- 1Department of Plant Breeding, Horticum, Swedish University of Agricultural Sciences, Lomma, Sweden
- 2Agrobiodiversity and Environment Research Centre, Malaysian Agricultural Research and Development Institute (MARDI), Serdang, Selangor, Malaysia
- 3Cell and Molecular Sciences, James Hutton Institute, Dundee, United Kingdom
The use of biocontrol agents with plant growth-promoting activity has emerged as an approach to support sustainable agriculture. During our field evaluation of potato plants treated with biocontrol rhizobacteria, four bacteria were associated with increased plant height. Using two important solanaceous crop plants, tomato and potato, we carried out a comparative analysis of the growth-promoting activity of the four bacterial strains: Pseudomonas fluorescens SLU99, Serratia plymuthica S412, S. rubidaea AV10, and S. rubidaea EV23. Greenhouse and in vitro experiments showed that P. fluorescens SLU99 promoted plant height, biomass accumulation, and yield of potato and tomato plants, while EV23 promoted growth in potato but not in tomato plants. SLU99 induced the expression of plant hormone-related genes in potato and tomato, especially those involved in maintaining homeostasis of auxin, cytokinin, gibberellic acid and ethylene. Our results reveal potential mechanisms underlying the growth promotion and biocontrol effects of these rhizobacteria and suggest which strains may be best deployed for sustainably improving crop yield.
1 Introduction
The plant rhizosphere is a significant carbon sink (Strand et al., 2008; Drigo et al., 2010), and represents an ecosystem hosting a diverse microbial community, including a variety of phytopathogens. The co-evolution of plants and microbial communities has led to the formation of complex symbiotic associations (Delaux and Schornack, 2021; Abdelfattah et al., 2022). Several studies have indicated that plants recruit specific microbial communities through root exudates, positively regulating the rhizosphere (Besserer et al., 2006; De Weert et al., 2007; Rudrappa et al., 2008). Through secreting secondary metabolites such as pyrrolnitrin and fusaricidin (Burkhead et al., 1994; Vater et al., 2017; Liu et al., 2020), outer membrane vesicles (McMillan et al., 2021) and phytohormones (Tsukanova et al., 2017), microbial communities assist plants in their defence against pathogens by priming plant defences while keeping severe immune responses to a minimum (Van Wees et al., 2008). Termed biological control agents (BCA), several bacterial genera including Pseudomonas, Serratia, Bacillus, and Azospirillum compete in the rhizosphere environment with pathogens through siderophore biosynthesis, antibiosis, lytic enzyme production and detoxification, thus protecting the plant (Loper, 1988; Loper and Henkels, 1999; Compant et al., 2005; Anzalone et al., 2021). Some species of rhizospheric BCA, known as plant growth-promoting rhizobacteria (PGPR), have long been known to enhance plant growth through the secretion of auxin (Přikryl et al., 1985), cytokinin (Tien et al., 1979; Timmusk et al., 1999), 1-aminocyclopropane-1-carboxylate (ACC) deaminase (Glick et al., 1994), nitrogen fixation (Bal et al., 2012), volatile organic compound (VOC) production (Tahir et al., 2017), and phosphate solubilization (Son et al., 2006; Olanrewaju et al., 2017).
Many PGPR modulate root architecture, promote shoot elongation, and increase chlorophyll content and photosynthetic efficiency (Diagne et al., 2020). One of the most common alterations induced by PGPR in the root system architecture is the increased formation of lateral roots (LR) and root hairs (RH), thus assisting in improved water and nutrient acquisition from the soil. Changes in root system architecture (RSA) could be caused by PGPR interference with the signaling in the main hormonal pathways regulating plant root development, namely auxin and cytokinin (Ali et al., 2009; Dodd et al., 2010). PGPRs also promote plant growth by reducing stress-related ethylene levels via ACC deaminase activity (Glick et al., 1998; Hardoim et al., 2008; Amna et al., 2019). PGPR have been demonstrated to be beneficial for several important crop species including tomato, apple, grapes, and cereals such as rice, wheat and maize (Kumari et al., 2019).
Pseudomonas spp. are Gram-negative, chemoheterotrophic, motile rod-shaped bacteria that are adapted to a wide range of ecological niches. Extensive research has shown that several Pseudomonas strains function as PGPR through diverse metabolic capabilities. P. putida isolate WCS358 (Zamioudis et al., 2013) and P. chlororaphis subsp. aurantiaca strain JD37 (Fang et al., 2013) isolated from potato rhizosphere promote plant growth in Arabidopsis thaliana and maize, respectively. P. putida isolate GR12-2 enhances root elongation through the ethylene pathway (Glick et al., 1994) and root branching by producing cyclodipeptides that modulate auxin responses (Ortiz-Castro et al., 2020). Furthermore, we have recently demonstrated that P. aeruginosa encodes genes for tryptophan biosynthesis and indole-3-acetic acid (IAA) synthesis, and promotes tomato growth (Ghadamgahi et al., 2022). P. fluorescens strain WCS365 has been shown to colonize both tomato and potato rhizospheres (Mercado-Blanco and Bakker, 2007). P. fluorescens strain WCS417 inhibits primary root elongation while stimulating LR and RH development through auxin signaling (Zamioudis et al., 2013).
Serratia sp. are Gram-negative bacteria belonging to the family Enterobacteriaceae. Several species of Serratia are reported to enhance plant growth, such as S. fonticola strain AU-P3 and Serratia sp. SY5 that promote growth in pea and maize plants, respectively (Koo and Cho, 2009; Devi et al., 2013). Recently, it was reported that Serratia rubidaea strain ED1 promotes seed germination in quinoa (Mahdi et al., 2021).
A shift in societal acceptance away from using harmful and often expensive chemical fertilizers to a safer and natural alternative, combined with the increasing challenges of climate change and the growing world population, has resulted in PGPR drawing increased attention. The effects of PGPR-plant interactions on molecular mechanisms are complex and may vary depending on the strain or consortia of strains, plant species, and receiving environment (Tsukanova et al., 2017). Understanding how a beneficial bacterium improves the growth and health of a specific crop in a specific environment is therefore critical.
The complex effects of PGPR on plant gene expression, including genes that play key roles in signal transduction, metabolism, and catabolism of plant hormones have been reported in several studies (Shen et al., 2013; Tsukanova et al., 2017; Wei et al., 2020). However, studies on global transcriptomic changes induced by PGPR treatment in the context of plant growth and development are limited. Moreover, to our knowledge, there is no report on the transcriptome profiling of potato plants under PGPR treatment. Understanding how plants including potato and tomato respond to PGPR treatment will greatly empower the usage of PGPR towards enhancing sustainable agriculture.
The aim of this study was to conduct a holistic study on the effect of PGPR on potato and tomato growth and select the best-performing PGPR to gain insights into their mechanism of action. The strains used in this study were isolated from potato and tomato rhizosphere soils. In vitro antagonistic analysis indicated a strong activity against plant pathogens, including P. colocasiae (Kelbessa et al., 2022), P. infestans and R. solani (unpublished). To that end, we carried out a field trial with six bacteria with biological control activity on potato plants in a high disease pressure P. infestans infested field. Selected strains associated with increased potato growth were then tested under greenhouse conditions for growth-promoting activity. Further, we carried out transcriptome profiling of potato and tomato roots and leaves treated with the bacterial supernatant to gain insight into the growth promotion mechanisms of P. fluorescens.
2 Materials and methods
2.1 Field trial
Field experiments were carried out in the summer of 2020 using the potato cultivar Kuras. A total of six bacterial isolates that displayed antagonistic activity against Phytophthora infestans under lab conditions were assessed for their plant growth promotion activity. These strains include Pseudomonas fluorescens SLU99, Serratia rubidaea EV23 and AV10, S. plymuthica S412 and AS13, and S. proteamaculans S4. A single colony of each bacterial strain was cultured in Luria-Bertani (LB) liquid for 16 h at 28°C. Then the cultures were centrifuged at 3000 × g for 10 min and the bacterial cells were resuspended in 5 L sterile water to a final OD600 0.2. The bacterial suspension was transferred to a 5 L pressure sprayer for field application, and water treatment was used as a control. The testing site was Mosslunda, near the city of Kristianstad, Sweden (55°58’00.3”N 14°07’03.0”E) where the average daily temperature ranged between 12 and 18°C and average monthly precipitation was 42-64 mm, with an average daylength was 17.5 hours. The experiment was carried out in a plot with four rows of 10 meters in width and 15 meters in length. The experimental design consisted of randomized block design with four blocks. Each block contained all eight treatments distributed randomly, with 10 plants per treatment. The potato plants were sprayed with six biocontrol bacteria (individual treatments) once every two weeks for a period of eight weeks. Statistical analysis of plant height was performed using JMPpro software.
2.2 Greenhouse and PGPR treatment
To evaluate the effects of selected rhizobacteria on tomato and potato plant growth, a pot experiment was conducted for each crop in two separate plant cultivation chambers (biotron). Nine and ten biological replicates were used for tomato and potato, respectively. The tomato seeds were surface sterilized using 3% sodium hypochlorite (v/v) followed by washing with sterile water three times before sowing in a 105-hole seed tray filled with nursery soil. At 25 days after sowing, uniform four-leaf stage seedlings were transplanted into 1 L pots containing 325 g of nursery soil (1 seedling/pot). For bacterial inoculations, strains SLU99, EV23, AV10 and S412 were cultured as described in the previous section. Thirty-five milliliters of bacterial suspensions at OD600 0.2 prepared with 1 × phosphate-buffered saline (PBS) (Thermo Scientific, Waltham, MA, USA) or only 1 × PBS (control treatment) was added to the roots of 25-day-old tomato seedlings. For potato, all seed tubers were first cleaned with water, treated with 100 ppm gibberellic acid, and dried for eight days to promote bud initiation. Prior to planting in 2 L pots (containing 650 g nursery soil), the seed tubers were inoculated with bacteria suspension at OD600 0.2 prepared with 1 × PBS or with only 1 × PBS for 15 min.
The inoculation was repeated twice, once at 10 and 30 days after transplanting (DAT) for tomato or days after planting (DAP) for potato with 35 ml bacterial suspension (OD600 0.2) per plant in both crops to ensure the growth of bacteria in the soil. The liquid was poured 2-3 cm from each plant’s base and 5 cm deep in the soil. For the duration of the experiments, the plants were grown in two separate growth chambers in 16 h light/8 h dark photoperiods supplemented with 250 µmol m-2 s-1 light, temperatures of 20-23°C and 60% humidity for 60 days. The plant positions within the growth chambers were randomly rearranged at least twice a week.
2.3 Phenotyping
To determine the effects of selected rhizobacteria on plant growth, parameters of plant height, total plant dry weight and yield were measured. Plant height was measured from the soil surface to the tip of the plant every ten days from planting until harvest. The yield of tomato and potato were measured by total fruit or tuber number and total fresh weight. Firstly, fruit or tubers were harvested, counted, and weighed. Then, the shoot and root systems were separated. Leaves were separated from the plant by cutting the leaf petiole, and the stems were cut. The number of leaves was counted and recorded. The intact roots were gently shaken to remove soil, briefly washed with water, and gently blotted dry using paper towels. Then, immediately the roots, leaves, stems, and fruits/tubers were separately placed in paper bags and oven-dried at 65°C until they reached constant weight. Finally, the dry weight was measured using a precision scale and recorded.
2.4 Chlorophyll content index measurement
The chlorophyll content of tomato and potato plants grown in the growth chamber were measured at harvest using the MC-100 Chlorophyll Concentration Meter (Apogee Instruments Inc., Logan, UT, USA). The MC-100 was calibrated to measure chlorophyll concentration with units of chlorophyll content index (CCI) and zeroed before commencing measurement according to the manufacturer’s instructions (Apogee Instruments, 2018). For tomato plants, the measurements were made by clipping the sensor onto the second terminal leaflet on the fifth fully expanded leaf from the top of each plant (Matsuda et al., 2014). For potato plants, the measurements were made on the top point of the top leaflet of the 4th compound leaf (Li et al., 2012).
2.5 Soil sampling and analysis
To evaluate the effects of rhizobacteria applications on soil nutrient content, rhizosphere soils were collected and pooled from all pots of each bacterial treatment for analysis. For the control treatment, soils were collected from eight randomly selected non-inoculated plants. 30g of dried soil samples were sent to the LMI AB testing laboratory (Helsingborg, Sweden) for analysis. The soils sampled before planting and at harvest were analyzed for pH, soil organic matter (SOM), electrical conductivity (EC), total nitrogen, available phosphorus (P), and available potassium (K).
2.6 In vitro growth conditions for plants
Tomato seeds or potato explants were grown in half-strength Murashige and Skoog (MS) culture medium (M0221; Duchefa Biochemie B.V., Haarlem, The Netherlands), pH 5.8, supplemented with 0.25% (w/v) Phytagel (Sigma-Aldrich Co., St. Louis, MO, United States), 0.05% (w/v) 2-(N-morpholino)ethanesulfonic acid (MES) and 1% sucrose (w/v) (Duchefa Biochemie B.V., Haarlem, The Netherlands). For SLU99 treatment, bacteria were cultured overnight in LB until an OD600 of 2 was reached. Cell-free supernatant was obtained by centrifuging at 3000 × g for 10 min, filtered through a 0.22-μm filter and mixed with ½ MS medium at 1:10 ratio before plating. For mock treatment, 0.22-μm sterile filtered liquid LB media was mixed with ½ MS media at 1:10 ratio before plating seeds or explants. Tomato var Moneymaker seeds were obtained from Plantagen AB and were surface sterilized with 3% (v/v) sodium hypochlorite for 5 min and washed three times with sterile water. Seeds were then plated either on control or SLU99 supernatant supplemented ½ MS plates and stratified for 48 h at 4°C. For potato plants, the explants from sterile potato plants were grown either on mock-treated or SLU99 supernatant supplemented ½ MS media in plant tissue culture boxes. The plants were grown in a growth chamber with 16 h light/8 h dark photoperiods and temperatures of 20-22°C.
