- State Key Laboratory of Crop Biology, College of Horticulture Science and Engineering, Shandong Agricultural University, Tai’an, Shandong, China
Introduction: Both nitrogen (N) and magnesium (Mg) play important roles in biochemical and physiological processes in plants. However, the application of excessive N and insufficient Mg may be the factor leading to low nitrogen utilization rate (NUE) and fruit quality degradation in apple production.
Methods: In this study, we analyzed the effects of different application rates of Mg (0, 50, 100, 150, 200 kg/ha) on the photosynthetic nitrogen use efficiency (PNUE), the accumulation and distribution of carbon (C), N metabolism, anthocyanin biosynthesis and fruit quality of the ‘Red Fuji’ apple in 2018 and 2019.
Results: The results showed that the application of Mg significantly increased the 15NUE and increased the allocation rate of 15N in the leaves whereas the 15N allocation rate in the perennial organs and fruits was decreased. With the increase in Mg supply, the activities of N metabolism enzymes (NiR, GS, and GOGAT) were significantly promoted and the content of intermediate products in N metabolism (, , and free amino acid) was significantly decreased. Furthermore, an appropriate rate of Mg significantly promoted the net photosynthetic rate (Pn) and photosynthetic nitrogen use efficiency (PNUE), enhanced the enzyme activities of C metabolism (SS, SPS, S6PDH), and increased the contents of sorbitol and sucrose in leaves. In addition, Mg upregulated the gene expression of sugar transporters (MdSOT1, MdSOT3, MdSUT1, and MdSUT4) in fruit stalk and fruit fresh; 13C isotope tracer technology also showed that Mg significantly increased the 13C allocation in the fruits. Mg also significantly increased the expression of anthocyanin biosynthesis genes (MdCHS and MdF3H) and transcription factors (MdMYB1 and MdbZIP44) and the content of anthocyanin in apple peel.
Conclusion: The comprehensive analysis showed that the appropriate application of Mg (150 kg/ha) promoted PNUE, C–N metabolism, and anthocyanin biosynthesis in apple trees.
Introduction
Mg is a vital macronutrient for plant growth and plays many important roles in biochemical and physiological processes in plants, including the synthesis of chlorophyll, nucleic acids, and proteins; the formation and utilization of ATP; and enzyme activation and participation in photosynthesis (Shaul, 2002; Marschner, 2012; Cakmak, 2013; Verbruggen and Hermans, 2013). An appropriate application of Mg can promote plant growth, increase photosynthetic efficiency (Tian et al., 2021), improve crop yield and quality (Orlovius and Mchoul, 2015; Yang et al., 2012), and also significantly promote the absorption of N, P, K, Ca, and other nutrients (Ding et al., 2012). However, the application of Mg is neglected by China’s apple industry, and the application rate of Mg fertilizer is almost negligible. The lack of Mg may not only inhibit plant growth and reduce the utilization rate of nutrients but also decrease fruit yield and quality. Therefore, Mg cannot be ignored in the management of apple nutrient resources.
Mg plays a key role in maintaining N homeostasis in plants (Peng et al., 2020b). Both Mg and N are components of chlorophyll, protein, and various structural substances in plants, playing an important role in photosynthesis and the formation of crop quality (Bloom and Arnold, 2015; Chen et al., 2018). Both N absorption and assimilation depend on H+-ATP for energy, whereas ATP synthesis depends heavily on the participation of Mg (Tang et al., 2012b; Tian et al., 2021). Studies have shown that Mg can promote N absorption and assimilation of crops. Ding et al. (2006) showed that there was a unimodal curve relationship between the activities of nitrate reductase (NR) and glutamine synthase (GS) and Mg content in rice leaves. Marschner (2012) found that Mg application was beneficial to leaf protein synthesis. Ding et al. (2012) found that the absorption of cabbage N and Mg can be significantly increased by the appropriate proportion of N and Mg. Grzebisz (2013) found that the application of Mg can achieve higher N utilization under the condition of low N application. In addition, Mg increased the nitrogen utilization rate (NUE) of maize, winter wheat, and sugar beet (Szulc et al., 2008; Potarzycki, 2011; Zhang et al., 2020b; Poglodzinski et al., 2021). It is seen that scientific application of Mg may have great potential to improve the NUE in the apple industry.
Scientific and effective supply of Mg not only is beneficial to N metabolism but also has a significant effect on the improvement of crop yield and quality. It had been reported in corn (Jezek et al., 2015), sunflower (Ertiftik and Zengin, 2016), radish (Kleiber et al., 2012), citrus (Tang et al., 2012a), potato (Orlovius and Mchoul, 2015), and other crops. Mg deficiency restricts the loading of carbohydrates in the phloem, resulting in obstructed transport of photosynthates to sink organs (Ishfaq et al., 2022). The accumulated carbohydrates in the source leaves reduce the photosynthetic efficiency through the negative feedback regulation of the photosynthetic mechanism (Pego et al., 2000), and the structure and function of the photosynthetic organs are also damaged, ultimately inhibiting the synthesis of photosynthates (Samborska et al., 2018). Peng et al. (2018) showed that low Mg treatment inhibited SWEET gene expression in soybean, thus obstructing the transport of sucrose to the roots. The decrease in root sucrose content further inhibited root growth and N uptake (Peng et al., 2020b). Hermans et al. (2005) suggested that after 11 days of the low-Mg stress, there is a huge reduction in the translocation of sucrose in sugar beet, and the sucrose transport rate could be restored to the Mg-sufficient level with resupplying Mg for 12–24 h transiently (Cakmak et al., 1994; Cakmak and Kirkby, 2008). The effect of Mg application on improving the quality of different crops is also different. Ceylan et al. (2016) showed that Mg application could promote the crude protein content and crude gluten content of wheat. Jezek et al. (2015) found that Mg application could improve the photosynthetic rate of maize and increase the content of soluble sugar and fat in maize. Chapagain and Wiesman (2004) showed that Mg application could increase the soluble solids and vitamin C content of tomatoes and also improve the appearance quality of tomatoes.
Anthocyanins not only determine the color of red-skinned apples and affect fruit appearance quality but also are a substance beneficial to human health. The biosynthesis of anthocyanin in peels is regulated by the content of N and sugar. Wang et al. (2020a) showed that the biosynthesis of anthocyanin was promoted with the reduction in N content in fruits. Kühn et al. (2011) also obtained similar results. In addition, the contents of sucrose, sorbitol, glucose, and fructose in fruits were significantly positively correlated with the content of anthocyanin, so the biosynthesis of anthocyanin was closely related to C and N metabolism (Jiang et al., 2020; Liao et al., 2022).
China is the world’s largest producer of apples, but it has not paid enough attention to the application of Mg. Currently, the reduction in fruit quality is the main problem in China’s apple industry, and the formation of fruit quality is directly related to C–N metabolism, whereas Mg plays a significant role in regulating C–N metabolism. Therefore, the deficiency of Mg may be a potential factor leading to the reduction in apple fruit quality. Therefore, in this study, the effects of Mg on apple C–N metabolism and fruit quality were investigated to provide a scientific basis for improving the fruit quality during apple production.
Materials and methods
Experimental materials
This study was conducted in an apple orchard at Laishan district, Yantai City, Shandong Province, China (121°42′55′′E, 37°49′58′′N), during the fruit expansion stage in 2018 and 2019. Thirty ‘Yanfu3’/M26/Malus hupehensis Rehd. apple trees were used as experimental material in this study. Trees were planted in the year 2013 in rows spaced 1.5 m apart with 4 m between the rows and were trained as a slender spindle. The density of the experimental site was 111 apple trees per 667 m2. The mean temperatures in August, September, and October were 27.3°C, 22.1°C, and 14.6°C in 2018 and 27.1°C, 21.8°C, and 14.3°C in 2019, respectively. The precipitation in August, September, and October was 126.6, 52.2, and 4.1 mm in 2018 and 124.2, 51.3, and 3.5 mm in 2019, respectively. The soil was brown loam, the pH of soil was 5.77, the soil organic matter content was 12.56 g/kg, and available potassium (K), available phosphorus (P), NO3-N, and were 211.23, 59.51, 45.73, and 29.31 mg/kg, respectively.
Experimental design
Thirty apple trees with the same crop loads (5.5–6.2 fruit per cm2 cross-sectional area of fruiting branch), developmental attributes, and number of autumn branches were selected to reduce the individual differences in the sources of photoassimilates in the fruits. In the experiment, there were five treatments, namely, CK, Mg50, Mg100, Mg150, and Mg200, which represented 0, 50, 100, 150, and 200 kg/ha of pure Mg application (equal to 0, 250, 500, 750, and 1,000 kg/ha of MgSO4), respectively. The date of flowering was 25th April, and the date of fruit maturity stage was 20th October.
To exclude the natural abundance of 13C and 15N in the apple trees affected by different Mg treatments, each treatment was divided into two groups of three replicates, the first group for isotope labeling and the second for the determination of natural abundance and other indicators. Fertilization treatment was conducted on 1st August (95 days after blooming) in 2018 and 2019. Each tree of group 1 was supplied with 100 g of common urea and 20 g of 15N-urea (abundance of 10.28%), each tree of group 2 was supplied with 120 g of common urea (200 kg/ha of pure N), and a circular ditch with a depth and width of 20 cm was dug 40 cm away from the center trunk when fertilizing. MgSO4, as the only Mg source, mixed with urea/(15N-urea) was evenly watered in the circular ditch after being dissolved in water. All apple trees were subjected to destructive sampling at the 20th October (180 days after blooming, the period of fruit maturity stage).
15N and 13C labeling method
15N labeling was performed on 1st August (95 days after blooming). Group 1 of each treatment was supplemented with 20 g of 15N-urea and 100 g of common urea, which were mixed and solutioned with MgSO4 and then fertilized to the soil. The whole plant was destructively sampled on 20th October (180 days after flowering), and then the indexes correlated with 15N were determined.
13C isotope labeling was performed on 17th October (177 days after flowering). Fans, a beaker with 8 g of Ba13CO3 and reduced iron powder, and the labeled whole apple tree were placed into a labeling chamber, which was composed of 0.1-mm-thick Mylar plastic bags and bracket. The light intensity inside was 90% of the natural light intensity. The 13C isotope labeling was performed at 8.30 a.m.; the fan was turned on, and the labeling room was sealed. To maintain a suitable temperature (25°C–35°C), an appropriate amount of ice was placed in the labeling room. To maintain a suitable concentration of CO2, we injected 1 ml hydrochloric acid into the labeling room by a syringe for every 30 min. The whole plant was destructively after 72 h (180 days after flowering), and then the indexes correlated with 13C were determined.
15N and 13C concentration determination
The apple trees were divided into different organs (fruits, roots, perennial branches, trunk, annual branches, and leaves) and the fresh weight weighed; partial samples were then taken with the fresh weight weighed, then washed with clear water and dried at 105 °C for 30 min and 80 °C for 72 h, and then the dry weight was weighed to calculate the water content of each organ; the dry weight of each organ = the fresh weight of each organ × the water content of each organ. Subsequently, the samples were crushed by an electric mill and passed through a 60-mesh sieve (Sha et al., 2020). The content of N and the abundance of 15N were determined with a ZHT-03 mass spectrometer (Beijing Analytical Instrument factory). The δ13C values were determined with a DELTA V advantage isotope ratio mass spectrometer (Thermo Fisher, China). Six biological replicates and three technical repetitions were conducted for each treatment.
Calculation of 15N
Calculation of 13C
Fnl: no 13C labeling, natural abundance of 13C of each organ
N and Mg concentration determination
The samples were weighed and wet digested in concentrated HNO3–H2O2 at 100°C, 140°C, and 160°C for 1.5 h each until no brown fume appeared, and then further digested at 200°C until the digest became clear. Mg content was analyzed by a spectrometer (ICP-MS systems, Agilent No. 7500 Series). The concentration of N was measured by the Tian et al. (2023) method. Thirty replicates were conducted for each treatment.
Photosynthetic parameter determination
The net photosynthetic rate (Pn), transpiration rate (Tr), stomatal conductance (Gs), and intercellular CO2 concentration (Ci) were measured by a Li-6400 photosynthetic instrument (LI-COR Inc., USA) from 8:30 to 10:30 a.m. under standardized climatic conditions; the light-saturation point was set to 1,200 μmol (photon)·mÀ 2·sÀ 1, the ambient temperature of the apple leaves was kept constant at 30°C, the CO2 concentration was 400 μmol (CO2)·molÀ 1, relative humidity was 60%–65%, and air flow was 500 μmol sÀ 1. The chlorophyll content was measured, as described by Wen et al. (2019). Rubisco activity was measured by the method described by Liu et al. (2013).
Determination of the concentrations of sorbitol, sucrose, fructose, and glucose
Sorbitol, sucrose, fructose, and glucose concentrations were determined, as described by Ma et al. (2019). The sample was weighed for 1 g and treated with 4 ml of 75% ethanol, then heated at 70°C for 12 min in a water bath; the mixed liquor was centrifuged at 5,500g for 8 min, the supernatants were taken out and then treated with 4 ml of 75% ethanol and centrifuged again. The supernatant from the two centrifugations was amalgamated and dried, and the residual was resuspended in 2.5 ml of distilled water and desalinized using an ion-exchange resin column (Selion SBA 2000). The sucrose, fructose, glucose, and sorbitol contents were determined by filtration using liquid chromatography methods (Filip et al., 2016).
Enzyme activity determination
Nitrate reductase (NR), glutamine synthetase (GS), and glutamate synthase (GOGAT) contents were determined following the method of Hu et al. (2016). Nitrite reductase (NiR) activity was assayed according to the method previously described (Seith et al., 1994).
