- Donald Danforth Plant Science Center, St. Louis, MO, United States
Introduction: Roots have a central role in plant resource capture and are the interface between the plant and the soil that affect multiple ecosystem processes. Field pennycress (Thlaspi arvense L.) is a diploid annual cover crop species that has potential utility for reducing soil erosion and nutrient losses; and has rich seeds (30-35% oil) amenable to biofuel production and as a protein animal feed. The objective of this research was to (1) precisely characterize root system architecture and development, (2) understand plastic responses of pennycress roots to nitrate nutrition, (3) and determine genotypic variance available in root development and nitrate plasticity.
Methods: Using a root imaging and analysis pipeline, the 4D architecture of the pennycress root system was characterized under four nitrate regimes, ranging from zero to high nitrate concentrations. These measurements were taken at four time points (days 5, 9, 13, and 17 after sowing).
Results: Significant nitrate condition response and genotype interactions were identified for many root traits, with the greatest impact observed on lateral root traits. In trace nitrate conditions, a greater lateral root count, length, density, and a steeper lateral root angle was observed compared to high nitrate conditions. Additionally, genotype-by-nitrate condition interaction was observed for root width, width:depth ratio, mean lateral root length, and lateral root density.
Discussion: These findings illustrate root trait variance among pennycress accessions. These traits could serve as targets for breeding programs aimed at developing improved cover crops that are responsive to nitrate, leading to enhanced productivity, resilience, and ecosystem service.
1 Introduction
Agriculture is a significant emitter of greenhouse gasses contributing 11.2% of all emissions in the US and 74.2% of all nitrous oxide emissions (EPA, 2022). The intensification of agricultural practices, including the use of chemical fertilizers, herbicides, and heavy machinery since the Green Revolution, has accelerated soil erosion, quality loss, and pollution (Kaspar and Singer, 2015). Adoption of sustainable agricultural practices are urgently needed for a 43% reduction in net greenhouse gas emissions by 2030 to limit atmospheric warming to 1.5°C (IPCC, 2022). Integration of cover crops into field management strategies is one avenue towards the needed shift to sustainable agriculture as they maintain important ecological processes year-round with active root systems (Langdale et al., 1991; Gyssels et al., 2005; Dunn et al., 2016; Han et al., 2021). Despite cover crops being a proximal and impactful solution to more sustainable agriculture practices, minimal crop breeding and optimization has occurred compared to cash crops as below-ground processes are challenging to measure (Griffiths et al., 2022a).
The root system of a plant is vital for capture of water and nutrients. The spatial and temporal organization of the root system, termed root system architecture, greatly affects plant access to these soil resources. Root system development is highly influenced by plant resource status and the environment and is the product of evolution and local plasticity. Root responses to nutrient stresses, especially to nitrogen deficiency have been observed in other crops such as Brassica napus and Zea mays (Gao et al., 2015; Guo et al., 2017). However, roots and belowground processes are inherently challenging to study as digging for roots is a destructive process with root losses during washing (Zhu et al., 2011; Freschet et al., 2021). Characterization and quantification of root development without disrupting the root system architecture is an additional challenge, especially in numbers large enough to query natural variation across a plant species. Therefore, various image-based high-throughput and non-destructive root phenotyping approaches have been developed for assessment of traits of large genetic populations with clear gel, growth-pouches, and rhizotrons (Clark et al., 2011; Topp et al., 2013; Atkinson et al., 2015; Seck et al., 2020). Leveraging these root phenotyping analysis pipelines to characterize and exploit the genetic diversity available in root system architecture is crucial for breeding more productive and resilient crops (Topp et al., 2016).
Field pennycress (Thlaspi arvense L.) is a diploid annual species that has promising application as a cash cover crop, which is a cover crop that can additionally provide a harvestable product (Dorn et al., 2015; Frels et al., 2019). Pennycress is found across a wide array of climatypes with most prevalence in temperate regions. It is winter hardy and is therefore an ideal candidate as a fall-planted cover crop between maize-soybean rotations in the US Midwest. The potential utility of pennycress is threefold: as a cover crop providing ecosystem services such as reduction in soil erosion and nutrient losses; and as an additional income source for farmers with production of rich oil seeds amenable as a biofuel (30-35% oil) and as a protein animal feed (Frels et al., 2019). Pennycress is potential model cover crop as it is diploid, has a small genome size (539 Mb), is a close relative to the model plant Arabidopsis thaliana, and has a fast generation time of approximately two to three months depending on vernalization requirements (Sedbrook et al., 2014). Recent work has shown that genome editing techniques could rapidly introduce desirable traits into this otherwise mostly wild species (Chopra et al., 2020a).
Genetic bottlenecks are a common circumstance in major crop species such as maize, wheat and rice. In these crops, above-ground agronomic traits and yield are typically the major selection criteria, often under high fertilizer and irrigated water regimes. In turn, root system architecture and performance, particularly under low input conditions, has been largely overlooked (Waines and Ehdaie, 2007; Eshel and Beeckman, 2013). Efforts to widen the genetic pool in these species are being made to introgress near- and distant- ancestral material back into modern crops (Schmidt et al., 2016; Grewal et al., 2018; Yang et al., 2019). In contrast to the conventional cash crops, pennycress remains undomesticated, offering a wide genetic pool that can potentially be selected from for traits including those for roots. So far, over 500 natural accessions of pennycress have been collected and sequenced for study in research trials (Frels et al., 2019).
To our knowledge, no study exists on the variation of pennycress root systems among collected accession panels. Here, we employed a 4D gel-based root imaging and analysis pipeline to reveal the root development of pennycress and its plastic response to nitrate nutrition in the seedling stage. Among a genetically and geographically diverse panel of 24 pennycress accessions, significant variation in root traits and correlation among traits was observed. A significant interaction between genotype and nitrate levels was also observed with common nitrate treatment responses for root traits.
2 Materials and methods
2.1 Plant materials
Twenty-four pennycress accessions, three spring ecotypes and 21 winter ecotypes, in which seed was available were used in this study. Latitudes of the original collection locations ranged from 61.6 to -51.73. For the original collection locations, 21 accessions were from North America, one accession from South America, and one accession from Europe. Included in the accessions tested is MN106, a winter North American line, which serves as the reference genome for pennycress. All accessions tested have also now been sequenced (Frels et al., 2019; Chopra et al., 2020b).
2.2 Experimental design and growth conditions
Pennycress seeds were surface sterilized with 70% ethanol, then 5% bleach (v/v) for 12 minutes, before being washed three times with double distilled water (ddH2O). Seeds were then transferred to sterile Whatman™ filter paper (Global Life Sciences Solutions Operations UK Ltd, Sheffield, UK) on plates moistened with 0.2 mM CaSO4. The seeds were then stratified at 4°C for 3 days in the dark. Stratified seeds were allowed to germinate in the dark at 21/18°C with a day:night cycle of 18/6 h. After 30 h, uniformly germinated seedlings, with a burst testa and maximum 1 mm emerged radicle, were used for the experiments.
Modified Hoagland’s solution imaging gels solidified with 5% Gelzan™ (Caisson Labs, UT, USA) were prepared for the root imaging experiments. For plant nutrition, nitrate concentration of the gels was varied between zero and 5 mM. The high nitrate solution was composed of (in µM) 500 KH2PO4, 5,000 KNO3, 2,000 CaCl2, 1,000 MgSO4, 46 H3BO3, 7 ZnSO4.7H2O, 9 MnCl2.4H2O, 0.32 CuSO4.5H2O, 0.114 (NH4)6Mo7O24.4H2O, and 150 Fe(III)-EDTA (C10H12N2NaFeO8). For a low, trace, and zero nitrate Hoagland’s solution, nitrate levels were modified with (in µM) 1,000, 100, and 0 KNO3 and replaced with 4,000, 4,900 and 5,000 KCl respectively. A 2.8 mM 2-(N-morpholino)ethanesulfonic acid (MES) buffer was added to the Hoagland’s solution and pH was adjusted to 6 using HCl to minimize pH fluctuation and preserve root membrane potential.
