- 1Department of GreenBio Science, Gyeongsang National University, Jinju, Republic of Korea
- 2Department of Plant and Biomaterials Science, Gyeongsang National University, Jinju, Republic of Korea
- 3Department of Food Science, Gyeongsang National University, Jinju, Republic of Korea
- 4Division of Horticultural Science, Gyeongsang National University, Jinju, Republic of Korea
Introduction: Limonium (L.) tetragonum (Thunb.) A. A. Bullock, a halophyte that grows all over the southwest coast of Korea, is a medicinal plant with various pharmacological effects. The salt defense mechanism stimulates the biosynthesis of various secondary metabolites and improves functional substances. In this study, we investigated the optimal NaCl concentration for the growth and enhancement of secondary metabolites in hydroponically grown L. tetragonum.
Methods: The seedlings grown for 3 weeks in a hydroponic cultivation system were treated with 0-, 25-, 50-, 75-, and 100-mM NaCl in Hoagland’s nutrient solution for 8 weeks. No significant effect on the growth and chlorophyll fluorescence was observed for the NaCl concentrations below 100-mM.
Results and discussions: The increase in the NaCl concentration resulted in the decrease in the water potential of the L. tetragonum leaves. The Na+ content accumulated in the aerial part increased rapidly and the content of K+, which acts as an antagonist, decreased with the increase in NaCl concentrations in hydroponics. The total amino acid content of L. tetragonum decreased compared to the 0-mM NaCl, and most of the amino acid content decreased as the NaCl concentration increased. In contrast, the content of urea, proline (Pro), β-alanine, ornithine, and arginine was increased with an increase in NaCl concentration. The Pro content at 100-mM NaCl accounted for 60% of the total amino acids and was found to be a major osmoregulator as an important component of the salt defense mechanisms. The top five compounds identified in the L. tetragonum were classified as flavonoids while the flavanone compound was detected only in the NaCl treatments. A total of four myricetin glycosides were increased in comparison to the 0-mM NaCl. Among the differentially expressed genes, a significantly large change in Gene ontology was seen in the circadian rhythm. NaCl treatment enhanced the flavonoid-based substances of L. tetragonum. The optimum NaCl concentration for the enhancement of secondary metabolites of the L. tetragonum in the vertical farm-hydroponic cultivation system was 75-mM NaCl.
1 Introduction
Limonium (L.) tetragonum (Thunb.) A. A. Bullock is a biennial, herbaceous, and dicotyledonous plant in the Plumbaginaceae family. It is a halophyte and inhabits the saline environment of Korea’s southwest coast, yet it may also grow in non-saline environments (Ihm et al., 2001). In addition, this plant is physiologically a recretohalophyte with salinity glands and a salt bladder on the leaves, which dilute the plant’s salinity through this structure (Min, 1998). Limonium tetragonum is rich in bioactive catechins and flavonoids with anticancerous (Kong et al., 2008), antioxidant (Lee et al., 2011), hepatoprotective (Kim et al., 2015), and antiobesity activities (Kim et al., 2017). The roots and young buds of L. tetragonum are widely used as edible vegetables in Korea (Kim et al., 2017). Lee et al. (2011) reported quercetin, myricetin (a natural bioflavonoid), and myricetin glycosides in L. tetragonum. Myricetin is also present in various plants such as vegetables, fruit, and medicinal crops (Hertog et al., 1993). Myricetin has the potential to be employed in the treatment of diabetes and cardiovascular disorders due to its antioxidant and pro-oxidative properties (Ong and Khoo, 1997).
Abnormal weather conditions, water scarcity, and reduced available land area due to salinity accumulation negatively impact the production of staple crops such as wheat (Deng et al., 2016). In saline soil, the concentration of ions such as Na+ and Cl−, which interfere with water absorption of the roots, is high and, thus, has adverse effects on plants such as damage to the chloroplast structure and reduction in plant yield (Soni et al., 2021; Zhu et al., 2022). Halophytes can complete their life cycle at NaCl concentrations above 200 mM (Flowers and Colmer, 2008). To adapt to salt stress, halophytes have morphological, anatomical, and physiological processes (Nikalje et al., 2018). Selective ion uptake by the roots, osmotic regulation, intracellular compartmentalization of toxic ions, and scavenging of reactive oxygen species (ROS) enable survival at high salinity (Meng et al., 2018; Nikalje et al., 2018). Salinity stress is detrimental to most plants, but some may experience eustress. The polyphenol content of safflower (Gengmao et al., 2015), mangroves (Parida et al., 2004), and rapeseed (Falcinelli et al., 2017) was increased with NaCl treatment, suggesting that salt stress at an appropriate concentration may stimulate defense mechanisms and improve physiological activity.
A vertical farm produces crops without time and space restrictions in controlled environmental factors such as light, carbon dioxide, temperature, airflow, humidity, and inorganic nutrients (Son, 2022). The vertical farm has a framework that makes the most of the available area by stacking tall panels (Despommier, 2010). Vertical farms mainly produce leafy vegetables containing various bioactive substances (Zhang et al., 2018; Matysiak and Kowalski, 2019). Leaf vegetables have low economic value but high yields as these crops can be grown year-round on vertical farms. High-value-added crops with several useful compounds are gaining popularity as a solution for the aforementioned problem. Medicinal crops such as ginseng (Park et al., 2019) and nasturtium (Xu et al., 2021) are grown in vertical farms to be used as high-value-added crops. Efforts have been made to increase economic value as well as the enhancement of secondary metabolites of plants such as sprout ginseng (Hwang et al., 2021) and coriander (Nguyen et al., 2019). Limonium tetragonum is a medicinal plant and can be used as a high-value-added crop. The vertical farm is suitable for its cultivation and the enhancement of secondary metabolites.
The habitat of L. tetragonum is flooded once or twice a month, and the seawater flows underground and is greatly affected by NaCl during the growing season (Min, 1998). Although many studies have been done on the physiological efficacy (Bae et al., 2016) and substance analysis (Kim et al., 2017) of L. tetragonum, there are few studies on the growth of this plant. Kim (2007) reported that when grown in a nutrient solution environment with different NaCl concentrations in hydroponics, this concentration did not affect the growth of mature L. tetragonum. Jeong (2017) reported that the optimal salinity concentration of L. tetragonum is 0%–1% and that of phenolic compounds is improved by 1%–2%. Although there are basic studies on the NaCl concentration of L. tetragonum, there are few studies on the relationship between NaCl concentration and growth and active substances. Therefore, to investigate the effect of saline stress on the growth, secondary metabolism, and transcriptome of L. tetragonum, it was grown at various NaCl concentrations using a hydroponics system on a vertical farm.
2 Materials and methods
2.1 Plant materials
The seeds of L. tetragonum were collected in August 2020 from Gohyeon-myeon, Namhae-gun, South Korea (34°52′07.6″N, 127°53′36.1″E) and stored at 4°C. The seeds were sterilized by immersion in benomyl and thiram wettable powder [benomyl (20%) + thiram (20%)] 0.25% (w/v) for 10 min and subsequently washed three times with sterile water.
2.2 Growth conditions
Limonium tetragonum seeds were sown in urethane sponge cubes (2 seeds per cell; W × L × H, 25 × 25 × 30 mm) in a cultivation room. After one plant per cell remained, the 3-week-old seedlings were planted in individual pots (ø 45 × height 35 mm). The seedlings per pot and 12 pots per culture panel (36 × 51 cm) were transplanted into a deep flow technique system (W × L × H, 51 × 36 × 7.5 cm). Each treatment was performed in three replicates. In total, 36 plants per treatment were placed in the cultivation room at a temperature of 24°C ± 3°C, relative humidity of 70% ± 10%, and photosynthetic photon flux density of 160 ± 10 µmol m−2 s−1 [white light-emitting diodes (LEDs), 12 h photoperiod]. Five LED tubes (LED T5, Samsung LED, Korea) were installed per zone, and the white LEDs include red and blue lights (Figure S1). The seedlings were given Hoagland’s nutrient solution (HNS; pH 6.5, EC 1.1 dS m−1) for 1 week, and then for 8 weeks, NaCl nutrient solution (NNS) of 0-, 25-, 50-, 75-, and 100-mM NaCl concentration prepared by mixing NaCl with HNS was provided (Tables S1, S2). Before this study, we performed a study and tested a wide range of NaCl concentrations (0-, 50-, 100-, 200-, and 400-mM NaCl). The growth suppression of L. tetragonum was observed in NaCl at a concentration of >200 mM (data not published). Excessive NaCl reduces the growth of L. tetragonum and is not economically efficient; thus, the purpose of the present study was to focus on the detailed concentrations to improve the growth and functional substances in vertical farms. The pH and electrical conductivity (EC) were measured weekly (Table S2) to confirm that the pH was constant, and the NNS was replaced with a fresh nutrient solution every 2 weeks during the experiment to avoid nutrient depletion in the nutrient solution.
2.3 Physiological analysis
2.3.1 Growth characteristics
The 8-week-old L. tetragonum seedlings were harvested, and 10 plants from each treatment were selected as biological replicates. To investigate the growth characteristics of L. tetragonum, fresh weight (FW), the number of leaves, and the total leaf area (LA) of the aerial part and root were measured, and dry weight (DW) was measured after oven-drying (WOF-155, Daihan, Wonju, Korea) at 50°C for 5 days. Samples used for the analysis were ground-dried aerial parts and stored at 4°C until further analysis. The specific leaf weight (SLW) was calculated based on the DW of the leaf per unit LA.
2.3.2 Chlorophyll fluorescence
Chl fluorescence was measured 1 day before harvest in the aerial part treated with different NaCl concentrations for 8 weeks. Four plants were selected from each treatment as biological replicates. The modulated Chl fluorescence parameters were obtained by selecting the broad leaf at the top and dark-adapted for 30 min, and then using a PAM-2100 Chl fluorometer (Heinz Walz GmbH, Effeltrich, Germany) with a saturation pulse (8,000 μmol·m−2·s−1), before (F0) and after (Fm) fluorescence were measured for 1 s. The maximum photochemical efficiency of photosystem II (PSII) [Fv/Fm = (Fm − F0)/Fm] was calculated according to the method of Genty et al. (1989).
