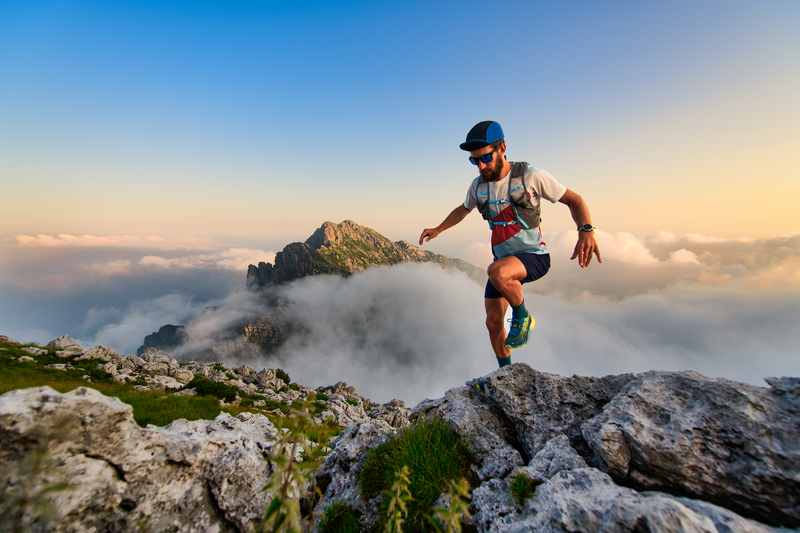
95% of researchers rate our articles as excellent or good
Learn more about the work of our research integrity team to safeguard the quality of each article we publish.
Find out more
ORIGINAL RESEARCH article
Front. Plant Sci. , 20 February 2023
Sec. Plant Symbiotic Interactions
Volume 14 - 2023 | https://doi.org/10.3389/fpls.2023.1103487
This article is part of the Research Topic Rhizospheric Interactions: Integrating Plant-Microbe Signaling During Stresses View all 9 articles
Beneficial microorganisms are an important strategy for sustainable plant production processes such as stimulate root exudation, stress tolerance, and yield improvement. This study investigated various microorganisms isolated from the rhizosphere of Oryza sativa L. in order to inhibit Magnaporthe oryzae cause of rice blast, by direct and indirect mode of action. The results indicated that Bacillus vallismortis strain TU–Orga21 significantly reduced M. oryzae mycelium growth and deformed the hyphal structures. The effects of biosurfactant TU–Orga21 was studied against M. oryzae spore development. The dose of ≥5% v/v biosurfactant significantly inhibited the germ tubes and appressoria formation. The biosurfactants were evaluated as surfactin and iturin A by Matrix-assisted laser desorption ionization dual time-of-flight tandem mass spectrometry. Under greenhouse conditions, priming the biosurfactant three times before M. oryzae infection significantly accumulated endogenous salicylic acid, phenolic compounds, and hydrogen peroxide (H2O2) during the infection process of M. oryzae. The SR-FT-IR spectral changes from the mesophyll revealed higher integral area groups of lipids, pectins, and proteins amide I and amide II in the elicitation sample. Furthermore, scanning electron microscope revealed appressorium and hyphal enlargement in un-elicitation leaves whereas appressorium formation and hyphal invasion were not found in biosurfactant-elicitation at 24 h post inoculation. The biosurfactant treatment significantly mitigated rice blast disease severity. Therefore, B. vallismortis can be a promising novel biocontrol agent which contains the preformed active metabolites for a rapid control of rice blast by a direct action against pathogen and by boosting plant immunity.
Rice (Oryza sativa L.) production is negatively affected by various factors, such as poor soil quality, mineral deficiency, pests, and diseases, among others, ultimately reducing rice yield (Timsina et al., 2018). One of the major diseases of rice is blast caused by Magnaporthe oryzae (Howard and Valent, 1996). The rice blast disease is widespread across Southeast Asia and Japan and is responsible for yield losses of 20%–50% on average and even up to 100% in severe cases (Saleh et al., 2014). In Thailand, rice blast is a serious threat to rice production systems because of favorable climatic conditions to disease spread (Kawasaki and Herath, 2018). Therefore, reducing the prevalence and severity of rice blast is a priority for improving the yield and reducing the losses in Thai rice production. At present, the major control methods for rice blast rely on the application of synthetic chemicals, such as captan, carbendazim, thiram, or tricyclazole (Kongcharoen et al., 2020). However, these components may negatively affect humans and the environment. Therefore, novel bioproducts must be explored to control rice blast.
Currently, biocontrol of rice diseases using microorganisms, biomass, or crude extracts of microorganisms is an exciting new avenue in the development of biofungicides, which are safe for humans, animals, and the environment. These agents hold the potential to control plant diseases through promoting antibiotic production, competition, parasitism, and plant health (Köhl et al., 2019; Khanna et al., 2019). In addition, synthesizing various antimicrobial agents or toxins in order to directly attack the pathogens also activates the systemic resistance mechanisms of plants and enhances the defense enzyme properties (Khanna et al., 2021; Abdelaziz et al., 2023).
Bacillus species are potential candidates for developing biofungicides as well as a method to combat bacterial rice pathogens since they produce multifarious active compounds and strong antimicrobial activity such as lipopeptides antibiotics (Hashizume and Nishimura, 2008; Sharma and Thakur, 2020; Ngalimat et al., 2021). Lipopeptides are a group of antimicrobial metabolites composed of surfactin, lichenycin, iturin, and fengycin. These substances affect cellular integrity and can destroy the fungal membrane (Romero et al., 2007). According to Zhang and Sun (2018), cyclic lipopeptides and fengycin produced by Bacillus subtilis 155 can damage rice membrane, inhibiting M. grisea hyphal growth (Zhang and Sun, 2018). Similarly, B. amyloliquefaciens S170 and B. pumilus S9 can colonize rice plants to protect them against M. oryzae by producing proteases, siderophores, cellulases, and volatile compounds (Sha et al., 2020).
Moreover, lipopeptide substances secreted by Bacillus sp. are involved in the elicitation of induced systemic resistance (ISR) to inhibit a wide range of plant pathogens such as fungi, bacteria, nematodes, insects, and viral vectors (Jourdan et al., 2009; Malviya et al., 2020). ISR is a systemic means of inducing preconditioned resistance through prior infection or biotic treatment. Preconditioning induces resistance, rendering the host less susceptible to subsequent infection and blocking the spread of infection in plant tissues (Romera et al., 2019). In the mechanism of ISR, small molecules and cellular tissues in the plant cell wall structure are modified, and antimicrobial compounds are synthesized through the salicylic acid (SA) pathway (Heil and Bostock, 2002; Deice Raasch-Fernandes et al., 2019). Following infection, endogenous levels of SA increase locally, thereby systemically enhancing plant defense mechanisms through the shikimic acid pathway and producing phenolic compounds in plant cell walls and lignin in response to a pathogen attack (Mandal et al., 2010). Moreover, according to Sedlářová and Lebeda (2001), rapid accumulation of phenolic compounds was associated with a hypersensitive response (HR) to downy mildew in lettuce (Sedlářová and Lebeda, 2001). Furthermore, in groundnut, plant growth-promoting rhizobacterial (PGPR) bioformulation enhanced the expression of defense enzymes and pathogenesis-related proteins against collar rot via augmenting the enzymatic activity of phenolics (Senthilraja et al., 2013)
Although beneficial microbes, such as Bacillus species, have been proven to effectively suppress M. oryzae, the biocontrol potential of their multiple modes of action against pathogens has not been fully exploited as yet. Therefore, the objective of the present study was to explore the potential benefits of microbes for the inhibition of M. oryzae, their antimicrobial compounds, and their direct inhibiting potential on M. oryzae spore development in parallel with stimulation of the plant defense intermedia including endogenous salicylic acid, phenolic compound, and H2O2 accumulation. Moreover, we also analyzed the biochemical changes including lipid, pectin, amides I, amides II, lignin, and polysaccharides in rice plants during M. oryzae infection.
