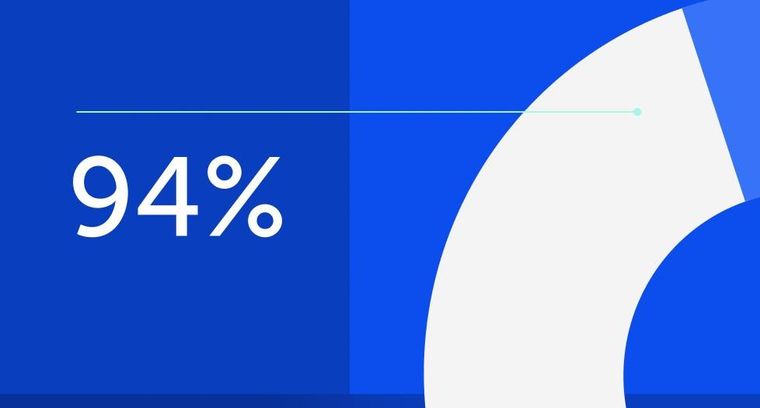
94% of researchers rate our articles as excellent or good
Learn more about the work of our research integrity team to safeguard the quality of each article we publish.
Find out more
REVIEW article
Front. Plant Sci., 20 February 2023
Sec. Plant Physiology
Volume 14 - 2023 | https://doi.org/10.3389/fpls.2023.1139744
This article is part of the Research TopicRole of Shoot-Derived Signals in Root Responses to Environmental ChangesView all 5 articles
High concentrations of heavy metals in the environment will cause serious harm to ecosystems and human health. It is urgent to develop effective methods to control soil heavy metal pollution. Phytoremediation has advantages and potential for soil heavy metal pollution control. However, the current hyperaccumulators have the disadvantages of poor environmental adaptability, single enrichment species and small biomass. Based on the concept of modularity, synthetic biology makes it possible to design a wide range of organisms. In this paper, a comprehensive strategy of “microbial biosensor detection - phytoremediation - heavy metal recovery” for soil heavy metal pollution control was proposed, and the required steps were modified by using synthetic biology methods. This paper summarizes the new experimental methods that promote the discovery of synthetic biological elements and the construction of circuits, and combs the methods of producing transgenic plants to facilitate the transformation of constructed synthetic biological vectors. Finally, the problems that should be paid more attention to in the remediation of soil heavy metal pollution based on synthetic biology were discussed.
The global soil heavy metal (HM) pollution is increasing (Outridge et al., 2018). Heavy metals in the environment enter organisms along the food chain, causing harm to organisms and human bodies (Moynihan et al., 2017). For example, “Minamata disease” in Japan is caused by Hg pollution (Eto, 2000). Both physical remediation and chemical remediation have the disadvantages of high treatment cost, large treatment project (Ferrucci et al., 2017; Mu'azu et al., 2018; Asadollahfardi et al., 2021), and disturbing the soil microenvironment (Zhou et al., 2015). Although microbial remediation can effectively deal with heavy metal pollution in soil, it also has some shortcomings such as demanding environmental conditions (such as specific pH, temperature, etc.) (Cabral et al., 2013). Nanomaterials have excellent adsorption properties for heavy metals (Marques Neto et al., 2019), but the interaction mechanism between them remains to be studied (Hizhnyi et al., 2017; Zhang et al., 2021), so there are limitations when they are used to remove heavy metals. It is urgent to find appropriate methods to control soil heavy metal pollution.
Phytoremediation has low cost and simple operation, which is suitable for dealing with heavy metal pollution in large areas of soil. It is expected to reduce the content of heavy metals in contaminated soil to a safe level for a long time, so as to eradicate the problem of heavy metal pollution in soil (Pilon-Smits, 2005). Hyperaccumulators are commonly used in phytoremediation (Li et al., 2018). However, the discovered hyperaccumulators have poor environmental adaptability and enrichment specificity and are lack of critical biomass for effective phytoremediation (Mijovilovich et al., 2009). Synthetic biological methods to improve plant tolerance and toxic metal accumulation have great potential in phytoremediation.
The behavior of these pathways in plants can be predicted, regulated and finally programmed (Schwille, 2011). Unlike traditional method, synthetic biology is a new way to build modules with new functions (Liu and Stewart, 2015). In this paper, the comprehensive process of soil heavy metal pollution control and the strategies of synthetic biology involved are proposed, and the latest progress of experimental technologies that contribute to synthetic biology and plant gene transformation is summarized. This review also discusses the potential challenges of applying synthetic biology to phytoremediation. Efforts should be made to formulate breeding plans to improve the characteristics of natural hyperaccumulators, and cultivate these characteristics into non-food, high accumulation, high biomass plants for phytoremediation of heavy metals.
Synthetic biology combines biotechnology and engineering ideas to connect genes into a network, enabling cells to complete various tasks of artificial design (Khalil and Collins, 2010; Cameron et al., 2014). Compared with bacteria, yeast and mammalian cells, plant synthetic biology is still in its infancy (Liu and Stewart, 2015). Moreover, phytoremediation of heavy metal contaminated soil also has some problems, such as mixed heavy metal pollution. Thlaspi caerulescens, a cadmium/zinc hyperaccumulation plant, is sensitive to Cu toxicity, which is a problem in the application of this plant to the remediation of cadmium/zinc contaminated soil with Cu (Mijovilovich et al., 2009).
Although plant sensors have been developed to use chlorophyll destruction to achieve visualization (Antunes et al., 2011), due to the limitations of plants, such as their bright colors and spontaneous fluorescence, and in view of the large variety of heavy metals, we propose to implement the strategy of “microbial biosensor detection-phytoremediation-heavy metal recovery” for soil heavy metal pollution (Figure 1). Synthetic biological elements are constantly added to the gene circuit of microbial biosensors and phytoremediation, so that their functions are more and more abundant and their performance is constantly improved.
Whole cell microbial biosensor refers to using microbial cells as sensing elements to convert the collected molecular information to light, electricity and other signals. The signal intensity is proportional to the content of the substance to be measured (Du et al., 2019), so as to achieve quantitative and qualitative dynamic monitoring of the substance to be measured. The diversification of detection targets is one of the development trends of microbial biosensors. In order to achieve functional integration of multi target detection, more gene elements must be installed in the gene loop of microbial biosensor, which leads to increasingly complex gene loop. Synthetic biology provides theoretical and technical support for the integration and optimization of gene circuits to ultimately achieve programmed/customized sensitivity, specificity, and dynamic ranges of sensors to meet their real world detection requirement (Figure 2).
The first reported gene engineering cell biosensor was responsive to aromatic hydrocarbon contamination (King et al., 1990). After that, many heavy metal microbial biosensors were developed. AND, OR, NOR, NAND, XOR and other complex modular logic gate structures are designed and constructed, and used for the development of multi-level gene circuit type whole cell microbial biosensors (Wang et al., 2013; Saltepe et al., 2018). The whole-cell-based biosensor consists of sensing elements and reporting elements, all of which are located in the chassis cell to realize the designed gene circuit (Harms et al., 2006).
The microbial biosensor uses autologous cells as sensing units to sense the measured objects and convert them into recognizable signals according to certain rules (Yagi, 2007). Due to the easy culture, rapid reproduction and relatively simple metabolism of microorganisms, microbial biosensors have natural advantages. However, the microorganisms commonly used to construct microbial biosensors are limited to bacteria, yeast, cyanobacteria, green algae and other microorganisms (Ma et al., 2022).
The sensing elements of heavy metal microbial biosensor mainly include heavy metal responsive transcription factor/transcription regulator (Brown et al., 2003; Busenlehner et al., 2003; Fang and Zhang, 2022), two-component system (Leonhartsberger et al., 2001) and riboswitch (Cromie et al., 2006). The regulatory structure of transcription factors/transcription regulators is the most widely studied and applied gene circuit sensing element at present. These proteins have two functional domains, namely ligand-binding domain (LBD) and DNA-binding domain (DBD). The LBD is the signal receiving module of the sensing element, which determines the specificity and diversity of the ligand. The DBD is a signal conversion module, which can specifically identify transcription factor/transcription regulator binding sites. Metal responsive transcription regulators (i.e. metalloregulators) have different families: ArsR/SmtB, MerR, CsoR/RcnR, CopY, DtxR, Fur, NikR, etc (Pennella and Giedroc, 2005; Osman and Cavet, 2010). At present, MerR family proteins and ArsR/SmtB family proteins are mainly used in the construction of microbial biosensors. Transcription factors/transcriptional regulators have clear functional domains, which can be separated and recombined in a modular way to a certain extent. Based on the modular structure of natural transcription factors/transcriptional regulators, artificial transcription factors/transcriptional regulators (ATF/ATR) can be designed and constructed. ATF/ATR integrates different LBDs and DBDs and directly targets key gene regulatory networks that govern intended downstream application (Tungtur et al., 2007). Promoters containing transcription factor binding sites are also the focus of research. The response performance can be adjusted by changing the strength of promoters (Xu et al., 2020), the location (Dabirian et al., 2019)and number (David et al., 2016) of transcription factor binding sites.
The report element is a report gene that can be monitored. At first, luciferase was used to construct the microbial biosensor. Later, fluorescent protein became the mainstream reporting element, and the programmable design of heavy metal microbial biosensor was realized. A series of constitutive promoters are used to regulate the expression level of MerR family proteins, which are used as regulators in the genetic circuit to regulate the detection sensitivity to control the expression of fluorescent protein eGFP. Based on this, a heavy metal microbial biosensor with adjustable sensitivity is designed (Guo et al., 2019). The concentration of heavy metal ions in the environment can be converted by measuring the fluorescence intensity of microbial biosensor cells (Du et al., 2019). However, as far as the sensitivity to heavy metals is concerned, the performance of luciferase is better than that of fluorescence (Huang et al., 2015). Pigment genes are also used to construct microbial biosensors (Fujimoto et al., 2006).
Integrated with micro/nano technology, some systems have been developed to optimize microbial biosensors and make them easy to use (Rothert et al., 2005; Buffi et al., 2011; Kim et al., 2015). With the discovery of new mechanisms of recognition and allosteric of metalloregulators (Liu et al., 2019; Fang et al., 2021), microbial biosensors are easy to use and have higher sensitivity and faster detection speed.
