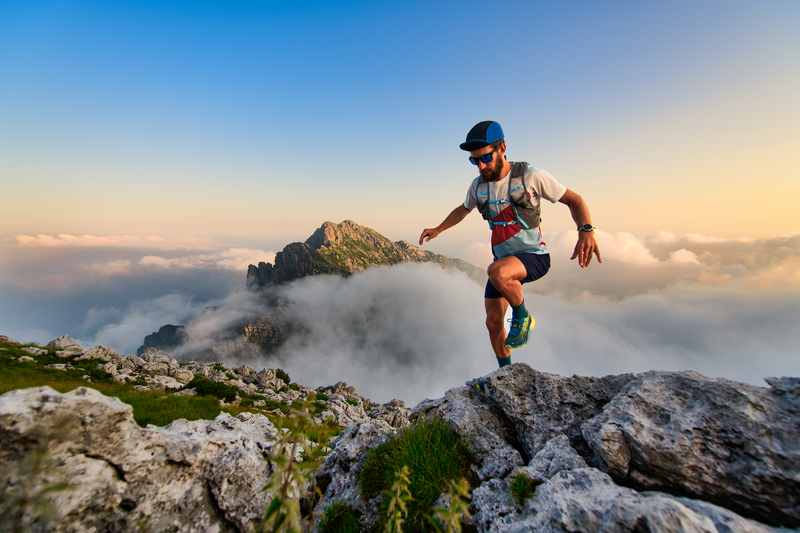
95% of researchers rate our articles as excellent or good
Learn more about the work of our research integrity team to safeguard the quality of each article we publish.
Find out more
ORIGINAL RESEARCH article
Front. Plant Sci. , 05 June 2023
Sec. Plant Pathogen Interactions
Volume 14 - 2023 | https://doi.org/10.3389/fpls.2023.1096831
This article is part of the Research Topic Molecular crosstalk between oilseed crops and pathogens View all 10 articles
Sclerotinia sclerotiorum, the causal agent of Sclerotinia stem rot (SSR) on more than 450 plant species, is a notorious fungal pathogen. Nitrate reductase (NR) is required for nitrate assimilation that mediates the reduction of nitrate to nitrite and is the major enzymatic source for NO production in fungi. To explore the possible effects of nitrate reductase SsNR on the development, stress response, and virulence of S. sclerotiorum, RNA interference (RNAi) of SsNR was performed. The results showed that SsNR-silenced mutants showed abnormity in mycelia growth, sclerotia formation, infection cushion formation, reduced virulence on rapeseed and soybean with decreased oxalic acid production. Furthermore SsNR-silenced mutants are more sensitive to abiotic stresses such as Congo Red, SDS, H2O2, and NaCl. Importantly, the expression levels of pathogenicity-related genes SsGgt1, SsSac1, and SsSmk3 are down-regulated in SsNR-silenced mutants, while SsCyp is up-regulated. In summary, phenotypic changes in the gene silenced mutants indicate that SsNR plays important roles in the mycelia growth, sclerotia development, stress response and fungal virulence of S. sclerotiorum.
Sclerotinia sclerotiorum (Lib.) de Bary is a necrotrophic pathogen that causes white mold disease on many crops, including rapeseed, soybean, sunflower, peanut, and other economically important crops (Bolton et al., 2006). Sclerotinia stem rot (SSR) is one of the most important diseases on rapeseed in China, which causes significant yield losses and economic damage. SSR accounts for an estimated 10–30% of yield losses and may reach 80% in certain years. This fungus produces black sclerotia to overcome cold winters and survival in the field for many years (Adams and Ayers, 1979). When the average temperature is cool (8–14°C) during the flowering period of rapeseed, the prolonged humid or wet conditions are conducive to carpogenic germination of sclerotia-producing apothecia to release ascospores for infection and favor disease development. Under the new policy guidelines and the increasingly negative effects of traditional chemical control, elucidating the molecular mechanism of pathogenesis of S. sclerotiorum may provide important information for the effective control SSR of oilseed.
Currently, most studies mainly focus on the mechanism of action of pathogenic factors, such as hydrolase and oxalic acid (OA) secreted by S. sclerotiorum during infection. Cutinases secreted by S. sclerotiorum degrade the cuticle of host plants, promoting penetrating the epidermis cells. SsCut, a cutinase produced by S. sclerotiorum, could cause plant cell necrosis and induce host plant resistance (Zhang et al., 2014). Many plant cell wall degradation enzymes (PCWDEs) and proteases, including cellulases, hemicellulose, pectinases, xylanases, and aspartyl protease secreted by S. sclerotiorum to degrade host cell wall, and pectinases have received attention, especially polygalacturonases (PGs).
S. sclerotiorum has 183 PCWDEs (including lignase), among which 33 are pectin degradation enzymes (Amselem et al., 2011; Lyu et al., 2015; Seifbarghi et al., 2017). In addition, SsXyl1 (endo-onosine-1, 4-xylanase) is involved in the pathogenesis (Yu et al., 2016). Aspartate protease SsAp1 was significantly expressed during the early stage of infection on Brassica napus and Phaseolus vulgaris (Oliveira et al., 2015; Seifbarghi et al., 2017). OA plays an important role in the process of infecting host plants; the oxaloacetic acid hydrolase (OAH1, EC 3.7.1.1) deletion mutant, although able to infect the host plants, only causes smaller necrotic leaf lesions (Liang et al., 2015b; Xu et al., 2015).
