- 1School of Plant and Environmental Sciences, Virginia Tech, Blacksburg, VA, United States
- 2Department of Biochemistry, Virginia Tech, Blacksburg, VA, United States
The digestibility of soybean meal can be severely impacted by trypsin inhibitor (TI), one of the most abundant anti-nutritional factors present in soybean seeds. TI can restrain the function of trypsin, a critical enzyme that breaks down proteins in the digestive tract. Soybean accessions with low TI content have been identified. However, it is challenging to breed the low TI trait into elite cultivars due to a lack of molecular markers associated with low TI traits. We identified Kunitz trypsin inhibitor 1 (KTI1, Gm01g095000) and KTI3 (Gm08g341500) as two seed-specific TI genes. Mutant kti1 and kti3 alleles carrying small deletions or insertions within the gene open reading frames were created in the soybean cultivar Glycine max cv. Williams 82 (WM82) using the CRISPR/Cas9-mediated genome editing approach. The KTI content and TI activity both remarkably reduced in kti1/3 mutants compared to the WM82 seeds. There was no significant difference in terms of plant growth or maturity days of kti1/3 transgenic and WM82 plants in greenhouse condition. We further identified a T1 line, #5-26, that carried double homozygous kti1/3 mutant alleles, but not the Cas9 transgene. Based on the sequences of kti1/3 mutant alleles in #5-26, we developed markers to co-select for these mutant alleles by using a gel-electrophoresis-free method. The kti1/3 mutant soybean line and associated selection markers will assist in accelerating the introduction of low TI trait into elite soybean cultivars in the future.
Introduction
Soybean meal provides an excellent source of protein in animal feed since it is rich in amino acids with a high nutritional profile (Cromwell et al., 1991). For instance, soy makes up 26% and 50% of swine and poultry feed, respectively (Gillman et al., 2015). However, reduced feed efficiency has been observed due to anti-nutritional and biologically active factors in raw soybean seeds (Liener, 1996). Among these factors, trypsin inhibitor (TI) accounts for a substantial amount of this effect that cannot be ignored (Hymowitz, 1986). TI restrains the activity of trypsin in monogastric animals. Because this enzyme is essential for optimal protein digestion, its restriction can lead to animal growth inhibition of 30-50% due to pancreatic hypertrophy/hyperplasia when raw soybeans are used in feed (Hymowitz, 1986; Cook et al., 1988; Liener, 1994). In soybean meal processing facilities, TI in soybean meal is deactivated via a heating process at 90.5°C-100°C with the presence of 1% NaOH (Chen et al., 2014). This process not only reduces the nutritional value of soybean meal due to thermal destruction of amino acids, but also increases the energy cost of meal production by 25% (Chang et al., 1987).
With the recent increase in feed price and shipping cost, livestock farmers and grain operations are reconsidering soybean varieties with low-TI or TI-free traits as a way to reduce farm expenses by using raw soybeans as feed. Raising low-TI or TI-free soybeans on farms creates a niche market for integrated crop and livestock farmers, increasing their farm’s profitability. The use of soybean lines with genetically reduced levels of TI has proven as an effective strategy for improving animal growth. For instance, chicks fed soy-based diets with raw, unprocessed, low-KTI soymeal had higher feed efficiency ratios than chicks fed diets containing raw, unprocessed, conventional soybean meal (Batal and Parsons, 2004). Thus, soybean cultivars with low TI content in the seeds is a long-term breeding goal for higher protein digestibility, better economic benefits, reduced environmental pollution caused by phosphorus, and the pursuit of sustainability for humanity and nature.
Plants have evolved a group of TI genes encoding proteins that can suppress the enzyme activities of proteases found in in plants, herbivores, animals and human beings (Jofuku and Goldberg, 1989; Schuler et al., 1999). The TIs in soybean can be classified into two families: the 21 kDa Kunitz trypsin inhibitor protein family (KTI) and the 7-8 kDa Bowman-Birk inhibitor protein family (BBTI) (Kunitz, 1945; Wei, 1983; Gillman et al., 2015). KTI proteins are thought to be largely specific for trypsin inhibition, while the major isoform of BBTI contains domains that interact with and inhibit both trypsin and chymotrypsin (Hwang et al., 1977; Gillman et al., 2015). Currently, only the KTI genes are targeted for selection of low-TI soybeans because KTI serves as the major contributor to trypsin inhibitor activity in soybeans. By far, the most significant success in reducing TI activity in soybean was the identification of a soybean accession (PI 157740) with dramatically reduced (∼40%) TI activity (Gillman et al., 2015). A frameshift mutation that results in premature termination during translation of KTI3 (Gm08g341500) was identified in PI 157740, which is responsible for the low TI phenotype (Jofuku et al., 1989). This frameshift mutation is caused by the alteration of three nucleotides located at positions +481, +482, and +486. PI 157740 has been used in feeding trials, and it was found that raw extruded protein meal with lower KTI3 protein is superior for animal weight gain when compared to raw soybean meal harboring functional KTI3 (Cook et al., 1988; Perez-Maldonado et al., 2003). However, weight gain for young animals fed with non-heat-treated soybean materials including nonfunctional KTI3 soybean materials is still inferior to those fed with heat-treated soybeans (Cook et al., 1988; Perez-Maldonado et al., 2003). Another soybean germplasm accession (PI 68679) was identified to carry a nonfunctional mutation on KTI1 (Gm01g095000) gene, where a single base deletion which introduces (GGG356 → 358GG, relative to start codon) a frameshift mutation (Gillman et al., 2015). KTI1 and KTI3 genes were determined to be synergistically controlling the TI content in soybean seeds (Gillman et al., 2015). Therefore, it is desirable to breed new soybean cultivars carrying both kti1 and kti3 mutant alleles. However, it is time-consuming to breed low TI soybean cultivars by selecting progenies derived from crosses between PI 157740, PI 68679, and elite varieties. Besides, the linkage drags associated with KTI1 and KTI3 may introduce undesirable agronomic traits, which could be difficult to remove by backcrossing. Although Kompetitive Allele Specific PCR (KASP) markers associated with KTI3 and its mutant allele with 86% efficiency are available (Rosso et al., 2021), attempts at developing molecular markers associated with KTI1 have not been successful (Gillman et al., 2015). Therefore, it is highly desirable to develop new KTI1 mutant alleles that can be tagged with convenient molecular markers.
CRISPR/Cas9 mediated genome editing employs a Cas9 endonuclease and an 18-22 bp small guide RNA (sgRNA) that have a region that is complementary to a target gene sequence. The sgRNA binds to Cas9 and recruits the complex to target a gene. The Cas9 endonuclease generates DNA breaks, leading to mis-repaired target genes that contain deletions or insertions that disrupt gene function. In addition, several sgRNAs can be co-expressed in a single cell with Cas9, which allows the multiplex mutations of different genes simultaneously (Liang et al., 2016). Because genome edited plants without transgenes are not considered as genetically modified organisms (GMO) (Kim and Kim, 2016), mutant plants can be either directly released for field test or served as valuable resources for further breeding selection. Thus far, the CRISPR/Cas9 mediated genome editing technology has been widely used for targeted gene mutagenesis in diverse crop plant species, including soybean (Haun et al., 2014; Cai et al., 2015; Jacobs et al., 2015), rice (Xu et al., 2015), wheat (Upadhyay et al., 2013), maize (Chen et al., 2018), tomato (Vu et al., 2020), cotton (Gao et al., 2017), citrus (Peng et al., 2017), apple (Osakabe et al., 2018), grape (Osakabe et al., 2018), potato (Nakayasu et al., 2018), and banana (Shao et al., 2020) to improve their agronomic performances. For example, Jacobs et al. (2015) reported the first targeted mutagenesis in soybean using the CRISPR/Cas9 technology (Jacobs et al., 2015). Haun et al. (2014) generated a high oleic acid content soybean variety without transgenic components and improved the quality of soybean (Haun et al., 2014). A soybean mutant with a late flowering phenotype was created using CRISPR/Cas9 technology to knock out the GmFT2a gene (Cai et al., 2018). Thus far, there is no research reporting an attempt to apply CRISPR/Cas9 to target anti-nutritional factors in soybean.
In this study, we aimed to (1) simultaneously knockout KTI1 and KTI3 genes in soybean cultivar Williams 82 via CRISPR/Cas9-mediated genome editing and (2) develop molecular markers associated with kti1 and kti3 mutant alleles that can be used for marker-assisted selection (MAS). We successfully recovered transgenic soybean plants that are carrying both kti1 and kti3 mutations. KTI content and trypsin inhibition activities (TIA) are dramatically decreased in the kti1 and kti3 mutant lines. In addition, we also developed molecular markers for co-selection of the new kti1 and kti3 mutant alleles. These kti1 and kti3 mutant lines and the newly developed selection markers have great potential for breeding the low TI trait into elite soybean varieties in the future.
Results
KTI gene family consists of multiple members with distinct expression patterns
Soybean KTI genes belong to a gene family with 38 members (Figure S1). As displayed in the genetic map, 38 KTI genes are located on 9 chromosomes (Chr) in the soybean genome. Specifically, Chr1 carries 2 KTI genes; Chr3 carries 1 KTI gene; Chr6 carries 1 KTI gene; Chr8 carries 13 KTI genes; Chr8 carries 10 KTI genes; Chr12 carries 1 KTI gene; Chr16 carries 8 KTI genes; Chr18 carries 1 KTI gene; and Chr19 carries 1 KTI gene (Figure S1).
To identify the KTI genes expressed in the seed, we analyzed the expression patterns of all KTI genes in cv. WM82 based on the expression data acquired through the Gene Networks in Seed Development database (http://seedgenenetwork.net/sequence) (Figure 1A). According to the expression patterns of KTI genes in various soybean tissues as displayed in Figure 1A, four KTI genes (Gm01g095000 (KTI1), Gm08g341000, Gm08g342300, and Gm08g341500 (KTI3)) were identified as seed-specific KTI genes. Soybean breeding lines V98-9005 (normal TI) and V03-5903 (low TI), presenting significantly different amounts of KTI concentration in seeds, were used to validate the tissue specific expressions of the four KTI genes by real-time PCR. Gm01g095000 (KTI1) and Gm08g341500 (KTI3) were predominately expressed in seeds compared to other tissues (Figure 1B). Both Gm08g341000 and Gm08g342300 had a relatively lower expression level in seeds than KTI1 and KTI3 but had higher expression in other tissue types (Figure 1B). Interestingly, both KTI1 and KTI3 had a relatively low expression level in the seeds of V03-5903 (low TI line), but higher expression in V98-9005 (normal TI line). Thus, we conclude that KTI1 and KTI3 are two major genes that may directly contribute to the TI contents in soybean seeds.