2.7 Transcriptome analysis
For RNA-seq analysis, leaf or root samples were collected for each treatment and frozen in liquid nitrogen. Total RNA was extracted from three biological replicates using the RNeasy Mini Kit (Qiagen). Each biological replicate represented a pool of leaves or roots from three individual plants. The quality and integrity of the RNA was measured using an Agilent Bioanalyzer 2100 (Agilent, California, USA). Library preparation was carried out using a TruSeq RNA poly-A selection kit (Illumina, Inc.). Sequencing was performed at National Genomics Infrastructure (NGI), Stockholm on an Illumina NovaSeq6000 S4 platform. Adapter sequences and poor quality reads (<Q30) were removed using BBduk (Bushnell et al., 2017). Next, cleaned data were fed into STAR (Dobin et al., 2013) for alignment against the reference genome. FeatureCounts (Liao et al., 2014) was used for the quantification of aligned reads. Finally, R package DEseq2 (Love et al., 2014) was used for the analysis of differential gene expression and normalization. For volcano plots, R package ggplot2 (Ito and Murphy, 2013) was used. Pathway and enrichment analysis of differentially expressed genes was carried out with the R package clusterProfiler (Wu et al., 2021).
2.8 RT-qPCR analysis
Total RNA (1 μg) extracted for the purpose of transcriptome analysis was used for reverse transcription using qScript cDNA synthesis kit (Quantabio, Beverly, MA) according to the manufacturer’s instructions. 4 μL of 10-fold diluted cDNA was used as a template for the real-time PCR. Quantitative PCR was performed using DyNAmo Flash SYBR green kit (Thermo Scientific, Waltham, MA) according to the manufacturer’s instructions with three biological replicates and three technical replicates for each biological replicate. Relative expression levels of each of the target genes were normalized to that of tomato tubulin (SlTub) gene for quantification using the ΔΔCT method (Livak and Schmittgen, 2001).
3 Results
3.1 Plant growth-promoting traits of P. fluorescens and Serratia species
We investigated six bacterial strains with biological control activity, P. fluorescens SLU99, Serratia rubidaea EV23, AV10, Serratia plymuthica AS13, S412, and Serratia proteamaculans S4. To evaluate the growth promotion activity of these bacteria, potato plants grown in field conditions were subjected to treatment with these bacteria. Four out of the six tested strains, SLU99, EV23, AV10 and S412 resulted in significantly increased plant height, compared to the water treated control (Figure 1).
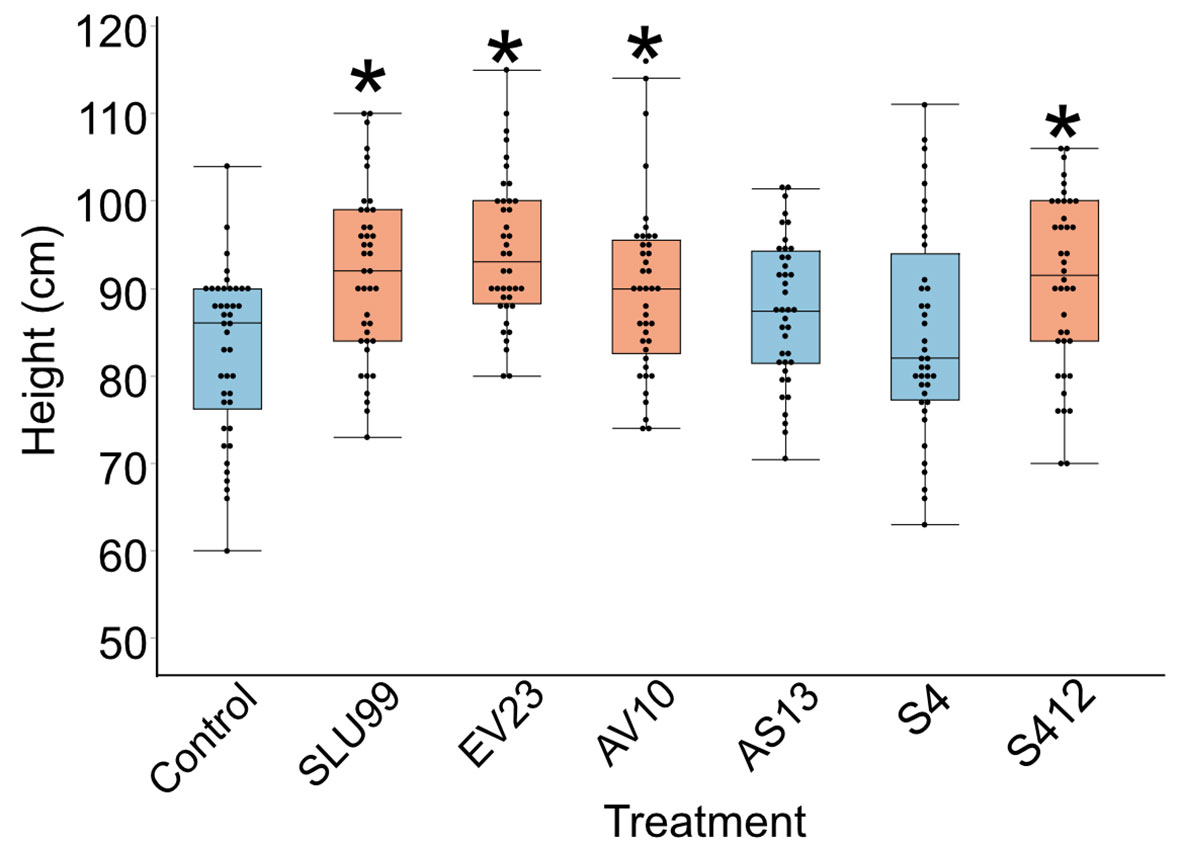
Figure 1 Effects of bacterial inoculations on the height of potato plants grown under field conditions. Strains used were Pseudomonas fluorescens SLU99, Serratia rubidaea EV23 and AV10, S. plymuthica S412 and AS13, and S. proteamaculans S4. Asterisks indicated statistical significance (* p < 0.001; Dunnett’s test, n=40 plants).
To examine the effectiveness of plant growth promotion, potato and tomato plants grown under greenhouse conditions were inoculated with the four selected strains. Inoculation with P. fluorescens SLU99 significantly increased plant height, total dry weight, and yield of tomato plants by 13.3%, 34.5%, and 183.9%, respectively, compared to non-inoculated plants. Meanwhile, S. rubidaea AV10-treated tomato plants had 12.3% greater total dry weight than non-inoculated plants. On the other hand, inoculation with S. rubidaea EV23 and S. plymuthica S412 had no significant effect on all morphological parameters tested on tomato plants (Table 1; Figures 2A–F). Furthermore, inoculation with both SLU99 and EV23, individually, significantly increased potato plant height, yield, and total plant dry weight (Table 1). Inoculation with AV10 promoted plant growth as total plant dry weight but not plant height, and vice versa for S412 on potato. Nevertheless, all inoculated plants had significantly higher tuber yields compared to the controls. The highest increase in yield for potato was recorded for EV23 (15%) and SLU99 (13.7%), followed by AV10 and S412 treated plants with 11.3%, and 10.7%, respectively (Table 1).
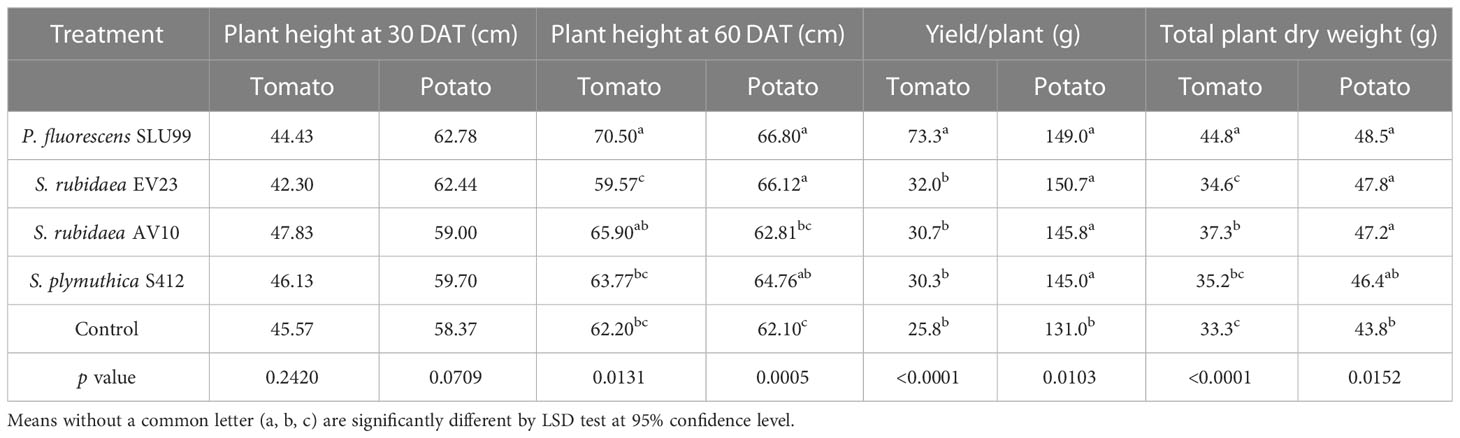
Table 1 Plant height at 30 and 60 days after transplanting, yield, and total dry weight of tomato and potato plants inoculated with P. fluorescens SLU99, Serratia rubidaea EV23, AV10, S. plymuthica S412 and control (mock inoculation) under greenhouse conditions.
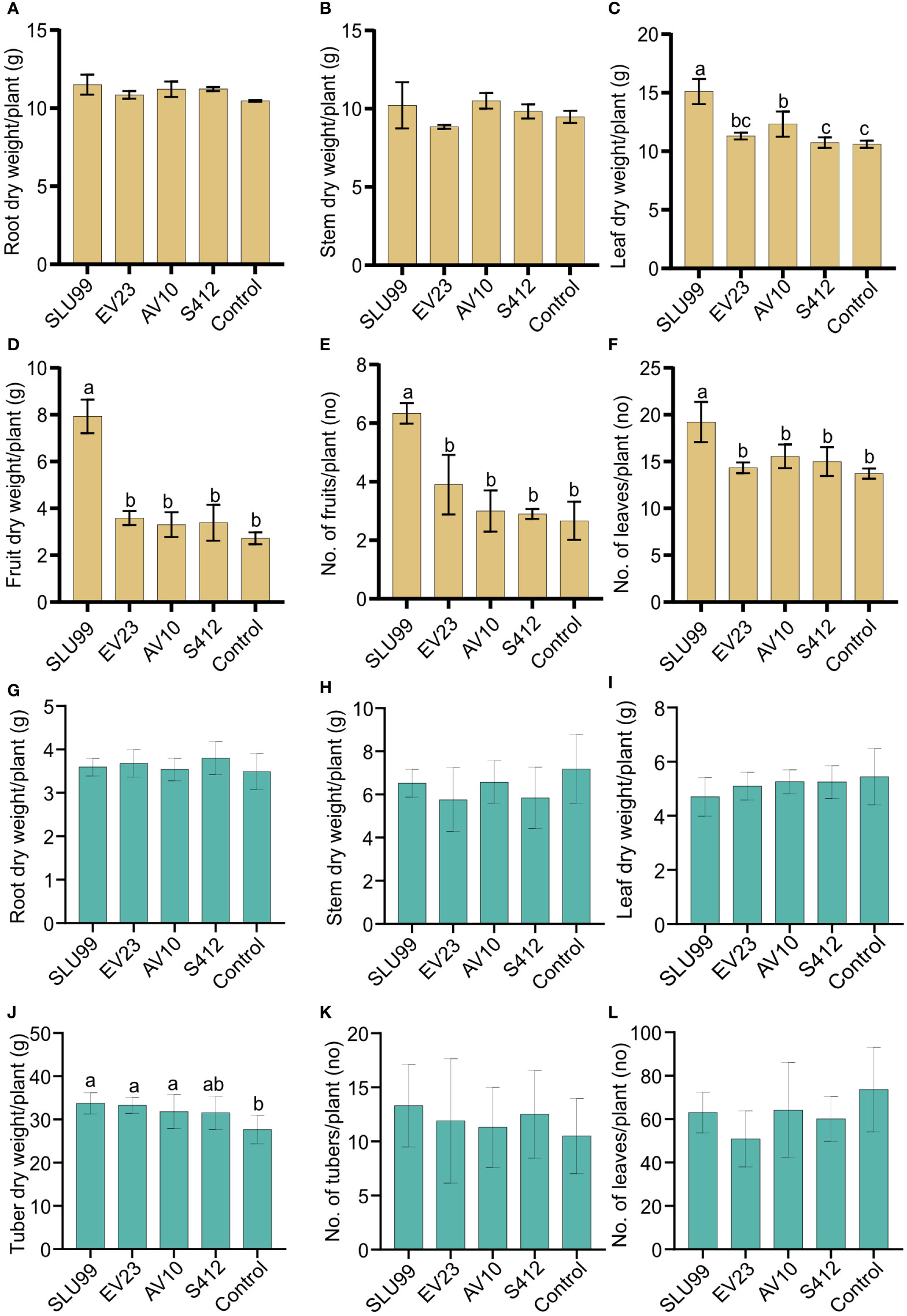
Figure 2 Effects of bacteria inoculations on the phenotypic characteristics of tomato and potato plants. Dry weight of different tomato (A–D) and potato (G–J) plant parts, and tomato fruit (E), potato tuber (K) and number of tomato and potato leaves (F, L) per plant. Yellow and green color bar plots represent measurements for tomato and potato, respectively. Means of root and stem dry weight were not significantly different among treatments. Strains used were Pseudomonas fluorescens SLU99, Serratia rubidaea EV23 and AV10, S. plymuthica S412. Means within the same bar graph without a common letter are significantly different by LSD’s test at 95% confidence level. Each value is the mean of nine and ten biological replicates per treatment for tomato and potato plants, respectively. Error bars represent standard deviations (SD).