Besides, many genes also participate in the pathogenesis of S. sclerotiorum, such as NADPH oxidase (SsNox1 & SsNox2) related to ROS production and the accumulation of oxalic acid (Kim et al., 2011). Ss-Ggt1 (γ-glutamyl transpeptidase) is involved in the formation of infection cushions (Li et al., 2012), Cu/Zn superoxide dismutase SsSOD1 deficient resulting in significantly decreased virulence (Veluchamy et al., 2012; Xu and Chen, 2013). Forkhead-box transcription factor SsFKH1 regulates the development of the infection cushion, and silenced mutants produce small disease spots on tomatoes (Fan et al., 2017; Cong et al., 2022). However, elicitor SsPemG1 negatively regulated the pathogenicity of S. sclerotiorum (Pan et al., 2015). Moreover, secreted proteins play important roles in the penetration and regulation of plant immunity response, including SsCm1, ssv263, SSITL, Ss-Caf1, SsCVNH, SsSSVP1, Ss-Rhs1, SsCP1, and SsCut1 (Djamei et al., 2011; Liang et al., 2013; Zhu et al., 2013; Xiao et al., 2014; Lyu et al., 2015; Lyu et al., 2016a; Yu et al., 2017; Pan et al., 2018; Yang et al., 2018; Gong et al., 2022). Furthermore, SsSSVP1 and SsCP1 have been identified as effectors and target host QCR8 and PR1, respectively (Lyu et al., 2016a; Yang et al., 2018).
Nitrogen metabolism in fungi is a strictly regulated process that allows fungi to have the ability to utilize other nitrogen sources when the desired substrate is insufficient (Bolton and Thomma, 2008). Nitrate reductase (NR) is required for nitrate assimilation in fungi that mediates the reduction of nitrate to nitrite and have been confirmed in Aspergillus nidulans, Neurospora crassa, and Aspergillus fumigatus (Johnstone et al., 1990; Okamoto et al., 1991; Amaar and Moore, 1998). NR is widely distributed in ectomycorrhizal (ECM) fungi to utilize nitrate as an N source even though the nitrate supply is very low (Nygren et al., 2008). While NR-silenced in mycorrhizal fungus Laccaria bicolor resulted in the inhibition of symbiosis with Populus (Kemppainen et al., 2009). The nitrate reductase (NR) gene (niaD) is required for nitric oxide (NO) production in A. nidulans during conidiation (Marcos et al., 2016; Franco-Cano et al., 2021), while nitrate reductase NIA1 is essential for nitrate assimilation and dispensable for pathogenicity in Magnaporthe oryzae (Samalova et al., 2013).
Here, we characterized an NR gene in S. sclerotiorum and applied RNAi technology to reveal the function of SsNR in S. sclerotiorum. We show that SsNR is associated with mycelium growth and pathogenesis and that SsNR knockdown results in defective infection cushion formation.
The Sclerotinia sclerotiorum wild-type strain FXGD2, preserved at the Fungus Laboratory of Anhui Agricultural University, was cultured on PDA. SsNR-silenced mutants were cultured on PDA containing 180 μg/mL hygromycin B at 25°C in the dark. S. sclerotiorum strains were cultured on PDA at 25°C for 3–5 days in the dark, and mycelium was collected to extract genomic DNA and RNA. The extraction procedure of total RNA of S. sclerotiorum was based on the instruction of E.Z.N.A.TM Total RNA Kit I from (Omega Bio-Tek, Atlanta, USA), and the extracted RNA was treated with RNAse-free DNAse I (TaKaRa Biotechnology, Dalian, China).
BLASTp searches were performed from the S. sclerotiorum genome database at National Center for Biotechnology Information (NCBI). A homolog of nitrate reductase (NR) was retrieved from the S. sclerotiorum genome and was named SsNR (SS1G_01885). The ORF of SsNR was amplified using primer SsNR-F/SsNR-R (Table S1), then cloned into pMD19-T for sequencing. ClustalX 2.1 (Larkin et al., 2007) was used for multiple sequence alignment of the SsNR and its homologs, and the phylogenetic tree was reconstructed by MEGA11 using maximum likelihood method (Tamura et al., 2021). The conserved motifs of the nitrate reductase family were analyzed at a web resource SMART (Letunic et al., 2021).
Total RNA was extracted from S. sclerotiorum strains according to the instruction of E.Z.N.A.TM Total RNA Kit I (Omega Bio-Tek, USA), and contaminant genomic DNA was removed with RNAse-free DNAse I (TaKaRa, Dalian, China). qRT-PCR was performed using SYBR Green RT-PCR Kit (TaKaRa, Dalian, China) to analyze the expression level of SsNR during mycelium growth and sclerotia formation and to confirm the SsNR-silenced mutants with house-keeping gene β-tubulin (SsTub, SS1G_04652) as reference gene. For gene expression of pathogenicity-related genes SsGgt1 (SS1G_14127), SsSmk3 (SS1G_05445), SsSac1 (SS1G_07715) and SsCyp (SS1G_06284) in SsNR-silenced mutants, total RNA was extracted from strains and transcribed into cDNA for qRT-PCR using CFX96 thermal cycler (Bio-Rad, CA, USA). For each gene, qRT-PCR was repeated at least twice with three biological replicates.
pSilent-1, which carries a hygromycin resistance cassette, was used for hairpin RNA expression of SsNR (Nakayashiki et al., 2005). Two fragments amplified from cDNA using SsNR-H-L/SsNR-X-R and SsNR-K-L/SsNR-S-R (Table S1) were ligated into pSilent-1 respectively, to generate pSilent-SsNR. Plasmids of pSilent-SsNR were transferred into protoplasts of FXGD2 with polyethylene glycol (PEG)-mediated transformation (Rollins, 2003). Hygromycin-resistant colonies on RM were transferred to new PDA plates containing 45 μg/mL hygromycin B, and transformants of pSilent-1 were used as control. To confirm all putative transformants, Primers Hyg-F and Hyg-R were used to amplify the partial sequence of the hygromycin resistance gene.
For mycelia growth and sclerotia formation, wild-type, SsNR-silenced transformants and mock strains were inoculated on PDA plates at 25°C for 2 d or 15 d. For infection cushion formation, agar plugs of all strains were inoculated onto the surfaces of glass slides and incubated at 25°C with 100% relative humidity, the number of infection cushion were counted at 24 h. All experiments were repeated three times independently.