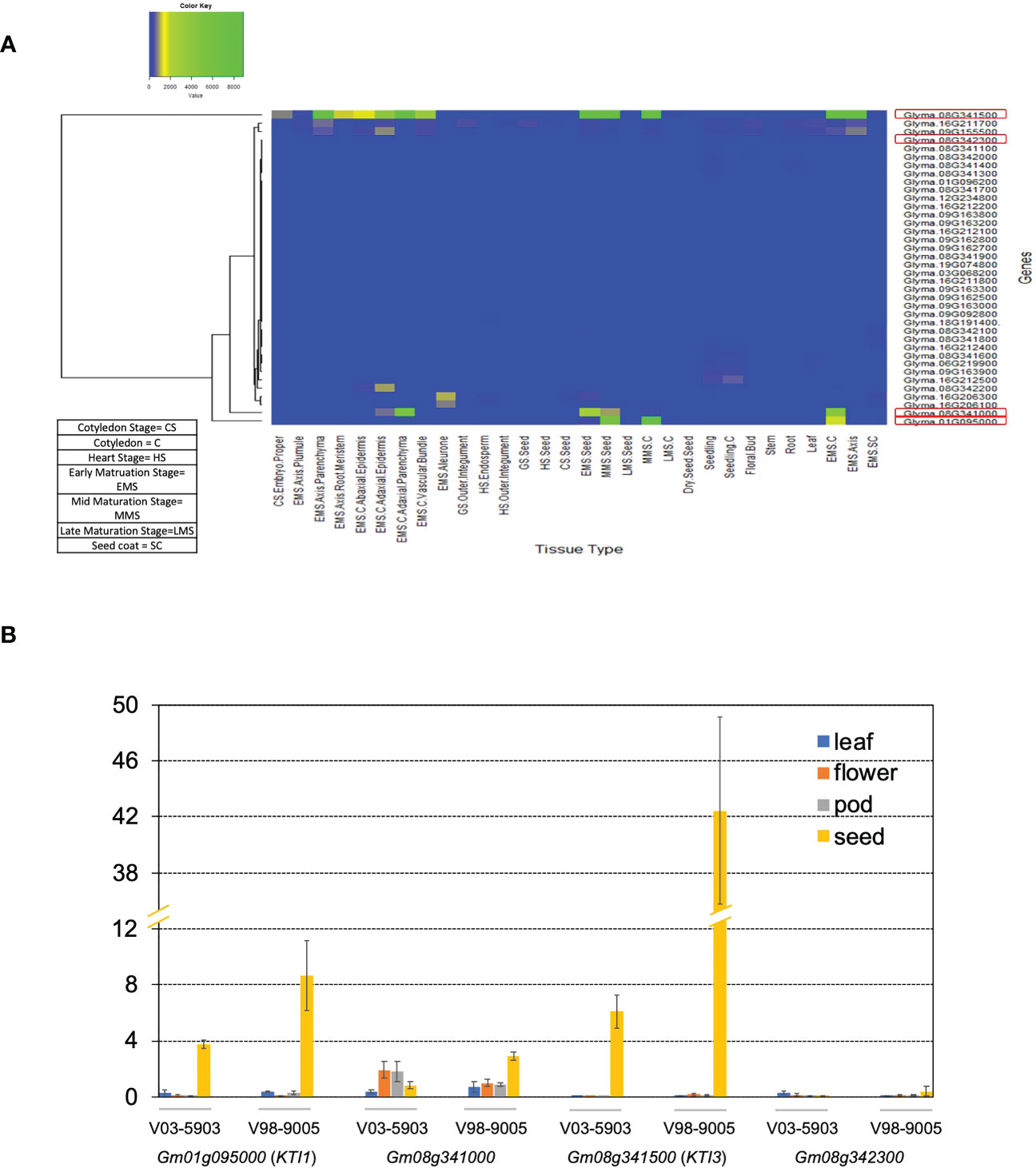
Figure 1 Expression levels of KTI genes in WM82. (A) RNA sequencing data of 38 KTI genes in 30 different tissue types of cv. Williams 82 acquired from Phytozome soybean database was used to construct the heatmap to visualize their expression patterns. (B) The expressions of four soybean KTI genes were monitored by real-time PCR. Samples of leaf, flower, pod, and seed tissues from 2 breeding lines, V98-9005 (normal-TI line) and V03-5903 (low-TI line), were collected for RNA extraction. After reverse transcription, real-time PCR was used to evaluate the expressions of 4 genes including Gm01g095000, Gm08g341000, Gm08g342300, and Gm08g341500 in different tissues with the ELF1B (Gm02g276600) as the reference gene. The expression data was normalized as ΔCT and shown as mean ± s.e. Experiments were repeated three times and obtained similar results.
Development of CRISPR/Cas9-based binary vector for genome-editing in soybean
To knock out the KTI1 and KTI3 genes from cv. WM82 genome and create a new soybean cultivar with low TI content in soybean seeds, we developed a CRISPR/Cas9 construct, pBAR-Cas9-kti13, where the nuclease gene Cas9 is expressed by Arabidopsis ubiquitin 10 (U10) promoter. A bar gene driven by a MAS (mannopine synthase) promoter was used for selection of the putative transformants with bialaphos or phosphinothricin (Figure 2A). A tandem array of two sgRNAs targeting KTI1 and one sgRNA targeting KTI3 was expressed by the U6 RNA promoter (Figure 2B).
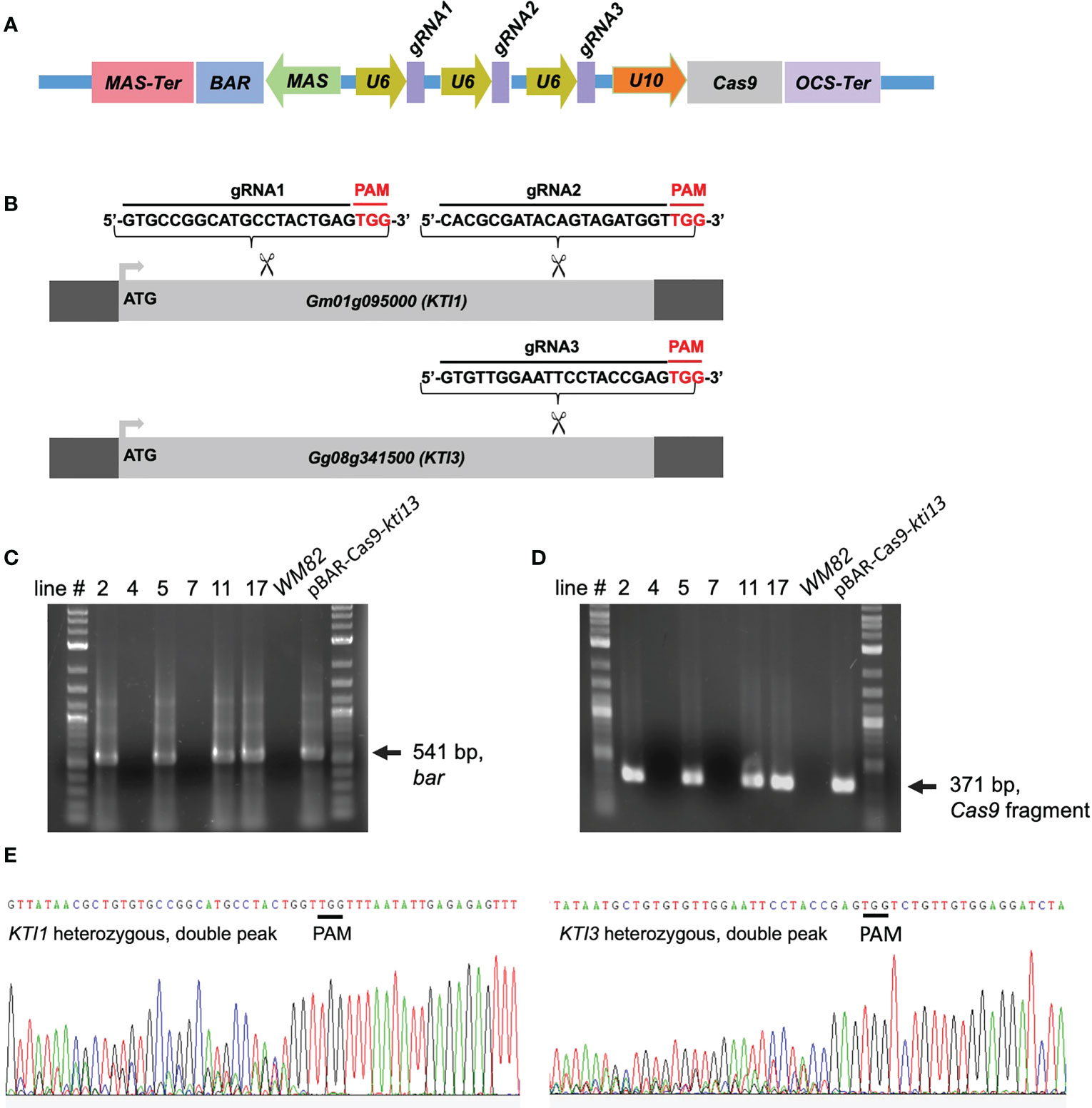
Figure 2 The scheme of binary vector used for CRISPR/Cas9 mediated gene editing on KTI1/KTI3, and transgenes and gene editing have been detected in the leaves of four T0 soybean plants. (A) The CRISPR/Cas9 construct harbors three necessary elements exhibited as below: the selection cassette consists of MAS promoter, Bar gene (soybean transformation selection marker), and MAS terminator; the Cas9 cassette consists of U10 promoter, Cas9 gene, and OCS terminator; three guide RNA cassettes and each of them consists of a U6 promoter, and one sgRNA. (B) The sequences of three sgRNAs is shown here. Two sgRNAs were designed, synthesized, and assembled to the plasmid to target on KTI1, while one sgRNA was designed, synthesized, assembled to the plasmid to target on KTI3. The fragments of two transgenes, (C) Cas9 and (D) Bar, have both been detected in lines #2, #5, #11, and #17 by PCR, but not lines #4 and #7. The WM82 gDNA serves as the template for negative control, while the plasmid DNA serves as the template for positive control. (E) The gene editing on KTI1 and KTI3 has also been observed in the leaf tissues of plants at T0 generation. The double peak sequence around the sgRNA region indicates the gene editing was ongoing but not completed.
KTI1 and KTI3 genes are knocked out by CRISPR/Cas9 mediated gene editing
pBAR-Cas9-kti1/kti3 was transformed into WM82 via Agrobacterium-mediated transformation (Plant Transformation Facility at Iowa State University). Seventeen putative transgenic shoots were regenerated. Six shoots elongated and were transferred to rooting mediums. After further selection, they were transplanted into soil. Four lines, No. #2, #5, #11 and #17 were confirmed to be true transformants by positive amplification of the bar gene and a part of the Cas9 gene (Figures 2C, D). The gene editing events in T0 plants were identified by amplification and sequencing of DNA fragments covering the sgRNA binding sites of KTI1 and KTI3. The double peaks in the sequencing chromatograms suggest that both KTI1 and KTI3 genes were mutated and resulted in heterozygous alleles in the edited plant cells (Figure 2E). T0 seeds were harvested from T0 lines #2, #5, #11 and #17. Four T0 seeds of each line were randomly picked for DNA extraction and genotyping of the KTI1 and KTI3 genes via PCR amplification and DNA sequencing. The KTI1 gene editing was completed and resulted in homozygous mutant alleles in all tested T0 seeds of the four lines. In addition, an identical gene editing pattern in KTI1 was detected in all tested T0 seeds, in which a small DNA fragment (66bp) between two sgRNAs was lost after the gene editing (Figures 3A–C). Homozygous KTI3 mutant alleles were only detected in T0 seeds from 2-3, #5-4, #11-2, and #11-4 (Figures 3D–G). The gene editing patterns in KTI3 included both small deletions and insertions that all resulted in frameshift mutations in KTI3.
