- Institut Sophia Agrobiotech, Institut National de Recherche pour l’Agriculture (INRAE), l’alimentation et l’Environnement, Université Côte d’Azur, Centre National de Recherche Scientifique (CNRS), Sophia-Antipolis, France
Symbiotic nodules formed on legume roots with rhizobia fix atmospheric N2. Bacteria reduce N2 to NH4+ that is assimilated into amino acids by the plant. In return, the plant provides photosynthates to fuel the symbiotic nitrogen fixation. Symbiosis is tightly adjusted to the whole plant nutritional demand and to the plant photosynthetic capacities, but regulatory circuits behind this control remain poorly understood. The use of split-root systems combined with biochemical, physiological, metabolomic, transcriptomic, and genetic approaches revealed that multiple pathways are acting in parallel. Systemic signaling mechanisms of the plant N demand are required for the control of nodule organogenesis, mature nodule functioning, and nodule senescence. N-satiety/N-deficit systemic signaling correlates with rapid variations of the nodules’ sugar levels, tuning symbiosis by C resources allocation. These mechanisms are responsible for the adjustment of plant symbiotic capacities to the mineral N resources. On the one hand, if mineral N can satisfy the plant N demand, nodule formation is inhibited, and nodule senescence is activated. On the other hand, local conditions (abiotic stresses) may impair symbiotic activity resulting in plant N limitation. In these conditions, systemic signaling may compensate the N deficit by stimulating symbiotic root N foraging. In the past decade, several molecular components of the systemic signaling pathways controlling nodule formation have been identified, but a major challenge remains, that is, to understand their specificity as compared to the mechanisms of non-symbiotic plants that control root development and how they contribute to the whole plant phenotypes. Less is known about the control of mature nodule development and functioning by N and C nutritional status of the plant, but a hypothetical model involving the sucrose allocation to the nodule as a systemic signaling process, the oxidative pentose phosphate pathway, and the redox status as potential effectors of this signaling is emerging. This work highlights the importance of organism integration in plant biology.
Introduction
Nitrate (NO3−) and ammonium (NH4+) are the major forms of inorganic nitrogen (N) in the soil. However, plant growth in terrestrial ecosystems is often limited by N availability (Verhoeven et al., 1996; Ågren et al., 2012). Approximately 65 million years ago, plants of the legume family (Fabaceae) and soil bacteria of the Rhizobia type gain the capacity to establish a symbiosis whose function is to reduce atmospheric nitrogen (N2) to ammonia (NH3/NH4+) within the bacteria, and then transfer the NH4+ to the plant when its N demand is not satisfied by mineral N present in the soil (Roy et al., 2020). Although atmospheric N2 is a non-limiting N resource, symbiotic nitrogen fixation (SNF) generally does not entirely meet the plant’s N requirements. Indeed, N acquisition through SNF generally does not reach the same level as the uptake of NO3− and NH4+ when these ions are present in non-limiting concentrations (Ruffel et al., 2008). However, symbiosis allows legume holobionts (i.e., plant in association with its symbiotic bacteria) to grow on poor soils lacking inorganic N. Nevertheless, when enough mineral N is present in soils, legume symbiosis is inhibited and plants satisfy their N demand by mineral N acquisition as non-symbiotic higher plants.
SNF takes place in a new organ, the nodule (Figure 1), in which the plant hosts and nourishes the bacteria (Oldroyd et al., 2011). After a stage of mutual recognition of the two partners, involving plant flavonoids and bacterial lipochito-oligosaccharides (the Nod factors), the bacteria penetrate inside the root hairs via a specific structure, the infection thread, while the root cortical cells divide to initiate nodule formation (Roy et al., 2020). The infection thread grows and crosses the root hair and then the cortical cells to reach the cells of the young growing nodule inside which the bacteria are released. In the nodule, the bacteria differentiate into bacteroids and acquire the ability to reduce N2 to NH3/NH4+ through the activity of a specific enzyme, the nitrogenase (Oldroyd and Downie, 2008). The nodules are of either indeterminate (clover, Medicago, alfalfa, and pea) or determinate (soybean, cowpea, and bean) type (Hirsch, 1992). Indeterminate nodules have a persistent meristem and are composed of four distinct zones (Figure 1): zone I (meristematic zone) where cells divide, zone II (infection zone) where bacteria infect cells of the plant and differentiate into bacteroids (i.e., specialized terminally differentiated bacteria unable to divide anymore), zone III (fixation zone) where the bacteroids reduce N2 to NH3/NH4+, and zone IV (senescence zone) where plant cells and bacteroids enter in senescence (Timmers et al., 2000). The determinate nodules have no persistent meristem and develop by cell expansion. Reduction of N2 by nitrogenase and subsequent transfer of NH4+ to the plant partner is the central process of symbiosis (Figure 2; Oldroyd and Downie, 2008). As nitrogenase is irreversibly inhibited by traces of oxygen (O2), the concentration of O2 inside the nodules is very low, approximately 10 to 40 nM (Appleby, 1992). Thus, the nodules must move from a normoxic environment, at the beginning of their development, to a microoxic one in the fixation zone of mature N2-fixing nodules. In exchange for reduced N, the plant provides carbon (C) in the form of dicarboxylic acids to the bacterial partner (Yurgel and Kahn, 2004; Udvardi and Poole, 2013). Terminally differentiated bacteroids display a metabolic specialization in nitrogen fixation. On the one hand, repression of NH4+ assimilation through the Glutamine synthetase/Glutamate synthase (GS/GOGAT) cycle makes the bacteroids dependent on amino acids supplied by the plant (Patriarca et al., 2002; Prell et al., 2009; Oldroyd et al., 2011). On the other hand, NH4+ produced by nitrogenase is exported outside of the bacteroid, acquired, and actively assimilated in surrounding plant cells in the presence of carbon skeleton acceptors derived from photosynthates translocated from shoots to roots and nodules (Figure 2).
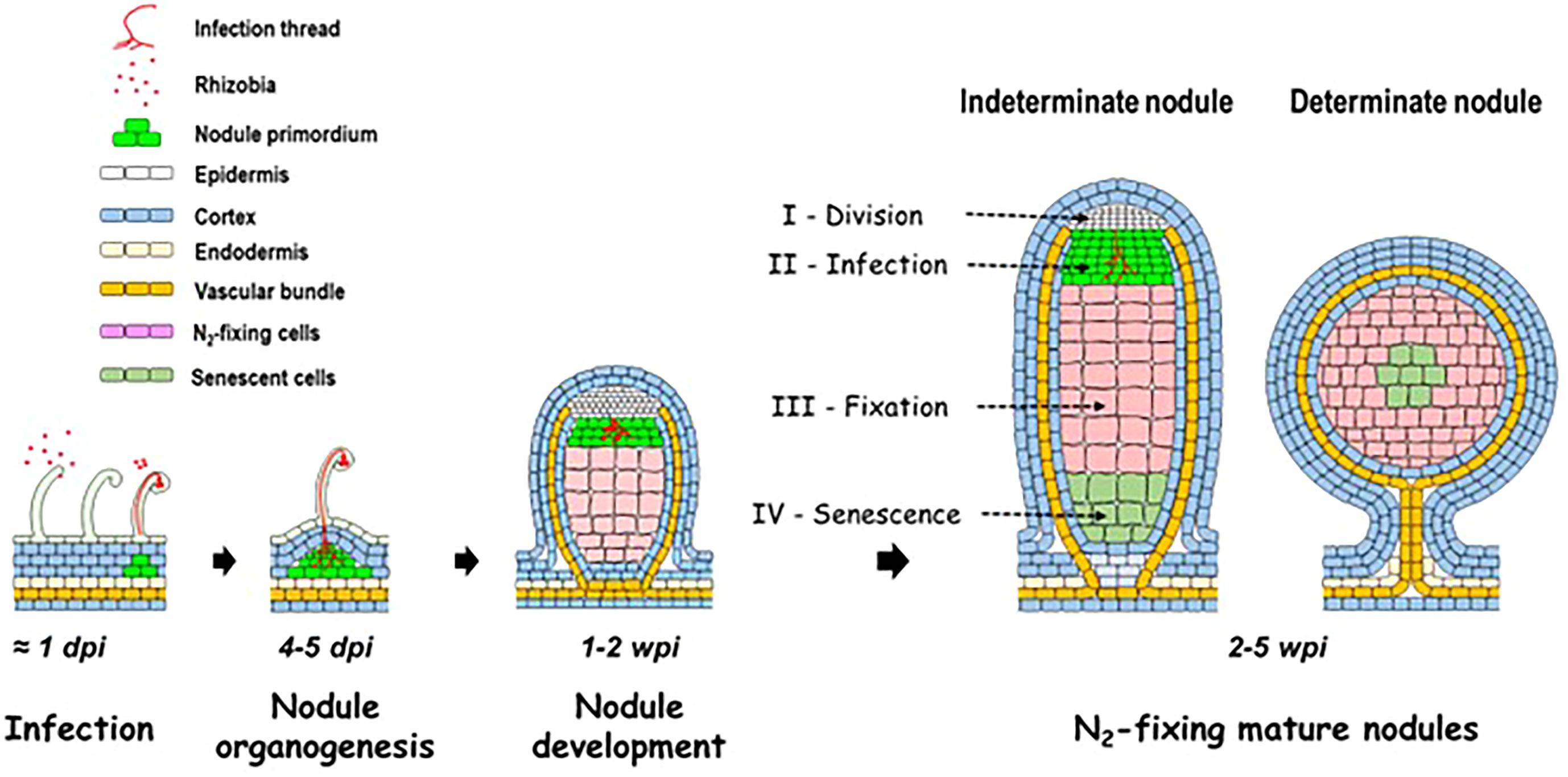
Figure 1 Schematic representation of the establishment and of the development of the legume–rhizobium symbiosis, and of the structure of mature indeterminate and determinate nodules. dpi, day post-inoculation; wpi, week post-inoculation.
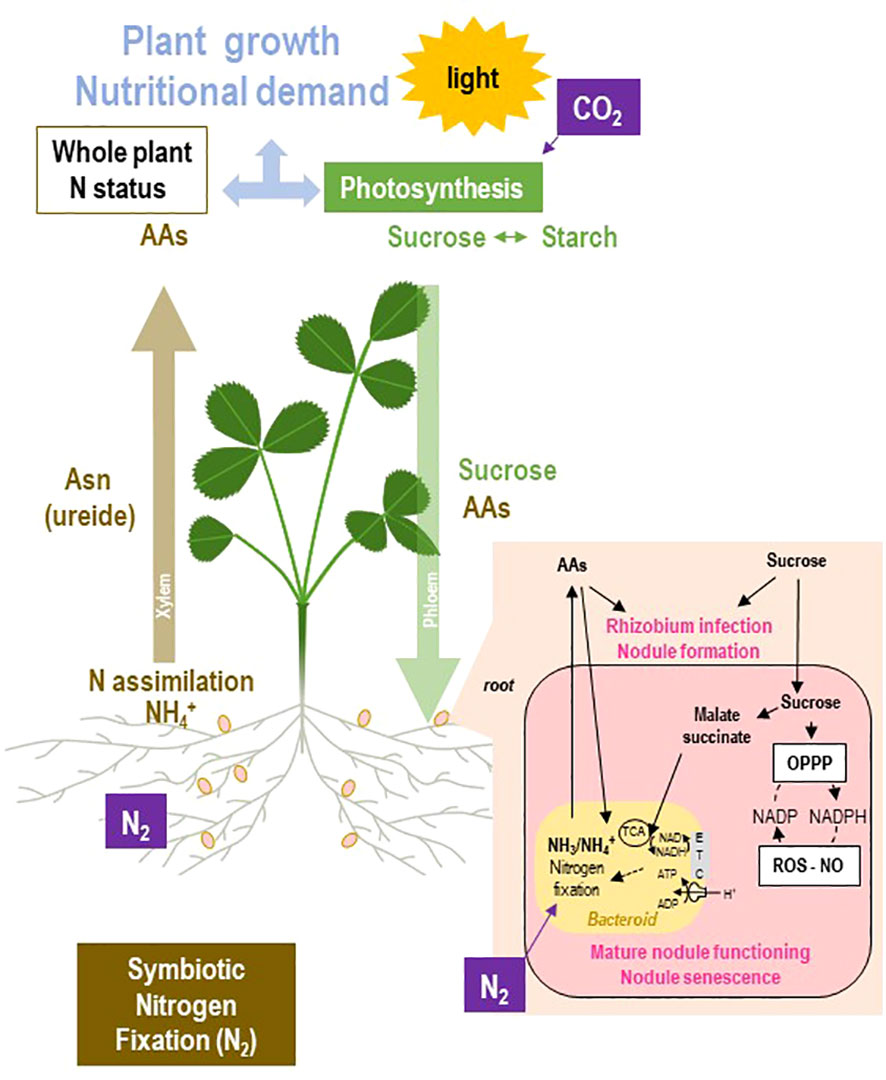
Figure 2 Overview of the N and C fluxes within the rhizobium–legume holobiont using symbiotic nitrogen fixation as the sole source of nitrogen. Atmospheric N2 is fixed into NH3/NH4+ by nitrogenase in the bacteroids of the root nodule. The resulting NH4+ is delivered to the plant cells where it is assimilated into amino acids (AAs). In temperate legumes, asparagine (Asn) is the main form of transport of nitrogen from the root to the shoot through the xylem flux, whereas in some tropical legumes, this transport involves ureides. Photosynthesis allows the plant to capture light and CO2 to produce sugars that are either distributed as sucrose within the plant through the phloem to the “sink” organs or stored as starch. Sucrose is delivered to the roots together with AAs enabling nodule development and symbiotic activity. Nitrogen fixation is energy costly and requires intense fueling by the plant to the symbiont. Malate and succinate are the main sources of carbon and energy provided by the plant to the bacteroid, where they are used through the tricarboxylic acid (TCA) cycle to generate reducing power and ATP. Despite the fact that nitrogen fixation produces large amounts of NH4+, the assimilation of NH4+ is repressed in bacteroids, and therefore amino acids are acquired by the bacteroid from the plant. Nodule sucrose also fuels the oxidative pentose phosphate pathway (OPPP) regenerating the reducing power required to maintain redox homeostasis under the microoxic conditions of nodules. The plant growth determines the N and C nutritional requirements of the plant. ETC, electron transfer chain; NO, nitric oxide; ROS, reactive oxygen species.
The N supply to the plant by nodules requires various regulatory mechanisms operating through the interplay between C and N metabolisms, the sensing of the whole plant N status, the adjustment of nodule capacity to the plant N demand, the supply of O2 to the nodules, and/or the redox homeostasis (Figure 2; Schulze, 2004; Schwember et al., 2019; Lindström and Mousavi, 2020; Chaulagain and Frugoli, 2021). Over the last 15 years, multiple regulatory mechanisms, acting either locally in response to the nodule environment or at the whole symbiotic plant level and involving systemic signaling, have been evidenced. This review aims to summarize our current knowledge on the regulation of the N-fixing symbiosis by the plant N and C nutritional status through the interplay of N, C, and energy metabolisms, and the diverse local and systemic signaling mechanisms. The current knowledge of the various pathways characterized at the molecular and genetic levels will be discussed regarding their impact on the whole plant phenotype. Finally, we will focus on the future challenges toward the understanding of the control of symbiosis by the plant nutritional demand and the attempt to understand crosstalk, interplays, and emerging properties of symbiotic holobionts in the context of a fluctuating environment. Oxygen plays a key role in regulating nitrogen fixation and nodule energy metabolism (Schulze, 2004; Schwember et al., 2019; Booth et al., 2021; Schulte et al., 2021). This topic is not exhaustively reviewed in this paper but will be occasionally mentioned when a crosstalk with regulation of symbiosis by the plant nutritional demand is suggested.
Symbiotic capacities are often limited by the environment or restricted by the plant
Rhizobium–legume symbiosis may be seen as an adaptive response of the legume–rhizobium holobiont to circumvent plant N deficit by activating a new N acquisition pathway from air, an unlimited N source (Figure 2). However, legume plants relying on N2 fixation are frequently N limited, indicating that symbiosis may not be sufficient to fulfill alone the N requirements for plant optimal nitrogen nutrition (Crozat et al., 1994; Gan et al., 2002; Moreau et al., 2008). Multiple factors contribute to the limitation of symbiotic capacity.
A major cause of this limitation is the carbon cost of the nitrogen fixation process (Minchin and Witty, 2005). It is generally observed that symbiotic development is tightly correlated to the plant capacity to supply the symbiotic structures with carbon (C) metabolites required for its formation, persistence, and functioning (Walsh et al., 1987; Voisin et al., 2003). Although nodules represent a small part of the plant mass, they can consume more than 25% of the products of photosynthesis for SNF (Schuize et al., 1999; Vance, 2008). The carbon cost per unit of fixed N (g C per g N fixed) was shown to vary widely with species, growth stage, and environmental conditions, ranging from 1.4 to 12 g C per gram fixed N (Schwember et al., 2019). The limitation of symbiosis by photosynthesis and the supply of carbon to the nodules has been a matter of debate. On one hand, some authors have argued that under normal growth conditions (non-limiting water and mineral supply, optimal photoperiod, and light intensity), the supply of sugars from photosynthesis to the nodules may not be limiting (Vance and Heichel, 1991; Schulze, 2004). On the other hand, under environmental stress, such as water deficiency, a reduced availability of C for bacteroid respiration and nitrogenase activity was associated with the decline in N2 fixation (Gordon et al., 1999; Baier et al., 2007). Furthermore, several studies have shown that elevated CO2 concentrations (eCO2) stimulate N2 fixation and plant biomass production, demonstrating that photosynthesis is effectively limiting symbiosis (Rogers et al., 2006; Sanz-Sáez et al., 2010; Lam et al., 2012; Li et al., 2017; Parvin et al., 2020). In M. truncatula under eCO2, nodule number and size are increased and most N2 fixation-related genes are upregulated (Guo et al., 2013). This was further confirmed by Parvin et al. (2020) who showed, either in normal growth condition or under hydric stress, that faba bean under eCO2 is only able to increase its C gain if nodule activity is maintained. This response of symbiotic legumes to eCO2 is original as compared to non-symbiotic C3 plants supplied by NO3− as N source displaying an eCO2 acclimation and a reduction of NO3− uptake and assimilation (Stitt and Krapp, 1999; Rogers et al., 2006; Guo et al., 2013). The causes of this acclimation to eCO2 are not yet understood, but several hypotheses have been recently raised, such as a lower NO3− concentration in most plant organs, a reduced NO3− acquisition due to a decreased leaf transpiration, an insufficient NADH power for NO3− reduction due to reduced photorespiration under eCO2, or the repression of most NO3− uptake and assimilation systems by eCO2 (Gojon et al., 2022).
