- 1Department of Microbial, Cellular and Molecular Biology, Addis Ababa University, Addis Ababa, Ethiopia
- 2Department of Biology, Bule Hora University, Bule Hora, Ethiopia
- 3Department of Chemistry, Addis Ababa University, Addis Ababa, Ethiopia
- 4Ethiopian Institute of Agricultural Research, Jimma Agricultural Research Center, Jimma, Ethiopia
- 5Department of Plant Breeding, Swedish University of Agricultural Sciences, Alnarp, Sweden
Coffee wilt disease (CWD) is a serious threat to the food security of small-scale farmers in Ethiopia, causing significant reductions in coffee yield. Currently, there are no effective control measures available against the causative agent of CWD, Fusarium xylarioides. The main objective of this study was therefore to develop, formulate, and evaluate a range of biofungicides against F. xylarioides, derived from Trichoderma species and tested under in vitro, greenhouse, and field conditions. In total, 175 Trichoderma isolates were screened as microbial biocontrol agents against F. xylarioides. The efficacy of two biofungicide formulations, wettable powder and water dispensable granules, were tested on the susceptible Geisha coffee variety in three different agro-ecological zones in southwestern Ethiopia over three years. The greenhouse experiments were set up using a complete block design, while in the field a randomized complete block design was used, with twice yearly applications of biofungicide. The test pathogen spore suspension was applied to the coffee seedlings by soil drenching, and the subsequent incidence and severity of CWD evaluated annually. The mycelial growth inhibition profiles of the Trichoderma isolates against F. xylarioides ranged from 44.5% to 84.8%. In vitro experiments revealed that T. asperelloides AU71, T. asperellum AU131 and T. longibrachiatum AU158 reduced the mycelial growth of F. xylarioides by over 80%. The greenhouse study indicated that wettable powder (WP) of T. asperellum AU131 had the highest biocontrol efficacy (84.3%), followed by T. longibrachiatum AU158 (77.9%) and T. asperelloides AU71 (71.2%); they also had a significant positive impact on plant growth. The pathogen-treated control plants had a disease severity index of 100% across all the field experiments, and of 76.7% in the greenhouse experiments. In comparison to untreated controls, the annual and cumulative disease incidence over the three years of the study period varied from 46.2 to 90%, 51.6 to 84.5%, and 58.2 to 91%, at the Teppi, Gera and Jimma field experimental locations. Overall, the greenhouse and field experiments and in vitro assays support the biocontrol potential of Trichoderma isolates, and T. asperellum AU131 and T. longibrachiatum AU158 in particular are recommended for the management of CWD under field conditions.
1 Introduction
East Africa is one of the most suitable regions for coffee production and export across the globe (International Coffee Organization (ICO), 2016). The center of origin and natural diversity of Coffea arabica L., the world’s most significant commercial coffee species, is in the Afromontane rainforests of southwestern Ethiopia, and Ethiopia has conducive temperature, rainfall, soil type, and pH levels at appropriate altitudes for successful coffee production (Alemu and Sokar, 2000; Woldetsadik and Kebede, 2000; Vega et al., 2009; Muleta et al., 2013; Nsabimana and Tirkaso, 2020). Coffee has played a key role for centuries in the Ethiopian economy, and represents the main cash crop cultivated by small-scale farmers, with social, economic, political and ecological benefits (Adugna et al., 2010; Jefuka et al., 2010; Getachew et al., 2012; Tefera et al., 2012). More than 25 million Ethiopians are engaged in the production, processing, and marketing of coffee crops, from which they derive a direct or indirect source of income (ICO, 2016). Overall, Ethiopia is Africa’s main exporter of coffee, but the sector is facing the combined threats of genetic erosion, market price fluctuations, climate change, displacement of coffee with other cash crops, and production constraints such as emerging fungal and bacterial diseases (Adugna et al., 2010; Avelino et al., 2011; Tsegaye, 2018).
One of the most economically significant diseases endangering Ethiopia’s Arabica coffee industry is coffee wilt disease (CWD), caused by the fungus Fusarium xylarioides (Adugna et al., 2010; Alemu, 2012; Getachew et al., 2012). Fusarium xylarioides is presumed to be a heterothallic ascomycete, with a natural sexual or teleomorphic state that produces fertile perithecia in dead coffee plants (Adugna et al., 2005). While the prevalence of CWD varies depending on the type of coffee production system (forest, semi-forest, garden, or plantation), Ethiopia’s overall 30–40% decline in coffee yield as a result of CWD presents a huge challenge for smallholder coffee producers. Coffee wilt disease is also threatening the global environmental benefit of Ethiopia’s coffee trees, which sequester over 200 million tons of carbon (Moat et al., 2017).
Current gaps in the development of management schedules for CWD include the lack of resistant coffee varieties, a lack of efficient chemical controls, a shortage of virulent biocontrol agents that may work at the field level, and an unclear mode of action. The ability of F. xylarioides to survive in the absence of coffee plants has substantial implications for managing the disease through chemical and cultural controls (Girma et al., 2009; Belachew et al., 2017a; Krishnan, 2017). The Ethiopian coffee industry is also being severely and negatively affected by climate change. According to Moat et al. (2017), depending on the emissions scenario, the bioclimatically suitable area for growing Arabica coffee could decrease 39–59% by the end of the 21st century. The rate of coffee plant death as a result of rapidly rising temperatures is alarming, particularly in the context of bacterial and fungal diseases (Moat et al., 2017). Additionally, the physiological and genetic variability of the pathogen is reducing the durability of resistance in coffee plants (Belete et al., 2014).
Ethiopia’s recent increase in CDW infections highlights the need for long-term integrated disease management (IDM) strategies. The evolution of fungicide-resistant strains of plant diseases, climate change and the growing global concern over chemical residues in the food chain have increased interest in developing biological solutions that are both effective and practical from both an economic and ecological standpoint. To date there are no effective control measures available for the management of CWD, but an eco-friendly and efficient biological solution is urgently required because of the destructiveness of the disease, the lack of resistant coffee cultivars, and the significance of coffee to the national economy.
Trichoderma species are already widely used to prevent plant pathogens and boost plant immunity in greenhouse and field conditions. However, while the potential of Trichoderma species for plant pathogen control is well recognized, this approach has been largely unexploited for many diseases of tropical perennial crops including coffee plants. The biocontrol potential of Trichoderma species is vast because of their beneficial interactions with plants via the production of cell wall-degrading enzymes (CWDEs), secondary metabolites, competition for nutrients and space, and antimicrobial compounds that are active against a wide range of plant pathogens, including Fusarium species (Saravanakumar et al., 2016b; Thambugala et al., 2020). Trichoderma species are found in Ethiopian coffee rhizosphere soils due to the diverse ecological features and climatic conditions of the country’s coffee-growing zones. Our previous study (Mulatu et al., 2022a) revealed that large Trichoderma species existed in native forest, semi-forest and garden coffee production systems, where CWD frequently found. Hence, using native and pathogen-specific microbial biocontrol agents (MBCAs) has the benefit of preventing a particular disease and improving the positive interaction between rhizosphere microbiome and plant immunity (Rao et al., 2016; Yu and Hochholdinger, 2018; Reghmit et al., 2021). However, there are only limited scientific studies on protecting coffee trees against F. xylarioides by using MBCAs belonging to the genus Trichoderma, under either greenhouse or field conditions. Thus, the application of a connventional biological control technique comprising the collection and release of possible Trichoderma species from the coffee arabica center of origin was examined in order to reduce the incidence and severity of CWD.
The development of new biocontrol agents to combat plant pathogens necessitates the screening of a large number of potential antagonists before formulating the agent in order to maximize its shelf life, facilitate delivery, and improve bioactivity (Montesinos, 2003; Kashyap et al., 2022; Manzar et al., 2022a; Mulatu et al., 2022a). A crucial element in the production of microbial biopesticides is the development of cutting-edge technologies for maximizing both quantity and quality. Trichoderma based formulation is considered as a stable and standardized combination of inert and active ingredients that produces a straightforward, secure, and highly effective solution to use against the target disease. Numerous formulation types, such as dusts, granules, pellets, wettable powders, capsules or beads, water-dispersible granules, or emulsifiable liquids, have been reported. Wettable powder (WP) and water dispensable granules (WDG) are two of these that are frequently used agricultural input formulations with good safety record. By assessing the effect of ingredients and processes on the physical characteristics, biological activity, storage stability, and field efficacy of selected biocontrol agents, formulation-based solutions to significant problems related to MBCA stability, efficacy, and toxicity have been addressed (Mulatu et al., 2021; Mulatu et al., 2022a; Mulatu et al., 2022b). Early screening processes have already evaluated coffee plants as a target crop, the CWD pathogen and the epidemiological stage of the disease, and the antagonistic efficacy of the proposed Trichoderma isolates, resulting in a pool of potential biocontrol candidates (Alemu and Kapoor, 2004; Tiru et al., 2013; Mulatu et al., 2022a; Manzar et al., 2022b).
To prove the value of biocontrol as a “best practice” for coffee farmers, the economic viability of optimal biocontrol systems was assessed. The study’s findings will significantly advance scientific understanding and provide useful information for making decisions. The planned research is helpful for reducing the effects of CWD and improving coffee production, which in turn improves Ethiopia’s ability to feed its people. The effective use and exploitation of these research findings will boost coffee crop yield as well as the nation’s foreign revenues. The findings can be applied to the development of decision-supporting tools to guarantee Ethiopia’s sustainable coffee producing system. It will contribute to safeguard the future of coffee production in Ethiopia and other East African countries, sustain a crucial source of income for resource-strapped farming communities, and stabilize the national economies by strengthening the ability of people battling the CWD. Therefore, the main objective of this study was to develop, formulate, and evaluate biofungicides against F. xylarioides from the pool of Trichoderma isolates, under in vitro, greenhouse, and field conditions. The efficacy of two biofungicide formulations, WDG and WP, were tested on the susceptible Geisha coffee variety in three different agro-ecological zones in southwestern Ethiopia over a period of three years.
2 Materials and methods
2.1 Biocontrol screening of Trichoderma isolates
2.1.1 Dual culture assay
A total of 175 Trichoderma isolates, previously identified from major coffee-growing regions of Ethiopia (Mulatu et al., 2022a), was evaluated against F. xylarioides following the method of Dennis and Webster (1971). Briefly, mycelial discs (5 mm in diameter) from 7-day-old growing edges of Trichoderma and F. xylarioides were placed on opposite sides of a potato dextrose agar (PDA) Petri dish (6 cm away from each other). Three replications of culture plates were used; control plates had no Trichoderma discs. The plates were incubated at 25°C with a 12-h photoperiod for 10 days. Isolate growth was monitored every 48 h, to determine the percentage inhibition (PI) of the pathogen by each Trichoderma isolate, using Szekeres et al.’s (2005) formula:
where R1 denotes the control pathogen’s radius, and R2 denotes the antagonistic pathogen’s radius. Following Matarese et al. (2012), a standard regression growth curve was created by measuring the radius of the F. xylarioides colony in each plate as well as the effectiveness of the Trichoderma isolates in inhibiting the mycelial growth of F. xylarioides (Dennis and Webster, 1971). Using the criteria established by Soytong (1988), the antagonistic activity of Trichoderma isolates was classified as follows: >75% extremely high antagonistic activity; 61–75% high antagonistic activity; 51–60% moderate antagonistic activity; 50% low antagonistic activity. To evaluate the biocontrol potential of Trichoderma species, F. xylarioides (DSM No. 62457, strain: IMB 11646) was used as a test pathogen.
2.1.2 Agar diffusion assay
Based on the in vitro antagonistic assay, 10 Trichoderma isolates, with a PI > 75%, were used for secondary metabolite extraction, as recommended by Saravanakumar et al. (2016a): T. viride AU23, T. longibrachiatum AU32, T. koningiopsis AU70, T. asperelloides AU71, T. asperellum AU97, T. harzianum AU105, T. aethiopicum AU106, T. asperellum AU131, T. longibrachiatum AU158 and T. asperellum AU171. In brief, 1 liter of potato dextrose broth (PDB) was injected with 1 × 107 spores/ml Trichoderma isolates, which were then cultivated for 21 days at 28°C. The mycelia were homogenized by addition of ethyl acetate, centrifuged at 5000 rpm for 20 min and filtered to remove the insoluble particles from the solutions. The culture filtrates were concentrated using rotary evaporator at 40°C. The concentrated extracts were then dissolved in methanol so that they could be partially purified in a Sephadex LH-20 column. The chromatographic fractions from the Sephadex column were tested against F. xylarioides using an agar diffusion assay on King B medium (20 g peptone, 1.5 g K2HPO4, 1.5 g MgSO4.7H2O, 10 ml glycerol, and 15 g agar-agar in distilled water at pH 7; Bano and Musarrat, 2004; Hernández-Rodríguez et al., 2008; Dhodary et al., 2018).
Every bioassay was carried out in triplicate and contrasted with similarly made solvent controls. The diameters of the inhibition zones were measured, and the PI determined. The plates were photographed using a Canon digital camera, and “Fiji ImageJ” used to analyze the images (GNU General Public License), so that the following equation could be applied (Khan et al., 2021):
where I denotes the initial hole diameter (5 mm), T denotes the diameter of treatment inhibition zones, and C denotes the diameter of solvent control inhibition zones.
2.1.3 Biocontrol-related enzymes
Based on the same dual culture results (PI >75%), the 10 Trichoderma isolates were tested for hydrolytic enzyme activity. The isolates were grown on 1/10th strength PDB for 48 h in a rotary shaker at 150 revolutions per minute (rpm) and 28°C. To induce enzyme production, basal salts of the following compositions were added: MgSO4.7H2O (0.3 g), (NH4)2SO4 (3 g), KH2PO4 (2 g), agar (15 g). They were augmented with 1% (w/v) colloidal chitin (Rojas-Avelizapa et al., 1999; El-Sobky et al., 2019), 1% (w/v) CMC, 1% (w/v) gelatin, and 2.0% (w/v) laminarin), to detect the enzymes chitinase, cellulase, protease and β-(1–3) glucanase (Nagpure et al., 2014; Putri et al., 2022), respectively. The incubation was continued for 24 h, and the culture filtrate separated by filtration and centrifugation (at 5000 rpm, 4°C, for 10 min) and used as the enzyme source (Maria et al., 2005; Agrawal and Kotasthane, 2012; Saravanakumar et al., 2017). Fusarium xylarioides was then inoculated in the center of Petri dishes and the following equation used to calculate the percentage enzyme activity (after Saravanakumar et al., 2016b):
2.2 Characterization of Trichoderma isolates for their plant growth-promoting attributes
2.2.1 Quantitative analysis of indole-3-acetic acid production
Trichoderma isolates were cultured in a rotary shaker for 4–5 days at 150 rpm and 25°C, in PDB supplemented with L-tryptophan (0.1g/L) (Bader et al., 2020). At the end of the incubation period, mycelia and debris were separated by filtration and centrifugation, respectively. After a 10-min incubation in the dark, Salkowski reagent (2% 0.5 M ferric chloride in 35% chloric acid) was added to 1 ml of the culture filtrate, so that a pink–red color could be seen. After 30 min, the optical density (OD) was assessed at 530 nm using a UV/VIS spectrophotometer (Mwajita, 2017; Kashyap et al., 2021). A calibration curve of standard IAA solutions was used to ascertain the IAA concentration, and an uninoculated PDB was maintained as a control. The experiment was carried out in triplicate, twice for each isolate.
