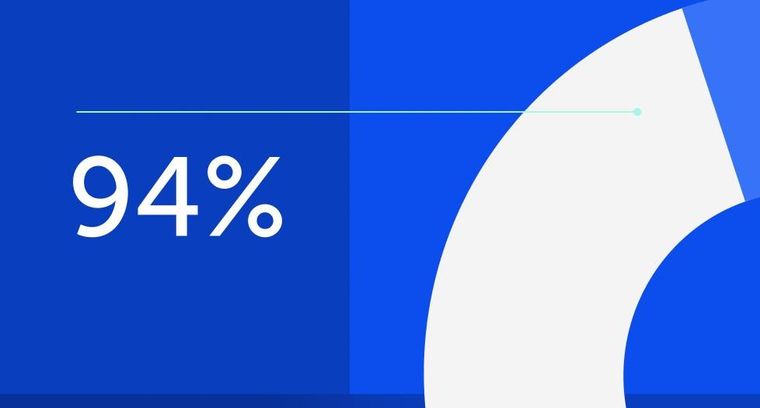
94% of researchers rate our articles as excellent or good
Learn more about the work of our research integrity team to safeguard the quality of each article we publish.
Find out more
ORIGINAL RESEARCH article
Front. Plant Sci., 17 April 2023
Sec. Plant Abiotic Stress
Volume 14 - 2023 | https://doi.org/10.3389/fpls.2023.1119530
This article is part of the Research TopicThe Interaction of Biotic and Abiotic StressesView all 5 articles
Devastating citrus disease Huanglongbing (HLB) is without existing cures. Herein, we present results demonstrating the possible mechanisms (hypoxia stress) behind HLB-triggered shoot dieback by comparing the transcriptomes, hormone profiles, and key enzyme activities in buds of severely and mildly symptomatic ‘Hamlin’ sweet orange (Citrus sinensis). Within six months (October – May) in field conditions, severe trees had 23% bud dieback, greater than mild trees (11%), with a concomitant reduction in canopy density. In February, differentially expressed genes (DEGs) associated with responses to osmotic stress, low oxygen levels, and cell death were upregulated, with those for photosynthesis and cell cycle downregulated in severe versus mild trees. For severe trees, not only were the key markers for hypoxia, including anaerobic fermentation, reactive oxygen species (ROS) production, and lipid oxidation, transcriptionally upregulated, but also alcohol dehydrogenase activity was significantly greater compared to mild trees, indicating a link between bud dieback and hypoxia. Tricarboxylic acid cycle revival, given the upregulation of glutamate dehydrogenase and alanine aminotransferase DEGs, suggests that ROS may also be generated during hypoxia-reoxygenation. Greater (hormonal) ratios of abscisic acid to cytokinins and jasmonates and upregulated DEGs encoding NADPH oxidases in severe versus mild trees indicate additional ROS production under limited oxygen availability due to stomata closure. Altogether, our results provided evidence that as HLB progresses, excessive ROS produced in response to hypoxia and during hypoxia-reoxygenation likely intensify the oxidative stress in buds leading to cell death, contributing to marked bud and shoot dieback and decline of the severely symptomatic sweet orange trees.
Huanglongbing (HLB; citrus greening disease) has become a significant threat to citriculture. HLB is presumably caused by a phloem-limited bacterium Candidatus Liberibacter asiaticus (CLas) and vectored by Asian citrus psyllid (Diaphorina citri Kuwayama) respectively (Bové, 2006). To date, there is no cure for HLB. Unfortunately, no known citrus germplasm is resistant to HLB. In Florida, citrus production has declined by 73% in the last fifteen years due to HLB (USDA, 2020). Following CLas-infection, dieback of the feeder roots occurs before the prominence of HLB symptoms on the aboveground tree canopy, limiting nutrients and water uptake (Johnson et al., 2014; Shahzad et al., 2020). With HLB progression, leaves show blotchy mottles, nutrient deficit, phloem plugging, and starch accumulation (Bové, 2006). Altogether, leaf drop and twig dieback result in canopy loss, leading to yield losses.
Canopy density is the key indicator to assess HLB severity; tree productivity and fruit drop have been directly correlated with the canopy density of HLB-affected trees (Tang and Vashisth, 2020). It is noteworthy that HLB severity increases over time, and affected trees become non-productive within seven years of infection (Stover et al., 2016). A thinner canopy causes more intense sunlight exposure on vegetative parts and to the ground; thus, an increase in temperature affects the respiration rate of the tree body (Griffin et al., 2002). Since shoot (bud) dieback signifies the first step in the rapid aboveground decline of affected trees, a better understanding of the underlying mechanism will help mitigate or decelerate the progression of HLB symptoms. The basic understanding behind HLB-triggered bud dieback in citrus trees still needs to be discovered. So this study aims to understand the underlying canopy bud dieback mechanism at the physiological, molecular, and hormonal levels in HLB-affected ‘Hamlin’ and ‘Valencia’ sweet orange trees.
Fifteen-year-old early-maturing ‘Hamlin’ and seventeen-year-old late-maturing ‘Valencia’ sweet orange trees grafted on ‘Swingle’ citrumelo (Citrus paradisi × Poncirus trifoliata) rootstock grown in an experimental citrus grove located at the University of Florida Citrus Research and Education Center, Lake Alfred were used in this study. Almost 100% of citrus trees are CLas-infected in Florida, so it is implausible to find HLB-free (healthy) citrus trees in the open field for comparison. Therefore, citrus trees (n = 4) exhibiting severe and mild visual symptoms of HLB, i.e., blotchy mottling on leaves and branch dieback (Slinski, 2016), were selected for comparison. Based on the amount of light passing through the canopy to estimate canopy density, photosynthetic photon flux density was higher in severe (625 µmol m-2 s-1) than mild trees (150 µmol m-2 s-1) at the start of experiment, indicating ≈ a 4-fold thinner canopy in severe trees.
