- 1Michigan State University-Department of Energy Plant Research Laboratory and Department of Plant Biology, Michigan State University, East Lansing, MI, United States
- 2Howard Hughes Medical Institute and Department of Biology, Duke University, Durham, NC, United States
To defend themselves in the face of biotic stresses, plants employ a sophisticated immune system that requires the coordination of other biological and metabolic pathways. Photorespiration, a byproduct pathway of oxygenic photosynthesis that spans multiple cellular compartments and links primary metabolisms, plays important roles in defense responses. Hydrogen peroxide, whose homeostasis is strongly impacted by photorespiration, is a crucial signaling molecule in plant immunity. Photorespiratory metabolites, interaction between photorespiration and defense hormone biosynthesis, and other mechanisms, are also implicated. An improved understanding of the relationship between plant immunity and photorespiration may provide a much-needed knowledge basis for crop engineering to maximize photosynthesis without negative tradeoffs in plant immunity, especially because the photorespiratory pathway has become a major target for genetic engineering with the goal to increase photosynthetic efficiency.
Introduction
In nature, plants are constantly exposed to a dynamic external biotic environment, which drives the development of the plant immune system. As the first layer of immunity, elicitors from pathogenic and nonpathogenic microbes, known as microbe-associated molecular patterns (MAMPs), are recognized by plasma membrane-localized receptors known as pattern recognition receptors (PRRs) to activate pattern-triggered immunity (PTI) (Yu et al., 2017). Flg22, a peptide from the conserved domain of the bacterial flagellin, is one of the MAMPs. PTI also comprises plant responses to plant-derived endogenous elicitors generated in response to wounding or infection, such as small peptides and nucleotides, which are called damage-associated molecular patterns (DAMPs) (Yu et al., 2017). During PTI, intracellular signaling, transcriptional reprogramming, and other physiological responses culminate to limit pathogen growth. These events include increases in cytosolic Ca2+ concentration, reactive oxygen species (ROS) burst, and biosynthesis of phytohormones such as salicylic acid (SA) and jasmonate (JA) (Yu et al., 2017). To infect successfully, most pathogens can secrete virulent effectors into plant cells to suppress plant defense (Deslandes and Rivas, 2012). As the second layer of immunity, plants use intracellular nucleotide-binding/leucine-rich-repeat (NLR) receptors to recognize effectors, either directly or indirectly, leading to the activation of effector-triggered immunity (ETI) (Cui et al., 2015). ETI responses are similar to, but stronger than, those of the PTI, and often cause local programmed cell death called the hypersensitive response (HR) (Cui et al., 2015) (Figure 1). Recent studies reveal that PTI and ETI are not simply two independent and distinct pathways but work together to regulate immune responses (Ngou et al., 2021; Pruitt et al., 2021; Yuan et al., 2021).
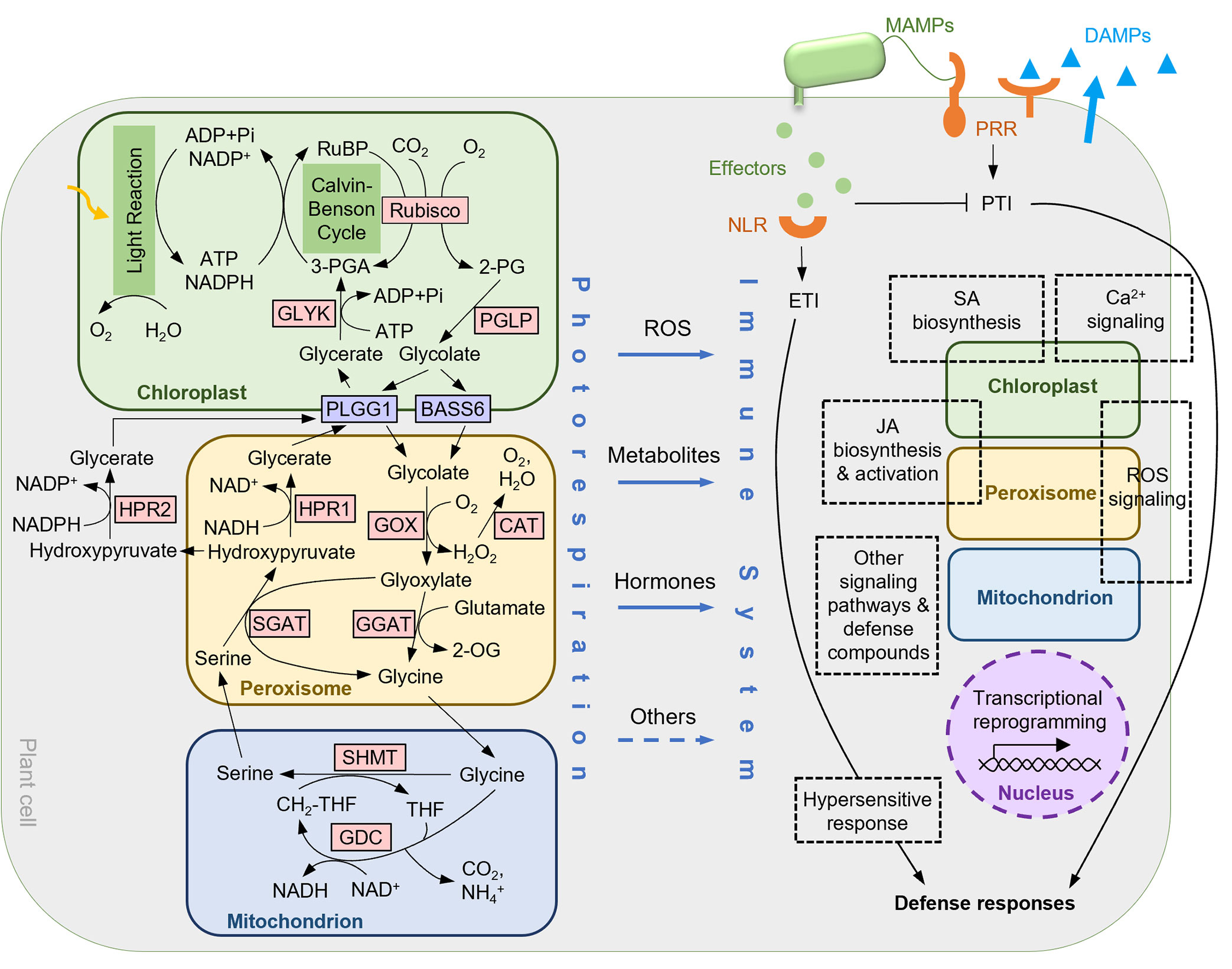
Figure 1 A working model for the connections between photorespiration and plant immunity. ROS, photorespiratory metabolites, defense hormones, and possibly other mechanisms connect the photorespiratory pathway to key components of the immune network. See main text for detailed information of the photorespiratory pathway and plant immunity, as well as mechanisms/potential mechanisms for their connections. Overlaps between some subcomponents of the immune response network and the photorespiratory organelles indicate the involvement of the particular organelles. 2-OG, 2-oxoglutarate; 2-PG, 2-phosphoglycolate; 3-PGA, 3-phosphoglycerate; BASS6, bile acid sodium symporter 6; CAT, catalase; GGAT, glutamate:glyoxylate aminotransferase; GDC, glycine decarboxylase complex; GLYK, glycerate kinase; GOX, glycolate oxidase; HPR, hydroxypyruvate reductase; PGLP, 2-PG phosphatase; PLGG1, plastidial glycolate/glycerate transporter 1; Rubisco, RuBP carboxylase/oxygenase; RuBP, ribulose-1,5-bisphosphate; SGAT, serine:glyoxylate aminotransferase; SHMT, serine hydroxymethyltransferase; THF, tetrahydrofolate; MAMP, microbe-associated molecular pattern; DAMP, damage-associated molecular pattern; PRR, pattern recognition receptor; NLR, nucleotide-binding/leucine-rich-repeat receptor; PTI, pattern-triggered immunity; ETI, effector-triggered immunity; ROS, reactive oxygen species; SA, salicylic acid; JA, jasmonate.
