- 1Department of Agroecology and Crop Production, Faculty of Agrobiology, Food and Natural Resources, Czech University of Life Sciences Prague, Prague, Czechia
- 2Excellent Team for Mitigation (E.T.M.), Faculty of Forestry and Wood Sciences, Czech University of Life Sciences Prague, Prague, Czechia
- 3Department of Crop Sciences and Agroforestry, The Faculty of Tropical AgriSciences, Czech University of Life Sciences Prague, Prague, Czechia
The continuous use of herbicides for controlling weeds has led to the evolution of resistance to all major herbicidal modes of action globally. Every year, new cases of herbicide resistance are reported. Resistance is still in progress in many species, which must be stopped before it becomes a worldwide concern. Several herbicides are known to cause stressful conditions that resemble plant abiotic stresses. Variation in intracellular calcium (Ca2+) concentration is a primary event in a wide range of biological processes in plants, including adaptation to various biotic and abiotic stresses. Ca2+ acts as a secondary messenger, connecting various environmental stimuli to different biological processes, especially during stress rejoindering in plants. Even though many studies involving Ca2+ signalling in plants have been published, there have been no studies on the roles of Ca2+ signalling in herbicide stress response. Hence, this mini-review will highlight the possible sensing and molecular communication via Ca2+ signals in weeds under herbicide stress. It will also discuss some critical points regarding integrating the sensing mechanisms of multiple stress conditions and subsequent molecular communication. These signalling responses must be addressed in the future, enabling researchers to discover new herbicidal targets.
1 Introduction
Plants cells are the depot for different ions, including calcium. Calcium (Ca+2) is an essential bivalent cation with varying plant utilities (Aldon et al., 2018; Yadav et al., 2022). Calcium ions (Ca2+) partake in several physiological parameters in plants, including cell division, cytoplasmic streaming, thigmotropism, photomorphogenesis, cell polarization, fruit development and ripening and plant microbe interaction (Gao et al., 2019; Furuichi, 2020; Eichstädt et al., 2021; Duo et al., 2022). Since the discovery of its effects on muscle contraction, the perception of calcium as a regulatory molecule in living organisms has gained wide-range recognition globally. Plant and animal cells contain a group of proteins with Ca2+-binding properties (Bose et al., 2011; Jia et al., 2022). These proteins alter their conformation upon stress by external stimulus, increasing the cytoplasmic Ca2+ ([Ca2+]cyt) concentration. Thereafter, the [Ca2+]cyt couples the extracellular stimuli to their distinctive intracellular responses and synchronize a wide range of endogenous processes. Besides increasing the concentration of the [Ca2+]cyt, sometimes these special Ca2+-binding proteins directly interact with other targets and allow them to execute their respective response (Batistič and Kudla, 2009). Several shreds of evidence have established the ubiquitous role of Ca2+ as an essential cellular second messenger (Marcec et al., 2019). The details on the characteristics and molecular mechanisms of calcium signalling can be found in Bickerton and Pittman, 2012 and Kudla et al., 2018.
The importance of herbicides to modern crop production is immutable. However, due to excessive application of synthetic herbicides, there has been a continuous rise in the number of cases of herbicide-resistant weeds. The evolution of herbicide resistance in weeds is an exceptional example of the adaptability of weed species to abiotic stress (Délye et al., 2013). An increase in cellular Ca2+ concentration is a primary signalling event when plants confront abiotic stimuli (Xu et al., 2022). Hence, assuming that calcium signalling might play a vital role in weeds toward herbicidal resistance/tolerance under herbicide stress would not be erroneous. However, to date, there have been no attempts to explore the plausible role of Ca2+ in this area (Supplementary Figure 1). Hence, in this perspective, we will discuss the plausible roles of Ca2+ signalling in conferring herbicide resistance. Because of space limitations, we will cover the main aspects of calcium as a signal transducer involved in herbicide stresses.
2 Ca2+ signalling in abiotic stresses in plants
Among the vital environmental stresses affecting agriculture, the most important are drought, salt, and temperature stresses (Hirayama and Shinozaki, 2010; Chaudhry and Sidhu, 2022; Zhang H. et al., 2022). To overcome these constraints, plants developed sophisticated mechanisms, including systematic regulation of Ca2+ ions within the cells, which might lead to intonations of gene expression (Reddy et al., 2011; Manishankar et al., 2018). Studies suggest Ca2+ plays a crucial role in regulating the physiological response to drought conditions by acting as a secondary messenger and transmitting drought signals (Hong-Bo et al., 2008; Pathak et al., 2020). It has also been elucidated that cytosolic-free calcium plays a pivotal role in stomatal movement, and changes in its concentration can regulate the opening and closing of the stomata (Wang et al., 2005; Wang et al., 2016; Thor et al., 2020). The movement of stomata regulates two of the key plant physiological processes, i.e., transpiration and stomatal conductance, which is closely related to water-use efficiency, a physiological trait of immense importance concerning drought stress. Previously, the close relationship between Ca2+ concentration and the degree of drought stress in wheat seedlings was examined, and it was observed that the concentration of free Ca2+ in the nucleus increases with increasing drought duration, indicating its potential role in maintaining nucleus structure and integrity (Hong-Bo et al., 2008; Song et al., 2008).
Ca2+ ions not only promote plant growth but also possess the potency to reverse the detrimental effects of salt stress (Roy et al., 2019; Borjigin et al., 2021; Lian et al., 2022). For instance, salinity causes a severe reduction of hydraulic conductivity in the primary roots of the plants. However, exogenous application of Ca2+ mitigated these effects in maize and other plants (Ahmad et al., 2018; Pathak et al., 2020; Lian et al., 2022). Evidence also suggests that the Ca2+ signalling network is closely associated with the SOS (salt overly sensitive) signal transduction pathway and regulates the homeostasis of cellular Na+ and K+ (Brini et al., 2007).
Apart from salt and drought stresses, there has been a significant increase in research correlating Ca2+ ions and temperature stress tolerance. Ca2+ has been found to play a vital role in plants’ adaptation to cold and heat stress conditions (Hong-Bo et al., 2008; Pathak et al., 2020). For instance, in an attempt to acclimatize in the alpine subnivean conditions, Chorispora bungeana accumulates Ca2+ among its various tissues and organs (Fu et al., 2006). Ca2+-responsive protein kinases such as soybean calmodulin (GmCaM4) and Ca‐dependent protein kinase 6 (CDPK6) in Arabidopsis were found to regulate the metabolism of plant cells positively and confer cold tolerance (Pathak et al., 2020). In addition, exogenous treatment of Ca2+ during cold stress enhances plant growth, development, morphology, and physiology by modulating the plant’s photosynthetic capacity, ROS metabolism, and nitrogen assimilation (Yuan et al., 2018; Singh et al., 2022). In acclamatory response to heat stress, Ca2+ signalling causes increased expression levels of the gene coding for an enzyme called desaturase, an essential enzyme responsible for maintaining membrane fluidity under temperature stress (Huda et al., 2013). Ca-mediated heat shock proteins (HSPs) are also induced during heat stress and provide necessary cellular homeostasis (Pathak et al., 2020). In a previous study on tobacco, the heat stress caused a decrease in various photosynthetic parameters such as net photosynthetic rate, apparent quantum yield and stomatal conductance. However, the exogenous application of CaCl2 mitigated these effects (Tan et al., 2011). In the same study, activities of ascorbate peroxidase, superoxide dismutase, catalase, and peroxidase also decreased under heat stress, but with CaCl2 pretreatment, the levels of these enzymes showed significant alterations (Tan et al., 2011). Hydrogen peroxide (H2O2) tends to accumulate in plants as a response to stress like high temperatures and has been extensively used as a physiological indicator to assess the intensity of stress felt by plants (Asaeda et al., 2022). Interestingly, the levels of H2O2 have also been found to decrease upon exogenous calcium chloride treatment indicating enfeebling effects of Ca2+ during stresses (Tan et al., 2011; Pathak et al., 2020). Table 1 lists some critical Ca2+ signalling in abiotic stress-specific research works identified in major plant species in the last few years.
