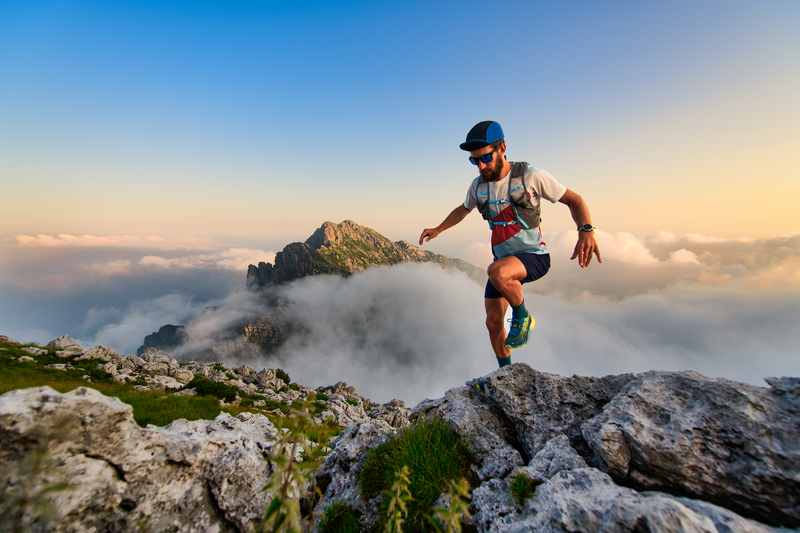
95% of researchers rate our articles as excellent or good
Learn more about the work of our research integrity team to safeguard the quality of each article we publish.
Find out more
REVIEW article
Front. Plant Sci. , 28 March 2023
Sec. Plant Biotechnology
Volume 14 - 2023 | https://doi.org/10.3389/fpls.2023.1170815
This article is part of the Research Topic The Utilization of Plants in Vaccine Research View all 9 articles
Plants provide not only food and feed, but also herbal medicines and various raw materials for industry. Moreover, plants can be green factories producing high value bioproducts such as biopharmaceuticals and vaccines. Advantages of plant-based production platforms include easy scale-up, cost effectiveness, and high safety as plants are not hosts for human and animal pathogens. Plant cells perform many post-translational modifications that are present in humans and animals and can be essential for biological activity of produced recombinant proteins. Stimulated by progress in plant transformation technologies, substantial efforts have been made in both the public and the private sectors to develop plant-based vaccine production platforms. Recent promising examples include plant-made vaccines against COVID-19 and Ebola. The COVIFENZ® COVID-19 vaccine produced in Nicotiana benthamiana has been approved in Canada, and several plant-made influenza vaccines have undergone clinical trials. In this review, we discuss the status of vaccine production in plants and the state of the art in downstream processing according to good manufacturing practice (GMP). We discuss different production approaches, including stable transgenic plants and transient expression technologies, and review selected applications in the area of human and veterinary vaccines. We also highlight specific challenges associated with viral vaccine production for different target organisms, including lower vertebrates (e.g., farmed fish), and discuss future perspectives for the field.
With global climate change, the risk for faster and wider geographical spread of infectious diseases in both human and animals is increasing. Vaccination has proven to be a highly effective measure for protecting humans and farm animals from infectious diseases. In general, vaccines are tightly controlled and thus considered as safe. In addition, they have minimal ecological impact, and are vital for human health and for the sustainability and expansion of global livestock production by providing efficient viral disease prophylaxis and disease control. Recombinant subunit vaccines offer particularly high safety as they cannot revert to virulent agents. They also offer high stability, are often easy to produce, and can have similarly high immunogenicity as full-fledged viral particles (Su and Su, 2018). Antigens, such as viral capsid and envelope proteins, are the key components of recombinant subunit vaccines, and can be expressed in various expression systems such as bacteria, yeast, transiently transformed plants and stable transgenic plants, insects, mammalian cell cultures, and cell-free systems. From these platforms, they can be isolated and formulated as vaccines.
With the continuing advancement of genetic engineering technologies, plants are increasingly used as bio-factories for the expression of antibodies as well as foreign antigens. Plants are advantageous as bio-factories, because they are inexpensive to grow at a large scale in greenhouses, bioreactors or open fields. Plants can express complex antigens while avoiding the risk of carrying human pathogens or endotoxins, which can contaminate viral, bacterial, and insect or mammalian cell expression systems (Aggarwal, 2009). In the present review, we discuss the status of vaccine production in plants using selected applications in human and veterinary vaccines as examples. We also highlight specific challenges associated with viral vaccine production for different target organisms, including lower vertebrates (e.g., farmed fish), and discuss future perspectives for the field. We argue that plant-based vaccines offer great promise in combating a range of viral diseases and can be a major avenue for low-cost and easily administered vaccines in the future.
Foreign proteins, including antigens, can be produced transiently in plants or stably in genetically modified (GM) plants. The underlying technologies have been established as an alternative option to mainstream production systems in microbes or animal cell cultures (Clarke et al., 2013; Bock, 2015; Dobrica et al., 2017; Castells-Graells and Lomonossoff, 2021). The feasibility, efficacy and safety of plant-based production of human and animal vaccines have been demonstrated by many successful examples and in numerous clinical studies (Takeyama et al., 2015; reviewed, e.g., in LeBlanc et al., 2020; Stander et al., 2022; Zahmanova et al., 2022). The strategy for vaccine production in plants is usually to test different expression vectors carrying the antigen-encoding foreign sequence. Common optimization steps include the test of different promoters, codon optimization of the transgene, test of different leader sequences to enhance translational efficiency, and targeting of the protein product to different subcellular locations (e.g., organelles) by appropriate transit or signal peptides (Joung et al., 2016).
Plant expression platforms provide a number of distinct advantages for the synthesis of recombinant subunit vaccines. Plant-made vaccines can substantially decrease the production costs and the energy input compared to other systems. Plants utilize solar energy and capture CO2, making them an environment friendly production system for recombinant proteins (Avesani et al., 2013). The low capital costs also make it easy to scale up plant biomass production for industrial utilization (Abiri et al., 2016). The post-translational modifications, folding and assembly of proteins produced in plant expression systems are often comparable to those in mammalian cells, which is often critical for immunogenicity (Margolin et al., 2020; Margolin et al., 2023). However, there are also some plant-specific features (e.g., in the protein glycosylation patterns) that need to be considered.
Many viral capsid proteins expressed or co-expressed in plants can self-assemble into viral-like particles (VLPs). VLPs don’t include nucleic acid, but have a similar structure, size and repetitive geometry as the wild-type virus, and therefore are ideal for inducing potent host immune responses (Marsian et al., 2017; Nooraei et al., 2021). Plant-made VLPs avoid the risk of insertion of viral nucleic acid sequences into the human genome and the associated potential oncogenesis (e.g., of DNA vaccines), do not trigger undesirable host responses to viral vector-derived vaccines, and do not have the danger of incomplete inactivation of attenuated viruses (Yang et al., 2017). As a subtype of VLP, recombinant plant virus particles (rPVPs) and genetically modified plant virus are increasingly studied as vectors for candidate vaccines (Lebel et al., 2015; Karpova and Nikitin, 2022). Many plant viruses such as the tobacco mosaic virus (TMV), cowpea mosaic virus (CPMV), potato virus X (PVX), alfalfa mosaic virus (AlMV), and papaya mosaic virus (PapMV) are currently used for the development of new vaccines. Specific modification of plant viruses allows dense expression of fused antigens, thereby contributing to the development of an effective immune response (Monreal-Escalante et al., 2022).
Plant-based expression platforms are roughly divided into three categories: transient expression systems, stable expression in the nuclear genome of transgenic plants or cell cultures, and stable expression in the plastid (chloroplast) genome of so-called transplastomic plants (Bock and Warzecha, 2010; Loüssl and Waheed, 2011; Clarke et al., 2013; Monreal-Escalante et al., 2022). Vaccines against viruses have been produced with all three platforms, which each have their specific advantages and limitations (Guan et al., 2013; He W. et al., 2021; Lobato Gómez et al., 2021). Plastid genome engineering in lettuce, tomato, potato, cabbage, and some other edible plants has been developed for recombinant protein production (Zhou et al., 2006; Cardi et al., 2010). The chloroplast expression system has also shown promising potential for oral vaccines (Davoodi-Semiromi et al., 2010). In particular, lettuce chloroplasts have been reported to express high levels of antigens for oral therapy in humans (Lakshmi et al., 2013; van Eerde et al., 2019).
Plant-based vaccines are especially suitable for oral immunization. Edible plants provide the advantage of convenient, needleless administration (Tacket, 2009). Orally administered vaccines can induce the host mucosal and systemic immunity after uptake by M cells in the follicle-associated epithelium in the gastrointestinal tract (Tatsuhiko et al., 2014). Orally delivered plant-produced vaccines can reduce the costs and the time involved in the purification of the antigens, by virtue of the consumed plant biomasses directly inducing immunity. Edible plant material can also be easily freeze-dried for long-term storage at low cost (Su et al., 2015; Rosales-Mendoza, 2020). When ingested as part of the diet, the plant-produced vaccines are protected from the acid environment of the stomach by the plant cell wall and are then slowly released in the gut. Therefore, cold chain facilities are not needed to stock the respective plant material, making plant vaccines more cost efficient and easier to distribute than conventional vaccines. Immune tolerance is considered as a potential barrier to the development of edible vaccines, because high-dosage oral administration can result in tolerance of antigen by the immune system. Therefore, the optimum antigen dosage needs to be carefully determined, and antigen purity and batch-to-batch consistency need to be verified (Jain et al., 2021).
Coronaviruses (CoVs), belonging to the family Coronaviridae, cause several respiratory diseases in humans and have become intensively studied since their unprecedented spread in the early 21st century (Song et al., 2019). Severe acute respiratory syndrome coronavirus (SARS-CoV) and Middle East respiratory syndrome coronavirus (MERS-CoV) are highly pathogenic beta-coronaviruses (β-CoVs) that pose a substantial threat to public health (Hahn et al., 2007). CoVs infect the human gastrointestinal, respiratory, hepatic and central nervous systems and also can infect a wide range of animals, including farm animals and wild birds, rodents, bats and others (Chen and Guo, 2016). Severe acute respiratory syndrome coronavirus 2 (SARS-CoV-2) emerged in December 2019, and since then, more than 659 million confirmed cases and over 6.6 million deaths have been reported up to January 11, 2023 (https://www.who.int/publications/m/item/weekly-epidemiological-update-on-covid-19—11-january-2023) (Rosales-Mendoza, 2020). Vaccination is one of the most effective strategies among all proposed therapeutic and prevention options for the control of the SARS-CoV-2 pandemic (LiDe and Clercq, 2020). SARS-CoV-2 is an enveloped beta-coronavirus with a positive-sense, single-stranded linear RNA genome, which is large at a size of 26-32 kb (Armbruster et al., 2019). In addition to envelope and capsid proteins, several non-structural proteins such as 3-chymotrypsin-like protease, papain-like protease, helicase, and RNA-dependent RNA polymerase have been considered as attractive targets for vaccine design and development of antiviral agents against CoVs (Li et al., 2020; Malik et al., 2020).
The spike glycoprotein (S) is most appealing because of its placement on the virion surface in the form of homotrimers that take the shape of the typical crown-like appearance of coronaviruses. The spike plays a crucial role in the interaction between the virion and the host cells during entry of viral particles into host cells, and is the major antigen for triggering host protective immune responses (Mahmood et al., 2021). Several vaccines have been developed expressing the S protein and have received local authorization (Funk and Ardakani, 2021). The U.S. Food and Drug Administration (FDA) has approved chewing gum containing clinical-grade plant material of the virus-trapping proteins CTB-ACE2 (angiotensin converting enzyme 2 fused to the non-toxic cholera toxin subunit B, CTB) expressed in chloroplasts of lettuce. Chewing gum with CTB-ACE2 provides an option to protect patients from infection in the oral cavity (Daniell et al., 2022).
New SARS-CoV-2 variants increase the demand for new vaccine expression systems that offer speedy and low-cost production to ensure rapid vaccine availability, especially in developing countries (Fuenmayor and Cervera, 2017; Williams and Burgers, 2021). There have been many successful cases of plant-expressed vaccines and pharmaceuticals developed and evaluated in clinical trials. A SARS-CoV-2 VLP was developed by Medicago (Table 1). Its design followed the well-established plant-based production of influenza virus proteins by introducing the corresponding genes into Nicotiana benthamiana via Agrobacterium-mediated transformation, leading to the production of influenza VLPs that were similar to the structure of native virions (Ward et al., 2014). Taking a similar approach, the gene for the S protein of SARS-CoV-2 was produced and the resulting candidate vaccine was successful in phase I human clinical trials and is currently undergoing phase 2 and 3 clinical trials (Rosales-Mendoza, 2020; Ward et al., 2021). There have been many attempts to produce SARS-CoV-2 vaccines in plant systems, and they are currently in different phases of clinical trials (Table 1). A plant-made SARS-CoV-2 vaccine now seems to be within reach (Table 1).
