- 1Independent Researcher, Hyderabad, India
- 2International Maize and Wheat Research Center, Centro Internacional de Mejoramiento de Maíz. y Trigo (CIMMYT), Nairobi, Kenya
- 3Department of Molecular Biology, College of Biotechnology, CCS Haryana Agricultural University, Hissar, India
- 4HarvestPlus Program, Alliance of Bioversity International and the International Center for Tropical Agriculture (CIAT), Cali, Colombia
- 5Swedish University of Agricultural Sciences, Lomma, Sweden
Malnutrition results in enormous socio-economic costs to the individual, their community, and the nation’s economy. The evidence suggests an overall negative impact of climate change on the agricultural productivity and nutritional quality of food crops. Producing more food with better nutritional quality, which is feasible, should be prioritized in crop improvement programs. Biofortification refers to developing micronutrient -dense cultivars through crossbreeding or genetic engineering. This review provides updates on nutrient acquisition, transport, and storage in plant organs; the cross-talk between macro- and micronutrients transport and signaling; nutrient profiling and spatial and temporal distribution; the putative and functionally characterized genes/single-nucleotide polymorphisms associated with Fe, Zn, and β-carotene; and global efforts to breed nutrient-dense crops and map adoption of such crops globally. This article also includes an overview on the bioavailability, bioaccessibility, and bioactivity of nutrients as well as the molecular basis of nutrient transport and absorption in human. Over 400 minerals (Fe, Zn) and provitamin A-rich cultivars have been released in the Global South. Approximately 4.6 million households currently cultivate Zn-rich rice and wheat, while ~3 million households in sub-Saharan Africa and Latin America benefit from Fe-rich beans, and 2.6 million people in sub-Saharan Africa and Brazil eat provitamin A-rich cassava. Furthermore, nutrient profiles can be improved through genetic engineering in an agronomically acceptable genetic background. The development of “Golden Rice” and provitamin A-rich dessert bananas and subsequent transfer of this trait into locally adapted cultivars are evident, with no significant change in nutritional profile, except for the trait incorporated. A greater understanding of nutrient transport and absorption may lead to the development of diet therapy for the betterment of human health.
Global impact of malnutrition
The availability of essential vitamins and minerals is a fundamental requirement for human wellbeing, especially during the early stage of life. The lack of the right amount of vitamins, minerals, and other nutrients leads to the development of malnutrition. Malnutrition has different forms including wasting (low weight for height), stunting (low height for age), and underweight (low weight for age). All these ensue from poor-quality diets and may lead to non-communicable diseases. More than 2 billion people across the globe suffer from one or more micronutrient malnutrition (Figure 1; Von Grebmer et al., 2022). As per estimates from the World Health Organization (WHO) and the Food and Agriculture Organization (FAO) of the United Nations, 149 million children under 5 are stunted, 47 million are wasted, and 462 million are underweight. About 50% of child deaths under the age of 5 in developing countries are linked to undernutrition (FAO et al., 2020). Therefore, malnutrition affects physical and mental development, immunity, and overall health, thereby hampering human life potential largely in low- and middle-income countries (LMICs). The magnitude of micronutrient deficiency is particularly alarming among children, women of reproductive age, and pregnant and nursing mothers. Increased availability, accessibility, and affordability of dietary diversity including animal and dairy products address this complex food-system-based health problem. Food production is meant for both food and nutrition security. This has been ignored in recent agricultural research for development undertakings. The agricultural sector gained momentum in producing high yields through the Green Revolution efforts, but ignoring crop-based essential nutrition. Alternative agriculture should emphasize the production of food crops with better nutritional quality to minimize the risk of malnutrition in developing countries.
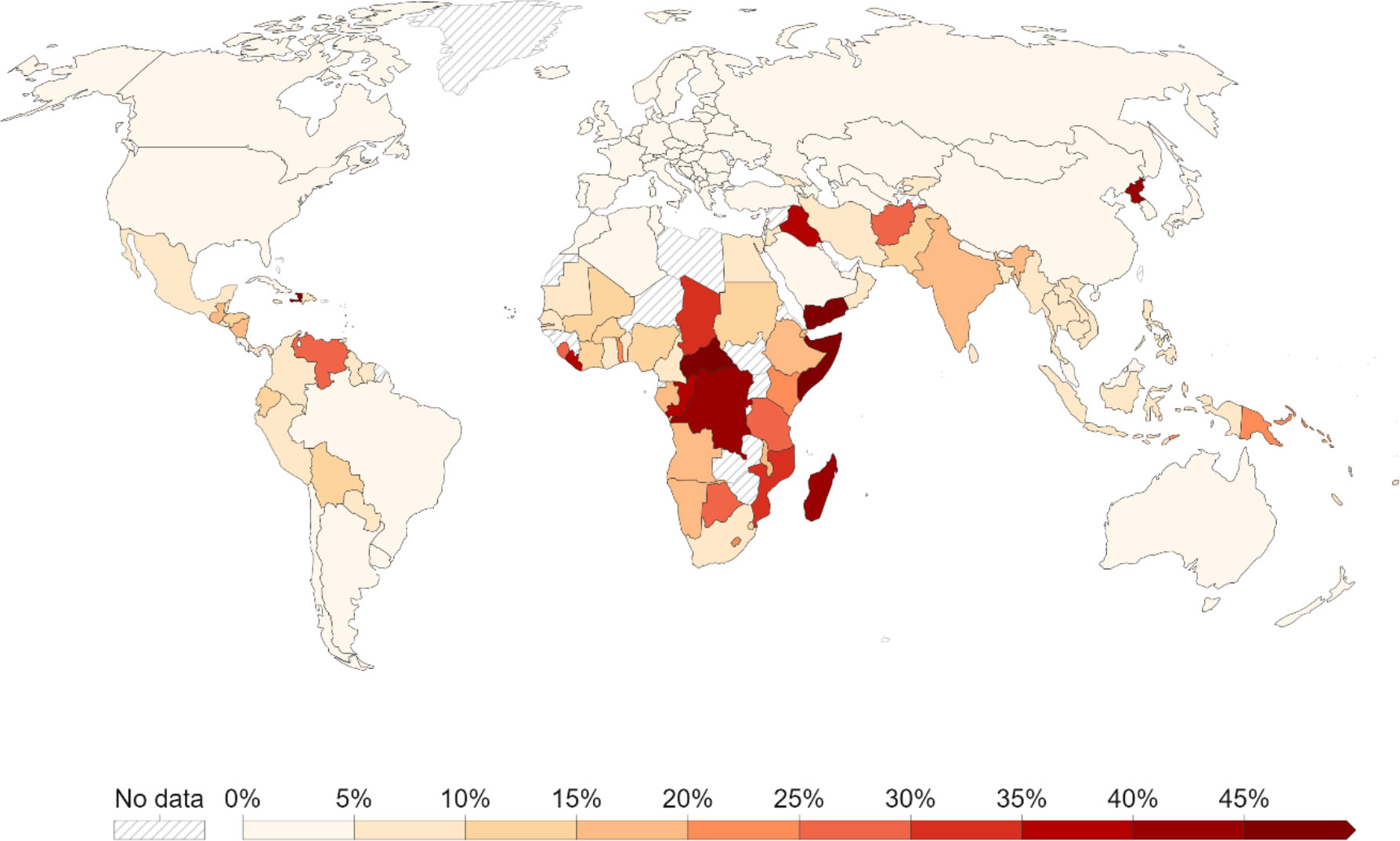
Figure 1 The prevalence of undernourishment (in percentage) in Global South (source: Asia, 2021; https://ourworldindata.org/hunger-and-undernourishment#long-term-decline-of-undernourishment).
Given its impact on health, education, and economic productivity, persistent undernutrition is a major obstacle to human development, impacting the country’s prospects for future economic growth. To overcome this devastating situation of malnutrition, long-term efforts include fostering nutritionally dense cultivars that are highly prioritized alongside increasing yields and reducing negative consequences affecting yields in specific conditions. Globally, tackling the burden of malnutrition in all its forms remains challenging. For instance, the staggering impact of malnutrition in developing countries, in terms of gross domestic product (GDP), is almost 11% in Asia and Africa. The World Bank (2020) estimated that loss in GDP due to hidden hunger is up to 12 billion for India alone. The highest nutrition priority for eradicating malnutrition and achieving optimum nutrition for all is embedded in the National Nutrition Policy (NNP) adopted in 1993 by the Government of India, which acknowledges high prevalence of malnutrition according to a national family health survey (NFHS-V, 2021).
Improving nutrient levels in the edible parts of staple crops, by either crossbreeding, biotechnology, or agronomy, through the application of soil and leaf fertilizers, are cost-effective and sustainable approaches to reducing the burden of micronutrient deficiencies in the developing world (Garg et al., 2018). The CGIAR HarvestPlus Program has led nutrition research for development since 2003, by focusing on the top three nutrients with the highest deficiency among populations: iron (Fe), zinc (Zn), and vitamin A (HarvestPlus, 2022). Iron deficiency consequences are anemia, fatigue, weakness, and impaired cognition. Zinc deficiency leads to stunting in children, susceptibility to infections, and cell damage. Vitamin A deficiency contributes to impaired vision, night blindness, a higher risk of infection/death, and poor pregnancy outcomes. Nutrition is always given less priority in core crop improvement programs in national and international research and investments, except in a few time-bound bilateral projects. Thus, reducing the magnitude of malnutrition, especially in rural households, remains a big challenge. The prevalence of malnutrition after the COVID-19 pandemic is expected to significantly increase. The malnutrition prevalence levels are unacceptable in the Global South. The cost of addressing malnutrition is increasing in LMICs. An estimated 10 billion vitamin A capsules have been distributed to preschool children over two decades in LMICs (Tam et al., 2020). The importance of food security and improved nutrition is highlighted in the Sustainable Development Goals (SDG 2), which aim to end all forms of hunger by 2030 (Jose et al., 2020). The ongoing transition in CGIAR crop breeding and modernization investments highlight that improving nutrition traits is a priority to achieve SDG2.
Global warming and elevated CO2 reduce the nutritional quality of food crops
Climate change and the quality of human diets are challenging issues and consequences for this century and upcoming ones. Increasing temperature and CO2 affects crop productivity, resilience, and nutritious quality. Semba et al. (2022) argued that climate change will affect micronutrient malnutrition by restricting the accessibility to micronutrient-rich crops. Furthermore, stoichiometric theory suggests that high CO2, as a rule, should alter the elemental plant composition (Loladze, 2002). The flour from cereals that were grown under elevated CO2 or using low nitrogen fertilizer amounts could lessen nutritional and processing quality and alter grain elemental composition (Erbs et al., 2010). It has also been noted that climate may reduce proteins (Jablonski et al., 2002; Stafford, 2007; Taub et al., 2008) and micronutrients in food crops (Stafford, 2007; Erbs et al., 2010; Scarpa et al., 2020). For example, as indicated by Hummel et al. (2018), elevated CO2 decreased iron intake by 4% for children below 5 years old and women of childbearing age, who eat common beans (Phaseolus vulgaris L.). Likewise, iron and zinc content in wheat (Triticum aestivum L.) grains were reduced by ca. 32% and 6%, respectively, under heat stress (Panigrahi et al., 2022). Loladze (2002) claimed that micronutrients may decline owing to “carbohydrate dilution” and reduce transpiration, thus affecting food quality and increasing micronutrient malnutrition, especially in the developing world.
Global warming lessens the uptake of micronutrients from the soil and the ensuing translocation within the plant (Maqbool et al., 2020). In this regard, high temperature negatively affects zinc acquisition in plants, thus resulting in poor nutritional quality. Ahmad et al. (2022) indicated that poor soil fertility management may further decrease the dietary supplementary supply of micronutrients. Likewise, owing to the ongoing COVID-19 pandemic, malnutrition has risen particularly in South Asia and sub-Saharan Africa (Sheoran et al., 2022). Hence, when it comes to biofortification, it must consider the target population of environments where the nutrient-dense bred germplasm will be further grown.
There are obvious interlinks between global warming, agri-food systems, and nutritional security (Bakker et al., 2021). Both agri-food systems and nutrition security are highly affected by climate variability and long-term climate change. The different types of foods consumed vary in their released greenhouse gases (GHGs), such as CO2 and NOx, and may promote change in land uses including an increase on clearing forests, draining wetlands, or tilling soil for agriculture, thereby contributing to climate change. This synergy steers different GHG emissions and distinct environmental footprints. A transdisciplinary approach should therefore be sought for developing an agri-food system that considers biofortification under rising temperatures, elevated CO2, and water stress, with the aim of ensuring food and nutrition security (Ebi et al., 2021). As shown by Huey et al. (2022), biofortification should be included in agri-food system interventions to overcome micronutrient deficiency in rural households. The microbiome may also provide a path towards nutrient-rich crops because of the known role of rhizospheres on crop nutrient dynamics (Tripathi et al., 2022).
Physiological and molecular basis of nutrient acquisition, transport, and storage
Besides three basic non-mineral elements [carbon (C), hydrogen (H), and oxygen (O)], 14 mineral elements [nitrogen (N), phosphorus (P), potassium (K), calcium (Ca), sulfur (S), magnesium (Mg), chloride (Cl), iron (Fe), boron (B), manganese (Mn), zinc (Zn), copper (Cu), nickel (Ni), and molybdenum (Mo)] are broadly accepted as essential for the normal growth and development of plants. Based on the relative amounts needed for plant growth processes, these essential mineral elements can be classified into macro nutrients (primary: N, P, and K; secondary: Ca, S, and Mg) and micronutrients (Cl, Fe, B, Mn, Zn, Cu, Ni, and Mo), which can be found in plants at concentrations of >0.1% and <0.01% of dry tissue weight, respectively (Grusak et al., 2016). Being sessile, plants have not only evolved various physiological and molecular strategies for the uptake of essential micronutrients (trace minerals), such as iron and zinc from soil, but also developed tightly regulated mechanisms through coordination of several processes for their optimal distribution in between different organs as excess concentrations of these essential trace metals are toxic for their normal growth and development (Garcia-Oliveira et al., 2018).
Primarily, rhizosphere conditions have a decisive role in the adequate supply of mineral elements to meet the metabolic requirements of plants. Iron is one of the most abundant metals in soils but mainly present in the form of ferric (hydro)oxides, which have extremely low solubility in soil, resulting in restricted availability to plants. Plants uptake iron from rhizosphere in the form of either ferrous Fe(II), as in the case of non-graminaceous plants by acidification–reduction strategy (Strategy I), or ferric Fe(III) by graminaceous plants using chelation strategy (Strategy II), or a combination thereof (Marschner et al., 1986; Ishimaru et al., 2006). Most of the plants (dicot and non-graminaceous) including the model plant Arabidopsis (Arabidopsis thaliana) acidify the rhizosphere through the proton pump, H+-ATPase 2 (AHA2), and reduce ferric chelates Fe(III) to Fe(II) by the ferric reductase oxidase 2 (FRO2) enzyme (Robinson et al., 1999), and then, consequently, Fe(II) is taken up from soil through iron regulated transporter 1 (IRT1) (Eide et al., 1996). Graminaceous plants such as rice (Oryza sativa L.), maize (Zea mays L.), and wheat (T. aestivum L.) secrete mugineic acid (MA) family phytosiderophores (PS), which are produced from nicotianamine (NA) and chelate Fe(III), and subsequently, the Fe(III)–PS complex is transported into the root cells by Yellow Stripe 1 (YS1) or YS1-Like (YSL) transporters (Curie et al., 2001; Curie et al., 2009).
Like iron, total zinc content is also typical and relatively high in soil but occurs in various discrete chemical forms, and their solubility and availability to plants are influenced by the composition as well the physicochemical properties of the soil and biological factors, resulting, therefore, in reduced availability of zinc for plant absorption (White and Broadley, 2011). Zinc is acquired by roots from the soil solution into rhizodermal cells as free Zn2+ ion through members of the Zn-regulated transporter (ZRT) and IRT-like protein (ZIP) transporters. Soil zinc, like iron, is also solubilized via acidification and secretion of organic chelators (e.g., citrate, malate, and oxalate) or PS such as deoxymugineic acid (DMA) in the rhizosphere (White and Broadley, 2011). The precise uptake of Zn-chelate is not yet fully understood; nonetheless, the role of the YSL protein transporter has been demonstrated in the uptake of Zn-PS complexes in maize (von Wirén et al., 1996).
Following the initial uptake of iron and zinc from the soil solution, these are transported radially across the concentric root cell layers of epidermis, cortex, and endodermis. Once Fe reaches the central vasculature, it is then unloaded into the xylem where it is chelated with organic acids, especially citrate and MAs, and translocated to the shoots for long-distance transport (Durrett et al., 2007). For root-to-shoot transport, a multidrug and toxic compound extrusion (MATE) family transporter ferric chelate reductase defective 3 (FRD3) and ferroportin 1 (FPN1), also known as iron regulated 1 (IREG1) in Arabidopsis, respectively, load citrate and iron into the xylem (Durrett et al., 2007; Morrissey et al., 2009). Similarly, FRD-3 like 1 (FRDL1) in rice and Al-activated citrate efflux transporter 1 (AACT1) in barley, orthologs of Arabidopsis FRD3, and transporter of MAs (TOM) are crucial in graminaceous plants for iron transport in the xylem through the efflux of citrate and MAs, respectively (Furukawa et al., 2007; Yokosho et al., 2016). Similar to Fe, and for long-distance transport, it is also necessary to load Zn2+ ions into xylem vessels for its transport from root to the plant aerial parts. This task is known to be performed by heavy metal P1B-ATPase subfamily transporter (HMA; HMA2 and HMA4) proteins, and the members of ZIP and YSL families are thought to be involved in zinc loading into phloem (Chen et al., 2018a; Zlobin, 2021).
In the arial plant parts, YSL2 plays an important role in the lateral transport of Fe-NA complexes from the xylem to neighboring cells (DiDonato et al., 2004). Following xylem loading, Fe can be transferred to phloem for further long-distance transport and remobilization towards the crucial sink organs, especially leaves and seeds. Based on the analysis on the Fe complexes in the phloem sap, Nishiyama et al. (2012) suggested that DMA is the main Fe-chelating agent in the rice phloem sap, which indicates that switching of chelators occurs once Fe is loaded onto the phloem. In leaves, Fe re-enters the symplast and is reduced to Fe(II), mainly by the action of FRO proteins and used later for photosynthesis. In Arabidopsis, this process is mediated by an OligoPeptide Transporter 3 (OPT3) family protein and might play an important role in the transfer of both iron and zinc from vegetative organs to seeds (Kim and Guerinot, 2007).
During seed development, plants remobilize and move Fe/Zn from vegetative source organs into seeds. Iron is loaded into seeds as Fe(II) complexes by sequestration into vacuoles by vacuolar iron transporters (VIT1 in Arabidopsis and VIT1/VIT2 in rice) (Kim et al., 2006; Zhang et al., 2012) and into ferritin (Connorton et al., 2017a). The proportion of iron in vacuoles and ferritin differs according to plant species (Zielińska-Dawidziak, 2015). For instance, iron is mainly stored in vacuoles in the aleurone layer of cereal grains such as rice and wheat in the form of Fe-phytate complexes that are notoriously poorly bioavailable. Ferritin is predominantly located in the plastids and constitutes a highly bioavailable source of Fe; thus, high concentrations of ferritin in legume seeds make them a novel alternative dietary iron source (Zhao, 2010).
However, relatively little is understood about the transporters involved in zinc loading in seed. Yet, YSL1 and YSL3 have been implicated in phloem unloading of Zn in seeds of Arabidopsis (Waters et al., 2006; Chu et al., 2010). It is worth noting that the roles of HMA transporters (HMA2 and HMA4) are not exclusive to zinc loading into xylem vessels, but also might be responsible for the efflux of Zn from leaves (Stanton et al., 2022). Members of the cation diffusion facilitator (CDF) family, such as orthologs of metal tolerance proteins (MTPs) and Mg2+/H+ antiporter (MHX) in Arabidopsis, are thought to be involved in zinc loading in developing seeds by sequestering zinc into the vacuole, and subsequently regulates accumulation in the aleurone (Desbrosses-Fonrouge et al., 2005). Besides iron, VIT might be responsible in regulating zinc distribution between the flag leaf and seed tissues (Zhang et al., 2012; Connorton et al., 2017a). Among seed tissues, embryo and aleurone are major sources for zinc storage in cereal grains, but phytate present in these tissues is bound to zinc, resulting in the lower bioavailability of zinc.
Despite iron and/or zinc homeostasis in plants, model plants such as Arabidopsis and food grain crops have improved over the past decades (Kim and Guerinot, 2007; Connorton et al., 2017a; Garcia-Oliveira et al., 2018; Zlobin, 2021; Murgia et al., 2022; Stanton et al., 2022); such information is very limited in tuber and root storage crops. From a biofortification point of view, the genes whose functionality is linked to Fe/Zn metabolism and has been validated in various crops could be utilized in root and tuber crops by either exploring the novel alleles of such genes in the germplasm or employing directly transgenic approaches. For instance, the co-overexpressing A. thaliana iron transporter IRT1 and ferritin FER1 transgenic cassava lines, compared with controls (non-transgenic) under field conditions, showed up to 18 and 10 times higher iron and zinc content, respectively (Narayanan et al., 2019).
Cross-talk between macro- and micronutrient transport and signaling in plants
In their normal life cycle, plants are frequently subjected to either deficiency or imbalance of nutrients, resulting in combined nutrient stresses. Various morphological, physiological, and agronomic studies clearly indicate the existence of mechanisms in plants that co-regulate these stresses. Nonetheless, metal nutrient cross-talk s at the molecular level have only been partly understood so far, and it will still take a long time to comprehensively understand the concept.
It is well established that interaction within or between macro- and micronutrients exists and can be grouped either as additive, antagonistic, or synergistic (Symeonidis and Karataglis, 1992). Antagonistic interactions between macro- and micronutrients (P and Fe, P and Zn) or within micronutrient (Fe and Zn, Fe and Mn, Zn and Cu) homeostasis have been reported at the physiological level. However, cross-talks between the signaling pathways integrating the homeostasis of these different essential mineral nutrients are still scarce.