Plant height increased rapidly during the vegetative stage until fruit set (40 DAT), or until the start of tuber initiation (30 DAP) for tomato and potato plants, respectively, and was not significantly different among treatments. Thereafter, during the fruit growth stage (50-60 DAT), a significant effect of bacteria inoculation on tomato plant height was observed (P=0.0431 and P=0.0131 at 50 and 60 DAT, respectively) (Table 1; Supplementary Figure 1B). In contrast, a 4.2% reduction in tomato plant height was observed when plants were treated with EV23 (Table 1). Applications of SLU99 resulted in increased tomato leaf (Figure 2E) and fruit number (Figure 2F) compared to other treatments. Consequently, SLU99-treated tomato plants had 39.7% and 191.2% more leaf and fruit dry weights, respectively, compared to non-inoculated plants.
For potato plants at 30 DAP, swelling stolon tips were observed in all treatments suggesting tuber initiation had started. The effect of bacteria inoculation on potato plant height was only significant during the tuber bulking stage (40-60 DAP) (Table 1; Supplementary Figure 1). In addition, all bacteria except S412 significantly increased tuber dry weight over control plants (P=0.0003) (Figure 2J). However, the dry weight of other plant parts was not enhanced by bacteria treatments (Figures 2G–I, K, L).
Relative chlorophyll content was significantly higher after SLU99 application in tomato (P=0.0188) and potato plants (P=0.0287) but was not elevated after EV23, AV10 and S412 treatments compared to the control (Figure 3).
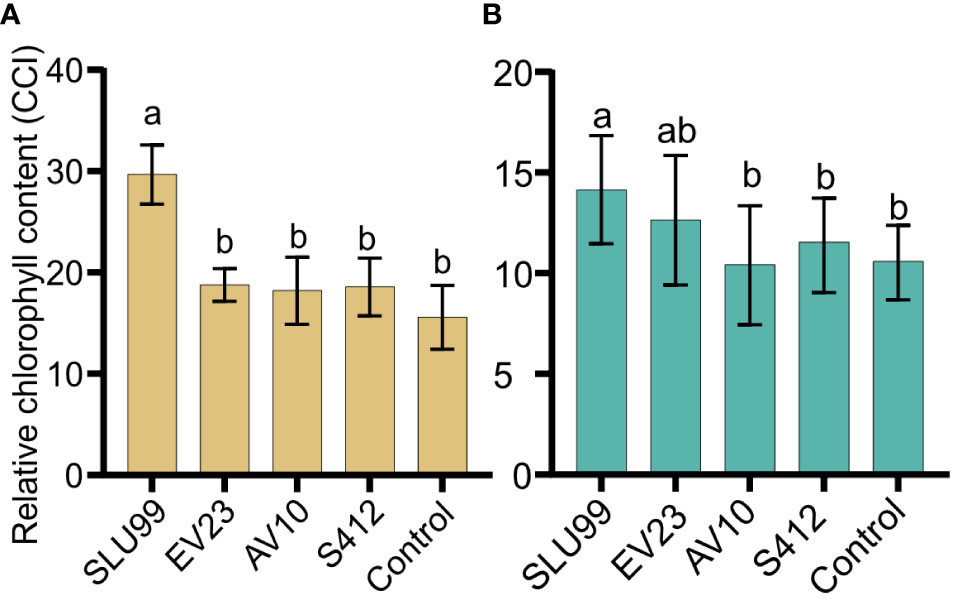
Figure 3 Relative chlorophyll content of tomato (A) and potato (B) plants inoculated with rhizobacteria. The values presented are mean values of relative chlorophyll content in the unit of Chlorophyll Content Index (CCI) measured using an Apogee MC-100 Chlorophyll Concentration Meter at harvest. Each value is the mean of 90 readings from nine biological replicates for tomato and 100 readings from ten biological replicates for potato per treatment. Means within the same bar graph without a common letter are significantly different by LSD’s test at 95% confidence level.
Additionally, at harvest, inoculation with SLU99 resulted in 3.4% higher total nitrogen (TN) in the tomato grown soil, while inoculation of EV23, AV10 and S412 resulted in 10.7%, 7.7% and 24.3% lower TN over control treatment, respectively (Supplementary Table 1). The elements P and K were slightly increased in tomato grown soil after SLU99 treatment, whereas EC and SOM were decreased. In potato grown soil, all bacterial treatments increased available K over the control by 22.2%-66.7%. TN in potato grown soil decreased in a small range after SLU99, EV23 and AV10 treatments (2.1%-6.2%). Meanwhile, the available P, pH, EC, and SOM at harvest of soils grown with potato were not affected by bacteria treatments (Supplementary Table 1). In summary, inoculation of SLU99 significantly promoted the growth of tomato and potato plants, and EV23 promoted the growth of potato plants grown in a controlled environment as reflected by high chlorophyll content, total plant biomass and yield.
3.2 Effects of rhizobacteria inoculation on growth of in vitro grown tomato and potato plants
To gain insights into the mechanisms behind growth promotion, tomato and potato plants were grown in MS media supplemented with the sterile-filtered bacterial culture supernatant. When compared to the mock treatment, tomato seeds grown in the SLU99-supernatant supplemented media showed a higher germination rate, increased root growth and shoot height, and enhanced number of lateral roots (Figures 4A, B). Potato explants grown in the media supplemented with SLU99 supernatant resulted in increased shoot height by 24.6% (p = 0.02) compared to the control (Figures 4C, D). SLU99 treatment also increased root growth and resulted in the formation of adventitious roots and secondary adventitious roots (SAR), which emerge from aerial parts (Figure 4D).
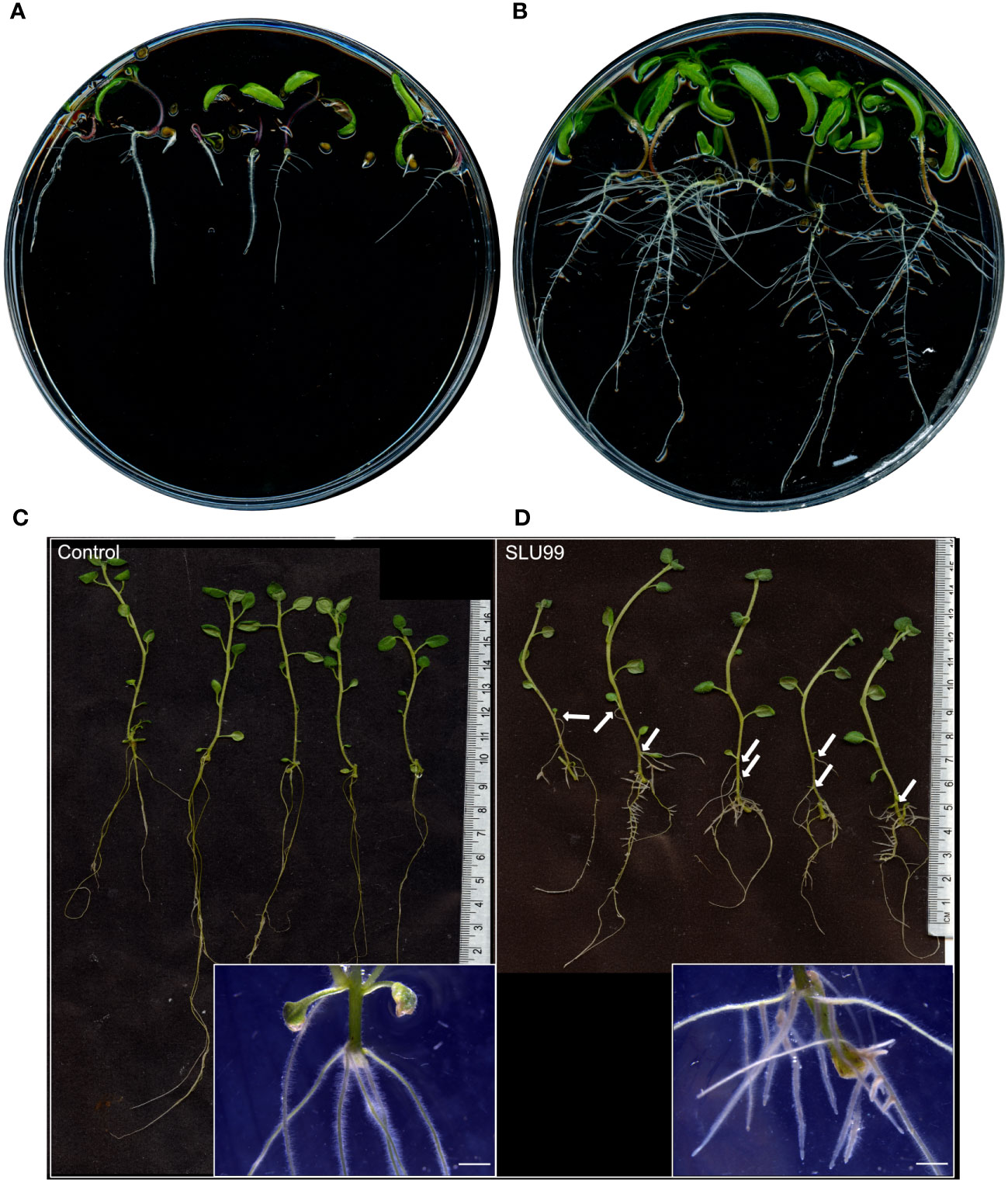
Figure 4 Effect of Pseudomonas fluorescens SLU99 on plants grown in vitro. Growth of tomato seedlings upon treatment with culture supernatant of SLU99 (B) compared to mock treatment (A). Growth of potato plants treated with P. fluorescens SLU99 (D) compared to control treatment (C). Arrows indicate secondary adventitious roots developed upon treatment with SLU99. Insets in (C, D) are representative stereo microscope images at root induction. The scale bar in the inset images represents 1 mm.
3.3 Differentially expressed genes (DEGs) and KEGG pathway enrichment analysis
To gain insights into the mechanisms of SLU99-mediated growth promotion in tomato and potato plants, RNA extracted from the roots and leaves grown in the media supplemented with the supernatant of SLU99 was subjected to transcriptome profiling. A total of 1193 and 2226 genes were differentially expressed (log2FC >1.5, p < 0.05) in the roots of tomato and potato, respectively, compared to the control samples (Table 2; q-value or padj value information can be found in Supplementary Excel files 1–4). Among them, 1076 and 996 genes were upregulated, and 117 and 1230 genes were downregulated in the respective samples (Table 2; Figures 5A, B). In tomato leaves, a total of 1732 DEGs were detected (log2FC >1.5, p < 0.05) with 1322 genes upregulated and 410 genes downregulated (Figure 5C). For potato leaves, we identified 959 (log2FC >1.5, p < 0.05) when treated with SLU99 compared to the control samples. Of the identified DEGs, 206 were upregulated, and 753 genes were downregulated (Table 2; Figure 5D).
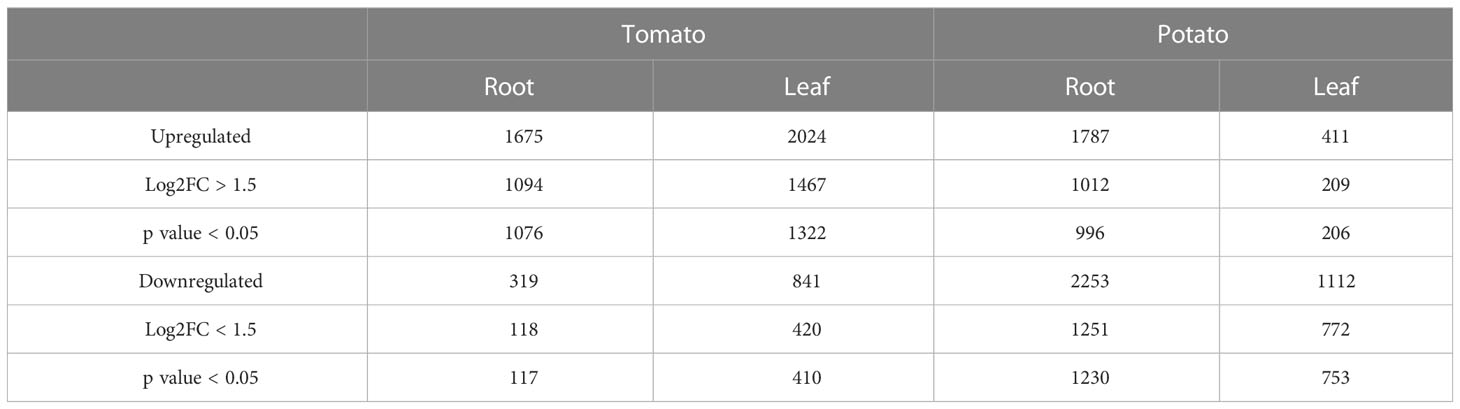
Table 2 Characteristics of the DEGs in tomato and potato plants treated with Pseudomonas fluorescens SLU99 culture supernatant.
