known as ETI (vanderHoorn and Kamoun, 2008; Win et al., 2012; Langner et al., 2018). In PTI, following PAMP perception of the viral pathogen through host plant PRRs, plants mediate an influx of extracellular Ca2+ in the cytosol, activating downstream immune responses (Ranf et al., 2011; Nomura et al., 2012; Bigeard et al., 2015; Jiang and Ding 2022), inducing reactive oxygen species (Chinchilla et al., 2007; Zhang et al., 2007), and activating various MAP kinase cascades (Zipfel et al., 2006; Bethke et al., 2012) that initiate transcriptional reprogramming of various TFs, such as WRKYs (Mao et al., 2011; Zhang et al., 2012) and downstream disease resistance gene(s). Viral pathogens deploy effector molecules to overcome host-deployed PTI. Once the host plant nucleotide-binding (NB) and leucine-rich-repeat (LRR)-containing receptors recognize these effectors, plants initiate ETI (Jones and Dangl, 2006; Peng et al., 2018; Alhoraibi et al., 2019). The recognition of pathogenic effectors by host NLRs triggers a hypersensitive response that mediates programmed cell death (Gao et al., 2017). The complex molecular mechanisms of plant resistance against viral disease resistance remain elusive. Thus, further investigations are needed to decipher the complete circuit networks and plant signaling pathways involved in response to viral pathogen attack and the pathways and mechanisms of plant immune response.
QTL mapping for various virus-disease-resistant grain legumes
Biparental QTL mapping is an important molecular approach for improving our understanding of the genetic control of viral disease resistance in grain legumes. Several QTL contributing to various virus diseases in grain legumes have been identified (Blair et al., 2007; Zheng et al., 2014; Ma et al., 2016; Mathivathana et al., 2019) (Figure 1). The previous section mentioned the resistance gene(s) controlling SMV; subsequent biparental QTL mapping helped discover the QTL controlling resistance against various strains of SMV (Wang et al., 2011; Zheng et al., 2014; Ma et al., 2016; Karthikeyan et al., 2017; Rui et al., 2017; Liu et al., 2019). Wang et al. (2011) reported that a single dominant gene on chromosome 2 flanked by BARCSOYSSR_02_0610 and BARCSOYSSR_02_0616 governed the genetics of SMV (SC18) resistance in a Kefeng No.1 × Nannong 1138-2 population. Further, QRT-PCR analysis identified five candidate genes. A single dominant allele Rsv1-h controlled resistance against multiple SMV strains in soybean cultivar Suweon 97 (Ma et al., 2016), but its chromosomal position was not detected. Subsequently, Zheng et al. (2014) revealed that a single dominant gene RSC3Q existing on chromosome 13 flanked by BARCSOYSSR_13_1114 and BARCSOYSSR_13_1136 with five underlying candidate genes Glyma13g25730, 25750, 25950, 25970, and 26000 controlled the genetics of resistance of SMV SC3 based on F1, F2, and F2:3 populations developed from Qihuang 1× Nannong 1138-2. Likewise, Ma et al. (2016) reported that a single dominant gene controlled the genetics of SMV SC6-N and SC7-N strains from Suweon 97 × Williams 82. Further SSR marker analysis in the F2 population mapped Rsv1-h to 97.5 kb in the Rsv1 locus on chromosome 13, with eight possible candidate genes disclosed in this genomic region. Two important genes (Glyma13g184800 and Glyma13g184900) encoding CC-NBS-LRR proteins were identified as candidate genes for Rsv1-h (Ma et al., 2016). Similarly, a novel locus Rsc15 conferring SMV (SC15) was mapped to a 95 kb genomic region on chromosome 6 flanked by SSR_06_17 and BARCSOYSSR_06_0835 markers with candidate genes Glyma.06g182600, Glyma.06g175100 and Glyma.06g184400 encoding receptor-like kinase, and Glyma.06g182900 and Glyma.06g183500 encoding serine/threonine kinase (Rui et al., 2017). For SMV (strain SC5) resistance, a combined approach of Mendelian genetics, biparental QTL mapping, fine mapping, and functional genomics was used (Karthikeyan et al., 2017). Mendelian genetics indicated that the disease resistance was dominant and controlled by a single dominant gene. Phenotyping and high-density linkage mapping in 427 recombinant lines positioned the resistant genomic region on chromosome 2 within a 500 kb interval with 11 putative candidate gene(s) (Karthikeyan et al., 2017). Functional validation of these candidate genes indicated Glyma02g13495 as the most resistant gene against the SC5 strain (Karthikeyan et al., 2017). Likewise, Karthikeyan et al. (2018) conducted combined classical genetics and biparental QTL mapping to unearth the genetic determinant controlling SMV (strain SC20) resistance. The classical genetics-based study indicated that a single dominant gene governed SMV SC20 resistance. Linkage mapping analysis identified the resistance genomic region on chromosome 13 flanked by BARCSOYSSR_13_1099 and BARCSOYSSR_13_1185 markers (see Table 3). The genomic region was narrowed to 79 kb with seven potential candidate genes, of which Glyma.13G194700 and Glyma.13G195100, encoding Toll Interleukin Receptor-nucleotide-binding-leucine-rich repeat resistance proteins, were the most likely candidate genes contributing to resistance. On chromosome 13, a QTL Rsc18 controlling resistance against SMV strain SC18 was mapped to a 415.357 kb region with three underlying candidate genes: one NBS-LRR type gene and two serine/threonine protein type genes (Liu et al., 2022a).
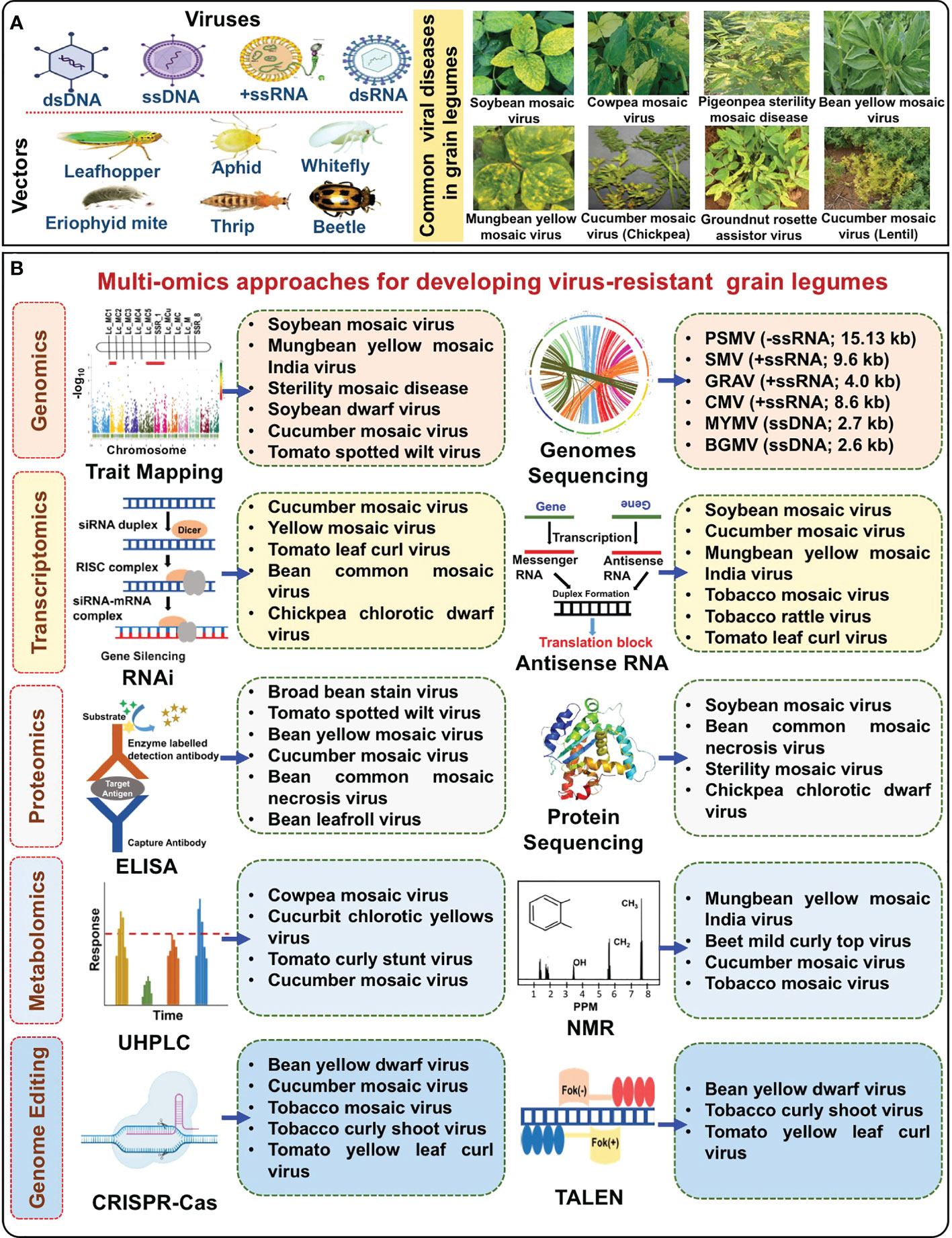
Figure 1 Multi-omics approaches for developing virus-resistant grain legumes. (A) Vectors like leaf hoppers, aphids, thrips, whiteflies, and eriophyid mites transmit five kinds of viruses, dsDNA, ssDNA, –ssRNA, +ssRNA, and dsRNA in grain legumes causing viral diseases such as soybean mosaic virus, cowpea mosaic virus, sterility mosaic disease, and rosette. (B) Approaches like genomics, transcriptomics, proteomics, metabolomics, and genome editing are used in different crops to develop resistance to viral diseases. Successful examples for each approach are in the boxes. Footnotes: ssDNA, Single-stranded DNA; ssRNA, Single-stranded RNA; PSMV, Pigeonpea sterility mosaic virus; SMV, Soybean mosaic virus; GRAV, Groundnut rosette assistor virus; CMV, Cucumber mosaic virus; MYMV, Mungbean yellow mosaic virus; BGMV, Bean golden mosaic virus; RNAi, RNA interference; ELISA, Enzyme-linked immunosorbent assay; UHPLC, Ultra-high performance liquid chromatography; NMR, Nuclear magnetic resonance spectroscopy; CRISPR-Cas, Clustered regularly interspaced short palindromic repeats-Cas; TALEN, Transcription activator-like effector nucleases.
Maroof et al. (2010) assessed two RIL-based mapping populations (D26 × Lee68 and V94-5152 × Lee68) to understand the molecular genetics of the Rsv 4 gene conferring SMV resistance. The soybean populations were genotyped with newly developed SSR and SNP markers from whole-genome shotgun sequencing, shortening the genetic interval containing Rsv4 to 0.7 cM and 1.3 cM in the V94-5152 × Lee68 and D26 × Lee68 mapping populations, respectively. The underlying candidate gene(s) encoded AGAMOUS-LIKE 28 TF and myb-like protein (Maroof et al., 2010). Subsequently, Ilut et al. (2016) fine-mapped this gene to a ~120 kb interval using a BC3F2 backcross population developed from Haman × Ilpumgeomjeong (see Table 3). Furthermore, haplotype analysis using SNP markers resolved the association signal to a ~94 kb region that contained two Rsv4 haplotypes. This Rsv4 locus was cloned via positional cloning, encoding an RNase H-family protein with dsRNA degradation (Ishibashi et al., 2019). Fine mapping of the genomic region within 186 kb flanked by SSR markers BS020610 and BS020620 on chromosome 2—conferring resistance against the SMV strain SC1 in the F2 population containing 218 individuals—identified 14 genes (Jin et al., 2022), of which the Rsv4 allele (designated Rsc1-DR) was accountable for resistance to SMV-SC1 (Jin et al., 2022). Likewise, cloning and functional analysis of MADS-box transcription factor GmCAL from soybean-resistant Kefeng-1 cultivar showed that overexpression of this gene conferred resistance against SMV-SC3, SMV-SC7, and SMV-SC8 in SMV-susceptible NN1138-2 soybean cultivar (Ren et al., 2022).
Lin et al. (2020) conducted genetic analysis in JD12 × HT F2 and recombinant inbred lines population to identify the QTL/genomic region controlling resistance against the novel recombinant SMV strain. They found that the resistant gene was dominant and governed by a single gene, with one QTL for resistance (qTsmv-13) and two QTL for tolerance (qTsmv-2 and qTsmv-3) against the novel recombinant SMV strain (see Table 3). Comparative analysis of known resistance genes indicated that qTsmv-13 and qTsmv-2 corresponded to Rsv1 and Rsv4, respectively. Map-based cloning of qTsmv-3 was delimited to 86 kb. Of the five identified candidate genes underlying the genomic region, Glyma.03G00550 (multidrug and toxic compound extrusion transporter gene) was a potential candidate gene for resistance against the disease. For combined resistance against SMV and BCMV in soybean, Wu et al. (2019) investigated the genetics of the gene controlling resistance against both diseases, reporting that a single dominant gene controlled each disease. Bulk segregation analysis indicated that the BCMV-resistance gene was linked to the SMV-resistant Rsv1 complex locus, with the SMV-resistant gene Rsv1-r flanked by BARCSOYSSR_13_1075 and BARCSOYSSR_13_1161 markers, and the BCMV-resistance gene flanked by BARCSOYSSR_13_1084 and BARCSOYSSR_13_1115 markers (Wu et al., 2019). Further, the authors narrowed the SMV- and BCMV-resistance genes to ~154.5 kb between two SNP markers (SNP-38 and SNP-50).
MYMIV is an important yield constraint in mungbean. To elucidate the QTL controlling MYMIV resistance in mungbean, an NM92 (MYMIV-tolerant cultivated line) × TC1966 RIL population was screened for MYMIV resistance under field conditions, with the population genotyped using RAPD, AFLP, SCAR, and CAP markers (Chen et al., 2012). Three QTL on LG9 (MYMIVr 9_6.4, MYMIVr 12.7, and MYMIVr 9_25) and MYMIVr 8_29.1 on LG8 contributed to MYMIV resistance (Chen et al., 2012) (see Table 3). MYMV is a major viral disease causing serious yield limitations in urdbean. Five QTL, including one major QTL qMYMV4-1 on chromosome 4, were identified in an interspecific cross Vigna radiata × V. umbellata using the genotyping-by-sequencing method and phenotyping the population under field conditions for two consecutive years (Mathivathana et al., 2019). Further, Subramaniyan et al. (2022) conducted phenotyping and QTL analysis of mapping population developed from MDU1× TU68 cross to uncover the genetic determinant/genomic region controlling MYMV resistance. Classical genetic analysis indicated inhibitory gene action with two genes controlling MYMV resistance, while QTL analysis suggested one major QTL qMYMVD_60 flanked by CEDG180 and CEDG116 marker at LG 10 controlling MYMV resistance (see Table 3). Recently, to introduce MYMIV resistance from Vigna umbellulata to urdbean, Dhaliwal et al. (2022) used a QTL-seq-based approach to identify qMYMIV6.1.1, a major QTL spanning 3.4 Mb on chromosome 6, contributing 70% phenotypic variation. Further, the authors elucidated three possible candidate genes (serine threonine kinase, UBE2D2, and BAK1/BRI1-ASSOCIATED RECEPTOR KINASE) underlying the identified genomic region.
BGYMV is an important disease causing significant yield losses in common bean. A SCAR marker SR2, linked to a bgm-1 resistance gene, was developed and mapped 7.8 cM from the resistance gene in a DOR476 × SEL1309 RIL population (Blair et al., 2007). The SR2 marker was located at the end of chromosome 5 in DOR364 × G19833 and BAT93 × Jalo EEP558 mapping populations. Notably, the bgm-1 resistance gene was closely related to the bc-1 resistance gene for BCMV (Blair et al., 2007). Thus, these genomic regions could be targeted for developing combined resistance against BGYMV and BCMV.
