- 1Ministry of Education Key Laboratory of Molecular and Cellular Biology, Hebei Research Center of the Basic Discipline of Cell Biology, Hebei Collaboration Innovation Center for Cell Signaling and Environmental Adaptation, Hebei Key Laboratory of Molecular and Cellular Biology, College of Life Sciences, Hebei Normal University, Shijiazhuang, China
- 2Grape Breeding, Shijiazhuang Institute of Pomology, Hebei Academy of Agriculture and Forestry Sciences, Shijiazhuang, China
Drought is a severe environmental condition that restricts the vegetative growth and reduces the yield of grapevine (Vitis vinifera L.). However, the mechanisms underlying grapevine response and adaptation to drought stress remain unclear. In the present study, we characterized an ANNEXIN gene, VvANN1, which plays a positive role in the drought stress response. The results indicated that VvANN1 was significantly induced by osmotic stress. Expression of VvANN1 in Arabidopsis thaliana enhanced osmotic and drought tolerance through modulating the level of MDA, H2O2, and O2·- at the seedling stage, implying that VvANN1 might be involved in the process of ROS homeostasis under drought or osmotic stress conditions. Moreover, we used yeast one-hybridization and chromatin immunoprecipitation assays to show that VvbZIP45 could regulate VvANN1 expression by directly binding to the promoter region of VvANN1 in response to drought stress. We also generated transgenic Arabidopsis that constitutively expressed the VvbZIP45 gene (35S::VvbZIP45) and further produced VvANN1Pro::GUS/35S::VvbZIP45 Arabidopsis plants via crossing. The genetic analysis results subsequently indicated that VvbZIP45 could enhance GUS expression in vivo under drought stress. Our findings suggest that VvbZIP45 may modulate VvANN1 expression in response to drought stress and reduce the impact of drought on fruit quality and yield.
Introduction
Drought stress is a major abiotic stress that reduces the yield and quality of plants during plant growth and development (Zhu, 2016). The expression of drought-related genes increased under drought stress, promoting a series of physiological, biochemical, and molecular reactions (Kooyers, 2015). Grapevine (Vitis vinifera L.) is an important fruit crop worldwide. Its fruit is used to produce wine, grape juice, and other foods (Ali et al., 2010; Liu et al., 2021a). However, the development of the grape industry and global climate change have led to grapevine cultivation lands being constantly subjected to drought, high temperature, cold, salt, and other abiotic stresses. Drought stress is a major constraint on grapevine productivity and quality (Hou et al., 2020; Li et al., 2021). Grapevines have evolved various mechanisms at the morphological, physiological, biochemical, and molecular levels in response to drought stress (Lovisolo et al., 2010; Romero et al., 2012). Moreover, several drought-related genes have been identified in grapevine. The expression of VaNAC17 was induced by drought stress and substantially enhanced drought tolerance in transgenic Arabidopsis thaliana (Su et al., 2020). Heterologous expressing of VaNAC26 in Arabidopsis improved drought tolerance by up-regulating drought stress-related genes and jasmonic acid (JA) signaling genes (Fang et al., 2016). Ectopic expression of VaCIPK02 in Arabidopsis enhanced drought resistance by regulating abscisic acid (ABA) signaling and production reactive oxygen species (ROS) (Xu et al., 2020). Identification and characterization of candidate genes associated with drought stress can be utilized to enhance drought stress response in grape varieties, thus improving grapevine yield.
Drought stress often leads to increased production of ROS, including hydrogen peroxide (H2O2), superoxide ions (O2·-), and hydroxyl radicals (OH•) (Cruz De Carvalho, 2008). High ROS concentrations could damage cellular compounds such as proteins, membranes, and cellular RNA and DNA (Apel and Hirt, 2004; Miller et al., 2010). Therefore, plants have evolved an enzymatic antioxidant defense system to maintain cellular ROS homeostasis under various stress conditions. The main plant ROS-scavenging enzymes include superoxide dismutase (SOD), ascorbate peroxidase (APX), catalase (CAT), glutathione peroxidase (GPX), and peroxiredoxin (PrxR) (Mittler et al., 2004). Previous studies have indicated that increased expression of ROS scavenging-related genes could increase tolerance to drought stress. For example, overexpression of OsLG3 increased drought stress tolerance in rice by inducing the expression of ROS scavenging genes (Xiong et al., 2018). Overexpression of SlbHLH22 improved tomato plant drought stress tolerance by improving ROS scavenging system (Waseem et al., 2019). VvWRKY13 negatively modulates plant drought tolerance through regulating the activities of CAT and SOD (Hou et al., 2020). Therefore, by studying ROS levels and antioxidant enzyme activities in plants under drought stress, we can deeply reveal the function of drought stress tolerance related genes.
Annexins are conserved Ca2+-dependent phospholipid-binding proteins that exist in plants, animals, and fungi (Rescher and Gerke, 2004; Mortimer et al., 2008). Previous studies have shown that, besides having peroxidase and ATPase/GTPase activities and responding to various abiotic stresses, annexins could mediate calcium transport in plants (Laohavisit and Davies, 2011; Clark et al., 2012; Demidchik et al., 2018; Saad et al., 2020). OsANN1 and OsANN10 conferred tolerance to abiotic stress in rice by modulating ROS levels (Qiao et al., 2015; Gao et al., 2020). AtANN1 regulated [Ca2+]cyt elevation in response to salinity stress and also participated in drought stress tolerance by regulating ROS production (Laohavisit et al., 2013). OsANN4 activated Ca2+ influx in response to ABA (Zhang et al., 2021). ZmANN33 and ZmANN35 were up-regulated and participated in plasma membrane (PM) recovery during seed germination. In addition, inhibiting the expression of ZmANN33 and ZmANN35 increased membrane damage under chilling stress (He et al., 2019). However, a few reports exist on the roles of ANNEXINs in grapevine under abiotic stresses (Jami et al., 2012; Briz-Cid et al., 2016). Investigation the role of grapevine ANNEXINs involvment in stress response will enrich the function of annexin in different species.
The dynamic balance between plant growth regulation and stress adaptive response is implicated by many regulatory proteins, among which transcription factors (TFs) are key components that modulate stress adaptation pathways in plant stress responses. The basic leucine zipper (bZIP) TFs belong to one of the largest transcription factor families and are characterized by a basic DNA-binding region with a specific motif (N-X7-R/K) at the N-terminus and leucine zipper region at the C-terminus. They play pivotal and diverse roles in plants under high-temperature, salt, and drought stress conditions (Corrêa et al., 2008; Nijhawan et al., 2008). OsbZIP62V significantly enhanced tolerance to drought and oxidative stress, and the osbzip62 mutants displayed reduced drought stress tolerance (Yang et al., 2019). Zea mays bZIP60 mediated the unfolded protein response during heat stress (Li et al., 2020). The wilting degree was noticeably lower in TabZIP15 overexpressing plants than in KN199 plants under salt treatment (Bi et al., 2021). HvbZIP21 play a key role in drought stress tolerance through modulating ROS scavenging (Pan et al., 2022). The bZIP TFs can bind to the core sequence (-ACGT-) in the promoter of downstream genes (e.g., the G-box, C-box, and A-box), thereby participating in the transcriptional regulation of plant responses to stress (Nijhawan et al., 2008). The maize bZIP TF bZIP68 acts as a negative regulator of cold tolerance and directly binds to the A-box/G-box in the DREB1.7 promoter, inhibiting the expression of the DREB1 gene (Li et al., 2022). To date, 55 bZIP genes have been identified in grapevines, of which 32 VvbZIP genes are widely involved in responding to drought stress (Liu et al., 2014). VvbZIP45, also named VvGRIP55 or VvABF2, could bind to the ABA-responsive element and play a positive role in response to drought stress (Nicolas et al., 2014; Liu et al., 2019).
In the present study, we aimed to isolate and characterize a grapevine putative annexin gene, VvANN1 (Vv18g03470). Our results showed that heterologous expression of grapevine VvANN1 improved drought stress tolerance in Arabidopsis via reducing malondialdehyde (MDA) and increasing the activities of SOD, POD, and CAT in leaves under drought conditions. In addition, we found that drought-responsive TF VvbZIP45 regulated the expression of VvANN1, thus improving drought resistance in grapevine. We reveal a working mechanism of VvbZIP45-mediated VvANN1 in response to drought stress that may reduce the impact of drought on fruit quality and yield.
Materials and methods
Plant materials and growth conditions
Grapevine (V. vinifera L. cv. ‘Summer Black’ and ‘Venus’) was used in this study. Plantlets were grown on solid Murashige and Skoog (MS) medium under a 16-h light/8-h dark cycle and 70% relative humidity at 25°C in the greenhouse.
All transgenic lines of VvANN1 were developed in the A. thaliana Columbia (Col-0) background. Col-0 plants were used as the wild type in the present study. Arabidopsis thaliana seedings were germinated on MS for 6 days and transferred to soil pots (7cm×7 cm). The seedlings were grown in a light incubator (22°C, 16-h day/8-h night cycle and 70% relative humidity).
Phenotypic analysis
Five-week-old plantlets were transferred to liquid MS medium for 2 days and then planted in a fresh liquid MS medium with 10% (w/v) PEG6000 (NO. A610432, Sangon Biotech, Shanghai, China) to evaluate osmotic stress tolerance. Stem apex samples were collected for quantitative real-time polymerase chain reaction (RT-qPCR) analysis.
Six-day-old VvANN1 transgenic and Col-0 seedlings were germinated on MS medium, transferred to soil pots (7 cm×7 cm) for 9 days with regular water. These were dried for 7 days and then allowed a 3-day recovery. The survival rates were recorded and the seedings were photographed.
Vector construction
The total cDNA of VvANN1 and a 1,538-bp fragment of the VvANN1 promoter were cloned from five-week-old grapevine ‘Summer Black’ plantlets. The VvANN1 coding region sequence (cDNA) was digested and ligated with pCAMBIA1301-HA, modified pMDC83, and pET32a vectors to obtain Ubi::VvANN1-HA, 35S::VvANN1-GFP, and VvANN1-His constructs, respectively.
The promoter region (1,538 bp) upstream of the VvANN1 start codon was amplified and cloned into the vector to produce transgenic VvANN1Pro::GUS lines. Genomic fragments (285 bp) upstream of VvANN1 were amplified and cloned into pAbAi and pGreenII 0800-LUC vectors to generate pAbAi-VvANN1Pro and pGreenII 0800-LUC-VvANN1Pro, respectively.
VvbZIP45 cDNA was amplified and cloned into pCAMBIA1301-HA, pGreenII 62-SK, pGADT7 and pMDC83 vectors to generate Ubi::VvbZIP45-HA, pGreenII 62-SK-VvbZIP45, AD-VvbZIP45 and 35S::VvbZIP45-GFP, respectively.
Related constructs were introduced into Agrobacterium tumefaciens cells (GV3101) and grown at 28°C for 3 days before being transformed into Col-0. The primers used to produce the constructs are listed in Supplemental Table S1.
RNA isolation and RT-qPCR analysis
Total RNA was extracted from grapevine stem apices and Arabidopsis leaves using TRIzol reagent (NO. B511311, Sangon Biotech, Shanghai, China). RNase-free DNase I (EN0521, Thermo Fisher Scientific, Waltham, MA, USA) was used to remove genomic DNA. RT-qPCR was performed using the ChamQ Universal SYBR® qPCR Master Mix Kit (Q711-02, Vazyme, Nanjing, China) using the QuantStudio Q5 thermal cycler (Thermo Fisher Scientific, Waltham, MA, USA). The experiment was performed using three biological replicates. Relative quantitative results were calculated by normalization to AtACTIN2 and VvACTIN7, the internal controls in Arabidopsis and grapevine, respectively. All primer sequences are listed in Supplemental Table S1.
