- 1Department of Chemistry and Biotechnology, Tallinn University of Technology, Tallinn, Estonia
- 2Department of Plant Sciences, Faculty of Biosciences, Norwegian University of Life Sciences (NMBU), Ås, Norway
- 3Department of Microbiology and Biotechnology, Faculty of Biology, University of Latvia, Riga, Latvia
- 4Laboratory of Genetics and Physiology, Institute of Agriculture, Lithuanian Research Centre for Agriculture and Forestry, Akademija, Lithuania
Due to an increase in the consumption of food, feed, and fuel and to meet global food security needs for the rapidly growing human population, there is a necessity to obtain high-yielding crops that can adapt to future climate changes. Currently, the main feed source used for ruminant livestock production is forage grasses. In temperate climate zones, perennial grasses grown for feed are widely distributed and tend to suffer under unfavorable environmental conditions. Genome editing has been shown to be an effective tool for the development of abiotic stress-resistant plants. The highly versatile CRISPR-Cas system enables increasingly complex modifications in genomes while maintaining precision and low off-target frequency mutations. In this review, we provide an overview of forage grass species that have been subjected to genome editing. We offer a perspective view on the generation of plants resilient to abiotic stresses. Due to the broad factors contributing to these stresses the review focuses on drought, salt, heat, and cold stresses. The application of new genomic techniques (e.g., CRISPR-Cas) allows addressing several challenges caused by climate change and abiotic stresses for developing forage grass cultivars with improved adaptation to the future climatic conditions. Genome editing will contribute towards developing safe and sustainable food systems.
1 Introduction
Grasses belong to the family of Poaceae, which constitutes the most economically important plant family (Lee et al., 2020; Huang et al., 2022). Grasslands and meadows extend over vast portions of the planet, on land, and even under the sea (Lopez et al., 2022; McSteen and Kellogg, 2022). Their importance in Earth’s ecosystems goes beyond their use in fields and pastures. Grassy biomes comprise more than one-quarter of the planet’s land area. Grasses not only provide food, shelter, and building materials for animals and humans, but they also generate oxygen and store carbon (Strömberg and Staver, 2022). This storage, mainly subterranean, contributes towards the fertilization of soils and makes grasslands valuable sinks of CO2 (Bengtsson et al., 2019; Terrer et al., 2021). Furthermore, grasses are considered more resilient to dryer and warmer conditions than trees. These facts suggest that in the climatic conditions predicted for the future, grasslands could be a better and more robust carbon sink than forests (Dass et al., 2018).
Grass crops provide the most essential dietary food sources globally. From these, forage grasses are the main component used to feed ruminant livestock (FAO, 2018; FAO, 2019). Grasses can be cultivated in less fertile lands compared to other crops. In these zones, normally associated with developing countries (Feller et al., 2012; Kwadzo and Quayson, 2021), animal husbandry and its derivates e.g., dairy production, remain essential (Capstaff and Miller, 2018; Moorby and Fraser, 2021). To cope with the predicted population growth and the consequential increase in food needs, high-yielding crops must be further developed (Raza et al., 2019). To reach food security, the strategies used must avoid causing negative environmental impacts. Synthetic nitrogen-based fertilizers have been important for reaching high yields, nevertheless, their production and usage are a source of massive generation and emission of greenhouse gases (GHGs) (Chai et al., 2019). It is well known that the high concentration of atmospheric GHGs is closely related to climate change. Therefore, the challenge is to increase farming efficiency while reducing the impact of agricultural activity on climate change (Rivero et al., 2021). Importantly, climate change not only directly affects crop productivity but also has indirect and socio-economic impacts, for instance soil fertility, need for irrigation, food demand, policy, rising costs (reviewed in (Raza et al., 2019)).
Grasses usage as forage and as reliable sinks of carbon emissions, call for an improvement in their biomass yield, and their resistance towards the new abiotic and biotic stresses caused by climate change (Giridhar and Samireddypalle, 2015). Especially, plants will have to cope with variations in temperature, water availability, and soil composition (Cushman et al., 2022). Said variations will generate stresses due to heat, cold, drought, and salinity conditions. A promising approach to provide grasses with stress resistance is using genome editing techniques (Bailey-Serres et al., 2019; Dhakate et al., 2022). The first attempts have been performed to use genome editing in forage grasses (Liu et al., 2020b; Weiss et al., 2020; Zhang et al., 2020; Cheng et al., 2021; Zhang et al., 2021; Zhu et al., 2021; Kumar et al., 2022; Wang et al., 2022). This is not an easy task due to their reproductive and genetic characteristics which are difficult to work with. The inability of forage grasses to self-pollinate hinders inbreeding. Additionally, forage grasses have high variability between the genetic background of different individuals. This provides them with a considerable gene pool, responsible for their adaptability and resilience towards environmental changes. Conversely, it creates difficulties for studies focused on identifying the genetic cause of traits or phenotypes of interest (Cropano et al., 2021; Muguerza et al., 2022). There are diverse ways of classifying grasses beyond their taxonomy. For instance, forage grasses can be divided into different types depending on their life cycle and ecotype. In the first case, according to the survival of the plant after going through its reproductive phase, grasses can be considered annual, biannual, or perennial. In terms of their ecotype, grasses can be separated into warm- or cool-season plants if their optimal growth happens during winter or summer, respectively. Importantly, warm-season grasses are C4 plants, while cool-season grasses are C3 plants (Moser and Hoveland, 1996; Moser et al., 2004).
In this review, we provide an overview of the main metabolic and molecular changes that plants suffer to cope with the effects of abiotic stress derived from climate change. Additionally, we summarize the actual state of genome editing applications in forage grasses. We propose how genome editing could be used to generate grass plants able to resist these abiotic stresses. Finally, we hypothesize how the new genetic resources and tools can be used to improve forage grass breeding that will help achieve food security in a sustainable way.
2 Cellular and molecular responses to cope with the main abiotic stresses
Extreme temperatures, uncommon precipitation patterns, and deterioration of soils are being observed due to climate change. These environmental consequences have a great impact on agriculture since plants are of sessile nature. The responses used by plants when encountering a stressor aim firstly to achieve acclimation to the new environment and later adaptation to it. Acclimation includes adjusting the physiology and metabolism of a plant to achieve a new state of homeostasis, while adaptation involves both phenotypic and genotypic alterations. Acclimation mediates quick responses to ensure the survival of a plant, whereas adaptation is considered an evolutionary and lengthy process whose goal is to preserve a population. Additionally, plants undergo epigenetic modifications when a stressing event happens (Guarino et al., 2022). Plants must cope with new and more extreme conditions, which lead to different abiotic and biotic stresses than those commonly present in their biomes (Sugimoto and Nowack, 2022). Abiotic stresses are those derived from the physical and chemical factors of an environment and are independent of living organisms (He et al., 2018). As a response to these environmental alterations, plants undergo morphological, metabolic, and physiological changes. In this review, we will focus on drought, salinity, cold, and heat stress responses at the cellular and molecular levels. These are not the only abiotic conditions that will vary due to climate change, but they represent some of the major alterations that will result from it (He et al., 2018; Villalobos-López et al., 2022). The stresses discussed in this review have a significant impact on the growth and development of plants, which is directly connected to crops’ yield and profitability (Bita and Gerats, 2013; Bulgari et al., 2019).
Even though the abiotic stresses will be described separately, in nature they tend to interact producing greater effects than individually. Therefore, plants normally must acclimate to a combination of stresses. This should not be ignored when designing strategies to improve crops’ tolerance to stress (Pascual et al., 2022).
2.1 Temperature conditions
One of the main effect of climate change is the alteration of temperature conditions (Pörtner et al., 2022). Temperature affects and limits plant growth and development directly (Loka et al., 2019). Therefore, it has a great impact on crop yield which is associated with food security (FAO, 2018; FAO, 2019; Pörtner et al., 2022). It is considered that there are two abiotic stresses derived from temperature variations: heat and cold stress.
2.1.1 Heat stress
As a direct consequence of climate change, global warming has led to steady and yearly temperature increase. Even in temperate zone it has become common to experience warmer seasons with particularly extreme temperatures during summer. Hence, heat waves have increased worldwide causing heat stress for plants (Jagadish et al., 2021). Heat stress appears with sudden increases in temperature, 10 or 15°C above usual conditions (Liu et al., 2020a), and its consequence depends on the plant genotype and ecotype, on the level of incremented temperature, and on the length of the stress (Hasanuzzaman et al., 2013; Wang et al., 2018). Plants may survive heat stress through heat-avoidance or heat-tolerance mechanisms (Aleem et al., 2020). The avoidance processes intend to ensure the survival of a plant, for example altering its leaf orientation or regulating its stomatal conductance, while heat-tolerance mechanisms are related to the plant’s ability to maintain its growth under heat stress. These processes involve the synthesis and regulation of different enzymes and other proteins (Hasanuzzaman et al., 2013). Plants primary sensing mechanism towards heat stress is located in the plasma membrane of cells. These membranes become more fluid and permeable under heat stress, which activates heat sensor proteins. It is believed that these heat sensors are, or interact, with calcium channels (Bourgine and Guihur, 2021). Calcium is known to be a key molecule involved in the activation of diverse stress responses mechanisms (Xu et al., 2022). Different transmembrane proteins related to calcium transport have been proposed to act as heat sensors. Members of the Annexin gene family, the protein Synaptotagmin A (SYTA) in Arabidospis thaliana (L.) Heynh. and the Cyclic Nucleotide-Gated Channels (CNGCs) are examples of heat sensor proteins from plants (DeFalco et al., 2016; Yan et al., 2017; Wu et al., 2022). The CNGCs are cation channels that regulate the entrance of ions, e.g., Ca2+, into the cytosol from the apoplast and have a calmodulin-binding domain in their cytosolic region. This suggests that increased levels of cytosolic Ca2+ trigger an unknown signaling cascade that mediates the accumulation of heat-shock proteins (HSPs) (Bourgine and Guihur, 2021). In rice, the induced loss of function of two of these CNGCs proteins, OsCNGC14 and OsCNGC16, showed that mutant plants exhibited reduced survival when exposed to both heat and cold stresses. This concurs with the observed role of CNGCs in heat stress signaling and shows that temperature stresses have overlapping signaling mechanisms (Cui et al., 2020). The abrupt changes derived from heat stress can degrade cellular components, altering the composition of membranes and denaturing proteins. Moreover, oxidative stress is also a common result of abiotic stresses. In consequence, the production of reactive oxygen species (ROS) increases. ROS can be generated in different cellular compartments, such as peroxisomes, mitochondria, and chloroplasts (Hasanuzzaman et al., 2020). These molecules are very toxic and can end up inducing cell death due to damage to proteins, cell membranes, and even DNA (Singh et al., 2019). To avoid drastic consequences, cells induce the synthesis of HSPs and heat-shock transcription factors (HSFs). In response to heat stress, these transcription factors bind the heat-shock elements (HSEs) that are conserved regions of the HSPs genes. This leads to increased levels of HSPs in the cells, which aims to preserve the integrity of cell proteins by preventing their misfolding and aggregation thanks to the chaperoning role of HSPs (Krishna, 2004). The overexpression of Lolium arundinaceum (Schreb.) Darbysh. heat stress transcription factor A2c (HsfA2c) produced plants tolerant to heat stress (Wang et al., 2017). In addition, to prevent damage from oxidative stress plants can use different antioxidant enzymes like peroxidase and catalase. The plant species and ecotype determine which enzymes will be responsible for coping with oxidative stress (Hasanuzzaman et al., 2020). Importantly, metabolic changes, like alterations in enzymes’ activity, also occur due to heat stress. In plants, for example, the oxygenase activity of rubisco rises, leading to more photorespiration and therefore reduced carbon fixation and photosynthesis. Furthermore, heat stress alters the degradation and synthesis of carotenoids and chlorophyll that causes a more pronounced decrease in photosynthetic activity (Loka et al., 2019).
2.1.2 Low temperature tolerance and winter hardiness
Winter survival of forage grasses is a very complex trait determined by the interaction of abiotic stresses like low temperature, frost, desiccation, water logging, ice-encasement and snow cover, which also can cause biotic stress by low-temperature fungi (Rognli, 2013). Winter hardiness, persistency and stable high yields are limiting factors for forage grass production in temperate regions. Short growing seasons with long days, the long winter with short days and low light intensity cause stressful conditions for perennial plants. Cold acclimation, tolerance to freezing and ice-encasement are crucial components of winter survival. Plant species from temperate climates, which are frequently exposed to sub-zero temperatures have developed advanced mechanisms to cope with extended periods of cold during winters. These plant species, when exposed to low but non-lethal temperatures, increase their freezing tolerance through a process called cold acclimation (Thomashow, 1999; Chinnusamy et al., 2007). Most forage grass species and winter-types of cereals need vernalization, i.e., the induction of flowering when exposed to low temperatures (Fjellheim et al., 2014). During autumn the plants produce only leaves until the vernalization requirement is met and the tillers switch from vegetative to generative growth. However, stem elongation and flowering need long days and normal growth temperatures and will not happen until spring (Heide, 1994).
Long duration of ice cover (ice-encasement) is the major cause of winter damage (Gudleifsson, 2009). Warm spells in winter cause snowmelt, which then form non-permeable ice layers when the temperature returns to below zero, causing anoxic conditions for plants (Larsen, 1994). Though freezing tolerance gives a good estimate for winter hardiness, the correlation between freezing tolerance and tolerance to ice-encasement is relatively less known (Andrews and Gudleifsson, 1983). Studies by Gudleifsson and colleagues showed a weak correlation (r=0.36) between freezing tolerance and ice-encasement (Gudleifsson et al., 1986).
Freezing tolerance is a complex dynamic trait which requires a fine-tuned coordinated response at the physiological and sub-cellular level in relation to environmental cues to induce physiological, biochemical, and metabolic changes (Maruyama et al., 2014; Nakaminami et al., 2014). Many of these resulting cold-associated changes are mainly due to changes in gene expression (Yamaguchi-Shinozaki and Shinozaki, 2006; Thomashow, 2010; Maruyama et al., 2014). Temperature, light, and a complex interaction of these two variables are key factors driving the process of cold acclimation and determining the extent of freezing tolerance acquired (Gray et al., 1997; Janda et al., 2014; Rapacz et al., 2014; Dalmannsdottir et al., 2017).
With the increase in autumn temperatures, cold acclimation will occur during late autumn or early winter under different irradiance levels than normal conditions (Dalmannsdottir et al., 2016; Dalmannsdottir et al., 2017). Water logging conditions as a result of the heavy precipitation in autumn during cold acclimation may also negatively affect cold acclimation and freezing tolerance (Jørgensen et al., 2020). Winter survival under novel climate conditions is likely to be determined by the ability to cold acclimate at low non-freezing temperatures, resist deacclimation during short warm spells in mid-winters and re-acclimation when the temperatures drop again after the warm spells (Kovi et al., 2016; Rapacz et al., 2017; Jaškūnė et al., 2022a).
The inducer of CBF expression (ICE), C-repeat binding factor (CBF) and cold-responsive (COR) genes are considered the master regulators of plants’ response to cold (Hwarari et al., 2022). They form the ICE-CBF-COR signaling cascade, which is known to play a key role in freezing tolerance and remains the best-characterized pathway to date (Thomashow, 2010; Ding et al., 2019b). CBF regulon consisting of genes CBF1, CBF2 and CBF3 amongst others contributes to acclimation to cold temperatures (Park et al., 2018). These genes were first studied in Arabidopsis and encode transcription factors that bind to dehydration responsive genes, as well as those with an early response to cold and dehydration (Galiba et al., 2009). Other important proteins contributing to winter survival are dehydrins (DHNs) or group 2 Late Embryogenesis Abundant (LEA) proteins. Many grass species are tolerant to freezing by upregulating DHN genes (Liu et al., 2017). Dehydrins are often regulated by CBF cold-responsive pathways. The C-repeat/dehydration-responsive element binding factors (CBF/DREB) are transcription factors that recognize and bind to the dehydration-responsive element/C-repeat (DRE/CRT) elements in the promoter of COR genes (Vazquez-Hernandez et al., 2017). The transcriptome analysis in Elymus nutans Griseb. showed that the genes encoding LEA14-A, cold-regulated plasma membrane protein COR413PM, cold-responsive protein COR14a and dehydrin COR410 had higher transcriptional abundance in a genotype with higher tolerance to cold (Fu et al., 2016). Further, quantitative trait loci (QTLs) for winter survival, frost and drought tolerance have been mapped in meadow fescue (Lolium pratense (Huds.) Darbysh.). Several of the QTLs were located in the same chromosomal regions as QTLs and genes in Triticeae species, notably DHNs, CBFs and vernalization response genes. The major frost tolerance/winter survival QTL co-located with the position of the CBF6 gene. Some of the winter survival QTLs co-located with frost tolerance QTLs, others with drought QTLs, while some were unique and most likely this was due to segregation for genes affecting seasonal adaptation, e.g., photoperiodic sensitivity (Alm et al., 2011).
