- 1Molecular Plant Sciences Program, Washington State University, Pullman, WA, United States
- 2Plant and Data Science, Heliponix, LLC, Evansville, IN, United States
- 3Dormancy and Adaptation Research Unit, RIKEN Center for Sustainable Resource Science, Tsurumi, Yokohama, Japan
- 4Wheat Health, Genetics, and Quality Research Unit, USDA-ARS, Pullman, WA, United States
- 5Department of Crop and Soil Science, Washington State University, Pullman, WA, United States
Introduction: The seeds of many plants are dormant and unable to germinate at maturity, but gain the ability to germinate through after-ripening during dry storage. The hormone abscisic acid (ABA) stimulates seed dormancy, whereas gibberellin A (GA) stimulates dormancy loss and germination.
Methods: To determine whether dry after-ripening alters the potential to accumulate ABA and GA, hormone levels were measured during an after-ripening time course in dry and imbibing ungerminated seeds of wildtype Landsberg erecta (Ler) and of the highly dormant GA-insensitive mutant sleepy1-2 (sly1-2).
Results: The elevated sly1-2 dormancy was associated with lower rather than higher ABA levels. Ler germination increased with 2-4 weeks of after-ripening whereas sly1-2 required 21 months to after-ripen. Increasing germination capacity with after-ripening was associated with increasing GA4 levels in imbibing sly1-2 and wild-type Ler seeds. During the same 12 hr imbibition period, after-ripening also resulted in increased ABA levels.
Discussion: The decreased ABA levels with after-ripening in other studies occurred later in imbibition, just before germination. This suggests a model where GA acts first, stimulating germination before ABA levels decline, and ABA acts as the final checkpoint preventing germination until processes essential to survival, like DNA repair and activation of respiration, are completed. Overexpression of the GA receptor GID1b (GA INSENSITIVE DWARF1b) was associated with increased germination of sly1-2 but decreased germination of wildtype Ler. This reduction of Ler germination was not associated with increased ABA levels. Apparently, GID1b is a positive regulator of germination in one context, but a negative regulator in the other.
1 Introduction
The regulation of seed germination is vital to plant and species survival. Seed dormancy prevents germination even under conditions suitable for germination in the short-term (Leubner-Metzger, 2005; Finch-Savage and Leubner-Metzger, 2006). Evolutionary benefits of seed dormancy include allowing time for seed dispersal, preventing germination out of season, and ensuring survival of natural disasters as seeds in the soil through a bet-hedging strategy (Koornneef et al., 2000; Venable, 2007; Poisot et al., 2011). Because of this, seed dormancy is more common in plants from areas with seasons (Baskin and Baskin, 1972). Dormancy can be lost through a period of dry storage called after-ripening. Depending on the species, dormancy can also be lost through scarification of the seed coat and water uptake in the cold called cold stratification. The precise control of physiological seed dormancy and dormancy loss are not fully understood, however the plant hormone abscisic acid (ABA) is known to promote dormancy whereas the hormone gibberellin A (GA) promotes seed germination (reviewed in Finkelstein et al., 2008). By examining changes in ABA and GA hormone levels at multiple after-ripening and imbibition timepoints, this study examined the timing of ABA and GA hormone changes in Arabidopsis seeds during after-ripening and imbibition before germination per se.
The process of seed germination in the strict sense begins with water uptake during imbibition and concludes with germination per se, the emergence of the radicle from the seed coat. The germination process involves the repair and activation of cellular systems needed for successful growth. The three phases of germination were defined by water uptake (reviewed in Bewley et al., 2013). Phase I involves the initial rapid uptake of water when the seed is first imbibed. Phase I processes include cellular rehydration, the initiation of DNA and mitochondrial repair, the translation of stored mRNAs, and the initiation of transcription. Dormancy may be imposed by repressors of these early cell processes. Seed moisture plateaus during Phase II. Phase II involves processes necessary for germination and post-germinative growth including: transcription and translation of new mRNAs, the completion of DNA repair, DNA synthesis, initial mobilization of stored reserves, and restoration of cellular integrity. The event of visible germination per se defines the end of Phase II and beginning of the post-germinative Phase III. The decision to germinate is likely tightly linked to regulation of Phase II processes since both dormant and non-dormant seeds enter Phase II, but only non-dormant seeds germinate (Nelson and Steber, 2017). Arabidopsis seeds do not germinate during cold stratification. However, cold stratification for short periods (3-5 d at 4°C) promotes germination, synchronizing seeds in early Phase II (Ogawa et al., 2003; Yamauchi et al., 2004; Cadman et al., 2006; Penfield and Springthorpe, 2012; Nelson and Steber, 2017). Longer cold stratification can induce secondary dormancy.
Dormancy loss involves a shift from dormancy-promotion by the sesquiterpene hormone ABA to germination-promotion by tetracyclic diterpene hormone GA. ABA levels peak during embryo maturation and remain high in mature dry seeds, consistent with its roles in establishment and maintenance of seed dormancy (Karssen et al., 1983; Lefebvre et al., 2006; Okamoto et al., 2006; Kanno et al., 2010; Lee et al., 2010). Mutants that reduce ABA biosynthesis or sensitivity cause decreased seed dormancy, while mutations in ABA turnover and hypersensitive mutants cause increased seed dormancy (reviewed in Finkelstein et al., 2002; Kushiro et al., 2004; Okamoto et al., 2006; Schramm et al., 2010; Schramm et al., 2013). Mutants that reduce GA biosynthesis or signaling increase seed dormancy or cause failure to germinate without exogenous GA application, whereas mutants with increased GA sensitivity are less dormant (Koornneef and van der Veen, 1980; Jacobsen and Olszewski, 1993; Steber et al., 1998; Olszewski et al., 2002; Willige et al., 2007). Furthermore, mature seed germination is inhibited by exogenous ABA application and stimulated by GA.
Several lines of evidence support the hormone balance theory suggestion that dormancy loss is correlated with a drop in endogenous ABA levels and a rise in GA levels (Karssen and Laçka, 1986). At timepoints late in imbibition, near the time of germination, ABA levels were higher in dormant than non-dormant Arabidopsis wild-type seeds, consistent with the idea that dormancy loss is associated with a decrease in ABA levels (Ali-Rachedi et al., 2004; Lee et al., 2010). Thus, dormancy appears to be associated with higher ABA levels during imbibition. Conversely, GA levels are very low in dry seeds and rise as seeds germinate (Jacobsen et al., 2002; Ogawa et al., 2003; Kanno et al., 2010). It is yet unclear, however, whether GA levels rise in response to dormancy loss before or after germination per se.
Detection of increasing bioactive GA prior to germination has been difficult because endogenous GA levels are much lower than ABA levels. In barley, an increase in bioactive GA1 was detected in after-ripened vs dormant embryos just as grains germinated (Jacobsen et al., 2002; Liu et al., 2013). Multiple imbibition timepoints from dry to 48 h imbibed barley indicated an increase in GA precursors with after-ripening that was strongest later in imbibition. However, some increase in GA biosynthetic precursors was also detected with after-ripening of dry seeds. To our knowledge, the only Arabidopsis data showing an increase in GA with after-ripening used the highly dormant GA-insensitive sly1-2 (sleepy1-2) mutant (Ariizumi et al., 2013). In that study, a decrease in ABA and increase in GA levels was seen with long after-ripening (19 months) following cold stratification for 4 days in the dark plus 12 h imbibition in the light at 22°C (late Phase II). Using a modification of the sensitive GA-optimized method (Varbanova et al., 2007) for measuring hormones, this study measured GA levels in dry seeds and in imbibing seeds before germination to determine if after-ripening increases germination potential through an increase in bioactive GA levels.
Mutations in genes encoding GA biosynthetic enzymes established that GAs stimulate seed germination, stem and cell elongation, fertility, and flowering time (reviewed in Sun and Gubler, 2004; Ueguchi-Tanaka et al., 2007b; Yamaguchi, 2008). While over 136 gibberellins have been identified, only a subset are bioactive including GA1, GA3, GA4, and GA7 (Hedden, 2020). GA1 is the major bioactive GA in monocots like rice and barley, whereas GA4 is the major bioactive GA in dicots like Arabidopsis (Ueguchi-Tanaka et al., 2007a). The GA biosynthetic pathway begins with synthesis of ent-kaurene from geranylgeranyl diphosphate in the plastid (Supplementary Figure 1; reviewed in Yamaguchi, 2008; Hedden, 2016). This leads to phenotypes that are rescued by GA application including failure to germinate, extreme dwarfism, and failure to transition to flowering. GA12 is synthesized in the endoplasmic reticulum and serves as the common precursor for both GA1 and GA4. In Arabidopsis, mutations in enzymes acting prior to synthesis of GA12 result in failure to germinate and severe dwarfism. The ga1-3 mutant largely blocks GA biosynthesis as a result of a deletion of the single copy of the early biosynthesis enzyme ent-copalyl diphosphate synthase (CPS) (Zeevaart and Talon, 1992). GA1 derives from hydroxylation of C-13 in GA12 to obtain GA53, whereas GA4 is derived from the non-13-hydroxylated GA15. The parallel non-13-hydroxylated and 13-hydroxylated synthesis of bioactive GA4 and GA1 are catalyzed by the P450 enzymes GA 20-oxidase (GA20ox) and GA 3-oxidase (GA3ox). Deactivation of bioactive GAs and their immediate precursors are catalyzed by GA 2-oxidase (GA2ox) activity. Mutations in GA20ox and GA3ox genes result in semi-dwarfs that are able to germinate without addition of GA, likely because they belong to multigene families. In Arabidopsis, there are five GA 20-oxidase genes, GA20ox1 to GA20ox5, four GA 3-oxidase genes, GA3ox1 to GA3ox4, and seven GA 2-oxidase genes, GA2ox1 to GA2ox8 (excluding GA2ox5, which is a pseudogene). Regulation of germination appears to largely depend on these late GA biosynthetic and turnover enzymes.
GA acts by lifting negative regulation GA responses by the DELLA (Asp-Glu-Leu-Leu-Ala) domain proteins (reviewed in Hauvermale et al., 2012). The absence of GA in ga1-3 seeds causes high levels of DELLA protein to repress germination. GA treatment of ga1-3 seeds triggers DELLA proteolysis and seed germination (Ariizumi and Steber, 2007). GA stimulates DELLA degradation through interaction with the GA receptor, GID1 (GA-INSENSITIVE DWARF1). In Arabidopsis there are three GID1 GA-receptor genes, GID1a, GID1b, and GID1c (Nakajima et al., 2006). GA binding by GID1 causes a conformational change that exposes DELLA-interacting residues, leading to formation of a GID1-GA-DELLA complex (Murase et al., 2008; Shimada et al., 2008; Ueguchi-Tanaka et al., 2008; Hirano et al., 2010). Formation of the GID1-GA-DELLA complex causes the SLEEPY1 (SLY1) F-box subunit of an SCF (Skp, Cullin, F-box) E3 ubiquitin ligase to bind to DELLA (Steber et al., 1998; McGinnis et al., 2003; Dill et al., 2004). SCFSLY1 catalyzes DELLA polyubiquitination and proteolysis by the 26S proteasome. DELLA destruction alleviates negative regulation of GA responses including seed germination.
DELLA proteins are nuclear-localized proteins believed to regulate GA responses through interaction with transcriptional regulators including INDETERMINATE DOMAIN (IDD) and PHYTOCRHOME-INTERACTING FACTORS PIF1, 3, and 4 (Silverstone et al., 1998; Itoh et al., 2002; Zentella et al., 2007; Bai et al., 2012; Hirano et al., 2012; Yoshida et al., 2014; Zhiponova et al., 2014). A limited number of DELLA-activated gene promoters have been identified such as the activator of GA responses SCARECROW-LIKE3 (SCL3), the GA biosynthesis enzymes GA20ox2 and GA3ox1, the GA receptors GID1a and GID1b, and the XERICO positive regulator of ABA biosynthesis (Zentella et al., 2007; Yoshida et al., 2014). Since DELLAs are negative regulators of GA responses, DELLA activation of GA biosynthesis and signaling genes are likely negative feedback mechanisms. However, XERICO is a putative E3 ubiquitin ligase that positively regulates ABA biosynthesis (Piskurewicz et al., 2008). DELLA activation of XERICO transcription may indicate that GA stimulates germination by reducing XERICO-stimulation of ABA biosynthesis.
In Arabidopsis, mutations in positive regulators of GA signaling lead to increased seed dormancy and the failure to germinate associated with overaccumulation of DELLA proteins. Both mutations in the single SLY1 F-box gene and the gid1a gid1b gid1c triple mutants result in a failure to germinate that cannot be rescued by GA (McGinnis et al., 2003; Griffiths et al., 2006; Ariizumi and Steber, 2007; Willige et al., 2007). Indeed, GA-insensitive mutants over-accumulate GA, likely as a feedback response to reduced signaling (Griffiths et al., 2006; Ariizumi et al., 2013). Mutations in SLY1 do not entirely block GA signaling due to a mechanism of GA signaling that does not require DELLA proteolysis but does require GA and GID1 protein (McGinnis et al., 2003; Ariizumi and Steber, 2007; Hauvermale et al., 2022). GA-insensitive sly1 mutants have elevated DELLA protein levels, a semi-dwarf stature, reduced male fertility, and increased seed dormancy. This study used the sly1-2 frame-shift mutation resulting in loss of the last 40 amino acids (26% of the protein), including the proposed DELLA binding site. sly1-2 seeds have a high degree of seed dormancy, requiring 1-3 years of after-ripening to reach 30-80% germination, whereas wild-type Landsberg erecta (Ler wt) germinates efficiently with 2 weeks of after-ripening. The sly1-2 germination phenotype is rescued both by long after-ripening and by overexpression of the GID1 receptor genes. However, neither of these rescue mechanisms result in reduced DELLA protein accumulation. Thus, examination of hormonal changes in after-ripening sly1-2 mutants will show changes that can occur without DELLA destruction. GID1a, GID1b, and GID1c overexpression (OE) all resulted in rescue of sly1-2 seed germination, but rescue by GID1b-OE was strongest (Ariizumi et al., 2013). This is likely due to the fact that only GID1b can bind DELLA to some degree in the absence of GA, and that GID1b has higher affinity for GA and DELLA than either GID1a or GID1c (Griffiths et al., 2006; Nakajima et al., 2006; Yamamoto et al., 2010; Nelson and Steber, 2016). In addition to germination, GID1 overexpression also caused partial rescue of other GA-regulated sly1 phenotypes including plant height and fertility (Ariizumi et al., 2008; Ariizumi et al., 2013). Increasing GA sensitivity is likely a fundamental mechanism associated with dormancy loss since after-ripening is associated with increased expression of both SLY1 mRNA and GID1 proteins (Lee et al., 2010; Hauvermale et al., 2015). It is possible that a relatively small increase in GA hormone level can stimulate germination as GA sensitivity increases with after-ripening or GID1 overexpression. This study examined the changes in GA and ABA hormone levels associated with sly1-2 germination-rescue by both long after-ripening and GID1b overexpression.
Seed dormancy is controlled through the balance between ABA and GA signaling. Many studies have examined changes in ABA levels with after-ripening, but few have investigated the corresponding changes in GA. This study examined the effects of after-ripening and GID1b-OE on wild-type and sly1-2 ABA and GA hormone levels. In a previous study, we examined ABA and GA levels in sly1-2 seeds at two after-ripening timepoints and an imbibition timepoint late in Phase II (Ariizumi et al., 2013). By taking a multidimensional approach to compare hormone levels across multiple after-ripening and imbibition timepoints, it was possible to ask novel questions about the changes in hormone levels associated with after-ripening and determine when in imbibition these changes can be observed. Furthermore, we demonstrated that loss of dormancy through after-ripening regulates the ability of cold stratification to stimulate an increase in GA during imbibition. By using a modification of the GA-optimized method of Plackett et al. (2012) for measuring hormone levels, we were able to detect GA in dry seeds and before germination across an after-ripening time course. Thus, we were able to show that GA hormone levels increase with after-ripening at a time in imbibition when ABA levels are high. This suggests that the role of GA in dormancy loss may precede that of ABA. We showed that GID1b-OE in wild-type resulted in decreased rather than increased germination as it did in sly1-2. Finally, we learned that the partial rescue for sly1-2 germination by after-ripening and GID1b-OE is not associated with a decrease in ABA levels, suggesting that other mechanisms result in dormancy-release.