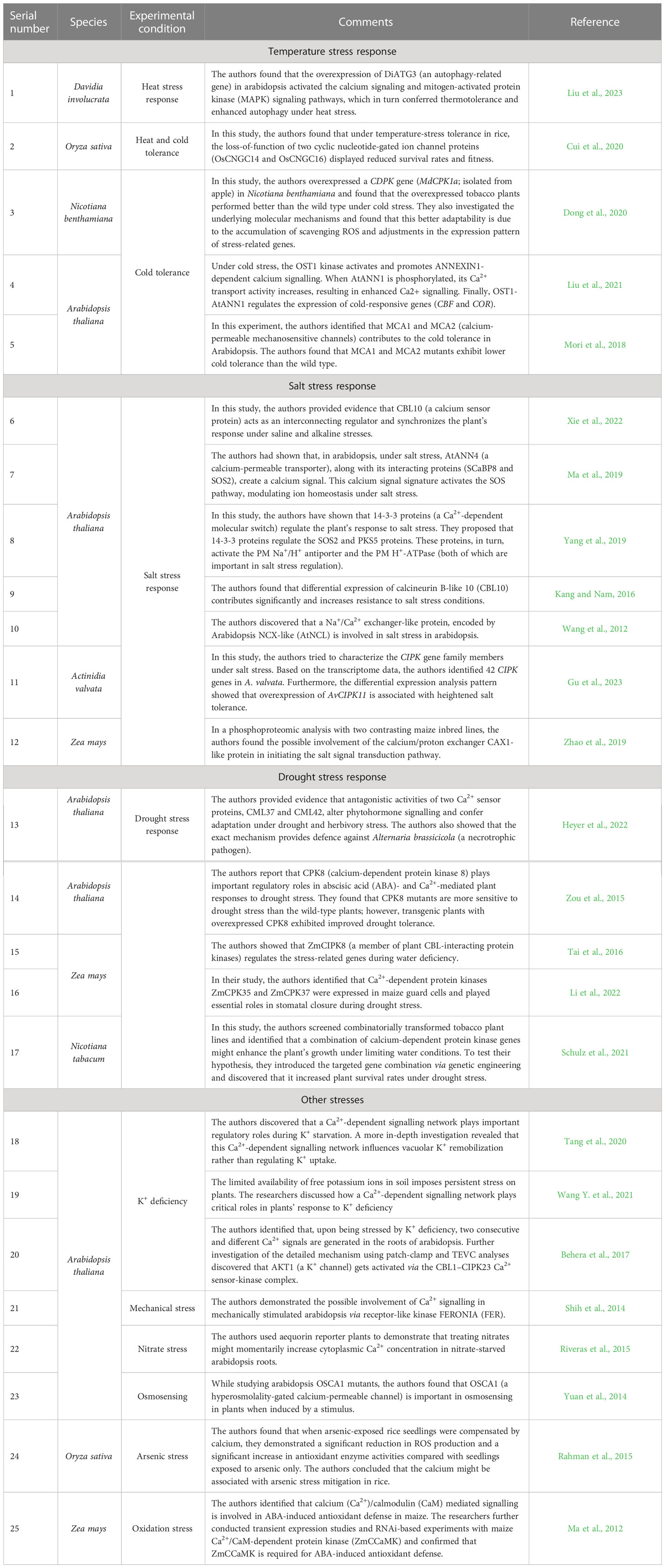
Table 1 Some important Ca2+ signalling in abiotic stress-specific research works identified in major plant species.
3 Ca2+ signalling might lead to transcriptional reprogramming during herbicide stress
The most basic molecular mechanisms of herbicide resistance include target-site resistance (TSR) and non-target-site resistance (NTSR). Among the TSR, genetic mutations and gene overexpression are the most prevalent mechanisms. NTSR mechanisms include enhanced metabolism, reduced absorption and translocation etc. (Gaines et al., 2020; Torra and Alcántara-de la Cruz, 2022). NTSR mechanisms are more complex than TSR mechanisms and involve transcriptional reprogramming of stress-related genes such as cytochrome P450s (CYP450s) and glutathione-S-transferases (GSTs). Hence, the development of herbicide resistance in grass weeds can be considered almost similar to other abiotic stresses such as temperature and drought. Weedy plants have developed several exciting mechanisms to adapt against herbicide stress. Although numerous mechanisms have been discovered, several such mechanisms, especially different signalling events, are yet to be discovered.
Ca2+ signalling events amend the expression patterns of several essential genes (mainly NTSR genes) in response to several biotic and abiotic stresses (Moscatiello et al., 2018; Yadav et al., 2022). Depending upon the activation mechanisms, Ca2+ influx transporter channels might translate a wide range of signals into diverse Ca2+ signatures. To date, five different families of Ca2+ channels have been recognized in higher plants. These include two-pore channels (TPCs), glutamate receptor-like channels (GLRs), Cyclic nucleotide-gated channels (CNGCs), mechanosensitive-like channels (MSLs) and the reduced hyperosmolality-induced [Ca2+]cyt increase (OSCAs) channels. Within the plant cells, these channels are maintained precisely in an extremely synergistic manner (Ghosh et al., 2022). Upon being stressed by herbicides, it could be assumed that these Ca2+ influx transporters might get triggered, and hence there might be a sudden influx of Ca2+, thereby creating herbicide stress-specific Ca2+ signatures. Thereafter, the diverse and extensive set of Ca2+ sensors (known as Ca2+ binding proteins) decodes and relays the signals for further processing (usually phosphorylation responses). These Ca2+ binding proteins can be classified into sensor relay proteins and sensor responder proteins. The sensor responder proteins include calcium-dependent protein kinases (CDPKs), which mainly bind to these Ca2+ ions and induce conformational changes (Hashimoto and Kudla, 2011). After that, the herbicide-stress-specific CDPKs decode and translate the message of elevated calcium concentration into enhanced protein kinase activity and subsequent downstream signalling events. Contrary to the responder proteins, the relay proteins lack effector domains and are not direct target proteins. The relay proteins include calmodulins (CaMs), and their primary function is to bind to these Ca2+ ions and induce conformational changes in the responder proteins (Hashimoto and Kudla, 2011).
Although the lack of higher-resolution genomic data and tools in weeds have limited our detailed knowledge of the CDPKs in these species, these proteins are well-characterized for the model plants such as A. thaliana (Shi et al., 2018). Additionally, molecular cloning and functional analysis studies have confirmed the essential roles of CPKs in abiotic stress tolerance in a wide range of plants (Atif et al., 2019). CDPKs contain a serine/threonine protein kinase domain, an autoinhibitory domain, and a CaM-like domain. The autoinhibitory domain plays a vital role in maintaining the activated state of the kinase domain. Once the cellular Ca2+ levels get elevated, the binding of Ca2+ to the CaM-like domain leads to a conformational change of the autoinhibitory domain and activates the kinase. In addition to the conformational changes of the CaM-like domain, auto-phosphorylation of these proteins further helps in the kinase activation process. Along with the CDPKs, plants contain two other sensor responder proteins: Ca2+/calmodulin-dependent protein kinases (CCaMKs) and CBL-interacting protein kinases (CIPKs). These kinases and their specific Ca2+ binding proteins form a complex cellular network in various cellular processes (Batistič and Kudla, 2009; Weinl and Kudla, 2009). Sometimes these complex cellular networks are stress-specific, and to the best of our knowledge, there are no reports or efforts to discover herbicide-stress-specific cellular signalling systems.
