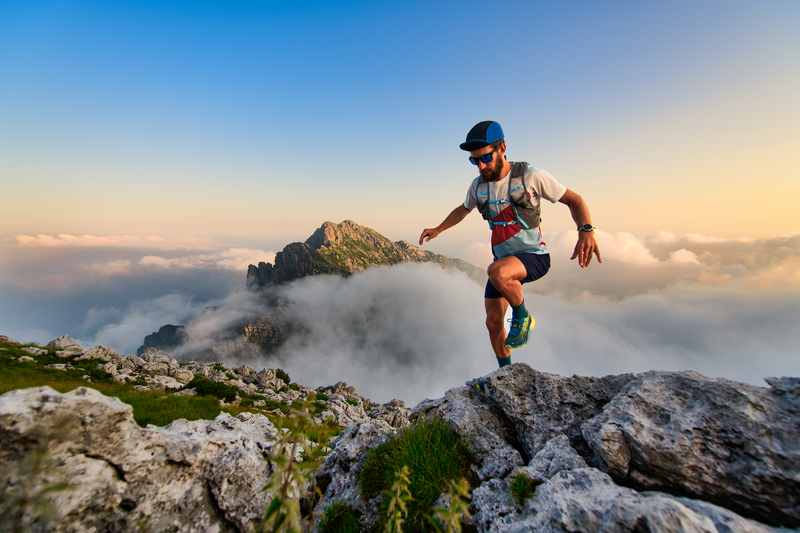
95% of researchers rate our articles as excellent or good
Learn more about the work of our research integrity team to safeguard the quality of each article we publish.
Find out more
REVIEW article
Front. Plant Sci. , 23 February 2023
Sec. Crop and Product Physiology
Volume 14 - 2023 | https://doi.org/10.3389/fpls.2023.1124335
This article is part of the Research Topic Environmental and Endogenous Signals: Crop Yield and Quality Regulation View all 7 articles
Climate change has led to the search for strategies to acclimatize plants to various abiotic stressors to ensure the production and quality of crops of commercial interest. Sorghum is the fifth most important cereal crop, providing several uses including human food, animal feed, bioenergy, or industrial applications. The crop has an excellent adaptation potential to different types of abiotic stresses, such as drought, high salinity, and high temperatures. However, it is susceptible to low temperatures compared with other monocotyledonous species. Here, we have reviewed and discussed some of the research results and advances that focused on the physiological, metabolic, and molecular mechanisms that determine sorghum cold tolerance to improve our understanding of the nature of such trait. Questions and opportunities for a comprehensive approach to clarify sorghum cold tolerance or susceptibility are also discussed.
Plants are sessile organisms that are continuously confronted with a variety of abiotic stresses (Kidokoro et al., 2021; Rawat et al., 2021; Li et al., 2022, Kidokoro et al., 2022; Huang et al., 2022). Cold is a limiting factor that has been considered among the main abiotic stresses that interfere with plant growth and development (Achard et al., 2008; Mehrotra et al., 2020). Cold temperatures affect critical cellular functions such as photosynthesis, respiration, and membrane permeability (Rawat et al., 2021; Daems et al., 2022; Li et al., 2022). Meanwhile, the process of cold acclimation involves many physiological, biochemical, metabolic, and molecular changes (Achard et al., 2008; Bu et al., 2021; Yu et al., 2022; Rani et al., 2021; Goswami et al., 2022; Hu et al., 2022; Liu et al., 2022; Zhang et al., 2022).
Therefore, understanding the cold stress response is crucial for improving crops against cold (Adhikari et al., 2022; Liu et al., 2022). Molecular, cellular, and physiological mechanisms activated to respond to cold stress determine the plant’s susceptibility or tolerance to the environment (Goswami et al., 2022; Liu et al., 2022; Zhang et al., 2022). Several studies have examined the cold response in different plants and tissues (Zhou et al., 2021; Adhikari et al., 2022; Huang et al., 2022; Khanthavong et al., 2022; (Kidokoro et al., 2022; Zhang et al., 2022). In this sense, sorghum has been identified as a susceptible crop at low temperatures (Burow et al., 2011; Chopra et al., 2015). Sorghum is a cold-sensitive crop, but some varieties are cold tolerant (Leon-Velasco et al., 2009; Mansour et al., 2021). Adverse effects in sorghum such as reduction in germination, plant growth, and development are evident by cold stress (Ercoli et al., 2004; Chinnusamy et al., 2007; Janmohammadi et al., 2015; Ortiz et al., 2017; Laza et al., 2022). Integration of signal perception and transduction, gene expression, and molecules produced in response to cold stress is essential to understand sorghum tolerance and acclimatization (Mehrotra et al., 2020). The purpose of this paper is to review and discuss some of the research results that focused on the physiological and gene regulation as well as signal transductions that determine sorghum cold tolerance to improve the understanding of the nature of such trait.
Cold is one of the main factors that determine the distribution of agricultural species worldwide, limiting their productivity and yield (Mehrotra et al., 2020). It is challenging to determine when and where the sorghum domestication occurred. However, it is assumed that it occurred in Northeastern Africa (Morris et al., 2013) under hot and dry field conditions (Jain et al., 2010; Laza et al., 2022). Sorghum has been well adapted to drought and high-temperature environments where the growth of other cereals crop may be challenged. However, sorghum is a susceptible crop at low temperatures compared with most other cereals (Jain et al., 2010; Chopra et al., 2015). Some varieties of sorghum have been developed in the cooler highland areas of China and some parts of Africa (Knoll et al., 2008; Maulana et al., 2017). These sorghums are cold tolerant and can be adapted and grown in highlands where rainfall is scarce and erratic or the growing season is usually less suitable for other sorghum varieties (Leon-Velasco et al., 2009). It is a crop adapted to warm areas (Vera-Hernández et al., 2018), where minimum average temperatures during the growing period are generally maintained above 18°C (Reshma et al., 2019). The gradual expansion to high-altitude regions has led to the development of genotypes that are adapted to cold climates. Its introduction in other regions of the world has resulted in more cold-tolerant varieties, as well as early maturation and insensitive photoperiod cultivars that are able to grow under these adverse conditions.
The term “cold tolerance or chilling tolerance” is used to describe the ability of sorghum to germinate, grow, and produce seed under conditions of low temperature but above freezing temperatures (Singh, 1985; Osuna-Ortega et al., 2003; Vera-Hernández et al., 2018; Emendack et al., 2021). Low temperature is one of the main abiotic stressors that affect plant growth and development and is a principal determinant of the geographical distribution of plants (Hurry et al., 2002; Mehrotra et al., 2020). The cold temperatures restrict sorghum production by reducing the window periods for cultivation in locations where the crop is already grown and by spatially limiting its growth in colder regions. A few varieties of sorghum have been developed in the cooler highland areas and can be adapted and grown in highlands where rainfall is scarce and erratic. The growing season is usually less suitable for other sorghum varieties (Leon-Velasco et al., 2009).
Higher survival under cold conditions (Bekele et al., 2014; Parra-Londono et al., 2018) as well as early vigor and germination in cold environments (Burow et al., 2011; Fiedler et al., 2012; Parra-Londono et al., 2018) have been determined in sorghum plants with tolerance to cold stress. Likewise, high chlorophyll content (Fiedler et al., 2014), enhanced transpiration, and affected stomatal conductance (Ortiz et al., 2017) under cold conditions have been identified in sorghum. Furthermore, an increase in anthocyanin levels (Chopra et al., 2017) has been suggested to have a protective function in chilling exposure (Krol et al., 1995). Other molecules, including genes and lipids, have been shown to change in response to cold stress (Marla et al., 2017). The effect of cold temperature on sorghum will depend on the stress intensity and duration, the developmental stage of the crop at the time at which low temperatures prevail (Emendack et al., 2021), and the variety of sorghum.
The molecular mechanisms that respond to cold temperatures are different from freezing (<0°C) in comparison with chilling stress (0°C to 15°C). During freezing, the physical state changes in membrane lipids, leading to cell or tissue injury, and this explain why cold-tolerant sorghum varieties are still affected by freezing temperatures (Palta and Weiss, 2018). Low temperatures decrease germination, emergence rate, seedling establishment, plant growth and development, root development, and biomass accumulation (Ercoli et al., 2004; Chinnusamy et al., 2007; Janmohammadi et al., 2015; Ortiz et al., 2017; Hu et al., 2022). The cold stress reaction in sorghum varies depending on the temperature level, duration, and phenological stage. In sorghum development, cold exposure could be in the early growth stages or in the late growth stages (Figure 1). The susceptible sorghum cultivars exposed to cold temperatures in early growth stages (i.e., when sorghum is sown at the beginning of the spring) show poor or no germination. Cold stress negatively affects germination, establishment, and growth, reducing sorghum biomass production and grain yield (Figure 1) (Knoll and Ejeta, 2008; Knoll et al., 2008; Maulana et al., 2017; Parra-Londono et al., 2018). Under continuous or late exposure to cold stress, susceptible genotypes can, sometimes, grow to vegetative phenological stages, but reproductive phases are affected. In some cases, flowering does not occur, and pollen production is reduced or even abolished (Osuna-Ortega et al., 2003). The young microspore stage is especially susceptible to cold, and male sterility is affected by cold, causing a massive grain yield loss. In addition, late or absent pollen production during flowering under cold conditions favors ergot (Claviceps africana) infection in cold-sensitive varieties (Hernández-Martinez et al., 2008).
Figure 1 Diagrammatic illustration of sorghum development and the cold exposure effect in sorghum. The principal growth stages are used to represent the effect in early, late, or any time of exposure to cold (Ostmeyer et al., 2022).
Low temperatures can lead to tissue dehydration and water deficit by reducing water uptake without reducing the leaf transpiration rate (Aroca et al., 2012; Bekele et al., 2014). The development of the root system can be influenced by low temperatures, which can affect water and mineral acquisition (Huang et al., 2005). Studies have shown that root elongation in sorghum seedlings is mostly determined by temperature and not by the diurnal cycle (Iijima et al., 1998). Some studies emphasize the role of root development in chilling survival in plants (Melkonian et al., 2004; Matsumoto et al., 2009). Bekele et al. (2014) found that root establishment was the most important factor, affecting plant survival under chilling stress. Under low-temperature conditions, extensive phenotyping of the root architecture of sorghum seedling showed that the root-to-shoot ratio, root biomass, and root length were correlated with the chlorophyll content (Bekele et al., 2014). Furthermore, it was found that root length and biomass developed at optimum temperature were correlated with chilling survival, reflecting the influence of root biomass on cold survival rather than only on primary root length (Bekele et al., 2014). Thus, root establishment was the most critical factor, affecting field establishment and survival under prolonged chilling stress conditions.
Sorghum–rhizosphere interactions can be a crucial component to consider when breeding this cultivar for cold stress tolerance. Numerous studies have focused on the effects of root exudates in determining the integration of the rhizosphere (Schlemper et al., 2017; Wang et al., 2021) and how these root microbiomes can mitigate different sorts of abiotic stresses (Wu et al., 2021; Yukun et al., 2021). Plant growth microorganisms that have potential to promote plant growth at low temperatures are a worldwide trend in the field of agricultural inoculation technology (Malusá et al., 2012; Pandey and Yarzábal, 2018). Cloutier et al. (2021) studied how the sorghum genotypic affected the root rhizosphere community and how these associations were affected by frost stress. They found that plant genotype influences root flavonoids and the rhizosphere community composition and that these relationships are affected by frost (Cloutier et al. 2021). The analysis of root hydraulic conductance and transpiration rate differences, as well as the analysis of varieties with different tolerance to cold stress, should provide a novel insight into the root contribution to chilling tolerance in sorghum and the genetic mechanisms implicated (Bekele et al., 2014).