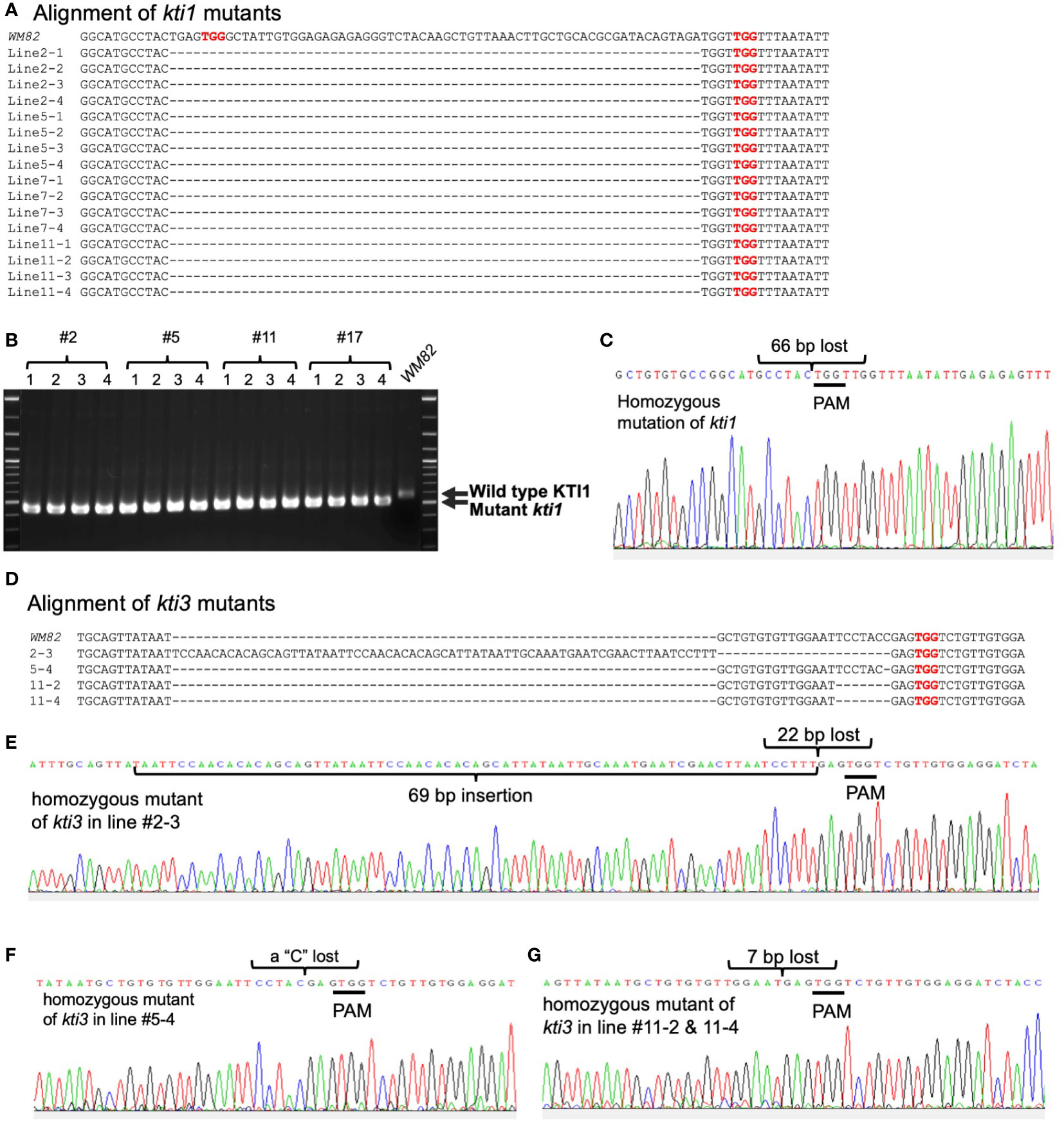
Figure 3 Gene editing on KTI1 has been completed for all seeds of T0 generation while it has been completed on KTI3 for some seeds of T0 generation. From each transgenic line (#2, #5, #11 and #17), four seeds of T0 generation were selected randomly for genotyping. (A) The gr of mutant kti1 in T0 seeds and T1 plant (#5-26) leaf, where the wild type KTI1 in WM82 was the control. (B) Gel electrophoresis of kti1 PCR products showed 16 seeds from line #2, #5, #11, and #17 had the same mutant on kti1, in which 66 nucleotides are lost between two sgRNAs. (C) Sanger sequencing result displayed the identical mutant kti1. (D) The alignment of mutant kti3 in T0 seeds (#2-3, #5-4, #5-26, #11-2 and #11-4) and T1 plant (#5-26) leaf, where the wild type KTI1 in WM82 was the control. (E–G) showed the sanger sequencing results of kti3 mutant in #2-3, #5-4, #11-2, #11-4, and #5-26.
TI content and activity dramatically declined in the edited soybean seeds
T0 seeds were also used for quantification of the KTI content by using a HPLC-based approach (Rosso et al., 2018). The tested seeds of #2-3, #5-4, #11-2, and #11-4, which carried mutations on both KTI1 and KTI3 genes had the lowest KTI content (Figure 4). The tested seeds of #2-1, #5-1, #11-1, and #17-1, with only the KTI1 mutation, also had lower KTI content than the wild-type WM82 seeds (Figure 4). The KTI content in other genotyped seeds with editing only on KTI1 was also lower than that in WM82 seeds (data not shown). We further tested the trypsin inhibition activity (TIA) using crude protein extracts from the T0 seeds. As shown in Figure 5, the crude proteins of seeds with mutant kti1 and kti3 had the lowest TIA. The seeds with mutant kti1 only also had reduced TIA (Figure 5) in comparison with WM82 and Glenn (a commercial soybean cultivar as a control). The KTI content and TIA were ranked in order as: kti1/3 double mutant < kti1 single mutant ≤ PI 547656 (low TI accession) < WM82 < Glenn. Taken together, we conclude that KTI1 and KTI3 are two major genes responsible for the KTI content and TIA in soybean seeds. Therefore, knockout of KTI1 and KTI3 reduced the KTI content and impaired the TIA in soybean seeds.
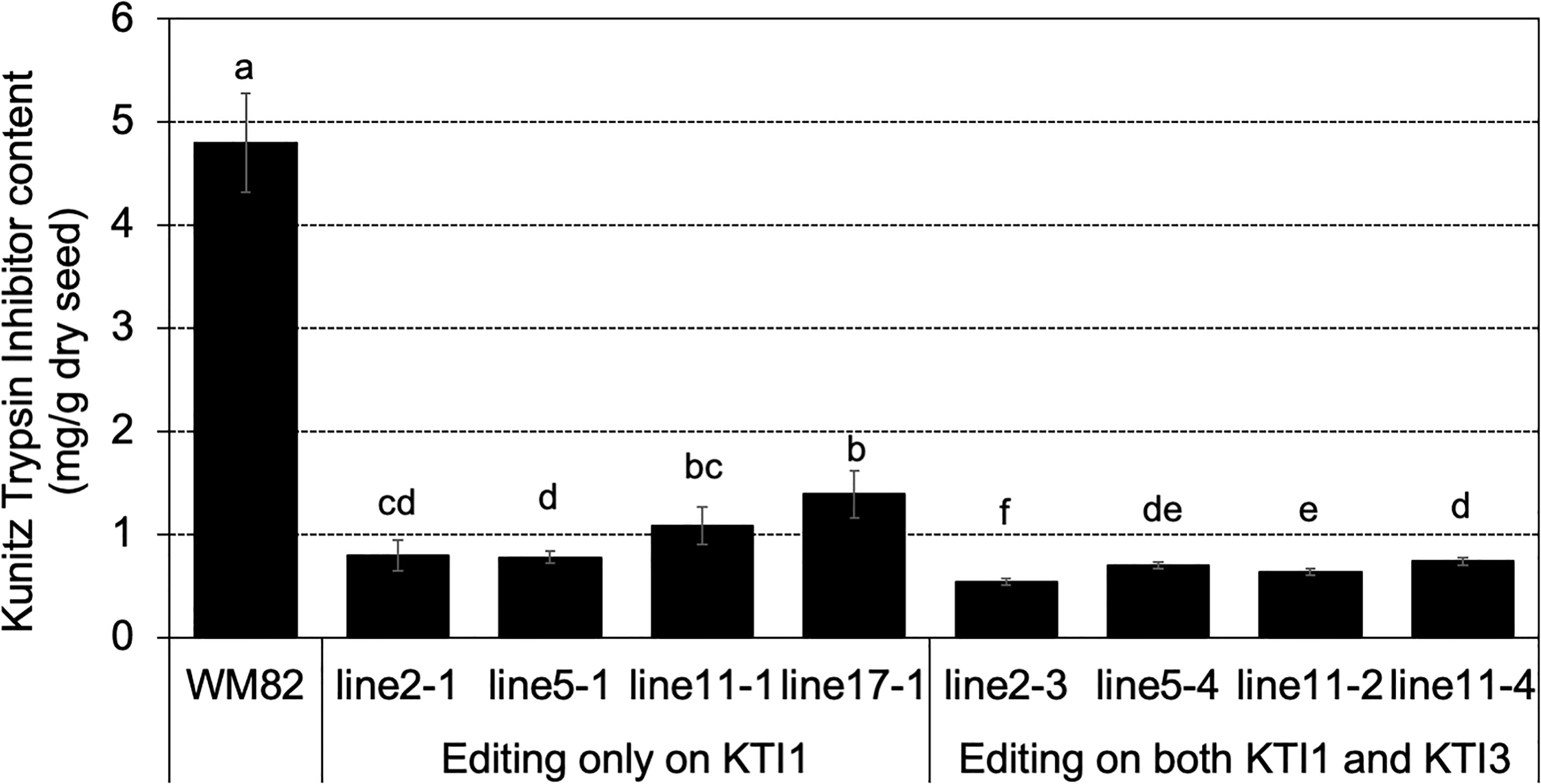
Figure 4 KTI content declined dramatically in gene-edited seeds. KTI content was measured in 4 double mutated seeds (#2-3, #5-4, #11-2, and #11-4) and 4 seeds with a single mutation on KTI1 (#2-1, #5-1, #11-1, and #17-1), where the KTI content in WM82 seed served as the control. Experiments were conducted with three technical replicates and showed comparable results, shown as mean ± s.e. Different letters indicate significant differences.