More than 350 million people are currently living with chronic HBV or HCV infections that can progress to liver fibrosis, cirrhosis and eventually hepatocellular carcinoma (HCC), the most prevalent form of liver cancer, with a poor survival rate (Llovet et al., 2021). HBV infection is not a curable disease today; current treatment relies on a combination of immunomodulators and direct acting antivirals (DAA), which significantly inhibit virus replication, but have no impact on the covalently closed circular DNA (cccDNA) pool responsible for HBV chronicity (Nassal, 2015). The recent development of orally administered antivirals that are able to cure the HCV infection in more than 90% of treated patients represents a significant breakthrough in HCV therapy. However, most patients in low/medium-income countries have little access to diagnosis and treatment (Quaranta et al, 2021). Prevention of the viral infection remains the most efficient way to reduce HBV/HCV-related morbidity and mortality. According to the World Health Organization (WHO), vaccination could avoid 4.5 million premature deaths caused by HBV/HCV-induced liver diseases by 2030 (WHO, 2022). This assessment urges development of a prophylactic HCV vaccine and improvement of currently available HBV vaccines to address non-responders.
The first attempt to produce a vaccine in plants was the production of HBV small (S) protein antigen (S-HBsAg) in Nicotiana tabaccum (Mason et al., 1992). The HBV small (S) envelope protein was the ideal model antigen to test the feasibility of using plants as a production platform for VLPs. Similar to the natural infection, when expressed in heterologous systems, the S protein retains the ability to self-assemble through complex disulfide linkages into spherical, 22-nm subviral particles that are secreted from cells (Eddleston, 1990). These complexes, known as the S-HBsAg, are highly immunogenic and the main component of the commercial HBV subunit vaccine produced in yeast (McAleer et al., 1984). The plant-made protein had similar physical properties to those of the antigen isolated from infected patients and was as immunogenic as the yeast-derived counterpart, confirming the suitability of plant cells for expression of functional, complex antigens (Thanavala et al., 1995). These seminal discoveries have attracted huge scientific interest, including the exploration of other plant species for the production of S-HBsAg, especially edible plants for potential oral administration (reviewed in Pantazica et al., 2021), and efforts to increase the repertoire of HBV-derived antigens in order to enhance the immune response. Consequently, successful production of the S-HBsAg was reported in a variety of plant species, such as potato (Ehsani et al., 1997; Richter et al., 2000);, lettuce (Pniewski et al., 2011), carrot (Imani et al., 2002), peanut (Chen et al., 2002), tomato (Gao et al., 2003; Srinivas et al., 2008), banana (Sunil Kumar et al., 2005), lupin (Pniewski et al., 2006), soybean (Ganapathi et al., 2007) and maize (Hayden et al., 2012), either in whole plants or cell cultures. The antigen expression levels varied significantly between hosts, ranging from a few nanograms to tens of micrograms per gram fresh weight of plant material, with higher yields obtained in transiently transformed plants and transgenic cell cultures (Joung et al., 2016).
In-depth characterization of the biochemical, antigenic and immunogenic properties of the S-HBsAg was performed in most of these preclinical studies, revealing appropriate folding and VLP formation, and induction of protective titers of anti-S antibodies (i.e., ≥10 mIU/mL after the last vaccination dose) (McMahon et al., 2009), depending on the quantity and purity of the administered antigen (Pniewski, 2013; Joung et al., 2016). The ability of the triggered antibodies to neutralize HBV infection in vitro, ultimately confirmed the quality of this immune response (Dobrica et al., 2017).
The great variation of the immunization approaches included oral, parenteral or mixed antigen administration regimes, and it appears that parenteral administration has the most encouraging prospects for vaccine development of plant-produced antigens, provided robust and sustainable production together with clinical-grade protein purification are achieved. Successful clinical trials, demonstrating high immunogenicity of M (middle) & L (large) proteins in non-responders to the standard vaccine, have led to the recent FDA approval of a three-antigen HBV vaccine containing the S, M and L proteins produced in mammalian cells (Vesikari et al., 2021). Owing to better immunogenic properties, expression of the two HBV envelope proteins M-HBsAg and L-HBsAg has also been attempted in several plants such as potato, tomato, and N. tabacum; however, antigen accumulation was significantly inferior to that of the S-HBsAg (Joung et al., 2016). To overcome drawbacks of expressing M-HBsAg and L-HBsAg alone, original antigens with relevant, virus-neutralizing epitopes from the L protein inserted in the antigenic loop of S were successfully produced in N. benthamiana and Lactuca sativa. These antigens assembled in VLPs and proved more immunogenic than the S-HBsAg in vaccinated mice, validating this approach as a promising strategy for further development of improved HBV antigens (Dobrica et al., 2017; Pantazica et al., 2022).
In striking contrast to the focus on production of HBV envelope proteins in plants, the suitability of this system to express the HCV envelope proteins E1 and E2 has only recently been addressed. HCV-E2 is the main target of broadly neutralizing antibodies and the major candidate for the development of a vaccine (Bailey et al., 2019). E1 and E2 are heavily glycosylated transmembrane proteins that undergo complex processing and folding to achieve their native conformation and do not assemble in VLPs, tentatively explaining the difficulty to produce them in recombinant systems. The E1E2 dimer and an N-glycosylation mutant, E1E2ΔN6, were first produced in lettuce, using a transient gene expression system, and were found to be correctly processed and glycosylated. Oral vaccination with the antigen-synthesizing lettuce induced vaccine-stimulated secretion of IgA (Immunoglobulin A) in mice (Clarke et al., 2017). This study provided compelling evidence that this complex viral antigen could be produced, processed, and functionally modified in edible plants. A full-length E2 was also obtained in N. benthamiana, with a similar N-glycosylation pattern to that observed in mammalian cells (Dobrica et al., 2021). Parenteral administration of the purified antigen triggered a significant humoral immune response in mice and antibodies with HCV-neutralizing activity, despite the low dose used for immunization. Interestingly, expression of E2-derived epitopes as fusions to the B subunit of cholera toxin (CTB) in N. benthamiana, followed by mucosal administration of crude protein extract in mice, failed to generate a protective immune response, indicating the importance of epitope presentation in the appropriate conformation (Nemchinov et al., 2000).
Vaccination with HBV/HCV envelope proteins targets mainly the humoral arm of the immune system, resulting in production of virus-neutralizing antibodies that efficiently prevent infection, but are not able to eliminate the virus from infected cells. Interestingly, antibodies to the viral capsid (core) are found in large amounts in patients with chronic HBV/HCV infection, suggesting a role in viral clearance, which predestines this antigen for development of therapeutic vaccines. For this reason, low-cost production of both HBV and HCV core proteins was attempted in plants. Very high levels of HBV core (HBcAg) of several milligrams per gram fresh weight were obtained in transiently transfected N. benthamiana. The antigen self-assembled into capsid-like particles (which likely explains the non-toxic accumulation of large quantities in the plant leaves), and proved highly immunogenic in mice (Huang et al., 2006). The conjugation of tandem Hepatitis B core (tHBcAg) VLPs and the GFP antigen was achieved in N. benthamiana without altering VLP formation or GFP fluorescence by SpyTag/SpyCatcher system (Peyret et al., 2020). In the case of HCV, core-derived peptides, rather than the full-length protein, were successfully expressed in tobacco plants, either alone (Madesis et al., 2010) or fused to the HBsAg in frame with other HCV-derived epitopes (Mohammadzadeh et al., 2016).
While there is still a long road ahead before a plant-made HBV/HCV vaccine will make it to the clinic, the intense research efforts made in the past two decades have unequivocally established that plants provide the appropriate environment for production of high-quality, fully functional HBV/HCV vaccine candidates. Future studies should focus on increasing the antigen yields and optimizing methods for large-scale production to make the plant expression platforms more competitive.
The Ebola virus (EBOV) belongs to the family Filoviridae, which also includes a number of other highly pathogenic viruses like Marburg, Diablo, Stria and Thamno (Zampieri et al., 2007; Hoenen et al., 2019). EBOV was first reported in 1976, in the northern Democratic Republic of the Congo (Bowen et al., 1977). Six different species of Ebola viruses are currently known: Zaire, Sudan, Reston, Bundibugyo, Tai Forest and Bombali ebolavirus (Miranda and Miranda, 2011). EBOV infects human and nonhuman primates causing severe hemorrhagic fever. The virus enters through mucosal surfaces or skin scratches. It may cause acute death within 7-16 days (Ksiazek et al., 1999). The initial symptoms are non-specific flu-like symptoms such as fever, headache, muscle ache, malaise, later developing to vascular, neurological, respiratory and gastrointestinal distress (Zaki and Goldsmith, 1999). When the viral load increases to a certain level, multiorgan necrosis and coagulation disorders will set in (Bwaka et al., 1999). The Zaire ebolavirus is the most virulent species with a mortality rate of 25% to 90% (Beeching et al., 2014), and is classified as a category A pathogen (Cenciarelli et al., 2015).
Ebola is an enveloped virus and has a negative-strand RNA genome of 19 kb (Zampieri et al., 2007). Due to its high virulence, research on EBOV can only be conducted in contained facilities of biosafety level 4, limiting vaccine development and drug discovery. The nucleoside analogues favipiravir (T-705) and BCX-4430, and an immunomodulatory CpG oligodeoxynucleotide (ODN) have been established to be effective in EBOV treatment, but they are forbidden because of their high teratogenicity and other side effects (Warren et al., 2014; Sissoko et al., 2016). Z-Mapp (Mapp Biopharmaceuticals), a mix of three monoclonal antibodies, has been reported to effectively neutralize EBOV (Qiu et al., 2014).
Some non-replicative vector-based vaccines derived from the adenovirus 5 replicon (Sullivan et al., 2000), or the Venezuelan equine encephalitis virus (VEEV) replicon (Herbert et al., 2013), replicative vector-based vaccines (recombinant vesicular stomatitis virus-Zaire Ebola virus rVSV-ZEBOV) and antigen-based vaccines against EBOV are currently under clinical trials, but concerns remain regarding pre-existing immunity against the vectors used, virulence relapse, side effects in humans, cost-efficiency and shelf life in developing countries (Rosales-Mendoza and Salazar-González, 2014).
Plant-made vaccines based on expressed viral protein antigens of EBOV represent an attractive alternative to currently available drugs and vector-based vaccines. Successful expression of two epitopes of the EBOV glycoprotein (GP) as fusions to the B subunit of the Escherichia coli heat-labile enterotoxin (LTB) in tobacco promises to provide a cost-effective candidate vaccine against EBOV in the near future (Ríos-Huerta et al., 2017). Furthermore, an antigen-antibody fusion was developed in N. benthamiana. It uses a geminiviral replicon system to express an Ebola immune complex consisting of the Ebola virus glycoprotein GP1 fused to the heavy chain of humanized 6D8 IgG monoclonal antibody, which specifically binds to an epitope of GP1 (6D8 IgG-GP1 fusion protein). It was reported that immunization of mice with the purified immune complex resulted in the induction of anti-Ebola virus antibodies at levels comparable to those obtained with GP1 VLPs, conferring 80% protection from fatal EBV infection (Phoolcharoen et al., 2011). In addition to vaccines, the humanized and glycoengineered monoclonal antibody cocktail against Ebola GP (MB-003) is produced in N. benthamiana (ZMapp) and demonstrated a 43-100% survival of challenged rhesus macaques, depending on the treatment time after infection with EBOV. This cocktail of three antibodies is currently the most efficient drug against EBOV and was approved by the WHO for Ebola disease treatment (Qiu et al., 2014).
Zika virus belongs to the genus Flavivirus (family Flaviviridae) and occurs in two genotypes, an African and Asian variant (Faye et al., 2014). The virus is primarily transmitted through the mosquito Aedes aegypti, but also through the related species A. africanus, A. albopictus and A. hensilli (Gorshkov et al., 2019). Sexual contact, blood transfusions, and transmission from mother to fetus during pregnancy are additional possible modes of Zika infection (Gregory et al., 2017). Infected humans are either asymptomatic or develop fever and cutaneous rash (Paniz-Mondolfi et al., 2019). Fetal microcephaly results from vertical transmission and is highly associated with Zika virus (Antoniou et al., 2020). Therefore, the WHO declared Zika virus a “public health emergency of international concern” (de Araújo et al., 2016).
