- 1Key Laboratory of Southern Vegetable Crop Genetic Improvement, Ministry of Agriculture, College of Horticulture, Nanjing Agricultural University, Nanjing, China
- 2Suqian Academy of Protected Horticulture, Nanjing Agricultural University, Suqian, China
As one of the key enzymes in the biosynthesis of polyamines, S-adenosylmethionine decarboxylase (SAMDC) plays an important role in plant stress resistance. In this study, four SAMDC genes (CsSAMDC1-4) were identified in cucumber (Cucumis sativus L.) and divided into three groups (I, II, and III) by phylogenetic analysis. Motif analysis suggested the existence of many conserved motifs, which is compatible with SAMDC protein classification. Gene structure analysis revealed that CsSAMDC2 and CsSAMDC3 in group I have no intron, which showed a similar response to salt stress by gene expression analysis. CsSAMDC3 responded differently to hormone and stress treatments, and was more susceptible to salt stress. Compared with wild-type (WT) tobacco, the activities of superoxide dismutase, peroxidase, and catalase were increased in CsSAMDC3-overexpressing tobacco under salt stress, but the content of electrolyte leakage, malondialdehyde, and hydrogen peroxide were decreased, which alleviated the inhibition of growth induced by salt stress. Under salt stress, overexpression of CsSAMDC3 in transgenic tobacco plants exhibited salt tolerance, mainly in the form of a significant increase in dry and fresh weight, the maximal quantum yield of PSII photochemistry, the net photosynthetic rate and the content of spermidine and spermine, while the content of putrescine was reduced. In addition, the expression levels of antioxidase-related coding genes (NtSOD, NtPOD, NtCAT) and PAs metabolism-related coding genes (NtSAMS, NtSPDS, NtSPMS, NtPAO) in transgentic plants was lower than WT under salt stress, which suggested that overexpression of CsSAMDC3 affected the expression of these genes. In summary, our results showed that CsSAMDC3 could be used as a potential candidate gene to improve salt tolerance of cucumber by regulating polyamine and antioxidant metabolism.
Introduction
Polyamines (PAs) are a class of low molecular weight aliphatic nitrogen compounds with strong biological activity produced during biological metabolism (Kuznetsov and Shevyakova, 2010). The main PAs in higher plants include putrescine (Put), spermidine (Spd), and spermine (Spm), which are widely involved in many aspects of plant growth and development. It includes root elongation (Tang and Newton, 2005), flower development and fruit ripening (Liu et al., 2006), leaf senescence (Mattoo and Sobieszczuk-Nowicka, 2019), cell division and differentiation (Masson et al., 2017), programmed cell death (Moschou and Roubelakis-Angelakis, 2014), transcription and translation (Tiburcio et al., 2014), DNA synthesis (Mustafavi et al., 2018), etc. In recent years, with the in-depth study of PAs, it has been found that there is an extraordinarily complex relationship between PAs and abiotic stress. On the one hand, PAs have cationic properties, they can bind directly to membrane phospholipids, proteins and nucleic acids to maintain functional stability under abiotic stresses (Mbarki et al., 2018). On the other hand, under abiotic stress, PAs can interact with abscisic acid (ABA) and nitric oxide (NO) to activate ion channels (Wimalasekera et al., 2011; Pottosin et al., 2012), as well as regulate stomatal and programmed cell death through signaling molecules such as hydrogen peroxide (H2O2) (Klingler et al., 2010; Tisi et al., 2011; Moschou et al., 2012), or modulate the expression of nucleoside diphosphate kinase to alter activities of antioxidant enzymes (Khare et al., 2018). In summary, PAs can directly or indirectly maintain cell ion balance, reactive oxygen species (ROS) stability, osmotic pressure balance, etc., and ultimately lead to enhanced plant abiotic stress tolerance.
S-adenosylmethionine decarboxylase (SAMDC) catalyzes the decarboxylation of S-adenosylmethionine (SAM) to produce decarboxylative S-adenosylmethionine (dcSAM), which provides the aminopropyl required for the synthesis of Spd and Spm from Put. It is one of the critical enzymes in the PAs biosynthesis pathway (Slocum et al., 1984). Mellidou et al. (2016) reported that tobacco plants with downregulated SAMDC exhibit reduced PAs synthesis and stress tolerance. Plants treated with methylglyoxal bis (guanylhydrazone), a SAMDC inhibitor, resulted in a reduction in the maximal quantum yield of PSII photochemistry (Fv/Fm) and effective PSII quantum yield (Y (II)), together with higher levels of lipid peroxidation and salt stress damage (Ikbal et al., 2014). In addition, many studies have found that overexpression of SAMDC could increase plant biomass and enhance plant resistance to extreme environmental stresses (Li and Chen, 2000; Zhao et al., 2010; Jiao et al., 2022). Under abiotic stress, upregulation of SAMDC can improve antioxidant protective enzyme activitives to protect plant cells from oxidative damage by scavenging ROS (Meng et al., 2021). Thu-Hang et al. (2002) demonstrated that heterologous expression of SAMDC in plants could increase the enzyme activity of SAMDC and lead to a significant accumulation of Spd and Spm in rice leaves. Overexpression of BvSAMDC in sugar beet BvM14 increases plant salt tolerance by enhancing antioxidant enzymes and reducing ROS production (Ji et al., 2019). Luo et al. (2017) showed that overexpression of CdSAMDC1 increased the synthesis of Spd and Spm in transgenic centipedegrass, causing an increase in polyamine oxidase activity to generate H2O2; elevated H2O2 increased nitrate reductase activity to produce NO, which in turn increased antioxidant enzyme activity and cold tolerance of transgenic plants. Therefore, the role of SAMDC under abiotic stress is complex and essential.
So far, genes encoding SAMDC have been identified in many plants, such as wheat (Zeng et al., 2011), soybean (Tian et al., 2004), sugarcane (Liu et al., 2010), navel orange (Wang et al., 2010), carnation flower (Lee et al., 1997), potato (Kumar et al., 1996), tomato (Kolotilin et al., 2011) and upland cotton (Tang et al., 2021). These studies have shown that SAMDC may be encoded by one or more SAMDC genes in different species and have different catalytic activity and expression characteristics. However, the in-depth study of cucumber SAMDCs has not been reported. In this study, we identified four cucumber SAMDC gene family members in the cucumber genome, named CsSAMDC1, CsSAMDC2, CsSAMDC3 and CsSAMDC4. Firstly, we analyzed the basic sequence information, structure, and sequence homology of these genes and detected the expression pattern of CsSAMDC1-4 in roots and leaves under salt stress. Secondly, the subcellular localization of CsSAMDC3 was observed, and its expression patterns in different tissues or under stress were detected. Finally, we further studied the role of CsSAMDC3 in salt stress response by overexpressing CsSAMDC3 in tobacco.
Materials and methods
Plant materials and stress treatment
Cucumber (Cucumis sativus L. cv. Jinyou No. 4) was used as experimental material. The seedlings were cultivated in half-strength Hoagland nutrient solution (pH 6.3 ± 0.2, EC=2.2 ± 0.2 mS·cm-1) and cultivated in the artificial climate chamber. The growth temperature was 25°C/16°C (day/night). The photoperiod was 14 h/10 h (day/night) with light intensity at 600 μmol·m-2·s-1, and relative humidity was stabilized at 60-75%. To analyze the effects of hormones on cucumber seedlings, 100 μM abscisic acid (ABA), 100 μM salicylic acid (SA), 100 μM ethylene (ETH), and 100 μM methyl jasmonate (MeJA) were sprayed on the leaves when the seedlings had two true leaves. To analyze the effects of abiotic stress on cucumber seedlings, 75 mM NaCl and 20% (w/v) polyethylene glycol (PEG) 6000 were added to the Hoagland nutrient solution of cultivated seedlings to simulate salt stress and drought stress, respectively. The seedlings were placed in a 4°C light incubator to simulate cold stress. Leaves and roots were collected at 0, 3, 6, 9, 12, 24, 48, and 72 h after treatment and stored at -80°C for use. To analyze the tissue-specific expression pattern, different tissues of the cucumber reproductive growth period were stored at -80°C.
Wild-type tobacco (Nicotiana tabacum L.) and transgenic tobacco (CsSAMDC3-overexpressing) were cultured in an artificial climate chamber. When the tobacco grew to four ture leaves, irrigated with 200 mM NaCl. After 5 days of treatment, the leaves and roots were stored at -80°C.
Identification and sequence analysis of cucumber SAMDC
The amino acid sequence of SAMDC was retrieved from the reported Cucurbit Genomics Database (http://cucurbitgenomics.org/) and verified in NCBI Database. The isoelectric point (pI) and molecular weight (MW) of the candidate SAMDC were calculated using ExPASy (https://www.expasy.org/). To study the evolutionary relationship of SAMDC between cucumber and other plant species, the SAMDC gene sequence and SAMDC protein sequence of 12 plants such as pumpkin, tobacco, and soybean were obtained by searching NCBI Genome Database. The SAMDC protein sequences of different species were compared by Clustal W, and the phylogenetic tree was constructed by NJ (neighbor-joining) method, Poisson correction, and 1000 bootstrap replicates in MEGA 11 software. The conserved motifs of different species and the conserved domains of SAMDC protein sequences were searched by the online MEME tool (https://meme-suite.org/meme/) and NCBI CDD (https://www.ncbi.nlm.nih.gov/cdd/), and visualized by TB Tools (Chen et al., 2020).
Genetic transformation and treatment of tobacco
To construct CsSAMDC3-overexpressing tobacco, the primer pair PAC019-CsSAMDC3-F/R (Supplemental Table 1) was designed. CsSAMDC3 was cloned from cucumber cDNA with PrimeSTAR® Max DNA Polymerase (Takara, China) following the instructions. The cucumber SAMDC3 gene fragment was ligated to the digested PAC019 vector using ClonExpress® II One Step Cloning Kit (Vazyme, China). The normal sequencing plasmid was transferred into Agrobacterium EHA105 by heat shock method. CsSAMDC3 was transformed into Nicotiana tabacum by Agrobacterium-mediated according to the previous research method (Wang et al., 2017). Homozygous transgenic plants were screened by kanamycin and identified by PCR analysis. Quantitative real-time PCR (qRT-PCR) analysis was also performed to validate the transformation of CsSAMDC3 further. T3 homozygous transgenic lines for further study.
RNA extraction, reverse transcription, and quantitative real-time PCR
RNA simple Total RNA Kit (Tiangen, China) was used to extract total RNA from samples. HiScript® III Q RT Super-Mix for qPCR Kit (Vazyme, China) reverse transcribed 1μg total RNA into cDNA for gene cloning and qPCR. Based on the selected gene sequence, the primer pair (Supplemental Table 1) was designed using Beacon Designer™ 8.10 (Premier Biosoft International, USA). qRT-PCR was conducted on Quant-Studio™ 5 Real-Time PCR System (Applied Biosystems) with ChamQ SYBR qPCR Master Mix (Vazyme, China), which included 10 μL of ChamQ SYBR qPCR Master Mix (2 ×), 0.4 μL of sense or anti-sense primer, 0.4 μL of ROX Reference dye (50 ×), 1 μL of cDNA and 7.8 μL of ddH2O in a total volume of 20 μL. The PCR program was as follows: 95°C for 30 s; 40 cycles of 95°C for 10 s, 58°C for 10 s; and finally, 72°C for 30 s.
The relative expression levels of the selected genes were calculated by 2-ΔΔCT method using cucumber or tobacco actin genes as internal controls (Livak and Schmittgen, 2001).
Electrolyte leakage, malondialdehyde, and hydrogen peroxide determination
The electrolyte leakage rate was measured according to the description of He et al. (2019). 0.5 g fresh sample was immersed in a test tube containing 20 mL deionized water. After shaken at room temperature for 4~5 h, the initial conductivity (EC1) was measured. Then, the sample was boiled at 95 °C for 20 min and cooled to room temperature, and the final conductivity (EC2) was measured in the bath. We also measured the conductivity of deionized water (EC0). EL (%) was calculated as [(EC1-EC0)/(EC2-EC0)] × 100.
