- 1Beijing Academy of Agriculture and Forestry Sciences, Beijing Academy of Forestry and Pomology Sciences, Beijing Engineering Research Center for Deciduous Fruit Trees, Key Laboratory of Biology and Genetic Improvement of Horticultural Crops, Ministry of Agriculture and Rural Affairs, Beijing, China
- 2College of Horticulture, China Agricultural University, Beijing, China
Potassium (K) is one of the most important macronutrients for plant development and growth. The influence mechanism of different potassium stresses on the molecular regulation and metabolites of apple remains largely unknown. In this research, physiological, transcriptome, and metabolite analyses were compared under different K conditions in apple seedlings. The results showed that K deficiency and excess conditions influenced apple phenotypic characteristics, soil plant analytical development (SPAD) values, and photosynthesis. Hydrogen peroxide (H2O2) content, peroxidase (POD) activity, catalase (CAT) activity, abscisic acid (ABA) content, and indoleacetic acid (IAA) content were regulated by different K stresses. Transcriptome analysis indicated that there were 2,409 and 778 differentially expressed genes (DEGs) in apple leaves and roots under K deficiency conditions in addition to 1,393 and 1,205 DEGs in apple leaves and roots under potassium excess conditions, respectively. Kyoto Encyclopedia of Genes and Genomes (KEGG) pathway enrichment showed that the DEGs were involved in flavonoid biosynthesis, photosynthesis, and plant hormone signal transduction metabolite biosynthetic processes in response to different K conditions. There were 527 and 166 differential metabolites (DMAs) in leaves and roots under low-K stress as well as 228 and 150 DMAs in apple leaves and roots under high-K stress, respectively. Apple plants regulate carbon metabolism and the flavonoid pathway to respond to low-K and high-K stresses. This study provides a basis for understanding the metabolic processes underlying different K responses and provides a foundation to improve the utilization efficiency of K in apples.
Introduction
Potassium (K) is one of the most essential macronutrients for plant growth and development, and it has essential physiological functions, such as plant osmoregulation, photosynthesis, protein synthesis, ion homeostasis, and enzyme activation (Kanai et al., 2011; Hafsi et al., 2014). Four forms of K exist in the soil, namely, exchangeable K, soluble K, lattice K, and fixed K. Only soluble K can be taken up by plants from the soil. The K concentration in the soil ranges from 0.1 to 6.0 mmol L−1 (Zeng et al., 2015). Too high or too low K concentrations in soil affect plant growth; in many regions, K concentrations are lower than 0.3 mmol L−1, and K deficiency limits plant growth (Schroeder et al., 1994). Under low-K conditions, the most common phenomena include stunted growth of plants, yellowing of leaf margins, and yield reduction (Hasanuzzaman et al., 2018). Excessive use of potassium fertilizer causes high-K stress, and excessive application of potassium fertilizer in soil causes soil and water pollution, reducing the productivity of crops. However, plants initiate a series of physiological processes as well as molecular and metabolite mechanisms to adapt to different levels of K stress. K deficiency and excess conditions are typical abiotic stress forms that induce a series of biological responses. Under different K stress conditions, reactive oxygen species (ROS) and phytohormones are affected (Ashley et al., 2006; Amtmann et al., 2008). Plant responses to different K conditions are also due to various complex gene regulatory networks that cause widespread changes in gene expression and metabolite contents (Liang et al., 2013).
Transcriptomes comprehensively and efficiently reveal gene expression, thereby allowing elucidation of the plant molecular mechanism response to different K stresses. In plants, many studies have focused on K uptake, loading, and transport mechanisms. Some related genes have been studied, such as the high-affinity K transporter/uptake transporter (HAK/KUP/KT) family, including AtHAK1/5, PpHAK2, AtHAK5, HvHAK1, OsHAK1, AtKUP3, AtKUP1, and OsHKT2, as well as shaker-like K channels (AKT), including OsAKT1 and AtAKT1/5 (Kim et al., 1998; Banuelos et al., 2002; Xu et al., 2006; Fulgenzi et al., 2008; Jung et al., 2009; Pyo et al., 2010; Kim et al., 2012; Oomen et al., 2012; Wu et al., 2019; Wang et al., 2021). These genes play a vital role in plants’ responses to different K conditions. Transcriptomic analysis of the response of Arabidopsis, rice, maize, soybean, sugarcane, and wild barley to K deficiency conditions has indicated that genes involved in metabolism, signal transduction, and ion transport are altered at the transcript level (Armengaud et al., 2004; Ma et al., 2012; Wang et al., 2012a; Zeng et al., 2014).
Metabolomics, known as qualitative and quantitative analysis of cellular metabolites, has become an important complementary tool for the study of plant functional genomics and systems biology (Weckwerth, 2003). Metabonomic analysis reflects the synthesis, decomposition, or transformation rules of some objects, all metabolites, or some metabolites in the tissue or cell (Hall, 2011). Abiotic stress causes changes in the expression of metabolic products, resulting in metabolite disorders in vivo (Meena et al., 2017). Many studies have reported the changes in small-molecule compounds in response to mineral nutritional stress. Sung et al. (2015) reported metabolic responses to deficiencies in nitrogen (N), phosphorus (P), and K, and they demonstrated that the lack of these elements decreases energy production and amino acid metabolism in tomato leaves and roots. Low-K stress increases monosaccharide, disaccharide, polysaccharide, and putrescine contents in barley (Zhao et al., 2021). The contents of putrescine, aconitate, citrate, malate, and fumarate increased in sunflower under low-K stress (Cui et al., 2019). Citric acid, arginine, and asparagine contents are upregulated under K deficiency in rapeseed leaves (Hu et al., 2021). The levels of glutamic acid and aspartic acid are decreased in peanut under low-K conditions, whereas the levels of histidine, lysine, and arginine are increased in peanut under low-K conditions (Patel et al., 2022). The amino acid contents are increased in both K-sensitive and K-tolerant genotypes of wheat roots under K starvation (Zhao et al., 2020).
Apple (Malus domestica) is one of the most important fruits in the world, and apple production and consumption are the highest in China. K fertilizer plays a key role in apple growth and ripening. When K deficiency occurs in apple trees, the middle and lower leaves of new shoots turn yellow. In severe cases, the leaves gradually show brown withered spots, resulting in a curly scorched appearance, and new shoots stop growing early, forming small flower buds and small fruits with a color difference and a decline in quality (Chang et al., 2014). High-K stress causes the occurrence of apple bitter pox, which reduces the absorption of cations, such as calcium and magnesium, by plants, thus affecting the yield of plants. Excessive application of potassium fertilizer causes soil environmental pollution and water pollution. Many studies have investigated the molecular mechanisms that occur under K deficiency in model plants, such as Arabidopsis, rice, and maize (Armengaud et al., 2004; Ma et al., 2012; Wang et al., 2012a). However, these mechanisms have rarely been reported in apple, especially under high-potassium stress. In the present study, we investigated the molecular response mechanism and metabolite changes of apple to low-potassium and high-potassium stresses, and we provided a theoretical basis for further study on the response mechanism of apple to potassium.
Materials and methods
Plant growth conditions
The experimental materials, namely, “CG-935” apple seedlings, were tissue cultured, after rooting, seeding, and transplantation, and the apple plants were then transported to the experimental field as previously reported by Sun et al. (2021).
Different potassium treatments
After 90 days, healthy apple seedlings of similar size (with 16–20 leaves) were transferred to a hydroponic slot (60 × 37 × 35 cm) containing 60 L of a 1/2-strength Hoagland nutrient solution (Hoagland and Arnon, 1950). Stress treatments were initiated after 10 days of precultivation. Apple seedlings were randomly divided into 3 groups with 54 plants per treatment, and there were three biological replicates for each stress treatment with 18 plants per replicate. The treatments were as follows: (1) control (CK), 1/2-strength Hoagland nutrient solution supplemented with 3 mM K2SO4; (2) low-K treatment (LK), 1/2-strength Hoagland nutrient solution with 50 μM K2SO4; and (3) high-K treatment (HK), 1/2-strength Hoagland nutrient solution with 15 mM K2SO4 (Chang et al., 2014). The solution was continuously aerated and refreshed every 3 days, and the experimental treatment lasted for 15 days. Plant roots and leaves were harvested for physiological, transcriptomic, and metabolomic analyses. The samples were designated as follows: the apple leaves and roots in the control condition were named CKL and CKR, respectively; the apple leaves and roots in the low-K condition were named LKL and LKR, respectively; and the apple leaves and roots in the high-K condition were named HKL and HKR, respectively.
Growth indices, photosynthetic indices, and nutrient concentration measurements
After 15 days of treatment, the plant heights, stem diameters, and dry weights (DWs) of the whole apple as well as the ratio of underground DW to aboveground DW (R/S) of the apple were calculated.
The net photosynthetic rate (Pn), transpiration rate (Tr), water use efficiency indicator (WUEi), stomatal conductance (Gs), and intercellular CO2 concentration (Ci) values of the apple leaves were measured by an LI-6800 portable photosynthesis system (LI-COR Inc., Lincoln, NE, USA) on sunny days.
The measurements of soil plant analytical development (SPAD) of apple leaves and the concentrations of N, P, and K in apple roots, apple stems, apple leaves, and whole apple were measured according to the method of Sun et al. (2021).
Determination of H2O2, enzyme activities, and phytohormones
For the determination of H2O2, enzyme activity, and phytohormones, nine apple seedlings were selected for each experimental replicate (n = 3) to provide an adequate amount of root and leaf tissue. The levels of hydrogen peroxide (H2O2, SO1300), and the activities of peroxidase (POD, KT5058), catalase (CAT, KT4957), abscisic acid (ABA, KT4924), and indoleacetic acid (IAA, NR, KT4992) were determined using commercial test kits purchased from Jiangsu Kete Biotechnology Co., Ltd. (Jiangsu, China). H2O2 was recorded on a UV-1750 spectrometer (Shimadzu, Japan). The enzyme activities were analyzed using an ELISA reader (Multiskan MS, Labsystems 325, Helsinki, Finland).