For stress tolerance assay, fresh mycelium plugs of wild-type, SsNR-silenced transformants and mock strains were cultured on MM medium containing Congo Red (2 mg/mL), SDS (0.01%), sorbitol (1.2 M), NaCl (1 M), and H2O2 (4 mM), respectively. Colony diameters were measured every 12 h, and sclerotia formation was observed at 7 days. Experiments were repeated three times with three biological replicates.
Strains were cultured on MM medium containing 0.01% bromophenol blue for qualitative analysis of oxalic acid production. For quantification of oxalic acid, mycelium plugs of different strains were cultured in 100 mL YSPU medium at 25°C for 5 days, and the content of oxalic acid secreted in YSPU was measured by KMnO4 titration method (Baker, 1952). This experiment was repeated three times.
Rapeseed and soybean plants were used for the pathogenicity assay of S. slcerotiorum wild-type, SsNR-silenced and mock strains. Detached leaves were inoculated with mycelia plugs (Φ=5 mm) from the margins of actively growing colonies on PDA in an incubator at 18°C and 100% relative humidity. Disease severity was calculated by lesion percentage of leaf and lesion size. These experiments were repeated three times, and each replicate was performed with three leaves.
All experiments were repeated three times. Microsoft Office 365 and SPSS v22.0 were used for statistical analysis. Statistical analysis was performed using Student’s t-test and one-way analysis of variance (ANOVA).
A nitrate reductase SsNR was identified from the S. scelrotiorum genome, and the ORF of SsNR is 2733 bp in length, encoding 910 amino acids. SsNR, like other nitrate reductases, has five functional domains: Oxidored_molyb structural domain (122-298 aa), Mo-co_dimer structural domain (326-475 aa), Cyt-b5 structural domain (541- 613 aa), FAD_binding_6 structural domain (645-751 aa) and NAD_binding_1 structural domain (771-895 aa) (Figure 1A). Phylogenetic analysis indicated that orthologs of SsNR are widely distributed in fungi and plant (Figure 1B).
Figure 1 Functional domain identification and phylogenetic tree reconstruction. (A) Functional domains of SsNR were identified by searching SMART containing an Oxidored_molyb, Mo-co_dimer, Cyt-b5 domain, FAD_binding_6 domain, and NAD_binding_1. (B) Phylogenetic tree reconstructed using the maximum likelihood (ML) method with 1,000 bootstraps.
qRT-PCR was used for SsNR expression analysis during hyphal growth and six stages of sclerotial formation: (S1) initiation, (S2) condensation, (S3) enlargement, (S4) consolidation, (S5) pigmentation, and (S6) maturation (Li and Rollins, 2009). Our qRT-PCR results showed that SsNR is highly expressed from S1 to S6. The transcript level increased by 1060%, 1124%, 717%, 96%, 98%, and 1888%, respectively, indicating that SsNR might be involved in the sclerotial development of S. sclerotiorum (Figure 2). We performed PEG-mediated transformation. We obtained 82 transformants that could grow on PDA-containing hygromycin. SsNR-silenced mutants were confirmed by qRT-PCR, and two mutants (NR12 and NR66) were chosen for analysis in which the expression of SsNR decreased by 76.5% and 66.3%, respectively (Figure 3A). SsNR-silenced mutants exhibited slower mycelial growth compared to wild-type and mock strain (Figure 3B). The wild-type and mock strain produced sclerotia in 6 days post-inoculation, but SsNR-silenced mutants produced few sclerotia or did not form scerotia, indicating that SsNR was involved in mycelium growth and sclerotial development (Figure 3B).
Figure 2 qRT-PCR analysis of the expression of SsNR in different stages of S. sclerotiorum. S0 = the hyphal stage of S. sclerotiorum; S1 = the initiation stage of sclerotial development; S2 = condensation stage; S3 = enlargement stage; S4 = consolidation stage; S5 = pigmentation stage; S6 = maturation stage. The expression level of SsNR cDNA measured by qRT-PCR was standardized with the housekeeping gene S. sclerotiorum β-tubulin. The abundance of cDNA from S0 samples was assigned a value of 1. Bars indicate standard error. Statistical significance is indicated: **, P < 0.01.
Figure 3 SsNR-silenced impaired hyphae growth and sclerotia development. (A) qRT-PCR analysis of the expression of SsNR in WT, SsNR-silenced mutants and mock. Statistical significance is indicated: **, P < 0.01. (B) Colony morphology of WT, SsNR-silenced mutants and mock cultured on PDA at 25°C. Photos were taken at 2 dpi, and the sclerotia of WT, SsNR-silenced mutants and mock cultured on PDA at 25°C. Photos were taken at 10 dpi.
To check the potential role of nitrate reductase in the cell wall and membrane integrity of S. sclerotiorum, SsNR-silenced mutants were cultured on MM containing Congo Red (2 mg/mL) and SDS (0.01%), respectively. Under Congo Red treatment, the colony diameter of SsNR-silenced mutants is about 1.85 and 2.72 cm, much smaller than the wild-type (4.93 cm) and mock strain (4.67 cm). Similarly, SsNR-silenced mutants grew much slower than wild-type and mock strain on an SDS-amended medium. The colony diameters of wild-type, mock strain and SsNR-silenced mutants (NR12 and NR66) were 5.75, 5.45, 3.3, and 4.1, respectively (Figure 4). These results showed that SsNR is required for cell wall integrity.
Figure 4 Tolerance of WT, SsNR-silenced mutants and mock to different chemicals. The phenotype of WT, SsNR-silenced mutants and mock grown on MM supplemented with 2 mg/mL Congo Red, 0.01% SDS, 1.2 M Sorbitol and 1 M NaCl and 4 mM H2O2. Photos were taken at 1 dpi, 5 dpi, 7 dpi, 2 dpi, and 7 dpi, respectively.