Histochemical β-glucuronidase (GUS) assay
GUS activity was detected via histochemical staining of tissues as previously described (Zhang et al., 2018a) but with slight modifications. All transgenic Arabidopsis tissues were incubated in GUS staining solution at 37°C with the corresponding time under dark conditions. All stained samples were washed with 70% ethanol to remove the residual dye and chlorophyll. Images were captured using a DVM6a 3D microscope (Leica, Wetzlar, Germany).
Subcellular localization of VvANN1
Transient expression assays were performed in grape protoplasts to determine the subcellular localization of VvANN1. Grape protoplasts were prepared, and transient expression assays were performed as described previously (Lee and Wetzstein, 1988; Wang et al., 2015). The recombinant plasmid 35S::VvANN1-GFP was introduced into grape protoplasts, followed by incubation at 28°C for 12 h. Fluorescence signals were observed using a Zeiss LSM710 laser scanning confocal microscope (Zeiss, Jena, Germany).
Recombinant VvANN1-His protein purification and Ca2+-binding activity
The Ca2+-binding assay was conducted by detecting fluorescence measurements of VvANN1 according to a method described previously (Qiao et al., 2015). The recombinant plasmid VvANN1-His was introduced into Escherichia coli (E. coli) strain BL21. Total VvANN1-His protein was induced by isopropyl β-D-thiogalactoside (IPTG; NO. A100487, Sangon Biotech, Shanghai, China) at 28°C for 12 h. E. coli cells were lysed via ultra-sonication, and the obtained samples were ultra-centrifuged at 12,000 ×g at 4°C for 15 min. The supernatant was collected and purified via affinity chromatography on Ni-agarose columns (Cat. No. 30210, Qiagen, Duesseldorf Germany). The assay media contained 2 μM VvANN1-His protein and 0 mM or 2 mM Ca2+. Fluorescence spectroscopy was carried out using a fluorescence spectrophotometer (F-4600; Hitachi, Tokyo, Japan).
Yeast one-hybrid assay
The pAbAi-VvANN1Pro (containing 285 bp partial promoter sequences with the ABRE element) vector was transformed into the yeast strain Y1HGold as bait. Positive yeast strains were diluted and spread onto selection medium (SD; Code No:630411, Clontech, Mountain View, CA, USA) lacking Ura containing various concentrations of Aureobasidin A (AbA; Code No.630466, Clontech, Mountain View, CA, USA) to screen for an appropriate concentration to eliminate self‐activation. AD-VvbZIP45 was transformed into Y1HGold with pAbAi-VvANN1Pro, and the pGADT7 plasmid was used as a negative control. Different experimental groups were cultured on SD/-Leu medium with or without 80 ng/mL AbA at 30°C for 3 days.
Luciferase reporter assays
A dual-luciferase reporter assay was conducted to test the transcriptional repression activity of VvbZIP45 in tobacco (Nicotiana tabacum) leaves. The pGreenII 62-SK and pGreenII 62-SK-VvbZIP45 were used as effector plasmids, and pGreenII 0800-LUC-VvANN1Pro was used as the reporter plasmid. The plasmids were mixed and expressed in tobacco leaves via A. tumefaciens GV3101 strain injection. After 2 days, total protein was extracted from infiltrated tobacco leaves, and the LUC/REN activity ratio was measured using the dual luciferase reporter assay system (E1960, Promega, Madison, WI, USA).
Chromatin Immunoprecipitation-qPCR assays
Approximately 1 g of plant tissue was harvested from two-week-old transgenic hybrid progeny seedlings that were germinated on MS plates in a light incubator. Samples were prepared according to previous reports (Yamaguchi et al., 2014; Zhao et al., 2022). ChIP experiments were performed using Abcam ChIP Kit - Plants (ab117137, Abcam, Cambridge, MA, USA) according to the manufacturer’s instructions. Chromatin was immunoprecipitated using anti-HA (ab9110, Abcam, Cambridge, MA, USA). Following immunoprecipitation, samples were analyzed by RT-qPCR. The specific primers used are listed in Supplemental Table S1.
Detection of H2O2 and O2·-in situ
Leaves were collected from VvANN1 transgenic Arabidopsis plants and Col-0 plants grown under normal conditions for 15 days, then grown with or without water for 5 days. H2O2 and O2·- levels were examined via histochemical staining with 3, 3′‐diaminobenzidine (DAB; CAS No: 91-95-2, Sigma-Aldrich, St. Louis, MO, USA) or nitro blue tetrazolium (NBT; CAS No: 298-83-9, Sigma-Aldrich, St. Louis, MO, USA), as previously described (Zhang et al., 2021). All measurements were determined using three independent biological replicates.
Measurement of antioxidant enzyme activity and MDA contents
Leaves were collected from VvANN1 transgenic Arabidopsis plants and Col-0 plants cultivated under normal conditions for 15 days (control), then without water for 5 days (drought-exposed).
Samples (each weighing 0.2 g) were homogenized in 1 mL of sodium phosphate buffer (50 mM phosphate, 1 mM EDTA-Na2, 1% (w/v) polyvinyl pyrrolidone; pH 7.4). Centrifugation was performed at 10,000 ×g at 4°C for 20 min, and the supernatant was used to detect antioxidant enzyme activity. The activities of SOD, POD, and CAT were determined according to methods described previously (Zhang et al., 2017).
Samples (each weighing 0.2 g) were homogenized in 2 mL of 10% thiobarbituric acid (TBA). Following centrifugation at 12,000 ×g at 4°C for 15 min, the MDA contents were detected according to the method described by Zhou et al. (2018).
Results
Characterization and expression of VvANN1
There are 14 predicted annexin genes in grapevine (Jami et al., 2012), however, the function of ANNEXIN family in grapevine remains unidentified. We isolated a putative grapevine annexin gene from Vitis vinifera via RT-PCR and named it VvANN1. Based on the results of bioinformatics analyses, the genomic sequence of VvANN1 is composed of five exons and four introns and produces a 930-bp coding sequence transcript encoding a protein of 309 amino acids with a molecular weight of 35 kDa (Figure 1A). This protein contains four annexin domain architectures (Figure 1B) with a type-II Ca2+ binding site in the first annexin conserved repeat domain.
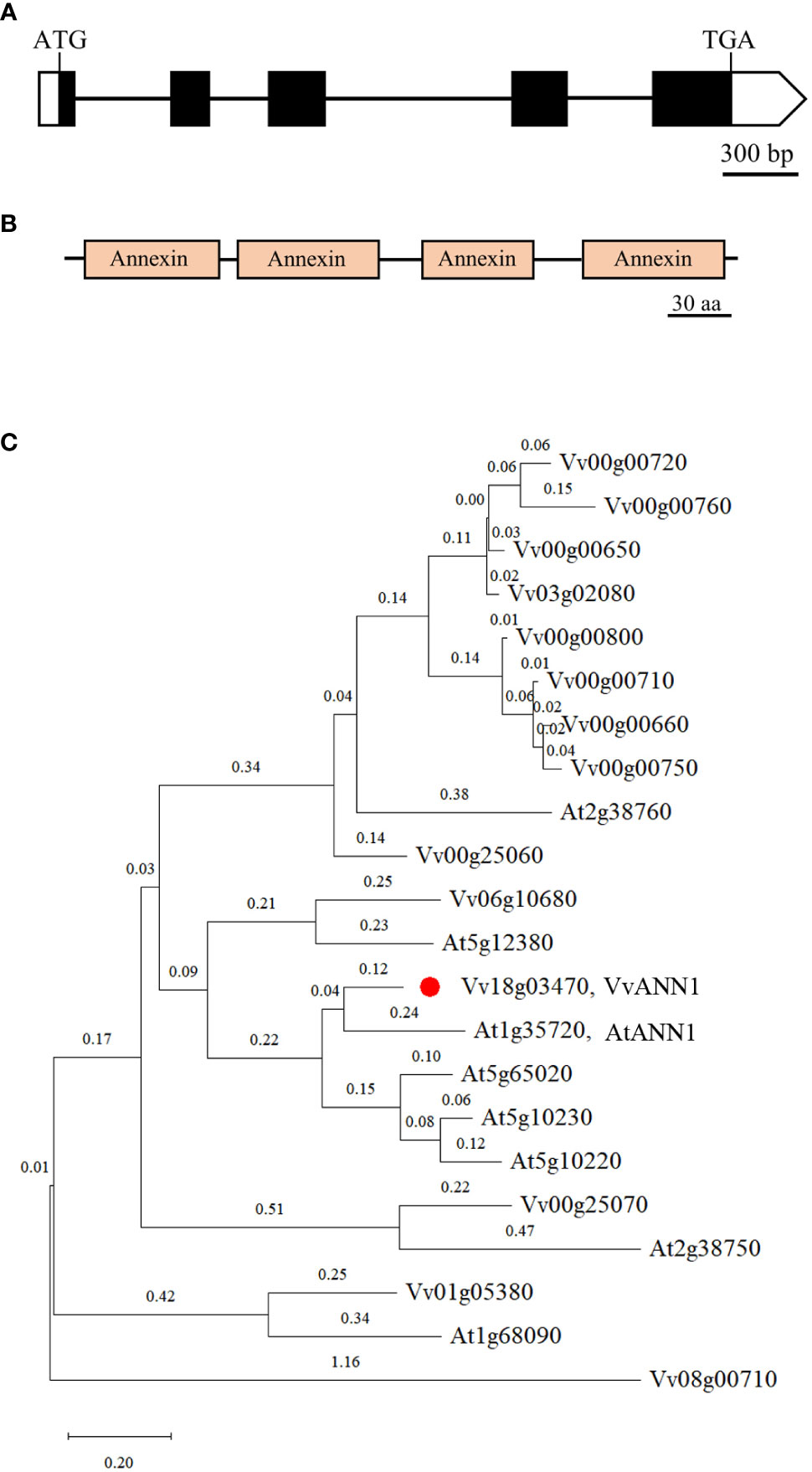
Figure 1 Characterization of VvANN1. (A, B) Schematic diagram showing the gene structure and protein domain of VvANN1. Exons are indicated by black boxes, introns are indicated by black lines between black boxes, non-translational regions are indicated by white boxes, and annexin domains are indicated by orange boxes. (C) Phylogenic tree of VvANNs and the Arabidopsis ortholog AtANNs. The phylogenetic trees were constructed with Mega X using the Neighbor-Joining method. Eight ANNEXINs family members from A thaliana including At1g35720 (AtANN1), At5g65020, At2g38760, At2g38750, At1g68090, At5g10220, At5g10230, At5g12380; fourteen ANNEXINs family members from V. vinifera including: Vv18g03470 (LOC100266093, VvANN1), Vv00g00650 (LOC100260243), Vv00g00660 (LOC100258538), Vv00g00710 (LOC100244780), Vv00g00720 (LOC100853064), Vv00g00750 (LOC100244780), Vv00g00760(LOC100256827), Vv00g00800 (LOC100260252), Vv00g25060 (LOC100256917), Vv00g25070 (LOC100253408), Vv01g05380 (LOC100250931), Vv03g02080 (LOC100243369), Vv06g10680(LOC100263694), Vv08g00710(LOC100240986), VvANN1 is marked with a red dot. The sequences of AtANNs are derived from NCBI, and the sequences of VvANNs are derived from Jami et al (2012).