Critical cellular functions such as photosynthesis, respiration, and membrane permeability are affected by cold temperatures (Rawat et al., 2021). Cold acclimation involves many physiological and biochemical changes, the principal changes being the reduction or cessation of growth (Achard et al., 2008). The reduced sorghum growth can be explained by the susceptibility of its C4 photosynthetic machinery to low temperatures, which, at the same time, can be modulated by the upregulation of C-repeat binding factor/dehydration-responsive element binding (CBF/DREB) that are required to activate cold-responsive genes, causing growth inhibition. These mechanisms have been studied in other plants, including Arabidopsis and rice (Ito et al., 2006). The mechanism of growth inhibition also involves the stimulation of gibberellin-inactivating enzymes in a process that promotes survival or escape from adverse environmental conditions (Achard et al., 2008). The reduction in growth could also be caused by an increase in reactive oxygen species (ROS) in plants, which affects growth and reduces crop yield (Devireddy et al., 2021; Wang et al., 2022).
Cold-sensitive plants usually have low leaf water potentials, whereas cold-tolerant plants preserve water potentials by closing their stomata and preventing water loss through transpiration during chilling (Wilkinson et al., 2001). Stomatal conductance and transpiration rates have been suggested as traits that can be used for marker-assisted selection in sorghum (Ortiz et al., 2017). The ability of the plants to maintain gas exchange is vital for biomass production (Khanthavong et al., 2022). Under waterlogging and low temperature, the environmental responses of stomatal conductance in sorghum showed a decrease in stomatal conductance, compared with the combination of moderated soil moisture or gradual soil drying with high temperature (Khanthavong et al., 2022).
Light and water use are more efficient in C4 plants than that in C3 plants (Zhu et al., 2008b). However, only a few C4 plants are able to maintain photosynthetically competent leaves at chilling temperatures (<15°C) (Du et al., 1999; Wang et al., 2008). The ability for active photosynthesis during exposure to cold is vital for sorghum or any other crop bred for cold tolerance (Hurry et al., 2002). The leaf greenness indicates photosynthetic activity, which is a necessary trait for selection of chilling tolerance.
Under cold conditions, pyruvate phosphate dikinase (PPDK) instability is associated with a reduced photosynthetic rate in leaves (Naidu et al., 2003; Wang and Li, 2010). PPDK is a cold-labile enzyme that plays a critical role in the carboxylation of pyruvate to phosphoenolpyruvate in C4 metabolism plants. However, some C4 plants such as Miscanthus giganteus are adapted to cold conditions (Naidu et al., 2003), which has a high level of expression of ribulose-1,5-bisphosphate carboxylase/oxygenase (Rubisco) and PPDK under cold stress, contrasting to maize and other cold susceptible C4 plants (Ortiz et al., 2017). The reduction in Rubisco activity under low temperatures is another limitation to carbon assimilation in C4 plants compared with the photosynthetic rates of C3 plants (Sage, 2002). The lower quantum yield of photosynthesis (ΦPSII) under cold stress indicates a reduction in the linear electron transport rate (Ortiz et al., 2017). Genetic variation in the photosynthetic response, the ability to recover, and the carbon fixation capacities of sorghum exposed to cold stress has been reported (Ortiz et al., 2017). Further analysis of sorghum varieties could include the selection of varieties with better carbon fixation under cold conditions, helping to improve yield and biomass.
The chloroplasts and peroxisomes are the primary sources of ROS generation in light exposition on the mitochondria in the darkness (Foyer and Noctor, 2003; Singh, 2010). The low efficiency of PSII affects excitation energy transfer, leading to photoinhibition and ROS production (Rochaix, 2014). Chilling stress and light can lead to photoinhibition, a phenomenon in which energy transfer from chlorophyll to oxygen is unbalanced. This can produce toxic ROS (Pimentel et al., 2005). During abiotic stress, ROS have a double role; they are toxic at high levels, but, at appropriate levels, it can activate plant defense systems, which are harmful to cells under cold stress (Nadarajah, 2020). ROS can cause membrane damage by lipid peroxidation, proteins, and DNA (Ahmad et al., 2008; Das and Roychoudhury, 2014). In intracellular signaling, ROS act as second messengers to elicit tolerance of abiotic and biotic stresses (Ben Rejeb et al., 2014).
Sorghum has developed mechanisms to prevent the production of ROS and, once formed, to detoxify and repair the damage (Mansour et al., 2021). Sorghum can reduce light absorption in two ways: photoreceptors can sense bright light and move the chloroplast away from the light (Jarillo et al., 2001; Kodama et al., 2008); and also some carotenoid, such as zeaxanthin and antheraxanthin, can dissipate the excess of absorbed light energy (Sharma and Hall, 1996) and inhibit lipid peroxidation (Niyogi et al., 1997). In addition, sorghum has antioxidant machinery that can scavenge ROS composed of enzymatic constituents such as superoxide dismutases (SODs), catalases, and glutathione reductases, as well as antioxidant compounds such as ascorbic acid and flavonoids (Dykes and Rooney, 2006). It has been reported that cold stress increases the activity of SODs and catalases (Petrić et al., 2013).
Cold acclimation comprises many biochemical changes, including the accumulation of cryoprotective molecules (Achard et al., 2008; Rani et al., 2021) such as several sugars, lipids, and nitrogenous compounds. These changes are the result of metabolic response (Figure 2) (Li et al., 2018; Vera-Hernández et al., 2018; Lin et al., 2019, Yu et al., 2022), which provides an excellent tool to improve the stress tolerance of this important crop.
Figure 2 Sugar, amino acid, and fatty acid metabolism response to cold stress. G6P, D-glucose-6-phosphate; G3P, D-glucose-3-phosphate; Tre6P, trehalose-6-phosphate; Tre, trehalose; TSP1, alpha-trehalose-phosphate synthase; COS, chitooligosaccharides; GSA, glutamatesemialdehyde; P5C, L-1-pyrroline-5-carboxylate; GABA, γ-aminobutyric acid; P5CR, P5C reductase; P5CS, glutamate 5-kinase; GDH, glutamate dehydrogenase; δOAT, ornithine aminotransferase; MGDG, monogalactosyldiacylglycerol; DGDG, digalactosyldiacylglycerol; GAlP, galactolipase; FA, fatty acid (Ostmeyer et al., 2022).
Cold stress enhance the accumulation of soluble sugars, which are originated from starch metabolism (Figure 2) (Lin et al., 2019; Shi et al., 2022). Sugars are an energy source, but they also have other functions as substrates for polymers, carbon precursors, storage as reserves, and signaling molecules during cold stress (Yamada and Osakabe, 2018). Trehalose and trehalose-6-phosphate are disaccharides that have a protective role in proteins and membranes during abiotic stress (Elbein et al., 2003; Iordachescu and Imai, 2008). Sorghum transgenic lines with overexpression of alpha-trehalose-phosphate synthase (TSP1) were able to tolerate high salinity and develop higher root growth and biomass (Yellisetty et al., 2015). Still, tolerance to cold stress has not been tested yet. Rice seedlings treated with chitooligosaccharides (COS) showed cold tolerance and increased contents of proline and glutamate, revealing that COS significantly induced genes associated with the glutamate and proline biosynthesis pathway (Zhang et al., 2019) (Figure 2). This suggests that COS might be a key regulator through the accumulations of glutamate and proline (Zhang et al., 2019) and that it could be explored in sorghum. Glucose and fructose levels increased under chilling in sorghum, and sucrose was slightly lower (Marla et al., 2017). The small starch content and the increase in simple sugars during chilling exposure to sorghum suggest an essential role for the B-amylases in cold acclimation (Marla et al., 2017).
Membrane lipids remodeling is key for cold tolerance. The fatty acid unsaturation helps preserve membrane integrity during chilling by lowering the melting temperature and increasing fluidity (Marla et al., 2017). The ability to adjust membrane fluidity to low temperature change is associated with regulating membrane fatty acid desaturation. The desaturation of both phospholipids and sphingolipids is essential for remodeling the plasma membrane under cold stress (Barrero-Sicilia et al., 2017). In chilling-sensitive plants at low temperatures, the galactolipase (GAlP) is significantly active, leading to an increase in free fatty acid in chloroplasts (Figure 2) (Kaniuga, 2008).
Chilling causes a decrease in the concentrations of mono-galactosyl-diacyl-glycerols (MGDGs) and sulpho-quinovosyl-diacyl-glycerols (Figure 2), which are significant lipids in the thylakoid (Marla et al., 2017; Liu et al., 2018). Lipid metabolism gene analysis in sorghum has identified lipids deregulated in response to chilling in sorghum seedlings that may cause chilling tolerance in this crop (Marla et al., 2017). Genes that are involved in fatty acid biosynthesis (i.e., acetyl coenzyme A (CoA) synthetase and acyl-Acetyl coenzyme A (CoA) oxidase 1), thylakoid lipid synthesis (MGDG synthase MGD2 and DGFGI synthase 1 DGD1), and phospholipids metabolism (G-3-phosphatase acyltransferase 5, phosphatidiylserine synthase, and phospholipase D delta) were upregulated in response to chilling (Marla et al., 2017). An increase in the unsaturation index of total phospholipid PC and LysoPC also has observed under chilling in sorghum (Marla et al., 2017).
During cold stress, certain amino acids and amine compounds adjust its metabolism, particularly those associated with proline biosynthesis (Figure 2) (Kaplan et al., 2004). The proline amino acid can act as a regulatory or signaling molecule capable of altering the expression of antioxidants-associated genes in plant response to environmental constraints (De Carvalho et al., 2013; Ben Rejeb et al., 2014). During dehydratation, proline accumulates and contributes to ROS formation in mitochondria, playing a role in the hypersensitive response in plants (Ben Rejeb et al., 2014). Proline is usually used as an osmolyte, but it can also function as a powerful antioxidant (Das and Roychoudhury, 2014). Proline accumulation is an adaptive mechanism that allow plants tolerate cold or chilling stress (Teh et al., 2016). Proline accumulation in stress situations will depend on both the activation of its biosynthesis and the inhibition of its degradation (Figure 2) (Ben Rejeb et al., 2014). In cold-tolerant sorghum plants, a higher proline accumulation is related to a better tolerance (Vera-Hernández et al., 2018). There are two biosynthesis pathways for proline, glutamate, and ornithine (Figure 2). The enzymes Δ1-pyrroline-5-carboxylate synthetase (P5CS), Δ1-pyrroline-5-carboxylate reductase (P5CR), and ornithine-δ-aminotransferase (δ-OAT) are involved in the proline biosynthesis (Figure 2) (Bagdi et al., 2015; Vera-Hernández et al., 2018). A transcription factor binding site analysis of these genes indicated the presence of a low-temperature response element in the promoter region of ɗ-OAT, suggesting that the proline accumulation in cold response in sorghum may be using the Orn pathway (Vera-Hernández et al., 2018). P5CS2 expression is associated with high proline levels in response to drought stress in sorghum, resulting in an exciting gene for cold tolerance analysis. γ-Aminobutyric acid (GABA) is another amine metabolite that can be rapidly accumulated in plant tissues and is associated with cryoprotection in monocots (Mazzucotelli et al., 2006), which could be involved in tolerance to chilling stress in sorghum (Figure 2).
