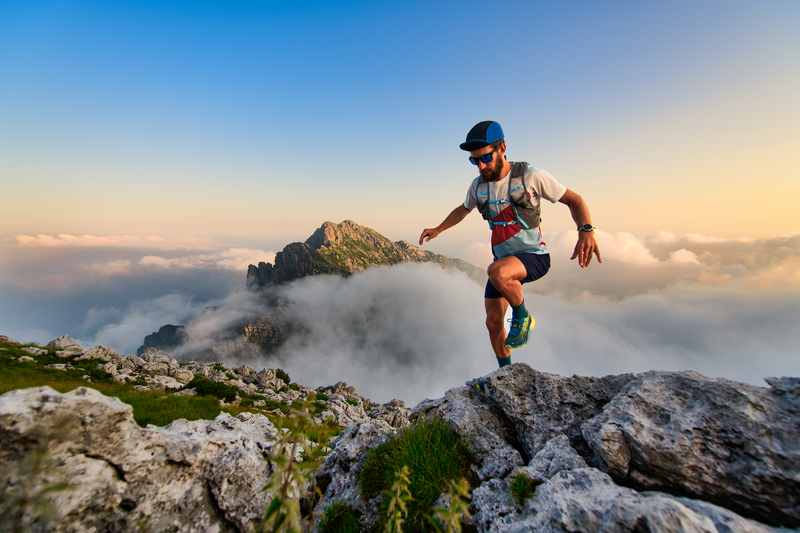
95% of researchers rate our articles as excellent or good
Learn more about the work of our research integrity team to safeguard the quality of each article we publish.
Find out more
REVIEW article
Front. Plant Sci. , 14 February 2023
Sec. Plant Abiotic Stress
Volume 14 - 2023 | https://doi.org/10.3389/fpls.2023.1108507
This article is part of the Research Topic The Potential Role of Melatonin in the Regulation of Abiotic Stress in Plants View all 10 articles
Melatonin is a pleiotropic signaling molecule that reduces the adverse effects of abiotic stresses, and enhances the growth and physiological function of many plant species. Several recent studies have demonstrated the pivotal role of melatonin in plant functions, specifically its regulation of crop growth and yield. However, a comprehensive understanding of melatonin, which regulates crop growth and yield under abiotic stress conditions, is not yet available. This review focuses on the progress of research on the biosynthesis, distribution, and metabolism of melatonin, and its multiple complex functions in plants and its role in the mechanisms of metabolism regulation in plants grown under abiotic stresses. In this review, we focused on the pivotal role of melatonin in the enhancement of plant growth and regulation of crop yield, and elucidated its interactions with nitric oxide (NO) and auxin (IAA, indole-3-acetic acid) when plants are grown under various abiotic stresses. The present review revealed that the endogenousapplication of melatonin to plants, and its interactions with NO and IAA, enhanced plant growth and yield under various abiotic stresses. The interaction of melatonin with NO regulated plant morphophysiological and biochemical activities, mediated by the G protein-coupled receptor and synthesis genes. The interaction of melatonin with IAA enhanced plant growth and physiological function by increasing the levels of IAA, synthesis, and polar transport. Our aim was to provide a comprehensive review of the performance of melatonin under various abiotic stresses, and, therefore, further explicate the mechanisms that plant hormones use to regulate plant growth and yield under abiotic stresses.
Abiotic stresses continuously reduce the growth and yield of different crops (Zhang et al., 2021a; Ahmad et al., 2022a). The growing of plants in altered environments often creates abiotic stresses such as salinity, drought, heat, cold, and heavy metals. The imposition of abiotic stresses can certainly affect plants’ morphophysiological, biochemical, and molecular activity, from seed germination to maturity, and, eventually, cause higher losses in plant yields (Rahman et al., 2022). It has been demonstrated that about 70% of staple food crop yields are adversely affected by abiotic stresses (Khan et al., 2015). These stresses induce numerous changes in the metabolism of plants by producing reactive oxygen species (ROS), which in turn disturb homeostasis and ion distribution in plants (Raza et al., 2022). Improving the response of plants to these stresses is particularly important for sustainable plant production (Gonzalez Guzman et al., 2022). Over the last few decades, tremendous efforts have been made by research scientists to enhance plant growth and yields via the extensive application of chemicals.
Melatonin (N-acetyl-5-methoxytryptamine) is an important bioactive compound in vascular plants, discovered in 1995 (Dubbels et al., 1995). Initially, it was regarded as a powerful antioxidant that had different beneficial roles in various stages of plant growth and development (Sheshadri et al., 2018), such as germination (Zhang et al., 2017), root elongation (Arnao and Hernández-Ruiz, 2019), photosynthesis (Li et al., 2017), and leaf senescence (Wang et al., 2022). It has also been a plant hormonewith an important role in enhancing the growth and regulation of plants (Arnao and Hernández-Ruiz, 2019). It is found in various plants tissues, such as the seeds, roots, leaves, and fruits (Zhang et al., 2017). Thepotential role melatonin could play in the enhancement of plant growth andregulation has been widely investigated by scientific researchers (Sun et al., 2020).
Recently, it has been reported that melatonin increases the fatty acid content and enhances the profile of alkaloids in coffee and soybean plants (Ramakrishna et al., 2012). However, the mechanism of enhanced fatty acid production via melatonin is far from clear and needs to be further investigated in different crops under various abiotic stresses. As a multiregulatory molecule, melatonin regulates the expression of genes involved in plant growth and development (Byeon and Back, 2014), redox reactions (Tomas and Montes, 2005), abiotic stress resistance (Boccalandro et al., 2011), sucrose metabolism [cell wall invertase (CWIN) and sucrose synthase (SUSY)] (Solfanelli et al., 2006; Dutta et al., 2013; Payyavula et al., 2013), and specialized metabolism [phenylpropanoid metabolism: phenylalanine ammonia lyase (PAL), chalcone synthase (CHS), chalcone isomerase (CHI), flavanone 3-hydroxylase (F3H), dihydroflavonol reductase (DFR), and anthocyanidin synthase (ANS)] (Weeda et al., 2014). The phytomelatonin receptor PMTR1 mediates the signaling of ROS, regulates homeostasis or, and delivers a dark indication to promote night stomatal closure (thus avoiding water loss during the night), thereby facilitating plant adaptation to dry land environments (Li et al., 2020). However, what genes participate in the signaling pathway to promote night stomatal closure, and how these genes evolved to facilitate plant adaptation to dry land environments, is still far from clear. In addition, because of the limitation of experimental methods, there is still no definitive evidence showing that melatonin function in plant organs is significantly enhanced at night as compared with the daytime (Van Tassel et al., 2001; Xie et al., 2022). The findings of various studies related to the role of phytomelatonin are of huge significance (Zhang et al., 2021b). Melatonin gives plants resistance to drought (Wang et al., 2014), salt (Hernández et al., 2015), osmotic stress (Zhang et al., 2013), high temperature (Byeon and Back, 2014), cold (Bajwa et al., 2014), and copper stress (Posmyk et al., 2009a).
It has been confirmed that the application of exogenous melatonin can mitigate the effects of abiotic stresses in various crops (Cao et al., 2019). Lower doses of melatonin (i.e., <10 μM) have been shown to promote seed germination and lateral root formation in cucumber plants under cold and drought stresses (Zhang et al., 2013; Simlat et al., 2018). In corn seedlings, melatonin increased drought resistance by alleviating oxidative damage and drought-induced photosynthetic inhibition (Ye et al., 2016). The pretreatment of melatonin also increased endogenous melatonin and inhibited the up-regulation of NCED1 genes, but selectively up-regulated catabolic genes, such as ABA80x1 and ABA80x3, and abscisic acid (ABA)-related synthesis genes, and decreased the accumulation of ABA and induced stomatal reopening in corn under drought stress (Li et al., 2021). In apple trees, melatonin maintained drought tolerance by regulating the concentrations of ABA metabolism and stomatal behavior (Li et al., 2015). In barley, the exogenous supply of melatonin increased photosynthetic carbon assimilation by improving the antioxidant defense of organelles under low temperature or drought stresses (Li et al., 2016). To date, most of the components in melatonin-related signaling pathways remain unclear and need to be further investigated, especially in plants under abiotic stresses (Zhou et al., 2020). In previous studies, melatonin has been shown to be present at high concentrations in several crops (e.g., wheat, rice, barley, corn, grape, oats, and tobacco), and in popular beverages (e.g., tea, coffee, and wine) (Arnao and Hernández-Ruiz, 2009; Ramakrishna et al., 2012; Arnao and Hernández-Ruiz, 2013; Shi et al., 2015a). However, it is still unknown if the response of melatonin in plants under various stresses is the same across different crops.
Therefore, in this manuscript we have aimed to provide a comprehensive review of advances in our knowledge of the roles, biosynthesis, distribution, metabolism, functions, and mechanisms of melatonin in regulating the growth and development of various crops under abiotic stresses. In addition, the interactions of melatonin with other phytohormones, such as nitric oxide (NO) and auxin (IAA, indole-3-acetic acid), are analyzed.