MDA content was determined according to the description of Dhindsa et al. (1981). The leaves were ground on ice with 5% TCA and centrifuged at 4000 g for 10 min. Then 2 mL of the supernatant was mixed with an equal amount of 0.67% thiobarbituric acid (TBA). After being heated in a boiling water bath for 30 min, the mixture was centrifuged at 3000 g for 15 min. The absorbance of the supernatant was read at 450, 532, and 600 nm and calculated. The MDA content is represented as nmol·g-1 FW.
The content of H2O2 was determined according to the description of Zhu et al. (2021). The 0.2 g leaf sample was ground on ice using 1.6 mL of pre-cooled 0.1% trichloroacetic acid (TCA) to a slurry and then centrifuged at 12000 g for 20 min. The 0.2 mL supernatant was mixed with 1 mL 1 M KI solution and 0.25 mL 0.1 M potassium phosphate buffer (pH 7.8) and placed in the dark for 1 h. The absorbance at 390 nm was read with 0.1% TCA as blank. The content of H2O2 in the sample was calculated according to the standard curve of known H2O2 concentration. The H2O2 content is expressed as μmol·g-1 FW.
Assay of antioxidant enzyme activity
The leaves were ground into a slurry in 50 mM precooled phosphate buffer (pH 7.8), transferred into a 2 mL centrifuge tube, and centrifuged at 4°C, 12000 g for 20 min. The resulting supernatant was the crude enzyme solution for determining antioxidant enzyme activity.
Superoxide dismutase (SOD) activity was determined with reference to the description of Giannopolitis and Ries (1977) with minor modifications. The NBT photoreduction method was used. The reaction mixture contained 50 mM PBS (pH 7.8), 30 μM EDTA-Na2, 14.5 mM methionine, 60 μM riboflavin and 2.25 mM nitroblue tetrazolium chloride (NBT). 3 mL reaction mixture was mixed with 40 μL crude enzyme solution, and the tubes were illuminated for 20 min. The absorbance was read at 560 nm. One unit of SOD activity was defined as the amount of enzyme required to cause 50% inhibition of NBT photoreduction.
Peroxidase (POD) and catalase (CAT) were determined according to the description of Wu et al. (2022). The enzyme extract was mixed with the reaction solution. The reaction solution to determine POD was 20 mM phosphate buffer (pH 6.0), 3.5 M guaiacol, and 30% H2O2. The reaction solution to determine CAT was 50 mM phosphate buffer (pH 7.0) and 30% H2O2. The absorbance changes at 470 or 240 nm within 40 s were measured. A unit of POD or CAT activity was expressed as a change of OD470 or OD240 value of 0.01 min-1.
Net photosynthetic rate and chlorophyll fluorescence parameters
The net photosynthetic rate (Pn) of tobacco leaves at the same leaf position was measured by portable photosynthesis system (Li-6400 XT, Li-COR, Lincoln, NE, USA). The light intensity, leaf temperature, relative humidity, and ambient CO2 concentration maintained at 800 μmol·m-2·s-1, 25°C, 70%, and 400 ± 10 μmol·mol-1, respectively. Chlorophyll fluorescence was measured by fluorescence imaging system (IMAGING-PAM, Heinz Walz, Effeltrich, Germany). The measured data and collected fluorescence images were analyzed by ImagingWin software (Heinz Walz, Effeltrich, Germany). The chlorophyll fluorescence parameters were measured according to the method of Shu et al. (2016), and the maximal quantum yield of PSII photochemistry (Fv/Fm) was calculated.
Measurement of PAs content
PAs were measured according to the description of Shu et al. (2012). The sample was ground into a slurry in 1.6 mL 5% precooled perchloric acid (PCA) and then centrifuged at 12000 g at 4°C for 20 min. The supernatant was collected to measure free and bound PAs, while the particles were used to measure bound PAs.
For free PAs, 0.7 mL of supernatant, 1.4 mL of 2 M NaOH, and 15 μL of benzoyl chloride were mixed, then vortexed for 20 s and kept at 30 °C for 30 min. Then, 2 mL saturated NaCl solution, and cold colddiehtyl ether were added to the mixed solution to extract benzoyl polyamines. After centrifugation at 4 °C for 12000 g for 5 min, 1 mL of ether phase was evaporated to dryness and redissolved in 1 mL of 64% (v/v) methanol.
For conjugated PAs, 0.7 mL of supernatant was mixed with 5 mL of 6 M HCl and sealed in an ampoule at 110 °C for 18 h to convert conjugated PAs into free PAs. The hydrolysate was evaporated at 70°C, and the resulting residue was re-suspended in 1.6 mL of 5% PCA. The following steps are the same as free PAs extraction.
For bound PAs, the particles were washed four times with 5% PCA and centrifuged at 3000 g for 5 min. The resulting particles were suspended in 5 mL 6 M HCl and then subjected to the same steps as conjugated PAs extraction.
Samples redissolved in methanol are stored at -20°C and filtered with a membrane (0.45 μm) before testing. UPLC system (Thermo, UltiMate 3000), including ACQUITY UPLC HSS T3 column, acetonitrile, and water (volume ratio of 44: 56) as the solvent, the flow rate of 0.45 mL min-1 for the detection of PAs content. The sum of three forms of PAs is the total amount of endogenous PAs.
Subcellular localization analysis
Subcellular localization prediction of CsSAMDC3 using the CELLO tool (http://cello.life.nctu.edu.tw/).
The agrobacterium strain EHA105 transformed with PAC019-CsSAMDC3 plasmid was transiently transformed into Nicotiana tabacum by injection infection method. A confocal laser scanning microscope (LSM 780, Zeiss, Germany) was used for imaging after incubation for 36-48 h under the light.
Statistical analysis
All data were analyzed by single factor analysis of variance (ANOVA) using IBM SPSS 26.0 software (SPSS Inc., Chicago, IL, USA). Duncan’s multiple comparison method was used to analyze the difference between different treatments at a P < 0.05 level of significance.
Result
Bioinformatics analysis of SAMDC family genes in cucumber
Four CsSAMDC gene sequences were identified by PCR amplification and sequencing, which were consistent with the search results of the database. As shown in Table 1, CsSAMDC1 and CsSAMDC3 are located on chromosome 3, while CsSAMDC2 and CsSAMDC4 are located on chromosome 6 and 2, respectively. The length of the CsSAMDC1-4 gene sequence is relatively close, all of which are about 1100 bp, the encoding amino acid (AA) number is 340-389, the encoding protein isoelectric point (pI) is 4.56-6.88, molecular weight is about 40 KDa, hydrophilic average coefficient (GRAVY) is close to 0, and it is inferred to be amphoteric protein.
Phylogenetic analysis of the full-length SAMDC protein sequences of 13 species, including cucumber, showed that these SAMDC proteins could be divided into 3 groups, of which CsSAMDC2 and CsSAMDC3 were in group 1, while CsSAMDC4 and CsSAMDC1 were in group 2 and group 3, respectively (Figure 1A). Conserved motif analysis of SAMDC protein sequence by the online MEME tool identified 16 conserved motifs. The distribution was consistent with the phylogenetic tree (Figure 1B). The conserved domain of the SAMDC protein sequence was analyzed by NCBI CDD. A typical SAMDC protease domain was found (Figure 1C). By searching NCBI Genome Database, the entire sequence of the SAMDC genes was obtained for visual analysis. The results showed that the SAMDC genes of group 1 basically did not contain introns, while the introns of group 2 and group 3 had different lengths (Figure 1D). In addition, the CDS regions of most SAMDC genes are continuous (Figure 1D).
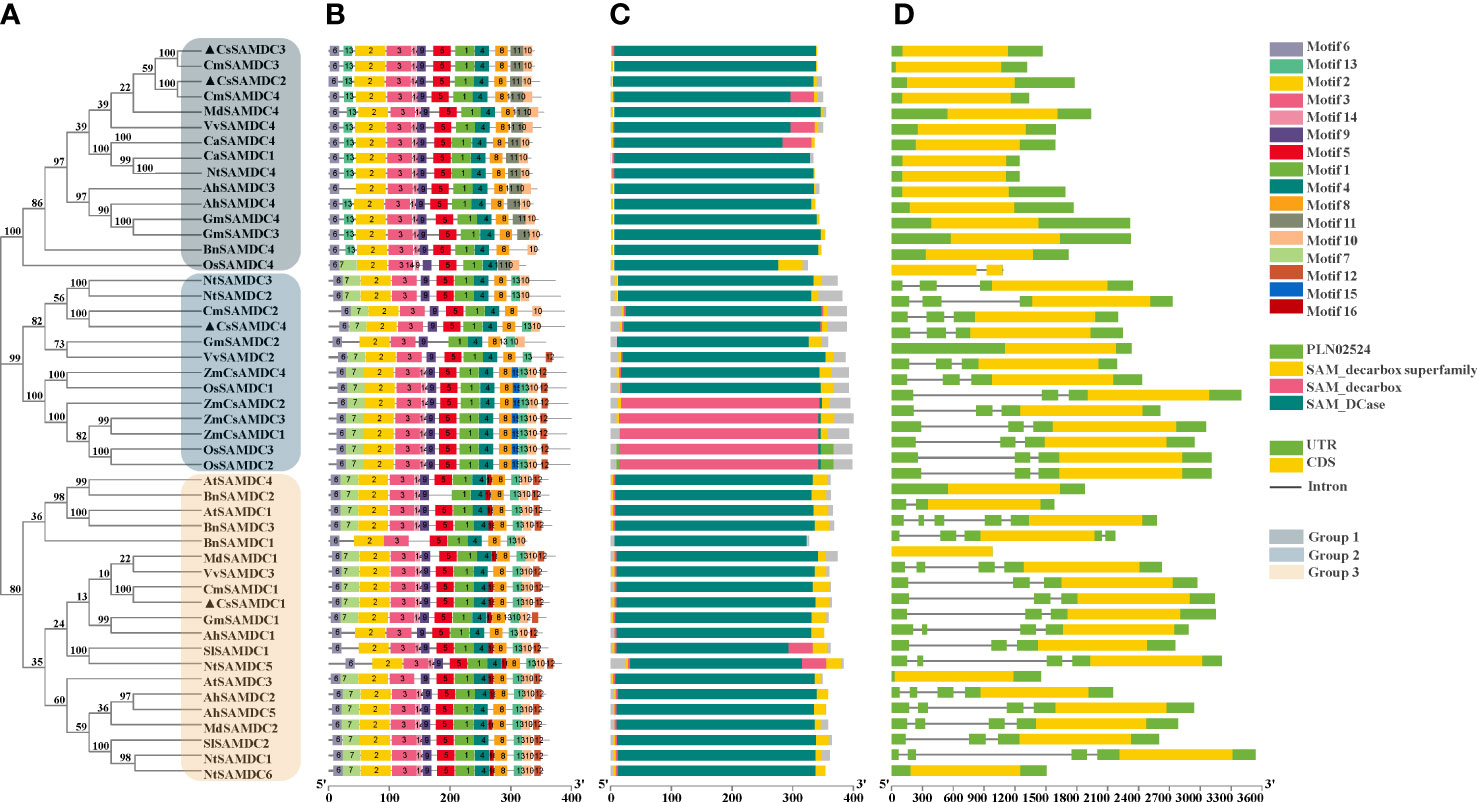
Figure 1 Bioinformatics analysis of SAMDC proteins and SAMDC genes from different plants. (A) Evolutionary relationships of SAMDC proteins. (B) Distribution of the 16 conserved motifs in the SAMDC proteins. (C) Protein domains of SAMDC proteins. (D) Gene structure of SAMDC genes. The accession numbers of SAMDC proteins and SAMDC genes used to construct the phylogenetic tree are listed in Supplemental Table 2. Motifs are colored by different boxes, and their sequences are listed in Supplemental Table 3.
Response of SAMDC family genes to salt stress in cucumber
The response of cucumber SAMDC family genes to salt stress was analyzed by qRT-PCR. As shown in Figure 2, there were differences in the expression patterns of SAMDC family genes in cucumber roots and leaves. The expression trends of CsSAMDC2 and CsSAMDC3 are similar, both of which firstly increased and then decreased. The expression levels of CsSAMDC2 and CsSAMDC3 in roots peaked after 9 h of salt treatment, which were 6 times and 8 times higher than that of the control, respectively. The expression of CsSAMDC2 in leaves also peaked at 9 h, which was 15 times higher than that of the control, while the expression of CsSAMDC3 peaked earlier at 6 h, which was 7 times higher than that of the control. It suggests that CsSAMDC3 is the most sensitive to salt stress and the first to respond to salt stress. In addition, the response of CsSAMDC1 to salt stress was relatively stable in leaves, while its expression level in roots was close to that of the control within 24 h of salt stress and then increased. The response of CsSAMDC4 to salt stress was more prominent in leaves, and its expression peaked at 48 h, which was 12 times higher than that of the control, while its expression in roots showed a trend of slight decrease and then increase. After that, we selected CsSAMDC3, which is sensitive to salt stress, as a typical case for further study.