Transcription factors (TFs) are a category of proteins that bind to cis-regulatory DNA sequences and are responsible for either positively or negatively affecting the transcription of certain genes. They determine whether a specific gene will be turned “on” or “off” (Philips and Hoopes, 2008). Currently, the plant’s most understood cold-signaling pathway is the CBF/DREB transcriptional regulatory cascade (Shi et al., 2015; Wang et al., 2022). The CBF genes respond rapidly to chilling temperatures and induce the expression of several proteins that protect plants from freezing temperatures. These proteins play a crucial role in cold acclimation (Agarwal et al., 2006; Nakashima and Yamaguchi-Shinozaki, 2006; Achard et al., 2008). SbCBF6 is highly expressed during chilling in tolerant sorghums (Chopra et al., 2015; Marla et al., 2017). CBF expression is controlled through jasmonic acid (JA) (Figure 3). In this sense, allene oxidase synthase and 12-oxoyphytodienic acid reductase, which are important genes in JA biosynthesis, are upregulated in chilling-tolerant sorghum. This suggests that JA biosynthesis contributes to chilling tolerance (Marla et al., 2017). DELLA proteins (a critical negative regulator of gibberellin signaling) may contribute to the CBF1-mediated freezing tolerance (Achard et al., 2008). DREBs (a significant subgroup of AP2/ERF TF) induce the expression of genes involved in abiotic stresses. Genes that code for DREB proteins regulate the transcription of many genes involved in the plant response to cold stress. Fifty-two ERF (ethylene response factor) genes encoding DREB proteins have been predicted in sorghum (Mathur et al., 2020). Bioinformatic analysis showed that the motifs within sorghum DREB promoters are principally involved in abscisic acid (ABA), light, and calcium-mediated regulation (Srivastav et al., 2010). However, the regulation of DREB genes is not well understood in sorghum. In sorghum, the CBF/DREB and ERF proteins have similar structures and evolutionary relationships (Mizoi et al., 2012; Rashid et al., 2012). In sorghum, CBF/DREB and ERF proteins have groups that contain an LWSY motif at the C-terminus, which respond to cold treatment, suggesting that such motif may be involved in cold plant tolerance (Yan et al., 2013). Sorghum genes that are predicted to be involved in ethylene biosynthesis, which regulates cold signaling pathways, were upregulated (Marla et al., 2017). Recently, it was indicated that TaMYC2 plays a critical role in cold stress in many plants and activates the ICE (inducer of CBF expression)–CBF-COR (cold responsive) cold resistance pathway through interaction with ICE in Arabidopsis (Wang et al., 2022), and some MYC2 (A/B/D) improved cold tolerance through ROS metabolic.
Figure 3 Schematic overview of the cold response and its overlapping dehydration response–mediated regulatory networks in Sorghum. Cold may be perceived by signaling receptor and the second messengers (IP3, Ca2+, and ROS), transducing signals through protein kinases or TF cascades. CBFs are activated by ICE1 and CAMTA TF. HOS1 and SIZ1 regulate ICE1 protein. Cold stress activates ICE1, and AREB is induced by ABA. The DRE element is recognized by CBF1 and control COR genes. MiR398, miR397, and miR408 regulate some COR genes. The JA signaling pathway activates MYC2, which can activate the expression of ICE in ICE-CBF-COR cold resistance pathway. ROS, reactive oxygen species; IP3, inositol 1,4,5-triphosphate; CPK, calcium-dependent protein kinase; MAPK Ras, mitogen-activated protein kinase; Pi, phosphoryl group; miR, microRNA; SOD, superoxide dismutase; GST, glutathione S-transferase; CBF1, C-repeat binding factors; Ub, ubiquitin; SUMO, small ubiquitin–related modifier. Modified by Shi and Yang (2014).
Another critical group of TF is NF-Ys (plant nuclear factors), which show differential stress response gene expression in several tissues and interact with abiotic stress signaling pathways to regulate the plant stress responses (Kavi et al., 2022). In sorghum, 24 NF-Y genes are expressed in cold stress (Maheshwari et al., 2019). Sixteen NF-Ys (NF-YA2/4/6/8, NF-YB2/7/10/11/12/14/16/17, and NF-YC4/6/12/13) are induced by both cold and high temperatures (Maheshwari et al., 2019). These suggest a crosstalk among both stresses and microRNA (miRNA) association in the regulation of SbNF-Ys (Maheshwari et al., 2019), denoting that miRNAs may also participate in gene networks controlled by TFs like NF-Ys (Maheshwari et al., 2019).
ABA is an important hormone implicated in the cold stress response by regulating specific stress-responsive genes (Xue-Xuan et al., 2010). The genes that are predicted to be involved in the biosynthesis of ABA were upregulated in sorghum during cold treatment (Marla et al., 2017). Regarding the amino acid sequences of ABA receptor (ABARs) family genes from Arabidopsis, eight candidate genes were identified in the sorghum genome (Dalal and Inupakutika, 2014). ABA binds to ABARs and brings conformational changes that facilitate ABA-mediated interaction of PYL with PP2C. Dalal and Inupakutika (2014) identified these eight genes as members of the PYL family in sorghum, namely, SbPYL1–SbPYL8. In addition, nine functional SbPP2C genes were predicted in the sorghum genome (Dalal and Inupakutika, 2014). Among ABARs receptors, the SbPYL4 gene was found to be specific for cold stress, with higher expression observed in leaf exposed to cold stress. PhyA and phyB genes have been reported to function antagonistically to regulate cold tolerance through ABA-dependent jasmonate signaling (Yang et al., 2019). In tomatoes, the genes HY3 and HY5 enhance cold tolerance by integrating myoinositol and light signaling (Yang et al., 2019). Lin et al. (2021) found that HY5 can be activated by photoreceptors to promote photomorphogenesis (Lin et al., 2021).
During chilling, genes encoding components of photosystem I (PSI/P700) and photosystem II (P680), responsible for the light reactions phase of photosynthesis, are downregulated (Marla et al., 2017). Sobic.003G370000, an ortholog of AtNPQ4 (nonphotochemical quenching), was increased under chilling and could be implicated in preventing damage to the photosynthetic apparatus due to photoinhibition (Marla et al., 2017), as was reported in Arabidopsis (Havaux et al., 2000). ROS detoxification in plants has been mediated by the ascorbate-glutathione cycle (Foyer and Noctor, 2011). Marla et al. (2017) showed that the monodehydroascorbate reductase 1 (Sobic.007G171000) and the ascorbate biosynthesis gene vitamin C defective 5 (VTC5, Sobic.008G064700) of the ascorbate–glutathione cycle were highly expressed during cold stress in sorghum. Similarly, some glutathione S-transferase (GST) enzymes have antioxidant properties. Some GST genes were highly upregulated in chilling-tolerant sorghum but not in chilling-sensitive sorghum in cold treatments. Other genes involved in sugar breakdown as hexokinase (Sobic.009G06980) and fructokinase (Sobic.003G386000) increased during cold in sorghum. Several starch synthase genes were induced, which correlated with the composition of the carbohydrate in sorghum during cold stress (Marla et al., 2017).
C2H2-type zinc finger proteins are one of the best-studied TF associated with abiotic stress in plants (Liu et al., 2022). In S. bicolor, 145 SbC2H2-ZFP members have been predicted (Cui et al., 2022). In sorghum, Sobic.005G121100 was significantly upregulated in cold stress, and Sobic.008G088842 was activated by cold and inhibited in drought in stems and leaves (Cui et al., 2022). In tomatoes, multiple B-box proteins (BBxs) play a role in responses to light quality and cold stress (Bu et al., 2021). In SlBBX7-, SlBBX9-, and SlBBX20-silenced tomato plants, cold tolerance was suppressed. Moreover, Bu et al. (2021) found a photosynthetic response instantly after cold stress by the impairment of non-photochemical quenching, and the consequent excess photon energy excited by low temperature is not consumed, leading to the over-reduction of electron carriers and damage of the photosystem (Bu et al., 2021). Twenty-four SbBBX genes have been identified in sorghum (Shalmani et al., 2019), suggesting that SbBBX studies in response to cold tolerance may improve the current understanding of this trait.
Differential expression of K+ transport genes might also be involved in sorghum’s cold response. Anil Kumar et al. (2022) found higher expression levels of potassium transport genes (SbAKT1, SbHAK7, SbHKT5, SbHAK25, and SbAKT7) in cold stress treatments. In this context, genes with differential expression could be proposed as good candidates for functional analysis and further use in genetic engineering, and traditional breeding programs increase the cold tolerance of sorghum.
Heat shock proteins (HSP) function as chaperons and protect proteins from the harmful effect of different types of abiotic stress besides heat, including cold (Sabehat et al., 1998; Swindell et al., 2007). Transcriptomic analysis showed that the HSP Sb03g027330 was highly abundant under cold stress (Chopra et al., 2015). GST enzymes have functions in detoxifying xenobiotic compounds and ROS and are abundant under cold stress in sorghum (Chopra et al., 2015). Late embryogenesis abundant proteins are essential for membrane stabilization when the cytoplasm becomes dehydrated (Hanin et al., 2011) and might be expressed under cold conditions by a crosstalk signaling process with ABA (Li et al., 2017). To understand better how cold stress signals are perceived and integrated into gene regulation by changes at multiple levels, it is necessary to know the TFs, miRNAs, and the expression of gene products that might be involved in different degrees of tolerance to low temperatures, resulting in a change in metabolites in plants.
miRNAs are small non-coding RNAs, approximately 18–24 nucleotides in length, that can control gene expression at the post-transcriptional level (Fire et al., 1998). miRNAs act as regulators of gene expression through the degradation or inhibition of target gene translation (Djami-Tchatchou et al., 2017). The upregulation of miRNAs is associated with reduced expression of its target gene (Megha et al., 2018). In contrast, the downregulation of a miRNA can increase its target genes expression. MiRNAs may mediate the gene regulation network under cold stress in two ways: regulating stress-related signal transduction pathways or modulating the expression of cold-responsive TFs (Huang et al., 2022). Target genes and miRNAs involved in cold stress responses as a complex gene network may influence cold tolerance.
Some miRNAs participate in plant responses to cold stress (Thiebaut et al., 2012; Liu et al., 2018; Martínez Núñez et al., 2021; Satyakam et al., 2022). MiR397 is involved in different abiotic stress, including cold (Huang et al., 2020). For example, under normal conditions, miR397 is expressed at high levels, altering the abundance of its target genes laccases, which play an essential role in anthocyanin biosynthesis and abiotic stress responses (Xu et al., 2022; Zaman et al., 2022). Under cold stress conditions, miR397 is downregulated, leading to the accumulation of laccases (Xu et al., 2022). This association between miR397 and its target genes under cold treatments has been observed in plants including wheat (Gupta et al., 2014) and grapevine (Sun et al., 2015). In addition, miR397 overexpression improved the tolerance to cold stress in Arabidopsis (Dong and Pei, 2014; Satyakam et al., 2022). The core regulator in cold acclimation ICE1 (Chinnusamy et al., 2007) was identified as a target of miR397 in cold adaption in wheat. In sorghum, a homolog of miR397 was identified (Dash et al., 2022).
MiR319 and its target genes have been analyzed in the monocot sugarcane under cold stress (Thiebaut et al., 2012). Thiebaut et al. (2012) found differences in the timing and intensity of regulation of miR319 and its target genes PCF5, PCF6, and GAMyb. The Myb family genes are known to be CBFs suppressors; therefore, the upregulation of miR319 can prevent its repression effect, leading to the expression of COR genes (Figure 3), possibly contributing to cold tolerance. The overexpression of miR319 can enhance cold tolerance, after chilling acclimation, in transgenic rice seedlings (Yang et al., 2013; Wang et al., 2014; Iqbal et al., 2021). Another example of this is the family of miR398; they control Cu/Zn SODs under oxidative stress caused accumulation of ROS during cold or some other kind of abiotic stress such as heat and salinity stress. SODs play an essential role in converting superoxide to H2O2 and molecular oxygen and thus reduce oxidative stress in plant cells. Downregulation of miR398 is vital for the SODs expression and ROS detoxifying. This association between miR398 and its target genes has been observed in cold-stress plants, including Arabidopsis (Sunkar and Zhu, 2004) and grapevine (Sun et al., 2015).
In sorghum, miRNA–target gene interactions generated with degradome analysis have been reported in cold response, and some interactions as miR169-NF-Y network were identified (Franke et al., 2018). Validation of miRNA–target gene networks will screen their roles in cold stress tolerance, which will also help develop plants with stress tolerance.