2 Materials and methods
2.1 Plant materials and growth conditions
Arabidopsis thaliana ecotype Landsberg erecta (Ler) wild-type (wt), Ler GID1b-OE, sly1-2, and sly1-2 GID1b-OE in the Ler background were previously described (Steber et al., 1998; Ariizumi et al., 2008; Ariizumi et al., 2013). The GID1b-OE allele is a translational fusion of HA : GID1b expressed on the 35S cauliflower mosaic virus promoter. Ler and sly1-2 contain independent single copy, homozygous transformation events that were selected for similar expression levels by RT-qPCR analysis. All plants used in this study were grown side-by-side in the same Conviron® growth chamber under fluorescent lights (200 µmol/m2/sec) according to McGinnis et al. (2003). The only exception to this was 21 month after-ripened (Long AR) sly1-2, which was grown under the same conditions in advance of the other seeds to allow capture of non-dormant sly1-2 seeds in our experiments. Only the Long AR sly1-2 seed batch was different from the batch used for 0, 2, and 4 week after-ripened sly1-2 seeds timepoints. All other hormone measurements for the same Arabidopsis genotype came from the same original seed batch, differing only by length of after-ripening and/or time of imbibition when seeds were collected for hormone measurements.
Seeds for hormone measurements were harvested at “physiological maturity” to obtain a dormant seed starting point for all lines including wild-type Ler, which is dormant at physiological maturity but loses dormancy rapidly through dry after-ripening (Russell et al., 2000). If Arabidopsis seeds are harvested after the plant has fully senesced and dried down, many seeds may have dry after-ripened for a few weeks since some siliques mature before others (Nelson et al., 2017). To obtain higher dormancy, Ler seeds were harvested when plants were partially yellow and partially green (Supplementary Figure 2A). Mature, yellow siliques shatter and release seeds readily whereas green siliques do not. Collection of only mature, brown, dry seeds was achieved by harvesting only seeds that freely fell from siliques. Supplementary Figure 2B shows that only brown, physiological mature seeds were collected. No green seeds were used in the experiments. After harvest, seeds were stored at room temperature and low humidity (≈15-30%) in open tubes for the specified period of dry after-ripening, then stored in a -20°C freezer to maintain dormancy status until use. Dormant 0 day/week after-ripened (0wkAR) seeds were stored overnight in open tubes. After-ripening timepoints used in this study were defined by additional storage for 1 d (1dAR), 2 d (2dAR), 2 weeks (2wkAR), 4 weeks (4wkAR), 5 weeks (5wkAR), or 21 months (Long AR).
2.2 Germination experiments
All germination screens were conducted with three replications of 60 to 100 seeds per replicate. Seeds were sterilized for 5 minutes in 70% ethanol and 0.01% SDS, followed by 10 minutes in 10% bleach and 0.01% SDS, washed thoroughly, and plated on 0.8% agar plates with 0.5× MS salts (Sigma-Aldrich) and 5 mM MES (2-(N-morpholino)ethanesulfonic acid), pH 5.5 (referred to as MS-agar plates). In most cases, seed germination is shown for both “Cold” and “No Cold” treatments. “Cold” seeds were cold stratified in the dark for 4 d at 4°C prior to scoring germination at 22°C in the light. Cold stratification was used to obtain synchronous seed germination in an effort to decrease variance in seed hormone measurements by capturing seeds at the same state. “No Cold” indicates germination directly at 22°C in the light. The 0h timepoint was collected immediately after cold stratification in the dark for 4 d at 4°C (Figure 1).
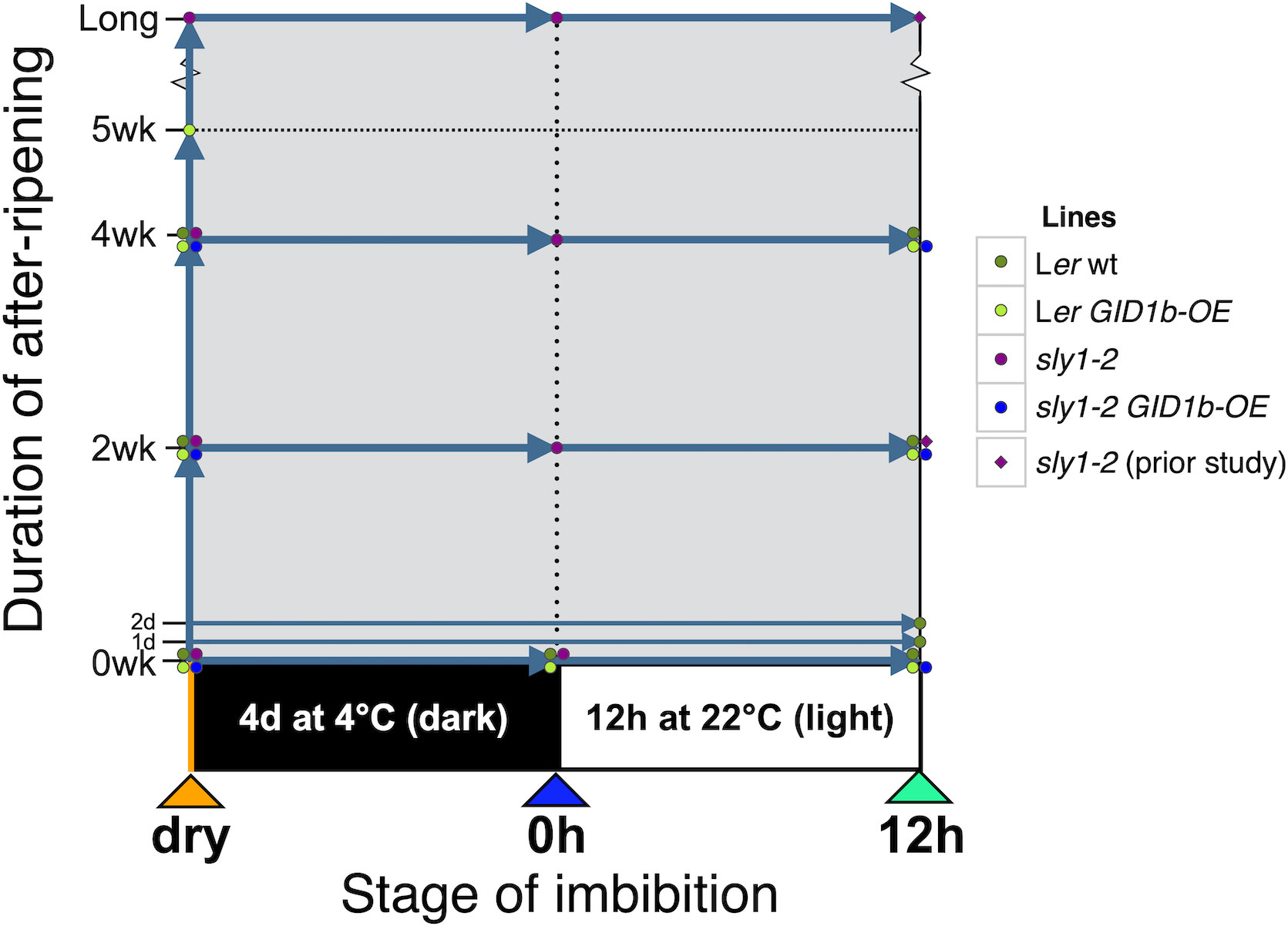
Figure 1 Diagram of sample timepoints in two dimensions: after-ripening (AR) and imbibition. Seeds are first stored for the specific duration of after-ripening and then samples were collected at the specified point in imbibition. After-ripening timepoints include: 0 days/weeks (d is day, wk is week), 1 d, 2 d, 2 wk, 4 wk, 5 wk, and long-after-ripened sly1-2 stored for 21 months (mo is months). Imbibition timepoints include: dry seed before the start of imbibition, “0h” seeds imbibed for 4 days at 4°C in the dark, and “12h” seeds imbibed for 4 days at 4°C and then moved to the light at 22°C for 12 hours. Data for sly1-2 at the 12h imbibition timepoint with 2 weeks or long after-ripening are from Ariizumi et al. (2013).
2.3 Hormone measurements
Hormone measurements were conducted for Ler wt, Ler GID1b-OE, sly1-2, and sly1-2 GID1b-OE imbibed seeds using 6 to 8 replicates of 100 to 200 mg of seed on a dry weight basis as outlined in Supplementary Figure 3. More seeds and replicates were required for timepoints with hormone levels close to the detection limit. Sampling was performed on dry seeds (dry), seeds after imbibition in the dark for 4 d at 4°C (0h), and seeds after imbibition in the dark for 4 d at 4°C plus 12 h in the light at 22°C (12h). Due to limited seed availability, not all genotypes were measured at all imbibition and after-ripening timepoints, see Supplementary Figure 3 for details. For dry seed samples, the mass of sample input was doubled to allow detection of very low GA levels. Dry samples included 3-4 replicates of 200 mg to 400 mg of seed. An initial experiment with 200 mg and 400 mg input of Columbia wild-type (Col wt) dry seeds indicated that only a minor increase in background resulted and did not obscure the locations of the ABA, GA4, or GA precursor peaks (Supplementary Figures 4A, B). Examples of GA peaks and associated background for Ler wt, sly1-2, and sly1-2 GID1b-OE are shown in Supplementary Figures 4C–E. Peaks for Ler GID1b-OE (not shown) were similar to Ler wt.
All samples were flash frozen in liquid N2 and lyophilized for 48 h. ABA, bioactive GA4, and GA precursors and catabolites were measured by liquid chromatography-electrospray ionization-tandem mass spectrometry (LC-ESI-MS/MS) with an Agilent 6410 triple quadrupole LC-MS using column purification to optimize for GA4 and GA precursor detection as described previously (Varbanova et al., 2007; Plackett et al., 2012) and for ABA as in Kanno et al. (2010). Hormone levels were quantified relative to internal standards for ABA, GA4, GA12, GA15, GA24, GA9, GA51, and GA34 obtained as described previously (Supplementary Figure 1; Varbanova et al., 2007; Plackett et al., 2012). The MS/MS parameters including retention times are shown in Supplementary Table 1. Statistical significance was determined by two-tailed Student’s t-tests performed using the R statistics package (R Core Team, 2010).
The dry and 0h imbibition timepoints were intended to determine ABA and GA changes with after-ripening earlier in imbibition than had previously been measured. The 12h timepoint was intended to allow comparison of wild-type Ler, Ler GID1b-OE, and sly1-2 GID1b-OE ABA and GA hormone changes with after-ripening to those of the 2 week after-ripened to 19 month (long after-ripened) sly1-2 from Ariizumi et al. (2013).
2.4 Reanalysis of microarray data
Microarray datasets from previous studies were reanalyzed to obtain insight into possible transcriptional regulation of changes observed in hormone levels. Our previous microarray experiments at the same “dry”, “0h”, and “12h” timepoints used in this study allowed comparisons of hormone and transcriptome data in the same lines at the same timepoints (Nelson and Steber, 2017; Nelson et al., 2017). The raw datasets from Carrera et al. (2007) were obtained from NASCarrays (http://affymetrix.arabidopsis.info/link_to_iplant.shtml). All microarray datasets were analyzed using Robust Multi-array Average (RMA) for background correction and normalization, with significance determined by False Discovery Rate (FDR) with α = 0.05 as described in Nelson and Steber (2017) (Benjamini and Hochberg, 1995; Irizarry et al., 2003). For references to differential regulation in A relative to B (AvsB), up in AvsB means up-regulated in A (or down-regulated in B), whereas down in AvsB means down-regulated in A (or up-regulated in B).
3 Results
3.1 Strategy for examining the seed ABA and GA hormone levels associated with degree of seed dormancy and stages in dormancy loss and germination
Dormancy loss and the germination process are complex multi-dimensional processes influenced by genotype, dry after-ripening time, cold stratification, and time during imbibition. To examine how ABA and GA hormones may regulate degree of seed dormancy and germination capacity, ABA and GA hormone levels were examined in Ler wt and in the highly dormant GA-insensitive line sly1-2 (Figure 1). Both after-ripening and GID1b overexpression can rescue sly1-2 dormancy. ABA and GA levels associated with dormancy-loss through dry after-ripening and due to overexpression of the GID1b GA receptor were examined to see if they were associated with similar hormonal changes. Hormonal differences associated with dormancy due to overaccumulation of DELLA repressor proteins in the sly1-2 mutant were examined by comparison to the corresponding wild-type, Ler. Hormone levels were measured in dry seeds (“dry” timepoint), in seeds harvested immediately after cold stratification for 4 d at 4°C in the dark (0h timepoint), and seeds harvested after 12 h imbibition at 22°C in the light following the cold stratification treatment (12h timepoint) for the genotypes indicated in Figure 1. Cold stratification was used to synchronize seeds at timepoints in imbibition as they approach germination per se, which reduced seed-to-seed variation in our analyses. Based on transcriptome data, the 0h timepoint corresponds to early Phase II and the 12h timepoint to late Phase II of imbibition (Nelson and Steber, 2017). Seeds were harvested at physiological maturity to capture a dormant state. After-ripening timepoints included: no after-ripening 0 week (0wkAR, 0dAR), 2 weeks (2wkAR), 4 weeks (4wkAR), 5 weeks (5wkAR), and 21 months (Long AR) of dry storage. The imbibition timepoints and some of the after-ripening timepoints corresponded with those used in two previous transcriptome studies, allowing comparison of hormone level differences with changes in the expression of GA biosynthesis and catabolic genes (Nelson and Steber, 2017; Nelson et al., 2017). As shown in those previous studies, the sly1-2 mutant exhibited little or no germination when freshly harvested, and only a mild increase in germination with 4 weeks dry after-ripening (Supplementary Figure 5). Long after-ripening, for 21 months, however, resulted in 82% germination in cold stratified seeds. The sly1-2 dormancy phenotype is also rescued by GID1b overexpression (Supplementary Figure 5; Figure 2E). Previous work showed little change in the transcriptome with GID1b-OE rescue of sly1-2 germination, suggesting that germination may result from non-transcriptional mechanisms, such as an altered hormone profile.
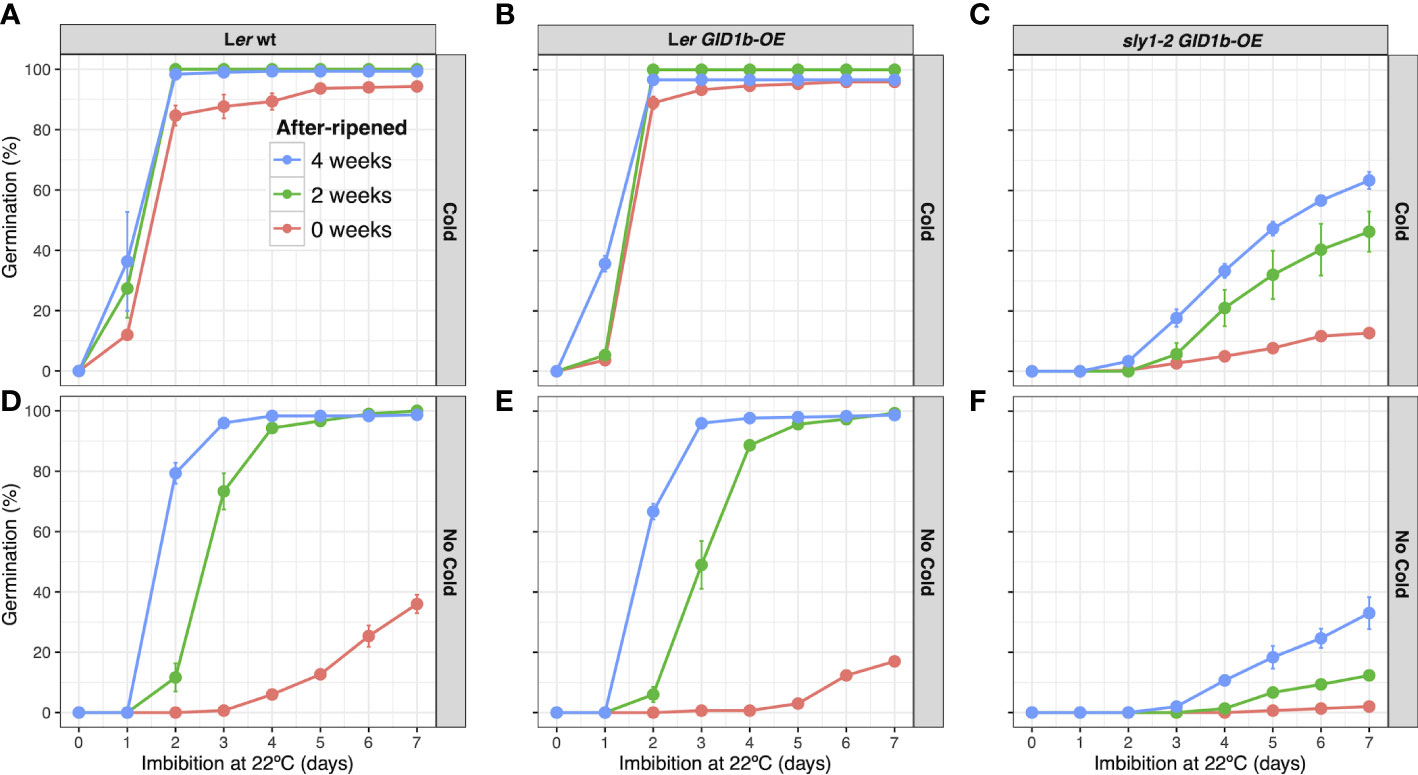
Figure 2 Germination of Ler wt, Ler GID1b-OE, and sly1-2 GID1b-OE over an after-ripening time course. Percent germination in the light at 22°C of Ler wt (A, B), Ler GID1b-OE (C), (D), and sly1-2 GID1b-OE (E, F), at 0, 2, and 4 weeks of after-ripening without cold stratification (Cold): (B, D, F), and after cold stratification for 4 d at 4°C in the dark (No Cold): (A, C, E). Error bars represent SE.