Hyperaccumulator is the basis of phytoremediation. Hyperaccumulator can grow normally in the soil with high concentration of heavy metals, and accumulate heavy metals in the aboveground parts with a concentration hundreds or even thousands of times as high as that of ordinary plants (Sytar et al., 2021).
In 2015, the online Global Hyperaccumulator Database (www.hyperaccumulators.org) was established (Reeves et al., 2018; Sytar et al., 2021). The word heavy metal hyperaccumulator first appeared in 1976, when Sebertia acuminata was discovered, which can absorb and enrich nickel (Ni) in soil (Jaffré et al., 1976). Later, many hyperaccumulator were found, such as Arabidopsis halleri (Briskine et al., 2017), Alfalfa (Wang et al., 2021), and Sedum alfredii (Qiong et al., 2021). Pteris. Vittata L is the first reported hyperaccumulator of As in the world, and also the first reported fern with super enrichment function. Unfortunately, the website does not provide services at present.
It is necessary to continue and accelerate the discovery of heavy metal hyperaccumulator. As heavy metal hyperaccumulators only exist or mainly exist on metal bearing soil, they are threatened by habitat loss with the reduction of mineral resources (Lange et al., 2017). Therefore, it is necessary to continue to identify hyperaccumulator species and other metal tolerant plants in order to study and utilize their unique physiological mechanisms and provide a basis for the practical application of phytoremediation technology. However, we advocate that native plant species should be used as much as possible to avoid the possible spread of invasive species.
Some crops can enrich heavy metals. As and Pb exceeding the standard were detected in the harvestable part of corn growing near the tailings (Armienta et al., 2020). Brassica juncea L. showed the ability to enrich copper, cadmium and lead from soil (Goswami and Das, 2015; Gonzaga et al., 2018; Gurajala et al., 2019). The edible part of rice contains a lot of arsenic (Meharg, 2004; Dolphen and Thiravetyan, 2019). When wheat and some vegetables are planted in arsenic contaminated farmland, a large amount of arsenic is accumulated in their edible parts (Saeed et al., 2021; Shukla et al., 2022). However, we emphasize that the synthetic biological chassis carrier for phytoremediation should not use existing crops to ensure that the products produced do not enter the food chain.
At present, more than 700 species of hyperaccumulators have been found, but the popularization of phytoremediation technology in practice is still restricted by many aspects. Each plant often accumulates only one or a few heavy metals, and shows some poisoning symptoms for other heavy metals with high concentrations in the soil. There are great limitations in the treatment of soil contaminated by multiple heavy metals (Wilson-Corral et al., 2012). The poor enrichment ability of heavy metals in soil, slow growth rate and small dry matter weight per plant bring great difficulties to the practical application of production (Bian et al., 2020).
The response of hyperaccumulators to heavy metals is closely related to a variety of genes, whose expression products mainly include metal transporter (Pence et al., 2000), phytochelatin synthase (PCS), metallothioneins (MTs) and metal reductase (Ellis et al., 2006). These proteins play an important role in the absorption, transport and partition of heavy metals in plants (Milner et al., 2013). The high level expression of TgMTP1 gene in the heavy metal hyperaccumulator Thlaspi goesingense is the reason for its strong ability to accumulate metal ions in vacuoles (Persans et al., 2001).
Genetic engineering tools have been successfully used to develop transgenic hyperaccumulators. By overexpressing metallothionein, phytochelatin, metal transporter and antioxidant enzymes in plants, it has been successfully demonstrated that the ability of phytoremediation is improved (Zanella et al., 2016; Balzano et al., 2020). Transferring genes responsible for hyperaccumulative phenotypes to plants with higher aboveground biomass is considered to be a feasible potential way to enhance phytoremediation (Brown et al., 1995; Rugh et al., 1998). However, the response of plants to heavy metals involves various proteins, amino acids, citric acid (Lu et al., 2013), etc. These substances are interrelated and interact to form a complex and huge signal network to regulate the whole growth and development process of plants. Only operating on individual genes can not create ideal hyperaccumulators, and even cause plants to be highly sensitive to heavy metals (Wojas et al., 2008). In addition, a single element can only enable transgenic organisms to obtain one of the functions of absorption, transport and transformation, while the synergy of multiple gene elements can obtain more potential repair species.
The most important core idea of synthetic biology is standardization. Different genes are designed into modular engineering elements. Through the design and assembly of the elements, a circuit that functions with time and space can be obtained (Endy, 2005). Synthetic biology of higher plants is emerging. The synthetic biology resource library iGEM (https://parts.igem.org/MainPage), SynbioML@TJU (http://www.synbioml.org/), Registry and database of bioparts for synthetic biology (https://www.biosino.org/rdbsb/), etc. constantly updated and supplemented the tested plant elements. SBOL has been updated to version 3.0 (Baig et al., 2020). Plant MoClo Syntax, a plant cloning system that can easily assemble complex vectors, has been established (Engler et al., 2014; Hussey et al., 2019).
Complex synthetic gene circuits have been achieved in plants. Recently, the first stable reprogramming synthetic gene circuit in plant cells has been realized. In this system, a series of key gene circuit functions were first established. Then, using recombinase and plant control elements, a series of operational logic gates were developed. The YES, OR and gates were used to activate transgenes, and the NOT, NOR and NAND gates were used to inhibit transgenes; A NIMPLY B gate combining activation and suppression is also realized. Through the use of gene recombination, these circuits have produced stable long-term changes in the expression and recording of past stimuli, proving the practicability of programmable manipulation of transcriptional activity in complex multicellular organisms (Lloyd et al., 2022). Gene circuits used to change root structure predictably have also been developed, which based on a series of synthetic transcriptional regulators developed for plants (Brophy et al., 2022). The application and development of hyperaccumulator in Phytoremediation will be promoted by using synthetic biological techniques to design and develop ideal hyperaccumulator with strong enrichment capacity.
Phytoremediation uses phytoextraction. If the plants that have absorbed heavy metals are not properly treated, the problem of environmental pollution still exists (Zhong et al., 2015). Research shows that plants are “bio factories” of metal nanoparticles. Brassica juncea can reduce silver ions and gold ions to form silver nanoparticles and gold nanoparticles with a particle size of 2~100 nm, and the output of the nanoparticles is affected by the amount of reducing sugar (Beattie and Haverkamp, 2011). After soybean (Glycine max) and rice (Oryza sativa L.) were exposed to silver ions, silver nanoparticles were also detected in plants, indicating that they were formed in vivo (Li et al., 2017). A variety of metal nanomaterials can be synthesized by genetically engineered microorganisms (Kang et al., 2008; Choi et al., 2018). MTs and PCS are commonly used in these modifications (Kang et al., 2008; Choi et al., 2018). Therefore, based on the development of sequencing technology and the application of synthetic biology in phytoremediation, it is possible to build a circuit in plants to generate metal nanoparticles at room temperature and pressure to achieve the classified recovery of heavy metals after adsorption.
Synthetic biology technology may strongly support the development of phytoremediation. There are a lot of undeveloped element resources in plants. At present, most of the regulatory elements verified by experiments and characterized by functions come from rice, Arabidopsis and other model plants. There are still a large number of regulatory elements in non model plants waiting for further exploration and development.
In the past decades, although some progresses have been made in studying the mechanism involved in the interaction between organisms and heavy metals using molecular biological techniques (Hu et al., 2005; He et al., 2011; Hossain and Komatsu, 2012; Muralidharan et al., 2012), the potential of phytoremediation has still not been fully exploited. Many emerging biotechnologies help to identify components and redesign circuits.
The third generation sequencing technology (TGS), also known as single molecule sequencing, has the advantages of long reading, single molecule and real-time sequencing (Schadt et al., 2010). At present, the mainstream platforms are single molecule real-time sequencing (SMRT-seq) and nanopore sequencing. Nanopore sequencing has been applied to DNA (Clarke et al., 2009; Manrao et al., 2012) and RNA (Wanunu et al., 2010) sequencing at the single molecular level, and has rapidly become the preferred technology for new genome assembly and structural variation identification (Schmidt et al., 2017; Fuselli et al., 2018; Tan et al., 2018). It can greatly improve the quality and integrity of sequencing data (Ding et al., 2020) and identify splice isoforms (Byrne et al., 2017; Depledge et al., 2019). The third generation of long reading sequencing enables us to obtain epigenome/epigenetic transcriptome data with single nucleotide resolution, which can be used to directly detect DNA and RNA modifications (Zhu et al., 2018; Tellgren-Roth and Couturier, 2022), and has become a common method to identify epigenetic modifications in plants (van Dijk et al., 2018; Zhao et al., 2020). This technology can accelerate the discovery of functional genes and can be used to manufacture biological components used in synthetic biology.
In the past ten years, the methods of single-cell omics have completely changed our understanding of the cell and molecular composition of life systems. The sequence and structure analysis of genome (Luo et al., 2019), transcriptome (Tang et al., 2009), epigenetic modification (Zhu et al., 2019; Luo et al., 2020), chromatin accessibility (Marand et al., 2021) and 3D genome structural characteristics (Zhou et al., 2019; Ulianov and Razin, 2022) under single cell resolution are helpful to explain biological development laws and physiological mechanisms. Smart-seq2 is one of the most widely used single cell full-length transcriptome sequencing technologies (Picelli et al., 2014). Combined with Nanopore long-read sequencing, scRNA-seq has been improved to flsnRNA-seq, which can analyze large-scale full-length RNA at a single-nucleus in a protoplasting-free manner (Long et al., 2021). Based on TGS platform, scNanoATAC-seq technology was developed, which is a long-read single-cell ATAC sequencing method on Nanopore sequencing platform for simultaneously detecting chromatin accessibility and genetic variation in a single cell (Hu et al., 2022). Live-seq technology enables a single cell to maintain cell viability after transcriptome sequencing, which is the first time to achieve continuous observation of the whole gene expression in living cells (Chen et al., 2022). Single cell multiomics sequencing directly relates different omics information at the same time, and further studies single cell status and molecular regulation mechanism (Guo et al., 2017; Lee et al., 2020; Thibivilliers and Libault, 2021).