Understanding the mechanisms of cold stress response at the molecular level is crucial for improving crops against stresses without affecting the yield (Adhikari et al., 2022). Integration of signal perception, signal transduction, gene expression, and molecules produced is essential to understand the cold stress response and cold plant acclimation. Several molecules, including calcium (Ca2+), receptors, ROS, and signaling proteins, have been identified in plants. TFs, miRNAs, and proteins also play an essential role, and several have been identified in sorghum’s cold response. In summary, an illustration of the cold response and its overlapping dehydration response–mediated regulatory networks in sorghum is proposed using the evidence previously described (Figure 3).
The mechanism of sensing low temperatures in plants is scarce, and it is still unknown whether there is a cold signaling receptor; possibly, with a similar complex as in rice (Ma et al., 2015), Chilling Tolerance Divergence1 (COLD1) encodes a regulator of G protein signaling coupled with rice G protein A subunit 1 that participates in cold stress signaling via Ca2+ signals (Ma et al., 2015; Zhu et al., 2022), regulating the cold stress–driven influx of intracellular Ca2+. Low temperatures can change the membrane fluidity; the potential sensors of cold include Ca2+ influx channels, transmembrane stress-sensing histidine kinases proteins (Fedurayev et al., 2018), and receptors associated with G proteins (Zhu et al., 2022), which could allow identify the low temperatures and induce a signal to subsequently transduce it to the nucleus (Chinnusamy et al., 2007). A decrease in cell membrane fluidity leads to conformational changes in membrane proteins and lipids, which generate second messengers IP3, Ca2+, and ROS, which are necessary to transduce signals through protein kinases or TF cascades (Figure 3). Ca2+ is the most common secondary messenger in plants. Within only a few seconds under low temperatures, cytosolic Ca2+ can liberate from vacuoles. The Ca2+ release is upstream to the expression of CBFs and COR genes in the cold signaling pathways (Hiraki et al., 2019); in the same manner, ROS and IP3 serve as second messengers to be integrated into a genetic response that initiates variations in gene expression, leading to physiological and metabolic changes in the cell, and culminates in response and tolerance (Pareek et al., 2017).
Plant hormone JA also affects the low-temperature stress response. The JA signaling pathway activates MYC2, which is involved in most JA-mediated responses; in the same way, MYC2 is capable of activating the expression of ICE in ICE-CBF-COR cold resistance pathway. CBFs and AREB TFs regulate COR genes containing CRT/DRE and ABRE motifs in their promoters, respectively (Figure 3). CBFs are activated by ICE1 and calmodulin-binding transcription activators (CAMTA) TFs but are suppressed by Myb family TFs. MiR319 can suppress TCP-like and MYB-like factors, stopping its repression effect and possibly contributing to cold tolerance. HOS1 and SIZ1 encode protein ligases that regulate the abundance of ICE1 protein (Zhou et al., 2011). Cold activates ICE1, and AREB is induced by ABA-mediated dehydration signaling. In response to cold stress, DRE element is recognized by CBF1 TFs, which control the expression of COR genes. At the same time, some COR genes are regulated by miRNAs, such as SODs by miR398, and laccases by miR397 and miR408. Consequently, the downregulation of these miRNAs is necessary for COR genes expression. There is a correct coordination between the different elements, and different elements are conserved in both biotic and abiotic stress responses. The more information that we have, the more precise the mechanisms of response and tolerance in sorghum will be.
The sorghum genome of ~730 Mb has been sequenced (Paterson et al., 2009). Quantitative trait locus (QTL) is the most comprehensive tool for marker‐assisted selection. In sorghum, co‐localization of different QTLs close to each other is known as hotspots, highly heritable genes map irregularly to the 10 sorghum chromosomes, and several are physically clustered together in chromosomes. In parallel, there are other vital tools, such as cold stress–induced transcriptome profiling (Chopra et al., 2015) and analysis of single-nucleotide polymorphisms (Chopra et al., 2015; Parra-Londono et al., 2018). Cold acclimatization is a process conserved between species where many genes are induced (Chinnusamy et al., 2007). Histone marks like acetylation and deacetylation are central for activation and repression during cold acclimation. The HOS15 gene product, which works as a histone deacetylation, interacts explicitly with histone H4 during cold acclimatization in Arabidopsis (Zhu et al., 2008a; Park et al., 2018). The chromatin remodeling derived from epigenetic changes during cold acclimatization has not been studied yet in sorghum. Nevertheless, there are some clues about epigenetic memory to cold stress; sorghum exposed to photoinhibition treatment changes the levels of the carotenoids and then restores during a recovery period, and, when photoinhibited, plants showed better protection (Sharma and Hall, 1996).
Some genetic sources of cold tolerance in sorghum have been identified (Singh, 2010; Burow et al., 2011; Woldesemayat et al., 2018). Genetic variability between sorghum genotypes for traits associated with early-stage chilling tolerance plays an essential role in future research (Rutayisire et al., 2021). One of the most studied sources of cold tolerances is Chinese landraces, showing a higher emergence and seedling vigor compared with commercial lines, under controlled and field cold conditions. Some undesirable characteristics are associated with cold-tolerant sorghums, particularly grain tannins and tall plants (Franks et al., 2006).
Cold tolerance in sorghum has been assessed by different traits such as germination (Tiryaki and Andrews, 2001) and seedling establishment and vigor (Upadhyaya et al., 2015) under low temperatures. The use of methods based on a rank summation index of traits such as emergence index, percentage, shoot and root dry weight, seedling height, and vigor score during growth under low temperature has been described (Yu et al., 2004; Rutayisire et al., 2021). Most of the research has focused on developing early-season cold-tolerant genotypes (Emendack et al., 2021). By planting early mature varieties and manipulating the sowing date, severe damage can be avoided in the early cold exposure conditions; however, the development of cold-tolerant varieties is necessary to deal with late or prolonged exposure to cold. Ideally, breeders will select individual plants with the same grain yield as the commercial hybrids to conserve the desired tolerance by using marker-assisted selection tools.
Selection for early and late cold tolerance under field conditions is often the most affordable way to select cold-tolerant genotypes, which can germinate, grow, and develop under challenging circumstances where sensitive genotypes cannot. In this sense, several sorghum hybrids have been developed under traditional breeding methods. These hybrids show a good adaptation to climatic conditions, where no other sorghum can grow and produce flowers, pollen, and seeds successfully (Osuna-Ortega et al., 2003; Leon-Velasco et al., 2009). However, variable climatic conditions might make a difficult selection for cold temperature stress. Furthermore, the multigenic nature of cold tolerance makes the evaluation difficult because of the genotype–environment interactions in the field. Controlled conditions are recommended for further research, which involves greenhouse screening for cold tolerance in combination with field evaluation in multi-environment conditions (Kapanigowda et al., 2013). For breeding programs, the application of short and intense chilling is more promising (Windpassinger et al., 2017). Germination tests at low temperatures have been proposed as a selection tool for early establishment in sorghum (Singh, 1985; Brar and Stewart, 1994), but there is a poor relationship association between germination in the laboratory and field selection for cold seedling tolerance because of its low heritability or probably low repeatability (Emendack et al., 2021). The flowering and maturity delay is observed when sorghum is subjected to cold temperatures after emergence (Kapanigowda et al., 2013; Maulana and Tesso, 2013), although a significant reduction of grain yield is not found (Maulana and Tesso, 2013; Windpassinger et al., 2017). A strong expression of heterosis (vigor hybrid) is desirable for yield and chilling tolerance in sorghum (Windpassinger et al., 2017). Sorghums with different degrees of cold tolerance represent an opportunity to know and integrate the physiological, metabolic, and molecular mechanisms associated with chilling stress tolerance and can help to identify the complex networks involved in plant tolerance to cold stress.
We have integrated the advances in the knowledge of sorghum in response to cold stress, describing the physiological, biochemical, metabolic, and molecular changes in response to cold stress in sorghum. This will improve the understanding and integration of the models involved, such as the ICE-CBF-COR model conserved in several plants.
The broadening and deepening of knowledge using different tools for massive sequencing of miRNAs, mRNAs, metabolomics, and proteomics will be key pieces that will help to better integrate the response effect of cold stress in sorghum. In this sense, the best networks could be proposed as good candidates for functional analysis for subsequent use in genetic engineering using tools such as the CRISPR-Cas system. Validation of the miRNA–target gene–protein networks will help to provide novel insights into the contribution to cold tolerance in sorghum and the genetic mechanisms involved, which will also help to develop stress tolerant plants. Upcoming efforts in the search for sorghum genes that are potentially able to confer cold tolerance will undoubtedly be more successful if high-throughput techniques, such as microarrays, next-generation sequencing, and proteomics, are more extensively used.
An essential part of the understanding of cold stress response in plants is influenced by epigenetics. Therefore, it will be relevant to consider sorghum varieties with different degrees of cold tolerance and multi-environment conditions for cold response. Moreover, it is important to consider that the rhizosphere microbiomes retain their potential to promote plant growth at low temperatures is a worldwide trend in the field of agricultural inoculation technology (Malusá et al., 2012; Pandey and Yarzábal, 2018). Therefore, the sorghum genotype–environment–rhizosphere microbiome interaction will be essential for selective breeding of sorghum for cold stress tolerance. This will provide an opportunity to study, understand, and integrate the mechanisms associated with cold stress. In the long term, the knowledge generated by breeders and plant biologists will help to understand the intricate molecular mechanisms governing stress response under challenging situations to develop more cold temperature–tolerant plants, allowing higher yields under difficult conditions to support profitable crops to feed the world’s growing population.
Comprehensive understanding of the physiological, biochemical, and molecular response mechanisms to low temperatures in sorghum can contribute to the selection of cold-tolerant genotypes and crop improvement. Here, we reviewed and discussed some of the research results that focused on these mechanisms and signal transductions and the molecules involved in sorghum cold stress, which will be essential information for future studies. Much advancement has been achieved in understanding cold tolerance in sorghum. Although there are interesting proposals for the function of some lipids, sugars, nitrogen compounds, TFs, and proteins in sorghum cold stress, it is crucial to elucidate the interactions between these elements, which could be essential to clarify the sorghum cold stress response. Still, reduced yield caused by low temperatures remains a problem, especially in high altitudes where sorghum is cultivated. In addition, we should consider that the incidence of extreme temperatures is expected to increase as part of general global warming, and losses caused by cold temperatures may increase (Raza et al., 2019). Cold-tolerant sorghum varieties will need to be bred, allowing the cultivar to tolerate, survive, and develop when exposed to cold. A strategy that considers breeding and genetic engineering tools confers cold tolerance in sorghum plants.
PH and FR contributed to the conception of the manuscript and drafted the manuscript. PH, LM and FR contributed to manuscript preparation and approved the final manuscript. All authors contributed to the article and approved the submitted version.
PH was supported by a Mexican National Council of Science and Technology (CONACyT) fellowship. This work was financed by CONACyT grant CB-2013-221522 and SIP grant 20221550. Additionally, we received partial support by the Instituto Politécnico Nacional for open-access publication.
The authors declare that the research was conducted in the absence of any commercial or financial relationships that could be construed as a potential conflict of interest.
All claims expressed in this article are solely those of the authors and do not necessarily represent those of their affiliated organizations, or those of the publisher, the editors and the reviewers. Any product that may be evaluated in this article, or claim that may be made by its manufacturer, is not guaranteed or endorsed by the publisher.