Five terminal buds of 20 non-bearing shoots (100 buds/tree) tagged in advance of the experiment were used to assess bud dieback over time; quiescent (dormant), viable (floral or vegetative), and dead buds were counted monthly from November 2018 through January 2019 and every two weeks from February 2019 through anthesis in March 2019. Given the correlation between canopy density and HLB severity (lower density indicating a greater extent of branch dieback because of HLB), canopy density is an important trait to represent tree health regarding HLB severity. To estimate canopy density, a line quantum meter with the detecting spectrum of photosynthetically active radiation (MQ-306; Apogee Instrument, Logan, UT) was used to quantify light interception by the canopy, expressed as photosynthetic photon flux density (PPFD), in the beginning, and end of the experiment (October 2018 and May 2019, respectively). Measurements were done at solar noon on a clear sky day with the line quantum meter placed underneath the canopy (10 cm above the ground) for both sides of trees; in-row readings (without tree shading) were taken at the same height following each tree measurement. The light interception was calculated by dividing the below-canopy reading by the in-row reading. The % change in PPFD is reported herein, showing the percent increase in light penetrating through the canopy between Oct 2018 and May 2019 measurement (means fewer leaves to intercept light, thinner canopy). Leaf nutrients analysis was performed on 30 leaves with intact petioles from non-bearing branches randomly selected per tree in January 2019, using the protocols described in Shahzad et al. (2020). Fruit yield was determined by hand harvesting ‘Hamlin’ and ‘Valencia’ trees on January 8 and March 10, 2019, respectively, and expressed as total weight (in kilograms) per tree.
Transcriptomic analysis and phytohormones quantification were performed only on ‘Hamlin’ trees exhibiting severe and mild HLB symptoms, and bud samples were collected at two-time points: November 2018, when the buds were dormant, and February 2019, when floral and vegetative buds started active growth. Five terminal buds from ten non-fruiting branches were collected from all four quadrants of the tree. Collected buds from each tree were pooled together and flash-frozen with liquid nitrogen and stored at −80 °C in the freezer until transcriptome, hormonal, and alcohol dehydrogenase enzyme (ADH) analyses.
Total RNA was extracted from ground bud tissues (100 mg) using the RNeasy Plant Mini Kit (Qiagen, Valencia, CA). RNA quantity and quality were determined using a spectrophotometer and denaturing formaldehyde agarose gels (1.2%) (Rio, 2015), respectively. Total RNA samples were sent to the Interdisciplinary Center for Biotechnology Research, University of Florida (Gainesville) for global transcriptome analyses. The Illumina TruSeq mRNA protocol (Illumina, San Diego, CA) was used for transcripts sequencing. The C. sinensis genome v1.1 (Wu et al., 2014) from the Phytozome database was used as the reference. Differential gene expression was analyzed using RSEM v1.2.31 (Li and Dewey, 2011) with a false discovery rate (FDR)-corrected probability value of less than 0.05 (as threshold cutoff). Multiple tools, including MapMan software version 3.5.1.R2 (Usadel et al., 2005), AgriGO in tandem with REVIGO (Tian et al., 2017), and Kyoto Encyclopedia of Genes and Genomes (KEGG) Mapper tool (Kanehisa et al., 2017) were used for the interpretation of the biological significance from RNA seq results as described in Shahzad et al. (2020)
Results obtained from RNA seq analysis were also validated using real-time quantitative PCR (qPCR) following the protocols described by Tang and Vashisth (2020), and gene-specific primers sequences are listed in Supplemental Table S1. Eight DEGs related to hormones and cell wall metabolism were analyzed with citrus actin and Thioredoxin-like protein YLS8 as reference genes (Tang and Vashisth, 2020).
The ground bud samples (also used for RNA seq analysis) were sent to the Proteomics and Metabolomics Facility, Nebraska Center for Biotechnology, the University of Nebraska-Lincoln for the phytohormonal profile of bud. Hormones and their derivatives, including cytokinins (cZ, tZ, cZR, tZR), auxins (IAA, IAA-Ala, IAA-Asp, Methyl-IAA, IAA-Trp), abscisic acid (ABA), jasmonates (OPDA, JA, JA-Ile), salicylic acid (SA), strigolactones, and gibberellins were extracted and analyzed following the protocols described in Hung et al. (2016). Hormonal ratios are presented herein indicate the combined effect between two hormones with antagonistic or mutually similar effects (either stimulating or suppressive).
ADH activity was quantified to assess the rate of alcoholic fermentation, a key marker for hypoxia stress. Buds collected monthly from November 2018 to February 2019 were used to determine ADH activity, following the protocols described by Gonçalves et al. (2010). The results for ADH activity presented herein are in µmol min-1 g-1 FW.
Physiological variables, phytohormone levels, and ADH activity were analyzed to determine the differences between mild and severe trees using t-test in Sigma Plot (version 12; Systat Software, San Jose, CA) with a significance level of α ≤ 0.05.
For both severe and mild ‘Hamlin’ sweet orange trees, shoots with zero dead buds were tagged before the beginning of the experiment (November 2018) (Figure 1). ‘Hamlin’ severe trees showed a progression in dead bud percentage starting a month later (December 2018), whereas mild trees did not show such a pattern until January 2019. Overall severe trees underwent significantly more bud dieback than mild trees. This increase in dead bud percentage in ‘Hamlin’ severe trees also coincided with more light penetrating through the canopy to the ground in May 2019, indicating sparse canopies compared to mild trees. Fruit yield was almost 50% lower in ‘Hamlin’ severe trees than in mild trees (Supplemental Figure S2A). No differences were found in leaf nutrients concentration in January 2019, floral and vegetative intensity per shoot (on each survey date) between severe and mild ‘Hamlin’ trees (Supplemental Figure S2B, C). A similar trend regarding physiological attributes was found in ‘Valencia’ trees exhibiting mild and severe trees (Supplemental Figure S3A, B, C).
Figure 1 Dead bud percentage (%) on individual survey days and % increase in light penetrating through the canopy (PPFD, µmole/m2s) at the end of the experiment in Huanglongbing (HLB)-affected ‘Hamlin’ sweet orange trees with severe and mild symptoms, respectively. Each point is the mean ± standard deviation of four biological replicates. Significant differences were considered when P < 0.05 using Tukey’s honestly significant difference (HSD) test. Different letters indicate significant differences in the canopy density between severe and mild trees.