One gram of soil sample in the rhizospheric region of rice plants was diluted into 9 mL of distilled water to obtain a serially diluted suspension at 10-8 g mL-1 for assays. Thereafter, 100 µL of the soil suspension was dropped on nutrient agar (NA) plates and spread over the surface of the agar with a sterile glass spreader; the plates were then incubated at 28°C for 48 h. A single colony of microorganisms was selected as the pure strain through the streak plate method for matrix-assisted laser desorption ionization dual time-of-flight tandem mass spectrometric (MALDI-TOF/TOF MS) identification and further experimentation.
To prepare the biosurfactant, the microorganisms were cultured in flasks containing 100 mL of lysogeny broth (LB) at 25°C ± 2°C and 180 rpm for 72 h. The culture was centrifuged (12,000 ×g, 25 min) to produce the biosurfactant without the cell biomass. The biosurfactant was flowed through a membrane filter with 0.45 µm pore size to remove the cellular residue and then preserved at 4°C for further experimentation.
Biosurfactant lipopeptides were precipitated by adding hydrochloric acid (3N) solution; the mixture was cooled down to 4°C to obtain the final pH of 2.0 and then placed in a fridge for 1 h. The precipitates were collected by centrifugation at 9,000 ×g for 25 min at 4°C and then dissolved in chloroform:methanol (2:1, v/v) before MALDI–TOF/TOF MS analysis.
A virulent strain of M. oryzae was provided by the Plant Pathology Section of Thammasat University. M. oryzae was grown on oatmeal–rice agar medium at 25°C ± 2°C for 10 days. The conidia were harvested using a loop via gentle scraping. The suspensions were filtered through layers of cheesecloth to obtain pure conidia, and their density was adjusted to approximately 1 × 105 conidia mL-1 using a hemocytometer.
Thai jasmine rice cultivar ‘Khao Dowk Mali 105’ (KDML 105), which is susceptible to blast disease, was used in the present experiment. Rice seeds were sterilized with 1% sodium hypochlorite solution for 1 min and then rinsed with sterile water three times. The seeds were soaked in water 24 h before planting. Ten seedlings were planted in 10–inch plant pots containing approximately 5 kg of clay soil. The pots were incubated in a greenhouse under 12 h of natural light at 28°C ± 4°C with 60%–75% humidity.
MALDI–TOF/TOF MS was performed at the Synchrotron Light Research Institute, Thailand, using Autoflex® maX (Bruker Daltonics, Bremen, Germany). Four to five pure single colonies (5–10 mg) of each microbial strain on NA medium were transferred to 1.5 mL microcentrifuge tubes and then mixed with 300 μL of HPLC–grade water. Next, 900 μL of ethanol was added, and the mixture was vortexed for 1 min. The sample solution was centrifuged at 13,500 ×g for 2 min two times. The cells were dried in a laminar air flow cabinet for 2 min, and then 5 µL of 70% formic acid was added. The sample was vortexed for 1 min. Afterwards, 5 µL of acetonitrile was added, and the solution was centrifuged at 13,000 ×g for 2 min. At this point, the clear solution was transferred to a fresh tube. Next, 1 μL of each sample was dropped on the MALDI–TOF/TOF MS target plate and left to dry; subsequently, 1 µL of HCCA matrix was dropped and left to dry. The target plates containing the samples were analyzed using MALDI–TOF/TOF MS (Autoflex® maX, Bruker Daltonics, Karlsruhe, Germany). The spectra were recorded in the positive linear mode at the laser frequency of 60 Hz and in the mass range of m/z 2,000–10,000 Dalton. Calibration was performed using the Escherichia coli strain DH5α, which presents ribosomal protein mass with RNA and myoglobin at peaks of 5096.8, 5381.4, 6255.4, 7274.5, 10,300.1, 13,683.2, and 16,952.3 m/z. Two–thousand shots of the mass spectrum profile data were collected from each sample. The mass spectrum profiles were processed with Flexcontrol v.3.4 (Karlsruhe, Germany). The protein mass fingerprints were analyzed at a molecular weight tolerance of 300 ppm. The resulting data were imported to MALDI BioTyper v.4.0 (Karlsruhe, Germany) to compare the sample mass fingerprints to the database (Elshafie et al., 2015).
Dual culture technique was used to determine the antagonistic activity of bacterial isolates against M. oryzae. M. oryzae fungal disks (8 mm in diameter) were placed at the center of 90 mm PDA plates, and five strains of beneficial microorganisms grown in Luria Bertani (LB) broth were streaked at the side of each plate using the dual culture method. LB broth media without the beneficial microorganism was used as the untreated control. The plates were incubated at 25°C ± 2°C. The best bacterial isolate for inhibition of mycelial growth was used for further analysis. Antagonistic activity was calculated based on the observation of mycelial growth at 10 days using formula (1), as follows:
Leaf segments the susceptible rice cultivar KDML 105 were used for evaluation. Briefly, 5–cm–long samples from the middle part of the leaves were extracted, placed on water agar (WA) plates, and sprayed with 1 × 105 conidia mL-1 suspension of M. oryzae. After 1 h of inoculation, the rice leaves were sprayed with the B. vallismortis biosurfactant at various concentrations (5%, 10%, 15%, 20%, 25%, and 30% v/v). LB broth without the biosurfactant was used as the negative control. All plates were incubated at 25°C ± 2°C in a growth chamber for 16 h. The leaf segments were stained with ethanol–lactophenol trypan blue (Koch and Slusarenko, 1990). Spore development and appressoria formation were monitored by microscopy. The percentage of spores, germ tubes, and appressorium and the percentage of inhibition of M. oryzae spore germination were calculated using formula (2) and (3) respectively as follows:
The lipopeptide biosurfactant was analyzed at the Synchrotron Light Research Institute, Thailand, with Autoflex® maX (Bruker Daltonics, Bremen, Germany) in the positive reflectron mode and the m/z range of 50–2,000 Dalton. The lipopeptide biosurfactant was mixed with an equal volume of matrix solution (30:70 v/v ACN : TFA 0.1% TFA), and then 1 μL of the lipopeptide sample was dropped on the plate and left to dry. The standard purified iturin A and surfactin (Sigma-Aldrich, USA) was used as a marker. The spectra were obtained using FlexControl v3.4 (Karlsruhe, Germany) and analyzed using FlexAnalysis v3.3 (Karlsruhe, Germany) (Elshafie et al., 2015).
The efficacy of the B. vallismortis biosurfactant as the sole regulator to induce systemic resistance was determined at concentrations of 20%, 25%, and 30% v/v. The evaluated treatments also included SA as the positive control and water as the negative control. Plants were sprayed with 30 mL of the biosurfactant solution at various concentrations and the corresponding control solutions once a week for 3 weeks from day 30 post–sowing. The rice plants were inoculated by spraying 1 × 105 conidia mL-1 suspension of M. oryzae and then covered with plastic bags for 12 h. Rice leaf samples (0.5 g) were collected from each treatment at 0, 12, 24, 36, 48, and 60 h post–inoculation (hpi) to examine endogenous SA. The leaf samples were soaked in liquid nitrogen (LN2) and homogenized with 1 mL of buffer solution containing 90% methanol, 9% glacial acetic acid, and 1% water by volume unit. The ground samples were centrifuged at 14,000 ×g for 10 min at 4°C. Next, 0.5 mL of the supernatant was transferred into an Eppendorf tube containing 0.5 mL of 0.02 M ferric ammonium sulfate, and the sample was incubated for 5 min at 30°C. Subsequently, 200 μL of each sample was transferred to a 96-well plate, and absorbance was measured at 530 nm using a Bio–Tek microplate reader (Winooski, VT, USA). Endogenous SA was calculated through comparison with the standard references (Udayashankar et al., 2011).