In addition, perennial grass species produce water soluble carbohydrates, such as fructans and raffinose family oligosaccharides during cold acclimation (Bhowmik et al., 2006; Abeynayake et al., 2015). Fructans are an important energy source found in temperate forage grasses. They are synthesized from sucrose and can be defined as storage carbohydrates that are non-structural (Waterhouse and Chatterton, 1993). Fructans are stored in vacuoles and will either have linear or branched fructose polymers with glycosidic bonds to sucrose (Valluru and Van den Ende, 2008). The linear polyfructose molecules tend to accumulate in plants either as an addition to or instead of starch (Chalmers et al., 2005). The levels of fructan in wintering plants are involved in freezing tolerance and they are important for survival during winter and regeneration or sprouting of tissues in spring, being an important sugar supply (Yoshida, 2021). Accumulation of fructans involves fructosyltransferases, invertases and fructan exohydrolases, which are regulated tightly and moreover, their genes have been characterized and isolated (Chalmers et al., 2005; Wu et al., 2021)
2.2 Drought
Drought is one of the main environmental factors limiting crop productivity and predicted climate change shifts in the future will result in temperature increase and change in precipitation patterns (Pörtner et al., 2022). In the semiarid regions, plants have evolved defense mechanisms allowing them to cope with stressful environments and survive prolonged desiccation. These mechanisms include an elaborated antioxidant defense system and complex gene expression programs, ensuring transcription and translation of LEA proteins, heat shock proteins, and other stress-responsive genes, as well as metabolic modulations consisting of various phytohormones and phytochemicals (Farrant et al., 2015; VanBuren et al., 2017; Hilhorst et al., 2018; Oliver et al., 2020). Annual crops escape the limited water conditions by completing their reproductive cycle producing seeds. While annuals can ensure the survival of species via seeds, perennial crops must cope with water shortage using drought tolerance and avoidance strategies (Kooyers, 2015; Loka et al., 2019). Plants avoid drought by reducing transpiration and maintaining or even increasing water uptake resulting in postponed tissue dehydration. In contrast, drought tolerant perennial crops experiencing stress survive by suspending shoot growth leading to leaf desiccation. However, the crowns of the plants stay vigorous and recover under adequate rainfall. The latter two strategies are of particular importance in forage crops because they are expected to be high yielding under mild stress and to quickly recover after it. Recent studies on vegetative desiccation tolerance have linked this mechanism to seed-development processes, by showing increased expression of seed-related genes in vegetative tissues during drying (Pardo et al., 2020). The finding suggests that desiccation and water-deficit tolerance mechanisms in grasses derive from an alternative use or “rewiring” of seed-development pathways. Unraveling the key players involved in this mechanism could be a significant step towards engineering the resurrection trait into drought tolerant forage crops.
Compared to semiarid regions, the typical mild summer drought of temperate zones does not threaten crop survival but causes a significant yield penalty (Moore and Lobell, 2015; Ergon et al., 2018). The strategies result in reduction of aboveground biomass growth and accumulation, which is one of the most agronomically important traits to achieve. Genotypes adapted to water deficit might maintain growth, and under temporary drought scenario they might be considered as competitive in terms of stable biomass accumulation (Jaškūnė et al., 2020). The limited water availability triggers responses at the whole-plant, tissue, cellular and molecular levels (Farooq et al., 2009; He et al., 2018). The perceived stress signal is converted to increased levels of abscisic acid (ABA) production and accumulation in stomatal guard cells which regulate transpiration through stomata closure and thus conserve water in tissues (Wilkinson and Davies, 2010; Lee and Luan, 2012). However, this type of water loss prevention negatively affects the photosynthetic activity and this in turn results in a slowdown of growth and, under prolonged water shortage, growth halt (Farooq et al., 2009). Although ABA negatively impacts the aboveground biomass accumulation, at the same time it has an opposite effect on growth and development of roots that largely help to overcome stress (Saab et al., 1990; Li et al., 2017; Khadka et al., 2019). Nevertheless, improving forage crops for superior yield through ABA-induced drought adaptation remains a great challenge because of ABA mediated stomatal closure leading to reduced carbon gain and ABA-induced senescence (Sah et al., 2016). Another consequence of drought stress in plants is overproduction of ROS causing an oxidative stress which in turn results in cellular membrane damage, imbalance of ions and oxidation of bioactive molecules (Hussain et al., 2016; Hussain et al., 2018).
ABA also plays an important role in inducing the protective role of DHNs. Dehydrins are a subfamily of group 2 LEA proteins that accumulate during late stages of seed development, when plant water content often decreases. In addition, DHNs accumulate in vegetative tissues that are exposed to various stress factors related to dehydration (drought, high salinity, low temperatures, wounding) (Svensson et al., 2002). Hundreds of DHN genes have been sequenced in both dicotyledonous and monocotyledonous plant species (Kosová et al., 2019). The regulation of these genes involves Ca2+ signaling pathways as well as ABA and mitogen-activated protein kinase (MAPK) cascades. Dehydrins help to detoxify ROS binding to metal ions and scavenging ROS through oxidative modification. Importantly, the characteristic lysine-rich K-segment of dehydrins displays high membrane affinity. DHNs are known to bind and to protect membranes and even DNA from potential damaging caused by adverse environment. It has been shown that DHNs interact with plasma membrane intrinsic proteins that are important members of the aquaporin family (Liu et al., 2017; Sun et al., 2021). The coordination of intracellular functions, including stress response, depends on the flow of information from the nucleus to cell organelles and back. The expression of many nuclear stress response genes is regulated by 3′-phosphoadenosine 5′-phosphate (PAP), known as a key player in chloroplast stress retrograde signaling, which accumulates during drought, salinity and intensive light stress (Pornsiriwong et al., 2017). The concentrations of PAP are regulated by phosphatase SAL1, which dephosphorylates PAP to Adenosine monophosphate (AMP) and thus reduces PAP levels (Estavillo et al., 2011). The studies on TaSal1 knockout wheat mutants obtained using CRISPR-Cas9 confirmed PAP accumulation, resulting in enhanced stress signaling and induced stomatal closure. Consequently, mutant plants had bent stem and rolled-leaf phenotype with better regulation of stomatal closure and seed germination (Abdallah et al., 2022).
2.3 Salinity
Salt stress is considered one of the most devastating environmental stresses that limits the productivity and quality of agricultural crops worldwide. Nowadays, over 20% of the world’s cultivable lands are affected by salinity stress and due to climate change, resulting in precipitation variation and temperature increase, these areas are continuously expanding (Qadir et al., 2014).
During the process of soil salinization, an excessive increase in water-soluble salts occurs. The most common cations found in saline soils are Na+, Ca2+, and Mg2+, whereas chloride, sulfates, and carbonates are the main source of anions. The high concentration of dissolved salts in the root zone reduces the osmotic potential difference between the soil and roots, which limits water uptake in plants, causing physiological water deficiency and malabsorption of essential elements (Farooq et al., 2022). The toxic effect of a high concentration of Na+ is the most prominent one – Na+ is not needed for plant metabolism, whereas it competes for binding sites with K+ that is essential for many cellular functions (Tester and Davenport, 2003).
In cells, exposition to salt stress primarily induces osmotic stress and ionic stress. Sensing salt ions and hyperosmolality triggers Ca2+ accumulation in the cytosol, activation of ROS signaling, and alteration of membrane phospholipid composition. These signals change phytohormone signaling, cytoskeleton dynamics, and the cell wall structure. Moreover, various physiological and molecular changes inhibit photosynthesis and alter sugar signaling, which may lead to plant growth retention (Zhao et al., 2021).
Several Na+-binding molecules have been demonstrated to act as sensors able to respond and signal an excess of Na+ (Shabala et al., 2015). The best-studied of them is the hyperosmolality-gated calcium-permeable channel family OSCA that has been identified in many species, including important cereals (Han et al., 2022b; She et al., 2022).
The environment-triggered Ca2+ influx signal in the cytoplasm is received by Ca2+-sensing proteins. Among those, calcineurin B-like proteins (CBLs) are responsible for maintaining the ion transport and homeostasis through interactions with the serine/threonine protein kinases (CIPKs) which activate Na+, K+, H+, NO3-, NH4+ and Mg2+ transporters located in different cellular membranes. In addition, regulation of ROS and ABA signaling is also modulated by CBL-CIPK complexes (Ma et al., 2020). Regulation of Na+ transport from cytosol to the apoplast is mediated by the salt overly sensitive (SOS) pathway where the specific complexes of CBLs-CIPKs interact with Na+/H+ antiporter SOS1 that removes excessive Na+. Another CBL-CIPK complex activates Na+/H+ exchange transporter 1 located in the vacuole tonoplast to transport the excess of Na+ to that organelle (Ma et al., 2020). The CBL and CIPK encoding genes seem to be conserved among dicots and monocots (Martínez-Atienza et al., 2007; Kanwar et al., 2014). Sequestering of the ions into vacuoles helps to avoid stress but needs the osmotic potential adjustment in the cytosol by the accumulation of osmotically active substances such as polyols, amides and amino acids, soluble carbohydrates, and quaternary ammonium compounds. The toxic and osmotic effects of salt ions in the cytoplasm are usually reached by scavenging ROS by antioxidant enzymes that also help to tolerate the toxic effects of salt ions (Flowers and Colmer, 2008).
Other early events in salt stress response include rise of cyclic nucleotides (e.g., cGMP) and ROS. The cGMP inhibits Na+ influx via non-selective ion channel. In addition, rise in cGMP and ROS induces transcriptional regulation that can activate MAPK cascades. Rise in expression of MAPKs leads to increased osmolyte synthesis to alleviate salt-induced osmotic stress. Osmolytes are also a signal for production of ABA, regulating stomatal closure and therefore osmotic homeostasis and water balance (Zhao et al., 2021). Salt stress-induced accumulation of ABA activates the sucrose non-fermenting-1 related protein kinases 2 (SnRK2s). In turn, activated MAPKs and SnRK2s transduce signals to downstream transcription factors to induce the expression of stress-responsive genes (Zhao et al., 2020).
The ability to resist saline environments differs remarkably among plants. Non-halophytic plants (i.e., glycophytes) are sensitive to salinity stress, and their growth and development are hampered by a salinized environment. However, glycophytes exhibit natural variation in their salinity tolerance. Such variation often relies on an allelic variation of genes involved in salinity stress response (Jamil et al., 2011). For example, it has been noticed that under salt treatment to reduce sodium influx in response to osmotic stress, an aquaporin, a cation antiporter, and a calcium-transporting ATPase were downregulated, while a manganese transporter and a vacuolar-type proton ATPase subunit were upregulated in the roots of a salt-tolerant accession of Poa pratensis L. when compared to a susceptible accession of P. pratensis (Bushman et al., 2016).
Halophytic plants have adapted to salinized environments and they show stimulation of growth enhancement and productivity at moderate salinity (50–250 mM NaCl) (Flowers and Colmer, 2008). These plant species have developed specific mechanisms that regulate internal salt load, e.g., many have developed specialized salt glands which excrete ions on the leaf surface. Such structures are mainly characteristic of C4 grasses belonging to the tribes Chlorideae, Sporoboleae and Aeluropodeae. Other halophytes, including as well C4 grasses (e.g., Paspalum vaginatum Sw.), use bladder-like protrusions from epidermal cells into which ions are sequestered and accumulated until these cells senesce and die (Chavarria et al., 2020; Spiekerman and Devos, 2020). The number and density of salt glands or salt bladders depends on salt concentration in the soil during plant growth indicating the dynamic adaptation to environmental conditions (Flowers and Colmer, 2008).
Identification of genetic components and their variance underlying salinity tolerance is a useful source for plant breeders (Zhai et al., 2020). The overexpression of several halophytic genes in glycophytic recipients has been demonstrated to enhance abiotic stress tolerance (Mishra and Tanna, 2017). An increasing number of transcriptomic studies from salt-tolerant non-halophytic and halophytic grasses grown under different salinity conditions will help to elucidate the gene networking process behind the effective salinity response (Xu et al., 2020; Mann et al., 2021; Vaziriyeganeh et al., 2021).
3 Genome editing: A tool for developing stress resistant forage grasses
The biggest challenge for agriculture nowadays is to obtain plants that are resilient to adverse environmental conditions, and at the same time provide enough yield to fulfill food and feed security in a sustainable way. In the case of perennial forage grasses, yield is determined by repeated harvesting of herbage over as many years as possible. Therefore, forage grass genotypes with improved survival and growth under abiotic stress conditions are needed.
Genome editing tools have proven to be useful for achieving such aims, especially the Nobel prize-winning discovery of application of RNA-directed Cas9 nuclease for genome editing (Gasiunas et al., 2012; Jinek et al., 2012) abbreviated as CRISPR-Cas9. Although this editing strategy was immediately applied in model and crop plants, almost ten years ago (Feng et al., 2013; Jiang et al., 2013; Li et al., 2013; Nekrasov et al., 2013; Shan et al., 2013), not much has been achieved in the forage grasses landscape. The European GMO database EUGENIUS lists only green foxtail (Setaria viridis (L.) P. Beauv.) line 193-31 that has been modified using CRISPR-Cas9 mediated mutagenesis. The expressed CRISPR-Cas9 system targeted the coding region of the S. viridis homolog of the Zea mays L. Indeterminate 1 (ID1) gene, which promotes flowering in maize. The deactivation of the homolog in S. viridis led to delayed flowering. In the knockout line 193-31, the CRISPR-Cas9 DNA construct was segregated away (GE Setaria viridis molecular characterization details, n.d).
To find out how many publications have been released showing edited genes in forage grasses, a search was carried out in the following databases: Scopus, Web of Science, Google scholar and PubMed. The search included the scientific or the common names of 47 grass species (Supplementary Table 1) or the name of each of the 12 subfamilies of Poaceae and, in addition, one of the following terms: “CRISPR”, “genome editing”, “genome editing”. The outcome of the search is shown in Table 1. The genome of only six species, three annual grasses and three perennial ones, all growing in temperate regions, has been targeted with CRISPR-Cas tools. Genome editing in S. viridis, a model plant for C4 grasses, has been reported three times. Most of the work has been done by knocking out a single gene using the easiest genome editing approach, i.e., CRISPR-Cas9.
CRISPR-Cas9 as a system for carrying out simple mutations (indels: insertions/deletions) that change the reading frame of a coding region and therefore generate knockouts, is straightforward and still mainly used for functional genomics. It consists of two main components: the Cas9 nuclease from Streptococcus pyogenes and the short guide RNA (gRNA) that targets the DNA sequence of interest. Designing the gRNA with precision enables the simultaneous mutations of all alleles of a gene in a polyploid plant, as it was the case for Panicum virgatum L. (tetraploid) and Lolium arundinaceum (allohexaploid, Table 1). Specific genes that have been knocked-out in forage grasses are related to flowering (phytochrome C—PHYC—of Setaria italica (L.) P.Beauv. and floral organ number 2—FON2—of S. viridis), tillering and branching (teosinte branched 1—tb1a and tb1b—of Panicum virgatum), meiosis (disrupted meitoic cDNA 1—DMC1—of Lolium multiflorum Lam.), haploid induction (matrilineal—MTL—of S. italica) and heat stress response (17.9 kDa class II heat shock protein—HSP17.9—of L. arundinaceum), apart from the phytoene desaturase (PDS) gene used as endogenous marker (Table 1 and references therein). In most of the cases the cited publications discuss the targeted mutagenesis method and results obtained, but the phenotypic characterization of the mutants is limited and far away from field trials. Interestingly, not only classical CRISPR-Cas9 system has been used, but also CRISPR-Cas12a in the case of L. arundinaceum (Zhang et al., 2021) and CRISPR-Cas9_Trex2 in the case of S. viridis (Weiss et al., 2020).
The toolkit of CRISPR-Cas applications has expanded to around twenty different techniques that allow diverse targeted modifications in the genome (Villalobos-López et al., 2022; Capdeville et al., 2023). On the one hand, Cas enzymes from different bacteria have been characterized and adopted for use. That is the case for Cas12a (former Cpf1), an enzyme from the Lachnospiraceae bacterium ND2006 that cuts DNA strands distal from the sequence recognized by the nuclease (the PAM site), generating 4-5 nucleotide overhangs that enable an easy insertion of donor DNA sequences (Zetsche et al., 2015; Moreno-Mateos et al., 2017). Other modifications of the CRISPR-Cas9 system imply the co-expression or the fusion of different proteins to the Cas9 nuclease, in its original or mutated versions. CRISPR-Cas9_Trex2, for example, has the Trex2 exonuclease co-expressed with Cas9 for increasing the mutation efficiency (Čermák et al., 2017; Weiss et al., 2020). Importantly, an enzymatically inactive variant of Cas9, called “dead Cas9” (dCas9) that maintains its specific DNA binding ability, can be fused to transcription activators or repressors to regulate transcriptional levels of endogenous genes (Ding et al., 2022). Therefore, CRISPR-Cas tools are not only meant to inactivate genes and create loss-of-function mutants, but also gain-of-function mutants can be obtained. In addition, thanks to the Super Nova Tag (SunTag) system, the transcriptional regulation can be potentiated. The SunTag contains peptide repeats that bind several transcription factors for cooperatively activating a target gene (Tanenbaum et al., 2014). Moreover, a gene of interest may also be up- or downregulated epigenetically. For instance, CRISPR-dCas9 linked to DRM methyltransferase catalytic domain targets methylation to specific loci and thereby inactivates the target gene (Papikian et al., 2019).