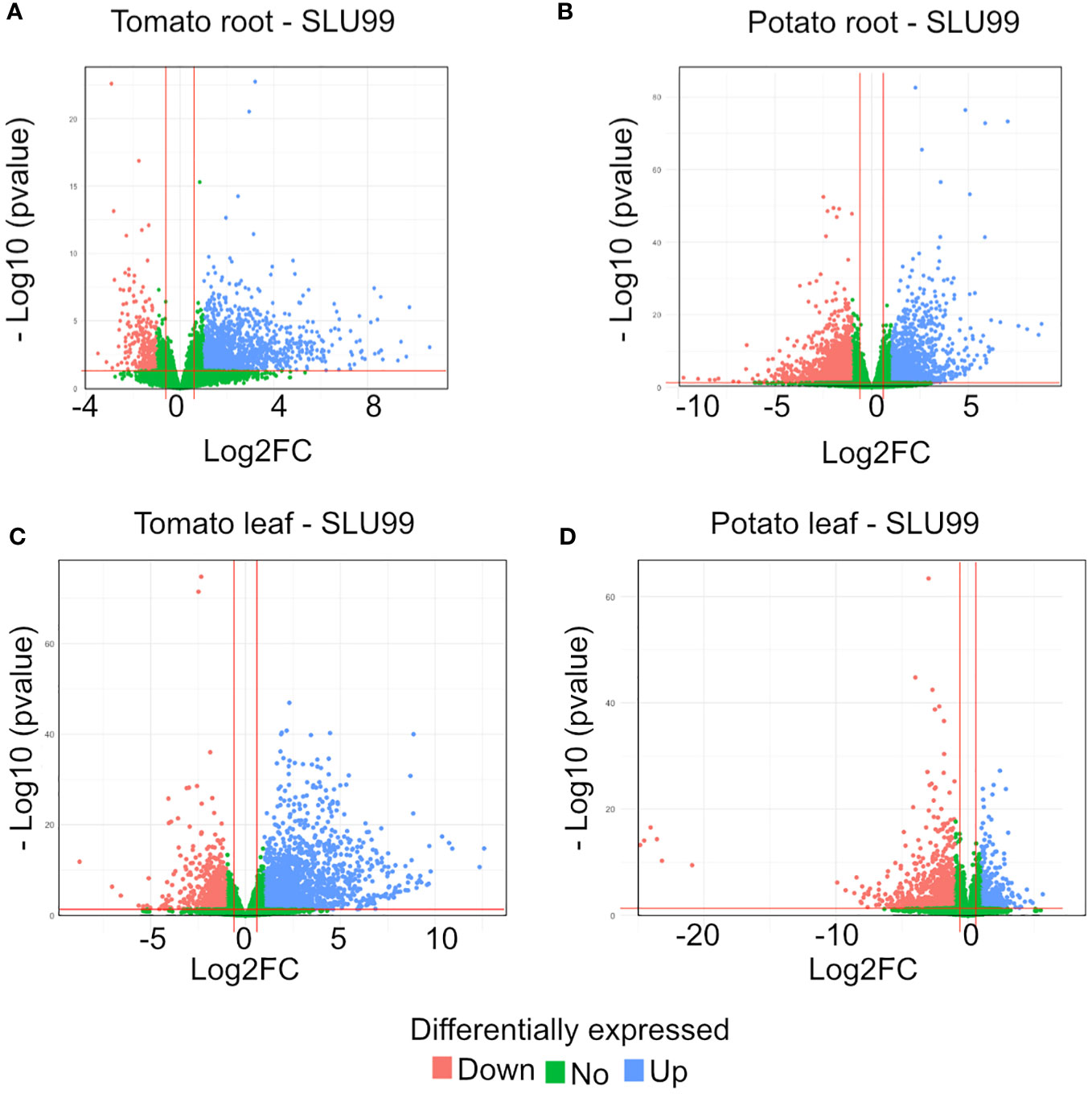
Figure 5 Volcano plot of differentially expressed genes (DEGs) for tomato and potato roots (A, B) and leaves (C, D) treated with the culture supernatant of Pseudomonas fluorescens SLU99, compared to the mock treatment. Upregulated genes are shown as blue dots at the right side of each plot; downregulated genes are shown as pink dots at the left side of each plot; non-differentially expressed genes are shown as green dots clustered at the centre (centred around Log2FC 0) of each plot.
We performed KEGG pathway enrichment analysis to gain a better understanding of the functional categories of the DEGs in response to the PGPR culture supernatant treatment. In the treated root samples, a total of 300 and 753 genes were grouped into enriched pathways in tomato and potato, respectively, and a total of 382 and 259 genes were grouped into enriched pathways in the treated leaves of tomato and potato, respectively. Treatment resulted in enrichment for genes involved in plant hormone signal transduction pathway (37 and 33 genes, respectively), zeatin biosynthesis (15 and 13 genes), plant-pathogen interactions (37 and 25 genes) and MAPK signaling pathway (30 and 19 genes) in both tomato and potato leaves. Additionally, a total of 40, 76, 44 and 25 genes related to the phenylpropanoid biosynthesis pathway were enriched in the roots and leaves of tomato and potato upon treatment with SLU99 culture supernatant (Figure 6).
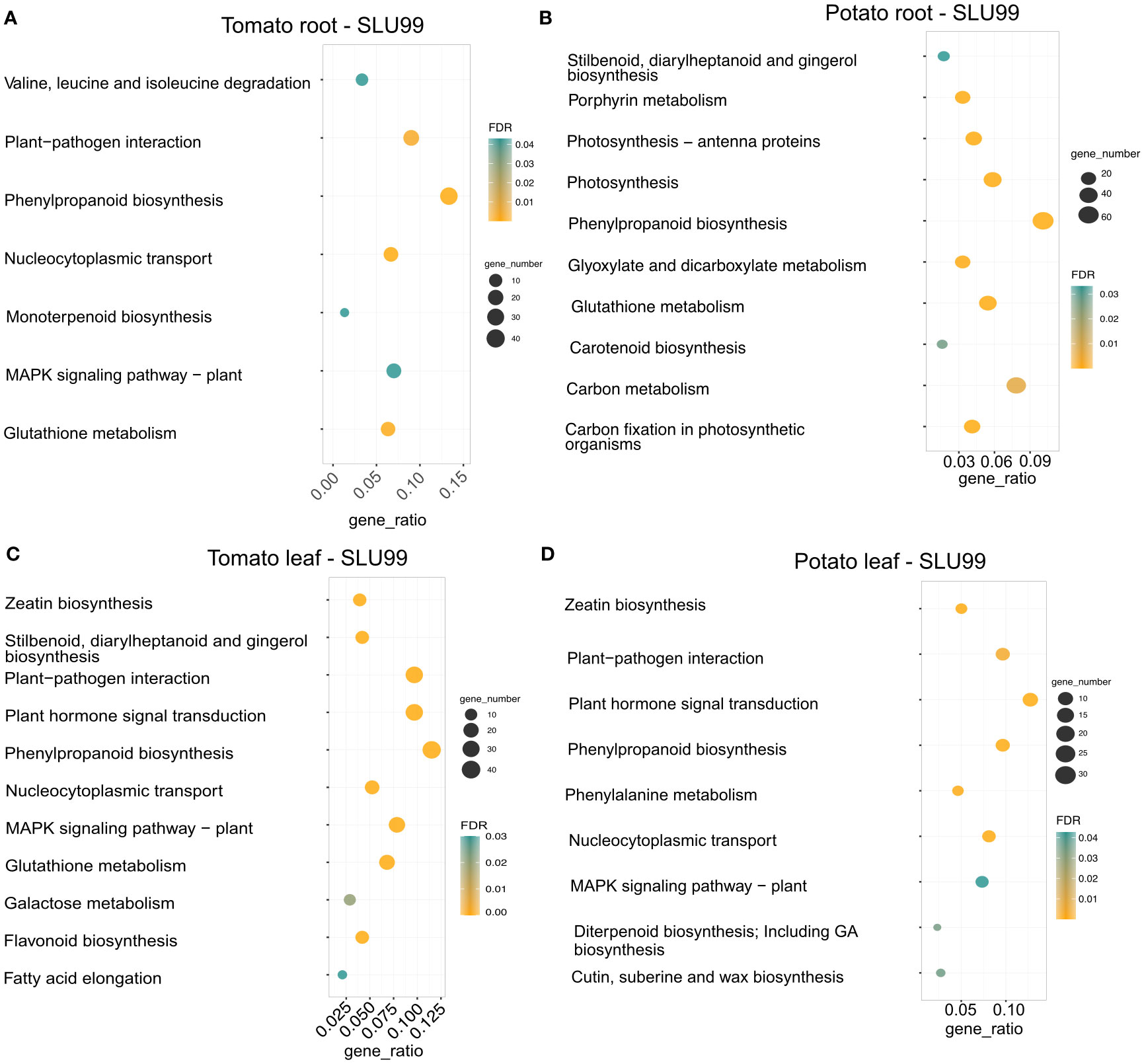
Figure 6 KEGG enrichment analysis of DEGs in the transcriptomes of tomato and potato roots (A, B) and leaves (C, D) treated with the culture supernatant of Pseudomonas fluorescens SLU99, compared to the mock treatment. The size of the circles in each plot represents the number of DEGs annotated for that pathway or process. The gene ratio on the x-axis represents the ratio of the count of core enriched genes to the count of pathway genes.
To compare the effects of the tomato and potato samples, we examined the overlap of the DEG products among the roots and leaves. The analysis showed an overlap of 164 gene products in the root samples, and 95 in the leaf samples upon SLU99 treatment in potato and tomato plants (Figures 7A, B). Within the species, there was an overlap of 515 gene products between root and leaf samples of tomato, while 265 similar gene products were responsive in the root and leaf samples of potato (Figures 7C, D).
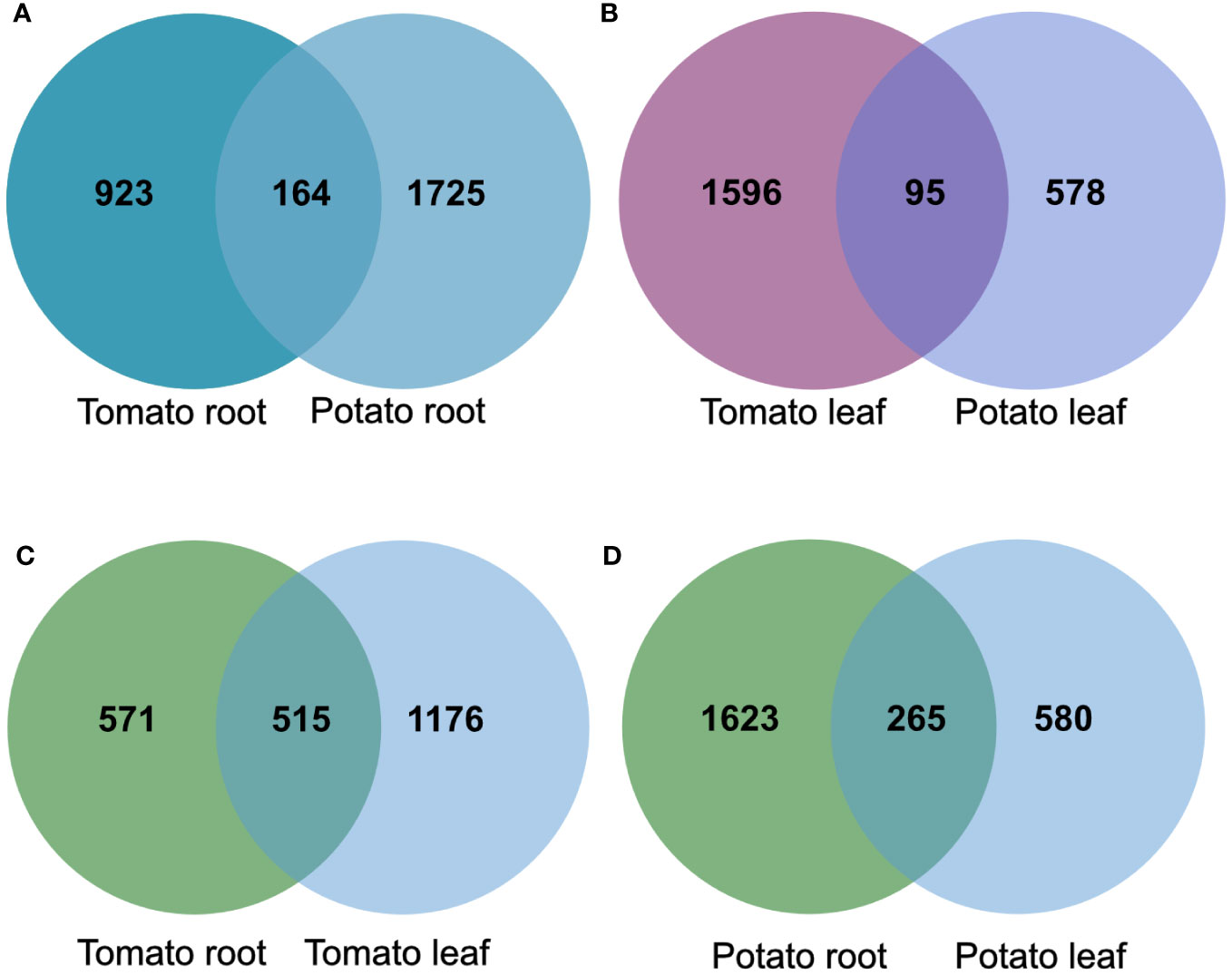
Figure 7 The overlap of products of differentially expressed genes (DEGs) in tomato and potato roots (A) and leaves (B), root and leaf samples of tomato (C) and potato (D) after treatments with Pseudomonas fluorescens SLU99 supernatant.