Nevertheless, C supply is not the only cause of the limitation of symbiotic capacities. Firstly, the nodule development process in N-limited plants requires several days to result to active SNF. Severe N deficit during this lag period is often detrimental for plant growth (especially if the N demand of the young plant is high) and may inhibit the process (Moreau et al., 2008). Secondly, as soon as the symbiosis is established, the SNF efficiency is frequently not at its maximum. Compatible rhizobia forming natural populations in the soil, able to form nodule with a legume host, may result in contrasted levels of SNF (Laguerre et al., 2012; Bourion et al., 2017; Boivin et al., 2020). Thirdly, symbiotic organs are highly sensitive to local environmental abiotic constraints such as drought, heavy metal, temperature, soil pH, or mineral deficiencies (phosphorus, sulfur) that may drastically inhibit SNF (Durand et al., 1987; Liu et al., 2011; Ferguson et al., 2013; Marino et al., 2013; Gil-Quintana et al., 2013b). The dynamics and the fluctuation of these constraints, as well as the time required to establish the new symbiotic structures necessary for the plant N limitation recovery, must also be considered. Fourthly, the nodule proliferation is tightly controlled and generally limited by the plant at multiple steps of the nodule development. Split-root studies have been used to characterize the systemic control of symbiosis by the whole plant (Figure 3). The autoregulation of nodule number (AON) mechanism limits the new infections by compatible rhizobia as soon as a first infection wave is progressing toward the formation of the symbiotic organ (Kosslak et al., 1983; Kosslak and Bohlool, 1984; Mathews et al., 1989; Olsson et al., 1989; Reid et al., 2011b; Kassaw et al., 2015; Ferguson et al., 2019). The inhibition occurs rapidly after infection before the completion of organogenesis and the activation of nitrogen fixation activity. The formation of spontaneous pseudo-nodules or nodules induced by R. meliloti mutants defective in their ability to invade and multiply within host tissues elicits the AON-related feedback suppression of nodule formation similarly to that elicited by the wild-type bacteria (Caetano-Anollés et al., 1990; Caetano-Anollés et al., 1991). Therefore, AON is related to developmental rather than to nutritional feedback. However, AON is also regulated by the plant N demand. In response to N deficit, the symbiotic plant releases the AON repression to increase its nodule number (Figure 3A; Jeudy et al., 2010; Laguerre et al., 2012). Fifthly, when the symbiotic organ is formed, its behavior remained tightly controlled by the plant. At the nodule level, the absence of N2 fixation triggers a local “plant sanction” response associated with the rapid arrest of nodule growth and the reduction of cultivable bacteria in the nodule (Kiers et al., 2003; Oono et al., 2009; Oono et al., 2011). Mature nodule development is tightly controlled by the systemic signaling of the plant nitrogen demand especially in indeterminate legumes (Figure 3B). On one hand, the plant N deficit stimulates the nodule expansion (as well as bacteroid differentiation), resulting in an increase in N2 fixation activity (Jeudy et al., 2010; Laguerre et al., 2012). On the other hand, the plant N satiety activates the destruction of bacteroids and the senescence of the organ (Pérez Guerra et al., 2010; Lambert et al., 2020).
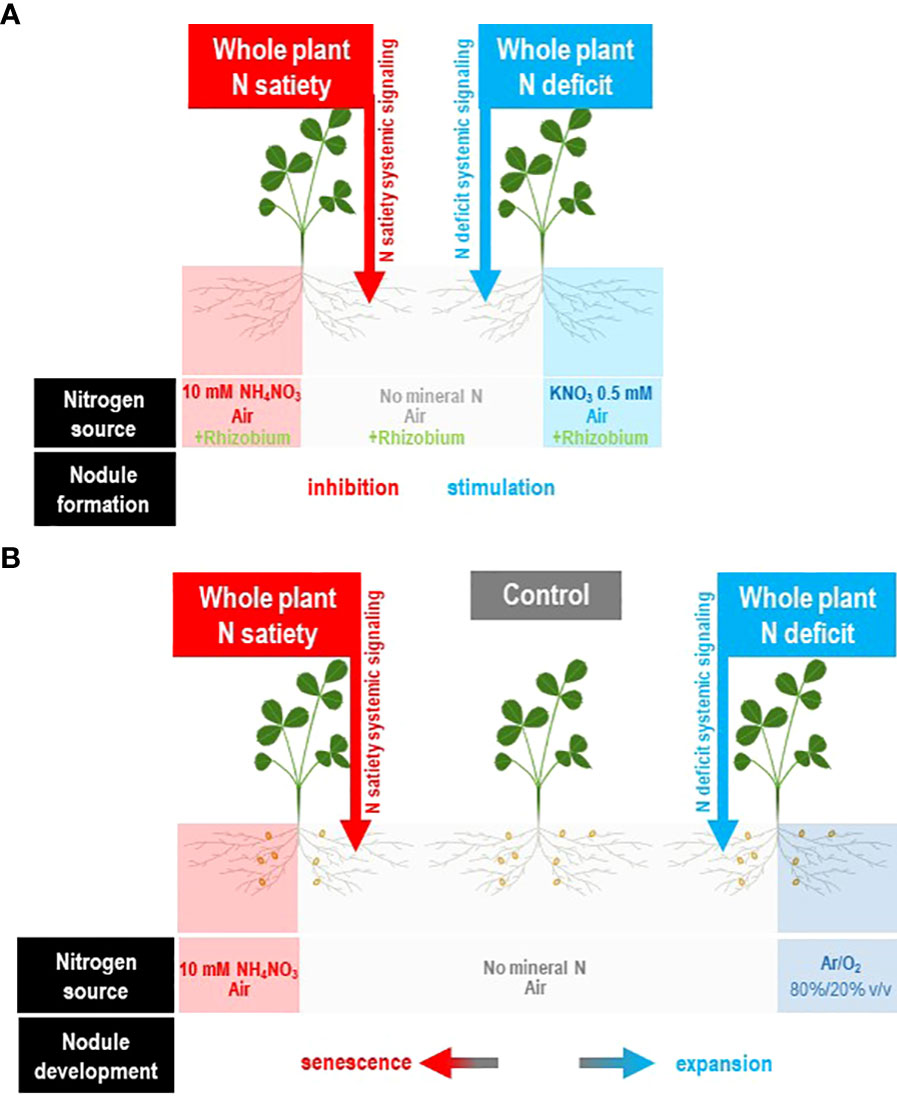
Figure 3 Split-root systems to characterize the control of nitrogen-fixing symbiosis by systemic N signaling of the plant N demand. The experimental systems are described in the original studies of Ruffel et al. (2008); Jeudy et al. (2010); Laguerre et al. (2012); Lambert et al. (2020), and Pervent et al. (2021). M. truncatula plants are cultivated hydroponically. The root system of each plant is separated into two compartments. Each compartment receives specific N supplies: either low level of mineral N supply (0.5 mM KNO3), high level of mineral N supply, air (N2/O2 80/20 v/v), or no gaseous N supply (Ar/O2 80/20 v/v). At the beginning of the experiment, a contrasted N supply is applied to a half-root system. The N treatment modifies the whole plant N status and the whole plant N demand without affecting the environment and the untreated half-root system. Response of the untreated roots to this treatment results necessarily from systemic signaling originated from the rest of plant to these roots. (A) Split root systems used to study the control of nodule formation by plant N demand (Pervent et al., 2021). Non symbiotic plants are used. Contrasted mineral N supply applied to half-root systems may either fully satisfy the plant N demand (N satiety) or results in plant N limitation (N deficit). The response to inoculation by rhizobium in untreated roots strongly relies on the level of N supply of the plant revealing the strong systemic control of nodule formation by systemic signaling of the plant N demand. (B) Split root systems used to study the control of mature nodules by the plant N demand (Jeudy et al., 2010; Laguerre et al., 2012; Lambert et al., 2020). Symbiotic plants supplied with air as a unique N source through the function of mature N2 fixing nodules are used. N treatment applied to a half-root system may either fully satisfy the plant N demand (N satiety) or result in plant N limitation (N deficit). Plant N satiety resulted in nodule senescence whereas plant N limitation stimulates the expansion of mature nodules. The development of the nodules in untreated roots is strongly dependent on the level of supply of the whole plant, revealing the control by the systemic signaling of the whole plant N demand.
Symbiotic development and function are tightly correlated to carbon allocation from the shoot to the symbiotic organs
Several 14CO2 pulse-chase studies have shown that sucrose from photosynthesis is delivered to the nodules via the phloem and then degraded in the cytosol of plant cells to produce organic acids (Gordon et al., 1985; Streeter and Wong, 1988; Rosendahl et al., 1990; Figure 4). Sucrose is first metabolized to UDP-glucose plus fructose, via sucrose synthase (SS), and then oxidized through glycolysis to phosphoenolpyruvate (PEP). PEP is then successively metabolized to oxaloacetate (OAA) and malate by PEP carboxylase (PEPC) and malate dehydrogenase (MDH), respectively. In the different types of nodules, determined or undetermined, the transport of sucrose and/or organic acids to infected cells is preferentially symplastic or apoplastic, or a combination of both (Booth et al., 2021). Thus, the presence of plasmodesmata in soybean (Brown et al., 1995), faba bean (Abd-Alla et al., 2000), and Medicago truncatula (Gaudioso-Pedraza et al., 2018) suggests symplastic transport of C metabolites. Furthermore, the high expression of SWEET-type transporters in vascular parenchyma cells of M. truncatula or Lotus japonicus (Kryvoruchko et al., 2016; Sugiyama et al., 2017) and ALMT (Takanashi et al., 2016) suggests that there is also an apoplastic pathway for the delivery of either sucrose or organic acids to infected cells. Analysis of the expression and activity of enzymes involved in the conversion of sugars to organic acids shows that both vascular parenchyma, non-infected and infected cells are involved in the process of degrading sucrose to malate, albeit in different ways depending on the nodule type (Vance, 2008; Booth et al., 2021). In the determinate nodules of soybean and chickpea, most of the carbon metabolism occurs in non-infected and vascular parenchyma cells (Kouchi et al., 1988; Day and Copeland, 1991), whereas in indeterminate nodules, such as in pea and M. sativa, or in L. japonicus, it is more evenly distributed between infected and non-infected cells and vascular parenchyma cells (Fedorova et al., 1999; Hohnjec et al., 2003; Takanashi et al., 2012). Microoxic conditions associated with O2 channeling by leghemoglobins prevail in central cells of the nodule as nitrogenase requires a very low O2 level to be active. In infected cells, some of the malate is transported to the mitochondria where it is used to regenerate ATP and produce carbon skeletons needed for the assimilation of the reduced nitrogen, i.e., NH4+, produced in the bacteroids (Gordon et al., 1985; Rosendahl et al., 1990; Smith et al., 2002). Nodule mitochondria are characterized by their ability to produce ATP more efficiently and at lower O2 levels than mitochondria in roots and other tissues (Booth et al., 2021). Furthermore, the operation of a phytoglobin-nitric oxide (Pgb-NO) respiration pathway, in which O2 is consumed by phytoglobins whose affinity for oxygen (Kd O2 ≈ 2–10 nM) is significantly higher than that of cytochrome oxidase (Kd O2 ≈ 50–200 nM), allows the innermost cells of nodules to regenerate ATP under the microoxic conditions prevailing in nodules (Berger et al., 2019; Berger et al., 2021). The question particularly arises as to what the O2 concentration is and what type of respiration is functioning in the infected and uninfected cells in the fixation zone. Indeed, measurements with microelectrodes in determinate nodules such as soybean (Tjepkema and Yocum, 1974) and L. japonicus (Denison, 1992), as well as in indeterminate nodules of M. sativa (Soupène et al., 1995), revealed a strong O2 gradient between the outside and inside of the nodules. However, to our knowledge, there is no direct way to investigate the difference in O2 concentration between infected and uninfected cells in vivo. Considering that leghemoglobins are exclusively localized in infected cells (Robertson et al., 1984), an O2 gradient between infected and uninfected cells could be hypothesized. O2 concentration in infected cells has been indirectly calculated by the fractional oxygenation of leghemoglobin (Denison and Layzell, 1991). In addition, several modeling studies have reported that pO2 ranges from 12 to 25 µm in the gas spaces surrounding infected and uninfected cells to 10–60 nM in infected cells (Thumfort et al., 1994; Thumfort et al., 1999; Thumfort et al., 2000). Some studies have indirectly addressed the issue of pO2 differences between infected and uninfected cells in alfalfa (Arrese-Igor et al., 1993), cowpea (Dakora and Atkins, 1990), or soybean (James et al., 1991) and led to the same conclusion of an O2 gradient within the fixation zone. Thus, it is very likely that depending on the local O2 concentration, the involvement of Pgb-NO respiration, alongside classical O2-dependent respiration, might be important for maintaining the energy state and the metabolism of cells (Berger et al., 2019; Berger et al., 2021). Mitochondria also have high MDH activity and low malic enzyme (ME) activity (Day and Mannix, 1988; Bryce and Day, 1990), which favors the reduction of malate to OAA for subsequent ammonia assimilation (Figure 4). Another part of the organic acids is transported in the bacteroids as dicarboxylate, mainly malate (Figure 4; Booth et al., 2021). The activity of the dicarboxylate transporter on the symbiosome membrane has been demonstrated, but the protein has not yet been identified. In contrast, the bacteroid membrane transporter, DctA, has been well characterized and identified in Rhizobium leguminosarum and Bradyrhizobium japonicum (Ronson et al., 1984; Pessi et al., 2007). DctA is upregulated and accounts for most of the carbon influx into the symbiosomes under symbiotic conditions. Once inside the bacteroid, malate is metabolized by the malic enzyme (ME) and MDH to produce pyruvate and OAA, respectively, which fuel the energy and carbon metabolism of the bacteroids (production of ATP and reducing power, storage of excess carbon in the form of carbon polymers, glycogen, and lipids), and allows the reduction of N2 to NH3 by nitrogenase (Lodwig et al., 2005; Terpolilli et al., 2016; Liu et al., 2018). Nitrogenase is the major bacteroid process of ATP and reducing power consumption (16 ATP and 8 e- per N2 fixed; Figure 4). A comprehensive study, combining experimental and metabolic modeling approaches, was recently conducted in R. leguminosarum and Azorhizobium caulinodans to explain the fundamental features of bacteroid metabolism (Schulte et al., 2021). The catabolism of dicarboxylates provides energy for N reduction and allows the synthesis of carbon polymers and alanine. Metabolic modeling of TCA cycle in the bacteroid suggests that catabolism of dicarboxylates induces a higher NADH/NAD ratio than it might have been if fueled by sugars (Schulte et al., 2021). In this study, the authors show that the entire process is dependent on the O2 concentration, the low O2 content of which protects nitrogenase from inhibition, reduces the assimilation of NH4+ into glutamate in the bacteroids, and promotes the export of NH4+ and alanine to the plant cell (Schulte et al., 2021). NH4+ exported by the bacteroid is transported into the cytosol of the infected host cells for its assimilation in amino acids, via the combined action of GS/GOGAT (Figure 4; Cordoba et al., 2003; Seabra et al., 2012). This assimilation process requires an additional flux of C skeleton (α-ketoglutarate) provided by photosynthesis to match the NH4+ flux. Assimilated N is then exported from the nodules to the leaves either as amino acids (mainly asparagine) in indeterminate nodules or as ureides in some determinate nodules (Vance, 2008; Sprent, 2009; Liu et al., 2018). The functioning of the SNF results in a combined action of C and N metabolisms, associated with a strong increase in the expression and activity of their primary enzymes as compared to roots (Vance, 2008), emphasizing the interrelation and co-regulation of the two metabolisms.
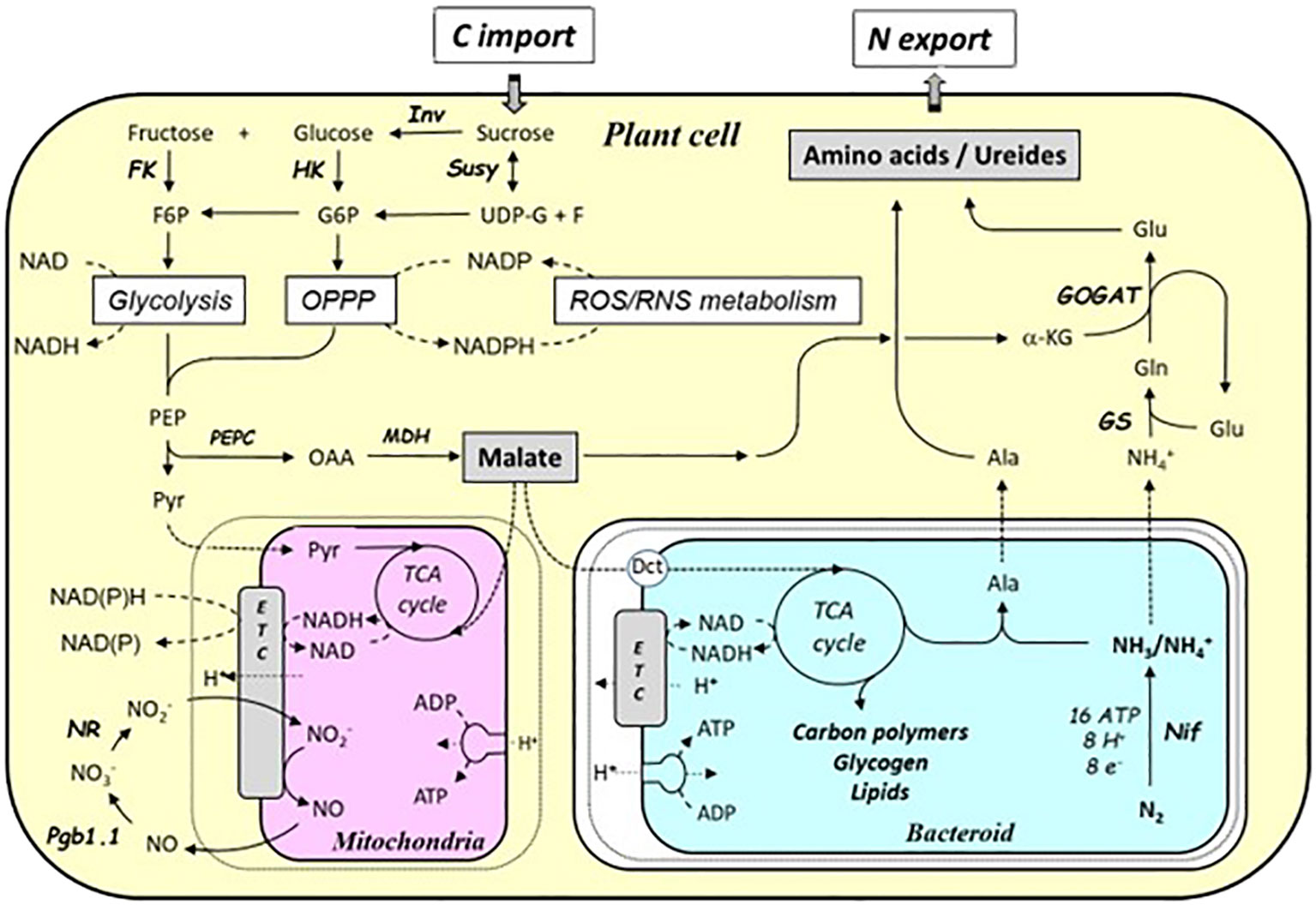
Figure 4 Schematic representation of the carbon and nitrogen metabolic pathways in mature nodules. Sucrose is first oxidized through glycolysis and oxidative pentose phosphate pathway to generate reducing power (NADH, NADPH) and phosphoenolpyruvate (PEP). PEP, on the one hand, supplies pyruvate to the mitochondria to fuel energy metabolism and, on the other hand, supplies the carbon skeletons to produce organic acids (malate, succinate). Part of the organic acids are supplied to the bacteroids and contribute to generating the reducing power and the energy necessary for the reduction of atmospheric nitrogen (N2) into ammonia (NH4+) by nitrogenase. Another part is used to produce the α-keto acids involved in the assimilation of NH4+ from bacteroids, via the glutamine synthetase/glutamate synthase pathway. Assimilated N is exported, as either amino acids (indetermined nodules) or ureides (determinate nodules), to the whole plant. Ala, alanine; Dct, dicarboxylate transporter; e-, electron; ETC, electron transfer chain; F6P, fructose-6-phosphate; FK, fructokinase; G6P, glucose-6-phosphate; GOGAT, glutamate synthase; Gln, glutamine; Glu, glutamate; GS, glutamine synthetase; H+, proton; HK, hexokinase; Inv, invertase; α-KG, α-ketoglutarate; MDH, malate dehydrogenase; N2, dinitrogen; NH4+, ammonium; Nif, nitrogenase; NO, nitric oxide; NO2−, nitrite; NO3−, nitrate; NR, nitrate reductase; OPPP, oxidative pentose phosphate pathway; PEP, phosphoenolpyruvate, PEPC, PEPC carboxylase; Pgb1.1, phytoglobin 1.1; Pyr, pyruvate; ROS/RNS, reactive oxygen species/reactive nitrogen species; Susy, sucrose synthase; TCA, tricarboxylic acid cycle; UDP-G, UDP glucose.