Photorespiration is one of the numerous cellular pathways shown to be involved in immune response. Closely linked to photosynthesis, photorespiration is initiated by the oxygenation of ribulose 1,5-bisphosphate (RuBP) catalyzed by ribulose 1,5-bisphosphate carboxylase-oxygenase (Rubisco), producing 2-phosphoglycolate (2-PG) which can inhibit cellular functions when accumulated. 2-PG is first dephosphorylated by 2-PG phosphatase (PGLP) to produce glycolate, which is then transported out of the chloroplast by plastidial glycolate/glycerate transporter 1 (PLGG1) and bile acid sodium symporter 6 (BASS6). Upon entering the peroxisome, glycolate is converted to glyoxylate by glycolate oxidase (GOX), producing H2O2 that is then scavenged by catalase (CAT). Both glutamate:glyoxylate aminotransferase (GGAT) and serine:glyoxylate aminotransferase (SGAT) catalyze the conversion of glyoxylate to glycine. After transporting to the mitochondrion, glycine is converted to serine by the glycine decarboxylase complex (GDC) and serine hydroxymethyltransferase (SHMT), accompanying the tetrahydrofolate (THF) cycle and releasing CO2 and NH3. Serine is then transported back to the peroxisome, converted to hydroxypyruvate by SGAT, and subsequently to glycerate by hydroxypyruvate reductase 1 (HPR1). HPR2 is an HPR isoform that can reduce hydroxypyruvate to glycerate in the cytosol. Finally, glycerate is imported into the chloroplast through PLGG1 and phosphorylated to 3-phosphoglycerate (3-PGA) by glycerate kinase (GLYK) to recycle back to the Calvin-Benson cycle. Photorespiration consumes ATP in the chloroplast and NAD(P)H in the peroxisome and the cytosol, and releases NADH in the mitochondrion (Eisenhut et al., 2019) (Figure 1).
Although photorespiration significantly reduces photosynthetic efficiency (Walker et al., 2016), it is essential to C3 plants and even vital for C4 plants such as maize (Zelitch et al., 2009) and Flaveria bidentis (Levey et al., 2019), highlighting its importance to plant survival. The plant immune system appears to take advantage of photorespiration as well. For example, tightly connected with plant primary metabolism (Shi and Bloom, 2021), photorespiration can provide signals, substrates, or energy for immunity in face of pathogen invasion. In addition, the coupled response of photorespiration to environmental signals like dynamic light intensities and stomatal conductance (Fu and Walker, 2023) may represent a way for immunity to integrate environmental cues for optimal response.
At present, no unequivocal conclusions have been drawn on how the level of photorespiratory enzymes is regulated in response to pathogen infections. Some studies show that photorespiratory genes are generally suppressed by pathogen infection (Zabala et al., 2015; Giraldo – González et al., 2021; Kalapos et al., 2021; Yue et al., 2021), whereas in other studies certain photorespiratory genes show increased expression instead (Mitsuya et al., 2009; Ahammed et al., 2018). At the protein level, both up- and down-regulation of the photorespiratory enzymes in presence of pathogens have been observed (Segarra et al., 2007; Zhao et al., 2013; Ma et al., 2020; He et al., 2021). These discrepancies are likely due to the different plant-pathogen systems used and may indicate the complex nature of the response of various photorespiratory genes to stress at the expression and protein levels.
Although the importance of photorespiratory ROS in defense is supported by abundant evidence (Sørhagen et al., 2013), how photorespiration fully participates in immunity remains an intriguing question. Evidence also exists to support the notion that photorespiration is involved in immunity via other mechanisms, such as through photorespiratory metabolites and defense hormone biosynthesis. Here, we summarize available evidence showing the connection between photorespiration and immunity and discuss current understanding of the underlying mechanisms.
Photorespiratory ROS: Important players in immune response
ROS such as H2O2 are crucial signaling molecules during plant-pathogen interactions (Camejo et al., 2016). Photorespiration is a major source of H2O2 in photosynthetic cells (Foyer et al., 2009), and photorespiratory organelles such as peroxisomes also contain H2O2-scavenging systems such as catalases (see below). Not surprisingly, studies of the roles of photorespiration in plant immunity have been mainly focused on H2O2.
GOX (Figure 1) contributes to disease resistance through its H2O2-producing capability. GOX-silenced tobacco plants show compromised non-host resistance to bacterial pathogens Pseudomonas syringae pv. tomato (Pst) strain T1, P. syringae pv. glycinea and Xanthomonas campestris pv. vesicatoria, as well as reduced ETI responses to the effector AvrPto (Rojas et al., 2012). Consistently, GOX-deficient Arabidopsis mutants show compromised non-host resistance to P. syringae pv. syringae strain B728A and P. syringae pv. tabaci, and reduced ETI responses to the effectors AvrB and AvrRps4 (Rojas et al., 2012). Null mutants of HAOX (hydroxy-acid oxidase), the enzyme that belongs to the same L-2-HAOX family as GOX (Esser et al., 2014), exhibit gox-like phenotypes in response to pathogens (Rojas et al., 2012). The Arabidopsis gox and hoax mutants also have decreased H2O2 levels after P. syringae pv. tabaci infection, which is independent of the H2O2-producing enzyme, NADPH oxidase (Rojas and Mysore, 2012; Rojas et al., 2012). In addition, reducing GOX2 expression in tomato lowers H2O2 levels in the leaf and increases plant susceptibility to the compatible pathogen Pst DC3000, a phenotype that can be rescued by H2O2 pre-treatment (Ahammed et al., 2018). Similarly, decreases in the level of H2O2 and increases in Pst DC3000 susceptibility were seen after application of isonicotinic acid hydrazide (INH), an inhibitor that blocks the conversion of glycine to serine in photorespiration and suppresses GOX activity (Ahammed et al., 2018). These results suggest that the H2O2 produced by GOX family members is important to immunity. However, silencing GOX1 in rice results in enhanced resistance to the compatible pathogen X. oryzae pv. oryzae (Chern et al., 2013). Additionally, three members from the tobacco GOX family contribute differently to H2O2 levels and defense (Xu et al., 2018), yet all five members of the Arabidopsis GOX family work additively to increase resistance (Rojas et al., 2012). These inconsistent results regarding the function of different GOX members may be due to distinct plant-pathogen systems utilized and the functional divergence of family members in different plant lineages.
