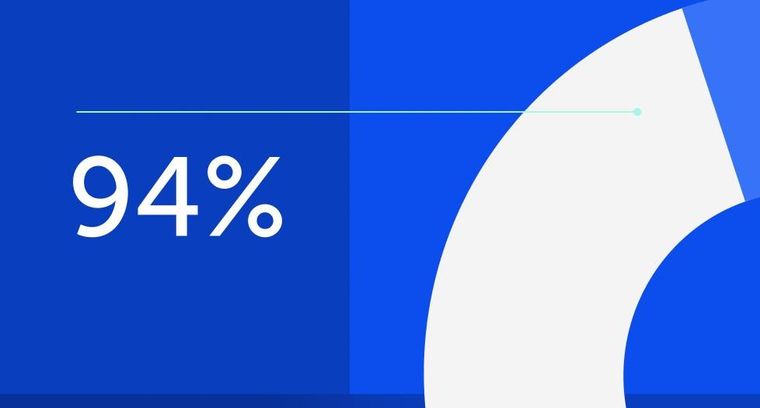
94% of researchers rate our articles as excellent or good
Learn more about the work of our research integrity team to safeguard the quality of each article we publish.
Find out more
ORIGINAL RESEARCH article
Front. Plant Sci., 08 May 2023
Sec. Plant Pathogen Interactions
Volume 14 - 2023 | https://doi.org/10.3389/fpls.2023.1154110
This article is part of the Research TopicGenome-wide Analyses of Pectobacterium and Dickeya Species, Volume IIView all 5 articles
Dickeya and Pectobacterium species are necrotrophic pathogens that macerate stems (blackleg disease) and tubers (soft rot disease) of Solanum tuberosum. They proliferate by exploiting plant cell remains. They also colonize roots, even if no symptoms are observed. The genes involved in pre-symptomatic root colonization are poorly understood. Here, transposon-sequencing (Tn-seq) analysis of Dickeya solani living in macerated tissues revealed 126 genes important for competitive colonization of tuber lesions and 207 for stem lesions, including 96 genes common to both conditions. Common genes included acr genes involved in the detoxification of plant defense phytoalexins and kduD, kduI, eda (=kdgA), gudD, garK, garL, and garR genes involved in the assimilation of pectin and galactarate. In root colonization, Tn-seq highlighted 83 genes, all different from those in stem and tuber lesion conditions. They encode the exploitation of organic and mineral nutrients (dpp, ddp, dctA, and pst) including glucuronate (kdgK and yeiQ) and synthesis of metabolites: cellulose (celY and bcs), aryl polyene (ape), and oocydin (ooc). We constructed in-frame deletion mutants of bcsA, ddpA, apeH, and pstA genes. All mutants were virulent in stem infection assays, but they were impaired in the competitive colonization of roots. In addition, the ΔpstA mutant was impaired in its capacity to colonize progeny tubers. Overall, this work distinguished two metabolic networks supporting either an oligotrophic lifestyle on roots or a copiotrophic lifestyle in lesions. This work revealed novel traits and pathways important for understanding how the D. solani pathogen efficiently survives on roots, persists in the environment, and colonizes progeny tubers.
Dickeya solani emerged in potato tuber cultivation (Solanum tuberosum) in Europe at the beginning of the 2000s (van der Wolf et al., 2014). A retrospective analysis of potato pathogen archives revealed that some D. solani had been isolated on rare occasions in Switzerland in the 1990s (Pédron et al., 2021). D. solani as well as other species of Dickeya and Pectobacterium genera (order of Enterobacterales) are the causative agents of soft rot disease on tubers and blackleg disease on stems of potato plants (van der Wolf et al., 2021). They also cause damage to a wide range of plants of agronomical interest worldwide (van der Wolf et al., 2021). The necrotrophic pathogens Dickeya and Pectobacterium secrete plant cell wall macerating enzymes as their main virulence factors and then proliferate in lesions (macerated tissues) by exploiting plant cell remains as nutrients (Van Gijsegem et al., 2021). How these pathogens persist and disseminate in agrosystems (soil, surface waters, plant reservoirs, insect vectors, and propagation via progeny tubers) and, more specifically, how they survive by colonizing and exploiting roots of their host (underground lifestyle) remain questions under investigation.
Comparative genomics has shown little genetic variation in D. solani isolates collected in Europe consistent with a bottleneck associated with a recent spread in potato and bulb plants (van der Wolf et al., 2014; Khayi et al., 2015; Golanowska et al., 2018; Blin P. et al., 2021). The primary host(s) of D. solani remain(s) uncertain. Horizontal gene transfer events have been observed in a few D. solani isolates, replacing some D. solani genes by their orthologous counterparts acquired from Dickeya dianthicola (Khayi et al., 2015). These transfer events suggest ecological promiscuity between the two species. Variation in the degree of symptoms caused to the potato host was also observed among D. solani isolates: these phenotypes were tentatively associated with single-nucleotide polymorphism in some genes (fliC, fliN, fhaB2, and vfmB) and the presence or absence of some other loci (arcZ, mtgA, and hrpQ) (Khayi et al., 2015; Golanowska et al., 2018; Blin P. et al., 2021). The involvement of these genetic variations in virulence remains to be confirmed by reverse genetics. Several studies compared D. solani, D. dadantii, and D. dianthicola, highlighting some distinctive traits in D. solani (Potrykus et al., 2015; Potrykus et al., 2018; Raoul des Essarts et al., 2019). Notably, some specific regulatory sequences trigger a higher expression of pelD and pelE genes, encoding macerating enzymes, in D. solani compared to D. dianthicola (Blin P. et al., 2021; Duprey et al., 2016). High expression of pelD and pelE would contribute to the capacity of D. solani to cause rotting at a lower bacterial inoculum than D. dianthicola (Blin P. et al., 2021; Raoul des Essarts et al., 2019). A comparative analysis of metabolic capabilities showed that D. solani exploited a wider range of compounds as nitrogen source than D. dianthicola (Raoul des Essarts et al., 2019). Transcriptomics of the pathogens recovered from tuber lesions pinpointed the higher expression of the glyoxylate shunt in D. solani than in D. dianthicola, a metabolic pathway that contributes to the exploitation of alternative sources of carbon when sugar is limiting (Raoul des Essarts et al., 2019). All these traits could facilitate the settlement of D. solani in the potato plant host.
The invasion success of D. solani could also be related to its capacity to survive under stressful conditions and to compete with the microbiota of the potato host. The D. solani genome contains several polyketide synthase (PKS) and non-ribosomal peptide synthetase (NRPS) clusters coding for different secondary metabolites: aryl-polyene, oocydin, zeamine, and solanimycin. Aryl-polyene synthesis is encoded by the ape genes that are widespread in β-proteobacteria and γ-proteobacteria, including several Enterobacterales such as D. solani and some strains of Escherichia coli (Schöner et al., 2016; Johnston et al., 2021). Aryl-polyenes are pigments anchored to the outer membrane of bacterial cells that may contribute to oxidative stress response and biofilm formation (Johnston et al., 2021). A recent study (Brual et al., 2021) highlighted three other PKS/NRPKS clusters coding for synthesis of oocydin, zeamine, and solanimycin, which are present in all published genomes of D. solani, but not all three together in the other Dickeya species. The ooc cluster is highly similar to the ooc genes coding for synthesis of oocydin A, a chlorinated macrolide present in some Serratia and Dickeya strains (Matilla et al., 2012). In different Dickeya and Serratia strains, the zms cluster is responsible for synthesis of zeamine, a cationic polyamine-polyketide (Zhou et al., 2011). The sol cluster is present in different Dickeya species and encodes solanimycin that is active against a broad range of micro-eukaryotes (Brual et al., 2021; Matilla et al., 2022). As compared to a wild-type strain of D. solani Ds0432-1, the constructed mutants ΔsolG, ΔzmsA, and ΔoocL were impaired in antibiosis against yeasts, Gram-positive bacteria, and Ascomyceta, respectively (Brual et al., 2021). The contribution of these secondary metabolites to plant colonization by D. solani is not known.
D. solani colonizes stems, xylem vessels, and leaves, as well as roots and stolons from which develop progeny tubers (Czajkowski et al., 2010). Under greenhouse conditions, when inoculated into pots, D. solani rapidly colonizes the roots (2 weeks post-inoculation of soil) and, to a lesser extent, stems even if no symptoms (blackleg lesions) were observed (Czajkowski et al., 2010). Several weeks later, blackleg symptoms develop in stems with an incidence mainly depending on pathogen abundance in the inoculum and wounding of roots; root wounding facilitates entry of pathogens (Czajkowski et al., 2010; Blin P. et al., 2021). At the end of the vegetative cycle, pathogens may cause soft rot in progeny tubers or may colonize them without symptoms. Transcriptomics revealed differentially expressed genes in D. solani living in soft rot lesions in potato tubers (Raoul des Essarts et al., 2019). In contrast, the lifestyle of D. solani and other pectinolytic Dickeya and Pectobacterium when they colonize roots remains poorly investigated. In a recent study, 10,000 Tn5-mutants carrying a promoterless gusA gene were screened to identify D. solani genes differentially expressed in the presence of leaves, stems, roots, and tubers of S. tuberosum (Czajkowski et al., 2020). A single gene (pstB) was identified as expressed in the presence of either root or tuber tissues, but not stem or leaf (Czajkowski et al., 2020). The pstABC genes encode a transporter involved in importing phosphate and phosphate-mediated gene regulation, including pathogeny, in different Enterobacterales (Daigle et al., 1995; Runyen-Janecky et al., 2005; Röder et al., 2021). Whether pst genes contribute to colonization and survival of D. solani on roots is not known.
