- State Key Laboratory of Marine Environmental Science, Key Laboratory of the Ministry of Education for Coastal and Wetland Ecosystem, College of the Environment and Ecology, Xiamen University, Xiamen, Fujian, China
As part of the plant water-use process, plant nocturnal sap flow (Qn) has been demonstrated to have important ecophysiological significance to compensate for water loss. The purpose of this study was to explore nocturnal water-use strategies to fill the knowledge gap in mangroves, by measuring three species co-occurring in a subtropical estuary. Sap flow was monitored over an entire year using thermal diffusive probes. Stem diameter and leaf-level gas exchange were measured in summer. The data were used to explore the different nocturnal water balance maintaining mechanisms among species. The Qn existed persistently and contributed markedly over 5.5%~24.0% of the daily sap flow (Q) across species, which was associated with two processes, nocturnal transpiration (En) and nocturnal stem water refilling (Rn). We found that the stem recharge of the Kandelia obovata and Aegiceras corniculatum occurred mainly after sunset and that the high salinity environment drove higher Qn while stem recharge of the Avicennia marina mainly occurred in the daytime and the high salinity environment inhibited the Qn. The diversity of stem recharge patterns and response to sap flow to high salinity conditions were the main reasons for the differences in Qn/Q among species. For Kandelia obovata and Aegiceras corniculatum, Rn was the main contributor to Qn, which was driven by the demands of stem water refilling after diurnal water depletion and high salt environment. Both of the species have a strict control over the stomata to reduce water loss at night. In contrast, Avicennia marina maintained a low Qn, driven by vapor pressure deficit, and the Qn mainly used for En, which adapts to high salinity conditions by limiting water dissipation at night. We conclude that the diverse ways Qn properties act as water-compensating strategies among the co-occurring mangrove species might help the trees to overcoming water scarcity.
Introduction
Daily water use strategy, reflected by both diurnal and nocturnal sap flow, is a critical component of plant water physiology (Steppe et al., 2006). Nocturnal sap flow is the night-time movement of fluid within the sapwood of root, stem or branch in trees (Forster, 2014). With the development of sap flow techniques, a substantial amount of evidence has shown that the nocturnal sap flow (Qn) occurs in a range of woody plants in different biomes and contributes significantly to daily sap flow (Q) (Dawson et al., 2007; Pfautsch et al., 2011; Forster, 2014). On a global scale, Qn contributed over 12% of Q among various woody plants in different biotas and the Qn/Q can reach up to 69% in some species (Forster, 2014). The ubiquity of Qn and its potentially high values have significant implications to model plant and ecosystem water and energy balances and need to be accurately quantified (Zeppel et al., 2010; Zeppel et al., 2014).
For terrestrial plants, Qn consists of two significant ecophysiological and ecohydrological components: transpiration of water from the canopy and stem water refilling at night (Daley & Phillips, 2006; Caird et al., 2007; Forster, 2014). Both processes influence forest water budgets and plant responses to water stress (Zeppel et al., 2014; Chen et al., 2020). For example, continuous nocturnal transpiration helps transports oxygen and nutrients into the thin-walled cells of the plant xylem, which maintains the oxygen and nutrient requirements of the plant cells (Daley and Phillips, 2006). Nocturnal stem water refilling contributed to removing embolisms and repairing cavitation in eight plant species in mesic and semi-arid environments (Zeppel et al., 2019). Previous research has also shown that Qn improved the pre-dawn disequilibrium relationship between soil and plant water potential in four temperate conifer species in northern Idaho (Kavanagh et al., 2007). The contribution of these two components to Qn varies widely among species. Liu et al. (2021) found that Qn in Platycladus orientalis and Quercus variabilis in a temperate forest consisted mainly of stem water refilling, which effectively maintained the stem water balance under drought stress. However, Zeppel et al. (2010) found that the nocturnal transpiration was the main component of Qn in two co-occurring evergreen forest species, Eucalyptus parramattensis and Angophora bakeri. The ecophysiological controls over Qn differed even under identical environmental conditions and different patterns of nocturnal water use also reflect habitat adaptation of species (Daley & Phillips, 2006). Therefore, quantifying the contributions of nocturnal transpiration and stem water refilling to Qn, has important implications in understanding nocturnal plant water-use strategies.
Growing evidence has suggested that in some plants the stomata remained open or partially open at night (Gindel, 1970; Caird et al., 2007; Fisher et al., 2007; Zhao et al., 2017), and that incomplete stomata closure provides the transpirational pull needed for nocturnal transpiration. Vapor pressure deficit (VPD) has been identified in some studies as the most crucial environmental driver of nocturnal transpiration (Dawson et al., 2007; Fisher et al., 2007; Zeppel et al., 2014; Wu et al., 2020). Other studies reported negative (Bucci et al., 2004) or negligible effects (Barbour et al., 2005) of VPD on nocturnal stomata. Wind speed affected the moisture movement around plant canopy (Fricke, 2019) and thus influenced on nocturnal water loss (Chen et al., 2020), which also could not be ignored. However, some studies discovered the opposite result that wind speed had little effects on the nocturnal water loss (Wang et al., 2018; Wu et al., 2020). Daytime water consumption during transpiration is also an important factor affecting Qn. Stem tissue water storage in is often depleted or partially depleted after intense transpiration during the daytime. This forces the roots to absorb water during the night to overcome the water deficit (Oren et al., 1999). At the end of the century, the predicted global average surface air temperature is projected to be 1.4 to 4.4°C higher than that in 1850–1900, with larger increases observed at night, especially in the tropics (IPCC, 2014; IPCC, 2021). Such a rise in temperature will result in an exponential climb in VPD, and more specifically in leaf-to-air VPD (Grossiord et al., 2020), which will affect plant transpiration during the day and enhance atmospheric evaporative demand at night (Zeppel et al., 2014). The processes of En and Rn will ultimately be affected. A better understanding of Qn will help to provide accurate predictions of plant adaptation in the face of climate change.
Mangroves are salt-tolerant plants that grow in the intertidal zones of tropical and subtropical latitudes around the world (Tomlinson, 2016). The strong osmotic gradient between the plant and saline growing environment and the highly negative water potentials of soil pore water, making water acquisition more energetically unfavorable than in non-saline soils (Reef and Lovelock, 2014; Barraclough et al., 2020) and eventually lead to physiological water shortage of mangroves (Saenger, 2002; Lambs & Saenger, 2011). Furthermore, nocturnal stem water refilling would be less likely to occur under these conditions because it would take more transpiration pull to overcome this osmotic water potential gradient. Therefore, maintaining water balance is always a challenge (Barraclough et al., 2020). Water-use strategies are generally considered to be a key factor in the adaptation of mangroves to high saline intertidal conditions (Muller et al., 2009). Recent stable isotope studies have shown that mangroves utilize less saline water sources when freshwater is available (Wei et al., 2013). Scholander et al. (1962) found that mangroves overcome the high osmotic potential of saline environments through both osmotic and hydrostatic potential in their xylem sap. Previous studies demonstrated that mangroves adopt a conservative water-use strategy and use water efficiently during photosynthesis (Krauss et al., 2008; Krauss et al., 2015). Monitoring has shown that mangroves maintain a certain amount of sap flow at night (Leng & Cao, 2020). Waisel et al. (1986) showed that mangroves maintain low hydrostatic potential during nighttime, which would allow mangroves to overcome osmotic potential of the seawater in the root environment. For those mangrove species with conservative nocturnal water use, Qn would be an adaptive strategy coping with temporary water deficit by effectively buffering stem water depletion after daytime transpiration. Mangroves grow in anoxic soil conditions (Lovelock et al., 2016), and En would also help maintaining cell oxygen. However, our knowledge of the nocturnal water use of mangroves is still limited. Understanding the physiological implications of Qn in mangroves is very important for exploring the adaptability of mangroves to special intertidal habitats.
To better understand the water-use strategies of mangrove species during the night, we measured sap flow in three co-occurring mangrove species, Kanderia obovata, Avicennia marina, and Aegiceras corniculatum, over an entire year (2019). The mangroves were located in a subtropical estuary mangrove forest in the Fujian Province of China. Meteorological factors were measured synchronously. Diurnal variations in stomatal conductance and stem diameter of the three species were also measured on typical clear days in summer. The objectives of this study were to (1) quantify the contribution of Qn to Q and its implication for water use, (2) explore the allocation and utilization patterns of Qn, and (3) identify the driving forces of Qn in three co-occurring mangrove species. We expected to reveal a new mechanism for how mangroves maintain water balance during the night after experiencing high water consumption by transpiration during the daytime, and to better understand the nocturnal water-use strategies and the adaptability to their unique habitats of mangroves.
Materials and methods
Site description
Field measurements were conducted for one entire year (2019) in the Zhangjiang Estuary Mangrove National Nature Reserve (23.9240°N, 117.4147°E) in the Fujian Province of China. The annual weather conditions in the estuary followed the patterns typical of a subtropical monsoon climate. Daily mean relative humidity (RH) ranged from 24.2% to 100% with a mean of 83.2%. Annual temperature extremes ranged from 9.35°C to 37.9°C with an annual mean air temperature of 23.7°C. The annual precipitation was 1714 mm with the peak in spring and summer. The estuary experiences a regular semidiurnal tide with an annual mean tidal range of 2.32 m. The sediment surface in mangrove forests is normally flooded with a maximum inundation depth of around 1.0 m. Salinity fluctuates seasonally, with measured minimum values of approximately 13-18 practical salinity units (PSU) during November-December and maximum values of 3-13 PSU during June-September. The mangrove forest naturally consists of three co-occurring species, Kandelia obovate (dominant), Avicennia marina, and Aegiceras corniculatum. Four trees of each species in close proximity to one another were randomly selected for sap flow monitoring and related measurements. The morphological features of the trees were measured before sap flow monitoring (Table 1).

Table 1 Tree height, diameter at breast height (DBH), Sapwood depth, canopy projection area and sensor type of the trees randomly selected for sap flow monitoring (Mean ± s.e., n=4).
The canopy projection area (m2) was calculated by an elliptic equation after measuring the canopy length (Cl) and canopy width (Cw) of each tree (Wang et al., 2018):
Environmental factor monitoring
To investigate plant physiological responses to the microclimate, a weather station was installed in the study area. A sensor of HOBO MX2301 (Onset Computer Corp., Bourne, MA, USA) was installed on an Eddy tower above the canopy to monitor air temperature (Ta, °C) and relative humidity (RH, %). Vapor pressure deficit (VPD, kPa) was calculated from Ta and RH. Photosynthetically active radiation (PAR) was measured from the data collected on an Eddy tower above the canopy using a PQS1 PAR Quantum sensor (Kipp & Zonen). Wind speed were measured using a 010C Wind speed Sensor (Met One Instruments, Inc) made above the mangrove canopy. Meteorological data were recorded every 30 s and stored at 10 min average in a data logger (CR1000 datalogger, Campbell Scientific, Inc., Logan, UT, USA). Tidal surface water salinity was also continuously measured and estimated from conductivity and temperature measurements using HOBO U24-002-C Conductivity Logger (Onset) deployed just above sediment surface. Raw 10-minute tidal data were consistently converted to half-hourly time series for data analysis.