The function of the H2O2-scavenging enzyme CAT (Figure 1) in immune response has been investigated extensively. Without pathogen infection, CAT-deficient mutants show SA accumulation, induced expression of the SA-pathway marker gene PR1 (pathogenesis-related 1), cell death, along with H2O2 accumulation in tobacco (Takahashi et al., 1997; Chamnongpol et al., 1998; Mittler et al., 1999) and Arabidopsis (Chaouch and Noctor, 2010; Chaouch et al., 2010). In addition, SA was found to bind to CAT and inhibit CAT activity to increase the level of H2O2 in a variety of plant species (Chen et al., 1993; Sánchez-Casas and Klessig, 1994). The inhibition of CAT activity by SA analogs correlates with the induction of the PR1 gene and plant resistance to tobacco mosaic virus (Conrath et al., 1995). Suppression of CAT2 by SA in Arabidopsis also leads to decreases in auxin and JA biosynthesis (Yuan et al., 2017). This is consistent with the increased biotroph resistance that is dependent on SA and repressed by auxin, and decreased JA-dependent necrotroph resistance in the cat2 mutant (Yuan et al., 2017). This data supports the role of CAT2 as a mediator between SA and auxin/JA signaling pathways in response to different pathogens. CAT2 also seems to connect Ca2+ signaling to the JA pathway, as the calmodulin-binding protein IQM1 (IQ-Motif Containing Protein 1) positively regulates JA biosynthesis by enhancing CAT2 function at both the transcription and enzymatic activity levels (Lv et al., 2019). The transcription factor GBF1 (G-box binding factor 1) downregulates CAT2 expression during pathogen response, leading to high H2O2 levels (Giri et al., 2017), reinforcing the view that photorespiratory H2O2, whose level is modulated by CATs, may act as a hub in coordinating defense responses.
Moreover, pathogens often target CAT to help with infection, which also suggests the importance of photorespiratory H2O2 in immunity. Effectors from the bacterial pathogen Ralstonia solanacearum (Sun et al., 2017) and the root-knot nematode Meloidogyne incognita (Zhao et al., 2021) inhibit CAT activity via physical interaction with the enzyme, and the 2b protein from the Cucumber mosaic virus induces CAT3 degradation in Arabidopsis (Murota et al., 2017). However, some pathogens seem to regulate the level of CAT positively. For example, the Pepino mosaic virus utilizes Triple Gene Block Protein 1 (TGBp1) to promote the activity of CAT1 and reduce H2O2 levels in tomato (Mathioudakis et al., 2013). Interestingly, the oomycete pathogen Phytophthora sojae has two effectors that interact with CATs and regulate H2O2 homeostasis in opposite directions (Zhang et al., 2015).
Evidence suggesting that CAT and GOX act together to regulate H2O2 homeostasis in defense has been reported. Under sub-ambient CO2 conditions, enhanced resistance to the biotrophic oomycete Hyaloperonospora arabidopsidis and high intracellular ROS content were observed in Arabidopsis (Williams et al., 2018). This resistant phenotype is abolished in the gox1 or haox1 mutants under the same low CO2 conditions after pathogen inoculation, and the CAT2 gene is down-regulated by infection (Williams et al., 2018), suggesting that both boosted GOX and suppressed CAT contribute to ROS accumulation. More direct evidence comes from rice, where SA treatment disrupts the physical interaction between GOX and CAT and induces H2O2 accumulation (Zhang et al., 2016). These results suggest that H2O2 homeostasis during plant-pathogen interaction is possibly regulated by the association and disassociation of GOX and CAT.
Besides peroxisomal H2O2, mitochondrial ROS can be influenced by photorespiration and involved in defense as well. The P-protein and H-protein of GDC, the mitochondrial multienzyme complex that catalyzes glycine decarboxylation (Figure 1), are repressed in activity by the victorin toxin produced by the fungus Cochliobolus victoriae (Navarre and Wolpert, 1995). Victorin treatment triggers mitochondrial ROS burst and subsequent apoptotic response in oat, a similar result to that caused by the GDC inhibitor aminoacetonitrile (AAN) (Yao et al., 2002). In addition, silencing GDC-T or GDC-P in tobacco suppresses victorin-triggered cell death and ETI response to the effector AvrPto (Gilbert and Wolpert, 2013). Furthermore, the bacterial elicitor harpin also inhibits GDC activity in Arabidopsis, resembling the inhibition by AAN treatment (Cristina Palmieri et al., 2010). Therefore, it is likely that GDC plays a role in reducing the level of ROS during plant-pathogen interaction to avoid damages caused by excess ROS.
The peroxisomal aminotransferase GGAT, which converts glyoxylate to glycine (Figure 1), is also connected with H2O2. Compared to wild-type plants, the Arabidopsis ggat1 mutant is more resistant to the necrotrophic fungal pathogen Botrytis cinerea and contains lower H2O2 concentrations upon infection, whereas a higher H2O2 level is observed when uninfected (González-lópez et al., 2021). How GGAT regulates H2O2 and whether this change in H2O2 levels imposes significant impacts on immune responses remains unknown.
The impact of photorespiration on ROS levels may differ among the three photorespiratory organelles during plant-pathogen interactions. In the chloroplast, photorespiration may actually prevent ROS production during plant immune response. As the major source of chloroplastic ROS, the photosynthetic electron transport chain produces excessive reducing equivalents and ATP under stress conditions (Voss et al., 2013). Therefore, photorespiration may function as an alternative sink for these reducing equivalents and ATP to decrease ROS accumulation in the chloroplast and protect photosystems from photodamage (Voss et al., 2013). Meanwhile, it is likely that the high photorespiratory rate under stress conditions enhances H2O2 production in the peroxisome, and increases NADH production by GDC in mitochondria to increase the level of mitochondrial ROS. Nonetheless, these hypotheses remain to be tested under pathogen defense conditions.
In conclusion, extensive evidence has demonstrated the key roles of ROS in plant immune response. The level of H2O2 is impacted by photorespiratory enzymes such as GOXs and CATs in peroxisomes and GDC in mitochondria, and potentially other photorespiratory proteins as well.