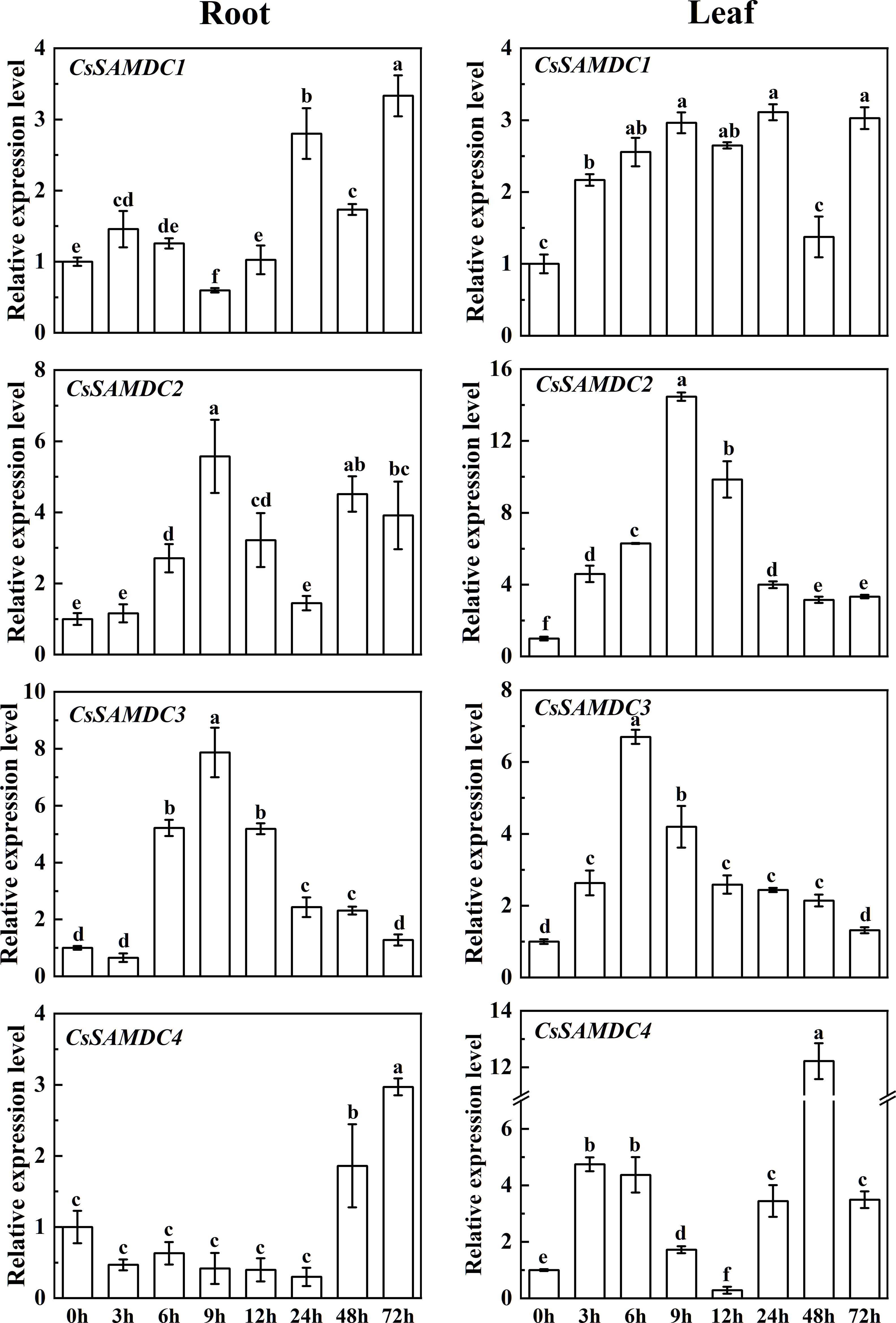
Figure 2 Responses of cucumber SAMDCs to salt stressEach value is shown as mean ± standard error of three biological replicates. Different letters indicate significant differences at P < 0.05, according to Duncan’s multiple range tests.
Tissue-specific expression and subcellular localization of CsSAMDC3
CsSAMDC3 was expressed in different tissues of cucumber. The expression level of CsSAMDC3 in fruit and flower was 5-8 times higher than that in leaves. In comparison, the expression level in the root, stem, and leaf was lower (Figure 3A), indicating that CsSAMDC3 was mainly related to flower organ development.
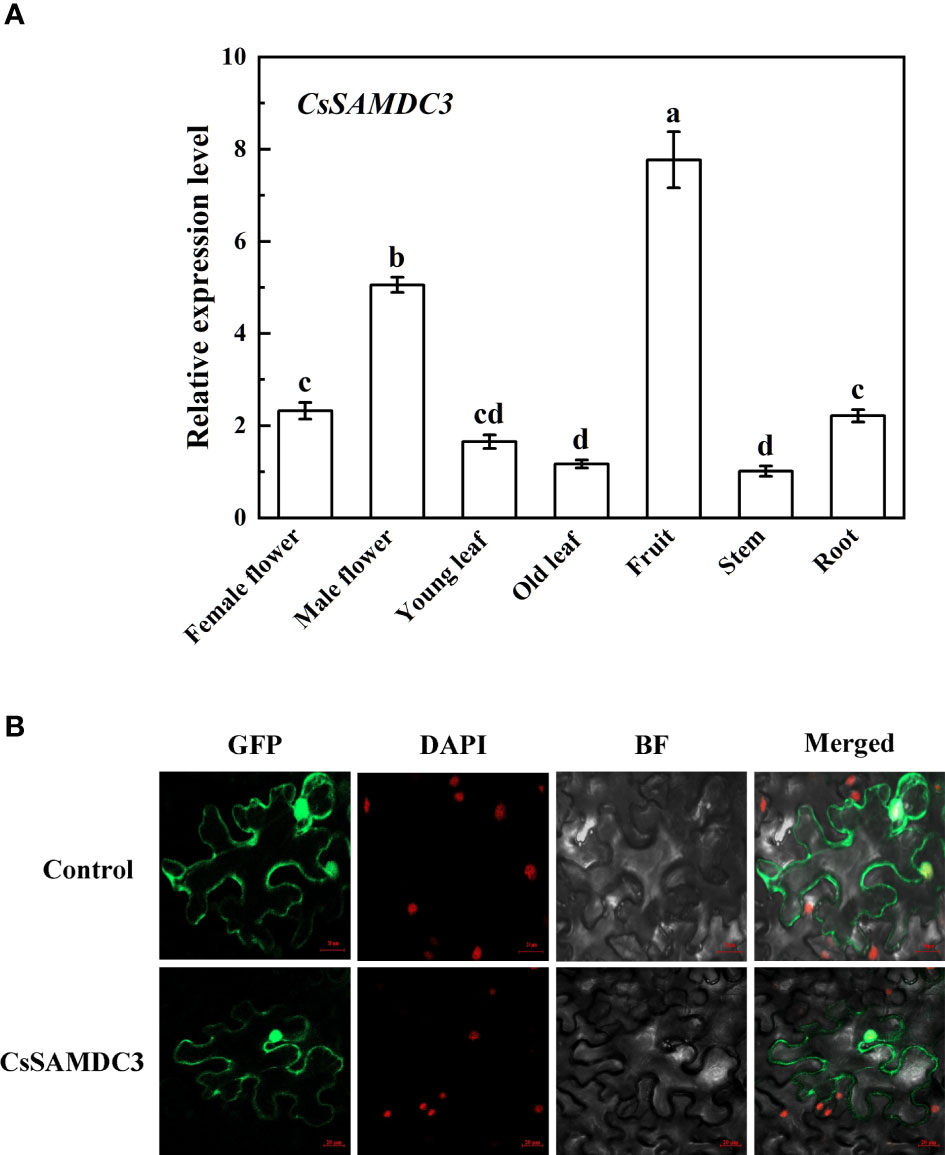
Figure 3 Tissue-specific expression of CsSAMDC3 (A) and subcellular localization (B) in cucumber. Expression level in stem is taken as 1. Each value is shown as mean ± standard error of three biological replicates. Different letters indicate significant differences at P < 0.05, according to Duncan’s multiple range tests.
Subcellular localization prediction of CsSAMDC3 showed that the protein was likely to be localized in the cell membrane and nucleus (Supplemental Figure 1). To verify the results of CELLO subcellular localization prediction, the green fluorescence signal of GFP-CsSAMDC3 fusion protein was detected by ultra-high resolution laser confocal microscopy. Figure 3B showed that the green fluorescence signal of the fusion protein was on the cell membrane and nucleus, indicating that CsSAMDC3 was located on the cell membrane and nucleus. The results were consistent with CELLO prediction.
Response of CsSAMDC3 to different hormone and abiotic stresses
As shown in Figure 4, under ABA treatment, the expression of CsSAMDC3 in cucumber leaves and roots increased and reached the peak value at 9 h, then decreased to the average level at 48 h. Under SA treatment, the expression of CsSAMDC3 in cucumber leaves fluctuated less compared with ABA treatment, and the peak value also appeared at 9 h, while the expression of CsSAMDC3 in cucumber roots reached the highest at 24 h, and the response was more intense. Under MeJA treatment, the expression of CsSAMDC3 in leaves increased at 3 h, decreased slightly, increased to the maximum at 12 h, and then decreased to the standard value. In roots, the response of CsSAMDC3 to MeJA was not strong, but the overall trend was upward. Under ETH treatment, the expression of CsSAMDC3 in leaves and roots was very prominent, reaching the peak at 24 h and 12 h, respectively, and the response of CsSAMDC3 to ETH was the strongest compared with other treatment groups. For 4 °C stress and PEG simulated drought stress, the overall response of CsSAMDC3 fluctuated less, especially in the leaves under cold stress and the roots under drought stress; The expression level has been maintained at a low level. Under salt stress, the expression levels of CsSAMDC3 in leaves and roots reached the peak at 6 h and 9 h, respectively, and the response in roots was more robust. Compared with other abiotic stress treatments, the expression of CsSAMDC3 reached the peak earlier under NaCl stress, indicating that CsSAMDC3 was more sensitive to salt stress.
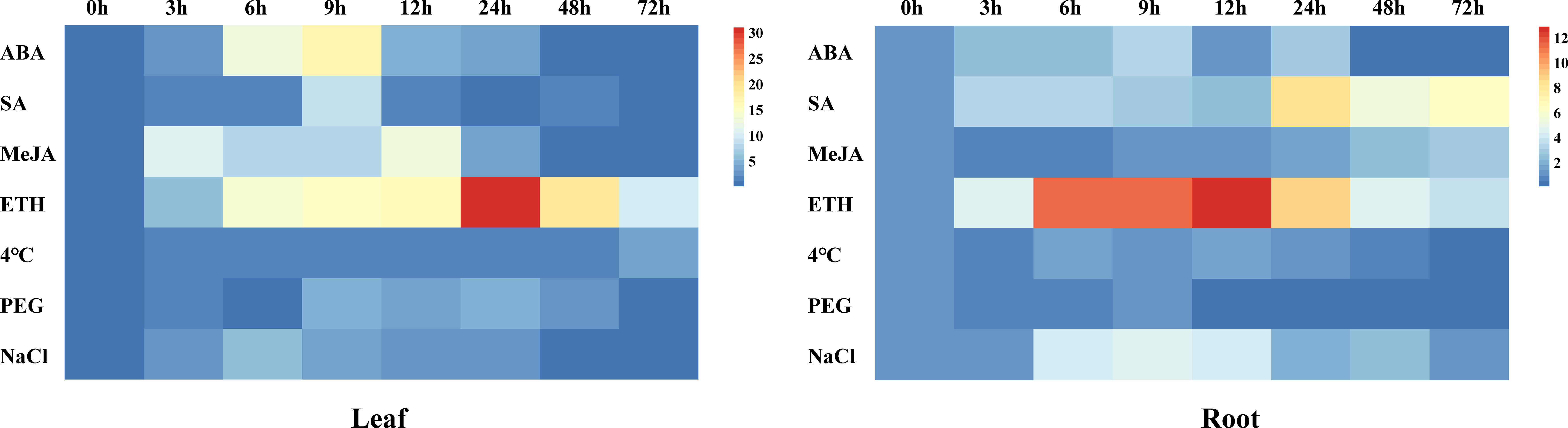
Figure 4 Heatmap illustrating gene expression changes of CsSAMDC3 in cucumber leaf and root under different hormones and stress treatments.
