- 1State Key Laboratory for Conservation and Utilization of Bio-Resources in Yunnan, College of Plant Protection, Yunnan Agricultural University, Kunming, China
- 2State Key Laboratory of Phytochemistry and Plant Resources in West China, Kunming Institute of Botany, Chinese Academy of Sciences, Kunming, China
- 3College of Water Conservancy, Yunnan Agricultural University, Kunming, China
Plant-derived natural products are important resources for pesticide discovery. Acetylcholinesterase (AChE) is a well-validated pesticide target, and inhibiting AChE proves fatal for insects. Recent studies have shown that the potential of various sesquiterpenoids as AChE inhibitors. However, few studies have been conducted with eudesmane-type sesquiterpenes with AChE inhibitory effects. Therefore, in this research, we isolated two new sesquiterpenes, laggeranines A (1) and B (2), along with six known eudesmane-type sesquiterpenes (3–8) from Laggera pterodonta, and characterized their structures and the inhibitory effect they exerted on AChE. The results showed that these compounds had certain inhibitory effects on AChE in a dose-dependent manner, of which compound 5 had the best inhibitory effect with IC50 of 437.33 ± 8.33 mM. As revealed by the Lineweaver-Burk and Dixon plots, compound 5 was observed to suppress AChE activity reversibly and competitively. Furthermore, all compounds exhibited certain toxicity levels on C. elegans. Meanwhile, these compounds had good ADMET properties. These results are significant for the discovery of new AChE targeting compounds, and also enrich the bioactivity activity repertoire of L. pterodonta.
1. Introduction
Acetylcholinesterase (AChE) is a critical enzyme performing important functions associated with nerve conduction, involving the catalysis of the degradation process of neurotransmitter acetylcholine and the subsequent termination of its stimulating effect on post-synaptic membrane excitation, through which the enzyme maintains normal nerve impulse transmission in organisms (Fournier and Mutero, 1994). In recent years, AChE has been studied in medicine, chemistry, pesticide and plant protection.
In pest control, AChE is a well-validated pesticide target, and inhibiting AChE proves fatal for insects (Rajashekar et al., 2014). Organophosphorus and carbamate insecticides are the most common AChE inhibitors (Fukuto, 1990). Although they play a great role in pest control, they can also cause harm to non-target organisms such as humans (Vale, 2015). In addition, due to the long-term use of the same insecticide, pests tend to develop resistance and make the inhibitors ineffective (Vaughan et al., 1997). So, we need to find new inhibitors to solve these problems. Many studies have shown that plant secondary metabolites are the main source of AChE inhibitors. In our previous study, 15 flavonoids isolated from Eupatorium adenophorum were discovered to suppress AChE in Spodoptera litura and C. elegans (Li et al., 2020), and 13 flavonoids isolated from Ginkgo biloba were found to inhibit AChE (Ding et al., 2013). Furthermore, we screened more than 200 compounds and the two sesquiterpenoids, parthenolide and tirotundin, extracted from Chrysanthemum parthenium and Tithonia diversifolia, respectively, elicited a strong inhibitory effect on nematode AChE (Lan et al., 2022). We think this work can provide some ideas for finding new inhibitors from natural products.
Laggera pterodonta (DC.) Benth. which grows in India, the Indochina Peninsula and tropical Africa (Gu et al., 2014), also extensively exist in southwestern China, particularly the Sichuan and Yunnan Provinces. This plant has long been used as folk medicine in China, and has been widely used clinically, with antioxidant, anti-tumor, antibacterial and analgesic effects. Previous studies on its constituents revealed that eudesmane-type sesquiterpene are one of the main secondary metabolites of this plant (Zhao et al., 1997; Yang et al., 2007; Lu et al., 2014; Xie et al., 2021). Sesquiterpenes are an important class of terpenoids with extensive biological activities, which have attracted our attention. A survey conducted by researchers found that 58 sesquiterpenes from various plants showed varying degrees of inhibitory activity against AChE in multiple studies over the past decade, and these findings shed light on the potential of sesquiterpenes to inhibit AChE (Arya et al., 2021). Therefore, we studied the chemical constituents of L. pterodonta and took the eudesmane-type sesquiterpene as the research object, trying to find the active compounds that inhibit AChE.
In this research, we isolated two new sesquiterpenes, laggeranines A (1) and B (2), along with six known sesquiterpenes (3–8), and characterized their structures and the inhibitory effect they exerted on AChE. At the same time, in order to understand the mechanism by which these compounds inhibit AChE, only compounds with strong activity levels were selected for kinetic studies. In addition, ADMET prediction is very important for early drug research and development. We also used the admeatSAR platform to make ADMET prediction for these compounds. This study provides experimental and theoretical foundations for novel acetylcholinesterase inhibitors in L. pterodonta.
2. Materials and methods
2.1. General experimental procedures
This study obtained chlorpyrifos (≥98% purity) from Sigma Chemical Co. (St. Louis, MO, USA), 5,5′-dithiobis-2-nitrobenzoic acid (DTNB, ≥98% purity) from Biological Engineering Co. (Huzhou, Zhejiang, China) and acetylcholine iodide (ATChI) (≥98% purity) from Fluka Chemical Co. (Milwaukee, WI, USA). Ultra-pure water (Milli-Q purification system, Millipore, MA) and acetonitrile (HPLC-grade, J.T. Baker, Phillipsburg, NJ) were utilized in semi-preparative HPLC. Additionally, petroleum ether, ethanol, acetone, ethyl acetate, methanol (MeOH), and chloroform of reagent grade were provided by Qingdao Marine Chemical Inc., China.
Bruker 500 and 600 MHz spectrometers were used to measure NMR spectra, using TMS as the endogenous reference. The BioRad FTS-135 spectrometer was utilized to survey IR spectra using KBr pellets, JASCO P-1020 digital polarimeter was employed for analyzing optical rotations, and the Shimadzu UV-2401A for recording UV spectra. The HR-ESI-MS were recorded on a triple quadrupole mass spectromete (Agilent, America). Furthermore, this study utilized silica gel (60–80, 200–300 and 300–400 mesh, Qingdao Marine Chemical Inc, China), SBC MCI gel (75–150 µm, Sci-Bio Chem Co. Ltd., Chengdu, China), Sephadex LH-20 (40–70 µm, Amersham Pharmacia Biotech AB) and silica gel H (10–40 µm, Qingdao Marine Chemical Inc, China) for Column Chromatography (CC). The YMC Luna C18 reversed-phase column (5 µm; 10 × 250 mm) was utilized for semi-preparative HPLC.