We also detect the response of different strains to other stress -including chemicals, such as sorbitol, NaCl, and H2O2. The results showed that colony morphology of SsNR-silenced mutants did not change on MM containing sorbitol and that the mycelium growth and sclerotial development were not affected, indicating that SsNR is not associated with osmotic pressure (Figure 4). NaCl at 1 M could significantly inhibit mycelium growth of SsNR-silenced mutants compared to wild-type and mock. Likewise, in SsNR-silenced mutants grown on MM containing H2O2, the diameter of the colony was much smaller (3.1 and 4.6 cm) compared to wild-type (6.0 cm), indicating that SsNR has the function of tolerance to H2O2 (Figure 4).
For SsNR-silenced mutants that exhibited abnormal colony morphology, we detected the hyphal tip under a microscope to determine whether SsNR affects hyphal growth (Figure 5A). The result showed that the hyphal branching of SsNR-silenced mutants increased in the number of tips, and the hyphal diaphragm was shortened compared to the wild-type. The infection cushions played important roles during penetration of S. sclerotiorum; we detected the number of infection cushions produced by different strains on hydrophobic surfaces that the SsNR-silenced mutants produce less and smaller infection cushions compared to WT (Figures 5B, C). These results indicated that SsNR plays an important role in infection cushions formation.
Figure 5 Mycelial growth and infection cushion formation of SsNR-silenced mutants. (A) Hyphal branching and tips stained of all strains were observed under a light microscope. (B) Infection cushions stained of all strains were observed under a light microscope. (C) Number of infection cushion produced by all strains. Bar = 100 μm, hyphae and infection cushions were by Calcofluor white (CFW) (10 μg/mL). Statistical significance is indicated: **, P < 0.01.
For SsNR is associated with mycelium growth, we also performed a pathogenicity assay on detached leaves of rapeseed and soybean plants. The results showed that SsNR-silenced mutants could produce lesions on leaves of both rapeseed and soybean (12.64%~13.12% and 44.36%~56.50%), but the lesion size of mutants was much smaller (3.28%~4.73% and 14.39%~15.72%) than wild-type and mock strains, indicating that SsNR is required for infection of S. sclerotiorum (Figure 6).
Figure 6 SsNR is required for virulence and accumulation of oxalic acid in S. sclerotiorum. (A) Morphology of WT, SsNR-silenced mutants and mock grown on MM supplemented with 0.01% bromophenol blue. Photos were taken at 2 dpi. (B) The diameters of the yellow zone produced by WT, SsNR-silenced mutants and mock (2 dpi). (C) Oxalic acid concentration of WT, SsNR-silenced mutants and mock cultured in liquid medium 5 days. (D) The percentages of lesion area produced by WT, SsNR-silenced mutants and mock-inoculated on detached leaves of oilseed rape and soybean (48 hpi). Statistical significance is indicated: **, P < 0.01.
Oxalic acid plays an important role in pathogenicity during infection. All strains were cultured on a medium containing bromophenol to determine the OA in wild-type and SsNR-silenced mutants. The diameters of the yellow zone produced by silenced transformants NR12 and NR66 were about 2.3 and 4.1 cm, respectively, which were significantly smaller than those of the wild-type (5.7 cm) and mock control (5.6 cm) (Figure 6). Also, the concentration OA of all strains were determined by KmnO4 titration, results showed that OA concentration of NR12 and NR66 were 16.65 mg and 36.9 mg in 100 ml medium, respectively, while those of wild-type and mock strain were 97.35 mg and 97.05 mg, respectively (Figure 6). These results showed that SsNR is important for OA production.
To investigate whether SsNR-silencing affects the transcriptional expression levels of other pathogenicity-related genes, Ggt1, Sac1, Smk3, and CYP, were analyzed by qRT-PCR. The results showed that the expression levels of Ggt1, Sac1, and Smk3 in SsNR-silenced mutants were decreased by 96%~98%, 65%~74%, and 56%~72%, respectively. At the same time, the expression level of CYP was increased by 230%~440% (Figure 7).
Figure 7 The expression of SsGgt1, SsSac1, SsSmk3, and SsCYP in different strains using β-tubulin as a reference gene. Statistical significance is indicated: **, P < 0.01.
Nitrate reductase is an enzyme that catalyzes the reduction of nitrate to nitrite and is the major enzymatic source for NO production in fungi (Cánovas et al., 2016). The nitrate reductase SsNR of S. sclerotiorum was studied using sequence analysis and RNAi technology which could be a good approach for gene function analysis in this fungus (Lyu et al., 2016a; Rana et al., 2021). SsNR-silenced mutants exhibited altered phenotypes, including hyphal growth and sclerotia development. We performed a pathogenicity assay on detached leaves of rapeseed and soybean plants; although the SsNR-silenced mutants could penetrate the host cell, they produced smaller lesions than WT.
SsNR-silenced mutants exhibited increased hyphal branching tips and shortened hyphal diaphragm, and diminished sclerotia development (Figure 3). These results indicated that mycelium growth might be related to sclerotia development, and these phenomena are also observed in knockout or knock down mutants of other genes, such as Sop1, Ss-Sl2, SsFkh1, SCD1, and THR1 (Yu et al., 2012; Lyu et al., 2016b; Fan et al., 2017; Yue et al., 2018). Also, endogenous small RNAs may regulate genes controlling sclerotial development (Xia et al., 2020). Nitrate reductase SsNR-silenced mutants may affect nitrogen metabolism and protein synthesis, which might cause abnormal hyphal growth and sclerotia formation. An infection cushion (or compound appressorium) is required for infection, which is the primary means of infection initiation by S. sclerotiorum to breach the cuticle layer of the host epidermal cell (Huang et al., 2008). SsNR-silenced mutants produced less infection cushion on parafilm. SsNR could regulate the development of infection cushion accomplished with decreased virulence (Figure 5). Several genes have been reported in regulating infection cushion development, such as Sac1, Ss-caf1, Ss-oah1, Ss-odc2, and Smk3 (Jurick and Rollins, 2007; Li et al., 2012; Xiao et al., 2014; Liang et al., 2015a; Liang et al., 2015b; Bashi et al., 2016), meanwhile Ggt1, Sac1, and Smk3 are downregulated in SsNR-silenced mutants, indicating that SsNR is involved in the expression of infection cushion development-related genes in fine-tuning the infection cushion formation process during penetration of S. sclerotiorum.