RNA isolation, qRT-PCR analysis, and transcriptome sequencing
Total RNA of apple leaves and roots was isolated using TRIzol Reagent (Invitrogen, Carlsbad, CA, USA) for quantitative real-time PCR (qRT-PCR) analysis and RNA sequencing (RNA-seq) analysis, and three biological replicates of each sample were sequenced. Quantitative real-time PCR (qRT-PCR) analysis was conducted according to Sun et al. (2021), and the primers used for qRT-PCR are listed in Table S1. Transcriptome analysis was performed by Wuhan MetWare Biotechnology Co., Ltd. (www.metware.cn, Wuhan, China). After rapid filtering (version 0.18.0) (Chen et al., 2018), the HISAT2.2.4 and Bowtie2 tools were used to compare clean reads with the apple genome (https://iris.angers.inra.fr/gddh13/index.html) (Langmead et al., 2009; Kim et al., 2015). The RESM software was used to calculate the values of fragment per kilobase of transcript per million mapped reads (FPKM) (Li and Dewey, 2011). Differentially expressed genes (DEGs) were determined according to cutoffs of log2(fold change) ≥ 1 and p ≤ 0.05. Kyoto Encyclopedia of Genes and Genomes (KEGG) and Gene Ontology (GO) tools were used to analyze the DEGs.
Metabolite analysis
Metabolites were extracted and analyzed at Wuhan MetWare Biotechnology Co., Ltd., Wuhan, China (www.metware.cn) (Zhang et al., 2019). The metabolite analysis was performed using a liquid chromatography–electrospray ionization–tandem mass spectrometry (LC-ESI-MS/MS) system (HPLC, Shim-pack UFLC SHIMADZU CBM30A system; MS, Applied Biosystems 6500 Q TRAP). Metabolite quantification was performed using multiple reaction monitoring (MRM) in triple quadrupole mass spectrometry (Chen et al., 2013). Metabolomic data analysis was performed according to previous methods (Zhu et al., 2018).
Statistical analysis
The statistical analysis of different plant treatments in triplicate (n = 3) was performed by one-way analysis of variance (ANOVA) using SPSS 20.0 software. A probability value of p < 0.05 was considered statistically significant. The data are presented as the mean ± standard deviation (SD) of three replicates.
Results
Potassium affects plant growth and mineral nutrients
After 15 days of treatment with 50 μM (LK treatment), 3 mM (CK treatment), or 15 mM (HK treatment) K2SO4 in hydroponic culture, the plant height, stem diameter, DW, and root/shoot ratio decreased under K stress. The plant height decreased more in LK (82.49%) than in HK (82.73%), and the stem diameter also showed a similar trend. The DW of LK-treated apple plants was 72.94% of that of CK plants, and the DW of HK-treated seedlings was 75.69% of that of CK seedlings. The root/shoot ratio significantly increased by 111.76% in LK but decreased by 65.5% in HK (Table 1).

Table 1 The growth indexes in apple under control (CK), low-potassium (LK), and high-potassium (HK) conditions.
The value of SPDA is a reliable indicator that can represent the content of chlorophyll (Tan et al., 2021). The SPDA values and photosynthetic characteristics were different under LK and HK stresses. The SPAD significantly decreased under LK, but there was no difference under HK. The Pn, Tr, WUEi, and Gs were significantly reduced under LK and HK, especially under K deficiency conditions (Table 2).

Table 2 The photosynthetic characteristics in apple under control (CK), low-potassium (LK), and high-potassium (HK) conditions.
Differences in K fertilization conditions were reflected in the apple root, stem, and leaf elemental N, P, and K mineral nutrients. The concentrations of K in apple trees were lower under LK stress but higher when more K was available, and the P and K concentrations were much higher in CK compared to HK and LK (Table S2).
H2O2 content, enzyme activities, and phytohormones
Plant biomass decreased under K stress, while root growth increased under LK stress (Figure 1A). The H2O2 content, superoxide dismutase (SOD) activity, and POD activity were affected by different K stresses. The H2O2 content increased in apple leaves and roots under the LK and HK treatments with greater increases under LK stress. Under different K conditions, the enzyme activities increased in both apple leaves and roots, but the range of increase varied. The activities of SOD and POD significantly increased under different K conditions. The ABA content increased by 1.13- and 1.29-fold in apple leaves under LK, and it increased by 1.14- and 1.25-fold in roots under HK (Figure 1E). The IAA content exhibited a similar trend (Figure 1F).
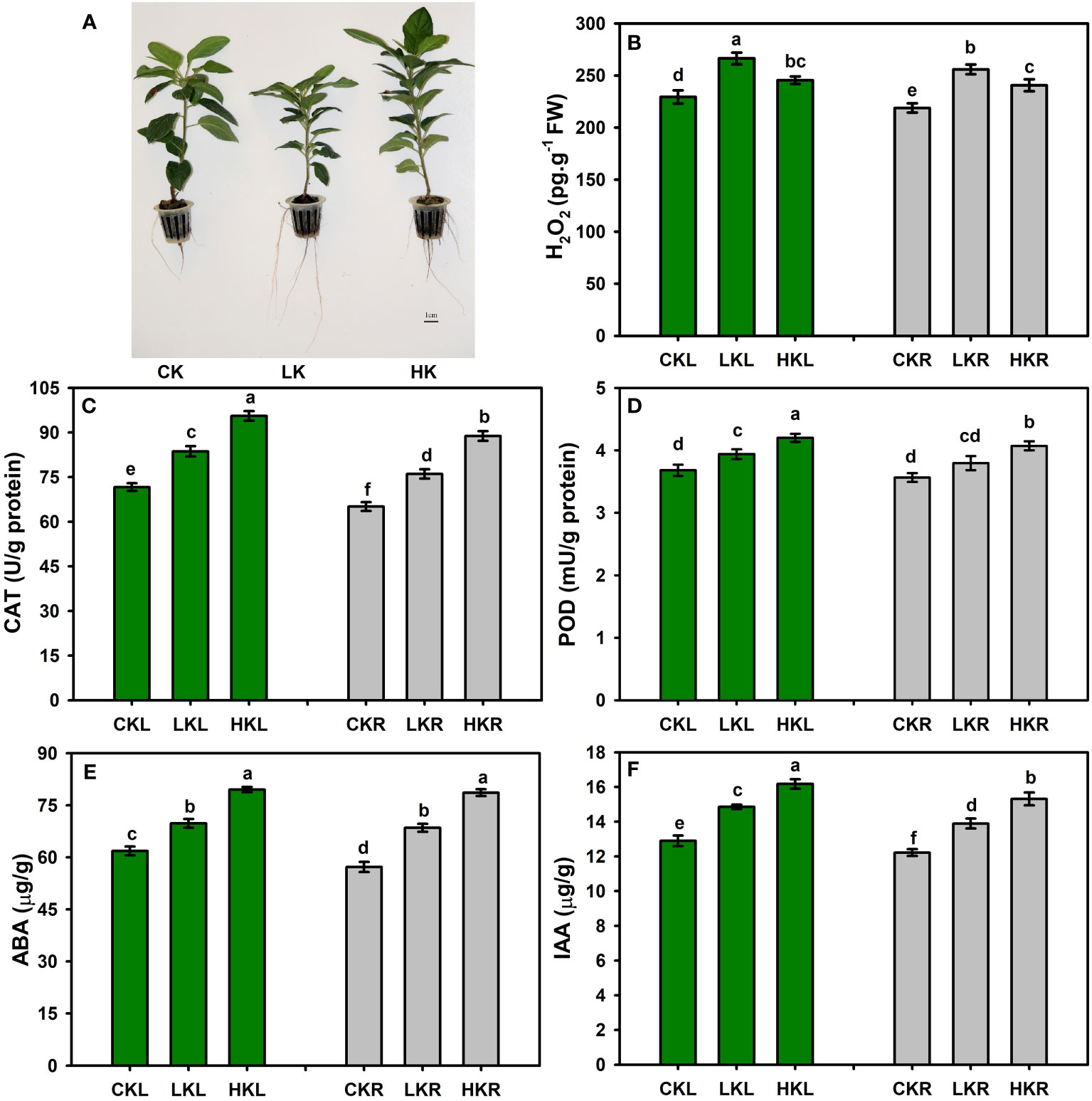
Figure 1 Measurement of H2O2, enzyme activities, and phytohormones in apple under different K treatments. (A) The phenotypic characteristics of apple. (B) The content of H2O2. The activities of CAT (C) and POD (D). The contents of ABA (E) and IAA (F). Different letters indicate significant differences according to Tukey’s multiple-range tests (p < 0.05).
Differential gene expression analysis
To obtain a global overview of the transcriptome responses to different K treatments in apple roots and leaves, four RNA-seq libraries were prepared, namely, HKL/CKL, HKL/CKL, LKR/CKR, and HKR/CKR, according to different K stress conditions in apple roots and leaves. The transcriptome data of the 18 samples described in the study have been deposited into the National Center for Biotechnology Information (NCBI) databases, and the bioproject accession number is PRJNA895870. The sequencing data are summarized in Table S3. The average for each sample of clean reads was approximately 4.5 × 107, and the sequence alignment efficiency ranged from 82.59% to 90.04%. The FPKM of HK was higher than those of CK and LK, and FPKM > 1 was used as the threshold to determine gene expression (Figure S1A). The Pearson correlations among the LK, HK, and CK replicates ranged from 0.97 to 1 in leaves and from 0.98 to l in roots (Figure S1B). Principal component analysis (PCA) indicated that the roots and leaves of LK, HK, and CK were clustered together, indicating significant differences in gene expression profiles. The LK, HK, and CK in leaf and root replicates were not tightly clustered, showing that inoculation occurred within the replicates (Figure S1C).
According to DESeq2 analysis using cutoffs of |log 2-fold change| ≥ 1 and false discovery rate (FDR) < 0.05, a total of 2,409 transcripts were differentially expressed in apple leaves under LK with 1,412 upregulated genes and 997 downregulated genes (Figure S2A). A total of 1,393 DEGs were detected under HK conditions with 829 upregulated DEGs and 564 downregulated DEGs in apple leaves under HK (Figure S2B). These observations suggested that under different K conditions, more DEGs were found under LK conditions than under HK conditions in apple leaves. A total of 778 DEGs were differentially expressed in apple roots under LK stress with 442 upregulated genes and 336 downregulated genes (Figure S2C), and a total of 1,205 DEGs were differentially expressed in apple roots under HK with 819 upregulated DEGs and 386 downregulated DEGs (Figure S2D). In terms of fold change gene expression values, the following maximum upregulation and maximum downregulation values were observed: 8.19 log2 FC and −8.38 log2 FC in leaves under LK, respectively; 7.92 and −7.42 log2 FC in leaves under HK, respectively; 7.59 and −13.15 log2 FC in roots under LK, respectively; and 8.24 and −4.64 log2 FC in roots under HK stresses, respectively (Figure S2E).
GO annotation analysis showed enrichment classifications according to biological processes (BPs), molecular functions (MFs), and cellular components (CCs) (Figure S3). Figure 2 shows the top 20 significantly enriched pathways under different K stresses in apple leaves and roots. KEGG pathway enrichment revealed that the vital biological pathways in response to different K conditions were involved in flavonoid biosynthesis, photosynthesis, plant hormone signal transduction, and biosynthesis of various plant secondary metabolite biosynthetic processes.