In buds of severe and mild ‘Hamlin’ trees collected in November 2018, there were only four differentially expressed genes (DEGs). Among these three DEGs [encoding LOB domain-containing protein 41 (orange1.1g022591m), B12D protein (orange1.1g048115m), and detoxification superfamily protein (orange1.1g033335m)] were upregulated, and one DEG encoding beta-glucosidase 11 (orange1.1g046009m) was downregulated in severe trees compared to mild trees (Table 1). These DEGs have been reported to be induced under low oxygen levels in plants (Liu et al., 2005; Lokdarshi, 2015).
Table 1 Differentially expressed genes (DEGs) in buds of severely symptomatic Huanglongbing (HLB)-affected ‘Hamlin’ sweet orange trees compared to mildly symptomatic trees in November.
In buds collected in February 2019, 2063 genes were differentially expressed between severe and mild trees. Among these, 982 and 1081 DEGs were downregulated and upregulated in the buds of severe trees compared to mild trees. There exists a significant and positive correlation (R = 0.78) between fold changes attained by RNA-seq analysis and relative gene expression levels found by qPCR (data not shown), indicating the validity of RNA-seq data.
MapMan enrichment analysis provides an overview of DEGs involved in metabolic and physiological processes in the bud of severe trees compared to mild trees in Feb 2019 (Figure 2A). A significant proportion of the DEGs (28%) came under the category with no assigned function (Bin 35), followed by RNA (BIN27; 13%), protein (BIN29; 12%), signaling (BIN30; 8%), miscellaneous enzyme families (BIN26; 6%), transport (BIN34; 6%), stress (BIN20; 5%), development (BIN33; 3%), DNA (BIN28; 3%), hormones (BIN17; 3%), secondary metabolism (BIN16, 2%), cell wall (BIN10, 2%), lipid metabolism (BIN11; 2%), photosynthesis (BIN1; 1%), and amino acid metabolism (BIN13; 1%), with the categories that comprise less than 1% of the DEGs.
Figure 2 Major functional categories (BINs) using the MapMan enrichment analysis (A); differentially expressed genes in pathogen/pest attack category (B), in buds of severely symptomatic Huanglongbing (HLB)-affected ‘Hamlin’ sweet orange trees compared to mildly symptomatic trees in February. This pathogen/pest attack category includes BINs related to hormones, redox reactions, defense genes, signaling, heat shock proteins, and biotic stress. Red and blue boxes represent upregulated and downregulated genes, respectively.
Using AgriGO and REViGO tools, gene ontology (GO) enrichment analysis was also performed with the downregulated and upregulated DEGs separately. Significant GO terms for biological processes in the bud of severe trees compared to mild trees (Table 2) and cellular processes and molecular functions are listed in Supplemental Table S2. In buds of severe versus mild trees for downregulated DEGs, top enriched GO terms were regulation of circadian rhythm (GO:0042752), response to blue light (GO:0009637), photosynthesis (GO:0015979), cell cycle (GO:0007049), cell division (GO:0051301), tissue development (GO:0009888), and auxin transport (GO:0060918) (Table 2; Supplemental Table S3). For the GO terms associated with molecular functions, chlorophyll binding (GO:0016168), ATP binding (GO:0005524), and carbohydrate phosphate activity (GO:0019203) were the most enriched in downregulated DEGs in severe trees compared to mild trees (Supplemental Table S2). For upregulated DEGs in severe versus mild trees, top enriched GO terms included abiotic stress [response to osmotic stress (GO:0006970), response to decreased oxygen levels (GO:0036293)], biotic stress [response to bacterium (GO:0009617), response to chitin (GO:0010200)], defense response, incompatible interaction (GO:0009814), oxidation-reduction process (GO:0055114), aging (GO:0007568), cell death (GO:0008219), hormones [ethylene-activated signaling pathway (GO:0009873) and jasmonic acid biosynthesis process (GO:0009695)] (Table 2). Considering molecular functions, dioxygenase activity (GO:0051213) and oxidoreductase activity (GO:0016491) were the most enriched terms in upregulated DEGs of severe trees than mild trees (Supplemental Table S2).
Table 2 A subset of GO categorization relating to the biological process of downregulated and upregulated differentially expressed genes (DEGs) in buds of severely symptomatic Huanglongbing (HLB)-affected ‘Hamlin’ sweet orange trees compared to mildly symptomatic trees in February.
In severe trees, 42 DEGs related to osmotic stress (GO:0006970) and 10 DEGs related to decreased oxygen levels (GO:0036293) were upregulated compared to mild trees (Table 2; Supplemental Table S4). DEGs known to be induced under hypoxia stress and in the hypoxia-reoxygenation phase are listed in Table 3. Anaerobic fermentation (ethanol) is the critical metabolic adaptation to confirm energy production in hypoxic conditions (Liu et al., 2005; Bui et al., 2015; León et al., 2021); DEG encoding pyruvate decarboxylase (orange1.1g007800m) and those encoding alcohol dehydrogenase (orange1.1g035170m, orange1.1g047713m) were upregulated in severe trees than mild trees. For the starch/sucrose utilization process, alpha-amylase-like 3 (orange1.1g002585m), hexokinase 2 (orange1.1g010895m), and phosphofructokinase 2 (orange1.1g042534m) genes were upregulated in severe trees than mild trees. Since hypoxia hampers the mitochondrial activity and plays a role in an anoxic burst of cytosolic calcium (Ca) (Subbaiah and Sachs, 2003; León et al., 2021), a DEG encoding autoinhibited Ca2+-ATPase 1 (orange1.1g016687m) was upregulated in severe trees than mild trees. Regarding low oxygen sensing mechanism, DEGs encoding group VII ethylene response factor family (ERFVIIs) (orange1.1g017035m, orange1.1g019887m) and two shikimate kinase genes (orange1.1g023118m, orange1.1g025114m) were upregulated in severe trees than mild trees. Ethylene-regulated DEGs encoding enzymes alanine aminotransferase (orange1.1g009517m) and glutamate dehydrogenase (orange1.1g012750m) were upregulated in severe trees compared to mild trees, indicating reoxygenation following hypoxia. Related to hormones, DEGs encoding cytokinin oxidase (orange1.1g009793m, orange1.1g008291m) and gibberellin 2-oxidase 1 (orange1.1g020152m) were upregulated in severe trees than mild trees. DEG encoding NADPH oxidases (orange1.1g002711m; responsible for ROS generation) were upregulated in severe trees compared to mild trees. During hypoxia, excessive production of ROS from apoplastic NADPH oxidases and then following reoxygenation cause oxidative stress via the electron transport chain from mitochondria and chloroplasts, resulting in autophagy (Chen et al., 2017; Qi et al., 2020; León et al., 2021; Foyer and Hanke, 2022). All six autophagy indicator genes were upregulated in severe trees than in mild trees.