At the molecular level, some mineral elements (divalent metals) compete for basic transporter proteins that have broad substrate specificity. For instance, IRT1 is the primary root iron transporter but also mediates the transport of Zn2+ and Mn2+, while NRAMPs support the transport of Fe2+ and Mn2+ (Rogers et al., 2000; Grotz and Guerinot, 2006). Similarly, ZIP and CDFs/MTP family proteins have also been reported to efflux Mn2+, Fe2+, and Zn2+ at the cellular level (Gustin et al., 2011). The transcription factor (leFER) that regulates the functions of Fe deficiency signaling was first identified in tomato (Solanum lycopersicum) plant (Ling et al., 2002). Subsequent studies identified FER-LIKE IRON DEFICIENCY INDUCED TRANSCRIPTION FACTOR (FIT) in Arabidopsis as the functional analog of FER, whose activity is regulated by a complex of the basic helix–loop–helix (bHLH) FIT, and at least one of the subgroup Ib bHLH proteins bHLH038, bHLH039, bHLH100, and bHLH101 (Gao and Dubos, 2021). FIT emerged as a central regulatory hub in root cells to steer and adjust the rate of Fe uptake and play an important role in fine-tuning Fe and Zn interactions (Chen et al., 2018b; Schwarz and Bauer, 2020). Recently, it has also been reported that FIT and bHLH Ib TFs, under iron deficiency conditions, do regulate the FRO4 and FRO5 genes involved in copper uptake (Cai et al., 2021).
From a better crop productivity perspective, P is considered one of the crucial macronutrients, but P in the form of Pi can continuously influence the bioavailability and mobility of Fe and Zn in soils (Briat et al., 2015; Xie et al., 2019). The transcription factor PHOSPHATE RESPONSE 1 (PHR1), originally found as a master regulator of P homeostasis in plants [which activates P deficiency response genes including transporters as well as regulatory RNA and proteins such as the micro-RNA miR399, IPS1, and the ubiquitin-conjugating E2 enzyme (PHO2)], also appeared as a regulator of the genes involved in the transport of both macronutrients such as S (sulfate transporters SULTR1;3, SULTR2;1, and SULTR3;4) and micronutrients including Fe (FER1 gene encoding the Fe storage protein ferritin) and Zn (ZIP2 and ZIP4 genes encoding zinc transporters) (Briat et al., 2015). Overall, the accumulation of omics data indicates that complex connections do exist between the various regulatory layers of the mineral nutrient homeostasis that balance the concentration of these essential micronutrients, at both the cellular and systemic levels.
Nutrient profiling, spatial and temporal distribution, and accumulation
Genetic variation
Plant genetic resources are the main source of variation from which researchers can draw functional allelic diversity for breeding cultivars showing a significant increase in nutrients that can bring health benefits. The greater emphasis in yield and uniformity led to the decline in crop diversity and the nutritional quality of food crops (Ortiz-Monasterio et al., 2007; Jacques and Jacques, 2012; Elgueta et al., 2021; Nicholson et al., 2021), whereas global warming may reduce the nutritional quality of food crops (Dwivedi et al., 2013; Scheelbeeka et al., 2018); Soares et al., 2019.
Substantial variation in cultivated gene pools for seed Fe and Zn concentrations in cereal and grain legume crops appears to be available (Tables 1, 2), while root and plantain crops also show sufficient variability for β-carotene (provitamin A) contents (Table 3). Landraces were shown to contain greater amounts of seed Fe and Zn than modern cultivars. To date, many germplasm lines surpass the target values fixed by HarvestPlus (https://www.harvestplus.org). As an example, Russian spring bread wheat germplasm “Novosibirskaya 16”, “Silach”, “Line 4-10-16”, “Element 22”, and “Lutescens 248/01” had seed Zn content in the range of 46 –49 mg kg−1 seed dry weight (SDW) (Shamanin et al., 2021), while the provitamin A content of orange maize landrace from Malawi met the target level (15 μg g−1 SDW) of biofortification (Hwang et al., 2016). Several maize inbreds containing a high amount of β-carotene were also above the HarvestPlus biofortification target (Ashokkumar et al., 2020; Azmach et al., 2021). Yet, and despite these progresses, the environment (E) and genotype × environment (G ×E) interaction are still a major obstacle in breeding for improved seed quality traits, including seed Fe, Zn, and β-carotene (Khokhar et al., 2018; Kumar et al., 2019; Mengesha et al., 2019; Gangashetty et al., 2021; Gasura et al., 2021; Shamanin et al., 2021). A multilocation evaluation will help in identifying the locations that are favorable for the production of nutrient-dense food crops with specific quality attributes and identification of potential lines for varietal release.
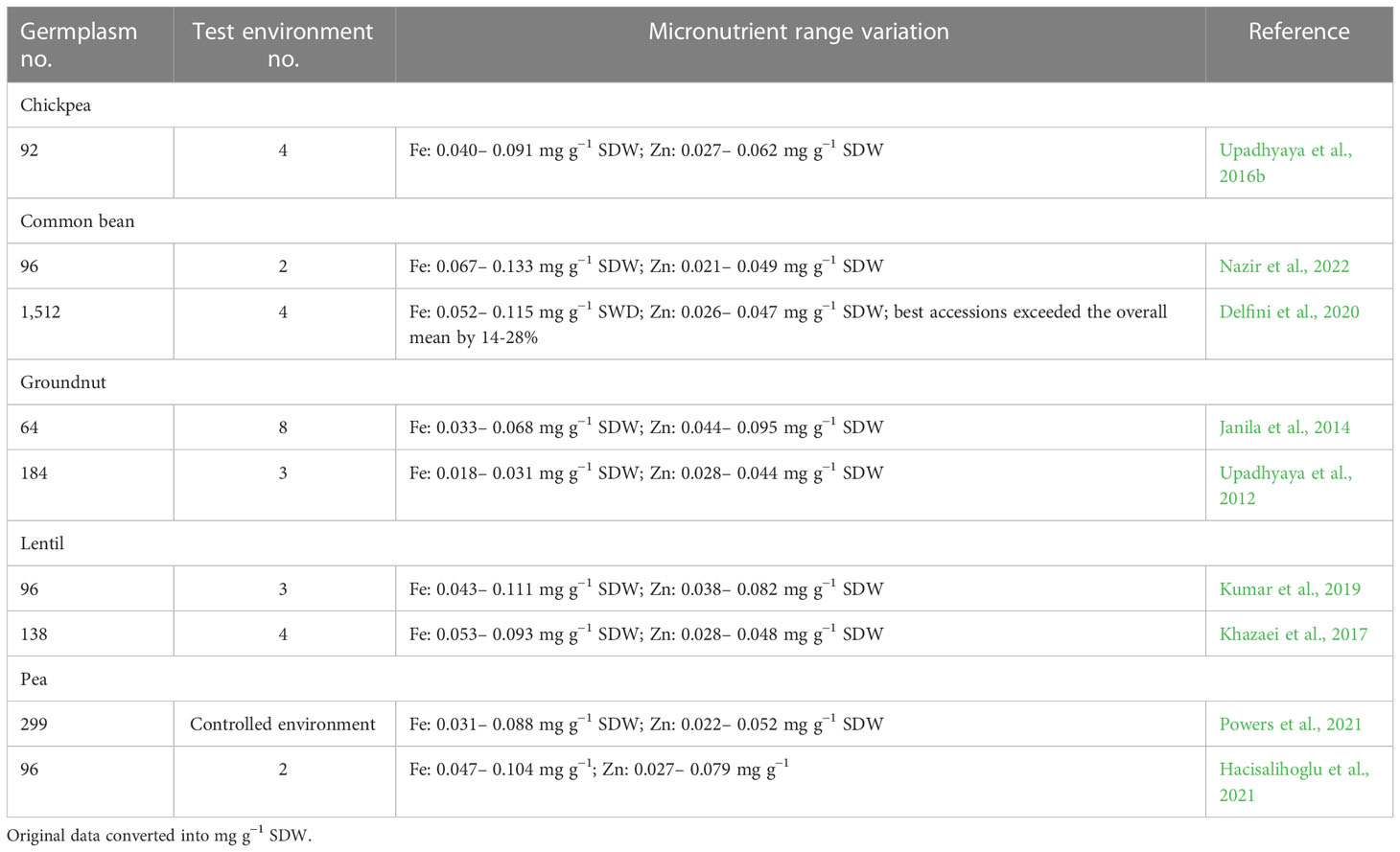
Table 2 Genetic variation for seed iron (Fe) and zinc (Zn) contents among food legume crop germplasm.
The development of mutant genetic resources, by either physical or chemical treatments, or targeted mutagenesis (TILLING, CASPR/Cas9 system), will allow the rapid detection of mutations in any gene of a genome (Holme et al., 2019). Knowing that the aleurone layer is the most nutritious part of cereal seeds, it is therefore desirable to seek targets to this seed portion to enhance the nutritional value of seeds. Concerning this, a rice mutant thick aleurone 2-1 (ta2-1) with thickened aleurone [an average of 4.8 aleurone cell layers and up to 10 cell layers in some region of the seed in comparison to single-cell layer aleurone in the wild type (WT)] was shown to enhance the levels of multiple micronutrients simultaneously. Yet, the increases of aleurone-associated nutrients may not be proportional to the increases in the aleurone thickness; as minerals uptake, transport and loading capacity possibly affect their final content in aleurone (Liu et al., 2018), or larger sink (i.e., lower capacity in extra aleurone to synthesize or store nutrients compared to WT aleurone or the aleurone development) does not synchronize with the nutrient accumulation during seed development required for multiple micronutrients (Yu et al., 2021). Recently, a mutant line with a thickened aleurone layer, associated with higher rice bran nutritional value, was isolated in rice cultivar “Mizuhochikara” (Nguyen et al., 2022).
Genetically stable (M7) mutants in spring wheat broaden the genetic variation for seed nutrients, with Fe and Zn content ranging from 40.9 to 89.0 and 22.2 to 89.6 mg kg−1 SDW, respectively. The mutants also varied in Phy : Fe (1.40–5.32) and Phy : Zn (1.78–11.78) molar ratio. The higher the molar ratio, the greater the bioavailable levels of micronutrients (Kenzhebayeva et al., 2019). Targeting Induced Local Lesions in Genomes (TILLING) is a powerful strategy to introduce knockout mutations on lcyE gene (McCallum et al., 2000). Numerous knockout mutants per gene in protein coding regions and their sequence data are publicly available to unlock previously hidden variation in durum and bread wheats (Krasileva et al., 2017). TILLING-induced null mutant in wheat revealed strong reduction in the expression of IcyE but with pleiotropic effects on β-ring hydroxylase enzyme acting downstream in the pathway. Biochemical profiling relative to WT had ~75% increased β-carotene in the grains of wheat mutant line (Sestili et al., 2019). In durum wheat (Triticum durum), the combination of lycopene ε-cyclase and β-carotene hydroxylase 2 homoeologs significantly increased the β-carotene accumulation in mutant lines (Yu et al., 2022), thus proving mutational breeding as a powerful non-transgenic approach to enrich β-carotene in wheat seeds and possibly in other crops.
Although crop wild relatives were mainly exploited for host plant resistance to stress (Mammadov et al., 2018), a few wild relatives in barley, chickpea, rice, and sorghum were additionally identified as a rich source of seed Fe and Zn (Neelamraju et al., 2012; Abdelhalim et al., 2019; Wiegmann et al., 2019; Sharma S. et al., 2021). The examples include high seed Fe and Zn in Oryza nivara and O. rufipogon in rice (Neelamraju et al., 2012) and introgressed lines involving wild barley with more than 50% higher Fe and Zn content over barley cultivar, Barke (Wiegmann et al., 2019). Sorghum wild relatives, “Almahkara” and “Abusabiba”, respectively, had in the seeds the highest concentrations of total and bioavailable iron, 3.17 mg 100 g−1 and 92.8 mg 100 g−1, while another wild sorghum “Adar Umbatikh” grain had a higher zinc content (Abdelhalim et al., 2019).
Einkorn (T. monococcum) wheat, the wild emmer (T. dicoccoides), diploid progenitors of hexaploid wheat (Aegilops tauschii), T. spelta, T. polonicum, and wheat landraces are the most promising sources of seed Fe and Zn (Cakmak et al., 2004; Chhuneja et al., 2006; Sharma V. et al., 2021). Resynthesized hexaploid wheat originating from crosses between T. durum or T. dicoccum and diverse sources of Ae. tauschii offer a large pool of variability for agronomic as well nutritional traits (Velu et al., 2016), while a few derivatives such as “WB02” and “Zinc-Shakti” have 20% to 40% higher Zn than local controls (Singh and Velu, 2017). Several lines in the wheat genetic background of “Pavon 76”, originating from crosses involving rye (Secale cereal) translocation and Ae Aegilops species, are reported to contain greater Fe and Zn compared to controls (Velu et al., 2019). The wheat tetraploid (2n = 4× = 28 chromosomes) species Triticum polonicum, grown on a limited acreage in Spain, southern Italy, Algeria, Ethiopia, and warm regions of Asia, is characterized by the significantly highest seed Fe (39.1 mg kg−1) and Zn (49.5 mg kg−1) content (Bieńkowska et al., 2019).
In many countries, milled rice is the predominant consumed staple food. However, milling substantially reduces nutrients in the consumed grains, including Fe and Zn. Research previously done indicates multifold differences in Fe and Zn content between brown rice (BR) and milled rice (MR) among diverse germplasm (Bollinedi et al., 2020; Madhu Babu et al., 2020). Such germplasm is an ideal genetic resource to utilize toward developing Fe and Zn biofortified rice cultivars. The bioavailability of Fe is an issue that should be factored in while developing Fe biofortified cultivars. Yet, increasing Fe content in wheat seeds seems to not correlate with higher Fe bioavailability and the underlying genetic regions controlling Fe content not found, yet, to colocalize with increased Fe absorption. In addition, phytate content also does not correlate with Fe bioavailability, leading to the conclusion that phytate binding may be insufficient to explain the lack of correlation between Fe bioavailability and Fe content (Wright et al., 2021). All these observations give hope that Fe content and bioavailability are independent and feasible to integrate the two traits simultaneously. This opens doors for more research on exploring such variability in the germplasm pools to identify genotypes with high nutrient content and presenting higher levels of nutrient bioavailability.
Diversity assessment
Assessing population structure and diversity among nutrient-dense germplasm offers researchers the opportunity to involve genetically diverse and nutritionally rich germplasm resources in crop breeding and genetics. A recent study involving advanced pearl millet breeding lines with threefold variation in panicle length, panicle girth, and 1,000-seed weight and large differences in seed Fe (35–116 mg kg−1 SDW) and Zn (21–80 mg kg−1 SDW) grouped 281 lines into 10 clusters, with cluster 5 showing the highest mean Fe, Zn, and 1,000-seed weight (Pujar et al., 2020). SNP-based diversity assessment differentiated 46 provitamin A maize inbred lines into two distinct clusters, with 0.60 average pairwise genetic distance and 0.359 average gene diversity (Kondwakwenda et al., 2020). The population structure of another set of 63 maize inbred lines bred for high levels of provitamin A revealed seven clusters with good agreement with Neighbor Joining clustering and was fairly correlated with pedigree and breeding origin (Sserumaga et al., 2019). The SSR-based diversity analysis of 24 maize inbred lines having wide variation in carotenoids, including β-carotene, containing crtRB1 gene unfolded wide gene diversity (0.08 to 0.79) and dissimilarity coefficient (0.28 to 0.84) and grouped the inbreds into three distinct clusters (Duo et al., 2021). Phenotypic and genotypic diversity assessment of provitamin A cassava germplasm grouped 188 accessions into six and nine distinct clusters, respectively (Kamanda et al., 2020). The key message is genetically diverse nutrient-dense and agronomically superior inbred lines should be used to harness heterosis for seed nutritional traits when developing hybrids.
Spatial and temporal distribution and accumulation of Fe and Zn
A large part of the population in less favored parts of the world is dependent on the consumption of grain-based food products for their calorie and nutrient need. Understanding the spatial distribution and localization of minerals within the edible parts of plant products may contribute to their nutritional improvement. A genetically biofortified wheat had a higher concentration of Fe and Zn in the seed. The location of the minerals is similar in both biofortified and non-biofortified wheat. Fe is abundant in the aleurone layer, while Zn is highly concentrated in the embryo. Developing seeds show a decreasing trend in concentration from the proximal to the distal ends. Phytic acid, present in these tissues, binds minerals as phytate. Minerals bound to phytate have poor bioavailability (Wan et al., 2022). Fe and Zn in maize primarily accumulate in the scutellum of the embryo during early seed development, with trace amounts detected in the aleurone layer at mature seed stage. P also accumulated in the scutellum, but no specific pattern was detected with Fe and Zn accumulation; Fe and Zn accumulated linearly in maize scutellum (Cheah et al., 2019).
Assessing the spatial patterns of Fe and Zn accumulation during grain development in barley lines with contrasting Zn concentrations revealed a gradual decrease in Zn concentrations from the aleurone to the endosperm, while Fe and P decreased sharply. Fe colocalized with P in the aleurone, and Zn with sulfur in the sub-aleurone. Thus, endosperm storage capacity largely influences differences in seed Zn concentrations (Detterbeck et al., 2020). A study of spatial distribution of minerals between and within the maternal and filial tissues in diverse wheat genotypes (cultivar, landrace, and wild species) revealed aleurone and scutellum as the major storage tissues for macro- and micronutrients. The genotypes showed distinct elemental distribution patterns, grouped into four distinct clusters. Wild relative (A. kotschyi) and landrace (IITR26) accumulated more Zn and Fe in scutellum and aleurone than wheat cultivars (WH291 and WL711). Other nutrients also showed a distinct distribution pattern (Singh et al., 2014).
In an experiment involving high yielding wheat cultivars but with contrasting seed Zn concentrations, Guo et al. (2021) noted 103%, 76%, 64%, 50%, and 33% higher Zn concentrations in the crease region, scutellum, endosperm, aleurone layer, and embryonic axis, respectively. Zn colocalized with P in the aleurone layer and the scutellum, but less colocalization of Zn with P and a much lower concentration of P:Zn ratio in the high-Zn cultivar. Thus, a lower proportion of P:Zn ratio in the high-Zn cultivar suggests the feasibility of combining high grain yield with high Zn content and high bioavailability.
Iron trafficking in plants is key to improving the nutritional quality of food crops. Sheraz et al. (2021) used iron-57 (57Fe) isotope labeling and NanoSIMS to visualize iron translocation between tissues and within cells in immature wheat seed between a TaVIT2 overexpressing line and WT. Fe transports from maternal tissues to the embryo through the different cell types and storage in vacuoles, with most of the Fe detected in intracellular bodies, indicating symplastic rather than apoplastic transport. The presence of highly enriched 57Fe in aleurone cells suggests iron being delivered to phytate globoids. This endosperm-specific expression of TaVIT2 may have relevance to developing new biofortification strategies in cereal crops.
How does Fe accumulate in embryo in Brassica species seeds? Perls/DAB staining is effective to localize iron at the cellular and subcellular levels. Brassica seed species, except for Vasconcellea pubescens, which accumulate and store Fe in cortex cell, accumulate Fe in nuclei in specific stages of embryo maturation before it is localized in vacuoles of cells surrounding provasculature in mature seeds, specific to Brassicales, not widely found in other Eudicotyledoneae (Ibeas et al., 2017; Ibeas et al., 2018). Surely, understanding where and how Fe and Zn accumulate in seed crops may assist in the development of biofortified staple crops with increased bioavailability of micronutrients.
Trait inheritance
Functionally characterized genes associated with Fe and Zn
Functionally characterized genes are those whose genetic functions have been determined either through transgenesis, RNAi approach, mutagenesis, or targeted gene editing (Alberts et al., 2002; Agrawal et al., 2003; Kamburova et al., 2017).
Cereal endosperm is a major source of dietary energy. It is well known that milling substantially reduces the nutritional quality of milled seeds or white flour. Enhancing the nutritional value of endosperm though biotechnological interventions may provide nutrient-dense seed or flour. Many functionally characterized genes are associated with seed Fe and Zn in cereals (Table 4). For example, barley transgenic lines containing HvMTP1 under the control of endosperm-specific promoter showed significant enhancement of Zn in the endosperm (Menguer et al., 2018). Transgenic rice expressing three (AtNRAMP3, AtNAS1, and PvFER) or two (AtNRAMP3 and PvFER) genes under the control of the endosperm-specific promoter increased Fe and Zn close to the recommended levels, with a maximum in milled grains with three genes (Wu et al., 2019). Wheat plants expressing OsNAS2 accumulated greater concentrations of Fe and Zn in endosperm and crease tissues (Beasley et al., 2019), while those expressing TaVIT2 enhanced Fe more than twofold in white flour fraction without an increase in phytate, with more bioavailable Fe (Connorton et al., 2017b).