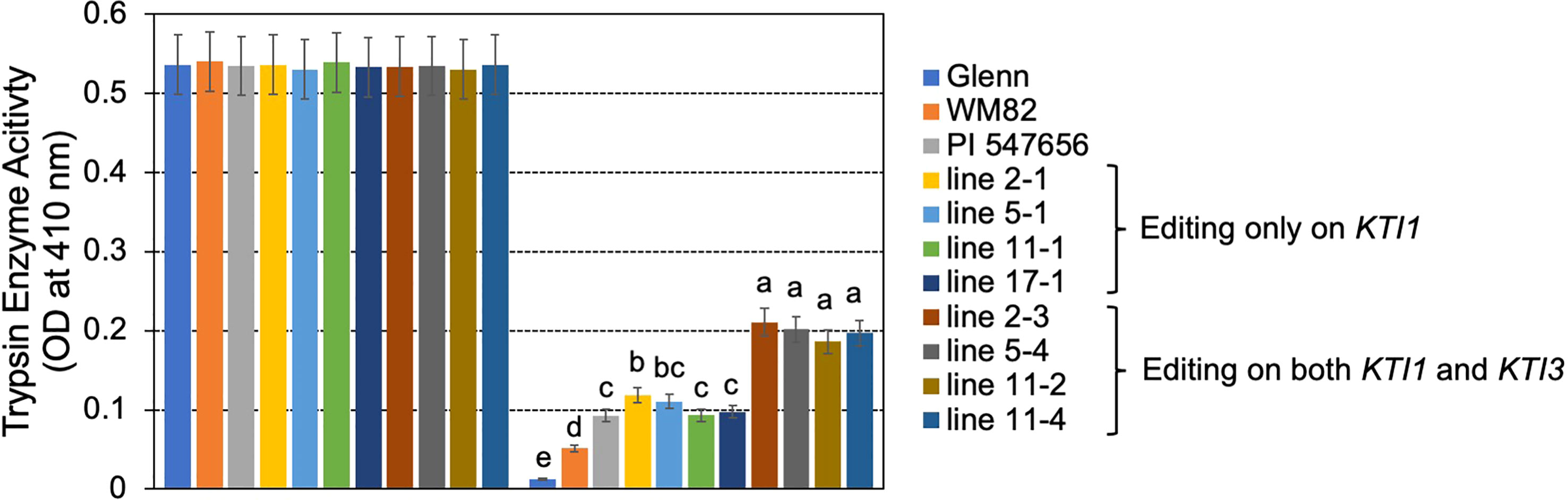
Figure 5 TIA declined dramatically in the gene-edited seeds. Bovine trypsin enzyme activities were measured using crude extracts of 4 double mutated seeds (#2-3, #5-4, #11-2, and #11-4), 4 seeds with a single mutation on KTI1 (#2-1, #5-1, #11-1, and #17-1), and WM82. Experiments were conducted with three technical replicates and showed comparable results, shown as mean ± s.e. Different letters indicate significant differences.
The edited KTI1 gene lost 66 bp that may result in mutant proteins with deletion of 22 amino acids. Truncated KTI1 may still possess some TIA. To rule out this possibility, we also tested the TIA of truncated KTI1Δ22aa protein in vitro. To this end, we cloned the open reading frames of KTI1Δ66bp and wild-type KTI1 and KTI3 into a protein expression vector, in which a 6xHis tag is fused to C-terminus of the expressed proteins. The purified proteins were subjected to a TIA assay which showed that while KTI1 and KTI3 both could inhibit trypsin activity, the truncated KTI1Δ22aa failed to suppress trypsin activity (Figures S2A, B). Therefore, the new kti1 allele (KTI1Δ66bp) encodes a truncated protein that loses its TI function.
Knockout KTI1 and KTI3 did not affect plant growth and maturity period days of soybean
To examine whether the mutant kti1/3 could significantly affect plant growth and maturity period days of soybean, we planted T0 seeds of line #2 and #5, and WM82 in a greenhouse. By bialaphos-mediated screening, we classified the T1 plants from line #2 and #5 as transgene-free plants or transgenic plants. We measured the agronomic traits of the transgenic plants including plant height, the number of main branches per plant, number of pods bearing branches, number of pods, leaf length, leaf width and petiole length. There was no significant difference in terms of all measured agronomic traits among the plants of WM82, Line 2 and Line 5 (Table 1). We also measured the maturity period days of the soybean plants by recording the dates from planting to beginning bloom (R1), to beginning pod (R3), to beginning seed (R5), to full seed (R6), to maturity (R8), and the total lifespan (from planting to maturity). There were no remarkable differences in terms of R1, R3, R5, R6, R8 and total life span among all tested plants (Table 1). Therefore, we conclude that knockout of KTI1 and KTI3 did not alter plant growth or the maturity period of soybean lines tested.
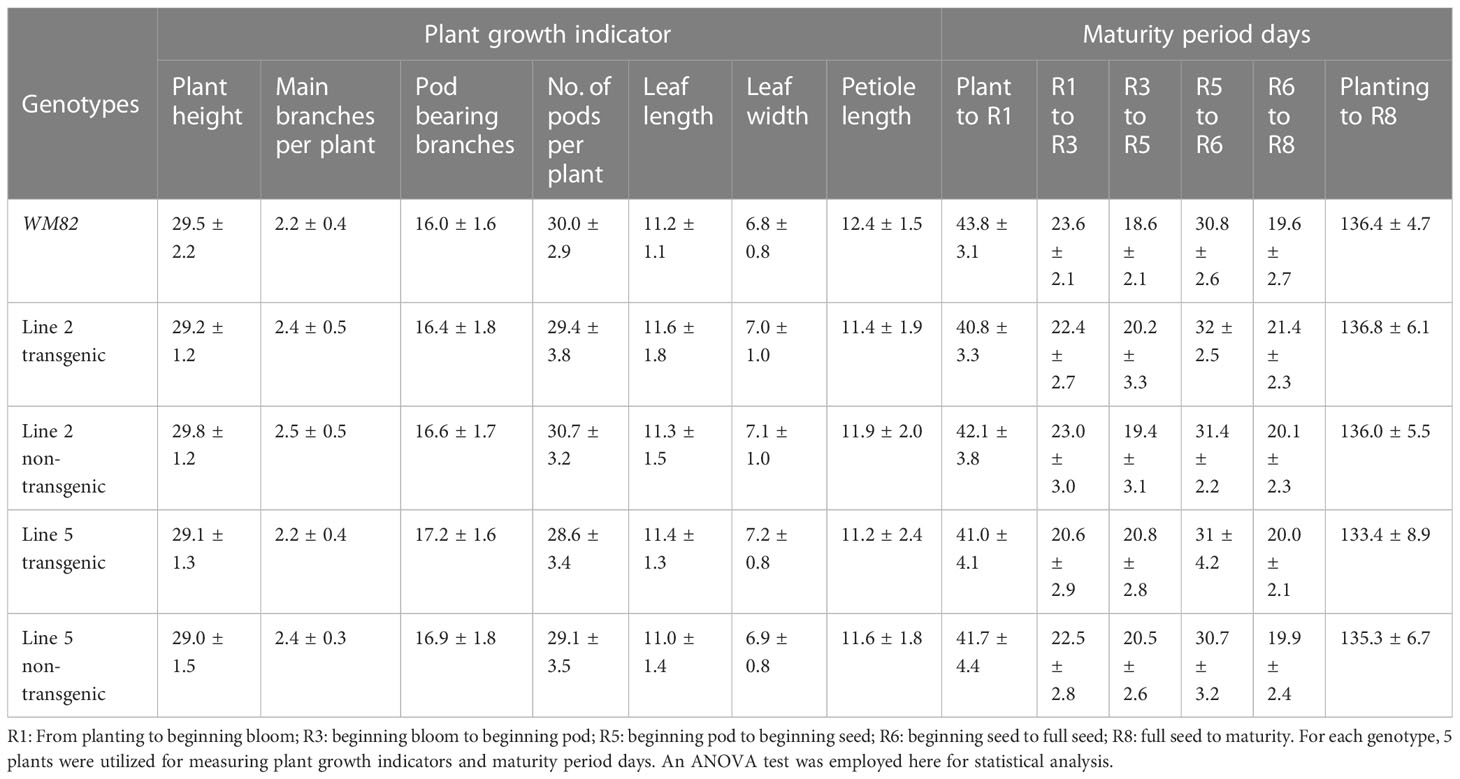
Table 1 Knockout of KTI1 and KTI3 does not alter the plant growth indicator and maturity period days of cv. WM82.
Development of molecular markers for selection of the kti1 and kti3 alleles
A double homozygous kti1 and kti3 mutant plant #5-26 that did not carry the Cas9 transgene was selected from T1 generation plants (Figures 3A, C, D). The ‘transgene-free’ soybean plants can be used to breed the low TI trait into other elite soybean cultivars. In order to co-select the kti1 and kti3 mutant alleles in the derived progenies, we attempted to develop co-dominate molecular markers that can distinguish between wild-type KTI1/KTI3 and mutant kti1/kti3 alleles.
In the genome of #5-26, the mutant allele of kti1 had a 66 bp deletion. We designed three PCR primers, ZW1, ZW2 and ZW3 (Figure 6A and Table S2). ZW1 was a common reverse primer that can bind to the same region in both KTI1 and kti1 alleles, while ZW2 and ZW3 were both forward primers binding to unique sequences of KTI1 and kti1 alleles, respectively (Figure 6A). Soybean cultivars that carry the wild-type KTI1 gene (WM82) amplified a 180 bp DNA fragment when hybridized with ZW1 and ZW2, but failed to amplify any fragments when hybridized with ZW1 and ZW3. On the contrary, the soybean lines carrying a homozygous kti1 mutant allele (#5-26) amplified a 134 bp DNA fragment with ZW1 and ZW3, but failed to amplify any fragments with ZW1 and ZW2 (Figure 6B).