2.3.3 Leaf water potential
The leaf water potential (LWP) was measured using a dew point water potential meter (WP4C, Meter Environment, Pullman, WA, USA) to investigate the osmotic properties of L. tetragonum leaves. The leaves were cleaned by gently rubbing them with distilled water and tissue before cutting them. The widest leaves (diameter, 3 cm) of each plant were collected and then immediately placed in a small steel chamber (diameter, 3.5 cm). The air temperature of the chamber was equilibrated between the inside and outside of the chamber at 24°C for 10 ± 5 min.
2.3.4 Mineral ion content
The mineral ion content in the aerial part of L. tetragonum which was grown with various NaCl concentrations was measured in five replicates. Approximately 0.1 g of dried aerial part was digested in 5 ml of 70% HNO3 at 125°C for 1.5 h. To stimulate digestion, 7.5 ml of H2O2 was added to the solution and heated to 123°C for 1 h. HCl (1 ml) was added to the solution again and heated to 200°C for 2 h. The resulting solution was then made up to the same volume (10 ml) using triple distilled water. The solution was filtered through quantitative filter paper (90 mm, Adcantec Co., Japan). The content of 12 elements (Ca, K, Mg, Na, Cd, Cr, Cu, Fe, Mn, Ni, Pb, and Zn) in the solution was determined using an ICP-OES spectrophotometer (Optima 8300 DV; Perkin Elmer, Waltham, MA, USA).
2.3.5 Free amino acids
The amino acid (AA) content was measured in the aerial part using an AA Analyzer(II)_PF (L-8900, Hitachi, Tokyo, Japan). Briefly, HPLC-grade water (5 ml) was added to 0.1 g of dry powdered sample, shaken, and reacted at 60°C in a heating block for 1 h. 5-Sulfosalicylic acid dihydrate 1 ml of 10% was added, left at 4°C for 2 h, and centrifuged for 3 min, and the supernatant was filtered. The supernatant was completely concentrated at 60°C with a vacuum concentrator. Lithium buffer (pH 2.2) was dissolved in a 2-ml solution. This buffer was analyzed after filtering with a membrane filter (pore size, 0.45 µm) in an LC vial, and the analysis was performed in triplicates.
2.4 LC-ESI-MS analysis of specific flavonoids in Limonium tetragonum
For LC-ESI-MS, an ABSciex 3200 QTRAP mass spectrometer (Applied Biosystems, MDS Sciex, Concord, Canada) was used as the MS system. In LC-ESI-MS, the HPLC system was a Waters ACQUITY UPLC system (Milford, MA, USA). MultiQuant MD software (Version 3.0.1) was used to process the data obtained using UPLC and MS.
LC-ESI-MS HPLC was performed using the method of Lee et al. (2011). The hot air-dried samples were powdered individually using a mortar and pestle and analyzed in five replicates per treatment. After adding 1 ml of methanol to 50 mg of L. tetragonum dry powder, ultrasonic extraction was performed for 2 h. Immediately after extraction, 700 μl of the supernatant obtained by separating the precipitate using a centrifuge was taken and filtered with a 0.45-μm PVDF filter. For LC-ESI-MS analysis, the extract was diluted 1,000 times with 50% acetonitrile solvent containing 0.05% formic acid and then injected into the LC.
Five index components were prepared at a concentration of 2,500 ppm using 50% acetonitrile containing 0.05% formic acid and then diluted stepwise. A calibration curve for five index components was prepared using peak area values according to the concentration range of 50 to 500 ppb. The linearity was determined using the correlation coefficient (r2).
As mobile phase solvents for LC analysis, 0.1% formic acid DW and 0.1% formic acid MeOH were used, and the analysis conditions are shown in Table S3. The column used for the analysis was Waters C18 (100 × 2 mm, 1.7 μm), and the flow rate was set to 0.4 ml/min.
To investigate the changes in secondary metabolite content between the 0-mM NaCl and the NaCl treatments, five major compounds, viz., myricetin-3-O-β-D-galactoside (1), myricetin-3-O-(3″-O-galloyl)-α-L-rhamnoside (2), myricetin-3-O-α-L-rhamnoside (3), myricetin-3-O-(2″-O-galloyl)-α-L-rhamnoside (4), and 5,7,2′,5′-tetrahydroxy flavanone-3-O-β-D-galactoside (5), identified by Lee et al. (2011) in L. tetragonum were analyzed. The structures of these five standard compounds were confirmed through NMR analysis after being separated from the extracts of L. tetragonum (Lee et al., 2017).
2.5 Transcriptome analysis
2.5.1 RNA isolation and sequencing
The L. tetragonum leaves treated with different NaCl concentrations for 8 weeks were harvested. Five new leaves with representative growth were selected from each treatment as biological replicates. Trimmomatic (v. 0.39) (Bolger et al., 2014) was used to remove the adapter sequence. Trimming and quality control used the SLIDINGWINDOW, LEADING, and TRAILING options according to the following conditions: 1) window size = 4, 2) mean quality 15, and 3) LEADING, TRAILING ≧3.
2.5.2 Transcriptome de novo assembly
De novo assembly of cleaned good quality reads was performed using the TRINITY (Grabherr et al., 2011) program. For indexing and mapping, a de novo assembled reference file provided by the client (TRINITY software) was utilized.
2.5.3 Enrichment analysis
The gene ontology (GO) of differentially expressed genes (DEGs) was defined using the DAVID tool and the GO consortium database. GO and Kyoto Encyclopedia of Genes and Genomes (KEGG) analyses were performed to obtain more detailed information about DEGs in GO terms (http://geneontology.org/) (Gene Ontology Consortium, 2001) and metabolic pathways (https://www.genome.jp/kegg/). The DEGs in the KEGG pathways and GO analysis were enriched by clusterProfiler R packages. The threshold (padj< 0.01) was determined by the significant enrichment of KEGG pathways and GO terms.
2.5.4 Differential expression analysis
Feature count was performed using the HiSeq-count, and DEGs were identified using the edgeR package tool (Varet et al., 2016). edgeR normalized the data using the truncated mean of the M-values calculated as the weighted average of the log ratios between this test and the baseline after excluding the most expressed genes and the genes with the largest log ratios.
The cutoff criteria established the DEG, which can be “log2 (fold change),” “false discovery rate [adjusted p-value (padj)],” and/or “read count.” Default criteria are “|log2(fold change)|>2,” “padj<0.05,” and “read count>1000” for the analyses. DEG enrichment analysis of data sets is performed using tools such as GO, the KEGG pathway, and the STRING database (Szklarczyk et al., 2021).
2.5.5 Annotation
TRINITY IDs were annotated using blastx (e-value ≤ 1e−10) against the database Viridiplantae of NCBI NR to understand the function of the gene. InterProScan was used for genome-scale protein function classification because it can perform fast sequence alignment and domain search against selected reference data sets. InterProScan’s settings have been set to default options (Jones et al., 2014).
2.6 Statistical analysis
Data were analyzed using Duncan’s multirange test. The one-way analysis of variance was applied using the NaCl concentrations as variables, and mean values were separated by Duncan’s multirange test (p< 0.05). All treatments comprised three replicates and each replicate consisted of 10 plants. Statistical analysis was performed using SAS software (version 9.4, SAS Institute Inc., Cary, NC, USA). The mean ± SE values are shown in the tables and figures.
3 Results and discussion
3.1 Physiological responses
3.1.1 Growth characteristics
The growth of L. tetragonum was not affected by the NaCl concentrations (100-mM NaCl) in the nutrient solution (Table 1 and Figure S2). The FW and DW of L. tetragonum were the highest at 75-mM NaCl, although there was no significant difference between the 0-mM NaCl and the NaCl treatments. The LA, leaf number, and SLW showed no significant differences between the 0-mM NaCl and the NaCl treatment. Jeong (2017) investigated the changes in plant height, leaf number, FW, and DW of L. tetragonum at various NaCl concentrations and reported that NaCl below 171 mM did not affect the growth of L. tetragonum. Our results also showed no significant change in growth below 100 mM of NaCl concentrations. Plants are classified into salt-tolerant “halophytes” and salt-tolerant “glycophytes” according to the degree of change in growth in a salinity environment (Flowers and Colmer, 2008). Dicotyledonous halophytes compartmentalize NaCl into vacuoles and store NaCl through the salt bladder and salt gland structures. In addition, halophytes reduce the cytoplasmic NaCl concentration by discharging them out of the leaf (Yuan et al., 2016). According to the method of classifying halophytes by Breckle (1986), L. tetragonum is classified as a recretohalophyte with a salt bladder and salt glands on the underside of leaves (Alhaddad et al., 2021). The dicotyledonous halophytes have no significant change in biomass production even at 200 mM of NaCl and have the genetic potential to resist various environmental stresses (O’Leary et al., 1985). The introduction of specific genes related to the resistance of halophytes is being studied as one of the methods to improve the stress tolerance of glycophytes (Himabindu et al., 2016).
3.1.2 Chlorophyll fluorescence
The Fv/Fm of L. tetragonum showed no significant difference between the 0-mM NaCl and the NaCl concentrations (Figure 1A). The ratio of Fv/Fm, which reflects a plant’s maximum photosynthetic capacity, is roughly 0.83 in healthy plants (Björkman and Demmig, 1987). The photochemical reaction was not affected by NaCl concentrations below 100 mM. The electron transfer rate (ETR) of L. tetragonum was not affected by NaCl treatment (Figure 1B). However, the ETR did not decrease at NaCl concentrations of >50 mM; thus, it was considered a temporary plant growth problem when measuring ETR.