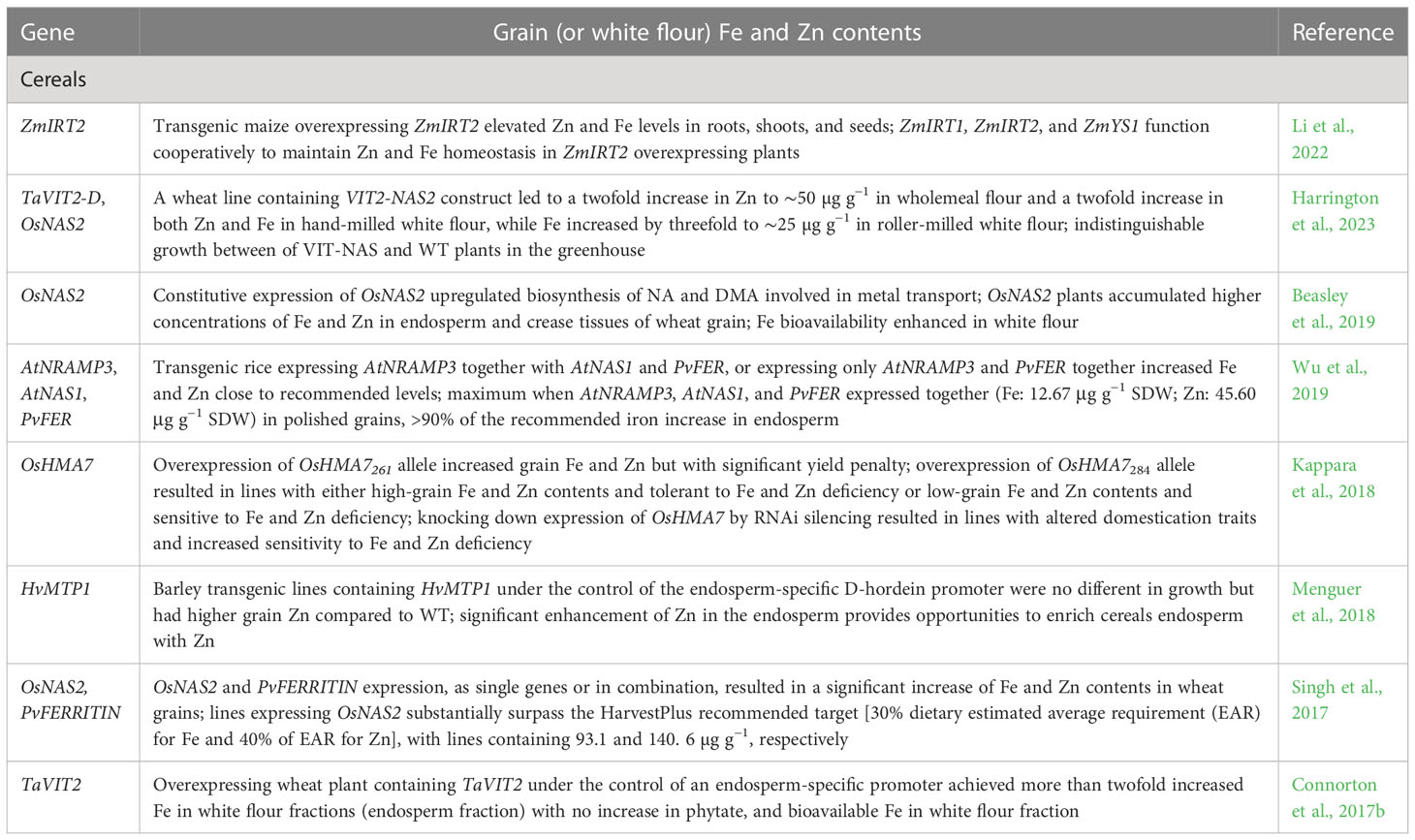
Table 4 Functionally characterized genes associated with increased seed Fe and Zn contents in cereal and food legume crops.
Quantitative trait loci and candidate genes associated with seed Fe and Zn
Advances in next-generation sequencing including genotype-by-sequencing (GBS) and development on bioinformatics resources to process large data sets, both genotyping and phenotyping, to store, retrieve, analyze, interpret, and share have facilitated researchers to identify abundant SNP-based markers, assess marker–trait associations (MTAs) through genome-wide association studies, develop high-density genetic maps, and fine-map to clone and functionally characterize genes. Diversity germplasm panels and advanced generations’ recombinant inbred lines (RILs) including those from multi-parent advanced generation inter-cross (MAGIC) and nested association mapping (NAM) panels in most crops serve as enduring resources to genetic analysis of complex traits (Perea et al., 2016; Arrones et al., 2020; Jan van Dijk et al., 2021; Wang et al., 2021; Zhao et al., 2021).
Numerous SNP-based significant MTAs, quantitative trait loci (QTLs), and candidate genes associated with seed Fe and Zn content are reported in cereal and legume crops, and a few QTL with major effects (Tables 5, 6). SNP was physically located within genes involved in seed Fe and Zn homeostasis (Thabet et al., 2022) or SNP in close vicinity to two yellow stripe-like (YSL) genes was associated with grain Zn (Detterbeck et al., 2019) in barley. QTL hotspots simultaneously affected multiple grain nutrients, and an exotic allele linked to HvGA20ox2 significantly increased multiple grain elements with no yield penalty in maize (Herzig et al., 2019), while in pearl millet, candidate genes related to Fe and Zn metabolism correlated with known QTL regions for grain Fe and Zn contents (Mahendrakar et al., 2020). qGZn9a linked to high-grain Zn in Oryza meridionalis also adversely affects fertility levels. One of the eight candidate genes in qGZn9a specifically expressed in the developing anther and possibly regulated anther dehiscence, which suggests that a balancing selection is needed to ensure simultaneous improvement in rice (Ogasawara et al., 2021), while haplotype-based association mapping involving 65 genes related to Zn responses on diverse germplasm panels and MAGIC populations revealed diversity panel- or population- specific candidate genes associated with high seed Zn content (Liu et al., 2021a). Many of the candidate genes associated with Fe and Zn uptake, transport, storage, and regulation in wheat were orthologs of known Arabidopsis and rice genes related to Fe and Zn homeostasis (Tong et al., 2022), while SNPs mapped on chromosome 1A and 2A in wheat genome significantly associated with seed Fe and Zn content had no adverse effects on seed weight (Liu et al., 2021b). CaqFe4.4, CaqFe4.5, and CaqZn4.1 associated with seed Fe and Zn collocated in the “QTL-hotspot” region on CaLG04 harboring several drought tolerance QTL, adding advantage to effect selection for greater Fe and Zn content combined with drought tolerance in chickpea (Sab et al., 2020). A critical follow-up step would be the functional validation of such putative candidate genes prior to these genes being directly deployed in crop improvement programs.
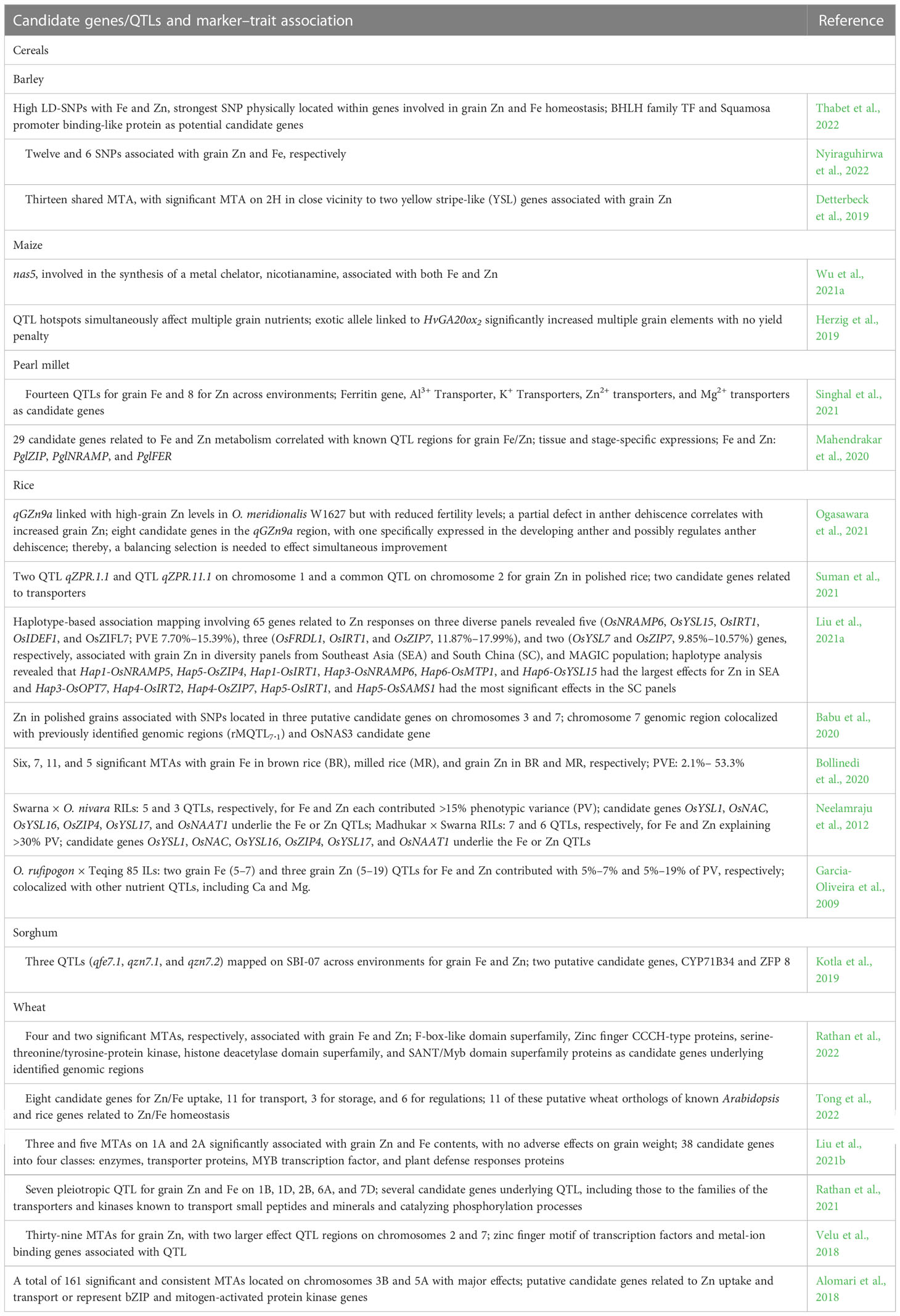
Table 5 Candidate genes/QTLs and marker–trait association (MTA) SNPs associated with increased grain Fe and Zn contents in cereal crops.
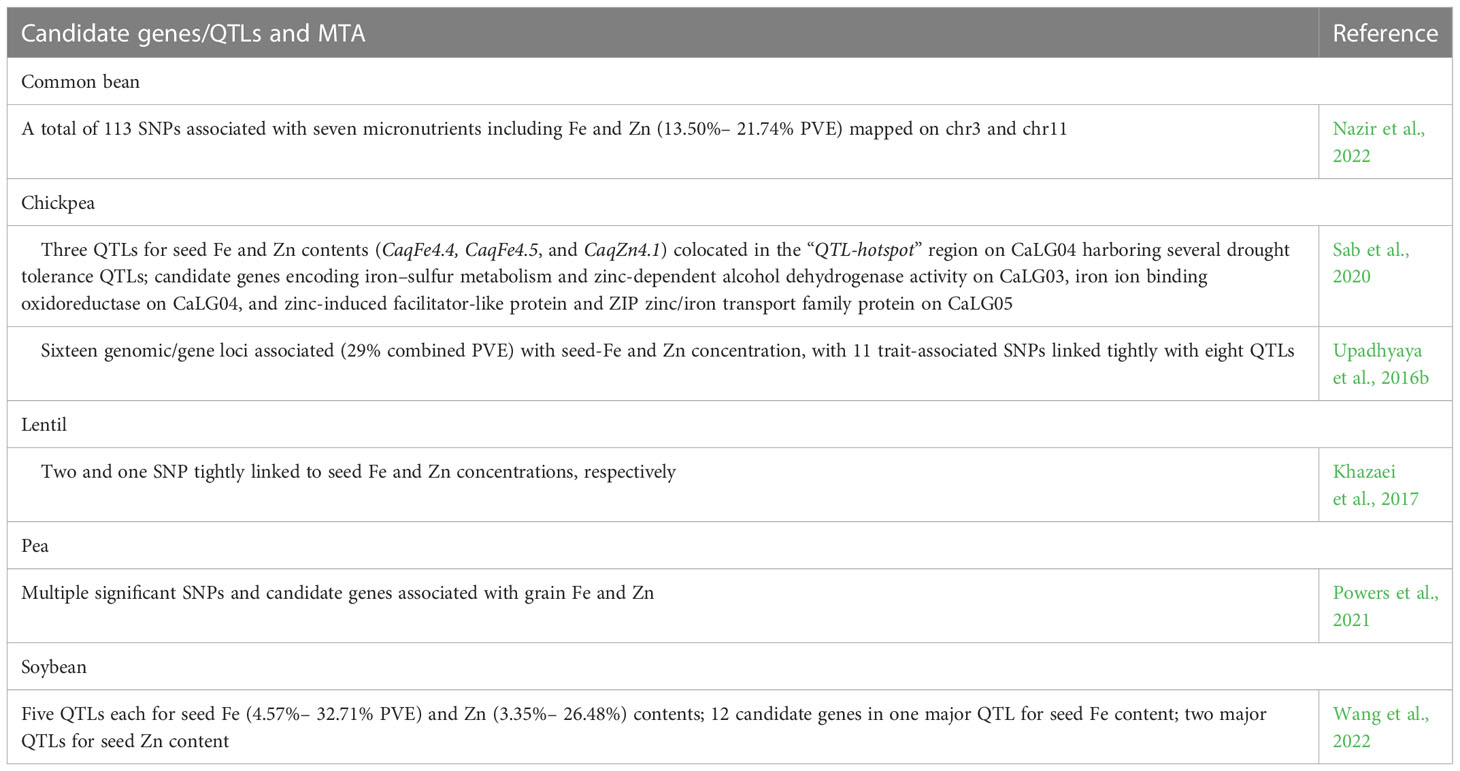
Table 6 Candidate genes/QTLs and marker–trait association (MTA) SNPs associated with increased seed Fe and Zn contents in food legume crops.
Carotenoid biosynthesis in plants
Carotenoids are synthesized in plant plastids and undergo a series of enzymatic reactions to produce carotenoids with varying color and composition that accumulate in a specialized organelle, the amyloplast, the major site for starch synthesis and storage in seed endosperm (Sun et al., 2018). Provitamin A, the intermediate product of biosynthetic pathway characterized by un-hydroxylated β-rings, is converted to retinol (or vitamin A) in the body via oxidative cleavage and storage in the lever (Stahl and Sies, 2005). Two retinyl groups of “β-carotene” and one retinyl group of “β-cryptoxanthin” and “α-carotene” are the most abundant provitamin A carotenoids in plant-based foods (Cazzonelli and Pogson, 2010). Carotenoid biosynthesis pathways and genes are highly conserved across plants, including the model plant Arabidopsis (Ruiz-Sola and Rodríguez-Concepción, 2012; Owens et al., 2014).
Phenotypic diversity for carotenoid profiles is extensively characterized in maize (Table 3). Yellow- and orange-colored seeds are the source of carotenoids. The first step in carotenoid biosynthesis is the five-carbon central intermediate isopentenyl pyrophosphate (IPP) produced by methyl-D-erythritol-4-phosphate (MEP) in the plastid. The condensation of two 20-carbon geranylgeranyl diphosphate (GDDP) molecules by the phytase synthase enzyme forms phytoene. Phytoene is sequentially desaturated and isomerized to produce lycopene. Lycopene is cyclized with two β-rings to form β-carotene or with one β-ring and one α-ring to form α-carotene. α-carotenes and β-carotenes are hydroxylated twice to form, respectively, lutein and zeaxanthin. Zeaxanthin is then modified to yield violaxanthin and neoxanthin (Diepenbrock et al., 2021). Eleven out of 44 QTLs were resolved to individual genes (vp5, lut1, im1, zep1, psy1, dxs2, lcyE, dxs3, ccd1, crtRB5, and crtRB1) regulating IPP biosynthesis and carotenoid biosynthesis and degradation. Six of these were correlated expression and effect QTLs (ceeQTLs), with strong correlation between RNA-seq expression abundances and QTL allelic effect estimates across six stages of seed development and largest PVE (%). Three to five loci captured the bulk of genetic variation for each trait. Most of these ceeQTLs had strongly correlated QTL allelic effect estimates across multiple carotenoid traits. A few genes (vp5, im1, dxs2, and dxs3) in the IP pathway and early steps of the carotenoid pathway that reside upstream of lycopene cyclization, not previously associated with natural variation in maize seed carotenoids (Harjes et al., 2008; Azmach et al., 2018; Baseggio et al., 2020; Diepenbrock et al., 2021), are the potential new targets in maize breeding. Thus, an in-depth genome-level understanding of the genetic and molecular control of carotenoids may provide a roadmap to accelerate breeding for provitamin A and other carotenoids in maize seed (Diepenbrock et al., 2021). An earlier study reported eQTLs for 4 of the 11 genes (lcyE, crtRB1, ccd1, and vp5), 2 of which (lcyE and crtRB1) are represented in the six ceeQTLs (Diepenbrock et al., 2021), and lcyE and crtRB1 expression significantly correlated with provitamin A concentrations (Fu et al., 2013).
Global efforts to breed nutrient-dense crops
Cross breeding and genomic-assisted breeding
Biofortification has been pursued actively in crop improvement programs of the Global South (Asia, 2021; Figure 1) in the last two decades. To demonstrate that biofortification breeding is successful in product delivery through regular testing and release networks, a strong breeding strategy was established by CGIAR HarvestPlus based on three stage-gate tasks such as discovery, development, and dissemination (Bouis et al., 2011; Saltzman et al., 2014; Bouis and Saltzman, 2017; Pfeiffer et al., 2018; Govindaraj et al., 2019).
Discovery is an initial stage to assess the biofortification breeding potential of each crop. The essential component includes identification of the target population and staple food (crop) consumption, establishing a breeding baseline and nutrient target levels, and screening and characterizing germplasm for target nutrients. The outcomes of the discovery phase will guide the product development stage for target nutrients in each crop. The essential stage is for developing biofortified cultivars with target levels. This development stage includes breeding pipelines, managing the genotype × environment (G × E) interaction on target nutrients, nutrition bioavailability assessment, and efficacy research involving human feeding trials. The delivery stage is for product release and assessing a farmer’s field performance and consumer preferences. The investment made in biofortification trait breeding and product development will be realized based on the final product release and incremental increase in the target minerals or vitamins over the regular or popular cultivars. Hence, key components are the release of biofortified products, dissemination, and market and consumer acceptance. The impact of biofortified crops can be further assessed by measuring the improved nutritional status of the target population after regular human consumption of their derived food.
The lack of precision phenotyping methods and accessibility for a variety of crops made the coordinated development of genomic markers for nutritional traits difficult. The preliminary list of usable DNA-validated markers is available elsewhere (https://excellenceinbreeding.org/toolbox/tools/kasp-low-density-genotyping-platform). This information supports the existing information about biofortified germplasm, varietal development, and releases (Andersson et al., 2017; Virk et al., 2021). Globally, more than 420 mineral- and vitamin-rich cultivars have been released to date (Figure 2). About 23 Zn-rich wheat cultivars were released in South Asia, with 2.2 million households currently growing these cultivars. Fifteen Zn rice cultivars are being cultivated by 2.4 million households across South Asia and Indonesia. For Zn maize, 11 cultivars are grown by 1 million in Colombia and Central America. Iron bean cultivars are the second highest in number (75) and reached about 3 million households in the sub-Saharan Africa and Latin America. About 10 iron pearl millet cultivars were released in Africa and India with 1 million households growing them nowadays. More than 20 provitamin A cassava cultivars were released and reached 2.6 million people in sub-Saharan Africa and Brazil. Sweet potato has the highest number of biofortified released cultivars in the Global South, reaching about 1.3 million people. The different cultivars reaching households may vary owing to its proportional farm adoption of nutrition-rich cultivars besides regular varieties and per-capita consumption in target populations (Figure 3).
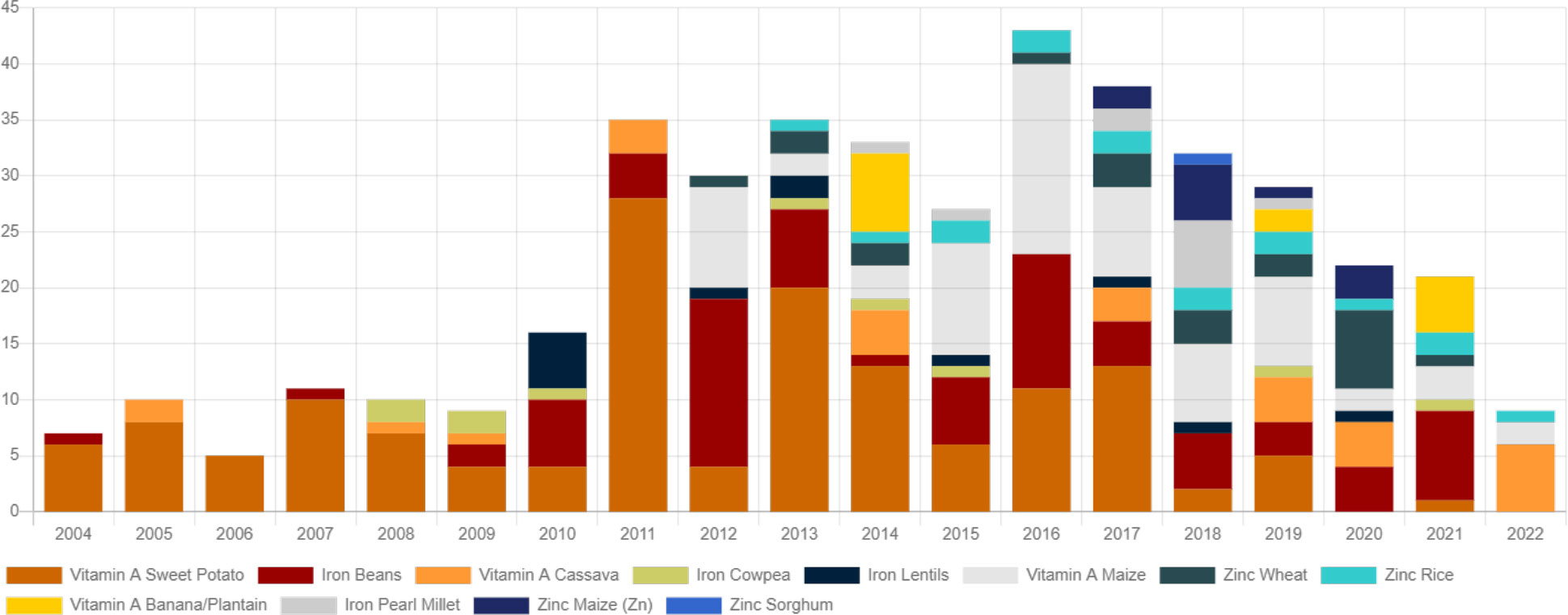
Figure 2 Nutrient-rich bred cultivars released in different crops for the last two decades (source: CGIAR HarvestPlus).