OA is important for infection of S. sclerotiorum primarily in acidifying the microenvironment in infection (Xu et al., 2018), in addition to other roles, including chelation with Ca2+, regulation stomatal closure, inhibition of reactive oxygen species (ROS) bursting, promotion of apoptosis, and repression autophagy of plant cell (Rollins and Dickman, 2001; Guimaraes and Stotz, 2004; Williams et al., 2011; Kabbage et al., 2013; Uloth et al., 2015; Derbyshire et al., 2022). OA production of SsNR-silenced mutants decreased compared to WT; we proposed that SsNR may play an important role in OA accumulation, implying that SsNR regulated the pathogenicity of S. sclerotiorum through oxalic acid secretion. For decreased OA concentration, SsNR-silenced mutants produced fewer infection cushions and impaired function of OA during plant-Sclerotinia interaction, causing debilitation in virulence. Moreover, cell wall integrity (CWI) is required in fungi to adapt to perturbing conditions, including osmotic pressure, heat, oxidative stress, and antifungals (Dichtl et al., 2016). SsNR-silenced mutants were more sensitive to Congo Red, SDS, and NaCl than the wild type, indicating impaired CWI. Reactive oxygen species (ROS) played important roles in plant immunity (Qi et al., 2017), while SsNR-silenced mutants showed more sensitivity to H2O2 compared to WT resulting in decreased virulence in plants (soybean and rapeseed). Similar results were obtained in the tea leaf spot which is caused by Didymella segeticola, the antimicrobial kasugamycin inhibits the pathogen by binding to NR, disturbing fungal metabolism with changes in hyphal growth and development (Jiang et al., 2022).
SsNR is essential for normal mycelium growth, sclerotia development, and virulence by regulating OA production and expression of pathogenesis-related genes involved in the infection of S. sclerotiorum.
The original contributions presented in the study are included in the article/Supplementary Material. Further inquiries can be directed to the corresponding authors.
ZG, GY, and YP designed the research. JW and CY conducted the experiments and data analysis. JW, CY, ZZ, ZG, GY, and YP wrote and revised the manuscript. All authors scrutinized and corrected the manuscript. All authors contributed to the article and approved the submitted version.
This research was supported by Anhui Provincial Natural Science Foundation (2008085MC76), the National Natural Science Foundation of China (31801676), the Natural Science Foundation of Anhui Higher Education Institutions of China (KJ2016A229), and The Research and Development Program of China (2018YFD0200900).
We thank Prof. Zhengguang Zhang of the Department of Plant Pathology, Nanjing Agricultural University, Nanjing, China, for the gift of the vector pSilent-1.
The authors declare that the research was conducted in the absence of any commercial or financial relationships that could be construed as a potential conflict of interest.
All claims expressed in this article are solely those of the authors and do not necessarily represent those of their affiliated organizations, or those of the publisher, the editors and the reviewers. Any product that may be evaluated in this article, or claim that may be made by its manufacturer, is not guaranteed or endorsed by the publisher.
The Supplementary Material for this article can be found online at: https://www.frontiersin.org/articles/10.3389/fpls.2023.1096831/full#supplementary-material
Adams, P. B., Ayers, W. A. (1979). Ecology of Sclerotinia species. Phytopathology 69 (8), 896–899. doi: 10.1094/Phyto-69-896
Amaar, Y. G., Moore, M. M. (1998). Mapping of the nitrate-assimilation gene cluster (crnA-niiA-niaD) and characterization of the nitrite reductase gene (niiA) in the opportunistic fungal pathogen Aspergillus fumigatus. Curr. Genet. 33 (3), 206–215. doi: 10.1007/s002940050328
Amselem, J., Cuomo, C. A., van Kan, J. A., Viaud, M., Benito, E. P., Couloux, A., et al. (2011). Genomic analysis of the necrotrophic fungal pathogens Sclerotinia sclerotiorum and Botrytis cinerea. PLoS Genet. 7 (8), e1002230. doi: 10.1371/journal.pgen.1002230
Baker, C. J. L. (1952). The determination of oxalates in fresh plant material. Analyst 77 (916), 340–344. doi: 10.1039/AN9527700340
Bashi, Z. D., Gyawali, S., Bekkaoui, D., Coutu, C., Lee, L., Poon, J., et al. (2016). The Sclerotinia sclerotiorum Slt2 mitogen-activated protein kinase ortholog, SMK3, is required for infection initiation but not lesion expansion. Can. J. Microbiol. 62 (10), 836–850. doi: 10.1139/cjm-2016-0091
Bolton, M. D., Thomma, B. P. H. J. (2008). The complexity of nitrogen metabolism and nitrogen-regulated gene expression in plant pathogenic fungi. Physiol. Mol. Plant Pathol. 72 (4), 104–110. doi: 10.1016/j.pmpp.2008.07.001
Bolton, M. D., Thomma, B. P. H. J., Nelson, B. D. (2006). Sclerotinia sclerotiorum (Lib.) de bary: biology and molecular traits of a cosmopolitan pathogen. Mol. Plant Pathol. 7 (1), 1–16. doi: 10.1111/j.1364-3703.2005.00316.x