Spatial transcriptomics uses in situ capture technology (Ståhl et al., 2016), which can reveal the spatial distribution of various cell types in tissues, the interaction between various cell populations, and map gene expression in different tissue regions (Chen et al., 2021; Liao et al., 2021). Stereo-seq is the technology with the highest spatial resolution at present (Chen et al., 2022). DBiT-seq realizes the joint measurement of spatially distributed mRNAs and proteins (Liu et al., 2020). Spatial profiling of chromatin accessibility (spatial-ATAC-seq) (Deng et al., 2022b) and histone modifications (Spatial-CUT&Tag) (Deng et al., 2022a) provides new opportunities for understanding life activities. In a word, the methods of single-cell omics enable people to accurately analyze various genetic variations and provides a basis for designing and manipulating various mechanisms. However, the application of these technologies in plants needs further exploration.
CRISPR–Cas-mediated genome editing efficiently and accurately simplifies, inserts or reconstructs the synthetic circuits and the genome of chassis organisms (Esvelt and Wang, 2013), providing strong support for the development of synthetic biology. It has been widely studied in many plants (Jiang et al., 2013; Li et al., 2013; Hu et al., 2017; Rodríguez-Leal et al., 2017). In addition, it has been able to replace large fragments of more than 100 kb (Wang et al., 2016) and knock out and knock in multiple genes at the same time at multiple targets (Jiang et al., 2016).
Based on dCas9 (Cas9 with H840A and D10A mutations) and different transcriptional regulatory domains, CRISPRi (CRISPR interference) and CRISPRa (CRISPR activation) achieve the goal of gene expression regulation without changing the target sequence (Maeder et al., 2013; Qi et al., 2013). dCas9 has also been developed as a tool for regulating gene expression at the level of epigenetic modification (Hilton et al., 2015). Based on the SunTag-dCas9-TET1cd system of Arabidopsis thaliana, an epigenetic editing system targeting the removal of rice genomic DNA methylation was constructed, which successfully reduced the DNA 5mC level of OsFIE1 gene and caused dwarfing phenotype (Tang et al., 2022).
Base editing technology allows the direct and stable conversion of target DNA or RNA bases into substitutes in a programmable manner, without the need for nucleic acid strand breaks and donor templates. The emergence of CBE (C•G to T•A base pair conversion) marks the birth of this technology, and it is found that the efficiency of nCas9(Cas9 with D10A mutation) is higher than that of dCas9, which is currently commonly used (Komor et al., 2016). ABE (adenine base editor) mediates the transformation from A•T to G•C in genomic DNA (Gaudelli et al., 2017). There are numerous SNP (Single Nucleotide Polymorphism) in plants, which are closely related to plant disease resistance and growth (Henry and Edwards, 2009; Malmberg et al., 2019). Plant base editing tools have been developed, and different CBEs may have different editing efficiency for the same region of the genome. Multiple CBE-ABE plant double base editors can edit different bases at the same time (Xiong et al., 2022). In plant epigenetics, APOBEC3BCtd-nCas9, a single base editor with high efficiency for editing methylcytosine, has been obtained (Liu et al., 2022).
At present, Prime Editor (PE) has realized free conversion of all 12 single bases and precise insertion/deletion of specific base sequences without relying on DNA templates (Anzalone et al., 2019). This method has been applied to some plants (Butt et al., 2020; Hua et al., 2020; Li et al., 2020; Lu et al., 2021), and has obtained tools with higher prime-editing efficiency (Jiang et al., 2020; Lin et al., 2021). The establishment of STEME (saturated targeted endogenous mutagenesis editor) has realized the directional evolution of OsACC gene in rice, thus obtaining herbicide resistance mutation, which provides the possibility for rapid acquisition of beneficial agronomic traits (Li et al., 2020).
The emergence of genome editing technology has accelerated the development of synthetic biology, but there are still some problems and room for improvement. At the same time, the application of this technology in the field of synthetic biology also needs further development.
A series of plant transformation systems have been developed: Agrobacterium-mediated method (Mayo et al., 2006; Zhang et al., 2006; Bahramnejad et al., 2019), particle bombardment (Ueki et al., 2009; Dong and Ronald, 2021), Electroporation (Furuhata et al., 2019) and Pollen-tube Pathway (Nagahara et al., 2021). However, these methods have some disadvantages, such as complex operation, long experiment period and few stable transformed species (Ramkumar et al., 2020). Due to the limitation of genotype, the use of gene editing is also limited for plants without a complete regeneration system. At present, most methods of plant genome modification involve tissue culture. The low transformation efficiency of plants is one of the bottlenecks in the development of phytoremediation.
Nanotechnology helps to efficiently and accurately deliver the required circuits to the chassis plants. Exogenous biomolecules can be internalized through the cell wall by nanomaterials without mechanical or external force assistance. Nanocarriers can effectively protect proteins, DNA, RNA and other biological molecules, and easily introduce target molecules into different tissues such as plant callus and endosperm. They have successfully mediated DNA transformation or delivered RNA to induce gene silencing in many plants (Pasupathy et al., 2008; Chang et al., 2013; Mitter et al., 2017; Kwak et al., 2019; Demirer et al., 2020; Lv et al., 2020; Schwartz et al., 2020; Zhang et al., 2021).
In the past ten years, through ectopic expression of developmental regulators (DR) such as BBM and Wus2, somatic embryo regeneration of some plants that cannot be transformed has been achieved to a certain extent (Boutilier et al., 2002; Passarinho et al., 2008; Che et al., 2022). Based on this, two methods based on Agrobacterium tumefaciens were established: Fast-TrACC (fast-treated Agrobacterium co-culture) and direct delivery (DD), which were used in dicotyledon plants to induce meristems by delivering Wus2 and BBM or other DRs involved in cytokinin synthesis to achieve genetic transformation. The operation of Fast-TrACC and DD is simple and time-consuming (Cody et al., 2022).
An extremely simple cut dip budding (CDB) system was created, which can easily and quickly obtain transgenic and genome editing plants without tissue culture under non sterile conditions. Based on root tillering, the system uses Agrobacterium rhizogenes to infect the cut root and stem junction to produce transformed roots, and then produces transformed buds through root transformation. This method realizes genetic transformation of multiple plant species. Moreover, this method has no genotype dependence.
The accumulation of heavy metals in phytoextraction mainly includes the following processes: absorption of heavy metal ions by roots, transportation by apoplast and symplast, loading from root cells to xylem, long-distance transportation of xylem, unloading from xylem and transmembrane transportation of cells. It has been found that many genes are involved in different processes of the interaction between plants and heavy metals, including Heavy Metal ATPase (Huang et al., 2016), CDF (Cation Diffusion Facilitator) protein family (Yuan et al., 2012), phytochelatin synthase, metallothionein (Cobbett and Goldsbrough, 2002), etc. Plants activate various signaling pathways in response to heavy metal hazards (Mourato et al., 2015). The coating protein complex component Sec24C mediates the localization of the transporter ABCC1/2 to the vacuole through a Golgi-independent pathway, enabling it to play the role of vacuolar compartmentalization, and enhancing the tolerance of plants to heavy metal cadmium and arsenic stress (Wu et al., 2011). At present, the detoxification mechanisms of hyperaccumulators to heavy metals generally include the chelation of heavy metals by cytoplasmic substances, the repair of stress damage and the compartmentalization of vacuolar (Hall, 2002), but the detoxification mechanisms need to be further explored. Moreover, plants such as Viola baoshanensis (Shu et al., 2019), Sedum alfredii (Chen et al., 2020; Niu et al., 2021), Leersia hexandra Swartz (Liu et al., 2011), and Pteris vittata (Han et al., 2022) have the ability to repair heavy metal pollution, but few studies have been done on their specific regulatory mechanisms. The response mechanism of plants to heavy metals is complex, so we should deeply study the uptake, translocation, chelation and other mechanisms of super enriched plants to further promote the development of phytoremediation.
At present, the understanding of the process of plant synthesis of metal nanoparticles is still limited. Most studies are based on plant extracts, that is, the preparation of metal nanoparticles uses the method of plant tissue homogenate reacting with metal ions under certain environmental conditions. The results showed that the main substances related to plant synthesis of metal nanoparticles are organic acids, reducing sugars (Beattie and Haverkamp, 2011), proteins (Xie et al., 2007), amino acids (Shankar and Rhim, 2015) and peptides (Tan et al., 2010). However, the formation mechanism of metal nanoparticles cannot be fully revealed at the level of living plants.
With the rapid development of synthetic biology, it is becoming easier and easier to artificially transform or create life systems, and the biosafety of artificial life systems has become increasingly prominent. It is necessary to inhibit the escape of natural environment and malignant rapid growth of synthetic organisms, avoid gene invasion caused by horizontal transfer of artificial biological elements, and prevent artificial biosynthesis of toxic metabolites. Therefore, it is urgent to strengthen the research on the safety prevention and control of synthetic biology, so as to realize the knowability and controllability of the whole process of artificial life system, and provide security guarantee for the application of synthetic biology in the environmental field. In order to avoid the risk of integration of foreign fragments into the genome, Cas9 protein and gRNA were assembled into a ribonucleoprotein (RNP) in vitro for DNA-free genome editing, which has been successfully tested in many plants (Metje-Sprink et al., 2018).
Phytoremediation is an effective method to control soil heavy metal pollution, but it is difficult to complete the remediation of complex pollution by a single plant at present. We propose that the remediation of soil heavy metal pollution should apply synthetic biology strategy, and the treatment process should use a comprehensive process of “microbial biosensor detection - phytoremediation - heavy metal recovery”. The heavy metal microbial biosensor should be integrated with microfluidic technology. The identification of hyperaccumulator species and other metal tolerant plants should continue, but local plant species should be used as much as possible to avoid the possible spread of invasive species. Moreover, chassis plants should be different from crops. The application of new experimental techniques will help to identify elements and redesign gene circuits.
The rapid development of synthetic biology has provided us with new technologies for creating modular and biological control systems. Accelerating the discovery of genetic elements and the artificial construction of gene circuits can improve the control effect of phytoremediation on soil heavy metal pollution.
SB and DF designed the project and wrote the manuscript. XH revised the manuscript. All authors contributed to the article and approved the submitted version.
The authors declare that the research was conducted in the absence of any commercial or financial relationships that could be construed as a potential conflict of interest.
All claims expressed in this article are solely those of the authors and do not necessarily represent those of their affiliated organizations, or those of the publisher, the editors and the reviewers. Any product that may be evaluated in this article, or claim that may be made by its manufacturer, is not guaranteed or endorsed by the publisher.