Bioavailability evidence for iron, zinc, and provitamin A
Bioavailability is the portion of a nutrient used or stored by the human body after the target food’s digestion and absorption. This is a very complex trait to measure because many factors affect this process including diet matrix, individual food habits, and other communicable and non-communicable diseases that can play a key role in nutrient absorption. For instance, fat in the food or meal improves provitamin A carotenoid absorption, while disease inhibits its absorption. The degree of specific nutrient absorption can intensely vary depending on the type or form of nutrients. For example, there are two forms of iron in diets, i.e., heme (in animal-derived food) and non-heme (in plant-derived food). The last form of Fe was present in nutrient-rich crops and biofortified cultivars. Non-heme Fe in grains potentially brings other possible inhibitors such as phytic acid, tannins, and polyphenols, which are found in cereal and legumes. They can affect Fe absorption besides having other biological benefits (Dasa and Tilahun, 2018). Hence, regular consumption of biofortified cultivars on nutrition and health outcomes is required to systematically assess the impact of consuming nutrient-dense crops. In such an assessment, the nutritional benefits of the given nutrient-rich cultivars of a given crop are always compared with a regular (low nutrient) cultivar. Several independent bioavailability studies were conducted in bean, cassava, maize, pearl millet, rice, sweet potato, and wheat for Fe, Zn, and provitamin A (PVA). Rigorous bioavailability and efficacy research provides evidence that additional Fe, Zn, and PVA in biofortified crops brought public health benefits (Virk et al., 2021). Figure 4 explains the potential target bioavailability in nutrient-rich crop cultivars to meet the recommended daily allowance (RDA) that has measurable human health and nutritional impacts. To date, about 15 bioavailability and efficacy randomized intervention trials were published (CAST, 2020). Briefly, Fe-rich pearl millet and bean cultivars contributed up to 80% of the daily requirement, while 40%, 50%, and 70% of daily Zn could be provided by Zn-rich maize, rice, and wheat, respectively. For PVA crops, up to 50% daily requirement could be met by consuming orange maize and 100% could be met from cassava and orange flesh sweet potato (Birol et al., 2021).
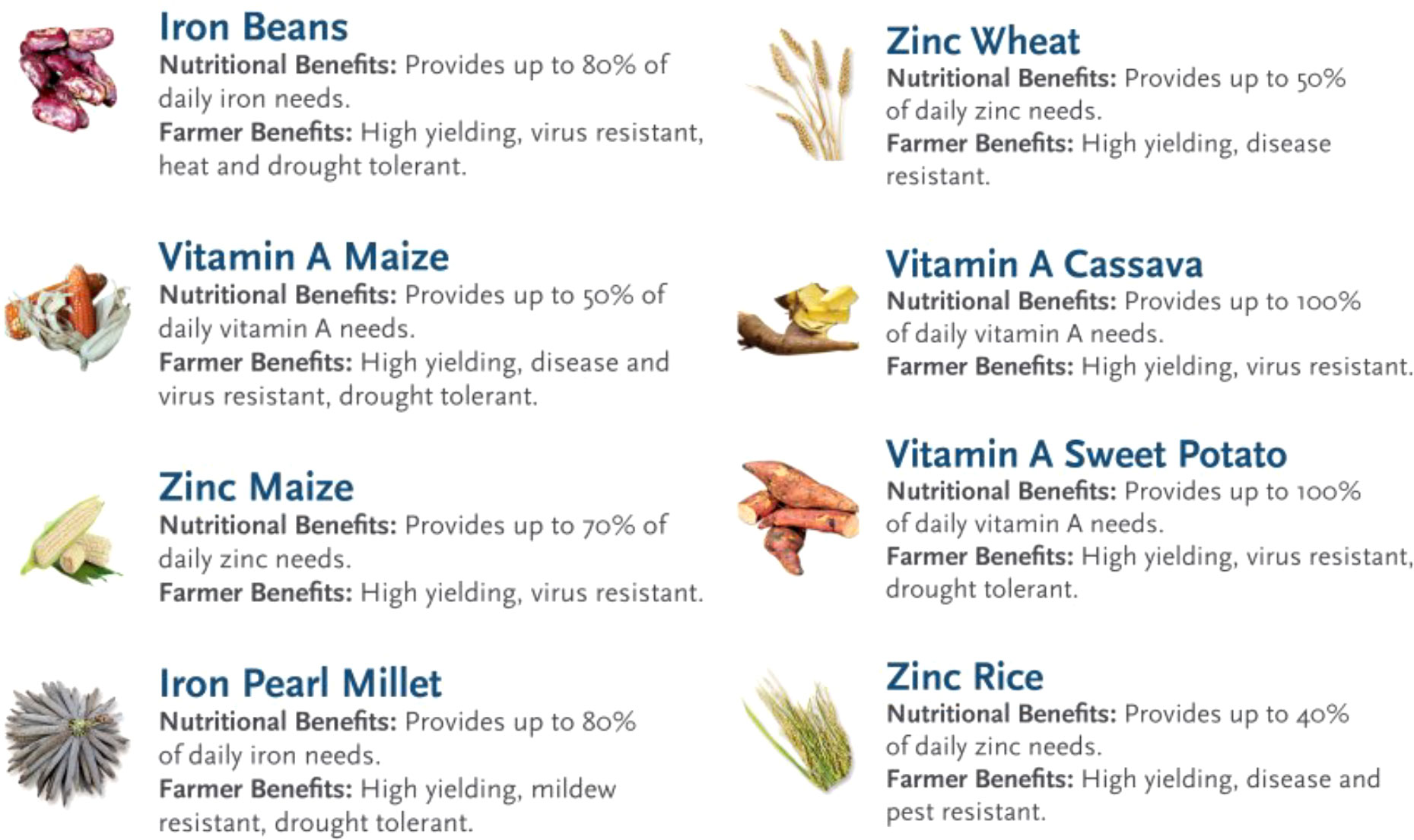
Figure 4 Detail of the nutrient-rich crops and their bioavailability potential and key farmer-preferred traits (source: CGIAR HarvestPlus).
Consuming Fe-rich beans for at least 4 months significantly enhanced the Fe levels (hemoglobin and total body Fe) among adolescents in Rwanda (Haas et al., 2016). Similar Fe improvement was noticed among children in Mexico after 6 months of consuming Fe beans (Finkelstein et al., 2019a; Finkelstein et al., 2019b). In India, students (12–16 years old) with Fe deficiency at the beginning of the study fed with Fe-rich pearl millet diet significantly improved iron level after 4 months compared with control pearl millet diet; thus, feeding Fe-rich pearl millet improves iron level in school-aged children (Finkelstein et al., 2015). Fe-rich pearl millet and bean cultivars also enhanced physical and cognizant activities among fed students compared to other cultivars of the same crops (Murray-Kolb, 2013; Murray-Kolb et al., 2017; Scott et al., 2018). Elsewhere, Fe bioavailability was comprehensively reviewed (La Frano et al., 2013; La Frano et al., 2014; Boy et al., 2017). Zn absorption from flour derived from Zn-wheat cultivar was 80% of the control post-harvest fortified Zn flour among healthy adult women (Signorell et al., 2019). Another study indicated that consuming biofortified cultivar Zn led to a 25% increase compared to using post-harvest fortified wheat flour for Zn (CAST, 2020). Similarly, it was estimated that 8% to 20% of the PVA in PVA-rich crops (cassava, maize, and sweet potato) are bioavailable.
Genetically modified Fe- and Zn-biofortified crops
High phytate content in the grains is a major barrier to Fe biofortification. Transgenic maize carrying endosperm-specific overexpression of soybean ferritin gene in the genetic background of low-phytate (lpa1-1) mutant accumulated up to 70 μg g−1 seed Fe and more than twofold improvement in bioavailable Fe. The level of bioavailable seed Fe greatly exceeded and closely approached values recommended to have a nutritional impact on target populations. Moreover, high-iron lpa1-1 seeds relative to WT seeds showed a higher germination rate and seedling vigor, a useful genetic resource in maize breeding (Aluru et al., 2011). Low-phytate mutants, with some exceptions (Bregitzer and Raboy, 2006; Yuan et al., 2007; Campion et al., 2009), show lower seed germination and seedling vigor, and yield low compared to WT (Meis et al., 2003; Pilu et al., 2003; Oltmans et al., 2005; Shi et al., 2007).
Field evaluation of genetically modified (GM) rice event (NASFer-274) containing OsNAS2 and SferH-1 genes and evaluated in two countries showed 15 μg g−1 Fe and 45.7 μg g−1 Zn in polished grain, which exceeded HarvestPlus’ breeding target of 13 μg g−1 Fe and 28 μg g−1 Zn (Bouis et al., 2011), without any yield penalty or altered seed quality and trait remaining stable across genetic backgrounds (Trijatmiko et al., 2016). Fe and Zn content in polished T1 seeds from transgenic rice overexpressing HvNAS1 increased by more than threefold and by twofold, respectively. Fe and Zn content also increased in both polished and brown T2 seeds (Masuda et al., 2009). Bioavailability of micronutrients is an issue for biofortification programs. Transgenic rice overexpressing OsNAS1 fused to a glutelin promoter increased Fe in brown and milled rice seeds, with twice more bioavailable Fe than WT seeds (Zheng et al., 2010). Transgenic rice expressing soybean ferritin gene under the control of the glutelin promoter, relative to WT, has increased Fe and Zn content in the polished grain in an elite indica line (Vasconcelos et al., 2003).
Genetically modified “provitamin A” biofortified crops
Grain crops
Transgenic approaches have been used as tools to complement conventional breeding techniques to enhance the nutritional quality of food crops. Overexpression of crtB and crtl under the control of an endosperm-specific promoter increased total carotenoids by up to 34-fold with a substantial accumulation of β-carotene in the maize endosperm, and high β-carotene transgenic events were stable across generations (Aluru et al., 2008). Overexpression of ibOr gene in maize inbred lines (H145 and H95) under the control of a seed-specific promoter significantly increased total carotenoid and β-carotene. The total carotenoid and β-carotene content in H145-IbOr.10 increased up to 10.36- and 15.11-fold, respectively, compared to the WT (H145-WT), while in H95-IbOr.6 relative to WT (H95-WT), total carotenoids and β-carotene respectively increased by 5.58- and 7.63-fold (Tran et al., 2017). Simultaneous modification of three separate metabolic pathways resulted in transgenic maize’s 169-, 6-, and 2-fold increase in the amount of β-carotene, ascorbate, and folate, respectively, and the levels of engineered vitamins remained stable at least through to the T3 homozygous generation (Naqvi et al., 2009).
Rice seed, unlike maize, is devoid of β-carotene. Transgenic “Golden Rice” was bred by introducing Zmpsy1 and crtl into Kaybonnet, an US temperate japonica rice (Ye et al., 2000; Paine et al., 2005). Thereafter, the “Golden Rice” (GR2E) characteristic was bred into Asian cultivars via marker-assisted backcross breeding (Mallikarjuna Swamy et al., 2021). The milled rice from GR2E contributed up to 89%–113% and 57%–99% of the estimated average requirement for vitamin A for preschool children in Bangladesh and the Philippines, respectively, according to efficacy trials (Mallikarjuna Swamy et al., 2019). A few Asian countries have already released Bt brinjal (eggplant, Solanum melongena) and GM maize for commercial production (Shelton et al., 2018; https://allianceforscience.cornell.edu/blog/2019/01/gmo-corn-transforming-farmers-lives-philippines/). The Philippine government has approved the release of provitamin A-rich (β-carotene) Golden Rice cultivar in their country (Steur et al., 2022; https://www.irri.org/news-and-events/news/philippines-becomes-first-country-approve-nutrient-enriched-golden-rice). “Golden Rice” has been judged as safe to eat by the US Food and Drug Administration (Owens, 2018).
Transgenic sorghum lines containing provitamin A genes (PSY1 + CRTI genes, +/− CRTB gene) and vitamin E (HGGT gene) from different genetic backgrounds revealed significantly greater provitamin A content (5.9–28.6 mg g−1 FW) compared to their respective null segregants (0.4 –1.2 mg g−1 FW) or WT (0.73 mg g−1 FW). A general increase in vitamin E content, 14.9 –36.2 mg g−1 FW in transgenic versus 14.2–32.4 mg g−1 FW in null/WT, was also noted. Fe and Zn accumulation among provitamin A biofortified sorghum events varied from 27.65–63.59 mg g−1 FW to 28.44–48.85 mg g−1 FW, respectively, with the highest levels achieved in the sorghum event, Tx430. The corresponding increase in vitamin E levels improved provitamin A stability over storage (Debelo et al., 2020). An earlier report also revealed that the HGGT gene stacked with carotenoid biosynthesis genes mitigated oxidation of β-carotene during storage (Che et al., 2016). More recently, porridges made from biofortified sorghum grains containing multiple transgenes (HGGT to increase vitamin E accumulation and stabilize provitamin A carotenoids during storage, CTR1 to increase provitamin A biosynthesis, PSY1 or CRTB to increase flux through the carotenoid pathway, and PhyA to decrease phytate) revealed greater amounts of carotenoids compared to the corresponding null segregants and WT control. Steeping prior to porridge production to pre-activate phytase substantially reduces phytate content, alters the profile of inositol phosphate conversion products, reduces phytate:Fe and phytate:Zn molar ratios, and enhances mineral bioavailability. Micellarization efficiency and the bioaccessible fraction of provitamin A carotenoids were 2,300% greater in transgenic events compared to the corresponding null segregants and WT and provided 53.7% of a 4- to 8-year-old child’s vitamin A estimated average requirement in a standard 200-g serving of porridge. Thus, it is feasible to enhance micronutrient content and bioaccessibility by adapting a combination of strategies (Dzakovich et al., 2022).
Transgenic soybean lines contain the β-conglycinin promoter:Phytoene synthase-2A-Carotene desaturase/t35S gene cassette into the genome of soybean cultivar. “Kwangan” enhances β-carotene productions that ranged from 170.47 to 213.58 μg g−1 (Qin et al., 2017). An earlier report of transgenic soybean lines carrying two carotenoid biosynthetic genes, Capsicum phytoene synthase and Pantoea carotene desaturase, accumulated 146 µg g−1 of total carotenoids, ~ 62-fold higher than non-transgenic seeds, of which 112 µg g−1 was β-carotene (Kim et al., 2012).
Root, tuber, and plantain crops
Provitamin A-biofortified transgenic Cavendish bananas containing MtPsy2a gene exceeded the target level of 20 μg g−1 with one line reaching 55 μg g−1 β-carotene equivalent fruit dry weight. The early activation of the rate-limiting enzyme in the carotenoid biosynthetic pathway and extended fruit maturation time are crucial factors to achieve optimal provitamin A concentrations in banana fruit (Paul et al., 2017). The project “Banana21” is currently underway to popularize Cavendish bananas in Uganda and surrounding countries through the generation of farmer- and consumer-acceptable edible bananas with significantly increased fruit levels of provitamin A (Paul et al., 2018).
BioCassava Plus (BC+) has developed transgenic cassava resistance to viral diseases, increased nutrients (Fe, Zn, vitamin A, protein) and shelf life, and reduced toxic cyanogenic glycosides to safe levels. Limited field trials in Puerto Rico and Nigeria and ex ante impact analyses demonstrate the efficacy of using transgenic technology for biofortification of cassava (Sayre et al., 2011). Roots harvested from transgenic cassava expressing DXS and crtB genes mediated by the patatin-type 1 promoter accumulated carotenoids up to ≤50 μg g−1 RDW, a 15- to 20-fold increase relative to non-transgenic cassava, ~85%–90% of which were β-carotene and display delayed onset of postharvest physiological deterioration. The inverse correlation between β-carotene and dry matter content, however, reduces 50%–60% dry matter in high carotenoid accumulating storage roots. This also resulted in reduced starch content but total fat, triacylglycerols, soluble sugars, and ascorbic acid levels increased, thus pinpointing the adverse effect of cassava biofortification on root dry weight and starch content (Beyene et al., 2018). Transgenic potato containing a bacterial mini-pathway (CrtB, CrtI, and CrtY) for β-carotene in a tuber-specific manner resulted in “golden” potato tubers that display a deep yellow to orange fleshed tuber phenotype containing β-carotene (> 3,000-fold over the WT), lutein (30-fold), β,β-xanthophylls (9-fold), and α-carotene (Diretto et al., 2007). Scaling up of provitamin A-rich banana, sweet potato, and cassava reached 8.5 million households in East Africa. More nutritious food systems supported by continued investment to enhance multiple nutrient targets into genetic background adapted to changing climates and increase investment in the inputs and marketing infrastructure are needed for popularization of nutrient-dense, vegetatively propagated crops (Low et al., 2022).
Overall, the proof of concept has demonstrated that it is achievable to develop GM crops with enhanced provitamin A. Equally important observations include the idea that seed chemistry was alike between GR2E and the near-isogenic control except for β-carotene and related carotenoids (Mallikarjuna Swamy et al., 2019), suggesting that golden rice cultivars are comparable to conventional rice for grain quality and nutritional traits and safe for human consumption. The drought-tolerant soybean (IND-ØØ41Ø-5) and wheat (IND-ØØ412-7) containing events HaHB4 were compositionally equivalent to their non-transgenic parental controls (Ayala et al., 2019; Chiozza et al., 2020). Furthermore, a 42-day broiler feeding trial involving isoenergetic diets containing 40% flour from transgenic wheat and its non-GM counterpart “Cadenza” support the nutritional equivalence of transgenic lines when compared with conventional wheat. A few significant differences noted with respect to a commercial variety were however associated with the genetic background (Miranda et al., 2022). Antinutrients such as phytic acid reduce seed minerals including Fe and Zn bioavailability. Yet, phytase seems to have no direct influence in grain mineral bioavailability. No significant differences in seed chemistry were noted between transgenic wheat containing phytase gene and its non-transgenic counterpart. However, a significant increase in phytase activity as well as iron and zinc bioaccessibility correlated well with a significant decrease in phytate (Akhtar et al., 2020). Argentina, Australia, Brazil, Colombia, New Zealand, Nigeria, and United States have recommended drought-tolerant GM wheat for food and feed uses (https://www.isaaa.org/gmapprovaldatabase/event/default.asp?EventID=574&=HB4%20Wheat). It therefore opens the way for the development of seed mineral (Fe and Zn)-dense and provitamin A-rich food crops to benefit the world’s poorest people. Bioavailability of nutrients is no longer an issue with the use of transgenic technologies.
Bioaccessability regulation and acceptability of genetically modified nutrient-dense crops
Genetic engineering and gene editing offer means to develop nutritious crops for healthy human diets. Examples include modifying fats in oilseeds and starch in roots and tubers; increasing micronutrients and carotenoids in cereals, roots, fruits, and oil crops, or minerals and antioxidants in some vegetables; and reducing glucosinates in Brassica species or flavonoids in vegetables (Winkler, 2011; Adenle et al., 2012; Hefferon, 2015; Hirschi, 2020). Although cultivars resulting from crossbreeding undergo a relatively simple regulation legal framework, the release of GM crops follow a lengthy and costly regulatory approval according to biosafety laws that align with the Cartagena Protocol of the Convention of Biological Diversity. The biosafety appraisals include the application of permits for field trials, the assessments of hazards for the environment (including biodiversity) and human/animal health (based on a nutritional study), and regulations for the marketing of GM crops and their derived food. As indicated by Turnbull et al. (2021), the laws should not be static and must adjust according to evolving science-led knowledge based on the years of cultivating GM crops elsewhere. Although omics technology could be incorporated into the strategy for evaluating GM biofortified crops, because it allows comparing it with a non-genetically engineered cultivar (Gould et al., 2022), the analyses should give priority to the nutrient composition of the edible parts. This product-based approach depends on characteristics that are measured by advances in molecular biology. For example, changes in DNA may be easily determined by comparing the sequences of a GM biofortified crop and the plant from which it derived, thus confirming that there are no unintended alterations. Omics technology may also be used for comparing the produce from a new GM biofortified crop with that of available cultivars grown by farmers. Nevertheless, any analysis should be relevant to the product safety rather than to changes in the RNA, proteins, or methylations that do not affect the phenotype.
Public controversy often slows the process of releasing GM biofortified crops (Baulcombe et al., 2014; Adeyeye and Idowu-Adebayo, 2019), mostly due to nonscience-based campaigns (often invoking the “precautionary principle) by those claiming unproven hazards to the environment and human health, e.g., GM mustard in India (Pulla, 2016; Jayaraman, 2017; Kumar, 2017) or “Golden Rice” (Mayer, 2005; Wu et al., 2021b). This calls for a more pragmatic view for this debate considering both the best available knowledge and the need to deliver zero hunger as stated in the UN agenda 2030 (Gbashi et al., 2021). Access to information and public participation will facilitate any citizen to exercise and protect their right to sufficient, affordable, safe, and nutritious food (Wolson, 2007). As noted by Goodman (2014), there is no science-based evidence that any of the approved GM crops poses any harm to humans or other animals, and they show consistency with the recommendations of the Codex Alimentarius. Moreover, the most appropriate approach for assessing GM crops for their use as food or feed source should be case-by-case (Nica-Badea and Balacescu, 2021). “Golden Rice” (GR2E event) provides an interesting case study for compositional analysis of its grains along with those from a non-GM near-isogenic line (Mallikarjuna Swamy et al., 2019). The results of this research demonstrated that their grains differ only in the levels of provitamin carotenoids (the target trait), while the other compositional parameters of GR2E were within the range noted in crossbred rice cultivars with a history of safe human consumption. Further molecular characterization and safety assessments provided substantiated evidence that provitamin A rice lacks any known hazards (Oliva et al., 2020); therefore, its grain is safe to use as food and as a potent cost-effective option for fighting malnutrition.
Modeling and food-based methods for assessing bioavailability and bioaccessibility of nutrients
Bioavailability, which is accepted as an important factor for health status, refers to the absorption level of an ingested nutrient from a given source that animals use in their metabolism. The different chemical forms of macro- and micronutrients affect their bioavailability. Furthermore, nutrients often interact among themselves or with other components of the diet at the absorption site, thus changing their bioavailability, resulting in a nil effect when enhancers and inhibitors cancel each other. Yates (2001) indicated that new bioavailability factors are defined as more chemical complexes of nutrients and food components are released into the market.