3.4 SLU99 regulates phytohormonal biosynthesis and signal transduction pathways
One of the major objectives of this study was to elucidate the changes in the host transcriptomes that are important for PGP activity. Phytohormones, as a result of their complex interaction and crosstalk, regulate various cellular processes involved in plant growth and development. The gibberellins (GAs) play an important role in several developmental processes including seed germination. Upon SLU99 treatment in tomato root, gibberellin 3-beta-dioxygenase 1-like (GA3OX1), a gene involved in the biosynthesis of GA was upregulated (log2FC 1.9, p<0.05). On the other hand, in tomato leaves, gibberellin 2-beta-dioxygenase 8 (GA2ox8), a gene involved in the deactivation of GA, was upregulated (log2FC 1.6, p<0.01) (Figure 8).
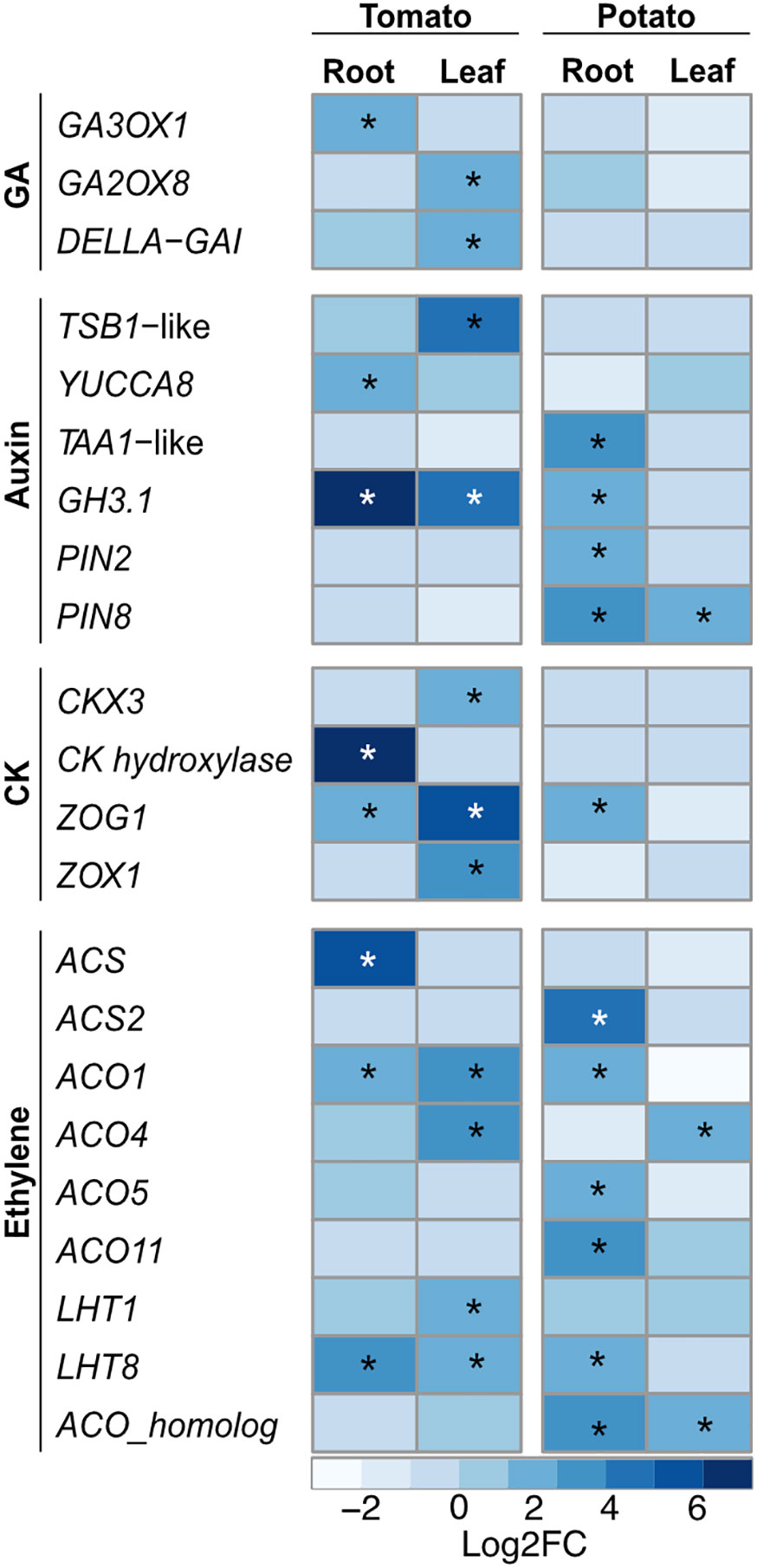
Figure 8 Heatmap of key differentially expressed genes (DEGs) involved in different phytohormonal pathways upon treatment with Pseudomonas fluorescens SLU99 culture supernatant. GA, gibberellic acid; CK, cytokinin. Asterisks indicated statistical significance (*p < 0.05).
The hormones auxin and CK are major players in the regulation of signaling pathways underlying plant growth and development. In tomato, tryptophan synthase beta subunit 1 (TSB1)-like, responsible for increased in tryptophan biosynthesis, and YUCCA8, responsible for the last enzymatic step from indole-3-pyruvic acid (IPA) to IAA were upregulated 16 and 3-fold (log2FC 4 and 1.6) in leaf and root samples, respectively. Additionally, in potato roots, SLU99 treatment resulted in increased expression (log2FC 3.3) of L-tryptophan-pyruvate aminotransferase 1 (TAA1)-like, which encodes an enzyme that converts L-tryptophan to IPA (Figure 8).
The activation of auxin biosynthesis genes and production of IAA by SLU99 (Kelbessa et al., 2022) prompted us to analyze the differences in the expression of genes involved in auxin signaling pathways. Major auxin-responsive genes such as Gretchen Hagen 3 (GH3) and small auxin up RNA (SAUR) are transcriptionally regulated by auxin at early stages of signal transduction. Following treatment with SLU99 culture supernatant, expression of the IAA-amido synthetase GH3.1 (GH3.1), important for maintaining auxin homeostasis increased significantly (log2FC 6.8, 4.6 and 1.6 in tomato root, tomato leaf and potato root, respectively) in comparison to the mock treatment. Within the SAUR family, five and seven genes were differentially expressed in the tomato and potato plants, respectively. Additionally, PIN2, a root-specific auxin transporter and PIN8, a constitutively active auxin transporter were also upregulated in the potato plants (Figure 8).
The gene encoding CK hydroxylase, an enzyme that catalyzes the biosynthesis of trans-zeatin (tZ), a biologically active CK, was strongly upregulated (log2FC 7.5) upon treatment with SLU99 in tomato roots. On the other hand, in tomato leaf CK dehydroxygenase 3 (CKX3), involved in the degradation of CK was upregulated 4-fold (log2FC 2) compared to the mock treatment. Furthermore, zeatin O-glycosyltransferase (ZOG1) and zeatin O-xylosyltransferase (ZOX1) were upregulated in tomato leaves (log2FC 5.4 and 3.5), while only ZOG1 was upregulated in the tomato and potato roots (log2FC 2.1 and 1.6). ZOG1 and ZOX1 are involved in the conversion of tZ to a stable and reversible O-glycosylzeatin and O-xylosylzeatin, respectively.
The PGP activity of bacteria is also attributed to the changes in the plant hormone ethylene (Poupin et al., 2016). Since SLU99 displayed ACC deaminase activity, we examined changes in the expression of genes encoding the enzymes of the ethylene biosynthesis pathway. 1-aminocyclopropane-1-carboxylate_synthase-like (ACS) involved in the synthesis of ACC, a direct precursor of ethylene, and 1-aminocyclopropane-1-carboxylate_oxidase (ACO) subsequently oxidizes ACC to ethylene. In tomato, ACS and ACO1 were upregulated in roots (Log2FC 5.1 and 1.8, respectively), while ACO1 and ACO4 were upregulated in leaves (Log2FC 3.2 and 2.6, respectively). In potato, ACS2 was upregulated in roots (log2FC 4.1), while ACS4 was upregulated in leaves (log2FC 2.2). ACO homologs were upregulated both in the roots and leaves (log2FC 2.9 and 1.7, respectively), while ACO1, ACO5 and ACO11 were upregulated in leaves (log2FC 2.1, 2.1 and 3.3, respectively). Lysine histidine transporters (LHT) are associated with the transportation of ACC. When treated with SLU99 supernatant, LHT8 was upregulated in both tomato and potato roots and tomato leaves by 3.6, 2.5 and 2.5-fold (log2FC), respectively, while LHT1 was upregulated only in tomato leaves (log2FC 1.6). To validate the observed gene expression differences in the NGS data we employed RT-qPCR. We analyzed three key genes involved in the ethylene pathway, SlACS, SlACO1, and SlLHT8 that showed increased gene expression following SLU99 treatment (Supplementary Figure 2), in agreement with the findings from RNA-seq. Taken together, these results indicate that treatment with the culture supernatant from SLU99 stimulates the accumulation of phytohormones auxin, CK and ethylene.
3.5 Reprogramming of host transcriptional networks
The discovery of several DEGs involved in signal transduction pathways in response to SLU99 treatment suggests strong regulation of host transcription factor networks. To investigate the interaction of SLU99 with the plant transcriptional network, DEGs encoding TFs were identified and assigned to families using the plant transcription factor database. In total, DEGs belonging to 24 different TF families were identified with the greatest number of DEGs identified in the root samples compared to the leaf samples (Supplementary Table 2). In the tomato and potato roots, a total of 112 and 182 TF encoding DEGs were found, whereas 62 to 92 DEGs were found in leaf samples, implying significant reprogramming of the host transcriptional network. The HD-ZIP TF genes were exclusively upregulated in tomato plants (11 upregulated and 2 downregulated), while 14 HD-ZIPs were downregulated in potato. Notably, the strongest upregulation was found for ATHB12 (log2FC 9.6) followed by ATHB40-like (log2FC 6.4), and ATHB7-like (log2FC 3.4) in tomato leaves. ATHB12 and ATHB7, considered paralogs, belong to the class-I HD-ZIP TF family and were also upregulated in root samples (log2FC 2.1 and 2.0, respectively). ATHB40, on the other hand, is a class-II HD-ZIP TF that was upregulated in the root (log2FC 3.7). ATHB12 and ATHB7 are associated with root elongation and leaf development, albeit at different stages (Ré et al., 2014; Hur et al., 2015). ATHB40 is a negative regulator of primary root development (Mora et al., 2022).
In addition to the PGP-related TF encoding DEGs, we also identified several DEGs encoding TFs involved in defence-related pathways, namely, WRKY, MYB, MYC, HSF, and NAC TFs. WRKY TFs are global regulators of host responses to phytopathogens. Treatment with SLU99 culture supernatant triggered differential expression of 14 WRKY genes in tomato and 11 genes in potato. Two of the WRKY TF encoding genes, WRKY30 and WRKY45, involved in defence against biotic and abiotic stresses, were upregulated in common between tomato and potato. WRKY6, WRKY55, and WRKY71 were uniquely upregulated in tomato leaf and, along with WRKY33B and WRKY75, are involved in defence against necrotrophic fungal pathogens. WRKY40 and WRKY41, genes involved in abiotic stress tolerance, were strongly upregulated in tomato leaves (log2FC 9.0 and 4.5, respectively), and were downregulated in potato leaves upon SLU99 treatment (log2FC -2.1 to -2.7). The MYC2 TF upregulated (log2FC 5.1) in tomato roots upon SLU99 treatment indicates a role in JA-mediated induced systemic resistance (ISR). Furthermore, treatment with SLU99 induced expression of several R-gene orthologs such as Cf-2,2-like (log2FC 2.2), Cf-9 (log2FC 3.4), RPM1 (log2FC 1.7), RPS5 (log2FC 4.1), CSA1 (log2FC 4.8) and Alternaria stem canker resistance (log2FC 1.6) in tomato leaves, LEAF RUST 10 DISEASE-RESISTANCE LOCUS RECEPTOR-LIKE PROTEIN KINASE-like 1.1 (LRK10) (log2FC 2.9 and 5.3 in root and leaf, respectively), TMV resistance protein N gene (log2FC 6.0) in tomato root and putative late blight resistance protein homolog R1A-10 (log2FC 1.6) and R1A-3 (log2FC 1.5) in potato leaves.
4 Discussion
Several soilborne rhizobacteria have long been known to promote plant growth across a wide range of plant species. In this study, six bacterial strains were chosen for their biocontrol potential and tested on potato plants in a late-blight hotspot field. Four of the strains that directly impacted the height of the potato plant in the field were chosen for detailed examination in a controlled environment to further evaluate their growth-promoting function. These strains included P. fluorescens strain SLU99, S. rubidaea strains EV23 and AV10, and S. plymuthica S412.
In the growth chamber, all four strains enhanced at least two growth variables in potato plants, indicating that all tested strains significantly impact potato growth, which is consistent with the field evaluation. However, the effect of these strains on the dry matter accumulation is only noticeable in the tuber, which accounts for more than 60% of the total dry weight, suggesting that PGPR treatment promotes photosynthate translocation into tubers. In contrast, growth-promotion activity in tomato plants is strain-dependent with SLU99 and AV10 improving plant height and total dry weight. Furthermore, SLU99 improved chlorophyll content, total leaf number and leaf area, suggesting enhanced light interception and photosynthesis rate explaining the obtained higher tomato fruit yield.