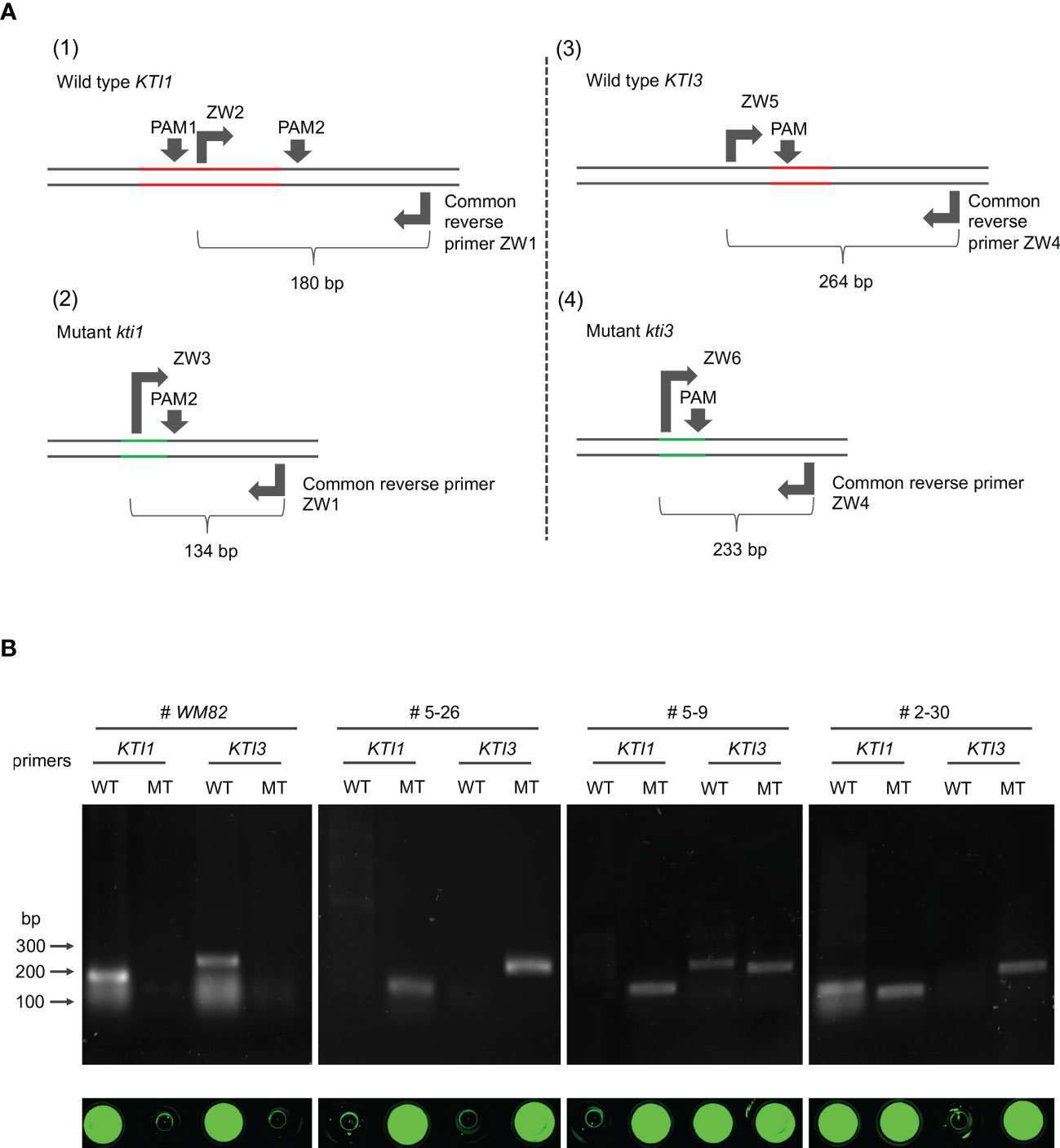
Figure 6 The development of selection markers for breeding low KTI soybean varieties based on the kti1 and kti3 mutants generated by CRISPR/Cas9-mediated gene editing. (A) Schematic development of primers for amplification of wild type KTI1 (1), mutant KTI1 (2), wild type KTI3 (3), and mutant KTI3 (4). The red lines indicate the lost fragment in KTI1 or KTI3 during gene editing. The green lines indicate new DNA regions in kti1 or kti3 generated by splicing two fragments. (B) The 4 pairs of primers in (A) were utilized to amplify the alleles of KTI1, kti1, KTI3, and kti3 with gDNA of four different soybean genotypes, including WM82, three transgenic lines #5-26, #5-9, and #2-30. Based on our genotyping data, #5-26 has homozygous mutations of kti1 and kti3; #5-9 only has a homozygous mutation of kti1 but carries the heterozygous mutation of kti3; #2-30 only has a homozygous mutation of kti3 but carries the heterozygous mutation of kti1. Thus, it was clear that the pair of ZW1/ZW2 can amplify wild type KTI1 from WM82 and #2-30 gDNA in PCR tests, while the pair of ZW1/ZW3 can amplify mutant kti1 from #5-9 and #5-26 gDNA. Also, the pair of ZW4/ZW5 can amplify wild type KTI3 from WM82 and #5-9 gDNA, while ZW4/ZW6 can amplify mutant kti3 from #2-30 and #5-26 gDNA. As shown in the bottom panel, only the positive PCR products incubated with the dye of sybrgreen at 75°C can display the fluorescent signals, suggesting the reliability of the developed gel-electrophoresis-free method for screening mutant alleles of kti1 and kti3.
In the genome of line #5-26, the mutant kti3 allele had a 38 bp deletion, which allowed us to design PCR primers ZW4, ZW5 and ZW6 (Figure 6A and Table S2). ZW4 was a common reverse primer for both KTI3 and kti3, while ZW5 and ZW6 were forward primers matched with unique sequences of KTI3 and kti3, respectively (Figure 6A). A soybean cultivar carrying the wild-type KTI3 gene (MW82) amplified a 264 bp DNA fragment with primers ZW4 and ZW5, but not with primers ZW4 and ZW6. In contrast, the soybean line carrying homozygous kti3 allele (#5-26) can amplify a 233 bp DNA fragment with primers ZW4 and ZW6, but not ZW4 and ZW5 (Figure 6B).
We further tested these PCR primers by amplifying DNA fragments from two T1 plants that were genotyped by DNA sequencing. Line #5-9 had homozygous kti1 alleles and heterozygous KTI3/kti3 alleles, where the kti3 allele was identical to the one in #5-26 (Figure S3). Line #2-30 had homozygous kti3 alleles and heterozygous KTI1/kti1 alleles, where the kti1 allele was identical to the one in #5-26 (Figure S3). As shown in Figure 6B, PCR amplification with the different combinations of ZW1, ZW2, ZW3, ZW4, ZW5 and ZW6 can accurately identify the KTI1/kti1 and KTI3/kti3 genotypes of #5-9 and #2-30 (Figure 6B). Therefore, we successfully developed molecular markers to select the kti1 and kti3 mutant alleles generated by CRISPR/Cas9 mediated mutagenesis. These molecular markers can assist in the breeding selection of low TI soybean plants harboring kti1/3.
To simplify the procedure of marker-aided selection, we tested a gel-electrophoresis-free protocol that can be implemented for high throughput screening of progenies derived from a cross between a soybean cultivar carrying wild-type KTI1/3 and one carrying the kti1/3 mutant. In brief, all PCR products as described above were mixed with 1X SYBR Green and heated at 75°C for 10 mins and then visualized under UV light. As shown in Figure 6B, the fluorescent signals were the indications of positive amplifications in WM82 with primers ZW1/ZW2 and ZW4/ZW5, while in #5-26, the fluorescent signals can only be observed with primers ZW1/ZW3 and ZW4/ZW6 (Hirotsu et al., 2010). Therefore, we identified the homozygous kti1 and kti3 alleles by directly staining the PCR products without the need of gel electrophoresis, which can significantly reduce the cost of labor and time.
Discussion
In this study, we optimized a CRISPR/cas9-vector for genome editing in soybean (Figure 2A). The modified vector allowed us to simultaneously knock out two seed specific KTI genes (KTI1 and KTI3). The kti1/3 mutant plants grew normally in greenhouse conditions, and the seeds of kti1/3 mutant had dramatically reduced KTI content and TI activities in comparison with wild type seed of WM82.
Soybean is one of the important sources of protein for animal and human consumption. However, in their evolution, soybeans have developed diverse defense components to protect seeds from being eaten by insects and animals including trypsin inhibitor, phytate acid, and raffinose family of oligosaccharides (RFOs). In the agricultural practice, the anti-nutritional and biologically active factors are responsible for reduced feed efficiency when raw soybeans are fed to animals. Therefore, it is of great significance to increase feed efficiency, especially the protein digestibility via assembling gene function exploration, application, and advanced genetically engineering together into the soybean industry. Proteinaceous plant trypsin inhibitors are a diverse family of (poly)peptides that play diverse roles in plant growth such as maintaining physiological homeostasis and serving the innate defense machinery (Li et al., 2008; Junker et al., 2012; Arnaiz et al., 2018; Zhao et al., 2019). Since TI proteins exert direct effects on pests and herbivores by interfering with their physiology, any food containing TI proteins will be avoided by these organisms. In alfalfa, the trypsin inhibitors Msti-94 and Msti-16 were demonstrated to act as a stomach poison, significantly reducing the survival and reproduction rates of aphid (Zhao et al., 2019). Mutant plants with reduced TI are usually more susceptible to pests. In wheat, α-amylase/trypsin inhibitors (ATIs) CM3 and 0.19 were identified as pest-resistance molecules, activating innate immune responses in monocytes, macrophages, and dendritic cells (Junker et al., 2012). For example, the Arabidopsis lines containing silenced atkti4 and atkti5 were found to have a higher susceptibility to T. urticae (Spider mite) than wild-type plants (Arnaiz et al., 2018). RNAi silencing of the AtKTI01 gene resulted in enhanced lesion development after infiltration of leaf tissue with the programmed cell death eliciting fungal toxin fumonisin B1 or the avirulent bacterial pathogen Pseudomonas syringae pv. tomato DC3000 carrying avrB (Li et al., 2008). Although similar defense functions have not been reported on TI genes in the soybean genome, it is reasonably suspected that certain members of the KTI gene family have comparable protecting roles for soybean plants.
As previously discussed, the high concentration of TI proteins in soybean meal restricts the function of trypsin, which causes low digestibility and reduces its nutritional value. Thus, cultivars have been developed by introgression of low TI traits into elite cultivars. We previously developed a low TI line via conventional breeding: V12-4590. During field trials in 2017 and 2018, we observed that this low TI line is indeed more susceptible to multiple phytopathogens such as: all races of Soybean Cyst Nematode (SCN) (Heterodera glycines), Stem Canker (Diaporthe aspalathi), Cercospora leaf blight (Cercospora kukuchii), Soybean vein necrosis virus, and Downy Mildew (Peronospora manshurica) (Zhang, unpublished data). This suggests that that the soybean KTI genes that are negatively selected do indeed have a role in plant immunity. It is also possible that some plant immunity related genes that genetically link with KTI genes are negatively selected during the breeding process. As shown in Figure S1, at least 13 KTI genes are clustered in a small region on chromosome 8. Interestingly, we also identified a putative TGACG-Binding (TGA) transcription factor (TF) that is tightly linked to the KTI gene cluster at Chr 8. Arabidopsis TGA TFs play a positive role in systemic acquired resistance (SAR) that is crucial in plant immunity (Hussain et al., 2018). Therefore, breeding of low TI soybean lines resulting in the loss or mutation of both of the KTI genes and the TGA TF gene, leading to an increased susceptibility of soybean challenged by phytopathogens.
The kti1/3 mutant soybean line generated via CRISPR/Cas9-mediated mutagenesis is an isogenic line of wild type WM82 (Table 1). Therefore, it will be an ideal test subject to see if KTI1/3 has a direct role in plant immunity. Since KTI1/3 were almost only expressed in seeds (Figure 1) (Gillman et al., 2015), the knockout of these two genes may not interfere with plant immunity in non-seed tissue, which deserves to be further investigated in the future.
In this study, we identified kti1/3 double homozygous mutants as well as kti1 single homozygous mutants. Our findings indicate that the kti1/3 double homozygous mutant exhibits the lowest levels of both KTI content and trypsin inhibition activity, as illustrated in Figures 4, 5. It is noteworthy that a significant reduction in KTI content was observed in seeds where only KTI1 was fully edited, implying that most of the wild-type KTI3 alleles in those seeds had been mutated. These results indicate that KTI1 and KTI3 act in synergy to contribute to the KTI content and TI activity in soy proteins. The previous report suggests that the soybean line carrying natural mutations of kti1 and kti3 has increased BBTI content (Gillman et al., 2015). It is unclear if the increased BBTI content is caused by un-intentional selection during the breeding process or if the expression of BBTI genes is increased because of the mutations of two KTI genes. Therefore, it will be interesting to test the BBTI content and activity in the seed proteins of the kti1/3 mutant generated in this study.
Despite the fact that the CRISPR/Cas9 technique has been successfully utilized to generate various soybean mutants, the current agrobacterium-mediated soybean transformation protocol is inefficient and genotype dependent. This limits the wide implementation of CRISPR/Cas9 technique in soybean breeding programs (Yamada et al., 2012). The soybean transformation protocol employs bialaphos as the selection agent (Luth et al., 2015). The Bar gene is used as the selection marker gene and encodes a phosphinothricin acetyltransferase protein that can confer the transformants’ resistance to bialaphos. It has been reported that the Bar gene expression must be fine-tuned in order to successfully select true transgenic plants (Testroet et al., 2017). The original CRISPR/Cas9 vector, pCut, has used a MAS promoter to express the Bar gene (Peterson et al., 2016). However, for unknown reasons, the vector does not work well, even in Arabidopsis thaliana (Liu and Zhao, unpublished data).