2.2.2 Siderophore production
Siderophore production by the Trichoderma isolates was determined using a modified chrome azurol S (CAS) agar test (Qi and Zhao, 2013). The isolates (5 mm) were inoculated onto CAS media and incubated for 5–7 days at 25°C. The development of a pink, yellow or orange color around or below the fungal colony indicated that the Trichoderma isolates had the ability to produce siderophores (Chen et al., 2019). Measurements were taken in triplicate.
2.3 In planta assay to evaluate bioefficacy against F. xylarioides under greenhouse and field conditions
2.3.1 Experimental sites
From January 2016 to June 2021, experiments were conducted in three different agro-ecological zones of southwestern Ethiopia, at the Teppi, Jimma, and Gera agricultural research centers (Supplementary Figure S1). These locations are within Ethiopia’s main coffee-growing area, and have documented repeated CWD infestations (Belachew et al., 2015; Teferi and Belachew, 2015; Belachew et al., 2017b). Climatic information from January 2018 to December 2021 was provided by the National Meteorology Agency of Ethiopia (Supplementary Table S1). The greenhouse trials were conducted at the Jimma Agricultural Research Center (JARC).
2.3.2 Preparation of F. xylarioides inoculum
Fresh coffee branches (twigs) were harvested from healthy trees and used to support the growth of F. xylarioides (Getachew et al., 2012; Mulatu et al., 2013; Tiru et al., 2013). The twigs were cut into small pieces (10 cm long) and lightly scraped to expose fresh wood. A Petri dish containing the twigs and a tiny roll of thoroughly wet cotton wool was sterilized in an autoclave. Each twig was then inoculated with 3 discs (5 mm) of F. xylarioides and incubated for 7–10 days at 22 ± 2°C (Adugna et al., 2005; Getachew et al., 2012). Twigs exhibiting healthy colony growth were completely rinsed off with sterile water to collect the conidia. The suspension was agitated with a magnetic stirrer and then filtered through two layers of cheese cloth. The spore concentration was adjusted with a hemocytometer (Neubauer chamber, Germany) to 2.3 × 106 conidia/ml and kept at 4°C until seedling inoculation (Adugna, 2004; Getachew et al., 2012).
2.3.3 Development and formulation of biofungicides
Two Trichoderma-based formulations, water dispensable granules (WDG) and wettable powder (WP), were used. To develop and formulate these products, Trichoderma isolates were cultured in PDB for 7–10 days, at 150 rpm and 25°C in an orbital shaker (Optima shaker OS-72, New Delhi, India). The broth was filtered via muslin cloth to eliminate the Trichoderma biomass, and the filtrate used to provide the active ingredients for the formulation of biofungicides (Mulatu et al., 2021; Vimala Devi et al., 2021). Talcum powder was used as the carrier substrate, glycerol as the dispersing agent, carboxymethyl cellulose (CMC) as the wetting agent, and a combination of white rice and wheat bran (1:2 w/w) as the bulking agent. WDG was developed using talcum powder as the carrier substrate and bulking agent, alginic acid as the dispersing agent, and CMC as the wetting agent (Jangir et al., 2021). The chemical reagents were selected according to the Collaborative International Pesticides Analytical Council’s (CIPAC) recommendations.
To formulate the WP, sterile talcum powder (500 g/kg) and dispersing agent (10 g/kg) were mixed with a substrate colonized by a Trichoderma isolate (440 g/kg), dried overnight, and then packed in polypropylene bags, sealed and kept at room temperature for later application in the greenhouse and field experiments (Ramanujam et al., 2010; Sachdev and Singh, 2016). To promote spore adhesion to the talcum powder, the pH of the Trichoderma colonized substrates was first brought to neutral by adding CaCO3 at a rate of 15 g/kg (Irfan et al., 2012).
To develop the WDG, the dispersion agent (10 g/kg), wetting agent (50 g/kg), and bulking agent (440 g/kg) were combined with adsorbed substrate (500 g/kg) (Jangir et al., 2021; Vimala Devi et al., 2021). After adding autoclaved water (15 to 20%), the product was mixed well, and the paste fed manually through an extruder machine using a 0.3-mm mesh screen. After air-drying overnight, the product was broken into granules and used for the greenhouse and field experiments. The spore concentration of the formulated biofungicides (WP and WDG) were adjusted to 109 spores/ml for greenhouse and field applications.
2.3.4 Bioefficacy of biofungicides under greenhouse conditions
Systematic pot culture experiments were carried out at JARC from January 2016 to April 2017. Normal sandy loam soil was taken from the coffee farm at JARC, passed through a 20-mm mesh, and sterilized in an autoclave at 121°C for 1 h. The experiments were performed in earthen plant pots with a capacity of 4 kg sandy loam soil (pH = 5.56, electronic conductivity (EC) 360 dS/m, organic carbon content (OC) 1.82%, and total nitrogen (TN) 0.45%; Table S2). A susceptible Geisha coffee variety was used to test the effectiveness of the variously prepared biofungicides (WDG and WP), and the following isolates were used for the treatments: T. viride AU23, T. longibrachiatum AU32, T. koningiopsis AU70, T. asperelloides AU71, T. asperellum AU97, T. harzianum AU105, T. aethiopicum AU106, T. asperellum AU131, T. longibrachiatum AU158, and T. asperellum AU171. Fusarium xylarioides alone was used as a positive control (PC), and no F. xylarioides or Trichoderma isolate was used as a negative control (NC). Before treatments were applied to the seedlings, the WDG product (5 g) was dissolved in water (20 ml) at room temperature for 24 h to initiate spore germination. Under greenhouse and field settings, control and biofungicides treatments were applied under the same conditions.
The treatments (control and biofungicides) were applied in a growth chamber at an optimum temperature of 22–25°C, and relative humidity (RH) above > 95%, to promote infection. After 10 days, the inoculated seedlings were moved and placed onto greenhouse benches. During the testing period, the greenhouse temperature fluctuated from 15 to 30°C, and the humidity between 65 and 85%. After 4 months, once two to three pairs of true leaves had developed, WDG was applied to the pots the following day by soil drenching at a concentration of 20 ml/seedling (Netsere et al., 2015). WP was applied to the pots by mixing the product (5 g/seedling) with the soil around the seedling rhizospheres. The seedlings were infected 7 days after WDG and WP treatment with 50 ml of the test pathogen’s spore suspension (2.3 × 106 conidia/ml) by soil drenching (10 ml/seedling) (Adugna, 2004; Getachew et al., 2012; Jangir et al., 2021).
Throughout the duration of the experiment, sufficient water was applied to maintain the growth of the seedlings in the greenhouse. The experiments were replicated three times, and the treatments were assigned using a completely randomized design (CRD) (Tiru et al., 2013). To maintain adequate populations of the antagonists in the soils, and so favor biological control as proposed by Brown et al. (2019), WDG and WP were applied twice a year, once at the beginning of the trial (May 2016) and again after six months. In order to determine the effectiveness of WDG and WP in controlling CWD, disease assessment evaluations were monitored periodically after 3 months of treatment application. Morpho-agronomic characteristics were also recorded to evaluate the effect of the treatments on seedling growth.
2.3.5 Bioefficacy of Trichoderma WP under field conditions
Based on the results of the greenhouse experiments, the WP from T. longibrachiatum AU32, T. asperellum AU71, T asperellum AU131, and T. longibrachiatum AU158 was chosen for the field experiments, which ran from January 2018 to September 2021 at Teppi, Jimma, and Gera agricultural research centers. Sick plots that had had a reported 50–100% incidence of CWD in previous years (Adugna et al., 2010; Getachew et al., 2012; Zeru et al., 2012; Belachew et al., 2015; Belachew et al., 2017b) were selected. The soil characteristics were a sandy loam, pH = 5.65, EC = 351.67 dS/m, and OC = 1.25% (Table S2). Four replicates of each treatment were used, in a randomized complete block design (RCBD). Each experimental site contained a total of 240 healthy Geisha coffee seedlings. In the first week of July 2018, six-month-old seedlings were transplanted into the field. Three months later, in the first week of October 2018, WP (10 g/seedling) was mixed into the soil surrounding the rhizosphere of the seedlings. A dual application of WP was made each year, the first in early October and the second in March (October 2018 to September 2021). Seven days after WP treatment, each seedling received 50 ml of pathogen spore suspension (106 spore/ml), using the soil drenching method (Adugna et al., 2010). To keep the soil moist and promote germination of Trichoderma in the field, sufficient water and mulching was applied. Treatments without the test pathogen and bioagents were used as negative controls.
2.3.6 Assessment of CWD under greenhouse and field conditions
In the greenhouse setting, the number of wilted seedlings exhibiting external symptoms was monitored and recorded periodically for one year, while in the field the plants were monitored for three consecutive years. Every two weeks following the commencement of disease, wilt symptoms were evaluated across all the exp erimental set-ups. The degree of vascular discoloration, and the presence and absence of internal symptoms, were examined on samples of seedlings without external symptoms. In order to reisolate the test pathogen, stem tissues from symptomatic and asymptomatic seedlings (n = 5) were plated on synthetic nutrient agar (SNA) media. In order to track the progression of CWD at the level of each pot, plot and seedling, the seedlings’ condition and symptoms were recorded periodically using an arbitrary 5-point scale of disease severity index (DSI), where 1 = no disease/symptoms, 2 = 1–25% curled leaves and stunted growth, 3 = 26–50% yellowing, leaf wilting and defoliation, 4 = 51–75% leaf necrosis, leaf wilting, and abscission, and 5 = 76–100% wilted leaves, defoliation, and total coffee plant death (Musoli et al., 2008; Adugna et al., 2010).
The formulae used by Deketelaere et al. (2017) and Di Marco et al. (2021) were adopted to determine yearly and cumulated disease incidence (DI, %), DSI (%), and biocontrol efficacy (BE, %):
a) annual CWD assessments
b) cumulated CWD assessments
The treatment DI was the incidence of disease in pathogen and biocontrol agent-inoculated seedlings, while the control DI was the incidence of disease in pathogen-only inoculated seedlings (Di Marco et al., 2021).
At the end of the greenhouse and field study experiments, for each year after treatment application, the growth of the coffee plants, using parameters such as plant height (cm) and girth (cm), was recorded and analyzed.
2.4 Statistical analysis
An analysis of variance (ANOVA) was used to evaluate the data from the in vitro bioassays, hydrolytic enzyme activity assays, and greenhouse and field experiments. Tukey’s honestly significant difference (HSD) test was used as a post hoc test, and the parameter values satisfied the requirements for normality and homogeneity of variances. All the ANOVA analyses were carried out using R Statistical Software (v4.1.2; R Core Team, 2021) running in RStudio 1.1, with the help of the packages “agricolae”, “smplot”, “supernova”, “tidyverse” “dplyr”, “reshape2”, “ggpubr”, “rstatix”, and “ggplot2” (https://CRAN.R-project.org). Differences were considered significant at p<0.05.
3 Results
3.1 Biocontrol screening of Trichoderma isolates
In the dual culture confrontation assays, F. xylarioides initially grew quickly, before stopping when it came into contact with the antagonist. All the Trichoderma isolates were able to inhibit the mycelial growth of F. xylarioides around the agar wells at the point of application. There was, however, significant differences between the isolates (p<0.05), with the percentage inhibition ranging from 44.5% to 84.8% (Table S3). Based on bioefficacy, 59 isolates showed moderate antagonistic activity, demonstrating between 51 and 60% inhibition of F. xylarioides, while another 96 isolates demonstrated high antagonistic activity, demonstrating 61–75% inhibition. Ten isolates demonstrated the highest levels of in vitro antagonistic activity against F. xylarioides, with mean inhibition values ranging from 75 to 84.8% (Figure 1). Extracts from T. asperellum AU71, T. asperellum AU131, and T. longibrachiatum AU158 displayed 83.5%, 86.7%, and 88.2% inhibition of F. xylarioides, respectively (Figure 1).
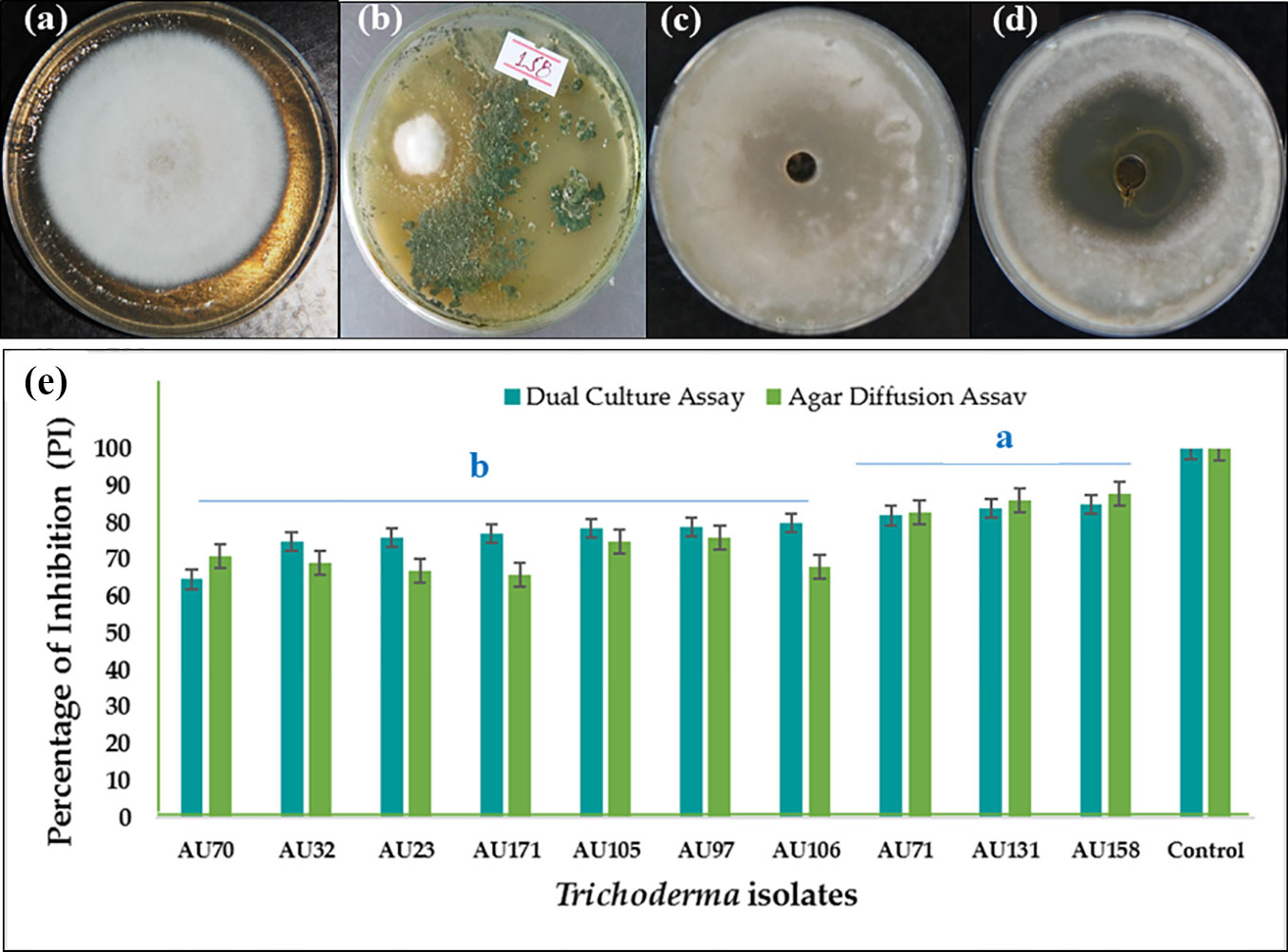
Figure 1 In vitro antagonistic activity of 10 Trichoderma isolates against F. xylarioides in dual culture and agar diffusion assays. (A) F. xylarioides control for dual culture assay, (B) in vitro dual culture assay showing inhibition of F. xylarioides by T. longibrachiatum AU158, (C) F. xylarioides control for agar diffusion assay, (D) agar diffusion assay showing inhibition of F. xylarioides by T. longibrachiatum AU158 and (E) bar graph for both dual culture and agar diffusion assay. Each value is the average of three duplicate samples with standard error, and various alphabets shown in superscript indicate mean treatments that are substantially different according to Tukey’s HSD posthoc test at p ≤ 0.05. Trichoderma isolates: T. viride AU23, T. longibrachiatum AU32, T. koningiopsis AU70, T. asperelloides AU71, T. asperellum AU97, T. harzianum AU105, T. aethiopicum AU106, T. asperellum AU131, T. longibrachiatum AU158 and T. asperellum AU171.