Transposon-sequencing (Tn-seq) identifies insertional mutations that confer an advantage or a defect under a given condition. In several plant pathogens, Tn-seq unveiled genes involved in the competitive colonization of lesions caused by necrotrophic and biotrophic pathogens (Duong et al., 2018; Helmann et al., 2019; Royet et al., 2019; Su et al., 2021; Torres et al., 2021; Helmann et al., 2022; Luneau et al., 2022; Morinière et al., 2022). Tn-seq analyses revealed a large set of genes associated with the colonization of lesions provoked by D. dadantii on chicory leaves and by D. dianthicola and D. dadantii on potato tubers (Royet et al., 2019; Helmann et al., 2022). Tn-seq also permitted discovery of genes associated with the competitive colonization of roots in pathogenic and beneficial bacteria, under gnotobiotic conditions (Cole et al., 2017; Liu et al., 2018; Sivakumar et al., 2019). To our knowledge, a unique Tn-seq study explored the root colonization of a plant pathogen under non-gnotobiotic conditions using a non-sterile substrate for plant growth (Torres et al., 2021). Identification of genes associated with an underground lifestyle of pathogens could be useful for preventing disease by targeting root colonization traits of pathogens.
In this work, we constructed a Tn-mutant library in the necrotroph D. solani RNS 08.23.3.1.A (=PRI3337) to identify genes involved in the colonization of S. tuberosum roots under a non-gnotobiotic condition. We also searched for genes associated with the colonization of the lesions of stems (blackleg disease) and tubers (soft rot disease). In addition, we identified the D. solani genes contributing to growth on different nutrients derived from plant cell wall—pectin, galacturonate, glucoronate, and galactarate—and compared them with those identified in lesions. Then, we focused on four genes bscA (cellulose synthesis), dppA (peptide transport), pstA (phosphate importation), and apeH (aryl polyene synthesis) that we identified by Tn-seq as important for the competitive colonization of roots. The dppA, pstA, bcsA, and apeH genes had been characterized in some Enterobacteriales, especially in E. coli and D. dadantii (Olson et al., 1991; Daigle et al., 1995; Mee-Ngan et al., 2005; Runyen-Janecky et al., 2005; Prigent-Combaret et al., 2012; Schöner et al., 2016; Johnston et al., 2021; Röder et al., 2021), but their role in root colonization is not known. We constructed in-frame deletions of these genes in D. solani, and we validated their implication in the underground lifestyle of D. solani. We showed that the pstA gene is also important for colonizing progeny tubers. Overall, this work highlights a novel landscape of genes and traits involved in D. solani–S. tuberosum interactions, especially those involved in the underground lifestyle of this plant pathogen.
Bacterial strains are described in Table 1 and culture conditions are discussed in the Supplementary Materials SI1. Tubers (G0 generation, caliber 35–45 mm) of S. tuberosum variety Bintje were provided by Comité Nord Plants de Pomme de Terre (CNPPT, Achicourt, France).
The genome of D. solani RNS 08.23.3.1A contains a circular chromosome of 4,922,468 bp in which 169,132 TA dinucleotides are all potential sites for the insertion of the himar1 mariner transposon (Figure S1). Among them, 132,173 TA sites are distributed in 99.5% of the 4,518 coding sequences. Only 22 genes do not contain any TA dinucleotide in their sequence and could not be considered in this study (listed in Table S1).
A library of Tn-mutants was constructed in a rifampicin-resistant (RifR) derivative of D. solani RNS 08.23.3.1A using the plasmid pSAM-Ec, which harbors a modified Himar1 mariner associated to a kanamycin (Km) resistance cassette (Wiles et al., 2013). Five hundred conjugation matings between E. coli MFDpir carrying pSAM-Ec and D. solani RNS 08.23.3.1A RifR were performed as described by Gonzalez-Mula et al. (2019) (experimental details in SI2). Approximately 1.8 × 107 independent Tn-mutant colonies were recovered on agar TY medium supplemented with Rif and Km. This corresponds to approximately 100 times the number of TA sites present in the genome of D. solani RNS 08.23.3.1A (169,132 TA sites). All the Tn-mutant colonies were collected, and the resulting Tn-mutant library was homogenized, aliquoted, and stored in 25% glycerol at −80°C. Each aliquot contained 2.3 × 1010 CFU (colony-forming units) of Tn-mutants.
An aliquot of the Tn-mutant library was thawed on ice and then incubated at 28°C for 4 h in 10 ml of TY rich medium in order to reactivate the bacterial cells. Library cells were centrifugated and washed with a solution at 0.8% NaCl, and used for inoculating M9 minimal medium and plant materials. Four revived aliquots in TY rich medium were used to analyze the quality of the Tn-mutant library: these four replicates correspond to the TY rich medium condition. In the in vitro culture tests, the Tn-mutant library was diluted to obtain a final OD600nm of 0.02 in 50 ml of liquid M9 medium. M9 medium was supplemented with different carbon sources at 2 g/L: sucrose (CAS 57-50-1), galacturonate (CAS 91510-62-2), glucuronate (CAS 6556-12-3), and galactarate (CAS 526-99-8), which were purchased from Sigma-Aldrich (Saint-Quentin-Fallavier, France), and pectin Dipecta AG366 (Agdia EMEA, Soisy sur Seine, France). This pectin is routinely used in crystal violet pectate medium for isolating Dickeya and Pectobacterium from environmental samples (Hélias et al., 2012). Cultures were incubated at 28°C for 24 h under shaking (200 rpm). Bacterial cells were centrifuged (4,000 rpm, 4°C, 15 min) and stored at −20°C before DNA extraction. Each culture condition was performed in four replicates.
In the in planta assays, the Tn-mutant library was inoculated in three conditions: roots and stems of entire plants and potato tubers. Potato plants were cultivated individually in 2-L pots containing horticultural compost (Floragard, Oldenburg, Germany) under non-gnotobiotic conditions in a greenhouse (16-h days and 8-h nights at 24°C). In the root colonization assay, 1 ml at 6 units OD600nm of the Tn-mutant library was inoculated by watering (5.5 × 109 CFU per pot). Forty plants were inoculated when the aerial part reached approximately 30 cm in height (4 weeks after tuber planting). In the stem infection assay, 50 µl at 1 unit OD600nm of the Tn-mutant library was injected into the stem in the axil of the fourth leaf (5 × 107 CFU per stem). These plants were developed from axillary buds of tubers and 140 stems were inoculated when they reached a size of approximately 20–30 cm in height. In the tuber infection assay, 10 µl at 1 unit OD600nm of the Tn-mutant library was injected into the potato tubers (107 CFU per tuber). Forty tubers were inoculated and then placed at 24°C in the dark. Plant and tuber assays were described previously (Blin P. et al., 2021).
Six weeks post-inoculation, the 40 root systems were collected and grouped into eight lots that we designated as the eight replicates of the root condition. Symptoms of maceration were not observed on root systems. Roots were crushed in 25 ml of a 0.8% NaCl solution during 1 min using a laboratory blender (Waring, Stamford, USA). The homogenate was filtered through miracloth (Millipore, Bedford, MA, USA) and then plated onto TY agar medium supplemented with Rif, Km, and cycloheximide. After 48 h at 28°C, the Tn-mutant colonies were collected and resuspended in 0.8% NaCl solution. For each replicate, 2 ml of bacterial suspension was pelleted and frozen prior to DNA extraction.
Five days post-inoculation, macerated tissues of the stems and tubers were collected and grouped into eight stem replicates (each from 15 stems) and four tuber replicates (each from 10 tubers). Each replicate was crushed in 10 ml of 0.8% NaCl solution and the Tn-mutant colonies were recovered using TY agar medium supplemented with Rif, Km, and cycloheximide, following the same protocol as for the root condition. For each replicate, 2 ml of bacterial suspension was centrifugated and frozen prior to DNA extraction.
Overall, 42 samples were analyzed. Four replicates were collected in the TY rich medium condition, in which the Tn-library was constructed. Four replicates were collected in M9 medium for each of the five carbon sources: sucrose, pectin, galacturonate, glucuronate, and galactarate. In addition, four replicates were collected in the macerated tuber condition, eight in the macerated stem condition, and eight in the root condition.