The control of symbiosis by plant N demand involves systemic signaling
Mineral nitrogen and particularly NO3− is known to control its root acquisition at multiple levels. These controls may be related to the ion itself or to the product of its assimilation (Ruffel et al., 2008; Ruffel et al., 2014). These controls are exerted at the level of the organ in presence of the ions and involve local signaling, or at the level of the whole plant and involves systemic signaling. Split-root systems have been used to discriminate between these local and systemic signaling (Figure 3). NO3− itself stimulates root development and induces root activities involved in its uptake and assimilation. It is a common feature of many NO3− transporters and enzymes involved in NO3− assimilation to be activated by the presence of the ion (Vidal et al., 2020). Root NO3− acquisition induction generally requires the presence of the ion at the site of response (local effect) and may occur in absence of assimilation of the ion (Zhang et al., 1999; Wang et al., 2004). At the whole plant level, the main nitrogen sources (NO3−, NH4+, N2, or amino acids) are finally assimilated into the same downstream N metabolites to fulfill the N requirement generated by plant growth and functioning. The concept of N demand refers to the balance between the N requirement to fulfill the plant growth potential and plant N acquisition capacity (Imsande and Touraine, 1994; Gojon et al., 2009). Plant N demand may be contrasted according to plant growth rates and/or plant N regimes. Plant N satiety is reached when there is full satisfaction of the N demand (excess of mineral nitrogen for example), whereas plant N deficit occurs when the N demand is not fully satisfied, and the N provision limits plant growth. Variation of the level of downstream N metabolites (namely, amino acids) of the shoots translocated in roots may be associated with this variation of N demand, consistent with the hypothesis of amino acids cycling through the phloem being a signal of plant N demand (Imsande, 1986; Muller and Touraine, 1992; Parsons et al., 1993; Tillard et al., 1998; Girin et al., 2010). The control of NO3− uptake by a systemic signaling of the plant N demand has been evidenced in several biological systems (Gansel et al., 2001; Girin et al., 2007; Ruffel et al., 2008). N-satiety signaling represses NO3− transporters, while N-deficit signaling upregulates them. Evidence of regulation of root development by similar systemic control has been also evidenced (Forde, 2002). Although mechanisms behind local and systemic N signaling can be discriminated, they share many targets and, at the whole plant level, generally coexist and interact actively. Without specific experimental designs such as plant cultivated in split-root systems, it is therefore difficult to discriminate between these two modes (Figure 3).
There is little evidence on the impact of the plant N status on the early interactions between rhizobium and legume roots (Grillo et al., 2016). However, following the early interaction, the development of symbiosis requires a whole plant N deficit and is suppressed when plant is supplied by high mineral N supply (Streeter and Wong, 1988; Pervent et al., 2021). However, the plant N deficit must not be too extreme because when the seed reserves are totally consumed, nitrogen and carbon metabolites are still needed to form the new symbiotic structures. This argues for the empiric practice used by legume growers of adding a little amount of mineral nitrogen fertilizer as a “starter” at sowing before symbiosis establishment (Imsande, 1986; Streeter and Wong, 1988). Nevertheless, if seed reserves are consumed and if the plant is not able to fulfill its N demand with the mineral N, active symbiosis may be established. The use of split-root systems demonstrated that the control of nodulation by N demand is mainly exerted at the whole plant level (Figure 3A). Nodule formation requires whole plant N-deficit systemic signaling (Streeter and Wong, 1988; Pervent et al., 2021). Split-root studies in M. truncatula did not argue for a strong local effect of NO3− itself on nodule formation (Pervent et al., 2021), suggesting that repression of nodulation by NO3− is mainly related to the downstream N-metabolite production in the whole plant rather than to its presence in the nodule environment. The responses of M. truncatula root to systemic N signaling during the interaction with rhizobium and the nodule formation process were characterized (Pervent et al., 2021). The accumulation of many transcripts associated with the transcriptome reprogramming in response to rhizobium requires systemic signaling of N deficit and is repressed by systemic N signaling of N satiety. However, it is likely that systemic N signaling tunes the progression of the process rather than determine a capacity of the plant to respond to rhizobium (Pervent et al., 2021). Globally, the impact of systemic N signaling is more pronounced during nodule organogenesis, bacteroid differentiation, and activation of nitrogen fixation than on early phases of the interaction.
Evidence for a local regulation of mature symbiotic organs by the efficiency of SNF has been reported. Suppressing nitrogen fixation by Ar/O2 treatments in split-root systems results locally in a rapid inhibition of nodule growth (Singleton and van Kessel, 1987; Kiers et al., 2003; Jeudy et al., 2010). The general small size and the early developmental arrest of nodules formed by fix− bacteria are globally in agreement with an inhibition of nodule development in the absence of N2 fixation (Laguerre et al., 2012). It was proposed that the plant develops a local nodule autonomous mechanism to restrict the development of nodules formed with ineffective bacteria (Kiers et al., 2003; Oono et al., 2011). Long-term Ar/O2 treatments of determinate nodules resulted in a decrease of bacteroid fitness in nodules, associated with early nodule senescence (Kiers et al., 2003; Oono et al., 2009). This result was interpreted as a “host sanction” toward the less beneficial partners. In an evolutionary point of view, “sanction” tends to limit ineffective rhizobia multiplication and dispersion when they are released in the soil. The concept has been extended to indeterminate nodules although bacteroids are terminally differentiated and only undifferentiated bacteria are able to multiply (Oono and Denison, 2010; Oono et al., 2011). Although several reports in soybean/Bradyrhizobium and Medicago/Sinorhizobium symbioses indicate that mutations suppressing N2 fixation do not necessarily impact the reproductive fitness of rhizobia present in nodule (Marco et al., 2009; Laguerre et al., 2012), evidence for a long-term stimulation of the reproductive fitness of fix+ rhizobia versus fix− rhizobia in co-infected root system of Mimosa pudica/Cupriavidus taiwanensis have been also reported (Daubech et al., 2017). Mechanisms related to nodule oxygen permeability or pH have been proposed to be instrumental in the local control of legume–rhizobium symbiotic organs (Hunt and Layzell, 1993; Kiers et al., 2003) but, to date, they remain to be precisely elucidated: whether they are the cause, or the consequence of nitrogen fixation inhibition remains unknown.
Mature nodules are also under the control of systemic signaling of the whole plant N demand (Figures 3, 5, 6). SNF is highly sensitive to abiotic stress that may locally suppress plant N acquisition capacity (Durand et al., 1987; Marino et al., 2013; Gil-Quintana et al., 2013a), resulting in whole plant N deficit (Figure 5). Local suppression of N2 fixation in split-root systems by Ar/O2 treatment or by inoculation with fix− bacteria of a fix− half-root system results in a compensatory response on the remaining fix+ half-root system (Figures 3B, 5; Jeudy et al., 2010; Laguerre et al., 2012). The stimulation of mature nodule expansion and the formation of new symbiotic organs are observed (Jeudy et al., 2010; Laguerre et al., 2012), both tending to increase the nitrogen fixation of the fix+ half-root system (Figures 3B, 5). This is of biological importance as plants are sessile organisms; they face soil conditions highly variable in time and space. This systemic mechanism contributes to adjust the root N2 fixation capacity to the whole plant N demand and to symbiotic root “foraging”. However, it operates probably at the root bundle rather than at the nodule level (Laguerre et al., 2012). Plant facing a uniform reduction of its symbiotic root capacity (fix+ and fix− nodules uniformly distributed on the root system) is unable to trigger the systemic response probably because the plant cannot discriminate between efficient and inefficient root bundles and allocate resources preferentially to the efficient ones (Laguerre et al., 2012). The counterpart of the systemic stimulation of symbiosis by plant N deficit is its systemic repression by plant N satiety (Figures 3, 6). The supply of high level of mineral to a half-root system of N2 fixing plants cultivated in a split-root system results in systemic N-satiety signaling, represses nitrogen fixation, and activates the senescence of the nodules and the degradation of nitrogen-fixing bacteroids (Figures 3, 6; Pérez Guerra et al., 2010; Lambert et al., 2020). A control of symbiotic activity by downstream N metabolites produced in the shoots and translocated to the roots by the phloem has been frequently suggested (Parsons et al., 1993; Imsande and Touraine, 1994; Bacanamwo and Harper, 1997; Neo and Layzell, 1997). Amino acid supply has indeed a strong inhibitory effect on symbiosis (Bacanamwo and Harper, 1997). However, because amino acids may also be a source of nitrogen metabolized by the plant roots, additional evidence is required to confirm this “feedback” model. Transcriptome analysis of M. truncatula plants cultivated in split-root systems revealed that N-demand systemic signaling is a major driver of nodule development and functioning (Figures 5, 6; Lambert et al., 2020). Although plant N satiety activates a general bacterial transcript breakdown associated with bacteroid lysis during nodule senescence, there is little evidence of a gene-specific regulatory effect of systemic signaling of plant N demand on bacteroid transcriptome. However, plant N satiety and plant N-deficit systemic signaling respectively activates and downregulates numerous plant transcripts involved in nodule senescence, while respectively inhibiting and activating transcript families involved in bacteroid differentiation, nodule meristematic cell division, leghemoglobin synthesis, and sucrose transport (Lambert et al., 2020). They are also associated with large reprogramming of hormonal and plant defense genes (Lambert et al., 2020).
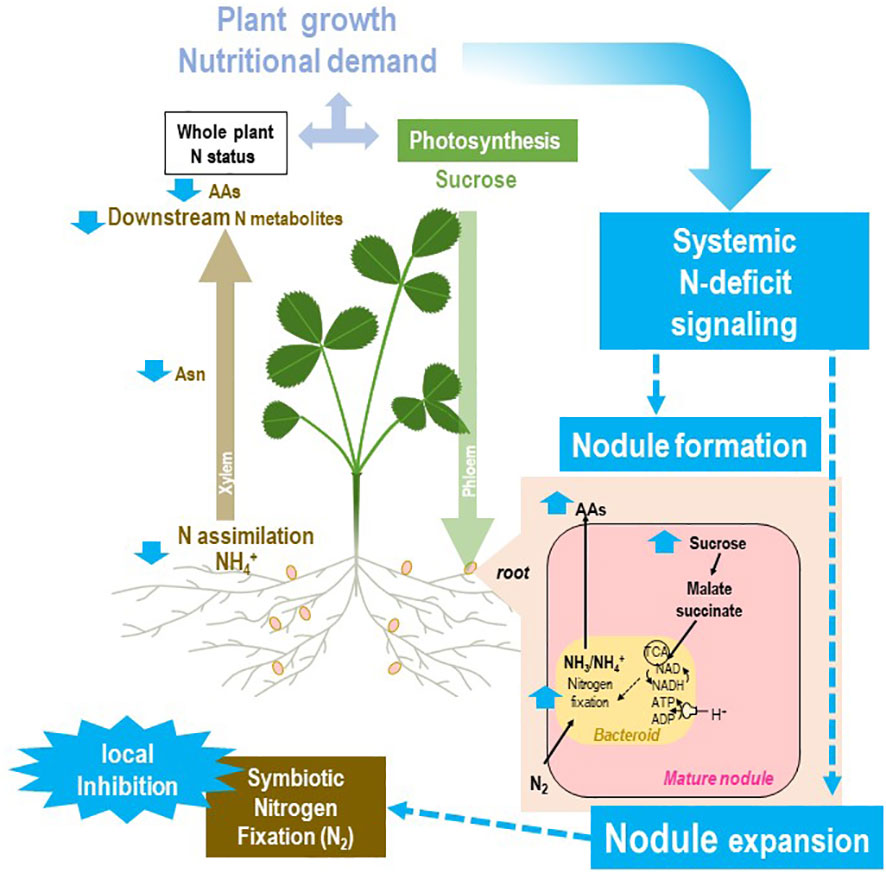
Figure 5 Systemic responses of the rhizobium–legume holobiont to plant N deficit. The general framework of the figure is described in Figure 2. Split-root systems used to characterize N-demand signaling are described in Figure 3. Various steps of the regulatory loop are indicated in blue. A local suppression of SNF may be obtained artificially (by replacing locally air by a mixture Ar/O2 80/20 v/v) or as the result of abiotic stresses. The local inhibition of symbiosis in the roots exposed to these conditions results in a partial decrease of the whole plant SNF. As the whole plant N demand is not fully satisfied, the systemic signaling promoting symbiosis is activated, resulting in the formation of new nodules on the other roots not exposed to the constraint. In mature nodules of these roots, the N-deficit systemic signaling results in a strong increase in nodule sucrose and organic acid levels associated with nodule expansion. This increase in nodule biomass is associated with higher levels of SNF in roots not exposed to the local constraint that may compensate the plant N deficit.
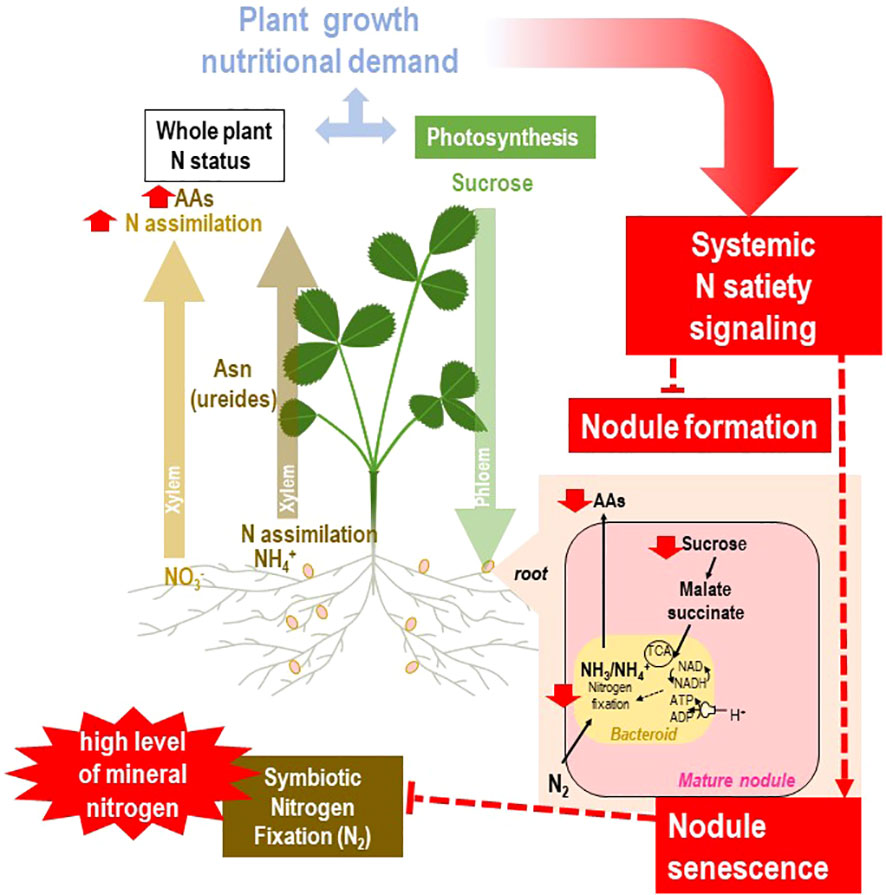
Figure 6 Systemic responses of the rhizobium–legume holobiont to plant N satiety. The general framework of the figure is described in Figure 2. Split-root systems used to characterize N-demand signaling are described in Figure 3. Various steps of the regulatory loop are indicated in red. The local availability of sufficient resources of mineral nitrogen fulfills the plant N demand. In agricultural aerated soils, NO3− is generally the main nitrogen source of annual crops. Assimilation of NO3− occurs mainly in shoots. Satisfaction of the whole N demand results in repressive systemic signaling, arresting nodule formation. In mature nodules, the N-satiety systemic signaling results in drastic reduction of nodule sucrose levels and rapid activation of nodule senescence and bacteroid proteolysis, associated with a sharp decrease in nitrogen fixation.
Mechanisms underlying the regulation of symbiosis by photosynthesis
Active nodules require a large flux of sucrose from the plant to fuel the N2 fixation in the bacteroids (energy and reducing power) and to assimilate the NH4+ released in plant cells. Despite numerous physiological evidence highlighting the tight coordination of photosynthesis and symbiotic activity, mechanisms responsible for the control of symbiosis by the plant C status remain unknown at the genetic and/or molecular levels. Both N-deficit and N-satiety signaling were associated with rapid variations of sucrose allocation from the plant to the active nodule (Lambert et al., 2020). This suggested the hypothesis that plant sucrose allocation is a systemic signal that modulates nodule activity as a function of plant N demand (Figure 7). Consistently with this model, sucrose, produced by shoot photosynthesis, is a major metabolite of phloem sap and its flux is expected to be correlated to the plant growth capacity. Interestingly, nodule Sweet sucrose transporters transcripts have been identified as potential targets of both N-satiety and N-deficit systemic signaling, supporting this hypothesis (Lambert et al., 2020). However, whether sucrose allocation variation is a signal by itself or the consequence of another signaling mechanism remains unknown. The central role of nodule sucrose in the control of symbiosis was already suggested by earlier studies in N-limited supply conditions and in response to drought (Baier et al., 2007). The drop in SNF in response to water stress correlated in several grain legume species (soybean, pea, and bean) to the rapid decline of Susy activity leading to sugar accumulation and organic acid depletion in the nodules (González et al., 1995; González et al., 1998; Gordon et al., 1999; Gálvez et al., 2005). This suggested a key role for Susy in the regulation of SNF by carbon. However, in M. sativa and M. truncatula, the drop in Susy activity only occurs after inhibition of SNF, which questions the possible role of the Susy in this regulation in forage legumes (Naya et al., 2007; Larrainzar et al., 2009).
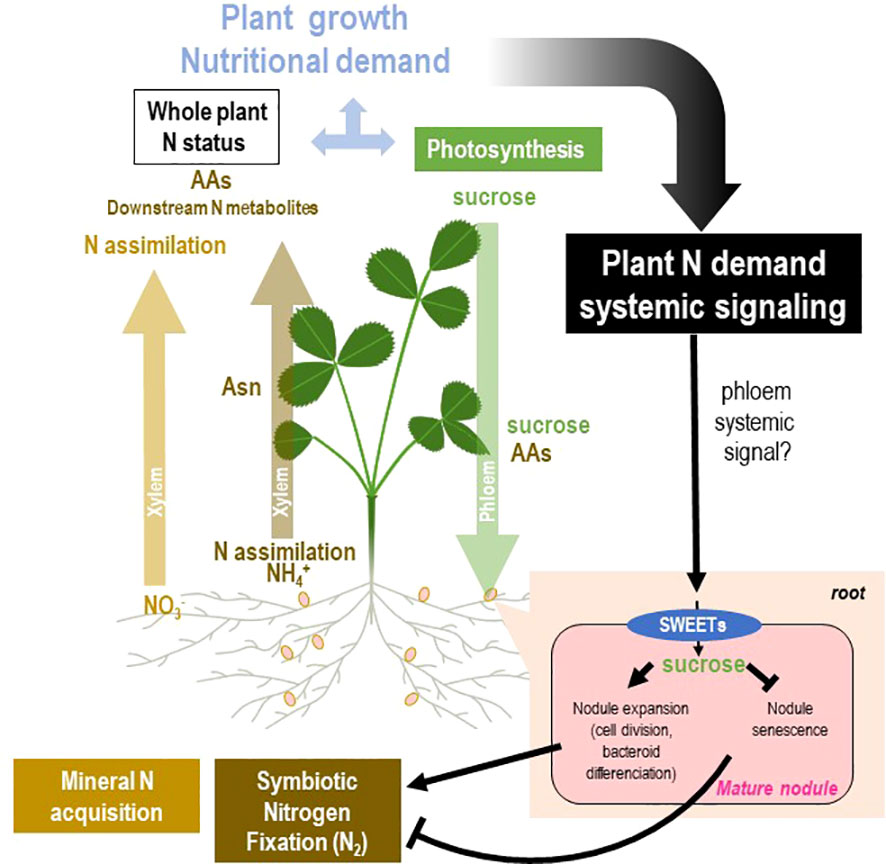
Figure 7 Model of systemic regulation of mature nodules by N demand through nodule sucrose allocation. The general framework of the figure is described in Figure 2. Split-root systems used to characterize N-demand signaling are described in Figure 3. Systemic control of mature nodule development and activity by the whole plant N demand is associated with variation of the allocation of sucrose produced by the photosynthesis to the mature nodule. The hypothetical systemic signal(s) translocated through the phloem remains unknown. On the one hand, the systemic N-satiety signaling lowers nodule sucrose levels and triggers nodule senescence. On the other hand, the systemic N-deficit signaling increases nodule sucrose levels and stimulates nodule expansion. This differential sucrose allocation associates with variations of the SWEET sucrose efflux transporter transcript levels in response to N-satiety and N-deficit systemic signaling.