2.2. Plant material
For this study, aerial parts of L. pterodonta were collected in July 2017 from Baoshan, Yunnan, China (25°5′N, 99°6′E). Prof. Hua Peng from the Kunming Institute of Botany, Chinese Academy of Sciences (CAS), identified each of our collected samples. Meanwhile, we deposited one voucher specimen (No. 1707016) at the State Key Laboratory of Phytochemistry and Plant Resource in West China, Kunming Institute of Botany, CAS.
2.3. Extraction and isolation
Ethanol was added to the extract, containing dried aerial L. pterodonta (5 kg), thrice under room temperature (RT), followed by solvent evaporation in a vacuum. The obtained products were then filtered and evaporated to obtain 8 L extracts, which were divided, using equivalent amounts of ethyl acetate and petroleum ether (thrice with each), to obtain ethyl acetate extracts (55 g) and petroleum ether extracts (68 g). Later, a silica gel column (10 × 100 cm) was used for the chromatography of EtOAc extracts and eluted using petroleum ether-acetone (100:1-1:1) to obtain 8 fractions (1–8).
MCI chromatography was conducted to purify fraction 3 (278 mg) and eluted using the MeOH–H2O mixed solution, which generated 4 fractions (3A–3D). We utilized silica gel to purify fraction 3B (102 mg) using petroleum ether-ethyl acetate (50:1–10:1), generating Fr.3B2 (14 mg), which was subsequently purified by semi-preparative HPLC (54% CH3CN within the water) to yield compound 1 (3.4 mg, tR = 15 min).
MCI chromatography was also utilized to purify fraction 4 (45.6 g), which was eluted using the MeOH-H2O mixed solution to yield eight corresponding fractions (4A–4H). Of these, we purified fraction 4D (10.5 g) using the silica gel column and performed the elution using petroleum ether-ethyl acetate (40:1–4:1, stepwise) to obtain another 5 fractions (4D1–4D5). Sephadex LH-20 CC was performed for the chromatography of Fr.4D2 (3.9 g) under MeOH elution, followed by semi-preparative HPLC, using the 58% acetonitrile solvent system (3 mL/min), to obtain compound 3 (226 mg, tR = 55 min), compound 4 (1.9 g, tR = 59 min) and compound 5 (213 mg, tR = 65 min). Similarly, we used the silica gel column to purify fraction 4E (221 mg), and elution was performed using petroleum ether-ethyl acetate (70:1–4:1, stepwise) to obtain three further fractions (4E1–4E3). Later, Sephadex LH-20 CC was employed to purify Fr.4E2 (46 mg), fraction 4F (2.1 g), and fraction 4G (104 mg) under MeOH elution, followed by semi-preparative HPLC, using the 55%, 47% and 45% acetonitrile solvent systems (3 mL/min), respectively, to yield compound 7 (10.5 mg, tR = 60 min), compound 6 (1.3 g) and compound 8 (76 mg, tR = 19 min), respectively.
The silica gel column was then utilized to purify fraction 7 (708 mg) and eluted using petroleum ether-ethyl acetate (40:1–5:1, stepwise) to generate fractions 7A-7G. Sephadex LH-20 CC was then performed to purify fraction 7C (56 mg) using a mobile phase of [dichloromethane-methanol (1:1)], which yielded two fractions (7C1–7C2). Semipreparative HPLC was adopted to purify Fr.7C2 (15 mg) with the 57% acetonitrile solvent system (3 mL/min) to yield compound 2 (2.8 mg, tR 45 min).
2.3.1. laggeranine A (1)
(c 0.16, MeOH); UV (MeOH) Λmax (log ε): 195 (4.17) nm; IR (KBr): 3429, 2930, 2874, 1695, 1623, 1461, 1434, 1384, 1264, 1189, 1151, 1081 cm-1; 1H and 13C NMR data, see Table 1. HR-ESI-MS: m/z 249.1495 [M-H]- (calcd for C15H22O3, 249.1496).
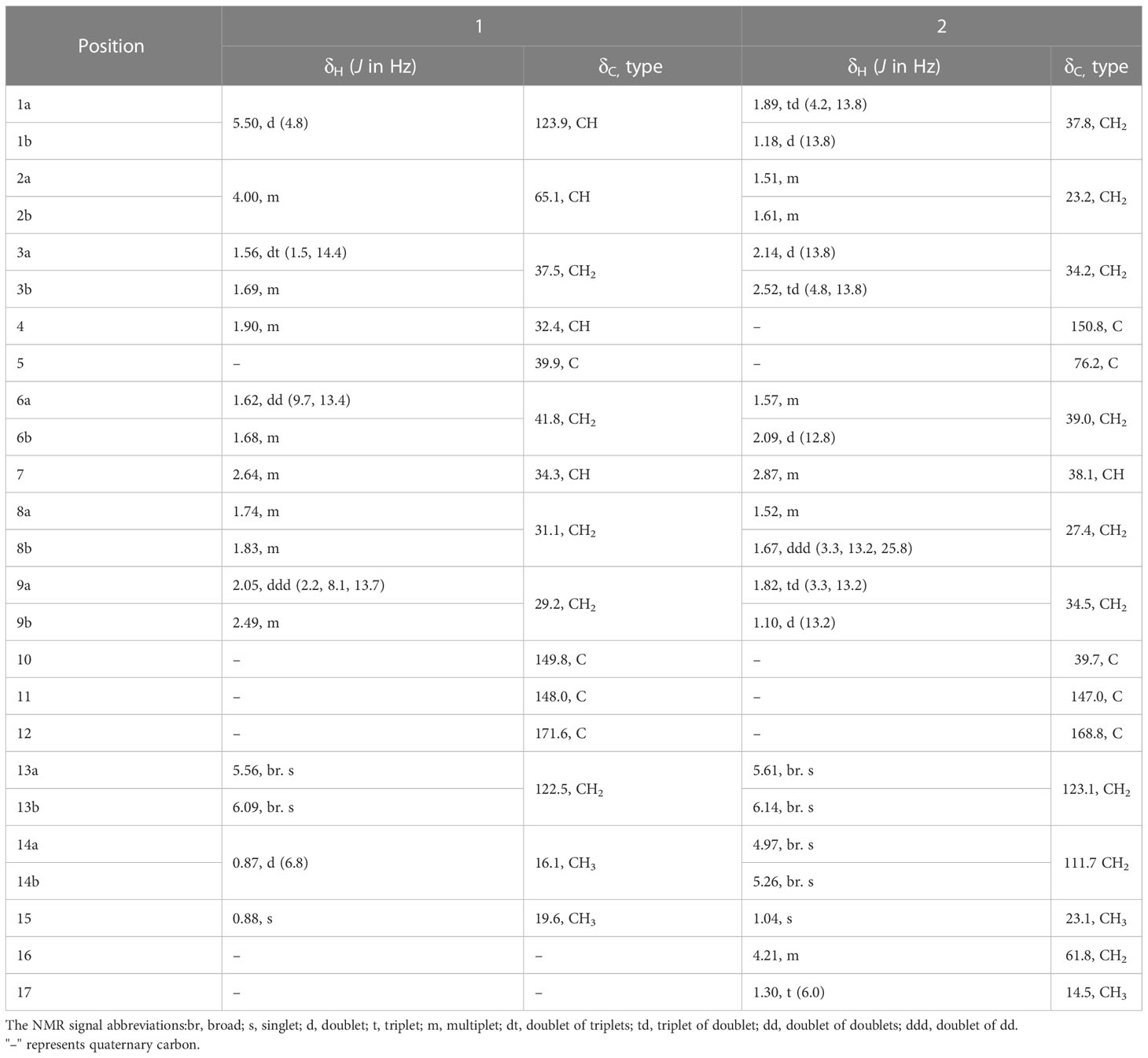
Table 1 1H and 13C NMR Data of Compounds 1 and 2 (δ in ppm, J in Hz, 600 MHz for 1H and 150 MHz for 13C, in Methanol-d4).