Achard, P., Gong, F., Cheminant, S., Alioua, M., Hedden, P., Genschika, P. (2008). The cold-inducible CBF1 factor-dependent signaling pathway modulates the accumulation of the growth-repressing DELLA proteins via its effect on gibberellin metabolism. Plant Cell. 20 (8), 2117–2129. doi: 10.1105/tpc.108.058941
Adhikari, L., Baral, R., Paudel, D., Min, D., Makaju, S. O., Poudel, H. P., et al. (2022). Cold stress in plants: Strategies to improve cold tolerance in forage species. Plant Stress. 4, 100081. doi: 10.1016/J.STRESS.2022.100081
Agarwal, M., Hao, Y., Kapoor, A., Dong, C. H., Fujii, H., Zheng, X., et al. (2006). A R2R3 type MYB transcription factor is involved in the cold regulation of CBF genes and in acquired freezing tolerance. J. Biol. Chem. 281, 37636–37645. doi: 10.1074/jbc.M605895200
Ahmad, P., Sarwat, M., Sharma, S. (2008). Reactive oxygen species, antioxidants and signaling in plants. J. Plant Biol. 51 (3), 167–173. doi: 10.1007/BF03030694
Anil Kumar, S., Hima Kumari, P., Nagaraju, M., Sudhakar Reddy, P., Durga Dheeraj, T., Mack, A., et al. (2022). Genome-wide identification and multiple abiotic stress transcript profiling of potassium transport gene homologs in sorghum bicolor. Front. Plant Sci. 2 (13). doi: 10.3389/FPLS.2022.965530
Aroca, R., Porcel, R., Ruiz-Lozano, J. M. (2012). Regulation of root water uptake under abiotic stress conditions. J. Exp. Bot. 63, 43–57. doi: 10.1093/JXB/ERR266
Bagdi, D., Shaw, B., Sahu, B., Purohit, G. (2015). Real time PCR expression analysis of gene encoding p5cs enzyme and proline metabolism under NaCI salinity in rice. Environ. Biol. 36 (4), 955–961.
Barrero-Sicilia, C., Silvestre, S., Haslam, R. P., Michaelson, L. V. (2017). Lipid remodelling: Unravelling the response to cold stress in arabidopsis and its extremophile relative eutrema salsugineum. Plant Sci. 263, 194–200. doi: 10.1016/J.PLANTSCI.2017.07.017
Bekele, W. A., Fiedler, K., Shiringani, A., Schnaubelt, D., Windpassinger, S., Uptmoor, R., et al. (2014). Unravelling the genetic complexity of sorghum seedling development under low-temperature conditions. Plant Cell Environ. 37 (3), 707–723. doi: 10.1111/pce.12189
Ben Rejeb, I., Pastor, V., Mauch-Mani, B. (2014). Plant responses to simultaneous biotic and abiotic stress: Molecular mechanisms. Plants 3, 458–475. doi: 10.3390/PLANTS3040458
Brar, G. S., Stewart, B. A. (1994). Germination under controlled temperature and field emergence of 13 sorghum cultivars. Crop Sci. 34, 1336–1340. doi: 10.2135/cropsci1994.0011183x003400050036x
Bu, X., Wang, X., Yan, J., Zhang, Y., Zhou, S., Sun, X., et al. (2021). Genome-wide characterization of b-box gene family and its roles in responses to light quality and cold stress in tomato. Front. Plant Sci. 5. doi: 10.3389/fpls.2021.698525
Burow, G., Burke, J. J., Xin, Z., Franks, C. D. (2011). Genetic dissection of early-season cold tolerance in sorghum (Sorghum bicolor (L.) moench). Mol. Breed. 28, 391–402. doi: 10.1007/s11032-010-9491-4
Chinnusamy, V., Zhu, J., Zhu, J. K. (2007). Cold stress regulation of gene expression in plants. Trends Plant Sci. 2 (10), 444–451. doi: 10.1016/j.tplants.2007.07.002
Chopra, R., Burow, G., Burke, J. J., Gladman, N., Xin, Z. (2017). Genome-wide association analysis of seedling traits in diverse sorghum germplasm under thermal stress. BMC Plant Biol. 17, 12. doi: 10.1186/s12870-016-0966-2
Chopra, R., Burow, G., Hayes, C., Emendack, Y., Xin, Z., Burke, J. (2015). Transcriptome profiling and validation of gene based single nucleotide polymorphisms (SNPs) in sorghum genotypes with contrasting responses to cold stress. BMC Genomics 9, 16:1040. doi: 10.1186/s12864-015-2268-8
Cloutier, M., Chatterjee, D., Elango, D., Cui, J., Bruns, M. A., Chopra, S. (2021). Sorghum root flavonoid chemistry, cultivar, and frost stress effects on rhizosphere bacteria and fungi. Phytobiomes J. 5, 39–50. doi: 10.1094/PBIOMES-01-20-0013-FI
Cui, H., Chen, J., Liu, M., Zhang, H., Zhang, S., Liu, D., et al. (2022). Genome-wide analysis of C2H2 zinc finger gene family and its response to cold and drought stress in sorghum [Sorghum bicolor (L.) moench]. Int. J. Mol. Sci. 23, 5571. doi: 10.3390/IJMS23105571/S1
Daems, S., Ceusters, N., Valcke, R., Ceusters, J. (2022). Effects of chilling on the photosynthetic performance of the CAM orchid phalaenopsis. Front. Plant Sci. 13. doi: 10.3389/fpls.2022.981581
Dalal, M., Inupakutika, M. (2014). Transcriptional regulation of ABA core signaling component genes in sorghum (Sorghum bicolor l. moench). Mol. Breed. 34, 1517–1525. doi: 10.1007/S11032-014-0114-3/FIGURES/3
Das, K., Roychoudhury, A. (2014). Reactive oxygen species (ROS) and response of antioxidants as ROS-scavengers during environmental stress in plants. Front. Environ. Sci. 2. doi: 10.3389/fenvs.2014.00053
Dash, P. K., Gupta, P., Pradhan, S. K., Shasany, A. K., Rai, R. (2022). Analysis of homologous regions of small RNAs MIR397 and MIR408 reveals the conservation of microsynteny among rice crop-wild relatives. Cells 11, 3461. doi: 10.3390/CELLS11213461/S1
De Carvalho, K., De Campos, M. K. F., Domingues, D. S., Pereira, L. F. P., Vieira, L. G. E. (2013). The accumulation of endogenous proline induces changes in gene expression of several antioxidant enzymes in leaves of transgenic swingle citrumelo. Mol. Biol. Rep. 40, 3269–3279. doi: 10.1007/S11033-012-2402-5/FIGURES/5
Devireddy, A. R., Tschaplinski, T. J., Tuskan, G. A., Muchero, W., Chen, J. G. (2021). Role of reactive oxygen species and hormones in plant responses to temperature changes. Int. J. Mol. Sci. 22, 8843. doi: 10.3390/IJMS22168843
Djami-Tchatchou, A. T., Sanan-Mishra, N., Ntushelo, K., Dubery, I. A. (2017). Functional roles of microRNAs in agronomically important plants-potential as targets for crop improvement and protection. Front. Plant Sci. 8. doi: 10.3389/FPLS.2017.00378/BIBTEX
Dong, C. H., Pei, H. (2014). Over-expression of miR397 improves plant tolerance to cold stress in arabidopsis thaliana. J. Plant Biol. 57, 209–217. doi: 10.1007/S12374-013-0490-Y
Du, Y. C., Nose, A., Wasano, K. (1999). Effects of chilling temperature on photosynthetic rates, photosynthetic enzyme activities and metabolite levels in leaves of three sugarcane species. Plant Cell Environ. 22 (3), 317–324. doi: 10.1046/j.1365-3040.1999.00415.x
Dykes, L., Rooney, L. W. (2006). Sorghum and millet phenols and antioxidants. J. Cereal Sci. 44, 236–251. doi: 10.1016/j.jcs.2006.06.007
Elbein, A. D., Pan, Y. T., Pastuszak, I., Carroll, D. (2003). New insights on trehalose: A multifunctional molecule. Glycobiology 3 (4), 17R–27R. doi: 10.1093/glycob/cwg047
Emendack, Y., Sanchez, J., Hayes, C., Nesbitt, M., Laza, H., Burke, J. (2021). Seed-to-seed early-season cold resiliency in sorghum. Sci. Rep. 11, 1–12. doi: 10.1038/s41598-021-87450-1
Ercoli, L., Mariotti, M., Masoni, A., Arduini, I. (2004). Growth responses of sorghum plants to chilling temperature and duration of exposure. Eur. J. Agron. 21, 93–103. doi: 10.1016/S1161-0301(03)00093-5
Fedurayev, P. V., Mironov, K. S., Gabrielyan, D. A., Bedbenov, V. S., Zorina, A. A., Shumskaya, M., et al. (2018). Hydrogen peroxide participates in perception and transduction of cold stress signal in synechocystis. Plant Cell Physiol. 59, 1255–1264. doi: 10.1093/PCP/PCY067
Fiedler, K., Bekele, W. A., Duensing, R., Gründig, S., Snowdon, R., Stützel, H., et al. (2014). Genetic dissection of temperature−dependent sorghum growth during juvenile development. Theor. Appl. Genet. 127 (9), 1935–1948. doi: 10.1007/s00122-014-2350-7
Fiedler, K., Bekele, W. A., Friedt, W., Snowdon, R., Stützel, H., Zacharias, A., et al. (2012). Genetic dissection of the temperature dependent emergence processes in sorghum using a cumulative emergence model and stability parameters. Theor. Appl. Genet. 1 25 (8), 1647–1661. doi: 10.1007/s00122-012-1941-4
Fire, A., Xu, S., Montgomery, M. K., Kostas, S. A., Driver, S. E., Mello, C. C. (1998). Potent and specific genetic interference by double-stranded RNA in caenorhabditis elegans. Nature 391, 806–811. doi: 10.1038/35888
Foyer, C. H., Noctor, G. (2003). Redox sensing and signalling associated with reactive oxygen in chloroplasts, peroxisomes and mitochondria. Physiol. Plant 119, 355–364. doi: 10.1034/J.1399-3054.2003.00223.X
Foyer, C. H., Noctor, G. (2011). Ascorbate and glutathione: The heart of the redox hub. Plant Physiol. 155, 2–18. doi: 10.1104/PP.110.167569
Franke, K. R., Schmidt, S. A., Park, S., Jeong, D. H., Accerbi, M., Green, P. J. (2018). Analysis of brachypodium miRNA targets: Evidence for diverse control during stress and conservation in bioenergy crops. BMC Genomics 19, 1–18. doi: 10.1186/S12864-018-4911-7/FIGURES/9
Franks, C. D., Burow, G. B., Burke, J. J. (2006). A comparison of U.S. and Chinese sorghum germplasm for early season cold tolerance. Crop Sci. 46 (3), 1371–1376. doi: 10.2135/cropsci2005.08-0279
Goswami, A. K., Maurya, N. K., Goswami, S., Bardhan, K., Singh, S. K., Prakash, J., et al. (2022). Physio-biochemical and molecular stress regulators and their crosstalk for low-temperature stress responses in fruit crops: A review. Front. Plant Sci. 13. doi: 10.3389/FPLS.2022.1022167
Gupta, O. P., Meena, N. L., Sharma, I., Sharma, P. (2014). Differential regulation of microRNAs in response to osmotic, salt and cold stresses in wheat. Mol. Biol. Rep. 41, 4623–4629. doi: 10.1007/S11033-014-3333-0
Hanin, M., Brini, F., Ebel, C., Toda, Y., Takeda, S., Masmoudi, K. (2011). Plant dehydrins and stress tolerance: Versatile proteins for complex mechanisms. Plant Signal. Behav. 6 (10), 1503–1509. doi: 10.4161/psb.6.10.17088