Cánovas, D., Marcos, J. F., Marcos, A. T., Strauss, J. (2016). Nitric oxide in fungi: is there NO light at the end of the tunnel? Curr. Genet. 62 (3), 513–518. doi: 10.1007/s00294-016-0574-6
Cong, J., Xiao, K., Jiao, W., Zhang, C., Zhang, X., Liu, J., et al. (2022). The coupling between cell wall integrity mediated by MAPK kinases and SsFkh1 is involved in sclerotia formation and pathogenicity of Sclerotinia sclerotiorum. Front. Microbiol. 13. doi: 10.3389/fmicb.2022.816091
Derbyshire, M. C., Newman, T. E., Khentry, Y., Taiwo, A. O. (2022). The evolutionary and molecular features of the broad-host-range plant pathogen sclerotinia sclerotiorum. Mol. Plant Pathol. 23 (8), 1075–1090. doi: 10.1111/mpp.13221
Dichtl, K., Samantaray, S., Wagener, J. (2016). Cell wall integrity signalling in human pathogenic fungi. Cell. Microbiol. 18 (9), 1228–1238. doi: 10.1111/cmi.12612
Djamei, A., Schipper, K., Rabe, F., Ghosh, A., Vincon, V., Kahnt, J., et al. (2011). Metabolic priming by a secreted fungal effector. Nature 478 (7369), 395–398. doi: 10.1038/nature10454
Fan, H., Yu, G., Liu, Y., Zhang, X., Liu, J., Zhang, Y., et al. (2017). An atypical forkhead-containing transcription factor SsFKH1 is involved in sclerotial formation and is essential for pathogenicity in Sclerotinia sclerotiorum. Mol. Plant Pathol. 18 (7), 963–975. doi: 10.1111/mpp.12453
Franco-Cano, A., Marcos, A. T., Strauss, J., Cánovas, D. (2021). Evidence for an arginine-dependent route for the synthesis of NO in the model filamentous fungus Aspergillus nidulans. Environ. Microbiol. 23 (11), 6924–6939. doi: 10.1111/1462-2920.15733
Gong, Y., Fu, Y., Xie, J., Li, B., Chen, T., Lin, Y., et al. (2022). Sclerotinia sclerotiorum SsCut1 modulates virulence and cutinase activity. J. Fungi 8 (5), 526. doi: 10.3390/jof8050526
Guimaraes, R. L., Stotz, H. U. (2004). Oxalate production by Sclerotinia sclerotiorum deregulates guard cells during infection. Plant Physiol. 136 (3), 3703–3711. doi: 10.1104/pp.104.049650
Huang, L., Buchenauer, H., Han, Q., Zhang, X., Kang, Z. (2008). Ultrastructural and cytochemical studies on the infection process of Sclerotinia sclerotiorum in oilseed rape. J. Plant Dis. Prot. 115 (1), 9–16. doi: 10.1007/BF03356233
Jiang, X., Jiang, S., Huang, H., Li, D., Yang, R., Yang, Y., et al. (2022). Multi-omics analysis reveals that the antimicrobial kasugamycin potential targets nitrate reductase in Didymella segeticola to achieve control of tea leaf spot. Phytopathology 112 (9), 1894–1906. doi: 10.1094/PHYTO-11-21-0457-R
Johnstone, I. L., McCabe, P. C., Greaves, P., Gurr, S. J., Cole, G. E., Brow, M. A. D., et al. (1990). Isolation and characterisation of the crnA-niiA-niaD gene cluster for nitrate assimilation in Aspergillus nidulans. Gene 90 (2), 181–192. doi: 10.1016/0378-1119(90)90178-T
Jurick, W. M., II, Rollins, J. A. (2007). Deletion of the adenylate cyclase (sac1) gene affects multiple developmental pathways and pathogenicity in Sclerotinia sclerotiorum. Fungal Genet. Biol. 44 (6), 521–530. doi: 10.1016/j.fgb.2006.11.005
Kabbage, M., Williams, B., Dickman, M. B. (2013). Cell death control: the interplay of apoptosis and autophagy in the pathogenicity of Sclerotinia sclerotiorum. PLoS Pathog. 9 (4), e1003287. doi: 10.1371/journal.ppat.1003287
Kemppainen, M., Duplessis, S., Martin, F., Pardo, A. G. (2009). RNA Silencing in the model mycorrhizal fungus Laccaria bicolor: gene knock-down of nitrate reductase results in inhibition of symbiosis with Populus. Environ. Microbiol. 11 (7), 1878–1896. doi: 10.1111/j.1462-2920.2009.01912.x
Kim, H.-j., Chen, C., Kabbage, M., Dickman, M. B. (2011). Identification and characterization of Sclerotinia sclerotiorum NADPH oxidases. Appl. Environ. Microbiol. 77 (21), 7721–7729. doi: 10.1128/aem.05472-11
Larkin, M. A., Blackshields, G., Brown, N. P., Chenna, R., McGettigan, P. A., McWilliam, H., et al. (2007). Clustal W and clustal X version 2.0. Bioinformatics 23 (21), 2947–2948. doi: 10.1093/bioinformatics/btm404
Letunic, I., Khedkar, S., Bork, P. (2021). SMART: recent updates, new developments and status in 2020. Nucleic Acids Res. 49 (D1), D458–D460. doi: 10.1093/nar/gkaa937
Li, M., Liang, X., Rollins, J. A. (2012). Sclerotinia sclerotiorum γ-glutamyl transpeptidase (Ss-Ggt1) is required for regulating glutathione accumulation and development of sclerotia and compound appressoria. Mol. Plant-Microbe Interact. 25 (3), 412–420. doi: 10.1094/mpmi-06-11-0159
Li, M., Rollins, J. A. (2009). The development-specific protein (Ssp1) from Sclerotinia sclerotiorum is encoded by a novel gene expressed exclusively in sclerotium tissues. Mycologia 101 (1), 34–43. doi: 10.3852/08-114
Liang, X., Liberti, D., Li, M., Kim, Y.-T., Hutchens, A., Wilson, R., et al. (2015b). Oxaloacetate acetylhydrolase gene mutants of Sclerotinia sclerotiorum do not accumulate oxalic acid, but do produce limited lesions on host plants. Mol. Plant Pathol. 16 (6), 559–571. doi: 10.1111/mpp.12211