The growth vessels were custom 2L ungraduated glass cylinders to which 1L of gel was added. A single germinated seedling was sown per gel onto the gel surface using sterile forceps. Plants were then transferred to the growth chamber with a day:night cycle of 16/8 h at 21/18°C at a photon flux density of 300 mmol m–2 s–1 PAR at canopy height. The plants were terminated once the roots reached the edge of the cylinder or imaging plane of view which was after 17 days. A complete randomized block design was used for all experiments.
Three experiments were conducted: the first was a nitrate response experiment with Spring32; the second experiment was a high and low nitrate experiment with Spring32, MN106 and ISU89; and the third experiment with 24 accessions all grown in high nitrate conditions.
2.3 Sample collection and harvest measures
For all experiments, root images were collected of each plant at four time points (days 5, 9, 13, and 17 after sowing). The imaging setup consisted of a computer interfacing with an Allied Vision Manta G-609 machine vision camera (Allied Vision Technologies GmbH, Stadtroda, Germany) with a Nikon 60mm f/2.8D lens (Nikon Inc., Melville, NY, USA) and an electronic turntable. The turntable operated in a water-filled tank to correct for light refraction when imaging the glass cylinders. The glass cylinders were partly submerged above the level of the gel when placed in the center of the turntable. A LED flat panel light was used as a backlight to produce grayscale images of the roots with a black silhouette of roots in the foreground against a white background. Root imaging took approximately 2 min per plant with 72 images collected over a 360-degree rotation. The imaging setup used was based on work by Clark et al. (2011).
After root imaging, roots were severed from the shoots and the shoot rosettes were immediately imaged using a flatbed scanner equipped with a transparency unit (Epson Expression 12000XL, Epson America Inc., CA, USA). Rosette size and leaf counts were determined using a modified PlantCV rosette imaging pipeline (Gehan et al., 2017). After shoot imaging, the root and shoot of each plant was dried separately at 60°C for 3 d at which a constant weight was reached with drying for determination of dry biomass. Plant measures and extracted traits are detailed in Supplementary Table 1.
2.4 3D root image processing
Collected gel images were reconstructed into 3D models, skeletonized, and traits extracted using GiARoots and DynamicRoots pipelines (Galkovskyi et al., 2012; Symonova et al., 2015). GiARoots was first used to scale, crop, threshold, and binarize the gel images. Source code for the pipeline used can be accessed at https://github.com/Topp-Roots-Lab/rsa-tools and https://github.com/Topp-Roots-Lab/Gia3D. For all roots in this study the RootWorkPerspective mode was used to downsample and reconstruct 36 of the 72 images into a 3D model with an octree node number of 100,000,000, Rotation Axis of -63, or 0, and a top line added to the top of the model. Root system reconstructions were visually inspected for completeness and artifacts. Seven root traits were then extracted from the 3D models using the GiaRoots3D measure function as detailed in Supplementary Table 1. A further 64 traits were extracted from the 3D point clouds using DynamicRoots with standard parameters (Supplementary Table 1).
2.5 Statistical analysis
Statistical analyses were conducted using R version 4.2.1 (R Core Team, 2022); the statistical analysis R codes including the packages needed are available https://doi.org/10.5281/zenodo.7536940. A total of 44 traits from GiaRoots3D, DynamicRoots, PlantCV, plus derived traits are described in Supplementary Table 1.
3 Results
3.1 Root nitrate response
In the first experiment, root growth in varying nitrate concentrations was evaluated across time. Spring32, a spring North American line, was used as it had seed readily available and represented a spring ecotype. Plants were grown under high N, low N, trace N, and zero N conditions. Root images were taken every 4 d with significant phenotypic variation observed across time and by N condition (Figure 1; Supplementary Table 2). Lateral roots started to appear between day 5 and day 9. Primary and lateral root total lengths were similar in length at day 9 (Figure 1A). By day 17, root elongation slowed in the zero N condition (Figure 1A). For all N conditions, lateral roots were the dominant root class for root length from day 13 (Figure 1A).
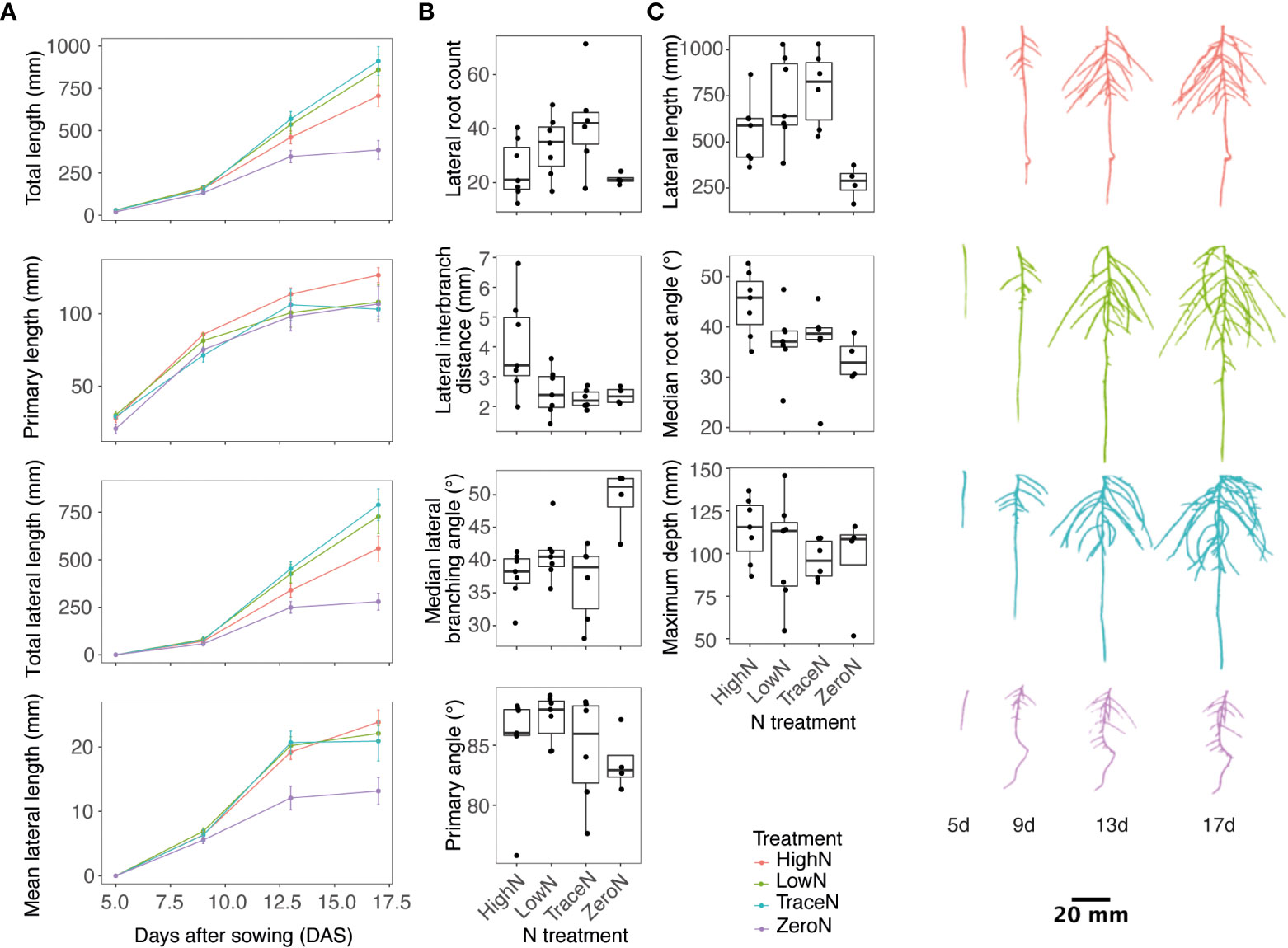
Figure 1 Pennycress root development response to varying nitrate concentration. (A) Line plots of root traits over time (B) Boxplots of root branching, angle, and distribution traits by varying nitrate concentration at day 17. (C) Representative images of 3D root system by age and nitrate treatment. High N is depicted as color red, low N as color green, trace N as color blue, and zero N as color purple.