Total DNA from each replicate was extracted and then the Tn insertion sites were amplified (Primers in Table S3) and sequenced as described previously (Gonzalez-Mula et al., 2019; Torres et al., 2021). Illumina NextSeq 500 instrument (Illumina, San Diego, USA) was used in a single read 75 run at the I2BC-sequencing platform (I2BC, Gif-sur-Yvette, France). Each population generated over 3 million reads after specific cleaning of the Tn-seq data: transposon trimming with Trimmomatic (Bolger et al., 2014) and then barcode removal.
The reads were mapped using Bowtie-1.1.2 (Langmead et al., 2009) to the D. solani RNS 08.23.3.1A genome (Khayi et al., 2018; GenBank CP016928.1). Genome annotation was generated at the LABGeM (CEA/Genoscope CNRS UMR8030, Evry, France). Correspondence between the Genoscope annotation and NCBI annotation (released in April 2022 https://www.ncbi.nlm.nih.gov/data-hub/genome/GCA_000511285.2/) is provided in Table S1.
The mapping results (.bam files) were analyzed by the ARTIST pipeline (Pritchard et al., 2014) using MATLAB software (MathWorks, Natick, USA). All.bam files of the 42 compared conditions were deposited as sequence read archives (SRA) in the bioproject PRJNA939571 at the National Center for Biotechnology Information (Table S4). When a high correlation (r² ≥ 0.80) was observed in distribution and relative abundance of Tn insertions between the replicates of the same culture medium condition, the sequencing data were pooled for further analyses using ARTIST. Two ARTIST analyses were performed with a p-value cutoff of p < 0.01 in the Mann–Whitney U test: EL-ARTIST and Con-ARTIST (Pritchard et al., 2014). By comparing the number of Tn sequences at each AT insertion site in a given culture condition, the EL-ARTIST pipeline predicts the genes that are “non-essential” because they still contain Tn insertions (class 1), “essential” (class 2) because they contain no Tn insertions, or an “essential domain” (class 3) because no Tn insertions are present in a part of the gene. The “essential” term is used in the ARTIST suite to indicate a decrease (p < 0.01) in the relative abundance of Tn-mutants of a considered gene in a given condition. The Con-ARTIST pipeline compares two conditions, a test condition versus a reference condition. By comparing the number of Tn sequences at each AT insertion site in the reference and test conditions, Con-ARTIST distinguishes the genes with a conditionally “essential domain” (class 1), conditionally “essential genes” (class 2), genes with a conditionally “enriched domain” (class 3), conditionally “enriched genes” (class 4), and genes conditionally “non-essential, not enriched” (class 5). In this work, the Con-ARTIST class 2 “essential genes” were retained as candidate genes that are important for growth and survival in a given growth condition as compared to a reference condition. In the case of in planta culture conditions (roots and macerated stems and tubers), each replicate was analyzed separately and compared to the reference condition (TY rich medium) to provide a list of fitness genes (class 2 “essential genes”). Then, those that had been assigned to Con-ARTIST class 2 “essential genes” in at least half of the replicates of a considered condition were retained in a final unique list of fitness genes, as described by Torres et al. (2021).
The identified genes were categorized using COG classification (Clusters of Orthologous Groups of proteins) by Galperin et al. (2015). Antismash was used for identifying secondary metabolite biosynthetic gene clusters (https://antismash.secondarymetabolites.org; Blin K. et al., 2021). Some genes were manually analyzed, such as those associated with carbon metabolism according to the published data in Enterobacteriales (Tervo and Reed, 2012; Bouvier et al., 2019; Kuivanen et al., 2019).
In-frame deletions were constructed in four genes for which Tn-seq analysis predicted a contribution to competitive fitness in root colonization: bcsA (=DS0823_v1_2374) involved in cellulose synthesis, dppA (=DS0823_v1_2392) involved in peptide transport, pstA (=DS0823_v1_2527) involved in phosphate transport, and apeH (=DS0823_v1_2513) involved in synthesis of an unknown aryl-polyene compound. The mutated genes were chosen within a cluster coding for the same function; therefore, the same behavior was expected, even in the case of a potential polar effect.
The D. solani RNS 08.23.3.1A RifR-GmR strain was obtained by integration of a Gm-resistance cassette at the unique attTn7 site of the D. solani genome, as described in SI3. The defective alleles were introduced in D. solani RNS 08.23.3.1A RifR-GmR by conjugation (Edwards et al., 1998; Brual et al., 2021). A detailed protocol is presented in SI4. To validate the in planta behavior of the mutants, two independent clones were retained per genotype and further analyzed in plant assays.
To measure the relative fitness of the four constructed deletion mutants (RifR GmR), root colonization assays were performed in the presence of the ancestral strain D. solani RNS 08.23.3.1A RifR. Competition between the ancestor D. solani RNS 08.23.3.1A RifR and its derivative D. solani RNS 08.23.3.1A RifR-GmR was used as control to evaluate the potential bias caused by the GmR cassette.
The D. solani RNS 08.23.3.1A RifR strain and its derivatives were grown overnight in TY medium. The cells were rinsed twice in a 0.8% NaCl solution and then their OD600nm was adjusted to 1 unit (109 CFU/ml). Each RifR GmR strain was mixed with the RifR strain at a 1:1 ratio. For each mutant construction, two independent clones were analyzed. Each clone was inoculated in 10 pots. Six weeks post-inoculation, roots of each pot were sampled and 10 g was crushed in 30 ml of 0.8% NaCl solution following the same procedure as described above for the Tn-infection assays. The homogenate was diluted and spread on TY Rif and TY Rif-Gm agar medium, both being supplemented with cycloheximide, to enumerate the total Dickeya population and the mutant population. For each replicate, a competitive index (CI) was calculated (Macho et al., 2010) following the formula presented in SI5. A CI value equal to one indicated an equal fitness between D. solani RNS 08.23.3.1A RifR and D. solani RNS 08.23.3.1A RifR-GmR (mutant strains), CI values greater than one indicated a fitness advantage of the RNS 08.23.3.1A RifR-GmR mutant strain, and CI values below one indicated a fitness advantage of RNS 08.23.3.1A RifR.
In addition to competition assays, each bacterial genotype was inoculated separately: in the first assay, pathogens were injected directly in stems, while in the second assay, pathogens were inoculated as described for the root colonization assay. For each mutant, two independent clones were analyzed. Each clone was inoculated on 10 plants. The strain RNS 08.23.3.1A RifR-GmR was used as a virulent reference and uninoculated plants were used as negative control. In the stem inoculation assay, the length of internal lesions was measured 5 days post-infection. Abundance of pathogens was quantified in lesions (CFU/g of stem fresh weight) using numerations on TY supplemented with Rif, Gm, and cycloheximide. In the root inoculation assay, 8 weeks post-infection, abundance of pathogens in pots was quantified by counting on TY agar medium supplemented with Rif, Gm, and cycloheximide. The number of asymptomatic and symptomatic progeny tubers was counted, and finally, pathogen acquisition in tuber progeny was evaluated by testing asymptomatic progeny tubers for the presence of D. solani using qPCR (Blin P. et al., 2021).
The correlation between the different replicates of the Tn-seq mapping results was analyzed by calculating the linear regression coefficient (r2). The traits measured in plants inoculated by the different bacterial genotypes (the CI values, length of the stem symptoms in cm, abundance of pathogens in rotten tissue or pots in CFU/g, and the number of symptomatic or asymptomatic plants) were compared by Kruskal–Wallis tests (α = 0.05). Then, a Dunn’s test was performed to compare traits of each constructed mutant with the strain RNS 08.23.3.1A RifR-GmR. For all statistical analyses and coefficient calculation, we used GraphPad Prism version 9.3.0, GraphPad Software (www.graphpad.com).
Using the himar1 mariner transposon (Tn), we constructed a library of Tn-mutants in a rifampicin-resistant derivative of D. solani RNS 08.23.3.1A (Table 1). To examine the distribution of Tn in the D. solani genome, we extracted total DNA from four Tn-library aliquots that were grown in TY rich medium. By comparing the relative abundance of the Tn insertions along the circular chromosome, a high correlation (r² > 0.98) was observed between the four replicates. Sequencing data from the replicates were pooled for further analysis. In the D. solani Tn-library, more than 70% of genes have at least 80% of their TA sites with a Tn insertion (Figure 1), revealing good coverage of the genome. In the next step, the EL-ARTIST analysis (Pritchard et al., 2014) pinpointed 521 genes (“essential genes” according to the EL-ARTIST classification) for which Tn-mutants had impaired growth in TY medium. These 521 genes represented 11.5% of the total genes of D. solani RNS 08.23.3.1A. A similar percentage had been observed in previous Tn-seq studies (Hooven et al., 2016; Gonzalez-Mula et al., 2019; Royet et al., 2019). According to the COG classification, these 521 genes are associated with four main functional categories: bacterial metabolism (31.9%), information storage and processing (29.4%), cellular processes and signaling (25.3%), and poorly characterized genes (13.4%) (Table S2). Most of them encompass genes encoding for cell processes that are essential for bacterial viability and optimal growth: for instance, the nuo cluster (aerobic respiration), atp cluster (synthesis of ATP), rpl genes (50S ribosomal protein), and dnaE (DNA polymerase) (Figure 1). The Tn-library was thereafter challenged to several growth conditions to unveil and compare the different gene repertoires supporting the D. solani lifestyles.