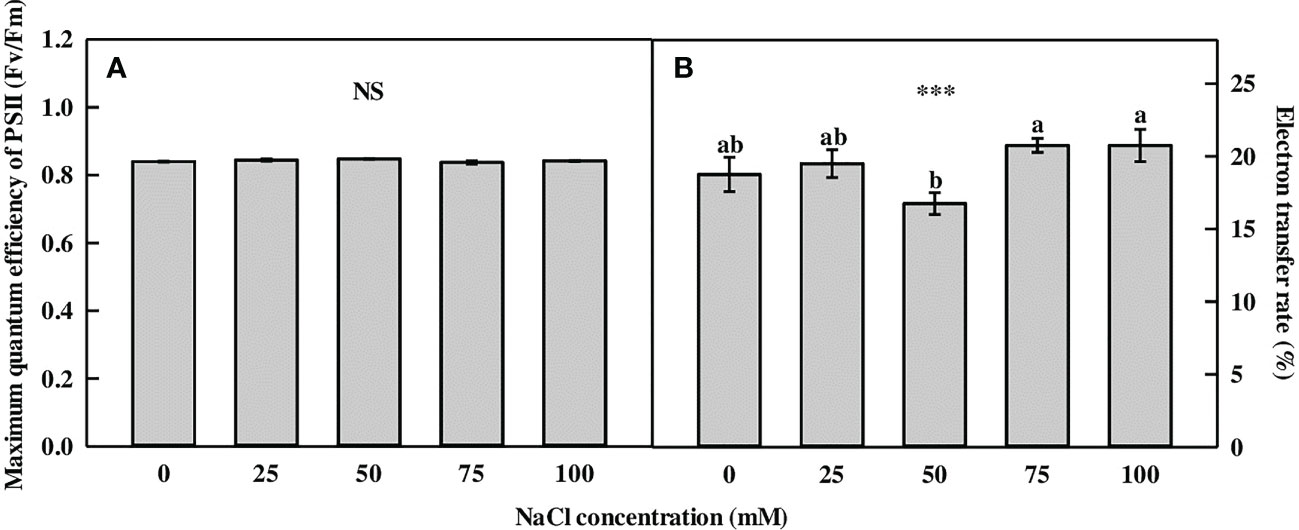
Figure 1 The chlorophyll fluorescence parameters of Limonium tetragonum under different NaCl concentrations (n = 4). (A) Maximum quantum efficiency of PSII (Fv/Fm) and (B) electron transfer rate. Statistical analysis was performed with Duncan’s multiple range test. NS, non-significant. ***p = 0.001.
Chl fluorescence has been used as an indicator to evaluate the response of plants to environmental stress (Sayed, 2003). Chl fluorescence was used to study the effect of salinity on barley (Belkhodja et al., 1999) and maize (Billah et al., 2017), which decreased at high salinity. However, our results did not show a decrease in Chl fluorescence. The Fv/Fm of the halophyte Lycium barbarum (Wei et al., 2006) and Panicum turgidum (Koyro et al., 2013) decreased at high salinity, but not at moderate salinity. This is consistent with the findings of the present study. ETR refers to the number of electrons passing through photosystem II, and high photosynthesis is influenced by ETR (Yao et al., 2017). Since the Fv/Fm and ETR of the NaCl-treated L. tetragonum did not differ from those of the 0-mM NaCl, it was our understanding that the 100-mM NaCl treatment did not affect the photosynthesis of L. tetragonum.
3.1.3 Leaf water potential
The leaves showed a significant difference in LWP in comparison with the 0-mM NaCl (Figure 2). The LWP of L. tetragonum was significantly higher in the 0-mM NaCl (−1.68 MPa) than in the NaCl treatments. The LWP of L. tetragonum grown under 25-, 50-, and 75-mM NaCl showed no significant difference, and the LWP of 100 mM of NaCl showed the lowest value (−3.01 MPa) compared with the other treatments. Water potential is a numerical value of the potential energy of water with respect to the content of the solute. The lower value of the water potential indicates a higher concentration of the solute compared with the solvent (Zhu et al., 2022). The amount of solutes accumulated in the leaves of L. tetragonum increased in the NaCl treatment in comparison with the 0-mM NaCl. The amount of NaCl accumulated in the leaves of L. tetragonum showed a tendency to increase at 100 mM compared with the NaCl accumulated at 75 mM. Salinity significantly affects the water potential of plants and soil. Excessive accumulation of salts in the soil increases the concentration of solutes, lowering the water potential. Water potential gradient differences between soil and plants reduce water uptake in plants such as tomatoes (Tanveer et al., 2020) and maize (Ali et al., 2021), which is the osmosis action of salt stress. However, dicotyledonous halophytes adapt to this osmotic stress by adjusting the NaCl concentration. Although a reduction in LWP was observed, growth remained unaffected with the increase in NaCl concentration; this indicated that the leaves of L. tetragonum were osmotically adjusted to survive in a saline environment.
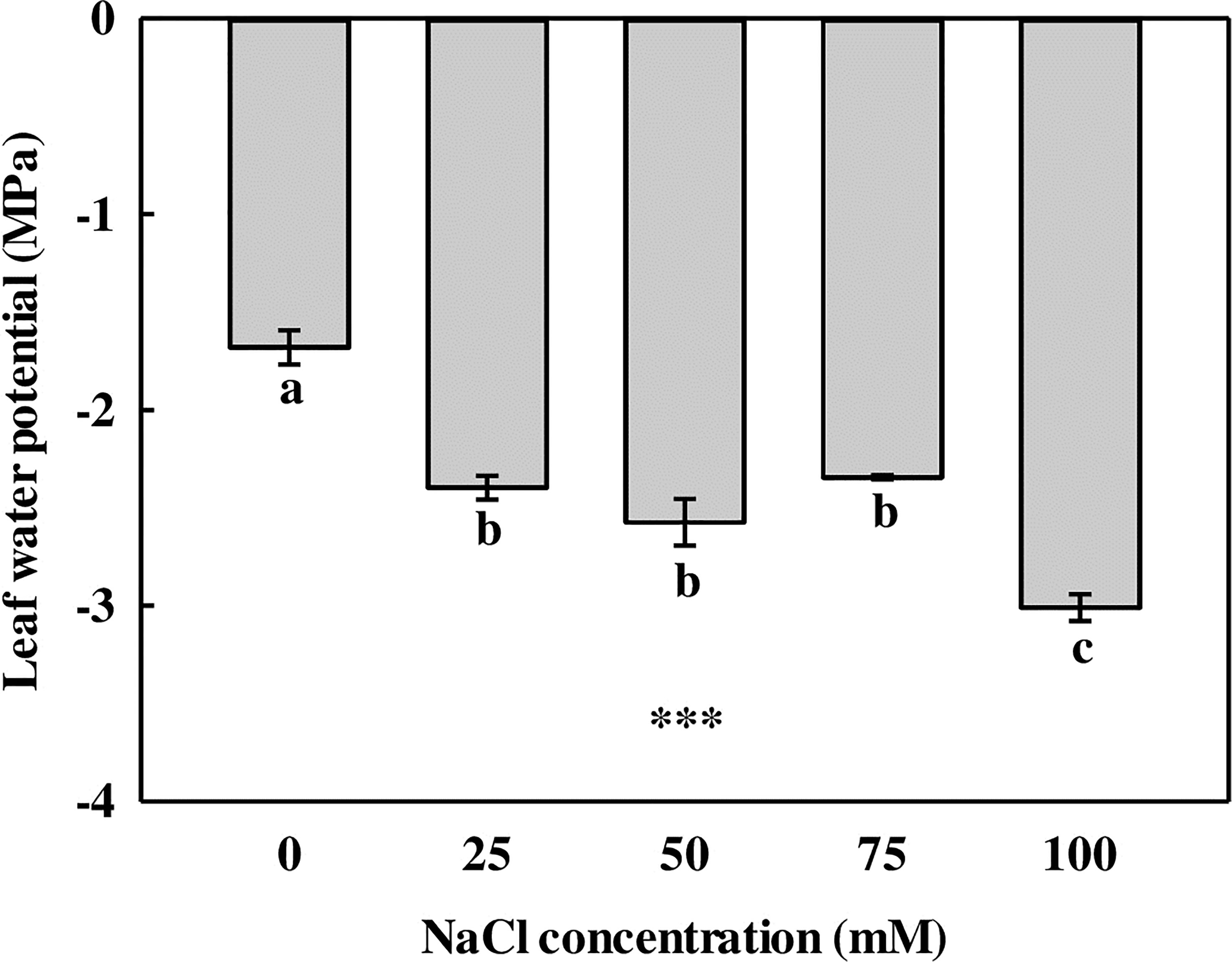
Figure 2 The leaf water potential of Limonium tetragonum under different NaCl concentrations (n = 4). Statistical analysis was performed with Duncan’s multiple range test. ***p = 0.001.
3.1.4 Mineral ion content
3.1.4.1 Macronutrients and Na+
The content of monovalent ions in the aerial part of L. tetragonum was changed significantly in accordance with the NaCl concentrations (Table 2). The K+ content was the highest in the 0-mM NaCl at 47.25 mg, and the content decreased significantly as the NaCl concentration increased. The 75-mM NaCl had the lowest K+ content (55.3% less in comparison with the 0-mM NaCl) among all the NaCl treatments. NaCl treatment significantly reduced the K+ content in the leaves of L. tetragonum. The Na+ content increased proportionally to the NaCl concentration, and there was a significant difference among all the NaCl concentrations. The Na+ content in the 25- and 50-mM NaCl increased approximately five times compared with the 0-mM NaCl and approximately 50 times at the 100-mM NaCl. A significant difference in K+/Na+ between different NaCl concentrations was observed. It showed the highest value at 59.55 mg in the 0-mM NaCl and was decreased by approximately 58 times or more in the NaCl treatments. The highest amount of Ca2+ (10.44 mg) and Mg2+ (9.9 mg) was observed at 25 mM.
K+ is an essential ion in plant physiology, which plays a vital role in osmotic pressure regulation, maintenance of swelling, and protein synthesis (Munns and Tester, 2008). However, absorption is inhibited by Na+, leading to a decrease in the concentration of K+ in plants (Qu et al., 2012), which leads to stomata closure and reduced photosynthetic rate and reduces plant yield (Siegel et al., 2009). The increase in NaCl concentration in the succulent stem of the perennial halophyte Sarcocornia quinqueflora decreased the K+ content. The K+ content showed a positive correlation with ascorbic acid and soluble sugar (Ahmed et al., 2021). The ability of the halophyte to accumulate salt varies from species to species. The NaCl content of the aerial part of T. decumbens increased approximately 7.9 times compared with the 0-mM NaCl (Sogoni et al., 2021). Accordingly, L. tetragonum showed excellent salt accumulation ability among halophytes. High concentrations of Na+ interfere with the synthesis of K+ transporter-associated KUP-HAK transporters, which leads to a decrease in K+ and, consequently, a decrease in K+/Na+ (Santa-María et al., 1997). However, halophytes have salt tolerance by self-regulating the NaCl concentration in the plant due to special organs (Yokoi et al., 2002). In halophytes, K+/Na+ is used as an indirect indicator of salt tolerance (Greenway and Munns, 1980). K+/Na+ decreased approximately 58-fold in the NaCl treatment in comparison with the 0-mM NaCl, indicating high salt tolerance of L. tetragonum. The leaves of L. tetragonum showed comparable accumulation tendencies for Ca2+ and Mg2+. The Limonium species showed a common decrease in Ca2+ and Mg2+ in all plants due to high NaCl concentrations, which was in line with our study (Al Hassan et al., 2017). Ca2+ is a substance involved in salinity and osmotic signaling that protects plants from ROS (Knight et al., 1997). Although 100 mM of NaCl reduced Ca2+ and K+ in the leaves of L. tetragonum, it does not affect plant growth due to the increased utilization efficiency of these minerals (Ghnaya et al., 2007).