An alternative way of inducing a change in the levels of expression of a gene is altering its promoter sequence. In fact, the promoter can be even swapped by another one that ensures e.g., higher levels of expression in a ubiquitous manner. Using CRISPR-Cas9 such a substitution is possible, as shown for the auxin-regulated gene involved in organ size 8 (ARGOS8) gene in maize, whose overexpression was associated with improved grain yield under field drought stress conditions (Shi et al., 2017).
It should be pointed out that yield and stress resistances are among the most difficult polygenic traits to improve through genetic engineering, but examples as the former one give hope that it can be achieved by CRISPR-Cas. Another example is the knockout via CRISPR-Cas9 of the main effect gene type-B response regulator 22 (OsRR22) that controls salt tolerance in rice. Obtained plants showed salt tolerance in growth chambers and no difference in agronomic traits compared to wild type plants in field trials under normal growth conditions (Zhang et al., 2019; Han et al., 2022a).
As explained in section 2, abiotic stress responses are complex, linked to different metabolic pathways and the genes involved in those mechanisms are mainly pleiotropic. Fishing out a specific key player, a master gene to be mutated, could be possible in some cases and it is worth trying. Since genome editing in grasses is in its early stages (Table 1), we selected specific genes related to the four abiotic stresses discussed in this review and figured out if those target genes would need to be overexpressed or downregulated to gain tolerance to specific stresses. The suggested genes can be found in Table 2. If a candidate gene was found in forage grasses or at least in a Poaceae species, that species was selected, but this was not possible in all cases. As shown in Table 2, there are genes that are related to more than one stress response. For simplicity, it is not shown that, e.g., DHN11 seems to be also involved in cold and drought stresses and COR410 appears to be related to drought stress as well.
Section 2 mentioned that plants detect an increase in temperature (in the soil or air) when the structure and fluidity of their cell membranes change. Heat stress tends to make membranes more fluid (Niu and Xiang, 2018), which activates pathways through heat sensors like the CNGCs. In theory, an increased expression of stress receptors can lead to an improved response to stress. Consequently, the genes involved in the heat stress response signaling pathway can be upregulated by overexpressing a heat sensor coding gene. In A. thaliana, an overexpression of the SYTA gene resulted in higher germination and seedlings survival rates than in wild-type and knockout lines after heat stress exposition. Moreover, the overexpression plants presented higher expression of both HSPs and HSFs, together with lower levels of membrane lipid peroxidation than in non-overexpression lines (Yan et al., 2017). All these changes provide evidence that upregulating a heat stress sensor can improve the stress tolerance of a plant. Therefore, overexpressing a similar gene in grasses, like a homologous of rice OsCNGC14 or OsCNGC16 gene, could result in forage species with higher tolerance to heat stress. A similar approach can be followed by upregulating proteins present in plants in a basal state that are involved in the responses to abiotic pressures (Figure 1). Kinase proteins are suitable for this goal since they are involved in most stress response pathways, regulating posttranslational modifications of other proteins as a response to both abiotic and biotic stress (Damaris and Yang, 2021). Therefore, overexpressing a gene from the SnRK2 family, a group of kinases specific to plants that have been shown to play important roles in abiotic stress regulation is an adequate approach (Zhang et al., 2016). The heterologous overexpression of the gene TaSnRK2.3 from wheat in Arabidopsis produced plants that had higher tolerance to drought conditions (Tian et al., 2013). Similarly, another study was able to overexpress the AcSnRK2.11 gene from Agropyron cristatum (L.) Gaertn., a forage grass species, in Nicotiana tabacum L. The overexpression plants had significantly higher survival rates than the wild-type ones after recovery periods from cold stress and presented significantly upregulated patterns of abiotic stress-related genes like dehydrins. Possibly, upregulation of these protein kinases could provide drought, cold and salinity stress tolerance to forage grasses plants.
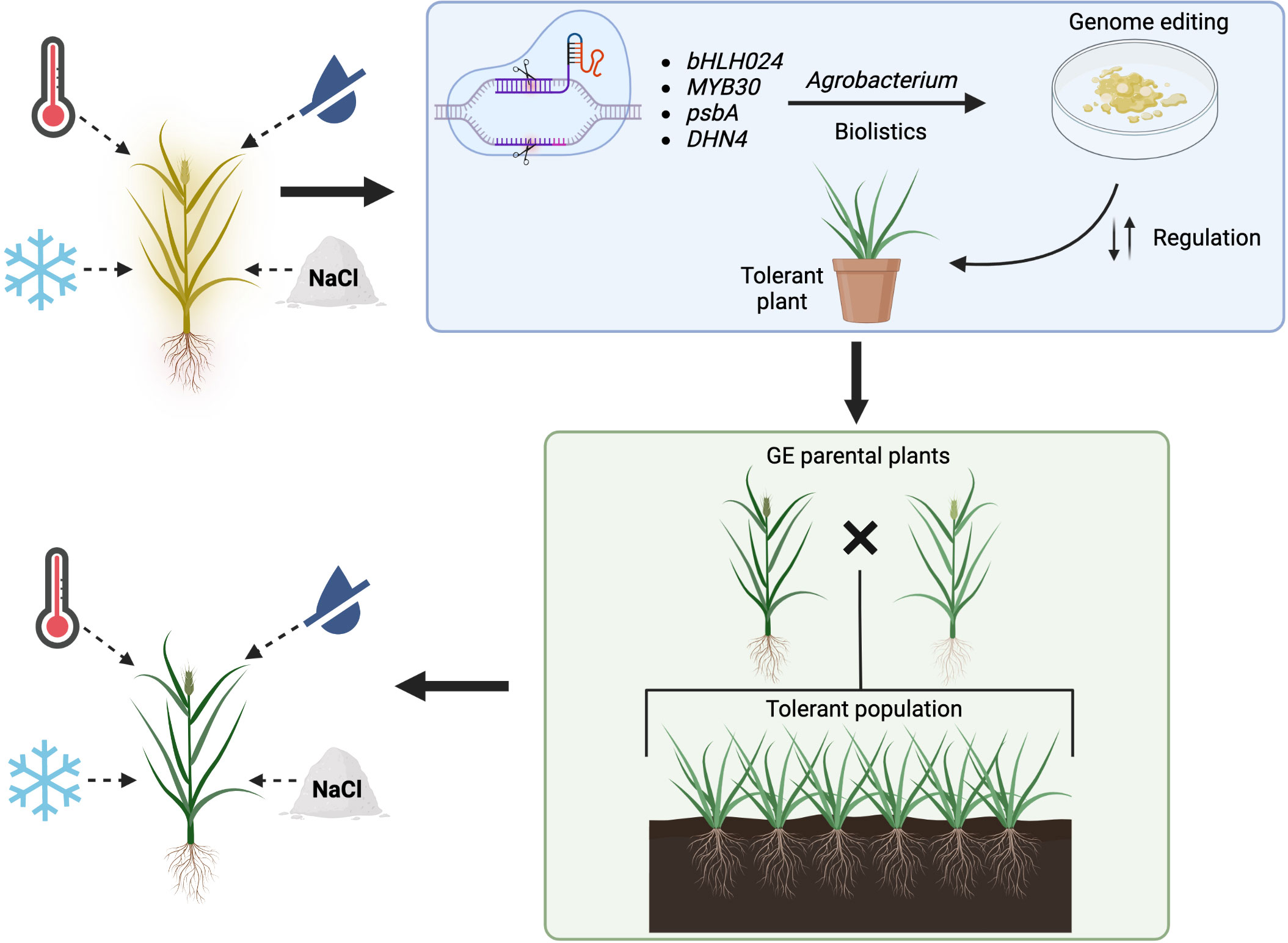
Figure 1 Proposed strategy for the improvement of abiotic stress tolerance in forage grasses using genome editing. Four abiotic stresses (heat, low temperature, drought, salinity) hinder the overall wellbeing of a non-tolerant grass (plant shown in yellow). Using the CRISPR-Cas system, different genes can be targeted. Agrobacterium-mediated transformation or biolistics are suitable delivery methods of the CRISPR-Cas+gRNAs complex for in-vitro culture modifications that lead towards the generation of abiotic stress tolerant plant (blue rectangle). Once tolerant parental plants are obtained (GE, gene editing), these can be crossed to produce a population able to overcome the effects of abiotic stress (green rectangle). The green plant on the bottom left represents a tolerant grass.
On the other hand, negative regulators of abiotic stress responses are also suitable targets for abiotic stress tolerance improvement by downregulating them via genome editing (Figure 1). Possible candidates for downregulation could be enzymes that degrade signaling molecules involved in stress response, like for example the inositol phosphatases (Jia et al., 2019). As previously mentioned in this review, the phosphatase SAL1 negatively regulates plants’ response to drought (Chan et al., 2016). Using the CRISPR-Cas9 system, scientists have already generated Tasal1 knockout mutant wheat with fewer and smaller stomata, that germinate and grow better under drought conditions (Abdallah et al., 2022). Likewise, modifying the expression of transcription factors related to abiotic stress is another alternative for producing tolerant plants. The transcription factors of the basic helix-loop-helix (bHLH) family have been shown to participate in abiotic stress regulation in different plant species (Guo et al., 2021). In rice, the OsbHLH024 gene seems to negatively regulate salinity tolerance. This was demonstrated by generating knockout plants using the CRISPR-Cas9 system. The mutated plants had an increased salinity tolerance when compared to the wild-type ones. Additionally, the knockout lines presented a reduced accumulation of sodium ions and ROS, but higher concentrations of potassium ions than the control plants. Finally, the expression of genes encoding ion transporter was upregulated in the knockout plants in comparison to the wild-type ones (Alam et al., 2022). All these variations suggest that the downregulation of homologues of the OsbHLH024 gene in grasses could provide them with salinity stress tolerance.
During the last years, innovative ways of inserting specific targeted mutations based on CRISPR-Cas have been developed, e.g., base- and prime editing and for now, some technical problems need to be overcome when applied to plants (Anzalone et al., 2020; Zhu et al., 2020; Hua et al., 2022). In these cases, the Cas9 nuclease is mutated in such a way that it acts as nickase, cutting only one strand of the targeted DNA. These strategies and the activation of homology- directed repair (HDR) instead of Non-Homologous End Joining (NHEJ), makes it possible to produce a wide range of mutations from single nucleotide changes and small indels to increasingly larger insertions and deletions, replacements or even to generate chromosomal rearrangements (Puchta et al., 2022; Villalobos-López et al., 2022).
The possibilities to induce targeted changes with CRISPR-Cas in the genome of crops, and specifically in forage grasses, are immense, not to mention the speed of obtaining the desired traits compared to conventional breeding techniques. In addition, genome editing can be easily multiplexed for targeting different sequences at one shot. Depending on the specific trait and species, there can be bottlenecks to be removed like specific ways of transforming a plant or availability and annotation quality of the reference genome. These obstacles are thought to be solved with technical advances, however in the case of grasses, important biological features need to be taken into consideration when aiming to combine genome editing with a breeding program. These challenges are elaborated in section 5. Here we briefly mention that also reproductive characteristics of grasses can be changed with genome editing.
Forage grasses have a strong gametophytic self-incompatibility (SI) system that makes inbreeding almost impossible. The two multi-allelic S and Z genes have since long been known to govern SI in grasses (Lundqvist, 1955; Cornish et al., 1979), and recently it was shown that two DUF247 genes are behind the S and Z loci (Manzanares et al., 2016; Herridge et al., 2022). With the sequences and molecular function of these genes known, they would be an obvious target for generating self-fertile knockout lines by genome editing. A similar approach has been used to develop self-compatibility in potato (Ye et al., 2018).
To obtain male sterile lines is also of importance in the case of forage grasses. The way has been paved by research in maize, where genes male sterility 1 (Ms1) and Ms45 have been targeted by CRISPR-Cas9 and male-sterile wheat lines for hybrid seed production have been obtained (Singh et al., 2018; Okada et al., 2019).
Fully homozygous doubled haploid lines can be generated by artificially inducing haploids with a knockout of MTL gene, as it has been done already in S. italica (Table 1) (Cheng et al., 2021).
Finally, apomixis is present in several grass species, e.g., Poa pratensis, a species used both in lawns, pastures, and leys. Inducing apomixis in other forage grasses would be of importance for genetic fixation of hybrid vigor of parental line. Some steps towards achieving this aim have been taken already in rice. Mutations using CRISPR-Cas of several genes related to the abolishment of meiotic steps produced clonal diploid gametes. Then, parthenogenesis was induced by ectopic expression in the egg cell of BABY BOOM1 and clonal progeny was obtained (Khanday et al., 2019; Zhu et al., 2020).
4 Genome editing versus traditional genetic modifications
Genetic variation is fundamental to crop improvement. Modern plant breeding started in the late 19th century with the advent of cross-breeding which still is the backbone of most plant breeding efforts (Hickey et al., 2019; Gao, 2021). After the discovery that physical and chemical factors can lead to heritable changes in genetic material, random mutagenesis became a valuable tool for plant breeding to increase genetic diversity and to develop specific traits. With the discovery of recombinant DNA technology in the 1970s, the development of new combinations of genetic elements by splicing genes and regulatory elements from different species became possible. The discovery of Agrobacterium-mediated transformation enabled scientists to introduce these novel combinations of genes into plant genomes to produce new traits (Gao, 2021). While the introduction of transgenes into plant genomes has contributed enormously to the understanding of gene functions in plants, the commercial applications have been limited to mostly herbicide tolerance and insect resistance, which provide obvious advantages for farmers, but little direct, tangible benefits for consumers in developed countries. Only a few commercial applications of transgenic plants with improved yield and abiotic stress resistance are known. Wheat expressing the sunflower transcription factor HomeoBox 4 (HaHB4) has been shown to provide improved water use efficiency resulting in higher grain production (González et al., 2019). Wheat HB4 marketed by the company Bioceres Crop Solutions has been authorized for food and feed uses in a number of countries, such as Argentina, Australia, Brazil and United States, but its cultivation is approved only in Argentina (Argentina First to market with drought-resistant GM wheat, 2021; HB4 Wheat| GM Approval Database- ISAAA.org, n.d.). Maize MON87403 contains the ARABIDOPSIS THALIANA HOMEOBOX 17 (ATHB17) gene from A. thaliana encoding a transcription factor of the HD-Zip II family with reported increase in ear biomass at the early reproductive phase (Rice et al., 2014), which may provide an opportunity for increased grain yield under field conditions (Leibman et al., 2014). Maize MON87460 expresses the Bacillus subtilis cold shock protein B (CspB) resulting in increased grain yield under drought conditions (Nemali et al., 2015). Both GMO events have been assessed by the European Food Safety Authority (EFSA) (EFSA Panel on Genetically Modified Organisms (GMO) et al., 2012b; EFSA Panel on Genetically Modified Organisms (GMO) et al., 2018), and MON87460 was authorized for food and feed uses in the EU. Transformation techniques have been developed for most of the economically important forage and turf grass (Wang and Ge, 2006), however, very few transgenic forage grasses have been registered for commercial cultivation. The ISAAA GMO approval database lists only one transgenic event in creeping bentgrass (Agrostis stolonifera L.) with tolerance to glyphosate (ASR368) (Creeping Bentgrass (Agrostis stolonifera) GM Events | GM Approval Database - ISAAA.org, n.d.).
Even though commercial cultivation of GM crops has brought clear benefits to farmers and more indirect benefits to environment through reduced land and pesticides use (Brookes, 2019; Brookes, 2022), cultivation and use of transgenic plants for food and feed have been controversial in many regions of the world, and especially in Europe. Agronomic, environmental, human health, social and economic effects of transgenic crops have been comprehensively reviewed by the US National Academies of Sciences in 2016 (National Academies of Sciences, Engineering, and Medicine et al., 2016).
Genome editing became possible with advances in protein engineering which allowed production of site-directed nucleases (SDNs), such as zinc finger nucleases (ZFNs) and transcription activator-like effector nucleases (TALENs) (Shukla et al., 2009; Urnov et al., 2010). As outlined in section 3, genome editing has several advantages over the transgenic techniques including precision, lower number of off-target effects, more streamlined production, multiplex possibility, as well as potential for modification of many more different traits. A few examples include lower gluten content in wheat through simultaneous editing of alpha-gliadin genes (Sánchez-León et al., 2018), increased production of gamma-aminobutyric acid in tomato (Li et al., 2018) or increased accumulation of provitamin D3 in tomato (Li et al., 2022). The maize with an increased expression of ARGOS8 gene, as detailed in section 3, contained no exogenous DNA sequences, thus, theoretically, it could be exempt from GMO regulation depending on country-specific policies.