Genome-wide association study capturing viral-disease-resistant genomic regions across the whole genome
The GWAS approach using marker-trait associations and various statistical models could identify the underlying candidate gene(s)/QTL/genomic regions controlling viral disease resistance in various legumes, overcoming the limitations of biparental QTL mapping (Huang and Han, 2014) (Figure 1). For example, a comprehensive GWAS for TRSV in a set of 19,652 soybean genotypes using the SoySNP50K iSelect BeadChip detected a single locus associated with TRSV sensitivity on chromosome 2 and predicted two leucine-rich repeat receptor-like kinase genes Glyma02g13460 and Glyma02g13470 underlying the locus (Chang et al., 2016). Similarly, Liu et al. (2019) conducted GWAS in two soybean populations containing 409 and 199 genotypes in a SoySNP50K assay to unearth significant marker-trait associations for SMV seed transmission rate, seed coat mottling, and seed yield loss due to SMV infection. The study identified a single locus contributing to SMV seed transmission rate on chromosome 9, loci for seed coat mottling on chromosome 3, and a single locus for seed yield loss due to SMV infection on chromosome 3 (Liu et al., 2019). An earlier GWAS investigating SMV(SC7) resistance in 165 soybean genotypes identified three genes, homologous to WRKY72, eEF1Bβ, and RLP9, conferring disease resistance in Arabidopsis on chromosomes 1, 3, and 9, respectively (Che et al., 2017). Combined recombinant inbred line based linkage and GWAS analysis allowed deciphering a major QTL qSMV13 on chromosome 13 conferring resistance against SMV (SC3 and SC7) strain explaining 71% and 76% PV, respectively (Chu et al., 2021).
A GWAS of 182 common bean genotypes belonging to the Durango diversity panel in association with 1.26 million SNPs detected significant marker-trait associations for resistance against BCMNV isolates NL-8 and NL-3 on PV03 and PV05 chromosomes that corresponded to bc-1 and bc-u resistance gene loci, respectively, elucidating two candidate genes for bc-1 (Phvul.003G038700 and Phvul.003G038800) and one bZIP protein gene for bc-u (Phvul.005G124100) (Soler-Garzón et al., 2021). Further advances in GWAS models could help minimize population-structure-related problems.
Progress of functional genomics: discovery of candidate resistance gene(s) with putative functions
Functional genomics is a powerful approach for discovering candidate gene(s) related to various disease resistance with putative functions, including viral diseases in grain legumes. Recent advances in RNA-seq-based transcriptome sequencing have offered novel insights into the molecular mechanisms of disease resistance and identified possible candidate gene(s) conferring resistance against various viral diseases in grain legumes (Martin et al., 2016; Dasgupta et al., 2021).
Yuan et al. (2020) used transcriptomic analysis of R and S isogenic lines developed from Qihuang-1 Nannong 1138-2 × Soybean cv. Qihuang-1 subjected to SMV infection at 6, 20, and 48 h post-inoculation (hpi) to elucidate the underlying candidate gene(s) conferring SMV resistance. A further DEGs analysis revealed the downregulation of Glyma03g28650, Glyma19g31395, and Glyma11g33790 encoding calmodulin-like protein in S lines and upregulation of jasmonic acid repressor genes (TIFY/JAZ) and abscisic-acid-induced genes (PP2C3a) in R lines in response to SMV infection. Likewise, Chen et al. (2022) deciphered the role of genes related to phytohormone-mediated SMV resistance using RNA-seq-based transcriptome analysis in contrasting soybean genotypes, Kefeng-1 (resistant) and NN1138-2 (susceptible). Using DEGs and gene ontology analyses and the functional validation of candidate genes revealed the downregulation of Glyma.11G239000 and Glyma.18G018400, belonging to ethylene-insensitive 3/ethylene-insensitive3-like (EIN3/EIL) protein family, in NN1138-2. At 48 hpi, jasmonic acid repressor genes (TIFY/JAZ) and NPR1 involved in the salicylic acid signaling were downregulated in NN1138-2 but upregulated in Kefeng-1 (Chen et al., 2022).
Sun et al. (2022) investigated the role of abundant H2O2 production in regulating callose deposition on plasmodesmata, mediating the blockage of intercellular transport of SMV infection in soybean. Two genes regulated by H2O2 (GmSEOB and GmPAP27) conferred resistance against SMV by positively regulating callose accumulation in response to SMV infection at the transcriptomic and VIGS levels.
Dasgupta et al. (2021) performed RNA-seq-based transcriptome analysis of PMR-1 (resistant) and Pusa Vishal (susceptible) mungbean genotypes, elucidating the participatory role of WRKY, NAC, and MYB transcription factors in mediating YMV resistance. A DEGs analysis revealed that PMR-1 upregulated peroxidase, (S)-2-hydroxy-acid oxidase, and classes of lipoxygenase and downregulated oxidoreductase, 2OG-Fe(II) oxygenase family protein, 4-coumarate:CoA ligase like, and O-methyltransferase encoding genes in response to YMV. Further, the authors validated 11 defense-related transcripts contributing to YMV resistance. In another study, RNA-seq of mungbean elucidated the role of various DEGs related to TFs, hormone signaling, receptor-like kinases, serine/threonine protein kinases, defense, and pathogenesis in mediating MYMV resistance (Sudha et al., 2022) (Figure 1). In addition, qRT-PCR analysis was used to functionally validate select candidate DEGs related to MYMV defense mechanisms (Vradi08g04110, Vradi09g06830, Vradi04g07450, Vradi06g13520, Vradi06g11500, and Vradi01g04820).
A PCR-based suppression subtractive hybridization technique identified 345 candidate genes differentially expressed in response to MYMIV infection in urdbean, contributing various cellular functions mediating resistance against MYMIV, including Ca2+-mediated signaling, reactive oxygen species generation, phenylpropanoids, and ubiquitin-proteasomal pathways (Kundu et al., 2015). Further, Kundu et al. (2019) performed transcriptome analysis of two contrasting urdbean genotypes, VM84 (resistant) and T9 (susceptible), to decipher the gene(s) and molecular mechanisms mediating MYMV resistance. They discovered 2,158 and 1,679 DEGs from VM84 and T9 in response to MYMV infection, of which NB-LRR, WRKY33, ankyrin, argonaute, and NAC TFs exhibited upregulatory responses in MYMV-resistant VM84, indicating their role in conferring disease resistance.
A transcriptome analysis of a BCMV-susceptible common cultivar (Stringless green refugee) in response to two BCMV infection isolates revealed upregulation of genes related to receptor-like protein kinase, pathogenesis-related proteins, and oxidative-stress-related genes and downregulation of genes related to photosynthetic machinery (Martin et al., 2016). Among the various TF genes, NAC and WRKY_Zn families had increased expression, and Myb_related and bHLH families had reduced expression in response to BCMV infection (Martin et al., 2016), improving our understanding of various gene networks that mediate resistance against BCMV in common bean.
Evidence of non-coding RNAs, including miRNAs, siRNAs, and long non-coding regulating disease resistance, is well established in plants (Jha et al., 2021; Song et al., 2021). Patwa et al. (2021) uncovered 422 differentially expressed miRNAs responding to MYMIV infection in P. vulgaris. Validation of selected candidate miRNAs revealed their role in regulating various TFs involved in MYMIV resistance. Illustrations of non-coding RNAs conferring viral disease resistance are limited in grain legumes, requiring further studies on their molecular mechanisms and corresponding target gene(s) conferring viral disease resistance.
Genetic engineering approach for designing virus-disease-resistant grain legumes
Genetic engineering is a powerful option for developing virus-resistant grain legumes (Bonfim et al., 2007; Zhang et al., 2012; Aragão et al., 2013; de Paula et al., 2015) (Figure 1), especially when there are limited options for host plant resistance. Different functional genomic approaches spanning transgenic to non-transgenic technologies can be used to design virus-resistant plants. Transgenic technologies are the primary initiative for plant virus management, with success stories in papaya and cucurbits (Kreuze and Valkonen, 2017) but limited application in grain legumes. There are only a few examples of RNAi-mediated transgenic legumes developed for virus resistance, mainly in cowpea, common bean, and soybean, with pathogen-derived resistance (expressing the virus gene/genome) adopted. An RNAi strategy was used to manage cowpea severe mosaic virus and CABMV in transgenic cowpea expressing proteinase cofactor and coat protein for the respective viruses (Cruz and Aragão, 2014). Similarly, enhanced resistance in cowpea against multiple Begomovirus infections was achieved through the expression of AC2 and AC4 and fusion of AC2 and AC4 gene-based hairpin constructs (Kumar et al., 2017). Further, an RNAi strategy was used in soybean to confer resistance against multiple potyviruses. The first SMV-resistant transgenic soybean lines were developed by integrating an inverted repeat sequence of HC-Pro of SMV (Gao et al., 2015). Likewise, introducing a 248 bp inverted repeat of the replicase (nuclear inclusion b, Nib) gene from the SMV SC3 strain into soybean resulted in transgenic soybean plants with stable resistance against five strains of SMV (SC3, SC7, SC15, SC18, and a recombinant SMV), BCMV, and watermelon mosaic virus (WMV) (Yang et al., 2017). Inserting a 302 bp inverted repeat of the P3 cistron, isolated from SMV strain SC3 resulted in RNAi-mediated genetically engineered soybean lines with stable resistance against multiple virus strains of SMV, BCMV, and WMV (Yang et al., 2018). RNAi strategies have also been used to combat bean golden mosaic virus (BGMV) in common bean by silencing the rep (AC1) viral gene (Bonfim et al., 2007; Aragão et al., 2013; de Paula et al., 2015) and to prevent whitefly (Bamicia tabaci) infection by suppressing the ATPase (Bt-vATPase) gene in B. tabaci (Ferreira et al., 2022). Thus, pathogen-derived transgenic resistance can be achieved using an RNAi strategy to develop virus resistance in legumes (see Figure 2).
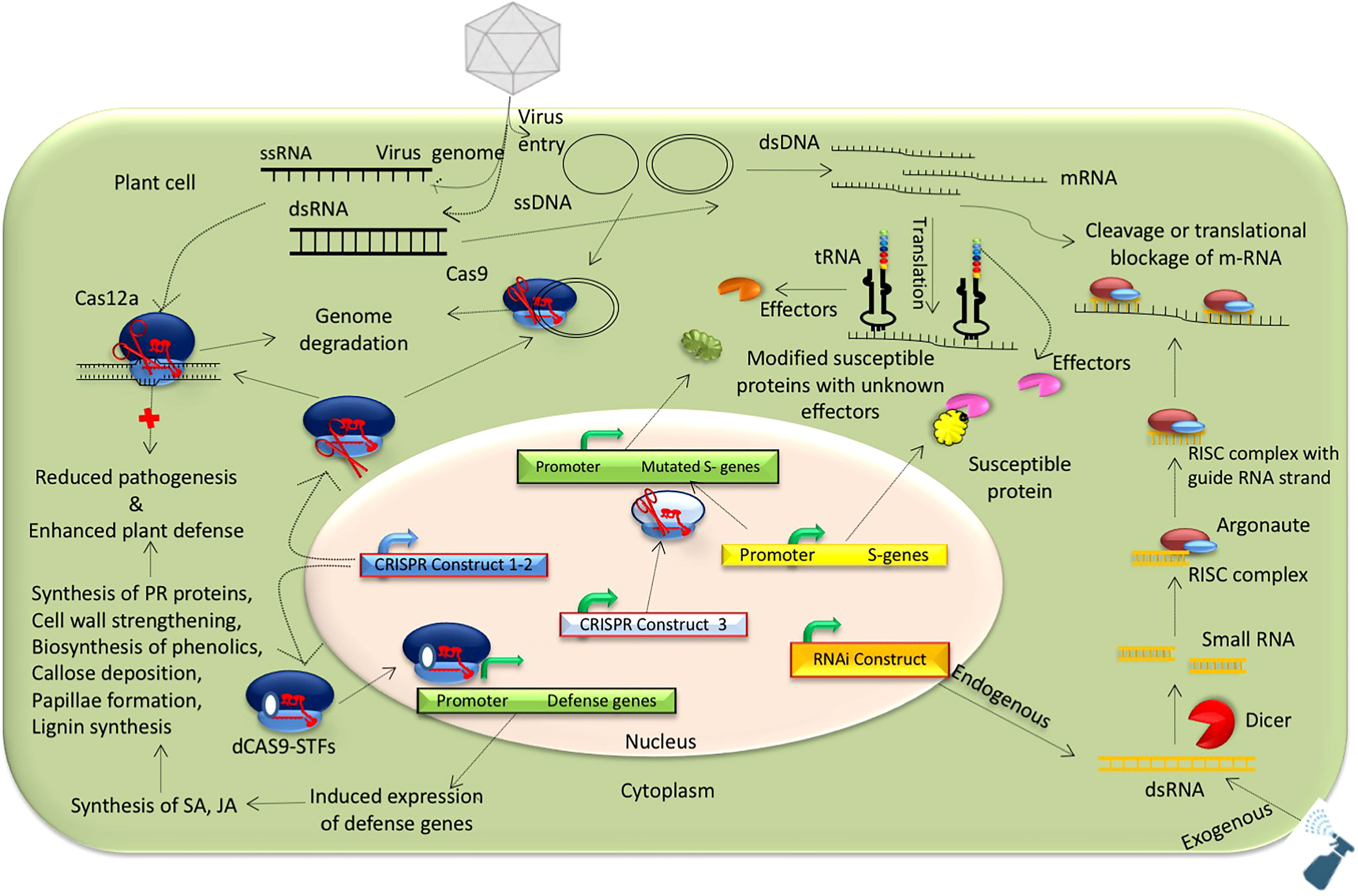
Figure 2 Possible mechanisms for developing virus-resistant grain legumes using RNAi and genome editing technologies.
The host-derived strategy (targeting host-susceptible genes) is another option for transgenic resistance against plant viruses in grain legumes. This strategy can be used to silence the host eukaryotic translation initiation factor to prevent pathogen replication or overexpress resistance gene(s) and transcription factors (Chattopadhyay et al., 2019). The silencing of eukaryotic translation initiation factor 4E (eIF4E) in soybean via RNAi technology resulted in broad-spectrum resistance against multiple potyviruses, including SMV strains (SC3/7/15/18 and SMV-R), BCMV, and WMV (Gao et al., 2020). In contrast, overexpression of GmKR3 (TIR-NBS-LRR type R gene) in transgenic soybean showed high resistance against SMV, BCMV, WMV, and secovirus BPMV by mediating ABA signaling (Xun et al., 2019) (Table 4). Similarly, the overexpression of GmAKT2 in transgenic soybean significantly increased K+ concentrations and enhanced SMV resistance (Zhou et al., 2014). Further, metabolic engineering of susceptible soybean lines enhanced SMV resistance by overexpressing a cinnamyl alcohol dehydrogenase-coding GsCAD1 gene (Xun et al., 2022) and purple acid phosphatase encoding GmPAP2.1 gene (Widyasari et al., 2022).
Non-transgenic approaches have become popular as they avoid time constraints and overcome transgenics-associated risks (Teli et al., 2020). Exogenous application of RNAi-inducing double-stranded RNA (dsRNA) molecules is an alternative approach to generating antiviral RNAi in plants (Mitter et al., 2017), especially when genetic transformation and regeneration are time-consuming. The successful delivery of dsRNA within host cells is crucial for executing RNAi-mediated plant defenses against viruses. In cowpea, the bioefficacy of dsRNAs targeting the potyviral nuclear inclusion b (NIb) protein and coat protein-encoding gene of BCMV was reported (Worrall et al., 2019). Similarly, direct foliar application of dsRNA derived from the full-length NSs gene of groundnut bud necrosis virus significantly reduced the virus infection in cowpea (Gupta et al., 2021). However, dsRNA delivery is an issue, particularly for field applications. Delivering dsRNA through layered double hydroxide nanoparticles prevented virus replication better than naked dsRNA as it facilitated the slow release of dsRNAs and long-term viral protection (Mitter et al., 2017). Furthermore, targeting vector transmission through exogenous dsRNA application could be an innovative option for developing a tailor-made bio-pesticide. In cowpea, bio-clay mediated application of dsRNA prevented aphid-mediated transmission of BCMV (Worrall et al., 2019). However, these strategies require testing under field conditions to predict possible environmental risks.