The total phenolic content of the leaves was determined following a slightly modified Folin–Ciocalteu assay (Lamuela-Raventós, 2018). Leaf samples (0.5 g) were soaked in LN2 and then ground with 2.5 mL of 80% methanol. The finely ground samples were centrifuged at 14,000 ×g for 10 min at 4°C. Next, 0.2 mL of the supernatant was transferred to an Eppendorf tube containing 0.2 mL of Folin–Ciocalteu reagent and mixed by vortexing. Then, 2 mL of 2% sodium carbonate was added prior to incubation for 20 min at 30°C in the dark. Subsequently, 200 μL of each sample was transferred to a 96-well plate, and absorbance was measured at 760 nm using a Bio–Tek microplate reader (Winooski, VT, USA). Gallic acid was used as the calibration standard, and the total phenolic content was expressed as microgram of gallic acid equivalent (g-1 FW) on a fresh weight basis (µg GAE g-1 FW).
Rice leaf samples from each treatment (0.5 g) were soaked in LN2, homogenized with 5 mL of 0.1% trichloroacetic acid, and centrifuged at 14,000 ×g and 4°C for 15 min. The samples (0.5 mL) were mixed with 0.5 mL of 10 mM potassium phosphate buffer (pH 7.0) and 1 mL of 1 M potassium iodide. Absorbance was measured at 390 nm using a Bio–Tek microplate reader (Winooski, VT, USA) (Sahebani and Hadavi, 2009).
Treated and untreated leaf samples were collected, fixed in the optimal cutting temperature compound, and frozen in LN2. The samples were transversely sectioned at 8 μm using a cryostat microtome (Wetzlar, Germany) and then laid on 13 × 2 mm slides. Spectral derivatives were generated using Beamline 4.1 at the Synchrotron Light Research Institute, Thailand. Measurements were performed by mapping at an aperture size of 10 × 10 μm and spectral resolution mode of 4 cm-1 with 64 scans. Spectral derivatives were generated using OPUS 7.2 (Hanau, Germany), The data and principal component analysis (PCA) were analyzed using Cytospec™ and UnscramblerX 10.0 (New Jersey, USA) (Thepbandit et al., 2021).
The M. oryzae infection process during plant defense response was analyzed using scanning electron microscopy (SEM). Rice plants were spayed for each elicitor three times and then inoculated with M. oryzae using 30 mL of the conidial suspension (1 × 105 conidia mL-1) per pot, as described above. Thereafter, 5–cm–long leaf samples were collected from different sets of treatments and soaked in phenol to stop the infection process. Finally, the samples were subjected to SEM.
Disease symptoms appearing on the tested rice leaves at 14 days post–inoculation (dpi) were analyzed based on the IRRI standard for rice blast disease scoring (IRRI, 2013), specified as follows:
The disease severity index and control efficacy were calculated using the formulas (4) and (5), respectively, as follows:
To ensure data consistency, the experiments were performed under greenhouse conditions and repeated three times. The obtained experimental data were analyzed using one–way ANOVA with SPSS 20 (SPSS Inc., Chicago, USA). Significant differences in means among groups were analyzed using Duncan’s multiple range test at p ≤ 0.05.
The spectra of five microbial strains analyzed with FlexAnalysis 3.4 and MALDI–BioTyper v.4.0 (Karlsruhe, Germany) were used to compare the mass fingerprints of samples with the library database. The analysis revealed that the microbial strains at the genus level presented log score values of >2.000, corresponding to species–level identification. Four species were identified as belonging to the genus Bacillus, and one was identified as Pseudomonas aeruginosa (Table 1). The characteristic spectra of the five microbial species are presented in Figure 1.
Figure 1 Characteristic MALDI–TOF/TOF MS spectra. Characteristic spectra of (A) Pseudomonas aeruginosa, (B) Bacillus cereus, (C) B cereus, (D) B vallismortis, and (E) B cereus generated using the Bruker BioTyper MALDI–TOF/TOF MS system. Absolute intensities of ions are shown on the y–axis, and the masses (m/z) of ions are shown on the x–axis. The m/z values represent the mass–to–charge ratios.
In the antagonist testing experiment, microbial biocontrol agents produced a strong effect against M. oryzae growth. Two Bacillus species showed comparable maximum percent inhibition of mycelial growth (Table 2). Specifically, B. vallismortis significantly suppressed M. oryzae growth by 77.03% (Table 2, Figures 2A–C). The hyphal morphology of M. oryzae was clearly affected by B. vallismortis under light microscopy. As such, the hyphae lost their normal growth and branching patterns, presenting wrinkled, twisted, and abnormal bending compared with hyphae in untreated samples (Figures 2D, E).
Table 2 Antagonistic activity of Bacillus sp. and Pseudomonas sp. against Magnaporthe oryzae causing rice blast, as assessed using the dual culture method.
Figure 2 Observations of the antagonist testing against Magnaporthe oryzae. (A) M. oryzae mycelium growth in an untreated control plate. (B) Inhibition by Bacillus vallismortis. (C) M. oryzae mycelium growth bar plot in an untreated control plate compared with that in a B vallismortis –treated plate (D) Normal hyphae of M. oryzae in an untreated control plate. (E) Damaged hyphae of M. oryzae in a B vallismortis –treated plate. Means in the graph followed by different letters are significantly different according to DMRT at p = 0.01.
To determine the efficacy of the B. vallismortis biosurfactant against the primary infection process of M. oryzae, the percentage of spore, germ tube and appressoria formed on rice leaves at different concentrations were calculated as spore number of each development stage divided by the total number of spores. The percentages of germ tubes and appressoria formed in the control samples (0% v/v of biosurfactant) were 19.75% and 68.75%, respectively whereas spore without germination was 11.30%. Appressoria formation decreased with increasing biosurfactant concentration (53.00%, 34.75%, 30.00%, 20.50%, 16.75%, and 12.00% at 5%, 10%, 15%, 20%, 25%, and 30%, respectively). Meanwhile, germ tube formation was higher when using 15% v/v of biosurfactant (51.25%), although the difference was non–significant compared with values at 10% and 20% v/v biosurfactant doses (49% and 46.50%, respectively), whereas at 25% and 30% v/v biosurfactant doses (35.00% and 32.50%, respectively) were shown significantly lower. However, spore germination including germ tube and appressorium for each of the treatment was converted to percent inhibition compared with the control sample. The increase of biosurfactant concentration effectively increased the inhibition of spore germination by 50% compared with the control (Figure 3).
Figure 3 Efficacy of the Bacillus vallismortis biosurfactant. (A) Percentage of spore, germ tube, and appressorium (left ordinate) and inhibition of spore germination compared with the control treatment (0%) at 16 hpi (right ordinate). (B) Spore and spore which produced germ tube on hydrophobic coverslips in B vallismortis biosurfactant-treated (C) Spore germination and appressorium formation on hydrophobic coverslips in control. (D) Spore tip mucilage and appressorium formation in control sample. (E) Spores without germination in 30% v/v of biosurfactant-treated sample. Values are presented as the mean of four replicates. Means in the graph followed by different letters is a are significantly different according to DMRT at p = 0.05.
The B. vallismortis biosurfactant was further analyzed using MALDI–TOF/TOF MS, and intense signals were detected in the m/z range of 600–1,200. Results of molecular mass–ion analysis showed that the samples obtained from the B. vallismortis biosurfactant contained three primary peaks at 1,066, 1,080, and 1,110 m/z (Figure 4A). These groups of peaks corresponded to iturin A at 1,044, 1,080, and 1,100 m/z (Figure 4B) and surfactin at 1,017 and 1,059 m/z (Figure 4C) according to their standards. Although the peaks detected at 1,066 m/z did not exactly match with the established standard, these readings can be assigned as surfactin species, as they are detected in the range of m/z 1,000–1,074 (Vater et al., 2002).