Across the root phenotypes measured, a significant day-by-nitrate condition interaction was observed for lateral root count, length, growth rate, distribution, and angle traits (Figure 1B; Supplementary Table 2). The greatest lateral root count was observed in the trace N condition with a 51.3% greater count than high N condition and a 65.6% greater count than the zero N condition (Figure 1B). Lateral root interbranch distance, a measure of lateral root density, was the smallest in the trace N and zero N conditions with an observed 56.3% and 51.9% smaller distance respectively to the high N conditions (Figure 1B). For the total lateral root length, the greatest length was observed in the trace N condition with a 34.2% greater length than the high N condition and 95.5% greater length than the zero N condition (Figure 1B). For root angle traits, the zero N condition had the steepest median lateral root angle at 33.7° with a 10.9° difference from the shallowest under high N conditions (Figure 1B). In addition, the zero N condition also had the greatest lateral root branching angle from the parent root with a 11.7° greater branching angle than the high N condition (Figure 1B). For the primary root traits, the differences were less, with the deepest roots observed in the high N condition with a 17% greater root depth than the zero N condition, and the primary root angle was shallowest in the zero N condition with a 3.7° shallower primary root than the low N condition (Figure 1B).
Next, we evaluated GxE responses to nitrate nutrition in the key lines Spring32, MN106, and ISU89, which are important lines as they represent spring, winter, and southern ecotype diversity, respectively. The three accessions were grown under high N and trace nitrate conditions. Root images were taken every 4 d with significant phenotypic variation observed by genotype and nitrate condition (Figure 2; Supplementary Table 3). All accessions had a greater lateral root length and count under trace N conditions compared to high N conditions (Figure 2A; Supplementary Table 3). For width:depth ratio a significant genotype-by-nitrate condition interaction was observed (Figure 2A; Supplementary Table 3). All accessions had a greater width:depth ratio in trace nitrate conditions over high nitrate with ISU89 having the greatest increase of 74.9%. MN106 had the smallest increase in width:depth ratio with a 25.9% increase in low nitrate conditions.
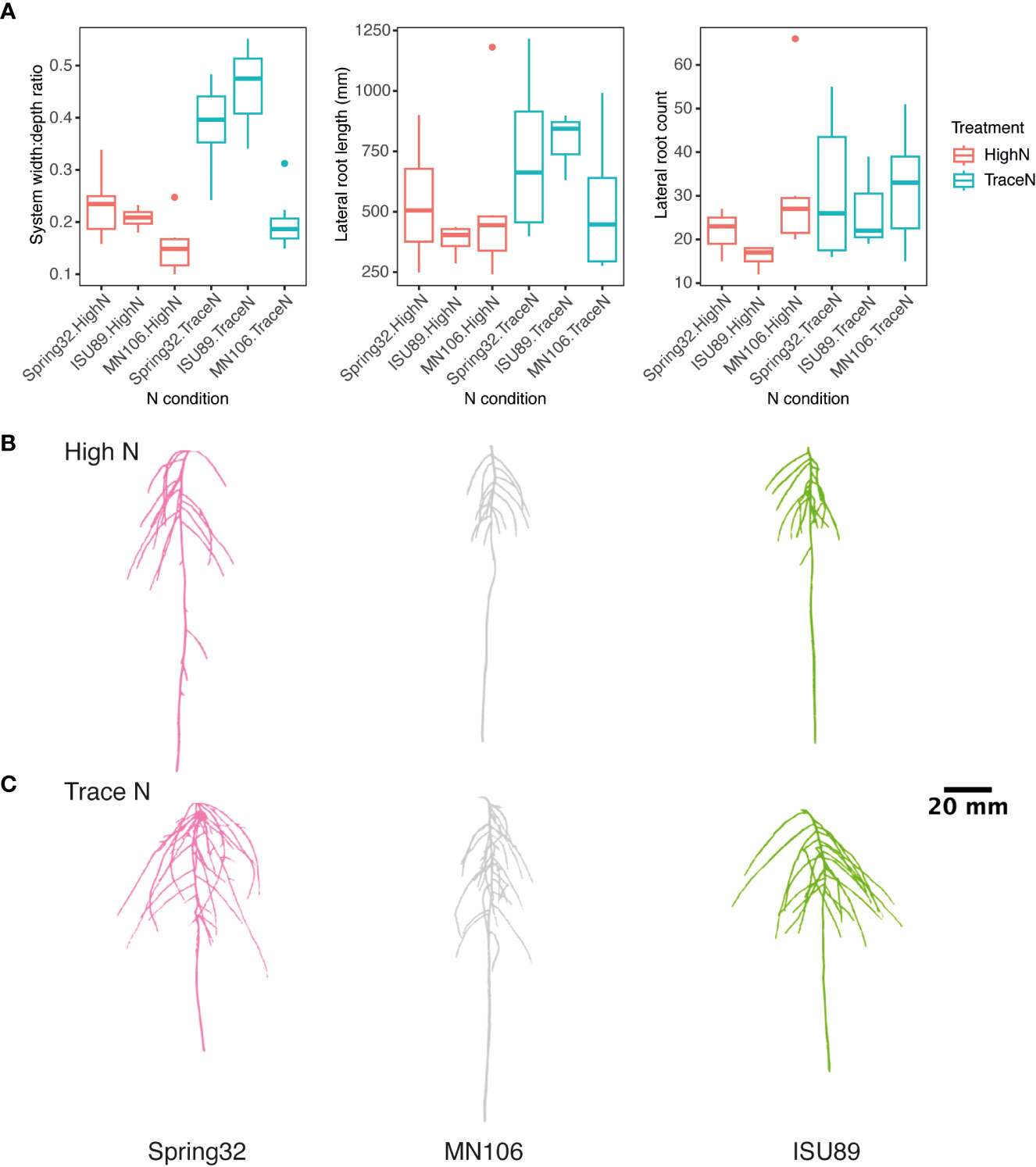
Figure 2 Genotypic and N condition variance in pennycress lateral root traits. (A) Boxplots of accession root traits by nitrate treatment. Representative images of 3D root systems under (B) high and (C) trace nitrate concentrations.
3.2 Phenotypic variation among genotypes
In the large experiment, 24 diverse accessions were grown in high N conditions. Root images were taken every 4 d with significant phenotypic variation and visual differences observed by genotype and across time (Figures 3, 4; Supplementary Table 4). For root size traits including length, radius, and volume; and root distribution traits including bushiness, convex hull volume, width, tortuosity, soil angle, and branching angle; a significant genotype-by-day interaction was observed (Supplementary Table 4). With a limited number of spring ecotypes tested, separation between spring and winter ecotypes was observed in a linear discriminant analysis with the spring ecotype in general larger than winter types (Figures 3A–C). The spring ecotypes had on average a 47.2% greater width:depth ratio, 43% greater lateral root length, and a 25.4% greater root count (Figures 3A, B).