Intriguingly, although all four strains are favorable to potato yield, only SLU99 is significantly beneficial to tomato yield. Previous research found that bacterial consortiums aid plant growth by enhancing stress tolerance (Silambarasan et al., 2019; Yang et al., 2021; Kelbessa et al., 2023). Recently, results from our group demonstrated that SLU99 is compatible with strains EV23 and AV10 (Kelbessa et al., 2022), implying that these bacteria could co-exist as a consortium in the rhizosphere. Considering that all these strains are potent biocontrol agents (Kelbessa et al., 2022), a bacterial consortium of these strains could act synergistically to contribute to reducing disease under natural conditions. Moreover, plant exudates attract rhizobacteria to colonize the roots (Bais et al., 2006). It has been demonstrated that the composition of root exudates varies between cultivars of the same species (Zhang et al., 2020) and thus cultivar-specific traits might have contributed to a negative regulatory effect of the colonization of Serratia species in the tomato variety used in this study.
PGPR also enhance the mobilization of locally available nutrients for plant uptake by solubilizing P, K, and Zn, and nitrogen fixation (Prasanna et al., 2016). N and K are the most abundant nutrients in plant tissues (Sardans and Peñuelas, 2015). Potato cultivation takes up K in large quantities and is very important to gain a higher yield of marketable tubers (Khan et al., 2012). Our results suggest increased K in the soil following the treatment with PGPR, consistent with a previous study that showed Enterobacter cloacae, a PGPR, led to a higher amount of K in the soil (Ghadam Khani et al., 2019). However, further research validation is required to assess the impact of PGPR on soil nutrient contents. On the other hand, in tomato, TN, available P and K were slightly increased after SLU99 treatments, indicating that this strain may have facilitated nutrient availability for plant uptake (Meena et al., 2014).
Phytohormone-mediated signal transduction and their interplay regulate several physiological processes in plant growth and development. Phytohormones also mediate cellular responses during abiotic and biotic stress. Rhizobacterial-stimulated plant growth is intrinsically linked to the production of phytohormones, siderophores, and secretion of ACC-deaminase that reduces ethylene biosynthesis (Yang et al., 2009). Accordingly, KEGG pathway enrichment analysis suggested that treatment with SLU99 culture supernatant resulted in significant differential expression of genes involved in plant hormone signal transduction. Genes involved in the biosynthesis of zeatin, a naturally occurring CK that promotes cell division in plants, were significantly differentially expressed in both potato and tomato leaves upon SLU99 treatment. Strain SLU99 enhanced the expression of CK hydroxylase in tomato root suggesting an increase in the biosynthesis of trans-zeatin, which is reported to be transported through the xylem (Osugi et al., 2017). However, excess CK needs to be stored to be protected against CK oxidases. Conversion of zeatin to O-glucosyl- and O-xylosyl-zeatin, a reversible process, is important for the storage of CK. Upregulation of ZOG1 and ZOX1 genes in the current study suggests the CK is synthesized in excess and is stored in roots and leaves, upon SLU99 treatment in tomato.
The increase in the germination of the tomato seeds requires specific reprogramming of the GA pathway (Groot and Karssen, 1987). Moreover, the presence of GA in the root meristem and elongation zone is necessary for the normal growth of the root. GA3OX encodes a key enzyme involved in the last step in the biosynthesis of the GA and is reported to be expressed in the root elongation zone (Barker et al., 2021). SLU99 culture supernatant treatment of tomato plants resulted in an increased expression level of GA3OX, suggesting an increase in the GA in the roots. GA homeostasis is necessary for plant growth and development (Richards et al., 2003). GA 2-oxidation mediated by the GA2ox gene family is reported to be a major GA inactivation pathway that functions throughout Arabidopsis development (Schomburg et al., 2003; Rieu et al., 2008) and is necessary for maintaining GA levels in peach (Cheng et al., 2021). Upregulation of GA2ox8 in tomato leaves suggests PGPR aid in maintaining GA levels. Our results of increased expression of genes encoding DELLA-GAI and a slight increase of AP2/ERF in tomato leaves are in line with reports showing lowered GA levels are also associated with improved stress tolerance through DELLA-mediated activation of AP2/ERF (Colebrook et al., 2014; Castro-Camba et al., 2022).
It is well-established that several auxin-producing PGPR regulate auxin localization and distribution in the plant (Tsukanova et al., 2017). Rhizobacterial-produced auxin has also been shown to be important for PGPR-mediated morphological changes in plants (Spaepen et al., 2014). Treatment with these PGPR also resulted in increased levels of endogenous auxin and is associated with root growth. Indeed, local auxin maxima in the pericycle is necessary for the formation of lateral root primordia and their subsequent development into lateral roots. However, the effect of exogenous auxin on the root is concentration dependent. At higher concentrations, exogenous auxin inhibits root growth (Ivanchenko et al., 2010). Consequently, large amounts of auxin generated by some rhizobacteria strains, such as Enterobacter (Park et al., 2015), P. fluorescens (Zamioudis et al., 2013) and P. syringae (Loper and Schroth, 1986) inhibit primary root elongation in lettuce, Arabidopsis, and sugar beet, respectively. In short, while auxin is an important contributing factor to root growth, regulating endogenous auxin levels is also crucial. GH3 genes, upon induction by IAA accumulation, encode enzymes involved in the conjugation of free IAA to amino acids thereby maintaining auxin homeostasis (Staswick et al., 2005). Our study is consistent with earlier reports of P. fluorescens producing auxin and with increased lateral root formation (Chu et al., 2020; Ortiz-Castro et al., 2020). Additionally, treatment with SLU99 also impaired primary root elongation in potato plants. Taken together, our results support the hypothesis that treatment with SLU99, while increasing the endogenous IAA levels through upregulation of YUCCA8, and utilizing necessary auxin for lateral root development, also aided in maintaining its homeostasis through GH3.1 mediated conjugation of free auxin (Figure 9).
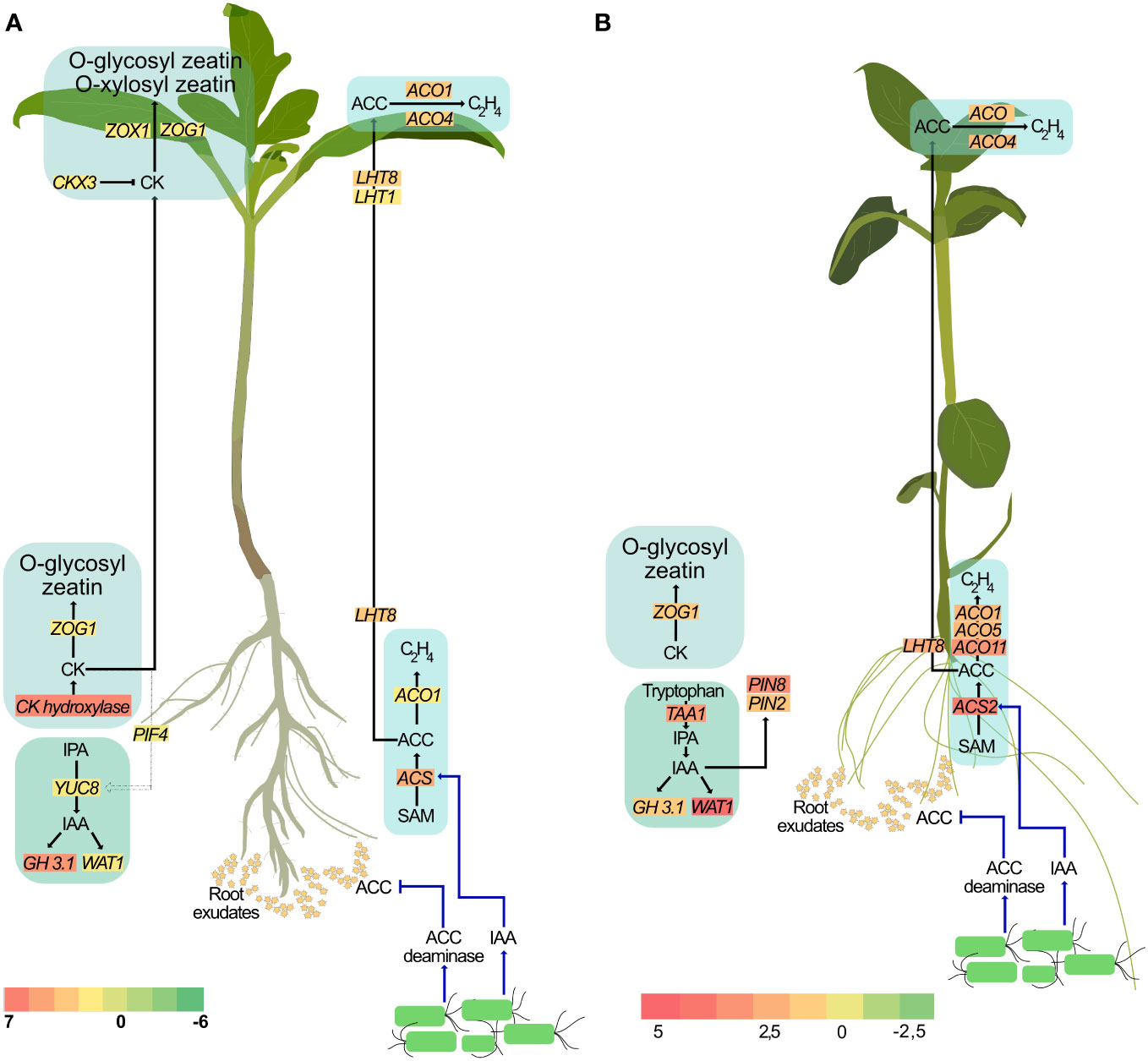
Figure 9 A proposed model illustrating the effect of P. fluorescens strain SLU99 on different phytohormonal pathways in tomato (A) and potato (B) plants.
Higher auxin levels are also associated with increased ethylene biosynthesis (Fa Yang and Hoffman, 1984). Regulating cellular ethylene content is a critical aspect of plant growth and development. Application of exogenous auxin is also reported to increase expression of ACS genes that encode enzymes involved in the conversion of S-adenosylmethionine to ACC, the ethylene precursor (Tsuchisaka and Theologis, 2004; Niu et al., 2022). Considering that PGPR produce auxin, it can be hypothesized that the auxin produced by PGPR result in increased expression of ACS, and thus might promote ACC production. Providing further evidence of ethylene synthesis, ACO1, which encodes ACC oxidase, the enzyme required for the synthesis of ethylene from ACC, is also upregulated upon SLU99 treatment. However, higher ethylene levels not only inhibit root development but also trigger an adaptive response such as growth inhibition and delayed flowering (Ravanbakhsh et al., 2018). PGPR belonging to diverse genera are reported to produce ACC deaminase, an enzyme that lowers host ACC levels (Glick, 2005). It is important to note that although bacteria produce ACC deaminase, due to the higher substrate affinity of ACC oxidase than ACC deaminase, the ethylene level in the plant cannot be totally eliminated (Glick et al., 1998). Intriguingly, two differentially expressed genes, LHT8 and LHT1, belonging to the lysine histidine transporter family that was previously linked to ACC transport (Shin et al., 2015; Vanderstraeten and van der Straeten, 2017) are upregulated in treated roots and leaves, respectively, suggesting that the excess ACC may be transported to the shoot (Figure 9). Further research using ethylene biosynthetic and signaling mutants is necessary to enhance our understanding of the involvement of PGPR in modulating ethylene levels during plant development.
Plant hormone homeostasis is critical in plant growth and development. Since PGPR can have a significant influence on hormone biosynthesis and signaling, plants need to cope with such differences. Upregulation of CKX3, GA2OX, and GH3 in PGPR-treated plants in our study might thus be an adaptation mechanism to increased hormone levels.
All of the rhizobacteria strains tested here have previously been shown to have biocontrol activity against the plant pathogen Phytophthora colocasiae (Kelbessa et al., 2022), both in culture and in planta. The activation of plant metabolite pathways (growth and defence) by the rhizobacteria, and their direct antagonistic activity against pathogens, suggests that these strains will have utility in sustainable agriculture by controlling serious crop diseases and boosting yield.
Data availability statement
The datasets presented in this study can be found in online repositories. The names of the repository/repositories and accession number(s) can be found below: Bioproject https://www.ncbi.nlm.nih.gov/bioproject/851815, accession https://www.ncbi.nlm.nih.gov/, SAMN29251990, SAMN29251991, SAMN29251992, SAMN29251993.
Author contributions
Conceptualization and designing the experiment: PK and RV. Methodology: NH, FG, RV, and PK. Data validation and analysis: NH, FG, SG, RV, and PK. Investigation: NH, PK, and FG. Resources: RV, RO, and PK. Writing - original draft preparation: NH and PK. Writing - review and editing: NH, RV, SW, SG, RO, and PK. Supervision and project administration: PK, RV, SW, and RO. Funding acquisition: PK, RV, and RO. All authors contributed to the article and approved the submitted version.
Funding
This research work was supported by FORMAS (2019-01316) and the Swedish Research Council (2019–04270), Novo Nordisk Fonden (0074727), Carl Tryggers Stiftelse (CTS 20:464), SLU’s Centre for Biological Control and Partneskap Alnarp (PA1365-2021). SW acknowledges funding from the Scottish Government Rural and Environment Science and Analytical Services (RESAS) Division.
Conflict of interest
The authors declare that the research was conducted in the absence of any commercial or financial relationships that could be construed as a potential conflict of interest.
