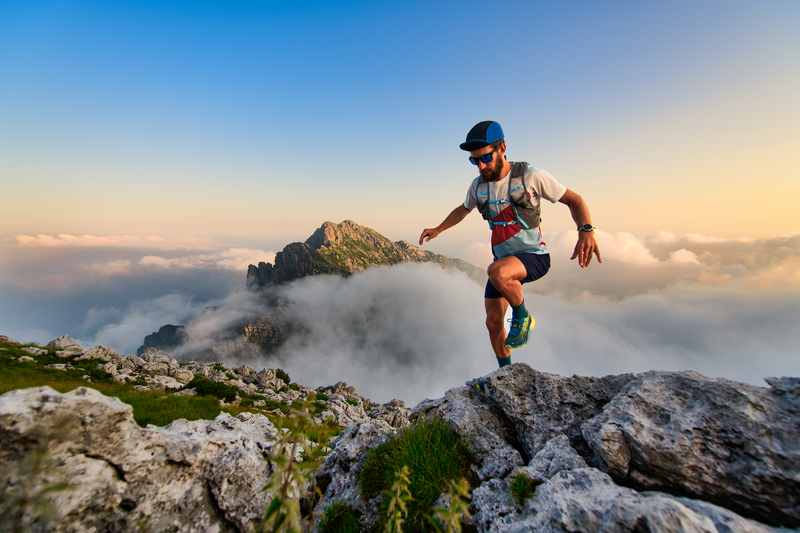
95% of researchers rate our articles as excellent or good
Learn more about the work of our research integrity team to safeguard the quality of each article we publish.
Find out more
REVIEW article
Front. Plant Sci. , 12 June 2023
Sec. Plant Pathogen Interactions
Volume 14 - 2023 | https://doi.org/10.3389/fpls.2023.1163270
This article is part of the Research Topic Innovative Strategies for Enhancing Crop Resilience Against Plant Viral Diseases View all 12 articles
This review analyzes methods for controlling plant viral infection. The high harmfulness of viral diseases and the peculiarities of viral pathogenesis impose special requirements regarding developing methods to prevent phytoviruses. The control of viral infection is complicated by the rapid evolution, variability of viruses, and the peculiarities of their pathogenesis. Viral infection in plants is a complex interdependent process. The creation of transgenic varieties has caused much hope in the fight against viral pathogens. The disadvantages of genetically engineered approaches include the fact that the resistance gained is often highly specific and short-lived, and there are bans in many countries on the use of transgenic varieties. Modern prevention methods, diagnosis, and recovery of planting material are at the forefront of the fight against viral infection. The main techniques used for the healing of virus-infected plants include the apical meristem method, which is combined with thermotherapy and chemotherapy. These methods represent a single biotechnological complex method of plant recovery from viruses in vitro culture. It widely uses this method for obtaining non-virus planting material for various crops. The disadvantages of the tissue culture-based method of health improvement include the possibility of self-clonal variations resulting from the long-term cultivation of plants under in vitro conditions. The possibilities of increasing plant resistance by stimulating their immune system have expanded, which results from the in-depth study of the molecular and genetic bases of plant resistance toward viruses and the investigation of the mechanisms of induction of protective reactions in the plant organism. The existing methods of phytovirus control are ambiguous and require additional research. Further study of the genetic, biochemical, and physiological features of viral pathogenesis and the development of a strategy to increase plant resistance to viruses will allow a new level of phytovirus infection control to be reached.
Viruses cause various pathological changes, which affect all aspects of plant life. Most viral diseases are characterized by systemic damage, in which the virus moves from the primary site of inoculation to other parts of the plant organism (Ershova et al., 2022; Wang et al., 2022). A virus is usually present until it dies off once the plant is infected, and it is passed to the offspring by vegetative propagation. The viral infection manifests itself in the appearance of plants.
The physiology and biochemistry of the host cells and tissues have changed internally and as a result of the virus (Hull, 2014). The properties of the host plant, the virulence and aggressiveness of the virus strain, the length of the infection, and the environmental factors all affect the disease’s diagnostic indications, which can vary greatly (Jones, 2009). These factors also determine the duration of the incubation period. The incubation period is usually a few days or weeks, which can be more than a year for herbaceous plants. Viral, viroid, and mycoplasma diseases are considered very harmful due to their chronic nature. They damage plant species, which leads to plant stress, death, and low crop yields (Table 1). They also change the chemical composition and deteriorate the quality of tubers (Adolf et al., 2020; Kreuze et al., 2020). Viral infection significantly changes the metabolism of plants, which includes a reduction in the photosynthetic activity of plants, which suppresses carbohydrates and other types of metabolism (Anikina and Seitzhanova, 2015). Chloroplasts are destroyed, changed, or aggregated due to viral infection. This leads to the destruction of chlorophyll or its non-participation in synthesis. The degree of photosynthetic suppression depends mainly on the disease development, the characteristics of the virus strain, the disease development phase, the virus strain–disease development phase’s characteristics, the host plant, and the environmental conditions (Table 2) (Chen et al., 2019; Akbar et al., 2021).
Almost all viral diseases are characterized by a decreased total carbohydrate content (Handford and Carr, 2007). This occurs due to the destruction of chlorophyll in the mosaic and jaundice lesions. The disruption of the transport of photosynthetic products from the leaves to other plant organs is also affected by viral infections (Akbar et al., 2021). The outflow of starch is disrupted when the phloem is affected, which overloads the parenchyma cells. As a result, the leaves become thicker, leathery, and brittle (Yu, 2015). Changes in the permeability of the parenchyma cell cytoplasm or the carbohydrase activity may also cause delayed starch outflow from the leaves. The study of the histological structure of plants revealed hypertrophy and hyperplasia, which resulted in the formation of tumors and enations. Many viruses affect the vascular system xylem and phloem, which causes the formation of tillers and cell death (Hull, 2014). As a result, the wilting of plants, the delayed outflow of the assimilates from the leaves, and the appearance of necroses on the vegetative plant in tubers and fruits are observed (Gupta N. et al., 2021). The phenomena of hypoplasia and metaplasia accompany the dwarfism of plants and the color changes in the case of virus infection. Many viruses cause changes in the fine structure of infected cells. Vesicles are formed at the periphery of the chloroplasts when they are under the influence of thymoviruses. Chloroplasts in tobacco mosaic virus (TMV)-infected tobacco cells become deformed and often degenerate. The formation of new chloroplasts is also inhibited in these types of cells (Bhattacharyya et al., 2015). These changes are responsible for the chlorosis and mosaic coloration of the affected leaves.
Viral infection causes metabolic abnormalities in the cells of the diseased plant. For example, the water regime is disturbed when it is infected with phytoviruses, which are accompanied by changes in the transpiration intensity and the water inflow due to changes in the vascular system (Anikina and Seitzhanova, 2015). The transpiration flow slows down, and the intensity of transpiration decreases, resulting from changes in the conductive system. In addition, there are changes in leaf transpiration surface due to the development of necroses (stomata malfunction) and the death of a part of the leaf apparatus. The metabolism of the affected plant changes, which can lead to its death due to the water balance disruption (Gupta N. et al., 2021). Almost all viral diseases are characterized by the disruption of nitrogen metabolism. Viruses have no enzymatic activity, but the essential role of the host plant’s enzymes is observed with the changes in the nitrogen-containing compounds (Hull, 2014). The proteolytic activity is significantly increased in potato leaves that are affected by the wrinkle mosaic, which can only be attributed to the proteinases of the potato itself. An increased soluble and nitrate nitrogen content is observed in the leaves and tubers of the potatoes that are affected by leaf curl, which is associated with the disruption of the nitrate restoration and protein synthesis processes. The total amount of nitrogen in the plant does not change when tobacco is infected with a mosaic pathogen. A significant portion of it still goes to virion building since the viral protein is formed at the expense of the host plant’s protein. The amount of non-protein nitrogen in infected tobacco is greatly reduced under nitrogen deficiency conditions. In contrast, the content of free amino acids increases with excess nitrogen probably due to the increased hydrolysis of the plant proteins (Dyakov et al., 2007).
Studies about the respiration of plants affected by viruses showed that viral infection stimulates the activity of dehydrases, which affect the initial phases of respiration, and the peroxidase activity of the affected plants increases at the same time (Hull, 2014). An increase in the respiration activity in tobacco plants during TMV infection was observed, whereas an increased oxidative activity after the influence of the beets and potatoes’ viral infection was also revealed. The activation of respiration is attributed to the protective reactions of the host plant. The peak of the respiration activity and the oxidative enzymes inhibit the reproduction of the virus at the beginning of the infection. The respiration intensity of the infected plant decreases, and the virion synthesis is activated. Many researchers show that viral diseases of plants are accompanied by dwarfism; the appearance of tumors and enations; changes in the shape of the leaves, flowers, and fruits; the formation of excessive buds; and the imbalance of the hormonal metabolism in plants under the influence of viral infection (Ma et al., 2022; Wang et al., 2022). This review aims to analyze and discuss contemporary methods for controlling viral infestation in plants by comparing various methods and taking into account the negative effects of viral diseases as well as the unique difficulties associated with viral pathogenesis. It also aims to identify efficient preventative measures to deal with phytoviruses by examining the molecular and genetic principles underpinning viral pathogenicity. As it focuses on creating strategies to control viral infections in plants, which is crucial for guaranteeing good crop production and addressing issues with food security, this evaluation helps to achieve Sustainable Development Goal 2: Zero Hunger by addressing these objectives.