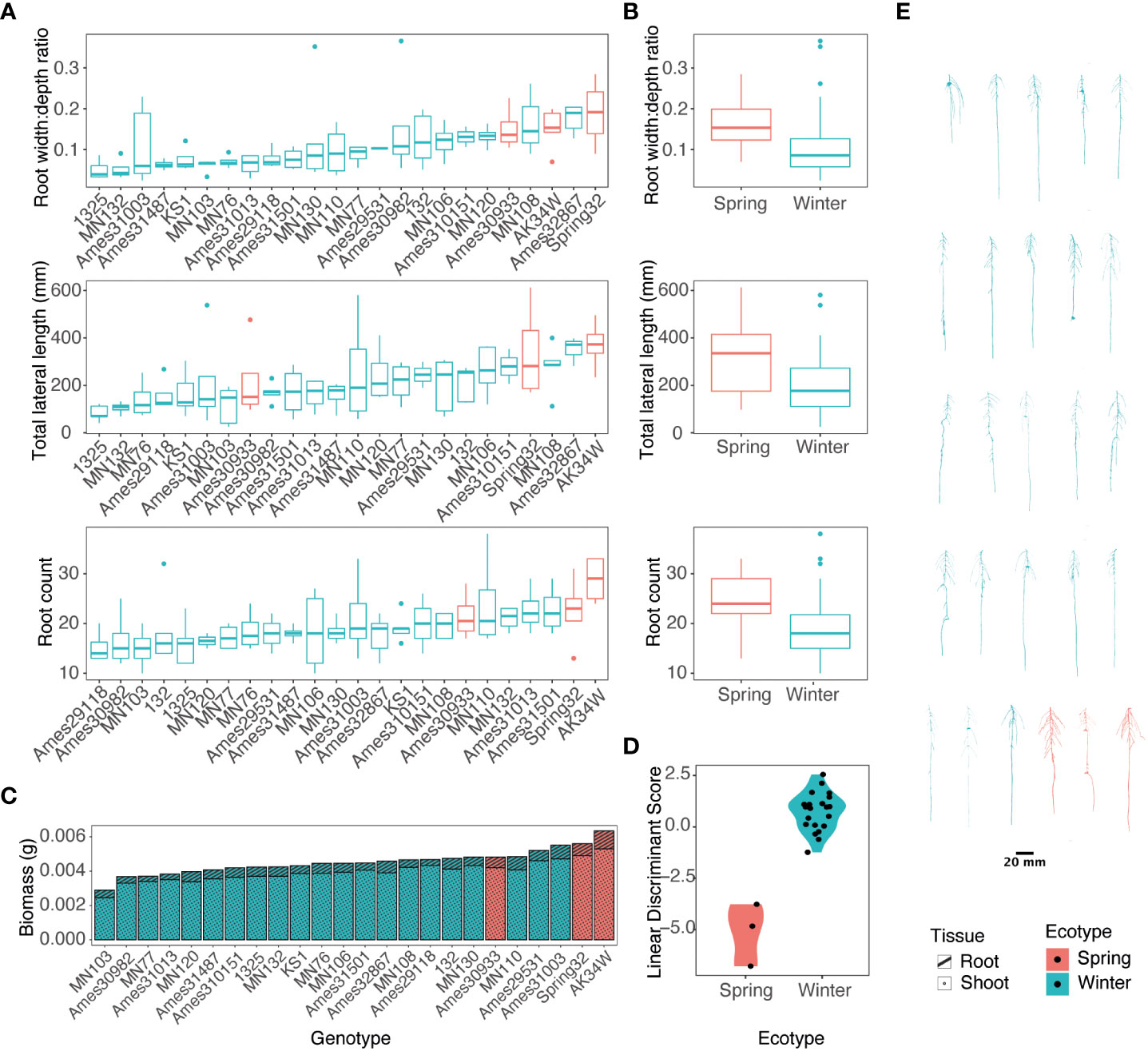
Figure 3 Variance of spring and winter pennycress ecotypes for root traits at 17 days after sowing. (A) Boxplots of root measures of individual accessions. (B) Means of individuals summarized by ecotype. (C) Stacked bar chart of mean root and shoot biomass allocation per accession. (D) Linear discriminant analysis between ecotypes. (E) Representative images of 3D root systems of pennycress accessions. Root images and plots are color coded by ecotype, spring as red and winter as green.
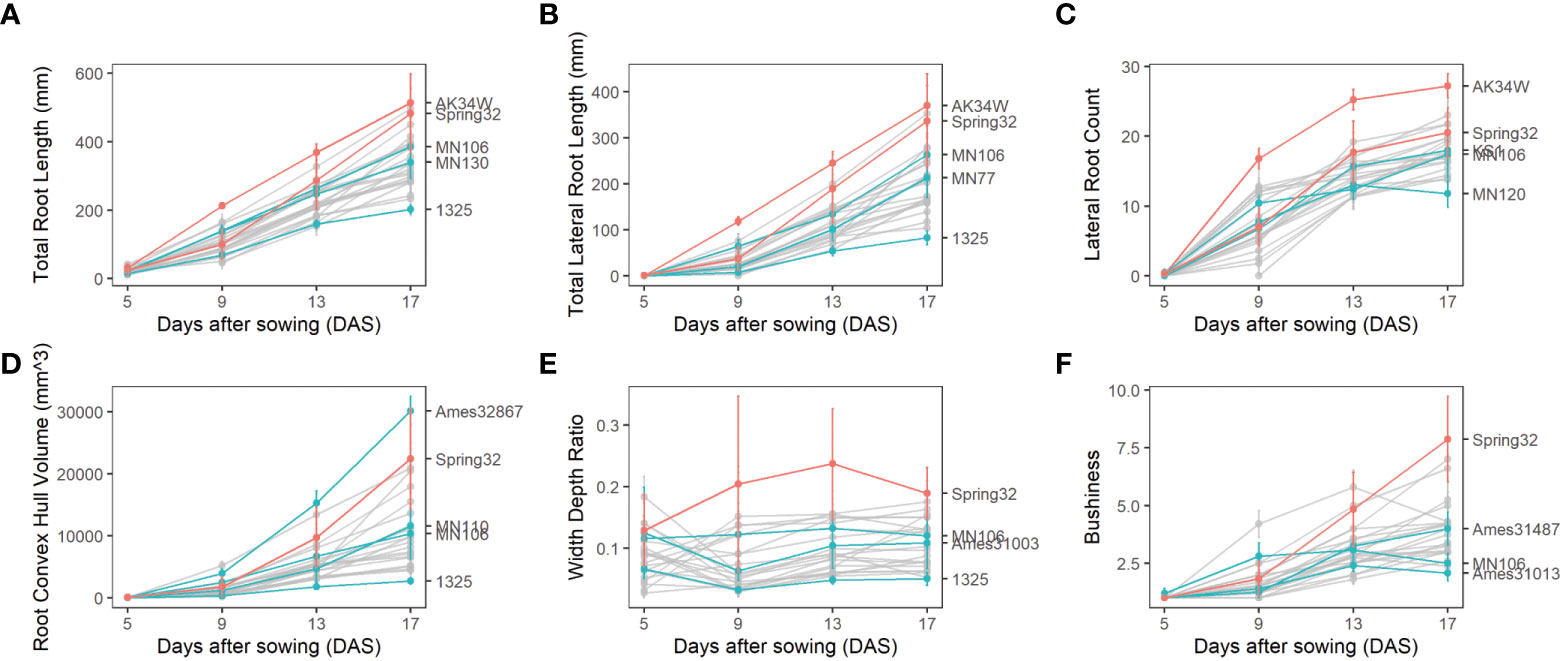
Figure 4 Root phenotypes of diverse pennycress accessions at 5,9, 13, and 17 days after sowing with mean and extreme genotypes highlighted along with Spring32 and MN106 reference lines. (A) Total Root Length. (B) Lateral Root Count. (C) Lateral Root Count. (D) Root Convex Hull. (E) Width Depth Ratio. (F) Bushiness.