Lessons may be learned from studies on the mechanisms regulating the acquisition of N by C in non-symbiotic plants (review by Chaput et al., 2020). Sugars from photosynthesis or storage organs are known to be regulators of plant metabolism and plant gene expression (Rolland et al., 2006; Eveland and Jackson, 2012). Sucrose, transported from source tissues to sink organs, is first hydrolyzed either by Susy (to UDP-glucose and fructose) or by invertase (to glucose and fructose) before entering cellular metabolism, to be ultimately oxidized to CO2 through respiration. Multiple levels of sugar sensing have been characterized: a sucrose sensing pathway via a yet unknown sensor (Vaughn et al., 2002); the hexokinase pathway, which, independently of its glucose phosphorylation activity, has glucose sensing activity (Jang et al., 1997; Granot et al., 2013); a hexokinase-independent pathway, probably linked to the regulator of G-protein signaling (RGS1) located on the plasma membrane (Grigston et al., 2008; Urano et al., 2012); a glycolysis-dependent pathway downstream of hexokinase (Xiao et al., 2000); a trehalose pathway (Lunn et al., 2014); and a pathway related to the supply of carbon substrates to mitochondrial respiration (Aubert et al., 1996). As regards N acquisition, several investigations carried out in Arabidopsis roots have shown that the expression of the NO3− transporter genes NRT2.1 and NPF6.3 was directly related to the concentration of glucose-6-phosphate in the roots (Lejay et al., 2003; Lejay et al., 2008). The use of 6-aminonicotinamide, an inhibitor of glucose-6-phosphate dehydrogenase (G6PDH) and 6-phosphogluconate dehydrogenase (6PGDH), two enzymes of the oxidative part of the pentose phosphate pathway (OPPP), as well as the use of a knockdown mutant for plastid 6-phosphogluconolactonase (PGL3), respectively made it possible to highlight the role of OPPP in the regulation of the expression of NRT2.1 and NPF6.3 (Lejay et al., 2008), as well as nitrate reductase (NR) and nitrite reductase (NiR; Bussell et al., 2013). Together with several studies highlighting the role of OPPP and sugars in the regulation of transporters involved in N acquisition (Oji et al., 1985; Bowsher et al., 1989; Bowsher et al., 1992; Neuhaus and Emes, 2000), these investigations supported the existence of an OPPP-dependent sugar signaling pathway for the regulation of plant N acquisition by roots. In plants, OPPP is the main NADPH regeneration pathway that helps maintain cellular redox balance, especially under oxidative stress. An increased flux through the OPPP results in an increased NADPH/NADP ratio and a better resistance to oxidative stress (Ralser et al., 2007), whereas the mutation of G6PDH, which determines the level of NADPH by controlling the flux of G6P that enters the OPPP, leads to a lower resistance to stress (Juhnke et al., 1996). In Arabidopsis, the recent demonstration of the regulation of AtNRT2.1 by the redox status (Bellegarde et al., 2019) suggests that OPPP, via the regeneration of NADPH, could be the intermediary in C signaling. The chromatin factor HIGH NITROGEN INSENSITIVE9 (HNI9), encoded by a genetically identified regulatory locus of AtNRT2.1, was found to reduce the ROS levels under high, but not low, N provision (Widiez et al., 2011; Bellegarde et al., 2019). Interestingly, in Arabidopsis, the bZIP transcription factor ELONGATED HYPOCOTYL5 (HY5) was shown to be a shoot-to-root mobile systemic signal that mediates light promotion of root growth and NO3− uptake via the activation of NRT2.1 (Chen et al., 2016). In the shoot, HY5 promotes indirectly carbon assimilation and translocation, whereas in the root, HY5-dependent upregulation of NRT2.1 and NO3− uptake are favored by an increase in photosynthesis-derived sugars. Together with HNI9, HY5 is required for activation of the detoxification ROS program under high N (Bellegarde et al., 2019). The ability of HY5 to bind the promoter G-box of ROS-responsive genes and regulate de-etiolation in response to light and ROS suggests that HY5 could be involved in the crosstalk between sugars and redox state for the regulation of NRT2.1 and several other NO3− transporter genes by C through the OPPP (Chen et al., 2016; Gangappa and Botto, 2016; Chaput et al., 2020). This knowledge acquired in Arabidopsis on the regulation of NO3− acquisition provides a basis to propose a hypothetical model for the regulation of symbiosis by photosynthesis (Figure 8). Interestingly, in soybean, the HY5 ortholog light-induced TGACG-motif binding factor 3/4 (GmSTF3/4) and FLOWERING LOCUS T (GmFTs) were shown to interdependently induce nodule organogenesis (Wang et al., 2021), supporting the idea that these transcription factors could also be part of the systemic regulation of symbiosis by C (Figure 8). OPPP also has a major role in symbiosis (Figure 8). NADPH is the primary redox cofactor that regulates the regeneration of glutathione and reduced ascorbate, which, in turn, act as secondary redox cofactors in the turnover, or even the detoxification, of reactive oxygen species (ROS) and reactive nitrogen species (RNS) (Noctor and Foyer, 1998; Apel and Hirt, 2004). Interestingly, ROS and nitric oxide (NO), as well as glutathione and homoglutathione, have been shown to be major regulators of symbiosis establishment and functioning (Pauly et al., 2006; Puppo et al., 2013; Berger et al., 2019). In mature nodules, NADPH oxidases (RBOHs) are major sources of H2O2 production, via superoxide anion dismutation (Marino et al., 2011; Arthikala et al., 2014). NR and electron transfer chains from both plant and bacterial partners significantly contribute to NO production in N2-fixing nodules (Sánchez et al., 2010; Horchani et al., 2011; Berger et al., 2021). The NO concentration is itself finely regulated by the phytoglobin Pgb1.1, whose function is to allow NO to exercise its signaling and metabolic intermediary functions at the different stages of the symbiosis without reaching toxic levels for the metabolism (Fukudome et al., 2016; Fukudome et al., 2019; Berger et al., 2020). In this reaction, NO is first oxidized to NO3− by oxyPgb, which is converted to metPgb. MetPgb is then reduced by a MetPgb reductase (MetPgb-R) at the expense of NAD(P)H reducing power (Igamberdiev et al., 2006). Both S-sulfenylated and S-nitrosylated proteins, resulting from post-translational modifications generated by H2O2 and NO, have been detected during early interaction and in functioning nodules, linking ROS/NO production to redox-based protein regulation (Puppo et al., 2013). Thus, considered together, many studies allow to make functional links between carbon metabolism, the regeneration of NADPH and the regulation of redox status by OPPP, and the regulation of SNF by ROS and NO in mature nodules (Figure 8). However, this model remains highly speculative and demonstration of such mechanisms remains to be done.
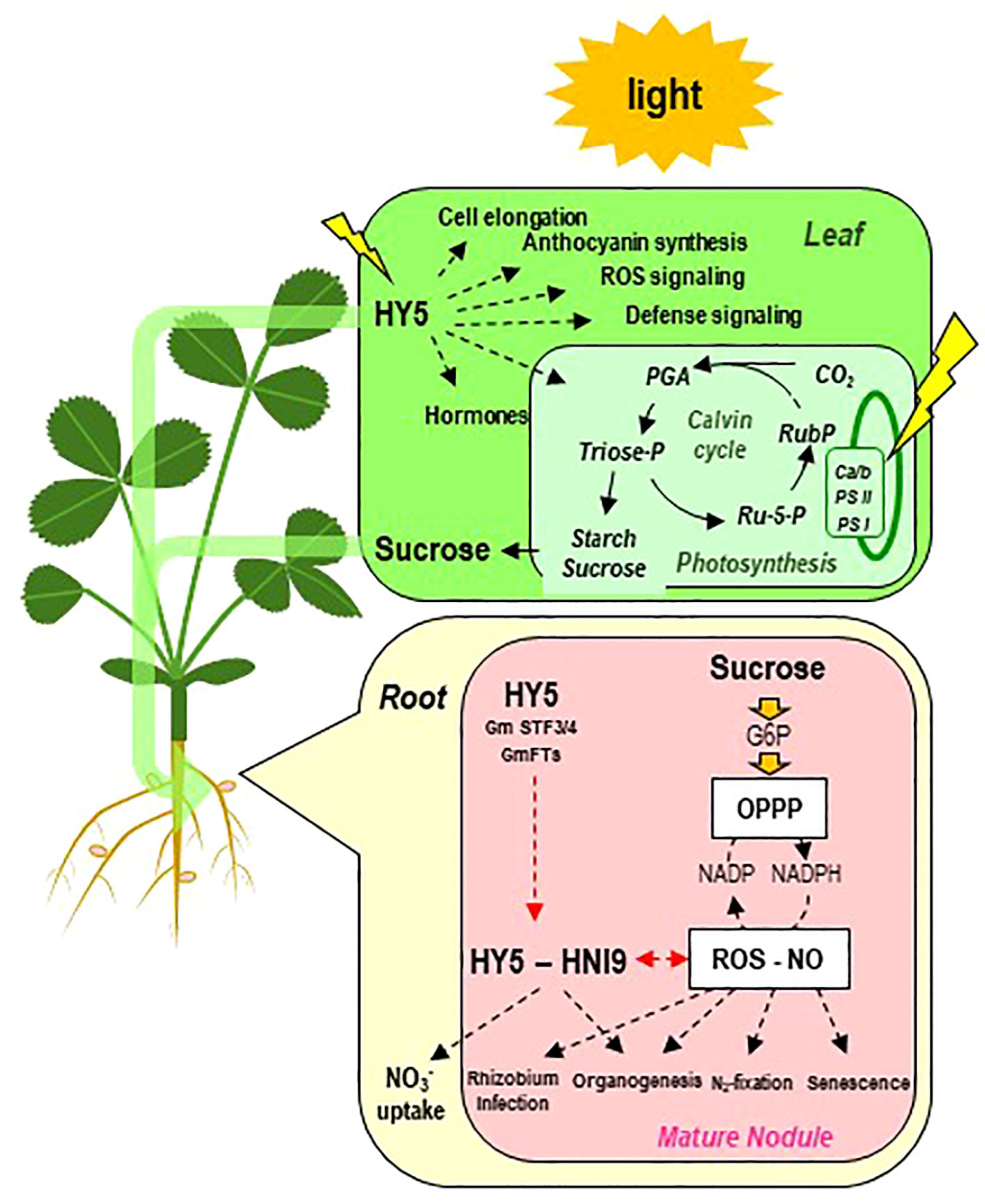
Figure 8 Schematic overview of the current knowledge of regulatory pathways potentially involved in the control of symbiosis by photosynthesis. On the one hand, sucrose resulting from photosynthesis is exported to the roots and the nodules. The fraction of sugars metabolized via the oxidative pentose phosphate pathway (OPPP) generates reducing power (via the NADP/NADPH ratio), controlling the cellular redox state (ROS-NO). Redox state is involved in the regulation of many aspects of the establishment and the functioning of symbiosis. In the root, the redox state and the OPPP are also implicated in the regulation of NO3− transporters. On the other hand, in the leaves, the b-ZIP transcription factor ELONGATED HYPOCOTHYL 5 (HY5) is activated by light and regulates the assimilation and export of carbon to the root system. HY5 may translocate from shoots to roots via the phloem. In roots, HY5 together with the nuclear factor HIGH NITROGEN INSENSITIVE 9 (HNI9) activates the ROS detoxification program in connection with the cellular redox state and regulates downstream NO3− transporters and nodule organogenesis (in soybean). Ca/b, chlorophyll a/b binding complex; PS, photosystem; G6P, glucose-6-phosphate; NO, nitric oxide; OPPP, oxidative pentose phosphate pathway; PGA, 3-phosphoglycerate; phosphoglycerate; ROS, reactive oxygen species; Ru5P, ribose-5-phosphate; RubP, ribulose-5-phosphate.
Multiple pathways are involved in the systemic control of symbiosis
In the last decade, significant discoveries allowed the characterization of receptors, peptides, and transduction pathways involved in the systemic control of nodule formation (Figure 9). Nevertheless, how these mechanisms are integrated at the whole plant level and contribute to the global phenotypes in response to variation of plant N demand and photosynthesis remains elusive. AON was shown to result in the inhibition of nodule formation by a pre-existing nodule (Kosslak et al., 1983; Kosslak and Bohlool, 1984; Mathews et al., 1989; Olsson et al., 1989; Kassaw et al., 2015). Evidenced by split-root experiments, this regulation involves systemic signaling between shoots and roots (Kosslak and Bohlool, 1984; Olsson et al., 1989; Kassaw et al., 2015). Pioneer genetic studies in several legume species allowed the identification of AON components (Caetano-Anollés and Gresshoff, 1991; Sagan et al., 1995; Wopereis et al., 2000; Chaulagain and Frugoli, 2021). AON mutants form generally more nodules than wild type and therefore display super/hyper nodulation phenotypes (Caetano-Anollés and Gresshoff, 1991; Sagan et al., 1995). Several recent reviews described in detail our current knowledge of the related molecular mechanisms (Figure 9; Chaulagain and Frugoli, 2021; Gautrat et al., 2021; Roy and Müller, 2022). AON involves CLV3-like 12-amino acid peptides (CLE) synthetized in roots, translocated by the xylem flux to the shoots, where they bind CLV3-like Leucine Rich Repeat Receptors Like Kinases LRR-RLK (Figure 9). SUNN, HAR1, and NARK loci encode these AON LRR-RLK in M. truncatula, L. japonicus, and soybean, respectively (Krusell et al., 2002; Nishimura et al., 2002; Searle et al., 2003; Schnabel et al., 2005). The receptors exist as homodimers or heterodimers formed with truncated co-receptors (LjCLV2, LjKLV in L. japonicus or MtCLV2 and MtCRN in M. truncatula; Miyazawa et al., 2010; Krusell et al., 2011; Crook et al., 2016). CLE peptides are encoded by large gene families in legumes and non-legume plants (Yamaguchi et al., 2016). The role of CLE peptides in the control of nodule number was demonstrated only for a few of them: MtCLE12, MtCLE13, and MtCLE35 in M. truncatula (Mortier et al., 2010; Mortier et al., 2012; Mens et al., 2020); LjCLE-RS1, LjCLE-RS2, and LjCLE-RS3 in L. japonicus (Okamoto et al., 2009; Nishida et al., 2016); and Gm-RIC1 and GmRIC2 in soybean (Reid et al., 2011a). The corresponding genes are upregulated in the roots in response to the rhizobium/nodule formation (Figure 9). The interaction of AON LRR-RLK receptor and CLE peptides activates the shoot-derived systemic inhibition of nodulation (Figure 8). A downstream component of AON LRR-RLK receptors is the small RNA miR2111 (Figure 9; Tsikou et al., 2018). miR2111 is synthetized as a precursor in the shoots, processed and translocated to the roots by the phloem. In the root, miR2111 post-transcriptionally represses TML1 and 2 genes, encoding ubiquitin ligases, resulting in the inhibition of nodule formation according to a still unknown mechanism (Figure 9; Magori et al., 2009; Takahara et al., 2013; Tsikou et al., 2018; Gautrat et al., 2019). Other signaling processes may also be implicated downstream of AON LRR-RLK receptors. In L. japonicus, the symbiosis establishment results in a HAR1-dependent upregulation of cytokinin synthesis in the shoots that is implicated in the regulation of nodule formation in roots (Sasaki et al., 2014). In addition, AON was associated in M. truncatula with a reduction of the shoot-to-root transport of auxin (van Noorden et al., 2006). Early studies revealed that AON mutants maintain the ability to form nodules under high NO3− supply, demonstrating the role of AON in the control of nodulation by the plant N status (Carroll et al., 1985; Sagan et al., 1995). The resistance of nodulation to NO3− in the sunn mutant of M. truncatula was related to a release of the N-satiety systemic repression (Jeudy et al., 2010). The response of sunn and wild-type roots to systemic N signaling during nodule formation was compared using split-root systems. A role of AON in the control of nodule formation by plant N demand was confirmed but AON-independent components were evidenced (Kassaw et al., 2015; Pervent et al., 2021). The role of AON in the regulation of nodulation by NO3− was also discussed in L. japonicus (Nishida et al., 2020). Some CLE genes encoding peptides were found to be upregulated by NO3− (MtCLE35, LjCLE-RS2, LjCLE-RS3, and Gm-NIC1) and to inhibit partial nodulation through the AON LRR-RLK receptor/miR2111/TML pathway (Figure 9; Okamoto et al., 2009; Nishida et al., 2016; Lebedeva et al., 2020; Mens et al., 2020; Moreau et al., 2021). In M. truncatula, only MtTML2 is downregulated in response to MtCLE35 overexpression, suggesting a specificity of the response to NO3− as compared to the response to rhizobium/nodule formation (Moreau et al., 2021). For decades, AON was the unique identified systemic pathway controlling symbiotic development. Discovery of M. truncatula TR185/cra2 mutants shaded the light on an additional pathway responsible for systemic activation of the root nodulation capacity (Bourion et al., 2014; Huault et al., 2014; Laffont et al., 2019). The mutants display highly branched root phenotype and modified responses to NO3− in non-symbiotic conditions (Bourion et al., 2014; Huault et al., 2014). Their capacity to form nodules with rhizobium is dramatically impaired due to the absence of a systemic signaling originated from the shoot (Huault et al., 2014; Laffont et al., 2019). The MtCRA2 gene, impaired in the mutants, encodes a Leucine-Rich Repeat Receptor-Like Kinase (LRR-RLK) present in shoots able to interact with peptides of the CEP family (C terminally encoded peptides; Figure 9). Both MtCEP1 and MtCEP7 peptides were found to activate the CRA2 systemic signaling, allowing nodulation (Laffont et al., 2019; Laffont et al., 2020). The two corresponding genes are upregulated in the root in response to the absence of mineral nitrogen and to rhizobium, suggesting a control of the pathway by both nitrogen status of the plant and infection by the bacteria. Intriguingly, MtCEP/MtCRA2 and the AON MtCLE/MtSUNN pathways share the downstream miR2111/TML component but act antagonistically on it (Figure 9). MtCEP/MtCRA2 stimulates the accumulation of miR2111 in the shoot to promote the cleavage MtTML transcript in the root, resulting in a stimulation of the root nodulation capacity (Gautrat et al., 2019; Gautrat et al., 2020). Nevertheless, the relative contribution of MtCEP/MtCRA2, MtCLE/MtSUNN, and possibly other unknown components in the control of nodulation by the N status of the plant is not well understood. Furthermore, the role, if any, of MtCEP/MtCRA2 on the response mature nodules to N demand and mineral N is not known because the mutant is impaired in nodule formation. Physiological and molecular characterization of TR185/cra2 plants in non-symbiotic conditions described a N-limitation phenotype and the effect of the mutation on plant NO3− acquisition and on root NO3− transporters’ gene expression. AtCEPR1/AtCEPR2 orthologs of MtCRA2 as well as CEP peptides were identified in Arabidopsis (Tabata et al., 2014). A root target of systemic action of AtCEP1/CEPR1 is the high-affinity NO3− transporter gene AtNRT2.1, known to be upregulated in response to the whole plant N deficiency. AtCEPD1 and AtCEPD2 (CEP downstream 1 and 2), two putative Class 3 glutaredoxins, might play the role of a systemic phloem signal from shoot to root to upregulate AtNRT2.1 (Ohkubo et al., 2017). High-affinity NO3− transporters are not the only targets of CEP/CEPR1 and CEP/CRA2 pathways both in Arabidopsis and in non-symbiotic M. truncatula plants, and root architecture was found to be strongly impaired in related mutants (Bourion et al., 2014; Delay et al., 2019; Chapman et al., 2020). How similar are the CEP receptors’ transduction pathways involved in the control of NO3− uptake and root development in non-symbiotic conditions and the control of nodule formation in symbiotic conditions is not clearly understood. The role of CEPD proteins in the systemic regulation of nodulation downstream of CRA2 in M. truncatula was questioned (Gautrat et al., 2020). Although it cannot be excluded that CRA2 might be a component of the same mechanism adjusting either nodule formation, NO3− acquisition, or root development to plant N demand, this remains to be demonstrated. Because the inactivation of CEP receptor genes has pleiotropic impacts on plant development and functioning, as well as mineral nitrogen acquisition, discriminating between direct and indirect impacts on the nodule formation phenotype in legumes is difficult. Nevertheless, the dramatic inhibition of nodulation observed in the cra2 mutant cannot be simply explained as the result of the N-deficiency phenotype because plant N deficit stimulates rather than inhibits nodulation. To our knowledge, there is little convincing evidence for an active role of bacteria in the regulation of nodules by the holobiont N status (Lambert et al., 2020). However, several reports indicate that GlnD and PII, two bacterial regulatory components controlling nitrogen metabolism in bacteria, are required for symbiosis functioning, suggesting that the question might deserve more investigation (Arcondéguy et al., 1997; Yurgel et al., 2012; D’Apuzzo et al., 2015).