Figure 1 Characteristics of the Tn-library in Dickeya solani. The position and abundance of Tn insertions in the circular chromosome of D. solani RNS 08.23.3.1A RifR under TY rich medium conditions are shown. (A) Number of genes according to percentage of mutated TA sites per gene. (B) From the outside to the inside, the tracks represent the following: forward and reverse coding sequences in blue color; Log10 of number of Tn insertions per TA site for each gene in black; EL-ARTIST analysis that classified genes in three categories: “non-essential genes” in red, “genes with an essential domain” in yellow, and “essential genes” in green. In the center, the circle chart shows the total number of “non-essential genes” (3,829), “essential genes” (521), and “genes with an essential domain” (146). Examples of “essential genes” are indicated.
Because D. solani secretes plant cell wall macerating enzymes, we searched for genes associated with growth of D. solani on nutrients derived from plant cell walls. The Tn-library was cultivated in M9 synthetic medium supplemented with different carbon sources: galacturonate, glucuronate, galactarate, and pectin. M9 medium with sucrose was used as a reference condition in comparative analyses. On average, 3 million reads were obtained and analyzed per replicate to determine the relative abundance of the Tn along the chromosome. We observed a good correlation between the four replicates of each growth condition: sucrose (r2 > 0.94), galacturonate (r2 > 0.98), glucuronate (r2 > 0.88), galactarate (r2 > 0.97), and pectin (r2 > 0.99). Hence, we pooled sequencing data for each carbon source for further comparisons.
Using EL-ARTIST, we analyzed the sequencing data obtained under sucrose growth conditions and then we used Con-ARTIST to compare the distribution of the Tn insertions in sucrose versus other conditions (Table S2). We focused on the “conditionally essential genes” (class 2) according to the Con-ARTIST analysis and we retained them as fitness genes. We identified 98 genes important for growth on pectin, 89 for growth on galacturonate, 96 for growth on glucoronate, and 87 for growth on galactarate (Table S2). Our Tn-seq experiments revealed some common and some nutrient-specific genes, which are important for the competitive growth of D. solani in the presence of plant cell wall components (Figure 2). Some common genes are involved in the pentose phosphate pathway and upper glycolysis/gluconeogenesis (tpiA, fbp, pgi, zwf, pgl, and gnd). Some nutrient-specific genes are associated with the importation and degradation of distinctive plant compounds: pectin (kduI and kduD), glucuronate (one unnamed MSF-transporter = DS0823_v1_1165, yeiQ, and uxuA), galacturonate (exuT, uxaB, and uxaA), and galactarate (gudP, gudD, gudX, and garL). The genes kdgK and eda (=kdgA) were important for fitness in growth on pectin, glucuronate, and galacturonate, but not galactarate. These genes reflect the metabolic pattern of D. solani in each of the tested growth conditions. Their identification was useful to interpret the fitness genes in complex environments such as roots and macerated stems and tubers.
Figure 2 Dickeya solani pathways associated with competitive growth on plant cell wall components. This scheme exemplifies fitness genes pinpointed by Tn-seq (a complete list is available in Table S2) when the D. solani Tn-library was grown in M9 minimal medium with distinctive plant cell wall components as carbon and energy sources. In the schematic pathways, enzymes encoded by the identified genes are indicated in red; in the red box, crosses indicate for each enzyme in which growth condition they were identified using Tn-seq: pectin, glucuronate, galacturonate, and galactarate in M9 minimal medium, as well as in planta conditions: roots and rotted tubers and stems. DGHD, 3-deoxy-D-glycero-hexo-2,5-diulosonate; KDPG, 2-keto-3-deoxy-6-phosphogluconate; KDG, 2-keto-3-deoxy-gluconate.
To identify the D. solani genes involved in proliferation in macerated plant tissues, we inoculated 107 CFU of the Tn-library on 40 tubers and 120 stems. After a 5-day incubation, D. solani reached 2.3 × 1010 ± 3.6 × 1010 CFU/g of lesions in tubers and 2.0 × 109 ± 5.5 × 108 CFU/g of lesions in stems. The macerated tubers and stems were assembled in four lots (each from 10 tubers) and eight lots (each from 15 stems), respectively. Each lot of macerated tissue is considered as a replicate. After DNA extraction and sequencing of Tn insertion sites, Con-ARTIST analysis was performed using TY medium as a reference condition. We identified 126 fitness genes important for the competitive colonization of tuber lesions and 207 for stem lesions, including 96 genes common to both conditions (Table S2). Most of these 237 genes are involved in metabolism (40%) and cellular processes and signaling (32%) categories according to the COG classification (Table S2). These genes are scattered along the D. solani genome (Figure 3). A noticeable exception is a large cluster of 39 genes coding for motility (fli, flg, and flh) and chemotaxis (che and chp), which were important for competitive fitness in stem lesions (Figure 3 and Table S2). Additional operons associated with fitness in stem lesions are involved in the synthesis of purines (purCDEHLM) and different amino acids such arginine (argABCEG), histidine (hisABCDFGHI), leucine (leuABCD), valine, and isoleucine (ilvCYDEM). Some remarkable genes were important for fitness in both conditions, tuber and stem lesions: this category includes genes involved in upper glycolysis/gluconeogenesis (fbp, zwf, and gnd) and assimilation of pectin (kduI, kduD, and eda) or galactarate (gudD, garL, garR, and garK) (Table S2). Other common genes between macerated tuber and stem conditions were associated with detoxification (acrAB genes coding for an efflux system) and aerobic respiration (cyoABCD encoding the cytochrome o ubiquinol oxidase).
Figure 3 Genome map of Dickeya solani genes associated with competitive growth on roots and lesions in stems and tubers of Solanum tuberosum. From the outside to the inside, the tracks represent the following: forward and reverse coding sequence (in blue) and fitness genes (complete lists available in Table S2) when D. solani grew in macerated stems (green circle), in macerated tubers (yellow circle), and on roots (orange circle). The Venn diagram represents a comparison of fitness genes between the three in planta conditions. Some examples of fitness genes are indicated, such as a large cluster of motility genes that are important under macerated stem conditions, arcAB and cyoABCDE genes under macerated stem and tuber conditions, and the four clusters associated to fitness in roots condition, including ddp, ape, pst, bcs, dpp, and ooc genes.
In the root colonization condition, no symptoms on roots were observed: pathogens reached 1.9 × 105 ± 1.6 × 105 CFU/g of roots. Forty root systems were grouped into eight lots that represent eight replicates. Tn-seq analysis revealed 83 genes (Table S2), which are clustered in four genomic regions (Figure 3). None of them was in common with those identified in the macerated stem and tuber conditions (Figure 3, 4). In the first identified cluster, 11 of 13 adjacent genes were predicted to be involved in the synthesis of oocydin (oocJKLMNRSTUVW). In the second cluster, 31 adjacent genes code for C4-dicarboxylic acid transporter (dctA), cellulose biosynthesis (celY-bcsABCDQO), a putative dipeptide ABC-transporter (dppABCDF), conversion of 2-keto-3-deoxy-gluconate (kdgK), and some other less characterized functions. In the third cluster (21 adjacent genes), some genes are associated with biosynthesis of an aryl polyene (apeDEFGHIJKLMNOPQR) and a phosphate ABC-transporter (pstABCS). In the fourth cluster, 18 adjacent genes code for another putative dipeptide ABC-transporter (ddpABC), conversion of fructuronate (yeiQ), and some other poorly characterized functions. Among the genes pinpointed by Tn-seq in the root condition, two genes (kdgK and yeiQ) were also important in M9 medium supplemented with glucuronate as a carbon source (Figure 2).
Figure 4 Dickeya solani pathways associated with competitive growth on roots and lesions in stems and tubers of Solanum tuberosum. From left to right, pictures and arrows indicate the plant tissues that are colonized by D. solani and analyzed by Tn-seq. For each condition, some genes and pathways are indicated (complete lists of fitness genes are available in Table S2). NA indicates that genes were not associated with a variation of fitness in a given condition according to Tn-seq analyses.