The activity of C-metabolizing enzymes was measured as follows. For the preparation of enzyme solution, 1 g of the fruit sample was ground into an ice bath in a precooled mortar; 100 mol·l-1 of 5 ml Tris–HCl (pH 7.0) buffer, containing 2% glycol, 2 mol·l-1 EDTA, 5 mol·l-1 MgCl2, 2% PVPP, 2% bovine serum protein (BSA), and 5 mmol·L-1 DTT, was added for fractional times. 3 ml of the supernatant was put into a dialysis bag after centrifugation at 4°C and 10,000 r·min-1 for 20 min. The extraction buffer diluted five times (removing PVPP) was used for dialysis for 15 to 24 h at low temperature (2°C–4°C). The enzyme solution after dialysis was used for determination of various enzyme activities. Sorbitol dehydrogenase (SDH) activity was determined as described by Rufly and Huber (1983). Sorbitol oxidase (SOX) activity was determined as described by Yamaki and Asakura (1991). Sucrose synthase decomposition direction activity (SS-c) was determined, as described by Huber (1983). Sucrose synthase (SS) and sucrose phosphate synthase (SPS) activities were determined as described by Xu et al. (2012). The activities of acid invertase (AI) and neutral invertase (NI) were determined as described by Merlo and Passera (1991).
The content of , free amino acid, and soluble protein determination
0.1 g of fresh samples was crushed in 1 ml deionized water; the homogenates were then incubated for 30 min in a boiling water bath. After cooling, they were centrifuged at 25°C, 12,000g, for 15 min, and the supernatant was taken to be tested. The content was measured by nitration of salicylic acid (Cataldo et al., 1975).
0.1 g of fresh samples was homogenized in an extraction solution (1 ml of 100 145 mM HCI and 500 µl of chloroform) and centrifuged at 12,000g at 4 °C for 10 min, and the supernatant was taken to be tested. The content was determined using the method of Brautigam et al. (2007). The content in roots and leaves was analyzed as described by Gou et al. (2020).
0.2 g of fresh samples was crushed in 5 ml cold phosphate buffer (50 mM of 150 KH2PO4, pH 7) and centrifuged at 12,000 g for 15 min. The supernatant was used for analysis. The free amino acid and soluble protein content were measured according to the method of Ruiz and Romero (2002).
RNA isolation and qRT-PCR analysis
According to the previous research and the purpose of our experiment (Peng et al., 2020a; Wang et al., 2020a; Sha et al., 2020), three MdSOTs, three MdSUTs, and seven genes related to anthocyanin biosynthesis were selected to determine the relative expression. Total RNA was extracted from around 60 mg of fresh leaves or roots tissues using RNAiso Plus (Takara, Otsu, Shiga, Japan). cDNA synthesis was performed using the ReverTra Ace® qPCR RT Master Mix with gDNA Remover (TOYOBO, Osaka, Japan). Gene relative expression was analyzed by RT-qPCR on a LightCycler 96 (Roche, Basel, Switzerland) using the TranStart Top Green qPCR SuperMix (TransGen Biotech). The actin gene was used as an internal control. Six biological replicates and three technical replicates were determined for each treatment. Expression was normalized by the 2-ΔΔCt method. The qRT-PCR primers are listed in Table S1.
Fruit quality determination
The concentration of soluble sugar in fruit was measured as described by Liu et al. (2018). The sample of apple flesh was placed in a test tube, then 5 ml of distilled water was added and mixed after cutting it into chunks. The supernatant was collected after 30 min of boiling water bath. This step was repeated twice, using distilled water to adjust the volume of the solution to 10 ml. The absorbance of the solution was measured at 630 nm after adding sulfuric acid and anthrone. Six biological replicates and three technical repetitions were conducted for each treatment.
The concentration of anthocyanin was measured as described by Zheng et al. (2021). The sample of fruit peel was ground with liquid nitrogen rapidly and then extracted with 1.5 ml of 1% (v/v) HCl methanol at 3°C for 30 h in darkness. The concentration of anthocyanin was measured by a multifunctional microplate reader (BioTek, USA). Six biological replicates and three technical repetitions were conducted for each treatment.
The concentration of titratable acid was measured by NaOH titration (Nie et al., 2012), and the fruit hardness was measured by a HP-230 hardness tester. The diameter of fruit was measured with a vernier caliper. Single fruit weight was measured by 1% precision electronic balance, and the average of 30 measured values was taken.
Statistical analysis
The figures were prepared using Origin 2019b (OriginLab Corporation, USA). The contents of N and Mg in fruits and leaves were determined for 30 biological replicates and three technical repetitions; other indexes were determined for six biological replicates and three technical repetitions. Data were analyzed with SPSS 17.0 (Statistics software, version 20.0, IBM, USA) using one-way analysis of variance (ANOVA). The significant differences were considered at a probability level of P ≤ 0.05.
Results
Effects of Mg application on fruit quality
As presented in Table 1, the application of Mg significantly improved the fruit yield and quality. Fruit yield increased with the increase in Mg application, compared with CK treatment; the yield in Mg150 treatment was increased by 6.86% and 7.29% in 2018 and 2019, respectively. In addition, with the increase in the application of Mg, the single fruit mass, transverse diameter, longitudinal diameter, soluble sugar, and sugar–acid ratio were all increased and reached the highest value at Mg150 treatment, which increased by 7.77%, 4.35%, 5.06%, 19.07%, and 19.05% (2018) and 7.29%, 6.93%, 4.48%, 16.89%, and 16.89% (2019) higher than those under CK treatment, respectively. However, there was no significant difference in fruit firmness and the content of titratable acid among different treatments.
Effects of Mg application on anthocyanin content and anthocyanin biosynthesis-related gene expression in the peel
As shown in Figure 1, we found that the application of Mg significantly promoted fruit coloring (Figure 1A). To further verify the discovery, we measured the expression of genes (MdCHS, MdCHI, MdF3H, MdDFR, and MdUFGT) and transcription factors (MdMYB1 and MdbZIP44) associated with anthocyanin synthesis in peel. The results showed that the application of Mg significantly upregulated the expression of MdCHS, MdF3H, MdMYB1, and MdbZIP44 (Figure 1C). The content of anthocyanin also increased with the increased application of Mg (Figure 1B) and reached the highest values at Mg150 and Mg200 treatments, which were 91.97% and 94.88% (2018) and 82.41% and 83.76% (2019) higher than that at CK treatment, respectively.
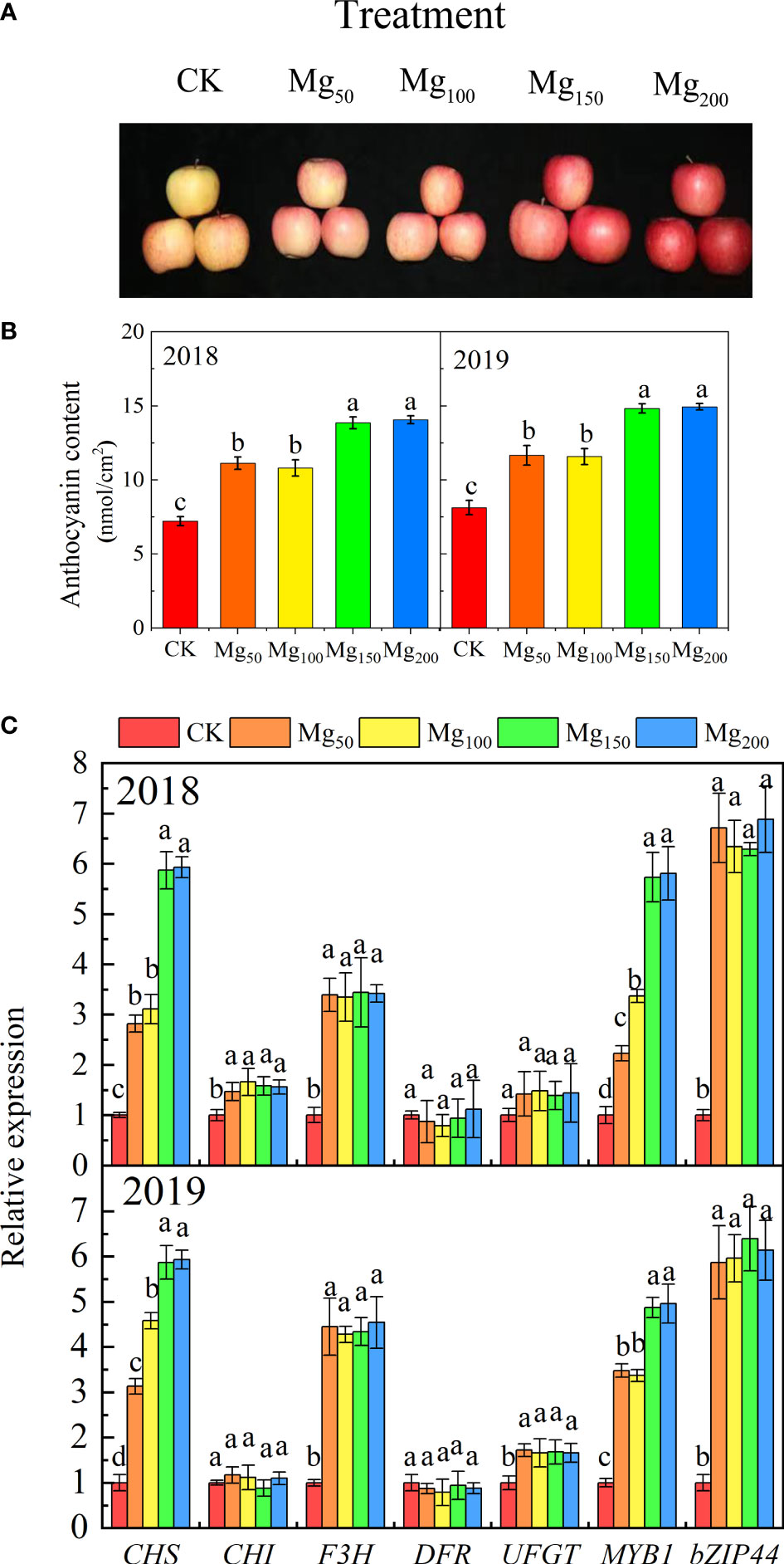
Figure 1 Effects of Mg application on fruit peel coloring. A representative photograph (A) shows the color of the fruit peel under different Mg treatments. Anthocyanin concentration (B) and anthocyanin biosynthesis-related gene expression (C) of fruit peel were measured in each treatment. The error bars indicate SD of six replications. Significant differences were detected by different letters at P ≤ 0.05.
Effects of Mg application on the content of N and Mg in leaves and fruits
Different Mg treatments significantly affected the contents of N and Mg in leaves and fruits. As shown in Figure 2, with the increase in Mg application, the content of Mg both in leaves and fruits showed a significant increase. The content of N was also increased significantly in leaves and reached the maximum value at Mg150 and Mg200 treatments, and there was no significant difference between Mg150 and Mg200 treatments. On the contrary, the N content of fruits decreased with the increase in Mg application.
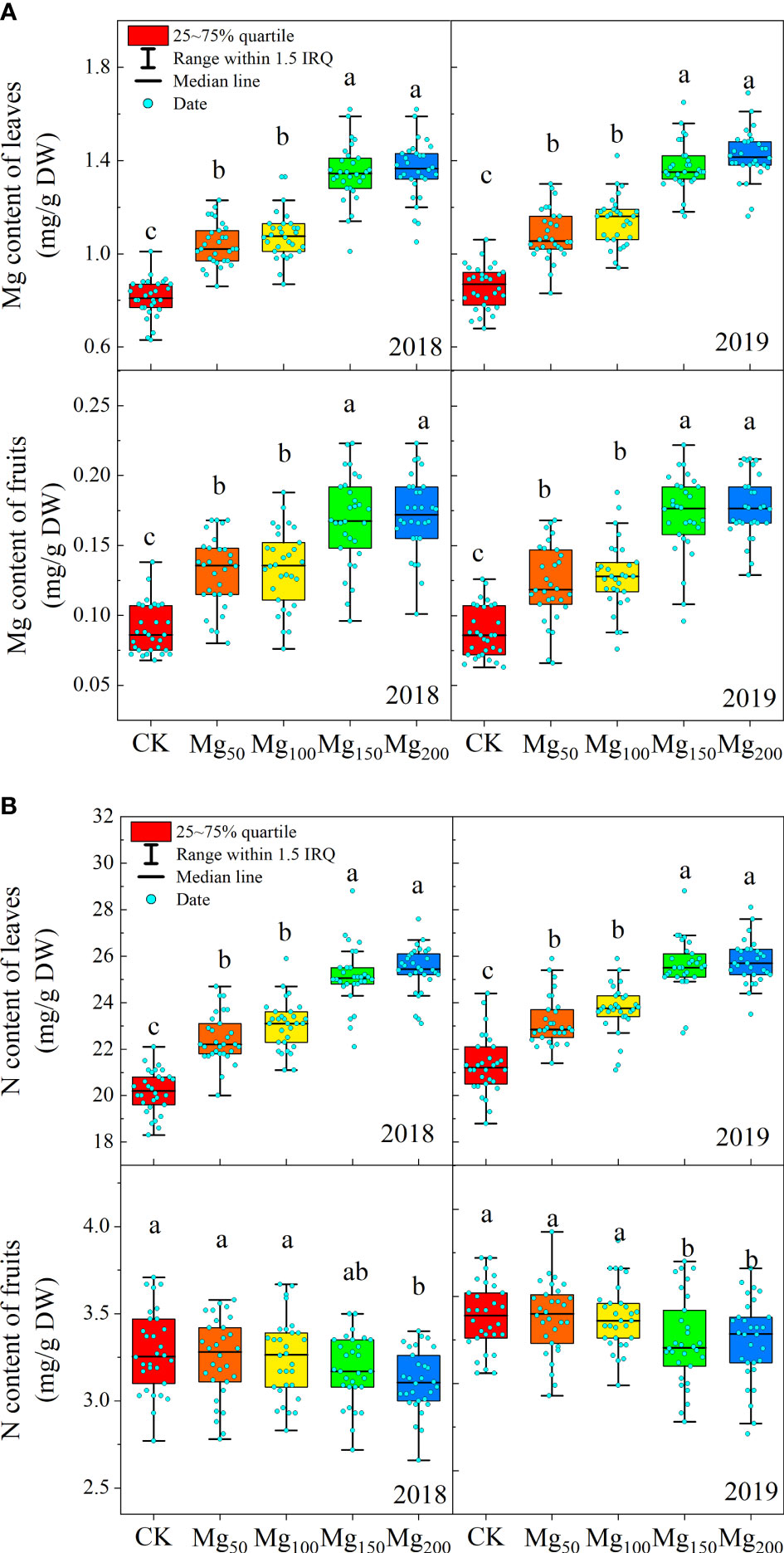
Figure 2 Effects of Mg application on the content of Mg (A) and N (B) in the leaves and fruits in 2018 and 2019. The bars indicate the range within 1.5 IRQ of 30 replications, the box represents 25 to 75% quartile, and the line in the box indicates the median line. Significant differences were detected by different letters at P ≤ 0.05.