The final step in calcium signalling under herbicide stress will be converting these complex signals into their respective transcriptional responses. The activation of the Ca2+ sensor kinases further induces phosphorylation events, thus leading to the transcriptional reprogramming of essential genes (Khare et al., 2020). Usually, these transcriptional reprogramming events are mediated by activating stress-specific transcription factors (Himanen and Sistonen, 2019). Ca2+/CaM-mediated transcription factors relay Ca2+ transients generated by herbicide stress to transcriptional reprogramming of CYP450s and GSTs. RNA-seq transcriptome profiling studies have provided shreds of evidence that upregulation of CYP450 isoforms is involved in the metabolism of different herbicides in several weed species, including Echinochloa sp. (Fang et al., 2019; Pan et al., 2022), Alopecurus sp. (Zhao et al., 2017; Franco-Ortega et al., 2021). However, in most of the cases, the mechanisms of the CYP450 upregulation were not investigated. Transcription factors are potentially involved in regulating the expression of the NTSR genes, such as CYP450s, GSTs and ABC transporters. A recent study by Zhang Y. et al. (2022) on Echinochloa spp. has shown that upregulation of the bZIP88 transcription factor confers resistance to three different herbicides (Zhang Y. et al., 2022). However, the molecular mechanisms behind the upregulation were not further investigated. bZIP transcription factors are known to regulate abiotic stress responses in many plants and are expected to play a regulatory role in NTSR (Century et al., 2008; Zhang H. et al., 2022). Investigations of the calcium signalling events during the herbicide treatment might provide new understandings to such unexplained questions. A schematic diagram showing the putative role of calcium signalling in weeds in herbicide resistance can be found in Figure 1.
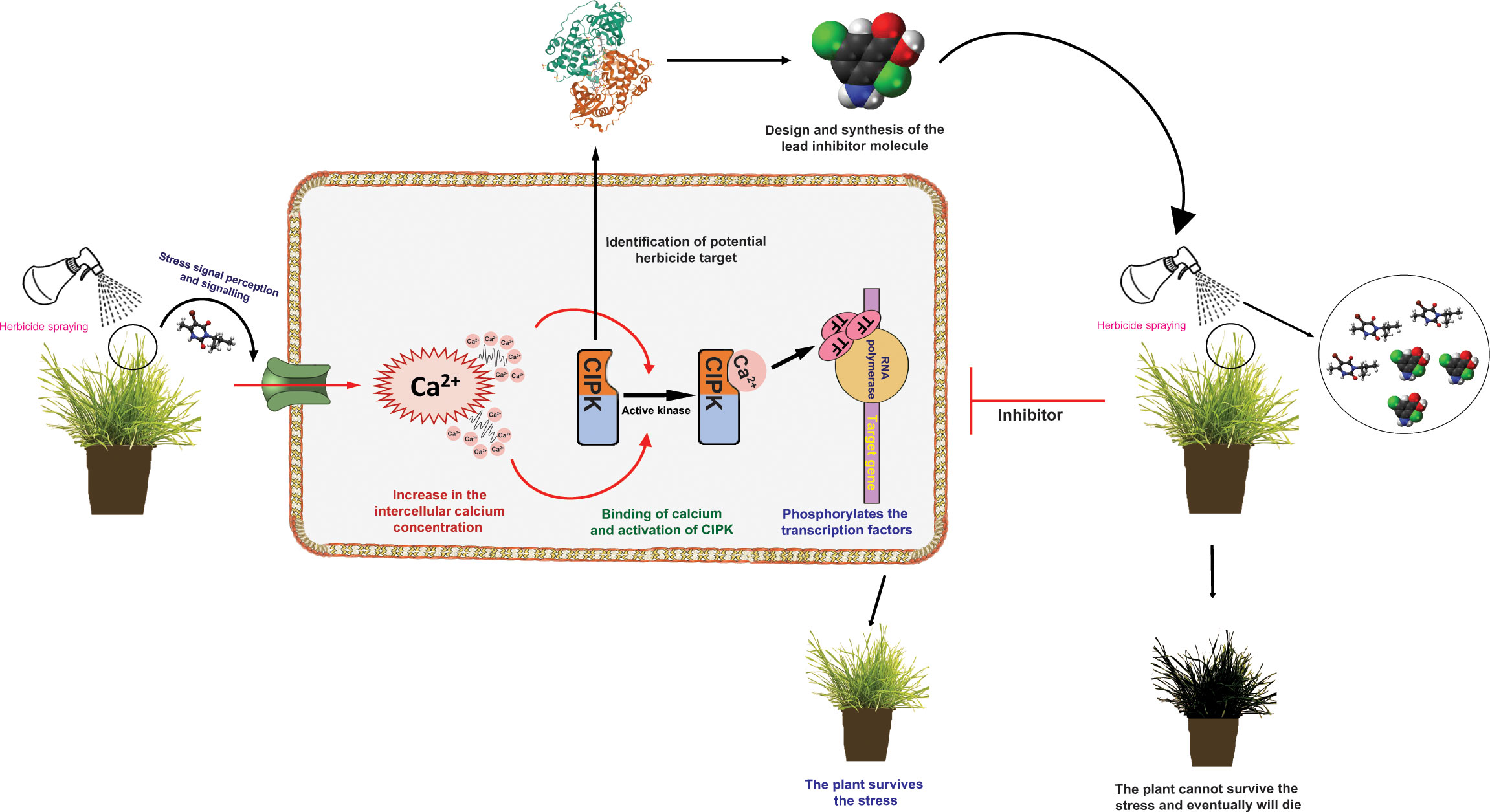
Figure 1 Schematic diagram showing the possible role of calcium (Ca2+) signalling in weeds in herbicide resistance. The resistant plant (R) survives the herbicide stress by target site and non-target site mechanisms. The hypothesis is that upon perceiving and transducing the herbicide stress signals, there might be an abnormal elevation of the Ca2+ ions within the cytoplasm of the cell. This initiates the calcium signalling pathways. The Ca2+ ions are thought to bind to the sensor responder proteins and activate their kinase activities. Instead, these kinases activate the transcription factors by phosphorylating them, thus leading to the overexpression of the stress-responsive metabolism-related genes. In turn, these genes enhance the herbicide metabolism process, conferring resistance in R plants. Following the identification and validation of the potential herbicidal targets (e.g., CIPK isoforms), computer-aided drug design can be used to identify and screen the new inhibitors, which might contribute to the novel herbicidal lead compound discovery.
Besides playing a role in conferring resistance against the herbicides, Ca2+ signalling might also play an essential role during stressful “herbicide-exposure memories” in weeds. Higher plants, including weeds, have developed different mechanisms (established over a long period of evolution) to respond and adapt to recurring stresses (Bhar et al., 2022). Among the two distinct mechanisms of herbicide resistance, the NTSR mechanisms play critical roles in integrating and coordinating whole plant herbicide stress responses. There is considerable evidence that [Ca2+]cyt signatures get modified by previous experience with an environmental challenge (Bruce et al., 2007). There is also evidence that prior exposure to contrasting stress can alter the Ca2+ signatures provoked by a particular stress (Tuteja and Mahajan, 2007). These indicate a cross-talk between the signalling cascades. Frequent exposure to herbicides might lead to an attenuated response of Ca2+ signalling pathways, and the cells might retain the previous information. Since maintaining a cellular [Ca2+] homeostasis is vital for any plant, this Ca2+ memory is significant for herbicide-resistant weeds. These weeds can thus quickly retort better to the herbicide stress without upsetting the delicate and sensitive balance of Ca2+.