Havaux, M., Bonfils, J. P., Lutz, C., Niyogi, K. K. (2000). Photodamage of the photosynthetic apparatus and its dependence on the leaf developmental stage in the npq1 arabidopsis mutant deficient in the xanthophyll cycle enzyme violaxanthin de-epoxidase. Plant Physiol. 124, 273–284. doi: 10.1104/PP.124.1.273
Hernández-Martinez, M., Mendoza-Onofre, L. E., Ramirez-Vallejo, P., Osada-Kawasoe, S., Cardenas-Soriano, E., Zavala-Garcia, F. (2008). “Response of sorghum b and r lines to ergot (Claviceps africana) at celaya, guanajuato, Mexico,” in Sorghum and millets diseases (Iowa, USA: Iowa Sate Press). doi: 10.1002/9780470384923.ch14
Hiraki, H., Uemura, M., Kawamura, Y. (2019). Calcium signaling-linked CBF/DREB1 gene expression was induced depending on the temperature fluctuation in the field: Views from the natural condition of cold acclimation. Plant Cell Physiol. 60, 303–317. doi: 10.1093/PCP/PCY210
Hu, Y., Zhang, H., Gu, B., Zhang, J. (2022). The transcription factor VaMYC2 from Chinese wild vitis amurensis enhances cold tolerance of grape (V. vinifera) by up-regulating VaCBF1 and VaP5CS. Plant Physiol. Biochem. 192, 218–229. doi: 10.1016/J.PLAPHY.2022.10.011
Huang, C., Cheng, P., Zhang, H., Jiang, C., Jin, L. (2022). Identification and differential expression of cold-stress-responsive microRNAs in cold-tolerant and -susceptible hemerocallis fulva varieties. New Z J. Crop Hort Sci 50, 1–15. doi: 10.1080/01140671.2021.2013260
Huang, S., Zhou, J., Gao, L., Tang, Y., Huang, S., Zhou, J., et al. (2020). Plant miR397 and its functions. Funct. Plant Biol. 48, 361–370. doi: 10.1071/FP20342
Huang, X., Lakso, A. N., Eissenstat, D. M. (2005). Interactive effects of soil temperature and moisture on Concord grape root respiration. J. Exp. Bot. 56 (420), 2651–2660. doi: 10.1093/jxb/eri258
Hurry, V., Druart, N., Cavaco, A., Gardeström, P., Strand, Å. (2002). Photosynthesis at low temperatures. Plant Cold Hardiness, 161–179. doi: 10.1007/978-1-4615-0711-6_12
Iijima, M., Oribe, Y., Horibe, Y., Kono, Y. (1998). Time lapse analysis of root elongation rates of rice and sorghum during the day and night. Ann. Bot. 81, 603–607. doi: 10.1006/ANBO.1998.0611
Iordachescu, M., Imai, R. (2008). Trehalose biosynthesis in response to abiotic stresses. J. Integr. Plant Biol. 50 (10), 1223–1229. doi: 10.1111/j.1744-7909.2008.00736.x
Iqbal, Z., Iqbal, M. S., Khan, M. I. R., Ansari, M. I. (2021). Toward integrated multi-omics intervention: Rice trait improvement and stress management. Front. Plant Sci. 12. doi: 10.3389/FPLS.2021.741419
Ito, Y., Katsura, K., Maruyama, K., Taji, T., Kobayashi, M., Seki, M., et al. (2006). Functional analysis of rice DREB1/CBF-type transcription factors involved in cold-responsive gene expression in transgenic rice. Plant Cell Physiol. 47 (1), 141–153. doi: 10.1093/pcp/pci230
Jain, M., Chourey, P. S., Boote, K. J., Allen, L. H. (2010). Short-term high temperature growth conditions during vegetative-to-reproductive phase transition irreversibly compromise cell wall invertase-mediated sucrose catalysis and microspore meiosis in grain sorghum (Sorghum bicolor). J. Plant Physiol. 167, 578–582. doi: 10.1016/J.JPLPH.2009.11.007
Janmohammadi, M., Zolla, L., Rinalducci, S. (2015). Low temperature tolerance in plants: Changes at the protein level. Phytochemistry 117, 76–89. doi: 10.1016/J.PHYTOCHEM.2015.06.003
Jarillo, J. A., Gabrys, H., Capel, J., Alonso, J. M., Ecker, J. R., Cashmore, A. R. (2001). Phototropin-related NPL1 controls chloroplast relocation induced by blue light. Nature 410, 952–954. doi: 10.1038/35073622
Kaniuga, Z. (2008). Chilling response of plants: importance of galactolipase, free fatty acids and free radicals*. Plant Biol. 10, 171–184. doi: 10.1111/J.1438-8677.2007.00019.X
Kapanigowda, M. H., Perumal, R., Aiken, R. M., Herald, T. J., Bean, S. R., Little, C. R. (2013). Analyses of sorghum (Sorghum bicolor (L.) moench) lines and hybrid in response to early season planting and cool conditions. Can. J. Plant Sci. 93, 773. doi: 10.4141/cjps2012-311
Kaplan, F., Kopka, J., Haskell, D. W., Zhao, W., Schiller, K. C., Gatzke, N., et al. (2004). Exploring the temperature-stress metabolome of arabidopsis. Plant Physiol. 136 (4), 4159–4168. doi: 10.1104/pp.104.052142
Kavi, K. P. B., Ganie, S. A., Wani, S. H., Guddimalli, R., Karumanchi, A. R., Edupuganti, S., et al. (2022). Nuclear factor-y (NF-y): Developmental and stress-responsive roles in the plant lineage. J. Plant Growth Regul. doi: 10.1007/s00344-022-10739-6
Khanthavong, P., Yabuta, S., Malik, A. I., Hossain, M. A., Akagi, I., Sakagami, J. I. (2022). Combinational variation temperature and soil water response of stomata and biomass production in maize, millet, sorghum and rice. Plants 11, 1039. doi: 10.3390/PLANTS11081039
Kidokoro, S., Hayashi, K., Haraguchi, H., Ishikawa, T., Soma, F., Konoura, I., et al. (2021). Posttranslational regulation of multiple clock-related transcription factors triggers cold-inducible gene expression in arabidopsis. Proc. Natl. Acad. Sci. U. S. A. 118, e2021048118. doi: 10.1073/PNAS.2021048118/SUPPL_FILE/PNAS.2021048118.SD01.XLSX
Kidokoro, S., Shinozaki, K., Yamaguchi-Shinozaki, K. (2022). Transcriptional regulatory network of plant cold-stress responses. Trends Plant Sci. 9, 922–935. doi: 10.1016/j.tplants.2022.01.008
Knoll, J., Ejeta, G. (2008). Marker-assisted selection for early-season cold tolerance in sorghum: QTL validation across populations and environments. Theor. Appl. Genet. 116, 541–553. doi: 10.1007/s00122-007-0689-8
Knoll, J., Gunaratna, N., Ejeta, G. (2008). QTL analysis of early-season cold tolerance in sorghum. Theor. Appl. Genet. .116, 577–587. doi: 10.1007/s00122-007-0692-0
Kodama, Y., Tsuboi, H., Kagawa, T., Wada, M. (2008). Low temperature-induced chloroplast relocation mediated by a blue light receptor, phototropin 2, in fern gametophytes. J. Plant Res. 121 (4), 441–448. doi: 10.1007/s10265-008-0165-9
Krol, M., Gray, G. R., Hurry, V. M., Oquist, G., Malek, L., Huner, N. P. (1995). Low-temperature stress and photoperiod affect an increased tolerance to photoinhibition in pinus banksiana seedlings. Can. J. Bot. Can. J. Bot. 73 (8), 1119–1127. doi: 10.1139/b95-122
Laza, H. E., Kaur-Kapoor, H., Xin, Z., Payton, P. R., Chen, J. (2022). Morphological analysis and stage determination of anther development in sorghum [Sorghum bicolor (L.) moench]. Planta 255, 1–12. doi: 10.1007/S00425-022-03853-Y/FIGURES/5
Leon-Velasco, H., Mendoza-Onofre, L., Castillo-Gonzalez, F., Cervantes-Santana, T., Martinez-Garza, A. (2009). Evaluation of two generations of cold tolerant sorghum hybrids and parental lines. ii: combining ability, heterosis and heterobeltiosis. Agrociencia 43, 609–623.
Li, Y., Wang, M., Guo, T., Li, S., Teng, K., Dong, D., et al. (2022). Overexpression of abscisic acid-insensitive gene ABI4 from Medicago truncatula, which could interact with ABA2, improved plant cold tolerance mediated by ABA signaling. Front. Plant Sci. 13. doi: 10.3389/FPLS.2022.982715
Li, S., Yu, X., Cheng, Z., Yu, X., Ruan, M., Li, W., et al. (2017). Global gene expression analysis reveals crosstalk between response mechanisms to cold and drought stresses in cassava seedlings. Front. Plant Sci. 18. doi: 10.3389/fpls.2017.01259
Li, S., Yang, Y., Zhang, Q., Liu, N., Xu, Q., Hu, L. (2018). Differential physiological and metabolic response to low temperature in two zoysiagrass genotypes native to high and low latitude. PloS One 13 (6), e0198885. doi: 10.1371/journal.pone.0198885
Lin, F., Cao, J., Yuan, J., Liang, Y., Li, J. (2021). Integration of light and brassinosteroid signaling during seedling establishment. Int. J. Mol. Sci. 22, 12971. doi: 10.3390/IJMS222312971
Lin, Q., Xie, Y., Guan, W., Duan, Y., Wang, Z., Sun, C. (2019). Combined transcriptomic and proteomic analysis of cold stress induced sugar accumulation and heat shock proteins expression during postharvest potato tuber storage. Food. Chem. 297, 124991. doi: 10.1016/J.FOODCHEM.2019.124991
Liu, Y., Khan, A. R., Gan, Y., Liu, Y., Khan, A. R., Gan, Y. (2022). C2H2 zinc finger proteins response to abiotic stress in plants. Int. J. Mol. Sci. 23, 1–15. doi: 10.3390/IJMS23052730
Liu, X., Zhou, Y., Xiao, J., Bao, F. (2018). Effects of chilling on the structure, function and development of chloroplasts. Front. Plant Sci. 22. doi: 10.3389/FPLS.2018.01715
Ma, Y., Dai, X., Xu, Y., Luo, W., Zheng, X., Zeng, D., et al. (2015). COLD1 confers chilling tolerance in rice. Cell 160, 1209–1221. doi: 10.1016/j.cell.2015.01.046
Maheshwari, P., Kummari, D., Palakolanu, S. R., Nagasai Tejaswi, U., Nagaraju, M., Rajasheker, G., et al. (2019). Genome-wide identification and expression profile analysis of nuclear factor y family genes in sorghum bicolor l. (Moench). PloS One 14, e0222203. doi: 10.1371/JOURNAL.PONE.0222203
Malusá, E., Sas-Paszt, L., Ciesielska, J. (2012). Technologies for beneficial microorganisms inocula used as biofertilizers. Sci. World J. 2012, 1–13. doi: 10.1100/2012/491206
Mansour, M. M. F., Emam, M. M., Salama, K. H. A., Morsy, A. A. (2021). Sorghum under saline conditions: responses, tolerance mechanisms, and management strategies. Planta 254, 1–38. doi: 10.1007/S00425-021-03671-8
Marla, S. R., Shiva, S., Welti, R., Liu, S., Burke, J. J., Morris, G. P., et al. (2017). Comparative transcriptome and lipidome analyses reveal molecular chilling responses in chilling-tolerant sorghums. Plant Genome. 10, 13. doi: 10.3835/PLANTGENOME2017.03.0025