There are guidance documents that describe how to obtain data regarding nutrient bioavailability from proposed new sources (ANS et al., 2018). Knowledge on the bioavailability of nutrients from whole foods helps design foods, meals, and diets, particularly for distinct dietary targets related to health outcomes (Melse-Boonstra, 2020). Research on bioavailability using humans or animals is costly and limited by the number of bioactive compounds in the food or feed source(s). In vitro digestion systems, modeling, and analytical methods may be used as alternatives, but each has its pros and cons (King et al., 2000; Wood and Tamura, 2001; Carbonell-Capella et al., 2014; Marze, 2017). Hence, as indicated by Wang et al. (2021), an ideal method for determining all micronutrients is not feasible but rather each method under appropriate procedure can be effective for minimizing the differences between simulated results and reality.
Bioaccessibility, which includes bioactivity, is another term used to find out the nutritional efficiency of food and food formulas aimed for the betterment of human health. It gives important information regarding the most appropriate dosage and source of food matrices that ensure nutritional efficacy (Fernández-García et al., 2009). Bioaccessibility refers to the fraction of a compound released from its matrix in the gastrointestinal tract and is therefore available for intestinal absorption, while bioactivity encompasses the events related to the transport of a bioactive compound until it reaches the target tissue, its interaction with other biomolecules, the metabolism it will go through, and the biomarker generation along with the ensuing physiologic response. In vitro approaches are used for early bioaccessibility screening and are complemented by in vivo research that remains as the main criterion for determining bioaccessibility of nutrients and bioactive compounds. Any health claims should therefore include measurements of bioavailability, bioaccessibility, and bioactivity to show their significance.
Genetic and molecular basis of nutrient transport and absorption in human
Multiple pathways affect the gene regulation of metabolism. Goodman (2010) indicated that the enzymes digesting basic carbohydrates, proteins, and fats in the gastrointestinal tract are well-known. Such a knowledge provides insights into the digestion and absorption of nutrients. The metabolism of carotenoid was also elucidated and biochemically characterized in the 2000s (von Lintig, 2010). The molecular basis for retinol esterification enzymatic activity was further revealed recently through studying mutations in selective proteins that influence vitamin A in human patients (Chelstowska et al., 2016).
Genetic variation among human beings determines nutrient efficacy and tolerance or intolerance, as well as affects the nutrient intake (Stover, 2007). SNPs modulate the inter-individual variation for the bioavailability of vitamins A, D, and E; lutein; lycopene; and phytosterols (Borel and Desmarchelier, 2018). These SNPs in genes are related to the intestinal uptake and transport of vitamins, carotenoids, and phytochemicals in humans. Although each SNP effect may be low, a combination of them accounts significantly for interindividual variability. Such an example includes the 28 SNPs from 11 genes that are involved in the modulation of the vitamin E bioavailability inter-individual variation.
The nutrients and genomes often interact with the former controlling distinct nutrient utilization and the latter modifying genome expression, stability, and viability (Stover, 2007). While nutrients alter human phenotypes by inducing gene expression, human gene variants affect important metabolic pathways and the nutrient ability to interact with them (Paoloni-Giacobino et al., 2003). A better understanding of both provides knowledge about how metabolic homeostasis is kept and how to develop a diet therapy for human health. In this regard, nutrition genetics has two main fields of study, namely, nutrigenetics and nutrigenomics (Carrillo-Espel et al., 2016). Nutrigenetics studies the interaction between genes and dietary nutrients, while nutrigenomics investigates how food compounds regulate genes. Both contribute to personalized diets informed by molecular, genetic, and epigenetics features, and aim to prevent metabolic diseases by knowing the patient’s genome. As noted by Uthpala et al. (2020), innovative functional foods may be based on genetic patterns, thereby enhancing personalized diets for human consumption. Both nutrigenetics and nutrigenomics are still on the development stage, and their further advancement will facilitate eating foods that are beneficial to human DNA.
Concluding remarks
Malnutrition, in all its forms, imposes a staggering cost to human welfare and the nation’s economy and is a major impediment to achieving Sustainable Development Goal 2 “Zero Hunger” by 2030. Crossbreeding and genetic engineering techniques have been successfully applied to develop nutrient-dense (Fe, Zn) and provitamin A-rich staple food crops. Fe- and Zn-rich beans, pearl millet, rice, wheat, cassava, and sweet potato cultivars, developed through cross breeding and selection, are grown and widely consumed in the Global South. Genetic engineering has been applied to develop provitamin A-rich “Golden Rice” and dessert bananas. The provitamin A trait used for developing “Golden Rice” has been successfully transferred into several locally adapted cultivars in Asia and released in the Philippines. Enhancing multiple nutrient targets through genetic engineering is now achievable as evidenced in maize grains rich in provitamin A, ascorbate, and folate and sorghum grains rich in both provitamin A and vitamin E.
Plants have developed tightly regulated mechanisms to achieve nutrient acquisition, transport, and storage in plant organs and relocation from source to sink organs at critical stages of plant growth and development. A comprehensive understanding of the cross-talk s between the signaling pathways integrating the homeostasis of essential mineral nutrients at the molecular level could allow researchers to develop a more efficient breeding strategy to enhance nutrient levels in plant edible parts.
Because of global warming, today’s food is less nutritious. Future research should focus on (i) identifying nutrient-dense germplasm, including sources from crop wild relatives, under elevated CO2, drought, and heat stress; (ii) assessing and identifying more efficient root microbiomes, rhizomes, and mycorrhiza fungi adapted to harsh environments; (iii) unlocking the complex connect between regulatory layers of homoeostasis of mineral nutrients balancing concentrations of essential micronutrients both at the cellular and systemic levels; (iv) understanding where and how micronutrients accumulate in seed crops, as well as managing variation for aleurone layers in germplasm; (v) identifying and exploiting genetic variability to reveal genomic regions controlling micronutrients that do not colocalize with increased nutrient absorption; (vi) integrating low-phytate germplasm with those high in essential micronutrients to develop biofortified cultivars; (vii) using genome-wide association genetics to reveal major QTLs, putative and functionally characterized genes, and associated SNPs to select for multiple elements with improved genetic background; (viii) identifying and exploiting positive pleiotropic loci impacting multiple target traits; (ix) unlocking genetic and molecular variation to reveal the inverse relationship between provitamin A, tuberous root dry weight, and starch content in root crops such as cassava; (x) enhancing the nutritional value of endosperm through biotechnological interventions to develop nutrient-dense grain or derived flour; (xi) using and promoting genetically engineered crops through multiple gene stacking for greater bioavailability and bioaccessibility of essential micronutrients; and (xii) unraveling the molecular basis of variation to avoid degradation of provitamin A during storage.
Author contributions
SD: conceptualization, investigation, writing—original draft, and writing—review and editing. AG-O: investigation, writing—original draft, and editing. MG: investigation and writing—original draft. RO: conceptualization, project administration, investigation, writing—original draft, and writing—review and editing. All authors contributed to the article and approved the manuscript for submission.
Acknowledgments
The support of the HarvestPlus Program to MG is gratefully acknowledged.
Conflict of interest
The authors declare that the research was conducted in the absence of any commercial or financial relationships that could be construed as a potential conflict of interest.
Publisher’s note
All claims expressed in this article are solely those of the authors and do not necessarily represent those of their affiliated organizations, or those of the publisher, the editors and the reviewers. Any product that may be evaluated in this article, or claim that may be made by its manufacturer, is not guaranteed or endorsed by the publisher.
References
Abdelhalim, T. S., Kamal, N. M., Hassan, A. B. (2019). Nutritional potential of wild sorghum: Grain quality of Sudanese wild sorghum genotypes (Sorghum bicolor l. moench). Food Sci. Nutr. 7, 1529–1539. doi: 10.1002/fsn3.1002
Adenle, A. A., Aworh, O. C., Akromah, R., Parayil, G. (2012). Developing GM super cassava for improved health and food security: future challenges in Africa. Agric. Food Sec. 1, 11. doi: 10.1186/2048-7010-1-11
Adeyeye, S. A. O., Idowu-Adebayo, F. (2019). Genetically modified and biofortified crops and food security in developing countries. Nutr. Food Sci. 49, 978–986. doi: 10.1108/NFS-12-2018-0335
Agrawal, N., Dasaradhi, P. V. N., Mohmmed, A., Malhotra, P., Bhatnagar, R. K., Mukherjee, S. K. (2003). RNA Interference: Biology, mechanism, and applications. Microbiol. Mol. Biol. Rev. 67, 657–685. doi: 10.1128/MMBR.67.4.657-685.2003
Ahmad, W., Ullah, N., Xu, L., El Sabagh, A. (2022). Global food and nutrition security under changing climates. Front. Agron. 3. doi: 10.3389/fagro.2021.799878
Akhtar, A., Rizvi, Z., Irfan, M., Maqbool, A., Bashir, A., Malik, K. A. (2020). Biochemical and morphological risk assessment of transgenic wheat with enhanced iron and zinc bioaccessibility. J. Cereal Sci. 61, 102881. doi: 10.1016/j.jcs.2019.102881
Alberts, B., Johnson, A., Lewis, J., Raff, M., Roberts, K., Walter, P. (2002). “Molecular biology of cell,” in Studying gene expression and function, 4th (New York, NY: Garland Science). Available at: https://ncbi.nlm.nih.gov/books/NBK26818/.
Alomari, D. Z., Eggert, K., von Wirén, N., Alqudah, A. M., Polley, A., Plieske, J., et al. (2018). Identifying candidate genes for enhancing grain zn concentration in wheat. Front. Plant Sci. 9. doi: 10.3389/fpls.2018.01313
Aluru, M. R., Rodermel, S. R., Reddy, M. B. (2011). Genetic modification of low phytic acid 1-1 maize to enhance iron content and bioavailability. J. Agric. Food. Chem. 59, 12954–12962. doi: 10.1021/jf203485a
Aluru, M., Xu, Y., Guo, R., Wang, Z., Li, S., White, W., et al. (2008). Generation of transgenic maize with enhanced provitamin a content. J. Exp. Bot. 59, 3551–3562. doi: 10.1093/jxb/ern212
Amah, D., Alamu, E., Adesokan, M., Biljon, A., Maziya-Dixon, B., Swennen, R., et al. (2019). Variability of carotenoids in a Musa germplasm collection and implications for provitamin a biofortification. Food Chem. 2, 100024. doi: 10.1016/j.fochx.2019.100024
Andersson, M., Saltzman, A., Virk, P., Pfeiffer, W. (2017). Progress update: crop development of biofortified staple food crops under HarvestPlus. Afr. J. Food Agric. Nutr. Dev. 17, 11905–11935. doi: 10.18697/ajfand.78.HarvestPlus05
ANS [EFSA Panel on Food Additives and Nutrient Sources added to Food], Younes, M., Aggett, P., Aguilar, F., Crebelli, R., Dusemund, B., et al. (2018). Guidance on safety evaluation of sources of nutrients and bioavailability of nutrient from the sources. EFSA J. 16, 5294. doi: 10.2903/j.efsa.2018.5294
Anuradha, N., Satyavathi, C. T., Bhardwaj, C., Napolean, T., Sankar, S. M., Singh, S. P., et al. (2017). Deciphering genomic regions for high grain iron and zinc content using association mapping in pearl millet. Front. Plant Sci. 8. doi: 10.3389/fpls.2017.00412
Arrones, A., Vilanova, S., Plazas, M., Mangino, G., Pascual, L., Díez, M. J., et al. (2020). The dawn of the age of multi-parent MAGIC populations in plant breeding: Novel powerful next-generation resources for genetic analysis and selection of recombinant elite material. Biol. (Basel) 9, 229. doi: 10.3390/biology9080229
Ashokkumar, K., Govindraj, M., Karthikeyan, A., Shobhana, V. G., Warkentin, T. W. (2020). Genomics-integrated breeding for carotenoids and folates in staple cereal grains to reduce malnutrition. Front. Genet. 11. doi: 10.3389/fgene.2020.00414
Asia, D. (2021). Funding agricultural innovation for the global south: Does it promote sustainable agricultural intensification? (Colombo, Sri Lanka: Commission on Sustainable Agriculture Intensification), 57p.
Ayala, F., Fedrigo, G. V., Burachik, M., Miranda, P. V. (2019). Compositional equivalence of event IND-ØØ412-7 to non-transgenic wheat. Transgenic Res. 28, 165–176. doi: 10.1007/s11248-019-00111-y
Azmach, G., Gedil, M., Spillane, C., Menkir, A. (2021). Combining ability and heterosis for endosperm carotenoids and agronomic traits in tropical maize lines. Front. Plant Sci. 12. doi: 10.3389/fpls.2021.674089
Azmach, G., Menkir, A., Gedil, M. (2018). Genetic loci controlling carotenoid biosynthesis in diverse tropical maize lines. G3 8, 1049–1065. doi: 10.1534/g3.117.300511
Babu, P. M., Neeraja, C. N., Rathod, S., Suman, K., Uttam, G. A. N., Chakravartty, N., et al. (2020). Stable SNP allele associations with high grain zinc content in polished rice (Oryza sativa l.) identified based on ddRAD sequencing. Front. Genet. 11. doi: 10.3389/fgene.2020.00763
Badakhshan, H., Moradi, N., Mohammadzadeh, H., Zaker, M. R. (2013). Genetic variability analysis of grains fe, zn and β-carotene concentration of prevalent what varieties in Iran. Int. J. Agric. Crop Sci. 6, 57–62.
Bakker, S., Macheka, L., Eunice, L., Koopmanschap, D., Bosch, D., Hennemann, I., et al. (2021). “Food-system interventions with climate change and nutrition co-benefits; a literature review,” in Wageningen centre for development innovation report WCDI-21-153 (Wageningen University & Research, Wageningen, The Netherlands).
Baseggio, M., Murray, M., Magallanes-Lundback, M., Kaczmar, N., Chamness, J., Buckler, E. S., et al. (2020). Natural variation for carotenoids in fresh kernels is controlled by uncommon variants in sweet corn. Plant Genome 13, https://doi.org/10.1002/tpg2.20008. doi: 10.1002/tpg2.20008
Baulcombe, D., Dunwell, J., Jones, J., Pickett, J., Puigdomenech, P. (2014). GM science update: A report to the council for science and technology (London. United Kingdom: Office of Science and Technology).
Beasley, J. T., Bonneau, J. P., Sánchez-Palacios, J. T., Moreno-Movano, L. T., Callahan, D. L., Taki, E., et al. (2019). Metabolic engineering of bread wheat improves grain iron concentration and bioavailability. Plant Biotechnol. J. 17, 1514–1526. doi: 10.1111/pbi.13074
Beyene, G., Solomon, F. R., Chauhan, R. D., Gaitán-Solis, E., Narayanan, N., Gehan, J., et al. (2018). Provitamin a biofortification of cassava enhances shelf life but reduces dry matter content of storage roots due to altered carbon partitioning into starch. Plant Biotechnol. J. 16, 1186–1200. doi: 10.1111/pbi.12862
Bieńkowska, T., Suchowilska, E., Kandler, W., Krska, R., Wiwart, M., Wiwart, M. (2019). Triticum polonicum l. as potential source material for the biofortification of wheat with essential micronutrients. Plant Genet. Resour. 17, 213–220. doi: 10.1017/S1479262118000394
Birol, E., Foley, F., Aytekin, D. (2021). Biofortification: The evidence (HarvestPlus publication), p14.
Blomme, G., Ocimati, W., Nabuuma, D., Sivirihauma, C., Davet, M., Buah, S., et al. (2020). Pro-vitamin a carotenoid content of 48 plantain (Musa AAB genome) cultivars sourced from eastern democratic republic of Congo. J. Sci. Food Agric. 100, 634–647. doi: 10.1002/jsfa.10058
Bollinedi, H., Yadav, A. K., Vinod, K. K., Krishnan, S. G., Bhowmick, P. K., Nagarajan, N., et al. (2020). Genome-wide association study reveals novel marker-trait associations (MTAs) governing the localization of fe and zn in the rice grain. Front. Genet. 11. doi: 10.3389/fgene.202000213
Borel, P., Desmarchelier, C. (2018). Bioavailability of fat-soluble vitamins and phytochemicals in humans: Effects of genetic variation. Annu. Rev. Nutr. 38, 69–96. doi: 10.1146/annurev-nutr-082117-051628
Bouis, H. E., Hotz, C., McClaferty, B., Meenakshi, J. V., Pfeiffe, W. H. (2011). Biofortification: A new tool to reduce micronutrient malnutrition. Food Nutr. Bull. 32, S31–S40. doi: 10.1177/15648265110321S105
Bouis, H. E., Saltzman, A. (2017). Improving nutrition through biofortification: A review of evidence from HarvestPlus 2003 through 2016. Glob Food Sec. 12, 49–58. doi: 10.1016/j.gfs.2017.01.009
Boy, E., Haas, J., Petry, N., Cercamondi, C. I., Gahutu, J., Mehta, S., et al. (2017). Efficacy of iron crops. Afr. J. Food Agric. Nutr. Dev. 17, 11879–11892. doi: 10.18697/ajfand.78.HarvestPlus03
Bregitzer, P., Raboy, V. (2006). Effect of four independent low-phytate mutations on barley agronomic performance. Crop Sci. 46, 1318–1322. doi: 10.2135/cropsci2005.09-0301
Briat, J. F., Rouached, H., Tissot, N., Gaymard, F., Dubos, C. (2015). Integration of p, s, fe, and zn nutrition signals in arabidopsis thaliana: Potential involvement of PHOSPHATE STARVATION RESPONSE 1 (PHR1). Front. Plant Sci. 6. doi: 10.3389/fpls.2015.00290
Cai, Y., Li, Y., Liang, G. (2021). FIT and bHLH ib transcription factors modulate iron and copper crosstalk in Arabidopsis. Plant Cell Environ. 44, 1679–1691. doi: 10.1111/pce.14000
Cakmak, I., Torun, A., Millet, E., Feldman, M., Fahima, T., Korol, A., et al. (2004). Triticum dicoccoides: An important genetic resource for increasing zinc and iron concentration in modern cultivated wheat. Soil Sci. Plant Nutr. 50, 1047–1054. doi: 10.1080/00380768.2004.10408573
Campion, B., Sparvoli, F., Doria, E., Tagliabue, G., Galasso, I., Fileppi, M., et al. (2009). Isolation and characterization of an lpa (low phytic acid) mutant in common bean (Phaseolus vulgaris l.). Theor. Appl. Genet. 118, 1211–1221. doi: 10.1007/s00122-009-0975-8
Carbonell-Capella, J. M., Buniowska, M., Barba, F. J., Esteve, M. J., Frígola, A. (2014). Analytical methods for determining bioavailability and bioaccessibility of bioactive compounds from fruits and vegetables. Compr. Rev. Food Sci. Food Saf. 13, 155–171. doi: 10.1111/1541-4337.12049
Cardoso, L. M., Pinheiro, S. S., Linhares da Silva, L., Menezes, C. B., Carvalho, C. W. P., Tardin, F. D., et al. (2015). Tocochromanols and carotenoids in sorghum (Sorghum bicolor l.): Diversity and stability to the heat treatment. Food Chem. 172, 900–908. doi: 10.1016/j.foodchem.2014.09.117
Carrillo-Espel, R., Carrillo-Córdova, C. A., García de Alba-Graue, P., Carrillo-Córdova, D. M. (2016). Molecular biology and genetics in nutrition. Cirugía Cirujanos 84 (Supl. 1), 25–30.