4 Ca2+signal-regulated alternative splicing in weeds
Alternative splicing (AS), in general, generates multiple mRNA isoforms from the same pre-mRNA and is alleged to contribute to increasing transcriptomic and proteomic diversity (Chaudhary et al., 2019; Mandadi et al., 2022; Tognacca et al., 2022). The putative roles of AS in a wide range of physiological processes (such as plant metabolism, plant immunity, plant disease resistance etc.) have been well explored in many plants. However, despite the evidence of multiple copies of important herbicide target genes (such as ALS, ACCase and EPSPS), the area of alternative splicing has remained unexplored in weedy plants (Xu et al., 2014; Iwakami et al., 2017; Yakimowski et al., 2021). Although no experiments have shown AS in weeds, NGS and omics approaches in plants have revealed that most of the plant multi-exon genes undergo AS (Chaudhary et al., 2019). We hypothesize that Ca2+-signalling might influence the alternative splicing of these herbicide target genes by controlling the relevant splicing factors. Earlier, we discussed that elevation of the intracellular Ca2+ level activates a variety of signalling kinases; after that, the Ca2+-binding sensor proteins trigger phosphorylation responses during abiotic stresses (Xu et al., 2022). So, it is possible that when exposed to herbicides, Ca2+-binding sensor proteins might stimulate the splicing factors via phosphorylation. Furthermore, Ca2+-signalling regulates the subcellular redistribution of splicing regulatory proteins (Razanau and Xie, 2013). For example, in rats, thapsigargin treatment triggered an increase in the intracellular calcium concentration, leading to hyperphosphorylation and further accretion of a splicing factor in the cytoplasm (Daoud et al., 2002). Moreover, polarization and depolarization might also significantly impact the chromatin accessibility of the variable exon by regulating the histone modifications (Razanau and Xie, 2013). As a result, Ca2+-signalling is thought to play an important role in mRNA isoform expression by controlling chromatin status. In summary, comprehensive experiments are needed to elucidate possible regulatory roles of Ca2+-signalling in alternative splicing in herbicide-resistant weeds.
5 Monitoring the Ca2+ signal patterns associated with herbicide stress response
Even though many indicators are available for plants, none of those have been used in weedy plants to understand the role of Ca2+ signalling in alleviating herbicide stress. Since changes in the intracellular and cytosolic Ca2+ concentration are stimulus-specific, Jaffe posited three critical criteria to ensure that a particular Ca2+ signal pattern is associated with a particular stress response. These criteria include: (a) inhibition of the levels of Ca2+ must also impede the physiological response; (b) an artificial increase in the Ca2+ levels must provoke the physiological response, even in the absence of the stimulus; and (c) an increase in the cytosolic Ca2+ concentration must either precede or accompany the response (Jaffe, 1980). The first two criteria can be accomplished by various chelators and ionophores, whereas the last criterion requires techniques that can measure intracellular resting and stimulated Ca2+ levels in weeds. Hence, monitoring the Ca2+ signal patterns associated with the herbicide stress response is crucial.
In the past years, Ca2+-selective microelectrodes were used to evaluate the modifications of the intracellular Ca2+ concentration. However, owing to their convenience, in recent years, these microelectrodes have been replaced by luminous Ca2+ indicators, such as Fura Red, Fluo-4, quin-2 and indo-1 (Bush and Jones, 1990; Kanchiswamy et al., 2014). Confocal laser scanning microscopy (CLSM) with these fluorescent Ca2+ indicators might be an excellent option for measuring Ca2+ in living plant cells. Besides CLSM, calcium imaging can also be done based on Förster Resonance Energy Transfer (FRET) and Selective Plane Illumination Microscopy (SPIM). Even though FRET has been used in Arabidopsis, to our knowledge, these techniques have not been used to understand calcium signalling in any weedy plants. In addition to these Ca2+ dyes, protein-based Ca2+ indicators (such as aequorin–based and GFP–based indicators) are also used to measure Ca2+ in living plant cells (Mithöfer and Mazars, 2002; Granatiero et al., 2014; Kanchiswamy et al., 2014). Aequorin is a calcium-activated photoprotein composed of apoaequorin (as apoprotein) and coelenterazine (a luciferin molecule, as a prosthetic group). When Ca2+ occupies the Ca2+-binding sites of the aequorin, the coelenterazine gets converted into coelenteramide and is released together with carbon dioxide. Thereafter, on returning to its ground state, blue light (λ = 469 nm) is emitted, which can be detected with a luminometer. Bioluminescent probes paired with Ca2+-sensitive aequorin can be used in the weeds for real-time measurement of the Ca2+ signal patterns linked with herbicide stress. In addition to the aequorin-based Ca2+ sensors, since their discovery in 1997, the GFP-based Ca2+ indicators have also gained the interest of plant biotechnologists (Kanchiswamy et al., 2014).
6 Current challenges in Ca2+ signalling in the weeds and the way forward
To understand the mechanisms of Ca2+-mediated molecular signalling processes, understanding the expression patterns and correlations with their biochemical activities is vital. Additionally, conducting these analyses in a spatiotemporal fashion will be better. Even though these Ca2+-induced changes are well documented in some model plants (Cui et al., 2020; Schulz et al., 2021; Wang J. et al., 2021; Gao et al., 2022; Li et al., 2022), reckonable data at high spatiotemporal resolution are still inadequate. In weeds, to date, there are no attempts to study both Ca2+-induced changes and high spatio-temporal resolute data. Elucidation of the molecular signalling mechanisms in model species is mainly based on high-resolution genomic analyses under controlled experimental conditions. Quite the reverse, the unattainability of high-resolution genomic data hampers the identification of the connection between various Ca2+ signatures with the phenotypic effects in non-model organisms such as weeds. Additionally, no practical tools for in-vivo Ca2+ quantification are available for the weed system. However, attempts must be made using existing tools available for Ca2+ quantification within the plant system. Additionally, In-silico analysis of the gene families involved in the Ca2+ signalling will help detect Ca2+ transporters in weeds (Goel et al., 2011; Mohanta et al., 2015; Yadav et al., 2022).
7 Conclusions and future perspectives
Despite extensive research on the mechanisms of herbicides in recent years, the mechanisms of herbicides and the herbicide stress signalling network have not been investigated and discovered. Much progress has been made in understanding several Ca2+-mediated signal networks in crops and plants under various environmental stresses such as cold, heat and drought (Kudla et al., 2018). Similar to plant abiotic stresses, herbicides are known to cause oxidative stress (Caverzan et al., 2019). Hence, the resistance against these chemicals involves the increased expression of several stress-responsive genes, such as CYP450s and GSTs (Délye et al., 2013). Several signal pathways might be involved during herbicide stress signalling (Alberto et al., 2016). In this perspective, we attempted to describe a possible hypothesis on how a Ca2+-mediated signal network might confer herbicide resistance. However, due to the lack of any solid experimental attempts evidence, we had to confine ourselves to the theoretical concept as discussed in Section 3 and Figure 1. Currently, research efforts are increasing to obtain reference weed genomes. In addition, bioinformatics-based modelling approaches, RNA-seq transcriptome and gene editing experiments (using RNAi and/or CRISPR/Cas systems) are needed to elucidate the exact roles of Ca2+ signalling in weeds under herbicide stress. Implementing the multi-omics data-based approaches (generated from genomics and transcriptomics) will be very useful in identifying the candidate Ca2+ transducing proteins, channels, exchangers and pumps involved in weed-herbicide interactions and other abiotic stress responses in weeds. Weeds have developed resistance against all the major herbicidal modes of action. Hence, further genetic engineering of these stress-specific Ca2+-signalling regulators might enable the herbicide industries to discover novel targets and use them for herbicide discovery. Research on weed molecular communication during herbicide stress (particularly Ca2+ signalling) is still in its infancy. This area of research should be an exciting area for future research in terms of novel and applied science.
Data availability statement
The original contributions presented in the study are included in the article/Supplementary Material. Further inquiries can be directed to the corresponding author.
Author contributions
MS and AR conceived the idea. MS, KH, RB and PK drafted the manuscript. MS and RAC designed and created the figure. AR and JS edited the manuscript MKS revised the manuscript. All authors contributed to the article and approved the submitted version.