Effects of Mg application on photosynthesis and C metabolism
Effects of Mg application on photosynthetic parameter
As presented in Table 2, 2 years of repeated experiments showed that different Mg treatments significantly affected the photosynthetic performance of leaves. The treatments of Mg150 and Mg200 enhanced the chlorophyll content and Rubisco activity to varying degrees, compared with CK, with increases of 79.77%–95.49% and 64.86%–69.44% in both years, respectively. In addition, the application of Mg also significantly increased the transpiration rate (Tr), stomatal conductance (Gs), and intercellular CO2 concentration (Ci). In addition, Pn and PNUE of leaves were also significantly changed by Mg (Figure 3). The values of Pn and PNUE under CK treatment were at the lowest level; compared with CK treatment, Pn significantly increased under Mg50 and Mg100 treatment, whereas PNUE had no significant difference. With further increase in Mg application (150–200 kg/ha), PNUE had significantly increased.
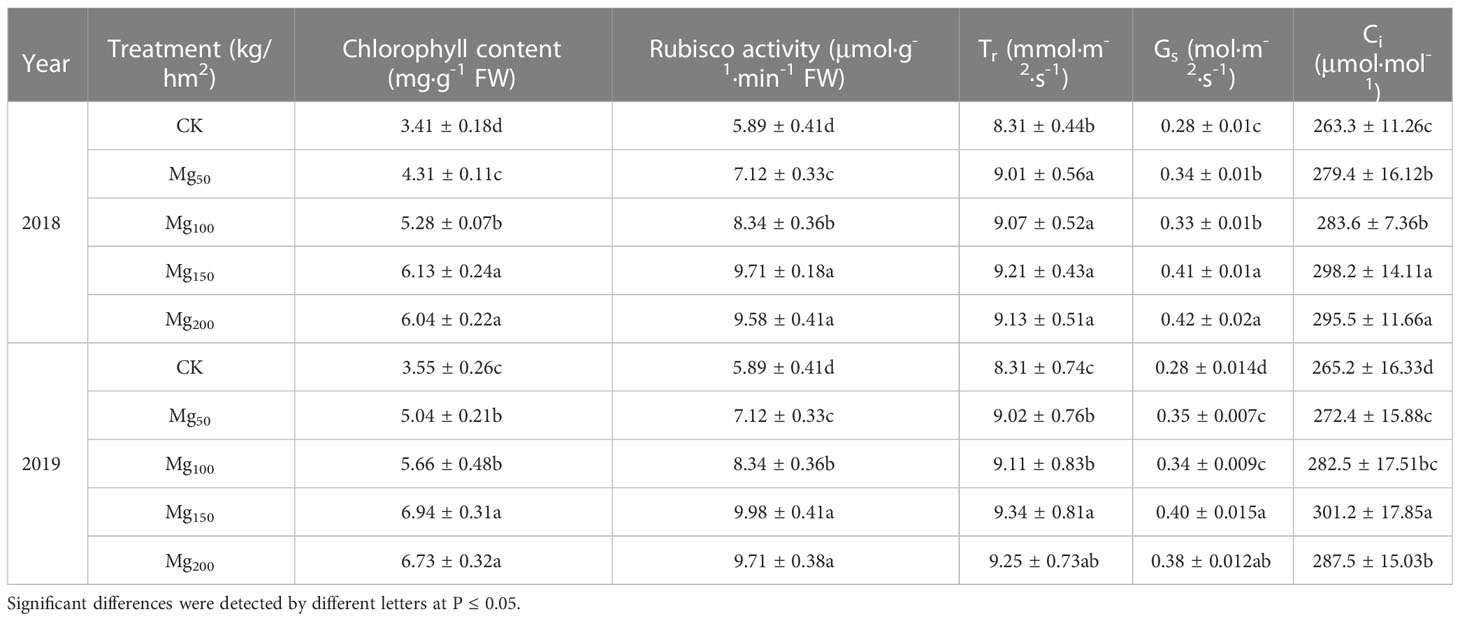
Table 2 Effects of Mg application on chlorophyll content, rubisco activity, transpiration rate (Tr), stomatal conductance (Gs), and intercellular CO2 concentration (Ci) in 2018 and 2019.
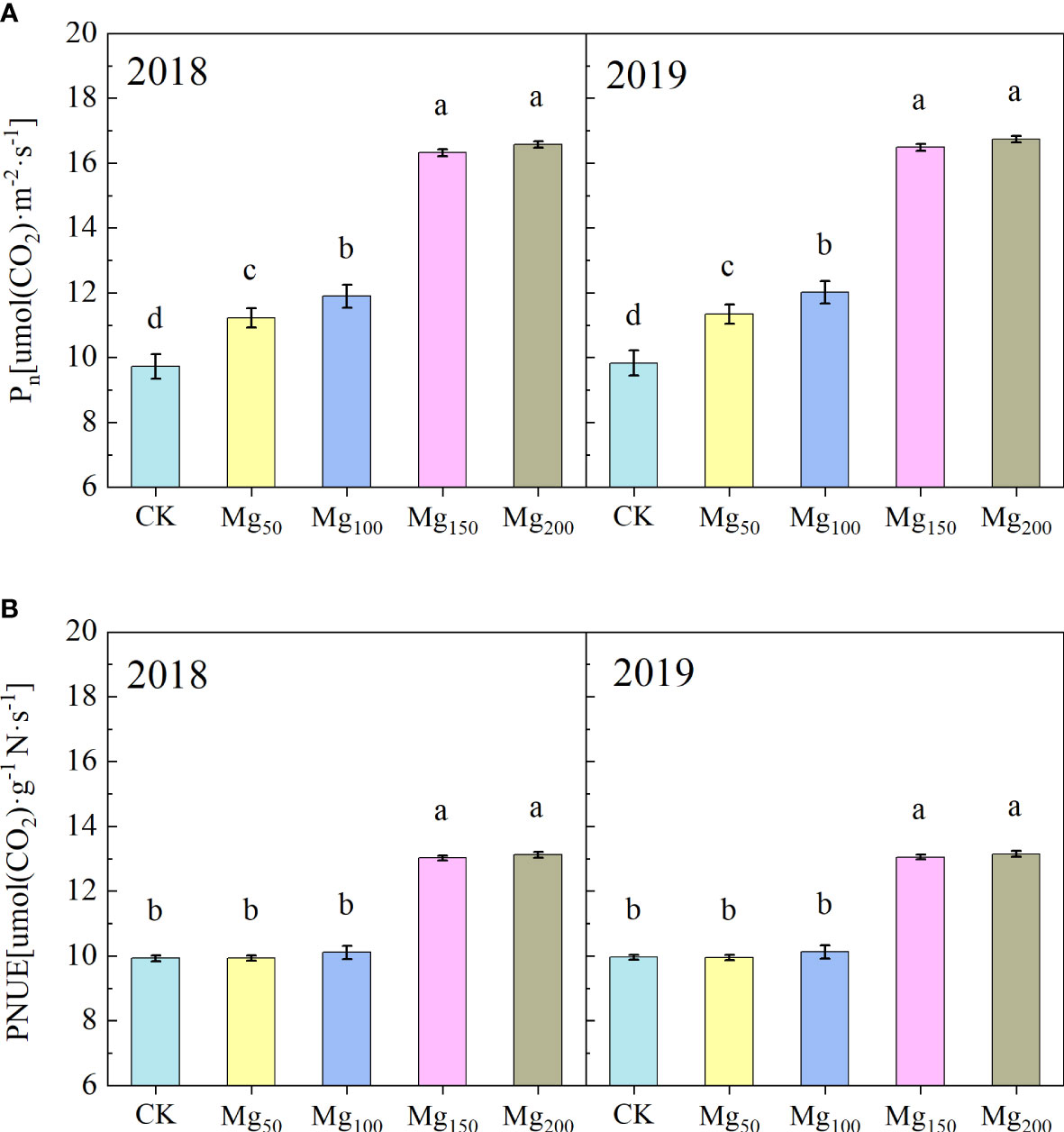
Figure 3 Effects of Mg application on the net photosynthetic rate (Pn) (A) and photosynthetic nitrogen use efficiency (PNUE) (B) in the leaves in 2018 and 2019. The bars represent mean ± SE, n = 6. Different letters on vertical bars indicate significant differences (P< 0.05).
Effects of Mg application on δ13C and 13C distribution rates
13C isotope tracer technology showed that different Mg applications changed the 13C accumulation of fruits (Figure 4). Under CK treatment, the δ13C values of fruits were at the lowest level, which were 35.88‰ (2018) and 37.24‰ (2019). With the application of Mg reaching 150–200 kg/ha, δ13C of fruits significantly increased by 43.2% (2018) and 40.4% (2019) compared with the CK treatment, respectively. In addition, the allocation of 13C in different organs was analyzed. We found that Mg application significantly increased the distribution of 13C in fruits and decreased in leaves but had no significant effect in other organs (roots, trunk, perennial branches, and annual branches), which showed that Mg application could promote the distribution of 13C from leaves to fruits.
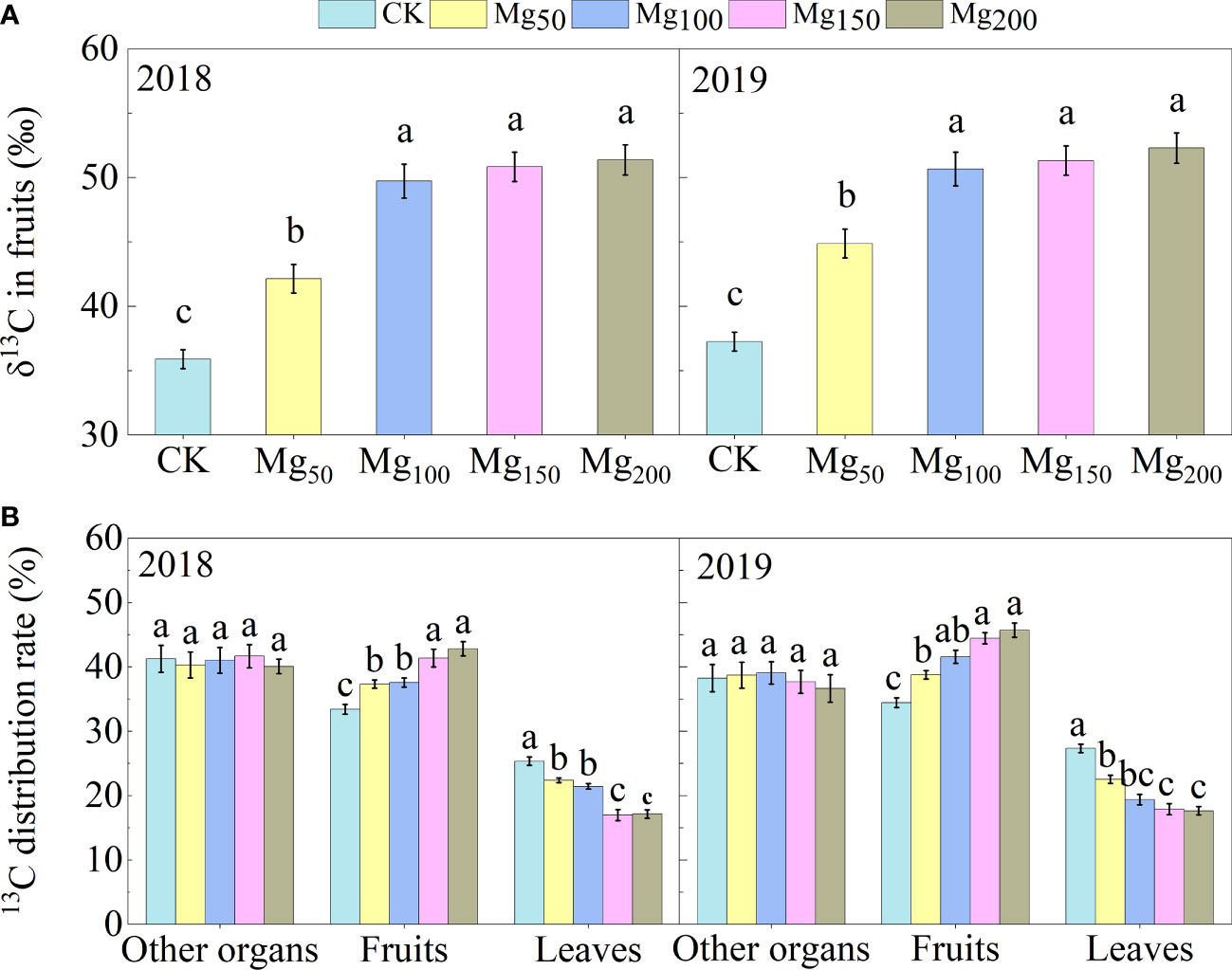
Figure 4 δ13C value in fruit (A) and 13C distribution rate in different organs (B) of the ‘Red Fuji’ apple tree were measured under Mg0, Mg50, Mg100, Mg150, and Mg200 treatments in 2018 and 2019. The bars represent mean ± SE, n = 6. Different letters on vertical bars indicate significant differences (P< 0.05).