With the intention of improving the transformation system, we modified the bialaphos selection vector in the pMU3T (Liu et al., 2016). Specifically, we replaced the Kanamycin selection marker gene with the Bar gene, whose expression was driven by a MAS promoter (Figure 2A). The MAS promoter is known to be most active in the roots of emerging seedlings and very active in the cotyledons and lower leaves (Langridge et al., 1989). Despite the MAS promoter having a lower level of expression than p35S, populations of transformants created with this promoter show normally distributed expression levels (Perez-Gonzalez and Caro, 2019). Thus, the MAS promoter can be used for functional screening of positive transformants in both of our shoot re-generation and rooting medium supplemented with bialaphos.
It is noteworthy that, before the initiation of stable transformation, we evaluated the effectiveness of gRNAs by using a convenient Agrobacterium-mediated transient assay method (Wang et al., 2023). As soybean plants have a long-life cycle (4-6 months), the estimation of the gRNAs’ effectiveness helps to avoid the waste of time and enhance the possibility of obtaining authentic gene-edited plants.
Although the soybean cultivars with natural variations on either KTI1 (PI 68679) or KTI3 (PI 542044) have been discovered, conventional breeding to develop new cultivars stacking with two mutant alleles via crossing will take a long time. In addition, linkage drag might lead to interference with the functions of genes located at the flanking sequences of mutant kti1 or kti3. The limited genetic background of natural kti1 and kti3 mutants may also reduce the genetic diversity of soybean breeding lines with low TI trait, and it can be difficult to stack low TI trait with a bundle of various, desirable traits. In the present study, the kti1/3 mutant line was created using cv. WM82, which has a genetic background distinct from accessions that harbor natural kti1 and/or kti3 mutations. Therefore, it offers a new recourse for breeding low TI traits in soybean practice.
Current soybean transformation protocol is genotype dependent, and only a few cultivars (WM82, Jack, Thorne, etc.) can be efficiently transformed (Yamada et al., 2012). A mutant allele must be created in those transformable cultivars and bred into other elite cultivars via marker-assisted selection (MAS). Thus, creating a mutant allele tagged with convenient molecular markers is essential for MAS (Hasan et al., 2021). CRIPSR/Cas9-based genome editing can introduce small deletions/insertions to targeted genes, enabling us to develop molecular markers based on the sequences of the insertion and deletion mutation regions. In this study, we tested using single sgRNA and two sgRNAs for generation of mutagenesis on KTI1 and KTI3, respectively (Figure 2B). Interestingly, we observed that all genotyped mutant lines carried an identical gene editing pattern of the kti1 gene, where 66 nucleotides between the two gRNAs were deleted. The homozygous kti1 allele can be identified in all tested seeds of the T0 generation, while homozygous kti3 alleles were identified in some of those genotyped T0 seeds (Figure 3). Therefore, it is possible that two sgRNAs are more efficient for triggering the gene editing events in early generations of transgenic plants.
MAS has been widely implemented in plant breeding including soybean programs (Hasan et al., 2021). The selection marker of kti3 has been developed based on its natural mutant allele, but the molecular marker for the natural mutation of kti1 in PI 68679 is still not available (Gillman et al., 2015). Therefore, it is challenging to breed the natural kti1/3 mutant alleles into a new cultivar via MAS. In this study, we created co-dominant markers that can distinguish between the wild and mutant alleles of KTI1/KTI3 and kti1/kti3 based on small deletions created by CRISPR/Cas9 machinery (Figure 6). In addition, a simple gel-electrophoresis-free method can be used to identify plants carrying mutant kti1 and kti3 alleles (Hirotsu et al., 2010). Thus, MAS makes it possible to effectively breed the new mutant kti1/3 alleles into other elite cultivars. Taken together, the whole experimental design may serve as a practical example of how to create and select mutant alleles in crop plants in the future.
Conclusions
The present study developed non-transgenic, low TI soybean mutant in cv. William 82. The mutant gene alleles are tagged with convenient molecular markers that are suitable for high throughput marker-aided selection. We expect the low TI soybean mutant will be widely used to breed low-TI or TI-free soybean cultivars for commercial production in value-added meal industry and for stacking with other valuable agronomic traits in the future.
Methods
Plant materials and growth conditions
Soybean plants were grown in 2.5-gallon pots using Miracle-Gro all-purpose potting soil mix in Keck Greenhouse at Virginia Tech (14h/10h light/dark cycle at 25°C/20°C) for the experiments described herein. The plants were watered by an automatic irrigation system. Soybean transformation was performed at the plant transformation facility at Iowa State University as previously described (Paz et al., 2006; Luth et al., 2015; Ge et al., 2016). The plant growth indicators and maturity period days of WM82 and progeny plants of the T1 generation derived from lines #2 and #5 were measured in the green house. The 4-week-old T1 soybean plants were used for genotyping. The seeds of T1 plants were used for seed weight analysis.
Constructing a soybean KTI gene map
The gene map showing locations of KTI genes on soybean chromosomes was made using MapInspect. Locations of all KTI genes were obtained from the Phytozome database (https://phytozome-next.jgi.doe.gov/) and plotted on their respective chromosomes.
Bacterial growth
E. coli strains DH5α and C41 (DE3) (Lucigen, Middleton, WI) were grown on Luria agar medium at 37°C. Agrobacterium tumefaciens (A. tumefaciens) EHA105 was grown on Luria agar medium at 28°C (Zhao et al., 2011; Traore et al., 2019). E. coli antibiotic selections used in this study were as follows: 50 μg/ml kanamycin, 100 μg/ml carbenicillin, 100 μg/ml spectinomycin. A. tumefaciens antibiotic selection were 100 μg/ml rifampicin, and/or 100 μg/ml spectinomycin.
Cloning
The open reading frames (ORFs) of KTI1 and KTI3, were amplified from the genomic DNA of WM82. The KTI1Δ66bp, truncated ORF of KTI1, was amplified from the genomic DNA of mutant soybean plant #2-1. All PCR primers with annotations are listed in Table S2. The genes/fragments were then cloned into a pDonr207 plasmid (Thermo Fisher Scientific) for future use.
T1 plant genomic DNA (gDNA) was used as the templates to amplify KTI1 and/or its mutant allele, and KTI3 and/or its mutant allele by PCR. The purified PCR fragments were used for genotyping by Sanger sequencing at Virginia Tech Genomic Sequencing Center and cloned to the PCR8/GW/TOPO vector by TA cloning (Invitrogen) for molecular marker tests.
In order to apply the CRISPR/Cas9 system to gene editing in soybean, we modified our current CRISPR/Cas9 construct (Liu et al., 2016). The cassette consists of a MAS promoter, the bialaphos resistant gene, and a MAS terminator that was amplified using plasmid DNA of pEarleyGate101 as the template. All PCR primers with annotations are listed in Table S2. The cassette was assembled to the backbone of CRISPR/Cas9 construct using Gibson Assembly® Cloning Kit (New England Biolabs Inc). Since 38 KTI genes in soybean share conserved sequences, an alignment was conducted for these genes to design gRNAs that can exclusively target on KTI1 and KTI3. The gRNAs were synthesized in one cassette at GenScript Biotech Corp. The backbone of the new CRISPR/Cas9 construct and the fragment of gRNAs were assembled together using Gibson Assembly® Cloning Kit.
Expression analysis of KTI genes in WM82
RNA sequencing data, in FPKM (fragments per kilobase of transcript per million fragments mapped), of 38 KTI genes in 30 different tissue types from Williams 82 were acquired through the Gene Networks in Seed Development database (http://seedgenenetwork.net/sequence). Construction of the heatmap to visualize expression data was done using the heatmap.2 function from the ggplot2 package in R. A green/blue color gradient was chosen to show expression with blue representing little to no expression and green representing high expression. The code for the heatmap is as follows:
heatmap.2(x=KTI Expression, main = “KTI Expression In Different Soybean Tissue”, notecol=“black”, density.info=“none”, trace=“none”, margins =c(12,9), col=my_palette, breaks=col_breaks, dendrogram=“row”,Colv=“NA”, ylab= “Genes”, xlab= “Tissue Type”, cexCol=.9,cexRow = .8)
RNA isolation and real-time PCR
All RNA was extracted from V98-9005 and V03-5903 seeds using TRIzol reagent (Thermo Fisher Scientific) according to the manufacturer’s instructions. Any DNA residue was eliminated by treating with UltraPure DNase I (Thermo Fisher Scientific). The integrity and quantity of total RNA were determined by electrophoresis in 1% agarose gel and a NanoDrop ND-1000 spectrophotometer (NanoDrop Technologies, Wilmington, DE). cDNA synthesis was performed using the SuperScript III First-Strand RT-PCR Kit (Thermo Fisher Scientific) with an oligo-dT primer based on the manufacturer’s instructions. Real-time PCR was conducted with cDNA as the template using the Quantitect SYBR Green PCR kit (Qiagen) according to the manufacturer’s protocol. Oligo primers are listed in Table S2. The soybean ELF1B gene (Gm02g276600) was used as reference gene, and data is presented as ΔCT (Jian et al., 2008).
Expression and purification of KTI1, KTI1Δ22aa, and KTI3 proteins
The KTI1, KTI1Δ66bp, and KTI3 genes in pDonr207 were subcloned into a Gateway compatible pET28a destination vector via a LR® Gateway cloning kit (Thermo Fisher Scientific) (Earley et al., 2006; Liu et al., 2016). The plasmids were transformed into E. coli C41 cells (Lucigen). KTI1, KTI1Δ22aa, and KTI3 proteins were expressed and purified following a procedure as previously described (Liu et al., 2020). Protein purity was evaluated by SDS-PAGE. The protein concentration was determined by a protein assay kit (Bio-Rad) using bovine serum albumin as standard (Han et al., 2015).
Standard bioassay to measure trypsin inhibitor activity
A TI activity bioassay was performed following American Association of Cereal Chemists Official Method 22-40 (AACC, 1999) with some modifications previously reported (Rosso et al., 2018). Briefly, 30 mg of finely ground soybean seed powder was mixed with 3 mL of 9 mM HCl (pH 2.0). The mixture was shaken for 1 h at room temperature. 2 mL of the extracts was centrifuged at 10,350 rpm for 20 min at room temperature, and the supernatant was diluted by 10 times with 9 mM HCl for measuring TI activity. A TI activity assay was performed in a 96-well plate format following the same steps described previously (Rosso et al., 2018). Each sample row was repeated three times. Portions of diluted HCl extracts (0, 20, 30, 40, and 60 µL) or 50 µg recombinant proteins of KTI1, KTI1Δ22aa, and KTI3 were pipetted into the microplate wells, and the volume was adjusted to 60 µL with 9 mM HCl. 60 µL of extractant was used as a sample blank and 60 µL of water were used as a substrate blank. 60 µL of trypsin (from bovine pancreas, Sigma-Aldrich T8003) solution was added to each sample well, and the microplates were placed in an oven at 37°C for 15 min. After the incubation, 150 µL of BAPNA substrate pre-warmed at 37°C was added to all wells, and the plates were incubated for exactly 10 min at 37°C. The reaction was stopped by adding 30 µL of acetic acid solution to all wells. The absorbance of each well was read on a plate reader (FLOUstar Omega, BMG Labtech) at 410 nm for 30 s after shaking at 700 rpm.