To analyze further some fitness genes associated with root colonization, we constructed in-frame deletion mutants of four genes: dppA (=DS0823_v1_2392), encoding an oligopeptide transporter; pstA (=DS0823_v1_2527), a phosphate transporter; bcsA (=DS0823_v1_2374), involved in cellulose synthesis; and apeH (=DS0823_v1_2513), involved in synthesis of an aryl-polyene.
We evaluated the capability of the constructed mutants in D. solani to provoke blackleg symptoms when injected directly in the stem of the potato plant. The mutants caused similar lesions to those of the wild-type strain (Figure 5A). Moreover, mutants and wild-type strains colonized stem lesions at a similar abundance (Figure 5B). Thus, a deletion of these genes did not abolish the virulence program of D. solani when injected directly in plant tissues.
Figure 5 Virulence of the Dickeya solani constructed mutants ΔdppA, ΔapeH, ΔpstA, and ΔbcsA. Five days after infection in S. tuberosum stems, we measured length of lesions in cm (A) and enumerated D. solani pathogens (CFU/g of macerated tissues) (B). Two independent mutants of each genotype ΔdppA, ΔapeH, ΔpstA, and ΔbcsA were analyzed (20 infected stems per genotype) and compared to D. solani RNS 08.23.3.1A RifR-GmR (10 infected stems). The strain D. solani RNS 08.23.3.1A RifR-GmR is indicated as WT Rif-Gm in the graph. Uninoculated stems (T-) did not develop symptoms. In A, Kruskal–Wallis test compared the six conditions (Kruskal–Wallis statistic = 50.52; p-value <0.0001; DF = 5) and then Dunn test compared the symptom severity of each mutant genotype with that of D. solani RNS 08.23.3.1A RifR-GmR. The p-value of the Dunn test is shown: all D. solani genotypes caused similar symptoms. In B, center lines show the medians; the crosses show the mean values; box limits are the 25th and 75th percentiles; whiskers extend 1.5 times the interquartile distance; outliers are represented by dots. The statistical analysis was carried out by a Kruskal–Wallis test (Kruskal–Wallis statistic = 54.20; p-value <0.0001; DF = 5) followed by a Dunn test to compare each mutant genotype with D. solani RNS 08.23.3.1A RifR-GmR. The p-values of the Dunn test are indicated on the graph: all D. solani genotypes reached a similar abundance in lesions.
Then, we evaluated the competitive fitness of these mutants in root colonization when they were challenged by their ancestor (RNS 08.23.3.1A RifR). A control competition between RNS 08.23.3.1A RifR-GmR and RNS 08.23.3.1A RifR was performed to evaluate a potential bias caused by the presence of a Gm-resistance cassette (strains are described in Table 1). Eight weeks post-inoculation, the pathogens living on roots were enumerated on selective media and a CI value was calculated for each replicate. CI values of the competitions between RNS 08.23.3.1A RifR and RNS 08.23.3.1A RifR-GmR did not differ from one (Dunn test, p-value >0.9999). By contrast, median CI of the ΔdppA, ΔapeH, and ΔpstA mutants was below one (0.002, 0.01, and 0.01, respectively), revealing a decrease in the competitive fitness of the constructed mutants (Figure 6). These CI values significantly differed from CI values of the reference competition (RNS 08.23.3.1A RifR-GmR versus RNS 08.23.3.1A RifR). In the case of the ΔbcsA mutant, the median CI was also below one (0.19), but the heterogeneity of the CI values between replicates compromised statistical significance (p-value = 0.503) when CI values were compared to those of the reference competition RNS 08.23.3.1A RifR-GmR versus RNS 08.23.3.1A RifR (Figure 6). This fitness variability suggests that multiple environmental parameters could influence the behavior of the ΔbcsA mutant during root colonization.
Figure 6 Competitivity of the D. solani constructed mutants ΔdppA, ΔapeH, ΔpstA, and ΔbcsA in root colonization assays. Two independent clones of each genotype ΔdppA, ΔapeH, ΔpstA, and ΔbcsA and D. solani RNS 08.23.3.1A RifR-GmR (indicated as WT Rif-Gm) were challenged with their ancestor D. solani RNS 08.23.3.1A RifR in root colonization assays. Competitive index (CI) values were determined in 10 inoculated plants for each constructed clone (hence 20 per mutant genotype) and 10 plants for the RNS 08.23.3.1A RifR-GmR. Center lines show the medians; crosses show the mean value; box limits indicate the 25th and 75th percentiles as determined by Prism software; whiskers extend from the minimum to maximum values. Statistical analysis was performed with the Kruskal–Wallis test (Kruskal–Wallis statistic = 37.64; p-value <0.0001; DF = 4) and then CI values of the mutants were compared with those of RNS 08.23.3.1A RifR-GmR (post-hoc Dunn’s multiple comparison tests).
We further investigated how a deletion in the bcsA, ddpA, apeH, and pstA genes could modify the underground behavior of D. solani. Each pathogen (the four mutants and strain RNS 08.23.3.1A RifRGmR used as a reference) was inoculated separately by watering the roots with 1010 CFU per pot. Eight weeks later, when tuber progeny had developed, we observed that potato plants produced a similar number of progeny tubers (mean value 10.0 tubers ± 0.4) when inoculated by the mutants and RNS 08.23.3.1A RifRGmR or not inoculated (Kruskal–Wallis test, statistic 7.635, p-value= 0.1775). However, the plants inoculated by ΔbcsA, ΔapeH, and ΔpstA mutants exhibited a lower soft-rot disease incidence (% of rotted progeny tubers) when compared to those inoculated by the RNS 08.23.3.1A RifRGmR strain (Dunn test, p-values <0.05; Figure 7A). In the same experiment, the ΔpstA mutant was significantly impaired in its capacity to colonize asymptomatic progeny tubers as compared to the RNS 08.23.3.1A RifRGmR strain (Dunn test, p-values <0.0005; Figure 7B) while the three other mutants did not show a difference compared to the parental strain. Finally, we decided to evaluate the ability of the mutants to survive in the compost at the vicinity of the roots: all four mutants were impaired in this trait, as compared to strain RNS 08.23.3.1A RifRGmR (Figure 7C).
Figure 7 Underground behavior of the Dickeya solani constructed mutants ΔdppA, ΔapeH, ΔpstA, and ΔbcsA. Two independent mutants of each genotype ΔdppA, ΔapeH, ΔpstA, and ΔbcsA and D. solani RNS 08.23.3.1A RifR-GmR (indicated as WT Rif-Gm) were inoculated into potato plant pots by watering. Eight weeks later, progeny tubers were harvested from 20 plants per mutant genotype and 10 plants inoculated by RNS 08.23.3.1A RifR-GmR. The percentage (%) of plants exhibiting blackleg symptoms was determined (A) as well as the abundance of pathogens in the asymptomatic progeny tuber (B). In C, pathogens were enumerated in compost in the vicinity of the roots. Statistical analyses were performed with the Kruskal–Wallis test: in A, Kruskal–Wallis statistic = 16.09; p-value = 0.0066; DF = 5; in B, Kruskal–Wallis statistic = 48.20; p-value <0.0001; DF = 5; in C, Kruskal–Wallis statistic = 48.97; p-value <0.0001; DF = 5. The p-values of the comparisons between each mutant genotype and RNS 08.23.3.1A RifR-GmR (post-hoc Dunn’s multiple comparison tests) are indicated on the graph.
In the pathogen D. solani, our Tn-seq analyses highlighted four genomic regions, grouping 13, 18, 21, and 31 adjacent genes, respectively, which may contribute to the pathogen’s capacity to colonize roots of potato plants grown under non-gnotobiotic conditions. These root colonization genes differed from those involved in the competitive proliferation in lesions of tubers (soft-rot disease) and stems (blackleg disease). Hence, this study revealed two contrasting lifestyles of D. solani colonizing a potato plant host: an oligotroph lifestyle on roots where it survived at 105 CFU/g of roots, and a copiotroph lifestyle in lesions where D. solani proliferation reached 109 to 1010 CFU/g of macerated tissues. D. solani used different gene repertoires to survive and proliferate under these contrasting conditions: roots and lesions. Because of some limitations in the Tn-seq approach, which we discussed in Torres et al. (2021), we could not exclude the fact that additional genes contribute to an efficient colonization of roots and lesions.