The fight against viral infection is complicated due to the rapid evolution of viruses and the obligate parasitic nature of viruses. They penetrate the nucleus of cells, where viruses use the internal reserves of the plant for their reproduction (Tian and Valkonen, 2013; Jones and Naidu, 2019). Promising measures against plant viruses include breeding programs in regard to creating virus-resistant forms, which are in particular based on the marker-assisted selection method to evaluate the genetic defenses of a variety as well as the creation of transgenic varieties with high resistances to viral diseases (Klimenko et al., 2019; Akhter et al., 2021). It has become possible to insert certain genes into the genome of a plant, which is based on genetic engineering, which has resulted in new protective proteins that determine that the resistance to viruses is synthesized in the plant cells. Plant varieties with genes of extreme resistance to certain viruses have already been obtained. For example, a variety of soybean L29 with exceptional resistance to isolates of G5, G6, G7, and G5H strains has been obtained (Tran et al., 2018). R-resistance genes are expressed in plant cells during infection. More than 200 R genes with plant resistance to viruses, bacteria, and fungi have been cloned (Sharipova et al., 2013). Potato R genes are responsible for plant resistance to the potato virus X (PVX). Tomato SW-5 gene resists tomato spotted wilt virus, and tomato Tm and Tm2 genes resist tomato mosaic virus. Mutational analysis showed that R genes encode translation initiation factors that lead to overcoming the RNA virus infection (Sharipova et al., 2013). Studies about the molecular mechanisms of extreme resistance and their relationship with hypersensitive response concluded that the same NLR genes can trigger both extreme resistance and hypersensitive response. Genes that generally provide the phenotype of extreme resistance can be stimulated in order to induce a hypersensitive response by experimentally increasing cellular levels of derived pathogen proteins (Ross et al., 2021).
Most plant NLR proteins consist of three primary domains, which include the N-terminal helix-helix domain, the Toll/interleukin-1 receptor or divergent helix-helix domain, the central nucleotide-binding domain, and the C-terminal domain rich in leucine repeats (LRR). These proteins function as intracellular immune receptors, and the nucleotide-binding state partially controls their ability to induce an immune response. Inactive NLR proteins are specifically bound to ADP, whereas the recognition and binding of the effector pathogen protein allow the NLR to switch to an active and ATP-bound state that can initiate an immune response (Lolle et al., 2020). A total of 30 genes that are responsible for the resistance of potato plants to viruses X, T, A, and M have currently been identified. Genetic silencing is the deactivation or reduction of one of the plant’s genes using RNA interference, which involves the suppression of the gene expression by double-stranded RNA and is also used in order to create genetically modified virus-resistant plants. RNA silencing is an essential antiviral mechanism (Li and Wang, 2022; Lu et al., 2022). A DNA fragment is isolated from the genome and placed in a genetic construct in an inverted (antisense) position to turn off the target gene. Synthesized RNA does not encode anything in this case, but it can bind to the target gene’s mRNA. Translation stops and mRNA destruction occurs, and the expression of the target gene is drastically reduced or even completely stopped (Calil and Fontes, 2017; Kochetov and Shumny, 2017). There are currently great expectations for studying the regulatory pathways of plant resistance formation. Signal transduction from external factors leads to the activation of serine/threonine protein kinases, which phosphorylate the threonine or tyrosine residues of other regulatory proteins in this group that is activated by phosphorylation. The coordinated interaction of regulatory signaling pathways occurs during the plant infection, which results in the expression of resistance genes and increased plant defense against pathogens. A study of plant antiviral defense mechanisms revealed that when attacked by an infection, they activate the genes encoding PR proteins, which are pathogenesis-related proteins.
The type and level of PR-protein accumulation depend on the nature and level of the plant damage. Some PR proteins, such as proteinases and β-1,3-glucanases, promote the virus infection of plants. Other PR proteins, such as proteinase inhibitors, ribonucleases, and peroxidases, effectively protect plants against viruses. Signaling systems regulate their coordinated accumulation in plants. Thus, it has been determined that the accumulation of PR-10 proteins around the pathogen introduction or wounding site indicates their participation in plant defense mechanisms (Prasad and Srivastava, 2017). Transgenic plants with increased RNase expression are more resistant to pathogens than the original plants (Kochetov and Shumny, 2017). The disadvantages of the genetically engineered approaches include that the acquired resistance is often specific and short-lived, and there is a gene silencing problem (Li and Wang, 2022). The negative factors of cultivating genetically modified plants resistant to certain viruses include the possible redistribution of the virus species structure, which can spread other harmful viral infections. There are restrictions on using genetically modified organisms (GMOs), such as genetically modified potato varieties not being used in Kazakhstan. Modern methods of prevention, as well as the diagnosis and recovery of planting material, are at the forefront of potato virus infection control (Mumford et al., 2016; Kesiraju and Sreevathsa, 2017; Wang et al., 2022).
Progress in the development of resistant varieties, including those based on transgenic methods, is slow due to the lack of host genes and the duration of the breeding process and the problems associated with the limited use of transgenic plants in many countries (Tiwari et al., 2022). Genome editing technology is a promising approach to control viral pathologies. Breakthroughs in genome editing technology have been made in functional genomics and crop improvement (Zhang et al., 2018; Hofvander et al., 2022). Zinc finger nucleases (ZFNs) and effector nucleases (TALEN) were used as the first available genome-editing tools. These nucleases are chimeric proteins created by fusing their respective DNA-binding domains (DBDs) with the DNA cleavage domain of Fok I restrictase. The method of genome editing based on ZFNs and TALENS has been used for several plant species despite being labor-intensive, and important achievements have been obtained (Shan et al., 2013). However, nowadays, a new genome editing platform has been successfully used, which has surpassed the efficiency of ZFNs and TALEN in plants. According to scientists, CRISPR/Cas is currently the most powerful biological tool for targeted genome modification (Silva and Fontes, 2022; Tiwari et al., 2022). Many researchers consider the CRISPR/Cas-mediated immunity mechanism based on regularly spaced short palindromic repeats (CRISPR) and CRISPR-associated (Cas) proteins crucial in the fight against pathogens. This type of immunity has evolved as an adaptive immune system in archaea and bacteria against an invasion of foreign nucleic acids from viral or plasmid pathogens (Nussenzweig and Marraffini, 2020; Nidhi et al., 2021).
In recent years, there has been growing interest in using various aspects of CRISPR/Cas technologies to create plants resistant to viral pathogens. There are two ways to use CRISPR/Cas systems: the first involves direct targeting of viral genomes, and the second involves introducing targeted mutations into specific host plant genes that encode proteins used by the virus for successful replication and spread in the plant. The first method is the most widespread (Kavuri et al., 2022). Cas class 1 system include types I, III, and IV with different variants and effector complexes, whereas Cas class 2 systems comprise types II, V, and VI with an effector module containing a single multifunctional protein (Kavuri et al., 2022). Because of their simpler organization, class 2 Cas systems are most widely used as genome-editing tools, with type II and V systems using Cas9 and Cas12 enzymes to edit DNA. Notably, the CRISPR/Cas9 type II class 2 protein is one of the first Cas proteins to be studied, leading to its widespread use for DNA editing in animals, plants, and bacteria (Kavuri et al., 2022). Since the discovery of the CRISPR/Cas9 system in Streptococcus pyogenes, related systems have been discovered in many bacterial and archaeal species (Robertson et al., 2022). CRISPR/Cas9 has now produced important advances in plant research because of its simplicity, multiplexing, cost-effectiveness, high efficiency, and minimal target bias. The practical application of the CRISPR/Cas technology faces several bottlenecks whose solution is crucial for plant gene editing, one of which, for example, is the method of delivering components of the Cas9 RNA editing complex into plants. Gene delivery using plasmid DNA and Agrobacterium transformation (the main method used) leads to transgenic plants, which is prohibited by the legislation of many countries. The preferred methods are direct (free-associated) delivery of kgRNA and Cas9 protein, which have been actively developed recently (Tiwari et al., 2022). Knowledge of all aspects of CRISPR/Cas systems is constantly expanding.