Table 3 Differentially expressed genes (DEGs) relating to biological processes induced by cellular hypoxia levels and during the hypoxia-reoxygenation phase in buds of severely symptomatic Huanglongbing (HLB)-affected ‘Hamlin’ sweet orange trees compared to mildly symptomatic trees in February.
GO terms related to stress (GO:0006950) and oxidation-reduction process (GO:0055114) were upregulated in severe trees than in mild trees (Table 2). MapMan category for tree-pathogen/pest interactions was highly enriched, which consists of redox, hormones metabolism, cell wall, pathogenesis-related (PR) proteins, secondary metabolism, transcription factors, and abiotic stresses (Figure 2B; Supplemental Table S5 and S6). In the case of the redox category, two and 11 DEGs related to glutathione peroxidase and glutathione S transferase, respectively, were upregulated in severe trees than in mild trees (Supplementary Table S5). Fatty acid beta-oxidation-related DEGs (11) were upregulated in severe trees compared to mild trees (Supplemental Table S6), indicating oxidative stress. Consistently, the GO term for cell death (GO:0008219) was upregulated in severe trees compared to mild trees (Supplemental Table S4). In addition, DEGs related to stress-induced hormones ABA and JA biosynthesis processes were upregulated in severe trees compared to mild trees (Supplemental Table S6).
In buds collected in February 2019, severe trees had higher ratios of ABA/cZR (2-fold), ABA/JA (2.5-fold), ABA/JA-Ile (3-fold), IAA/cZR (1.8-fold), IAA/JA (2.3-fold), and IAA/JA-Ile (2.5-fold) in comparison to mild trees (Figure 3). The SA/JA (2.3-fold), SA/JA-Ile (2.9-fold), and SA/cZR (3-fold) ratios were also higher in severe trees than in mild trees. No such differences were found in the phytohormones ratios in the bud of severe and mild trees in November 2018 (data not shown). Furthermore, gibberellins and strigolactones were not detected in bud samples at any time.
Figure 3 Phytohormones ratios (ABA, IAA, JA, JA/Ile, SA, and cZR) in buds of Huanglongbing (HLB)-affected ‘Hamlin’ trees. Data are presented as means ± standard deviation (n=4). Different letters marked on bars indicate significant differences among severe and mild trees. Grey and black colors represent mild and severe trees, respectively. ABA, abscisic acid; JA, jasmonic acid; JA-Ile, jasmonic acid iso leucine; IAA, auxins; SA, salicylic acid; cZR, cytokinins.
In December 2018, both mild and severe trees had the maximum ADH activity (Figure 4). In January 2019, ADH activity decreased rapidly in mild trees (when active growth started). In contrast, severe trees retained high ADH activity similar to the mild trees’ level in December 2018. A similar pattern for ADH activity between mild and severe trees was found in February 2019. Overall, severe trees had higher ADH activities than mild trees at all-time points.
Figure 4 Alcohol dehydrogenase enzyme activity (µmol min-1 g-1 FW) in buds of Huanglongbing (HLB)-affected ‘Hamlin’ trees. Data are presented as means ± standard deviation (n=4). Different letters indicate significant differences among severe and mild trees. Grey and black colors represent mild and severe trees, respectively.
This study presents multiple lines of evidence regarding physiochemical and transcriptional regulation for bud dieback in HLB-affected sweet orange trees for the first time. Following CLas-infection, branches of citrus trees start to die back, leading to low productivity in a few years (Stover et al., 2016). Our results showed that both ‘Hamlin’ and ‘Valencia’ displaying severe HLB symptoms undergo higher bud dieback than mild trees. Additionally, the upregulation of DEGs related to osmotic stress in severe trees than in mild trees indicated limited nutrient and water uptake, which is a hallmark of HLB-affected trees as a result of the reduction in root-to-shoot ratio, root longevity, leaf area, and stomatal conductance compared with healthy trees (Hamido et al., 2019; Shahzad et al., 2020; Silva et al., 2021). Reduced photosynthesis and increased respiration have been reported in HLB-affected trees compared to healthy trees (Silva et al., 2021). Moreover, HLB-affected leaves have lower predawn and midday stem water potential (by 13%) due to less stomatal conductance than healthy trees, directly influencing the stomatal conductivity (Hamido et al., 2019; Silva et al., 2021), thus, gas exchange and water uptake efficiency.
Stomatal oscillations determine transpiration and are modulated by a network of phytohormones (Song et al., 2006; Melotto et al., 2017). Our results showed higher ABA/cZR, ABA/JA, IAA/cZR, and IAA/JA ratios in buds of severe trees than in mild trees in February. Water deficit results in ABA accumulation which initiates signals for stomatal closure resulting in decreased water conductivity and transpiration (Stoll et al., 2000; Tanaka et al., 2006). Water deficit also inhibits cytokinin synthesis in roots and its translocation in the shoot system, whereas higher cytokinins can suppress stomatal closure induced by ABA and help to regulate transpiration (Stoll et al., 2000; Song et al., 2006). Auxin typically stimulates stomatal opening and suppresses ABA-induced stomatal closure (Daszkowska-Golec and Szarejko, 2013); however, excessive auxin accumulation can result in stomatal closure (Qi et al., 2020), as high auxin accumulation occurs under drought/hypoxia stress (León et al., 2021). A similar trend of high IAA and ABA ratios to other hormones was found in severe trees compared to mild trees. HLB-affected trees have been reported to have higher SA accumulation (Nehela et al., 2018); in this study, severe trees also had higher SA/cZR, SA/JA, and SA/JA-Ile ratios than those mild trees. SA stimulates stomatal closure, while cZR and JA induce stomatal opening (Song et al., 2006). Hormone-dependent stomatal oscillation plays a role in pathogen-associated molecular patterns (PAMPs) in Arabidopsis. According to a PAMP model by Melotto et al. (2017), in the first phase of PAMPs, stomata close regarding changes in ABA levels within an hour to limit pathogen entry. In the second phase, stomata open after 3 to 4 hours in a JA-dependent manner. Due to the recognition of bacterial pathogens, the third phase starts when stomata close again in association with SA. Similarly, alterations in hormone balances have been documented in ‘Valencia’ sweet orange upon CLas infection (Martinelli et al., 2013; Nehela et al., 2018). Taken together, it is likely that the changes in hormone ratios (high SA, ABA, and auxin and low cZR and JA) observed in buds of severe trees contributed to a greater extent of stomatal closure compared with mild trees. All the DEGs involved in the JA biosynthesis process were upregulated in severe trees compared to mild trees (Supplementary Table 5). However, the ratios of JA and its derivatives (JA-Ile) were lower in severe trees. A possible explanation of such discrepancy is that the JA biosynthesis process occurs in different cell parts involving chloroplast, peroxisome, and cytoplasm (Supplemental Figure S4) (Miersch et al., 2008). In later synthesis stages, JA in the cytoplasm is converted into the derivative compounds [Me-JA, JA-Ile, and 12-hydroxyjasmonic acid (12-OH-JA)]. In our study, 3 DEGs encoding the enzyme for 12-OH-JA were upregulated in severe trees than mild trees, which is an inactive form of JA and responsible for negative feedback mechanism through activation of jasmonate ZIM domain (JAZ) repressor genes by JA (Miersch et al., 2008). Finally, a possible switch-off mechanism behind upregulated genes for JA signaling coincides with the hydroxylation of JA.