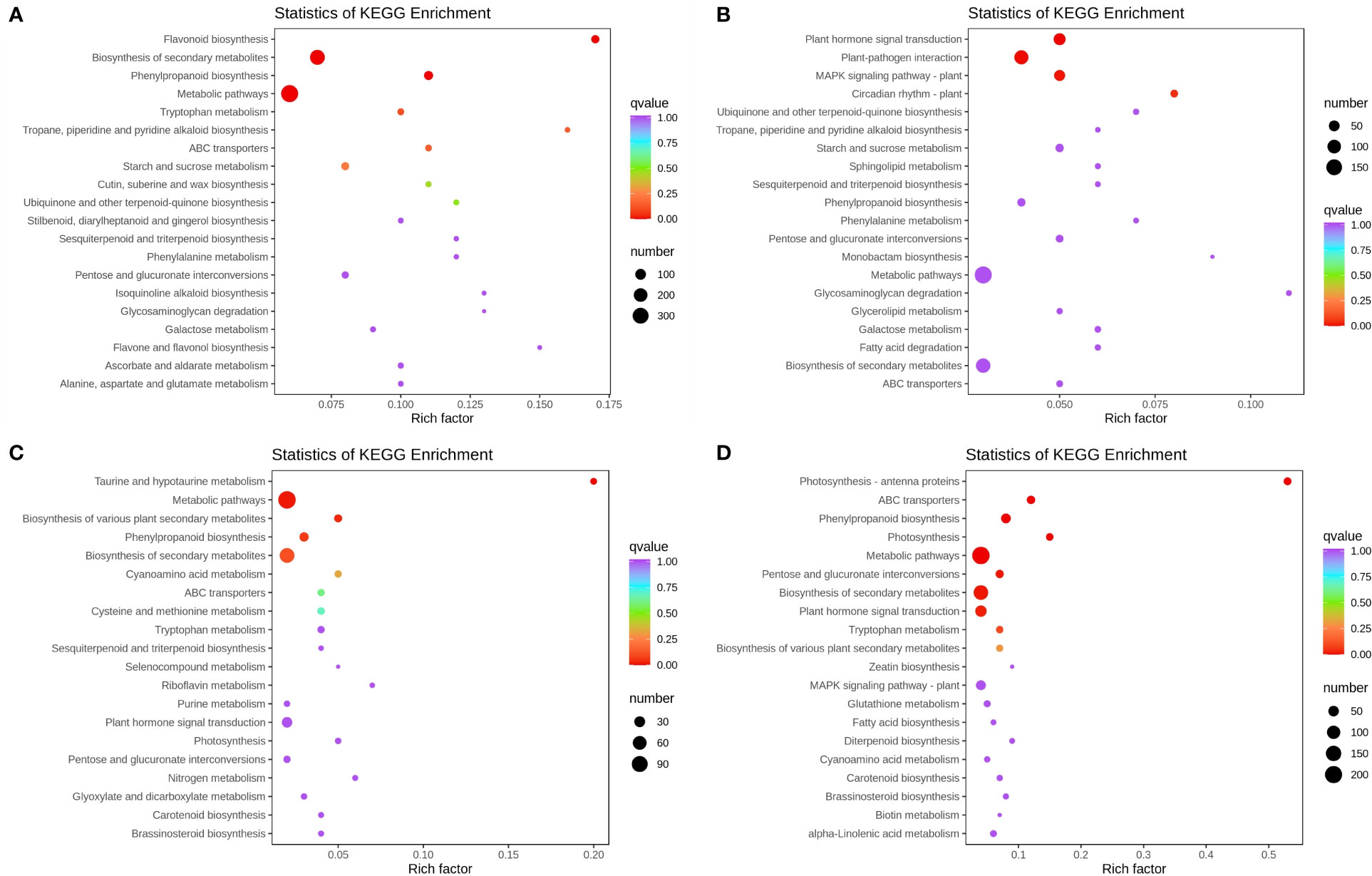
Figure 2 Statistics of KEGG enrichment under different K conditions in apple leaves and roots. (A) Statistics of KEGG enrichment under low-K conditions in apple leaves. (B) Statistics of KEGG enrichment under high K conditions in apple leaves. (C) Statistics of KEGG enrichment under low-K conditions in apple roots. (D) Statistics of KEGG enrichment under high K conditions in apple roots.
The DEGs involved in potassium metabolism were then thoroughly analyzed. The DEGs associated with potassium uptake, loading, and transport processes were detected in four pairs of libraries (Table 3). Most of the potassium transporter gene family members were increased in the apple seedlings.
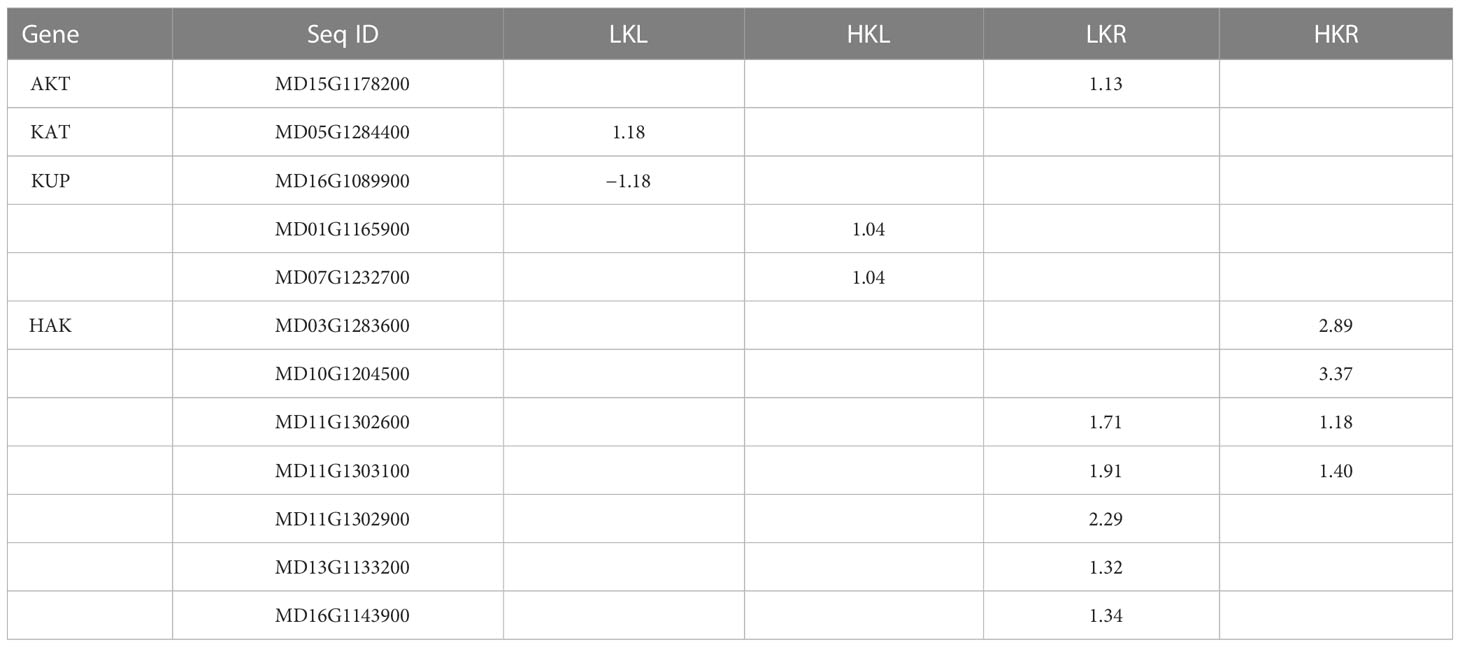
Table 3 Genes encoding transporters showed differential expression in response to different K stresses.
qRT-PCR validation of the DEGs
Ten apple genes were detected by qRT-PCR for expression analysis to validate the RNA-seq results. The RT-qPCR analysis results were not significantly different from the RNA-Seq data, and similar trends were found in the up- and downregulated genes (Figure S4).
Metabolomic response to different K treatments in apple
The metabolome of apple seedlings was analyzed using four different pairs of libraries, namely, LKL/CKL, HKL/CKL, LKR/CKR, and HKR/CKR. Overall, 527 DAMs were quantified and identified in LKL/CKL with 246 upregulated DAMs and 122 downregulated DAMs. For HKL/CKL, 228 DAMs were significantly upregulated, and 129 DAMs were downregulated. For LKR/CKR, 166 DAMs were identified with 74 upregulated DAMs and 92 downregulated DAMs. For HKR/CKR, 150 DAMs were identified with 92 upregulated DAMs and 74 downregulated DAMs (Table S4). The heatmaps of the differences in metabolites among the four combinations show the above trends (Figure 3).