Likewise, genome editing has emerged as a versatile tool for designing viral-disease-resistant crop plants, including grain legumes (Langner et al., 2018), and overcoming public and scientific concerns related to the development end release of transgenic plants. Genome editing technologies have been used for genome engineering in many crop plants, but there is limited evidence in grain legumes (Gayen and Karmakar, 2021), except for cowpea (Ji et al., 2019; Juranić et al., 2020; Che et al., 2021), chickpea (Badhan et al., 2021), and soybean (Al Amin et al., 2019; Bao et al., 2019; Bao et al., 2020), due to hurdles related to in vitro gene transfer and poor regeneration (Bhowmik et al., 2021). Despite that, gene-editing technologies have opened up new opportunities for multiple trait improvement in grain legumes, especially for plant virus resistance. So far, CRISPR-Cas9-mediated genome editing has been used to manage different virus pathogens, including Merremia mosaic virus (Ali et al., 2016), tobacco rattle virus (Ali et al., 2015), beet necrotic yellow vein virus, pea early browning virus (Ali et al., 2018), tobacco mosaic virus (Cody et al., 2017), beet yellow dwarf virus (Liang et al., 2016), and TYLCV (Tashkandi et al., 2018). However, notable examples are only available for soybean and mungbean. CRISPR/Cas9-mediated multiplex gene-editing technology was used to increase isoflavone content for enhancing SMV resistance in soybean by editing two flavanone‐3‐hydroxylase genes (GmF3H1, GmF3H2) and one flavone synthase (GmFNSII-1) gene (Zhang et al., 2020). Thus, CRISPR/Cas-mediated metabolic engineering could be an option for virus resistance in legumes. Further, manipulation of host-susceptible factors, viz., eukaryotic translation initiation factor 4E (eIF4E), could help achieve potyvirus resistance in different legume crops. CRISPR/Cas9-mediated editing of virus genomes can also be used to protect plants from virus infection (see Figure 2). The CRISPR/Cas9 system with two guide RNA cassettes was used to edit the MYMV genome in mung bean, targeting the replicase enzyme (AC1) and coat protein (AV1) encoding genes (Talakayala et al., 2022). Such multiplex strategies assembling different gRNA cassettes for several viral genomes could be suitable for managing multiple plant viruses simultaneously.
Emerging breeding tools to design grain legumes with viral disease resistance
Genomics selection facilitates the selection of genotypes with high genetic merit by calculating the genomic-estimated breeding value of individuals based on various prediction models using genotypic and phenotypic information from the ‘training population’ (Meuwissen et al., 2001; Lorenz et al., 2011). Thus, this breeding tool can be used to select superior progenies or genotypes from large germplasm sets without needing phenotypic evaluation. For example, a genomic prediction model incorporating GAPIT and rrBLUP was used to predict TRSV sensitivity in soybean, with 140 soybean PIs used as the validation population and 557 soybean PIs used as the training population (Chang et al., 2016). An additional 55 soybean accessions were evaluated for TRSV sensitivity to assess the model’s prediction accuracy. The results revealed a correlation of 0.67 (P < 0.01) between actual and predicted severities of TRSV (Chang et al., 2016). More recently, a smart breeding scheme, ‘integrated genomic–enviromic prediction’, or an advanced form of genomic prediction constituting multi-omics data, big data, and artificial intelligence, was proposed for prediction-based crop redesign (Xu et al., 2022). This high throughput technology could open up avenues for designing viral-disease-resistant grain legumes.
‘Rapid generation advancement’ protocols have been developed in various crop plants, including grain legumes, shortening the breeding cycle and advancing mapping populations (Watson et al., 2018; Hickey et al., 2019). These protocols have optimized photoperiods, daylengths, and temperatures in soybean, cowpea, chickpea, and pigeon pea by growing them in regulated growth chambers (Samineni et al., 2019; Saxena et al., 2019; Fang et al., 2021; Edet and Ishii, 2022). Thus, harnessing the potential of this technique could help develop viral disease resistance in grain legumes.
Advances in next-generation sequencing-based approaches and bioinformatic analyses have facilitated the identification of target genomic regions conferring disease resistance in various crops, including grain legumes (Thudi et al., 2020; Gangurde et al., 2022). Further, these technologies have enabled WGRS of large-scale global germplasm and pangenome construction for various legumes, including soybean (Li et al., 2014), chickpea (Varshney et al., 2021), pigeon pea (Zhao et al., 2020), cowpea (Liang et al., 2022), and mungbean (Liu et al., 2022b). Thus, these genomic resources could help underpin novel presence/absence variations contributing to various viral-disease-resistance genes and pathogenesis genes for developing next-generation viral-disease-resistant grain legumes.
Furthermore, rapid developments in synthetic biology have enabled desirable crop designs. Synthetic biology approaches involve designing genetic circuits, synthetic promoters (SPs), and synthetic transcription factors (STFs) that facilitate the modification of crop plants by remodeling the gene/genome structure, reprogramming gene function, and engineering existing metabolic pathways (Liu and Stewart, 2016). Adequate advances have been made in designing SPs and STFs for regulating specific genes involved in multiple trait improvement in crop plants, including biotic and stress tolerance (Dey et al., 2015; Yasmeen et al., 2023). Synthetic promoters are designed rationally and constructed for specific binding to upstream motifs or native core promoter sites (Khan et al., 2023) so that external stimuli can regulate gene expression (Rushton et al., 2002). This strategy is mostly suitable for enhancing biotic stress tolerance when pathogen-inducible SPs can be employed against diverse pathogens (Khan et al., 2023), as exemplified against Ascochyta rabiei (Shokouhifar et al., 2019). Similarly, STFs can be constructed by fusing various DNA binding domains (DBDs) with an activator/repressor domain and nuclear localization signals for regulated gene expression in plants. Nowadays, synthetic DBDs based on C2H2 zinc-finger (ZF) proteins, transcription activator-like effectors (TALEs), and CRISPR/dCas9 can be designed easily for specific binding to promoter sequences of endogenous genes or transgenes (Liu and Stewart, 2016). These STFs, viz., ZF-TFs, TALE-TFs, and dCas9-TFs, can regulate endogenous genes or transgenes to confer defense against broad-spectrum pathogens such as plant viruses. Sometimes, SPs are not adequate for obtaining higher expression levels of transgenes. In such situations, SFs can be delivered with SPs to maximize gene expression at full strength and specificity. Hence, simultaneous expression of SPs with corresponding synthetic TFs is necessary for transgene activation in legumes targeted against plant viruses. Therefore, synthetic biology tools like SPs and STFs can provide tremendous advantages over their natural counterparts for designing legumes resistant to multiple viruses.
Likewise, to overcome transgenics-related issues, CRISPR/Cas9-based genome editing has emerged as a versatile tool for designing viral-disease-resistant crop plants, including grain legumes (Langner et al., 2018). Notable examples of viral diseases include Merremia mosaic virus (Ali et al., 2016), tobacco rattle virus (Ali et al., 2015), beet necrotic yellow vein virus, and pea early browning virus (Ali et al., 2018), tobacco mosaic virus (Cody et al., 2017), beet yellow dwarf virus (Liang et al., 2016), and TYLCV (Tashkandi et al., 2018). Similarly, a CRISPR/Cas9-mediated approach was used to manipulate single-stranded DNA-A of AC1 (rep protein) and AV1 (coat protein) for developing MYMV-resistant mungbean (Talakayala et al., 2022). However, the development of viral-resistant grain legumes using genome editing tools remains low. Hence, optimizing the transformation protocol and genome editing tools could help develop grain legumes with virus resistance.
Integration of “OMICS” technologies with plant breeding for uncovering the virus resistance gene(s)/QTLs and deciphering the complex gene networks of controlling virus resistance in grain legumes
To understand the molecular mechanism of plant - virus interaction, resistance mechanism and complex gene networks controlling virus resistance in plant including grain legume, a multi-layered scientific approach is needed (Fernie and Schauer, 2009; Weckwerth, 2011). Plant ‘omics’ encompassing genomics, transcriptomics, proteomics, metabolomics, epigenomics, and phenomics and genetic engineering including RNAi and synthetic biology in concert with classical and emerging plant breeding approaches could greatly assist in elucidating the candidate gene(s) conferring virus resistance along with their precise function (Fernie and Schauer, 2009; Weckwerth, 2011; Ghatak et al., 2017a, Ghatak et al., 2017b; Ghatak et al., 2018; Weckwerth et al., 2020).
Limitations of breeding and biotechnological tools for developing virus-resistant legumes
Plant breeding is a practical approach for developing virus-resistant grain legumes. However, transferring disease-resistant gene(s)/QTL is time-consuming, involving the hybridization and selection of disease-resistant plants. Marker-assisted plant breeding can sometimes support the process, but the poor associations between markers and traits make the markers ‘breeder unfriendly,’ which may not transfer well between populations. Biotechnological and molecular biological strategies for developing virus-resistant legumes also face limitations. The scarcity of genomic information for different legumes adds to the difficulty. As grain legumes are recalcitrant to transformation, a precise and robust protocol should be necessary to deliver the genome engineering machinery into germline cells for developing genome-edited plants (Zaidi et al., 2020). Currently, the delivery of CRISPR/Cas cassettes into plant cells occurs through Agrobacterium-mediated transformation, particle bombardment, or protoplast transfection strategy (Zaidi et al., 2020), all of which involve tedious tissue culture procedures. To bypass the complexity of plant tissue culture, plant virus-based vectors can be used for the successful delivery of CRISPR/Cas reagents, such as CRISPR/Cas proteins, guide RNA (gRNA), and ribonucleoproteins (RNP), into plant cells (Zhang et al., 2022). The virus‐inducible genome editing (VIGE) system based on plant virus vectors has been applied successfully for genome editing and antiviral breeding (Ji et al., 2018). VIGE is highly suitable for precise genome editing, minimizing ‘off-target mutations’ common in ‘normal’ genome editing (Hahn and Nekrasov, 2019). Off-target mutations are highly desirable when developing CRISPR/Cas9-based genome editing systems against plant DNA viruses infecting grain legumes.
The design of virus-resistant legumes via genome editing targets the virus genome by cleaving through CRISPR/Cas9, which can exert natural selection pressure on the virus population, leading to virus evolution through sequence variation in the virus genome and the emergence of recombinant virus species/strains (especially begomoviruses) resistant to Cas9 cleavage (Cao et al., 2020; Mushtaq et al., 2020). Further, knocking out host susceptibility factors, such as eIF4E, is a common practice for designing potyvirus-resistant plants, but the loss-of-function of the host factor may inhibit plant growth (Gauffier et al., 2016). This problem can be avoided by introducing point mutation (base editing) in the gene to prevent plant virus infection without impairing plant growth (Bastet et al., 2018). Furthermore, the CRISPR-Cas9 edited virus-resistant plant should be rigorously tested in the field under multiple environments to ensure durable resistance against plant viruses (Robertson et al., 2022). A proper regulatory framework should be established before the public release of genome-edited plants. Their classification as ‘GMOs (genetically modified organisms)’ or non-GMOs should also be clarified. Controversy and confusion persist around the GMO and non-GMO classification of genome-edited plants, which could be simply defined based on the presence or absence of foreign DNA. Usually, transgenic expression of the CRISPR-gRNA construct is needed for successful genome editing. However, avoiding transgenic approaches through the transient delivery of CRISPR/Cas and gRNA constructs via the VIGE system could make it possible to generate plant genomes free from foreign DNA. Finally, legumes are highly susceptible to infection by multiple viruses, often leading to co-infections. To combat this, a multiplexed CRISPR strategy could be used to design plants resistant to multiple viruses. However, the large construct size required could reduce the efficiency of this strategy. These limitations of biotechnological tools impede the development of virus-resistant legumes, highlighting the need for continued research to overcome these challenges and improve the efficiency of genome-editing techniques.
Outstanding questions stimulating further research in this area
Several outstanding questions remain regarding the development of virus-resistant legumes. The ever-emerging population of plant viruses poses a major challenge, as virus evolution and the emergence of new virus strains infecting legumes is constant. With the increasing host range of legume-infecting viruses and their co-infections, tackling the problem of emerging virus strains becomes even more difficult in the global warming and climate change scenario. The durability of host resistance is thus always in question. Further, the expression of plant resistance often results in energy loss and yield costs, which should be addressed through advanced breeding and biotechnological approaches.
Conclusion and future perspective
Global climate change has caused significant challenges for agroecosystems and global food security (Schmidhuber and Tubiello, 2007). The increasing incidence of various diseases, including viral disease, challenges grain legume yields. Moreover, the growing global human population, estimated to reach 9–10 billion by 2030, further pressures global food security (Pérez-Escamilla, 2017). Consequently, food production needs to double to sustainably meet the rising demand for food supply. Harnessing the global genetic diversity of grain legumes with resistance to various viral diseases is a promising and sustainable approach to developing climate-resilient grain legumes with improved viral disease resistance. Pre-breeding approaches and marker-assisted breeding schemes can help transfer gene(s)/QTL conferring resistance against viral diseases to high-yielding grain legumes. Unprecedented advances in sequencing technologies have enabled access to genomic information related to disease resistance and virulence gene(s)/genomic regions across the whole-genome level for designing virus-resistant crop plants. GWAS, WGRS, and pangenome approaches have further underpinned novel resistance R genes and effector encoding genes at the whole-genome level. Furthermore, functional genomics, including transcriptomics, has assisted in the discovery of novel candidate gene(s) conferring disease resistance and the molecular mechanisms of host plant disease resistance. Advances in genetic engineering techniques, such as RNAi technology, can be used to unravel virus-resistance gene function and design virus-resistant grain legume genotypes. However, progress is limited in legumes, which could be accelerated by applying powerful transgene-free, genome-editing technologies targeting viral genomes (e.g., replicase enzyme (AC1) and coat protein (AV1)-encoding genes) and the plant S gene accounting for viral disease development (Zaidi et al., 2020). Thus, integration of various ‘omics’technologies (Fernie and Schauer, 2009; Weckwerth, 2011; Ghatak et al., 2017a; Ghatak et al., 2017b; Ghatak et al., 2018; Weckwerth et al., 2020) and genetic engineering approaches including RNAi and synthetic biology with advanced plant breeding tools including speed breeding, genomic selection, ‘integrated genomic–enviromic prediction,’ and genome editing tools could help develop grain legumes with high viral disease resistance to meet the rising demand for food supply.
Author contributions
UJ and KS conceptualized the idea and wrote the manuscript. HN, MT, RB, AL, SG, GD, and PP wrote the genomics section. YN and AC drew the figures and wrote the transgenic section. All authors contributed to the article and approved the submitted version.
Acknowledgments
UJ acknowledges support from ICAR, New Delhi, India.
Conflict of interest
The authors declare that the research was conducted in the absence of any commercial or financial relationships that could be construed as a potential conflict of interest.
Publisher’s note
All claims expressed in this article are solely those of the authors and do not necessarily represent those of their affiliated organizations, or those of the publisher, the editors and the reviewers. Any product that may be evaluated in this article, or claim that may be made by its manufacturer, is not guaranteed or endorsed by the publisher.
References
Abraham, A., Vetten, H. J. (2022). Chickpea chlorotic stunt virus: a threat to cool-season food legumes. Arch. Virol. 167, 21–30. doi: 10.1007/s00705-021-05288-4
Agarwal, G., Clevenger, J., Kale, S. M., Wang, H., Pandey, M. K., Choudhary, D., et al. (2019). A recombination bin-map identified a major QTL for resistance to tomato spotted wilt virus in peanut (Arachis hypogaea). Sci. Rep. 9, 18246. doi: 10.1038/s41598-019-54747-1
Akbar, W., Akhtar, M. A., Murtaza, G., Hussain, A., Javed, H. M., Arshad, M., et al. (2019). Mungbean yellow mosaic disease and its management. JAB S 4, 34–44.
Al Amin, N., Ahmad, N., Wu, N., Pu, X., Ma, T., Du, Y., et al. (2019). CRISPR-Cas9 mediated targeted disruption of FAD2–2 microsomal omega-6 desaturase in soybean (Glycine max. l). BMC Biotechnol. 19 (1), 1–10. doi: 10.1186/s12896-019-0501-2
Alhoraibi, H., Bigeard, J., Rayapuram, N., Colcombet, J., Hirt, H. (2019). Plant immunity: the MTI-ETI model and beyond. Curr. Issues Mol. Biol. 30 (1), 39–58. doi: 10.21775/cimb.030.039
Ali, M. A. (1950). Genetics of resistance to the common bean mosaic virus in the bean (Phaseolus vulgaris l). Phytopathol 40, 69–79.
Ali, Z., Abulfaraj, A., Idris, A., Ali, S., Tashkandi, M., Mahfouz, M. M. (2015). CRISPR/Cas9-mediated viral interference in plants. Genome Biol. 16, 1–11. doi: 10.1186/s13059-015-0799-6
Ali, Z., Ali, S., Tashkandi, M., Zaidi, S. S. E. A., Mahfouz, M. M. (2016). CRISPR/Cas9-mediated immunity to geminiviruses: differential interference and evasion. Sci. Rep. 6, 1–13. doi: 10.1038/srep26912
Ali, Z., Eid, A., Ali, S., Mahfouz, M. M. (2018). Pea early-browning virus-mediated genome editing via the CRISPR/Cas9 system in Nicotiana benthamiana and Arabidopsis. Virus Res. 244, 333–337. doi: 10.1016/j.virusres.2017.10.009
Aragão, F. J., Faria, J. C. (2009). First transgenic geminivirus-resistant plant in the field. Nat. Biotechnol. 27 (12), 1086–1088. doi: 10.1038/nbt1209-1086
Aragão, F. J., Nogueira, E. O., Tinoco, M. L. P., Faria, J. C. (2013). Molecular characterization of the first commercial transgenic common bean immune to the bean golden mosaic virus. J. Biotechnol. 166, 42–50. doi: 10.1016/j.jbiotec.2013.04.009
Ashfaq, M., Khan, M. A., Mughal, S. M., Javed, N., Mukhtar, T., Bashir, M. (2007). Evaluation of urdbean germplasm for resistance against urdbean leaf crinkle virus. Pak. J. Bot. 39, 2103–2111.