Construction of tobacco overexpressing CsSAMDC3
To further study the function of CsSAMDC3 under salt stress, the genetic transformation of tobacco was carried out by the Agrobacterium-mediated leaf disc method. Positive plants were screened by kanamycin, and DNA was extracted and verified by PCR with PAC019-F/PAC019-CsSAMDC3-R (Supplemental Table 1) (Figure 5A). The expression of CsSAMDC3 gene in two lines with good seed quality was detected by qRT-PCR. It can be seen from Figure 5B that the expression level of CsSAMDC3 in the transgenic line OE-1# was 19 times higher than that in the transgenic line OE-2#.
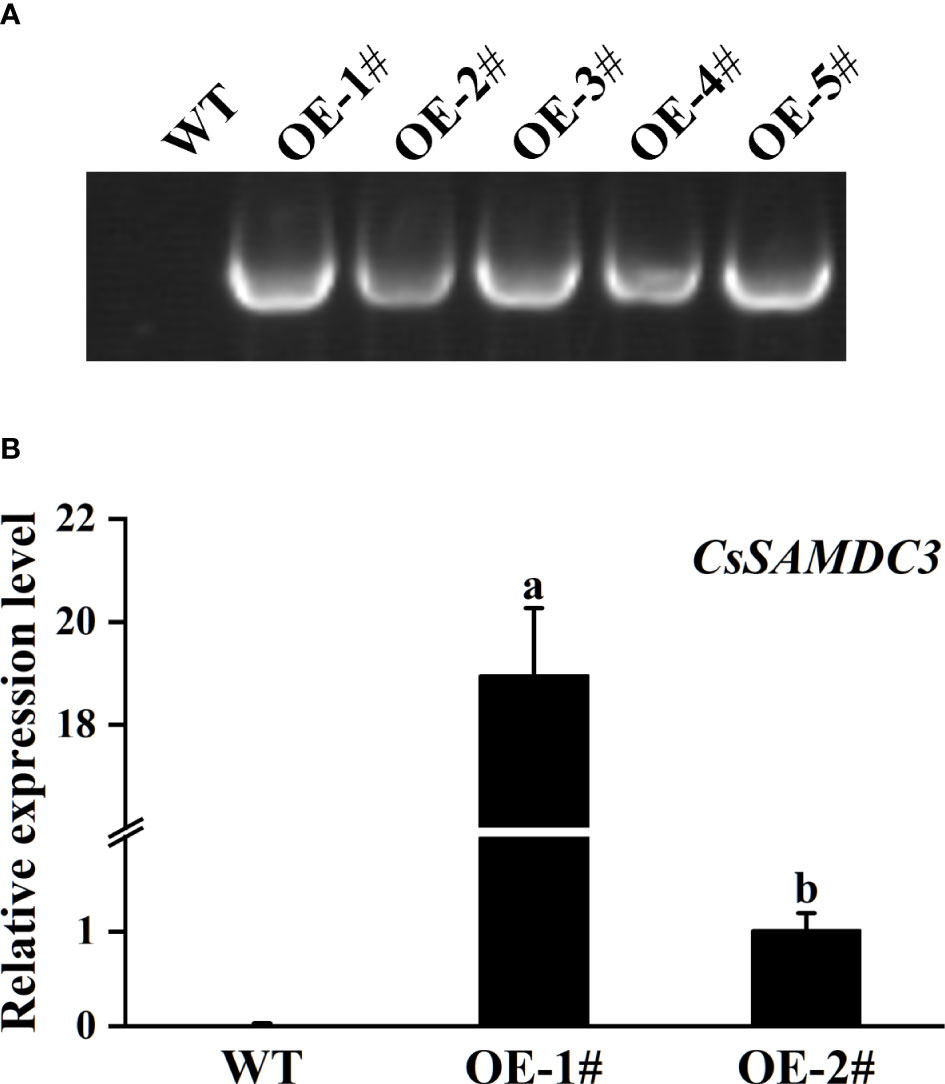
Figure 5 Identification of the CsSAMDC3-overexpressing transgenic tobacco. (A) Genomic DNA amplification in wild-type and overexpression transgenic plants. (B) qRT-PCR tested in transgenic lines and wild type. Expression level in OE-2# is taken as 1. Each value is shown as the mean ± standard error of three biological replicates. Different letters indicate significant differences at P < 0.05, according to Duncan’s multiple range tests.
Effects of overexpression of CsSAMDC3 on biomass and photosynthetic capacity of tobacco under salt stress
As shown in Figures 6A, B, after 5 days of 200 mM NaCl treatment, the growth of wild-type and overexpressed CsSAMDC3 tobacco was inhibited, showed different degrees of salt damage: compared with overexpressed plants, wild-type plants were shorter and smaller, and root growth was more significantly inhibited. Among them, the fresh and dry weight of the overexpression lines OE-1# and OE-2# decreased by 32.73%, 39.83% and 29.78%, 31.00%, respectively, compared with the control group. In contrast, the wild type decreased more significantly, 53.71% and 57.74%, respectively (Figures 6D, E). In addition, the Fv/Fm and Pn values of wild-type tobacco after salt treatment were significantly lower than those of CsSAMDC3-overexpressing tobacco, which were 36.17% and 13.00% lower, respectively (Figures 6C, F, G). The above results showed that the growth and ФPSII of wild-type tobacco were more severely inhibited under salt stress, and overexpression of CsSAMDC3 alleviated the growth inhibition induced by salt stress.
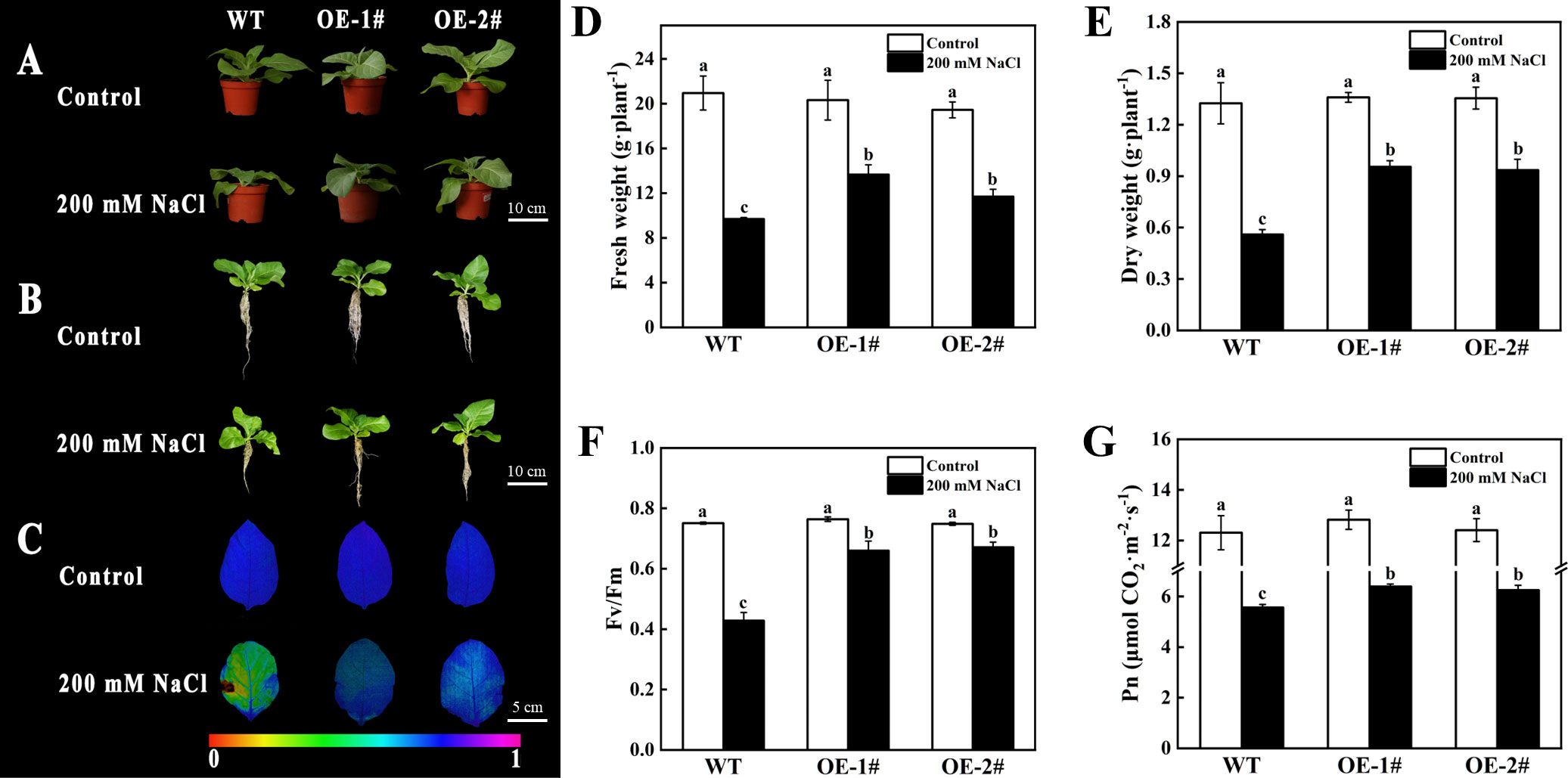
Figure 6 Phenotype and photosynthetic capacity analysis of the wild type and CsSAMDC3-overexpressing transgenic lines under salt stress. (A, B) Phenotype. (C, F) maximal photochemical efficiency. (D) Fresh weight. (E) Dry weight. (G) Net photosynthetic rate. Each value is shown as the mean ± standard error of three biological replicates. Different letters indicate significant differences at P < 0.05, according to Duncan’s multiple range tests.
Effects of overexpression of CsSAMDC3 on antioxidant capacity of tobacco under salt stress
To verify the role of CsSAMDC3 in salt stress, we further analyzed the oxidative indexes of wild-type and CsSAMDC3-overexpressing tobacco under salt stress. As shown in Figure 7, the electrical conductivity, MDA content, and H2O2 content of overexpressing plants after salt treatment were significantly lower than those of wild-type plants in both leaves and roots. Among them, the electrical conductivity of OE-1# and OE-2# decreased more in leaves, which were 19.75% and 21.99%, respectively (Figure 7A). MDA content decreased more in roots, 39.24% and 45.16%, respectively (Figure 7D). H2O2 content in leaves decreased by 27.64% and 11.91%, respectively (Figure 7E). In addition, we determined the activities of antioxidant enzymes in leaves and roots of wild-type and CsSAMDC3-overexpressing tobacco under salt stress. The results showed that for SOD, the activity of CsSAMDC3-overexpressing tobacco in roots was substantially higher than that of wild-type tobacco, whereas the activity in leaves was comparable to that of wild-type tobacco (Figures 8A, B). For POD, the activity of CsSAMDC3-overexpressing tobacco in roots and leaves was significantly higher than that of wild-type tobacco, and the activity of OE-1# in leaves and roots was 81.79% and 223.00% higher than that of WT, respectively (Figures 8C, D); For CAT, the activities of OE-1# and OE-2# in leaves were 29.44% and 22.38% higher than WT, respectively (Figure 8E). The activities of OE-1# and OE-2# in roots were 20.53% and 9.47% higher than WT, respectively (Figure 8F). Overall, overexpression of CsSAMDC3 in tobacco can reduce MDA and H2O2 content by increasing antioxidant enzyme activity, thereby enhancing the tolerance of tobacco to salt stress.