There are certain limitations in DNA editing using CRISPR, such as the protospacer adjacent motif (PAM) site requirement, non-targeted mutations, and low efficacy against viruses (Ahmad et al., 2020). The recently identified CRISPR/Cas type VI systems can overcome some of these limitations, which use the Cas13 protein to provide sequence-specific cleavage of single-stranded RNA (ssRNA) molecules (Wolter and Puchta, 2018; Kavuri et al., 2022). The discovery of Cas endonucleases targeting RNA molecules marked a new turn in developing CRISPR/Cas technologies. One of the best known is the Cas13 class 2, type VI protein (including Cas13a and Cas13b). Unlike most CRISPR/Cas systems, Cas13 lacks a DNAase motif but contains two RNAase domains (HEPN). RNA manipulations are advantageous over DNA editing because they prevent unwanted pleiotropic effects, and RNA products can be precisely and spatially regulated (Robertson et al., 2022; Sharma et al., 2022). According to the Abudayyeh et al. research, Cas13 cleaves only target RNA molecules and can cleave ssRNA molecules containing sites homologous to cRNA, while, like Cas12a, Cas13 does not need tracrRNA and depends only on cRNA (Abudayyeh et al., 2017). Since most plant viruses contain RNAi genomes, this research opens up new technological possibilities for combating plant viruses. Another widely used RNA editing nuclease is FnCas9 from Francisella novicida (Price et al., 2015). This enzyme also works with guide 23 RNA and targets endogenous RNA in vivo. However, unlike Cas13, this protein does not contain HEPN domains. It has been suggested that FnCas9 may either recruit endogenous RNAases to cleave the target RNA or possess alternative domains with endonucleolytic activity (Robertson et al., 2022). Like Cas13, FnCas9 has been reprogrammed to target and inhibit RNA-containing human and plant viruses by blocking viral RNA translation and replication (Price et al., 2015; Zhang et al., 2018). Most research on the direct use of the CRISPR/Cas system has been performed on viruses belonging to the family Geminiviridae, which cause significant yield losses in economically important crops. The first experiments on CRISPR-mediated resistance to geminiviruses were carried out on the beet severe curly top virus (BSCTV, genus Curtovirus) and the bean yellow dwarf virus (BeYDV; genus Mastervirus) in Nicotiana benthamiana and Arabidopsis thaliana plants (Baltes et al., 2014). CRISPR/Cas9 constructs (including kgRNA and Cas9) were targeted to cut coding (such as the replication-related protein gene [Rep]) and non-coding regions in viral genomes (such as Rep binding sites) and an invariant non-coding sequence, the nanonucleotide [TAATATTAC], common to all geminiviruses, contained in the intergenic region [IR]. Plants were transfected with the obtained constructs, and it was shown that the transgenic plants showed a high level of resistance to the target virus, which is manifested by a decrease (up to 87%) in virus accumulation and a reduction of symptom manifestation (Baltes et al., 2014). Zhan et al. showed that the CRISPR/Cas13a system effectively provides a wide range of resistance in transgenic potato plants to potato virus Y (PVY) strains (Zhan et al., 2019). Also, studies by Zhao showed that the LshCas13a system can degrade viral RNA genomes and confer resistance to the RNA virus in monocotyledonous grain plants (Zhao et al., 2020). Transgenic rice plants carrying the CRISPR/Cas13a system were created with three sgRNAs, each targeting the RNA genomes of southern rice black-streaked dwarf virus (SRBSDV) and rice stripe mosaic virus (RSMV). The study confirmed the suppression of viral infection in transgenic rice plants, indicating that CRISPR/Cas13a can also effectively target viral RNA in monocotyledonous plants.
The advantages of this technology include that by working precisely at the gene level, it is possible to bypass the problems of “genetic modification” because genome editing occurs without integrating foreign DNA or RNA into the host genome. Moreover, this method is simple and versatile when compared to other modern breeding technologies (Robertson et al., 2022). Unlike genetically modified organisms, CRISPR/Cas changes the existing genome without introducing foreign genes, particularly site-directed nucleases (SDN1 and SDN2). Consequently, varieties obtained using CRISPR/Cas are expected to be transgenic-free, and biosafety issues will be eliminated. The creation of next-generation systems characterizes the current stage of development of CRISPR methods such as CRISPR-MAD7 and CAS12A nucleases, increasing accuracy, range of possibilities, and applications. The efficiency of the CRISPR-MAD7 system has been proven on mutant rice and wheat plants at 65.6% (Silva and Fontes, 2022). Using MAD7 expands the CRISPR genome-editing toolkit because of its highly efficient target for gene disruption and insertion. According to (Silva and Fontes, 2022), using the new CRISPR/CAS systems (CAS3, CAS12, CAS13, and CAS14) as multiplexed SGRNAs by targeting them to different sites is a new and more effective strategy for increasing resistance to a wide range of viral infections and disease control in the field.
One of the main methods used for recovering virus-infected plants is the method of apical meristems. This method uses the apical virus-free zone to obtain an initial healthy plant, which serves as the progenitor of the starting material for primary potato seed production. The effectiveness of this method has been repeatedly confirmed for many plant crops (Bi et al., 2018; Zhang et al., 2019). Many valuable potato varieties have been renewed and used in production for a long time. Potato yields have increased by more than 42% with the implementation of this method (Galeev et al., 2018). An apical meristem is a group of meristematic (formative) cells organized into a growth center, which occupy the terminal position in a shoot or root and form all organs and primary tissues. The upper part of the apical meristem is represented by initials, which are a single cell in horsetails, many ferns, and a multicellular structure in seed plants. The nearest derivatives of the initial cells are often distinguished in the protomeristem zone. There are currently several hypotheses about the reasons for the absence of viral infection in the apical meristem, which are provided below.
1. The absence of a conductive system in the apex slows the spread of viruses from cell to cell. The growth of the apical meristem is faster than viral propagation.
2. The high concentration of auxins in the apex excludes the possibility of virus replication.
3. There are mechanical barriers to the viral infection advancement into the meristematic zone due to the small size of the plasmodesmata.
The apical meristems of the seedlings are isolated at 12–13 plastochrone, which is the time interval between the initiations of two leaf tubercles. Isolated meristems are cultured under aseptic conditions on nutrient media rich in macro-salts and micro-salts with an increased concentration of cytokinins (6-BAP 2 mg/L). The temperature was maintained at 25°C ± 2°C in an air-conditioned culture room, which included 70% humidity, 5 klx illumination, and a photoperiod of 16 h. A small part of the 0.15- to 0.5-mm meristem is usually planted on the nutrient media. The general pattern is provided next. The smaller the size of the meristem, the more likely virus-free plants are obtained. It is isolated under sterile conditions of a laminar box under the magnification of a binocular microscope. On average, 30–45 days pass from planting the meristem on a medium to forming seedlings with five to six leaves, which sometimes takes 2 to 8 months. The media are renewed as they are depleted, and the seedlings are periodically transplanted to new media under sterile conditions. The disadvantages of this method include the difficulty of obtaining the initial virus-free material. The peculiarities of the apical meristem method consist of the complexity of the regeneration of meristems that are 0.1 mm in size. Their engraftment and regenerative ability increase when the size of the meristems increases, but the risk of viruses being present also increases (Moses et al., 2017). It is necessary at this stage to increase the method’s effectiveness and suppress the viral pathogenesis in a larger area of the meristem. According to some experts, it is also advisable to use additional procedures, such as treatment with UHF rays with a narrow-band laser as well as thermotherapy, cryotherapy, and chemotherapy (Zhao et al., 2018; Wang et al., 2021; Bettoni et al., 2022).
The thermotherapy method, which includes heating without lighting, was conducted on the potato tubers and microplants. Exposure modes may vary depending on the varietal characteristics. The efficiency of rehabilitation during the thermal treatment of the potato microplants is 2.4 times higher than for the same method for tubers (Oves and Gaytova, 2016). According to the research by Wang et al. (2021), in vitro cultured shallot shoots infected with onion yellow dwarf virus (OYDV) and shallot latent virus (SLV) were thermo-treated at a constant temperature of 36°C for 0, 2, and 4 weeks. The meristems (0.5 mm) that contain one to two leaf primordia were then excised and cultured for shoot regrowth. The meristem culture with thermotherapy produced much higher virus-free plants, which included 70% for OYDV, 80% for SLV, and 50% for both viruses (Wang et al., 2021). Bi et al. (2018) described a droplet-vitrification cryotherapy method for eradicating grapevine leafroll-associated virus-3 (GLRaV-3) from Vitis plants’ diseased in vitro shoots. All the plants recovered after cryotherapy and were free of GLRaV-3 in two wines, which included one table and one rootstock cultivar Vitis spp (Bi et al., 2018).
The chemotherapy method is based on adding broad-spectrum antiviral drugs, such as virazole, to the nutrient medium where the plant explants are cultivated. The experimental results showed the effectiveness of virazole and amixin for inhibition (78.2%). The high antiviral activity of this drug was found in different cultures, such as against Odonotoglossum cymbidium ringspot virus and rose mosaic viruses, but its pronounced toxic effect, which inhibits the differentiation and proliferation processes in plant tissue culture in vitro, was also revealed. Substances that are capable of inactivating several viruses have been identified. Particular success in this direction was obtained on potatoes, tobacco, tomatoes, narcissus, and tulips (Sochacki and Podwyszyńska, 2012; Bettoni et al., 2022). Ryabtseva et al. (2015) discovered that for the recovery of the potatoes’ in vitro culture, the following virus inhibitors showed a high efficiency, which included chitosan with a dose between 0.1% and 0.01%, interferon with a dose between 0.05% and 0.1%, and virazole with a dose of 0.01%. The percentage of the plants that recovered from viruses was from 25% to 100%, which depended on the variety (Ryabtseva et al., 2015).
The yield of healthy plant regenerants compared to the control was higher by 14.3%–50.0% when antiviral medications, such as interferon, Kagocel, and Arbidol, were used in the nutrient medium in the amount of 50 mg/L. This was revealed in a study on the effect of antiviral drugs in in vitro culture on the yield of viable plant explants (Yalovik et al., 2019). Virus inhibitors include chemical nature malonic, oxalic, ascorbic, nucleic acids, antibiotics, gibberellin, heteroauxin, malachite green dye, methylene blue dye, safronin dye, alkalis, formaldehyde, urea, and the salts of heavy metals. The inhibitors of plant viruses were also found in the leaves of currants, forest strawberries, raspberries, pelargonium, parsley, wormwood, apples, cherries, maple, linden, and beets (Anikina and Seitzhanova, 2015). Several groups of synthetic antiviral compounds have also been identified. Most of them are analogs of bases and nucleosides, and their metabolites, such as the purine base derivative 8-azaguanine, which inhibits TMV, PVX, PVY, and 1-β-d-ribofuranosyl-1,2,4-triazole-3-carboxamide (ribavirin), are also active against numerous plant viruses, such as TMV, PVX, and CMV. Their action is based on the inhibition of viral genome replication. Representatives of pyrimidine-like compounds include 2-thiouracil and 5-azadihydrouracil. They inhibit the reproduction of viruses TMV, PVX, PVY, and CMV by inhibiting the biosynthesis of uridine-5-phosphate from orotidine-5′-phosphate by decarboxylase inhibition (Kumar et al., 2001; Sharipova et al., 2013).