3.1.4.2 Micronutrients
The salinity changed the content of trace elements in the aerial part of L. tetragonum (Table 2). Cr and Fe increased in the NaCl treatments than in the 0-mM NaCl, and Mn and Zn2+ decreased in the NaCl treatments. Cu2+ and Pb2+ showed no significant change between the 0-mM NaCl and the NaCl treatment, and neither Cd2+ nor Ni was detected. Fe showed the lowest value at 100 mM of NaCl, and it decreased by 20% compared with the 0-mM NaCl. Mn showed the lowest content at 25 and 75 mM of NaCl and decreased by approximately 65% in comparison with the 0-mM NaCl. Zn2+ showed the lowest content at 75 mM of NaCl and decreased by 18% in comparison with the 0-mM NaCl.
Micronutrients such as iron, zinc, or copper are necessary nutrients for all living organisms (Waters and Sankaran, 2011). Depending on the type of plant, the effects of salt stress on micronutrients varied. Appropriate NaCl concentration increased micronutrients in zucchini (Víllora et al., 2000).
3.2 Free amino acids
The free AAs accumulated in the aerial part of L. tetragonum showed apparent differences according to the NaCl concentrations (Table 3). The free AA content changed proportionally with the NaCl concentration. Treating L. tetragonum with NaCl led to an increase in the non-essential AAs—urea, proline (Pro), β-alanine (β-Ala), ornithine (Orn), and arginine (Arg)—and a decrease in the remaining non-essential AAs. There was an overall decrease in essential AAs in NaCl treatment. Alanine accounted for 25% of the non-essential AAs and showed the highest content in the 0-mM NaCl. In contrast, Pro accounted for 60% of the total non-essential AAs in the NaCl treatment, and the total non-essential AA content of the NaCl treatment decreased compared with that of the 0-mM NaCl. The total AA content was maintained due to Pro. The accumulated Pro in the aerial part of L. tetragonum increased rapidly as the NaCl concentration increased and was found to be a major AA constituting most of the total AAs.
Various AAs such as proline, glutamic acid, glycine, alanine, and arginine are used as indicators of plant stress tolerance (Younis et al., 2009). Pro is an AA that controls osmosis together with glycine, betaine, and total soluble sugar. Pro acts as an antioxidant and is involved in the antioxidant system of halophytes. Pro is an osmotic substance that is synthesized to stabilize the intracellular osmotic balance. Most halophytes accumulate Na+ in vacuoles in cases of excessive salt absorption. Increased solute concentration in the vacuole leads to an increase in the Pro content to adjust the osmotic pressure outside the vacuole (Szabados and Savouré, 2010). Similarly, in this study, the Pro content of the leaves increased with increasing NaCl concentration. Salt stress is accompanied by water stress, and Pro is easily synthesized from glutamate, ornithine, and arginine in Cyclotella cryptica (Liu and Hellebust, 1976). In the salt-treated L. tetragonum, glutamate appears to be mostly converted to Pro and reduced. Arg is one of the AAs that serves as a precursor to proteins as well as nitric oxide, urea, proline, and glutamate (Wu and Morris, 1998). Orn is the major metabolite of Arg in the urea cycle and shares many pharmacological effects with Arg (Endo et al., 2004). Orn has a wound-healing effect (Shi et al., 2002). A small amount of Orn was detected in the salt-treated L. tetragonum, but both Orn and Arg were increased compared with the 0-mM NaCl, suggesting the possibility that salt stress can improve the wound-healing effect of L. tetragonum. β-Ala is present in all living organisms and is required for plant growth as it is a precursor to vitamin B5 and pantothenate (Raman and Rathinasabapathi, 2004). In most plants of the Plumbaginaceae family, β-Ala is converted to β-Ala betaine, an osmoprotectant, which is involved in salt tolerance (Rathinasabapathi et al., 2001; Raman and Rathinasabapathi, 2003). The content of β-Ala was increased by various stresses (Parthasarathy et al, 2019). The β-Ala content increases along with other non-essential AAs under slight salt stress caused by low and medium concentrations of NaCl.
3.3 Contents of specific flavonoid compounds
The five compounds (1–5) contained in L. tetragonum were measured via liquid chromatography-electrospray ionization-mass spectrometry high-performance liquid chromatography (LC-ESI-MS HPLC). The metabolite concentration accumulated in the aerial part of L. tetragonum was significantly increased by NaCl treatment (Figure 3). Myricetin-3-O-β-D-galactoside (compound 1) was found to be 66.2 ppm in the 0-mM NaCl, and it increased in response to NaCl treatment (Figure 3A). The concentration of “compound 1” was higher at 25 mM (192.8 ppm) and 75 mM (193.7 ppm) of NaCl compared with the 0-mM NaCl. Among the NaCl treatments, 50- and 100-mM NaCl tended to decrease the “compound 1” content compared with the other NaCl treatments. Myricetin-3-O-(3″-O-galloyl)-α-L-rhamnoside (compound 2) gradually increased with NaCl concentration. The lowest concentration (41 ppm) was observed in the 0-mM NaCl, and it significantly increased in all NaCl treatments (Figure 3B). The concentration of “compound 2” was the highest at 100-mM NaCl (154.1 ppm), but there was no significant difference between 50- and 75-mM NaCl, and these concentrations increased by 254.1%–275.8% compared with the 0-mM NaCl. Although 25-mM NaCl (115 ppm) showed the lowest value among the NaCl treatments, it increased by 180.4% compared with the 0-mM NaCl. Myricetin-3-O-α-L-rhamnoside (compound 3) gradually increased with NaCl concentration (Figure 3C). Compound 3 in the 0-mM NaCl (21.66 ppm) was the lowest, and it significantly increased in all NaCl treatments compared with the 0-mM NaCl. It was the highest at 100 mM of NaCl, but there was no significant difference compared with 75-mM NaCl, and it increased by 114.4% and 110.4%, respectively, compared with the 0-mM NaCl. Myricetin-3-O-(2″-O-galloyl)-α-L-rhamnoside (compound 4) was found to be 51.6 ppm in the 0-mM NaCl and was increased in all NaCl treatments compared with the 0-mM NaCl (Figure 3D). A tendency to increase at NaCl concentrations above 50 mM was observed, but there was no significant difference among the treatments. The concentration of “compound 4” was the lowest at 25-mM NaCl compared with the other NaCl treatments but increased by 95.8% compared with the 0-mM NaCl. 5,7,2′,5′-Tetrahydroxy flavanone-3-O-β-D-galactoside (compound 5) was not detected in the 0-mM NaCl but was found only in small amounts in the NaCl treatment (Figure 3E). At 75-mM NaCl (13ppm), it showed the highest value among the other NaCl treatments. It was found that the concentration of “compound 5” was the lowest at 50- and 100-mM NaCl.
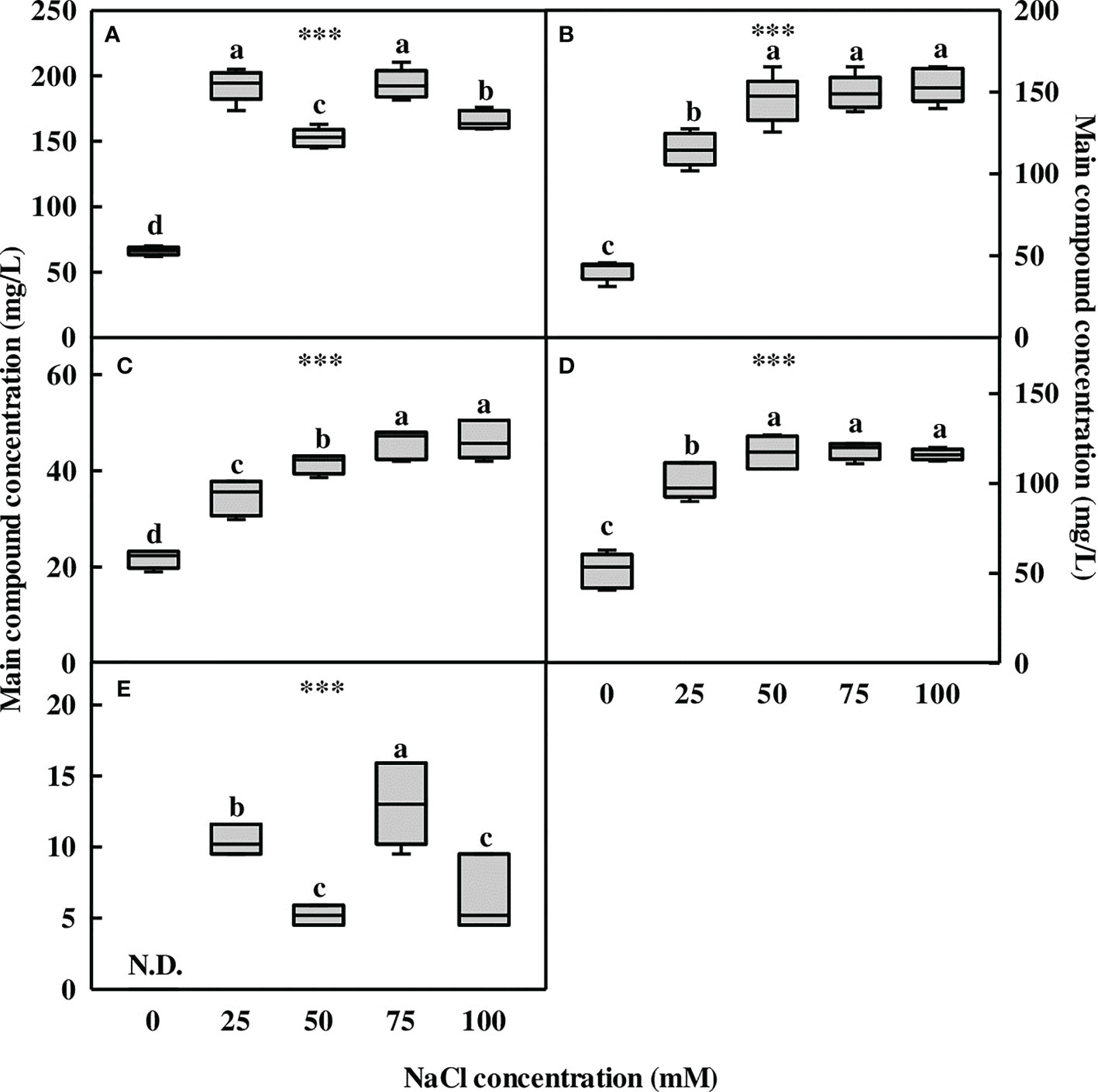
Figure 3 The concentration of the major compounds of Limonium tetragonum under different NaCl concentrations analyzed using LC-ESI-MS (n = 5). (A) Compound 1 (myricetin-3-O-β-D-galactoside), (B) compound 2, (C) compound 3, (D) compound 4, and (E) compound 5. Statistical analysis was performed with Duncan’s multiple range test. ***p = 0.001. N.D., non-detected.