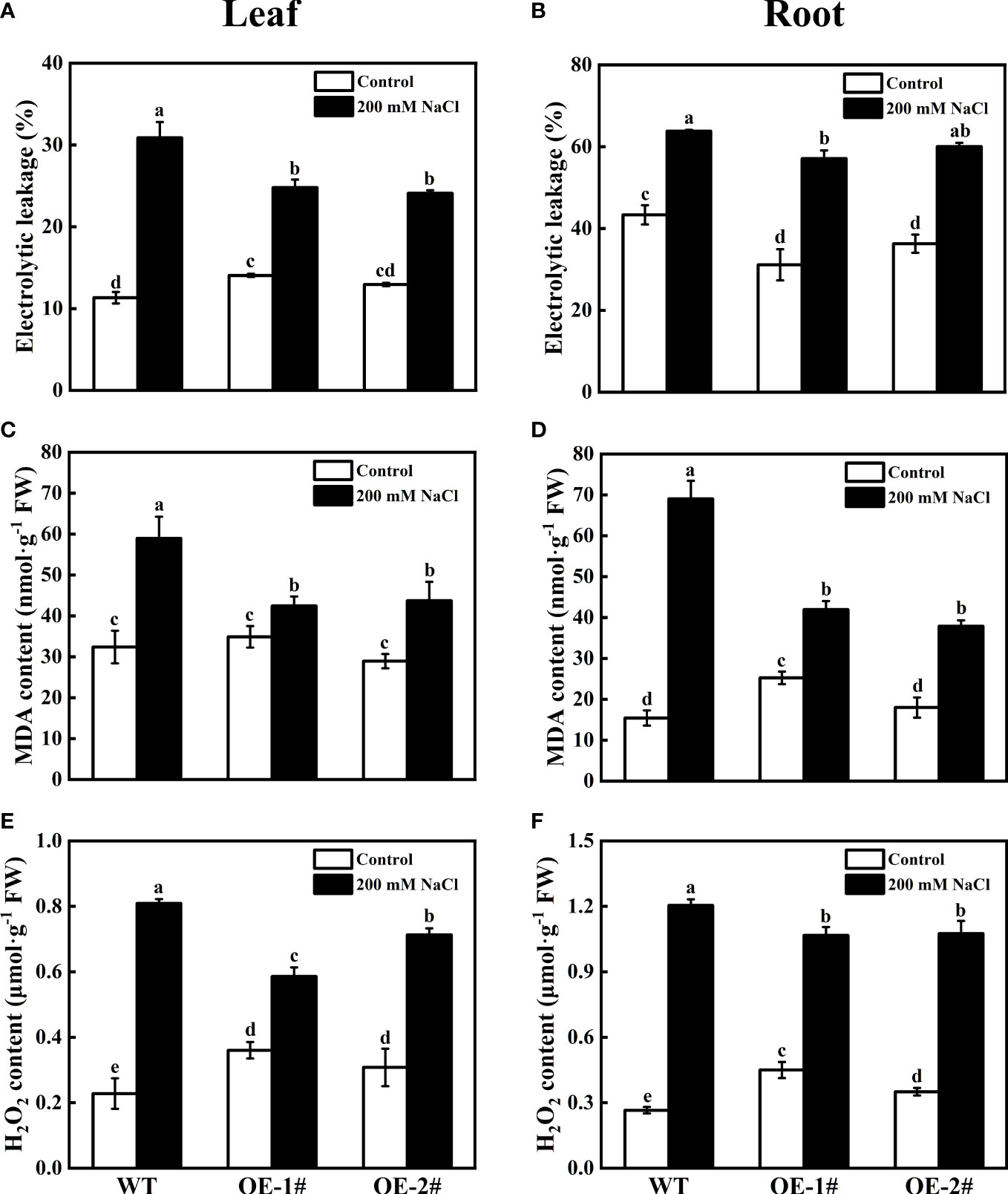
Figure 7 Electrolyte leakage, MDA, and H2O2 content analysis of wild type and CsSAMDC3-overexpressing transgenic lines under salt stress. (A, B) Electrolyte leakage. (C, D) MDA content. (E, F) H2O2 content. Each value is shown as the mean ± standard error of three biological replicates. Different letters indicate significant differences at P < 0.05, according to Duncan’s multiple range tests.
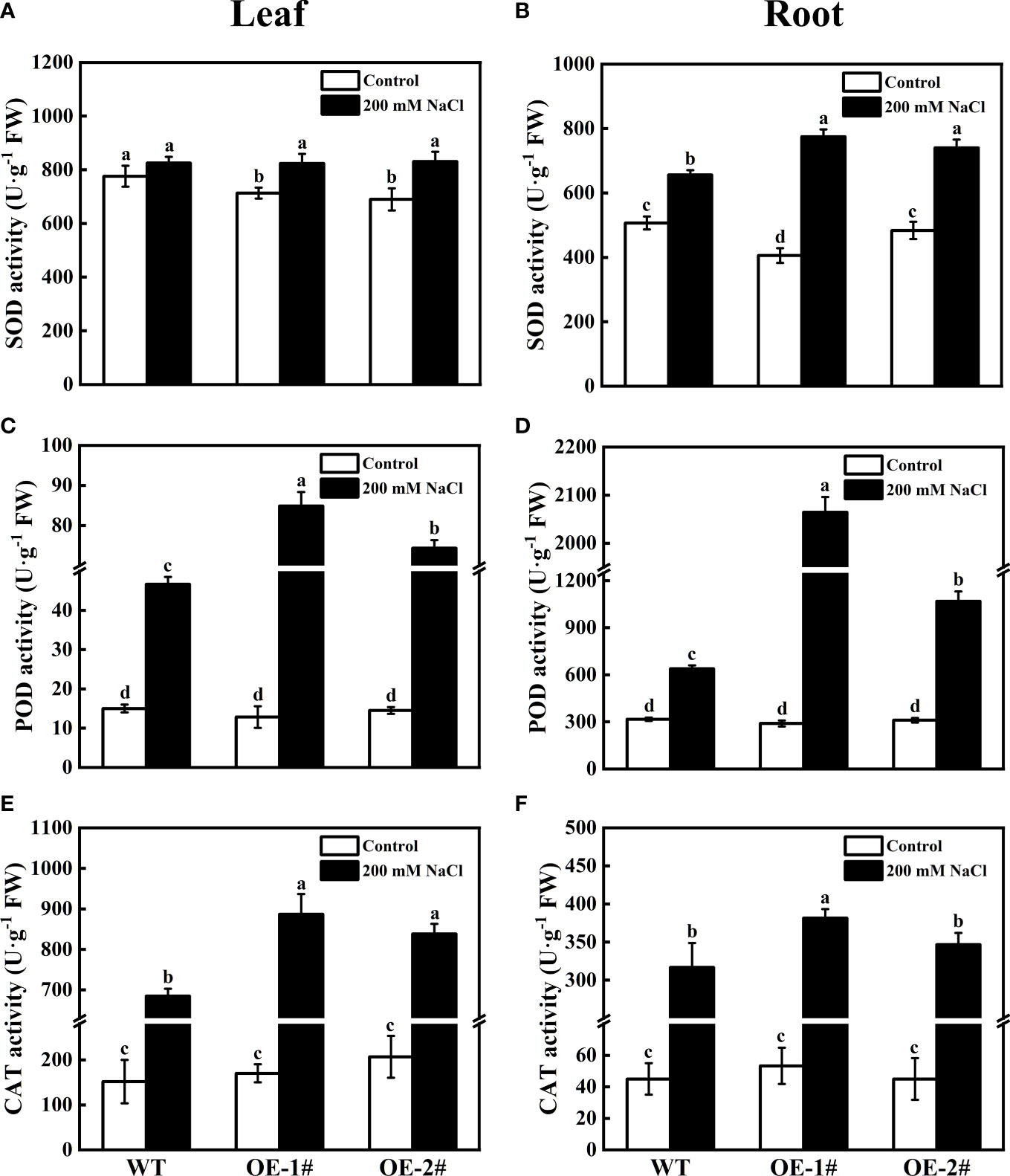
Figure 8 Antioxidant enzyme activity of wild-type and CsSAMDC3-overexpressing transgenic lines under salt stress. (A, B) SOD activity. (C, D) POD activity. (E, F) CAT activity. Each value is shown as the mean ± standard error of three biological replicates. Different letters indicate significant differences at P < 0.05, according to Duncan’s multiple range tests.
The expression levels of antioxidase-related coding genes (NtSOD, NtPOD, NtCAT) were detected. As shown in Figure 9, compared with WT, the expression levels of NtSOD, NtPOD, and NtCAT in OE-1# and OE-2# were significantly decreased under salt stress. Among them, the expression of NtSOD was the most obvious difference, and OE-1# and OE-2# were 66.92% and 68.26% lower than WT, respectively (Figure 9A). Under the control conditions, except NtPOD, the expression difference of other antioxidant enzyme related coding genes was not significant between transgenic tobacco and wild type.
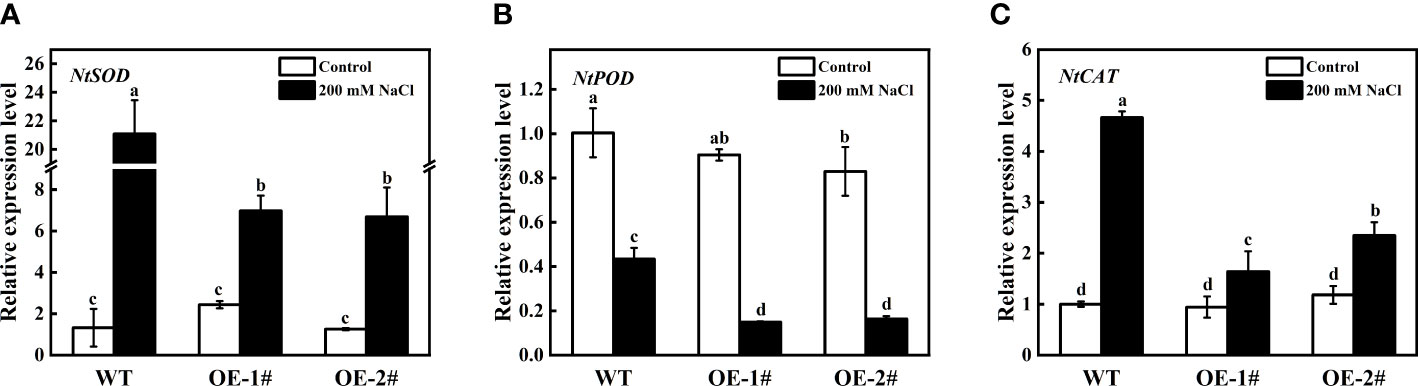
Figure 9 Effects of salt stress on relative gene expression of NtSOD (A), NtPOD (B), NtCAT (C) of wild-type and CsSAMDC3-overexpressing transgenic tobacco seedling roots. Each value is shown as the mean ± standard error of three biological replicates. Different letters indicate significant differences at P < 0.05, according to Duncan’s multiple range tests.
Effects of salt stress on polyamine metabolism in CsSAMDC3-overexpressing tobacco
As shown in Figure 10, transgenic tobacco plants had lower Put content and higher Spd and Spm content compared to wild type under the control conditions. Compared with WT, the Spd and Spm content in OE-1# increased by 12.78% and 42.21% respectively, while the Put content decreased by 29.78%; the Spd and Spm content in OE-2# increased by 4.40% and 33.98% respectively, while the Put content decreased by 18.88%. However, under salt stress, the content of Put, Spd, and Spm in OE-1# decreased by 16.71%, increased by 28.81%, and increased by 45.15% compared with WT, respectively. The content of Put, Spd, and Spm in OE-2# decreased by 2.87%, increased by 21.25% and 51.68%, respectively, compared with WT. The difference between Spd and Spm content of CsSAMDC3-overexpressing tobacco and wild type under salt stress was further widened (Figures 10B, C). The values of (Spd + Spm)/Put in OE-1# and OE-2# were increased under salt stress compared with WT (Figure 10D). This indicates that overexpression of CsSAMDC3 under salt stress can promote the synthesis of more Spd and Spm in transgenic tobacco.