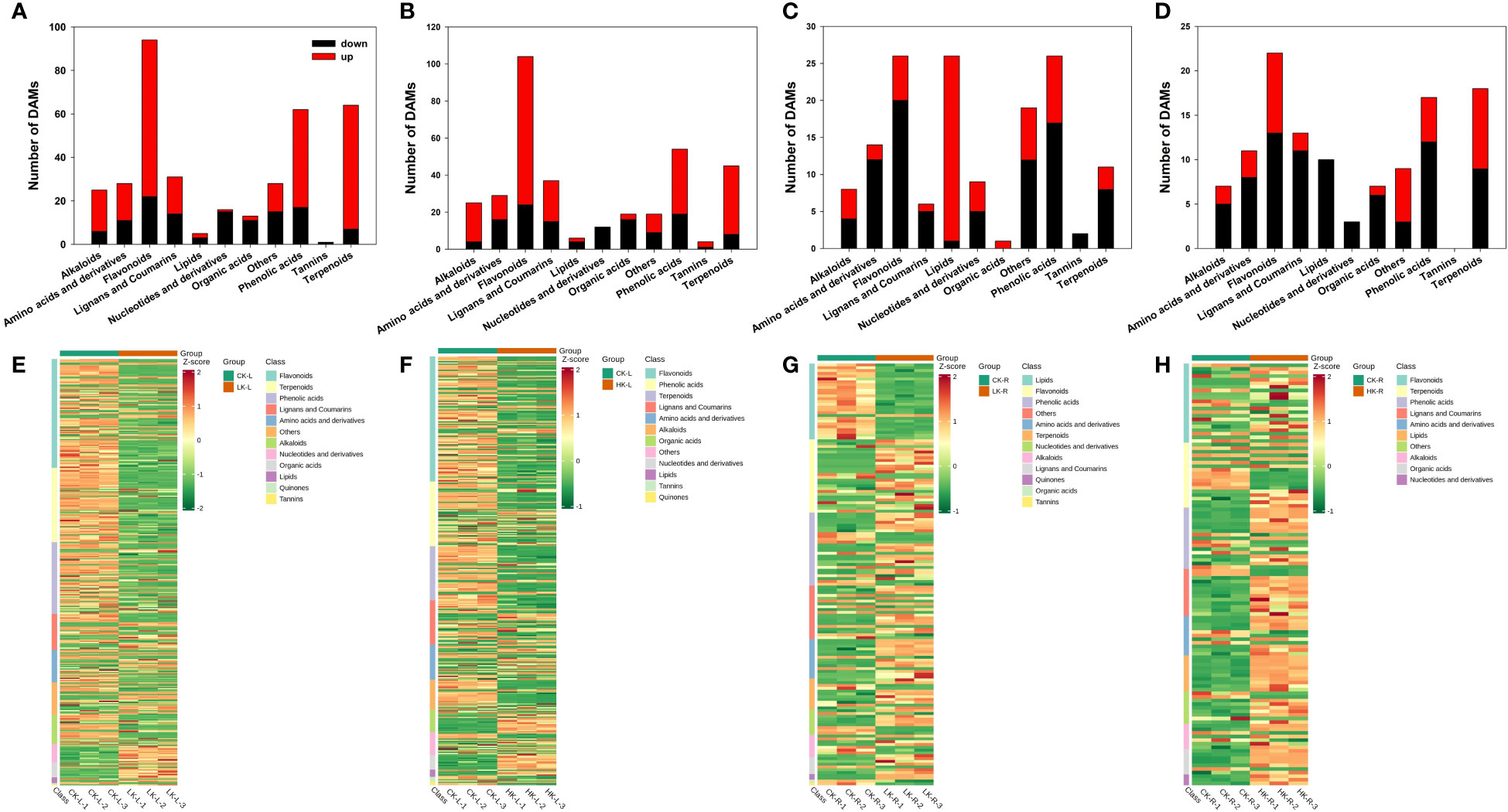
Figure 3 Differentially accumulated metabolites among different potassium treatments in apple. (A, E) Major classes of detected metabolites and heatmaps of differential metabolites between LKL/CKL. (B, F) Major classes of detected metabolites and heatmaps of differential metabolites between HKL/CKL. (C, G) Major classes of detected metabolites and heatmaps of differential metabolites between LKR/CKR. (D, H) Major classes of detected metabolites and heatmaps of differential metabolites between HKR/CKR. Three independent replicates of each stage are also displayed in the heatmap.
Under different K stresses, the contents of amino acids, amino acid derivatives, organic acids, carbohydrates, flavonoids, and lipids changed. Under LK conditions, most lipids, flavonoids, and phenolic acids increased in apple roots. The metabolites of apple leaves and roots under different potassium stress conditions are presented in Figure 3. In apple leaves, the content of amino acids, amino acid derivatives, phenolic acids, terpenoids, and flavonoids increased under different K stresses, while nucleotides and nucleotide derivatives decreased. Under LK conditions, the content of lipids was upregulated in apple roots.
The co-joint KEGG enrichment analysis determined the co-mapped pathways in apple leaves and roots under K deficiency and excess conditions (Figures 4A, D, G, J). Of the metabolic pathways, the co-mapped pathways, namely, flavonoid biosynthesis, carbon metabolism, biosynthesis of secondary metabolites, glycerolipid biosynthesis, and phenylpropanoid biosynthesis, were the significantly enriched pathways under different K stresses. The Pearson correlation coefficients for the nine quadrants are shown in Figures 4B, E, H, K. In the third and seventh quadrants, the gene and metabolite differential expression patterns were consistent; the genes were positively correlated with the regulation of metabolites, and the changes in metabolites were positively regulated by the genes. The DEGs and DAMs with Pearson correlation coefficients (PCCs) higher than 0.8 were further selected and represented by heatmaps (Figures 4C, F, I, L).
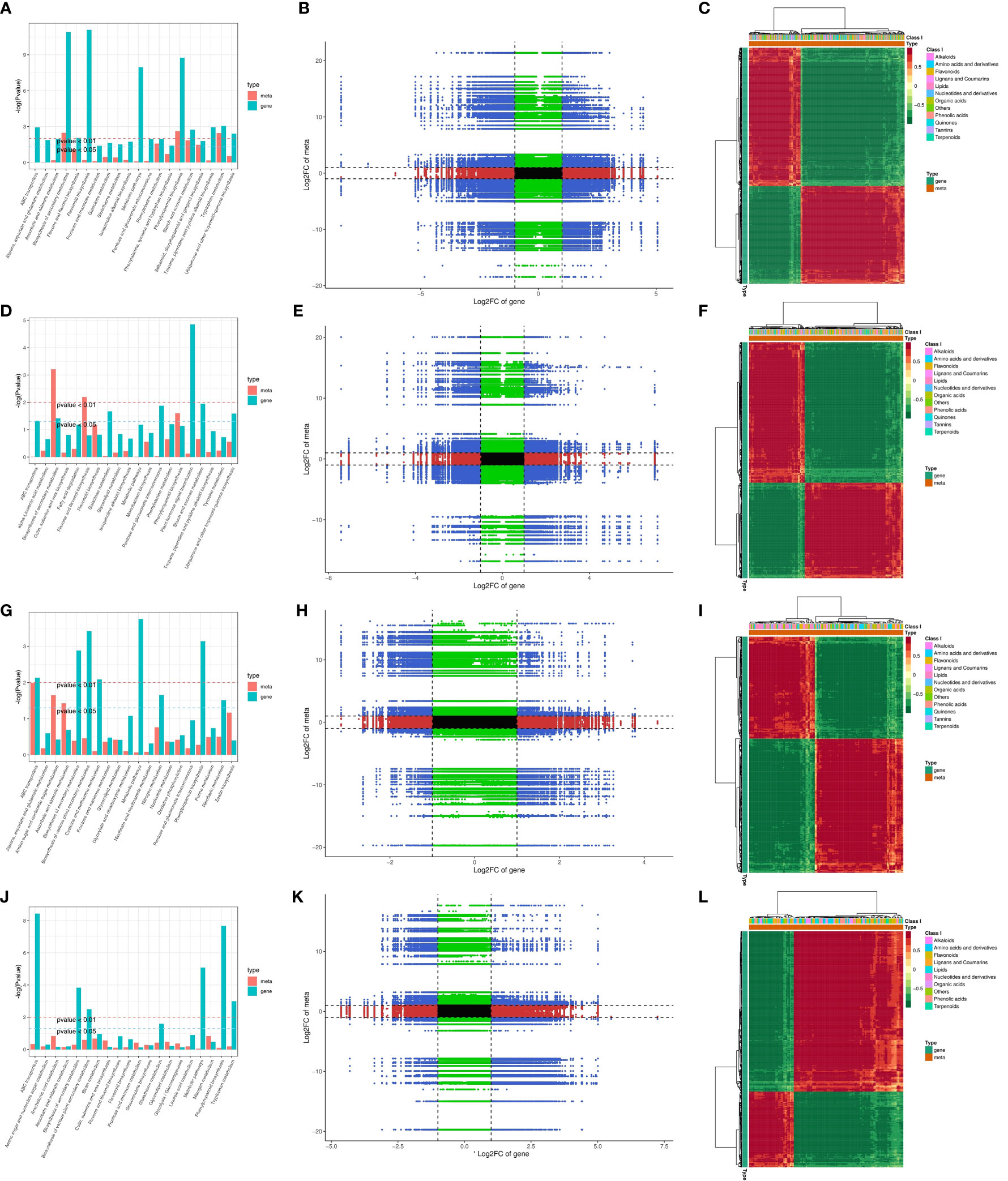
Figure 4 (A, D, G, J) Histograms of joint KEGG enrichment p-values, and (B, E, H, K) the associations of transcriptomic and metabolomic variation quadrant diagrams in LKL/CKL, HKL/CKL, LKR/CKR, and HKR/CKR; the black dotted lines indicate the differential thresholds. Outside the threshold lines, there were significant differences in the gene/metabolites, and within the threshold lines are shown the unchanged gene/metabolites. Each point represents a gene/metabolite. Black dots, green dots, red dots, and blue dots indicate unchanged genes/metabolites, differentially accumulated metabolites with unchanged genes, differentially expressed genes with unchanged metabolites, and both differentially expressed genes and differentially accumulated metabolites, respectively. (C, F, I, L) Heatmaps of the correlation coefficient clusters (>0.8), p-values < 0.05.
Responses of carbon metabolite and flavonoid metabolites in apple to different potassium conditions
The concentrations of glucose, glycerate-3P, and succinate, which are involved in carbon metabolism, particularly glycolysis and the tricarboxylic acid (TCA) cycle, were increased in apple leaves under LK. The glucose content was upregulated in LKR/CKR and HKL/CKL, whereas glycerate-3P was increased in HKR/CKR (Figure 5). Under LK stress, the following changes were observed: the expression of PFK (MD05G13633600), CS (MD13G1111200), FUM (MD03G1292300), and IDH (MD09G1029200) was decreased in leaves; the expression of PFK (MD01G107500 and MD07G1144100) and CS (MD13G1153500) was increased in leaves; and the expression of PFK (MD 05G1363600) and PPDK (MD16G1179400) was decreased in roots. Under HP conditions, the expression of PFK (MD 05G1363600) and PPDK (MD16G1179400) was downregulated in leaves; the expression of PFK (MD05G1363600) was increased in roots; and the expression of AD (MD11G1038900) and PPCK (MD01G1046200) was decreased in roots (Figure 5).