Martínez Núñez, M., Ruíz Rivas, M., Gregorio Jorge, J., Hernández, P. F. V., Luna Suárez, S., de Folter, S., et al. (2021). Identification of genuine and novel miRNAs in amaranthus hypochondriacus from high-throughput sequencing data. Genomics 113, 88–103. doi: 10.1016/J.YGENO.2020.11.027
Mathur, S., Priyadarshini, S. S., Singh, V., Vashisht, I., Jung, K. H., Sharma, R., et al. (2020). Comprehensive phylogenomic analysis of ERF genes in sorghum provides clues to the evolution of gene functions and redundancy among gene family members. 3 Biotech. 10 (3), 139. doi: 10.1007/S13205-020-2120-Y
Matsumoto, T., Lian, H. L., Su, W. A., Tanaka, D., Liu, C. W., Iwasaki, I., et al. (2009). Role of the aquaporin PIP1 subfamily in the chilling tolerance of rice. Plant Cell Physiol. 50, 216–229. doi: 10.1093/PCP/PCN190
Maulana, F., Tesso, T. T. (2013). Cold temperature episode at seedling and flowering stages reduces growth and yield components in sorghum. Crop Sci. 53, 564–574. doi: 10.2135/cropsci2011.12.0649
Maulana, F., Weerasooriya, D., Tesso, T. (2017). Sorghum landrace collections from cooler regions of the world exhibit magnificent genetic differentiation and early season cold tolerance. Front. Plant Sci. 8. doi: 10.3389/fpls.2017.00756
Mazzucotelli, E., Tartari, A., Cattivelli, L., Forlani, G. (2006). Metabolism of γ-aminobutyric acid during cold acclimation and freezing and its relationship to frost tolerance in barley and wheat. J. Exp. Bot. 57 (14), 3755–3766. doi: 10.1093/jxb/erl141
Megha, S., Basu, U., Kav, N. N. V. (2018). Regulation of low temperature stress in plants by microRNAs. Plant Cell Environ. 41 (1), 1–15. doi: 10.1111/pce.12956
Mehrotra, S., Verma, S., Kumar, S., Kumari, S., Mishra, B. N. (2020). Transcriptional regulation and signalling of cold stress response in plants: An overview of current understanding. Environ. Exp. Bot. 180, 104243. doi: 10.1016/J.ENVEXPBOT.2020.104243
Melkonian, J., Yu, L. X., Setter, T. L. (2004). Chilling responses of maize (Zea mays l.) seedlings: root hydraulic conductance, abscisic acid, and stomatal conductance. J. Exp. Bot. 55, 1751–1760. doi: 10.1093/JXB/ERH215
Mizoi, J., Shinozaki, K., Yamaguchi-Shinozaki, K. (2012). AP2/ERF family transcription factors in plant abiotic stress responses. Biochim. Biophys. Acta - Gene Regul. Mech. 1819 (2), 86–96. doi: 10.1016/j.bbagrm.2011.08.004
Morris, G. P., Ramu, P., Deshpande, S. P., Hash, C. T., Shah, T., Upadhyaya, H. D., et al. (2013). Population genomic and genome-wide association studies of agroclimatic traits in sorghum. Proc. Natl. Acad. Sci. U. S. A. 8, 110(2):453–8. doi: 10.1073/pnas.1215985110
Nadarajah, K. K. (2020). ROS homeostasis in abiotic stress tolerance in plants. Int. J. Mol. Sci. 21, 5208. doi: 10.3390/IJMS21155208
Naidu, S. L., Moose, S. P., AK, A. L.-S., Raines, C. A., Long, S. P. (2003). Cold tolerance of C4 photosynthesis in miscanthus x giganteus: adaptation in amounts and sequence of C4 photosynthetic enzymes. Plant Physiol. 132, 1688–1697. doi: 10.1104/pp.103.021790
Nakashima, K., Yamaguchi-Shinozaki, K. (2006). Regulons involved in osmotic stress-responsive and cold stress-responsive gene expression in plants. Physiol. Plant 126, 62–71. doi: 10.1111/J.1399-3054.2005.00592.X
Niyogi, K. K., Björkman, O., Grossman, A. R. (1997). The roles of specific xanthophylls in photoprotection. Proc. Natl. Acad. Sci. U. S. A. 94 (25), 14162–14167. doi: 10.1073/pnas.94.25.14162
Ortiz, D., Hu, J., Salas Fernandez, M. G. (2017). Genetic architecture of photosynthesis in sorghum bicolor under non-stress and cold conditions. J. Exp. Bot. 68, 4545–4557. doi: 10.1093/jxb/erx276
Ostmeyer, T. J., Bahuguna, R. N., Kirkham, M. B., Bean, S., Jagadish, S. V. K. (2022). Enhancing Sorghum yield through efficient use of nitrogen – Challenges and opportunities. Front. Plant Sci. 13, 845443. doi: 10.3389/fpls.2022.845443
Osuna-Ortega, J., Mendoza-Castillo, M. D. C., Mendoza-Onofre, L. E. (2003). Sorghum cold tolerance, pollen production, and seed yield in the central high valleys of Mexico. Maydica 48, 125–132.
Palta, J. P., Weiss, L. S. (2018). Ice formation and freezing injury: an overview on the survival mechanisms and molecular aspects of injury and cold acclimation in herbaceous plants. Adv. Plant Cold Hardiness, 143–176. doi: 10.1201/9781351069526-11
Pandey, A., Yarzábal, L. A. (2018). Bioprospecting cold-adapted plant growth promoting microorganisms from mountain environments. Appl. Microbiol. Biotechnol. 103, 643–657. doi: 10.1007/S00253-018-9515-2
Pareek, A., Khurana, A., Sharma, A. K., Kumar, R. (2017). An overview of signaling regulons during cold stress tolerance in plants. Curr. Genomics 18 (6), 498–511. doi: 10.2174/1389202918666170228141345
Park, J., Lim, C. J., Shen, M., Park, H. J., Cha, J. Y., Iniesto, E., et al. (2018). Epigenetic switch from repressive to permissive chromatin in response to cold stress. Proc. Natl. Acad. Sci. U. S. A. 115 (23), E5400–E5409. doi: 10.1073/pnas.1721241115
Parra-Londono, S., Fiedler, K., Kavka, M., Samans, B., Wieckhorst, S., Zacharias, A., et al. (2018). Genetic dissection of early-season cold tolerance in sorghum: genome-wide association studies for seedling emergence and survival under field and controlled environment conditions. Theor. Appl. Genet. 131, 581–595. doi: 10.1007/s00122-017-3021-2
Paterson, A. H., Bowers, J. E., Bruggmann, R., Dubchak, I., Grimwood, J., Gundlach, H., et al. (2009). The sorghum bicolor genome and the diversification of grasses. Nature 457 (7229), 551–556. doi: 10.1038/nature07723
Petrić, M., Jevremović, S., Trifunović, M., Tadić, V., Milošević, S., Dragićević, M., et al. (2013). The effect of low temperature and GA3 treatments on dormancy breaking and activity of antioxidant enzymes in fritillaria meleagris bulblets cultured in vitro. Acta Physiol. Plant 35, 3223–3236. doi: 10.1007/S11738-013-1357-Z/FIGURES/7
Philips, T., Hoopes, L. (2008). Transcription factors and transcriptional control in eukaryotic cells. Nat. Educ. 1 (1), 119.
Pimentel, C., Davey, P. A., Juvik, J. A., Long, S. P. (2005). Gene loci in maize influencing susceptibility to chilling dependent photoinhibition of photosynthesis. Photosynth. Res. 85 (3), 319–326. doi: 10.1007/s11120-005-5738-z
Rani, A., Kiran, A., Sharma, K. D., Vara Prasad, P. V., Jha, U. C., Siddique, K. H. M., et al. (2021). Cold tolerance during the reproductive phase in chickpea (Cicer arietinum l.) is associated with superior cold acclimation ability involving antioxidants and cryoprotective solutes in anthers and ovules. Antioxidants 10, 1693. doi: 10.3390/ANTIOX10111693
Rashid, M., Guangyuan, H., Guangxiao, Y., Hussain, J., Xu, Y. (2012). AP2/ERF transcription factor in rice: Genome-wide anvas and yntenic relationships between monocots and udicots. Evol. Bioinforma. 8, 321–355. doi: 10.4137/EBO.S9369
Rawat, N., Singla-Pareek, S. L., Pareek, A. (2021). Membrane dynamics during individual and combined abiotic stresses in plants and tools to study the same. Physiol. Plant 171, 653–676. doi: 10.1111/PPL.13217
Raza, A., Razzaq, A., Mehmood, S. S., Zou, X., Zhang, X., Lv, Y., et al. (2019). Impact of climate change on crops adaptation and strategies to tackle its outcome: A review. Plants 8, 34. doi: 10.3390/PLANTS8020034
Reshma, M. A., Kirkham, M. B., Timothy, T. C., Scott, R. B., D. Wilson, J., R. Armstrong, P., et al. (2019). Low-temperature tolerance of maize and sorghum seedlings grown under the same environmental conditions. J. Crop Improvement. 33 (3), 287–305. doi: 10.1080/15427528.2019.1579139
Rochaix, J. D. (2014). Regulation and dynamics of the light-harvesting system some of the authors of this publication are also working on these related projects: state transition view project psaA trans-splicing view project. Artic. Annu. Rev. Plant Biol. 65, 287–309. doi: 10.1146/annurev-arplant-050213-040226
Rutayisire, A., Lubadde, G., Mukayiranga, A., Edema, R. (2021). Response of sorghum to cold stress at early developmental stage. Int. J. Agron. 2021, 1–10. doi: 10.1155/2021/8875205
Sabehat, A., Lurie, S., Weiss, D. (1998). Expression of small heat-shock proteins at low temperatures: A possible role in protecting against chilling injuries. Plant Physiol. 117 (2), 651–658. doi: 10.1104/pp.117.2.651
Sage, R. F. (2002). Variation in the kcat of rubisco in C3 and C4 plants and some implications for photosynthetic performance at high and low temperature. J. Exp. Bot. 53 (369), 609–620. doi: 10.1093/jexbot/53.369.609
Satyakam, Zinta, G., Singh, R. K., Kumar, R. (2022). Cold adaptation strategies in plants-an emerging role of epigenetics and antifreeze proteins to engineer cold resilient plants. Front. Genet. 13. doi: 10.3389/fgene.2022.909007
Schlemper, T. R., Leite, M. F. A., Lucheta, A. R., Shimels, M., Bouwmeester, H. J., van Veen, J. A., et al. (2017). Rhizobacterial community structure differences among sorghum cultivars in different growth stages and soils. FEMS Microbiol. Ecol. 93, 1–11. doi: 10.1093/FEMSEC/FIX096
Shalmani, A., Jing, X. Q., Shi, Y., Muhammad, I., Zhou, M. R., Wei, X. Y., et al. (2019). Characterization of B-BOX gene family and their expression profiles under hormonal, abiotic and metal stresses in poaceae plants. BMC Genomics 20, 27. doi: 10.1186/s12864-018-5336-z
Sharma, P. K., Hall, D. O. (1996). Effect of photoinhibition and temperature on carotenoids in sorghum leaves. Indian J. Biochem. Biophys. 33 (6), 471–477.