Myricetin derivatives and their glycosides were identified as the major compounds contained in L. tetragonum (Lee et al., 2011). Of the five major flavonoids in L. tetragonum, the concentration of myricetin glycosides (1–4) was increased in the NaCl treatment, and the flavanone compound (5) was detected only in the NaCl treatments. In addition, the concentration of the five compounds increased to the maximum in the 75-mM NaCl treatment than in the 0-mM NaCl treatment. Myricetin glycosides and flavanone compounds are in the flavonoid family and are the most abundant substances in L. tetragonum. Flavonoids and Pro are essential protective substances with antioxidative properties (Zhu, 2001). Many studies have reported that salt stress affects the flavonoid biosynthetic pathway in plants, leading to an increase in flavonoids (Ben Abdallah et al., 2016). Based on the above results, it can be inferred that the flavonoid biosynthetic pathway is stimulated by salt stress.
3.4 Transcriptome analysis
3.4.1 Differentially expressed genes
The difference in the transcriptional characteristics expressed in the leaves of L. tetragonum in response to the NaCl concentration is shown in Table 4 and Figures 4–6. Hierarchical clustering showed a clear separation between groups, and the principal component analysis (PCA) plots suggest that each sample was inherently different from the NaCl non-treated sample.
Hierarchical clustering using heatmaps revealed differences between the 0-mM NaCl and the 50- and 100-mM NaCl treatments (Figure 4). The THI42_VITVI (TV) was upregulated in the NaCl treatment compared with the 0-mM NaCl. The expression of genes in plants changes under the influence of salinity, and their expression profiles have been studied in different plant species and at different stages of development in response to salinity stress (Jamil et al., 2011). The transcriptome analysis revealed differences in gene expression between the L. tetragonum NaCl treatment and the 0-mM NaCl. Some major salt-related components and DEGs were identified. The results of the present study can be used as clues for the analysis of the salinity response and salinity resistance in L. tetragonum.
TV, a protein-coding factor, is a thiamine thiazole synthase and was upregulated by NaCl treatment. Thiamine is an essential water-soluble vitamin for all organisms, found in high amounts in green vegetables, beans, cereal germs, and nuts (Goyer, 2010). NaCl treatment of the shiitake plant has the potential to enhance its thiamine content.
3.4.2 Principal component analysis
The PCA revealed that the 0-mM NaCl and the NaCl treatments were clearly distinguished (Figure 5). In addition to the difference between the 0-mM NaCl and the NaCl treatments, the dispersion distribution was displayed in accordance with the NaCl concentration, suggesting that the greater the NaCl concentration, the greater the differential expression in L. tetragonum.
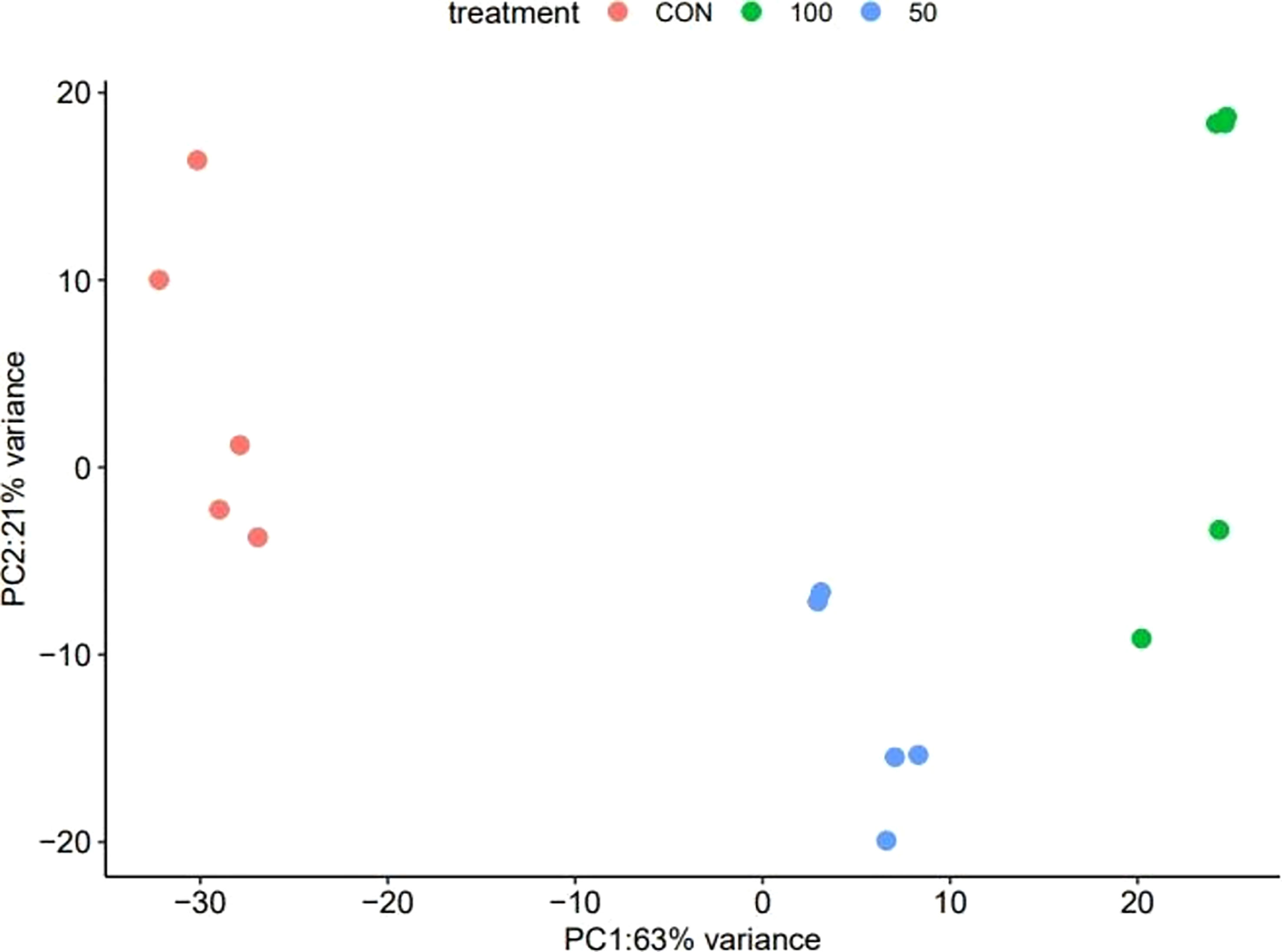
Figure 5 The principal component analysis of Limonium tetragonum under different NaCl concentrations (n = 5).
3.4.3 Gene ontology
The biological processes of L. tetragonum were changed according to the NaCl concentration (Figure 6). The most abundant GO associated with biological processes in the 50-mM NaCl/0-mM NaCl was circadian rhythm (50%). Circadian rhythms are a subset of biological rhythms with cycles defined as the time to complete one circadian cycle (Dunlap and Loros, 2004). The transcription rate and transcriptional accumulation of Arabidopsis LHCB (Millar and Kay, 1991) and many other genes (McClung and Kay, 1994) are associated with circadian regulation (McClung, 2006). Even in the 100-mM NaCl/0-mM NaCl, circadian rhythms accounted for a large portion and may be a major contributor to salt tolerance.
3.4.4 KEGG pathway
The KEGG analysis revealed common pathways, viz., “biosynthesis of secondary metabolites,” “tryptophan metabolism,” and “circadian rhythm: plant” in the 0-mM NaCl and the 50- and 100-mM NaCl (Table 4). There were no significantly enriched molecular function terms in DEG, but the 100-mM NaCl enriched several cellular component terms, i.e., the chloroplast and plasma membrane. The KEGG pathway analysis results were similar to the GO “biological processes” terms. At 50-mM NaCl, “tryptophan metabolism” was found to be the most enriched pathway, followed by “circadian rhythm-plant,” “MAPK signaling pathway-plant,” and “biosynthesis of secondary metabolites.” However, “biosynthesis of secondary metabolites” and “tryptophan metabolism” were not statistically significant pathways at 100-mM NaCl.