The biosynthetic pathway of melatonin in plants is well documented (Park et al., 2012; Kang et al., 2013). The concept of plant-synthesized melatonin was first introduced in an isotope tracer study (Murch et al., 2000). The biosynthetic pathway of phytomelatonin in vascular plants is thought to be similar to that in animals, although there is much debate surrounding this (Murch et al., 2000; Tan et al., 2013; Zhao et al., 2019). Based on a number of findings, tryptophan is considered as the initial substrate of melatonin synthesis and is involved in four enzymatic steps catalyzed by at least six enzymes, including tryptophan decarboxylase (TDC), tryptophan hydroxylase (TPH), tryptamine 5‐hydroxylase (T5H), serotonin N‐acetyltransferase (SNAT), N‐acetylserotonin methyltransferase (ASMT), and caffeic acid O‐methyl transferase (COMT) (Back et al., 2016; Sun et al., 2021) (Figure 1). The two required processes that contribute to tryptophan are hydroxylation and decarboxylation for melatonin biosynthesis. They have been identified in herbivorous plants (Park et al., 2012). Auxin [indole-3-acetic acid (IAA)], which occurs naturally in plants, is biosynthesized from tryptophan via four proposed routes, that is, indole-3-acetaldoxime (IAOx), indole-3-pyruvic acid (IPyA), indole-3-acetamide (IAM), and tryptamine (TAM). The biosynthesis pathway of auxin from tryptophan is still unknown and needs to be further investigated in different crops under abiotic stresses. Serotonin is catalyzed via SNATs to form N-acetylserotonin, which is then methoxylated by ASMTs to form melatonin (Wang et al., 2017). Serotonin performs various important functions in plants, such as growth regulation and stress defense (Figure 1). Currently, the presence and function of serotonin in plants is an increasingly popular research area, but to date, there are only minor studies available about the functions of serotonin under different abiotic stresses. It has been shown that in rice TDC-catalyzed decarboxylation of tryptophan is the first step in melatonin biosynthesis, followed by T5H‐catalyzed hydroxylation (Park et al., 2012). The T5H gene is considered an essential gene for serotonin biosynthesis. It has been found that suppression of the T5H gene in transgenic rice increases the melatonin concentration, suggesting that melatonin concentration in plants is not proportional to serotonin concentration (Sun et al., 2021). The increase in melatonin concentration and the up-regulation of the T5H gene for serotonin biosynthesis under abiotic stresses in other crops are still far from being clearly understood.
Figure 1 The regulatory role of biosynthetic melatonin under stress conditions. Tryptophan is the initial substrate of melatonin synthesis and is divided into four enzymatic steps catalyzed by six enzymes: tryptophan decarboxylase (TDC), tryptophan hydroxylase (TPH), tryptamine 5‐hydroxylase (T5H), serotonin N‐acetyltransferase (SNAT), N‐acetylserotonin methyltransferase (ASMT), and caffeic acid O‐methyl transferase (COMT). Serotonin is catalyzed via SNAT to form N-acetylserotonin, which is further methoxylated by ASMTs to form melatonin and acts as a growth regulator stress defense molecule. The T5H gene improves serotonin biosynthesis. TDC catalyzes decarboxylation of tryptophan, and it is considered the first step of melatonin biosynthesis. Suppression of the T5H gene increases the concentration of melatonin in rice plants and increases yield. Auxin produced naturally in plants is biosynthesized from tryptophan in four ways: indole-3-acetaldoxime (IAOx), indole-3-pyruvic acid (IPyA), indole-3-acetamide (IAM), and tryptamine (TAM).
The immunohistochemical localization of melatonin has demonstrated that the compound is present in the primary roots and seeds of sunflower and Arabidopsis seedlings (Pelagio-Flores et al., 2012; Mukherjee et al., 2014; Figure 2). The accumulation of melatonin was observed in the oily body of plants, including in the cotyledon cells of both control and salt-treated seedlings, thus showing the effect of long-distance signaling, induced by sodium chloride (NaCl) stress, from roots to cotyledons (Mukherjee et al., 2014). A study found that NaCl stress induced slower mobilization in the cotyledons of sunflower seedlings (David et al., 2010). NaCl stress caused melatonin accumulation in seedling cotyledons, and, as a result, reduced degradation of the oily body. The mobilization of the oily body and the activity of fatty acid-metabolizing enzymes are considered to mitigate the effects of salt stress (David et al., 2010). The accumulation of melatonin in cotyledons played a positive antioxidative role, in that it maintained the activity of the enzymes required for lipid mobilization during seedling growth (David et al., 2010). The accumulation mechanism of melatonin in the oily body of plants, including in the cotyledon cells of control and salt-treated seedlings, is less well documented, and further studies are required so that, ultimately, antioxidant defense systems of various crops can be improved.
Figure 2 The distribution and regulatory roles of an exogenous supply of melatonin in mitigating abiotic stresses are divided into three parts. (i) Melatonin distributed in the roots and seeds. Sodium chloride (NaCl) induces slow mobilization of enzymes and reduces the enzymes’ activity in the oily body of plants in seedlings and roots and, as a result, alters salt stress. The supply of exogenous melatonin enhances the level of plant antioxidants and enzyme activities. (ii) Salt stress reduces chlorophyll content, but exogenous melatonin improves chlorophyll content, chloroplast gene expression, and protein content, and, as a result, enhances photosynthesis activity and chlorophyll accumulation. In addition, it enhances plant Pn and CE and, as a result, inhibits chlorophyll degradation. The improvement of all these traits enhances plant growth and metabolism. (iii) Drought stress causes ROS in plants, which induces Ch degradation and Ch reduction. The application of endogenous melatonin during drought reduces ROS and O2– content and, as a result, increases chlorophyll content and plant growth physiological function. CE, carboxylation efficiency; Ch, chlorophyll; Pn, net photosynthetic rate; ROS, reactive oxygen species.
Moreover, melatonin can trigger the accumulation of nitric oxide via its up-regulation of nitrate reductase expression and down-regulation of S-nitrosoglutathione reductase (GSNOR) expression. The application of melatonin can alter the levels of NO in plants, and, as a result, affect the level of endogenous melatonin. The molecular interaction mechanisms of melatonin and NO are indispensable to different physiological activitiesin plants. However, the molecular interaction mechanisms of melatonin with NO in plants is still far from clear (He and He, 2020). Melatonin can mediate the crosstalk between NO and ethylene and regulate the ripening of fruits via N-nitrosomelatonin (NOMela) signaling (Mukherjee, 2019). In pear fruits, for example, melatonin reduced ethylene production and delayed post-harvest senescence by regulating NO synthesis (Liu et al., 2019). A recent discovery identified that the interaction between melatonin and NO resulted in the formation of NOMela (i.e., N-nitrosomelatonin), and the promised roles in plant morphophysiological activity (Martínez-Lorente et al., 2022). However, owing to the limited available knowledge on melatonin, the interaction of these two compounds and fruit ripening occurring via NOMela in various plants are poorly understood when these plants are under abiotic stresses. In addition, melatonin induces the accumulation of IAA via NO and, as a result, affects the formation of adventitious roots in tomato seedlings (Xie et al., 2022). Melatonin also regulates the transport and distribution of auxin, in turn promoting the formation of adventitious roots in tomato plants (Wen et al., 2016).
Melatonin has been widely shown to promote plant growth and photosynthetic activity under salt stress (Wang et al., 2016; Figure 2). Melatonin greatly reduced the decrease in chlorophyll a (Chl a), chlorophyll b (Chl b), and total chlorophyll (Chls) contents caused by salt stress, and promoted the net photosynthetic rate (Pn) and carboxylation efficiency (CE), showing that it can alleviate chlorophyll degradation caused by salt stress in plants (Kudoh and Sonoike, 2002; Yin et al., 2019). Previous studies found that the contents of Chl a, Chl b, and Chls were much higher in plants treated with melatonin than in untreated plants, indicating that melatonin facilitated both chloroplast gene expression and protein content turnover to promote the accumulation of chlorophyll content (Suo et al., 2015; Siddiqui et al., 2019). Melatonin can act as an antioxidant agent, reducing ROS activity and, as a result, inhibits chlorophyll degradation (Ma et al., 2018; Figure 2). There are studies that indicate that melatonin reduces the degradation of chlorophyll by down-regulating the expression of chlorophyll degradation-related genes during methyl jasmonate-induced senescence (Wang et al., 2019). However, more studies are needed to identify the various genes and measure gene expression involved in reducing chlorophyll degradation in various plants under abiotic stresses.
It is essential to study the physiological responses of crops regulated by the interactions between melatonin and NO to ensure higher yields of these crops. Melatonin and NO affect several physiological processes, such as root growth, mitigation of iron deficiency, and aging (Kaya et al., 2020; Figure 3). The interactions between the two compounds regulate many genes involved in hormone synthesis and, as a result, change the levels of phytohormones (Zhu et al., 2019; Singhal et al., 2021). Interactions between melatonin and NO have recently been identified under conditions of plant stress (Arnao and Hernández-Ruiz, 2018). A previous study demonstrated that melatonin triggers the endogenous accumulation and synthesis of NO, which acts as an antioxidant and regulates other plant defense mechanisms (Okant and Kaya, 2019). The G protein-coupled receptor, as a melatonin receptor, mediates hydrogen peroxide (H2O2) signaling transduction, which is in turn involved in melatonin-induced stomatal closure in Arabidopsis plants (Wei et al., 2018; He and He, 2020). An example of this is melatonin promoting the production of NO in tomato plants when they were exposed to alkaline stress. In this situation, NO could be a downstream signal that plays an crucial role in the tolerance enhanced by melatonin in tomato plants grown under alkaline stress (Liu et al., 2015). Melatonin, together with NO, promotes plant growth and physiological function. The current review suggests that the mechanism of melatonin’s interaction with NO in plants under abiotic stress is still not clearly understood, and the various genes activated as a result of that interaction have not yet been identified.
Figure 3 The interactive role of melatonin with nitric oxide (NO) in mitigating abiotic stress. The application of melatonin in tomato plants enhances NO content. NO further triggers antioxidant enzymes activity in plants, which shows resistance to abiotic stress and enhances root growth, iron deficiency, aging, and the expression of synthesis genes. In addition, G protein as a melatonin receptor enhances root growth, iron deficiency, aging, and the expression of synthesis genes mediates hydrogen peroxide (H2O2) signaling transduction that is involved in a melatonin-induced stomatal closure in Arabidopsis.
Previous studies of the relationship between melatonin and auxin have focused on their chemical similarity (Arnao and Hernández-Ruiz, 2021). Melatonin promotes growth by increasing the concentration of IAA, synthesis of IAA, and polar IAA transport (Wang et al., 2016; Figure 4). Various studies have also identified the ability of melatonin and auxin to regulate root and shoot growth and to promote photosynthesis in a similar way (Tan et al., 2019; Mao et al., 2020). A study of plants under drought stress showed that melatonin encouraged the plants to produce more IAA, which helped to increase plant growth and yield. During the maturity stage, the concentration of melatonin decreased, and the increase in IAA concentration was negligible (Ahmad et al., 2022). This decrease in IAA concentration seen in plants in the later growth stages is due to the decreased demand for IAA (Jia et al., 2020). Another similar study showed that the content of IAA decreased from the early growth to the maturity stages in plants under drought stress. Thus, it is conceivable that plants need higher levels of IAA during seedling growth. Plants require certain hormones during their growth and development. Melatonin boosts the IAA levels in plants, and IAA plays an indispensable role in the growth of plants and their development from germination to maturity.