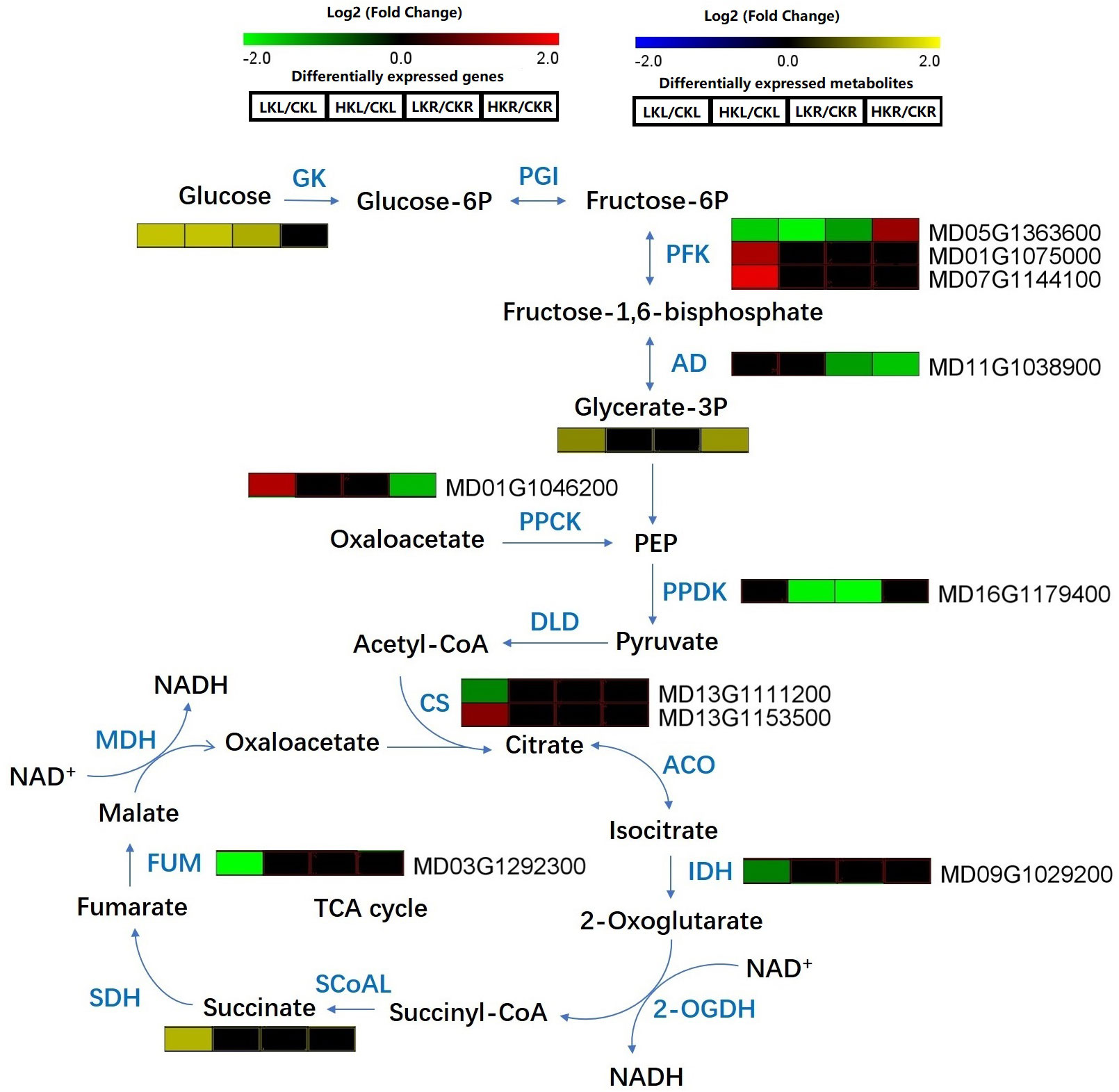
Figure 5 Carbon metabolites in apple leaves and roots under different K stresses. The boxes in the pathway represent DEGs or DAMs. Red and green represent upregulated and downregulated genes, respectively. Yellow and blue represent upregulated and downregulated metabolites, respectively.
The level of naringenin chalcone, which is involved in flavonoid metabolites, was increased under LK conditions in apple leaves and roots. The phenylalanine content was decreased in apple leaves under different K conditions. The gene expression of PAL in leaves was increased under LK stress but decreased under HK conditions. The C4H, 4CL, CHS, F3H, ANS, CHI, and DFR genes were upregulated under LK stress, whereas the UGT gene was decreased under LK stress. Under HK conditions, the PAL, UGT, CHI (MD01G1118300), and DFR (MD11G1229100) genes were downregulated in leaves (Figure 6).
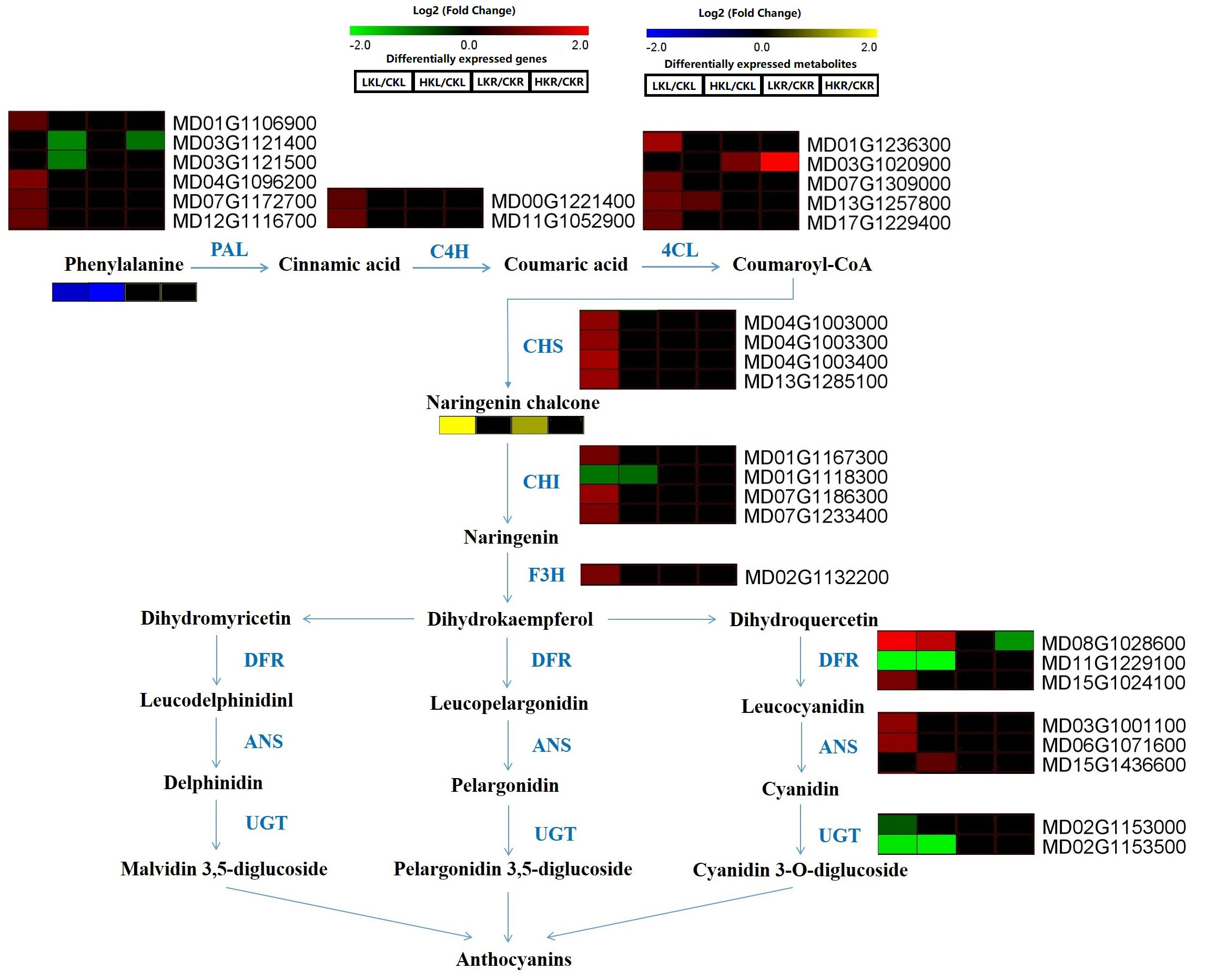
Figure 6 Flavonoid metabolites in apple leaves and roots under different K stresses. The boxes in the pathway represent DEGs or DAMs. Red and green represent upregulated and downregulated genes, respectively. Yellow and blue represent upregulated and downregulated metabolites, respectively.
Discussion
K plays essential roles in many physiological and biochemical processes in the plants, such as ion homeostasis, enzyme activation, osmoregulation, and protein synthesis (Kanai et al., 2007; Ma et al., 2020). K stress affects the normal growth of plants; the scarcity of K slows plant growth, reduces plant height, reduces stem diameter (Tester and Blatt, 1989), and decreases photosynthesis (Kanai et al., 2011). The plant height, stem diameter, plant DW, and photosynthesis were decreased under different LK and HK stresses in apple (Tables 1, 2). Trankner et al. (2018) revealed that K deficiency also reduces photosynthetic CO2 fixation, as well as the transportation and consumption of photoassimilates, thus damaging plant membranes and chlorophyll under low-K conditions. In the present research, the SPDA value and photosynthetic characteristics decreased under LK stress in apple (Table 2). Xu et al. (2020) reported that an adequate supply of K increased the photoassimilate transportation rate from apple leaves to roots as well as increased nutrient use efficiency by influencing photosynthesis. The SPAD value of apple plants was slightly increased under HK stress, while the photosynthetic index decreased to a lesser extent under HK stress compared to LK stress (Table 2); it means that apple seedlings under low-potassium stress are more damaged than those under high-potassium stress. In the present study, RNA-Seq and KEGG enrichment analysis indicated that a different potassium environment had an effect on plant photosynthesis. Combined transcriptome metabolome analysis showed that the DEGs and DAMs were associated with biological processes, such as carbohydrate metabolism and photosynthesis. Hafsi et al. (2014) revealed that K stress limits plant leaf growth, which may be due to sugar starvation in stems and leaves. In the present study, genes involved in the TCA cycle, such as CS, IDH, and TCA, were downregulated in apple leaves under LK (Figure 5), which may induce apple plant growth restriction.
Plants under K stress conditions increase ROS production, resulting in oxidative stress (Hernandez et al., 2012). The accumulation of higher K in plant cells restores oxidative stress by increasing the activity of antioxidant enzymes, such as glutathione reductase (GPX), dehydroascorbate reductase (DHAR), ascorbate peroxidase (APX), CAT, SOD, and POD (García-Martí et al., 2019). The H2O2 content, SOD activity, and POD activity were affected by different K stresses in apple seedlings. The SOD and POD activities were significantly increased to combat different K conditions (Figures 1B–D). Under HK stress, the enzyme activities increased more significantly than under LK stress, which indicated that LK had a greater effect on apple plants. A previously metabolome analysis has revealed that the glutathione content is increased in roots in low-K-tolerant KN9204 wheat but not in low-K-sensitive BN207 wheat (Zhao et al., 2020). Under low potassium, the content of S-(methyl)glutathione in apple leaves and roots was significantly increased in the present study (Table S4). Thus, these findings indicated that glutathione is an important metabolite for plant adaptation to K deficiency.