Funding
This work was supported by grant from the Long-term conceptual development of research organization [The Ministry of Education, Youth and Sports (Czech Republic)]. The salary for AR is obtained from grant “EXTEMIT - K,” No. CZ.02.1.01/0.0/0.0/15_003/0000433 and EVA 4.0,” No. CZ.02.1.01/0.0/0.0/16 019/0000803 supported by OP RDE.
Conflict of interest
The authors declare that the research was conducted in the absence of any commercial or financial relationships that could be construed as a potential conflict of interest.
Publisher’s note
All claims expressed in this article are solely those of the authors and do not necessarily represent those of their affiliated organizations, or those of the publisher, the editors and the reviewers. Any product that may be evaluated in this article, or claim that may be made by its manufacturer, is not guaranteed or endorsed by the publisher.
Supplementary material
The Supplementary Material for this article can be found online at: https://www.frontiersin.org/articles/10.3389/fpls.2023.1135845/full#supplementary-material
Supplementary Figure 1 | Number of calcium signalling-related publications (2010–2022) retrieved from Web of Science (https://www.webofscience.com/wos/woscc/basic-search). The searches were done by using the keywords “calcium signalling”, “calcium signalling in animals”, “calcium signalling in plants” and “calcium signalling in weedy plants” (done in 10th February, 2023).
References
Ahmad, P., Alyemeni, M. N., Abass Ahanger, M., Wijaya, L., Alam, P., Kumar, A., et al. (2018). Upregulation of antioxidant and glyoxalase systems mitigates NaCl stress in Brassica juncea by supplementation of zinc and calcium. J. Plant Interact. 13, 151–162. doi: 10.1080/17429145.2018.1441452
Alberto, D., Serra, A.-A., Sulmon, C., Gouesbet, G., Couée, I. (2016). Herbicide-related signaling in plants reveals novel insights for herbicide use strategies, environmental risk assessment and global change assessment challenges. Sci. Total Environ. 569–570, 1618–1628. doi: 10.1016/j.scitotenv.2016.06.064
Aldon, D., Mbengue, M., Mazars, C., Galaud, J.-P. (2018). Calcium signalling in plant biotic interactions. Int. J. Mol. Sci. 19, 665. doi: 10.3390/ijms19030665
Asaeda, T., Rahman, M., Vamsi-Krishna, L., Schoelynck, J., Rashid, M. H. (2022). Measurement of foliar H2O2 concentration can be an indicator of riparian vegetation management. Sci. Rep. 12, 13803. doi: 10.1038/s41598-022-17658-2
Atif, R. M., Shahid, L., Waqas, M., Ali, B., Rashid, M. A. R., Azeem, F., et al. (2019). Insights on calcium-dependent protein kinases (CPKs) signaling for abiotic stress tolerance in plants. Int. J. Mol. Sci. 20, 5298. doi: 10.3390/ijms20215298
Batistič, O., Kudla, J. (2009). Plant calcineurin b-like proteins and their interacting protein kinases. Biochim. Biophys. Acta (BBA) - Mol. Cell Res. 1793, 985–992. doi: 10.1016/j.bbamcr.2008.10.006
Behera, S., Long, Y., Schmitz-Thom, I., Wang, X. P., Zhang, C., Li, H., et al. (2017). Two spatially and temporally distinct Ca2+ signals convey Arabidopsis thaliana responses to k+ deficiency. New Phytol. 213 (2), 739–750. doi: 10.1111/nph.14145
Bhar, A., Chakraborty, A., Roy, A. (2022). Plant responses to biotic stress: Old memories matter. Plants 11, 84. doi: 10.3390/plants11010084
Bickerton, P. D., Pittman, J. K. (2012). Calcium signalling in plants. eLS. doi: 10.1002/9780470015902.a0023722
Borjigin, C., Schilling, R. K., Jewell, N., Brien, C., Sanchez-Ferrero, J. C., Eckermann, P. J., et al. (2021). Identifying the genetic control of salinity tolerance in the bread wheat landrace mocho de espiga branca. Funct. Plant Biol. 48, 1148–1160. doi: 10.1071/FP21140
Bose, J., Pottosin, I. I., Shabala, S. S., Palmgren, M. G., Shabala, S. (2011). Calcium efflux systems in stress signaling and adaptation in plants. Front. Plant Sci. 2. doi: 10.3389/fpls.2011.00085
Brini, F., Hanin, M., Mezghani, I., Berkowitz, G. A., Masmoudi, K. (2007). Overexpression of wheat Na+/H+ antiporter TNHX1 and h+-pyrophosphatase TVP1 improve salt- and drought-stress tolerance in Arabidopsis thaliana plants. J. Exp. Bot. 58, 301–308. doi: 10.1093/jxb/erl251
Bruce, T. J. A., Matthes, M. C., Napier, J. A., Pickett, J. A. (2007). Stressful “memories” of plants: Evidence and possible mechanisms. Plant Sci. 173, 603–608. doi: 10.1016/j.plantsci.2007.09.002
Bush, D. S., Jones, R. L. (1990). Measuring intracellular Ca2+ levels in plant cells using the fluorescent probes, indo-1 and fura-2: progress and prospects. Plant Physiol. 93 (3), 841–845. doi: 10.1104/pp.93.3.841
Caverzan, A., Piasecki, C., Chavarria, G., Stewart, C. N., Vargas, L. (2019). Defenses against ROS in crops and weeds: The effects of interference and herbicides. Int. J. Mol. Sci. 20, 1086. doi: 10.3390/ijms20051086
Century, K., Reuber, T. L., Ratcliffe, O. J. (2008). Regulating the regulators: The future prospects for transcription-Factor-Based agricultural biotechnology products. Plant Physiol. 147, 20–29. doi: 10.1104/pp.108.117887
Chaudhary, S., Khokhar, W., Jabre, I., Reddy, A. S., Byrne, L. J., Wilson, C. M., et al. (2019). Alternative splicing and protein diversity: Plants versus animals. Front. Plant Sci. 10. doi: 10.3389/fpls.2019.00708
Chaudhry, S., Sidhu, G. P. S. (2022). Climate change regulated abiotic stress mechanisms in plants: A comprehensive review. Plant Cell Rep. 41, 1–31. doi: 10.1007/s00299-021-02759-5
Cui, Y., Lu, S., Li, Z., Cheng, J., Hu, P., Zhu, T., et al. (2020). CYCLIC NUCLEOTIDE-GATED ION CHANNELs 14 and 16 promote tolerance to heat and chilling in rice. Plant Physiol. 183, 1794–1808. doi: 10.1104/pp.20.00591
Daoud, R., Mies, G., Smialowska, A., Olah, L., Hossmann, K. A., Stamm, S. (2002). Ischemia induces a translocation of the splicing factor tra2-β1 and changes alternative splicing patterns in the brain. J. Neurosci. 22 (14), 5889–5899. doi: 10.1523/JNEUROSCI.22-14-05889.2002
Délye, C., Jasieniuk, M., Le Corre, V. (2013). Deciphering the evolution of herbicide resistance in weeds. Trends Genet. 29, 649–658. doi: 10.1016/j.tig.2013.06.001
Dong, H., Wu, C., Luo, C., Wei, M., Qu, S., Wang, S. (2020). Overexpression of MdCPK1a gene, a calcium dependent protein kinase in apple, increase tobacco cold tolerance via scavenging ROS accumulation. PloS One 15, e0242139. doi: 10.1371/journal.pone.0242139
Duo, H., Yu, H., Sun, E., Zhao, D., Zuo, C. (2022). RNA Sequencing reveals that cell wall, Ca2+, hypersensitive response and salicylic acid signals are involved in pear suspension cells responses to Valsa pyri infection. Scientia Hortic. 305, 111422. doi: 10.1016/j.scienta.2022.111422
Eichstädt, B., Lederer, S., Trempel, F., Jiang, X., Guerra, T., Waadt, R., et al. (2021). Plant immune memory in systemic tissue does not involve changes in rapid calcium signaling. Front. Plant Sci. 12. doi: 10.3389/fpls.2021.798230
Fang, J., Zhang, Y., Liu, T., Yan, B., Li, J., Dong, L. (2019). Target-site and metabolic resistance mechanisms to penoxsulam in barnyardgrass (Echinochloa crus-galli (L.) p. beauv). J. Agric. Food Chem. 67, 8085–8095. doi: 10.1021/acs.jafc.9b01641
Franco-Ortega, S., Goldberg-Cavalleri, A., Walker, A., Brazier-Hicks, M., Onkokesung, N., Edwards, R. (2021). Non-target site herbicide resistance is conferred by two distinct mechanisms in black-grass (Alopecurus myosuroides). Front. Plant Sci. 12. doi: 10.3389/fpls.2021.636652
Fu, X., Chang, J., An, L., Zhang, M., Xu, S., Chen, T., et al. (2006). Association of the cold-hardiness of Chorispora bungeana with the distribution and accumulation of calcium in the cells and tissues. Environ. Exp. Bot. 55, 282–293. doi: 10.1016/j.envexpbot.2004.11.009
Furuichi, N. (2020). Effector signaling in hypersensitive response of plant microbe interaction: Single-Molecule-Signaling of suppressor from Phytophthora infestans and host selective toxin of Alternaria solani on Ca2+-dependent protein-kinase (CDPK). Insights Biotechnol. Bioinforma 1 (1), 1003.