Figure 4 The interactive role of melatonin with auxin (IAA, indole-3-acetic acid) in mitigating the effects of drought stress. The application of melatonin increases IAA content in plants. Melatonin and IAA promote plant photosynthetic activity in the same way. When plants are under drought stress, melatonin interacts with IAA at the maturity stage, and increases plant growth and yield.
Publishedexamples of melatonin mitigating abiotic stresses in various species of cropplants are shown in Table 1. Salt stress decreased chlorophyll content and photosynthetic activity and enhanced ROS activity and photoperiod regulation (Yin et al., 2019). Melatonin improved thegrowth of green bean seedlings under salt stress, increased photosynthetic activity, and mitigated the oxidative damage caused by ROS by improving antioxidant defense systems in plants (Hasanuzzaman et al., 2020; Elsayed et al., 2021). Multiple studies indicate that melatonin plays a vital role in adaptive responses to salt stress in various plant species (Chen et al., 2018; Liang et al., 2018). However, most of these studies are observational and the findings have not been supported by physiological and molecular research (Liu et al., 2020). In rice, melatonin enhanced salt stress by enabling K+ retention (a vital component of plant tissue tolerance mechanisms) in the roots of plants, and by enabling the process that required Oryza sativa (OS) respiratory burstoxidase homolog F (OsRBOHF)-dependent ROS signaling to trigger stress-responsive genes, which in turn increased the expression of K+ uptake transporters (particularly OsHAK5) in the tips of roots (Liu et al., 2020). Potassium is an essential element for plant growth and development, and its reduction has been observed under salt stress (Chen et al., 2018; Liu et al., 2019). These results correspond with the findings of Huang et al. (2019), who reported that NaCl-induced respiratory burst oxidase homolog (RBOH)-mediated production of H2O2 may be essential for stress signaling and plant adaptation to saline stress. However, studies on the role of OsRBOHF-dependent ROS signaling in the activation of stress-responsive genes and increased expression of K+ uptake transporters in the root tip of plants are lacking, as they have not been conducted on a large variety of plants under abiotic stresses. In addition, further research should focus on identifying responsive genes from OsRBOHF-dependent ROS signaling to increase the uptake of K+ transporter ions in the root tips of different crops under various stresses (Yu et al., 2018).
Melatonin also promotes ethylene biosynthesis, and the application of melatonin was found to strongly induce MYB108A and ACS1 genes during grape berry ripening (Xu et al., 2017). The MYB108A and ACS1 genes, which perform their function as transcription and essential genes that participate in the production of ethylene, were induced by the application of melatonin (Dong et al., 2011). ACS genes are considered a significant target under abiotic stresses to regulate ethylene production in plants. The salt-responsive gene VviACS1 has been identified as being responsible for ethylene production in plants (Xu et al., 2019). In addition, the ACSa and ACS1 genes are significant in that they are considered a primary target for salt tolerance in corn and tobacco (Cao et al., 2006; Lee and Back, 2016). Melatonin, combined with 1-aminocyclopropane-1-carboxylic acid (ACC, an ethylene precursor), improved salt tolerance in grapevine plants. In addition, ethylene production was involved in melatonin-induced salt tolerance (Xu et al., 2019). The mechanism and function of MYB108A, ACS1, ACSa, and VviACS1 genes in ethylene production due to the melatonin induction under abiotic stresses in different plants is largely unknown.
Drought stress negatively affects plants’ morphophysiological and biochemical activity, leading to a decrease in crop yields (Singh et al., 2015; Chen et al., 2019). Drought stress is the cause of oxidative stress and damages plant cells, and, via the higher accumulation of ROS, decreases stomatal closure and photosynthetic activity, and results in a deterioration of antioxidant defense systems. The accumulation of ROS is considered a threat to the survival of plant cells as it leads to electron leakage, lipid peroxidation, and subsequent membrane injury, as well as damaged protein and nucleic acid contents (Maksup et al., 2014). To prevent this damage, plants have developed various strategies to regulate their growth under different environmental stresses (Kim and Kim, 2020). As a new plant growth regulator, melatonin is thought to be involved in drought stress responses (Zhang et al., 2015; Li et al., 2021). Drought stress reduced morphological activity in plants, including that pertaining to leaf size and the relative water conductivity of corn seedlings. Meanwhile, both leaf size and relative water conductivity were significantly enhanced by the application of melatonin (Li et al., 2021). A similar result was revealed by Ye et al. (2016), who reported that melatonin improved the shoot dry weight and leaf size of corn seedlings. In plants, physiological processes in leaves, such as photosynthesis, respiration, and transpiration, are maintained by stomata, the opening and closing of which are controlled by complex signal transduction pathways and water balance. In the presence of drought stress, plants regulate their cellular moisture content by regulating stomatal closure and reducing their transpiration rate. However, the density of stomata significantly increases with the contraction of guard cells, and deteriorates under drought stress (Xue et al., 2021). In general, the application of melatonin has shown resistance against the deterioration of stomata cells and increased its length and width under drought stress in corn (Li et al., 2021). The contrasting results in the study by Li et al. (2015), however, demonstrated that drought stress did not reduce stomatal cell density in apples. Nevertheless, the exogenous supply of melatonin maintained high turgor pressure and kept the stomata open. The difference in the findings might be because of the differences in the regulatory mechanism of melatonin in different plant species (Li et al., 2021). The present review demonstrates that melatonin’s quantity, performance, and mechanisms of action differ from plant species to plant species, but fewer morphophysiological responses have been documented under drought stress in different plants.
High levels of heat stress increase endogenous melatonin concentrations and, thereby, enhance thermotolerance, because of the potent antioxidant capacity of melatonin in plants (Liang et al., 2018; Ahammed et al., 2019). A previous study on Arabidopsis plants demonstrated that melatonin increased the seed germination rate from approximately 30% to 39% under heat stress (Hernández et al., 2015). It has been confirmed by the correlation between the synthesis of phytomelatonin and seed germination that phytomelatonin is synthesized during the germination of cucumber seeds, and that its synthesis peaks 14 hours after germination (Zhang et al., 2014). Nevertheless, further research on various crops is still needed. Melatonin improved germination capability by promoting soluble sugar utilization and synthesis of new proteins, and increased amylase and α-amylase activities in melon and Limonium bicolor seeds (Castañares and Bouzo, 2019; Li et al., 2019). Recent research has revealed the mechanisms by which melatonin significantly mitigates the effects of heat stress on plant seeds. First, because of the high potency of melatonin, it maintained high viability and germination capacity (Hernández et al., 2015). When plants are exposed to high levels of heat stress, the activities of antioxidant enzymes, such as superoxide dismutase (SOD), peroxidase (POD), and catalase (CAT), are increased (Wang et al., 2022), and melatonin inhibits the accumulation of H2O2 (Marta et al., 2016). Melatonin treatment up-regulates genes, such as GA20ox and GA3ox, which are involved in gibberellin (GA) biosynthesis. The content of GA, particularly GA4, is also increased by melatonin. However, unfortunately, melatonin down-regulates the expression of the essential gene NECD2, which is mainly involved in ABA biosynthesis (Zhang et al., 2014; Li et al., 2019). The mechanisms of the up-regulation and down-regulation of gene expression should be studied further in plant cells under heat stress.
Furthermore, heat stress can deteriorate the balance between antioxidants, resulting in ROS accumulation and causing peroxidative damage to cell membranes (Sun et al., 2021). The exogenous application of melatonin in tomatoes and rice reversed the adverse effects of heat stress on plant shoot and root growth (Wang et al., 2018). Melatonin also reduced the damage caused by heat stress by regulating redox homeostasis, and modulating NO and polyamine biosynthesis in tomato seedlings (Jahan et al., 2019). In Arabidopsis plants, the heat shock protein HSP90 and heat shock factors (i.e., HSFA2 and HSFA32) contributed to the alleviation of melatonin-mediated heat stress (Shi et al., 2015a). A study demonstrated that HSPs prevented the cellular proteins of tomatoes, grown under heat stress, from refolding or degrading denatured proteins (Xu et al., 2016). Heat shock proteins (HSPs) can isolate and store unfolded proteins. In addition, HSPs can act as chaperones by protecting cells against stresses that can induce protein denaturation and block protein aggregation, and by enhancing the survival of cells and, in turn, of the cellular activity during high levels of heat stress. However, our current understanding of how heat shock proteins and heat shock factors relate to melatonin-mediated heat stress is limited, and in need of further investigation.
Cold stress is one of the major abiotic stresses that reduces crop growth and yield, especially in temperate zones and highly elevated areas (Bhat et al., 2022). Plants exposed to cold stress experience changes in various physiological, molecular, metabolic, and biochemical activities. Examples include variations in membrane fluidity, metabolism homeostasis, and enzyme activity (Wu et al., 2022). Photosynthesis is a pivotal plant metabolism process, and one that is highly sensitive to cold stress. This is because low temperature hinders many major components of photosynthesis (Dahal et al., 2012). Chlorophyll content decreases under cold stress, leading to chlorosis in leaves (Kaura et al., 2022). The chlorophyll content of leaves provides important information about the effectiveness of physiological processes in plants in plants (Gitelson et al., 2003). Plants treated with melatonin had a higher concentration of chlorophyll than non-treated plants under cold stress (Yang et al., 2022). Plant growth at low temperatures induces the excessive production or inefficient deactivation of ROS, such as H2O2, superoxide anions (i.e., O2–), and hydroxyl radicals (i.e., OH), which in turn can cause injury to plants (Ghaderian et al., 2015). In addition, ROS accumulation causes the oxidation of proteins and peroxidation of lipids within plant cells, resulting in reduced plant growth (Nahar et al., 2015). For self-defense against oxidative injury caused by ROS, plants have evolved effective antioxidant systems to scavenge ROS, such as SOD, POD, and CAT, as well as non-enzymatic antioxidants, including proline and glutathione (Erdal et al., 2015; Ghaderian et al., 2015). Several studies have demonstrated that exogenous melatonin can stimulate plant growth in various plants, such as corn, and can promote the germination of cucumber seeds under cold stress (Posmyk et al., 2009b; Kolodziejczyk et al., 2016). In Arabidopsis plants, melatonin modulates leaf senescence against cold stress (Shi et al., 2015b). Melatonin applications enhance the resistance of Bermuda grass to cold stress by improving cell membrane stability, and by regulating photosynthesis and metabolic activity (Khalid et al., 2022). Melatonin played a role as both a first-line defense and internal sensor of oxidative stress in a study of different species of plants (Iqbal and Khan, 2022). For example, in barley, exogenous melatonin can enhance photosynthetic carbon assimilation by improving the plant antioxidant defense systems of organelles under cold stress (Li et al., 2016). Therefore, the improved performance of primed seeds in terms of seedling growth and germination might be the result of improved antioxidant defense systems under cold stress (Cao et al., 2022). However, an understanding of the growth of waxy corn and other crop seeds primed with melatonin in response to cold stress is still limited (Cao et al., 2022).