Publisher’s note
All claims expressed in this article are solely those of the authors and do not necessarily represent those of their affiliated organizations, or those of the publisher, the editors and the reviewers. Any product that may be evaluated in this article, or claim that may be made by its manufacturer, is not guaranteed or endorsed by the publisher.
Supplementary material
The Supplementary Material for this article can be found online at: https://www.frontiersin.org/articles/10.3389/fpls.2023.1141692/full#supplementary-material
References
Abdelfattah, A., Tack, A. J. M., Wasserman, B., Liu, J., Berg, G., Norelli, J., et al. (2022). Evidence for host–microbiome co-evolution in apple. New Phytol. 234, 2088–2100. doi: 10.1111/NPH.17820
Ali, B., Sabri, A. N., Ljung, K., Hasnain, S. (2009). Auxin production by plant associated bacteria: impact on endogenous IAA content and growth of triticum aestivum l. Lett. Appl. Microbiol. 48, 542–547. doi: 10.1111/J.1472-765X.2009.02565.X
Amna, Din, B. U., Sarfraz, S., Xia, Y., Kamran, M. A., Javed, M. T., et al. (2019). Mechanistic elucidation of germination potential and growth of wheat inoculated with exopolysaccharide and ACC- deaminase producing bacillus strains under induced salinity stress. Ecotoxicol. Environ. Saf. 183. doi: 10.1016/J.ECOENV.2019.109466
Anzalone, A., Di Guardo, M., Bella, P., Ghadamgahi, F., Dimaria, G., Zago, R., et al. (2021). Bioprospecting of beneficial bacteria traits associated with tomato root in greenhouse environment reveals that sampling sites impact more than the root compartment. Front. Plant Sci. 12. doi: 10.3389/fpls.2021.637582
Bais, H. P., Weir, T. L., Perry, L. G., Gilroy, S., Vivanco, J. M. (2006). The role of root exudates in rhizosphere interactions with plants and other organisms. Annu. Rev. Plant Biol. 57, 233–266. doi: 10.1146/ANNUREV.ARPLANT.57.032905.105159
Bal, A., Chanway, Christopher, P. (2012). Evidence of nitrogen fixation in lodgepole pine inoculated with diazotrophic paenibacillus polymyxa. Botany 90, 891–896. doi: 10.1139/b2012-044
Barker, R., Fernandez Garcia, M. N., Powers, S. J., Vaughan, S., Bennett, M. J., Phillips, A. L., et al. (2021). Mapping sites of gibberellin biosynthesis in the arabidopsis root tip. New Phytol. 229, 1521–1534. doi: 10.1111/nph.16967
Besserer, A., Puech-Pagès, V., Kiefer, P., Gomez-Roldan, V., Jauneau, A., Roy, S., et al. (2006). Strigolactones stimulate arbuscular mycorrhizal fungi by activating mitochondria. PloS Biol. 4, 1239–1247. doi: 10.1371/JOURNAL.PBIO.0040226
Burkhead, K. D., Schisler, D. A., Slininger, P. J. (1994). Pyrrolnitrin production by biological control agent pseudomonas cepacia B37w in culture and in colonized wounds of potatoes. Appl. Environ. Microbiol. 60, 2031. doi: 10.1128/AEM.60.6.2031-2039.1994
Bushnell, B., Rood, J., Singer, E. (2017). BBMerge - accurate paired shotgun read merging via overlap. PloS One 12. doi: 10.1371/JOURNAL.PONE.0185056
Castro-Camba, R., Sánchez, C., Vidal, N., Vielba, J. M. (2022). Interactions of gibberellins with phytohormones and their role in stress responses. Horticulturae 8 (3), 241. doi: 10.3390/horticulturae8030241
Cheng, J., Ma, J., Zheng, X., Lv, H., Zhang, M., Tan, B., et al. (2021). Functional analysis of the gibberellin 2-oxidase gene family in peach. Front. Plant Sci. 12. doi: 10.3389/fpls.2021.619158
Chu, T. N., Bui, L. V., Hoang, M. T. T. (2020). Pseudomonas PS01 isolated from maize rhizosphere alters root system architecture and promotes plant growth. Microorganisms 8 (4), 471. doi: 10.3390/microorganisms8040471
Colebrook, E. H., Thomas, S. G., Phillips, A. L., Hedden, P., Davies, S. A., Dow, J. A. T., et al. (2014). The role of gibberellin signalling in plant responses to abiotic stress. J. Exp. Biol. 217, 67–75. doi: 10.1242/JEB.089938
Compant, S., Duffy, B., Nowak, J., Clément, C., Barka, E. A. (2005). Use of plant growth-promoting bacteria for biocontrol of plant diseases: principles, mechanisms of action, and future prospects. Appl. Environ. Microbiol. 71, 4951–4959. doi: 10.1128/AEM.71.9.4951-4959.2005
Delaux, P. M., Schornack, S. (2021). Plant evolution driven by interactions with symbiotic and pathogenic microbes. Science 371, eaba6605. doi: 10.1126/SCIENCE.ABA6605
Devi, U., Khatri, I., Kumar, N., Kumar, L., Sharma, D., Subramanian, S., et al. (2013). Draft genome sequence of a plant growth-promoting rhizobacterium, serratia fonticola strain AU-P3(3). Genome Announc. 1 (6), e00946-13. doi: 10.1128/GENOMEA.00946-13
De Weert, S., Vermeiren, H., Mulders, I. H. M., Kuiper, I., Hendrickx, N., Bloemberg, G. V., et al. (2007). Flagella-driven chemotaxis towards exudate components is an important trait for tomato root colonization by pseudomonas fluorescens. Mol. Plant Microbe Interact. 15, 1173–1180. doi: 10.1094/MPMI.2002.15.11.1173
Diagne, N., Ndour, M., Djighaly, P. I., Ngom, D., Ngom, M. C. N., Ndong, G., et al. (2020). Effect of plant growth promoting rhizobacteria (PGPR) and arbuscular mycorrhizal fungi (AMF) on salt stress tolerance of casuarina obesa (Miq.). Front. Sustain. Food Syst. 4. doi: 10.3389/FSUFS.2020.601004
Dobin, A., Davis, C. A., Schlesinger, F., Drenkow, J., Zaleski, C., Jha, S., et al. (2013). STAR: ultrafast universal RNA-seq aligner. Bioinformatics 29, 15–21. doi: 10.1093/BIOINFORMATICS/BTS635
Dodd, I. C., Zinovkina, N. Y., Safronova, V. I., Belimov, A. A. (2010). Rhizobacterial mediation of plant hormone status. Ann. Appl. Biol. 157, 361–379. doi: 10.1111/J.1744-7348.2010.00439.X
Drigo, B., Pijl, A. S., Duyts, H., Kielak, A. M., Gamper, H. A., Houtekamer, M. J., et al. (2010). Shifting carbon flow from roots into associated microbial communities in response to elevated atmospheric CO2. Proc. Natl. Acad. Sci. U. S. A. 107, 10938–10942. doi: 10.1073/PNAS.0912421107/SUPPL_FILE/PNAS.200912421SI.PDF
Fang, R., Lin, J., Yao, S., Wang, Y., Wang, J., Zhou, C., et al. (2013). Promotion of plant growth, biological control and induced systemic resistance in maize by pseudomonas aurantiaca JD37. Ann. Microbiol. 63, 1177–1185. doi: 10.1007/s13213-012-0576-7
Fa Yang, S., Hoffman, N. E. (1984). Ethylene biosynthesis and its regulation in higher plants. Ann. Rev. Plant Physiol. 35, 155–189. doi: 10.1146/annurev.pp.35.060184.001103
Ghadamgahi, F., Tarighi, S., Taheri, P., Saripella, G. V., Anzalone, A., Kalyandurg, P. B., et al. (2022). Plant growth-promoting activity of pseudomonas aeruginosa FG106 and its ability to act as a biocontrol agent against potato, tomato and taro pathogens. Biology 11 (1), 140. doi: 10.3390/biology11010140
Ghadam Khani, A., Enayatizamir, N., Norouzi Masir, M. (2019). Impact of plant growth promoting rhizobacteria on different forms of soil potassium under wheat cultivation. Lett. Appl. Microbiol. 68, 514–521. doi: 10.1111/lam.13132
Glick, B. R. (2005). Modulation of plant ethylene levels by the bacterial enzyme ACC deaminase. FEMS Microbiol. Lett. 251, 1–7. doi: 10.1016/J.FEMSLE.2005.07.030
Glick, B. R., Jacobson, C. B., Schwarze, M. K., Pasternak, J. J. (1994). 1-Aminocyclopropane-1-carboxylic acid deaminase mutants of the plant growth promoting rhizobacterium pseudomonas putida GR12-2 do not stimulate canola root elongation. Can. J. Microbiol. 40, 911–915. doi: 10.1139/M94-146
Glick, B. R., Penrose, D. M., Li, J. (1998). A model for the lowering of plant ethylene concentrations by plant growth-promoting bacteria. J. Theor. Biol. 190, 63–68. doi: 10.1006/jtbi.1997.0532
Groot, S. P. C., Karssen, C. M. (1987). Gibberellins regulate seed germination in tomato by endosperm weakening: a study with gibberellin-deficient mutants. Planta 171, 525–531.
Hardoim, P. R., van Overbeek, L. S., van Elsas, J. D. (2008). Properties of bacterial endophytes and their proposed role in plant growth. Trends Microbiol. 16, 463–471. doi: 10.1016/j.tim.2008.07.008
Hur, Y. S., Um, J. H., Kim, S., Kim, K., Park, H. J., Lim, J. S., et al. (2015). Arabidopsis thaliana homeobox 12 (ATHB12), a homeodomain-leucine zipper protein, regulates leaf growth by promoting cell expansion and endoreduplication. New Phytol. 205, 316–328. doi: 10.1111/NPH.12998
Ito, K., Murphy, D. (2013). Application of ggplot2 to pharmacometric graphics. CPT pharmacometrics Syst. Pharmacol. 2, e79. doi: 10.1038/PSP.2013.56
Ivanchenko, M. G., Napsucialy-Mendivil, S., Dubrovsky, J. G. (2010). Auxin-induced inhibition of lateral root initiation contributes to root system shaping in arabidopsis thaliana. Plant J. 64, 740–752. doi: 10.1111/J.1365-313X.2010.04365.X
Kelbessa, B. G., Dubey, M., Catara, V., Ghadamgahi, F., Ortiz, R., Vetukuri, R. R. (2023). Potential of plant growth-promoting rhizobacteria to improve crop productivity and adaptation to a changing climate. CABI Reviews 1–14. doi: 10.1079/cabireviews.2023.0001
Kelbessa, B. G., Ghadamgahi, F., Bhattacharjee, R., Vetukuri, R. R. (2022). Antagonistic and plant growth promotion of rhizobacteria against phytophthora colocasiae in taro. Front. Plant Sci. 13, 1–17. doi: 10.3389/fpls.2022.1035549
Khan, M. Z., Akhtar, M. E., Mahmood-ul-Hassan, M., Mahmood, M. M., Safdar, M. N. (2012). Potato tuber yield and quality as affected by rates and sources of potassium fertilizer. J. Plant Nutr. 35, 664–677. doi: 10.1080/01904167.2012.653072
Koo, S.-Y., Cho, K.-S. (2009). Isolation and characterization of a plant growth-promoting rhizobacterium, serratia sp. SY5. J. Microbiol. Biotechnol. 19, 1431–1438.
Kumari, B., Mallick, M. A., Solanki, M. K., Solanki, A. C., Hora, A., Guo, W. (2019). Plant growth promoting rhizobacteria (PGPR): modern prospects for sustainable agriculture. Plant Heal. Under Biot. Stress, 109–127. doi: 10.1007/978-981-13-6040-4_6
Li, L., Qin, Y., Liu, Y., Hu, Y., Fan, M. (2012). Leaf positions of potato suitable for determination of nitrogen content with a SPAD meter. Plant Prod. Sci. 15, 317–322. doi: 10.1626/pps.15.317
Liao, Y., Smyth, G. K., Shi, W. (2014). featureCounts: an efficient general purpose program for assigning sequence reads to genomic features. Bioinformatics 30, 923–930. doi: 10.1093/BIOINFORMATICS/BTT656
Liu, H., Wang, J., Sun, H., Han, X., Peng, Y., Liu, J., et al. (2020). Transcriptome profiles reveal the growth-promoting mechanisms of paenibacillus polymyxa YC0136 on tobacco (Nicotiana tabacum l.). Front. Microbiol. 11. doi: 10.3389/fmicb.2020.584174
Livak, K. J., Schmittgen, T. D. (2001). Analysis of relative gene expression data using real-time quantitative PCR and the 2–ΔΔCT method. Methods 25, 402–408.