A phylogenetic tree generated using the ANNEXINs of A. thaliana and V. vinifera suggests that VvANN1 has the maximum homology with AtANN1 of A. thaliana, so the gene was designated as VvANN1. This finding implies that VvANN1 proteins may regulate stress response processes similar to its homologous AtANN1 (Figure 1C).
Transcript levels in different organs of the grapevine ‘Venus’ were performed via RT-qPCR to analyze the tissue-specific expression of VvANN1. The expression of VvANN1 was higher in the flower, stem, and tendril than in the root, fruit, and leaf (Figure 2A). Furthermore, we also generated Arabidopsis transgenic lines (#1, #4, #7 and #9) containing the VvANN1Pro::GUS, and detected GUS activities in seedlings, flowers, which was consistent with the findings shown by RT-qPCR (Figure 2B). Moreover, important discrepancies were noted when comparing the GUS staining observed in different VvANN1Pro::GUS transgenic lines, such as #1 and #9. This result suggested the cassette VvANN1::GUS might be inserted various sites in different Arabidopsis transgenic lines and resulted in different GUS expression level based on the position effect, for example, there might be diverse enhancers near to the insertion sites.
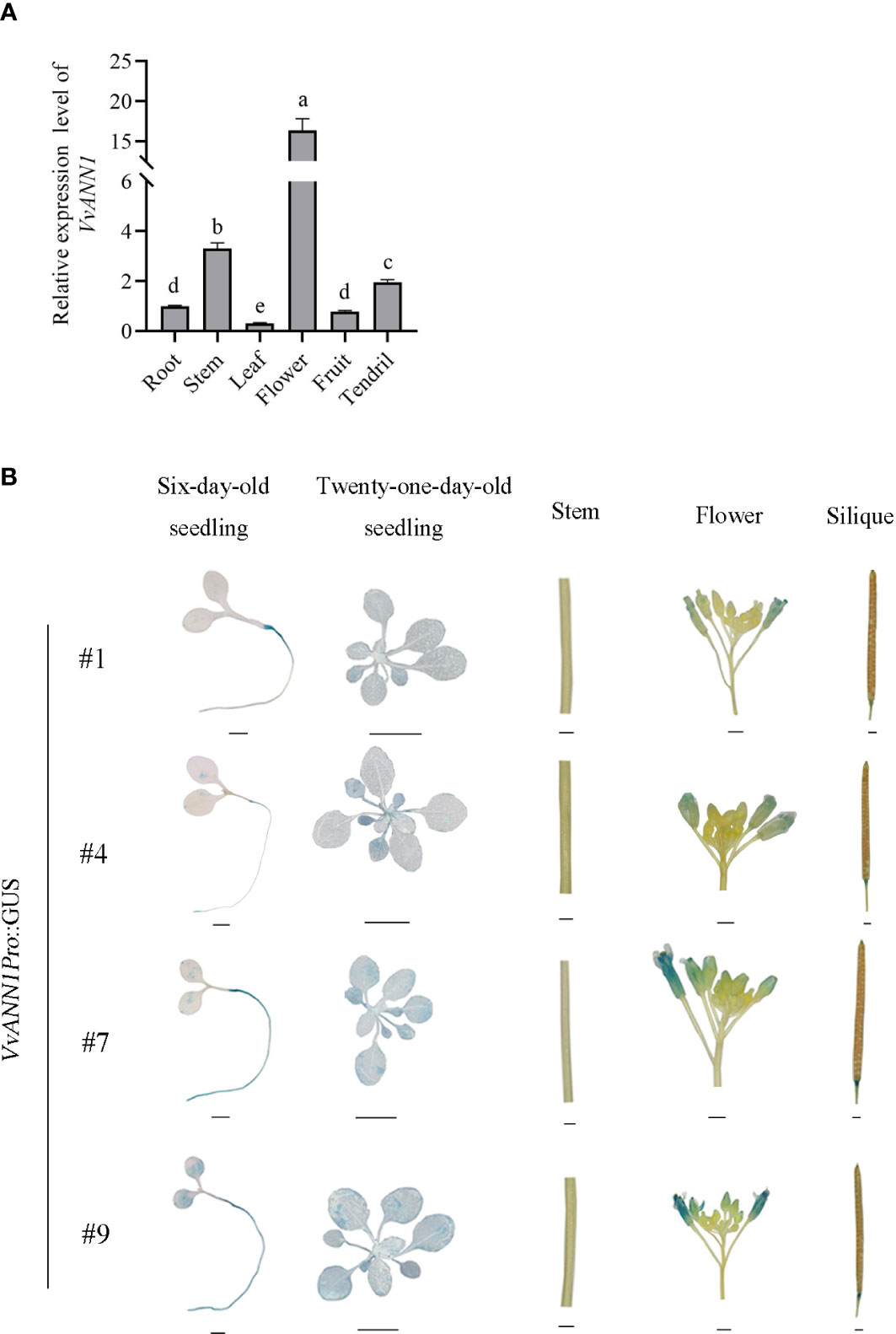
Figure 2 Tissue expression pattern analysis of VvANN1. (A) Expression analysis of VvANN1 in different tissues of Vitis spp. cv ‘Venus’ via RT-qPCR. VvACTIN7 was used as an internal control and compared to expression in root. Data are presented as mean ± SD (n = 3). Statistical significance was determined via one-way ANOVA; P < 0.05. (B) GUS histochemical staining of different tissues of VvANN1Pro::GUS transgenic Arabidopsis lines. Scale bars are 1 mm for six-day-old seedling, flower, silique, and stem images and 1 cm for 21-day-old seedling images.
We transformed the 35S::VvANN1-GFP vector transiently into grapevine protoplasts to investigate the subcellular localization of VvANN1. VvANN1-GFP signal could be detected in cytoplasm, whereas GFP signals were ubiquitously distributed in the grapevine protoplast (Supplemental Figure S1).
Furthermore, we carried out a VvANN1-His recombinant protein fluorescence experiment to verify the Ca2+ binding ability of VvANN1. First, we transformed the VvANN1-His plasmid into E. coli strain BL21. The VvANN1-His recombinant protein was successfully expressed in BL21 by adding IPTG, purified via Ni-NTA affinity chromatography, and further detected via SDS-PAGE (Supplemental Figure S2). Next, a UV spectrophotometer was used to measure the fluorescence intensity of the VvANN1-His recombinant protein when incubated with or without Ca2+. The highest fluorescence intensity was approximately 300 A.U. at 390 nm. By contrast, fluorescence intensity was reduced to 150 A.U. at 390 nm in the presence of Ca2+ (Supplemental Figure S3), indicating that VvANN1 might have Ca2+-binding capacity.
VvANN1 is responsive to osmotic stress and drought stress
AtANN1 is up-regulated in a Ca2+-dependent manner to regulate drought stress responses synergistically. Here, we investigated whether VvANN1 was responsive to drought stress. We performed RT-qPCR to detect the VvANN1 expression pattern in plantlets (Vitis spp. cv ‘Summer Black’ and ‘Venus’) treated with or without 10% PEG6000. The results showed that the expression of VvANN1 in ‘Summer Black’ and ‘Venus’ was significantly induced by PEG treatment up to 11-fold and 4-fold, respectively, at 24 h (Supplemental Figure S4A).
GUS staining was performed to determine further the expression of VvANN1 in six-day-old transgenic VvANN1Pro::GUS plants under PEG treatment. Histochemical staining revealed that, VvANN1Pro::GUS signals were mainly expressed in the vascular tissues of roots under normal conditions. This expression pattern of GUS activity was increased following treatment with 10% PEG6000 for 12 h (Supplemental Figure S4B), suggesting that VvANN1 expression may be induced by PEG treatment.
To further determine the role of VvANN1 in modulating plant osmotic stress, we further generated 3 independent transgenic Arabidopsis lines driven by the CaMV35S promoter (L2, L3 and L4) and 3 independent transgenic Arabidopsis lines driven by the Ubi promoter (L5, L6 and L7). The transcript level of VvANN1 was analyzed with RT-qPCR and three homozygous transgenic Arabidopsis lines (L2, L3 and L6) were used in subsequent experiments (Supplemental Figure S5). VvANN1 transgenic and Col-0 seedlings were cultivated on MS medium with 0 mM, 250 mM or 300 mM mannitol. The germination rates showed no apparent difference between VvANN1 transgenic and Col-0 seedlings in MS medium after 84 h. Conversely, all VvANN1 transgenic seedlings showed higher germinating rates than Col-0 in the presence of mannitol (Figures 3A, B), suggesting that, during the germination stage, VvANN1 transgenic seedlings were less sensitive to osmotic stress than Col-0 seedlings. Based on these results, we concluded that VvANN1 might positively regulate osmotic stress in Arabidopsis.
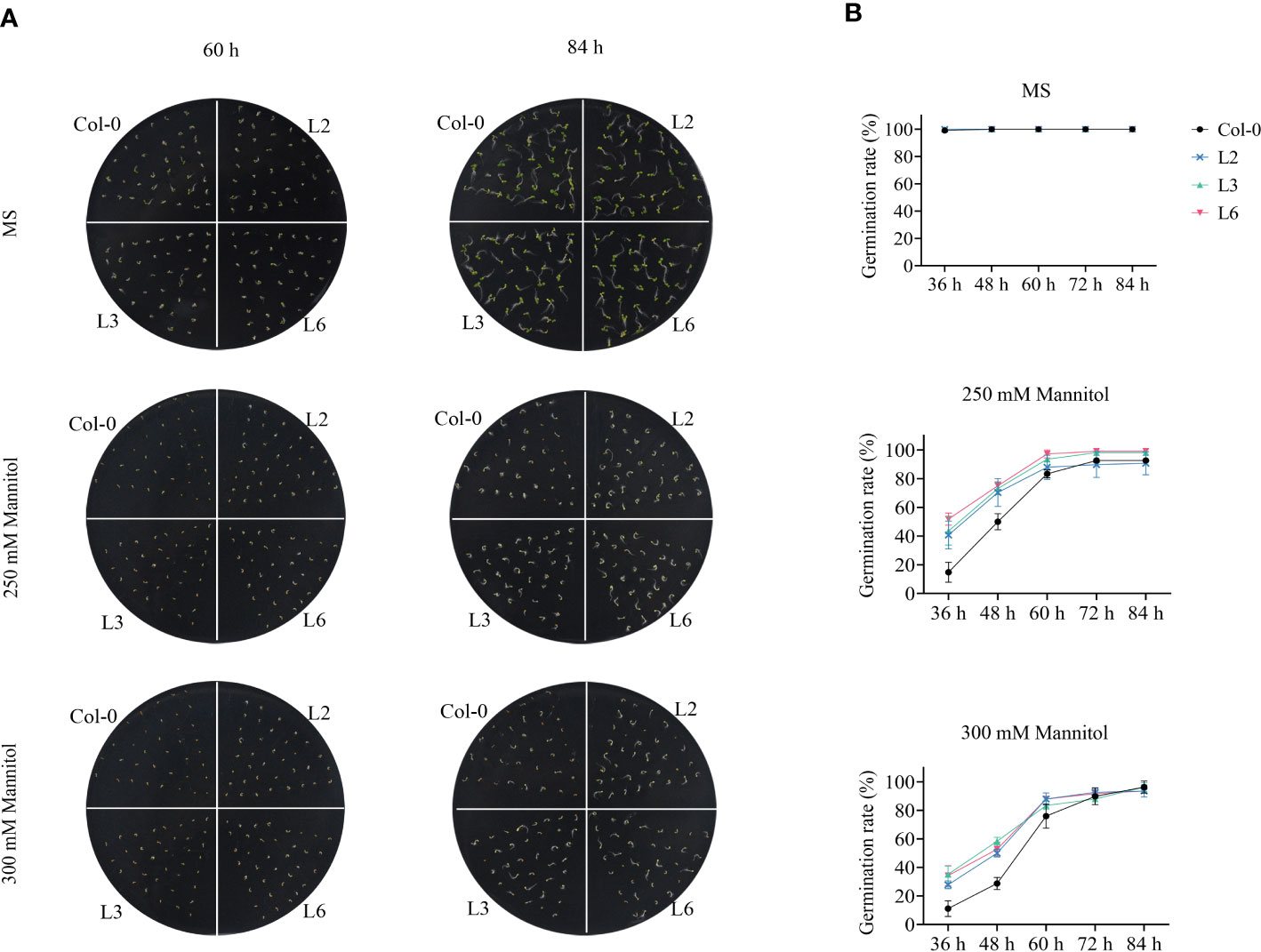
Figure 3 VvANN1 positively regulates osmotic stress at germination in Arabidopsis. (A) Seedings of Col-0 and VvANN1 transgenic Arabidopsis were stratified at 4°C for 2 days and plated on MS medium supplemented with 0 mM, 250 mM or 300 mM mannitol. Photographs were captured at 60 h and 84 h after germination. (B) Germination rates of seedings were determined with respect to radicle emergence when supplemented with 0 mM, 250 mM or 300 mM mannitol at the 84-h time point.