Effects of Mg application on enzyme activities of C metabolism in leaves and fruits
As shown in Table 3, with the increase in Mg addition, the activities of sucrose synthase (SS), sucrose phosphate synthase (SPS), and sorbitol 6-phosphate dehydrogenase (S6PDH) in leaves were significantly promoted and Mg could also significantly improve the activities of sorbitol dehydrogenase (NAD+-SDH), sucrose synthase cleavage direction (SS-c), neutral invertase (NI), and acid invertase (AI) in fruits. However, the activities of sorbitol oxidase (SOX) in leaves had no change with Mg application, indicating that Mg could promote the synthesis of sucrose and sorbitol in leaves and the decomposition of sorbitol and sucrose in fruits.
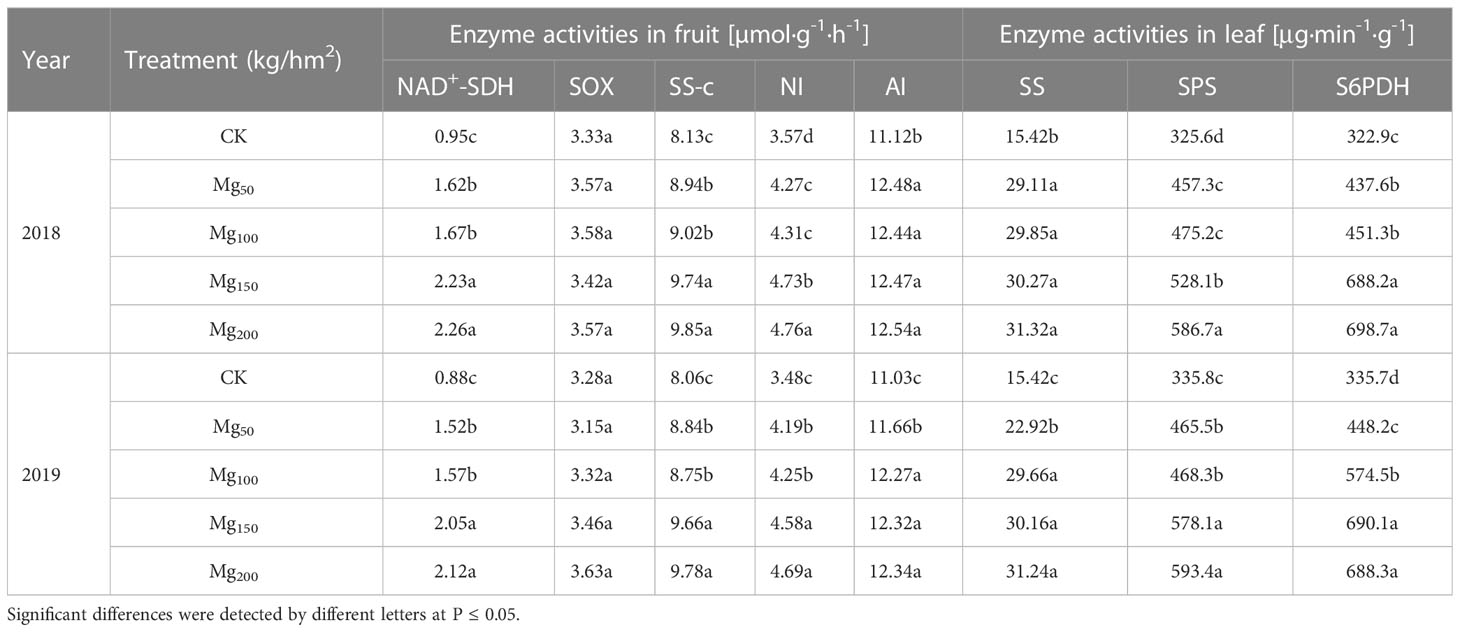
Table 3 Effects of Mg application on enzyme activities of C metabolism in fruit (NAD+-SDH, SOX, SS-c, NI and AI) and leaf (SS, SPS, S6PDH).
Effects of Mg application on the content of soluble sugar in leaves and fruits
The concentrations of sucrose, sorbitol, fructose, and glucose showed to be different in fruits and leaves (Figure 5). Under CK treatment, the content of sorbitol was the highest in leaves, followed by sucrose, whereas fructose and glucose contents were at the lowest level. On the contrary, the fructose content in fruits was the highest, followed by glucose and sucrose, and finally sorbitol. Different Mg treatments changed the sugar content in leaves and fruits; with the increase in Mg application, the contents of sorbitol and sucrose in leaves were significantly enhanced whereas there was no change in the contents of fructose and glucose. Compared with the CK treatment, the concentration of sorbitol and sucrose increased at 28.67%–31.53% and 155.34%–238.77% under Mg150 and Mg200 treatments for both years, respectively. Moreover, the application of Mg had no change in sorbitol concentration, and the treatments of Mg150 and Mg200 enhanced the sucrose, fructose, and glucose concentrations to varying degrees, compared with CK, with increases of 12.89%–37.87%, 22.42%–25.27%, and 31%–33.81% in both years, respectively.
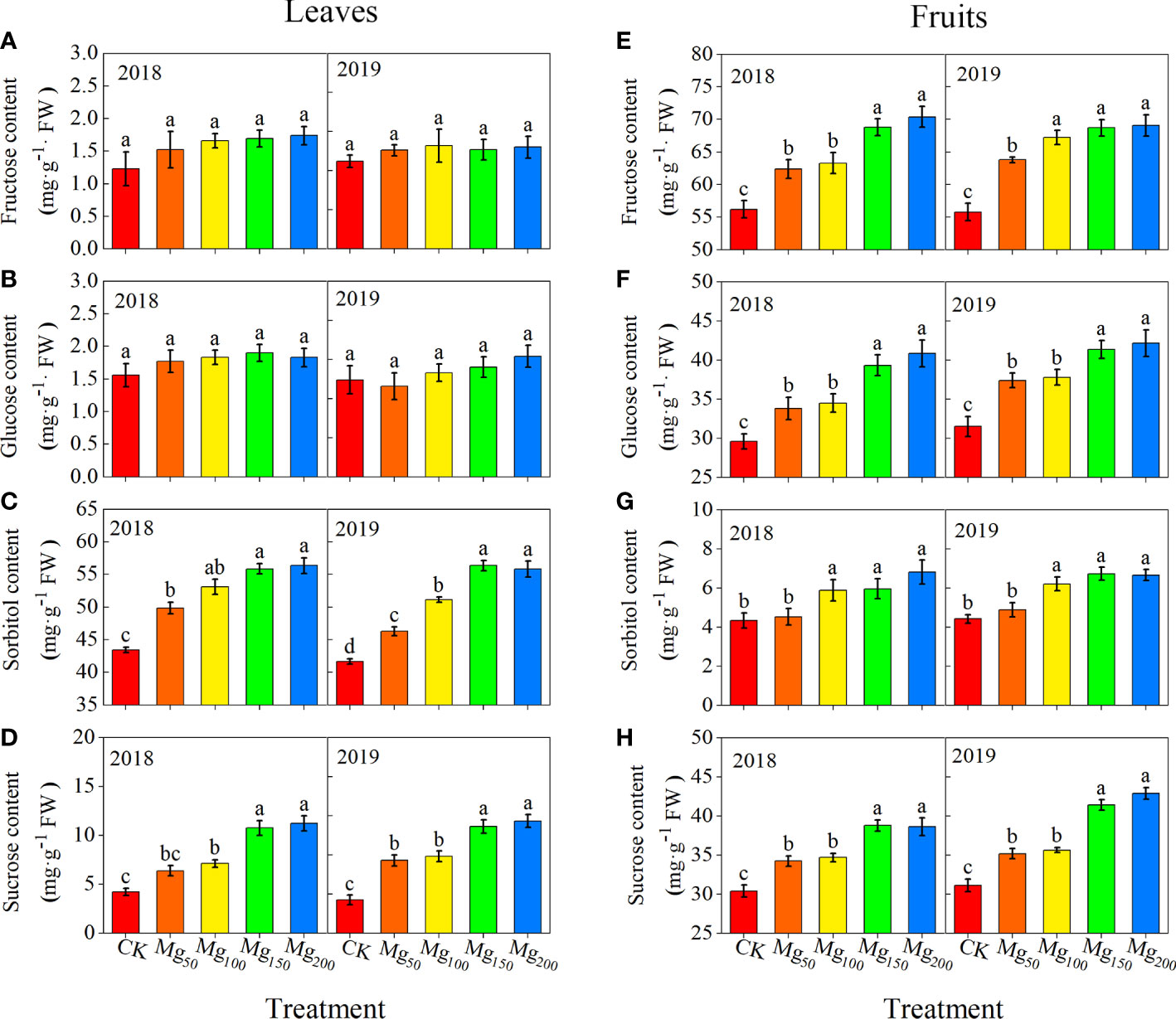
Figure 5 Effects of Mg application on the content of fructose (A, E), glucose (B, F), sorbitol (C, G), and sucrose (D, H) in leaf (A-D) and fruit (E-H) in 2018 and 2019. The error bars indicate SD of six replications. Significant differences were detected by different letters at P ≤ 0.05.
Effects of Mg application on the gene expression of sugar transporter
To further investigate the effect of Mg application on sorbitol and sucrose transport to fruits, we determined the expression of genes related to sugar transport (Figure 6), and the results showed that MdSOT1/3 and MdSUT1/4 were significantly upregulated by Mg under Mg150 and Mg200 treatments. The results indicated that sufficient Mg promoted sorbitol transport to fruits by inducing MgSOT1/3 expression and promoted sucrose transport to fruits by upregulating MdSUT1/4. These results may explain the distribution of 13C between leaves and fruits to some extent.
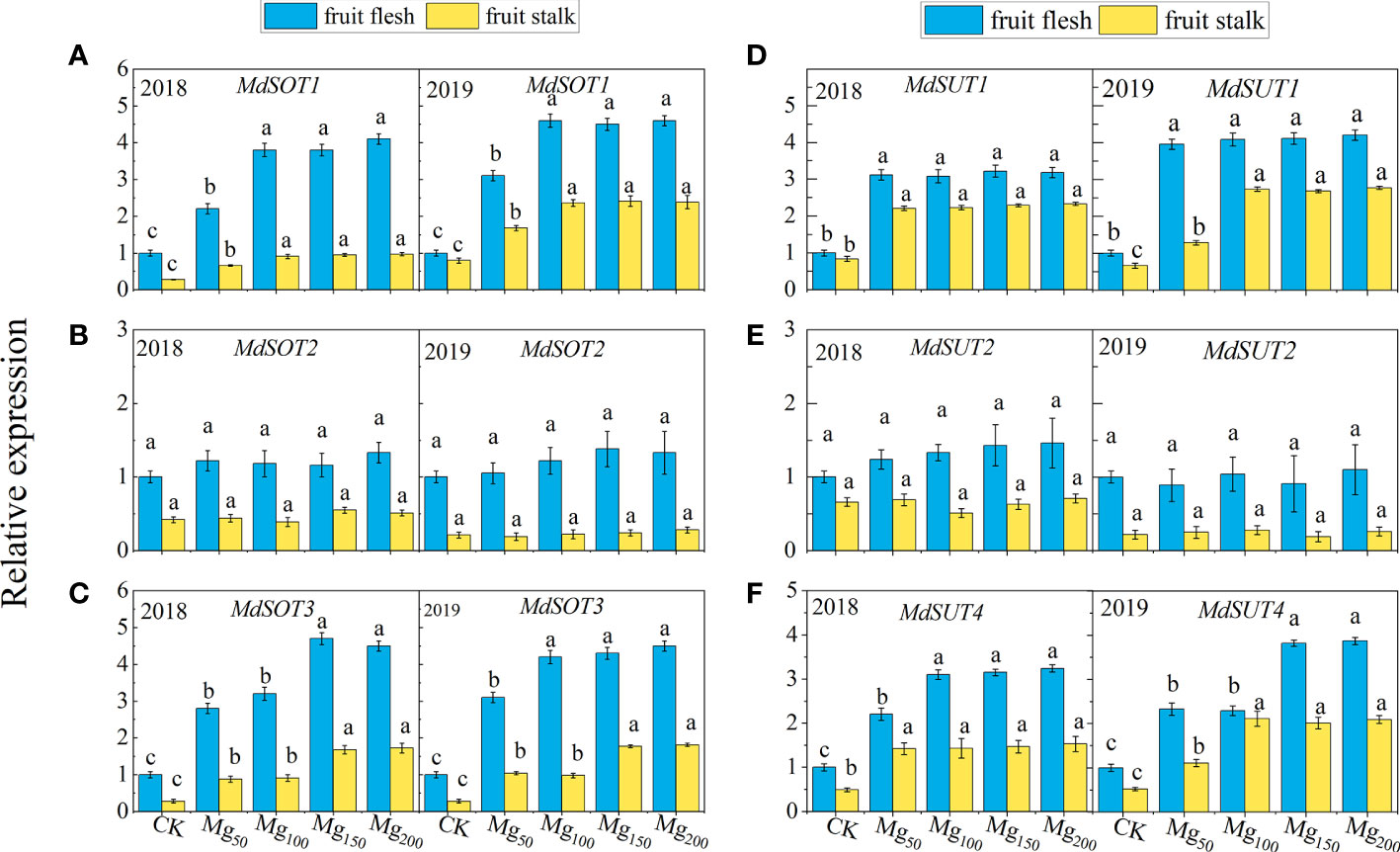
Figure 6 Effects of Mg application on the relative expression of MdSOT1 (A), MdSOT2 (B), MdSOT3 (C), MdSUT1 (D), MdSUT2 (E), and MdSUT4 (F) in fruit stalk and fruit flesh in 2018 and 2019. The bars represent mean ± SE, n = 6. Different letters on vertical bars indicate significant differences (P< 0.05).