Reduced photosynthesis in HLB-affected trees (Silva et al., 2021) and hormonal-induced stomatal closure are indicators of the reduced availability of oxygen at the cellular level. Trees uptake mineral nutrients and oxygen from the soil via roots (Cannon, 1932). Oxygen availability provides the most energy in plants and animals, which is crucial for the sustainability of most life forms (Schmidt-Rohr, 2020). Hypoxia act as a double-edged sword. Developmental hypoxia is critical during dormancy to preserve genome integrity by maintaining quiescence within meristems (Considine et al., 2017). On the other hand, hypoxia stress can occur during growth and development phases; metabolically active cells such as developing meristems, including shoots or root apical zones, are partially liable to oxygen deficiency due to higher oxygen demand and less intracellular spaces to conduct oxygen via gas diffusion (Drew, 1977). Hypoxia cores can develop when internal oxygen concentrations fall short of the ambient oxygen levels, even though oxygen is available externally (Subbaiah and Sachs, 2003). It is also noteworthy that within all bacteria, including gram-negative CLas, energy acquisition by the electron transport chain and ATP synthase for multiplication relies on oxygen (Boyer, 1997). So, it is possible that severely HLB-affected trees would have more oxygen demand than available supply resulting in the development of more hypoxia cores within the plant, thus, intensifying HLB severity.
Transcriptomic results and ADH activity also indicate that severe trees were likely undergoing hypoxia stress. Under hypoxia, the mitochondrial respiratory activity is hampered, and the resulting low ATP level triggers the upregulation of genes responsible for energy production, including fermentation, regulation of metabolic processes, and transcription factors, to cope with the accumulation of toxic products (lactate, or acetaldehyde/ethanol) and ROS (Liu et al., 2005; Bui et al., 2015; León et al., 2021). ROS production and fermentation are key indicators of hypoxia stress (León et al., 2021). NADPH oxidases (also known as respiratory burst oxidase homologs; RBOHs) generated ROS in plant apoplasts as documented in different crops (Hong et al., 2020), including HLB-affected sweet orange (Ma et al., 2022) and increased H2O2 production in response to hypoxia (Mori et al., 2001; Pasternak et al., 2005; Hong et al., 2020). Ethanol fermentation ensures energy production under low oxygen conditions. In the current study, DEGs encoding alcohol dehydrogenase and pyruvate decarboxylase were upregulated in severe trees compared to mild trees. Concomitantly, higher alcohol dehydrogenase (ADH) enzyme activity was seen in severe trees compared to mild trees. ADH activity increases to meet the energy demand through ATP synthesis under hypoxic as documented in different crops (Sousa and Sodek, 2002; Gonçalves et al., 2010). In citrus, an ADH gene was upregulated in HLB-affected trees compared to healthy trees (Zhu et al., 2020), suggesting CLas-infection responses involving ADH. In Arabidopsis, hypoxia causes an anoxia burst of cytosolic Ca, which acts as a messenger to regulate ion homeostasis activating the downstream signaling pathway following low oxygen conditions (Subbaiah and Sachs, 2003; León et al., 2021). Hypoxia also hampers the aquaporin’s activity by lowering the cytosolic pH and downregulating DEGs encoding lateral organ boundary domain protein (LBD) that are important for communication between the cells within meristem regions (Liu et al., 2005). Sensitivity to low oxygen levels relies on the stability of the group VII ethylene response factor family (ERFVII), which are oxygen sensors in plants by the N-end rule pathway for protein degradation (Sasidharan and Mustroph, 2011; Bui et al., 2015), and their expression decreases with reoxygenation. The involvement of ERFVIIs with N terminal met-Cys motif (SK1) induces hypoxia tolerance (León et al., 2021), and these DEGs (orange1.1g023118m, orange1.1g025114m) were upregulated in severe trees than mild trees. Furthermore, alternative oxidase (AOX) is essential to protect lipids from oxidative stress (e.g., nitric oxide and superoxide) under the hypoxia-reoxygenation phase (Jayawardhane et al., 2020), and our results demonstrated the downregulation of AOX in severe in comparison to mild trees, suggesting severe trees underwent a greater level of oxidative stress.