Sap flow measurements
Sap flow was measured using Granier-type thermal dissipation probes as per Zhao et al. (2018) (Figure 1). In order not to damage the sample trees, we selected 4 trees of different diameter classes in each of the three species to establish the relationship between diameter at breast height and sapwood depth, and the operation method referred to the sapwood measurement method mentioned by Leng & Cao, 2020. Two different size probes (TDP10 with a probe length of 10 mm, and TDP30 with a length of 30 mm) were used to fit different sapwood widths. Each probe was installed at 1.3 m above the sediment surface and on the north-facing side of the stem to avoid the effects of sunlight. The probes were covered with reflective aluminum sheets and wrapped with a plastic tarp to protect them from solar irradiation and rain damage. Each probe consisted of two needles with thermocouples and a heater. The upper needle was continuously heated with a constant power of 0.2 W, while the lower needle stayed at the temperature of the unheated sapwood. The temperature difference between the two probes was measured at 30 s intervals, and the averaged values for each 10 min were recorded with a data logger (CR1000, Campbell Scientific Inc., Logan, UT, USA). The monitoring period was from January 1 to December 31, 2019. The sap flux density (SFD, g cm−2 s−1) was calculated based on the differential temperature (ΔT, °C) between the heated and reference probes following an empirical equation (Granier, 1987):
where ΔT is the measured differential temperature, and ΔTm represents the ΔT when Sap flow density is zero.
The xylem wood of the three species in this study is diffuse porous (Lin et al., 1998). According to previous studies, the radial sap flow density is not significantly different in diffuse-porous wood (Phillips et al., 1996; Clearwater et al., 1999). Therefore, the daily sap flow (Q, kg d−1) for each sampled tree was calculated as follows:
where As is the cross-sectional sapwood area of an individual tree, and t is 10 min because the data are being stored as 10 min averages.
Differentiation of En and Rn
To quantify the contribution of the two components (En and Rn) to the Qn, the sap flow of three sample trees of each species was monitored at breast height and the base of the live crown (Figure 1) (Daley & Phillips, 2006). In this analysis, the 24-h sum of sap flow at the base of the live crown was assumed to be equal to the 24-h sum of sap flow at breast height (Goldstein et al., 1998; Daley & Phillips, 2006). We normalized the 24-h sum of sap flow at the base of the live crown in each sample tree so that it equaled the 24-h sum of the sap flow at breast height. Specifically, 10-min sap flow at the base of the live crown multiply the ratio of the 24-h sum of sap flow at breast height to 24-h sum of sap flow at the base of the live crown. This is similar to the approach described by Goldstein et al. (1998). Previous studies showed lower water storage in the crown than in the stem (Waring et al., 1979), so we assumed the normalized sap flow at the base of the live crown could represent the total transpiration (Daley & Phillips, 2006). We calculated the difference between the sap flow rates at the stem breast height and mean transpiration at 10-min intervals. The positive values represented stem water refilling, whereas the negative values represented stem water storage was used by transpiration (Goldstein et al., 1998; Daley & Phillips, 2006; Zeppel et al., 2010).
Stem diameter variations measurements
Since their first appearance in 1970, point or ring dendrometers have been increasingly used for the measurement of stem diameter variation. These first observations suggest a close link between diurnal variation in plant water status and diurnal variation in stem thickness. Radial movement of water back and forth through the storage tissue in the stem leads to measurable changes in stem diameter (Barraclough et al., 2020). The stem diameter variations were measured with precision dendrometers (Figure 1). To explore the radial movement of water in the stem, electronic point dendrometers (RRDIM10, Rainroot, China) were installed at the breast height of the main stem on two of the four sampled trees of each species. The resolution of the electronic point dendrometers was 10 μm. Data were obtained every 15 s and instruments were logged at 5 min intervals with a data logger (RR-1004, Rainroot, China). To get the largest diurnal variations of stem diameter during the same measuring period, stem diameter was measured during seven typical clear days in summer (from August 22 to August 28, 2019). We took the stem diameter value at 0:00 on a day after the equipment was stabilized as the stable point of reference.
Leaf gas exchange measurements
Leaf transpiration (Tr) and stomatal conductance (gs) from three trees of each species were measured on a typical sunny day in summer (August 26, 2019). Three to five mature, healthy, and fully expanded leaves on a south-facing branch were randomly selected from lower and upper crown locations for leaf gas exchange measurement using a portable photosynthesis system (Li-6400xt, Li-cor, USA) (Figure 1). Measurements were taken at 2-hr intervals for 24 hours from 10:00 on the first day to 10:00 on the following morning. During the measurements, PAR, Ta, RH, and CO2 concentrations in the leaf chamber were set to match ambient environmental conditions. The measurement chamber was not illuminated at night.
Data analysis and statistics
Night was defined as the period from 18:00 to 6:00 on the following day with PAR lower than 20 μmol m−2 s−1 (Daley & Phillips, 2006; Wang et al., 2011; Siddiq & Cao, 2018). We subdivided night into a first part (18:00 to 24:00) and second part (0:00 to 6:00). To calculate the amount of stem expansion and shrinkage during the night, we averaged stem diameter data every 10 minutes (Di, where 1 ≤ i ≤ 72). When Di+1 - Di> 0, the stem was expanding and when Di+1 - Di< 0, the stem was shrinking. Thus, the amount of stem expansion and shrinkage during the nighttime was calculated as follows:
where Di is the mean stem diameter at the ith 10 minutes, and Di+1is the mean stem diameter value at the (i+1)th 10 minutes. De is the cumulative amount of stem expansion and Ds is the cumulative amount of stem shrinkage.
The vapor pressure deficit (VPD, kPa) was determined using measured atmospheric temperature (Ta) and relative humidity (RH), and the calculation formula is as follows (Buck, 1981):
Since the sap flow data for the entire year did not have a normal distribution, we used the nonparametric Kruskal-Wallis test to analyze the difference between Q, Qn, and the contribution of Qn to Q (Qn/Q) among the three mangrove species. An independent sample t-test was conducted to test whether there were significant differences between the mean cumulative amount of stem expansion and the mean cumulative amount of stem shrinkage for each species. The general linear regression and curvilinear model regression were fitted to identify the relationships between (1) salinity and sap flow (daily sap flow and nocturnal sap flow), (2) sap flow density (SFD) (daytime SFD, nocturnal SFD, SFD in the first part of the night and SFD in the second part of the night) and VPD, (3) wind speed sap flow (daily sap flow and nocturnal sap flow), (4) Qn and Q. At the same time, the analysis of Covariance (ANCOVA) was used to test whether there were significant differences in the slopes of fitted linear relationships for different species within the same salinity environment. The Gompertz models (three parameters) was used to test the relationship between the Qn/Q of different tree species and latitude. The Qn/Q data (n=56) for different tree species were based on data provided by Forster (2014). The significance level was set at P< 0.05. All data were analyzed using SPSS 25.0 (SPSS, Chicago, Illinois, USA).
Results
Magnitude of nocturnal sap flow in mangrove forests
For the species Kandelia obovata, Avicennia marina, and Aegiceras corniculatum, the annual mean Q per sapwood area ranged from 162.0 Kg m−2 d−1 to 348.1 Kg m−2 d−1 (Figure 2A). The magnitude of the annual mean Qn per sapwood area varied from 16.9 ± 0.7 Kg m−2 d−1 to 35.2 ± 1.1 Kg m−2 d−1 (Figure 2B). The annual mean Qn/Q was 24.0 ± 0.6%, 5.5 ± 0.2%, and 12.1 ± 0.4% for Kandelia obovata, Avicennia marina, and Aegiceras corniculatum, respectively. These values were up to 45.6%, 17.9% and 32.0%, respectively, higher on some days (Figure 2C). For Q, Qn, and Qn/Q, significant differences were observed among the three species. The Q of Avicennia marina was significantly higher than the Q of the other two species, while the Qn of Avicennia marina was significant lower than the Qn of the other two species (Figures 2A–C).
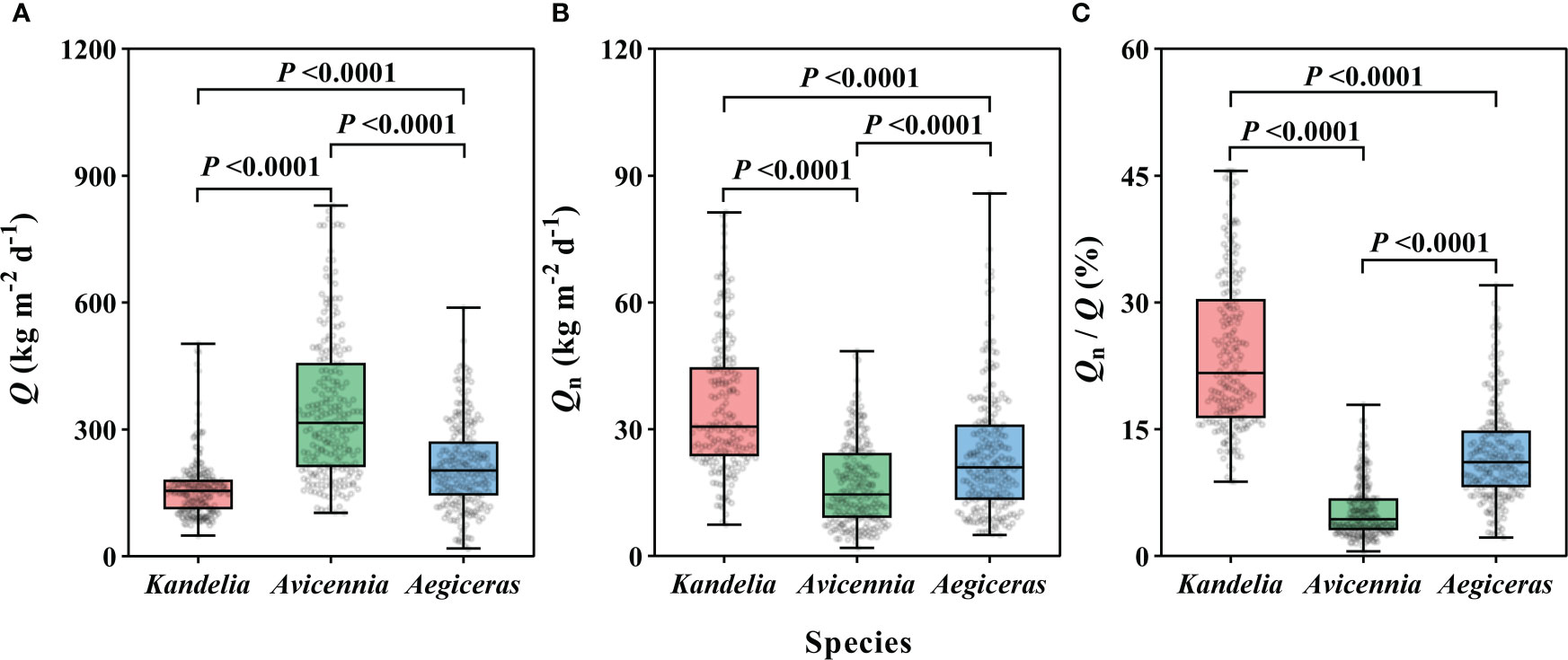
Figure 2 The daily sap flow (Q) in per sapwood area (A), the nocturnal sap flow (Qn) (B), and the proportion of Qn/Q (C) of each species from January to December, 2019. Data of the three species were retrieved from the same five consecutive typical sunny days per month. Statistical results from Kruskal-Wallis test in nonparametric tests among the three species were shown. For each species, the values reported were from four replicated trees. Significance level was set at P< 0.05.