Certain heavy metals, such as zinc (Zn), cadmium (Cd), iron (Fe), and copper (Cu), are essential for plant growth and metabolism, but their accumulation to higher levels can negatively affect plant growth and yield. Heavy metal stress inhibits plant photosynthetic activity, the activity of enzymes involved in the Calvin cycle, and carbohydrate metabolism (Hasan et al., 2019). In addition, higher levels of ROS accumulation during heavy metal stress inhibit root growth and promote leaf senescence in turn, chloroplasts (Zeng et al., 2022). Previous studies have demonstrated that plants treated with melatonin can improve their growth and yield by improving their morphophysiological activities under heavy metal stress conditions. The production of endogenous melatonin in plants can be triggered by the application of exogenous melatonin which builds up heavy metal tolerance (Menhas et al., 2022). Melatonin enhanced plant metabolism and antioxidant enzymes activity, and triggered the ascorbate–glutathione cycle to counteract the effects of heavy metal stress (Moustafa-Farag et al., 2020). In wheat seedlings, exogenous melatonin increased endogenous melatonin and, as a result, enhanced root and shoot growth under cadmium (Cd) toxicity (Zeng et al., 2022). The increase of endogenous melatonin mitigates cadmium toxicity by balancing H2O2 homeostasis and activating antioxidant defense systems in wheat (Ni et al., 2018). Melatonin effectively mitigated Cd toxicity by improving H+-ATPase activity and phytochelatin and glutathione content, and by facilitating Cd sequestration in tomato plant cells (Hasan et al., 2015). Melatonin impacted sulfur metabolism, which plays an important role in plant tolerance against Cd stress (Menhas et al., 2022). In tomatoes, melatonin deficiency reduced the sulfur concentration and increased the accumulation of cadmium (Hasan et al., 2019). The overexpression of melatonin biosynthetic enzymes genes improved heavy metal stress in Arabidopsis plants (Yin et al., 2022). Similarly, in rice, various gene families, such as NRAMP, HMA, MTP, YSL, and ZIP, are involved in heavy metal stress (Maharajan et al., 2022). These genes reduced the uptake of heavy metals and accumulation in rice grains (Peris-Peris et al., 2017). Several studies investigating the role of melatonin in plant morphophysiological activity, antioxidant capacity, and biosynthetic genes in various crops have recently been undertaken. However, the role of melatonin in improving these activities, as well as the transduction pathways of different genes in cotton, rice, and other crops under heavy metal stress, is still unknown.
Ultraviolet (UV) radiations negatively effects plant growth and development, andtheir intensity continuously increasing caused by rapid ozone layer depletion. The higher levels of UV radiation can substantially reduce crop productivity by hindering plant PSII, photosynthetic activity, nucleic acids, and biomass accumulation and portioning (Bera et al., 2022). Plants exposed to higher levels of UV radiation have reduced expression and synthesis of key photosynthetic proteins, such as chlorophyll a/b binding proteins (Khudyakova et al., 2019). Melatonin plays a vital role in mitigating the negative effects of UV radiation on crop productivity. It has been demonstrated that exogenous melatonin in Malus hupehensis and Nicotiana sylvestris plants facilitates the UV-induced damage to DNA and UV radiation induced by ROS (Wei et al., 2019). Melatonin is considered a potent antioxidant that protects plants against UV radiation; it regulates the expression of various UV signaling pathways, such as transcription factors RUP1/2, HY5, and HYH, and the ubiquitin-degrading enzyme COP1 (Yao et al., 2021). Exogenous melatonin improved the expression of RUP1/1, HY5, HYH, and COP1, which perform a key role in the protection against UV radiation (Hassan et al., 2022). Hence, melatonin regulates antioxidant defense systems to prevent plants from the negative impacts of UV stress (Yao et al., 2021). Endogenous melatonin is substantially increased in Glycyrrhiza uralensis plants when their roots are exposed to UV radiation, and as a result plant DNA damage is reduced (Wei et al., 2019). Similarly, the accumulation of endogenous melatonin induces a tolerance response to UV stress in Mediterranean and alpine species of plants (Nawaz et al., 2022). Although limited research has demonstrated a role for melatonin in UV stress tolerance (Hassan et al., 2022), more studies are required to investigate the role of melatonin in regulating various UV signaling pathways that are involved in mitigating the negative effects of UV radiation in various crops.
The impact of abiotic stresses on plant development is considered a significant threat to agricultural productivity. Plants adopt different physiological, biochemical, and molecular responses to overcome the negative effects of abiotic stresses (Ahmad et al., 2022b). Phytomelatonin has potential to be used as a tool for reducing or alleviating the adverse effects of abiotic stresses in various crops. The exogenous application of melatonin is essential for plant growth and development under abiotic stresses. Phytomelatonin plays a key role in plant metabolism and the complex mechanism of plant function; however, the role that melatonin plays in the underlying mechanisms in plants grown under abiotic stress is still poorly understood. Moreover, the interaction of melatonin with NO and with IAA/auxin, and their responses to abiotic stresses, make for attractive targets in molecular research. The relationship between melatonin and NO regulates morphophysiological and biochemical activities by way of the G protein-coupled receptor and synthesis genes. Furthermore, the mechanism by which G protein regulates the morphophysiological activity and the different genes involved in the regulation by melatonin are still unclear. In addition, the interaction of melatonin with auxin enhanced growth and physiological function by increasing the levels of auxin, synthesis, and polar transport. In the later growth stage, the content of auxin is decreased because of the decreased melatonin concentrations in plants. To grow and achieve a higher yield, plants need a continuous supply of IAA from sowing to maturity. In the lateral growth stage, the effects of an exogenous supply of melatonin, and the mechanism by which melatonin boosts IAA levels in various crops, are still unknown.
Melatonin has an important role in regulating plant metabolism and increasing yield under various abiotic stresses. In addition, the OsRBOHF-dependent ROS signaling that activated stress-responsive genes in plants grown under abiotic stress enhanced the uptake of potassium (K+) transporter (OsHAK5) in the roots. The potassium transporter OsHAK5 plays a vital role in potassium acquisition and transport from root tissue to the shoots, especially in plants exposed to low potassium concentrations, enhancing plant metabolism and physiological function under salt stress. The mechanism of the K+ transporter OsHAK5 and the activation of gene identification, which are due to the OsRBOHF-dependent ROS signaling in various crops under abiotic stresses, however, is poorly understood.
Ethylene in plants is considered a multifunctional phytohormone that significantly improves plant growth and senescence. However, the role of genes such as MYB108A, ACS1, ACSa, and VviACS1 in ethylene production in different plants under various stresses is still poorly understood.
In addition, serotonin plays a vital function in plants, acting as a growth regulator and as a stress defense molecule. The relationship of melatonin with T5H genes in serotonin biosynthesis under abiotic stress is still unknown in many plants.
Stomata density is closely associated with plant growth properties, and photosynthetic activity is improved by melatonin application. Stomatal cells undergo deterioration when plants are exposed to drought stress (Salehi-Lisar and Bakhshayeshan-Agdam, 2016). In contrast, no adverse effects on stomatal density were observed in apple plants grown under drought stress (Li et al., 2015). The reason for these different results might be that there are different signaling pathways in different crops. The mechanisms of this phenomenon in various crops are still poorly understood. These mechanisms need to be further investigated under various stresses because the amount performance, and mechanism of action of melatonin vary among plant species.
It has been confirmed that, melatonin up-regulates GA20ox and GA3ox, genes that are involved in GA biosynthesis and result in increased GA4 while down-regulating the NECD2, which is involved in ABA biosynthesis. The role of melatonin in the up- and down-regulation of genes involved in the biosynthesis of GA and ABA under various abiotic stresses remains unclear.
The heat shock proteins of tomatoes protect the plants’ cellular protein against heat stress due to refolding or degradation of denatured proteins. However, the response and activity of heat shock proteins and heat factors in plants with a melatonin supply under abiotic stresses in different crops is still poorly understood.
IA was involved in the conceptualization, writing/reviewing, and editing the original draft. GuiZ was involved in supervision. MI and GuaZ were involved in collection of the literature. YJ and XS contributed to the writing of the manuscript. AA and MY eliminated grammatical errors. All authors contributed to the article and approved the submitted version.
The research was financially supported by National Key Research and Development Project of China (2022YFE0113400).
The authors declare that the research was conducted in the absence of any commercial or financial relationships that could be construed as a potential conflict of interest.
All claims expressed in this article are solely those of the authors and do not necessarily represent those of their affiliated organizations, or those of the publisher, the editors and the reviewers. Any product that may be evaluated in this article, or claim that may be made by its manufacturer, is not guaranteed or endorsed by the publisher.