ROS plays a vital role in stress response (biotic and abiotic) along with the developmental process by establishing communication among cells and transducing signals over long distances (Foyer and Hanke, 2022). While hypoxia-reoxygenation mechanisms about ROS production and regulation of transcription factors have been well studied in animals (Mallet et al., 2018), little is known in plants. Plants produce ROS through non-enzymatic (the electron transport chain in chloroplasts and mitochondria) and enzymatic pathways (photorespiration in peroxisomes and plasma membrane-localized NADPH oxidases) (Foyer and Hanke, 2022). Our results demonstrated that the upregulation of DEGs encoding alanine aminotransferase and glutamate dehydrogenase enzymes played a critical role in the tricarboxylic acid cycle revival upon reoxygenation following hypoxia (Tsai et al., 2016), resulting in additional ROS formation (Yeung et al., 2019). Furthermore, in plants, the retrieval process from hypoxia also involves a darkness-to-light transition along with reoxygenation which causes a light-induced reduction in photosynthesis capacity followed by photoinhibition, altogether resulting in excessive ROS production (Yeung et al., 2019). Another source of ROS and its accumulation is the plasma membrane-localized NADPH oxidases (RBOHs) which can be activated with higher ABA, auxin, and SA accumulation (Mori et al., 2001; Pasternak et al., 2005). Our results show that severe trees had higher ratios of ABA, auxin, and SA to other hormones and upregulated DEG encoding RBOH compared to mild trees. ROS generated through simultaneous reoxygenation, darkness-to-light transition, and oxidative stresses in the hypoxia-reaeration phase can cause chlorosis due to accelerated chlorophyll degradation (Yeung et al., 2019), a typical HLB symptom that was observed in severe trees in this study. Under stress conditions, excessive ROS accumulation can be detrimental to cells. A recent report also suggests that HLB results in excessive ROS production causing cellular damage in citrus (Ma et al., 2022). Taken together, hypoxia and hypoxia-reoxygenation phases produce and accumulate excessive ROS resulting in oxidative stress and induced cell death in the severe trees.
Our results provided evidence suggesting that buds of severely affected HLB trees underwent hypoxia, resulting in ≈ 50% greater dieback over time than mild trees. Bud from severe trees had higher ABA/cZR and ABA/JA ratios than mild trees, which likely induced stomatal closure and accentuated osmotic stress. It is possible that the reduction in feeder root biomass, photosynthesis, and transpiration, which corresponded to the severe HLB symptoms at the canopy level, in severe trees, limited oxygen availability at the cellular level than mild trees. Following CLas-infection and HLB progression, a high energy demand (for defense and growth) is being compensated by attenuating respiration, a continuous process all day and night. Thus, to efficiently generate and preserve energy, trees switch to anaerobic respiration under low oxygen conditions, but in the long run, it is a waste of available carbohydrate sources, along with excessive ROS production and toxins accumulation (acetaldehyde/ethanol or lactate) as indicated by more dead buds in severe trees than mild trees. Further, severe trees were undergoing hypoxia stress as indicated by a variety of adaptive responses that include shifting to alternate pathways for ATP production and efficient utilization, phytohormones regulation, and antioxidants mechanism to cope with ostensibly higher ROS accumulation. In the hypoxia recovery phase, reoxygenation involves excessive ROS production leading to oxidative stress, resulting in cell death that may be responsible for rapid bud dieback in severe HLB-affected trees compared to mild trees. It should be noted that before the appearance of aboveground HLB symptoms, dieback occurs first at the feeder roots, which are prone to develop hypoxia cores due to highly active metabolism but little internal air space for oxygen diffusion. However, whether there is a direct connection between hypoxia and CLas-caused initial root dieback remains unclear. Therefore, further research is needed to explore this relationship as well as the bases for the hypoxia localized in specific tree organs (feeder roots, developing leaves and fruit, etc.) with HLB progression.
The datasets presented in this study can be found in onlinerepositories. The names of the repository/repositories and accessionnumber(s) can be found below: NCBI, GSE228890.
FS executed the experiment, analyzed the data, and wrote the manuscript. LT executed experiments in the field, collected samples, assisted in writing manuscript. TV supervised the study, developed a research goal and experimental plan, provided intellectual support. All authors contributed to the article and approved the submitted version.
This research was funded by the Florida State Legislature for UF/IFAS Citrus Initiative.
The authors declare that the research was conducted in the absence of any commercial or financial relationships that could be construed as a potential conflict of interest.
All claims expressed in this article are solely those of the authors and do not necessarily represent those of their affiliated organizations, or those of the publisher, the editors and the reviewers. Any product that may be evaluated in this article, or claim that may be made by its manufacturer, is not guaranteed or endorsed by the publisher.
The Supplementary Material for this article can be found online at: https://www.frontiersin.org/articles/10.3389/fpls.2023.1119530/full#supplementary-material
Bové, J. M. (2006). Huanglongbing: a destructive, newly-emerging, century-old disease of citrus. J. Plant Pathol., 7–37.