Involvement of photorespiratory metabolites in immunity
Photorespiration involves a variety of metabolites connected to several primary metabolic pathways, including photosynthesis, C1 metabolism, amino acid metabolism, and nitrogen assimilation (Hodges et al., 2016). Metabolite analysis of Arabidopsis suspension cultured cells in which immunity was activated by Pst DC3000, mutant Pst DC3000 (D28E), or flg22, revealed large-scale metabolic changes, including the glyoxylate and dicarboxylate metabolism and the amino acid metabolism that partially overlap with the photorespiratory pathway (Misra et al., 2016). In cucumber, nitrate-induced resistance to the fungus Fusarium oxysporum f. sp. cucumerinum (FOC), along with the accumulation of most of the photorespiratory intermediates except serine, was observed (Sun et al., 2021). As discussed below, specific photorespiratory metabolites have also been shown to be involved in plant-pathogen interactions.
Catalyzing the bidirectional conversion of serine and THF to glycine and 5,10-methylene-THF, the photorespiratory enzyme SHMT (Figure 1) is also a crucial enzyme in C1 metabolism (Hanson and Roje, 2001). GmSHMT08c, which encodes a cytosolic SHMT in soybean, was identified to be a resistant gene to the soybean cyst nematode (Heterodera glycines, SCN) (Liu et al., 2012; Kandoth et al., 2017). The resistance is resulted from two amino acid substitutions in the GmSHMT08c protein that impede THF binding and reduce catalytic activity of the enzyme (Liu et al., 2012; Korasick et al., 2020). GmSHMT08c confers SCN-resistance in soybean roots (Liu et al., 2012), so it is less likely that photorespiration is involved in this resistance. Other members of the GmSHMT family do not seem to function in SCN resistance individually (Lakhssassi et al., 2019). However, considering the probable functional redundancy of the five mitochondrial GmSHMT members, folate metabolism is a possible point at which photorespiration affects plant immunity. Moreover, the Arabidopsis shmt1 mutant exhibits compromised defense responses to both biotrophic and necrotrophic pathogens (Moreno et al., 2005). Silencing tomato SHMT1 dampens resistance to P. syringae independent of H2O2, whereas overexpressing the gene enhances the resistance (Ahammed et al., 2018). Further, Arabidopsis SHMT4 binds to SA (Manohar et al., 2015), and rice SHMT1 interacts with the disease-resistance protein RPM1 (Wang et al., 2021), although their roles in immunity in these contexts have not been shown. Taken together, SHMT plays a role in defense response in several plant species. Except for the potential connection to folate metabolism in soybean, the underlying mechanisms are still unknown in most species.
The peroxisomal HPR enzyme that converts hydroxypyruvate to glycerate (Figure 1) engages in immunity through photorespiratory metabolites. A soybean HPR interacts with P34, the receptor of the P. syringae elicitor syringolide, and applying glycerate and 3-PGA, products of the HPR-catalyzed reaction and the downstream step, respectively, restrains syringolide-triggered HR (Okinaka et al., 2002). Additionally, the cytosolic Arabidopsis HPR2 protein binds to SA, but evidence for its role in immunity is lacking (Manohar et al., 2015).
The role of photorespiration-associated amino acids in plant immunity has been illustrated in several studies. In rice, 18 different amino acids, among which glutamate, glycine and serine are photorespiratory intermediates (Figure 1), can induce systemic resistance against rice blast when individually applied to roots (Kadotani et al., 2016). Soaking tomato fruits in glutamate solution reduces colonization of the fungal pathogen Alternaria alternata and activates several primary metabolic pathways such as nitrogen metabolism, the γ-aminobutyric acid shunt, and SA signaling (Yang et al., 2017). Consistently, glutamate can serve as a DAMP to induce Ca2+ signaling and thereafter defense responses in plants (Toyota et al., 2018).
Taken together, current data provide evidence for the influence of photorespiratory metabolites on plant defense response. Further and in-depth studies are needed to elucidate the underlying mechanisms.
Influence of photorespiration on the biosynthesis of defense hormones
SA and JA are the two major phytohormones in plant defense (Pieterse et al., 2012). SA is synthesized in plastids and in the cytosol (Lefevere et al., 2020), and the biosynthesis and activation of JA involve plastids, peroxisomes and the cytosol (Wasternack and Song, 2017). Recently, CAT2-promoted JA biosynthesis in Arabidopsis was shown to be achieved by the direct interaction between the N-terminus of CAT2 and the JA biosynthetic enzymes acyl-CoA oxidase 2 (ACX2) and ACX3, without the requirement of H2O2 (Zhang et al., 2021). Another study demonstrated that the JA-activated defense to the necrotrophic pathogen Erwinia amylovora is partially dependent on GOX2 and does not involve obvious changes to the level of H2O2 (Launay et al., 2022), indicating that other mechanisms independent of H2O2 may exist in this immune response. Given the overlap of the locations for photorespiration and defense hormone biosynthesis in several subcellular compartments, it is possible that one or multiple photorespiratory enzymes or metabolites serve as mediators or signals in the biosynthesis of SA and JA. Although evidence for the connection between photorespiration and defense hormone biosynthesis is still scarce, it is a promising research direction that merits further investigations.
Other photorespiratory components involved in defense
A few other photorespiratory enzymes are also involved in immunity, yet the mechanisms behind are inconclusive.
In a Pseudoperonospora cubensis-resistant melon cultivar, genes encoding two aminotransferases — homologs of the Arabidopsis peroxisomal aminotransferase SGAT, which converts glyoxylate to glycine and serine to hydroxypyruvate (Figure 1), were found among the resistance genes (Taler et al., 2004). Overexpressing either gene confers resistance to the pathogen in the susceptible cultivar (Benjamin et al., 2009). That the resistant melon cultivar also exhibits high GOX activities indicates that this SGAT-regulated resistance may be attributed to high H2O2 levels (Taler et al., 2004). However, the positive role of SGAT in plant resistance to P. syringae in tomato was shown to be independent of H2O2 (Ahammed et al., 2018). Additionally, Arabidopsis SGAT was identified as an SA-binding protein, with unknown consequences in defense (Manohar et al., 2015). Further studies are needed to dissect the precise mechanism of the role of SGAT in immunity.
The chloroplast photorespiratory kinase GLYK, which phosphorylates glycerate to make 3-PGA (Figure 1), appears to play a positive role in immunity at multiple levels. Full-length GLYK in potato is a target for the Irish potato famine pathogen Phytophthora infestans effector protein AVRvnt1 through protein binding, resulting in the impediment of GLYK trafficking into chloroplasts and enhancement of GLYK degradation, as well as the activation of the ETI response mediated by Rpi-vnt1.1, the NLR that recognizes AVRvnt1 (Gao et al., 2020). GLYK silencing results in increased plant susceptibility to P. infestans lacking AVRvnt1 via an unknown mechanism (Gao et al., 2020). Interestingly, the full-length GLYK protein is mainly produced under the light (Gao et al., 2020), when photorespiration operates, indicating that the function of GLYK in immunity likely depends on photorespiration.