The inhibition of F. xylarioides’ radial growth in the agar diffusion assays was attributed to the production of secondary metabolites by each Trichoderma isolate, and the isolates had an impact on the pathogen’s development rate as early as the first day (Figure 2A). The levels of inhibition were negatively correlated with incubation duration (days), with a correlation coefficient (R2) of 0.013 and a significance level of p<0.001 (Figure 2B).
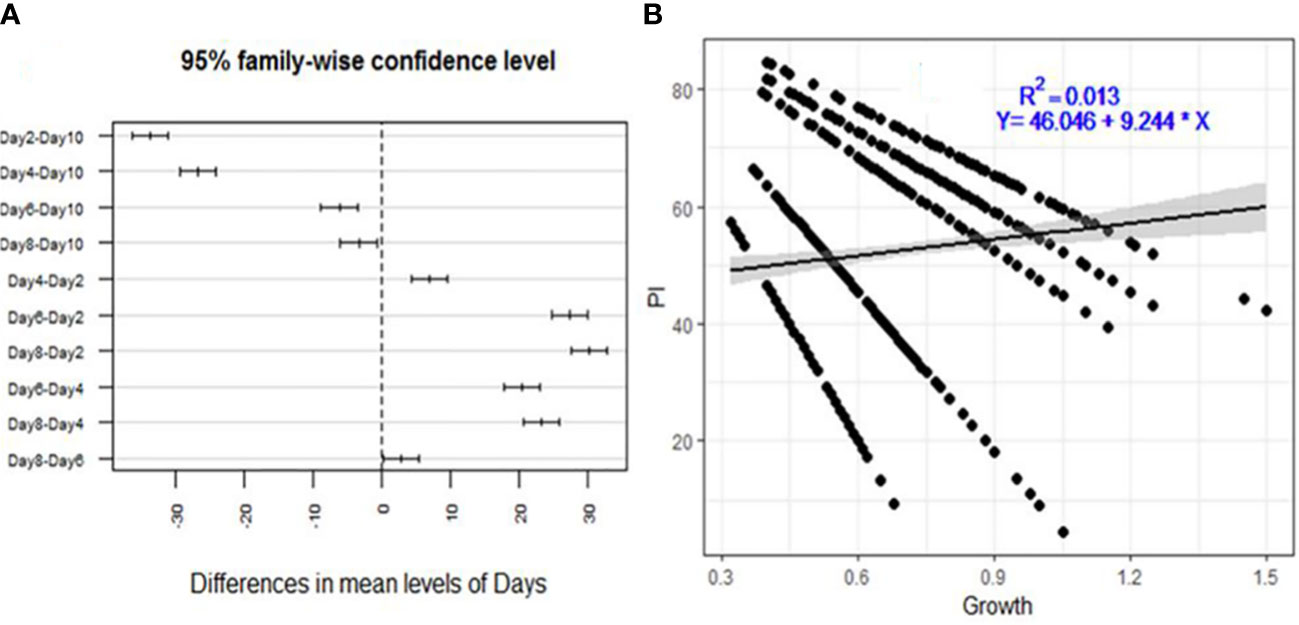
Figure 2 Descriptive analysis of percentage inhibition (PI) for the 175 Trichoderma isolates tested against F. xylarioides. (A) A pair-wise comparison of incubation period (days) and (B) Regression analysis between mycelial growth. PI, Percentage of Inhibition.
3.2 Hydrolytic enzyme activity of Trichoderma isolates
Trichoderma longibrachiatum AU158 had higher enzyme activity levels for chitinase (76.9%), protease (64.9%), β-(1-3) glucanase (51.5%), and cellulase (44.9%) than the other isolates tested. Trichoderma koningiopsis AU70 had a low hydrolytic activity for chitinase (5.5%), while T. aethiopicum AU106 had a low protease activity (23.0%), T. longibrachiatum AU32 had a low cellulase activity (5.3%), and T. harzianum AU105 had a low β-(1-3) glucanase activity (4.2%) (Figure 3). There was a positive correlation between the hydrolytic and antagonist activity of the Trichoderma isolates (Figure 4). In general, chitinase, cellulase, and protease had moderate to high antagonistic activity against F. xylarioides mycelial growth, whereas β-(1-3) glucanase had low antagonistic activity (Figure 4).
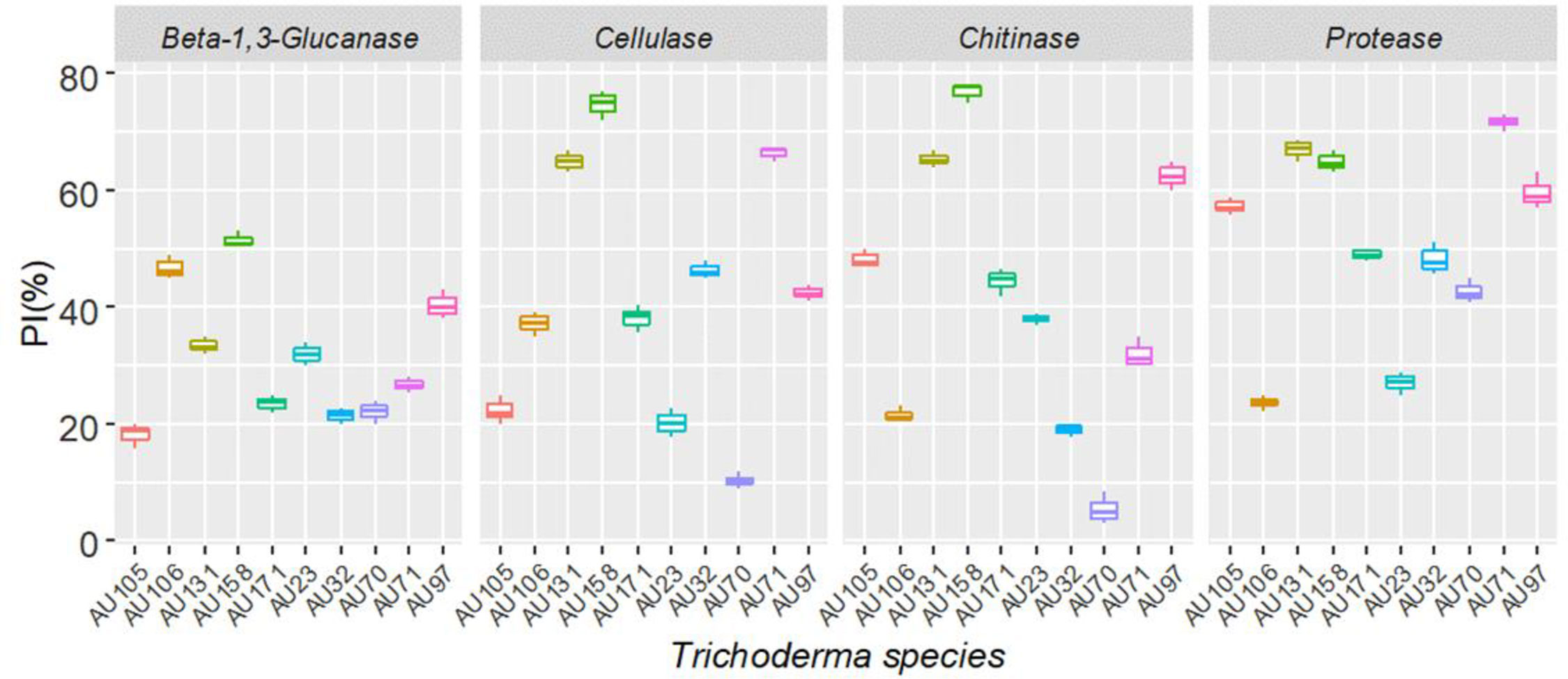
Figure 3 Boxplots of the hydrolytic enzyme activity of 10 Trichoderma isolates against F. xylarioides. Trichoderma isolates: T. viride AU23, T. longibrachiatum AU32, T. koningiopsis AU70, T. asperelloides AU71, T. asperellum AU97, T. harzianum AU105, T. aethiopicum AU106, T. asperellum AU131, T. longibrachiatum AU158 and T. asperellum AU171. The boundaries of the boxplots indicate the 25th and 75th percentiles, and the solid line within the boxes indicates the median value. PI, Percentage of Inhibition.
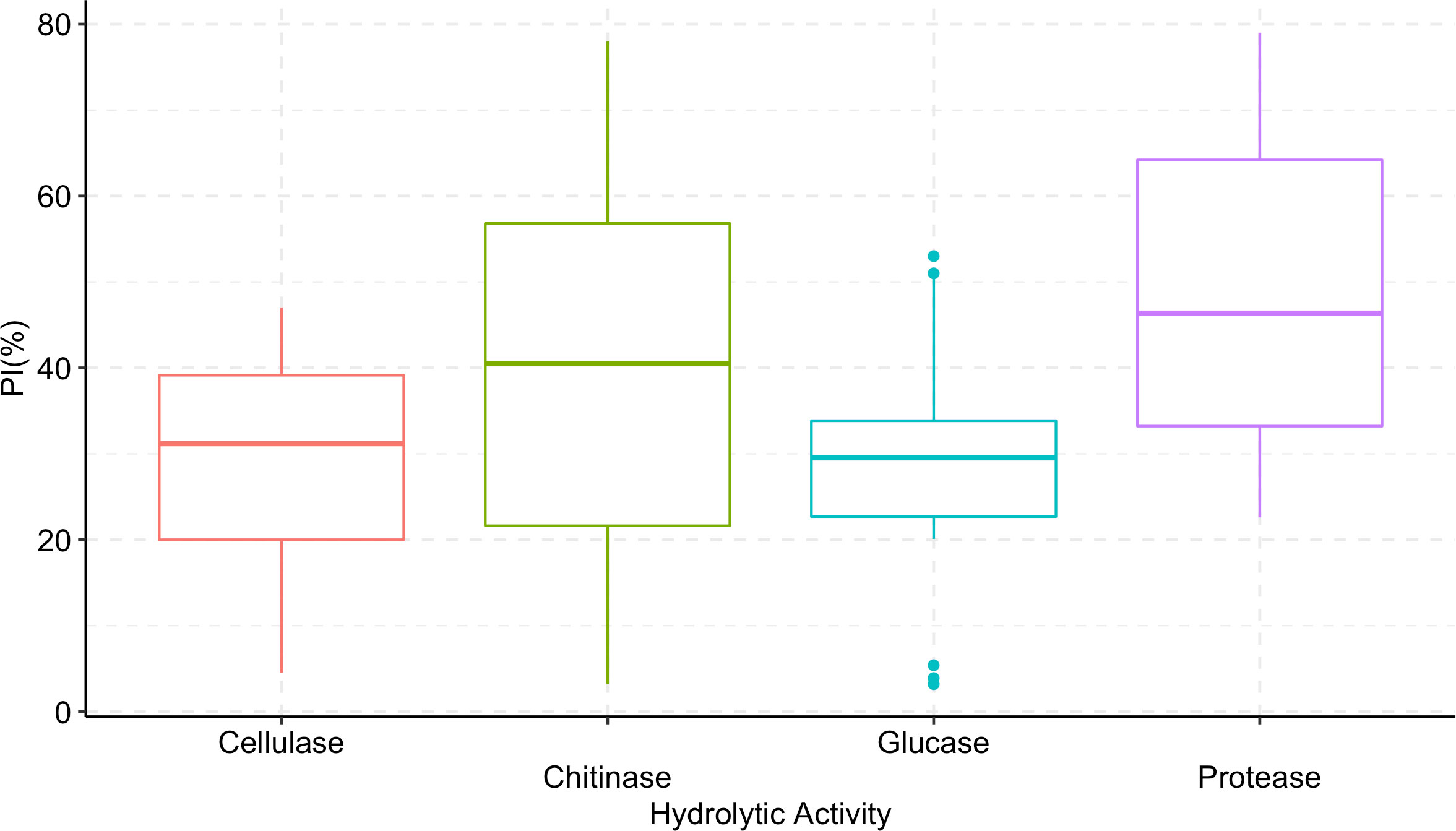
Figure 4 Boxplots showing the correlation between hydrolytic enzyme activity of Trichoderma isolates and percentage inhibition (PI) of F. xylarioides mycelial growth. The boundaries of the boxplots indicate the 25th and 75th percentiles, and the solid lines within the boxes indicate the median value. PI, Percentage of Inhibition.
3.3 Potential growth-promoting attributes of Trichoderma isolates
The plant growth-promoting attributes of Trichoderma differed significantly (p<0.05) between isolates. All of the isolates tested positive for IAA production, but T. longibrachiatum AU158, T. asperellum AU131, and T. asperellum AU171 displayed the highest levels (Figure 5), as quantified by spectrophotometry (Figure S2). The levels overall ranged from 8 to 44.5 μg/ml. All of the Trichoderma isolates were also able to synthesize siderophore units, with a range of 41.2 to 96.7%, as indicated by orange halos around the colonies on the blue CAS agar plate (Figure S2). The varied colors of the medium indicated that the synthesized siderophores differed structurally. After 6 days of incubation, T. longibrachiatum AU158, T. asperelloides AU71, and T. asperellum AU97 showed maximum siderophore units of 95.2%, 92.6%, and 84.2%, respectively. Trichoderma aethiopicum AU106 produced the fewest siderophore units (41%), while other Trichoderma isolates produced moderate levels of siderophore units, as shown in Figure 5.