Boyer, P. D. (1997). The ATP synthase–a splendid molecular machine. Annu. Rev. Biochem. 66 (1), 717–749. doi: 10.1146/annurev.biochem.66.1.717
Bui, L. T., Giuntoli, B., Kosmacz, M., Parlanti, S., Licausi, F. (2015). Constitutively expressed ERF-VII transcription factors redundantly activate the core anaerobic response in Arabidopsis thaliana. Plant Sci. 236, 37–43. doi: 10.1016/j.plantsci.2015.03.008
Cannon, W. A. (1932). Absorption of oxygen by roots when the shoot is in darkness or in light. Plant Physiol. 7 (4), 673. doi: 10.1104/pp.7.4.673
Chen, L., Su, Z. Z., Huang, L., Xia, F. N., Qi, H., Xie, L. J., et al. (2017). The AMP-activated protein kinase KIN10 is involved in the regulation of autophagy in Arabidopsis. Front. Plant Sci. 8, 1201. doi: 10.3389/fpls.2017.01201
Considine, M. J., Diaz-Vivancos, P., Kerchev, P., Signorelli, S., Agudelo-Romero, P., Gibbs, D. J., et al. (2017). Learning to breathe: developmental phase transitions in oxygen status. Trends Plant Sci. 22 (2), 140–153. doi: 10.1016/j.tplants.2016.11.013
Daszkowska-Golec, A., Szarejko, I. (2013). Open or close the gate–stomata action under the control of phytohormones in drought stress conditions. Front. Plant Sci. 4, 138. doi: 10.3389/fpls.2013.00138
Drew, M. C. (1997). Oxygen deficiency and root metabolism: injury and acclimation under hypoxia and anoxia. Annu. Rev. Plant Physiol. 48 (1), 223–250. doi: 10.1146/annurev.arplant.48.1.223
Foyer, C. H., Hanke, G. (2022). ROS production and signalling in chloroplasts: cornerstones and evolving concepts. Plant J. 111 (3), 642–661. doi: 10.1111/tpj.15856
Gonçalves, J. F. D. C., Lima, R. B. S., Fernandes, A. V., Borges, E. E. D. L., Buckeridge, M. S. (2010). Physiological and biochemical characterization of the assai palm (Euterpe oleracea mart.) during seed germination and seedling growth under aerobic and anaerobic conditions. Rev. Arvore. 34, 1045–4053. doi: 10.1590/S0100-67622010000600010
Griffin, K. L., Turnbull, M., Murthy, R. (2002). Canopy position affects the temperature response of leaf respiration in Populus deltoides. New Phytol. 154 (3), 609–619. doi: 10.1046/j.1469-8137.2002.00410.x
Hamido, S. A., Ebel, R. C., Morgan, K. T. (2019). Interaction of huanglongbing and foliar applications of copper on water relations of Citrus sinensis cv. Valencia. Plants 8 (9), 298. doi: 10.3390/plants8090298
Hong, C. P., Wang, M. C., Yang, C. Y. (2020). NADPH oxidase RbohD and ethylene signaling are involved in modulating seedling growth and survival under submergence stress. Plants 9 (4), 471. doi: 10.3390/plants9040471
Hung, C. Y., Qiu, J., Sun, Y. H., Chen, J., Kittur, F. S., Henny, R. J., et al. (2016). Gibberellin deficiency is responsible for shy-flowering nature of Epipremnum aureum. Sci. Rep. 6 (1), 1–11. doi: 10.1038/srep28598
Jayawardhane, J., Cochrane, D. W., Vyas, P., Bykova, N. V., Vanlerberghe, G. C., Igamberdiev, A. U. (2020). Roles for plant mitochondrial alternative oxidase under normoxia, hypoxia, and reoxygenation conditions. Front. Plant Sci. 11, 566. doi: 10.3389/fpls.2020.00566
Johnson, E. G., Wu, J., Bright, D. B., Graham, J. H. (2014). Association of ‘Candidatus liberibacter asiaticus’ root infection, but not phloem plugging with root loss on huanglongbing-affected trees prior to appearance of foliar symptoms. Plant Pathol. 63 (2), 290–298. doi: 10.1111/ppa.12109
Kanehisa, M., Furumichi, M., Tanabe, M., Sato, Y., Morishima, K. (2017). KEGG: new perspectives on genomes, pathways, diseases and drugs. Nucleic Acids Res. 45 (D1), D353–D361. doi: 10.1093/nar/gkw1092
León, J., Castillo, M. C., Gayubas, B. (2021). The hypoxia–reoxygenation stress in plants. J. Exp. Bot. 72 (16), 5841–5856. doi: 10.1093/jxb/eraa591
Li, B., Dewey, C. N. (2011). RSEM: accurate transcript quantification from RNA-seq data with or without a reference genome. BMC Bioinform. 12 (1), 1–16. doi: 10.1186/1471-2105-12-323
Liu, F., VanToai, T., Moy, L. P., Bock, G., Linford, L. D., Quackenbush, J. (2005). Global transcription profiling reveals comprehensive insights into hypoxic response in arabidopsis. Plant Physiol. 137 (3), 1115–1129. doi: 10.1104/pp.104.055475
Lokdarshi, A. (2015). Calmodulin-like protein 38: a component of ribonucleoprotein particles during hypoxic stress responses in arabidopsis.
Ma, W., Pang, Z., Huang, X., Xu, J., Pandey, S. S., Li, J., et al. (2022). Citrus huanglongbing is a pathogen-triggered immune disease that can be mitigated with antioxidants and gibberellin. Nat. Commun. 13 (1), 1–13. doi: 10.1038/s41467-022-28189-9
Mallet, R. T., Manukhina, E. B., Ruelas, S. S., Caffrey, J. L., Downey, H. F. (2018). Cardioprotection by intermittent hypoxia conditioning: evidence, mechanisms, and therapeutic potential. Am. J. Physiol. Heart Circ. 315 (2), H216–H232. doi: 10.1152/ajpheart.00060.2018
Martinelli, F., Reagan, R. L., Uratsu, S. L., Phu, M. L., Albrecht, U., Zhao, W., et al. (2013). Gene regulatory networks elucidating huanglongbing disease mechanisms. PloS One 8 (9), e74256. doi: 10.1371/journal.pone.0074256
Melotto, M., Zhang, L., Oblessuc, P. R., He, S. Y. (2017). Stomatal defense a decade later. Plant Physiol. 174 (2), 561–571. doi: 10.1104/pp.16.01853
Miersch, O., Neumerkel, J., Dippe, M., Stenzel, I., Wasternack, C. (2008). Hydroxylated jasmonates are commonly occurring metabolites of jasmonic acid and contribute to a partial switch-off in jasmonate signaling. New Phytol. 177 (1), 114–127. doi: 10.1111/j.1469-8137.2007.02252.x
Mori, I. C., Pinontoan, R., Kawano, T., Muto, S. (2001). Involvement of superoxide generation in salicylic acid-induced stomatal closure in Vicia faba. Plant Cell Physiol. 42 (12), 1383–1388. doi: 10.1093/pcp/pce176
Nehela, Y., Hijaz, F., Elzaawely, A. A., El-Zahaby, H. M., Killiny, N. (2018). Citrus phytohormonal response to Candidatus liberibacter asiaticus and its vector Diaphorina citri. Physiol. Mol. Plant Pathol. 102, 24–35. doi: 10.1016/j.pmpp.2017.11.004