Antunes, M., Morey, K., Smith, J., Albrecht, K., Bowen, T., Zdunek, J., et al. (2011). Programmable ligand detection system in plants through a synthetic signal transduction pathway. PloS One 6 (1), e16292. doi: 10.1371/journal.pone.0016292
Anzalone, A., Randolph, P., Davis, J., Sousa, A., Koblan, L., Levy, J., et al. (2019). Search-and-Replace genome editing without double-strand breaks or donor DNA. Nature 576 (7785), 149–157. doi: 10.1038/s41586-019-1711-4
Armienta, M., Beltrán, M., Martínez, S., Labastida, I. (2020). Heavy metal assimilation in maize (Zea mays l.) plants growing near mine tailings. Environ. geochemistry Health 42 (8), 2361–2375. doi: 10.1007/s10653-019-00424-1
Asadollahfardi, G., Sarmadi, M., Rezaee, M., Khodadadi-Darban, A., Yazdani, M., Paz-Garcia, J. (2021). Comparison of different extracting agents for the recovery of Pb and zn through electrokinetic remediation of mine tailings. J. Environ. Manage. 279, 111728. doi: 10.1016/j.jenvman.2020.111728
Bahramnejad, B., Naji, M., Bose, R., Jha, S. (2019). A critical review on use of agrobacterium rhizogenes and their associated binary vectors for plant transformation. Biotechnol. Adv. 37 (7), 107405. doi: 10.1016/j.biotechadv.2019.06.004
Baig, H., Fontanarrosa, P., Kulkarni, V., McLaughlin, J., Vaidyanathan, P., Bartley, B., et al. (2020). Synthetic biology open language (Sbol) version 3.0.0. J. Integr. Bioinf. 17, 2–3. doi: 10.1515/jib-2020-0017
Balzano, S., Sardo, A., Blasio, M., Chahine, T., Dell'Anno, F., Sansone, C., et al. (2020). Microalgal metallothioneins and phytochelatins and their potential use in bioremediation. Front. Microbiol. 11. doi: 10.3389/fmicb.2020.00517
Beattie, I., Haverkamp, R. (2011). Silver and gold nanoparticles in plants: Sites for the reduction to metal. Metallomics integrated biometal Sci. 3 (6), 628–632. doi: 10.1039/c1mt00044f
Bian, F., Zhong, Z., Zhang, X., Yang, C., Gai, X. (2020). Bamboo - an untapped plant resource for the phytoremediation of heavy metal contaminated soils. Chemosphere 246, 125750. doi: 10.1016/j.chemosphere.2019.125750
Boutilier, K., Offringa, R., Sharma, V., Kieft, H., Ouellet, T., Zhang, L., et al. (2002). Ectopic expression of baby boom triggers a conversion from vegetative to embryonic growth. Plant Cell 14 (8), 1737–1749. doi: 10.1105/tpc.001941
Briskine, R., Paape, T., Shimizu-Inatsugi, R., Nishiyama, T., Akama, S., Sese, J., et al. (2017). Genome assembly and annotation of arabidopsis halleri, a model for heavy metal hyperaccumulation and evolutionary ecology. Mol. Ecol. Resour. 17 (5), 1025–1036. doi: 10.1111/1755-0998.12604
Brophy, J., Magallon, K., Duan, L., Zhong, V., Ramachandran, P., Kniazev, K., et al. (2022). Synthetic genetic circuits as a means of reprogramming plant roots. Sci. (New York NY) 377 (6607), 747–751. doi: 10.1126/science.abo4326
Brown, S., Chaney, R., Angle, J., Baker, A. (1995). Zinc and cadmium uptake by hyperaccumulator thlaspi caerulescens and metal tolerant silene vulgaris grown on sludge-amended soils. Environ. Sci. Technol. 29 (6), 1581–1585. doi: 10.1021/es00006a022
Brown, N., Stoyanov, J., Kidd, S., Hobman, J. (2003). The merr family of transcriptional regulators. FEMS Microbiol. Rev. 27, 145–163. doi: 10.1016/s0168-6445(03)00051-2
Buffi, N., Merulla, D., Beutier, J., Barbaud, F., Beggah, S., van Lintel, H., et al. (2011). Development of a microfluidics biosensor for agarose-bead immobilized escherichia coli bioreporter cells for arsenite detection in aqueous samples. Lab. chip 11 (14), 2369–2377. doi: 10.1039/c1lc20274j
Busenlehner, L., Pennella, M., Giedroc, D. (2003). The Smtb/Arsr family of metalloregulatory transcriptional repressors: Structural insights into prokaryotic metal resistance. FEMS Microbiol. Rev. 27, 131–143. doi: 10.1016/s0168-6445(03)00054-8
Butt, H., Rao, G., Sedeek, K., Aman, R., Kamel, R., Mahfouz, M. (2020). Engineering herbicide resistance Via prime editing in rice. Plant Biotechnol. J. 18 (12), 2370–2372. doi: 10.1111/pbi.13399
Byrne, A., Beaudin, A., Olsen, H., Jain, M., Cole, C., Palmer, T., et al. (2017). Nanopore long-read rnaseq reveals widespread transcriptional variation among the surface receptors of individual b cells. Nat. Commun. 8, 16027. doi: 10.1038/ncomms16027
Cabral, L., Giovanella, P., Gianello, C., Bento, F., Andreazza, R., Camargo, F. (2013). Isolation and characterization of bacteria from mercury contaminated sites in Rio grande do sul, Brazil, and assessment of methylmercury removal capability of a pseudomonas putida V1 strain. Biodegradation 24 (3), 319–331. doi: 10.1007/s10532-012-9588-z
Cameron, D., Bashor, C., Collins, J. (2014). A brief history of synthetic biology. Nat. Rev. Microbiol. 12 (5), 381–390. doi: 10.1038/nrmicro3239
Chang, F., Kuang, L., Huang, C., Jane, W., Hung, Y., Hsing, Y., et al. (2013). A simple plant gene delivery system using mesoporous silica nanoparticles as carriers. J. materials Chem. B 1 (39), 5279–5287. doi: 10.1039/c3tb20529k
Che, P., Wu, E., Simon, M., Anand, A., Lowe, K., Gao, H., et al. (2022). Wuschel2 enables highly efficient Crispr/Cas-targeted genome editing during rapid De novo shoot regeneration in sorghum. Commun. Biol. 5 (1), 344. doi: 10.1038/s42003-022-03308-w
Chen, W., Guillaume-Gentil, O., Rainer, P., Gäbelein, C., Saelens, W., Gardeux, V., et al. (2022). Live-seq enables temporal transcriptomic recording of single cells. Nature 608 (7924), 733–740. doi: 10.1038/s41586-022-05046-9
Chen, A., Liao, S., Cheng, M., Ma, K., Wu, L., Lai, Y., et al. (2022). Spatiotemporal transcriptomic atlas of mouse organogenesis using DNA nanoball-patterned arrays. Cell 185 (10), 1777–92.e21. doi: 10.1016/j.cell.2022.04.003
Chen, Y., Qian, W., Lin, L., Cai, L., Yin, K., Jiang, S., et al. (2021). Mapping gene expression in the spatial dimension. Small Methods 5 (11), e2100722. doi: 10.1002/smtd.202100722
Chen, S., Yu, M., Li, H., Wang, Y., Lu, Z., Zhang, Y., et al. (2020). Sahsfa4c from hance enhances cadmium tolerance by regulating ros-scavenger activities and heat shock proteins expression. Front. Plant Sci. 11, 142. doi: 10.3389/fpls.2020.00142
Choi, Y., Park, T., Lee, D., Lee, S. (2018). Escherichia colirecombinant as a biofactory for various single- and multi-element nanomaterials. Proc. Natl. Acad. Sci. United States America 115 (23), 5944–5949. doi: 10.1073/pnas.1804543115
Clarke, J., Wu, H., Jayasinghe, L., Patel, A., Reid, S., Bayley, H. (2009). Continuous base identification for single-molecule nanopore DNA sequencing. Nat. nanotechnol 4 (4), 265–270. doi: 10.1038/nnano.2009.12
Cobbett, C., Goldsbrough, P. (2002). Phytochelatins and metallothioneins: Roles in heavy metal detoxification and homeostasis. Annu. Rev. Plant Biol. 53, 159–182. doi: 10.1146/annurev.arplant.53.100301.135154
Cody, J., Maher, M., Nasti, R., Starker, C., Chamness, J., Voytas, D. (2022). Direct delivery and fast-treated agrobacterium Co-culture (Fast-tracc) plant transformation methods for nicotiana benthamiana. Nat. Protoc. 1, 81–107. doi: 10.1038/s41596-022-00749-9
Cromie, M., Shi, Y., Latifi, T., Groisman, E. (2006). An rna sensor for intracellular Mg(2+). Cell 125 (1), 71–84. doi: 10.1016/j.cell.2006.01.043
Dabirian, Y., Li, X., Chen, Y., David, F., Nielsen, J., Siewers, V. (2019). Saccharomyces cerevisiaeexpanding the dynamic range of a transcription factor-based biosensor in. ACS synthetic Biol. 8 (9), 1968–1975. doi: 10.1021/acssynbio.9b00144
David, F., Nielsen, J., Siewers, V. (2016). Flux control at the malonyl-coa node through hierarchical dynamic pathway regulation in saccharomyces cerevisiae. ACS synthetic Biol. 5 (3), 224–233. doi: 10.1021/acssynbio.5b00161
Demirer, G., Zhang, H., Goh, N., Pinals, R., Chang, R., Landry, M. (2020). Carbon nanocarriers deliver sirna to intact plant cells for efficient gene knockdown. Sci. Adv. 6 (26), eaaz0495. doi: 10.1126/sciadv.aaz0495
Deng, Y., Bartosovic, M., Kukanja, P., Zhang, D., Liu, Y., Su, G., et al. (2022a). Spatial-Cut&Tag: Spatially resolved chromatin modification profiling at the cellular level. Sci. (New York NY) 375 (6581), 681–686. doi: 10.1126/science.abg7216
Deng, Y., Bartosovic, M., Ma, S., Zhang, D., Kukanja, P., Xiao, Y., et al. (2022b). Spatial profiling of chromatin accessibility in mouse and human tissues. Nature 609 (7926), 375–383. doi: 10.1038/s41586-022-05094-1