Nocturnal leaf stomatal conductance and transpiration rate
A substantial amount of SFD and leaf Tr was detected during the night. For all three species, SFD, leaf Tr and gs decreased sharply after 16:00 and then remained steady until sunrise on the following day (Figures 3A, C, E). During the night, Kandelia obovata and Aegiceras corniculatum maintained low gs, less than 0.02 mol H2O m−2 s−1. These values decreased to about 9% and 6%, respectively, of day values. Tr was also low, below 0.30 mmol H2O m−2 s−1 (Figures 3C, E). There was no significant difference in gs and Trbetween Kandelia obovata and Aegiceras corniculatum (Figures 3D, F). Although the nocturnal SFD of the Avicennia marina was significantly lower than that of the other two species, the gs and Tr were significantly higher (Figures 3B, D, F). The mean nocturnal gs of Avicennia marina was 0.05 mol H2O m−2 s−1, and the value decreased to 16% of day value. The mean nocturnal Trof Avicennia marina was 1.09 mmol H2O m−2 s−1 (Figures 3C, E).
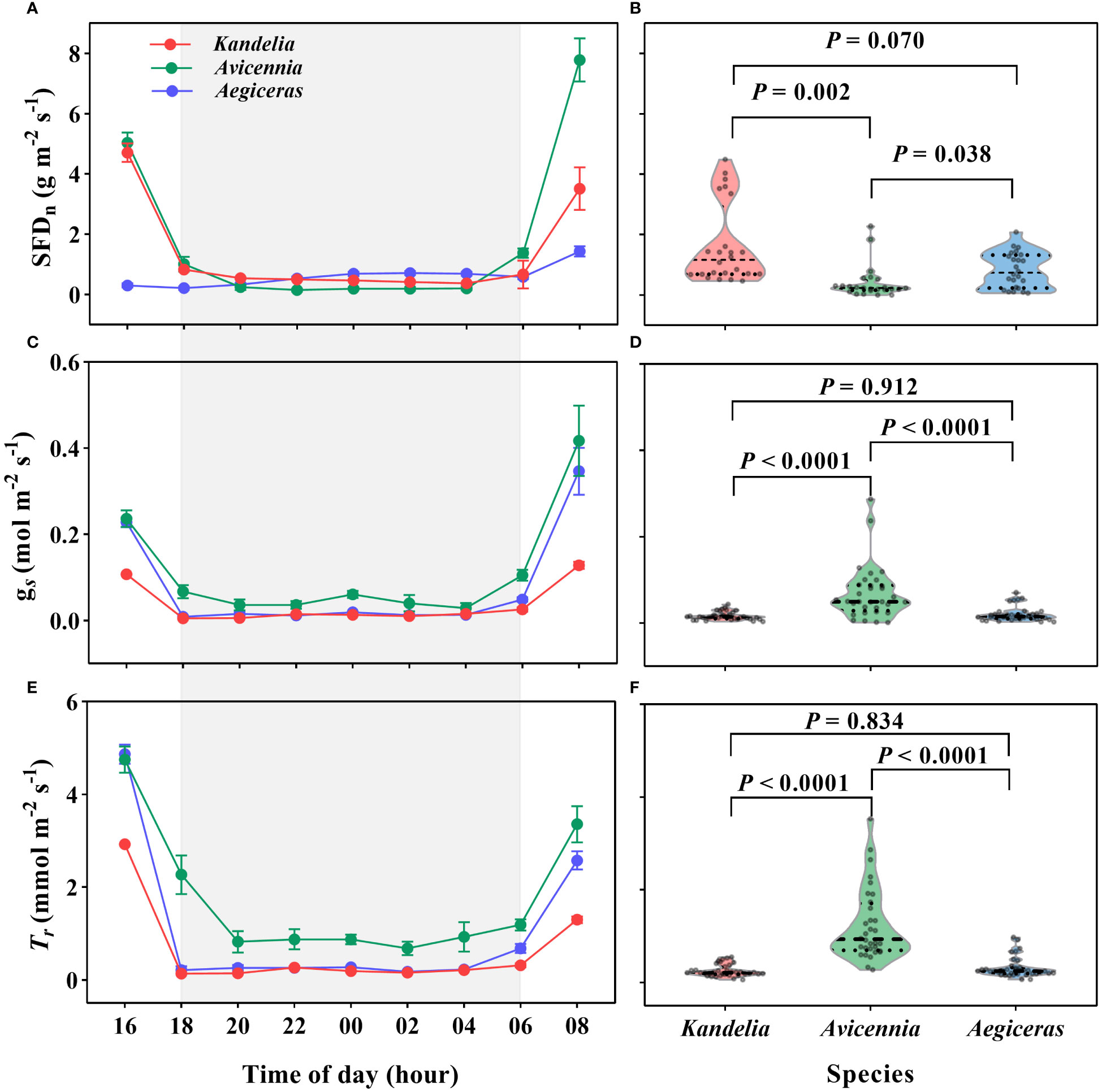
Figure 3 The variation patterns of the nocturnal sap flow density (SFDn) at breast height (A, B), leaf-level stomatal conductance [gs, (C, D)] and leaf-level transpiration rate (Tr, E and R) of the three species during the nighttime on a typical sunny day (26 August 2019). Statistical results from One-Way ANOVA among the three mangrove species were shown. Each SFD values for each species were the mean of four replicated trees. Significance level was set at P< 0.05. The shaded area in (A, C, E) represented the nighttime.
Nocturnal transpiration and stem water refilling
Early in the morning (about 6:00-10:00), sap flow at breast height was lower than sap flow at the base of the live crown, indicating that the three mangrove species utilized water stored in the stem for transpiration at sunrise (Figures 4A–C). Stem water refilling and stem water storage withdrawal during the night occurred in all three species, but stem water refilling occurred most of the night for Kandelia obovata and Aegiceras corniculatum, while Avicennia marina experienced mainly stem water storage withdrawal (Figures 4D–F). The contribution of En and Rn to Qn in Kandelia obovata was 43.7% and 56.3%, respectively. Similar to Kandelia obovata, the contribution of En and Rn to Qn in Aegiceras corniculatum was 32.7% and 67.3%, respectively. In contrast, the Qn of Avicennia marina consisted mainly of En and the En exceeded the Qn (En accounted for 103% of Qn). In Avicennia marina, stem water refilling occurred during the day and stem water storage was partially depleted at night during the nocturnal transpiration process (Figure 4E).
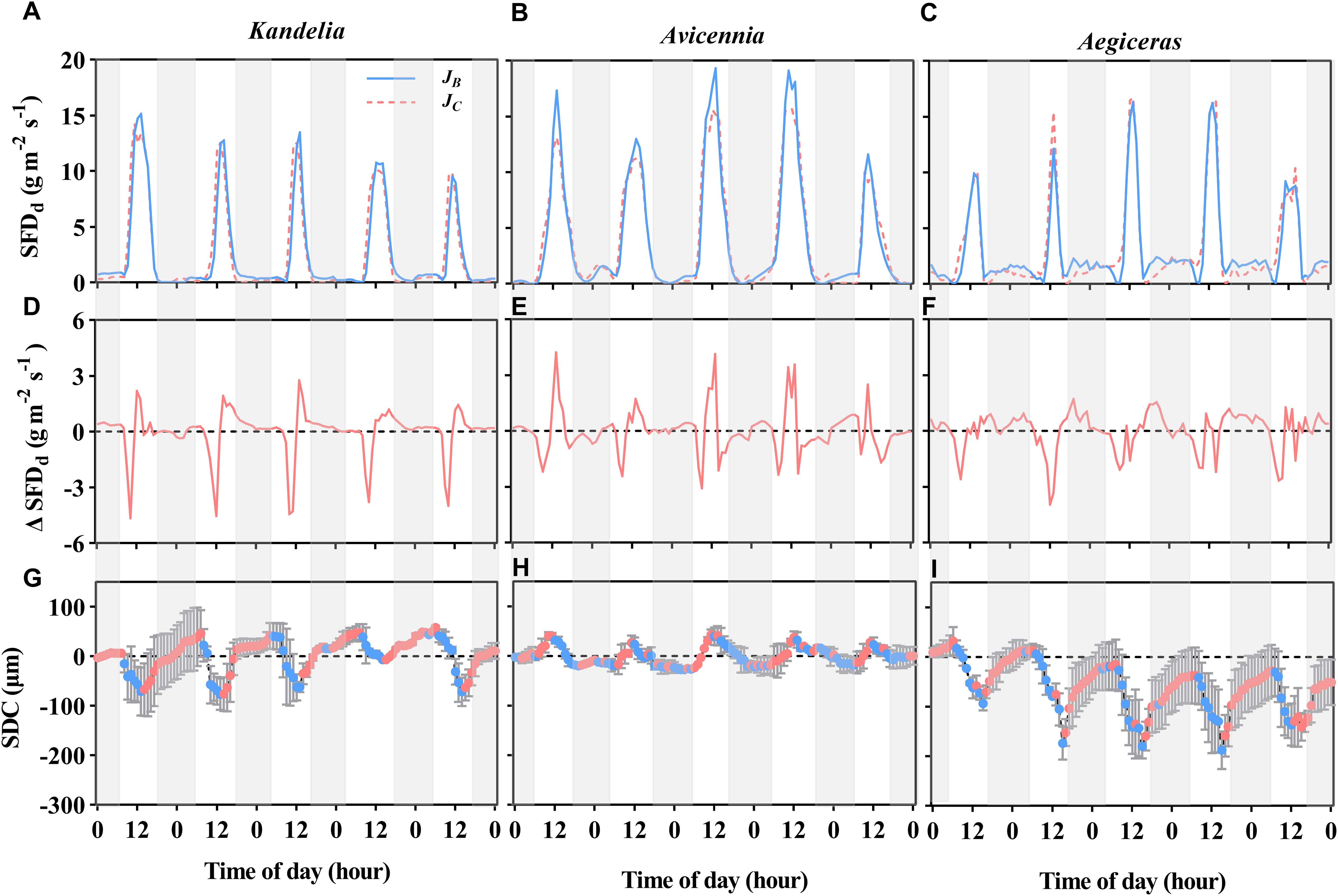
Figure 4 The variation patterns of the diurnal sap flow density (SFDd) of three mangrove species (A–C) within the same five consecutive typical sunny days (from Aug. 24 to Aug. 28 in 2019), and the difference between breast height sap flow density (JB) and the normalized sap flow density at the base of the live crown (JC) [Δ SFDd, (D–F)]. Positive value in the (D–F) indicate time periods when stem water recharge, and negative values indicate time periods when canopy transpiration was preferentially stem water storage. The diurnal patterns of stem diameter change (SDC) for each species [(G–I), the red circles represent stem expansion and the blue circles represent stem shrinkage]. The shaded area represents nighttime.