HPLC method to quantify Kunitz trypsin inhibitor
The HPLC method to quantify KTI was performed following the method developed previously (Rosso et al., 2018). Briefly, 10 mg of finely ground soybean seed powder was mixed with 1.5 mL of 0.1 M sodium acetate buffer (pH 4.5). Samples were vortexed and shaken for 1 h at room temperature. The sample was centrifuged at 12,000 rpm for 15 min. 1mL of the supernatant was filtered through a syringe with an IC Millex-LG 13-mm mounted 0.2-mm low protein binding hydrophilic millipore (polytetrafluoroethylene [PTFE]) membrane filter (Millipore Ireland). The KTI in solution was separated on an Agilent 1260 Infinity series (Agilent Technologies) equipped with a guard column (4.6 x 5 mm) packed with POROS R2 10-mm Self Pack Media and a Poros R2/H perfusion analytical column (2.1 x 100 mm, 10 um). The mobile Phase A consisted of 0.01% (v/v) trifluoroacetic acid in Milli-Q water, and the mobile Phase B was 0.085% (v/v) trifluoroacetic acid in acetonitrile. The injection volume was 10 uL and the detection wavelength was 220 nm.
Development of molecular selection markers with a gel electrophoresis free method for high throughput screening
The transgene free and double homozygous mutant line, #5-26, was selected for the development of molecular selection markers. Based on the genotyping data of #5-26, two pairs of markers were designed: ZW1 with ZW2 or ZW3. ZW1 is the common reverse primer for both KTI1 and kti1, while ZW2 and ZW3 are two reverse primers matched with unique sequences in KTI1 and kti1, respectively. Similarly, two pairs of molecular markers, ZW4 with ZW5 or ZW6, were designed. ZW4 is the common reverse primer for both KTI3 and kti3, while ZW5 and ZW6 are two reverse primers matched with unique sequences in KTI3 and kti3, respectively. The gDNA of WM82, #5-26 (homozygous mutants of both kti1 and kti3), #5-9 (homozygous mutant of kti1 while heterozygous mutant of kti3) and #2-30 (homozygous mutant of kti3 while heterozygous mutant of kti1) were used as templates to test the efficiency and reliability of these markers in PCR.
PCR amplifications were performed in a total volume of 20 μl containing 50 ng of gDNA, 0.5 μM each of forward and reverse primers (Table S2), 10 μl 2X BioMix Red (Bioline) and ddH2O. The PCR program was set to be 95°C for 5 min for pre-denature, followed by 35 cycles of denaturation at 95°C for 30 s, annealing at 55°C for 30 s, extension at 72°C for 30 s, followed by final extension at 72°C for 5 min.
In order to screen the large-scale progenies derived from crosses between soybean elite cultivars carrying wild type KTI1/3 and the newly developed mutant plant carrying kti1/3, a simple gel electrophoresis-free method was designed. 1X sybrgreen dye (Thermo Fisher) was added to complete PCR reactions, and the solution was incubated for 10 mins at 75°C before placing in the gel doc (Biorad) for imaging the fluorescent signals. Only the positive PCR products with the dye will display fluorescent signals while the failed PCR will not show signals.
Statistical data analysis
Analytical experiments were performed with at least three technical replicates. Statistical significance was based on one-way ANOVA test for multiple comparisons. Data was analyzed using JMP Pro14. Values of P<0.05 were considered significant.
Data availability statement
The datasets presented in this study can be found in online repositories. The names of the repository/repositories and accession number(s) can be found in the article/Supplementary Material.
Author contributions
BYZ and ZW designed the experiments and analyzed data. ZW performed experiments, analyzed data, and wrote the manuscript. ZS, LR, CS, JL, and PB also performed experiments. BYZ, BZ, ZS, LR, and PB edited the manuscript. BZ supervised the project. All authors contributed to the article and approved the submitted version.
Funding
This work was supported by the Virginia Soybean Board (#467059) and a seed grant from the Translational Plant Science Center at Virginia Tech. An integrated internal competitive grant from the College of Agriculture and Life Sciences at Virginia Tech and Virginia Agricultural Experiment Station (VA160144).
Conflict of interest
The authors declare that the research was conducted in the absence of any commercial or financial relationships that could be construed as a potential conflict of interest.
Publisher’s note
All claims expressed in this article are solely those of the authors and do not necessarily represent those of their affiliated organizations, or those of the publisher, the editors and the reviewers. Any product that may be evaluated in this article, or claim that may be made by its manufacturer, is not guaranteed or endorsed by the publisher.
Supplementary material
The Supplementary Material for this article can be found online at: https://www.frontiersin.org/articles/10.3389/fpls.2023.1111680/full#supplementary-material
Supplementary Figure 1 | Physical mapping of 38 GmKTI genes on soybean 20 chromosomes. The gene map showing locations of KTI genes on soybean chromosomes was made using MapInspect. As displayed in the map, 38 KTI genes are located on 9 out of 20 chromosomes.
Supplementary Figure 2 | SDS-PAGE of purified recombinant proteins and in-frame mutated protein of KTI1Δ22aa nearly lost the TIA. (A) SDS-PAGE was used to assess the purity of three recombinant proteins, KTI1, KTI3, and KTI1Δ22aa. (B) Purified proteins of KTI1 and KTI3, but not KTI1Δ22aa were able to inhibit the trypsin activity in vivo. Experiments were conducted with three technical replicates and obtained similar results.
Supplementary Figure 3 | Sequence information of KTI1 and/or KTI3 in the T1 plants used for the development of selection markers. (A) The alignment of mutant kti1 in T1 plant leaves (#5-9 and #5-26), where the wild type KTI1 in WM82 was the control. (B) The alignment of mutant kti3 in T1 plant leaves (#2-30 and #5-26), where the wild type KTI1 in WM82 was the control.
References
Arnaiz, A., Talavera-Mateo, L., Gonzalez-Melendi, P., Martinez, M., Diaz, I., Santamaria, M. E. (2018). Arabidopsis kunitz trypsin inhibitors in defense against spider mites. Front. Plant Sci. 9, 986. doi: 10.3389/fpls.2018.00986
Batal, A. B., Parsons, C. M. (2004). Utilization of various carbohydrate sources as affected by age in the chick. Poultry Sci. 83, 1140–1147. doi: 10.1093/ps/83.7.1140
Cai, Y., Chen, L., Liu, X., Guo, C., Sun, S., Wu, C., et al. (2018). CRISPR/Cas9-mediated targeted mutagenesis of GmFT2a delays flowering time in soya bean. Plant Biotechnol. J. 16, 176–185. doi: 10.1111/pbi.12758
Cai, Y., Chen, L., Liu, X., Sun, S., Wu, C., Jiang, B., et al. (2015). CRISPR/Cas9-mediated genome editing in soybean hairy roots. PloS One 10, e0136064. doi: 10.1371/journal.pone.0136064
Chang, C. J., Tanksley, T. D., Knabe, D. A., Jr., Zebrowska, T. (1987). Effects of different heat treatments during processing on nutrient digestibility of soybean meal in growing swine. J. Anim. Sci. 65, 1273–1282. doi: 10.2527/jas1987.6551273x
Chen, R., Xu, Q., Liu, Y., Zhang, J., Ren, D., Wang, G., et al. (2018). Generation of transgene-free maize Male sterile lines using the CRISPR/Cas9 system. Front. Plant Sci. 9, 1180. doi: 10.3389/fpls.2018.01180
Chen, Y., Xu, Z., Zhang, C., Kong, X., Hua, Y. (2014). Heat-induced inactivation mechanisms of kunitz trypsin inhibitor and bowman-birk inhibitor in soymilk processing. Food Chem. 154, 108–116. doi: 10.1016/j.foodchem.2013.12.092
Cook, D. A., Jensen, A. H., Fraley, J. R., Hymowitz, T. (1988). Utilization by growing and finishing pigs of raw soybeans of low kunitz trypsin inhibitor content. J. Anim. Sci. 66, 1686–1691. doi: 10.2527/jas1988.6671686x
Cromwell, G. L., Stahly, T. S., Monegue, H. J. (1991). Amino acid supplementation of meat meal in lysine-fortified, corn-based diets for growing-finishing pigs. J. Anim. Sci. 69, 4898–4906. doi: 10.2527/1991.69124898x
Earley, K. W., Haag, J. R., Pontes, O., Opper, K., Juehne, T., Song, K., et al. (2006). Gateway-compatible vectors for plant functional genomics and proteomics. Plant J. 45, 616–629. doi: 10.1111/j.1365-313X.2005.02617.x
Gao, W., Long, L., Tian, X., Xu, F., Liu, J., Singh, P. K., et al. (2017). Genome editing in cotton with the CRISPR/Cas9 system. Front. Plant Sci. 8, 1364. doi: 10.3389/fpls.2017.01364
Ge, L., Yu, J., Wang, H., Luth, D., Bai, G., Wang, K., et al. (2016). Increasing seed size and quality by manipulating BIG SEEDS1 in legume species. Proc. Natl. Acad. Sci. United States America 113, 12414–12419. doi: 10.1073/pnas.1611763113
Gillman, J. D., Kim, W. S., Krishnan, H. B. (2015). Identification of a new soybean kunitz trypsin inhibitor mutation and its effect on bowman-birk protease inhibitor content in soybean seed. J. Agric. Food Chem. 63, 1352–1359. doi: 10.1021/jf505220p
Han, Q., Zhou, C., Wu, S., Liu, Y., Triplett, L., Miao, J., et al. (2015). Crystal structure of xanthomonas AvrRxo1-ORF1, a type III effector with a polynucleotide kinase domain, and its interactor AvrRxo1-ORF2. Structure 23, 1900–1909. doi: 10.1016/j.str.2015.06.030
Hasan, N., Choudhary, S., Naaz, N., Sharma, N., Laskar, R. A. (2021). Recent advancements in molecular marker-assisted selection and applications in plant breeding programmes. J. Genet. Eng. Biotechnol. 19, 128. doi: 10.1186/s43141-021-00231-1
Haun, W., Coffman, A., Clasen, B. M., Demorest, Z. L., Lowy, A., Ray, E., et al. (2014). Improved soybean oil quality by targeted mutagenesis of the fatty acid desaturase 2 gene family. Plant Biotechnol. J. 12, 934–940. doi: 10.1111/pbi.12201
Hirotsu, N., Murakami, N., Kashiwagi, T., Ujiie, K., Ishimaru, K. (2010). Protocol: a simple gel-free method for SNP genotyping using allele-specific primers in rice and other plant species. Plant Methods 6, 12. doi: 10.1186/1746-4811-6-12
Hussain, R. M. F., Sheikh, A. H., Haider, I., Quareshy, M., Linthorst, H. J. M. (2018). Arabidopsis WRKY50 and TGA transcription factors synergistically activate expression of PR1. Front. Plant Sci. 9. doi: 10.3389/fpls.2018.00930
Hwang, D. L., Foard, D. E., Wei, C. H. (1977). A soybean trypsin inhibitor. crystallization and x-ray crystallographic study. J. Biol. Chem. 252, 1099–1101. doi: 10.1016/S0021-9258(19)75211-9
Hymowitz, T. (1986). Genetics and breeding of soybeans lacking the kunitz trypsin inhibitor. Adv. Exp. Med. Biol. 199, 291–298. doi: 10.1007/978-1-4757-0022-0_18
Jacobs, T. B., Lafayette, P. R., Schmitz, R. J., Parrott, W. A. (2015). Targeted genome modifications in soybean with CRISPR/Cas9. BMC Biotechnol. 15, 16. doi: 10.1186/s12896-015-0131-2
Jian, B., Liu, B., Bi, Y., Hou, W., Wu, C., Han, T. (2008). Validation of internal control for gene expression study in soybean by quantitative real-time PCR. BMC Mol. Biol. 9, 59. doi: 10.1186/1471-2199-9-59
Jofuku, K. D., Goldberg, R. B. (1989). Kunitz trypsin inhibitor genes are differentially expressed during the soybean life cycle and in transformed tobacco plants. Plant Cell 1, 1079–1093. doi: 10.1105/tpc.1.11.1079
Jofuku, K. D., Schipper, R. D., Goldberg, R. B. (1989). A frameshift mutation prevents kunitz trypsin inhibitor mRNA accumulation in soybean embryos. Plant Cell 1, 567. doi: 10.1105/tpc.1.4.427
Junker, Y., Zeissig, S., Kim, S. J., Barisani, D., Wieser, H., Leffler, D. A., et al. (2012). Wheat amylase trypsin inhibitors drive intestinal inflammation via activation of toll-like receptor 4. J. Exp. Med. 209, 2395–2408. doi: 10.1084/jem.20102660
Kim, J., Kim, J. S. (2016). Bypassing GMO regulations with CRISPR gene editing. Nat. Biotechnol. 34, 1014–1015. doi: 10.1038/nbt.3680
Kunitz, M. (1945). Crystallization of a trypsin inhibitor from soybean. Science 101, 668–669. doi: 10.1126/science.101.2635.668
Langridge, W. H., Fitzgerald, K. J., Koncz, C., Schell, J., Szalay, A. A. (1989). Dual promoter of agrobacterium tumefaciens mannopine synthase genes is regulated by plant growth hormones. Proc. Natl. Acad. Sci. United States America 86, 3219–3223. doi: 10.1073/pnas.86.9.3219
Li, J., Brader, G., Palva, E. T. (2008). Kunitz trypsin inhibitor: an antagonist of cell death triggered by phytopathogens and fumonisin b1 in arabidopsis. Mol. Plant 1, 482–495. doi: 10.1093/mp/ssn013
Liang, G., Zhang, H., Lou, D., Yu, D. (2016). Selection of highly efficient sgRNAs for CRISPR/Cas9-based plant genome editing. Sci. Rep. 6, 21451. doi: 10.1038/srep21451
Liener, I. E. (1994). Implications of antinutritional components in soybean foods. Crit. Rev. Food Sci. Nutr. 34, 31–67. doi: 10.1080/10408399409527649
Liener, I. E. (1996). Effects of processing on antinutritional factors in legumes: the soybean case. Archivos Latinoamericanos Nutricion 44, 48S–54S.