Gaines, T. A., Duke, S. O., Morran, S., Rigon, C. A. G., Tranel, P. J., Küpper, A., et al. (2020). Mechanisms of evolved herbicide resistance. J. Biol. Chem. 295, jbc.REV120.013572. doi: 10.1074/jbc.REV120.013572
Gao, Q., Wang, C., Xi, Y., Shao, Q., Li, L., Luan, S. (2022). A receptor–channel trio conducts Ca2+ signalling for pollen tube reception. Nature 607, 534–539. doi: 10.1038/s41586-022-04923-7
Gao, Q., Xiong, T., Li, X., Chen, W., Zhu, X. (2019). Calcium and calcium sensors in fruit development and ripening. Scientia Hortic. 253, 412–421. doi: 10.1016/j.scienta.2019.04.069
Ghosh, S., Bheri, M., Bisht, D., Pandey, G. K. (2022). Calcium signaling and transport machinery: Potential for development of stress tolerance in plants. Curr. Plant Biol. 29, 100235. doi: 10.1016/j.cpb.2022.100235
Goel, A., Taj, G., Pandey, D., Gupta, S., Kumar, A. (2011). Genome-wide comparative in silico analysis of calcium transporters of rice and sorghum. Genomics Proteomics Bioinf. 9, 138–150. doi: 10.1016/S1672-0229(11)60017-X
Granatiero, V., Patron, M., Tosatto, A., Merli, G., Rizzuto, R. (2014). The use of aequorin and its variants for Ca2+ measurements. Cold Spring Harbor Protoc. 2014 (1), pdb–top066118. doi: 10.1101/pdb.top066118
Gu, S., Abid, M., Bai, D., Chen, C., Sun, L., Qi, X., et al. (2023). Transcriptome-wide identification and functional characterization of CIPK gene family members in Actinidia valvata under salt stress. Int. J. Mol. Sci. 24 (1), 805. doi: 10.3390/ijms24010805
Hashimoto, K., Kudla, J. (2011). Calcium decoding mechanisms in plants. Biochimie 93, 2054–2059. doi: 10.1016/j.biochi.2011.05.019
Heyer, M., Scholz, S. S., Reichelt, M., Kunert, G., Oelmüller, R., Mithöfer, A. (2022). The Ca2+ sensor proteins CML37 and CML42 antagonistically regulate plant stress responses by altering phytohormone signals. Plant Mol. Biol. 109, 611–625. doi: 10.1007/s11103-021-01184-2
Himanen, S. V., Sistonen, L. (2019). New insights into transcriptional reprogramming during cellular stress. J. Cell Sci. 132, jcs238402. doi: 10.1242/jcs.238402
Hirayama, T., Shinozaki, K. (2010). Research on plant abiotic stress responses in the post-genome era: past, present and future. Plant J. 61, 1041–1052. doi: 10.1111/j.1365-313X.2010.04124.x
Hong-Bo, S., Li-Ye, C., Ming-An, S., Shi-Qing, L., Ji-Cheng, Y. (2008). Bioengineering plant resistance to abiotic stresses by the global calcium signal system. Biotechnol. Adv. 26, 503–510. doi: 10.1016/j.biotechadv.2008.04.004
Huda, K., Md, K., Mst, S. A., Tuteja, R., Tuteja, N. (2013). Global calcium transducer p-type Ca2+-ATPases open new avenues for agriculture by regulating stress signalling. J. Exp. Bot. 64, 3099–3109. doi: 10.1093/jxb/ert182
Iwakami, S., Shimono, Y., Manabe, Y., Endo, M., Shibaike, H., Uchino, A., et al. (2017). Copy number variation in acetolactate synthase genes of thifensulfuron-methyl resistant alopecurus aequalis (shortawn foxtail) accessions in Japan. Front. Plant Sci. 8. doi: 10.3389/fpls.2017.00254
Jaffe, L. F. (1980). Calcium explosions as triggers of development. Ann. New York Acad. Sci. 339 (1), 86–101. doi: 10.1111/j.1749-6632.1980.tb15971.x
Jia, B., Li, Y., Sun, X., Sun, M. (2022). Structure, function, and applications of soybean calcium transporters. Int. J. Mol. Sci. 23, 14220. doi: 10.3390/ijms232214220
Kanchiswamy, C. N., Malnoy, M., Occhipinti, A., Maffei, M. E. (2014). Calcium imaging perspectives in plants. Int. J. Mol. Sci. 15 (3), 3842–3859. doi: 10.3390/ijms15033842
Kang, H. K., Nam, K. H. (2016). Reverse function of ROS-induced CBL10 during salt and drought stress responses. Plant Sci. 243, 49–55. doi: 10.1016/j.plantsci.2015.11.006
Khare, T., Srivastav, A., Kumar, V. (2020). “Calcium/calmodulin activated protein kinases in stress signaling in plants,” in Protein Kinases and Stress Signaling in Plants. Ed. G. K. Pandey (Chichester, UK: John Wiley & Sons, Ltd), 266–280. doi: 10.1002/9781119541578.ch11
Kudla, J., Becker, D., Grill, E., Hedrich, R., Hippler, M., Kummer, U., et al. (2018). Advances and current challenges in calcium signaling. New Phytol. 218, 414–431. doi: 10.1111/nph.14966
Li, X.-D., Gao, Y.-Q., Wu, W.-H., Chen, L.-M., Wang, Y. (2022). Two calcium-dependent protein kinases enhance maize drought tolerance by activating anion channel ZmSLAC1 in guard cells. Plant Biotechnol. J. 20, 143–157. doi: 10.1111/pbi.13701
Lian, H., Qin, C., Zhao, Q., Begum, N., Zhang, S. (2022). Exogenous calcium promotes growth of adzuki bean (Vigna angularis willd.) seedlings under nitrogen limitation through the regulation of nitrogen metabolism. Plant Physiol. Biochem. 190, 90–100. doi: 10.1016/j.plaphy.2022.08.028
Liu, Q., Ding, Y., Shi, Y., Ma, L., Wang, Y., Song, C., et al. (2021). The calcium transporter ANNEXIN1 mediates cold-induced calcium signaling and freezing tolerance in plants. EMBO J. 40, e104559. doi: 10.15252/embj.2020104559
Liu, Q., Xu, L., Li, Y., Xu, W., Vetukuri, R. R., Xu, X. (2023). Overexpression of an autophagy-related gene DiATG3 from Davidia involucrata improves plant thermotolerance by enhancing the accumulation of polyamines and regulating genes in calcium and MAPK signaling pathways. Environ. Exp. Bot. 105235. doi: 10.1016/j.envexpbot.2023.105235
Ma, F. F., Lu, R., Liu, H. Y., Shi, B., Zhang, J. H., Tan, M. P., et al. (2012). Nitric oxide-activated calcium/calmodulin-dependent protein kinase regulates the abscisic acid-induced antioxidant defence in maize. J. Exp. Bot. 63, 4835–4847. doi: 10.1093/jxb/ers161
Ma, L., Ye, J., Yang, Y., Lin, H., Yue, L., Luo, J., et al. (2019). The SOS2-SCaBP8 complex generates and fine-tunes an AtANN4-dependent calcium signature under salt stress. Dev. Cell 48, 697–709.e5. doi: 10.1016/j.devcel.2019.02.010