Stem diameter change patterns
For Kandelia obovata and Aegiceras corniculatum, stem diameter decreased in the morning and started to increase again in the afternoon. Although the increasing trend of stem diameter in Kandelia obovata and Aegiceras corniculatum at night was weaker than the increasing trend before the sunset, the stem diameter of these two species remained in an expanded state at night (Figures 4G, I). However, the stem diameter changes of Avicennia marina showed different diurnal patterns from the former two species, with an increase during the morning and a decrease in the afternoon, which follow the variation of the SFD (Figure 4H). Unlike Kandelia obovata and Aegiceras corniculatum, little the cumulative amount of stem expansion can be detected during certain nighttime periods in Avicennia marina. By the independent sample t-test analysis, the cumulative amount of stem expansion was significantly higher than the cumulative amount of stem shrinkage in Kandelia obovata and Aegiceras corniculatum. However, the cumulative amount of stem expansion was significantly lower than the cumulative amount of stem shrinkage in Avicennia marina (Figure 5).
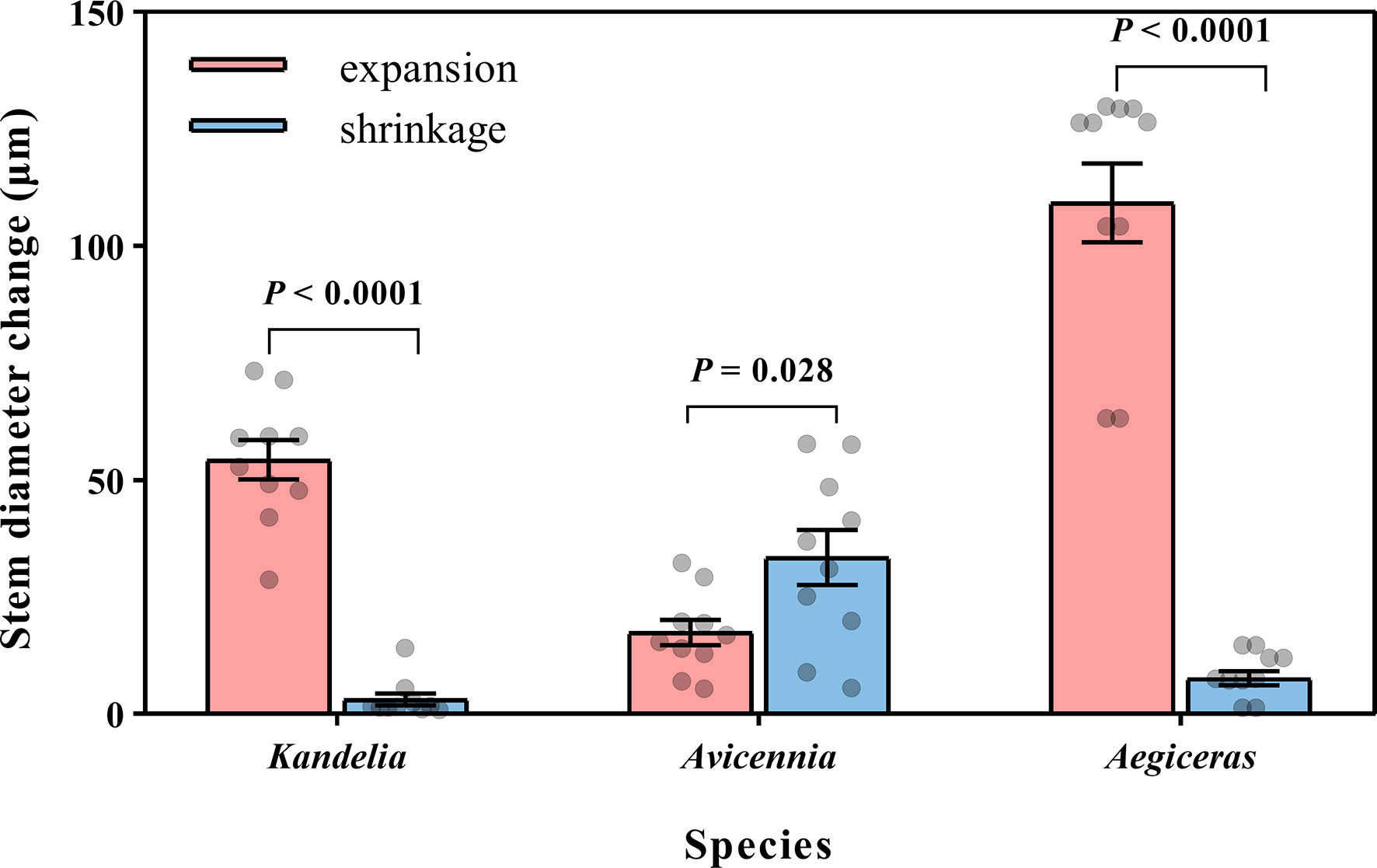
Figure 5 Mean night-time cumulative amount of stem expansion and shrinkage of three mangrove species within the same five consecutive typical sunny days (from Aug. 24 to Aug. 28 in 2019). Significant differences between expansion and shrinkage was analyzed by independent sample t-test. For each species, the values reported were from two replicated trees. Significance level was set at P< 0.05.
Relationships between salinity and sap flow
There was a significant negative linear relationship between the salinity and the daily sap flow of the three species (Figure 6). The slopes of fitted linear relationships between salinity and daily sap flow of Kandelia obovata was significantly lower than that of Aegiceras corniculatum and Avicennia marina. When the ambient salinity increased from 5 PSU to 15 PSU, the daily sap flow of Kandelia obovata, Avicennia marina and Aegiceras corniculatum decreased to 26.3%, 48.3% and 50.6%, respectively. During the night, different species showed different relationship between salinity and sap flow. There was a positive linear relationship between salinity and sap flow of Kandelia obovata while a negative linear relationship between salinity and sap flow of Avicennia marina (Figures 6A, B). For Aegiceras corniculatum, there was a non-linear relationship between salinity and sap flow (Figure 6C).
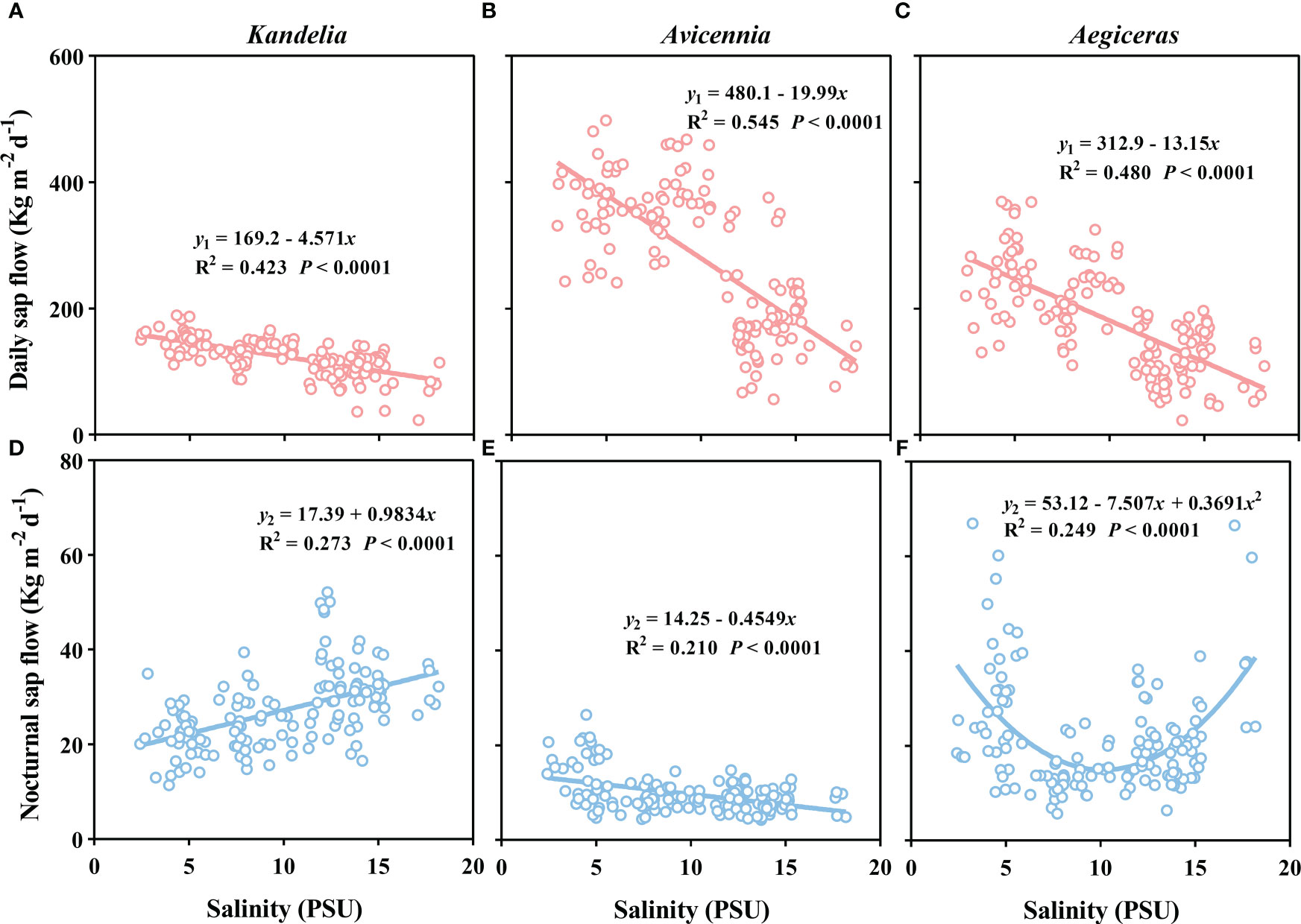
Figure 6 The relationship between salinity and daily sap flow (A–C), and the relationship between salinity and nocturnal sap flow (D–F) in per sapwood area for Kandelia, Avicennia and Aegiceras from August to December.
Relationships between wind speed, VPD, and sap flow
As can be seen from Figure S1, wind speed had no significant effect on the daily sap flow and nocturnal sap flow. The relationships between SFD and VPD differed between day and night. During the day, there was an exponential relationship between SFD and VPD (SFD = i + a · (1 - e-bVPD)), and the VPD explained 57.0%–60.4% SFD variations in three mangrove species. During the night, a weak generally linear relationship (SFD = i + a · VPD) existed (Figure 7). Furthermore, the correlation coefficient (R2) differed among the three mangrove species (Figure 7). For Avicennia marina, the VPD explained 27.3% of the nocturnal SFD variation, whereas for Kandelia obovata and Aegiceras corniculatum, it explained only 9.0% and 12.6%, respectively (Figures 7A–C). SFD presented a sharp decreasing trend after sunset in Kandelia obovata and Avicennia marina, which was similar to the change of VPD. The SFD of Kandelia obovata showed a slow declining trend after 20:00 and maintained a high SFD until sunrise of the next day. After a sharp decline, the SFD of Avicennia marina stabilized and remained low in the second part of the night (Ns). In contrast, the SFD of Aegiceras corniculatum showed an increasing trend after a brief decline at night, and then maintained a steady-state with high SFD in Ns. The variation in nocturnal SFD of Aegiceras corniculatum was inconsistent with the variation of VPD (Figure S2). For Kandelia obovata and Avicennia marina, the responses of the nocturnal SFD to VPD occurred in the first part of the night (Nf). VPD explained 21.1% and 50.3% of the nocturnal SFD variation, respectively. Interestingly, there was a significant negative correlation between the nocturnal SFD and VPD of Aegiceras corniculatum (Table 2).