Fifteen-day-old transgenic Arabidopsis and Col-0 plants were subjected to a drought treatment to evaluate the function of VvANN1 in drought tolerance. Once watering was stopped for 7 days, VvANN1 transgenic plants exhibited less wilting than Col-0 plants (Figure 4A). After rewatering, VvANN1 transgenic plants had higher survival rates (58%, 54% and 60% for L2, L3 and L6, respectively) than Col-0 plants (36%) (Figure 4B), indicating that VvANN1 transgenic plants significantly improved drought tolerance in Arabidopsis.
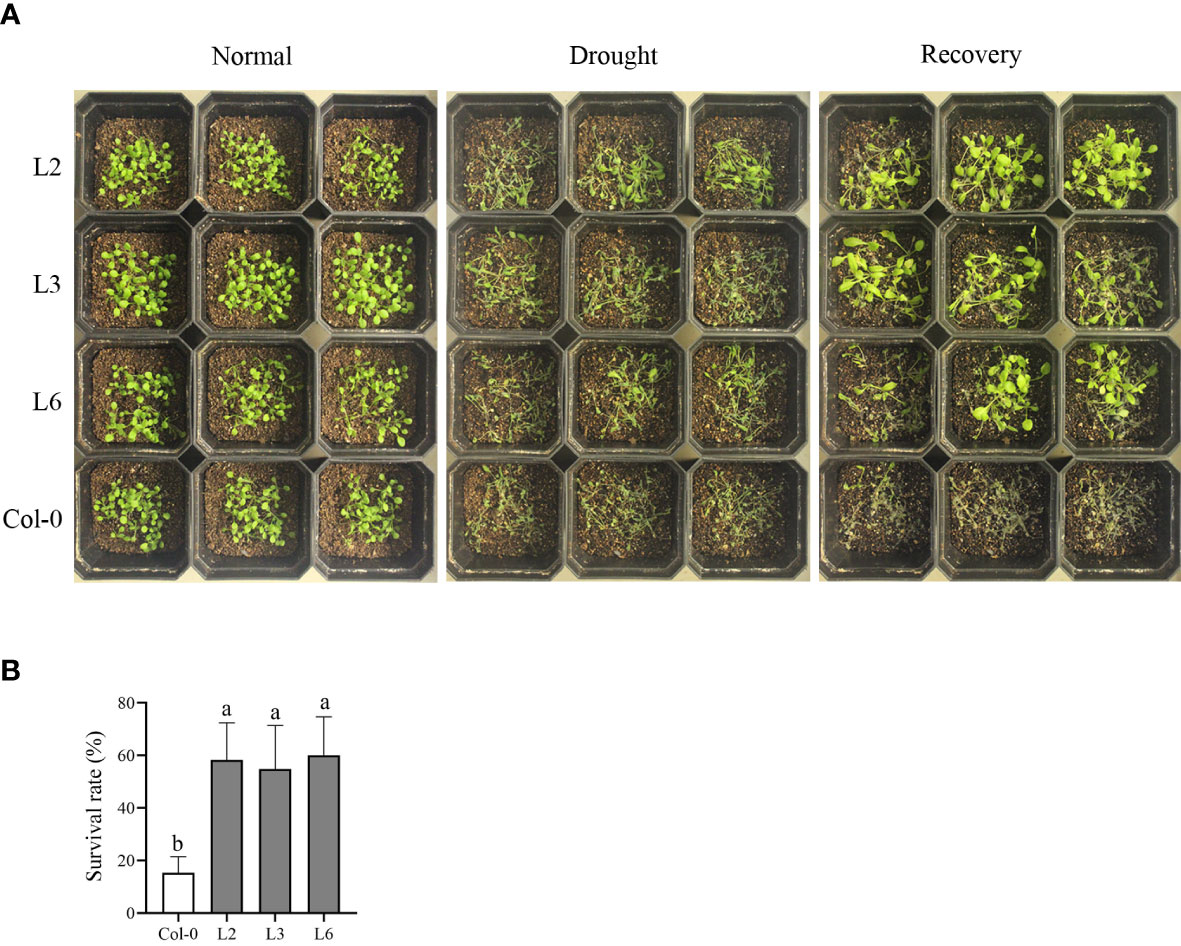
Figure 4 Heterologous expression of VvANN1 increases resistance to drought stress in Arabidopsis. (A) Performance of VvANN1 transgenic plants and Col-0 plants subjected to soil drought stress without watering for 7 days, followed by recovery for 3 days. (B) Survival rates of VvANN1 transgenic plants and Col-0 plants after rewatering for 3 days. Values represent the means ± SD from three independent repeats (n = 48), and different letters indicate significant differences (one-way ANOVA, P < 0.05).
VvbZIP45 binds to the promoter of VvANN1 and activates its expression
We analyzed the VvANN1 promoter using the PlantCARE database (http://bioinformatics.psb.ugent.be/webtools/plantcare) to gain further insights into the regulatory mechanism of VvANN1 and found several stress-responsive cis-elements (e.g., MYC, MYB and ABRE). Among these are two ABRE cis-elements in the 0- to 300-bp region of the VvANN1 promoter. Studies have shown that VvbZIP45 can bind to the ABRE element in the promoter region of the target gene and enhance drought stress tolerance in Arabidopsis (Liu et al., 2014; Nicolas et al., 2014; Liu et al., 2019). Thus, we hypothesized that VvbZIP45 could bind to the promoter region of VvANN1 and regulate its expression.
We performed a Y1H assay, where the VvANN1 promoter sequence (from -1 to -285 bp) containing two ABRE motifs was first constructed into the pAbAi vector (pAbAi-VvANN1Pro). Next, this bait plasmid was transformed into yeast strain Y1HGold. Finally, the pGADT7-VvbZIP45 and pGADT7 vectors were transformed into the yeast Y1HGold carrying the bait plasmid. The results showed that Y1HGold carrying pAbAi-VvANN1Pro and transformed with the pGADT7-VvbZIP45 plasmid grew on SD/-Leu medium containing 80 ng/mL AbA, whereas yeast cells carrying the pGADT7 vector did not (Figure 5A). This result showed that VvbZIP45 could bind to the promoter region of VvANN1.
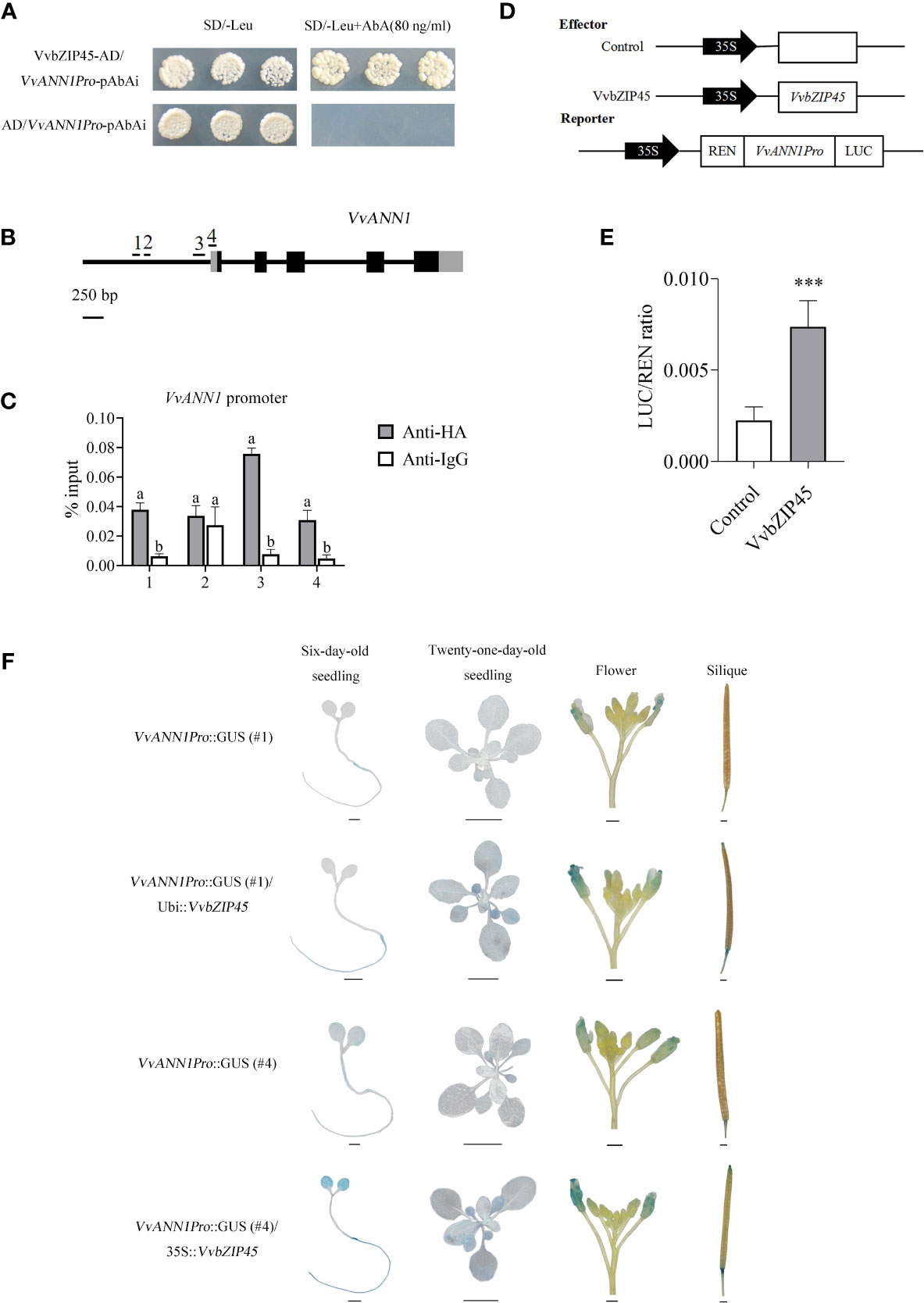
Figure 5 VvbZIP45 positively regulates the transcription of VvANN1 by binding to its promoter. (A) Y1H assay showing the interaction between VvbZIP45 and the VvANN1 promoter. (B, C) ChIP-qPCR assays showing the binding of VvbZIP45 to the promoter of VvANN1 in vivo. ChIP-qPCR analysis using VvANN1Pro::GUS/UBI::VvbZIP45-HA and Col-0 plants with anti-HA and anti-IgG, respectively. Immunoprecipitated DNA samples were quantified by qPCR using primers specific to regions within the VvANN1 promoter (1-4). Relative enrichment is represented as input (%). Values represent the means ± SD from three independent repeats, and different letters indicate significant differences (one-way ANOVA, P < 0.05). (D) Reporter and effector used in the dual-luciferase reporter assay. (E) Activation of the VvANN1 promoter by VvbZIP45. The 35S promoter was used as a negative control (Student’s t-test, ***P < 0.001). (F) Tissue expression patterns of VvANN1 in the presence of VvbZIP45 using VvbZIP45 as the effector and VvANN1Pro::GUS as the reporter. GUS expression was visualized in different tissues of Arabidopsis transformed with effector and reporter constructs. Scale bars are 1 mm for six-day-old seedling, flower, and silique images and 1cm for 21-day-old seedling images.