Ahammed, G. J., Xu, W., Liu, A., Chen, S. (2019). Endogenous melatonin deficiency aggravates high temperature-induced oxidative stress in solanum lycopersicum l. Environ. Exp. Bot. 161, 303–311. doi: 10.1016/j.envexpbot.2018.06.006
Ahmad, S., Wang, G. Y., Muhammad, I., Farooq, S., Kamran, M., Ahmad, I., et al. (2022). Application of melatonin-mediated modulation of drought tolerance by regulating photosynthetic efficiency, chloroplast ultrastructure, and endogenous hormones in maize. Chem. Biol. Technol. Agric. 9, 1–14. doi: 10.1186/s40538-021-00272-1
Ahmad, I., Zhu, G., Zhou, G., Song, X., Hussein Ibrahim, M. E., Ibrahim Salih, E. G. (2022a). Effect of n on growth, antioxidant capacity, and chlorophyll content of sorghum. Agronomy 12, 501. doi: 10.3390/agronomy12020501
Ahmad, I., Zhu, G., Zhou, G., Song, X., Hussein Ibrahim, M. E., Ibrahim Salih, E. G., et al. (2022b). Pivotal role of phytohormones and their responsive genes in plant growth and their signaling and transduction pathway under salt stress in cotton. Int. J. Mol. Sci. 23, 7339. doi: 10.3390/ijms23137339
Arnao, M. B., Hernández-Ruiz, J. (2009). Chemical stress by different agents affects the melatonin content of barley roots. J. Pineal Res. 46, 295–299. doi: 10.1111/j.1600-079X.2008.00660.x
Arnao, M. B., Hernández-Ruiz, J. (2013). Growth conditions determine different melatonin levels in l upinus albus l. J. Pineal Res. 55, 149–155. doi: 10.1111/jpi.12055
Arnao, M. B., Hernández-Ruiz, J. (2018). Melatonin and its relationship to plant hormones. Ann. Bot. 121, 195–207. doi: 10.1093/aob/mcx114
Arnao, M. B., Hernández-Ruiz, J. (2019). Melatonin: A new plant hormone and/or a plant master regulator? Trends Plant Sci. 24, 38–48. doi: 10.1016/j.tplants.2018.10.010
Arnao, M., Hernández-Ruiz, J. (2021). Melatonin as a regulatory hub of plant hormone levels and action in stress situations. Plant Biol. 23, 7–19. doi: 10.1111/plb.13202
Back, K., Tan, D. X., Reiter, R. J. (2016). Melatonin biosynthesis in plants: multiple pathways catalyze tryptophan to melatonin in the cytoplasm or chloroplasts. J. Pineal Res. 61, 426–437. doi: 10.1111/jpi.12364
Bajwa, V. S., Shukla, M. R., Sherif, S. M., Murch, S. J., Saxena, P. K. (2014). Role of melatonin in alleviating cold stress in a rabidopsis thaliana. J. Pineal Res. 56, 238–245. doi: 10.1111/jpi.12115
Bera, K., Ball, K., Ghosh, S., Sadhukhan, S., Dutta, P. (2022). UV Radiation: plant responses and an in-depth mechanism of sustainability under climatic extremities. doi: 10.21203/rs.3.rs-2219238/v1
Bhat, K. A., Mahajan, R., Pakhtoon, M. M., Urwat, U., Bashir, Z., Shah, A. A., et al. (2022). Low temperature stress tolerance: An insight into the omics approaches for legume crops. Front. Plant Sci. 13. doi: 10.3389/fpls.2022.888710
Boccalandro, H. E., González, C. V., Wunderlin, D. A., Silva, M. F. (2011). Melatonin levels, determined by LC-ESI-MS/MS, fluctuate during the day/night cycle in vitis vinifera cv Malbec: evidence of its antioxidant role in fruits. J. Pineal Res. 51, 226–232. doi: 10.1111/j.1600-079X.2011.00884.x
Byeon, Y., Back, K. (2014). Melatonin synthesis in rice seedlings in vivo is enhanced at high temperatures and under dark conditions due to increased serotonin n-acetyltransferase and n-acetylserotonin methyltransferase activities. J. Pineal Res. 56, 189–195. doi: 10.1111/jpi.12111
Cao, Q., Li, G., Cui, Z., Yang, F., Jiang, X., Diallo, L., et al. (2019). Seed priming with melatonin improves the seed germination of waxy maize under chilling stress via promoting the antioxidant system and starch metabolism. Sci. Rep. 9, 1–12. doi: 10.1038/s41598-019-51122-y
Cao, W. H., Liu, J., Zhou, Q. Y., Cao, Y. R., Zheng, S. F., Du, B. X., et al. (2006). Expression of tobacco ethylene receptor NTHK1 alters plant responses to salt stress. Plant Cell Environ. 29, 1210–1219. doi: 10.1111/j.1365-3040.2006.01501.x
Cao, Q., Wang, W., Akhtar, S. S., Yang, F., Kong, F., Cui, Z., et al. (2022). Exogenous foliar spray of selenium (Se) alleviates cold stress by promoting photosynthesis and antioxidant defense in waxy maize. doi: 10.21203/rs.3.rs-1719103/v1
Castañares, J. L., Bouzo, C. A. (2019). Effect of exogenous melatonin on seed germination and seedling growth in melon (Cucumis melo l.) under salt stress. Hortic. Plant J. 5, 79–87. doi: 10.1016/j.hpj.2019.01.002
Chen, J., Li, Y., Luo, Y., Tu, W., Wan, T. (2019). Drought differently affects growth properties, leaf ultrastructure, nitrogen absorption and metabolism of two dominant species of hippophae in Tibet plateau. Acta Physiol. Plant 41, 1–12. doi: 10.1007/s11738-018-2785-6
Chen, G., Liu, C., Gao, Z., Zhang, Y., Zhang, A., Zhu, L., et al. (2018). Variation in the abundance of OsHAK1 transcript underlies the differential salinity tolerance of an indica and a japonica rice cultivar. Front. Plant Sci. 8, 2216. doi: 10.3389/fpls.2017.02216
Dahal, K., Kane, K., Gadapati, W., Webb, E., Savitch, L. V., Singh, J., et al. (2012). The effects of phenotypic plasticity on photosynthetic performance in winter rye, winter wheat and brassica napus. Physiol. Plant 144, 169–188. doi: 10.1111/j.1399-3054.2011.01513.x
David, A., Yadav, S., Bhatla, S. C. (2010). Sodium chloride stress induces nitric oxide accumulation in root tips and oil body surface accompanying slower oleosin degradation in sunflower seedlings. Physiol. Plant 140, 342–354. doi: 10.1111/j.1399-3054.2010.01408.x
Dong, H., Zhen, Z., Peng, J., Chang, L., Gong, Q., Wang, N. N. (2011). Loss of ACS7 confers abiotic stress tolerance by modulating ABA sensitivity and accumulation in arabidopsis. J. Exp. Bot. 62, 4875–4887. doi: 10.1093/jxb/err143
Dubbels, R., Reiter, R., Klenke, E., Goebel, A., Schnakenberg, E., Ehlers, C., et al. (1995). Melatonin in edible plants identified by radioimmunoassay and by high performance liquid chromatography-mass spectrometry. J. Pineal Res. 18, 28–31. doi: 10.1111/j.1600-079X.1995.tb00136.x
Dutta, A., Sen, J., Deswal, R. (2013). New evidences about strictosidine synthase (Str) regulation by salinity, cold stress and nitric oxide in catharanthus roseus. J. Plant Biochem. 22, 124–131. doi: 10.1007/s13562-012-0118-1
Elsayed, A. I., Rafudeen, M. S., Gomaa, A. M., Hasanuzzaman, M. (2021). Exogenous melatonin enhances the reactive oxygen species metabolism, antioxidant defense-related gene expression, and photosynthetic capacity of phaseolus vulgaris l. @ to confer salt stress tolerance. Physiol. Plant 173, 1369–1381. doi: 10.1111/ppl.13372
Erdal, S., Genisel, M., Turk, H., Dumlupinar, R., Demir, Y. (2015). Modulation of alternative oxidase to enhance tolerance against cold stress of chickpea by chemical treatments. J. Plant Physiol. 175, 95–101. doi: 10.1016/j.jplph.2014.10.014
Ghaderian, S. M., Ghasemi, R., Heidari, H., Vazirifar, S. (2015). Effects of Ni on superoxide dismutase and glutathione reductase activities and thiol groups: a comparative study between alyssum hyperaccumulator and non-accumulator species. Aust. J. Bot. 63, 65–71. doi: 10.1071/BT14282
Gitelson, A. A., Gritz, Y., Merzlyak, M. N. (2003). Relationships between leaf chlorophyll content and spectral reflectance and algorithms for non-destructive chlorophyll assessment in higher plant leaves. J. Plant Physiol. 160, 271–282. doi: 10.1078/0176-1617-00887
Gonzalez Guzman, M., Cellini, F., Fotopoulos, V., Balestrini, R., Arbona, V. (2022). New approaches to improve crop tolerance to biotic and abiotic stresses. Physiol. Plant 174, e13547. doi: 10.1111/ppl.13547
Hasan, M. K., Ahammed, G. J., Sun, S., Li, M., Yin, H., Zhou, J. (2019). Melatonin inhibits cadmium translocation and enhances plant tolerance by regulating sulfur uptake and assimilation in Solanum lycopersicum l. J. Agric. Food Chem. 67, 10563–10576. doi: 10.1021/acs.jafc.9b02404
Hasan, M. K., Ahammed, G. J., Yin, L., Shi, K., Xia, X., Zhou, Y., et al. (2015). Melatonin mitigates cadmium phytotoxicity through modulation of phytochelatins biosynthesis, vacuolar sequestration, and antioxidant potential in solanum lycopersicum l. Front. Plant Sci. 6. doi: 10.3389/fpls.2015.00601
Hasanuzzaman, M., Bhuyan, M., Zulfiqar, F., Raza, A., Mohsin, S. M., Mahmud, J. A., et al. (2020). Reactive oxygen species and antioxidant defense in plants under abiotic stress: Revisiting the crucial role of a universal defense regulator. Antioxidants 9, 681. doi: 10.3390/antiox9080681
Hassan, M. U., Mahmood, A., Awan, M. I., Maqbool, R., Aamer, M., Alhaithloul, H. A., et al. (2022). Melatonin-induced protection against plant abiotic stress: mechanisms and prospects. Front. Plant Sci. 13. doi: 10.3389/fpls.2022.902694