Depledge, D., Srinivas, K., Sadaoka, T., Bready, D., Mori, Y., Placantonakis, D., et al. (2019). Direct rna sequencing on nanopore arrays redefines the transcriptional complexity of a viral pathogen. Nat. Commun. 10 (1), 754. doi: 10.1038/s41467-019-08734-9
Ding, T., Yang, J., Pan, V., Zhao, N., Lu, Z., Ke, Y., et al. (2020). DNA Nanotechnology assisted nanopore-based analysis. Nucleic Acids Res. 48 (6), 2791–2806. doi: 10.1093/nar/gkaa095
Dolphen, R., Thiravetyan, P. (2019). Reducing arsenic in rice grains by leonardite and arsenic-resistant endophytic bacteria. Chemosphere 223, 448–454. doi: 10.1016/j.chemosphere.2019.02.054
Dong, O., Ronald, P. (2021). Targeted DNA insertion in plants. Proc. Natl. Acad. Sci. United States America 118 (22), e2004834117. doi: 10.1073/pnas.2004834117
Du, R., Guo, M., He, X., Huang, K., Luo, Y., Xu, W. (2019). Feedback regulation mode of gene circuits directly affects the detection range and sensitivity of lead and mercury microbial biosensors. Analytica chimica Acta 1084, 85–92. doi: 10.1016/j.aca.2019.08.006
Ellis, D., Gumaelius, L., Indriolo, E., Pickering, I., Banks, J., Salt, D. (2006). A novel arsenate reductase from the arsenic hyperaccumulating fern pteris vittata. Plant Physiol. 141 (4), 1544–1554. doi: 10.1104/pp.106.084079
Endy, D. (2005). Foundations for engineering biology. Nature 438 (7067), 449–453. doi: 10.1038/nature04342
Engler, C., Youles, M., Gruetzner, R., Ehnert, T., Werner, S., Jones, J., et al. (2014). A golden gate modular cloning toolbox for plants. ACS synthetic Biol. 3 (11), 839–843. doi: 10.1021/sb4001504
Esvelt, K., Wang, H. (2013). Genome-scale engineering for systems and synthetic biology. Mol. Syst. Biol. 9, 641. doi: 10.1038/msb.2012.66
Eto, K. (2000). Minamata disease. Neuropathology. 20 (1), S14–S19. doi: 10.1046/j.1440-1789.2000.00295.x
Fang, C., Philips, S., Wu, X., Chen, K., Shi, J., Shen, L., et al. (2021). Cuer activates transcription through a DNA distortion mechanism. Nat. Chem. Biol. 17 (1), 57–64. doi: 10.1038/s41589-020-00653-x
Fang, C., Zhang, Y. (2022). Bacterial merr family transcription regulators: Activationby distortion. Acta Biochim. Biophys. Sin. 54 (1), 25–36. doi: 10.3724/abbs.2021003
Ferrucci, A., Vocciante, M., Bagatin, R., Ferro, S. (2017). Electrokinetic remediation of soils contaminated by potentially toxic metals: Dedicated analytical tools for assessing the contamination baseline in a complex scenario. J. Environ. Manage. 203, 1163–1168. doi: 10.1016/j.jenvman.2017.02.037
Fujimoto, H., Wakabayashi, M., Yamashiro, H., Maeda, I., Isoda, K., Kondoh, M., et al. (2006). Whole-cell arsenite biosensor using photosynthetic bacterium rhodovulum sulfidophilum. rhodovulum sulfidophilum as an arsenite biosensor. Appl. Microbiol. Biotechnol. 73 (2), 332–338. doi: 10.1007/s00253-006-0483-6
Furuhata, Y., Sakai, A., Murakami, T., Morikawa, M., Nakamura, C., Yoshizumi, T., et al. (2019). A method using electroporation for the protein delivery of cre recombinase into cultured arabidopsis cells with an intact cell wall. Sci. Rep. 9 (1), 2163. doi: 10.1038/s41598-018-38119-9
Fuselli, S., Baptista, R., Panziera, A., Magi, A., Guglielmi, S., Tonin, R., et al. (2018). A new hybrid approach for mhc genotyping: High-throughput ngs and long read minion nanopore sequencing, with application to the non-model vertebrate alpine chamois (Rupicapra rupicapra). Heredity 121 (4), 293–303. doi: 10.1038/s41437-018-0070-5
Gaudelli, N., Komor, A., Rees, H., Packer, M., Badran, A., Bryson, D., et al. (2017). Programmable base editing of a•T to G•C in genomic DNA without DNA cleavage. Nature 551 (7681), 464–471. doi: 10.1038/nature24644
Gonzaga, M., Mackowiak, C., Quintão de Almeida, A., Wisniewski, A., Figueiredo de Souza, D., da Silva Lima, I., et al. (2018). Assessing biochar applications and repeated brassica juncea l. production cycles to remediate Cu contaminated soil. Chemosphere 201, 278–285. doi: 10.1016/j.chemosphere.2018.03.038
Goswami, S., Das, S. (2015). A study on cadmium phytoremediation potential of Indian mustard, brassica juncea. Int. J. phytoremediation 17, 583–588. doi: 10.1080/15226514.2014.935289
Guo, M., Du, R., Xie, Z., He, X., Huang, K., Luo, Y., et al. (2019). Using the promoters of merr family proteins as "Rheostats" to engineer whole-cell heavy metal biosensors with adjustable sensitivity. J. Biol. Eng. 13, 70. doi: 10.1186/s13036-019-0202-3
Guo, F., Li, L., Li, J., Wu, X., Hu, B., Zhu, P., et al. (2017). Single-cell multi-omics sequencing of mouse early embryos and embryonic stem cells. Cell Res. 27 (8), 967–988. doi: 10.1038/cr.2017.82
Gurajala, H., Cao, X., Tang, L., Ramesh, T., Lu, M., Yang, X. (2019). Comparative assessment of Indian mustard (Brassica juncea l.) genotypes for phytoremediation of cd and Pb contaminated soils. Environ. pollut. (Barking Essex 1987) 254, 113085. doi: 10.1016/j.envpol.2019.113085
Hall, J. (2002). Cellular mechanisms for heavy metal detoxification and tolerance. J. Exp. Bot. 53 (366), 1–11. doi: 10.1093/jxb/53.366.1
Han, R., Chen, J., He, S., Dai, Z., Liu, X., Cao, Y., et al. (2022). Arsenic-induced up-regulation of p transporters Pvpht1;3-1;4 enhances both as and p uptake in as-hyperaccumulator pteris vittata. J. hazardous materials 438, 129430. doi: 10.1016/j.jhazmat.2022.129430
Harms, H., Wells, M., van der Meer, J. (2006). Whole-cell living biosensors–are they ready for environmental application? Appl. Microbiol. Biotechnol. 70 (3), 273–280. doi: 10.1007/s00253-006-0319-4
He, M., Li, X., Liu, H., Miller, S., Wang, G., Rensing, C. (2011). Characterization and genomic analysis of a highly chromate resistant and reducing bacterial strain lysinibacillus fusiformis Zc1. J. hazardous materials 185, 682–688. doi: 10.1016/j.jhazmat.2010.09.072
Henry, R., Edwards, K. (2009). New tools for single nucleotide polymorphism (Snp) discovery and analysis accelerating plant biotechnology. Plant Biotechnol. J. 7 (4), 311. doi: 10.1111/j.1467-7652.2009.00417.x
Hilton, I., D'Ippolito, A., Vockley, C., Thakore, P., Crawford, G., Reddy, T., et al. (2015). Epigenome editing by a crispr-Cas9-Based acetyltransferase activates genes from promoters and enhancers. Nat. Biotechnol. 33 (5), 510–517. doi: 10.1038/nbt.3199
Hizhnyi, Y., Nedilko, S., Borysiuk, V., Shyichuk, A. (2017). Ab initio computational study of chromate molecular anion adsorption on the surfaces of pristine and b- or n-doped carbon nanotubes and graphene. Nanoscale Res. Lett. 12 (1), 71. doi: 10.1186/s11671-017-1846-x
Hossain, Z., Komatsu, S. (2012). Contribution of proteomic studies towards understanding plant heavy metal stress response. Front. Plant Sci. 3, 310. doi: 10.3389/fpls.2012.00310
Hu, P., Brodie, E., Suzuki, Y., McAdams, H., Andersen, G. (2005). Whole-genome transcriptional analysis of heavy metal stresses in caulobacter crescentus. J. bacteriol 187 (24), 8437–8449. doi: 10.1128/jb.187.24.8437-8449.2005
Hu, Y., Jiang, Z., Chen, K., Zhou, Z., Zhou, X., Wang, Y., et al. (2022). Scnanoatac-seq: A long-read single-cell atac sequencing method to detect chromatin accessibility and genetic variants simultaneously within an individual cell. Cell Res. 33 (1), 83–86. doi: 10.1038/s41422-022-00730-x
Hu, B., Li, D., Liu, X., Qi, J., Gao, D., Zhao, S., et al. (2017). Engineering non-transgenic gynoecious cucumber using an improved transformation protocol and optimized Crispr/Cas9 system. Mol. Plant 10 (12), 1575–1578. doi: 10.1016/j.molp.2017.09.005
Hua, K., Jiang, Y., Tao, X., Zhu, J. (2020). Precision genome engineering in rice using prime editing system. Plant Biotechnol. J. 18 (11), 2167–2169. doi: 10.1111/pbi.13395
Huang, X., Deng, F., Yamaji, N., Pinson, S., Fujii-Kashino, M., Danku, J., et al. (2016). A heavy metal p-type atpase Oshma4 prevents copper accumulation in rice grain. Nat. Commun. 7, 12138. doi: 10.1038/ncomms12138
Huang, C., Yang, S., Sun, M., Liao, V. (2015). Development of a set of bacterial biosensors for simultaneously detecting arsenic and mercury in groundwater. Environ. Sci. pollut. Res. Int. 22 (13), 10206–10213. doi: 10.1007/s11356-015-4216-1
Hussey, S., Grima-Pettenati, J., Myburg, A., Mizrachi, E., Brady, S., Yoshikuni, Y., et al. (2019). A standardized synthetic eucalyptus transcription factor and promoter panel for re-engineering secondary cell wall regulation in biomass and bioenergy crops. ACS synthetic Biol. 8 (2), 463–465. doi: 10.1021/acssynbio.8b00440
Jaffré, T., Brooks, R., Lee, J., Reeves, R. (1976). Sebertia acuminata: A hyperaccumulator of nickel from new Caledonia. Sci. (New York NY) 193 (4253), 579–580. doi: 10.1126/science.193.4253.579