Figure 4 MALDI-TOF/TOF mass spectra of lipopeptides detected the range of 600–1,200 m/z. (A) Iturin A and surfactin in biosurfactant produced by Bacillus vallismortis assigned. (B) Iturin A standard. (C) Surfactin standard.
The effects of the B. vallismortis biosurfactant on the endogenous SA content were evaluated at pre– and post–inoculation time points. Sprayed SA and biosurfactant doses on the rice plants increased endogenous SA content at 12, 24, and 36 hpi, but the values dropped at 48 hpi. In contrast, endogenous SA content in control samples (0% v/v biosurfactant) increased at 24 hpi and dropped at 36 hpi. Regarding relative increase, the highest endogenous SA content was recorded in SA treatment (160%), followed by that in the 20% v/v biosurfactant treatment (140%) (Figure 5).
Figure 5 Endogenous salicylic acid (SA) activity in ‘KDML 105’ treated with biosurfactant following Magnaporthe oryzae infection. (A) Endogenous SA content and (B) relative increase in endogenous SA content.
Both biosurfactant and SA treatments elevated total phenolic content post–inoculation, with a faster increase from 12 to 48 hpi, whereas total phenolic content in the control treatment was only slightly increased at 24 hpi. The highest value of total phenolic content (48%) in rice leaves was recorded at 48 hpi following the 20% v/v biosurfactant treatment. On the contrary, phenolic content (8.5%) in the control treatment (0% v/v biosurfactant) showed lower percentage increase at 24 hpi (Figure 6).
Figure 6 Total phenolic compound activity in ‘KDML 105’ treated with biosurfactant following Magnaporthe oryzae infection. (A) total phenolic compound content and (B) relative increase in total phenolic compound content.
H2O2 levels in rice plants started increasing at 6 hpi but decreased at 24 hpi in all treatments. H2O2 levels in rice plants treated with SA showed the highest increase (63%), followed by those in rice plants treated with the biosurfactant (~58%–60%) at 12 hpi; increase in the control treatment was only 25% (Figure 7).
Figure 7 The hydrogen peroxide activity in ‘KDML 105’ treated with biosurfactant following Magnaporthe oryzae infection. (A) hydrogen peroxide content and (B) relative increase in hydrogen peroxide content.
SR–FT–IR spectra were used for monitoring changes in rice biochemical composition corresponding to rice leaf mesophyll following treatment with the B. vallismortis surfactant compared with composition in the positive (SA) and negative (water–mock) control treatments. Three clearly distinguishable groups were noted: B. vallismortis, SA, and water–mock. Clusters of samples and PCA biplots were described by the first (PC1, 19%) and second (PC2, 7%) principal component (Figure 8A). PC1 showed high positive loadings at 2,859, 1,346, 1,141, and 1,103 cm-1, related to the positive score plots of SA–treated samples (positive controls). PC1 showed high negative loadings at 1,604, 1,160, 946, and 921 cm-1, related to the negative score plots of water–treated samples (negative controls) (Figure 8B). PC2 high positive loadings at 1,741, 1,654, 1,546, and 1,182 cm-1, related to the positive score plots of B. vallismortis–treated samples (Figure 8C). Spectral band variability through loading plots associated with the second derivative of the average spectra in the mesophyll showed different regions of the samples. The spectral region at 3,000–2,800 cm-1 from samples revealed peaks at 2,960, 2,923, and 2,859 cm-1, corresponding to the CH2 and CH3 parts of lipid groups. The region at 1,700–1,500 cm-1, corresponding to proteins and peptides, revealed peaks at 1,737, 1,654, 1,604, 1,546, and 1,513. The region at 1,300–900 cm-1, corresponding to polysaccharides and carbohydrates, revealed peaks at 1,160, 1,103, 1,033, 993, 946, and 921 cm-1 (Figure 8D). All these spectral averages were used to quantitatively identify the molecular structures of biochemical compounds and to obtain the integral areas of the biochemical segments of samples. From the integral area, the amounts of Amide I and Amide II were significantly increased but the amounts of polysaccharide were significantly decreased in B. vallismortis –treated samples compared with those in negative controls (p< 0.05) (Figure 8E).
Figure 8 SR-FT-IR spectra of mesophyll in rice leaf tissue. (A) Principal component analysis (PCA). (B) Loading plots from PCA analysis (PC1). (C) Loading plots from PCA analysis (PC2). (D) Overlay of the average second derivative spectrum. (E) Integral areas of absorbance between 3,000 and 900 cm-1 Means in the graph followed by different letters are significantly different according to DMRT at p = 0.05. BA, Bacillus vallismortis –treated sample; SA, salicylic acid–treated sample; CT, control water–treated sample.
Observations of the infection process of M. oryzae at 24 hpi revealed that M. oryzae successfully infected and colonized untreated leaves, presenting appressorium formation and hyphal development. During the same period, treatment with the B. vallismortis biosurfactant as an inducer prevented the hyphal invasion in rice tissue by M. oryzae (Figure 9).
Figure 9 Scanning electron micrograph of Magnaporthe oryzae on detached rice leaves at 24 hpi. (A) M. oryzae successfully established appressoria and hyphae on the leaves of the untreated rice plant. (B) M. oryzae conidia adhesion on the leaves of Bacillus vallismortis –treated rice plant.
The efficacy of the B. vallismortis biosurfactant at a range of concentrations, including 20%, 25%, and 30% v/v, against rice blast was evaluated under greenhouse conditions. SA as the inducer was used as the positive control and 0% v/v biosurfactant as the negative control. Biosurfactant treatment before inoculation reduced disease severity compared with both positive and negative control treatments, as expressed by higher disease reduction percentages. The initial disease symptoms appeared at 14 dpi. Common rice blast symptoms were detected as elliptical lesions or white–grey spots surrounded by necrotic brown edges. At 21 dpi, disease severity was respectively 67.59% and 37.96% in negative and positive control samples. Treatment with 30% v/v biosurfactant showed the lowest disease severity (19.44%), followed by treatments with 25% and 20% v/v biosurfactant (24.07% and 31.48%, respectively); these values were significantly lower than that in the positive control treatment (37.96%) (Figure 10).
Figure 10 Effectiveness of the Bacillus vallismortis biosurfactant against rice blast caused by Magnaporthe oryzae in ‘KDML 105’. (A) Disease severity and disease reduction. (B) Visible symptoms on treated leaves at 21 days post-inoculation. Means in the graph followed by different letters are significantly different according to DMRT at p = 0.05.
Beneficial microorganisms have been considerably characterized for their antagonism against common agricultural pathogens. Moreover, such microorganisms have been exploited as eco–friendly substitutes for pesticides, as they produce antimicrobial compounds and activate plant immunity to combat a broad range of plant pathogens. Most studies addressing beneficial microorganisms have explored their antimicrobial activities or plant defense induction potential. Therefore, in the present study, we analyzed the control ability of five representative microorganisms isolates from paddy fields near the rhizospheric region against rice blast through both direct and indirect antagonism.