Freshly harvested wild type Ler (0wkAR) showed mild seed dormancy that was additively relieved by cold stratification and dry after-ripening (Figures 2A, B). Whereas GID1b-OE resulted in increased sly1-2 germination (Supplementary Figure 5; Figures 2E, F), GID1b-OE resulted in decreased germination of wild-type Ler at 0 wk AR (Figures 2A–D; Supplementary Figure 6). At 0wkAR, Ler was dormant reaching 27% germination, whereas Ler GID1b-OE reached only 17% germination by 7 d of imbibition. This negative effect on germination decreased with 2 and 4 weeks of after-ripening, although the percent germination remained lower than untransformed wild type at multiple imbibition timepoints. Although cold stratification is a dormancy-breaking treatment, cold stratification and after-ripening had additive effects (Figure 2). This is consistent with the fact that dormant sly1-2 cannot germinate following cold stratification, but can germinate with both long after-ripening and cold stratification (Supplementary Figure 5; Ariizumi and Steber, 2007; Ariizumi et al., 2013).
3.2 Hormone changes associated with wild-type Landsberg erecta seed imbibition and dormancy loss through dry after-ripening
To examine if there are changes in ABA and GA levels with imbibition of dormant wild-type Ler, hormone levels were compared with 0wkAR in dry seeds, at 0h, and 12h imbibition timepoints (Figure 3A). In dormant seeds, the high ABA levels in dry seeds decreased strongly during cold stratification (dry vs 0h, -114.80 ng/g DW, p = 6.7 x 10-14), and also decreased from 0h to 12h of imbibition (-23.73 ng/g DW, p = 1.6 x 10-10). GA4 levels increased with cold stratification (dry vs 0h, +0.34 ng/g DW, p = 5.9 x 10-3), but showed a much stronger increase over Phase II of imbibition (0h vs 12h,+2.09 ng/g DW, p = 9.4 x 10-10). Thus, ABA decreases and GA increases with imbibition even in seeds that are not after-ripened. These results are similar to a previous study examining non-dormant Ler wt seeds imbibed without cold stratification that found high ABA levels in dry seeds that rapidly declined within the first 8 hours of imbibition, and found low GA4 levels in dry seeds that did not rise until 24 h of imbibition (Ogawa et al., 2003).
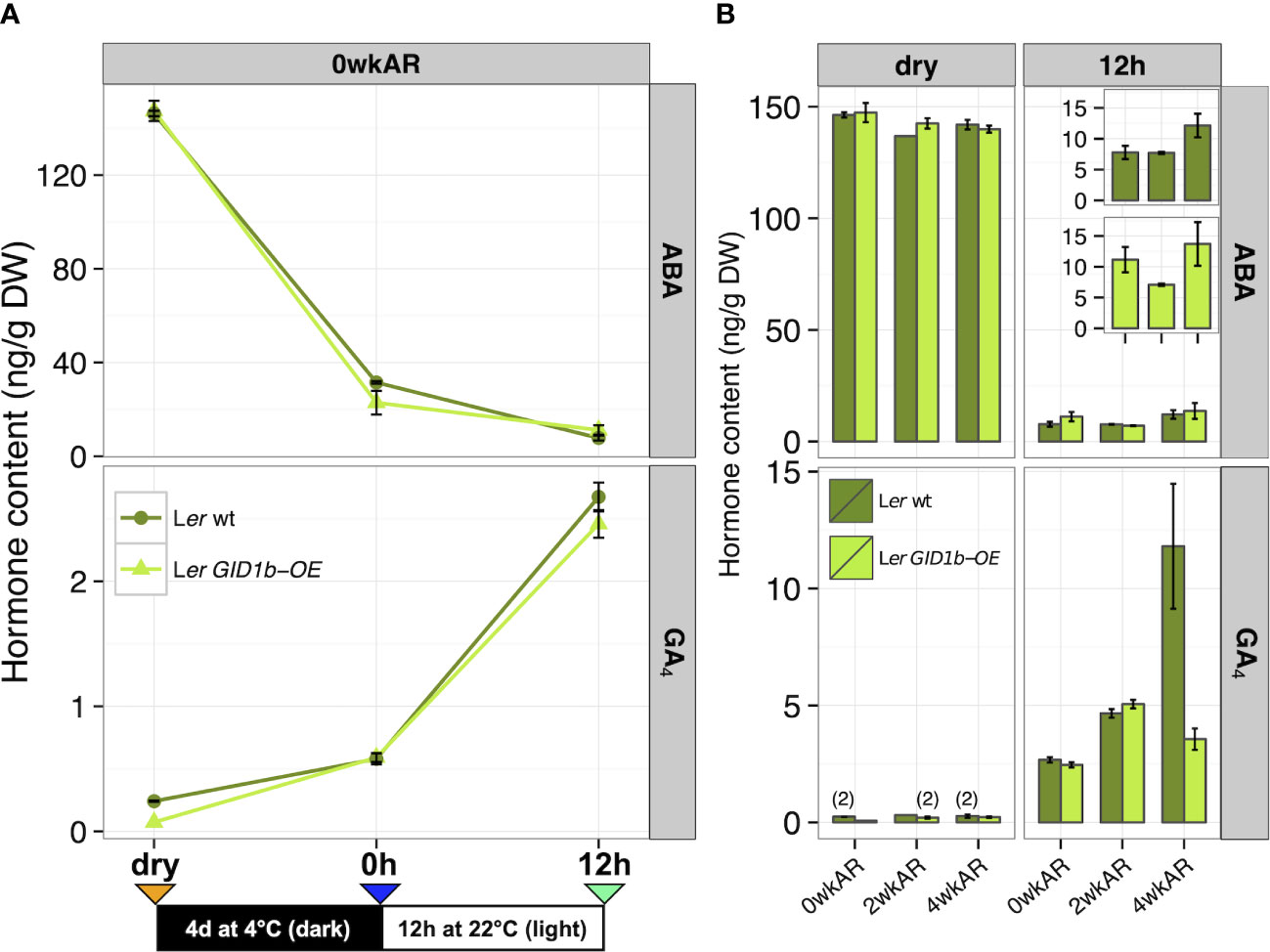
Figure 3 ABA and GA hormone measurements for Ler (dark green) and Ler GID1b-OE (light green). (A) Measurements across imbibition: dry, 0h, and 12h levels of ABA and GA in dormant 0 week after-ripened (0wkAR) seeds. (B) Measurements at a fixed imbibition timepoint (dry or 12h), for comparison of hormone changes with after-ripening for 0, 2, or 4 weeks (0, 2, 4wkAR). Error bars represent SE. “(2)” indicates that endogenous levels were only detected twice, and bars without error bars were only detected once of total replicates assayed.
To examine changes in ABA and GA levels with after-ripening, hormone levels were measured at 0, 2, and 4wkAR in both dry and 12h imbibed Ler seeds (Figure 3B). Interestingly, there was no significant decrease in ABA with after-ripening either in the dry seed or the 12h timepoint. In fact, there was a significant increase in ABA content from 2wkAR to 4wkAR in late Phase II (12h) seeds (+4.45 ng/g DW, p = 0.03). No statistically significant changes in GA4 levels were detected with after-ripening of dry Ler wt seeds, due to the fact that GA4 levels were close to the detection limit (Figure 3B; Supplementary Figure 7A; Supplementary Figure 4C). In Ler WT At 12h of imbibition, there was a significant increase in GA4 hormone levels between 0wkAR and 2wkAR (+1.99 ng/g DW, p = 9.3 x 10-7), and between 2wkAR and 4wkAR seeds (+7.15 ng/g DW, p = 9.3 x 10-3) (Figure 3B). Thus, the increase in germination potential was associated with an increase in GA4 levels with early after-ripening, and associated with an increase instead of a decrease in ABA levels from 2wk to 4wk AR at 12h imbibition. Previous work by Ali-Rachedi et al. (2004) in Cvi showed that after-ripened seeds had higher ABA levels than dormant at 12h imbibition, but lower ABA levels than dormant at 24h imbibition, just before seeds germinated. Carrera et al. (2007) made similar observations in ecotype C24. While no measurements was taken at 24h imbibition in the current study, current and previous observations together suggest a model where GA4 levels increase with after-ripening at 12h imbibition, before an after-ripening-dependent decrease in ABA levels would likely have been detected at 24h imbibition.
To determine whether the rapid after-ripening of Ler wt seeds is associated with rapid changes in hormone content, ABA and GA4 levels were measured with 1 and 2 days of dry storage at the 12h imbibition timepoint (1dAR and 2dAR in Supplementary Figure 7B). The only significant change observed was a slight decrease in GA4 levels from 0dAR to 2dAR (-0.41 ng/g DW, p = 0.03) that was not associated with a significant change in percent germination.
3.3 The effect of GID1b-OE on ABA and GA hormone levels in Ler
While GID1b-OE stimulates sly1-2 seed germination, it appeared to slow or inhibit the germination of Ler wt (Figure 2; Supplementary Figure 6; Ariizumi et al., 2013). To examine whether this reduction in germination potential was associated with altered ABA and GA levels, hormone levels were examined over imbibition and after-ripening (Figures 3A, B). In almost every comparison, Ler wt and Ler GID1b-OE ABA and GA4 hormone levels were indistinguishable. Like Ler wt, there was no statistically significant decrease in ABA levels with 2wkAR and 4wkAR in Ler GID1b-OE (p ≥ 0.07). ABA levels appeared slightly higher in the GID1b-OE than wt at the 0wkAR 12h timepoint, however this difference was not statistically significant. Whereas Ler wt GA4 hormone levels increased from 0 to 2wkAR and from 2 to 4wkAR, the Ler GID1b-OE GA4 levels increased from 0 to 2wkAR but then decreased from 2 to 4wkAR at 12h imbibition (Figures 3B, 4B). This led to lower GA4 levels at 4wkAR in the GID1b-OE line. However this seems unlikely to influence germination given that both GID1b and GA levels are elevated. Thus, the suppressive effect of GID1b-OE on wild-type seed germination cannot be well explained by differences in GA or ABA hormone levels.
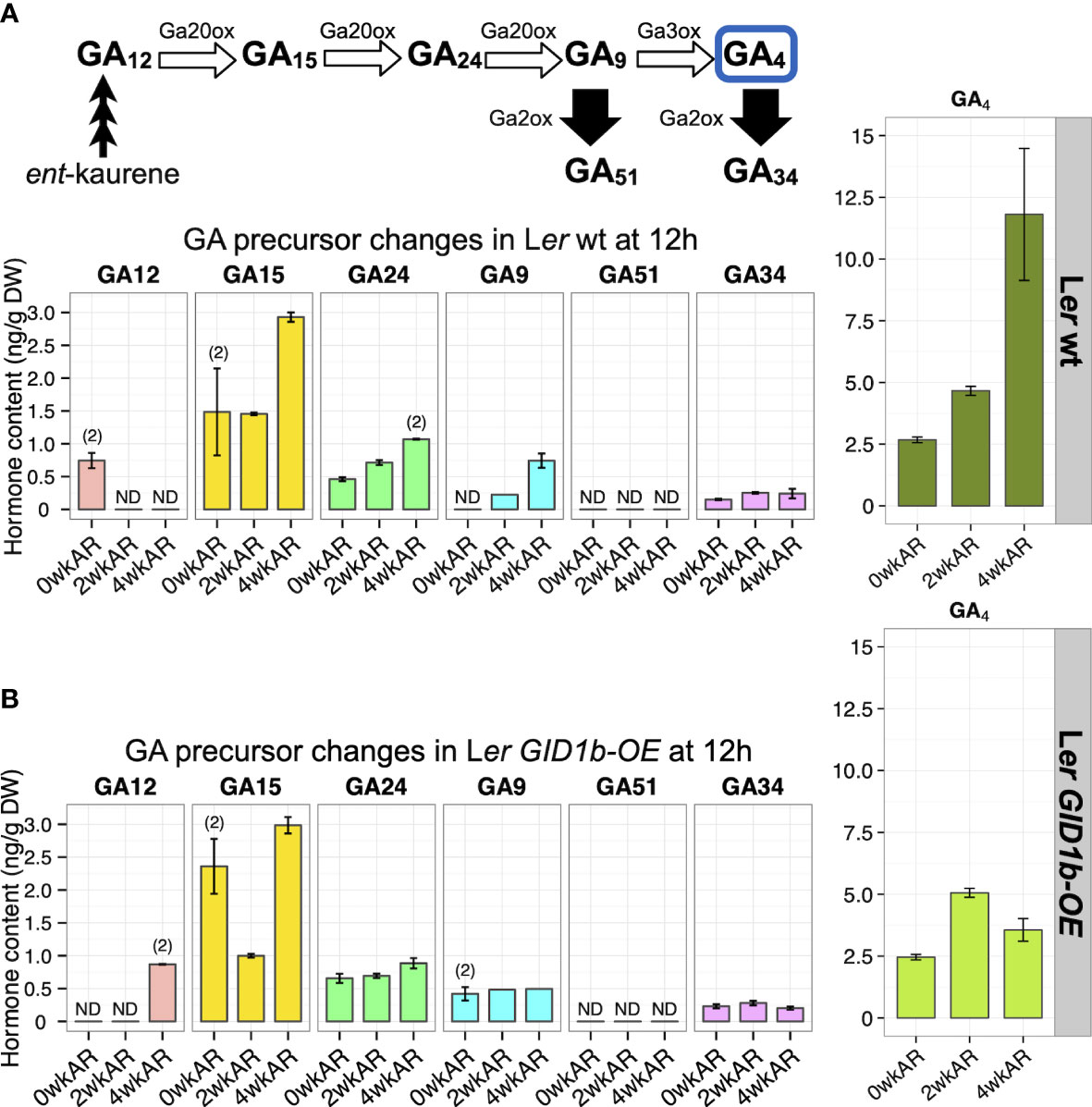
Figure 4 The impact of after-ripening and GID1b-over-expression on GA and GA precursor levels in the wild-type background at late Phase II of imbibition. GA precursor levels at 12h of imbibition for (A) Ler wt, and (B) Ler GID1b-OE, at 0, 2, and 4 weeks of after-ripening. Bioactive GA4 levels are shown for comparison. For all plots error bars represent SE. “(2)” indicates that endogenous levels were detected twice of 6-7 replicates assayed. Bars without error bars were only detected in one replicate. ND indicates levels were not detectable.