We performed a ChIP-qPCR assay to investigate whether VvbZIP45 could bind directly to the ABRE cis-element in the promoter region of VvANN1. Ubi::VvbZIP45-HA transgenic Arabidopsis plants were crossed with VvANN1Pro::GUS transgenic plant, and hybrid transgenic A. thaliana seedlings were verified via PCR. Four fragments spanning different regions of the VvANN1 promoter with or without the ABRE motif were selected for qPCR analysis (Figure 5B). Figure 5C shows that 1, 3 and 4 fragments of the VvANN1 promoter were markedly enriched in ChIP-qPCR assay with anti-HA compared with anti-IgG.
We used a luciferase reporting system to determine whether VvbZIP45 could activate VvANN1 expression in vivo (Figures 5D, E). A pGreenII 0800 vector harboring a dual-luciferase reporter gene driven by the VvANN1 promoter was co-transformed into tobacco leaves with pGreenII 62-SK or pGreenII 62-SK-VvbZIP45. The results showed that tobacco plants expressing pGreenII 62-SK-VvbZIP45 exhibited significantly higher LUC/REN activity than control plants.
To further assure the regulation of VvbZIP45 in VvANN1 expression in vivo, we also generated transgenic Arabidopsis that constitutively expressed the VvbZIP45 gene (35S::VvbZIP45) and further produced VvANN1Pro::GUS/35S::VvbZIP45 Arabidopsis plants via crossing. The lower GUS expression level VvANN1Pro::GUS lines #1 and #4 (as shown in Figure 2C) were used as the male parents, 35S::VvbZIP45 Arabidopsis as female parent. The results of histochemical GUS assays showed that staining intensity was significantly higher in hybrid plants (VvANN1Pro::GUS/VvbZIP45) than in VvANN1Pro::GUS transgenic plants (Figure 5F). Interestingly, 10% PEG6000 treatment could be resulted in much more strong GUS staining in VvANN1Pro::GUS/35S::VvbZIP45 hybrid Arabidopsis plants comparing to VvANN1Pro::GUS transgenic plants itself (Figure 6). These results indicated that VvbZIP45 could act as a transcription factor to promote VvANN1 expression under normal or drought stress conditions.
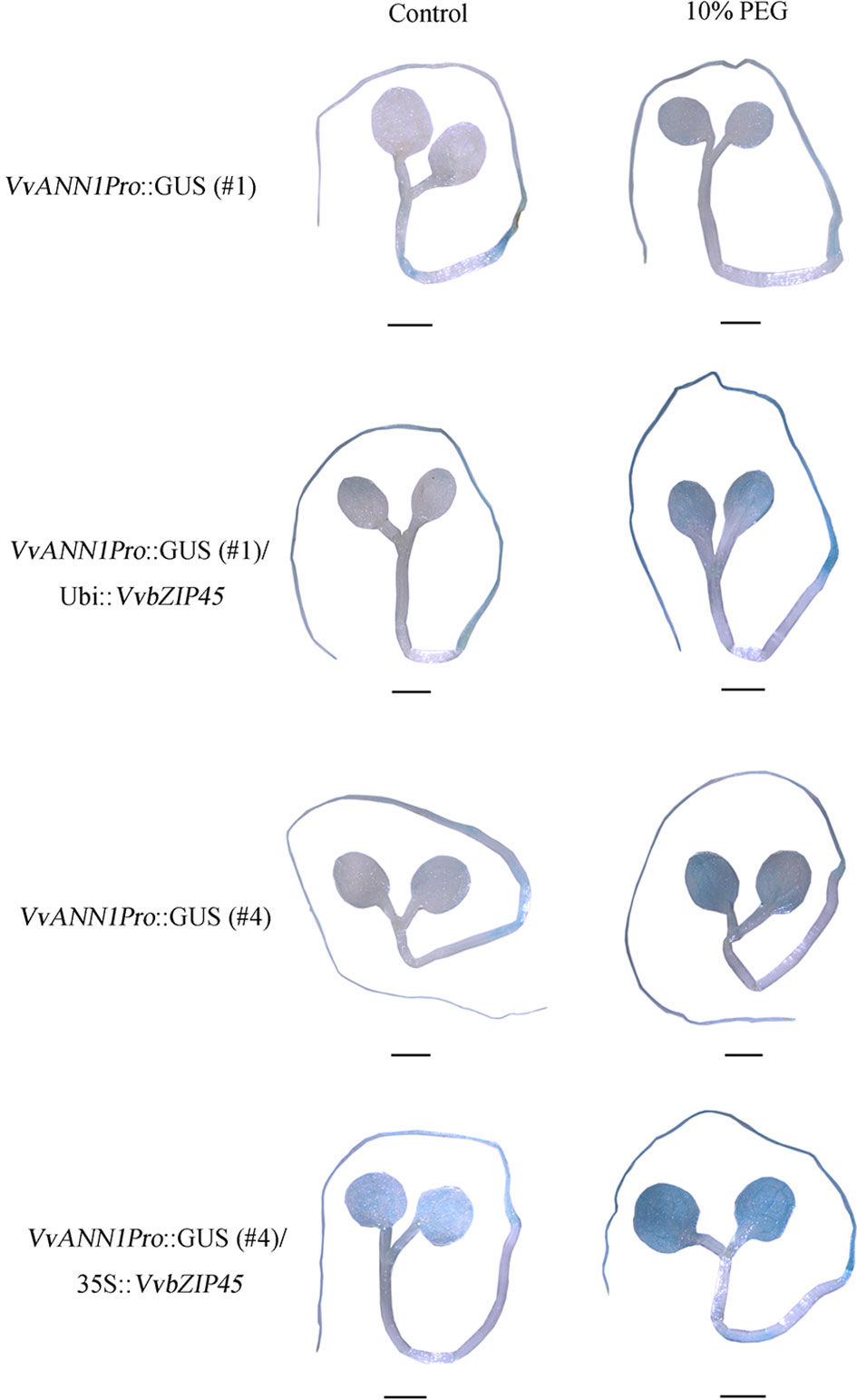
Figure 6 VvbZIP45 enhanced VvANN1 expression under PEG treatment. GUS staining of six-day-old VvANN1Pro::GUS/35S::VvbZIP45 and VvANN1Pro::GUS transgenic Arabidopsis seedlings under normal and PEG treatment. Scale bars=1 mm.
Further examination of the expression level of VvbZIP45 in five-week-old grapevine plantlets under osmotic stress showed that VvbZIP45 expression was rapidly activated by PEG treatment and reached the highest level at 12 h (Supplemental Figure S6). This result suggests that VvbZIP45 could be up-regulated under drought stress.
VvANN1 improved the ROS scavenging ability of transgenic Arabidopsis under drought stress
In plants, ROS serve as signal molecules at low levels but can cause cell damage at extreme doses. Drought stress could lead to ROS accumulation. Here, H2O2 accumulation was detected via DAB staining. The results showed no difference in DAB staining of VvANN1 transgenic plants and Col-0 plants under normal conditions. However, after 5 days of drought treatment, weaker staining was observed in VvANN1 transgenic leaves than in Col-0 leaves (Figure 7A). The accumulation of O2.- detected via NBT staining showed similar results under drought stress treatment (Figure 7B). These results indicated that less H2O2 and O2.- were produced in VvANN1 transgenic plants than in Col-0 plants under drought stress.
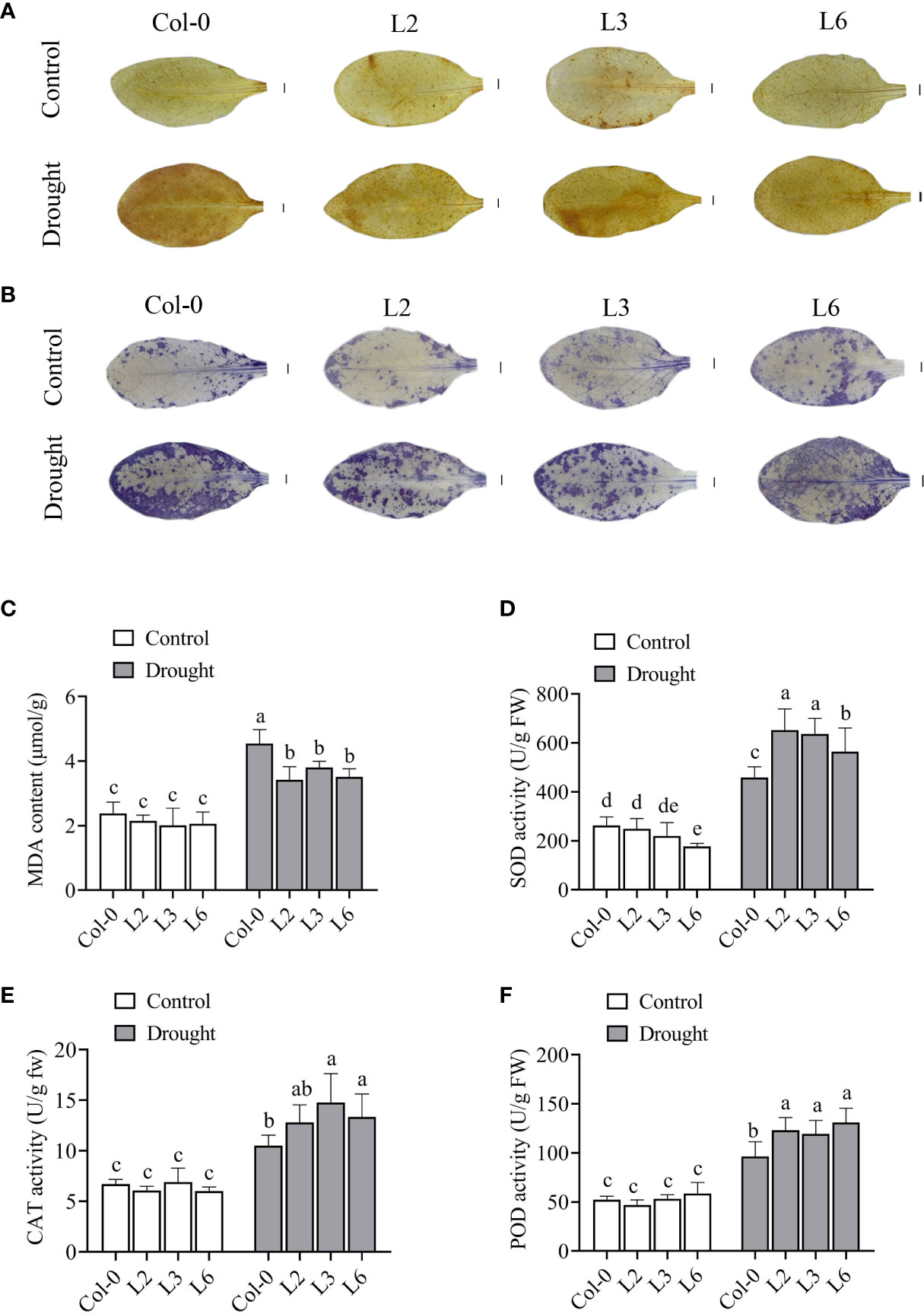
Figure 7 VvANN1 modulates ROS production, lipid peroxidation, and antioxidase activity under drought stress in Arabidopsis. (A, B) DAB and NBT staining of leaves in VvANN1 transgenic plants and Col-0 plants under normal and drought stress conditions. Scale bars = 1 mm. (C) MDA content in VvANN1 transgenic plants and Col-0 plants under normal and drought stress conditions. (D–F) Activities of SOD, CAT, and POD in VvANN1 transgenic plants and Col-0 plants under normal and drought stress conditions. Values represent the means ± SD from three independent repeats, and different letters indicate significant differences (one-way ANOVA, P < 0.05).