By imaging roots over a time course, a diversity of changes in root traits between genotypes over time was quantified. Over time, total root length increased at a constant rate, lateral root production decreased, root convex hull volume increased at an accelerated rate, and width:depth ratio stayed mostly constant. Genotypes that were the extremes of some traits tended to be extreme in other traits. For example, the genotype AK34W demonstrated larger root systems when measured across several traits including total root length, total lateral root length, and lateral root count (Figures 4A–C). Likewise, the genotype 1325 demonstrated a smaller root system based on total root length, total lateral root length, convex hull, and width depth (Figures 4A, B, D, E). For traits where AK34W and 1325 were not the most extreme, the genotypes were still among the most extreme for the respective traits (Figures 4C–F).
3.3 Correlation among traits
To determine relationships among pennycress plant traits correlation and principal component analysis (PCA) were conducted using root phenotyping data of 24 diverse pennycress accession lines. A correlation analysis showed a strong positive correlation among root and shoot size traits (Figure 5A). For root system size and distribution traits, a positive correlation was observed for convex hull volume, root width:depth ratio, and root length (Figure 5A). Root mean radius was positively correlated with total volume, width:depth ratio, and interbranch distance which may be driven by variation in the primary root. Interbranch mean distance was positively correlated with root length distribution. For root depth, a positive correlation was observed between root median lateral root branching angle and a negative correlation with root solidity and root mean radius. For the distribution traits, there was no significant correlation observed between root system width and root system depth (Figure 5B). A positive correlation was observed between root system width and lateral root length (Figure 5C).
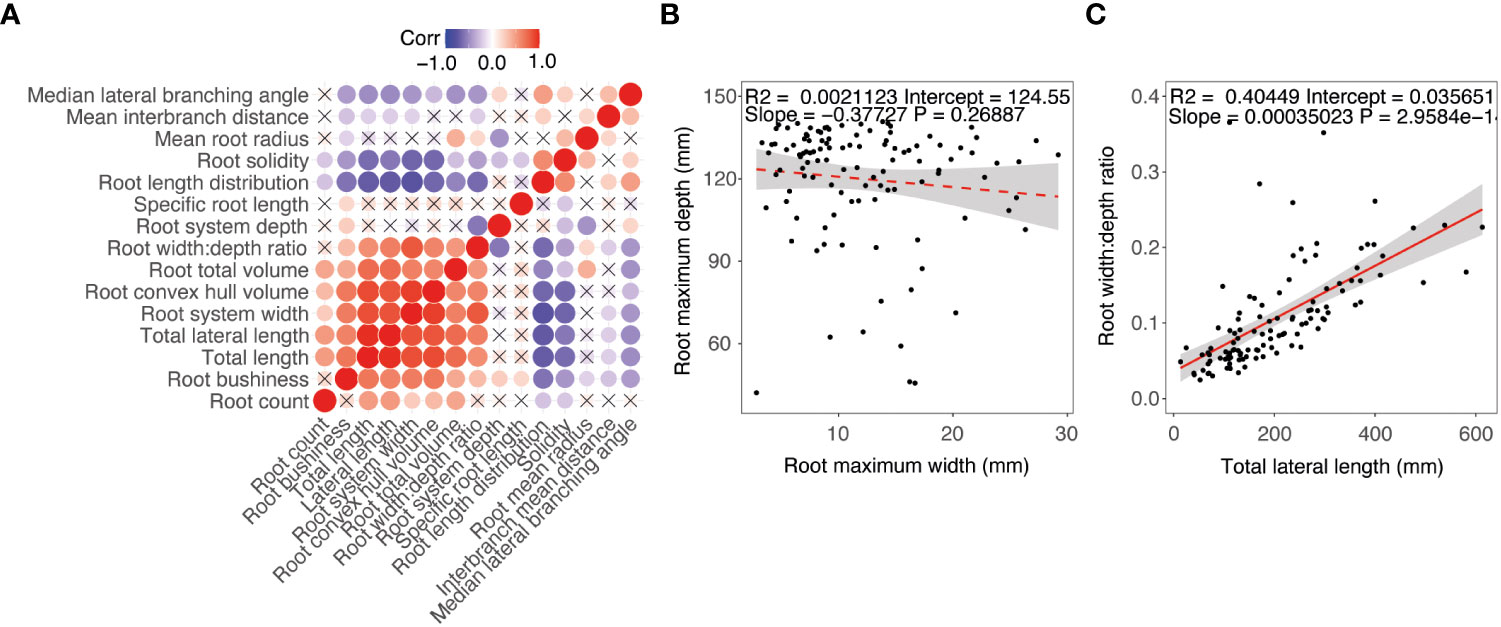
Figure 5 Phenotype correlation between diverse pennycress accessions at 17 days after sowing. (A) Correlation matrix. (B) Regression between maximum root system width and depth. (C) Regression between root with:depth ration and total lateral root length.
Principal component analysis was used to explore relationships among the plant traits and to explore if there was clustering by pennycress population structure traits (Figures 6A, B). With a subset of traits selected to avoid collinearity, the first two principal components explained 60.2% of the trait variation observed (Figure 6A). Over 75% of the trait variation could be explained by the first four principal components. The loadings for PC1 were mostly root size and width traits including root system and lateral root length, root system maximum width, bushiness, and convex hull volume (Figure 6C). Root depth, width:depth ratio, and root mean radius, and solidity were the main traits contributing to PC2 (Figure 6D). A PCA individuals plot was conducted with clustering based on ecotype (Figure 6B). Based on the phenotypes collected at this seedling stage minor separation in clustering was observed between spring or winter ecotypes.
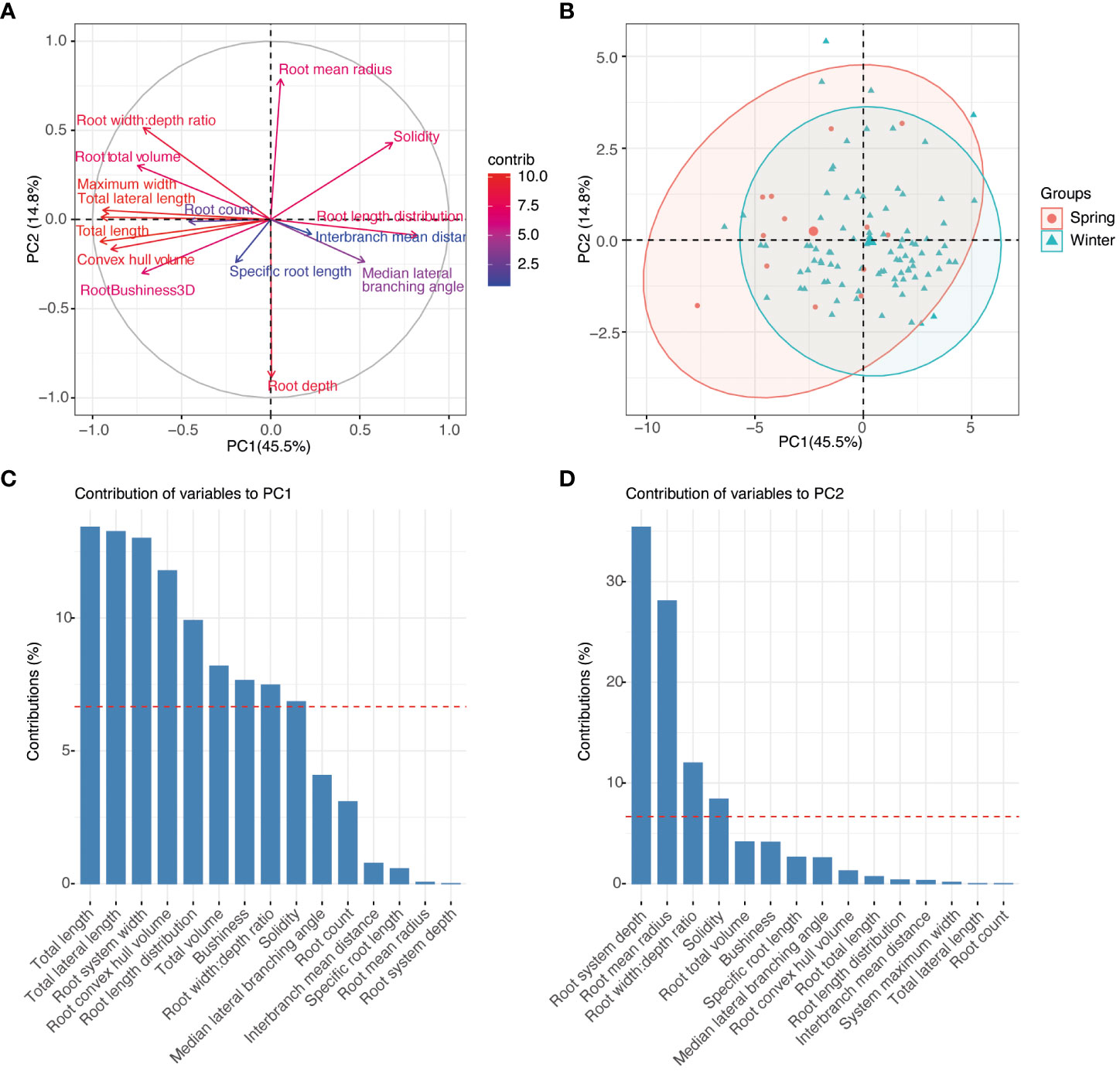
Figure 6 Phenotype association between diverse pennycress accessions at 17 days after sowing. (A) PCA plot of variables. (B) PCA biplot of individuals with ecotype clustering. (C) PCA contribution plot for PC1. (D) PCA contribution plot for PC2.