In stem and tuber lesions, carbon metabolism of D. solani is oriented towards exploitation of plant cell debris. Comparison of the Tn-seq data acquired in planta and in culture media showed that carbon metabolism in planta resembled but was not identical to that observed in the presence of pectin and galactarate (Figure 2). Notably, kduD, involved in the degradation of pectin, was also identified by Tn-seq as a fitness gene in D. dianthicola and D. dadantii colonizing potato tubers (Helmann et al., 2022). Catabolism of these cell wall derivatives is connected to the upper part of glycolysis/gluconeogenesis (connecting glucose and glyceraldehyde-3P) and to the pentose phosphate pathway (Figure 2). In the lower part of glycolysis, the Pta-AckA pathway was also important under maceration conditions (Table S2). In E. coli, this pathway is involved in ATP production from acetyl-CoA with acetate as a by-product (Schütze et al., 2020). This pathway was also pinpointed in a Tn-seq analysis of D. dadantii macerating chicory leaves (Royet et al., 2019). We observed that survival of D. solani in lesions is also dependent on its capacity to detoxify plant compounds using the Acr and Sap systems. The Sap transporter is involved in resistance to snakin-1, which is the most abundant antimicrobial peptide in potato tubers (López-Solanilla et al., 1998). In Erwinia amylovora, acr genes are involved in the resistance to several plant phytoalexins and antibiotics (Al-Karablieh et al., 2009). The Acr efflux pump is also important for the colonization of chicory leaves by D. dadantii (Royet et al., 2019). In D. dadantii, this efflux system is involved in resistance to a wide spectrum of toxic compounds including the plant antimicrobial peptide thionin (Valecillos et al., 2006). Two important characteristics of the D. solani behavior in stem lesions are motility and synthesis of several amino acids (Figure 4). Motility may be connected to propagation of the pathogen in plant vessels causing a progressive lesion along the stem. Requirement of amino acid synthesis would reflect an imbalance of carbon and nitrogen resources in cell wall remains. Motility and amino acid synthesis were also highlighted in Tn-seq analyses of D. dadantii and D. dianthicola proliferating in progressive lesions of chicory leaves and in tubers (Royet et al., 2019; Helmann et al., 2022).
In the root colonization condition, Tn-seq data revealed that D. solani coped with another pattern of environmental constraints. Some oligopeptides, organic acids, and mineral phosphate constitute important resources for nutrients that could be imported by the dpp, ddp, dctA, and pst transporters (Figures 3, 4). Some sugars (glucoronate and its by-products) would also be assimilated (Figure 2). S. tuberosum exudates contain a wide variety of molecules, among which glucuronate represents approximately 20% of total sugars (Koroney et al., 2016). D. solani reallocated part of its resources to synthesize carbon-rich molecules: cellulose (celY and bcs genes), antibiotic oocydin A (ooc genes), and an aryl polyene (ape genes). This suggests a trade-off between proliferation and response to biotic and abiotic stresses. In D. dadantii, cellulose synthesis is associated with the formation of biofilm at the air–liquid interface in a carbon-rich culture medium (Mee-Ngan et al., 2005). On roots, the formation of a cellulose biofilm, hence the fitness associated to bcs genes, would strongly depend on the abundance of carbon resources and moisture. Variations in these environmental parameters could explain heterogeneity in CI values that we observed when the ΔbcsA mutant challenged a wild-type strain (Figure 6). In D. solani Ds0432.1, ooc genes are involved in antibiosis against several Ascomyceta: Botrytis cinerea, Magnaporthe oryzae, and Sclerotinia sclerotiorum (Brual et al., 2021). Aryl polyenes are present in numerous bacterial genera throughout the Proteobacteria and Bacteroidetes, often in host-associated bacteria (Schöner et al., 2016). Aryl polyenes share a remarkably similar chemical scaffold, consisting of an aryl head group conjugated to a polyene carboxylic acid tail. The biosynthetic pathway is well characterized in several genera, including Escherichia, Vibrio, and Xenorhabdus (Schöner et al., 2016; Johnston et al., 2021). This pigment molecule is exported to the outer membrane. In E. coli, it contributes to oxidative stress resistance (H2O2) and biofilm formation.
The role of pst genes is remarkable. In D. solani, we observed that a ΔapeH mutant was impaired in the competitive colonization of roots and persistence in horticultural compost (Figures 6, 7). In addition, we observed that this locus was important for the colonization of progeny tubers and hence vertical transmission of the pathogens from plant host to its progeny (Figure 7). In different pathogens, pst genes are involved in phosphate importation as well as in several phosphate-regulated processes including virulence and adherence to surfaces (Daigle et al., 1995; Runyen-Janecky et al., 2005; Röder et al., 2021). In D. solani IPO2222, pstB was shown to be expressed in the presence of pieces of roots and tubers (Czajkowski et al., 2020), in agreement with an important role in the underground lifestyle of this pathogen.
Overall, this work highlights the versatile lifestyle of D. solani through its interaction with the potato host plant: a copiotrophic lifestyle in plant lesions exploits abundant carbon sources and faces plant defense compounds while an oligotrophic lifestyle on roots exploits less abundant, but more diverse carbon resources and competes with microbiota. Several traits and genes related to the underground behavior and proliferation in lesions remain to be investigated further (using complementation of mutants, gene expression monitoring, or structure–function analysis of the encoded proteins) to understand how D. solani pathogens efficiently survive on roots, persist in the environment, and colonize progeny tubers.
The original contributions presented in the study are publicly available. This data can be found here: NCBI Sequence Read Archives, accession PRJNA939571.
DF and KR conceived experiments; KR constructed the Tn-library in D. solani and prepared the DNA samples that were sequenced by DN at the I2BC platform; KR, GE, and EG constructed the deletion mutants; KR performed inoculation of Tn-library and deletion mutants in tuber, root, and culture medium conditions; KR and EM performed inoculation of Tn-library and deletion mutants in the stem condition. KR performed statistical analyses; KR and DF analyzed the list fitness genes and wrote the manuscript. All authors contributed to the article and approved the submitted version.
KR was supported by PhD and post-PhD grants from FN3PT/inov3P. This work has benefited from the facilities and expertise of the high-throughput sequencing platform and the plant culture facilities at I2BC (Gif-sur-Yvette). This work was supported by CNRS (SE2016-2017-2018-2019), the French Federation of Seed Potato Growers (FN3PT/inov3PT), and LabEx Saclay Plant Sciences-SPS (ANR-10-LABX-0040-SPS). The LABGeM (CEA/Genoscope & CNRS UMR8030), the France Génomique, and French Bioinformatics Institute national infrastructures (funded as part of Investissement d’Avenir program managed by Agence Nationale pour la Recherche, contracts ANR-10-INBS-09 and ANR-11-INBS-0013) are acknowledged for support within the MicroScope annotation platform. The funders had no role in study design, data collection and analysis, decision to publish, or preparation of the manuscript.
The authors declare that the research was conducted in the absence of any commercial or financial relationships that could be construed as a potential conflict of interest.
All claims expressed in this article are solely those of the authors and do not necessarily represent those of their affiliated organizations, or those of the publisher, the editors and the reviewers. Any product that may be evaluated in this article, or claim that may be made by its manufacturer, is not guaranteed or endorsed by the publisher.