Mandadi, K. K., Petrillo, E., Dubrovina, A. S., Kiselev, K. V. (2022). Regulation of alternative splicing in plant stress responses. Front. Plant Sci. 13. doi: 10.3389/fpls.2022.1120961
Manishankar, P., Wang, N., Köster, P., Alatar, A. A., Kudla, J. (2018). Calcium signaling during salt stress and in the regulation of ion homeostasis. J. Exp. Bot. 69, 4215–4226. doi: 10.1093/jxb/ery201
Marcec, M. J., Gilroy, S., Poovaiah, B. W., Tanaka, K. (2019). Mutual interplay of Ca2+ and ROS signaling in plant immune response. Plant Sci. 283, 343–354. doi: 10.1016/j.plantsci.2019.03.004
Mithöfer, A., Mazars, C. (2002). Aequorin-based measurements of intracellular Ca2+-signatures in plant cells. Biol. Procedures Online 4, 105–118. doi: 10.1251/bpo40
Mohanta, T. K., Mohanta, N., Mohanta, Y. K., Parida, P., Bae, H. (2015). Genome-wide identification of calcineurin b-like (CBL) gene family of plants reveals novel conserved motifs and evolutionary aspects in calcium signaling events. BMC Plant Biol. 15, 189. doi: 10.1186/s12870-015-0543-0
Mori, K., Renhu, N., Naito, M., Nakamura, A., Shiba, H., Yamamoto, T., et al. (2018). Ca2+-permeable mechanosensitive channels MCA1 and MCA2 mediate cold-induced cytosolic Ca2+ increase and cold tolerance in arabidopsis. Sci. Rep. 8 (1), 550. doi: 10.1038/s41598-017-17483-y
Moscatiello, R., Sello, S., Ruocco, M., Barbulova, A., Cortese, E., Nigris, S., et al. (2018). The hydrophobin HYTLO1 secreted by the biocontrol fungus trichoderma longibrachiatum triggers a NAADP-mediated calcium signalling pathway in Lotus japonicus. Int. J. Mol. Sci. 19, 2596. doi: 10.3390/ijms19092596
Pan, L., Guo, Q., Wang, J., Shi, L., Yang, X., Zhou, Y., et al. (2022). CYP81A68 confers metabolic resistance to ALS and ACCase-inhibiting herbicides and its epigenetic regulation in Echinochloa crus-galli. J. Hazardous Materials 428, 128225. doi: 10.1016/j.jhazmat.2022.128225
Pathak, J., Ahmed, H., Kumari, N., Pandey, A., Rajneesh, Sinha, R. P. (2020). “Role of calcium and potassium in amelioration of environmental stress in plant,” in Protective chemical agents in the amelioration of plant abiotic stress (Chichester, UK: John Wiley & Sons, Ltd), 535–562. doi: 10.1002/9781119552154.ch27
Rahman, A., Mostofa, M. G., Alam, M., Nahar, K., Hasanuzzaman, M., Fujita, M. (2015). Calcium mitigates arsenic toxicity in rice seedlings by reducing arsenic uptake and modulating the antioxidant defense and glyoxalase systems and stress markers. BioMed. Res. Int. 2015. doi: 10.1155/2015/340812
Razanau, A., Xie, J. (2013). Emerging mechanisms and consequences of calcium regulation of alternative splicing in neurons and endocrine cells. Cell. Mol. Life Sci. 70, 4527–4536. doi: 10.1007/s00018-013-1390-5
Reddy, A. S. N., Ali, G. S., Celesnik, H., Day, I. S. (2011). Coping with stresses: Roles of calcium- and Calcium/Calmodulin-regulated gene expression. Plant Cell 23, 2010–2032. doi: 10.1105/tpc.111.084988
Riveras, E., Alvarez, J. M., Vidal, E. A., Oses, C., Vega, A., Gutiérrez, R. A. (2015). The calcium ion is a second messenger in the nitrate signaling pathway of Arabidopsis. Plant Physiol. 169 (2), 1397–1404. doi: 10.1104/pp.15.00961
Roy, P. R., Tahjib-Ul-Arif, M., Polash, M. A. S., Hossen, M. Z., Hossain, M. A. (2019). Physiological mechanisms of exogenous calcium on alleviating salinity-induced stress in rice (Oryza sativa l.). Physiol. Mol. Biol. Plants 25, 611–624. doi: 10.1007/s12298-019-00654-8
Schulz, P., Piepenburg, K., Lintermann, R., Herde, M., Schöttler, M. A., Schmidt, L. K., et al. (2021). Improving plant drought tolerance and growth under water limitation through combinatorial engineering of signalling networks. Plant Biotechnol. J. 19, 74–86. doi: 10.1111/pbi.13441
Shi, S., Li, S., Asim, M., Mao, J., Xu, D., Ullah, Z., et al. (2018). The Arabidopsis calcium-dependent protein kinases (CDPKs) and their roles in plant growth regulation and abiotic stress responses. Int. J. Mol. Sci. 19, 1900. doi: 10.3390/ijms19071900
Shih, H.-W., Miller, N. D., Dai, C., Spalding, E. P., Monshausen, G. B. (2014). The receptor-like kinase FERONIA is required for mechanical signal transduction in arabidopsis seedlings. Curr. Biol. 24, 1887–1892. doi: 10.1016/j.cub.2014.06.064
Singh, A., Mehta, S., Yadav, S., Nagar, G., Ghosh, R., Roy, A., et al. (2022). How to cope with the challenges of environmental stresses in the era of global climate change: An update on ROS stave off in plants. Int. J. Mol. Sci. 23, 1995. doi: 10.3390/ijms23041995
Song, W. Y., Zhang, Z. B., Shao, H. B., Guo, X. L., Cao, H. X., Zhao, H. B., et al. (2008). Relationship between calcium decoding elements and plant abiotic-stress resistance. Int. J. Biol. Sci. 4 (2), 116–125. doi: 10.7150/ijbs.4.116
Tai, F., Yuan, Z., Li, S. P., Wang, Q., Liu, F., Wang, W. (2016). ZmCIPK8, a CBL-interacting protein kinase, regulates maize response to drought stress. Plant Cell Tiss. Org. Cul. 124, 459–469. doi: 10.1007/s11240-015-0906-0
Tan, W., Meng, Q., Brestic, M., Olsovska, K., Yang, X. (2011). Photosynthesis is improved by exogenous calcium in heat-stressed tobacco plants. J. Plant Physiol. 168, 2063–2071. doi: 10.1016/j.jplph.2011.06.009
Tang, R. J., Zhao, F. G., Yang, Y., Wang, C., Li, K., Kleist, T. J., et al. (2020). A calcium signalling network activates vacuolar k+ remobilization to enable plant adaptation to low-K environments. Nat. Plants 6 (4), 384–393. doi: 10.1038/s41477-020-0621-7
Thor, K., Jiang, S., Michard, E., George, J., Scherzer, S., Huang, S., et al. (2020). The calcium-permeable channel OSCA1.3 regulates plant stomatal immunity. Nature 585, 569–573. doi: 10.1038/s41586-020-2702-1
Tognacca, R. S., Rodríguez, F. S., Aballay, F. E., Cartagena, C. M., Servi, L., Petrillo, E. (2022). Alternative splicing in plants: Current knowledge and future directions for assessing the biological relevance of splice variants. J. Exp. Botany. doi: 10.1093/jxb/erac431