Badhan, S., Ball, A. S., Mantri, N. (2021). First report of CRISPR/Cas9 mediated DNA-free editing of 4CL and RVE7 genes in chickpea protoplasts. Int. J. Mol. Sci. 22 (1), 396. doi: 10.3390/ijms22010396
Bag, M. K., Gautam, N. K., Prasad, T. V., Pandey, S., Dutta, M., Roy, A. (2014). Evaluation of an Indian collection of black gram germplasm and identification of resistance sources to mungbean yellow mosaic virus. Crop Prot. 61, 92–101. doi: 10.1016/j.cropro.2014.03.021
Bao, A., Chen, H., Chen, L., Chen, S., Hao, Q., Guo, W., et al. (2019). CRISPR/Cas9-mediated targeted mutagenesis of GmSPL9 genes alters plant architecture in soybean. BMC Plant Biol. 19 (1), 1–12. doi: 10.1186/s12870-019-1746-6
Bao, D., Ganbaatar, O., Cui, X., Yu, R., Bao, W., Falk, B. W., et al. (2018). Down-regulation of genes coding for core RNAi components and disease resistance proteins via corresponding microRNAs might be correlated with successful soybean mosaic virus infection in soybean. Mol. Plant Pathol. 19 (4), 948–960. doi: 10.1111/mpp.12581
Bao, A., Zhang, C., Huang, Y., Chen, H., Zhou, X., Cao, D. (2020). Genome editing technology and application in soybean improvement. Oil Crop Sci. 5 (1), 31–40. doi: 10.1016/j.ocsci.2020.03.001
Basak, J., Kundagrami, S., Ghose, T. K., Pal, A. (2005). Development of yellow mosaic virus (YMV) resistance linked DNA marker in Vigna mungo from populations segregating for YMV-reaction. Mol. Breed. 14, 375–383. doi: 10.1007/s11032-005-0238-6
Basavaraj, S., Padmaja, A. S., Nagaraju, N., Ramesh, S. (2019). Identification of stable sources of resistance to mungbean yellow mosaic virus (MYMV) disease in mungbean [Vigna radiata (L.) Wilczek]. Plant Genet. Resour. 17, 362–370.
Bashir, M., Ahmad, Z., Ghafoor, A. (2002). Cowpea aphid-borne mosaic potyvirus: a review. Int. J. Pest Manage. 48, 155–168. doi: 10.1080/09670870110118722
Bashir, M., Ahmad, Z., Mansoor, S. (2006). Occurrence and distribution of viral diseases of mungbean and mashbean in punjab, Pakistan. Pak. J. Bot. 38, 1341–1351.
Bashir, M., Mughal, S. M., Malik, B. A. (1991). Assessment of yield losses due to leaf crinkle virus in urdbean (Vigna mungo (L) hepper). Pak. J. Bot. 23, 140–142.
Bastet, A., Lederer, B., Giovinazzo, N., Arnoux, X., German-Retana, S., Reinbold, C., et al. (2018). Trans-species synthetic gene design allows resistance pyramiding and broad-spectrum engineering of virus resistance in plants. Plant Biotechnol. J. 16, 1569–1581. doi: 10.1111/pbi.12896
Beniwal, S. P. S., Bharathan, N. (1980). Beetle transmission of urdbean leaf crinkle virus. Indian Phytopathol. 33, 600–601.
Bethke, G., Pecher, P., Eschen-Lippold, L., Tsuda, K., Katagiri, F., Glazebrook, J., et al. (2012). Activation of the Arabidopsis thaliana mitogen-activated protein kinase MPK11 by the flagellin-derived elicitor peptide, flg22. Mol. Plant-Microbe Interact. 25, 471–480. doi: 10.1094/MPMI-11-11-0281
Bhowmik, P., Konkin, D., Polowick, P., Hodgins, C. L., Subedi, M., Xiang, D., et al. (2021). CRISPR/Cas9 gene editing in legume crops: opportunities and challenges. Legume Sci. 3 (3), e96. doi: 10.1002/leg3.96
Bianchini, A. (1999). Resistance to bean golden mosaic virus in bean genotypes. Plant Dis. 83, 615–620. doi: 10.1094/PDIS.1999.83.7.615
Bigeard, J., Colcombet, J., Hirt, H. (2015). Signaling mechanisms in pattern-triggered immunity (PTI). Mol. Plant 8, 521–539. doi: 10.1016/j.molp.2014.12.022
Binyamin, R., Khan, M. A., Khan, A. I., Khan, M. A., Awan, F. S., Khan, N. A. (2011). Molecular characterization of urdbean (Vigna mungo) germplasm related to resistance against urdbean leaf crinkle virus. Genet. Mol. Res. 10 (3), 1681–1688. doi: 10.4238/vol10-3gmr1446
Biswas, K. K., Tarafdar, A., Biswas, K. (2012). “Viral diseases and its mixed infection in mungbean and urd bean: major biotic constraints in production of food pulses in India,” in Modern trends in microbial biodiversity of natural ecosystem. biotech books. Eds. Sinhamn, A., Sharma, B. K., Srivastava, M.(New Delhi: Biotech Books), 301–319.
Blair, M. W., Morales, F. J. (2008). Geminivirus resistance breeding in common bean. CAB Rev. Perspect. Agric. Vet. Sci. Nutr. Nat. Resour. 3, 1–15.
Blair, M. W., Rodriguez, L. M., Pedraza, F., Morales, F., Beebe, S. (2007). Genetic mapping of the bean golden yellow mosaic geminivirus resistance gene bgm-1 and linkage with potyvirus resistance in common bean (Phaseolus vulgaris l.). Theor. Appl. Genet. 114, 261–271. doi: 10.1007/s00122-006-0428-6
Bonfim, K., Faria, J. C., Nogueira, E. O., Mendes, É.A., Aragão, F. J. (2007). RNAi-mediated resistance to bean golden mosaic virus in genetically engineered common bean (Phaseolus vulgaris). Mol. Plant-Microbe Interact. 20, 717–726. doi: 10.1094/MPMI-20-6-0717
Boukar, O., Bhattacharjee, R., Fatokun, C., Kumar, P. L., Gueye, B. (2013). “Chapter 6: Cowpea,” in Genetic and Genomic Resources of Grain Legume Improvement. eds. Singh, M., Upadhyaya, H. D., Bisht, I. S. (Oxford: Elsevier), 137–156.
Bowers, G. R., Goodman, R. M. (1979). Soybean mosaic virus: infection of soybean seed parts and seed transmission. Phytopathol 69, 569–572. doi: 10.1094/Phyto-69-569
Buss, G. R., Ma, G., Chen, P., Tolin, S. A. (1997). Registration of V94-5152 soybean germplasm resistant to soybean mosaic potyvirus. Crop Sci. 37, 1987–1988. doi: 10.2135/cropsci1997.0011183X003700060068x
Buss, G. R., Ma, G., Kristipati, S., Chen, P., Tolin, S. A. (1999). “A new allele at the Rsv3 locus for resistance to soybean mosaic virus,” in Proc. world soybean res. conf. VI. Ed. Kaufman, H. E.(Chicago: Champaign, IL: Superior Printing), 490.
Buzzell, R. I., Tu, J. C. (1989). Inheritance of a soybean stem-tip necrosis reaction to soybean mosaic virus. J. Hered. 80, 400–401. doi: 10.1093/oxfordjournals.jhered.a110882
Cao, Y., Zhou, H., Zhou, X., Li, F. (2020). Control of plant viruses by CRISPR/Cas system-mediated adaptive immunity. Front. Microbiol. 11, 593700. doi: 10.3389/fmicb.2020.593700
Chang, H. X., Brown, P. J., Lipka, A. E., Domier, L. L., Hartman, G. L. (2016). Genome-wide association and genomic prediction identifies associated loci and predicts the sensitivity of Tobacco ringspot virus in soybean plant introductions. BMC Genomics 17, 153. doi: 10.1186/s12864-016-2487-7
Chattopadhyay, A., Purohit, J., Tiwari, K. K., Deshmukh, R. (2019). Targeting transcription factors for plant disease resistance: shifting paradigm. Curr. Sci. 117, 1598–1607. doi: 10.18520/cs/v117/i10/1598-1607
Che, P., Chang, S., Simon, M. K., Zhang, Z., Shaharyar, A., Ourada, J., et al. (2021). Developing a rapid and highly efficient cowpea regeneration, transformation and genome editing system using embryonic axis explants. Plant J. 106 (3), 817–830. doi: 10.1111/tpj.15202
Che, Z., Liu, H., Yi, F., Cheng, H., Yang, Y., Wang, L., et al. (2017). Genome-wide association study reveals novel loci for SC7 resistance in a soybean mutant panel. Front. Plant Sci. 8, 1771. doi: 10.3389/fpls.2017.01771
Chen, P., Buss, G. R., Roane, C. W., Tolin, S. A. (1991). Allelism among genes for resistance to soybean mosaic virus in strain differential soybean cultivars. Crop Sci. 31, 305–309. doi: 10.2135/cropsci1991.0011183X003100020015x
Chen, P., Buss, G. R., Roane, C. W., Tolin, S. A. (1994). Inheritance in soybean of resistant and necrotic reactions to soybean mosaic virus strains. Crop Sci. 34, 414–422. doi: 10.2135/cropsci1994.0011183X003400020021x
Chen, P., Buss, G. R., Tolin, S. A. (1993). Resistance to soybean mosaic virus conferred by two independent dominant genes in PI 486355. J. Hered. 84, 25–28. doi: 10.1093/oxfordjournals.jhered.a111272
Chen, P., Buss, G. R., Tolin, S. A., Gunduz, I., Cicek, M. (2002). A valuable gene in suweon 97 soybean for resistance to soybean mosaic virus. Crop Sci. 42, 333–337. doi: 10.2135/cropsci2002.3330
Chen, H. M., Ku, H. M., Schafleitner, R., Bains, T. S., George Kuo, C., Liu, C. A., et al. (2013). The major quantitative trait locus for mungbean yellow mosaic Indian virus resistance is tightly linked in repulsion phase to the major bruchid resistance locus in a cross between mungbean [Vigna radiata (L.) wilczek] and its wild relative vigna radiata ssp. sublobata. Euphytica 192, 205–216. doi: 10.1007/s10681-012-0831-9
Chen, P., Ma, G., Buss, G. R., Gunduz, I., Roane, C. W., Tolin, S. A. (2001). Inheritance and allelism tests of raiden soybean for resistance to soybean mosaic virus. J. Hered. 92, 51–55. doi: 10.1093/jhered/92.1.51
Chen, Y., Shen, Y., Chen, B., Xie, L., Xiao, Y., Chong, Z., et al. (2022). Comparative transcriptome analyses between resistant and susceptible varieties in response to soybean mosaic virus infection. Agronomy 12, 1785. doi: 10.3390/agronomy12081785
Chinchilla, D., Zipfel, C., Robatzek, S., Kemmerling, B., Nurnberger, T., Jones, J. D., et al. (2007). A flagellin-induced complex of the receptor FLS2 and BAK1 initiates plant defence. Nature 448, 497–500. doi: 10.1038/nature05999
Cho, E. K., Choi, S. H., Cho, W. T. (1983). Newly recognized soybean mosaic virus mutants and sources of resistance in soybeans. Res. Rep. ORD 25, 18–22.
Cho, E. K., Chung, B. J., Lee, S. H. (1977). Studies of identification and classification of soybean virus diseases in korea. II. etiology of necrotic disease of Glycine max. Plant Dis. Rep. 61, 313–317.
Cho, E. K., Goodman, R. M. (1979). Strains of soybean mosaic virus: classification based on virulence in resistant soybean cultivars. Phytopathol 69, 467–470. doi: 10.1094/Phyto-69-467
Cho, E. K., Goodman, R. M. (1982). Evaluation of resistance in soybeans to soybean mosaic virus strains. Crop Sci. 22, 1133–1136. doi: 10.2135/cropsci1982.0011183X002200060012x
Chu, J., Li, W., Piao, D., Lin, F., Huo, X., Zhang, H., et al. (2021). Identification of a major QTL related to resistance to soybean mosaic virus in diverse soybean genetic populations. Euphytica 217, 176. doi: 10.1007/s10681-021-02907-8
Cody, W. B., Scholthof, H. B., Mirkov, T. E. (2017). Multiplexed gene editing and protein overexpression using a tobacco mosaic virus viral vector. Plant Physiol. 175, 23–35. doi: 10.1104/pp.17.00411
Crittenden, H. W., Hastings, K. M., Moore, G. M. (1966). Soybean losses caused by tobacco ringspot virus. Plant Dis. Rep. 50, 910–913.
Cruz, A. R. R., Aragão, F. J. L. (2014). RNAi-based enhanced resistance to c owpea severe mosaic virus and c owpea aphid-borne mosaic virus in transgenic cowpea. Plant Pathol. 63 (4), 831–837. doi: 10.1111/ppa.12178
Das, R. A., Ray, D. D., Bhattacharyya, P. K. (2017). Field evaluation of soybean varieties for resistance to yellow mosaic virus (YMV) in the lower gangetic plains of West Bengal, India. J. Mycopathol. Res. 55, 265–268.
Dasgupta, U., Mishra, G. P., Dikshit, H. K., Mishra, D. C., Bosamia, T., Roy, A., et al. (2021). Comparative RNA-seq analysis unfolds a complex regulatory network imparting yellow mosaic disease resistance in mungbean [Vigna radiata (L.) r. wilczek]. PloS One 16, e0244593. doi: 10.1371/journal.pone.0244593
Daspute, A., Fakrudin, B., Bhairappanavar, S. B., Kavil, S. P., Narayana, Y. D., Kaumar, A., et al. (2014). Inheritance of pigeonpea sterility mosaic disease resistance in pigeonpea. Plant Pathol. J. 30, 188. doi: 10.5423/PPJ.NT.10.2013.0104
de Paula, N. T., de Faria, J. C., Aragão, F. J. (2015). Reduction of viral load in whitefly (Bemisia tabaci gen.) feeding on RNAi-mediated bean golden mosaic virus resistant transgenic bean plants. Virus Res. 210, 245–247. doi: 10.1016/j.virusres.2015.08.012
Dey, N., Sarkar, S., Acharya, S., Maiti, I. B. (2015). Synthetic promoters in planta. Planta 242 (5), 1077–1094. doi: 10.1007/s00425-015-2377-2
Dhaliwal, S. K., Gill, R. K., Sharma, A., Kaur, A., Bhatia, D., Kaur, S. A. (2022). Large-effect QTL introgressed from ricebean imparts resistance to mungbean yellow mosaic India virus in blackgram (Vigna mungo (L.) hepper). Theor. Appl. Genet. 135, 4495–4506. doi: 10.1007/s00122-022-04234-5
Dhole, V. J., Reddy, K. (2012). Genetic analysis of resistance to mungbean yellow mosaic virus in mungbean (Vigna radiata). Plant Breed. 131, 414–417. doi: 10.1111/j.1439-0523.2012.01964.x
Dickson, M. H., Natti, J. J. (1968). Inheritance of resistance of Phaseolus vulgaris to bean yellow mosaic virus. Phytopathol 58 (1450), 846.
Dodds, P. N., Rathjen, J. P. (2010). Plant immunity: towards an integrated view of plant pathogen interactions. Nat. Rev. Genet. 11, 539–548. doi: 10.1038/nrg2812
Drijfhout, E. (1978). “Genetic interaction between phaseolus vulgaris and bean common mosaic virus with implications for strain identification and breeding for resistance,” in Agric. res. rep. cent. agric. publ. doc, vol. 872. (Netherlands: Wageningen), 1–98.
Drijfhout, E., Morales, F. (2005). “Bean common mosaic,” in Compendium of bean diseases, 2nd ed. Eds. Schwartz, H. F., Steadman, J. R., Hall, R., Forster, R. L. (St. Paul, MN: American Phytopathological Society), 60–62.