2.3.2. laggeranine B (2)
− 4.76 (c 0.14, MeOH); UV (MeOH) Λmax (log ε): 195 (3.64) nm; IR (KBr): 3434, 2927, 2855, 1714, 1627, 1448, 1383, 1263, 1171, 1123, 1047 cm-1; 1H and 13C NMR data, see Table 1. HR-ESI-MS: m/z 301.1773 [M + Na] + (calcd for C17H26O3, 301.1774).
2.4. Nematode
This investigation acquired C. elegans from the Insect Toxicology Laboratory of Yunnan Agricultural University, Kunming, Yunnan, China, and cultivated them using an oat medium under RT.
2.5. Determination of IC50 of compounds to AChE
Third-instar juvenile stage samples of C. elegans were thoroughly ground using a glass homogenizer. The homogenate was then dissolved in PBS (pH 7.0, which contained 0.1% Triton X-100) using a suitable volume, followed by a 30-min centrifugation at 6000 r/min under 4°C to harvest supernatants to analyze the enzymes (Ellman et al., 1961; Zhao et al., 2018) [Nematode (3rd instar): 4000 individuals/mL].
After dissolving the eight compounds and chlorpyrifos in acetone, multiple dilutions were made to obtain test concentrations at 5 levels. Thereafter, the test solution of every level (4 µL) was blended with the enzyme solution (96 µL), followed by 2-h incubation at 37°C in a 96-well plate. The final concentrations of compounds were 29.25, 58.50, 117.00, 234.00, and 468.00 µg/mL, respectively. Thereafter, 1.5 mM acetylthiocholine iodide (ATCHI) (50 µL) was added for a further 0.5-h incubation at 37°C. The reaction was finally terminated by adding 0.3 mM 5,5′- dithiobis (2 - nitrobenzoic acid) (DTNB) (50 µL). Afterward, the residual activity of acetylcholinesterase was measured with a microplate reader at 405 nm. Corresponding treatments were then applied to different groups as given below:
1. Treatment group:
2. Compound control group:
3. Substrate control group:
4. PBS control group:
The inhibition rate (%) was calculated follows:
2.6. Kinetic study on the inhibitory effect of AChE by compound
This study prepared the enzyme solution at multiple gradients (0.125, 0.25, 0.5, and 1.0 U/mL). After adding compound 5 (29.25, 58.50, 117.00, 234.00, and 468.00 µg/mL), the mixed sample was then subjected to a 1-h incubation on a 96-well plate at 37°C (the buffer was used as a substitute for the control group). Later, 1.5 mM ATCHI (50 µL) was added and incubated for a 30-min period, followed by an addition of the 0.3 mM DTNB (50 µL). After 30 s, a microplate reader was utilized to record the OD value at 405 nm (five times at 1-min intervals). The change in the OD value every minute was used to calculate the reaction rate (ΔA/min). Later, the reaction rate curve, as a function of enzyme concentration, was plotted to compare reaction rates (v) among diverse test compound levels. Associations between diverse enzyme levels were utilized to evaluate the inhibitory effect of the compound on nematode AChE (Xiong et al., 2016). In addition, the Lineweaver-Burk double reciprocal graph, demonstrating the reaction rate as a function of enzyme level, was plotted to infer the inhibition type (Guo et al., 2018).
2.7. Toxic effects of compounds on C. elegans
In the toxicity analysis, 95 µL of the nematode solution (containing approximately 80 C. elegans) along with 5 µL of each compound was added to a 96-well plate. The final concentration of each compound was 0.5 mg/mL, and chlorpyrifos (20 µg/mL) was used as the positive control. The samples were mixed sufficiently, followed by 48-h incubation of plates under RT, and subsequently, the dead nematodes were counted to determine the lethality rate (Roh and Choi, 2008).
2.8. ADMET prediction of compounds
Will compound SMILES format into admetSAR web site (http://lmmd.ecust.edu.cn/admetsar1) prediction module, the small molecule ADMET forecast information can be obtained by clicking on Predict.
2.9. Data analysis
GraphPad Prism 8 was employed for kinetics analysis and IC50 value determination of AChE, while SPSS18 was employed for calculating the statistical significance. Data in the bar graph are represented as the mean ± SD. Duncan’s new multiple range analysis was conducted to compare and analyze the significance of the difference, where p<0.05 indicated for a significant difference. Each experiment was conducted in three independent replicates.
3. Results and discussion
3.1. Structural elucidation of compounds 1-8
This study identified compound 1 as a colorless oily substance, whose molecular formula was determined to be C15H22O3 based on HR-ESI-MS at m/z 249.1495 ([M-H] −, calcd, 249.1496). Besides, its IR absorption bands were detected at 3429 cm–1 and 1695 cm–1, implying the presence of one conjugated carboxylic acid group. As observed from the 1H NMR spectrum (Table 1), there were signals for three olefinic protons (δH 5.50, 5.56, and 6.09) and two methyls groups (δH 0.87, 0.88). Both DEPT and 13C NMR spectroscopy analyses (Table 1) identified 15 carbon signals, which included two methyl (δC 16.1 and 19.6), five methylenes (δC 29.2, 31.1, 37.5, 41.8, and 122.5), four methines (δC 32.4, 34.3, 65.1, and 123.9) and four quaternary carbons (δC 39.9, 148.0, 149.8, and 171.6). Correlations in the 1H-1H HSQC and COSY diagrams suggested that there were 2 proton-bearing fragments, CHCHCH2CHMe (a) and CH2CHCH2CH2 (b) (Figure 1). In the HMBC spectrum, 2 methyl groups [δH 0.87 (3H, d, J = 6.8 Hz) and δH 0.88 (3H, s)] exhibited HMBC associations with C-4 and C-5, which indicated their positions within nearby carbons. Meanwhile, the HMBC associations between H3–14 and C-3 as well as H3–15 with C-6 revealed the C-14/C-4/C-5(C-15)/C-6 association. Besides, HMBC associations between H3–15 and C-10; H-1 and C-9, C-10; H-9 and C-7, C-8, revealed the C-1/C-10(C-5)/C-9 connection. Therefore, as per the associations of H-6 with C-7 and C-11, and H-13 with C-7, C-11, as well as C-12, we determined the compound skeleton. (Figure 1).