Effects of Mg application on N metabolism
Effects of Mg application on 15N utilization and distribution
As shown in Figure 7, the 15N isotope tracer technique showed that the application of Mg could significantly increase the 15N utilization rate of the whole apple tree. Compared with CK treatment, the 15N utilization rate under Mg150 and Mg200 treatments increased by 69.87%–70.99% (2018) and 67.67%–69.01% (2019), respectively. The results showed that the application of Mg increased the absorption of N by apple trees. However, the 15N allocation rate showed to be different in organs. The 15N allocation rate of leaves increased with the increase in Mg application. On the contrary, the 15N allocation rate of fruits and other organs showed a downward trend, which indicated that Mg was conducive to the transfer of 15N from other organs to leaves.
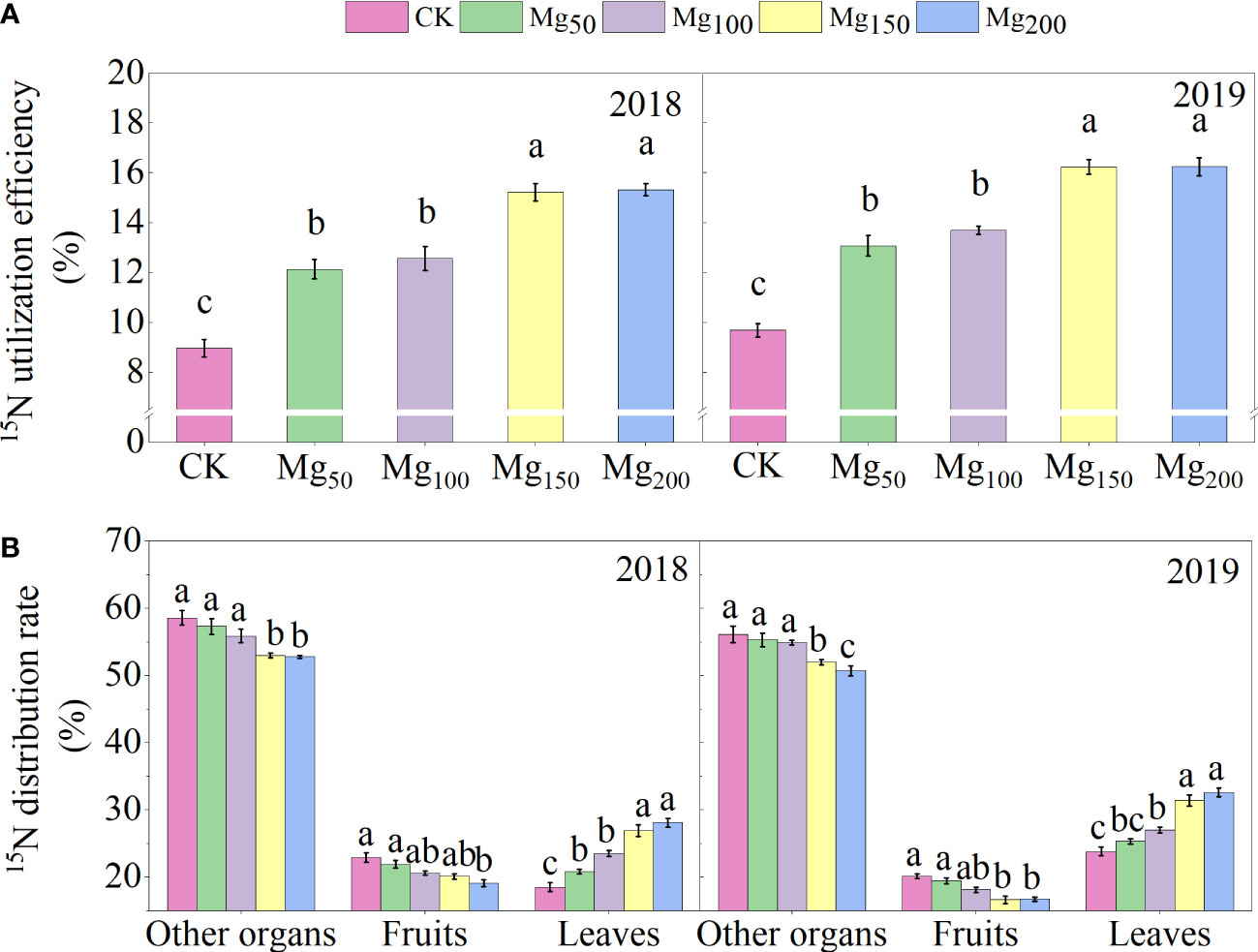
Figure 7 Effects of Mg application on 15NUE (A) and 15N distribution rate (B) in different organs of the ‘Red Fuji’ apple tree in 2018 and 2019. The error bars indicate SD of six replications. Significant differences were detected by different letters at P ≤ 0.05.
Effects of Mg application on enzyme activities of N metabolism
Nitrate absorbed by plant roots is reduced into ammonium by nitrate reductase (NR), which is partly carried out in the roots and partly in the leaves. Apples are perennial fruit trees, and nitrate reduction is mostly carried out before being transferred to the leaves, so the NR activity in the leaves is extremely low. As shown in Figure 8, different Mg treatments significantly affected the enzyme activities of N metabolism. The enzyme activities of each treatment showed the lowest level with 0 Mg application and increased to the highest level under the application of 150–200 kg/ha Mg, and there was no significant difference between the two treatments. Different enzymes were affected to different degrees, among which glutamine synthase (GS) was the most promoted by 150–200 kg/ha Mg application, and the highest value was 1.84 times (2018) and 1.77 times (2019) of the CK treatment.
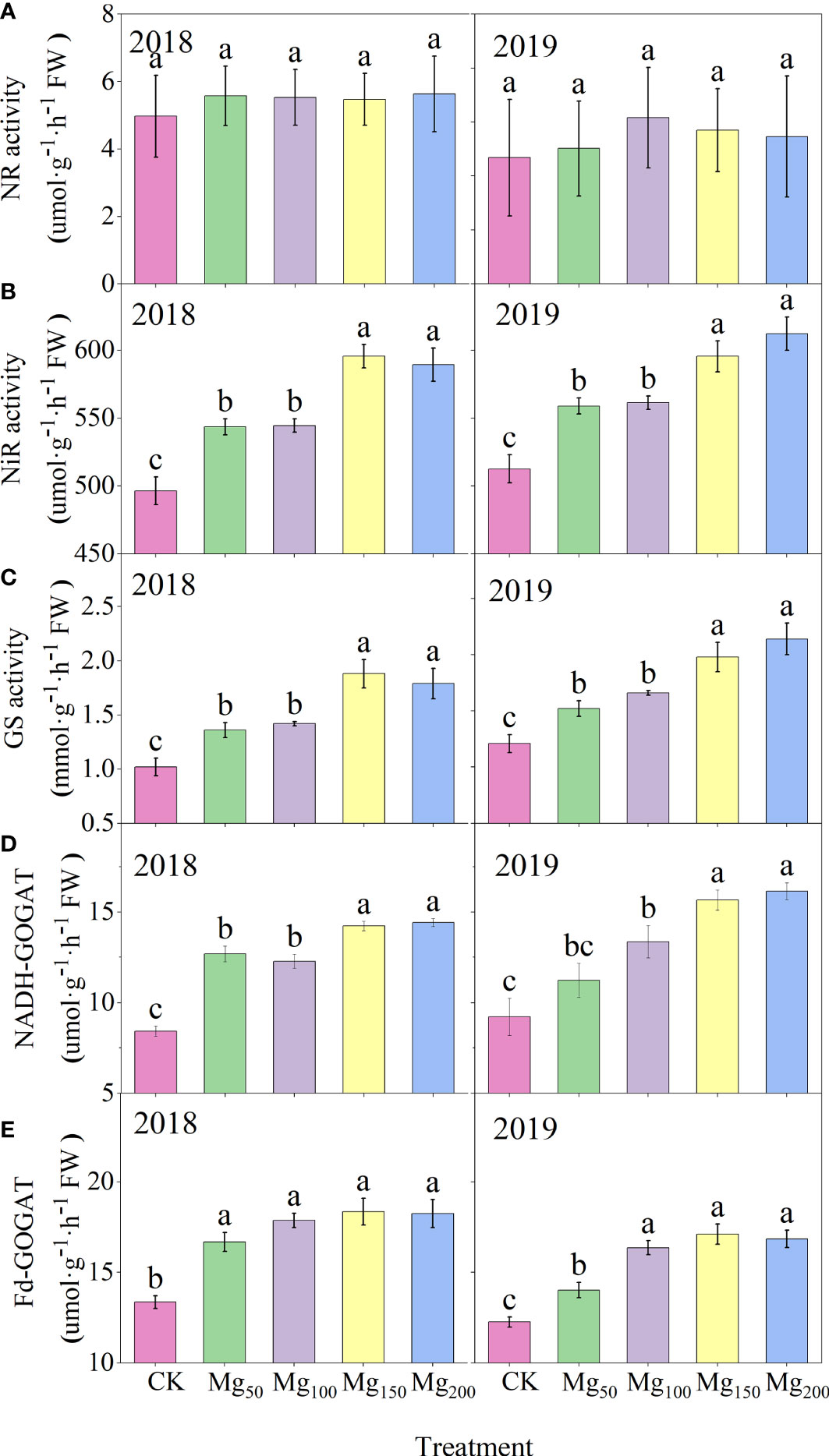
Figure 8 Effects of Mg application on the NR (A), NiR (B), GS (C), NADH-GOGAT (D), and Fd-GOGAT (E) activities in leaves in 2018 and 2019. The error bars indicate SD of six replications. Significant differences were detected by different letters at P ≤ 0.05.
Effects of Mg application on the content of intermediate products in N metabolism
As presented in Figure 9, Mg supply had no significant effect on content in leaves, but it greatly reduced the contents of and , due to the fact that Mg improved the activities of NiR and GS and promoted the further transformation of and . Glutamine is an important form of N storage in plants; under Mg-deficiency treatment, the concentration in leaves was higher and the GS activity was improved with the increase in Mg, which promoted the synthesis of into glutamine in large quantities and effectively prevented the accumulation of poisoning. In addition, under CK treatment, the content of free amino acid in leaves was the highest, whereas the content of soluble protein was the lowest. The addition of Mg could significantly reduce the content of free amino acid and increase the content of soluble protein.
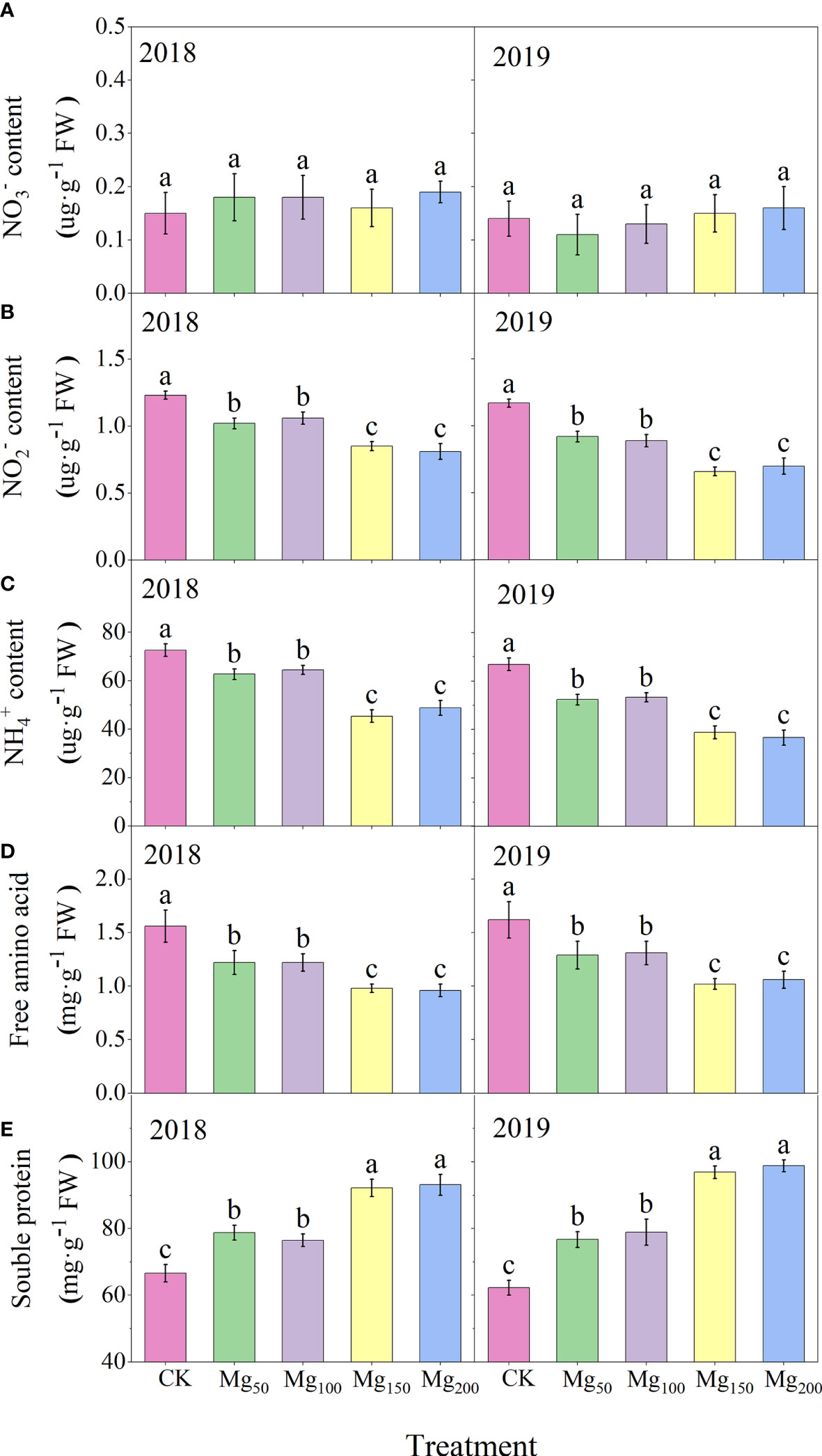
Figure 9 Effects of Mg application on the content of (A), (B), (C), free amino acid (D), and soluble protein (E), in leaves in 2018 and 2019. The error bars indicate SD of six replications. Significant differences were detected by different letters at P ≤ 0.05.