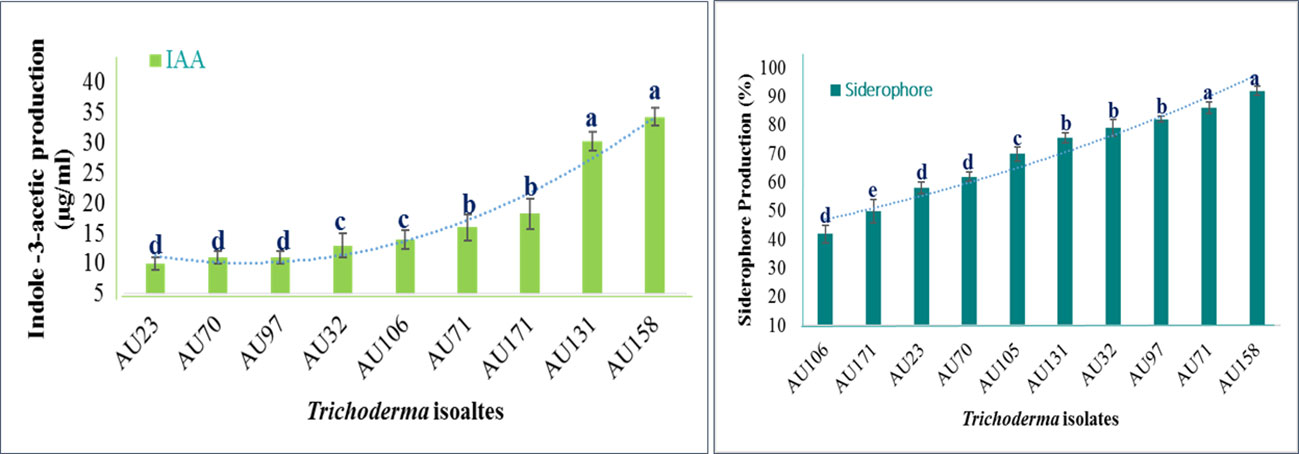
Figure 5 Quantitative estimates of the plant growth-promoting attributes of Trichoderma isolates Trichoderma isolates: T. viride AU23, T. longibrachiatum AU32, T. koningiopsis AU70, T. asperelloides AU71, T. asperellum AU97, T. aethiopicum AU106, T. asperellum AU131, T. longibrachiatum AU158 and T. asperellum AU171. Each value is the average of three duplicate samples with standard error, and various alphabets shown indicated mean treatments that are substantially different according to Tukey’s HSD posthoc test at p ≤ 0.05.
3.4 Bioefficacy of biofungicides under greenhouse conditions
Greenhouse experiments were conducted to evaluate the biocontrol efficiency of selected Trichoderma isolates against F. xylarioides using Geisha coffee seedlings. CWD infection was found in all replicates of pots treated with the pathogen. Seedlings treated with only the F. xylarioides spore suspension had the highest percentage incidence of CWD (92.5%) and DSI (76.7%) (Figure 6). After three months, typical partial wilting symptoms appeared in the positive control treatments (Figure 7B). However, compared to positive control treatments, the application of WDG and WP significantly (p<0.05) reduced the DI and DSI (Figure 7C). The DI of seedlings treated with T. asperellum AU131 WP was significantly reduced (15.7%), followed by T. longibrachiatum AU158 WP (22.1%). Trichoderma asperellum AU131 WP had the highest BE (84.3%) for reducing CWD, followed by T. longibrachiatum AU158 WP (77.9%) and T. asperelloides AU71 WP (71.2%) (Figure 6). The highest DI was observed in seedlings inoculated with T. aethiopicum AU106 (73.7%) WDG, T. koningiopsis AU70 WP (67.8%), T. asperellum AU97 WDG (63.4%), and T. asperellum AU171 WDG (53.5%). The highest DSI was observed with T. aethiopicum AU106 WDG (56.3%), while the lowest DSI was observed with T. longibrachiatum AU158 WP (8.3%). The distilled water-inoculated seedlings did not exhibit any disease symptoms (Figure 7A). In general, WP formulations caused a statistically significant reduction in CWD DI and DSI in the treated pots compared to WDG (Figure 6). As a result, WP-based formulations were chosen for the field experiments.
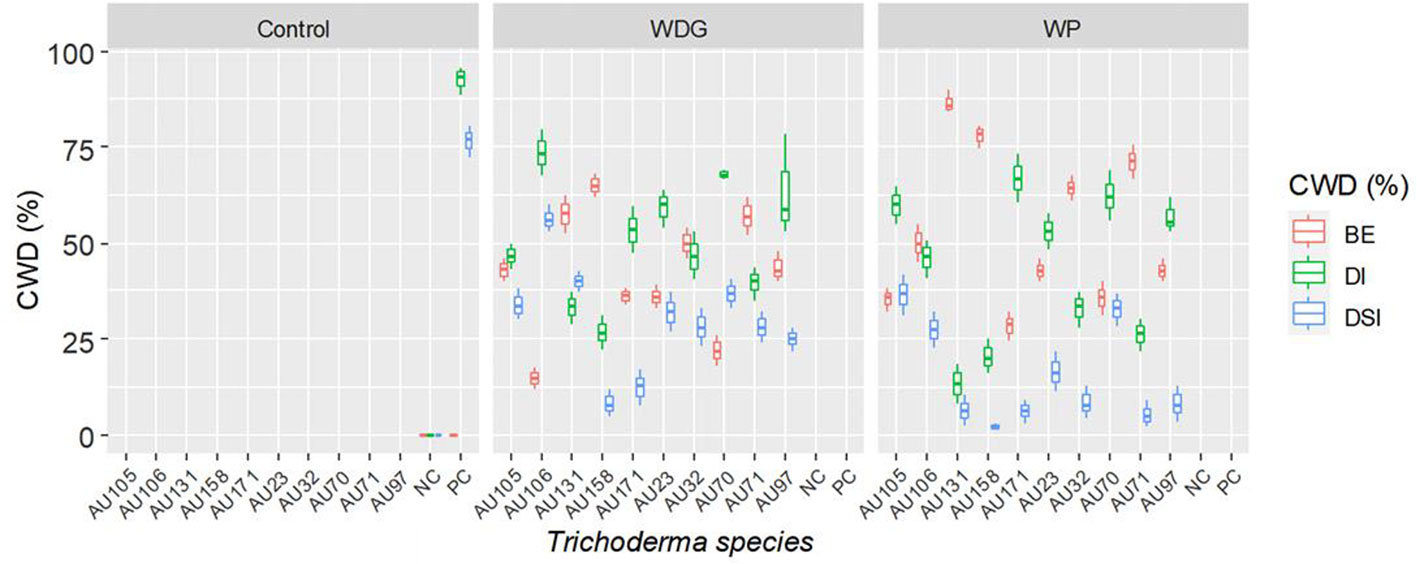
Figure 6 Boxplots of the antagonistic effectiveness of Trichoderma isolate WDG and WP products against F. xylarioides on coffee seedlings under greenhouse conditions. Trichoderma isolates: T. viride AU23, T. longibrachiatum AU32, T. koningiopsis AU70, T. asperelloides AU71, T. asperellum AU97, T. harzianum AU105, T. aethiopicum AU106, T. asperellum AU131, T. longibrachiatum AU158 and T. asperellum AU171. CWD, Coffee wilt disease; DI, Disease Incidence; DSI, Disease Severity Index; BE, Biocontrol Efficacy; PC, Positive control; NC, Negative control; WP, Wettable Powder and WDG , Water Dispensable Granules. The boundaries of the boxplots indicate the 25th and 75th percentiles, and the solid line within the boxes indicates the median value.
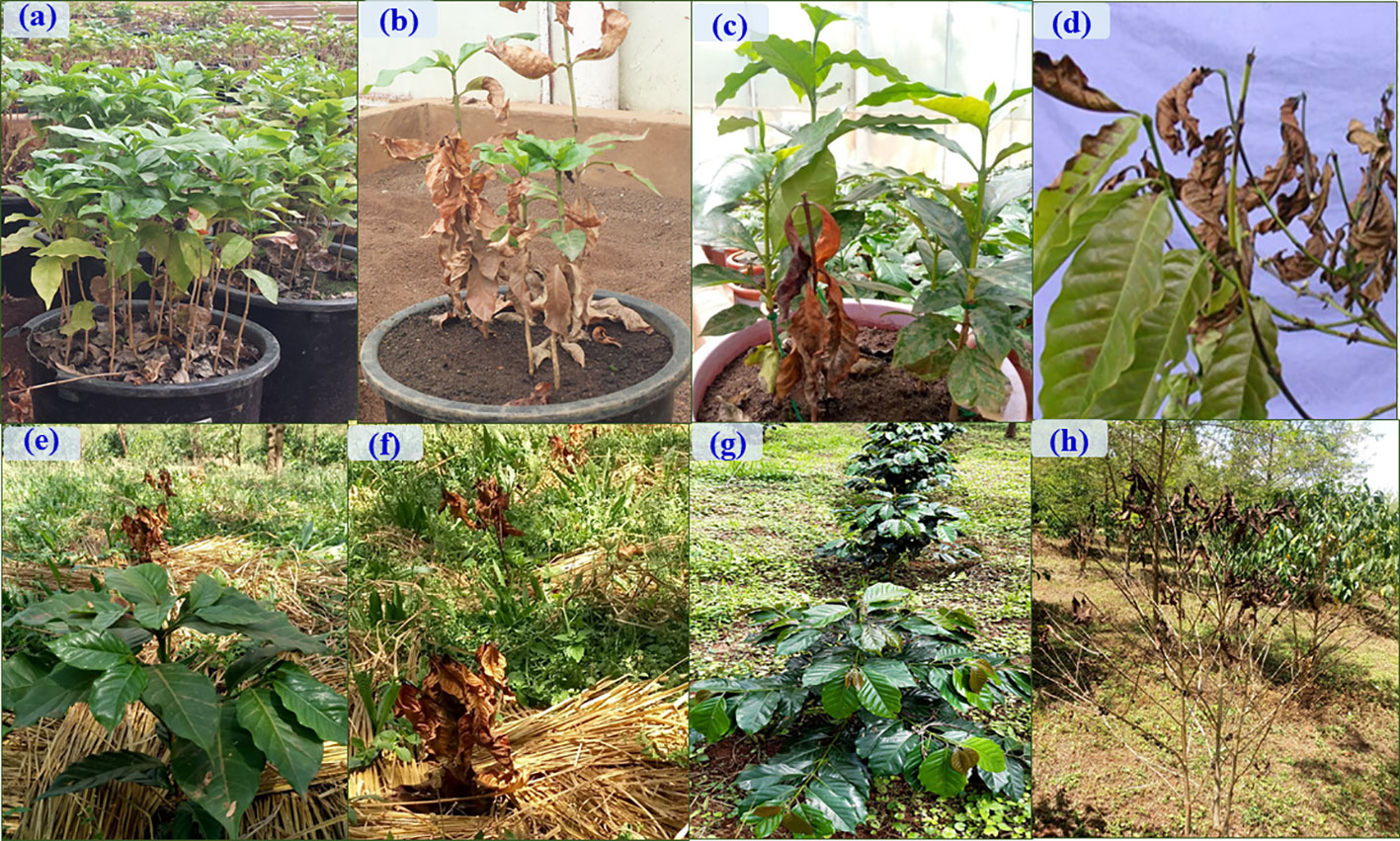
Figure 7 Characterization and evaluation of Trichoderma isolates as a biocontrol agent for against F. xylarioides on coffee seedlings under greenhouse and field conditions. Greenhouse experiment: (A) Negative control (F. xylarioides and biofungicides were not applied), (B) Positive control (F. xylarioides was applied to Geisha coffee seedlings) and (C) Geisha coffee seedlings treated with biofungicides (WDG and WP). Field experiment: (E) Negative control (F. xylarioides and biofungicides were not applied), (F) Positive control (F. xylarioides was applied to Geisha coffee plants) and (G) Geisha coffee seedlings treated with Wettable powder (WP) biofungicide. Typical symptoms: (D) and (H) are characteristic wilting symptoms manifested in Geisha coffee plants. The coffee plants treated with biofungicides and challenged with the pathogen showed healthy growth indicating the protection offered by Trichoderma species as a biocontrol agent.
Phenological indices revealed that seedlings treated with Trichoderma formulations grew significantly faster than untreated control seedlings (Figure 8). Overall, seedlings treated with T. asperellum AU97 and T. asperellum AU131 WP and WDG had higher plant heights and girths than untreated and F. xylarioides-treated seedlings. Thus, the use of WP and WDG formulations not only had an antifungal effect in controlling CWD, but also a growth-promoting effect (Figure 8).
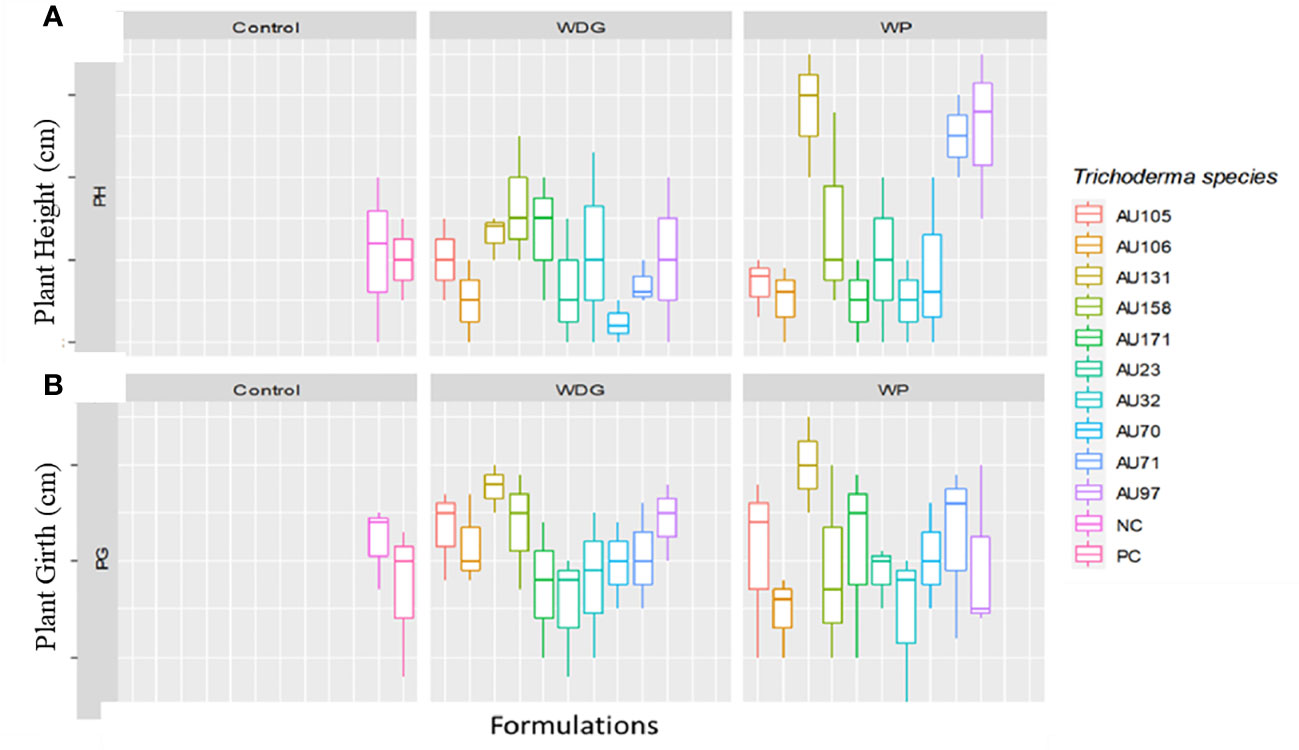
Figure 8 Boxplots of plant growth parameters for coffee seedlings treated with Trichoderma bioformulations. Trichoderma isolates: T. viride AU23, T. longibrachiatum AU32, T. koningiopsis AU70, T. asperelloides AU71, T. asperellum AU97, T. harzianum AU105, T. aethiopicum AU106, T. asperellum AU131, T. longibrachiatum AU158 and T. asperellum AU171. (A) Plant height and (B) plant girth. WP, Wettable Powder; WDG, Water Dispensable Granules; PC, Positive control and NC, Negative control. The boundaries of the boxplots indicate the 25th and 75th percentiles, and the solid line within the boxes indicates the median value.