Liang, X., Moomaw, E. W., Rollins, J. A. (2015a). Fungal oxalate decarboxylase activity contributes to Sclerotinia sclerotiorum early infection by affecting both compound appressoria development and function. Mol. Plant Pathol. 16 (8), 825–836. doi: 10.1111/mpp.12239
Liang, Y., Yajima, W., Davis, M. R., Kav, N. N. V., Strelkov, S. E. (2013). Disruption of a gene encoding a hypothetical secreted protein from Sclerotinia sclerotiorum reduces its virulence on canola (Brassica napus). Can. J. Plant Pathol. 35 (1), 46–55. doi: 10.1080/07060661.2012.745904
Lyu, X., Shen, C., Fu, Y., Xie, J., Jiang, D., Li, G., et al. (2015). Comparative genomic and transcriptional analyses of the carbohydrate-active enzymes and secretomes of phytopathogenic fungi reveal their significant roles during infection and development. Sci. Rep. 5, 15565. doi: 10.1038/srep15565
Lyu, X., Shen, C., Fu, Y., Xie, J., Jiang, D., Li, G., et al. (2016a). A small secreted virulence-related protein is essential for the necrotrophic interactions of Sclerotinia sclerotiorum with its host plants. PLoS Pathog. 12 (2), e1005435. doi: 10.1371/journal.ppat.1005435
Lyu, X., Shen, C., Fu, Y., Xie, J., Jiang, D., Li, G., et al. (2016b). The microbial opsin homolog Sop1 is involved in Sclerotinia sclerotiorum development and environmental stress response. Front. Microbiol. 6 (1504). doi: 10.3389/fmicb.2015.01504
Marcos, A. T., Ramos, M. S., Marcos, J. F., Carmona, L., Strauss, J., Cánovas, D. (2016). Nitric oxide synthesis by nitrate reductase is regulated during development in Aspergillus. Mol. Microbiol. 99 (1), 15–33. doi: 10.1111/mmi.13211
Nakayashiki, H., Hanada, S., Quoc, N. B., Kadotani, N., Tosa, Y., Mayama, S. (2005). RNA Silencing as a tool for exploring gene function in ascomycete fungi. Fungal Genet. Biol. 42 (4), 275–283. doi: 10.1016/j.fgb.2005.01.002
Nygren, C. M. R., Eberhardt, U., Karlsson, M., Parrent, J. L., Lindahl, B. D., Taylor, A. F. S. (2008). Growth on nitrate and occurrence of nitrate reductase-encoding genes in a phylogenetically diverse range of ectomycorrhizal fungi. New Phytol. 180 (4), 875–889. doi: 10.1111/j.1469-8137.2008.02618.x
Okamoto, P. M., Fu, Y.-H., Marzluf, G. A. (1991). Nit-3, the structural gene of nitrate reductase in Neurospora crassa: nucleotide sequence and regulation of mRNA synthesis and turnover. Mol. Gen. Genet. 227 (2), 213–223. doi: 10.1007/bf00259673
Oliveira, M. B., de Andrade, R. V., Grossi-de-Sá, M. F., Petrofeza, S. (2015). Analysis of genes that are differentially expressed during the Sclerotinia sclerotiorum–Phaseolus vulgaris interaction. Front. Microbiol. 6. doi: 10.3389/fmicb.2015.01162
Pan, Y., Wei, J., Yao, C., Reng, H., Gao, Z. (2018). SsSm1, a cerato-platanin family protein, is involved in the hyphal development and pathogenic process of Sclerotinia sclerotiorum. Plant Sci. 270, 37–46. doi: 10.1016/j.plantsci.2018.02.001
Pan, Y., Xu, Y., Li, X., Yao, C., Gao, Z. (2015). SsPemG1 encodes an elicitor-homologous protein and regulates pathogenicity in Sclerotinia sclerotiorum. Physiol. Mol. Plant Pathol. 92, 70–78. doi: 10.1016/j.pmpp.2015.08.010
Qi, J., Wang, J., Gong, Z., Zhou, J.-M. (2017). Apoplastic ROS signaling in plant immunity. Curr. Opin. Plant Biol. 38, 92–100. doi: 10.1016/j.pbi.2017.04.022
Rana, K., Ding, Y., Banga, S. S., Liao, H., Zhao, S., Yu, Y., et al. (2021). Sclerotinia sclerotiorum Thioredoxin1 (SsTrx1) is required for pathogenicity and oxidative stress tolerance. Mol. Plant Pathol. 22 (11), 1413–1426. doi: 10.1111/mpp.13127
Rollins, J. A. (2003). The Sclerotinia sclerotiorum pac1 gene is required for sclerotial development and virulence. Mol. Plant-Microbe Interact. 16 (9), 785–795. doi: 10.1094/Mpmi.2003.16.9.785
Rollins, J. A., Dickman, M. B. (2001). pH signaling in Sclerotinia sclerotiorum: identification of a pacC/RIM1 homolog. Appl. Environ. Microbiol. 67 (1), 75–81. doi: 10.1128/aem.67.1.75-81.2001
Samalova, M., Johnson, J., Illes, M., Kelly, S., Fricker, M., Gurr, S. (2013). Nitric oxide generated by the rice blast fungus Magnaporthe oryzae drives plant infection. New Phytol. 197 (1), 207–222. doi: 10.1111/j.1469-8137.2012.04368.x
Seifbarghi, S., Borhan, M. H., Wei, Y., Coutu, C., Robinson, S. J., Hegedus, D. D. (2017). Changes in the Sclerotinia sclerotiorum transcriptome during infection of Brassica napus. BMC Genomics 18 (1), 266. doi: 10.1186/s12864-017-3642-5
Tamura, K., Stecher, G., Kumar, S. (2021). MEGA11: molecular evolutionary genetics analysis version 11. Mol. Biol. Evol. 38 (7), 3022–3027. doi: 10.1093/molbev/msab120
Uloth, M. B., Clode, P. L., You, M. P., Barbetti, M. J. (2015). Calcium oxalate crystals: an integral component of the Sclerotinia sclerotiorum/Brassica carinata pathosystem. PLoS One 10 (3), e0122362. doi: 10.1371/journal.pone.0122362