Previous studies have shown that ROS accumulation affects intracellular environment stability and severely damages the plant cell membrane (Zhang et al., 2018b). The level of MDA is an important indicators of cell membrane damage (Gao et al., 2020). In the present study, the MDA levels in VvANN1 transgenic plants were similar to Col-0 plants under normal conditions. Following drought treatment for 5 days, three VvANN1 transgenic plants had significantly lower MDA content (3.42, 3.80 and 3.51 μmol/g) than Col-0 (4.54 μmol/g) (Figure 7C). These results suggest that VvANN1 plays a vital role in the reduced accumulation and damage of ROS in cells under drought stress.
The activities of ROS scavenging enzymes (SOD, POD and CAT) were examined to analyze the mechanism of VvANN1 function in regulating the level of ROS (Figures 7D–F). The activities of SOD, POD, and CAT were not significantly different in both VvANN1 transgenic plants and Col-0 plants under normal conditions. However, after drought stress treatment, the enzymatic activities of SOD, POD, and CAT were significantly higher in VvANN1 transgenic plants than in Col-0 plants. These results suggested that the expression of VvANN1 driven by CaMV35S or Ubi promoter in Arabidopsis could improve the activity of SOD, POD and CAT to eliminate excess ROS, subsequently assisting in the maintenance of plasma membrane integrity and improving drought stress tolerance.
Discussion
Over the past few decades, the role of annexin in regulating abiotic and biotic stresses have been extensively studied in plants, especially in fruits and vegetables. For instance, FaAnn5s and FaAnn8 were important in regulating plant hormone signaling during the growth and maturing of strawberry fruit (Chen et al., 2016). Furthermore, overexpression of RsANN1a in Arabidopsis enhanced heat tolerance, suggesting a key role in the heat stress response of radish (Shen et al., 2021). Additionally, BnaANN genes played important roles in JA signaling and multiple stress responses in Brassica napus (He et al., 2020). However, the biological functions and regulatory mechanisms of ANNEXINs in grapevine remain unclear. This study will shed light on the roles of VvANN1 in drought stress in grapevine.
In the present study, we identified an annexin gene from V. vinifera and named it VvANN1. Sequence analysis and phylogenetic analysis showed that this protein contains four annexin domain architectures and has close homology with ANN1 in A. thaliana (Figures 1A–C). Therefore, it is speculated that VvANN1 and AtANN1 might have similar functions. Furthermore, the different expression levels of VvANN1 in grapevine tissues and ectopic expression VvANN1 in Arabidopsis indicate that VvANN1 may have distinct functions (Figure 2), so we firstly verified the ability of VvANN1 to response to drought stress. As expected, the expression of VvANN1 was induced by osmotic stress, and VvANN1 transgenic plants showed higher germinating rates than Col-0 plants under osmotic stress (Supplemental Figure S4 and Figure 3). In addition, overexpression of VvANN1 enhanced drought tolerance in A. thaliana (Figure 4).
The bZIP TFs are widely distributed across several plant species and are involved in many responses to abiotic stresses, such as drought, salt, and low temperature (Wei et al., 2012; Liu et al., 2014; Li et al., 2015). For example, OsbZIP23 positively regulates drought and high-salinity stress responses by modulating the expression of stress-related genes in rice (Xiang et al., 2008). ANAC096 interacts with AtABF2 and further regulates the expression of ABA-inducible genes, enhancing dehydration and osmotic stress tolerance in Arabidopsis (Xu et al., 2013). VvbZIP45 transgenic Arabidopsis plants exhibited more tolerance to osmotic stress compared to WT (Liu et al., 2019). However, studies on the function of VvbZIP45 in regulating the expression of grapevine annexin genes have not been reported. Our results indicated that VvbZIP45 expression in grapevine ‘Summer Black’ was significantly induced by PEG treatment (Supplemental Figure S6). Transient expression assays, Y1H assays, genetics investigations and ChIP-qPCR assays all showed that VvbZIP45 could bind to the promoter of VvANN1 and activate its expression (Figures 5A–E).
As shown in Figure 2C, various VvANN1Pro::GUS lines showed differently level of GUS staining (strong or weak). This result implied that the cassette VvANN1 Pro::GUS might be inserted in various sites of chromosomes in Arabidopsis, and resulted in different expression level of GUS based on the position effect, for example, there might be diverse enhancers near to the insertion sites of T-DNA, and also reflected that there might be a certain range of regulation levels of VvANN1 promoter by TFs in Arabidopsis. Therefore, the VvANN1Pro::GUS/35S::VvbZIP45 hybrid lines showed more strong GUS staining intensity than that of the original VvANN1Pro::GUS transgenic lines (Figure 5F). In addition, the genetic analysis also demonstrated that VvbZIP45 could enhance the expression of VvANN1 under PEG treatment (Figure 6). Taken together, these results indicated that VvbZIP45 could bind to the promoter of VvANN1 and further active its expression under drought stress.
Drought stress often results in excessive ROS accumulation (Mahmood et al., 2019). A low ROS concentration serves as a signal in regulating plant growth and stress responses; however, excessive ROS accumulation can destroy cellular compounds. The antioxidant defense system is a crucial way to balance excess ROS in plants (You and Chan, 2015; Hussain et al., 2019). Plant annexins have been shown to exhibit the ability to respond to abiotic stresses by modulating ROS formation. For instance, overexpression of OsANN1 in rice exposed to heat stress conditions enhanced the activities of SOD and CAT, decreased the content of H2O2, and improved the plant’s tolerance to heat (Qiao et al., 2015). Treatment with exogenous ABA showed significantly higher levels of O2.– and H2O2 in the mesophyll cells of OsANN4-RNAi lines than in the WT (Zhang et al., 2021). Our results were broadly in line with those of previous studies; for example, the contents of O2.– and H2O2 in VvANN1 transgenic plants were lower than Col-0 plants under drought stress (Figures 7A, B). The activities of SOD, POD and CAT in VvANN1 transgenic plants were significantly higher than in Col-0 (Figures 7C–F). Therefore, we hypothesize that VvANN1 responds to drought stress, at least in part by modulating ROS accumulation. In this study, we have not identified a direct relationship between H2O2 content and the function of VvANN1. However, our results imply that VvANN1 may play crucial role on regulation the intracellular level of H2O2.
Ca2+ acts as a second messenger in plants and regulates the activation of a wide range of downstream processes in response to environmental and developmental stimuli (Xi et al., 2017; Tong et al., 2021). Ca2+ influx is primarily dependent on ion channels, such as the cyclic nucleotide-gated channel (CNGC) or glutamate receptor-like (GLR). Annexins were shown to function as Ca2+-permeable transporters based on their Ca2+-binding ability. AtANN1 plays a positive regulatory role in response to cold stress by mediating cold-triggered Ca2+ influx; moreover, the [Ca2+]cyt elevation was reduced in atann1 mutants (Liu et al., 2021b). MYB30 regulates the oxidative and heat stress responses through AtANN1 and AtANN4 by mediating Ca2+ signals (Liao et al., 2017). ZmANN33 and ZmANN35 were involved in Ca2+ signaling transduction processes under chilling stress (He et al., 2019). Although we found that VvANN1 was capable of Ca2+-binding activity (Supplemental Figure S3), the exact mechanism underlying how VvANN1 regulates Ca2+ under drought stress remains to be further explored.
We propose a hypothetical model depicting the role of VvANN1 in response to drought stress based on our findings (Figure 8). The ABRE binding protein VvbZIP45 directly binds to the promoter region of VvANN1 and activates its expression, thus further modulating ROS to alleviate the damage caused by drought stress. Our study provides insights into the roles of ANNEXINs in regulating drought responses in grapevine.
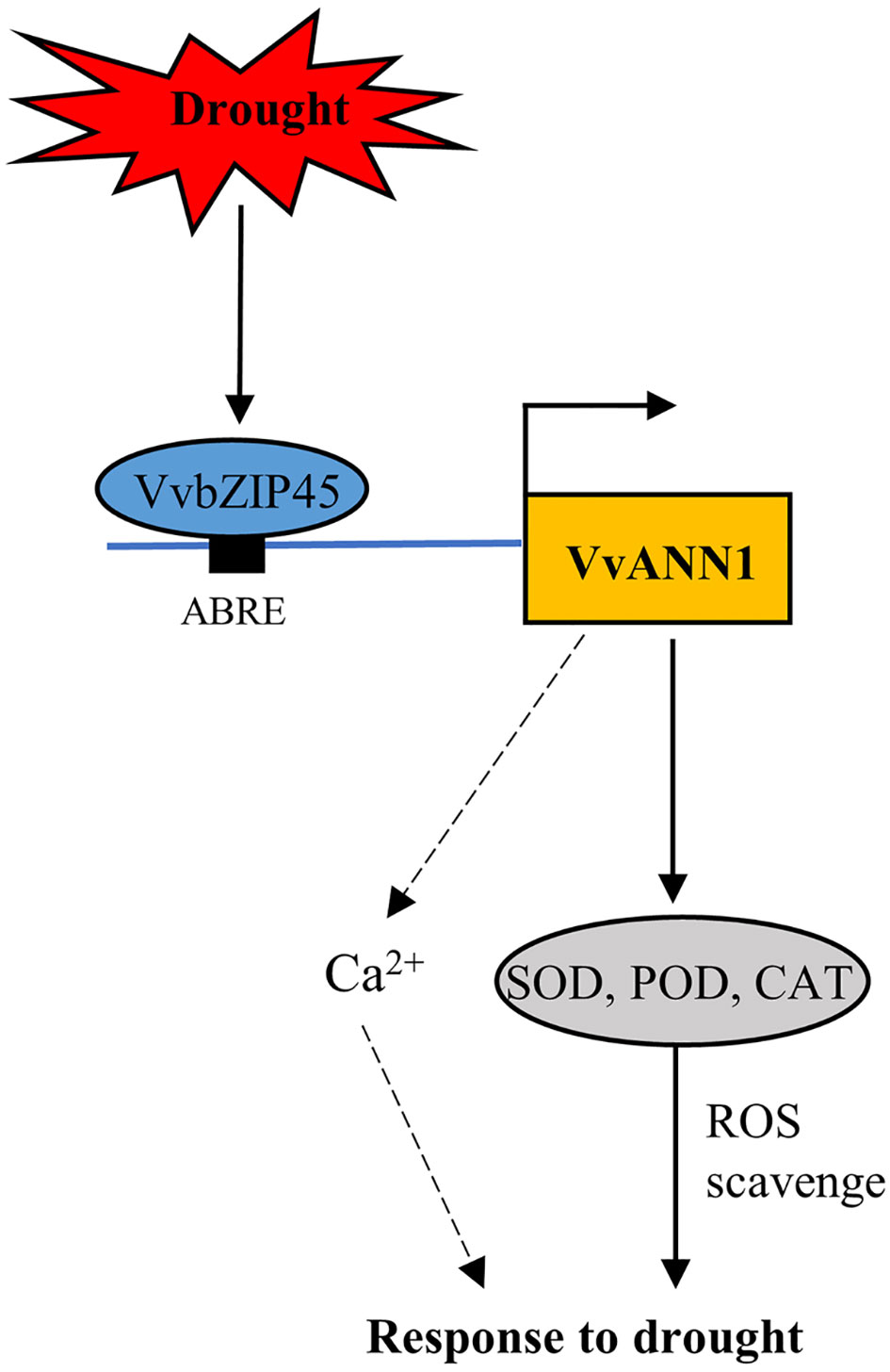
Figure 8 A hypothetical working model of VvbZIP45 modulates VvANN1 response to drought stress. Drought stress induces the expression of VvbZIP45 in grapevine, which then positively regulates the transcription of VvANN1. VvANN1 enhances the activity of SOD, POD, and CAT to scavenge excess ROS. VvANN1 may also participate in Ca2+ signaling transduction in grapevine under drought stress.