Drijfhout, E., Silbernagel, M. J., Burke, D. W. (1978). Differentiation of strains of bean common mosaic virus. Neth. J. Plant Pathol. 84, 13–26. doi: 10.1007/BF01978099
Edet, O. U., Ishii, T. (2022). Cowpea speed breeding using regulated growth chamber conditions and seeds of oven-dried immature pods potentially accommodates eight generations per year. Plant Methods 18 (1), 106. doi: 10.1186/s13007-022-00938-3
Fajinmi, A. A. (2019). Interactive effect of blackeye cowpea mosaic virus and cucumber mosaic virus on Vigna unguiculata. Hortic. Plant J. 5, 88–92. doi: 10.1016/j.hpj.2019.01.001
Fang, Y., Wang, L., Sapey, E., Fu, S., Wu, T., Zeng, H., et al. (2021). Speed-breeding system in soybean: integrating off-site generation advancement, fresh seeding, and marker-assisted selection. Front. Plant Sci. 12, 717077. doi: 10.3389/fpls.2021.717077
Feng, X., Myers, J. R., Karasev, A. V. (2015). Bean common mosaic virus isolate exhibits a novel pathogenicity profile in common bean, overcoming the bc-3 resistance allele coding for the mutated eIF4E translation initiation factor. Phytopathol 105, 1487–1495. doi: 10.1094/PHYTO-04-15-0108-R
Feng, X., Orellana, G. E., Myers, J. R., Karasev, A. V. (2018). Recessive resistance to bean common mosaic virus conferred by the bc-1 and bc-2 genes in common bean (Phaseolus vulgaris) affects long-distance movement of the virus. Phytopathol 108, 1011–1018. doi: 10.1094/PHYTO-01-18-0021-R
Fernie, A. R., Schauer, N. (2009). Metabolomics-assisted breeding: a viable option for crop improvement? Trends Genet. 25, 39–48. doi: 10.1016/j.tig.2008.10.010
Ferreira, A. L., Faria, J. C., Moura, M. C., Zaidem, A. L. M., Pizetta, C. S. R., Freitas, E. O., et al. (2022). White y-tolerant transgenic common bean (Phaseolus vulgaris) line. Front. Plant Sci. 13, 984804. doi: 10.3389/fpls.2022.984804
Flores-Estévez, N., Acosta-Gallegos, J. A., Silva-Rosales, L. (2003). Bean common mosaic virus and bean common mosaic necrosis virus in Mexico. Plant Dis. 87, 21–25. doi: 10.1094/PDIS.2003.87.1.21
Gálvez, G. E., Morales, F. J. (1989). “Whitefly-transmitted viruses,” in Bean production problems in the tropics. Eds. Schwartz, H. F., Pastor- Corrales, M. A. (Cali: CIAT), 379–408.
Gangurde, S. S., Xavier, A., Naik, Y. D., Jha, U. C., Rangari, S. K., Kumar, R., et al. (2022). Two decades of association mapping: insights on disease resistance in major crops. Front. Plant Sci. 13. doi: 10.3389/fpls.2022.1064059
Gao, L., Ding, X., Li, K., Liao, W., Zhong, Y., Ren, R., et al. (2015). Characterization of soybean mosaic virus resistance derived from inverted repeat-SMV-HC-Pro genes in multiple soybean cultivars. Theor. Appl. Genet. 128, 1489–1505. doi: 10.1007/s00122-015-2522-0
Gao, L., Luo, J., Ding, X., Wang, T., Hu, T., Song, P., et al. (2020). Soybean RNA interference lines silenced for eIF4E show broad potyvirus resistance. Mol. Plant Pathol. 21, 303–317. doi: 10.1111/mpp.12897
Gao, Y., Wu, Y., Du, J., Zhan, Y., Sun, D., Zhao, J., et al. (2017). Both light-induced SA accumulation and ETI mediators contribute to the cell death regulated by BAK1 and BKK1. Front. Plant Sci. 8, 622. doi: 10.3389/fpls.2017.00622
Gauffier, C., Lebaron, C., Moretti, A., Constant, C., Moquet, F., Bonnet, G., et al. (2016). A TILLING approach to generate broad-spectrum resistance to potyviruses in tomato is hampered by eIF4E gene redundancy. Plant J. 85, 717–729. doi: 10.1111/tpj.13136
Gayen, D., Karmakar, S. (2021). Designing, performing, and analyzing CRISPR-Cas9-Mediated genome editing experiments in leguminous plants. CRISPR-Cas Methods 2, 103–122. doi: 10.1007/978-1-0716-1657-4_8
Ghatak, A., Chaturvedi, P., Paul, P., Agrawal, G. K., Rakwal, R., Kim, S. T., et al. (2017a). Proteomics survey of solanaceae family: current status and challenges ahead. J. Proteomics 169, 41–57. doi: 10.1016/j.jprot.2017.05.016
Ghatak, A., Chaturvedi, P., Weckwerth, W. (2017b). Cereal crop proteomics: systemic analysis of crop drought stress responses towards marker-assisted selection breeding. Front. Plant Sci. 8, 757. doi: 10.3389/fpls.2017.00757
Ghatak, A., Chaturvedi, P., Weckwerth, W. (2018). Metabolomics in plant stress physiology. Plant Genet. Mol. Biol. 164, 187–236.
Gillaspie, J. A. G. (2001). Resistance to cucumber mosaic virus in cowpea and implications for control of cowpea stunt disease. Plant Dis. 85, 1004–1005.
Gillaspie, J. A. G. (2002). Registration of GC-86L-98 cowpea germplasm resistant to cucumber mosaic virus and blackeye cowpea mosaic virus. (Registrations of germplasm). Crop Sci. 42, 1385–1386. doi: 10.2135/cropsci2002.1385
Gnanesh, B. N., Bohra, A., Sharma, M., Byregowda, M., Pande, S., Wesley, V., et al. (2011). Genetic mapping and quantitative trait locus analysis of resistance to sterility mosaic disease in pigeonpea [Cajanus cajan (L.) millsp.]. Field Crops Res. 123, 53–61. doi: 10.1016/j.fcr.2011.04.011
Graham, P. H., Vance, C. P. (2003). Legumes: importance and constraints to greater use. Plant Physiol. 131, 872–877. doi: 10.1104/pp.017004
Grewal, J. S. (1978). Diseases of mungbean in India (Los Banos, Philippines: International Mungbean Symposium).
Gumedzoe, M. Y., Rossel, H. W., Thottappilly, G., Asselin, A., Huguenot, C. (1998). Reaction of cowpea (Vigna unguiculata l. walp.) to six isolates of blackeye cowpea mosaic virus (BlCMV) and cowpea aphid-borne mosaic virus (CAMV), two potyviruses infecting cowpea in Nigeria. Int. J. Pest Manage. 44, 11–16. doi: 10.1080/096708798228464
Gunduz, I., Buss, G. R., Chen, P., Tolin, S. A. (2002). Characterization of SMV resistance genes in tousan 140 and hourei soybean. Crop Sci. 42, 90–95. doi: 10.2135/cropsci2002.9000
Gunduz, I., Buss, G. R., Chen, P., Tolin, S. A. (2004). Genetic and phenotypic analysis of Soybean mosaic virus resistance in PI 88788 soybean. Phytopathol 94, 687–692. doi: 10.1094/PHYTO.2004.94.7.687
Gunduz, I., Buss, G. R., Ma, G., Chen, P., Tolin, S. A. (2001). Genetic analysis of resistance to soybean mosaic virus in OX670 and harosoy soybean. Crop Sci. 41, 1785–1791. doi: 10.2135/cropsci2001.1785
Guo, D., Zhi, H., Wang, Y. (2005). Identification and distribution of soybean mosaic virus strains in middle and northern Huang huai region of China. Chin. J. Oil Crop Sci. 27, 64–68.
Gupta, D., Singh, O. W., Basavaraj, Y. B., Roy, A., Mukherjee, S. K., Mandal, B. (2021). Direct foliar application of dsRNA derived from the full-length gene of NSs of groundnut bud necrosis virus limits virus accumulation and symptom expression. Front. Plant Sci. 12, 734618. doi: 10.3389/fpls.2021.734618
Ha, J., Lee, S. H. (2020). Updates on legume genome sequencing. Methods Mol. Biol. 2107, 1–18. doi: 10.1007/978-1-0716-0235-5_1
Hagedorn, D., Inglis, D. (1986). Handbook of bean diseases (Madison, Wisconsin: University of Wisconsin).
Hahn, F., Nekrasov, V. (2019). CRISPR/Cas precision: do we need to worry about off-targeting in plants? Plant Cell Reps 38, 437–441. doi: 10.1007/s00299-018-2355-9
Hampton, R., Thottappilly, G., Rossel, H. (1997). “Viral diseases of cowpea and their control by resistance conferring genes,” in Advances in cowpea research. Eds. Singh, B. B., Mohan Raj, D. R., Dashiell, K. E., Jackai, L. E. N. (Ibadan: International Institute of Tropical Agriculture), 159–175.
Hart, J. P., Griffiths, P. D. (2013). A series of eIF4E alleles at the bc-3 locus are associated with recessive resistance to clover yellow vein virus in common bean. Theor. Appl. Genet. 126, 2849–2863. doi: 10.1007/s00122-013-2176-8
Hart, J. P., Griffiths, P. D. (2015). Genotyping-by-sequencing enabled mapping and marker development for the by-2 potyvirus resistance allele in common bean. Plant Genome 8 (1), 1–14. doi: 10.3835/plantgenome2014.09.0058
Hartman, G. L., Domier, L. L. (2015). “Tobacco ringspot virus,” in Compendium of soybean diseases and pests. Eds. Hartman, G. L., Rupe, J. C., Sikora, E. F., Domier, L. L., Davis, J. A., Steffey, K. L. (St. Paul: American Phytopathological Society), 121–122.
Hayes, A., Jeong, S., Gore, M., Yu, Y., Buss, G., Tolin, S., et al. (2004). Recombination within a nucleotide-binding-site/leucine-rich-repeat gene cluster produces new variants conditioning resistance to soybean mosaic virus in soybeans. Genetics 166, 493–503. doi: 10.1534/genetics.166.1.493
Hayes, A. J., Ma, G., Buss, G. R., Saghai-Maroof, M. A. (2000). Molecular marker mapping of Rsv4, a gene conferring resistance to all known strains of soybean mosaic virus. Crop Sci. 40, 1434–1437. doi: 10.2135/cropsci2000.4051434x
Hedesh, R. M., Shams-Bakhsh, M., Mozafari, J. (2011). Evaluation of common bean lines for their reaction to tomato yellow leaf curl virus-Ir2. Crop Prot. 30, 163–167. doi: 10.1016/j.cropro.2010.10.009
Hickey, L. T., Hafeez, N. A., Robinson, H., Jackson, S. A., Leal-Bertioli, S. C. M., Tester, M., et al. (2019). Breeding crops to feed 10 billion. Nat. Biotechnol. 37, 744–754. doi: 10.1038/s41587-019-0152-9
Hilaire, J., Tindale, S., Jones, G., Pingarron-Cardenas, G., Bačnik, K., Ojo, M., et al. (2022). Risk perception associated with an emerging agri-food risk in Europe: plant viruses in agriculture. Agric. Food Secur. 11, 21. doi: 10.1186/s40066-022-00366-5
Hill, J. H. (1999). “Soybean mosaic virus,” in Compendium of soybean diseases. Eds. Hartman, G. L., Sinclair, J. B., Rupe, J. C. (St. Paul, MN, USA: American Phytopathology Society Press), 70–71.
Huang, X., Han, B. (2014). Natural variations and genome-wide association studies in crop plants. Annu. Rev. Plant Biol. 65, 531–551. doi: 10.1146/annurev-arplant-050213-035715
Hughes, Jd’A., Naidu, R. A., Shoyinka, S. A. (2003). “Future of plant virus disease research in sub-sahara African agriculture,” in Proceeding of a conference plant virology in Sub-Sahara Africa. Eds. Hughes, J.d’A, Du, B. O. O. (Nigeria: IITA), 589.
Hussain, M., Qazi, J., Mansoor, S., Iram, S., Bashir, M., Zafar, Y. (2004). First report of mungbean yellow mosaic India virus on mungbean in Pakistan. Plant Pathol. 53, 518. doi: 10.1111/j.1365-3059.2004.01037.x
Ilut, D. C., Lipka, A. E., Jeong, N., Bae, D. N., Kim, D. H., Kim, J. H., et al. (2016). Identification of haplotypes at the Rsv4 genomic region in soybean associated with durable resistance to soybean mosaic virus. Theor. Appl. Genet. 129, 453–468. doi: 10.1007/s00122-015-2640-8
Ilyas, M., Qazi, J., Mansoor, S., Briddon, R. W. (2009). Molecular characterization and infectivity of a "legumovirus" (genus begomovirus: family geminiviridae) infecting the leguminous weed rhynchosia minima in Pakistan. Virus Res. 145, 279–284. doi: 10.1016/j.virusres.2009.07.018
Iqbal, U., Iqbal, S. M., Afzal, R., Jamal, A., Farooq, M. A., Zahid, A. (2011). Screening of mungbean germplasm against mung bean yellow mosaic virus (MYMV) under field conditions. Pak. J. Phytopathol. 23, 48–51.
Ishibashi, K., Saruta, M., Shimizu, T., Shu, M., Anai, T., Komatsu, K., et al. (2019). Soybean antiviral immunity conferred by dsRNase targets the viral replication complex. Nat. Commun. 10, 1–10. doi: 10.1038/s41467-019-12052-5
Jeong, S. C., Kristipati, S., Hayes, A. J., Maughan, P. J., Noffsinger, S. L., Gunduz, I., et al. (2002). Genetic and sequence analysis of markers tightly linked to the soybean mosaic virus resistance gene, Rsv3. Crop Sci. 42, 265–270.
Jha, U. C., Bohra, A., Pandey, S., Parida, S. (2020). Breeding, genetics and genomics approaches for improving fusarium wilt resistance in major grain legumes. Front. Genet. 11, 1001. doi: 10.3389/fgene.2020.01001
Jha, U. C., Nayyar, H., Mantri, N., Siddique, K. H. M. (2021). Non-coding RNAs in legumes: their emerging roles in regulating Biotic/Abiotic stress responses and plant growth and development. Cells 10, 1674. doi: 10.3390/cells10071674
Jha, U. C., Nayyar, H., von Wettberg, E. J. B., Naik, Y. D., Thudi, M., Siddique, K. H.M. (2022b). Legume pangenome: status and scope for crop improvement. Plants 22, 3041. doi: 10.3390/plants11223041
Jha, R., Yadav, H. K., Raiya, R., Singh, R. K., Jha, U. C., Sathee, L., et al. (2022a). Integrated breeding approaches to enhance the nutritional quality of food legumes. Front. Plant Sci. 13, 984700. doi: 10.3389/fpls.2022.984700
Ji, X., Si, X., Zhang, Y., Zhang, H., Zhang, F., Gao, C. (2018). Conferring DNA virus resistance with high specificity in plants using virus-inducible genome-editing system. Genome Biol. 19, 197. doi: 10.1186/s13059-018-1580-4
Ji, J., Zhang, C., Sun, Z., Wang, L., Duanmu, D., Fan, Q. (2019). Genome editing in cowpea vigna unguiculata using CRISPR-Cas9. Int. J. Mol. Sci. 20 (10), 2471. doi: 10.3390/ijms20102471
Jiang, Y., Ding, P. (2022). Calcium signaling in plant immunity: a spatiotemporally controlled symphony. Trends Plant Sci. 28, 74–89. doi: 10.1016/j.tplants.2022.11.001
Jin, T., Karthikeyan, A., Wang, L., Zong, T., Wang, T., Yin, J., et al. (2022). Digs out and characterization of the resistance gene accountable to soybean mosaic virus in soybean (Glycine max (L.) Merrill). Theor. Appl. Genet. 16, 1–6. doi: 10.1007/s00122-022-04213-w
Jones, R. A. C., Coutts, B. A., Latham, L. J., McKirdy, S. J. (2008). Cucumber mosaic virus infection of chickpea stands: temporal and spatial patterns of spread and yield-limiting potential. Plant Pathol. 57, 842–853. doi: 10.1111/j.1365-3059.2008.01838.x
Jones, J. D., Vance, R. E., Dangl, J. L. (2016). Intracellular innate immune surveillance devices in plants and animals. Science 354, aaf6395. doi: 10.1126/science.aaf6395
Juranić, M., Nagahatenna, D. S., Salinas-Gamboa, R., Hand, M. L., Sánchez-León, N., Leong, W. H., et al. (2020). A detached leaf assay for testing transient gene expression and gene editing in cowpea (Vigna unguiculata [L.] walp.). Plant Methods 16 (1), 1–17. doi: 10.1186/s13007-020-00630-4
Kahraman, A., Pandey, A., Khan, M. K., Lindsay, D., Moenga, S., Vance, L., et al. (2017). Distinct subgroups of cicer echinospermum are associated with hybrid sterility and breakdown in interspecific crosses with cultivated chickpea. Crop Sci. 57, 3101–3111. doi: 10.2135/cropsci2017.06.0335
Kaiser, W. J., Hannan, R. M. (1983). Additional hosts of alfalfa mosaic virus and its seed transmission in tumble pigweed and bean. Plant Dis. Reptr. 59, 770. doi: 10.1094/PD-67-1354
Kanakala, S., Kuria, P. (2018). Chickpea chlorotic dwarf virus: an emerging monopartite dicot infecting mastrevirus. Viruses 11 (1), 5. doi: 10.3390/v11010005
Karthikeyan, A., Li, K., Jiang, H., Ren, R., Li, C., Zhi, H., et al. (2017). Inheritance, fine-mapping, and candidate gene analyses of resistance to soybean mosaic virus strain SC5 in soybean. Mol. Genet. Genom. 292, 811–822. doi: 10.1007/s00438-017-1310-8
Karthikeyan, A., Li, K., Li, C., Yin, J., Li, N., Yang, Y., et al. (2018). Fine-mapping and identifying candidate genes conferring resistance to soybean mosaic virus strain SC20 in soybean. Theor. Appl. Genet. 131, 461–476. doi: 10.1007/s00122-017-3014-1
Kato, S., Takada, Y., Shimamura, S., Hirata, K., Sayama, T., Taguchi Shiobara, F., et al. (2016). Transfer of the Rsv3 locus from “Harosoy” for resistance to soybean mosaic virus strains c and d in Japan. Breed. Sci. 66, 319–327. doi: 10.1270/jsbbs.66.319
Kelly, J. D., Afanador, L., Haley, S. D. (1995). Pyramiding genes for resistance to bean common mosaic virus. Euphytica 82, 207–212. doi: 10.1007/BF00029562
Khan, A., Nasim, N., Pudhuvai, B., Koul, B., Upadhyay, S. K., Sethi, L., et al. (2023). Plant synthetic promoters: advancement and prospective. Agriculture 13, 298. doi: 10.3390/agriculture13020298
Khattak, G. S. S., Haq, M. A., Ashraf, M., Elahi, T. (2000). Genetics of mungbean yellow mosaic virus (MYMV) in mungbean (Vigna radiata (L.) wilczek). J. Genet. Breed. 54, 237–243.