The increased precision, low off-target potential and the absence of exogenous DNA in some of the genome-edited plants suggested that genome editing would not be regulated similarly to GMOs. For example, in Japan Sanatech Seed has commercialized high gamma-aminobutyric acid tomato (Waltz, 2021). In the EU, however, the Court of Justice of the European Union (CJEU, case C-528/16) ruled that organisms resulting from mutagenesis techniques in legal aspects are GMOs and are subject to the regulations laid down by the Directive 2001/18/EC. This applies to mutagenesis techniques introduced since 2001, when the GMO Directive was adopted. Site-directed nucleases can modify plant genomes according to three scenarios, SDN-1, SDN-2 and SDN-3 (EFSA Panel on Genetically Modified Organisms (GMO) et al., 2012a), where only SDN-3 scenario results in transgenic plants, while under SDN-1 and SDN-2 scenarios no exogenous DNA is inserted into the genome. However, under the CJEU ruling, also the SDN-1 and SDN-2 techniques, including CRISPR-Cas fall under the GMO Directive, while chemical and radiation random mutagenesis remains exempt according to Annex IB of the Directive 2001/18/EC. The ruling provoked a strong response from both academia and biotech industry, which stressed that from a scientific point of view the application of GMO Directive to products created by a much more precise technique than random mutagenesis and transgenesis results in a disproportionate regulatory burden (Purnhagen et al., 2018; Urnov et al., 2018; Christiansen et al., 2019; Wasmer, 2019; Schulman et al., 2020). It was also noted that this ruling leads to a situation when two identical products with the same mutation resulting in, e.g., herbicide tolerance trait could be regulated in different ways. In addition, it would create an unsustainable situation with detection, since no technology can determine the origin of simple mutations, such as single nucleotide polymorphisms. Consequently, reliable detection methods for SDN-1 and SDN-2 products are problematic (European Network of GMO Laboratories (ENGL), 2019). This legal uncertainty makes genome-editing research in the EU less appealing, as seeking regulatory approval for gene-edited products would involve the same cumbersome procedure as for GMOs. So far there are no applications for regulatory approval involving gene-editing, although a few applications for authorization of products obtained with CRISPR-Cas9 in SDN-3 scenario, e.g., maize DP-915635-4 have been submitted to member states and are currently under review by EFSA (Maize DP-915635-4, n.d.).
According to the EU (Council Decision (EU) 2019/1904), the European Commission (EC) conducted a study involving input from the Member States and different stakeholders regarding the status of new genomic techniques (NGTs) including genome editing. Within this framework, the EC mandated EFSA to issue a scientific opinion on the risk assessment of plants produced by the SDN-1, SDN-2, and oligonucleotide-directed mutagenesis techniques. EFSA has assessed the safety of plants developed using SDN-1 and SDN-2 techniques and did not identify new hazards specifically linked to these techniques compared to both SDN-3 and conventional breeding. In addition, EFSA concluded that the existing Guidance for risk assessment of food and feed from GM plants and the Guidance on the environmental risk assessment of genetically modified plants are sufficient, but only partially applicable, to plants generated via SDN-1 and SDN-2 (EFSA Panel on Genetically Modified Organisms (GMO) et al., 2020; Rostoks, 2021). As part of the ongoing effort to update the EU GMO legislation upon EC request, EFSA recently produced an updated scientific opinion on cisgenesis and intragenesis (EFSA Panel on Genetically Modified Organisms (GMO) et al., 2022b). The EFSA scientific opinion concluded that no new risks were identified in cisgenic and intragenic plants obtained with NGTs, as compared with those already considered for plants obtained with conventional breeding and established genomic techniques, although only limited information on such plants was available. EFSA determined that the use of NGTs reduces the risks associated with potential unintended modifications of the host genome resulting in fewer requirements for the assessment of cisgenic and intragenic plants, due to site-specific integration of the added genetic material. However, there was no legal necessity to overhaul the GMO legislation, since the EFSA concluded that the current guidelines were partially applicable and sufficient. Importantly, the data requirements could be reduced on a case-by-case basis for the risk assessment of cisgenic or intragenic plants obtained through NGTs. While cisgenesis and intragenesis is just one of the possible approaches for forage grass breeding, EFSA also recently issued a statement on criteria for risk assessment of plants produced by targeted mutagenesis, cisgenesis and intragenesis (EFSA Panel on Genetically Modified Organisms (GMO) et al., 2022a). These criteria could be used by policy makers to design a more flexible and proportionate risk assessment framework for gene edited plants. Recently, several regulatory options have been proposed (Bratlie et al., 2019; Kearns et al., 2021; Gould et al., 2022). They range from maintaining the status quo (full risk assessment of genome edited organisms as GMOs) to product-based regulation or regulation based on the presence/absence of foreign DNA in the genome. These two options would be preferable for commercial deployment of genome edited crops, but they would require substantial reexamination of GMO Directive and authorization procedure. The EC is expected to present a new policy and/or legal proposal by the second quarter of 2023. Meanwhile, other jurisdictions around the world have already developed legal framework for genome edited plants, e.g., under Argentina NBT Resolution N° 21/2021, if a product (plant, animal or microorganism) does not have a new combination of genetic material, the product is non-GM and considered as conventional product (Goberna et al., 2022). Different regulatory approaches are summarized in a recent review (Entine et al., 2021).
Interestingly, the “EU GMO database of Deliberate Release into the environment of plants GMOs for any other purposes than placing on the market (experimental releases)” lists over 900 applications for field trials registered by the Member States since 2002 (Experimental releases of GM Plants, n.d.). Among those there is only one application for field trial of high fructan transgenic ryegrass in 2006, and there are no applications for field trials of genome edited forage grasses, although at least 14 field trials of plants edited with CRISPR-Cas9 have been authorized.
In conclusion, while there are a few basic studies on gene function in forage grasses using genome editing technique as described in section 3 of this review, these are yet to see commercial application. The main limiting factor for the investment in research and development of genome edited forage grasses is probably the regulatory uncertainty, especially in the EU. Although edited plants without foreign DNA in the genome are expected to receive the least amount of regulatory scrutiny, they are also less prone to show major changes in relevant traits. This is because gene knockouts or simple gene edits are unlikely to result in complex phenotypes, such as enhanced abiotic stress tolerance, higher yield or improved nutritional composition, especially considering the genetic complexity that has hindered progress in characterization of the genes underlying such traits in forage grasses. Nevertheless, as recent years have witnessed a dynamic development of genome editing tools and genotype-independent transformation approaches along with increasing genomic resources, the manipulation of plant responses may become possible to overcome abiotic stresses when combining modern techniques and good breeding management strategies.
5 Breeding grasses in the genome editing era
Forage grasses are outbreeding species and highly heterozygous due to the strong gametophytic SI system. Inbred line development is thus very difficult with strong inbreeding depression as a result. Therefore, cultivars of forage grasses are usually synthetic populations (Posselt, 2010). Forage grass breeders usually start by phenotypic selection of superior candidate genotypes for traits with high heritability, e.g., heading date and disease resistance, among a large number of spaced plants. However, forage grasses are sown in swards and because yield and other traits will be affected by competition in the swards, such traits cannot be selected on single spaced plants. The candidate genotypes are therefore put in some form of progeny testing system, e.g., polycross to produce half-sib (HS) families or bi-parental crosses producing full-sib (FS) families, and selection for yield and forage quality traits are based the performance of such families in swards (genotypic selection). Synthetic populations/cultivars are constructed by crossing the best genotypes based on their performance in the progeny test or by mixing HS or FS families. The synthetic populations are further multiplied to obtain enough seed for establishing sward plots for testing in multi-location-year trials before the best candidate cultivars are being submitted to official variety testing. A typical breeding cycle will take 10-15 years before synthetic cultivars are available for farmers. With the advent of high-throughput molecular markers, whole-genome sequences, and genomic selection methods, the breeding cycle can be shortened (Rognli et al., 2021; Barre et al., 2022). Specifically, if genome editing is used for specific reproductive traits, like breaking down self-incompatibility, the forage grass breeding cycle could be shortened according to us by 4-5 years.
The success of a breeding program is very much dependent on the genetic variation present in the initial breeding material. Many agronomically important traits, like yield and adaptability to biotic and abiotic stresses, have been partly fixed within elite germplasm, however, they still exhibit large genetic variation and are thus of primary importance in breeding programs (Meyer et al., 2012; Swinnen et al., 2016). This variation might be employed for future improvements of crop productivity and tolerance to stress; however, landraces, closely related species and wild relatives can offer much wider and unexploited germplasm resources (Jonavičienė et al., 2009; Brozynska et al., 2016). Extensive studies of perennial ryegrass diversity among modern European cultivars revealed that modern cultivars are mostly related to ecotypes from north-western Europe (Blanco-Pastor et al., 2019), while most of the natural genetic variation remains unexploited. Later studies on the genetic structure of geographically diverse perennial ryegrass collection supported these findings and in addition showed that latitude was a prominent force shaping the diversity of wild-growing perennial ryegrass populations (Jaškūnė et al., 2020). Furthermore, the ecotypes exhibit biomass and seed yielding potential similar to cultivars (Bachmann-Pfabe et al., 2018; Jaškūnė et al., 2022b), suggesting that ecotypes could serve as valuable trait donors in breeding programs. Field testing of many L. perenne ecotypes and cultivars at several Nordic and Baltic locations identified tetraploid Baltic breeding lines and diploid ecotypes from Eastern Europe as being most winter hardy with stable performances across environments (Gylstrøm, 2020). None of the cultivars were among the most stable entries, and diploid ecotypes displayed a larger variation in heading date, regrowth, and winter survival than the cultivars. Thus, there is ample genetic variation still to be exploited within the genetic resources of perennial ryegrass. Induced polyploidization is also widely exploited in forage crop breeding as one of unconventional techniques to develop new superior yielding and abiotic stress tolerant breeding material (Akinroluyo et al., 2019; Akinroluyo et al., 2020; Rauf et al., 2021).
To utilize transgenes or gene-edits in grass breeding, first, efficient methods for introduction and regeneration in vitro need to be available in a range of independent genotypes. In principle, introgression of new genes can either be introduced into the parental clones of already existing varieties (variety-parent approach) or transferred into a new base population (population approach) (Posselt, 2010). Repeated backcrossing and an efficient selection system is needed to bring transgenes/gene-edits to homozygosity in the parental clones. A side-effect of this could be increased inbreeding depression due to linkage drags creating longer homozygous chromosomal segments. Traditional random insertion of transgenes in several genotypes that are intercrossed to construct synthetic cultivars is problematic due to the presence of multiple insertion sites, silencing and variable expression levels. The availability of complete genome sequences also of forage grass species, notably L. perenne (Nagy et al., 2022), and genome editing technologies, makes it possible to induce precise genome alterations. This will make it easier to develop synthetic cultivars of outbreeding crops like forage grasses with stable expression of genetic modifications.
Integration of transgenic traits in perennial grasses and the challenges associated with deployment and management of transgenic cultivars has been discussed by Badenhorst and colleagues as well as by Smith and Spangenberg (Badenhorst et al., 2016; Smith and Spangenberg, 2016). Using gene-drive technologies (Bier, 2022) would in principle be an efficient method for spreading gene-edits through breeding populations of grasses. However, the risk of gene flow between cultivars and to feral populations is high and would probably preclude practical use of such technologies.
A pertinent question is what the most important targets for genetic engineering in forage grasses would be. Genetic gain for yield has been modest due to the long breeding cycles and extensive field testing (Sampoux et al., 2011; McDonagh et al., 2016). The potential heterosis is only partially exploited in synthetic cultivars, and it is expected that great yield increased could be achieved if F1 hybrids, which has been very successfully exploited in maize, could be developed (Herridge et al., 2020). Self-incompatibility, inbreeding depression, and the lack of male-sterile lines for making hybrids are major obstacles for developing F1 hybrids. Inbreeding depression needs to be tackled to implement self-fertile lines in forage breeding programs. By generating a large number of self-fertile plants with diverse genetic backgrounds by gene-editing, and selecting genotypes with good seed set, the prospects of developing inbred lines in forage grasses have never been better. These lines could be used for F1 hybrid production and would also be very useful for functional studies. Other methods for capturing heterosis would be the development of facultative apomixis. The evolution of apomixis in natural populations and the challenges of utilizing apomixis in breeding has been reviewed recently (Hojsgaard and Hörandl, 2019).
6 Conclusion
In the current review, we focus on possible improvements of abiotic stress tolerance in forage grasses using new genome editing tools. The potential impact of climate change is described in relation to forage grass tolerance to four important abiotic stresses, such as heat, low temperature, drought and salinity. We propose approaches for editing the genome of grasses to regulate stress responses. Furthermore, we discuss the latest developments in the regulatory framework for genome editing, especially with regard to the EU, and identify factors affecting the application of genome editing techniques for the improvement of grasses. Finally, we address breeding strategies specific to the reproductive biology of forage grasses and identify how genome editing could be used to facilitate breeding and achieve food security in a sustainable way. In conclusion, we describe pathways for developing abiotic stress tolerance in forage grasses under climate change using genome editing technologies, provided that an appropriate legal framework is developed.
Author contributions
Conceptualization, FS-S, CS. writing—manuscript preparation, FS-S, NR, KJ, MS, OAR, MRK, GS and CS. writing—review and editing, FS-S and CS. funding acquisition, KJ, NR, OAR and CS. All authors have read and agreed to the published version of the manuscript. All authors contributed to the article and approved the submitted version.
Funding
This work was supported by the project “Improving adaptability and resilience of perennial ryegrass for safe and sustainable food systems through CRISPR-Cas9 technology – EditGrass4Food”, ID No. EEA-RESEARCH-64, Contract No. EEZ/BPP/VIAA/2021/4 is financially supported by the European Economic Area (EEA) grants.
Acknowledgments
The authors are thankful to students and technicians who have contributed to the EditGrass4Food project. Figure 1 was created with BioRender.com. This review is accessible as a preprint (doi: 10.20944/preprints202212.0184.v1).
Conflict of interest
The authors declare that the research was conducted in the absence of any commercial or financial relationships that could be construed as a potential conflict of interest.
Publisher’s note
All claims expressed in this article are solely those of the authors and do not necessarily represent those of their affiliated organizations, or those of the publisher, the editors and the reviewers. Any product that may be evaluated in this article, or claim that may be made by its manufacturer, is not guaranteed or endorsed by the publisher.
Supplementary material
The Supplementary Material for this article can be found online at: https://www.frontiersin.org/articles/10.3389/fpls.2023.1127532/full#supplementary-material
References
Abdallah, N. A., Elsharawy, H., Abulela, H. A., Thilmony, R., Abdelhadi, A. A., Elarabi, N. I. (2022). Multiplex CRISPR/Cas9-mediated genome editing to address drought tolerance in wheat. GM Crops Food 0, 1–17. doi: 10.1080/21645698.2022.2120313
Abdellatif, I. M. Y., Yuan, S., Na, R., Yoshihara, S., Hamada, H., Suzaki, T., et al. (2022). Functional characterization of tomato phytochrome a and B1B2 mutants in response to heat stress. Int. J. Mol. Sci. 23, 1681. doi: 10.3390/ijms23031681
Abeynayake, S. W., Etzerodt, T. P., Jonavičienė, K., Byrne, S., Asp, T., Boelt, B. (2015). Fructan metabolism and changes in fructan composition during cold acclimation in perennial ryegrass. Front. Plant Sci. 6. doi: 10.3389/fpls.2015.00329
Akinroluyo, O. K., Jaškūnė, K., Kemešytė, V., Statkevičiutė, G. (2020). Drought stress response of westerwolths ryegrass (Lolium multiflorum ssp. multiflorum) cultivars differing in their ploidy level. Žemdirb. Agric. 107, 161–170. doi: 10.13080/z-a.2020.107.021
Akinroluyo, O. K., Urbanavičiūtė, I., Jaškūnė, K., Kemešytė, V., Statkevičiūtė, G. (2019). Differences in salt tolerance between diploid and autotetraploid lines of lolium multiflorum at the germination and vegetative stages. Žemdirb. Agric. 106, 329–336. doi: 10.13080/z-a.2019.106.042
Alam, M. S., Kong, J., Tao, R., Ahmed, T., Alamin, M., Alotaibi, S. S., et al. (2022). CRISPR/Cas9 mediated knockout of the OsbHLH024 transcription factor improves salt stress resistance in rice (Oryza sativa l.). Plants 11, 1184. doi: 10.3390/plants11091184
Aleem, S., Sharif, I., Amin, E., Tahir, M., Parveen, N., Aslam, R., et al. (2020). Heat tolerance in vegetables in the current genomic era: an overview. Plant Growth Regul. 92, 497–516. doi: 10.1007/s10725-020-00658-5
Alm, V., Busso, C. S., Ergon, Å., Rudi, H., Larsen, A., Humphreys, M. W., et al. (2011). QTL analyses and comparative genetic mapping of frost tolerance, winter survival and drought tolerance in meadow fescue (Festuca pratensis huds.). Theor. Appl. Genet. 123, 369–382. doi: 10.1007/s00122-011-1590-z
Andrews, C. J., Gudleifsson, B. E. (1983). A comparison of cold hardiness and ice encasement tolerance of timothy grass and winter wheat. Can. J. Plant Sci. 63, 429–435. doi: 10.4141/cjps83-049
Anzalone, A. V., Koblan, L. W., Liu, D. R. (2020). Genome editing with CRISPR–cas nucleases, base editors, transposases and prime editors. Nat. Biotechnol. 38, 824–844. doi: 10.1038/s41587-020-0561-9
Argentina First to market with drought-resistant GM wheat. In: Nat. Biotechnol. Available at: https://www.nature.com/articles/s41587-021-00963-y (Accessed 2021).