Measurement of photorespiration rate in defense response
Measuring physiological parameters of photorespiration in plants after pathogen infection provides new perspectives in dissecting the relationship between photorespiration and defense. Photorespiration rate, which can be estimated by the difference of net CO2 assimilation rate between 2% and 21% O2, is increased upon Pst DC3000 infection, whereas INH, the inhibitor that blocks the conversion of glycine to serine in photorespiration and suppresses GOX activity, suppresses this increase (Ahammed et al., 2018). Other indicators of photorespiration rate used in the measurements include the photorespiratory CO2 compensation point (Γ*) and the ratio of glycine to serine (Gly/Ser). FOC-inoculated banana seedlings contain higher Γ* than untreated plants (Dong et al., 2016). In nitrate-induced FOC resistance cucumber plants, both Γ* and Gly/Ser are increased (Sun et al., 2021). Further studies are needed to determine whether the increased photorespiration rate reported in these studies contributes to defense responses. This quantitative approach may also be extended to additional studies aimed at dissecting the interplay between photorespiration and immunity.
Discussion
The photorespiratory pathway has become a major target for genetic engineering with the goal to increase photosynthetic efficiency (Betti et al., 2016). Therefore, a more precise understanding of the contribution of photorespiration to plant physiology and plant interaction with the environment is vital for such efforts. Studies demonstrate the key role of photorespiration in plant immunity through changes in ROS homeostasis, while other mechanisms such as the participation of photorespiratory metabolites, the direct impact of photorespiration on defense hormone biosynthesis, and etc, are also emerging (Table 1). Considering the complexity of both the photorespiratory pathway and immune responses, large-scale and systematic approaches involving simultaneous measurements of photorespiration and immune response will be required in order to obtain a comprehensive view of the interplay between these two systems under different conditions. Such knowledge is highly needed for the rational design of a new generation of crop plants to feed the growing human population. Engineering photorespiration to manipulate ROS levels or the pool size of some metabolites may be a promising approach to enhance crop resistance.
Author contributions
XJ and JH co-conceptualized this review. XJ, BW, SH, and JH co-wrote the manuscript. All authors contributed to the article and approved the submitted version.
Funding
This work was supported by the Chemical Sciences, Geoscience and Biosciences Division, Office of Basic Energy Sciences, Office of Science, U.S. Department of Energy (award number DE-FG02-91ER20021) to JH.
Acknowledgments
The authors thank the former and current members of the Hu, He, and Walker labs for their contribution to this research and helpful discussions.
Conflict of interest
The authors declare that the research was conducted in the absence of any commercial or financial relationships that could be construed as a potential conflict of interest.
Publisher’s note
All claims expressed in this article are solely those of the authors and do not necessarily represent those of their affiliated organizations, or those of the publisher, the editors and the reviewers. Any product that may be evaluated in this article, or claim that may be made by its manufacturer, is not guaranteed or endorsed by the publisher.
References
Ahammed, G. J., Li, X., Zhang, G., Zhang, H., Shi, J., Pan, C., et al. (2018). Tomato photorespiratory glycolate-oxidase-derived H2O2 production contributes to basal defence against pseudomonas syringae. Plant Cell Environ. 41, 1126–1138. doi: 10.1111/pce.12932
Benjamin, I., Kenigsbuch, D., Galperin, M., Abrameto, J. A., Cohen, Y. (2009). Cisgenic melons over expressing glyoxylate-aminotransferase are resistant to downy mildew. Eur. J. Plant Pathol. 125, 355–365. doi: 10.1007/s10658-009-9485-4
Betti, M., Bauwe, H., Busch, F. A., Fernie, A. R., Keech, O., Levey, M., et al. (2016). Manipulating photorespiration to increase plant productivity: Recent advances and perspectives for crop improvement. J. Exp. Bot. 67, 2977–2988. doi: 10.1093/jxb/erw076
Camejo, D., Guzmán-Cedeño, Á., Moreno, A. (2016). Reactive oxygen species, essential molecules, during plant-pathogen interactions. Plant Physiol. Biochem. 103, 10–23. doi: 10.1016/j.plaphy.2016.02.035
Chamnongpol, S., Willekens, H., Moeder, W., Langebartels, C., Sandermann, H., Van Montagu, M., et al. (1998). Defense activation and enhanced pathogen tolerance induced by H2O2 in transgenic tobacco. Proc. Natl. Acad. Sci. U. S. A. 95, 5818–5823. doi: 10.1073/pnas.95.10.5818
Chaouch, S., Noctor, G. (2010). Myo-inositol abolishes salicylic acid-dependent cell death and pathogen defence responses triggered by peroxisomal hydrogen peroxide. New Phytol. 188, 711–718. doi: 10.1111/j.1469-8137.2010.03453.x
Chaouch, S., Queval, G., Vanderauwera, S., Mhamdi, A., Vandorpe, M., Langlois-Meurinne, M., et al. (2010). Peroxisomal hydrogen peroxide is coupled to biotic defense responses by ISOCHORISMATE SYNTHASE1 in a daylength-related manner. Plant Physiol. 153, 1692–1705. doi: 10.1104/pp.110.153957
Chen, Z., Silva, H., Klessig, D. F. (1993). Active oxygen species in the induction of plant systemic acquired resistance by salicylic acid. Sci. (80-. ). 262, 1883–1886. doi: 10.1126/science.8266079
Chern, M., Bai, W., Chen, X., Canlas, P. E., Ronald, P. C. (2013). Reduced expression of glycolate oxidase leads to enhanced disease resistance in rice. PeerJ 2013, 1–16. doi: 10.7717/peerj.28