As indicated by further HMBC analyses, 1 had a close structural resemblance to tessaric acid, a known eremophilane sesquiterpene (Marcela et al., 1997). The only major discrepancy was the replacement of C=0 group of tessaric acid, with a hydroxyl group of 1, which was inferred from H-1, H-3, H-4 with C-2 cross-peaks in HMBC. The ROESY spectrum (Figure 2) was used to assign the relative configuration for 1, where H-2/H-4 and H-4/H-7 associations suggested that they were co-facial with an α-orientation. Since 1 had a specific rotation ( –159.1), similar to that of tessaric acid ( –156.2). The specific rotation of tessaric acid was determined by X-ray single-crystal diffraction (Zheng et al., 2003), and the absolute configuration of compound 1 was be the same as that of tessaric acid. Therefore, the structure of compound 1 could be inferred through the above rationale (Figure 3).
Compound 2 was also identified as a colorless oily substance. Based on HR-ESI-MS at m/z 301.1773 ([M + Na] +, calcd, 301.1774), we determined the molecular formula to be C17H26O3. As revealed by the IR spectrum, the typical absorption bands were observed for hydroxy (3434 cm−1) and ester carbonyl (1714 cm−1) functional groups. Signals for four olefinic protons (δH 4.97, 5.26, 5.61, and 6.14) and two methyls (δH 1.04 and 1.30) were observed from the 1H NMR spectrum (Table 1). Besides, 17 carbon signals, which included 2 methyl (δC 14.5, 23.1), nine methylenes (δC 23.2, 27.4, 34.2, 34.5, 37.8, 39.0, 61.8, 111.7, and 123.1), one methine (δC 38.0) and five quaternary carbons (δC 39.7, 76.2, 147.0, 150.8, and 168.8), were observed from DEPT and 13C NMR spectroscopy (Table 1). Besides, 1H along with 13C NMR spectroscopy for 2 (Table 1) displayed close resemblance to 5α-hydroxycostic acid (Sanz et al., 1990), but with an additional C2H5O group (δC 61.8 and δC 14.5). 2D NMR data was utilized for subsequent analysis. According to 1H-1H COSY associations, 3 fragments: a (C-1–C-3), b (C-8/C-9), and c (C-16-C17), were present (Figure 1). Moreover, the C2H5O group existed in carboxyl (C-12), according to the HMBC association of H-13 with C-7, C-11, C-12; H3–17 with C-16, as well as H-16 with C-12. The structure of 2 was thereby characterized this way (Figure 3).
For known compounds, 1H NMR and 13C NMR spectra were determined and compared with the data in the literature, the structures were determined to be eudesma-5,12-dien-13-oic acid (3) (Xu et al., 2006), isocostic acid (4) (Cruz and Martinez, 1982), costic acid (5) (Bawdekar and Kelkar, 1965), 5α-hydroxy-4α,15-dihydrocostic acid (6) (Xie et al., 2011), 5α-hydroxycostic acid (7) (Xie et al., 2011), and 3-oxo-di-nor-eudesma-4-en-11-oic acid (8) (Wang et al., 2013). 1H NMR and 13C NMR spectra can be found in supporting materials.
3.2. Determination of IC50 of compounds to AChE
To evaluate the inhibitory activity of these compounds on AChE activity, we measured their IC50 values with respect to C. elegans AChE (chlorpyrifos being utilized as the positive control). IC50 represents the compound dose required for 50% inhibition of AChE activity, where a lower value would indicate a more potent inhibition on AChE (Wang et al., 2021). The results showed that those eight compounds inhibited C. elegans AChE in a dose-dependent manner, such as compound 5 (Figure 4), and the most effective inhibitory effects were compound 5 (IC50 = 437.33 ± 8.33 μM), followed by compound 3 (IC50 = 464.0 ± 14.74 μM), compound 6 (IC50 = 470.33 ± 15.57 μM), compound 7 (IC50 = 485.0 ± 11.53 μM), compound 1, compound 4 (IC50 < 530 μM), and compounds 2 and 8 (IC50 < 710 μM) (Table 2).