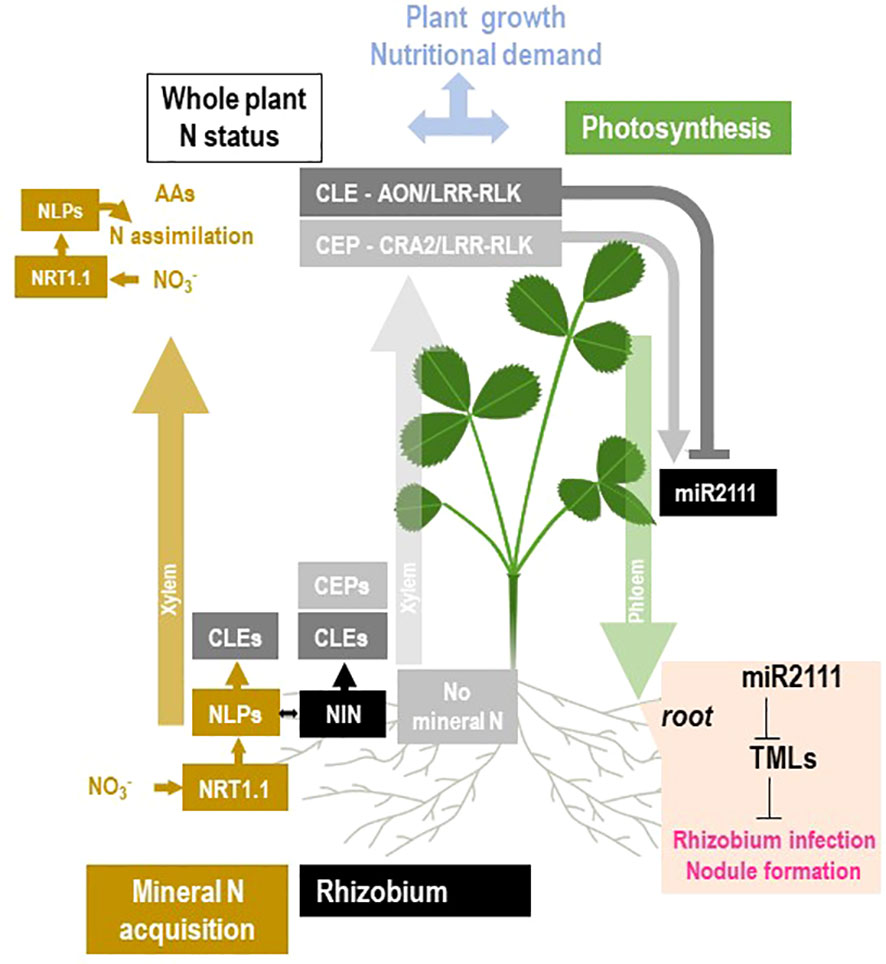
Figure 9 Schematic overview of the current knowledge of the molecular mechanisms potentially involved in the systemic regulation of nodule formation by the plant N status. The general framework of the figure is described in Figure 2. These mechanisms were initially related to the plant response to NO3− (NRT1.1/NLPs module; in brown) or to the systemic control of nodule formation by the plant (CLE-AON/LRR-RLK and CEP-CRA2/LRR-RLK modules; respectively in dark and light gray). The mechanism related to the NO3− response and to NO3− assimilation is in brown. NO3− fuels N assimilation and the production of amino acids. It also activates, through the action of the NO3− transceptor NRT1.1 (NPF6.3/CHL1) and NLP transcription factors (MtNLP1 and LjNRSYM1), the accumulation of NO3−-responsive CLE peptides (MtCLE35, LjCLE-RS2, LjCLE-RS2, and Gm NIC1), as well as the genes encoding the enzyme of NO3− assimilation (including NR and NiR). Rhizobium interaction triggers the accumulation of the NIN transcription factor, also related to NLP family, involved in the activation of CLE peptides in response to rhizobium (MtCLE12, MtCLE13). CLE peptides may be transported from the roots to the shoots through the xylem flow. In the shoots, the CLE peptide activation of AON/LRR-RLK receptors (MtSUNN, LjHAR1 forming possibly heterodimers with MtCLV2 and MtCRN or LjCLV2, and LjKLV) results in the downregulation of the level of miR2111 circulating in the phloem between shoot and roots. In the absence of mineral nitrogen, CEP peptides accumulate in the roots (MtCEP1 and MtCEP7) and are translocated to the shoot by the xylem. In the shoots, the CEP peptide activation of the CRA2/LRR-RLK receptor stimulates the miR2111 levels, antagonistically regulated by CLE-AON/LRR-RLK and CEP-CRA2/LRR-RLK pathways. In the roots, the miR2111 inhibits TML (by mRNA cleavage) that is actively repressing nodule formation. Consequently, CLE-AON/LRR-RLK and CEP-CRA2/LRR-RLK pathways respectively inhibits or promotes nodule formation.
Does the local sensing of NO3− contribute to the control of symbiosis by plant N demand?
In addition to its role as a resource for downstream N-metabolite synthesis, NO3− itself plays a role of signaling molecule in plant organs (review by Maghiaoui et al., 2020). In Arabidopsis, the use of null NR mutants’ NO3−-specific effects is independent of its reduction (Zhang et al., 1999; Wang et al., 2004). The complex mechanisms related to root NO3− sensing begin to unravel in Arabidopsis (Maghiaoui et al., 2020). The NO3− transporter AtNRT1.1 (NPF6.3/CHL1) plays the role of sensor and governs a wide range of response to NO3− independently of its transport activity and NO3− assimilation (Ho et al., 2009; Krouk et al., 2010; Bouguyon et al., 2015; Riveras et al., 2015; Maghiaoui et al., 2020). Other central players are some NLP transcription factors required for the induction of many target genes by NO3− including those responsible for its reduction and assimilation (Castaings et al., 2009; Marchive et al., 2013; Guan et al., 2017; Liu et al., 2017; Liu et al., 2022). Several studies described the regulation in legumes of both nodule formation and functioning by NO3− through the action of NLP proteins (Figure 9; Lin et al., 2018; Nishida et al., 2018; Moreau et al., 2021). In M. truncatula, MtNLP1, activated in response to NO3−, and MtNIN, required for nodulation in the presence of rhizobium, were shown to antagonistically interact for the transcriptional activation of key genes (Figure 9; Lin et al., 2018). NLP proteins (MtNLP1 and LjNRSYM1) were implicated in the transcriptional activation of NO3−-responsive genes, including MtCLE35 and LjCLE-RS2, as well as NR and NiR genes (Nishida et al., 2018; Moreau et al., 2021). This local activation of CLE genes was shown to be associated with activating the LjHAR1/MtSUNN AON LRR-RLK-dependent systemic inhibition of nodulation in model legumes (Figure 9). Nevertheless, the biological significance of the hypothesis of systemic inhibition of nodulation activated locally by NO3− remains elusive because (1) split-root studies rather suggest a regulation of nodulation by N demand related to downstream N-metabolite production at the whole plant level (Pervent et al., 2021) and (2) such mechanism does not explain inhibition of symbiosis by other N sources such as amino acids or NH4+ (Yamashita et al., 2019). Furthermore, because of their roles in the activation of NO3− assimilation, an indirect impact of NLPs on downstream N-metabolite synthesis cannot be ruled out. The use of legume mutant background impaired in NO3− reduction such as null NR mutants may unequivocally discriminate between a role of these NLPs in the inhibition of nodulation by NO3− itself or by products of its assimilation. Finally, there are intriguing reports showing that, in some conditions, NO3− may be required for optimal nitrogen fixation in mature nodules of L. japonicus through the action of specific nodule NO3− transporters (Valkov et al., 2017; Valkov et al., 2020). This raised the hypothesis of a control of symbiotic activity by the NO3− flux fueling the Pgb-NO respiration known to be active in the microoxic conditions of mature nodules (Horchani et al., 2011).
Perspectives
The last decade yielded important knowledge on multiple mechanisms involved in the adjustment of the symbiotic capacity to the plant nutritional demand as a function of the plant environment. Because symbiosis allows the plant to acquire N from air at the expense of photosynthates, the plant N and C status are major drivers of these mechanisms. Local environmental conditions are tuning the adjustment of symbiosis activity to the whole plant nutritional status not only through the availability of N and C resources (mineral nitrogen, light, and CO2) but also by allowing or inhibiting the development and/or functioning of symbiotic organs. Plants continuously adapt to these conditions that are frequently heterogeneous in space and time. The nutritional demand is therefore necessarily integrated at the level of the whole plant, resulting in foraging responses either by stimulating symbiotic capacity (under N-deficit or eCO2 conditions) or by inhibiting it (under N-satiety or low-light conditions). These responses are activated by both systemic and local signaling pathways. The discovery of multiple pathways, acting simultaneously and targeting almost all aspects of nodule development and functioning, revealed not only the central role of the adjustment of the symbiotic capacity to the plant nutritional demand, but also its extraordinary complexity. However, the biological impact of these pathways and their relative role in the whole plant phenotype as a function of the environment is far to be understood.
Up to now, most investigations mainly focused on regulatory circuits controlling early plant–rhizobium interaction and nodule formation. Because the nodule formation process is associated with the activation of a large set of specific genes, earlier studies have predicted that specific symbiotic mechanisms may be operating in this control (Ruffel et al., 2008). However, the current knowledge prompted us to modulate this interpretation. Although the MtCEP/MtCRA2, the AON MtCLE/MtSUNN, or the NLP-related NO3−-responsive pathways target many specific symbiotic genes and development processes in legume plants, there is increasing evidence indicating that (1) legume mutants impaired in these pathways display non-symbiotic phenotypes often related to N nutrition, and (2) these pathways belong to families of pathways present in non-legumes regulating root development and mineral nitrogen acquisition in response to NO3−. The HY5 pathway was found to be important not only for the systemic regulation by light of NO3− acquisition under non-symbiotic conditions but also for nodule formation under symbiotic conditions. A major challenge for future studies will be to revisit the plant phenotypes and discriminate between the pleiotropic consequences of mutations impairing the functions of these pathways. How much the impact of the Mtcra2 mutation on non-symbiotic functions (nitrogen limitation and root development) might influence the nodule formation phenotype of the mutant remains an open question. We do not know if, in NLP mutants (LjNRsym1 and Mtnlp1), the reduced activation by NO3− of the NO3− assimilation pathway, which is expected to lower the levels of downstream N metabolites, contributes to reduce the response of nodulation to NO3−. Discriminating between the effects of NO3− itself and its assimilation on the regulation of nodulation will require appropriate strategies (NR mutants, for example). Although many progresses have been made in our understanding of AON, several studies suggest that some pieces of the puzzle in the control nodule formation by systemic signaling N demand remain unknown, particularly the mechanisms involved in the bacteroid differentiation and in the activation of nitrogen fixation in newly formed nodules (Pervent et al., 2021). Furthermore, although the last decade yields the discoveries of CLE and CEP peptides as well as miR2111, playing the role of signal molecules between root and shoot, the role of the other plant hormones in the systemic control of nodule formation by plant N demand remains to be clarified. Although ethylene has been implicated in the control of nodulation (particularly infection), its role in the plant response to N and C status through systemic signaling regulation deserves further investigation (Penmetsa et al., 2003; Prayitno et al., 2006; Zhu et al., 2020).
Less attention was made on the control of mature nodule development and functioning by the whole plant nutritional status. However, the N and C status of the plant may strongly determine mature nodule behavior, either by stimulating nodule expansion or by activating nodule senescence. Consistently with the tight integration of N and C signaling, the regulation of sucrose allocation to the nodule was associated with N-satiety and N-deficit systemic signaling, suggesting that the fueling symbiosis by C metabolites may contribute to a systemic N-demand signaling process. (Jeudy et al., 2010; Lambert et al., 2020). Supporting this hypothesis, transcripts encoding sucrose transporters likely responsible for the nodule acquisition of sucrose are targets of the N-demand systemic signaling pathway (Lambert et al., 2020). However, further studies are required to validate this model and its biological relevance. More globally, there is a lack of knowledge on mechanisms responsible for the coordination of symbiotic activity and photosynthesis. The last decade yielded the discovery of the role of the HY5 pathway in the control of nodule formation (Chen et al., 2016; Wang et al., 2021). However, questions related to biological significance and physiological impact of this pathway in the control of symbiosis deserve further investigations. The HY5 pathway was identified as a response to light, whereas most of the physiological data suggest a control of symbiosis by photosynthates allocated from the shoots to the roots. Furthermore, eCO2 stimulates symbiosis without any change in light and, therefore, independently of light-induced regulation of HY5. Up to now, most of the reported functions of HY5 relate to nodule formation and little is known about its role in mature nodule functioning. Whether additional function of HY5, or other mechanisms, is involved in the regulation of the symbiosis by photosynthesis and sucrose allocation by the plant remains to be further investigated. The discovery of the role of OPPP and the redox status in the regulation of plant NO3− acquisition by photosynthesis suggests evaluating its role in the control of symbiosis in legumes. Although the critical role of the redox status (ROS and NOS) in symbiotic development and functioning has been clearly demonstrated, the possible role of the C metabolites’ allocation and the metabolites’ flux through the OPPP (providing reducing power necessary for the control of redox status) is an attracting hypothesis in the context of the regulation of symbiosis by the whole plant nutritional status. One of the major lessons of the last decade is our need to analyze integrated phenotypes of mutants impaired in regulatory components of symbiosis taking into account not only specific symbiotic function but also their possible interactions with whole holobiont development, and considering C and N economy as well as their impact on metabolic fluxes. Several efforts in this direction have been made: (1) modeling C and N exchanges as well as metabolic flux in the context of the symbiotic plant (Salon et al., 2009; Moreau et al., 2012; diCenzo et al., 2016; Schulte et al., 2021) and (2) investigating these regulations using split-root systems, which allows discrimination between local and inter-organ signaling (Ruffel et al., 2008; Jeudy et al., 2010; Laguerre et al., 2012; Lambert et al., 2020; Pervent et al., 2021).
A major biological role of these mechanisms relates to the whole symbiotic plant adaptation to local conditions impairing symbiotic activity (such as drought, salt, temperature, heavy metals, and flooding). As a sessile organism, the holobiont adapts to local stress and circumvents the plant N deficit resulting from local inhibition of SNF by stimulating nodule development in the root, allowing efficient symbiosis (Jeudy et al., 2010; Laguerre et al., 2012). This adaptation to satisfy the N demand is made by allocating preferentially C resources to more efficient roots at the expense of inefficient ones. In the context of climate change, soil conditions will be more heterogeneous and fluctuating than ever, resulting more frequently in local stresses (Dusenge et al., 2019). In addition, atmospheric ambient CO2 will increase, potentially modifying the conditions of plant C acquisition. A major challenge for plant science is to design strategies to select genotypes better adapted to these new conditions. The potential of legumes in this context has been highlighted because, unlike cereals acquiring mainly NO3− as a N source, symbiotic N2-fixing legumes can benefit from elevated CO2 (Rogers et al., 2009). Despite the major threat of climate change in agriculture, changes in the equilibrium of C/N trade-offs in symbiotic crop legumes may be an opportunity for the selection of new genotypes able to better adapt to soil local constraints and display better competitivity as compared to other C3 non-symbiotic plants.
Author contributions
All authors listed have made a substantial, direct, and intellectual contribution to the work and approved it for publication.
Funding
ML and RB were supported by the ANR grant Psyche (ANR-16-CE20-0009-02) and the LABEX SIGNALIFE program (ANR-11-LABX-0028-01).
Conflict of interest
The authors declare that the research was conducted in the absence of any commercial or financial relationships that could be construed as a potential conflict of interest.
Publisher’s note
All claims expressed in this article are solely those of the authors and do not necessarily represent those of their affiliated organizations, or those of the publisher, the editors and the reviewers. Any product that may be evaluated in this article, or claim that may be made by its manufacturer, is not guaranteed or endorsed by the publisher.
References
Abd-Alla, M. H., Koyro, H.-W., Yan, F., Schubert, S., Peiter, E. (2000). Functional structure of the indeterminate vicia faba l. root nodule: Implications for metabolite transport. J. Plant Physiol. 157, 335–343. doi: 10.1016/S0176-1617(00)80056-5
Ågren, G. I., Wetterstedt, J.Å.M., Billberger, M. F. K. (2012). Nutrient limitation on terrestrial plant growth – modeling the interaction between nitrogen and phosphorus. New Phytol. 194, 953–960. doi: 10.1111/j.1469-8137.2012.04116.x
Apel, K., Hirt, H. (2004). Reactive oxygen species: Metabolism, oxidative stress, and signal transduction. Annu. Rev. Plant Biol. 55, 373–399. doi: 10.1146/annurev.arplant.55.031903.141701
Appleby, C. A. (1992) The origin and functions of haemoglobin in plants. Sci. Progress Oxford (Sage Publications, Ltd.) 76, 365–398. Available at: https://www.jstor.org/stable/43421309.