Next we examined changes in GA precursor and catabolite levels with Ler wt after-ripening at 12h imbibition (Figure 4A). The significant increase in GA4 with 2wk and 4wk after-ripening was associated with increases in the precursors GA15, GA24, and GA9. But there was no increase in catabolites GA51 and GA34. This suggests that increases in GA4 are resulting from increasing biosynthesis with little or no increase in turnover. Reanalysis of Ler wt after-ripened vs dormant transcriptomics data from Carrera et al., 2007 suggests that this pattern may result from significant Phase II increases in the expression of GA20ox1, GA20ox2, GA3ox1, and GA3ox2, associated with a small but significant decrease in the levels of the GA turnover enzyme GA2ox6 (Supplementary Figure 8). In Ler GID1b-OE at 12h, GA4 levels increase at 2wkAR but decrease at 4wkAR (Figure 3B). The increase in GA4 at 2wkAR is associated only with a decrease in precursor GA15 levels (Figure 4B). The depletion of GA15 and lack of increase in other precursors may explain why there is no sustained increase in GA4 levels in the Ler GID1b-OE line. In dry seeds, there was no significant increase in GA4 levels in dry Ler wt seeds (Supplementary Figure 7A), but there was a strong decrease in precursor GA15 with Ler wt after-ripening from 0wk to 2wk and from 2wk to 4wk after-ripening, and a small increase in the penultimate precursor GA9 between 0wk and 2 wk after-ripening (Supplementary Figure 7C). It is unclear if the increase in GA9 is in preparation leads to increased GA4 synthesis upon imbibition. In dry Ler GID1b-OE seeds, there are no statistically significant changes in GA precursors with after-ripening (Supplementary Figure 7D).
3.4 Differences in ABA and GA levels with after-ripening and imbibition of sly1-2 and sly1-2 GID1b-OE seeds
The sly1-2 mutant has higher seed dormancy than wild-type Ler that is reduced either by long after-ripening or by overexpression of GA receptors including GID1b (Ariizumi and Steber, 2007; Ariizumi et al., 2013; Nelson et al., 2017). The sly1-2 mutant showed 0-2% germination and sly1-2 GID1b-OE showed 63% germination with cold stratification and 4 weeks of after-ripening at 7 d of imbibition (Supplementary Figure 5; Figures 2E, F). Since cold stratified sly1-2 seeds did not germinate well by 4wkAR (8%), hormone measurements were also performed in sly1-2 seeds following 21 months of dry after-ripening (“Long AR” timepoint, 78% germination). By harvesting sly1-2 GID1b-OE at physiological maturity, highly dormant seeds were recovered at 0wkAR (2% germination), and dormancy loss was observed over 2 and 4wkAR (Figures 2E, F; Supplementary Figure 5). After-ripening and cold stratification acted additively to increase sly1-2 GID1b-OE germination potential, reaching 13% at 0wkAR, 48% 2wkAR, and 63% 4wkAR germination at 7 d imbibition. In order to better understand sly1-2 seed dormancy and dormancy loss, hormone measurements were performed over more detailed after-ripening and imbibition time courses.
To determine whether rescue of sly1-2 seed germination by GID1b-OE (48% versus 0% germination in sly1-2 after 7d imbibition) is associated with decreased ABA and increased GA4 levels, hormone levels were measured over an imbibition time course at 2wkAR (Figures 2E, F; Supplementary Figure 5). For this experiment, sly1-2 hormone measurements at dry and 0h timepoints were compared to the previously published 12h imbibition timepoint (Figure 5; Ariizumi et al., 2013). The sly1-2 GID1b-OE hormone levels were measured in dry seeds and at 12h, but not at the 0h timepoint. ABA levels were very similar in sly1-2 and sly1-2 GID1b-OE, and decreased 117-124 ng/g DW from dry seed to 12h of imbibition. Thus the higher germination potential in sly1-2 GID1b-OE cannot be explained by a decrease in ABA accumulation. GA4 levels were higher in dry seeds of sly1-2 GID1b-OE than in sly1-2, but only sly1-2 GA levels increased significantly with 12h of imbibition. Thus, in spite of the fact that sly1-2 GID1b-OE has higher germination potential at 2 weeks of after-ripening than sly1-2, its GA4 levels do not increase with cold stratification and 12h imbibition. This suggests that GID1b overexpression rescues sly1-2 germination by a mechanism other than decreasing ABA, possibly due to the higher starting GA4 content in dry seeds.
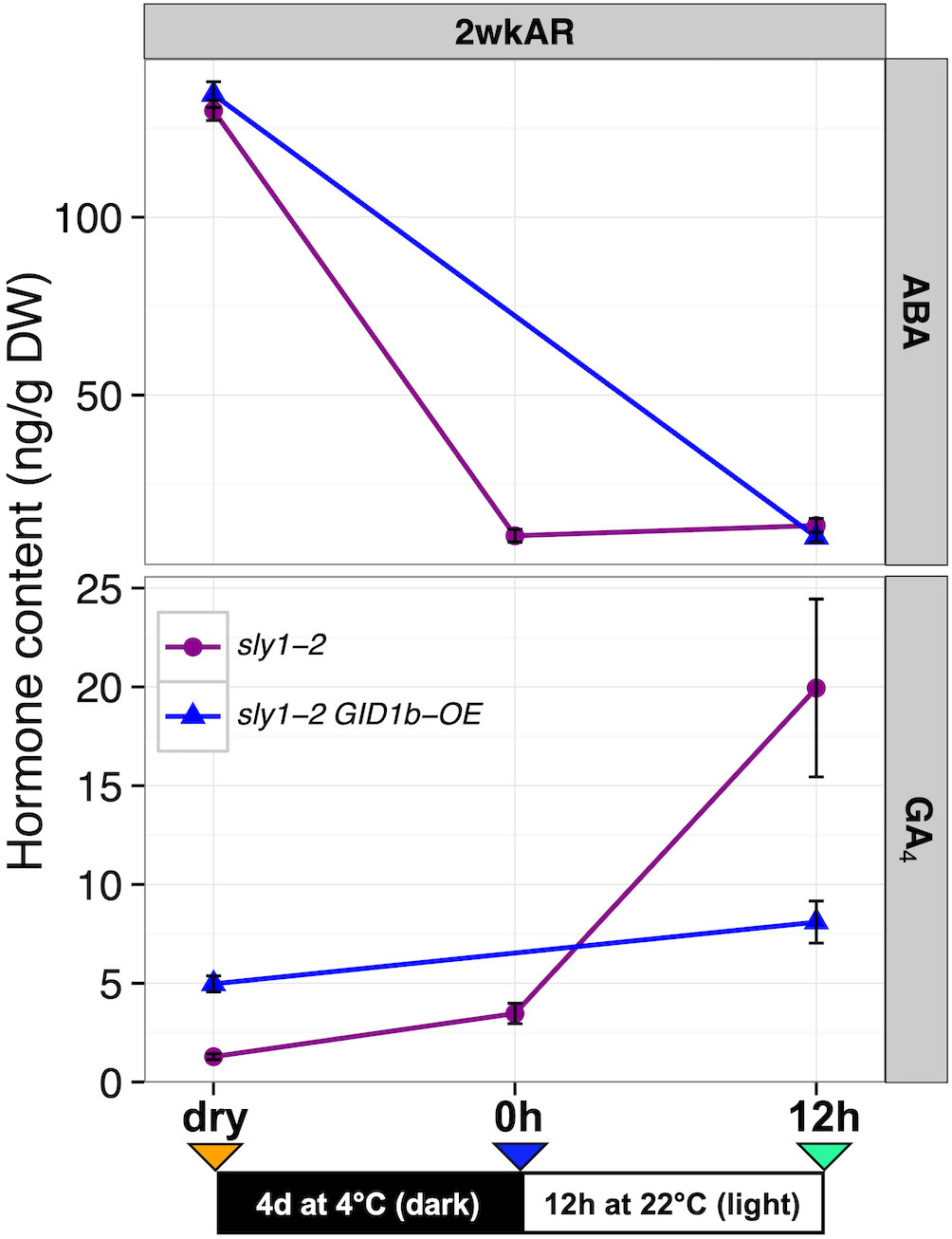
Figure 5 Changes in ABA and GA hormone levels during imbibition in sly1-2 background lines. Comparison of sly1-2 (purple) and sly1-2 GID1b-OE (blue) ABA and GA hormone levels at the dry, 0h, and 12h imbibition timepoints in 2 week after-ripened (2wkAR) seeds. sly1-2 GID1b-OE was not measured at the 0h timepoint. Data for sly1-2 at 12h was reported in Ariizumi et al., 2013. Error bars represent SE.
While after-ripening caused gradual increases in dry sly1-2 seed ABA hormone levels over time, there were tremendous changes upon cold imbibition (Figure 6). When sly1-2 ABA levels across an after-ripening time course were compared between dry and cold stratified (dry vs 0h) seeds, cold stratification was associated with clear decreases in ABA levels at all after-ripening timepoints (from -108.61 to -143.43 ng/g DW). Looking at the 0h timepoint, there was an initial significant decrease in ABA levels from 0 to 2wkAR (p = 1.2 x 10-3), but no significant change from 2 to 4wkAR. Interestingly, ABA levels increased with long after-ripening (21 months) of sly1-2 both in dry and in 0h imbibed seeds (2wkAR vs Long AR, dry: p = 0.03, 0h: p = 5.5 x 10-3). Thus, while comparison of 2 week to 19 month after-ripened sly1-2 seed at 12h showed a significant decrease in ABA levels in a previous study, we saw no decrease from 2 weeks to 21 months of after-ripening at the dry or 0h timepoint.
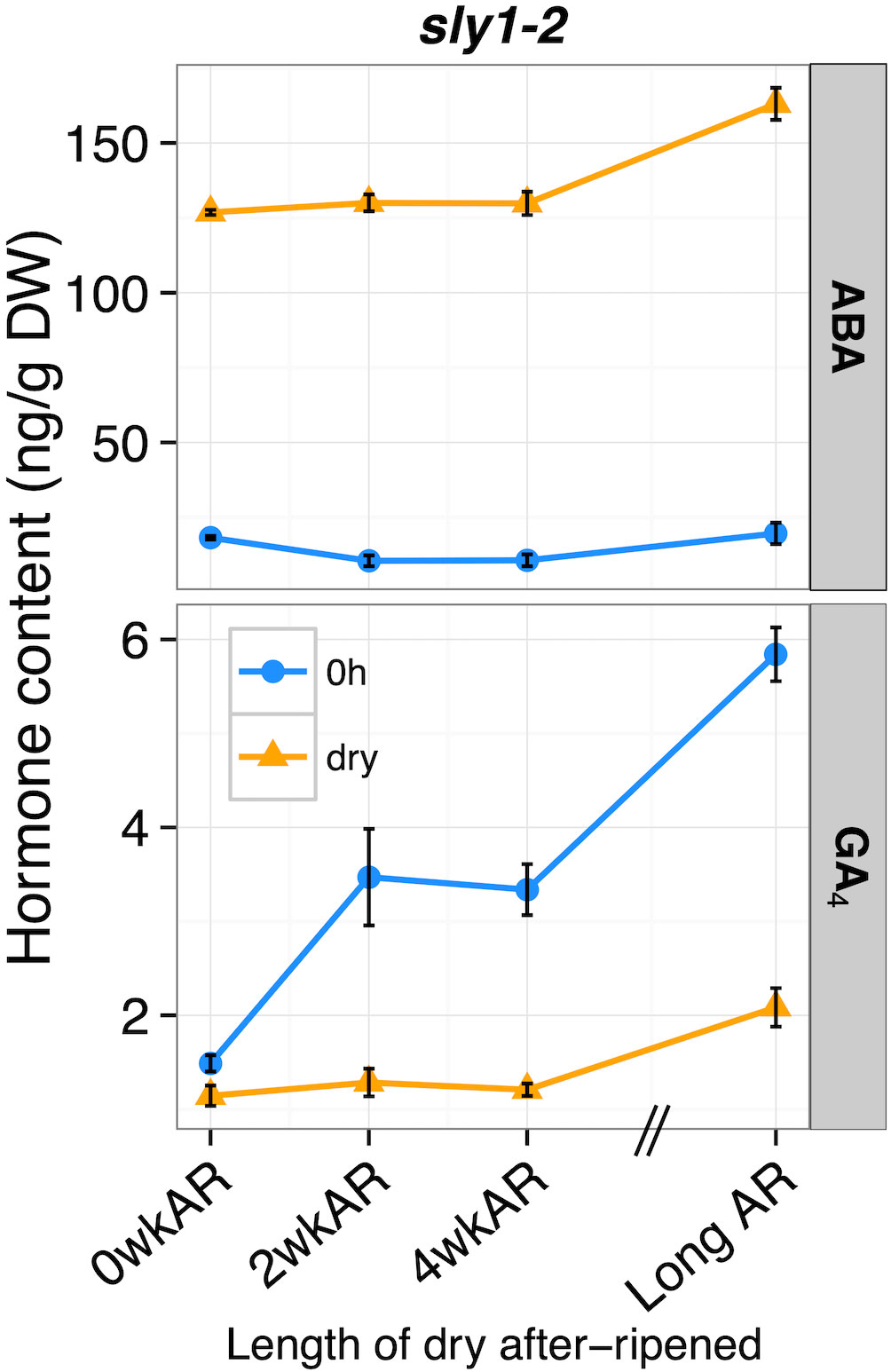
Figure 6 The effect of cold stratification on hormone levels across an after-ripening timecourse in sly1-2 seeds. ABA and GA4 levels of sly1-2 seeds at dry (orange) and 0h imbibed (light blue) timepoints across after-ripening for 0, 2, 4 weeks (0, 2, 4wkAR), and 21 months (Long AR) of after-ripening. 0h seeds were sampled after cold stratification for 4 d at 4°C in the dark. Error bars represent SE.
Next we reanalyzed previously published transcriptomic data to examine which ABA biosynthesis and turnover genes are differentially expressed in dormant sly1-2 seeds versus sly1-2 seeds whose germination was rescued by long AR or GID1b overexpression (Nelson and Steber, 2017; Nelson et al., 2017). ABA levels were higher in dry than in imbibed seeds of all genotypes (Figures 3, 5, 6). This change was not associated with changes in ABA biosynthesis and turnover gene expression in sly1-2 seeds (Supplementary Figure 9). In fact, ABA biosynthesis genes ZEP, NCED9, ABAO, and MoCo were upregulated in 0h and 12h imbibed seeds compared to dry suggesting that active ABA biosynthesis is needed to maintain ABA levels in imbibing seeds. This ABA turnover may result from stored hydrolytic enzyme activity. The increase in ABA levels at 12h imbibition, however, was associated with increased expression of the ABA biosynthesis genes NCED9, ABAO, and MoCo.
After-ripening increased the effectiveness of cold stratification in stimulating GA4 accumulation in sly1-2 seeds (Figure 6). In sly1-2, the four day cold stratification treatment (0h vs dry timepoint), caused a small increase in GA4 levels in seeds without after-ripening at 0wkAR (+0.34 ng/g DW, p = 0.04). However, cold stratification (0h vs dry) of sly1-2 was associated with significant increases in GA4 levels in 2wkAR (+2.19 ng/g DW), 4wkAR (+2.13 ng/g DW), and Long AR (+3.77 ng/g DW) seeds. At the 0h imbibition timepoint, after-ripening was associated with significant increases in GA4 levels from 0 to 2wkAR (p = 8.6 x 10-4) and from 4wkAR to LongAR (p = 4.2 x 10-5). Long after-ripening was associated with increased GA4 levels in dry seeds as well. The increasing germination potential of sly1-2 GID1b-OE seed with 2wk dry after-ripening was also associated with a strong increase in GA4 levels in dry seeds (Supplementary Figure 10; Figure 2F). However, this was followed by a decrease in GA4 at 4wkAR. In sly1-2 GID1b-OE, GA4 levels increased with 12h imbibition compared to dry seeds with 2wk and 4wkAR. Examination of dry seed GA precursors did not clearly explain why GA4 levels increased with after-ripening of dry sly1-2 and sly1-2 GID1b-OE seeds (Supplementary Figure 11). There was, however, an increase in GA12 with long after-ripening of sly1-2 at 0h of imbibition (Supplementary Figure 12).
The transcripts of several GA biosynthesis genes showed increasing expression in sly1-2 relative to wild-type with imbibition time including CPS1, KS1, KO1, GA3ox1, GA3ox4, and GA20ox1, 2, and 3 (Figure 7). The only strongly upregulated GA biosynthetic transcript with sly1-2 after-ripening was GA3ox4 at 12h inhibition. GA3ox4 is upregulated, but less strongly, with GID1b-OE. This is consistent with the increase in GA4 levels with sly1-2 after-ripening at the 12h timepoint, but does not provide an explanation for increase in GA4 with sly1-2 after-ripening at the 0h timepoint (Supplementary Figure 8). At the 0h timepoint, GA4 levels increased rapidly from 0 to 2wkAR, but were similar from 2 to 4wkAR. By 21 months of after-ripening a significant increase in GA4 levels could be observed, suggesting that after-ripening may cause an initial strong increase followed by a gradual increase in GA4 levels. Investigation of GA precursors did not provide a clear indication of the mechanisms leading to increasing GA4 levels with after-ripening at 0h (Supplementary Figure 12).