B. vallismortis exhibited potent antagonistic activity against M. oryzae (>50%), as determined based on the production of antimicrobial substances. Furthermore, we investigated the ability of the B. vallismortis biosurfactant to inhibit M. oryzae development and observed that at high concentrations, the biosurfactant can effectively suppress spore germination and appressorium development in M. oryzae. These findings are consistent with previous reports that lipopeptide biosurfactants from B. subtilis inhibited the germination of M. oryzae—the causal agent of wheat blast (Chakraborty et al., 2020). In previous studies, different Bacillus strains, including B. subtilis, B. cereus, B. amyloliquefaciens, B. licheniformis, and B. pumilus, have been applied as biocontrol agents against rice blast for organic and sustainable farming. For instance, B. vallismortis R2 could be used as an alternative to combat several plant pathogenic fungi, including Alternaria sp., Rhizoctonia oryzae, Fusarium sp., Colletotrichum sp., Helminthosporium sp., and Magnaporthe grisea (Kaur et al., 2015; Sha et al., 2020). In addition, B. vallismortis, which produce numerous antimicrobial bioactive metabolites, such as thermostable alkalophilic cellulose, bacillomycin D, surfactins, iturins, and fengycins, has been tested for its biocontrol properties (Zhao et al., 2010; Kaur et al., 2017).
Our MALDI–TOF/TOF MS analysis of the B. vallismortis biosurfactant revealed the production of two major classes of lipopeptide antibiotics based on secondary metabolites, including iturin A and surfactin. The groups of peaks at m/z values between 1,000 and 1,074 correspond to surfactins and those between 1,076 and 1,150 correspond to iturins (Vater et al., 2002). Iturin A peak in the sample was detected at 1,080 m/z, consistent with its standard (1,080 m/z). Surfactin peak in the sample was detected at 1,066 m/z, close to its standard (1,059 m/z). The surfactin peak was different from its standard, although it was still in the range of surfactin species (Vater et al., 2002; Hoefler et al., 2012).
Iturins have been identified as antifungal substances that degrade the cell wall, inhibit substance exchange and metabolic processes, and lower viscoelastic fluid pressure in fungal cells (Jiang et al., 2020). The antifungal mechanism of iturin lipopeptides is associated with their interaction in the cytoplasmic membrane, which increases potassium permeability (Maget-Dana and Peypoux, 1994). Furthermore, surfactins destroy the internal structure of cells through digesting the cytoplasm and organelles (Xiao et al., 2021). Iturins and surfactins not only exhibit a strong antifungal activity but also act as bioactive molecules to induce plant defense (Raaijmakers et al., 2010). Several studies have reported that lipopeptides, in addition to iturins, surfactins, and fengycins, activate plant ISR, through which the gene expression of defense proteins, including phenylalanine lyase, peroxidases peroxidases, and other pathogenesis–related (PR) proteins, is promoted. This mechanism is essentially responsible for the innate immunization to inhibit pathogens in different plants. Previous studies have demonstrated that lipopeptides, as products of bacterial biosurfactants, activate specific plant defense pathways against pathogens, such as pathways against Colletotrichum gloeosporioides in strawberry, pathways against Sclerotinia sclerotiorum in tomato, and pathways against M. oryzae in rice (Yamamoto et al., 2015; Farzand et al., 2019; Lam et al., 2021).
The pivotal roles of the B. vallismortis biosurfactant in plant immune interactions was further investigated in the present study by analyzing the changes in host defense intermediate biochemicals related to enhanced resistance against M. oryzae. Spraying the B. vallismortis biosurfactant three times before pathogen inoculation resulted in the establishment of plant immunological parameters, including increases in endogenous SA and phenolic levels (p< 0.05).
SA, jasmonic acid (JA), and ethylene (ET) are important hormonal signal molecules involved in biotic stress responses during plant–pathogen interactions. Typically, SA–mediated defense responses play a central role in local and systemic–acquired resistance against biotrophic and hemi–biotrophic pathogens, whereas ET/JA–mediated responses contribute to defense against necrotrophic pathogens (Bari and Jones, 2009; D. Tamaoki et al., 2013). M. oryzae is a hemi–biotrophic fungus that causes rice blast; it assumes an initial biotrophic stage during which the plant immune system is suppressed, followed by the necrotrophic stage that promotes plant cell death (Fernandez and Orth, 2018).
The SA pathway is the dominant signaling cascade under pathogen attacks due to the presence of communication channels that can promote generation of energy and synthesis of potential intermediates and enzymes to inhibit pathogens (Koo et al., 2020). Studies on defense responses of multiple plant species, including rice, soybean, and tobacco, have reported that pathogen infection leads to SA accumulation (Yalpani et al., 1993; Bawa et al., 2019; Liang et al., 2022). At the early stages of pathogen infection, plants recognize and activate the defense signals as well as induce damage–associated molecular patterns (DAMPs), including extracellular protein fragments, peptides, nucleotides, and amino acids (Hou et al., 2019). The transcription factors for PR genes activate SA to mediate host response in infected plant cells (Hou et al., 2019). Kawagoe et al. (2015) characterized the signaling pathways of ISR stimulated by lipopeptides in Arabidopsis through the SA and JA signaling pathways, which activated the transcription of defense genes (Kawagoe et al., 2015). Moreover, SA accumulated around the infected area generates HRs at the initial infection site (Daisuke Wildermuth et al., 2001; Tamaoki et al., 2013). Lakkis et al. (2019) reported that Pseudomonas fluorescens is an inducer of ISR in grapevine, which activates both immune response and priming phenomenon under Botrytis cinerea infection; this event is related to the constitutively enhanced expression of SA–related genes and increased total phenolic content (Lakkis et al., 2019).
The involvement of phenolic compounds in the defense reaction is a crucial physiological function in several plants, such as lettuce, banana, and rice plants, to offer resistance against multiple biotic stressors (Sedlářová and Lebeda, 2001; Dallagnol et al., 2011; Fortunato et al., 2012; Pratyusha, 2022). According to our results, the total phenolic content showed the highest increase in the 20% v/v biosurfactant treatment. In contrast, in the 30% v/v biosurfactant treatment, the magnitude of increase was lower. This may be because B. vallismortis produces iturin A and surfactin in the biosurfactant, which produce a direct antimicrobial effect against the pathogen; thus, the inoculum should have a lower density than in the other treatments. Similarly, H2O2 did not produce the strongest effect as it is a defense intermediary contributing to program cell death (PCD) under pathogen attack. These observations are consistent with previous reports that phenolic production is linked to SA–induced resistance responses, which are often augmented after a pathogen attack leading to HR and contributing to subsequent cell death (Wallis and Galarneau, 2020). Betsuyaku et al. (2018) reported higher levels of SA in concentric patterns of cellular responses in effector–triggered immunity. PAMP–triggered immunity induces an HR and localized PCD to inhibit pathogen spread, which effectively protects the plant tissues from disease severity (Betsuyaku et al., 2018; Radojičić et al., 2018). In the present study, the comparison of H2O2–mediated PCD and initiation of defense responses against M. oryzae infection between treated and untreated samples revealed significant temporal differences in the accumulation of H2O2. This result confirms that in the present experiment, plant defense activity exclusively occurred after M. oryzae infection. The higher H2O2 activity suggests that reactive oxygen species (ROS) contribute to the disruption of the electron transport chain at the early stages of infection (6–12 hpi) produced by elicitation with biosurfactant. The higher H2O2 levels may enhance the ability of cells to detoxify H2O2, which may generate damage–related signals and activate transcriptional regulators of PCD–related genes. PCD under host–pathogen interactions in the elicitation affects the rapid inhibition of pathogen development. These results are consistent with the plant timing defense initiation theory and corroborate the observations of Ye et al. (2021), who reported increased ROS concentrations after pathogen infection, leading to a change in the antioxidant status of cells and induction of plant responses in terms of immunity–related cell death (Ye et al., 2021).