The effectiveness of virocides has also been proven with outdoor plant treatments. The limiting factor is the phytotoxicity of virocides and the teratogenic effect of some virocides (Maksimov et al., 2019). In addition, there are still questions about the mutagenic effect of antiviral drugs, and their use also requires control of the identity of the material (Ryabtseva et al., 2015). Both thermotherapy and chemotherapy methods are used as auxiliary tools of the apical meristem method in in vitro culture. These methods essentially represent a single biotechnological complex method of plant virus recovery. This method is widely used worldwide, and different plant species have been revitalized with its help (Alam et al., 2013; Moses et al., 2017; Wang et al., 2021). Significant disadvantages of the tissue culture-based rehabilitation method include the possibility of self-clonal variations. These types of plants can deviate from the original variety with their morphological traits and properties, which means that they lose varietal individuality. This is a serious risk for primary seed production because the valuable economic traits of the cultivated variety are still under consideration, and they obtain mutants that they may not have (Oves and Gaytova, 2016; Antonova et al., 2017; Kesiraju and Sreevathsa, 2017). The researchers concluded that regular control of the material for identity is required during the long-term cultivation of plants in the tissue culture. The search for a solution to this problem has led some researchers to propose complementing the apical meristem method with the clonal selection or verifying the genetic identity of the regenerated plants in vitro using random amplification of polymorphic DNA (RAPD) (Evstratova et al., 2018). In addition, the cured potatoes can quickly be reinfected in the field.
Virus reservoirs can be weeds along the field edges, birds and insects that travel long distances, and viruses, which can also be transmitted via service aggregates. Preventive measures, such as spatial isolation and insecticidal and stimulant treatments, are essential to controlling the spread of plant viruses (Tian and Valkonen, 2013; Wasilewska-Nascimento et al., 2020). These medicines have become important economic factors in regard to increasing the profitability and eco-friendliness of production. They have a beneficial effect on plant product growth, development, yield, and quality, but they are also inducers of resistance to abiotic stress factors and various phytopathogens, which include viruses (Palukaitis et al., 2017). The research for biosafe means of controlling phytopathogens is relevant in light of the increased attention to the ecologization of agricultural crop production. The search for opportunities to stimulate the immune system of plants and build a plant protection system based on pesticide application as well as on the reserve possibilities of the organism itself is essential, which open up new prospects for the development of biotechnology (Maksimov et al., 2019).
Nucleotide-binding proteins with leucine-rich repeats (NLRs), which recognize intracellular proteins of pathogenic origin, often control immune responses to pathogens in plant organisms. Genetic resistance to plant viruses is often phenotypically characterized by programmed cell death at or near the site of infection, which is a hypersensitive reaction. New approaches in order to control viral pathogens are urgently needed (Akhter et al., 2021). One type of approach is plant immunization, which is a process of activating the body’s natural defense systems. The possibilities of increasing plant resistance by stimulating their immune system have expanded, which results from the in-depth study of the molecular and genetic bases of plant resistance to viruses and the investigation of the mechanisms of induction of the defense reactions in the plant organism. The key role in the induction of immunity belongs to different substances, which are called elicitors.
The biological immunization of plants involves treating them with weakened cultures of pathogens, non-pathogens, or their metabolites (Kothari and Patel, 2004; Dyakov et al., 2007). Chemical immunization is based on using substances called elicitors, resistance inducers, activators, or immunomodulators, which activate the defense reactions. A cascade of defense reactions is triggered in plants under the action of these drugs, and chemical and physical barriers are formed that prevent pathogen development. Inducers typically send excitatory signals to plant defense genes, which activate a cascade of defense responses and ultimately induced systemic resistance (Calil and Fontes, 2017; Beris et al., 2018). Elicitors were associated with the induction of phytoalexin synthesis at the beginning of these types of studies, but further studies revealed other protective responses of plants that expanded the list of compounds under the influence of how plants trigger their defense mechanisms (Poliksenova, 2009). Biologically active substances have different characteristics, and many of them are plant growth regulators, which act as elicitors.
Zircon is among the medicines with high elicitor activity, which is obtained based on the plant material of Echinacea purpurea. Zircon’s efficiency has been proven both as a growth stimulator and as a resistance inducer against various types of diseases, which include viral diseases (Malevannay, 2001). The zircon phytoregulator exhibited an antiviral effect on potato in vitro plants when introduced into a Murashige and Skoog culture medium. The application of a zircon phytoregulator at a dose of 0.25 ml/L on potato in vitro plants reduced the development of the PVY virus by 10%–70%, which depended on the variety. We show that chlormequat chloride in the Murashige and Skoog culture medium at a dose of 0.3 ml/L exhibited antiviral activity when the antiviral action of the growth regulator chlormequat chloride (C5H13Cl2N) was analyzed, which belongs to the group of retardants. The percentage of regenerants that are affected by the potato Y virus decreased from 14% to 37.5%, which depended on the variety (Anikina and Seitzhanova, 2015).
The study of the action of plant polyphenols and flavonoids revealed their high effectiveness in suppressing plant virus infection, particularly with the tobacco mosaic virus, the apple stem borer virus, the tomato spot nepovirus, and the potato X-virus (Chojnacka et al., 2021). Evstratova et al. (2018) showed the high elicitor activity of chitosan-based medicine against the potato Y virus (Evstratova et al., 2018). The search for biologically active substances (BASs) that are capable of stimulating plant immune system mechanisms has significantly increased (Prasad and Srivastava, 2017; Gupta AK. et al., 2021). Mandal (2010) investigated the resistance of eggplant under the action of four elicitors, which included salicylic acid, chitosan, methyl salicylate, and methyl jasmonate. The effect of the elicitors resulted in a threefold to fivefold increase in the accumulation of phenols and lignin in the tissues in addition to an increase in the activity level of the main protective enzymes phenylalanine ammonia-lyase peroxidase, polyphenol oxidase, cinnamic alcohol dehydrogenase, and catalase several times, which certainly contribute to the plant’s resistance to pathogens (Mandal, 2010). The effectiveness of the steroid glycoside compounds against the tobacco mosaic virus has in particular been illustrated. It was discovered that steroidal glycosides act comprehensively, and under their action in vitro, the infectivity of the virus is reduced, and the particle structure and antigenic properties are not violated. They have an elicitor effect on the host plant metabolism. At the same time, RNAase is activated, the formation of new proteins is induced, and the cell ultrastructure is stabilized. Other researchers also confirmed the effectiveness of steroidal glycosides as immunomodulators that increase plant resistance to phytopathogenic viruses (Poliksenova, 2009).
The use of microbial inoculants to induce systemic resistance against viral diseases is of great interest. The researchers showed the high bioprotective potential of microbial inoculants, which included Pseudomonas fluorescens and Bacillus sp. isolated from banana roots, against the banana virus disease, which was caused by the banana bunchy top virus (BBTV). The banana plants were inoculated with P. fluorescens Pf1 and CHA0 strains in combination with endophytic bacterial strains EPB5 and EPB22 (Pf1 + CHA0EP + B5 + EPB22). These plants showed a 60% reduction in the BBTV virus infection compared to untreated plants when grown in soil (Nowak and Shulaev, 2003).
Maksimov et al. (2019) showed that the isolates of Pseudomonas spp., which included P. fluorescens, Pseudomonas putida, Pseudomonas aeruginosa, Pseudomonas taiwanensis, and Bacillus spp., contributed to the protection of papaya plants against the ringspot virus (PRSV). According to studies by Maksimov et al. (2019), the preparation of Phytosporin-M based on Bacillus subtilis 26D also showed a high efficiency for plant protection against viral diseases, and the activity of these microorganisms was confirmed against a wide range of diseases, which included bacterial, fungal, and viral ones.
According to Maximov, the biocidal activity of rhizosphere and endophytic bacteria suggests that it indirectly exhibits antiviral activity due to their production of antibiotic substances. Hence, phytopathogenic bacteria, fungi, nematodes, and insect pests can be vectors-transporters of many plant viral infections. One promising approach to creating new antiviral drugs is to use strains of microorganisms with obvious insecticidal or other biocidal effects to control virus vectors. An isolate of B. subtilis BS3A25 and its culture filtrate inhibited the development of cucumber mosaic virus in tomato plants by inhibiting the development of the melon aphid Aphis gossypii, which is a vector of this disease that may be related to oxidation of surfactants that are produced by the bacteria (Rodríguez et al., 2018) and to the activation of the plant defense mechanisms against the insect and/or the virus that is under the influence of the bacteria (Maksimov et al., 2019). The colonization of internal onion tissues by the endophytic fungus Hypocrea lixii (F3ST1) significantly reduced the titer of iris yellow spot virus (IYSV) particles in plants, which reduced their damage by its main transmitter, which is called Thrips tabaci Lind (Muvea et al., 2018).
Bettoni et al. (2022) showed high effectiveness in regard to treating potatoes with arachidonic acid against Phytophthora and scab as well as against viruses X and M. It is promising to use preparations that are based on bacterial enzymes as elicitors. They became available after the success of genetic engineering in regard to creating highly productive strain producers. Diener (1961) established the antiviral activity of ribonucleases against RNA viruses and showed that the treatment of potato regenerants with RNase resulted in the inactivation of potato virus X and the tobacco mosaic virus in cucumbers.