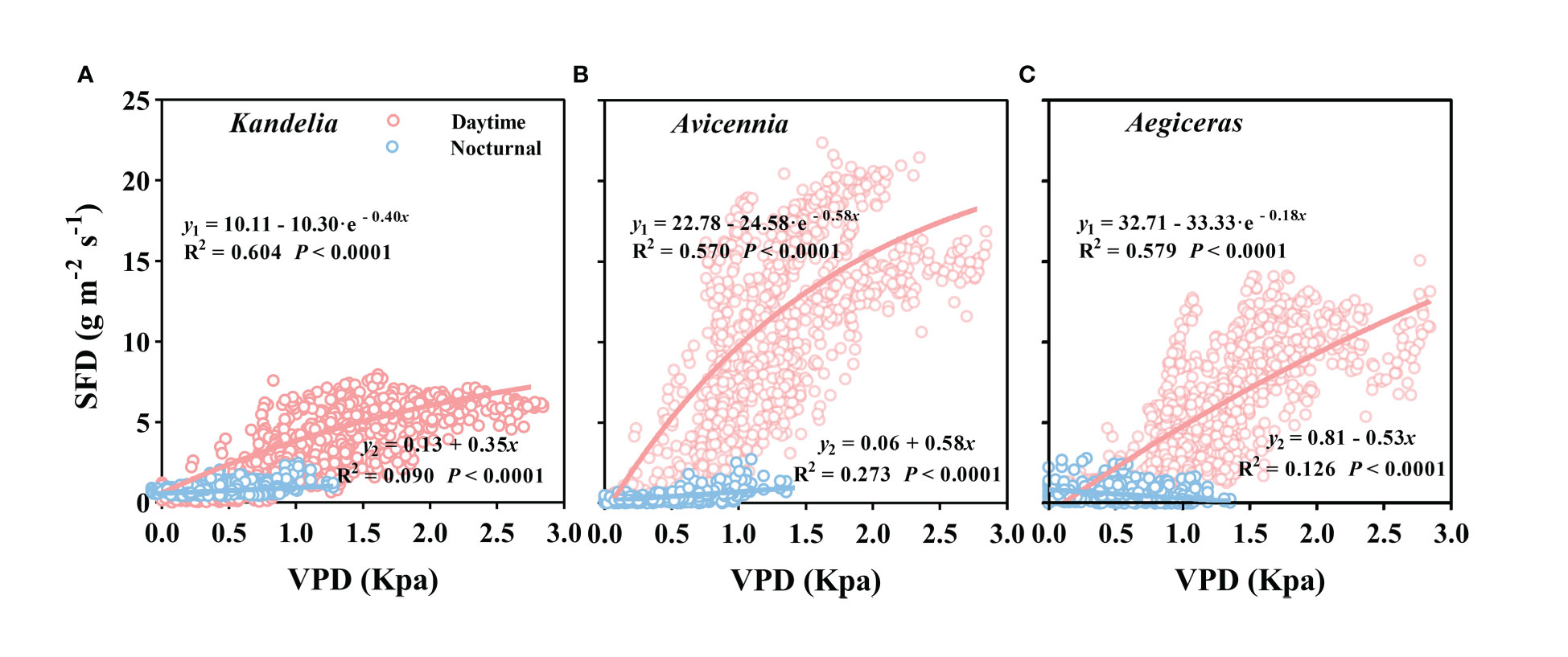
Figure 7 The relationship between vapor pressure deficit (VPD) and mean hourly sap flow density (SFD) for Kandelia (A), Avicennia (B) and Aegiceras (C) from January to December. y1 and y2 represented daytime SFD and nocturnal SFD respectively. Data of each species were retrieved from the typical sunny days per month. The best fit exponential relationship (y = i + a(1-e-bVPD)) is shown for daytime (red line) and linear relationship (y = i + a·VPD) was shown for nocturnal (blue line).
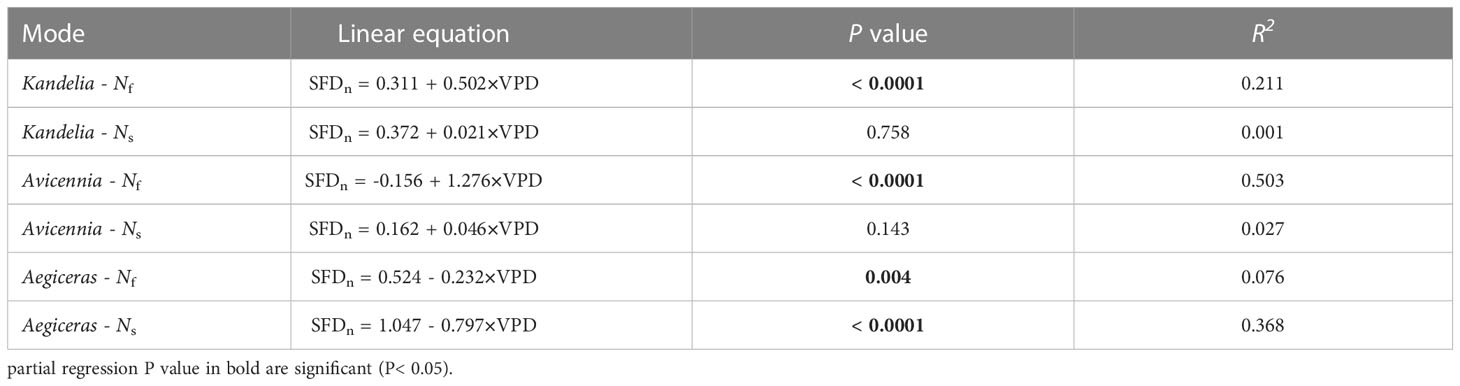
Table 2 Liner regressions of nocturnal sap flow density (SFDn, g m-2 s-1) at breast height and vapor pressure deficit (VPD, Kpa) during the first part of the nighttime (Nf, 18:00 - 24:00) and second part of the nighttime (Ns, 0:00 - 6:00), respectively.
Relationships between Qn and Q
The general linear regression analysis showed a significant and positive relationship between Qn and Q in Kandelia obovata (y = 4.718 + 0.215x, R2 = 0.539, P< 0.0001) and Aegiceras corniculatum (y = 3.543 + 0.096x, R2 = 0.573, P< 0.0001). These results indicate that the Qn increased with the increasing water lost from transpiration during the day. However, this linear relationship was not found between Qn and Q of Avicennia marina (R2 = 0.062 P = 0.056) (Figure 8), and the Qn of Avicennia marina was almost not affected by the water lost from transpiration during the day.
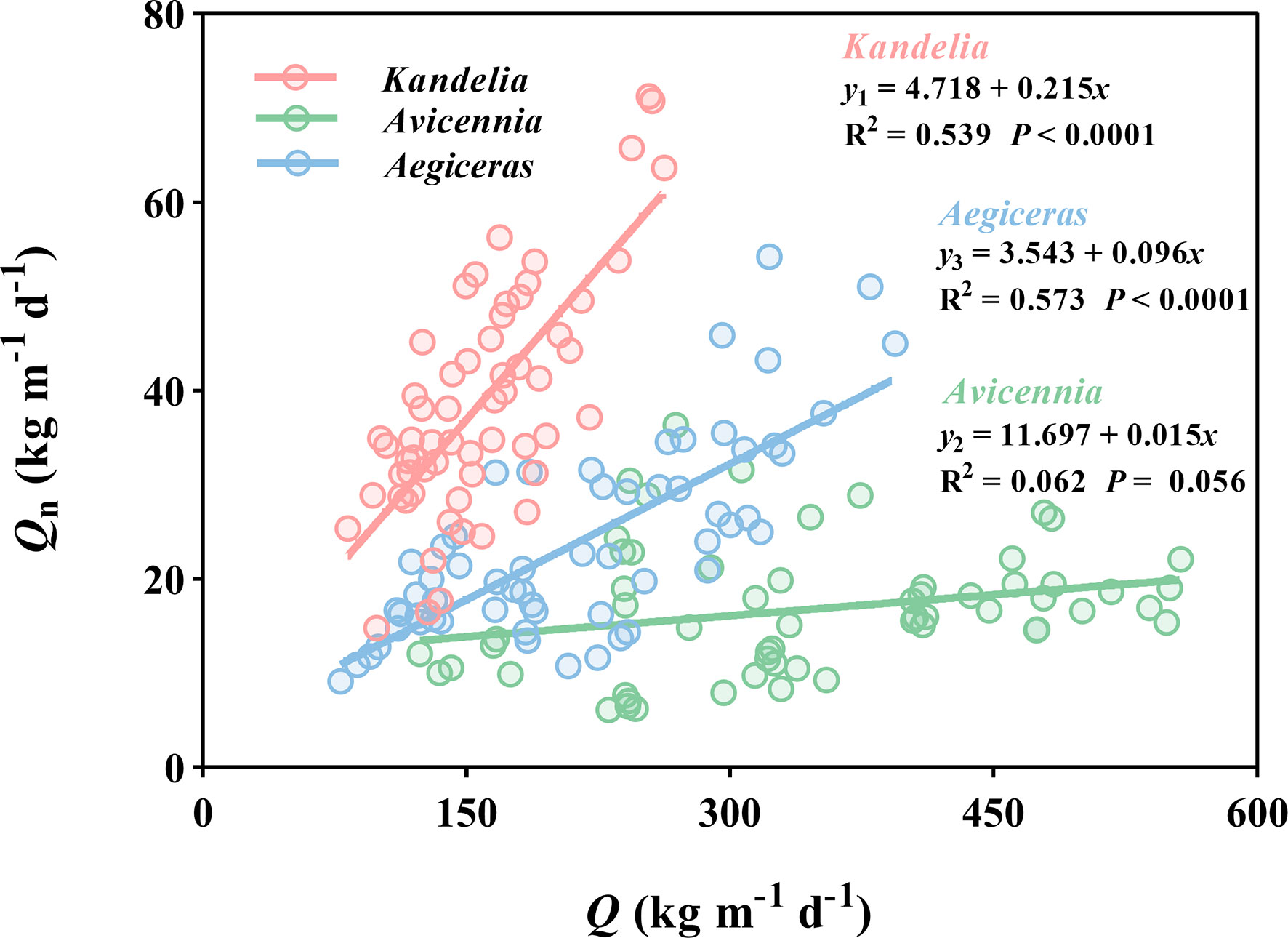
Figure 8 Linear relationships of daily sap flow (Q) and nocturnal sap flow (Qn) of the three mangrove species of the whole year. Data of each species were retrieved from the same five consecutive typical sunny days per month. For each species, the sap flow values reported were the mean of four replicated trees. y1, y2 and y3 represented the Qn of Kandelia, Avicennia and Aegiceras respectively.