Discussion
The appropriate application of Mg promoted N metabolism
N is an essential nutrient for the growth and development of apple trees. It has an irreplaceable effect on the organ construction, material metabolism, physiological and biochemical processes, and formation of fruit yield and quality (Liu et al., 2022b). The fruit expansion stage is the period when the fruit has the strongest nutrient demand. Insufficient N supply reduces the precursor substances of C metabolism, which is not conducive to tree development and fruit quality. Previous studies have shown that Mg addition can improve the N absorption in tea plants (Jayaganesh and Venkatesan, 2010), rice (Ding et al., 2006), and maize (Szulc et al., 2008). Our research on apples has achieved similar results that appropriate application of Mg significantly increased the Mg and N content of leaves (Figure 2), and the 15N utilization rate also increased with the increase in Mg application (Figure 7), indicating that the application of Mg could increase the absorption of N and improve the NUE in apple trees.
Nitrate reductase (NR), nitrite reductase, (NiR) glutamine synthetase (GS), and glutamate synthase (GOGAT) are key enzymes in the process of N assimilation, and their activity can regulate the key steps of N assimilation. The absorbed by plants first reduced to under the action of NR, and this process is carried out in roots or leaves. In our study, both the NR activity and content of leaves were at a low level (Figures 8A, 9A), and the difference between treatments was not significant, indicating that most had been reduced to before being transported to the leaves. Under the action of NiR and GS, synthesizes and glutamine, which is then decomposed into glutamate by GOGAT and finally metabolized into other amino acids and N-containing compounds. We found that with the increase in Mg application, the activities of NiR, GS, and GOGAT in leaves increased significantly (Figures 8B–E), whereas the contents of N-metabolizing intermediates such as and decreased significantly (Figures 9B–E), indicating that Mg improved the activity of N-metabolizing enzymes to promote N metabolism and continued to consume N-metabolizing intermediates to promote the conversion of N to amino acids and N compounds. Previous studies also found that Mg can improve the activity of N metabolism enzymes in black tea (Jayaganesh and Venkatesan, 2010) and rice (Ding et al., 2006), which is consistent with our study. Marschner (2012) showed that when the supply of Mg was interrupted, protein synthesis stopped immediately and the process resumed quickly after the resumption of Mg supply, indicating that Mg had a significant effect on protein synthesis. We also found that under adequate Mg supply (Mg150–Mg200), free amino acid content decreased and soluble protein content increased in leaves (Figures 9D, E), indicating that Mg promoted the conversion of free amino acid into soluble protein. Soluble protein is an important component of protoplasts, most of which are key enzymes involved in physiological metabolism. Therefore, the application of Mg promoted N metabolism and increased the synthesis of soluble protein in leaves, which not only promoted the activity of various physiological and biochemical reactions in leaves but also enhanced the demand for N in leaves, thus further enhancing the requisition and allocation of N in leaves. Further analysis showed that the 15N allocation rate of leaves increased with the increase in Mg application (Figure 7B), whereas the 15N allocation rate of perennial organs and fruits decreased, indicating that Mg application effectively promoted the transfer of N from other organs to leaves. In general, the fruit expansion stage is the period of maximum efficiency of N accumulation by fruits; however, it is also the period of the fastest accumulation of fruit biomass. The appropriate application of Mg increased the competition for N in leaves, so the newly absorbed 15N allocated to fruits was relatively reduced, which caused N allocated to fruits to not match the rapid growth of fruits; therefore, the 15N distribution in fruits (Figure 4B) and the N content of fruits (Figure 2B) all showed a significant decrease. More N distribution to fruits can decrease the enzyme activities of sugar metabolizing in fruits and decrease fruit quality (Kühn et al., 2011; Sha et al., 2020; Wang et al., 2020a). Therefore, in order to decrease the accumulation of N in fruits, scholars applied nitrification inhibitors 3,4-dimethylpyrazole phosphate (Wang et al., 2020a), abscisic acid (Wang et al., 2020b), and paclobutrazol (Sha et al., 2021) to limit N transport to fruits. We provided a new idea: Mg significantly decreased 15N allocated to fruits, which was beneficial to fruit quality to a certain extent. Meanwhile, the allocation of N in various organs also affects the N absorption and assimilation. Mg enhanced the allocation of N to leaves, which is conducive to various physiological and biochemical reactions. The less N allocation in other organs may also further promote the absorption of N through signal stimulation (Chen et al., 2020).
Mg promoted C metabolism and the transport of photosynthates from leaves to fruits
Photosynthesis realizes the assimilation and distribution of C in plants by using CO2 as raw material. In horticultural plants, the accumulation and distribution of photosynthates in different organs affect the fruit quality directly. Compared with Mg deficiency, Mg150 and Mg200 treatments significantly improved the chlorophyll content, the activity of Rubisco enzymes, Pn, and PNUE of leaves (Table 2; Figures 3), thus accelerating the synthesis and accumulation of photosynthates. In addition, Mg150 and Mg200 treatments significantly increased the enzyme activities of SS, SPS, and S6PDH in leaves (Table 3) and the contents of sucrose and sorbitol in leaves also increased by sufficient Mg addition (Figures 5C, D), which also meant that Mg promoted the synthesis of sorbitol and sucrose in leaves. Relevant conclusions have been found in soybean (Peng et al., 2018), sugar beet (Poglodzinski et al., 2021), watermelon (Huang et al., 2016), and other crops; these studies are consistent with our results.
The synthesis and transport of photosynthates from leaves to fruits is a very complicated process. CO2 is assimilated into propanose phosphate in chloroplasts and then transported into the cytoplasm by related transporters and enzymes; after synthesis of sorbitol and sucrose in the cytoplasm, they are transported over long distances and unloaded into the fruits (Desnoues et al., 2018). Therefore, the effective transfer and distribution of photosynthates to fruits is a key factor affecting fruit growth and development (Katz et al., 2007; Álvaro et al., 2008). Previous studies have shown that Mg facilitates the transport of photosynthates in beet (Hermans et al., 2005), beans (Cakmak and Kirkby, 2008), tea plants (Ruan et al., 2012), and wax gourd (Zhang et al., 2020a). Sucrose is the main photosynthate of most plants, but there is some difference in apple plants; compared with sucrose, sorbitol is the main photosynthate of apples, which accounts for 80% and sucrose only 20% (Chong and Taper, 1971). Although different crops are associated with different photosynthates, Mg has a certain similarity in regulating their transport. The results of 13C isotope tracer technology showed that Mg significantly increased the δ13C value and 13C allocation rate of fruits (Figure 4), whereas the 13C allocation of leaves decreased, indicating that Mg application promoted the transport of 13C photosynthates from leaves to fruits. Subsequently, we determined the expression of key genes involved in sorbitol and sucrose loading and unloading in fruit stalk and fruit flesh. The results showed that adequate application of Mg upregulated the expression of MdSOT1/3 and MdSUT1/4 and promoted the loading and unloading of sucrose and sorbitol in phloem (Figure 6) and thus promoted the transport efficiency of sugar. After sucrose and sorbitol are transported to the fruits, they are rapidly decomposed into fructose and glucose under the action of related enzymes. We found that appropriate application of Mg could significantly improve the activities of sucrose decomposition enzymes (SS-c, AI, NI) and sorbitol decomposition enzyme (NAD+-SDH) in fruits (Table 3), indicating that Mg promotes the decomposition of sucrose and sorbitol in the fruits. Therefore, the content of fructose and glucose in fruits also significantly increased in fruits (Figures 5E, F). These results are consistent with those of 13C isotopic labeling. The application of Mg had not changed SOX activity, but the decomposition of sorbitol had no effect on fruits. It was due to the fact that more than 80% of SOX existed in bound form and its activity was only one-fifth of NAD+-SDH, so it had little effect on sorbitol metabolism (Yamaki and Asakura, 1991).
There are two ways of loading and unloading sugar in phloem, namely, the symplastic pathway and the apoplastic pathway (Rennie and Turgeon, 2009). The symplastic pathway refers to the concentration gradient at source and sink organ, and the sugar enters the sink organ through the intercellular desmodesmata between the sieve tube and the companion cell. This process does not involve transmembrane transport and is not limited by energy and transporters (Tegeder and Hammes, 2018). The apoplastic pathway refers to the transmembrane transport of assimilates by sugar transporters, which consumed ATP. Our study suggests that the application of Mg may enhance both pathways and facilitate the transport of sugars from source to sink. 1) The application of Mg significantly promoted the synthesis of sorbitol and sucrose in leaves, increased the content of sugar, and thus increased the source strength (Figures 5C, D). Meanwhile, Mg significantly promoted the decomposition of sucrose and sorbitol into glucose and fructose in the sink organ (Table 3; Figure 5), which not only reduced the osmotic potential in the vacuoles but also increased the concentration gradient of sorbitol at both source and sink organ, and sorbitol ceaselessly entered the sink organ through the cytoplasmic desmata (Eom et al., 2012). Ultimately, the transport efficiency of sorbitol in the symplastic pathway was improved. 2) Different from sorbitol, we found that the sucrose content was higher in the fruits than in the leaves. Previous studies found that this difference was more obvious in the fruit expansion stage (Yang et al., 2019; Sha et al., 2020). Therefore, sucrose transport from leaves to fruits is an inverse concentration gradient process, indicating that sucrose transport depends on energy and sucrose transporters. Mg significantly induced the expression of sugar transporters MdSOT1/3 and MdSUT1/4 in fruit stalk and flesh (Figure 6), thus promoting the transport of sorbitol and sucrose in the phloem extracellular pathway. Previous studies have shown that low Mg inhibits the transport of carbohydrates from source to sink by downregulating the expression of BvSUT1 and SWEET genes (Hermans et al., 2005; Peng et al., 2018); this is consistent with our results. In addition, the substrate of ATPase is the Mg–ATP complex; the supply of Mg effectively promotes ATP synthesis and provides energy for the loading and unloading of sugars in the apoplastic pathway (Shaul, 2002). Therefore, sufficient Mg promotes the transport of sorbitol and sucrose in the symplastic pathway and also facilitates the transport of sorbitol in the apoplastic pathway.
Mg improved the anthocyanin biosynthesis and fruit quality
An appropriate application of Mg can increase the yield of corn (Jezek et al., 2015), citrus (Tang et al., 2012b), and potato (Orlovius and Mchoul, 2015). Consistent with previous studies, Mg significantly improved the single fruit weight and transverse and longitudinal diameters of apples (Table 1), and the apple fruit yield was also increased with the increase in application of Mg; the highest yield was 2,802 kg/667 m2 and 2839.5 kg/667 m2 in 2018 and 2019, which were increased by 6.86% and 7.29% than CK treatment, respectively. In addition to fruit yield, Mg also significantly improved the fruit quality. There are many indicators to evaluate the quality of fruit, and consumers prefer apples with a large fruit size, a full red color, and a suitable sugar–acid ratio. High firmness means better storage and transportation, but the application of Mg did not seem to change firmness in our study. The appropriate sugar–acid ratio can increase the flavor of apple, but the titratable acid content has no significant response to Mg, so the sugar–acid ratio is mainly regulated by the soluble sugar content in fruits. The key to the increase in soluble sugar content lies in the efficiency of synthesizing photosynthates and transporting to the fruits. In our study, Mg obviously promoted this process. We observed that Mg150 and Mg200 treatment promoted the synthesis of sucrose and sorbitol in leaves by improving photosynthetic efficiency and enzyme activity of SS, SPS, and S6PDH (Table 2; Table 3). Meanwhile, Mg promoted the transport of sorbitol and sucrose in the apoplastic pathway by upregulating the expression of MdSOT1/3 and MdSUT1/4 (Figure 6) and promoted the decomposition of sorbitol in fruits by increasing the activity of NAD+-SDH (Table 3), thus significantly increasing the contents of glucose and fructose (Figure 5). This not only effectively improves the sugar–acid ratio (Table 1) but also increases the pressure potential difference between the source and the sink by reducing the osmotic potential in the vacuoles, thus further promoting the transport of sorbitol through the symplastic pathway.