Using elicitors to control viruses in agricultural plantings is a promising, affordable, and environmentally friendly method of viral disease control. Induced systemic resistance solves many problems with agricultural crop production. It is environmentally friendly and biosafe compared to biocides because it activates the natural plant’s defense mechanisms and is simultaneously effective against different phytopathogens under field conditions (Maksimov et al., 2019). The attractiveness of this direction is obvious because preparations with bioregulatory activity are used as immunomodulators, which induce the protective responses of the plant organism as well as positively affect the yield and quality indicators of plants. The search for virus inhibitors among the preparations with biological activity is of particular practical interest for potato producers because it will greatly simplify the task of obtaining healthy material for seed production (Acharya, 2013; Calil and Fontes, 2017; Somalraju et al., 2022). The proposed approaches for plant protection from phytoviruses are generalized in Table 3.
Viral infection in plants is a complex and interdependent process. Viruses are breeding programs to create virus-resistant forms based on genome editing, using the marker-mediated selection method, which makes it possible to evaluate the genetic protection of a variety and use RNA silencing as an important antiviral mechanism. The disadvantages of genetically engineered approaches include that the gained resistance is often specific and short-lived, and there is a problem with “gene silencing”. A negative factor of growing genetically changed plants resistant to certain viruses is the possible redistribution of virus species structure, which can provoke the spread of other harmful viral infections. The apical meristem method, combined with other auxiliary methods (thermotherapy and chemotherapy) during the last 50 years, has been the basis for controlling harmful plant viruses. Significant disadvantages of the practice of health improvement based on tissue culture include the possibility of auto-clonal variations because of long-term cultivation of plants under in vitro conditions, which are mutant plants genetically different from the original variety. In addition, this technique does not guarantee against rapid reinfection of diseased material under field conditions.
The use of virus inhibitors and elicitors is most justified in the field. At present, several groups of synthetic antiviral compounds have been identified. The efficacy of virocides has been proven in outdoor plant treatments. A limiting factor is the phytotoxicity of virocides, and also the teratogenic effect of some virocides concerning humans has been revealed. Using elicitors to control viruses in agricultural plantings is a promising, affordable, and environmentally friendly approach to preventing viral diseases. Induced systemic resistance is environmentally friendly and biosafe when compared to biocides because it activates natural plant defense mechanisms and is effective simultaneously against different phytopathogens under field conditions. The disadvantages of the method include insufficiently high efficiency. Vector control is a well-known method for preventing the spread of viral infection. The limitations, in this case, are low efficiency and high costs. In addition, considerable damage has been inflicted to the ecology. An alternative direction is the agroecosystem approach based on the study of aspects of multitrophic interactions where the activity of microbial strains with clear insecticidal or other biocidal effects is used to control vector-borne viruses. The antiviral effectiveness of drugs based on microbial enzymes has also been shown. The disadvantages of using microbial preparations include low efficacy. As shown in the literature review, the existing methods of phytovirus control are ambiguous. Further research is needed into plant resistance mechanisms, including the molecular interaction between the host and viral factors. Further study of genetic, biochemical, and physiological features of viral pathogenesis and the development of a strategy for increasing plant resistance to viruses will allow a new level of control of phytovirus infection to be achieved.
All authors listed have made a substantial, direct, and intellectual contribution to the work and approved it for publication.
This research was funded by Project Erasmus+ (no. 609563-EPP-1-2019-1-DE-EPPKA2-CBHE-JP).
The authors declare that the research was conducted in the absence of any commercial or financial relationships that could be construed as a potential conflict of interest.
All claims expressed in this article are solely those of the authors and do not necessarily represent those of their affiliated organizations, or those of the publisher, the editors and the reviewers. Any product that may be evaluated in this article, or claim that may be made by its manufacturer, is not guaranteed or endorsed by the publisher.
Abudayyeh, O. O., Gootenberg, J. S., Essletzbichler, P., Han, S., Joung, J., Belanto, J. J., et al. (2017). RNA Targeting with CRISPR–Cas13. Nature 550 (7675), 280–284. doi: 10.1038/nature24049
Acharya, S. (2013). Control of the potato virus X through application of root extracts of chlorophytum nepalense to potato plants and tubers. Potato Res. 56 (1), 1–10. doi: 10.1007/s11540-012-9227-4
Adolf, B., Andrade-Piedra, J., Bittara Molina, F., Przetakiewicz, J., Hausladen, H., Kromann, P., et al. (2020). “Fungal, oomycete, and plasmodiophorid diseases of potato,” in The potato crop (Cham: Springer), 307–350.
Ahmad, S., Wei, X., Sheng, Z., Hu, P., Tang, S. (2020). CRISPR/Cas9 for development of disease resistance in plants: recent progress, limitations and future prospects. Briefings Funct. Genomics 19 (1), 26–39. doi: 10.1093/bfgp/elz041
Akbar, S., Yao, W., Qin, L., Yuan, Y., Powell, C. A., Chen, B., et al. (2021). Comparative analysis of sugar metabolites and their transporters in sugarcane following sugarcane mosaic virus (SCMV) infection. Int. J. Mol. Sci. 22 (24), 13574. doi: 10.3390/ijms222413574
Akhter, M., Nakahara, K. S., Masuta, C. (2021). Resistance induction based on the understanding of molecular interactions between plant viruses and host plants. Virol. J. 18 (1), 1–12. doi: 10.1186/s12985-021-01647-4
Alam, I., Sharmin, S. A., Naher, M., Alam, M., Anisuzzaman, M., Alam, M. F. (2013). Elimination and detection of viruses in meristem-derived plantlets of sweetpotato as a low-cost option toward commercialization. 3 Biotech. 3 (2), 153–164. doi: 10.1007/s13205-012-0080-6
Alazem, M., Lin, N.-S. (2017). Antiviral roles of abscisic acid in plants [Review]. Front. Plant Sci. 8. doi: 10.3389/fpls.2017.01760
AlMaarri, K., Massa, R., AlBiski, F. (2012). Evaluation of some therapies and meristem culture to eliminate potato y potyvirus from infected potato plants. Plant Biotechnol. 29 (3), 237–243. doi: 10.5511/plantbiotechnology.12.0215a
Alonso-Prados, J., Fraile, A., Garcia-Arenal, F. (1997). Impact of cucumber mosaic virus and watermelon mosaic virus 2 infection on melon production in central Spain. J. Plant Pathol. 79 (2), 131–134. Available at: https://www.jstor.org/stable/41997879.
Antonova, O. Y., Apalikova, O., Ukhatova, Y. V., Krylova, E., Shuvalov, O. Y., Shuvalova, A., et al. (2017). Eradication of viruses in microplants of three cultivated potato species (Solanum tuberosum l., s. phureja juz. & buk., s. stenotomum juz. & buk.) using combined thermo-chemotherapy method. Agric. Biol. 52 (1), 95–104. doi: 10.15389/agrobiology.2017.1.95rus
Baltes, N. J., Gil-Humanes, J., Cermak, T., Atkins, P. A., Voytas, D. F. (2014). DNA Replicons for plant genome engineering. Plant Cell. 26 (1), 151–163. doi: 10.1105/tpc.113.119792
Bari, R., Jones, J. D. (2009). Role of plant hormones in plant defence responses. Plant Mol. Biol. 69, 473–488. doi: 10.1007/s11103-008-9435-0
Batuman, O., Turini, T. A., LeStrange, M., Stoddard, S., Miyao, G., Aegerter, B. J., et al. (2020). Development of an IPM strategy for thrips and tomato spotted wilt virus in processing tomatoes in the central valley of California. Pathogens 9 (8), 636. doi: 10.3390/pathogens9080636
Beris, D., Theologidis, I., Skandalis, N., Vassilakos, N. (2018). Bacillus amyloliquefaciens strain MBI600 induces salicylic acid dependent resistance in tomato plants against tomato spotted wilt virus and potato virus y. Sci. Rep. 8 (1), 1–11. doi: 10.1038/s41598-018-28677-3