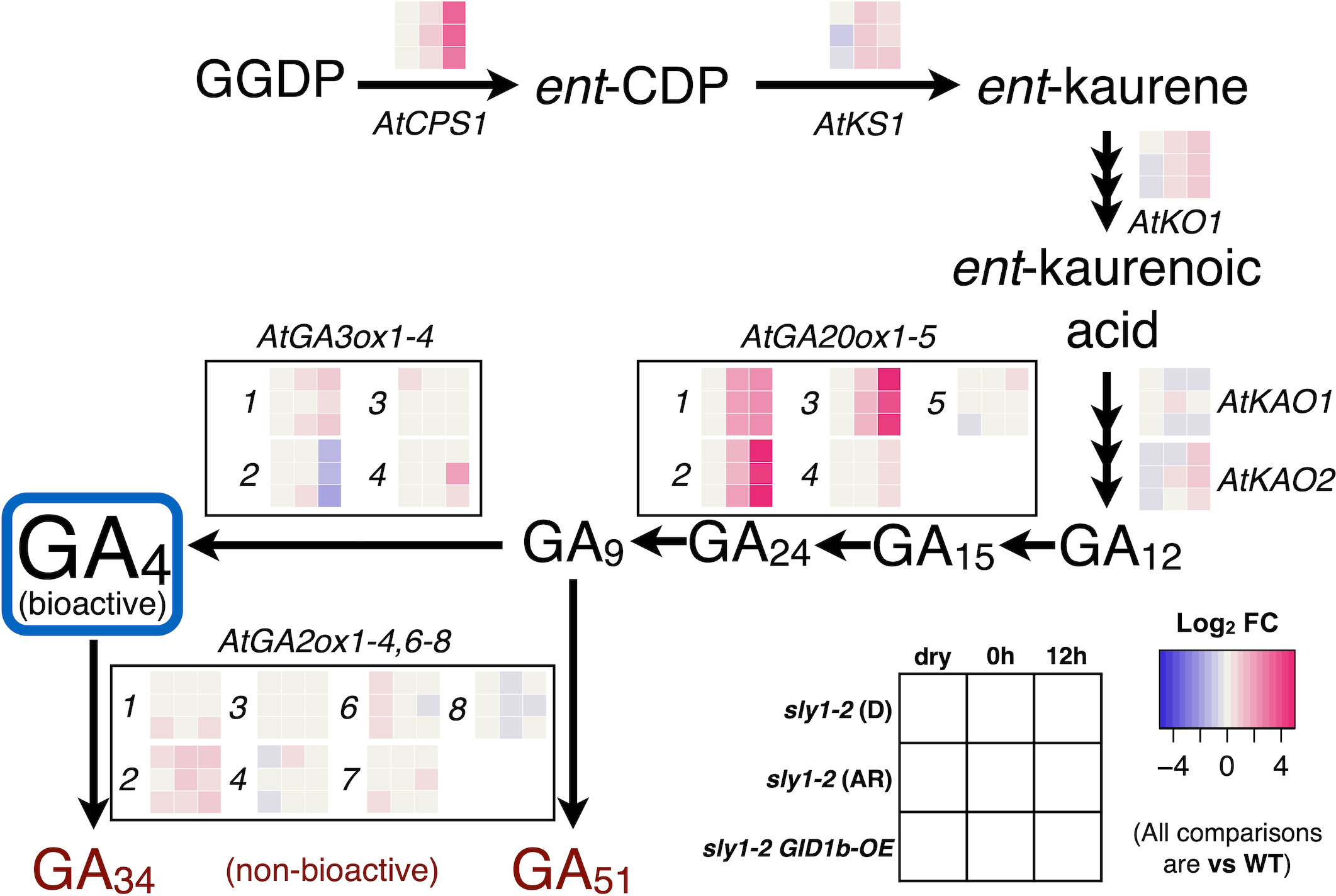
Figure 7 Transcriptional changes in the GA biosynthesis pathway. Transcript levels of GA biosynthesis and catabolic enzymes in dormant (D) 2wk AR sly1-2, 19 mo after-ripened (AR, or long AR) sly1-2, and in sly1-2 GID1b-OE are shown in heat maps relative to levels in Ler wt (WT) at 2wkAR at dry, 0h, and 12h imbibition timepoints. Magenta indicates a positive and blue indicates a negative fold change (FC) relative to WT. GA34 and GA51 are products of bioactive GA4 turnover. Data come from the reanalysis of microarray datasets from Nelson and Steber (2017) and Nelson et al. (2017). Previous reports did not compare dry and imbibed seed transcript levels.
Next we examined whether the increasing germination potential of sly1-2 GID1b-OE with after-ripening was associated with either decreasing ABA or increasing GA4 levels at the 12h imbibition timepoint (Figures 2E, 8). The non-significant downward trend in ABA levels from 0 to 2wkAR was followed by a large significant increase in ABA levels from 2 to 4wkAR (+19.15 ng/g DW, p = 9.6 x 10-5). Thus, the increase in sly1-2 GID1b-OE seed germination was not associated with a decrease in ABA levels. Increasing germination potential was associated with increasing GA4 levels with after-ripening of sly1-2 GID1b-OE late in Phase II (12h) of imbibition. There was a significant increase in GA4 levels from 0 to 2wkAR (4.41 ng/g DW, p = 9.1 x 10-3), followed by a larger increase from 2 to 4wkAR (9.51 ng/g DW, p = 1.5 x 10-5). The effect of after-ripening on GA precursors and catabolites was also examined in sly1-2 GID1b-OE at 12h of imbibition (Supplementary Figure 13). The precursor GA9 showed increasing accumulation with after-ripening, similar to GA4. The GA9 catabolite, GA51, also increased with 4wkAR. The precursors GA15 and GA24 levels were highest at 0wkAR, and decreased with after-ripening as GA9 and GA4 levels increased.
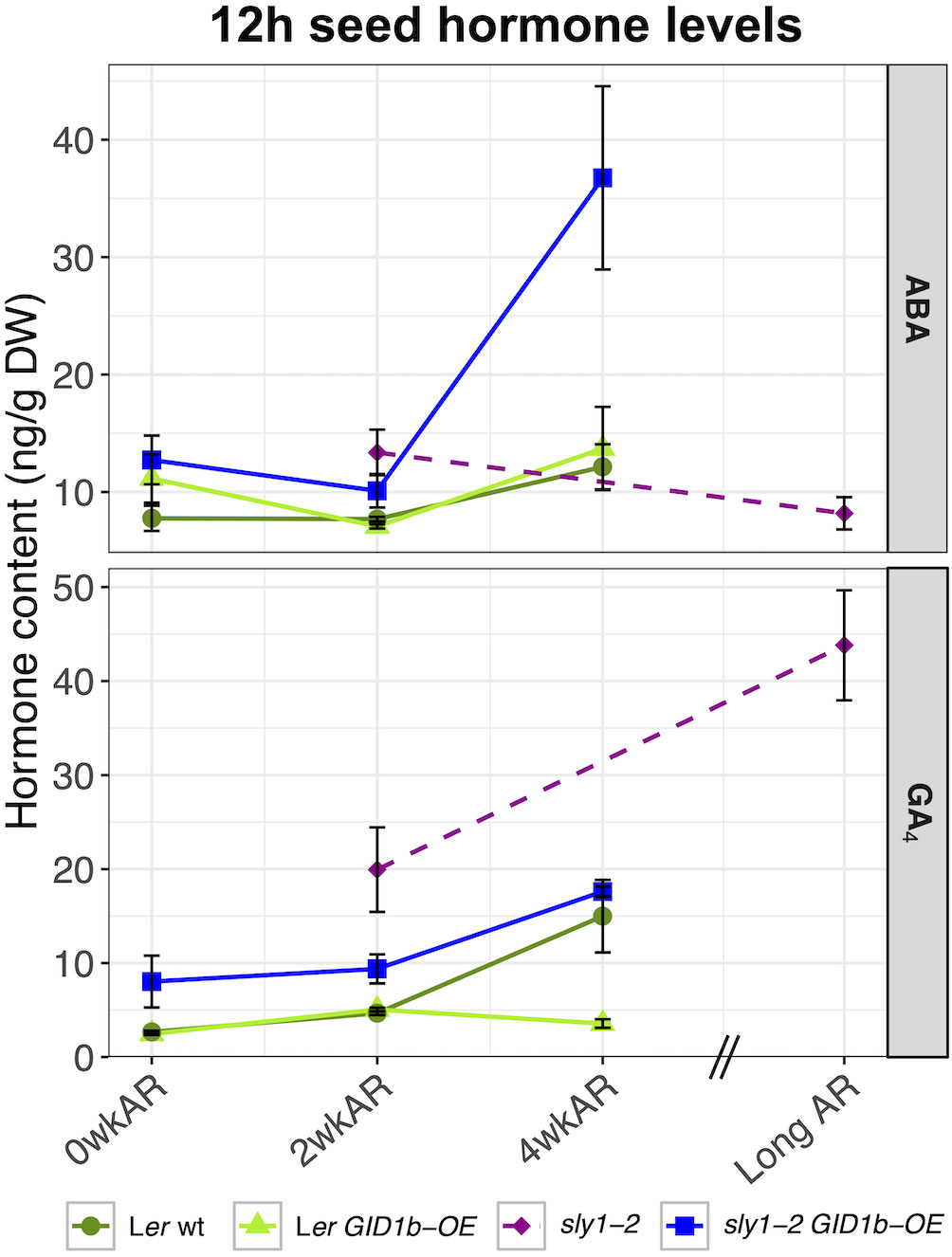
Figure 8 Comparison of ABA and GA changes with after-ripening at 12h of imbibition. Ler wt (dark green), Ler GID1b-OE (light green), and sly1-2 GID1b-OE (blue) were collected at 0wk, 2wk, and 4wk of after-ripening. The sly1-2 (purple) measurements at 2wk and 19 months after-ripening from Ariizumi et al. (2013) are plotted for side-by-side comparison with other genotypes. Error bars represent SE.
3.5 Associations between hormone levels and changes in transcript levels
The sly1-2 mutant background allows us to examine changes in hormone levels during after-ripening when DELLA protein levels are elevated (Ariizumi and Steber, 2007; Ariizumi et al., 2013). Reanalysis of previously published transcriptomics data enabled us to examine whether changes in hormone levels were associated with the expression of DELLA-regulated genes previously identified in Zentella et al. (2007). The GA 20-oxidase genes were the most prominently sly1-upregulated in all three comparisons, dormant sly1-2 vs Ler WT (DvsWT), long after-ripened sly1-2 vs Ler WT (ARvsWT), and sly1-2 GID1b-OE vs Ler WT (GIDvsWT), at both 0h and 12h timepoints, suggesting that GA 20-oxidase activity is responsible for the high GA levels in sly1-2 background lines (Supplementary Figure 14; Nelson and Steber, 2017). While all of the DELLA-induced regulatory transcripts showed increasing expression with imbibition time, few showed differences in expression in dormant versus after-ripened sly1-2 (Supplementary Figure 15). The exceptions included increased expression of the RING and XERICO transcripts and decreased expression of the Calmodulin-binding protein CaM-BP with sly1-2 after-ripening.
3.6 Comparisons of ABA and GA levels across all genotypes with dry seed after-ripening
To examine the effects of sly1-2 seed dormancy and of dormancy loss on dry seed ABA and GA levels, hormone levels were compared in all four genotypes over an after-ripening time course (Figure 9). If higher ABA levels in dry seeds leads to higher initial seed dormancy, then we would expect higher ABA levels in sly1-2 seeds. Interestingly, dry seed ABA levels in sly1-2 and sly1-2 GID1b-OE were lower than in Ler and Ler GID1b-OE at all measured after-ripening timepoints. The sly1-2 mutant may have either less ABA biosynthesis or more ABA turnover during seed development (Kanno et al., 2010). ABA did not decline significantly with after-ripening of dry seeds for any of the four genotypes, but did show a downward trend in Ler and Ler GID1b-OE from 0 to 4wkAR. Long after-ripening was associated with increasing ABA levels in dry sly1-2 seeds (4wkAR vs Long AR, +33.23 ng/g DW, p = 7.4 x 10-3). Thus in dry seeds, the higher seed dormancy of sly1-2 seeds at 0wkAR was associated with lower ABA levels than wild-type, whereas loss of sly1-2 seed dormancy was associated with increasing ABA levels. Thus, elevated sly1-2 dormancy levels do not appear to be the direct result of elevated dry seed ABA levels.
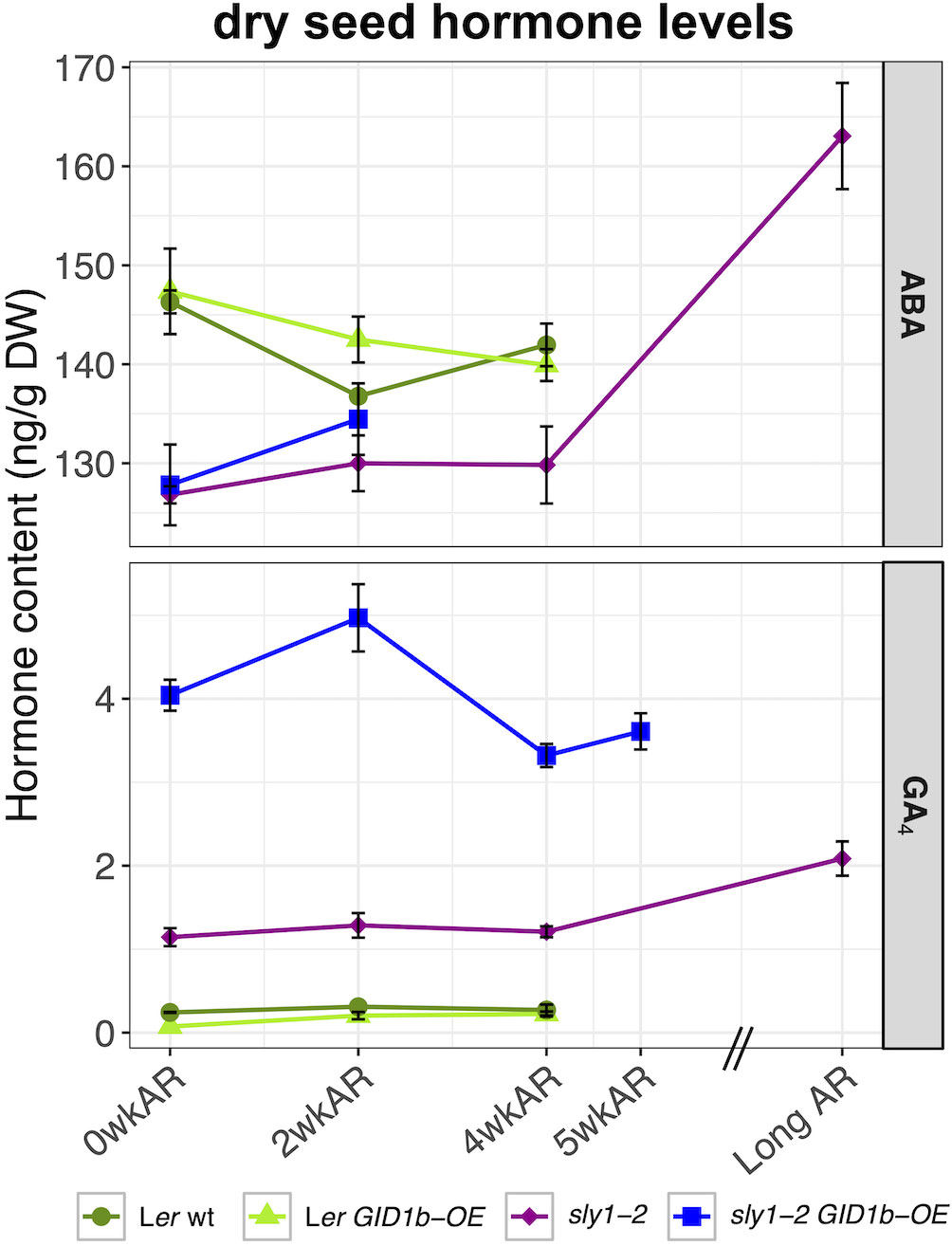
Figure 9 Comparison of dry seed hormone measurements in all genotypes. ABA and GA were measured in Ler wt (dark green), Ler GID1b-OE (light green), sly1-2 (purple), and sly1-2 GID1b-OE (blue) across after-ripening timepoints. Error bars represent SE.