Scientific and effective N metabolism is conducive to the formation of fruit quality, and the lack of N will affect the synthesis and distribution of C assimilates and thus affect fruit quality. In our study, the application of Mg promoted the utilization and assimilation of N (Figures 7A; 8), which enhanced the physiological and biochemical reactions and promoted the synthesis and transportation of photosynthate. The fruit expansion stage is the most critical period of fruit growth and development. Excessive N distribution in fruits reduces fruit quality (Wang et al., 2020a). However, our study showed that Mg application significantly improved the requisition and distribution of N in leaves and reduced the allocation of N to fruits (Figures 2B, 7B). Therefore, appropriate application of Mg could also improve fruit quality by reducing N accumulation in fruits. Wang et al. (2020b) believed that less N distribution in fruits would also lead to reproductive growth restriction. In our study, appropriate application of Mg increased single fruit weight and fruit yield (Table 1), indicating that it did not lead to reproductive growth restriction. In fact, there is also an interaction between C and N metabolism. N metabolism promotes C metabolism by providing more photozyme and related pigments. Not only does C metabolism provides C skeleton for N metabolism but also products of C metabolism act as signaling substances to stimulate N uptake by plants (Peng et al., 2020b; Liu et al., 2022a). Therefore, the appropriate application of Mg can promote the C–N metabolism of trees and the C–N metabolism could promote each other and ultimately improve the fruit quality in common.
In addition to intrinsic quality, fruit color is also an economic trait. A higher anthocyanin content in peel is not only beneficial to apple skin coloring but also beneficial to human health. Wang et al. (2020b) showed that anthocyanins are synthesized through the flavonoid pathway and regulated by the expression of two types of genes, including structural genes encoding related synthases and transcription factors regulating structural genes, which included chalcone synthase (MdCHS), chalcone isomerase (MdCHI), flavanone 3-hydroxylase (MdF3H), dihydro flavonol reductase (MdDFR), and UDP-flavonoid dallyl transferase (MdUFGT), MdMYB1 gene, and the MdbZIP44 gene. Compared with CK treatment, Mg150 and Mg200 treatments could significantly upregulate the expression of MdCHS, MdF3H, MdMYB1, and MdBZIP44, and the anthocyanin content and fruit coloring were also improved (Figure 1).
Sugar is the precursor substance of anthocyanin synthesis, not only as an energy source but also as an osmoregulatory substance to promote anthocyanin synthesis through signal stimulation (Smeekens, 2000; Solfanelli et al., 2006). Loreti et al. (2008) showed that sucrose can upregulate the expression of related genes to promote anthocyanin biosynthesis in Arabidopsis. The contents of glucose and fructose in fruits were positively correlated with anthocyanin content (Huang et al., 2019). In addition, high N content in fruits would inhibit anthocyanin synthesis (Kühn et al., 2011). In our study, the application of Mg significantly decreased the concentration of N and significantly increased the concentration of glucose and fructose in fruits (Figures 2B, 5E, F). Therefore, Mg150 and Mg200 treatments may regulate the expression of anthocyanin synthesis genes and transcription factors by changing the N signal and sugar signal, and the specific mechanism needs to be further studied.
Conclusion
An appropriate application of Mg (150 kg/ha) increased NUE, promoted N assimilation, and decreased the allocation of N to fruits. Moreover, Mg promoted the synthesis of sucrose and sorbitol in leaves by improving the photosynthetic N use efficiency (PNUE) and enzyme activity of SS, SPS, and S6PDH. Meanwhile, Mg promoted the transport of sorbitol and sucrose to fruits by upregulating the expression of MdSOT1/3 and MdSUT1/4. Subsequently, the decrease in N content and increase in sugar content in fruits may further stimulate the expression of anthocyanin synthesis genes to induce anthocyanin synthesis in the peel.
Data availability statement
The original contributions presented in the study are included in the article/Supplementary Material. Further inquiries can be directed to the corresponding authors.
Author contributions
SG and GT conceived and designed the experiments. GT and XX performed the experiments. CL, HQ and YX provided technical assistance. GT, JL, ML and ZF analyzed the data. GT wrote the manuscript. HJ, YJ and ZZ provided critical comments and revisions to the paper. All authors contributed to the article and approved the submitted version.
Funding
This work was supported by the Special Fund for the Natural Science Foundation of Shandong Province (ZR2021MC093), the earmarked fund for the China Agriculture Research System (CARS-27), the Taishan Scholar Assistance Program from Shandong Provincial Government (TSPD20181206), and the Xinlianxin Innovation Center for Efficient Use of Nitrogen Fertilizer (2020-apple).
Conflict of interest
The authors declare that the research was conducted in the absence of any commercial or financial relationships that could be construed as a potential conflict of interest.
Publisher’s note
All claims expressed in this article are solely those of the authors and do not necessarily represent those of their affiliated organizations, or those of the publisher, the editors and the reviewers. Any product that may be evaluated in this article, or claim that may be made by its manufacturer, is not guaranteed or endorsed by the publisher.
Supplementary material
The Supplementary Material for this article can be found online at: https://www.frontiersin.org/articles/10.3389/fpls.2023.1136179/full#supplementary-material
References
Álvaro, F., Royo, C., García del Moral, L. F., Villegas, D. (2008). Grain filling and dry matter translocation responses to source-sink modifications in a historical series of durum wheat. Crop Sci. 48, 1523–1531. doi: 10.2135/cropsci2007.10.0545
Bloom, Arnold, J. (2015). Photorespiration and nitrate assimilation: a major intersection between plant carbon and nitrogen. Photosynth. Res. 123 (2), 117–128. doi: 10.1007/s11120-014-0056-y
Brautigam, A., Gagneul, D., Weber, A. P. (2007). High-throughput colorimetric method for the parallel assay of glyoxylic acid and ammonium in a single extract. Anal. Bio. Chem. 362, 151–153. doi: 10.1016/j.ab.2006.12.033
Cakmak, I. (2013). Magnesium in crop production, food quality and human health. Plant Soil 368, 1–4. doi: 10.1071/cpv66n12_fo
Cakmak, I., Hengeler, C., Marschner, H. (1994). Changes in phloem export of sucrose in leaves in response to phosphorus, potassium and magnesium-deficiency in bean-plants. J. Exp. Bot. 45, 1251–1257. doi: 10.1093/jxb/45.9.1251
Cakmak, I., Kirkby, E. A. (2008). Role of magnesium in carbon partitioning and alleviating photooxidative damage. Physiol. Plant 133, 692–704. doi: 10.1111/J.1399-3054.2007.01042
Cataldo, D. A., Haroon, M., Schrader, L. E., Youngs, V. L. (1975). Rapid colorimetric determination of nitrate in plant tissues by nitration of salicylic acid. Commun. Soil Sci. Plant Anal. 6, 71–80. doi: 10.1080/00103627509366547
Ceylan, Y., Kutman, U. B., Mengutay, M. (2016). Magnesium applications to growth medium and foliage affect the starch distribution, increase the grain size and improve the seed germination in wheat. Plant Soil 406, 145–156. doi: 10.1007/s11104-016-2871-8
Chapagain, B. P., Wiesman, Z. (2004). Effect of nutrition peak foliar spray on plant development, yield, and fruit quality in greenhouse tomatoes. entia Hortic. 102 (2), 177–188. doi: 10.1016/j.scienta.2003.12.010
Chen, K. E., Chen, H. Y., Tseng, C. S. (2020). Improving nitrogen use efficiency by manipulating nitrate remobilization in plants. Nat. Plants 6, 1126–1135. doi: 10.1038/s41477-020-00758-0
Chen, Z. C., Peng, W. T., Li, J., Liao, H. (2018). Functional dissection and transport mechanism of magnesium in plants. Semin. Cell Dev. Biol. 74, 142–152. doi: 10.1016/j.semcdb.2017.08.005
Chong, C., Taper, C. D. (1971). Daily variation of sorbitol and related carbohydrates in malus leaves. Can. J. Bot. 49 (1), 173–177. doi: 10.1139/b71-029
Desnoues, E., Génard, M., Baldazzi, V. (2018). A kinetic model of sugar metabolism in peach fruit reveals a functional hypothesis of markedly low fructose-to-glucose ratio phenotype. Plant J. 94 (4), 685–698. doi: 10.1111/tpj.13890
Ding, Y. C., Jiao, X. Y., Nie, D., Li, J., Huang, M. J. (2012). Effects of combined application of different nitrogen sources and magnesium fertilizers on cabbage yield, quality and nutrient uptake. Chin. J. OF ECO-AGRICULTURE 20 (8), 996–1002. doi: 10.3724/SP.J.1011.2012.00996
Ding, Y., Luo, W., Xu, G. (2006). Characterisation of magnesium nutrition and interaction of magnesium and potassium in rice. Ann. Appl. Biol. 149, 111–123. doi: 10.1111/j.1744-7348.2006.00080.x
Eom, J. S., Choi, S. B., Ward, J. M., Jeon, J. S. (2012). The mechanism of phloem loading in rice (Oryza sativa). Mol. Cells 33 (5), 431–438. doi: 10.1007/s00425-007-0545-8
Ertiftik, H., Zengin, M. (2016). Response of sunflower to potassium and magnesium fertilizers in calcerous soils in central anatolia of turkey. J. Plant Nutr. 39, 1734–1744. doi: 10.1080/01904167.2016.1187741
Filip, M., Vlassa, M., Coman, V., Halmagyi, A. (2016). Simultaneous determination of glucose, fructose, sucrose and sorbitol in the leaf and fruit peel of different apple cultivars by the HPLC–RI optimized method. Food Chem. 199, 653–659. doi: 10.1016/j.foodchem.2015.12.060
Gou, T., Yang, L., Hu, W., Chen, X., Zhu, Y., Guo, J., et al. (2020). Silicon improves the growth of cucumber under excess nitrate stress by enhancing nitrogen assimilation and chlorophyll synthesis. Plant Physiol. Biochem. 152, 608 53–608 61. doi: 10.1016/j.plaphy.2020.04.031
Grzebisz, W. (2013). Crop response to magnesium fertilization as affected by nitrogen supply. Plant Soil. 368 (1-2), 23–39. doi: 10.1007/s11104-012-1574-z
Hermans, C., Bourgis, F., Faucher, M., Strasser, R. J., Delrot, S., Verbruggen, N. (2005). Magnesium deficiency in sugar beets alters sugar partitioning and phloem loading in young mature leaves. Planta 220 (4), 541–549. doi: 10.1007/s00425-004-1376-5
Hu, W., Zhao, W., Yang, J., Oosterhuis, D. M., Loka, D. A., Zhou, Z. G. (2016). Relationship between potassium fertilization and nitrogen metabolism in the leaf subtending the cotton (Gossypium hirsutum l.) boll during the boll development stage. Plant Physiol. Bioch. 101, 113–123. doi: 10.1016/j.plaphy.2016.01.019
Huang, Y., Jiao, Y., Nawaz, M. A., Chen, C., Liu, L., Lu, Z., et al. (2016). Improving magnesium uptake, photosynthesis and antioxidant enzyme activities of watermelon by grafting onto pumpkin rootstock under low magnesium. Plant Soil. 409 (1-2), 1–18. doi: 10.1007/s11104-016-2965-3
Huang, Z., Wang, Q., Xia, L., Hui, J., Li, J., Feng, Y. (2019). Preliminarily exploring of the association between sugars and anthocyanin accumulation in apricot fruit during ripening. Sci. Hortic. 248, 112–117. doi: 10.1016/j.scienta.2019.01.012
Huber, S. C. (1983). Role of sucrose-phosphate synthase in partitioning of carbon in leaves. Plant Physiol. 71 (4), 818–821. doi: 10.1104/pp.71.4.818
Ishfaq, M., Wang, Y., Yan, M., Wang, Z., Wu, L., Li, C., et al. (2022). Physiological essence of magnesium in plants and its widespread deficiency in the farming system of China. Front. Plant Sci. 13. doi: 10.3389/fpls.2022.802274
Jayaganesh, S., Venkatesan, S. (2010). Impact of magnesium sulphate on biochemical and quality constituents of black tea. Am. J. Food Technol. 5 (1), 31–39. doi: 10.3923/ajft.2010.31.39
Jezek, M., Geilfus, C. M., Bayer, A., MüHling, K. H. (2015). Photosynthetic capacity, nutrient status, and growth of maize (zea mays l.) upon mgso4 leaf-application. Front. Plant Science. 5. doi: 10.3389/fpls.2014.00781