Phytohormones are active substances that widely exist in plants to regulate their physiological metabolism, affect plant development, affect plant growth, and play a regulatory role in stress conditions. Different potassium environments influence phytohormones in plants, such as brassinosteroids, IAA, ABA, and jasmonic acid (JA) (Ahanger et al., 2018; Ahanger et al., 2020; Yang et al., 2020). After 15 days of different K stress treatments, ABA and IAA contents increased in both apple leaves and roots (Figures 1E, F). The concentration of ABA in peanut leaves also increases under low-K stress (Patel et al., 2022). Therefore, considering the importance of phytohormones in plant growth, these findings indicated that ABA, JA, SA, and other phytohormones are important molecules in plant resistance to K stress.
Potassium uptake and absorption are mainly accomplished through potassium transporters and potassium channels in the plasma membrane (Ashley et al., 2006). K transporters and channels play vital roles in translocation and cell growth in various plants (Wang and Wu, 2013). Under the condition of K deficiency, the expression levels of HAK1 and HAK5 in maize are upregulated, and AtHAK5, OsHAK1, and HvHAK1 are also induced by K-limited conditions (Santa-María et al., 1997; Banuelos et al., 2002; Ahn et al., 2004; Gierth et al., 2005; Fulgenzi et al., 2008; Qin et al., 2019). In addition, transcriptome analysis of rice roots under LK stress has revealed that the OsHAK1, OsHAK7, OsHAK11, and OsHKT2;1 genes are upregulated; in addition, potassium channel genes, such as OsAKT1, OsAKT2/3, and OsKCO1, are also increased in response to low-K conditions (Ma et al., 2012). In tobacco seedlings, the KUP3 K transporter and the SKOR K channel are increased under LK stress (Lu et al., 2015). In apple seedlings, the AKT (MD15G1178200) and HAK (MD11G1302600, MD11G1303100, MD11G1302900, MD13G1133200, and MD16G1143900) genes were upregulated in roots under KL. Moreover, the KAT (MD05G1284400) and KUP (MD01G1165900 and MD07G1232700) genes were upregulated in apple leaves, whereas HAK (MD03G1283600, MD10G1204500, MD11G1302600, and MD11G1303100) was increased in apple roots (Table 3). These results were consistent with previous studies, showing that a common regulatory mechanism exists across plant species whereby the transcription of genes encoding K transporters and channels increases, which may be an efficient strategy to increase potassium uptake in plants under a K-deficient environment. Therefore, these findings suggested that apple AKT, HAK, KUP, and HAK are key genes involved in potassium channels and transporters, which play an important role in coping with low- and high-potassium stresses in apple seedlings.
Under potassium-deficient conditions, the amino acid content increases in cotton (Wang et al., 2012b) and in roots of the K-tolerant genotype of wheat (Zhao et al., 2020), and lysine, histidine, and arginine accumulated in peanut leaves and roots (Patel et al., 2022). In addition, the citric acid, arginine, and asparagine contents increase under LK in rapeseed (Hu et al., 2021), and most amino acids increase in tomato roots under LK (Sung et al., 2015). Armengaud et al. (2009) found that the selective reduction of acidic amino acids contributes to maintaining charge balance in response to potassium-deficient conditions. Under high-N conditions, most amino acids decreased in apple leaves, and under HK conditions, most amino acids decrease in apple (Sun et al., 2021). In plants, the accumulation of free amino acids has been reported under N and P deficiency conditions (Hernández et al., 2007; Pant et al., 2015; Mo et al., 2019; Ding et al., 2021). In this study, we found that the synthesis of most amino acids was increased in apple leaves under LK stress, especially in ornithine and arginine (Figure 5; Table S4). These results show that the deficiency of macronutrients affects the accumulation of amino acids in plants.
Carbohydrate metabolism plays core roles in plant metabolism, providing energy for plant growth and development, and it acts as a bridge in the communication of proteins, lipids, and metabolism (Rolland et al., 2006). The increased content of soluble sugars, including glucose, sucrose, and fructose, in plants is a typical response to different stresses (Armengaud et al., 2004; Rosa et al., 2009; Carvalhais et al., 2011; Wang et al., 2012b; Tang et al., 2015). Zeng et al. (2018) found that most sugars are significantly upregulated under a K-deficient environment in barley roots and leaves. Under LK and HK stresses, the glucose and glycerate-3P contents also increased in apple, suggesting that increased accumulation of sugar may be one of the physiological characteristics for different K stress adaptations in plants. Sugar and potassium have a common function in regulating osmotic potential. We also identified DEGs involved in carbohydrate metabolism, especially those related to glycolysis and the TCA cycle, which were differentially expressed in response to different K stresses. Glycolysis is a process of glucose breakdown to form pyruvate (Fernie et al., 2004). Changes in the levels of gene transcripts in the glycolytic pathway, such as phosphofruckinase-1 (PFK), aldolase (AD), pyruvate-phosphate kinase (PPDK), and phosphoenolpyruvate carboxylase kinase (PPCK), were found under LK and HK conditions in apple. PFK catalyzes a reversible reaction in glycolysis and regulates the glycolysis pathway (Schaeffer et al., 1996; Mustroph et al., 2013). It has been reported that the PFK gene is upregulated in barley under LK (Ye et al., 2022). In this study, the expression of PFK (MD01G107500 and MD07G1144100) was increased in apple leaves, which may have induced an increase the glycerate-3P content in apple leaves under LK (Figure 5). Li et al. (2018) revealed that the TCA cycle of two soybean genotypes is inhibited in leaves and roots under low-N stress. We have previously reported that the TCA cycle is also decreased under N-deficient stress in apple leaves (Sun et al., 2021). The DEGs involved in the TCA cycle, namely, fumarase (FUM), isocitrate dehydrogenase (IDH), and citrate synthase (CS), were also downregulated in apple leaves under LK (Figure 5), indicating that LK stress caused greater damage to apple leaves. Carbohydrate metabolism enzymes, particularly those involved in glycolysis and the TCA cycle, may be indispensable for plant survival under low nutrient conditions (Zeng et al., 2015).
Combined KEGG enrichment analysis of these pathways showed that the biosynthesis of flavonoids was a significantly enriched pathway under different K stresses (Figure 6). Flavonoids are secondary metabolites with low molecular weights, and they are widely found in plant communities and are closely related to the UV protection, flower color formation, plant growth regulation, and pathogen resistance. Many studies have found that flavonoids are related to macronutrients in plants, such as N, P, and K. In rapeseed, nitrogen deficiency enhances ANS and DFR gene expression (Koeslin-Findeklee et al., 2015). The expression of the PAL5, CHS2, F3’H, and F3’5’H genes is significantly increased in tomato leaves under N deficiency stress (Løvdal et al., 2010). Luo et al. (2019) reported that flavonoids are significantly decreased under low P in maize. In the present study, the DEGs and DAMs involved in the flavonoid pathway also changed in apple under different K conditions (Figure 6). The level of naringenin chalcone in apple leaves and roots was increased under LK conditions. Moreover, the phenylalanine content decreased in apple leaves under different K conditions. The PAL gene in apple leaves was upregulated under LK stress but downregulated under HK conditions. The C4H, 4CL, CHS, F3H, ANS, CHI, and DFR genes were upregulated under LK stress, but the UGT gene was downregulated under LK stress. Under HK conditions, the PAL, UGT, CHI (MD01G1118300), and DFR (MD11G1229100) genes were downregulated in leaves. Together, these results indicated that the flavonoid pathway plays an important role in the apple response to different potassium stresses.
Data availability statement
The datasets presented in this study can be found in online repositories. The names of the repository/repositories and accession number(s) can be found in the article/Supplementary Material.
Author contributions
BZ and QW designed the experiments. TS prepared the plant samples. TS, JKZ, and QZ conducted the experiments and analyzed the data. TS, BZ, and QW wrote the manuscript, with the help of XL, ML, YY, and JZ. All authors contributed to the article and approved the submitted version.
Funding
This work was supported by the National Key Research and Development Project of China (2020YFD1000201) and the National Natural Science Foundation of China (31901965).
Conflict of interest
The authors declare that the research was conducted in the absence of any commercial or financial relationships that could be construed as a potential conflict of interest.
Publisher’s note
All claims expressed in this article are solely those of the authors and do not necessarily represent those of their affiliated organizations, or those of the publisher, the editors and the reviewers. Any product that may be evaluated in this article, or claim that may be made by its manufacturer, is not guaranteed or endorsed by the publisher.