Bettoni, J. C., Mathew, L., Pathirana, R., Wiedow, C., Hunter, D. A., McLachlan, A., et al. (2022). Eradication of potato virus s, potato virus a, and potato virus m from infected in vitro-grown potato shoots using in vitro therapies. Front. Plant Sci. 13, 878733. doi: 10.3389/fpls.2022.878733
Bhattacharyya, D., Gnanasekaran, P., Kumar, R. K., Kushwaha, N. K., Sharma, V. K., Yusuf, M. A., et al. (2015). A geminivirus betasatellite damages the structural and functional integrity of chloroplasts leading to symptom formation and inhibition of photosynthesis. J. Exp. botany. 66 (19), 5881–5895. doi: 10.1093/jxb/erv299
Bi, W. L., Hao, X. Y., Cui, Z. H., Pathirana, R., Volk, G. M., Wang, Q. C. (2018). Shoot tip cryotherapy for efficient eradication of grapevine leafroll-associated virus-3 from diseased grapevine in vitro plants. Ann. Appl. Biol. 173 (3), 261–270. doi: 10.1111/aab.12459
Bwalya, J., Alazem, M., Kim, K.-H. (2022). Photosynthesis-related genes induce resistance against soybean mosaic virus: evidence for involvement of the RNA silencing pathway. Mol. Plant Pathology. 23 (4), 543–560. doi: 10.1111/mpp.13177
Calil, I. P., Fontes, E. P. (2017). Plant immunity against viruses: antiviral immune receptors in focus. Ann. Botany. 119 (5), 711–723. doi: 10.1093/aob/mcw200
Chen, S., Li, W., Huang, X., Chen, B., Zhang, T., Zhou, G. (2019). Symptoms and yield loss caused by rice stripe mosaic virus. Virol. J. 16 (1), 1–10. doi: 10.1186/s12985-019-1240-7
Chojnacka, K., Skrzypczak, D., Izydorczyk, G., Mikula, K., Szopa, D., Witek-Krowiak, A. (2021). Antiviral properties of polyphenols from plants. Foods 10 (10), 2277. doi: 10.3390/foods10102277
Cisar, G., Brown, C., Jedlinski, H. (1982). Effect of fall or spring infection and sources of tolerance of barley yellow dwarf of winter wheat 1. Crop Science. 22 (3), 474–478. doi: 10.2135/cropsci1982.0011183X002200030009x
Clark, C. A., Davis, J. A., Abad, J. A., Cuellar, W. J., Fuentes, S., Kreuze, J. F., et al. (2012). Sweetpotato viruses: 15 years of progress on understanding and managing complex diseases. Plant disease. 96 (2), 168–185. doi: 10.1094/PDIS-07-11-0550
Dhankhar, S. K. (2016). Genetic improvement of okra cultivars for Yellow vein mosaic virus disease resistance using a wild Abelmoschus species. Acta Hortic. 1127, 71–74. doi: 10.17660/ActaHortic.2016.1127.13
Dhital, S. P., Sakha, B. M., Lim, H. T. (2007). Utilization of shoot cuttings for elimination of PLRV and PVY by thermotherapy and chemotherapy from potato (Solanum tuberosum l.). Nepal J. Sci. Technol. 7 (0), 1–6. doi: 10.3126/njst.v7i0.528
Diener, T. O. (1961). Virus infection and other factors affecting ribonuclease activity of plant leaves. Virology 14 (2), 177–189. doi: 10.1016/0042-6822(61)90193-3
Dyakov, Y. T., Dzhavakhiya, V., Korpela, T. (2007). Molecular basis of plant immunization. Compr. Mol. Phytopathology. Elsevier;, 423–438. doi: 10.1016/B978-044452132-3/50020-1
Ershova, N., Sheshukova, E., Kamarova, K., Arifulin, E., Tashlitsky, V., Serebryakova, M., et al. (2022). Nicotiana benthamiana kunitz peptidase inhibitor-like protein involved in chloroplast-to-nucleus regulatory pathway in plant-virus interaction. Front. Plant Sci. 13, 1041867. doi: 10.3389/fpls.2022.1041867
Evstratova, L., Kuznetsova, L., Nikolaeva, E., Evstratov, I. (2018). Combination of apical meristem and clonal selection in original potato seed production. Sci. Notes Petrozavodsk State University. 8 (177), 23–26. doi: 10.15393/uchz.art.2018.245
Farooq, T., Adeel, M., He, Z., Umar, M., Shakoor, N., da Silva, W., et al. (2021). Nanotechnology and plant viruses: an emerging disease management approach for resistant pathogens. ACS Nano. 15 (4), 6030–6037. doi: 10.1021/acsnano.0c10910
Fletcher, P. J., Fletcher, J. D., Cross, R. J. (1998). Potato germplasm: in vitro storage and virus reduction. New Z. J. Crop Hortic. Sci. 26 (3), 249–252. doi: 10.1080/01140671.1998.9514060
French, C. J., Towers, G. H. N. (1992). Inhibition of infectivity of potato virus X by flavonoids. Phytochemistry 31 (9), 3017–3020. doi: 10.1016/0031-9422(92)83438-5
Galeev, R. R., Vyshegurov, S. K., Demshina, V. S., Shulga, M. S., Gumel, M. A. (2018). Efficiency of regenerating potato varieties by the apical meristem method. J. Pharm. Sci. Res. 10 (1), 124–128.
Gong, H., Igiraneza, C., Dusengemungu, L. (2019). Major in vitro techniques for potato virus elimination and post eradication detection methods. a review. Am. J. potato Res. 96, 379–389. doi: 10.1007/s12230-019-09720-z
Guo, Z., Li, Y., Ding, S.-W. (2019). Small RNA-based antimicrobial immunity. Nat. Rev. Immunol. 19 (1), 31–44. doi: 10.1038/s41577-018-0071-x
Gupta, N., Reddy, K., Bhattacharyya, D. (2021). Plant responses to geminivirus infection: guardians of the plant immunity. Virol. J. 18 (1), 1–25. doi: 10.1186/s12985-021-01612-1
Gupta, A. K., Verma, J., Srivastava, A., Srivastava, S., Prasad, V. (2021). A comparison of induced antiviral resistance by the phytoprotein CAP-34 and isolate P1f of the rhizobacterium pseudomonas putida. 3 Biotech. 11 (12), 1–13. doi: 10.1007/s13205-021-03057-3
Handford, M. G., Carr, J. P. (2007). A defect in carbohydrate metabolism ameliorates symptom severity in virus-infected arabidopsis thaliana. J. Gen. Virol. 88 (1), 337–341. doi: 10.1099/vir.0.82376-0
Hofvander, P., Andreasson, E., Andersson, M. (2022). Potato trait development going fast-forward with genome editing. Trends Genet. 38 (3), 218–221. doi: 10.1016/j.tig.2021.10.004
Hong, J. S., Ju, H. J. (2017). The plant cellular systems for plant virus movement. Plant Pathol. J. 33 (3), 213–228. doi: 10.5423/PPJ.RW.09.2016.0198
Hossain, M., Akanda, A., Hossain, M. (2011). Effect of tomato yellow leaf curl virus (tylcv) on plant growth and yield contributing characters of sixsteen tomato varieteis. Arab. J. Plant Prot. 39 (1), 79–83. doi: 10.22268/AJPP-039.1.079083
Hull, R. (2014). Plant virology. 5th Edition (London: Academic Press), 854. Available at: https://www.elsevier.com/books/plant-virology/hull/978-0-12-384871-0.
Islam, W., Naveed, H., Zaynab, M., Huang, Z., Chen, H. Y. (2019). Plant defense against virus diseases; growth hormones in highlights. Plant Signal. Behav. 14 (6), 1596719. doi: 10.1080/15592324.2019.1596719
Jin, L., Qin, Q., Wang, Y., Pu, Y., Liu, L., Wen, X., et al. (2016). Rice dwarf virus P2 protein hijacks auxin signaling by directly targeting the rice OsIAA10 protein, enhancing viral infection and disease development. PloS Pathog. 12 (9), e1005847. doi: 10.1371/journal.ppat.1005847
Jones, R. A. (2009). Plant virus emergence and evolution: origins, new encounter scenarios, factors driving emergence, effects of changing world conditions, and prospects for control. Virus Res. 141 (2), 113–130. doi: 10.1016/j.virusres.2008.07.028
Jones, R. A., Naidu, R. A. (2019). Global dimensions of plant virus diseases: current status and future perspectives. Annu. Rev. Virol. 6, 387–409. doi: 10.1146/annurev-virology-092818-015606
Kavuri, N. R., Ramasamy, M., Qi, Y., Mandadi, K. (2022). Applications of CRISPR/Cas13-based RNA editing in plants. Cells 11 (17), 2665. doi: 10.3390/cells11172665
Kesiraju, K., Sreevathsa, R. (2017). Apical meristem-targeted in planta transformation strategy: an overview on its utility in crop improvement. Agri Res. Technol. Open Access J. 8 (555734), 19080. doi: 10.19080/ARTOAJ.2017.08.555734
Klimenko, N., Antonova, O. Y., Zheltova, V., Fomina, N., Kostina, L., Mamadbokirova, F., et al. (2019). Screening of Russian potato cultivars (Solanum tuberosum l.) with DNA markers linked to the genes conferring extreme resistance to potato virus y. Sel'skokhozyaistvennaya Biologiya 54 (5), 958–969. doi: 10.15389/agrobiology.2019.5.958eng
Kochetov, A., Shumny, V. (2017). Transgenic plants as genetic models for studying functions of plant genes. Russian J. Genetics: Appl. Res. 7 (4), 421–427. doi: 10.1134/S2079059717040050
Kothari, I. L., Patel, M. (2004). Plant immunization [Review]. Indian J. Exp. Biol. 42 (3), 244–252. Available at: http://nopr.niscpr.res.in/handle/123456789/23379.
Kourelis, J., van der Hoorn, R. A. L. (2018). Defended to the nines: 25 years of resistance gene cloning identifies nine mechanisms for r protein function. Plant Cell. 30 (2), 285–299. doi: 10.1105/tpc.17.00579
Kreuze, J. F., Souza-Dias, J. A. C., Jeevalatha, A., Figueira, A. R., Valkonen, J. P. T., Jones, R. A. C. (2020). “Viral diseases in potato,” in The potato crop: its agricultural, nutritional and social contribution to humankind. Eds. Campos, H., Ortiz, O. (Cham: Springer International Publishing), 389–430.