Jiang, N., Gutierrez-Diaz, A., Mukundi, E. (2020). Synergy between the anthocyanin and RDR6/SGS3/DCL4 siRNA pathways expose hidden features of arabidopsis carbon metabolism. Nat. Commun. 11, 2456. doi: 10.1038/s41467-020-16289-3
Katz, E., Fon, M., Lee, Y. J. (2007). The citrus fruit proteome: insights into citrus fruit metabolism. Planta 226, 989–1005. doi: 10.1007/s00425-007-0545-8
Kleiber, T., Golcz, A., Krzesiński, W. (2012). Effect of magnesium nutrition of onion (Allium cepa l.). part i. yielding and nutrient status. Ecol. Chem. Eng. S. 19, 97–105. doi: 10.2478/v10216-011-0010-2
Kühn, B. F., Marianne, B., Sørensen, L. (2011). Optimising quality-parametersof apple cv. ‘pigeon’ by adjustment of nitrogen. Sci. Hortic. 129, 369–375. doi: 10.1016/j.scienta
Liao, H. S., Yang, C. C., Hsieh, M. H. (2022). Nitrogen deficiency and sucrose-induced anthocyanin biosynthesis is modulated by HISTONE DEACETYLASE15 in arabidopsis. J. Exp. Botany. 73, 3726–3742. doi: 10.1093/jxb/erac067
Liu, H., Fu, Y., Hu, D., Yu, J., Liu, H. (2018). Effect of green, yellow and purple radiation on biomass, photosynthesis, morphology and soluble sugar content of leafy lettuce via spectral wavebands “knock”out. Sci. Hortic. 236, 10–17. doi: 10.1016/j.scienta.2018.03.027
Liu, J., Lyu, M., Xu, X., Liu, C., Qin, H., Tian, G. (2022a). Exogenous sucrose promotes the growth of apple rootstocks under high nitrate supply by modulating carbon and nitrogen metabolism. Plant Physiol. Bioch. 192, 196–206. doi: 10.1016/j.plaphy.2022.10.005
Liu, J. R., Ma, Y. N., Lyu, F. J., Chen, J., Zhou, Z. G., Wang, Y. H., et al. (2013). Changes of sucrose metabolism in leaf subtending to cotton boll under cool temperature due to late planting. Field Crop Res. 144, 200–211. doi: 10.1016/j.fcr.2013.02.003
Liu, Q., Wu, K., Song, W., Zhong, N., Wu, Y., Fu, X. (2022b). Improving crop nitrogen use efficiency toward sustainable green revolution. Annu. Rev. Plant Biol. 73, 523–551. doi: 10.1146/annurev-arplant-070121-015752
Loreti, E., Povero, G., Novi, G., Solfanelli, C., Alpi, A., Perata, P. (2008). Gibberellins, jasmonate and abscisic acid modulate the sucrose-induced expression of anthocyanin biosynthetic genes in arabidopsis. New Phytol. 179, 1004–1016. doi: 10.1111/j.1469-8137.2008.02511.x
Ma, Q. J., Sun, M. H., Kang, H., Lu, J., You, C. X., Hao, Y. J. (2019). A CIPK protein kinase targets sucrose transporter MdSUT2.2 at Ser254 for phosphorylation to enhance salt tolerance. Plant Cell Environ. 42, 918–930. doi: 10.1111/pce.13349
Marschner (2012). Marschner’s mineral nutrition of higher plants. 3rd edition., (2011). Exp. Agric. 48 (2), 305–305. doi: 10.1017/S001447971100130X
Merlo, L., Passera, C. (1991). Changes in carbohydrate and enzyme levels during development of leaves of prunus persica, a sorbitol synthesizing species. Plant Physiol. 83 (4), 621–626. doi: 10.1111/j.1399-3054.1991.tb02478.x
Nie, J., Li, H., Li, J., Wang, K., Li, Z., Wu, Y. (2012). Studies on taste evaluation indices for fresh apple juice based on cultivars. Acta Hortic. Sin. 39, 1999–2008. doi: 10.16420/j.issn.0513-353x.2012.10.020
Orlovius, K., Mchoul, J. (2015). Effect of two magnesium fertilizers on leaf magnesium concentration, yield, and quality of potato and sugar beet. J. Plant Nutr. 38, 2044–2054. doi: 10.1080/01904167.2014.958167
Pego, J. V., Kortstee, A. J., Huijser, C. (2000). Photosynthesis, sugars and the regulation of gene expression. J. Exp. Bot. 51, 407–416. doi: 10.1093/jexbot/51.suppl_1.407
Peng, Q., Cai, Y., Lai, E., Nakamura, M., Liao, L., Zheng, B., et al. (2020a). The sucrose transporter MdSUT4.1 participates in the regulation of fruit sugar accumulation in apple. BMC Plant Biol. 20, 191. doi: 10.1186/s12870-020-02406-3
Peng, W. T., Qi, W. L., Nie, M. M. (2020b). Magnesium supports nitrogen uptake through regulating NRT2.1/2.2 in soybean. Plant Soil. 457, 97–111. doi: 10.1007/s11104-019-04157-z
Peng, W. T., Zhang, L. D., Zhou, Z., Fu, C., Chen, Z. C., Liao, H. (2018). Magnesium promotes root nodulation through facilitation of carbohydrate allocation in soybean. Physiol. Plant. 163 (3), 372–385. doi: 10.1111/ppl.12730
Poglodzinski, R., Barlog, P., Grzbisz, W. (2021). Effect of nitrogen and magnesium sulfate application on sugar beet yield and quality. Plant Soil Environ. 67, 507–513. doi: 10.17221/336/2021-PSE
Potarzycki, J. (2011). Effect of magnesium or zinc supplementation at the background of nitrogen rate on nitrogen management by maize canopy cultivated in monoculture. Plant Soil Environ. 57 (1), 19–25. doi: 10.1080/00103624.2011.552658
Rennie, E. A., Turgeon, R. (2009). A comprehensive picture of phloem loading strategies. Proc. Natl. Acad. Sci. U.S.A 106 (33), 14162–14167. doi: 10.1073/pnas.0902279106
Ruan, J. Y., Ma, L. F., Yang, Y. J. (2012). Magnesium nutrition on accumulation and transport of amino acids in tea plants. J. Sci. Food Agric. 92, 1375–1383. doi: 10.1002/jsfa.4709
Rufly, T. W., Huber, S. C. (1983). Changes in starch formation and activities of sucrose phosphate synthase and cytoplasmic fructose-1,6-biosphatase in response to source-sink alteration. Plant Physiol. 72 (2), 474–478. doi: 10.1104/pp.72.2.474
Ruiz, J., Romero, L. (2002). Relationship between potassium fertilization and nitrate assimilation in leaves and fruits of cucumber (Cucumis sativus) plants. Ann. Appl. Biol. 140, 241–245. doi: 10.1111/j.1744-7348.2002.tb00177.x
Samborska, I. A., Kalaji, H. M., Sieczko, L., Goltsev, V., Borucki, W., Jajoo, A. (2018). Structural and functional disorder in the photosynthetic apparatus of radish plants under magnesium deficiency. Funct. Plant Biol. 45, 668–679. doi: 10.1071/fp17241
Seith, B., Setzer, B., Flaig, H., Mohr, H. (1994). Appearance of nitrate reductase, nitrite reductase and glutamine synthetase in different organs of the scots pine (Pinus sylvestris) seedling as affected by light, nitrate and ammonium. Physiol. Plant 91, 419–426. doi: 10.1111/j.1399-3054.1994.tb02969.x
Sha, J., Ge, S., Zhu, Z., Du, X., Jiang, Y. (2021). Paclobutrazol regulates hormone and carbon-nitrogen nutrition of autumn branches, improves fruit quality and enhances storage nutrition in ‘fuji’ apple. Sci. Hortic. 282, 110022. doi: 10.1016/j.scienta.2021.110022
Sha, J., Wang, F., Xu, X., Chen, Q., Zhu, Z., Jiang, Y., et al. (2020). Studies on the translocation characteristics of 13C-photoassimilates to fruit during the fruit development stage in ‘fuji’ apple-sciencedirect. Plant Physiol. Biochem. 154, 636–645. doi: 10.1016/j.plaphy.2020.06.044
Shaul, O. (2002). Magnesium transport and function in plants: the tip of the iceberg. Biometals 15 (3), 307–321. doi: 10.1023/A:1016091118585
Smeekens, S. (2000). Sugar-induced signal transduction in plants. annu. rev. Plant Physiol. Plant Mol. Biol. 51, 49–81. doi: 10.1146/annurev.arplant.51.1.49
Solfanelli, C., Poggi, A., Loreti, E., Alpi, A., Perata, P. (2006). Sucrose-specific induction of the anthocyanin biosynthetic pathway in arabidopsis. Plant Physiol. 140, 637–646. doi: 10.1104/pp.105.072579
Szulc, P., Waligóra, H., Skrzypczak, W. (2008). Better effectiveness of maize fertilization with nitrogen through additional application of magnesium and sulphur. Nauka Przyr Technol. 2 (3), 1–9. doi: 10.4028/www.scientific.net/MSF.269-272.981
Tang, Z., Fan, X., Li, Q., Feng, H., Miller, A. J., Shen, Q. (2012b). Knockdown of a rice stelar nitrate transporter alters long-distance translocation but not root influx. Plant Physiol. 160 (4), 2052–2063. doi: 10.1104/pp.112.204461
Tang, N., Li, Y., Chen, L. S. (2012a). Magnesium deficiency–induced impairment of photosynthesis in leaves of fruiting citrus reticulata trees accompanied by up-regulation of antioxidant metabolism to avoid photo-oxidative damage. J. Plant Nutr. Soil Sci. 175 (5), 784–793. doi: 10.1002/jpln.201100329
Tegeder, M., Hammes, U. Z. (2018). The way out and in: phloem loading and unloading of amino acids. Curr. Opin. Plant Biol. 43, 16–21. doi: 10.1016/j.pbi.2017.12.002
Tian, X. Y., Bai, S., Chen, Z. C., He, D. D., Wang, Z., Wu, L. Q., et al. (2021). Physiological and molecular advances in magnesium nutrition of plants. Plant Soil 468, 1–17. doi: 10.1007/s11104-021-05139-w
Tian, G., Liu, C., Xu, X., Xing, Y., Liu, J., Lyu, M., et al. (2023). Effects of magnesium on nitrate uptake and sorbitol synthesis and translocation in apple seedlings. Plant Physiol. Bioch. 196, 139–151. doi: 10.1016/j.plaphy.2023.01.033
Verbruggen, N., Hermans, C. (2013). Physiological and molecular responses to magnesium nutritional imbalance in plants. Plant Soil 368, 87–99. doi: 10.1007/s11104-013-1589-0
Wang, F., Sha, J. C., Chen, Q., Xu, X. X., Zhu, Z. L., Ge, S. F., et al. (2020a). Exogenous abscisic acid regulates distribution of 13C and 15N and anthocyanin synthesis in ‘Red fuji’ apple fruit under high nitrogen supply. Front. Plant Sci. 10. doi: 10.3389/fpls.2019.01738
Wang, F., Xu, X., Jia, Z., Hou, X., Chen, Q., Sha, J., et al. (2020b). Nitrification Inhibitor3,4-dimethylpyrazole phosphate application during the later stage of apple fruit expansion regulates soil mineral nitrogen and tree carbon–nitrogen nutrition, and improves fruit quality. Front. Plant Sci. 11. doi: 10.3389/fpls.2020.00764
Wen, B. B., Li, C., Fu, X. L., Li, D. M., Gao, D. S. (2019). Effects of nitrate deficiency on nitrate assimilation and chlorophyll synthesis of detached apple leaves. Plant Physiol. Bioch. 142, 363–371. doi: 10.1016/j.plaphy.2019.07.007
Xu, G. H., Fan, X. R., Miller, A. J. (2012). Plant nitrogen assimilation and use efficiency. Annu. Rev. Plant Biol. 63, 153–182. doi: 10.1146/annurev-arplant-042811-105532
Yamaki, S., Asakura, T. (1991). Stimulation of the uptake of sorbitol into vacuoles from apple fruit flesh by abscisic acid and into protoplasts by indoleacetic acid. Plant Cell Physiol. 32, 315–318. doi: 10.1093/oxfordjournals.pcp.a078082
Yang, G. H., Yang, L. T., Jiang, H. X. (2012). Physiological impacts of magnesium-deficiency in citrus seedlings: photosynthesis, antioxidant system and carbohydrates. Trees 26, 1237–1250. doi: 10.1007/s00468-012-0699-2
Yang, J. J., Zhang, C. X., Wang, Z. Y., Sun, S., Zhan, R., Zhao, Y. (2019). Melatonin-mediated sugar accumulation and growth inhibition in apple plants involves down-regulation of fructokinase expression and activity. Front. Plant Sci. 10. doi: 10.3389/fpls.2019.00150
Zhang, B., Cakmak, I., Feng, J., Yu, C., He, Y. (2020a). Magnesium deficiency reduced the yield and seed germination in wax gourd by affecting the carbohydrate translocation. Front. Plant Science. 11. doi: 10.3389/fpls.2020.00797
Zhang, M., Geng, Y., Cao, G., Zou, X., Stephano, M. F. (2020b). Effect of magnesium fertilizer combined with straw return on grain yield and nitrogen use efficiency. Agron. J. 113 (1), 345–357. doi: 10.1002/agj2.20483
Keywords: apple, Mg, 13C, 15N, sugar, anthocyanin, gene expression
Citation: Tian G, Qin H, Liu C, Xing Y, Feng Z, Xu X, Liu J, Lyu M, Jiang H, Zhu Z, Jiang Y and Ge S (2023) Magnesium improved fruit quality by regulating photosynthetic nitrogen use efficiency, carbon–nitrogen metabolism, and anthocyanin biosynthesis in ‘Red Fuji’ apple. Front. Plant Sci. 14:1136179. doi: 10.3389/fpls.2023.1136179
Received: 02 January 2023; Accepted: 07 February 2023;
Published: 23 February 2023.
Edited by:
Shenghui Jiang, Qingdao Agricultural University, ChinaReviewed by:
Liu Songzhong, Beijing Academy of Agriculture and Forestry Sciences, ChinaMingjun Li, Northwest A&F University, China
Copyright © 2023 Tian, Qin, Liu, Xing, Feng, Xu, Liu, Lyu, Jiang, Zhu, Jiang and Ge. This is an open-access article distributed under the terms of the Creative Commons Attribution License (CC BY). The use, distribution or reproduction in other forums is permitted, provided the original author(s) and the copyright owner(s) are credited and that the original publication in this journal is cited, in accordance with accepted academic practice. No use, distribution or reproduction is permitted which does not comply with these terms.
*Correspondence: Zhanling Zhu, emhsemhAc2RhdS5lZHUuY24=; Yuanmao Jiang, eW1qaWFuZ0BzZGF1LmVkdS5jbg==; Shunfeng Ge, Z2VzaHVuZmVuZzIxMEAxMjYuY29t