Jiang, Y., Chai, Y., Lu, M., Han, X., Lin, Q., Zhang, Y., et al. (2020). Prime editing efficiently generates W542l and S621i double mutations in two als genes in maize. Genome Biol. 21 (1), 257. doi: 10.1186/s13059-020-02170-5
Jiang, Y., Chen, B., Duan, C., Sun, B., Yang, J., Yang, S. (2016). Erratum for jiang et al., multigene editing in the escherichia coli genome Via the crispr-Cas9 system. Appl. Environ. Microbiol. 82 (12), 3693. doi: 10.1128/aem.01181-16
Jiang, W., Zhou, H., Bi, H., Fromm, M., Yang, B., Weeks, D. (2013). Demonstration of Crispr/Cas9/Sgrna-mediated targeted gene modification in arabidopsis, tobacco, sorghum and rice. Nucleic Acids Res. 41 (20), e188. doi: 10.1093/nar/gkt780
Kang, S., Bozhilov, K., Myung, N., Mulchandani, A., Chen, W. (2008). Microbial synthesis of cds nanocrystals in genetically engineered e. coli. Angewandte Chemie (International ed English) 47 (28), 5186–5189. doi: 10.1002/anie.200705806
Khalil, A., Collins, J. (2010). Synthetic biology: Applications come of age. Nat. Rev. Genet. 11 (5), 367–379. doi: 10.1038/nrg2775
Kim, M., Lim, J., Kim, H., Lee, S., Lee, S., Kim, T. (2015). Chemostat-like microfluidic platform for highly sensitive detection of heavy metal ions using microbial biosensors. Biosensors bioelectronics 65, 257–264. doi: 10.1016/j.bios.2014.10.028
King, J., Digrazia, P., Applegate, B., Burlage, R., Sanseverino, J., Dunbar, P., et al. (1990). Rapid, sensitive bioluminescent reporter technology for naphthalene exposure and biodegradation. Sci. (New York NY) 249 (4970), 778–781. doi: 10.1126/science.249.4970.778
Komor, A., Kim, Y., Packer, M., Zuris, J., Liu, D. (2016). Programmable editing of a target base in genomic DNA without double-stranded DNA cleavage. Nature 533 (7603), 420–424. doi: 10.1038/nature17946
Kwak, S., Lew, T., Sweeney, C., Koman, V., Wong, M., Bohmert-Tatarev, K., et al. (2019). Chloroplast-selective gene delivery and expression in planta using chitosan-complexed single-walled carbon nanotube carriers. Nat. nanotechnol 14 (5), 447–455. doi: 10.1038/s41565-019-0375-4
Lange, B., van der Ent, A., Baker, A., Echevarria, G., Mahy, G., Malaisse, F., et al. (2017). Copper and cobalt accumulation in plants: A critical assessment of the current state of knowledge. New Phytol. 213 (2), 537–551. doi: 10.1111/nph.14175
Lee, J., Hyeon, D., Hwang, D. (2020). Single-cell multiomics: Technologies and data analysis methods. Exp. Mol. Med. 52 (9), 1428–1442. doi: 10.1038/s12276-020-0420-2
Leonhartsberger, S., Huber, A., Lottspeich, F., Böck, A. (2001). The Hydh/G genes from escherichia coli code for a zinc and lead responsive two-component regulatory system. J. Mol. Biol. 307 (1), 93–105. doi: 10.1006/jmbi.2000.4451
Li, C., Dang, F., Li, M., Zhu, M., Zhong, H., Hintelmann, H., et al. (2017). Effects of exposure pathways on the accumulation and phytotoxicity of silver nanoparticles in soybean and rice. Nanotoxicology 11 (5), 699–709. doi: 10.1080/17435390.2017.1344740
Li, J., Gurajala, H., Wu, L., van der Ent, A., Qiu, R., Baker, A., et al. (2018). Hyperaccumulator plants from China: A synthesis of the current state of knowledge. Environ. Sci. Technol. 52 (21), 11980–11994. doi: 10.1021/acs.est.8b01060
Li, H., Li, J., Chen, J., Yan, L., Xia, L. (2020). Precise modifications of both exogenous and endogenous genes in rice by prime editing. Mol. Plant 13 (5), 671–674. doi: 10.1016/j.molp.2020.03.011
Li, J., Norville, J., Aach, J., McCormack, M., Zhang, D., Bush, J., et al. (2013). Multiplex and homologous recombination-mediated genome editing in arabidopsis and nicotiana benthamiana using guide rna and Cas9. Nat. Biotechnol. 31 (8), 688–691. doi: 10.1038/nbt.2654
Li, C., Zhang, R., Meng, X., Chen, S., Zong, Y., Lu, C., et al. (2020). Targeted, random mutagenesis of plant genes with dual cytosine and adenine base editors. Nat. Biotechnol. 38 (7), 875–882. doi: 10.1038/s41587-019-0393-7
Liao, J., Lu, X., Shao, X., Zhu, L., Fan, X. (2021). Uncovering an organ's molecular architecture at single-cell resolution by spatially resolved transcriptomics. Trends Biotechnol. 39 (1), 43–58. doi: 10.1016/j.tibtech.2020.05.006
Lin, Q., Jin, S., Zong, Y., Yu, H., Zhu, Z., Liu, G., et al. (2021). High-efficiency prime editing with optimized, paired pegrnas in plants. Nat. Biotechnol. 39 (8), 923–927. doi: 10.1038/s41587-021-00868-w
Liu, J., Duan, C., Zhang, X., Zhu, Y., Lu, X. (2011). Potential of leersia hexandra Swartz for phytoextraction of cr from soil. J. hazardous materials 188, 85–91. doi: 10.1016/j.jhazmat.2011.01.066
Liu, X., Hu, Q., Yang, J., Huang, S., Wei, T., Chen, W., et al. (2019). Selective cadmium regulation mediated by a cooperative binding mechanism in cadr. Proc. Natl. Acad. Sci. United States America 116 (41), 20398–20403. doi: 10.1073/pnas.1908610116
Liu, W., Stewart, C. (2015). Plant synthetic biology. Trends Plant Sci. 20 (5), 309–317. doi: 10.1016/j.tplants.2015.02.004
Liu, Z., Tang, S., Hu, W., Lv, R., Mei, H., Yang, R., et al. (2022). Precise editing of methylated cytosine in arabidopsis thaliana using a human Apobec3bctd-Cas9 fusion. Sci. China Life Sci. 65 (1), 219–222. doi: 10.1007/s11427-021-1970-x
Liu, Y., Yang, M., Deng, Y., Su, G., Enninful, A., Guo, C., et al. (2020). High-Spatial-Resolution multi-omics sequencing Via deterministic barcoding in tissue. Cell 183 (6), 1665–81.e18. doi: 10.1016/j.cell.2020.10.026
Lloyd, J., Ly, F., Gong, P., Pflueger, J., Swain, T., Pflueger, C., et al. (2022). Synthetic memory circuits for stable cell reprogramming in plants. Nat. Biotechnol. 40 (12), 1862–1872. doi: 10.1038/s41587-022-01383-2
Long, Y., Liu, Z., Jia, J., Mo, W., Fang, L., Lu, D., et al. (2021). Flsnrna-seq: Protoplasting-free full-length single-nucleus rna profiling in plants. Genome Biol. 22 (1), 66. doi: 10.1186/s13059-021-02288-0
Lu, Y., Tian, Y., Shen, R., Yao, Q., Zhong, D., Zhang, X., et al. (2021). Precise genome modification in tomato using an improved prime editing system. Plant Biotechnol. J. 19 (3), 415–417. doi: 10.1111/pbi.13497
Lu, L., Tian, S., Zhang, J., Yang, X., Labavitch, J., Webb, S., et al. (2013). Efficient xylem transport and phloem remobilization of zn in the hyperaccumulator plant species sedum alfredii. New Phytol. 198 (3), 721–731. doi: 10.1111/nph.12168
Luo, C., Fernie, A., Yan, J. (2020). Single-cell genomics and epigenomics: Technologies and applications in plants. Trends Plant Sci. 25 (10), 1030–1040. doi: 10.1016/j.tplants.2020.04.016
Luo, C., Li, X., Zhang, Q., Yan, J. (2019). Single gametophyte sequencing reveals that crossover events differ between sexes in maize. Nat. Commun. 10 (1), 785. doi: 10.1038/s41467-019-08786-x
Lv, Z., Jiang, R., Chen, J., Chen, W. (2020). Nanoparticle-mediated gene transformation strategies for plant genetic engineering. Plant J. Cell Mol. Biol. 104 (4), 880–891. doi: 10.1111/tpj.14973
Ma, Z., Meliana, C., Munawaroh, H., Karaman, C., Karimi-Maleh, H., Low, S., et al. (2022). Recent advances in the analytical strategies of microbial biosensor for detection of pollutants. Chemosphere 306, 135515. doi: 10.1016/j.chemosphere.2022.135515
Maeder, M., Linder, S., Cascio, V., Fu, Y., Ho, Q., Joung, J. (2013). Crispr rna-guided activation of endogenous human genes. Nat. Methods 10 (10), 977–979. doi: 10.1038/nmeth.2598
Malmberg, M., Spangenberg, G., Daetwyler, H., Cogan, N. (2019). Assessment of low-coverage nanopore long read sequencing for snp genotyping in doubled haploid canola (Brassica napus l.). Sci. Rep. 9 (1), 8688. doi: 10.1038/s41598-019-45131-0
Manrao, E., Derrington, I., Laszlo, A., Langford, K., Hopper, M., Gillgren, N., et al. (2012). Reading DNA at single-nucleotide resolution with a mutant mspa nanopore and Phi29 DNA polymerase. Nat. Biotechnol. 30 (4), 349–353. doi: 10.1038/nbt.2171
Marand, A., Chen, Z., Gallavotti, A., Schmitz, R. (2021). A cis-regulatory atlas in maize at single-cell resolution. Cell 184 (11), 3041–55.e21. doi: 10.1016/j.cell.2021.04.014
Marques Neto, J., Bellato, C., Silva, D. (2019). Iron Oxide/Carbon Nanotubes/Chitosan magnetic composite film for chromium species removal. Chemosphere 218, 391–401. doi: 10.1016/j.chemosphere.2018.11.080
Mayo, K., Gonzales, B., Mason, H. (2006). Genetic transformation of tobacco Nt1 cells with agrobacterium tumefaciens. Nat. Protoc. 1 (3), 1105–1111. doi: 10.1038/nprot.2006.176