Kumar, R., Sharma, N., Nath, M., Saffran, H. A., Tyrrell, D. L. J. (2001). Synthesis and antiviral activity of novel acyclic nucleoside analogues of 5-(1-azido-2-haloethyl) uracils. J. medicinal Chem. 44 (24), 4225–4229. doi: 10.1021/jm010227k
Li, F., Wang, A. (2022). Transient expression–mediated gene silencing in plants and suppression of gene silencing with viral suppressors. Plant Virol. Springer; 2400, 33–41. doi: 10.1007/978-1-0716-1835-6_4
Liu, J.-Z., Li, F., Liu, Y. (2017). Editorial: plant immunity against viruses [Editorial]. Front. Microbiol. 8. doi: 10.3389/fmicb.2017.00520
Liu, W., Zhang, Z., Li, Y., Zhu, L., Jiang, L. (2023). Efficient production of d-tagatose via DNA scaffold mediated oxidoreductases assembly in vivo from whey powder. Food Res. Int. 166, 112637. doi: 10.1016/j.foodres.2023.112637
Lolle, S., Stevens, D., Coaker, G. (2020). Plant NLR-triggered immunity: from receptor activation to downstream signaling. Curr. Opin. Immunol. 62, 99–105. doi: 10.1016/j.coi.2019.12.007
Lopez-Gomollon, S., Baulcombe, D. C. (2022). Roles of RNA silencing in viral and non-viral plant immunity and in the crosstalk between disease resistance systems. Nat. Rev. Mol. Cell Biol. 23 (10), 645–662. doi: 10.1038/s41580-022-00496-5
Lu, L., Zhai, X., Li, X., Wang, S., Zhang, L., Wang, L., et al (2022). Met1-specific motifs conserved in OTUB subfamily of green plants enable rice OTUB1 to hydrolyse Met1 ubiquitin chains. Nat. Commun. 13 (1), 4672. doi: 10.1038/s41467-022-32364-3
Ma, S., Wang, R., Gao, R., Wang, X., Liu, Y., Li, Y, et al. (2022). Antiviral spirooliganones C and D with a unique spiro [bicyclo [2.2. 2] octane-2, 2′-bicyclo [3.1. 0] hexane] carbon skeleton from the roots of Illicium oligandrum. Chin. Chem. Lett. 33 (9), 4248–4252. doi: 10.1016/j.cclet.2022.02.050
Maksimov, I., Sorokan, A., Burkhanova, G., Veselova, S., Alekseev, V., Shein, M., et al. (2019). Mechanisms of plant tolerance to RNA viruses induced by plant-growth-promoting microorganisms. Plants 8 (12), 575. doi: 10.3390/plants8120575
Malevannay, N. (2001). The explosive temperament of zircon in the service of plants. New Gardener Farmer. 1, 45.
Malhotra, B., Onyilagha, J. C., Bohm, B. A., Towers, G. H. N., James, D., Harborne, J. B., et al. (1996). Inhibition of tomato ringspot virus by flavonoids. Phytochemistry 43 (6), 1271–1276. doi: 10.1016/S0031-9422(95)00522-6
Mandal, S. (2010). Induction of phenolics, lignin and key defense enzymes in eggplant (Solanum melongena l.) roots in response to elicitors. Afr. J. Biotechnol. 9 (47), 8038–8047. doi: 10.5897/AJB10.984
Mishra, GP, Singh, B, Seth, T, Singh, AK, Halder, J, Krishnan, N, et al (2017). Biotechnological Advancements and Begomovirus Management in Okra (Abelmoschus esculentus L.): Status and Perspectives. Front. Plant Sci. 8, 360. doi: 10.3389/fpls.2017.00360
Moses, W., Rogers, K., Mildred, O.-S. (2017). Effect of thermotherapy duration, virus type and cultivar interactions on elimination of potato viruses X and s in infected seed stocks. Afr. J. Plant Sci. 11 (3), 61–70. doi: 10.5897/AJPS2016.1497
Mumford, R., Macarthur, R., Boonham, N. (2016). The role and challenges of new diagnostic technology in plant biosecurity. Food Secur. 8 (1), 103–109. doi: 10.1007/s12571-015-0533-y
Musidlak, O., Nawrot, R., Goździcka-Józefiak, A. (2017). Which plant proteins are involved in antiviral defense? review on In vivo and In vitro activities of selected plant proteins against viruses. Int. J. Mol. Sci. 18 (11), 2300. doi: 10.3390/ijms18112300
Muvea, A. M., Subramanian, S., Maniania, N. K., Poehling, H.-M., Ekesi, S., Meyhöfer, R. (2018). Endophytic colonization of onions induces resistance against viruliferous thrips and virus replication. Front. Plant Sci. 9. doi: 10.3389/fpls.2018.01785
Mwanga, R., Moyer, J., Zhang, D., Carey, E., Yencho, G. (2001). Nature of resistance of sweetpotato to sweetpotato virus disease. I international conference on sweetpotato. Food Health Future 583, 113–119. doi: 10.17660/ActaHortic.2002.583.12
Nasir, I. A., Tabassum, B., Latif, Z., Javed, M. A., Haider, M. S., Javed, M. A., et al. (2010). Strategies to control potato virus y under in vitro conditions. Pakistan J. Phytopathol. 22 (1), 63–70.
Nidhi, S., Anand, U., Oleksak, P., Tripathi, P., Lal, J. A., Thomas, G., et al. (2021). Novel CRISPR–cas systems: an updated review of the current achievements, applications, and future research perspectives. Int. J. Mol. Sci. 22 (7), 3327. doi: 10.3390/ijms22073327
Nowak, J., Shulaev, V. (2003). Priming for transplant stress resistance in in vitro propagation. In Vitro Cell. Dev. Biol. - Plant 39 (2), 107–124. doi: 10.1079/ivp2002403
Nussenzweig, P. M., Marraffini, L. A. (2020). Molecular mechanisms of CRISPR-cas immunity in bacteria. Annu. Rev. Genet. 54, 93–120. doi: 10.1146/annurev-genet-022120-112523
Orazov, O., Nikitina, V. (2009). Development of the cucumber plants infected by tobacco mosaic virus after their treatment by flavonoid-bearing concentrates. Agric. Biol. 1, 93–98.
Oves, E., Gaytova, N. (2016). New elements in technology of improvement and obtaining of basic potato clones of promising cultivars and hybrids. Achievements Sci. Technol. Agro - Ind. Complex. 30 (11), 60–62.
Palukaitis, P., Yoon, J.-Y., Choi, S.-K., Carr, J. P. (2017). Manipulation of induced resistance to viruses. Curr. Opin. Virol. 26, 141–148. doi: 10.1016/j.coviro.2017.08.001
Perry, K. L., Kolb, F. L., Sammons, B., Lawson, C., Cisar, G., Ohm, H. (2000). Yield effects of barley yellow dwarf virus in soft red winter wheat. Phytopathology 90 (9), 1043–1048. doi: 10.1094/PHYTO.2000.90.9.1043
Poliksenova, V. (2009). Induced plant stability to pathogens and abiotic stress factors. Bull. BSU. 2 (1), 48–60.
Prasad, V., Srivastava, S. (2017). Phytoproteins and induced antiviral defence in susceptible plants: the Indian context. A century Plant Virol. India. Springer; p, 689–728. doi: 10.1007/978-981-10-5672-7_28
Price, A. A., Sampson, T. R., Ratner, H. K., Grakoui, A., Weiss, D. S. (2015). Cas9-mediated targeting of viral RNA in eukaryotic cells. Proc. Natl. Acad. Sci. 112 (19), 6164–6169. doi: 10.1073/pnas.1422340112
Robertson, G., Burger, J., Campa, M. (2022). CRISPR/Cas-based tools for the targeted control of plant viruses. Mol. Plant Pathol. 23 (11), 1701–1718. doi: 10.1111/mpp.13252
Rodríguez, M., Marín, A., Torres, M., Béjar, V., Campos, M., Sampedro, I. (2018). Aphicidal activity of surfactants produced by bacillus atrophaeus L193. Front. Microbiol. 9, 3114. doi: 10.3389/fmicb.2018.03114
Rodríguez-Negrete, E. A., Carrillo-Tripp, J., Rivera-Bustamante, R. F. (2009). RNA Silencing against geminivirus: complementary action of posttranscriptional gene silencing and transcriptional gene silencing in host recovery. J. Virology. 83 (3), 1332–1340. doi: 10.1128/JVI.01474-08
Ross, B. T., Zidack, N. K., Flenniken, M. L. (2021). Extreme resistance to viruses in potato and soybean. Front. Plant Science. 12, 658981. doi: 10.3389/fpls.2021.658981
Ryabtseva, T. V., Kulikova, V. I., Ilkevich, O. G. (2015). Improvement of potatoes by the chemotherapy method in culture of in vitro. Int. Sci. Res. J. 10 (41), 66–68. doi: 10.18454/IRJ.2015.41.127
Sáez, C., Flores-León, A., Montero-Pau, J., Sifres, A., Dhillon, N. P. S., López, C., et al. (2022). RNA-Seq transcriptome analysis provides candidate genes for resistance to tomato leaf curl new Delhi virus in melon. Front. Plant Sci. 12. doi: 10.3389/fpls.2021.798858
Sevik, M. A., Arli-Sokmen, M. (2012). Estimation of the effect of tomato spotted wilt virus (TSWV) infection on some yield components of tomato. Phytoparasitica 40, 87–93. doi: 10.1007/s12600-011-0192-2
Shan, Q., Wang, Y., Chen, K., Liang, Z., Li, J., Zhang, Y., et al. (2013). Rapid and efficient gene modification in rice and brachypodium using TALENs. Mol. Plant 6 (4), 1365–1368. doi: 10.1093/mp/sss162
Sharipova, M., Balaban, N., Mardanova, A., Valeeva, L. (2013). Mechanisms of plant resistance to infections. Sci. Notes Kazan Univ. Natural Sci. Series. 155 (4), 28–58. Available at: https://repo.btu.kharkov.ua//handle/123456789/1573.