Like other Flaviviruses, Zika virus is enveloped, icosahedral and has a non-segmented, single-stranded, positive-sense RNA genome (of ~10 kb). Genetic similarity between strains can cause antibody-dependent enhancement of infection (ADE), posing an additional challenge to vaccine development (Valiant et al., 2018; Pierson and Diamond, 2020). Glycoprotein E with its DIII domain (zEDIII) is a principal candidate for development of a subunit vaccine due to its roles in virus attachment to cellular receptors, membrane binding and fusion for viral entry, its involvement in mediating viral assembly and its potential to induce potent neutralizing antibodies (Chambers and Monath, 2003; Wang et al., 2019). The ZIKV envelope protein E forms a heterodimer with another structural protein, precursor membrane protein (prM). When the two proteins were synthesized in N. benthamiana by transient expression, low protein accumulation and limited VLP formation were observed (Peyret et al., 2020). However, ZIKV E transiently expressed in N. benthamiana showed potent activation of E protein-specific antibody production and cellular immune responses in mice (Yang et al., 2018). When expressed in N. benthamiana, VLPs consisting of the HBV capsid protein HBcAg and presenting zEDIII also had the ability to trigger humoral and cellular immune responses in mice (Yang et al., 2017). zEDIII also could be fused to the IgG heavy chain to form recombinant immune complexes (N-RICs), which improved immunogenicity and led to up to 150 folds higher titers of Zika-specific antibodies in mice than administration of zEDIII alone (Diamos et al., 2020a). Moreover, joint administration of plant produced HBcAg VLP displaying ZE3 and RIC zEDIII RICs had a synergistic effect and triggered enhanced immune responses (Diamos et al., 2020b).
Dengue virus (DENV), another flavivirus in the family Flaviviridae, is distributed in tropical and subtropical areas and transmitted by mosquitos (A. aegypti and A. albopictus). The WHO estimates that over 3.6 billion people are under risk of infection, and more than 200 million are infected, with 21,000 deaths occurring, every year (Murugesan and Manoharan, 2020). DENV infection may proceed asymptomatically, or result in self-limited dengue fever, which may progress to severe dengue haemorrhagic fever and dengue shock syndrome (Bäck and Lundkvist, 2013). The DENV genome has a single reading frame encoding three structural proteins (capsid protein, precursor membrane protein and envelope proteins) and at least seven non-structural (NS) proteins, all of which are produced from a single large precursor protein by proteolytic cleavage (Zhang et al., 2021).
The dengue DIII domain of the E protein (dEDIII) is considered as a primary target for vaccine development due to its potential to activate a neutralizing antibody responses (Leng et al., 2009; Chen et al., 2013). dEDIII and the premembrane protein (prM) have been successfully expressed in cowpea, lettuce chloroplasts, N. benthamiana and tobacco chloroplasts (Martínez et al., 2010; Kanagarajan et al, 2012; Carlos Marques et al., 2014; Gottschamel et al., 2016; van Eerde et al., 2019). For example, dEDIII was expressed in N. tabacum by stable nuclear transformation (Kim et al, 2015), chloroplast transformation (Gottschamel et al., 2016), or transient expression in N. benthamiana, with the latter approach leading to higher production levels (Chen et al., 2013; Kim et al., 2015; Gottschamel et al., 2016). dEDIII produced in lettuce chloroplasts for oral vaccination showed immunogenicity in rabbits (van Eerde et al., 2019). To display the antigen on the surface of HBcAg capsid-like particles (CLP), dEDIII was inserted into the immunodominant c/e1 loop region of tandem HBcAg, leading to the formation of chimeric VLPs in N. benthamiana (Saejung et al., 2007; Martínez et al., 2010). DENV VLPs were also produced by transient co-expression of DENV structural proteins, including the capsid (C), the precursor membrane (prM) and the envelope (E) protein, and a truncated version of the non-structural proteins (NS2B-NS3) in N. benthamiana. The immunogenicity was evaluated and high antibody responses in mice were found (Ponndorf et al., 2021). Finally, a chimeric Dengue-Ebola recombinant immune complex was developed with dEDIII fused to the heavy chain of the 6D8 monoclonal antibody (Dengue RIC technology, DERIC; Phoolcharoen et al, 2011). This approach led to enhanced anti-cEDIII immune responses, suggesting its promise for the development of a new candidate vaccine (Kim et al., 2015).
There have been many reports on attempts to develop plant-made vaccines against zoonotic viruses, including yellow fever virus (YFV), west Nile virus (WNV), rabies virus (RABV), Japanese encephalitis virus (JEV), hantaviruses (HVs), rift valley fever virus (RVFV), crimean-congo haemorrhagic fever virus (CCHFV), chikungunya virus (CHIKV), and hepatitis E virus (HEV) (Table 2). However, there are still many diseases caused by zoonotic viruses for which therapeutic options are completely lacking such as Marburg virus (MV), Hendra virus (HeV), Nipah virus (NiV), tick-borne encephalitis virus (TBEV) and others.
Avian influenza is caused by influenza A virus (IAV) belonging to the genus Alphainfluenzavirus, family Orthomyxoviridae (International Committee on Taxonomy of Viruses, ICTV). AIV particles are spherical, approximately 100 nm in diameter, or filamentous, about 300 nm in length, and contain an eighth-segmented, negative ssRNA genome (Palese, 2007). The glycoprotein spikes of haemagglutinin (HA) represent nearly 80% of the total surface proteins, neuraminidase (NA) represents 17%, and the ion channel matrix protein 2 (M2) is another major surface protein. The M1 protein surrounds the core virus particle. AIVs are divided into serotypes of 16 HA and 9 NA variants, giving rise to many different combinations (named, e.g., H5N1). During infection of the same cell with two or more serotypes, reassortment event (i.e., exchange of genomic segments) can take place and produce new serotypes (referred to as antigenic shift). Frequent appearance of point mutations (due to lack of proofreading activity of the genome-replicating RNA-dependent RNA polymerase) leads to antigenic drift, which may further contribute to escape from host immune responses (Harder et al., 2016). Low-pathogenicity avian influenza (LPAI) may cause mild to intense respiratory disease symptoms and a mortality of up to 20% in chickens (Pusch and Suarez, 2018). Highly pathogenic avian influenza (HPAI), often characterized by a polybasic cleavage site in HA (of variants H5 and H7), causes intense symptoms and can induce peracute death without clinical signs, thus leading to high mortality in chickens and turkeys (Swayne and Sims, 2021).
HPAI H5 and H7, and LPAI H9N2, have been widely used as prototypical antigens for plant made AIV vaccine preparation for application in poultry (Pusch and Suarez, 2018). There have been many attempts to express AIV antigens in a variety of plant species such as N. benthamiana, alfalfa, soybean, lettuce, Arabidopsis thaliana, N. tabacum and duckweed. For example, the H7 variant of the HA antigen was transiently expressed in N. benthamiana (Kanagarajan et al., 2012). Lettuce showed the lowest antigen production upon comparison of alfalfa, lettuce and soybean (Farsad et al., 2016). To improve protein accumulation, different signal peptides targeting the antigen protein to the ER, the apoplast or protein bodies were introduced and protein accumulation was compared in alfalfa. A signal peptide targeting the antigen to the apoplast yielded the highest protein accumulation level. Furthermore, full-length HA performed best when targeted to the apoplast, whereas a truncated HA form accumulated to higher levels in the ER (Mortimer et al., 2012).
In an alternative approach, the ectodomain of the H5 variant of HA was fused to the intrinsic trigger motif of GCN4 (general control transcription factor 4), which promotes protein folding, was expressed in N. benthamiana. Plant extracts containing HA oligomers induced higher titers of neutralizing antibodies than extracts containing HA trimers in immunized mice (Phan et al., 2020). Similar results have been obtained in chickens (Mokoena et al., 2019). HA can self-assemble to produce VLPs, but co-transformation of HA and M2 improved the efficiency of VLP production (Smith et al., 2020). Engineered N. benthamania with reduced xylosylated and/or core alpha1,3-fucosylated glycan structures made the N-glycosylation patterns more similar to that in vertebrates (Major et al., 2015). AIV VLPs formulated with the Montanide adjuvant elicited strong immune responses, and provided better protection against viral challenge in chickens. It was estimated that 1 kg of plant material is sufficient to provide vaccine for up to 30,000 chickens, suggesting high cost-efficiency of the plant-made antigen and suitability of the vaccine also for use in resource-poor areas. The HA variant H5 expressed in transgenic A. thaliana triggered robust immune responses and provided protection from lethal challenge with AIV when freeze-dried leaf powder was orally administrated in mice (Lee et al., 2015). Similarly, mice and ferrets immunized with a truncated H5 protein produced in N. benthamiana could be protected from AIV infection (Major et al., 2015).
The extracellular domain of M2 (of 23 amino acids) is highly conserved in all AIV variants, thus making vaccines developed from M2 potentially effective against all subtypes. The M2e peptide, when expressed in duckweed, provided protection in mice after immunization by oral administration (Firsov et al., 2018).
Newcastle disease virus (NDV) infects birds through oropharyngeal secretions and faecal matter, or inhalation of contaminated dust or aerosols containing the virus. There are four pathotypes that have been classified according to the disease severity they cause in chicken: asymptomatic enteric, lentogenic, mesogenic and velogenic. The strongest velogenic strains cause acute lethal infection, occasional haemorrhagic lesions in the intestine, and neurological and respiratory disorders leading to morbidity and mortality of up to 100% (Aldous and Alexander, 2001).
NDV is a paramyxovirus, also known as avian orthoavulavirus 1 (AOaV-1), that belongs to the genus Orthoavulavirus and the subfamily Avulavirinae. It is an enveloped virus with a non-segmented negative-sense RNA genome encoding six structural proteins: nucleoprotein (NP), phosphoprotein (P), matrix protein (M), fusion protein (F), haemagglutinin-neuraminidase (HN), and large polymerase (L). The HN, F and M proteins are related to the viral envelope. HN and F mediate viral entry and are the main targets of host neutralizing antibodies (Maclachlan et al., 2010).
Among these viral proteins, the glycoproteins F and HN have been expressed in various plants such as N. benthamiana, N. tabacum, Zea mays, Solanum tuberosum and Oryza sativa. The expression levels obtained suggested great potential for further development of these antigens as candidate NDV vaccine. The plant-produced glycoproteins were folded properly and correctly post-translationally modified (Margolin et al., 2020). As early as in 2006, recombinant NDV HN protein produced in transgenic tobacco-derived suspension cell cultures was found to give 90% protection against lethal NDV challenge in chickens after three doses of subcutaneous injection (Yusibov et al., 2011). NDV HN gene was also expressed in tobacco by transient expression. Six-week-old chickens fed with transgenic tobacco expressing HN of NDV with sterilized PBS did not show a specific immune response (Hahn et al., 2007). However, recombinant HN and F proteins expressed in transgenic potato could stimulate mucosal immune responses in mice upon oral immunization (Gomez et al., 2008). Transgenic corn expressing the F and HN proteins also induced production of locally secreted IgY (the avian IgG equivalent) in orally immunized chickens (Shahid et al., 2020).
Immune tolerance in poultry is much less common than in laboratory animals like rats and mice (Friedman, 2008). Therefore, application of transgenic plants as edible vaccines has also been explored for ND prevention. The NDV F protein expressed in staple crops like transgenic rice and corn was reported to induce the production of neutralizing antibodies in chickens challenged with a velogenic strain and provide similar survival rates as commercial LaSota vaccine based on the attenuated virus (Guerrero-Andrade et al., 2006; Ma et al., 2020). Recombinant concatenated F and HN proteins were expressed in transgenic tobacco but unfortunately induced unideal production of antibodies in rabbits (Shahriari et al., 2019).
Over 40 farmed marine fish species are susceptible to viral nervous necrosis (VNN) caused by the nervous necrosis virus (NNV) (Lin et al., 2007; Walker and Winton, 2010). The disease is also called viral encephalopathy and retinopathy (VER), and its symptoms include skin darkening, abnormal swimming behaviour (Grotmol et al., 1997; Bovo et al., 1999; Thiery et al., 1999) and a mortality rate of up to 100% in larvae and early juvenile fish (Lin et al., 2007). NNV belongs to the betanodaviruses (Mori et al., 1992) in the family Nodaviridae. There are different genotypes of NNV such as striped jack NNV (SJNNV), tiger puffer NNV (TPNNV), barfin flounder NNV (BFNNV), redspotted grouper NNV (RGNNV) (Nishizawa et al., 1997), turbot NNV (TNNV) (Johansen et al., 2004) and three unclassified genotypes (Sahul Hameed et al., 2019). NNV particles are non-enveloped, and 25-30 nm in diameter. NNV contains two positive-sense RNAs, RNA1 encoding the RNA-dependent RNA polymerase and the non-structural protein B, and RNA2, encoding the coat protein precursor alpha, with RNA2 regulating RNA3 synthesis (Friesen and Rueckert, 1984; Mori et al., 1992).