Bachmann-Pfabe, S., Willner, E., Dehmer, K. J. (2018). Ex-situ evaluations of lolium perenne l. ecotypes collected in Bulgaria, Croatia, Spain and Ireland reveal valuable breeding material. Genet. Resour. Crop Evol. 65, 1423–1439. doi: 10.1007/s10722-018-0623-8
Badenhorst, P. E., Smith, K. F., Spangenberg, G. (2016). Development of a molecular breeding strategy for the integration of transgenic traits in outcrossing perennial grasses. Agronomy 6, 56. doi: 10.3390/agronomy6040056
Bailey-Serres, J., Parker, J. E., Ainsworth, E. A., Oldroyd, G. E. D., Schroeder, J. I. (2019). Genetic strategies for improving crop yields. Nature 575, 109–118. doi: 10.1038/s41586-019-1679-0
Barre, P., Asp, T., Byrne, S., Casler, M., Faville, M., Rognli, O. A., et al. (2022). “Genomic prediction of complex TraitsComplex traits in forage plants species: Perennial grasses case,” in Genomic prediction of complex traits: Methods and protocols methods in molecular biology. Eds. Ahmadi, N., Bartholomé, J. (New York, NY: Springer US), 521–541. doi: 10.1007/978-1-0716-2205-6_19
Bengtsson, J., Bullock, J. M., Egoh, B., Everson, C., Everson, T., O’Connor, T., et al. (2019). Grasslands–more important for ecosystem services than you might think. Ecosphere 10, e02582. doi: 10.1002/ecs2.2582
Bhowmik, P. K., Tamura, K., Sanada, Y., Tase, K., Yamada, T. (2006). Sucrose metabolism of perennial ryegrass in relation to cold acclimation. Z. Für Naturforschung C 61, 99–104. doi: 10.1515/znc-2006-1-218
Bier, E. (2022). Gene drives gaining speed. Nat. Rev. Genet. 23, 5–22. doi: 10.1038/s41576-021-00386-0
Bita, C., Gerats, T. (2013). Plant tolerance to high temperature in a changing environment: scientific fundamentals and production of heat stress-tolerant crops. Front. Plant Sci. 4. doi: 10.3389/fpls.2013.00273
Blanco-Pastor, J. L., Manel, S., Barre, P., Roschanski, A. M., Willner, E., Dehmer, K. J., et al. (2019). Pleistocene climate changes, and not agricultural spread, accounts for range expansion and admixture in the dominant grassland species lolium perenne l. J. Biogeogr. 46, 1451–1465. doi: 10.1111/jbi.13587
Bourgine, B., Guihur, A. (2021). Heat shock signaling in land plants: From plasma membrane sensing to the transcription of small heat shock proteins. Front. Plant Sci. 12. doi: 10.3389/fpls.2021.710801
Bratlie, S., Halvorsen, K., Myskja, B. K., Mellegård, H., Bjorvatn, C., Frost, P., et al. (2019). A novel governance framework for GMO. EMBO Rep. 20, e47812. doi: 10.15252/embr.201947812
Brookes, G. (2019). Twenty-one years of using insect resistant (GM) maize in Spain and Portugal: farm-level economic and environmental contributions. GM Crops Food 10, 90–101. doi: 10.1080/21645698.2019.1614393
Brookes, G. (2022). Farm income and production impacts from the use of genetically modified (GM) crop technology 1996-2020. GM Crops Food 13, 171–195. doi: 10.1080/21645698.2022.2105626
Brozynska, M., Furtado, A., Henry, R. J. (2016). Genomics of crop wild relatives: expanding the gene pool for crop improvement. Plant Biotechnol. J. 14, 1070–1085. doi: 10.1111/pbi.12454
Bulgari, R., Franzoni, G., Ferrante, A. (2019). Biostimulants application in horticultural crops under abiotic stress conditions. Agronomy 9, 306. doi: 10.3390/agronomy9060306
Bushman, B. S., Amundsen, K. L., Warnke, S. E., Robins, J. G., Johnson, P. G. (2016). Transcriptome profiling of Kentucky bluegrass (Poa pratensis l.) accessions in response to salt stress. BMC Genomics 17, 48. doi: 10.1186/s12864-016-2379-x
Capdeville, N., Schindele, P., Puchta, H. (2023). Getting better all the time — recent progress in the development of CRISPR/Cas-based tools for plant genome engineering. Curr. Opin. Biotechnol. 79, 102854. doi: 10.1016/j.copbio.2022.102854
Capstaff, N. M., Miller, A. J. (2018). Improving the yield and nutritional quality of forage crops. Front. Plant Sci. 9. doi: 10.3389/fpls.2018.00535
Čermák, T., Curtin, S. J., Gil-Humanes, J., Čegan, R., Kono, T. J. Y., Konečná, E., et al. (2017). A multipurpose toolkit to enable advanced genome engineering in plants. Plant Cell 29, 1196–1217. doi: 10.1105/tpc.16.00922
Chai, R., Ye, X., Ma, C., Wang, Q., Tu, R., Zhang, L., et al. (2019). Greenhouse gas emissions from synthetic nitrogen manufacture and fertilization for main upland crops in China. Carbon Balance Manage. 14, 20. doi: 10.1186/s13021-019-0133-9
Chalmers, J., Lidgett, A., Cummings, N., Cao, Y., Forster, J., Spangenberg, G. (2005). Molecular genetics of fructan metabolism in perennial ryegrass. Plant Biotechnol. J. 3, 459–474. doi: 10.1111/j.1467-7652.2005.00148.x
Chan, K. X., Mabbitt, P. D., Phua, S. Y., Mueller, J. W., Nisar, N., Gigolashvili, T., et al. (2016). Sensing and signaling of oxidative stress in chloroplasts by inactivation of the SAL1 phosphoadenosine phosphatase. Proc. Natl. Acad. Sci. 113, E4567–E4576. doi: 10.1073/pnas.1604936113
Chavarria, M. R., Wherley, B., Jessup, R., Chandra, A. (2020). Leaf anatomical responses and chemical composition of warm-season turfgrasses to increasing salinity. Curr. Plant Biol. 22, 100147. doi: 10.1016/j.cpb.2020.100147
Chen, J.-H., Chen, S.-T., He, N.-Y., Wang, Q.-L., Zhao, Y., Gao, W., et al. (2020). Nuclear-encoded synthesis of the D1 subunit of photosystem II increases photosynthetic efficiency and crop yield. Nat. Plants 6, 570–580. doi: 10.1038/s41477-020-0629-z
Cheng, Z., Sun, Y., Yang, S., Zhi, H., Yin, T., Ma, X., et al. (2021). Establishing in planta haploid inducer line by edited SiMTL in foxtail millet (Setaria italica). Plant Biotechnol. J. 19, 1089–1091. doi: 10.1111/pbi.13584
Chinnusamy, V., Zhu, J., Zhu, J.-K. (2007). Cold stress regulation of gene expression in plants. Trends Plant Sci. 12, 444–451. doi: 10.1016/j.tplants.2007.07.002
Christiansen, A. T., Andersen, M. M., Kappel, K. (2019). Are current EU policies on GMOs justified? Transgenic Res. 28, 267–286. doi: 10.1007/s11248-019-00120-x
Cornish, M. A., Hayward, M. D., Lawrence, M. J. (1979). Self-incompatibility in ryegrass. Heredity 43, 95–106. doi: 10.1038/hdy.1979.63
Creeping bentgrass (Agrostis stolonifera) GM events. In: GM approval database - ISAAA.org (n.d.). Available at: https://www.isaaa.org/gmapprovaldatabase/crop/default.asp?CropID=8&Crop=Creeping%20Bentgrass (Accessed December 2, 2022).
Cropano, C., Manzanares, C., Yates, S., Copetti, D., Do Canto, J., Lübberstedt, T., et al. (2021). Identification of candidate genes for self-compatibility in perennial ryegrass (Lolium perenne l.). Front. Plant Sci. 12. doi: 10.3389/fpls.2021.707901
Cui, Y., Lu, S., Li, Z., Cheng, J., Hu, P., Zhu, T., et al. (2020). CYCLIC NUCLEOTIDE-GATED ION CHANNELs 14 and 16 promote tolerance to heat and chilling in rice. Plant Physiol. 183, 1794–1808. doi: 10.1104/pp.20.00591
Cushman, J. C., Denby, K. J., Mittler, R. (2022). Plant responses and adaptations to a changing climate. Plant J. 109, 319–322. doi: 10.1111/tpj.15641
Dalmannsdottir, S., Jørgensen, M., Rapacz, M., Østrem, L., Larsen, A., Rødven, R., et al. (2017). Cold acclimation in warmer extended autumns impairs freezing tolerance of perennial ryegrass (Lolium perenne) and timothy (Phleum pratense). Physiol. Plant 160, 266–281. doi: 10.1111/ppl.12548
Dalmannsdottir, S., Rapacz, M., Jørgensen, M., Østrem, L., Larsen, A., Rødven, R., et al. (2016). Temperature before cold acclimation affects cold tolerance and photoacclimation in Timothy (Phleum pratense l.), perennial ryegrass (Lolium perenne l.) and red clover (Trifolium pratense l.). J. Agron. Crop Sci. 202, 320–330. doi: 10.1111/jac.12149
Damaris, R. N., Yang, P. (2021). “Protein phosphorylation response to abiotic stress in plants,” in Plant phosphoproteomics: Methods and protocols methods in molecular biology. Ed. Wu, X. N. (New York, NY: Springer US), 17–43. doi: 10.1007/978-1-0716-1625-3_2
Dass, P., Houlton, B. Z., Wang, Y., Warlind, D. (2018). Grasslands may be more reliable carbon sinks than forests in California. Environ. Res. Lett. 13, 074027. doi: 10.1088/1748-9326/aacb39
Daszkowska-Golec, A., Karcz, J., Plociniczak, T., Sitko, K., Szarejko, I. (2020). Cuticular waxes–a shield of barley mutant in CBP20 (Cap-binding protein 20) gene when struggling with drought stress. Plant Sci. 300, 110593. doi: 10.1016/j.plantsci.2020.110593
DeFalco, T. A., Moeder, W., Yoshioka, K. (2016). Opening the gates: Insights into cyclic nucleotide-gated channel-mediated signaling. Trends Plant Sci. 21, 903–906. doi: 10.1016/j.tplants.2016.08.011
Dhakate, P., Sehgal, D., Vaishnavi, S., Chandra, A., Singh, A., Raina, S., et al. (2022). Comprehending the evolution of gene editing platforms for crop trait improvement. Front. Genet 13. doi: 10.3389/fgene.2022.876987
Ding, Y., Lv, J., Shi, Y., Gao, J., Hua, J., Song, C., et al. (2019a). EGR2 phosphatase regulates OST1 kinase activity and freezing tolerance in arabidopsis. EMBO J. 38, e99819. doi: 10.15252/embj.201899819
Ding, Y., Shi, Y., Yang, S. (2019b). Advances and challenges in uncovering cold tolerance regulatory mechanisms in plants. New Phytol. 222, 1690–1704. doi: 10.1111/nph.15696
Ding, X., Yu, L., Chen, L., Li, Y., Zhang, J., Sheng, H., et al. (2022). Recent progress and future prospect of CRISPR/Cas-derived transcription activation (CRISPRa) system in plants. Cells 11, 3045. doi: 10.3390/cells11193045
EFSA Panel on Genetically Modified Organisms (GMO), Arpaia, S., Birch, A. N., Chesson, A., du Jardin, P., Gathmann, A., et al. (2012a). Scientific opinion addressing the safety assessment of plants developed using zinc finger nuclease 3 and other site-directed nucleases with similar function. EFSA J. 10, 2943. doi: 10.2903/j.efsa.2012.2943
EFSA Panel on Genetically Modified Organisms (GMO), Arpaia, S., Birch, A. N., Chesson, A., du Jardin, P., Gathmann, A., et al. (2012b). Scientific opinion on an application (EFSA-GMO-NL-2009-70) for the placing on the market of genetically modified drought tolerant maize MON 87460 for food and feed uses, import and processing under regulation (EC) no 1829/2003 from Monsanto. EFSA J. 10, 2936. doi: 10.2903/j.efsa.2012.2936
EFSA Panel on Genetically Modified Organisms (GMO), Mullins, E., Bresson, J.-L., Dalmay, T., Dewhurst, I. C., Epstein, M. M., et al. (2022a). Criteria for risk assessment of plants produced by targeted mutagenesis, cisgenesis and intragenesis. EFSA J. 20, e07618. doi: 10.2903/j.efsa.2022.7618
EFSA Panel on Genetically Modified Organisms (GMO), Mullins, E., Bresson, J.-L., Dalmay, T., Dewhurst, I. C., Epstein, M. M., et al. (2022b). Updated scientific opinion on plants developed through cisgenesis and intragenesis. EFSA J. 20, e07621. doi: 10.2903/j.efsa.2022.7621
EFSA Panel on Genetically Modified Organisms (GMO), Naegeli, H., Birch, A. N., Casacuberta, J., De Schrijver, A., Gralak, M. A., et al. (2018). Assessment of genetically modified maize MON 87411 for food and feed uses, import and processing, under regulation (EC) no 1829/2003 (application EFSA-GMO-NL-2015-124). EFSA J. 16, e05310. doi: 10.2903/j.efsa.2018.5310
EFSA Panel on Genetically Modified Organisms (GMO), Naegeli, H., Bresson, J.-L., Dalmay, T., Dewhurst, I. C., Epstein, M. M., et al. (2020). Applicability of the EFSA opinion on site-directed nucleases type 3 for the safety assessment of plants developed using site-directed nucleases type 1 and 2 and oligonucleotide-directed mutagenesis. EFSA J. 18, e06299. doi: 10.2903/j.efsa.2020.6299
Entine, J., Felipe, M. S. S., Groenewald, J.-H., Kershen, D. L., Lema, M., McHughen, A., et al. (2021). Regulatory approaches for genome edited agricultural plants in select countries and jurisdictions around the world. Transgenic Res. 30, 551–584. doi: 10.1007/s11248-021-00257-8
Ergon, Å., Seddaiu, G., Korhonen, P., Virkajärvi, P., Bellocchi, G., Jørgensen, M., et al. (2018). How can forage production in Nordic and Mediterranean Europe adapt to the challenges and opportunities arising from climate change? Eur. J. Agron. 92, 97–106. doi: 10.1016/j.eja.2017.09.016
Estavillo, G. M., Crisp, P. A., Pornsiriwong, W., Wirtz, M., Collinge, D., Carrie, C., et al. (2011). Evidence for a SAL1-PAP chloroplast retrograde pathway that functions in drought and high light signaling in arabidopsis. Plant Cell 23, 3992–4012. doi: 10.1105/tpc.111.091033
European Network of GMO Laboratories (ENGL) (2019) Detection of food and feed plant products obtained by new mutagenesis techniques. Available at: https://gmwatch.org/en/67-uncategorised/18923-detection-of-food-and-feed-obtained-by-new-plant-mutagenesis-techniques (Accessed November 15, 2022).
Experimental releases of GM plants. Available at: https://webgate.ec.europa.eu/fip/GMO_Registers/GMO_Part_B_Plants.php (Accessed November 24, 2022).
FAO (2019). Moving forward on food loss and waste reduction (Rome: Food and Agriculture Organization of the United Nations).