Pasternak, T., Potters, G., Caubergs, R., Jansen, M. A. (2005). Complementary interactions between oxidative stress and auxins control plant growth responses at plant, organ, and cellular level. J. Exp. Bot. 56 (418), 1991–2001. doi: 10.1093/jxb/eri196
Qi, H., Li, J., Xia, F. N., Chen, J. Y., Lei, X., Han, M. Q., et al. (2020). Arabidopsis SINAT proteins control autophagy by mediating ubiquitylation and degradation of ATG13. Plant Cell Rep. 32 (1), 263–284. doi: 10.1105/tpc.19.00413
Rio, D. C. (2015). Denaturation and electrophoresis of RNA with formaldehyde. Cold Spring Harb. Protoc. 2015 (2), pdb–prot080994. doi: 10.1101/pdb.prot080994
Sasidharan, R., Mustroph, A. (2011). Plant oxygen sensing is mediated by the n-end rule pathway: a milestone in plant anaerobiosis. Plant Cell. 23 (12), 4173–4183. doi: 10.1105/tpc.111.093880
Schmidt-Rohr, K. (2020). Oxygen is the high-energy molecule powering complex multicellular life: Fundamental corrections to traditional bioenergetics. ACS omega 5 (5), 2221–2233. doi: 10.1021/acsomega.9b03352
Shahzad, F., Chun, C., Schumann, A., Vashisth, T. (2020). Nutrient uptake in huanglongbing-affected sweet orange: Transcriptomic and physiological analysis. J. Am. Soc Hortic. 145 (6), 349–362. doi: 10.21273/JASHS04929-20
Silva, J. R. D., Boaretto, R. M., Lavorenti, J. A. L., Dos Santos, B. C. F., Coletta-Filho, H. D., Mattos, D., Jr. (2021). Effects of deficit irrigation and huanglongbing on sweet orange trees. Front. Plant Sci., 2228. doi: 10.3389/fpls.2021.731314
Song, X. G., She, X. P., He, J. M., Huang, C., Song, T. S. (2006). Cytokinin-and auxin-induced stomatal opening involves a decrease in levels of hydrogen peroxide in guard cells of Vicia faba. Funct. Plant Biol. 33 (6), 573–583. doi: 10.1071/FP05232
Sousa, C. A. F. D., Sodek, L. (2002). The metabolic response of plants to oxygen deficiency. Braz. J. Plant Physiol. 14, 83–94. doi: 10.1590/S1677-04202002000200002
Stoll, M., Loveys, B., Dry, P. (2000). Hormonal changes induced by partial rootzone drying of irrigated grapevine. J. Exp. Bot. 51 (350), 1627–1634. doi: 10.1093/jexbot/51.350.1627
Stover, E., Inch, S., Richardson, M. L., Hall, D. G. (2016). Conventional citrus of some scion/rootstock combinations show field tolerance under high huanglongbing disease pressure. HortScience 51 (2), 127–132. doi: 10.21273/HORTSCI.51.2.127
Subbaiah, C. C., Sachs, M. M. (2003). Calcium-mediated responses of maize to oxygen deprivation. Russ. J. Plant Physiol. 50 (6), 752–761. doi: 10.1023/B:RUPP.0000003273.44823.cd
Tanaka, Y., Sano, T., Tamaoki, M., Nakajima, N., Kondo, N., Hasezawa, S. (2006). Cytokinin and auxin inhibit abscisic acid-induced stomatal closure by enhancing ethylene production in Arabidopsis. J. Exp. Bot. 57 (10), 2259–2266. doi: 10.1093/jxb/erj193
Tang, L., Vashisth, T. (2020). New insight in huanglongbing-associated mature fruit drop in citrus and its link to oxidative stress. Sci. Hortic. 265, 109246. doi: 10.1016/j.scienta.2020.109246
Tian, T., Liu, Y., Yan, H., You, Q., Yi, X., Du, Z., et al. (2017). agriGO v2. 0: a GO analysis toolkit for the agricultural community 2017 update. Nucleic Acids Res. 45 (W1), W122–W129.
Tsai, K. J., Lin, C. Y., Ting, C. Y., Shih, M. C. (2016). Ethylene-regulated glutamate dehydrogenase fine-tunes metabolism during anoxia-reoxygenation. Plant Physiol. 172 (3), 1548–1562. doi: 10.1104/pp.16.00985
Usadel, B., Nagel, A., Thimm, O., Redestig, H., Blaesing, O. E., Palacios-Rojas, N., et al. (2005). Extension of the visualization tool MapMan to allow statistical analysis of arrays, display of coresponding genes, and comparison with known responses. Plant Physiol. 138 (3), 1195–1204. doi: 10.1104/pp.105.060459
USDA (2020). Florida Citrus statistics 2018–2019 (Florida Department of Agriculture and Consumer Services), 1–114. Available at: https://www.nass.usda.gov/Publications/Todays_Reports/reports/cfrt0820.pdf.
Wu, G. A., Prochnik, S., Jenkins, J., Salse, J., Hellsten, U., Murat, F., et al. (2014). Sequencing of diverse mandarin, pummelo and orange genomes reveals complex history of admixture during citrus domestication. Nat. Biotechnol. 32 (7), 656–662. doi: 10.1038/nbt.2906
Yeung, E., Bailey-Serres, J., Sasidharan, R. (2019). After the deluge: plant revival post-flooding. Trends Plant Sci. 24 (5), 443–454. doi: 10.1016/j.tplants.2019.02.007
Keywords: citrus greening, hypoxia, hormones, oxidative stress, ROS production
Citation: Shahzad F, Tang L and Vashisth T (2023) Unraveling the mystery of canopy dieback caused by citrus disease Huanglongbing and its link to hypoxia stress. Front. Plant Sci. 14:1119530. doi: 10.3389/fpls.2023.1119530
Received: 08 December 2022; Accepted: 15 February 2023;
Published: 17 April 2023.
Edited by:
Prachi Pandey, National Institute of Plant Genome Research (NIPGR), IndiaReviewed by:
Xiaochun Zhao, Southwest University, ChinaCopyright © 2023 Shahzad, Tang and Vashisth. This is an open-access article distributed under the terms of the Creative Commons Attribution License (CC BY). The use, distribution or reproduction in other forums is permitted, provided the original author(s) and the copyright owner(s) are credited and that the original publication in this journal is cited, in accordance with accepted academic practice. No use, distribution or reproduction is permitted which does not comply with these terms.
*Correspondence: Tripti Vashisth, dHZhc2hpc3RoQHVmbC5lZHU=
Disclaimer: All claims expressed in this article are solely those of the authors and do not necessarily represent those of their affiliated organizations, or those of the publisher, the editors and the reviewers. Any product that may be evaluated in this article or claim that may be made by its manufacturer is not guaranteed or endorsed by the publisher.
Research integrity at Frontiers
Learn more about the work of our research integrity team to safeguard the quality of each article we publish.