3.5 Bioefficacy of Trichoderma WP under field conditions
WP formulations were applied to the susceptible Geisha coffee variety in the field to test the biocontrol potential of the chosen Trichoderma isolates in an ecologically relevant habitat. Six months after F. xylarioides application, the first symptoms of CWD were observed. The wilting began in the upper branches and spread to the entire tree canopy, resulting in a generalized green leaf defoliation (Figures 7D, H). Throughout the study period, all plants treated with Trichoderma isolates showed a significant reduction in DI and DSI compared to the positive controls (p<0.05) (Figures 7F, G, 9). In the first (2018/19), second (2019/20), and third (2020/21) years, the percentage of DI in plants treated with F. xylarioides alone was 60.5, 98, and 100% at Jimma, 65, 96.5, and 100% at Teppi, and 77.3, 98.4 and 100% at Gera, respectively. The CWD DI and DSI was higher at Gera than at the other field sites. From the second year (2019/20), untreated negative control coffee plants also exhibited a lower DI and DSI (Figure 7E), as a result of the presence of natural inoculum in the field experimental sites (Figure 9).
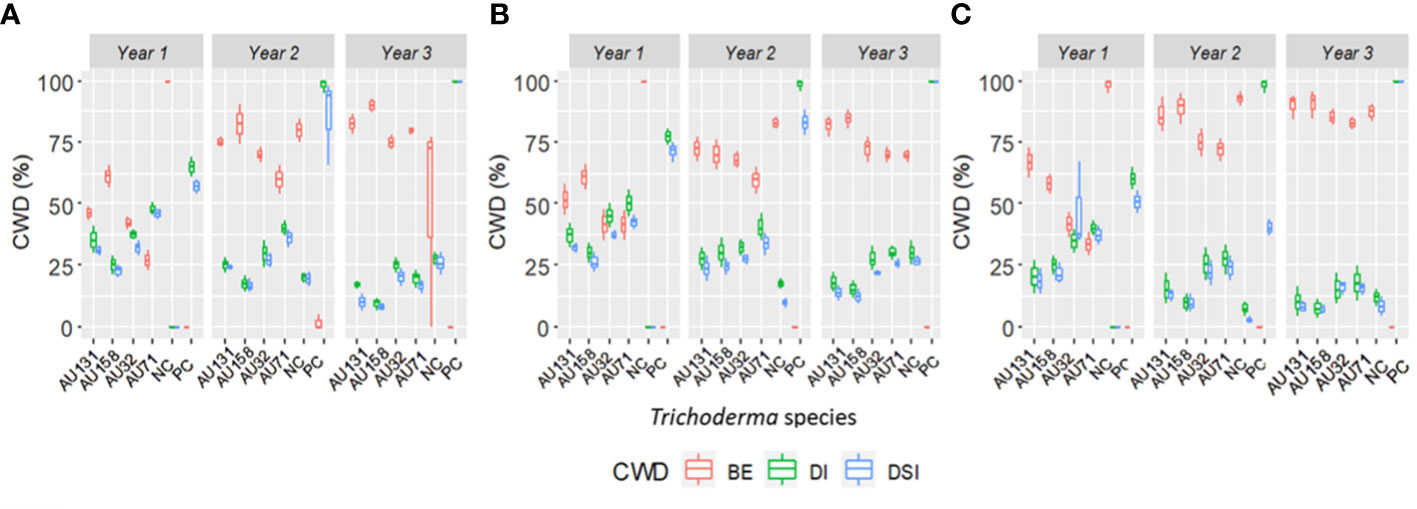
Figure 9 Boxplots representing the annual distribution of CWD for three consecutive years. (A) Teppi field site, (B) Gera field site and (C) Jimma field site. Trichoderma isolates: T. longibrachiatum AU32, T. asperellum AU71, T. asperellum AU131, T. longibrachiatum AU158. PC, Positive Control; NC, Negative Control; CWD, Coffee Wilt Disease; BE, Biocontrol Efficacy; DI, Disease Incidence and DSI, Disease Severity Index. Year 1 = 2018/19, Year 2 = 2019/20 and Year 3 = 2020/21. The boundaries of the boxplots indicate the 25th and 75th percentiles, and the solid line within the boxes indicates the median value.
A reduction in annual CWD DI and DSI was seen in all the experimental fields over the study period, although it varied significantly (p<0.05) from year to year (Figure 9). There were no significant differences in DI between the treatments in Gera and Teppi during the first year (2018/19), and the positive control had a significantly higher DI (Figures 8A, B). Over the three years, at all the experimental sites all plants treated with Trichoderma isolates showed a significant (p<0.05) reduction in DI and DSI compared to the positive controls. Trichoderma asperellum AU131 and T. longibrachiatum AU158 gave the best results, with a maximum BE at Gera of 81.8 and 84.5%, at Teppi of 82.5 and 90%, and at Jimma of 90 and 91%, respectively (Figure 9). The CWD pressure on the plants was reduced by all Trichoderma treatments, and the BE was different across all the field sites over the three years. However, only moderate disease reduction was seen with T. asperellum AU71 and T. longibrachiatum AU32 across all sites.
Overall, the three-year preventive applications demonstrated a very high reduction in CWD development, plant death, and cumulative incidence compared to untreated controls, with BE ranging from 46.2 to 90% at Teppi, 51.6 to 84.5% at Gera, and 58.2 to 91% at Jimma (Figure 10). There was a statistically significant reduction in the cumulative incidence of disease in the treated plots after just one year of treatment, but there was a difference in the level of disease reduction between the isolates. However, consistent BE effects were observed at all the sites over the three consecutive years (Figures 10, 11). By the end of the third year, the cumulative BE of Trichoderma isolates was 67.7% at Gera, 71.4% at Teppi, and 80.5% at Jimma, respectively (Figure 11B). In the presence of natural inoculum of the test pathogen, the Trichoderma isolates appeared to provide efficient, sustainable, environmentally friendly, and long-lasting protection (Figure 7F).
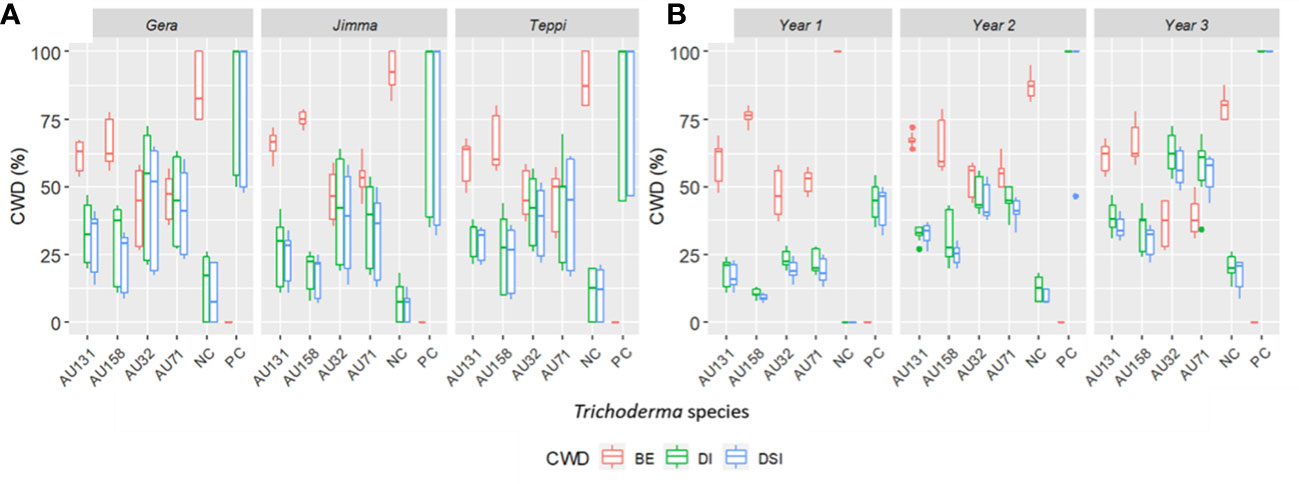
Figure 10 Boxplots showing cumulated incidence and severity of CWD, and biocontrol efficacy (BE) of Trichoderma formulations. (A) Effect of Trichoderma treatments against F. xylarioides at three field experimental sites and (B) Cumulated incidence and severity of CWD surveyed over three years. Trichoderma isolates: T. longibrachiatum AU32, T. asperellum AU71, T. asperellum AU131, T. longibrachiatum AU158. PC, Positive Control; NC, Negative Control; CWD, Coffee Wilt Disease; BE, Biocontrol Efficacy; DI, Disease Incidence and DSI, Disease Severity Index. Year 1 = 2018/19, Year 2 = 2019/20 and Year 3 = 2020/21. The boundaries of the boxplots indicate the 25th and 75th percentiles, and the solid line within the boxes indicates the median value.
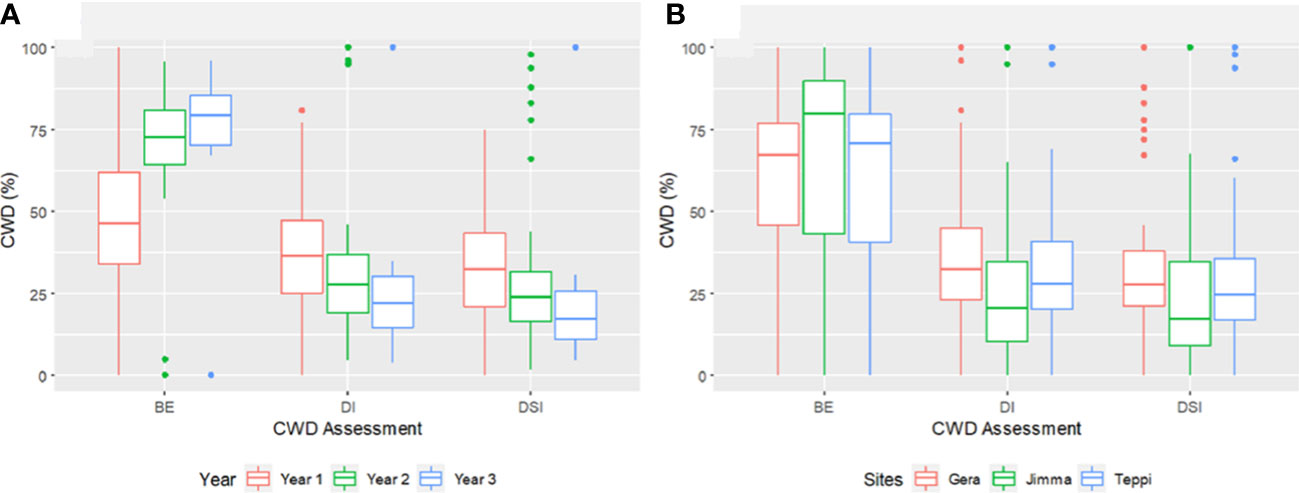
Figure 11 Boxplots summarizing the cumulated CWD assessments (A) across three years and (B) at different experimental field sites. CWD, Coffee Wilt Disease; BE, Biocontrol Efficacy; DI, Disease Incidence and DSI, Disease Severity Index. Year 1 = 2018/19, Year 2 = 2019/20 and Year 3 = 2020/21. The boundaries of the boxplot indicate the 25th and 75th percentiles, and the solid line within the boxes indicates the median value.
In general, a significant (p<0.05) promotion of plant growth was indicated by Trichoderma treatments under field conditions, compared to untreated controls (Figure 12). However, the annual plant height and girth values varied significantly (p<0.05) between individual Trichoderma isolates and experimental sites. At all sites, plants treated with T. asperellum AU71 and AU131, and T. longibrachiatum AU158, had higher plant height and girth values than untreated controls, while there was no significant difference in plant height or girth between the untreated controls and T. longibrachiatum AU32-treated plants (Figure 12).
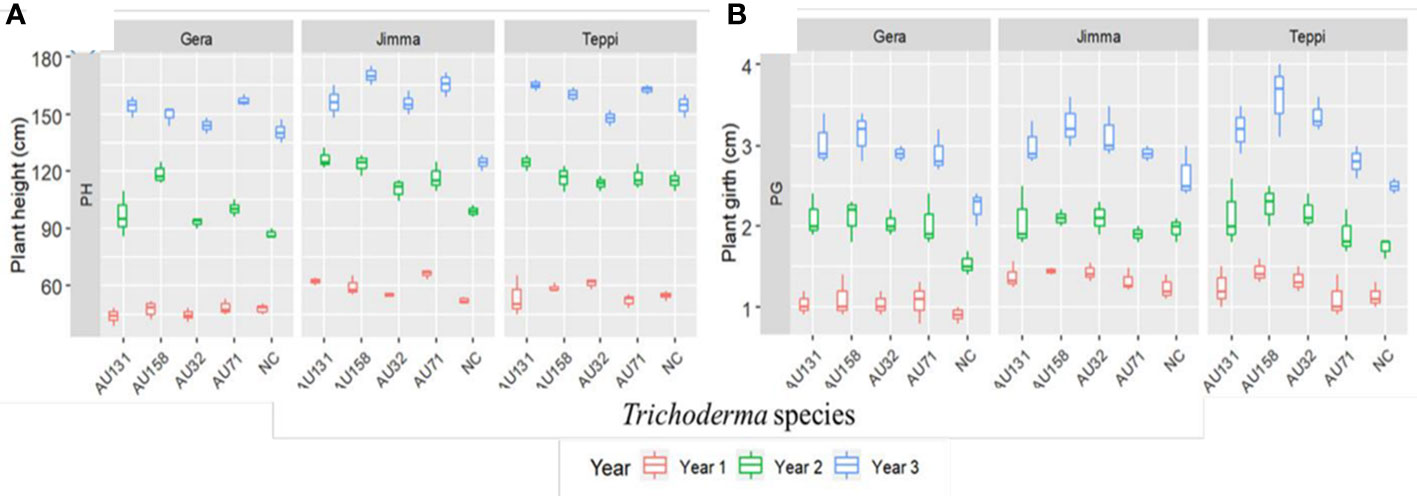
Figure 12 Boxplots of Geisha coffee plant growth after treatment with Trichoderma WP under field conditions. (A) Plant height and (B) Plant girth. Trichoderma isolates: T. longibrachiatum AU32, T. asperellum AU71, T. asperellum AU131, T. longibrachiatum AU158, NC, Negative Control; Year 1 = 2018/19, Year 2 = 2019/20 and Year 3 = 2020/21. The boundaries of the boxplot indicate the 25th and 75th percentiles, and the solid line within the boxes indicates the median value.