The coat protein precursor can self-assemble into provirions (Gallagher and Rueckert, 1988; Cheng et al., 1994). NNV VLPs have the potential to be used for vaccine development. Dragon grouper NNV (DGNNV) VLPs and grouper NNV (GNNV) VLPs expressed in E. coli inhibited viral attachment to the surface of fish nerve cells and exhibited immune stimulatory activity in vaccinated fish (Lu et al., 2003; Lai et al., 2014), as well as stimulation of the complement system, an important component in teleost innate immunity (Lai et al., 2014). Plant-produced vaccines and especially VLPs, are potentially cost-efficient, which is particularly important in aquaculture (Marsian et al., 2019). The coat protein of Atlantic cod NNV (ACNNV) was expressed in N. benthamiana leaves and transgenic tobacco BY-2 cells, producing VLPs with similar structure to previously reported GNNV particles. The VLPs provided partial protection against ACNNV in intraperitoneally or intramuscularly immunized sea bass (Dicentrarchus labrax) (Marsian et al., 2019).
The cardiomyopathy syndrome (CMS) has been reported in both farmed and wild Atlantic salmon (Salmo salar) since the 1980s (Amin and Trasti, 1988; Poppe and Seierstad, 2003; Haugland et al., 2011) It leads to infiltration of mononuclear cells in the subendocardium, and degeneration and necrosis of the spongious myocardium (Bruno et al., 2013), which in turn can result in rupture of the atrium wall or sinus venosus and consequently sudden death (Bruno and Poppe, 1996). The causative pathogen, piscine myocarditis virus (PMCV), tentatively assigned to the Totiviridae family, is a small, non-enveloped icosahedral virus. Its single, linear double-stranded RNA genome encodes three open reading frames (ORF1-3), encoding a capsid protein and an RNA-dependent RNA polymerase. A subunit vaccine produced in plants has been established as an economical approach to combat PMCV infections (Clarke et al., 2017; van Eerde et al., 2019). There is currently no commercial vaccine available against PMCV, but the putative capsid protein of PMCV has the potential to be developed as an effective vaccine. The protein was transiently expressed in N. benthamiana and shown to form VLPs. The VLPs triggered innate immune responses, resulting in viral load inhibition in several tissues and reduction of lesions in the heart, as evaluated by a newly established PMCV infection model (Su et al., 2021). Plant-made VLP antigens against PMCV are worthy of further study towards providing protection from CMS in the future.
Vaccines against viral diseases in humans and animals based on plant expression platforms have been developed in many plant species, including the grain crops rice and maize, and vegetable crops such as banana and tomato. Compared to traditional vaccines, the long shelf-life, the potential delivery as raw plant material, and the superior temperature stability (bypassing the necessity to maintain an uninterrupted cooling chain) are key attractions of plant-based edible vaccines (Yang et al., 2017). Although requiring more labour and longer time to produce, plant-made vaccines don’t have the strict limitations of instability and low temperature storage of mRNA-based vaccines. Nevertheless, plant made vaccines have a high requirement for costly infrastructure for downstream processing and purification as well as the necessary clinical trials. Compared to microbes and mammalian cells which require expensive fermenters and sterile conditions to ensure compliance with pharmaceutical Good Manufacturing Practice (GMP), plants cultivated in greenhouses or open fields are less expensive and easily scalable, but there are challenges in controlling quality and safety, as well as costly extraction and purification (Schillberg and Finnern, 2021). General factors that need to be addressed with plant-produced vaccines include batch-to-batch consistency, requirement for dosage standardization, dependence of product yields on environmental factors, plant development and plant health, and immunogenicity after downstream processing and (for oral vaccines) after food processing (e.g., cooking or drying) (Masavuli et al., 2017). Uncertainty in the business world is a further challenge, exemplified by the closure of Medicago (producer of the SARS-CoV-2 VLP vaccine) by its parent company, Mitsubishi.
Plant-based vaccines are subject to strict regulatory requirements, especially when produced in genetically modified plants. The use of transgenic technologies adds further issues that need to be dealt with, including biosafety concerns, people’s acceptance, the risk of contaminating the food chain, plant-specific post-translational modifications, and the possible development of oral tolerance to edible vaccines (Kirk et al., 2005). Nevertheless, proper regulatory measures, appropriate monitoring and risk management upon production and distribution should reduce these potential risks to an acceptable level.
To date, the successful application and potential for large-scale production of plant based VLP vaccines have been demonstrated for several viral diseases, including HBV, HPV, HIV and AIV. Furthermore, the plant-based production technologies have also been applied to antibody production for oral, topical or parenteral administration (Venkataraman et al., 2021; Monreal-Escalante et al., 2022).
Future research should contribute to overcoming the limitations and regulatory hurdles of plant-made viral vaccines such as low productivity, costs of downstream processing and slow translation to applications (reviewed by Schillberg and Finnern, 2021). To exploit the advantages of plant-produced viral vaccines and overcome their limitations will make plant molecular farming an important source of vaccines against viral diseases.
In summary, plant-made vaccines against viral diseases in humans or farm animals offer great potential in disease prevention and antiviral therapy. Many plant produced vaccines under clinical trials are likely to provide more evidence of safety and efficacy for future applications. It can be expected that the rapidly progressing field of plant-derived vaccines and biopharmaceuticals will be able to provide fast, safe, and cost-effective solutions for disease control and prevention, with substantial benefits especially to the developing world. As more and more products enter the market, a proper and, ideally, internationally harmonized regulatory framework for the production of recombinant vaccine proteins in (transgenic) plants should be developed and regularly reviewed to ensure that it remains fit for purpose.
HS and JC initiated and designed the overall concept and wrote the manuscript. All authors contributed to the article and approved the submitted version.
This work was supported by the EEA Grants 2014-2021, SmartVac project no. 1SEE/2019, the Research Council of Norway and National Natural Science Foundation of China co-funded Feed2Food project Grant no. 319693, and NIBIO core funding to China collaboration (Project No. 51133).
The authors declare that the research was conducted in the absence of any commercial or financial relationships that could be construed as a potential conflict of interest.
All claims expressed in this article are solely those of the authors and do not necessarily represent those of their affiliated organizations, or those of the publisher, the editors and the reviewers. Any product that may be evaluated in this article, or claim that may be made by its manufacturer, is not guaranteed or endorsed by the publisher.
Abiri, R., Valdiani, A., Maziah, M., Shaharuddin, N. A., Sahebi, M., Yusof, Z. N. B., et al. (2016). A critical review of the concept of transgenic plants: insights into pharmaceutical biotechnology and molecular farming. Curr. Issues Mol. Biol. 18, 21–42. doi: 10.21775/cimb.018.021
Aggarwal, S. (2009). What’s fueling the biotech engine-2008. Nat. Biotechnol. 27, 987–993. doi: 10.1038/nbt1109-987
Aldous, E. W., Alexander, D. J. (2001). Detection and differentiation of Newcastle disease virus (avian paramyxovirus type 1). Avian. Pathol. 30, 117–128. doi: 10.1080/03079450120044515
Amin, A., Trasti, J. (1988). Endomyocarditis in Atlantic salmon in Norwegian seafarms. B. Eur. Assoc. Fish Pat. 8, 70–71.
Antoniou, E., Orovou, E., Sarella, A., Iliadou, M., Rigas, N., Palaska, E., et al. (2020). Zika virus and the risk of developing microcephaly in infants: A systematic review. Int. J. Environ. Res. Public Health 17, 3806. doi: 10.3390/ijerph17113806
Armbruster, N., Jasny, E., Petsch, B. (2019). Advances in RNA vaccines for preventive indications: a case study of a vaccine against rabies. Vaccines 7, 132. doi: 10.3390/vaccines7040132
Ashraf, S., Singh, P. K., Yadav, D. K., Shahnawaz, M., Mishra, S., Sawant, S. V., et al. (2005). High level expression of surface glycoprotein of rabies virus in tobacco leaves and its immunoprotective activity in mice. J. Biotechnol. 119, 1–14. doi: 10.1016/j.jbiotec.2005.06.009
Avesani, L., Merlin, M., Gecchele, E., Capaldi, S., Pezzotti, M. (2013). Comparative analysis of different biofactories for the production of a major diabetes autoantigen. Transgenic Res. 23, 281–291. doi: 10.1007/s11248-013-9749-9
Bäck, A. T., Lundkvist, A. (2013). Dengue viruses-an overview. Infect. Ecol. Epidemiol. 3, 19839. doi: 10.3402/iee.v3i0.19839
Bailey, J. R., Barnes, E., Cox, A. L. (2019). Approaches, progress, and challenges to hepatitis c vaccine development. Gastroenterology 156, 418–430. doi: 10.1053/j.gastro.2018.08.060
Beeching, N. J., Fenech, M., Houlihan, C. F. (2014). Ebola Virus disease. BMJ Dec 10 349, 7348. doi: 10.1136/bmj.g7348
Bock, R. (2015). Engineering plastid genomes: methods, tools, and applications in basic research and biotechnology. Annu. Rev. Plant Biol. 66, 211–241. doi: 10.1146/annurev-arplant-050213-040212
Bock, R., Warzecha, H. (2010). Solar-powered factories for new vaccines and antibiotics. Trends Biotechnol. 28, 246–252. doi: 10.1016/j.tibtech.2010.01.006
Bovo, G., Nishizawa, T., Maltese, C., Borghesan, F., Mutinelli, F., Montesi, F., et al. (1999). Viral encephalopathy and retinopathy of farmed marine fish species in Italy. Virus Res. 63, 143–146. doi: 10.1016/s0168-1702(99)00068-4
Bowen, E. T., Platt, G. S., Lloyd, G., Baskerville, A., Harris, W. J., Vella, E. E. (1977). Viral haemorrhagic fever in southern Sudan and northern zaire. preliminary studies on the aetiological agent. Lancet, 571–573.
Bruno, D. W., Noguera, P. A., Poppe, T. T. (2013). “Viral diseases: piscine myocardiatis virus (cardiomyopathy syndrome),” in A colour atlas of salmonid diseases (Dordrecht: Springer), 67–72. doi: 10.1007/978-94-007-2010-7
Bruno, D., Poppe, T. (1996). “Diseases of uncertain aetiology,” in Cardiac myopathy syndrome. a colour atlas of salmonid diseases (London: Academic Press), 140–141.
Bwaka, M. A., Bonnet, M. J., Calain, P., Colebunders, R., De Roo, A., Guimard, Y., et al. (1999). Ebola Hemorrhagic fever in kikwit, democratic republic of the Congo: clinical observations in 103 patients. J. Infect. Dis. 1999 Feb 1 179, S1–S7. doi: 10.1086/514308
Cardi, T., Lenzi, P., Maliga, P. (2010). Chloroplasts as expression platforms for plant-produced vaccines. Expert Rev. Vaccines 9, 893–911. doi: 10.1586/erv.10.78
Carlos Marques, L. É., da Silva, B. B., Lisboa Magalhães, Marques Mendes, I. C., de Almeida, M. M., Florindo Guedes, L. M. (2014). M. i. production of dengue 2 envelope domain III in plant using CPMV-based vector system. BMC Proc. 8, P80. doi: 10.1186/1753-6561-8-S4-P80
Castells-Graells, R., Lomonossoff, G. P. (2021). Plant-based production can result in covalent cross-linking of proteins. Plant Biotechnol. J. 19, 1095–1097. doi: 10.1111/pbi.13598
Cenciarelli, O., Gabbarini, V., Pietropaoli, S., Malizia, A., Tamburrini, A., Ludovici, G. M. (2015). Viral bioterrorism: learning the lesson of Ebola virus in West Africa 2013-2015. Virus Res. 210, 318–326. doi: 10.1016/j.virusres.2015.09.002
Chambers, T., Monath, T. (2003). The flaviviruses: structure, replication and evolution. 1st ed (Cambridge, MA, USA: Academic Press), ISBN 9780080493817.
Chen, Y., Guo, D. (2016). Molecular mechanisms of coronavirus RNA capping and methylation. Virol. Sin. 31, 3–11. doi: 10.1007/s12250-016-3726-4
Chen, T. H., Hu, C. C., Liao, J. T., Lee, Y. L., Huang, Y. W., Lin, N. S., et al. (2017). Production of Japanese encephalitis virus antigens in plants using bamboo mosaic virus-based vector. Front. Microbiol. 8. doi: 10.3389/fmicb.2017.00788
Chen, H. W., Liu, S. J., Li, Y. S., Liu, H. H., Tsai, J. P., Chiang, C. Y., et al. (2013). A consensus envelope protein domain III can induce neutralizing antibody responses against serotype 2 of dengue virus in non-human primates. Arch. Virol. 158, 1523–1531. doi: 10.1007/s00705-013-1639-1
Chen, H. Y., Zhang, J., Gao, Y., Du, H. L., Ma, Y., Zheng, W. Z., et al. (2002). Transforming HBsAg into peanut and detection of its immunogenecity. Lett. Biotechnol. 4, 245–250.