Farooq, M., Asif, S., Jang, Y.-H., Park, J.-R., Zhao, D.-D., Kim, E.-G., et al. (2022). Effect of different salts on nutrients uptake, gene expression, antioxidant, and growth pattern of selected rice genotypes. Front. Plant Sci. 13. doi: 10.3389/fpls.2022.895282
Farooq, M., Wahid, A., Kobayashi, N., Fujita, D., Basra, S. M. A. (2009). “Plant drought stress: Effects, mechanisms and management,” in Sustainable agriculture. Eds. Lichtfouse, E., Navarrete, M., Debaeke, P., Véronique, S., Alberola, C. (Dordrecht: Springer Netherlands), 153–188. doi: 10.1007/978-90-481-2666-8_12
Farrant, J. M., Cooper, K., Hilgart, A., Abdalla, K. O., Bentley, J., Thomson, J. A., et al. (2015). A molecular physiological review of vegetative desiccation tolerance in the resurrection plant xerophyta viscosa (Baker). Planta 242, 407–426. doi: 10.1007/s00425-015-2320-6
Feller, C., Blanchart, E., Bernoux, M., Lal, R., Manlay, R. (2012). Soil fertility concepts over the past two centuries: the importance attributed to soil organic matter in developed and developing countries. Arch. Agron. Soil Sci. 58, S3–S21. doi: 10.1080/03650340.2012.693598
Feng, Z., Zhang, B., Ding, W., Liu, X., Yang, D.-L., Wei, P., et al. (2013). Efficient genome editing in plants using a CRISPR/Cas system. Cell Res. 23, 1229–1232. doi: 10.1038/cr.2013.114
Fjellheim, S., Boden, S., Trevaskis, B. (2014). The role of seasonal flowering responses in adaptation of grasses to temperate climates. Front. Plant Sci. 5. doi: 10.3389/fpls.2014.00431
Flowers, T. J., Colmer, T. D. (2008). Salinity tolerance in halophytes. New Phytol. 179, 945–963. doi: 10.1111/j.1469-8137.2008.02531.x
Fu, J., Miao, Y., Shao, L., Hu, T., Yang, P. (2016). De novo transcriptome sequencing and gene expression profiling of elymus nutans under cold stress. BMC Genomics 17, 870. doi: 10.1186/s12864-016-3222-0
Galiba, G., Vágújfalvi, A., Li, C., Soltész, A., Dubcovsky, J. (2009). Regulatory genes involved in the determination of frost tolerance in temperate cereals. Plant Sci. 176, 12–19. doi: 10.1016/j.plantsci.2008.09.016
Gao, C. (2021). Genome engineering for crop improvement and future agriculture. Cell 184, 1621–1635. doi: 10.1016/j.cell.2021.01.005
Gasiunas, G., Barrangou, R., Horvath, P., Siksnys, V. (2012). Cas9–crRNA ribonucleoprotein complex mediates specific DNA cleavage for adaptive immunity in bacteria. Proc. Natl. Acad. Sci. 109, E2579–E2586. doi: 10.1073/pnas.1208507109
Giridhar, K., Samireddypalle, A. (2015). “Impact of climate change on forage availability for livestock,” in Climate change impact on livestock: Adaptation and mitigation. Eds. Sejian, V., Gaughan, J., Baumgard, L., Prasad, C. (New Delhi: Springer India), 97–112. doi: 10.1007/978-81-322-2265-1_7
Goberna, M. F., Whelan, A. I., Godoy, P., Lewi, D. M. (2022). Genomic editing: The evolution in regulatory management accompanying scientific progress. Front. Bioeng. Biotechnol. 10. doi: 10.3389/fbioe.2022.835378
González, F. G., Capella, M., Ribichich, K. F., Curín, F., Giacomelli, J. I., Ayala, F., et al. (2019). Field-grown transgenic wheat expressing the sunflower gene HaHB4 significantly outyields the wild type. J. Exp. Bot. 70, 1669–1681. doi: 10.1093/jxb/erz037
Gould, F., Amasino, R. M., Brossard, D., Buell, C. R., Dixon, R. A., Falck-Zepeda, J. B., et al. (2022). Toward product-based regulation of crops. Science 377, 1051–1053. doi: 10.1126/science.abo3034
Gray, G. R., Chauvin, L. P., Sarhan, F., Huner, N. P. A. (1997). Cold acclimation and freezing tolerance (A complex interaction of light and temperature). Plant Physiol. 114, 467–474. doi: 10.1104/pp.114.2.467
Guarino, F., Cicatelli, A., Castiglione, S., Agius, D. R., Orhun, G. E., Fragkostefanakis, S., et al. (2022). An epigenetic alphabet of crop adaptation to climate change. Front. Genet. 13. doi: 10.3389/fgene.2022.818727
Gudleifsson, B. E. (2009)Ice encasement damage on grass crops and alpine plants in Iceland - impact of climate change. In: Plant cold hardiness: from the laboratory to the field (CABI). Available at: https://www.cabdirect.org/cabdirect/abstract/20103094813 (Accessed November 30, 2022).
Gudleifsson, B. E., Andrews, C. J., Bjornsson, H. (1986). Cold hardiness and ice tolerance of pasture grasses grown and tested in controlled environments. Can. J. Plant Sci. 66, 601–608. doi: 10.4141/cjps86-080
Guo, J., Sun, B., He, H., Zhang, Y., Tian, H., Wang, B. (2021). Current understanding of bHLH transcription factors in plant abiotic stress tolerance. Int. J. Mol. Sci. 22, 4921. doi: 10.3390/ijms22094921
Gylstrøm, K. H. (2020). Available at: https://nmbu.brage.unit.no/nmbu-xmlui/handle/11250/2682676 (Accessed November 30, 2022).
Han, X., Chen, Z., Li, P., Xu, H., Liu, K., Zha, W., et al. (2022a). Development of novel rice germplasm for salt-tolerance at seedling stage using CRISPR-Cas9. Sustainability 14, 2621. doi: 10.3390/su14052621
Han, Y., Wang, Y., Zhai, Y., Wen, Z., Liu, J., Xi, C., et al. (2022b). OsOSCA1.1 mediates hyperosmolality and salt stress sensing in oryza sativa. Biology 11, 678. doi: 10.3390/biology11050678
Hasanuzzaman, M., Bhuyan, M. H. M. B., Zulfiqar, F., Raza, A., Mohsin, S. M., Mahmud, J. A., et al. (2020). Reactive oxygen species and antioxidant defense in plants under abiotic stress: Revisiting the crucial role of a universal defense regulator. Antioxidants 9, 681. doi: 10.3390/antiox9080681
Hasanuzzaman, M., Nahar, K., Alam, M., Roychowdhury, R., Fujita, M. (2013). Physiological, biochemical, and molecular mechanisms of heat stress tolerance in plants. Int. J. Mol. Sci. 14, 9643–9684. doi: 10.3390/ijms14059643
HB4 wheat. In: GM approval database- ISAAA.org (n.d.). Available at: https://www.isaaa.org/gmapprovaldatabase/event/default.asp?EventID=574 (Accessed November 30, 2022).
He, M., He, C.-Q., Ding, N.-Z. (2018). Abiotic stresses: General defenses of land plants and chances for engineering multistress tolerance. Front. Plant Sci. 9. doi: 10.3389/fpls.2018.01771
Heide, O. M. (1994). Control of flowering and reproduction in temperate grasses. New Phytol. 128, 347–362. doi: 10.1111/j.1469-8137.1994.tb04019.x
Herridge, R. P., Macknight, R. C., Brownfield, L. R. (2020). Prospects for F1 hybrid production in ryegrass. N. Z. J. Agric. Res. 63, 405–415. doi: 10.1080/00288233.2018.1559867
Herridge, R., McCourt, T., Jacobs, J. M. E., Mace, P., Brownfield, L., Macknight, R. (2022). Identification of the genes at s and z reveals the molecular basis and evolution of grass self-incompatibility. Front. Plant Sci. 13. doi: 10.3389/fpls.2022.1011299
Hickey, L. T., Hafeez, N. A., Robinson, H., Jackson, S. A., Leal-Bertioli, S. C. M., Tester, M., et al. (2019). Breeding crops to feed 10 billion. Nat. Biotechnol. 37, 744–754. doi: 10.1038/s41587-019-0152-9
Hilhorst, H. W. M., Costa, M.-C. D., Farrant, J. M. (2018). A footprint of plant desiccation tolerance. does it exist? Mol. Plant 11, 1003–1005. doi: 10.1016/j.molp.2018.07.001
Hojsgaard, D., Hörandl, E. (2019). The rise of apomixis in natural plant populations. Front. Plant Sci. 10. doi: 10.3389/fpls.2019.00358
Hua, K., Han, P., Zhu, J.-K. (2022). Improvement of base editors and prime editors advances precision genome engineering in plants. Plant Physiol. 188, 1795–1810. doi: 10.1093/plphys/kiab591
Huang, W., Zhang, L., Columbus, J. T., Hu, Y., Zhao, Y., Tang, L., et al. (2022). A well-supported nuclear phylogeny of poaceae and implications for the evolution of C4 photosynthesis. Mol. Plant 15, 755–777. doi: 10.1016/j.molp.2022.01.015
Hussain, M., Farooq, S., Hasan, W., Ul-Allah, S., Tanveer, M., Farooq, M., et al. (2018). Drought stress in sunflower: Physiological effects and its management through breeding and agronomic alternatives. Agric. Water Manage. 201, 152–166. doi: 10.1016/j.agwat.2018.01.028
Hussain, S., Khan, F., Cao, W., Wu, L., Geng, M. (2016). Seed priming alters the production and detoxification of reactive oxygen intermediates in rice seedlings grown under Sub-optimal temperature and nutrient supply. Front. Plant Sci. 7. doi: 10.3389/fpls.2016.00439
Hwarari, D., Guan, Y., Ahmad, B., Movahedi, A., Min, T., Hao, Z., et al. (2022). ICE-CBF-COR signaling cascade and its regulation in plants responding to cold stress. Int. J. Mol. Sci. 23, 1549. doi: 10.3390/ijms23031549
Jørgensen, M., Torp, T., Mølmann, J. A. B. (2020). Impact of waterlogging and temperature on autumn growth, hardening and freezing tolerance of timothy (Phleum pratense). J. Agron. Crop Sci. 206, 242–251. doi: 10.1111/jac.12385
Jagadish, S. V. K., Way, D. A., Sharkey, T. D. (2021). Plant heat stress: Concepts directing future research. Plant Cell Environ. 44, 1992–2005. doi: 10.1111/pce.14050
Jamil, A., Riaz, S., Ashraf, M., Foolad, M. R. (2011). Gene expression profiling of plants under salt stress. Crit. Rev. Plant Sci. 30, 435–458. doi: 10.1080/07352689.2011.605739
Janda, T., Majláth, I., Szalai, G. (2014). Interaction of temperature and light in the development of freezing tolerance in plants. J. Plant Growth Regul. 33, 460–469. doi: 10.1007/s00344-013-9381-1
Jaškūnė, K., Aleliūnas, A., Statkevičiūtė, G., Kemešytė, V., Studer, B., Yates, S. (2020). Genome-wide association study to identify candidate loci for biomass formation under water deficit in perennial ryegrass. Front. Plant Sci. 11. doi: 10.3389/fpls.2020.570204
Jaškūnė, K., Armonienė, R., Liatukas, Ž., Statkevičiūtė, G., Cesevičienė, J., Brazauskas, G. (2022a). Relationship between freezing tolerance and leaf growth during acclimation in winter wheat. Agronomy 12, 859. doi: 10.3390/agronomy12040859
Jaškūnė, K., Kemešytė, V., Aleliūnas, A., Statkevičiūtė, G. (2022b). Genome-wide markers for seed yield and disease resistance in perennial ryegrass. Crop J. 10, 508–514. doi: 10.1016/j.cj.2021.07.005
Jia, Q., Kong, D., Li, Q., Sun, S., Song, J., Zhu, Y., et al. (2019). The function of inositol phosphatases in plant tolerance to abiotic stress. Int. J. Mol. Sci. 20, 3999. doi: 10.3390/ijms20163999
Jiang, W., Zhou, H., Bi, H., Fromm, M., Yang, B., Weeks, D. P. (2013). Demonstration of CRISPR/Cas9/sgRNA-mediated targeted gene modification in arabidopsis, tobacco, sorghum and rice. Nucleic Acids Res. 41, e188. doi: 10.1093/nar/gkt780
Jinek, M., Chylinski, K., Fonfara, I., Hauer, M., Doudna, J. A., Charpentier, E. (2012). A programmable Dual-RNA–guided DNA endonuclease in adaptive bacterial immunity. Science 337, 816–821. doi: 10.1126/science.1225829
Jonavičienė, K., Paplauskienė, V., Brazauskas, G. (2009). Isozymes and ISSR markers as a tool for the assessment of genetic diversity in phleum spp. Žemdirb. Agric. 96, 47–57.
Ju, H., Li, D., Li, D., Yang, X., Liu, Y. (2021). Overexpression of ZmDHN11 could enhance transgenic yeast and tobacco tolerance to osmotic stress. Plant Cell Rep. 40, 1723–1733. doi: 10.1007/s00299-021-02734-0
Kanwar, P., Sanyal, S. K., Tokas, I., Yadav, A. K., Pandey, A., Kapoor, S., et al. (2014). Comprehensive structural, interaction and expression analysis of CBL and CIPK complement during abiotic stresses and development in rice. Cell Calcium 56, 81–95. doi: 10.1016/j.ceca.2014.05.003
Kearns, P. W. E., Kleter, G. A., Bergmans, H. E. N., Kuiper, H. A. (2021). Biotechnology and biosafety policy at OECD: Future trends. Trends Biotechnol. 39, 965–969. doi: 10.1016/j.tibtech.2021.03.001
Khadka, V. S., Vaughn, K., Xie, J., Swaminathan, P., Ma, Q., Cramer, G. R., et al. (2019). Transcriptomic response is more sensitive to water deficit in shoots than roots of vitis riparia (Michx.). BMC Plant Biol. 19, 72. doi: 10.1186/s12870-019-1664-7
Khanday, I., Skinner, D., Yang, B., Mercier, R., Sundaresan, V. (2019). A male-expressed rice embryogenic trigger redirected for asexual propagation through seeds. Nature 565, 91–95. doi: 10.1038/s41586-018-0785-8
Kooyers, N. J. (2015). The evolution of drought escape and avoidance in natural herbaceous populations. Plant Sci. Int. J. Exp. Plant Biol. 234, 155–162. doi: 10.1016/j.plantsci.2015.02.012
Kosová, K., Prášil, I. T., Vítámvás, P. (2019). “Role of dehydrins in plant stress response,” in Handbook of plant and crop stress, fourth edition (CRC Press). eBook ISBN9781351104609.