The genes involved in secondary metabolite biosynthesis pathways are shown in Table 4. Serine dehydratase regulator 1 (SDR1), dominant cataract 2 (CAT2), Aldolase superfamily protein (AT2G26800), GDP-L-galactose phosphorylase 1 (VTC2), O-methyltransferase 1 (OMT1), and NPC intracellular cholesterol transporter 1 (NPC1) are genes that are commonly upregulated in the NaCl treatments compared to the 0-mM NaCl. GLN phosphoribosyl pyrophosphate amidotransferase 2 (ASE2) was downregulated in the NaCl treatment compared with the 0-mM NaCl. Specifically, peroxidase superfamily protein (PRX52) and nine-cis-epoxycarotenoid dioxygenase 4 (NCED4) showed differential expression patterns according to the NaCl concentration. PRX52 was upregulated compared with the 0-mM NaCl at 50-mM NaCl but downregulated at higher NaCl concentrations. NCED4 was downregulated at 50-mM NaCl but upregulated at higher NaCl concentrations.
SDR1 is a gene involved in sugar sensing and signaling essential for many metabolic processes such as germination and growth in Arabidopsis (Rolland et al., 2002). SDR1 was found to be upregulated with increasing NaCl concentration. CAT catalyzes the breakdown of H2O2 into water and oxygen (Willekens et al., 1997). CAT expression/activity was found to be higher in halophytes than in glycophytes during salinity stress, suggesting that CAT helps in salt tolerance by scavenging H2O2 (Bose et al., 2014). In halophytes, salt stress increases the production of the phenylpropanoid genes PRX52 and OMT1 (Gao et al., 2019).
Genes belonging to the tryptophan metabolism pathway were flavin-containing monooxygenase family protein (YUC10), catalase-like (LOC110894758), and OMT1. The relative expression of YUC10 was upregulated at 50 mM of NaCl but downregulated at 100 mM of NaCl. LOC110894758 and OMT1 were upregulated in the NaCl treatment. OMT1 was commonly observed in secondary metabolite biosynthesis and tryptophan metabolism.
The YUC gene induces the biosynthesis of auxin and is a developmental induction gene necessary for the development of embryogenesis, seedlings, and flowers. YUC10 is an essential gene for the embryogenesis development and leaf formation of Arabidopsis and induces plant development (Cheng et al., 2007). In the leaves of L. tetragonum grown with 50-mM NaCl, YUC10 was upregulated, indicating its role in the growth and development of L. tetragonum in a saline environment. The KEGG pathway analysis revealed that “biosynthesis of secondary metabolites” and “tryptophan metabolism” were consistently enriched pathways in the database, requiring further investigation with respect to secondary metabolites present in L. tetragonum.
The protein–protein interaction network analysis using the STRING database showed similar results as the KEGG pathway analysis. The molecules related to the “circadian rhythm” are well clustered in the network. The circadian rhythm, which accounted for the largest proportion in the enriched GO term, was upregulated in the NaCl treatment, and a significant difference was observed at different NaCl concentrations. Homeodomain-like superfamily protein (LHY) was upregulated in the NaCl treatment, with the highest expression at 50 mM of NaCl. E74-like ETS transcription factor 3 (ELF3) and PRR5 (proline-rich 5) were downregulated at 50-mM NaCl but upregulated at 100-mM NaCl.
LHY is one of the Arabidopsis homologs and is a gene involved in flowering, stomatal development, and stress regulation in the succulent Suaeda fruticosa (Diray-Arce et al., 2019). Arabidopsis Early Flowing 3 (AELF3) is a gene associated with a circadian clock that physically interacts with photoreceptors (Liu et al., 2001) and induces proper expression of plant growth promoters in Arabidopsis (Nusinow et al., 2011).
4 Conclusion
Our results showed the same results as the studies on the NaCl concentration of L. tetragonum. NaCl treatment at less than 100 mM in a hydroponics system did not affect the growth and Chl fluorescence of L. tetragonum, indicating that L. tetragonum was tolerant to the saline environment. The water potential of the leaves of L. tetragonum decreased as NaCl concentration increased, indicating that solutes accumulated in the leaves increased. The leaves of Limonium tetragonum accumulated minerals and Na+ was found to have the highest concentration. The K+ content decreased in inverse proportion to the Na+. NaCl treatment increased some of the AAs of the leaves of L. tetragonum, and among them, the proline content increased significantly. The chemical profiling was done using LC-ESI-MS HPLC. In this study, the concentration of the four myricetin glycosides and the flavanone compound was significantly increased by NaCl treatment. Flavanone was found in the form of 5,7,2′,5′-tetrahydroxy flavanone-3-O-β-D-galactoside in L. tetragonum, and its content improved in all the NaCl treatments. Transcriptome analysis of the aerial part of L. tetragonum showed clear differences in the secondary metabolite biosynthetic pathway, tryptophan metabolome, and circadian rhythm between the 0-mM NaCl and the NaCl treatments. The NaCl concentrations of 100 mM or less did not affect the growth of L. tetragonum. However, a number of DEGs identified in the transcriptome analysis of plants grown in NaCl treatment confirmed differences in the regulatory machinery under NaCl conditions. This study describes a methodology to improve the functional materials in the NaCl range that do not reduce the growth of L. tetragonum in vertical farms. In addition, this study revealed that NaCl treatment was necessary to improve the flavonoid myricetin glycoside. The optimal NaCl concentration for the enhancement of the secondary metabolites of L. tetragonum in the present study is 75-mM NaCl.
Data availability statement
The original contributions presented in the study are publicly available. This data can be found here: https://www.ncbi.nlm.nih.gov/bioproject/929564.
Author contributions
K-HS and EJ designed the experiments. K-HS, KC, and JY organized the project. S-NJ, M-JK, and YK performed the experiments. S-NJ wrote the manuscript. All authors contributed to the article and approved the submitted version.
Funding
This work was supported by a National Research Foundation of Korea (NRF) grant funded by the Korean government (MSIT) (no. 2021R1F1A1047454) and the Korea Institute of Planning and Evaluation for Technology in Food, Agriculture and Forestry (IPET), through the Technology Commercialization Support Program, funded by the Ministry of Agriculture, Food and Rural Affairs (MAFRA) (821037031HD020).
Acknowledgments
We are grateful for the contributions of and discussions conducted with the members of Jeong’s laboratory.
Conflict of interest
The authors declare that the research was conducted in the absence of any commercial or financial relationships that could be construed as a potential conflict of interest.
Publisher’s note
All claims expressed in this article are solely those of the authors and do not necessarily represent those of their affiliated organizations, or those of the publisher, the editors and the reviewers. Any product that may be evaluated in this article, or claim that may be made by its manufacturer, is not guaranteed or endorsed by the publisher.
Supplementary material
The Supplementary Material for this article can be found online at: https://www.frontiersin.org/articles/10.3389/fpls.2023.1159625/full#supplementary-material
Supplementary Figure 1 | The photon flux density for the wavelength of the LED.
Supplementary Figure 2 | The growth of L. tetragonum under different NaCl concentrations.
Supplementary Table 1 | Composition of Hoagland’s nutrient solution used in the cultivation of Limonium tetragonum.
Supplementary Table 2 | NaCl components and chemical property for L. tetragonum under different NaCl concentrations used in this study.
References
Ahmed, H. A. I., Shabala, L., Shabala, S. (2021). Tissue-specificity of ROS-induced k+ and Ca2+ fluxes in succulent stems of the perennial halophyte Sarcocornia quinqueflora in the context of salinity stress tolerance. Plant Physiol. Biochem. 166, 1022–1031. doi: 10.1016/j.plaphy.2021.07.006
Alhaddad, F. A., Abu-Dieyeh, M. H., ElAzazi, E. S. M., Ahmed, T. A. (2021). Salt tolerance of selected halophytes at the two initial growth stages for future management options. Sci. Rep. 11, 10194. doi: 10.1038/s41598-021-89462-3
Al Hassan, M., Estrelles, E., Soriano, P., López-Gresa, M. P., Bellés, J. M., Boscaiu, M., et al. (2017). Unraveling salt tolerance mechanisms in halophytes: a comparative study on four Mediterranean Limonium species with different geographic distribution patterns. Front. Plant Sci. 8, 1438. doi: 10.3389/fpls.2017.01438
Ali, M., Afzal, S., Parveen, A., Kamran, M., Javed, M. R., Abbasi, G. H., et al. (2021). Silicon mediated improvement in the growth and ion homeostasis by decreasing na+ uptake in maize (Zea mays l.) cultivars exposed to salinity stress. Plant Physiol. Biochem. 158, 208–218. doi: 10.1016/j.plaphy.2020.10.040
Bae, M. J., Karadeniz, F., Lee, S. G., Seo, Y., Kong, C. S. (2016). Inhibition of MMP-2 and MMP-9 activities by Limonium tetragonum extract. Prev. Nutr. Food Sci. 21, 38–43. doi: 10.3746/pnf.2016.21.1.38
Belkhodja, R., Morales, F., Abadía, A., Medrano, H., Abadía, J. (1999). Effects of salinity on chlorophyll fluorescence and photosynthesis of barley (Hordeum vulgare l.) grown under a triple-line-source sprinkler system in the field. Photosynthetica 36, 375–387. doi: 10.1023/A:1007019918225
Ben Abdallah, S., Aung, B., Amyot, L., Lalin, I., Lachâal, M., Karray-Bouraoui, N., et al. (2016). Salt stress (NaCl) affects plant growth and branch pathways of carotenoid and flavonoid biosyntheses in Solanum nigrum. Acta Physiol. Plant 38, 1–13. doi: 10.1007/s11738-016-2096-8
Billah, M., Rohman, M. M., Hossain, N., Uddin, M. S. (2017). Exogenous ascorbic acid improved tolerance in maize (Zea mays l.) by increasing antioxidant activity under salinity stress. Afr. J. Agric. Res. 12 (17), 1437–1446. doi: 10.5897/AJAR2017.12295
Björkman, O., Demmig, B. (1987). Photon yield of O2 evolution and chlorophyll fluorescence characteristics at 77 K among vascular plants of diverse origins. Planta 170, 489–504. doi: 10.1007/BF00402983
Bolger, A. M., Lohse, M., Usadel, B. (2014). Trimmomatic: A flexible trimmer for illumina sequence data. Bioinformatics 30, 2114–2120. doi: 10.1093/bioinformatics/btu170
Bose, J., Rodrigo-Moreno, A., Shabala, S. (2014). ROS homeostasis in halophytes in the context of salinity stress tolerance. J. Exp. Bot. 65, 1241–1257. doi: 10.1093/jxb/ert430
Breckle, S. W. (1986). Studies on halophytes from Iran and Afghanistan. Proc. R. Soc Edinb. B Biol. Sci. 89, 203–215. doi: 10.1017/S0269727000009039
Cheng, Y., Dai, X., Zhao, Y. (2007). Auxin synthesized by the YUCCA flavin monooxygenases is essential for embryogenesis and leaf formation in arabidopsis. Plant Cell 19, 2430–2439. doi: 10.1105/tpc.107.053009
Deng, Y. Q., Bao, J., Yuan, F., Liang, X., Feng, Z. T., Wang, B. S. (2016). Exogenous hydrogen sulfide alleviates salt stress in wheat seedlings by decreasing na+ content. Plant Growth Regul. 79, 391–399. doi: 10.1007/s10725-015-0143-x
Despommier, D. (2010). The vertical farm: Feeding the world in the 21st century (Providence, RI: Macmillan).