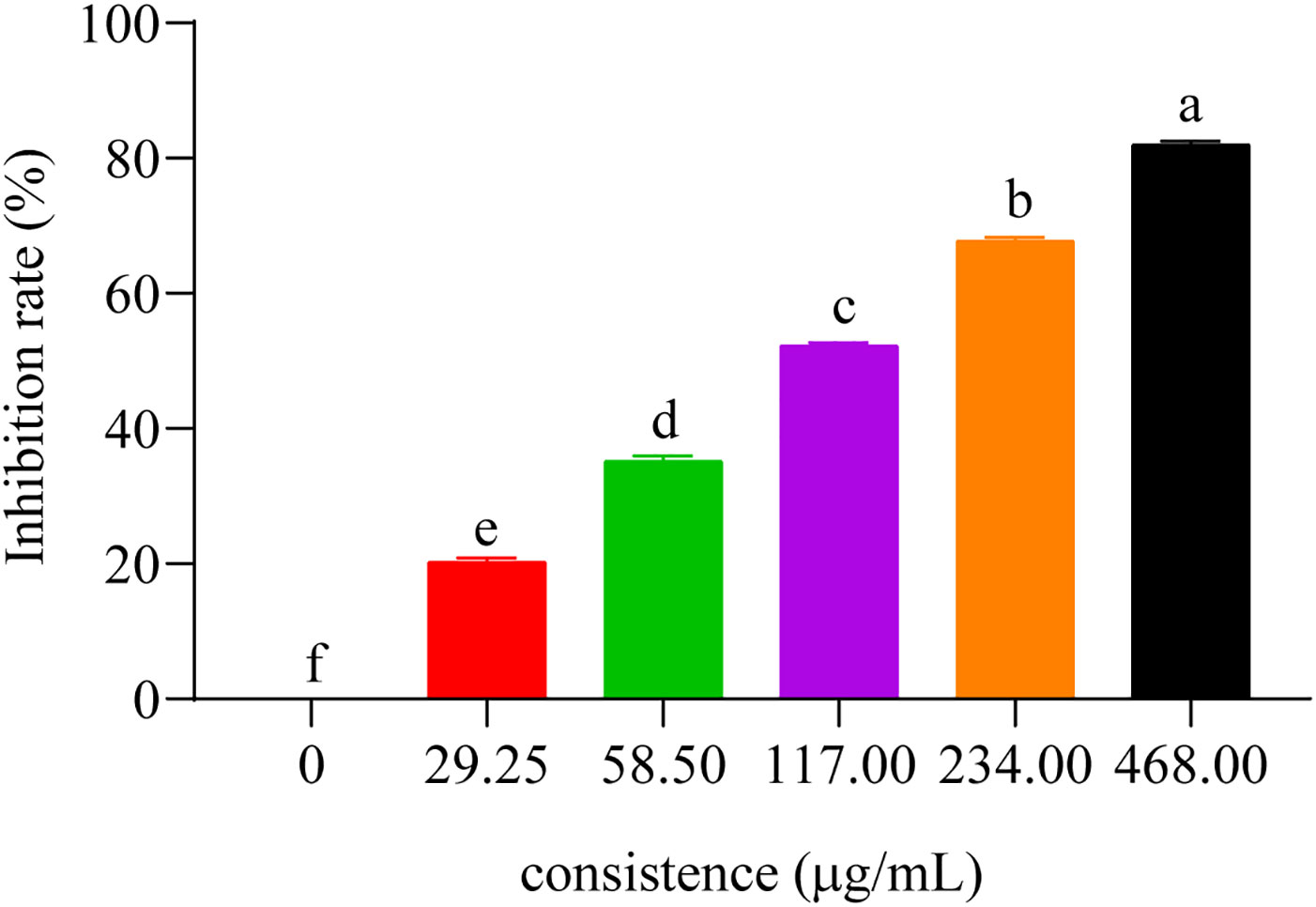
Figure 4 The inhibitory effect of compound 5 on the AChE. Concentrations of compound 5 (29.25, 58.50, 117.00, 234.00, and 468.00 μg/mL) for curves are indicated in the column.
Among the 8 compounds, compound 2 showed the worst inhibitory activity, and except compound 2, all the other compounds were eudesmane-type sesquiterpene acids. Therefore, we hypothesized that the carboxyl group in the compound should increase the activity. Compounds 3, 4, and 5 are isomers, while compound 5 shows better activity. Before this, the cytotoxic activity of compounds 4 and 5 against SF9 was also reported, and the same compound 5 showed better activity (Azucena et al., 2005). The difference in their structure lies in the different positions of a double bond. Therefore, we believe that the change of the position of the double bond has certain influence on the activity, and the terminal double bond of the compound should have better activity. The only structural difference between compound 4 and compound 8 is that the C-3 of compound 8 is a carbonyl group, but the activity of compound 4 is significantly higher than that of compound 8. In addition, we found that the hydroxyl group is connected to the skeletons of compounds 1, 6 and 7, but their activities are also not ideal. Can the presence of hydroxyl and carbonyl groups on the eudesmane-type sesquiterpene acid skeleton reduce the inhibitory activity of the compound against AChE? For this purpose, we reviewed studies in the last decade on the inhibition of AChE activity by sesquiterpenoids. However, prior to this study, there were no studies on the inhibition of AChE by the eudesmane-type sesquiterpene acid. Therefore, we hypothesized that the addition of hydroxyl or carbonyl groups to the backbone of eudesmane-type sesquiterpene acid may weaken the inhibitory effect of the compounds on AChE, but this needs to be verified by more experiments.
Compared with various sesquiterpenoids with acetylcholinesterase inhibitory activities reported in the literature, 8 compounds in this study showed unsatisfactory inhibitory activities. Meanwhile, we found that other eudesmane-type sesquiterpenes and their derivatives reported in the literature also showed poor inhibitory activity against AChE, while among the various constituents with AChE inhibitory activity, various sesquiterpene lactones seemed to be the most promising AChE inhibitors.
3.3. Kinetic study on the inhibitory effect of AChE by compound
To further explore the inhibition mechanism against AChE, we conducted a kinetics analysis on compound 5. The relationship between the maximal reaction initial speed (v) and diverse enzyme levels was analyzed by evaluating the reversible inhibitory effect exerted by compound 5 (Figure 5A). According to Figure 5A, the fitting curve indicated the original rate under diverse levels of compound 5 and a straight line was observed for the enzyme level. Moreover, every straight line intersected at the origin, with the slope dropping as the compound level increased, implying that compound 5 was a reversible inhibitor of AChE.
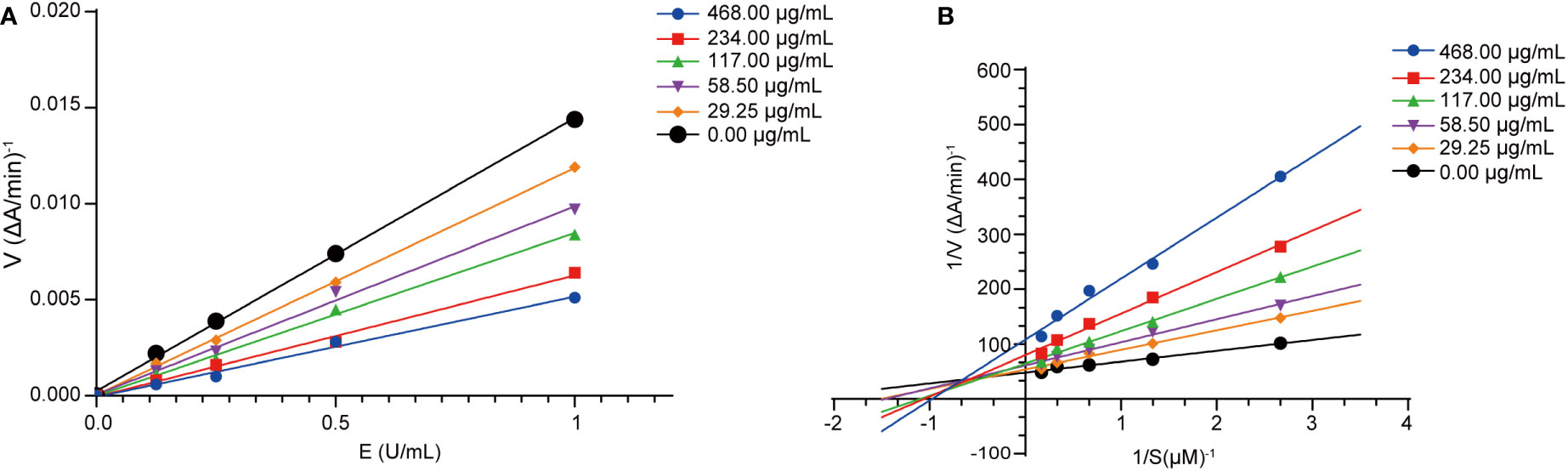
Figure 5 Acetylcholinesterase inhibition kinetics analysis of compound 5. (A) Hydrolytic activity of acetylcholinesterase concentration under the action of different concentrations of compound 5 (29.25, 58.50, 117.00, 234.00, and 468.00 μg/mL). (B) Lineweaver-Burk plots for the inhibition of compound 5 (29.25, 58.50, 117.00, 234.00, and 468.00 μg/mL).