4 Discussion
A large number of Trichoderma isolates from the rhizosphere of C. arabica trees have been screened (Mulatu et al., 2022a) and evaluated under in vitro, greenhouse, and field conditions with F. xylarioides, in order to identify potential native biocontrol agents. As a result of the stepwise multiple screening, several isolates have emerged as viable candidates for biocontrol agents in the management of CWD. All of the candidate isolates displayed strong antagonistic activity, and successfully inhibited the mycelial growth of F. xylarioides, with mean PIs ranging from 44.4 to 84.8% (Table S3). However, there was significant variation across the isolates in the strength of the antagonistic response, which could have been the result of various factors.
According to Filizola et al. (2019), some species prevent the growth of phytopathogens by hyper-parasitism, while others achieve it through antibiosis or competition for nutrients and space. Trichoderma species have been shown to proliferate more quickly than competing phytopathogens as a result of their more efficient use of food sources (Marik et al., 2019). Additionally, harzianolide, peptaibols, pyrones, and other secondary metabolites generated by Trichoderma species have been described as having antimicrobial potential and acting as plant growth promoters (Hermosa et al., 2014; Manzar et al., 2021; Zhang et al., 2021). The number of extracellular lytic enzymes and secondary metabolites produced by each species of Trichoderma work in concert to promote a synergistic effect of their many mechanisms of action (Hermosa et al., 2014).
Trichoderma species are known to produce a broad spectrum of bioactive secondary metabolites that can affect a wide range of phytopathogens, including Alternaria alternata, Botrytis cinerea, Fusarium species, Pythium species, Rhizoctonia solani, Sclerotinia sclerotiorum, and Ustilago maydis (Hyder et al., 2017; Sood et al., 2020). A number of commercial Trichoderma-based biopesticides have already been developed, and T. asperellum, T. asperelloides, T. harzianum, T. longibrachiatum, and T. viride are known to be effective against a variety of Fusarium species (Kari et al., 2012; Temesgen Belayneh et al., 2013; Błaszczyk et al., 2017; Mulatu et al., 2022a). Several studies have also demonstrated the effectiveness of T. asperellum, T. asperelloides, and T. longibrachiatum against different pathogens using a variety of modes of action, including antibiosis, mycoparasitism, competition for nutrients, and inducing resistance (Wu et al., 2017; Shrestha, 2019; Wei et al., 2019). Compared to pathogenic microbes, the ability of Trichoderma species to proliferate quickly offers them a notable advantage in the competition for nutrients, space, and dominance over their prey host, which are all equally significant and interconnected (Kari et al., 2012).
In the present study, in vitro experiments identified the 10 Trichoderma isolates with the most significant PI of the test pathogen. It appeared that the production of bioactive secondary metabolites, cell wall-degrading enzymes, IAA, and siderophores gave the isolates their antifungal properties, and the variation in their mycelial growth inhibition rates suggested that their antagonistic activity had various modes of action. In order for antibiotics or antifungal compounds to enter plant pathogens, hydrolytic enzymes are necessary (Strakowska et al., 2014; Saravanakumar et al., 2016b), and the isolates used in this study were able to produce chitinase, cellulase, protease, and β-(1,3)-glucanase. Previous studies indicating that chitinases are involved in triggering a plant’s defense mechanisms corroborate the current results (Baek et al., 1999; Gueye et al., 2020). The current results are also supported by studies that have found that the hydrolytic enzymes (cellulase, chitinase, protease, and β-(1,3)-glucanases) produced by T. asperellum, T. harzianum, and T. longibrachiatum have a high level of antagonistic activity against F. oxysporum (Schirmböck et al., 1994; Putri et al., 2022). These enzymes play a key role in the breakdown of the pathogen’s cell walls, which facilitates simple invasion and colonization by the biocontrol agents, induces a defense response and slows the spread of the pathogen while also indirectly promoting plant growth and development (Qualhato et al., 2013; Cherkupally et al., 2017). Overall, T. asperellum AU131 and T. longibrachiatum AU158 isolates appear to be the most promising biological control agents against F. xylarioides, primarily because of the synergistic effect of various mechanisms of action, including mycoparasitism, competition, production of bioactive secondary metabolites, and induction of systematic resistance (Aita et al., 2019; Mulatu et al., 2022b).
All the Trichoderma isolates tested led to a considerable reduction in CWD and promoted the growth of coffee seedlings under greenhouse conditions. Trichoderma asperellum AU131 WP displayed the best BE (84.3%) for reducing CWD, followed by T. longibrachiatum AU158 (77.9%) and T. asperelloides AU71 (71.2%). Similar findings have been seen with the application of a rhizosphere Trichoderma species under greenhouse conditions, which led to a 100% reduction in Fusarium wilt disease and boosted plant growth metrics by 250% (Thangavelu and Gopi, 2015). The current results demonstrate that Trichoderma isolates can promote the growth of coffee plants, which may be related to their capacity to produce IAA and siderophores, and engage in biocontrol activities by secreting hydrolytic enzymes. This supports the work of Singh et al. (2013), who discovered that the production of siderophore and hydrolytic enzymes inhibited a number of plant diseases, and elsewhere Trichoderma species have been shown to produce siderophores and IAA, and promote plant growth (Saber et al., 2017; Bader et al., 2020).
The experimental field sites were chosen based on their edaphic and climatic characteristics, crop history and level of F. xylarioides inoculum in soil, in order to evaluate selected formulations in a variety of real-life scenarios within a coffee-growing region. A previous study has shown that T. asperellum, T. asperelloides, and T. longibrachiatum are the three most dominant and prevalent Trichoderma species in Ethiopia’s major coffee-growing regions (Mulatu et al., 2022a), and the adaptability and versatility of Trichoderma species enables them to display antimicrobial activity under a wide range of climatic and environmental conditions. The current field study provides a fresh perspective on how these species could function as preventative biocontrol agents. At Gera, Teppi, and Jimma, the mean cumulative BE demonstrated by Trichoderma isolates for 2020/21 was 67.7%, 71.4%, and 80.5%, respectively. The lower efficacy at Gera could be explained by the levels of natural soil pathogen inoculum, which were much higher than at Jimma and Teppi. Additionally, the high altitude and low temperatures, below 15°C, at Gera are favorable for pathogen growth (Table S1). However, from the first year of field studies, the effectiveness of each Trichoderma isolate as a biocontrol agent was evident. The Trichoderma treatments show good and cumulative efficacy in reducing disease pressure over time, giving rise to the theory that a decline in annual incidence may be associated with improved plant defensive responses, as proposed by Di Marco et al. (2021). These findings are encouraging because they suggest that Trichoderma species can be used in the coffee ecosystem under various agro-climatic conditions.
The spread of CWD is aided by agronomic practices such as replanting, slashing, and close spacing, as well as transporting diseased trees from one location to another (Adugna et al., 2010; Tsegaye, 2018). As a result, affected coffee plants are typically uprooted and burned to control the spread of the disease. However, a socioeconomic survey revealed that 60% of Ethiopian farmers use the wood for fences, 26% build dwellings and animal shelters with the wood, 10% give their neighbors any leftover wilting trees for firewood, and 2% actually sell the trees (Adugna et al., 2010). The incidence of CWD is negatively impacted by these agronomic methods, with rates in garden and plantation coffee plots being noticeably higher. The repeated cycles of infection increased CWD’s effects on farmers. Coffee farming is no longer a reliable source of revenue for many farmers, who had lost their livelihoods. The fact that coffee prices were declining on international markets only made things worse. But for many, CWD had the most apparent direct impact. It was difficult to afford the inputs required to manage plots and try to recover output due to lower incomes. Di Marco et al. (2021) suggest that the best way to reduce the damage caused by grapevine leaf stripe disease is to apply formulated products to pruning wounds early and regularly. Di Marco et al. (2021) also reported on preventive applications of T. asperellum strain ICC 012 and T. gamsii strain ICC 080 against diseases of grapevine trunk (Esca Complex) over 9 years. A large reduction in symptom development was seen in the treated vines, as well as a reduction in annual and cumulative incidence and vine death, with overall disease reduction ranging from 66 to almost 90%. Young coffee plants, that have not yet been affected by the disease, should therefore be the perfect target for preventative protection against CWD, by using Trichoderma-based biofungicides before the occurrence and spread of the disease. The current field results suggest that it is crucial to apply Trichoderma WP as soon as the coffee seedlings are transplanted, in order to boost the effectiveness of the biocontrol agents and provide long-term protection.
Thangavelu and Gopi (2015) have shown that Fusarium wilt disease is significantly reduced by a combined application of rhizosphere Trichoderma species NRCB under field conditions, 15 days prior to planting, and 2 and 4 months after planting. This indicates that prolonged and repeated application of MBCAs in the field is necessary for continued effectiveness. A protocol of applying treatments twice a year to protect coffee plants from CWD is therefore suggested, based on the sanitary measures used in the current study. Coffee growers require IDM of CWD using coffee varieties that have some resistance, and disease treatments for both old and new plantings as a means of good sanitary measures. The field investigations unequivocally demonstrated that dual therapy applications were effective during Ethiopia’s short rainy seasons from September to October and February to May. However, in comparison to field treatments, applying biofungicides at the nursery stage may be more advantageous from a practical, financial, and efficiency point of view. In order to manage CWD effectively, realistic management techniques such as biocontrol need to eliminate microsclerotia or stop their germination in soil (Tesfaye et al., 2020). This strategy can only be achieved in field conditions by applying MBCAs to the planting holes, to inhibit microsclerotia viability around the rhizosphere and protect the coffee plants during the first year of planting (Flood et al., 2016).
Many researchers have reported that Trichoderma species are antagonistic against a variety of phytopathogens in dual culture tests, but not so far in the field, largely because field conditions are significantly different from in vitro conditions (Zamanizadeh et al., 2011; Kari et al., 2012). However, the present study has revealed T. asperellum AU131 and T. longibrachiatum AU158 to be promising biocontrol agents against F. xylarioides, based on a stepwise protocol from in vitro, to greenhouse, to field experiments. The WP formulation consistently reduced the DI and DSI of CWD and enhanced the growth of susceptible Geisha coffee plants in three different agro-climatic zones and years. These findings demonstrate that Trichoderma isolates can provide preventative protection for the management of CWD. The ability of T. asperellum AU131 and T. longibrachiatum AU158 to inhibit the mycelial growth of F. xylarioides under in vitro, greenhouse, and field conditions, as well as to improve the growth of coffee plants, means that they offer the most potential for reducing CWD DI and DSI, and this study provides a practical basis for the development of eco-friendly treatments and a useful methodology for developing preventative tools within an IDM strategy against CWD.
Soil samples from Ethiopia’s coffee rhizosphere have previously been used to examine the biodiversity and distribution status of Trichoderma species (Mulatu et al., 2022a). A variety of parameters, such as the type of coffee production system, pH, water availability, temperature, altitude, pesticides, and other biotic and abiotic stress conditions, appear to influence the distribution and ecological preferences of Trichoderma species. The three dominant species in Ethiopia’s coffee ecosystem, T. asperellum, T. asperelloides, and T. longibrachiatum, can be found in all of the country’s major coffee-growing regions and districts (Mulatu et al., 2022a). The data presented here have generated the hypothesis that the coexistence of T. asperellum AU131 and T. longibrachiatum AU158 in coffee plant rhizospheres may retard or even prevent the development of CWD. Furthermore, field testing of T. asperellum AU131 and T. longibrachiatum AU158 WP in sick plots in a hot-spot area clearly demonstrated that the pathogen and disease could be successfully controlled and managed, as evidenced by the decreased incidence and severity of disease. To the best of our knowledge, this is the first report of the technological development of T. asperellum AU131 and T. longibrachiatum AU158 from the laboratory to the field, resulting in effective application of a biocontrol-based management strategy for CWD in Ethiopia.
The secondary metabolites from these two Trichoderma isolates have been profiled, characterized, and elucidated using gas chromatography (GC-MS) and untargeted liquid chromatography-high resolution mass spectrometry (LC-HRMS), and their toxicological effects investigated using Swiss albino mice (Mulatu et al., 2022b). GC-MS identified the substances in the ethyl acetate extracts as alcohols, benzene acids, sesquiterpenes, phenols, ketones, and aromatic chemicals. At various concentrations, neither the examined spore suspensions nor the methanol extracts of these isolates were harmful or pathogenic to Swiss albino mice (Mulatu et al., 2022b). As the Trichoderma formulations developed here promote the biological control of the plant pathogen for long-term CWD management, T. asperellum AU131 and T. longibrachiatum AU158 are recommended for use by small-and large-scale coffee producers.
In-depth study of the molecular mechanisms governing plant-microbe interactions is essential for the development of novel bio-formulations since it may reveal interacting mechanisms involved in the rhizosphere (Vishwakarma et al., 2020). The molecular elements that control plant-Trichoderma interactions in perennial crops like coffee plants are little known, despite the significance of these interactions. Additionally, research into the mechanism of action against F. xylarioides as well as the population dynamics of Trichoderma species under field circumstances following treatment is ongoing. To determine the potential mechanisms and how Trichoderma species are behaving in the field, these areas of the study have been initiated and will be thoroughly explored. It is also being studied how the genes for the defense enzymes expressed by Trichoderma isolates function.
5 Conclusions
Based on their mycelial growth inhibition profiles, the two isolates with the highest levels of in vitro antagonistic activity against F. xylarioides were T. asperellum AU131 and T. longibrachiatum AU158. The WP of T. asperellum AU131 demonstrated the greatest reduction in CWD incidence (84.3%), followed by the WP of T. longibrachiatum AU158 (77.9%), and both also had a significant positive effect on coffee plant growth under greenhouse and field conditions. In comparison to untreated controls, the preventive applications of WP of both MBCAs over a three-year period led to a significant decrease in annual and cumulative DI, with the BE ranging from 46.2 to 90% at Teppi, 51.6 to 84.5% at Gera, and 58.2 to 91% at Jimma. After 3 years, the cumulative BE of Trichoderma isolates was 67.7% at Gera, 71.4% at Teppi, and 80.5% at Jimma.
To the best of our knowledge, this is the first report of a biological solution, taken from the laboratory into the field, that can effectively manage CWD. The significant reduction in DI and DSI observed over three years clearly demonstrates the preventive outcome of treating the coffee plant’s rhizosphere. This study also clearly demonstrates how a preventive approach can be used to control CWD within natural ecosystems. Disease pressure was significantly reduced across all the experimental sites, highlighting the critical role of bioformulated biofungicides in CWD management. The biocontrol potential of T. asperellum AU131 and T. longibrachiatum AU158, as well as their exudates, is supported by in vitro tests, and greenhouse and field studies. Small- and large-scale coffee farmers are advised to use these two bioagents in the treatment of CWD.
Data availability statement
The original contributions presented in the study are included in the article/Supplementary Material. Further inquiries can be directed to the corresponding author.