4 Discussion
The spatial and temporal arrangement of root systems are critical for their ability to capture transient and unequally distributed soil resources needed for plant growth and productivity. In turn, root systems are a primary input of soil carbon and drive biological, chemical, and physical changes to the soil which have major ecological implications. Evaluation of in-situ root development at high precision is challenging and requires specialized growth, imaging, and analysis approaches (Jiang et al., 2019). In this study, we employed a 4D gel-based root imaging platform to assess root system development, architecture, and response to nitrate availability across diverse pennycress accessions.
The primary reasons for adoption of cover crops are to prevent soil erosion and provide other ecosystem services, most of which are directly related to root system architecture and function (Griffiths et al., 2022a). Despite this, little is known about the root system development of most cover crops and their impact on the environment. Evaluating root traits in pennycress can provide valuable insights into functional mechanisms and may provide opportunities for enhancing ecosystem services and yield. Pennycress is a tap-rooted plant and here we show that the lateral root system was the dominant root class after 13 days of growth. Pennycress seedlings were grown under varying nitrate concentrations and the lateral root system was most responsive, a phenomenon also observed in Arabidopsis, wheat, barley, maize, rice, and others (Drew and Saker, 1975; Trachsel et al., 2013; Yu et al., 2014; De Pessemier et al., 2022; Griffiths et al., 2022b). In pennycress, three distinguishable nitrate plasticity responses were observed; (1) normal root development under sufficient or excess available nitrate, (2) intense root foraging to increase access to nitrate for stress avoidance in nitrate limited conditions, and (3) root growth arrest when nitrate became critically insufficient. As nitrogen is heterogeneously distributed in agricultural soil, both spatially and temporally, these plastic responses in root system architecture can facilitate greater uptake with responsive root exploration combined with expression of nitrate transporters (Yu et al., 2014; Melino et al., 2015).
Under trace nitrate conditions an intensive root foraging strategy was observed with greater lateral root count, lateral root length, smaller interbranch distance, and steep lateral root angles. Similar root angle and biomass allocation responses have been reported in various studies under nitrogen stress, and they are positively correlated with deep nitrate uptake (Trachsel et al., 2013; Griffiths et al., 2022c). Deep rooting is an ideotype proposed for more nitrate stress tolerant crops and could in turn be used for reducing nitrate runoff as an ecosystem service (Trachsel et al., 2013; Griffiths et al., 2022a). However, root development has a cost and there must be enough available nitrate for roots to continue development. In the trace nitrate conditions, more nitrate was required for adequate development yet enough was available to facilitate the extra root development. Before day 13, root development was similar across each nitrate condition, however as seen in the zero-nitrate treatment the seed nitrate reserves were likely expired after 13 days as evidenced by root growth arrest. In the high nitrate conditions, a lower lateral root length and interbranch distance was observed compared to the trace nitrate conditions. This indicates that sufficient nitrate was available to the plant and available in the local root zone. Therefore, extra roots were not needed or had diminishing returns with resources likely allocated for other developmental processes.
Pennycress has a wide geographic distribution with collections mainly from temperate regions in the northern hemisphere. The accessions evaluated in this study represent a small proportion of the natural variation available for breeding. Here among a subset of the accessions it was demonstrated that there is common root plastic responses to nitrate concentration. Common responses include changes in root distribution traits including depth, root length distribution, lateral root angle, convex hull, and solidity. For width:depth ratio of the root system a genotype-by-nitrate condition interaction was observed. All accessions tested had a greater width:depth ratio under low nitrate attributed to the greater lateral root length, but the magnitude of change differed between genotypes. These differential responses to nitrate may indicate variations in stress tolerance and nitrate capture effectiveness. Harnessing plasticity of yield impacting traits is important for developing generalist genotypes that produce well matched phenotypes to the environment or specialist genotypes that are stable in a trait of interest. Generalist genotypes will outperform specialist genotypes in variable environments and specialist genotypes outperform in more stable environments (Wuest et al., 2021; Schneider, 2022). Measurement of nitrate uptake performance with isotopes and extending the study to the full lifecycle would provide insight into how these root responses affect plant uptake performance and yield traits.
Comparing root phenotypes of 24 pennycress accessions originally collected from locations in North America, South America, and Europe, significant variance was observed for most measured traits. These differences observed likely represent local adaptation to environments in which they were collected. Significant root trait differences were observed for most root traits with exception of primary root angle. Despite a limited sample size of spring lines available for this study, the spring lines grown at 21/18°C had an overall greater lateral root length and exploration compared to the winter lines. This indicates that the spring lines may have greater seedling vigor facilitating a shorter lifecycle without a vernalization period requirement, i.e. completing growth and reproduction in the spring only. Roots have a central role in multiple ecosystem processes and the variance observed in pennycress root traits will likely affect such ecosystem service performance. A correlation matrix and PCA analysis using root phenotypic data from all accessions revealed two root trait spectra for breeding: (1) root size, density, and width traits and (2) root depth and lateral root angle traits. Denser rooting is one such trait that has desirable application for sustainable agriculture by reducing soil erosion rate (Langdale et al., 1991; Gyssels et al., 2005) Lateral roots are important for nutrient foraging and were shown here to greatly contribute to the dicot root system width (Wang et al., 2002; Bao et al., 2014). Deeper and more extensive rooting of pennycress will be important to exploit for mobile resource capture and indirectly benefit agricultural systems by making biopores for subsequent crop roots to follow (Huang et al., 2020). At the seedling stage analyzed in this study no tradeoff was observed in having both a wide and deep root system with seedling vigor a promising seedling target for appropriate establishment (Pace et al., 2014; Atkinson et al., 2015). Lateral roots are important for nutrient foraging and were shown here to greatly contribute to the dicot root system width (Wang et al., 2002; Bao et al., 2014). Harnessing and matching these diverse root phenotypes to the growth environment and desired ecosystem function may be possible. This would be aided by characterization of a mapping population for genetic mapping studies that will allow functional genomic analysis of root traits and identify loci for crop improvement. Admixture of divergent genomes is expected to enhance climate adaptation and yield improvement (Lovell et al., 2021).
Data availability statement
The datasets presented in this study can be found in online repositories. The names of the repository/repositories and accession number(s) can be found below: https://doi.org/10.5281/zenodo.7536940.