Data availability statement
The raw data supporting the conclusions of this article will be made available by the authors, without undue reservation.
Author contributions
ZZ and SN designed this study. SN performed most of the experiments. XG, QZ, XT, ZC, JL, XW, CY, ZL, and XW assisted in some experiments. ZZ, SN, and XG wrote the manuscript. All authors contributed to the article and approved the submitted version.
Funding
This work was supported by Natural Science Foundation of Hebei Province of China (C2020205019), the National Natural Science Foundation of China (31571638, 31340046).
Conflict of interest
The authors declare that the research was conducted in the absence of any commercial or financial relationships that could be construed as a potential conflict of interest.
Publisher’s note
All claims expressed in this article are solely those of the authors and do not necessarily represent those of their affiliated organizations, or those of the publisher, the editors and the reviewers. Any product that may be evaluated in this article, or claim that may be made by its manufacturer, is not guaranteed or endorsed by the publisher.
Supplementary material
The Supplementary Material for this article can be found online at: https://www.frontiersin.org/articles/10.3389/fpls.2023.1128002/full#supplementary-material
Supplementary Figure 1 | Subcellular localization of VvANN1-GFP. Subcellular localization of VvANN1-GFP in grape protoplasts. Protoplasts transformed with 35S::GFP were used as control. Scale bars=20 μm.
Supplementary Figure 2 | Affinity purification of VvANN1-His protein. Purification of VvANN1-His recombinant protein was performed with 0.2 mL elution buffer containing 500 mM imidazole (lane 1), lane 2 shows the molecular weight marker.
Supplementary Figure 3 | The Ca2+-binding capacity of VvANN1-His. Fluorescence intensity of VvANN1-His without (black curve) or with (grey curve) the addition of 2 mM CaCl2.
Supplementary Figure 4 | Expression patterns of VvANN1 under PEG treatment. (A) RT-qPCR analysis of VvANN1 expression in the five-week-old Vitis spp. cv ‘Summer Black’ and ‘Venus’ plantlets after 10% PEG6000 treatment. VvACTIN7 was used as an internal control and compared to expression in 0 h. Values represent the means ± SD from three independent repeats, and different letters indicate significant differences (one-way ANOVA, P<0.05). (B) GUS staining of six-day-old VvANN1Pro::GUS transgenic Arabidopsis seedlings under normal and PEG treatment. Scale bars=1 mm.
Supplementary Figure 5 | RT-qPCR analysis of VvANN1 expression in different VvANN1 transgenic Arabidopsis lines. AtACTIN2 was used as an internal control. Values represent the means ± SD from three independent repeats.
Supplementary Figure 6 | VvbZIP45 transcript expression levels under PEG treatment in five-week-old Vitis spp. cv ‘Venus’. VvACTIN7 was used as an internal control and compared to expression in 0 h. Values represent the means ± SD from three independent repeats, and different letters indicate significant differences (one-way ANOVA, P < 0.05).
References
Ali, K., Maltese, F., Choi, Y. H., Verpoorte, R. (2010). Metabolic constituents of grapevine and grape-derived products. Phytochem. Rev. 9, 357–378. doi: 10.1007/s11101-009-9158-0
Apel, K., Hirt, H. (2004). Reactive oxygen species: metabolism, oxidative stress, and signal transduction. Annu. Rev. Plant Biol. 55, 373–399. doi: 10.1146/annurev.arplant.55.031903.141701
Bi, C., Yu, Y., Dong, C., Yang, Y., Zhai, Y., Du, F., et al. (2021). The bZIP transcription factor TabZIP15 improves salt stress tolerance in wheat. Plant Biotechnol. J. 19, 209–211. doi: 10.1111/pbi.13453
Briz-Cid, N., Pose-Juan, E., Rial-Otero, R., Simal-Gándara, J. (2016). Proteome changes in garnacha tintorera red grapes during post-harvest drying. LWT-Food Sci. Technol. 69, 608–613. doi: 10.1016/j.lwt.2016.02.026
Chen, J., Mao, L., Mi, H., Lu, W., Ying, T., Luo, Z. (2016). Involvement of three annexin genes in the ripening of strawberry fruit regulated by phytohormone and calcium signal transduction. Plant Cell Rep. 35, 733–743. doi: 10.1007/s00299-015-1915-5
Clark, G. B., Morgan, R. O., Fernandez, M. P., Roux, S. J. (2012). Evolutionary adaptation of plant annexins has diversified their molecular structures, interactions and functional roles. New Phytol. 196, 695–712. doi: 10.1111/j.1469-8137.2012.04308.x
Corrêa, L. G., Riaño-Pachón, D. M., Schrago, C. G., Dos Santos, R. V., Mueller-Roeber, B., Vincentz, M. (2008). The role of bZIP transcription factors in green plant evolution: adaptive features emerging from four founder genes. PloS One 3, e2944. doi: 10.1371/journal.pone.0002944
Cruz De Carvalho, M. H. (2008). Drought stress and reactive oxygen species: Production, scavenging and signaling. Plant Signal Behav. 3, 156–165. doi: 10.4161/psb.3.3.5536
Demidchik, V., Shabala, S., Isayenkov, S., Cuin, T. A., Pottosin, I. (2018). Calcium transport across plant membranes: mechanisms and functions. New Phytol. 220, 49–69. doi: 10.1111/nph.15266
Fang, L., Su, L., Sun, X., Li, X., Sun, M., Karungo, S. K., et al. (2016). Expression of Vitis amurensis NAC26 in Arabidopsis enhances drought tolerance by modulating jasmonic acid synthesis. J. Exp. Bot. 67, 2829–2845. doi: 10.1093/jxb/erw122
Gao, S., Song, T., Han, J., He, M., Zhang, Q., Zhu, Y., et al. (2020). A calcium-dependent lipid binding protein, OsANN10, is a negative regulator of osmotic stress tolerance in rice. Plant Sci. 293, 110420. doi: 10.1016/j.plantsci.2020.110420
He, F., Gao, C., Guo, G., Liu, J., Gao, Y., Pan, R., et al. (2019). Maize annexin genes ZmANN33 and ZmANN35 encode proteins that function in cell membrane recovery during seed germination. J. Exp. Bot. 70, 1183–1195. doi: 10.1093/jxb/ery452
He, X., Liao, L., Xie, S., Yao, M., Xie, P., Liu, W., et al. (2020). Comprehensive analyses of the annexin (ANN) gene family in Brassica rapa, Brassica oleracea and Brassica napus reveals their roles in stress response. Sci. Rep. 10, 4295. doi: 10.1038/s41598-020-59953-w
Hou, L., Fan, X., Hao, J., Liu, G., Zhang, Z., Liu, X. (2020). Negative regulation by transcription factor VvWRKY13 in drought stress of Vitis vinifera l. Plant Physiol. Bioch. 148, 114–121. doi: 10.1016/j.plaphy.2020.01.008
Hussain, H. A., Men, S., Hussain, S., Chen, Y., Ali, S., Zhang, S., et al. (2019). Interactive effects of drought and heat stresses on morpho-physiological attributes, yield, nutrient uptake and oxidative status in maize hybrids. Sci. Rep. 9, 3890. doi: 10.1038/s41598-019-40362-7
Jami, S. K., Clark, G. B., Ayele, B. T., Ashe, P., Kirti, P. B. (2012). Genome-wide comparative analysis of annexin superfamily in plants. PloS One 7, e47801. doi: 10.1371/journal.pone.0047801
Kooyers, N. J. (2015). The evolution of drought escape and avoidance in natural herbaceous populations. Plant Sci. 234, 155–162. doi: 10.1016/j.plantsci.2015.02.012
Laohavisit, A., Davies, J. M. (2011). Annexins. New Phytol. 189, 40–53. doi: 10.1111/j.1469-8137.2010.03533.x
Laohavisit, A., Richards, S. L., Shabala, L., Chen, C., Colaço, R. D., Swarbreck, S. M., et al. (2013). Salinity-induced calcium signaling and root adaptation in Arabidopsis require the calcium regulatory protein annexin1. Plant Physiol. 163, 253–262. doi: 10.1104/pp.113.217810
Lee, N., Wetzstein, H. Y. (1988). Protoplast isolation and callus production from leaves of tissue-cultured Vitis spp. Plant Cell Rep. 7, 531–534. doi: 10.1007/bf00272749
Li, Z., Fu, D., Wang, X., Zeng, R., Zhang, X., Tian, J., et al. (2022). The transcription factor bZIP68 negatively regulates cold tolerance in maize. Plant Cell. 34, 2833–2851. doi: 10.1093/plcell/koac137
Li, D., Fu, F., Zhang, H., Song, F. (2015). Genome-wide systematic characterization of the bZIP transcriptional factor family in tomato (Solanum lycopersicum l.). BMC Genom. 16, 771. doi: 10.1186/s12864-015-1990-6
Li, Z., Tang, J., Srivastava, R., Bassham, D. C., Howell, S. H. (2020). The transcription factor bZIP60 links the unfolded protein response to the heat stress response in maize. Plant Cell. 32, 3559–3575. doi: 10.1105/tpc.20.00260
Li, G., Xu, W., Jing, P., Hou, X., Fan, X. (2021). Overexpression of VyDOF8, a Chinese wild grapevine transcription factor gene, enhances drought tolerance in transgenic tobacco. Environ. Exp. Bot. 190, 104592. doi: 10.1016/j.envexpbot.2021.104592
Liao, C., Zheng, Y., Guo, Y. (2017). MYB30 transcription factor regulates oxidative and heat stress responses through ANNEXIN-mediated cytosolic calcium signaling in Arabidopsis. New Phytol. 216, 163–177. doi: 10.1111/nph.14679
Liu, J., Chen, N., Chen, F., Cai, B., Dal Santo, S., Tornielli, G. B., et al. (2014). Genome-wide analysis and expression profile of the bZIP transcription factor gene family in grapevine (Vitis vinifera). BMC Genom. 15, 281. doi: 10.1186/1471-2164-15-281
Liu, J., Chu, J., Ma, C., Jiang, Y., Ma, Y., Xiong, J., et al. (2019). Overexpression of an ABA-dependent grapevine bZIP transcription factor, VvABF2, enhances osmotic stress in Arabidopsis. Plant Cell Rep. 38, 587–596. doi: 10.1007/s00299-019-02389-y
Liu, Q., Ding, Y., Shi, Y., Ma, L., Wang, Y., Song, C., et al. (2021b). The calcium transporter ANNEXIN1 mediates cold-induced calcium signaling and freezing tolerance in plants. EMBO J. 40, e104559. doi: 10.15252/embj.2020104559