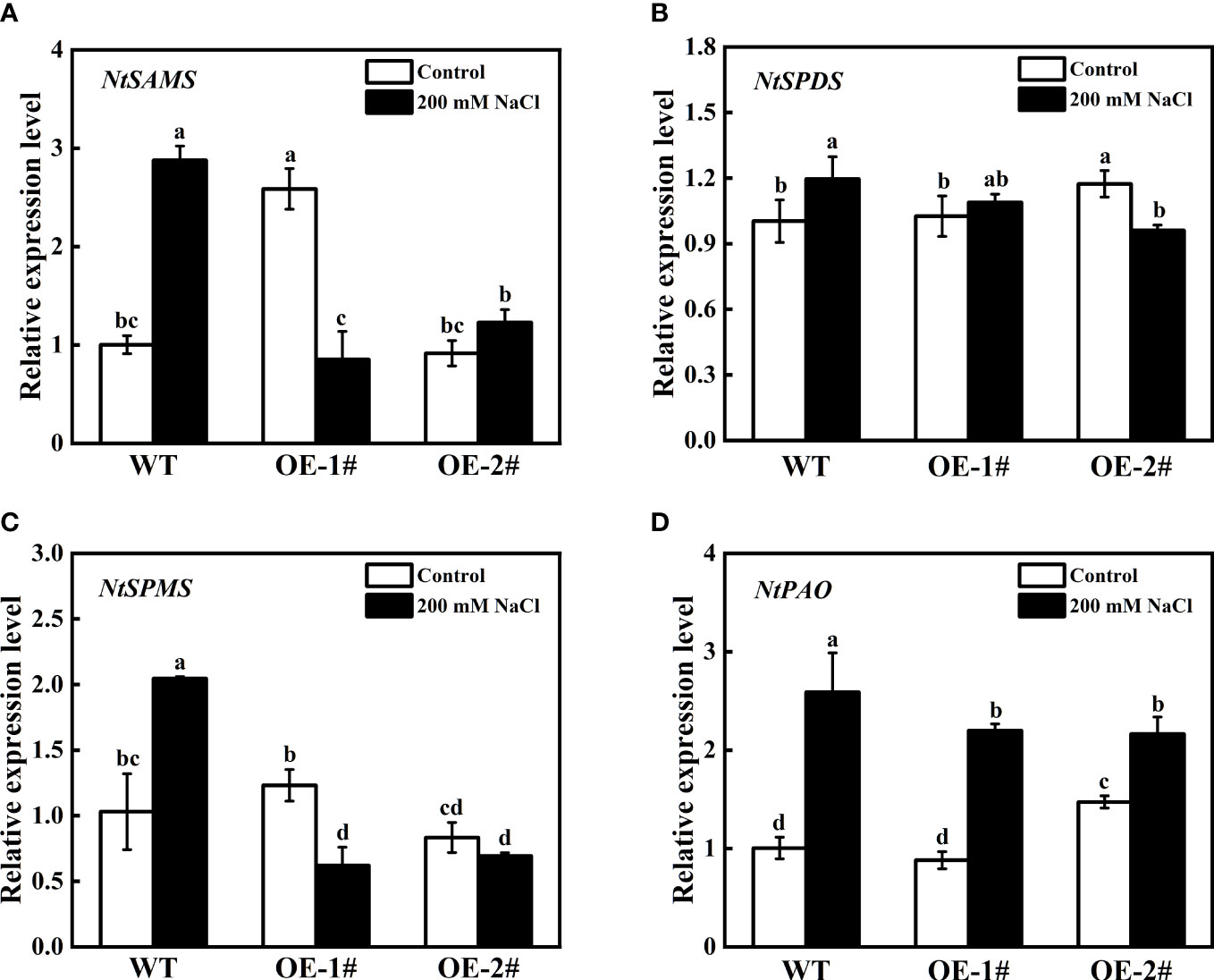
Figure 10 Total polyamine content of wild-type and CsSAMDC3-overexpressing transgenic lines under salt stress. (A) Put. (B) Spd. (C) Spm. (D) The value of (Spd + Spm)/Put. Each value is shown as the mean ± standard error of three biological replicates. Different letters indicate significant differences at P < 0.05, according to Duncan’s multiple range tests.
As shown in Figure 11, under control conditions, the expression levels of NtSAMS and NtSPMS in OE-1# were significantly higher than those in WT and OE-2#, and the expression levels of NtSPDS and NtPAO in OE-2# were significantly higher than those in WT and OE-1#. However, under salt stress, compared with WT, the expression levels of PAs metabolism-related coding genes (NtSAMS, NtSPDS, NtSPMS, NtPAO) in OE-1# and OE-2# were decreased.
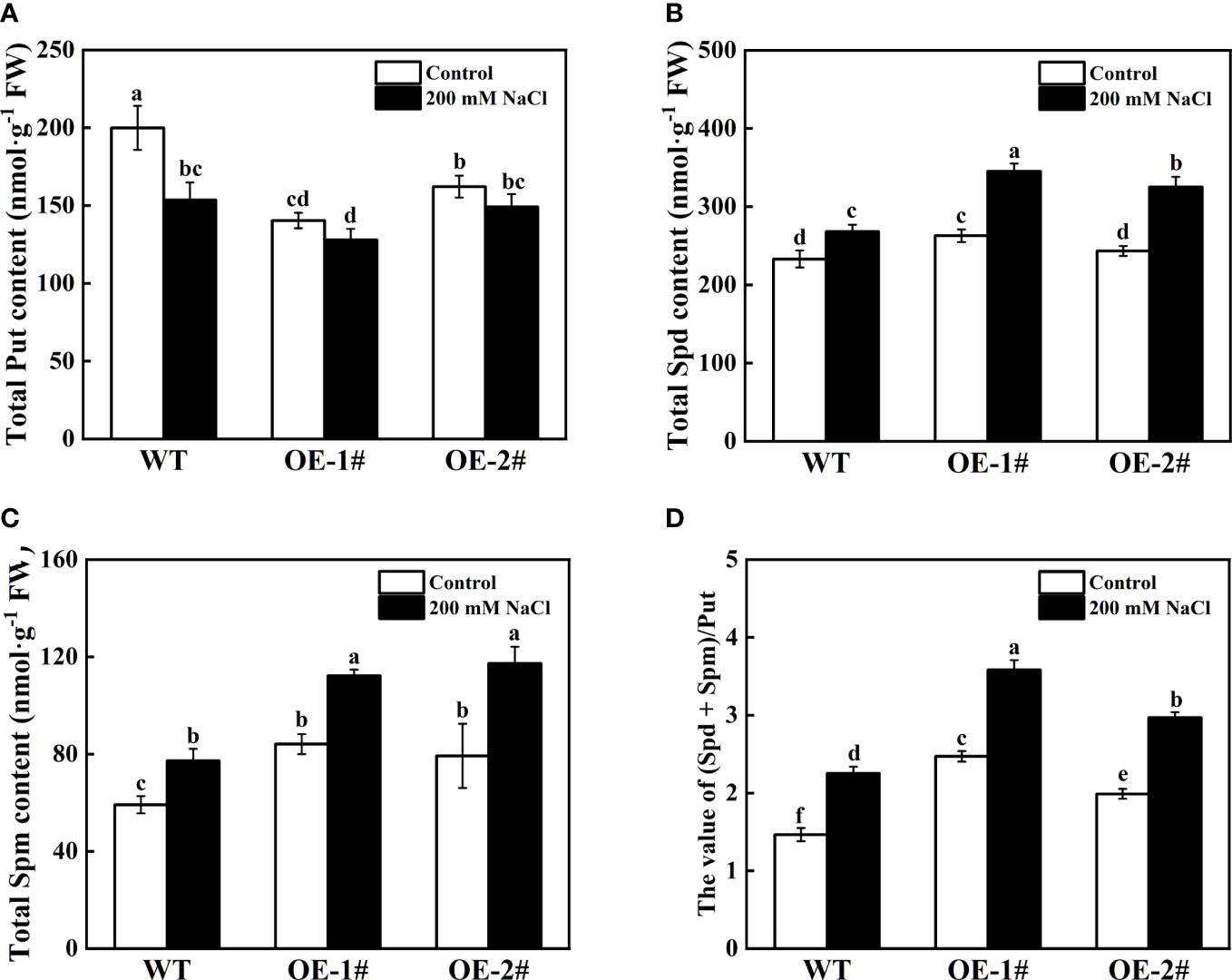
Figure 11 Effects of salt stress on relative gene expression of NtSAMS (A), NtSPDS (B), NtSPMS (C), and NtPAO (D) of wild-type and CsSAMDC3-overexpressing transgenic tobacco seedling roots. Each value is shown as the mean ± standard error of three biological replicates. Different letters indicate significant differences at P < 0.05, according to Duncan’s multiple range tests.
Discussion
High salinity can cause osmotic and ion stress, interfering with plant growth and metabolism (Wang et al., 2022). PAs play a critical role in enhancing plant resistance. Changes in endogenous PAs content or morphology affect plant tolerance to abiotic stresses (Roy and Ghosh, 1996; Bouchereau et al., 1999; Tassoni et al., 2008; Alcázar et al., 2010). As one of the main rate-limiting enzymes in polyamine synthesis and metabolism, S-adenosylmethionine decarboxylase (SAMDC) inevitably affects the changes of endogenous PAs in plants. However, reports on cucumber SAMDC are still rare, especially at the genetic level. Therefore, it is of great significance to study the mechanism of cucumber SAMDC in salt stress from the gene perspective.
In this study, we first analyzed and identified the cucumber SAMDCs gene sequences by bioinformatics and studied the response of the cucumber SAMDCs gene to salt stress, which laid a research foundation. The cucumber SAMDCs gene family has four homologous genes (CsSAMDC1-4), and the molecular weight of the encoded protein is similar to many species, about 40 KDa (Mad Arif et al., 1994; Li and Chen, 2000; Tian et al., 2004; Zhao et al., 2017). Analysis of the full-length SAMDC protein sequences from 13 species revealed that CsSAMDC2 and CsSAMDC3 were in the same evolutionary group (Figure 1A), but they were located on different chromosomes (Table 1). Furthermore, SAMDC proteins are highly conserved in evolution and have a typical SAMDC protease domain (Figure 1C), which is close to the molecular weight of SAMDC proteins in many species. The response of different cucumber SAMDCs genes to salt stress was also different. The expression levels of CsSAMDC2 and CsSAMDC3 increased first and then decreased (Figure 2), which was consistent with the response patterns of other plant SAMDCs to salt stress (Peng et al., 2013; Ji et al., 2019). In addition, their expression levels peaked earlier than CsSAMDC1 and CsSAMDC4, so we infer that they are more sensitive to salt stress.
Then we chose the CsSAMDC3 gene for further study. Tissue-specific expression analysis showed that the CsSAMDC3 gene was strongly expressed in cucumber fruits and flowers (Figure 3A), indicating that the expression of CsSAMDC3 was related to flower organ development. Falasca et al. (2010) reported that the SAMDC gene was expressed in germinated pollen and played an essential role in pollen maturation. Subcellular localization showed that CsSAMDC3 was localized on the cell membrane and nucleus (Figure 3B). Through different hormone treatments, we found that CsSAMDC3 had different degrees of response to ABA, SA, MeJA, and ETH treatments (Figure 4), indicating that CsSAMDC3 may be involved in its signaling pathway. Among them, the response of CsSAMDC3 to ETH is very intense, which indicates that excessive exogenous ETH can promote the expression of the CsSAMDC3 gene, so that the common precursor SAM of PAs and ethylene biosynthesis pathway is more inclined to PAs synthesis and metabolism, and the accumulation of PAs will interfere with the biosynthesis of ethylene and reduce the ethylene content in plants (Bregoli et al., 2002; Madhulatha et al., 2014). In addition, through different abiotic stress treatments, we found that the response of CsSAMDC3 to cold and drought stress was not prominent, but it was more sensitive to salt stress.