He, H., He, L. F. (2020). Crosstalk between melatonin and nitric oxide in plant development and stress responses. Physiol. Plant 170, 218–226. doi: 10.1111/ppl.13143
Hernández, I. G., Gomez, F. J. V., Cerutti, S., Arana, M. V., Silva, M. F. (2015). Melatonin in arabidopsis thaliana acts as plant growth regulator at low concentrations and preserves seed viability at high concentrations. Plant Physiol. Biochem. 94, 191–196. doi: 10.1016/j.plaphy.2015.06.011
Huang, Y., Cao, H., Yang, L., Chen, C., Shabala, L., Xiong, M., et al. (2019). Tissue-specific respiratory burst oxidase homolog-dependent H2O2 signaling to the plasma membrane h+-ATPase confers potassium uptake and salinity tolerance in cucurbitaceae. J. Exp. Bot. 70, 5879–5893. doi: 10.1093/jxb/erz328
Iqbal, R., Khan, T. (2022). Application of exogenous melatonin in vitro and in planta: a review of its effects and mechanisms of action. Biotechnol. Lett. 1-18. doi: 10.1007/s10529-022-03270-x
Jahan, M. S., Shu, S., Wang, Y., Chen, Z., He, M., Tao, M., et al. (2019). Melatonin alleviates heat-induced damage of tomato seedlings by balancing redox homeostasis and modulating polyamine and nitric oxide biosynthesis. BMC Plant Biol. 19, 1–16. doi: 10.1186/s12870-019-1992-7
Jia, C., Yu, X., Zhang, M., Liu, Z., Zou, P., Ma, J., et al. (2020). Application of melatonin-enhanced tolerance to high-temperature stress in cherry radish (Raphanus sativus l. var. radculus pers). J. Plant Growth Regul. 39, 631–640. doi: 10.1007/s00344-019-10006-1
Kang, K., Lee, K., Park, S., Byeon, Y., Back, K. (2013). Molecular cloning of rice serotonin n-acetyltransferase, the penultimate gene in plant melatonin biosynthesis. J. Pineal Res. 55, 7–13. doi: 10.1111/jpi.12011
Kaura, V., Malhotra, P., Mittal, A., Sanghera, G., Kaur, N., Bhardwaj, R., et al. (2022). Physiological, biochemical, and gene expression responses of sugarcane under cold, drought and salt stresses. J. Plant Growth Regul., 1–10. doi: 10.1007/s00344-022-10850-8
Kaya, C., Ashraf, M., Alyemeni, M. N., Ahmad, P. (2020). Responses of nitric oxide and hydrogen sulfide in regulating oxidative defence system in wheat plants grown under cadmium stress. Physiol. Plant 168, 345–360. doi: 10.1111/ppl.13012
Khalid, M., Rehman, H. M., Ahmed, N., Nawaz, S., Saleem, F., Ahmad, S., et al. (2022). Using exogenous melatonin, glutathione, proline, and glycine betaine treatments to combat abiotic stresses in crops. Int. J. Mol. Sci. 23, 12913. doi: 10.3390/ijms232112913
Khan, M. I. R., Fatma, M., Per, T. S., Anjum, N. A., Khan, N. A. (2015). Salicylic acid-induced abiotic stress tolerance and underlying mechanisms in plants. Front. Plant Sci. 6. doi: 10.3389/fpls.2015.00462
Khudyakova, A. Y., Kreslavski, V. D., Shmarev, A. N., Lyubimov, V. Y., Shirshikova, G. N., Pashkovskiy, P. P., et al. (2019). Impact of UV-b radiation on the photosystem II activity, pro-/antioxidant balance and expression of light-activated genes in arabidopsis thaliana hy4 mutants grown under light of different spectral composition. J. Photochem. Photobiol. 194, 14–20. doi: 10.1016/j.jphotobiol.2019.02.003
Kim, S., Kim, T.-H. (2020). Alternative splicing for improving abiotic stress tolerance and agronomic traits in crop plants. J. Plant Biol. 63, 409–420. doi: 10.1007/s12374-020-09282-2
Kołodziejczyk, I., Dzitko, K., Szewczyk, R., Posmyk, M. M. (2016). Exogenous melatonin improves corn (Zea mays l.) embryo proteome in seeds subjected to chilling stress. J. Plant Physiol. 193, 47–56. doi: 10.1016/j.jplph.2016.01.012
Kudoh, H., Sonoike, K. (2002). Irreversible damage to photosystem I by chilling in the light: Cause of the degradation of chlorophyll after returning to normal growth temperature. Planta 215, 541–548. doi: 10.1007/s00425-002-0790-9
Lee, H. J., Back, K. (2016). 2-hydroxymelatonin promotes the resistance of rice plant to multiple simultaneous abiotic stresses (combined cold and drought). J. Pineal Res. 61, 303–316. doi: 10.1111/jpi.12347
Liang, D., Gao, F., Ni, Z., Lin, L., Deng, Q., Tang, Y., et al. (2018). Melatonin improves heat tolerance in kiwifruit seedlings through promoting antioxidant enzymatic activity and glutathione s-transferase transcription. Molecules 23, 584. doi: 10.3390/molecules23030584
Li, H., Chang, J., Chen, H., Wang, Z., Gu, X., Wei, C., et al. (2017). Exogenous melatonin confers salt stress tolerance to watermelon by improving photosynthesis and redox homeostasis. Front. Plant Sci. 8. doi: 10.3389/fpls.2017.00295
Li, Z., Su, X., Chen, Y., Fan, X., He, L., Guo, J., et al. (2021). Melatonin improves drought resistance in maize seedlings by enhancing the antioxidant system and regulating abscisic acid metabolism to maintain stomatal opening under PEG-induced drought. J. Plant Biol. 64, 299–312. doi: 10.1007/s12374-021-09297-3
Li, X., Tan, D. X., Jiang, D., Liu, F. (2016a). Melatonin enhances cold tolerance in drought-primed wild-type and abscisic acid-deficient mutant barley. J. Pineal Res. 61, 328–339. doi: 10.1111/jpi.12350
Li, X., Tan, D. X., Jiang, D., Liu, F. (2016b). Melatonin enhances cold tolerance in drought-primed wild-type and abscisic acid-deficient mutant barley. J. Pineal Res. 61, 328–339. doi: 10.1111/jpi.12350
Li, C., Tan, D.-X., Liang, D., Chang, C., Jia, D., Ma, F. (2015). Melatonin mediates the regulation of ABA metabolism, free-radical scavenging, and stomatal behaviour in two malus species under drought stress. J. Exp. Bot. 66, 669–680. doi: 10.1093/jxb/eru476
Liu, N., Gong, B., Jin, Z., Wang, X., Wei, M., Yang, F., et al. (2015). Sodic alkaline stress mitigation by exogenous melatonin in tomato needs nitric oxide as a downstream signal. J. Plant Physiol. 186, 68–77. doi: 10.1016/j.jplph.2015.07.012
Liu, J., Shabala, S., Shabala, L., Zhou, M., Meinke, H., Venkataraman, G., et al. (2019). Tissue-specific regulation of na+ and k+ transporters explains genotypic differences in salinity stress tolerance in rice. Front. Plant Sci. 10. doi: 10.3389/fpls.2019.01361
Liu, J., Shabala, S., Zhang, J., Ma, G., Chen, D., Shabala, L., et al. (2020). Melatonin improves rice salinity stress tolerance by NADPH oxidase-dependent control of the plasma membrane k+ transporters and k+ homeostasis. Plant Cell Environ. 43, 2591–2605. doi: 10.1111/pce.13759
Li, D., Wei, J., Peng, Z., Ma, W., Yang, Q., Song, Z., et al. (2020). Daily rhythms of phytomelatonin signaling modulate diurnal stomatal closure via regulating reactive oxygen species dynamics in arabidopsis. J. pineal Res. 68, e12640. doi: 10.1111/jpi.12640
Li, J., Zhao, C., Zhang, M., Yuan, F., Chen, M. (2019). Exogenous melatonin improves seed germination in limonium bicolor under salt stress. Plant Signal. 14, 1659705. doi: 10.1080/15592324.2019.1659705
Maharajan, T., Chellasamy, G., Tp, A. K., Ceasar, S. A., Yun, K. (2022). The role of metal transporters in phytoremediation: A closer look at Arabidopsis. Chemosphere 136881. doi: 10.1016/j.chemosphere.2022.136881
Maksup, S., Roytrakul, S., Supaibulwatana, K. (2014). Physiological and comparative proteomic analyses of Thai jasmine rice and two check cultivars in response to drought stress. J. Plant Interact. 9, 43–55. doi: 10.1080/17429145.2012.752042
Mao, J., Niu, C., Li, K., Chen, S., Tahir, M. M., Han, M., et al. (2020). Melatonin promotes adventitious root formation in apple by promoting the function of MdWOX11. BMC Plant Biol. 20, 1–11. doi: 10.1186/s12870-020-02747-z
Marta, B., Szafrańska, K., Posmyk, M. M. (2016). Exogenous melatonin improves antioxidant defense in cucumber seeds (Cucumis sativus l.) germinated under chilling stress. Front. Plant Sci. 7. doi: 10.3389/fpls.2016.00575
Martínez-Lorente, S. E., Pardo-Hernández, M., Martí-Guillén, J. M., López-Delacalle, M., Rivero, R. M. (2022). Interaction between melatonin and no: action mechanisms, main targets, and putative roles of the emerging molecule NOMela. Int. J. Mol. Sci. 23, 6646. doi: 10.3390/ijms23126646
Ma, X., Zhang, J., Burgess, P., Rossi, S., Huang, B. (2018). Interactive effects of melatonin and cytokinin on alleviating drought-induced leaf senescence in creeping bentgrass (Agrostis stolonifera). Environ. Exp. Bot. 145, 1–11. doi: 10.1016/j.envexpbot.2017.10.010
Menhas, S., Yang, X., Hayat, K., Aftab, T., Bundschuh, J., Arnao, M. B., et al. (2022). Exogenous melatonin enhances cd tolerance and phytoremediation efficiency by ameliorating cd-induced stress in oilseed crops: A review. J. Plant Growth Regul. 41, 922–935. doi: 10.1007/s00344-021-10349-8