This indirect inhibitory effect of the biosurfactant causes a shift in intracellular biochemical elements, which were detected using SR–FT–IR microspectroscopy. The biomolecule–related intensities from average spectra revealed significantly higher agglomeration of Amide I (1,600–1,800 cm-1) and Amide II (1,470–1,570 cm-1) proteins. A plant protein peptide bond is an amide group as well as a type of endogenous factor in disease resistance to encourage plant–microbe interaction (Liu et al., 2022). Plant peptides induce immune responses that are established at the receptor level of plant peptides in response to pathogen invasion, including the induction of defense–related phytoalexin, lignification, and PR proteins (Hu et al., 2018; Kumar et al., 2018). Moreover, significantly less polysaccharides (1,200–900 cm-1), such as carbohydrates and sugar, were accumulated in biosurfactant–treated samples. This may be because during the infection process, plants adapt or alter their energy storage to trigger defense responses against pathogens (Tauzin and Giardina, 2014). We further examined the M. oryzae infection process on leaves using SEM. Elicitation of rice plants with the biosurfactant disrupted the development process of M. oryzae, confirming that the biosurfactant produced an indirect inhibitory effect on host plant infection (Figure 11).
Figure 11 A proposed schematic model of systemic resistance in rice plant against Magnaporthe oryzae after being treated with Bacillus vallismortis biosurfactant.
The results of the present study confirmed that the B. vallismortis biosurfactant exhibits antibacterial activity against M. oryzae–the causative agent of rice blast–through both direct and indirect inhibitory effects. Moreover, experimental data confirmed that the biosurfactant, as an elicitor, effectively reduced rice blast severity through the production of antibiotic substances, including iturin A and surfactin. In addition, levels of endogenous SA, phenolics, H2O2, amide I and, amide II, all of which are defense intermediaries, were increased, contributing to against M. oryzae during pathogenesis.
The original contributions presented in the study are included in the article/supplementary material. Further inquiries can be directed to the corresponding author.
WT and DA: Conceptualization and design. WT, AS, SS, and SN: Implementation, investigation, and analyzed the data. WT and SS: Arranged the results, discussion, and writing the original draft. WT, SS, and DA: Revised the manuscript. All authors contributed to the article and approved the submitted version.
This study was supported by Thammasat Postdoctoral Fellowship Grant Number TUPD6/2564, the Thammasat University Center of Excellence in Agriculture Innovation Centre through Supply Chain and Value Chain, and the Thailand Science Research and Innovation Fundamental Fund.
The authors would like to thank the Synchrotron Light Research Institute (SLRI) in Thailand, which provided the FTIR instruments and the beamline 4.1 Infrared Spectroscopy and Imaging systems. We would like to thank Editage (www.editage.com) for English language editing.
The authors declare that the research was conducted in the absence of any commercial or financial relationships that could be construed as a potential conflict of interest.
All claims expressed in this article are solely those of the authors and do not necessarily represent those of their affiliated organizations, or those of the publisher, the editors and the reviewers. Any product that may be evaluated in this article, or claim that may be made by its manufacturer, is not guaranteed or endorsed by the publisher.
Abdelaziz, A. M., Hashem, A. H., El-Sayyad, G. S., El-Wakil, D. A., Selim, S., Alkhalifah, D. H. M., et al. (2023). Biocontrol of soil borne diseases by plant growth promoting rhizobacteria. Trop. Plant Pathol. doi: 10.1007/s40858-022-00544-7
Bari, R., Jones, J. D. (2009). Role of plant hormones in plant defence responses. Plant Mol. Biol. 69, 473–488. doi: 10.1007/s11103-008-9435-0
Bawa, G., Feng, L., Yan, L., Du, Y., Shang, J., Sun, X., et al. (2019). Pre-treatment of salicylic acid enhances resistance of soybean seedlings to fusarium solani. Plant Mol. Biol. 101, 315–323. doi: 10.1007/s11103-019-00906-x
Betsuyaku, S., Katou, S., Takebayashi, Y., Sakakibara, H., Nomura, N., Fukuda, H. (2018). Salicylic acid and jasmonic acid pathways are activated in spatially different domains around the infection site during effector-triggered immunity in arabidopsis thaliana. Plant Cell Physiol. 59, 8–16. doi: 10.1093/pcp/pcx181
Chakraborty, M., Mahmud, N. U., Gupta, D. R., Tareq, F. S., Shin, H. J., Islam, T. (2020). Inhibitory effects of linear lipopeptides from a marine bacillus subtilis on the wheat blast fungus magnaporthe oryzae triticum [Original research]. Front. Microbiol. 11. doi: 10.3389/fmicb.2020.00665
Dallagnol, L. J., Rodrigues, F. A., DaMatta, F. M., Mielli, M. V. B., Pereira, S. C. (2011). Deficiency in silicon uptake affects cytological, physiological, and biochemical events in the rice–bipolaris oryzae interaction. Phytopathology® 101, 92–104. doi: 10.1094/phyto-04-10-0105
Deice Raasch-Fernandes, L., Bonaldo, S. M., de Jesus Rodrigues, D., Magela Vieira-Junior, G., Regina Freitas Schwan-Estrada, K., Rocco da Silva, C., et al. (2019). Induction of phytoalexins and proteins related to pathogenesis in plants treated with extracts of cutaneous secretions of southern amazonian bufonidae amphibians. PloS One 14, e0211020. doi: 10.1371/journal.pone.0211020
Elshafie, A. E., Joshi, S. J., Al-Wahaibi, Y. M., Al-Bemani, A. S., Al-Bahry, S. N., Al-Maqbali, D. A., et al. (2015). Sophorolipids production by candida bombicola atcc 22214 and its potential application in microbial enhanced oil recovery [Original research]. Front. Microbiol. 6. doi: 10.3389/fmicb.2015.01324
Farzand, A., Moosa, A., Zubair, M., Khan, A. R., Massawe, V. C., Tahir, H. A. S., et al. (2019). Suppression of sclerotinia sclerotiorum by the induction of systemic resistance and regulation of antioxidant pathways in tomato using fengycin produced by bacillus amyloliquefaciens fzb42. Biomolecules 9, 613. doi: 10.3390/biom9100613
Fernandez, J., Orth, K. (2018). Rise of a cereal killer: The biology of magnaporthe oryzae biotrophic growth. Trends Microbiol. 26, 582–597. doi: 10.1016/j.tim.2017.12.007
Fortunato, A. A., Rodrigues, F., do Nascimento, K. J. (2012). Physiological and biochemical aspects of the resistance of banana plants to fusarium wilt potentiated by silicon. Phytopathology 102, 957–966. doi: 10.1094/phyto-02-12-0037-r
Hashizume, H., Nishimura, Y. (2008). “Cyclic lipopeptide antibiotics,” in Studies in natural products chemistry, vol. 35 . Ed. Atta ur, R. (Cham, Switzerland: Elsevier), 693–751. doi: 10.1016/S1572-5995(08)80016-6
Heil, M., Bostock, R. M. (2002). Induced systemic resistance (isr) against pathogens in the context of induced plant defences. Ann. Bot. 89, 503–512. doi: 10.1093/aob/mcf076
Hoefler, B. C., Gorzelnik, K. V., Yang, J. Y., Hendricks, N., Dorrestein, P. C., Straight, P. D. (2012). Enzymatic resistance to the lipopeptide surfactin as identified through imaging mass spectrometry of bacterial competition. Proc. Natl. Acad. Sci. U.S.A. 109, 13082–13087. doi: 10.1073/pnas.1205586109
Hou, S., Liu, Z., Shen, H., Wu, D. (2019). Damage-associated molecular pattern-triggered immunity in plants. Front. Plant Sci. 10. doi: 10.3389/fpls.2019.00646
Howard, R. J., Valent, B. (1996). Breaking and entering: Host penetration by the fungal rice blast pathogen magnaporthe grisea. Annu. Rev. Microbiol. 50, 491–512. doi: 10.1146/annurev.micro.50.1.491
Hu, Z., Zhang, H., Shi, K. (2018). Plant peptides in plant defense responses. Plant Signal Behav. 13, e1475175. doi: 10.1080/15592324.2018.1475175
IRRI: International Rice Research Institute, Calabarzon, Philippines (2013). Standardization evaluation system for rice.