Author contributions
The study was conceptualized, designed, planned, and carried out by AM, TA, RV and DT. AM, TA, DT, NM, and RV analyzed, validated, and curated the data. AM wrote and prepared the original manuscript. TA, DT, NM, and RV reviewed and edited the manuscript. TA and RV handled the funding’s acquisition and administration. All authors read and agreed to the published version of the manuscript. All authors contributed to the article and approved the submitted version.
Funding
The EBTin, which administers the Biotechnology Product Development Research Grant, provided funding for this study. We also acknowledge the support from RRV research group. RV received funding from the Swedish Research Council (VR) (grant number 2019-04270) and the Swedish Research Council’s (FORMAS) (grant number 2019-01316), Novo NordiskFonden (0074727), SLU Partnerskap Alnarp and the SLU Centre for Biological Control.
Acknowledgments
We appreciate the funding provided for this study by the Ethiopian Bio and Emerging Technology Institute (EBTin) under the theme “Bio-fungicide production for CWD control in Ethiopia”. The laboratory facilities were provided by the Department of Microbial, Cellular, and Molecular Biology, Addis Ababa University (AAU), Ethiopia. The Ethiopian Institute of Agricultural Research (EIAR) Centers (Jimma, Teppi, and Gera Agricultural Research Centers) are acknowledged for providing the Geisha coffee variety and field experimental sites. We particularly want to express our gratitude to the Department of Plant Breeding at the Swedish University of Agricultural Sciences (SLU), Sweden, for their invaluable assistance with our research endeavor.
Conflict of interest
The authors declare that the research was conducted in the absence of any commercial or financial relationships that could be construed as a potential conflict of interest.
Publisher’s note
All claims expressed in this article are solely those of the authors and do not necessarily represent those of their affiliated organizations, or those of the publisher, the editors and the reviewers. Any product that may be evaluated in this article, or claim that may be made by its manufacturer, is not guaranteed or endorsed by the publisher.
Supplementary material
The Supplementary Material for this article can be found online at: https://www.frontiersin.org/articles/10.3389/fpls.2023.1113949/full#supplementary-material
References
Adugna, G. (2004). Diversity in pathogenicity and genetics of gibberella xylarioides (Fusarium xylarioides) populations and resistance of coffee species. Ph.D dissertation (Bonn, Germany: University of Bonn).
Adugna, G., Hindorf, H., Steiner, U., Nirenberg, H., Dehne, H.-W., Schellander, K. (2005). Genetic diversity in the coffee wilt pathogen (Gibberella xylarioides) populations: Differentiation by host specialization and RAPD analysis/Genetische diversität in der population des erregers der kaffeewelke (Gibberella xylarioides): Differenzierung durch wirtsspezifität und RAPD analyse. J. Plant Dis. Protec. 14, 134–145.
Adugna, G., Million, A., Hindorf, H., Zeru, A., Teferi, D., Jefuka, C. (2010). Coffee wilt disease in Ethiopia (London, UK: CAB International).
Agrawal, T., Kotasthane, A. S. (2012). Chitinolytic assay of indigenous Trichoderma isolates collected from different geographical locations of chhattisgarh in central India. SpringerPlus 1, 1–10. doi: 10.1186/2193-1801-1-73
Aita, B. C., Spannemberg, S. S., Schmaltz, S., Zabot, G. L., Tres, M. V., Kuhn, R. C., et al. (2019). Production of cell-wall degrading enzymes by solid-state fermentation using agroindustrial residues as substrates. J. Environ. Chem. Eng. 7, 103193. doi: 10.1016/j.jece.2019.103193
Alemu, T. (2012). A review of coffee wilt disease, Gibberella xylarioides (Fusarium xylarioides) in Africa with special reference to Ethiopia. Ethiop. J. Biol. Sci. 11, 65–103.
Alemu, T., Kapoor, I. (2004). In vitro evaluation of Trichoderma and Gliocladium species against botrytis corm rot of Gladiolus. Pest Manage. J. Ethiop. 8, 97–103.
Alemu, T., Sokar, I. (2000). The status of coffee berry disease in minor coffee growing regions. Ethiopian Agricultural Research Organization, Addis Ababa, Ethiopia.
Avelino, J., Ten Hoopen, G. M., Declerck, F. A. (2011). ““Ecological mechanisms for pest and disease control in coffee and cacao agroecosystems of the neotropics,”,” in Ecosystem services from agriculture and agroforestry measurement and payment (London, UK: Earthscan), 91–117.
Bader, A. N., Salerno, G. L., Covacevich, F., Consolo, V. F. (2020). Native Trichoderma harzianum strains from Argentina produce indole-3 acetic acid and phosphorus solubilization, promote growth and control wilt disease on tomato (Solanum lycopersicum l.). J. King Saud Uni-Sci. 32, 867–873. doi: 10.1016/j.jksus.2019.04.002
Baek, J.-M., Howell, C. R., Kenerley, C. M. (1999). The role of an extracellular chitinase from Trichoderma virens Gv29-8 in the biocontrol of Rhizoctonia solani. Curr. Gen. 35, 41–50. doi: 10.1007/s002940050431
Bano, N., Musarrat, J. (2004). Characterization of a novel carbofuran degrading Pseudomonas sp. with collateral biocontrol and plant growth promoting potential. FEMS Microbiol. Lett. 231, 13–17. doi: 10.1016/S0378-1097(03)00894-2
Belachew, K., Teferi, D., Gidisa, G. (2015). Screening of some coffee arabica genotypes against coffee wilt diseases (Gibberella xylarioides heim and saccus) at jimma, southwest Ethiopia. Int. J. Sustan. Agric. Res. 2, 66–76. doi: 10.18488/journal.70/2015.2.3/70.3.66.76
Belachew, K., Teferi, D., Gidisa, G. (2017a). “Study on coffee wilt diseases (Gibberella xylarioides heim and saccus) resistant coffee genotypes at Jimma, southwest Ethiopia” in Proceedings of Ethiopian coffee science society (ECSS) inaugural conference, ECSS, Jimma, Ethiopia.
Belachew, K., Teferi, D., Hagos, L. (2017b). Current Status of Re-Emerging Coffee Diseases in Ethiopia, the Case of Coffee Thread Blight (Corticium koleroga). ECSS, Jimma, Ethiopia.
Belete, Y., Belachew, B., Fininsa, C. (2014). Performance evaluation of indigenous arabica coffee genotypes across different environments. J. Plant Breed. Crop Sci. 6, 171–178. doi: 10.5897/JPBCS2014.0447
Błaszczyk, L., Basińska-Barczak, A., Ćwiek-Kupczyńska, H., Gromadzka, K., Popiel, D., Stępień, Ł. (2017). Suppressive effect of Trichoderma species on toxigenic Fusarium species. Polish J. Microbiol. 66, 85–100. doi: 10.5604/17331331.1234997
Brown, M. S., Baysal-Gurel, F., Oliver, J. B., Addesso, K. M. (2019). Comparative performance of fungicides, biofungicides, and host plant defense inducers in suppression of Phytophthora root rot in flowering dogwood during simulated root flooding events. Plant Dis. 103, 1703–1711. doi: 10.1094/PDIS-09-18-1597-RE
Chen, L., Bóka, B., Kedves, O., Nagy, V. D., Szűcs, A., Champramary, S., et al. (2019). Towards the biological control of devastating forest pathogens from the genus Armillaria. Forests 10, 1013. doi: 10.3390/f10111013
Cherkupally, R., Amballa, H., Reddy, B. N. (2017). In vitro screening for enzymatic activity of Trichoderma species for biocontrol potential. Ann. Plant Sci. 6, 1784–1789. doi: 10.21746/aps.2017.6.11.11
Deketelaere, S., Tyvaert, L., França, S. C., Höfte, M. (2017). Desirable traits of a good biocontrol agent against Verticillium wilt. Front. Microbiol. 8, 1186. doi: 10.3389/fmicb.2017.01186
Dennis, C., Webster, J. (1971). Antagonistic properties of species-groups of Trichoderma: II. production of volatile antibiotics. Trans. Brit. Mycol. Soc 57, 41–IN44.
Dhodary, B., Schilg, M., Wirth, R., Spiteller, D. (2018). Secondary metabolites from Escovopsis weberi and their role in attacking the garden fungus of leaf-cutting ants. Chem. A Europ. J. 24, 4445–4452. doi: 10.1002/chem.201706071
Di Marco, S., Metruccio, E. G., Moretti, S., Nocentini, M., Carella, G., Pacetti, A., et al. (2021). Activity of Trichoderma asperellum strain ICC 012 and Trichoderma gamsii strain ICC 080 toward diseases of esca complex and associated pathogens. Front. Microbiol. 12, 813410. doi: 10.3389/fmicb.2021.813410
El-Sobky, M., Fahmi, A., Eissa, R., El-Zanaty, A. (2019). Genetic characterization of Trichoderma spp. isolated from different locations of menoufia Egypt and assessment of their antagonistic ability. J. Microb. Biochem. Technol. 11, 9–23. doi: 10.4172/1948-5948.1000409
Filizola, P. R. B., Luna, M. A. C., De Souza, A. F., Coelho, I. L., Laranjeira, D., Campos-Takaki, G. M. (2019). Biodiversity and phylogeny of novel Trichoderma isolates from mangrove sediments and potential of biocontrol against Fusarium strains. J. Microb. Cell Factor. 18, 89–99. doi: 10.1186/s12934-019-1108-y
Flood, J., Ten Hoopen, G. M., Krauss, U., Akrofi, A. (2016). “Root-infecting fungi attacking theobroma cacao,” in Cacao diseases (Gewerbestrasse, Switzerland: Springer), 449–480.
Getachew, S., Adugna, G., Lemessa, F., Hindorf, H. (2012). Coffee wilt disease (Gibberella xylarioides heim and saccas) in forest coffee systems of southwest and southeast Ethiopia. Plant Pathol. J. 11, 10–17. doi: 10.3923/ppj.2012.10.17
Girma, A., Million, A., Hindorf, H., Arega, Z., Teferi, D., Jefuka, C. (2009). Coffee wilt disease in Ethiopia. (UK: CABI), 50–68. doi: 10.1079/9781845936419.00
Gueye, N., Kumar, G. K., Ndiaye, M., Sall, S. D., Ndiaye, M. A. F., Diop, T. A., et al. (2020). Factors affecting the chitinase activity of Trichoderma asperellum isolated from agriculture field soils. J. Appl. Biol. Biotechnol. 8, 41–44. doi: 10.7324/JABB.2020.80207
Hermosa, R., Cardoza, R. E., Rubio, M. B., Gutiérrez, S., Monte, E. (2014). “Secondary metabolism and antimicrobial metabolites of trichoderma,” in Biotechnology and biology of trichoderma (Amesterdam, Netherland: Elsevier), 125–137.
Hernández-Rodríguez, A., Heydrich-Pérez, M., Acebo-Guerrero, Y., Velazquez-Del Valle, M. G., Hernandez-Lauzardo, A. N. (2008). Antagonistic activity of Cuban native rhizobacteria against Fusarium verticillioides (Sacc.) nirenb. in maize (Zea mays l.). Appl. Soil Ecol. 39, 180–186. doi: 10.1016/j.apsoil.2007.12.008
Hyder, S., Inam-Ul-Haq, M., Bibi, S., Humayun, A., Ghuffar, S., Iqbal, S. (2017). Novel potential of trichoderma spp. as biocontrol agent. J. Entomol. Zool. Stud. 5, 214–222.
ICO (2016). Total production by all exporting countries (London, UK: International Coffee Organization).
Irfan, M., Nadeem, M., Syed, Q. (2012). Influence of nutritional conditions for endoglucanase production by Trichoderma viride in SSF. Glob. J. Biotechnol. Biochem. 7, 7–12. doi: doi: 10.5829/idosi.gjbb.2012.7.1.6210
Jangir, M., Sharma, S., Sharma, S. (2021). Development of next-generation formulation against Fusarium oxysporum and unraveling bioactive antifungal metabolites of biocontrol agents. Sci. Rep. 11, 1–15. doi: 10.1038/s41598-021-02284-1
Jefuka, C., Fininsa, C., Adugna, G., Hindorf, H. (2010). Coffee leaf rust epidemics (Hemileia vastatrix) in montane coffee (Coffea arabica l.) forests in southwestern Ethiopia. East Afri. J. Sci. 4, 86–95.
Kari, D., Mohammed, G., Dalalpour, M., Rabiei, M., Rohani, N., Varma, A. (2012). Biocontrol potential of root endophytic fungi and Trichoderma species against Fusarium wilt of lentil under in vitro and greenhouse conditions. J. Agr. Sci. Techn. 14, 407–420.
Kashyap, A. S., Manzar, N., Nebapure, S. M., Rajawat, M. V. S., Deo, M. M., Singh, J. P., et al. (2022). Unraveling microbial volatile elicitors using a transparent methodology for induction of systemic resistance and regulation of antioxidant genes at expression levels in chili against bacterial wilt disease. Antioxidants 11 (2), 404. doi: 10.3390/antiox11020404
Kashyap, A. S., Manzar, N., Rajawat, M. V. S., Kesharwani, A. K., Singh, R. P., Dubey, S. C., et al. (2021). Screening and biocontrol potential of rhizobacteria native to gangetic plains and hilly regions to induce systemic resistance and promote plant growth in chilli against bacterial wilt disease. Plants 10, 2125. doi: 10.3390/plants10102125
Khan, M. R., Chonhenchob, V., Huang, C., Suwanamornlert, P. (2021). Antifungal activity of propyl disulfide from neem (Azadirachta indica) in vapor and agar diffusion assays against anthracnose pathogens (Colletotrichum gloeosporioides and Colletotrichum acutatum) in mango fruit. Microorganisms 9, 48–62. doi: 10.3390/microorganisms9040839
Manzar, N., Kashyap, A. S., Goutam, R. S., Rajawat, M. V. S., Sharma, P. K., Sharma, S. K., et al. (2022b). Trichoderma: Advent of versatile biocontrol agent, its secrets and insights into mechanism of biocontrol potential. Sustainability 14 (19), 12786. doi: 10.3390/su141912786
Manzar, N., Kashyap, A. S., Maurya, A., Rajawat, M. V. S., Sharma, P. K., Srivastava, A. K., et al. (2022a). Multi-gene phylogenetic approach for identification and diversity analysis of Bipolaris maydis and Curvularia lunata isolates causing foliar blight of zea mays. J. Fungi 8 (8), 802. doi: 10.3390/jof8080802
Manzar, N., Singh, Y., Kashyap, A. S., Sahu, P. K., Rajawat, M. V. S., Bhowmik, A., et al. (2021). Biocontrol potential of native Trichoderma spp. against anthracnose of great millet (Sorghum bicolour l.) from tarai and hill regions of India. Biol. Contr. 152, 104474. doi: 10.3390/su141912786
Maria, G., Sridhar, K., Raviraja, N. (2005). Antimicrobial and enzyme activity of mangrove endophytic fungi of southwest coast of India. J. Agric. Technol. 1, 67–80.