Conrath, U., Chen, Z., Ricigliano, J. R., Klessig, D. F. (1995). Two inducers of plant defense responses, 2,6-dichloroisonicotinic acid and salicylic acid, inhibit catalase activity in tobacco. Proc. Natl. Acad. Sci. U. S. A. 92, 7143–7147. doi: 10.1073/pnas.92.16.7143
Cristina Palmieri, M., Lindermayr, C., Bauwe, H., Steinhauser, C., Durner, J. (2010). Regulation of plant glycine decarboxylase by s-nitrosylation and glutathionylation. Plant Physiol. 152, 1514–1528. doi: 10.1104/pp.109.152579
Cui, H., Tsuda, K., Parker, J. E. (2015). Effector-triggered immunity: From pathogen perception to robust defense. Annu. Rev. Plant Biol. 66, 487–511. doi: 10.1146/annurev-arplant-050213-040012
Deslandes, L., Rivas, S. (2012). Catch me if you can: Bacterial effectors and plant targets. Trends Plant Sci. 17, 644–655. doi: 10.1016/j.tplants.2012.06.011
Dong, X., Wang, M., Ling, N., Shen, Q., Guo, S. (2016). Potential role of photosynthesis-related factors in banana metabolism and defense against Fusarium oxysporum f. sp. cubense. Environ. Exp. Bot. 129, 4–12. doi: 10.1016/j.envexpbot.2016.01.005
Eisenhut, M., Roell, M. S., Weber, A. P. M. (2019). Mechanistic understanding of photorespiration paves the way to a new green revolution. New Phytol. 223, 1762–1769. doi: 10.1111/nph.15872
Esser, C., Kuhn, A., Groth, G., Lercher, M. J., Maurino, V. G. (2014). Plant and animal glycolate oxidases have a common eukaryotic ancestor and convergently duplicated to evolve long-chain 2-hydroxy acid oxidases. Mol. Biol. Evol. 31, 1089–1101. doi: 10.1093/molbev/msu041
Foyer, C. H., Bloom, A. J., Queval, G., Noctor, G. (2009). Photorespiratory metabolism: Genes, mutants, energetics, and redox signaling. Annu. Rev. Plant Biol. 60, 455–484. doi: 10.1146/annurev.arplant.043008.091948
Fu, X., Walker, B. J. (2023). Dynamic response of photorespiration in fluctuating light environments. J. Exp. Bot. 74, 600–611. doi: 10.1093/jxb/erac335
Gao, C., Xu, H., Huang, J., Sun, B., Zhang, F., Savage, Z., et al. (2020). Pathogen manipulation of chloroplast function triggers a light-dependent immune recognition. Proc. Natl. Acad. Sci. U. S. A. 117, 9613–9620. doi: 10.1073/pnas.2002759117
Gilbert, B. M., Wolpert, T. J. (2013). Characterization of the LOV1-mediated, victorin-induced, cell-death response with virus-induced gene silencing. Mol. Plant-Microbe Interact. 26, 903–917. doi: 10.1094/mpmi-01-13-0014-r
Giraldo – González, J. J., de Souza Carvalho, F. M., Ferro, J. A., Herai, R. H., Chaves Bedoya, G., Rodas Mendoza, E. F. (2021). Transcriptional changes involved in kumquat (Fortunella spp) defense response to Xanthomonas citri subsp. citri in early stages of infection. Physiol. Mol. Plant Pathol. 116, 101729. doi: 10.1016/j.pmpp.2021.101729
Giri, M. K., Singh, N., Banday, Z. Z., Singh, V., Ram, H., Singh, D., et al. (2017). GBF1 differentially regulates CAT2 and PAD4 transcription to promote pathogen defense in Arabidopsis thaliana. Plant J. 91, 802–815. doi: 10.1111/tpj.13608
González-lópez, M. D. C., Jijón-moreno, S., Dautt-castro, M., Ovando-vázquez, C., Ziv, T., Horwitz, B. A., et al. (2021). Secretome analysis of Arabidopsis–Trichoderma atroviride interaction unveils new roles for the plant glutamate: Glyoxylate aminotransferase ggat1 in plant growth induced by the fungus and resistance against Botrytis cinerea. Int. J. Mol. Sci. 22, 1–28. doi: 10.3390/ijms22136804
Hanson, A. D., Roje, S. (2001). One-carbon metabolism in higher plants. Annu. Rev. Plant Physiol. Plant Mol. Biol. 52, 119–137. doi: 10.1146/annurev.arplant.52.1.119
He, L., Jin, P., Chen, X., Zhang, T. Y., Zhong, K. L., Liu, P., et al. (2021). Comparative proteomic analysis of Nicotiana benthamiana plants under Chinese wheat mosaic virus infection. BMC Plant Biol. 21, 1–17. doi: 10.1186/s12870-021-02826-9
Hodges, M., Dellero, Y., Keech, O., Betti, M., Raghavendra, A. S., Sage, R., et al. (2016). Perspectives for a better understanding of the metabolic integration of photorespiration within a complex plant primary metabolism network. J. Exp. Bot. 67, 3015–3026. doi: 10.1093/jxb/erw145
Kadotani, N., Akagi, A., Takatsuji, H., Miwa, T., Igarashi, D. (2016). Exogenous proteinogenic amino acids induce systemic resistance in rice. BMC Plant Biol. 16, 1–10. doi: 10.1186/s12870-016-0748-x
Kalapos, B., Juhász, C., Balogh, E., Kocsy, G., Tóbiás, I., Gullner, G. (2021). Transcriptome profiling of pepper leaves by RNA-seq during an incompatible and a compatible pepper-tobamovirus interaction. Sci. Rep. 11, 1–19. doi: 10.1038/s41598-021-00002-5
Kandoth, P. K., Liu, S., Prenger, E., Ludwig, A., Lakhssassi, N., Heinz, R., et al. (2017). Systematic mutagenesis of serine hydroxymethyltransferase reveals an essential role in nematode resistance. Plant Physiol. 175, 1370–1380. doi: 10.1104/pp.17.00553
Korasick, D. A., Kandoth, P. K., Tanner, J. J., Mitchum, M. G., Beamer, L. J. (2020). Impaired folate binding of serine hydroxymethyltransferase 8 from soybean underlies resistance to the soybean cyst nematode. J. Biol. Chem. 295, 3708–3718. doi: 10.1074/jbc.RA119.012256
Lakhssassi, N., Patil, G., Piya, S., Zhou, Z., Baharlouei, A., Kassem, M. A., et al. (2019). Genome reorganization of the GmSHMT gene family in soybean showed a lack of functional redundancy in resistance to soybean cyst nematode. Sci. Rep. 9, 1–16. doi: 10.1038/s41598-018-37815-w
Launay, A., Jolivet, S., Clément, G., Zarattini, M., Dellero, Y., Le Hir, R., et al. (2022). DspA/E-triggered non-host resistance against E. amylovora depends on the Arabidopsis GLYCOLATE OXIDASE 2 gene. Int. J. Mol. Sci. 23, 4224. doi: 10.3390/ijms23084224
Lefevere, H., Bauters, L., Gheysen, G. (2020). Salicylic acid biosynthesis in plants. Front. Plant Sci. 11. doi: 10.3389/fpls.2020.00338