Arcondéguy, T., Huez, I., Tillard, P., Gangneux, C., de Billy, F., Gojon, A., et al. (1997). The rhizobium meliloti PII protein, which controls bacterial nitrogen metabolism, affects alfalfa nodule development. Genes Dev. 11, 1194–1206. doi: 10.1101/gad.11.9.1194
Arrese-Igor, C., Royuela, M., de Lorenzo, C., de Felipe, M. R., Aparicio-Tejo, P. M. (1993). Effect of low rhizosphere oxygen on growth, nitrogen fixation and nodule morphology in lucerne. Physiologia Plantarum 89, 55–63. doi: 10.1111/j.1399-3054.1993.tb01786.x
Arthikala, M.-K., Sánchez-López, R., Nava, N., Santana, O., Cárdenas, L., Quinto, C. (2014). RbohB, a phaseolus vulgaris NADPH oxidase gene, enhances symbiosome number, bacteroid size, and nitrogen fixation in nodules and impairs mycorrhizal colonization. New Phytol. 202, 886–900. doi: 10.1111/nph.12714
Aubert, S., Gout, E., Bligny, R., Marty-Mazars, D., Barrieu, F., Alabouvette, J., et al. (1996). Ultrastructural and biochemical characterization of autophagy in higher plant cells subjected to carbon deprivation: Control by the supply of mitochondria with respiratory substrates. J. Cell Biol. 133, 1251–1263. doi: 10.1083/jcb.133.6.1251
Bacanamwo, M., Harper, J. E. (1997). The feedback mechanism of NO3- inhibition of nitrogenase activity in soybean may involve asparagine and/or products of its metabolism. Physiologia Plantarum 100, 371–377. doi: 10.1111/j.1399-3054.1997.tb04795.x
Baier, M. C., Barsch, A., Küster, H., Hohnjec, N. (2007). Antisense repression of the medicago truncatula nodule-enhanced sucrose synthase leads to a handicapped nitrogen fixation mirrored by specific alterations in the symbiotic transcriptome and metabolome. Plant Physiol. 145, 1600–1618. doi: 10.1104/pp.107.106955
Bellegarde, F., Maghiaoui, A., Boucherez, J., Krouk, G., Lejay, L., Bach, L., et al. (2019). The chromatin factor HNI9 and ELONGATED HYPOCOTYL5 maintain ROS homeostasis under high nitrogen provision. Plant Physiol. 180, 582–592. doi: 10.1104/pp.18.01473
Berger, A., Boscari, A., Frendo, P., Brouquisse, R. (2019). Nitric oxide signaling, metabolism and toxicity in nitrogen-fixing symbiosis. J. Exp. Bot. 70, 4505–4520. doi: 10.1093/jxb/erz159
Berger, A., Boscari, A., Puppo, A., Brouquisse, R. (2021). NO3- reductases and hemoglobins control nitrogen-fixing symbiosis by regulating nitric oxide accumulation. J. Exp. Bot. 72, 873–884. doi: 10.1093/jxb/eraa403
Berger, A., Guinand, S., Boscari, A., Puppo, A., Brouquisse, R. (2020). Medicago truncatula phytoglobin 1.1 controls symbiotic nodulation and nitrogen fixation via the regulation of nitric oxide concentration. New Phytol. 227, 84–98. doi: 10.1111/nph.16462
Boivin, S., Ait Lahmidi, N., Sherlock, D., Bonhomme, M., Dijon, D., Heulin-Gotty, K., et al. (2020). Host-specific competitiveness to form nodules in rhizobium leguminosarum symbiovar viciae. New Phytol. 226, 555–568. doi: 10.1111/nph.16392
Booth, N. J., Smith, P. M. C., Ramesh, S. A., Day, D. A. (2021). Malate transport and metabolism in nitrogen-fixing legume nodules. Molecules 26, 6876. doi: 10.3390/molecules26226876
Bouguyon, E., Brun, F., Meynard, D., Kubeš, M., Pervent, M., Leran, S., et al. (2015). Multiple mechanisms of NO3- sensing by arabidopsis NO3- transceptor NRT1.1. Nat. Plants 1, 15015. doi: 10.1038/nplants.2015.15
Bourion, V., Heulin-Gotty, K., Aubert, V., Tisseyre, P., Chabert-Martinello, M., Pervent, M., et al. (2017). Co-Inoculation of a pea core-collection with diverse rhizobial strains shows competitiveness for nodulation and efficiency of nitrogen fixation are distinct traits in the interaction. Front. Plant Sci. 8. doi: 10.3389/fpls.2017.02249
Bourion, V., Martin, C., de Larambergue, H., Jacquin, F., Aubert, G., Martin-Magniette, M.-L., et al. (2014). Unexpectedly low nitrogen acquisition and absence of root architecture adaptation to NO3- supply in a medicago truncatula highly branched root mutant. J. Exp. Bot. 65, 2365–2380. doi: 10.1093/jxb/eru124
Bowsher, C. G., Boulton, E. L., Rose, J., Nayagam, S., Emes, M. J. (1992). Reductant for glutamate synthase in generated by the oxidative pentose phosphate pathway in non-photosynthetic root plastids. Plant J. 2, 893–898. doi: 10.1111/j.1365-313X.1992.00893.x
Bowsher, C. G., Hucklesby, D. P., Emes, M. J. (1989). Nitrite reduction and carbohydrate metabolism in plastids purified from roots of pisum sativum l. Planta 177, 359–366. doi: 10.1007/BF00403594
Brown, S. M., Oparka, K. J., Sprent, J. I., Walsh, K. B. (1995). Symplastic transport in soybean root nodules. Soil Biol. Biochem. 27, 387–399. doi: 10.1016/0038-0717(95)98609-R
Bryce, J. H., Day, D. A. (1990). Tricarboxylic acid cycle activity in mitochondria from soybean nodules and cotyledons. J. Exp. Bot. 41, 961–967. doi: 10.1093/jxb/41.8.961
Bussell, J. D., Keech, O., Fenske, R., Smith, S. M. (2013). Requirement for the plastidial oxidative pentose phosphate pathway for NO3- assimilation in arabidopsis. Plant J. 75, 578–591. doi: 10.1111/tpj.12222
Caetano-Anollés, G., Gresshoff, P. M. (1991). Plant genetic control of nodulation. Annu. Rev. Microbiol. 45, 345–382. doi: 10.1146/annurev.mi.45.100191.002021
Caetano-Anollés, G., Joshi, P. A., Gresshoff, P. M. (1991). Spontaneous nodules induce feedback suppression of nodulation in alfalfa. Planta 183, 77–82. doi: 10.1007/BF00197570
Caetano-Anollés, G., Lagares, A., Bauer, W. D. (1990). Rhizobium meliloti exopolysaccharide mutants elicit feedback regulation of nodule formation in alfalfa. Plant Physiol. 92, 368–374. doi: 10.1104/pp.92.2.368
Carroll, B. J., McNeil, D. L., Gresshoff, P. M. (1985). A supernodulation and NO3–Tolerant symbiotic (nts) soybean mutant. Plant Physiol. 78, 34–40. doi: 10.1104/pp.78.1.34
Castaings, L., Camargo, A., Pocholle, D., Gaudon, V., Texier, Y., Boutet-Mercey, S., et al. (2009). The nodule inception-like protein 7 modulates NO3- sensing and metabolism in arabidopsis. Plant J. 57, 426–435. doi: 10.1111/j.1365-313X.2008.03695.x
Chapman, K., Ivanovici, A., Taleski, M., Sturrock, C. J., Ng, J. L. P., Mohd-Radzman, N. A., et al. (2020). CEP receptor signalling controls root system architecture in arabidopsis and medicago. New Phytol. 226, 1809–1821. doi: 10.1111/nph.16483
Chaput, V., Martin, A., Lejay, L. (2020). Redox metabolism: the hidden player in carbon and nitrogen signaling? J. Exp. Bot. 71, 3816–3826. doi: 10.1093/jxb/eraa078
Chaulagain, D., Frugoli, J. (2021). The regulation of nodule number in legumes is a balance of three signal transduction pathways. Int. J. Mol. Sci. 22, 1117. doi: 10.3390/ijms22031117
Chen, X., Yao, Q., Gao, X., Jiang, C., Harberd, N. P., Fu, X. (2016). Shoot-to-Root mobile transcription factor HY5 coordinates plant carbon and nitrogen acquisition. Curr. Biol. 26, 640–646. doi: 10.1016/j.cub.2015.12.066
Cordoba, E., Shishkova, S., Vance, C. P., Hernández, G. (2003). Antisense inhibition of NADH glutamate synthase impairs carbon/nitrogen assimilation in nodules of alfalfa (Medicago sativa l.). Plant J. 33, 1037–1049. doi: 10.1046/j.1365-313X.2003.01686.x
Crook, A. D., Schnabel, E. L., Frugoli, J. A. (2016). The systemic nodule number regulation kinase SUNN in medicago truncatula interacts with MtCLV2 and MtCRN. Plant J. 88, 108–119. doi: 10.1111/tpj.13234
Crozat, Y., Aveline, A., Coste, F., Gillet, J. P., Domenach, A. M. (1994). Yield performance and seed production pattern of field-grown pea and soybean in relation to n nutrition. Eur. J. Agron. 3, 135–144. doi: 10.1016/S1161-0301(14)80119-6
Dakora, F. D., Atkins, C. A. (1990). Morphological and structural adaptation of nodules of cowpea to functioning under sub- and supra-ambient oxygen pressure. Planta 182, 572–582. doi:10.1007
D’Apuzzo, E., Valkov, V. T., Parlati, A., Omrane, S., Barbulova, A., Sainz, M. M., et al. (2015). PII overexpression in lotus japonicus affects nodule activity in permissive low-nitrogen conditions and increases nodule numbers in high nitrogen treated plants. MPMI 28, 432–442. doi: 10.1094/MPMI-09-14-0285-R
Daubech, B., Remigi, P., Doin de Moura, G., Marchetti, M., Pouzet, C., Auriac, M.-C., et al. (2017). Spatio-temporal control of mutualism in legumes helps spread symbiotic nitrogen fixation. Elife 6, e28683. doi: 10.7554/eLife.28683
Day, D. A., Copeland, L. (1991). Carbon metabolism and compartmentation in nitrogen-fixing legume nodules. Plant Physiol. Biochem. 29, 185–201.
Day, D. A., Mannix, M. (1988). Malate oxidation by soybean nodule mitochondria and the possible consequences for nitrogen fixation. Plant Physiol. Biochem. 26, 567–573.
Delay, C., Chapman, K., Taleski, M., Wang, Y., Tyagi, S., Xiong, Y., et al. (2019). CEP3 levels affect starvation-related growth responses of the primary root. J. Exp. Bot. 70, 4763–4774. doi: 10.1093/jxb/erz270
Denison, R. F. (1992). Mathematical modeling of oxygen diffusion and respiration in legume root nodules. Plant Physiol. 98, 901–907. doi: 10.1104/pp.98.3.901
Denison, R. F., Layzell, D. B. (1991). Measurement of legume nodule respiration and O2 permeability by noninvasive spectrophotometry of leghemoglobin. Plant Physiol. 96, 137–143. doi: 10.1104/pp.96.1.137
diCenzo, G. C., Checcucci, A., Bazzicalupo, M., Mengoni, A., Viti, C., Dziewit, L., et al. (2016). Metabolic modelling reveals the specialization of secondary replicons for niche adaptation in sinorhizobium meliloti. Nat. Commun. 7, 12219. doi: 10.1038/ncomms12219
Durand, J. L., Sheehy, J. E., Minchin, F. R. (1987). Nitrogenase activity, photosynthesis and nodule water potential in soyabean plants experiencing water deprivation. J. Exp. Bot. 38, 311–321. doi: 10.1093/jxb/38.2.311
Dusenge, M. E., Duarte, A. G., Way, D. A. (2019). Plant carbon metabolism and climate change: elevated CO2 and temperature impacts on photosynthesis, photorespiration and respiration. New Phytologist 221, 32–49. doi: 10.1111/nph.15283
Eveland, A. L., Jackson, D. P. (2012). Sugars, signalling, and plant development. J. Exp. Bot. 63, 3367–3377. doi: 10.1093/jxb/err379
Fedorova, M., Tikhonovich, I. A., Vance, C. P. (1999). Expression of c-assimilating enzymes in pea (Pisum sativum l.) root nodules. In situ localization in effective nodules. Plant Cell Environ. 22, 1249–1262. doi: 10.1046/j.1365-3040.1999.00490.x
Ferguson, B., Lin, M.-H., Gresshoff, P. M. (2013). Regulation of legume nodulation by acidic growth conditions. Plant Signaling Behav. 8, e23426. doi: 10.4161/psb.23426
Ferguson, B. J., Mens, C., Hastwell, A. H., Zhang, M., Su, H., Jones, C. H., et al. (2019). Legume nodulation: The host controls the party. Plant Cell Environ. 42, 41–51. doi: 10.1111/pce.13348
Forde, B. G. (2002). Local and long-range signaling pathways regulating plant responses to NO3-. Annu. Rev. Plant Biol. 53, 203–224. doi: 10.1146/annurev.arplant.53.100301.135256
Fukudome, M., Calvo-Begueria, L., Kado, T., Osuki, K., Rubio, M. C., Murakami, E., et al. (2016). Hemoglobin LjGlb1-1 is involved in nodulation and regulates the level of nitric oxide in the lotus japonicus–mesorhizobium loti symbiosis. J. Exp. Bot. 67, 5275–5283. doi: 10.1093/jxb/erw290
Fukudome, M., Watanabe, E., Osuki, K.-I., Imaizumi, R., Aoki, T., Becana, M., et al. (2019). Stably transformed lotus japonicus plants overexpressing phytoglobin LjGlb1-1 show decreased nitric oxide levels in roots and nodules as well as delayed nodule senescence. Plant Cell Physiol. 60, 816–825. doi: 10.1093/pcp/pcy245
Gálvez, L., González, E. M., Arrese-Igor, C. (2005). Evidence for carbon flux shortage and strong carbon/nitrogen interactions in pea nodules at early stages of water stress. J. Exp. Bot. 56, 2551–2561. doi: 10.1093/jxb/eri249
Gan, Y., Stulen, I., Van Keulen, H., Kuiper, P. J. C. (2002). Physiological changes in soybean (Glycine max) Wuyin9 in response to n and p nutrition. Ann. Appl. Biol. 140, 319–329. doi: 10.1111/j.1744-7348.2002.tb00188.x
Gangappa, S. N., Botto, J. F. (2016). The multifaceted roles of HY5 in plant growth and development. Mol. Plant 9, 1353–1365. doi: 10.1016/j.molp.2016.07.002
Gansel, X., Muños, S., Tillard, P., Gojon, A. (2001). Differential regulation of the NO3- and NH4+ transporter genes AtNrt2.1 and AtAmt1.1 in arabidopsis: Relation with long-distance and local controls by n status of the plant. Plant J. 26, 143–155. doi: 10.1046/j.1365-313x.2001.01016.x
Gaudioso-Pedraza, R., Beck, M., Frances, L., Kirk, P., Ripodas, C., Niebel, A., et al. (2018). Callose-regulated symplastic communication coordinates symbiotic root nodule development. Curr. Biol. 28, 3562–3577.e6. doi: 10.1016/j.cub.2018.09.031
Gautrat, P., Laffont, C., Frugier, F. (2020). Compact root architecture 2 promotes root competence for nodulation through the miR2111 systemic effector. Curr. Biol. 30, 1339–1345.e3. doi: 10.1016/j.cub.2020.01.084
Gautrat, P., Laffont, C., Frugier, F., Ruffel, S. (2021). Nitrogen systemic signaling: From symbiotic nodulation to root acquisition. Trends Plant Sci. 26, 392–406. doi: 10.1016/j.tplants.2020.11.009
Gautrat, P., Mortier, V., Laffont, C., De Keyser, A., Fromentin, J., Frugier, F., et al. (2019). Unraveling new molecular players involved in the autoregulation of nodulation in medicago truncatula. J. Exp. Bot. 70, 1407–1417. doi: 10.1093/jxb/ery465
Gil-Quintana, E., Larrainzar, E., Arrese-Igor, C., González, E. M. (2013a). Is n-feedback involved in the inhibition of nitrogen fixation in drought-stressed medicago truncatula? J. Exp. Bot. 64, 281–292. doi: 10.1093/jxb/ers334
Gil-Quintana, E., Larrainzar, E., Seminario, A., Díaz-Leal, J. L., Alamillo, J. M., Pineda, M., et al. (2013b). Local inhibition of nitrogen fixation and nodule metabolism in drought-stressed soybean. J. Exp. Bot. 64, 2171–2182. doi: 10.1093/jxb/ert074
Girin, T., El-Kafafi, E.-S., Widiez, T., Erban, A., Hubberten, H.-M., Kopka, J., et al. (2010). Identification of arabidopsis mutants impaired in the systemic regulation of root NO3- uptake by the nitrogen status of the plant. Plant Physiol. 153, 1250–1260. doi: 10.1104/pp.110.157354
Girin, T., Lejay, L., Wirth, J., Widiez, T., Palenchar, P. M., Nazoa, P., et al. (2007). Identification of a 150 bp cis-acting element of the AtNRT2.1 promoter involved in the regulation of gene expression by the n and c status of the plant. Plant Cell Environ. 30, 1366–1380. doi: 10.1111/j.1365-3040.2007.01712.x
Gojon, A., Cassan, O., Bach, L., Lejay, L., Martin, A. (2022). The decline of plant mineral nutrition under rising CO2: physiological and molecular aspects of a bad deal. Trends Plant Sci. 28, 185–198. doi: 10.1016/j.tplants.2022.09.002
Gojon, A., Nacry, P., Davidian, J.-C. (2009). Root uptake regulation: A central process for NPS homeostasis in plants. Curr. Opin. Plant Biol. 12, 328–338. doi: 10.1016/j.pbi.2009.04.015
González, E. M., Aparicio-Tejo, P. M., Gordon, A. J., Minchin, F. R., Royuela, M., Arrese-Igor, C. (1998). Water-deficit effects on carbon and nitrogen metabolism of pea nodules. J. Exp. Bot. 49, 1705–1714. doi: 10.1093/jxb/49.327.1705
González, E. M., Gordon, A. J., James, C. L., Arrese-lgor, C. (1995). The role of sucrose synthase in the response of soybean nodules to drought. J. Exp. Bot. 46, 1515–1523. doi: 10.1093/jxb/46.10.1515
Gordon, A. J., Minchin, F. R., James, C. L., Komina, O. (1999). Sucrose synthase in legume nodules is essential for nitrogen fixation. Plant Physiol. 120, 867–878. doi: 10.1104/pp.120.3.867
Gordon, A. J., Ryle, G. J. A., Mitchell, D. F., Powell, D. C. E. (1985). The flux of 14C-labelled photosynthate through soyabean root nodules during N2 fixation. J. Exp. Bot. 36, 756–769. doi: 10.1093/jxb/36.5.756
Granot, D., David-Schwartz, R., Kelly, G. (2013). Hexose kinases and their role in sugar-sensing and plant development. Front. Plant Sci. 4. doi: 10.3389/fpls.2013.00044
Grigston, J. C., Osuna, D., Scheible, W.-R., Liu, C., Stitt, M., Jones, A. M. (2008). D-glucose sensing by a plasma membrane regulator of G signaling protein, AtRGS1. FEBS Lett. 582, 3577–3584. doi: 10.1016/j.febslet.2008.08.038
Grillo, M. A., Stinchcombe, J. R., Heath, K. D. (2016). Nitrogen addition does not influence pre-infection partner choice in the legume-rhizobium symbiosis. Am. J. Bot. 103, 1763–1770. doi: 10.3732/ajb.1600090
Guan, P., Ripoll, J.-J., Wang, R., Vuong, L., Bailey-Steinitz, L. J., Ye, D., et al. (2017). Interacting TCP and NLP transcription factors control plant responses to NO3- availability. Proc. Natl. Acad. Sci. 114, 2419–2424. doi: 10.1073/pnas.1615676114
Guo, H., Sun, Y., Li, Y., Liu, X., Ren, Q., Zhu-Salzman, K., et al. (2013). Elevated CO2 modifies n acquisition of medicago truncatula by enhancing n fixation and reducing NO3- uptake from soil. PloS One 8, e81373. doi: 10.1371/journal.pone.0081373
Hirsch, A. M. (1992). Developmental biology of legume nodulation. New Phytol. 122, 211–237. doi: 10.1111/j.1469-8137.1992.tb04227.x
Ho, C.-H., Lin, S.-H., Hu, H.-C., Tsay, Y.-F. (2009). CHL1 functions as a NO3- sensor in plants. Cell 138, 1184–1194. doi: 10.1016/j.cell.2009.07.004
Hohnjec, N., Perlick, A. M., Pühler, A., Küster, H. (2003). The medicago truncatula sucrose synthase gene MtSucS1 is activated both in the infected region of root nodules and in the cortex of roots colonized by arbuscular mycorrhizal fungi. MPMI 16, 903–915. doi: 10.1094/MPMI.2003.16.10.903