Supplementary material
The Supplementary Material for this article can be found online at: https://www.frontiersin.org/articles/10.3389/fpls.2023.1131708/full#supplementary-material
References
Ahanger, M., Ashraf, M., Bajguz, A., Ahmad, P. (2018). Brassinosteroids regulate growth in plants under stressful environments and crosstalk with other potential phytohormones. J. Plant Growth Regul. 37, 1007–1024. doi: 10.1007/s00344-018-9855-2
Ahanger, M. A., Mir, R. A., Alyemeni, M. N., Ahmad, P. (2020). Combined effects of brassinosteroid and kinetin mitigates salinity stress in tomato through the modulation of antioxidant and osmolyte metabolism. Plant Physiol. Biochem. 147, 31–42. doi: 10.1016/j.plaphy.2019.12.007
Ahn, S. J., Shin, R., Schachtman, D. P. (2004). Expression of KT/KUP genes in Arabidopsis and the role of root hairs in k+ uptake. Plant Physiol. 134, 1135–1145. doi: 10.1104/pp.103.034660
Amtmann, A., Troufflard, S., Armengaud, P. (2008). The effect of potassium nutrition on pest and disease resistance in plants. Physiol. Plant 133, 682–691. doi: 10.1111/j.1399-3054.2008.01075.x
Armengaud, P., Breitling, R., Amtmann, A. (2004). The potassium-dependent transcriptome of arabidopsis reveals a prominent role of jasmonic acid in nutrient signaling. Plant Physiol. 136, 2556–2576. doi: 10.1104/pp.104.046482
Armengaud, P., Sulpice, R., Miller, A. J., Stitt, M., Amtmann, A., Gibon, Y. (2009). Multilevel analysis of primary metabolism provides new insights into the role of potassium nutrition for glycolysis and nitrogen assimilation in Arabidopsis roots. Plant Physiol. 150, 772–785. doi: 10.1104/pp.108.133629
Ashley, M. K., Grant, M., Grabov, A. (2006). Plant responses to potassium deficiencies: A role for potassium transport proteins. J. Exp. Bot. 57, 425–436. doi: 10.1093/jxb/erj034
Banuelos, M. A., Garciadeblas, B., Cubero, B., Rodriguez-Navarro, A. (2002). Inventory and functional characterization of the HAK potassium transporters of rice. Plant Physiol. 130, 784–795. doi: 10.1104/pp.007781
Carvalhais, L., Fedoseyenko, D., Haijirezai, M., Borriss, R., Vonwiren, N. (2011). Root exudation of sugars, amino acids, and organic acids by maize as affected by nitrogen, phosphorous, potassium, iron deficiency. J. Plant Nutr. Soil Sci. 174, 3–11. doi: 10.1002/jpln.201000085
Chang, C., Li, C., Li, C. Y., Kang, X. Y., Zou, Y. J., Ma, F. W. (2014). Differences in the efficiency of potassium (K) uptake and use in five apple rootstock genotypes. J. Integr. Agric. 13, 1934–1942. doi: 10.1016/S2095-3119(14)60839-X
Chen, W., Gong, L., Guo, Z., Wang, W., Zhang, H., Liu, X., et al. (2013). A novel integrated method for large-scale detection, identification, and quantification of widely targeted metabolites: application in the study of rice metabolomics. Mol. Plant 6, 1769–1780. doi: 10.1093/mp/sst080
Chen, S., Zhou, Y., Chen, Y., Gu, J. (2018). Fastp: An ultra-fast all-in-one FASTQ preprocessor. Bioinformatics 34, i884–i890. doi: 10.1093/bioinformatics/bty560
Cui, J., Abadie, C., Carroll, A., Lamade, E., Tcherkez, G. (2019). Responses to K deficiency and waterlogging interact via respiratory and nitrogen metabolism. Plant Cell Environ. 42, 647–658. doi: 10.1111/pce.13450
Ding, N., Huertas, R., Torres-Jerez, I., Liu, W., Watson, B., Scheible, W. R., et al. (2021). Transcriptional, metabolic, physiological and developmental responses of switchgrass to phosphorus limitation. Plant Cell Environ. 44, 186–202. doi: 10.1111/pce.13872
Fernie, A. R., Carrari, F., Sweetlove, L. J. (2004). Respiratory metabolism: Glycolysis, the TCA cycle and mitochondrial electron transport. Curr. Opin. Plant Biol. 7, 254–261. doi: 10.1016/j.pbi.2004.03.007
Fulgenzi, F. R., Peralta, M. A. L., Mangano, S., Danna, C. H., Vallejo, A. J., Puigdomenech, P., et al. (2008). The ionic environment controls the contribution of the barley HvHAK1 transporter to potassium acquisition. Plant Physiol. 147, 252–262. doi: 10.1104/pp.107.114546
García-Martí, M., Piñero, M. C., García-Sanchez, F., Mestre, T. C., López-Delacalle, M., Martínez, V., et al. (2019). Amelioration of the oxidative stress generated by simple or combined abiotic stress through the K⁺ and Ca2+ supplementation in tomato plants. Antioxidants (Basel) 8 (4), 81. doi: 10.3390/antiox8040081
Gierth, M., Mäser, P., Schroeder, J. I. (2005). The potassium transporter AtHAK5 functions in k+ deprivation-induced high-affinity k+ uptake and AKT1 k+ channel contribution to k+ uptake kinetics in Arabidopsis roots. Plant Physiol. 137, 1105–1114. doi: 10.1104/pp.104.057216
Hafsi, C., Debez, A., Abdelly, C. (2014). Potassium deficiency in plants: Effects and signaling cascades. Acta Physiologiae Plantarum 36, 1055–1070. doi: 10.1007/s11738-014-1491-2
Hall, R. D. (2011). Plant metabolomics in a nutshell: Potential and future challenges. Biolioy Plant metabolomics 43, 1–24. doi: 10.1002/9781444339956.ch1
Hasanuzzaman, M., Bhuyan, M. H. M. B., Nahar, K., Hossain, M. S., Mahmud, J. A., Hossen, M. S., et al. (2018). Potassium: A vital regulator of plant responses and tolerance to abiotic stresses. Agronomy 8, 31. doi: 10.3390/agronomy8030031
Hernandez, M., Fernandez-Garcia, N., Garcia-Garma, J., Rubio-Asensio, J. S., Rubio, F., Olmos, E. (2012). Potassium starvation induces oxidative stress in Solanum lycopersicum l. roots. J. Plant Physiol. 169, 1366–1374. doi: 10.1016/j.jplph.2012.05.015
Hernández, G., Ramírez, M., Valdés-López, O., Tesfaye, M., Graham, M. A., Czechowski, T., et al. (2007). Phosphorus stress in common bean: Root transcript and metabolic responses. Plant Physiol. 144, 752–767. doi: 10.1104/pp.107.096958
Hoagland, D. R., Arnon, D. I. (1950). The water-culture method for growing plants without soil. Cal. Agric. Exp. St. Circ. 347.
Hu, W., Lu, Z., Meng, F., Li, X., Cong, R., Ren, T., et al. (2021). Potassium modulates central carbon metabolism to participate in regulating CO2 transport and assimilation in Brassica napus leaves. Plant Sci. 307, 110891. doi: 10.1016/j.plantsci.2021.110891
Jung, J. Y., Shin, R., Schachtman, D. (2009). Ethylene mediates response and tolerance to potassium deprivation in Arabidopsis. Plant Cell 21, 607–621. doi: 10.1105/tpc.108.063099
Kanai, S., Moghaieb, R. E., El-Shemy, H. A., Panigrahi, R., Mohapatra, P. K., Ito, J., et al. (2011). Potassium deficiency affects water status and photosynthetic rate of the vegetative sink in green house tomato prior to its effects on source activity. Plant Sci. 180, 368–374. doi: 10.1016/j.plantsci.2010.10.011
Kanai, S., Ohkura, K., Adu-Gyamfi, J. J., Mohapatra, P. K., Nguyen, N. T., Saneoka, H., et al. (2007). Depression of sink activity precedes the inhibition of biomass production in tomato plants subjected to potassium deficiency stress. J. Exp. Bot. 58, 2917–2928. doi: 10.1093/jxb/erm149
Kim, E. J., Kwak, J. M., Uozumi, N., Schroeder, J. I. (1998). AtKUP1: An Arabidopsis gene encoding high-affinity potassium transport activity. Plant Cell 10, 51–62. doi: 10.1105/tpc.10.1.51
Kim, D., Langmead, B., Salzberg, S. L. (2015). HISAT: A fast spliced aligner with low memory requirements. Nat. Methods 12, 357–360. doi: 10.1038/nmeth.3317
Kim, M. J., Ruzicka, D., Shin, R., Schachtman, D. P. (2012). The Arabidopsis AP2/ERF transcription factor RAP2.11 modulates plant response to low-potassium conditions. Mol. Plant 5, 1042–1057. doi: 10.1093/mp/sss003
Koeslin-Findeklee, F., Rizi, V. S., Becker, M. A., Parra-Londono, S., Arif, M., Balazadeh, S., et al. (2015). Transcriptomic analysis of nitrogen starvation- and cultivar-specific leaf senescence in winter oilseed rape (Brassica napus l.). Plant Sci. 233, 174–185. doi: 10.1016/j.plantsci.2014.11.018
Løvdal, T., Olsen, K. M., Slimestad, R., Verheul, M., Lillo, C. (2010). Synergetic effects of nitrogen depletion, temperature, and light on the content of phenolic compounds and gene expression in leaves of tomato. Phytochemistry 71, 605–613. doi: 10.1016/j.phytochem.2009.12.014
Langmead, B., Trapnell, C., Pop, M., Salzberg, S. L. (2009). Ultrafast and memory-efficient alignment of short DNA sequences to the human genome. Genome Biol. 10, 25. doi: 10.1186/gb-2009-10-3-r25
Li, B., Dewey, C. N. (2011). RSEM: Accurate transcript quantification from RNA-seq data with or without a reference genome. BMC Bioinf. 12, 323. doi: 10.1186/1471-2105-12-323
Li, M., Xu, J., Wang, X., Fu, H., Zhao, M., Wang, H., et al. (2018). Photosynthetic characteristics and metabolic analyses of two soybean genotypes revealed adaptive strategies to low-nitrogen stress. J. Plant Physiol. 229, 132–141. doi: 10.1016/j.jplph.2018.07.009
Liang, C., Tian, J., Liao, H. (2013). Proteomics dissection of plant responses to mineral nutrient deficiency. Proteomics 13, 624–636. doi: 10.1002/pmic.201200263
Lu, L., Chen, Y., Lu, L., Lu, Y., Li, L. (2015). Transcriptome analysis reveals dynamic changes in the gene expression of tobacco seedlings under low potassium stress. J. Genet. 94, 397–406. doi: 10.1007/s12041-015-0532-y
Luo, B., Ma, P., Nie, Z., Zhang, X., He, X., Ding, X., et al. (2019). Metabolite profiling and genome-wide association studies reveal response mechanisms of phosphorus deficiency in maize seedling. Plant J. 97, 947–969. doi: 10.1111/tpj.14160
Ma, N., Dong, L., Lü, W., Lü, J., Meng, Q., Liu, P. (2020). Transcriptome analysis of maize seedling roots in response to nitrogen-, phosphorus-, and potassium deficiency. Plant Soil 447, 637–658. doi: 10.1007/s11104-019-04385-3
Ma, T. L., Wu, W. H., Wang, Y. (2012). Transcriptome analysis of rice root responses to potassium deficiency. BMC Plant Biol. 12, 161. doi: 10.1186/1471-2229-12-161