Moustafa-Farag, M., Mahmoud, A., Arnao, M. B., Sheteiwy, M. S., Dafea, M., Soltan, M., et al. (2020). Melatonin-induced water stress tolerance in plants: Recent advances. Antioxidants 9, 809. doi: 10.3390/antiox9090809
Mukherjee, S. (2019). Insights into nitric oxide–melatonin crosstalk and n-nitrosomelatonin functioning in plants. J. Exp. Bot. 70, 6035–6047. doi: 10.1093/jxb/erz375
Mukherjee, S., David, A., Yadav, S., Baluška, F., Bhatla, S. C. (2014). Salt stress-induced seedling growth inhibition coincides with differential distribution of serotonin and melatonin in sunflower seedling roots and cotyledons. Physiol. Plant 152, 714–728. doi: 10.1111/ppl.12218
Murch, S., Krishnaraj, S., Saxena, P. (2000). Tryptophan is a precursor for melatonin and serotonin biosynthesis in in vitro regenerated st. john's wort (Hypericum perforatum l. cv. anthos) plants. Plant Cell Rep. 19, 698–704. doi: 10.1007/s002990000206
Nahar, K., Hasanuzzaman, M., Alam, M., Fujita, M. (2015). Roles of exogenous glutathione in antioxidant defense system and methylglyoxal detoxification during salt stress in mung bean. Biol. Plant 59, 745–756. doi: 10.1007/s10535-015-0542-x
Nawaz, K., Chaudhary, R., Sarwar, A., Ahmad, B., Gul, A., Hano, C., et al. (2020). Melatonin as master regulator in plant growth, development and stress alleviator for sustainable agricultural production: Current status and future perspectives. Sustainability 13, 294. doi: 10.3390/su13010294
Ni, J., Wang, Q., Shah, F. A., Liu, W., Wang, D., Huang, S., et al. (2018). Exogenous melatonin confers cadmium tolerance by counterbalancing the hydrogen peroxide homeostasis in wheat seedlings. Molecules 23, 799. doi: 10.3390/molecules23040799
Okant, M., Kaya, C. (2019). The role of endogenous nitric oxide in melatonin-improved tolerance to lead toxicity in maize plants. Environ. Sci. pollut. Res. 26, 11864–11874. doi: 10.1007/s11356-019-04517-3
Park, S., Lee, K., Kim, Y. S., Back, K. (2012). Tryptamine 5-hydroxylase-deficient sekiguchi rice induces synthesis of 5-hydroxytryptophan and n-acetyltryptamine but decreases melatonin biosynthesis during senescence process of detached leaves. J. Pineal Res. 52, 211–216. doi: 10.1111/j.1600-079X.2011.00930.x
Payyavula, R. S., Singh, R. K., Navarre, D. A. (2013). Transcription factors, sucrose, and sucrose metabolic genes interact to regulate potato phenylpropanoid metabolism. J. Exp. Bot. 64, 5115–5131. doi: 10.1093/jxb/ert303
Pelagio-Flores, R., Muñoz-Parra, E., Ortiz-Castro, R., López-Bucio, J. (2012). Melatonin regulates arabidopsis root system architecture likely acting independently of auxin signaling. J. Pineal Res. 53, 279–288. doi: 10.1111/j.1600-079X.2012.00996.x
Peris-Peris, C., Serra-Cardona, A., Sánchez-Sanuy, F., Campo, S., Ariño, J., San Segundo, B. (2017). Two NRAMP6 isoforms function as iron and manganese transporters and contribute to disease resistance in rice. Mol. Plant Microbe Interact. 30, 385–398. doi: 10.1094/MPMI-01-17-0005-R
Posmyk, M. M., Bałabusta, M., Wieczorek, M., Sliwinska, E., Janas, K. (2009a). Melatonin applied to cucumber (Cucumis sativus l.) seeds improves germination during chilling stress. J. Pineal Res. 46, 214–223. doi: 10.1111/j.1600-079X.2008.00652.x
Posmyk, M. M., Janas, K. M., Kontek, R. (2009). Red cabbage anthocyanin extract alleviates copper-induced cytological disturbances in plant meristematic tissue and human lymphocytes. Biometals 22, 479–490. doi: 10.1007/s10534-009-9205-8
Rahman, M. M., Ibrahim, M., Muktadir, M. A., Sadeque, A., Abdel Latef, A., Ashrafuzzaman, M. (2022). “Rice: Role and responses under abiotic stress,” in Sustainable remedies for abiotic stress in cereals (Springer), 125–147. doi: 10.1007/978-981-19-5121-3_6
Ramakrishna, A., Giridhar, P., Sankar, K. U., Ravishankar, G. A. (2012). Melatonin and serotonin profiles in beans of coffea species. J. Pineal Res. 52, 470–476. doi: 10.1111/j.1600-079X.2011.00964.x
Raza, A., Salehi, H., Rahman, M. A., Zahid, Z., Madadkar Haghjou, M., Najafi-Kakavand, S., et al. (2022). Plant hormones and neurotransmitter interactions mediate antioxidant defenses under induced oxidative stress in plants. Front. Plant Sci. 13. doi: 10.3389/fpls.2022.961872
Salehi-Lisar, S. Y., Bakhshayeshan-Agdam, H. (2016). “Drought stress in plants: Causes, consequences, and tolerance,” in Drought stress tolerance in plants, vol. 1. (Springer), 1–16. doi: 10.1007/978-3-319-28899-4_1
Sheshadri, S., Nishanth, M., Yamine, V., Simon, B. (2018). Effect of melatonin on the stability and expression of reference genes in catharanthus roseus. Sci. Rep. 8, 1–11. doi: 10.1038/s41598-018-20474-2
Shi, H., Jiang, C., Ye, T., Tan, D.-X., Reiter, R. J., Zhang, H., et al. (2015b). Comparative physiological, metabolomic, and transcriptomic analyses reveal mechanisms of improved abiotic stress resistance in bermudagrass [Cynodon dactylon (L). pers.] by exogenous melatonin. J. Exp. Bot. 66, 681–694. doi: 10.1093/jxb/eru373
Shi, H., Reiter, R. J., Tan, D. X., Chan, Z. (2015a). Indole-3-acetic acid inducible 17 positively modulates natural leaf senescence through melatonin-mediated pathway in Arabidopsis. J. Pineal Res. 58, 26–33. doi: 10.1111/jpi.12188
Siddiqui, M. H., Alamri, S., Al-Khaishany, M. Y., Khan, M. N., Al-Amri, A., Ali, H. M., et al. (2019). Exogenous melatonin counteracts NaCl-induced damage by regulating the antioxidant system, proline and carbohydrates metabolism in tomato seedlings. Int. J. Mol. Sci. 20, 353. doi: 10.3390/ijms20020353
Simlat, M., Ptak, A., Skrzypek, E., Warchoł, M., Morańska, E., Piórkowska, E. (2018). Melatonin significantly influences seed germination and seedling growth of stevia rebaudiana bertoni. PeerJ 6, e5009. doi: 10.7717/peerj.5009
Singhal, R. K., Jatav, H. S., Aftab, T., Pandey, S., Mishra, U. N., Chauhan, J., et al. (2021). Roles of nitric oxide in conferring multiple abiotic stress tolerance in plants and crosstalk with other plant growth regulators. J. Plant Growth Regul. 40, 2303–2328. doi: 10.1007/s00344-021-10446-8
Singh, R., Mishra, A., Dhawan, S. S., Shirke, P. A., Gupta, M. M., Sharma, A. (2015). Physiological performance, secondary metabolite and expression profiling of genes associated with drought tolerance in withania somnifera. Protoplasma 252, 1439–1450. doi: 10.1007/s00709-015-0771-z
Solfanelli, C., Poggi, A., Loreti, E., Alpi, A., Perata, P. (2006). Sucrose-specific induction of the anthocyanin biosynthetic pathway in arabidopsis. Plant Physiol. 140, 637–646. doi: 10.1104/pp.105.072579
Sun, Y.-D., Guo, D.-L., Yang, S.-D., Zhang, H.-C., Wang, L.-L., Yu, Y.-H. (2020). Melatonin treatment improves the shelf-life and postharvest quality of table grape (Vitis labrusca l. cv.’Fengzao’). J. Berry Res. 10, 665–676. doi: 10.3233/JBR-200569
Sun, C., Liu, L., Wang, L., Li, B., Jin, C., Lin, X. (2021). Melatonin: A master regulator of plant development and stress responses. J. Integr. Plant Biol. 63, 126–145. doi: 10.1111/jipb.12993
Suo, J., Zhao, Q., Zhang, Z., Chen, S., Liu, G., Wei, X., et al. (2015). Cytological and proteomic analyses of osmunda cinnamomea germinating spores reveal characteristics of fern spore germination and rhizoid tip growth. Mol. Cell. Proteomics 14, 2510–2534. doi: 10.1074/mcp.M114.047225
Tan, X. L., Fan, Z. Q., Kuang, J. F., Lu, W. J., Reiter, R. J., Lakshmanan, P., et al. (2019). Melatonin delays leaf senescence of Chinese flowering cabbage by suppressing ABFs-mediated abscisic acid biosynthesis and chlorophyll degradation. J. Pineal Res. 67, e12570. doi: 10.1111/jpi.12570
Tan, D. X., Manchester, L. C., Liu, X., Rosales-Corral, S. A., Acuna-Castroviejo, D., Reiter, R. J. (2013). Mitochondria and chloroplasts as the original sites of melatonin synthesis: a hypothesis related to melatonin's primary function and evolution in eukaryotes. J. Pineal Res. 54, 127–138. doi: 10.1111/jpi.12026
Tomas, C., Montes, A. (2005). A proposed mechanism to explain the stimulatory effect of melatonin on antioxidantive en-zymes. J. Pineal Res. 39, 99–104. doi: 10.1111/j.1600-079X.2005.00248.x
Van Tassel, D. L., Roberts, N., Lewy, A., O'neill, S. D. (2001). Melatonin in plant organs. J. Pineal Res. 31, 8–15. doi: 10.1034/j.1600-079X.2001.310102.x
Wang, Q., An, B., Wei, Y., Reiter, R. J., Shi, H., Luo, H., et al. (2016). Melatonin regulates root meristem by repressing auxin synthesis and polar auxin transport in arabidopsis. Front. Plant Sci. 7. doi: 10.3389/fpls.2016.01882