Torra, J., Alcántara-de la Cruz, R. (2022). Molecular mechanisms of herbicide resistance in weeds. Genes 13, 2025. doi: 10.3390/genes13112025
Tuteja, N., Mahajan, S. (2007). Calcium signaling network in plants: An overview. Plant Signal Behav. 2, 79–85. doi: 10.4161/psb.2.2.4176
Wang, W.-H., He, E.-M., Guo, Y., Tong, Q.-X., Zheng, H.-L. (2016). Chloroplast calcium and ROS signaling networks potentially facilitate the primed state for stomatal closure under multiple stresses. Environ. Exp. Bot. 122, 85–93. doi: 10.1016/j.envexpbot.2015.09.008
Wang, P., Li, Z. W., Wei, J. S., Zhao, Z. L., Sun, D. Y., Cui, S. J. (2012). A Na+/Ca2+ exchanger-like protein (AtNCL) involved in salt stress in arabidopsis. J. Biol. Chem. 287, 44062–44070. doi: 10.1074/jbc.M112.351643
Wang, J., Song, J., Wu, X.-B., Deng, Q.-Q., Zhu, Z.-Y., Ren, M.-J., et al. (2021). Seed priming with calcium chloride enhances wheat resistance against wheat aphid schizaphis graminum rondani. Pest Manage. Sci. 77, 4709–4718. doi: 10.1002/ps.6513
Wang, Y., Dai, X., Xu, G., Dai, Z., Chen, P., Zhang, T., et al. (2021). The Ca2+-CaM signaling pathway mediates potassium uptake by regulating reactive oxygen species homeostasis in tobacco roots under low-K+ stress. Front. Plant Sci. 12, 658609
Wang, Y.-J., Yu, J.-N., Chen, T., Zhang, Z.-G., Hao, Y.-J., Zhang, J.-S., et al. (2005). Functional analysis of a putative Ca2+ channel gene TaTPC1 from wheat. J. Exp. Bot. 56, 3051–3060. doi: 10.1093/jxb/eri302
Weinl, S., Kudla, J. (2009). The CBL–CIPK Ca2+-decoding signaling network: function and perspectives. New Phytol. 184, 517–528. doi: 10.1111/j.1469-8137.2009.02938.x
Xie, Q., Yang, Y., Wang, Y., Pan, C., Hong, S., Wu, Z., et al. (2022). The calcium sensor CBL10 negatively regulates plasma membrane h+-ATPase activity and alkaline stress response in arabidopsis. Environ. Exp. Bot. 194, 104752. doi: 10.1016/j.envexpbot.2021.104752
Xu, T., Niu, J., Jiang, Z. (2022). Sensing mechanisms: Calcium signaling mediated abiotic stress in plants. Front. Plant Sci. 13. doi: 10.3389/fpls.2022.925863
Xu, H., Zhang, W., Zhang, T., Li, J., Wu, X., Dong, L. (2014). Determination of ploidy level and isolation of genes encoding acetyl-CoA carboxylase in Japanese foxtail (Alopecurus japonicus). PloS One 9 (12), e114712. doi: 10.1371/journal.pone.0114712
Yadav, M., Pandey, J., Chakraborty, A., Hassan, M. I., Kundu, J. K., Roy, A., et al. (2022). A comprehensive analysis of calmodulin-like proteins of glycine max indicates their role in calcium signaling and plant defense against insect attack. Front. Plant Sci. 13. doi: 10.3389/fpls.2022.817950
Yakimowski, S. B., Teitel, Z., Caruso, C. M. (2021). Defence by duplication: The relation between phenotypic glyphosate resistance and EPSPS gene copy number variation in Amaranthus palmeri. Mol. Ecol. 30 (21), 5328–5342. doi: 10.1111/mec.16231
Yang, Z., Wang, C., Xue, Y., Liu, X., Chen, S., Song, C., et al. (2019). Calcium-activated 14-3-3 proteins as a molecular switch in salt stress tolerance. Nat. Commun. 10, 1199. doi: 10.1038/s41467-019-09181-2
Yuan, P., Yang, T., Poovaiah, B. W. (2018). Calcium signaling-mediated plant response to cold stress. Int. J. Mol. Sci. 19, 3896. doi: 10.3390/ijms19123896
Yuan, F., Yang, H., Xue, Y., Kong, D., Ye, R., Li, C., et al. (2014). OSCA1 mediates osmotic-stress-evoked Ca2+ increases vital for osmosensing in arabidopsis. Nature 514 (7522), 367–371. doi: 10.1038/nature13593
Zhang, Y., Gao, H., Fang, J., Wang, H., Chen, J., Li, J., et al. (2022). Up-regulation of bZIP88 transcription factor is involved in resistance to three different herbicides in both Echinochloa crus-galli and E. glabrescens. J. Exp. Bot. 73, 6916–6930. doi: 10.1093/jxb/erac319
Zhang, H., Zhu, J., Gong, Z., Zhu, J.-K. (2022). Abiotic stress responses in plants. Nat. Rev. Genet. 23, 104–119. doi: 10.1038/s41576-021-00413-0
Zhao, X., Bai, X., Jiang, C., Li, Z. (2019). Phosphoproteomic analysis of two contrasting maize inbred lines provides insights into the mechanism of salt-stress tolerance. Int. J. Mol. Sci. 20, 1886. doi: 10.3390/ijms20081886
Zhao, N., Li, W., Bai, S., Guo, W., Yuan, G., Wang, F., et al. (2017). Transcriptome profiling to identify genes involved in mesosulfuron-methyl resistance in Alopecurus aequalis. Front. Plant Sci. 8. doi: 10.3389/fpls.2017.01391
Zou, J. J., Li, X. D., Ratnasekera, D., Wang, C., Liu, W. X., Song, L. F., et al. (2015). Arabidopsis CALCIUM-DEPENDENT PROTEIN KINASE8 and CATALASE3 function in abscisic acid-mediated signaling and H2O2 homeostasis in stomatal guard cells under drought stress. Plant Cell 27 (5), 1445–1460. doi: 10.1105/tpc.15.00144
Keywords: abiotic stress, calcium signalling, food security, herbicide resistance, weeds
Citation: Hamouzová K, Sen MK, Bharati R, Košnarová P, Chawdhery MRA, Roy A and Soukup J (2023) Calcium signalling in weeds under herbicide stress: An outlook. Front. Plant Sci. 14:1135845. doi: 10.3389/fpls.2023.1135845
Received: 01 January 2023; Accepted: 06 March 2023;
Published: 24 March 2023.
Edited by:
Indrakant K. Singh, University of Delhi, IndiaReviewed by:
Prachi Pandey, National Institute of Plant Genome Research (NIPGR), IndiaCopyright © 2023 Hamouzová, Sen, Bharati, Košnarová, Chawdhery, Roy and Soukup. This is an open-access article distributed under the terms of the Creative Commons Attribution License (CC BY). The use, distribution or reproduction in other forums is permitted, provided the original author(s) and the copyright owner(s) are credited and that the original publication in this journal is cited, in accordance with accepted academic practice. No use, distribution or reproduction is permitted which does not comply with these terms.
*Correspondence: Madhab Kumar Sen, c2VubUBhZi5jenUuY3o=
†These authors have contributed equally to this work