Author contributions
MG, AL, and CT conceptualization. MG, AL, SG, NM, and EM experimentation. MG, AL, and TP image processing; MG and AL statistical analysis. All authors contributed to the article and approved the submitted version.
Funding
This study was supported by the U.S. Department of Energy, Office of Science, Office of Biological and Environmental Research, Genomic Science Program grant no. DE-SC0021286 to CT.
Acknowledgments
The authors would like to thank Tim Parker for computation assistance; Ni Jiang for DynamicRoot guidance; Ratan Chopra, John C. Sedbrook, Winthrop B. Phippen, and David Marks for providing seeds used in this study; and all iPREP project members for guidance.
Conflict of interest
The authors declare that the research was conducted in the absence of any commercial or financial relationships that could be construed as a potential conflict of interest.
Publisher’s note
All claims expressed in this article are solely those of the authors and do not necessarily represent those of their affiliated organizations, or those of the publisher, the editors and the reviewers. Any product that may be evaluated in this article, or claim that may be made by its manufacturer, is not guaranteed or endorsed by the publisher.
Supplementary material
The Supplementary Material for this article can be found online at: https://www.frontiersin.org/articles/10.3389/fpls.2023.1145389/full#supplementary-material
References
Atkinson, J. A., Wingen, L. U., Griffiths, M., Pound, M. P., Gaju, O., John Foulkes, M., et al. (2015). Phenotyping pipeline reveals major seedling root growth QTL in hexaploid wheat. J. Exp. Bot. 66 (8), 2283–2292. doi: 10.1093/jxb/erv006
Bao, Y., Aggarwal, P., Robbins, N. E., 2nd, Sturrock, C. J., Thompson, M. C., Tan, H. Q., et al. (2014). Plant roots use a patterning mechanism to position lateral root branches toward available water. Proc. Natl. Acad. Sci. U. S. A. 111 (25), 9319–9324. doi: 10.1073/pnas.1400966111
Chopra, R., Johnson, E. B., Emenecker, R., Cahoon, E. B., Lyons, J., Kliebenstein, D. J., et al. (2020a). Identification and stacking of crucial traits required for the domestication of pennycress. Nat. Food 1 (1), 84–91. doi: 10.1038/s43016-019-0007-z
Chopra, R., Marks, M., Sedbrook, J., Frels, K. (2020b). Pennycress - a solution for global food security, renewable energy and ecosystem benefits (Boca Raton, FL: CRC Press), Vol. 2020. doi: 10.46936/10.25585/60001359
Clark, R. T., MacCurdy, R. B., Jung, J. K., Shaff, J. E., McCouch, S. R., Aneshansley, D. J., et al. (2011). Three-dimensional root phenotyping with a novel imaging and software platform. Plant Physiol. 156 (2), 455–655. doi: 10.1104/pp.110.169102
De Pessemier, J., Moturu, T. R., Nacry, P., Ebert, R., De Gernier, H., Tillard, P., et al. (2022). Root system size and root hair length are key phenes for nitrate acquisition and biomass production across natural variation in arabidopsis. J. Exp. Bot. 73 (11), 3569–3583. doi: 10.1093/jxb/erac118
Dorn, K. M., Fankhauser, J. D., Wyse, D. L., David Marks, M. (2015). A draft genome of field pennycress (Thlaspi arvense) provides tools for the domestication of a new winter biofuel crop. DNA Res.: Int. J. Rapid Publ. Rep. Genes Genomes 22 (2), 121–315. doi: 10.1093/dnares/dsu045
Drew, M. C., Saker, L. R. (1975). Nutrient supply and the growth of the seminal root system in barley. J. Exp. Bot 26 (1), 79–90. doi: 10.1093/jxb/26.1.79
Dunn, M., Ulrich-Schad, J. D., Prokopy, L. S., Myers, R. L., Watts, C. R., Scanlon, K. (2016). Perceptions and use of cover crops among early adopters: findings from a national survey. J. Soil Water Conserv. 71 (1), 29–40. doi: 10.2489/jswc.71.1.29
EPA (2022) Inventory of U.S. greenhouse gas emissions and sinks 1990 to 2020. Available at: https://www.epa.gov/system/files/documents/2022-04/us-ghg-inventory-2022-main-text.pdf.
Frels, K., Chopra, R., Dorn, K. M., Wyse, D. L., David Marks, M., Anderson, J. A. (2019). Genetic diversity of field pennycress (Thlaspi arvense) reveals untapped variability and paths toward selection for domestication. Agronomy 9 (6), 3025. doi: 10.3390/agronomy9060302
Freschet, G. T., Pagès, L., Iversen, C. M., Comas, L. H., Rewald, B., Roumet, C., et al. (2021). A starting guide to root ecology: strengthening ecological concepts and standardising root classification, sampling, processing and trait measurements. New Phytol. 232 (3), 973–1122. doi: 10.1111/nph.17572
Galkovskyi, T., Mileyko, Y., Bucksch, A., Moore, B., Symonova, O., Price, C. A., et al. (2012). GiA roots: software for the high throughput analysis of plant root system architecture. BMC Plant Biol. 12 (July), 116. doi: 10.1186/1471-2229-12-116
Gao, K., Chen, F., Yuan, L., Zhang, F., Mi, G. (2015). A comprehensive analysis of root morphological changes and nitrogen allocation in maize in response to low nitrogen stress. Plant. Cell Environ. 38 (4), 740–505. doi: 10.1111/pce.12439
Gehan, M. A., Fahlgren, N., Abbasi, A., Berry, J. C., Callen, S. T., Chavez, L., et al. (2017). PlantCV v2: image analysis software for high-throughput plant phenotyping. PeerJ 5 (December), e4088. doi: 10.7717/peerj.4088
Grewal, S., Hubbart-Edwards, S., Yang, C., Scholefield, D., Ashling, S., Burridge, A., et al. (2018). Detection of t. urartu introgressions in wheat and development of a panel of interspecific introgression lines. Front. Plant Sci. 9 (October), 1565. doi: 10.3389/fpls.2018.01565
Griffiths, M., Delory, B. M., Jawahir, V., Wong, K. M., Bagnall, G.C., Dowd, T. G., et al. (2022a). Optimisation of root traits to provide enhanced ecosystem services in agricultural systems: a focus on cover crops. Plant. Cell Environ. 45 (3), 751–705. doi: 10.1111/pce.14247
Griffiths, M., Mellor, N., Sturrock, C. J., Atkinson, B. S., Johnson, J., Mairhofer, S., et al. (2022b). X-Ray CT reveals 4D root system development and lateral root responses to nitrate in soil. Plant Phenome. J. 5 (1), e20036. doi: 10.1002/ppj2.20036
Griffiths, M., Wang, X., Dhakal, K., Guo, H., Seethepalli, A., Kang, Y., et al. (2022c). Interactions among rooting traits for deep water and nitrogen uptake in upland and lowland ecotypes of switchgrass (Panicum virgatum l.). J. Exp. Bot. 73 (3), 967–795. doi: 10.1093/jxb/erab437
Guo, Q., Love, J., Roche, J., Song, J., Turnbull, M. H., Jameson, P. E. (2017). A RootNav analysis of morphological changes in brassica napus l. roots in response to different nitrogen forms. Plant Growth Regul. 83 (1), 83–925. doi: 10.1007/s10725-017-0285-0
Gyssels, G., Poesen, J., Bochet, E., Li, Y. (2005). Impact of plant roots on the resistance of soils to erosion by water: a review. Prog. Phys. Geography.: Earth Environ. 29 (2), 189–217. doi: 10.1191/0309133305pp443ra
Han, E., Li, F., Perkons, U., Küpper, P. M., Bauke, S. L., Athmann, M., et al. (2021). Can precrops uplift subsoil nutrients to topsoil? Plant Soil 463, 329–345. doi: 10.1007/s11104-021-04910-3
Huang, N., Athmann, M., Han, E. (2020). Biopore-induced deep root traits of two winter crops. Collection. FAO.: Agric. 10 (12), 6345. doi: 10.3390/agriculture10120634
IPCC (2022). Summary for Policymakers. In Climate Change 2022: Mitigation of Climate Change. Contribution of Working Group III to the Sixth Assessment Report of the Intergovernmental Panel on Climate Change Shukla, P.R., Skea, J., Slade, R., Khourdajie Al, A., Diemen van, R., McCollum, D., et al (Cambridge, UK and New York, NY, USA: IPCC Press Release). doi: 10.1017/9781009157926.001
Jiang, N., Floro, E., Adam, L., Laws, B. B., Duncan, K. E., Topp, C. N. (2019). Three-dimensional time-lapse analysis reveals multiscale relationships in maize root systems with contrasting architectures. Plant Cell 31 (8), 1708–1225. doi: 10.1105/tpc.19.00015
Kaspar, T. C., Singer, J. W. (2015). “The use of cover crops to manage soil,” in Soil management: building a stable base for agriculture (Madison, WI, USA: Soil Science Society of America), 321–337.