The Supplementary Material for this article can be found online at: https://www.frontiersin.org/articles/10.3389/fpls.2023.1154110/full#supplementary-material
Al-Karablieh, N., Weingart, H., Ullrich, M. S. (2009). The outer membrane protein TolC is required for phytoalexin resistance and virulence of the fire blight pathogen Erwinia amylovora. Microb. Biotechnol. 2, 465–475. doi: 10.1111/j.1751-7915.2009.00095.x
Blin, P., Robic, K., Khayi., S., Cigna, J., Munier, E., Dewaegeneire, P., et al. (2021). Pattern and causes of the establishment of the invasive bacterial potato pathogen Dickeya solani and of the maintenance of the resident pathogen d. dianthicola. Mol. Ecol. 30, 608–624. doi: 10.1111/mec.15751
Blin, K., Shaw, S., Kloosterman, A. M., Charlop-Powers, Z., Wezel, G. P. V., Medema, M. H., et al. (2021). AntiSMASH 6.0: improving cluster detection and comparison capabilities. Nucleic Acids Res. 49, W29–W35. doi: 10.1093/nar/gkab335
Bolger, A. M., Lohse, M., Usadel, B. (2014). Trimmomatic: a flexible trimmer for illumina sequence data. Bioinformatics 30, 2114–2120. doi: 10.1093/bioinformatics/btu170
Bouvier, J. T., Sernova, N. V., Ghasempur, S., Rodionova, I. A., Vetting, M. W., Al-Obaidi, N. F., et al. (2019). Novel metabolic pathways and regulons for hexuronate utilization in proteobacteria. J. Bacteriol. 201 (2), e00431–e00418. doi: 10.1128/JB.00628-18
Brual, T., Effantin, G., Baltenneck, J., Attaiech, L., Groisbois, C., Royer, M., et al. (2021). A natural single nucleotide mutation in the small regulatory RNA ArcZ of Dickeya solani switches off the antimicrobial activities against yeast and bacteria. bioRχiv. doi: 10.1101/2021.07.19.452942
Cole, B. J., Feltcher, M. E., Waters, R. J., Wetmore, K. M., Mucyn, T. S., Ryan, E. M., et al. (2017). Genome-wide identification of bacterial plant colonization genes. PloS Biol. 15, 1–24. doi: 10.1371/journal.pbio.2002860
Czajkowski, R., de Boer, W. J., Velvis, H., van der Wolf, J. M. (2010). Systemic colonization of potato plants by a soilborne, green fluorescent protein-tagged strain of dickeya sp. biovar 3. Phytopathology 100, 134–142. doi: 10.1094/PHYTO-100-2-0134
Czajkowski, R., Fikowicz-Krosko, J., Maciag., T., Rabalski, L., Czaplewska, P., Jafra, S., et al. (2020). Genome-wide identification of Dickeya solani transcriptional units up-regulated in response to plant tissues from a crop-host Solanum tuberosum and a weed-host Solanum dulcamara. Front. Plant Sci. 11. doi: 10.3389/fpls.2020.580330
Daigle, F., Fairbrother, J. M., Harel, J. (1995). Identification of a mutation in the pst-phoU operon that reduces pathogenicity of an Escherichia coli strain causing septicemia in pigs. Infect. Immun. 63, 4924–4927. doi: 10.1128/iai.63.12.4924-4927
Duong, D. A., Jensen, R. V., Stevens, A. M. (2018). Discovery of pantoea stewartii ssp. stewartii genes important for survival in corn xylem through a tn-seq analysis. Mol. Plant Pathol. 19, 1929–1941. doi: 10.1111/mpp.12669
Duprey, A., Nasser, W., Léonard, S., Brochier-Armanet, C., Reverchon, S. (2016). Transcriptional start site turnover in the evolution of bacterial paralogous genes - the pelE-pelD virulence genes in Dickeya. FEBS J. 283, 4192–4207. doi: 10.1111/febs.13921
Edwards, R. A., Keller, L. H., Schifferli, D. M. (1998). Improved allelic exchange vectors and their use to analyze 987P fimbria gene expression. Gene 207, 149–157. doi: 10.1016/S0378-1119(97)00619-7
Galperin, M. Y., Makarova, K. S., Wolf, Y. I., Koonin, E. V. (2015). Expanded microbial genome coverage and improved protein family annotation in the COG database. Nucleic Acids Res. 43, D261–D269. doi: 10.1093/nar/gku1223
Golanowska, M., Potrykus, M., Motyka-Pomagruk, A., Kabza, M., Bacci, G., Galardini, M., et al. (2018). Comparison of highly and weakly virulent Dickeya solani strains, with a view on the pangenome and panregulon of this species. Front. Microbiol. 9. doi: 10.3389/fmicb.2018.01940
Gonzalez-Mula, A., Lachat, J., Mathias, L., Naquin, D., Lamouche, F., Mergaert, P., et al. (2019). The biotroph Agrobacterium tumefaciens thrives in tumors by exploiting a wide spectrum of plant host metabolites. New Phytol. 222, 455–467. doi: 10.1111/nph.15598
Hélias, V., Hamon, P., Huchet, E., Wolf, J. V. D., Andrivon, D. (2012). Two new effective semiselective crystal violet pectate media for isolation of Pectobacterium and Dickeya. Plant Pathol. 61, 339–345. doi: 10.1111/j.1365-3059.2011.02508.x
Helmann, T. C., Deutschbauer, A. M., Lindow, S. E. (2019). Genome-wide identification of Pseudomonas syringae genes required for fitness during colonization of the leaf surface and apoplast. Proc. Natl. Acad. Sci. U.S.A. 116, 18900–18910. doi: 10.1073/pnas.1908858116
Helmann, T. C., Filiatrault, M. J., Stodghill, P. V. (2022). Genome-wide identification of genes important for growth of Dickeya dadantii and Dickeya dianthicola in potato (Solanum tuberosum) tubers. Front. Microbiol. 13. doi: 10.3389/fmicb.2022.778927
Hooven, T. A., Catomeris, A. J., Akabas, L. H., Randis, T. M., Maskell, D. J., Peters, S. E., et al. (2016). The essential genome of Streptococcus agalactiae. BMC Genomics 17, 406. doi: 10.1186/s12864-016-2741-z
Jackson, S. A., Fellows, B. J., Fineran, P. C. (2020). Complete genome sequences of the Escherichia coli donor strains ST18 and MFD pir. Microbiol. Resour Announc. 9, e01014-20. doi: 10.1128/MRA.01014-20
Johnston, I., Osborn, L. J., Markley, R. L., McManus, E. A., Kadam, A., Schultz, K. B., et al. (2021). Identification of essential genes for Escherichia coli aryl polyene biosynthesis and function in biofilm formation. Biofilms Microbiomes 7, 56. doi: 10.1038/s41522-021-00226-3
Khayi, S., Blin, P., Chong, T. M., Robic, K., Chan, K. G., Faure, D. (2018). Complete genome sequences of the plant pathogens Dickeya solani RNS 08.23.3.1.A and Dickeya dianthicola RNS04.9. Genome Announcements 6 (4), e01447–e01417. doi: 10.1128/genomeA.01447-17
Khayi, S., Blin, P., Pédron, J., Chong, T. M., Chan, K. G., Moumni, M., et al. (2015). Population genomics reveals additive and replacing horizontal gene transfers in the emerging pathogen Dickeya solani. BMC Genomics 16, 788. doi: 10.1186/s12864-015-1997-z
Koroney, A. S., Plasson, C., Pawlak, B., Sidikou, R., Driouich, A., Menu-Bouaouiche, L., et al. (2016). Root exudate of Solanum tuberosum is enriched in galactose-containing molecules and impacts the growth of Pectobacterium atrosepticum. Ann. Bot. 118, 797–808. doi: 10.1093/aob/mcw128
Kuivanen, J., Biz, A., Richard, P. (2019). Microbial hexuronate catabolism in biotechnology. AMB Express. 9 (1), 16. doi: 10.1186/s13568-019-0737-1
Langmead, B., Trapnell, C., Pop, M., Salzberg, S. L. (2009). Ultrafast and memory-efficient alignment of short DNA sequences to the human genome. Genome Biol. 10 (3), R25. doi: 10.1186/gb-2009-10-3-r25
Liu, Z., Beskrovnaya, P., Melnyk, R. A., Hossain, S. S., Khorasani, S., O’Sullivan, L. R., et al. (2018). A genome-wide screen identifies genes in rhizosphere-associated Pseudomonas required to evade plant defenses. mBio 9 (6), e00433–e00418. doi: 10.1128/mBio.00433-18
López-Solanilla, E., García-Olmedo, F., Rodríguez-Palenzuela, P. (1998). Inactivation of the sapA to sapF locus of Erwinia chrysanthemi reveals common features in plant and animal bacterial pathogenesis. Plant Cell 10, 917–924. doi: 10.1105/tpc.10.6.917
Luneau, J. S., Baudin, M., Quiroz-Monnens, T., Carrère, S., Bouchez, O., Jardinaud, F., et al. (2022). Genome-wide identification of fitness determinants in the Xanthomonas campestris bacterial pathogen during early stages of plant infection. New Phytol. 236, 235–248. doi: 10.1111/nph.18313
Macho, A. P., Guidot, A., Barberis, P., Beuzón, C. R., Genin, S. (2010). A competitive index assay identifies several Ralstonia solanacearum type III effector mutant strains with reduced fitness in host plants. Mol. Plant-Microbe Interact. 23, 1197–1205. doi: 10.1094/MPMI-23-9-1197
Matilla, M. A., Monson, R. E., Murphy, A., Schicketanz, M., Rawlinson, A., Duncan, C., et al. (2022). Solanimycin: biosynthesis and distribution of a new antifungal antibiotic regulated by two quorum-sensing systems. mBio 13 (6), e0247222. doi: 10.1128/mbio.02472-22
Matilla, M. A., Stöckmann, H., Leeper, F. J., Salmond, G. P. C. (2012). Bacterial biosynthetic gene clusters encoding the anti-cancer haterumalide class of molecules. J. Biol. Chem. 287, 39125–39138. doi: 10.1074/jbc.M112.401026