Under the scenario of compound 5 being identified as a reversible inhibitor, we adopted the double-reciprocal graph (Lineweaver-Burk) to model the maximal original velocity and substrate level to explore the inhibition patterns. According to Figure 5B, each straight line had a unique intercept and slope but intersected within the second quadrant in the Lineweaver-Burk diagram. Meanwhile, as the compound level increased, fitted curves had elevated X-intercepts (-1/Km), Y-intercept (1/Vmax) and slopes, which indicated an increase in the Michaelis constant Km, but a decrease in Vmax. Consequently, compound 5 inhibited AChE in a mixed-type competitive manner, and could be an AChE inhibitor with dual binding sites (Shaik et al., 2019; Tang et al., 2019).
The Dixon plot was made to ascertain the AChE inhibition pattern as well as the dissociation constant (Ki) of our tested compounds. Typically, the Ki value represents the dissociation constant of the enzyme-inhibitor complex, where a lower value indicates a higher affinity of the compound to AChE. Based on the Dixon plot (Figure 6) and the slope in the double reciprocal graph (regarding compound 5 level with maximal original velocity), compound 5 had a Ki value of 24.668 µg/mL (Figure 6).
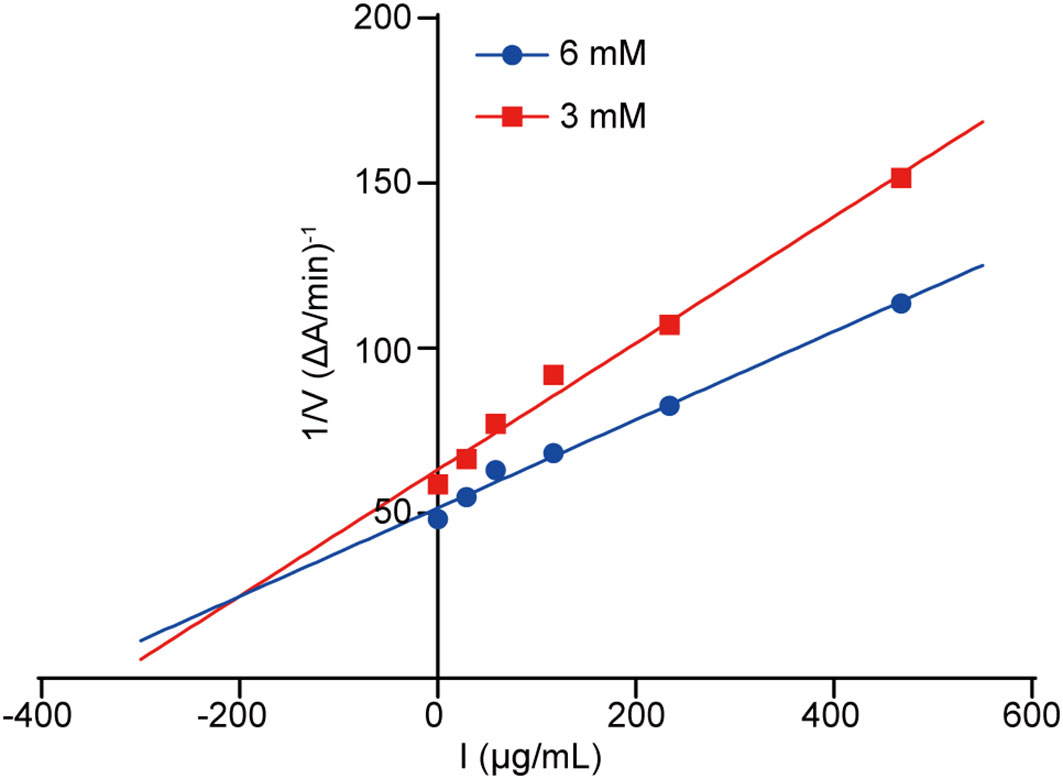
Figure 6 Dixon diagram. Dixon plot showing the inhibitory activities of compound 5 against AChE in the presence of different concentrations of substrate. Red and blue lines indicate concentrations of 3 and 6 mM, respectively.
3.4. Toxic effects of compounds on C. elegans
To evaluate the level of toxicity on C. elegans, we chose eight compounds for analysis. According to the results, the compounds had a potential toxicity level with respect to C. elegans at the experimental concentration, where compounds 3 and 5 were highly toxic, with median lethality rates of 60.83 ± 2.6% and 72.92 ± 3.15%, respectively (Table 3). Similarly, compound 2 still showed the lowest toxicity level, which further proved that the carboxyl group in the compound could increase the activity. Compounds 3, 4, and 5 as isomers also showed different toxicity levels, which further confirmed that the change of double bond position had a certain effect on the activity, and the terminal double bond of the compound should have a better activity. At the same time, the toxicity of compounds 1, 6, 7, and 8 with hydroxyl or carbonyl groups in the skeleton is still not ideal, which increases the possibility that the addition of hydroxyl or carbonyl groups to the main chain of the eudesmane-type sesquiterpene acid may reduce the toxicity of the compounds.
The in vivo toxicological results of these compounds were similar to those of AChE inhibition experiments, suggesting that the toxic effects of these compounds on C. elegans may be related to their inhibitory activity on AChE to some extent. However, this is not enough to conclude that there was a causal relationship between them. Previous studies have shown that drug toxicity to nematodes occurs in multiple biological tissues (Roh and Choi, 2008). To understand the relationship between AChE activity and toxicity, further experiments are needed for verification.
3.5. ADMET prediction of compounds
The main indexes of ADMET prediction were Blood Brain Barrier (BBB), Human Intestinal Absorption (HIA), Caco-2 permeability (CCP) and Ames mutagenesis (ATT), Carcinogenicity and cytochrome CYP2D6 are shown in Table 4. The 8 compounds isolated from L. pterodonta were easy to be absorbed or assimilated by the human intestine and could penetrate human intestinal cell lines without mutagenic toxicity or carcinogenicity. This indicates shown that these compounds have good ADMET properties. In addition, except for compounds 1, 6, and 7, which could not easily cross the blood-brain barrier, all other compounds could easily cross the blood-brain barrier. Comparing the structural differences between compounds 1, 6, and 7 and other compounds, it was found that this might be related to the hydroxyl group connected to the skeleton of these three compounds.