Marik, T., Tyagi, C., Balázs, D., Urbán, P., Szepesi, Á., Bakacsy, L., et al. (2019). Structural diversity and bioactivities of peptaibol compounds from the Longibrachiatum clade of the filamentous fungal genus Trichoderma. Front. Microbiol. 10, 44–62. doi: 10.3389/fmicb.2019.01434
Matarese, F., Sarrocco, S., Gruber, S., Seidl-Seiboth, V., Vannacci, G. (2012). Biocontrol of Fusarium head blight: interactions between Trichoderma and mycotoxigenic Fusarium. Microbiol. 158, 98–106. doi: 10.1099/mic.0.052639-0
Moat, J., Williams, J., Baena, S., Wilkinson, T., Gole, T. W., Challa, Z. K., et al. (2017). Resilience potential of the Ethiopian coffee sector under climate change. Nat. Plants 3, 1–14. doi: 10.1038/nplants.2017.81
Montesinos, E. (2003). Development, registration and commercialization of microbial pesticides for plant protection. Inter. Microbiol. 6, 245–252. doi: 10.1007/s10123-003-0144-x
Mulatu, A., Alemu, T., Megersa, N., Vetukuri, R. R. (2021). Optimization of culture conditions and production of bio-fungicides from Trichoderma species under solid-state fermentation using mathematical modeling. Microorganisms 9, 1675–1700. doi: 10.3390/microorganisms9081675
Mulatu, A., Megersa, N., Abena, T., Kanagarajan, S., Liu, Q., Alemu, T. T., et al. (2022a). Biodiversity of the genus Trichoderma in the rhizosphere of coffee (Coffea arabica) plants in Ethiopia and their potential use in biocontrol of coffee wilt disease. Crops 2, 120–141. doi: 10.3390/crops2020010
Mulatu, A., Megersa, N., Alemu, T. (2013). Characterization of fungal extracts from Trichoderma isolates: their effects against coffee wilt pathogen (Gibberella xylarioides). SINET: Ethiop. J. Sci. 36, 81–92.
Mulatu, A., Megersa, N., Tolcha, T., Alemu, T., Vetukuri, R. R. (2022b). Antifungal compounds, GC-MS analysis and toxicity assessment of methanolic extracts of Trichoderma species in an animal model. PloS One 17, e0274062. doi: 10.1371/journal.pone.0274062
Muleta, D., Assefa, F., Börjesson, E., Granhall, U. (2013). Phosphate-solubilising rhizobacteria associated with Coffea arabica l. in natural coffee forests of southwestern Ethiopia. J. Saudi Societ. Agric. Sci. 12, 73–84. doi: 10.1016/j.jssas.2012.07.002
Musoli, C. P., Pinard, F., Charrier, A., Kangire, A., Ten Hoopen, G. M., Kabole, C., et al. (2008). Spatial and temporal analysis of coffee wilt disease caused by Fusarium xylarioides in Coffea canephora. Europ. J. Plant Pathol. 122, 451–460. doi: 10.1007/s10658-008-9310-5
Mwajita, M. R. (2017). Isolation and rice plants response to bio-fertilizing and bio-enhancing isolates from Kenyan coastal, central and Western regions.Ph.D thesis (Nairobi, Kenya: Jomo Kenyata University of Agriculture and Technology).
Nagpure, A., Choudhary, B., Gupta, R. K. (2014). Mycolytic enzymes produced by Streptomyces violaceusniger and their role in antagonism towards wood-rotting fungi. J. Basic Microbiol. 54, 397–407. doi: 10.1002/jobm.201200474
Netsere, A., Kufa, T., Shimber, T. (2015). Review of arabica coffee nursery management research in Ethiopia. J. Biol. Agric. Healthcare 13, 2224–3208.
Nsabimana, A., Tirkaso, W. T. (2020). Examining coffee export performance in Eastern and southern African countries: do bilateral trade relations matter? Agrekon 59, 46–64. doi: 10.1080/03031853.2019.1631864
Putri, N., Sulistyowati, L., Aini, L., Muhibuddin, A., Trianti, I. (2022). Screening of endophytic fungi as potential antagonistic agents of Pyricularia oryzae and evaluation of their ability in producing hydrolytic enzymes. Biodiv. J. Biol. Div. 23, 55–69. doi: 10.13057/biodiv/d230248
Qi, W., Zhao, L. (2013). Study of the siderophore-producing Trichoderma asperellum Q1 on cucumber growth promotion under salt stress. J. Basic Microbiol. 53, 355–364. doi: 10.1002/jobm.201200031
Qualhato, T. F., Lopes, F. A. C., Steindorff, A. S., Brandao, R. S., Jesuino, R. S. A., Ulhoa, C. J. (2013). Mycoparasitism studies of Trichoderma species against three phytopathogenic fungi: evaluation of antagonism and hydrolytic enzyme production. Biotechnol. Lett. 35, 1461–1468. doi: 10.1007/s10529-013-1225-3
R Core Team. (2021). R: A language and environment for statistical computing. R Foundation for Statistical Computing, Vienna, Austria. Available at: https://www.R-project.org/.
Ramanujam, B., Prasad, R., Sriram, S., Rangeswaran, R. (2010). Mass production, formulation, quality control and delivery of Trichoderma for plant disease management. J. Plant Protect. Sci. 2, 1–8.
Rao, K., Raju, K. S., Ravisankar, H. (2016). Cultural conditions on the production of extracellular enzymes by Trichoderma isolates from tobacco rhizosphere. Braz. J. Microbiol. 47, 25–32. doi: 10.1016/j.bjm.2015.11.007
Reghmit, A., Benzina-Tihar, F., López Escudero, F. J., Halouane-Sahir, F., Oukali, Z., Bensmail, S., et al. (2021). Trichoderma species isolates from the rhizosphere of healthy olive trees in northern Algeria and their biocontrol potentials against the olive wilt pathogen, Verticillium dahliae. Org. Agric. 11, 639–657. doi: 10.1007/s13165-021-00371-1
Rojas-Avelizapa, L. I., Cruz-Camarillo, R., Guerrero, M., Rodríguez-Vázquez, R., Ibarra, J. (1999). Selection and characterization of a proteo-chitinolytic strain of Bacillus thuringiensis, able to grow in shrimp waste media. World J. Microbiol. Biotechnol. 15, 299–308. doi: 10.1023/A:1008947029713
Saber, W. I., Ghoneem, K. M., Rashad, Y. M., Al-Askar, A. A. (2017). Trichoderma harzianum WKY1: an indole acetic acid producer for growth improvement and anthracnose disease control in sorghum. Biocont. Sci. Technol. 27, 654–676. doi: 10.1080/09583157.2017.1321733
Sachdev, S., Singh, R. P. (2016). Current challenges, constraints and future strategies for development of successful market for biopesticides. Clim. Change Environ. Sustain. 4, 129–136. doi: 10.5958/2320-642X.2016.00014.4
Saravanakumar, K., Li, Y., Yu, C., Wang, Q.-Q., Wang, M., Sun, J., et al. (2017). Effect of Trichoderma harzianum on maize rhizosphere microbiome and biocontrol of Fusarium stalk rot. Sci. Rep. 7, 1–13. doi: 10.1038/s41598-017-01680-w
Saravanakumar, K., Yu, C., Dou, K., Wang, M., Li, Y., Chen, J. (2016a). Biodiversity of Trichoderma community in the tidal flats and wetland of southeastern China. PloS One 11, e0168020. doi: 10.1371/journal.pone.0168020
Saravanakumar, K., Yu, C., Dou, K., Wang, M., Li, Y., Chen, J. (2016b). Synergistic effect of Trichoderma-derived antifungal metabolites and cell wall degrading enzymes on enhanced biocontrol of Fusarium oxysporum f. sp. cucumerinum. Biol. Cont. 94, 37–46. doi: 10.1016/j.biocontrol.2015.12.001
Schirmböck, M., Lorito, M., Wang, Y.-L., Hayes, C. K., Arisan-Atac, I., Scala, F., et al. (1994). Parallel formation and synergism of hydrolytic enzymes and peptaibol antibiotics, molecular mechanisms involved in the antagonistic action of Trichoderma harzianum against phytopathogenic fungi. Appl. Environ. Microbiol. 60, 4364–4370. doi: 10.1128/aem.60.12.4364-4370.1994
Shrestha, R. (2019). Evaluation of trichoderma harzianum as a biocontrol agent on fusarium wilt of tomato grown in Eastern Nepal (Tribhuvan, Nepal: Ph.D Dissertation, Central Campus of Technology).
Singh, H., Singh, B. N., Singh, S., Sarma, B. (2013). Exploring different avenues of Trichoderma as a potent bio-fungicidal and plant growth promoting candidate-an overview. Ann. Rev. Plant Pathol. 15, 315–321. doi: 10.3390/microorganisms9081675
Sood, M., Kapoor, D., Kumar, V., Sheteiwy, M. S., Ramakrishnan, M., Landi, M., et al. (2020). Trichoderma: The “Secrets” of a multitalented biocontrol agent. Plants 9, 762–787. doi: 10.3390/plants9060762
Soytong, K. (1988). Identification of species of chaetomium in the Philippines and screening for their biocontrol properties against seed borne fungi of rice. MSc thesis (University of the Philippines, Manila, Philippines).
Strakowska, J., Błaszczyk, L., Chełkowski, J. (2014). The significance of cellulolytic enzymes produced by Trichoderma in opportunistic lifestyle of this fungus. J. Basic Microbiol. 54, 2–13. doi: 10.1002/jobm.201300821
Szekeres, A., Leitgeb, B., Kredics, L., Antal, Z., Hatvani, L., Manczinger, L., et al. (2005). Peptaibols and related peptaibiotics of Trichoderma. Acta Microbiol. Immunol. Hung. 52, 137–168. doi: 10.1556/AMicr.52.2005.2.2
Tefera, A., Tefera, T., Gray, Q. (2012). Coffee annual report (J. Global Agri. Info. Network (USAID), Addis Ababa, Ethiopia).
Teferi, D., Belachew, K. (2015). Evaluation of released arabica coffee varieties (Coffea arabica l) for major coffee diseases with especial emphasis to coffee wilt disease (Gibberella xylarioides) at jimma, Ethiopia. J. Biol. Agric. Healthcare 5, 81–86.
Temesgen Belayneh, M., Druzhinina, I. S., Kubicek, C. P., Atanasova, L. (2013). Novel endophytic Trichoderma spp. isolated from healthy Coffea arabica roots are capable of controlling coffee tracheomycosis. Diversity 5, 750–766. doi: 10.3390/d5040750
Tesfaye, T., Bizuayehu, T., Girma, A. (2020). Coffee production constraints and opportunities at major growing districts of southern Ethiopia. Cogent Food Agric. 6, 1741982. doi: 10.1080/23311932.2020.1741982
Thambugala, K. M., Daranagama, D. A., Phillips, A. J., Kannangara, S. D., Promputtha, I. (2020). Fungi vs. fungi in biocontrol: An overview of fungal antagonists applied against fungal plant pathogens. Front. Cell. Infect. Microbiol. 718. doi: 10.3389/fcimb.2020.604923
Thangavelu, R., Gopi, M. (2015). Combined application of native Trichoderma isolates possessing multiple functions for the control of Fusarium wilt disease in banana cv. grand naine. Biocont. Sci. Technol. 25, 1147–1164. doi: 10.1080/09583157.2015.1036727
Tiru, M., Muleta, D., Berecha, G., Adugna, G. (2013). Antagonistic effect of rhizobacteria against coffee wilt disease caused by Gibberella xylarioides. Asian J. Plant Pathol. 7, 109–122. doi: 10.3923/ajppaj.2013.109.122
Tsegaye, B. (2018). “Coffee production and climate change in Ethiopia,” in Sustainable agriculture reviews. Ed. Lichtfouse, E. (Berlin, Germany: Springer), 99–113.
Vega, F. E., Infante, F., Castillo, A., Jaramillo, J. (2009). The coffee berry borer, Hypothenemus hampei (Ferrari)(Coleoptera: Curculionidae): a short review, with recent findings and future research directions. Terr. Arthrop. Rev. 2, 129–147. doi: 10.1163/187498209X12525675906031
Vimala Devi, P., Duraimurugan, P., Chandrika, K., Vineela, V. (2021). Development of a water dispersible granule (WDG) formulation of Bacillus thuringiensis for the management of s (Fab.). Biocont. Sci. Technol. 31, 850–864. doi: 10.1080/09583157.2021.1895073
Vishwakarma, K, Kumar, N, Shandilya, C, Mohapatra, S, Bhayana, S, Varma, A (2020). Revisiting plant-microbe interactions and microbial consortia application for enhancing sustainable agriculture: a review.Front. Microbiol 11, 560406. doi: 10.3389/fmicb.2020.560406
Wei, F., Zhang, Y., Shi, Y., Feng, H., Zhao, L., Feng, Z., et al. (2019). Evaluation of the biocontrol potential of endophytic fungus Fusarium solani CEF559 against Verticillium dahliae in cotton plant. Biomed. Res. Intern. 1, 1–12. doi: 10.1155/2019/3187943
Woldetsadik, W., Kebede, K. (2000). Coffee production systems in Ethiopia. (Addis Ababa, Ethiopia: Ethiopian Agricultural Research Organization, Addis Ababa, Ethiopia).
Wu, Q., Sun, R., Ni, M., Yu, J., Li, Y., Yu, C., et al. (2017). Identification of a novel fungus, Trichoderma asperellum and comprehensive evaluation of its biocontrol efficacy. PloS One 12, e0179957. doi: 10.1371/journal.pone.0179957
Yu, P., Hochholdinger, F. (2018). The role of host genetic signatures on root–microbe interactions in the rhizosphere and endosphere. Front. Plant Sci. 9, 1896–1901. doi: 10.3389/fpls.2018.01896
Zamanizadeh, H. R., Hatami, N., Aminaee, M. M., Rakhshandehroo, F. (2011). Application of biofungicides in control of damping disease off in greenhouse crops as a possible substitute to synthetic fungicides. Inter. J. Environ. Sci. Technol. 8, 129–136. doi: 10.1007/BF03326202
Zeru, A., Assefa, F., Adugna, G., Hindorf, H. (2012). Occurrence of fungal diseases of Coffea arabica l. in montane rainforests of Ethiopia. J. Appl. Bot. Food Qual. 82, 148–151.
Keywords: bioassays, biocontrol agents, field evaluation, formulation, Trichoderma isolates
Citation: Mulatu A, Megersa N, Teferi D, Alemu T and Vetukuri RR (2023) Biological management of coffee wilt disease (Fusarium xylarioides) using antagonistic Trichoderma isolates. Front. Plant Sci. 14:1113949. doi: 10.3389/fpls.2023.1113949
Received: 01 December 2022; Accepted: 27 February 2023;
Published: 17 March 2023.
Edited by:
Islam A. Abd El-Daim, Agricultural Research Center (Egypt), EgyptReviewed by:
Abhijeet Shankar Kashyap, National Bureau of Agriculturally Important Microorganisms (ICAR), IndiaElsherbiny A. Elsherbiny, Mansoura University, Egypt
Copyright © 2023 Mulatu, Megersa, Teferi, Alemu and Vetukuri. This is an open-access article distributed under the terms of the Creative Commons Attribution License (CC BY). The use, distribution or reproduction in other forums is permitted, provided the original author(s) and the copyright owner(s) are credited and that the original publication in this journal is cited, in accordance with accepted academic practice. No use, distribution or reproduction is permitted which does not comply with these terms.
*Correspondence: Ramesh Raju Vetukuri, cmFtZXNoLnZldHVrdXJpQHNsdS5zZQ==