Shi, W., Ma, Q., Yin, W., Liu, T., Song, Y., Chen, Y., et al. (2022). The transcription factor StTINY3 enhances cold-induced sweetening resistance by coordinating starch resynthesis and sucrose hydrolysis in potato. J. Exp. Bot. 73, 4968–4980. doi: 10.1093/JXB/ERAC171
Shi, Y., Yang, S. (2014). “ABA regulation of the cold stress response in plants,” in Abscisic acid: Metabolism, transport and signaling. Ed. Zhang, D. P. (Dordrecht: Springer), 337–363. doi: 10.1007/978-94-017-9424-4_17
Shi, Y., Ding, Y., Yang, S. (2015). Cold signal transduction and its interplay with phytohormones during cold acclimation. Plant Cell Physiol. 1, 7–15. doi: 10.1093/pcp/pcu115
Singh, S. P. (1985). Sources of cold tolerance in grain sorghum. Can. J. Plant Sci. 65, 251–257. doi: 10.4141/cjps85-037
Singh, S. P. (2010). Sources of cold tolerance in grain sorghum. Can. J. Plant Sci. 65, 251–257. doi: 10.4141/cjps85-037
Srivastav, A., Mehta, S., Lindlof, A., Bhargava, S. (2010). Over-represented promoter motifs in abiotic stress-induced DREB genes of rice and sorghum and their probable role in regulation of gene expression. Plant Signal. Behav. 5:7, 775–784. doi: 10.4161/psb.5.7.11769
Sun, X., Fan, G., Su, L., Wang, W., Liang, Z., Li, S., et al. (2015). Identification of cold-inducible microRNAs in grapevine. Front. Plant Sci. 6. doi: 10.3389/FPLS.2015.00595
Sunkar, R., Zhu, J. K. (2004). Novel and stress-regulated MicroRNAs and other small RNAs from arabidopsis. Plant Cell. 16, 2001. doi: 10.1105/TPC.104.022830
Swindell, W. R., Huebner, M., Weber, A. P. (2007). Transcriptional profiling of arabidopsis heat shock proteins and transcription factors reveals extensive overlap between heat and non-heat stress response pathways. BMC Genomics 8, 125. doi: 10.1186/1471-2164-8-125
Teh, C. Y., Shaharuddin, N. A., Ho, C. L., Mahmood, M. (2016). Exogenous proline significantly affects the plant growth and nitrogen assimilation enzymes activities in rice (Oryza sativa) under salt stress. Acta Physiol. Plant 38, 1–10. doi: 10.1007/S11738-016-2163-1/FIGURES/5
Thiebaut, F., Rojas, C. A., Almeida, K. L., Grativol, C., Domiciano, G. C., Lamb, C. R. C., et al. (2012). Regulation of miR319 during cold stress in sugarcane. Plant Cell Environ. 35, 502–512. doi: 10.1111/J.1365-3040.2011.02430.X
Tiryaki, I., Andrews, D. J. (2001). Germination and seedling cold tolerance in sorghum: II. parental lines and hybrids. Agron. J. 93, 1386–1391. doi: 10.2134/AGRONJ2001.1386
Upadhyaya, H. D., Wang, Y. H., Sastry, D. V. S. S. R., Dwivedi, S. L., Prasad, P. V. V., Burrell, A. M., et al. (2015). Association mapping of germinability and seedling vigor in sorghum under controlled low-temperature conditions. Genome 59 (2), 137–145. doi: 10.1139/gen-2015-0122
Vera-Hernández, P., Ortega-Ramírez, M. A., Martínez Nuñez, M., Ruiz-Rivas, M., Rosas-Cárdenas, F. F. (2018). Proline as a probable biomarker of cold stress tolerance in sorghum (Sorghum bicolor). Mex. J. Biotechnol. 3 (3), 77–86. doi: 10.29267/mxjb.2018.3.3.77
Wang, P., Chai, Y. N., Roston, R., Dayan, F. E., Schachtman, D. P. (2021). The sorghum bicolor root exudate sorgoleone shapes bacterial communities and delays network formation. mSystems 6, e00749-20. doi: 10.1128/mSystems.00749-20
Wang, J., Li, R. (2010). Integration of C4-specific ppdk gene of echinochloa to C3 upland rice and its photosynthesis characteristics analysis. Afr. J. Biotechnol. 7, 783–787. doi: 10.4314/ajb.v7i6.58529
Wang, S. T., Sun, X. L., Hoshino, Y., Yu, Y., Jia, B., Sun, Z. W., et al. (2014). MicroRNA319 positively regulates cold tolerance by targeting OsPCF6 and OsTCP21 in rice (Oryza sativa l.). PloS One 9 (3), e91357. doi: 10.1371/journal.pone.0091357
Wang, R., Yu, M., Xia, J., Xing, J., Fan, X., Xu, Q., et al. (2022). Overexpression of TaMYC2 confers freeze tolerance by ICE-CBF-COR module in arabidopsis thaliana. Front. Plant Sci. 13. doi: 10.3389/FPLS.2022.1042889
Wang, D., Portis, A. R., Jr., Moose, S. P., Long, S. P. (2008). Cool C4 photosynthesis: pyruvate pi dikinase expression and activity corresponds to the exceptional cold tolerance of carbon assimilation in miscanthus x giganteus. Plant Physiol. 1, 557–567. doi: 10.1104/pp.108.120709
Wilkinson, S., Clephan, A. L., Davies, W. J. (2001). Rapid low temperature-induced stomatal closure occurs in cold-tolerant commelina communis leaves but not in cold-sensitive tobacco leaves, via a mechanism that involves apoplastic calcium but not abscisic acid. Plant Physiol. 126 (4), 1566–1578. doi: 10.1104/pp.126.4.1566
Windpassinger, S., Friedt, W., Deppé, I., Werner, C., Snowdon, R., Wittkop, B. (2017). Towards enhancement of early-stage chilling tolerance and root development in sorghum F1 hybrids. J. Agron. Crop Sci. 203, 146–160. doi: 10.1111/JAC.12171
Woldesemayat, A. A., Modise, D. M., Gemeildien, J., Ndimba, B. K., Christoffels, A. (2018). Cross-species multiple environmental stress responses: An integrated approach to identify candidate genes for multiple stress tolerance in sorghum (Sorghum bicolor (L.) moench) and related model species. PloS One 13 (3), e0192678. doi: 10.1371/journal.pone.0192678
Wu, A. L., Jiao, X. Y., Wang, J. S., Dong, E. W., Guo, J., Wang, L. G., et al. (2021). Sorghum rhizosphere effects reduced soil bacterial diversity by recruiting specific bacterial species under low nitrogen stress. Sci. Tot. Environ. 770, 144742. doi: 10.1016/J.SCITOTENV.2020.144742
Xu, X., Zhang, Y., Liang, M., Kong, W., Liu, J. (2022). The citrus laccase gene CsLAC18 contributes to cold tolerance. Int. J. Mol. Sci. 23, 14509. doi: 10.3390/IJMS232314509
Xue-Xuan, X., Hong-Bo, S., Yuan-Yuan, M., Gang, X., Jun-Na, S., Dong-Gang, G., et al. (2010). Biotechnological implications from abscisic acid (ABA) roles in cold stress and leaf senescence as an important signal for improving plant sustainable survival under abiotic-stressed conditions. Crit. Rev. Biotechnol. 30, 222–230. doi: 10.3109/07388551.2010.487186
Yamada, K., Osakabe, Y. (2018). Sugar compartmentation as an environmental stress adaptation strategy in plants. Semin. Cell Dev. Biol. 83, 106–114. doi: 10.1016/J.SEMCDB.2017.12.015
Yan, H. W., Hong, L., Zhou, Y. Q., Jiang, H. Y., Zhu, S. W., Fan, J., et al. (2013). A genome-wide analysis of the ERF gene family in sorghum. Genet. Mol. Res. 12 (2), 2038–2055. doi: 10.4238/2013.May.13.1
Yang, J., Duan, G., Li, C., Liu, L., Han, G., Zhang, Y., et al. (2019). The crosstalks between jasmonic acid and other plant hormone signaling highlight the involvement of jasmonic acid as a core component in plant response to biotic and abiotic stresses. Front. Plant Sci. 10. doi: 10.3389/fpls.2019.01349
Yang, C., Li, D., Mao, D., Liu, X., Ji, C., Li, X., et al. (2013). Overexpression of microRNA319 impacts leaf morphogenesis and leads to enhanced cold tolerance in rice (Oryza sativa l.). Plant Cell Environ. 36, 2207–2218. doi: 10.1111/PCE.12130
Yellisetty, V., Reddy, L. A., Mandapaka, M. (2015). In planta transformation of sorghum (Sorghum bicolor (L.) moench) using TPS1 gene for enhancing tolerance to abiotic stresses. J. Genet. 4 (3), 425–434. doi: 10.1007/s12041-015-0540-y
Yu, J., Tuinstra, M. R., Claassen, M. M., Gordon, W. B., Witt, M. D. (2004). Analysis of cold tolerance in sorghum under controlled environment conditions. F. Crop Res. 85 (1), 21–30. doi: 10.1016/S0378-4290(03)00125-4
Yu, T., Zhang, J., Cao, J., Li, X., Li, S., Liu, C., et al. (2022). Metabolic insight into cold stress response in two contrasting maize lines. Life (Basel) 12 (2), 282. doi: 10.3390/life12020282
Yukun, G., Jianghui, C., Genzeng, R., Shilin, W., Puyuan, Y., Congpei, Y., et al. (2021). Changes in the root-associated bacteria of sorghum are driven by the combined effects of salt and sorghum development. Environ. Microbiomes 16, 1–15. doi: 10.1186/S40793-021-00383-0/FIGURES/6
Zaman, F., Zhang, M., Liu, Y., Wang, Z., Xu, L., Guo, D., et al. (2022). DkmiR397 regulates proanthocyanidin biosynthesis via negative modulating DkLAC2 in Chinese PCNA persimmon. Int. J. Mol. Sci. 23, 3200. doi: 10.3390/IJMS23063200/S1
Zhang, Y., Fan, L., Zhao, M., Chen, Q., Qin, Z., Feng, Z., et al. (2019). Chitooligosaccharide plays essential roles in regulating proline metabolism and cold stress tolerance in rice seedlings. Acta Physiol. Plant 41, 1–11. doi: 10.1007/s11738-019-2864-3
Zhang, X., Sun, Y., Qiu, X., Lu, H., Hwang, I., Wang, T. (2022). Tolerant mechanism of model legume plant medicago truncatula to drought, salt, and cold stresses. Front. Plant Sci. 13. doi: 10.3389/FPLS.2022.847166
Zhou, M. Q., Shen, C., Wu, L. H., Tang, K. X., Lin, J. (2011). CBF-dependent signaling pathway: A key responder to low temperature stress in plants. Crit. Rev. Biotechnol. 2, 186–192. doi: 10.3109/07388551.2010.505910
Zhou, Y., Sommer, M. L., Hochholdinger, F. (2021). Cold response and tolerance in cereal roots. J. Exp. Bot. 72, 7474–7481. doi: 10.1093/JXB/ERAB334
Zhu, Y., Furuichi, T., Liu, J., Shariq Iqbal, M., Iqbal, Z., Memon, A. G., et al. (2022). Calcium mediated cold acclimation in plants: Underlying signaling and molecular mechanisms. Front. Plant Sci. 13. doi: 10.3389/FPLS.2022.855559
Zhu, J., Jae, C. J., Zhu, Y., Sokolchik, I., Miyazaki, S., Zhu, J. K., et al. (2008a). Involvement of arabidopsis HOS15 in histone deacetylation and cold tolerance. Proc. Natl. Acad. Sci. U. S. A. 105 (12), 4945–4950. doi: 10.1073/pnas.0801029105
Keywords: sorghum, chilling, molecular breeding, genes, cold tolerance
Citation: Vera Hernández PF, Mendoza Onofre LE and Rosas Cárdenas FF (2023) Responses of sorghum to cold stress: A review focused on molecular breeding. Front. Plant Sci. 14:1124335. doi: 10.3389/fpls.2023.1124335
Received: 15 December 2022; Accepted: 30 January 2023;
Published: 23 February 2023.
Edited by:
Prashant Vikram, Shriram Bioseed Genetics, IndiaReviewed by:
Swati Puranik, Czech Academy of Sciences, CzechiaCopyright © 2023 Vera Hernández, Mendoza Onofre and Rosas Cárdenas. This is an open-access article distributed under the terms of the Creative Commons Attribution License (CC BY). The use, distribution or reproduction in other forums is permitted, provided the original author(s) and the copyright owner(s) are credited and that the original publication in this journal is cited, in accordance with accepted academic practice. No use, distribution or reproduction is permitted which does not comply with these terms.
*Correspondence: Flor de Fátima Rosas Cárdenas, ZnJvc2FzY0BpcG4ubXg=
Disclaimer: All claims expressed in this article are solely those of the authors and do not necessarily represent those of their affiliated organizations, or those of the publisher, the editors and the reviewers. Any product that may be evaluated in this article or claim that may be made by its manufacturer is not guaranteed or endorsed by the publisher.
Research integrity at Frontiers
Learn more about the work of our research integrity team to safeguard the quality of each article we publish.