Levey, M., Timm, S., Mettler-Altmann, T., Borghi, G. L., Koczor, M., Arrivault, S., et al. (2019). Efficient 2-phosphoglycolate degradation is required to maintain carbon assimilation and allocation in the C4 plant Flaveria bidentis. J. Exp. Bot. 70, 575–587. doi: 10.1093/jxb/ery370
Liu, S., Kandoth, P. K., Warren, S. D., Yeckel, G., Heinz, R., Alden, J., et al. (2012). A soybean cyst nematode resistance gene points to a new mechanism of plant resistance to pathogens. Nature 492, 256–260. doi: 10.1038/nature11651
Lv, T., Li, X., Fan, T., Luo, H., Xie, C., Zhou, Y., et al. (2019). The calmodulin-binding protein IQM1 interacts with CATALASE2 to affect pathogen defense. Plant Physiol. 181, 1314–1327. doi: 10.1104/pp.19.01060
Manohar, M., Tian, M., Moreau, M., Park, S. W., Choi, H. W., Fei, Z., et al. (2015). Identification of multiple salicylic acid-binding proteins using two high throughput screens. Front. Plant Sci. 5. doi: 10.3389/fpls.2014.00777
Ma, H., Sheng, C., Qiao, L., Zhao, H., Niu, D. (2020). A comparative proteomic approach to identify defence-related proteins between resistant and susceptible rice cultivars challenged with the fungal pathogen Rhizoctonia solani. Plant Growth Regul. 90, 73–88. doi: 10.1007/s10725-019-00551-w
Mathioudakis, M. M., Veiga, R. S. L., Canto, T., Medina, V., Mossialos, D., Makris, A. M., et al. (2013). Pepino mosaic virus triple gene block protein 1 (TGBp1) interacts with and increases tomato catalase 1 activity to enhance virus accumulation. Mol. Plant Pathol. 14, 589–601. doi: 10.1111/mpp.12034
Misra, B. B., de Armas, E., Chen, S. (2016). Differential metabolomic responses of PAMP-triggered immunity and effector-triggered immunity in Arabidopsis suspension cells. Metabolomics 12, 1–15. doi: 10.1007/s11306-016-0984-y
Mitsuya, Y., Takahashi, Y., Berberich, T., Miyazaki, A., Matsumura, H., Takahashi, H., et al. (2009). Spermine signaling plays a significant role in the defense response of Arabidopsis thaliana to cucumber mosaic virus. J. Plant Physiol. 166, 626–643. doi: 10.1016/j.jplph.2008.08.006
Mittler, R., Herr, E. H., Orvar, B. L., Van Camp, W., Willekens, H., Inzé, D., et al. (1999). Transgenic tobacco plants with reduced capability to detoxify reactive oxygen intermediates are hyperresponsive to pathogen infection. Proc. Natl. Acad. Sci. U. S. A. 96, 14165–14170. doi: 10.1073/pnas.96.24.14165
Moreno, J. I., Martín, R., Castresana, C. (2005). Arabidopsis SHMT1, a serine hydroxymethyltransferase that functions in the photorespiratory pathway influences resistance to biotic and abiotic stress. Plant J. 41, 451–463. doi: 10.1111/j.1365-313X.2004.02311.x
Murota, K., Shimura, H., Takeshita, M., Masuta, C. (2017). Interaction between Cucumber mosaic virus 2b protein and plant catalase induces a specific necrosis in association with proteasome activity. Plant Cell Rep. 36, 37–47. doi: 10.1007/s00299-016-2055-2
Navarre, D. A., Wolpert, T. J. (1995). Inhibition of the glycine decarboxylase multienzyme complex by the host-selective toxin victorin. Plant Cell 7, 463–471. doi: 10.1105/tpc.7.4.463
Ngou, B. P. M., Ahn, H. K., Ding, P., Jones, J. D. G. (2021). Mutual potentiation of plant immunity by cell-surface and intracellular receptors. Nature 592, 110–115. doi: 10.1038/s41586-021-03315-7
Okinaka, Y., Yang, C.-H., Herman, E., Kinney, A., Keen, N. T. (2002). The P34 syringolide elicitor receptor interacts with a soybean photorespiration enzyme, NADH-dependent hydroxypyruvate reductase. Mol. Plant-Microbe Interact. 15, 1213–1218. doi: 10.1094/MPMI.2002.15.12.1213
Pieterse, C. M. J., van der Does, D., Zamioudis, C., Leon-Reyes, A., Van Wees, S. C. M. (2012). Hormonal modulation of plant immunity. Annu. Rev. Cell Dev. Biol. 28, 489–521. doi: 10.1146/annurev-cellbio-092910-154055
Pruitt, R. N., Locci, F., Wanke, F., Zhang, L., Saile, S. C., Joe, A., et al. (2021). The EDS1–PAD4–ADR1 node mediates Arabidopsis pattern-triggered immunity. Nature 598, 495–499. doi: 10.1038/s41586-021-03829-0
Rojas, C. M., Mysore, K. S. (2012). Glycolate oxidase is an alternative source for H2O2 production during plant defense responses and functions independently from NADPH oxidase. Plant Signal. Behav. 7, 752–755. doi: 10.4161/psb.20429
Rojas, C. M., Senthil-Kumar, M., Wang, K., Ryu, C.-M., Kaundal, A., Mysore, K. S. (2012). Glycolate oxidase modulates reactive oxygen species-mediated signal transduction during nonhost resistance in Nicotiana benthamiana and Arabidopsis. Plant Cell 24, 336–352. doi: 10.1105/tpc.111.093245
Sørhagen, K., Laxa, M., Peterhänsel, C., Reumann, S. (2013). The emerging role of photorespiration and non-photorespiratory peroxisomal metabolism in pathogen defence. Plant Biol. 15, 723–736. doi: 10.1111/j.1438-8677.2012.00723.x
Sánchez-Casas, P., Klessig, D. F. (1994). A salicylic acid-binding activity and a salicylic acid-inhibitable catalase activity are present in a variety of plant species. Plant Physiol. 106, 1675–1679. doi: 10.1104/pp.106.4.1675
Segarra, G., Casanova, E., Bellido, D., Odena, M. A., Oliveira, E., Trillas, I. (2007). Proteome, salicylic acid, and jasmonic acid changes in cucumber plants inoculated with Trichoderma asperellum strain T34. Proteomics 7, 3943–3952. doi: 10.1002/pmic.200700173
Shi, X., Bloom, A. (2021). Photorespiration: The futile cycle? Plants 10, 908. doi: 10.3390/plants10050908
Sun, Y., Li, P., Deng, M., Shen, D., Dai, G., Yao, N., et al. (2017). The Ralstonia solanacearum effector RipAK suppresses plant hypersensitive response by inhibiting the activity of host catalases. Cell. Microbiol. 19, e12736. doi: 10.1111/cmi.12736
Sun, Y., Li, Y., Li, Y., Wang, M., Mur, L. A. J., Shen, Q., et al. (2021). Nitrate mediated resistance against Fusarium infection in cucumber plants acts via photorespiration. Plant Cell Environ. 44, 3412–3431. doi: 10.1111/pce.14140