4. Conclusions
This study tested novel sesquiterpenes and six known eudesmane-type sesquiterpene acid isolated from L. pterodonta, to analyze their inhibitory activity on C. elegans AChE. The results showed that all these compounds had certain inhibitory effects on AChE in a dose-dependent manner, of which compound 5 had the best inhibitory effect with IC50 of 437.33 ± 8.33 μM. Meanwhile, as revealed by the Lineweaver-Burk and Dixon plots, compound 5 was observed to suppress AChE activity reversibly and competitively. Furthermore, all 8 compounds exhibited certain toxicity levels on C. elegans. Finally, the ADMET prediction was carried out for these 8 compounds, and it was found that all compounds had good ADMET properties. Collectively, we believe that these results are significant for the discovery of new AChE targeting compounds, and also enrich the bioactivity activity repertoire of L. pterodonta.
Data availability statement
The raw data supporting the conclusions of this article will be made available by the authors, without undue reservation.
Author contributions
JL, XQ, and XD conceived and designed the experiments. JL and FL performed the experiments and analyzed the data. XQ, XD, GW, FG, HL, LX, YZ and XH contributed reagents, materials, and analysis tools. All authors contributed to the article and approved the submitted version.
Funding
This research was supported financially by grants from the Natural Science Foundation of Yunnan Province (202001AT070053, 202001AT070055, and 2019FY003003), grants of State Key Laboratory of Phytochemistry and Plant Resources in West China (P2019-ZZ06 and P2021-ZZ04), and the National Key R&D Program of China (2021YFD1400701). This study received funding from Science and Technology Planning Project of Yunnan Province of China National Tobacco Corporation (2021530000242030). The funder was not involved in the study design, collection, analysis, interpretation of data, the writing of this article or the decision to submit it for publication.
Acknowledgments
We thank Bo Li, Yineng He (Kunming Institute of Botany) for technical assistances.
Conflict of interest
The authors declare that the research was conducted in the absence of any commercial or financial relationships that could be construed as a potential conflict of interest.
This study received funding from the Science and Technology Planning Project of Yunnan Province of China National Tobacco Corporation 2021530000242030. The funder was not involved in the study design, collection, analysis, interpretation of data, the writing of this article or the decision to submit it for publication.
Publisher’s note
All claims expressed in this article are solely those of the authors and do not necessarily represent those of their affiliated organizations, or those of the publisher, the editors and the reviewers. Any product that may be evaluated in this article, or claim that may be made by its manufacturer, is not guaranteed or endorsed by the publisher.
Supplementary material
The Supplementary Material for this article can be found online at: https://www.frontiersin.org/articles/10.3389/fpls.2023.1074184/full#supplementary-material
References
Arya, A., Chahal, R., Rao, R., Rahman, M. H., Kaushik, D., Akhtar, M. F., et al (2021). Acetylcholinesterase inhibitory potential of various sesquiterpene analogues for Alzheimer's disease therapy. Biomolecules 11, 350. doi: 10.3390/BIOM11030350
Azucena, G. C., Ana, G., Carlos, E. T., Marta, E. S. (2005). Antifeedant/Insecticidal terpenes from Asteraceae and Labiatae species native to argentinean semi-arid lands. Z. Naturforsch. C. 60, 855–861. doi: 10.1515/znc-2005-11-1207
Bawdekar, A. S., Kelkar, G. R. (1965). Terpenoids−LXVIII structure and absolute configuration of costic acid− a new sesquiterpenic acid from costus root oil. Tetrahedron 21, 1521–1528. doi: 10.1016/s0040-4020(01)98315-2
Cruz, R., Martinez, R. M. (1982). Stereoselective total synthesis of (±) - isocostic and (±)-3-Oxoisocostic acids. Aust. J. Chem. 35, 451–456. doi: 10.1071/CH9820451
Ding, X., Ouyang, M. A., Liu, X., Wang, R. Z. (2013). Acetylcholinesterase inhibitory activities of flavonoids from the leaves of ginkgo biloba against brown planthopper. J. Chem. 2013, 645086. doi: 10.1155/2013/645086
Ellman, G. L., Courtney, K. D., Andres, V., Featherstone, R. M. (1961). A new and rapid colorimetric determination of acetylcholinesterase activity. Biochem. Pharmacol. 7, 88–95. doi: 10.1016/0006-2952(61)90145-9
Fournier, D., Mutero, D. (1994). Modification of acetylcholinesterase as a mechanism of resistance to insecticides. Comp. Biochem. Physiol. 108, 19–31. doi: 10.1016/1367-8280(94)90084-1
Fukuto, T. R. (1990). Mechanism of action of organophosphorus and carbamate insecticides. Environ. Health Persp. 87, 245–254. doi: 10.1289/ehp.9087245
Gu, J. L., Li, Z. J., Zhang, H. X., Du, Z. Z. (2014). Fragrant volatile sesquiterpenoids isolated from the essential oil of Laggera pterodonta by using olfactory-guided fractionation. Chem. Biodivers. 11, 1398–1405. doi: 10.1002/cbdv.201400051
Guo, Y. Q., Tang, G. H., Lou, L. L., Li, W., Zhang, B., Liu, B., et al. (2018). Prenylated flavonoids as potent phosphodiesterase-4 inhibitors from Morus alba: Isolation, modification, and structure-activity relationship study. Eur. J. Med. Chem. 144, 758–766. doi: 10.1016/j.ejmech.2017.12.057
Lan, M. X., Gao, X., Duan, X., Li, H. M., Yu, H., Li, J. L., et al. (2022). Nematicidal activity of tirotundin and parthenolide isolated from Tithonia diversifolia and Chrysanthemum parthenium. J. Environ. Sci. Health B. 57, 54–61. doi: 10.1080/03601234.2021.2022945