Kovi, M. R., Ergon, Å., Rognli, O. A. (2016). Freezing tolerance revisited–effects of variable temperatures on gene regulation in temperate grasses and legumes. Curr. Opin. Plant Biol. 33, 140–146. doi: 10.1016/j.pbi.2016.07.006
Krishna, P. (2004). “Plant responses to heat stress,” in Plant responses to abiotic stress topics in current genetics. Eds. Hirt, H., Shinozaki, K. (Berlin, Heidelberg: Springer), 73–101. doi: 10.1007/978-3-540-39402-0_4
Kumar, R., Kamuda, T., Budhathoki, R., Tang, D., Yer, H., Zhao, Y., et al. (2022). Agrobacterium- and a single Cas9-sgRNA transcript system-mediated high efficiency gene editing in perennial ryegrass. Front. Genome Ed. 4. doi: 10.3389/fgeed.2022.960414
Kwadzo, M., Quayson, E. (2021). Factors influencing adoption of integrated soil fertility management technologies by smallholder farmers in Ghana. Heliyon 7, e07589. doi: 10.1016/j.heliyon.2021.e07589
Larsen, A. (1994). “Breeding winter hardy grasses,” in Breeding fodder crops for marginal conditions: Proceedings of the 18th eucarpia fodder crops section meeting, loen, Norway, 25–28 august 1993 developments in plant breeding. Eds. Rognli, O. A., Solberg, E., Schjelderup, I. (Dordrecht: Springer Netherlands), 149–158. doi: 10.1007/978-94-011-0966-6_18
Lee, S., Choi, S., Jeon, D., Kang, Y., Kim, C. (2020). Evolutionary impact of whole genome duplication in poaceae family. J. Crop Sci. Biotechnol. 23, 413–425. doi: 10.1007/s12892-020-00049-2
Lee, S. C., Luan, S. (2012). ABA signal transduction at the crossroad of biotic and abiotic stress responses. Plant Cell Environ. 35, 53–60. doi: 10.1111/j.1365-3040.2011.02426.x
Leibman, M., Shryock, J. J., Clements, M. J., Hall, M. A., Loida, P. J., McClerren, A. L., et al. (2014). Comparative analysis of maize (Zea mays) crop performance: natural variation, incremental improvements and economic impacts. Plant Biotechnol. J. 12, 941–950. doi: 10.1111/pbi.12202
Li, R., Li, R., Li, X., Fu, D., Zhu, B., Tian, H., et al. (2018). Multiplexed CRISPR/Cas9-mediated metabolic engineering of γ-aminobutyric acid levels in solanum lycopersicum. Plant Biotechnol. J. 16, 415–427. doi: 10.1111/pbi.12781
Li, J.-F., Norville, J. E., Aach, J., McCormack, M., Zhang, D., Bush, J., et al. (2013). Multiplex and homologous recombination–mediated genome editing in arabidopsis and nicotiana benthamiana using guide RNA and Cas9. Nat. Biotechnol. 31, 688–691. doi: 10.1038/nbt.2654
Li, J., Scarano, A., Gonzalez, N. M., D’Orso, F., Yue, Y., Nemeth, K., et al. (2022). Biofortified tomatoes provide a new route to vitamin d sufficiency. Nat. Plants 8, 611–616. doi: 10.1038/s41477-022-01154-6
Liu, Z., Jia, Y., Ding, Y., Shi, Y., Li, Z., Guo, Y., et al. (2017c). Plasma membrane CRPK1-mediated phosphorylation of 14-3-3 proteins induces their nuclear import to fine-tune CBF signaling during cold response. Mol. Cell 66, 117–128.e5. doi: 10.1016/j.molcel.2017.02.016
Liu, X., Meng, P., Yang, G., Zhang, M., Peng, S., Zhai, M. Z. (2020a). Genome-wide identification and transcript profiles of walnut heat stress transcription factor involved in abiotic stress. BMC Genomics 21, 474. doi: 10.1186/s12864-020-06879-2
Liu, Y., Song, Q., Li, D., Yang, X., Li, D. (2017). Multifunctional roles of plant dehydrins in response to environmental stresses. Front. Plant Sci. 8. doi: 10.3389/fpls.2017.01018
Liu, Y., Wang, W., Yang, B., Currey, C., Fei, S. (2020b). Functional analysis of the teosinte branched 1 gene in the tetraploid switchgrass (Panicum virgatum l.) by CRISPR/Cas9-directed mutagenesis. Front. Plant Sci. 11. doi: 10.3389/fpls.2020.572193
Li, X., Wilkinson, S., Shen, J., Forde, B. G., Davies, W. J. (2017). Stomatal and growth responses to hydraulic and chemical changes induced by progressive soil drying. J. Exp. Bot. 68, 5883–5894. doi: 10.1093/jxb/erx381
Loka, D., Harper, J., Humphreys, M., Gasior, D., Wootton-Beard, P., Gwynn-Jones, D., et al. (2019). Impacts of abiotic stresses on the physiology and metabolism of cool-season grasses: A review. Food Energy Secur. 8, e00152. doi: 10.1002/fes3.152
Lopez, B., Hines, P. J., Ash, C. (2022). The unrecognized value of grass. Science 377, 590–591. doi: 10.1126/science.add6362
Lundqvist, A. (1955). Genetics of self-incompatibility in festuca pratensis huds. Hereditas 41, 518–520. doi: 10.1111/j.1601-5223.1955.tb03007.x
Lv, A., Fan, N., Xie, J., Yuan, S., An, Y., Zhou, P. (2017). Expression of CdDHN4, a novel YSK2-type dehydrin gene from bermudagrass, responses to drought stress through the ABA-dependent signal pathway. Front. Plant Sci. 8. doi: 10.3389/fpls.2017.00748
Ma, Y., Dai, X., Xu, Y., Luo, W., Zheng, X., Zeng, D., et al. (2015). COLD1 confers chilling tolerance in rice. Cell 160, 1209–1221. doi: 10.1016/j.cell.2015.01.046
Ma, Y., Dias, M. C., Freitas, H. (2020). Drought and salinity stress responses and microbe-induced tolerance in plants. Front. Plant Sci. 11. doi: 10.3389/fpls.2020.591911
Maize DP-915635-4 (n.d.). Available at: https://open.efsa.europa.eu (Accessed December 5, 2022).
Mann, A., Kumar, N., Kumar, A., Lata, C., Kumar, A., Meena, B. L., et al. (2021). de novo transcriptomic profiling of differentially expressed genes in grass halophyte urochondra setulosa under high salinity. Sci. Rep. 11, 5548. doi: 10.1038/s41598-021-85220-7
Manzanares, C., Barth, S., Thorogood, D., Byrne, S. L., Yates, S., Czaban, A., et al. (2016). A gene encoding a DUF247 domain protein cosegregates with the s self-incompatibility locus in perennial ryegrass. Mol. Biol. Evol. 33, 870–884. doi: 10.1093/molbev/msv335
Martínez-Atienza, J., Jiang, X., Garciadeblas, B., Mendoza, I., Zhu, J.-K., Pardo, J. M., et al. (2007). Conservation of the salt overly sensitive pathway in rice. Plant Physiol. 143, 1001–1012. doi: 10.1104/pp.106.092635
Maruyama, K., Urano, K., Yoshiwara, K., Morishita, Y., Sakurai, N., Suzuki, H., et al. (2014). Integrated analysis of the effects of cold and dehydration on rice metabolites, phytohormones, and gene transcripts. Plant Physiol. 164, 1759–1771. doi: 10.1104/pp.113.231720
McDonagh, J., O’Donovan, M., McEvoy, M., Gilliland, T. J. (2016). Genetic gain in perennial ryegrass (Lolium perenne) varieties 1973 to 2013. Euphytica 212, 187–199. doi: 10.1007/s10681-016-1754-7
McSteen, P., Kellogg, E. A. (2022). Molecular, cellular, and developmental foundations of grass diversity. Science 377, 599–602. doi: 10.1126/science.abo5035
Meyer, R. S., DuVal, A. E., Jensen, H. R. (2012). Patterns and processes in crop domestication: an historical review and quantitative analysis of 203 global food crops. New Phytol. 196, 29–48. doi: 10.1111/j.1469-8137.2012.04253.x
Miao, C., Xiao, L., Hua, K., Zou, C., Zhao, Y., Bressan, R. A., et al. (2018). Mutations in a subfamily of abscisic acid receptor genes promote rice growth and productivity. Proc. Natl. Acad. Sci. 115, 6058–6063. doi: 10.1073/pnas.1804774115
Mishra, A., Tanna, B. (2017). Halophytes: Potential resources for salt stress tolerance genes and promoters. Front. Plant Sci. 8. doi: 10.3389/fpls.2017.00829
Moorby, J. M., Fraser, M. D. (2021). Review: New feeds and new feeding systems in intensive and semi-intensive forage-fed ruminant livestock systems. Animal 15, 100297. doi: 10.1016/j.animal.2021.100297
Moore, F. C., Lobell, D. B. (2015). The fingerprint of climate trends on European crop yields. Proc. Natl. Acad. Sci. 112, 2670–2675. doi: 10.1073/pnas.1409606112
Moreno-Mateos, M. A., Fernandez, J. P., Rouet, R., Vejnar, C. E., Lane, M. A., Mis, E., et al. (2017). CRISPR-Cpf1 mediates efficient homology-directed repair and temperature-controlled genome editing. Nat. Commun. 8, 2024. doi: 10.1038/s41467-017-01836-2
Moser, L. E., Burson, B. L., Sollenberger, L. E. (2004). Warm-season (C4) grass overview. In Warm-season (C4) grasses. Eds. Moser Burson, L. E. , Burson, B. L., Sollenberger, L. E.. doi: 10.2134/agronmonogr45.c1
Moser, L. E., Hoveland, C.S. (1996). Cool-Season Grass Overview. In Cool-Season Forage Grasses Eds Moser, L.E., Buxton, D.R., Casler, M.D.. doi: 10.2134/agronmonogr34.c1
Muguerza, M. B., Gondo, T., Ishigaki, G., Shimamoto, Y., Umami, N., Nitthaisong, P., et al. (2022). Tissue culture and somatic embryogenesis in warm-season grasses–current status and its applications: A review. Plants 11, 1263. doi: 10.3390/plants11091263
Nagy, I., Veeckman, E., Liu, C., Bel, M. V., Vandepoele, K., Jensen, C. S., et al. (2022). Chromosome-scale assembly and annotation of the perennial ryegrass genome. BMC Genomics 23, 505. doi: 10.1186/s12864-022-08697-0
Nakaminami, K., Matsui, A., Nakagami, H., Minami, A., Nomura, Y., Tanaka, M., et al. (2014). Analysis of differential expression patterns of mRNA and protein during cold-acclimation and de-acclimation in arabidopsis. Mol. Cell. Proteomics 13, 3602–3611. doi: 10.1074/mcp.M114.039081
National Academies of Sciences, Engineering, and Medicine, Division on Earth and Life Studies, Board on Agriculture and Natural Resources, Committee on Genetically Engineered Crops: Past Experience and Future Prospects (2016) Genetically engineered crops: Experiences and prospects (Washington (DC: National Academies Press (US). Available at: http://www.ncbi.nlm.nih.gov/books/NBK424543/ (Accessed November 11, 2022).
Nekrasov, V., Staskawicz, B., Weigel, D., Jones, J. D. G., Kamoun, S. (2013). Targeted mutagenesis in the model plant nicotiana benthamiana using Cas9 RNA-guided endonuclease. Nat. Biotechnol. 31, 691–693. doi: 10.1038/nbt.2655
Nemali, K. S., Bonin, C., Dohleman, F. G., Stephens, M., Reeves, W. R., Nelson, D. E., et al. (2015). Physiological responses related to increased grain yield under drought in the first biotechnology-derived drought-tolerant maize. Plant Cell Environ. 38, 1866–1880. doi: 10.1111/pce.12446
Niu, Y., Xiang, Y. (2018). An overview of biomembrane functions in plant responses to high-temperature stress. Front. Plant Sci. 9. doi: 10.3389/fpls.2018.00915
Okada, A., Arndell, T., Borisjuk, N., Sharma, N., Watson-Haigh, N. S., Tucker, E. J., et al. (2019). CRISPR/Cas9-mediated knockout of Ms1 enables the rapid generation of male-sterile hexaploid wheat lines for use in hybrid seed production. Plant Biotechnol. J. 17, 1905–1913. doi: 10.1111/pbi.13106
Oliver, M. J., Farrant, J. M., Hilhorst, H. W. M., Mundree, S., Williams, B., Bewley, J. D. (2020). Desiccation tolerance: Avoiding cellular damage during drying and rehydration. Annu. Rev. Plant Biol. 71, 435–460. doi: 10.1146/annurev-arplant-071219-105542
Papikian, A., Liu, W., Gallego-Bartolomé, J., Jacobsen, S. E. (2019). Site-specific manipulation of arabidopsis loci using CRISPR-Cas9 SunTag systems. Nat. Commun. 10, 729. doi: 10.1038/s41467-019-08736-7
Pardo, J., Man Wai, C., Chay, H., Madden, C. F., Hilhorst, H. W. M., Farrant, J. M., et al. (2020). Intertwined signatures of desiccation and drought tolerance in grasses. Proc. Natl. Acad. Sci. 117, 10079–10088. doi: 10.1073/pnas.2001928117
Park, S., Gilmour, S. J., Grumet, R., Thomashow, M. F. (2018). CBF-dependent and CBF-independent regulatory pathways contribute to the differences in freezing tolerance and cold-regulated gene expression of two arabidopsis ecotypes locally adapted to sites in Sweden and Italy. PloS One 13, e0207723. doi: 10.1371/journal.pone.0207723
Pascual, L. S., Segarra-Medina, C., Gómez-Cadenas, A., López-Climent, M. F., Vives-Peris, V., Zandalinas, S. I. (2022). Climate change-associated multifactorial stress combination: A present challenge for our ecosystems. J. Plant Physiol 276. doi: 10.1016/j.jplph.2022.153764
Pornsiriwong, W., Estavillo, G. M., Chan, K. X., Tee, E. E., Ganguly, D., Crisp, P. A., et al. (2017). A chloroplast retrograde signal, 3’-phosphoadenosine 5’-phosphate, acts as a secondary messenger in abscisic acid signaling in stomatal closure and germination. eLife 6, e23361. doi: 10.7554/eLife.23361
Posselt, U. K. (2010). “Breeding methods in cross-pollinated species,” in Fodder crops and amenity grasses handbook of plant breeding. Eds. Boller, B., Posselt, U. K., Veronesi, F. (New York, NY: Springer), 39–87. doi: 10.1007/978-1-4419-0760-8_3
Pörtner, H. O., Roberts, D. C., Adams, H., Adler, C., Aldunce, P., Ali, E., et al (2022). Climate change 2022: Impacts, adaptation and vulnerability. 3056 Geneva, Switzerland: IPCC. doi: 10.1017/9781009325844
Puchta, H., Jiang, J., Wang, K., Zhao, Y. (2022). Updates on gene editing and its applications. Plant Physiol. 188, 1725–1730. doi: 10.1093/plphys/kiac032
Purnhagen, K. P., Kok, E., Kleter, G., Schebesta, H., Visser, R. G. F., Wesseler, J. (2018). EU Court casts new plant breeding techniques into regulatory limbo. Nat. Biotechnol. 36, 799–800. doi: 10.1038/nbt.4251
Qadir, M., Quillérou, E., Nangia, V., Murtaza, G., Singh, M., Thomas, R. j., et al. (2014). Economics of salt-induced land degradation and restoration. Nat. Resour. Forum 38, 282–295. doi: 10.1111/1477-8947.12054
Rapacz, M., Ergon, Å., Höglind, M., Jørgensen, M., Jurczyk, B., Østrem, L., et al. (2014). Overwintering of herbaceous plants in a changing climate. still more questions than answers. Plant Sci. 225, 34–44. doi: 10.1016/j.plantsci.2014.05.009
Rapacz, M., Jurczyk, B., Sasal, M. (2017). Deacclimation may be crucial for winter survival of cereals under warming climate. Plant Sci. 256, 5–15. doi: 10.1016/j.plantsci.2016.11.007
Rauf, S., Ortiz, R., Malinowski, D. P., Clarindo, W. R., Kainat, W., Shehzad, M., et al. (2021). Induced polyploidy: A tool for forage species improvement. Agriculture 11, 210. doi: 10.3390/agriculture11030210
Raza, A., Razzaq, A., Mehmood, S., Zou, X., Zhang, X., Lv, Y., et al. (2019). Impact of climate change on crops adaptation and strategies to tackle its outcome: A review. Plants 8, 34. doi: 10.3390/plants8020034
Rice, E. A., Khandelwal, A., Creelman, R. A., Griffith, C., Ahrens, J. E., Taylor, J. P., et al. (2014). Expression of a truncated ATHB17 protein in maize increases ear weight at silking. PloS One 9, e94238. doi: 10.1371/journal.pone.0094238
Rivero, R. M., Mittler, R., Blumwald, E., Zandalinas, S. I. (2021). Developing climate-resilient crops: improving plant tolerance to stress combination. Plant J 109 (2). doi: 10.1111/tpj.15483
Rognli, O. A. (2013). “Breeding for improved winter survival in forage grasses,” in Plant and microbe adaptations to cold in a changing world. Eds. Imai, R., Yoshida, M., Matsumoto, N. (New York, NY: Springer), 197–208. doi: 10.1007/978-1-4614-8253-6_17
Rognli, O. A., Pecetti, L., Kovi, M. R., Annicchiarico, P. (2021). Grass and legume breeding matching the future needs of European grassland farming. Grass Forage Sci. 76, 175–185. doi: 10.1111/gfs.12535
Rostoks, N. (2021). Implications of the EFSA scientific opinion on site directed nucleases 1 and 2 for risk assessment of genome-edited plants in the EU. Agronomy 11, 572. doi: 10.3390/agronomy11030572
Saab, I. N., Sharp, R. E., Pritchard, J., Voetberg, G. S. (1990). Increased endogenous abscisic acid maintains primary root growth and inhibits shoot growth of maize seedlings at low water potentials 1. Plant Physiol. 93, 1329–1336. doi: 10.1104/pp.93.4.1329