Loper, J. E. (1988). Role of fluorescent siderophore production in biological control of pythium ultimum by a pseudomonas fluorescens strain. Phytopathology 78, 166. doi: 10.1094/PHYTO-78-166
Loper, J. E., Henkels, M. D. (1999). Utilization of heterologous siderophores enhances levels of iron available to pseudomonas putida in the rhizosphere. Appl. Environ. Microbiol. 65, 5357–5363. doi: 10.1128/AEM.65.12.5357-5363.1999
Loper, J. E., Schroth, M. N. (1986). Influence of bacterial sources of indole-3-acetic acid on root elongation of sugar beet. Phytopathology 76, 386–389. doi: 10.1094/Phyto-76-386
Love, M. I., Huber, W., Anders, S. (2014). Moderated estimation of fold change and dispersion for RNA-seq data with DESeq2. Genome Biol. 15, 550. doi: 10.1186/S13059-014-0550-8
Mahdi, I., Hafidi, M., Allaoui, A., Biskri, L. (2021). Halotolerant endophytic bacterium serratia rubidaea ED1 enhances phosphate solubilization and promotes seed germination. Agric. 11, 224. doi: 10.3390/AGRICULTURE11030224
Matsuda, R., Ozawa, N., Fujiwara, K. (2014). Leaf photosynthesis, plant growth, and carbohydrate accumulation of tomato under different photoperiods and diurnal temperature differences. Sci. Hortic. 170, 150–158. doi: 10.1016/j.scienta.2014.03.014
McMillan, H. M., Zebell, S. G., Ristaino, J. B., Dong, X., Kuehn, M. J. (2021). Protective plant immune responses are elicited by bacterial outer membrane vesicles. Cell Rep. 34, 108645. doi: 10.1016/J.CELREP.2020.108645
Meena, V. S., Maurya, B. R., Verma, J. P. (2014). Does a rhizospheric microorganism enhance k+ availability in agricultural soils? Microbiol. Res. 169, 337–347. doi: 10.1016/J.MICRES.2013.09.003
Mercado-Blanco, J., Bakker, P. A. H. M. (2007). Interactions between plants and beneficial pseudomonas spp.: exploiting bacterial traits for crop protection. Antonie van Leeuwenhoek 92, 367–389. doi: 10.1007/S10482-007-9167-1
Mora, C. C., Perotti, M. F., González-Grandío, E., Ribone, P. A., Cubas, P., Chan, R. L. (2022). AtHB40 modulates primary root length and gravitropism involving CYCLINB and auxin transporters. Plant Sci. 324, 111421. doi: 10.1016/J.PLANTSCI.2022.111421
Niu, H., Wang, H., Zhao, B., He, J., Yang, L., Ma, X., et al. (2022). Exogenous auxin-induced ENHANCER OF SHOOT REGENERATION 2 (ESR2) enhances femaleness of cucumber by activating the CsACS2 gene. Hortic. Res. 9, uhab085. doi: 10.1093/HR/UHAB085
Olanrewaju, O. S., Glick, B. R., Babalola, O. O. (2017). Mechanisms of action of plant growth promoting bacteria. World J. Microbiol. Biotechnol. 33, 1–16. doi: 10.1007/s11274-017-2364-9
Ortiz-Castro, R., Campos-García, J., López-Bucio, J. (2020). Pseudomonas putida and pseudomonas fluorescens influence arabidopsis root system architecture through an auxin response mediated by bioactive cyclodipeptides. J. Plant Growth Regul. 39, 254–265. doi: 10.1007/s00344-019-09979-w
Osugi, A., Kojima, M., Takebayashi, Y., Ueda, N., Kiba, T., Sakakibara, H. (2017). Systemic transport of trans-zeatin and its precursor have differing roles in arabidopsis shoots. Nat. Plants 3, 1–6. doi: 10.1038/nplants.2017.112
Park, J. M., Radhakrishnan, R., Kang, S. M., Lee, I. J. (2015). IAA producing enterobacter sp. I-3 as a potent bio-herbicide candidate for weed control: a special reference with lettuce growth inhibition. Indian J. Microbiol. 55, 207–212. doi: 10.1007/S12088-015-0515-Y
Poupin, M. J., Greve, M., Carmona, V., Pinedo, I. (2016). A complex molecular interplay of auxin and ethylene signaling pathways is involved in arabidopsis growth promotion by burkholderia phytofirmans. Front. Plant Sci. 7, 492. doi: 10.3389/fpls.2016.00492
Prasanna, R., Nain, L., Rana, A., Shivay, Y. S. (2016). Biofortification with microorganisms: present status and future challenges. Biofortification Food Crop, 249–262. doi: 10.1007/978-81-322-2716-8_19
Přikryl, Z., Vančura, V., Wurst, M. (1985). Auxin formation by rhizosphere bacteria as a factor of root growth. Biol. Plant 27, 159–163. doi: 10.1007/BF02902155
Ravanbakhsh, M., Sasidharan, R., Voesenek, L. A. C. J., Kowalchuk, G. A., Jousset, A. (2018). Microbial modulation of plant ethylene signaling: ecological and evolutionary consequences. Microbiome 6, 52. doi: 10.1186/s40168-018-0436-1
Ré, D. A., Capella, M., Bonaventure, G., Chan, R. L. (2014). Arabidopsis AtHB7 and AtHB12 evolved divergently to fine tune processes associated with growth and responses to water stress. BMC Plant Biol. 14, 1–14. doi: 10.1186/1471-2229-14-150
Richards, D. E., King, K. E., Ait-ali, T., Harberd, N. P. (2003). HOW GIBBERELLIN REGULATES PLANT GROWTH AND DEVELOPMENT: a molecular genetic analysis of gibberellin signaling. Annu. Rev. Plant Physiol. Plant Mol. Biol. 52, 67–88. doi: 10.1146/ANNUREV.ARPLANT.52.1.67
Rieu, I., Eriksson, S., Powers, S. J., Gong, F., Griffiths, J., Woolley, L., et al. (2008). Genetic analysis reveals that C19-GA 2-oxidation is a major gibberellin inactivation pathway in arabidopsis. Plant Cell 20, 2420–2436. doi: 10.1105/TPC.108.058818
Rudrappa, T., Czymmek, K. J., Paré, P. W., Bais, H. P. (2008). Root-secreted malic acid recruits beneficial soil bacteria. Plant Physiol. 148, 1547–1556. doi: 10.1104/PP.108.127613
Sardans, J., Peñuelas, J. (2015). Potassium: a neglected nutrient in global change. Glob. Ecol. Biogeogr. 24, 261–275. doi: 10.1111/GEB.12259
Schomburg, F. M., Bizzell, C. M., Lee, D. J., Zeevaart, J. A. D., Amasino, R. M. (2003). Overexpression of a novel class of gibberellin 2-oxidases decreases gibberellin levels and creates dwarf plants. Plant Cell 15, 151–163. doi: 10.1105/TPC.005975
Shen, X., Hu, H., Peng, H., Wang, W., Zhang, X. (2013). Comparative genomic analysis of four representative plant growth-promoting rhizobacteria in pseudomonas. BMC Genomics 14, 1–20. doi: 10.1186/1471-2164-14-271
Shin, K., Lee, S., Song, W. Y., Lee, R. A., Lee, I., Ha, K., et al. (2015). Genetic identification of ACC-RESISTANT2 reveals involvement of LYSINE HISTIDINE TRANSPORTER1 in the uptake of 1-Aminocyclopropane-1-Carboxylic acid in arabidopsis thaliana. Plant Cell Physiol. 56, 572–582. doi: 10.1093/PCP/PCU201
Silambarasan, S., Logeswari, P., Cornejo, P., Kannan, V. R. (2019). Role of plant growth–promoting rhizobacterial consortium in improving the vigna radiata growth and alleviation of aluminum and drought stresses. Environ. Sci. pollut. Res. 26, 27647–27659. doi: 10.1007/S11356-019-05939-9
Son, H. J., Park, G. T., Cha, M. S., Heo, M. S. (2006). Solubilization of insoluble inorganic phosphates by a novel salt- and pH-tolerant pantoea agglomerans r-42 isolated from soybean rhizosphere. Bioresour. Technol. 97, 204–210. doi: 10.1016/J.BIORTECH.2005.02.021
Spaepen, S., Bossuyt, S., Engelen, K., Marchal, K., Vanderleyden, J. (2014). Phenotypical and molecular responses of arabidopsis thaliana roots as a result of inoculation with the auxin-producing bacterium azospirillum brasilense. New Phytol. 201, 850–861. doi: 10.1111/NPH.12590
Staswick, P. E., Serban, B., Rowe, M., Tiryaki, I., Maldonado, M. T., Maldonado, M. C., et al. (2005). Characterization of an arabidopsis enzyme family that conjugates amino acids to indole-3-Acetic acid. Plant Cell 17, 616–627. doi: 10.1105/TPC.104.026690
Strand, A. E., Pritchard, S. G., McCormack, M. L., Davis, M. A., Oren, R. (2008). Irreconcilable differences: fine-root life spans and soil carbon persistence. Science. 319, 456–458. doi: 10.1126/SCIENCE.1151382
Tahir, H. A. S., Gu, Q., Wu, H., Raza, W., Hanif, A., Wu, L., et al. (2017). Plant growth promotion by volatile organic compounds produced by bacillus subtilis SYST2. Front. Microbiol. 8. doi: 10.3389/FMICB.2017.00171
Tien, T. M., Gaskins, M. H., Hubbell, D. H. (1979). Plant growth substances produced by azospirillum brasilense and their effect on the growth of pearl millet (Pennisetum americanum l.). Appl. Environ. Microbiol. 37, 1016. doi: 10.1128/AEM.37.5.1016-1024.1979
Timmusk, S., Nicander, B., Granhall, U., Tillberg, E. (1999). Cytokinin production by paenibacillus polymyxa. Soil Biol. Biochem. 31, 1847–1852. doi: 10.1016/S0038-0717(99)00113-3
Tsuchisaka, A., Theologis, A. (2004). Unique and overlapping expression patterns among the arabidopsis 1-Amino-Cyclopropane-1-Carboxylate synthase gene family members. Plant Physiol. 136, 2982. doi: 10.1104/PP.104.049999
Tsukanova, K. A., Сhеbоtаr, V., Meyer, J. J. M., Bibikova, T. N. (2017). Effect of plant growth-promoting rhizobacteria on plant hormone homeostasis. South Afr. J. Bot. 113, 91–102. doi: 10.1016/J.SAJB.2017.07.007
Vanderstraeten, L., van der Straeten, D. (2017). Accumulation and transport of 1-aminocyclopropane-1-carboxylic acid (ACC) in plants: current status, considerations for future research and agronomic applications. Front. Plant Sci. 8. doi: 10.3389/fpls.2017.00038
Van Wees, S. C., van der Ent, S., Pieterse, C. M. (2008). Plant immune responses triggered by beneficial microbes. Curr. Opin. Plant Biol. 11, 443–448. doi: 10.1016/J.PBI.2008.05.005
Vater, J., Herfort, S., Doellinger, J., Weydmann, M., Dietel, K., Faetke, S., et al. (2017). Fusaricidins from paenibacillus polymyxa m-1, a family of lipohexapeptides of unusual complexity–a mass spectrometric study. J. Mass Spectrom. 52, 7–15. doi: 10.1002/JMS.3891
Wei, H.-L., Khan, N., Preet Padda, K., Wang, C., Liu, H., Wang, J., et al. (2020). Transcriptome profiles reveal the growth-promoting mechanisms of paenibacillus polymyxa YC0136 on tobacco (Nicotiana tabacum l.). Front. Microbiol. 11, 584174 doi: 10.3389/fmicb.2020.584174
Wu, T., Hu, E., Xu, S., Chen, M., Guo, P., Dai, Z., et al. (2021). clusterProfiler 4.0: a universal enrichment tool for interpreting omics data. Innov. (Camb) 2 (3), 100141. doi: 10.1016/J.XINN.2021.100141
Yang, J., Kloepper, J. W., Ryu, C. M. (2009). Rhizosphere bacteria help plants tolerate abiotic stress. Trends Plant Sci. 14, 1–4. doi: 10.1016/J.TPLANTS.2008.10.004
Yang, N., Nesme, J., Røder, H. L., Li, X., Zuo, Z., Petersen, M., et al. (2021). Emergent bacterial community properties induce enhanced drought tolerance in arabidopsis. NPJ Biofilms Microbiomes 7, 1–11. doi: 10.1038/s41522-021-00253-0
Zamioudis, C., Mastranesti, P., Dhonukshe, P., Blilou, I., Pieterse, C. M. J. (2013). Unraveling root developmental programs initiated by beneficial pseudomonas spp. bacteria. Plant Physiol. 162, 304–318. doi: 10.1104/pp.112.212597
Keywords: PGPR - plant growth-promoting rhizobacteria, Pseudomonas fluorescens, beneficial bacteria, IAA, ethylene, Serratia, transcriptome (RNA-seq)
Citation: Hanifah NASb, Ghadamgahi F, Ghosh S, Ortiz R, Whisson SC, Vetukuri RR and Kalyandurg PB (2023) Comparative transcriptome profiling provides insights into the growth promotion activity of Pseudomonas fluorescens strain SLU99 in tomato and potato plants. Front. Plant Sci. 14:1141692. doi: 10.3389/fpls.2023.1141692
Received: 10 January 2023; Accepted: 03 April 2023;
Published: 18 July 2023.
Edited by:
Islam A. Abd El-Daim, Agricultural Research Center, EgyptReviewed by:
Ho Won Jung, Dong-A University, Republic of KoreaAlfonso Méndez-Bravo, Morelia Unit, National Autonomous University of Mexico, Mexico
Copyright © 2023 Hanifah, Ghadamgahi, Ghosh, Ortiz, Whisson, Vetukuri and Kalyandurg. This is an open-access article distributed under the terms of the Creative Commons Attribution License (CC BY). The use, distribution or reproduction in other forums is permitted, provided the original author(s) and the copyright owner(s) are credited and that the original publication in this journal is cited, in accordance with accepted academic practice. No use, distribution or reproduction is permitted which does not comply with these terms.
*Correspondence: Pruthvi B. Kalyandurg, cHJ1dGh2aS5iYWxhY2hhbmRyYUBzbHUuc2U=; Ramesh R. Vetukuri, cmFtZXNoLnZldHVrdXJpQHNsdS5zZQ==