Liu, C., Kang, H., Wang, Y., Yao, Y., Gao, Z., Du, Y. (2021a). Melatonin relieves ozone stress in grape leaves by inhibiting ethylene biosynthesis. Front. Plant Sci. 12. doi: 10.3389/fpls.2021.702874
Lovisolo, C., Perrone, I., Carra, A., Ferrandino, A., Flexas, J., Medrano, H., et al. (2010). Drought-induced changes in development and function of grapevine (Vitis spp.) organs and in their hydraulic and non-hydraulic interactions at the whole-plant level: A physiological and molecular update. Funct. Plant Biol. 37, 98–116. doi: 10.1071/FP09191
Mahmood, T., Khalid, S., Abdullah, M., Ahmed, Z., Shah, M. K. N., Ghafoor, A., et al. (2019). Insights into drought stress signaling in plants and the molecular genetic basis of cotton drought tolerance. Cells. 9, 105. doi: 10.3390/cells9010105
Miller, G., Suzuki, N., Ciftci-Yilmaz, S., Mittler, R. (2010). Reactive oxygen species homeostasis and signalling during drought and salinity stresses. Plant Cell Environ. 33, 453–467. doi: 10.1111/j.1365-3040.2009.02041.x
Mittler, R., Vanderauwera, S., Gollery, M., Van Breusegem, F. (2004). Reactive oxygen gene network of plants. Trends Plant Sci. 9, 490–498. doi: 10.1016/j.tplants.2004.08.009
Mortimer, J. C., Laohavisit, A., Macpherson, N., Webb, A., Brownlee, C., Battey, N. H., et al. (2008). Annexins: multifunctional components of growth and adaptation. J. Exp. Bot. 59, 533–544. doi: 10.1093/jxb/erm344
Nicolas, P., Lecourieux, D., Kappel, C., Cluzet, S., Cramer, G., Delrot, S., et al. (2014). The basic leucine zipper transcription factor ABSCISIC ACID RESPONSE ELEMENT-BINDING FACTOR2 is an important transcriptional regulator of abscisic acid-dependent grape berry ripening processes. Plant Physiol. 164, 365–383. doi: 10.1104/pp.113.231977
Nijhawan, A., Jain, M., Tyagi, A. K., Khurana, J. P. (2008). Genomic survey and gene expression analysis of the basic leucine zipper transcription factor family in rice. Plant Physiol. 146, 333–350. doi: 10.1104/pp.107.112821
Pan, R., Buitrago, S., Feng, Z., Abou-Elwafa, S. F., Xu, L., Li, C., et al. (2022). HvbZIP21, a novel transcription factor from wild barley confers drought tolerance by modulating ROS scavenging. Front. Plant Sci. 13. doi: 10.3389/fpls.2022.878459
Qiao, B., Zhang, Q., Liu, D., Wang, H., Yin, J., Wang, R., et al. (2015). A calcium-binding protein, rice annexin OsANN1, enhances heat stress tolerance by modulating the production of H2O2. J. Exp. Bot. 66, 5853–5866. doi: 10.1093/jxb/erv294
Rescher, U., Gerke, V. (2004). Annexins–unique membrane binding proteins with diverse functions. J. Cell Sci. 117, 2631–2639. doi: 10.1242/jcs.01245
Romero, P., Dodd, I. C., Martinez-Cutillas, A. (2012). Contrasting physiological effects of partial root zone drying in field-grown grapevine (Vitis vinifera l. cv. monastrell) according to total soil water availability. J. Exp. Bot. 63, 4071–4083. doi: 10.1093/jxb/ers088
Saad, R. B., Ben Romdhane, W., Ben Hsouna, A., Mihoubi, W., Harbaoui, M., Brini, F. (2020). Insights into plant annexins function in abiotic and biotic stress tolerance. Plant Signal Behav. 15, 1699264. doi: 10.1080/15592324.2019.1699264
Shen, F., Ying, J., Xu, L., Sun, X., Wang, J., Wang, Y., et al. (2021). Characterization of annexin gene family and functional analysis of RsANN1a involved in heat tolerance in radish (Raphanus sativus l.). Physiol. Mol. Biol. Plants 27, 2027–2041. doi: 10.1007/s12298-021-01056-5
Su, L., Fang, L., Zhu, Z., Zhang, L., Sun, X., Wang, Y., et al. (2020). The transcription factor VaNAC17 from grapevine (Vitis amurensis) enhances drought tolerance by modulating jasmonic acid biosynthesis in transgenic Arabidopsis. Plant Cell Rep. 39, 621–634. doi: 10.1007/s00299-020-02519-x
Tong, T., Li, Q., Jiang, W., Chen, G., Xue, D., Deng, F., et al. (2021). Molecular evolution of calcium signaling and transport in plant adaptation to abiotic stress. Int. J. Mol. Sci. 22, 22. doi: 10.3390/ijms222212308
Wang, H., Wang, W., Zhan, J., Huang, W., Xu, H. J. S. H. (2015). An efficient PEG-mediated transient gene expression system in grape protoplasts and its application in subcellular localization studies of flavonoids biosynthesis enzymes. Sci. Hortic. 191, 82–89. doi: 10.1016/j.scienta.2015.04.039
Waseem, M., Rong, X., Li, Z. (2019). Dissecting the role of a basic helix-loop-helix transcription factor, SlbHLH22, under salt and drought stresses in transgenic Solanum lycopersicum l. Front. Plant Sci. 10. doi: 10.3389/fpls.2019.00734
Wei, K., Chen, J., Wang, Y., Chen, Y., Chen, S., Lin, Y., et al. (2012). Genome-wide analysis of bZIP-encoding genes in maize. DNA Res. 19, 463–476. doi: 10.1093/dnares/dss026
Xi, Y., Liu, J., Dong, C., Cheng, Z. M. (2017). The CBL and CIPK gene family in grapevine (Vitis vinifera): Genome-wide analysis and expression profiles in response to various abiotic stresses. Front. Plant Sci. 8. doi: 10.3389/fpls.2017.00978
Xiang, Y., Tang, N., Du, H., Ye, H., Xiong, L. (2008). Characterization of OsbZIP23 as a key player of the basic leucine zipper transcription factor family for conferring abscisic acid sensitivity and salinity and drought tolerance in rice. Plant Physiol. 148, 1938–1952. doi: 10.1104/pp.108.128199
Xiong, H., Yu, J., Miao, J., Li, J., Zhang, H., Wang, X., et al. (2018). Natural variation in OsLG3 increases drought tolerance in rice by inducing ROS scavenging. Plant Physiol. 178, 451–467. doi: 10.1104/pp.17.01492
Xu, Z. Y., Kim, S. Y., Hyeon Do, Y., Kim, D. H., Dong, T., Park, Y., et al. (2013). The Arabidopsis NAC transcription factor ANAC096 cooperates with bZIP-type transcription factors in dehydration and osmotic stress responses. Plant Cell. 25, 4708–4724. doi: 10.1105/tpc.113.119099
Xu, W., Shen, W., Ma, J., Ya, R., Zheng, Q., Wu, N., et al. (2020). Role of an amur grape CBL-interacting protein kinase VaCIPK02 in drought tolerance by modulating ABA signaling and ROS production. Environ. Exp. Bot. 172, 103999. doi: 10.1016/j.envexpbot.2020.103999
Yamaguchi, N., Winter, C. M., Wu, M. F., Kwon, C. S., William, D. A., Wagner, D. (2014). PROTOCOLS: Chromatin immunoprecipitation from Arabidopsis tissues. Arabidopsis Book. 12, e0170. doi: 10.1199/tab.0170
Yang, S., Xu, K., Chen, S., Li, T., Xia, H., Chen, L., et al. (2019). A stress-responsive bZIP transcription factor OsbZIP62 improves drought and oxidative tolerance in rice. BMC Plant Biol. 19, 260. doi: 10.1186/s12870-019-1872-1
You, J., Chan, Z. (2015). ROS regulation during abiotic stress responses in crop plants. Front. Plant Sci. 6. doi: 10.3389/fpls.2015.01092
Zhang, Q., Song, T., Guan, C., Gao, Y., Ma, J., Gu, X., et al. (2021). OsANN4 modulates ROS production and mediates Ca(2+) influx in response to ABA. BMC Plant Biol. 21, 474. doi: 10.1186/s12870-021-03248-3
Zhang, B., Zhang, C., Liu, C., Jing, Y., Wang, Y., Jin, L., et al. (2018a). Inner envelope CHLOROPLAST MANGANESE TRANSPORTER 1 supports manganese homeostasis and phototrophic growth in Arabidopsis. Mol. Plant 11, 943–954. doi: 10.1016/j.molp.2018.04.007
Zhang, G., Zhang, M., Zhao, Z., Ren, Y., Li, Q., Wang, W. (2017). Wheat TaPUB1 modulates plant drought stress resistance by improving antioxidant capability. Sci. Rep. 7, 7549. doi: 10.1038/s41598-017-08181-w
Zhang, Y., Zhao, H., Zhou, S., He, Y., Luo, Q., Zhang, F., et al. (2018b). Expression of TaGF14b, a 14-3-3 adaptor protein gene from wheat, enhances drought and salt tolerance in transgenic tobacco. Planta 248, 117–137. doi: 10.1007/s00425-018-2887-9
Zhao, W., Wang, X., Zhang, Q., Zheng, Q., Yao, H., Gu, X., et al. (2022). H3K36 demethylase JMJ710 negatively regulates drought tolerance by suppressing MYB48-1 expression in rice. Plant Physiol. 189, 1050–1064. doi: 10.1093/plphys/kiac095
Zhou, Y. B., Liu, C., Tang, D. Y., Yan, L., Wang, D., Yang, Y. Z., et al. (2018). The receptor-like cytoplasmic kinase STRK1 phosphorylates and activates CatC, thereby regulating H2O2 homeostasis and improving salt tolerance in rice. Plant Cell. 30, 1100–1118. doi: 10.1105/tpc.17.01000
Keywords: grapevine, annexin, bZIP transcription factor, drought stress, ROS
Citation: Niu S, Gu X, Zhang Q, Tian X, Chen Z, Liu J, Wei X, Yan C, Liu Z, Wang X and Zhu Z (2023) Grapevine bZIP transcription factor bZIP45 regulates VvANN1 and confers drought tolerance in Arabidopsis. Front. Plant Sci. 14:1128002. doi: 10.3389/fpls.2023.1128002
Received: 20 December 2022; Accepted: 30 January 2023;
Published: 09 February 2023.
Edited by:
Ravinder Kumar, Central Potato Research Institute (ICAR), IndiaReviewed by:
Alessandra Ruggiero, National Research Council (CNR), ItalyPrafull Salvi, National Agri-Food Biotechnology Institute, India
Ujjal Jyoti Phukan, University of Arizona, United States
Alok Ranjan, Indian Institute of Agricultural Biotechnology (ICAR), India
Copyright © 2023 Niu, Gu, Zhang, Tian, Chen, Liu, Wei, Yan, Liu, Wang and Zhu. This is an open-access article distributed under the terms of the Creative Commons Attribution License (CC BY). The use, distribution or reproduction in other forums is permitted, provided the original author(s) and the copyright owner(s) are credited and that the original publication in this journal is cited, in accordance with accepted academic practice. No use, distribution or reproduction is permitted which does not comply with these terms.
*Correspondence: Zhengge Zhu, emh1emhlbmdnZUBoZWJ0dS5lZHUuY24=