Sharma, V. K., Marla, S., Zheng, W., Mishra, D., Huang, J., Zhang, W., et al. (2022). CRISPR guides induce gene silencing in plants in the absence of cas. Genome Biol. 23, 1–24. doi: 10.1186/s13059-021-02586-7
Silva, F. D., Fontes, E. P. (2022). Clustered regularly interspaced short palindromic repeats-associated protein system for resistance against plant viruses: applications and perspectives. Front. Plant Sci. 13. doi: 10.3389/fpls.2022.904829
Sochacki, D., Podwyszyńska, M. (2012). Virus eradication in narcissus and tulip by chemotherapy. Floriculture Ornam. Biotechnol. 6, 114–121.
Somalraju, A., Mccallum, J. L., Main, D., Peters, R. D., Fofana, B. (2022). Foliar selenium application reduces late blight severity and incidence in potato and acts as a pathogen growth inhibitor and elicitor of induced plant defence. Can. J. Plant Pathol. 44 (1), 39–55. doi: 10.1080/07060661.2021.1954093
Tian, Y.-P., Valkonen, J. P. (2013). Genetic determinants of potato virus y required to overcome or trigger hypersensitive resistance to PVY strain group O controlled by the gene ny in potato. Mol. Plant-Microbe Interact. 26 (3), 297–305. doi: 10.1094/MPMI-09-12-0219-R
Tiwari, J. K., Tuteja, N., Khurana, S. P. (2022). Genome editing (CRISPR-cas)-mediated virus resistance in potato (Solanum tuberosum l.). Mol. Biol. Rep., 1–11. doi: 10.1007/s11033-022-07704-7
Tolkach, V. F., Nadezhda, K., Volkov, Y., Shchelkanov, M. (2019). “Viruses and their vectors of the vegetable cultures in the russian far east,” in AI Kurentsov's Annual Memorial Meetings. 200–210. doi: 10.25221/kurentzov.30.19
Tran, P.-T., Widyasari, K., Seo, J.-K., Kim, K.-H. (2018). Isolation and validation of a candidate Rsv3 gene from a soybean genotype that confers strain-specific resistance to soybean mosaic virus. Virology 513, 153–159. doi: 10.1016/j.virol.2017.10.014
Wang, M. R., Hamborg, Z., Blystad, D. R., Wang, Q. C. (2021). Combining thermotherapy with meristem culture for improved eradication of onion yellow dwarf virus and shallot latent virus from infected in vitro-cultured shallot shoots. Ann. Appl. Biol. 178 (3), 442–449. doi: 10.1111/aab.12646
Wang, P., Liu, J., Lyu, Y., Huang, Z., Zhang, X., Sun, B., et al. (2022). A review of vector-borne rice viruses. Viruses 14 (10), 2258. doi: 10.3390/v14102258
Wang, Q., Liu, Y., Xie, Y., You, M. (2006). Cryotherapy of potato shoot tips for efficient elimination of potato leafroll virus (PLRV) and potato virus y (PVY). Potato Res. 49 (2), 119–129. doi: 10.1007/s11540-006-9011-4
Wasilewska-Nascimento, B., Boguszewska-Mańkowska, D., Zarzyńska, K. (2020). Challenges in the production of high-quality seed potatoes (Solanum tuberosum l.) in the tropics and subtropics. Agronomy 10 (2), 260. doi: 10.3390/agronomy10020260
Wei, J., Jia, D., Mao, Q., Zhang, X., Chen, Q., Wu, W., et al. (2018). Complex interactions between insect-borne rice viruses and their vectors. Curr. Opin. Virol. 33, 18–23. doi: 10.1016/j.coviro.2018.07.005
Wolter, F., Puchta, H. (2018). The CRISPR/Cas revolution reaches the RNA world: Cas13, a new Swiss army knife for plant biologists. Plant J. 94 (5), 767–775. doi: 10.1111/tpj.13899
Wu, J., Yang, Z., Wang, Y., Zheng, L., Ye, R., Ji, Y., et al. (2015). Viral-inducible Argonaute18 confers broad-spectrum virus resistance in rice by sequestering a host microRNA. eLife 4, e05733. doi: 10.7554/eLife.05733
Xu, Y., Kang, D., Shi, Z., Shen, H., Wehner, T. (2004). Inheritance of resistance to zucchini yellow mosaic virus and watermelon mosaic virus in watermelon. J. Heredity. 95 (6), 498–502. doi: 10.1093/jhered/esh076
Yalovik, A., Fedorova, Y., Yalovik, L. (2019). The effect of chemotherapy method effect on in vitro potato plants. Bull. Altai State Agrarian University. 10 (180), 28–32.
Yang, Z., Li, Y. (2018). Dissection of RNAi-based antiviral immunity in plants. Curr. Opin. Virol. 32, 88–99. doi: 10.1016/j.coviro.2018.08.003
Yi, J.-Y., Lee, G.-A., Jeong, J.-W., Lee, S.-Y., Lee, Y.-G. (2014). Eliminating potato virus y (PVY) and potato leaf roll virus (PLRV) using cryotherapy of in vitro-grown potato shoot tips. Korean J. Crop Science. 4 (59), 498–504. doi: 10.7740/kjcs.2014.59.4.498
Yi Lan, F., Shambbu Prasad, D., Kui Hua, L., Dong Man, K., Hae Young, K., Ye Su, S., et al. (2005). Utilization of single nodal cuttings and therapies for eradicating double-infected potato virus (PLRV, PVY) from In vitro plantlets of potato (Solanum tuberosum. ). HORTICULTURE Environ. Biotechnol. 46 (2), 119–125.
Yu, H. (2015). Discussion on the occurrence and control countermeasures of rice yellow dwarf virus. J. Guangxi Agric. 30, 15–17. doi: 10.16872/j.cnki.1671-4652.1983.04.008
Zhan, X., Zhang, F., Zhong, Z., Chen, R., Wang, Y., Chang, L., et al. (2019). Generation of virus-resistant potato plants by RNA genome targeting. Plant Biotechnol. J. 17 (9), 1814–1822. doi: 10.1111/pbi.13102
Zhang, Z., Wang, Q.-C., Spetz, C., Blystad, D.-R. (2019). In vitro therapies for virus elimination of potato-valuable germplasm in Norway. Sci. Hortic. 249, 7–14. doi: 10.1016/j.scienta.2019.01.027
Zhang, T., Zheng, Q., Yi, X., An, H., Zhao, Y., Ma, S., et al. (2018). Establishing RNA virus resistance in plants by harnessing CRISPR immune system. Plant Biotechnol. J. 16 (8), 1415–1423. doi: 10.1111/pbi.12881
Zhao, L., Wang, M.-R., Cui, Z.-H., Chen, L., Volk, G. M., Wang, Q.-C. (2018). Combining thermotherapy with cryotherapy for efficient eradication of apple stem grooving virus from infected in-vitro-cultured apple shoots. Plant Dis. 102 (8), 1574–1580. doi: 10.1094/PDIS-11-17-1753-RE
Zhao, S., Wu, Y., Wu, J. (2021). Arms race between rice and viruses: a review of viral and host factors. Curr. Opin. Virol. 47, 38–44. doi: 10.1016/j.coviro.2021.01.002
Zhao, Y., Yang, X., Zhou, G., Zhang, T. (2020). Engineering plant virus resistance: from RNA silencing to genome editing strategies. Plant Biotechnol. J. 18 (2), 328–336. doi: 10.1111/pbi.13278
Zhu-Jun, W., Qiang, Z., Xin-Huai, Z. (2011). Induced-differentiation of two flavones and two flavonols on a human esophageal squamous cell carcinoma cell line (KYSE-510). J. Medicinal Plants Res. 5 (24), 5677–5691. Available at: http://www.academicjournals.org/JMPR.
Keywords: plant virus, resistance genes, PR-proteins, elicitors, method of apical meristems
Citation: Anikina I, Kamarova A, Issayeva K, Issakhanova S, Mustafayeva N, Insebayeva M, Mukhamedzhanova A, Khan SM, Ahmad Z, Lho LH, Han H and Raposo A (2023) Plant protection from virus: a review of different approaches. Front. Plant Sci. 14:1163270. doi: 10.3389/fpls.2023.1163270
Received: 10 February 2023; Accepted: 25 May 2023;
Published: 12 June 2023.
Edited by:
Chellappan Padmanabhan, USDA APHIS PPQ Science and Technology, United StatesReviewed by:
Kathleen L Hefferon, Cornell University, United StatesCopyright © 2023 Anikina, Kamarova, Issayeva, Issakhanova, Mustafayeva, Insebayeva, Mukhamedzhanova, Khan, Ahmad, Lho, Han and Raposo. This is an open-access article distributed under the terms of the Creative Commons Attribution License (CC BY). The use, distribution or reproduction in other forums is permitted, provided the original author(s) and the copyright owner(s) are credited and that the original publication in this journal is cited, in accordance with accepted academic practice. No use, distribution or reproduction is permitted which does not comply with these terms.
*Correspondence: Shujaul Mulk Khan, c2h1amE2MEBnbWFpbC5jb20=; Linda Heejung Lho, aGVlZWxob0BnbWFpbC5jb20=; Heesup Han, aGVlc3VwLmhhbkBnbWFpbC5jb20=
Disclaimer: All claims expressed in this article are solely those of the authors and do not necessarily represent those of their affiliated organizations, or those of the publisher, the editors and the reviewers. Any product that may be evaluated in this article or claim that may be made by its manufacturer is not guaranteed or endorsed by the publisher.
Research integrity at Frontiers
Learn more about the work of our research integrity team to safeguard the quality of each article we publish.