Horchani, F., Prévot, M., Boscari, A., Evangelisti, E., Meilhoc, E., Bruand, C., et al. (2011). Both plant and bacterial NO3- reductases contribute to nitric oxide production in medicago truncatula nitrogen-fixing nodules. Plant Physiol. 155, 1023–1036. doi: 10.1104/pp.110.166140
Huault, E., Laffont, C., Wen, J., Mysore, K. S., Ratet, P., Duc, G., et al. (2014). Local and systemic regulation of plant root system architecture and symbiotic nodulation by a receptor-like kinase. PLoS Genet. 10, e1004891. doi: 10.1371/journal.pgen.1004891
Hunt, S., Layzell, D. B. (1993). Gas exchange of legume nodules and the regulation of nitrogenase activity. Annu. Rev. Plant Physiol. Plant Mol. Biol. 44, 483–511. doi: 10.1146/annurev.pp.44.060193.002411
Igamberdiev, A. U., Bykova, N. V., Hill, R. D. (2006). Nitric oxide scavenging by barley hemoglobin is facilitated by a monodehydroascorbate reductase-mediated ascorbate reduction of methemoglobin. Planta 223, 1033–1040. doi: 10.1007/s00425-005-0146-3
Imsande, J. (1986). Inhibition of nodule development in soybean by NO3- or reduced nitrogen. J. Exp. Bot. 37, 348–355. doi: 10.1093/jxb/37.3.348
Imsande, J., Touraine, B. (1994). N demand and the regulation of NO3- uptake. Plant Physiol. 105, 3–7. doi: 10.1104/pp.105.1.3
James, E. K., Sprent, J. I., Minchin, F. R., Brewin, N. J. (1991). Intercellular location of glycoprotein in soybean nodules: effect of altered rhizosphere oxygen concentration. Plant Cell Environ. 14, 467–476. doi: 10.1111/j.1365-3040.1991.tb01516.x
Jang, J. C., León, P., Zhou, L., Sheen, J. (1997). Hexokinase as a sugar sensor in higher plants. Plant Cell 9, 5–19. doi: 10.1105/tpc.9.1.5
Jeudy, C., Ruffel, S., Freixes, S., Tillard, P., Santoni, A. L., Morel, S., et al. (2010). Adaptation of medicago truncatula to nitrogen limitation is modulated via local and systemic nodule developmental responses. New Phytol. 185, 817–828. doi: 10.1111/j.1469-8137.2009.03103.x
Juhnke, H., Krems, B., Kötter, P., Entian, K.-D. (1996). Mutants that show increased sensitivity to hydrogen peroxide reveal an important role for the pentose phosphate pathway in protection of yeast against oxidative stress. Molec. Gen. Genet. 252, 456–464. doi: 10.1007/BF02173011
Kassaw, T., Bridges, W., Frugoli, J. (2015). Multiple autoregulation of nodulation (AON) signals identified through split-root analysis of medicago truncatula sunn and rdn1 mutants. Plants (Basel) 4, 209–224. doi: 10.3390/plants4020209
Kiers, E. T., Rousseau, R. A., West, S. A., Denison, R. F. (2003). Host sanctions and the legume-rhizobium mutualism. Nature 425, 78–81. doi: 10.1038/nature01931
Kosslak, R. M., Bohlool, B. B. (1984). Suppression of nodule development of one side of a split-root system of soybeans caused by prior inoculation of the other side. Plant Physiol. 75, 125–130. doi: 10.1104/pp.75.1.125
Kosslak, R. M., Bohlool, B. B., Dowdle, S., Sadowsky, M. J. (1983). Competition of rhizobium japonicum strains in early stages of soybean nodulation. Appl. Environ. Microbiol. 46, 870–873. doi: 10.1128/aem.46.4.870-873.1983
Kouchi, H., Fukai, K., Katagiri, H., Minamisawa, K., Tajima, S. (1988). Isolation and enzymological characterization of infected and uninfected cell protoplasts from root nodules of glycine max. Physiologia Plantarum 73, 327–334. doi: 10.1111/j.1399-3054.1988.tb00606.x
Krouk, G., Lacombe, B., Bielach, A., Perrine-Walker, F., Malinska, K., Mounier, E., et al. (2010). NO3–regulated auxin transport by NRT1.1 defines a mechanism for nutrient sensing in plants. Dev. Cell 18, 927–937. doi: 10.1016/j.devcel.2010.05.008
Krusell, L., Madsen, L. H., Sato, S., Aubert, G., Genua, A., Szczyglowski, K., et al. (2002). Shoot control of root development and nodulation is mediated by a receptor-like kinase. Nature 420, 422–426. doi: 10.1038/nature01207
Krusell, L., Sato, N., Fukuhara, I., Koch, B. E. V., Grossmann, C., Okamoto, S., et al. (2011). The Clavata2 genes of pea and lotus japonicus affect autoregulation of nodulation. Plant J. 65, 861–871. doi: 10.1111/j.1365-313X.2010.04474.x
Kryvoruchko, I. S., Sinharoy, S., Torres-Jerez, I., Sosso, D., Pislariu, C. I., Guan, D., et al. (2016). MtSWEET11, a nodule-specific sucrose transporter of medicago truncatula1[OPEN]. Plant Physiol. 171, 554–565. doi: 10.1104/pp.15.01910
Laffont, C., Huault, E., Gautrat, P., Endre, G., Kalo, P., Bourion, V., et al. (2019). Independent regulation of symbiotic nodulation by the SUNN negative and CRA2 positive systemic pathways. Plant Physiol. 180, 559–570. doi: 10.1104/pp.18.01588
Laffont, C., Ivanovici, A., Gautrat, P., Brault, M., Djordjevic, M. A., Frugier, F., et al. (2020). The NIN transcription factor coordinates CEP and CLE signaling peptides that regulate nodulation antagonistically. Nat. Commun. 11, 3167. doi: 10.1038/s41467-020-16968-1
Laguerre, G., Heulin-Gotty, K., Brunel, B., Klonowska, A., Le Quéré, A., Tillard, P., et al. (2012). Local and systemic n signaling are involved in medicago truncatula preference for the most efficient sinorhizobium symbiotic partners. New Phytol. 195, 437–449. doi: 10.1111/j.1469-8137.2012.04159.x
Lam, S. K., Chen, D., Norton, R., Armstrong, R., Mosier, A. R. (2012). Nitrogen dynamics in grain crop and legume pasture systems under elevated atmospheric carbon dioxide concentration: A meta-analysis. Global Change Biol. 18, 2853–2859. doi: 10.1111/j.1365-2486.2012.02758.x
Lambert, I., Pervent, M., Le Queré, A., Clément, G., Tauzin, M., Severac, D., et al. (2020)a. Responses of mature symbiotic nodules to the whole-plant systemic nitrogen signaling. J. Exp. Bot. 71, 5039–5052. doi: 10.1093/jxb/eraa221
Larrainzar, E., Wienkoop, S., Scherling, C., Kempa, S., Ladrera, R., Arrese-Igor, C., et al. (2009). Carbon metabolism and bacteroid functioning are involved in the regulation of nitrogen fixation in medicago truncatula under drought and recovery. MPMI 22, 1565–1576. doi: 10.1094/MPMI-22-12-1565
Lebedeva, M., Azarakhsh, M., Yashenkova, Y., Lutova, L. (2020). NO3–induced CLE peptide systemically inhibits nodulation in medicago truncatula. Plants (Basel) 9, E1456. doi: 10.3390/plants9111456
Lejay, L., Gansel, X., Cerezo, M., Tillard, P., Müller, C., Krapp, A., et al. (2003). Regulation of root ion transporters by photosynthesis: functional importance and relation with hexokinase. Plant Cell 15, 2218–2232. doi: 10.1105/tpc.013516
Lejay, L., Wirth, J., Pervent, M., Cross, J. M.-F., Tillard, P., Gojon, A. (2008). Oxidative pentose phosphate pathway-dependent sugar sensing as a mechanism for regulation of root ion transporters by photosynthesis. Plant Physiol. 146, 2036–2053. doi: 10.1104/pp.107.114710
Li, Y., Yu, Z., Liu, X., Mathesius, U., Wang, G., Tang, C., et al. (2017). Elevated CO2 increases nitrogen fixation at the reproductive phase contributing to various yield responses of soybean cultivars. Front. Plant Sci. 8. doi: 10.3389/fpls.2017.01546
Lin, J., Li, X., Luo, Z., Mysore, K. S., Wen, J., Xie, F. (2018). NIN interacts with NLPs to mediate NO3- inhibition of nodulation in medicago truncatula. Nat. Plants 4, 942–952. doi: 10.1038/s41477-018-0261-3
Lindström, K., Mousavi, S. A. (2020). Effectiveness of nitrogen fixation in rhizobia. Microb. Biotechnol. 13, 1314–1335. doi: 10.1111/1751-7915.13517
Liu, A., Contador, C. A., Fan, K., Lam, H.-M. (2018). Interaction and regulation of carbon, nitrogen, and phosphorus metabolisms in root nodules of legumes. Front. Plant Sci. 9. doi: 10.3389/fpls.2018.01860
Liu, K.-H., Liu, M., Lin, Z., Wang, Z.-F., Chen, B., Liu, C., et al. (2022). NIN-like protein 7 transcription factor is a plant NO3- sensor. Science 377, 1419–1425. doi: 10.1126/science.add1104
Liu, K., Niu, Y., Konishi, M., Wu, Y., Du, H., Sun Chung, H., et al. (2017). Discovery of NO3–CPK-NLP signalling in central nutrient-growth networks. Nature 545, 311–316. doi: 10.1038/nature22077
Liu, Y., Wu, L., Baddeley, J. A., Watson, C. A. (2011). Models of biological nitrogen fixation of legumes. a review. Agron. Sust. Developm. 31, 155–172. doi: 10.1051/agro/2010008
Lodwig, E. M., Leonard, M., Marroqui, S., Wheeler, T. R., Findlay, K., Downie, J. A., et al. (2005). Role of polyhydroxybutyrate and glycogen as carbon storage compounds in pea and bean bacteroids. MPMI 18, 67–74. doi: 10.1094/MPMI-18-0067
Lunn, J. E., Delorge, I., Figueroa, C. M., Van Dijck, P., Stitt, M. (2014). Trehalose metabolism in plants. Plant J. 79, 544–567. doi: 10.1111/tpj.12509
Maghiaoui, A., Gojon, A., Bach, L. (2020). NRT1.1-centered NO3- signaling in plants. J. Exp. Bot. 71, 6226–6237. doi: 10.1093/jxb/eraa361
Magori, S., Oka-Kira, E., Shibata, S., Umehara, Y., Kouchi, H., Hase, Y., et al. (2009). Too much love, a root regulator associated with the long-distance control of nodulation in lotus japonicus. Mol. Plant Microbe Interact. 22, 259–268. doi: 10.1094/MPMI-22-3-0259
Marchive, C., Roudier, F., Castaings, L., Bréhaut, V., Blondet, E., Colot, V., et al. (2013). Nuclear retention of the transcription factor NLP7 orchestrates the early response to NO3- in plants. Nat. Commun. 4, 1713. doi: 10.1038/ncomms2650
Marco, D. E., Carbajal, J. P., Cannas, S., Pérez-Arnedo, R., Hidalgo-Perea, A., Olivares, J., et al. (2009). An experimental and modelling exploration of the host-sanction hypothesis in legume-rhizobia mutualism. J. Theor. Biol. 259, 423–433. doi: 10.1016/j.jtbi.2009.03.033
Marino, D., Andrio, E., Danchin, E. G. J., Oger, E., Gucciardo, S., Lambert, A., et al. (2011). A medicago truncatula NADPH oxidase is involved in symbiotic nodule functioning. New Phytol. 189, 580–592. doi: 10.1111/j.1469-8137.2010.03509.x
Marino, D., Damiani, I., Gucciardo, S., Mijangos, I., Pauly, N., Puppo, A. (2013). Inhibition of nitrogen fixation in symbiotic medicago truncatula upon cd exposure is a local process involving leghaemoglobin. J. Exp. Bot. 64, 5651–5660. doi: 10.1093/jxb/ert334
Mathews, A., Carroll, B. J., Gresshoff, P. M. (1989). Development ofBradyrhizobium infections in supernodulating and non-nodulating mutants of soybean (Glycine max [L.] Merrill). Protoplasma 150, 40–47. doi: 10.1007/BF01352919
Mens, C., Hastwell, A. H., Su, H., Gresshoff, P. M., Mathesius, U., Ferguson, B. J. (2020). Characterisation of medicago truncatula CLE34 and CLE35 in NO3- and rhizobia regulation of nodulation. New Phytol 229, 2525–2534. doi: 10.1111/nph.17010
Minchin, F. R., Witty, J. F. (2005). “Respiratory/Carbon costs of symbiotic nitrogen fixation in legumes,” in Plant respiration: From cell to ecosystem advances in photosynthesis and respiration. Eds. Lambers, H., Ribas-Carbo, M. (Dordrecht: Springer Netherlands), 195–205. doi: 10.1007/1-4020-3589-6_11
Miyazawa, H., Oka-Kira, E., Sato, N., Takahashi, H., Wu, G.-J., Sato, S., et al. (2010). The receptor-like kinase KLAVIER mediates systemic regulation of nodulation and non-symbiotic shoot development in lotus japonicus. Development 137, 4317–4325. doi: 10.1242/dev.058891
Moreau, D., Burstin, J., Aubert, G., Huguet, T., Ben, C., Prosperi, J.-M., et al. (2012). Using a physiological framework for improving the detection of quantitative trait loci related to nitrogen nutrition in medicago truncatula. Theor. Appl. Genet. 124, 755–768. doi: 10.1007/s00122-011-1744-z
Moreau, C., Gautrat, P., Frugier, F. (2021). NO3–induced CLE35 signaling peptides inhibit nodulation through the SUNN receptor and miR2111 repression. Plant Physiol. 185, 1216–1228. doi: 10.1093/plphys/kiaa094
Moreau, D., Voisin, A.-S., Salon, C., Munier-Jolain, N. (2008). The model symbiotic association between medicago truncatula cv. jemalong and rhizobium meliloti strain 2011 leads to n-stressed plants when symbiotic N2 fixation is the main n source for plant growth. J. Exp. Bot. 59, 3509–3522. doi: 10.1093/jxb/ern203
Mortier, V., Den Herder, G., Whitford, R., Van de Velde, W., Rombauts, S., D’Haeseleer, K., et al. (2010). CLE peptides control medicago truncatula nodulation locally and systemically. Plant Physiol. 153, 222–237. doi: 10.1104/pp.110.153718
Muller, B., Touraine, B. (1992). Inhibition of NO⊟3 uptake by various phloem-translocated amino acids in soybean seedlings. J. Exp. Bot. 43, 617–623. doi: 10.1093/jxb/43.5.617
Naya, L., Ladrera, R., Ramos, J., González, E. M., Arrese-Igor, C., Minchin, F. R., et al. (2007). The response of carbon metabolism and antioxidant defenses of alfalfa nodules to drought stress and to the subsequent recovery of plants. Plant Physiol. 144, 1104–1114. doi: 10.1104/pp.107.099648
Neo, H. H., Layzell, D. B. (1997). Phloem glutamine and the regulation of O2 diffusion in legume nodules. Plant Physiol. 113, 259–267. doi: 10.1104/pp.113.1.259
Neuhaus, H. E., Emes, M. J. (2000). Nonphotosynthetic metabolism in plastids. Annu. Rev. Plant Physiol. Plant Mol. Biol. 51, 111–140. doi: 10.1146/annurev.arplant.51.1.111
Nishida, H., Handa, Y., Tanaka, S., Suzaki, T., Kawaguchi, M. (2016). Expression of the CLE-RS3 gene suppresses root nodulation in lotus japonicus. J. Plant Res. 129, 909–919. doi: 10.1007/s10265-016-0842-z
Nishida, H., Ito, M., Miura, K., Kawaguchi, M., Suzaki, T. (2020). Autoregulation of nodulation pathway is dispensable for NO3–induced control of rhizobial infection. Plant Signal Behav. 15, 1733814. doi: 10.1080/15592324.2020.1733814
Nishida, H., Tanaka, S., Handa, Y., Ito, M., Sakamoto, Y., Matsunaga, S., et al. (2018). A NIN-LIKE PROTEIN mediates NO3–induced control of root nodule symbiosis in lotus japonicus. Nat. Commun. 9, 499. doi: 10.1038/s41467-018-02831-x
Nishimura, R., Hayashi, M., Wu, G.-J., Kouchi, H., Imaizumi-Anraku, H., Murakami, Y., et al. (2002). HAR1 mediates systemic regulation of symbiotic organ development. Nature 420, 426–429. doi: 10.1038/nature01231
Noctor, G., Foyer, C. H. (1998). ASCORBATE AND GLUTATHIONE: Keeping active oxygen under control. Annu. Rev. Plant Physiol. Plant Mol. Biol. 49, 249–279. doi: 10.1146/annurev.arplant.49.1.249
Ohkubo, Y., Tanaka, M., Tabata, R., Ogawa-Ohnishi, M., Matsubayashi, Y. (2017). Shoot-to-root mobile polypeptides involved in systemic regulation of nitrogen acquisition. Nat. Plants 3, 17029. doi: 10.1038/nplants.2017.29
Oji, Y., Watanabe, M., Wakiuchi, N., Okamoto, S. (1985). Nitrite reduction in barley-root plastids: Dependence on NADPH coupled with glucose-6-phosphate and 6-phosphogluconate dehydrogenases, and possible involvement of an electron carrier and a diaphorase. Planta 165, 85–90. doi: 10.1007/BF00392215
Okamoto, S., Ohnishi, E., Sato, S., Takahashi, H., Nakazono, M., Tabata, S., et al. (2009). Nod factor/NO3–induced CLE genes that drive HAR1-mediated systemic regulation of nodulation. Plant Cell Physiol. 50, 67–77. doi: 10.1093/pcp/pcn194
Oldroyd, G. E. D., Downie, J. A. (2008). Coordinating nodule morphogenesis with rhizobial infection in legumes. Annu. Rev. Plant Biol. 59, 519–546. doi: 10.1146/annurev.arplant.59.032607.092839
Oldroyd, G. E. D., Murray, J. D., Poole, P. S., Downie, J. A. (2011). The rules of engagement in the legume-rhizobial symbiosis. Annu. Rev. Genet. 45, 119–144. doi: 10.1146/annurev-genet-110410-132549
Olsson, J. E., Nakao, P., Bohlool, B. B., Gresshoff, P. M. (1989). Lack of systemic suppression of nodulation in split-root systems of supernodulating soybean (Glycine max [L.] merr.) mutants. Plant Physiol. 90, 1347–1352. doi: 10.1104/pp.90.4.1347
Oono, R., Anderson, C. G., Denison, R. F. (2011). Failure to fix nitrogen by non-reproductive symbiotic rhizobia triggers host sanctions that reduce fitness of their reproductive clonemates. Proc. Biol. Sci. 278, 2698–2703. doi: 10.1098/rspb.2010.2193
Oono, R., Denison, R. F. (2010). Comparing symbiotic efficiency between swollen versus nonswollen rhizobial bacteroids. Plant Physiol. 154, 1541–1548. doi: 10.1104/pp.110.163436
Oono, R., Denison, R. F., Kiers, E. T. (2009). Controlling the reproductive fate of rhizobia: how universal are legume sanctions? New Phytol. 183, 967–979. doi: 10.1111/j.1469-8137.2009.02941.x
Parsons, R., Stanforth, A., Raven, J. A., Sprent, J. I. (1993). Nodule growth and activity may be regulated by a feedback mechanism involving phloem nitrogen. Plant Cell Environ. 16, 125–136. doi: 10.1111/j.1365-3040.1993.tb00854.x
Parvin, S., Uddin, S., Tausz-Posch, S., Armstrong, R., Tausz, M. (2020). Carbon sink strength of nodules but not other organs modulates photosynthesis of faba bean (Vicia faba) grown under elevated [CO2] and different water supply. New Phytol. 227, 132–145. doi: 10.1111/nph.16520
Patriarca, E. J., Tatè, R., Iaccarino, M. (2002). Key role of bacterial NH4+ metabolism in rhizobium-plant symbiosis. Microbiol. Mol. Biol. Rev. 66, 203–222. doi: 10.1128/MMBR.66.2.203-222.2002
Pauly, N., Pucciariello, C., Mandon, K., Innocenti, G., Jamet, A., Baudouin, E., et al. (2006). Reactive oxygen and nitrogen species and glutathione: Key players in the legume-rhizobium symbiosis. J. Exp. Bot. 57, 1769–1776. doi: 10.1093/jxb/erj184
Penmetsa, R. V., Frugoli, J. A., Smith, L. S., Long, S. R., Cook, D. R. (2003). Dual genetic pathways controlling nodule number in medicago truncatula. Plant Physiol. 131, 998–1008. doi: 10.1104/pp.015677
Pérez Guerra, J. C., Coussens, G., De Keyser, A., De Rycke, R., De Bodt, S., Van De Velde, W., et al. (2010). Comparison of developmental and stress-induced nodule senescence in medicago truncatula. Plant Physiol. 152, 1574–1584. doi: 10.1104/pp.109.151399