Plasma membrane damage induced by salt stress is directly related to the increase of highly toxic oxygen free radicals (Hernandez et al., 1993), resulting in MDA accumulation, lipid peroxidation, and electrolyte leakage (Lutts et al., 1996; Liang et al., 2003; Mansour, 2013). High concentration of salt will destroy plant photosynthetic system and reduce the photosynthetic rate to inhibit plant growth (Shu et al., 2013; Zahra et al., 2022). In tobacco, overexpression of CsSAMDC3 increased the activity of antioxidant enzymes (Figure 8), alleviated the oxidative damage caused by salt stress (Figure 7), and enhanced the salt tolerance of tobacco in photosynthesis and growth (Figure 6). In addition, the accumulation of ROS in CsSAMDC3-overexpressing tobacco decreased under salt stress, which may be related to the high content of polyamines, especially Spd and Spm (Figure 10). Studies have shown that the ratio of (Spd + Spm)/Put in plants increases with environmental salinity (Zapata et al., 2004). Fan et al. (2013) found that high (Spd + Spm)/Put ratio and Spm accumulation were beneficial to improve the salt tolerance of cucumber seedlings. In this study, overexpression of CsSAMDC3 resulted in the accumulation of Spd and Spm and the reduction t of Put in tobacco (Figure 10), thereby improving the salt tolerance of tobacco. Jia et al. (2021), who overexpressed TrSAMDC1 in Arabidopsis thaliana, found that overexpression of TrSAMDC1 can enhance the tolerance of Arabidopsis thaliana to salt and drought stress by increasing endogenous PAs levels and antioxidant enzyme activity. Similarly, Wi et al. (2014) obtained a similar conclusion by overexpressing CaSAMDC in Arabidopsis thaliana. Interestingly, we determined the expression levels of antioxidant enzymes and polyamine metabolism-related genes in tobacco and found that they were negatively correlated with antioxidant enzyme activity or polyamine accumulation (Figures 9, 11). This suggests that enhanced antioxidant enzyme activity or polyamine accumulation may negatively regulate the expression of related genes, thereby maintaining a relatively stable dynamic balance in plants (Xu et al., 1999; Michelet et al., 2011).
Conclusion
In summary, we identified four SAMDC genes (CsSAMDC1-4) in cucumber and divided them into three groups. The CsSAMDC2 and CsSAMDC3 encoding genes in the same group 1 showed similar salt stress response patterns. CsSAMDC3 is highly expressed in flowers and fruits, indicating that it plays a vital role in reproductive growth.Overexpression of CsSAMDC3 in tobacco confirmed that CsSAMDC3 could increase Spd and Spm content, increase antioxidant enzyme activity, enhance plant salt tolerance by scavenging reactive oxygen species, and is a candidate gene for improving plant salt tolerance. The results of this study laid a foundation for further study on the mechanism of polyamines regulating the salt tolerance of cucumber at the molecular level. In the future, more exploration and evidence are needed to clarify the role and relationship of CsSAMDCs in plant stress resistance.
Data availability statement
The datasets presented in this study can be found in online repositories. The names of the repository/repositories and accession number(s) can be found in the article/Supplementary material.
Author contributions
SS designed the experimental research content. MZ performed the experiments and wrote the manuscript. GC provided some technical assistance for experiments about the genetic transformation of tobacco. JQW, JW, YW and SG modified the manuscript. All authors contributed to the article and approved the submitted version.
Funding
This work was supported by the National Natural Science Foundation of China (No. 32072649; 32272793), sponsored by the Independent Innovation Fund of Ningxia Hui Autonomous Region Agricultural Science and Technology (NGSB-2021-8-02) and the Fundamental Research Funds for the Central Universities (KYGL2022004).
Conflict of interest
The authors declare that the research was conducted in the absence of any commercial or financial relationships that could be construed as a potential conflict of interest.
Publisher’s note
All claims expressed in this article are solely those of the authors and do not necessarily represent those of their affiliated organizations, or those of the publisher, the editors and the reviewers. Any product that may be evaluated in this article, or claim that may be made by its manufacturer, is not guaranteed or endorsed by the publisher.
Supplementary material
The Supplementary Material for this article can be found online at: https://www.frontiersin.org/articles/10.3389/fpls.2023.1076153/full#supplementary-material
References
Alcázar, R., Altabella, T., Marco, F., Bortolotti, C., Reymond, M., Koncz, C., et al. (2010). Polyamines: molecules with regulatory functions in plant abiotic stress tolerance. Planta 231, 1237–1249. doi: 10.1007/s00425-010-1130-0
Bouchereau, A., Aziz, A., Larher, F., Martin-Tanguy, J. (1999). Polyamines and environmental challenges: recent development. Plant Sci. 140, 103–125. doi: 10.1016/S0168-9452(98)00218-0
Bregoli, A. M., Scaramagli, S., Costa, G., Sabatini, E., Ziosi, V., Biondi, S., et al. (2002). Peach (Prunus persica) fruit ripening: aminoethoxyvinylglycine (AVG) and exogenous polyamines affect ethylene emission and flesh firmness. Physiologia Plantarum 114, 472–481. doi: 10.1034/j.1399-3054.2002.1140317.x
Chen, C. J., Chen, H., Zhang, Y., Thomas, H. R., Frank, M. H., He, Y. H., et al. (2020). TBtools: an integrative toolkit developed for interactive analyses of big biological data. Mol. Plant 13, 1194–1202. doi: 10.1016/j.molp.2020.06.009
Dhindsa, R. S., Plumb-Dhindsa, P., Thorpe, T. A. (1981). Leaf senescence: correlated with increased levels of membrane permeability and lipid peroxidation, and decreased levels of superoxide dismutase and catalase. J. Exp. Bot. 32, 93–101. doi: 10.1093/jxb/32.1.93
Falasca, G., Franceschetti, M., Bagni, N., Altamura, M., Biasi, R. (2010). Polyamine biosynthesis and control of the development of functional pollen in kiwifruit. Plant Physiol. Biochem. 48, 565–573. doi: 10.1016/j.plaphy.2010.02.013
Fan, H. F., Du, C. X., Guo, S. R. (2013). Nitric oxide enhances salt tolerance in cucumber seedlings by regulating free polyamine content. Environ. Exp. Bot. 86, 52–59. doi: 10.1016/j.envexpbot.2010.09.007
Giannopolitis, C. N., Ries, S. K. (1977). Superoxide dismutases: I. occurrence in higher plants. Plant Physiol. 59, 309–314. doi: 10.1104/pp.59.2.309
He, M. W., Wang, Y., Wu, J. Q., Shu, S., Sun, J., Guo, S. R. (2019). Isolation and characterization of s-adenosylmethionine synthase gene from cucumber and responsive to abiotic stress. Plant Physiol. Biochem. 141, 431–445. doi: 10.1016/j.plaphy.2019.06.006
Hernandez, J. A., Corpas, F. J., Gomez, M., Del Rio, L. A., Sevilla, F. (1993). Salt-induced oxidative stress mediated by activated oxygen species in pea leaf mitochondria. Physiologia Plantarum 89, 103–110. doi: 10.1111/j.1399-3054.1993.tb01792.x
Ikbal, F. E., Hernández, J. A., Barba-Espín, G., Koussa, T., Aziz, A., Faize, M., et al. (2014). Enhanced salt-induced antioxidative responses involve a contribution of polyamine biosynthesis in grapevine plants. J. Plant Physiol. 171, 779–788. doi: 10.1016/j.jplph.2014.02.006
Ji, M. C., Wang, K., Wang, L., Chen, S. X., Li, H. Y., Ma, C. Q., et al. (2019). Overexpression of a s-adenosylmethionine decarboxylase from sugar beet M14 increased Araidopsis salt tolerance. Int. J. Mol. Sci. 20, 1990. doi: 10.3390/ijms20081990
Jia, T., Hou, J. R., Iqbal, M. Z., Zhang, Y. Z., Cheng, B. Z., Feng, H. H., et al. (2021). Overexpression of the white clover TrSAMDC1 gene enhanced salt and drought resistance in Arabidopsis thaliana. Plant Physiol. Biochem. 165, 147–160. doi: 10.1016/j.plaphy.2021.05.018
Jiao, P., Jin, S. Y., Chen, N. N., Wang, C. L., Liu, S. Y., Qu, J., et al. (2022). Improvement of cold tolerance in maize (Zea mays l.) using Agrobacterium-mediated transformation of ZmSAMDC gene. GM Crops Food 13, 131–141. doi: 10.1080/21645698.2022.2097831
Khare, T., Srivastav, A., Shaikh, S., Kumar, V. (2018). “Polyamines and their metabolic engineering for plant salinity stress tolerance,” in Salinity responses and tolerance in plants, Volume 1: Targeting Sensory, Transport and Signaling Mechanisms, eds. Kumar, V., Wani, S. H., Suprasanna, P., Tran, L.-S. P. (Cham: Springer International Publishing), 339–358. doi: 10.1007/978-3-319-75671-4_13
Klingler, J. P., Batelli, G., Zhu, J.-K. (2010). ABA receptors: the START of a new paradigm in phytohormone signalling. J. Exp. Bot. 61, 3199–3210. doi: 10.1093/jxb/erq151
Kolotilin, I., Koltai, H., Bar-Or, C., Chen, L., Nahon, S., Shlomo, H., et al. (2011). Expressing yeast SAMdc gene confers broad changes in gene expression and alters fatty acid composition in tomato fruit. Physiologia Plantarum 142, 211–223. doi: 10.1111/j.1399-3054.2011.01458.x
Kumar, A., Taylor, M. A., Arif, S. A. M., Davies, H. V. (1996). Potato plants expressing antisense and sense s-adenosylmethionine decarboxylase (SAMDC) transgenes show altered levels of polyamines and ethylene: antisense plants display abnormal phenotypes. Plant J. 9, 147–158. doi: 10.1046/j.1365-313X.1996.09020147.x
Kuznetsov, V. V., Shevyakova, N. I. (2010). “Polyamines and plant adaptation to saline environments,” in Desert Plants: Biology and Biotechnology, ed. K.G. Ramawat. (Berlin, Heidelberg: Springer), 261–298. doi: 10.1007/978-3-642-02550-1_13
Lee, M. M., Lee, S. H., Park, K. Y. (1997). Characterization and expression of two members of the s-adenosylmethionine decarboxylase gene family in carnation flower. Plant Mol. Biol. 34, 371–382. doi: 10.1023/A:1005811229988
Li, Z. Y., Chen, S. Y. (2000). Isolation and characterization of a salt-and drought-inducible gene for s-adenosylmethionine decarboxylase from wheat (Triticum aestivum l.). J. Plant Physiol. 156, 386–393. doi: 10.1016/S0176-1617(00)80078-4
Liang, Y. C., Chen, Q., Liu, Q., Zhang, W. H., Ding, R. X. (2003). Exogenous silicon (Si) increases antioxidant enzyme activity and reduces lipid peroxidation in roots of salt-stressed barley (Hordeum vulgare l.). J. Plant Physiol. 160, 1157–1164. doi: 10.1078/0176-1617-01065
Liu, J. H., Honda, C., Moriguchi, T. (2006). Involvement of polyamine in floral and fruit development. Japan Agric. Res. Quarterly: JARQ 40, 51–58. doi: 10.6090/jarq.40.51
Liu, J. X., Que, Y. X., Guo, J. L., Xu, L. P., Xu, J. S., Chen, R. K. (2010). Molecular cloning of sugarcane s-adenosylmethionine decarboxylase gene (Sc-SAMDC) and its expression analysis. Scientia Agricultura Sin. 43, 1448–1457.