Cheng, R. H., Reddy, V. S., Olson, N. H., Fisher, A. J., Baker, T. S., Johnson, J. E. (1994). Functional implications of quasi-equivalence in a T = 3 icosahedral animal virus established by cryo-electron microscopy and X-ray crystallography. Structure 2, 271–282. doi: 10.1016/s0969-2126(00)00029-0
Clarke, J. L., Paruch, L., Dobrica, M. O., Caras, I., Tucureanu, C., Onu, A., et al. (2017). Lettuce- produced hepatitis c virus E1E2 heterodimer triggers immune responses in mice and antibody production after oral vaccination. Plant Biotechnol. J. 15, 1611–1621. doi: 10.1111/pbi.12743
Clarke, J. L., Waheed, M. T., Loüssl, A. G. (2013). How can plant genetic engineering contribute to cost-effective fish vaccine development for promoting sustainable aquaculture? Plant Mol. Biol. 83, 33–40. doi: 10.1007/s11103-013-0081-9
Daniell, H., Nair, S. K., Esmaeili, N., Wakade, G., Shahid, N., Ganesan, P. K., et al. (2022). Debulking SARS-CoV-2 in saliva using angiotensin converting enzyme 2 in chewing gum to decrease oral virus transmission and infection. Mol. Ther. 30, 1966–1978. doi: 10.1016/j.ymthe.2021.11.008
Davoodi‐Semiromi, A., Schreiber, M., Nalapalli, S., Verma, D., Singh, N. D., Banks, R. K. (2010). Chloroplast‐derived vaccine antigens confer dual immunity against cholera and malaria by oral or injectable delivery. Plant biotechnology journal 8, 223–242. doi: 10.1111/j.1467-7652.2009.00479.x
de Araújo, T. V. B., Rodrigues, L. C., de Alencar Ximenes, R. A., de Barros Miranda-Filho, D., Montarroyos, U. R., de Melo, A. P. L., et al. (2016). Association between zika virus infection and microcephaly in Brazil, January to may 2016: Preliminary report of a case-control study. Lancet Infect. Dis. 16, 1356–1363. doi: 10.1016/S1473-3099(16)30318-8
Diamos, A. G., Pardhe, M. D., Sun, H., Hunter, J. G. L., Kilbourne, J. (2020a). A highly expressing, soluble, and stable plant-made IgG fusion vaccine strategy enhances antigen immunogenicity in mice without adjuvant. Front. Immunol. 11. doi: 10.3389/fimmu.2020.576012
Diamos, A. G., Pardhe, M. D., Sun, H., Hunter, J. G., Mor, T., Meador, L., et al. (2020b). Codelivery of improved immune complex and virus-like particle vaccines containing zika virus envelope domain III synergistically enhances immunogenicity. Vaccine 38, 3455–3463. doi: 10.1016/j.vaccine.2020.02.089
Dobrica, M. O., Lazar, C., Paruch, L., Skomedal, H., Steen, H., Haugslien, S., et al. (2017). A novel chimeric hepatitis b virus S/preS1 antigen produced in mammalian and plant cells elicits stronger humoral and cellular immune response than the standard vaccine-constituent. s protein. Antivir. Res. 144, 256–265. doi: 10.1016/j.antiviral.2017.06.017
Dobrica, M. O., van Eerde, A., Tucureanu, C., Onu, A., Paruch, L., Caras, I., et al. (2021). Hepatitis c virus E2 envelope glycoprotein produced in Nicotiana benthamiana triggers humoral response with virus-neutralizing activity in vaccinated mice. Plant Biotechnol. J. 19, 2027–2039. doi: 10.1111/pbi.13631
Eddleston, A. (1990). Modern vaccines. hepatitis. Lancet 335, 1142–1145. doi: 10.1016/0140-6736(90)91138-Z
Ehsani, P., Khabiri, A., Domansky, N. N. (1997). Polypeptides of hepatitis b surface antigen produced in transgenic potato. Gene 190, 107–111. doi: 10.1016/S0378-1119(96)00647-6
Farsad, A. S., Malek Zadeh, S., Moshtaqi, N., Fotouhi, F., Zibaei, S. (2016). Transient expression of HA1 antigen of avian influenza virus (H5N1) in alfalfa, soybean and lettuce leaves by agroinfiltration. Agric. Biotechnol. J. 8, 61–80. doi: 10.22103/JAB.2016.1419
Faye, O., Freire, C. C. M., Iamarino, A., Faye, O., de Oliveira, J. V. C., Diallo, M., et al. (2014). Molecular evolution of zika virus during its emergence in the 20th century. PloS Negl. Trop. Dis. 8, e2636. doi: 10.1371/journal.pntd.0002636
Firsov, A., Tarasenko, I., Mitiouchkina, T., Shaloiko, L., Kozlov, O., Vinokurov, L., et al. (2018). Expression and immunogenicity of M2e peptide of avian influenza virus H5N1 fused to ricin toxin b chain produced in duckweed plants. Front. Chem. 6. doi: 10.3389/fchem.2018.00022
Friedman, A. (2008). Oral tolerance in birds and mammals: Digestive tract development determines the strategy. J. Appl. Poult. Res. 17, 168–173. doi: 10.3382/japr.2007-00099
Friesen, P. D., Rueckert, R. R. (1984). Early and late functions in a bipartite RNA virus: evidence for translational control by competition between viral mRNAs. J. Virol. 49, 116–124. doi: 10.1128/jvi.49.1.116-124.1984
Fuenmayor, J., Cervera, L. (2017). Production of virus like particles for vaccines. New Biotechnol. 39, 174–180. doi: 10.1016/j.nbt.2017.07.010
Funk, C. D., Ardakani, A. (2021). Target product profile analysis of COVID-19 vaccines in phase III clinical trials and beyond: an early 2021 perspective. Viruses 13, 418. doi: 10.3390/v13030418
Gallagher, T. M., Rueckert, R. R. (1988). Assembly-dependent maturation cleavage in provirions of a small icosahedral insect ribovirus. J. Virol. 62, 3399–3406. doi: 10.1128/jvi.62.9.3399-3406.1988
Ganapathi, T. R., Sunil Kumar, G. B., Srinivas, L., Revathi, C. J., Bapat, V. A. (2007). Analysis of the limitations of hepatitis b surface antigen expression in soybean cell suspension cultures. Plant Cell Rep. 26, 1575–1584. doi: 10.1007/s00299-007-0379-7
Gao, Y., Ma, Y., Li, M., Cheng, T., Li, S. W., Zhang, J., et al. (2003). Oral immunization of animals with transgenic cherry tomatillo expressing HBsAg. World J. Gastroenterol. 9, 996–1002. doi: 10.3748/wjg.v9.i5.996
Ghiasi, S. M., Salmanian, A. H., Chinikar, S., Zakeri, S. (2011). Mice orally immunized with a transgenic plant expressing the glycoprotein of Crimean-Congo hemorrhagic fever virus. Clin. Vaccine Immunol. 18, 2031–2037. doi: 10.1128/CVI.05352-11
Gobeil, P., Pillet, S., Séguin, A., Boulay, I., Mahmood, A., Vinh, D. C., et al. (2021). Interim report of a phase 2 randomized trial of a plant-produced virus-like particle vaccine for covid-19 in healthy adults aged 18*64 and older adults aged 65 and older. medRxiv 2021.
Gomez, E., Chimeno Zoth, S., Carrillo, E., Estela Roux, M., Berinstein, A. (2008). Mucosal immunity induced by orally administered transgenic plants. Immunobiology 213, 671–675. doi: 10.1016/j.imbio.2008.02.002
Gonzalez-Rabade, N., McGowan, E. G., Zhou, F., McCabe, M. S., Bock, R., Dix, P. J., et al. (2011). Immunogenicity of chloroplast-derived HIV-1 p24 and a p24-nef fusion protein following subcutaneous and oral administration in mice. Plant Biotechnol. J. 9, 629–638. doi: 10.1111/j.1467-7652.2011.00609.x
Gorshkov, K., Shiryaev, S. A., Fertel, S., Lin, Y. W., Huang, C. T., Pinto, A., et al. (2019). Zika virus: origins, pathological action, and treatment strategies. Front. Microbiol. 9. doi: 10.3389/fmicb.2018.03252
Gottschamel, J., Lössl, A., Ruf, S., Wang, Y., Skaugen, M., Bock, R., et al. (2016). Production of dengue virus envelope protein domain III-based antigens in tobacco chloroplasts ising inducible and constitutive expression systems. Plant Mol. Biol. 91, 497–512. doi: 10.1007/s11103-016-0484-5
Govea-Alonso, D. O., Gómez-Cardona, E. E., Rubio-Infante, N., García-Hernández, A. L., Varona-Santos, J. T., Salgado-Bustamante, M., et al. (2013). Production of an antigenic C4(V3)6 multiepitopic HIV protein in bacterial and plant systems. Plant Cell Tissue Organ Cult. 113, 73–79. doi: 10.1007/s11240-012-0252-4
Gregory, C. J., Oduyebo, T., Brault, A. C., Brooks, J. T., Chung, K. W., Hills, S., et al. (2017). Modes of transmission of zika virus. J. Infect. Dis. 216, S875–S883. doi: 10.1093/infdis/jix396
Grotmol, S., Totland, G. K., Thorud, K., Hjeltnes, B. K. (1997). Vacuolating encephalopathy and retinopathy associated with a nodavirus-like agent: a probable cause of mass mortality of cultured larval. Dis. Aquat. Org 29, 85–97. doi: 10.3354/dao029085
Guan, Z. J., Guo, B., Huo, Y. L., Guan, Z. P., Dai, J. K., Wei, Y. H. (2013). Recent advances and safety issues of transgenic plant-derived vaccines. appl. Microbiol. Biotechnol. 97, 2817–2840. doi: 10.1007/s00253-012-4566-2
Guerrero-Andrade, O., Loza-Rubio, E., Olivera-Flores, T., Fehervari-Bone, T., Gomez-Lim, M. A. (2006). Expression of the Newcastle disease virus fusion protein in transgenic maize and immunological studies. Transgenic Res. 15, 455–463. doi: 10.1007/s11248-006-0017-0
Hahn, B. S., Jeon, I. S., Jung, Y. J., Kim, J. B., Parl, J. S., Ha, S. H., et al. (2007). Expression of hemagglutinin-neuraminidase protein of Newcastle disease virus in transgenic tobacco. Plant Biotechnol. Rep. 1, 85–92. doi: 10.1007/s11816-007-0012-9
Harder, T. C., Buda, S., Hengel, H., Beer, M., Mettenleiter, T. C. (2016). Poultry food products-a source of avian influenza virus transmission to humans? Clin. Microbiol. Infect. 22, 141–146. doi: 10.1016/j.cmi.2015.11.015
Haugland, O., Mikalsen, A. B., Nilsen, P., Lindmo, K., Thu, B. J., Eliassen, T. M., et al. (2011). Cardiomyopathy syndrome of Atlantic salmon (Salmo salar l.) is caused by a double-stranded RNA virus of the totiviridae family. J. Virol. 85, 5275–5286. doi: 10.1128/JVI.02154-10
Hayden, C. A., Egelkrout, E. M., Moscoso, A. M., Enrique, C., Keener, T. K., Jimenez-Flores, R., et al. (2012). Production of highly concentrated, heat-stable hepatitis b surface antigen in maize. Plant Biotechnol. J. 10, 979–984. doi: 10.1111/j.1467-7652.2012.00727.x
He, J., Lai, H., Brock, C., Chen, Q. (2011). A novel system for rapid and cost-effective production of detection and diagnostic reagents ofWest Nile virus in plants. J. Biomed. Biotechnol. 2012, e106783. doi: 10.1155/2012/106783
He, J., Lai, H., Esqueda, A., Chen, Q. (2021). Plant-produced antigen displaying virus-like particles evokes potent antibody responses against West Nile virus in mice. Vaccines 9, 60. doi: 10.3390/vaccines9010060
He, W., Baysal, C., Lobato Gómez, M., Huang, X., Alvarez, D., Zhu, C., et al. (2021). Contributions of the international plant science community to the fight against infectious diseases in humans-part 2: Affordable drugs in edible plants for endemic and re-emerging diseases. Plant Biotechnol. J. 19, 1921–1936. doi: 10.1111/pbi.13658
He, J., Peng, L., Lai, H., Hurtado, J., Stahnke, J., Chen, Q. (2014). A plant-produced antigen elicits potent immune responses against West Nile virus in mice. BioMed. Res. Int. 2014, e952865. doi: 10.1155/2014/952865
Herbert, A. S., Kuehnek, A. I., Barth, J. F., Ortiz, R. A., Nichols, D. K., Zak, S. E. (2013). Venezuelan Equine encephalitis virus replicon particle vaccine protects non-human primates from intramuscular and aerosol challenge with ebolavirus. J. Virol. 87, 4952–4964. doi: 10.1128/JVI.03361-12