Jiang, C., Li, Z., Shi, Y., Guo, D., Pang, B., Chen, X., et al. (2020). Bacillus subtilis inhibits aspergillus carbonarius by producing iturin a, which disturbs the transport, energy metabolism, and osmotic pressure of fungal cells as revealed by transcriptomics analysis. Int. J. Food Microbiol. 330, 108783. doi: 10.1016/j.ijfoodmicro.2020.108783
Jourdan, E., Henry, G., Duby, F., Dommes, J., Barthélemy, J. P., Thonart, P., et al. (2009). Insights into the defense-related events occurring in plant cells following perception of surfactin-type lipopeptide from bacillus subtilis. Mol. Plant Microbe Interact. 22, 456–468. doi: 10.1094/mpmi-22-4-0456
Kaur, P. K., Joshi, N., Singh, I. P., Saini, H. S. (2017). Identification of cyclic lipopeptides produced by bacillus vallismortis r2 and their antifungal activity against alternaria alternata. J. Appl. Microbiol. 122, 139–152. doi: 10.1111/jam.13303
Kaur, P., kaur, J., Saini, H. (2015). Antifungal potential of bacillus vallismortis r2 against different phytopathogenic fungi. Spanish J. Agric. Res. 13, 1004. doi: 10.5424/sjar/2015132-6620
Kawagoe, Y., Shiraishi, S., Kondo, H., Yamamoto, S., Aoki, Y., Suzuki, S. (2015). Cyclic lipopeptide iturin a structure-dependently induces defense response in arabidopsis plants by activating sa and ja signaling pathways. Biochem. Biophys. Res. Commun. 460:1015–1020. doi: 10.1016/j.bbrc.2015.03.143
Kawasaki, J., Herath, S. (2018). Impact assessment of climate change on rice production in khon kaen province, thailand. Environmental Science Journal of International Society for Southeast Asian Agricultural SciencesJ. ISSAAS Vol. 17, No. 2:14-28 (2011).
Khanna, K., Jamwal, V. L., Kohli, S. K., Gandhi, S. G., Ohri, P., Bhardwaj, R., et al. (2019). Role of plant growth promoting bacteria (pgprs) as biocontrol agents of meloidogyne incognita through improved plant defense of lycopersicon esculentum. Plant Soil 436, 325–345. doi: 10.1007/s11104-019-03932-2
Khanna, K., Kohli, S. K., Sharma, P., Kour, J., Singh, A. D., Sharma, N., et al. (2021). “Antioxidant potential of plant growth-promoting rhizobacteria (pgpr) in agricultural crops infected with root-knot nematodes,” in Antioxidants in plant-microbe interaction. Eds. Singh, H. B., Vaishnav, A., Sayyed, R. Z. (Singapore: Springer), 339–379. doi: 10.1007/978-981-16-1350-0_16
Koch, E., Slusarenko, A. (1990). Arabidopsis is susceptible to infection by a downy mildew fungus. Plant Cell 2, 437–445. doi: 10.1105/tpc.2.5.437
Köhl, J., Kolnaar, R., Ravensberg, W. J. (2019). Mode of action of microbial biological control agents against plant diseases: Relevance beyond efficacy [Review]. Front. Plant Sci. 10. doi: 10.3389/fpls.2019.00845
Kongcharoen, N., Kaewsalong, N., Dethoup, T. (2020). Efficacy of fungicides in controlling rice blast and dirty panicle diseases in thailand. Sci. Rep. 10, 16233–16233. doi: 10.1038/s41598-020-73222-w
Koo, Y. M., Heo, A. Y., Choi, H. W. (2020). Salicylic acid as a safe plant protector and growth regulator. Plant Pathol. J. 36, 1–10. doi: 10.5423/ppj.Rw.12.2019.0295
Kumar, V., Bhatt, V., Kumar, N. (2018). “Chapter 9 - amides from plants: Structures and biological importance,” in Studies in natural products chemistry, vol. 56 . Ed. Atta ur, R. (Amsterdam Netherlands: Elsevier), 287–333. doi: 10.1016/B978-0-444-64058-1.00009-1
Lakkis, S., Trotel-Aziz, P., Rabenoelina, F., Schwarzenberg, A., Nguema-Ona, E., Clément, C., et al. (2019). Strengthening grapevine resistance by pseudomonas fluorescens pta-ct2 relies on distinct defense pathways in susceptible and partially resistant genotypes to downy mildew and gray mold diseases [Original research]. Front. Plant Sci. 10. doi: 10.3389/fpls.2019.01112
Lam, V. B., Meyer, T., Arias, A. A., Ongena, M., Oni, F. E., Höfte, M. (2021). Bacillus cyclic lipopeptides iturin and fengycin control rice blast caused by pyricularia oryzae in potting and acid sulfate soils by direct antagonism and induced systemic resistance. Microorganisms 9, 1441. doi: 10.3390/microorganisms9071441
Lamuela-Raventós, R. M. (2018). “Folin–ciocalteu method for the measurement of total phenolic content and antioxidant capacity,” in Measurement of antioxidant activity & capacity, John Wiley & Sons Ltd. UK 107–115. doi: 10.1002/9781119135388.ch6
Liang, B., Wang, H., Yang, C., Wang, L., Qi, L., Guo, Z., et al. (2022). Salicylic acid is required for broad-spectrum disease resistance in rice. Int. J. Mol. Sci. 23, 1354. doi: 10.3390/ijms23031354
Liu, S., Jiang, J., Ma, Z., Xiao, M., Yang, L., Tian, B., et al. (2022). The role of hydroxycinnamic acid amide pathway in plant immunity. Front. Plant Sci. 13. doi: 10.3389/fpls.2022.922119
Maget-Dana, R., Peypoux, F. (1994). Iturins, a special class of pore-forming lipopeptides: Biological and physicochemical properties. Toxicology 87, 151–174. doi: 10.1016/0300-483x(94)90159-7
Malviya, D., Sahu, P. K., Singh, U. B., Paul, S., Gupta, A., Gupta, A. R., et al. (2020). Lesson from ecotoxicity: Revisiting the microbial lipopeptides for the management of emerging diseases for crop protection. Int. J. Environ. Res. Public Health 17, 1434. doi: 10.3390/ijerph17041434
Mandal, S. M., Chakraborty, D., Dey, S. (2010). Phenolic acids act as signaling molecules in plant-microbe symbioses. Plant Signal Behav. 5, 359–368. doi: 10.4161/psb.5.4.10871
Ngalimat, M. S., Mohd Hata, E., Zulperi, D., Ismail, S. I., Ismail, M. R., Mohd Zainudin, N. A. I., et al. (2021). Plant growth-promoting bacteria as an emerging tool to manage bacterial rice pathogens. Microorganisms 9, 682. doi: 10.3390/microorganisms9040682
Pratyusha, S. (2022). Phenolic compounds in the plant development and defense: An overview intechopen, UK. doi: 10.5772/intechopen.102873
Raaijmakers, J. M., De Bruijn, I., Nybroe, O., Ongena, M. (2010). Natural functions of lipopeptides from bacillus and pseudomonas: More than surfactants and antibiotics. FEMS Microbiol. Rev. 34, 1037–1062. doi: 10.1111/j.1574-6976.2010.00221.x
Radojičić, A., Li, X., Zhang, Y. (2018). Salicylic acid: A double-edged sword for programed cell death in plants [Perspective]. Front. Plant Sci. 9. doi: 10.3389/fpls.2018.01133
Romera, F. J., García, M. J., Lucena, C., Martínez-Medina, A., Aparicio, M. A., Ramos, J., et al. (2019). Induced systemic resistance (isr) and fe deficiency responses in dicot plants [Review]. Front. Plant Sci. 10. doi: 10.3389/fpls.2019.00287
Romero, D., Vicente, A., Olmos, J. L., Dávila, J. C., Pérez-García, A. (2007). Effect of lipopeptides of antagonistic strains of bacillus subtilis on the morphology and ultrastructure of the cucurbit fungal pathogen podosphaera fusca. J. Appl. Microbiol. 103, 969–976. doi: 10.1111/j.1365-2672.2007.03323.x