Langdale, G. W., Blevins, R. L., Karlen, D. L., McCool, D. K., Nearing, M. A., Skidmore, E. L., et al. (1991). “Cover crop effects on soil erosion by wind and water,” in Cover crops for clean water(Ankeny, Iowa: Soil and Water Conservation Society), 15–21.
Lovell, J. T., MacQueen, A. H., Mamidi, S., Bonnette, J., Jenkins, J., Napier, J. D., et al. (2021). “Genomic mechanisms of climate adaptation in polyploid bioenergy switchgrass.” Nature 590 (7846), 438–444. doi: 10.1038/s41586-020-03127-1
Melino, V. J., Fiene, G., Enju, A., Cai, J., Buchner, P., Heuer, S. (2015). Genetic diversity for root plasticity and nitrogen uptake in wheat seedlings. Funct. Plant Biol.: FPB. 42 (10), 942–565. doi: 10.1071/FP15041
Pace, J., Lee, N., Naik, H. S., Ganapathysubramanian, B., Lübberstedt, T. (2014). Analysis of maize (Zea mays l.) seedling roots with the high-throughput image analysis tool ARIA (Automatic root image analysis). PloS One 9 (9), e1082555. doi: 10.1371/journal.pone.0108255
R Core Team (2022). R: a language and environment for statistical computing (Vienna, Austria: R Foundation for Statistical Computing). Available at: https://www.R-project.org/.
Schmidt, J. E., Bowles, T. M., Gaudin, A.C.M. (2016). Using ancient traits to convert soil health into crop yield: impact of selection on maize root and rhizosphere function. Front. Plant Sci. 7 (March), 373. doi: 10.3389/fpls.2016.00373
Schneider, H. M. (2022). Characterization, costs, cues and future perspectives of phenotypic plasticity. Ann. Bot. 130 (2), 131–148. doi: 10.1093/aob/mcac087
Seck, W., Torkamaneh, D., Belzile, F. (2020). Comprehensive genome-wide association analysis reveals the genetic basis of root system architecture in soybean. Front. Plant Sci. 11 (December), 590740. doi: 10.3389/fpls.2020.590740
Sedbrook, J. C., Phippen, W. B., Marks, M.D. (2014). New approaches to facilitate rapid domestication of a wild plant to an oilseed crop: example pennycress (Thlaspi arvense l.). Plant Sci.: Int. J. Exp. Plant Biol. 227 (October), 122–132. doi: 10.1016/j.plantsci.2014.07.008
Symonova, O., Topp, C. N., Edelsbrunner, H. (2015). DynamicRoots: a software platform for the reconstruction and analysis of growing plant roots. PloS One 10 (6), e01276575. doi: 10.1371/journal.pone.0127657
Topp, C. N., Bray, A. L., Ellis, N. A., Liu, Z. (2016). How can we harness quantitative genetic variation in crop root systems for agricultural improvement? J. Integr. Plant Biol. 58 (3), 213–255. doi: 10.1111/jipb.12470
Topp, C. N., Iyer-Pascuzzi, A. S., Anderson, J. T., Lee, C.-R., Zurek, P. R., Symonova, O., et al. (2013). 3D phenotyping and quantitative trait locus mapping identify core regions of the rice genome controlling root architecture. Proc. Natl. Acad. Sci. U. S. A. 110 (18), E1695–E1704. doi: 10.1073/pnas.1304354110
Trachsel, S., Kaeppler, S. M., Brown, K. M., Lynch, J. P. (2013). Maize root growth angles become steeper under low n conditions. Field Crops Res. 140 (January), 18–31. doi: 10.1016/j.fcr.2012.09.010
Waines, J.G., Ehdaie, B. (2007). Domestication and crop physiology: roots of green-revolution wheat. Ann. Bot. 100 (5), 991–985. doi: 10.1093/aob/mcm180
Wang, X.-B., Wu, P., Bin, H., Chen, Q.-S. (2002). Effects of nitrate on the growth of lateral root and nitrogen absorption in rice. Acta Bot. Sin. 44, 678–683. https://www.jipb.net/EN/Y2002/V44/I6/678
Wuest, S. E., Peter, R., Niklaus, P. A. (2021). Ecological and evolutionary approaches to improving crop variety mixtures.” Nat. Ecol. Evol. 5 (8), 1068–1775. doi: 10.1038/s41559-021-01497-x
Yang, C. J., Samayoa, L. F., Bradbury, P. J., Olukolu, B. A., Xue, W., York, A. M., et al. (2019). The genetic architecture of teosinte catalyzed and constrained maize domestication. Proc. Natl. Acad. Sci. U. S. A. 116 (12), 5643–5652. doi: 10.1073/pnas.1820997116
Yu, P., Li, X., Yuan, L., Li, C. (2014). A novel morphological response of maize (Zea mays) adult roots to heterogeneous nitrate supply revealed by a split-root experiment. Physiol. Plantarum. 150 (1), 133–445. doi: 10.1111/ppl.12075
Keywords: root system architecture, nitrate, plasticity, abiotic stress, phenotyping, cover crop, field pennycress, ecosystem service
Citation: Griffiths M, Liu AE, Gunn SL, Mutan NM, Morales EY and Topp CN (2023) A temporal analysis and response to nitrate availability of 3D root system architecture in diverse pennycress (Thlaspi arvense L.) accessions. Front. Plant Sci. 14:1145389. doi: 10.3389/fpls.2023.1145389
Received: 16 January 2023; Accepted: 23 May 2023;
Published: 22 June 2023.
Edited by:
Yinglong Chen, University of Western Australia, AustraliaReviewed by:
Peng Yu, University of Bonn, GermanyDarlene L. Sanchez, Texas A&M AgriLife Research and Extension Center at Beaumont, United States
Copyright © 2023 Griffiths, Liu, Gunn, Mutan, Morales and Topp. This is an open-access article distributed under the terms of the Creative Commons Attribution License (CC BY). The use, distribution or reproduction in other forums is permitted, provided the original author(s) and the copyright owner(s) are credited and that the original publication in this journal is cited, in accordance with accepted academic practice. No use, distribution or reproduction is permitted which does not comply with these terms.
*Correspondence: Christopher N. Topp, ctopp@danforthcenter.org
†These authors have contributed equally to this work
‡ORCID: Marcus Griffiths, orcid.org/0000-0003-2349-8967
Alexander E. Liu, orcid.org/0000-0002-3836-6556
Shayla L. Gunn, orcid.org/0000-0003-2299-7035
Nida M. Mutan, orcid.org/0000-0003-3999-835X
Elisa Y. Morales, orcid.org/0000-0002-9070-7813
Christopher N Topp, orcid.org/0000-0001-9228-6752