Wang, L., Feng, C., Zheng, X., Guo, Y., Zhou, F., Shan, D., et al. (2017). Plant mitochondria synthesize melatonin and enhance the tolerance of plants to drought stress. J. Pineal Res. 63, e12429. doi: 10.1111/jpi.12429
Wang, Y., Reiter, R. J., Chan, Z. (2018). Phytomelatonin: A universal abiotic stress regulator. J. Exp. Bot. 69, 963–974. doi: 10.1093/jxb/erx473
Wang, K., Xing, Q., Ahammed, G. J., Zhou, J. (2022). Functions and prospects of melatonin in plant growth, yield and quality. J. Exp. Bot. doi: 10.1093/jxb/erac233
Wang, M., Zhang, T., Ding, F. (2019). Exogenous melatonin delays methyl jasmonate-triggered senescence in tomato leaves. Agronomy 9, 795. doi: 10.3390/agronomy9120795
Wang, L., Zhao, Y., Reiter, R. J., He, C., Liu, G., Lei, Q., et al. (2014). Changes in melatonin levels in transgenic ‘Micro-tom’tomato overexpressing ovine AANAT and ovine HIOMT genes. J. Pineal Res. 56, 134–142. doi: 10.1111/jpi.12105
Weeda, S., Zhang, N., Zhao, X., Ndip, G., Guo, Y., Buck, G. A., et al. (2014). Arabidopsis transcriptome analysis reveals key roles of melatonin in plant defense systems. PLoS One 9, e93462. doi: 10.1371/journal.pone.0093462
Wei, Z., Li, C., Gao, T., Zhang, Z., Liang, B., Lv, Z., et al. (2019). Melatonin increases the performance of malus hupehensis after UV-b exposure. Plant Physiol. Biochem. 139, 630–641. doi: 10.1016/j.plaphy.2019.04.026
Wei, J., Li, D. X., Zhang, J. R., Shan, C., Rengel, Z., Song, Z. B., et al. (2018). Phytomelatonin receptor PMTR 1-mediated signaling regulates stomatal closure in arabidopsis thaliana. J. Pineal Res. 65, e12500. doi: 10.1111/jpi.12500
Wen, D., Gong, B., Sun, S., Liu, S., Wang, X., Wei, M., et al. (2016). Promoting roles of melatonin in adventitious root development of solanum lycopersicum l. by regulating auxin and nitric oxide signaling. Front. Plant Sci. 7. doi: 10.3389/fpls.2016.00718
Wu, J., Nadeem, M., Galagedara, L., Thomas, R., Cheema, M. (2022). Recent insights into cell responses to cold stress in plants: Signaling, defence, and potential functions of phosphatidic acid. Environ. Exp. Bot., 105068. doi: 10.1016/j.envexpbot.2022.105068
Xie, Q., Zhang, Y., Cheng, Y., Tian, Y., Luo, J., Hu, Z., et al. (2022). The role of melatonin in tomato stress response, growth and development. Plant Cell Rep., 1–20. doi: 10.1007/s00299-022-02876-9
Xu, W., Cai, S. Y., Zhang, Y., Wang, Y., Ahammed, G. J., Xia, X. J., et al. (2016). Melatonin enhances thermotolerance by promoting cellular protein protection in tomato plants. J. Pineal Res. 61, 457–469. doi: 10.1111/jpi.12359
Xue, F., Liu, W., Cao, H., Song, L., Ji, S., Tong, L., et al. (2021). Stomatal conductance of tomato leaves is regulated by both abscisic acid and leaf water potential under combined water and salt stress. Physiol. Plant 172, 2070–2078. doi: 10.1111/ppl.13441
Xu, L., Xiang, G., Sun, Q., Ni, Y., Jin, Z., Gao, S., et al. (2019). Melatonin enhances salt tolerance by promoting MYB108A-mediated ethylene biosynthesis in grapevines. Hortic. Res. 6. doi: 10.1038/s41438-019-0197-4
Xu, L., Yue, Q., Bian, F. E., Sun, H., Zhai, H., Yao, Y. (2017). Melatonin enhances phenolics accumulation partially via ethylene signaling and resulted in high antioxidant capacity in grape berries. Front. Plant Sci. 8. doi: 10.3389/fpls.2017.01426
Yang, X., Ren, J., Li, J., Lin, X., Xia, X., Yan, W., et al. (2022). Meta-analysis of the effect of melatonin application on abiotic stress tolerance in plants. Plant Biotechnol. Rep., 1–14. doi: 10.1007/s11816-022-00770-0
Yao, J. W., Ma, Z., Ma, Y. Q., Zhu, Y., Lei, M. Q., Hao, C. Y., et al. (2021). Role of melatonin in UV-b signaling pathway and UV-b stress resistance in Arabidopsis thaliana. Plant Cell Environ. 44, 114–129. doi: 10.1111/pce.13879
Ye, J., Wang, S., Deng, X., Yin, L., Xiong, B., Wang, X. (2016). Melatonin increased maize (Zea mays l.) seedling drought tolerance by alleviating drought-induced photosynthetic inhibition and oxidative damage. Acta Physiol. Plant 38, 1–13. doi: 10.1007/s11738-015-2045-y
Yin, X., Bai, Y. L., Gong, C., Song, W., Wu, Y., Ye, T., et al. (2022). The phytomelatonin receptor PMTR1 regulates seed development and germination by modulating abscisic acid homeostasis in Arabidopsis thaliana. J. Pineal Res. 72, e12797. doi: 10.1111/jpi.12797
Yin, Z., Lu, J., Meng, S., Liu, Y., Mostafa, I., Qi, M., et al. (2019). Exogenous melatonin improves salt tolerance in tomato by regulating photosynthetic electron flux and the ascorbate–glutathione cycle. J. Plant Interact. 14, 453–463. doi: 10.1080/17429145.2019.1645895
Yu, Y., Lv, Y., Shi, Y., Li, T., Chen, Y., Zhao, D., et al. (2018). The role of phyto-melatonin and related metabolites in response to stress. Molecules 23, 1887. doi: 10.3390/molecules23081887
Zeng, W., Mostafa, S., Lu, Z., Jin, B. (2022). Melatonin-mediated abiotic stress tolerance in plants. Front. Plant Sci. 13. doi: 10.3389/fpls.2022.847175
Zhang, N., Sun, Q., Zhang, H., Cao, Y., Weeda, S., Ren, S., et al. (2015). Roles of melatonin in abiotic stress resistance in plants. J. Exp. Bot. 66, 647–656. doi: 10.1093/jxb/eru336
Zhang, Y., Wang, J., Chen, X., Lu, X., Wang, D., Wang, J., et al. (2021b). Genome-wide identification and characteristic analysis of the downstream melatonin metabolism gene GhM2H in Gossypium hirsutum l. Biol. Res. 54, 1–17. doi: 10.1186/s40659-021-00358-y
Zhang, T., Wang, J., Sun, Y., Zhang, L., Zheng, S. (2021a). Versatile roles of melatonin in growth and stress tolerance in plants. J. Plant Growth Regul., 1–17. doi: 10.1007/s00344-021-10317-2
Zhang, N., Zhang, H.-J., Sun, Q.-Q., Cao, Y.-Y., Li, X., Zhao, B., et al. (2017). Proteomic analysis reveals a role of melatonin in promoting cucumber seed germination under high salinity by regulating energy production. Sci. Rep. 7, 1–15. doi: 10.1038/s41598-017-00566-1
Zhang, H. J., Zhang, N., Yang, R. C., Wang, L., Sun, Q. Q., Li, D. B., et al. (2014). Melatonin promotes seed germination under high salinity by regulating antioxidant systems, ABA and GA 4 interaction in cucumber (C ucumis sativus l.). J. Pineal Res. 57, 269–279. doi: 10.1111/jpi.12167
Zhang, N., Zhao, B., Zhang, H. J., Weeda, S., Yang, C., Yang, Z. C., et al. (2013). Melatonin promotes water-stress tolerance, lateral root formation, and seed germination in cucumber (Cucumis sativus l.). J. Pineal Res. 54, 15–23. doi: 10.1111/j.1600-079X.2012.01015.x
Zhao, D., Yu, Y., Shen, Y., Liu, Q., Zhao, Z., Sharma, R., et al. (2019). Melatonin synthesis and function: evolutionary history in animals and plants. Front. Endocrinol. 10. doi: 10.3389/fendo.2019.00249
Zhou, Y., Chen, M., Guo, J., Wang, Y., Min, D., Jiang, Q., et al. (2020). Overexpression of soybean DREB1 enhances drought stress tolerance of transgenic wheat in the field. J. Exp. Bot. 71, 1842–1857. doi: 10.1093/jxb/erz569
Keywords: abiotic stresses, auxin, nitric oxide, phytomelatonin, plant growth and metabolism
Citation: Ahmad I, Song X, Hussein Ibrahim ME, Jamal Y, Younas MU, Zhu G, Zhou G and Adam Ali AY (2023) The role of melatonin in plant growth and metabolism, and its interplay with nitric oxide and auxin in plants under different types of abiotic stress. Front. Plant Sci. 14:1108507. doi: 10.3389/fpls.2023.1108507
Received: 26 November 2022; Accepted: 09 January 2023;
Published: 14 February 2023.
Edited by:
Muhammad Ahsan Altaf, Hainan University, ChinaReviewed by:
Shahbaz Atta Tung, Pir Mehr Ali Shah Arid Agriculture University, PakistanCopyright © 2023 Ahmad, Song, Hussein Ibrahim, Jamal, Younas, Zhu, Zhou and Adam Ali. This is an open-access article distributed under the terms of the Creative Commons Attribution License (CC BY). The use, distribution or reproduction in other forums is permitted, provided the original author(s) and the copyright owner(s) are credited and that the original publication in this journal is cited, in accordance with accepted academic practice. No use, distribution or reproduction is permitted which does not comply with these terms.
*Correspondence: Guisheng Zhou, Z3N6aG91QHl6dS5lZHUuY24=
Disclaimer: All claims expressed in this article are solely those of the authors and do not necessarily represent those of their affiliated organizations, or those of the publisher, the editors and the reviewers. Any product that may be evaluated in this article or claim that may be made by its manufacturer is not guaranteed or endorsed by the publisher.
Research integrity at Frontiers
Learn more about the work of our research integrity team to safeguard the quality of each article we publish.