While GA levels were previously known to increase with cold stratification and during seed germination, the effect of dry seed after-ripening was unknown (Yamauchi et al., 2004). Dry seed GA4 levels were considerably higher in sly1-2 GID1b-OE than in sly1-2 seeds, and higher in sly1-2 than in Ler and Ler GID1b-OE seeds at 0, 2, and 4wkAR (Figure 9). This is consistent with the notion that increased GA4 levels result as a feedback effect of a GA-insensitive phenotype. Analysis of sly1-2 and sly1-2 GID1b-OE GA precursors and catabolites did not provide a clear explanation for the highly elevated GA4 levels in sly1-2 GID1b-OE relative to sly1-2 and Ler wt (Supplementary Figure 11A). Precursor GA15 and catabolite GA51 levels were elevated in both sly1-2 and sly1-2 GID1b-OE dry seeds, however the GA15 peak was difficult to detect and measure, thus GA15 bars are often derived from a single measurement. Long after-ripening (21 mo) of dry sly1-2 seeds was associated with a significant increase in dry seed GA4 levels (+0.88 ng/g DW, p = 1.5 x 10-2), as well as with increased levels of the GA precursors GA12 and GA9, and of the GA catabolites GA51 and GA34 (Supplementary Figure 11B). This suggests that sly1-2 dry seed after-ripening can result in increased bioactive GA levels through an unknown mechanism. In dry sly1-2 GID1b-OE, GA4 levels showed a non-significant increase from 0 to 2wkAR, but then decreased significantly from 2 to 4wkAR (p = 0.02), and finally showed no significant increase between 4 and 5wkAR (Figure 9).
3.7 Comparisons of ABA and GA levels across all genotypes at 12h of imbibition
The effects of after-ripening on ABA and GA levels at 12 h of imbibition were compared in Ler wt, Ler GID1b-OE, sly1-2, and in sly1-2 GID1b-OE by including sly1-2 and sly 1-2 GID1b-OE data from Ariizumi et al. (2013) (Figure 8). ABA levels increased from 2wk to 4wk AR in Ler wt, Ler GID1b-OE, and in sly1-2 GID1b-OE. The ABA increase was strongest in the sly1-2 GID1b-OE. In contrast, ABA levels decreased in long AR versus 2 wkAR in sly1-2. We cannot rule out the possibility that longer AR would have a similar effect on the other genotypes. Rescue of sly1-2 germination by GID1b-OE at 2wkAR was associated with a small decrease in ABA compared to sly1-2, but no corresponding increase in GA4 at 12h. Thus at 12h imbibition, decreasing ABA levels may contribute to rescue of sly1-2 germination by after-ripening and GID1b overexpression. Examination of changes in GA4 levels with after-ripening of sly1-2 GID1b-OE seeds at 12h showed a significant increase from 2 to 4 weeks of after-ripening (+11.31 ng/g DW, p = 2.92 x 10-5) (Figure 8). When 12h changes in GA4 levels from 2 to 4 weeks of after-ripening for Ler, Ler GID1b-OE, and sly1-2 GID1b-OE were compared to the previously published changes in 12h GA levels of dormant and long after-ripened sly1-2, all lines showed increasing GA4 levels with after-ripening, with the exception of the previously discussed decrease in Ler GID1b-OE GA levels (Figure 8). This suggests that an increase in GA4 levels by late in Phase II of imbibition is a key part of after-ripening in Arabidopsis. For sly1-2, a decrease in ABA levels at 12 hr imbibition but not in dry seeds was associated with increasing germination potential with after-ripening and GID1b overexpression.
4 Discussion
The antagonism between ABA and GA in the regulation of seed germination is well known, but key questions regarding their relative roles in dormancy loss remain. Previous work found that ABA levels declined at or near the time of seed germination with after-ripening of Arabidopsis, barley, and wheat seeds, apparently due to increasing turnover due to the expression of the ABA catabolic enzyme ABA 8’ hydroxylase (Ali-Rachedi et al., 2004; Millar et al., 2006; Okamoto et al., 2006; Barrero et al., 2009; Liu et al., 2013). In this study we examined the effect of after-ripening on ABA and GA accumulation in dry seeds, and in early and late Phase II of imbibition. This study found that GA4 levels increase with after-ripening in dry sly1-2 seeds or early in imbibition of wild-type seeds, suggesting a role for GA in early dormancy release (Figures 3, 6, 8, 9). While decreasing ABA levels were previously associated with germination of after-ripened seeds, we found that elevated ABA levels during Phase II of imbibition in after-ripened seeds, suggesting a role of ABA in preventing premature germination (Figure 8). Finally, we learned that dormancy in sly1-2 is associated with low ABA and elevated GA levels in dry seeds, suggesting that initial sly1-2 dormancy may result from an ABA-independent mechanism (Figure 9).
The fact that after-ripening is correlated with a drop in ABA levels during seed imbibition led to the previous model in which a drop in ABA levels is the primary trigger of seed dormancy loss (Karssen and Laçka, 1986; Ali-Rachedi et al., 2004; Piskurewicz et al., 2008; Barrero et al., 2009). While it was clear that GA is required for Arabidopsis seed germination, the importance of GA in dormancy loss was unclear because bioactive GAs were virtually undetectable until seeds were on the verge of germination (Kanno et al., 2010). The caveat was that researchers could not judge whether bioactive GA levels are undetected because GA is absent or because levels were below the detection threshold. Detection might be challenging because GA synthesis can be restricted to a small number of cells in either shoot or root primordia (Hay et al., 2004). It is possible, for example, that a small increase in bioactive GA accumulation can have a strong effect in seeds poised to respond strongly due to the increased expression of SLY1 and the GID1 receptors with after-ripening (Lee et al., 2010; Hauvermale et al., 2015). In fact, GID1b mRNA and protein levels were shown to increase before ABA hormone levels decreased. Moreover, research in barley showed that after-ripening was associated with increased accumulation of GA precursors before imbibition, suggesting that after-ripening increases the potential to synthesize bioactive GA (Jacobsen et al., 2002). In a previous study, we showed that GA4 levels increased and ABA decreased in late Phase II of imbibition with after-ripening of the highly dormant sly1-2 mutant (Ariizumi et al., 2013). In the current study, we detected increased GA4 accumulation associated not with decreased, but with increased ABA content as a result of dry seed after-ripening at 12h imbibition in Ler WT and in sly1-2 GID1b-OE (Figure 3; Figure 8); and in dry seeds of sly1-2 with long AR (Figure 6; Figure 9). The only instance where a decrease in ABA levels was seen together with an increase in GA4 was at the 12h imbibition timepoint with long AR of sly1-2 (Figure 9; Ariizumi et al., 2013). Previously published work in ecotypes Cvi and C24, showed a decline in ABA levels in after-ripened seeds just as seeds were about to germinate at 24h imbibition (Ali-Rachedi et al., 2004; Carrera et al., 2007). Taken together these observations suggest a model where after-ripening increases germination potential through an increase in GA4 levels at 12h imbibition. This is associated with an increase in ABA levels needed to temporarily prevent germination as the seeds complete cellular repair in Phase II at 12h imbibition. ABA levels likely decrease at 24h inhibition enabling normal germination of after-ripened seeds. Future work will need to confirm this model using later imbibition timepoints and possibly longer after-ripening of wild-type seeds.
4.1 The relative roles of ABA and GA in controlling dormancy release
ABA is a potent inhibitor of seed germination that establishes seed dormancy during seed maturation and serves as a barrier to germination and embryo growth in mature seeds (Finkelstein et al., 2008; Lee et al., 2010). Based on this, we expected ABA levels to be negatively correlated with germination capacity at each after-ripening timepoint. In contrast, dry seed ABA levels increased as the germination capacity of sly1-2 seeds increased with long after-ripening for 21 months (Figure 6). While sly1-2 ABA levels decreased with after-ripening at 12h, ABA levels increased from 2 wk to 4 wk of after-ripening in Ler wt, Ler GID1-OE, and sly1-2 GID1b-OE (Figure 8). A similar increase in dry seed ABA content was observed with after-ripening of highly dormant Arabidopsis ecotype Cape Verde Islands (Cvi) at around 6hr of imbibition, followed by a decrease at 12h of imbibition (Ali-Rachedi et al., 2004). Thus, there are other genotypes where increased ABA levels were associated with increasing germination capacity prior to germination per se.
Previous studies did not observe a decrease in ABA content with after-ripening until seeds were closer to germination at 12-24 hr of imbibition in Arabidopsis ecotypes Cvi and C24 (Ali-Rachedi et al., 2004; Lee et al., 2010). Thus, it appears that ABA levels do not decrease in after-ripened seeds until the seeds are on the verge of germination. Many critical processes must be completed in Phase II of imbibition before a seed can safely germinate, including DNA repair, membrane repair, mitochondrial repair, the initiation of transcription and translation, and the initiation of stored reserve mobilization (reviewed in Bewley et al., 2013). It is possible that ABA serves as a final “checkpoint” to block germination in non-dormant seeds until essential Phase II processes are completed, enabling a successful seed-to-seedling transition. This proposed function is consistent with previous research showing that ABA can serve as a checkpoint blocking the growth, not only of seeds, but of early seedlings when they experience premature drying after germination (Lopez-Molina et al., 2001).
This raises the question of how the seed knows it is ready to complete Phase II and germinate. Future research will need to discover the mechanisms controlling the ABA checkpoint in non-dormant/after-ripened seeds. Previous transcriptional research has implicated the ABA turnover enzyme ABA 8’ hydroxylase (Ali-Rachedi et al., 2004; Millar et al., 2006; Okamoto et al., 2006; Carrera et al., 2007; Barrero et al., 2009; Liu et al., 2013). Research should examine the potential role of ABA 8’ hydroxylase or of ABA glucosyltransferases in lifting ABA repression of the final decision to germinate (Liu et al., 2013; Dong and Hwang, 2014). GA may also be involved in recognizing the safe point for germination. The germination of the ga1-3 GA biosynthesis mutant can be partly rescued by overexpressing the GID1 GA receptors (Hauvermale and Steber, 2020). The resulting seedlings die, however, because they are apparently unable to grow and complete development. It is possible GID1-OE-rescue of ga1-3 results in germination before the completion of Phase II repair processes, resulting in death. Future work will need to examine if a similar “ghost” seedling phenotype can be induced by blocking ABA synthesis during Phase II of wildtype germination.
This study provides evidence that GA4 may stimulate dormancy loss through after-ripening before ABA levels decline (Figure 3B). For sly1-2, there is evidence that this increase occurs as early as the dry seed stage and is clearly observable at the 0h timepoint (Figure 6; Figure 9). Furthermore, in Ler wt the increase in GA4 with after-ripening was observed at 12h of imbibition, when a corresponding decrease in ABA was not yet observed (Figure 3B). Thus, it appears that increasing GA4 levels may be an early signal triggering dormancy loss through dry after-ripening. ABA levels do not rise during Phase II of dormant seeds, likely because dormant seeds are unable to germinate either because GA4 levels are low or for more fundamental reasons, making the ABA checkpoint unnecessary.
The model we propose is that after-ripening results in increasing GA sensitivity as a result of increasing expression of GA signaling proteins such as SLY1 and GID1 (Lee et al., 2010; Hauvermale et al., 2015). This enables seeds to respond to small increases in GA4 levels with after-ripening and cold stratification thereby triggering germination-promoting processes. Once this happens, ABA levels increase during early to mid-Phase II to prevent germination until the completion of processes needed to safely undergo the seed to seedling transition, such as DNA repair, restoration of membrane integrity and activation of respiration, transcription and translation. Upon completion of Phade II, ABA levels decline and seeds germinate.
4.2 The effects of GID1b-overexpression in wild-type Ler and sly1-2
Overexpression of GID1b in the sly1-2 background led to an increase in seed germination, so it was expected that it would have a similar effect in Ler wt. Interestingly, when germination of Ler GID1b-OE was compared to that of Ler wt at three after-ripening timepoints, Ler GID1b-OE consistently germinated less efficiently than untransformed wild-type (Figures 2A–D; Supplementary Figure 6). This is consistent with previous observations that GID1b can act as a negative regulator of GA signaling. Mutations in GID1b can increase stem elongation or increase seed germination in the dark or in under dim lighting suggesting that GID1b negatively regulates GA signaling under some conditions (Griffiths et al., 2006; Ge and Steber, 2018; Hauvermale and Steber, 2020). Future work will need to investigate whether GID1b negatively regulates germination and plant growth via DELLA repressors or via the recently identified GID1-interacting protein PUX1 (Plant UBX domain containing protein 1) (Hauvermale et al., 2022).
It appears that repression of Ler seed germination by GID1b overexpression does not result from hormonal changes because ABA and GA hormone levels were very similar in Ler wt and Ler GID1b-OE (Figure 3). The only exception was that from 2 to 4 weeks of after-ripening in late Phase II the GA4 hormone levels of Ler wt increased greatly, while the levels of Ler GID1b-OE decreased. Examination of Ler GID1b-OE GA precursor levels in late Phase II were also dissimilar from Ler wt levels, suggesting that the difference in GA levels is regulated through the biosynthetic pathway (Supplementary Figures 7C, D). The GID1b GA receptor is highly sensitive to GA and can bind DELLAs to a certain degree even in the absence of GA (Nakajima et al., 2006; Yamamoto et al., 2010). Thus, one possible model for the decrease in GA4 levels with longer after-ripening of Ler wt is that GID1b-OE causes negative feedback regulation of GA biosynthesis. If GA sensitivity increases with after-ripening, and GID1b feedback regulation of GA biosynthesis is a GA signaling response, then once sensitivity reaches a high enough level, elevated GID1b levels lead to a decrease in GA production. In sly1-2, where DELLA-proteolysis dependent GA signaling cannot occur, the feedback down-regulation of GA may not be possible. The slightly reduced germination of Ler GID1b-OE could be due to a decrease in GA levels, although only the 4wkAR GA4 levels were lower for Ler GID1b-OE than Ler wt at the 12h imbibition timepoint. Further work will need to investigate changes in GA hormone levels at the 0h timepoint or other timepoints earlier in imbibition to determine if GID1b-OE inhibits GA accumulation at these timepoints.
4.3 High GA levels in sly1-2 background lines during early and late phase II of imbibition
The sly1-2 mutation leads to an overaccumulation of GA that is highest in long after-ripened seeds, but still apparent in 2-week-after-ripened dormant sly1-2 and sly1-2 GID1b-OE seeds at late Phase II of imbibition (Ariizumi et al., 2013). It was postulated that the heightened level of GA in sly1-2 background lines is due to inability to degrade DELLA through the SCFSLY1 ubiquitin proteasome pathway. In this model, DELLAs induce GA production as a mechanism of negative self-regulation, but since GA cannot stimulate DELLA-proteolysis in sly1-2, both DELLAs and GA accumulate in a feedback loop. The present work investigated bioactive GA4 levels in dry and 0h imbibed seeds to determine if sly1-2 lines had elevated GA levels throughout imbibition. Indeed, GA4 levels in all sly1-2 background lines analyzed in this study were higher than in wild-type in dry, 0h, and in 12h imbibed seeds regardless of after-ripening time (Figures 3, 6, 8, 9). We used GA metabolite levels and gene expression data for genes in the GA biosynthesis pathway to determine which steps of the GA biosynthesis pathway and which GA biosynthetic genes are likely responsible for the increased GA accumulation in sly1-2 background lines (Figure 7; Supplementary Figures 11-13; Nelson and Steber, 2017; Nelson et al., 2017). Transcripts that are likely important points of regulation during after-ripening of sly1-2 include KAO1 (ENT-KAURENOIC ACID OXIDASE1) and KAO2, and GA3ox4 (Figure 7; Supplementary Figure 8). Indeed, long after-ripening stimulated an increase in levels of GA12, the GA precursor directly downstream of KAO1 and KAO2, in 0h imbibed sly1-2 seeds (Supplementary Figure 12). However, not all changes in ABA and GA4 hormone levels observed corresponded to a transcriptional change suggesting that some of the regulation may be posttranscriptional.