Relationships between Qn/Q and latitude
The curvilinear model regression analysis showed a significant and positive relationship between the Qn/Q of different tree species and latitudes (y = 6.203 · 39.518 ^ e(-0.0634x), R2 = 0.693, P< 0.0001) (Figure 9).
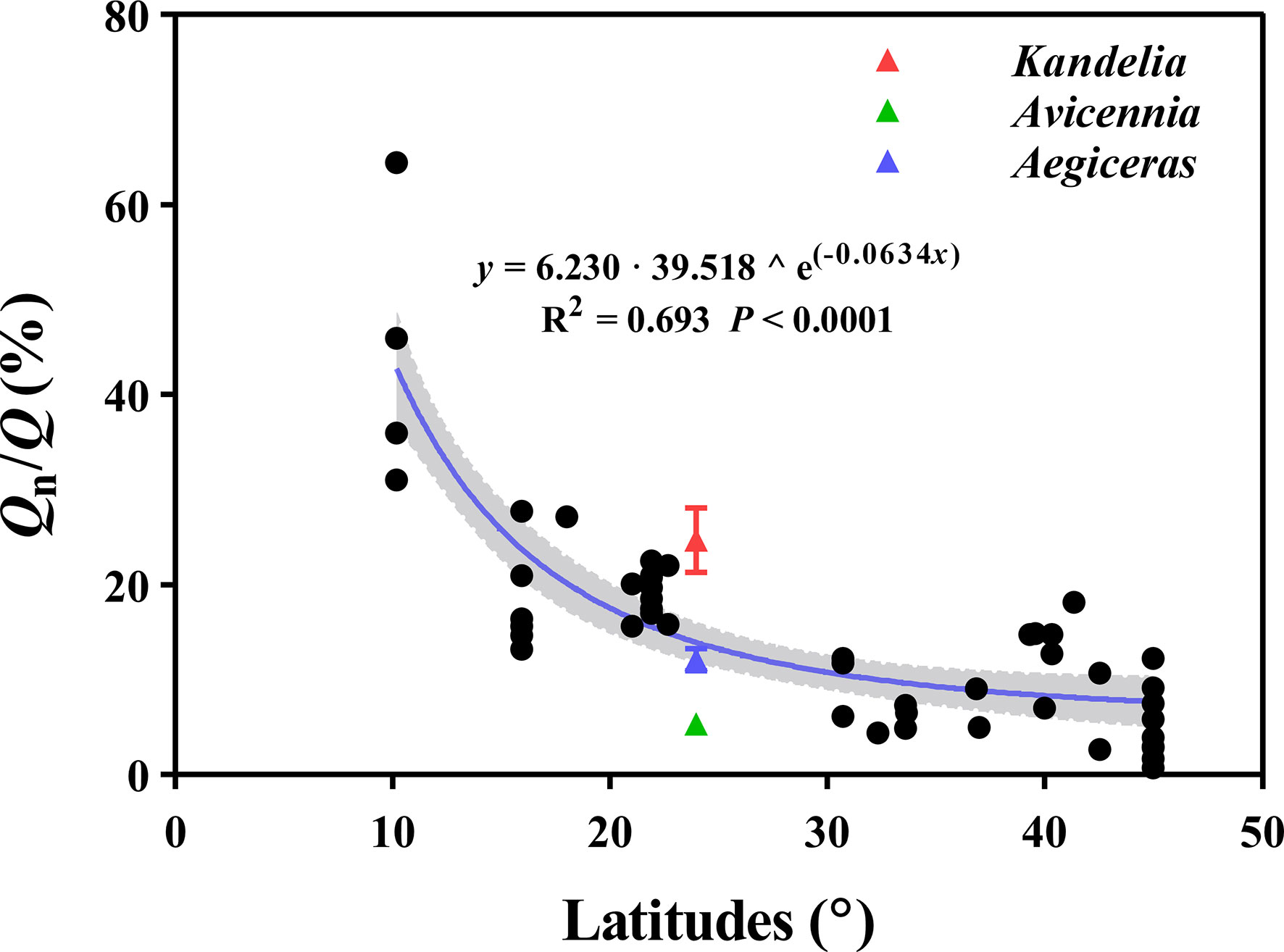
Figure 9 The relation between the Qn/Q of different tree species and latitudes. The data (n=56) of Qn/Q are mainly based on the data provided by Forster (2014). The red, green, and blue triangles represent Kandelia, Avicennia and Aegiceras in this study respectively. The shaded areas are represented by 95% confidence interval.
Discussion
Qn/Q for mangrove species
Previous studies confirmed that Qn occurs in species across all vegetation types and biomes, making a significant contribution to Q (Daley & Phillips, 2006; Zeppel et al., 2010; Forster, 2014). By analyzing 98 tree species from different biomes, Forster (2014) reported that Qn/Q was 7.6%, 16.5%, and 29.1% for forests in warm temperate, tropical, and equatorial, respectively. Our results demonstrated that Qn occurred in each of the three mangrove species during most of the night. Qn/Q ranged from 5.5% to 24.0% of these subtropical mangrove species (Figure 2C). In the dataset of Forster (2014), a significant negative relationship (y = 6.230 · 39.518 ^ e(−0.0634x)) was observed between Qn/Q and latitude (Figure 9). The highest Qn/Q occurred in equatorial and tropical biomes, under conditions of high atmospheric evaporative demand, suggesting that tree species growing at lower latitudes would have higher Qn/Q (Figure 9). We predict that mangrove species growing in equatorial and tropical regions have even greater Qn, which has significant implications for the water balance between soil and plant and hydrology.
This study is one of the few to quantify inter-species differences of Qn among the co-occurring species. Previous studies showed that Qn rates varied among plant species because of different adaptive strategies (Phillips et al., 2010; Zeppel et al., 2010). In this study, significant differences were observed in the Qn/Q between species (Figure 2C), and this result supports the conclusion that different adaptive strategies lead to Qn/Q diversity. The relatively high average Qn/Q of Kandelia obovata and Aegiceras corniculatum in this study, indicating that the two species were highly dependent on Qn and Qn was important for maintaining water balance in these species. In contrast, Avicennia marina had little dependence on Qn. The reason for the difference in Qn/Q between species may be that stem water refilling of Kandelia obovata and Aegiceras corniculatum occurred mainly after sunset while the stem water refilling of Avicennia marina occurred during the daytime (Figures 4D, E, F). Due to the special habitat of mangrove species, we had to consider the effect of salinity changes on sap flow. For the Q of the three mangrove species, high salt conditions contribute to more conservative water use in mangroves (Figure 6). During the nighttime, high salt conditions drove high Qn of Kandelia obovata and Aegiceras corniculatum while the high salt conditions inhibited the Qn of Avicennia marina. The high salt conditions drove high Qn may be related to mangrove species overcoming the high osmotic potential of saline environments through enhance both osmotic and hydrostatic potential in their xylem sap during the nighttime. The various responses of different species to salinity at night were also a reason for their different Qn/Q.
Allocation and utilization patterns of Qn for mangrove species
Qn was associated with two physiological processes, En and Rn. Direct evidence for En comes from leaf gas exchange measurements (Daley & Phillips, 2006). A previous study showed that weak stomatal conductance at night might result from slight En (Rosado et al., 2012). However, some reported not low levels of Qn with incomplete stomatal closure during the night in many plant species (Snyder et al., 2003; Dawson et al., 2007). For Kandelia obovata and Aegiceras corniculatum, the SFD at night remained at a relatively high level with a low gsand Tr, indicating that both species occurred weak En and had strict control over their stomata to reduce the water loss at night. In contrast, the gsand Tr of Avicennia marina at night were significantly higher with lower SFD than the other two species (Figures 3B, D, F), indicating that a large part of the Qn of Avicennia marina was dissipated into the atmosphere through the stomata. These different controls of leaf stoma in co-occurring species of the same environment were also reported in a mixed deciduous forest (Daley & Phillips, 2006).
The water-use pattern in which trees use stem stored water during the day to buffer water deficits and refill the stem water during the night time when evaporative demand is low, has been observed in different tree species (Goldstein et al., 1998; Phillips et al., 2003). Here, we found that in Kandelia obovata and Aegiceras corniculatum, Rn was a major component (over 60%) of Qn. The high ratio of Rn to Qn indicates a strong reliance of these plants on stem storage water, which helps them to maintain a balance between water supply and water demand during periods with high transpiration demands (Scholz et al., 2011; McCulloh et al., 2014). High salinity conditions promote more conserved daytime water use in Kandelia obovata and Aegiceras corniculatum and drive higher levels of Qn, which maintains water balance in mangroves in highly negative water potential environments in the form of Rn. Therefore, we deemed Qn to be an effective water-use strategy to avoid physiological dehydration and adapt to high salt environments in some mangrove species. Unlike Kandelia obovata and Aegiceras corniculatum, the Qn of Avicennia marina was mainly used for En, and the stored water was released from the stem to supply the water needed for En, indicating that the water consumption during transpiration at night was higher than the water absorbed by the roots from the soil. This leads to the question of how Avicennia marina maintained water balance at night after experiencing high water consumption by transpiration during the day. Different from Kandelia obovata and Aegiceras corniculatum, Avicennia marina is able to maintain water refilling while transpiring and uptake water from the saline soil more efficiently during daytime (Figure 4E). This process enables the water storage tissue to retain sufficient water after the water loss during the day and beneficial for maintaining stem water balance at night. Furthermore, previous studies showed that Avicennia marina had unique wood anatomy in which successive or multiple cambia resulted in consecutive bands of xylem interspersed with internal secondary phloem strands connected by parenchyma tissue (Schmitz et al., 2008; Robert et al., 2011). This anatomy is conducive to internal water storage and security and is deemed to be an adaptive trait to deal with physiological drought (Robert et al., 2011). At the same time, our results showed that Avicennia marina would limit Qn under high salt conditions to reduce water loss at night, which was also a strategy to overcome physiological dehydration. In addition, Coopman et al. (2021) found that Avicennia marina can absorb atmospheric moisture through deliquescence of salt on leaf surfaces, driving top-down sap flow and replenishes the water needs of the stem. This may be one of the mechanisms for maintaining the water balance of Avicennia marina at night. Due to technical limitations, however, the thermal dissipation probe could not detect this part of the reverse flow. The neglect of reverse flow leads to an underestimation of its nocturnal sap flow and this will be a limitation of the experiment.
The changing patterns of stem diameter indirectly support our findings. From hourly to daily scale, the fluctuations in stem diameter reflected changes in the elastic tissue water content (Zweifel et al., 2001; Pfautsch et al., 2015). The cumulative amount of stem expansion was significantly higher than the cumulative amount of stem shrinkage in Kandelia obovata and Aegiceras corniculatum (Figure 5), which indicated that Rn occurred more frequently than stem water storage withdrawal at night. In contrast, the cumulative amount of stem expansion was significantly lower than the cumulative amount of stem shrinkage in Avicennia marina (Figure 5), indicating that relatively large of the stored water in the stems was released into the xylem flow to participate in the En. The unusual stem variation pattern of Avicennia marina has also been reported by Barraclough et al. (2020).