Sah, S. K., Reddy, K. R., Li, J. (2016). Abscisic acid and abiotic stress tolerance in crop plants. Front. Plant Sci. 7. doi: 10.3389/fpls.2016.00571
Sampoux, J.-P., Baudouin, P., Bayle, B., Béguier, V., Bourdon, P., Chosson, J.-F., et al. (2011). Breeding perennial grasses for forage usage: An experimental assessment of trait changes in diploid perennial ryegrass (Lolium perenne l.) cultivars released in the last four decades. Field Crops Res. 123, 117–129. doi: 10.1016/j.fcr.2011.05.007
Sánchez-León, S., Gil-Humanes, J., Ozuna, C. V., Giménez, M. J., Sousa, C., Voytas, D. F., et al. (2018). Low-gluten, nontransgenic wheat engineered with CRISPR/Cas9. Plant Biotechnol. J. 16, 902–910. doi: 10.1111/pbi.12837
Santosh Kumar, V. V., Verma, R. K., Yadav, S. K., Yadav, P., Watts, A., Rao, M. V., et al. (2020). CRISPR-Cas9 mediated genome editing of drought and salt tolerance (OsDST) gene in indica mega rice cultivar MTU1010. Physiol. Mol. Biol. Plants 26, 1099–1110. doi: 10.1007/s12298-020-00819-w
Schulman, A. H., Oksman-Caldentey, K., Teeri, T. H. (2020). European Court of justice delivers no justice to Europe on genome-edited crops. Plant Biotechnol. J. 18, 8–10. doi: 10.1111/pbi.13200
Shabala, S., Wu, H., Bose, J. (2015). Salt stress sensing and early signalling events in plant roots: Current knowledge and hypothesis. Plant Sci. 241, 109–119. doi: 10.1016/j.plantsci.2015.10.003
Shanmugam, S., Boyett, V. A., Khodakovskaya, M. (2021). Enhancement of drought tolerance in rice by silencing of the OsSYT-5 gene. PloS One 16, e0258171. doi: 10.1371/journal.pone.0258171
Shan, Q., Wang, Y., Li, J., Zhang, Y., Chen, K., Liang, Z., et al. (2013). Targeted genome modification of crop plants using a CRISPR-cas system. Nat. Biotechnol. 31, 686–688. doi: 10.1038/nbt.2650
She, K., Pan, W., Yan, Y., Shi, T., Chu, Y., Cheng, Y., et al. (2022). Genome-wide identification, evolution and expressional analysis of OSCA gene family in barley (Hordeum vulgare l.). Int. J. Mol. Sci. 23, 13027. doi: 10.3390/ijms232113027
Shi, J., Gao, H., Wang, H., Lafitte, H. R., Archibald, R. L., Yang, M., et al. (2017). ARGOS8 variants generated by CRISPR-Cas9 improve maize grain yield under field drought stress conditions. Plant Biotechnol. J. 15, 207–216. doi: 10.1111/pbi.12603
Shukla, V. K., Doyon, Y., Miller, J. C., DeKelver, R. C., Moehle, E. A., Worden, S. E., et al. (2009). Precise genome modification in the crop species zea mays using zinc-finger nucleases. Nature 459, 437–441. doi: 10.1038/nature07992
Singh, M., Kumar, M., Albertsen, M. C., Young, J. K., Cigan, A. M. (2018). Concurrent modifications in the three homeologs of Ms45 gene with CRISPR-Cas9 lead to rapid generation of male sterile bread wheat (Triticum aestivum l.). Plant Mol. Biol. 97, 371–383. doi: 10.1007/s11103-018-0749-2
Singh, A., Kumar, A., Yadav, S., Singh, I. K. (2019). Reactive oxygen species-mediated signaling during abiotic stress. Plant Gene 18, 100173. doi: 10.1016/j.plgene.2019.100173
Smith, K. F., Spangenberg, G. (2016). Considerations for managing agricultural Co-existence between transgenic and non-transgenic cultivars of outcrossing perennial forage plants in dairy pastures. Agronomy 6, 59. doi: 10.3390/agronomy6040059
Spiekerman, J. J., Devos, K. M. (2020). The halophyte seashore paspalum uses adaxial leaf papillae for sodium sequestration. Plant Physiol. 184, 2107–2119. doi: 10.1104/pp.20.00796
Strömberg, C. A. E., Staver, A. C. (2022). The history and challenge of grassy biomes. Science 377, 592–593. doi: 10.1126/science.add1347
Sugimoto, K., Nowack, M. K. (2022). Plant development meets climate emergency – it’s time to plant an apple tree. Curr. Opin. Plant Biol. 65, 102175. doi: 10.1016/j.pbi.2022.102175
Sun, Z., Li, S., Chen, W., Zhang, J., Zhang, L., Sun, W., et al. (2021). Plant dehydrins: Expression, regulatory networks, and protective roles in plants challenged by abiotic stress. Int. J. Mol. Sci. 22, 12619. doi: 10.3390/ijms222312619
Svensson, J., Ismail, A. M., Tapio Palva, E., Close, T. J. (2002). “Chapter 11 - dehydrins,” in Cell and molecular response to stress sensing, signaling and cell adaptation. Eds. Storey, K. B., Storey, J. M. (Elsevier), 155–171. doi: 10.1016/S1568-1254(02)80013-4
Swinnen, G., Goossens, A., Pauwels, L. (2016). Lessons from domestication: Targeting cis-regulatory elements for crop improvement. Trends Plant Sci. 21, 506–515. doi: 10.1016/j.tplants.2016.01.014
Tanenbaum, M. E., Gilbert, L. A., Qi, L. S., Weissman, J. S., Vale, R. D. (2014). A protein-tagging system for signal amplification in gene expression and fluorescence imaging. Cell 159, 635–646. doi: 10.1016/j.cell.2014.09.039
Terrer, C., Phillips, R. P., Hungate, B. A., Rosende, J., Pett-Ridge, J., Craig, M. E., et al. (2021). A trade-off between plant and soil carbon storage under elevated CO2. Nature 591, 599–603. doi: 10.1038/s41586-021-03306-8
Tester, M., Davenport, R. (2003). Na+ tolerance and na+ transport in higher plants. Ann. Bot. 91, 503–527. doi: 10.1093/aob/mcg058
Thomashow, M. F. (1999). PLANT COLD ACCLIMATION: Freezing tolerance genes and regulatory mechanisms. Annu. Rev. Plant Physiol. Plant Mol. Biol. 50, 571–599. doi: 10.1146/annurev.arplant.50.1.571
Thomashow, M. F. (2010). Molecular basis of plant cold acclimation: Insights gained from studying the CBF cold response pathway. Plant Physiol. 154, 571–577. doi: 10.1104/pp.110.161794
Tian, S., Mao, X., Zhang, H., Chen, S., Zhai, C., Yang, S., et al. (2013). Cloning and characterization of TaSnRK2.3, a novel SnRK2 gene in common wheat. J. Exp. Bot. 64, 2063–2080. doi: 10.1093/jxb/ert072
Urnov, F. D., Rebar, E. J., Holmes, M. C., Zhang, H. S., Gregory, P. D. (2010). Genome editing with engineered zinc finger nucleases. Nat. Rev. Genet. 11, 636–646. doi: 10.1038/nrg2842
Urnov, F. D., Ronald, P. C., Carroll, D. (2018). A call for science-based review of the European court’s decision on gene-edited crops. Nat. Biotechnol. 36, 800–802. doi: 10.1038/nbt.4252
Valluru, R., Van den Ende, W. (2008). Plant fructans in stress environments: emerging concepts and future prospects. J. Exp. Bot. 59, 2905–2916. doi: 10.1093/jxb/ern164
VanBuren, R., Wai, C. M., Zhang, Q., Song, X., Edger, P. P., Bryant, D., et al. (2017). Seed desiccation mechanisms co-opted for vegetative desiccation in the resurrection grass oropetium thomaeum. Plant Cell Environ. 40, 2292–2306. doi: 10.1111/pce.13027
Vaziriyeganeh, M., Khan, S., Zwiazek, J. J. (2021). Transcriptome and metabolome analyses reveal potential salt tolerance mechanisms contributing to maintenance of water balance by the halophytic grass puccinellia nuttalliana. Front. Plant Sci. 12. doi: 10.3389/fpls.2021.760863
Vazquez-Hernandez, M., Romero, I., Escribano, M. I., Merodio, C., Sanchez-Ballesta, M. T. (2017). Deciphering the role of CBF/DREB transcription factors and dehydrins in maintaining the quality of table grapes cv. autumn royal treated with high CO2 levels and stored at 0°C. Front. Plant Sci. 8. doi: 10.3389/fpls.2017.01591
Villalobos-López, M. A., Arroyo-Becerra, A., Quintero-Jiménez, A., Iturriaga, G. (2022). Biotechnological advances to improve abiotic stress tolerance in crops. Int. J. Mol. Sci. 23, 12053. doi: 10.3390/ijms231912053
Vlčko, T., Ohnoutková, L. (2020). Allelic variants of CRISPR/Cas9 induced mutation in an inositol trisphosphate 5/6 kinase gene manifest different phenotypes in barley. Plants 9, 195. doi: 10.3390/plants9020195
Waltz, E. (2021). GABA-enriched tomato is first CRISPR-edited food to enter market. Nat. Biotechnol. 40, 9–11. doi: 10.1038/d41587-021-00026-2
Wang, Z.-Y., Ge, Y. (2006). Recent advances in genetic transformation of forage and turf grasses. Vitro Cell. Dev. Biol. - Plant 42, 1–18. doi: 10.1079/IVP2005726
Wang, X., Huang, W., Liu, J., Yang, Z., Huang, B. (2017). Molecular regulation and physiological functions of a novel FaHsfA2c cloned from tall fescue conferring plant tolerance to heat stress. Plant Biotechnol. J. 15, 237–248. doi: 10.1111/pbi.12609
Wang, H., Jia, G., Zhang, N., Zhi, H., Xing, L., Zhang, H., et al. (2022). Domestication-associated PHYTOCHROME c is a flowering time repressor and a key factor determining setaria as a short-day plant. New Phytol. 236, 1809–1823. doi: 10.1111/nph.18493
Wang, J., Vanga, S. K., Saxena, R., Orsat, V., Raghavan, V. (2018). Effect of climate change on the yield of cereal crops: A review. Climate 6, 41. doi: 10.3390/cli6020041
Wasmer, M. (2019). Roads forward for European GMO policy–uncertainties in wake of ECJ judgment have to be mitigated by regulatory reform. Front. Bioeng. Biotechnol. 7. doi: 10.3389/fbioe.2019.00132
Waterhouse, A. L., Chatterton, N. J. (1993). “Glossary of fructan terms,” in Science and technology of fructans (FL: CRC Press Boca Raton), 1–7.
Weiss, T., Wang, C., Kang, X., Zhao, H., Elena Gamo, M., Starker, C. G., et al. (2020). Optimization of multiplexed CRISPR/Cas9 system for highly efficient genome editing in setaria viridis. Plant J. 104, 828–838. doi: 10.1111/tpj.14949
Wilkinson, S., Davies, W. J. (2010). Drought, ozone, ABA and ethylene: new insights from cell to plant to community. Plant Cell Environ. 33, 510–525. doi: 10.1111/j.1365-3040.2009.02052.x
Wu, S., Greiner, S., Ma, C., Zhong, J., Huang, X., Rausch, T., et al. (2021). A fructan exohydrolase from maize degrades both inulin and levan and Co-exists with 1-kestotriose in maize. Int. J. Mol. Sci. 22, 5149. doi: 10.3390/ijms22105149
Wu, X., Wang, Y., Bian, Y., Ren, Y., Xu, X., Zhou, F., et al. (2022). A critical review on plant annexin: Structure, function, and mechanism. Plant Physiol. Biochem. 190, 81–89. doi: 10.1016/j.plaphy.2022.08.019
Xiang, D.-J., Man, L.-L., Cao, S., Liu, P., Li, Z.-G., Wang, X.-D. (2020). Heterologous expression of an agropyron cristatum SnRK2 protein kinase gene (AcSnRK2.11) increases freezing tolerance in transgenic yeast and tobacco. 3 Biotech. 10, 209. doi: 10.1007/s13205-020-02203-7
Xu, H.-S., Guo, S.-M., Zhu, L., Xing, J.-C. (2020). Growth, physiological and transcriptomic analysis of the perennial ryegrass lolium perenne in response to saline stress. R. Soc Open Sci. 7, 200637. doi: 10.1098/rsos.200637
Xu, T., Niu, J., Jiang, Z. (2022). Sensing mechanisms: Calcium signaling mediated abiotic stress in plants. Front. Plant Sci. 13. doi: 10.3389/fpls.2022.925863
Yamaguchi-Shinozaki, K., Shinozaki, K. (2006). Transcriptional regulatory networks in cellular responses and tolerance to dehydration and cold stresses. Annu. Rev. Plant Biol. 57, 781–803. doi: 10.1146/annurev.arplant.57.032905.105444
Yan, Q., Huang, Q., Chen, J., Li, J., Liu, Z., Yang, Y., et al. (2017). SYTA has positive effects on the heat resistance of arabidopsis. Plant Growth Regul. 81, 467–476. doi: 10.1007/s10725-016-0224-5
Ye, M., Peng, Z., Tang, D., Yang, Z., Li, D., Xu, Y., et al. (2018). Generation of self-compatible diploid potato by knockout of s-RNase. Nat. Plants 4, 651–654. doi: 10.1038/s41477-018-0218-6
Yoshida, M. (2021). Fructan structure and metabolism in overwintering plants. Plants 10, 933. doi: 10.3390/plants10050933
Yu, W., Wang, L., Zhao, R., Sheng, J., Zhang, S., Li, R., et al. (2019). Knockout of SlMAPK3 enhances tolerance to heat stress involving ROS homeostasis in tomato plants. BMC Plant Biol. 19, 354. doi: 10.1186/s12870-019-1939-z
Zeng, Y., Wen, J., Zhao, W., Wang, Q., Huang, W. (2020). Rational improvement of rice yield and cold tolerance by editing the three genes OsPIN5b, GS3, and OsMYB30 with the CRISPR–Cas9 system. Front. Plant Sci. 10. doi: 10.3389/fpls.2019.01663
Zetsche, B., Gootenberg, J. S., Abudayyeh, O. O., Slaymaker, I. M., Makarova, K. S., Essletzbichler, P., et al. (2015). Cpf1 is a single RNA-guided endonuclease of a class 2 CRISPR-cas system. Cell 163, 759–771. doi: 10.1016/j.cell.2015.09.038
Zhai, Y., Wen, Z., Han, Y., Zhuo, W., Wang, F., Xi, C., et al. (2020). Heterogeneous expression of plasma-membrane-localised OsOSCA1.4 complements osmotic sensing based on hyperosmolality and salt stress in arabidopsis osca1 mutant. Cell Calcium 91, 102261. doi: 10.1016/j.ceca.2020.102261
Zhang, H., Li, W., Mao, X., Jing, R., Jia, H. (2016). Differential activation of the wheat SnRK2 family by abiotic stresses. Front. Plant Sci. 7. doi: 10.3389/fpls.2016.00420
Zhang, A., Liu, Y., Wang, F., Li, T., Chen, Z., Kong, D., et al. (2019). Enhanced rice salinity tolerance via CRISPR/Cas9-targeted mutagenesis of the OsRR22 gene. Mol. Breed. 39, 47. doi: 10.1007/s11032-019-0954-y
Zhang, Y., Ran, Y., Nagy, I., Lenk, I., Qiu, J.-L., Asp, T., et al. (2020). Targeted mutagenesis in ryegrass (Lolium spp.) using the CRISPR/Cas9 system. Plant Biotechnol. J. 18, 1854–1856. doi: 10.1111/pbi.13359
Zhang, L., Wang, T., Wang, G., Bi, A., Wassie, M., Xie, Y., et al. (2021). Simultaneous gene editing of three homoeoalleles in self-incompatible allohexaploid grasses. J. Integr. Plant Biol. 63, 1410–1415. doi: 10.1111/jipb.13101
Zhang, W., Zhi, H., Tang, S., Zhang, H., Sui, Y., Jia, G., et al. (2021b). Identification of no pollen 1 provides a candidate gene for heterosis utilization in foxtail millet (Setaria italica l.). Crop J. 9, 1309–1319. doi: 10.1016/j.cj.2021.03.018
Zhao, S., Zhang, Q., Liu, M., Zhou, H., Ma, C., Wang, P. (2021). Regulation of plant responses to salt stress. Int. J. Mol. Sci. 22, 4609. doi: 10.3390/ijms22094609
Zhao, C., Zhang, H., Song, C., Zhu, J.-K., Shabala, S. (2020). Mechanisms of plant responses and adaptation to soil salinity. Innovation 1, 100017. doi: 10.1016/j.xinn.2020.100017
Zhu, H., Li, C., Gao, C. (2020). Applications of CRISPR–cas in agriculture and plant biotechnology. Nat. Rev. Mol. Cell Biol. 21, 661–677. doi: 10.1038/s41580-020-00288-9
Keywords: CRISPR, genome editing, gene editing, forage grass, abiotic stress, plant, plant breeding
Citation: Sustek-Sánchez F, Rognli OA, Rostoks N, Sõmera M, Jaškūnė K, Kovi MR, Statkevičiūtė G and Sarmiento C (2023) Improving abiotic stress tolerance of forage grasses – prospects of using genome editing. Front. Plant Sci. 14:1127532. doi: 10.3389/fpls.2023.1127532
Received: 19 December 2022; Accepted: 24 January 2023;
Published: 07 February 2023.
Edited by:
Davinder Singh, The University of Sydney, AustraliaReviewed by:
Gothandapani Sellamuthu, Czech University of Life Sciences Prague, CzechiaFrank Hartung, Julius Kühn-Institut, Germany
Copyright © 2023 Sustek-Sánchez, Rognli, Rostoks, Sõmera, Jaškūnė, Kovi, Statkevičiūtė and Sarmiento. This is an open-access article distributed under the terms of the Creative Commons Attribution License (CC BY). The use, distribution or reproduction in other forums is permitted, provided the original author(s) and the copyright owner(s) are credited and that the original publication in this journal is cited, in accordance with accepted academic practice. No use, distribution or reproduction is permitted which does not comply with these terms.
*Correspondence: Cecilia Sarmiento, Y2VjaWxpYS5zYXJtaWVudG9AdGFsdGVjaC5lZQ==