Sahebani, N., Hadavi, N. (2009). Induction of h2o2 and related enzymes in tomato roots infected with root knot nematode (m. javanica) by several chemical and microbial elicitors. Biocontrol Sci. Technol. 19, 301–313. doi: 10.1080/09583150902752012
Saleh, D., Milazzo, J., Adreit, H., Fournier, E., Tharreau, D. (2014). South-east asia is the center of origin, diversity and dispersion of the rice blast fungus, magnaporthe oryzae. New Phytol. 201, 1440–1456. doi: 10.1111/nph.12627
Sedlářová, M., Lebeda, A. (2001). Histochemical detection and role of phenolic compounds in the defense response of lactuca spp. to lettuce downy mildew (bremia lactucae). J. Phytopathol. 149, 693–697. doi: 10.1046/j.1439-0434.2001.00698.x
Senthilraja, G., Anand, T., Kennedy, J. S., Raguchander, T., Samiyappan, R. (2013). Plant growth promoting rhizobacteria (pgpr) and entomopathogenic fungus bioformulation enhance the expression of defense enzymes and pathogenesis-related proteins in groundnut plants against leafminer insect and collar rot pathogen. Physiol. Mol. Plant Pathol. 82, 10–19. doi: 10.1016/j.pmpp.2012.12.002
Sha, Y., Zeng, Q., Sui, S. (2020). Screening and application of bacillus strains isolated from nonrhizospheric rice soil for the biocontrol of rice blast. Plant Pathol. J. 36, 231–243. doi: 10.5423/PPJ.OA.02.2020.0028
Sharma, P., Thakur, D. (2020). Antimicrobial biosynthetic potential and diversity of culturable soil actinobacteria from forest ecosystems of northeast india. Sci. Rep. 10, 4104. doi: 10.1038/s41598-020-60968-6
Tamaoki, D., Seo, S., Yamada, S., Kano, A., Miyamoto, A., Shishido, H., et al. (2013). Jasmonic acid and salicylic acid activate a common defense system in rice. Plant Signal Behav. 8, e24260. doi: 10.4161/psb.24260
Tauzin, A. S., Giardina, T. (2014). Sucrose and invertases, a part of the plant defense response to the biotic stresses. Front. Plant Sci. 5. doi: 10.3389/fpls.2014.00293
Thepbandit, W., Papathoti, N. K., Daddam, J. R., Thumanu, K., Siriwong, S., Thanh, T. L., et al. (2021). Identification of salicylic acid mechanism against leaf blight disease in oryza sativa by sr-ftir microspectroscopic and docking studies. Pathogens 10, 652. doi: 10.3390/pathogens10060652
Timsina, J., Wolf, J., Guilpart, N., van Bussel, L. G. J., Grassini, P., van Wart, J., et al. (2018). Can bangladesh produce enough cereals to meet future demand? Agric. Sys. 163, 36–44. doi: 10.1016/j.agsy.2016.11.003
Udayashankar, A., Nayaka, S. C., Reddy, M., Srinivas, C. (2011). Plant growth-promoting rhizobacteria mediate induced systemic resistance in rice against bacterial leaf blight caused by xanthomonas oryzae pv. oryzae. Biol. Control 59, 114–122. doi: 10.1016/j.biocontrol.2011.06.010
Vater, J., Kablitz, B., Wilde, C., Franke, P., Mehta, N., Cameotra, S. S. (2002). Matrix-assisted laser desorption ionization–time of flight mass spectrometry of lipopeptide biosurfactants in whole cells and culture filtrates of bacillus subtilis c-1 isolated from petroleum sludge. Appl. Environ. Microbiol. 68, 6210–6219. doi: 10.1128/aem.68.12.6210-6219.2002
Wallis, C. M., Galarneau, E. R. A. (2020). Phenolic compound induction in plant-microbe and plant-insect interactions: A meta-analysis. Front. Plant Sci. 11. doi: 10.3389/fpls.2020.580753
Wildermuth, M. C., Dewdney, J., Wu, G., Ausubel, F. M. (2001). Isochorismate synthase is required to synthesize salicylic acid for plant defence. Nature 414, 562–565. doi: 10.1038/35107108
Xiao, J., Guo, X., Qiao, X., Zhang, X., Chen, X., Zhang, D. (2021). Activity of fengycin and iturin a isolated from bacillus subtilis z-14 on gaeumannomyces graminis var. tritici and soil microbial diversity. Front. Microbiol. 12. doi: 10.3389/fmicb.2021.682437
Yalpani, N., Leon, J., Lawton, M. A., Raskin, I. (1993). Pathway of salicylic acid biosynthesis in healthy and virus-inoculated tobacco. Plant Physiol. 103, 315–321. doi: 10.1104/pp.103.2.315
Yamamoto, S., Shiraishi, S., Suzuki, S. (2015). Are cyclic lipopeptides produced by bacillus amyloliquefaciens s13-3 responsible for the plant defence response in strawberry against colletotrichum gloeosporioides? Lett. Appl. Microbiol. 60, 379–386. doi: 10.1111/lam.12382
Ye, C., Zheng, S., Jiang, D., Lu, J., Huang, Z., Liu, Z., et al. (2021). Initiation and execution of programmed cell death and regulation of reactive oxygen species in plants. Int. J. Mol. Sci. 22, 12942. doi: 10.3390/ijms222312942
Zhang, L., Sun, C. (2018). Fengycins, cyclic lipopeptides from marine bacillus subtilis strains, kill the plant-pathogenic fungus magnaporthe grisea by inducing reactive oxygen species production and chromatin condensation. Appl. Environ. Microbiol. 84. doi: 10.1128/aem.00445-18
Keywords: plant health, lipopeptide biosurfactant, biotic stress, phytohormone, plant-immunity, sustainable agriculture, plant–microbe interaction, rice disease
Citation: Thepbandit W, Srisuwan A, Siriwong S, Nawong S and Athinuwat D (2023) Bacillus vallismortis TU-Orga21 blocks rice blast through both direct effect and stimulation of plant defense. Front. Plant Sci. 14:1103487. doi: 10.3389/fpls.2023.1103487
Received: 20 November 2022; Accepted: 07 February 2023;
Published: 20 February 2023.
Edited by:
Kanika Khanna, Guru Nanak Dev University, IndiaReviewed by:
Jiaoyu Wang, Zhejiang Academy of Agricultural Sciences, ChinaCopyright © 2023 Thepbandit, Srisuwan, Siriwong, Nawong and Athinuwat. This is an open-access article distributed under the terms of the Creative Commons Attribution License (CC BY). The use, distribution or reproduction in other forums is permitted, provided the original author(s) and the copyright owner(s) are credited and that the original publication in this journal is cited, in accordance with accepted academic practice. No use, distribution or reproduction is permitted which does not comply with these terms.
*Correspondence: Dusit Athinuwat, YWR1c2l0QHN0YWZmLnR1LmFjLnRo
Disclaimer: All claims expressed in this article are solely those of the authors and do not necessarily represent those of their affiliated organizations, or those of the publisher, the editors and the reviewers. Any product that may be evaluated in this article or claim that may be made by its manufacturer is not guaranteed or endorsed by the publisher.
Research integrity at Frontiers
Learn more about the work of our research integrity team to safeguard the quality of each article we publish.