The partitioning method used in this study had its limitations because the sap flow in terminal branches could not be measured, the same as the previous study (Daley & Phillips, 2006). For Kandelia obovata and Aegiceras corniculatum, the nocturnal gsand Tr were low (Figures 3D, F), and SFD was low sensitive to VPD at night (Figure 7, Table 2), leading to low En. Therefore, the contribution of En to Qn may have been overestimated in these two species. For Avicennia marina, the sap flow at the base of the live crown exceeded which at breast height (Figures 4B, E), indicating that part of the stem storage water was used for En, while there was no Rn in the terminal branches. Thus, we can reliably estimate the contribution of En to Qn in Avicennia marina.
In the water stress environment, mangrove species developed water-saving mechanisms to reduce water loss via transportation during the day (Ball, 1988). Our results indicated that a significant water compensation mechanism used by Kandelia obovata and Aegiceras corniculatum operated at night. Thus the more water was lost owing to diurnal transpiration, the higher the Qn was at night to make up for the lost water. This newly found stratagem in mangrove species provides evidence of a nocturnal water recharging mechanism in addition to their diurnal water-saving strategies. We suggest that mangrove species with nocturnal water recharging mechanisms and tight control of stomata will be better adapted to future environmental conditions of enhanced atmospheric evaporation demand than species without these capabilities. As for the Avicennia marina, its unique daytime hydration and timely restriction of water loss under high salt conditions provides its ability to survive under conditions of limited water.
Driving force of nocturnal sap flow in mangroves
Qn is driven by environmental factors and biotic factors, separately or synergistically. Previous studies suggested that VPD was the main environmental factor driving En because VPD between the leaf surface and the atmosphere provided conditions for stomata to open or partially open at night (Caird et al., 2007; Dawson et al., 2007; Zeppel et al., 2010). Wind speed affected the moisture movement around plant canopy (Fricke, 2019) and thus influenced on nocturnal water loss (Chen et al., 2020), which also could not be ignored. In this study, we found that wind speed was not a driving force in sap flow, whether during the daytime or nighttime, which is consistent with the result that wind speed had little effects on the nocturnal water loss by Wang et al., 2018 and Wu et al., 2020. Rosado et al. (2012) found strong positive relationships between VPD and Qn in woody species in rain forest and indicated that the En was driven by atmospheric demand for water. Wang et al. (2018) concluded that VPD had little effect on Qn because the correlation coefficient was low (R2 = 0.100, P< 0.0001). Similarly, our findings also showed that VPD had only a slight effect on the Qn of Kandelia obovata and Aegiceras corniculatum, indicating that VPD was not the main driver of Qn. In addition, there was a significant positive correlation between Q and Qn in Kandelia obovata and Aegiceras corniculatum. Based on the principle of water balance, Qn of these two species was thus mainly driven by the demands of stem water refilling after diurnal water depletion. In addition, high salinity conditions were also a driver of Qn in Kandelia obovata and Aegiceras corniculatum, and the high salinity environment prompts both of the species to refilling more water at night. However, the Qn of Avicennia marina occurred mainly in the early night and was driven by VPD (R2 = 50.3%) (Figure S2, Table 2). Our results suggest that Qn is driven by VPD, high salinity conditions or the demand for stem rehydration, separately or synergistically. The main driving force differs among species.
Conclusions
The Qn of three co-occurring intertidal mangrove species (Kandelia obovata, Avicennia marina, and Aegiceras corniculatum) contributed markedly to daily total sap flow (5.5%–24.0%) across species during the study period. The diversity of stem recharge patterns and response to sap flow to high salinity conditions are the main reasons for the differences in Qn/Q among species. Different degrees of control of leaf stoma at night were found. Kandelia obovata and Aegiceras corniculatum exhibited strict control over their stomata to reduce water loss. In contrast, a large part of the Qn of Avicennia marina was dissipated into the atmosphere through the stomata of the leaves. Qn of three co-occurring mangrove species consisted of En and Rn. Rn was the main contributor to the Qn in Kandelia obovata and Aegiceras corniculatum (56.3% and 67.3%, respectively), which was an effective water-use strategy for avoiding physiological dehydration and xylem embolism. However, the Qn of Avicennia marina was mainly used for En (En accounted for 103% of Qn), and the stem water storage was partially depleted at night during the nocturnal transpiration process. The unique daytime hydration and timely restriction of water loss under high salt conditions allow the Avicennia marina to adapt to harsh habitats. The major driver of Qn in Kandelia obovata and Aegiceras corniculatum was the demand for stem water refilling after diurnal water depletion and high salinity, while that of Avicennia marina was VPD. Our findings highlight the diversity of nocturnal water use strategies in co-occurring mangrove species and the conservative nocturnal water use in mangrove species. The diverse ways Qn properties act as water-compensating strategies among the co-occurring mangrove species will help mangrove species to adapt to future climate conditions.
Data availability statement
The raw data supporting the conclusions of this article will be made available by the authors, without undue reservation.
Author contributions
SW, XG, YZ, and LC planned and designed the research. SW, XG, and YZ performed experiments and conducted fieldwork. SW analyzed data. SW, XG, and LC contributed to writing the manuscript. All authors contributed to the article and approved the submitted version.
Funding
This study was supported by the National Natural Science Foundation of China [42076176], Natural Science Foundation of Fujian Province of China [2020J01048], and the Fundamental Research Funds for the Central Universities of China [20720210075] supported LC.
Conflict of interest
The authors declare that the research was conducted in the absence of any commercial or financial relationships that could be construed as a potential conflict of interest.
Publisher’s note
All claims expressed in this article are solely those of the authors and do not necessarily represent those of their affiliated organizations, or those of the publisher, the editors and the reviewers. Any product that may be evaluated in this article, or claim that may be made by its manufacturer, is not guaranteed or endorsed by the publisher.
Supplementary material
The Supplementary Material for this article can be found online at: https://www.frontiersin.org/articles/10.3389/fpls.2023.1118970/full#supplementary-material
References
Barbour, M. M., Cernusak, L. A., Whitehead, D., Griffin, K. L., Turnbull, M. H., Tissue, D. T., et al. (2005). Nocturnal stomatal conductance and implications for modelling δ 18O of leaf-respired CO2 in temperate tree species. Funct. Plant Biol. 32, 1107–1121. doi: 10.1071/FP05118
Barraclough, A. D., Cusens, J., Zweifel, R., Leuzinger, S. (2020). Environmental drivers of stem radius change and heterogeneity of stem radial water storage in the mangrove Avicennia marina (Forssk.) vierh. Agric. For Meteorol 280, 0168–1923. doi: 10.1016/j.agrformet.2019.107764
Bucci, S. J., Scholz, F. G., Goldstein, G., Meinzer, F. C., Hinojosa, J. A., Hoffmann, W. A., et al. (2004). Processes preventing nocturnal equilibration between leaf and soil water potential in tropical savanna woody species. Tree Physiol. 24, 1119–1127. doi: 10.1093/treephys/24.10.1119
Buck, A. L. (1981). New equations for computing vapor-pressure and enhancement factor. J. Appl. Meteorol 20, 1527–1532. doi: 10.1175/1520-0450(1981)020<1527:NEFCVP>2.0.CO;2
Caird, M. A., Richards, J. H., Donovan, L. A. (2007). Nighttime stomatal conductance and transpiration in c-3 and c-4 plants. Plant Physiol. 143, 4–10. doi: 10.1104/pp.106.092940
Chen, Z., Zhang, Z. Q., Sun, G., Chen, L., Xu, H., Chen, S. (2020). Biophysical controls on nocturnal sap flow in plantation forests in a semi-arid region of northern China. Agric. For Meteorol 284, 0168–1923. doi: 10.1016/j.agrformet.2020.107904
Clearwater, M. J., Meinzer, F. C., Andrade, J. L., Goldstein, G., Holbrook, N. M. (1999). Potential errors in measurement of nonuniform sap flow using heat dissipation probes. Tree Physiol. 19, 681–687. doi: 10.1093/treephys/19.10.681
Coopman, R. E., Nguyen, H. T., Mencuccini, M., Oliveira, R. S., Sack, L., Lovelock, C. E., et al. (2021). Harvesting water from unsaturated atmospheres: deliquescence of salt secreted onto leaf surfaces drives reverse sap flow in a dominant arid climate mangrove, Avicennia marina. New Phytol. 231, 1401–1414. doi: 10.1111/nph.17461
Daley, M. J., Phillips, N. G. (2006). Interspecific variation in nighttime transpiration and stomatal conductance in a mixed new England deciduous forest. Tree Physiol. 26, 411–419. doi: 10.1093/treephys/26.4.411
Dawson, T. E., Burgess, S. S. O., Tu, K. P., Oliveira, R. S., Santiago, L. S., Fisher, J. B., et al. (2007). Nighttime transpiration in woody plants from contrasting ecosystems. Tree Physiol. 27, 561–575. doi: 10.1093/treephys/27.4.561
Fisher, J. B., Baldocchi, D. D., Misson, L., Dawson, T. E., Goldstein, A. H. (2007). What the towers don't see at night: nocturnal sap flow in trees and shrubs at two ameriflux sites in California. Tree Physiol. 27, 597–610. doi: 10.1093/treephys/27.4.597
Forster, M. A. (2014). How significant is nocturnal sap flow? Tree Physiol. 34, 757–765. doi: 10.1093/treephys/tpu051
Fricke, W. (2019). Night-time transpiration - favouring growth? Trends Plant Sci. 24, 311–317. doi: 10.1016/j.tplants.2019.01.007
Gindel, I. (1970). Nocturnal behaviour of xerophytes grown under arid conditions. New Phytol. 69, 399–404. doi: 10.1111/j.1469-8137.1970.tb02438.x
Goldstein, G., Andrade, J. L., Meinzer, F. C., Holbrook, N. M., Cavalier, J., Jackson, P., et al. (1998). Stem water storage and diurnal patterns of water use in tropical forest canopy trees. Plant Cell Environ. 21, 397–406. doi: 10.1046/j.1365-3040.1998.00273.x
Granier, A. (1987). Evaluation of transpiration in a Douglas-fir stand by means of sap flow measurements. Tree Physiol. 3 (4), 309–320. doi: 10.1093/treephys/3.4.309
Grossiord, C., Buckley, T., Cernusak, L., Novick, K., Poulter, B. J. M., Siegwolf, R., et al. (2020). Plant responses to rising vapor pressure deficit. New Phytol. 226, 1550–1566. doi: 10.1111/nph.16485
IPCC (2014). “Climate change 2014: impacts, adaptation, and vulnerability,” in Part a: global and sectoral aspects. contribution of working group II to the fifth assessment report of the intergovernmental panel on climate change (Cambridge, UK, and New York, NY, USA: Cambridge University Press).
IPCC (2021). Climate change 2021: the physical science basis, contribution of working group I to the sixth assessment report of the intergovernmental panel on climate change (Cambridge, United Kingdom: Cambridge University Press).