Liu, Y., Miao, J., Traore, S., Kong, D., Liu, Y., Zhang, X., et al. (2016). SacB-SacR gene cassette as the negative selection marker to suppress agrobacterium overgrowth in agrobacterium-mediated plant transformation. Front. Mol. Biosci. 3, 70. doi: 10.3389/fmolb.2016.00070
Liu, Y., Wang, K., Cheng, Q., Kong, D., Zhang, X., Wang, Z., et al. (2020). Cysteine protease RD21A regulated by E3 ligase SINAT4 is required for drought-induced resistance to pseudomonas syringae in arabidopsis. J. Exp. Bot. 71, 5562–5576. doi: 10.1093/jxb/eraa255
Luth, D., Warnberg, K., Wang, K. (2015). Soybean [Glycine max (L.) merr]. Methods Mol. Biol. 1223, 275–284. doi: 10.1007/978-1-4939-1695-5_22
Nakayasu, M., Akiyama, R., Lee, H. J., Osakabe, K., Osakabe, Y., Watanabe, B., et al. (2018). Generation of alpha-solanine-free hairy roots of potato by CRISPR/Cas9 mediated genome editing of the St16DOX gene. Plant Physiol. Biochem. 131, 70–77. doi: 10.1016/j.plaphy.2018.04.026
Osakabe, Y., Liang, Z., Ren, C., Nishitani, C., Osakabe, K., Wada, M., et al. (2018). CRISPR-Cas9-mediated genome editing in apple and grapevine. Nat. Protoc. 13, 2844–2863. doi: 10.1038/s41596-018-0067-9
Paz, M. M., Martinez, J. C., Kalvig, A. B., Fonger, T. M., Wang, K. (2006). Improved cotyledonary node method using an alternative explant derived from mature seed for efficient agrobacterium-mediated soybean transformation. Plant Cell Rep. 25, 206–213. doi: 10.1007/s00299-005-0048-7
Peng, A., Chen, S., Lei, T., Xu, L., He, Y., Wu, L., et al. (2017). Engineering canker-resistant plants through CRISPR/Cas9-targeted editing of the susceptibility gene CsLOB1 promoter in citrus. Plant Biotechnol. J. 15, 1509–1519. doi: 10.1111/pbi.12733
Perez-Gonzalez, A., Caro, E. (2019). Benefits of using genomic insulators flanking transgenes to increase expression and avoid positional effects. Sci. Rep. 9, 8474. doi: 10.1038/s41598-019-44836-6
Perez-Maldonado, R. A., Mannion, P. F., Farrell, D. J. (2003). Effects of heat treatment on the nutritional value of raw soybean selected for low trypsin inhibitor activity. Br. Poultry Sci. 44, 299–308. doi: 10.1080/0007166031000085463
Peterson, B. A., Haak, D. C., Nishimura, M. T., Teixeira, P. J., James, S. R., Dangl, J. L., et al. (2016). Genome-wide assessment of efficiency and specificity in CRISPR/Cas9 mediated multiple site targeting in arabidopsis. PloS One 11, e0162169. doi: 10.1371/journal.pone.0162169
Rosso, M. L., Shang, C., Correa, E., Zhang, B. (2018). An efficient HPLC approach to quantify kunitz trypsin inhibitor in soybean seeds. Crop Sci. 58, 1616–1623. doi: 10.2135/cropsci2018.01.0061
Rosso, M. L., Shang, C., Song, Q., Escamilla, D., Gillenwater, J., Zhang, B. (2021). Development of breeder-friendly KASP markers for low concentration of kunitz trypsin inhibitor in soybean seeds. Int. J. Mol. Sci. 22(5): 2675. doi: 10.3390/ijms22052675
Schuler, T. H., Poppy, G. M., Kerry, B. R., Denholm, I. (1999). Potential side effects of insect-resistant transgenic plants on arthropod natural enemies. Trends Biotechnol. 17, 210–216. doi: 10.1016/S0167-7799(98)01298-0
Shao, X., Wu, S., Dou, T., Zhu, H., Hu, C., Huo, H., et al. (2020). Using CRISPR/Cas9 genome editing system to create MaGA20ox2 gene-modified semi-dwarf banana. Plant Biotechnol. J. 18, 17–19. doi: 10.1111/pbi.13216
Testroet, A., Lee, K., Luth, D., Wang, K. (2017). Comparison of transformation frequency using the bar gene regulated by the CaMV 35S or NOS promoter in agrobacterium-mediated soybean (Glycine max l.) transformation. In Vitro Cell. Dev. Biol. - Plant 53, 188–199. doi: 10.1007/s11627-017-9810-0
Traore, S. M., Eckshtain-Levi, N., Miao, J., Castro sparks, A., Wang, Z., Wang, K., et al. (2019). Nicotiana species as surrogate host for studying the pathogenicity of acidovorax citrulli, the causal agent of bacterial fruit blotch of cucurbits. Mol. Plant Pathol. 20, 800–814. doi: 10.1111/mpp.12792
Upadhyay, S. K., Kumar, J., Alok, A., Tuli, R. (2013). RNA-Guided genome editing for target gene mutations in wheat. G3 3, 2233–2238. doi: 10.1534/g3.113.008847
Vu, T. V., Sivankalyani, V., Kim, E. J., Doan, D. T. H., Tran, M. T., Kim, J., et al. (2020). Highly efficient homology-directed repair using CRISPR/Cpf1-geminiviral replicon in tomato. Plant Biotechnol. J 18(10): 2133–2143. doi: 10.1111/pbi.13373
Wang, Z., Shea, Z., Li, Q., Wang, K., Mills, K., Zhang, B., et al. (2023). Evaluate the guide RNA effectiveness via agrobacterium-mediated transient assays in nicotiana benthamiana. Front. Plant Sci. 14, 1111683. doi: 10.3389/fpls.2023.1111683
Wei, C. H. (1983). Crystallization of two cubic forms of soybean trypsin inhibitor e-I, a member of the bowman-birk inhibitor family. J. Biol. Chem. 258, 9357–9359. doi: 10.1016/S0021-9258(17)44675-8
Xu, R. F., Li, H., Qin, R. Y., Li, J., Qiu, C. H., Yang, Y. C., et al. (2015). Generation of inheritable and "transgene clean" targeted genome-modified rice in later generations using the CRISPR/Cas9 system. Sci. Rep. 5, 11491. doi: 10.1038/srep11491
Yamada, T., Takagi, K., Ishimoto, M. (2012). Recent advances in soybean transformation and their application to molecular breeding and genomic analysis. Breed. Sci. 61, 480–494. doi: 10.1270/jsbbs.61.480
Zhao, B., Dahlbeck, D., Krasileva, K. V., Fong, R. W., Staskawicz, B. J. (2011). Computational and biochemical analysis of the xanthomonas effector AvrBs2 and its role in the modulation of xanthomonas type three effector delivery. PloS Pathog. 7, e1002408. doi: 10.1371/journal.ppat.1002408
Keywords: soybean, anti-nutritional factor, Kunitz trypsin inhibitor (KTI), KTI1, KTI3, CRISPR/Cas9, allele-based selection marker
Citation: Wang Z, Shea Z, Rosso L, Shang C, Li J, Bewick P, Li Q, Zhao B and Zhang B (2023) Development of new mutant alleles and markers for KTI1 and KTI3 via CRISPR/Cas9-mediated mutagenesis to reduce trypsin inhibitor content and activity in soybean seeds. Front. Plant Sci. 14:1111680. doi: 10.3389/fpls.2023.1111680
Received: 29 November 2022; Accepted: 31 March 2023;
Published: 08 May 2023.
Edited by:
Hairul Roslan, Universiti Malaysia Sarawak, MalaysiaReviewed by:
Tetsuya Yamada, Hokkaido University, JapanPankaj Kumar Bhowmik, National Research Council Canada (NRC), Canada
Raj Kumar Joshi, Rama Devi Women's University, India
Copyright © 2023 Wang, Shea, Rosso, Shang, Li, Bewick, Li, Zhao and Zhang. This is an open-access article distributed under the terms of the Creative Commons Attribution License (CC BY). The use, distribution or reproduction in other forums is permitted, provided the original author(s) and the copyright owner(s) are credited and that the original publication in this journal is cited, in accordance with accepted academic practice. No use, distribution or reproduction is permitted which does not comply with these terms.
*Correspondence: Bingyu Zhao, YnpoYW8wN0B2dC5lZHU=; Bo Zhang, emhhbmc3NkB2dC5lZHU=
†These authors have contributed equally to this work
‡ORCID: Luciana Rosso, orcid.org/0000-0002-6059-8767
Bingyu Zhao, orcid.org/0000-0002-5392-0279
Bo Zhang, orcid.org/0000-0002-0155-385X