Kiihl, R. A. S., Hartwig, E. E. (1979). Inheritance of reaction to soy- bean mosaic virus in soybeans. Crop Sci. 20, 403–404.
Kitsanachandee, R., Somta, P., Chatchawankanphanich, O., Akhtar, K. P., Shah, T. M., Nair, R. M., et al. (2013). Detection of quantitative trait loci for mungbean yellow mosaic India virus (MYMIV) resistance in mungbean (Vigna radiata (L.) wilczek) in India and Pakistan. Breed Sci. 63, 367–373. doi: 10.1270/jsbbs.63.367
Klepadlo, M., Chen, P., Shi, A., Mason, R. E., Korth, K. L., Srivastava, V., et al. (2017). Two tightly linked genes for soybean mosaic virus resistance in soybean. Crop Sci. 57, 1844–1853. doi: 10.2135/cropsci2016.05.0290
Kreuze, J. F., Valkonen, J. P. (2017). Utilization of engineered resistance to viruses in crops of the developing world, with emphasis on sub-Saharan Africa. Curr. Opin. Virol. 26, 90–97. doi: 10.1016/j.coviro.2017.07.022
Kumar, P. L., Latha, T. K., Kulkarni, N. K., Raghavendra, N., Saxena, K. B., Waliyar, F., et al. (2005). Broad-based resistance to pigeonpea sterility mosaic disease in wild relatives of pigeonpea (Cajanus: phaseoleae). Ann. Appl. Biol. 146, 371–379. doi: 10.1111/j.1744-7348.2005.040091.x
Kumar, S., Tanti, B., Patil, B. L., Mukherjee, S. K., Sahoo, L. (2017). RNAi-derived transgenic resistance to mungbean yellow mosaic India virus in cowpea. PloS One 12, e0186786. doi: 10.1371/journal.pone.0186786
Kundu, A., Pal, A. (2012). Identification and characterization of elite inbred lines with MYMIV-resistance in vigna mungo. Field Crops Res. 135, 116–125. doi: 10.1016/j.fcr.2012.07.006
Kundu, A., Patel, A., Paul, S., Pal, A. (2015). Transcript dynamics at early stages of molecular interactions of MYMIV with resistant and susceptible genotypes of the leguminous host, Vigna mungo. PloS One 10 (4), e0124687. doi: 10.1371/journal.pone.0124687
Kundu, A., Singh, P. K., Dey, A., Ganguli, S., Pal, A. (2019). Complex molecular mechanisms underlying MYMIV-resistance in vigna mungo revealed by comparative transcriptome profiling. Sci. Reps. 9, 1–3. doi: 10.1038/s41598-019-45383-w
Kyle, M. M., Dickson, M. H., Provvidenti, R. (1986). Linkage analysis of hypersensitive resistance to four viruses in phaseolus vulgaris. Bean Improv. Coop. 29, 80–81.
Kyle, M. M., Provvidenti, R. (1987). Inheritance of resistance to potato y viruses in phaseolus vulgaris l. Theor. Appl. Genet. 74, 595–600. doi: 10.1007/BF00288858
Langner, T., Kamoun, S., Belhaj, K. (2018). CRISPR crops: plant genome editing toward disease resistance. Annu. Rev. Phytopathol. 56, 479–512. doi: 10.1146/annurev-phyto-080417-050158
Levy, A., Tzfira, T. (2010). Bean dwarf mosaic virus: a model system for the study of viral movement. Mol. Plant Pathol. 11, 451–461. doi: 10.1111/j.1364-3703.2010.00619.x
Li, D., Chen, P., Alloatti, J., Shi, A., Chen, Y. F. (2010). Identification of new alleles for resistance to soybean mosaic virus in soybean. Crop Sci. 50, 649–655. doi: 10.2135/cropsci2009.06.0302
Li, Y. H., Zhou, G., Ma, J., Jiang, W., Jin, L. G., Zhang, Z., et al. (2014). De novo assembly of soybean wild relatives for pan-genome analysis of diversity and agronomic traits. Nat. Biotechnol. 32, 1045–1052. doi: 10.1038/nbt.2979
Liang, Q., Muñoz-Amatriain, M., Shu, S., Lo, S., Wu, X., Carlson, J. W., et al. (2022). A view of the pan-genome of domesticated cowpea (Vigna unguiculata [L.] walp.). bioRxiv 2022-08. doi: 10.1101/2022.08.22.504811
Liang, G., Zhang, H., Lou, D., Yu, D. (2016). Selection of highly efficient sgRNAs for CRISPR/Cas9-based plant genome editing. Sci. Rep. 6, 1–8. doi: 10.1038/srep21451
Liao, L., Chen, P., Buss, G. R., Yang, Q., Tolin, A. (2002). Inheritance and allelism of resistance to soybean mosaic virus in Zao18 soybean from China. J. Hered. 93, 447–452. doi: 10.1093/jhered/93.6.447
Liao, L., Chen, P., Rajcan, I., Buss, G. R., Tolin, S. A. (2011). Genetic analysis of “8101” soybean containing three genes for resistance to soybean mosaic virus. Crop Sci. 51, 503–511. doi: 10.2135/cropsci2010.02.0113
Lima, J. A., Silva, A. K., Aragão, M. D., Ferreira, N. R., Teófilo, E. M. (2011). Simple and multiple resistances to viruses in cowpea genotypes. Pesquisa Agropecuária Brasileira. 46, 1432–1438. doi: 10.1590/S0100-204X2011001100003
Lima, J. A., Sittolin, I. M., Lima, R. C., Freire Filho, F., Lima, J. (2005). Diagnose e estratégias de controle de doenças ocasionadas pexilrus. feijão caupi: avanços tecnológicos. Brasília: Embrapa Informação Tecnológica, 404–459.
Lin, J., Lan, Z., Hou, W., Yang, C., Wang, D., Zhang, M., et al. (2020). Identification and fine-mapping of a genetic locus underlying soybean tolerance to SMV infections. Plant Sci. 292, 110367. doi: 10.1016/j.plantsci.2019.110367
Liu, S. L., Cheng, Y. B., Mu, L. I., Jiang, Z., Xia, Q. J., Hai, N. I. (2022a). Fine mapping and genetic analysis of resistance genes, Rsc18, against soybean mosaic virus. J. Integr. Agric. 21, 644–653. doi: 10.1016/S2095-3119(20)63569-9
Liu, Q., Hobbs, H. A., Domier, L. L. (2019). Genome-wide association study of the seed transmission rate of soybean mosaic virus and associated traits using two diverse population panels. Theor. Appl. Genet. 132, 3413–3424. doi: 10.1007/s00122-019-03434-w
Liu, W., Stewart, C. N., Jr. (2016). Plant synthetic promoters and transcription factors. Curr. Opin. Biotechnol. 37, 36–44. doi: 10.1016/j.copbio.2015.10.001
Liu, C., Wang, Y., Peng, J., Fan, B., Xu, D., Wu, J., et al. (2022b). High-quality genome assembly and pan-genome studies facilitate genetic discovery in mung bean and its improvement. Plant Commun. 26, 100352. doi: 10.1016/j.xplc.2022.100352
Lorenz, A. J., Chao, S., Asoro, F. G., Heffner, E. L., Hayashi, T., Iwata, H., et al. (2011). Genomic selection in plant breeding: knowledge and prospects. Adv. Agron. 110, 77–123. doi: 10.1016/B978-0-12-385531-2.00002-5
Ma, G., Chen, P., Buss, G. R., Tolin, S. A. (1995). Genetic characteristics of two genes for resistance to soybean mosaic virus in PI 486355 soybean. Theor. Appl. Genet. 91, 907–914. doi: 10.1007/BF00223899
Ma, G., Chen, P., Buss, G. R., Tolin, S. A. (2002). Complementary action of two independent dominant genes in Columbia soybean for resistance to soybean mosaic virus. J. Hered. 93, 179–184. doi: 10.1093/jhered/93.3.179
Ma, G., Chen, P., Buss, G. R., Tolin, S. A. (2003). Genetic study of a lethal necrosis to soybean mosaic virus in PI 507389 soybean. J. Hered. 94, 205–211. doi: 10.1093/jhered/esg059
Ma, F. F., Wu, X. Y., Chen, Y. X., Liu, Y. N., Shao, Z. Q., Wu, P., et al. (2016). Fine mapping of the Rsv1-h gene in the soybean cultivar suweon 97 that confers resistance to two Chinese strains of the soybean mosaic virus. Theor. Appl. Genet. 129, 2227–2236. doi: 10.1007/s00122-016-2769-0
Maiti, S., Basak, J., Kundagrami, S., Kundu, A., Pal, A. (2011). Molecular marker-assisted genotyping of mungbean yellow mosaic India virus resistant germplasms of mungbean and urdbean. Mol. Biotechnol. 47, 95–104. doi: 10.1007/s12033-010-9314-1
Makkouk, K., Pappu, H., Kumari, S. G. (2012). “Virus diseases of peas, beans, and faba bean in the Mediterranean region,” in Advances in virus research, vol. 84. (London: Academic Press), 367–402.
Malathi, V. C., John, P. (2009). “Mungbean yellow mosaic viruses,” in Desk encyclopedia of plant and fungal virology. Eds. Van Regenmortal, M., Mahy, B. (London: Academic Press), 217–226.
Mallikarjuna, N., Saxena, K. B., Jadhav, D. R. (2011). “Cajanus,” in Wild crop relatives: genomic and breeding resources, legume crops and forages. Ed. Kole, C. (Berlin, Heidelberg: Springer-Verlag), 21–33.
Mao, G., Meng, X., Liu, Y., Zheng, Z., Chen, Z., Zhang, S. (2011). Phosphorylation of a WRKY transcription factor by two pathogen-responsive MAPKs drives phytoalexin biosynthesis in arabidopsis. Plant Cell 23, 1639–1653. doi: 10.1105/tpc.111.084996
Maroof, M. A. S., Tucker, D. M., Skoneczka, J. A., Bowman, B. C., Tripathy, S., Tolin, S. A. (2010). Fine mapping and candidate gene discovery of the Soybean mosaic virus resistance gene, Rsv4. Plant Genome. 3, 14. doi: 10.3835/plantgenome2009.07.0020
Martin, K., Singh, J., Hill, J. H., Whitham, S. A., Cannon, S. B. (2016). Dynamic transcriptome profiling of bean common mosaic virus (BCMV) infection in common bean (Phaseolus vulgaris l.). BMC Genome 1, 1–9. doi: 10.1186/s12864-016-2976-8
Mathivathana, M. K., Murukarthick, J., Karthikeyan, A., Jang, W., Dhasarathan, M., Jagadeeshselvam, N., et al. (2019). Detection of QTLs associated with mungbean yellow mosaic virus (MYMV) resistance using the interspecific cross of Vigna radiata × Vigna exillateata. J. Appl. Genet. 60, 255–268. doi: 10.1007/s13353-019-00506-x
Mayo, M. A. (2005). Changes to virus taxonomy 2004. Arch. Virol. 150, 189–198. doi: 10.1007/s00705-004-0429-1
McKern, N. M., Mink, G. I., Barnett, O. W., Mishra, A., Whittaker, L. A., Silbernagel, M. J., et al. (1992). Isolates of bean common mosaic virus comprising two distinct potyviruses. Phytopathol 82, 923–929. doi: 10.1094/Phyto-82-923
Meuwissen, T. H. E., Hayes, B. J., Goddard, M. E. (2001). Prediction of total genetic value using genome-wide dense marker maps. Genetics 157, 1819–1829. doi: 10.1093/genetics/157.4.1819
Meziadi, C., Blanchet, S., Geffroy, V., Pflieger, S. (2017). Genetic resistance against viruses in phaseolus vulgaris l.: state of the art and future prospects. Plant Sci. 265, 39–50. doi: 10.1016/j.plantsci.2017.08.009
Miklas, P. N., Larsen, R. C., Riley, R., Kelly, J. D. (2000). Potential marker-assisted selection for bc-1 2 resistance to bean common mosaic potyvirus in common bean. Euphytica 116, 211–219. doi: 10.1023/A:1004006514814
Miklas, P. N., Seo, Y., Gilbertson, R. L. (2009). Quantitative resistance to bean dwarf mosaic virus in common bean is associated with the bct gene for resistance to beet curly top virus. Plant Dis. 93, 645–648. doi: 10.1094/PDIS-93-6-0645
Mitter, N., Worrall, E. A., Robinson, K. E., Xu, Z. P., Carroll, B. J. (2017). Induction of virus resistance by exogenous application of double-stranded RNA. Curr. Opin. Virol. 26, 49–55. doi: 10.1016/j.coviro.2017.07.009
Monci, F., García-Andrés, S., Maldonado, J. A., Moriones, E. (2005). Resistance to monopartite begomoviruses associated with the bean leaf crumple disease in phaseolus vulgaris controlled by a single dominant gene. Phytopathol 95, 819–826. doi: 10.1094/PHYTO-95-0819
Moon, J. K., Jeong, S. C., Van, K., Maroof, M. A. S., Lee, S. H. (2009). Marker-assisted identification of resistance genes to soybean mosaic virus in soybean lines. Euphytica 169, 375–385. doi: 10.1007/s10681-009-9970-z
Morales, F. J. (2006). “Common beans,” in Nat. resist. mech. plants to viruses. Eds. Loebenstein, G., Carr, J. P. (The Netherlands: Springer, Dordrecht), 367–382.