Li, M. Y., Gao, X., Lan, M. X., Liao, X. B., Su, F. W., Fan, L. M., et al. (2020). Inhibitory activities of flavonoids from Eupatorium adenophorum against acetylcholinesterase. Pestic. Biochem. Phys. 170, 104701. doi: 10.1016/j.pestbp.2020.104701
Lu, P., Wu, J. M., Chen, L. J., Li, W. (2014). Chemical constituents from Laggera pterodonta. Chin. Med. Mat. 5, 816–819. doi: 10.13863/j.issn1001-4454.2014.05.022
Marcela, B., Sanz, K., Donadel, O. J., Rossomando, P. C., Tonn, E. (1997). Sesquiterpenes from Tessaria absinthioides. Phytochemistry 44, 897–900. doi: 10.1016/s0031-9422(96)00590-0
Rajashekar, Y., Raghavendra, A., Bakthavatsalam, N. (2014). Acetylcholinesterase inhibition by biofumigant (Coumaran) from leaves of Lantana camara in stored grain and household insect pests. Biomed. Res. Int. 2014, 187019. doi: 10.1155/2014/187019
Roh, J. Y., Choi, J. (2008). Ecotoxicological evaluation of chlorpyrifos exposure on the nematode Caenorhabditis elegans. Ecotoxicol. Environ. Safe. 71, 483–489. doi: 10.1016/j.ecoenv.2007.11.007
Sanz, J. F., Falcó, E., Marco, J. A. (1990). Further new sesquiterpene lactones from Artemisia herba-alba subsp. J. Nat. Prod. 53, 940–945. doi: 10.1021/np50070a024
Shaik, J. B., Yeggoni, D. P., Kandrakonda, Y. R., Penumala, M., Zinka, R. B., Kotapati, K. V., et al. (2019). Synthesis and biological evaluation of flavone-8-acrylamide derivatives as potential multi-target-directed anti Alzheimer agents and investigation of binding mechanism with acetylcholinesterase. Bioorg. Chem. 88, 102960. doi: 10.1016/j.bioorg.2019.102960
Tang, H. J., Song, P., Li, J., Zhao, D. S. (2019). Effect of salvia miltiorrhiza on acetylcholinesterase: Enzyme kinetics and interaction mechanism merging with molecular docking analysis. Int. J. Biol. Macromol. 135, 303–313. doi: 10.1016/j.ijbiomac.2019.05.132
Vale, A. (2015). Organophosphorus and carbamate insecticide poisoning. Handb. Clin. Neurol. 131, 149–168. doi: 10.1016/B978-0-444-62627-1.00010-X
Vaughan, A., Rocheleau, T., ffrench-Constant, R. (1997). Site-directed mutagenesis of an acetylcholinesterase gene from the yellow fever mosquito Aedes aegypti confers insecticide insensitivity. Exp. Parasitol. 87, 237–244. doi: 10.1006/expr.1997.4244
Wang, G. C., Li, G. Q., Geng, H. W., Li, T., Xu, J. J., Ma, F., et al. (2013). Eudesmane-type sesquiterpene derivatives from Laggera alata. Phytochemistry 96, 201–207. doi: 10.1016/j.phytochem.2013.07.014
Wang, Y., Yuan, F. J., Zhang, M., Yu, L., Liu, W. Y., Wu, H. Y., et al. (2021). Acetylcholinesterase inhibition effect of flavonoids from Flemigia philippinensis. Sci. Technol. Food. Ind. 42, 118–124. doi: 10.13386/j.issn1002-0306.2021040243
Xie, Y. Q., Fan, W. F., Chen, X. Q., Li, R. T., Zhang, Z. J. (2021). Chemical constituents from Laggera pterodonta. Biochem. Syst. Ecol. 94, 104222. doi: 10.1016/J.BSE.2020.104222
Xie, W. D., Weng, C. W., Shen, T., Gao, X. (2011). Sesquiterpenoids from Aster himalaicus. Chem. Nat. Compd+. 47, 309–310. doi: 10.1007/s10600-011-9916-2
Xiong, Z. Q., Liu, W., Zhou, L., Zou, L. Q., Chen, J. (2016). Mushroom (Agaricus bisporus) polyphenoloxidase inhibited by apigenin: multi-spectroscopic analyses and computational docking simulation. Food. Chem. 203, 430–439. doi: 10.1016/j.foodchem.2016.02.045
Xu, Y. Q., Lv., Y. D., Quan, Y. L. (2006). Eudesma-5,12-dien-13-oic acid from Laggera pterodonta. Acta Cryst. E. 62, 1844–1845. doi: 10.1107/S1600536806012529
Yang, G. Z., Li, Y. F., Yu, X., Mei, Z. N. (2007). Terpenoids and flavonoids from Laggera pterodonta. Acta Pharm. Sin. 42, 511–515. doi: 10.16438/j.0513-4870.2007.05.011
Zhao, Y., Kongstad, K. T., Jäger, A. K., Nielsen, J., Staerk, D. (2018). Quadruple high-resolution α-glucosidase/α-amylase/PTP1B/radical scavenging profiling combined with high-performance liquid chromatography-high-resolution mass spectrometry-solid-phase extraction-nuclear magnetic resonance spectroscopy for identification of antidiabetic constituents in crude root bark of Morus alba l. J. Chromatogr. A. 1556, 55–63. doi: 10.1016/j.chroma.2018.04.041
Zhao, Y., Yue, J. M., Lin, Z. W., Ding, J. K., Sun, H. D. (1997). Eudesmane sesquiterpenes from Laggera pterodonta. Phytochemistry 44, 459–464. doi: 10.1016/S0031-9422(96)00521-3
Keywords: Laggera pterodonta, sesquiterpenes, acetylcholinesterase, enzyme kinetics, toxic effects
Citation: Li J, Li F, Wu G, Gui F, Li H, Xu L, Hao X, Zhao Y, Ding X and Qin X (2023) Acetylcholinesterase inhibitory activity of sesquiterpenoids isolated from Laggera pterodonta. Front. Plant Sci. 14:1074184. doi: 10.3389/fpls.2023.1074184
Received: 19 October 2022; Accepted: 30 January 2023;
Published: 10 February 2023.
Edited by:
Minmin LI, Institute of Food Science and Technology, Chinese Academy of Agricultural Sciences, ChinaReviewed by:
Lucas Campos Curcino Vieira, Federal University of Mato Grosso, BrazilMarcus Scotti, Federal University of Paraíba, Brazil
Copyright © 2023 Li, Li, Wu, Gui, Li, Xu, Hao, Zhao, Ding and Qin. This is an open-access article distributed under the terms of the Creative Commons Attribution License (CC BY). The use, distribution or reproduction in other forums is permitted, provided the original author(s) and the copyright owner(s) are credited and that the original publication in this journal is cited, in accordance with accepted academic practice. No use, distribution or reproduction is permitted which does not comply with these terms.
*Correspondence: Yuhan Zhao, emhhb3l1aGFuQG1haWwua2liLmFjLmNu; Xiao Ding, ZGluZ3hpYW9AbWFpbC5raWIuYWMuY24=; Xiaoping Qin, cXhwOTlAMTYzLmNvbQ==
†These authors have contributed equally to this work and share first authorship