Takahashi, H., Chen, Z., Du, H., Liu, Y., Klessig, D. F. (1997). Development of necrosis and activation of disease resistance in transgenic tobacco plants with severely reduced catalase levels. Plant J. 11, 993–1005. doi: 10.1046/j.1365-313X.1997.11050993.x
Taler, D., Galperin, M., Benjamin, I., Cohen, Y., Kenigsbuch, D. (2004). Plant eR genes that encode photorespiratory enzymes confer resistance against disease. Plant Cell 16, 172–184. doi: 10.1105/tpc.016352
Toyota, M., Spencer, D., Sawai-Toyota, S., Jiaqi, W., Zhang, T., Koo, A. J., et al. (2018). Glutamate triggers long-distance, calcium-based plant defense signaling. Sci. (80-. ). 361, 1112–1115. doi: 10.1126/science.aat7744
Voss, I., Sunil, B., Scheibe, R., Raghavendra, A. S. (2013). Emerging concept for the role of photorespiration as an important part of abiotic stress response. Plant Biol. 15, 713–722. doi: 10.1111/j.1438-8677.2012.00710.x
Walker, B. J., VanLoocke, A., Bernacchi, C. J., Ort, D. R. (2016). The costs of photorespiration to food production now and in the future. Annu. Rev. Plant Biol. 67, 107–129. doi: 10.1146/annurev-arplant-043015-111709
Wang, A., Shu, X., Jing, X., Jiao, C., Chen, L., Zhang, J., et al. (2021). Identification of rice (Oryza sativa L.) genes involved in sheath blight resistance via a genome-wide association study. Plant Biotechnol. J. 19, 1553–1566. doi: 10.1111/pbi.13569
Wasternack, C., Song, S. (2017). Jasmonates: Biosynthesis, metabolism, and signaling by proteins activating and repressing transcription. J. Exp. Bot. 68, 1303–1321. doi: 10.1093/jxb/erw443
Williams, A., Pétriacq, P., Schwarzenbacher, R. E., Beerling, D. J., Ton, J. (2018). Mechanisms of glacial-to-future atmospheric CO2 effects on plant immunity. New Phytol. 218, 752–761. doi: 10.1111/nph.15018
Xu, Y. P., Yang, J., Cai, X. Z. (2018). Glycolate oxidase gene family in Nicotiana benthamiana: Genomewide identification and functional analyses in disease resistance. Sci. Rep. 8, 1–11. doi: 10.1038/s41598-018-27000-4
Yang, J., Sun, C., Fu, D., Yu, T. (2017). Test for L-glutamate inhibition of growth of Alternaria alternata by inducing resistance in tomato fruit. Food Chem. 230, 145–153. doi: 10.1016/j.foodchem.2017.03.033
Yao, N., Tada, Y., Sakamoto, M., Nakayashiki, H., Park, P., Tosa, Y., et al. (2002). Mitochondrial oxidative burst involved in apoptotic response in oats. Plant J. 30, 567–579. doi: 10.1046/j.1365-313X.2002.01314.x
Yuan, M., Jiang, Z., Bi, G., Nomura, K., Liu, M., Wang, Y., et al. (2021). Pattern-recognition receptors are required for NLR-mediated plant immunity. Nature 592, 105–109. doi: 10.1038/s41586-021-03316-6
Yuan, H. M., Liu, W. C., Lu, Y. T. (2017). CATALASE2 coordinates SA-mediated repression of both auxin accumulation and JA biosynthesis in plant defenses. Cell Host Microbe 21, 143–155. doi: 10.1016/j.chom.2017.01.007
Yue, H., Huang, L. P., Lu, D. Y. H., Zhang, Z. H., Zhang, Z., Zhang, D. Y., et al. (2021). Integrated analysis of microRNA and mRNA transcriptome reveals the molecular mechanism of Solanum lycopersicum response to Bemisia tabaci and Tomato chlorosis virus. Front. Microbiol. 12. doi: 10.3389/fmicb.2021.693574
Yu, X., Feng, B., He, P., Shan, L. (2017). From chaos to harmony: Responses and signaling upon microbial pattern recognition. Annu. Rev. Phytopathol. 55, 109–137. doi: 10.1146/annurev-phyto-080516-035649
Zabala, M., de, T., Littlejohn, G., Jayaraman, S., Studholme, D., Bailey, T., et al. (2015). Chloroplasts play a central role in plant defence and are targeted by pathogen effectors. Nat. Plants 1, 1–14. doi: 10.1038/NPLANTS.2015.74
Zelitch, I., Schultes, N. P., Peterson, R. B., Brown, P., Brutnell, T. P. (2009). High glycolate oxidase activity is required for survival of maize in normal air. Plant Physiol. 149, 195–204. doi: 10.1104/pp.108.128439
Zhang, M., Li, Q., Liu, T., Liu, L., Shen, D., Zhu, Y., et al. (2015). Two cytoplasmic effectors of Phytophthora sojae regulate plant cell death via interactions with plant catalases. Plant Physiol. 167, 164–175. doi: 10.1104/pp.114.252437
Zhang, Y., Song, R., Yuan, H., Li, T., Wang, L., Lu, K., et al. (2021). Overexpressing the N-terminus of CATALASE2 enhances plant jasmonic acid biosynthesis and resistance to necrotrophic pathogen Botrytis cinerea B05.10. Mol. Plant Pathol. 22, 1226–1238. doi: 10.1111/mpp.13106
Zhang, Z., Xu, Y., Xie, Z., Li, X., He, Z. H., Peng, X. X. (2016). Association-dissociation of glycolate oxidase with catalase in rice: A potential switch to modulate intracellular H2O2 levels. Mol. Plant 9, 737–748. doi: 10.1016/j.molp.2016.02.002
Zhao, J., Sun, Q., Quentin, M., Ling, J., Abad, P., Zhang, X., et al. (2021). A Meloidogyne incognita C-type lectin effector targets plant catalases to promote parasitism. New Phytol. 232, 2124–2137. doi: 10.1111/nph.17690
Keywords: photorespiration, immunity, reactive oxygen species, photorespiratory metabolites, defense hormones
Citation: Jiang X, Walker BJ, He SY and Hu J (2023) The role of photorespiration in plant immunity. Front. Plant Sci. 14:1125945. doi: 10.3389/fpls.2023.1125945
Received: 16 December 2022; Accepted: 19 January 2023;
Published: 01 February 2023.
Edited by:
Caiji Gao, South China Normal University, ChinaReviewed by:
Lijing Liu, Shandong University, ChinaMo Wang, Fujian Agriculture and Forestry University, China
Copyright © 2023 Jiang, Walker, He and Hu. This is an open-access article distributed under the terms of the Creative Commons Attribution License (CC BY). The use, distribution or reproduction in other forums is permitted, provided the original author(s) and the copyright owner(s) are credited and that the original publication in this journal is cited, in accordance with accepted academic practice. No use, distribution or reproduction is permitted which does not comply with these terms.
*Correspondence: Jianping Hu, aHVqaUBtc3UuZWR1