Morales, F. J., Anderson, P. K. (2001). The emergence and dissemination of whitefly-transmitted geminiviruses in Latin America. Arch. Virol. 146, 415–441. doi: 10.1007/s007050170153
Mukeshimana, G., Paneda, A., Rodríguez-Suárez, C., Ferreira, J. J., Giraldez, R., Kelly, J. D. (2005). Markers linked to the bc-3 gene conditioning resistance to bean common mosaic potyviruses in common bean. Euphytica 144, 291–299. doi: 10.1007/s10681-005-7397-8
Mushtaq, M., Mukhtar, S., Sakina, A., Dar, A. A., Bhat, R., Deshmukh, R., et al. (2020). Tweaking genome-editing approaches for virus interference in crop plants. Plant Physiol. Biochem. 147, 242–250. doi: 10.1016/j.plaphy.2019.12.022
Nagaraj, Basavaraj, S., Padmaja, A. S., Nagaraju, N., Ramesh, S. (2019). Identification of stable sources of resistance to mungbean yellow mosaic virus (MYMV) disease in mungbean [Vigna radiata (L.) wilczek]. Plant Genet. Resour. 17, 362–370. doi: 10.1017/S1479262119000121
Nagaraj, K. M., Kulkarni, R. S. (2004). Inheritance of resistance to sterility mosaic virus in pigeonpea (Cajanus cajan (L.) millsp.). Indian J. Genet. Plant Breed. 64, 118–120.
Nair, R. M., Götz, M., Winter, S., Giri, R. R., Boddepalli, V. N., Sirari, A., et al. (2017). Identification of mungbean lines with tolerance or resistance to yellow mosaic in fields in India where different begomovirus species and different Bemisia tabaci cryptic species predominate. Eur. J. Plant Pathol. 149, 349–365. doi: 10.1007/s10658-017-1187-8
Nene, Y. L. (1972). A survey of viral diseases of pulse crops in uttar pradesh. G. B. Pant Univ. Agric. Tech. Res. Bull. 4, 191.
Nene, Y. L., Reddy, M. V., Haware, M. P., Ghanekar, A. M., Amin, K. S., Pande, S., et al. (2012). Field diagnosis of chickpea diseases and their control. information bulletin no. 28 (revised) (Patancheru: International Crops Research Institute for the Semi-Arid Tropics).
Nomura, H., Komori, T., Uemura, S., Kanda, Y., Shimotani, K., Nakai, K., et al. (2012). Chloroplast-mediated activation of plant immune signalling in arabidopsis. Nat. Commun. 3, 926. doi: 10.1038/ncomms1926
Ogundiwin, E. A., Thottappilly, G., AkenOva, M. E., Ekpo, E. J. A., Fatokun, C. A. (2002). Resistance to cowpea mottle carmovirus in Vigna exillateata. Plant Breed. 121 (6), 517–520. doi: 10.1046/j.1439-0523.2002.00769.x
Ogunsola, K. E., Ilori, C., Fatokun, C. A., Boukar, O., Ogunsanya, P., Kumar, P. L. (2021). Disease incidence and severity in cowpea lines evaluated for resistance to single and multiple infections of endemic viruses in Nigeria. J. Crop Improvement. 35, 427–452. doi: 10.1080/15427528.2020.1824952
Pandey, M. K., Wang, H., Khera, P., Vishwakarma, M. K., Kale, S. M., Culbreath, A. K., et al. (2017). Genetic dissection of novel QTLs for resistance to leaf spots and tomato spotted wilt virus in peanut (Arachis hypogaea l.). Front. Plant Sci. 8, 25. doi: 10.3389/fpls.2017.00025
Patil, B. L., Kumar, P. L. (2015). Pigeonpea sterility mosaic virus: a legume-infecting emaravirus from south Asia. Mol. Plant Pathol. 16, 775–786. doi: 10.1111/mpp.12238
Patwa, N., Chatterjee, C., Basak, J. (2020). Differential responses of phaseolus vulgaris cultivars following mungbean yellow mosaic India virus infection. Physiol. Mol. Biol. Plants. 4, 817–828. doi: 10.1007/s12298-019-00741-w
Peng, Y., van Wersch, R., Zhang, Y. (2018). Convergent and divergent signaling in PAMP-triggered immunity and effector-triggered immunity. Mol. Plant Microbe Interact. 31, 403–409. doi: 10.1094/MPMI-06-17-0145-CR
Pérez-Escamilla, R. (2017). Food security and the 2015–2030 sustainable development goals: from human to planetary health: perspectives and opinions. Curr. Develops Nutri. 1, e000513. doi: 10.3945/cdn.117.000513
Pflieger, S., Blanchet, S., Meziadi, C., Richard, M., Thareau, V., Mary, F., et al. (2014). The "one-step" bean pod mottle virus (BPMV)-derived vector is a functional genomics tool for efficient overexpression of heterologous protein, virus-induced gene silencing and genetic mapping of BPMV r-gene in common bean (Phaseolus vulgaris l.). BMC Plant Biol. 14, 1–16. doi: 10.1186/s12870-014-0232-4
Pompeu, A. S., Kranz, W. M. (1977). Linhagens de feijoeiro (Phaseolus vulgaris l.) resistentes ao virus do mosaico do feijoeiro. Summa Phytopathol. 3, 162–163.
Prasada Rao, R. D. V. J., Reddy, D. V. R., Nigam, S. N., Reddy, A. S., Waliyar, F., Reddy, T. Y., et al. (2003). Peanut stem necrosis: a new disease of groundnut in india. information bulletin no. 67 (Patancheru, 502 324, Andhra Pradesh, India: International Crops Research Institute for Semi-Arid Tropics), 16.
Provvidenti, R. (1974). Inheritance of resistance to watermelon mosaic virus 2 in phaseolus vulgaris. Phytopathol 64, 1448–1450. doi: 10.1094/Phyto-64-1448
Radhakrishnan, T., Thirumalaisamy, P. P., Vemana, K., Kumar, A., Rathnakumar, A. L. (2016). “Major virus diseases of groundnut in India and their management,” in Plant viruses: evolution and management. Eds. Gaur, R., Petrov, N., Patil, B., Stoyanova, M. (Singapore: Springer).
Rajpal, V. R., Singh, A., Kathpalia, R., Thakur, R. K., Khan, M., Pandey, A., et al. (2023). The prospects of gene introgression from crop wild relatives into cultivated lentil for climate change mitigation. Front. Plant Sci. 14, 800. doi: 10.3389/fpls.2023.1127239
Ranf, S., Eschen-Lippold, L., Pecher, P., Lee, J., Scheel, D. (2011). Interplay between calcium signalling and early signalling elements during defence responses to microbe- or damage-associated molecular patterns. Plant J. 68, 100–113. doi: 10.1111/j.1365-313X.2011.04671.x
Ren, Q., Jiang, H., Xiang, W., Nie, Y., Xue, S., Zhi, H., et al. (2022). A MADS-box gene is involved in soybean resistance to multiple soybean mosaic virus strains. Crop J. 10, 802–808. doi: 10.1016/j.cj.2021.10.003
Robertson, G., Burger, J., Campa, M. (2022). CRISPR/Cas-based tools for the targeted control of plant viruses. Mol. Plant Pathol. 23 (11), 1701–1718. doi: 10.1111/mpp.13252
Román, M. A., Castañeda, A. M., Sánchez, J. C. A., Muñoz, C. G., Beaver, J. S. (2004). Inheritance of normal pod development in bean golden yellow mosaic resistant common bean. J. Amer. Soc Hortic. Sci. 129, 549–552. doi: 10.21273/JASHS.129.4.0549
Ross, J. P. (1968). Effect of single and double infections of soybean mosaic and bean pod mottle viruses on soybean yield and seed characters. Plant Dis. Rep. 52, 344–348.
Rui, R., Liu, S., Karthikeyan, A., Wang, T., Niu, H., Yin, J., et al. (2017). Fine-mapping and identification of a novel locus Rsc15 underlying soybean resistance to soybean mosaic virus. Theor. Appl. Genet. 130, 2395–2410. doi: 10.1007/s00122-017-2966-5
Rushton, P. J., Reinstädler, A., Lipka, V., Lippok, B., Somssich, I. E. (2002). Synthetic plant promoters containing defined regulatory elements provide novel insights into pathogen- and wound-induced signaling. Plant Cell. 14, 749–762. doi: 10.1105/tpc.010412
Samineni, S., Sen, M., Sajja, S. B., Gaur, P. M. (2019). Rapid generation advance (RGA) in chickpea to produce up to seven generations per year and enable speed breeding. Crop J. 8, 164–169. doi: 10.1016/j.cj.2019.08.003
Saxena, R. K., Kale, S. M., Kumar, V., Parupali, S., Joshi, S., Singh, V., et al. (2017). Genotyping-by-sequencing of three mapping populations for identification of candidate genomic regions for resistance to sterility mosaic disease in pigeonpea. Sci. Reps 7 (1), 1813. doi: 10.1038/s41598-017-01535-4
Saxena, K. B., Lava Kumar, P., Kulkarni, N. K., Jones, A. T., Muniyappa, V., Waliyar, F. (2004). Sterility mosaic disease the green plague of pigeonpea. Plant Dis. 88, 436–445.
Saxena, K. B., Saxena, R. K., Hickey, L. T., Varshney, R. K. (2019). Can a speed breeding approach accelerate genetic gain in pigeonpea? Euphytica 215, 202. doi: 10.1007/s10681-019-2520-4
Schmidhuber, J., Tubiello, F. N. (2007). Global food security under climate change. Proc. Nat. Acad. Sci. 104 (50), 19703–19708. doi: 10.1073/pnas.0701976104
Schroeder, W. T., Provvidenti, R. (1968). Resistance of bean (Phaseolus vulgaris) to PV2 strain of bean yellow mosaic virus conditioned by single dominant gene by. Phytopathol 58 (12), 1710.
Selvi, R., Muthiah, A. R., Manivannan, N. (2006). Tagging of RAPD marker for MYMV resistance in mungbean (Vigna radiata (L.) wilczek). Asian J. Plant Sci. 5, 277–280. doi: 10.3923/ajps.2006.277.280
Seo, Y. S., Gepts, P., Gilbertson, R. L. (2004). Genetics of resistance to the geminivirus, bean dwarf mosaic virus, and the role of the hypersensitive response in common bean. Theor. Appl. Genet. 108, 786–793. doi: 10.1007/s00122-003-1504-9
Seo, J.-K., Lee, H.-G., Choi, H.-S., Lee, S.-H., Kim, K.-H. (2009). Infectious in vivo transcripts from a full-length clone of soybean mosaic virus strain G5H. Plant Pathol. J. 25, 54–61. doi: 10.5423/PPJ.2009.25.1.054
Seo, Y. S., Rojas, M. R., Lee, J. Y., Lee, S. W., Jeon, J. S., Ronald, P., et al. (2006). A viral resistance gene from common bean functions across plant families and is up- regulated in a non-virus-specific manner. Proc. Natl. Acad. Sci. 103, 11856–11861. doi: 10.1073/pnas.0604815103
Sharma, D., Gupta, S. C., Rai, G. S., Reddy, M. V. (1984). Inheritance of resistance to sterility mosaic disease in pigeonpea I. Indian J. Genet. 44, 84–90.
Sharma, M., Rathore, A., Mangala, U. N., Ghosh, R., Sharma, S., Upadhyay, H., et al. (2012). New sources of resistance to fusarium wilt and sterility mosaic disease in a mini-core collection of pigeonpea germplasm. Eur. J. Plant Pathol. 133, 707–714. doi: 10.1007/s10658-012-9949-9
Shi, A., Chen, P., Li, D. X., Zheng, C., Hou, A., Zhang, B. (2008). Genetic confirmation of 2 independent genes for resistance to soybean mosaic virus in J05 soybean using SSR markers. J. Hered. 99, 598–603. doi: 10.1093/jhered/esn035
Shokouhifar, F., Bahrabadi, M., Bagheri, A., Mamarabadi, M. (2019). Transient expression analysis of synthetic promoters containing f and d cis-acting elements in response to ascochyta rabiei and two plant defense hormones. AMB Express. 9 (1), 195. doi: 10.1186/s13568-019-0919-x
Singh, D. P. (1980). Inheritance of resistance to yellow mosaic virus in blackgram (Vigna mungo (L.) hepper). Theor. Appl. Genet. 57, 233–235. doi: 10.1007/BF00264676
Singh, B. V., Ahuja, M. R. (1977). Phaseolus sublobatus roxb. a source of resistance to yellow mosaic virus for cultivated mung. Indian J. Genet. Plant Breed. 37, 130–132.
Singh, V. K., Khan, A. W., Saxena, R. K., Kumar, V., Kale, S. M., Sinha, P., et al. (2016). Next generation sequencing for identification of candidate genes for fusarium wilt and sterility mosaic disease in pigeonpea (Cajanus cajan). Plant Biotechnol. J. 4, 1183–1194. doi: 10.1111/pbi.12470
Singh, B. V., Pandya, B. P., Gautam, P. L., Beniwal, S. P. S., Pandey, M. P. (1983). Inheritance of resistance to sterility mosaic virus in pigeonpea. Indian J. Genet. 43, 487–493.
Soler-Garzón, A., Oladzad, A., Beaver, J., Beebe, S., Lee, R., Lobaton, J. D., et al. (2021). NAC candidate gene marker for bgm-1 and interaction with QTL for resistance to bean golden yellow mosaic virus in common bean. Front. Plant Sci. 12, 628443. doi: 10.3389/fpls.2021.628443
Song, L., Fang, Y., Chen, L., Wang, J., Chen, X. (2021). Role of non-coding RNAs in plant immunity. Plant Communs. 2, 100180. doi: 10.1016/j.xplc.2021.100180
Sravika, A., Kennedy, J. S., Rajabaskar, D., Rajeswari, E. (2019). Field screening of greengram (Vigna radiataL.) genotypes for resistance against urdbean leaf crinkle virus. Indian J. Agric. Res. 53, 458–462.
Srinivas, T., Reddy, M. V., Jain, K. C., Reddy, M. S. S. (1997a). Studies on inheritance of resistance and allelic relationships for strain-2 of pigeonpea sterility mosaic pathogen. Ann. Appl. Biol. 130, 105–110. doi: 10.1111/j.1744-7348.1997.tb05786.x
Srinivas, T., Reddy, M. V., Jain, K. C., Reddy, M. S. S. (1997b). Inheritance of resistance to two isolates of sterility mosaic pathogen in pigeonpea (Cajanus cajan (L.) millsp.). Euphytica 97, 45–52.
Strausbaugh, C. A., Myers, J. R., Forster, R. L., McClean, P. E. (1999). Bc-1 and bc-u–two loci controlling bean common mosaic virus resistance in common bean are linked. J. Amer Soc Hortic. Sci. 124, 644–648. doi: 10.21273/JASHS.124.6.644
Subramaniyan, R., Narayana, M., Krishnamoorthy, I., Natarajan, G., Gandhi, K. (2022). Novel and stable QTL regions conferring resistance to MYMV disease and its inheritance in blackgram (Vigna mungo (L.) hepper). J. Genet. 101, 1–7. doi: 10.1007/s12041-022-01359-w
Subramanya, K. S (2013). Viruses and sub-viral agents In: Plant Virus and Viroid Diseases in the Tropics. (Berlin: Springer)pp. 11–97.
Sudha, M., Karthikeyan, A., Madhumitha, B., Veera Ranjani, R., Kanimoli Mathivathana, M., Dhasarathan, M., et al. (2022). Dynamic transcriptome profiling of mungbean genotypes unveil the genes respond to the infection of mungbean yellow mosaic virus. Pathogens 11, 190. doi: 10.3390/pathogens11020190
Suh, S. J., Bowman, B. C., Jeong, N., Yang, K., Kastl, C., Tolin, S. A., et al. (2011). The Rsv3 locus conferring resistance to soybean mosaic virus is associated with a cluster of coiled-coil nucleotide-binding leucine-rich repeat genes. Plant Genome 4, 55–64. doi: 10.3835/plantgenome2010.11.0024
Sun, T., Sun, X., Li, F., Ma, N., Wang, M., Chen, Y., et al. (2022). H2O2 mediates transcriptome reprogramming during soybean mosaic virus-induced callose deposition in soybean. Crop J. 10, 262–272. doi: 10.1016/j.cj.2021.04.005
Swenson, K. (1968). Relation of environment and nutrition to plant susceptibility to bean yellow mosaic virus by aphid transmission, Oregon agr, exp. sta. tech. bull 106, 23.
Takahashi, K., Tanaka, T., Lida, W. (1963). Occurrence of strains of soybean mosaic and dwarf virus. Annu. Phytopathol. Soc Jpn. 28, 87.
Takahashi, K., Tanaka, T., Lida, W., Tsuda, Y. (1980). Studies on virus diseases and causal viruses of soybean in Japan. Bull. Tohoku Natl. Agric. Exp. Stn. 62, 1–130.