Hoenen, T., Groseth, A., Feldmann, H. (2019). Therapeutic strategies to target the Ebola virus life cycle. Nat. Rev. Microbiol. 17, 593–606. doi: 10.1038/s41579-019-0233-2
Huang, Z., Santi, L., LePore, K., Kilbourne, J., Arntzen, C. J., Mason, H. S. (2006). Rapid, high-level production of hepatitis b core antigen in plant leaf and its immunogenicity in mice. Vaccine 24, 2506–2513. doi: 10.1016/j.vaccine.2005.12.024
Imani, J., Berting, A., Nitsche, S., Schaefer, S., Gerlich, W. H., Neumann, K. H. (2002). The integration of a major hepatitis b virus gene into cell-cycle synchronized carrot cell suspension cultures and its expression in regenerated carrot plants. Plant Cell Tiss. Org. Cult 71, 157–164. doi: 10.1023/A:1019903216459
Jain, I. R., Ansari, N. M., Shingi, R. S., Walode, S. G., Chatur, V. M. (2021). Plant based edible vaccines: A review. World J. Pharmaceut. Res. 10, 378–392. doi: 10.20959/wjpr202114-22267
Johansen, R., Sommerset, I., Torud, B., Korsnes, K., Hjortaas, M. J., Nilsen, F., et al. (2004). Characterization of nodavirus and viral encephalopathy and retinopathy in farmed turbot, Scophthalmus maximus (L.). J. Fish Dis. 27, 591–601. doi: 10.1111/j.1365-2761.2004.00581.x
Joung, Y. H., Park, S. H., Moon, K. B., Jeon, J. H., Cho, H. S., Kim, H. S. (2016). The last ten years of advancements in plant-derived recombinant vaccines against hepatitis b. Int. J. Mol. Sci. 17, 1715. doi: 10.3390/ijms17101715
Kalbina, I., Lagerqvist, N., Moiane, B., Ahlm, C., Andersson, S., Strid, Å., et al. (2016). Arabidopsis thaliana plants expressing rift valley fever virus antigens: Mice exhibit systemic immune responses as the result of oral administration of the transgenic plants. Protein Expr. Purif. 127, 61–67. doi: 10.1016/j.pep.2016.07.003
Kanagarajan, S., Tolf, C., Lundgren, A., Waldenström, J., Brodelius, P. E. (2012). Transient expression of hemagglutinin antigen from low pathogenic avian influenza a (H7N7) in nicotiana benthamiana. PloS One 7, e33010. doi: 10.1371/journal.pone.0033010
Karpova, O. V., Nikitin, N. A. (2022). Plant viruses: New opportunities under the pandemic. Her. Russ. Acad. Sci. 92, 464–469. doi: 10.1134/S1019331622040153
Khattak, S., Darai, G., Süle, S., Rösen-Wolff, A. (2002). Characterization of expression of puumala virus nucleocapsid protein in transgenic plants. Intervirology 45, 334–339. doi: 10.1159/000067926
Kim, M. Y., Reljic, R., Kilbourne, J., Ceballos-Olvera, I., Yang, M. S., Reyes-del Valle, J., et al. (2015). Novel vaccination approach for dengue infection based on recombinant immune complex universal platform. Vaccine 33, 1830–1838. doi: 10.1016/j.vaccine.2015.02.036
Kirk, D. D., McIntosh, K., Walmsley, A. M., Peterson, R. K. D. (2005). Risk analysis for plant-made vaccines. Transgenic Res. 14, 449–462. doi: 10.1007/s11248-005-5697-3
Ksiazek, T. G., Rollin, P. E., Williams, A. J., Bressler, D. S., Martin, M. L., Swanepoel, R., et al. (1999). Clinical virology of Ebola hemorrhagic fever (EHF): virus, virus antigen, and IgG and IgM antibody findings among EHF patients in kikwit, democratic republic of the Cong. J. Infect. Dis. 179, S177–S187. doi: 10.1086/514321
Lai, Y. X., Jin, B. L., Xua, Y., Huanga, L. J., Huanga, R. Q., Zhanga, Y., et al. (2014). Immune responses of orange-spotted grouper, epinephelus coioides, against virus-like particles of betanodavirus produced in escherichia coli. Vet. Immunol. Immunopathol. 157, 87–96. doi: 10.1016/j.vetimm.2013.10.003
Lai, H., Paul, A. M., Sun, H., He, J., Yang, M., Bai, F., et al. (2018). A plant-produced vaccine protects mice against lethal West Nile virus infection without enhancing zika or dengue virus infectivity. Vaccine 36, 1846–1852. doi: 10.1016/j.vaccine.2018.02.073
Lakshmi, P. S., Verma, D., Yang, X., Lloyd, B., Daniell, H., Cardona, P. J. (2013). Low cost tuberculosis vaccine antigens in capsules: expression in chloroplasts, bio-encapsulation, stability and functional evaluation in vitro. PloS One 8, e54708. doi: 10.1371/journal.pone.0054708
Lebel, M., Chartrand, K., Leclerc, D., Lamarre, A. (2015). Plant viruses as nanoparticle-based vaccines and adjuvants. Vaccines 3, 620–637. doi: 10.3390/vaccines3030620
LeBlanc, Z., Waterhouse, P., Bally, J. (2020). Plant-based vaccines: The way ahead? Viruses 13, 5. doi: 10.3390/v13010005
Lee, G., Na, Y. J., Yang, B. G., Choi, J. P., Seo, Y. B., Hong, C. P., et al. (2015). Oral immunization of haemaggulutinin H5 expressed in plant endoplasmic reticulum with adjuvant saponin protects mice against highly pathogenic avian influenza a virus infection. Plant Biotechnol. J. 13, 62–72. doi: 10.1111/pbi.12235
Leng, C. H., Liu, S. J., Tsai, J. P., Li, Y. S., Chen, M. Y., Liu, H. H., et al. (2009). A novel dengue vaccine candidate that induces cross-neutralizing antibodies and memory immunity. Microbes Infect. 11, 288–295. doi: 10.1016/j.micinf.2008.12.004
Li, G., De Clercq, ,. E. (2020). Therapeutic options for the 2019 novel coronaviru-nCoV) (Berlin: Nature Publishing Group). doi: 10.1038/d41573-020-00016-0
Li, G., Fan, Y., Lai, Y., Han, T., Li, Z., Zhou, P., et al. (2020). Coronavirus infections and immune responses. J. Med. Virol. 92, 424–432. doi: 10.1002/jmv.25685
Lin, C. C., Lin, J. H. Y., Chen, M. S., Yang, H. L. (2007). An oral nervous necrosis virus vaccine that induces protective immunity in larvae of grouper (Epinephelus coioides). Aquaculture 268, 265–273. doi: 10.1016/j.aquaculture.2007.04.066
Llovet, J. M., Kelley, R. K., Villanueva, A., Singal, A. G., Pikarsky, E., Roayaie, S., et al. (2021). Hepatocellular carcinoma. Nat. Rev. Dis. Primers 7, 6. doi: 10.1038/s41572-020-00240-3
Loüssl, A. G., Waheed, M. T. (2011). Chloroplast-derived vaccines against human diseases: achievements, challenges and scopes. Plant Biotechnol. J. 9, 527–539. doi: 10.1111/j.1467-7652.2011.00615.x
Lobato Gómez, M., Huang, X., Alvarez, D., He, W., Baysal, C., Zhu, C., et al. (2021). Contributions of the international plant science community to the fight against human infectious diseases-part 1: epidemic and pandemic diseases. Plant Biotechnol. J. 19, 1901–1920. doi: 10.1111/pbi.13657
Loza-Rubio, E., Rojas-Anaya, E., López, J., Olivera-Flores, M. T., Gómez-Lim, M., Tapia-Pérez, G. (2012). Induction of a protective immune response to rabies virus in sheep after oral immunization with transgenic maize, expressing the rabies virus glycoprotein. Vaccine 30, 5551–5556. doi: 10.1016/j.vaccine.2012.06.039
Lu, M. W., Liu, W., Lin, C. S. (2003). Infection competition against grouper nervous necrosis virus by virus-like particles produced in escherichia coli. J. Gen. Virol. 84, 1577–1582. doi: 10.1099/vir.0.18649-0
Ma, F., Zhang, E., Li, Q., Xu, Q., Ou, J., Yin, H., et al. (2020). A plant-produced recombinant fusion protein-based Newcastle disease subunit vaccine and rapid differential diagnosis platform. Vaccines 8, 122. doi: 10.3390/vaccines8010122
Maclachlan, N. J., Dubovi, Fenner, E. J. (2010). Veterinary virology. 5th ed (Cambridge, MA, USA: Academic Press).
Madesis, P., Osathanunkul, M., Georgopoulou, U., Gisby, M. F., Mudd, E. A., Nianiou, I. (2010). A hepatitis C virus core polypeptide expressed in chloroplasts detects anti-core antibodies in infected human sera. J. Biotechnol. 145, 377–386. doi: 10.1016/j.jbiotec.2009.12.001
Maharjan, P. M., Choe, S. (2021). Plant-based COVID-19 vaccines: Current status, design, and development strategies of candidate vaccines. Vaccines 9, 992. doi: 10.3390/vaccines9090992
Mahmood, N., Nasir, S. B., Hefferon, K. (2021). Plant-based drugs and vaccines for COVID-19. Vaccines 9, 15. doi: 10.3390/vaccines9010015
Major, D., Chichester, J. A., Pathirana, R. D., Guilfoyle, K., Shoji, Y., Guzman, C. A., et al. (2015). Intranasal vaccination with a plant-derived H5 HA vaccine protects mice and ferrets against highly pathogenic avian influenza virus challenge. Hum. Vaccines Immunother. 11, 1235–1243. doi: 10.4161/21645515.2014.988554
Malik, Y. S., Sircar, S., Bhat, S., Sharun, K., Dhama, K., Dadar, D. (2020). Emerging novel coronaviru-nCoV)-current scenario, evolutionary perspective based on genome analysis and recent developments. Vet. Q. 40, 68–76. doi: 10.1080/01652176.2020.1727993
Mardanova, E., Takova, K., Toneva, V., Zahmanova, G., Tsybalova, L., Ravin, N. (2020). A plant-based transient expression system for the rapid production of highly immunogenic hepatitis e virus-like particles. Biotechnol. Lett. 42, 2441–2446. doi: 10.1007/s10529-020-02995-x
Margolin, E., Crispin, M., Meyers, A., Chapman, R., Rybicki, E. P. (2020). A roadmap for the molecular farming of viral glycoprotein vaccines: engineering glycosylation and glycosylation-directed folding. Front. Plant Sci. 11. doi: 10.3389/fpls.2020.609207
Margolin, E., Schafer, G., Allen, J., Gers, S., Woodward, J., Sutherland, A. D., et al. (2023). A plant-produced SARS-CoV-2 spike protein elicits heterologous immunity in hamsters. Front. Plant Sci. 14. doi: 10.3389/fpls.2023.1146234
Marsian, J., Fox, H., Bahar, M. W., Kotecha, A., Fry, E. E., Stuart, D. I., et al. (2017). Plant-made polio type 3 stabilized VLPs-a candidate synthetic polio vaccine. Nat. Commun. 8, 245. doi: 10.1038/s41467-017-00090-w
Marsian, J., Hurdiss, D. L., Ranson, N. A., Ritala, A., Paley, R., Cano, I., et al. (2019). Plant-made nervous necrosis virus-like particles protect fish against disease. Front. Plant Sci. 10. doi: 10.3389/fpls.2019.00880
Martínez, C. A., Topal, E., Giulietti, A. M., Talou, J. R., Mason, H. (2010). Exploring different strategies to express dengue virus envelope protein in a plant system. Biotechnol. Lett. 32, 867–875. doi: 10.1007/s10529-010-0236-6
Masavuli, M. G., Wijesundara, D. K., Torresi, J., Gowans, E. J., Grubor-Bauk, B. (2017). Preclinical development and production of virus-like particles as vaccine candidates for hepatitis c. Front. Microbiol. 8. doi: 10.3389/fmicb.2017.02413
Mason, H. S., Man-Kit, L. D., Arntzen, C. J. (1992). Expression of hepatitis b surface antigen in transgenic plants. Proc. Nati. Acad. Sci. U.S.A. 89, 11745–11749. doi: 10.1073/pnas.89.24.11745
Matoba, N., Magérus, A., Geyer, B. C., Zhang, Y., Muralidharan, M., Alfsen, A., et al. (2004). A mucosally targeted subunit vaccine candidate eliciting HIV-1 transcytosis-blocking abs. Proc. Natl. Acad. Sci. U.S.A. 101, 13584–13589. doi: 10.1073/pnas.040529710
Mbewana, S., Meyers, A. E., Rybicki, E. P. (2019). Chimaeric rift valley fever virus-like particle vaccine candidate production in Nicotiana benthamiana. Biotechnol. J. 14, e1800238. doi: 10.1002/biot.201800238