Carvalho, L. J. C. B., Agustini, M. A. V., Anderson, J. V., Vieira, E. A., de Souza, C. R. B., Chen, S., et al. (2016). Natural variation in expression of genes associated with carotenoid biosynthesis and accumulation in cassava (Manihot esculenta crantz) storage root. BMC Plant Biol. 16, 133. doi: 10.1186/s12870-016-0826-0
Cazzonelli, C. I., Pogson, B. J. (2010). Source to sink: Regulation of carotenoid biosynthesis in plants. Trends Plant Sci. 15, 266–274. doi: 10.1016/j.tplants.2010.02.003
Cheah, Z. X., Kopittke, P. M., Harper, S. M., OˈHare, T. J., Wang, P., Paterson, D. J., et al. (2019). In situ analyses of inorganic nutrient distribution in sweetcorn and maize kernels using synchrotron-based X-ray fluorescence microscopy. Ann. Bot. 123, 543–556. doi: 10.1093/aob/mcy189
Chelstowska, S., Widjaja-Adhi, M. A. K., Silvaroli, J. A., Golczak, M. (2016). Molecular basis for vitamin a uptake and storage in vertebrates. Nutrients 8, 676. doi: 10.3390/nu8110676
Chen, C. L., Cui, Y., Cui, M., Zhou, W. J., Wu, H. L., Ling, H. Q. (2018a). A FIT-binding protein is involved in modulating iron and zinc homeostasis in Arabidopsis. Plant Cell Environ. 41, 1698–1714. doi: 10.1111/pce.13321
Chen, Z. R., Kuang, L., Gao, Y. Q., Wang, Y. L., Salt, D. E., Chao, D. Y. (2018b). AtHMA4 drives natural variation in leaf zn concentration of Arabidopsis thaliana. Front. Plant Sci. 9. doi: 10.3389/fpls.2018.00270
Che, P., Zhao, Z.-Y., Glassman, K., Dolde, D., Hu, T. X., Jones, T. J., et al. (2016). Elevated vitamin e content improves all-trans β-carotene accumulation and stability in biofortified sorghum. Proc. Natl. Acad. Sci. U.S.A. 113, 11040–11045. doi: 10.1073/pnas.1605689113
Chhuneja, P., Dhaliwal, H. S., Bains, N. S., Singh, K. (2006). Aegilops kotschyi and Aegilops tauschii as sources for higher levels of grain iron and zinc. Plant Breed. 125, 529–531. doi: 10.1111/j.1439-0523.2006.01223.x
Chiozza, M. V., Burachik, M., Miranda, P. V. (2020). Compositional analysis of soybean event IND-ØØ41Ø-5. GM Crops Food 11, 154–163. doi: 10.1080/21645698.2020.1742040
Chu, H.-H., Chiecko, J., Punshon, T., Lanzirotti, A., Lahner, B., Salt, D. E., et al. (2010). Successful reproduction requires the function of arabidopsis YELLOW STRIPE-LIKE1 and YELLOW STRIPE-LIKE3 metal-nicotianamine transporters in both vegetative and reproductive structures. Plant Physiol. 154, 197–210. doi: 10.1104/pp.110.159103
Connorton, J. M., Balk, J., Rodríguez-Celma, J. (2017a). Iron homeostasis in plants–a brief overview. Metallomics 9, 813–823. doi: 10.1039/c7mt00136c
Connorton, J. M., Jones, E. R., Rodríguez-Ramiro, I., Fairweather-Tait, S., Uauy, C., Balk, J. (2017b). Wheat vacuolar iron transporter TaVIT2 transports fe and Mn and is effective for biofortification. Plant Physiol. 174, 2434–2444. doi: 10.1104/pp.17.00672
CAST (2020). Food biofortification: Reaping the benefits of science to overcome hidden hunger: A paper in the series on the need for agricultural innovation to sustainably feed the world by 2050. CAST Issue Paper 69. https://www.cast-science.org/wp-content/uploads/2020/10/CAST_IP69_Biofortification-1.pdf
Cruet-Burgos, C., Cox, S., Loerger, B. P., Perumal, R., Hu, Z., Herald, T. J., et al. (2020). Advancing provitamin a biofortification in sorghum: Genome-wide association studies of grain carotenoids in global germplasm. Plant Genome 13, e20013. doi: 10.1002/tpg2.20013
Cruet-Burgos, C., Morris, G. P., Rhodes, D. H. (2022). Characterization of grain carotenoids in global sorghum germplasm to guide genomics-assisted breeding strategies. doi: 10.21203/rs.3.rs-1774418/v1
Curie, C., Cassin, G., Couch, D., Divol, F., Higuchi, K., Le Jean, M., et al. (2009). Metal movement within the plant: contribution of nicotianamine and yellow stripe 1-like transporters. Ann. Bot. 103, 1–11. doi: 10.1093/aob/mcn207
Curie, C., Panaviene, Z., Loulergue, C., Dellaporta, S. L., Briat, J. F., Walker, E. L. (2001). Maize yellow stripe1 encodes a membrane protein directly involved in Fe(III) uptake. Nature 409, 346–349. doi: 10.1038/35053080
Dasa, F., Tilahun, A. (2018). Factors affecting iron absorption and mitigation mechanisms: A review. Int. J. Agric. Sci. Food Technol. 4, 024–030. doi: 10.17352/2455-815X.000033
Debelo, H., Albertsen, M., Simon, M., Che, P., Ferruzi, M. (2020). Identification and characterization of carotenoids, vitamin e and minerals of biofortified sorghum. Curr. Dev. Nutr. 4 (Suppl 2), 1792. doi: 10.1093/cdn/nzaa067_019
Delfini, J., Moda-Cirino, V., dos Santos Neto, J., Buratto, J. S., cio Ruas, P. M., Gonçalves, L. S. A. (2020). Diversity of nutritional content in seeds of Brazilian common bean germplasm. PloS One 15, e0239263. doi: 10.1371/journal.pone.0239263
Desbrosses-Fonrouge, A. G., Voigt, K., Schröder, A., Arrivault, S., Thomine, S., Krämer, U. (2005). Arabidopsis thaliana MTP1 is a zn transporter in the vacuolar membrane which mediates zn detoxification and drives leaf zn accumulation. FEBS Lett. 579, 4165–4174. doi: 10.1016/j.febslet.2005.06.046
Detterbeck, A., Nagel, M., Rensch, S., Weber, M., Böner, A., Persson, D. P., et al. (2019). The search for candidate genes associated with natural variation of grain zn accumulation in barley. Biochem. J. 476, 1889–1909. doi: 10.1042/BCJ20190181
Detterbeck, A., Pongrac, P., Persson, D. P., Vogel-Mikuš, K., Kelemen, M., Vavpetič, P., et al. (2020). Temporal and spatial patterns of zinc and iron accumulation during barley (Hordeum vulgare l.) grain development. J. Agric. Food Chem. 68, 12229–12240. doi: 10.1021/acs.jafc.0c04833
DiDonato, R. J., Roberts, L. A., Sanderson, T., Eisley, R. B., Walker, E. L. (2004). Arabidopsis yellow stripe-Like2 (YSL2): a metal-regulated gene encoding a plasma membrane transporter of nicotianamine-metal complexes. Plant J. 39, 403–414. doi: 10.1111/j.1365-313X.2004.02128.x
Diepenbrock, C. H., Ilut, D. C., Magallanes-Lundback, M., Kandianis, C. B., Lipka, A. E., Bradbury, P. J., et al. (2021). Eleven biosynthetic genes explain the majority of natural variation in carotenoid levels in maize grain. Plant Cell 33, 882–900. doi: 10.1093/plcell/koab032
Diretto, G., Al-Babili, S., Tavazza, R., Papacchioli, V., Beyer, P., Giuliano, G. (2007). Metabolic engineering of potato carotenoid content through tuber-specific overexpression of a bacterial mini-pathway. PloS One 2, e350. doi: 10.1371/journal.pone.0000350
Duo, H., Hossain, F., Muthusamy, V., Zuniare, R. U., Goswami, R., Chand, G., et al. (2021). Development of sub-tropically adapted diverse provitamin-a rich maize inbreds through marker-assisted pedigree selection, their characterization and utilization in hybrid breeding. PloS One 16, e0245497. doi: 10.1371/journal.pone.0245497
Durrett, T. P., Gassmann, W., Rogers, E. E. (2007). The FRD3-mediated efflux of citrate into the root vasculature is necessary for efficient iron translocation. Plant Physiol. 144, 197–205. doi: 10.1104/pp.107.097162
Dwivedi, S., Sahrawat, K., Upadhyaya, H., Ortiz, R. (2013). Food, nutrition and agrobiodiversity under global climate change. Adv. Agron. 120, 1–128. doi: 10.1016/B978-0-12-407686-0.00001-4
Dzakovich, M., Debelo, H., Albertsen, M., Che, P., Jones, T., Simon, M., et al (2022). Trait stacking simultaneously enhances mineral and provitamin a carotenoid bioaccessibility in biofortified Sorghum bicolor. Curr. Develop. Nutr. 6 (Supplement_1), 1179. doi: 10.1093/cdn/nzac074.008
Ebi, K. L., Anderson, C. L., Hess, J. J., Kim, S.-H., Loladze, I., Neumann, R. B., et al. (2021). Nutritional quality of crops in a high CO2 world: an agenda for research and technology development. Environ. Res. Lett. 16, 064045. doi: 10.1088/1748-9326/abfcfa
Eide, D., Broderius, M., Fett, J., Guerinot, M. L. (1996). A novel iron regulated metal transporter from plants identified by functional expression in yeast. Proc. Natl. Acad. Sci. U.S.A. 93, 5624–5628. doi: 10.1073/pnas.93.11.5624
Elgueta, A. V., Navarro, N., Uribe, M., Robe, K., Gaymard, F., Dubos, C., et al. (2021). 2000 years of agriculture in the atacama desert lead to changes in the distribution and concentration of iron in maize. Sci. Rep. 11, 17322. doi: 10.1038/s41598-021-96819-1
Erbs, M., Manderscheid, R., Jansen, G., Seddig, S., Pacholski, A., Weigel, H.-J. (2010). Effects of free-air CO2 enrichment and nitrogen supply on grain quality parameters and elemental composition of wheat and barley grown in a crop rotation. Agric. Ecosyst. Env. 136, 59–68. doi: 10.1016/j.agee.2009.11.009
FAO, IFAD, UNICEF, WFP, WHO (2020). The state of food security and nutrition in the world 2020. transforming food systems for affordable healthy diets (Rome: FAO), 320, ISBN: ISBN: 978-92-5-132901-6.
Fernández-García, E., Carvajal-Lérida, I., Pérez-Gálvez, A. (2009). In vitro bioaccessibility assessment as a prediction tool of nutritional efficiency. Nutr. Res. 29, 751–760. doi: 10.1016/j.nutres.2009.09.016
Finkelstein, J. L., Fothergill, A., Hackl, L. S., Haas, J. D., Mehta, S. (2019a). Iron biofortification interventions to improve iron status and functional outcomes. Proc. Nutr. Soc 78, 197–207. doi: 10.1017/S0029665118002847
Finkelstein, J. L., Mehta, S., Udipi, S. A., Ghungre, P. S., Luna, S. V., Wenger, M. J., et al. (2015). A randomized trial of iron-biofortified pearl millet in school children in India. J. Nutr. 145, 1576–1581. doi: 10.3945/jn.114.208009
Finkelstein, J. L., Mehta, S., Villalpando, S., Mundo-Rosas, V., Luna, S. V., Rahn, M., et al. (2019b). A randomized feeding trial of iron-biofortified beans on school children in Mexico. Nutrients 11, 381. doi: 10.3390/nu11020381
Fu, J., Cheng, Y., Linghu, J., Yang, X., Kang, L., Zhang, Z., et al. (2013). RNA Sequencing reveals the complex regulatory network in the maize kernel. Nat. Commun. 4, 2832. doi: 10.1038/ncomms3832
Furukawa, J., Yamaji, N., Wang, H., Mitani, N., Murata, Y., Sato, K., et al. (2007). An aluminum-activated citrate transporter in barley. Plant Cell Physiol. 48, 1081–1091. doi: 10.1093/pcp/pcm091
Gangashetty, P. I., Riyazuddin, M., Sanogo, M. D., Inousa, D., Issoufou, K. A., Asungre, P. A., et al. (2021). Identification of high-yielding iron-biofortified open-pollinated varieties of pearl millet in West Africa. Front. Plant Sci. 12. doi: 10.3389/fpls.2021.688937
Gao, F., Dubos, C. (2021). Transcriptional integration of plant responses to iron availability. J. Exp. Bot. 72, 2056–2070. doi: 10.1093/jxb/eraa556
Garcia-Oliveira, A. L., Chander, S., Ortiz, R., Menkir, A., Gedil, M. (2018). Genetic basis and breeding perspectives of grain iron and zinc enrichment in cereals. Front. Plant Sci. 9. doi: 10.3389/fpls.2018.00937
Garcia-Oliveira, A. L., Tan, L., Fu, Y., Sun, C. (2009). Genetic identification of quantitative trait loci for contents of mineral nutrients in rice grain. J. Integr. Plant Biol. 51, 84–92. doi: 10.1111/j.1744-7909.2008.00730.x
Garg, M., Sharma, N., Sharma, S., Kapoor, P., Kumar, A., Chunduri, V., et al. (2018). Biofortified crops generated by breeding, agronomy, and transgenic approaches are improving lives of millions of people around the world. Front. Nutr. 5. doi: 10.3389/fnut.2018.00012
Gasura, E., Matsaure, F., Setimela, P. S., Rugare, J. T., Nyakurwa, C. S., Andrade, M. (2021). Performance, variance components, and acceptability of pro-vitamin a-biofortified sweetpotato in southern Africa and implications in future breeding. Front. Plant Sci. 12. doi: 10.3389/fpls.2021.696738
Gbashi, S., Adebo, O., Adebiyi, J. A., Targuma, S., Tebele, S., Areo, O. M., et al. (2021). Food safety, food security and genetically modified organisms in Africa: a current perspective. Biotechnol. Genet. Eng. Rev. 37, 30–63. doi: 10.1080/02648725.2021.1940735
Goodman, B. E. (2010). Insights into digestion and absorption of major nutrients in humans. Adv. Physiol. Educ. 34, 44–53. doi: 10.1152/advan.00094.2009
Goodman, R. E. (2014). Biosafety: Evaluation and regulation of genetically modified (GM) crops in the USA. J. Huazhong Agric. Univ. 33, 85–114. http://digitalcommons.unl.edu/foodsciefacpub/145
Gould, F., Amasino, R. M., Brossard, D., Buell, C. R., Dixon, R.-A., Falck-Zepeda, J. B., et al. (2022). Toward product-based regulation of crops. Science 377, 1051–1053. doi: 10.1126/science.abo3034
Govindaraj, M., Rai, K. N., Cherian, B., Pfeiffer, W. H., Kanatti, A., Shivade, H. (2019). Breeding biofortified pearl millet varieties and hybrids to enhance millet markets for human nutrition. Agriculture 9, 106. doi: 10.3390/agriculture9050106
Govindraj, M., Kanatti, A., Rai, K. N., Pfeiffer, W. H., Shivade, H. (2022). Association of grain iron and zinc content with other nutrients in pearl millet germplasm, breeding lines, and hybrids. Front. Nutr. 8. doi: 10.3389/fnut.2021.746625
Grotz, N., Guerinot, M. L. (2006). Molecular aspects of Cu, fe and zn homeostasis in plants. Biochim. Biophys. Acta Mol. Cell Res. 1763, 595–608. doi: 10.1016/j.bbamcr.2006.05.014
Grusak, M. A., Broadley, M. R., White, P. J. (2016). Plant macro- and micronutrient minerals. Encyclopedia Life Sci. 1–5. doi: 10.1002/9780470015902.a0001306.pub2
Guo, Z., Zhang, X., Wang, L., Wang, X., Wang, R., Hui, X., et al. (2021). Selecting high zinc wheat cultivars increases grain zinc bioavailability. J. Agric. Food Chem. 69, 38. doi: 10.1021/acs.jafc.1c03166
Gustin, J. L., Zanis, M. J., Salt, D. E. (2011). Structure and evolution of the plant cation diffusion facilitator family of ion transporters. BMC Evol. Biol. 11, 1–13. doi: 10.1186/1471-2148-11-76
Haas, J. D., Luna, S. V., Lung’aho, M. G., Wenger, M. J., Murray-Kolb, L. E., Beebe, S., et al. (2016). Consuming iron biofortified beans increases iron status in Rwandan women after 128 days in a randomized controlled feeding trial. J. Nutr. 146, 1586–1592. doi: 10.3945/jn.115.224741
Hacisalihoglu, G., Beisel, N. S., Settles, A. M. (2021). Characterization of pea seed nutritional value within a diverse population of Pisum sativum. PloS One 16, e0259565. doi: 10.1371/journal.pone.0259565
Harjes, C. E., Rocheford, T. R., Bai, L., Brutnell, T. P., Kandianis, C. B., Sowinski, S. G., et al. (2008). Natural genetic variation in lycopene epsilon cyclase tapped for maize biofortification. Science 319, 330–333. doi: 10.1126/science.1150255
Harrington, S. A., Connorton, J. M., Nyangoma, N. I. M., McNelly, R., Morgan, Y. M. L., Aslam, M. F., et al. (2023). A two-gene strategy increases the iron and zinc concentration of wheat flour and improves mineral bioaccesibility for human nutrition. Plant Physiol. 191, 528–541. doi: 10.1093/plphys/kiac499
HarvestPlus. (2022). Responding to crisis, building resilience: HarvestPlus 2021 annual report. HarvestPlus Annual Report 11. Washington, DC: International Food Policy Research Institute (IFPRI). https://ebrary.ifpri.org/cdm/ref/collection/p15738coll2/id/136342
Hefferon, K.-L. (2015). Nutritionally enhanced food crops; progress and perspectives. Int. J. Mol. Sci. 16, 3895–3914. doi: 10.3390/ijms16023895
Herzig, P., Backhaus, A., Seiffert, U., Wirén, N., Pillen, K., Maurer, A. (2019). Genetic dissection of grain elements predicted by hyperspectral imaging associated with yield-related traits in a wild barley NAM population. Plant Sci. 289, 151–164. doi: 10.1016/j.plantsci.2019.05.008
Hirschi, K. D. (2020). Genetically modified plants: nutritious sustainable yet underrated. J. Nutr. 150, 2628–2634. doi: 10.1093/jn/nxaa220
Holme, I. B., Gregersen, P. L., Brinch-Pedersen, H. (2019). Induced genetic variation in crop plants by random or targeted mutagenesis: Convergence and differences. Front. Plant Sci. 10. doi: 10.3389/fpls.2019.01468
Huey, S. L., Krisher, J. T., Bhargava, A., Friesen, V. M., Konieczynski, E. M., Mbuya, M. N. N., et al. (2022). Review of the impact pathways of biofortified foods and food products. Nutrients 14, 1200. doi: 10.3390/nu14061200
Hummel, M., Hallahan, B. F., Brychkova, G., Ramirez-Villegas, J., Guwela, V., Chataika, B., et al. (2018). Reduction in nutritional quality and growing area suitability of common bean under climate change induced drought stress in Africa. Sci. Rep. 8, 16187. doi: 10.1038/s41598-018-33952-4
Hwang, T., Ndolo, V. U., Katundu, M., Nyirenda, B., Bezner-Kerr, R., Arntfield, S., et al. (2016). Provitamin a potential of landrace orange maize variety (Zea mays l.) grown in different geographical locations of central Malawi. Food Chem. 196, 1315–1324. doi: 10.1016/j.foodchem.2015.10.067
Ibeas, M. A., Grant-Grant, S., Coronas, M. F., Vargas-Pérez, J. I., Navarro, N., Abreu, I., et al. (2018). The diverse iron distribution in eudicotyledoneae seeds: From arabidopsis to quinoa. Front. Plant Sci. 9. doi: 10.3389/fpls.2018.01985
Ibeas, M. A., Grant-Grant, S., Navarro, N., Perez, M. F., Roschzttardtz, H. (2017). Dynamic subcellular localization of iron during embryo development in brassicaceae seeds. Front. Plant Sci. 8. doi: 10.3389/fpls.2017.02186
Ishimaru, Y., Suzuki, M., Tsukamoto, T., Suzuki, K., Nakazono, M., Kobayashi, T., et al. (2006). Rice plants take up iron as an Fe3+-phytosiderophore and as Fe2+. Plant J. 45, 335–346. doi: 10.1111/j.1365-313X.2005.02624.x
Jablonski, L., Wang, X., Curtis, P. (2002). Plant reproduction under elevated CO2 conditions: a meta-analysis of reports on 79 crop and wild species. New Phytol. 156, 9–26. doi: 10.1046/j.1469-8137.2002.00494.x
Jacques, P., Jacques, J. R. (2012). Monocropping cultures into ruin: The loss of food varieties and cultural diversity. Sustainability 4, 2970–2997. doi: 10.3390/su4112970
Janila, P., Nigam, S. N., Rathor, R., Kumar, A., Manohar, S. S., Venuprasad, R. (2014). Iron and zinc concentrations in peanut (Arachis hypogaea l.) seeds and their relationship with other nutritional and yield parameters. J. Agric. Sci. 153, 975–994. doi: 10.1017/S0021859614000525
Jan van Dijk, A. D., Kootstra, G., Kruijer, W., de Ridder, D. (2021). Machine learning in plant science and plant breeding. iScience 24, 101890. doi: 10.1016/j.isci.2020.101890
Jayaraman, K. (2017). Activists bury india’s GM mustard hopes. Nat. Biotechnol. 35, 1124. doi: 10.1038/nbt1217-1124a
Jose, S., Gulati, A., Khurana, K. (2020). Achieving nutritional security in India: vision 2030 Indian council for research on international economic relations (ICRIER) (NABARD Research Study-9), ISBN: ISBN 978-81-937769-4-0.