Livak, K. J., Schmittgen, T. D. (2001). Analysis of relative gene expression data using real-time quantitative PCR and the 2–ΔΔCT method. methods 25, 402–408. doi: 10.1006/meth.2001.1262
Luo, J. H., Liu, M. X., Zhang, C. D., Zhang, P. P., Chen, J. J., Guo, Z. F., et al. (2017). Transgenic centipedegrass (Eremochloa ophiuroides [Munro] hack.) overexpressing s-adenosylmethionine decarboxylase (SAMDC) gene for improved cold tolerance through involvement of H2O2 and NO signaling. Front. Plant Sci. 8. doi: 10.3389/fpls.2017.01655
Lutts, S., Kinet, J., Bouharmont, J. (1996). NaCl-Induced senescence in leaves of rice (Oryza sativa l.) cultivars differing in salinity resistance. Ann. Bot. 78, 389–398. doi: 10.1006/anbo.1996.0134
Mad Arif, S. A., Taylor, M. A., George, L. A., Butler, A. R., Burch, L. R., Davies, H. V., et al. (1994). Characterisation of the s-adenosylmethionine decarboxylase (SAMDC) gene of potato. Plant Mol. Biol. 26, 327–338. doi: 10.1007/BF00039543
Madhulatha, P., Gupta, A., Gupta, S., Kumar, A., Pal, R., Rajam, M. (2014). Fruit-specific over-expression of human s-adenosylmethionine decarboxylase gene results in polyamine accumulation and affects diverse aspects of tomato fruit development and quality. J. Plant Biochem. Biotechnol. 23, 151–160. doi: 10.1007/s13562-013-0194-x
Mansour, M. M. F. (2013). Plasma membrane permeability as an indicator of salt tolerance in plants. Biol. Plantarum 57, 1–10. doi: 10.1007/s10535-012-0144-9
Masson, P. H., Takahashi, T., Angelini, R. (2017). “Molecular mechanisms underlying polyamine functions in plants”. Front. Plant Sci. 8. doi: 10.3389/fpls.2017.00014
Mattoo, A. K., Sobieszczuk-Nowicka, E. (2019). Polyamine as signaling molecules and leaf senescence. Senescence signalling and control in Plants. (Elsevier: Academic Press) 125–138. doi: 10.1016/B978-0-12-813187-9.00008-1
Mbarki, S., Sytar, O., Cerda, A., Zivcak, M., Rastogi, A., He, X., et al. (2018). “Strategies to mitigate the salt stress effects on photosynthetic apparatus and productivity of crop plants,” in Salinity responses and tolerance in plants, vol. volume 1. (cham: Springer), 85–136. doi: 10.1007/978-3-319-75671-4_4
Mellidou, I., Moschou, P. N., Ioannidis, N. E., Pankou, C., Gėmes, K., Valassakis, C., et al. (2016). Silencing s-Adenosyl-L-Methionine decarboxylase (SAMDC) in nicotiana tabacum points at a polyamine-dependent trade-off between growth and tolerance responses. Front. Plant Sci. 7. doi: 10.3389/fpls.2016.00379
Meng, D. Y., Yang, S., Xing, J. Y., Ma, N. N., Wang, B. Z., Qiu, F. T., et al. (2021). Peanut (Arachis hypogaea l.) s-adenosylmethionine decarboxylase confers transgenic tobacco with elevated tolerance to salt stress. Plant Biol. 23, 341–350. doi: 10.1111/plb.13173
Michelet, L., Lefebvre-Legendre, L., Burr, S. E., Rochaix, J. D., Goldschmidt-Clermont, M. (2011). Enhanced chloroplast transgene expression in a nuclear mutant of Chlamydomonas. Plant Biotechnol. J. 9, 565–574. doi: 10.1111/j.1467-7652.2010.00564.x
Moschou, P. N., Roubelakis-Angelakis, K. A. (2014). Polyamines and programmed cell death. J. Exp. Bot. 65, 1285–1296. doi: 10.1093/jxb/ert373
Moschou, P., Wu, J., Cona, A., Tavladoraki, P., Angelini, R., Roubelakis-Angelakis, K. (2012). The polyamines and their catabolic products are significant players in the turnover of nitrogenous molecules in plants. J. Exp. Bot. 63, 5003–5015. doi: 10.1093/jxb/ers202
Mustafavi, S. H., Naghdi Badi, H., Sękara, A., Mehrafarin, A., Janda, T., Ghorbanpour, M., et al. (2018). Polyamines and their possible mechanisms involved in plant physiological processes and elicitation of secondary metabolites. Acta Physiologiae Plantarum 40, 1–19. doi: 10.1007/s11738-018-2671-2
Peng, X. J., Zhang, L. X., Zhang, L. X., Liu, Z., Cheng, L. Q., Yang, Y., et al. (2013). The transcriptional factor LcDREB2 cooperates with LcSAMDC2 to contribute to salt tolerance in Leymus chinensis. Plant Cell Tissue Organ Culture (PCTOC) 113, 245–256. doi: 10.1007/s11240-012-0264-0
Pottosin, I., Velarde-Buendía, A.-M., Zepeda-Jazo, I., Dobrovinskaya, O., Shabala, S. (2012). Synergism between polyamines and ROS in the induction of Ca2+ and k+ fluxes in roots. Plant Signaling Behav. 7, 1084–1087. doi: 10.4161/psb.21185
Roy, M., Ghosh, B. (1996). Polyamines, both common and uncommon, under heat stress in rice (Oryza sativa) callus. Physiologia Plantarum 98, 196–200. doi: 10.1111/j.1399-3054.1996.tb00692.x
Shu, S., Guo, S. R., Sun, J., Yuan, L. Y. (2012). Effects of salt stress on the structure and function of the photosynthetic apparatus in Cucumis sativus and its protection by exogenous putrescine. Physiologia plantarum 146, 285–296. doi: 10.1111/j.1399-3054.2012.01623.x
Shu, S., Tang, Y. Y., Yuan, Y. H., Sun, J., Zhong, M., Guo, S. R. (2016). The role of 24-epibrassinolide in the regulation of photosynthetic characteristics and nitrogen metabolism of tomato seedlings under a combined low temperature and weak light stress. Plant Physiol. Biochem. 107, 344–353. doi: 10.1016/j.plaphy.2016.06.021
Shu, S., Yuan, L. Y., Guo, S. R., Sun, J., Yuan, Y. H. (2013). Effects of exogenous spermine on chlorophyll fluorescence, antioxidant system and ultrastructure of chloroplasts in Cucumis sativus l. under salt stress. Plant Physiol. Biochem. 63, 209–216. doi: 10.1016/j.plaphy.2012.11.028
Slocum, R. D., Kaur-Sawhney, R., Galston, A. W. (1984). The physiology and biochemistry of polyamines in plants. Arch. Biochem. Biophysics 235, 283–303. doi: 10.1016/0003-9861(84)90201-7
Tang, W., Newton, R. J. (2005). Polyamines promote root elongation and growth by increasing root cell division in regenerated Virginia pine (Pinus virginiana mill.) plantlets. Plant Cell Rep. 24, 581–589. doi: 10.1007/s00299-005-0021-5
Tang, X. X., Wu, L., Wang, F. L., Tian, W. G., Hu, X. M., Jin, S. X., et al. (2021). Ectopic expression of GhSAMDC3 enhanced salt tolerance due to accumulated spd content and activation of salt tolerance-related genes in Arabidopsis thaliana. DNA Cell Biol. 40, 1144–1157. doi: 10.1089/dna.2020.6064
Tassoni, A., Franceschetti, M., Bagni, N. (2008). Polyamines and salt stress response and tolerance in Arabidopsis thaliana flowers. Plant Physiol. Biochem. 46, 607–613. doi: 10.1016/j.plaphy.2008.02.005
Thu-Hang, P., Bassie, L., Safwat, G., Trung-Nghia, P., Christou, P., Capell, T. (2002). Expression of a heterologous s-adenosylmethionine decarboxylase cDNA in plants demonstrates that changes in s-adenosyl-L-methionine decarboxylase activity determine levels of the higher polyamines spermidine and spermine. Plant Physiol. 129, 1744–1754. doi: 10.1104/pp.010966
Tian, A. G., Zhao, J. Y., Zhang, J. S., Gai, J. Y., Chen, S. Y. (2004). Genomic characterization of the s-adenosylmethionine decarboxylase genes from soybean. Theor. Appl. Genet. 108, 842–850. doi: 10.1007/s00122-003-1507-6
Tiburcio, A. F., Altabella, T., Bitrián, M., Alcázar, R. (2014). The roles of polyamines during the lifespan of plants: from development to stress. Planta 240, 1–18. doi: 10.1007/s00425-014-2055-9
Tisi, A., Federico, R., Moreno, S., Lucretti, S., Moschou, P. N., Roubelakis-Angelakis, K. A., et al. (2011). Perturbation of polyamine catabolism can strongly affect root development and xylem differentiation. Plant Physiol. 157, 200–215. doi: 10.1104/pp.111.173153
Wang, C. F., Han, G. L., Yang, Z. R., Li, Y. X., Wang, B. S. (2022). Plant salinity sensors: Current understanding and future directions. Front. Plant Sci. 13. doi: 10.3389/fpls.2022.859224
Wang, L. W., He, M. W., Guo, S. R., Zhong, M., Shu, S., Sun, J. (2017). NaCl Stress induces CsSAMs gene expression in cucumis sativus by mediating the binding of CsGT-3b to the GT-1 element within the CsSAMs promoter. Planta 245, 889–908. doi: 10.1007/s00425-017-2650-7
Wang, J., Liu, J. H., Kurosawa, T., Nada, K., Ban, Y., Moriguchi, T. (2010). Cloning, biochemical identification, and expression analysis of a gene encoding S-adenosylmethionine decarboxylase in navel orange (Citrus sinensis osbeck). J. Hortic. Sci. Biotechnol. 85, 219–226. doi: 10.1080/14620316.2010.11512658
Wi, S. J., Kim, S. J., Kim, W. T., Park, K. Y. (2014). Constitutive s-adenosylmethionine decarboxylase gene expression increases drought tolerance through inhibition of reactive oxygen species accumulation in arabidopsis. Planta 239, 979–988. doi: 10.1007/s00425-014-2027-0
Wimalasekera, R., Tebartz, F., Scherer, G. F. (2011). Polyamines, polyamine oxidases and nitric oxide in development, abiotic and biotic stresses. Plant Sci. 181, 593–603. doi: 10.1016/j.plantsci.2011.04.002
Wu, J. Q., Liu, W. K., Jahan, M. S., Shu, S., Sun, J., Guo, S. R. (2022). Characterization of polyamine oxidase genes in cucumber and roles of CsPAO3 in response to salt stress. Environ. Exp. Bot. 194, 104696. doi: 10.1016/j.envexpbot.2021.104696
Xu, Y. L., Li, L., Gage, D. A., Zeevaart, J. A. (1999). Feedback regulation of GA5 expression and metabolic engineering of gibberellin levels in arabidopsis. Plant Cell 11, 927–935. doi: 10.1105/tpc.11.5.927
Zahra, N., Al Hinai, M. S., Hafeez, M. B., Rehman, A., Wahid, A., Siddique, K. H., et al. (2022). Regulation of photosynthesis under salt stress and associated tolerance mechanisms. Plant Physiol. Biochem. 178, 55–69. doi: 10.1016/j.plaphy.2022.03.003
Zapata, P. J., Serrano, M. A., Pretel, M. T., Amorós, A., Botella, M.Á. (2004). Polyamines and ethylene changes during germination of different plant species under salinity. Plant Sci. 167, 781–788. doi: 10.1016/j.plantsci.2004.05.014
Zeng, X. Q., Wang, C. Y., Zhang, H., Wei, Z. X., Liu, X. L., Wang, Y. J., et al. (2011). Cloning and characterization of an up-regulated s-adenosylmethionine decarboxylase (SAMDC) gene induced by stripe rust in wheat. J. Triticeae Crops 31, 811–818.
Zhao, M. M., Liu, H., Deng, Z., Chen, J. S., Yang, H., Li, H. P., et al. (2017). Molecular cloning and characterization of s-adenosylmethionine decarboxylase gene in rubber tree (Hevea brasiliensis). Physiol. Mol. Biol. Plants 23, 281–290. doi: 10.1007/s12298-017-0417-z
Zhao, L. L., Song, L. Q., You, C. X., Moriguchi, T., Hao, Y. J. (2010). Functional characterization of the apple MdSAMDC2 gene by ectopic promoter analysis and over-expression in tobacco. Biol. plantarum 54, 631–638. doi: 10.1007/s10535-010-0113-0
Keywords: antioxidant metabolism, cucumber, CsSAMDC3, polyamines, salt stress
Citation: Zhu M, Chen G, Wu J, Wang J, Wang Y, Guo S and Shu S (2023) Identification of cucumber S-adenosylmethionine decarboxylase genes and functional analysis of CsSAMDC3 in salt tolerance. Front. Plant Sci. 14:1076153. doi: 10.3389/fpls.2023.1076153
Received: 21 October 2022; Accepted: 31 January 2023;
Published: 21 April 2023.
Edited by:
Shifeng Cao, Zhejiang Wanli University, ChinaReviewed by:
Min Zhong, South China Agricultural University, ChinaLingyun Yuan, Anhui Agricultural University, China
Copyright © 2023 Zhu, Chen, Wu, Wang, Wang, Guo and Shu. This is an open-access article distributed under the terms of the Creative Commons Attribution License (CC BY). The use, distribution or reproduction in other forums is permitted, provided the original author(s) and the copyright owner(s) are credited and that the original publication in this journal is cited, in accordance with accepted academic practice. No use, distribution or reproduction is permitted which does not comply with these terms.
*Correspondence: Sheng Shu, c2h1c2hlbmdAbmphdS5lZHUuY24=