Meena, K. K., Sorty, A. M., Bitla, U. M., Choudhary, K., Gupta, P., Pareek, A., et al. (2017). Abiotic stress responses and microbe-mediated mitigation in plants: The omics strategies. Front. Plant Sci. 8, 172. doi: 10.3389/fpls.2017.00172
Mo, X., Zhang, M., Liang, C., Cai, L., Tian, J. (2019). Integration of metabolome and transcriptome analyses highlights soybean roots responding to phosphorus deficiency by modulating phosphorylated metabolite processes. Plant Physiol. Biochem. 139, 697–706. doi: 10.1016/j.plaphy.2019.04.033
Mustroph, A., Stock, J., Hess, N., Aldous, S., Dreilich, A., Grimm, B. (2013). Characterization of the phosphofructokinase gene family in rice and its expression under oxygen deficiency stress. Front. Plant Sci. 4, 125. doi: 10.3389/fpls.2013.00125
Oomen, R. J. F. J., Benito, B., Sentenac, H., Rodríguez-Navarro, A., Talón, M., Véry, A.-A., et al. (2012). HKT2;2/1, a k+-permeable transporter identified in a salt-tolerant rice cultivar through surveys of natural genetic polymorphism. Plant J. 71, 750–762. doi: 10.1111/j.1365-313X.2012.05031.x
Pant, B. D., Pant, P., Erban, A., Huhman, D., Kopka, J., Scheible, W. R. (2015). Identification of primary and secondary metabolites with phosphorus status-dependent abundance in Arabidopsis, and of the transcription factor PHR1 as a major regulator of metabolic changes during phosphorus limitation. Plant Cell Environ. 38, 172–187. doi: 10.1111/pce.12378
Patel, M., Fatnani, D., Parida, A. K. (2022). Potassium deficiency stress tolerance in peanut (Arachis hypogaea) through ion homeostasis, activation of antioxidant defense, and metabolic dynamics: Alleviatory role of silicon supplementation. Plant Physiol. Biochem. 182, 55–75. doi: 10.1016/j.plaphy.2022.04.013
Pyo, Y. J., Gierth, M., Schroeder, J. I., Cho, M. H. (2010). High-affinity k(+) transport in Arabidopsis: AtHAK5 and AKT1 are vital for seedling establishment and postgermination growth under low-potassium conditions. Plant Physiol. 153, 863–875. doi: 10.1104/pp.110.154369
Qin, Y. J., Wu, W. H., Wang, Y. (2019). ZmHAK5 and ZmHAK1 function in k+ uptake and distribution in maize under low k+ conditions. J. Integr. Plant Biol. 61, 691–705. doi: 10.1111/jipb.12756
Rolland, F., Baena-Gonzalez, E., Sheen, J. (2006). Sugar sensing and signaling in plants: Conserved and novel mechanisms. Annu. Rev. Plant Biol. 57, 675–709. doi: 10.1146/annurev.arplant.57.032905.105441
Rosa, M., Prado, C., Podazza, G., Interdonato, R., Gonzalez, J. A., Hilal, M., et al. (2009). Soluble sugars–metabolism, sensing and abiotic stress: A complex network in the life of plants. Plant Signal Behav. 4, 388–393. doi: 10.4161/psb.4.5.8294
Santa-María, G. E., Rubio, F., Dubcovsky, J., Rodríguez-Navarro, A. (1997). The HAK1 gene of barley is a member of a large gene family and encodes a high-affinity potassium transporter. Plant Cell 9, 2281–2289. doi: 10.1105/tpc.9.12.2281
Schaeffer, C., Johann, P., Nehls, U., Hampp, R. (1996). Evidence for an up-regulation of the host and a down-regulation of the fungal phosphofructokinase activity in ectomycorrhizas of Norway spruce and fly agaric. New Phytol. 134, 697–702. doi: 10.1111/j.1469-8137.1996.tb04935.x
Schroeder, J. I., Ward, J. M., Gassmann, W. (1994). Perspectives on the physiology and structure of inward-rectifying k+ channels in higher plants: Biophysical implications for k+ uptake. Annu. Rev. Biophys. Biomol Struct. 23, 441–471. doi: 10.1146/annurev.bb.23.060194.002301
Sun, T., Zhang, J., Zhang, Q., Li, X., Li, M., Yang, Y., et al. (2021). Integrative physiological, transcriptome, and metabolome analysis reveals the effects of nitrogen sufficiency and deficiency conditions in apple leaves and roots. Environ. Exp. Bot. 192, 104633. doi: 10.1016/j.envexpbot.2021.104633
Sung, J., Lee, S., Lee, Y., Ha, S., Song, B., Kim, T., et al. (2015). Metabolomic profiling from leaves and roots of tomato (Solanum lycopersicum l.) plants grown under nitrogen, phosphorus or potassium-deficient condition. Plant Sci. 241, 55–64. doi: 10.1016/j.plantsci.2015.09.027
Tan, L., Zhou, L., Zhao, N., He, Y., Qiu, Z. (2021). Development of a low-cost portable device for pixel-wise leaf spad estimation and blade-level SPAD distribution visualization using color sensing. Comput. Electron. Agriculture. 190, 106487. doi: 10.1016/j.compag.2021.106487
Tang, Z.-H., Zhang, A.-J., Wei, M., Chen, X.-G., Liu, Z.-H., Li, H.-M., et al. (2015). Physiological response to potassium deficiency in three sweet potato (Ipomoea batatas lam.) genotypes differing in potassium utilization efficiency. Acta Physiol Plant 37, 184. doi: 10.1007/s11738-015-1901-0
Tester, M., Blatt, M. R. (1989). Direct measurement of k channels in thylakoid membranes by incorporation of vesicles into planar lipid bilayers. Plant Physiol. 91, 249–252. doi: 10.1104/pp.91.1.249
Trankner, M., Tavakol, E., Jakli, B. (2018). Functioning of potassium and magnesium in photosynthesis, photosynthate translocation and photoprotection. Physiol. Plant 163, 3. doi: 10.1111/ppl.12747
Wang, C., Chen, H., Hao, Q., Sha, A., Shan, Z., Chen, L., et al. (2012a). Transcript profile of the response of two soybean genotypes to potassium deficiency. PloS One 7, e39856. doi: 10.1371/journal.pone.0039856
Wang, N., Hua, H., Eneji, A. E., Li, Z., Duan, L., Tian, X. (2012b). Genotypic variations in photosynthetic and physiological adjustment to potassium deficiency in cotton (Gossypium hirsutum). J. Photochem. Photobiol. B 110, 1–8. doi: 10.1016/j.jphotobiol.2012.02.002
Wang, Y., Wu, W. H. (2013). Potassium transport and signaling in higher plants. Annu. Rev. Plant Biol. 64, 451–476. doi: 10.1146/annurev-arplant-050312-120153
Wang, X., Zhao, J., Fang, Q., Chang, X., Sun, M., Li, W., et al. (2021). GmAKT1 is involved in k+ uptake and Na+/K+ homeostasis in Arabidopsis and soybean plants. Plant Sci. 304, 110736. doi: 10.1016/j.plantsci.2020.110736
Weckwerth, W. (2003). Metabolomics in systems biology. Annu. Rev. Plant Biol. 54, 669–689. doi: 10.1146/annurev.arplant.54.031902.135014
Wu, L. B., Holtkamp, F., Wairich, A., Frei, M. (2019). Potassium ion channel gene OsAKT1 affects iron translocation in rice plants exposed to iron toxicity. Front. Plant Sci. 10, 579. doi: 10.3389/fpls.2019.00579
Xu, X., Du, X., Wang, F., Sha, J., Chen, Q., Tian, G., et al. (2020). Effects of potassium levels on plant growth, accumulation and distribution of carbon, and nitrate metabolism in apple dwarf rootstock seedlings. Front. Plant Sci. 11, 904. doi: 10.3389/fpls.2020.00904
Xu, J., Li, H. D., Chen, L. Q., Wang, Y., Liu, L. L., He, L., et al. (2006). A protein kinase, interacting with two calcineurin b-like proteins, regulates k+ transporter AKT1 in Arabidopsis. Cell 125, 1347–1360. doi: 10.1016/j.cell.2006.06.011
Yang, T., Feng, H., Zhang, S., Xiao, H., Hu, Q., Chen, G., et al. (2020). The potassium transporter OsHAK5 alters rice architecture via ATP-dependent transmembrane auxin fluxes. Plant Commun. 1, 100052. doi: 10.1016/j.xplc.2020.100052
Ye, Z., He, X., Liu, C. (2022). Comparative analysis of transcriptome profiles reveals the mechanisms in the difference of low potassium tolerance among cultivated and tibetan wild barleys. Agronomy 12, 1094. doi: 10.3390/agronomy12051094
Zeng, J., He, X., Wu, D., Zhu, B., Cai, S., Nadira, U. A., et al. (2014). Comparative transcriptome profiling of two Tibetan wild barley genotypes in responses to low potassium. PloS One 9, e100567. doi: 10.1371/journal.pone.0100567
Zeng, Q., Ling, Q., Fan, L., Li, Y., Hu, F., Chen, J., et al. (2015). Transcriptome profiling of sugarcane roots in response to low potassium stress. PloS One 10, e0126306. doi: 10.1371/journal.pone.0126306
Zeng, J., Quan, X., He, X., Cai, S., Ye, Z., Chen, G., et al. (2018). Root and leaf metabolite profiles analysis reveals the adaptive strategies to low potassium stress in barley. BMC Plant Biol. 18, 187. doi: 10.1186/s12870-018-1404-4
Zhang, S., Ying, H., Pingcuo, G., Wang, S., Zhao, F., Cui, Y., et al. (2019). Identification of potential metabolites mediating bird's selective feeding on Prunus mira flowers. BioMed. Res. Int. 2019, 1395480. doi: 10.1155/2019/1395480
Zhao, H., Ni, S., Cai, S., Zhang, G. (2021). Comprehensive dissection of primary metabolites in response to diverse abiotic stress in barley at seedling stage. Plant Physiol. Biochem. 161, 54–64. doi: 10.1016/j.plaphy.2021.01.048
Zhao, Y., Sun, R., Liu, H., Liu, X., Xu, K., Xiao, K., et al. (2020). Multi-omics analyses reveal the molecular mechanisms underlying the adaptation of wheat (Triticum aestivum l.) to potassium deprivation. Front. Plant Sci. 11, 588994. doi: 10.3389/fpls.2020.588994
Keywords: apple, potassium deficiency, potassium excess, transcriptome analysis, metabolome analysis
Citation: Sun T, Zhang J, Zhang Q, Li X, Li M, Yang Y, Zhou J, Wei Q and Zhou B (2023) Transcriptional and metabolic responses of apple to different potassium environments. Front. Plant Sci. 14:1131708. doi: 10.3389/fpls.2023.1131708
Received: 26 December 2022; Accepted: 17 February 2023;
Published: 10 March 2023.
Edited by:
Chao Li, Northwest A&F University, ChinaReviewed by:
Zhengyang Wang, Northwest A&F University, ChinaWang Hua, Huazhong Agricultural University, China
Copyright © 2023 Sun, Zhang, Zhang, Li, Li, Yang, Zhou, Wei and Zhou. This is an open-access article distributed under the terms of the Creative Commons Attribution License (CC BY). The use, distribution or reproduction in other forums is permitted, provided the original author(s) and the copyright owner(s) are credited and that the original publication in this journal is cited, in accordance with accepted academic practice. No use, distribution or reproduction is permitted which does not comply with these terms.
*Correspondence: Beibei Zhou, YmVpYmVpX3pob3VAMTI2LmNvbQ==; Qinping Wei, cXB3ZWlAc2luYS5jb20=