Kamanda, I., Blay, E. T., Asante, I. K., Danquah, A., Ifie, B. E., Parkes, E., et al. (2020). Genetic diversity of provitamin-a cassava (Manihot esculenta crantz) in Sierra Leone. Genet. Resour. Crop Evol. 67, 1193–1208. doi: 10.1007/s10722-020-00905-8
Kamburova, V. S., Nikitina, E. V., Shermatove, S. E., Buriev, Z. T., Kumpatla, S. P., Emani, C., et al. (2017). Genome editing in plants: an overview of tools and applications. Int. J. Agron. 2017, 7315351. doi: 10.1155/2017/7315351
Kappara, S., Neelamraju, S., Ramanan, R. (2018). Down regulation of a heavy metal transporter gene influences several domestication traits and grain fe and zn content in rice. Plant Sci. 276, 208–219. doi: 10.1016/j.plantsci.2018.09.003
Kenzhebayeva, S., Abekova, A., Atabayeva, S., Yernazarova, G., Omirbekowa, N., Zhang, G., et al. (2019). Mutant lines of spring wheat with increased iron, zinc, and micronutrients in grains and enhanced bioavailability for human health. Biomed. Res. Int. 2019, 9692053. doi: 10.1155/2019/9692053
Khazaei, H., Podder, R., Caron, C. T., Kundu, S. S., Diapari, M., Vandenberg, A., et al. (2017). Marker-trait association analysis of iron and zinc concentration in lentil (Lens culinaris medik.) seeds. Plant Genome 10. doi: 10.3835/plantgenome2017.02.0007
Khokhar, J. S., King, J., King, I. P., Young, S. D., Foulkes, M. J., De Silva, J., et al. (2020). Novel sources of variation in grain zinc (Zn) concentration in bread wheat germplasm derived from Watkins landraces. PloS One 15, e0229107. doi: 10.1371/journal.pone.0229107
Khokhar, J. S., Sareen, S., Tyagi, B. S., Singh, G., Wilson, L., King, I. P., et al. (2018). Variation in grain zn concentration, and the grain ionome, in field-grown Indian wheat. PloS One 13, e0192026. doi: 10.1371/journal.pone.0192026
Kim, S. A., Guerinot, M. L. (2007). Mining iron: iron uptake and transport in plants. FEBS Lett. 581, 2273–2280. doi: 10.1016/j.febslet.2007.04.043
Kim, M.-J., Kim, J. K., Kim, H. J., Pak, J. H., Lee, J. H., Kim, D. H., et al. (2012). Genetic modification of the soybean to enhance the β-carotene content through seed-specific expression. PloS One 7, e48287. doi: 10.1371/journal.pone.0048287
Kim, S. A., Punshon, T., Lanzirotti, A., Li, L., Alonso, J. M., Ecker, J. R., et al. (2006). Localization of iron in Arabidopsis seed requires the vacuolar membrane transporter VIT1. Science 314, 1295–1298. doi: 10.1126/science.1132563
King, J. C., Donangelo, C. M., Woodhouse, L. R., Mertz, S. D., Shames, D. M., Viteri, F. E., et al. (2000). Measuring iron and zinc bioavailability in humans. Food Nutr. Bull. 21, 434–439. doi: 10.1177/156482650002100418
Kondwakwenda, A., Sibiya, J., Amelework, A. B., Zengeni, R. (2020). Diversity analysis of provitamin a maize inbred lines using single nucleotide polymorphism markers. Acta Agriculturae Scandinavica Section B — Soil Plant Sci. 70, 265–271. doi: 10.1080/09064710.2020.1718198
Kotla, A., Phuke, R., Hariprasanna, K., Mehtre, S. P., Rathore, A., Gorthy, S., et al. (2019). Identification of QTLs and candidate genes for high grain fe and zn concentration in sorghum [Sorghum bicolor (L.)Moench]. J. Cereal Sci. 90, 102850. doi: 10.1016/j.jcs.2019.102850
Krasileva, K. V., Vasquez-Gross, H. A., Howell, T., Bailey, P., Paraiso, F., Clissold, L., et al. (2017). Uncovering hidden variation in polyploid wheat. Proc. Natl. Acad. Sci. U.S.A. 114, E913–E921. doi: 10.1073/pnas.1619268114
Kumar, H., Singh, A., Dikshit, H. K., Mishra, G. P., Aski, M., Meena, M. C., et al. (2019). Genetic dissection of grain iron and zinc concentrations in lentil (Lens culinaris medik.). J. Genet. 98, 66. doi: 10.1007/s12041-019-1112-3
La Frano, M. R., de Moura, F. F., Boy, E., Lönnerdal, B., Burri, B. J. (2014). Bioavailability of iron, zinc, and provitamin a carotenoids in biofortified staple crops. Nutr. Rev. 72, 289–307. doi: 10.1111/nure.12108
La Frano, M. R., Woodhouse, L. R., Burnett, D. J., Burri, B. J. (2013). Biofortified cassava increases betacarotene and vitamin a concentrations in the TAG-rich plasma layer of American women. Br. J. Nutr. 110, 310–320. doi: 10.1017/S0007114512005004
Li-na, J., Jing-li, M. A., Xiao-jie, W., Gang-gang, I. I. U., Zhao-long, Z. H. U., Chen-yang, Q. I., et al. (2022). Grain zinc and iron concentrations of Chinese wheat landraces and cultivars and their responses to foliar micronutrient applications. J. Integr. Agric. 21, 532–541. doi: 10.1016/S2095-3119(21)63614-6
Ling, H. Q., Bauer, P., Bereczky, Z., Keller, B., Ganal, M. (2002). The tomato fer gene encoding a bHLH protein controls iron-uptake responses in roots. Proc. Natl. Acad. Sci. U.S.A. 99, 13938–13943. doi: 10.1073/pnas.212448699
Li, S., Song, Z., Liu, X., Zhou, X., Yang, W., Chen, J., et al. (2022). Mediation of zinc and iron accumulation in maize by ZmIRT2, a novel iron-regulated transporter. Plant Cell Physiol. 63, 521–534. doi: 10.1093/pcp/pcab177
Liu, J., Huang, L., Li, T., Liu, Y., Yan, Z., Tang, G., et al. (2021b). Genome-wide association study for grain micronutrient concentrations in wheat advanced lines derived from wild emmer. Front. Plant Sci. 12. doi: 10.3389/fpls.2021.651283
Liu, J., Wu, X., Yao, X., Yu, R., Larkin, P. J., Liu, C.-M. (2018). Mutations in the DNA demethylase OsROS1 result in a thickened aleurone and improved nutritional value in rice grains. Proc. Natl. Acad. Sci. U.S.A. 115, 11327–11332. doi: 10.1073/pnas.1806304115
Liu, J., Zhan, J., Chen, J., Lu, X., Zhi, S., Ye, G. (2021a). Validation of genes affecting rice grain zinc content through candidate gene-based association analysis. Front. Genet. 12. doi: 10.3389/fgene.2021.701658
Loladze, I. (2002). Rising atmospheric CO2 and human nutrition: Toward globally imbalanced plant stoichiometry? Trends Ecol. Evol. 17, 457–461. doi: 10.1016/S0169-5347(02)02587-9
Low, J., Ball, A.-M., Ilona, P., Ekesa, B., Heck, S., Pfeiffer, W. (2022). “Scaling readiness of biofortified root, tuber, and banana crops for Africa,” in Root, tuber and banana food system innovations. Eds. Thiele, G., Friedmann, M., Campos, H., Polar, V., Bentley, J. W. (Cham: Springer). doi: 10.1007/978-3-030-92022-7_17
Madhu Babu, P., Neeraja, C. N., Rathod, S., Suman, K., Uttam, A. G., et al. (2020). Stable SNP allele associations with high grain zinc content in polished rice (Oryza sativa l.) identified based on ddRAD sequencing. Front. Genet. 11. doi: 10.3389/fgene.2020.00763
Mahendrakar, M. D., Parveda, M., Kishor, P. B. K., Srivastava, R. K. (2020). Discovery and validation of candidate genes for grain iron and zinc metabolism in pearl millet [Pennisetum glaucum (L.) r. br.]. Sci. Rep. 10, 16562. doi: 10.1038/s41598-020-73241-7
Mallikarjuna Swamy, B. P., Marundan, S., Jr., Samia, M., Ordonio, R. L., Rebond, D. B., Ordonio, R. L., et al. (2021). Development and characterization of R2E golden rice introgression lies. Sci. Rep. 11, 2496. doi: 10.1038/s41598-021-82001-0
Mallikarjuna Swamy, B. P., Samia, M., Boncodin, R., Marundan, S., Jr., Rebond, D. B., Ordonio, R. L., et al. (2019). Compositional analysis of genetically engineered GR2E “Golden rice” in comparison to that of conventional rice. J. Agric. Food Chem. 67, 7986–7994. doi: 10.1021/acs.jafc.9b01524
Mammadov, J., Buyyarapu, R., Guttikonda, S. K., Parliament, K., Abdurakhmonov, I. Y., Kumpatla, S. P. (2018). Wild relatives of maize, rice, cotton, and soybean: Treasure troves for tolerance to biotic and abiotic stresses. Front. Plant Sci. 9. doi: 10.3389/fpls.2018.00886
Maqbool, A., Abrar, M., Bakhsh, A., Çalışkan, S., Khan, H. Z., Aslam, M., et al. (2020). “Biofortification under climate change: The fight between quality and quantity,” in Environment, climate, plant and vegetation growth. Eds. Fahad, S., Hasanuzzaman, M., Alam, M., Ullah, H., Saeed, M., Khan, I. A. (Springer, Cham, Switzerland). doi: 10.1007/978-3-030-49732-3_9
Marschner, H., Römheld, V., Kissel, M. (1986). Different strategies in higher plants in mobilization and uptake of iron. J. Plant Nutr. 9 (3-7), 695–713. doi: 10.1080/01904168609363475
Marze, S. (2017). Bioavailability of nutrients and micronutrients: Advances in modeling and in vitro approaches. Annu. Rev. Food Sci. Technol. 8, 35–55. doi: 10.1146/annurev-food-030216-030055
Masuda, H., Usuda, K., Kobayashi, T., Ishimaru, Y., Kakei, Y., Takahashi, M., et al. (2009). Overexpression of the barley nicotianamine synthase gene HvNAS1 increases iron and zinc concentrations in rice grains. Rice 2, 155–166. doi: 10.1007/s12284-009-9031-1
Mayer, J. E. (2005). The golden rice controversy: Useless science or unfounded criticism? BioScience 55, 726–727. doi: 10.1641/0006-3568(2005)055[0726:TGRCUS]2.0.CO;2
Mbabazi, R., Harding, R., Khanna, H., Namanya, P., Arinaitwe, G., Tushemereirwe, W., et al. (2019). Provitamin a carotenoids in East African highland banana and other musa cultivars grown in Uganda. Food Sci. Nutr. 8, 311–321. doi: 10.1002/fsn3.1308
McCallum, C. M., Comai, L., Greene, E. A., Henikoff, S. (2000). Targeting induced local lesions IN genomes (TILLING) for plant functional genomics. Plant Physiol. 123, 439–442. doi: 10.1104/pp.123.2.439
Meis, S. J., Fehr, W. R., Schnebly, S. R. (2003). Seed source effect on field emergence of soybean lines with low phytate. Crop Sci. 43, 1336–1339. doi: 10.2135/cropsci2003.1336
Melse-Boonstra, A. (2020). Bioavailability of micronutrients from nutrient-dense whole foods: Zooming in on dairy, vegetables, and fruits. Front. Nutr. 7. doi: 10.3389/fnut.2020.00101
Mengesha, W., Menkir, A., Meseka, S., Bossey, B., Afolabi, A., Burgueno, J., et al. (2019). Factor analysis to investigate genotype and genotype × environment interaction effects on pro-vitamin a content and yield in maize synthetics. Euphytica 215, 180. doi: 10.1007/s10681-019-2505-3
Menguer, P. K., Vincent, T., Miller, A. J., Brown, J. K. M., Vincze, E., Borg, S., et al. (2018). Improving zinc accumulation in cereal endosperm using HvMTP1, a transition metal transporter. Plant Biotechnol. J. 16, 63–71. doi: 10.1111/pbi.12749
Menkir, A., Liu, W., White, W. S., Maziya-Dixon, B., Rocheford, T. (2008). Carotenoid diversity in tropical-adapted yellow maize inbred lines. Food Chem. 109, 521–529. doi: 10.1016/j.foodchem.2008.01.002
Menkir, A., Rocheford, T., Mazia-Dixon, B., Tanumihardio, S. (2015). Exploiting natural variation in exotic germplasm for increasing provitamin-a carotenoids in tropical maize. Euphytica 205, 203–217. doi: 10.1007/s10681-015-1426-z
Miranda, P. V., Iglesias, B. F., Charriere, M. V., Burachik, M. (2022). Drought tolerant wheat IND-ØØ412-7 is nutritionally equivalent to its non-transgenic comparator. GM Crops Food 13, 119–125. doi: 10.1080/21645698.2022.2079179
Morrissey, J., Baxter, I. R., Lee, J., Li, L., Lahner, B., Grotz, N., et al. (2009). The ferroportin metal efflux proteins function in iron and cobalt homeostasis in Arabidopsis. Plant Cell 21, 3326–3338. doi: 10.1105/tpc.109.069401
Murgia, I., Marzorati, F., Vigani, G., Morandini, P. (2022). Plant iron nutrition: The long road from soil to seeds. J. Exp. Bot. 73, 1809–1824. doi: 10.1093/jxb/erab531
Murray-Kolb, L. E. (2013). Iron and brain functions. Curr. Opin. Clin. Nutr. Metab. Care 16, 703–707. doi: 10.1097/MCO.0b013e3283653ef8
Murray-Kolb, L. E., Wenger, M. J., Scott, S. P., Rhoten, S. E., Lung’aho, M. G., Haas, J. D. (2017). Consumption of iron-biofortified beans positively affects cognitive performance in 18- to 27-year-old Rwandan female college students in an 18-week randomized controlled efficacy trial. J. Nutr. 147, 2109–2117. doi: 10.3945/jn.117.255356
Muthusamy, V., Hossain, F., Thirunavukkarasu, N., Saha, S., Agrawal, P. K., Guleria, S. K., et al. (2015). Genetic variability and interrelationship of kernel carotenoids among indigenous and exotic maize (Zea mays l.) inbreds. Cereals Res. Commun. 43, 567–578. doi: 10.1556/0806.43.2015.012
Naqvi, S., Zhu, C., Farre, G., Ramessar, K., Bassie, L., Breitenbach, J., et al. (2009). Transgenic multivitamin corn through biofortification of endosperm with three vitamins representing three distinct metabolic pathways. Proc. Natl. Acad. Sci. U.S.A. 106, 7762–7767. doi: 10.1073/pnas.0901412106
Narayanan, N., Beyene, G., Chauhan, R. D., Gaitán-Solís, E., Gehan, J., Butts, P., et al. (2019). Biofortification of field-grown cassava by engineering expression of an iron transporter and ferritin. Nat. Biotechnol. 37, 144–151. doi: 10.1038/s41587-018-0002-1
Natesan, S., Singh, T. S., Duraisamy, T., Chandrasekharan, N., Chandran, S., Adhimoolam, K., et al. (2020). Characterization of crtRB1 gene polymorphism and β-carotene content in maize landraces originated from northeastern Himalayan region (NEHR) of India. Front. Sustain. Food Syst. 4. doi: 10.3389/fsuf.2020.00078
NFSH 5 (2021). Compendium of nutritional fact sheets., (2019-21) - international institute for population sciences (IIPS) and ICF (Ministry of Health and Family Welfare is a Ministry under the Government of India), p734. Available at: https://www.iipsindia.ac.in/content/nfhs-project.
Nazir, M., Mahajan, R., Mansoor, S., Rasool, S., Mir, R. A., Sing, R., et al. (2022). Identification of QTLs/candidate genes for seed mineral contents in common bean (Phaseolus vulgaris l.) through genotyping-by-sequencing. Front. Genet. 13. doi: 10.3389/fgene.2022.750814
Neelamraju, S., Mallikarjuna Swamy, B. P., Kaladhar, K., Aunradha, K., Venkateswar Rao, Y., Batchu, A. K., et al. (2012). Increasing iron and zinc in rice grains using deep water rices and wild species – identifying genomic segments and candidate genes. special issue: Ist ICC India grains conf. in partnership with ICRISAT. quality assessment and safety crops and food. Eds. van der Kamp, J. W., Taylor, J. R. N., 138–138. doi: 10.1111/j.1757-837X.2012.00142.x
Nguyen, T. M. P., Abiko, T., Nakamura, T., Mochizuki, T. (2022). Development and application of a plate method for visualizing aleurone layers in mature rice grains. Plant Prod. Sci. 25, 260–267. doi: 10.1080/1343943X.2022.2030239
Nica-Badea, D., Balacescu, A. (2021). Biosafety assessment of food products derived from genetically modified plant crops in relation to allergenic potential. ann. constantin brancusi univ. Targu Jiu Eng. Ser. 3, 79–786.
Nicholson, C. C., Emery, B. F., Niles, M. T. (2021). Global relationships between crop diversity and nutritional stability. Nat. Commun. 12, 5310. doi: 10.1038/s41467-021-25615-2
Nishiyama, R., Kato, M., Nagata, S., Yanagisawa, S., Yoneyama, T. (2012). Identification of zn–nicotianamine and fe–2′-deoxymugineic acid in the phloem sap from rice plants (Oryza sativa l.). Plant Cell Physiol. 53, 381–390. doi: 10.1093/pcp/pcr188
Nyiraguhirwa, S., Grana, Z., Ouabbbou, H., Iraqi, D., Ibriz, M., Mamidi, S., et al. (2022). A genome-wide association study identifying single-nucleotide polymorphisms for iron and zinc biofortification in a worldwide barley collection. Plants 11, 1349. doi: 10.3390/plants11101349
Ogasawara, M., Miyazaki, N., Monden, G., Taniko, K., Lim, S., Iwata, M., et al. (2021). Role of qGZn9a in controlling grain zinc concentration in rice, Oryza sativa l. Theor. Appl. Genet. 134, 3013–3022. doi: 10.1007/s00122-021-03873-4
Olayide, P., Large, A., Stridh, L., Rabbi, I., Baldermann, S., Stavolone, L., et al. (2020). Gene expression and metabolite profiling of thirteen Nigerian cassava landraces to elucidate starch and carotenoid composition. Agronomy 10, 424. doi: 10.3390/agronomy10030424
Oliva, N., Florida Cueto-Reaño, M., Trijatmiko, K. R., Samia, M., Welsch, R., Schaub, P., et al. (2020). Molecular characterization and safety assessment of biofortified provitamin a rice. Sci. Rep. 10, 1376. doi: 10.1038/s41598-020-57669-5
Oltmans, S. E., Fehr, W. R., Welke, G. A., Cianzio, S. R. (2005). Agronomic and seed traits of soybean lines with low phytate. Crop Sci. 45, 593–598. doi: 10.2135/cropsci2005.0593
Ortiz-Monasterio, I., Palacios-Rojas, N., Meng, E., Pixley, K., Trethowan, R., Pena, R. J. (2007). Enhancing the mineral and vitamin content of wheat and maize through plant breeding. J. Cereal Sci. 46, 293–307. doi: 10.1016/j.jcs.2007.06.005
Owens, B. (2018). Golden rice is safe to eat, says FDA. Nat. Biotechnol. 36, 559–560. doi: 10.1038/nbt0718-559a
Owens, B. F., Lipka, A. E., Magallanes-Lundback, M., Tiede, T., Diepenbrock, C., Kandianis, C. B., et al. (2014). A foundation for provitamin a biofortification of maize: Genome-wide association and genomic prediction models of carotenoid levels. Genetics 198, 1699–1716. doi: 10.1534/genetics.114.169979
Paine, J. A., Shipton, C. A., Chaggar, S., Howells, R. M., Kennedy, M. J., Vernon, G., et al. (2005). Improving the nutritional value of golden rice through increased provitamin a content. Nat. Biotechnol. 23, 482–487. doi: 10.1038/nbt1082
Panigrahi, S., Pamkaj, Y. K., Kumar, V., Kumar, R., Singh, S. K. (2022). Studies on effects of terminal heat stress on yield stability, grain iron and zinc contents in wheat (Triticum aestivum l.). Indian J. Genet. 82, 289–298. doi: 10.31742/ISGPB.82.3.3
Paoloni-Giacobino, A., Grimble, R., Pichard, C. (2003). Genetics and nutrition. Clin. Nutr. 22, 429–435. doi: 10.1016/S0261-5614(03)00064-5
Paul, J.-Y., Harding, R., Tushemeirwe, W., Dale, J. (2018). Banana21: From gene discovery to deregulated golden bananas. Front. Plant Sci. 9. doi: 10.3389/fpls.2018.00558
Paul, J.-Y., Khanna, H., Kleidon, J., Hoang, P., Geijskes, J., Daniells, J., et al. (2017). Golden bananas in the field: Elevated fruit pro-vitamin a from the expression of a single banana transgene. Plant Biotechnol. J. 15, 520–532. doi: 10.1111/pbi.12650
Perea, C., de la Hoz, J. F., Cruz, D. F., Lobaton, J. D., Izquierdo, P., Quintero, J. C., et al. (2016). Bioinformatic analysis of genotype by sequencing (GBS) data with NGSEP. BMC Genom. 17, 498. doi: 10.1186/s12864-016-2827-7
Pfeiffer, W., Andersson, M., Govindaraj, M., Parminder, V., Cherian, B., Illona, P., et al. (2018). “Biofortification in underutilized staple crops for nutrition in Asia and Africa,” in Regional expert consultation on underutilized crops for food and nutritional security in Asia and the pacific – thematic, strategic papers and country status reports (Asia-Pacific Association of Agricultural Research Institutions (APAARI), Bangkok, Thailand), 70–81, ISBN: ISBN 978-616-7101-10-1.