McAleer, W., Buynak, E., Maigetter, R., Wampler, D. E., Miller, W. J., Hilleman, M. R. (1984). Human hepatitis b vaccine from recombinant yeast. Nature 307, 178–180. doi: 10.1038/307178a0
McCabe, M. S., Klaas, M., Gonzalez-Rabade, N., Poage, M., Badillo-Corona, J. A., Zhou, F., et al. (2008). Plastid transformation of high-biomass tobacco variety Maryland mammoth for production of human immunodeficiency virus type 1 (HIV-1) p24 antigen. Plant Biotechnol. J. 6, 914–929. doi: 10.1111/j.1467-7652.2008.00365.x
McLain, L., Durrani, Z., Wisniewski, L. A., Porta, C., Lomonossoff, G. P., Dimmock, N. J. (1996). Stimulation of neutralizing antibodies to human immunodeficiency virus type 1 in three strains of mice immunized with a 22 amino acid peptide of Gp41 expressed on the surface of a plant virus. Vaccine 14, 799–810. doi: 10.1016/0264-410X(95)00229-T
McLain, L., Porta, C., Lomonossoff, G. P., Durrani, Z., Dimmock, N. J. (1995). Human immunodeficiency virus type 1-neutralizing antibodies raised to a glycoprotein 41 peptide expressed on the surface of a plant virus. AIDS Res. Hum. Retrovir. 11, 327–334. doi: 10.1089/aid.1995.11.327
McMahon, B. J., Dentinger, C. M., Bruden, D., Zanis, C., Peters, H., Hurlburt, D. (2009). Antibody levels and protection after hepatitis b vaccine: results of a 22-year follow-up study and response to a booster dose. J. Infect. Dis. 200, 1390–1396. doi: 10.1086/606119
Miranda, M. E., Miranda, N. L. (2011). Reston ebolavirus in humans and animals in the Philippines: a review. J. Infect. Dis. 204, S757–S760. doi: 10.1093/infdis/jir296
Mohammadzadeh, S., Roohvand, F., Memarnejadian, A., Jafari, A., Ajdary, S., Salmanian, A. H., et al. (2016). Co-Expression of hepatitis c virus polytope-HBsAg and p19-silencing suppressor protein in tobacco leaves. Pharm. Biol. 54, 465–473. doi: 10.3109/13880209.2015.1048371
Mokoena, N. B., Moetlhoa, B., Rutkowska, D. A., Mamputha, S., Dibakwane, V. S., Tsekoa, T. L., et al. (2019). Plant-produced bluetongue chimaeric VLP vaccine candidates elicit serotype-specific immunity in sheep. Vaccine 37, 6068–6075. doi: 10.1016/j.vaccine.2019.08.042
Monreal-Escalante, E., Ramos-Vega, A., Angulo, C., Bañuelos-Hernández, B. (2022). Plant-based vaccines: Antigen design, diversity, and strategies for high level production. Vaccines 10, 100. doi: 10.3390/vaccines10010100
Mori, K., Nakai, T., Muroga, K., Arimoto, M., Mushiake, K., Furusawa, I. (1992). Properties of a new virus belonging to nodaviridae found in larval striped jack (Pseudocaranx dentex) with nervous necrosis. Virology 187368-, 371. doi: 10.1016/0042-6822(92)90329-n
Mortimer, E., Maclean, J. M., Mbewana, S., Buys, A., Williamson, A. L., Hitzeroth, I. I., et al. (2012). Setting up a platform for plant-based influenza virus vaccine production in south Africa. BMC Biotechnol. 12, 14. doi: 10.1186/1472-6750-12-14
Murugesan, A., Manoharan, M. (2020). “Chapter 16-dengue virus,” in Emerging and reemerging viral pathogens. Ed. Ennaji, M. M. (Cambridge, MA, USA: Academic Press), 281–359. doi: 10.3390/life12020156
Nassal, M. (2015). HBV cccDNA: viral persistence reservoir and key obstacle for a cure of chronic hepatitis b. Gut 64, 1972–1984. doi: 10.1136/gutjnl-2015-309809
Nemchinov, L. G., Liang, T. J., Rifaat, M. M., Mazyad, H. M., Hadidi, A., Keith, J. M. (2000). Development of a plant-derived subunit vaccine candidate against hepatitis c virus. Arch. Virol. 145, 2557–2573. doi: 10.1007/s007050070008
Nishizawa, T., Furuhashi, M., Nagai, T., Nakai, T., Muroga, K. (1997). Genomic classification of fish nodaviruses by molecular phylogenetic analysis of the coat protein gene. Appl. Environ. Microbiol. 63, 1633–1636. doi: 10.1128/aem.63.4.1633-1636.1997
Nooraei, S., Bahrulolum, H., Hoseini, Z. S., Katalani, C., Hajizade, A., Easton, A. J. (2021). Virus-like particles: preparation, immunogenicity and their roles as nanovaccines and drug nanocarriers. J.Nanobiotech. 19, 1–27. doi: 10.1186/s12951-021-00806-7
Ortega-Berlanga, B., Pniewski, T. (2022). Plant-based vaccines in combat against coronavirus diseases. Vaccines 10, 138. doi: 10.3390/vaccines10020138
Palese, P. (2007). Orthomyxoviridae: The viruses and their replication. In Fields Virology, 5th ed.; Fields, B.N., Knipe, D.M., Howley, P.M., Eds.; Wolters Kluwer Health/Lippincott Williams & Wilkins: Philadelphia, PA, USA, 2007. 1647–1689.
Paniz-Mondolfi, A. E., Blohm, G. M., Hernandez-Perez, M., Larrazabal, A., Moya, D., Marquez, M., et al. (2019). Cutaneous features of zika virus infection: A clinicopathological overview. Clin. Exp. Dermatol. 44, 13–19. doi: 10.1111/ced.13793
Pantazica, A.-M. M., Cucos, L. M., Stavaru, C., Clarke, J. C., Branza-Nichita, N. (2021). Challenges and prospects of plant-derived oral vaccines against hepatitis b and c viruses. Plants 10, 2037. doi: 10.3390/plants10102037
Pantazica, A. M., Dobrica, M. O., Lazar, C., Scurtu, C., Tucureanu, C., Caras, I., et al. (2022). Efficient cellular and humoral immune response and production of virus- neutralizing antibodies by the hepatitis b virus S/preS116-42 antigen. Front. Immunol. 13, 941243. doi: 10.3389/fimmu.2022.941243
Perea Arango, I., Loza Rubio, E., Rojas Anaya, E., Olivera Flores, T., de la Vara, L. G., Gómez Lim, M. A. (2008). Expression of the rabies virus nucleoprotein in plants at high-levels and evaluation of immune responses in mice. Plant Cell Rep. 27, 677–685. doi: 10.1007/s00299-007-0324-9
Peyret, H., Ponndorf, D., Meshcheriakova, Y., Richardson, J., Lomonossoff, G. P. (2020). Covalent protein display on hepatitis b core-like particles in plants through the in vivo use of the SpyTag/SpyCatcher system. Sci. Rep. 10, 17095. doi: 10.1038/s41598-020-74105-w
Phan, H. T., Pham, V. T., Ho, T. T., Pham, N. B., Chu, H. H., Vu, T. H., et al. (2020). Immunization with plant-derived multimeric H5 hemagglutinins protect chicken against highly pathogenic avian influenza virus H5N1. Vaccines 8, 593. doi: 10.3390/vaccines8040593
Phoolcharoen, W., Bhoo, S. H., Lai, H., Ma, J., Arntzen, C. J., Chen, Q., et al. (2011). Expression of an immunogenic Ebola immune complex in nicotiana benthamiana. Plant Biotechnol. J. 9, 807–816. doi: 10.1111/j.1467-7652.2011.00593.x
Pierson, T. C., Diamond, M. S. (2020). The continued threat of emerging flaviviruses. Nat. Microbiol. 5, 796–812. doi: 10.1038/s41564-020-0714-0
Pniewski, T. (2013). The twenty-year story of a plant-based vaccine against hepatitis b: Stagnation or promising prospects? Int. J. Mol. Sci. 14, 1978–1998. doi: 10.3390/ijms14011978
Pniewski, T., Kapusta, J., Bociąg, P., Wojciechowicz, J., Kostrzak, A., Gdula, M., et al. (2011). Low-dose oral immunization with lyophilized tissue of herbicide-resistant lettuce expressing hepatitis b surface antigen for prototype plant-derived vaccine tablet formulation. J. Appl. Genet. 52, 125–136. doi: 10.1007/s13353-010-0001-5
Pniewski, T., Kapusta, J., Płucienniczak, A. (2006). Agrobacterium tumefaciens-mediated transformation of yellow lupin to generate callus tissue producing surface antigen of HBV in a long-term culture. J. Appl. Genet. 47, 309–318. doi: 10.1007/BF03194640
Ponndorf, D., Meshcheriakova, Y., Thuenemann, E. C., Dobon Alonso, A., Overman, R., Holton, N., et al. (2021). Plant-made dengue virus-like particles produced by Co-expression of structural and non-structural proteins induce a humoral immune response in mice. Plant Biotechnol. J. 19, 745–756. doi: 10.1111/pbi.13501
Poppe, T. T., Seierstad, S. L. (2003). First description of cardiomyopathy syndrome (CMS)- related lesions in wild Atlantic salmon salmo salar in Norway. Dis. Aquat. Org. 56, 87–88. doi: 10.3354/dao056087
Porta, C., Spall, V. E., Loveland, J., Johnson, J. E., Barker, P. J., Lomonossoff, G. P. (1994). Development of cowpea mosaic virus as a high-yielding system for the presentation of foreign peptides. Virology 202, 949*955. doi: 10.1006/viro.1994.1417
Pusch, E. A., Suarez, D. L. (2018). The multifaceted zoonotic risk of H9N2 avian influenza. Vet. Sci. 5, 82. doi: 10.3390/vetsci5040082
Qiu, X., Wong, G., Audet, J., Bello, A., Fernando, L., Alimonti, J. B., et al. (2014). Reversion of advanced Ebola virus disease in nonhuman primates with ZMapp. Nature 514, 47–53. doi: 10.1038/nature13777
Quaranta, M. G., Ferrigno, L., Tata, X., D’Angelo, F., Coppola, C., Ciancio, A. (2021). Liver function following hepatitis c virus eradication by direct acting antivirals in patients with liver cirrhosis: data from the PITER cohort. BMC Infect. Dis. 21, 413. doi: 10.1186/s12879-021-06053-3
Ramírez, Y. J. P., Tasciotti, E., Gutierrez-Ortega, A., Torres, A. J. D., Flores, M. T. O., Giacca, M., et al. (2007). Fruit-specific expression of the human immunodeficiency virus type 1 tat gene in tomato plants and its immunogenic potential in mice. Clin. Vaccine Immunol. 14, 685–692. doi: 10.1128/CVI.00028-07
Richter, L. J., Thanavala, Y., Arntzen, C. J., Mason, H. S. (2000). Production of hepatitis b surface antigen in transgenic plants for oral immunization. Nat. Biotechnol. 18, 1167–1171. doi: 10.1038/81153
Ríos-Huerta, R., Monreal-Escalante, E., Govea-Alonso, D. O., Angulo, C., Rosales-Mendoza, S. (2017). Expression of an immunogenic LTB-based chimeric protein targeting Zaire ebolavirus epitopes from GP1 in plant cells. Plant Cell Rep. 36, 355–365. doi: 10.1007/s00299-016-2088-6
Rosales-Mendoza, S. (2020). Will plant-made biopharmaceuticals play a role in the fight against COVID-19? Expert. Opin. Biol. Ther. 20, 545–548. doi: 10.1080/14712598.2020.1752177
Rosales-Mendoza, S., Salazar-González, J. A. (2014). Immunological aspects of using plant cells as delivery vehicles for oral vaccines. Expert Rev. Vaccines 13, 737–749. doi: 10.1586/14760584.2014.913483
Saejung, W., Fujiyama, K., Takasaki, T., Ito, M., Hori, K., Malasit, P., et al. (2007). Production of dengue 2 envelope domain III in plant using TMV-based vector system. Vaccine 25, 6646–6654. doi: 10.1016/j.vaccine.2007.06.029
Sahul Hameed, A. S., Ninawe, A. S., Naka, T., Chi, S. C., Johnson, K. L., ICTV Report Consortium (2019). ICTV virus taxonomy profile: nodaviridae. J. Gen. Virol. 100, 3–4. doi: 10.1099/jgv.0.001170
Schillberg, S., Finnern, R. (2021). Plant molecular farming for the production of valuable proteins–critical evaluation of achievements and future challenges. J. Plant Physiol. 258, 153359. doi: 10.1016/j.jplph.2020.153359