Kavanagh, K. L., Pangle, R., Schotzko, A. D. (2007). Nocturnal transpiration causing disequilibrium between soil and stem predawn water potential in mixed conifer forests of Idaho. Tree Physiol. 27, 621–629. doi: 10.1093/treephys/27.4.621
Krauss, K. W., Barr, J. G., Engel, V., Fuentesd, J. D., Wang, H. Q. (2015). Approximations of stand water use versus evapotranspiration from three mangrove forests in southwest Florida USA. Agric. For Meteorol 213, 291–303. doi: 10.1016/j.agrformet.2014.11.014
Krauss, K. W., Lovelock, C. E., McKee, K. L., López-Hoffman, L., Ewe, S. M. L., Sousa, W. P. (2008). Environmental drivers in mangrove establishment and early development: a review. Aquat. Bot. 89, 105127. doi: 10.1016/j.aquabot.2007.12.014
Lambs, L., Saenger, A. (2011). Sap flow measurements of Ceriops tagal and Rhizophora mucronata mangrove trees by deuterium tracing and lysimetry. Rapid Commun. Mass Spectrom 25, 2741–2748. doi: 10.1002/rcm.5098
Leng, B., Cao, K. F. (2020). The sap flow of six tree species and stand water use of a mangrove forest in hainan, China. Glob Ecol. Conserv. 24, e01233. doi: 10.1016/j.gecco.2020.e01233
Lin, P., Lin, Y. M., Lin, J. H. (1998). Structure and evolution of secondary xylem in mangrove species. Acta Oceanologica Sin. 20, 97–102.
Liu, Z. Q., Liu, Q. Q., Wei, Z. J., Yu, X. X., Jia, G. D., Jiang, J. (2021). Partitioning tree water usage into storage and transpiration in a mixed forest. For Ecosyst. 8, 72. doi: 10.1186/s40663-021-00353-5
Lovelock, C. E., Krauss, K. W., Osland, M. J., Reef, R., Ball, M. C. (2016). The physiology of mangrove trees with changing climate (Cham, Switzerland: Springer).
McCulloh, K. A., Johnson, D. M., Meinzer, F. C., Woodruff, D. R. (2014). The dynamic pipeline: hydraulic capacitance and xylem hydraulic safety in four tall conifer species. Plant Cell Environ. 37, 1171–1183. doi: 10.1111/pce.12225
Muller, E., Lambs, L., Fromard, F. (2009). Variations in water use by a mature mangrove of Avicennia germinans, French Guiana. Ann. For Sci. 66, 803. doi: 10.1051/forest/2009079
Oren, R., Phillips, N., Ewers, B. E., Pataki, D. E., Megonigal, J. P. (1999). Sap-flux-scaled transpiration responses to light, vapor pressure deficit, and leaf area reduction in a flooded taxodium distichum forest. Tree Physiol. 19, 337–347. doi: 10.1093/treephys/19.6.337
Pfautsch, S., Hölttä, T., Mencuccini, M. (2015). Hydraulic functioning of tree stems: fusing ray anatomy, radial transfer and capacitance. Tree Physiol. 35, 706–722. doi: 10.1093/treephys/tpv058
Pfautsch, S., Keitel, C., Turnbull, T. L., Braimbridge, M. J., Wright, T. E., Simpson, R. R., et al. (2011). Diurnal patterns of water use in eucalyptus victrix indicate pronounced desiccation-rehydration cycles despite unlimited water supply. Tree Physiol. 31, 1041–1051. doi: 10.1093/treephys/tpr082
Phillips, N. G., Lewis, J. D., Logan, B. A., Tissue, D. T. (2010). Inter-and intra-specific variation in nocturnal water transport in eucalyptus. Tree Physiol. 30, 586–596. doi: 10.1093/treephys/tpq009
Phillips, N. G., Oren, R., Zimmermann, R. (1996). Radial patterns of xylem sap flow in non-, diffuse- and ring- porous tree species. Plant Cell Environ. 19, 983–990. doi: 10.1111/j.1365-3040.1996.tb00463.x
Phillips, N. G., Ryan, M. G., Bond, B. J., McDowell, N. G., Hinckley, T. M., Cermák, J. (2003). Reliance on stored water increases with tree size in three species in the pacific Northwest. Tree Physiol. 23, 237–245. doi: 10.1093/treephys/23.4.237
Reef, R., Lovelock, C. E. (2014). Regulation of water balance in mangroves. Ann. Bot. 115 (3), 385–395. doi: 10.1093/aob/mcu174
Robert, E. M. R., Schmitz, N., Okello, J. A., Boeren, I., Beeckman, H., Koedam, N. (2011). Mangrove growth rings: fact or fiction? Trees 25, 49–58. doi: 10.1007/s00468-010-0487-9
Rosado, B. H. P., Oliveira, R. S., Joly, C. A., Aidar, M. P. M., Burgess, S. S. O. (2012). Diversity in nighttime transpiration behavior of woody species of the Atlantic rain forest, Brazil. Agric. For Meteorol 158, 13–20. doi: 10.1016/j.agrformet.2012.02.002
Schmitz, N., Robert, E. M., Verheyden, A., Kairo, J. G., Beeckman, H., Koedam, N. (2008). A patchy growth via successive and simultaneous cambia: key to success of the most widespread mangrove species avicennia marina? Ann. Bot. 101, 49–58. doi: 10.1093/aob/mcm280
Scholander, P. F., Hammel, H. T., Hemmingsen, E., Garey, W. (1962). Salt balance in mangroves. Plant Physiol. 37, 722–729. doi: 10.1104/pp.37.6.722
Scholz, F. G., Phillips, N. G., Bucci, S. J., Meinzer, F. C., Goldstein, G. (2011). Hydraulic capacitance: biophysics and functional significance of internal water sources in relation to tree size (Dordrecht, Netherlands: Springer).
Siddiq, Z., Cao, K. F. (2018). Nocturnal transpiration in 18 broadleaf timber species under a tropical seasonal climate. For Ecol. Manag 418, 47–54. doi: 10.1016/j.foreco.2017.12.043
Snyder, K. A., Richards, J. H., Donovan, L. A. (2003). Night-time conductance in C3 and C4 species: do plants lose water at night? For Ecol. Manag 54, 861–865. doi: 10.1093/jxb/erg082
Steppe, K., Pauw, D., Lemeur, R., Vanrolleghem, P. A. (2006). A mathematical model linking tree sap flow dynamics to daily stem diameter fluctuations and radial stem growth. Tree Physiol. 26, 257–273. doi: 10.1093/treephys/26.3.257
Tomlinson, P. B. (2016). The botany of mangroves, 2nd edn (Cambridge: Cambridge University Press). doi: 10.1017/CBO9781139946575
Waisel, Y., Eshel, A., Agami, M. (1986). Salt balance of leaves of the mangrove Avicennia marina. Physiol. Plant 67, 67–72. doi: 10.1111/j.1399-3054.1986.tb01264.x
Wang, Q., Gao, J. G., Zhao, P., Zhu, L. W., Ouyang, L., Ni, G. Y., et al. (2018). Biotic- and abiotic-driven variations of the night-time sap flux of three co-occurring tree species in a low subtropical secondary broadleaf forest. AoB Plants 10, 2041–2851. doi: 10.1093/aobpla/ply025
Wang, H., Zhao, P., Holscher, D., Wang, Q., Lu, P., Cai, X. A., et al. (2011). Nighttime sap flow of Acacia mangium and its implications for nighttime transpiration and stem water storage. J. Plant Ecol. 5, 294–304. doi: 10.1093/jpe/rtr025
Waring, R., Whitehead, D., Jarvis, P. (1979). The contribution of stored water to transpiration in scots pine. Plant Cell Environ. 2, 309–318. doi: 10.1111/j.1365-3040.1979.tb00085.x
Wei, L., Lockington, D. A., Poh, S. C., Gasparon, M., Lovelock, C. E. (2013). Water use patterns of estuarine vegetation in a tidal creek system. Oecologia 172, 485–494. doi: 10.1007/s00442-012-2495-5
Wu, J., Liu, H., Zhu, J., Gong, L., Xu, C., Jina, G. X., et al. (2020). Nocturnal sap flow is mainly caused by stem refilling rather than nocturnal transpiration for Acer truncatum in urban environment. Urban For Urban Green 56, 1618–8667. doi: 10.1016/j.ufug.2020.126800
Zeppel, M. J. B., Anderegg, W. R. L., Adams, H. D., Hudson, P., Cook, A., Rumman, R., et al. (2019). Embolism recovery strategies and nocturnal water loss across species influenced by biogeographic origin. Ecol. Evol. 9, 5348–5361. doi: 10.1002/ece3.5126
Zeppel, M. J. B., Lewis, J. D., Phillips, N. G., Tissue, D. T. (2014). Consequences of nocturnal water loss: a synthesis of regulating factors and implications for capacitance, embolism and use in models. Tree Physiol. 34, 1047–1055. doi: 10.1093/treephys/tpu089
Zeppel, M. J. B., Tissue, D., Taylor, D., MacInnis-Ng, C., Eamus, D. (2010). Rates of nocturnal transpiration in two evergreen temperate woodland species with differing water-use strategies. Tree Physiol. 30, 988–1000. doi: 10.1093/treephys/tpq053
Zhao, C. Y., Si, J. H., Feng, Q., Yu, T. F., Du, L. P. (2017). Comparative study of daytime and nighttime sap flow of Populus euphratica. Plant Growth Regul. 82, 353–362. doi: 10.1007/s10725-017-0263-6
Zhao, H. W., Yang, S. C., Guo, X. D., Peng, C. J., Gu, X. X., Deng, C. Y., et al. (2018). Anatomical explanations for acute depressions in radial pattern of axial sap flow in two diffuse-porous mangrove species: implications for water use. Tree Physiol. 38, 276–286. doi: 10.1093/treephys/tpx172
Keywords: mangrove, nocturnal sap flow, stem diameter change, stem water refilling, transpiration, vapor pressure deficit, water-saving mechanism, water-use strategy
Citation: Wu S, Gu X, Zheng Y and Chen L (2023) Nocturnal sap flow as compensation for water deficits: an implicit water-saving strategy used by mangroves in stressful environments. Front. Plant Sci. 14:1118970. doi: 10.3389/fpls.2023.1118970
Received: 08 December 2022; Accepted: 11 April 2023;
Published: 08 May 2023.
Edited by:
David Rosenthal, Ohio University, United StatesReviewed by:
Kelsey R. Carter, Los Alamos National Laboratory (DOE), United StatesXin Song, Shenzhen University, China
Copyright © 2023 Wu, Gu, Zheng and Chen. This is an open-access article distributed under the terms of the Creative Commons Attribution License (CC BY). The use, distribution or reproduction in other forums is permitted, provided the original author(s) and the copyright owner(s) are credited and that the original publication in this journal is cited, in accordance with accepted academic practice. No use, distribution or reproduction is permitted which does not comply with these terms.
*Correspondence: Luzhen Chen, bHV6aGVuY2hlbkB4bXUuZWR1LmNu
†ORCID: Sipan Wu, orcid.org/0000-0002-8245-7172
Xiaoxuan Gu, orcid.org/0000-0002-5238-9017
Yanghang Zheng, orcid.org/0000-0001-6702-1429
Luzhen Chen, orcid.org/0000-0002-0673-8538