Talakayala, A., Mekala, G. K., Reddy, M. K., Ankanagari, S., Garladinne, M. (2022). Manipulating resistance to mungbean yellow mosaic virus in greengram (Vigna radiata l): through CRISPR/Cas9 mediated editing of the viral genome. Front. Sustain. Food Syst. 6, 911574. doi: 10.3389/fsufs.2022.911574
Tashkandi, M., Ali, Z., Aljedaani, F. R., Shami, A., Mahfouz, M. M. (2018). Engineering resistance against tomato yellow leaf curl virus via the CRISPR/Cas9 system in tomato. Plant Signal. Behav. 13, e1525996. doi: 10.1080/15592324.2018.1525996
Teli, B., Purohit, J., Rashid, M. M., Jailani, A. A. K., Chattopadhyay, A. (2020). Omics insight on fusarium head blight of wheat for translational research perspective. Curr. Genom. 21, 411–428. doi: 10.2174/1389202921999200620222631
Thomma, B. P., Nurnberger, T., Joosten, M. H. (2011). Of PAMPs and effectors: the blurred PTI-ETI dichotomy. Plant Cell 23, 4–15. doi: 10.1105/tpc.110.082602
Thudi, M., Palakurthi, R., Schnable, J. C., Chitikineni, A., Dreisigacker, S., Mace, E., et al. (2020). Genomic resources in plant breeding for sustainable agriculture. J. Plant Physiol. 2020, 153351. doi: 10.1016/j.jplph.2020.153351
Tolin, S. A. (1999). Diseases caused by viruses: compendium of soybean diseases Vol. 1999 (St. Paul, MN, USA: APS Press).
Tracy, S. L., Frenkel, M. J., Gough, K. H., Hanna, P. J., Shukla, D. D. (1992). Bean yellow mosaic, clover yellow vein, and pea mosaic are distinct potyviruses: evidence from coat protein gene sequences and molecular hybridization involving the 3′ non-coding regions. Arch. Virol. 122, 249–261.
Tsai, W. S., Shih, S. L., Rauf, A., Safitri, R., Hidayati, N., Huyen, B. T. T., et al. (2013). Genetic diversity of legume yellow mosaic begomoviruses in Indonesia and Vietnam. Ann. Appl. Biol. 163, 367–377.
Tu, J. C. (1992). Symptom severity, yield, seed mottling and seed transmission of soybean mosaic virus in susceptible and resistant soybean: the influence of infection stage and growth temperature. J. Phytopathol. 135, 28–36.
Umaharan, P., Ariyanayagam, R. P., Haque, S. Q. (1997a). Resistance to cowpea severe mosaic virus, determined by three dosage dependent genes in Vigna unguiculata l. walp. Euphytica 95, 49–55.
Umaharan, P., Haque, S. Q., Ariyananyagam, R. P. (1997b). Identification of resistance to cowpea severe mosaic virus (Trinidad isolate) in cowpea [Vigna unguiculata (L.) walp.]. Trop. Agric. (Trinidad) 74, 324–328.
Urrea, C. A., Miklas, P. N., Beaver, J. S., Riley, R. H. (1996). A codominant randomly amplified polymorphic DNA (RAPD) marker useful for indirect selection of bean golden mosaic virus resistance in common bean. J. Amer Soc. Hortic. Sci. 121, 1035–1039. doi: 10.21273/JASHS.121.6.1035
Usovsky, M., Chen, P., Li, D., Wang, A., Shi, A., Zheng, C., et al. (2022). Decades of genetic research on Soybean mosaic virus resistance in soybean. Viruses 14, 1122. doi: 10.3390/v14061122
Vahabi, N., Michailidis, G. (2022). Unsupervised multi-omics data integration methods: a comprehensive review. Front. Genet. 13. doi: 10.3389/fgene.2022.854752
vanderHoorn, R. A., Kamoun, S. (2008). From guard to decoy: a new model for perception of plant pathogen effectors. Plant Cell 20, 2009–2017. doi: 10.1105/tpc.108.060194
Varshney, R. K., Roorkiwal, M., Sun, S., Bajaj, P., Chitikineni, A., Thudi, M., et al. (2021). A chickpea genetic variation map based on the sequencing of 3,366 genomes. Nature 599, 622–627. doi: 10.1038/s41586-021-04066-1
Velez, J. J., Bassett, M. J., Beaver, J. S., Molina, A. (1998). Inheritance of resistance to bean golden mosaic virus in common bean. J. Amer. Soc Hortic. Sci. 123, 628–631. doi: 10.21273/JASHS.123.4.628
Vemana, K., Md Khureshi, C. S., Padma, J. G., Shabbir, S., Seshadri Goud, T. E., Anthony Johnson, A. M., et al. (2015). First report of Groundnut bud necrosis virus infecting Parthenium hysterophorus in India. Plant Dis. 99, PDIS-02-15-0162. doi: 10.1094/PDIS-02-15-0162-PDN
Verma, R. P., Singh, D. P. (1986). The allelic relationship of genes giving resistance to mungbean yellow mosaic virus in blackgram. Theor. Appl. Genet. 72, 737–738. doi: 10.1007/BF00266537
Wang, Y., Hobbs, H. A., Bowen, C. R., Bernard, R. L., Hill, C. B., Haudenshield, J. S., et al. (2006b). Evaluation of soybean cultivars, ‘Williams’ isogenic lines, and other selected soybean lines for resistance to two soybean mosaic virus strains. Crop Sci. 46, 2649–2653. doi: 10.2135/cropsci2006.04.0267
Wang, R. Y., Kritzman, A., Hershman, D. E., Ghabrial, S. A. (2006a). Aphis glycines as a vector of persistently and nonpersistently transmitted viruses and potential risks for soybean and other crops. Plant Dis. 90, 920–926. doi: 10.1094/PD-90-0920
Wang, D., Ma, Y., Yang, Y., Liu, N., Li, C., Song, Y., et al. (2011). Fine mapping and analyses of r SC8 resistance candidate genes to soybean mosaic virus in soybean. Theor. Appl. Genet. 122, 555–565. doi: 10.1007/s00122-010-1469-4
Wang, D., Tian, Z., Li, K., Li, H., Huang, Z., Hu, G., et al. (2013). Identification and variation analysis of soybean mosaic virus strains in Shandong, henan and anhui provinces of China. Soybean Sci. 32, 806–809.
Watson, A., Ghosh, S., Williams, M. J., Cuddy, W. S., Simmonds, J., Rey, M. D., et al. (2018). Speed breeding is a powerful tool to accelerate crop research and breeding. Nat. Plants 4, 23–29. doi: 10.1038/s41477-017-0083-8
Weckwerth, W. (2011). Green systems biology–from single genomes, proteomes and metabolomes to ecosystems research and biotechnology. J. Proteomics 75, 284–305. doi: 10.1016/j.jprot.2011.07.010
Weckwerth, W., Ghatak, A., Bellaire, A., Chaturvedi, P., Varshney, R. K. (2020). PANOMICS meets germplasm. Plant Biotechnol. J. 18, 1507–1525. doi: 10.1111/pbi.13372
Wen, R. H., Khatabi, B., Ashfield, T., Maroof, M. A. S., Hajimorad, M. R. (2013). The HC pro and P3 cistrons of an avirulent soybean mosaic virus are recognized by different resistance genes at the complex Rsv1 locus. Mol. Plant-Microbe Interact. 26, 203–215. doi: 10.1094/MPMI-06-12-0156-R
Widyasari, K., Tran, P. T., Shin, J., Son, H., Kim, K. H. (2022). Overexpression of purple acid phosphatase GmPAP2. 1 confers resistance to soybean mosaic virus in a susceptible soybean cultivar. J. Expt. Bot. 73 (5), 1623–1642. doi: 10.1093/jxb/erab496
Wilcox, J. R., Laviolette, F. A. (1968). Seedcoat mottling response of soybean genotypes to infection with soybean mosaic virus. Phytopathol 58, 1446–1447.
Win, J., Chaparro-Garcia, A., Belhaj, K., Saunders, D. G., Yoshida, K., Dong, S., et al. (2012). Effector biology of plant associated organisms: concepts and perspectives. Cold Spring Harb. Symp. Quant. Biol. 77, 235–247. doi: 10.1101/sqb.2012.77.015933
Worrall, E. A., Bravo-Cazar, A., Nilon, A. T., Fletcher, S. J., Robinson, K. E., Carr, J. P., et al. (2019). Exogenous application of RNAi-inducing double-stranded RNA inhibits aphid-mediated transmission of a plant virus. Front. Plant Sci. 10, 265. doi: 10.3389/fpls.2019.00265
Worrall, E. A., Wamonje, F. O., Mukeshimana, G., Harvey, J. J., Carr, J. P., Mitter, N. (2015). Bean common mosaic virus and bean common mosaic necrosis virus: relationships, biology, and prospects for control. Adv. Virus Res. 93, 1–46. doi: 10.1016/bs.aivir.2015.04.002
Wu, M., Liu, Y. N., Zhang, C., Liu, X. T., Liu, C. C., Guo, R., et al. (2019). Molecular mapping of the gene (s) conferring resistance to soybean mosaic virus and bean common mosaic virus in the soybean cultivar raiden. Theor. Appl. Genet. 132, 3101–3114. doi: 10.1007/s00122-019-03409-x
Xu, Y., Zhang, X., Li, H., Zheng, H., Zhang, J., Olsen, M. S., et al. (2022). Smart breeding driven by big data, artificial intelligence and integrated genomic-enviromic prediction. Mol. Plant. 15, 1664–1695. doi: 10.1016/j.molp.2022.09.001
Xun, H., Qian, X., Wang, M., Yu, J., Zhang, X., Pang, J., et al. (2022). Overexpression of a cinnamyl alcohol dehydrogenase-coding gene, GsCAD1, from wild soybean enhances resistance to soybean mosaic virus. Int. J. Mol. Sci. 23 (23), 15206. doi: 10.3390/ijms232315206
Xun, H., Yang, X., He, H., Wang, M., Guo, P., Wang, Y., et al. (2019). Over-expression of GmKR3, a TIR–NBS–LRR type r gene, confers resistance to multiple viruses in soybean. Plant Mol. Biol. 99, 95–111. doi: 10.1007/s11103-018-0804-z
Yan, H., Wang, H., Cheng, H., Hu, Z., Chu, S., Zhang, G., et al. (2015). Detection and fine-mapping of SC7 resistance genes via linkage and association analysis in soybean. J. Integr. Plant Biol. 57, 722–729. doi: 10.1111/jipb.12323
Yang, X., Niu, L., Zhang, W., He, H., Yang, J., Xing, G., et al. (2017). Robust RNAi-mediated resistance to infection of seven potyvirids in soybean expressing an intron hairpin NIb RNA. Transgenic Res. 26, 665–676. doi: 10.1007/s11248-017-0041-2
Yang, X., Niu, L., Zhang, W., He, H., Yang, J., Xing, G., et al. (2019). Increased multiple virus resistance in transgenic soybean overexpressing the double-strand RNA-specific ribonuclease gene PAC1. Transgenic Res. 28, 129–140. doi: 10.1007/s11248-018-0108-8
Yang, X., Niu, L., Zhang, W., Yang, J., Xing, G., He, H., et al. (2018). RNAi-mediated SMV P3 cistron silencing confers significantly enhanced resistance to multiple potyvirus strains and isolates in transgenic soybean. Plant Cell Reps. 37, 103–114. doi: 10.1007/s00299-017-2186-0
Yasmeen, E., Wang, J., Riaz, M., Zhang, L., Zuo, K. (2023). Designing artificial synthetic promoters for accurate, smart and versatile gene expression in plants. Plant Commun. 8, 100558. doi: 10.1016/j.xplc.2023.100558
Yu, Y. G., Buss, G. R., Saghai-Maroof, M. A. (1996). Isolation of a superfamily of candidate disease-resistance genes in soybean based on a conserved nucleotide-binding site. Proc. Natl. Acad. Sci. U.S.A. 93, 11751–11756. doi: 10.1073/pnas.93.21.11751
Yu, Y. G., Saghai Maroof, M. A., Buss, G. R., Maughan, P. J., Tolin, S. A. (1994). RFLP and microsatellite mapping of a gene for soybean mosaic virus resistance. Phytopathol 84, 60–64. doi: 10.1094/Phyto-84-60
Yuan, Y., Yang, Y., Yin, J., Shen, Y., Li, B., Wang, L., et al. (2020). Transcriptome-based discovery of genes and networks related to RSC3Q-mediated resistance to soybean mosaic virus in soybean. Crop Pasture Sci. 71, 987–995. doi: 10.1071/CP20253
Zaidi, S. S. E. A., Mahas, A., Vanderschuren, H., Mahfouz, M. M. (2020). Engineering crops of the future: CRISPR approaches to develop climate-resilient and disease-resistant plants. Genome Biol. 21, 1–19. doi: 10.1186/s13059-020-02204-y
Zhang, P., Du, H., Wang, J., Pu, Y., Yang, C., Yan, R., et al. (2020). Multiplex CRISPR/Cas9-mediated metabolic engineering increases soya bean isoflavone content and resistance to soya bean mosaic virus. Plant Biotechnol. J. 18 (6), 1384–1395. doi: 10.1111/pbi.13302
Zhang, C., Grosic, S., Whitham, S. A., Hill, J. H. (2012). The requirement of multiple defense genes in soybean Rsv1–mediated extreme resistance to soybean mosaic virus. Mol. Plant Microbe Interact. 25, 1307–1313. doi: 10.1094/MPMI-02-12-0046-R
Zhang, C., Liu, S., Li, X., Zhang, R., Li, J. (2022). Virus-induced gene editing and its applications in plants. Int. J. Mol. Sci. 23 (18), 10202. doi: 10.3390/ijms231810202
Zhang, J., Shao, F., Li, Y., Cui, H., Chen, L., Li, H., et al. (2007). A Pseudomonas syringae effector inactivates MAPKs to suppress PAMP- induced immunity in plants. Cell Host Microbe 1, 175–185. doi: 10.1016/j.chom.2007.03.006
Zhao, J., Bayer, P. E., Ruperao, P., Saxena, R. K., Khan, A. W., Golicz, A. A., et al. (2020). Trait associations in the pangenome of pigeon pea (Cajanus cajan). Plant Biotechnol. J. 18, 1946–1954. doi: 10.1111/pbi.13354
Zheng, C., Chen, P., Gergerich, R. (2005). Characterization of resistance to soybean mosaic virus in diverse soybean germplasm. Crop Sci. 45, 2503–2509. doi: 10.2135/cropsci2005.0114
Zheng, C., Chen, P., Gergerich, R. (2006). Genetic analysis of resistance to soybean mosaic virus in J05 soybean. J. Hered. 97, 429–437. doi: 10.1093/jhered/esl024
Zheng, G. J., Yang, Y. Q., Ying, M. A., Yang, X. F., Chen, S. Y., Rui, R. E. N., et al. (2014). Fine mapping and candidate gene analysis of resistance gene RSC3Q to soybean mosaic virus in qihuang 1. J. Integr. Agric. 13 (12), 2608–2615. doi: 10.1016/S2095-3119(13)60738-8
Zhou, L., He, H., Liu, R., Han, Q., Shou, H., Liu, B. (2014). Overexpression of GmAKT2potassium channel enhances resistance to soybean mosaic virus. BMC Plant Biol. 14 (1), 1–11. doi: 10.1186/1471-2229-14-154
Keywords: Grain legume, disease, virus, QTL, genome sequence, PTI, ETI
Citation: Jha UC, Nayyar H, Chattopadhyay A, Beena R, Lone AA, Naik YD, Thudi M, Prasad PVV, Gupta S, Dixit GP and Siddique KHM (2023) Major viral diseases in grain legumes: designing disease resistant legumes from plant breeding and OMICS integration. Front. Plant Sci. 14:1183505. doi: 10.3389/fpls.2023.1183505
Received: 10 March 2023; Accepted: 05 April 2023;
Published: 09 May 2023.
Edited by:
Palak Chaturvedi, University of Vienna, AustriaReviewed by:
Arindam Ghatak, University of Vienna, AustriaAshish Prasad, Kurukshetra University, India
Mohd. Kamran Khan, Selcuk University, Türkiye
Copyright © 2023 Jha, Nayyar, Chattopadhyay, Beena, Lone, Naik, Thudi, Prasad, Gupta, Dixit and Siddique. This is an open-access article distributed under the terms of the Creative Commons Attribution License (CC BY). The use, distribution or reproduction in other forums is permitted, provided the original author(s) and the copyright owner(s) are credited and that the original publication in this journal is cited, in accordance with accepted academic practice. No use, distribution or reproduction is permitted which does not comply with these terms.
*Correspondence: Uday Chand Jha, dTk4MTE5ODFAZ21haWwuY29t; Kadambot H. M. Siddique, a2FkYW1ib3Quc2lkZGlxdWVAdXdhLmVkdS5hdQ==