If DELLA overaccumulation in sly1-2 leads to up-regulation of GA20ox DELLA targets in imbibed seeds, it is likely that other putative DELLA target genes may show a similar pattern of up-regulation in sly1-2 vs WT (Nelson et al., 2017). In addition to GA20ox2, 17 other putative DELLA targets were defined in Zentella et al. (2007), 10 of which showed a similar pattern of up-regulation to GA20ox2 in comparisons of sly1-2 to wild-type Ler at 0h and 12h of imbibition (Supplementary Figure 15). This up-regulation compared to WT was seen even when sly1-2 germination was rescued by GID1b-OE or by after-ripening, suggesting that this increase in expression is due to DELLA overaccumulation. This is consistent with the idea that DELLA overaccumulation in sly1-2 caused increased GA accumulation through positive transcriptional regulation of DELLA targets in the GA biosynthesis pathway.
Analysis of DELLA-regulated gene expression also helps to understand why ABA levels are high in sly1-2 mutants. The XERICO gene is a DELLA-upregulated positive regulator of ABA biosynthesis (Zentella et al., 2007; Piskurewicz et al., 2008). A previous study found that XERICO mRNA levels were slightly down-regulated with after-ripening of sly1-2, consistent with a decrease in ABA levels at 12h of imbibition (Ariizumi et al., 2013). Based on transcriptome data, XERICO mRNA levels were elevated in sly1-2 compared to WT at both 0h and 12h of imbibition, even when sly1-2 germination is rescued by after-ripening and GID1-OE (Supplementary Figure 15). This is consistent with the observation that ABA levels were higher in imbibing seeds of sly1-2 lines compared to wild-type Ler lines at 12h imbibition and 2wkAR(Figure 8). In contrast, sly1-2 ABA levels were lower than WT in dry seeds (Figure 9). However, not all changes in ABA hormone levels were associated with the expected changes in XERICO expression, again implying that other regulatory mechanisms exist.
4.4 The effect of dormancy on induction of GA4 during cold stratification
The germination promoting effects of cold stratification are associated with an increase in GA4 hormone levels (Derkx et al., 1994). While short periods of cold during imbibition promote germination in a number of Arabidopsis ecotypes, longer periods of cold treatment inhibit germination through the induction of secondary dormancy (Penfield and Springthorpe, 2012). In this study, cold stratification led to increased GA4 accumulation in Ler, Ler GID1b-OE, and sly1-2 seeds. Dormant Ler wt seeds at 0wkAR showed only a small increase in GA4 content with cold stratification (Figure 3A). A study using long after-ripened Ler seeds showed GA4 levels close to 4 ng/g DW after 4 d at 4°C, much higher than the GA induction that we observed in dormant seeds (Yamauchi et al., 2004). This suggests that dormancy loss through dry after-ripening potentiates the ability of cold stratification to induce GA production. Consistent with this idea, cold stratification at 0wkAR sly1-2 also showed the least increase in GA4 levels (dry to 0h imbibed seed +0.34 ng/g DW, p = 0.04), while long AR sly1-2 showed the greatest increase in GA4 (dry to 0h seeds +3.76 ng/g DW, p = 6.0 x 10-5) (Figure 6).
4.5 Potential mechanisms for dry after-ripening of seeds
Long after-ripening of sly1-2 was associated with increased ABA and increased GA4 levels in dry seeds (Figure 9). Because there is little metabolism in a dry seed, it is hard to conclude that increasing GA levels are due to an active biological mechanism. However, they may be functionally relevant upon imbibition. It is possible that similar changes occur in wild-type seeds, but remain undetected due to the fact the wild-type GA levels are much lower than those in sly1-2. This observation of altered hormone levels in dry seeds with after-ripening is not without precedent, however. Increased levels of GA precursors were detected with after-ripening of dry barley grain (Jacobsen et al., 2002), and increased ABA levels were detected with after-ripening of dry Cvi and C24 Arabidopsis ecotypes (Ali-Rachedi et al., 2004; Lee et al., 2010). ABA levels were consistently high in dry seeds, and decreased strongly with cold stratification and a further 12h imbibition of sly1-2 and wild-type Ler, both with and without GID1b-OE. This is consistent with previous work showing that ABA levels declined with cold stratification of Ler wt following a pulse of far-red light to inhibit germination (Yamauchi et al., 2004).
How can a dry seed acquire the ability to germinate at moisture levels (5-10%) too low from most biological processes? A promising theory is that dry after-ripening leads to the gradual destruction of negative regulators of germination (Bazin et al., 2011; Nelson and Steber, 2017; Bailly, 2019). Gradual damage to negative regulators of germination by oxidation from exposure to air is one way to create a clock allowing time to pass before a dry seed acquires the ability to germinate. Given that the decision to germinate is governed by the balance between ABA and GA hormone signaling, these hormone signaling pathways seem likely targets of such regulation. Bazin et al. (2011) identified fourteen mRNAs that were impacted by oxidation during dry after-ripening. While some of these genes may have been involved in hormone signaling, none encoded hormone biosynthesis or turnover enzymes. However, regulation may not necessarily be at the level of mRNA stability. Potential targets include the hormones themselves, the enzymes involved in hormone biosynthesis or turnover, their transcripts, transcription factors regulating these enzymes, or elements of the signaling pathways.
Key hormone biosynthesis and catabolic enzymes showing regulation by after-ripening are good candidates for such regulation by destruction during after-ripening. Examples of good candidates include genes showing upregulation with sly1-2 after-ripening including the GA biosynthesis gene GA3ox4 and the ABA turnover gene CYP707A3 encoding ABA 8’-hydroxylase (Figure 7; Supplementary Figure 9). Future work could examine if transcription factors regulating these genes are regulated by oxidation or after-ripening. Reactive oxygen species have been linked to ABA and GA signaling in seeds. For example, ROS have been reported to stimulate GA biosynthesis through transcriptional effects, and H2O2 in germinating seeds was associated with ABA degradation, possibly through increased ABA catabolism by ABA8’ hydroxylase (Ishibashi et al., 2015; Li et al., 2018; Bailly, 2019; Jurdak et al., 2022).
Data availability statement
The original contributions presented in the study are included in the article/Supplementary Material. Further inquiries can be directed to the corresponding author.
Author contributions
CS provided the initial research design and obtained funding. SN, MS, YK, and CS designed the experiments and the approach to analysis After-ripening and germination experiments were performed by SN. Hormone measurements were performed in the lab of MS by SN and YK. SN performed hormone extractions and YK performed LCMS runs. SN performed remaining experiments and analyses. CS and SN wrote and edited the article together with YK and MS. All authors contributed to the article and approved the submitted version.
Funding
This research was funded by National Science Foundation (NSF) Award 0850981 and the USDA-ARS (to CS) and by the JSPS/NSF-EAPSI program (to SN).
Acknowledgments
We would like to thank all members of the M. Seo lab at RIKEN Plant Science Center, Yokohama, Japan, for training and assistance with hormone measurements. We thank the members of the Steber lab, A. Hauvermale, S. Martinez, K. Tuttle, and T. Harris, for helpful suggestions about the research and manuscript. Thanks are also due to M. Neff, H. Hellmann, A. McCubbin, and P. Okubara for helpful comments on the manuscript.
Conflict of interest
Author SN was employed by Heliponix, LLC.
The remaining authors declare that the research was conducted in the absence of any commercial or financial relationships that could be construed as a potential conflict of interest.
Publisher’s note
All claims expressed in this article are solely those of the authors and do not necessarily represent those of their affiliated organizations, or those of the publisher, the editors and the reviewers. Any product that may be evaluated in this article, or claim that may be made by its manufacturer, is not guaranteed or endorsed by the publisher.
Supplementary material
The Supplementary Material for this article can be found online at: https://www.frontiersin.org/articles/10.3389/fpls.2023.1145414/full#supplementary-material
References
Ali-Rachedi, S., Bouinot, D., Wagner, M.-H., Bonnet, M., Sotta, B., Grappin, P., et al. (2004). Changes in endogenous abscisic acid levels during dormancy release and maintenance of mature seeds: studies with the cape Verde islands ecotype, the dormant model of Arabidopsis thaliana. Planta 219, 479–488. doi: 10.1007/s00425-004-1251-4
Ariizumi, T., Hauvermale, A. L., Nelson, S. K., Hanada, A., Yamaguchi, S., Steber, C. M. (2013). Lifting della repression of arabidopsis seed germination by nonproteolytic gibberellin signaling. Plant Physiol. 162, 2125–2139. doi: 10.1104/pp.113.219451
Ariizumi, T., Murase, K., Sun, T., Steber, C. M. (2008). Proteolysis-independent downregulation of DELLA repression in arabidopsis by the gibberellin receptor GIBBERELLIN INSENSITIVE DWARF1. Plant Cell 20, 2447–2459. doi: 10.1105/tpc.108.058487
Ariizumi, T., Steber, C. M. (2007). Seed germination of GA-insensitive sleepy1 mutants does not require RGL2 protein disappearance in arabidopsis. Plant Cell 19, 791–804. doi: 10.1105/tpc.106.048009
Bai, M.-Y., Shang, J.-X., Oh, E., Fan, M., Bai, Y., Zentella, R., et al. (2012). Brassinosteroid, gibberellin and phytochrome impinge on a common transcription module in arabidopsis. Nat. Cell Biol. 14, 810–817. doi: 10.1038/ncb2546
Bailly, C. (2019). The signalling role of ROS in the regulation of seed germination and dormancy. Biochem. J. 476, 3019–3032. doi: 10.1042/BCJ20190159
Barrero, J. M., Talbot, M. J., White, R. G., Jacobsen, J. V., Gubler, F. (2009). Anatomical and transcriptomic studies of the coleorhiza reveal the importance of this tissue in regulating dormancy in barley. Plant Physiol. 150, 1006–1021. doi: 10.1104/pp.109.137901
Baskin, J. M., Baskin, C. C. (1972). Ecological life cycle and physiological ecology of seed germination of Arabidopsis thaliana. Can. J. Bot 50 (2), 353–360. doi: 10.1139/b72-049
Bazin, J., Langlade, N., Vincourt, P., Arribat, S., Balzergue, S., El-Maarouf-Bouteau, H., et al. (2011). Targeted mRNA oxidation regulates sunflower seed dormancy alleviation during dry after-ripening. Plant Cell 23, 2196–2208. doi: 10.1105/tpc.111.086694
Benjamini, Y., Hochberg, Y. (1995). Controlling the false discovery rate: a practical and powerful approach to multiple testing. J. R. Stat. Soc Ser. B Methodol. 57, 289–300. doi: 10.1111/j.2517-6161.1995.tb02031.x
Bewley, J. D., Bradford, K. J., Hilhorst, H. W. M., Nonogaki, H. (2013). Seeds : physiology of development, germination and dormancy (New York, NY: Springer). Available at: http://site.ebrary.com/id/10656390.
Cadman, C. S., Toorop, P. E., Hilhorst, H. W., Finch-Savage, W. E. (2006). Gene expression profiles of arabidopsis cvi seeds during dormancy cycling indicate a common underlying dormancy control mechanism. Plant J. 46, 805–822. doi: 10.1111/j.1365-313X.2006.02738.x
Carrera, E., Holman, T., Medhurst, A., Peer, W., Schmuths, H., Footitt, S., et al. (2007). Gene expression profiling reveals defined functions of the ATP-binding cassette transporter COMATOSE late in phase II of germination. Plant Physiol. 143, 1669–1679. doi: 10.1104/pp.107.096057
Derkx, M. P. M., Vermeer, E., Karssen, C. M. (1994). Gibberellins in seeds of Arabidopsis thaliana: biological activities, identification and effects of light and chilling on endogenous levels. Plant Growth Regul. 15, 223–234. doi: 10.1007/BF00029895
Dill, A., Thomas, S. G., Hu, J., Steber, C. M., Sun, T. (2004). The arabidopsis f-box protein SLEEPY1 targets gibberellin signaling repressors for gibberellin-induced degradation. Plant Cell 16, 1392–1405. doi: 10.1105/tpc.020958
Dong, T., Hwang, I. (2014). Contribution of ABA UDP-glucosyltransferases in coordination of ABA biosynthesis and catabolism for ABA homeostasis. Plant Signal. Behav. 9, e28888. doi: 10.4161/psb.28888
Finch-Savage, W. E., Leubner-Metzger, G. (2006). Seed dormancy and the control of germination. New Phytol. 171, 501–523. doi: 10.1111/j.1469-8137.2006.01787.x
Finkelstein, R. R., Gampala, S. S. L., Rock, C. D. (2002). Abscisic acid signaling in seeds and seedlings. Plant Cell 14 Suppl, S15–S45. doi: 10.1105/tpc.010441
Finkelstein, R., Reeves, W., Ariizumi, T., Steber, C. M. (2008). Molecular aspects of seed dormancy. Annu. Rev. Plant Biol. 59, 387–415. doi: 10.1146/annurev.arplant.59.032607.092740
Ge, W., Steber, C. M. (2018). Positive and negative regulation of seed germination by the arabidopsis GA hormone receptors, GID1a , b , and c. Plant Direct 2, e00083. doi: 10.1002/pld3.83
Griffiths, J., Murase, K., Rieu, I., Zentella, R., Zhang, Z.-L., Powers, S. J., et al. (2006). Genetic characterization and functional analysis of the GID1 gibberellin receptors in arabidopsis. Plant Cell 18, 3399–3414. doi: 10.1105/tpc.106.047415
Hauvermale, A. L., Ariizumi, T., Steber, C. M. (2012). Gibberellin signaling: a theme and variations on DELLA repression. Plant Physiol. 160, 83–92. doi: 10.1104/pp.112.200956
Hauvermale, A. L., Cárdenas, J. J., Bednarek, S. Y., Steber, C. M. (2022). GA signaling expands: the plant UBX domain-containing protein 1 is a binding partner for the GA receptor. Plant Physiol. 190, 2651–2670. doi: 10.1093/plphys/kiac406
Hauvermale, A. L., Steber, C. M. (2020). GA signaling is essential for the embryo-to-seedling transition during arabidopsis seed germination, a ghost story. Plant Signal. Behav. 15, 1705028. doi: 10.1080/15592324.2019.1705028
Hauvermale, A. L., Tuttle, K. M., Takebayashi, Y., Seo, M., Steber, C. M. (2015). Loss of Arabidopsis thaliana seed dormancy is associated with increased accumulation of the GID1 GA hormone receptors. Plant Cell Physiol. 56, 1773–1785. doi: 10.1093/pcp/pcv084
Hay, A., Barkoulas, M., Tsiantis, M. (2004). PINning down the connections: transcription factors and hormones in leaf morphogenesis. Curr. Opin. Plant Biol. 7, 575–581. doi: 10.1016/j.pbi.2004.07.007
Hedden, P. (2016). “Gibberellin biosynthesis in higher plants,” in Annual plant reviews, vol. 49. (Chichester, UK: John Wiley & Sons, Ltd), 37–72. doi: 10.1002/9781119210436.ch2
Hedden, P. (2020). The current status of research on gibberellin biosynthesis. Plant Cell Physiol 61 (11), 1832–1849. doi: 10.1093/pcp/pcaa092
Hirano, K., Asano, K., Tsuji, H., Kawamura, M., Mori, H., Kitano, H., et al. (2010). Characterization of the molecular mechanism underlying gibberellin perception complex formation in rice. Plant Cell 22, 2680–2696. doi: 10.1105/tpc.110.075549
Hirano, K., Kouketu, E., Katoh, H., Aya, K., Ueguchi-Tanaka, M., Matsuoka, M. (2012). The suppressive function of the rice DELLA protein SLR1 is dependent on its transcriptional activation activity. Plant J. 71, 443–453. doi: 10.1111/j.1365-313X.2012.05000.x
Irizarry, R. A., Hobbs, B., Collin, F., Beazer-Barclay, Y. D., Antonellis, K. J., Scherf, U., et al. (2003). Exploration, normalization, and summaries of high density oligonucleotide array probe level data. Biostat. Oxf. Engl. 4, 249–264. doi: 10.1093/biostatistics/4.2.249
Ishibashi, Y., Kasa, S., Sakamoto, M., Aoki, N., Kai, K., Yuasa, T., et al. (2015). A role for reactive oxygen species produced by NADPH oxidases in the embryo and aleurone cells in barley seed germination. PLoS One 10, e0143173. doi: 10.1371/journal.pone.0143173
Itoh, H., Ueguchi-Tanaka, M., Sato, Y., Ashikari, M., Matsuoka, M. (2002). The gibberellin signaling pathway is regulated by the appearance and disappearance of SLENDER RICE1 in nuclei. Plant Cell 14, 57–70. doi: 10.1105/tpc.010319
Jacobsen, S. E., Olszewski, N. E. (1993). Mutations at the SPINDLY locus of arabidopsis alter gibberellin signal transduction. Plant Cell 5, 887–896. doi: 10.1105/tpc.5.8.887
Jacobsen, J. V., Pearce, D. W., Poole, A. T., Pharis, R. P., Mander, L. N. (2002). Abscisic acid, phaseic acid and gibberellin contents associated with dormancy and germination in barley. Physiol. Plant 115, 428–441. doi: 10.1034/j.1399-3054.2002.1150313.x
Jurdak, R., Rodrigues, G., de, A. G., Chaumont, N., Schivre, G., Bourbousse, C., et al. (2022). Intracellular reactive oxygen species trafficking participates in seed dormancy alleviation in arabidopsis seeds. New Phytol. 234, 850–866. doi: 10.1111/nph.18038
Kanno, Y., Jikumaru, Y., Hanada, A., Nambara, E., Abrams, S. R., Kamiya, Y., et al. (2010). Comprehensive hormone profiling in developing arabidopsis seeds: examination of the site of ABA biosynthesis, ABA transport and hormone interactions. Plant Cell Physiol. 51, 1988–2001. doi: 10.1093/pcp/pcq158
Karssen, C. M., Brinkhorst-Van Der Swan, D. L. C., Breekland, A. E., Koornneef, M. (1983). Induction of dormancy during seed development by endogenous abscisic acid: studies on abscisic acid deficient genotypes of Arabidopsis thaliana (L.) heynh. Planta 157, 158–165. doi: 10.1007/BF00393650
Karssen, C. M., Laçka, E. (1986). “A revision of the hormone balance theory of seed dormancy: studies on gibberellin and/or abscisic acid-deficient mutants of Arabidopsis thaliana,” in Plant growth substances 1985 (Berlin, Heidelberg: Springer Berlin Heidelberg), 315–323. doi: 10.1007/978-3-642-71018-6_41
Koornneef, M., Alonso-Blanco, C., Bentsink, L., Blankestijn-de Vries, H., Debeaujon, I., Hanhart, C. J., et al. (2000). The genetics of seed dormancy in Arabidopsis thaliana. Dormancy in plants: from whole plant behaviour to cellular control (Wallingford UK: CABI Publishing), 365–373. Available at: https://www.cabidigitallibrary.org/doi/10.1079/9780851994475.0365.