Diray-Arce, J., Knowles, A., Suvorov, A., O’Brien, J., Hansen, C., Bybee, S. M., et al. (2019). Identification and evolutionary characterization of salt-responsive transcription factors in the succulent halophyte Suaeda fruticosa. PloS One 14, e0222940. doi: 10.1371/journal.pone.0222940
Dunlap, J. C., Loros, J. J. (2004). The neurospora circadian system. J. Biol. Rhythms 19, 414–424. doi: 10.1177/0748730404269116
Endo, F., Matsuura, T., Yanagita, K., Matsuda, I. (2004). Clinical manifestations of inborn errors of the urea cycle and related metabolic disorders during childhood. J. Nutr. 134, 1605S–1609S. doi: 10.1093/jn/134.6.1605S
Falcinelli, B., Sileoni, V., Marconi, O., Perretti, G., Quinet, M., Lutts, S., et al. (2017). Germination under moderate salinity increases phenolic content and antioxidant activity in rapeseed (Brassica napus var oleifera Del.) sprouts. Molecules 22 (8), 1377.
Flowers, T. J., Colmer, T. D. (2008). Salinity tolerance in halophytes. New Phytol. 179, 945–963. doi: 10.1111/j.1469-8137.2008.02531.x
Gao, Y., Long, R., Kang, J., Wang, Z., Zhang, T., Sun, H., et al. (2019). Comparative proteomic analysis reveals that antioxidant system and soluble sugar metabolism contribute to salt tolerance in alfalfa (Medicago sativa l.) leaves. J. Proteome Res. 18, 191–203. doi: 10.1021/acs.jproteome.8b00521
Gene Ontology Consortium (2001). Creating the gene ontology resource: design and implementation. Genome Res. 11 (8), 1425–1433. doi: 10.1101/gr.180801
Gengmao, Z., Yu, H., Xing, S., Shihui, L., Quanmei, S., Changhai, W. (2015). Salinity stress increases secondary metabolites and enzyme activity in safflower. ind. Crops Prod. 64, 175–181. doi: 10.1016/j.indcrop.2014.10.058
Genty, B., Briantais, J. M., Baker, N. R. (1989). The relationship between the quantum yield of photosynthetic electron transport and quenching of chlorophyll fluorescence. BBA Gen. Subj. 990, 87–92. doi: 10.1016/S0304-4165(89)80016-9
Ghnaya, T., Slama, I., Messedi, D., Grignon, C., Ghorbel, M. H., Abdelly, C. (2007). Effects of Cd2+ on k+, Ca2+ and n uptake in two halophytes sesuvium portulacastrum and mesembryanthemum crystallinum: consequences on growth. Chemosphere 67, 72–79. doi: 10.1016/j.chemosphere.2006.09.064
Goyer, A. (2010). Thiamine in plants: aspects of its metabolism and functions. Phytochemistry 71, 1615–1624. doi: 10.1016/j.phytochem.2010.06.022
Grabherr, M. G., Haas, B. J., Yassour, M., Levin, J. Z., Thompson, D. A., Amit, I., et al. (2011). Full-length transcriptome assembly from RNA-seq data without a reference genome. Nat. Biotechnol. 15;29 (7), 644–652. doi: 10.1038/nbt.1883
Greenway, H., Munns, R. (1980). Mechanisms of salt tolerance in nonhalophytes. Annu. Rev. Plant Physiol. 31, 149–190. doi: 10.1146/annurev.pp.31.060180.001053
Hertog, M. G., Feskens, E. J., Hollman, P. C., Katan, M. B., Kromhout, D. (1993). Dietary antioxidant flavonoids and risk of coronary heart disease: the zutphen elderly study. Lancet 342, 1007–1011. doi: 10.1016/0140-6736(93)92876-U
Himabindu, Y., Chakradhar, T., Reddy, M. C., Kanygin, A., Redding, K. E., Chandrasekhar, T. (2016). Salt-tolerant genes from halophytes are potential key players of salt tolerance in glycophytes. Environ. Exp. Bot. 124, 39–63. doi: 10.1016/j.envexpbot.2015.11.010
Hwang, S. H., Kim, S. C., Seong, J. A., Lee, H. Y., Cho, D. Y., Kim, M. J., et al. (2021). Comparison of ginsenoside contents and antioxidant activity according to the size of ginseng sprout has produced in a plant factory. Appl. Biol. Chem. 64, 253–261. doi: 10.3839/jabc.2021.035
Ihm, B. S., Lee, J. S., Kim, J. W. (2001). Coastal vegetation on the western, southern, and eastern coasts of south Korea. J. Plant Biol. 44, 163–167. doi: 10.1007/BF03030234
Jamil, A., Riaz, S., Ashraf, M., Foolad, M. R. (2011). Gene expression profiling of plants under salt stress. Crit. Rev. Plant Sci. 30, 435–458. doi: 10.1080/07352689.2011.605739
Jeong, J. H. (2017). Seed germination, plant growth and antioxidant capacity of Limonium tetragonum under different salt concentrations. Korean J. Plant Res. 30, 364–371. doi: 10.7732/kjpr.2017.30.4.364
Jones, P., Binns, D., Chang, H. Y., Fraser, M., Li, W., McAnulla, C., et al. (2014). InterProScan 5: genome-scale protein function classification. Bioinformatics 30 (9), 1236–1240. doi: 10.1093/bioinformatics/btu031
Kim, M. H. (2007). Effects of NaCl concentration on the growth of limonium tetragonum in deep flow culture (Korea: Sangmyung university graduate school). Available at: http://www.riss.kr/link?id=T11208974.
Kim, N. H., Heo, J. D., Rho, J. R., Yang, M. H., Jeong, E. J. (2017). Anti-obesity effect of halophyte crop, Limonium tetragonum in high-fat diet-induced obese mice and 3T3-L1 adipocytes. Biol. Pharm. Bull. 40, 1856–1865. doi: 10.1248/bpb.b17-00296
Kim, N. H., Sung, S. H., Heo, J. D., Jeong, E. J. (2015). The extract of Limonium tetragonum protected liver against acute alcohol toxicity by enhancing ethanol metabolism and antioxidant enzyme activities. Nat. Prod. Sci. 21, 54–58.
Knight, H., Trewavas, A. J., Knight, M. R. (1997). Calcium signalling in Arabidopsis thaliana responding to drought and salinity. Plant J. 12, 1067–1078. doi: 10.1046/j.1365-313X.1997.12051067.x
Kong, C. S., Um, Y. R., Lee, J. I., Kim, Y. A., Lee, J. S., Seo, Y. (2008). Inhibition effects of extracts and its solvent fractions isolated from Limonium tetragonum on growth of human cancer cells. KSBB J. 23, 177–182.
Koyro, H. W., Hussain, T., Huchzermeyer, B., Khan, M. A. (2013). Photosynthetic and growth responses of a perennial halophytic grass Panicum turgidum to increasing NaCl concentrations. Environ. Exp. Bot. 91, 22–29. doi: 10.1016/j.envexpbot.2013.02.007
Lee, J. S., Kim, Y. N., Kim, N. H., Heo, J. D., Yang, M. H., Rho, J. R., et al. (2017). Identification of hepatoprotective constituents in Limonium tetragonum and development of simultaneous analysis method using high-performance liquid chromatography. Pharmacogn Mag. 13, 535. doi: 10.4103/pm.pm_477_16
Lee, J. I., Kong, C. S., Jung, M. E., Hong, J. W., Lim, S. Y., Seo, Y. (2011). Antioxidant activity of the halophyte Limonium tetragonum and its major active components. Biotechnol. Bioproc. E. 16, 992–999. doi: 10.1007/s12257-011-0213-5
Liu, X. L., Covington, M. F., Fankhauser, C., Chory, J., Wagner, D. R. (2001). ELF3 encodes a circadian clock–regulated nuclear protein that functions in an Arabidopsis PHYB signal transduction pathway. Plant Cell 13, 1293–1304. doi: 10.1105/TPC.000475
Liu, M. S., Hellebust, J. A. (1976). Effects of salinity and osmolarity of the medium on amino acid metabolism in Cyclotella cryptica. Can. J. Bot. 54, 938–948. doi: 10.1139/b76-098
Matysiak, B., Kowalski, A. (2019). White, blue and red LED lighting on growth, morphology and accumulation of flavonoid compounds in leafy greens. Zemdirbyste 106, 281–286. doi: 10.13080/z-a.2019.106.036
McClung, C. R., Kay, S. A. (1994). Circadian rhythms in Arabidopsis thaliana. cold spring harb. Monogr> S. 27, 615–615.