Meharg, A. (2004). Arsenic in rice–understanding a new disaster for south-East Asia. Trends Plant Sci. 9 (9), 415–417. doi: 10.1016/j.tplants.2004.07.002
Metje-Sprink, J., Menz, J., Modrzejewski, D., Sprink, T. (2018). DNA-Free genome editing: Past, present and future. Front. Plant Sci. 9, 1957. doi: 10.3389/fpls.2018.01957
Mijovilovich, A., Leitenmaier, B., Meyer-Klaucke, W., Kroneck, P., Götz, B., Küpper, H. (2009). Complexation and toxicity of copper in higher plants. ii. different mechanisms for copper versus cadmium detoxification in the copper-sensitive Cadmium/Zinc hyperaccumulator thlaspi caerulescens (Ganges ecotype). Plant Physiol. 151 (2), 715–731. doi: 10.1104/pp.109.144675
Milner, M., Seamon, J., Craft, E., Kochian, L. (2013). Transport properties of members of the zip family in plants and their role in zn and Mn homeostasis. J. Exp. Bot. 64 (1), 369–381. doi: 10.1093/jxb/ers315
Mitter, N., Worrall, E., Robinson, K., Li, P., Jain, R., Taochy, C., et al. (2017). Clay nanosheets for topical delivery of rnai for sustained protection against plant viruses. Nat. Plants 3, 16207. doi: 10.1038/nplants.2016.207
Mourato, M., Moreira, I., Leitão, I., Pinto, F., Sales, J., Martins, L. (2015). Effect of heavy metals in plants of the genus brassica. Int. J. Mol. Sci. 16 (8), 17975–17998. doi: 10.3390/ijms160817975
Moynihan, M., Peterson, K., Cantoral, A., Song, P., Jones, A., Solano-González, M., et al. (2017). Dietary predictors of urinary cadmium among pregnant women and children. Sci. total Environ. 575, 1255–1262. doi: 10.1016/j.scitotenv.2016.09.204
Mu'azu, N., Haladu, S., Jarrah, N., Zubair, M., Essa, M., Ali, S. (2018). Polyaspartate extraction of cadmium ions from contaminated soil: Evaluation and optimization using central composite design. J. hazardous materials 342, 58–68. doi: 10.1016/j.jhazmat.2017.08.013
Muralidharan, S., Thompson, E., Raftos, D., Birch, G., Haynes, P. (2012). Quantitative proteomics of heavy metal stress responses in Sydney rock oysters. Proteomics 12 (6), 906–921. doi: 10.1002/pmic.201100417
Nagahara, S., Higashiyama, T., Mizuta, Y. (2021). Detection of a biolistic delivery of fluorescent markers and Crispr/Cas9 to the pollen tube. Plant Reprod. 34 (3), 191–205. doi: 10.1007/s00497-021-00418-z
Niu, H., Wu, H., Chen, K., Sun, J., Cao, M., Luo, J. (2021). Effects of decapitated and root-pruned sedum alfredii on the characterization of dissolved organic matter and enzymatic activity in rhizosphere soil during cd phytoremediation. J. hazardous materials 417, 125977. doi: 10.1016/j.jhazmat.2021.125977
Osman, D., Cavet, J. (2010). Bacterial metal-sensing proteins exemplified by arsr-smtb family repressors. Natural product Rep. 27 (5), 668–680. doi: 10.1039/b906682a
Outridge, P., Mason, R., Wang, F., Guerrero, S., Heimbürger-Boavida, L. (2018). Updated global and oceanic mercury budgets for the united nations global mercury assessment 2018. Environ. Sci. Technol. 52 (20), 11466–11477. doi: 10.1021/acs.est.8b01246
Passarinho, P., Ketelaar, T., Xing, M., van Arkel, J., Maliepaard, C., Hendriks, M., et al. (2008). Baby boom target genes provide diverse entry points into cell proliferation and cell growth pathways. Plant Mol. Biol. 68 (3), 225–237. doi: 10.1007/s11103-008-9364-y
Pasupathy, K., Lin, S., Hu, Q., Luo, H., Ke, P. (2008). Direct plant gene delivery with a Poly(Amidoamine) dendrimer. Biotechnol. J. 3 (8), 1078–1082. doi: 10.1002/biot.200800021
Pence, N., Larsen, P., Ebbs, S., Letham, D., Lasat, M., Garvin, D., et al. (2000). The molecular physiology of heavy metal transport in the Zn/Cd hyperaccumulator thlaspi caerulescens. Proc. Natl. Acad. Sci. United States America 97 (9), 4956–4960. doi: 10.1073/pnas.97.9.4956
Pennella, M., Giedroc, D. (2005). Structural determinants of metal selectivity in prokaryotic metal-responsive transcriptional regulators. Biometals 18 (4), 413–428. doi: 10.1007/s10534-005-3716-8
Persans, M., Nieman, K., Salt, D. (2001). Functional activity and role of cation-efflux family members in Ni hyperaccumulation in thlaspi goesingense. Proc. Natl. Acad. Sci. United States America 98 (17), 9995–10000. doi: 10.1073/pnas.171039798
Picelli, S., Faridani, O., Björklund, A., Winberg, G., Sagasser, S., Sandberg, R. (2014). Full-length rna-seq from single cells using smart-Seq2. Nat. Protoc. 9 (1), 171–181. doi: 10.1038/nprot.2014.006
Pilon-Smits, E. (2005). Phytoremediation. Annu. Rev. Plant Biol. 56, 15–39. doi: 10.1146/annurev.arplant.56.032604.144214
Qi, L., Larson, M., Gilbert, L., Doudna, J., Weissman, J., Arkin, A., et al. (2013). Repurposing crispr as an rna-guided platform for sequence-specific control of gene expression. Cell 152 (5), 1173–1183. doi: 10.1016/j.cell.2013.02.022
Qiong, W., Fengshan, P., Xiaomeng, X., Rafiq, M., Xiao'e, Y., Bao, C., et al. (2021). Cadmium level and soil type played a selective role in the endophytic bacterial community of hyperaccumulator sedum alfredii hance. Chemosphere 263, 127986. doi: 10.1016/j.chemosphere.2020.127986
Ramkumar, T., Lenka, S., Arya, S., Bansal, K. (2020). A short history and perspectives on plant genetic transformation. Methods Mol. Biol. (Clifton NJ) 2124, 39–68. doi: 10.1007/978-1-0716-0356-7_3
Reeves, R., Baker, A., Jaffré, T., Erskine, P., Echevarria, G., van der Ent, A. (2018). A global database for plants that hyperaccumulate metal and metalloid trace elements. New Phytol. 218 (2), 407–411. doi: 10.1111/nph.14907
Rodríguez-Leal, D., Lemmon, Z., Man, J., Bartlett, M., Lippman, Z. (2017). Engineering quantitative trait variation for crop improvement by genome editing. Cell 171 (2), 470–80.e8. doi: 10.1016/j.cell.2017.08.030
Rothert, A., Deo, S., Millner, L., Puckett, L., Madou, M., Daunert, S. (2005). Whole-Cell-Reporter-Gene-Based biosensing systems on a compact disk microfluidics platform. Analytic Biochem. 342 (1), 11–19. doi: 10.1016/j.ab.2004.10.048
Rugh, C., Senecoff, J., Meagher, R., Merkle, S. (1998). Development of transgenic yellow poplar for mercury phytoremediation. Nat. Biotechnol. 16 (10), 925–928. doi: 10.1038/nbt1098-925
Saeed, M., Masood Quraishi, U., Naseem Malik, R. (2021). Arsenic uptake and toxicity in wheat (Triticum aestivum l.): A review of multi-omics approaches to identify tolerance mechanisms. Food Chem. 355, 129607. doi: 10.1016/j.foodchem.2021.129607
Saltepe, B., Kehribar, E., Su Yirmibeşoğlu, S., Şafak Şeker, U. (2018). Cellular biosensors with engineered genetic circuits. ACS sensors 3 (1), 13–26. doi: 10.1021/acssensors.7b00728
Schadt, E., Turner, S., Kasarskis, A. (2010). A window into third-generation sequencing. Hum. Mol. Genet. 19, R227–R240. doi: 10.1093/hmg/ddq416
Schmidt, M., Vogel, A., Denton, A., Istace, B., Wormit, A., van de Geest, H., et al. (2017). Solanum pennelliide Novo assembly of a new accession using nanopore sequencing. Plant Cell 29 (10), 2336–2348. doi: 10.1105/tpc.17.00521
Schwartz, S., Hendrix, B., Hoffer, P., Sanders, R., Zheng, W. (2020). Carbon dots for efficient small interfering rna delivery and gene silencing in plants. Plant Physiol. 184 (2), 647–657. doi: 10.1104/pp.20.00733
Schwille, P. (2011). Bottom-up synthetic biology: Engineering in a tinkerer's world. Sci. (New York NY) 333 (6047), 1252–1254. doi: 10.1126/science.1211701
Shankar, S., Rhim, J. (2015). Amino acid mediated synthesis of silver nanoparticles and preparation of antimicrobial Agar/Silver nanoparticles composite films. Carbohydr. polymers 130, 353–363. doi: 10.1016/j.carbpol.2015.05.018
Shu, H., Zhang, J., Liu, F., Bian, C., Liang, J., Liang, J., et al. (2019). Viola baoshanensiscomparative transcriptomic studies on a cadmium hyperaccumulator and its non-tolerant counterpart. Int. J. Mol. Sci. 20 (8), 1906. doi: 10.3390/ijms20081906
Shukla, J., Mohd, S., Kushwaha, A., Narayan, S., Saxena, P., Bahadur, L., et al. (2022). Endophytic fungus serendipita indica reduces arsenic mobilization from root to fruit in colonized tomato plant. Environ. pollut. (Barking Essex 1987) 298, 118830. doi: 10.1016/j.envpol.2022.118830
Ståhl, P., Salmén, F., Vickovic, S., Lundmark, A., Navarro, J., Magnusson, J., et al. (2016). Visualization and analysis of gene expression in tissue sections by spatial transcriptomics. Sci. (New York NY) 353 (6294), 78–82. doi: 10.1126/science.aaf2403
Sytar, O., Ghosh, S., Malinska, H., Zivcak, M., Brestic, M. (2021). Physiological and molecular mechanisms of metal accumulation in hyperaccumulator plants. Physiologia plantarum 173 (1), 148–166. doi: 10.1111/ppl.13285