Koornneef, M., van der Veen, J. H. (1980). Induction and analysis of gibberellin sensitive mutants in Arabidopsis thaliana (L.) heynh. Theor. Appl. Genet. 58, 257–263. doi: 10.1007/BF00265176
Kushiro, T., Okamoto, M., Nakabayashi, K., Yamagishi, K., Kitamura, S., Asami, T., et al. (2004). The arabidopsis cytochrome P450 CYP707A encodes ABA 8’-hydroxylases: key enzymes in ABA catabolism. EMBO J. 23, 1647–1656. doi: 10.1038/sj.emboj.7600121
Lee, K. P., Piskurewicz, U., Turecková, V., Strnad, M., Lopez-Molina, L. (2010). A seed coat bedding assay shows that RGL2-dependent release of abscisic acid by the endosperm controls embryo growth in arabidopsis dormant seeds. Proceedings of the National Academy of Sciences 107, 19108–19113. doi: 10.1073/pnas.1012896107
Lefebvre, V., North, H., Frey, A., Sotta, B., Seo, M., Okamoto, M., et al. (2006). Functional analysis of arabidopsis NCED6 and NCED9 genes indicates that ABA synthesized in the endosperm is involved in the induction of seed dormancy. Plant J. 45, 309–319. doi: 10.1111/j.1365-313X.2005.02622.x
Leubner-Metzger, G. (2005). Beta-1,3-Glucanase gene expression in low-hydrated seeds as a mechanism for dormancy release during tobacco after-ripening. Plant J. 41, 133–145. doi: 10.1111/j.1365-313X.2004.02284.x
Li, Z., Gao, Y., Zhang, Y., Lin, C., Gong, D., Guan, Y., et al. (2018). Reactive oxygen species and gibberellin acid mutual induction to regulate tobacco seed germination. Front. Plant Sci. 9. doi: 10.3389/fpls.2018.01279
Liu, A., Gao, F., Kanno, Y., Jordan, M. C., Kamiya, Y., Seo, M., et al. (2013). Regulation of wheat seed dormancy by after-ripening is mediated by specific transcriptional switches that induce changes in seed hormone metabolism and signaling. PLoS One 8, e56570. doi: 10.1371/journal.pone.0056570
Lopez-Molina, L., Mongrand, S., Chua, N. H. (2001). A postgermination developmental arrest checkpoint is mediated by abscisic acid and requires the ABI5 transcription factor in arabidopsis. Proc. Natl. Acad. Sci. U. S. A. 98, 4782–4787. doi: 10.1073/pnas.081594298
McGinnis, K. M., Thomas, S. G., Soule, J. D., Strader, L. C., Zale, J. M., Sun, T., et al. (2003). The arabidopsis SLEEPY1 gene encodes a putative f-box subunit of an SCF E3 ubiquitin ligase. Plant Cell 15, 1120–1130. doi: 10.1105/tpc.010827
Millar, A. A., Jacobsen, J. V., Ross, J. J., Helliwell, C. A., Poole, A. T., Scofield, G., et al. (2006). Seed dormancy and ABA metabolism in arabidopsis and barley: the role of ABA 8’-hydroxylase. Plant J. 45, 942–954. doi: 10.1111/j.1365-313X.2006.02659.x
Murase, K., Hirano, Y., Sun, T., Hakoshima, T. (2008). Gibberellin-induced DELLA recognition by the gibberellin receptor GID1. Nature 456, 459–463. doi: 10.1038/nature07519
Nakajima, M., Shimada, A., Takashi, Y., Kim, Y.-C., Park, S.-H., Ueguchi-Tanaka, M., et al. (2006). Identification and characterization of arabidopsis gibberellin receptors. Plant J. 46, 880–889. doi: 10.1111/j.1365-313X.2006.02748.x
Nelson, S. K., Ariizumi, T., Steber, C. M. (2017). Biology in the dry seed: transcriptome changes associated with dry seed dormancy and dormancy loss in the arabidopsis GA-insensitive sleepy1-2 mutant. Front. Plant Sci. 8. doi: 10.3389/fpls.2017.02158
Nelson, S. K., Steber, C. M. (2016). “Gibberellin hormone signal perception: down-regulating DELLA repressors of plant growth and development,” in Annual plant reviews, vol. 49. (Chichester, UK: John Wiley & Sons, Ltd), 153–188. doi: 10.1002/9781119210436.ch6
Nelson, S. K., Steber, C. M. (2017). Transcriptional mechanisms associated with seed dormancy and dormancy loss in the gibberellin-insensitive sly1-2 mutant of Arabidopsis thaliana. PLoS One 12, e0179143. doi: 10.1371/journal.pone.0179143
Ogawa, M., Hanada, A., Yamauchi, Y., Kuwahara, A., Kamiya, Y., Yamaguchi, S. (2003). Gibberellin biosynthesis and response during arabidopsis seed germination. Plant Cell 15, 1591–1604. doi: 10.1105/tpc.011650
Okamoto, M., Kuwahara, A., Seo, M., Kushiro, T., Asami, T., Hirai, N., et al. (2006). CYP707A1 and CYP707A2, which encode abscisic acid 8’-hydroxylases, are indispensable for proper control of seed dormancy and germination in arabidopsis. Plant Physiol. 141, 97–107. doi: 10.1104/pp.106.079475
Olszewski, N., Sun, T., Gubler, F. (2002). Gibberellin signaling: biosynthesis, catabolism, and response pathways. Plant Cell 14 Suppl, S61–S80. doi: 10.1105/tpc.010476
Penfield, S., Springthorpe, V. (2012). Understanding chilling responses in arabidopsis seeds and their contribution to life history. Philos. Trans. B 367, 291–297. doi: 10.1098/rstb.2011.0186
Piskurewicz, U., Jikumaru, Y., Kinoshita, N., Nambara, E., Kamiya, Y., Lopez-Molina, L. (2008). The gibberellic acid signaling repressor RGL2 inhibits arabidopsis seed germination by stimulating abscisic acid synthesis and ABI5 activity. Plant Cell 20, 2729–2745. doi: 10.1105/tpc.108.061515
Plackett, A. R. G., Powers, S. J., Fernandez-Garcia, N., Urbanova, T., Takebayashi, Y., Seo, M., et al. (2012). Analysis of the developmental roles of the Arabidopsis gibberellin 20-oxidases demonstrates that GA20ox1 , -2 , and -3 are the dominant paralogs. Plant Cell 24, 941–960. doi: 10.1105/tpc.111.095109
Poisot, T., Bever, J. D., Nemri, A., Thrall, P. H., Hochberg, M. E. (2011). A conceptual framework for the evolution of ecological specialisation. Ecol. Lett. 14, 841–851. doi: 10.1111/j.1461-0248.2011.01645.x
R Core Team (2000). R: A language and environment for statistical computing. R Foundation for Statistical Computing(Vienna, Austria). https://www.R-project.org/
Russell, L., Larner, V., Kurup, S., Bougourd, S., Holdsworth, M. (2000). The arabidopsis COMATOSE locus regulates germination potential. Development 127, 3759–3767.
Schramm, E. C., Nelson, S. K., Kidwell, K. K., Steber, C. M. (2013). Increased ABA sensitivity results in higher seed dormancy in soft white spring wheat cultivar “Zak”. Theor. Appl. Genet. 126, 791–803. doi: 10.1007/s00122-012-2018-0
Schramm, E., Schramm, E. C., Abellera, J. C., Abellera, J., Strader, L., Strader, L. C., et al. (2010). Isolation of ABA-responsive mutants in allohexaploid bread wheat (Triticum aestivum l.): drawing connections to grain dormancy, preharvest sprouting, and drought tolerance. Plant Sci. 179, 620–629. doi: 10.1016/j.plantsci.2010.06.004
Shimada, A., Ueguchi-Tanaka, M., Nakatsu, T., Nakajima, M., Naoe, Y., Ohmiya, H., et al. (2008). Structural basis for gibberellin recognition by its receptor GID1. Nature 456, 520–523. doi: 10.1038/nature07546
Silverstone, A. L., Ciampaglio, C. N., Sun, T. (1998). The arabidopsis RGA gene encodes a transcriptional regulator repressing the gibberellin signal transduction pathway. Plant Cell 10, 155–169. doi: 10.1105/tpc.10.2.155
Steber, C. M., Cooney, S. E., McCourt, P. (1998). Isolation of the GA-response mutant sly1 as a suppressor of ABI1-1 in Arabidopsis thaliana. Genetics 149, 509–521. doi: 10.1093/genetics/149.2.509
Sun, T., Gubler, F. (2004). Molecular mechanism of gibberellin signaling in plants. Annu. Rev. Plant Biol. 55, 197–223. doi: 10.1146/annurev.arplant.55.031903.141753
Ueguchi-Tanaka, M., Hirano, K., Hasegawa, Y., Kitano, H., Matsuoka, M. (2008). Release of the repressive activity of rice DELLA protein SLR1 by gibberellin does not require SLR1 degradation in the gid2 mutant. Plant Cell 20, 2437–2446. doi: 10.1105/tpc.108.061648
Ueguchi-Tanaka, M., Nakajima, M., Katoh, E., Ohmiya, H., Asano, K., Saji, S., et al. (2007a). Molecular interactions of a soluble gibberellin receptor, GID1, with a rice DELLA protein, SLR1, and gibberellin. Plant Cell 19, 2140–2155. doi: 10.1105/tpc.106.043729
Ueguchi-Tanaka, M., Nakajima, M., Motoyuki, A., Matsuoka, M. (2007b). Gibberellin receptor and its role in gibberellin signaling in plants. Annu. Rev. Plant Biol. 58, 183–198. doi: 10.1146/annurev.arplant.58.032806.103830
Varbanova, M., Yamaguchi, S., Yang, Y., McKelvey, K., Hanada, A., Borochov, R., et al. (2007). Methylation of gibberellins by arabidopsis GAMT1 and GAMT2. Plant Cell 19, 32–45. doi: 10.1105/tpc.106.044602
Venable, D. L. (2007). Bet hedging in a guild of desert annuals. Ecology 88, 1086–1090. doi: 10.1890/06-1495
Willige, B. C., Ghosh, S., Nill, C., Zourelidou, M., Dohmann, E. M. N., Maier, A., et al. (2007). The DELLA domain of GA INSENSITIVE mediates the interaction with the GA INSENSITIVE DWARF1A gibberellin receptor of arabidopsis. Plant Cell 19, 1209–1220. doi: 10.1105/tpc.107.051441
Yamaguchi, S. (2008). Gibberellin metabolism and its regulation. Annu. Rev. Plant Biol. 59, 225–251. doi: 10.1146/annurev.arplant.59.032607.092804
Yamamoto, Y., Hirai, T., Yamamoto, E., Kawamura, M., Sato, T., Kitano, H., et al. (2010). A rice gid1 suppressor mutant reveals that gibberellin is not always required for interaction between its receptor, GID1, and DELLA proteins. Plant Cell 22, 3589–3602. doi: 10.1105/tpc.110.074542
Yamauchi, Y., Ogawa, M., Kuwahara, A., Hanada, A., Kamiya, Y., Yamaguchi, S. (2004). Activation of gibberellin biosynthesis and response pathways by low temperature during imbibition of Arabidopsis thaliana seeds. Plant Cell 16, 367–378. doi: 10.1105/tpc.018143
Yoshida, H., Hirano, K., Sato, T., Mitsuda, N., Nomoto, M., Maeo, K., et al. (2014). DELLA protein functions as a transcriptional activator through the DNA binding of the INDETERMINATE DOMAIN family proteins. Proc. Natl. Acad. Sci. 111, 7861–7866. doi: 10.1073/pnas.1321669111
Zeevaart, J. A., Talon, M. (1992). “Gibberellin mutants in Arabidopsis thaliana,” in Progress in plant growth regulation (Springer), 34–42.
Zentella, R., Zhang, Z.-L., Park, M., Thomas, S. G., Endo, A., Murase, K., et al. (2007). Global analysis of DELLA direct targets in early gibberellin signaling in arabidopsis. Plant Cell 19, 3037–3057. doi: 10.1105/tpc.107.054999
Zhiponova, M. K., Morohashi, K., Vanhoutte, I., Machemer-Noonan, K., Revalska, M., Van Montagu, M., et al. (2014). Helix-loop-helix/basic helix-loop-helix transcription factor network represses cell elongation in arabidopsis through an apparent incoherent feed-forward loop. Proceedings of the National Academy of Sciences 111, 2824–2829. doi: 10.1073/pnas.1400203111
Keywords: SLY1, Arabidopsis, dormancy, dry after-ripening, germination, seeds, gibberellin, abscisic acid
Citation: Nelson SK, Kanno Y, Seo M and Steber CM (2023) Seed dormancy loss from dry after-ripening is associated with increasing gibberellin hormone levels in Arabidopsis thaliana. Front. Plant Sci. 14:1145414. doi: 10.3389/fpls.2023.1145414
Received: 16 January 2023; Accepted: 20 April 2023;
Published: 18 May 2023.
Edited by:
Shingo Nakamura, Institute of Crop Science (NARO), JapanReviewed by:
Xingyou Gu, South Dakota State University, United StatesShigeko Utsugi, Okayama University, Japan
Copyright © 2023 Nelson, Kanno, Seo and Steber. This is an open-access article distributed under the terms of the Creative Commons Attribution License (CC BY). The use, distribution or reproduction in other forums is permitted, provided the original author(s) and the copyright owner(s) are credited and that the original publication in this journal is cited, in accordance with accepted academic practice. No use, distribution or reproduction is permitted which does not comply with these terms.
*Correspondence: Camille M. Steber, Y2FtaWxsZS5zdGViZXJAdXNkYS5nb3Y=
†These authors have contributed equally to this work