Pervent, M., Lambert, I., Tauzin, M., Karouani, A., Nigg, M., Jardinaud, M.-F., et al. (2021). Systemic control of nodule formation by plant nitrogen demand requires autoregulation-dependent and independent mechanisms. J. Exp. Bot. 72, 7942–7956. doi: 10.1093/jxb/erab374
Pessi, G., Ahrens, C. H., Rehrauer, H., Lindemann, A., Hauser, F., Fischer, H.-M., et al. (2007). Genome-wide transcript analysis of bradyrhizobium japonicum bacteroids in soybean root nodules. MPMI 20, 1353–1363. doi: 10.1094/MPMI-20-11-1353
Prayitno, J., Rolfe, B. G., Mathesius, U. (2006). The ethylene-insensitive sickle mutant of medicago truncatula shows altered auxin transport regulation during nodulation. Plant Physiol. 142, 168–180. doi: 10.1104/pp.106.080093
Prell, J., White, J. P., Bourdes, A., Bunnewell, S., Bongaerts, R. J., Poole, P. S. (2009). Legumes regulate rhizobium bacteroid development and persistence by the supply of branched-chain amino acids. Proc. Natl. Acad. Sci. U.S.A. 106, 12477–12482. doi: 10.1073/pnas.0903653106
Puppo, A., Pauly, N., Boscari, A., Mandon, K., Brouquisse, R. (2013). Hydrogen peroxide and nitric oxide: key regulators of the legume-rhizobium and mycorrhizal symbioses. Antioxid Redox Signal 18, 2202–2219. doi: 10.1089/ars.2012.5136
Ralser, M., Wamelink, M. M., Kowald, A., Gerisch, B., Heeren, G., Struys, E. A., et al. (2007). Dynamic rerouting of the carbohydrate flux is key to counteracting oxidative stress. J. Biol. 6, 10. doi: 10.1186/jbiol61
Reid, D. E., Ferguson, B. J., Gresshoff, P. M. (2011a). Inoculation- and NO3–induced CLE peptides of soybean control NARK-dependent nodule formation. Mol. Plant Microbe Interact. 24, 606–618. doi: 10.1094/MPMI-09-10-0207
Reid, D. E., Ferguson, B. J., Hayashi, S., Lin, Y.-H., Gresshoff, P. M. (2011b). Molecular mechanisms controlling legume autoregulation of nodulation. Ann. Bot. 108, 789–795. doi: 10.1093/aob/mcr205
Riveras, E., Alvarez, J. M., Vidal, E. A., Oses, C., Vega, A., Gutiérrez, R. A. (2015). The calcium ion is a second messenger in the NO3- signaling pathway of arabidopsis. Plant Physiol. 169, 1397–1404. doi: 10.1104/pp.15.00961
Robertson, J. G., Wells, B., Bisseling, T., Farnden, K. J. F., Johnston, A. W. B. (1984). Immuno-gold localization of leghaemoglobin in cytoplasm in nitrogen-fixing root nodules of pea. Nature 311, 254–256. doi: 10.1038/311254a0
Rogers, A., Gibon, Y., Stitt, M., Morgan, P. B., Bernacchi, C. J., Ort, D. R., et al. (2006). Increased c availability at elevated carbon dioxide concentration improves n assimilation in a legume. Plant Cell Environ. 29, 1651–1658. doi: 10.1111/j.1365-3040.2006.01549.x
Rogers, A., Ainsworth, E. A., Leakey, A. D. B. (2009). Will elevated carbon dioxide concentration amplify the benefits of nitrogen fixation in legumes? Plant Physiol. 151, 1009–1016. doi: 10.1104/pp.109.144113
Rolland, F., Baena-Gonzalez, E., Sheen, J. (2006). Sugar sensing and signaling in plants: conserved and novel mechanisms. Annu. Rev. Plant Biol. 57, 675–709. doi: 10.1146/annurev.arplant.57.032905.105441
Ronson, C. W., Astwood, P. M., Downie, J. A. (1984). Molecular cloning and genetic organization of C4-dicarboxylate transport genes from rhizobium leguminosarum. J. Bacteriol 160, 903–909. doi: 10.1128/jb.160.3.903-909.1984
Rosendahl, L., Vance, C. P., Pedersen, W. B. (1990). Products of dark CO2 fixation in pea root nodules support bacteroid metabolism 1. Plant Physiol. 93, 12–19. doi: 10.1104/pp.93.1.12
Roy, S., Liu, W., Nandety, R. S., Crook, A., Mysore, K. S., Pislariu, C. I., et al. (2020). Celebrating 20 years of genetic discoveries in legume nodulation and symbiotic nitrogen fixation. Plant Cell 32, 15–41. doi: 10.1105/tpc.19.00279
Roy, S., Müller, L. M. (2022). A rulebook for peptide control of legume-microbe endosymbioses. Trends Plant Sci. 27, 870–889. doi: 10.1016/j.tplants.2022.02.002
Ruffel, S., Freixes, S., Balzergue, S., Tillard, P., Jeudy, C., Martin-Magniette, M. L., et al. (2008). Systemic signaling of the plant nitrogen status triggers specific transcriptome responses depending on the nitrogen source in medicago truncatula. Plant Physiol. 146, 2020–2035. doi: 10.1104/pp.107.115667
Ruffel, S., Gojon, A., Lejay, L. (2014). Signal interactions in the regulation of root NO3- uptake. J. Exp. Bot. 65, 5509–5517. doi: 10.1093/jxb/eru321
Sagan, M., Morandi, D., Tarenghi, E., Duc, G. (1995). Selection of nodulation and mycorrhizal mutants in the model plant medicago truncatula (Gaertn.) after γ-ray mutagenesis. Plant Sci. 111, 63–71. doi: 10.1016/0168-9452(95)04229-N
Salon, C., Lepetit, M., Gamas, P., Jeudy, C., Moreau, S., Moreau, D., et al. (2009). Analysis and modeling of the integrative response of medicago truncatula to nitrogen constraints. C. R. Biol. 332, 1022–1033. doi: 10.1016/j.crvi.2009.09.009
Sánchez, C., Gates, A. J., Meakin, G. E., Uchiumi, T., Girard, L., Richardson, D. J., et al. (2010). Production of nitric oxide and nitrosylleghemoglobin complexes in soybean nodules in response to flooding. MPMI 23, 702–711. doi: 10.1094/MPMI-23-5-0702
Sanz-Sáez, Á., Erice, G., Aranjuelo, I., Nogués, S., Irigoyen, J. J., Sánchez-Díaz, M. (2010). Photosynthetic down-regulation under elevated CO2 exposure can be prevented by nitrogen supply in nodulated alfalfa. J. Plant Physiol. 167, 1558–1565. doi: 10.1016/j.jplph.2010.06.015
Sasaki, T., Suzaki, T., Soyano, T., Kojima, M., Sakakibara, H., Kawaguchi, M. (2014). Shoot-derived cytokinins systemically regulate root nodulation. Nat. Commun. 5, 4983. doi: 10.1038/ncomms5983
Schnabel, E., Journet, E.-P., de Carvalho-Niebel, F., Duc, G., Frugoli, J. (2005). The medicago truncatula SUNN gene encodes a CLV1-likeLeucine-rich repeat receptor kinase that regulates nodule number and root length. Plant Mol. Biol. 58, 809–822. doi: 10.1007/s11103-005-8102-y
Schuize, J., Adgo, E., Merbach, W. (1999). Carbon costs associated with N2 fixation in vicia faba l and pisum sativum 1. over a 14-day period. Plant Biol. 1, 625–631. doi: 10.1111/j.1438-8677.1999.tb00273.x
Schulte, C. C. M., Borah, K., Wheatley, R. M., Terpolilli, J. J., Saalbach, G., Crang, N., et al. (2021). Metabolic control of nitrogen fixation in rhizobium-legume symbioses. Sci. Adv. 7, eabh2433. doi: 10.1126/sciadv.abh2433
Schulze, J. (2004). How are nitrogen fixation rates regulated in legumes? J. Plant Nutr. Soil Sci. 167, 125–137. doi: 10.1002/jpln.200320358
Schwember, A. R., Schulze, J., del Pozo, A., Cabeza, R. A. (2019). Regulation of symbiotic nitrogen fixation in legume root nodules. Plants 8, 333. doi: 10.3390/plants8090333
Seabra, A. R., Pereira, P. A., Becker, J. D., Carvalho, H. G. (2012). Inhibition of glutamine synthetase by phosphinothricin leads to transcriptome reprograming in root nodules of medicago truncatula. Mol. Plant Microbe Interact. 25, 976–992. doi: 10.1094/MPMI-12-11-0322
Searle, I. R., Men, A. E., Laniya, T. S., Buzas, D. M., Iturbe-Ormaetxe, I., Carroll, B. J., et al. (2003). Long-distance signaling in nodulation directed by a CLAVATA1-like receptor kinase. Science 299, 109–112. doi: 10.1126/science.1077937
Singleton, P. W., van Kessel, C. (1987). Effect of localized nitrogen availability to soybean half-root systems on photosynthate partitioning to roots and nodules. Plant Physiol. 83, 552–556. doi: 10.1104/pp.83.3.552
Smith, P. M. C., Winter, H., Storer, P. J., Bussell, J. D., Schuller, K. A., Atkins, C. A. (2002). Effect of short-term N2 deficiency on expression of the ureide pathway in cowpea root nodules. Plant Physiol. 129, 1216–1221. doi: 10.1104/pp.010714
Soupène, E., Foussard, M., Boistard, P., Truchet, G., Batut, J. (1995). Oxygen as a key developmental regulator of rhizobium meliloti N2-fixation gene expression within the alfalfa root nodule. Proc. Natl. Acad. Sci. U.S.A. 92, 3759–3763. doi: 10.1073/pnas.92.9.3759
Sprent, J. I. (2009). “Development and functioning of nodules,” in Legume nodulation (John Wiley & Sons, Ltd), 79–95. doi: 10.1002/9781444316384.ch5
Stitt, M., Krapp, A. (1999). The interaction between elevated carbon dioxide and nitrogen nutrition: the physiological and molecular background. Plant Cell Environ. 22, 583–621. doi: 10.1046/j.1365-3040.1999.00386.x
Streeter, J., Wong, P. P. (1988). Inhibition of legume nodule formation and N2 fixation by NO3-. Crit. Rev. Plant Sci. 7, 1–23. doi: 10.1080/07352688809382257
Sugiyama, A., Saida, Y., Yoshimizu, M., Takanashi, K., Sosso, D., Frommer, W. B., et al. (2017). Molecular characterization of LjSWEET3, a sugar transporter in nodules of lotus japonicus. Plant Cell Physiol. 58, 298–306. doi: 10.1093/pcp/pcw190
Tabata, R., Sumida, K., Yoshii, T., Ohyama, K., Shinohara, H., Matsubayashi, Y. (2014). Perception of root-derived peptides by shoot LRR-RKs mediates systemic n-demand signaling. Science 346, 343–346. doi: 10.1126/science.1257800
Takahara, M., Magori, S., Soyano, T., Okamoto, S., Yoshida, C., Yano, K., et al. (2013). Too much love, a novel kelch repeat-containing f-box protein, functions in the long-distance regulation of the legume-rhizobium symbiosis. Plant Cell Physiol. 54, 433–447. doi: 10.1093/pcp/pct022
Takanashi, K., Sasaki, T., Kan, T., Saida, Y., Sugiyama, A., Yamamoto, Y., et al. (2016). A dicarboxylate transporter, LjALMT4, mainly expressed in nodules of lotus japonicus. MPMI 29, 584–592. doi: 10.1094/MPMI-04-16-0071-R
Takanashi, K., Takahashi, H., Sakurai, N., Sugiyama, A., Suzuki, H., Shibata, D., et al. (2012). Tissue-specific transcriptome analysis in nodules of lotus japonicus. MPMI 25, 869–876. doi: 10.1094/MPMI-01-12-0011-R
Terpolilli, J. J., Masakapalli, S. K., Karunakaran, R., Webb, I. U. C., Green, R., Watmough, N. J., et al. (2016). Lipogenesis and redox balance in nitrogen-fixing pea bacteroids. J. Bacteriol 198, 2864–2875. doi: 10.1128/JB.00451-16
Thumfort, P. P., Atkins, C. A., Layzell, D. B. (1994). A re-evaluation of the role of the infected cell in the control of O2 diffusion inlegume nodules. Plant Physiol. 105, 1321–1333. doi: 10.1104/pp.105.4.1321
Thumfort, P. P., Layzell, D. B., Atkins, C. A. (1999). Diffusion and reaction of oxygen in the central tissue ofureide-producing legume nodules. Plant Cell Environ. 22, 1351–1363. doi: 10.1046/j.1365-3040.1999.00498.x
Thumfort, P. P., Layzell, D. B., Atkins, C. A. (2000). A simplified approach for modeling diffusion into cells. J. Theor. Biol. 204, 47–65. doi: 10.1006/jtbi.2000.1071
Tillard, P., Passama, L., Gojon, A. (1998). Are phloem amino acids involved in the shoot to root control of NO-3 uptake in ricinus communis plants? J. Exp. Bot. 49, 1371–1379. doi: 10.1093/jxb/49.325.1371
Timmers, A. C., Soupène, E., Auriac, M. C., de Billy, F., Vasse, J., Boistard, P., et al. (2000). Saprophytic intracellular rhizobia in alfalfa nodules. Mol. Plant Microbe Interact. 13, 1204–1213. doi: 10.1094/MPMI.2000.13.11.1204
Tjepkema, J. D., Yocum, C. S. (1974). Measurement of oxygen partial pressure within soybean nodules by oxygen microelectrodes. Planta 119, 351–360. doi: 10.1007/BF00388335
Tsikou, D., Yan, Z., Holt, D. B., Abel, N. B., Reid, D. E., Madsen, L. H., et al. (2018). Systemic control of legume susceptibility to rhizobial infection by a mobile microRNA. Science 362, 233–236. doi: 10.1126/science.aat6907
Udvardi, M., Poole, P. S. (2013). Transport and metabolism in legume-rhizobia symbioses. Annu. Rev. Plant Biol. 64, 781–805. doi: 10.1146/annurev-arplant-050312-120235
Urano, D., Phan, N., Jones, J. C., Yang, J., Huang, J., Grigston, J., et al. (2012). Endocytosis of the seven-transmembrane RGS1 protein activates G-protein-coupled signalling in arabidopsis. Nat. Cell Biol. 14, 1079–1088. doi: 10.1038/ncb2568
Valkov, V. T., Rogato, A., Alves, L. M., Sol, S., Noguero, M., Léran, S., et al. (2017). The nitrate transporter family protein LjNPF8.6 controls the n-fixing nodule activity. Plant Physiol 175, 1269–1282. doi: 10.1104/pp.17.01187
Valkov, V. T., Sol, S., Rogato, A., Chiurazzi, M. (2020). The functional characterization of LjNRT2.4 indicates a novel, positive role of nitrate for an efficient nodule N2 -fixation activity. New Phytol. 228, 682–696. doi: 10.1111/nph.16728
Vance, C. P., Heichel, G. H. (1991). Carbon in N2 fixation: Limitation or exquisite adaptation. Annu. Rev. Plant Physiol. Plant Mol. Biol. 42, 373–390. doi: 10.1146/annurev.pp.42.060191.002105
Vance, C. P. (2008). Carbon and Nitrogen Metabolism in Legume Nodules. in Nitrogen-fixing Leguminous Symbioses Nitrogen Fixation: Origins, Applications, and Research Progress. Dilworth, M. J., James, E. K., Sprent, J. I., Newton, W. E. (Dordrecht: Springer Netherlands), 293–320. doi: 10.1007/978-1-4020-3548-7_10
van Noorden, G. E., Ross, J. J., Reid, J. B., Rolfe, B. G., Mathesius, U. (2006). Defective long-distance auxin transport regulation in the medicago truncatula super numeric nodules mutant. Plant Physiol. 140, 1494–1506. doi: 10.1104/pp.105.075879
Vaughn, M. W., Harrington, G. N., Bush, D. R. (2002). Sucrose-mediated transcriptional regulation of sucrose symporter activity in the phloem. Proc. Natl. Acad. Sci. 99, 10876–10880. doi: 10.1073/pnas.172198599
Verhoeven, J. T. A., Koerselman, W., Meuleman, A. F. M. (1996). Nitrogen- or phosphorus-limited growth in herbaceous, wet vegetation: Relations with atmospheric inputs and management regimes. Trends Ecol. Evol. 11, 494–497. doi: 10.1016/S0169-5347(96)10055-0
Vidal, E. A., Alvarez, J. M., Araus, V., Riveras, E., Brooks, M. D., Krouk, G., et al. (2020). Nitrate in 2020: Thirty years from transport to signaling networks. Plant Cell 32, 2094–2119. doi: 10.1105/tpc.19.00748
Voisin, A. S., Salon, C., Jeudy, C., Warembourg, F. R. (2003). Symbiotic N2 fixation activity in relation to c economy of pisum sativum l. as a function of plant phenology. J. Exp. Bot. 54, 2733–2744. doi: 10.1093/jxb/erg290
Walsh, K. B., Vessey, J. K., Layzell, D. B. (1987). Carbohydrate supply and N2 fixation in soybean 1. Plant Physiol. 85, 137–144. doi: 10.1104/pp.85.1.137
Wang, T., Guo, J., Peng, Y., Lyu, X., Liu, B., Sun, S., et al. (2021). Light-induced mobile factors from shoots regulate rhizobium-triggered soybean root nodulation. Science 374, 65–71. doi: 10.1126/science.abh2890
Wang, R., Tischner, R., Gutiérrez, R. A., Hoffman, M., Xing, X., Chen, M., et al. (2004). Genomic analysis of the nitrate response using a nitrate reductase-null mutant of arabidopsis. Plant Physiol. 136, 2512–2522. doi: 10.1104/pp.104.044610
Widiez, T., El Kafafi, E. S., Girin, T., Berr, A., Ruffel, S., Krouk, G., et al. (2011). High nitrogen insensitive 9 (HNI9)-mediated systemic repression of root NO3- uptake is associated with changes in histone methylation. Proc. Natl. Acad. Sci. U.S.A. 108, 13329–13334. doi: 10.1073/pnas.1017863108
Wopereis, J., Pajuelo, E., Dazzo, F. B., Jiang, Q., Gresshoff, P. M., De Bruijn, F. J., et al. (2000). Short root mutant of lotus japonicus with a dramatically altered symbiotic phenotype. Plant J. 23, 97–114. doi: 10.1046/j.1365-313x.2000.00799.x
Xiao, W., Sheen, J., Jang, J. C.. (2000). The role of hexokinase in plant sugar signal transduction and growth and development. Plant Mol. Biol. 44, 451–61. doi: 10.1023/a:1026501430422
Yamaguchi, Y. L., Ishida, T., Sawa, S. (2016). CLE peptides and their signaling pathways in plant development. J. Exp. Bot. 67, 4813–4826. doi: 10.1093/jxb/erw208
Yamashita, N., Tanabata, S., Ohtake, N., Sueyoshi, K., Sato, T., Higuchi, K., et al. (2019). Effects of different chemical forms of nitrogen on the quick and reversible inhibition of soybean nodule growth and nitrogen fixation activity. Front. Plant Sci. 10. doi: 10.3389/fpls.2019.00131
Yurgel, S. N., Kahn, M. L. (2004). Dicarboxylate transport by rhizobia. FEMS Microbiol. Rev. 28, 489–501. doi: 10.1016/j.femsre.2004.04.002
Yurgel, S. N., Rice, J., Kahn, M. L. (2012). Nitrogen metabolism in sinorhizobium meliloti–alfalfa symbiosis: Dissecting the role of GlnD and PII proteins. MPMI 25, 355–362. doi: 10.1094/MPMI-09-11-0249
Zhang, H., Jennings, A., Barlow, P. W., Forde, B. G. (1999). Dual pathways for regulation of root branching by nitrate. Proc. Natl. Acad. Sci. 96, 6529–6534. doi: 10.1073/pnas.96.11.6529
Keywords: rhizobium, legumes, symbiosis, nitrogen, photosynthesis, carbon, systemic signaling, plant nutrition
Citation: Lepetit M and Brouquisse R (2023) Control of the rhizobium–legume symbiosis by the plant nitrogen demand is tightly integrated at the whole plant level and requires inter-organ systemic signaling. Front. Plant Sci. 14:1114840. doi: 10.3389/fpls.2023.1114840
Received: 02 December 2022; Accepted: 16 February 2023;
Published: 09 March 2023.
Edited by:
Anis M. Limami, Université d’Angers, FranceReviewed by:
Maurizio Chiurazzi, National Research Council (CNR), ItalyCesar Arrese-Igor, Public University of Navarre, Spain
Copyright © 2023 Lepetit and Brouquisse. This is an open-access article distributed under the terms of the Creative Commons Attribution License (CC BY). The use, distribution or reproduction in other forums is permitted, provided the original author(s) and the copyright owner(s) are credited and that the original publication in this journal is cited, in accordance with accepted academic practice. No use, distribution or reproduction is permitted which does not comply with these terms.
*Correspondence: Marc Lepetit, marc.lepetit@inrae.fr