Shahid, N., Samiullah, T. R., Shakoor, S., Latif, A., Yasmeen, A., Azam, S., et al. (2020). Early stage development of a Newcastle disease vaccine candidate in corn. Front. Vet. Sci. 7. doi: 10.3389/fvets.2020.00499
Shahriari, A. G., Bagheri, A., Afsharifar, A., Habibi-Pirkoohi, M. (2019). Induction of immune response in animal model using recombinant anti-NDV vaccine. Iran. J. Biotechnol. 17, e2215. doi: 10.21859/IJB.2215
Sissoko, D., Laouenan, C., Folkesson, E., M’lebing, A. B., Beavogui, A. H., Baize, S., et al. (2016). Experimental treatment with favipiravir for Ebola virus disease (the JIKI trial): a historically controlled, single-arm proof-of-concept trial in Guinea. PloS Med. 13, e1001967. doi: 10.1371/journal.pmed.1001967
Smith, T., O’Kennedy, M. M., Wandrag, D. B. R., Adeyemi, M., Abolnik, C. (2020). Efficacy of a plant-produced virus-like particle vaccine in chickens challenged with influenza a H6N2 virus. Plant Biotechnol. J. 18, 502–512. doi: 10.1111/pbi.13219
Song, Z., Xu, Y., Bao, L., Zhang, L., Yu, P., Qu, Y., et al. (2019). Thrusting coronaviruses into the spotlight. Viruses 11, 59. doi: 10.3390/v11010059
Srinivas, L., Sunil Kumar, G. B., Ganapathi, T. R., Revathi, C. J., Bapat, V. A. (2008). Transient and stable expression of hepatitis b surface antigen in tomato (Lycopersicon esculentum l.). Plant Biotechnol. Rep. 2, 1–6. doi: 10.1007/s11816-008-0041-z
Stander, J., Mbewana, S., Meyers, A. E. (2022). Plant-derived human vaccines: Recent developments. BioDrugs 36, 573–589. doi: 10.1007/s40259-022-00544-8
Su, H., Su, J. G. (2018). Cyprinid viral diseases and vaccine development. Fish Shellfish Immunol. 83, 84–95. doi: 10.1016/j.fsi.2018.09.003
Su, H., van Eerde, A., Steen, H. S., Heldal, I., Haugslien, S., Ørpetveit, I., et al. (2021). Establishment of a piscine myocarditis virus (PMCV) challenge model and testing of a plant-produced subunit vaccine candidate against cardiomyopathy syndrome (CMS) in Atlantic salmon Salmo salar. Aquaculture 541, 736806. doi: 10.1016/j.aquaculture.2021.736806
Su, J., Zhu, L., Sherman, A., Wang, X., Lin, S., Kamesh, A., et al. (2015). Low cost industrial production of coagulation factor IX bioencapsulated in lettuce cells for oral tolerance induction in hemophilia b. Biomaterials 70, 84–93. doi: 10.1016/j.biomaterials.2015.08.004
Sullivan, N. J., Sanchez, A., Rollin, P. E., Yang, Z. Y., Nabel, G. J. (2000). Development of a preventive vaccine for Ebola virus infection in primates. Nature 408, 605–609. doi: 10.1038/35046108
Sunil Kumar, G. B., Ganapathi, T. R., Revathi, C. J., Srinivas, L., Bapat, V. A. (2005). Expression of hepatitis b surface antigen in transgenic banana plants. Planta 222, 484–493. doi: 10.1007/s00425-005-1556-y
Swayne, D. E., Sims, L. (2021). “Avian influenza,” in Veterinary vaccines: Principles and applications. Eds. Metwally, S., El Idrissi, M., Viljoen, G. (Chichester, UK: Wiley-Blackwell).
Tacket, C. O. (2009). Plant-based oral vaccines: results of human trials. Curr. Top. Microbiol. Immunol. 332, 103–117. doi: 10.1007/978-3-540-70868-1_6
Takeyama, N., Kiyono, H., Yuki, Y. (2015). Plant-based vaccines for animals and humans: recent advances in technology and clinical trials. Therapeutic advances in vaccines 3, 139–154. doi: 10.1177/2051013615613272
Tatsuhiko, A., Yoshikazu, Y., Hiroshi, K. (2014). Challenges in mucosal vaccines for the control of infectious diseases. Int. Immunol. 26, 517. doi: 10.1093/intimm/dxu063
Thanavala, Y., Yang, Y. F., Lyons, P., Mason, H. S., Arntzen, C. (1995). Immunogenicity of transgenic plant-derived hepatitis b surface antigen. Proc. Natl. Acad. Sci. U.S.A. 92, 3358–3361. doi: 10.1073/pnas.92.8.3358
Thiery, R., Raymond, J. C., Castric, J. (1999). Natural outbreak of viral encephalopathy and retinopathy in juvenile sea bass, Dicentrarchus labrax: study by nested reverse transcriptase-polymerase chain reaction. Virus Res. 63, 11–17. doi: 10.1016/s0168-1702(99)00053-2
Tottey, S., Shoji, Y., Jones, R. M., Chichester, J. A., Green, B. J., Musiychuk, K., et al. (2017). Plant-produced subunit vaccine candidates against yellow fever induce virus neutralizing antibodies and confer protection against viral challenge in animal models. Am. J. Trop. Med. Hyg. 98, 420–431. doi: 10.4269/ajtmh.16-0293
Valiant, W. G., Huang, Y.-J. S., Vanlandingham, D. L., Higgs, S., Lewis, M. G., Mattapallil, J. J. (2018). Zika convalescent macaques display delayed induction of anamnestic cross-neutralizing antibody responses after dengue infection. Emerg. Microbes Infect. 7, 1–11. doi: 10.1038/s41426-018-0132-z
van Eerde, A., Gottschamel, J., Bock, R., Hansen, K. E. A., Munang’andu, H. M., Daniell, H., et al. (2019). Production of tetravalent dengue virus envelope protein domain III based antigens in lettuce chloroplasts and immunologic analysis for future oral vaccine development. Plant Biotechnol. J. 17, 1408–1417. doi: 10.1111/pbi.13065
Venkataraman, S., Hefferon, K., Makhzoum, A., Abouhaidar, M. (2021). Combating human viral diseases: will plant-based vaccines be the answer? Vaccines 9, 761. doi: 10.3390/vaccines9070761
Vesikari, T., Langley, J. M., Segall, N., Ward, B. J., Cooper, C., Poliquin, G., et al. (2021). Immunogenicity and safety of a tri-antigenic versus a mono-antigenic hepatitis b vaccine in adults (Protect): A randomised, double-blind, phase 3 trial. Lancet Infect. Dis. 21, 1271–1281. doi: 10.1016/S1473-3099(20)30780-5
Walker, P. J., Winton, J. R. (2010). Emerging viral diseases of fish and shrimp. Vet. Res. 41, 51. doi: 10.1051/vetres/2010022
Wang, Y., Deng, H., Zhang, X., Xiao, H., Jiang, Y., Song, Y., et al. (2009). Generation and immunogenicity of Japanese encephalitis virus envelope protein expressed in transgenic rice. Biochem. Biophys. Res. Commun. 380, 292–297. doi: 10.1016/j.bbrc.2009.01.061
Wang, L., Wang, R., Wang, L., Ben, H., Yu, L., Gao, F., et al. (2019). Structural basis for neutralization and protection by a zika virus-specific human antibody. Cell Rep. 26, 3360–3368. doi: 10.1016/j.celrep.2019.02.062
Ward, B. J., Gobeil, P., Se´guin, A., Atkins, J., Boulay, I., Charbonneau, P.-Y., et al. (2021). Phase 1 randomized trial of a plant-derived virus-like particle vaccine for COVID-19. Nat. Med. 27, 1071–1078. doi: 10.1038/s41591-021-01370-1
Ward, B. J., Landry, N., Tre´panier, S., Mercierb, G., Dargis, M., Couture, M., et al. (2014). Human antibody response to n-glycans present on plant-made influenza virus-like particle (VLP) vaccines. Vaccine 32, 6098–6106. doi: 10.1016/j.vaccine.2014.08.079
Warren, T. K., Wells, J., Panchal, R. G., Stuthman, K. S., Garza, N. L., Van Tongeren, S. A., et al. (2014). Protection against filovirus diseases by a novel broad-spectrum nucleoside analogue BCX4430. Nature 508, 402–405. doi: 10.1038/nature13027
Williams, T. C., Burgers, W. A. (2021). SARS-CoV-2 evolution and vaccines: cause for concern? Lancet Respir. Med. 9, 333–335. doi: 10.1016/S2213-2600(21)00075-8
Yang, M., Lai, H., Sun, H., Chen, Q. (2017). Virus-like particles that display zika virus envelope protein domain III induce potent neutralizing immune responses in mice. Sci. Rep. 7, 7679. doi: 10.1038/s41598-017-08247-9
Yang, M., Sun, H., Lai, H., Hurtado, J., Chen, Q. (2018). Plant-produced zika virus envelope protein elicits neutralizing immune responses that correlate with protective immunity against zika virus in mice. Plant Biotechnol. J. 16, 572–580. doi: 10.1111/pbi.12796
Yusibov, V., Hooper, D. C., Spitsin, S. V., Fleysh, N., Kean, R. B., Mikheeva, T., et al. (2002). Expression in plants and immunogenicity of plant virus-based experimental rabies vaccine. Vaccine 20, 3155–3164. doi: 10.1016/S0264-410X(02)00260-8
Yusibov, V., Streatfield, S. J., Kushnir, N. (2011). Clinical development of plant-produced recombinant pharmaceuticals: Vaccines, antibodies and beyond. hum. Vaccine 7, 313–321. doi: 10.4161/hv.7.3.14207
Zahmanova, G., Mazalovska, M., Takova, K., Toneva, V., Minkov, I., Peyret, H. (2021). Efficient production of chimeric hepatitis b virus-like particles bearing an epitope of hepatitis e virus capsid by transient expression in Nicotiana benthamiana. Life 11, 64. doi: 10.3390/life11010064
Zahmanova, G., Takova, K., Valkova, R., Toneva, V., Minkov, I., Andonov, A., et al. (2022). Plant-derived recombinant vaccines against zoonotic viruses. Life 12, 156. doi: 10.3390/life12020156
Zaki, S. R., Goldsmith, C. S. (1999). Pathologic features of filovirus infections in humans. Curr. Top. Microbiol. Immunol. 235, 97–116. doi: 10.1007/978-3-642-59949-1_7
Zampieri, C. A., Sullivan, N. J., Nabel, G. J. (2007). Immunopathology of highly virulent pathogens: insights from Ebola virus. Nat. Immunol. 8, 1159–1164. doi: 10.1038/ni1519
Zhang, X., Zhang, Y., Jia, R., Wang, M., Yin, Z., Cheng, A. (2021). Structure and function of capsid protein in flavivirus infection and its applications in the development of vaccines and therapeutics. Vet. Res. 52, 98. doi: 10.1186/s13567-021-00966-2
Keywords: plant-made vaccines, viral diseases, humans, farm animals, molecular farming, plant transformation
Citation: Su H, van Eerde A, Rimstad E, Bock R, Branza-Nichita N, Yakovlev IA and Clarke JL (2023) Plant-made vaccines against viral diseases in humans and farm animals. Front. Plant Sci. 14:1170815. doi: 10.3389/fpls.2023.1170815
Received: 21 February 2023; Accepted: 14 March 2023;
Published: 28 March 2023.
Edited by:
Sezer Okay, Hacettepe University, TürkiyeReviewed by:
Andrew G. Diamos, Arizona State University, United StatesCopyright © 2023 Su, van Eerde, Rimstad, Bock, Branza-Nichita, Yakovlev and Clarke. This is an open-access article distributed under the terms of the Creative Commons Attribution License (CC BY). The use, distribution or reproduction in other forums is permitted, provided the original author(s) and the copyright owner(s) are credited and that the original publication in this journal is cited, in accordance with accepted academic practice. No use, distribution or reproduction is permitted which does not comply with these terms.
*Correspondence: Jihong Liu Clarke, amlob25nLmxpdS1jbGFya2VAbmliaW8ubm8=
Disclaimer: All claims expressed in this article are solely those of the authors and do not necessarily represent those of their affiliated organizations, or those of the publisher, the editors and the reviewers. Any product that may be evaluated in this article or claim that may be made by its manufacturer is not guaranteed or endorsed by the publisher.
Research integrity at Frontiers
Learn more about the work of our research integrity team to safeguard the quality of each article we publish.