Mee-Ngan, Y., Yang, C. H., Barak, J. D., Jahn, C. E., Charkowski, A. O. (2005). The Erwinia chrysanthemi type III secretion system is required for multicellular behavior. J. Bacteriol. 187, 639–648. doi: 10.1128/JB.187.2.639-648
Morinière, L., Mirabel, L., Gueguen, E., Bertolla, F. (2022). A comprehensive overview of the genes and functions required for lettuce infection by the hemibiotrophic phytopathogen Xanthomonas hortorum pv. vitians. mSystems 7, e01290–e01221. doi: 10.1128/msystems.01290-21
Olson, E. R., Dunyak, D. S., Jurss, L. M. (1991). Identification and characterization of dppA, an Escherichia coli gene encoding a periplasmic dipeptide transport protein. J. Bacteriol. 173 (1), 234–244. doi: 10.1128/jb.173.1.234-244.1991
Pédron, J., Schaerer, S., Kellenberger, I., Van Gijsegem, F. (2021). Early emergence of Dickeya solani revealed by analysis of Dickeya diversity of potato blackleg and soft rot causing pathogens in switzerland. Microorganisms 9, 1187. doi: 10.3390/microorganisms9061187
Peters, J. M., Koo, B. M., Patino, R., Heussler, G. E., Hearne, C. C., Qu, J., et al. (2019). Enabling genetic analysis of diverse bacteria with mobile-CRISPRi. Nat. Microbiol. 4, 244–250. doi: 10.1038/s41564-018-0327-z
Potrykus, M., Golanowska, M., Hugouvieux-Cotte-Pattat, N., Lojkowska, E. (2015). Regulators involved in Dickeya solani virulence, genetic conservation, and functional variability. Mol. Plant Microbe Interact. 1, 57–68. doi: 10.1094/MPMI-99-99-0003-R
Potrykus, M., Hugouvieux-Cotte-Pattat, N., Lojkowska, E. (2018). Interplay of classic exp and specific vfm quorum sensing systems on the phenotypic features of Dickeya solani strains exhibiting different virulence levels. Mol. Plant Pathol. 19, 1238–1251. doi: 10.1111/mpp.12614
Prigent-Combaret, C., Zghidi-Abouzid, O., Effantin, G., Lejeune, P., Reverchon, S., Nasser, W. (2012). The nucleoid-associated protein Fis directly modulates the synthesis of cellulose, an essential component of pellicle–biofilms in the phytopathogenic bacterium dickeya dadantii. Mol. Microbiol. 86, 172–186. doi: 10.1111/j.1365-2958.2012.08182.x
Pritchard, J. R., Chao, M. C., Abel, S., Davis, B. M., Baranowski, C., Zhang, Y. J., et al. (2014). ARTIST: high-resolution genome-wide assessment of fitness using transposon-insertion sequencing. PloS Genet. 10, e1004782. doi: 10.1371/journal.pgen.1004782
Raoul des Essarts, Y., Pédron, J., Blin, P., Van Dijk, E., Faure, D., Van Gijsegem, F. (2019). Common and distinctive adaptive traits expressed in Dickeya dianthicola and Dickeya solani pathogens when exploiting potato plant host. Environ. Microbiol. 21, 1004–1018. doi: 10.1111/1462-2920.14519
Röder, J., Felgner, P., Hensel, M. (2021). Single-cell analyses reveal phosphate availability as critical factor for nutrition of Salmonella enterica within mammalian host cells. Cell. Microbiol. 10, e13374. doi: 10.1111/cmi.13374
Royet, K., Parisot, N., Rodrigue, A., Gueguen, E., Condemine, G. (2019). Identification by tn-seq of Dickeya dadantii genes required for survival in chicory plants. Mol. Plant Pathol. 20, 287–306. doi: 10.1111/mpp.12754
Runyen-Janecky, L. J., Boyle, A. M., Kizzee, A., Liefer, L., Payne, S. M. (2005). Role of the pst system in plaque formation by the intracellular pathogen Shigella flexneri. Infect. Immun. 73, 1404–1410. doi: 10.1128/IAI.73.3.1404-1410
Schöner, T. A., Gassel, S., Osawa, A., Tobias, N. J., Okuno, Y., Sakakibara, Y., et al. (2016). Aryl polyenes, a highly abundant class of bacterial natural products, are functionally related to antioxidative carotenoids. Chem Bio Chem 17, 247–253. doi: 10.1002/cbic.201500474
Schütze, A., Benndorf, D., Püttker, S., Kohrs, F., Bettenbrock, K. (2020). The impact of ackA, pta, and ackA-pta mutations on growth, gene expression and protein acetylation in Escherichia coli K-12. Front. Microbiol. 11. doi: 10.3389/fmicb.2020.00233
Sivakumar, R., Ranjani, J., Vishnu, U. S., Jayashree, S., Lozano, G. L., Miles, J., et al. (2019). Evaluation of INSeq to identify genes essential for Pseudomonas aeruginosa PGPR2 corn root colonization. G3 (Bethesda) 9, 651–661. doi: 10.1534/g3.118.200928
Su, Y., Xu, Y., Liang, H., Yuan, G., Wu, X., Zheng, D. (2021). Genome-wide identification of Ralstonia solanacearum genes required for survival in tomato plants. mSystems 6, e00838–e00821. doi: 10.1128/mSystems.00838-21
Tervo, C. J., Reed, J. L. (2012). FOCAL: an experimental design tool for systematizing metabolic discoveries and model development. Genome Biol. 13, R116. doi: 10.1186/gb-2012-13-12-r116
Torres, M., Jiquel, A., Jeanne, E., Naquin, D., Dessaux, Y., Faure, D. (2021). Agrobacterium tumefaciens Fitness genes involved in the colonization of plant tumors and roots. New Phytol. 233, 905–918. doi: 10.1111/nph.17810
Valecillos, A. M., Palenzuela, P. R., López-Solanilla, E. (2006). The role of several multidrug resistance systems in Erwinia chrysanthemi pathogensis. Mol. Plant-Microbe Interact. 19, 607–613. doi: 10.1094/MPMI-19-0607
van der Wolf, J. M., Acuña, I., De Boer, S. H., Brurberg, M. B., Cahill, G., Charkowski, A. O., et al. (2021). “Diseases caused by pectobacterium and dickeya species around the world,” in Plant diseases caused by dickeya and pectobacterium species. Eds. Van Gijsegem, F., van der Wolf, J. M., Toth, I. K. (Cham: Springer International Publishing), 215–261. doi: 10.1007/978-3-030-61459-1_7
van der Wolf, J. M., Nijhuis, E. H., Kowalewska, M. J., Saddler, G. S., Parkinson, N., Elphinstone, J. G., et al. (2014). Dickeya solani Sp. nov., a pectinolytic plant-pathogenic bacterium isolated from potato (Solanum tuberosum). Int. J. Syst. Evol. Microbiol. 64, 768–774. doi: 10.1099/ijs.0.052944-0
Van Gijsegem, F., Hugouvieux-Cotte-Pattat, N., Kraepiel, Y., Lojkowska, E., Moleleki, L. N., Gorshkov, V., et al. (2021). “Molecular interactions of pectobacterium and dickeya with plants,” in Plant diseases caused by dickeya and pectobacterium species. Eds. Van Gijsegem, F., van der Wolf, J. M., Toth, I. K. (Cham: Springer International Publishing), 85–147. doi: 10.1007/978-3-030-61459-1_4
Wiles, T. J., Norton, J. P., Russell, C. W., Dalley, B. K., Fischer, K. F., Mulvey, M. A. (2013). Combining quantitative genetic footprinting and trait enrichment analysis to identify fitness determinants of a bacterial pathogen. PloS Genet. 9 (8), e1003716. doi: 10.1371/journal.pgen.1003716
Zhou, J., Zhang, H., Wu, J., Liu, Q., Xi, P., Lee, J., et al. (2011). A novel multidomain polyketide synthase is essential for zeamine production and the virulence of Dickeya zeae. Mol. Plant-Microbe Interact. 24, 1156–1164. doi: 10.1094/MPMI-04-11-0087
Keywords: blackleg disease, Tn-seq, soft rot, Solanum tuberosrum, root colonization
Citation: Robic K, Munier E, Effantin G, Lachat J, Naquin D, Gueguen E and Faure D (2023) Dissimilar gene repertoires of Dickeya solani involved in the colonization of lesions and roots of Solanum tuberosum. Front. Plant Sci. 14:1154110. doi: 10.3389/fpls.2023.1154110
Received: 30 January 2023; Accepted: 12 April 2023;
Published: 08 May 2023.
Edited by:
Mohammad Arif, University of Hawaii at Manoa, United StatesReviewed by:
Lucy N Moleleki, University of Pretoria, South AfricaCopyright © 2023 Robic, Munier, Effantin, Lachat, Naquin, Gueguen and Faure. This is an open-access article distributed under the terms of the Creative Commons Attribution License (CC BY). The use, distribution or reproduction in other forums is permitted, provided the original author(s) and the copyright owner(s) are credited and that the original publication in this journal is cited, in accordance with accepted academic practice. No use, distribution or reproduction is permitted which does not comply with these terms.
*Correspondence: Denis Faure, ZGVuaXMuZmF1cmVAaTJiYy5wYXJpcy1zYWNsYXkuZnI=
†ORCID: Delphine Naquin, orcid.org/0000-0001-9174-4686
Erwan Gueguen, orcid.org/0000-0001-8784-1491
Denis Faure, orcid.org/0000-0002-5379-8867
Disclaimer: All claims expressed in this article are solely those of the authors and do not necessarily represent those of their affiliated organizations, or those of the publisher, the editors and the reviewers. Any product that may be evaluated in this article or claim that may be made by its manufacturer is not guaranteed or endorsed by the publisher.
Research integrity at Frontiers
Learn more about the work of our research integrity team to safeguard the quality of each article we publish.