Pilu, R., Panzeri, D., Gavazzi, G., Rasmussen, S. K., Consonni, G., Nelsen, E. (2003). Phenotypic, genetic and molecular characterization of a maize low phytic acid mutant (lpa241). Theor. Appl. Genet. 107, 980–987. doi: 10.1007/s00122-003-1316-y
Pinson, S. R. M., Tarpley, L., Yan, W., Yeater, K., Lahner, B., Yakubova, E., et al. (2015). Worldwide genetic diversity for mineral element concentrations in rice grain. Crop Sci. 55, 294 –311. doi: 10.2135/cropsci2013.10.0656
Powers, S., Boatwright, J. L., Thavarajah, D. (2021). Genome-wide association studies of mineral and phytic acid concentrations in pea (Pisum sativum l.) to evaluate biofortification potential. G3 11, jkab227. doi: 10.1093/g3journal/jkab227
Pujar, M., Govindraj, M., Gangaprasad, S., Kanatti, A., Shivade, H. (2020). Genetic variation and diversity for grain iron, zinc, protein and agronomic traits in advanced breeding lines of pearl millet [Pennisetum glaucum (L.) r, br] for biofortification breeding. Genet. Resour. Crop Evol. 67, 2009–2022. doi: 10.1007/s10722-020-00956-x
Pulla, P. (2016). India Nears putting GM mustard on the table. Science 352, 1043. doi: 10.1126/science.352.6289.1043
Qin, Y., Park, S.-Y., Oh, S.-W., Lim, M.-H., Shin, K.-S., Cho, S.-H., et al. (2017). Nutritional composition analysis for beta-carotene-enhanced transgenic soybeans (Glycine max l.). Appl. Biol. Chem. 60, 299–309. doi: 10.1007/s13765-017-0282-z
Rabbi, I. Y., Udoh, L. I., Wolfe, M., Parkes, E. Y., Gedil, M. A., Dixon, A., et al. (2017). Genome-wide association mapping of correlated traits in cassava: Dry matter and total carotenoid content. Plant Genome 10, 10.3835. doi: 10.3835/plantgenome2016.09.0094
Rathan, N. D., Krishna, H., Elluer, R. K., Sehgal, D., Govindan, V., Ahlawat, A. K., et al. (2022). Genome-wide association study identifies loci and candidate genes for grain micronutrients and quality traits in wheat (Triticum aestivum l.). Sci. Rep. 12, 7037. doi: 10.1038/s41598-022-10618-w
Rathan, N. D., Sehgal, D., Thiyagarajan, K., Singh, R., Singh, A. M., Govindan, V. (2021). Identification of genetic loci and candidate genes related to grain zinc and iron concentration using a zinc-enriched wheat ‘Zinc-shakti’. Front. Genet. 12. doi: 10.3389/fgene.2021.652653
Robinson, N. J., Procter, C. M., Connolly, E. L., Guerinot, M. L. (1999). A ferric-chelate reductase for iron uptake from soils. Nature 397, 694–697. doi: 10.1038/17800
Rogers, E. E., Eide, D. J., Guerinot, M. L. (2000). Altered selectivity in an Arabidopsis metal transporter. Proc. Natl. Acad. Sci. U.S.A. 97, 12356–12360. doi: 10.1073/pnas.210214197
Ruiz-Sola, M. A., Rodríguez-Concepción, M. (2012). Carotenoid biosynthesis in arabidopsis: A colorful pathway. Arabidopsis Book 10 (2012), e0158. doi: 10.1199/tab.0158
Sab, S., Lokesha, R., Mannur, D. M., Somasekhar, Jadhav, K., Mallikarjuna, B. P., et al. (2020). Genomewide SNP discovery and mapping QTLs for seed iron and zinc concentrations in chickpea (Cicer arietinum l.). Front. Nutr. 7. doi: 10.3389/fnut.2020.559120
Saltzman, A., Birol, E., Bouis, H. E., Boy, E., De Moura, F. F., Islam, Y., et al. (2014). Biofortification: progress toward a more nourishing future. Glob. Food Sec. 2, 9–17. doi: 10.1016/j.gfs.2012.12.003
Sayre, R., Beeching, J. R., Cahoon, E. B., Egesi, C., Fauquet, C., Fellman, J., et al. (2011). The BioCassava plus program: Biofortification of cassava for Sub-Saharan Africa. Annu. Rev. Plant Biol. 62, 251–272. doi: 10.1146/annurev-arplant-042110-103751
Scarpa, G., Berrang-Ford, L., Zavaleta-Cortijo, C., Marshall, L., Sherilee, L. H., Cade, J. E. (2020). The effect of climatic factors on nutrients in foods: evidence from a systematic map. Environ. Res. Lett. 15, 113002. doi: 10.1088/1748-9326/abafd4
Scheelbeeka, P. F. D., Birda, F. A., Tuomistob, H. L., Greena, R., Harria, F. B., Joyan, E. J. M., et al. (2018). Effect of environmental changes on vegetable and legume yields and nutritional quality. Proc. Natl. Acad. Sci. U.S.A. 115, 6804–6809. doi: 10.1073/pnas.1800442115
Schwarz, B., Bauer, P. (2020). FIT, a regulatory hub for iron deficiency and stress signaling in roots, and FIT-dependent and-independent gene signatures. J. Exp. Bot. 71, 1694–1705. doi: 10.1093/jxb/eraa012
Scott, S. P., Murray-Kolb, L. E., Wenger, M. J., Udipi, S. A., Ghugre, P. S., Boy, E., et al. (2018). Cognitive performance in Indian school-going adolescents is positively affected by consumption of iron-biofortified pearl millet: A 6-month randomized controlled efficacy trial. J. Nutr. 148, 1462–1471. doi: 10.1093/jn/nxy113
Semba, R. D., Askari, S., Gibson, S., Bloem, M. W., Kraemer, K. (2022). The potential impact of climate change on the micronutrient-rich food supply. Adv. Nutr. 13, 80–100. doi: 10.1093/advances/nmab104
Sestili, F., Garcia-Molina, M. D., Gambacorta, G., Beleggia, R., Botticela, E., De Vita, P., et al. (2019). Provitamin a biofortification of durum wheat through a TILLING approach. Int. J. Mol. Sci. 20, 5703. doi: 10.3390/ijms20225703
Shamanin, V. P., Fli, P., Savin, T. V., Shepelev, S. S., Kuzmin, O. G., Chursin, A. S., et al. (2021). Genotypic and ecological variability of zinc content in the grain of spring bread wheat varieties in the international nursery KASIB. Vavilov J. Genet. Breed. 25, 543–551. doi: 10.18699/VJ21.061
Sharma, V., Choudhary, M., Kumar, P., Choudhary, J. R., Khokhar, J. S., Kaushik, P., et al. (2021). Harnessing the wild relatives and landraces for fe and zn biofortification in wheat through genetic interventions–a review. Sustainability 13, 12975. doi: 10.3390/su132312975
Sharma, S., Lavale, S. A., Nimje, C., Singh, S. (2021). Characterization and identification of annual wild Cicer species for seed protein and mineral concentrations for chickpea improvement. Crop Sci. 61, 305–319. doi: 10.1002/csc2.20413
Shelton, A. M., Hossain, M. J., Paranjape, M. J., Azad, A. K., Rahman, M. L., Khan, A.S.M.M. R. L., et al. (2018). Bt eggplant project in Bangladesh: History, present status, and future direction. Front. Bioeng. Biotechnol. 6. doi: 10.3389/fbioe.2018.00106
Sheoran, S., Kumar, S., Ramtekey, V., Kar, P., Meena, R. S., Jangir, C. K. (2022). Current status and potential of biofortification to enhance crop nutritional quality: an overview. Sustainability 14, 3301. doi: 10.3390/su14063301
Sheraz, S., Wan, Y., Venter, E., Verma, S. K., Xiong, Q., Waites, J., et al. (2021). Subcellular dynamics studies of iron reveal how tissue-specific distribution patterns are established in developing wheat grains. New Phytol. 231, 1644–1657. doi: 10.1111/nph.17440
Shi, J. R., Wang, H. Y., Schelin, K., Li, B., Faller, M., Stoop, J. M., et al. (2007). Embryo-specific silencing of a transporter reduces phytic acid content of maize and soybean seeds. Nat. Biotechnol. 25, 930–937. doi: 10.1038/nbt1322
Signorell, C., Zimmermann, M. B., Cakmak, I., Wegmüller, R., Zeder, C., Hurrell, R., et al. (2019). Zinc absorption from agronomically biofortified wheat is similar to post-harvest fortified wheat and is a substantial source of bioavailable zinc in humans. J. Nutr. 149, 840–846. doi: 10.1093/jn/nxy328
Singhal, T., Satyavathi, C. T., Singh, S. P., Kumar, A., Sankar, S. M., Bhardwaj, C., et al. (2021). Multi-environment quantitative trait loci mapping for grain iron and zinc content using bi-parental recombinant inbred line mapping population in pearl millet. Front. Plant Sci. 12. doi: 10.3389/fpls.2021.659789
Singh, S. P., Keller, B., Gruissem, W., Bhulla, N. K. (2017). Rice NICOTIANAMINE SYNTHASE 2 expression improves dietary iron and zinc levels in wheat. Theor. Appl. Genet. 130, 283–292. doi: 10.1007/s00122-016-2808-x
Singh, R. P., Govindan, V. (2017). Zinc-Biofortified Wheat: Harnessing Genetic Diversity for Improved Nutritional Quality. Science Brief: Biofortification No. 1 (May 2017). CIMMYT, HarvestPlus, and the Global Crop Diversity Trust. Bonn, Germany.
Singh, S. P., Vogel-Mikuš, K., Vavpwtič, P., Jeromal, L., Pelicon, P., Kumar, J., et al. (2014). Spatial X-ray fluorescence micro-imaging of minerals in grain tissues of wheat and related genotypes. Planta 240, 277–289. doi: 10.1007/s00425-014-2084-4
Soares, J. C., Santos, C. S., Carvalho, S. M. P., Pintado, M. M., Vasconcelos, M. W. (2019). Preserving the nutritional quality of crop plants under a changing climate: importance and strategies. Plant Soils 443, 1–26. doi: 10.1007/s11104-019-04229-0
Sserumaga, J. P., Makumbi, D., Warburton, M. L., Opiyo, S. O., Asea, G., Muwonge, A., et al. (2019). Genetic diversity among tropical provitamin a maize inbred lines and implications for a biofortification program. Cereal Res. Commun. 47, 134–144. doi: 10.1556/0806.46.2018.066
Stahl, W., Sies, H. (2005). Bioactivity and protective effects of natural carotenoids. Biochim. Biophys. Acta 1740, 101–107. doi: 10.1016/j.bbadis.2004.12.006
Stanton, C., Sanders, D., Krämer, U., Podar, D. (2022). Zinc in plants: Integrating homeostasis and biofortification. Mol. Plant 15, 65–85. doi: 10.1016/j.molp.2021.12.008
Steur, H. D., Stein, A. J., Demont, M. (2022). From golden rice to golden diets: How to turn its recent approval into practice. Glob. Food Sec. 32, 100596. doi: 10.1016/j.gfs.2021.100596
Stover, P. J. (2007). Human nutrition and genetic variation. Food Nutr. Bull. 28, S101–S115. doi: 10.1177/15648265070281S109
Suman, K., Neeraja, C. N., Madhubabu, P., Rathod, S., Bej, S., Jadhav, K. P., et al. (2021). Identification of promising RILs for high grain zinc through genotype × environment analysis and stable grain zinc QTL using SSRs and SNPs in rice (Oryza sativa l.). Front. Plant Sci. 12. doi: 10.3389/fpls.2021.587482
Sun, T., Yuan, H., Cao, H., Yazdani, M., Tadmor, Y., Li, L. (2018). Carotenoid metabolism in plants: The role of plastids. Mol. Plant 11, 58–74. doi: 10.1016/j.molp.2017.09.010
Symeonidis, L., Karataglis, S. (1992). Interactive effects of cadmium, lead and zinc on root growth of two metal tolerant genotypes of Holcus lanatus l. Biometals 5, 173–178. doi: 10.1007/BF01061325
Tam, E., Keats, E. C., Rind, F., Das, J. K., Bhutta, Z. A. (2020). Micronutrient supplementation and fortification interventions on health and development outcomes among children under-five in low- and middle-income countries: A systematic review and meta-analysis. Nutrients 12, 289. doi: 10.3390/nu12020289
Taub, D. R., Miller, B., Allen, H. (2008). Effects of elevated CO2 on the protein concentration of food crops: a meta-analysis. Global Change Biol. 14, 565–575. doi: 10.1111/j.1365-2486.2007.01511.x
Thabet, S. G., Alomari, D. Z., Brinch-Pedersen, H., Alqudah, A. M. (2022). Genetic analysis toward more nutritious barley grains for a food secure world. Botanical Stud. 63, 6. doi: 10.1186/s40529-022-00334-z
Tong, J., Zhao, C., Sun, M., Fu, L., Song, J., Liu, D., et al. (2022). High resolution genome wide association studies reveal rich genetic architectures of grain zinc and iron in common wheat (Triticum aestivum l.). Front. Plant Sci. 13. doi: 10.3389/fpls.2022.840614
Tran, T.-L., Ho, T.-H., Nguyen, D.-H. (2017). Overexpression of the IbOr gene from sweet potato (Ipomea batatas ‘Hoang long’) in maize increases total carotenoid and β-carotene contents. Turk. J. Biol. 41, 1003–1010. doi: 10.3906/biy-1708-87
Trijatmiko, K. R., Dueñas, C., Tsakirpaloglou, N., Torrizo, L., Arines, F. M., Adeva, C., et al. (2016). Biofortified indica rice attains iron and zinc nutrition dietary targets in the field. Sci. Rep. 6, 19792. doi: 10.1038/srep19792
Tripathi, S., Bahuguna, R. N., Shrivastava, N., Singh, S., Chatterjee, A., Varma, A., et al. (2022). Microbial biofortification: A sustainable route to grow nutrient-rich crops under changing climate. Field Crops Res. 287, 108662. doi: 10.1016/j.fcr.2022.108662
Turnbull, C., Lillemo, M., Hvoslef-Eide, T. A. K. (2021). Global regulation of genetically modified crops amid the gene edited crop boom – a review. Front. Plant Sci. 12. doi: 10.3389/fpls.2021.630396
Upadhyaya, H. D., Bajaj, D., Das, S., Kumar, V., Gowda, C. L. L., Sharma, S., et al. (2016b). Genetic dissection of seed-iron and zinc concentrations in chickpea. Sci. Rep. 6, 24050. doi: 10.1038/srep24050
Upadhyaya, H. D., Dronavalli, N., Singh, S., Dwivedi, S. L. (2012). Variability and stability for kernel iron and zinc contents in the ICRISAT mini core collection of peanut. Crop Sci. 52, 2628–2637. doi: 10.2135/cropsci2012.05.0306
Upadhyaya, H. D., Dwivedi, S. L., Singh, S., Sahrawat, K. L., Singh, S. K. (2016a). Genetic variation and postflowering drought effects on seed iron and zinc in ICRISAT sorghum mini core collection. Crop Sci. 56, 374–384. doi: 10.2135/cropsci2015.05.0308
Uthpala, T. G. G., Fernando, H. N., Thibbotuwawa, A., Jayasinghe, M. (2020). Importance of nutrigenomics and nutrigenetics in food science. MOJ Food Process Technol. 8, 114–119. doi: 10.15406/mojfpt.2020.08.00250
Vasconcelos, V., Datta, K., Oliva, N., Khalekuzzaman, M., Torrizo, L., Krishnan, S., et al. (2003). Enhanced iron and zinc accumulation in transgenic rice with the ferritin gene. Plant Sci. 164, 371–378. doi: 10.1016/S0168-9452(02)00421-1
Velu, G., Guzman, C., Mondal, S., Autrique, J. E., Huerta, J., Singh, R. P. (2016). Effect of drought and elevated temperature on grain zinc and iron concentrations in CIMMYT spring wheat. J. Cereal Sci. 69, 182–186. doi: 10.1016/j.jcs.2016.03.006
Velu, G., Herrera, L. C., Guzman, C., Huerta, J., Payne, T., Singh, R. P. (2019). Assessing genetic diversity to breed competitive biofortified wheat with enhanced grain zn and fe concentrations. Front. Plant Sci. 9. doi: 10.3389/fpls.2018.01971
Velu, G., Singh, R. P., Crespo-Herrera, L., Juliana, P., Dreisigacker, S., Valluru, R., et al. (2018). Genetic dissection of grain zinc concentration in spring wheat for mainstreaming biofortification in CIMMYT wheat breeding. Sci. Rep. 18, 13526. doi: 10.1038/s41598-018-31951-z
Virk, P. S., Andersson, M. S., Arcos, J., Govindaraj, M., Pfeiffer, W. H. (2021). Transition from targeted breeding to mainstreaming of biofortification traits in crop improvement programs. Front. Plant Sci. 12. doi: 10.3389/fpls.2021.703990
Von Grebmer, K., Bernstein, J., Resnick, D., Wiemers, M., Reiner, L., Bachmeier, M., et al. (2022) Global hunger index: Food systems transformation and local governance (Bonn: Welthungerhilfe; and Dublin: Concern Worldwide). Available at: https://www.globalhungerindex.org/issues-in-focus/2022.html (Accessed Assessed on Oct 15, 2022).
von Lintig, J. (2010). Colors with functions: Elucidating the biochemical and molecular basis of carotenoid metabolism. Annu. Rev. Nutr. 30, 35–56. doi: 10.1146/annurev-nutr-080508-141027
von Wirén, N., Marschner, H., Romheld, V. (1996). Roots of iron-efficient maize also absorb phytosiderophore-chelated zinc. Plant Physiol. 111, 1119–1125. doi: 10.1104/pp.111.4.1119
Wang, X., He, Y., Gao, Q., Yang, D., Liang, J. (2021). Approaches to evaluate nutrition of minerals in food. Food Sci. Hum. Wellness 10, 141–148. doi: 10.1016/j.fshw.2021.02.002
Wang, H., Jia, J., Cai, Z., Duan, M., Jiang, Z., Xia, Q., et al. (2022). Identification of quantitative trait loci (QTLs) and candidate genes of seed iron and zinc content in soybean [Glycine max (L.) merr.]. BMC Genom. 23, 146. doi: 10.1186/s12864-022-08313-1
Wan, Y., Stewart, T., Amrahli, M., Evans, J., Sharp, P., Govindan, V., et al. (2022). Localisation of iron and zinc in grain of biofortified wheat. J. Cereal Sci. 105, 103470. doi: 10.1016/j.jcs.2022.103470
Waters, B. M., Chu, H. H., DiDonato, R. J., Roberts, L. A., Eisley, R. B., Lahner, B., et al. (2006). Mutations in Arabidopsis yellow stripe-like1 and yellow stripe-like3 reveal their roles in metal ion homeostasis and loading of metal ions in seeds. Plant Physiol. 141, 1446–1458. doi: 10.1104/pp.106.082586
White, P. J., Broadley, M. R. (2011). Physiological limits to zinc biofortification of edible crops. Front. Plant Sci. 2. doi: 10.3389/fpls.2011.00080
Wiegmann, M., Thomas, W. T. B., Bull, H. J., Flavell, A. J., Zeyner, A., Peiter, E., et al. (2019). Wild barley serves as a source for biofortification of barley grains. Plant Sci. 283, 83–94. doi: 10.1016/j.plantsci.2018.12.030
Winkler, J. T. (2011). “Biofortification: improving the nutritional quality of staple crops,” in Access not excess. Ed. Pasternak, C. (Smith-Gordon Publishing, Cambridgeshire, United Kingdom), 100–112.
Wolson, R. (2007). Assessing the prospects for adoption of biofortified crops in south Africa. AgBioForum 10, 184–191.
Wood, R. J., Tamura, T. (2001). Methodological issues in assessing bioavailability of nutrients and other bioactive substances in dietary supplements. J. Nutr. 131, 1396S–1398S. doi: 10.1093/jn/131.4.1396S
World Bank. (2020). “The crisis of malnutrition in India,” in World bank report 2020 (The World Bank 1818 H Street, NW Washington, DC 20433). Available at: https://web.worldbank.org/archive/website01394/WEB/IMAGES/INDIA.PDF.
Wright, T. I. C., Gardner, K. A., Glahn, R. P., Milner, M. J. (2021). Genetic control of iron bioavailability is independent from iron concentration in a diverse winter wheat mapping population. BMC Plant Biol. 21, 212. doi: 10.1186/s12870-021-02996-6
Wu, T.-Y., Gruissem, W., Bhullar, N. K. (2019). Targeting intracellular transport combined with efficient uptake and storage significantly increases grain iron and zinc levels in rice. Plant Biotechnol. J. 17, 9–20. doi: 10.1111/pbi.12943
Wu, D., Tanaka, R., Li, X., Ramstein, G. P., Cu, S., Hamilton, J. P., et al. (2021a). High-resolution genome-wide association study pinpoints metal transporter and chelator genes involved in the genetic control of element levels in maize grain. G3 11, jkab059. doi: 10.1093/g3journal/jkab059
Wu, D., Wesselerc, J., Zilbermand, D., Russelle, R. M., Chena, C., Dubockh, A. C. (2021b). Allow golden rice to save lives. Proc. Natl. Acad. Sci. U.S.A. 118, e2120901118. doi: 10.1073/pnas.2120901118
Xie, X., Hu, W., Fan, X., Chen, H., Tang, M. (2019). Interactions between phosphorus, zinc, and iron homeostasis in nonmycorrhizal and mycorrhizal plants. Front. Plant Sci. 10. doi: 10.3389/fpls.2019.01172
Yates, A. A. (2001). National nutrition and public health policies: Issues related to bioavailability of nutrients when developing dietary reference intakes. J. Nutr. 131, 1331S–1334S. doi: 10.1093/jn/131.4.1331S
Ye, X., Al-Babili, S., Klöti, A., Zhang, J., Lucca, P., Beyer, P., et al. (2000). Engineering the provitamin a (beta-carotene) biosynthetic pathway into (carotenoid-free) rice endosperm. Science 287, 303–305. doi: 10.1126/science.287.5451.303
Yokosho, K., Yamaji, N., Ma, J. F. (2016). OsFRDL1 expressed in nodes is required for distribution of iron to grains in rice. J. Exp. Bot. 67, 5485–5494. doi: 10.1093/jxb/erw314
Yuan, F. J., Zhao, H. J., Ren, X. L., Zhu, S. L., Fu, X. J., Shu, K. Y. (2007). Generation and characterization of two novel low phytate mutations in soybean (Glycine max l. merr.). Theor. Appl. Genet. 115, 945–957. doi: 10.1007/s00122-007-0621-2
Yu, S., Li, M., Dubcovsky, J., Tian, L. (2022). Mutant combinations of lycopene ε-cyclase and β-carotene hydroxylase 2 homoeologs increased β-carotene accumulation in endosperm of tetraploid wheat (Triticum turgidum l.) grains. Plant Biotechnol. J. 20, 564–576. doi: 10.1111/pbi.13738
Yu, R., Wu, X., Liu, J., Howitt, C. A., Bird, A. R., Liu, C.-M., et al. (2021). Rice with multilayer aleurone: A larger sink for multiple micronutrients. Rice 14, 102. doi: 10.1186/s12284-021-00543-3
Zhao, Y., Thorwarth, P., Jiang, Y., Schulthess, N. P. A. W., Boeven, M. G. P. H. G., Schacht, F. H. L. J., et al. (2021). Unlocking big data doubled the accuracy in predicting the grain yield in hybrid wheat. Sci. Adv. 7, eabf9106. doi: 10.1126/sciadv.abf9106
Zhang, Y., Xu, Y. H., Yi, H. Y., Gong, J. M. (2012). Vacuolar membrane transporters OsVIT1 and OsVIT2 modulate iron translocation between flag leaves and seeds in rice. Plant J. 72, 400–410. doi: 10.1111/j.1365-313X.2012.05088.x
Zhao, G. (2010). Phytoferritin and its implications for human health and nutrition. Biochim. Biophys. Acta - Gen. Subj 1800 (8), 815–823. doi: 10.1016/j.bbagen.2010.01.009
Zheng, L., Cheng, Z., Ai, C., Jiang, X., Bei, X., Zheng, Y., et al. (2010). Nicotianamine, a novel enhancer of rice iron bioavailability to humans. PloS One 5, e10190. doi: 10.1371/journal.pone.0010190
Zielińska-Dawidziak, M. (2015). Plant ferritin–a source of iron to prevent its deficiency. Nutrients 7, 1184–1201. doi: 10.3390/nu7021184
Keywords: bioavailability, bioaccessibility and absorption, biofortified crop cultivars, climate change, genes and genetic markers, genetic engineering, nutrient acquisition, transport and storage
Citation: Dwivedi SL, Garcia-Oliveira AL, Govindaraj M and Ortiz R (2023) Biofortification to avoid malnutrition in humans in a changing climate: Enhancing micronutrient bioavailability in seed, tuber, and storage roots. Front. Plant Sci. 14:1119148. doi: 10.3389/fpls.2023.1119148
Received: 08 December 2022; Accepted: 12 January 2023;
Published: 30 January 2023.
Edited by:
Owen A Hoekenga, Cayuga Genetics Consulting Group LLC, United StatesReviewed by:
Philip Kear, International Potato Center, ChinaKurniawan Rudi Trijatmiko, International Rice Research Institute (IRRI), Philippines
Copyright © 2023 Dwivedi, Garcia-Oliveira, Govindaraj and Ortiz. This is an open-access article distributed under the terms of the Creative Commons Attribution License (CC BY). The use, distribution or reproduction in other forums is permitted, provided the original author(s) and the copyright owner(s) are credited and that the original publication in this journal is cited, in accordance with accepted academic practice. No use, distribution or reproduction is permitted which does not comply with these terms.
*Correspondence: Rodomiro Ortiz, cm9kb21pcm8ub3J0aXpAc2x1LnNl