Veluchamy, S., Williams, B., Kim, K., Dickman, M. B. (2012). The CuZn superoxide dismutase from Sclerotinia sclerotiorum is involved with oxidative stress tolerance, virulence, and oxalate production. Physiol. Mol. Plant Pathol. 78, 14–23. doi: 10.1016/j.pmpp.2011.12.005
Williams, B., Kabbage, M., Kim, H.-J., Britt, R., Dickman, M. B. (2011). Tipping the balance: Sclerotinia sclerotiorum secreted oxalic acid suppresses host defenses by manipulating the host redox environment. PLoS Pathog. 7 (6), e1002107. doi: 10.1371/journal.ppat.1002107
Xia, Z., Wang, Z., Kav, N. N. V., Ding, C., Liang, Y. (2020). Characterization of microRNA-like RNAs associated with sclerotial development in Sclerotinia sclerotiorum. Fungal Genet. Biol. 144, 103471. doi: 10.1016/j.fgb.2020.103471
Xiao, X., Xie, J., Cheng, J., Li, G., Yi, X., Jiang, D., et al. (2014). Novel secretory protein ss-Caf1 of the plant-pathogenic fungus Sclerotinia sclerotiorum is required for host penetration and normal sclerotial development. Mol. Plant-Microbe Interact. 27 (1), 40–55. doi: 10.1094/MPMI-05-13-0145-R
Xu, L., Chen, W. (2013). Random T-DNA mutagenesis identifies a Cu/Zn superoxide dismutase gene as a virulence factor of Sclerotinia sclerotiorum. Mol. Plant-Microbe Interact. 26 (4), 431–441. doi: 10.1094/MPMI-07-12-0177-R
Xu, L., Liu, G., Jiang, D., Chen, W. (2018). Sclerotinia sclerotiorum: an evaluation of virulence theories. Annu. Rev. Phytopathol. 56 (1), 311–318. doi: 10.1146/annurev-phyto-080417-050052
Xu, L., Xiang, M., White, D., Chen, W. (2015). pH dependency of sclerotial development and pathogenicity revealed by using genetically defined oxalate-minus mutants of Sclerotinia sclerotiorum. Environ. Microbiol. 17 (8), 2896–2909. doi: 10.1111/1462-2920.12818
Yang, G., Tang, L., Gong, Y., Xie, J., Fu, Y., Jiang, D., et al. (2018). A cerato-platanin protein SsCP1 targets plant PR1 and contributes to virulence of Sclerotinia sclerotiorum. New Phytol. 217 (2), 739–755. doi: 10.1111/nph.14842
Yu, Y., Jiang, D., Xie, J., Cheng, J., Li, G., Yi, X., et al. (2012). Ss-Sl2, a novel cell wall protein with PAN modules, is essential for sclerotial development and cellular integrity of Sclerotinia sclerotiorum. PLoS One 7 (4), e34962. doi: 10.1371/journal.pone.0034962
Yu, Y., Xiao, J., Du, J., Yang, Y., Bi, C., Qing, L. (2016). Disruption of the gene encoding endo-β-1, 4-xylanase affects the growth and virulence of Sclerotinia sclerotiorum. Front. Microbiol. 7. doi: 10.3389/fmicb.2016.01787
Yu, Y., Xiao, J., Zhu, W., Yang, Y., Mei, J., Bi, C., et al. (2017). Ss-Rhs1, a secretory rhs repeat-containing protein, is required for the virulence of Sclerotinia sclerotiorum. Mol. Plant Pathol. 18 (8), 1052–1061. doi: 10.1111/mpp.12459
Yue, L., Wei, X., Siegrid, S., Jie, F. (2018). Deficiency of the melanin biosynthesis genes SCD1 and THR1 affects sclerotial development and vegetative growth, but not pathogenicity, in Sclerotinia sclerotiorum. Mol. Plant Pathol. 19 (6), 1444–1453. doi: 10.1111/mpp.12627
Zhang, H., Wu, Q., Cao, S., Zhao, T., Chen, L., Zhuang, P., et al. (2014). A novel protein elicitor (SsCut) from Sclerotinia sclerotiorum induces multiple defense responses in plants. Plant Mol. Biol. 86 (4-5), 495–511. doi: 10.1007/s11103-014-0244-3
Keywords: Sclerotinia sclerotiorum, nitrate reductase, SsNR, sclerotial development, infection cushion, virulence
Citation: Wei J, Yao C, Zhu Z, Gao Z, Yang G and Pan Y (2023) Nitrate reductase is required for sclerotial development and virulence of Sclerotinia sclerotiorum. Front. Plant Sci. 14:1096831. doi: 10.3389/fpls.2023.1096831
Received: 12 November 2022; Accepted: 02 May 2023;
Published: 05 June 2023.
Edited by:
Qi Peng, Jiangsu Academy of Agricultural Sciences (JAAS), ChinaReviewed by:
Wenjun Zhu, Wuhan Polytechnic University, ChinaCopyright © 2023 Wei, Yao, Zhu, Gao, Yang and Pan. This is an open-access article distributed under the terms of the Creative Commons Attribution License (CC BY). The use, distribution or reproduction in other forums is permitted, provided the original author(s) and the copyright owner(s) are credited and that the original publication in this journal is cited, in accordance with accepted academic practice. No use, distribution or reproduction is permitted which does not comply with these terms.
*Correspondence: Guogen Yang, eWFuZ2d1b2dlbkBhaGF1LmVkdS5jbg==; Yuemin Pan, cGFueXVlbWluMjAwOEAxNjMuY29t
†These authors have contributed equally to this work
Disclaimer: All claims expressed in this article are solely those of the authors and do not necessarily represent those of their affiliated organizations, or those of the publisher, the editors and the reviewers. Any product that may be evaluated in this article or claim that may be made by its manufacturer is not guaranteed or endorsed by the publisher.
Research integrity at Frontiers
Learn more about the work of our research integrity team to safeguard the quality of each article we publish.