Meng, X., Zhou, J., Sui, N. (2018). Mechanisms of salt tolerance in halophytes: current understanding and recent advances. Open Life Sci. 13, 149–154. doi: 10.1515/biol-2018-0020
Millar, A. J., Kay, S. A. (1991). Circadian control of cab gene transcription and mRNA accumulation in Arabidopsis. Plant Cell 3, 541–550. doi: 10.2307/3869359
Munns, R., Tester, M. (2008). Mechanisms of salinity tolerance. Annu. Rev. Plant Biol. 59, 651–681. doi: 10.1146/annurev.arplant.59.032607.092911
Nguyen, D. T., Lu, N., Kagawa, N., Takagaki, M. (2019). Optimization of photosynthetic photon flux density and root-zone temperature for enhancing secondary metabolite accumulation and production of coriander in plant factory. Agronomy 9, 224. doi: 10.3390/agronomy9050224
Nikalje, G. C., Srivastava, A. K., Pandey, G. K., Suprasanna, P. (2018). Halophytes in biosaline agriculture: mechanism, utilization, and value addition. Land Degrad. Dev. 29, 1081–1095. doi: 10.1002/ldr.2819
Nusinow, D. A., Helfer, A., Hamilton, E. E., King, J. J., Imaizumi, T., Schultz, T. F., et al. (2011). The ELF4–ELF3–LUX complex links the circadian clock to diurnal control of hypocotyl growth. Nature 475, 398–402. doi: 10.1038/nature10182
O’Leary, J. W., Glenn, E. P., Watson, M. C. (1985). Agricultural production of halophytes irrigated with seawater. Plant Soil 89, 311–321. doi: 10.1007/BF02182250
Ong, K. C., Khoo, H. E. (1997). Biological effects of myricetin. Gen. Pharmacol. 29, 121–126. doi: 10.1016/S0306-3623(96)00421-1
Parida, A. K., Das, A. B., Sanada, Y., Mohanty, P. (2004). Effects of salinity on biochemical components of the mangrove, aegiceras corniculatum. Aquat. Bot. 80, 77–87. doi: 10.1016/j.aquabot.2004.07.005
Park, J. E., Kim, H., Kim, J., Choi, S. J., Ham, J., Nho, C. W., et al. (2019). A comparative study of ginseng berry production in a vertical farm and an open field. Ind. Crops Prod. 140, 111612. doi: 10.1016/j.indcrop.2019.111612
Parthasarathy, A., Savka, M. A., Hudson, A. O. (2019). The synthesis and role of β-alanine in plants. Front. Plant Sci. 10, 921. doi: 10.3389/fpls.2019.00921
Qu, C., Liu, C., Gong, X., Li, C., Hong, M., Wang, L., et al. (2012). Impairment of maize seedling photosynthesis caused by a combination of potassium deficiency and salt stress. Environ. Exp. Bot. 75, 134–141. doi: 10.1016/j.envexpbot.2011.08.019
Raman, S. B., Rathinasabapathi, B. (2003). B-alanine n-methyltransferase of Limonium latifolium. cDNA cloning and functional expression of a novel n-methyltransferase implicated in the synthesis of the osmoprotectant b-alanine betaine. Plant Physiol. 132, 1642–1651. doi: 10.1104/pp.103.020453
Raman, S. B., Rathinasabapathi, B. (2004). Pantothenate synthesis in plants. Plant Sci. 167, 961–968. doi: 10.1016/j.plantsci.2004.06.019
Rathinasabapathi, B., Fouad, W. M., Sigua, C. A. (2001). B-alanine betaine synthesis in the plumbaginaceae: purification and characterization of a trifunctional, s-adenosyl-L-methionine-dependent n-methyltransferase from Limonium latifolium leaves. Plant Physiol. 126, 1241–1249. doi: 10.1104/pp.126.3.1241
Rolland, F., Moore, B., Sheen, J. (2002). Sugar sensing and signaling in plants. Plant Cell 14 (suppl_1), S185–S205. doi: 10.1105/tpc.010455
Santa-María, G. E., Rubio, F., Dubcovsky, J., Rodríguez-Navarro, A. (1997). The HAK1 gene of barley is a member of a large gene family and encodes a high-affinity potassium transporter. Plant Cell 9, 2281–2289. doi: 10.1105/tpc.9.12.2281
Sayed, O. H. (2003). Chlorophyll fluorescence as a tool in cereal crop research. Photosynthetica 41, 321–330. doi: 10.1023/B:PHOT.0000015454.36367.e2
Shi, H. P., Fishel, R. S., Efron, D. T., Williams, J. Z., Fishel, M. H., Barbul, A. (2002). Effect of supplemental ornithine on wound healing. J. Surg. Res. 106, 299–302. doi: 10.1006/jsre.2002.6471
Siegel, R. S., Xue, S., Murata, Y., Yang, Y., Nishimura, N., Wang, A., et al. (2009). Calcium elevation-dependent and attenuated resting calcium-dependent abscisic acid induction of stomatal closure and abscisic acid-induced enhancement of calcium sensitivities of s-type anion and inward-rectifying k+ channels in arabidopsis guard cells. Plant J. 59, 207–220. doi: 10.1111/j.1365-313X.2009.03872.x
Sogoni, A., Jimoh, M. O., Kambizi, L., Laubscher, C. P. (2021). The impact of salt stress on plant growth, mineral composition, and antioxidant activity in tetragonia decumbens mill.: An underutilized edible halophyte in south Africa. Horticulturae> 7, 140. doi: 10.3390/horticulturae7060140
Soni, S., Kumar, A., Sehrawat, N., Kumar, A., Kumar, N., Lata, C., et al. (2021). Effect of saline irrigation on plant water traits, photosynthesis and ionic balance in durum wheat genotypes. Saudi J. Biol. Sci. 28, 2510–2517. doi: 10.1016/j.sjbs.2021.01.052
Szabados, L., Savouré, A. (2010). Proline: a multifunctional amino acid. Trends Plant Sci. 15, 89–97. doi: 10.1016/j.tplants.2009.11.009
Szklarczyk, D., Gable, A. L., Nastou, K. C., Lyon, D., Kirsch, R., Pyysalo, S., et al. (2021). The STRING database in 2021: customizable protein–protein networks, and functional characterization of user-uploaded gene/measurement sets. Nucleic Acids Res. 49, D605–D612. doi: 10.1093/nar/gkab835
Tanveer, K., Gilani, S., Hussain, Z., Ishaq, R., Adeel, M., Ilyas, N. (2020). Effect of salt stress on tomato plant and the role of calcium. J. Plant Nutr. 43 (1), 28–35. doi: 10.1080/01904167.2019.1659324
Varet, H., Brillet-Guéguen, L., Coppée, J. Y., Dillies, M. A. (2016). SARTools: a DESeq2-and EdgeR-based r pipeline for comprehensive differential analysis of RNA-seq data. PloS One 11, e0157022. doi: 10.1371/journal.pone.0157022
Víllora, G., Moreno, D. A., Pulgar, G., Romero, L. (2000). Yield improvement in zucchini under salt stress: determining micronutrient balance. Sci. Hortic. 86, 175–183. doi: 10.1016/S0304-4238(00)00149-7
Waters, B. M., Sankaran, R. P. (2011). Moving micronutrients from the soil to the seeds: genes and physiological processes from a biofortification perspective. Plant Sci. 180, 562–574. doi: 10.1016/j.plantsci.2010.12.003
Wei, Y., Xu, X., Tao, H., Wang, P. (2006). Growth performance and physiological response in the halophyte Lycium barbarum grown at salt-affected soil. Ann. Appl. Biol. 149, 263–269. doi: 10.1111/j.1744-7348.2006.00092.x
Willekens, H., Chamnongpol, S., Davey, M., Schraudner, M., Langebartels, C., Van Montagu, M., et al. (1997). Catalase is a sink for H2O2 and is indispensable for stress defence in C3 plants. Eng. Med. 16, 4806–4816. doi: 10.1093/emboj/16.16.4806
Wu, G., Morris, S. M. (1998). Arginine metabolism: nitric oxide and beyond. Biochem. J. 336, 1–17. doi: 10.1042/bj3360001
Xu, W., Lu, N., Kikuchi, M., Takagaki, M. (2021). Continuous lighting and high daily light integral enhance yield and quality of mass-produced nasturtium (Tropaeolum majus l.) in plant factories. Plants (Basel) 10, 1203. doi: 10.3390/plants10061203
Yao, X., Li, C., Li, S., Zhu, Q., Zhang, H., Wang, H., et al. (2017). Effect of shade on leaf photosynthetic capacity, light-intercepting, electron transfer and energy distribution of soybeans. Plant Growth Regul. 83, 409–416. doi: 10.1007/s10725-017-0307-y
Yokoi, S., Bressan, R. A., Hasegawa, P. M. (2002). Salt stress tolerance of plants. JIRCAS Work. Rep. 23, 25–33.
Younis, M. E., Hasaneen, M. N. A., Kazamel, A. M. S. (2009). Plant growth, metabolism and adaptation in relation to stress conditions. XXVII. can ascorbic acid modify the adverse effects of NaCl and mannitol on amino acids, nucleic acids and protein patterns in vicia faba seedlings? Protoplasma 235, 37–47.
Yuan, F., Leng, B., Wang, B. (2016). Progress in studying salt secretion from the salt glands in recretohalophytes: how do plants secrete salt? Front. Plant Sci. 7. doi: 10.3389/fpls.2016.00977
Zhang, X., He, D., Niu, G., Yan, Z., Song, J. (2018). Effects of environment lighting on the growth, photosynthesis, and quality of hydroponic lettuce in a plant factory. Int. J. Agric. Biol. 11, 33–40. doi: 10.25165/j.ijabe.20181102.3240
Zhu, J. K. (2001). Plant salt tolerance. Trends Plant Sci. 6, 66–71. doi: 10.1016/S1360-1385(00)01838-0
Keywords: halophyte, Limonium tetragonum, salt stress, myricetin, hydroponics
Citation: Jang S-N, Kang M-J, Kim YN, Jeong EJ, Cho KM, Yun JG and Son K-H (2023) Physiological and biochemical responses of Limonium tetragonum to NaCl concentrations in hydroponic solution. Front. Plant Sci. 14:1159625. doi: 10.3389/fpls.2023.1159625
Received: 06 February 2023; Accepted: 27 March 2023;
Published: 26 April 2023.
Edited by:
Md Asaduzzaman, Bangladesh Agricultural Research Institute, BangladeshReviewed by:
Mohamed Magdy F. Mansour, Ain Sham University, EgyptMarco Santin, University of Pisa, Italy
Copyright © 2023 Jang, Kang, Kim, Jeong, Cho, Yun and Son. This is an open-access article distributed under the terms of the Creative Commons Attribution License (CC BY). The use, distribution or reproduction in other forums is permitted, provided the original author(s) and the copyright owner(s) are credited and that the original publication in this journal is cited, in accordance with accepted academic practice. No use, distribution or reproduction is permitted which does not comply with these terms.
*Correspondence: Ki-Ho Son, c29ua2hAZ251LmFjLmty