Tan, M., Austin, C., Hammer, M., Lee, Y., Croft, L., Gan, H. (2018). Finding nemo: Hybrid assembly with Oxford nanopore and illumina reads greatly improves the clownfish (Amphiprion ocellaris) genome assembly. GigaScience 7 (3), 1–6. doi: 10.1093/gigascience/gix137
Tan, Y., Lee, J., Wang, D. (2010). Uncovering the design rules for peptide synthesis of metal nanoparticles. J. Am. Chem. Soc. 132 (16), 5677–5686. doi: 10.1021/ja907454f
Tang, F., Barbacioru, C., Wang, Y., Nordman, E., Lee, C., Xu, N., et al. (2009). Mrna-seq whole-transcriptome analysis of a single cell. Nat. Methods 6 (5), 377–382. doi: 10.1038/nmeth.1315
Tang, S., Yang, C., Wang, D., Deng, X., Cao, X., Song, X. (2022). Targeted DNA demethylation produces heritable epialleles in rice. Sci. China Life Sci. 65 (4), 753–756. doi: 10.1007/s11427-021-1974-7
Tellgren-Roth, C., Couturier, M. (2022). Detecting DNA methylations in the hyperthermoacidophilic crenarchaeon sulfolobus acidocaldarius using smrt sequencing. Methods Mol. Biol. (Clifton NJ) 2516, 39–50. doi: 10.1007/978-1-0716-2413-5_3
Thibivilliers, S., Libault, M. (2021). Plant single-cell multiomics: Cracking the molecular profiles of plant cells. Trends Plant Sci. 26 (6), 662–663. doi: 10.1016/j.tplants.2021.03.001
Tungtur, S., Egan, S., Swint-Kruse, L. (2007). Functional consequences of exchanging domains between laci and purr are mediated by the intervening linker sequence. Proteins 68 (1), 375–388. doi: 10.1002/prot.21412
Ueki, S., Lacroix, B., Krichevsky, A., Lazarowitz, S., Citovsky, V. (2009). Functional transient genetic transformation of arabidopsis leaves by biolistic bombardment. Nat. Protoc. 4 (1), 71–77. doi: 10.1038/nprot.2008.217
Ulianov, S., Razin, S. (2022). The two waves in single-cell 3d genomics. Semin. Cell Dev. Biol. 121, 143–152. doi: 10.1016/j.semcdb.2021.05.021
van Dijk, E., Jaszczyszyn, Y., Naquin, D., Thermes, C. (2018). The third revolution in sequencing technology. Trends Genet. TIG 34 (9), 666–681. doi: 10.1016/j.tig.2018.05.008
Wang, B., Barahona, M., Buck, M. (2013). A modular cell-based biosensor using engineered genetic logic circuits to detect and integrate multiple environmental signals. Biosensors bioelectronics 40 (1), 368–376. doi: 10.1016/j.bios.2012.08.011
Wang, K., Fredens, J., Brunner, S., Kim, S., Chia, T., Chin, J. (2016). Defining synonymous codon compression schemes by genome recoding. Nature 539 (7627), 59–64. doi: 10.1038/nature20124
Wang, Q., Wei, N., Jin, X., Min, X., Ma, Y., Liu, W. (2021). Molecular characterization of the Copt/Ctr-type copper transporter family under heavy metal stress in alfalfa. Int. J. Biol. macromolecules 181, 644–652. doi: 10.1016/j.ijbiomac.2021.03.173
Wanunu, M., Dadosh, T., Ray, V., Jin, J., McReynolds, L., Drndić, M. (2010). Rapid electronic detection of probe-specific micrornas using thin nanopore sensors. Nat. nanotechnol 5 (11), 807–814. doi: 10.1038/nnano.2010.202
Wilson-Corral, V., Anderson, C., Rodriguez-Lopez, M. (2012). Gold phytomining. a review of the relevance of this technology to mineral extraction in the 21st century. J. Environ. Manage. 111, 249–257. doi: 10.1016/j.jenvman.2012.07.037
Wojas, S., Clemens, S., Hennig, J., Sklodowska, A., Kopera, E., Schat, H., et al. (2008). Overexpression of phytochelatin synthase in tobacco: Distinctive effects of Atpcs1 and cepcs genes on plant response to cadmium. J. Exp. Bot. 59 (8), 2205–2219. doi: 10.1093/jxb/ern092
Wu, Q., Shigaki, T., Williams, K., Han, J., Kim, C., Hirschi, K., et al. (2011). Expression of an arabidopsis Ca2+/H+ antiporter Cax1 variant in petunia enhances cadmium tolerance and accumulation. J. Plant Physiol. 168 (2), 167–173. doi: 10.1016/j.jplph.2010.06.005
Xie, J., Lee, J., Wang, D., Ting, Y. (2007). Identification of active biomolecules in the high-yield synthesis of single-crystalline gold nanoplates in algal solutions. Small (Weinheim an der Bergstrasse Germany) 3 (4), 672–682. doi: 10.1002/smll.200600612
Xiong, X., Li, Z., Liang, J., Liu, K., Li, C., Li, J. (2022). A cytosine base Editor toolkit with varying activity windows and target scopes for versatile gene manipulation in plants. Nucleic Acids Res. 50 (6), 3565–3580. doi: 10.1093/nar/gkac166
Xu, X., Li, X., Liu, Y., Zhu, Y., Li, J., Du, G., et al. (2020). Pyruvate-responsive genetic circuits for dynamic control of central metabolism. Nat. Chem. Biol. 16 (11), 1261–1268. doi: 10.1038/s41589-020-0637-3
Yagi, K. (2007). Applications of whole-cell bacterial sensors in biotechnology and environmental science. Appl. Microbiol. Biotechnol. 73 (6), 1251–1258. doi: 10.1007/s00253-006-0718-6
Yuan, L., Yang, S., Liu, B., Zhang, M., Wu, K. (2012). Molecular characterization of a rice metal tolerance protein, Osmtp1. Plant Cell Rep. 31 (1), 67–79. doi: 10.1007/s00299-011-1140-9
Zanella, L., Fattorini, L., Brunetti, P., Roccotiello, E., Cornara, L., D'Angeli, S., et al. (2016). Overexpression of Atpcs1 in tobacco increases arsenic and arsenic plus cadmium accumulation and detoxification. Planta 243 (3), 605–622. doi: 10.1007/s00425-015-2428-8
Zhang, H., Cao, Y., Xu, D., Goh, N., Demirer, G., Cestellos-Blanco, S., et al. (2021). Gold-Nanocluster-Mediated delivery of sirna to intact plant cells for efficient gene knockdown. Nano Lett. 21 (13), 5859–5866. doi: 10.1021/acs.nanolett.1c01792
Zhang, X., Henriques, R., Lin, S., Niu, Q., Chua, N. (2006). Agrobacterium-mediated transformation of arabidopsis thaliana using the floral dip method. Nat. Protoc. 1 (2), 641–646. doi: 10.1038/nprot.2006.97
Zhang, J., Li, T., Li, X., Liu, Y., Li, N., Wang, Y., et al. (2021). A key role of inner-Cation-Π interaction in adsorption of Pb(Ii) on carbon nanotubes: Experimental and dft studies. J. hazardous materials 412, 125187. doi: 10.1016/j.jhazmat.2021.125187
Zhao, L., Song, J., Liu, Y., Song, C., Yi, C. (2020). Mapping the epigenetic modifications of DNA and rna. Protein Cell 11 (11), 792–808. doi: 10.1007/s13238-020-00733-7
Zhong, D., Zhong, Z., Wu, L., Xue, H., Song, Z., Luo, Y. (2015). Thermal characteristics of hyperaccumulator and fate of heavy metals during thermal treatment of sedum plumbizincicola. Int. J. phytoremediation 17 (8), 766–776. doi: 10.1080/15226514.2014.987373
Zhou, S., Jiang, W., Zhao, Y., Zhou, D. (2019). Single-cell three-dimensional genome structures of rice gametes and unicellular zygotes. Nat. Plants 5 (8), 795–800. doi: 10.1038/s41477-019-0471-3
Zhou, M., Wang, H., Zhu, S., Liu, Y., Xu, J. (2015). Electrokinetic remediation of fluorine-contaminated soil and its impact on soil fertility. Environ. Sci. pollut. Res. Int. 22 (21), 16907–16913. doi: 10.1007/s11356-015-4909-5
Zhu, S., Beaulaurier, J., Deikus, G., Wu, T., Strahl, M., Hao, Z., et al. (2018). Mapping and characterizing N6-methyladenine in eukaryotic genomes using single-molecule real-time sequencing. Genome Res. 28 (7), 1067–1078. doi: 10.1101/gr.231068.117
Keywords: root, shoot, phytoremediation, heavy metal, soil
Citation: Bai S, Han X and Feng D (2023) Shoot-root signal circuit: Phytoremediation of heavy metal contaminated soil. Front. Plant Sci. 14:1139744. doi: 10.3389/fpls.2023.1139744
Received: 07 January 2023; Accepted: 08 February 2023;
Published: 20 February 2023.
Edited by:
Wenna Zhang, China Agricultural University, ChinaReviewed by:
Yang Li, Huazhong Agricultural University, ChinaCopyright © 2023 Bai, Han and Feng. This is an open-access article distributed under the terms of the Creative Commons Attribution License (CC BY). The use, distribution or reproduction in other forums is permitted, provided the original author(s) and the copyright owner(s) are credited and that the original publication in this journal is cited, in accordance with accepted academic practice. No use, distribution or reproduction is permitted which does not comply with these terms.
*Correspondence: Dan Feng, Znd4eWRsQDEyNi5jb20=
†These authors have contributed equally to this work
Disclaimer: All claims expressed in this article are solely those of the authors and do not necessarily represent those of their affiliated organizations, or those of the publisher, the editors and the reviewers. Any product that may be evaluated in this article or claim that may be made by its manufacturer is not guaranteed or endorsed by the publisher.
Research integrity at Frontiers
Learn more about the work of our research integrity team to safeguard the quality of each article we publish.